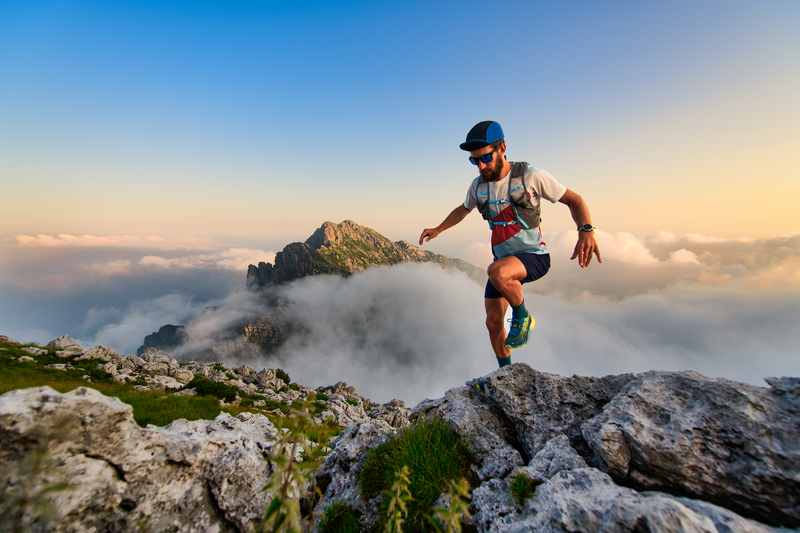
94% of researchers rate our articles as excellent or good
Learn more about the work of our research integrity team to safeguard the quality of each article we publish.
Find out more
ORIGINAL RESEARCH article
Front. Neuroanat. , 15 December 2020
Volume 14 - 2020 | https://doi.org/10.3389/fnana.2020.605029
This article is part of the Research Topic The Earliest-Born Cortical Neurons as Multi-Tasking Pioneers: Expanding Roles for Subplate Neurons in Cerebral Cortex Organization and Function, Volume II View all 5 articles
Increased white matter neuron density has been associated with neuropsychiatric disorders including schizophrenia. However, the pathogenic features of these neurons are still largely unknown. Subplate neurons, the earliest generated neurons in the developing cortex have also been associated with schizophrenia and autism. The link between these neurons and mental disorders is also not well established. Since cortical layer VIb neurons are believed to be the remnant of subplate neurons in the adult rodent brain, in this study, we aimed to examine the cytoarchitecture of neurons in cortical layer VIb and the underlying white matter in heterozygous Disc1 mutant (Het) mice, a mouse model of schizophrenia. In the white matter, the number of NeuN-positive neurons was quite low in the external capsule; however, the density of these cells was found increased (54%) in Het mice compared with wildtype (WT) littermates. The density of PV-positive neurons was unchanged in the mutants. In the cortical layer VIb, the density of CTGF-positive neurons increased (21.5%) in Het mice, whereas the number of Cplx3-positive cells reduced (16.1%) in these mutants, compared with WT mice. Layer VIb neurons can be classified by their morphological characters. The morphology of Type I pyramidal neurons was comparable between genotypes while the dendritic length and complexity of Type II multipolar neurons were significantly reduced in Het mice. White matter neurons and layer VIb neurons receive synaptic inputs and modulate the process of sensory information and sleep/arousal pattern. Aberrances of these neurons in Disc1 mutants implies altered brain functions in these mice.
Schizophrenia is a major chronic mental disorder that occurs in about 1% of the general population (McGrath et al., 2004). It is diagnosed by clinical signs and syndromes, including hallucinations, delusions, disorganized thought and speech, flattening effect, social withdrawal alogia, and deficits in cognitive functions. While the pathogenic mechanisms of schizophrenia are still not fully understood, both genetic and environmental factors are thought to play important roles in triggering the disease (van Os et al., 2010).
Disrupted-In-Schizophrenia 1 (DISC1) is one of the susceptible genes of schizophrenia. A defective variant of the DISC1 gene first discovered from a Scottish pedigree, and carried a balanced translocation (1;11; q42.1; q14.3) that was strongly associated with major mental illness such as schizophrenia, depression, and bipolar disorder (St Clair et al., 1990; Millar et al., 2000). The linkage between DISC1 gene abnormalities and schizophrenia has also been found in Taiwanese families (Hwu et al., 2003; Liu et al., 2009). DISC1 proteins are expressed in the cerebral cortex, hippocampus, hypothalamus, cerebellum, and brainstem in humans (Kirkpatrick et al., 2006); while in rodents, the expression of Disc1 has also been explored. Disc1 protein is detected in the cerebrum, cerebellum, brainstem, and various internal organs of mice as early as embryonic day 13. In the cerebrum, the expression of Disc1 increases during the perinatal period with a peak during the first postnatal week and gradually declines thereafter (Kuroda et al., 2011). The expression profile suggests a role of Disc1 in brain development. In addition to neurons, DISC1 is also expressed in astrocytes, oligodendrocytes, and microglia (Seshadri et al., 2010). DISC1 protein contains the N-terminal globular head domain and the coiled-helical coil-containing C-terminal tail domain. It has been demonstrated to interact with a great number of proteins; therefore, it is suggested to be a hub for multiple signaling pathways (Brandon and Sawa, 2011). DISC1 and its binding partners have been shown to regulate several cellular functions, such as neuronal migration, axon extension, dendritic differentiation, mitochondria motility, cargo transport, and synaptic plasticity. A variety of psychiatric phenotypes may be derived if DISC1 protein is disrupted (Brandon and Sawa, 2011; Bradshaw and Porteous, 2012; Lipina and Roder, 2014; Shao et al., 2017; Tropea et al., 2018).
Schizophrenia is a human-specific mental disorder; however, animal models could be used to replicate some aspects of the disease, especially the impact of certain susceptibility genes (Dahoun et al., 2017). Several mouse models have been made to mimic the disruption in the DISC1 gene (Jaaro-Peled, 2009; Tomoda et al., 2016). We had established a Disc1 mutant mouse model, in which a 25-bp deletion variant of 129S6/SvEv Disc1 gene was brought to the C57BL/6J background by generations of backcrossing (Juan et al., 2014). Compared with other Disc1 mutant mice, our model exhibits relatively moderate abnormalities, suggesting that these mice are in a prodromal status (Juan et al., 2014; Baskaran et al., 2020). This model is therefore suitable for studies concerning the impact of environmental risk factors. It has been suggested that structural abnormalities in the brain during neurodevelopmental mainly attributed to a gene mutation such as DISC1 deficiency may pave a way for functional impairments elicited by later environmental insults that promote the manifestation of psychotic symptoms (Narayan et al., 2013).
The cortical subplate zone is located between the developing cortical plate and the axons of afferent nerves (Allendoerfer and Shatz, 1994) and contains cells that are among the earliest generated neurons in the developing cortex and play important roles in cortical development (Kostovic and Rakic, 1980; Chun and Shatz, 1989; Bayer and Altman, 1990; McQuillen and Ferriero, 2005; Bystron et al., 2008; Judaš et al., 2010; Kanold and Luhmann, 2010; Hoerder-Suabedissen and Molnár, 2013; Ohtaka-Maruyama et al., 2018; Ohtaka-Maruyama, 2020). For example, these neurons set up the first connection between the thalamus and cortex that play crucial roles in the maturation of the inhibitory circuit and the organization of the neocortex (Kanold and Shatz, 2006; Kanold and Luhmann, 2010; Hoerder-Suabedissen and Molnár, 2015). Many subplate neurons go through programmed cell death after birth. The number of subplate neurons declines and remains as white matter and layer VIb neurons (Chun and Shatz, 1989; García-Marín et al., 2010; Judaš et al., 2010; Marx et al., 2017; Hoerder-Suabedissen et al., 2018). A genetic study showed the expression profile of mouse subplate neurons associated with autism and schizophrenia (Hoerder-Suabedissen et al., 2013). Abnormalities in subplate or white matter neurons have been linked to mental disorders such as schizophrenia (Duchatel et al., 2016, 2019; Serati et al., 2019; Kubo, 2020); however, the pathogenic role of remnant subplate neurons is still largely unknown (Judaš et al., 2010).
