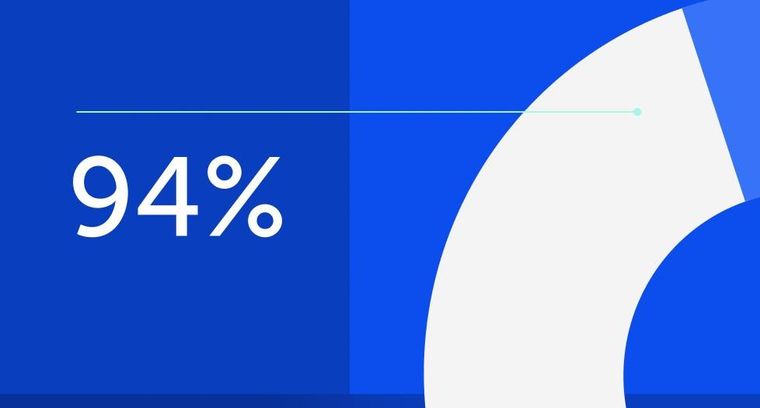
94% of researchers rate our articles as excellent or good
Learn more about the work of our research integrity team to safeguard the quality of each article we publish.
Find out more
REVIEW article
Front. Neuroanat., 09 July 2024
Volume 18 - 2024 | https://doi.org/10.3389/fnana.2024.1398400
This article is part of the Research TopicRecent Advances in the Anatomy, Physiology, And Pathophysiology of the Peripheral Nervous SystemView all 9 articles
Peripheral nerve damage often leads to the onset of neuropathic pain (NeuP). This condition afflicts millions of people, significantly burdening healthcare systems and putting strain on families’ financial well-being. Here, we will focus on the role of peripheral sensory neurons, specifically the Dorsal Root Ganglia neurons (DRG neurons) in the development of NeuP. After axotomy, DRG neurons activate regenerative signals of axons-soma communication to promote a gene program that activates an axonal branching and elongation processes. The results of a neuronal morphological cytoskeleton change are not always associated with functional recovery. Moreover, any axonal miss-targeting may contribute to NeuP development. In this review, we will explore the epidemiology of NeuP and its molecular causes at the level of the peripheral nervous system and the target organs, with major focus on the neuronal cross-talk between intrinsic and extrinsic factors. Specifically, we will describe how failures in the neuronal regenerative program can exacerbate NeuP.
With the term “pain” we refer to an “unpleasant sensory and emotional experience that is associated with or resemble actual or potential tissue damage,” as defined by the International Association for the Study of Pain (IASP; Raja et al., 2020). We consider pain a debilitating condition, but in reality this is an evolutionary conserved protective response to harmful stimuli, such as excessive cold/heat, chemical irritants and dangerous mechanical forces (Testa et al., 2021). Indeed, patients with congenital insensitivity to pain suffer from multiple lesions, untreated bone fractures and severe complications (Phatarakijnirund et al., 2016; Wang et al., 2016; Hartono et al., 2020). Nonetheless, excessive pain is detrimental and pharmacological treatments are necessary to abate it.
We distinguish two phases of pain: acute and chronic. Acute pain arises from chemical exposure (acetone, capsaicin), temperature (heat and cold) and mechanical stimuli (Fernandez Rojas et al., 2023). Acute pain is the first response to damaged tissue, followed by inflammation that triggers swelling of the area and promotes tissue repair (Rabiller et al., 2021; Parisien et al., 2022). However, if the pain persists for more than 3 months, it is defined as chronic and it becomes a pathological condition in itself (Treede et al., 2019). A temporal parameter is used to differentiate between acute and chronic pain due to the lack of consistent biomarkers that could be applied in the clinical setting.
Pain is also classified according to its origin as nociceptive (when tissues are injured), neuropathic (if nerves are damaged) or nociplastic (when the nervous system is sensitized, while no damages are observed on tissues and/or peripheral nerves; Fitzcharles et al., 2021). In the clinical practice, it may be difficult to separate the types of pain and most of the conditions may present a mixed phenotype such as neuropathic and nociplastic (Caraceni and Shkodra, 2019).
Neuropathic pain (NeuP) can arise because of lesions or diseases (genetic or acquired) affecting the somatosensory nervous system (SNS). The SNS is called spinothalamic tract ascending pathways formed by the synapses of three order of neurons: primary neurons (housed in dorsal root ganglia), secondary neurons (located in the spinal cord) and tertiary neurons (present in the thalamus; Viswanath et al., 2020; LRM et al., 2021). This system is responsible for the perception of crude touch, pain, temperature, as it integrates information from external stimuli and conveys them from the periphery to the cerebral cortex. Any damage of this pathway disrupts the signal transmission and can results in pain (Colloca et al., 2017).
While acute trauma is a common trigger, NeuP can develop from non-traumatic conditions that affect the nervous system. These conditions may be: (a) genetic mutations or polymorphisms, (b) acquired afflictions, like infections or injuries, or (c) medical treatments or drugs.
In humans, mutations of certain genes, such as PMP22, GJB1, MPZ and GDAP1, cause Charcot–Marie–Tooth disease, a group of inherited disorders characterized by nerve damage with painful motor and sensory neuropathy (Liu et al., 2020). People suffering from erythromelalgia and paroxysmal extreme pain disorder (Ahn et al., 2013; Goodwin and McMahon, 2021), generally called idiopathic painful small fiber neuropathies, present gain-of-function mutations in sodium voltage-gated channel encoding NaV1.7, NaV1.8, and NaV1.9. Other mutation in TRPA1, TRPV1, α-galactosidase and KIF5A (Biegstraaten et al., 2012; Boukalova et al., 2014; Rinaldi et al., 2015) are responsible for sensory neurons hyperexcitability that clinically manifests as sudden bouts of pain propagating inward from the extremities. Mutations of SPTLC1, a serine palmitoyltransferase, cause a form of hereditary sensory neuropathy with early sensory loss and later “lightning” or “shooting” pains (Lorenzoni et al., 2023). More gene variations have been associated to the development of painful syndromes, as reported in the DOLORisk study1 (Pascal et al., 2019) and the Human Pain Genetics Database (HPGDB; humanpaingeneticsdb.ca; Meloto et al., 2018).
A plethora of acquired afflictions can damage nerves and provoke NeuP. This is the case of spinal cord injury (Shiao and Lee-Kubli, 2018), diabetes (Feldman et al., 2019), herpes zoster infection (Kinchington and Goins, 2011), HIV infection (Laast et al., 2011), Lyme disease (Karri and Bruel, 2021) and also COVID infection (Fernández-De-las-peñas et al., 2022). Cancer may induce NeuP by compressing the surrounding nerves while growing or by inducing fibrosis, both of which cause pain fibers hypersensitivity (Oh and Yoon, 2018). Moreover, the pro-inflammatory cytokines released by the immune cells recruited in the tumor microenvironment may increase pain perception and hyperalgesia (Bennett et al., 2012; Caraceni and Shkodra, 2019).
People suffering from painful conditions often turn to surgical or pharmacological treatments, but they may not always find relief. Surgical operations cause additional nerve damage, which can evolve in persistent Surgically-Induced Neuropathic Pain (SNPP; Borsook et al., 2013). Drugs used to treat pain, such as psychotropic and anticonvulsants (e.g., gabapentin; Jones et al., 2019), can trigger Drug Induced Peripheral Neuropathy (DIPN). Chemotherapy-induced peripheral neuropathy (CIPN) can cause irreversible nerve damage with pain that cannot be relieved even after the end of the treatment (Zhang et al., 2016; Bjornard et al., 2018; Eldridge et al., 2021). In particular, CIPN patients present altered activity and expression of voltage-gated ion channels (i.e., neurotransmission) and loss of intraepidermal nerve fibers and Meissner’s corpuscles in the skin (Boyette-Davis et al., 2015). Understanding the molecular basis of neuropathic pain to develop targeted analgesic could be incredibly beneficial for all these patients.
It is estimated that between 6.9% and 10% of the world general population suffers from chronic NeuP (Van Hecke et al., 2014). The prevalence reported in population studies varies between 3.2% and 14.5%, likely due to differences in evaluation methods, language barriers, sample recruitment processes, and patient self-reported information employed in the data collection (Figure 1).
Figure 1. The world map represents the prevalence of NeuP in different countries in the general population. A color gradient was used for those countries with published data. Gray color was used for countries were no studies were found. The figure was created using Datawrapper, and is also available online at the following link https://datawrapper.dwcdn.net/kgAz0/1/. Additional information on the studies used to compile this graph is available in the Supplementary Table 1.
Clinical diagnosis (e.g., documented neurological lesion) is rarely used in the census studies due to the difficulties in the patients’ recruitment process. The majority of the epidemiologic investigations employ one of three screening questionnaires for NeuP assessment: PainDETECT, LANSS (Leeds Assessment of Neuropathic Symptoms and Signs), or DN4 (Douleur neuropathique 4). Even though their results do not completely overlap (VanDenKerkhof et al., 2016; Attal et al., 2018), these questionnaires are a useful tool to identify the classical symptoms of NeuP, specifically allodynia (i.e., pain by a stimulus that should not be causing discomfort), hypersensitivity, shooting pain, numbness, burning and tingling sensations (Truini et al., 2013).
NeuP symptoms greatly affect the quality of life of the people and increase the individual healthcare cost. Looking at five European countries (Italy, Spain, France, the UK and Germany), the average annual spending healthcare-related per patient ranged from €1,939 to €3,131, when adjusted to 2012 prices (Liedgens et al., 2016). Additionally, psychological factors (such as stress, anxiety, and depression) can worsen and, at the same time, be worsened by neuropathic pain (Breivik et al., 2013; Meng et al., 2020; Roughan et al., 2021). This psychological spiral is usually attenuated when the patients have a support system around them to help dealing with pain (Cohen et al., 2021).
Consumption of western-style high-fat diet, excessive alcohol and sedentariness are on the rise worldwide, and unfortunately they are also correlated with an increased risk of developing NeuP (Brandão et al., 2020; Dudek et al., 2020; Smith et al., 2020; Tanaka et al., 2023). Therefore, there is an urgent need for proper unbiased pain biomarkers to be employed in the clinics to diagnosis and then alleviate pain in the sufferers.
It is widely known that pain perception varies according to age, sex, and ethnic group (Mills et al., 2019; Chang et al., 2022). Females more than males suffer from NeuP, a phenomenon observed in both rodents and humans (Szabo-Pardi et al., 2021; Elliott et al., 2024). Sex hormones are known to influence pain perception, as both estrogen and testosterone receptors are expressed in sensory neurons. In particular in peripheral nociceptors, 17-β-estradiol increase sensitivity to mechanical and thermal pain (Patrone et al., 1999; Deng et al., 2017), while testosterone, binding to TRPM8, dampens pain perception (Barbosa Neto et al., 2019). The molecular bases of sex-dimorphism in NeuP are still unclear, but it has been speculated that sex steroids might influence specific protective or detrimental gene expression for pain perception (Stephens et al., 2019) and axonal regeneration (Ward et al., 2021).
Population studies indicate that NeuP is prevalent in the elders. This does not mean that young people are exempt from nerve damage. In both humans and animals, the nerve damage occurring at an early age will trigger NeuP only in late childhood and adolescence (Walco et al., 2010). In rats, specifically, nerve injuries before P28 will develop into NeuP only after 3 weeks, a time that corresponds to the animal’s adolescence (Fitzgerald and McKelvey, 2016). This phenomenon occurs because before P28 the neuroimmune response is skewed toward anti-inflammation, which suppresses nociceptors excitability and prevents NeuP. As the rodent grows, the neuroimmune signature shifts toward pro-inflammation, which uncovers the latent pain response to early trauma (McKelvey et al., 2015).
In general, with age there are increased number of abnormal or degenerating neuronal fibers, slower conduction speed, altered endogenous inhibition and decreased function of neurotransmitters, all of which favor NeuP development (Giovannini et al., 2021). Nociceptor gene expression also changes with age. Aged murine models (18–24 months) have increased pain sensitization (Tac1 and Calca) and stress (Atf3) markers in DRGs, and also elevated levels of neurotrophic factor Bdnf (Vincent et al., 2020).
The described physiological variability renders pain detection and analgesic development incredibly challenging. It will be difficult to develop an all-encompassing wonder drug to resolve NeuP in all the conditions for all type of patients. Pharmacological studies, especially, will have to be even more attentive in subject clustering to properly identify drug candidates.
Animals perceive pain, defined as intense above threshold thermal, mechanical or chemical stimuli, via a subpopulation of peripheral nerve fibers called nociceptors (Basbaum et al., 2009), that are to the primary order neurons mentioned previously. These nociceptors have their soma situated in the dorsal root ganglia (DRG), bilateral structures that reside inside the intervertebral foramina. Therefore, DRGs are functional centers for sensory transduction and modulation, but also for pain transmission and maintenance of pain states (Berger et al., 2021). The neurons residing in the DRG structures are a population heterogeneous in size and function. In the same DRG it is possible to recognize nociceptors’, mechanoceptors’ and propioceptors’ cell bodies (Belmonte and Viana, 2008), which present diverse gene expression profiles. Through single cell-sequencing, several researchers could even obtain the transcriptome signature of the different DRG nociceptors, a throve of information available online in several databases (Table 1).
Nociceptors have their soma enveloped by satellite glial cells (Avraham et al., 2022; Mapps et al., 2022), that are multipotent glial precursors implicated in pain transmission. The axons of these sensory neurons are in close association with myelinating or non-myelinating Schwann cells (Harty and Monk, 2017). Nociceptors with myelinated axonal projections are termed Aδ-fibers (1-5 μm diameter), while those lacking myelin wrapping are C-fibers (0.2–1.5 μm diameter). In the distal peripheral nerve, C-fibers are closely associated with non-myelinating Schwann cells, forming Remak bundles, that are structures crucial for neuronal repair after peripheral nerve injury (Harty and Monk, 2017). The two types of fibers serve different functions: Aδ-nociceptors elicit fast, sharp pain (“first pain”) after mechanical and chemical stimuli; C-nociceptors transmit slow, aching dull pain (“second/slow pain”) following an ample range of stressors (i.e., polymodal function; Basbaum et al., 2009).
