- Department of Animal Physiology and Molecular Biomedicine, Institute of Animal Physiology, Justus-Liebig-University Gießen, Gießen, Germany
Microtubules are essential components of the cytoskeleton of all eukaryotic cells and consist of α- and β-tubulin heterodimers. Several tissue-specific isotypes of α- and β-tubulins, encoded by distinct genes, have been described in vertebrates. In the African clawed frog (Xenopus laevis), class II β-tubulin (tubb2b) is expressed exclusively in neurons, and its promoter is used to establish different transgenic frog lines. However, a thorough investigation of the expression pattern of tubb2b has not been carried out yet. In this study, we describe the expression of tubb2b-dependent Katushka fluorescence in the forebrain of premetamorphic Xenopus laevis at cellular resolution. To determine the exact location of Katushka-positive neurons in the forebrain nuclei and to verify the extent of neuronal Katushka expression, we used a transgenic frog line and performed several additional antibody stainings. We found tubb2b-dependent fluorescence throughout the Xenopus forebrain, but not in all neurons. In the olfactory bulb, tubb2b-dependent fluorescence is present in axonal projections from the olfactory epithelium, cells in the mitral cell layer, and fibers of the extrabulbar system, but not in interneurons. We also detected tubb2b-dependent fluorescence in parts of the basal ganglia, the amygdaloid complex, the pallium, the optic nerve, the preoptic area, and the hypothalamus. In the diencephalon, tubb2b-dependent fluorescence occurred mainly in the prethalamus and thalamus. As in the olfactory system, not all neurons of these forebrain regions exhibited tubb2b-dependent fluorescence. Together, our results present a detailed overview of the distribution of tubb2b-dependent fluorescence in neurons of the forebrain of larval Xenopus laevis and clearly show that tubb2b-dependent fluorescence cannot be used as a pan-neuronal marker.
Introduction
Microtubules, dynamic hollow cylinders composed of α- and β-tubulin heterodimers (Ludueña et al., 1977; Sullivan, 1988; Ludueña and Banerjee, 2008b; Knossow et al., 2020), are essential constituents of the cytoskeleton of all eukaryotic cells (Wade, 2009; Kapitein and Hoogenraad, 2015). They are involved in several fundamental cellular processes, including the maintenance of cell shape, the division of cells during mitosis and meiosis, ciliary and flagellar motion, and various forms of intracellular transport (Ludueña et al., 1977; Nogales, 2000; Muroyama and Lechler, 2017). In developing neurons, microtubules play an essential role in forming the accurate structure of axons, dendrites, and synapses. These functions persist into adulthood to maintain the neurite structure and enable the trafficking of vesicles between organelles (Lasser et al., 2018). A varying number of α- and β-tubulin isotypes have been described in different vertebrate species (Ludueña and Banerjee, 2008a). The analysis of their occurrence in various cell types and tissues has demonstrated a complex pattern of differential distribution of the multiple isotypes (Lewis et al., 1987; Ludueña, 1998; Katsetos et al., 2003). In the brain of many vertebrates, class III β-tubulin is specifically expressed in neurons (Moody, 1989; Lee et al., 1990a,b; Katsetos et al., 1993, 2003; Moody et al., 1996; Latremoliere et al., 2018). However, the African clawed frog (Xenopus laevis) steps out of the line as class III β-tubulin could not be detected in the nervous system (Moody et al., 1996). In larval Xenopus, another β-tubulin isotype, class II β-tubulin, has been shown to be specially expressed in neurons (Richter et al., 1988; Oschwald et al., 1991). Therefore, the tubb2b promoter has been used to establish transgenic frog lines, for example, Xla.Tg(tubb2b:Katushka;cryga:Venus)EXRC (Love et al., 2011) or Xla.Tg(tubb2b:GCaMP6s;Rno.elas:GFP)NXR (Horb et al., 2019; Offner et al., 2020), where transgenes are specifically expressed in neurons. Although the tubb2b promoter is used in many transgenic frog lines, a detailed investigation of its activity pattern at cellular resolution has not been performed yet. In the present study, we analyzed the tubb2b-dependent fluorescence expression pattern in the forebrain of larval Xenopus laevis on a cellular level. We found tubb2b-dependent fluorescence in neurons throughout the Xenopus forebrain, that is, the olfactory bulb (OB), parts of the basal ganglia, the pallium, the amygdaloid complex, the optic nerve (OpN), the preoptic area (POA), the prethalamus (PTh), the thalamus (Th), and the hypothalamus (Hyp). Still, clearly, not all neurons in these areas were fluorescently labeled. Together, the results of this work present a detailed overview of the distribution of tubb2b-dependent fluorescence in forebrain neurons and show that tubb2b-driven fluorescent expression cannot be used as a pan-neuronal marker in the brain of larval Xenopus laevis.
Materials and Methods
Animals
Transgenic NBT-Katushka γ-cry-Venus [Xla.Tg(tubb2b:Katu shka;cryga:Venus)EXRC; Love et al., 2011] and custom-bred NBT-Katushka pax6-GFP [Xla.Tg(tubb2b:Katushka;cryga:Ven us;pax6:GFP;CMV:DsRED)Manzini] larval Xenopus laevis were raised in the animal husbandry facility at the Institute of Animal Physiology of the Justus-Liebig-University of Gießen, Germany. Transgenic NBT-Katushka pax6-GFP frogs were naturally bred from transgenic NBT-Katushka γ-cry-Venus and pax6-GFP CMV-DsRED [Xla.Tg(pax6:GFP;CMV:DsRED)Papal; Hartley et al., 2001] Xenopus laevis. Animals were kept in water tanks of 1.8–7.5 l at a water temperature of 19–22°C and fed with a mix of spirulina and chlorella algae (MS-Tierbedarf). For all experiments, tadpoles of stages 48–52 were used as a representative of the premetamorphic developmental phase (Nieuwkoop and Faber, 1994). All animal procedures were performed following the guidelines of Laboratory Animal Research of the Institutional Care and Use Committee of the Justus-Liebig-University of Gießen (649_M).
Whole Mount Preparations and Tissue Slicing
All animals were anesthetized in 0.02% MS-222 solution (ethyl 3-aminobenzoate methanesulfonate; TCI Germany), dissolved in tap water, and killed by severing the spinal cord at the level of the brainstem. Tissue blocks containing the whole brain and spinal cord were dissected. Palatial tissue covering the brain was removed, and the blocks were collected in frog Ringer solution (98 mM NaCl, 2 mM KCl, 1 mM CaCl2, 2 mM MgCl, 5 mM Na-pyruvate, 5 mM glucose, 10 mM HEPES, pH 7.8, osmolarity of 230 mOsmol/l). For fixation, the samples were immersed in 4% formaldehyde for 1 h and washed with PBS (137 mM NaCl, 2.7 mM KCl, 8 mM Na2HPO4, 1.4 mM KH2PO4, dissolved in purified water, pH 7.4). Several blocks were then embedded in 5% of low-melting point agarose (Sigma-Aldrich) and cut into 300-μm-thick coronal slices using a half-automatic vibratome (Leica VT1200S; Leica Biosystems).
Immunohistochemistry
To help localize neurons with tubb2b-dependent fluorescence in the larval forebrain, we performed immunohistochemical stainings against Pax7, calretinin (CR), and tyrosine hydroxylase (TH). All tissue slices were permeabilized using PBST (PBS containing 0.2% Triton-X100; Carl Roth). Unspecific binding sites were blocked with PBST with 2% normal goat serum (NGS; MP Biomedicals) for 1 h at room temperature. The slices were incubated with primary antibodies (1:100) in PBST and 2% NGS at 4°C for 3 days. Katushka was enhanced with anti-tRFP (AB233, polyclonal, derived from rabbit; BioCat) in combination with anti-HuC/D (A-21271, monoclonal, derived from mouse; Thermo Fisher Scientific), anti-calretinin (6B3, monoclonal, derived from mouse; Swant), anti-Pax7 (PAX7, monoclonal, derived from mouse), or anti-tyrosine hydroxylase (22941, monoclonal, derived from mouse; Immunostar). PAX7 was deposited to the DSHB by Kawakami, A. (DSHB Hybridoma Product PAX7). GFP was enhanced with anti-GFP (ab1218, monoclonal, derived from mouse; Abcam). The primary antibodies were washed off with PBS, and the samples were incubated with the secondary antibodies Alexa Fluor 594 goat anti-rabbit (Invitrogen, Thermo Fisher Scientific; 1:100) and Alexa Fluor 488 goat anti-mouse (Invitrogen, Thermo Fisher Scientific; 1:100) in PBS with 2% NGS at 4°C for 3 days. After several washing steps with PBS, the samples were transferred to a recording chamber for multiphoton microscopy (A1R MP; Nikon).
Image Processing and Data Analysis
All images were recorded at a z-resolution of 1 μm at an excitation wavelength of 780 nm. Image processing was performed using open-source processing software ImageJ (Schindelin et al., 2012).1 The raw image data were acquired as multidimensional image stacks. Images obtained from whole mount preparations are shown as maximum projections of all planes of the recorded z-stacks. Images obtained from the coronal slices are shown as individual z-planes. Due to the size of the samples, multiple image stacks were stitched together to show larger brain areas in one picture (Preibisch et al., 2009). Some images were cropped to show details. Brightness and contrast were linearly adjusted in all images. Labeled cells were manually counted using ImageJ ROI Manager in the OB as an example brain region. Mean counts are presented with standard deviation.
Results
In this study, we characterized the activity pattern of the neuronal β-tubulin (tubb2b) promoter in the forebrain of premetamorphic Xenopus laevis. We used whole-brain preparations and coronal slices of transgenic tadpoles: NBT-Katushka γ-cry-Venus (n = 21 whole brains) and NBT-Katushka pax6-GFP (n = 13). Additionally, we performed antibody stainings against Pax7 (n = 6), TH (n = 8), and CR (n = 15) to have landmarks of the main forebrain regions and to describe the precise location of neurons in which the promoter tubb2b is active. To gain information about the identity of tubb2b-positive cells, CR, TH, and pax6 were used to differentiate types of interneurons within the OB. Since pax6 is established as a marker for the main pallial regions of the telencephalon (Stoykova and Gruss, 1994; Puelles et al., 2000; Moreno et al., 2008), we used the NBT-Katushka pax6-GFP transgenic Xenopus line to visualize the main parts of the pallium and subpallium. CR is known to be expressed in the central thalamic nucleus and fibers of the lateral forebrain bundle (Morona and González, 2013). We used CR to visualize the boundary between the thalamic nuclei of the diencephalon and the telencephalic hypothalamic areas, which are located ventrally. Within the diencephalon, Pax7 was used to differentiate prosomeres 1–3 (p1–3) as Pax7 expression has been found in p3 and the epiphysis which is part of p2 (Bandín et al., 2013). tubb2b-driven fluorescence was found mainly in somata. In some cases, we found additional tubb2b-dependent fluorescence in fibers and fiber terminals. We present our results in a rostral–caudal axis, starting from the OB and terminating in the thalamus and hypothalamus. We used the following publications as references to evaluate the expression in specific brain areas: olfactory bulb: Nezlin and Schild, 2000; Pinelli et al., 2004; Gaudin and Gascuel, 2005; Weiss et al., 2020; telencephalon and diencephalon: González et al., 1994; Marín et al., 1997a,b; Moreno and González, 2003, 2004, 2005; Morona and González, 2013; Bandín et al., 2014.
