- 1Retina Group, Instituto Universitario de Oftalmobiología Aplicada (IOBA), Universidad de Valladolid, Valladolid, Spain
- 2Postgraduate Unit, Faculty of Biological Sciences, National University of San Marcos, Lima, Peru
- 3Group for Advanced Materials and Nanobiotechnology (GIR BIOFORGE), CIBER-BBN, Edificio LUCIA, Universidad de Valladolid, Valladolid, Spain
- 4Centro en Red de Medicina Regenerativa y Terapia Celular de Castilla y León, Valladolid, Spain
- 5Molecular Medicine Unit, Department of Medicine, University of Salamanca, Salamanca, Spain
- 6Institute of Biomedical Research of Salamanca (IBSAL), Salamanca, Spain
- 7Institute of Molecular and Cellular Biology of Cancer (IBMCC), University of Salamanca-CSIC, Salamanca, Spain
- 8Red Temática de Investigación Cooperativa en Salud (RETICS), Oftared, Instituto de Salud Carlos III, Valladolid, Spain
- 9RetiBrain (RED2018-102499-T), Ministerio de Ciencia, Innovación y Universidades, Valladolid, Spain
Retinal neurodegenerative diseases are the leading causes of visual impairment and irreversible blindness worldwide. Although the retinal response to injury remains closely similar between different retinal neurodegenerative diseases, available therapeutic alternatives are only palliative, too expensive, or very specific, such as gene therapy. In that sense, the development of broad-spectrum neuroprotective therapies seems to be an excellent option. In this regard, it is essential to identify molecular targets involved in retinal degeneration, such as cell death mechanisms. Apoptosis has been considered as the primary cell death mechanism during retinal degeneration; however, recent studies have demonstrated that the only use of anti-apoptotic drugs is not enough to confer good neuroprotection in terms of cell viability and preservation. For that reason, the interrelationship that exists between apoptosis and other cell death mechanisms needs to be characterized deeply to design future therapeutic options that simultaneously block the main cell death pathways. In that sense, the study aimed to characterize the programmed cell death (in terms of apoptosis and necroptosis) and autophagy response and modulation in retinal neurodegenerative diseases, using an in vitro model of spontaneous retinal neurodegeneration. For that purpose, we measured the mRNA relative expression through qPCR of a selected pool of genes involved in apoptosis (BAX, BCL2, CASP3, CASP8, and CASP9), necroptosis (MLKL, RIPK1, and RIPK3), and autophagy (ATG7, BCLIN1, LC3B, mTOR, and SQSTM1); besides, the immunoexpression of their encoding proteins (Casp3, MLKL, RIPK1, LC3B, and p62) were analyzed using immunohistochemistry. Our results showed an increase of pro-apoptotic and pro-necroptotic related genes and proteins during in vitro retinal neurodegeneration. Besides, we describe for the first time the modulation between programmed cell death mechanisms and autophagy in an in vitro retinal neurodegeneration model. This study reinforces the idea that cell death mechanisms are closely interconnected and provides new information about molecular signaling and autophagy along the retinal degeneration process.
Introduction
Retinal neurodegenerative diseases (RND) are the leading causes of irreversible blindness worldwide. The most representative RND is Age-related Macular Degeneration (AMD), which affects up to 8.7% of the global population over 65 years-old being the third cause of visual impairment globally (Wong et al., 2014; Bourne et al., 2021; Steinmetz et al., 2021). RND causes a severe and irreversible impact on the quality of life of the patients and an enormous financial global burden (Bourne et al., 2021; Steinmetz et al., 2021). At present, there is no curative treatment available for RND apart from costly gene therapy for very selective mutations (Russell et al., 2017; Cehajic-Kapetanovic et al., 2020). However, despite the etiology, retinal response to injury in terms of cellular signalization pathways remains closely similar for most RND (Cuenca et al., 2014). In that sense, looking for common therapeutic molecular targets through the characterization of the different cell death mechanisms could be an excellent therapeutic option for many of these diseases.
Retinal response to injury includes promoting inflammatory response, activation of the antioxidant machinery, and programmed cell death mechanisms (Cuenca et al., 2014). Cell death events during retinal degeneration have been associated predominantly with the activation of apoptosis as the primary programmed cell death mechanism (Barber et al., 1998; Choudhury et al., 2013; Comitato et al., 2014; Radi et al., 2014). However, recent studies have highlighted the importance of other programmed cell death mechanisms, such as necroptosis. Besides, the modulation of autophagy, an essential physiological catabolic mechanism, has also been associated with retinal neurodegeneration (Murakami et al., 2013; Newton and Megaw, 2020). The importance and predominance of these mechanisms vary according to the RND. For instance, novel studies have highlighted the importance of necroptosis during the pathophysiology of the Retinitis Pigmentosa (RP) (Arango-Gonzalez et al., 2014; Viringipurampeer et al., 2019) and autophagy in AMD (Mitter et al., 2012; Kaarniranta et al., 2017). The importance of non-apoptotic cell death mechanisms and autophagy have been confirmed in vivo, in which molecular targeting studies have failed to prevent cell death by blocking only the apoptotic pathways (Hisatomi et al., 2001; Zacks et al., 2003; Trichonas et al., 2010). This background suggests a complex interrelationship between these mechanisms during retinal degeneration. However, the implications of these interactions remain unclear (Newton and Megaw, 2020).
In vitro retinal explant cultures are helpful tools for studying neuroretinal degeneration processes because they replicate, with in vitro limitations, the cellular and molecular changes that occur in retina degeneration (Li et al., 2018; Murali et al., 2019; Fernandez-Bueno and Usategui-Martin, 2021). Although this model does not mimic a specific retinal disease, it resembles neurodegeneration, a joint event in most RND (Cuenca et al., 2014).
Describing the programmed cell death mechanisms and other cell processes involved in retinal neurodegeneration is essential to identify critical molecular targets to develop unspecific neuroprotective therapies for RND. In this scenario, this study aimed to characterize the programmed cell death and autophagy mechanisms in neuroretina using an in vitro spontaneous neuroretinal degeneration model.
Materials and Methods
In vitro Model of Spontaneous Neuroretinal Degeneration
Organ neuroretinal explants cultures were used as a spontaneous neuroretinal degeneration model described by Fernandez-Bueno and Usategui-Martin (2021). As a summary, we used fresh eyes extracted from pigs 6 to 8 months derived from the local slaughterhouse. Two neuroretinal explants (5 × 5 mm) derived from the area centralis (cone-enriched visual streak without blood vessels) were extracted from each eye (Supplementary Figure 1) and placed over the porous membrane of a Transwell plate (24 mm diameter, 0.4 mm pore; Corning Life Sciences, Corning, NY, United States) with the photoreceptor layer facing the membrane.
Cultures were maintained in 1.5 ml of a medium composed by Neurobasal A supplemented with 10% fetal bovine serum (FBS), 1% antibiotic-antifungal mixture, 2% B-27, and 1% L-glutamine (Gibco, Invitrogen, Paisley, United Kingdom) under standard conditions (37°C, 5% CO2 atmosphere) for 1, 3, 6, and 9 days. In parallel, neuroretinal explants were extracted and processed directly as negative control (fresh neuroretina). Experimental conditions were determined as fresh neuroretina, 1, 3, 6, and 9 days of neuroretinal degeneration. We ran experiments in triplicate; three retinal explants were used for each experimental condition, and thus, we used a total number of 15 retinal explants.
RNA Extraction, Reverse Transcription, and Real-Time Quantitative PCR
Neuroretinas of all experimental conditions were submerged in RNA stabilizing solution (RNAlater, Invitrogen, CA, United States) and stored at −80°C until processing. RNA extraction was performed using Trizol reagent (Invitrogen, CA, United States) following the manufacturer’s instructions. The purity and quantity of the RNA were determined using a spectrophotometer (NanoDrop 2000, Thermo Fisher, MA, United States).
According to the manufacturer manual, the synthesis of the complementary DNA (cDNA) was performed through reverse transcription of the extracted mRNA using the High-Capacity cDNA Reverse Transcription Kit (Applied Biosystems, CA, United States). The relative quantitative real-time polymerase chain reaction (qPCR) was performed using the SYBR Green PCR master mix (Applied Biosystems, CA, United States) in the Applied Biosystems 7500 Real-Time PCR System (Applied Biosystems, CA, United States). Specific porcine primers were used to analyze the relative mRNA expression for apoptosis-related genes (Pseudokinase mixed lineage kinase domain-like, MLKL; receptor-interacting serine/threonine-protein kinase 1 and 3, RIPK1 and RIPK3) necroptosis-related genes (BCL2 associated X, BAX; B-cell lymphoma 2, BCL2; cleaved caspase 3, 8 and 9, CASP3, CASP8, and CASP9) and autophagy-related genes (mammalian Target of Rapamycin, mTOR; moesin-like BCL2 interacting protein, BCLIN1; microtubule-associated protein 1A/1B-light chain 3, LC3B; autophagy-related 7, ATG7; sequestosome1, SQSTM1) (Table 1). The following cycle conditions were applied: 95°C for 10 mins, 40 cycles of 95°C for 15 s, 60°C for 1 min, and including a final melting curve step. GAPDH was used as a housekeeping gene to normalize the expression level of mRNA. Finally, we determined the threshold cycle for each reaction, and gene expression was quantified using the 2–ΔΔCt method (Livak and Schmittgen, 2001). We performed all the qPCR assays in triplicate for each experimental condition.
Immunohistochemical Characterization of Reactive Gliosis, Programmed Cell Death, and Autophagy
Neuroretinal explants were fixed with 4% paraformaldehyde (Panreac Quimica, Barcelona, Spain) for 24 h at 4°C. Then, they were subjected to a sucrose concentration gradient starting from 15, 20, and 30% of sucrose diluted in phosphatase-buffered saline (PBS) for 2 h at 4°C each step. Finally, explants were embedded in the Tissue-Tek OCT compound (Sakura Finetek Europe, Alphen, Netherlands) and cut into 5 μm cryosections using a cryostat (CM1900, Leica Microsystems Wetzlar, Germany).