DRG neurons possess a peculiar morphology: in vivo they are bipolar in shape during the embryonic stage, while upon maturation they become pseudo-unipolar (Nascimento et al., 2018), with a single axon—the stem axon—that bifurcates (Figure 2). The peripheral branch innervates skin, muscle and viscera and acts as the afferent portion of the system, while the central branch reaches the dorsal horn of spinal cord (laminae I and II) where it synapses with second-order neurons (Basbaum et al., 2009; Nascimento et al., 2018). These spinal neurons project via the spinothalamic tract to upper brain structures (like the cerebral cortex) to transmit noxious stimuli and information about intensity and location. Some of the secondary order neurons project to the cingulate and insular cortex via the connections in the parabrachial nucleus and the amygdala, contributing to the pain experience (Yam et al., 2018).
Figure 2. This illustration depicts key components involved in pain signaling within a dorsal root ganglion (DRG) neuron. Sensory channels located in the peripheral axon detect mechanical, thermal (heat/cold), and chemical stimuli. Resident macrophages and Schwann cells near the neuron release neurotrophins, cytokines, and growth factors, which support cell survival. Following axotomy, calcium ions enter the neuron, initiating an injury signal. This signal activates sodium and potassium channels, which transmit the signal towards the nucleus. Transcription factors, such as AP-1, bind importin α3 and are transported into the nucleus, where they induce the expression of genes associated with pain and axonal regeneration. Additionally, the injury signal is conveyed to the central nervous system via saltatory conduction. At the synapse with the second-order neuron, the calcium influx in the sensory neuron triggers the release of substance P and glutamate. Activated pro-inflammatory microglia in the surrounding region amplify mechanical hypersensitivity and pain. This image was created with BioRender.
The DRG structure contains other non-neuronal cells, such as macrophages and T-lymphocytes and a small number of B-lymphocytes (Laast et al., 2011; Makker et al., 2017; Zhou et al., 2022; Feng et al., 2023). Endothelial and smooth muscle cells are also present, as fenestrated capillaries directly irrorate the DRGs to release oxygen and blood borne molecules that interact with the neuronal cells (Jimenez-Andrade et al., 2008). These surrounding cells and their released factors directly influence the functions of the sensory neurons.
In general, NeuP arises when nerves are damaged. Rupturing the nociceptor plasma membrane triggers signaling cascades that alter the expression and function of ion channels. This causes a change in the electrical signal transmission, which the nervous system processes and perceives as pain.
When the nociceptor peripheral branch undergoes axotomy (Figure 2), the distal axon is separated from the cell body and is subjected to Wallerian degeneration, an active process that disrupt the axolemma. At the same time, the proximal axon is exposed to inflammatory cytokines and trophic factors from the surrounding cells (e.g., Schwann cells, macrophages; Campbell and Meyer, 2006; Rotshenker, 2011), which activate signaling cascades. Axotomy does not activate ion channels like TRPV1 (heat), TRPM8 (cold), ASICs (acidic milieu), TRPA1 (chemical irritant), KCNK2/TREK-1 (mechanical stimuli) and Piezo1 (mechanical stimuli; Wang and Woolf, 2005; Patapoutian et al., 2009; Coste et al., 2010; Wemmie et al., 2013; Djillani et al., 2019).
The plasma membrane rupture leads to ionic influx, elevated intracellular calcium levels, and cytoskeleton disruption through calpain activation (George et al., 1995; Zang et al., 2015). Apart from calpain activity, the axotomy causes actomyosin contraction, which makes the sensory neurons shrink. This is necessary to eliminate water via aquaporin channels and to prevent excessive swelling that may lead to cell death (Aydın et al., 2023). The calcium wave moves toward the soma to trigger epigenetic changes and regeneration-associated genes (RAGs) expression. Slow motor-based retrograde complexes deliver injury signaling (such as ERK) to the nucleus (Puttagunta et al., 2014). Axon injury activate other molecular pathways such as cAMP/PKA, PTEN/mTOR, gp130/Jak and DLK/JNK (Zigmond, 2012; Li et al., 2015; Valakh et al., 2015; Chen et al., 2016; Alber et al., 2023), all of which target transcription factors (such as ATF3, CREB, STAT3, and c-Jun) to promote regeneration (Bareyre et al., 2011; Moore and Goldberg, 2011). Gene inactivation mediated by DNA methylation as well as gene downregulation by non-coding RNA transcripts (miRNAs, siRNAs, lncRNAs) are involved in the control of the axon regeneration program (Oh et al., 2018; Han et al., 2022). Interestingly, these regenerative programs are sexually dimorphic in the early phases (Chernov and Shubayev, 2022). Several alterations in gene expression post-nerve injury have been reported in a number of studies, some of which are reported in Table 1.
As mentioned, the calcium influx is necessary to induce the initial neuronal survival program. However, if the calcium influx persists, it lowers the threshold for action potentials, making the DRG neurons hyperexcitable, which favors NeuP development (Chung and Chung, 2002). Even sodium and potassium ionic currents, propagating along the axons through specific channels, are involved in pain signaling. In sensory neurons, the main sodium channels are NaV1.7, NaV1.8 and NaV1.9, while the potassium ones are Kv1.2, TRAAK and TREK-1: improper activities of these channels can lead to either hyperalgesia or analgesia (Tsantoulas and McMahon, 2014; Goodwin and McMahon, 2021). Ionic currents travel toward the central axonal branch that forms a synapse with the second-order neurons in the dorsal horn of the spinal cord (Todd, 2010). Here, the calcium influx triggers the release of neurotransmitters and neuropeptides (such as glutamate, substance P and CGRP), that will be captured by the spinal cord neurons and transmitted to the CNS (Gross and Üçeyler, 2020).
When nociceptor activation is persistent, neural circuits undergo rearrangements. Changes have been observed in genes and proteins expression which affect neuronal excitability and transmission (i.e., functional plasticity), in the spines morphology (i.e., structural remodeling) and in the neural connectivity (Tracey et al., 2019; Fiore et al., 2023). The result of these alterations is sensitization to pain, either at the peripheral or central levels, which can be aggravated by the pro-inflammatory products released by surrounding cells (Woller et al., 2017; Rosenbaum et al., 2022).
As mentioned, damaged sensory axons activate two response phases: an early one, mediated by ion influxes (mainly calcium), and a late one, characterized by slower signals conveyed through molecular motors. These molecular motors travel on microtubules and move vesicles, organelles, proteins, and RNA granules containing snRNP along the axons (Rishal and Fainzilber, 2014; Saito and Cavalli, 2016; Smith et al., 2020). There are two type of motor proteins: the plus-end directed kinesins, and the minus-end directed dynein. In neurons, dynein exclusively moves cargo from pre-synapses back to the soma (Terenzio et al., 2020), a process called “retrograde transport.” Retrograde transport is essential for regulating cell homeostasis, neurotrophic factor signaling, autophagy–lysosomal degradation, nerve injury response and pain signaling (Rishal et al., 2012; Rishal and Fainzilber, 2014; Prior et al., 2017; Mao et al., 2019; Marvaldi et al., 2020). Indeed, reduced expression of the dynein heavy chain 1 (Dync1h1) in sensory and motor neurons causes accelerated axonal outgrowth and delayed recovery after injury (Di Pizio et al., 2023).
Protein kinase signaling pathways and post-translational microtubule modifications regulate the efficiency of retrograde transport (Barlan and Gelfand, 2017; Brady and Morfini, 2017). To properly function, retrograde axonal transport requires the interaction between the dynein motor and its cargo, which is usually mediated by adaptor proteins. Adaptor/scaffold proteins dictate the specificity of the cargoes to be shuttled. Any deregulation caused by modifications of key adaptors and scaffolds could result in neuropathic pain. Indeed, some forms of hereditary Charcot–Marie–Tooth have mutations that compromise retrograde transport (Markworth et al., 2021).
Importins are a family of adaptor proteins involved in retrograde transport (Figures 2, 3). These proteins, classified as karyopherins, are divided into ɑ and β subunits. To be functional, importins form heterodimers, where β interacts directly with dynein while ɑ binds the nuclear localization signals (NLS) of cargo proteins (Panayotis et al., 2015). Moreover, importin β mediates the docking of the importin/substrate assembly to the nuclear pore complex (NPC) through binding to nucleoporin FxFG repeats (Lott and Cingolani, 2011).
Figure 3. Following axotomy, the fate of nociceptors is influenced by both extrinsic and intrinsic factors. After axonal injury, pro-survival factors such as neurotrophins are released by surrounding cells. Concurrently, specific molecular cascades are activated within the damaged neurons. These signals must be transported from the periphery to the nucleus to initiate pain and axonal regeneration signaling. This retrograde transport is facilitated by the motor protein dynein and its adaptor importin, which are localized along the axons and shuttle cargoes to the nucleus of nociceptors. Any impairment in this retrograde transport can affect both pain perception and axonal recovery. This image was created with BioRender.
Mouse and human have, respectively, six and seven isoforms of importin ɑ, with specific tissue expression profiles and cargo-binding selectivity. For example, importin ɑ5 directly binds and regulates nuclear import of MeCP2, which affects anxiety levels (Panayotis et al., 2018). Importins ɑ1 and ɑ5 were also found to bind viral proteins to aid viral replication of herpes simplex virus and Newcastle disease virus, respectively (Döhner et al., 2018; Duan et al., 2018). Interestingly, mutant importin ɑ4 can cause Infantile-Onset Hereditary Spastic Paraplegia, though the molecular mechanism is unclear (Schob et al., 2021). Importin ɑ3 was recently found to be relevant for persistence of chronic NeuP (Marvaldi et al., 2020).
In both naive and injured sciatic nerve, importin α-s are in axons constitutively associated with dynein, while importin β1 protein assumes axonal localization only after an injury occurred (Hanz et al., 2003; Alber et al., 2023). Moreover, only upon damaged importin β undergoes local axonal translation and forms α/β functional heterodimers to accelerate the retrograde transport of cargo (Perlson et al., 2005).
What are the cargoes that are retrogradely transported after axonal injury? Transcription factors (TF), such as ATFs and STATs, have been found to used importin-based nucleocytoplasmic transport (Lindwall and Kanje, 2005; Michaelevski et al., 2010). Members of AP-1 family of TF, which have roles in neuronal activation and axonal regeneration, also bind importins (Raivich et al., 2004). In particular c-FOS, a member of the AP-1 group, binds importin α3-β complex, which results in its nuclear import and the expression of downstream genes that regulate pain (Manassero et al., 2012; Marvaldi et al., 2020). Mice injected with AAV9 vector [that specifically targets sensory neurons (Chan et al., 2017)], carrying importin α3 shRNA had reduced pain perception in the acute and chronic pain response. Coherently, blocking the nuclear import of AP-1 factors was sufficient to reduce pain. This effect was even reproduced pharmacologically with the use of two non-analgesic FDA-approved drugs (sulmazole and sulfamethizole), identified via cMAP screening analysis (https://www.broadinstitute.org/connectivity-map-cmap Connectivity Map (CMAP) | Broad Institute). Further analysis showed that indeed these two drugs reduced pain by blocking c-Fos nuclear import (Marvaldi et al., 2020).
STAT3 is another TF that not only is locally translated in the axon and activated upon injury, but also retrogradely transported by dynein-importin α5. This modulates survival of sensory neurons in vivo by acting as an anti-apoptotic factor (Ben-Yaakov et al., 2012). Experimental evidence suggests that even members of the Myc/Max, PPAR and Smad families may undergo the same dynein-mediated transport in rodents after sciatic nerve injury (Ben-Yaakov et al., 2012), though additional biochemical assay will be required to obtain a full picture of the phenomenon.
Signaling endosomes are another kind of cargoes retrogradely transported after injury via dynein motors. The maturation and movement of these endosomes are regulated by Rab5/Rab7 and Erk1/2 (Deinhardt et al., 2006; Ito and Enomoto, 2016). Specifically, Rab5 is found associated with stationary organelles, while Rab7 is present in the moving endosomes. In sensory neurons, the tethering of the signaling endosomes to dynein motor protein is mediated by retrolinkin (a membrane endosomal protein) that directly binds BPAG1n4, which in turn is associated with dynactin/dynein (Liu et al., 2003, 2007).
As they originate from the plasma membrane, the signaling endosomes are responsible for endocytosis of ligand and their receptors, such as P2X3, NaV1.7 and Trk receptors (Chen et al., 2012; Higerd-Rusli et al., 2023). Interestingly, P2X3, a ATP-receptor highly expressed in DRG nociceptors, has been associated with neuropathic pain and its pharmacological downregulation has showed analgesic effects in rat (Dan et al., 2021). TrkA-NGF complexes are endocytosed and retrogradely transported together with CREB TF (that was locally translated) and other signaling molecules like MEK, ERK, PLCγ and PI3K (Cosker et al., 2008; Marlin and Li, 2015; Crerar et al., 2019). By doing so, the signaling endosomes effectively become platforms for the propagation of molecular cascades that got activated a the nerve terminal. The CREB TF contained in the vesicles, once delivered in the proximity of the nucleus, activates genes for neuronal survival (Cox et al., 2008; Melemedjian et al., 2014). Alteration of this signaling pathway was observed in Charcot–Marie–Tooth mice models carrying Gars mutations and, as expected, these mice also display sensory defects (Sleigh et al., 2017).
In DRG neurons, other neurotrophins-receptor complexes, such as BDNF-TrkB (Vermehren-Schmaedick et al., 2022) undergo similar retrograde transport prompting the expression of anti-apoptotic/pro-survival genes that prevent nerve degeneration.
The injury signals delivered through retrograde transport induce alterations of the cytoskeletal architecture and of the gene expression profile (Renthal et al., 2020). These rearrangements require epigenetic changes dependent on the activity of MeCP2, DNMTs, and on the export of HDAC5 (Cho et al., 2013; Penas and Navarro, 2018).
The nuclear import of transcription factors [e.g., Jun, ELK1, STAT3, SMAD (Doron-Mandel et al., 2015)] promotes the expression of several genes associated with regeneration, such as Atf3, Sprr1a, Gap43, Sox11, Gadd45a, Smad1 and NPY (Jang et al., 2021). Gap43, a well-known protein involved in axonal growth, also increases in the axons following the local translation of mTOR (Terenzio et al., 2018). Axonal regeneration is promoted by reduced levels of molecules, such as Spry2, Sarm1, Gas5 and DRAK2 kinase, that regulate the activity of growth factor receptors and inflammatory pathways (Marvaldi et al., 2014, 2015; Thongrong et al., 2016; Han et al., 2022; Park et al., 2023). In the growth cone, the axonal elongation is at the same time stabilized by p110δ PI 3-kinase and destabilized by RhoA/ROCK (Eickholt et al., 2007), while the branching relies MAP7 and Sema3A signaling (Terenzio et al., 2018; Hu et al., 2021). The directionality of the axonal growth is controlled by gradients of cytokines and growth factors (released by the surrounding cells; Turney et al., 2016; Grasman and Kaplan, 2017), that regulate Slit/Robo and Netrin/DCC signaling pathways (Yi et al., 2006; Webber et al., 2011).