In this study, we examined the cytoarchitecture of neurons in cortical layer VIb and the underlying white matter in heterozygous Disc1 mutant (Het) mice. If the abnormalities of these neurons found in this genetic mouse model of schizophrenia could replicate the findings in patients with schizophrenia (Duchatel et al., 2019; Serati et al., 2019; Kubo, 2020), then we may have a promising rodent model for investigating molecular and cellular events which might contribute to the pathogenesis of schizophrenia. Our results showed alterations in the density of layer VIb and white matter neurons as well as the morphology of layer VIb neurons in Disc1 mutant mice and suggested a pathogenic feature of neuropsychiatric disorders.
Mice were housed in the Laboratory Animal Center of the College of Medicine, National Taiwan University (NTU) approved by AAALAC, under a 12:12 light-dark cycle with free access to food and water. Young adult (10–12 weeks old) male wild-type (WT) and heterozygous Disc1 (Het) mice were used in this study. Mice were genotyped using genomic DNA isolated from the toes and PCR-mediated methods as previously described (Juan et al., 2014). Animal experiments were performed following the guideline of the Institutional Animal Care and Use Committee of the College of Medicine, NTU. Efforts were constantly made to minimize animal discomfort and the number of mice used.
WT and Het male mice were anesthetized and transcardially perfused with 0.1 M phosphate-buffered saline (PBS; pH 7.4) followed by 4% paraformaldehyde in 0.1 M PBS. Whole brains were removed and post-fixed in the same fixation overnight. Then, the brains were stored in 0.1% NaN3 in 0.1 M PBS.
Brain samples were sectioned coronally at 30 μm with a vibratome (VT1000S, Leica Biosystems, Wetzlar, Germany) and one out of 12 sections which ranged from Bregma 1.00 mm to 0.00 mm was used for immunohistochemistry. The free-floating sections were first bleached in 0.1% H2O2 in 0.1 M PBS for 15 min to suppress endogenous peroxidase activity and washed in 0.1 M PBS. Then, the sections were incubated in the blocking solution containing 4% normal goat serum, 1% bovine serum albumin, and 0.4% Triton X-100 in 0.1 M PBS for 2 h to prevent non-specific binding. Afterward, the sections were incubated overnight with primary antibodies in diluted blocking solution on a shaker. The following primary antibodies were used: mouse monoclonal anti-NeuN (1:1,000, RRID: AB_177621; MAB377B; Millipore, Merck KGaA, Darmstadt, Germany), mouse polyclonal anti-Parvalbumin (PV; 1:3,000, RRID: AB_477329; P3088; Sigma–Aldrich, Merck KGaA), goat polyclonal anti-CTGF (1:1,000; RRID: AB_638805; sc-14939; Santa Cruz, CA, USA), mouse polyclonal anti-Nurr1 (1:250, RRID: AB_2153894; AF2156; R&D Systems, Minneapolis, MN, USA) and rabbit polyclonal anti-Cplx3 (1:2,000, RRID: AB_2281240, Cat# 122 302; Synaptic Systems, Göttingen, Germany). After washed with 0.1 M PBS, the sections were incubated in biotinylated secondary antibodies in 0.4% Triton X-100 for 1 h. The following secondary antibodies were used: biotinylated goat anti-mouse, biotinylated goat anti-rabbit, and biotinylated rabbit anti-goat (1:500, Jackson ImmunoResearch, West Grove, PA, USA). After washes, the sections were incubated with the reagents of the Vectastin (ABC kit, Vector Laboratories, Burlingame, CA, USA) for 1 h. Finally, sections were reacted with 2 mg/ml of 3′,3′-diaminobenzidine with 0.01% H2O2 in PBS, washed in PBS, and mounted.
Images of immunostained coronal sections were taken from the somatosensory cortex. Counting squares of 100 × 100 μm were used to estimate the densities of NeuN- and PV-immunopositive signals in the upper, middle, and lower regions of the cortex, while a frame of 25 × 100 μm was used to count CTGF-, Cplx3-, and Nurr1-positive cells in the cortical layer VIb. To evaluate the density of cells in the white matter, the areas of cingulum (cg) and external capsule (ec) underneath the somatosensory cortex were measured according to a mouse brain atlas (Paxinos and Franklin, 2008). The junction between gray matter and white matter was determined based on the immunostainings of NeuN. The numbers of NeuN- and PV-positive neurons in the cg and ec were counted. To measure the distribution of cortical neurons, the thickness of the cortex was equally subdivided into 20 counting bins, starting from the pia surface to the border of white matter. Due to the different densities of immunopositive signals, the width of the counting bin was set 50 μm for NeuN-positive and 100 μm for PV-positive cells, respectively. The numbers of cells were totaled and the proportion of cells in each bin was calculated and represented as a percentage across all 20 counting bins. In this counting system, bins 1–2 roughly correspond to cortical layer I, bins 3–6 for layer II/III, bin 7–8 for layer IV, bins 9–14 for layer V, bins 15–19 for layer VIa, and bin 20 for layer VIb, respectively.
Brain samples were collected and bathed in an impregnation solution (FD Rapid GolgiStain kit, NeuroTechnologies, Ellicott City, MD, USA) at room temperature for 3 weeks. Impregnated samples were transferred to ddH2O for at least 1 day and then serially sectioned at 150 μm using a vibratome (Leica). The sections were bathed in a mixture of developer and fixer solution from the same kit, then washed, and mounted. The images of subplate neurons in layer VIb of the somatosensory cortex were collected under a light microscope (Olympus, Tokyo, Japan) with a 20× objective lens for dendritic analysis. The stacks of images were obtained using the StereoInvestigator system (MicroBrightField Bioscience, Williston, VT, USA) and the morphology of subplate neurons was reconstructed with Neurolucida software (MicroBrightField). The Sholl and branched structure analyses provided in Neurolucida Explorer software were used to quantify the topological parameters such as the number of primary dendrites and branching nodes and size-related parameters, including soma size, convex hull volume, dendritic length, as well as intersections and nodes in relation to the distance from the soma.
Quantitative data of distribution, cell density, thickness, and morphometric parameters were measured blindly to the genotype of mice. The data were analyzed by two-tailed Student’s t-test and were presented as mean ± SEM. p < 0.05 was considered significance (*p < 0.05, **p < 0.01, ***p < 0.001). Statistics of the dendritic polar plot were done using R version 3.4.3 (RCoreTeam, 2017) and the ggplot2 (Wickham, 2016) package.
Disc1 has been suggested to play a role in cortical neuron migration (Narayan et al., 2013; Muraki and Tanigaki, 2015). We first examined the density and distribution of cortical neurons in the somatosensory cortex (Figure 1A) of WT and Disc1 Het mice. Neurons in the somatosensory cortex were immunostained with a pan-neuronal marker, NeuN (Figure 1B), and a marker for inhibitory interneurons, parvalbumin (PV, Figure 1C), and then quantified by counting of immunolabeled cells. The densities of NeuN-positive cells measured in upper, middle, and lower cortical regions were comparable between WT and Disc1 Het mice (Figure 1D). To measure the distribution of cortical neurons, the thickness of the cortex was divided into 20 counting bins and the counted neurons were totaled and converted into the proportion of cells in each bin (Yu et al., 2019). We regarded bins 1–2 as the layer I of the cerebral cortex; bins 3–6 as layers II/III; bins 7–8 as layer IV; bins 9–14 as layer V, bins 15–19 as layer VIa and bin 20 as layer VIb (Figure 1B). The distribution of NeuN-positive neurons was similar between genotypes in most counting bins, except for a difference in bin 8, the layer IV, between WT and Het groups (Figure 1E). PV was used as a marker for inhibitory neurons (Figure 1C), which make up about 40% of the interneurons in the somatosensory cortex (Rudy et al., 2011). The densities of PV-positive cortical neurons in the upper, middle, and lower regions were comparable between WT and Disc1 Het mice (Figure 1F). The distributions of PV-expressing neurons were also similar between groups (Figure 1G). These results indicated that the Disc1 haploinsufficiency does not significantly affect the density of cortical neurons while only a subtle difference in the distribution of layer IV neurons was noted.