Cryosections were washed in PBS and blocked [4% goat serum (Sigma-Aldrich) and 0.3% of Triton X-100 (Sigma-Aldrich) in PBS] for 2 h at room temperature (RT). Then, primary antibodies were incubated as described in Table 2. Molecular markers related to reactive gliosis [Glial fibrillary acidic protein (GFAP)], apoptosis [cleaved caspase-3, (Casp3)], necroptosis [Mixed lineage kinase domain-like protein (MLKL), Receptor-interacting serine/threonine-protein kinase 1 (RIPK1)] and autophagy [Nucleoporine P62 (p62), Microtubule-associated protein 1A/1B-light chain 3 (LC3B)] were evaluated. Afterward, species-specific secondary antibodies anti-rabbit IgG Alexa Fluor™ 568 goat (Thermo Fisher, MA, United States) and anti-mouse IgG Alexa Fluor™ 488 goat (Thermo Fisher, MA, United States) were incubated for 2 h at RT. Then, immunostaining with DAPI (4′,6-diamidino-2-fenilindol) was performed to visualize the nuclei. Subsequently, the samples were mounted using a fluorescent mounting medium (Dako, Glostrup, Denmark) and a coverslipped. All the immunohistochemical analyzes were performed in triplicate for each experimental condition, and control slides in which primary antibodies were omitted were processed in parallel.
TdT-Mediated dUTP Nick-End Labeling Immunohistochemical Analysis and Quantification
The TdT-mediated dUTP nick-end labeling (TUNEL) kit for detecting apoptosis at the single-cell level is based on labeling the DNA strand breaks. It was performed using a direct immunofluorescence protocol described in the manufacturer instructions. Cryosections were washed in PBS and blocked [4% goat serum (Sigma-Aldrich), 0.3% of Triton X-100 (Sigma-Aldrich) in PBS] for 2 h at RT. Then, TUNEL was incubated under the conditions detailed in Table 2. Afterward, DAPI immunostaining was used to visualize nuclei. We performed TUNEL analysis in triplicate for each experimental condition and control slides in which primary antibodies were omitted processed in parallel.
To quantify TUNEL immunoexpression, immunofluorescence micrographs (40× images; n = 12 sections per sample) were acquired at the same exposure, intensity, and gain levels. Afterward, each nuclear layer manually counted the TUNEL-stained nuclei using the software ImageJ (1.49 version, National Institutes of Health, Bethesda, MD, United States). Finally, the TUNEL labeled nuclei were correlated with the total DAPI-stained nuclei to obtain quantifiable results expressed as arbitrary units (AU).
Immunofluorescence Micrographs Imaging
Immunofluorescence micrographs were captured using a LEICA TCS SP8 LIGHTNING confocal microscope (Leica Microsystems, Wetzlar, Germany) and analyzed with LEICA LAS AF software. Finally, processing and designing of figures were performed using the Pixelmator 3.8.2 Phoenix (Pixelmator Team, Vilnius, Lithuania).
Statistical Analysis
Descriptive statistical analysis presented continuous variables in terms of mean and standard deviation (SD). Sample normality was evaluated using the Kolmogorov-Smirnov test. The parametric variables were analyzed using a t-test in the case of two groups and analysis of variance (ANOVA) for more than two. Meanwhile, non-parametric variables were analyzed using the Mann-Whitney U test for two groups and the Kruskal-Wallis test in the case of more than two groups. A confidence interval of 95% (p < 0.05) was considered to conclude significant differences between variables. Finally, the statistical analysis was performed using the SPSS version 22.0 statistical package (SPSS, Chicago, IL, United States).
Results
Reactive Gliosis
As a sign of retinal neurodegeneration (Cuenca et al., 2014), reactive gliosis was assessed using GFAP immunoexpression, upregulated in Müller cells during retinal degeneration (Barber et al., 1998). GFAP immunoexpression in fresh neuroretina was mainly restricted to the inner neuroretina; then, increasing cultivation time reached the outer retina, being detectable in all neuroretinal layers from day 6. Besides, nuclei organization and distribution became more heterogeneous from days 1 to 9, retinal layers were hard to differentiate since day 6 (Figure 1).
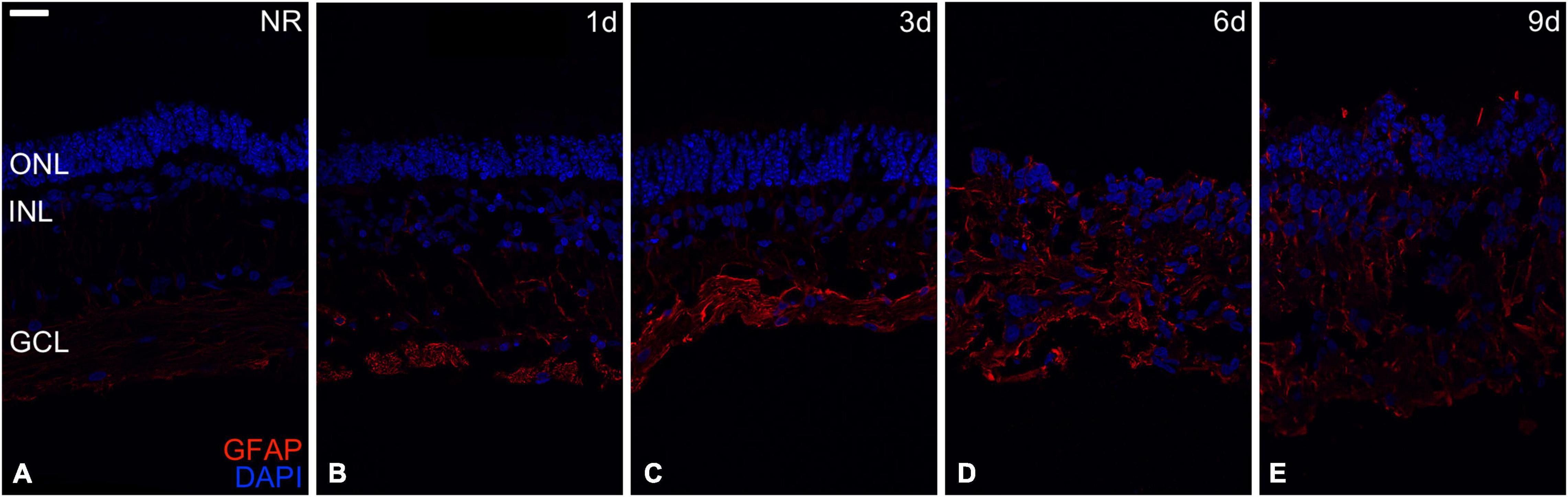
Figure 1. Reactive gliosis evaluation. Reactive gliosis analysis in the Glial fibrillary acidic protein (GFAP) immunoexpression during days 0, 1, 3, 6, and 9 of neuroretinal degeneration (A–E). GFAP immunoexpression for Fresh neuroretina (A), day 1 (B), day 3 (C), day 6 (D), and day 9 of neuroretinal degeneration (E). Scale bar: 25 μm.
Apoptosis Analysis
The relative mRNA expression of the apoptosis-related genes BAX, BCL2, CASP3, CASP8, and CASP9 was analyzed to study the events associated with the apoptosis process during spontaneous retinal degeneration. In addition, the immunoexpression of the Casp3 protein and quantification of the TUNEL apoptosis detection kit was performed.
Relative mRNA expression of the BAX, CASP3, CASP8, and CASP9 genes was significantly higher in all the experimental conditions than fresh neuroretina. BAX, CASP3, and CASP9 mRNA relative expression remained at the same level from fresh to day 1 and increased significantly from day 1 to 3. From day 3 to 6, the relative RNA expression of CASP3 increased significantly, and CASP8 maintained the same expression. From day 6 to 9, CASP 8 decreased significantly, and CASP3 remained the same. In the case of CASP9 mRNA relative expression, there were no significant differences after day 1. Besides, the relative mRNA expression of BCL2 was significantly increased in fresh and day 1 compared to all the other experimental conditions; then, it remained the same after day 3 (Figure 2).
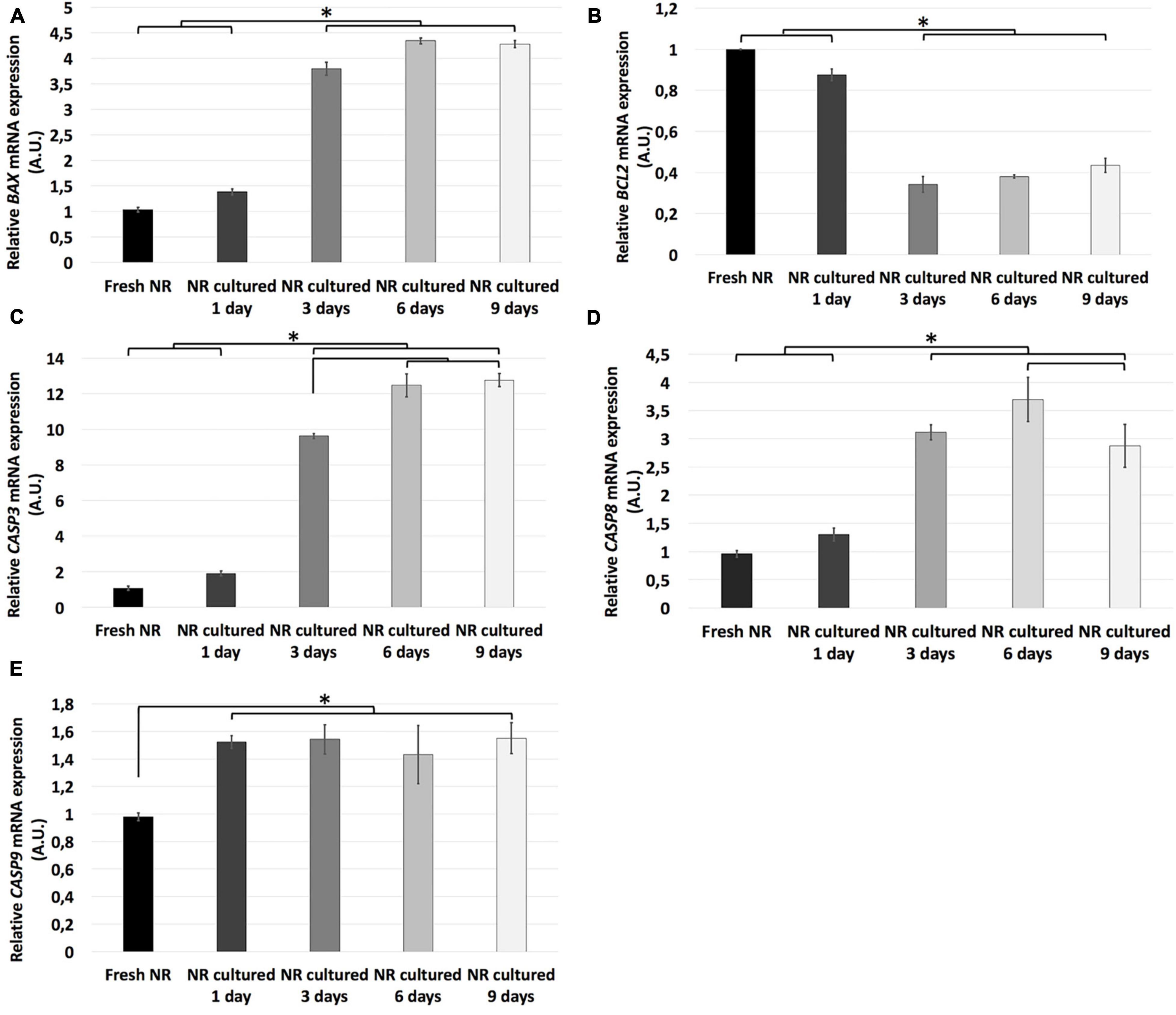
Figure 2. Relative mRNA expression of apoptosis-related genes during neuroretinal degeneration. Relative mRNA expression of the apoptosis-related genes: BAX (A), BCL2 (B), CASP3 (C), CASP8 (D), and CASP9 (E) during days 0, 1, 3, 6, and 9 of neuroretinal degeneration. *p < 0.05, AU, arbitrary units.