Axonal regeneration requires all these steps and more, however actual functional recovery is slow, often incomplete and accompanied by NeuP. Moreover, some transcription factors that promote axon growth also contribute to NeuP development. Among these TFs are listed the previously mentioned Jun/Fos, but also the upregulated OCT1 and the downregulated EBF1 and NRF2 (Yuan et al., 2019; Vasavda et al., 2022; Liang et al., 2024). Perturbation of guidance molecules gradients and altered axonal sprouting, which lead to impaired pathfinding and tissue mistargeting, can cause NeuP (Xie et al., 2017; Gangadharan et al., 2022). Painful neuromas are one of the most common clinical manifestation of erroneous target innervation (Shamoun et al., 2022).
The retrogradely-transported transcription factors are not the only elements that perturb gene expressions in sensory neurons after injury. Altered levels of non-coding RNA (ncRNAs), mainly miRNA and lncRNA, have been associated with neuropathic pain. Some ncRNAs have been even proposed as NeuP biomarkers, but significative differences were observed between in vivo and in vitro experiments (Hu et al., 2021), invalidating their widespread use.
Functionally, ncRNAs expressed by sensory neurons act at the post-transcriptional level to modulate the expression of proteins involved in the injury/regenerative response. For example, miR-21 and miR-222, which are found elevated in rat DRG post sciatic nerve injury, downregulate TIMP3, a pro-apoptotic protein, and promote neuronal viability (Strickland et al., 2011; Zhou et al., 2015). Few miRNAs have been identified to affect DRG neurons, by either favoring or impairing the axonal elongation. Among these figure miR-132, that by targeting RASA1 promote axonal extension (Hancock et al., 2014), and miR-138, which is downregulated in injured DRG neurons as it suppress axonal growth by targeting SIRT1 (Liu and Wang, 2013). Even lncRNAs found in DRG post nerve injury, such as lncRNA BC089918, were found to affect neuronal growth (Yu et al., 2013).
Few ncRNAs have been found deregulated in murine models of NeuP. In particular, in rat DRG the expression of several potassium channels was compromised by the upregulation of miR-18a, miR-19a, miR-19b, and miR-92a (Sakai et al., 2017). Both miR-30b and miR-182, highly expressed in NeuP developed post nerve injury, could reduce the amount of NaV1.7 and alleviate NeuP (Shao et al., 2016; Cai et al., 2018). Ion channels are not the only targets of ncRNA in NeuP conditions. In rat with constricted nerves, miR-206 favors analgesia by physiologically reducing the levels of BDNF (Sun et al., 2017). On the other hand, lncRNA LINC01119, upregulated in NeuP conditions, binds BDNF mRNA and stabilizes it, promoting hypersensitivity (Zhang et al., 2021).
Human pathologies with NeuP symptoms display altered expression of ncRNAs. For examples, in the patients’ blood miR-34a and miR-101 were downregulated, while miR-199a-3p and miR-455-3p were upregulated (Shenoda et al., 2016; Li et al., 2017; Asahchop et al., 2018; Liu et al., 2019). Interestingly, reduced levels of miR-101 correspond to an increase of importin β protein (which is the miRNA direct target) and to the activation NF-κB signaling, which contributes to NeuP development (Liu et al., 2019).
After peripheral nerve injury, the surrounding cells (i.e., glial, immune, and tissue cells) undergo changes to promote neuronal regeneration. Notably, Schwann cells organize themselves in Büngner bands to serve as guideposts for sprouting axons (Ribeiro-Resende et al., 2009). Meanwhile, perineuronal satellite cells and resident macrophages proliferate to support regeneration (Lindborg et al., 2018; Feng et al., 2023; Konnova et al., 2023).
All these cells release cytokines (e.g., gp130, IL-6, TGFβ), neurotrophins (e.g., FGF-2, NT-3, NGF and GDNF) and other mediators. These released factors on one hand dampen pain perception, on the other sensitize cells to fire action potentials, promoting peripheral/central sensitization and chronic NeuP (Figures 2, 3; Krames, 2014). Notably, trophic factors like NGF have peculiar mechanisms of action on DRG neurons, as they regulate development, plasticity, cell death, and survival (Lykissas et al., 2007; Khan and Smith, 2015). However, excessive NGF sensitize nociceptors and cause hyperalgesia and/or allodynia in both human and murine models by eliciting pro-inflammatory responses and by increasing the expression of voltage-gated sodium channels (Barker et al., 2020).
The contribution of glial cells to NeuP is extensively studied. In mice models, Schwann cells promote an inflammatory response by releasing ATP through the Panx1 channels and by recruiting T-cells through the expression of MHC II (Hartlehnert et al., 2017; Wang et al., 2022). In rodents, satellite glial cells also release ATP and potassium, which increase neuronal excitability and promote peripheral sensitization (i.e., hyperalgesia; McGinnis and Ji, 2023).
Macrophages phagocyte the endosomes released by damaged DRG neurons and, in response, secrete pro-inflammatory cytokines and NGF, giving rise to and sustaining mechanical allodynia (Simeoli et al., 2017; Green et al., 2019; Yu et al., 2020). Indeed, the DRG-resident macrophages are critical contributors to both the initiation and maintenance of NeuP in rodents (Yu et al., 2020). Upon peripheral nerve injury, these macrophages assume M1 phenotype to produce pro-inflammatory peptides (e.g., IL6, IL-1β, TNF-α, IGF-1) that exacerbate NeuP by increasing the nociceptors excitability (Zhao et al., 2023). In the late stage of nerve damage, regulatory T cells influence the M1/M2 polarization of the macrophages through the release of cytokines. This promotes a shift toward the M2 macrophage phenotype, which alleviates pain and favors axon outgrowth in rats (Chen et al., 2022). Indeed, the anti-inflammatory M2 macrophages secrete high amount of opioid peptides (such as β-endorphin, Met-enkephalin, and dynorphin A) that reduce allodynia in mice (Labuz et al., 2009; Pannell et al., 2016).
Cells localized in the innervated tissue can also affect NeuP, though the studies are limited in number. Murine and human fibroblasts and keratinocytes release NGF, IL-6 and ATP to alter neuronal activity and promote NeuP (Baumbauer et al., 2015; Shinotsuka and Denk, 2022; Xu et al., 2022). In mice, fibroblasts secrete SMOC2, a component of basement membrane, that is necessary for basal mechanical nociceptive threshold in the DRG. By interacting with P2X7 receptor expressed on satellite glial cells, SMOC2 inhibits the coupled activation of adjacent DRG neurons, which in turn suppresses the nociceptive signaling (Zhang et al., 2022). Peripheral inflammation actually causes SMOC2 downregulation in DRG, which exacerbates mechanical allodynia. Fibroblasts also release Protease Inhibitor (PI)16 that that promotes NeuP development by altering the blood-nerve barrier permeability and the leukocyte infiltration (Singhmar et al., 2020; Garrity et al., 2023). PI6 may be an optimal target for new analgesics as (a) it has a limited distribution and (b) in its absence mice are protected from NeuP development (Singhmar et al., 2020). Even adipocytes can influence pain. In mice with nerve damage, adipocytes release adipokine leptin that not only causes allodynia by activating macrophages, but also promotes Schwann cell metabolic adaptation to favor nerve repair (Maeda et al., 2009; Sundaram et al., 2023).
The extracellular matrix (ECM) provides structural support and maintenance of cellular regulation. In particular, ECM influences differentiation, survival, growth and migration. Neurons, like other cells, have receptors on their plasma membrane to interact with ECM components. These are principally glycoproteins (both collagenous and non-collagenous proteins) and proteoglycans secreted by cells in the vicinity. In the case of human and murine DRGs, the ECM elements are principally produced by fibroblasts and neuronal cells (Vroman et al., 2023).
ECM mechanical properties, such as substrate stiffness, module sensory neuron axonal outgrowth and morphology (Roumazeilles et al., 2018). DRG neurons are mechanosensitive cells and their morphology varies according to the stiffness of the substrate (Rosso et al., 2017). The stiffness is perceived through the activation of Piezo1 channel, which induces a calcium influx that regulates E-cadherin and integrin-β1 functions to modify the neuronal cytoskeleton (Lei et al., 2023). Softer substrates actually favor the neurite branching of DRG neurons (Koch et al., 2012) by contrasting the effect of Sema3a, a guidance cue that induce growth cone collapse. In fact, the expression of Sema3a receptors Nrp1 and Plxna4 is controlled by stiffness: stiffer substrates increase Nrp1 mRNA levels while reducing the amounts of Plxna4 mRNA (Vela-Alcatara et al., 2022).
While in normal conditions, the ECM environment support nerve maintenance, when an injury occurs the ECM shifts toward a pro-regenerative status to favor axonal sprouting. In vitro studies highlighted how collagen, fibronectin and laminin can differentially affect the neurite outgrowth of sensory neurons and their remyelination post-injury (Baron-Van Evercooren et al., 1982; Deister et al., 2007; Yu et al., 2023). Interestingly, combining ECM components with neurotrophins promotes sensory axons regeneration and target reinnervation. Indeed, treating rats after sciatic nerve injury with a combination of collagen, laminin matrix and NGF/NT3 could regenerate sensory neurons and improve sensory functional recovery (Santos et al., 2017). Notably, chicken DRG in vitro culture manifested differences in growth as a response of either NGF or NT3 treatment, depending on the ECM substrate composition they were cultivated on (Guan et al., 2003).
There are increasing evidences that alterations in ECM molecules/pathways are associated with painful conditions. For example, in CIPN models (specifically Drosophila and murine sensory neurons) nociceptive neurons showed altered branching pattern as a result of integrins overexpression (Shin et al., 2021). In addition, after peripheral nerve injury, some types of collagen (i.e., col4α5, col18α1, col19α1) are found upregulated at the damaged site (Roumazeilles et al., 2018). Interestingly, even samples of people suffering from NeuP presented dysregulation of these ECM-genes (Vroman et al., 2023).
The pursuit of new drugs for NeuP poses significant challenges, considering the complexities of pain mechanisms and the limitations of existing treatments. Pain-suppressing agents like gabapentin and pregabalin, that block ion channels, can have adverse effects such as somnolence and nausea (Attal, 2019). Opioids, while effective, are associated with addiction and mortality concerns (Neuman et al., 2019; Campbell et al., 2020). The economic burden of pain management is substantial, amounting to $18.3 billion for prescription analgesics and $2.6 billion for non-prescription analgesics in the US only (Turk and Patel, 2022). Finding safer and more effective alternatives is a priority for the pharmaceutical industry.
New approaches to block NeuP at the injury sites are being tested, taking into consideration the recent advances in the field. In a few trials to impair signaling transmission, botulinum toxin A was injected and the patients reported analgesic effects (Attal et al., 2016). Local DRG stimulation with electrodes has also been tested to block pain signaling, but at the moment there is not enough evidence to support its efficacy as a treatment (Knotkova et al., 2021). The VX-548 drug, a NaV1.8 channel inhibitor acting on the PNS, is showing promising results in the clinical trials (Jones et al., 2023). Gene therapies and cellular reprogramming approaches have been tested as a way to achieve analgesia and to promote nerve regeneration, with mixed results (Carvalho et al., 2019; Park et al., 2019). In mice, the targeted ubiquitination of a calcium channel, achieved by viral delivery of a genetically modified protein in DRG neurons, could actually abate hyperalgesia in response to nerve injury (Sun et al., 2022).
Even modulation of the growth factors signaling has been explored as a possible therapeutic method (Li et al., 2020). Tanezumab, an inhibitor of NGF, could reduce lower back pain and diabetic neuropathy, however it was not effective in treating postherpetic neuropathy (Patel et al., 2018). In preliminary studies, neurotrophic factors combined with ECM components were able to enhance sensory axons regeneration and promote appropriate target reinnervation in rat (Santos et al., 2017). Decellularized ECM-structures without growth factors are being tested in rodents to ameliorate the recovery post nerve injury. The results vary, as some boosted neovascularization but not axonal regrowth, while others improved electrophysiologic response and axon counts (Ren et al., 2018; Meder et al., 2021).
More technological approaches are being experimented to alleviate NeuP, such as 3D-bioprinted implantable devices to promote nerve guidance (Sanchez Rezza et al., 2022). Combined expertise of biomechanics, biology and bioengineering will be crucial to develop new implants and achieve complete functional recovery.
Millions of people worldwide suffer from neuropathic pain (NeuP), which has a huge cost on the healthcare systems and reduces the quality of life and the lifespan of the individuals. This problem is also underestimated as there are not many studies that take into consideration the differences in pain perception between man and women and the effect of aging.
Even though pain perception involves both central and peripheral nervous system, in this review we focalized only on the latter. In particular, we explored what happens after damage of the axons innervating tissue and viscera, while only briefly mentioning the signaling in the spinal cord region.
Peripheral sensory neurons, have a crucial role in pain perception as the initiators of the injury signal. These cells are heavily influenced by extrinsic factors released by neighboring cells (i.e., immune, glial, tissue cells) and by the activation of intrinsic elements (e.g., signaling cascades, axonal-soma communication). The cross-talk between intrinsic and extrinsic factors dictate the outcome of the regenerative program after nerve injury. Any alteration can lead to failure of organ innervation and functional recovery, giving rise to neuropathic pain. The ability to control axonal growth and directionality, while limiting the firing potential (that causes the release of painful stimuli), could be highly beneficial for patients suffering from chronic pain. Discovering new drugs that specifically target the peripheral nervous system should be a priority, as this approach may help manage pain more effectively without affecting central nervous system functions. Such targeted therapies could provide relief by modulating the peripheral mechanisms of pain without the side effects associated with broader systemic treatments.
LT: Data curation, Investigation, Methodology, Writing – original draft, Writing – review & editing. SD: Data curation, Investigation, Writing – review & editing. LM: Conceptualization, Data curation, Funding acquisition, Investigation, Methodology, Supervision, Writing – original draft, Writing – review & editing. AV: Writing – original draft.
