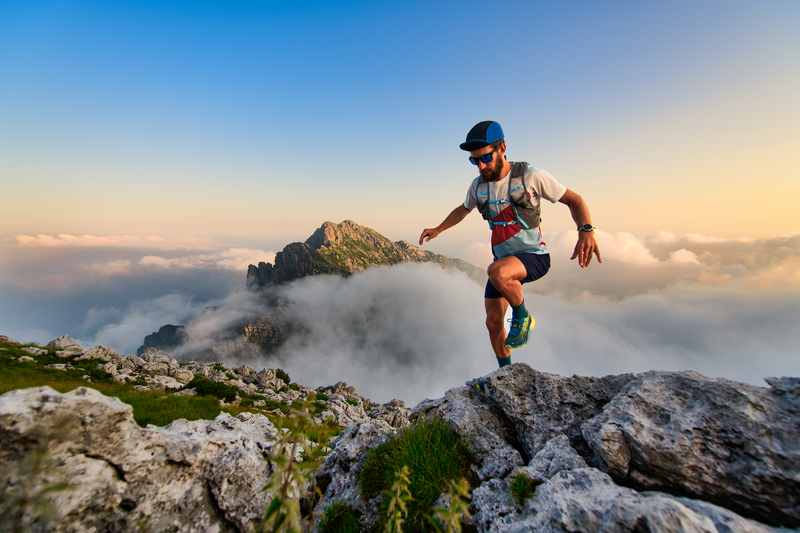
95% of researchers rate our articles as excellent or good
Learn more about the work of our research integrity team to safeguard the quality of each article we publish.
Find out more
ORIGINAL RESEARCH article
Front. Neuroanat. , 20 November 2020
Volume 14 - 2020 | https://doi.org/10.3389/fnana.2020.600555
Objective: This study aimed to investigate the direct monosynaptic projections from cortical functional regions to the cerebrospinal fluid (CSF)-contacting nucleus for understanding the functions of the CSF-contacting nucleus.
Methods: The Sprague–Dawley rats received cholera toxin B subunit (CB) injections into the CSF-contacting nucleus. After 7–10 days of survival time, the rats were perfused, and the whole brain and spinal cord were sliced under a freezing microtome at 40 μm. All sections were treated with the CB immunofluorescence reaction. The retrogradely labeled neurons in different cortical areas were revealed under a confocal microscope. The distribution features were further illustrated under 3D reconstruction.
Results: The retrogradely labeled neurons were identified in the olfactory, orbital, cingulate, insula, retrosplenial, somatosensory, motor, visual, auditory, association, rhinal, and parietal cortical areas. A total of 12 functional areas and 34 functional subregions showed projections to the CSF-contacting nucleus in different cell intensities.
Conclusion: According to the connectivity patterns, we conclude that the CSF-contacting nucleus participates in cognition, emotion, pain, visceral activity, etc. The present study firstly reveals the cerebral cortex→CSF-contacting nucleus connections, which implies the multiple functions of this special nucleus in neural and body fluid regulations.
The cerebrospinal fluid (CSF)-contacting nucleus is a special nucleus identified by our group in the brain (Wang and Zhang, 1992; Zhang et al., 1994, 2003; Lu et al., 2008; Song et al., 2019). It is “rivet”-like shape located in the brainstem caudal to the dorsal raphe nucleus (DR) (Song et al., 2019). The neural somata of this nucleus are located in the brain parenchyma, but the axons pass across the brain-CSF barrier and stretch directly into the CSF (Song and Zhang, 2018; Song et al., 2019). Morphological experiments have confirmed that the CSF-contacting nucleus has broad connections with non-CSF-contacting cells, blood vessels, and CSF (Zhang et al., 2003). The CSF-contacting nucleus is regarded as a pivotal structure bridging and facilitating communications between the nerves and body fluids (CSF and plasma) (Song and Zhang, 2018; Song et al., 2019). Many biological substances existed in the CSF-contacting nucleus as revealed by the combination of specific labeling (Lu et al., 2008) and immunofluorescence double-staining technique (Lu et al., 2011; Wang et al., 2014; Liu et al., 2017). The involvements of the CSF-contacting nucleus in sodium appetite, pain, morphine dependence and withdrawal, and stress have been discussed (Lu et al., 2011; Wu et al., 2015; Xing et al., 2015; Zhou et al., 2017). However, the anatomical pathways and mechanisms of this nucleus in different biological activities have not been clarified yet.
The cerebral cortex is one of the most complicated and top-level regions of the central nervous system (CNS). It is the primary organ modulating the functioning of the whole body and occupies the peak position in the motor and sensory system. It is the largest region of the cerebrum in the mammalian brain and has important roles in memory, attention, perception, cognition, awareness, consciousness, etc. (Kandel, 2013). On the basis of morphology, neuronal cell types, and connections, the cortical neurons can be divided into different layers, which have different functions and connections with other cortical and subcortical areas (Mountcastle, 1997; Katzel et al., 2011). The allocortex (consisting of the paleo- and archicortex) has three layers, and the neocortex has six layers (Posimo et al., 2013). On the basis of the basic functions of the cortex, it can be divided into multiple functional regions that drive cognition, emotion, somatosensory, motor, visual, etc.
The main aim of neuroscience research is to discuss the neural connection networks between different brain regions and thereby understand the brain functions (Watabe-Uchida et al., 2012). We have already illustrated the diencephalon (Song et al., 2020b), brainstem, and spinal cord anatomical projections to the CSF-contacting nucleus (Song et al., 2020a). The cerebral cortex is regarded as the core of the brain’s cognitive system (Bota et al., 2015), and it is also important to identify the cortex→CSF-contacting nucleus. In this study, we planned to inject the retrograde tracer into the CSF-contacting nucleus, the anatomical cortex→CSF-contacting nucleus projections can be observed by using an immunofluorescence technique, and the possible functional significance of the nucleus can be determined on the basis of the projection relationships, which will lay the foundation for further deeper research.