Casp3 is the primary execution apoptotic protein common for all the apoptosis pathways (Cuenca et al., 2014); meanwhile, the TUNEL detection kit labels DNA strand breaks in cells that undergo apoptosis. Both were mostly restricted to the GCL on day 1, reached the INL on day 3, and the ONL on days 6 and 9. However, TUNEL seemed to be mostly restricted to ONL at day 9; meanwhile, Casp 3 were distributed through the whole neuroretina (Figures 3A–J). The TUNEL quantification showed that the apoptosis rate in the total retina and all nuclear retinal layers, in an independent way (GCL, INL, and ONL), increased progressively from fresh to day 6. From day 6 to 9, the apoptosis rate in the total retina, GCL, and ONL remained the same and raised in the case of INL. The apoptosis peak in the whole retina, GCL, and ONL were at day 6; meanwhile, in the INL, the apoptosis rate peak was at day 9 (Figures 3K–N).
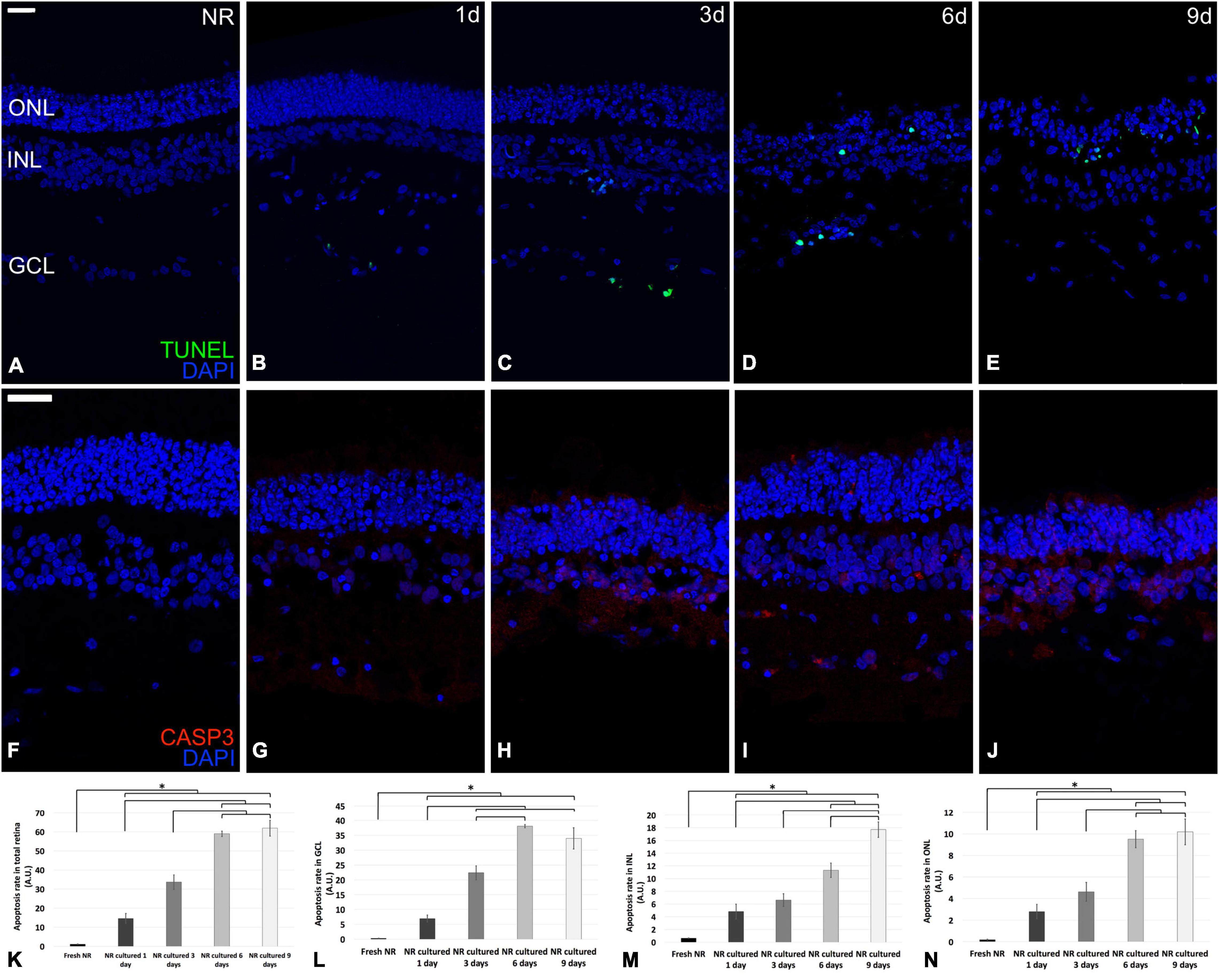
Figure 3. Immunohistochemistry analysis and quantification of apoptosis-related molecular markers during neuroretinal degeneration. Immunoexpression of the apoptosis-related protein cleaved caspase-3 and the molecular marker TUNEL during days 0, 1, 3, 6, and 9 of neuroretinal degeneration and quantification of the TUNEL labeled nuclei for each retinal nuclear layer. TUNEL immunoexpression for Fresh neuroretina (A), day 1 (B), day 3 (C), day 6 (D), and day 9 of neuroretinal degeneration (E). Casp3 immunoexpression for Fresh neuroretina (F), day 1 (G), day 3 (H), day 6 (I), and day 9 of neuroretinal degeneration (J). Quantification of TUNEL labeled nuclei for total retina (K), GCL (L), INL (M), and ONL (N). *p < 0.05, AU, arbitrary units; GCL, ganglion cell layer; INL, inner nuclear layer; ONL, outer nuclear layer. Scale bar: 25 μm.
Necroptosis Analysis
We assessed the necroptotic events by analyzing the relative mRNA expression of the MLKL, RIPK1, and RIPK3 genes and the immunoexpression of the proteins MLKL and RIPK1.
The results showed that relative mRNA expression of necroptosis-related genes studied (MLKL, RIPK1, and RIPK3) remained the same from fresh to day 1 and significantly increased from day 1 to 3. In the case of MLKL and RIPK1 genes, its relative mRNA expression significantly increased from day 3 to 6, and both decreased from day 6 to 9. Meanwhile, the relative mRNA expression of RIPK3 remained the same since days 3 to 9 (Figure 4).
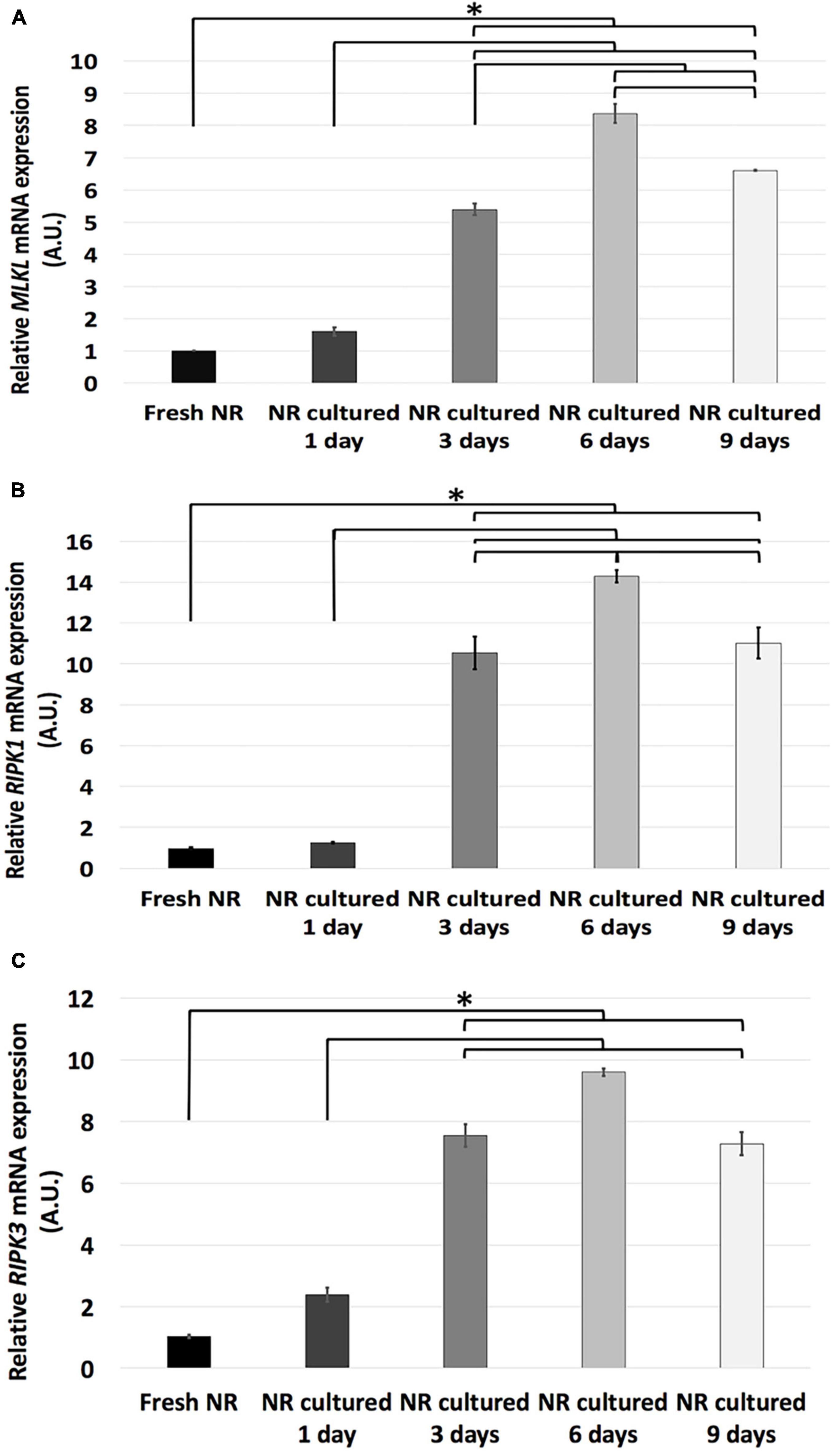
Figure 4. Relative mRNA expression of necroptosis-related genes during neuroretinal degeneration. Relative mRNA expression of the necroptosis-related genes: MLKL (A), RIPK1 (B), RIPK3 (C) during days 0, 1, 3, 6, and 9 of neuroretinal degeneration. *p < 0.05, AU, arbitrary units.