The author(s) declare that financial support was received for the research, authorship, and/or publication of this article. Our research on these topics has been generously supported by the Rita Levi Montalcini 2021 Grant (MIUR, Italy). This research was also funded by Ministero dell’Istruzione dell’Università e della Ricerca MIUR project “Dipartimenti di Eccellenza 2023–2027” to Department of Neuroscience “Rita Levi Montalcini.”
The authors thank Christian Oliver Pritz and Ida Rishal for the critical comments.
The authors declare that the research was conducted in the absence of any commercial or financial relationships that could be construed as a potential conflict of interest.
All claims expressed in this article are solely those of the authors and do not necessarily represent those of their affiliated organizations, or those of the publisher, the editors and the reviewers. Any product that may be evaluated in this article, or claim that may be made by its manufacturer, is not guaranteed or endorsed by the publisher.
The Supplementary material for this article can be found online at: https://www.frontiersin.org/articles/10.3389/fnana.2024.1398400/full#supplementary-material
SUPPLEMENTARY TABLE 1 | Information on the NeuP population studies. Here we report the studies per country used to create Figure 1. We were able to find only nineteen studies in the literature that investigated the prevalence of NeuP in the general population. These studies greatly varied in the number of cases examined, the use of controls, and the way the NeuP was evaluated. Moreover, different types of questionnaire to define NeuP were used, such as LANSS, DN4, PainDETECT. While all the questionnaires are extensively used as diagnostic tools, they don’t measure the same parameters. All these factors may partially explain the great variation in NeuP prevalence between countries.
Ahn, H. S., Vasylyev, D. V., Estacion, M., MacAla, L. J., Shah, P., Faber, C. G., et al. (2013). Differential effect of D623N variant and wild-type Nav1.7 sodium channels on resting potential and interspike membrane potential of dorsal root ganglion neurons. Brain Res. 1529, 165–177. doi: 10.1016/j.brainres.2013.07.005
Alber, S., Di Matteo, P., Zdradzinski, M. D., Costa, I. D., Medzihradszky, K. F., Kawaguchi, R., et al. (2023). PTBP1 regulates injury responses and sensory pathways in adult peripheral neurons. Sci. Adv. 9:eadi0286. doi: 10.1126/sciadv.adi0286
Asahchop, E. L., Branton, W. G., Krishnan, A., Chen, P. A., Yang, D., Kong, L., et al. (2018). HIV-associated sensory polyneuropathy and neuronal injury are associated with miRNA-455-3p induction. JCI Insight 3:3. doi: 10.1172/jci.insight.122450
Attal, N. (2019). Pharmacological treatments of neuropathic pain: the latest recommendations. Rev. Neurol. 175, 46–50. doi: 10.1016/j.neurol.2018.08.005
Attal, N., Bouhassira, D., and Baron, R. (2018). Diagnosis and assessment of neuropathic pain through questionnaires. Lancet Neurol. 17, 456–466. doi: 10.1016/S1474-4422(18)30071-1
Attal, N., de Andrade, D. C., Adam, F., Ranoux, D., Teixeira, M. J., Galhardoni, R., et al. (2016). Safety and efficacy of repeated injections of botulinum toxin a in peripheral neuropathic pain (BOTNEP): a randomised, double-blind, placebo-controlled trial. Lancet Neurol. 15, 555–565. doi: 10.1016/S1474-4422(16)00017-X
Avraham, O., Chamessian, A., Feng, R., Yang, L., Halevi, A. E., Moore, A. M., et al. (2022). Profiling the molecular signature of satellite glial cells at the single cell level reveals high similarities between rodents and humans. Pain 163, 2348–2364. doi: 10.1097/j.pain.0000000000002628
Aydın, M. Ş., Bay, S., Yiğit, E. N., Özgül, C., Oğuz, E. K., Konuk, E. Y., et al. (2023). Active shrinkage protects neurons following axonal transection. iScience 26:107715. doi: 10.1016/j.isci.2023.107715
Barbosa Neto, J. O., Garcia, J. B. S., Cartágenes, M. D. S. D. S., Amaral, A. G., Onuchic, L. F., and Ashmawi, H. A. (2019). Influence of androgenic blockade with flutamide on pain behaviour and expression of the genes that encode the NaV1.7 and NaV1.8 voltage-dependent sodium channels in a rat model of postoperative pain. J. Transl. Med. 17:287. doi: 10.1186/s12967-019-2031-z
Bareyre, F. M., Garzorz, N., Lang, C., Misgeld, T., Büning, H., and Kerschensteiner, M. (2011). In vivo imaging reveals a phase-specific role of stat3 during central and peripheral nervous system axon regeneration. Proc. Natl. Acad. Sci. U. S. A. 108, 6282–6287. doi: 10.1073/pnas.1015239108
Barker, P. A., Mantyh, P., Arendt-Nielsen, L., Viktrup, L., and Tive, L. (2020). Nerve growth factor signaling and its contribution to pain. J. Pain Res. 13, 1223–1241. doi: 10.2147/JPR.S247472
Barlan, K., and Gelfand, V. I. (2017). Microtubule-based transport and the distribution, tethering, and organization of organelles. Cold Spring Harb. Perspect. Biol. 9:a025817. doi: 10.1101/cshperspect.a025817
Baron-Van Evercooren, A., Kleinman, H. K., Ohno, S., Marangos, P., Schwartz, J. P., and Dubois-Dalcq, M. E. (1982). Nerve growth factor, laminin, and fibronectin promote neurite growth in human fetal sensory ganglia cultures. J. Neurosci. Res. 8, 179–193. doi: 10.1002/jnr.490080208
Basbaum, A. I., Bautista, D. M., Scherrer, G., and Julius, D. (2009). Cellular and molecular mechanisms of pain. Cell 139, 267–284. doi: 10.1016/j.cell.2009.09.028
Baumbauer, K. M., Deberry, J. J., Adelman, P. C., Miller, R. H., Hachisuka, J., Lee, K. H., et al. (2015). Keratinocytes can modulate and directly initiate nociceptive responses. eLife 4:674. doi: 10.7554/eLife.09674
Belmonte, C., and Viana, F. (2008). Molecular and cellular limits to somatosensory specificity. Mol. Pain 4:14. doi: 10.1186/1744-8069-4-14
Bennett, M. I., Rayment, C., Hjermstad, M., Aass, N., Caraceni, A., and Kaasa, S. (2012). Prevalence and aetiology of neuropathic pain in cancer patients: a systematic review. Pain 153, 359–365. doi: 10.1016/j.pain.2011.10.028
Ben-Yaakov, K., Dagan, S. Y., Segal-Ruder, Y., Shalem, O., Vuppalanchi, D., Willis, D. E., et al. (2012). Axonal transcription factors signal retrogradely in lesioned peripheral nerve. EMBO J. 31, 1350–1363. doi: 10.1038/emboj.2011.494
Berger, A. A., Liu, Y., Possoit, H., Rogers, A. C., Moore, W., Gress, K., et al. (2021). Dorsal root ganglion (Drg) and chronic pain. Anesthesiol. Pain Med. 11:e113020. doi: 10.5812/aapm.113020
Biegstraaten, M., Hollak, C. E. M., Bakkers, M., Faber, C. G., Aerts, J. M. F. G., and van Schaik, I. N. (2012). Small fiber neuropathy in Fabry disease. Mol. Genet. Metab. 106, 135–141. doi: 10.1016/J.YMGME.2012.03.010
Bhuiyan, S. A., Xu, M., Yang, L., Semizoglou, E., Bhatia, P., Pantaleo, K. I., et al. (2023). Harmonized cross-species cell atlases of trigeminal and dorsal root ganglia.. Prepr Serv Biol. doi: 10.1101/2023.07.04.547740
Bjornard, K. L., Gilchrist, L. S., Inaba, H., Diouf, B., Hockenberry, M. J., Kadan-Lottick, N. S., et al. (2018). Peripheral neuropathy in children and adolescents treated for cancer. Lancet Child Adolesc Heal 2, 744–754. doi: 10.1016/S2352-4642(18)30236-0
Borsook, D., Kussman, B. D., George, E., Becerra, L. R., and Burke, D. W. (2013). Surgically induced neuropathic pain: understanding the perioperative process. Ann. Surg. 257, 403–412. doi: 10.1097/SLA.0b013e3182701a7b
Boukalova, S., Touska, F., Marsakova, L., Hynkova, A., Sura, L., Chvojka, S., et al. (2014). Gain-of-function mutations in the transient receptor potential channels TRPV1 and TRPA1: how painful? Physiol. Res. 63, S205–S213. doi: 10.33549/physiolres.932658
Boyette-Davis, J. A., Walters, E. T., and Dougherty, P. M. (2015). Mechanisms involved in the development of chemotherapy-induced neuropathy. Pain Manag 5, 285–296. doi: 10.2217/pmt.15.19
Brady, S. T., and Morfini, G. A. (2017). Regulation of motor proteins, axonal transport deficits and adult-onset neurodegenerative diseases. Neurobiol. Dis. 105, 273–282. doi: 10.1016/j.nbd.2017.04.010
Brandão, A. F., Bonet, I. J. M., Pagliusi, M., Zanetti, G. G., Pho, N., Tambeli, C. H., et al. (2020). Physical activity induces nucleus Accumbens genes expression changes preventing chronic pain susceptibility promoted by high-fat diet and sedentary behavior in mice. Front. Neurosci. 13, 1–17. doi: 10.3389/fnins.2019.01453
Breivik, H., Eisenberg, E., and O’Brien, T. (2013). The individual and societal burden of chronic pain in Europe: the case for strategic prioritisation and action to improve knowledge and availability of appropriate care. BMC Public Health 13:1229. doi: 10.1186/1471-2458-13-1229
Cai, W., Zhao, Q., Shao, J., Zhang, J., Li, L., Ren, X., et al. (2018). MicroRNA-182 alleviates neuropathic pain by regulating Nav1.7 following spared nerve injury in rats. Sci. Rep. 8:16750. doi: 10.1038/s41598-018-34755-3
Campbell, J. N., and Meyer, R. A. (2006). Mechanisms of neuropathic pain. Neuron 52, 77–92. doi: 10.1016/j.neuron.2006.09.021
Campbell, G., Noghrehchi, F., Nielsen, S., Clare, P., Bruno, R., Lintzeris, N., et al. (2020). Risk factors for indicators of opioid-related harms amongst people living with chronic non-cancer pain: findings from a 5-year prospective cohort study. EClinicalMedicine 28:100592. doi: 10.1016/j.eclinm.2020.100592
Caraceni, A., and Shkodra, M. (2019). Cancer pain assessment and classification. Cancers 11:510. doi: 10.3390/cancers11040510
Carvalho, C. R., Oliveira, J. M., and Reis, R. L. (2019). Modern trends for peripheral nerve repair and regeneration: beyond the hollow nerve guidance conduit. Front. Bioeng. Biotechnol. 7:337. doi: 10.3389/fbioe.2019.00337
Chan, K. Y., Jang, M. J., Yoo, B. B., Greenbaum, A., Ravi, N., Wu, W. L., et al. (2017). Engineered AAVs for efficient noninvasive gene delivery to the central and peripheral nervous systems. Nat. Neurosci. 20, 1172–1179. doi: 10.1038/nn.4593
Chang, S. J., Kim, H. J., Juon, H. S., Park, H., Choi, S. W., Eun, L. K., et al. (2022). A comparison of the influencing factors of chronic pain and quality of life between older Koreans and Korean–Americans with chronic pain: a correlational study. Qual. Life Res. 31, 1179–1189. doi: 10.1007/s11136-021-02983-2
Chen, H., Jiang, L., Zhang, D., Chen, J., Luo, X., Xie, Y., et al. (2022). Exploring the correlation between the regulation of macrophages by regulatory T cells and peripheral neuropathic pain. Front. Neurosci. 16:751. doi: 10.3389/fnins.2022.813751
Chen, W., Lu, N., Ding, Y., Wang, Y., Chan, L. T., Wang, X., et al. (2016). Rapamycin-resistant mTOR activity is required for sensory axon regeneration induced by a conditioning lesion. eNeuro 3, ENEURO.0358–ENEU16.2016. doi: 10.1523/ENEURO.0358-16.2016
Chen, X. Q., Wang, B., Wu, C., Pan, J., Yuan, B., Su, Y. Y., et al. (2012). Endosome-mediated retrograde axonal transport of P2X3 receptor signals in primary sensory neurons. Cell Res. 22, 677–696. doi: 10.1038/cr.2011.197
Chernov, A. V., and Shubayev, V. I. (2022). Sexually dimorphic transcriptional programs of early-phase response in regenerating peripheral nerves. Front. Mol. Neurosci. 15:568. doi: 10.3389/fnmol.2022.958568
Cho, Y., Sloutsky, R., Naegle, K. M., and Cavalli, V. (2013). Injury-induced HDAC5 nuclear export is essential for axon regeneration. Cell 155, 894–908. doi: 10.1016/j.cell.2013.10.004
Chung, J. M., and Chung, K. (2002). Importance of Hyperexcitability of DRG neurons in neuropathic pain. Pain Pract. 2, 87–97. doi: 10.1046/j.1533-2500.2002.02011.x
Cohen, S. P., Vase, L., and Hooten, W. M. (2021). Chronic pain: an update on burden, best practices, and new advances. Lancet 397, 2082–2097. doi: 10.1016/S0140-6736(21)00393-7
Colloca, L., Ludman, T., Bouhassira, D., Baron, R., Dickenson, A. H., Yarnitsky, D., et al. (2017). Neuropathic pain. Nat. Rev. Dis. Primers. 3, 17002–17020. doi: 10.1038/nrdp.2017.2