Figure 1. Density and distribution of neurons in the somatosensory cortex. The region of interest was labeled by a red rectangle in the somatosensory cortex (A). Cortical neurons were immunostained with NeuN (B), or Parvalbumin (PV, C). The densities of NeuN-positive cortical neurons in counting boxes (blue squares in B) in the upper, middle, and lower regions were measured (D). The distribution of NeuN-positive cortical neurons was measured in 20 counting bins with the width of 50 μm (blue bins in B), the counted neurons in each bin were converted into the proportion of total cells (E). The densities of PV-positive cortical neurons in the upper, middle, and lower regions were measured (F). The distribution of PV-positive cortical neurons was measured using 20 counting bins with a width of 100 μm (blue bins in C) (G). Scale bar = 100 μm in panel (C). N = 8 wildtype (WT) and 7 Het mice. Results are means ± SEM. *p < 0.05.
Defects of neuronal migration might be manifested as altered white matter neuron density (Akbarian et al., 1996), we then examined the neurons in the white matter of Het mice (Figure 2). NeuN-positive neurons were evident in the cingulum and external capsule (Figure 2A). The density of NeuN-positive neurons was comparable between genotypes in the cingulum (Figure 2B); whereas in the external capsule, the density of NeuN-positive neurons was significantly greater in Het mice (Figure 2C). PV-positive neurons were sparsely distributed in the cingulum and external capsule (Figure 2D) and the densities of PV-positive neurons in WT and Het mice were similar in both areas (Figures 2E,F). Abnormalities in white matter neurons have been linked to mental disorders such as schizophrenia (Duchatel et al., 2016, 2019; Serati et al., 2019; Kubo, 2020), these results demonstrated, for the first time, an increased density of white matter neurons in a mouse model of schizophrenia.
Figure 2. The density of white matter neurons in WT and Disc1 Het mice. NeuN-positive neurons in the white matter including the cingulum (cg) and external capsule (ec) were identified (A) and measured (B,C). PV-positive white matter neurons (D) were also measured (E,F). Scale bar = 100 μm in (A,D). N = 8 WT and 7 Het mice. Results are means ± SEM. ***p < 0.001. cc, corpus callosum; LV, lateral ventricle; cg, cingulum; ec, external capsule.
During cortical development, most subplate neurons go through programmed cell death, and only a few neurons are left in the adult brain. Cortical layer VIb neurons are believed to be the remnant of subplate neurons in the adult rodent brain. There are several markers expressed in subplate neurons during the embryonic stage, and also expressed in adult layer VIb, such as connective tissue growth factor (CTGF), complexin-3 (Cplx3), and nuclear receptor-related 1 protein (Nurr1). It has been shown that these markers were not simultaneously expressed in the same subplate neuron population yet some overlapping still occurs (Mikhailova et al., 2017). In the present study, we picked these three markers to label subplate neurons in the adult brains (Figure 3), to examine if Disc1 mutation affects the fate of subplate neurons. CTGF was expressed in cortical VIb of both WT and Het mice (Figure 3A), roughly composed 4% of the cortical thickness and this value was comparable between genotypes (Figure 3B). Notably, the density of CTGF-positive neurons in layer VIb was greater in the Het mice (Figure 3C). Cplx3 was also expressed in layer VIb (Figure 3D). Cplx3-positive zone composed about 4.2% of the cortical thickness in both genotypes (Figure 3E). The density of Cplx3-positive neurons in the layer VIb was lower in Het mice (Figure 3F). Nurr1 was expressed in the nuclei of some layer VIb cells (Figure 3G) and composed about 3.3% of the thickness of the cortex (Figure 3H). The relative thickness and density of Nurr1-positive neurons were similar between genotypes (Figures 3H,I). Here we reported the relative thickness of layer VIb was comparable between WT and Het mice; whereas the densities of some layer VIb neuron subtypes were altered in Het mice.
Figure 3. Expression of subplate-specific markers in cortical layer VIb. CTGF is expressed by the subplate neurons in cortical layer VIb of both WT and Het mice (A). The relative thickness and density of CTGF-positive cells were measured and compared between genotypes (B,C). Cplx3 is expressed by the subplate neurons in layer VIb (D). The relative thickness of Cplx3-expressing cells was determined (E); the density of these cells was measured and compared (F). Nurr1 is evident in the nuclei of subplate neurons in layer VIb (G). The relative thickness of Nurr1-positive signals was determined (H); the density of these cells was measured and compared (I). Scale bar = 200 μm in panels (A,D,G) and 50 μm in the inserts. N = 8 WT and 7 Het mice. Results are means ± SEM. *p < 0.05, ***p < 0.001.
Subplate neurons have a large variety in cell morphology and a diversity in molecular marker expression (Kanold and Luhmann, 2010; Hoerder-Suabedissen and Molnár, 2013). In postnatal rodents, pyramidal-like, multipolar, horizontal, tangentially, and inverted subplate neurons have been discovered in the somatosensory cortex (Marx et al., 2017). In this study, Golgi-stained subplate neurons in layer VIb of the somatosensory cortex were collected from WT and Het mice and 3D reconstructed using Neurolucida software. The morphology of these neurons was classified based on quantitative analyses of their dendritic morphology. According to previously published reports (Marx and Feldmeyer, 2013; Marx et al., 2017), we classified the layer VIb neurons into pyramidal (Type I), multipolar (Type II), and horizontal (Type III) neurons. Few inverted (Type IV) subplate neurons were also identified. Different types of subplate neurons were demonstrated in Figure 4. In total, 72 and 77 subplate neurons were collected from WT and Disc1 Het mice, respectively. The proportions of cell types were comparable between WT and Het groups (Table 1).
Figure 4. Classification of neurons in the layer VIb. Golgi-Cox impregnated layer VIb neurons were collected from WT and Het mice and reconstructed. Dendritic polar plots were also generated. Based on their morphological features, cells were classified as Type I pyramidal neurons (A), Type II multipolar neurons (B), Type III horizontal neurons (C), and Type IV inverted neurons (D) of each type. Scale bar = 50 μm.
Parameters including soma size, primary dendrites, branch nodes, highest order, segment length, total dendritic length, and convex hull volume were measured, segment number and Sholl analyses (the numbers of intersections and branching nodes in relation to the distance from the soma) were conducted to evaluate the complexity of the dendritic trees.
Pyramidal subplate neurons showed featured with the long and thick apical dendrite which branches several times towards the terminal and projects vertically to the cortical surface (Figure 4A). However, the actual length of the apical dendrite might be underestimated due to the limitation of the Golgi stain. A few short and thin basal dendrites emerged from the base of soma projected radially. The morphological features of the pyramidal subplate neurons in WT and Het groups were similar. No significant difference was noted between groups (Figure 5).
Figure 5. Morphometric analysis of Type I layer VIb neurons. Somatodendritic features of pyramidal layer VIb neurons including the soma size (A), number of primary dendrites (B), number of branch nodes (C), highest order (D), length of dendritic segments (E), total dendritic length (F) and the convex hull volume (G) were measured. The complexity of the dendritic arbor was estimated by plotting the number of segments against the dendritic order (H) and using the concentric method of Sholl: the numbers of intersections (I) and nodes (J) were counted and plotted along the distance from the soma. Results are means ± SEM.