The protein RIPK1 induces phosphorylation of the MLKL, which produces the formation of cell membrane pores leading to cell death (Vandenabeele et al., 2010). The immunoexpression of both proteins was not detectable in fresh neuroretinas. The immunoexpression distribution of the MLKL protein begins at the CGL and then proliferates to the most external parts, reaching the whole neuroretina at day. Meanwhile, the immunoexpression of the RIPK1 protein started its proliferation from the photoreceptors’ outer segments heading toward the most internal parts, reaching the whole neuroretina at day 3 (Figure 5).
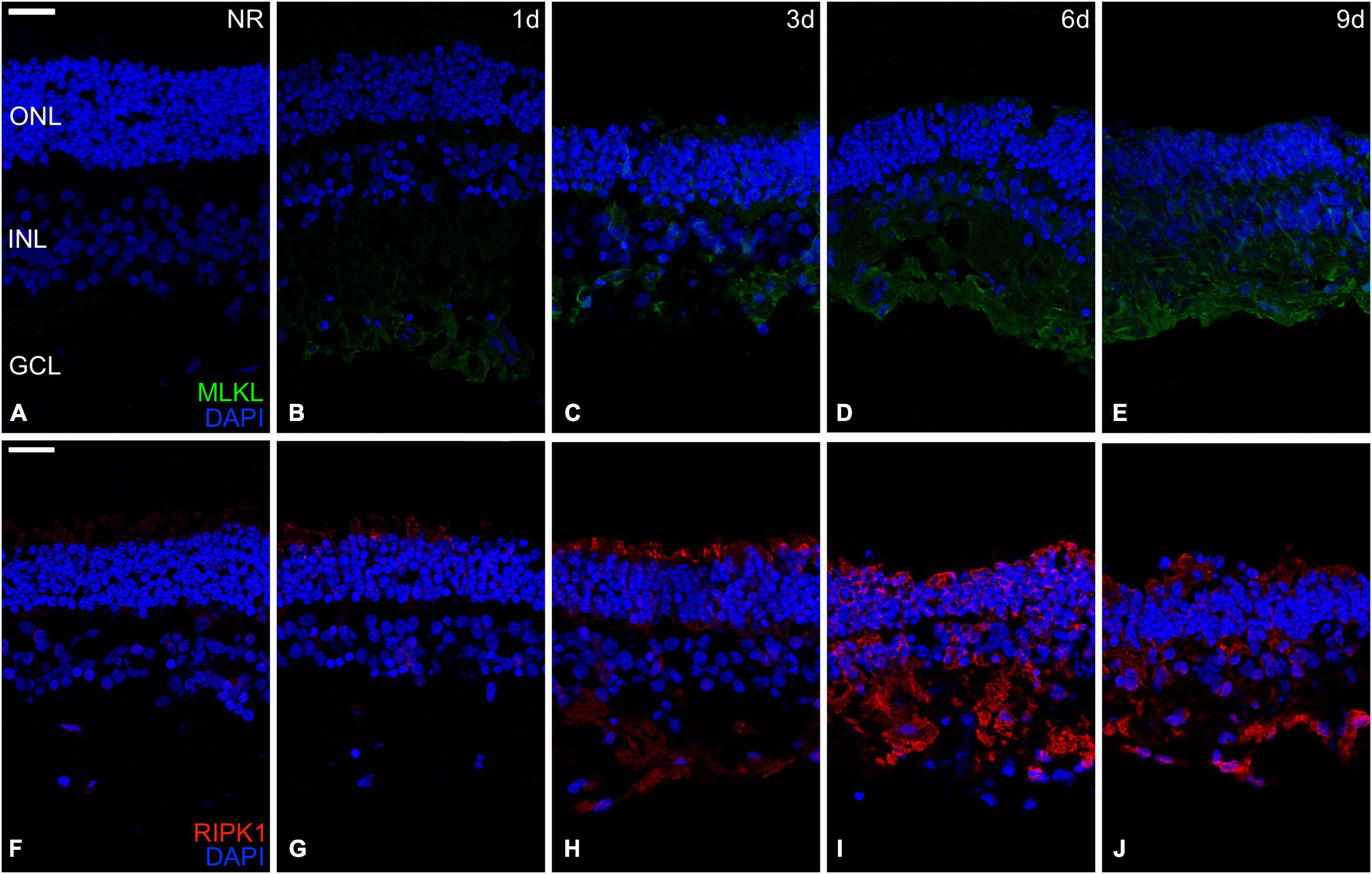
Figure 5. Immunohistochemistry analysis of necroptosis-related proteins during neuroretinal degeneration. Immunoexpression of the apoptosis-related proteins Mixed lineage kinase domain-like protein (MLKL) and Receptor-interacting serine/threonine-protein kinase 1 (RIPK1) during days 0, 1, 3, 6, and 9 of neuroretinal degeneration. MLKL immunoexpression for Fresh neuroretina (A), day 1 (B), day 3 (C), day 6 (D), and day 9 of neuroretinal degeneration (E). RIPK1 immunoexpression for Fresh neuroretina (F), day 1 (G), day 3 (H), day 6 (I), and day 9 of neuroretinal degeneration (J). Scale bar: 25 μm.
Autophagy Analysis
Regarding autophagy, the relative mRNA expression of ATG7, BCLIN1, LC3B, mTOR, and SQSTM1 genes and the immunoexpression of the LC3B and p62 proteins were analyzed.
Relative mRNA expression of mTOR, LC3B, and SQSTM1 genes was significantly higher in fresh neuroretinas than in any other experimental conditions, where there were no significant differences between mTOR and SQSTM1 genes. In the case of LC3B mRNA relative expression, it increased progressively from day 1 to 6 and remained stable from day 6 to 9. In contrast, the relative mRNA expression of ATG7 and BCLIN1 genes significantly increase from fresh to day 1, where it reaches its expression peak. The mRNA relative expression of BCLIN1 remained the same from day 1 to 3 and progressively decreased from day 3 to 9. Finally, ATG7 reduced gradually from day 1 to 9 (Figure 6).
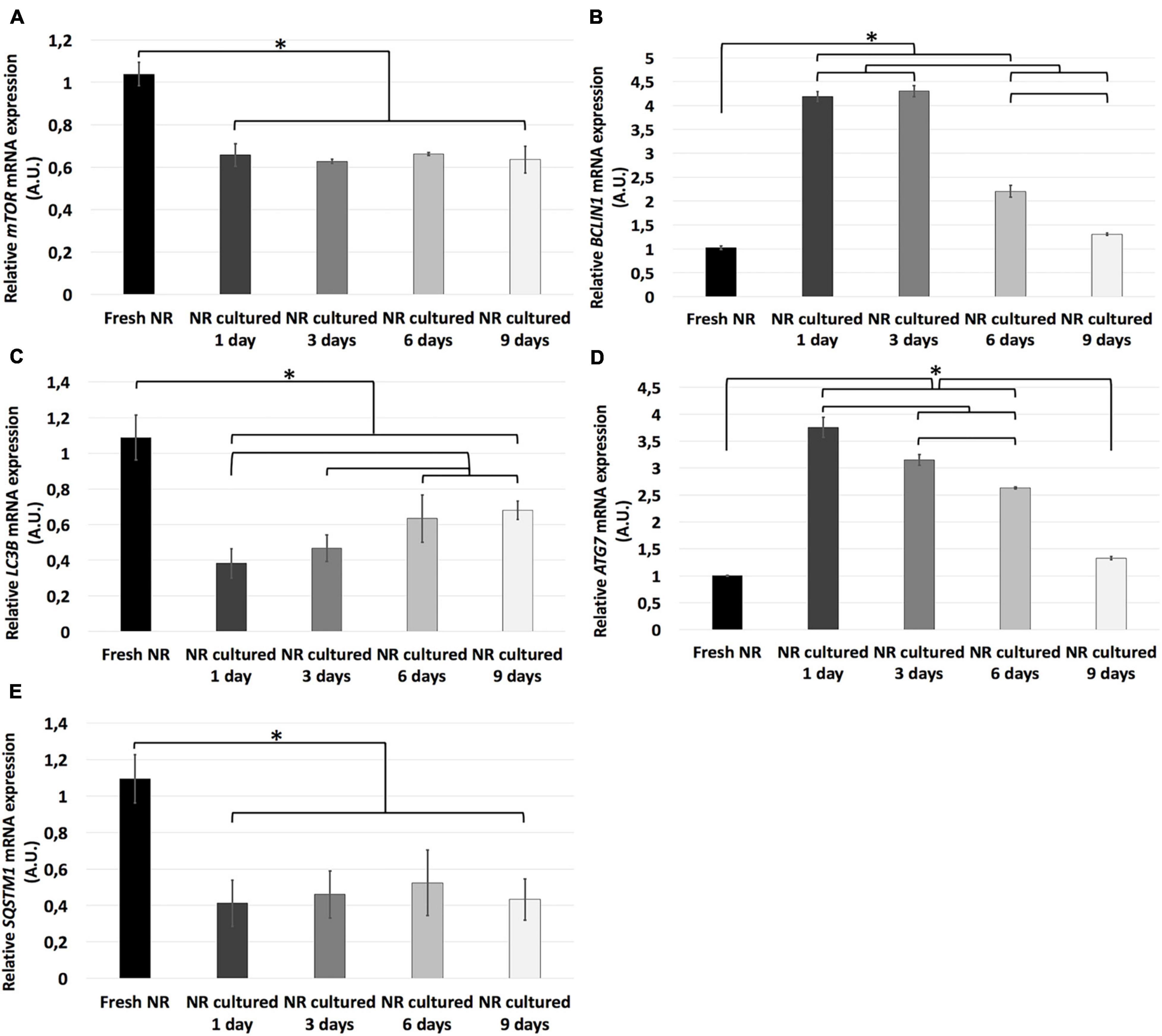
Figure 6. Relative mRNA expression of autophagy-related genes during neuroretinal degeneration. Relative mRNA expression of the autophagy-related genes: LC3B (A), BCLIN1 (B), LC3B (C), ATG7 (D), and SQSTM1 (E) during days 0, 1, 3, 6, and 9 of neuroretinal degeneration. *p < 0.05, AU, arbitrary units.
