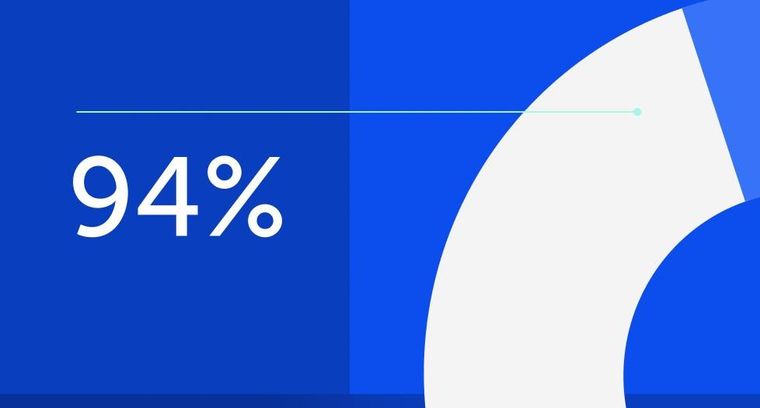
94% of researchers rate our articles as excellent or good
Learn more about the work of our research integrity team to safeguard the quality of each article we publish.
Find out more
REVIEW article
Front. Neuroanat., 12 February 2025
Volume 19 - 2025 | https://doi.org/10.3389/fnana.2025.1504065
The microbiota-gut-brain axis (MGBA) plays a significant role in the maintenance of brain structure and function. The MGBA serves as a conduit between the CNS and the ENS, facilitating communication between the emotional and cognitive centers of the brain via diverse pathways. In the initial stages of this review, we will examine the way how MGBA affects neurogenesis, neuronal dendritic morphology, axonal myelination, microglia structure, brain blood barrier (BBB) structure and permeability, and synaptic structure. Furthermore, we will review the potential mechanistic pathways of neuroplasticity through MGBA influence. The short-chain fatty acids (SCFAs) play a pivotal role in the MGBA, where they can modify the BBB. We will therefore discuss how SCFAs can influence microglia, neuronal, and astrocyte function, as well as their role in brain disorders such as Alzheimer’s disease (AD), and Parkinson’s disease (PD). Subsequently, we will examine the technical strategies employed to study MGBA interactions, including using germ-free (GF) animals, probiotics, fecal microbiota transplantation (FMT), and antibiotics-induced dysbiosis. Finally, we will examine how particular bacterial strains can affect brain structure and function. By gaining a deeper understanding of the MGBA, it may be possible to facilitate research into microbial-based pharmacological interventions and therapeutic strategies for neurological diseases.
The gut microbiota refers to the collective of microorganisms that inhabit the gastrointestinal tract (GI). The gut microbiota constitutes approximately 1–2 kg of the adult human body (Forsythe and Kunze, 2013; Toda et al., 2019), which is equivalent in weight to that of a normal adult brain. A multitude of physiological processes within the human body can be ascribed to the gut microbiota, with the maturation and development of the central nervous system (CNS) representing a particularly pivotal role (Liang et al., 2018; Cryan et al., 2019; Socała et al., 2021; Loh et al., 2024), as well as the development and modulation of the immune response (Zhang et al., 2021; Castillo-Álvarez and Marzo-Sola, 2022). In contrast to the brain, the gut microbiota is susceptible to direct intervention through the administration of prebiotics, probiotics, synbiotics, and antibiotics, and is amenable to modification by lifestyle factors. A substantial body of research has indicated that the microbiota may be involved in regulating brain morphology. For example, germ-free (GF) animals have demonstrated brain abnormalities in the absence of microbiota, as evidenced by studies (Sudo et al., 2004; Gareau et al., 2011; Heijtz et al., 2011; Neufeld et al., 2011; Clarke et al., 2013). Moreover, alterations in behaviour have been observed in animals administered specific strains of bacteria (Bercik et al., 2011; Bravo et al., 2011; Savignac et al., 2014; Desbonnet et al., 2015; Jarosz et al., 2024; Wlaź et al., 2024). Moreover, evidence indicates that exposure to a single microbial strain can confer protection against certain neurological disorders and systemic immune alterations. The findings of this study lend support to the hypothesis that microbe-based interventions may prove beneficial in the treatment of neurological disorders.
A variety of pathways facilitate the transmission of signals generated in the gut to the brain. The microbiota in the gut can communicate with the brain in several ways. Nevertheless, further research is necessary to gain a comprehensive understanding of the influence that bacteria in the GI tract exert on the brain and behaviour (Guzzetta et al., 2022; Kasarello et al., 2023). The release of cytokines by immune cells into the circulation represents the primary mode of immune communication. Moreover, pathogen-associated or damage-associated molecular patterns may enter the circulation and affect the functioning of internal organs and the gut microbiota. Since both pathogen-associated molecular patterns (PAMPs), derived from microorganisms, and damage-associated molecular patterns (DAMPs), released from stressed host cells, are identified by the immune system through pattern recognition receptors (PRRs) present by dendritic cells and macrophages. Such recognition contributes to immune dysregulation and intestinal permeability, which might collectively impact the gut microbiota by reducing microbial diversity, encouraging detrimental pathobionts, and hindering the microbiota’s capability to effectively regulate immune responses (Stringer, 2024). Endocrine communication represents the most expansive form of communication, encompassing the hypothalamic–pituitary–adrenal axis (HPA). The primary mode of neural communication is through direct anatomical connections established by the vagus nerve or indirect connections facilitated by the enteric nervous system (ENS). The development of novel techniques for studying the microbiome has enabled a more profound comprehension of the interrelationship between neurological disorders and the gut microbiota (Zhu et al., 2021; Loh et al., 2024). A variety of techniques have been developed for the study of MGBA. These include the GF mouse model, antibiotic-induced dysbiosis, fecal microbiota transplantation (FMT), probiotics, prebiotics, and synbiotics. There is substantial evidence indicating that microbes within the gut microbiome play a role in alterations to brain morphology. MGBA has been demonstrated to exert influence over several processes within the CNS, including neurogenesis, the growth of neuronal and microglia dendrites, axon growth, myelination, the structure and permeability of the blood–brain barrier (BBB), and the structure and function of synapses. These topics will be discussed in detail in this review.
The microbiota exerts an influence on the brain by producing short-chain fatty acids (SCFAs). The primary metabolites generated in the colon through bacterial fermentation of dietary fibres and resistant starch are acetate, propionate, and butyrate. Additionally, minor metabolites such as lactate, valerate, and formate are produced (Pascale et al., 2018). There is a considerable body of evidence that the MGBA can influence neuroplasticity in the brain (Leung and Thuret, 2015; Murciano-Brea et al., 2021; Tang et al., 2021; Salami and Soheili, 2022; Sarubbo et al., 2022; Damiani et al., 2023; Lu et al., 2024). The gut microbiota plays a significant role in the conversion of ingested food into nutrients. Consequently, the microbiota may be regarded as a “filter” and a “sensor” for exogenous compounds that enter the body (Clarke et al., 2014). The impact of microbiota alterations on brain plasticity can be exerted through a multitude of mechanisms, including the regulation of gene expression, the production of neuroactive molecules, and the modulation of microglial activity. This review will examine the modulation of brain plasticity by gut microbiota through neurotrophic factors and the impact of aging-related alterations in gut microbiota on the elderly. The objective of this review is to provide a comprehensive overview of how the field of MGBA has increased understanding of the influence of MGBA on brain structure and function. This is crucial for advancing research into microbial-based interventions and therapeutic strategies for neurological diseases.
The blood–brain barrier (BBB) is a structural and biochemical barrier that protects the brain by regulating which metabolites and nutrients can enter the brain from the blood or exit into circulation (Schiera et al., 2024). Its genesis is controlled by brain cells that interact with brain capillary endothelial cells, including pericytes, glial cells, neurons, and astrocytes (Schiera et al., 2024). Both astrocytes and microglia support synaptic development and remodelling in a healthy brain (Vainchtein and Molofsky, 2020). However, they differ in their physiological functions. Astrocytes surround most neuronal synapses, contributing to the formation of the brain’s borders and vasculature. Meanwhile, the role of microglia during development appears to be phagocytic. They engulf apoptotic neuronal corpses and phagocytose synapses (Sofroniew, 2014). Following an injury, astrocytes enlarge to rebuild the BBB, and maintain its structural integrity, while microglia boost their phagocytic activity to clear debris (Peri and Nüsslein-Volhard, 2008). Also, microglia are essential for normal brain myelination. Despite being the most abundant glial cell type in CNS, less is known about astrocytes’ role in this process (Molina-Gonzalez and Miron, 2019). Another open question is whether transplanting astrocytes can restore healthy myelination in progressive diseases. The examples above represent a small subset of astrocyte and microglia roles, including myelination formation, angiogenesis, and BBB regulation. Future research focusing on structural and functional changes in astrocytes and microglia caused by disruptions in the gut microbiome may reveal novel therapeutic interventions for brain disorders.
Accumulating evidence suggests that microbes within the gut microbiome are involved in brain morphology alterations. First, research in GF animals demonstrated that the brain morphology is impacted when the microbiota is absent (Hegstrand and Hine, 1986; Sudo et al., 2004; Gareau et al., 2011; Heijtz et al., 2011; Neufeld et al., 2011; Clarke et al., 2013). Secondly, animals that received particular strains of bacteria observed changes in different brain regions (Mckernan et al., 2010; Bercik et al., 2011; Savignac et al., 2014; Desbonnet et al., 2015). Also, human genomic studies on these strains and the brain validated the possible applicability of the findings (Tillisch et al., 2013; Allen et al., 2016; Pinto-Sanchez et al., 2017). Furthermore, population-based research on individuals affected by infection, particularly in Canada, has shown changes in brain structure and overall microbiota composition (Thabane et al., 2010). Finally, preclinical studies have demonstrated long-term impacts on the brain, spinal cord, and ENS from antibiotic exposure or chronic bacterial infection during early life or adulthood as a result of gut dysbiosis (Verdu et al., 2008; O’Mahony et al., 2014). The gut microbiota can influence neurogenesis, myelination, dendritic morphology, microglia morphology, BBB structure and permeability, synapse structure and function (Figure 1).
Figure 1. Gut-Brain axis association with brain morphology alterations. Green highlighted bacteria have shown the ability to ameliorate DG neurogenesis, CA3-CA1 synaptic activity, hippocampal BDNF–TrkB signalling, improve hippocampus neurogenesis, myelination gene regulation, maintain dendritic spine density and branching. Red highlighted bacteria were reported to reduce synaptic strength and plasticity, induce neuroinflammation, dendritic spine loss, and decreased dendritic arborization or branching. Created with BioRender.com.
Hippocampal neurogenesis is decreased significantly in the GF mice model or dysbiosis induced by vancomycin in C57BL/6 mice (Möhle et al., 2016; Sawada et al., 2018). However, Lactobacillus casei has been demonstrated to ameliorate dentate gyrus (DG) neurogenesis, CA3-CA1 synaptic activity, and hippocampal brain-derived neurotrophic factor-Tropomyosin receptor kinase B (BDNF–TrkB) signaling when impaired by dysbiosis of two-week antibiotic cocktail administration (Guida et al., 2018) and increase serotonin mRNA levels in juvenile rats DG (Barrera-Bugueño et al., 2017). While, colonization of GF mice with control mice microbiota has demonstrated increased levels of neurogenesis, interestingly, the same colonization of the GF mice lacking TPH1, has eliminated serotonin synthesis and decreased Nestin+ neural precursors, hence reducing levels of neurogenesis (De Vadder et al., 2018). In addition, adult neurogenesis is significantly influenced by the microbiome (Ogbonnaya et al., 2015; Sawada et al., 2018). Butyrate, a metabolite produced by gut bacteria, has been shown to elevate hippocampal neurogenesis in pigs. It is therefore hypothesized that butyrate producing bacteria such as Faecalibacterium prausnitzii, Anaerobutyricum hallii, [Eubacterium] rectale, Lactobacillus casei, Coprococcus eutactus, Coprococcus comes, [butyricicoccus] pullicaecorum, and Clostridium butyricum can influence neurogenesis via gut-brain axis (Hébuterne, 2003; Miquel et al., 2013; Martín et al., 2018; Fusco et al., 2023).
Myelination is the formation of the myelin sheath surrounding the nerve, which enhances its conductivity (Hughes and Appel, 2016). Emerging evidence supports the link between intestinal microbiota and myelin formation, as GF C57BL/6 mice have displayed decreased expression of myelin basic protein (Lu et al., 2018), while another animal study has reported hypermyelination, resulting in thicker myelin sheaths in the prefrontal cortex (PFC) and axon functional impairment (Hoban et al., 2016). These findings suggest the importance of intestinal bacteria in myelination gene regulation. The findings are supported by evidence from a mouse model of Huntington’s disease. When compared with a control group an abnormal increase in axon myelin thickness and impaired white matter integrity were recorded in the corpus callosum, as well as downregulation of myelin-related proteins and mature oligodendrocytes (Radulescu et al., 2019). Furthermore, GF B6-deficient Wistar rats exhibited a significantly higher incidence of irregular myelin splitting when compared with conventional B6-deficient rats in the peripheral nervous system (Sumi et al., 1977). The abundance of the Akkermansia genus has been found to be correlated with autoimmune encephalomyelitis and myelin damage in a mouse model of multiple sclerosis (MS) (Lee et al., 2011). These findings are consistent with those of a study that observed a similar pattern in MS patients. Specifically, the study found that MS patients exhibited a high abundance of Akkermansia muciniphila, Acinetobacter calcoaceticus, and low levels of Parabacteroides distasonis, a strain linked with anti-inflammatory activity. Indeed, these results were compared with those of healthy human samples (Cekanaviciute et al., 2017).
During early development, dendritic growth is regulated by both cell-intrinsic programs and extrinsic factors that regulate various aspects of dendritic development. It was always believed that dendritic growth is intrinsically determined. However, over the past 2 decades, many studies have shown that the dendritic growth process is remarkably responsive to extrinsic factors, influencing local and global mechanisms of dendrite development (Valnegri et al., 2015; Hamad et al., 2023). The extrinsic factors include neurotransmitters and neurotrophins. Morphological modifications of dendrites could significantly influence their signal integration, neuronal stimulation, and their overall function (Cline, 2001; Inglis et al., 2002; Jan and Jan, 2003; Sirzen-Zelenskaya et al., 2006; Hamad et al., 2011, 2014, 2021, 2024; Rajan et al., 2021). Moreover, the microbiome exerts a distinct influence on dendritic structure, either by preserving or altering it. In line with previous findings, the administration of galacto-oligosaccharides (GOS), a well-studied prebiotic, for 40 days has been shown to increase dendritic spine density in rats, which is a reliable indicator of hippocampal excitatory synapses (Waworuntu et al., 2016). In contrast, GF mice exhibited aberrant mushroom-shaped dendrites that were thinner, shorter, and shrunken compared with control mice. This reduced synaptic strength and plasticity, despite an increase in dendritic branching (Ferrante et al., 2013). Additionally, the mode of delivery affects the composition of the microbiota, which may influence dendritic arborization. Studies have shown that mice and rats delivered by caesarean section (C-section) have exhibited decreased dendritic arborization or branching (Juárez et al., 2008; Chiesa et al., 2019). The transplantation of microbiota from aged experimental animals to young recipients has been observed to result in a reduction in dendritic spines in the hippocampus and PFC as well as impaired memory performance and altered neuron plasticity protein expression (D’Amato et al., 2020; Li et al., 2020). The collective evidence indicates that the gut-brain axis can influence the morphology of distinct types of neurons. Consequently, supplementation of a mouse model of amyotrophic lateral sclerosis (ALS) with GOS for 74 days has resulted in an increased abundance of Bifidobacterium and Lactobacillus genus, thereby reducing motor neuron death and spinal cord inflammation when compared with the untreated group (Song et al., 2013). In contrast, GF mice had pyramidal neuron atrophy, and a reduction in the branching of these cells in the hippocampal DG and amygdala (London et al., 2013).
Microglia regulate synaptic plasticity, and phagocytosis, promoting the survival of neurons and neural progenitors by releasing growth factors that maintain neurons’ homeostasis and function (Tay et al., 2017). The interactions between the microbiota, the immune system, and the brain are currently being recognized as crucial mechanisms that shape the maturation, activation, and morphology of microglia (Rea et al., 2016; Vuong et al., 2017). This hypothesis is supported by the observation that GF mice exhibit immature microglia in the corpus callosum, cortex, hippocampus, olfactory bulb, and cerebellum, accompanied by an abnormal density and morphology. However, these abnormalities were reversed by microbiota colonization with SCFAs (Erny et al., 2015). In contrast, a stroke model of GF mice exhibited a reduction in the number of microglia (Singh et al., 2018). The administration of an antibiotic cocktail to C57BL/6 mice resulted in the induction of gut dysbiosis, which was accompanied by abnormal activation of microglia and astrocytes, indicative of inflammation in the hippocampus (Guida et al., 2018). Moreover, the induction of gut perturbations in the mouse model of Parkinson’s disease (PD) resulted in a reduction of microglial diameter and overall size in the caudate-putamen and substantia nigra, thereby impairing their function (Sampson et al., 2016). It is evident that Bacteria species such as Lactobacillus helveticus, which is known as Lactobacillus acidophilus in natural human inhabitants, as well as Bifidobacterium longum, can regulate microglia and synaptogenesis in the hypothalamus in response to induced stress (Ait-Belgnaoui et al., 2014). Lactobacillus delbrueckii, Lactobacillus casei, Lactobacillus acidophilus, and exogenous Lactobacillus plantarum have been demonstrated to regulate microglial activation in aged Wistar rats’ hippocampus (Distrutti et al., 2014). It has been demonstrated that in an obese-insulin-resistant rat model, Lactobacillus casei supplementation for 12 weeks was able to restore microglia function by regulating their activation (Chunchai et al., 2018). The use of antibiotics was found to result in a reduction in the process of microglia-mediated synapse engulfment, which is associated with a reduction in synapse density observed in postmortem cortical tissue of individuals with schizophrenia (SCZ) (Sellgren et al., 2019).These findings indicate that a healthy and diverse GI microbiome is essential for maintaining healthy microglia and optimal cognitive function (Cryan and Dinan, 2015; Thion et al., 2018).
The BBB is a complex structure comprising endothelial cells, which are held together by tight junctions and adherens junctions, and interact with pericytes, the basement membrane of capillaries, microglia, and astrocytes (Ary et al., 2023). The primary function of the BBB is to safeguard the brain from toxic substances, facilitate their removal from the brain to the bloodstream, and provide essential nutrients to brain tissue. Consequently, the BBB is subject to rigorous regulation, and most neurological disorders are characterized by elevated permeability. A growing body of evidence from clinical and experimental studies supports the role of the gut-brain axis in regulating the integrity of BBB (Esposito et al., 2002; Braniste et al., 2014; Montagne et al., 2015; Thevaranjan et al., 2017; Dhaliwal et al., 2018; Li et al., 2018). Mice with normal microbiota develop a BBB around postnatal day 14. At postnatal day 15, the permeability of the BBB begins to decrease, while GF mice exhibit increased BBB permeability and decreased endothelial tight junction proteins at postnatal day 16. This suggests that gut bacteria play a role in maintaining the integrity of the BBB, as colonization with bacteria restores its function and normal selectivity (Braniste et al., 2014). Lactobacillus plantarum, a Gram-positive lactic acid bacterium that is frequently present in fermented food products, has been demonstrated to increase the integrity of the BBB in Swiss albino mice when supplemented for 28 days in rats (Dhaliwal et al., 2018). Moreover, administration of Clostridium butyricum 14 days prior to and following traumatic brain injury in mice has been demonstrated to mitigate neuronal degeneration and BBB permeability (Li et al., 2018). Clinical studies have indicated that both age and stress can compromise the integrity and function of the GI barrier because of microbial dysbiosis, which in turn impacts the permeability of the BBB, thereby hastening the process of inflammation associated with aging. These findings are corroborated by the observation that bacterial metabolites, such as butyrate and propionate, enhance the integrity of the epithelial barrier by facilitating the assembly of tight junctions (Peng et al., 2009; Tong et al., 2016).
Synapses are the points of contact between neurons, where connections are established, and signals are transmitted between them. The number of synaptic connections possessed by neurons may vary, ranging from a few to hundreds of thousands. These connections may link to the neuron itself, adjacent neurons, or neurons in different brain areas (Caire et al., 2021). Synaptic plasticity is evaluated by the capability of neurons to adjust the intensity of their connections, which plays a crucial role in the formation and reconstruction of brain networks following disruption (Stampanoni Bassi et al., 2019). A measure of synaptic plasticity that controls the strength of neuro-connectivity is long-term potentiation (LTP) and long-term depression. These are persistent alterations in synaptic strength (Bliss and Cooke, 2011) which have been demonstrated to be influenced by gut microbes (Maren and Quirk, 2004). Synaptic plasticity in the hippocampus is primarily regulated by glucocorticoids, which are metabolized by microbiome bacteria such as Eggerthella lenta, [Clostridium] scindens 1, and [Clostridium] scindens 2. These bacteria influence changes in synaptic function and excess neuronal injury (Lupien et al., 2005; Chen et al., 2006; Ridlon et al., 2013; Morris and Ridlon, 2017), which were not observed in GF mice (Bokkenheuser et al., 1984). Another study of GF mice in a model of Huntington’s disease demonstrated alterations in plasticity (Radulescu et al., 2019). Moreover, Lactobacillus casei has been linked to the regulation of synaptogenesis, synaptic refinement, and pruning in the hippocampus, which is crucial in the context of brain disorders involving neurodegeneration (Ogbonnaya et al., 2015; Guida et al., 2018; Sawada et al., 2018). A novel probiotic composition, comprising bifidobacteria (Bifidobacterium longum, Bifidobacterium breve, Bifidobacterium infantis), lactobacilli (Lactobacillus acidophilus, Lactobacillus plantarum, Lactobacillus casei, Lactobacillus delbrueckii subsp. bulgaricus), and Streptococcus thermophilus, has been demonstrated to enhance neuroplasticity in a mouse model of Alzheimer’s disease (AD), effectively reversing deficits in LTP (Bonfili et al., 2017). Another study has demonstrated the pivotal role of Lactobacillus reuteri in rectifying deficits in synaptic plasticity, which resulted in the amelioration of social behaviors (Zhu et al., 2017). The combination of a probiotic with a prebiotic (synbiotic), including Lactobacillus casei with inulin, was found to significantly mitigate synaptic plasticity and increase 5-HT1A in the hippocampus region, particularly in the CA1 and DG of healthy juvenile rats (Barrera-Bugueño et al., 2017). Despite the adversities in quality control and manufacturing of synbiotics, they have been used in microbiome-based therapies for AD, which influenced desirable insulin regulation and amyloid plaque reduction (Nakhal et al., 2024). The findings highlight the importance of the connections between the MGBA and brain morphology, as outlined in Table 1.
The accumulating evidence linking bacteria in the gut and neurons in the brain has prompted a paradigm shift in neurosciences. Neuroplasticity is the brain’s ability to reorganize itself through the formation of new neural connections and the construction of novel networks in response to learning, experience, or injury. This process, known as neurogenesis, is a fundamental aspect of brain development. This phenomenon allows the brain to adapt and change throughout life, influencing various behaviours. Conversely, the gut microbiota comprises a varied population of microorganisms inhabiting the digestive system, which is pivotal for digestion, and immune response. The link between neuroplasticity and the gut microbiota stems from the microbiota’s ability to affect brain function and behaviour via multiple pathways, including neurotransmitter production, immune system modulation, and inflammation regulation. Consequently, this interplay may influence both cognitive functions and mental health. Maintaining a balanced gut microbiota is crucial for proper gut physiology and signaling of the MGBA (Figure 2). When there is an imbalance in the microbiota or its functions, known as dysbiosis, it can have adverse effects on various systems, including the GI tract and the CNS. Thus, the understanding of the mechanisms of synaptic plasticity may provide essential insights into the pathophysiological nature of neuropsychiatric disorders, such as Attention-deficit/hyperactivity disorder (ADHD), and neurological disorders, including epileptic seizures, pointing to new therapeutic interventions.