Multipolar subplate neurons had multiple dendrites with varied orientations but no apical-like thick dendrite (Figure 4B). The soma size, numbers of primary dendrites and branch nodes as well as the highest order were not different between genotypes (Figures 6A–D). The length of internodal segments was significantly shorter in the Disc1 Het group than in the WT group (Figure 6E), resulting in shorter total dendritic length (Figure 6F) and smaller convex hull volume in neurons collected from these mutant mice (Figure 6G). The numbers of segments were not changed in Het mice (Figure 6H). Sholl analysis showed reduced intersections and branch nodes in neurons of the Disc1 Het group (Figures 6I,J), indicating reduced dendritic complexity and branching deficiencies in type II subplate neurons in Het mice.
Figure 6. Morphometric analysis of Type II layer VIb neurons. Somatodendritic characteristics of multipolar layer VIb neurons including the soma size (A), number of primary dendrites (B), number of branch nodes (C), highest order (D), length of dendritic segments (E), total dendritic length (F) and the convex hull volume (G) were measured. The number of segments was plotted against the dendritic order (H). The numbers of intersections (I) and nodes (J) were counted and plotted along the distance from the soma. Results are means ± SEM. *p < 0.05, **p < 0.01.
The neurons in this category had a thick apical-like main dendrite extending. However, the directions of the main dendrites were not towards the cortical surface but mainly towards the horizontal orientation, which paralleled the white matter beneath the cortex, and some oriented obliquely from the soma. The other dendrites had no particular orientations (Figure 4C). The number of primary orders was lower in the Het group compared to the WT control (Figures 7B,H), however, other morphological characteristics were comparable between groups (Figure 7).
Figure 7. Morphometric analysis of Type III layer VIb neurons. Morphological features of horizontal layer VIb neurons including the soma size (A), number of primary dendrites (B), number of branch nodes (C), highest order (D), length of dendritic segments (E), total dendritic length (F) and the convex hull volume (G) were measured. The numbers of segments (H), intersections (I), and nodes (J) were counted and plotted. Results are means ± SEM. *p < 0.05, **p < 0.01.
Few neurons have an atypically oriented thick apical-like main dendrite extended from the base of soma and projected vertically towards the white matter (Figure 4D). Because of the rarity of this type of subplate neurons, we did not measure and compare these cells.
Together, our morphological study revealed a change in layer VIb subplate neurons, particularly the type II and type III subplate neurons, in Het mice.
Increased interstitial white matter neurons have been associated with psychiatric disorders including schizophrenia and autism (Duchatel et al., 2019; Serati et al., 2019; Kubo, 2020). The pathogenic role of aberrant subplate neuron remnants had been suggested (Judaš et al., 2010; Kostović et al., 2011). In the present study, we used a mouse model of schizophrenia, heterozygous Disc1 mutant mice, to test this hypothesis. We observed increased white matter neurons, altered CTGF- and Cplx3-positive layer VIb neuron densities as well as reduced dendritic complexity in layer VIb neurons. Increased white matter neurons and aberrant subplate neuron remnants in Disc1 mutants might contribute to altered brain functions in these mice.
Schizophrenia has been long recognized as dysfunctions in higher cognitive domains in humans. However, since the core symptoms include hallucination, defects in the sensory aspects might be relevant (Vukadinovic, 2012; Weilnhammer et al., 2020). Deficits in primary visual, auditory, and somatosensory cortices in schizophrenia patients have been reported (Fletcher and Frith, 2009; Huang et al., 2010, 2019; Molnár et al., 2014; Bordier et al., 2018; Daskalakis et al., 2020). We, therefore, in this study, decided to examine the properties of subplate neurons in the somatosensory cortex, which occupies a great cortical area with a substantial white matter thickness, in the rodent brain, for a proof of concept exploration.
In the present study, we showed an increased density of NeuN-positive neurons in the white matter of Disc1 Het mice, a haploinsufficiency model of schizophrenia (Juan et al., 2014; Baskaran et al., 2020). This finding was in line with previous reports of human studies: increased densities of white matter neurons in the postmortem brain tissues from patients with schizophrenia (Anderson et al., 1996; Kirkpatrick et al., 1999, 2003, 2006; Yang et al., 2011; Joshi et al., 2012; for review, see Duchatel et al., 2019; Serati et al., 2019; Kubo, 2020). We acknowledged that in these studies, the increased densities of white matter neurons were found in the dorsolateral prefrontal, orbitofrontal, parietal, anterior cingulate, and superior temporal regions. However, less attention had been paid to the primary sensory regions. Sensory processing defects were prominent in patients with schizophrenia (Javitt, 2009), cytoarchitectural elevations of the white matter in the sensory areas are encouraged. The density of white matter PV-positive neurons was very low and not changed in our model. However, in a rat model of schizophrenia, maternal immune activation paradigm, increased white matter somatostatin-positive inhibitory neurons have been noticed (Duchatel et al., 2016). Therefore, the role of inhibitory neurons in white matter is unneglectable (Joshi et al., 2012).
Altered white matter neurons might be resulted from impaired neuronal migration or programmed cell death during brain development (Akbarian et al., 1996; Kubo, 2020). Defects of neuronal migration are linked with brain disorders and Disc1 has been suggested to play a role in this process (Tomita et al., 2011; Narayan et al., 2013; Muraki and Tanigaki, 2015). In the present study, the increased NeuN-positive white matter neurons in adult Disc1 mutant mice might reflect a Disc1-mediated defect in cortical neuron migration. In our model, there is a 25-bp deletion in exon 6 of the Disc1 gene (Juan et al., 2014) which leads to a frameshift in the rest sequences including a great portion of the tail domain. LIS1 and NDEL1, components of the dynein complex that are associated with the DISC1 tail domain, are involved in the dynamics of the cytoskeleton and neuronal migration (Kamiya et al., 2005). However, in our Disc1 Het mice, the impact size was minimal, not as significant as other Disc1 knockdown models (Kamiya et al., 2005; Kubo et al., 2010; Steinecke et al., 2014). Compensatory responses might be activated to ensure proper cortical development in our and other Disc1 mutant mice (Koike et al., 2006; Kuroda et al., 2011). Nevertheless, the defects may still occur in the white matter, during an earlier period.
White matter neurons receive glutamatergic thalamic inputs and could be modulated by orexin, neurotensin, and dopamine, suggesting a role of white matter neurons in sleep/arousal state control (Case et al., 2017). Increased density of white matter neurons might thus be relevant to dysregulation of sleep. Sleep/circadian rhythm disturbances have been considered as environmental risk factors related to various psychiatric disorders (Wulff et al., 2010; Jagannath et al., 2013). Such disturbances might interact with genetic risk factors and contribute to the manifestation of psychiatric disorders.
Most subplate neurons go through programmed cell death with only 10–20% of neurons remaining in adulthood (Torres-Reveron and Friedlander, 2007; Luhmann et al., 2018). In rodents, most remaining subplate cells compose cortical layer VIb (Marx et al., 2017) and still receive both excitatory and inhibitory inputs and project to different cortex layers and thalamus (Torres-Reveron and Friedlander, 2007; Liao and Lee, 2012; Marx and Feldmeyer, 2013; Viswanathan et al., 2017; Hoerder-Suabedissen et al., 2018; Zolnik et al., 2020). The relative thickness of layer VIb in the cerebral cortex was comparable between genotypes; however, the densities of some subset subplate neurons were differentially altered in Disc1 Het mice.