The proteins LC3B and p62 are essential for autophagosome formation, which delivers cell components to lysosomal degradation (Levine and Kroemer, 2008). The immunoexpression of both proteins was present in fresh days 3, 6, and 9, being almost undetectable at day 1 (Figure 7).
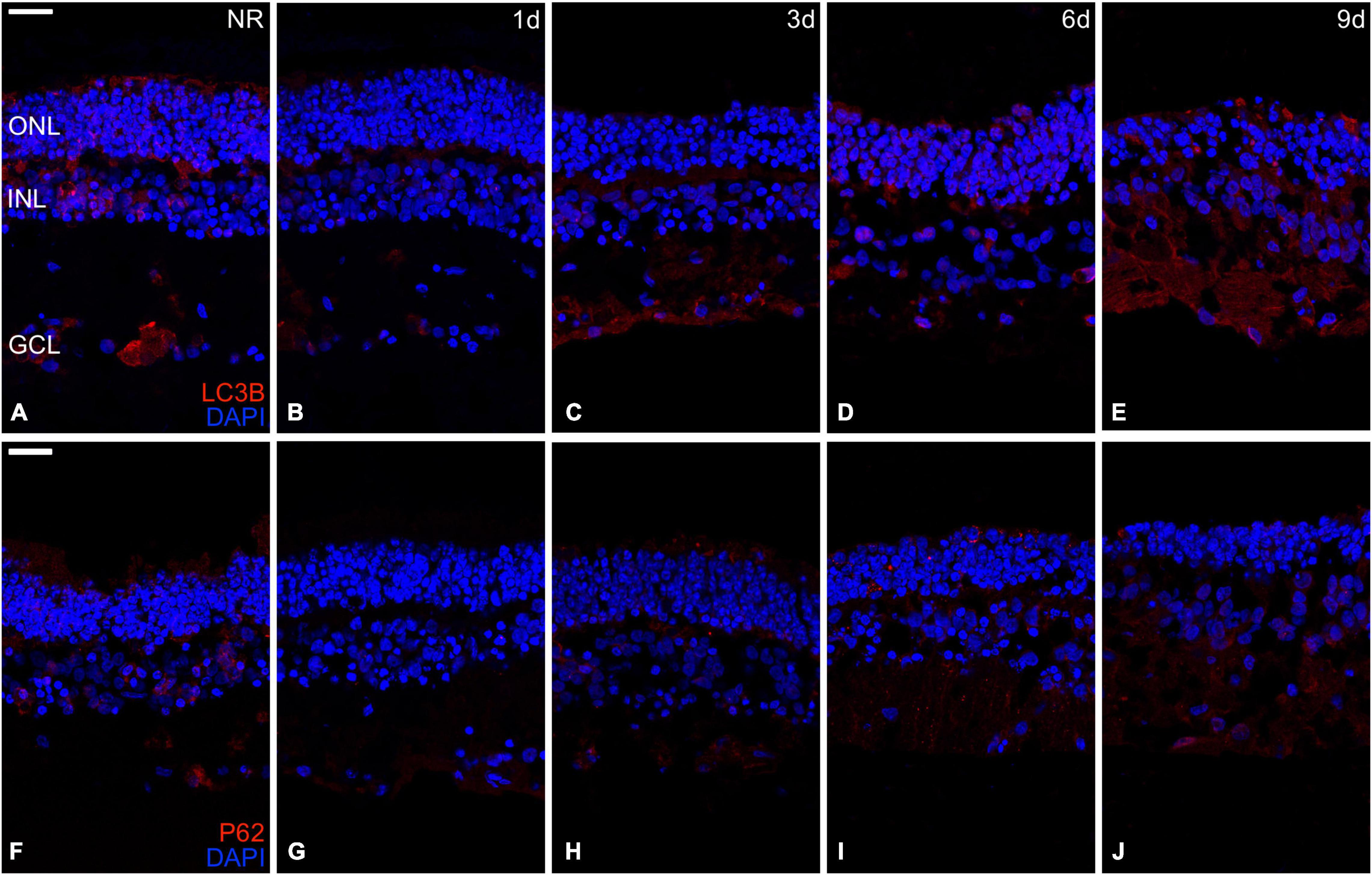
Figure 7. Immunohistochemistry analysis of autophagy-related proteins during neuroretinal degeneration. Immunoexpression of the autophagy-related proteins Microtubule-associated protein 1A/1B-light chain 3 (LC3B) and Nucleoporine P62 (p62) during days 0, 1, 3, 6, and 9 of neuroretinal degeneration. LC3B immunoexpression for Fresh neuroretina (A), day 1 (B), day 3 (C), day 6 (D), and day 9 of neuroretinal degeneration (E). P62 immunoexpression for Fresh neuroretina (F), day 1 (G), day 3 (H), day 6 (I), and day 9 of neuroretinal degeneration (J). Scale bar: 25 μm.
Discussion
As mentioned, RND is the leading cause of irreversible blindness worldwide, and current therapeutic options are only palliative or too specific and expensive. But this group of diseases, independently of its etiology, have closely related pathophysiology at the cellular and molecular level (Cuenca et al., 2014). In that sense, unspecific neuroprotective therapies to stop or slow down their progressions, such as targeting pathophysiological molecular mechanisms related to cell death and autophagy, seem to be an attractive therapeutic alternative. Hence, it is necessary to characterize as profoundly as possible the molecular pathways that converge during retinal degeneration to target the most relevant molecules during the development of therapeutic options. For the first time, our study describes and provides a comprehensive evaluation of the central programmed cell death and autophagy mechanisms during in vitro neuroretinal degeneration. The in vitro study presented here is the first step for future in vivo studies that focuses on the molecular pathways analyzed here for subsequent translation to clinical practice.
However, this study incurs certain limitations. Firstly, our in vitro retinal neurodegeneration model lacks an RPE layer, vascularization, and the RGC were axotomized. Secondly, spontaneous neuroretinal degeneration does not represent a retinal disease from a clinical point of view. However, even with these limitations, this work contributes significantly to understanding the crucial role of programmed cell death and autophagy during retinal neurodegeneration.
The retinal spontaneous degeneration model used for this study is an excellent alternative to study the molecular and cellular changes that occur during retinal degenerative diseases since they retain the complex neuroretinal architecture, interactions of neuroretinal cells, and mimic the in situ responses under controlled conditions (Li et al., 2018; Murali et al., 2019; Fernandez-Bueno and Usategui-Martin, 2021). However, it presents the drawback of lacking both retinal and choroidal vascularization, the axotomy of retinal ganglion cells, and the absence of retinal pigmented epithelium, for that reason, this can only resemble the degeneration of the neuroretina, a joint event in most retinal neurodegenerative diseases.
One of the most representative structural changes during retinal degeneration is reactive gliosis, which means the proliferation of glial cells to neuroprotect retinal neurons, which can be detrimental if the cause is not eliminated (Cuenca et al., 2014). The upregulation of GFAP in Müller cells has been previously described in animal models of diabetic retinopathy (Barber et al., 1998), RP (Fernandez-Sanchez et al., 2010), and glaucoma (Fernandez-Sanchez et al., 2013), and in vitro by our research group (Fernandez-Bueno et al., 2008, 2012, 2013; Di Lauro et al., 2016; Labrador-Velandia et al., 2019; Usategui-Martín et al., 2020) by using the same organotypic neuroretina model, our results presented here to add more evidence to this premise.
The primary programmed cell death mechanism during retinal neurodegeneration is apoptosis. However, recent studies have proved and highlighted the interrelationship between apoptosis, other cell death mechanisms, and autophagy (Newton and Megaw, 2020). Apoptosis is a programmed cell death mechanism that removes potentially dangerous cells without damaging the surrounding tissues. It can be mediated through the caspase-dependent or -independent pathways (Doonan et al., 2005; Kroemer and Martin, 2005; Murakami et al., 2013). This study evaluated apoptosis-related genes implicated in the caspase-dependent pathway. They play primary roles in the extrinsic (Casp8), intrinsic (BAX, BCL2, and Casp9), and execution (Casp3) pathways (Cuenca et al., 2014). Additionally, the balance between pro-apoptotic (Bax) and anti-apoptotic (BCL2) genes was evaluated (Singh et al., 2019). Our results showed that until day 1 of retinal spontaneous in vitro neurodegeneration, the relative mRNA expression of the anti-apoptotic BCL2 gene was predominant; however, since day 3, the anti-apoptotic mechanisms are inhibited both extrinsic and intrinsic caspase-dependent pathways are activated. Then, apoptosis-related genes increased progressively until day 9, even when most of the retinal architecture had been lost. At the protein level, we corroborated this analysis with the immunoexpression curve of TUNEL and Casp3, which progressed simultaneously to the activation of the apoptosis-related genes. Besides, TUNEL quantification showed that apoptosis seems to be proportionally activated in all retinal nuclear layers.