Telencephalon
In anurans, the OB is the most anterior telencephalic region (Figure 1L) and is structured in six distinct layers (Byrd and Burd, 1991; Manzini and Schild, 2010; Manzini et al., 2022). The OB, which is connected to the olfactory epithelium via the olfactory nerve (ON), is the first relay station of the olfactory system (Manzini and Schild, 2010). We found tubb2b-dependent fluorescence in cell bodies of olfactory receptor neurons (ORNs) in the MOE (Figures 1A–C) and associated axons within the ON (Figures 1I–K). Terminals of these axons in the glomerular layer of the main and accessory OB also show tubb2b-dependent fluorescence (projection fields 1–9, α, β, γ, and δ; Figures 1D–G, 2A–D). Within the OB of larval Xenopus laevis, axon terminals of ORNs form nine large and four small projection fields, which are highly conserved among anurans (Gaudin and Gascuel, 2005; Weiss et al., 2020, 2021). We detected tubb2b-dependent fluorescence in axon terminals innervating all of these regions (projection fields 1–9, α, β, γ, and δ; Figures 1D–G, 2A–D) and in a bundle of fibers that project through the OB toward more caudal brain regions (Figure 1I; white arrow).
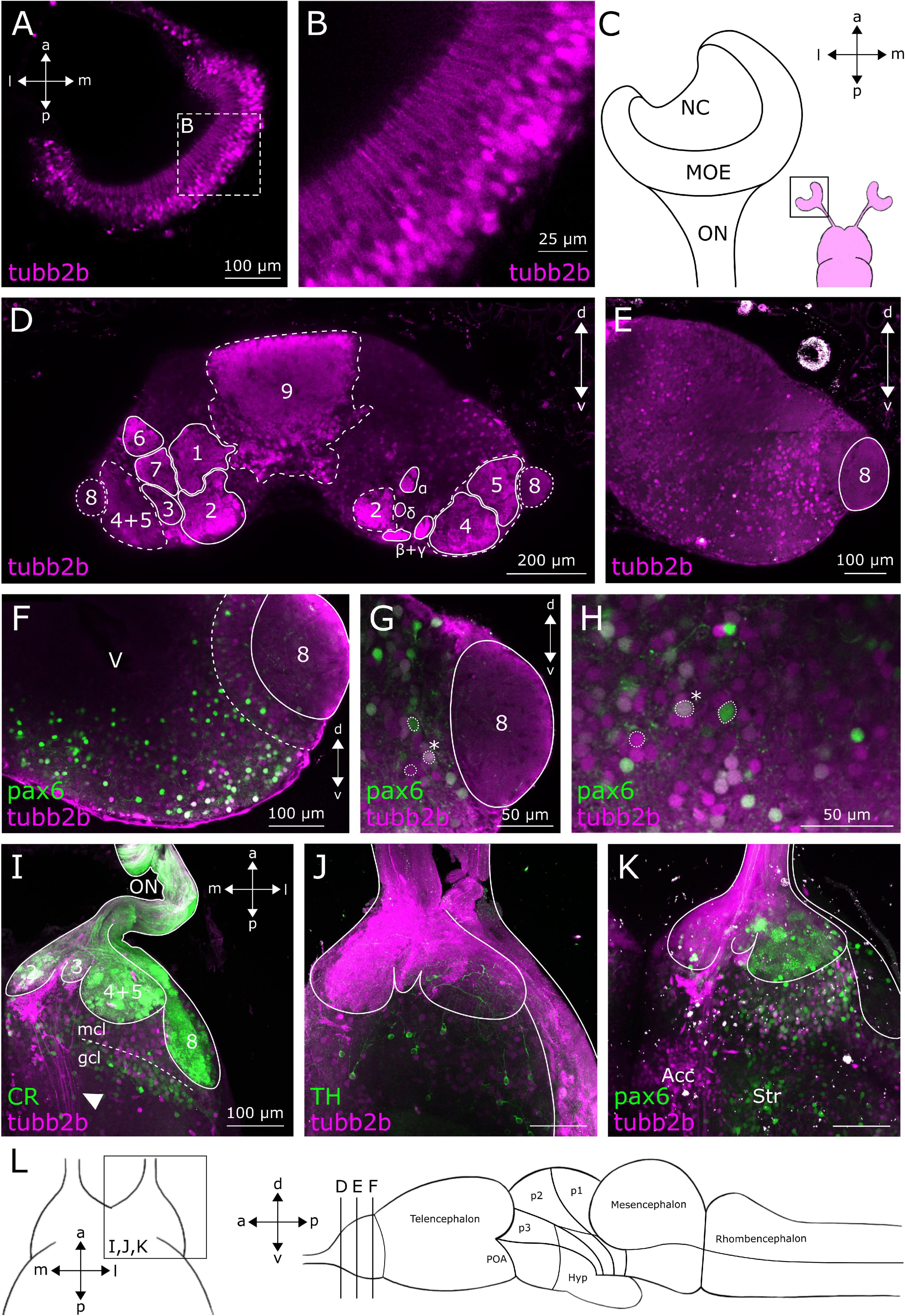
Figure 1. tubb2b-dependent fluorescence within the MOE and OB of larval Xenopus laevis. (A) Dorsal view of a single focal plane of the left main olfactory epithelium (MOE). tubb2b-dependent fluorescence (magenta) is present in olfactory receptor neurons. (B) Close-up of tubb2b-positive olfactory receptor neurons of the MOE. (C) Schematic overview of the olfactory organs (magenta). Close-up of the left MOE. (D) Coronal slice of the OB (left and right) of a transgenic NBT-Katushka γ-cry-Venus tadpole. tubb2b-dependent fluorescence (magenta) is present in axons of ORNs terminating in all known axonal projection fields of the OB (projection fields 1–9 and projection fields α, β, γ, and δ; see Gaudin and Gascuel, 2005). (E) Coronal slice of the OB (one side only) of transgenic NBT-Katushka γ-cry-Venus tadpoles and tubb2b-dependent fluorescence in cells of the mitral cell layer of the main and accessory (projection field 8) olfactory bulb. (F–H) Coronal slices of the OB (one side only) of transgenic NBT-Katushka pax6-GFP tadpoles. (F) tubb2b- (magenta) and pax6-dependent fluorescence (green) in cells in the mitral cell layer. (G,H) Close-ups of the mitral cell layer of the main and accessory OB showing tubb2b-dependent fluorescence and pax6-dependent fluorescence. Examples of cells featuring tubb2b-dependent fluorescence only and pax6-dependent fluorescence only, and double-fluorescent cells (*) are encircled. (I–K) Maximum projection of the whole ventral OB of one brain hemisphere. (I) tubb2b-dependent fluorescence has not been detected in calretinin (CR; green)-positive cells. tubb2b-positive fibers that bypass the OB were found in the intermediate OB (white arrow). (J) tubb2b-dependent fluorescence has not been detected in tyrosine hydroxylase (TH; green)-positive cells. (K) tubb2b-dependent fluorescence and pax6-dependent fluorescence have been detected in cells of the mitral cell layer. tubb2b-dependent fluorescence was also found in the nucleus accumbens (Acc) and pax6-dependent fluorescence in the anterior part of the striatum (Str). (L) Scheme of the larval brain showing the approximate positions (lines) of the sections shown in (A–L). POA, preoptic area; Hyp, hypothalamus; NC, nasal cavity; ON, olfactory nerve; mcl, mitral cell layer; gcl, granule cell layer; V, ventricle; d, dorsal; v, ventral; a, anterior; p, posterior; m, medial; l, lateral.
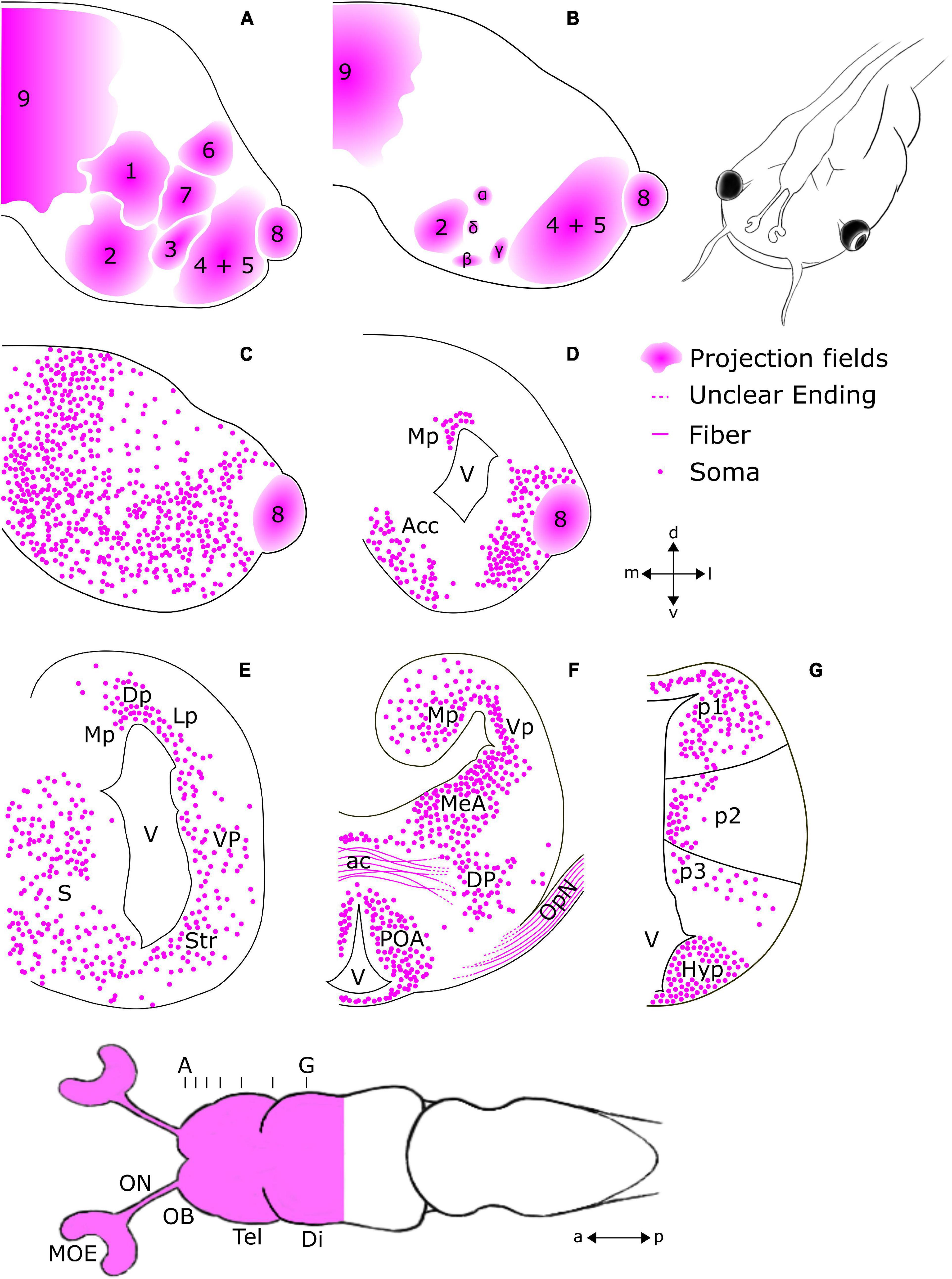
Figure 2. Schematic overview of regions featuring tubb2b-dependent fluorescence in the forebrain of premetamorphic Xenopus laevis. Ventral view of the larval forebrain and transverse sections of the olfactory bulb (OB; A–C), telencephalon (Tel; A–F), diencephalon (Di), and hypothalamus (G). The position and density of the cells shown for each region are presented based on observation and not via quantitative analysis. (A,B) tubb2b-dependent fluorescence is present in projection fields of axons arriving from the olfactory epithelium (1–9 + α, β, γ, δ; Gaudin and Gascuel, 2005). (C,D) tubb2b-dependent fluorescence in cells of the mitral cell layer of the main and accessory olfactory bulbs, the nucleus accumbens (Acc), and pallium. (E) tubb2b-dependent fluorescence in the medial pallium (Mp), lateral pallium (Lp), dorsal pallium (Dp), ventral pallium (Vp), striatum (Str), and septum (S). (F) tubb2b-dependent fluorescence in the anterior commissure (ac), medial amygdala (MeA), optic nerve (OpN), dorsal pallidum (DP), and preoptic area (POA). (G) tubb2b-dependent fluorescence in prosomeres 1–3 (p1–3). Hyp, Hypothalamus; lfb, lateral forebrain bundle; V, ventricle; MOE, main olfactory epithelium; ON, olfactory nerve; a, anterior; p, posterior; d, dorsal; v, ventral; l, lateral; m, medial.