Cosker, K. E., Courchesne, S. L., and Segal, R. A. (2008). Action in the axon: generation and transport of signaling endosomes. Curr. Opin. Neurobiol. 18, 270–275. doi: 10.1016/j.conb.2008.08.005
Coste, B., Mathur, J., Schmidt, M., Earley, T. J., Ranade, S., Petrus, M. J., et al. (2010). Piezo1 and Piezo2 are essential components of distinct mechanically activated cation channels. Science 330, 55–60. doi: 10.1126/science.1193270
Cox, L. J., Hengst, U., Gurskaya, N. G., Lukyanov, K. A., and Jaffrey, S. R. (2008). Intra-axonal translation and retrograde trafficking of CREB promotes neuronal survival. Nat. Cell Biol. 10, 149–159. doi: 10.1038/ncb1677
Crerar, H., Scott-Solomon, E., Bodkin-Clarke, C., Andreassi, C., Hazbon, M., Logie, E., et al. (2019). Regulation of NGF signaling by an axonal untranslated mRNA. Neuron 102, 553–563.e8. doi: 10.1016/j.neuron.2019.02.011
Dan, Y., Guo, H., Zheng, C., Wu, B., Guo, J., and Li, G. (2021). Neferine alleviates P2X3 receptor in rat dorsal root ganglia mediated neuropathic pain. Neurosci. Res. 170, 265–272. doi: 10.1016/j.neures.2020.08.004
Deinhardt, K., Salinas, S., Verastegui, C., Watson, R., Worth, D., Hanrahan, S., et al. (2006). Rab5 and Rab7 control endocytic sorting along the axonal retrograde transport pathway. Neuron 52, 293–305. doi: 10.1016/j.neuron.2006.08.018
Deister, C., Aljabari, S., and Schmidt, C. E. (2007). Effects of collagen 1, fibronectin, laminin and hyaluronic acid concentration in multi-component gels on neurite extension. J. Biomater. Sci. Polym. Ed. 18, 983–997. doi: 10.1163/156856207781494377
Deng, C., Gu, Y. J., Zhang, H., and Zhang, J. (2017). Estrogen affects neuropathic pain through upregulating N-methyl-D-aspartate acid receptor 1 expression in the dorsal root ganglion of rats. Neural Regen. Res. 12, 464–469. doi: 10.4103/1673-5374.202925
Di Pizio, A., Marvaldi, L., Birling, M. C., Okladnikov, N., Dupuis, L., Fainzilber, M., et al. (2023). A conditional null allele of Dync1h1 enables targeted analyses of dynein roles in neuronal length sensing. J. Cell Sci. 136:220. doi: 10.1242/jcs.260220
Djillani, A., Mazella, J., Heurteaux, C., and Borsotto, M. (2019). Role of TREK-1 in health and disease, focus on the central nervous system. Front. Pharmacol. 10:379. doi: 10.3389/fphar.2019.00379
Döhner, K., Ramos-Nascimento, A., Bialy, D., Anderson, F., Hickford-Martinez, A., Rother, F., et al. (2018). Importin α1 is required for nuclear import of herpes simplex virus proteins and capsid assembly in fibroblasts and neurons. PLoS Pathog. 14:e1006823. doi: 10.1371/journal.ppat.1006823
Doron-Mandel, E., Fainzilber, M., and Terenzio, M. (2015). Growth control mechanisms in neuronal regeneration. FEBS Lett. 589, 1669–1677. doi: 10.1016/j.febslet.2015.04.046
Duan, Z., Xu, H., Ji, X., Zhao, J., Xu, H., Hu, Y., et al. (2018). Importin α5 negatively regulates importin β1-mediated nuclear import of Newcastle disease virus matrix protein and viral replication and pathogenicity in chicken fibroblasts. Virulence 9, 783–803. doi: 10.1080/21505594.2018.1449507
Dudek, I., Hajduga, D., Sieńko, C., Maani, A., Sitarz, E., Sitarz, M., et al. (2020). Alcohol-induced neuropathy in chronic alcoholism: causes, pathophysiology, diagnosis, and treatment options. Curr. Pathobiol. Rep. 8, 87–97. doi: 10.1007/s40139-020-00214-w
Eickholt, B. J., Ahmed, A. I., Davies, M., Papakonstanti, E. A., Pearce, W., Starkey, M. L., et al. (2007). Control of axonal growth and regeneration of sensory neurons by the p110δ PI 3-kinase. PLoS One 2:869. doi: 10.1371/journal.pone.0000869
Eldridge, S., Scuteri, A., Jones, E. M. C., Cavaletti, G., Guo, L., and Glaze, E. (2021). Considerations for a reliable in vitro model of chemotherapy-induced peripheral neuropathy. Toxics 9:300. doi: 10.3390/toxics9110300
Elliott, J., Sloan, G., Stevens, L., Selvarajah, D., Cruccu, G., Gandhi, R. A., et al. (2024). Female sex is a risk factor for painful diabetic peripheral neuropathy: the EURODIAB prospective diabetes complications study. Diabetologia 67, 190–198. doi: 10.1007/s00125-023-06025-z
Feldman, E. L., Callaghan, B. C., Pop-Busui, R., Zochodne, D. W., Wright, D. E., Bennett, D. L., et al. (2019). Diabetic neuropathy. Nat. Rev. Dis. Primers. 5:41. doi: 10.1038/s41572-019-0092-1
Feng, R., Saraswathy, V. M., Mokalled, M. H., and Cavalli, V. (2023). Self-renewing macrophages in dorsal root ganglia contribute to promote nerve regeneration. Proc. Natl. Acad. Sci. U. S. A. 120:6120. doi: 10.1073/pnas.2215906120
Fernandez Rojas, R., Brown, N., Waddington, G., and Goecke, R. (2023). A systematic review of neurophysiological sensing for the assessment of acute pain. NPJ Digit Med 6, 1–25. doi: 10.1038/s41746-023-00810-1
Fernández-De-las-peñas, C., Herrero-Montes, M., Cancela-Cilleruelo, I., Rodríguez-Jiménez, J., Parás-Bravo, P., Varol, U., et al. (2022). Understanding sensitization, cognitive and neuropathic associated mechanisms behind post-COVID pain: a network analysis. Diagnostics 12:1538. doi: 10.3390/diagnostics12071538
Fiore, N. T., Debs, S. R., Hayes, J. P., Duffy, S. S., and Moalem-Taylor, G. (2023). Pain-resolving immune mechanisms in neuropathic pain. Nat. Rev. Neurol. 19, 199–220. doi: 10.1038/s41582-023-00777-3
Fitzcharles, M. A., Cohen, S. P., Clauw, D. J., Littlejohn, G., Usui, C., and Häuser, W. (2021). Nociplastic pain: towards an understanding of prevalent pain conditions. Lancet 397, 2098–2110. doi: 10.1016/S0140-6736(21)00392-5
Fitzgerald, M., and McKelvey, R. (2016). Nerve injury and neuropathic pain—a question of age. Exp. Neurol. 275, 296–302. doi: 10.1016/j.expneurol.2015.07.013
Gangadharan, V., Zheng, H., Taberner, F. J., Landry, J., Nees, T. A., Pistolic, J., et al. (2022). Neuropathic pain caused by miswiring and abnormal end organ targeting. Nature 606, 137–145. doi: 10.1038/s41586-022-04777-z
Garrity, R., Arora, N., Haque, M. A., Weis, D., Trinh, R. T., Neerukonda, S. V., et al. (2023). Fibroblast-derived PI16 sustains inflammatory pain via regulation of CD206+ myeloid cells. Brain Behav. Immun. 112, 220–234. doi: 10.1016/j.bbi.2023.06.011
George, E. B., Glass, J. D., and Griffin, J. W. (1995). Axotomy-induced axonal degeneration is mediated by calcium influx through ion-specific channels. J. Neurosci. 15, 6445–6452. doi: 10.1523/JNEUROSCI.15-10-06445.1995
Giovannini, S., Coraci, D., Brau, F., Galluzzo, V., Loreti, C., Caliandro, P., et al. (2021). Neuropathic pain in the elderly. Diagnostics 11:613. doi: 10.3390/diagnostics11040613
Goodwin, G., and McMahon, S. B. (2021). The physiological function of different voltage-gated sodium channels in pain. Nat. Rev. Neurosci. 22, 263–274. doi: 10.1038/s41583-021-00444-w
Grasman, J. M., and Kaplan, D. L. (2017). Human endothelial cells secrete neurotropic factors to direct axonal growth of peripheral nerves. Sci. Rep. 7:4092. doi: 10.1038/s41598-017-04460-8
Green, D. P., Limjunyawong, N., Gour, N., Pundir, P., and Dong, X. (2019). A mast-cell-specific receptor mediates neurogenic inflammation and pain. Neuron 101, 412–420.e3. doi: 10.1016/j.neuron.2019.01.012
Gross, F., and Üçeyler, N. (2020). Mechanisms of small nerve fiber pathology. Neurosci. Lett. 737:135316. doi: 10.1016/j.neulet.2020.135316
Guan, W., Puthenveedu, M. A., and Condic, M. L. (2003). Sensory neuron subtypes have unique substratum preference and receptor expression before target innervation. J. Neurosci. 23, 1781–1791. doi: 10.1523/JNEUROSCI.23-05-01781.2003
Han, X., Xu, J., Chen, Z., Li, P., Zhao, L., Tao, J., et al. (2022). Gas5 inhibition promotes the axon regeneration in the adult mammalian nervous system. Exp. Neurol. 356:114157. doi: 10.1016/j.expneurol.2022.114157
Hancock, M. L., Preitner, N., Quan, J., and Flanagan, J. G. (2014). MicroRNA-132 is enriched in developing axons, locally regulates Rasa1 mRNA, and promotes axon extension. J. Neurosci. 34, 66–78. doi: 10.1523/JNEUROSCI.3371-13.2014
Hanz, S., Perlson, E., Willis, D., Zheng, J. Q., Massarwa, R., Huerta, J. J., et al. (2003). Axoplasmic importins enable retrograde injury signaling in lesioned nerve. Neuron 40, 1095–1104. doi: 10.1016/S0896-6273(03)00770-0
Hartlehnert, M., Derksen, A., Hagenacker, T., Kindermann, D., Schäfers, M., Pawlak, M., et al. (2017). Schwann cells promote post-traumatic nerve inflammation and neuropathic pain through MHC class II. Sci. Rep. 7:12518. doi: 10.1038/s41598-017-12744-2
Hartono, F., Tanjung, C., Besinga K, E., Marpaung, D., Ananditya, T., and Budisantoso, A. B. (2020). Catastrophic results due to unrecognizing of congenital insensitivity to pain with anhidrosis in children with multiple long bones fractures: a case report of 27 years follow-up of two siblings. Int. J. Surg. Case Rep. 73, 213–217. doi: 10.1016/j.ijscr.2020.07.010
Harty, B. L., and Monk, K. R. (2017). Unwrapping the unappreciated: recent progress in Remak Schwann cell biology. Curr. Opin. Neurobiol. 47, 131–137. doi: 10.1016/j.conb.2017.10.003
Higerd-Rusli, G. P., Tyagi, S., Liu, S., Dib-Hajj, F. B., Waxman, S. G., and Dib-Hajj, S. D. (2023). The fates of internalized NaV1.7 channels in sensory neurons: retrograde cotransport with other ion channels, axon-specific recycling, and degradation. J. Biol. Chem. 299:102816. doi: 10.1016/j.jbc.2022.102816
Hu, C., He, M., Xu, Q., and Tian, W. (2021). Advances with non-coding RNAs in neuropathic pain. Front. Neurosci. 15:760936. doi: 10.3389/fnins.2021.760936
Ito, K., and Enomoto, H. (2016). Retrograde transport of neurotrophic factor signaling: implications in neuronal development and pathogenesis. J. Biochem. 160, 77–85. doi: 10.1093/JB/MVW037
Jang, E. H., Bae, Y. H., Yang, E. M., Gim, Y., Suh, H. J., Kim, S., et al. (2021). Comparing axon regeneration in male and female mice after peripheral nerve injury. J. Neurosci. Res. 99, 2874–2887. doi: 10.1002/jnr.24955
Jimenez-Andrade, J. M., Herrera, M. B., Ghilardi, J. R., Vardanyan, M., Melemedjian, O. K., and Mantyh, P. W. (2008). Vascularization of the dorsal root ganglia and peripheral nerve of the mouse: implications for chemical-induced peripheral sensory neuropathies. Mol. Pain 4:1744-8069-4-10. doi: 10.1186/1744-8069-4-10
Jones, J., Correll, D. J., Lechner, S. M., Jazic, I., Miao, X., Shaw, D., et al. (2023). Selective inhibition of Na V 1.8 with VX-548 for acute pain. N. Engl. J. Med. 389, 393–405. doi: 10.1056/NEJMoa2209870
Jones, M. R., Urits, I., Wolf, J., Corrigan, D., Colburn, L., Peterson, E., et al. (2019). Drug-induced peripheral neuropathy: a narrative review. Curr. Clin. Pharmacol. 15, 38–48. doi: 10.2174/1574884714666190121154813
Jung, M., Dourado, M., Maksymetz, J., Jacobson, A., Laufer, B. I., Baca, M., et al. (2023). Cross-species transcriptomic atlas of dorsal root ganglia reveals species-specific programs for sensory function. Nat. Commun. 14:366. doi: 10.1038/s41467-023-36014-0
Karri, J., and Bruel, B. (2021). Dorsal root ganglion stimulation for post-Lyme disease chronic peripheral neuropathic pain. Neuromodulation 24, 794–795. doi: 10.1111/ner.13136
Khan, N., and Smith, M. T. (2015). Neurotrophins and neuropathic pain: role in pathobiology. Molecules 20, 10657–10688. doi: 10.3390/molecules200610657
Kinchington, P. R., and Goins, W. F. (2011). Varicella zoster virus-induced pain and post-herpetic neuralgia in the human host and in rodent animal models. J. Neurovirol. 17, 590–599. doi: 10.1007/s13365-011-0069-7
Knotkova, H., Hamani, C., Sivanesan, E., Le Beuffe, M. F. E., Moon, J. Y., Cohen, S. P., et al. (2021). Neuromodulation for chronic pain. Lancet 397, 2111–2124. doi: 10.1016/S0140-6736(21)00794-7
Koch, D., Rosoff, W. J., Jiang, J., Geller, H. M., and Urbach, J. S. (2012). Strength in the periphery: growth cone biomechanics and substrate rigidity response in peripheral and central nervous system neurons. Biophys. J. 102, 452–460. doi: 10.1016/j.bpj.2011.12.025