Specific pathogen-free (SPF)-grade Sprague–Dawley rats weighing 250 ± 50 g were acquired from the Experimental Animal Center of Xuzhou Medical University. Six rats (n = 6) successfully injected with a tracer into the CSF-contacting nucleus were used for analysis. All experiments were approved by the Committee for Ethical Use of Laboratory Animals of Xuzhou Medical University and were carried out according to the Guidelines for the Care and Use of Laboratory Animals.
As described previously (Song et al., 2020a, b), rats were anesthetized with pentobarbital sodium (40 mg/kg, i.p.). The heads were fixed on the stereotaxic instrument (Stoelting 51700, United States). The 1% cholera toxin B subunit (CB) solution (0.2 μL; Sigma, United States, Cat#SAE0069) was injected into the CSF-contacting nucleus according to the stereotaxic coordinates provided by Song et al. (2019) by using the Hamilton syringe (33 Gauge, Hamilton Company, Switzerland). Microinfusion pump (KD Scientific, United States) was applied for the injections over 30 min periods. After injection, the microsyringe was left for 10–15 min before retraction.
After 7–10 days, the animals were perfused as described previously (Song et al., 2020a). The whole brain and spinal cord were isolated and placed in the same 4% paraformaldehyde solution for postfixation at 4°C overnight. After fixation, the brain and spinal cord were immersed in 30% sucrose solution until sinking to the bottom. Then, serial coronal sections at 40 μm thickness were made on a cryostat (Leica CM1900, Germany). In this study, only the cortical areas were analyzed.
The staining and counting steps were performed following previous methods (Song et al., 2020a, b). All the sections were incubated with rabbit anti-CB primary antibody (diluted in 1:600, Abcam, Cat#ab34992) at 4°C overnight. After washing in 0.01 M PBS for three times, the sections were incubated with donkey anti-rabbit Alexa Fluor 488 secondary antibody (diluted in 1:200, Life Technologies, Cat#A-21206) at room temperature for 2 h. Then the sections were mounted on the slides in sequence and coverslipped. The results were observed and captured under a confocal microscope (Zeiss, Germany). The cell density (cell number/0.2 mm2 area) of CB-positive neurons was calculated by using Image-Pro Plus 7.0 software, and the density of >10, 6–10, and <5 positive neurons were classified as dense, moderate, and sparse distributions, respectively.
The CB retrogradely labeled neurons in the cortex were registered into the rat reference atlas (Paxinos and Watson, 2007). The Imaris software version 8.4.1 (Bitplane, United States) was used for three-dimensional (3D) surface rendering of cortex connections. The color codes representing the connection intensity was the same as previous studies (Song et al., 2020a, b).
The retrograde tracer CB injection into the CSF-contacting nucleus showed dense positive staining (green). The injection needle tract was located within the boundary of the CSF-contacting nucleus (Figure 1).
Figure 1. The injection site of the retrograde tracer CB and representative section of the cerebrospinal fluid (CSF)-contacting nucleus. (A,B) The injection site. (C,D) CSF-contacting nucleus representative coronal section. Aq, aqueduct. Bar = 100 μm in panel (B). Bar = 70 μm in panel (C). Bar = 40 μm in panel (D).
After the tracer CB is injected, it transports retrogradely via axoplasmic transport, and neural somata of cortex→CSF-contacting nucleus projections can be detected.
In the olfactory bulb, the retrogradely labeled neurons appear round or fusiform. The sizes of the neurons are not identical, and the dendrites are sparse and short. In the medial orbital cortex, the labeled neurons are mainly located in layers V and VI. The CB-positive neurons in layer V are mainly pyramidal neurons, which have many processes. Among them, one to two processes are longer and reach toward the superficial region of the cortex. In other parts of the cortex, the labeled neurons are mainly pyramidal neurons in layer V (Figure 2).
Figure 2. The neural morphology of positive cells. (A1–D1) Positive neurons in the olfactory bulb, orbital cortex, somatosensory cortex, and piriform cortex, respectively. (A2–D2) The corresponding sections in Nissl staining in A1-D1. “↑” is the mitral cell layer in the olfactory bulb. Bar = 100 μm.
All the cortex→CSF-contacting nucleus connections are shown by positively labeled neurons. In olfactory regions, CB retrogradely labeled neurons are found in the olfactory bulb (OB), accessory olfactory bulb (AOB), and piriform cortex (Pir). The OB sends sparse connections, and the CB-labeled neurons are mainly located in the mitral cell layer. The AOB sends moderate projections to the CSF-contacting nucleus, while Pir sends strong and dense connections. The positive neurons are obvious throughout the Pir and are mainly located at the pyramidal layer (Figure 3).
Figure 3. The CB retrogradely labeled neuron distribution in the olfactory region. (A) Olfactory bulb (OB). (B) Accessory olfactory bulb (AOB). (C) Piriform cortex (Pir). Bar = 100 μm.
In the orbital cortex, the CB-positive neurons can be found in eight areas. Among these, the prelimbic cortex (PrL), infralimbic cortex (IL), medial orbital cortex (MO), dorsal peduncular cortex (DP), and tenia tecta (TT) have dense projections to the CSF-contacting nucleus. The ventral orbital cortex (VO) and lateral orbital cortex (LO) have moderate connections. The dorsolateral orbital cortex (DLO) has sparse connections (Figure 4).
Figure 4. The CB retrogradely labeled neuron distribution in the orbital cortex (A–H). PrL, prelimbic cortex; IL, infralimbic cortex; MO, medial orbital cortex; DP, dorsal peduncular cortex; VO, ventral orbital cortex; LO, lateral orbital cortex; DLO, dorsolateral orbital cortex. Bar = 100 μm.