Subplate neurons that express CTGF are non-pyramidal layer VIb neurons (Zolnik et al., 2020) and the density of this subset was found to increase in Het mice. CTGF is a secreted extracellular matrix-associated protein that plays various roles in regulating cellular functions (Malik et al., 2015; Ramazani et al., 2018). Previous studies indicate an inhibitory role of CTGF in regulating the differentiation of oligodendrocytes (Stritt et al., 2009; Ercan et al., 2017). We showed an increased density of oligodendrocytes in the external capsule of forebrain-specific CTGF knockout mice (Yu et al., 2019), demonstrating a paracrine function of subplate neuron-derived CTGF. In this regard, increased CTGF-positive neurons in layer VIb might influence the maturation and function of oligodendrocytes in adjacent white matter. Pathological features in the white matter have been noticed in patients with schizophrenia (Chen et al., 2018; Raabe et al., 2019), and the role of DISC1 has been addressed (Miyata et al., 2015; Vasistha et al., 2019). Here, we provided evidence that supernumerary CTGF-positive subplate neurons in Disc1 mutant mice might be a pathogenic sign leading to white matter dysfunction. However, the causal relationship between Disc1 haploinsufficiency and CTGF expression in the layer VIb is still unknown.
Another subset of subplate neurons express Cplx3 (Hoerder-Suabedissen et al., 2013) that project to the septal region of layer IV and medial posterior thalamic nucleus. These Cplx3-expressing subplate neurons are thus positioned to integrate thalamocortical and corticothalamic circuits (Viswanathan et al., 2017). In this point of view, the reduction of Cplx3-positive layer VIb neurons might affect the processing of sensory information in Disc1 Het mice which might model the sensory processing deficiency in patients with schizophrenia (Javitt, 2009).
The mechanisms underlying the differential changes among the subsets of layer VIb neurons in Het mice are not known. Since we only quantified the densities of layer VIb neurons at a single time point, the developmental trajectories of different subset neurons are not clear. Since most subplate neurons are removed during cortical development, the differential changes among CTGF-, Cplx3- and Nurr1-positive cells might be attributed to cell-type-specific survival mechanisms (Pfisterer and Khodosevich, 2017). We should examine the early signs of programmed cell death (Yamaguchi and Miura, 2015) at different time points in a subset-specific manner. The role of Disc1 in these processes still awaits to be explored.
Type I pyramidal subplate neurons, like the pyramidal neurons in layer VIa in morphology and function, have corticothalamic and corticocortical projections (Thomson, 2010; Marx and Feldmeyer, 2013). A recent study revealed that pyramidal layer VIb subplate neurons are Drd1a-positive and project to layers I and V and high order thalamus (Zolnik et al., 2020). The dendritic morphology of Type I subplate neurons was similar between WT and Het mice, implying that the function of these neurons might be spared in the mutants. However, due to the limitation of Gogi-stain and sample collection, the morphometric features of the apical dendrites might be underestimated. Besides, the detailed dendritic features such as dendritic diameter, density, and shape of dendritic spines, and ultrastructural characters have not been tested. We are not able to conclude this notion.
The multipolar and horizontal (Types II and III) subplate neurons are non-pyramidal layer VIb neurons that receive inputs from various cortical and subcortical sources (Zolnik et al., 2020). Some of these cells, especially the Type II cells, might be CTGF-positive layer VIb neurons. The reduced dendritic field in these cells explained the increased density of CTGF-positive neurons in mutant mice while the thickness of the CTGF-positive layer was comparable to that in WT mice. The dendritic length, complexity, and volume of the convex hull in Type II multipolar subplate neurons and the number of primary dendrites in Type III horizontal neurons were reduced in Het mice, indicating a reduced receptive field of synaptic transmission between layer VIb neurons and other cortical/subcortical neurons in Disc1 mutants. Future studies involving the projections of neurons and synaptic connectivity are required to clarify the functional roles of subplate neurons in different subpopulations. Subplate neurons in younger mice should be identified to advance our knowledge of Disc1-mediated dendritic growth or pruning. Further, white matter neurons can be classified and compared with layer VIb neurons.
In the somatosensory cortex, the densities and distributions of NeuN- and PV-positive neurons were similar between WT and Het mice, except for a subtle increase in the proportion of NeuN-positive neurons in bin 8, the cortical layer IV, of Disc1 Het mice. Layer IV neurons are the major recipients of thalamic and cortical inputs and play a critical role in sensory processing. Altered neuronal distribution in layer IV together with the changes of layer VIb subplate neurons might suggest a defect in sensory function. We should elaborate on this possibility using tactile-related behavioral tests (Lee, 2009; Arakawa et al., 2014) in Disc1 Het mice.
Subplate neurons regulate the radial migration of excitatory neurons in the cerebral cortex (Ohtaka-Maruyama et al., 2018) and modulate the thalamocortical and corticothalamic circuits that have been associated with cognitive functions. The subplate-specific gene expression profile is related to mental disorders including autism and schizophrenia (Hoerder-Suabedissen et al., 2013). Our results showed Disc1 haploinsufficiency-mediated pathogenic features in the white matter and layer VIb that can be associated with schizophrenia. This mouse model should be used to explore the developmental trajectory to replicate the at-risk subjects of developmental neuropsychiatric disorders and develop a preventive strategy. Our pilot study should also encourage the exploration of subplate-specific gene and network study during cortical development.
The raw data supporting the conclusions of this article will be made available by the authors, without undue reservation.
The animal study was reviewed and approved by Institutional Animal Care and Use Committee of the College of Medicine, National Taiwan University.
S-HT and C-YT conducted the experiments. S-HT analyzed the data and composed the draft of the manuscript. L-JL finalized the manuscript. All authors contributed to the article and approved the submitted version.
This work was supported by the Ministry of Science and Technology of the Republic of China; Grant numbers: 105-2628-B-002-004-MY3 and 108-2320-B-002-066-MY3 (to L-JL).
The authors declare that the research was conducted in the absence of any commercial or financial relationships that could be construed as a potential conflict of interest.