Figure 2. The impact of gut microbiota on brain development. The figure illustrates the correlation between alterations in gut microbiota and hippocampal neurogenesis, as well as the association between these changes and the development of neurological disorders. The gut microbiota plays a crucial role in brain development, including neuroplasticity, by regulating neurogenesis, microglial maturation, the HPA axis, and CNS myelination. These processes may be influenced by the gut microbiota, either positively or negatively, resulting in the promotion or disruption of their development. Created with BioRender.com.
Plasticity involves a variety of mechanisms, including synaptic plasticity, neurogenesis, and changes in glial cell function, which enable neurons to form new connections, strengthen existing ones, and weaken others (Dzyubenko and Hermann, 2023). One research group demonstrated that the calcium-binding protein S100B in the cytoplasm and nucleus of astrocytes significantly modulates long-term synaptic plasticity (Nishiyama et al., 2002). Astrocytes release glutamate through a natural increase in their internal calcium levels, subsequently inducing significant glutamatergic activity in nearby neurons (Parpura and Haydon, 2000). Oligodendrocyte precursor cells receive glutamatergic signals directly from hippocampal pyramidal neurons (Bergles et al., 2000). These findings suggest that bidirectional communication between glial cells and neurons may contribute to synaptic plasticity. Another study showed that mice lacking S100B mutants grew normally and had no observable abnormalities in brain cytoarchitecture. However, these mutants displayed enhanced synaptic plasticity, as evidenced by increased LTP in the hippocampal CA1 region. Remarkably, it’s evident that a glial protein has been shown to modulate neuronal synaptic plasticity, working memory, and learning (Nishiyama et al., 2002). This offers a hallmark feature for the understanding of numerous diseases, including the neurodegenerative ones, and their association with the dysbiosis induced by astrocytic dysfunction. In terms of cognitive aspects, neural plasticity can be defined as the ability to modify the functioning of neural circuits based on experience, thus influencing thoughts, feelings, and behavior (Citri and Malenka, 2008). The neuroplasticity that involves changes in the strength and number of synapses between neurons over time is called synaptic plasticity.
There are two main forms of synaptic plasticity: Hebbian and homeostatic plasticity (Galanis and Vlachos, 2020). Hebbian plasticity involves a change in synaptic strength mediated by increasing or decreasing neuronal activity after the onset of stimulation and is involved in lifelong changes (Vitureira and Goda, 2013). It plays a critical role in learning and memory. In 1966, Terje Lømo and Tim Bliss studied the effects of activating the perforant path to dentate granule cells in the hippocampus of anesthetized rabbits, and they observed that brief trains of stimuli resulted in increased efficiency of transmission at the perforant path-granule cell synapses that could last for hours. When they applied a burst of tetanic stimulus to the perforant path fibers, it resulted in a dramatic and long-lasting enhancement in the post-synaptic response of cells in the dentate gyrus. In 1968, both scientists proposed the role of the hippocampus in certain forms of memory (Lømo, 2003).
Homeostatic plasticity constitutes a negative feedback loop in response to increased neuronal activity. It involves the regulation of neuronal excitability or the stabilization of overall synaptic strength (von Bernhardi et al., 2017). Also, it involves coordinated changes among distinct parts of the neuron, such as the synapses, the cell body, and the axon. White matter plasticity or myelin plasticity offers a way in which the structure of white matter can be altered by experience (Sampaio-Baptista et al., 2020). Despite the importance of myelination in circuit activity, there remains a lack of understanding of how neuronal activity and brain plasticity might impact myelination. Myelin, which is formed and wrapped around axons by oligodendrocytes, originates from embryonic neural progenitors, progressing through stages of OL precursor or progenitor cells before maturing into oligodendrocytes. Apart from direct alterations to existing myelin sheaths, an essential mechanism regulating myelin plasticity involves the genesis, differentiation, and proliferation of myelinating glia (Chorghay et al., 2018). Receptors located in axons and cells originating from the oligodendrocytes lineage detect neuronal activity. These cells utilize the metabolites derived from this activity to either maintain the myelin sheaths, such as through lipid synthesis, or to offer metabolic support by transporting lactate (Chorghay et al., 2018). As myelination progresses rapidly in early life, perturbations in the neonatal gut microbiota during initial colonization could disrupt proper myelination by insidiously affecting the immune responses and neuronal differentiation. The effects of neonatal antibiotic-induced dysbiosis on the development of the MGBA, including aspects such as myelination and behaviour, remain unclear. Neonatal antibiotic-induced dysbiosis affects myelination and behaviour in mice, and it was hypothesized that neonatal antibiotic-induced dysbiosis disrupts host–microbe interactions, resulting in impaired myelination in the brain and changes to the MGBA (Keogh et al., 2021). The introduction of the SCFA butyrate helped reverse myelination deficits in the PFC. The presence or absence of certain bacterial taxa, such as Bacteroidaceae and Coriobacteriaceae, is known to be important for short-chain fatty acid production and is reduced in certain conditions like MS, suggesting an indirect link between gut dysbiosis and altered myelination (Yan and Charles, 2018). The study highlighted a persistent effect of neonatal antibiotic treatment on the MGBA, impacting myelin regulation in the PFC, and reducing cognitive function. Furthermore, bacterial metabolites were found to effectively reverse this altered phenotype, and the gut microbiota showed a critical role in mediating these effects (Keogh et al., 2021).
BDNF plays various roles in brain physiology. It is involved in LTP and synaptic plasticity, where it shapes the morphology of mature neurons by promoting axonal outgrowth and pruning which is a natural process that removes excess or unused synapses, allowing the brain to optimize its efficiency and function. The gut microbiota can control the expression of a variety of neurotrophic factors, such as BDNF and glial cell line-derived neurotrophic factor. The glial cell line-derived neurotrophic factor was initially thought to be able to regulate the growth, survival, and differentiation of neural-derived cell types. However, these factors and their receptors are also widely found to be expressed across many different neurodegenerative diseases, like AD, and PD (Azman and Zakaria, 2022), epilepsy (Leifeld et al., 2022) as well as psychiatric disorders, such as major depressive disorder (MDD), and autism spectrum disorder (ASD). Several communication pathways connecting the gut microbiota and the brain have been delineated. These include neural pathways through the vagus nerve and the ENS, immune signaling mediated by cytokines due to the high concentration of immune cells in the gut, and hormonal modulation via the HPA axis, leading to changes in glucocorticoid levels. The vagus nerve relays vital information between the brain and the GI, respiratory, and cardiovascular systems (Figure 3).
Figure 3. The connection between gut bacteria and the brain and factors influencing hippocampal neurogenesis. The GI tract is connected to the brain through an important nerve called the vagus nerve. The vagus nerve originates in the brain stem and extends through the neck, chest, and abdomen. In the human body, the nervous system and another axis and the HPA axis are the main systems that respond to stress. Prolonged stress can adversely affect gut bacteria due to the interconnectedness of the HPA axis with the gut-brain axis: Furthermore, alterations in the gut microbiota can also influence the brain, potentially increasing susceptibility to brain conditions like depression. Regular physical activity regularly, a nutrient-rich diet, and the prescription of antidepressants are key factors that have a direct impact on our functional microbiota-gut-brain axis, resulting in a healthy immune system and an increased neurogenesis in the adult hippocampus. However, following an unhealthy diet with a high concentration in saturated fat, overuse of antibiotics, and being under stress are other markers that abnormally influence the physiological processes of the immune and endocrine systems, resulting in a decreased neurogenesis in the adult hippocampus, and disruption of the gut microbiome. The human gut is composed of dominant phyla, Bacteroidetes, and Firmicutes, and the activity and electrical properties of enteric neurons can be influenced by gut bacteria through actions on ion channels, thus affecting their adaptability. Created with BioRender.com.
The impact of the microbiota on brain plasticity in the elderly extends to exploring its potential implications for neurological health and age-related cognitive decline. One study found that GF mice exhibited decreased levels of BDNF expression in both the cortex and hippocampus, as well as reduced expression of the NR2B subunit of the N-methyl-D-aspartate (NMDA) receptor. Moreover, NMDA receptors contribute to memory formation by modulating synaptic plasticity (Li and Tsien, 2009). Furthermore, there is a decrease in adult hippocampal neurogenesis in the elderly, which parallels a decline in cognitive function and synaptic plasticity. Therefore, it could be inferred that the pathophysiological effects of microbiota are not recognized until old age. More specifically, as individuals age, there are physiological changes that affect metabolic, genomic, and immunological functions, leading to an increased susceptibility to infections and diseases. These changes can result in a condition known as inflammaging, which is a chronic, low-grade inflammation associated with age-related diseases.
Witte et al. (2009) conducted the initial study showing the beneficial effects of calorie restriction on memory performance in an elderly group. Similarly, intermittent fasting in mice led to increased markers of brain plasticity (Singh et al., 2012). Exploring the complex relationship between gut microbiota and synaptic plasticity in the elderly offers the potential to develop innovative therapeutic approaches to mitigate age-related cognitive decline.
There are a variety of tools and techniques which has been recently introduced to study the MGBA, paving the way for researchers to narrow the gaps in understanding of the MGBA. These include the GF mouse model, antibiotic-induced dysbiosis, FMT, probiotics, and prebiotics. In this chapter, we will focus on the GF mice as a tool to study the MGBA.
The GF mice, which have been exposed to no microbial agents, serve as a model for investigating the intricate interplay between the gut microbiota and the gut-brain axis. Microbiological evaluation of these mice reveals the complete lack of growth for anaerobic, aerobic, and mycotic bacteria, confirming their GF status. Studies utilizing GF mice have yielded compelling evidence for the involvement of gut microbiota in the gut-brain axis.
Several studies have demonstrated that GF mice, exhibit differences in microglial gene expression and morphology compared to mice with normal gut bacteria (Abdel-Haq et al., 2019; Huang et al., 2023). In contrast to mice with normal gut bacteria, GF mice have a greater number of microglia in their brains. However, these cells exhibit abnormal shapes and a reduced ability to respond to infection (Erny et al., 2015; Zhang et al., 2022). Similarly, antibiotic treatment in normal mice also results in analogous microglial alterations, thereby indicating that gut bacteria are of pivotal importance for microglial development and functionality (Çalışkan et al., 2022). The reintroduction of a complex mixture of gut bacteria or SCFAs, produced by gut bacteria, was found to restore normal microglial function in GF mice (Erny et al., 2015). Moreover, a recent study demonstrated that the gut microbiota exerts a significant influence on age-related changes in microglial function. By comparing microglial gene expression in young-adult and aged mice under GF and specific pathogen-free conditions, the authors reported that the absence of gut microbiota in GF mice reduced oxidative stress and improved mitochondrial function in aged microglia (Mossad et al., 2022). Moreover, they also reported that metabolomic analyses revealed that N6-carboxymethyllysine accumulated in aging brains of specific pathogen-free mice, impairing mitochondrial activity and increasing oxidative stress. This age-related increase in N6-carboxymethyllysine was validated in both mice and human samples and linked to microbiota-induced intestinal permeability, thus highlighting the gut-brain axis’s role in microglial aging (Mossad et al., 2022).
Astrocytes, the primary glial cells in the brain, play a central role in a multitude of metabolic interactions with neurons, regulation of blood flow, homeostasis of extracellular fluid, ions, and transmitters, energy provision, regulation of synapse function, and synaptic remodelling (Gradisnik and Velnar, 2023). Astrocytes provide fuel for neuronal activity by releasing lactate in response to synaptic glutamate release. This process is designated as the astrocyte-neuron lactate shuttle. Recent research has identified a critical influence of the gut microbiota on the modulation of genes involved in the astrocyte-neuron lactate shuttle, a key component of brain energy metabolism. In a 2020 study, Margineanu and colleagues demonstrated that microbial colonization of GF mice led to the upregulation of Atp1a2 (encoding the ATPase, Na+/K+ transporting, alpha 2 sub-unit) and Pfkfb3 (encoding 6-phosphofructo-2-kinase/fructose-2,6-biphosphatase 3), which is predominantly expressed in astrocytes and is crucial for their metabolic functions (Margineanu et al., 2020). ATP1A2 is essential for maintaining ionic balance and membrane potential in astrocytes, while PFKFB3 plays a key role in glycolysis and lactate production. Specifically, the mRNA levels of Atp1a2 and Pfkfb3 were elevated in the hippocampus of mice colonized with microbes for 24 h, in comparison to conventionally raised mice (Margineanu et al., 2020). Furthermore, Pfkfb3 was upregulated in GF mice, while the increase in Atp1a2 was confirmed at the protein level by Western blot analysis. Furthermore, in an animal model of chronic psychosocial stress, 6-week dietary supplementation with prebiotics (fructo-and galacto-oligosaccharides) also upregulated Atp1a2 and Pfkfb3 mRNA expression in the hippocampus (Margineanu et al., 2020). These findings indicate that the gut microbiota and prebiotics can significantly influence the metabolic coupling between neurons and astrocytes by modulating the expression of key genes, including Atp1a2, Pfkfb3, lactate dehydrogenase A, lactate dehydrogenase B, monocarboxylate transporter 1, and glycogen synthase 1.
A study by Gong et al. (2024) investigated the impact of gut microbiota dysbiosis on BBB integrity using GF mice that underwent FMT from patients with anti-(NMDA) receptor encephalitis. The dysbiotic microbiota exhibited a low short-chain fatty acid content and an altered bacterial composition, including a decrease in Lachnospiraceae and an increase in Verrucomicrobiota, Akkermansia, Parabacteroides, and Oscillospirales (Gong et al., 2024). Following FMT, the mice exhibited increased susceptibility to an encephalitis-like phenotype, characterized by behavioural deficits, and elevated T2-weighted image hyperintensities (Gong et al., 2024). The study demonstrated that dysbiosis resulted in impaired BBB integrity, as evidenced by increased Evan’s blue dye extravasation and reduced expression of tight junction proteins ZO-1 and claudin-5. Furthermore, proinflammatory cytokines (IL-1, IL-6, IL-17, TNF-α, and LPS) were elevated, contributing to a pro-inflammatory state and significant brain inflammation, particularly in the hippocampus and cortex, with decreased NMDA receptor expression (Gong et al., 2024). These findings underscore the crucial role of the gut microbiota in maintaining the integrity of the BBB and suggest that dysbiosis may exacerbate susceptibility to neuroinflammatory conditions such as NMDAR-excitotoxicity.
A further study conducted in 2022 on GF mice demonstrated that the absence of gut microbiota leads to increased BBB permeability. This is evidenced by a reduction in the expression of tight junction proteins, including claudin-5 and occludin, which are essential for maintaining the integrity of the BBB (Park and Im, 2022). Consequently, GF mice exhibit greater cognitive disabilities than mice with a normal microbiota. Moreover, the administration of specific probiotics and microbiota-derived metabolites, such as SCFAs, has been demonstrated to protect and enhance BBB integrity (Park and Im, 2022). This underscores the pivotal role of the gut microbiota in regulating BBB permeability and safeguarding against neuroinflammation and cognitive impairments.
When the BBB is compromised, it negatively impacts neurogenesis during early development by exposing the brain to excessive glucocorticoid molecules (Figure 4A). Consequently, cognitive deficits and neuropsychological symptoms are highly prevalent during the long-term effects of glucocorticoids. However, a large body of evidence suggests that gut microorganisms may regulate cell junctions in the BBB structure. GF mice treated with either Clostridium tyrobutyricum (Braniste et al., 2014), a rod-shaped Gram-positive bacterium that grows under anaerobic conditions, produces butyric acid, decreases the expression of pro-inflammatory cytokines, and increases the diversity of the gut microbiota, or Bacteroides thetaiotaomicron, which processes various polysaccharides and interacts with other gut microbes (Durant et al., 2020; Rutsch et al., 2020), showed an improvement in the integrity of the BBB (Figure 4B). Identical effects were observed microscopically when GF mice were directly treated with butyrate, which enhances intestinal barrier function and mucosal immunity.
Figure 4. The BBB is not fully developed in GF mice. This barrier controls the biological substances essential for brain metabolic activity and neuronal function. Thus, the functional and structural integrity of the BBB is critical for preserving molecules circulating in the blood system and maintaining homeostasis of the brain microenvironment. When the BBB is compromised, it negatively impacts neurogenesis during early development by exposing the brain to excessive glucocorticoid molecules (A). Consequently, cognitive deficits and neuropsychological symptoms are highly prevalent during the long-term effects of glucocorticoids. However, a large body of evidence suggests that gut microorganisms may regulate cell junctions in the blood–brain barrier structure. GF mice treated with either Clostridium tyrobutyricum, a rod-shaped Gram-positive bacterium that grows under anaerobic conditions, produces butyric acid, decreases the expression of pro-inflammatory cytokines, and increases the diversity of the gut microbiota, or Bacteroides thetaiotaomicron, which processes various polysaccharides and interacts with other gut microbes, showed an improvement in the integrity of the BBB (B). Identical effects were observed microscopically when GF mice were directly treated with butyrate, which enhances intestinal barrier function and mucosal immunity, highlighting its important impact on the MGBA. Created with BioRender.com.
A growing body of evidence indicates that neurogenesis in the hippocampus, is influenced by the gut microbiota. In GF mice, the survival of newly generated neurons in the subgranular zone of the hippocampus is increased, which is associated with spatial memory. Nevertheless, cell proliferation remains unaltered (Guzzetta et al., 2022). This enhancement in neurogenesis may be linked to the observed deficits in spatial learning and memory in GF mice. More recent studies have highlighted the significant impact of the gut microbiota on neurogenesis. Kundu et al. (2019) further expanded upon these findings by demonstrating that young GF mice that received gut microbiota transplants from older mice exhibited increased hippocampal neurogenesis and intestinal growth. The transplanted microbiota led to an enrichment of butyrate-producing microbes, resulting in elevated levels of fibroblast growth factor 21 (Kundu et al., 2019). This increase in fibroblast growth factor 21 correlated with enhanced activation of AMPK and SIRT-1, along with reduced mTOR signaling, suggesting a mechanistic link between gut-derived metabolites and brain health (Kundu et al., 2019). The findings indicate the significance of the microbiota in brain morphology using GF mice, which are summarized in Table 2.
Recent studies have demonstrated that the gut microbiota can influence brain function and behaviour, with the potential to contribute to neurodegenerative diseases, such as PD. The degeneration of dopaminergic neurons is a hallmark of PD, and there is evidence that changes in the composition of the gut microbiota can lead to alterations in the brain’s dopaminergic systems. A recent study has demonstrated that GF mice exhibited significant dopaminergic dysfunction in comparison to specific pathogen-free mice, suggesting an increased susceptibility to PD (Wang et al., 2023). The GF mice exhibited lower dopamine levels in the frontal cortex, striatum, and hippocampus, as well as altered tyrosine hydroxylase (TH) expression, with decreased TH mRNA in the cerebellum and reduced TH protein in the striatum (Wang et al., 2023). This highlights the critical role of gut microbiota in maintaining dopaminergic function and its involvement in PD pathology.
In a study conducted by Berding and Donovan (2019), researchers examined the effects of gut microbiota on ASD by transplanting fecal microbiota from children with ASD into GF mice. The study revealed that the recipient mice exhibited behavioural changes and GI alterations analogous to those observed in individuals with ASD, including increased anxiety-like behaviors and disrupted gut function (Berding and Donovan, 2019). These changes were associated with alterations in the expression of several genes involved in immune function and neural signalling, including those related to synaptic plasticity and inflammation. Notably, the ASD microbiota promoted extensive alternative splicing of ASD-relevant genes in the brain, particularly affecting genes such as Cntnap2, Shank3, and Gabrb3, which are implicated in ASD pathology (Berding and Donovan, 2019). In contrast, other studies claimed that the absence of microbiota, results in various ASD like behaviors and neurodevelopmental changes, such as increased anxiety, reduced sociability, and neurodevelopmental alterations, that mimics ASD symptoms (Desbonnet et al., 2014; Arentsen et al., 2015). The specific bacterial taxa involved included a reduction in the levels of Bifidobacterium and an increase in the levels of Lactobacillus and Desulfovibrio. Although the transplanted microbiota did not result in the development of autism in the mice, the findings indicate that the composition of the gut microbiota plays a pivotal role in influencing ASD-related symptoms. This underscores the potential for gut microbiota-targeted therapies in managing ASD symptoms.
A recent study revealed that the transplantation of microbiota from patients with CD to GF mice resulted in the development of colitis (Sheikh et al., 2024). To investigate the potential association between CD and the gut microbiota in the context of the gut-brain axis, researchers collected fecal samples from healthy controls and CD patients. GF mice were inoculated with these samples, resulting in distinct transcriptomic profiles in the colon. GF mice with CD microbiota exhibited increased susceptibility to Crohn’s-like symptoms, as evidenced by histopathology and immunohistochemistry (Sheikh et al., 2024). Principal component analysis revealed distinct clustering, with inflammatory pathways such as chemokine signalling and leukocyte transmigration significantly enriched. The expression of CD74, TNF, and cytokine receptors was found to be elevated in GF mice with CD microbiota, indicating an augmented immune response. The GF mice exhibited a greater susceptibility to CD compared to those with a healthy microbiota, as evidenced by significant inflammation and immune cell infiltration (Sheikh et al., 2024). This suggests that the gut microbiota plays a significant role in the pathogenesis of CD.
In their 2021 study, Kamimura and colleagues investigated the behavioural and neurobiological consequences of microbiota deficiency in GF mice. The results indicated that GF mice exhibited increased anxiety-like behaviours compared to specific pathogen-free mice, as evidenced by increased avoidance in open field tests (Kamimura et al., 2021). Neurobiologically, GF mice exhibited altered expression of brain-derived neurotrophic factor (BDNF) and ΔFosB in the prefrontal cortex (PFC), a region critical for stress and emotional regulation, thereby affecting the gut-brain axis (Kamimura et al., 2021). Cohabitation with specific pathogen-free mice resulted in the normalization of these behaviours, indicating a pivotal role for the gut microbiota in regulating anxiety and related neurobiological pathways (Kamimura et al., 2021).
Recent studies have highlighted the significant role of gut microbiota in modulating AD pathologies and cognitive disorders through neuroinflammation associated with polyunsaturated fatty acids (PUFAs). A study conducted on GF mice, which were recolonized with fecal samples from both AD patients and healthy donors, revealed a substantial reduction in cerebral amyloid-β plaques and neurofibrillary tangles when compared to specific-pathogen-free mice (Chen et al., 2022). This underscores the importance of a complex gut microbiome for the emergence of behavioural abnormalities and AD pathologies. The study identified an enrichment of bacteroides in the gut microbiota composition, which mediates proinflammatory PUFA metabolism, thereby activating microglia and promoting neuroinflammation in the brain. Metabolomic analysis revealed elevated levels of PUFA metabolites and oxidative enzymes, including cyclooxygenases (COX-1, COX-2) and 5-lipoxygenase, which are linked to inflammation and cognitive impairments (Chen et al., 2022). The activation of the C/EBPβ/asparagine endopeptidase (AEP) pathway was notably observed, further associating gut dysbiosis with exacerbated AD pathology and cognitive dysfunctions (Chen et al., 2022). To achieve a near-germ-free status, researchers administered a short-term antibiotic to deplete the gut microbiota in APPSWE/PS1ΔE9 transgenic mice (Peng et al., 2009; Tong et al., 2016). This was followed by FMT from aged AD mice donors. The study revealed that this microbiota reconstitution resulted in increased amyloid-beta plaque formation and altered astrocyte activation around the plaques, rather than affecting microglia. The study identified several key biomolecular changes, including the suppression of astrocytic complement component C3 and alterations in astrocyte morphology (Wang et al., 2021). The findings indicate the significance of the microbiota in Neuroimmune and neuroinflammatory, which are summarized in Table 3.