Dense positive neurons are found in Cg1 and Cg2 of the cingulate cortex. Moderately positive neurons are seen in the M1 and M2 of the motor cortex. In the somatosensory cortex, sparse positive neurons are seen in the S1 and moderate positive neurons are located in S2 (Figure 5).
Figure 5. The CB retrogradely labeled neuron distribution in the cingulate, motor, and somatosensory cortices (A–F). Cg1, cingulate cortex, area 1; Cg2, cingulate cortex, area 2; M1, primary motor cortex; M2, secondary motor cortex; S1, primary somatosensory cortex; S2, secondary somatosensory cortex. Bar = 100 μm.
In the insular cortex, dense CB-positive neurons are located in the dysgranular insular (DI) part of the insular cortex and moderate positive neurons can be seen in the granular insular (GI) and agranular insular (AI) parts (Figure 6).
Figure 6. The CB retrogradely labeled neurons distribution in the insular cortex (A–E). GI, granular insular cortex; DI, dysgranular insular cortex; AI, agranular insular cortex. Bar = 100 μm.
In the retrosplenial cortex (RSC), moderate positive neurons are found in both the retrosplenial dysgranular (RSD) and retrosplenial granular (RSG) regions (Figure 7).
Figure 7. The CB retrogradely labeled neuron distribution in the retrosplenial cortex (A–C). RSD, retrosplenial dysgranular cortex; RSG, retrosplenial granular cortex. Bar = 100 μm.
In the association cortex, dense positive neurons are found in medial parietal association cortex (MPtA) and temporal association cortex (TeA) and moderate positive neurons are found in lateral parietal association cortex (LPtA). Sparse positive neurons are found in the posterior area of the parietal cortex (PtP) (Figure 8).
Figure 8. The CB retrogradely labeled neuron distribution in the association cortex and PtP (A–D). MPtA, medial parietal association cortex; LPtA, lateral parietal association cortex; TeA, temporal association cortex; PtP, parietal cortex, posterior area. Bar = 100 μm.
In the visual cortex, sparse positive neurons are seen in the primary visual cortex (V1) and moderate positive neurons are seen in the secondary visual cortex (V2). In the auditory cortex, moderate positive neurons are found in the primary auditory cortex (Au1), secondary auditory cortex, dorsal area (AuD), and secondary auditory cortex, ventral area (AuV). In the rhinal cortex, strong and dense projections are found in the ectorhinal cortex (Ect), and moderate positive projections are found in the entorhinal cortex (Ent) and perirhinal cortex (PRh) (Figure 9).
Figure 9. The CB retrogradely labeled neuron distribution in the visual, auditory, and rhinal cortices (A–K). V1, primary visual cortex; V2, secondary visual cortex; Au1, primary auditory cortex; AuD, secondary auditory cortex, dorsal area; AuV, secondary auditory cortex, ventral area; Ent, entorhinal cortex; Ect, ectorhinal cortex; PRh, perirhinal cortex. Bar = 100 μm.
In summary, the retrogradely labeled neurons in the cortex are distributed in 12 cortical areas (34 functional sub-regions), ranged from sparse to dense. The CB-positive neurons do not exist in other parts of the cortex.
The retrograde labeling neurons throughout the cortical areas are reconstructed and visualized in 3D. The red areas represent dense connections (PrL, IL, MO, DP, TT, Pir, Cg1, Cg2, DI, MPtA, TeA, and Ect); the green areas show moderate connections (VO, LO, AOB, GI, AI, RSD, RSG, S2, M1, M2, V2, Au1, AuD, AuV, LPtA, Ent, and PRh); and the blue areas indicate sparse connections (DLO, OB, S1, V1, and PtP) (Figure 10).
Figure 10. 3D structure of the positive neuron distribution in the cortical areas from different perspectives (A–D). The red, green, and blue areas represent dense, moderate, and weak cortex→CSF-contacting nucleus connections, respectively.
In the 12 functional areas of the cortex, the retrogradely labeled neurons are located in 34 subregions. The amount of the cortical projections is shown in Figure 11.
Figure 11. Whole statistics of cortex→CSF-contacting nucleus retrogradely labeled neurons (A–L) (mean ± SD, n = 6).
The CSF-contacting nucleus is special compared with other already known nuclei in the brain (Figure 12). This article systematically presents comprehensive and standardized monosynaptic input to the CSF-contacting nucleus from different cortical zones. Broad projections from 12 cortical functional areas send direct input to the CSF-contacting nucleus (Figure 13). The role of CSF-contacting nucleus in different functional modulations can be speculated depending on the connections.
Figure 12. The schematic diagram of the synapse connections between CSF-contacting neurons and general neurons and non-synapse between CSF-contacting neurons and vessels and CSF.
Figure 13. The schematic diagram of the cortex→CSF-contacting nucleus projections (including the number of subregions).
The CSF-contacting nucleus receives input from the orbital cortex, Ent, PRh, and RSC, which might participate in cognition.
In the orbital cortex, the medial prefrontal cortex (mPFC) is associated with higher-order cognitive functions, including decision-making, attention, working memory, and goal-directed behavior (Metz et al., 2009). In rodent physiological studies, prefrontal cortex (PFC) activity was shown to be likely to track sustained attention across the session, and the reduction of PFC activity was required in reducing the effort (Passetti et al., 2000; Dalley et al., 2001). The orbital cortex was shown to be involved in mental exertion in human imaging studies (Schmidt et al., 2012). Individual variations in the medial and lateral orbital cortices are correlated with the differences in effortful choice (McGuire and Botvinick, 2010; Treadway et al., 2012).
The Ent and PRh are primarily associated with declarative memory (Squire and Zola-Morgan, 1991) and process non-spatial and spatial information to the hippocampus (Knierim et al., 2014). Moreover, PRh has extensively been related to recognition memory, the ability to determine that an event has been experienced previously (Brown and Aggleton, 2001).