Akbarian, S., Kim, J. J., Potkin, S. G., Hetrick, W. P., Bunney, W. E. Jr., and Jones, E. G. (1996). Maldistribution of interstitial neurons in prefrontal white matter of the brains of schizophrenic patients. Arch. Gen. Psychiatry 53, 425–436. doi: 10.1001/archpsyc.1996.01830050061010
Allendoerfer, K. L., and Shatz, C. J. (1994). The subplate, a transient neocortical structure: its role in the development of connections between thalamus and cortex. Annu. Rev. Neurosci. 17, 185–218. doi: 10.1146/annurev.ne.17.030194.001153
Anderson, S. A., Volk, D. W., and Lewis, D. A. (1996). Increased density of microtubule associated protein 2-immunoreactive neurons in the prefrontal white matter of schizophrenic subjects. Schizophr Res. 19, 111–119. doi: 10.1016/0920-9964(96)88521-5
Arakawa, H., Akkentli, F., and Erzurumlu, R. S. (2014). Region-specific disruption of adenylate cyclase type 1 gene differentially affects somatosensorimotor behaviors in mice. eNeuro. 1:ENEURO.0007-14.2014. doi: 10.1523/ENEURO.0007-14.2014
Baskaran, R., Lai, C., Li, W., Tuan, L., Wang, C., Lee, L., et al. (2020). Characterization of striatal phenotypes in heterozygous Disc1 mutant mice, a model of haploinsufficiency. J. Comp. Neurol. 528, 1157–1172. doi: 10.1002/cne.24813
Bayer, S. A., and Altman, J. (1990). Development of layer I and the subplate in the rat neocortex. Exp. Neurol. 107, 48–62. doi: 10.1016/0014-4886(90)90062-w
Bordier, C., Nicolini, C., Forcellini, G., and Bifone, A. (2018). Disrupted modular organization of primary sensory brain areas in schizophrenia. NeuroImage Clin. 18, 682–693. doi: 10.1016/j.nicl.2018.02.035
Bradshaw, N. J., and Porteous, D. J. (2012). DISC1-binding proteins in neural development, signalling and schizophrenia. Neuropharmacology 62, 1230–1241. doi: 10.1016/j.neuropharm.2010.12.027
Brandon, N. J., and Sawa, A. (2011). Linking neurodevelopmental and synaptic theories of mental illness through DISC1. Nat. Rev. Neurosci. 12, 707–722. doi: 10.1038/nrn3120
Bystron, I., Blakemore, C., and Rakic, P. (2008). Development of the human cerebral cortex: Boulder Committee revisited. Nat. Rev. Neurosci. 9, 110–122. doi: 10.1038/nrn2252
Case, L., Lyons, D. J., and Broberger, C. (2017). Desynchronization of the rat cortical network and excitation of white matter neurons by neurotensin. Cereb. Cortex 27, 2671–2685. doi: 10.1093/cercor/bhw100
Chen, X., Duan, H., Xiao, L., and Gan, J. (2018). Genetic and epigenetic alterations underlie oligodendroglia susceptibility and white matter etiology in psychiatric disorders. Front. Genet. 9:565. doi: 10.3389/fgene.2018.00565
Chun, J. J., and Shatz, C. J. (1989). Interstitial cells of the adult neocortical white matter are the remnant of the early generated subplate neuron population. J. Comp. Neurol. 282, 555–569. doi: 10.1002/cne.902820407
Dahoun, T., Trossbach, S. V., Brandon, N. J., Korth, C., and Howes, O. D. (2017). The impact of disrupted-in-Schizophrenia 1 (DISC1) on the dopaminergic system: a systematic review. Transl. Psychiatry 7:e1015. doi: 10.1038/tp.2016.282
Daskalakis, A. A., Zomorrodi, R., Blumberger, D. M., and Rajji, T. K. (2020). Evidence for prefrontal cortex hypofunctioning in schizophrenia through somatosensory evoked potentials. Schizophr. Res. 215, 197–203. doi: 10.1016/j.schres.2019.10.030
Duchatel, R. J., Jobling, P., Graham, B. A., Harms, L. R., Michie, P. T., Hodgson, D. M., et al. (2016). Increased white matter neuron density in a rat model of maternal immune activation—implications for schizophrenia. Prog. Neuro-Psychopharmacol. Biol. Psychiatry 65, 118–126. doi: 10.1016/j.pnpbp.2015.09.006
Duchatel, R. J., Shannon Weickert, C., and Tooney, P. A. (2019). White matter neuron biology and neuropathology in schizophrenia. NPJ Schizophr. 5:10. doi: 10.1038/s41537-019-0078-8
Ercan, E., Han, J. M., DiNardo, A., Winden, K., Han, M. J., Hoyo, L., et al. (2017). Neuronal CTGF/CCN2 negatively regulates myelination in a mouse model of tuberous sclerosis complex. J. Exp. Med. 214, 681–697. doi: 10.1084/jem.20160446
Fletcher, P. C., and Frith, C. D. (2009). Perceiving is believing: a Bayesian approach to explaining the positive symptoms of schizophrenia. Nat. Rev. Neurosci. 10, 48–58. doi: 10.1038/nrn2536
García-Marín, V., Blazquez-Llorca, L., Rodriguez, J. R., Gonzalez-Soriano, J., and DeFelipe, J. (2010). Differential distribution of neurons in the gyral white matter of the human cerebral cortex. J. Comp. Neurol. 518, 4740–4759. doi: 10.1002/cne.22485
Hoerder-Suabedissen, A., and Molnár, Z. (2013). Molecular diversity of early-born subplate neurons. Cereb. Cortex 23, 1473–1483. doi: 10.1093/cercor/bhs137
Hoerder-Suabedissen, A., and Molnár, Z. (2015). Development, evolution and pathology of neocortical subplate neurons. Nat. Rev. Neurosci. 16, 133–146. doi: 10.1038/nrn3915
Hoerder-Suabedissen, A., Hayashi, S., Upton, L., Nolan, Z., Casas-Torremocha, D., Grant, E., et al. (2018). Subset of cortical layer 6b neurons selectively innervates higher order thalamic nuclei in mice. Cereb. Cortex 28, 1882–1897. doi: 10.1093/cercor/bhy036
Hoerder-Suabedissen, A., Oeschger, F. M., Krishnan, M. L., Belgard, T. G., Wang, W. Z., Lee, S., et al. (2013). Expression profiling of mouse subplate reveals a dynamic gene network and disease association with autism and schizophrenia. Proc. Natl. Acad. Sci. U S A 110, 3555–3560. doi: 10.1073/pnas.1218510110
Huang, J., Zhuo, C., Xu, Y., and Lin, X. (2019). Auditory verbal hallucination and the auditory network: from molecules to connectivity. Neuroscience 410, 59–67. doi: 10.1016/j.neuroscience.2019.04.051
Huang, M. X., Lee, R. R., Gaa, K. M., Song, T., Harrington, D. L., Loh, C., et al. (2010). Somatosensory system deficits in schizophrenia revealed by MEG during a median-nerve oddball task. Brain Topogr. 23, 82–104. doi: 10.1007/s10548-009-0122-5
Hwu, H., Liu, C., Fann, C., Ou-Yang, W., and Lee, S. (2003). Linkage of schizophrenia with chromosome 1q loci in Taiwanese families. Mol. Psychiatry 8, 445–452. doi: 10.1038/sj.mp.4001235
Jaaro-Peled, H. (2009). Gene models of schizophrenia: DISC1 mouse models. Prog. Brain Res. 179, 75–86. doi: 10.1016/S0079-6123(09)17909-8
Jagannath, A., Peirson, S. N., and Foster, R. G. (2013). Sleep and circadian rhythm disruption in neuropsychiatric illness. Curr. Opin. Neurobiol. 23, 888–894. doi: 10.1016/j.conb.2013.03.008
Javitt, D. C. (2009). Sensory processing in schizophrenia: neither simple nor intact. Schizophr. Bull. 35, 1059–1064. doi: 10.1093/schbul/sbp110
Joshi, D., Fung, S. J., Rothwell, A., and Weickert, C. S. (2012). Higher gamma-aminobutyric acid neuron density in the white matter of orbital frontal cortex in schizophrenia. Biol. Psychiatry 72, 725–733. doi: 10.1016/j.biopsych.2012.06.021
Juan, L., Liao, C., Lai, W., Chang, C., Pei, J., Wong, W., et al. (2014). Phenotypic characterization of C57BL/6J mice carrying the Disc1 gene from the 129S6/SvEv strain. Brain Struct. Funct. 219, 1417–1431. doi: 10.1007/s00429-013-0577-8