Creutzfeldt–Jakob disease (CJD) is a neurologic disorder caused by the buildup of misfolded prion proteins (PrPSc) in the brain, resulting in spongiform degeneration, and astrogliosis. Common symptoms of CJD include rapidly worsening dementia, myoclonus, visual impairments, and ataxia (Nakhal et al., 2024). In prion disease patients, there is a reduction in SCFAs due to decreased Prevotellaceae (Guo et al., 2022; Salim et al., 2023). Increased levels of Fusobacteria have also been observed in CJD patients (Sparks Stein et al., 2012; Bhattacharyya et al., 2016). A study reported that the absence of the commensal gut microbiota in germ-free mice did not affect the susceptibility to prion disease (Bradford et al., 2017). The distribution of neuropathological hallmarks of terminal prion disease, such as spongiform pathology, accumulation of PrPSc, astrogliosis, and microglial activation, were similar in both conventionally housed mice with a typical microbiota composition and germ-free mice (Bradford et al., 2017). During CNS prion disease, microglial activation is anti-inflammatory, aiding in prion clearance. The study found that microbiota absence minimally affected microglial abundance or complexity, indicating their limited role in microglial behavior during prion disease (Bradford et al., 2017). The influence of the microbiota on microglial function may depend on their activation status and phenotype.
The precise methods of communication between the gut microbiota and the CNS remain elusive. Nevertheless, several potential mechanisms have been proposed through which the bacteria in the gut may influence brain function (Borre et al., 2014). One pathway stands out significantly, namely the production of SCFAs with neuroactive characteristics (Tan et al., 2014; Fung et al., 2017; Dalile et al., 2019). The primary metabolites generated in the colon through bacterial fermentation of dietary fibers and resistant starch are acetate, propionate, and butyrate, along with additional minor metabolites such as lactate, valerate, and formate (Pascale et al., 2018). In addition to their established roles in energy provision and T regulatory cell regulation, there is mounting evidence suggesting that SCFAs play a pivotal role in influencing the physiological functions of the brain (Stilling et al., 2016; Dalile et al., 2019) (Figure 5). A number of studies in animals and humans have proposed the concept of manipulating the microbiota and administering SCFAs as potential key candidate treatments for neurological disorders such as depression, AD, PD, and ASD (Stilling et al., 2016; Zhang et al., 2017; Deng et al., 2019; Sharon et al., 2019).
Figure 5. The role of SCFAs produced by gut bacteria in overall brain structure and function. (A) The primary metabolites generated in the colon through bacterial fermentation of dietary fibers and resistant starch are acetate, propionate, and butyrate. (B) SCFAs are absorbed by colon cells, primarily via MCTs and SMCTs. This process allows for the direct influence of SCFAs on the brain through the vagus nerve. SCFAs that are not utilized by colon cells are transported into the. Moreover, SCFAs interact with GPCRs. Well-researched SCFA receptors include FFAR2 and FFAR3, as well as HCAR2 and GPR164. These receptors are expressed in a variety of tissues, including the GI mucosa, the CNS, and the immune system. SCFAs can influence the brain indirectly by binding to their receptors on enteroendocrine cells, thereby stimulating the production of GLP-1 and PYY. However, when binding to β-pancreatic cells, it causes an increase in insulin output. Furthermore, SCFAs can reduce inflammatory signalling in the CNS by influencing the immune system at the cellular level. This is achieved by regulating overall systemic functions and inhibiting the activity of HDAC, which leads to the acetylation of lysine residues on nucleosomal histones and the release of IL-4, IL-6, IL-10, and IL-11, furthermore, it has been well-documented that butyrate can induce regulatory T cell (Treg) differentiation and regulate inflammation. (C) SCFAs improve BBB selectivity, microglia maturation while reducing microglial activation and the release of pro-inflammatory cytokines by lowering the production of IL-1β, IL-6, and TNF-α, as well as the phosphorylation of p38 MAPK, JNK, and NF-κB. Acetate can regulate pro-inflammatory pathways, inflammatory cytokines signalling, activation, and proliferation of primary astrocytes. SCFAs enhance neuroplasticity and neurogenesis in the brain. Created with BioRender.com.
Following the production of SCFAs by microbial bacteria (Figure 5A), they are absorbed by colon cells, primarily via H+ − dependent (MCTs) or sodium-dependent monocarboxylate transporters. This process allows for the direct influence of SCFAs on the brain through the vagus nerve (Vijay and Morris, 2014). SCFAs that are not utilized by colon cells are transported into the portal vein and serve as an energy source for liver cells, with the exception of acetate, which is not metabolized in hepatocytes (Schönfeld and Wojtczak, 2016). Moreover, SCFAs interact with G protein-coupled receptors. Well-researched SCFA receptors include free fatty acid receptor (FFAR2) and FFAR3, as well as GPR109a/HCAR2 (hydrocarboxylic acid receptor) and GPR164 (Figure 5B). These receptors are expressed in a variety of tissues, including the GI mucosa, the CNS, and the immune system (Bolognini et al., 2016; Mohajeri et al., 2018). The outcome of stimulating such receptors varies considerably depending on the location of expression. For instance, SCFAs can influence the brain indirectly by binding to their receptors on enteroendocrine cells, thereby stimulating the production of glucagon-like peptide 1 and peptide YY (Cherbut et al., 1998). However, when binding to β-pancreatic cells, it causes an increase in insulin output (Puddu et al., 2014). Furthermore, SCFAs can reduce inflammatory signalling in the CNS by influencing the immune system at the cellular level. This is achieved by regulating overall systemic functions and inhibiting the activity of histone deacetylase, which leads to the acetylation of lysine residues on nucleosomal histones and the release of interleukins such as IL-4, IL-6, IL-10, and IL-11, furthermore, it has been well-documented that butyrate has the ability to induce regulatory T cell differentiation and regulate inflammation (Arpaia et al., 2013; Smith et al., 2013; Haghikia et al., 2015; Fung et al., 2017).
SCFAs have been linked with an essential role in the communication between the microbiota and the brain. This is evidenced by the fact that all three main metabolites (acetate, propionate, and butyrate) can be identified in human cerebrospinal fluid (Vijay and Morris, 2014). Acetate concentrations typically range from 0 to 171 μM, propionate from 0 to 6 μM, and butyrate from 0 to 2.8 μM, with a molar ratio of approximately 60:20:20 (Human Metabolome Database, 2023). Animal studies in rats and mice have demonstrated that the levels of butyrate in the brain increase significantly when supplemented with live bacteria such as Clostridium butyricum. Moreover, the high levels of monocarboxylate transporters in endothelial cells facilitate the crossing of SCFAs across the BBB, as evidenced by previous studies in which rats absorbed 14C-SCFAs injected into the carotid artery (Oldendorf, 1973; Kekuda et al., 2013; Liu et al., 2015; Sun et al., 2016). As demonstrated in (Figure 5), the role of bacteria-produced SCFAs is further elucidated.
SCFAs not only penetrate the BBB, but they also help maintain its integrity, which is crucial for regulating the transport of substances and nutrients from the bloodstream to the brain. This process is essential for brain growth and maintaining the balance of the CNS (Silva et al., 2020). This claim was supported by GF mice, which exhibited decreased levels of tight junction proteins, including claudin and occludin, resulting in elevated permeability of the BBB throughout prenatal development to adolescence. Nevertheless, the BBB quality was retrieved after recolonization with SCFAs-producing bacteria. Given that most neurological diseases are linked with BBB disruption and decreased selectivity, butyrate has been shown to attenuate the behavioral assessment results of neurological disorders by improving BBB selectivity (Downs et al., 2014).
Microglial cells have been demonstrated to play a pivotal role in the removal of superfluous or unnecessary synaptic connections, which is vital for the development of interconnections in the nervous system (Liu et al., 2015; Wilton et al., 2019). Consequently, it appears that gut microbiota bacteria exert a pivotal influence on the formation and functionality of the innate immune system within the CNS, through their impact on the maturation and functionality of microglial cells (Erny et al., 2015). This was corroborated by the observation that GF animals exhibited abnormalities in microglia, including changes in cell morphology and an immature phenotype, which resulted in compromised innate immune reactions. Supplementation of acetate, propionate, and butyrate to GF mice resulted in reversal of microglial characteristics and responsiveness, accompanied by improvement in maturation (Stilling et al., 2016; D’Alessandro et al., 2022). The hypothesis is that the pathway of microglial maturation through SCFAs produced by gut bacteria is through the activation of FFAR2, which results in the inhibition of Histone deacetylases (HDACs). This is based on the observation that mice lacking FFAR2 receptors in the GI mucosa showed similar microglial malfunction and phenotype to those in GF mice (Gautier et al., 2012). Subsequently, antibiotics have been shown to induce depletion of the gut microbiota in experimental animals, resulting in increased neurological inflammation and changes in microglial morphology towards a pro-inflammatory state (Minter et al., 2017; Jang et al., 2018). However, it is noteworthy that sodium butyrate has been demonstrated to possess the capacity to reduce microglial activation and the release of pro-inflammatory cytokines in a range of neurological disorders (Wang et al., 2015; Patnala et al., 2017; Yamawaki et al., 2018). Similarly, similar results were observed in other SCFAs, such as acetate. Microglial culture treatment with acetate has been shown to decrease the expression of inflammatory signals by lowering the production of IL-1β, IL-6, and TNF-α, as well as the phosphorylation of p38 MAPK, JNK, and NF-κB (Soliman et al., 2012).
Microbial metabolites contribute to neuronal function, as evidenced by the reported regulation of neurotransmitter and neurotrophic factor levels by SCFAs through the HPA axis (Silva et al., 2020). For example, acetate has been shown to alter the levels of neurotransmitters, including glutamate, glutamine, and GABA, in the hypothalamus, and to enhance the expression of anorexigenic neuropeptides (Frost et al., 2014). It has been demonstrated that propionate and butyrate modulate intracellular potassium levels, thereby suggesting that SCFAs are involved in cell signalling systems (Oleskin and Shenderov, 2016). SCFAs exert their influence on the brain neurons through a variety of mechanisms. For instance, they regulate the levels of tryptophan 5-hydroxylase 1, an enzyme involved in serotonin production, and tyrosine hydroxylase, which is essential for the synthesis of dopamine, noradrenaline, and adrenaline. This enables them to influence cerebral neurochemistry (Clarke et al., 2014; Nankova et al., 2014; Reigstad et al., 2015; Yano et al., 2015). Additionally, SCFAs have been demonstrated to modulate neurotrophic factors, including nerve growth factor, glial cell line-derived neurotrophic factor, and BDNF. These factors are involved in the development, viability, proliferation, and differentiation of neurons and synapses in the brain (Intlekofer et al., 2013; Savignac et al., 2013; Barichello et al., 2015; Varela et al., 2015). In a corresponding manner, all three SCFAs were observed to enhance the survival and growth of human brain progenitor cells and to promote mitosis. These findings provide insights into the potential role of SCFAs in regulating early nervous system development and hippocampal neurogenesis (Levenson et al., 2004; Yang et al., 2020). The significance of SCFAs in shaping the CNS development is well established. Emerging evidence indicates that SCFAs can attenuate long-term memory, cognition, and anxiety in various neurodevelopmental and neurodegenerative diseases (Wang et al., 2012; Dinan and Cryan, 2017; Kelly et al., 2017; Ho et al., 2018; Skonieczna-Żydecka et al., 2018).
Astrocytes are the predominant cells in the CNS of humans, considered as a subtype of glial cells (Parpura et al., 2012). They provide reduction against glutamate toxicity, glucose induced stress, and redox stress (Bolaños, 2016). It is well documented that astrocytes lose their homeostatic functions and gain toxic functions in neurodegenerative diseases (Valori et al., 2019). Nonetheless acetate has shown the ability to regulate pro-inflammatory pathways, inflammatory cytokines signalling, activation, and proliferation of primary astrocytes, which elucidates the attenuating effects of experimental models induced neurological disorders when supplemented with acetate (Soliman et al., 2013). In contrast, there is a lack of evidence to the role of propionate and butyrate in modulating astrocyte’s function.
A number of studies have demonstrated that the composition of the gut microbiome and metabolome is altered in individuals with various brain disorders (Hill et al., 2014; Li et al., 2016; Unger et al., 2016; He et al., 2018; Zhai et al., 2019). Consequently, the targeting of gut bacteria metabolites for the prevention or mitigation of CNS pathologies progression represents a promising avenue for future research (Wang et al., 2012; Dinan and Cryan, 2017; Kelly et al., 2017; He et al., 2018; Skonieczna-Żydecka et al., 2018). In accordance with this hypothesis, clinical studies have indicated that the diagnosis and severity of ASD are correlated with the composition of SCFAs-producing bacteria in human stool. Specifically, butyrate-producing bacteria levels were found to be considerably low, while propionate-producing bacteria were increased (Finegold et al., 2010; Finegold, 2011). Consequently, one of the validated experimental animal models of autism is the induction of autism by propionate. The administration of elevated amounts of propionate to different animal models via the subcutaneous, intragastric, intraperitoneal, or intracerebroventricular routes has been shown to initiate microglia abnormal stimulation, neurotoxic cytokine output, genetic expression modifications, atypical hippocampal histology, and ASD-like behaviours, including repetitive movement, increased anxiety, and impaired social cooperation (Choi et al., 2018). Conversely, butyrate has been shown to mitigate the behavioural outcomes of Black and Tan BRachyury (BTBR) mice with ASD-like symptoms (Kratsman et al., 2016). The aforementioned improvements may result from HDAC inhibition, which leads to epigenetic changes and regulates the transcription of inhibitory neurotransmitters in the brain’s PFC (Kratsman et al., 2016). Additionally, enhancing BBB integrity may contribute to these improvements (Delgado, 2000). Moreover, depression is a highly prevalent mood disorder that is characterized by social impairments and a high risk of mortality. It is typically associated with a deficiency of monoamines, disturbances in neurogenesis, and elevated levels of inflammatory biomarkers in the brain (Miller and Raison, 2016; Szczesniak et al., 2016). In accordance with these findings, clinical reports have illustrated that stool SCFAs levels were significantly lower in patients diagnosed with depression in comparison with healthy controls (Valvassori et al., 2016; Skonieczna-Żydecka et al., 2018). Other animal models of depression exhibited a similar fecal composition and low SCFAs levels (Deng et al., 2019). SCFAs have also been associated with improving behavioural assessment of the mouse model of depression. This is evidenced by the induction of antidepressant-like benefits, higher energy levels, decreased anhedonia, improved sociability, and cognitive functions (Wei et al., 2015; Burokas et al., 2017). SCFAs have demonstrated efficacy in the treatment of mood disorders, with evidence suggesting their ability to reverse social impairment and reduce stress-induced corticosterone release (Van De Wouw et al., 2018). Additionally, they have been shown to decrease manic attacks and depressive-like characteristics in rats (Resende et al., 2013). Butyrate demonstrated anti-manic properties in a rat model of bipolar disorder induced by ouabain (Valvassori et al., 2016). Conversely, a clinical study of schizophrenic patients has documented elevated levels of SCFA-producing bacteria in their microbiome composition, despite direct evaluation of metabolite levels or the presence of metabolite imbalances (He et al., 2018).
AD is the most prevalent form of dementia. It is characterized by a progressive decline in cognitive function (Prince et al., 2016). Several studies have demonstrated the benefits of maintaining a balanced microbiome in slowing the progression of AD. There is also a growing body of evidence indicating a link between dysbiosis and the increasing severity of the disease (Cryan and Dinan, 2012; Hill et al., 2014; Hoffman et al., 2019). In this context, an animal microbiome study reported a decline in SCFAs levels in a mouse model of AD (Zhang et al., 2017). SCFAs act by interrupting the main synapse dysfunction and cognitive impairment toxin known as amyloid-β peptides, which disrupt their progression into neurotoxic molecules (Ho et al., 2019). This notion is consistent with previous research demonstrating the positive impacts of butyrate and probiotic therapy on cognitive function and memory in D-galactose aging mice and rats models, which are associated with the development and advancement of AD (Garcez et al., 2018; Ho et al., 2019). Finally, butyrate supplementation improved memory function and elevated the expression of genes associated with cognitive learning in the APP/PS1 mice model of AD by inhibiting HDAC (Govindarajan et al., 2011).
The role of SCFAs in PD is a topic of contention. PD is a complex, multifactorial disorder that presents with tremors, stiffened muscles, bradykinesia, and distorted movement. These symptoms result from α-synuclein (αSyn) aggregation, which downregulates dopaminergic neurons. The sequencing results of the stool microbiota of PD patients indicated a reduced population of propionate-producing bacteria species, as well as increased amounts of Enterobacteriaceae, a family known to deplete SCFAs production when compared with healthy controls (Unger et al., 2016). Nevertheless, the presence of gut microbiota is of substantial importance for αSyn abnormal expression, as evidenced by the findings of gut dysbiosis models, which demonstrated an improvement in disease progression. The intricate composition of the microbiome is either dominated by bacterial strains that ferment fibre and provide SCFAs, which ameliorate PD pathophysiology, or by bacterial strains that degrade fibre, thereby exacerbating PD progression and development due to excessive synthesis of endotoxins and neurotoxins (Li et al., 2017). In accordance with these reports, the administration of butyrate to animal models of Parkinson’s disease has been observed to enhance motor function and alleviate dopamine depletion (Laurent et al., 2013; Sharma et al., 2015; Liu et al., 2017; Paiva et al., 2017).
The pivotal role of these biochemical messengers produced by intestinal bacteria in CNS development and homeostasis has been established (Silva et al., 2020). Whole-genome sequencing and metabolomics have identified some of the bacteria that produce SCFAs. The production of SCFAs can occur directly from bacterial fermentation or by cross-feeding from other SCFAs, such as acetate, which assists the growth of propionate (Fusco et al., 2023). The primary species responsible for butyrate production are Lachnospiraceae and Ruminococcaceae, as well as other families such as Lactobacillaceae, Unclassified Clostridiales 5, and Clostridiaceae, which are predominantly classified to the Firmicutes phylum (Singh et al., 2023). Nevertheless, a few species belonging to Actinobacteria, Fusobacteria, and Proteobacteria are also capable of producing butyrate (Vital et al., 2014; Anand et al., 2016). The Ruminococcaceae family contains Faecalibacterium prausnitzii, which constitutes between 5 and 17% of the microbiota found in the feces of healthy adults. This bacterium is one of the most prevalent in the production of butyrate (Miquel et al., 2013; Martín et al., 2018). Other species, including Anaerobutyricum hallii, Eubacterium rectale, Lactobacillus casei, Coprococcus eutactus, Coprococcus comes, Butyricicoccus pullicaecorum, Roseburia intestinalis, and Clostridium butyricum, were also found to produce butyrate in healthy individuals (Fusco et al., 2023). An example of cross-feeding is observed when Akkermansia muciniphila stimulates the release of oligosaccharides and acetate to feed Anaerobutyricum hallii, thereby generating propionate and butyrate (Belzer et al., 2017; Geerlings et al., 2018; Paone and Cani, 2020). Additionally, increased butyrate production through cross-feeding is observed among Faecalibacterium prausnitzii and Bifidobacterium adolescentis (Fusco et al., 2023). Moreover, genomic studies in animal models and healthy humans have indicated that an increase in Bifidobacteria is associated with elevated SCFAs levels (Wang et al., 2014; Hor et al., 2019; Pérez-Burillo et al., 2020). In particular, B. bifidum, B. infantis, and B. breve are the primary acetate producers within the Bifidobacteriaceae family (Matsuki et al., 2016; Tsukuda et al., 2021), in addition to Dialister invisus and Akkermansia muciniphila, which are capable of producing both acetate and propionate (Nagao-Kitamoto and Kamada, 2017; Rodrigues et al., 2022). Other documented propionate-producing bacteria include Phascolarctobacterium succinatutens, Anaerobutyricum hallii, Anaerostipes caccae, Coprococcus eutactus, Coprococcus comes, and Prevotella spp. (Watanabe et al., 2012; Reichardt et al., 2014; Salonen et al., 2014; De Vadder et al., 2016).
The abundance of Acinetobacter calcoaceticus in patients with MS was found to be higher than in healthy participants (Cekanaviciute et al., 2017). The mechanism of action of Acinetobacter calcoaceticus has been demonstrated to promote MS by inducing pro-inflammatory cytokines and Th1, while blocking T regulatory cells in humans and mice (Chatterjee and Banerjee, 2020). Conversely, not all species from the Actinobacteria phylum are linked with deteriorating effects. For instance, Bifidobacterium bifidum (Martínez Pizarro, 2022), Bifidobacterium longum (Pinto-Sanchez et al., 2017), and Bifidobacterium breve (Głaz et al., 2023), it was demonstrated that this bacterium decreases depression scores and mitigates major depression disorder by modulating the gut flora and tryptophan metabolism. This could be a potential target for depression treatment or prevention (Li et al., 2023). Furthermore, a reduction in the population of gut microbiota belonging to the Bifidobacterium spp. was observed in AD patients when compared to healthy controls (Chandra et al., 2023). A taxonomic analysis of the Actinobacteria phylum was conducted in schizophrenic patients, revealing an increase in the abundance of Bifidobacterium dentium, Collinsella aerofaciens, and Bifidobacterium adolescentis, while Bifidobacterium bifidum, Bifidobacterium longum, and Bifidobacterium breve exhibited a decrease (Kraeuter et al., 2020). In accordance with previous studies, two-year-old mice exhibited a reduction in beneficial bifidobacteria (Bifidobacterium bifidum, Bifidobacterium breve, Bifidobacterium infantis, Bifidobacterium longum) in comparison with their fecal composition at a younger age. Furthermore, colonization with a mixture of infant-type bifidobacterium strains has been shown to reverse cognitive deficits in GF mice (Luk et al., 2018). Their abundance is negatively correlated with pro-inflammatory cytokines in humans (Hébuterne, 2003). In a mouse model of AD, supplementation with SLAB51, which includes Bifidobacterium longum, Bifidobacterium breve, and Bifidobacterium infantis, restored neuroplasticity by acting on neuronal proteolytic pathways, such as autophagy and the ubiquitin-proteasome system. Additionally, it reduced oxidative stress biomarkers in the brain (Bonfili et al., 2017). In humans, supplementation with Bifidobacterium animalis has been linked with reduced psychiatric symptoms and anxiety occurrence (Severance et al., 2017). Furthermore, an fMRI study demonstrated that Bifidobacterium longum administration resulted in a reduction in the reactivity to negative emotional stimuli in various brain regions, including the amygdala and fronto-limbic areas, when compared to the placebo group (Pinto-Sanchez et al., 2017). In a similar vein, the administration of Bifidobacterium longum has been observed to upregulate hypothalamic BDNF, which in turn promotes neural plasticity and neurogenesis in a brain stress-induced model of mice (Ait-Belgnaoui et al., 2014). Consequently, the brain alterations associated with Bifidobacterium longum have been demonstrated to result in behavioural and cognitive ameliorations in clinical and experimental studies. These include stress attenuation, anxiety reduction, and improved memory performance in healthy humans and AD patients (Allen et al., 2016). Additionally, elevated fear learning and short-term memory have been observed in anxious adult mice (Savignac et al., 2015) It was demonstrated that Bifidobacterium longum could improve the behavioural symptoms of autistic children (Grimaldi et al., 2018). Furthermore, it has been shown to decrease depression scores in individuals with mild to moderate MDD (Kazemi et al., 2019) and IBS patients (Pinto-Sanchez et al., 2017). Finally, Bifidobacterium animalis has been shown to restore cognition and memory in an AD rat model (Athari Nik Azm et al., 2018). The anxiolytic impact observed in this study could be attributed to the ability of the compound to regulate the HPA axis response, as evidenced by previous studies in mice, rats, and humans (Messaoudi et al., 2011; Ait-Belgnaoui et al., 2014). This effect involves the regulation of CNS and ENS hormone secretion homeostasis. Inulin and omega-3 have both been demonstrated to enhance brain function, and there is also evidence that they can increase the population of bifidobacteria in the microbiome (De Preter et al., 2008; Robertson et al., 2017). In a study conducted by Luk et al. (2018), it was observed that colonization of bifidobacteria in adult mice resulted in enhanced anxiety, cognition, locomotion, and memory in infants (Luk et al., 2018). This evidence suggests that the microbiome plays a pivotal role in the process of aging. The Human Microbiome Project has proposed that the microbiome found in faeces has the ability to generate glutamate decarboxylase, an enzyme that transforms glutamic acid into gamma-aminobutyric acid (GABA) (Pokusaeva et al., 2017). Similarly, a study reported that Bifidobacterium adolescentis from the human gut (Barrett et al., 2012) was able to convert monosodium glutamate into GABA. This process was also observed in rats’ hippocampus after gut dysbiosis caused by antibiotics (Liang et al., 2017). Bifidobacterium adolescentis has been linked with the reduction of depression, anxiety, and improvement of sleep quality in humans (Lee et al., 2021). These findings show varying effects of Actinobacteria on the brain, with species-specific variations in benefits and detriments.