Apoptosis and necrosis are two primary cell death mechanisms (Kroemer et al., 2009). Necrosis has been considered an unregulated form of cell death; however, recent evidence suggests that it can be induced by regulated signal transduction pathways (Festjens et al., 2006; Golstein and Kroemer, 2007). Regulated necrosis includes several cell-death modalities, although necroptosis is the best characterized. Necroptosis is a programmed cell death mechanism that implies disruption of the cell membrane, causing liberation of damage-associated molecular patterns that induce inflammation (Pasparakis and Vandenabeele, 2015). Necroptosis is mainly regulated by the three genes evaluated in this study (RIPK1, RIPK3, and MLKL). The interaction between RIPK1 and RIPK3 activates the phosphorylation and oligomerization of the MLKL that induce the formation of membrane pores, resulting in the loss of membrane integrity, causing cell death (Degterev et al., 2005; Vandenabeele et al., 2010; Dondelinger et al., 2014). Our results showed that necroptosis-related proteins immunoexpression could be detected since day 1; however, there has been a substantial increase in relative mRNA expression and protein distribution of necroptosis since day 3.
The pro-apoptotic and pro-necroptotic proteins’ upregulation during neuroretinal degeneration is correlated with the alteration in nuclei organization and distribution observed from days 1 to 9. Besides, a reduced number of nuclei and an increment of TUNEL labeled nuclei during neuroretinal degeneration is apparently, suggesting that the up-regulation of these proteins could be associated with retinal cells loss; however, to confirm this, it would be more accurate to count manually the number of nuclei per μm2 in every nuclear layer individually on hematoxylin-eosin stained neuroretinal non-serial sections, as in our previous work (Usategui-Martín et al., 2020).
The interrelationship between necroptosis and apoptosis has been described in vivo in a retinal detachment model. It exhibited overexpression of necroptosis as a compensatory mechanism when apoptosis is inhibited. Besides, it has been demonstrated that necroptosis is an effective cell death mechanism involving rod and cone degeneration during RP pathogenesis using in vivo animal models (Murakami et al., 2012; Sato et al., 2013; Yang et al., 2018; Viringipurampeer et al., 2019). Our results showed that the activation of necroptosis occurs parallel to apoptosis in vitro after the anti-apoptotic mechanisms were suppressed, having a substantial increase by day 3 and continue growing progressively until day 9. This simultaneous activation of apoptosis and necroptosis in vitro reinforces the hypothesized interrelationship between them during retinal neurodegeneration.
Autophagy is a lysosome-mediated pathway that degenerates and recycles cells’ defective proteins and organelles. It is essential for survival, differentiation, development, and homeostasis (Levine and Kroemer, 2008; Newton and Megaw, 2020). For that reason, it occurs at low basal levels in virtually any cell; however, it could be rapidly upregulated when the cell needs to generate intracellular energy to undergo structural remodeling, induced by oxidative stress or protein aggregate accumulation, events that are crucial in retinal degeneration (Klionsky, 2007; Maiuri et al., 2007; Mizushima and Klionsky, 2007; Rubinsztein et al., 2007; Cuenca et al., 2014). Autophagy requires the formation of a double-membrane vesicle where the target is encapsulated, called the autophagosome, and its subsequent fusion with the lysosomes degrades the target (Levine and Kroemer, 2008). The genes selected for this study is associated with crucial phases of autophagy, including the isolation of the future membrane of the autophagosome (BCLN1), elongation and formation of the membrane (LC3B and SQSTM1), and ATG7 by the activation of LC3B; besides, we evaluated the primary inhibitory signal that shuts off autophagy in the presence of growth factors or nutrients (mTOR) (Klionsky, 2007; Mizushima and Klionsky, 2007; Liu et al., 2013). Our results revealed how autophagy processes are modulated during in vitro neuroretinal degeneration, supporting the hypothesis that autophagy may play an essential role in the RND pathophysiology.
P62/SQTM1 plays a fundamental role in activating and organizing autophagy (Wang et al., 2014). Furthermore, P62 and LC3B, both components of the autophagosome membrane, are decreased through autophagy-mediated degradation during the process (Viiri et al., 2013). Our results are highly correlated with this premise. Both P62 and LC3B proteins can be detected through immunostaining in fresh neuroretinas, but not at day 1, then both recover their immunoreactivity since day 3. Besides, the anti-autophagic signal mediated by the mTOR gene is higher in fresh neuroretinas and decreased since day 1. These results suggest that, unlike apoptosis and necroptosis, the inhibition of anti-autophagy factors may start since day 1 of spontaneous in vitro neuroretinal degeneration. That autophagy could reach its maximum expression by day 1, before apoptosis and necroptosis (by day 3). These findings correlate with the previous idea that autophagy leads to apoptosis when severe cellular injury (Mukhopadhyay et al., 2001). Besides, the role of autophagy during in vitro RND has been tested in vivo, specifically in models of RP (Rodríguez-Muela et al., 2015; Yao et al., 2018; Li et al., 2019; Qiu et al., 2019) and AMD (Mitter et al., 2012; Kaarniranta et al., 2017). In that sense, autophagy plays a leading role in the early stages of in vitro neuroretinal degeneration.
The mechanism described in this study may cause changes in retinal cells’ morphology and connectivity, leading to retinal remodeling, which is a joint event in many RND (Cuenca et al., 2014). The up-regulation of apoptosis has been associated with atrophic and neovascular forms of AMD (Ramírez et al., 2001; Dunaief et al., 2002; Bhutto and Lutty, 2012), RP (Comitato et al., 2014), and diabetic retinopathy (Martin et al., 2004). Besides, over-expression of necroptosis has been implicated in models of RP (Murakami et al., 2012; Sato et al., 2013; Yang et al., 2017; Viringipurampeer et al., 2019), retinal detachment (Dong and Sun, 2011), retinal ischemia-reperfusion injury (Rosenbaum et al., 2010), and achromatopsia (Viringipurampeer et al., 2014). On the other hand, the curve modulation of autophagy described by our results may be consistent with the association of the down-regulation of the mTOR pathway and retinal cell death through secondary activation of apoptosis, suggested in RP models (Newton and Megaw, 2020).
Our results support that non-apoptotic cell death mechanisms are essential during neuroretinal degeneration. Besides, the time course modulation of the three mechanisms described could help target a specific cell death mechanism depending on the disease progression. Moreover, this study provides new information about the modulation of autophagy during retinal neurodegeneration; it has been demonstrated that autophagy has significant implications during retinal at the therapeutic level using drugs such as rapamycin, an autophagy agonist (Rubinsztein et al., 2007) or Rasagiline, anti-apoptotic and autophagy modulating medication (Eigeldinger-Berthou et al., 2012).
In conclusion, this study provides new information about molecular signaling of cell death mechanisms along the neuroretinal degeneration process and reinforces the idea that cell death mechanisms could be interconnected. This information is relevant to the future development of an integral neuroprotective therapy that thoroughly covers the cell death process during neurodegenerative retinal diseases. Finally, we consider that effective neuroprotective treatment must simultaneously prevent the activation of main cell death mechanisms to be effective, as observed in this study.
Data Availability Statement
The raw data supporting the conclusions of this article will be made available by the authors, without undue reservation.
Author Contributions
RU-M and IF-B designed the study. IF-B coordinated the study. RU-M, KP-N, NG-C, LH-R, FG-P, and IF-B generated research data. KP-N, RU-M, JP JR-C, RG-S, and IF-B analyzed and discussed the results. KP-N and IF-B wrote the manuscript. All authors reviewed the manuscript, contributed to discussion and approved the final version of the manuscript.
Funding
The authors are grateful for the funding from the Spanish Government (PID2019-110709RB-100, RED2018-102417-T, FPU16/04015, PID2020-114585RA-I00, and PID2020-118860RB-I00), Junta de Castilla y León (VA317P18, Infrared2018-UVA06), Interreg V España Portugal POCTEP (0624_2IQBIONEURO_6_E) and Centro en Red de Medicina Regenerativa y Terapia Celular de Castilla y León. KP-N was funded by Fundación Carolina, the call for predoctoral contracts UVa2020 (co-funded by Santander Bank) and predoctoral contracts of the Junta de Castilla y Leon 2021.
Conflict of Interest
The authors declare that the research was conducted in the absence of any commercial or financial relationships that could be construed as a potential conflict of interest.
Publisher’s Note
All claims expressed in this article are solely those of the authors and do not necessarily represent those of their affiliated organizations, or those of the publisher, the editors and the reviewers. Any product that may be evaluated in this article, or claim that may be made by its manufacturer, is not guaranteed or endorsed by the publisher.
Acknowledgments
The authors acknowledge the staff of Justino Gutierrez S. L. slaughterhouse (Valladolid, Spain) for providing the porcine eye globes used in this work.
Supplementary Material
The Supplementary Material for this article can be found online at: https://www.frontiersin.org/articles/10.3389/fnana.2022.812487/full#supplementary-material
Supplementary Figure 1 | Dissected areas from pig retinas. Area centralis (AC) and optic nerve (OP) in (A) dissected posterior segment in the porcine globe; (B) dissected porcine neuroretina in Petri dish; and (C) neuroretina schematic drawing. AC: area centralis; ON: optic nerve.