Konnova, E. A., Deftu, A. F., Chu Sin Chung, P., Pertin, M., Kirschmann, G., Decosterd, I., et al. (2023). Characterisation of GFAP-expressing glial cells in the dorsal root ganglion after spared nerve injury. Int. J. Mol. Sci. 24:15559. doi: 10.3390/ijms242115559
Krames, E. S. (2014). The role of the dorsal root ganglion in the development of neuropathic pain. Pain Med. 15, 1669–1685. doi: 10.1111/pme.12413
Laast, V. A., Shim, B., Johanek, L. M., Dorsey, J. L., Hauer, P. E., Tarwater, P. M., et al. (2011). Macrophage-mediated dorsal root ganglion damage precedes altered nerve conduction in SIV-infected macaques. Am. J. Pathol. 179, 2337–2345. doi: 10.1016/j.ajpath.2011.07.047
Labuz, D., Schmidt, Y., Schreiter, A., Rittner, H. L., Mousa, S. A., and Machelska, H. (2009). Immune cell-derived opioids protect against neuropathic pain in mice. J. Clin. Invest. 119:1051. doi: 10.1172/JCI36246C1
Lei, M., Wang, W., Zhang, H., Gong, J., Wang, Z., Cai, H., et al. (2023). Cell-cell and cell-matrix adhesion regulated by Piezo1 is critical for stiffness-dependent DRG neuron aggregation. Cell Rep. 42:113522. doi: 10.1016/j.celrep.2023.113522
Li, C., Hisamoto, N., and Matsumoto, K. (2015). Axon regeneration is regulated by Ets–C/EBP transcription complexes generated by activation of the cAMP/Ca2+signaling pathways. PLoS Genet. 11:e1005603. doi: 10.1371/journal.pgen.1005603
Li, R., Hui, L. D., Yu, Z. H., Wang, J., Kun, L. X., and Xiao, J. (2020). Growth factors-based therapeutic strategies and their underlying signaling mechanisms for peripheral nerve regeneration. Acta Pharmacol. Sin. 41, 1289–1300. doi: 10.1038/s41401-019-0338-1
Li, Y. B., Wu, Q., Liu, J., Fan, Y. Z., Yu, K. F., and Cai, Y. (2017). miR-199a-3p is involved in the pathogenesis and progression of diabetic neuropathy through downregulation of SerpinE2. Mol. Med. Rep. 16, 2417–2424. doi: 10.3892/mmr.2017.6874
Liang, Z., Hore, Z., Harley, P., Uchenna Stanley, F., Michrowska, A., Dahiya, M., et al. (2020). A transcriptional toolbox for exploring peripheral neuroimmune interactions. Pain 161, 2089–2106. doi: 10.1097/j.pain.0000000000001914
Liang, Y., Sharma, D., Wang, B., Wang, H., Feng, X., Ma, R., et al. (2024). Transcription factor EBF1 mitigates neuropathic pain by rescuing Kv1.2 expression in primary sensory neurons. Transl. Res. 263, 15–27. doi: 10.1016/j.trsl.2023.08.002
Liedgens, H., Obradovic, M., De Courcy, J., Holbrook, T., and Jakubanis, R. (2016). A burden of illness study for neuropathic pain in Europe. Clin Outcomes Res 8, 113–126. doi: 10.2147/CEOR.S81396
Lindborg, J. A., Niemi, J. P., Howarth, M. A., Liu, K. W., Moore, C. Z., Mahajan, D., et al. (2018). Molecular and cellular identification of the immune response in peripheral ganglia following nerve injury. J. Neuroinflammation 15, 1–17. doi: 10.1186/S12974-018-1222-5/FIGURES/8
Lindwall, C., and Kanje, M. (2005). Retrograde axonal transport of JNK signaling molecules influence injury induced nuclear changes in p-c-Jun and ATF3 in adult rat sensory neurons. Mol. Cell. Neurosci. 29, 269–282. doi: 10.1016/j.mcn.2005.03.002
Liu, J. J., Ding, J., Kowal, A. S., Nardine, T., Allen, E., Delcroix, J. D., et al. (2003). BPAG1n4 is essential for retrograde axonal transport in sensory neurons. J. Cell Biol. 163, 223–229. doi: 10.1083/jcb.200306075
Liu, J. J., Ding, J., Wu, C., Bhagavatula, P., Cui, B., Chu, S., et al. (2007). Retrolinkin, a membrane protein, plays an important role in retrograde axonal transport. Proc. Natl. Acad. Sci. USA. 104, 2223–2228. doi: 10.1073/pnas.0602222104
Liu, X., Duan, X., Zhang, Y., Sun, A., and Fan, D. (2020). Molecular analysis and clinical diversity of distal hereditary motor neuropathy. Eur. J. Neurol. 27, 1319–1326. doi: 10.1111/ene.14260
Liu, C. M., and Wang, R. Y. (2013). Saijilafu, Jiao ZX, Zhang BY, Zhou FQ. MicroRNA-138 and SIRT1 form a mutual negative feedback loop to regulate mammalian axon regeneration. Genes Dev. 27, 1473–1483. doi: 10.1101/gad.209619.112
Liu, J. C., Xue, D. F., Wang, X. Q., Bin, A. D., and Qin, P. J. (2019). MIR-101 relates to chronic peripheral neuropathic pain through targeting KPNB1 and regulating NF-κb signaling. Kaohsiung J. Med. Sci. 35, 139–145. doi: 10.1002/kjm2.12025
Lopes, D. M., Malek, N., Edye, M., Jager, S. B., McMurray, S., McMahon, S. B., et al. (2017). Sex differences in peripheral not central immune responses to pain-inducing injury. Sci. Rep. 7:16460. doi: 10.1038/s41598-017-16664-z
Lorenzoni, P. J., Bayer, D. L., Ducci, R. D. P., Fustes, O. J. H., Do Vale Pascoal Rodrigues, P. R., Werneck, L. C., et al. (2023). Spectrum of SPTLC1-related disorders: a novel case of ‘Ser331 syndrome’ that expand the phenotype of hereditary sensory and autonomic neuropathy type 1A and motor neuron diseases. Neurol. Sci. 44, 2551–2554. doi: 10.1007/s10072-023-06763-3
Lott, K., and Cingolani, G. (2011). The importin β binding domain as a master regulator of nucleocytoplasmic transport. Biochim. Biophys. Acta, Mol. Cell Res. 1813, 1578–1592. doi: 10.1016/j.bbamcr.2010.10.012
LRM, R., OJM, V., Veltkamp, M., Van Swol, F. P., and Grutters, J. C. (2021). Current view of diagnosing small Fiber neuropathy. J Neuromuscul Dis 8, 185–207. doi: 10.3233/JND-200490
Lykissas, M., Batistatou, A., Charalabopoulos, K., and Beris, A. (2007). The role of Neurotrophins in axonal growth, guidance, and regeneration. Curr. Neurovasc. Res. 4, 143–151. doi: 10.2174/156720207780637216
Maeda, T., Kiguchi, N., Kobayashi, Y., Ikuta, T., Ozaki, M., and Kishioka, S. (2009). Leptin derived from adipocytes in injured peripheral nerves facilitates development of neuropathic pain via macrophage stimulation. Proc. Natl. Acad. Sci. U. S. A. 106, 13076–13081. doi: 10.1073/pnas.0903524106
Makker, P. G. S., Duffy, S. S., Lees, J. G., Perera, C. J., Tonkin, R. S., Butovsky, O., et al. (2017). Characterisation of immune and neuroinflammatory changes associated with chemotherapy-induced peripheral neuropathy. PLoS One 12:e0170814. doi: 10.1371/journal.pone.0170814
Manassero, G., Repetto, I. E., Cobianchi, S., Valsecchi, V., Bonny, C., Rossi, F., et al. (2012). Role of JNK isoforms in the development of neuropathic pain following sciatic nerve transection in the mouse. Mol. Pain 8:1744-8069-8-39. doi: 10.1186/1744-8069-8-39
Mao, S., Huang, T., Chen, Y., Shen, L., Zhou, S., Zhang, S., et al. (2019). Circ-Spidr enhances axon regeneration after peripheral nerve injury. Cell Death Dis. 10:787. doi: 10.1038/s41419-019-2027-x
Mapps, A. A., Thomsen, M. B., Boehm, E., Zhao, H., Hattar, S., and Kuruvilla, R. (2022). Diversity of satellite glia in sympathetic and sensory ganglia. Cell Rep. 38:110328. doi: 10.1016/j.celrep.2022.110328
Markworth, R., Bähr, M., and Burk, K. (2021). Held up in traffic—defects in the trafficking machinery in Charcot-Marie-tooth disease. Front. Mol. Neurosci. 14:5294. doi: 10.3389/fnmol.2021.695294
Marlin, M. C., and Li, G. (2015). Biogenesis and function of the NGF/TrkA signaling endosome. Int. Rev. Cell Mol. Biol. 314:2. doi: 10.1016/bs.ircmb.2014.10.002
Marvaldi, L., Hausott, B., Auer, M., Leban, J., and Klimaschewski, L. (2014). A novel DRAK inhibitor, SC82510, promotes axon branching of adult sensory neurons in vitro. Neurochem. Res. 39, 403–407. doi: 10.1007/s11064-014-1238-x
Marvaldi, L., Panayotis, N., Alber, S., Daga, S. Y., Okladnikov, N., Koppel, I., et al. (2020). Importin a3 regulates chronic pain pathways in peripheral sensory neurons. Science 369, 842–846. doi: 10.1126/science.aaz5875
Marvaldi, L., Thongrong, S., Kozłowska, A., Irschick, R., Pritz, C. O., Bäumer, B., et al. (2015). Enhanced axon outgrowth and improved long-distance axon regeneration in sprouty2 deficient mice. Dev. Neurobiol. 75, 217–231. doi: 10.1002/dneu.22224
McGinnis, A., and Ji, R. R. (2023). The similar and distinct roles of satellite glial cells and spinal astrocytes in neuropathic pain. Cells 12:965. doi: 10.3390/cells12060965
McKelvey, R., Berta, T., Old, E., Ji, R. R., and Fitzgerald, M. (2015). Neuropathic pain is constitutively suppressed in early life by anti-inflammatory neuroimmune regulation. J. Neurosci. 35, 457–466. doi: 10.1523/JNEUROSCI.2315-14.2015
Meder, T., Prest, T., Skillen, C., Marchal, L., Yupanqui, V. T., Soletti, L., et al. (2021). Nerve-specific extracellular matrix hydrogel promotes functional regeneration following nerve gap injury. NPJ Regen Med 6:69. doi: 10.1038/s41536-021-00174-8
Melemedjian, O. K., Tillu, D. V., Moy, J. K., Asiedu, M. N., Mandell, E. K., Ghosh, S., et al. (2014). Local translation and retrograde axonal transport of CREB regulates IL-6-induced nociceptive plasticity. Mol. Pain 10:45. doi: 10.1186/1744-8069-10-45
Meloto, C. B., Benavides, R., Lichtenwalter, R. N., Wen, X., Tugarinov, N., Zorina-Lichtenwalter, K., et al. (2018). Human pain genetics database: a resource dedicated to human pain genetics research. Pain 159, 749–763. doi: 10.1097/j.pain.0000000000001135
Meng, W., Adams, M. J., Reel, P., Rajendrakumar, A., Huang, Y., Deary, I. J., et al. (2020). Genetic correlations between pain phenotypes and depression and neuroticism. Eur. J. Hum. Genet. 28, 358–366. doi: 10.1038/s41431-019-0530-2
Michaelevski, I., Segal-Ruder, Y., Rozenbaum, M., Medzihradszky, K. F., Shalem, O., Coppola, G., et al. (2010). Signaling to transcription networks in the neuronal retrograde injury response. Sci. Signal. 3:ra53. doi: 10.1126/scisignal.2000952
Mills, S. E. E., Nicolson, K. P., and Smith, B. H. (2019). Chronic pain: a review of its epidemiology and associated factors in population-based studies. Br. J. Anaesth. 123, e273–e283. doi: 10.1016/j.bja.2019.03.023
Moore, D. L., and Goldberg, J. L. (2011). Multiple transcription factor families regulate axon growth and regeneration. Dev. Neurobiol. 71, 1186–1211. doi: 10.1002/dneu.20934
Nascimento, A. I., Mar, F. M., and Sousa, M. M. (2018). The intriguing nature of dorsal root ganglion neurons: linking structure with polarity and function. Prog. Neurobiol. 168, 86–103. doi: 10.1016/j.pneurobio.2018.05.002
Neuman, M. D., Bateman, B. T., and Wunsch, H. (2019). Inappropriate opioid prescription after surgery. Lancet 393, 1547–1557. doi: 10.1016/S0140-6736(19)30428-3
Nguyen, M. Q., von Buchholtz, L. J., Reker, A. N., Ryba, N. J. P., and Davidson, S. (2021). Single-nucleus transcriptomic analysis of human dorsal root ganglion neurons. eLife 10:752. doi: 10.7554/eLife.71752
Oh, Y. M., Mahar, M., Ewan, E. E., Leahy, K. M., Zhao, G., and Cavalli, V. (2018). Epigenetic regulator UHRF1 inactivates REST and growth suppressor gene expression via DNA methylation to promote axon regeneration. Proc. Natl. Acad. Sci. U. S. A. 115:8115. doi: 10.1073/pnas.1812518115
Oh, J., and Yoon, S. Y. (2018). Neuropathic cancer pain: prevalence, pathophysi-ology, and management. Korean J. Intern. Med. 33, 1058–1069. doi: 10.3904/kjim.2018.162
Panayotis, N., Karpova, A., Kreutz, M. R., and Fainzilber, M. (2015). Macromolecular transport in synapse to nucleus communication. Trends Neurosci. 38, 108–116. doi: 10.1016/j.tins.2014.12.001
Panayotis, N., Sheinin, A., Dagan, S. Y., Tsoory, M. M., Rother, F., Vadhvani, M., et al. (2018). Importin α5 regulates anxiety through MeCP2 and sphingosine kinase 1. Cell Rep. 25, 3169–3179.e7. doi: 10.1016/j.celrep.2018.11.066
Pannell, M., Labuz, D., Celik, M., Keye, J., Batra, A., Siegmund, B., et al. (2016). Adoptive transfer of M2 macrophages reduces neuropathic pain via opioid peptides. J. Neuroinflammation 13:262. doi: 10.1186/s12974-016-0735-z
Parisien, M., Lima, L. V., Dagostino, C., El-Hachem, N., Drury, G. L., Grant, A. V., et al. (2022). Acute inflammatory response via neutrophil activation protects against the development of chronic pain. Sci. Transl. Med. 14, 1–12. doi: 10.1126/scitranslmed.abj9954