Faecalibacterium prausnitzii has been demonstrated to exhibit anti-inflammatory, anxiolytic, and antidepressant therapeutic benefits in rats. These benefits are achieved by increasing butyrate production and IL-10 while reducing corticosterone and IL-6 (Hao et al., 2019). Inulin administration has been shown to enhance Faecalibacterium prausnitzii growth (Ramirez-Farias et al., 2008). Furthermore, a clinical study involving over 1,000 participants demonstrated that individuals with depression and low quality of life exhibited a lower abundance of species belonging to the Faecalibacterium and Coprococcus genera when compared with healthy controls (Valles-Colomer et al., 2019). An analysis of fecal microbiota revealed reduced levels of Firmicutes, particularly Faecalibacterium, in individuals with bipolar disorder (Evans et al., 2017). In a separate study, fecal samples from Chinese patients with transient ischemic attacks exhibited significantly reduced levels of Faecalibacterium in their fecal samples (Yin et al., 2015). The levels of Faecalibacterium prausnitzii are negatively correlated with aging, as this bacterium has been associated with reducing frailty in geriatrics (Bartosch et al., 2004; Van Tongeren et al., 2005). The abundance of Faecalibacterium prausnitzii is reduced in individuals with mild cognitive impairment, and specific strains of Faecalibacterium prausnitzii obtained from healthy volunteers have been demonstrated to enhance cognitive function in mice with cognitive impairment. A comprehensive metagenome and whole-genome analysis has identified these strains as potential candidates for interventions targeting the gut microbiome in AD. It can be seen that there is a difference in the levels of Clostridium coccoides present in the stool samples of patients with PD and those of healthy controls (Ueda et al., 2021). This is in accordance with the findings of Hasegawa et al., 2015, where the abundance of Clostridium coccoides was found to be significantly lower in the stool samples of patients with PD than in those of healthy controls (Hasegawa et al., 2015). The colonization of Clostridium coccoides into GF mice resulted in a decrease in anxiety levels, while having no significant impact on locomotor activity (Nishino et al., 2013). Such behavioural modifications may be attributed to the enzymatic activity of beta glucuronidase, which enables Clostridium coccoides and Clostridium leptum to alter dopamine into its active form (Asano et al., 2012).
Some species from the Firmicutes phylum exhibit both commensal and pathogenic characteristics. Clostridioides difficile, for instance, is known to induce mental confusion and brain fog as a result of dopamine metabolism dysregulation (Vinithakumari et al., 2021). This bacterium is prevalent in autistic children, although its presence has been associated with the use of intense antibiotic regimens that destroy beneficial bacteria and allow Clostridioides difficile to flourish (Kakabadze et al., 2022). The expression of IL-10 in mice was found to be associated with a higher presence of Clostridium spp., suggesting that there may be a collaborative relationship between the gut bacteria, the adaptive immune system, and the CNS (Smith et al., 2014). Conversely, certain species belonging to the Blautia genus, including Blautia coccoides and Blautia hydrogenotrophica, which are responsible for the production of propionate and acetate, respectively, have been linked to 5-HT production. In a mouse model of autism, these bacteria have been observed to display pro-5-HT activity (Golubeva et al., 2017). In addition to the modulation of 5-HT production in humans and rodents by Clostridium perfringens (Beaver and Wostmann, 1962). Clostridium butyricum has been demonstrated to confer anti-depressive benefits in a chronic stress mouse model (Sun et al., 2018). A taxonomic analysis revealed a decline in lactobacillus species with age, suggesting a potential correlation with age-related neurodegenerative diseases (Hébuterne, 2003). Lactobacillus reuteri has been shown to improve behavioural examination results in a mouse model of ASD, including sociability, social novelty, and reciprocal social interaction (Sgritta et al., 2019).
Lactobacillus acidophilus, Lactobacillus reuteri, Lactobacillus casei, Lactobacillus delbrueckii subsp. bulgaricus, and Lactobacillus brevis have been demonstrated to produce GABA and glucagon-like peptide-1. However, GF mice exhibited lower levels of these metabolites when compared with control groups (Li et al., 2008; Zareian et al., 2012; Simon et al., 2015; Panwar et al., 2016). In accordance with this hypothesis, Lactobacillus strains have been extensively employed for this purpose as probiotic treatments. A variety of strains from the Lactobacillus family have been demonstrated to improve object recognition memory impairments caused by continuous restraint stress (Liang et al., 2015), as well as to avoid these impairments in GF mice (Gareau et al., 2011), a mouse model of colitis (Emge et al., 2016), diabetic mice (Davari et al., 2013), and immunodeficient mice (Smith et al., 2014). It is noteworthy that the administration of lactobacillus strains has been shown to improve cognitive test results in healthy adults (Chung et al., 2014) and AD patients (Akbari et al., 2016), in comparison with a placebo group. Conversely, a high abundance of lactobacillus has been observed in PD patients (Hasegawa et al., 2015). The strains were successful in correcting spatial memory impairments caused by numerous factors in mice. Furthermore, the depletion of species from the Lachnospiraceae and Lactobacillus families has been observed in human MDD (Naseribafrouei et al., 2014; Aizawa et al., 2016). Lactobacillus farciminis and Lactobacillus salivarius have been observed to inhibit the overstimulation of the HPA axis during acute stress in rats. This effect may be attributed to their ability to prevent excessive permeability of the gut, which has been linked to benefits in the reduction of aggression, anxiety, and depression-related disorders (Ait-Belgnaoui et al., 2012; Bagga et al., 2018). Lactobacillus acidophilus, Lactobacillus plantarum, Lactobacillus casei, and Lactobacillus delbrueckii subspecies Bulgaricus have been demonstrated to improve age-related impairments in LTP, upregulate hippocampal BDNF, and downregulate pro-inflammatory cytokines, thereby improving neuroplasticity and neurogenesis in aged rats (Distrutti et al., 2014). The administration of Lacticaseibacillus rhamnosus during pregnancy has been observed to markedly reduce the incidence of postnatal depression and anxiety in humans (Slykerman et al., 2017). This effect has also been demonstrated in the context of obesity (Sanchez et al., 2017). The administration of a probiotic formulation comprising Lacticaseibacillus rhamnosus and Lactobacillus helveticus has been demonstrated to mitigate the impact of early-life stress on fear behavior and its protective effects on brain networks during development. This explains their benefits in neurodevelopmental diseases such as ASD (Callaghan et al., 2016; Cowan et al., 2016). Experimental and clinical studies have demonstrated that Lacticaseibacillus rhamnosus functions via a G protein-coupled receptor-mediated pathway (Mao et al., 2013). This results in increased vagal and spinal cord nerve bundle firing patterns (Perez-Burgos et al., 2013), which have been observed to have beneficial effects, including the alleviation of anxiety in an impaired adaptive immune system mouse model It was demonstrated that Lacticaseibacillus rhamnosus reversed the reduction in GABA levels induced by antibiotics in the hippocampus of juvenile rats (Smith et al., 2014; Liang et al., 2017). Furthermore, in 2011, it was shown that Lacticaseibacillus rhamnosus prevented memory dysfunction induced by Citrobacter rodentium by regulating the c-fos gene (Gareau et al., 2011). Additionally, it was observed that Lacticaseibacillus rhamnosus normalized fear behavior in maternally deprived pups (Cowan et al., 2016). Finally, in 2017, it was demonstrated that Lacticaseibacillus rhamnosus improved psychiatric symptoms in humans and males, particularly (Severance et al., 2017). The supplementation of Lactobacillus reuteri, initially known as Lactobacillus fermentum, has been demonstrated to upregulate NMDAR expression in the CA1 and DG of the rat hippocampus, thereby enhancing memory (Wang et al., 2015). The abundance of the Roseburia genus is indicative of a healthy microbiota, given its anti-inflammatory and immune homeostasis properties. It has been demonstrated that characteristics such as abundance are associated with the consumption of healthy unsaturated fatty acids (Devillard et al., 2007). In particular, the Eubacterium rectale bacteria from the Roseburia genus have been observed to increase with exercise in rats, and they are considered to be a component of a healthy microbiome (Queipo-Ortuño et al., 2013). Furthermore, a reduction in species belonging to the Vellionella genus was observed in a genetic model of autism in mice (Tabouy et al., 2018). Some species from the Firmicutes phylum have been identified as harmful bacteria, including Megasphaera spp., which has been observed to be present in patients with transient ischemic attacks (Yin et al., 2015). A multitude of studies have demonstrated the effect of species from the Lachnospiraceae family on the brain of humans and experimental animals. Lachnospiraceae levels were found to be significantly lower in human depression (Naseribafrouei et al., 2014) and bipolar patients (Flowers et al., 2017), but increased in sleep fragmentation of mice (Poroyko et al., 2016). Nevertheless, atypical antipsychotics have been shown to significantly increase Lachnospiraceae levels in bipolar cohorts’ microbiomes (Flowers et al., 2017). Such influence may be attributed to SCFAs production (Duncan et al., 2002) and the regulation of brain reactivity to fear in the PFC of humans (Callaghan et al., 2020).
It is well established that protein-rich diets and the Mediterranean diet elevate levels of Bacteroides and Parabacteroides in humans and other experimental models (Wu and Hui, 2011; Marlow et al., 2013; Olson et al., 2018). It has been demonstrated that Bacteroides fragilis can influence brain functions through its capsular exopolysaccharide at the cell surface, which affects the ENS (Mao et al., 2013). The Mullen Scales of Early Learning indicate that one-year-old toddlers with higher levels of GM Bacteroides were less likely to be born by C-section and exhibited significantly better cognitive abilities (Carlson et al., 2018). Clinical and experimental studies have indicated that Bacteroides may play a role, particularly Bacteroides fragilis, in ameliorating brain reactivity to fear in the PFC of children (Callaghan et al., 2020), reducing anxiety and stress responses in mice (Hsiao et al., 2013), without improving sociability. A taxonomic analysis of neurodegenerative diseases such as MS, AD, and PD revealed significantly reduced levels of Parabacteroides distasonis (Cekanaviciute et al., 2017) and Bacteroides fragilis (Hasegawa et al., 2015) when compared with healthy controls. This suggests that these bacteria may have anti-inflammatory benefits in various brain regions. Despite the benefits of Parabacteroides distasonis and Bacteroides fragilis, a cohort study of 37 depressed patients revealed that the overall Bacteroidales were relatively higher when compared with healthy controls (Naseribafrouei et al., 2014). VPA-exposed experimental models are known to develop ASD-like symptoms (Nakhal et al., 2023), accompanied by disruption of the GI microbiota, which is characterized by alterations in the Firmicutes: Bacteroidetes ratio (De Vadder et al., 2014). The microbiome of patients with PD exhibited a striking reduction in species belonging to the Prevotellaceae family (Keshavarzian et al., 2015). Furthermore, a reduction in species belonging to the Prevotella genus was observed in a genetic mouse model of autism (Tabouy et al., 2018). Porphyromonas spp. have been linked to cognitive decline in humans (Bajaj et al., 2012; Collins et al., 2012), as well as aging and increased anxiety in mice (Scott et al., 2017).
A significant prevalence of sulphate-reducing bacteria species from the Desulfovibrio genus, including Desulfovibrio desulfuricans and Desulfovibrio desulfuricans 2, has been observed in children with ASD compared to healthy controls (Karnachuk et al., 2021). In another study, species from the Enterobacteriaceae family were found to be increased in PD cohorts and their levels were positively correlated with postural instability and dynamic (Keshavarzian et al., 2015). In accordance with these findings, Enterobacter and Desulfovibrio spp. were prevalent in Chinese patients with transient ischemic attack (Yin et al., 2015). AdditionallyEscherichia coli are known to produce biogenic amines, which are frequently observed in neurological disorders (Pugin et al., 2017).
The prevention of sudden epileptic seizures was found to be modulated by microbiota changes resulting from the ketogenic diet, with an increase in Erysipelotrichaceae levels (Olson et al., 2018). It is noteworthy that newly diagnosed patients with PD who had not yet received levodopa displayed low levels of Erysipelotrichaceae in their microbiota (Bedarf et al., 2017).
Bacteria from the Verrucomicrobia phylum have been demonstrated to possess pro-inflammatory characteristics in clinical studies (Keshavarzian et al., 2015). Moreover, the Verrucomicrobia phylum is present in small quantities in the typical flora of healthy individuals (Eckburg et al., 2005). However, alterations in abundance were observed in aged adults associated with low cognition scores (Manderino et al., 2017), and presented in high abundance in PD patients (Keshavarzian et al., 2015). Akkermansia muciniphila was found to be highly abundant in fecal samples of MS patients (Cekanaviciute et al., 2017), which could be linked with outcomes such as autoimmune encephalomyelitis and myelin damage in a mouse model of MS (Lee et al., 2011). In contrast, semi-supercentenarians with an age between 105 and 109 exhibited increased Akkermansia at such an advanced age, prompting further investigation into the potential role of this bacterium in healthy longevity (Biagi et al., 2016; van der Lugt et al., 2018).
Probiotic supplementation improves the gut microbiota composition in healthy adults (Khalesi et al., 2019; Li et al., 2021). Common probiotics include Lactobacillus, Bifidobacterium, Enterococcus, and Bacillus. Probiotics can increase the levels of tryptophan-derived neurotrophic factors, and aid in either preventing or treating cognitive dysfunction (Bhattacharjee and Lukiw, 2013). Certain Lactobacillus and Bifidobacterium strains produce neurotransmitters (GABA, acetylcholine, dopamine, 5-HT) that support cognition, and neural excitatory-inhibitory balance (O’Mahony et al., 2015). Prebiotics like FOS and GOS stimulate the growth of some beneficial gut bacteria growth (Duncan and Flint, 2013).
FMT involves transferring functional bacteria from the faeces of healthy individuals into the gastrointestinal tract of patients to rebuild the gut microbiota (Li et al., 2021). Sun et al. showed that FMT from healthy mice improved motor function in PD mice by mitigating intestinal inflammation and increasing dopamine levels. The anti-inflammatory effects were achieved by reduced activity of microglia and astrocytes and increased producing SCFAs (Sun et al., 2018). Huang et al. reported that three FMT sessions improved constipation and motor symptoms in a PD patient, though tremors recurred after two months, whereas constipation was relieved even after three months (Huang et al., 2019). Additionally, FMT from healthy hamsters alleviated the ASD-like symptoms in the autism hamster model by alleviating the brain oxidative stress response. In a clinical trial with 18 children with ASD, 7–8 weeks of FMT significantly improved gastrointestinal problems and behavioural symptoms (Kang et al., 2019). Also, FMT improved the bacterial diversity by increasing the abundance of Bifidobacterium, Desulfovibrio, and Prevotella.
In contrast to the brain, the gut microbiota is accessible to direct interventions, such as prebiotics, probiotics, and antibiotics, and can be influenced by lifestyle. The concept of the MGBA emerged from extensive research that clearly demonstrated a connection between the gut and the brain. The MGBA has been associated with several neurological disorders. The advent of novel techniques for studying the microbiome has facilitated a deeper comprehension of the interrelationship between neurological disorders and the gut microbiota. The evidence is accumulating that the gut microbiome affects brain morphology, function, and behaviour, including depression, psychosis, and neurological disorders. While there is a consensus on the influence of the gut microbiota on neuroplasticity and behaviour, several questions remain enigmatic. These include the following: It would be advantageous to determine which types of neurons are influenced by signals from the gut microbiota. What is the impact of the gut microbiota on the excitation/inhibition balance in the brain? Does antibiotic-induced dysbiosis affect memory storage? A deeper comprehension of the MGBA may facilitate research into microbial-based interventions and therapeutic strategies for neurological diseases.
LY: Conceptualization, Investigation, Resources, Validation, Writing – original draft, Writing – review & editing. MN: Conceptualization, Investigation, Validation, Writing – original draft. AlA: Conceptualization, Investigation, Resources, Writing – original draft. AfA: Conceptualization, Data curation, Investigation, Resources, Validation, Writing – original draft. AM: Conceptualization, Investigation, Writing – original draft. AmA: Investigation, Resources, Validation, Writing – original draft, Writing – review & editing. MH: Conceptualization, Data curation, Funding acquisition, Investigation, Project administration, Resources, Supervision, Validation, Visualization, Writing – original draft, Writing – review & editing.
The author(s) declare that financial support was received for the research, authorship, and/or publication of this article. This work was supported by the United Arab Emirates University startup grant numbers 12 M142 and 31 M525 to MH, the UAEU UPAR grant number 12 M159 to MH.
The authors declare that the research was conducted in the absence of any commercial or financial relationships that could be construed as a potential conflict of interest.
The authors declare that no Generative AI was used in the creation of this manuscript.
All claims expressed in this article are solely those of the authors and do not necessarily represent those of their affiliated organizations, or those of the publisher, the editors and the reviewers. Any product that may be evaluated in this article, or claim that may be made by its manufacturer, is not guaranteed or endorsed by the publisher.
Abdel-Haq, R., Schlachetzki, J. C. M., Glass, C. K., and Mazmanian, S. K. (2019). Microbiome–microglia connections via the gut–brain axis. J. Exp. Med. 216, 41–59. doi: 10.1084/jem.20180794
Ait-Belgnaoui, A., Colom, A., Braniste, V., Ramalho, L., Marrot, A., Cartier, C., et al. (2014). Probiotic gut effect prevents the chronic psychological stress-induced brain activity abnormality in mice. Neurogastroenterol. Motil. 26, 510–520. doi: 10.1111/nmo.12295
Ait-Belgnaoui, A., Durand, H., Cartier, C., Chaumaz, G., Eutamene, H., Ferrier, L., et al. (2012). Prevention of gut leakiness by a probiotic treatment leads to attenuated HPA response to an acute psychological stress in rats. Psychoneuroendocrinology 37, 1885–1895. doi: 10.1016/j.psyneuen.2012.03.024
Aizawa, E., Tsuji, H., Asahara, T., Takahashi, T., Teraishi, T., Yoshida, S., et al. (2016). Possible association of Bifidobacterium and Lactobacillus in the gut microbiota of patients with major depressive disorder. J. Affect. Disord. 202, 254–257. doi: 10.1016/j.jad.2016.05.038
Akbari, E., Asemi, Z., Daneshvar Kakhaki, R., Bahmani, F., Kouchaki, E., Tamtaji, O. R., et al. (2016). Effect of probiotic supplementation on cognitive function and metabolic status in Alzheimer’s disease: a randomized, double-blind and controlled trial. Front. Aging Neurosci. 8:256. doi: 10.3389/fnagi.2016.00256
Allen, A. P., Hutch, W., Borre, Y. E., Kennedy, P. J., Temko, A., Boylan, G., et al. (2016). Bifidobacterium longum 1714 as a translational psychobiotic: modulation of stress, electrophysiology and neurocognition in healthy volunteers. Transl. Psychiatry 6:e939. doi: 10.1038/tp.2016.191
Anand, S., Kaur, H., and Mande, S. S. (2016). Comparative in silico analysis of butyrate production pathways in gut commensals and pathogens. Front. Microbiol. 7:1945. doi: 10.3389/fmicb.2016.01945
Arentsen, T., Raith, H., Qian, Y., Forssberg, H., and Heijtz, R. D. (2015). Host microbiota modulates development of social preference in mice. Microb. Ecol. Health Disease 26:29719. doi: 10.3402/mehd.v26.29719
Arpaia, N., Campbell, C., Fan, X., Dikiy, S., Van Der Veeken, J., de Roos, P., et al. (2013). Metabolites produced by commensal bacteria promote peripheral regulatory T-cell generation. Nature 504, 451–455. doi: 10.1038/nature12726
Ary, K., Dotiwala, A.K., McCausland, C., and Samra, N.S. (2023). Anatomy, head and neck, blood brain barrier. Available at: https://pubmed.ncbi.nlm.nih.gov/30137840/
Asano, Y., Hiramoto, T., Nishino, R., Aiba, Y., Kimura, T., Yoshihara, K., et al. (2012). Critical role of gut microbiota in the production of biologically active, free catecholamines in the gut lumen of mice. Am. J. Physiol. Gastrointest. Liver Physiol. 303, G1288–G1295. doi: 10.1152/ajpgi.00341.2012
Athari Nik Azm, S., Djazayeri, A., Safa, M., Azami, K., Ahmadvand, B., Sabbaghziarani, F., et al. (2018). Lactobacilli and bifidobacteria ameliorate memory and learning deficits and oxidative stress in β-amyloid (1–42) injected rats. Appl. Physiol. Nutr. Metab. 43, 718–726. doi: 10.1139/apnm-2017-0648
Azman, K. F., and Zakaria, R. (2022). Recent advances on the role of brain-derived neurotrophic factor (BDNF) in neurodegenerative diseases. Int. J. Mol. Sci. 23:6827. doi: 10.3390/ijms23126827
Bagga, D., Reichert, J. L., Koschutnig, K., Aigner, C. S., Holzer, P., Koskinen, K., et al. (2018). Probiotics drive gut microbiome triggering emotional brain signatures. Gut Microbes 9, 1–11. doi: 10.1080/19490976.2018.1460015
Bajaj, J. S., Ridlon, J. M., Hylemon, P. B., Thacker, L. R., Heuman, D. M., Smith, S., et al. (2012). Linkage of gut microbiome with cognition in hepatic encephalopathy. American journal of physiology-gastrointestinal and liver. Physiology 302, G168–G175. doi: 10.1152/ajpgi.00190.2011
Barichello, T., Generoso, J. S., Simões, L. R., Faller, C. J., Ceretta, R. A., Petronilho, F., et al. (2015). Sodium butyrate prevents memory impairment by re-establishing BDNF and GDNF expression in experimental pneumococcal meningitis. Mol. Neurobiol. 52, 734–740. doi: 10.1007/s12035-014-8914-3
Barrera-Bugueño, C., Realini, O., Escobar-Luna, J., Sotomayor-Zárate, R., Gotteland, M., Julio-Pieper, M., et al. (2017). Anxiogenic effects of a Lactobacillus, inulin and the synbiotic on healthy juvenile rats. Neuroscience 359, 18–29. doi: 10.1016/j.neuroscience.2017.06.064
Barrett, E., Ross, R. P., O’Toole, P. W., Fitzgerald, G. F., and Stanton, C. (2012). γ-Aminobutyric acid production by culturable bacteria from the human intestine. J. Appl. Microbiol. 113, 411–417. doi: 10.1111/j.1365-2672.2012.05344.x
Bartosch, S., Fite, A., Macfarlane, G. T., and McMurdo, M. E. T. (2004). Characterization of bacterial communities in feces from healthy elderly volunteers and hospitalized elderly patients by using real-time PCR and effects of antibiotic treatment on the fecal microbiota. Appl. Environ. Microbiol. 70, 3575–3581. doi: 10.1128/AEM.70.6.3575-3581.2004
Beaver, M. H., and Wostmann, B. S. (1962). Histamine and 5-HYDROXYTRYPTAMINE in the intestinal tract of germ-free animals, animals HARBOURING one microbial species and conventional animals. Br. J. Pharmacol. Chemother. 19, 385–393. doi: 10.1111/j.1476-5381.1962.tb01443.x
Bedarf, J. R., Hildebrand, F., Coelho, L. P., Sunagawa, S., Bahram, M., Goeser, F., et al. (2017). Erratum to: functional implications of microbial and viral gut metagenome changes in early stage L-DOPA-naïve Parkinson’s disease patients. Genome Med. 9:61. doi: 10.1186/s13073-017-0451-z
Belzer, C., Chia, L. W., Aalvink, S., Chamlagain, B., Piironen, V., Knol, J., et al. (2017). Microbial metabolic networks at the mucus layer Lead to diet-independent butyrate and vitamin B 12 production by intestinal symbionts. MBio 8, e00770–e00717. doi: 10.1128/mBio.00770-17
Bercik, P., Denou, E., Collins, J., Jackson, W., Lu, J., Jury, J., et al. (2011). The intestinal microbiota affect central levels of brain-derived neurotropic factor and behavior in mice. Gastroenterology 141, 599–609.e3. doi: 10.1053/j.gastro.2011.04.052
Berding, K., and Donovan, S. M. (2019). Dietary patterns impact temporal dynamics of fecal microbiota composition in children with autism Spectrum disorder. Front. Nutr. 6:193. doi: 10.3389/fnut.2019.00193
Bergles, D. E., Roberts, J. D., Somogyi, P., and Jahr, C. E. (2000). Glutamatergic synapses on oligodendrocyte precursor cells in the hippocampus. Nature 405, 187–191. doi: 10.1038/35012083
Bhattacharjee, S., and Lukiw, W. J. (2013). Alzheimer’s disease and the microbiome. Front. Cell. Neurosci. 7:153. doi: 10.3389/fncel.2013.00153
Bhattacharyya, S., Ghosh, S. K., Shokeen, B., Eapan, B., Lux, R., Kiselar, J., et al. (2016). FAD-I, a Fusobacterium nucleatum Cell Wall-associated Diacylated lipoprotein that mediates human Beta Defensin 2 induction through toll-like Receptor-1/2 (TLR-1/2) and TLR-2/6. Infect. Immun. 84, 1446–1456. doi: 10.1128/IAI.01311-15
Biagi, E., Franceschi, C., Rampelli, S., Severgnini, M., Ostan, R., Turroni, S., et al. (2016). Gut microbiota and extreme longevity. Curr. Biol. 26, 1480–1485. doi: 10.1016/j.cub.2016.04.016
Bliss, T. V. P., and Cooke, S. F. (2011). Long-term potentiation and long-term depression: a clinical perspective. Clinics 66, 3–17. doi: 10.1590/S1807-59322011001300002
Bokkenheuser, V. D., Morris, G. N., Ritchie, A. E., Holdeman, L. V., and Winter, J. (1984). Biosynthesis of androgen from cortisol by a species of Clostridium recovered from human fecal Flora. J. Infect. Dis. 149, 489–494. doi: 10.1093/infdis/149.4.489
Bolaños, J. P. (2016). Bioenergetics and redox adaptations of astrocytes to neuronal activity. J. Neurochem. 139, 115–125. doi: 10.1111/jnc.13486
Bolognini, D., Tobin, A. B., Milligan, G., and Moss, C. E. (2016). The pharmacology and function of receptors for short-chain fatty acids. Mol. Pharmacol. 89, 388–398. doi: 10.1124/mol.115.102301
Bonfili, L., Cecarini, V., Berardi, S., Scarpona, S., Suchodolski, J. S., Nasuti, C., et al. (2017). Microbiota modulation counteracts Alzheimer’s disease progression influencing neuronal proteolysis and gut hormones plasma levels. Sci. Rep. 7:2426. doi: 10.1038/s41598-017-02587-2
Borre, Y. E., O’Keeffe, G. W., Clarke, G., Stanton, C., Dinan, T. G., and Cryan, J. F. (2014). Microbiota and neurodevelopmental windows: implications for brain disorders. Trends Mol. Med. 20, 509–518. doi: 10.1016/j.molmed.2014.05.002
Bradford, B. M., Tetlow, L., and Mabbott, N. A. (2017). Prion disease pathogenesis in the absence of the commensal microbiota. J. Gen. Virol. 98, 1943–1952. doi: 10.1099/jgv.0.000860
Braniste, V., al-Asmakh, M., Kowal, C., Anuar, F., Abbaspour, A., Tóth, M., et al. (2014). The gut microbiota influences blood-brain barrier permeability in mice. Sci. Transl. Med. 6:263ra158. doi: 10.1126/scitranslmed.3009759
Bravo, J. A., Forsythe, P., Chew, M. V., Escaravage, E., Savignac, H. M., Dinan, T. G., et al. (2011). Ingestion of Lactobacillus strain regulates emotional behavior and central GABA receptor expression in a mouse via the vagus nerve. Proc. Natl. Acad. Sci. USA 108, 16050–16055. doi: 10.1073/pnas.1102999108
Burokas, A., Arboleya, S., Moloney, R. D., Peterson, V. L., Murphy, K., Clarke, G., et al. (2017). Targeting the microbiota-gut-brain Axis: prebiotics have anxiolytic and antidepressant-like effects and reverse the impact of chronic stress in mice. Biol. Psychiatry 82, 472–487. doi: 10.1016/j.biopsych.2016.12.031
Caire, M. J., Reddy, V., and Varcallo, M. (2021). Physiology, Synapse. Treasure Island, FL: StatPearls Publishing.