The RSC is involved in hippocampal-dependent contextual and spatial learning and memory (Burgess, 2008), possibly by affecting hippocampal information processing (Sugar et al., 2011). Lesions of the RSC that occur either before or after conditioning impair contextual fear memory in rats (Keene and Bucci, 2008).
The CSF-contacting nucleus receives extensive inputs from the orbital cortex and cingulate cortex, which may participate in emotional modulation. The PrL and IL in the orbital cortex in both rodents and humans play important roles in emotional modulation (Farrell et al., 2010; Rive et al., 2013). For example, the PrL mainly participates in the emergence of fear, while the IL is engaged in fear extinction (Vidal-Gonzalez et al., 2006; Corcoran and Quirk, 2007; Burgos-Robles et al., 2009). Using the optogenetic method to activate IL mediates rapid and persistent anti-depressive effects (Fuchikami et al., 2015). MO is closely related to the emergence of anxiety-like behavior (Shi et al., 2017). In the cingulate cortex, the anterior cingulate cortex (ACC) participates in fear behaviors (Shackman et al., 2011). Electrical stimulation of the cingulate cortex causes significant emotional effects (Caruana et al., 2018).
The CSF-contacting nucleus receives input from S1, S2, PFC, ACC, and insular cortex projections, which may participate in pain modulation.
The classical spino-thalamic-cortex pathway sends nociceptive signals to the primary (S1) and secondary (S2) somatosensory cortices, which represents aspects of localization and intensity and modality discrimination of nociception (Pedersen et al., 2007).
Recently, the PFC, ACC, and insular cortex were considered to form the medial nociceptive system, which mainly mediates the emotional–affective and cognitive components of pain (Rainville et al., 1997; Pedersen et al., 2007; Linnman et al., 2010). Under chronic pain conditions, morphological alterations take place within the mPFC (Gundel et al., 2008). Moreover, the mPFC is considered to mediate placebo analgesia, which means that expectations and beliefs shape reality by affecting pain perception and influencing pain-related behavior (Krummenacher et al., 2010; Petrovic et al., 2010). The role of ACC in pain is also related to the emotional–affective profile confirmed by using neuroimaging techniques, and the ACC was shown to be positively correlated to the patient’s feelings of unpleasantness (Rainville et al., 1997). In addition to its emotional–affective functions, the ACC also participates in modulating the sensory component of pain (Sikes et al., 2008; Wei and Zhuo, 2008; Wu et al., 2008; Xu et al., 2008), which is supposed to be associated with the descending facilitatory system (Xu et al., 2008). In the insular cortex, rostral lesions result in diminishing the inflammatory and neuropathic pain-related behaviors (Coffeen et al., 2011), while caudal lesions before or after neuropathic pain result in the alleviation of allodynia without affecting normal mechanical thresholds (Benison et al., 2011).
The orbital cortex has been confirmed to remarkably influence visceral/autonomic activity. It is viewed as the “visceral cortex” (Hurley-Gius and Neafsey, 1986; Neafsey, 1990; Hassan et al., 2013) and has extensive projections with autonomic structures (Hurley et al., 1991). Acute rectal stimulation in rats induced activation of the orbital cortex (Wang et al., 2008). In addition, stimulation or lesions of this region can cause a variety of autonomic responses (Panteleev and Grundy, 2000).
The ACC spontaneous activity is enhanced in viscerally hypersensitive rats. These rats show a reduction in the colorectal distention pressure threshold and an increased ACC neuronal response to visceral stimulation (Gao et al., 2010). The ACC participates in a functional circuit in modulating different processes of chronic visceral hypersensitivity (Cao et al., 2008) and produces emotional and motivational responses after visceral stimulation (Zhang et al., 2013).
The insular cortex is involved in viscero-autonomic functions, as revealed by fMRI findings in humans and neuronal level data in primates (Rolls, 2016). The visceral organs transmit information throughout different parts of the insular cortex (Sticht et al., 2015).
Apart from the brain regions’ input to the CSF-contacting nucleus, the nucleus also receives inputs from the olfactory cortex, visual cortex, auditory cortex, and motor cortex. Odors are registered at the main olfactory epithelium, then processed at the main OB, AOB, and the Pir (Manella et al., 2017; Tsuji et al., 2017). The visual cortex (V1 and V2) participates in coding and intergrading of visual messages or multisensory convergence (Hirokawa et al., 2008; Ajina and Bridge, 2018; Scheyltjens et al., 2018). The auditory cortex modulates auditory information (Kwon et al., 2012; Gao and Wang, 2018). The motor cortex is known for motor control (Green et al., 2018; Wei et al., 2018).
In the present study, the conventional tract-tracing method is applied to illustrate the projection patterns to the CSF-contacting nucleus from cortical areas. The neurons of the CSF-contacting nucleus in the brain parenchyma receive the inputs from the abovementioned cortical areas and form different cortex→CSF-contacting nucleus circuits, while the axons have different synaptic and non-synaptic connections with other functional structures in modulating the life activities via the neuron-neuron crosstalk and neuron-body fluids interactions (Song and Zhang, 2018; Song et al., 2019). Moreover, different cell types including the excitatory or inhibitory neurons have been identified in the cerebral cortex according to their chemical properties and firing patterns (Morishima et al., 2017; Nixima et al., 2017; Chistiakova et al., 2019). The projections from different neurons to the CSF-contacting nucleus might form a complex neural circuit and mediate different life activities. On the basis of the connection regularities of CSF-contacting nucleus from the cortex, we conclude that the CSF-contacting nucleus is involved in cognition, emotion, pain, visceral regulation, other sensory activities (smell, vision, and auditory sensation), motor function, etc. These findings provide neuroanatomical evidence for further assessment of the unique function of the CSF-contacting nucleus.
The original contributions presented in the study are included in the article/supplementary material, further inquiries can be directed to the corresponding author.
The animal study was reviewed and approved by Committee for Ethical Use of Laboratory Animals of Xuzhou Medical University.