To visualize all mature neurons of the OB and compare the pattern of tubb2b-expressing cells, antibody stainings against HuC/D were done in NBT-Katushka γ-cry-Venus transgenic tadpoles (Figures 3A–C). HuC/D expression was detected in all cell layers of the whole OB (Figures 3A,B; green). We found that tubb2b is not expressed in all neurons and surprisingly just in a limited number of cells (Figures 3A,C; magenta). Unlike HuC/D, tubb2b-positive cells were found only in the mitral cell layer and close to projection fields 1–9 (Figures 3A,C). For the whole OB, we counted an average number of 6,517 ± 210 HuC/D-positive cells (Figure 3D; green; n = 3). In comparison, we calculated an average number of 1,588 ± 191 tubb2b-positive cells (Figure 3D; magenta; n = 10). As the overall density of tubb2b-positive cells compared to HuC/D-positive cells, we calculated an average of 24.4% (Figure 3D). To assign tubb2b-dependent fluorescence to the specific bulbar cell types, we performed antibody stainings against CR and TH in transgenic NBT-Katushka γ-cry-Venus tadpoles and used transgenic NBT-Katushka pax6-GFP tadpoles. CR-positive cells were predominantly located in the granule cell layer of the OB (Figure 1I; green) and, to a lesser extent, in the mitral cell layer. All CR-positive cells did not show tubb2b-dependent fluorescence (Figure 1I; magenta). TH-positive cells were located in both the mitral cell layer and the periglomerular layer of the OB (Figure 1J; green), but not in the granule cell layer. Again, no cells exhibiting both TH-staining and tubb2b-dependent fluorescence were identified (Figure 1J; magenta). tubb2b-dependent fluorescence was exclusively located in some, but not all, cells of the mitral cell layer of the main and the accessory OB (Figures 1E–H, 2C,D, 3A,C, 4A; magenta). In this layer, we also found pax6-positive cells (Figures 1F–H,K; green), some of them also having tubb2b-dependent fluorescence (Figures 1G,H; asterisk). Additionally, a group of cells that show tubb2b-dependent fluorescence was located in the nucleus accumbens (Acc; Figures 1K, 2D). This group of cells is also visible in Figure 4A. Laterally, adjacent to Acc, we found pax6-positive cells (green) and cells with tubb2b-dependent fluorescence (magenta) in the striatum (Str; Figure 1K).
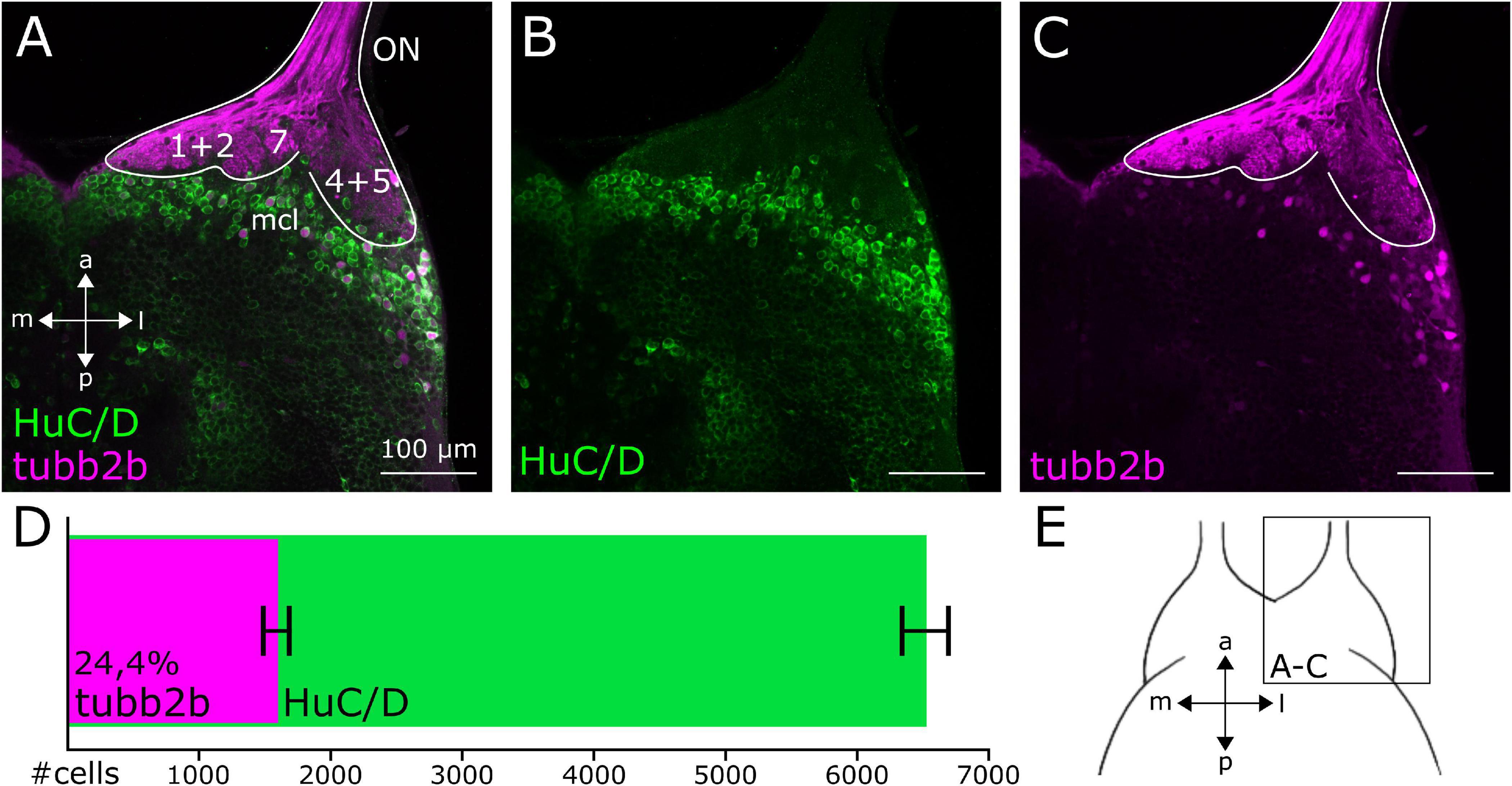
Figure 3. Cell density of tubb2b-positive neurons in the OB of premetamorphic Xenopus larvae. (A–C) Ventral view of a single focal plane of the OB of one brain hemisphere. (A) HuC/D-positive cells (green) are present throughout the whole OB. Few HuC/D-positive cells are also tubb2b-positive (magenta). (B) Pattern of HuC/D-positive cells within the OB. (C) tubb2b-positive cells are located in the mitral cell layer (mcl) of the OB. (D) Number of tubb2b-positive cells in comparison to HuC/D-positive cells of the whole OB. Average number of tubb2b-positive cells: 1,588 ± 191 (n = 10). Average number of HuC/D-positive cells: 6,517 ± 210 (n = 3). Cell density of tubb2b-positive cells compared to HuC/D-positive cells: 24,4%. (E) Scheme of the ventral OB showing the position of the images (A–C). ON, olfactory nerve; a, anterior; p, posterior; m, medial; l, lateral.