Judaš, M., Sedmak, G., and Pletikos, M. (2010). Early history of subplate and interstitial neurons: from Theodor Meynert (1867) to the discovery of the subplate zone (1974). J. Anat. 217, 344–367. doi: 10.1111/j.1469-7580.2010.01283.x
Kamiya, A., Kubo, K., Tomoda, T., Takaki, M., Youn, R., Ozeki, Y., et al. (2005). A schizophrenia-associated mutation of DISC1 perturbs cerebral cortex development. Nat. Cell Biol. 7, 1167–1178. doi: 10.1038/ncb1328
Kanold, P. O., and Shatz, C. J. (2006). Subplate neurons regulate maturation of cortical inhibition and outcome of ocular dominance plasticity. Neuron 51, 627–638. doi: 10.1016/j.neuron.2006.07.008
Kanold, P. O., and Luhmann, H. J. (2010). The subplate and early cortical circuits. Annu. Rev. Neurosci. 33, 23–48. doi: 10.1146/annurev-neuro-060909-153244
Kirkpatrick, B., Conley, R. C., Kakoyannis, A., Reep, R. L., and Roberts, R. C. (1999). Interstitial cells of the white matter in the inferior parietal cortex in schizophrenia: an unbiased cell-counting study. Synapse 34:95–102. doi: 10.1002/(SICI)1098-2396(199911)34:2<95::AID-SYN2>3.0.CO;2-I
Kirkpatrick, B., Messias, N. C., and Conley, R. R., and Roberts, R. C. (2003). Interstitial cells of the white matter in the dorsolateral prefrontal cortex in deficit and nondeficit schizophrenia. J. Nerv. Ment. Dis. 191, 563–567. doi: 10.1097/01.nmd.0000087181.61164.e1
Kirkpatrick, B., Xu, L., Cascella, N., Ozeki, Y., and Sawa, A., and Roberts, R. C. (2006). DISC1 immunoreactivity at the light and ultrastructural level in the human neocortex. J. Comp. Neurol. 497, 436–450. doi: 10.1002/cne.21007
Koike, H., Arguello, P. A., Kvajo, M., Karayiorgou, M., and Gogos, J. A. (2006). Disc1 is mutated in the 129S6/SvEv strain and modulates working memory in mice. Proc. Natl. Acad. Sci. U S A 103, 3693–3697. doi: 10.1073/pnas.0511189103
Kostovic, I., and Rakic, P. (1980). Cytology and time of origin of interstitial neurons in the white matter in infant and adult human and monkey telencephalon. J. Neurocytol. 9, 219–242. doi: 10.1007/BF01205159
Kostović, I., Judaš, M., and Sedmak, G. (2011). Developmental history of the subplate zone, subplate neurons and interstitial white matter neurons: relevance for schizophrenia. Int. J. Dev. Neurosci. 29, 193–205. doi: 10.1016/j.ijdevneu.2010.09.005
Kubo, K. I. (2020). Increased densities of white matter neurons as a cross-disease feature of neuropsychiatric disorders. Psychiatry Clin. Neurosci. 74, 166–175. doi: 10.1111/pcn.12962
Kubo, K., Tomita, K., Uto, A., Kuroda, K., Seshadri, S., Cohen, J., et al. (2010). Migration defects by DISC1 knockdown in C57BL/6, 129X1/SvJ and ICR strains via in utero gene transfer and virus-mediated RNAi. Biochem. Biophys. Res. Commun. 400, 631–637. doi: 10.1016/j.bbrc.2010.08.117
Kuroda, K., Yamada, S., Tanaka, M., Iizuka, M., Yano, H., Mori, D., et al. (2011). Behavioral alterations associated with targeted disruption of exons 2 and 3 of the Disc1 gene in the mouse. Hum. Mol. Genet. 20, 4666–4683. doi: 10.1093/hmg/ddr400
Lee, L. (2009). Neonatal fluoxetine exposure affects the neuronal structure in the somatosensory cortex and somatosensory-related behaviors in adolescent rats. Neurotox. Res. 15, 212–223. doi: 10.1007/s12640-009-9022-4
Liao, C., and Lee, L. (2012). Evidence for structural and functional changes of subplate neurons in developing rat barrel cortex. Brain Struct. Funct. 217, 275–292. doi: 10.1007/s00429-011-0354-5
Lipina, T. V., and Roder, J. C. (2014). Disrupted-In-Schizophrenia-1 (DISC1) interactome and mental disorders: impact of mouse models. Neurosci. Biobehav. Rev. 45, 271–294. doi: 10.1016/j.neubiorev.2014.07.001
Liu, Y., Liu, C., Tien, H., and Hwu, H. (2009). Construction of balanced translocation t(1;11) (q42.1;q14.3) probe and screening application in genomic samples in Taiwan. J. Formos. Med. Assoc. 108, 587–591. doi: 10.1016/S0929-6646(09)60377-6
Luhmann, H. J., Kirischuk, S., and Kilb, W. (2018). The superior function of the subplate in early neocortical development. Front. Neuroanat. 12:97. doi: 10.3389/fnana.2018.00097
Malik, A. R., Liszewska, E., and Jaworski, J. (2015). Matricellular proteins of the Cyr61/CTGF/NOV (CCN) family and the nervous system. Front. Cell Neurosci. 9:237.doi: 10.3389/fncel.2015.00237
Marx, M., and Feldmeyer, D. (2013). Morphology and physiology of excitatory neurons in layer 6b of the somatosensory rat barrel cortex. Cereb. Cortex 23, 2803–2817. doi: 10.1093/cercor/bhs254
Marx, M., Qi, G., Hanganu-Opatz, I. L., Kilb, W., Luhmann, H. J., and Feldmeyer, D. (2017). Neocortical layer 6B as a remnant of the subplate—a morphological comparison. Cereb. Cortex 27, 1011–1026. doi: 10.1093/cercor/bhv279
McGrath, J., Saha, S., Welham, J., El Saadi, O., MacCauley, C., and Chant, D. (2004). A systematic review of the incidence of schizophrenia: the distribution of rates and the influence of sex, urbanicity, migrant status and methodology. BMC Med. 2:13. doi: 10.1186/1741-7015-2-13
McQuillen, P. S., and Ferriero, D. M. (2005). Perinatal subplate neuron injury: implications for cortical development and plasticity. Brain Pathol. 15, 250–260. doi: 10.1111/j.1750-3639.2005.tb00528.x
Mikhailova, A., Sunkara, N., and McQuillen, P. S. (2017). Unbiased quantification of subplate neuron loss following neonatal hypoxia-ischemia in a rat model. Dev. Neurosci. 39, 171–181. doi: 10.1159/000460815
Millar, J. K., Wilson-Annan, J. C., Anderson, S., Christie, S., Taylor, M. S., Semple, C. A., et al. (2000). Disruption of two novel genes by a translocation co-segregating with schizophrenia. Hum. Mol. Genet. 9, 1415–1423. doi: 10.1093/hmg/9.9.1415
Miyata, S., Hattori, T., Shimizu, S., Ito, A., and Tohyama, M. (2015). Disturbance of oligodendrocyte function plays a key role in the pathogenesis of schizophrenia and major depressive disorder. Biomed. Res. Int. 2015:492367. doi: 10.1155/2015/492367
Molnár, Z., Kaas, J. H., de Carlos, J. A., Hevner, R. F., Lein, E., and Němec, P. (2014). Evolution and development of the mammalian cerebral cortex. Brain Behav. Evol. 83, 126–139. doi: 10.1016/j.urolonc.2020.01.018
Muraki, K., and Tanigaki, K. (2015). Neuronal migration abnormalities and its possible implications for schizophrenia. Front. Neurosci. 9:74. doi: 10.3389/fnins.2015.00074
Narayan, S., Nakajima, K., and Sawa, A. (2013). DISC1: a key lead in studying cortical development and associated brain disorders. Neuroscientist 19, 451–464. doi: 10.1177/1073858412470168
Ohtaka-Maruyama, C. (2020). Subplate neurons as an organizer of mammalian neocortical development. Front. Neuroanat. 14:8. doi: 10.3389/fnana.2020.00008
Ohtaka-Maruyama, C., Okamoto, M., Endo, K., Oshima, M., Kaneko, N., Yura, K., et al. (2018). Synaptic transmission from subplate neurons controls radial migration of neocortical neurons. Science 360, 313–317. doi: 10.1126/science.aar2866
Paxinos, G., and Franklin, K. B. (2008). The Mouse Brain in Stereotaxic Coordinates. San Diego, CA: Elsevier Academic Press.