References
Arango-Gonzalez, B., Trifunović, D., Sahaboglu, A., Kranz, K., Michalakis, S., Farinelli, P., et al. (2014). Identification of a Common Non-Apoptotic Cell Death Mechanism in Hereditary Retinal Degeneration. PLoS One 9:e112142. doi: 10.1371/journal.pone.0112142
Barber, A. J., Lieth, E., Khin, S. A., Antonetti, D. A., Buchanan, A. G., and Gardner, T. W. (1998). Neural apoptosis in the retina during experimental and human diabetes. Early onset and effect of insulin. J. Clin. Invest. 102, 783–791. doi: 10.1172/JCI2425
Bhutto, I., and Lutty, G. (2012). Understanding age-related macular degeneration (AMD): relationships between the photoreceptor/retinal pigment epithelium/Bruch’s membrane/choriocapillaris complex. Mol. Aspects Med. 33, 295–317. doi: 10.1016/j.mam.2012.04.005
Bourne, R., Steinmetz, J. D., Flaxman, S., Briant, P. S., Taylor, H. R., Resnikoff, S., et al. (2021). Trends in prevalence of blindness and distance and near vision impairment over 30 years: an analysis for the Global Burden of Disease Study. Lancet Glob. Health 9, e130–e143. doi: 10.1016/S2214-109X(20)30425-3
Cehajic-Kapetanovic, J., Xue, K., Martinez-Fernandez de la Camara, C., Nanda, A., Davies, A., and Wood, L. J. (2020). Initial results from a first-in-human gene therapy trial on X-linked retinitis pigmentosa caused by mutations in RPGR. Nat. Med. 26, 354–359. doi: 10.1038/s41591-020-0763-1
Choudhury, S., Bhootada, Y., Gorbatyuk, O., and Gorbatyuk, M. (2013). Caspase-7 ablation modulates UPR, reprograms TRAF2-JNK apoptosis and protects T17M rhodopsin mice from severe retinal degeneration. Cell Death Dis. 4:e528. doi: 10.1038/cddis.2013.34
Comitato, A., Sanges, D., Rossi, A., Humphries, M. M., and Marigo, V. (2014). Activation of Bax in Three Models of Retinitis Pigmentosa. Invest. Ophthalmol. Vis. Sci. 55, 3555–3561. doi: 10.1167/iovs.14-13917
Cuenca, N., Fernández-Sánchez, L., Campello, L., Maneu, V., De la Villa, P., and Lax, P. (2014). Cellular responses following retinal injuries and therapeutic approaches for neurodegenerative diseases. Prog. Retin. Eye Res. 43, 17–75. doi: 10.1016/j.preteyeres.2014.07.001
Degterev, A., Huang, Z., Boyce, M., Li, Y., Jagtap, P., Mizushima, N., et al. (2005). Chemical inhibitor of non-apoptotic cell death with therapeutic potential for ischemic brain injury. Nat. Chem. Biol. 1, 112–119. doi: 10.1038/nchembio711
Di Lauro, S., Rodriguez-Crespo, D., Gayoso, M. J., Garcia-Gutierrez, M. T., Pastor, J. C., Srivastava, G. K., et al. (2016). A novel coculture model of porcine central neuroretina explants and retinal pigment epithelium cells. Mol. Vis. 22, 243–253.
Dondelinger, Y., Declercq, W., Montessuit, S., Roelandt, R., Goncalves, A., Bruggeman, I., et al. (2014). MLKL compromises plasma membrane integrity by binding to phosphatidylinositol phosphates. Cell Rep. 7, 971–981. doi: 10.1016/j.celrep.2014.04.026
Dong, K., and Sun, X. (2011). Targeting death receptor induced apoptosis and necroptosis: a novel therapeutic strategy to prevent neuronal damage in retinal detachment. Med. Hypotheses 77, 144–146. doi: 10.1016/j.mehy.2011.03.049
Doonan, F., Donovan, M., and Cotter, T. G. (2005). Activation of Multiple Pathways during Photoreceptor Apoptosis in the rd Mouse. Invest. Ophthalmol. Vis. Sci. 46, 3530–3538. doi: 10.1167/iovs.05-0248
Dunaief, J. L., Dentchev, T., Ying, G.-S., and Milam, A. H. (2002). The role of apoptosis in age-related macular degeneration. Arch. Ophthalmol. 120, 1435–1442. doi: 10.1001/archopht.120.11.1435
Eigeldinger-Berthou, S., Meier, C., Zulliger, R., Lecaudé, S., Enzmann, V., and Sarra, G.-M. (2012). Rasagiline interferes with neurodegeneration in the Prph2/rds mouse. Retina 32, 617–628. doi: 10.1097/IAE.0b013e31821e2070
Fernandez-Bueno, I., Fernández-Sánchez, L., Gayoso, M. J., García-Gutierrez, M. T., Pastor, J. C., and Cuenca, N. (2012). Time course modifications in organotypic culture of human neuroretina. Exp. Eye Res. 104, 26–38. doi: 10.1016/j.exer.2012.08.012
Fernandez-Bueno, I., Garcia-Gutierrez, M. T., Srivastava, G. K., Gayoso, M. J., Gonzalo-Orden, J. M., and Pastor, J. C. (2013). Adalimumab (tumor necrosis factor-blocker) reduces the expression of glial fibrillary acidic protein immunoreactivity increased by exogenous tumor necrosis factor alpha in an organotypic culture of porcine neuroretina. Mol. Vis. 19, 894–903.
Fernandez-Bueno, I., Pastor, J. C., Gayoso, M. J., Alcalde, I., and Garcia, M. T. M. (2008). üller and macrophage-like cell interactions in an organotypic culture of porcine neuroretina. Mol. Vis. 14, 2148–2156.
Fernandez-Bueno, I., and Usategui-Martin, R. (2021). Ex Vivo Model of Spontaneous Neuroretinal Degeneration for Evaluating Stem Cells’ Paracrine Properties. Methods Mol. Biol. 2269, 125–137. doi: 10.1007/978-1-0716-1225-5_9
Fernandez-Sanchez, L., Esquiva, G., Pinilla, I., Martín-Nieto, J., and Cuenca, N. (2010). The Antiapoptotic TUDCA Protects Against Mitochondrial Dysfunction, Glial Cell Changes and Loss of the Capillary Network in the Transgenic Rat Model of Retinitis Pigmentosa P23H. Invest. Ophthalmol. Vis. Sci. 51:3721.
Fernandez-Sanchez, L., Perez de Sevilla Muller, L., Brecha, N., and Cuenca, N. (2013). Morphological and Functional Study of Retinal Astrocytes in DBA/2J Mice. Invest. Ophthalmol. Vis. Sci. 54:5096.
Festjens, N., Vanden Berghe, T., and Vandenabeele, P. (2006). Necrosis, a well-orchestrated form of cell demise: signalling cascades, important mediators and concomitant immune response. Biochim. Biophys. Acta 1757, 1371–1387. doi: 10.1016/j.bbabio.2006.06.014
Golstein, P., and Kroemer, G. (2007). Cell death by necrosis: towards a molecular definition. Trends Biochem. Sci. 32, 37–43. doi: 10.1016/j.tibs.2006.11.001
Hisatomi, T., Sakamoto, T., Murata, T., Yamanaka, I., Oshima, Y., Hata, Y., et al. (2001). Relocalization of apoptosis-inducing factor in photoreceptor apoptosis induced by retinal detachment in vivo. Am. J. Pathol. 158, 1271–1278. doi: 10.1016/S0002-9440(10)64078-3
Kaarniranta, K., Tokarz, P., Koskela, A., Paterno, J., and Blasiak, J. (2017). Autophagy regulates death of retinal pigment epithelium cells in age-related macular degeneration. Cell Biol. Toxicol. 33, 113–128. doi: 10.1007/s10565-016-9371-8
Klionsky, D. J. (2007). Autophagy: from phenomenology to molecular understanding in less than a decade. Nat. Rev. Mol. Cell Biol. 8, 931–937. doi: 10.1038/nrm2245
Kroemer, G., Galluzzi, L., Vandenabeele, P., Abrams, J., Alnemri, E. S., Baehrecke, E. H., et al. (2009). Classification of cell death: recommendations of the Nomenclature Committee on Cell Death 2009. Cell Death Differ. 16, 3–11. doi: 10.1038/cdd.2008.150
Labrador-Velandia, S., Alonso-Alonso, M. L., Di Lauro, S., García-Gutierrez, M. T., Srivastava, G. K., Pastor, J. C., et al. (2019). Mesenchymal stem cells provide paracrine neuroprotective resources that delay degeneration of co-cultured organotypic neuroretinal cultures. Exp. Eye Res. 185:107671. doi: 10.1016/j.exer.2019.05.011
Li, Y., Wang, C., Liu, Y., You, J., and Su, G. (2019). Autophagy, lysosome dysfunction and mTOR inhibition in MNU-induced photoreceptor cell damage. Tissue Cell 61, 98–108. doi: 10.1016/j.tice.2019.09.008
Li, Y., Zhang, Y., Qi, S., and Su, G. (2018). Retinal organotypic culture – A candidate for research on retinas. Tissue Cell 51, 1–7. doi: 10.1016/j.tice.2018.01.005
Liu, Y., Shi, S., Gu, Z., Du, Y., Liu, M., Yan, S., et al. (2013). Impaired autophagic function in rat islets with aging. Age 35, 1531–1544. doi: 10.1007/s11357-012-9456-0
Livak, K. J., and Schmittgen, T. D. (2001). Analysis of relative gene expression data using real-time quantitative PCR and the 2(-Delta Delta C(T)) Method. Methods 25, 402–408. doi: 10.1006/meth.2001.1262
Maiuri, M. C., Zalckvar, E., Kimchi, A., and Kroemer, G. (2007). Self-eating and self-killing: crosstalk between autophagy and apoptosis. Nat. Rev. Mol. Cell Biol. 8, 741–752. doi: 10.1038/nrm2239
Martin, P. M., Roon, P., Van Ells, T. K., Ganapathy, V., and Smith, S. B. (2004). Death of retinal neurons in streptozotocin-induced diabetic mice. Invest. Ophthalmol. Vis. Sci. 45, 3330–3336. doi: 10.1167/iovs.04-0247
Mitter, S. K., Rao, H. V., Qi, X., Cai, J., Sugrue, A., Dunn, W. A., et al. (2012). Autophagy in the retina: a potential role in age-related macular degeneration. Adv. Exp. Med. Biol. 723, 83–90. doi: 10.1007/978-1-4614-0631-0_12
Mizushima, N., and Klionsky, D. J. (2007). Protein turnover via autophagy: implications for metabolism. Annu. Rev. Nutr. 27, 19–40. doi: 10.1146/annurev.nutr.27.061406.093749
Mukhopadhyay, M., Shtrom, S., Rodriguez-Esteban, C., Chen, L., Tsukui, T., Gomer, L., et al. (2001). Dickkopf1 is required for embryonic head induction and limb morphogenesis in the mouse. Dev. Cell 1, 423–434. doi: 10.1016/s1534-5807(01)00041-7
Murakami, Y., Matsumoto, H., Roh, M., Suzuki, J., Hisatomi, T., Ikeda, Y., et al. (2012). Receptor interacting protein kinase mediates necrotic cone but not rod cell death in a mouse model of inherited degeneration. Proc. Natl. Acad. Sci. U. S. A. 109, 14598–14603. doi: 10.1073/pnas.1206937109
Murakami, Y., Notomi, S., Hisatomi, T., Nakazawa, T., Ishibashi, T., Miller, J. W., et al. (2013). Photoreceptor cell death and rescue in retinal detachment and degenerations. Prog. Retin. Eye Res. 37, 114–140. doi: 10.1016/j.preteyeres.2013.08.001
Murali, A., Ramlogan-Steel, C. A., Andrzejewski, S., Steel, J. C., and Layton, C. J. (2019). Retinal explant culture: a platform to investigate human neuro-retina. Clin. Exp. Ophthalmol. 47, 274–285. doi: 10.1111/ceo.13434
Newton, F., and Megaw, R. (2020). Mechanisms of Photoreceptor Death in Retinitis Pigmentosa. Genes 11:1120. doi: 10.3390/genes11101120
Pasparakis, M., and Vandenabeele, P. (2015). Necroptosis and its role in inflammation. Nature 517, 311–320. doi: 10.1038/nature14191
Qiu, Y., Yao, J., Jia, L., Thompson, D. A., and Zacks, D. N. (2019). Shifting the balance of autophagy and proteasome activation reduces proteotoxic cell death: a novel therapeutic approach for restoring photoreceptor homeostasis. Cell Death Dis. 10:547. doi: 10.1038/s41419-019-1780-1
Radi, E., Formichi, P., Battisti, C., and Federico, A. (2014). Apoptosis and Oxidative Stress in Neurodegenerative Diseases. J. Alzheimers Dis. 42, S125–S152.