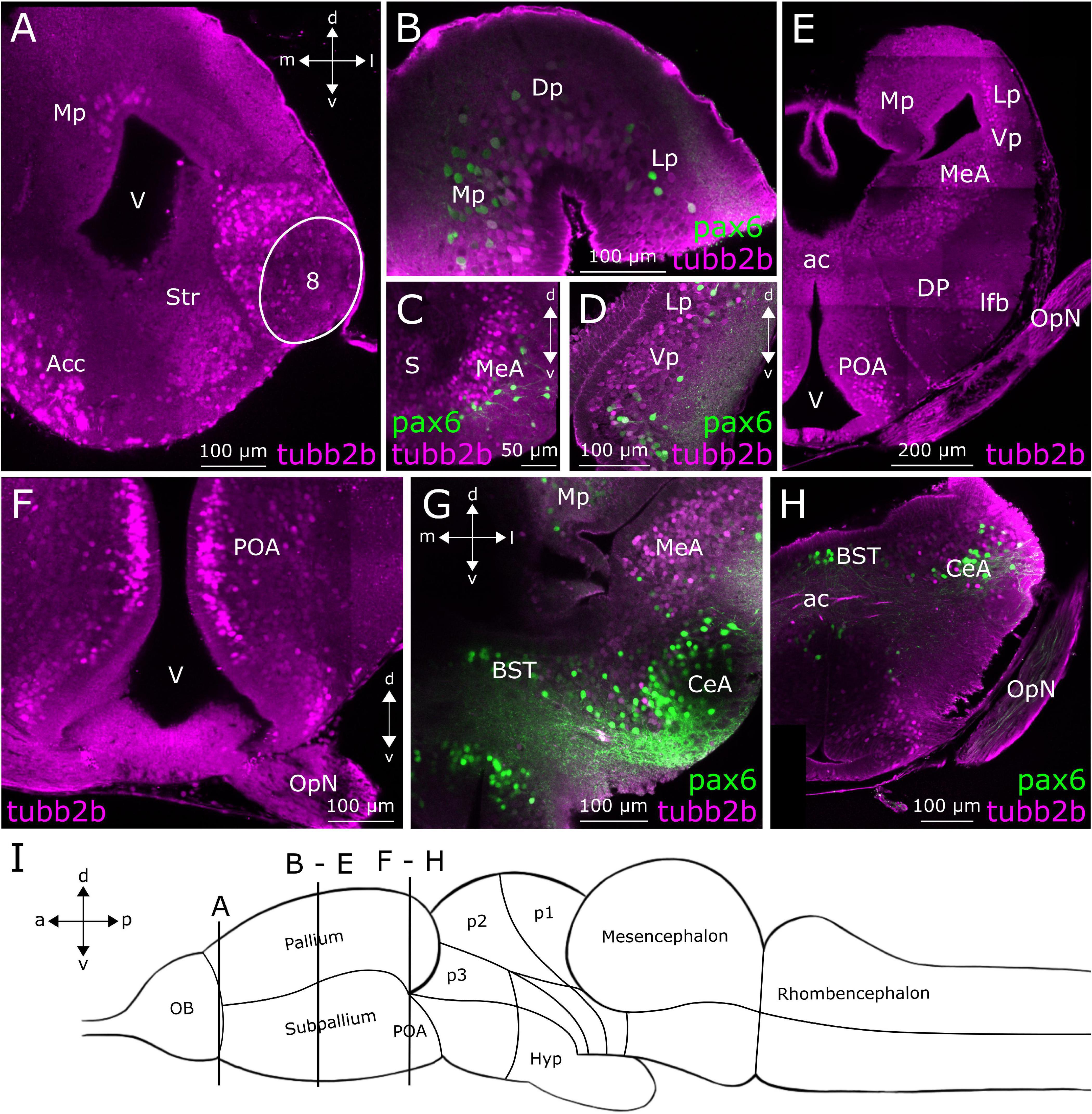
Figure 4. tubb2b-dependent fluorescence in the telencephalon of larval Xenopus laevis. Coronal sections through the telencephalon of NBT-Katushka γ-cry-Venus (A,E,F) and NBT-Katushka pax6-GFP (B–D,G,H) transgenic tadpoles. (A) tubb2b-dependent fluorescence (magenta) in the anterior telencephalon. Stained cells could be detected in the nucleus accumbens (Acc), striatum (Str), accessory OB (projection field 8), and pallium. (B–D) Close-ups of various zones of the anterior telencephalon showing tubb2b-dependent fluorescence in cells of the dorsal pallium (Dp), ventral pallium (Vp), medial pallium (Mp), lateral pallium (Lp), septum (S), and medial amygdala (MeA). (E) Sections through the posterior telencephalon showing tubb2b-dependent fluorescence in cells of the Mp, Lp, Vp, MeA, dorsal pallidum (DP), preoptic area (POA), and optic nerve (OpN). (F–H) Close-ups of various zones of the ventral posterior telencephalon. (F) tubb2b-dependent fluorescence in cells of the POA. (G,H) No overlap was detected between tubb2b- and pax6-dependent fluorescence (green) in the POA, ac, and central amygdala (CeA). (I) Scheme of the larval brain showing the approximate positions (dashed rectangles) of the sections shown in (A–H). Hyp, hypothalamus; OB, olfactory bulb; BST, bed nucleus of the stria terminalis; V, ventricle; d, dorsal; v, ventral; a, anterior; p, posterior; m, medial; l, lateral.
Figure 4A shows a coronal slice at a posterior position of the anterior telencephalon. At this level in the anterior–posterior axis, we found tubb2b-dependent fluorescence (magenta) in cells of the Str, Acc, and accessory OB (projection field 8). First, cells show tubb2b-dependent fluorescence in the pallium close to the lateral ventricle (Figures 2D, 4A). A little farther back in the telencephalon, tubb2b-dependent fluorescence is located in cells of the medial pallium (Mp; Figures 2E,F, 4B,E), lateral pallium (Lp; Figures 2E, 4B,D,E), and ventral pallium (Vp; Figures 2E,F, 4D,E). tubb2b-dependent fluorescence is also present in cells of the medial amygdala (MeA; Figures 2F, 4C,E,G), the central amygdala (CeA; Figures 4G,H), and the septum (S; Figure 4C). Several regions with tubb2b-dependent fluorescence also show pax6-dependent fluorescence (Figures 4B–D,G,H; green). Thereby, the most prominent group of cells that shows tubb2b-dependent fluorescence was present at the level of the CeA (Figures 4G,H). An additional group of cells with tubb2b-dependent fluorescence was found close to the dorsal pallidum (DP) and at the position of the lateral forebrain bundle (lfb; Figures 2F, 4E). tubb2b-dependent fluorescence-positive fibers were additionally observed in the optic nerve (OpN; Figures 2F, 4E,F,H) and cell bodies in the preoptic area (POA) close to the lateral ventricle (V; Figures 2F, 4E,F). At the level of the anterior commissure (ac), we found tubb2b-dependent fluorescence in fibers that project to the contralateral brain hemisphere (Figures 2F, 4H). Moreover, we found that cells of the hypothalamus show strong tubb2b-dependent fluorescence (Hyp; Figures 2G, 5B–D).
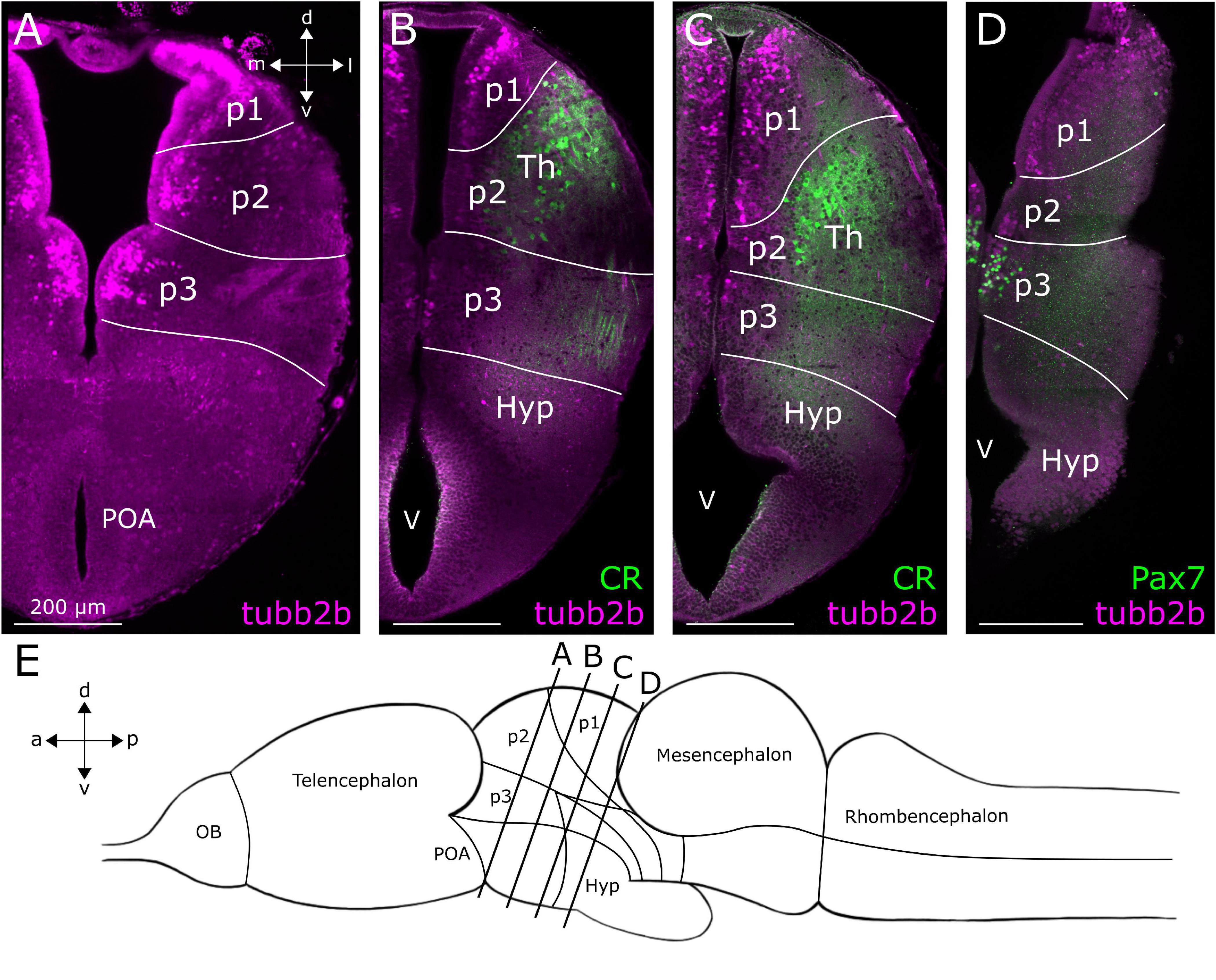
Figure 5. Location of tubb2b-dependent fluorescence in the diencephalon of larval Xenopus laevis. Coronal sections through the diencephalon of NBT-Katushka γ-cry-Venus transgenic tadpoles. (A) tubb2b-dependent fluorescence (magenta) in cells of prosomeres 1–3 (p1–3). (B) No tubb2b-dependent fluorescence could be detected in calretinin-positive cell bodies and fibers (CR; green) of the thalamus (Th). (C) tubb2b-dependent fluorescence-positive cell bodies in the area of the Th. CR-positive cells and fibers were not tubb2b-positive. (D) Cells in prosomere 3 (p3) did not show tubb2b-dependent fluorescence but could be stained with an antibody against Pax7 (green). (E) Approximate positions of the sliced regions are shown in a schematic image of the larval brain (lines). OB, olfactory bulb; Hyp, hypothalamus; POA, preoptic area; V, ventricle; d, dorsal; v, ventral; a, anterior; p, posterior; m, medial; l, lateral.