Çalışkan, G., French, T., Enrile, S., del, M., Steffen, J., Heimesaat, M. M., et al. (2022). Antibiotic-induced gut dysbiosis leads to activation of microglia and impairment of cholinergic gamma oscillations in the hippocampus. Brain Behav. Immun. 99, 203–217. doi: 10.1016/j.bbi.2021.10.007
Callaghan, B. L., Cowan, C. S. M., and Richardson, R. (2016). Treating generational stress: effect of paternal stress on development of memory and extinction in offspring is reversed by probiotic treatment. Psychol. Sci. 27, 1171–1180. doi: 10.1177/0956797616653103
Callaghan, B. L., Fields, A., Gee, D. G., Gabard-Durnam, L., Caldera, C., Humphreys, K. L., et al. (2020). Mind and gut: associations between mood and gastrointestinal distress in children exposed to adversity. Dev. Psychopathol. 32, 309–328. doi: 10.1017/S0954579419000087
Carlson, A. L., Xia, K., Azcarate-Peril, M. A., Goldman, B. D., Ahn, M., Styner, M. A., et al. (2018). Infant gut microbiome associated with cognitive development. Biol. Psychiatry 83, 148–159. doi: 10.1016/j.biopsych.2017.06.021
Castillo-Álvarez, F., and Marzo-Sola, M. E. (2022). Role of the gut microbiota in the development of various neurological diseases. Neurologia 37, 492–498. doi: 10.1016/j.nrl.2019.03.017
Cekanaviciute, E., Yoo, B. B., Runia, T. F., Debelius, J. W., Singh, S., Nelson, C. A., et al. (2017). Gut bacteria from multiple sclerosis patients modulate human T cells and exacerbate symptoms in mouse models. Proc. Natl. Acad. Sci. USA 114, 10713–10718. doi: 10.1073/pnas.1711235114
Chandra, S., Sisodia, S. S., and Vassar, R. J. (2023). The gut microbiome in Alzheimer’s disease: what we know and what remains to be explored. Mol. Neurodegener. 18:9. doi: 10.1186/s13024-023-00595-7
Chatterjee, K., and Banerjee, S. (2020). Microbiome and motor neuron diseases. Microbiome 176, 111–122. doi: 10.1016/bs.pmbts.2020.08.010
Chen, Y., Fenoglio, K. A., Dubé, C. M., Grigoriadis, D. E., and Baram, T. Z. (2006). Cellular and molecular mechanisms of hippocampal activation by acute stress are age-dependent. Mol. Psychiatry 11, 992–1002. doi: 10.1038/sj.mp.4001863
Chen, C., Liao, J., Xia, Y., Liu, X., Jones, R., Haran, J., et al. (2022). Gut microbiota regulate Alzheimer’s disease pathologies and cognitive disorders via PUFA-associated neuroinflammation. Gut 71, 2233–2252. doi: 10.1136/gutjnl-2021-326269
Cherbut, C., Ferrier, L., Rozé, C., Anini, Y., Blottière, H., Lecannu, G., et al. (1998). Short-chain fatty acids modify colonic motility through nerves and polypeptide YY release in the rat. American journal of physiology-gastrointestinal and liver. Physiology 275, G1415–G1422. doi: 10.1152/ajpgi.1998.275.6.G1415
Chiesa, M., Guimond, D., Tyzio, R., Pons-Bennaceur, A., Lozovaya, N., Burnashev, N., et al. (2019). Term or preterm cesarean section delivery does not Lead to long-term detrimental consequences in mice. Cereb. Cortex 29, 2424–2436. doi: 10.1093/cercor/bhy112
Choi, J., Lee, S., Won, J., Jin, Y., Hong, Y., Hur, T.-Y., et al. (2018). Pathophysiological and neurobehavioral characteristics of a propionic acid-mediated autism-like rat model. PLoS One 13:e0192925. doi: 10.1371/journal.pone.0192925
Chorghay, Z., Káradóttir, R. T., and Ruthazer, E. S. (2018). White matter plasticity keeps the brain in tune: axons conduct while glia wrap. Front. Cell. Neurosci. 12:428. doi: 10.3389/fncel.2018.00428
Chunchai, T., Thunapong, W., Yasom, S., Wanchai, K., Eaimworawuthikul, S., Metzler, G., et al. (2018). Decreased microglial activation through gut-brain axis by prebiotics, probiotics, or synbiotics effectively restored cognitive function in obese-insulin resistant rats. J. Neuroinflammation 15:11. doi: 10.1186/s12974-018-1055-2
Chung, Y.-C., Jin, H.-M., Cui, Y., Kim, D. S., Jung, J. M., Park, J.-I., et al. (2014). Fermented milk of Lactobacillus helveticus IDCC3801 improves cognitive functioning during cognitive fatigue tests in healthy older adults. J. Funct. Foods 10, 465–474. doi: 10.1016/j.jff.2014.07.007
Citri, A., and Malenka, R. C. (2008). Synaptic plasticity: multiple forms, functions, and mechanisms. Neuropsychopharmacology 33, 18–41. doi: 10.1038/sj.npp.1301559
Clarke, G., Grenham, S., Scully, P., Fitzgerald, P., Moloney, R. D., Shanahan, F., et al. (2013). The microbiome-gut-brain axis during early life regulates the hippocampal serotonergic system in a sex-dependent manner. Mol. Psychiatry 18, 666–673. doi: 10.1038/mp.2012.77
Clarke, G., Stilling, R. M., Kennedy, P. J., Stanton, C., Cryan, J. F., and Dinan, T. G. (2014). Minireview: gut microbiota: the neglected endocrine organ. Mol. Endocrinol. 28, 1221–1238. doi: 10.1210/me.2014-1108
Cline, H. T. (2001). Dendritic arbor development and synaptogenesis. Curr. Opin. Neurobiol. 11, 118–126. doi: 10.1016/s0959-4388(00)00182-3
Collins, S. M., Surette, M., and Bercik, P. (2012). The interplay between the intestinal microbiota and the brain. Nat. Rev. Microbiol. 10, 735–742. doi: 10.1038/nrmicro2876
Cowan, C. S. M., Callaghan, B. L., and Richardson, R. (2016). The effects of a probiotic formulation (Lactobacillus rhamnosus and L. helveticus) on developmental trajectories of emotional learning in stressed infant rats. Transl. Psychiatry 6:e823. doi: 10.1038/tp.2016.94
Cryan, J. F., and Dinan, T. G. (2012). Mind-altering microorganisms: the impact of the gut microbiota on brain and behaviour. Nat. Rev. Neurosci. 13, 701–712. doi: 10.1038/nrn3346
Cryan, J. F., and Dinan, T. G. (2015). Microbiota and neuroimmune signalling—Metchnikoff to microglia. Nat. Rev. Gastroenterol. Hepatol. 12, 494–496. doi: 10.1038/nrgastro.2015.127
Cryan, J. F., O’Riordan, K. J., Cowan, C. S. M., Sandhu, K. V., Bastiaanssen, T. F. S., Boehme, M., et al. (2019). The microbiota-gut-brain Axis. Physiol. Rev. 99, 1877–2013. doi: 10.1152/physrev.00018.2018
D’Alessandro, G., Marrocco, F., and Limatola, C. (2022). Microglial cells: sensors for neuronal activity and microbiota-derived molecules. Front. Immunol. 13:1011129. doi: 10.3389/fimmu.2022.1011129
D’Amato, A., Mannelli, L. D. C., Lucarini, E., Man, A. L., Le Gall, G., Branca, J. J. V., et al. (2020). Faecal microbiota transplant from aged donor mice affects spatial learning and memory via modulating hippocampal synaptic plasticity-and neurotransmission-related proteins in young recipients. Microbiome 8:140. doi: 10.1186/s40168-020-00914-w
Dalile, B., Van Oudenhove, L., Vervliet, B., and Verbeke, K. (2019). The role of short-chain fatty acids in microbiota–gut–brain communication. Nat. Rev. Gastroenterol. Hepatol. 16, 461–478. doi: 10.1038/s41575-019-0157-3
Damiani, F., Cornuti, S., and Tognini, P. (2023). The gut-brain connection: exploring the influence of the gut microbiota on neuroplasticity and neurodevelopmental disorders. Neuropharmacology 231:109491. doi: 10.1016/j.neuropharm.2023.109491
Davari, S., Talaei, S. A., Alaei, H., and Salami, M. (2013). Probiotics treatment improves diabetes-induced impairment of synaptic activity and cognitive function: behavioral and electrophysiological proofs for microbiome–gut–brain axis. Neuroscience 240, 287–296. doi: 10.1016/j.neuroscience.2013.02.055
De Preter, V., Vanhoutte, T., Huys, G., Swings, J., Rutgeerts, P., and Verbeke, K. (2008). Baseline microbiota activity and initial bifidobacteria counts influence responses to prebiotic dosing in healthy subjects. Aliment. Pharmacol. Ther. 27, 504–513. doi: 10.1111/j.1365-2036.2007.03588.x
De Vadder, F., Grasset, E., Mannerås, L., Karsenty, G., Macpherson, A. J., Olofsson, L. E., et al. (2018). Gut microbiota regulates maturation of the adult enteric nervous system via enteric serotonin networks. Proc. Natl. Acad. Sci. USA 115, 6458–6463. doi: 10.1073/pnas.1720017115
De Vadder, F., Kovatcheva-Datchary, P., Zitoun, C., Duchampt, A., Bäckhed, F., and Mithieux, G. (2016). Microbiota-Produced Succinate Improves Glucose Homeostasis via Intestinal Gluconeogenesis. Cell Metab. 24, 151–157. doi: 10.1016/j.cmet.2016.06.013
de, C., Wopereis, H., Ramadan, M., van, T., Lambert, J., Knol, J., et al. (2014). Altered gut microbiota and activity in a murine model of autism spectrum disorders. Brain Behav. Immun. 37, 197–206. doi: 10.1016/j.bbi.2013.12.005
Delgado, P. L. (2000). Depression: the case for a monoamine deficiency. J. Clin. Psychiatry 61, 7–11.
Deng, F.-L., Pan, J.-X., Zheng, P., Xia, J.-J., Yin, B.-M., Liang, W.-W., et al. (2019). Metabonomics reveals peripheral and central short-chain fatty acid and amino acid dysfunction in a naturally occurring depressive model of macaques. NDT 15, 1077–1088. doi: 10.2147/NDT.S186071
Desbonnet, L., Clarke, G., Shanahan, F., Dinan, T. G., and Cryan, J. F. (2014). Microbiota is essential for social development in the mouse. Mol. Psychiatry 19, 146–148. doi: 10.1038/mp.2013.65
Desbonnet, L., Clarke, G., Traplin, A., O’Sullivan, O., Crispie, F., Moloney, R. D., et al. (2015). Gut microbiota depletion from early adolescence in mice: implications for brain and behaviour. Brain Behav. Immun. 48, 165–173. doi: 10.1016/j.bbi.2015.04.004
Devillard, E., McIntosh, F. M., Duncan, S. H., and Wallace, R. J. (2007). Metabolism of linoleic acid by human gut Bacteria: different routes for biosynthesis of conjugated linoleic acid. J. Bacteriol. 189, 2566–2570. doi: 10.1128/JB.01359-06
Dhaliwal, J., Singh, D. P., Singh, S., Pinnaka, A. K., Boparai, R. K., Bishnoi, M., et al. (2018). Lactobacillus plantarum MTCC 9510 supplementation protects from chronic unpredictable and sleep deprivation-induced behaviour, biochemical and selected gut microbial aberrations in mice. J. Appl. Microbiol. 125, 257–269. doi: 10.1111/jam.13765
Dinan, T. G., and Cryan, J. F. (2017). The microbiome-gut-brain Axis in health and disease. Gastroenterol. Clin. N. Am. 46, 77–89. doi: 10.1016/j.gtc.2016.09.007
Distrutti, E., O’Reilly, J.-A., McDonald, C., Cipriani, S., Renga, B., Lynch, M. A., et al. (2014). Modulation of intestinal microbiota by the probiotic VSL#3 resets brain gene expression and ameliorates the age-related deficit in LTP. PLoS One 9:e106503. doi: 10.1371/journal.pone.0106503
Downs, R., Perna, J., Vitelli, A., Cook, D., and Dhurjati, P. (2014). Model-based hypothesis of gut microbe populations and gut/brain barrier permeabilities in the development of regressive autism. Med. Hypotheses 83, 649–655. doi: 10.1016/j.mehy.2014.09.005
Duncan, S. H., Barcenilla, A., Stewart, C. S., Pryde, S. E., and Flint, H. J. (2002). Acetate utilization and Butyryl coenzyme a (CoA):acetate-CoA transferase in butyrate-producing Bacteria from the human large intestine. Appl. Environ. Microbiol. 68, 5186–5190. doi: 10.1128/AEM.68.10.5186-5190.2002
Duncan, S. H., and Flint, H. J. (2013). Probiotics and prebiotics and health in ageing populations. Maturitas 75, 44–50. doi: 10.1016/j.maturitas.2013.02.004
Durant, L., Stentz, R., Noble, A., Brooks, J., Gicheva, N., Reddi, D., et al. (2020). Bacteroides thetaiotaomicron-derived outer membrane vesicles promote regulatory dendritic cell responses in health but not in inflammatory bowel disease. Microbiome 8:88. doi: 10.1186/s40168-020-00868-z
Dzyubenko, E., and Hermann, D. M. (2023). Role of glia and extracellular matrix in controlling neuroplasticity in the central nervous system. Semin. Immunopathol. 45, 377–387. doi: 10.1007/s00281-023-00989-1
Eckburg, P. B., Bik, E. M., Bernstein, C. N., Purdom, E., Dethlefsen, L., Sargent, M., et al. (2005). Diversity of the human intestinal microbial Flora. Science 308, 1635–1638. doi: 10.1126/science.1110591
Emge, J. R., Huynh, K., Miller, E. N., Kaur, M., Reardon, C., Barrett, K. E., et al. (2016). Modulation of the microbiota-gut-brain axis by probiotics in a murine model of inflammatory bowel disease. American journal of physiology-gastrointestinal and liver. Physiology 310, G989–G998. doi: 10.1152/ajpgi.00086.2016
Erny, D., Hrabě, A., Jaitin, D., Wieghofer, P., Staszewski, O., David, E., et al. (2015). Host microbiota constantly control maturation and function of microglia in the CNS. Nat. Neurosci. 18, 965–977. doi: 10.1038/nn.4030
Esposito, P., Chandler, N., Kandere, K., Basu, S., Jacobson, S., Connolly, R., et al. (2002). Corticotropin-releasing hormone and brain mast cells regulate blood-brain-barrier permeability induced by acute stress. J. Pharmacol. Exp. Ther. 303, 1061–1066. doi: 10.1124/jpet.102.038497
Evans, S. J., Bassis, C. M., Hein, R., Assari, S., Flowers, S. A., Kelly, M. B., et al. (2017). The gut microbiome composition associates with bipolar disorder and illness severity. J. Psychiatr. Res. 87, 23–29. doi: 10.1016/j.jpsychires.2016.12.007
Ferrante, M., Migliore, M., and Ascoli, G. A. (2013). Functional impact of dendritic branch-point morphology. J. Neurosci. 33, 2156–2165. doi: 10.1523/JNEUROSCI.3495-12.2013
Finegold, S. M. (2011). Desulfovibrio species are potentially important in regressive autism. Med. Hypotheses 77, 270–274. doi: 10.1016/j.mehy.2011.04.032
Finegold, S. M., Dowd, S. E., Gontcharova, V., Liu, C., Henley, K. E., Wolcott, R. D., et al. (2010). Pyrosequencing study of fecal microflora of autistic and control children. Anaerobe 16, 444–453. doi: 10.1016/j.anaerobe.2010.06.008
Flowers, S. A., Evans, S. J., Ward, K. M., McInnis, M. G., and Ellingrod, V. L. (2017). Interaction between atypical antipsychotics and the gut microbiome in a bipolar disease cohort. Pharmacotherapy 37, 261–267. doi: 10.1002/phar.1890
Forsythe, P., and Kunze, W. A. (2013). Voices from within: gut microbes and the CNS. Cell. Mol. Life Sci. 70, 55–69. doi: 10.1007/s00018-012-1028-z
Frost, G., Sleeth, M. L., Sahuri-Arisoylu, M., Lizarbe, B., Cerdan, S., Brody, L., et al. (2014). The short-chain fatty acid acetate reduces appetite via a central homeostatic mechanism. Nat. Commun. 5:3611. doi: 10.1038/ncomms4611
Fung, T. C., Olson, C. A., and Hsiao, E. Y. (2017). Interactions between the microbiota, immune and nervous systems in health and disease. Nat. Neurosci. 20, 145–155. doi: 10.1038/nn.4476
Fusco, W., Lorenzo, M. B., Cintoni, M., Porcari, S., Rinninella, E., Kaitsas, F., et al. (2023). Short-chain fatty-acid-producing Bacteria: key components of the human gut microbiota. Nutrients 15:2211. doi: 10.3390/nu15092211
Galanis, C., and Vlachos, A. (2020). Hebbian and homeostatic synaptic plasticity-do alterations of one reflect enhancement of the other? Front. Cell. Neurosci. 14:50. doi: 10.3389/fncel.2020.00050
Garcez, M. L., de, C., Mina, F., Bellettini-Santos, T., Schiavo, G. L., da, S., et al. (2018). Sodium butyrate improves memory and modulates the activity of histone deacetylases in aged rats after the administration of d-galactose. Exp. Gerontol. 113, 209–217. doi: 10.1016/j.exger.2018.10.005
Gareau, M. G., Wine, E., Rodrigues, D. M., Cho, J. H., Whary, M. T., Philpott, D. J., et al. (2011). Bacterial infection causes stress-induced memory dysfunction in mice. Gut 60, 307–317. doi: 10.1136/gut.2009.202515
Gautier, E. L., Shay, T., Miller, J., Greter, M., Jakubzick, C., et al. (2012). Gene-expression profiles and transcriptional regulatory pathways that underlie the identity and diversity of mouse tissue macrophages. Nat. Immunol. 13, 1118–1128. doi: 10.1038/ni.2419
Geerlings, S., Kostopoulos, I., De Vos, W., and Belzer, C. (2018). Akkermansia muciniphila in the human gastrointestinal tract: when, where, and How? Microorganisms 6:75. doi: 10.3390/microorganisms6030075
Głaz, A., Knop-Chodyła, K., Kochanowska-Mazurek, A., Wach, D., Piasecka, Z., Wesołek, E., et al. (2023). The role of probiotics in the treatment of depressive disorders. A critical review. J. Educ. Health Sport 48, 41–53. doi: 10.12775/JEHS.2023.48.01.003
Golubeva, A. V., Joyce, S. A., Moloney, G., Burokas, A., Sherwin, E., Arboleya, S., et al. (2017). Microbiota-related changes in Bile Acid & Tryptophan Metabolism are associated with gastrointestinal dysfunction in a mouse model of autism. EBioMedicine 24, 166–178. doi: 10.1016/j.ebiom.2017.09.020
Gong, X., Ma, Y., Deng, X., Li, A., Li, X., Kong, X., et al. (2024). Intestinal dysbiosis exacerbates susceptibility to the anti-NMDA receptor encephalitis-like phenotype by changing blood brain barrier permeability and immune homeostasis. Brain Behav. Immun. 116, 34–51. doi: 10.1016/j.bbi.2023.11.030
Govindarajan, N., Agis-Balboa, R. C., Walter, J., Sananbenesi, F., and Fischer, A. (2011). Sodium butyrate improves memory function in an Alzheimer’s disease mouse model when administered at an advanced stage of disease progression. JAD 26, 187–197. doi: 10.3233/JAD-2011-110080
Gradisnik, L., and Velnar, T. (2023). Astrocytes in the central nervous system and their functions in health and disease: a review. World J. Clin. Cases 11, 3385–3394. doi: 10.12998/wjcc.v11.i15.3385
Grimaldi, R., Gibson, G. R., Vulevic, J., Giallourou, N., Castro-Mejía, J. L., Hansen, L. H., et al. (2018). A prebiotic intervention study in children with autism spectrum disorders (ASDs). Microbiome 6:133. doi: 10.1186/s40168-018-0523-3
Guida, F., Turco, F., Iannotta, M., de, D., Palumbo, I., Sarnelli, G., et al. (2018). Antibiotic-induced microbiota perturbation causes gut endocannabinoidome changes, hippocampal neuroglial reorganization and depression in mice. Brain Behav. Immun. 67, 230–245. doi: 10.1016/j.bbi.2017.09.001
Guo, C., Huo, Y.-J., Li, Y., Han, Y., and Zhou, D. (2022). Gut-brain axis: focus on gut metabolites short-chain fatty acids. WJCC 10, 1754–1763. doi: 10.12998/wjcc.v10.i6.1754
Guzzetta, K. E., Cryan, J. F., and O’Leary, O. F. (2022). Microbiota-gut-brain Axis regulation of adult hippocampal neurogenesis. Brain Plast 8, 97–119. doi: 10.3233/BPL-220141
Haghikia, A., Jörg, S., Duscha, A., Berg, J., Manzel, A., Waschbisch, A., et al. (2015). Dietary fatty acids directly impact central nervous system autoimmunity via the small intestine. Immunity 43, 817–829. doi: 10.1016/j.immuni.2015.09.007
Hamad, M. I. K., Daoud, S., Petrova, P., Rabaya, O., Jbara, A., Al Houqani, S., et al. (2024). Reelin differentially shapes dendrite morphology of medial entorhinal cortical ocean and island cells. Development 151. doi: 10.1242/dev.202449
Hamad, M. I. K., Emerald, B. S., Kumar, K. K., Ibrahim, M. F., Ali, B. R., and Bataineh, M. F. (2023). Extracellular molecular signals shaping dendrite architecture during brain development. Front. Cell Dev. Biol. 11:1254589. doi: 10.3389/fcell.2023.1254589
Hamad, M. I. K., Jack, A., Klatt, O., Lorkowski, M., Strasdeit, T., Kott, S., et al. (2014). Type I TARPs promote dendritic growth of early postnatal neocortical pyramidal cells in organotypic cultures. Development 141, 1737–1748. doi: 10.1242/dev.099697
Hamad, M. I. K., Ma-Högemeier, Z.-L., Riedel, C., Conrads, C., Veitinger, T., Habijan, T., et al. (2011). Cell class-specific regulation of neocortical dendrite and spine growth by AMPA receptor splice and editing variants. Development 138, 4301–4313. doi: 10.1242/dev.071076
Hamad, M. I. K., Petrova, P., Daoud, S., Rabaya, O., Jbara, A., Melliti, N., et al. (2021). Reelin restricts dendritic growth of interneurons in the neocortex. Development 148:dev199718. doi: 10.1242/dev.199718