Ramírez, J. M., Ramírez, A. I., Salazar, J. J., de Hoz, R., and Triviño, A. (2001). Changes of Astrocytes in Retinal Ageing and Age-related Macular Degeneration. Exp. Eye Res. 73, 601–615. doi: 10.1006/exer.2001.1061
Rodríguez-Muela, N., Hernández-Pinto, A. M., Serrano-Puebla, A., García-Ledo, L., Latorre, S. H., and de la Rosa, E. J. (2015). Lysosomal membrane permeabilization and autophagy blockade contribute to photoreceptor cell death in a mouse model of retinitis pigmentosa. Cell Death Differ. 22, 476–487. doi: 10.1038/cdd.2014.203
Rosenbaum, D. M., Degterev, A., David, J., Rosenbaum, P. S., Roth, S., Grotta, J. C., et al. (2010). Necroptosis, a novel form of caspase-independent cell death, contributes to neuronal damage in a retinal ischemia-reperfusion injury model. J. Neurosci. Res. 88, 1569–1576. doi: 10.1002/jnr.22314
Rubinsztein, D. C., Gestwicki, J. E., Murphy, L. O., and Klionsky, D. J. (2007). Potential therapeutic applications of autophagy. Nat. Rev. Drug Discov. 6, 304–312.
Russell, S., Bennett, J., Wellman, J. A., Chung, D. C., Yu, Z.-F., Tillman, A., et al. (2017). Efficacy and safety of voretigene neparvovec (AAV2-hRPE65v2) in patients with RPE65-mediated inherited retinal dystrophy: a randomised, controlled, open-label, phase 3 trial. Lancet 390, 849–860. doi: 10.1016/S0140-6736(17)31868-8
Sato, K., Li, S., Gordon, W. C., He, J., Liou, G. I., Hill, J. M., et al. (2013). Receptor Interacting Protein Kinase-Mediated Necrosis Contributes to Cone and Rod Photoreceptor Degeneration in the Retina Lacking Interphotoreceptor Retinoid-Binding Protein. J. Neurosci. 33, 17458–17468. doi: 10.1523/JNEUROSCI.1380-13.2013
Singh, R., Letai, A., and Sarosiek, K. (2019). Regulation of apoptosis in health and disease: the balancing act of BCL-2 family proteins. Nat. Rev. Mol. Cell Biol. 20, 175–193. doi: 10.1038/s41580-018-0089-8
Steinmetz, J. D., Bourne, R. R. A., Briant, P. S., Flaxman, S. R., Taylor, H. R. B., Jonas, J. B., et al. (2021). Causes of blindness and vision impairment in 2020 and trends over 30 years, and prevalence of avoidable blindness in relation to VISION 2020: the Right to Sight: an analysis for the Global Burden of Disease Study. Lancet Glob. Health 9, e144–e160. doi: 10.1016/S2214-109X(20)30489-7
Trichonas, G., Murakami, Y., Thanos, A., Morizane, Y., Kayama, M., Debouck, C. M., et al. (2010). Receptor interacting protein kinases mediate retinal detachment-induced photoreceptor necrosis and compensate for inhibition of apoptosis. Proc. Natl. Acad. Sci. U. S. A. 107, 21695–21700. doi: 10.1073/pnas.1009179107
Usategui-Martín, R., Puertas-Neyra, K., García-Gutiérrez, M.-T., Fuentes, M., Pastor, J. C., and Fernandez-Bueno, I. (2020). Human Mesenchymal Stem Cell Secretome Exhibits a Neuroprotective Effect over In Vitro Retinal Photoreceptor Degeneration. Mol. Ther. Methods Clin. Dev. 17, 1155–1166. doi: 10.1016/j.omtm.2020.05.003
Vandenabeele, P., Galluzzi, L., Vanden Berghe, T., and Kroemer, G. (2010). Molecular mechanisms of necroptosis: an ordered cellular explosion. Nat. Rev. Mol. Cell Biol. 11, 700–714. doi: 10.1038/nrm2970
Viiri, J., Amadio, M., Marchesi, N., Hyttinen, J. M. T., Kivinen, N., Sironen, R., et al. (2013). Autophagy Activation Clears ELAVL1/HuR-Mediated Accumulation of SQSTM1/p62 during Proteasomal Inhibition in Human Retinal Pigment Epithelial Cells. PLoS One 8:e69563. doi: 10.1371/journal.pone.0069563
Viringipurampeer, I. A., Gregory-Evans, C. Y., Metcalfe, A. L., Bashar, E., Moritz, O. L., and Gregory-Evans, K. (2019). Cell Death Pathways in Mutant Rhodopsin Rat Models Identifies Genotype-Specific Targets Controlling Retinal Degeneration. Mol. Neurobiol. 56, 1637–1652. doi: 10.1007/s12035-018-1192-8
Viringipurampeer, I. A., Shan, X., Gregory-Evans, K., Zhang, J. P., Mohammadi, Z., and Gregory-Evans, C. Y. (2014). Rip3 knockdown rescues photoreceptor cell death in blind pde6c zebrafish. Cell Death Differ. 21, 665–675. doi: 10.1038/cdd.2013.191
Wang, L., Cano, M., and Handa, J. T. (2014). p62 provides dual cytoprotection against oxidative stress in the retinal pigment epithelium. Biochim. Biophys. Acta 1843, 1248–1258. doi: 10.1016/j.bbamcr.2014.03.016
Wong, W. L., Su, X., Li, X., Cheung, C. M. G., Klein, R., Cheng, C.-Y., et al. (2014). Global prevalence of age-related macular degeneration and disease burden projection for 2020 and 2040: a systematic review and meta-analysis. Lancet Glob. Health 2, e106–e116. doi: 10.1016/S2214-109X(13)70145-1
Yang, H., Fu, Y., Liu, X., Shahi, P. K., Mavlyutov, T. A., Li, J., et al. (2017). Role of the sigma-1 receptor chaperone in rod and cone photoreceptor degenerations in a mouse model of retinitis pigmentosa. Mol. Neurodegener. 12:68. doi: 10.1186/s13024-017-0202-z
Yang, Y. J., Peng, J., Ying, D., and Peng, Q. H. A. (2018). Brief Review on the Pathological Role of Decreased Blood Flow Affected in Retinitis Pigmentosa. J. Ophthalmol. 2018, 1–7. doi: 10.1155/2018/3249064
Yao, J., Qiu, Y., Frontera, E., Jia, L., Khan, N. W., Klionsky, D. J., et al. (2018). Inhibiting autophagy reduces retinal degeneration caused by protein misfolding. Autophagy 14, 1226–1238. doi: 10.1080/15548627.2018.1463121
Keywords: retina, neurodegeneration, autophagy, apoptosis, necroptosis
Citation: Puertas-Neyra K, Galindo-Cabello N, Hernández-Rodríguez LA, González-Pérez F, Rodríguez-Cabello JC, González-Sarmiento R, Pastor JC, Usategui-Martín R and Fernandez-Bueno I (2022) Programmed Cell Death and Autophagy in an in vitro Model of Spontaneous Neuroretinal Degeneration. Front. Neuroanat. 16:812487. doi: 10.3389/fnana.2022.812487
Received: 10 November 2021; Accepted: 18 January 2022;
Published: 11 February 2022.
Edited by:
Diego García-Ayuso, University of Murcia, SpainReviewed by:
Laura Fernández-Sánchez, University of Alicante, SpainMichela Ferrucci, University of Pisa, Italy
Copyright © 2022 Puertas-Neyra, Galindo-Cabello, Hernández-Rodríguez, González-Pérez, Rodríguez-Cabello, González-Sarmiento, Pastor, Usategui-Martín and Fernandez-Bueno. This is an open-access article distributed under the terms of the Creative Commons Attribution License (CC BY). The use, distribution or reproduction in other forums is permitted, provided the original author(s) and the copyright owner(s) are credited and that the original publication in this journal is cited, in accordance with accepted academic practice. No use, distribution or reproduction is permitted which does not comply with these terms.
*Correspondence: Ivan Fernandez-Bueno, aWZlcm5hbmRlemJAaW9iYS5tZWQudXZhLmVz; Ricardo Usategui-Martín, cnVzYXRlZ3VpbUBpb2JhLm1lZC51dmEuZXM=
†These authors have contributed equally to this work