Diencephalon
In the diencephalon (Figure 5E), we found tubb2b-dependent fluorescence in the subventricular zone of prosomeres 1–3 (p1–3; Figures 2G, 5A–D). In p2, some cells were found in the Th (Figures 5B,C). Similar to the observations made in the telencephalon, tubb2b-dependent fluorescence was not found in all neurons of the diencephalon. For instance, at the level of the prethalamus and Th, we found CR-positive fibers that clearly did not show tubb2b-dependent fluorescence (Figures 5B,C; green). Additionally, no tubb2b expression was seen in CR-positive cell bodies of the Th (Figures 5B,C; green). Moreover, a group of Pax7-positive cells (green) in prosomere 3 (p3) were tubb2b negative (Figure 5D).
Discussion
tubb2b-Dependent Fluorescence Is Not Present in All Neurons of the Xenopus Forebrain
β-Tubulin isotypes can occur in different tissues and different subtypes of cells from the same tissue (Eddé et al., 1981; Moura Neto et al., 1983; Sullivan and Cleveland, 1986). In the African clawed frog (Xenopus laevis), class II β-tubulin protein is exclusively and widely expressed in the nervous system (Dworkin-Rastl et al., 1986; Oschwald et al., 1991; Bieker and Yazdani-Buicky, 1992) and found to be specially expressed in neurons (Moody et al., 1996). In this study, we monitored the activity of class II β-tubulin tubb2b promoter in the forebrain of premetamorphic Xenopus. We confirm that it is active in neurons in most main forebrain regions, but we unambiguously found that it is not active in all neurons of these regions. In the OB, tubb2b-dependent fluorescence is absent from TH- and CR-immunoreactive interneurons, and only located in the mitral cell layer and periglomerular layer. Cell counts of tubb2b-positive cells in comparison to postmitotic HuC/D-positive cells of the OB show that tubb2b is only active in 24.4% of all mature neurons in this region. In more posterior areas of the telencephalon, the expression of tubb2b is lacking in pax6-positive neurons of the CeA, as well as the Mp and Lp. Within the diencephalon, we did not find tubb2b-dependent fluorescence in CR-positive neurons and fibers of the Th. Pax7-positive neurons in prosomere 3 also lacked tubb2b-dependent fluorescence. The results of the present study show that class II β-tubulin promoter is not active in all forebrain neurons. According to our observations made in the transgenic lines we used, β-tubulin class II cannot be considered a pan-neuronal marker in Xenopus laevis.
Polyploidy is a common condition in frogs, and Xenopus laevis is one of the few allotetraploid species with four sets of chromosomes (Bisbee et al., 1977; Kobel and Du Pasquier, 1986; Evans et al., 2004; Session et al., 2016). Thus, we cannot fully exclude a mosaic expression of the genome-inserted transgenes in the Xenopus lines we investigated. Also, expression patterns of genes and proteins can vary during the development of the animal. In zebrafish (Danio rerio), the expression of another tubulin isotype, class I β-tubulin (zβ1), is restricted to the nervous system (Oehlmann et al., 2004). In larvae, zβ1 is expressed in defined peripheral and central nervous system zones that comprise early differentiating neurons. During development, zβ1 decreases, and in adults, its expression is limited to some proliferative brain zones (including the OB) and the olfactory epithelium (Oehlmann et al., 2004). Similarly, tubb2b-dependent fluorescence in Xenopus larvae is also present in brain regions with proliferative activity, such as the Acc, Lp, Mp, Dp, the ventral POA, and the ventral Hyp (D’Amico et al., 2011). In contrast to the zβ1 expression in zebrafish, in larval Xenopus, tubb2b-dependent fluorescence was also present in brain regions not described to have proliferative activity, for example, the DP and Th (D’Amico et al., 2011). This characteristic expression pattern suggests that in premetamorphic Xenopus, β-tubulin class II could play a role in neurogenesis and have other functions in mature neurons. Because in this study, we focus on premetamorphic Xenopus larvae, it must be considered that the tubb2b expression in other developmental tadpole stages and in the adult frog could be different. Not only the expression patterns within the same tissue change but also the activity within individual neurons might be different. It has been shown that the expression of β-tubulin isotypes in single mammalian cells can change during development (Guo et al., 2011). In larval Xenopus laevis, like in mice, class II β-tubulin is involved in axonal growth and elongation (Moody et al., 1996). In mice, β-tubulins I, II, and III are expressed in neurons of the adult brain (Guo et al., 2011). In cultured neurons of newborn mice, β-tubulin II is no longer expressed in some parts of the cell bodies after differentiation and starts being expressed in neurites, where it might contribute to the regulation of neurite outgrowth (Guo et al., 2011). In the present study, we found that in premetamorphic Xenopus laevis, tubb2b-dependent fluorescence is mainly located in cell bodies. We observed a very strong tubb2b-dependent fluorescence in axons of the ON and OpN. Additionally, we found fibers of the ON that project toward the midbrain and the contralateral brain hemisphere without terminating in the OB. All three structures have their axons cover a relatively long distance, and their cell bodies are located in peripheral organs (Cima and Grant, 1982; Burd, 1991; Pinelli et al., 2004). Because we did not find tubb2b-positive neurites of intracerebral neurons, we assume that the expression of tubb2b in fibers from the periphery could play a special role and might be important to enable efficient intracellular transport over long distances.
tubb2b-Dependent Fluorescence in Projections From the Olfactory Organs to the Forebrain
In vertebrates, the olfactory system has an exceptional regenerative ability (Graziadei and Dehan, 1973; Graziadei and Monti Graziadei, 1983; Steuer et al., 2014; Yu and Wu, 2017; Calvo-Ochoa et al., 2021). Within the olfactory epithelium, olfactory sensory neurons undergo a constant natural turnover (Graziadei and Monti Graziadei, 1983; Higgs and Burd, 2001; Brann and Firestein, 2014) and have the ability to regrow after a lesion (Schwob, 2002). In premetamorphic Xenopus laevis, 7 weeks after ON injury, newborn olfactory sensory neuron axons fully reinnervate the OB (Hawkins et al., 2017). It has been shown that newly formed glomerular clusters and second-order neurons respond to olfactory stimuli during functional calcium imaging (Hawkins et al., 2017). In mammals, class II β-tubulin is associated with axonal growth and elongation (Joshi and Cleveland, 1989). Thus, it might also be involved in the regeneration of ON axons. In Xenopus embryos, class II β-tubulin has already been found during the differentiation of pioneering neurons (Moody et al., 1996); moreover, our results show that tubb2b-dependent fluorescence is present in the vast majority of axonal projections from the olfactory organs to the OB in premetamorphic Xenopus.
Therefore, we observed that some of these axonal projections bypass the OB and terminate in higher brain regions. Most likely, these fibers belong to the EBOS, which has been described in many aquatic vertebrates (Demski and Northcutt, 1983; Schmidt et al., 1988; Von Bartheld and Meyer, 1988; Hofmann and Meyer, 1989; Pinelli et al., 2004). Studies conducted in goldfish (Carassius auratus) and salamander (Triturus alpestris and Salamandra salamandra) show that EBOS fibers target regions like the hypothalamus and are potentially involved in the perception of pheromones (Demski and Northcutt, 1983; Schmidt et al., 1988). In larval Xenopus laevis, it has been shown that a particular glomerulus, the so-called γ-glomerulus, is innervated by ipsilateral and contralateral axonal projections originating in the olfactory epithelium (Kludt et al., 2015). These fibers bypass the ipsilateral OB, cross the brain midline via the anterior commissure, and project toward the contralateral OB (Kludt et al., 2015). The γ-glomerulus is particular in the sense that it is not innervated by axons of odorant-sensitive receptor neurons, but instead by temperature-sensitive fibers (Kludt et al., 2015). In the present study, we also observed tubb2b-dependent fluorescence in fibers projecting through the anterior commissure. Consequently, some of the tubb2b-dependent fibers detected in the OB possibly belong to the previously described temperature-sensitive system.
tubb2b-Dependent Fluorescence in Projection Neurons of the Olfactory Bulb
A large number of cells in the mitral cell layer of the OB show tubb2b-dependent fluorescence. In the vertebrate OB, the mitral cell layer and the external plexiform layer are populated with cell bodies of projection neurons, the so-called mitral and tufted cells (Nagayama et al., 2014; Kosaka and Kosaka, 2016). The somata of the various interneurons of the OB, for example, juxtaglomerular cells and granule cells, are, respectively, located in the glomerular layer and the granule cell layer (Nagayama et al., 2014; Manzini et al., 2022). In larval Xenopus laevis, like in other amphibians (Hoffman, 1963), the mitral cell layer and the external plexiform layer are not clearly disjunct, and thus, an unambiguous assignment of the different bulbar neuron types to the various layers of the OB is not easy (Scalia et al., 1991; Nezlin and Schild, 2000). To overcome this limitation, in the present study, we employed TH and CR immunostainings in the OB of larval NBT-Katushka γ-cry-Venus Xenopus to get information about the cell type of cells featuring tubb2b-dependent fluorescence. In the OB of larval Xenopus, TH antibodies have been shown to stain dopaminergic interneurons in the glomerular and external plexiform layers (González et al., 1994; Boyd and Delaney, 2002), and antibodies against CR have been shown to stain a subset of mitral cells in the mitral cell layer and interneurons (granule cells) in the granule cell layer (Porteros et al., 1997; Castro et al., 2008; Morona and González, 2013). In the present study, we did not find a single TH-positive cell that also shows tubb2b-dependent fluorescence. This shows that cells exhibiting tubb2b-dependent fluorescence are not dopaminergic TH-immunoreactive interneurons. Similarly, we did not detect a single CR-positive cell featuring tubb2b-dependent fluorescence. This rules out that granule cells in larval Xenopus express tubb2b-dependent fluorescence.