Pfisterer, U., and Khodosevich, K. (2017). Neuronal survival in the brain: neuron type-specific mechanisms. Cell Death Dis. 8:e2643. doi: 10.1038/cddis.2017.64
Raabe, F. J., Slapakova, L., Rossner, M. J., Cantuti-Castelvetri, L., Simons, M., Falkai, P. G., et al. (2019). Oligodendrocytes as a new therapeutic target in schizophrenia: from histopathological findings to neuron-oligodendrocyte interaction. Cells 8:1496. doi: 10.3390/cells8121496
Ramazani, Y., Knops, N., Elmonem, M. A., Nguyen, T. Q., Arcolino, F. O., van den Heuvel, L., et al. (2018). Connective tissue growth factor (CTGF) from basics to clinics. Matrix Biol. 68–69, 44–66. doi: 10.1016/j.matbio.2018.03.007
RCoreTeam. (2017). R: A Language and Environment for Statistical Computing. Vienna, Austria: R Foundation for Statistical Computing. Available online at: https://www.R-project.org/.
Rudy, B., Fishell, G., Lee, S., and Hjerling-Leffler, J. (2011). Three groups of interneurons account for nearly 100% of neocortical GABAergic neurons. Dev. Neurobiol. 71, 45–61. doi: 10.1002/dneu.20853
Serati, M., Delvecchio, G., Orsenigo, G., Mandolini, G. M., Lazzaretti, M., Scola, E., et al. (2019). The role of the subplate in schizophrenia and autism: a systematic review. Neuroscience 408, 58–67. doi: 10.1016/j.neuroscience.2019.03.049
Seshadri, S., Kamiya, A., Yokota, Y., Prikulis, I., Kano, S., Hayashi-Takagi, A., et al. (2010). Disrupted-in-schizophrenia-1 expression is regulated by beta-site amyloid precursor protein cleaving enzyme-1-neuregulin cascade. Proc. Natl. Acad. Sci. U S A 7, 5622–5627. doi: 10.1073/pnas.0909284107
Shao, L., Lu, B., Wen, Z., Teng, S., Wang, L., Zhao, Y., et al. (2017). Disrupted-in-schizophrenia-1 (DISC1) protein disturbs neural function in multiple disease-risk pathways. Hum. Mol. Genet. 26, 2634–2648. doi: 10.1093/hmg/ddx147
St Clair, D., Blackwood, D., Muir, W., Carothers, A., Walker, M., Spowart, G., et al. (1990). Association within a family of a balanced autosomal translocation with major mental illness. Lancet 336, 13–16. doi: 10.1016/0140-6736(90)91520-k
Steinecke, A., Gampe, C., Nitzsche, F., and Bolz, J. (2014). DISC1 knockdown impairs the tangential migration of cortical interneurons by affecting the actin cytoskeleton. Front. Cell Neurosci. 8:190. doi: 10.3389/fncel.2014.00190
Stritt, C., Stern, S., Harting, K., Manke, T., Sinske, D., Schwarz, H., et al. (2009). Paracrine control of oligodendrocyte differentiation by SRF-directed neuronal gene expression. Nat. Neurosci. 12, 418–427. doi: 10.1038/nn.2280
Thomson, A. M. (2010). Neocortical layer 6, a review. Front. Neuroanat. 4:13. doi: 10.3389/fnana.2010.00013
Tomita, K., Kubo, K., Ishii, K., and Nakajima, K. (2011). Disrupted-in-schizophrenia-1 (Disc1) is necessary for migration of the pyramidal neurons during mouse hippocampal development. Hum. Mol. Genet. 20, 2834–2845. doi: 10.1093/hmg/ddr194
Tomoda, T., Sumitomo, A., Jaaro-Peled, H., and Sawa, A. (2016). Utility and validity of DISC1 mouse models in biological psychiatry. Neuroscience. 321, 99–107. doi: 10.1016/j.neuroscience.2015.12.061
Torres-Reveron, J., and Friedlander, M. J. (2007). Properties of persistent postnatal cortical subplate neurons. J Neurosci. 27, 9962–9974. doi: 10.1523/JNEUROSCI.1536-07.2007
Tropea, D., Hardingham, N., Millar, K., and Fox, K. (2018). Mechanisms underlying the role of DISC1 in synaptic plasticity. J. Physiol. 596, 2747–2771. doi: 10.1113/JP274330
van Os, J., Kenis, G., and Rutten, B. P. (2010). The environment and schizophrenia. Nature 468, 203–212. doi: 10.1038/nature09563
Vasistha, N. A., Johnstone, M., Barton, S. K., Mayerl, S. E., Thangaraj Selvaraj, B., Thomson, P. A., et al. (2019). Familial t(1;11) translocation is associated with disruption of white matter structural integrity and oligodendrocyte-myelin dysfunction. Mol. Psychiatry 24, 1641–1654. doi: 10.1038/s41380-019-0505-2
Viswanathan, S., Sheikh, A., Looger, L. L., and Kanold, P. O. (2017). Molecularly defined subplate neurons project both to thalamocortical recipient layers and thalamus. Cereb. Cortex 27, 4759–4768. doi: 10.1093/cercor/bhw271
Vukadinovic, Z. (2012). Schizophrenia as a disturbance of cortical sensory maps. Transl. Neurosci. 3, 388–398. doi: 10.2478/s13380-012-0043-8
Weilnhammer, V., Röd, L., Eckert, A. L., Stuke, H., Heinz, A., and Sterzer, P. (2020). Psychotic experiences in schizophrenia and sensitivity to sensory evidence. Schizophr. Bull. 46, 927–936. doi: 10.1093/schbul/sbaa003
Wulff, K., Gatti, S., Wettstein, J. G., and Foster, R. G. (2010). Sleep and circadian rhythm disruption in psychiatric and neurodegenerative disease. Nat. Rev. Neurosci. 11, 589–599. doi: 10.1038/nrn2868
Yamaguchi, Y., and Miura, M. (2015). Programmed cell death in neurodevelopment. Dev. Cell 32, 478–490. doi: 10.1016/j.devcel.2015.01.019
Yang, Y., Fung, S. J., Rothwell, A., Tianmei, S., and Weickert, C. S. (2011). Increased interstitial white matter neuron density in the dorsolateral prefrontal cortex of people with schizophrenia. Biol. Psychiatry 69, 63–70. doi: 10.1016/j.biopsych.2010.08.020
Yu, I., Chang, H., Chen, K., Lu, Y., Shy, H., Chen, C., et al. (2019). Genetic elimination of connective tissue growth factor in the forebrain affects subplate neurons in the cortex and oligodendrocytes in the underlying white matter. Front. Neuroanat. 13:16. doi: 10.3389/fnana.2019.00016
Keywords: animal model, layer VIb, CTGF, subplate neuron, white matter neuron, neuron morphology
Citation: Tsai S-H, Tsao C-Y and Lee L-J (2020) Altered White Matter and Layer VIb Neurons in Heterozygous Disc1 Mutant, a Mouse Model of Schizophrenia. Front. Neuroanat. 14:605029. doi: 10.3389/fnana.2020.605029
Received: 11 September 2020; Accepted: 24 November 2020;
Published: 15 December 2020.
Edited by:
Zoltan Molnar, University of Oxford, United KingdomReviewed by:
Gavin John Clowry, Newcastle University, United KingdomCopyright © 2020 Tsai, Tsao and Lee. This is an open-access article distributed under the terms of the Creative Commons Attribution License (CC BY). The use, distribution or reproduction in other forums is permitted, provided the original author(s) and the copyright owner(s) are credited and that the original publication in this journal is cited, in accordance with accepted academic practice. No use, distribution or reproduction is permitted which does not comply with these terms.
*Correspondence: Li-Jen Lee, bGpsZWVAbnR1LmVkdS50dw==
Disclaimer: All claims expressed in this article are solely those of the authors and do not necessarily represent those of their affiliated organizations, or those of the publisher, the editors and the reviewers. Any product that may be evaluated in this article or claim that may be made by its manufacturer is not guaranteed or endorsed by the publisher.
Research integrity at Frontiers
Learn more about the work of our research integrity team to safeguard the quality of each article we publish.