Park, S. B., Cetinkaya-Fisgin, A., Argyriou, A. A., Höke, A., Cavaletti, G., and Alberti, P. (2023). Axonal degeneration in chemotherapy-induced peripheral neurotoxicity: clinical and experimental evidence. J. Neurol. Neurosurg. Psychiatry 94, 962–972. doi: 10.1136/jnnp-2021-328323
Park, J., Zhang, Y., Saito, E., Gurczynski, S. J., Moore, B. B., Cummings, B. J., et al. (2019). Intravascular innate immune cells reprogrammed via intravenous nanoparticles to promote functional recovery after spinal cord injury. Proc. Natl. Acad. Sci. U. S. A. 116, 14947–14954. doi: 10.1073/pnas.1820276116
Pascal, M. M. V., Themistocleous, A. C., Baron, R., Binder, A., Bouhassira, D., Crombez, G., et al. (2019). DOLORisk: study protocol for a multi-Centre observational study to understand the risk factors and determinants of neuropathic pain version 2; referees: 2 approved. Wellcome Open Res 3:63. doi: 10.12688/wellcomeopenres.14576.2
Patapoutian, A., Tate, S., and Woolf, C. J. (2009). Transient receptor potential channels: targeting pain at the source. Nat. Rev. Drug Discov. 8, 55–68. doi: 10.1038/nrd2757
Patel, M. K., Kaye, A. D., and Urman, R. D. (2018). Tanezumab: therapy targeting nerve growth factor in pain pathogenesis. J. Anaesthesiol. Clin. Pharmacol. 34, 111–116. doi: 10.4103/joacp.JOACP_389_15
Patrone, C., Andersson, S., Korhonen, L., and Lindholm, D. (1999). Estrogen receptor-dependent regulation of sensory neuron survival in developing dorsal root ganglion. Proc. Natl. Acad. Sci. U. S. A. 96, 10905–10910. doi: 10.1073/pnas.96.19.10905
Penas, C., and Navarro, X. (2018). Epigenetic modifications associated to neuroinflammation and neuropathic pain after neural trauma. Front. Cell. Neurosci. 12:158. doi: 10.3389/fncel.2018.00158
Perlson, E., Hanz, S., Ben-Yaakov, K., Segal-Ruder, Y., Seger, R., and Fainzilber, M. (2005). Vimentin-dependent spatial translocation of an activated MAP kinase in injured nerve. Neuron 45, 715–726. doi: 10.1016/j.neuron.2005.01.023
Phatarakijnirund, V., Mumm, S., McAlister, W. H., Novack, D. V., Wenkert, D., Clements, K. L., et al. (2016). Congenital insensitivity to pain: fracturing without apparent skeletal pathobiology caused by an autosomal dominant, second mutation in SCN11A encoding voltage-gated sodium channel 1.9. Bone 84, 289–298. doi: 10.1016/j.bone.2015.11.022
Prior, R., Van Helleputte, L., Benoy, V., and Van Den Bosch, L. (2017). Defective axonal transport: a common pathological mechanism in inherited and acquired peripheral neuropathies. Neurobiol. Dis. 105, 300–320. doi: 10.1016/j.nbd.2017.02.009
Puttagunta, R., Tedeschi, A., Sória, M. G., Hervera, A., Lindner, R., Rathore, K. I., et al. (2014). PCAF-dependent epigenetic changes promote axonal regeneration in the central nervous system. Nat. Commun. 5:3527. doi: 10.1038/ncomms4527
Rabiller, L., Labit, E., Guissard, C., Gilardi, S., Guiard, B. P., Moulédous, L., et al. (2021). Pain sensing neurons promote tissue regeneration in adult mice. NPJ Regen Med 6, 1–9. doi: 10.1038/s41536-021-00175-7
Raivich, G., Bohatschek, M., Da Costa, C., Iwata, O., Galiano, M., Hristova, M., et al. (2004). The AP-1 transcription factor c-Jun is required for efficient axonal regeneration. Neuron 43, 57–67. doi: 10.1016/j.neuron.2004.06.005
Raja, S. N., Carr, D. B., Cohen, M., Finnerup, N. B., Flor, H., Gibson, S., et al. (2020). The revised International Association for the Study of Pain definition of pain: concepts, challenges, and compromises. Pain 161, 1976–1982. doi: 10.1097/j.pain.0000000000001939
Ren, T., Faust, A., Van Der Merwe, Y., Xiao, B., Johnson, S., Kandakatla, A., et al. (2018). Fetal extracellular matrix nerve wraps locally improve peripheral nerve remodeling after complete transection and direct repair in rat. Sci. Rep. 8:4474. doi: 10.1038/s41598-018-22628-8
Renthal, W., Tochitsky, I., Yang, L., Cheng, Y. C., Li, E., Kawaguchi, R., et al. (2020). Transcriptional reprogramming of distinct peripheral sensory neuron subtypes after axonal injury. Neuron 108, 128–144.e9. doi: 10.1016/j.neuron.2020.07.026
Ribeiro-Resende, V. T., Koenig, B., Nichterwitz, S., Oberhoffner, S., and Schlosshauer, B. (2009). Strategies for inducing the formation of bands of Büngner in peripheral nerve regeneration. Biomaterials 30, 5251–5259. doi: 10.1016/j.biomaterials.2009.07.007
Rinaldi, F., Bassi, M. T., Todeschini, A., Rota, S., Arnoldi, A., Padovani, A., et al. (2015). A novel mutation in motor domain of KIF5A associated with an HSP/axonal neuropathy phenotype. J. Clin. Neuromuscul. Dis. 16, 153–158. doi: 10.1097/CND.0000000000000063
Rishal, I., and Fainzilber, M. (2014). Axon-soma communication in neuronal injury. Nat. Rev. Neurosci. 15, 32–42. doi: 10.1038/nrn3609
Rishal, I., Kam, N., Perry, R. B. T., Shinder, V., Fisher, E. M. C., Schiavo, G., et al. (2012). A motor-driven mechanism for cell-length sensing. Cell Rep. 1, 608–616. doi: 10.1016/j.celrep.2012.05.013
Rosenbaum, T., Morales-Lázaro, S. L., and Islas, L. D. (2022). TRP channels: a journey towards a molecular understanding of pain. Nat. Rev. Neurosci. 23, 596–610. doi: 10.1038/s41583-022-00611-7
Rosso, G., Young, P., and Shahin, V. (2017). Mechanosensitivity of embryonic neurites promotes their directional extension and Schwann cells progenitors migration. Cell. Physiol. Biochem. 44, 1263–1270. doi: 10.1159/000485485
Rotshenker, S. (2011). Wallerian degeneration: the innate-immune response to traumatic nerve injury. J. Neuroinflammation 8:109. doi: 10.1186/1742-2094-8-109
Roughan, W. H., Campos, A. I., García-Marín, L. M., Cuéllar-Partida, G., Lupton, M. K., Hickie, I. B., et al. (2021). Comorbid chronic pain and depression: shared risk factors and differential antidepressant effectiveness. Front. Psych. 12:643609. doi: 10.3389/fpsyt.2021.643609
Roumazeilles, L., Dokalis, N., Kaulich, E., and Lelievre, V. (2018). It is all about the support — the role of the extracellular matrix in regenerating axon guidance. Cell Adh. Migr. 12, 1–6. doi: 10.1080/19336918.2017.1291481
Russ, D. E., Cross, R. B. P., Li, L., Koch, S. C., Matson, K. J. E., Yadav, A., et al. (2021). A harmonized atlas of mouse spinal cord cell types and their spatial organization. Nat. Commun. 12:5722. doi: 10.1038/s41467-021-25125-1
Saito, A., and Cavalli, V. (2016). Signaling over distances. Mol. Cell. Proteomics 15, 382–393. doi: 10.1074/mcp.R115.052753
Sakai, A., Saitow, F., Maruyama, M., Miyake, N., Miyake, K., Shimada, T., et al. (2017). MicroRNA cluster miR-17-92 regulates multiple functionally related voltage-gated potassium channels in chronic neuropathic pain. Nat. Commun. 8:79. doi: 10.1038/ncomms16079
Sanchez Rezza, A., Kulahci, Y., Gorantla, V. S., Zor, F., and Drzeniek, N. M. (2022). Implantable biomaterials for peripheral nerve regeneration–technology trends and translational tribulations. Front. Bioeng. Biotechnol. 10:863969. doi: 10.3389/fbioe.2022.863969
Santos, D., González-Pérez, F., Giudetti, G., Micera, S., Udina, E., Del Valle, J., et al. (2017). Preferential enhancement of sensory and motor axon regeneration by combining extracellular matrix components with neurotrophic factors. Int. J. Mol. Sci. 18:65. doi: 10.3390/ijms18010065
Schob, C., Hempel, M., Safka Brozkova, D., Jiang, H., Kim, S. Y., Batzir, N. A., et al. (2021). Dominant KPNA3 mutations cause infantile-onset hereditary spastic paraplegia. Ann. Neurol. 90, 738–750. doi: 10.1002/ana.26228
Shamoun, F., Shamoun, V., Akhavan, A., and Tuffaha, S. H. (2022). Target receptors of regenerating nerves: neuroma formation and current treatment options. Front. Mol. Neurosci. 15:221. doi: 10.3389/fnmol.2022.859221
Shao, J., Cao, J., Wang, J., Ren, X., Su, S., Li, M., et al. (2016). MicroRNA-30b regulates expression of the sodium channel Nav1.7 in nerve injury-induced neuropathic pain in the rat. Mol. Pain 12:174480691667152. doi: 10.1177/1744806916671523
Shenoda, B. B., Alexander, G. M., and Ajit, S. K. (2016). Hsa-miR-34a mediated repression of corticotrophin releasing hormone receptor 1 regulates pro-opiomelanocortin expression in patients with complex regional pain syndrome. J. Transl. Med. 14:64. doi: 10.1186/s12967-016-0820-1
Shiao, R., and Lee-Kubli, C. A. (2018). Neuropathic pain after spinal cord injury: challenges and research perspectives. Neurotherapeutics 15, 635–653. doi: 10.1007/s13311-018-0633-4
Shin, G. J. E., Pero, M. E., Hammond, L. A., Burgos, A., Kumar, A., Galindo, S. E., et al. (2021). Integrins protect sensory neurons in models of paclitaxel-induced peripheral sensory neuropathy. Proc. Natl. Acad. Sci. U. S. A. 118:50118. doi: 10.1073/pnas.2006050118
Shinotsuka, N., and Denk, F. (2022). Fibroblasts: the neglected cell type in peripheral sensitisation and chronic pain? A review based on a systematic search of the literature. BMJ Open Sci 6:e100235. doi: 10.1136/bmjos-2021-100235
Simeoli, R., Montague, K., Jones, H. R., Castaldi, L., Chambers, D., Kelleher, J. H., et al. (2017). Exosomal cargo including microRNA regulates sensory neuron to macrophage communication after nerve trauma. Nat. Commun. 8:1778. doi: 10.1038/s41467-017-01841-5
Singhmar, P., Trinh, R. T. P., Ma, J., Huo, X. J., Peng, B., Heijnen, C. J., et al. (2020). The fibroblast-derived protein PI16 controls neuropathic pain. Proc. Natl. Acad. Sci. U. S. A. 117, 5463–5471. doi: 10.1073/pnas.1913444117
Sleigh, J. N., Dawes, J. M., West, S. J., Wei, N., Spaulding, E. L., Gómez-Martín, A., et al. (2017). Trk receptor signaling and sensory neuron fate are perturbed in human neuropathy caused by gars mutations. Proc. Natl. Acad. Sci. U. S. A. 114, E3324–E3333. doi: 10.1073/pnas.1614557114
Smith, B. H., Hébert, H. L., and Veluchamy, A. (2020). Neuropathic pain in the community: prevalence, impact, and risk factors. Pain 161, S127–S137. doi: 10.1097/j.pain.0000000000001824
Stephens, K. E., Zhou, W., Ji, Z., Chen, Z., He, S., Ji, H., et al. (2019). Sex differences in gene regulation in the dorsal root ganglion after nerve injury. BMC Genomics 20:147. doi: 10.1186/s12864-019-5512-9
Strickland, I. T., Richards, L., Holmes, F. E., Wynick, D., Uney, J. B., and Wong, L. F. (2011). Axotomy-induced miR-21 promotes axon growth in adult dorsal root ganglion neurons. PLoS One 6:e23423. doi: 10.1371/JOURNAL.PONE.0023423
Sun, L., Tong, C.-K., Morgenstern, T. J., Zhou, H., Yang, G., Colecraft, H. M., et al. (2022). Targeted ubiquitination of sensory neuron calcium channels reduces the development of neuropathic pain. Proc. Natl. Acad. Sci. U. S. A. 119:e2118129119. doi: 10.1073/pnas.2118129119
Sun, W., Zhang, L., and Li, R. (2017). Overexpression of miR-206 ameliorates chronic constriction injury-induced neuropathic pain in rats via the MEK/ERK pathway by targeting brain-derived neurotrophic factor. Neurosci. Lett. 646, 68–74. doi: 10.1016/j.neulet.2016.12.047
Sundaram, V. K., Schütza, V., Schröter, N. H., Backhaus, A., Bilsing, A., Joneck, L., et al. (2023). Adipo-glial signaling mediates metabolic adaptation in peripheral nerve regeneration. Cell Metab. 35, 2136–2152.e9. doi: 10.1016/j.cmet.2023.10.017
Szabo-Pardi, T. A., Barron, L. R., Lenert, M. E., and Burton, M. D. (2021). Sensory neuron TLR4 mediates the development of nerve-injury induced mechanical hypersensitivity in female mice. Brain Behav. Immun. 97, 42–60. doi: 10.1016/j.bbi.2021.06.011
Tanaka, K., Kuzumaki, N., Hamada, Y., Suda, Y., Mori, T., Nagumo, Y., et al. (2023). Elucidation of the mechanisms of exercise-induced hypoalgesia and pain prolongation due to physical stress and the restriction of movement. Neurobiol Pain 14:100133. doi: 10.1016/j.ynpai.2023.100133
Tavares-Ferreira, D., Shiers, S., Ray, P. R., Wangzhou, A., Jeevakumar, V., Sankaranarayanan, I., et al. (2022). Spatial transcriptomics of dorsal root ganglia identifies molecular signatures of human nociceptors. Sci. Transl. Med. 14, 1–18. doi: 10.1126/scitranslmed.abj8186