Another study conducted in Xenopus identified that Pax6 is expressed in cells of the periglomerular and internal granule cell layers (Bandín et al., 2014). Within the periglomerular layer, Pax6 is present in a subset of dopaminergic interneurons that also express in the TH (Bandín et al., 2014). In this study, we also found that pax6 promoter-driven fluorescence occurs in cells throughout the glomerular layer, which suggests that these cells might also be dopaminergic neurons. More posteriorly, we found pax6-dependent fluorescence in the mitral cell layer of the OB and the accessory OB of larval Xenopus, but not in the granule cell layer. In the mitral cell layer, we also found tubb2b-dependent fluorescence and a subset of cells featuring both pax6- and tubb2b-dependent fluorescence. Due to the location of the tubb2b-positive cell bodies and the observation that TH- and CR-immunoreactive interneurons are tubb2b-negative, we assume tubb2b is exclusively expressed in projection neurons. Additionally, the presence of double-positive pax6 and tubb2b cells could be an indication that pax6 is located in a subset of projection neurons as well. If this is the case, it remains the question of what distinguishes the tubb2b from the pax6 projection neuron population and what role double-positive tubb2b and pax6 cells play in the OB.
tubb2b-Dependent Fluorescence in Higher Olfactory Brain Regions
In Xenopus laevis, very little is known about the terminal processing centers of the olfactory system. However, potential target regions of OB neurons, like the Acc and MeA, have been reported in adult Xenopus laevis (Marín et al., 1997a,b; Moreno and González, 2003; Moreno et al., 2005). Our study shows that cells expressing tubb2b are present in all potential target regions of OB neurons. In the ventral telencephalon, we found tubb2b-dependent fluorescence in cell bodies of the Acc, bed nucleus of the stria terminalis (BST), the MeA, and axons of the OpN. The Acc is a main component of the basal ganglia (Marín et al., 1997a,b). Retrograde tracing studies in adult Xenopus have shown that the Acc receives input from neurons of the OB, and thus, it might be involved in olfactory information processing (Marín et al., 1997a,b). The BST, which is part of the extended amygdala (Alheid et al., 1995; Moreno et al., 2012), and the MeA are also considered part of the olfactory cortex (Swanson and Petrovich, 1998; Moreno and González, 2003; Moreno et al., 2005). Both might have a similar function as the teleost habenula, which is involved in the control of fear responses (Agetsuma et al., 2010; Lee et al., 2010; Miyasaka et al., 2014). Additionally, we also found tubb2b-dependent fluorescence in the CeA, which is the main component of the amygdaloid complex for integration and control of cardiovascular, respiratory, and gastrointestinal functions (Jolkkonen and Pitkänen, 1998; Moreno and González, 2005). Posteriorly in the telencephalon, we have detected tubb2b-dependent fluorescence in cells of the pallium, including the LA, which is part of the Vp (Moreno and González, 2004). In teleost fish, the dorsal part of the pallium is homolog to the olfactory cortex of mammals (Wullimann and Mueller, 2004; Miyasaka et al., 2014), and it was shown that in adult Xenopus laevis, the pallium is a target of neurons originating from the OB (Moreno et al., 2005). Similar to the MeA, the LA is also a terminal processing center of the olfactory system (Moreno and González, 2004; Moreno et al., 2005). Due to the wiring pattern of the LA, it is discussed to be a homolog of the mammalian basolateral complex (Moreno et al., 2005).
In addition to higher olfactory processing centers, we found that other main forebrain regions also show tubb2b-dependent fluorescence. In the telencephalon, tubb2b-positive cells are located in the Str, DP, POA, and Hyp. The DP and posterior tuberculum, together with the Str, are considered a homolog of the mammalian nigrostriatal pathway and to regulate different motor functions of the frog (Hoke et al., 2007). The Hyp serves as a control unit for endocrine mechanisms and regulates general functions of the body and behavioral patterns (Bruce, 2009; Domínguez et al., 2013, 2014). In the diencephalon, we observed tubb2b-dependent fluorescence in cells of p1–p3. A small number of tubb2b-positive cells were visible in the Th of p2. Although the thalamus serves as a relay station for sensory information, it does not process olfactory input (Jones, 2007; Bandín et al., 2015). Although tubb2b-dependent fluorescence has been detected in several regions that might be involved in olfactory information processing, we observed that it is clearly not restricted to it. We also found expression in fibers of the OpN, cell bodies in the area of the BST, the S, the POA, and Hyp.
In the past years, the promoter of class II β-tubulin (tubb2b) has been used as a tool to create transgenic Xenopus lines, like the tubb2b-GCaMP6s Rno.elas-GFP (NXR; Cat# 0.0107; Horb et al., 2019), to drive the expression of the genetically encoded calcium indicator GCaMP6s in the nervous system (Offner et al., 2020). Measurements of neuronal activity have been already possible in the OB and the olfactory amygdala (Offner et al., 2020) and could be performed in all regions (cells) featuring tubb2b-dependent fluorescence shown in the present study. Not only the tubb2b GCaMP6s transgenic line is a great advantage for functional studies but also our custom-bred NBT-Katushka pax6-GFP line is a great tool to mark and characterize a broad field of brain regions in larval Xenopus.
Data Availability Statement
The raw data supporting the conclusions of this article will be made available by the authors, without undue reservation.
Ethics Statement
The animal study was reviewed and approved by the Institutional Care and Use Committee of the Justus-Liebig-University of Giessen.
Author Contributions
DD, TH, and IM conceptualized the study. DD contributed to investigation, formal analysis, visualization, and writing of the original draft. TO provided the NBT-Katushka pax6-GFP transgenic Xenopus laevis line. DD, TO, TH, and IM helped with writing – review and editing the manuscript. TH and IM assisted with funding acquisition and resources, and supervision of the work. All authors contributed to the article and approved the submitted version.
Funding
This work was supported by DFG (Grant 4113/4-1).
Conflict of Interest
The authors declare that the research was conducted in the absence of any commercial or financial relationships that could be construed as a potential conflict of interest.
Publisher’s Note
All claims expressed in this article are solely those of the authors and do not necessarily represent those of their affiliated organizations, or those of the publisher, the editors and the reviewers. Any product that may be evaluated in this article, or claim that may be made by its manufacturer, is not guaranteed or endorsed by the publisher.
Abbreviations
1–9, α, β, γ, δ projection fields of axons from the olfactory organ; Acc, nucleus accumbens; ac, anterior commissure; BST, bed nucleus of the stria terminalis; CeA, central amygdala; DP, dorsal pallidum; Dp, dorsal pallium; EBOS, extrabulbar olfactory system; Hyp, hypothalamus; LA, lateral amygdala; lfb, lateral forebrain bundle; Lp, lateral pallium; MeA, medial amygdala; MOE, main olfactory epithelium; Mp, medial pallium; OB, olfactory bulb; ON, olfactory nerve; OpN, optic nerve; p 1–3, prosomeres 1–3; POA, preoptic area; Str, striatum; Th, thalamus; V, ventricle; Vp, ventral pallium.
Footnotes
References
Agetsuma, M., Aizawa, H., Aoki, T., Nakayama, R., Takahoko, M., Goto, M., et al. (2010). The habenula is crucial for experience-dependent modification of fear responses in zebrafish. Nat. Neurosci. 13, 1354–1356. doi: 10.1038/nn.2654
Alheid, G., de Olmos, J. S., and Beltramino, C. A. (1995). “Amygdala and extended amygdala,” in The Rat Nervous System, ed. G. Paxinos (San Diego: Academic Press), 495–578.
Bandín, S., Morona, R., and González, A. (2015). Prepatterning and patterning of the thalamus along embryonic development of Xenopus laevis. Front. Neuroanat. 9:107. doi: 10.3389/fnana.2015.00107
Bandín, S., Morona, R., López, J. M., Moreno, N., and González, A. (2014). Immunohistochemical analysis of Pax6 and Pax7 expression in the CNS of adult Xenopus laevis. J. Chem. Neuroanat. 5, 24–41. doi: 10.1016/j.jchemneu.2014.03.006
Bandín, S., Morona, R., Moreno, N., and González, A. (2013). Regional expression of Pax7 in the brain of Xenopus laevis during embryonic and larval development. Front. Neuroanat. 7:48. doi: 10.3389/fnana.2013.00048
Bieker, J. J., and Yazdani-Buicky, M. (1992). The multiple β-tubulin genes of Xenopus: isolation and developmental expression of a germ-cell isotype β-tubulin gene. Differentiation 50, 15–23. doi: 10.1111/j.1432-0436.1992.tb00481.x
Bisbee, C. A., Baker, M. A., Wilson, A. C., Hadji-Azimi, I., and Fischberg, M. (1977). Albumin phylogeny for clawed frogs (Xenopus). Science 195, 785–787. doi: 10.1126/science.65013
Boyd, J. D., and Delaney, K. R. (2002). Tyrosine hydroxylase-immunoreactive interneurons in the olfactory bulb of the frogs Rana pipiens and Xenopus laevis. J. Comp. Neurol. 454, 42–57. doi: 10.1002/cne.10428
Brann, J. H., and Firestein, S. J. (2014). A lifetime of neurogenesis in the olfactory system. Front. Neurosci. 8:182. doi: 10.3389/fnins.2014.00182
Bruce, L. L. (2009). “Evolution of the hypothalamus in amniotes,” in Encyclopedia of Neuroscience, eds M. D. Binder, N. Hirokawa, and U. Windhorst (Berlin: Springer), 1363–1367. doi: 10.1007/978-3-540-29678-2
Burd, G. D. (1991). Development of the olfactory nerve in the African clawed frog, Xenopus laevis I. Normal Dev. 304, 123–134. doi: 10.1002/cne.903040109
Byrd, C. A., and Burd, G. D. (1991). Development of the olfactory bulb in the clawed frog, Xenopus laevis: a morphological and quantitative analysis. J. Comp. Neurol. 314, 79–90. doi: 10.1002/cne.903140108
Calvo-Ochoa, E., Byrd-Jacobs, C. A., and Fuss, S. H. (2021). Diving into the streams and waves of constitutive and regenerative olfactory neurogenesis: insights from zebrafish. Cell Tissue Res. 383, 227–253. doi: 10.1007/s00441-020-03334-2
Castro, A., Becerra, M., Anadón, R., and Manso, M. J. (2008). Distribution of calretinin during development of the olfactory system in the brown trout, Salmo trutta fario: comparison with other immunohistochemical markers. J. Chem. Neuroanat. 35, 306–316. doi: 10.1016/j.jchemneu.2008.03.005
Cima, C., and Grant, P. (1982). Development of the optic nerve in Xenopus laevis. I. Early development and organization. J. Embryol. Exp. Morphol. 72, 225–249.
D’Amico, L. A., Boujard, D., and Coumailleau, P. (2011). Proliferation, migration and differentiation in juvenile and adult Xenopus laevis brains. Brain Res. 1405, 31–48. doi: 10.1016/j.brainres.2011.06.032
Demski, L. S., and Northcutt, R. G. (1983). The terminal nerve: a new chemosensory system in vertebrates? Science 220, 435–437. doi: 10.1126/science.6836287
Domínguez, L., González, A., and Moreno, N. (2014). Characterization of the hypothalamus of Xenopus laevis during development. II. The basal regions. J. Comp. Neurol. 522, 1102–1131. doi: 10.1002/cne.23471
Domínguez, L., Morona, R., González, A., and Moreno, N. (2013). Characterization of the hypothalamus of Xenopus laevis during development. I. The alar regions. J. Comp. Neurol. 521, 725–759. doi: 10.1002/cne.23222
Dworkin-Rastl, E., Kelley, D. B., and Dworkin, M. B. (1986). Localization of specific mRNA sequences in Xenopus laevis embryos by in situ hybridization. J. Embryol. Exp. Morphol. 91, 153–168.