Hao, Z., Wang, W., Guo, R., and Liu, H. (2019). Faecalibacterium prausnitzii (ATCC 27766) has preventive and therapeutic effects on chronic unpredictable mild stress-induced depression-like and anxiety-like behavior in rats. Psychoneuroendocrinology 104, 132–142. doi: 10.1016/j.psyneuen.2019.02.025
Hasegawa, S., Goto, S., Tsuji, H., Okuno, T., Asahara, T., Nomoto, K., et al. (2015). Intestinal Dysbiosis and lowered serum lipopolysaccharide-binding protein in Parkinson’s disease. PLoS One 10:e0142164. doi: 10.1371/journal.pone.0142164
He, Y., Kosciolek, T., Tang, J., Zhou, Y., Li, Z., Ma, X., et al. (2018). Gut microbiome and magnetic resonance spectroscopy study of subjects at ultra-high risk for psychosis may support the membrane hypothesis. Eur. Psychiatry 53, 37–45. doi: 10.1016/j.eurpsy.2018.05.011
Hébuterne, X. (2003). Gut changes attributed to ageing: effects on intestinal microflora. Curr. Opin. Clin. Nutr. Metab. Care 6, 49–54. doi: 10.1097/00075197-200301000-00008
Hegstrand, L. R., and Hine, R. J. (1986). Variations of brain histamine levels in germ-free and nephrectomized rats. Neurochem. Res. 11, 185–191. doi: 10.1007/BF00967967
Heijtz, R. D., Wang, S., Anuar, F., Qian, Y., Björkholm, B., Samuelsson, A., et al. (2011). Normal gut microbiota modulates brain development and behavior. Proc. Natl. Acad. Sci. USA 108, 3047–3052. doi: 10.1073/pnas.1010529108
Hill, J. M., Bhattacharjee, S., Pogue, A. I., and Lukiw, W. J. (2014). The gastrointestinal tract microbiome and potential link to Alzheimer’s disease. Front. Neurol. 5:43. doi: 10.3389/fneur.2014.00043
Ho, S.-T., Hsieh, Y.-T., Wang, S.-Y., and Chen, M.-J. (2019). Improving effect of a probiotic mixture on memory and learning abilities in d-galactose–treated aging mice. J. Dairy Sci. 102, 1901–1909. doi: 10.3168/jds.2018-15811
Ho, L., Ono, K., Tsuji, M., Mazzola, P., Singh, R., and Pasinetti, G. M. (2018). Protective roles of intestinal microbiota derived short chain fatty acids in Alzheimer’s disease-type beta-amyloid neuropathological mechanisms. Expert. Rev. Neurother. 18, 83–90. doi: 10.1080/14737175.2018.1400909
Hoban, A. E., Stilling, R. M., Ryan, F. J., Shanahan, F., Dinan, T. G., Claesson, M. J., et al. (2016). Regulation of prefrontal cortex myelination by the microbiota. Transl. Psychiatry 6:e774. doi: 10.1038/tp.2016.42
Hoffman, J. D., Yanckello, L. M., Chlipala, G., Hammond, T. C., McCulloch, S. D., Parikh, I., et al. (2019). Dietary inulin alters the gut microbiome, enhances systemic metabolism and reduces neuroinflammation in an APOE4 mouse model. PLoS One 14:e0221828. doi: 10.1371/journal.pone.0221828
Hor, Y.-Y., Lew, L.-C., Jaafar, M. H., Lau, A. S.-Y., Ong, J.-S., Kato, T., et al. (2019). Lactobacillus sp. improved microbiota and metabolite profiles of aging rats. Pharmacol. Res. 146:104312. doi: 10.1016/j.phrs.2019.104312
Hsiao, E. Y., McBride, S. W., Hsien, S., Sharon, G., Hyde, E. R., McCue, T., et al. (2013). Microbiota modulate behavioral and physiological abnormalities associated with neurodevelopmental disorders. Cell 155, 1451–1463. doi: 10.1016/j.cell.2013.11.024
Huang, Y., Wu, J., Zhang, H., Li, Y., Wen, L., Tan, X., et al. (2023). The gut microbiome modulates the transformation of microglial subtypes. Mol. Psychiatry 28, 1611–1621. doi: 10.1038/s41380-023-02017-y
Huang, H., Xu, H., Luo, Q., He, J., Li, M., Chen, H., et al. (2019). Fecal microbiota transplantation to treat Parkinson’s disease with constipation: a case report. Medicine 98:e16163. doi: 10.1097/MD.0000000000016163
Hughes, E. G., and Appel, B. (2016). The cell biology of CNS myelination. Curr. Opin. Neurobiol. 39, 93–100. doi: 10.1016/j.conb.2016.04.013
Human Metabolome Database (2023). Human Metabolome Database. Available at: http://www.hmdb.ca/ (Accessed December 27, 2023).
Inglis, F. M., Crockett, R., Korada, S., Abraham, W. C., Hollmann, M., and Kalb, R. G. (2002). The AMPA receptor subunit GluR1 regulates dendritic architecture of motor neurons. J. Neurosci. 22, 8042–8051. doi: 10.1523/JNEUROSCI.22-18-08042.2002
Intlekofer, K. A., Berchtold, N. C., Malvaez, M., Carlos, A. J., McQuown, S. C., Cunningham, M. J., et al. (2013). Exercise and sodium butyrate transform a subthreshold learning event into long-term memory via a brain-derived neurotrophic factor-dependent mechanism. Neuropsychopharmacology 38, 2027–2034. doi: 10.1038/npp.2013.104
Jan, Y.-N., and Jan, L. Y. (2003). The control of dendrite development. Neuron 40, 229–242. doi: 10.1016/s0896-6273(03)00631-7
Jang, H.-M., Lee, H.-J., Jang, S.-E., Han, M. J., and Kim, D.-H. (2018). Evidence for interplay among antibacterial-induced gut microbiota disturbance, neuro-inflammation, and anxiety in mice. Mucosal Immunol. 11, 1386–1397. doi: 10.1038/s41385-018-0042-3
Jarosz, Ł. S., Socała, K., Michalak, K., Wiater, A., Ciszewski, A., Majewska, M., et al. (2024). The effect of psychoactive bacteria, Bifidobacterium longum Rosell®-175 and Lactobacillus rhamnosus JB-1, on brain proteome profiles in mice. Psychopharmacology 241, 925–945. doi: 10.1007/s00213-023-06519-z
Juárez, I., Gratton, A., and Flores, G. (2008). Ontogeny of altered dendritic morphology in the rat prefrontal cortex, hippocampus, and nucleus accumbens following cesarean delivery and birth anoxia. J. Comp. Neurol. 507, 1734–1747. doi: 10.1002/cne.21651
Kakabadze, E., Makalatia, K., Bakuradze, N., Grdzelishvili, N., Kereselidze, S., Ediberidze, T., et al. (2022). Gut health of children with autism spectrum disorder. World Acad. Sci. J. 4:29. doi: 10.3892/wasj.2022.164
Kamimura, I., Kaneko, R., Morita, H., Mogi, K., and Kikusui, T. (2021). Microbial colonization history modulates anxiety-like and complex social behavior in mice. Neurosci. Res. 168, 64–75. doi: 10.1016/j.neures.2020.01.001
Kang, D.-W., Adams, J. B., Coleman, D. M., Pollard, E. L., Maldonado, J., McDonough-Means, S., et al. (2019). Long-term benefit of microbiota transfer therapy on autism symptoms and gut microbiota. Sci. Rep. 9:5821. doi: 10.1038/s41598-019-42183-0
Karnachuk, O. V., Ikkert, O. P., Avakyan, M. R., Knyazev, Y. V., Volochaev, M. N., Zyusman, V. S., et al. (2021). Desulfovibrio desulfuricans AY5 isolated from a patient with autism Spectrum disorder binds Iron in Low-soluble Greigite and pyrite. Microorganisms 9:2558. doi: 10.3390/microorganisms9122558
Kasarello, K., Cudnoch-Jedrzejewska, A., and Czarzasta, K. (2023). Communication of gut microbiota and brain via immune and neuroendocrine signaling. Front. Microbiol. 14:1118529. doi: 10.3389/fmicb.2023.1118529
Kazemi, A., Noorbala, A. A., Azam, K., Eskandari, M. H., and Djafarian, K. (2019). Effect of probiotic and prebiotic vs placebo on psychological outcomes in patients with major depressive disorder: a randomized clinical trial. Clin. Nutr. 38, 522–528. doi: 10.1016/j.clnu.2018.04.010
Kekuda, R., Manoharan, P., Baseler, W., and Sundaram, U. (2013). Monocarboxylate 4 mediated butyrate transport in a rat intestinal epithelial cell line. Dig. Dis. Sci. 58, 660–667. doi: 10.1007/s10620-012-2407-x
Kelly, J. R., Minuto, C., Cryan, J. F., Clarke, G., and Dinan, T. G. (2017). Cross talk: the microbiota and neurodevelopmental disorders. Front. Neurosci. 11:490. doi: 10.3389/fnins.2017.00490
Keogh, C. E., Kim, D. H. J., Pusceddu, M. M., Knotts, T. A., Rabasa, G., Sladek, J. A., et al. (2021). Myelin as a regulator of development of the microbiota-gut-brain axis. Brain Behav. Immun. 91, 437–450. doi: 10.1016/j.bbi.2020.11.001
Keshavarzian, A., Green, S. J., Engen, P. A., Voigt, R. M., Naqib, A., Forsyth, C. B., et al. (2015). Colonic bacterial composition in Parkinson’s disease. Mov. Disord. 30, 1351–1360. doi: 10.1002/mds.26307
Khalesi, S., Bellissimo, N., Vandelanotte, C., Williams, S., Stanley, D., and Irwin, C. (2019). A review of probiotic supplementation in healthy adults: helpful or hype? Eur. J. Clin. Nutr. 73, 24–37. doi: 10.1038/s41430-018-0135-9
Kraeuter, A.-K., Phillips, R., and Sarnyai, Z. (2020). The gut microbiome in psychosis from mice to men: a systematic review of preclinical and clinical studies. Front. Psych. 11:799. doi: 10.3389/fpsyt.2020.00799
Kratsman, N., Getselter, D., and Elliott, E. (2016). Sodium butyrate attenuates social behavior deficits and modifies the transcription of inhibitory/excitatory genes in the frontal cortex of an autism model. Neuropharmacology 102, 136–145. doi: 10.1016/j.neuropharm.2015.11.003
Kundu, P., Lee, H. U., Garcia-Perez, I., Tay, E. X. Y., Kim, H., Faylon, L. E., et al. (2019). Neurogenesis and prolongevity signaling in young germ-free mice transplanted with the gut microbiota of old mice. Sci. Transl. Med. 11:eaau4760. doi: 10.1126/scitranslmed.aau4760
Laurent, R. S., O’Brien, L. M., and Ahmad, S. T. (2013). Sodium butyrate improves locomotor impairment and early mortality in a rotenone-induced Drosophila model of Parkinson’s disease. Neuroscience 246, 382–390. doi: 10.1016/j.neuroscience.2013.04.037
Lee, H. J., Hong, J. K., Kim, J.-K., Kim, D.-H., Jang, S. W., Han, S.-W., et al. (2021). Effects of probiotic NVP-1704 on mental health and sleep in healthy adults: An 8-week randomized, double-blind, Placebo-Controlled Trial. Nutrients 13:2660. doi: 10.3390/nu13082660
Lee, Y. K., Menezes, J. S., Umesaki, Y., and Mazmanian, S. K. (2011). Proinflammatory T-cell responses to gut microbiota promote experimental autoimmune encephalomyelitis. Proc. Natl. Acad. Sci. USA 108, 4615–4622. doi: 10.1073/pnas.1000082107
Leifeld, J., Förster, E., Reiss, G., and Hamad, M. I. K. (2022). Considering the role of extracellular matrix molecules, in particular Reelin, in granule cell dispersion related to temporal lobe epilepsy. Front. Cell Dev. Biol. 10:917575. doi: 10.3389/fcell.2022.917575
Leung, K., and Thuret, S. (2015). Gut microbiota: a modulator of brain plasticity and cognitive function in ageing. Healthcare 3, 898–916. doi: 10.3390/healthcare3040898
Levenson, J. M., O’Riordan, K. J., Brown, K. D., Trinh, M. A., Molfese, D. L., and Sweatt, J. D. (2004). Regulation of histone acetylation during memory formation in the Hippocampus. J. Biol. Chem. 279, 40545–40559. doi: 10.1074/jbc.M402229200
Li, H., Gao, D., Cao, Y., and Xu, H. (2008). A high γ-aminobutyric acid-Producinglactobacillus brevis isolated from Chinese traditionalpaocai. Ann. Microbiol. 58, 649–653. doi: 10.1007/BF03175570
Li, H., Ni, J., and Qing, H. (2021). Gut microbiota: critical controller and intervention target in brain aging and cognitive impairment. Front. Aging Neurosci. 13:671142. doi: 10.3389/fnagi.2021.671142
Li, Y., Ning, L., Yin, Y., Wang, R., Zhang, Z., Hao, L., et al. (2020). Age-related shifts in gut microbiota contribute to cognitive decline in aged rats. Aging 12, 7801–7817. doi: 10.18632/aging.103093
Li, H., Sun, J., du, J., Wang, F., Fang, R., Yu, C., et al. (2018). Clostridium butyricum exerts a neuroprotective effect in a mouse model of traumatic brain injury via the gut-brain axis. Neurogastroenterol. Motil. 30:e13260. doi: 10.1111/nmo.13260
Li, H., Sun, J., Wang, F., Ding, G., Chen, W., Fang, R., et al. (2016). Sodium butyrate exerts neuroprotective effects by restoring the blood-brain barrier in traumatic brain injury mice. Brain Res. 1642, 70–78. doi: 10.1016/j.brainres.2016.03.031
Li, F., and Tsien, J. Z. (2009). Memory and the NMDA receptors. N. Engl. J. Med. 361, 302–303. doi: 10.1056/NEJMcibr0902052
Li, J., Wang, J., Wang, M., Zheng, L., Cen, Q., Wang, F., et al. (2023). Bifidobacterium: a probiotic for the prevention and treatment of depression. Front. Microbiol. 14:1174800. doi: 10.3389/fmicb.2023.1174800
Li, W., Wu, X., Hu, X., Wang, T., Liang, S., Duan, Y., et al. (2017). Structural changes of gut microbiota in Parkinson’s disease and its correlation with clinical features. Sci. China Life Sci. 60, 1223–1233. doi: 10.1007/s11427-016-9001-4
Liang, S., Wang, T., Hu, X., Luo, J., Li, W., Wu, X., et al. (2015). Administration of Lactobacillus helveticus NS8 improves behavioral, cognitive, and biochemical aberrations caused by chronic restraint stress. Neuroscience 310, 561–577. doi: 10.1016/j.neuroscience.2015.09.033
Liang, S., Wu, X., and Jin, F. (2018). Gut-brain psychology: rethinking psychology from the microbiota-gut-brain Axis. Front. Integr. Neurosci. 12:33. doi: 10.3389/fnint.2018.00033
Liang, L., Zhou, H., Zhang, S., Yuan, J., and Wu, H. (2017). Effects of gut microbiota disturbance induced in early life on the expression of extrasynaptic GABA-A receptor α5 and δ subunits in the hippocampus of adult rats. Brain Res. Bull. 135, 113–119. doi: 10.1016/j.brainresbull.2017.09.014
Liu, H.-Y., Huang, C.-M., Hung, Y.-F., and Hsueh, Y.-P. (2015). The microRNAs Let7c and miR21 are recognized by neuronal toll-like receptor 7 to restrict dendritic growth of neurons. Exp. Neurol. 269, 202–212. doi: 10.1016/j.expneurol.2015.04.011
Liu, J., Wang, F., Liu, S., du, J., Hu, X., Xiong, J., et al. (2017). Sodium butyrate exerts protective effect against Parkinson’s disease in mice via stimulation of glucagon like peptide-1. J. Neurol. Sci. 381, 176–181. doi: 10.1016/j.jns.2017.08.3235
Loh, J. S., Mak, W. Q., Tan, L. K. S., Ng, C. X., Chan, H. H., Yeow, S. H., et al. (2024). Microbiota-gut-brain axis and its therapeutic applications in neurodegenerative diseases. Signal Transduct. Target. Ther. 9:37. doi: 10.1038/s41392-024-01743-1
Lømo, T. (2003). The discovery of long-term potentiation. Philos. Trans. R. Soc. Lond. Ser. B Biol. Sci. 358, 617–620. doi: 10.1098/rstb.2002.1226
London, A., Cohen, M., and Schwartz, M. (2013). Microglia and monocyte-derived macrophages: functionally distinct populations that act in concert in CNS plasticity and repair. Front. Cell. Neurosci. 7:34. doi: 10.3389/fncel.2013.00034
Lu, J., Lu, L., Yu, Y., Cluette-Brown, J., Martin, C. R., and Claud, E. C. (2018). Effects of intestinal microbiota on brain development in humanized Gnotobiotic mice. Sci. Rep. 8:5443. doi: 10.1038/s41598-018-23692-w
Lu, Y., Yu, X., Wang, Z., Kong, L., Jiang, Z., Shang, R., et al. (2024). Microbiota-gut-brain axis: natural antidepressants molecular mechanism. Phytomedicine 134:156012. doi: 10.1016/j.phymed.2024.156012
Luczynski, P., Whelan, S. O., O’Sullivan, C., Clarke, G., Shanahan, F., Dinan, T. G., et al. (2016). Adult microbiota-deficient mice have distinct dendritic morphological changes: differential effects in the amygdala and hippocampus. Eur. J. Neurosci. 44, 2654–2666. doi: 10.1111/ejn.13291
Luk, B., Veeraragavan, S., Engevik, M., Balderas, M., Major, A., Runge, J., et al. (2018). Postnatal colonization with human “infant-type” Bifidobacterium species alters behavior of adult gnotobiotic mice. PLoS One 13:e0196510. doi: 10.1371/journal.pone.0196510
Lupien, S. J., Fiocco, A., Wan, N., Maheu, F., Lord, C., Schramek, T., et al. (2005). Stress hormones and human memory function across the lifespan. Psychoneuroendocrinology 30, 225–242. doi: 10.1016/j.psyneuen.2004.08.003
Manderino, L., Carroll, I., Azcarate-Peril, M. A., Rochette, A., Heinberg, L., Peat, C., et al. (2017). Preliminary evidence for an association between the composition of the gut microbiome and cognitive function in neurologically healthy older adults. J. Int. Neuropsychol. Soc. 23, 700–705. doi: 10.1017/S1355617717000492
Mao, Y.-K., Kasper, D. L., Wang, B., Forsythe, P., Bienenstock, J., and Kunze, W. A. (2013). Bacteroides fragilis polysaccharide a is necessary and sufficient for acute activation of intestinal sensory neurons. Nat. Commun. 4:1465. doi: 10.1038/ncomms2478
Maren, S., and Quirk, G. J. (2004). Neuronal signalling of fear memory. Nat. Rev. Neurosci. 5, 844–852. doi: 10.1038/nrn1535
Margineanu, M. B., Sherwin, E., Golubeva, A., Peterson, V., Hoban, A., Fiumelli, H., et al. (2020). Gut microbiota modulates expression of genes involved in the astrocyte-neuron lactate shuttle in the hippocampus. Eur. Neuropsychopharmacol. 41, 152–159. doi: 10.1016/j.euroneuro.2020.11.006
Marlow, G., Ellett, S., Ferguson, I. R., Zhu, S., Karunasinghe, N., Jesuthasan, A. C., et al. (2013). Transcriptomics to study the effect of a Mediterranean-inspired diet on inflammation in Crohn’s disease patients. Hum. Genomics 7:24. doi: 10.1186/1479-7364-7-24
Martín, R., Bermúdez-Humarán, L. G., and Langella, P. (2018). Searching for the bacterial effector: the example of the multi-skilled commensal bacterium Faecalibacterium prausnitzii. Front. Microbiol. 9:346. doi: 10.3389/fmicb.2018.00346
Martínez Pizarro, S. (2022). Probiotics in the treatment of depression. Revista Colombiana de Psiquiatría 51, 6–7. doi: 10.1016/j.rcpeng.2020.05.004
Matsuki, T., Yahagi, K., Mori, H., Matsumoto, H., Hara, T., Tajima, S., et al. (2016). A key genetic factor for fucosyllactose utilization affects infant gut microbiota development. Nat. Commun. 7:11939. doi: 10.1038/ncomms11939
Mckernan, D. P., Fitzgerald, P., Dinan, T. G., and Cryan, J. F. (2010). The probiotic Bifidobacterium infantis 35624 displays visceral antinociceptive effects in the rat. Neurogastroenterol. Motil. 22:1029. doi: 10.1111/j.1365-2982.2010.01520.x
Messaoudi, M., Lalonde, R., Violle, N., Javelot, H., Desor, D., Nejdi, A., et al. (2011). Assessment of psychotropic-like properties of a probiotic formulation (Lactobacillus helveticus R0052 and Bifidobacterium longum R0175) in rats and human subjects. Br. J. Nutr. 105, 755–764. doi: 10.1017/S0007114510004319
Miller, A. H., and Raison, C. L. (2016). The role of inflammation in depression: from evolutionary imperative to modern treatment target. Nat. Rev. Immunol. 16, 22–34. doi: 10.1038/nri.2015.5
Minter, M. R., Hinterleitner, R., Meisel, M., Zhang, C., Leone, V., Zhang, X., et al. (2017). Antibiotic-induced perturbations in microbial diversity during post-natal development alters amyloid pathology in an aged APPSWE/PS1ΔE9 murine model of Alzheimer’s disease. Sci. Rep. 7:10411. doi: 10.1038/s41598-017-11047-w
Miquel, S., Martín, R., Rossi, O., Bermúdez-Humarán, L., Chatel, J., Sokol, H., et al. (2013). Faecalibacterium prausnitzii and human intestinal health. Curr. Opin. Microbiol. 16, 255–261. doi: 10.1016/j.mib.2013.06.003
Mohajeri, M. H., Brummer, R. J. M., Rastall, R. A., Weersma, R. K., Harmsen, H. J. M., Faas, M., et al. (2018). The role of the microbiome for human health: from basic science to clinical applications. Eur. J. Nutr. 57, 1–14. doi: 10.1007/s00394-018-1703-4
Möhle, L., Mattei, D., Heimesaat, M. M., Bereswill, S., Fischer, A., Alutis, M., et al. (2016). Ly6Chi monocytes provide a link between antibiotic-induced changes in gut microbiota and adult hippocampal neurogenesis. Cell Rep. 15, 1945–1956. doi: 10.1016/j.celrep.2016.04.074
Molina-Gonzalez, I., and Miron, V. E. (2019). Astrocytes in myelination and remyelination. Neurosci. Lett. 713:134532. doi: 10.1016/j.neulet.2019.134532
Montagne, A., Barnes, S. R., Sweeney, M. D., Halliday, M. R., Sagare, A. P., Zhao, Z., et al. (2015). Blood-brain barrier breakdown in the aging human Hippocampus. Neuron 85, 296–302. doi: 10.1016/j.neuron.2014.12.032
Morris, D. J., and Ridlon, J. M. (2017). Glucocorticoids and gut bacteria: “the GALF hypothesis” in the metagenomic era. Steroids 125, 1–13. doi: 10.1016/j.steroids.2017.06.002