S-YS and L-CZ designed the study and drafted the manuscript. S-YS, X-MZ, J-HD, and L-LL made the tracer injections. S-YS, C-JS, JH, and J-LC conducted the immunofluorescence. S-YS did the 3D reconstruction experiment. All authors approved the final manuscript.
This research is funded by grants from the National Natural Science Foundation of China (Grant Nos. 81371243 and 81901131), the Natural Science Foundation of Jiangsu Province (Grant No. BK20190987), and China Postdoctoral Science Foundation (Grant No. 2018 M642328).
The authors declare that the research was conducted in the absence of any commercial or financial relationships that could be construed as a potential conflict of interest.
We thank all members of L.Z. group for their contributions to this study.
Ajina, S., and Bridge, H. (2018). Subcortical pathways to extrastriate visual cortex underlie residual vision following bilateral damage to V1. Neuropsychologia. 128:140–149. doi: 10.1016/j.neuropsychologia.2018.01.007
Benison, A. M., Chumachenko, S., Harrison, J. A., Maier, S. F., Falci, S. P., Watkins, L. R., et al. (2011). Caudal granular insular cortex is sufficient and necessary for the long-term maintenance of allodynic behavior in the rat attributable to mononeuropathy. J. Neurosci. 31, 6317–6328. doi: 10.1523/jneurosci.0076-11.2011
Bota, M., Sporns, O., and Swanson, L. W. (2015). Architecture of the cerebral cortical association connectome underlying cognition. Proc. Natl. Acad. Sci. USA 112, E2093–E2101.
Brown, M. W., and Aggleton, J. P. (2001). Recognition memory: what are the roles of the perirhinal cortex and hippocampus? Nat. Rev. Neurosci. 2, 51–61. doi: 10.1038/35049064
Burgos-Robles, A., Vidal-Gonzalez, I., and Quirk, G. J. (2009). Sustained conditioned responses in prelimbic prefrontal neurons are correlated with fear expression and extinction failure. J. Neurosci. 29, 8474–8482. doi: 10.1523/jneurosci.0378-09.2009
Cao, Z., Wu, X., Chen, S., Fan, J., Zhang, R., Owyang, C., et al. (2008). Anterior Cingulate Cortex Modulates Visceral Pain as Measured by Visceromotor Responses in Viscerally Hypersensitive Rats. Gastroenterology 134, 535–543. doi: 10.1053/j.gastro.2007.11.057
Caruana, F., Gerbella, M., Avanzini, P., Gozzo, F., Pelliccia, V., Mai, R., et al. (2018). Motor and emotional behaviours elicited by electrical stimulation of the human cingulate cortex. Brain 141, 3035–3051. doi: 10.1093/brain/awy219
Chistiakova, M., Ilin, V., Roshchin, M., Bannon, N., Malyshev, A., Kisvarday, Z., et al. (2019). Distinct Heterosynaptic Plasticity in Fast Spiking and Non-Fast-Spiking Inhibitory Neurons in Rat Visual Cortex. J. Neurosci. 39, 6865–6878. doi: 10.1523/jneurosci.3039-18.2019
Coffeen, U., Manuel Ortega-Legaspi, J., Lopez-Munoz, F. J., Simon-Arceo, K., Jaimes, O., and Pellicer, F. (2011). Insular cortex lesion diminishes neuropathic and inflammatory pain-like behaviours. Eur. J. Pain 15, 132–138. doi: 10.1016/j.ejpain.2010.06.007
Corcoran, K. A., and Quirk, G. J. (2007). Activity in prelimbic cortex is necessary for the expression of learned, but not innate, fears. J. Neurosci. 27, 840–844. doi: 10.1523/jneurosci.5327-06.2007
Dalley, J. W., McGaughy, J., O’Connell, M. T., Cardinal, R. N., Levita, L., and Robbins, T. W. (2001). Distinct changes in cortical acetylcholine and noradrenaline efflux during contingent and noncontingent performance of a visual attentional task. J. Neurosci. 21, 4908–4914. doi: 10.1523/jneurosci.21-13-04908.2001
Farrell, M. R., Sayed, J. A., Underwood, A. R., and Wellman, C. L. (2010). Lesion of infralimbic cortex occludes stress effects on retrieval of extinction but not fear conditioning. Neurobiol. Learn. Mem. 94, 240–246. doi: 10.1016/j.nlm.2010.06.001
Fuchikami, M., Thomas, A., Liu, R., Wohleb, E. S., Land, B. B., Dileone, R. J., et al. (2015). Optogenetic stimulation of infralimbic PFC reproduces ketamine’s rapid and sustained antidepressant actions. Proc. Natl. Acad. Sci. USA 112, 8106–8111. doi: 10.1073/pnas.1414728112
Gao, J., Wu, X., Owyang, C., and Li, Y. (2010). Enhanced responses of the anterior cingulate cortex neurones to colonic distension in viscerally hypersensitive rats. J. Physiol. 570, 169–183. doi: 10.1113/jphysiol.2005.096073
Gao, L., and Wang, X. (2018). Subthreshold Activity Underlying the Diversity and Selectivity of the Primary Auditory Cortex Studied by Intracellular Recordings in Awake Marmosets. Cereb. Cortex 29, 994–1005. doi: 10.1093/cercor/bhy006
Green, P. E., Ridding, M. C., Hill, K. D., Semmler, J. G., Drummond, P. D., and Vallence, A. M. (2018). Supplementary motor area-primary motor cortex facilitation in younger but not older adults. Neurobiol. Aging 64:85. doi: 10.1016/j.neurobiolaging.2017.12.016
Gundel, H., Valet, M., Sorg, C., Huber, D., Zimmer, C., Sprenger, T., et al. (2008). Altered cerebral response to noxious heat stimulation in patients with somatoform pain disorder. Pain 137, 413–421. doi: 10.1016/j.pain.2007.10.003