Eddé, B., Jeantet, C., and Gros, F. (1981). One β−tubulin subunit accumulates during neurite outgrowth in mouse neuroblastoma cells. Biomed. Biophys. Res. Commun. 103, 1035–1043. doi: 10.1016/0006-291x(81)90913-x
Evans, B. J., Kelley, D. B., Tinsley, R. C., Melnick, D. J., and Cannatella, D. C. (2004). A mitochondrial DNA phylogeny of African clawed frogs: phylogeography and implications for polyploid evolution. Mol. Phylogenet. Evol. 33, 197–213. doi: 10.1016/j.ympev.2004.04.018
Gaudin, A., and Gascuel, J. (2005). 3D atlas describing the ontogenic evolution of the primary olfactory projections in the olfactory bulb of Xenopus laevis. J. Comp. Neurol. 489, 403–424. doi: 10.1002/cne.20655
González, A., Marín, O., Tuinhof, R., and Smeets, W. J. A. J. (1994). Ontogeny of catecholamine systems in the central nervous system of anuran amphibians: an immunohistochemical study with antibodies against tyrosine hydroxylase and dopamine. J. Comp. Neurol. 346, 63–79. doi: 10.1002/cne.903460105
Graziadei, P. P. C., and Dehan, R. S. (1973). Neuronal regeneration in frog olfactory system. J. Cell Biol. 59, 525–530. doi: 10.1083/jcb.59.2.525
Graziadei, P. P. C., and Monti Graziadei, A. G. (1983). Regeneration in the olfactory system of vertebrates. Am. J. Otolaryngol. Neck Med. Surg. 4, 228–233. doi: 10.1016/S0196-0709(83)80063-5
Guo, J., Qiang, M., and Ludueña, R. F. (2011). The distribution of β-tubulin isotypes in cultured neurons from embryonic, newborn, and adult mouse brains. Brain Res. 1420, 8–18. doi: 10.1016/j.brainres.2011.08.066
Hartley, K. O., Hardcastle, Z., Friday, R. V., Amaya, E., and Papalopulu, N. (2001). Transgenic Xenopus embryos reveal that anterior neural development requires continued suppression of BMP signaling after gastrulation. Dev. Biol. 238, 168–184. doi: 10.1006/dbio.2001.0398
Hawkins, S. J., Weiss, L., Offner, T., Dittrich, K., Hassenklöver, T., and Manzini, I. (2017). Functional reintegration of sensory neurons and transitional dendritic reduction of mitral/tufted cells during injury-induced recovery of the larval Xenopus olfactory circuit. Front. Cell. Neurosci. 11:380. doi: 10.3389/fncel.2017.00380
Higgs, D. M., and Burd, G. D. (2001). Neuronal turnover in the Xenopus laevis olfactory epithelium during metamorphosis. J. Comp. Neurol. 433, 124–130. doi: 10.1002/cne.1130
Hoffman, H. H. (1963). The olfactory bulb, accessory olfactory bulb, and hemisphere of some anurans. J. Comp. Neurol. 120, 317–368. doi: 10.1002/cne.901200208
Hofmann, M. H., and Meyer, D. L. (1989). Central projections of the nervus terminalis in four species of amphibians. Brain. Behav. Evol. 34, 301–307. doi: 10.1159/000116515
Hoke, K. L., Ryan, M. J., and Wilczynski, W. (2007). Functional coupling between substantia nigra and basal ganglia homologues in amphibians. Behav. Neurosci. 121, 1393–1399. doi: 10.1037/0735-7044.121.6.1393
Horb, M., Wlizla, M., Abu-Daya, A., McNamara, S., Gajdasik, D., Igawa, T., et al. (2019). Xenopus resources: transgenic, inbred and mutant animals, training opportunities, and web-based support. Front. Physiol. 10:387. doi: 10.3389/fphys.2019.00387
Jolkkonen, E., and Pitkänen, A. (1998). Intrinsic connections of the rat amygdaloid complex: projections originating in the central nucleus. J. Comp. Neurol. 395, 53–72. doi: 10.1002/(SICI)1096-9861(19980525)395:1<53::AID-CNE5<3.0.CO;2-G
Joshi, H. C., and Cleveland, D. W. (1989). Differential utilization of β-tubulin isotypes in differentiating neurites. J. Cell Biol. 109, 663–673. doi: 10.1083/jcb.109.2.663
Kapitein, L. C., and Hoogenraad, C. C. (2015). Building the neuronal microtubule cytoskeleton. Neuron 87, 492–506. doi: 10.1016/j.neuron.2015.05.046
Katsetos, C. D., Frankfurter, A., Christakos, S., Mancall, E. L., Vlachos, I. N., and Urich, H. (1993). Differential localization of class III, beta-tubulin isotype and calbindin-D28k defines distinct neuronal types in the developing human cerebellar cortex. J. Neuropathol. Exp. Neurol. 52, 655–666. doi: 10.1097/00005072-199311000-00013
Katsetos, C. D., Herman, M. M., and Mörk, S. J. (2003). Class III β-tubulin in human development and cancer. Cell Motil. Cytoskelet. 55, 77–96. doi: 10.1002/cm.10116
Kludt, E., Okom, C., Brinkmann, A., and Schild, D. (2015). Integrating temperature with odor processing in the olfactory bulb. J. Neurosci. 35, 7892–7902. doi: 10.1523/JNEUROSCI.0571-15.2015
Knossow, M., Campanacci, V., Khodja, L. A., and Gigant, B. (2020). The mechanism of tubulin assembly into microtubules: insights from structural studies. iScience 23:101511. doi: 10.1016/j.isci.2020.101511
Kobel, H. R., and Du Pasquier, L. (1986). Genetics of polyploid Xenopus. Trends Genet. 2, 310–315. doi: 10.1016/0168-9525(86)90286-6
Kosaka, T., and Kosaka, K. (2016). Neuronal organization of the main olfactory bulb revisited. Anat. Sci. Int. 91, 115–127. doi: 10.1007/s12565-015-0309-7
Lasser, M., Tiber, J., and Lowery, L. A. (2018). The role of the microtubule cytoskeleton in neurodevelopmental disorders. Front. Cell. Neurosci. 12:165. doi: 10.3389/fncel.2018.00165
Latremoliere, A., Cheng, L., DeLisle, M., Wu, C., Chew, S., Hutchinson, E. B., et al. (2018). Neuronal-specific TUBB3 is not required for normal neuronal function but is essential for timely axon regeneration. Cell Rep. 24, 1865–1879. doi: 10.1016/j.cel.rep.2018.07.029
Lee, A., Mathuru, A. S., Teh, C., Kibat, C., Korzh, V., Penney, T. B., et al. (2010). The habenula prevents helpless behavior in larval zebrafish. Curr. Biol. 20, 2211–2216. doi: 10.1016/j.cub.2010.11.025
Lee, M. K., Rebhun, L. I., and Frankfurter, A. (1990a). Posttranslational modification of class III β-tubulin. Proc. Natl. Acad. Sci. U.S.A. 87, 7195–7199. doi: 10.1073/pnas.87.18.7195
Lee, M. K., Tuttle, J. B., Rebhun, L. I., Cleveland, D. W., and Frankfurter, A. (1990b). The expression and posttranslational modification of a neuron-specific β-tubulin isotype during chick embryogenesis. Cell Motil. Cytoskelet. 17, 118–132. doi: 10.1002/cm.970170207
Lewis, S. A., Gu, W., and Cowan, N. J. (1987). Free intermingling of mammalian β-tubulin isotypes among functionally distinct microtubules. Cell 49, 539–548. doi: 10.1016/0092-8674(87)90456-9
Love, N. R., Thuret, R., Chen, Y., Ishibashi, S., Sabherwal, N., Paredes, R., et al. (2011). pTransgenesis: a cross-species, modular transgenesis resource. Development 138, 5451–5458. doi: 10.1242/dev.066498
Ludueña, R. F. (1998). Multiple forms of tubulin: different gene products and covalent modifications. Int. Rev. Cytol. 178, 207–275. doi: 10.1016/s0074-7696(08)62138-5
Ludueña, R. F., and Banerjee, A. (2008b). “The tubulin superfamily,” in Cancer Drug Discovery And Development: The Role Of Microtubules In Cell Biology, Neurobiology, And Oncology, ed. T. Fojo (Totowa: Humana Press), 177–191. doi: 10.1002/3527603808.ch2
Ludueña, R. F., and Banerjee, A. (2008a). “The isotypes of tubulin,” in Cancer Drug Discovery And Development: The Role Of Microtubules In Cell Biology, Neurobiology, And Oncology, ed. T. Fojo (Totowa: Humana Press), 123–175. doi: 10.1007/978-1-59745-336-3_6
Ludueña, R. F., Shooter, E. M., and Wilson, L. (1977). Structure of the tubulin dimer. J. Biol. Chem. 252, 7006–7014.
Manzini, I., and Schild, D. (2010). “Olfactory coding in larvae of the African clawed frog Xenopus laevis,” in The Neurobiology of Olfaction, ed. A. Menini (Boca Raton (FL): Taylor & Francis).