Terenzio, M., Di Pizio, A., Rishal, I., Marvaldi, L., Di Matteo, P., Kawaguchi, R., et al. (2020). DYNLRB1 is essential for dynein mediated transport and neuronal survival. Neurobiol. Dis. 140:104816. doi: 10.1016/j.nbd.2020.104816
Terenzio, M., Koley, S., Samra, N., Rishal, I., Zhao, Q., Sahoo, P. K., et al. (2018). Locally translated mTOR controls axonal local translation in nerve injury. Science 359, 1416–1421. doi: 10.1126/science.aan1053
Testa, G., Cattaneo, A., and Capsoni, S. (2021). Understanding pain perception through genetic painlessness diseases: the role of NGF and proNGF. Pharmacol. Res. 169:105662. doi: 10.1016/j.phrs.2021.105662
Thongrong, S., Hausott, B., Marvaldi, L., Agostinho, A. S., Zangrandi, L., Burtscher, J., et al. (2016). Sprouty2 and −4 hypomorphism promotes neuronal survival and astrocytosis in a mouse model of kainic acid induced neuronal damage. Hippocampus 26, 658–667. doi: 10.1002/hipo.22549
Todd, A. J. (2010). Neuronal circuitry for pain processing in the dorsal horn. Nat. Rev. Neurosci. 11, 823–836. doi: 10.1038/nrn2947
Tracey, I., Woolf, C. J., and Andrews, N. A. (2019). Composite pain biomarker signatures for objective assessment and effective treatment. Neuron 101, 783–800. doi: 10.1016/j.neuron.2019.02.019
Treede, R. D., Rief, W., Barke, A., Aziz, Q., Bennett, M. I., Benoliel, R., et al. (2019). Chronic pain as a symptom or a disease: the IASP classification of chronic pain for the international classification of diseases (ICD-11). Pain 160, 19–27. doi: 10.1097/j.pain.0000000000001384
Truini, A., Garcia-Larrea, L., and Cruccu, G. (2013). Reappraising neuropathic pain in humans—how symptoms help disclose mechanisms. Nat. Rev. Neurol. 9, 572–582. doi: 10.1038/nrneurol.2013.180
Tsantoulas, C., and McMahon, S. B. (2014). Opening paths to novel analgesics: the role of potassium channels in chronic pain. Trends Neurosci. 37, 146–158. doi: 10.1016/j.tins.2013.12.002
Turk, D. C., and Patel, K. V. (2022). Epidemiology and economics of chronic and recurrent pain. Clinical Pain Management: A Practical Guide, Second Edition. Chapter 2 Eds. Mary E. Lynch, Kenneth D. Craig, and Philip W. Peng. John Wiley & Sons Ltd, 6–24. doi: 10.1002/9781119701170.ch2
Turney, S. G., Ahmed, M., Chandrasekar, I., Wysolmerski, R. B., Goeckeler, Z. M., Rioux, R. M., et al. (2016). Nerve growth factor stimulates axon outgrowth through negative regulation of growth cone actomyosin restraint of microtubule advance. Mol. Biol. Cell 27, 500–517. doi: 10.1091/mbc.e15-09-0636
Tuttle, A., Drerup, C. M., Marra, M., McGraw, H., and Nechiporuk, A. V. (2019). Retrograde ret signaling controls sensory pioneer axon outgrowth. eLife 8:92. doi: 10.7554/eLife.46092
Valakh, V., Frey, E., Babetto, E., Walker, L. J., and DiAntonio, A. (2015). Cytoskeletal disruption activates the DLK/JNK pathway, which promotes axonal regeneration and mimics a preconditioning injury. Neurobiol. Dis. 77, 13–25. doi: 10.1016/j.nbd.2015.02.014
Van Hecke, O., Austin, S. K., Khan, R. A., Smith, B. H., and Torrance, N. (2014). Neuropathic pain in the general population: a systematic review of epidemiological studies. Pain 155, 654–662. doi: 10.1016/j.pain.2013.11.013
VanDenKerkhof, E. G., Mann, E. G., Torrance, N., Smith, B. H., Johnson, A., and Gilron, I. (2016). An epidemiological study of neuropathic pain symptoms in Canadian adults. Pain Res. Manag. 2016, 1–13. doi: 10.1155/2016/9815750
Vasavda, C., Xu, R., Liew, J., Kothari, R., Dhindsa, R. S., Semenza, E. R., et al. (2022). Identification of the NRF2 transcriptional network as a therapeutic target for trigeminal neuropathic pain. Sci. Adv. 8:5633. doi: 10.1126/sciadv.abo5633
Vela-Alcatara, A. M., Rios-Ramirez, A., Santiago-Garcia, J., Rodriguez-Alba, J. C., and Tamariz, D. E. (2022). Modulation of DRG neurons response to semaphorin 3A via substrate stiffness. Cells Dev 171:203800. doi: 10.1016/j.cdev.2022.203800
Vermehren-Schmaedick, A., Olah, M. J., Ramunno-Johnson, D., Lidke, K. A., Cohen, M. S., and Vu, T. Q. (2022). Molecular-scale dynamics of long range retrograde brain-derived neurotrophic factor transport shaped by cellular spatial context. Front. Neurosci. 16:815. doi: 10.3389/fnins.2022.835815
Vincent, K., Dona, C. P. G., Albert, T. J., and Dahia, C. L. (2020). Age-related molecular changes in the lumbar dorsal root ganglia of mice: signs of sensitization, and inflammatory response. JOR Spine 3:e1124. doi: 10.1002/jsp2.1124
Viswanath, O., Urits, I., Burns, J., Charipova, K., Gress, K., McNally, A., et al. (2020). Central neuropathic mechanisms in pain signaling pathways: current evidence and recommendations. Adv. Ther. 37, 1946–1959. doi: 10.1007/s12325-020-01334-w
Vroman, R., Hunter, R. S., Wood, M. J., Davis, O. C., Malfait, Z., George, D. S., et al. (2023). Analysis of matrisome expression patterns in murine and human dorsal root ganglia. Front. Mol. Neurosci. 16:447. doi: 10.3389/fnmol.2023.1232447
Walco, G. A., Dworkin, R. H., Krane, E. J., LeBel, A. A., and Treede, R. D. (2010). Neuropathic pain in children: special considerations. Mayo Clin. Proc. 85, S33–S41. doi: 10.4065/mcp.2009.0647
Wang, Q. L., Guo, S., Duan, G., Ying, Y., Huang, P., Liu, J. Y., et al. (2016). Phenotypes and genotypes in five children with congenital insensitivity to pain with Anhidrosis. Pediatr. Neurol. 61, 63–69. doi: 10.1016/j.pediatrneurol.2016.04.006
Wang, Q., Yang, L. H., Min, L. Z., Chen, G., and Wei, Z. Y. (2022). Inhibition of Schwann cell pannexin 1 attenuates neuropathic pain through the suppression of inflammatory responses. J. Neuroinflammation 19:244. doi: 10.1186/s12974-022-02603-x
Ward, P. J., Davey, R. A., Zajac, J. D., and English, A. W. (2021). Neuronal androgen receptor is required for activity dependent enhancement of peripheral nerve regeneration. Dev. Neurobiol. 81, 411–423. doi: 10.1002/dneu.22826
Webber, C. A., Christie, K. J., Cheng, C., Martinez, J. A., Singh, B., Singh, V., et al. (2011). Schwann cells direct peripheral nerve regeneration through the Netrin-1 receptors, DCC and Unc5H2. Glia 59, 1503–1517. doi: 10.1002/glia.21194
Wemmie, J. A., Taugher, R. J., and Kreple, C. J. (2013). Acid-sensing ion channels in pain and disease. Nat. Rev. Neurosci. 14, 461–471. doi: 10.1038/nrn3529
Woller, S. A., Eddinger, K. A., Corr, M., and Yaksh, T. L. (2017). An overview of pathways encoding nociception. Clin. Exp. Rheumatol. 35, S40–S46.
Xie, W., Strong, J. A., and Zhang, J. M. (2017). Active nerve regeneration with failed target reinnervation drives persistent neuropathic pain. eNeuro 4, ENEURO.0008–ENEU17.2017. doi: 10.1523/ENEURO.0008-17.2017
Xu, X., Yu, C., Xu, L., and Xu, J. (2022). Emerging roles of keratinocytes in nociceptive transduction and regulation. Front. Mol. Neurosci. 15:2202. doi: 10.3389/fnmol.2022.982202
Yam, M. F., Loh, Y. C., Tan, C. S., Adam, S. K., Manan, N. A., and Basir, R. (2018). General pathways of pain sensation and the major neurotransmitters involved in pain regulation. Int. J. Mol. Sci. 19:2164. doi: 10.3390/ijms19082164
Yi, X. N., Zheng, L. F., Zhang, J. W., Zhang, L. Z., Xu, Y. Z., Luo, G., et al. (2006). Dynamic changes in Robo2 and Slit1 expression in adult rat dorsal root ganglion and sciatic nerve after peripheral and central axonal injury. Neurosci. Res. 56, 314–321. doi: 10.1016/j.neures.2006.07.014
Yu, X., Liu, H., Hamel, K. A., Morvan, M. G., Yu, S., Leff, J., et al. (2020). Dorsal root ganglion macrophages contribute to both the initiation and persistence of neuropathic pain. Nat. Commun. 11, 264–212. doi: 10.1038/s41467-019-13839-2
Yu, P., Zhang, G., Hou, B., Song, E., Wen, J., Ba, Y., et al. (2023). Effects of ECM proteins (laminin, fibronectin, and type IV collagen) on the biological behavior of Schwann cells and their roles in the process of remyelination after peripheral nerve injury. Front. Bioeng. Biotechnol. 11:1133718. doi: 10.3389/fbioe.2023.1133718
Yu, B., Zhou, S., Hu, W., Qian, T., Gao, R., Ding, G., et al. (2013). Altered long noncoding RNA expressions in dorsal root ganglion after rat sciatic nerve injury. Neurosci. Lett. 534, 117–122. doi: 10.1016/j.neulet.2012.12.014
Yuan, J., Wen, J., Wu, S., Mao, Y., Mo, K., Li, Z., et al. (2019). Contribution of dorsal root ganglion octamer transcription factor 1 to neuropathic pain after peripheral nerve injury. Pain 160, 375–384. doi: 10.1097/j.pain.0000000000001405
Zang, Y., Chen, S. X., Liao, G. J., Zhu, H. Q., Hong, W. X., Cui, Y., et al. (2015). Calpain-2 contributes to neuropathic pain following motor nerve injury via up-regulating interleukin-6 in DRG neurons. Brain Behav. Immun. 44, 37–47. doi: 10.1016/j.bbi.2014.08.003
Zeidler, M., Tavares-Ferreira, D., Brougher, J., Price, T. J., and Kress, M. (2023). NOCICEPTRA2.0—a comprehensive ncRNA atlas of human native and iPSC-derived sensory neurons. iScience 26:108525. doi: 10.1016/J.ISCI.2023.108525
Zhang, S., Cai, B., Li, Z., Wang, K., Bao, L., Li, C., et al. (2022). Fibroblastic SMOC2 suppresses mechanical nociception by inhibiting coupled activation of primary sensory neurons. J. Neurosci. 42, 4069–4086. doi: 10.1523/JNEUROSCI.2132-21.2022
Zhang, L., Feng, H., Jin, Y., Zhan, Y., Han, Q., Zhao, X., et al. (2021). Long non-coding RNA LINC01119 promotes neuropathic pain by stabilizing BDNF transcript. Front. Mol. Neurosci. 14:669. doi: 10.3389/fnmol.2021.673669
Zhang, H., Li, Y., De Carvalho-Barbosa, M., Kavelaars, A., Heijnen, C. J., Albrecht, P. J., et al. (2016). Dorsal root ganglion infiltration by macrophages contributes to paclitaxel chemotherapy-induced peripheral neuropathy. J. Pain 17, 775–786. doi: 10.1016/j.jpain.2016.02.011
Zhao, W., Ma, L., Deng, D., Zhang, T., Han, L., Xu, F., et al. (2023). M2 macrophage polarization: a potential target in pain relief. Front. Immunol. 14:149. doi: 10.3389/fimmu.2023.1243149
Zhou, L., Kong, G., Palmisano, I., Cencioni, M. T., Danzi, M., De Virgiliis, F., et al. (2022). Reversible CD8 T cell–neuron cross-talk causes aging-dependent neuronal regenerative decline. Science 376:eabd5926. doi: 10.1126/science.abd5926
Zhou, S., Zhang, S., Wang, Y., Yi, S., Zhao, L., Tang, X., et al. (2015). MiR-21 and miR-222 inhibit apoptosis of adult dorsal root ganglion neurons by repressing TIMP3 following sciatic nerve injury. Neurosci. Lett. 586, 43–49. doi: 10.1016/j.neulet.2014.12.006
Keywords: neuropathic pain, peripheral nerve injury, neurogenetics, axonal signaling, dorsal root ganglia, axonal regeneration, nerve regeneration
Citation: Testa L, Dotta S, Vercelli A and Marvaldi L (2024) Communicating pain: emerging axonal signaling in peripheral neuropathic pain. Front. Neuroanat. 18:1398400. doi: 10.3389/fnana.2024.1398400
Received: 09 March 2024; Accepted: 21 May 2024;
Published: 09 July 2024.
Edited by:
Michele Fornaro, Midwestern University, United StatesReviewed by:
Rainer Viktor Haberberger, University of Adelaide, AustraliaCopyright © 2024 Testa, Dotta, Vercelli and Marvaldi. This is an open-access article distributed under the terms of the Creative Commons Attribution License (CC BY). The use, distribution or reproduction in other forums is permitted, provided the original author(s) and the copyright owner(s) are credited and that the original publication in this journal is cited, in accordance with accepted academic practice. No use, distribution or reproduction is permitted which does not comply with these terms.
*Correspondence: Letizia Marvaldi, bGV0aXppYS5tYXJ2YWxkaUB1bml0by5pdA==
Disclaimer: All claims expressed in this article are solely those of the authors and do not necessarily represent those of their affiliated organizations, or those of the publisher, the editors and the reviewers. Any product that may be evaluated in this article or claim that may be made by its manufacturer is not guaranteed or endorsed by the publisher.
Research integrity at Frontiers
Learn more about the work of our research integrity team to safeguard the quality of each article we publish.