Mossad, O., Batut, B., Yilmaz, B., Dokalis, N., Mezö, C., Nent, E., et al. (2022). Gut microbiota drives age-related oxidative stress and mitochondrial damage in microglia via the metabolite N6-carboxymethyllysine. Nat. Neurosci. 25, 295–305. doi: 10.1038/s41593-022-01027-3
Murciano-Brea, J., Garcia-Montes, M., Geuna, S., and Herrera-Rincon, C. (2021). Gut microbiota and neuroplasticity. Cells 10:2084. doi: 10.3390/cells10082084
Nagao-Kitamoto, H., and Kamada, N. (2017). Host-microbial cross-talk in inflammatory bowel disease. Immune Netw. 17, 1–12. doi: 10.4110/in.2017.17.1.1
Nakhal, M. M., Jayaprakash, P., Aburuz, S., Sadek, B., and Akour, A. (2023). Canagliflozin ameliorates oxidative stress and autistic-like features in Valproic-acid-induced autism in rats: comparison with aripiprazole action. Pharmaceuticals 16:769. doi: 10.3390/ph16050769
Nakhal, M. M., Yassin, L. K., Alyaqoubi, R., Saeed, S., Alderei, A., Alhammadi, A., et al. (2024). The microbiota–gut–brain Axis and neurological disorders: a comprehensive review. Life 14:1234. doi: 10.3390/life14101234
Nankova, B. B., Agarwal, R., MacFabe, D. F., and La Gamma, E. F. (2014). Enteric bacterial metabolites propionic and butyric acid modulate gene expression, including CREB-dependent Catecholaminergic neurotransmission, in PC12 cells - possible relevance to autism Spectrum disorders. PLoS One 9:e103740. doi: 10.1371/journal.pone.0103740
Naseribafrouei, A., Hestad, K., Avershina, E., Sekelja, M., Linløkken, A., Wilson, R., et al. (2014). Correlation between the human fecal microbiota and depression. Neurogastroenterol. Motil. 26, 1155–1162. doi: 10.1111/nmo.12378
Neufeld, K. M., Kang, N., Bienenstock, J., and Foster, J. A. (2011). Reduced anxiety-like behavior and central neurochemical change in germ-free mice: behavior in germ-free mice. Neurogastroenterol. Motil. 23, 255–e119. doi: 10.1111/j.1365-2982.2010.01620.x
Nishino, R., Mikami, K., Takahashi, H., Tomonaga, S., Furuse, M., Hiramoto, T., et al. (2013). Commensal microbiota modulate murine behaviors in a strictly contamination-free environment confirmed by culture-based methods. Neurogastroenterol. Motil. 25, 521–528. doi: 10.1111/nmo.12110
Nishiyama, H., Knopfel, T., Endo, S., and Itohara, S. (2002). Glial protein S100B modulates long-term neuronal synaptic plasticity. Proc. Natl. Acad. Sci. USA 99, 4037–4042. doi: 10.1073/pnas.052020999
O’Mahony, S. M., Clarke, G., Borre, Y. E., Dinan, T. G., and Cryan, J. F. (2015). Serotonin, tryptophan metabolism and the brain-gut-microbiome axis. Behav. Brain Res. 277, 32–48. doi: 10.1016/j.bbr.2014.07.027
O’Mahony, S. M., Felice, V. D., Nally, K., Savignac, H. M., Claesson, M. J., Scully, P., et al. (2014). Disturbance of the gut microbiota in early-life selectively affects visceral pain in adulthood without impacting cognitive or anxiety-related behaviors in male rats. Neuroscience 277, 885–901. doi: 10.1016/j.neuroscience.2014.07.054
Ogbonnaya, E. S., Clarke, G., Shanahan, F., Dinan, T. G., Cryan, J. F., and O’Leary, O. F. (2015). Adult hippocampal neurogenesis is regulated by the microbiome. Biol. Psychiatry 78, e7–e9. doi: 10.1016/j.biopsych.2014.12.023
Oldendorf, W. (1973). Carrier-mediated blood-brain barrier transport of short-chain monocarboxylic organic acids. Amer. J. Physiol. Legacy Content 224, 1450–1453. doi: 10.1152/ajplegacy.1973.224.6.1450
Oleskin, A. V., and Shenderov, B. A. (2016). Neuromodulatory effects and targets of the SCFAs and gasotransmitters produced by the human symbiotic microbiota. Microb. Ecol. Health Disease 27:30971. doi: 10.3402/mehd.v27.30971
Olson, C. A., Vuong, H. E., Yano, J. M., Liang, Q. Y., Nusbaum, D. J., and Hsiao, E. Y. (2018). The gut microbiota mediates the anti-seizure effects of the ketogenic diet. Cell 173, 1728–1741.e13. doi: 10.1016/j.cell.2018.04.027
Paiva, I., Pinho, R., Pavlou, M. A., Hennion, M., Wales, P., Schütz, A.-L., et al. (2017). Sodium butyrate rescues dopaminergic cells from alpha-synuclein-induced transcriptional deregulation and DNA damage. Hum. Mol. Genet. 26, 2231–2246. doi: 10.1093/hmg/ddx114
Panwar, H., Calderwood, D., Gillespie, A. L., Wylie, A. R., Graham, S. F., Grant, I. R., et al. (2016). Identification of lactic acid bacteria strains modulating incretin hormone secretion and gene expression in enteroendocrine cells. J. Funct. Foods 23, 348–358. doi: 10.1016/j.jff.2016.02.040
Paone, P., and Cani, P. D. (2020). Mucus barrier, mucins and gut microbiota: the expected slimy partners? Gut 69, 2232–2243. doi: 10.1136/gutjnl-2020-322260
Park, J. C., and Im, S.-H. (2022). The gut-immune-brain axis in neurodevelopment and neurological disorders. Microbiome Res. Rep. 1:23. doi: 10.20517/mrr.2022.11
Parpura, V., and Haydon, P. G. (2000). Physiological astrocytic calcium levels stimulate glutamate release to modulate adjacent neurons. Proc. Natl. Acad. Sci. USA 97, 8629–8634. doi: 10.1073/pnas.97.15.8629
Parpura, V., Heneka, M. T., Montana, V., Oliet, S. H. R., Schousboe, A., Haydon, P. G., et al. (2012). Glial cells in (patho)physiology. J. Neurochem. 121, 4–27. doi: 10.1111/j.1471-4159.2012.07664.x
Pascale, A., Marchesi, N., Marelli, C., Coppola, A., Luzi, L., Govoni, S., et al. (2018). Microbiota and metabolic diseases. Endocrine 61, 357–371. doi: 10.1007/s12020-018-1605-5
Patnala, R., Arumugam, T. V., Gupta, N., and Dheen, S. T. (2017). HDAC inhibitor sodium butyrate-mediated epigenetic regulation enhances neuroprotective function of microglia during ischemic stroke. Mol. Neurobiol. 54, 6391–6411. doi: 10.1007/s12035-016-0149-z
Peng, L., Li, Z.-R., Green, R. S., Holzmanr, I. R., and Lin, J. (2009). Butyrate enhances the intestinal barrier by facilitating tight junction assembly via activation of AMP-activated protein kinase in Caco-2 cell monolayers. J. Nutr. 139, 1619–1625. doi: 10.3945/jn.109.104638
Perez-Burgos, A., Wang, B., Mao, Y.-K., Mistry, B., Neufeld, K.-A. M., Bienenstock, J., et al. (2013). Psychoactive bacteria Lactobacillus rhamnosus (JB-1) elicits rapid frequency facilitation in vagal afferents. American journal of physiology-gastrointestinal and liver. Physiology 304, G211–G220. doi: 10.1152/ajpgi.00128.2012
Pérez-Burillo, S., Pastoriza, S., Gironés, A., Avellaneda, A., Pilar Francino, M., and Rufián-Henares, J. A. (2020). Potential probiotic salami with dietary fiber modulates metabolism and gut microbiota in a human intervention study. J. Funct. Foods 66:103790. doi: 10.1016/j.jff.2020.103790
Peri, F., and Nüsslein-Volhard, C. (2008). Live imaging of neuronal degradation by microglia reveals a role for v0-ATPase a1 in Phagosomal fusion in vivo. Cell 133, 916–927. doi: 10.1016/j.cell.2008.04.037
Pinto-Sanchez, M. I., Hall, G. B., Ghajar, K., Nardelli, A., Bolino, C., Lau, J. T., et al. (2017). Probiotic Bifidobacterium longum NCC3001 reduces depression scores and alters brain activity: a pilot study in patients with irritable bowel syndrome. Gastroenterology 153, 448–459.e8. doi: 10.1053/j.gastro.2017.05.003
Pokusaeva, K., Johnson, C., Luk, B., Uribe, G., Fu, Y., Oezguen, N., et al. (2017). GABA-producing Bifidobacterium dentium modulates visceral sensitivity in the intestine. Neurogastroenterol. Motil. 29:e12904. doi: 10.1111/nmo.12904
Poroyko, V. A., Carreras, A., Khalyfa, A., Khalyfa, A. A., Leone, V., Peris, E., et al. (2016). Chronic sleep disruption alters gut microbiota, induces systemic and adipose tissue inflammation and insulin resistance in mice. Sci. Rep. 6:35405. doi: 10.1038/srep35405
Prince, M., Herrera, A., Knapp, M., and Karagiannidou, M. (2016). World Alzheimer report 2016. Improving healthcare for people living with dementia: Coverage, quality and costs now and in the future (doctoral dissertation, Alzheimer’s disease international).
Puddu, A., Sanguineti, R., Montecucco, F., and Viviani, G. L. (2014). Evidence for the gut microbiota short-chain fatty acids as key pathophysiological molecules improving diabetes. Mediat. Inflamm. 2014, 1–9. doi: 10.1155/2014/162021
Pugin, B., Barcik, W., Westermann, P., Heider, A., Wawrzyniak, M., Hellings, P., et al. (2017). A wide diversity of bacteria from the human gut produces and degrades biogenic amines. Microb. Ecol. Health Dis. 28:1353881. doi: 10.1080/16512235.2017.1353881
Queipo-Ortuño, M. I., Seoane, L. M., Murri, M., Pardo, M., Gomez-Zumaquero, J. M., Cardona, F., et al. (2013). Gut microbiota composition in male rat models under different nutritional status and physical activity and its association with serum leptin and ghrelin levels. PLoS One 8:e65465. doi: 10.1371/journal.pone.0065465
Radulescu, C. I., Garcia-Miralles, M., Sidik, H., Bardile, C. F., Yusof, N. A. B. M., Lee, H. U., et al. (2019). Manipulation of microbiota reveals altered callosal myelination and white matter plasticity in a model of Huntington disease. Neurobiol. Dis. 127, 65–75. doi: 10.1016/j.nbd.2019.02.011
Rajan, K. B., Weuve, J., Barnes, L. L., McAninch, E. A., Wilson, R. S., and Evans, D. A. (2021). Population estimate of people with clinical Alzheimer’s disease and mild cognitive impairment in the United States (2020-2060). Alzheimers Dement. 17, 1966–1975. doi: 10.1002/alz.12362
Ramirez-Farias, C., Slezak, K., Fuller, Z., Duncan, A., Holtrop, G., and Louis, P. (2008). Effect of inulin on the human gut microbiota: stimulation of Bifidobacterium adolescentis and Faecalibacterium prausnitzii. Br. J. Nutr. 101, 541–550. doi: 10.1017/S0007114508019880
Rea, K., Dinan, T. G., and Cryan, J. F. (2016). The microbiome: a key regulator of stress and neuroinflammation. Neurobiol. Stress 4, 23–33. doi: 10.1016/j.ynstr.2016.03.001
Reichardt, N., Duncan, S. H., Young, P., Belenguer, A., McWilliam Leitch, C., Scott, K. P., et al. (2014). Phylogenetic distribution of three pathways for propionate production within the human gut microbiota. ISME J. 8, 1323–1335. doi: 10.1038/ismej.2014.14
Reigstad, C. S., Salmonson, C. E., Rainey III, J. F., Szurszewski, J. H., Linden, D. R., Sonnenburg, J. L., et al. (2015). Gut microbes promote colonic serotonin production through an effect of short-chain fatty acids on enterochromaffin cells. FASEB J. 29, 1395–1403. doi: 10.1096/fj.14-259598
Resende, W. R., Valvassori, S. S., Réus, G. Z., Varela, R. B., Arent, C. O., Ribeiro, K. F., et al. (2013). Effects of sodium butyrate in animal models of mania and depression: implications as a new mood stabilizer. Behav. Pharmacol. 24, 569–579. doi: 10.1097/FBP.0b013e32836546fc
Ridlon, J. M., Ikegawa, S., Alves, J. M. P., Zhou, B., Kobayashi, A., Iida, T., et al. (2013). Clostridium scindens: a human gut microbe with a high potential to convert glucocorticoids into androgens. J. Lipid Res. 54, 2437–2449. doi: 10.1194/jlr.M038869
Robertson, R. C., Seira Oriach, C., Murphy, K., Moloney, G. M., Cryan, J. F., Dinan, T. G., et al. (2017). Omega-3 polyunsaturated fatty acids critically regulate behaviour and gut microbiota development in adolescence and adulthood. Brain Behav. Immun. 59, 21–37. doi: 10.1016/j.bbi.2016.07.145
Rodrigues, V. F., Elias-Oliveira, J., Pereira, Í. S., Pereira, J. A., Barbosa, S. C., Machado, M. S. G., et al. (2022). Akkermansia muciniphila and gut immune system: a good friendship that attenuates inflammatory bowel disease, obesity, and diabetes. Front. Immunol. 13:934695. doi: 10.3389/fimmu.2022.934695
Rutsch, A., Kantsjö, J. B., and Ronchi, F. (2020). The gut-brain Axis: How microbiota and host Inflammasome influence brain physiology and pathology. Front. Immunol. 11:604179. doi: 10.3389/fimmu.2020.604179
Salami, M., and Soheili, M. (2022). The microbiota-gut-hippocampus axis, The microbiota-gut- hippocampus axis. Front. Neurosci. 16:1065995. doi: 10.3389/fnins.2022.1065995
Salim, S., Ahmad, F., Banu, A., and Mohammad, F. (2023). Gut microbiome and Parkinson’s disease: perspective on pathogenesis and treatment. J. Adv. Res. 50, 83–105. doi: 10.1016/j.jare.2022.10.013
Salonen, A., Lahti, L., Salojärvi, J., Holtrop, G., Korpela, K., Duncan, S. H., et al. (2014). Impact of diet and individual variation on intestinal microbiota composition and fermentation products in obese men. ISME J. 8, 2218–2230. doi: 10.1038/ismej.2014.63
Sampaio-Baptista, C., Vallès, A., Khrapitchev, A. A., Akkermans, G., Winkler, A. M., Foxley, S., et al. (2020). White matter structure and myelin-related gene expression alterations with experience in adult rats. Prog. Neurobiol. 187:101770. doi: 10.1016/j.pneurobio.2020.101770
Sampson, T. R., Debelius, J. W., Thron, T., Janssen, S., Shastri, G. G., Ilhan, Z. E., et al. (2016). Gut microbiota regulate motor deficits and Neuroinflammation in a model of Parkinson’s disease. Cell 167, 1469–1480.e12. doi: 10.1016/j.cell.2016.11.018
Sanchez, M., Darimont, C., Panahi, S., Drapeau, V., Marette, A., Taylor, V., et al. (2017). Effects of a diet-based weight-reducing program with probiotic supplementation on satiety efficiency, eating behaviour traits, and psychosocial Behaviours in obese individuals. Nutrients 9:284. doi: 10.3390/nu9030284
Sarubbo, F., Cavallucci, V., and Pani, G. (2022). The influence of gut microbiota on neurogenesis: evidence and hopes. Cells 11:382. doi: 10.3390/cells11030382
Savignac, H. M., Corona, G., Mills, H., Chen, L., Spencer, J. P. E., Tzortzis, G., et al. (2013). Prebiotic feeding elevates central brain derived neurotrophic factor, N-methyl-d-aspartate receptor subunits and d-serine. Neurochem. Int. 63, 756–764. doi: 10.1016/j.neuint.2013.10.006
Savignac, H. M., Kiely, B., Dinan, T. G., and Cryan, J. F. (2014). B ifidobacteria exert strain-specific effects on stress-related behavior and physiology in BALB /c mice. Neurogastroenterol. Motil. 26, 1615–1627. doi: 10.1111/nmo.12427
Savignac, H. M., Tramullas, M., Kiely, B., Dinan, T. G., and Cryan, J. F. (2015). Bifidobacteria modulate cognitive processes in an anxious mouse strain. Behav. Brain Res. 287, 59–72. doi: 10.1016/j.bbr.2015.02.044
Sawada, N., Kotani, T., Konno, T., Setiawan, J., Nishigaito, Y., Saito, Y., et al. (2018). Regulation by commensal bacteria of neurogenesis in the subventricular zone of adult mouse brain. Biochem. Biophys. Res. Commun. 498, 824–829. doi: 10.1016/j.bbrc.2018.03.064
Schiera, G., Di Liegro, C. M., Schirò, G., Sorbello, G., and Di Liegro, I. (2024). Involvement of astrocytes in the formation, maintenance, and function of the blood-brain barrier. Cells 13:150. doi: 10.3390/cells13020150
Schönfeld, P., and Wojtczak, L. (2016). Short-and medium-chain fatty acids in energy metabolism: the cellular perspective. J. Lipid Res. 57, 943–954. doi: 10.1194/jlr.R067629
Scott, K. A., Ida, M., Peterson, V. L., Prenderville, J. A., Moloney, G. M., Izumo, T., et al. (2017). Revisiting Metchnikoff: age-related alterations in microbiota-gut-brain axis in the mouse. Brain Behav. Immun. 65, 20–32. doi: 10.1016/j.bbi.2017.02.004
Sellgren, C. M., Gracias, J., Watmuff, B., Biag, J. D., Thanos, J. M., Whittredge, P. B., et al. (2019). Increased synapse elimination by microglia in schizophrenia patient-derived models of synaptic pruning. Nat. Neurosci. 22, 374–385. doi: 10.1038/s41593-018-0334-7
Severance, E. G., Gressitt, K. L., Stallings, C. R., Katsafanas, E., Schweinfurth, L. A., Savage, C. L. G., et al. (2017). Probiotic normalization of Candida albicans in schizophrenia: a randomized, placebo-controlled, longitudinal pilot study. Brain Behav. Immun. 62, 41–45. doi: 10.1016/j.bbi.2016.11.019
Sgritta, M., Dooling, S. W., Buffington, S. A., Momin, E. N., Francis, M. B., Britton, R. A., et al. (2019). Mechanisms underlying microbial-mediated changes in social behavior in mouse models of autism Spectrum disorder. Neuron 101, 246–259.e6. doi: 10.1016/j.neuron.2018.11.018
Sharma, S., Taliyan, R., and Singh, S. (2015). Beneficial effects of sodium butyrate in 6-OHDA induced neurotoxicity and behavioral abnormalities: modulation of histone deacetylase activity. Behav. Brain Res. 291, 306–314. doi: 10.1016/j.bbr.2015.05.052
Sharon, G., Cruz, N. J., Kang, D.-W., Gandal, M. J., Wang, B., Kim, Y.-M., et al. (2019). Human gut microbiota from autism Spectrum disorder promote behavioral symptoms in mice. Cell 177, 1600–1618.e17. doi: 10.1016/j.cell.2019.05.004
Sheikh, I. A., Bianchi-Smak, J., Laubitz, D., Schiro, G., Midura-Kiela, M. T., Besselsen, D. G., et al. (2024). Transplant of microbiota from Crohn’s disease patients to germ-free mice results in colitis. Gut Microbes 16:2333483. doi: 10.1080/19490976.2024.2333483
Silva, Y. P., Bernardi, A., and Frozza, R. L. (2020). The role of short-chain fatty acids from gut microbiota in gut-brain communication. Front. Endocrinol. 11:25. doi: 10.3389/fendo.2020.00025
Simon, M.-C., Strassburger, K., Nowotny, B., Kolb, H., Nowotny, P., Burkart, V., et al. (2015). Intake of Lactobacillus reuteri improves Incretin and insulin secretion in glucose-tolerant humans: a proof of concept. Diabetes Care 38, 1827–1834. doi: 10.2337/dc14-2690
Singh, R., Lakhanpal, D., Kumar, S., Sharma, S., Kataria, H., Kaur, M., et al. (2012). Late-onset intermittent fasting dietary restriction as a potential intervention to retard age-associated brain function impairments in male rats. Age (Dordr.) 34, 917–933. doi: 10.1007/s11357-011-9289-2
Singh, V., Lee, G., Son, H., Koh, H., Kim, E. S., Unno, T., et al. (2023). Butyrate producers, “the sentinel of gut”: their intestinal significance with and beyond butyrate, and prospective use as microbial therapeutics. Front. Microbiol. 13:1103836. doi: 10.3389/fmicb.2022.1103836
Singh, V., Sadler, R., Heindl, S., Llovera, G., Roth, S., Benakis, C., et al. (2018). The gut microbiome primes a cerebroprotective immune response after stroke. J. Cereb. Blood Flow Metab. 38, 1293–1298. doi: 10.1177/0271678X18780130
Sirzen-Zelenskaya, A., Zeyse, J., and Kapfhammer, J. P. (2006). Activation of class I metabotropic glutamate receptors limits dendritic growth of Purkinje cells in organotypic slice cultures. Eur. J. Neurosci. 24, 2978–2986. doi: 10.1111/j.1460-9568.2006.05196.x
Skonieczna-Żydecka, K., Grochans, E., Maciejewska, D., Szkup, M., Schneider-Matyka, D., Jurczak, A., et al. (2018). Faecal short chain fatty acids profile is changed in polish depressive women. Nutrients 10:1939. doi: 10.3390/nu10121939
Slykerman, R. F., Hood, F., Wickens, K., Thompson, J. M. D., Barthow, C., Murphy, R., et al. (2017). Effect of Lactobacillus rhamnosus HN001 in pregnancy on postpartum symptoms of depression and anxiety: a randomised double-blind placebo-controlled trial. EBioMedicine 24, 159–165. doi: 10.1016/j.ebiom.2017.09.013
Smith, C. J., Emge, J. R., Berzins, K., Lung, L., Khamishon, R., Shah, P., et al. (2014). Probiotics normalize the gut-brain-microbiota axis in immunodeficient mice. American journal of physiology-gastrointestinal and liver. Physiology 307, G793–G802. doi: 10.1152/ajpgi.00238.2014
Smith, P. M., Howitt, M. R., Panikov, N., Michaud, M., Gallini, C. A., Bohlooly-Y, M., et al. (2013). The microbial metabolites, short-chain fatty acids, regulate colonic T reg cell homeostasis. Science 341, 569–573. doi: 10.1126/science.1241165