Hassan, S. F., Cornish, J. L., and Goodchild, A. K. (2013). Respiratory, metabolic and cardiac functions are altered by disinhibition of subregions of the medial prefrontal cortex. J. Physiol. 591, 6069–6088. doi: 10.1113/jphysiol.2013.262071
Hirokawa, J., Bosch, M., Sakata, S., Sakurai, Y., and Yamamori, T. (2008). Functional role of the secondary visual cortex in multisensory facilitation in rats. Neuroscience 153, 1402–1417. doi: 10.1016/j.neuroscience.2008.01.011
Hurley-Gius, K. M., and Neafsey, E. J. (1986). The medial frontal cortex and gastric motility: microstimulation results and their possible significance for the overall pattern of organization of rat frontal and parietal cortex. Brain Res. 365, 241–248. doi: 10.1016/0006-8993(86)91635-5
Hurley, K. M., Herbert, H., Moga, M. M., and Saper, C. B. (1991). Efferent projections of the infralimbic cortex of the rat. J. Comp. Neurol. 308, 249–276. doi: 10.1002/cne.903080210
Katzel, D., Zemelman, B. V., Buetfering, C., Wolfel, M., and Miesenbock, G. (2011). The columnar and laminar organization of inhibitory connections to neocortical excitatory cells. Nat. Neurosci. 14, 100–107. doi: 10.1038/nn.2687
Keene, C. S., and Bucci, D. J. (2008). Neurotoxic lesions of retrosplenial cortex disrupt signaled and unsignaled contextual fear conditioning. Behav. Neurosci. 122, 1070. doi: 10.1037/a0012895
Knierim, J. J., Neunuebel, J. P., and Deshmukh, S. S. (2014). Functional correlates of the lateral and medial entorhinal cortex: objects, path integration and local-global reference frames. Philos. Transac. R. Soc. Lond. B Biol. Sci. 369:20130369. doi: 10.1098/rstb.2013.0369
Krummenacher, P., Candia, V., Folkers, G., Schedlowski, M., and Schonbachler, G. (2010). Prefrontal cortex modulates placebo analgesia. Pain 148, 368–374. doi: 10.1016/j.pain.2009.09.033
Kwon, J. T., Jhang, J., Kim, H. S., Lee, S., and Han, J. H. (2012). Brain region-specific activity patterns after recent or remote memory retrieval of auditory conditioned fear. Learn. Memory 19, 487–494. doi: 10.1101/lm.025502.112
Linnman, C., Appel, L., Furmark, T., Söderlund, A., Gordh, T., Långström, B., et al. (2010). Ventromedial prefrontal neurokinin 1 receptor availability is reduced in chronic pain. Pain 149, 64–70. doi: 10.1016/j.pain.2010.01.008
Liu, P. F., Fang, H. Z., Yang, Y., Zhang, Q. Q., Zhou, Q. Q., Chen, S. S., et al. (2017). Activation of P2X3 receptors in the cerebrospinal fluid-contacting nucleus neurons reduces formalin-induced pain behavior via PAG in a rat model. Neuroscience 358, 93–102. doi: 10.1016/j.neuroscience.2017.06.036
Lu, X., Geng, X., Zhang, L., and Zeng, Y. (2008). The methodology for labeling the distal cerebrospinal fluid-contacting neurons in rats. J. Neurosci. Methods 168, 98–103. doi: 10.1016/j.jneumeth.2007.09.033
Lu, X. F., Li, Y. Y., Wang, C. G., Wei, J. Q., Ye, Y., Zhang, L. C., et al. (2011). Substance P in the cerebrospinal fluid-contacting nucleus contributes to morphine physical dependence in rats. Neurosci. Lett. 488, 188–192. doi: 10.1016/j.neulet.2010.11.026
Manella, L. C., Petersen, N., and Linster, C. (2017). Stimulation of the locus coeruleus modulates signal-to-noise ratio in the olfactory bulb. J. Neurosci. 37, 2026–2017.
McGuire, J. T., and Botvinick, M. M. (2010). Prefrontal cortex, cognitive control, and the registration of decision costs. Proc. Natl. Acad. Sci. USA 107, 7922–7926. doi: 10.1073/pnas.0910662107
Metz, A. E., Yau, H. J., Centeno, M. V., Apkarian, A. V., and Martina, M. (2009). Morphological and functional reorganization of rat medial prefrontal cortex in neuropathic pain. Proc. Natl. Acad. Sci. USA 106, 2423–2428. doi: 10.1073/pnas.0809897106
Morishima, M., Kobayashi, K., Kato, S., Kobayashi, K., and Kawaguchi, Y. (2017). Segregated Excitatory-Inhibitory Recurrent Subnetworks in Layer 5 of the Rat Frontal Cortex. Cereb. Cortex 27, 5846–5857. doi: 10.1093/cercor/bhx276
Mountcastle, V. B. (1997). The columnar organization of the neocortex. Brain 120(Pt 4), 701–722. doi: 10.1093/brain/120.4.701
Neafsey, E. J. (1990). Prefrontal cortical control of the autonomic nervous system: anatomical and physiological observations. Prog. Brain Res. 85, 147–165. doi: 10.1016/s0079-6123(08)62679-5
Nixima, K., Okanoya, K., Ichinohe, N., and Kurotani, T. (2017). Fast voltage-sensitive dye imaging of excitatory and inhibitory synaptic transmission in the rat granular retrosplenial cortex. J. Neurophysiol. 118, 1784–1799. doi: 10.1152/jn.00734.2016
Panteleev, S., and Grundy, D. (2000). Descending influences from the infralimbic cortex on vago-vagal reflex control of gastric motor activity in the rat. Autonom. Neurosci. Basic Clin. 86, 78–83. doi: 10.1016/s1566-0702(00)00249-6
Passetti, F., Dalley, J. W., O’Connell, M. T., Everitt, B. J., and Robbins, T. W. (2000). Increased acetylcholine release in the rat medial prefrontal cortex during performance of a visual attentional task. Eur. J. Neurosci. 12, 3051–3058. doi: 10.1046/j.1460-9568.2000.00183.x
Paxinos, G., and Watson, C. (2007). The rat brain in stereotaxic coordinates, 6th Edn. Cambridge, MA: Academic Press/Elsevier.