Manzini, I., Schild, D., and Di Natale, C. (2022). Principles of odor coding in vertebrates and artificial chemosensory systems. Physiol. Rev. 102, 61–154. doi: 10.1152/PHYSREV.00036.2020
Marín, O., Smeets, W. J. A. J., and González, A. (1997b). Basal ganglia organization in amphibians: catecholaminergic innervation of the striatum and the nucleus accumbens. J. Comp. Neurol. 378, 50–69. doi: 10.1002/(sici)1096-9861(19970203)378:1<50::aid-cne3<3.0.co;2-j
Marín, O., González, A., and Smeets, W. J. A. J. (1997a). Basal ganglia organization in amphibians: afferent connections to the striatum and the nucleus accumbens. J. Comp. Neurol. 378, 16–49. doi: 10.1002/(sici)1096-9861(19970203)378:1<16::aid-cne2<3.0.co;2-n
Miyasaka, N., Arganda-Carreras, I., Wakisaka, N., Masuda, M., Sümbül, U., Seung, H. S., et al. (2014). Olfactory projectome in the zebrafish forebrain revealed by genetic single-neuron labelling. Nat. Commun. 5:3639. doi: 10.1038/ncomms4639
Moody, S. A. (1989). Quantitative lineage analysis of the origin of frog primary motor and sensory neurons from cleavage stage blastomeres. J. Neurosci. 9, 2919–2930. doi: 10.1523/jneurosci.09-08-02919.1989
Moody, S. A., Miller, V., Spanos, A., and Frankfurter, A. (1996). Developmental expression of a neuron-specific β-tubulin in frog (Xenopus laevis): a marker for growing axons during the embryonic period. J. Comp. Neurol. 364, 219–230. doi: 10.1002/(SICI)1096-9861(19960108)364:2<219::AID-CNE3<3.0.CO;2-8
Moreno, N., and González, A. (2003). Hodological characterization of the medial amygdala in anuran amphibians. J. Comp. Neurol. 466, 389–408. doi: 10.1002/cne.10887
Moreno, N., and González, A. (2004). Localization and connectivity of the lateral amygdala in anuran amphibians. J. Comp. Neurol. 479, 130–148. doi: 10.1002/cne.20298
Moreno, N., and González, A. (2005). Central amygdala in anuran amphibians: neurochemical organization and connectivity. J. Comp. Neurol. 489, 69–91. doi: 10.1002/cne.20611
Moreno, N., Morona, R., López, J. M., Domínguez, L., Joven, A., Bandín, S., et al. (2012). Characterization of the bed nucleus of the stria terminalis in the forebrain of anuran amphibians. J. Comp. Neurol. 520, 330–363. doi: 10.1002/cne.22694
Moreno, N., Morona, R., López, J. M., Muñoz, M., and González, A. (2005). Lateral and medial amygdala of anuran amphibians and their relation to olfactory and vomeronasal information. Brain Res. Bull. 66, 332–336. doi: 10.1016/j.brainresbull.2005.05.017
Moreno, N., Rétaux, S., and González, A. (2008). Spatio-temporal expression of Pax6 in Xenopus forebrain. Brain Res. 1239, 92–99. doi: 10.1016/j.brainres.2008.08.052
Morona, R., and González, A. (2013). Pattern of calbindin-D28k and calretinin immunoreactivity in the brain of Xenopus laevis during embryonic and larval development. J. Comp. Neurol. 521, 79–108. doi: 10.1002/cne.23163
Moura Neto, V., Mallat, M., Jeantet, C., and Prochiantz, A. (1983). Microheterogeneity of tubulin proteins in neuronal and glial cells from the mouse brain in culture. EMBO J. 2, 1243–1248. doi: 10.1002/j.1460-2075.1983.tb01576.x
Muroyama, A., and Lechler, T. (2017). Microtubule organization, dynamics and functions in differentiated cells. Development 144, 3012–3021. doi: 10.1242/dev.153171
Nagayama, S., Homma, R., and Imamura, F. (2014). Neuronal organization of olfactory bulb circuits. Front. Neural Circuits 8:89. doi: 10.3389/fncir.2014.00098
Nezlin, L. P., and Schild, D. (2000). Structure of the olfactory bulb in tadpoles of Xenopus laevis. Cell Tissue Res. 302, 21–29. doi: 10.1007/s004410000208
Nieuwkoop, P. D., and Faber, J. (1994). Normal table of Xenopus laevis (Daudin): a systematical and chronological survey of the development from the fertilized egg till the end of metamorphosis. New York, NY: Garland Science.
Nogales, E. (2000). Structural insights into microtubule function. Annu. Rev. Biochem. 69, 277–302. doi: 10.1146/annurev.biochem.69.1.277
Oehlmann, V. D., Berger, S., Sterner, C., and Korsching, S. I. (2004). Zebrafish beta tubulin 1 expression is limited to the nervous system throughout development, and in the adult brain is restricted to a subset of proliferative regions. Gene Expr. Patterns 4, 191–198. doi: 10.1016/j.modgep.2003.09.001
Offner, T., Daume, D., Weiss, L., Hassenklöver, T., and Manzini, I. (2020). Whole-brain calcium imaging in larval Xenopus. Cold Spring Harb. Protoc. 2020:pdb.err107425. doi: 10.1101/pdb.prot106815
Oschwald, R., Richter, K., and Grunz, H. (1991). Localization of a nervous system-specific class II β-tubulin gene in Xenopus laevis embryos by whole-mount in situ hybridization. Int. J. Dev. Biol. 35, 399–405.
Pinelli, C., D’Aniello, B., Polese, G., and Rastogi, R. K. (2004). Extrabulbar olfactory system and nervus terminalis FMRFamide immunoreactive components in Xenopus laevis ontogenesis. J. Chem. Neuroanat. 28, 37–46. doi: 10.1016/j.jchemneu.2004.06.001
Porteros, A., Arévalo, R., Weruaga, E., Crespo, C., Briñón, J. G., Alonso, J. R., et al. (1997). Calretinin immunoreactivity in the developing olfactory system of the rainbow trout. Dev. Brain Res. 100, 101–109. doi: 10.1016/S0165-3806(97)00037-0
Preibisch, S., Saalfeld, S., and Tomancak, P. (2009). Globally optimal stitching of tiled 3D microscopic image acquisitions. Bioinformatics 25, 1463–1465. doi: 10.1093/bioinformatics/btp184
Puelles, L., Kuwana, E., Puelles, E., Bulfone, A., Shimamura, K., Keleher, J., et al. (2000). Pallial and subpallial derivatives in the embryonic chick and mouse telencephalon, traced by the expression of the genes Dlx-2, Emx-1, Nkx-2.1, Pax-6, and Tbr-1. J. Comp. Neurol. 424, 409–438. doi: 10.1002/1096-9861(20000828)424:3<409::AID-CNE3<3.0.CO;2-7
Richter, K., Grunz, H., and Dawid, I. B. (1988). Gene expression in the embryonic nervous system of Xenopus laevis. Proc. Natl. Acad. Sci. U.S.A. 85, 8086–8090. doi: 10.1073/pnas.85.21.8086
Scalia, F., Gallousis, G., and Roca, S. (1991). A note on the organization of the amphibian olfactory bulb. J. Comp. Neurol. 305, 435–442. doi: 10.1002/cne.903050307
Schindelin, J., Arganda-Carrera, I., Frise, E., Verena, K., Mark, L., Tobias, P., et al. (2012). Fiji: an open-source platform for biological-image analysis. Nat. Methods 9, 676–682. doi: 10.1038/nmeth.2019.Fiji
Schmidt, A., Naujoks-Manteuffel, C., and Roth, G. (1988). Olfactory and vomeronasal projections and the pathway of nervus terminalis in ten species of salamanders. Cell Tissue Res. 251, 45–50.
Schwob, J. E. (2002). Neural regeneration and the peripheral olfactory system. Anat. Rec. 269, 33–49. doi: 10.1002/ar.10047
Session, A. M., Uno, Y., Kwon, T., Chapman, J. A., Toyoda, A., Takahashi, S., et al. (2016). Genome evolution in the allotetraploid frog Xenopus laevis. Nature 538, 336–343. doi: 10.1038/nature19840
Steuer, E., Schaefer, M. L., and Belluscio, L. (2014). Using the olfactory system as an in vivo model to study traumatic brain injury and repair. J. Neurotrauma 31, 1277–1291. doi: 10.1089/neu.2013.3296
Stoykova, A., and Gruss, P. (1994). Roles of Pax-genes in developing and adult brain as suggested by expression patterns. J. Neurosci. 14, 1395–1412. doi: 10.1523/jneurosci.14-03-01395.1994
Sullivan, K. F. (1988). Structure and utilization of tubulin isotypes. Ann. Rev. Cell Biol. 4, 687–716. doi: 10.1146/annurev.cb.04.110188.003351
Sullivan, K. F., and Cleveland, D. W. (1986). Identification of conserved isotype-defining variable region sequences for four vertebrate β tubulin polypeptide classes. Proc. Natl. Acad. Sci. U.S.A. 83, 4327–4331. doi: 10.1073/pnas.83.12.4327
Swanson, L., and Petrovich, G. (1998). What is the amygdala? Trends Neurosci. 21, 323–331. doi: 10.1016/s0166-2236(98)01265-x
Von Bartheld, C. S., and Meyer, D. L. (1988). Central projections of the nervus terminalis in lampreys, lungfishes, and bichirs. Brain. Behav. Evol. 32, 151–159. doi: 10.1159/000116542
Wade, R. H. (2009). On and around microtubules: an overview. Mol. Biotechnol. 43, 177–191. doi: 10.1007/s12033-009-9193-5
Weiss, L., Jungblut, L. D., Pozzi, A. G., O’Connell, L. A., Hassenklöver, T., and Manzini, I. (2020). Conservation of glomerular organization in the main olfactory bulb of anuran larvae. Front. Neuroanat. 14:44. doi: 10.3389/fnana.2020.00044
Weiss, L., Manzini, I., and Hassenklöver, T. (2021). Olfaction across the water–air interface in anuran amphibians. Cell Tissue Res. 383, 301–325. doi: 10.1007/s00441-020-03377-5
Wullimann, M. F., and Mueller, T. (2004). Teleostean and mammalian forebrains contrasted: evidence from genes to behavior. J. Comp. Neurol. 475, 143–162. doi: 10.1002/cne.20183
Keywords: neuronal β-tubulin, class II β-tubulin, NBT, olfactory bulb, higher olfactory centers
Citation: Daume D, Offner T, Hassenklöver T and Manzini I (2022) Patterns of tubb2b Promoter-Driven Fluorescence in the Forebrain of Larval Xenopus laevis. Front. Neuroanat. 16:914281. doi: 10.3389/fnana.2022.914281
Received: 06 April 2022; Accepted: 20 June 2022;
Published: 08 July 2022.
Edited by:
Luis Puelles, University of Murcia, SpainReviewed by:
Ruth Morona, Complutense University of Madrid, SpainMarc Spehr, RWTH Aachen University, Germany
Florence Kermen, University of Copenhagen, Denmark
Darcy Brisbane Kelley, Columbia University, United States
Marissa Fabrezi, CONICET Instituto de Bio y Geociencias del NOA (IBIGEO), Argentina
Copyright © 2022 Daume, Offner, Hassenklöver and Manzini. This is an open-access article distributed under the terms of the Creative Commons Attribution License (CC BY). The use, distribution or reproduction in other forums is permitted, provided the original author(s) and the copyright owner(s) are credited and that the original publication in this journal is cited, in accordance with accepted academic practice. No use, distribution or reproduction is permitted which does not comply with these terms.
*Correspondence: Daniela Daume, ZGFuaWVsYS5kYXVtZUBwaHlzem9vbC5iaW8udW5pLWdpZXNzZW4uZGU=; Ivan Manzini, aXZhbi5tYW56aW5pQHBoeXN6b29sLmJpby51bmktZ2llc3Nlbi5kZQ==
†ORCID: Daniela Daume, orcid.org/0000-0002-5912-5663; Thomas Offner, orcid.org/0000-0002-0228-2545; Thomas Hassenklöver, orcid.org/0000-0002-9895-1263; Ivan Manzini, orcid.org/0000-0002-3575-9637
‡Present address: Thomas Offner, Max Planck Institute for Biological Intelligence, Research Group Olfactory Memory, Martinsried, Germany