Socała, K., Doboszewska, U., Szopa, A., Serefko, A., Włodarczyk, M., Zielińska, A., et al. (2021). The role of microbiota-gut-brain axis in neuropsychiatric and neurological disorders. Pharmacol. Res. 172:105840. doi: 10.1016/j.phrs.2021.105840
Sofroniew, M. V. (2014). Multiple roles for astrocytes as effectors of cytokines and inflammatory mediators. Neuroscientist 20, 160–172. doi: 10.1177/1073858413504466
Soliman, M. L., Combs, C. K., and Rosenberger, T. A. (2013). Modulation of inflammatory cytokines and mitogen-activated protein kinases by acetate in primary astrocytes. J. Neuroimmune Pharmacol. 8, 287–300. doi: 10.1007/s11481-012-9426-4
Soliman, M. L., Puig, K. L., Combs, C. K., and Rosenberger, T. A. (2012). Acetate reduces microglia inflammatory signaling in vitro. J. Neurochem. 123, 555–567. doi: 10.1111/j.1471-4159.2012.07955.x
Song, L., Gao, Y., Zhang, X., and Le, W. (2013). Galactooligosaccharide improves the animal survival and alleviates motor neuron death in SOD1G93A mouse model of amyotrophic lateral sclerosis. Neuroscience 246, 281–290. doi: 10.1016/j.neuroscience.2013.05.002
Sparks Stein, P., Steffen, M. J., Smith, C., Jicha, G., Ebersole, J. L., Abner, E., et al. (2012). Serum antibodies to periodontal pathogens are a risk factor for Alzheimer’s disease. Alzheimer’s Dementia 8, 196–203. doi: 10.1016/j.jalz.2011.04.006
Stampanoni Bassi, M., Iezzi, E., Gilio, L., Centonze, D., and Buttari, F. (2019). Synaptic plasticity shapes brain connectivity: implications for network topology. IJMS 20:6193. doi: 10.3390/ijms20246193
Stilling, R. M., Van De Wouw, M., Clarke, G., Stanton, C., Dinan, T. G., and Cryan, J. F. (2016). The neuropharmacology of butyrate: the bread and butter of the microbiota-gut-brain axis? Neurochem. Int. 99, 110–132. doi: 10.1016/j.neuint.2016.06.011
Stringer, A. (2024). The systemic outcomes of gastrointestinal mucositis: host–microbe interaction leading to systemic inflammatory responses. Current Opinion Supportive Palliative Care 18, 71–72. doi: 10.1097/SPC.0000000000000704
Sudo, N., Chida, Y., Aiba, Y., Sonoda, J., Oyama, N., Yu, X., et al. (2004). Postnatal microbial colonization programs the hypothalamic–pituitary–adrenal system for stress response in mice. J. Physiol. 558, 263–275. doi: 10.1113/jphysiol.2004.063388
Sumi, Y., Miyakawa, M., Kanzaki, M., and Kotake, Y. (1977). Vitamin B-6 deficiency in germfree rats. J. Nutr. 107, 1707–1714. doi: 10.1093/jn/107.9.1707
Sun, J., Ling, Z., Wang, F., Chen, W., Li, H., Jin, J., et al. (2016). Clostridium butyricum pretreatment attenuates cerebral ischemia/reperfusion injury in mice via anti-oxidation and anti-apoptosis. Neurosci. Lett. 613, 30–35. doi: 10.1016/j.neulet.2015.12.047
Sun, M.-F., Zhu, Y.-L., Zhou, Z.-L., Jia, X.-B., Xu, Y.-D., Yang, Q., et al. (2018). Neuroprotective effects of fecal microbiota transplantation on MPTP-induced Parkinson’s disease mice: gut microbiota, glial reaction and TLR4/TNF-α signaling pathway. Brain Behav. Immun. 70, 48–60. doi: 10.1016/j.bbi.2018.02.005
Szczesniak, O., Hestad, K. A., Hanssen, J. F., and Rudi, K. (2016). Isovaleric acid in stool correlates with human depression. Nutr. Neurosci. 19, 279–283. doi: 10.1179/1476830515Y.0000000007
Tabouy, L., Getselter, D., Ziv, O., Karpuj, M., Tabouy, T., Lukic, I., et al. (2018). Dysbiosis of microbiome and probiotic treatment in a genetic model of autism spectrum disorders. Brain Behav. Immun. 73, 310–319. doi: 10.1016/j.bbi.2018.05.015
Tan, J., McKenzie, C., Potamitis, M., Thorburn, A. N., Mackay, C. R., and Macia, L. (2014). The role of short-chain fatty acids in health and disease. Adv. Immunol. 121, 91–119. doi: 10.1016/B978-0-12-800100-4.00003-9
Tang, W., Meng, Z., Li, N., Liu, Y., Li, L., Chen, D., et al. (2021). Roles of gut microbiota in the regulation of hippocampal plasticity, inflammation, and Hippocampus-dependent behaviors. Front. Cell. Infect. Microbiol. 10:611014. doi: 10.3389/fcimb.2020.611014
Tay, T. L., Savage, J. C., Hui, C. W., Bisht, K., and Tremblay, M. (2017). Microglia across the lifespan: from origin to function in brain development, plasticity and cognition. J. Physiol. 595, 1929–1945. doi: 10.1113/JP272134
Thabane, M., Simunovic, M., Akhtar-Danesh, N., Garg, A. X., Clark, W. F., Collins, S. M., et al. (2010). An outbreak of acute bacterial gastroenteritis is associated with an increased incidence of irritable bowel syndrome in children. Am. J. Gastroenterol. 105, 933–939. doi: 10.1038/ajg.2010.74
Thevaranjan, N., Puchta, A., Schulz, C., Naidoo, A., Szamosi, J. C., Verschoor, C. P., et al. (2017). Age-associated microbial Dysbiosis promotes intestinal permeability, systemic inflammation, and macrophage dysfunction. Cell Host Microbe 21, 455–466.e4. doi: 10.1016/j.chom.2017.03.002
Thion, M. S., Low, D., Silvin, A., Chen, J., Grisel, P., Schulte-Schrepping, J., et al. (2018). Microbiome influences prenatal and adult microglia in a sex-specific manner. Cell 172, 500–516.e16. doi: 10.1016/j.cell.2017.11.042
Tillisch, K., Labus, J., Kilpatrick, L., Jiang, Z., Stains, J., Ebrat, B., et al. (2013). Consumption of fermented Milk product with probiotic modulates brain activity. Gastroenterology 144, 1394–1401.e4. doi: 10.1053/j.gastro.2013.02.043
Toda, T., Parylak, S. L., Linker, S. B., and Gage, F. H. (2019). The role of adult hippocampal neurogenesis in brain health and disease. Mol. Psychiatry 24, 67–87. doi: 10.1038/s41380-018-0036-2
Tong, L., Wang, Y., Wang, Z., Liu, W., Sun, S., Li, L., et al. (2016). Propionate ameliorates dextran sodium sulfate-induced colitis by improving intestinal barrier function and reducing inflammation and oxidative stress. Front. Pharmacol. 7:253. doi: 10.3389/fphar.2016.00253
Tsukuda, N., Yahagi, K., Hara, T., Watanabe, Y., Matsumoto, H., Mori, H., et al. (2021). Key bacterial taxa and metabolic pathways affecting gut short-chain fatty acid profiles in early life. ISME J. 15, 2574–2590. doi: 10.1038/s41396-021-00937-7
Ueda, A., Shinkai, S., Shiroma, H., Taniguchi, Y., Tsuchida, S., Kariya, T., et al. (2021). Identification of Faecalibacterium prausnitzii strains for gut microbiome-based intervention in Alzheimer’s-type dementia. Cell Rep. Med. 2:100398. doi: 10.1016/j.xcrm.2021.100398
Unger, M. M., Spiegel, J., Dillmann, K.-U., Grundmann, D., Philippeit, H., Bürmann, J., et al. (2016). Short chain fatty acids and gut microbiota differ between patients with Parkinson’s disease and age-matched controls. Parkinsonism Relat. Disord. 32, 66–72. doi: 10.1016/j.parkreldis.2016.08.019
Vainchtein, I. D., and Molofsky, A. V. (2020). Astrocytes and microglia: in sickness and in health. Trends Neurosci. 43, 144–154. doi: 10.1016/j.tins.2020.01.003
Valles-Colomer, M., Falony, G., Darzi, Y., Tigchelaar, E. F., Wang, J., Tito, R. Y., et al. (2019). The neuroactive potential of the human gut microbiota in quality of life and depression. Nat. Microbiol. 4, 623–632. doi: 10.1038/s41564-018-0337-x
Valnegri, P., Puram, S. V., and Bonni, A. (2015). Regulation of dendrite morphogenesis by extrinsic cues. Trends Neurosci. 38, 439–447. doi: 10.1016/j.tins.2015.05.003
Valori, C. F., Guidotti, G., Brambilla, L., and Rossi, D. (2019). Astrocytes: emerging therapeutic targets in neurological disorders. Trends Mol. Med. 25, 750–759. doi: 10.1016/j.molmed.2019.04.010
Valvassori, S. S., Dal-Pont, G. C., Steckert, A. V., Varela, R. B., Lopes-Borges, J., Mariot, E., et al. (2016). Sodium butyrate has an antimanic effect and protects the brain against oxidative stress in an animal model of mania induced by ouabain. Psychiatry Res. 235, 154–159. doi: 10.1016/j.psychres.2015.11.017
Van De Wouw, M., Boehme, M., Lyte, J. M., Wiley, N., Strain, C., O’Sullivan, O., et al. (2018). Short-chain fatty acids: microbial metabolites that alleviate stress-induced brain–gut axis alterations. J. Physiol. 596, 4923–4944. doi: 10.1113/JP276431
van der Lugt, B., Rusli, F., Lute, C., Lamprakis, A., Salazar, E., Boekschoten, M. V., et al. (2018). Integrative analysis of gut microbiota composition, host colonic gene expression and intraluminal metabolites in aging C57BL/6J mice. Aging 10, 930–950. doi: 10.18632/aging.101439
Van Tongeren, S. P., Slaets, J. P. J., Harmsen, H. J. M., and Welling, G. W. (2005). Fecal microbiota composition and frailty. Appl. Environ. Microbiol. 71, 6438–6442. doi: 10.1128/AEM.71.10.6438-6442.2005
Varela, R. B., Valvassori, S. S., Lopes-Borges, J., Mariot, E., Dal-Pont, G. C., Amboni, R. T., et al. (2015). Sodium butyrate and mood stabilizers block ouabain-induced hyperlocomotion and increase BDNF, NGF and GDNF levels in brain of Wistar rats. J. Psychiatr. Res. 61, 114–121. doi: 10.1016/j.jpsychires.2014.11.003
Verdu, E. F., Bercik, P., Huang, X. X., Lu, J., al-Mutawaly, N., Sakai, H., et al. (2008). The role of luminal factors in the recovery of gastric function and behavioral changes after chronicHelicobacter pyloriinfection. Physiology 295, G664–G670. doi: 10.1152/ajpgi.90316.2008
Vijay, N., and Morris, M. (2014). Role of Monocarboxylate transporters in drug delivery to the brain. CPD 20, 1487–1498. doi: 10.2174/13816128113199990462
Vinithakumari, A. A., Padhi, P., Hernandez, B., Je-Han Lin, S., Dunkerson-Kurzhumov, A., Showman, L., et al. (2021). Clostridioides difficile infection increases circulating p-cresol levels and dysregulates brain dopamine metabolism: linking gut-brain axis to autism spectrum disorders? Neuroscience. doi: 10.1101/2021.10.22.465382
Vital, M., Howe, A. C., and Tiedje, J. M. (2014). Revealing the bacterial butyrate synthesis pathways by analyzing (Meta)genomic data. MBio 5, e00889–e00814. doi: 10.1128/mBio.00889-14
Vitureira, N., and Goda, Y. (2013). Cell biology in neuroscience: the interplay between Hebbian and homeostatic synaptic plasticity. J. Cell Biol. 203, 175–186. doi: 10.1083/jcb.201306030
von Bernhardi, R., Bernhardi, L. E., and Eugenín, J. (2017). What is neural plasticity? Adv. Exp. Med. Biol. 1015, 1–15. doi: 10.1007/978-3-319-62817-2_1
Vuong, H. E., Yano, J. M., Fung, T. C., and Hsiao, E. Y. (2017). The microbiome and host behavior. Annu. Rev. Neurosci. 40, 21–49. doi: 10.1146/annurev-neuro-072116-031347
Wang, M., Cao, J., Gong, C., Amakye, W. K., Yao, M., and Ren, J. (2021). Exploring the microbiota-Alzheimer’s disease linkage using short-term antibiotic treatment followed by fecal microbiota transplantation. Brain Behav. Immun. 96, 227–238. doi: 10.1016/j.bbi.2021.06.003
Wang, L., Christophersen, C. T., Sorich, M. J., Gerber, J. P., Angley, M. T., and Conlon, M. A. (2012). Elevated fecal short chain fatty acid and Ammonia concentrations in children with autism Spectrum disorder. Dig. Dis. Sci. 57, 2096–2102. doi: 10.1007/s10620-012-2167-7
Wang, T., Hu, X., Liang, S., Li, W., Wu, X., Wang, L., et al. (2015). Lactobacillus fermentum NS9 restores the antibiotic induced physiological and psychological abnormalities in rats. Benef. Microbes 6, 707–717. doi: 10.3920/BM2014.0177
Wang, Y., Qiao, H., and Zhang, Y. (2023). Changes of dopamine and tyrosine hydroxylase levels in the brain of germ-free mice. Iran. J. Biotechnol. 21:e2798. doi: 10.30498/ijb.2022.236732.2798
Wang, L., Zhang, J., Guo, Z., Kwok, L., Ma, C., Zhang, W., et al. (2014). Effect of oral consumption of probiotic Lactobacillus planatarum P-8 on fecal microbiota, SIgA, SCFAs, and TBAs of adults of different ages. Nutrition 30, 776–783.e1. doi: 10.1016/j.nut.2013.11.018
Watanabe, Y., Nagai, F., and Morotomi, M. (2012). Characterization of Phascolarctobacterium succinatutens sp. nov., an Asaccharolytic, succinate-utilizing bacterium isolated from human feces. Appl. Environ. Microbiol. 78, 511–518. doi: 10.1128/AEM.06035-11
Waworuntu, R. V., Hanania, T., Boikess, S. R., Rex, C. S., and Berg, B. M. (2016). Early life diet containing prebiotics and bioactive whey protein fractions increased dendritic spine density of rat hippocampal neurons. Int. J. Dev. Neurosci. 55, 28–33. doi: 10.1016/j.ijdevneu.2016.09.001
Wei, Y. B., Melas, P. A., Wegener, G., Mathe, A. A., and Lavebratt, C. (2015). Antidepressant-like effect of sodium butyrate is associated with an increase in TET1 and in 5-Hydroxymethylation levels in the Bdnf gene. Int. J. Neuropsychopharmacol. 18:pyu032–pyu032. doi: 10.1093/ijnp/pyu032
Wilton, D. K., Dissing-Olesen, L., and Stevens, B. (2019). Neuron-Glia Signaling in Synapse Elimination. Annu. Rev. Neurosci. 42, 107–127. doi: 10.1146/annurev-neuro-070918-050306
Witte, A. V., Fobker, M., Gellner, R., Knecht, S., and Flöel, A. (2009). Caloric restriction improves memory in elderly humans. Proc. Natl. Acad. Sci. USA 106, 1255–1260. doi: 10.1073/pnas.0808587106
Wlaź, P., Wiater, A., Majewska, M., Wyska, E., Grąz, M., Śliwa-Dominiak, J., et al. (2024). Effect of dietary supplementation with Lactobacillus helveticus R0052 on seizure thresholds and antiseizure potency of sodium valproate in mice. Psychopharmacology 241, 327–340. doi: 10.1007/s00213-023-06489-2
Wu, S. V., and Hui, H. (2011). Treat your bug right. Front. Physiol. 2:9. doi: 10.3389/fphys.2011.00009
Yamawaki, Y., Yoshioka, N., Nozaki, K., Ito, H., Oda, K., Harada, K., et al. (2018). Sodium butyrate abolishes lipopolysaccharide-induced depression-like behaviors and hippocampal microglial activation in mice. Brain Res. 1680, 13–38. doi: 10.1016/j.brainres.2017.12.004
Yan, J., and Charles, J. F. (2018). Gut microbiota and IGF-1. Calcif. Tissue Int. 102, 406–414. doi: 10.1007/s00223-018-0395-3
Yang, L. L., Millischer, V., Rodin, S., MacFabe, D. F., Villaescusa, J. C., and Lavebratt, C. (2020). Enteric short-chain fatty acids promote proliferation of human neural progenitor cells. J. Neurochem. 154, 635–646. doi: 10.1111/jnc.14928
Yano, J. M., Yu, K., Donaldson, G. P., Shastri, G. G., Ann, P., Ma, L., et al. (2015). Indigenous Bacteria from the gut microbiota regulate host serotonin biosynthesis. Cell 161, 264–276. doi: 10.1016/j.cell.2015.02.047
Yin, J., Liao, S., He, Y., Wang, S., Xia, G., Liu, F., et al. (2015). Dysbiosis of gut microbiota with reduced trimethylamine-N-oxide level in patients with large-artery atherosclerotic stroke or transient ischemic attack. JAHA 4:e002699. doi: 10.1161/JAHA.115.002699
Zareian, M., Ebrahimpour, A., Bakar, F. A., Mohamed, A. K. S., Forghani, B., Ab-Kadir, M. S. B., et al. (2012). A glutamic acid-producing lactic acid Bacteria isolated from Malaysian fermented foods. IJMS 13, 5482–5497. doi: 10.3390/ijms13055482
Zhai, C.-D., Zheng, J.-J., An, B.-C., Huang, H.-F., and Tan, Z.-C. (2019). Intestinal microbiota composition in patients with amyotrophic lateral sclerosis: establishment of bacterial and archaeal communities analyses. Chin. Med. J. 132, 1815–1822. doi: 10.1097/CM9.0000000000000351
Zhang, H., Chen, Y., Wang, Z., Xie, G., Liu, M., Yuan, B., et al. (2022). Implications of gut microbiota in neurodegenerative diseases. Front. Immunol. 13:785644. doi: 10.3389/fimmu.2022.785644
Zhang, Y., Wang, Z., Peng, J., Gerner, S. T., Yin, S., and Jiang, Y. (2021). Gut microbiota-brain interaction: An emerging immunotherapy for traumatic brain injury. Exp. Neurol. 337:113585. doi: 10.1016/j.expneurol.2020.113585
Zhang, L., Wang, Y., Xiayu, X., Shi, C., Chen, W., Song, N., et al. (2017). Altered gut microbiota in a mouse model of Alzheimer’s disease. JAD 60, 1241–1257. doi: 10.3233/JAD-170020
Zhu, X., Han, Y., Du, J., Liu, R., Jin, K., and Yi, W. (2017). Microbiota-gut-brain axis and the central nervous system. Oncotarget 8, 53829–53838. doi: 10.18632/oncotarget.17754
Zhu, X., Li, B., Lou, P., Dai, T., Chen, Y., Zhuge, A., et al. (2021). The relationship between the gut microbiome and neurodegenerative diseases. Neurosci. Bull. 37, 1510–1522. doi: 10.1007/s12264-021-00730-8
Aβ – Amyloid-beta
AChE – Acetylcholinesterase
AD – Alzheimer’s disease
ADHD – Attention-deficit/hyperactivity disorder
AEP – Asparagine endopeptidase
ALS – Amyotrophic lateral sclerosis
AMPK – Adenosine monophosphate-activated protein kinase
ASD – Autism spectrum disorder
BBB – Blood–brain barrier
BDNF – Brain-derived neurotrophic factor
CD74 – Cluster of Differentiation 74
CJD – Creutzfeldt–Jakob disease
CML – N6-carboxymethyllysine
Cntnap2 – Contactin-associated protein-like 2 protein
CNS – Central nervous system
COX-1 – Cyclooxygenase-1
CD – Crohn’s disease
DG – Dentate gyrus
ΔFosB – Protein fosB
ENS – Enteric nervous system
FFAR – Free fatty acid receptor
FGF21 – Fibroblast growth factor-21
FMT – Fecal microbiota transplantation
GF – Germ-free
GI – Gastrointestinal tract
GOS – Galacto-oligosaccharides
GYS1 – Glycogen synthase 1
HDACs – Histone deacetylases
HPA – Hypothalamic–pituitary–adrenal axis
IL – Interleukins
5-LOX – 5-lipoxygenase
LDHA, LDHB – Lactate dehydrogenase-A or B
LPS – Lipopolysaccharide
LTP – Long-term potentiation
MCT1 – Monocarboxylate transporter 1
MDD – Major depressive disorder
MGBA – Microbiota-gut-brain axis
MS – Multiple sclerosis
mTOR – Mammalian target of rapamycin
NMDA – N-methyl-D-aspartate
NMDAR – Anti-N-methyl-D-aspartate receptor
OLs – Oligodendrocytes
PFC – Prefrontal cortex
PFKFB3 – 6-phosphofructo-2-kinase/fructose-2,6-biphosphatase 3
PrPSc – Scrapie prion protein
PUFAs – Polyunsaturated fatty acids
SCFAs – Short-chain fatty acids
SCZ – Schizophrenia
SGZ – Subgranular zone
Shank3 – SH3 and multiple ankyrin repeat domains 3
SIRT-1 – Sirtuin 1
TH – Tyrosine hydroxylase
TNF-α – Tumor necrosis factor-alpha
ZO-1 – Zonula occludens-1
Keywords: microbiota-gut-brain axis, short-chain fatty acid, blood–brain barrier, brain morphology, microbial interventions, neuroplasticity
Citation: Yassin LK, Nakhal MM, Alderei A, Almehairbi A, Mydeen AB, Akour A and Hamad MIK (2025) Exploring the microbiota-gut-brain axis: impact on brain structure and function. Front. Neuroanat. 19:1504065. doi: 10.3389/fnana.2025.1504065
Received: 30 September 2024; Accepted: 30 January 2025;
Published: 12 February 2025.
Edited by:
Cinzia Fabrizi, Sapienza University of Rome, ItalyReviewed by:
Valentina Caputi, University College Cork, IrelandCopyright © 2025 Yassin, Nakhal, Alderei, Almehairbi, Mydeen, Akour and Hamad. This is an open-access article distributed under the terms of the Creative Commons Attribution License (CC BY). The use, distribution or reproduction in other forums is permitted, provided the original author(s) and the copyright owner(s) are credited and that the original publication in this journal is cited, in accordance with accepted academic practice. No use, distribution or reproduction is permitted which does not comply with these terms.
*Correspondence: Mohammad I. K. Hamad, bS5oYW1hZEB1YWV1LmFjLmFl
Disclaimer: All claims expressed in this article are solely those of the authors and do not necessarily represent those of their affiliated organizations, or those of the publisher, the editors and the reviewers. Any product that may be evaluated in this article or claim that may be made by its manufacturer is not guaranteed or endorsed by the publisher.
Research integrity at Frontiers
Learn more about the work of our research integrity team to safeguard the quality of each article we publish.