Pedersen, L. H., Scheel-Kruger, J., and Blackburn-Munro, G. (2007). Amygdala GABA-A receptor involvement in mediating sensory-discriminative and affective-motivational pain responses in a rat model of peripheral nerve injury. Pain 127, 17–26. doi: 10.1016/j.pain.2006.06.036
Petrovic, P., Kalso, E., Petersson, K. M., Andersson, J., Fransson, P., and Ingvar, M. (2010). A prefrontal non-opioid mechanism in placebo analgesia. Pain 150, 59–65. doi: 10.1016/j.pain.2010.03.011
Posimo, J. M., Titler, A. M., Choi, H. J., Unnithan, A. S., and Leak, R. K. (2013). Neocortex and allocortex respond differentially to cellular stress in vitro and aging in vivo. PLoS One 8:e58596. doi: 10.1371/journal.pone.0058596
Rainville, P., Duncan, G. H., Price, D. D., Carrier, B. T., and Bushnell, M. C. (1997). Pain Affect Encoded in Human Anterior Cingulate But Not Somatosensory Cortex. Science 277, 968–971. doi: 10.1126/science.277.5328.968
Rive, M. M., van Rooijen, G., Veltman, D. J., Phillips, M. L., Schene, A. H., and Ruhe, H. G. (2013). Neural correlates of dysfunctional emotion regulation in major depressive disorder. A systematic review of neuroimaging studies. Neurosci. Biobehav. Rev. 37, 2529–2553. doi: 10.1016/j.neubiorev.2013.07.018
Rolls, E. T. (2016). Functions of the anterior insula in taste, autonomic, and related functions. Brain Cogn. 110, 4–19. doi: 10.1016/j.bandc.2015.07.002
Scheyltjens, I., Vreysen, S., Van den Haute, C., Sabanov, V., Balschun, D., Baekelandt, V., et al. (2018). Transient and localized optogenetic activation of somatostatin-interneurons in mouse visual cortex abolishes long-term cortical plasticity due to vision loss. Brain Struct. Funct. 223, 2073–2095. doi: 10.1007/s00429-018-1611-7
Schmidt, L., Lebreton, M., Clery-Melin, M. L., Daunizeau, J., and Pessiglione, M. (2012). Neural mechanisms underlying motivation of mental versus physical effort. PLoS Biol. 10:e1001266. doi: 10.1371/journal.pbio.1001266
Shackman, A. J., Salomons, T. V., Slagter, H. A., Fox, A. S., Winter, J. J., and Davidson, R. J. (2011). The integration of negative affect, pain and cognitive control in the cingulate cortex. Nat. Rev. Neurosci. 12, 154–167. doi: 10.1038/nrn2994
Shi, Q. X., Yang, L. K., Shi, W. L., Wang, L., Zhou, S. M., Guan, S. Y., et al. (2017). The novel cannabinoid receptor GPR55 mediates anxiolytic-like effects in the medial orbital cortex of mice with acute stress. Mol.Brain 10:38.
Sikes, R. W., Vogt, L. J., and Vogt, B. A. (2008). Distribution and properties of visceral nociceptive neurons in rabbit cingulate cortex. Pain 135, 160–174. doi: 10.1016/j.pain.2007.09.024
Song, S.-Y., Li, Y.-H., Bao, C.-Y., Li, Y., Yin, P.-C., Hong, J., et al. (2019). Stereotaxic Coordinates and Morphological Characterization of a Unique Nucleus (CSF-Contacting Nucleus) in Rat. Front.Neuroanat. 13:47. doi: 10.3389/fnana.2019.00047
Song, S. Y., Li, Y., Zhai, X. M., Li, Y. H., Bao, C. Y., Shan, C. J., et al. (2020a). Connection Input Mapping and 3D Reconstruction of the Brainstem and Spinal Cord Projections to the CSF-Contacting Nucleus. Front. Neural Circuits 14:11. doi: 10.3389/fncir.2020.00011
Song, S. Y., Li, Y., Zhai, X. M., Li, Y. H., Bao, C. Y., Shan, C. J., et al. (2020b). Monosynaptic Input Mapping of Diencephalic Projections to the Cerebrospinal Fluid-Contacting Nucleus in the Rat. Front.Neuroanat. 14:7. doi: 10.3389/fnana.2020.00007
Song, S. Y., and Zhang, L. C. (2018). The Establishment of a CSF-Contacting Nucleus “Knockout” Model Animal. Front. Neuroanat. 12:22. doi: 10.3389/fnana.2018.00022
Squire, L. R., and Zola-Morgan, S. (1991). The medial temporal lobe memory system. Science 253, 1380–1386. doi: 10.1126/science.1896849
Sticht, M. A., Limebeer, C. L., Rafla, B. R., and Parker, L. A. (2015). Intra-visceral insular cortex 2-arachidonoylglycerol, but not N-arachidonoylethanolamide, suppresses acute nausea-induced conditioned gaping in rats. Neuroscience 286, 338–344. doi: 10.1016/j.neuroscience.2014.11.058
Sugar, J., Witter, M. P., van Strien, N. M., and Cappaert, N. L. (2011). The retrosplenial cortex: intrinsic connectivity and connections with the (para)hippocampal region in the rat. An interactive connectome. Front.Neuroinform. 5:7. doi: 10.3389/fninf.2011.00007
Treadway, M. T., Buckholtz, J. W., Cowan, R. L., Woodward, N. D., Li, R., Ansari, M. S., et al. (2012). Dopaminergic mechanisms of individual differences in human effort-based decision-making. J.Neurosci. 32, 6170–6176. doi: 10.1523/jneurosci.6459-11.2012
Tsuji, C., Tsuji, T., Allchorne, A., Leng, G., and Ludwig, M. (2017). Effects of lateral olfactory tract stimulation on Fos immunoreactivity in vasopressin neurons of the rat piriform cortex. J. Neuroendocrinol. 29:12531
Vidal-Gonzalez, I., Vidal-Gonzalez, B., Rauch, S. L., and Quirk, G. J. (2006). Microstimulation reveals opposing influences of prelimbic and infralimbic cortex on the expression of conditioned fear. Learn. Memory 13, 728–733. doi: 10.1101/lm.306106
Wang, M. S., and Zhang, L. C. (1992). A comparison of HRP vs CB-HRP for labelling CSF contacting neurons. Acta Acad. Med. 12, 286–288.
Wang, X. Y., Yan, W. W., Zhang, X. L., Liu, H., and Zhang, L. C. (2014). ASIC3 in the cerebrospinal fluid-contacting nucleus of brain parenchyma contributes to inflammatory pain in rats. Neurol. Res. 36, 270–275. doi: 10.1179/1743132813y.0000000297
Wang, Z., Bradesi, S., Maarek, J. M., Lee, K., Winchester, W. J., Mayer, E. A., et al. (2008). Regional brain activation in conscious, nonrestrained rats in response to noxious visceral stimulation. Pain 138, 233–243. doi: 10.1016/j.pain.2008.04.018
Watabe-Uchida, M., Zhu, L., Ogawa, S. K., Vamanrao, A., and Uchida, N. (2012). Whole-brain mapping of direct inputs to midbrain dopamine neurons. Neuron 74, 858–873. doi: 10.1016/j.neuron.2012.03.017
Wei, F., and Zhuo, M. (2008). Activation of Erk in the anterior cingulate cortex during the induction and expression of chronic pain. Mole. Pain 4:28.
Wei, P., Bao, R., Lv, Z., and Jing, B. (2018). Weak but Critical Links between Primary Somatosensory Centers and Motor Cortex during Movement. Front. Hum. Neurosci. 12:1. doi: 10.3389/fnhum.2018.00001
Wu, X., Gao, J., Yan, J., Fan, J., Owyang, C., and Li, Y. (2008). Role for NMDA receptors in visceral nociceptive transmission in the anterior cingulate cortex of viscerally hypersensitive rats. Am. J. Physiol. 294, 918–927.
Wu, Y. H., Song, S. Y., Liu, H., Xing, D., Wang, X., Fei, Y., et al. (2015). Role of adrenomedullin in the cerebrospinal fluid-contacting nucleus in the modulation of immobilization stress. Neuropeptides 51, 43–54. doi: 10.1016/j.npep.2015.03.007
Xing, D., Wu, Y., Li, G., Song, S., Liu, Y., Liu, H., et al. (2015). Role of cerebrospinal fluid-contacting nucleus in sodium sensing and sodium appetite. Physiol. Behav. 147, 291–299. doi: 10.1016/j.physbeh.2015.04.034
Xu, H., Wu, L. J., Wang, H., Zhang, X., Vadakkan, K. I., Kim, S. S., et al. (2008). Presynaptic and postsynaptic amplifications of neuropathic pain in the anterior cingulate cortex. J. Neurosci. 28, 7445–7453. doi: 10.1523/jneurosci.1812-08.2008
Zhang, L. C., Wang, M., and Zhang, F. (1994). A tracing study on neuron contacting cerebrospinal fluid in rat. Chin. J. Anat. 1, 37–40.
Zhang, L. C., Zeng, Y. M., Ting, J., Cao, J. P., and Wang, M. S. (2003). The distributions and signaling directions of the cerebrospinal fluid contacting neurons in the parenchyma of a rat brain. Brain Res. 989, 1–8. doi: 10.1016/s0006-8993(03)03123-8
Zhang, X., Cao, B., Yan, N., Liu, J., Wang, J., Tung, V. O., et al. (2013). Vagus nerve stimulation modulates visceral pain-related affective memory. Behav. Brain Res. 236, 8–15. doi: 10.1016/j.bbr.2012.08.027
Keywords: CSF-contacting nucleus, projection, cerebral cortex, retrograde trace, 3D reconstruction
Citation: Song S-Y, Zhai X-M, Dai J-H, Lu L-L, Shan C-J, Hong J, Cao J-L and Zhang L-C (2020) The CSF-Contacting Nucleus Receives Anatomical Inputs From the Cerebral Cortex: A Combination of Retrograde Tracing and 3D Reconstruction Study in Rat. Front. Neuroanat. 14:600555. doi: 10.3389/fnana.2020.600555
Received: 30 August 2020; Accepted: 22 October 2020;
Published: 20 November 2020.
Edited by:
Yun-Qing Li, Fourth Military Medical University, ChinaReviewed by:
Weifeng Yu, Shanghai Jiao Tong University School of Medicine, ChinaCopyright © 2020 Song, Zhai, Dai, Lu, Shan, Hong, Cao and Zhang. This is an open-access article distributed under the terms of the Creative Commons Attribution License (CC BY). The use, distribution or reproduction in other forums is permitted, provided the original author(s) and the copyright owner(s) are credited and that the original publication in this journal is cited, in accordance with accepted academic practice. No use, distribution or reproduction is permitted which does not comply with these terms.
*Correspondence: Li-Cai Zhang, bGljYWl6aGFuZzAwMUAxNjMuY29t
Disclaimer: All claims expressed in this article are solely those of the authors and do not necessarily represent those of their affiliated organizations, or those of the publisher, the editors and the reviewers. Any product that may be evaluated in this article or claim that may be made by its manufacturer is not guaranteed or endorsed by the publisher.
Research integrity at Frontiers
Learn more about the work of our research integrity team to safeguard the quality of each article we publish.