- 1Department of Molecular and Cellular Mechanisms of Neurodegeneration, Paul Flechsig Institute for Brain Research, University of Leipzig, Leipzig, Germany
- 2Department of Neuropathology, University of Leipzig, Leipzig, Germany
Reversible formation of PHF-like phosphorylated tau, an early feature of Alzheimer’s disease (AD) was previously shown to occur in torpor during hibernation in the Golden hamster (Syrian hamster, Mesocricetus auratus). Here, we tackled the question to what extent hibernating Golden hamsters can serve as a model for the early stage of AD. During early AD, anosmia, the loss of olfactory function, is a common and typical feature. We, thus, investigated tau phosphorylation, synaptic plasticity and behavioral physiology of the olfactory system during hibernation. Tau was phosphorylated on several AD-relevant epitopes, and distribution of PHF-like phosphorylated tau in the olfactory bulb was quite similar to what is seen in AD. Tau phosphorylation was not associated with a destabilization of microtubules and did not lead to fibril formation. Previously, we observed a transient spine reduction in pyramidal cells in the hippocampus, which is correlated with the distribution of phosphorylated tau. Here we show that granule cells in the olfactory bulb are devoid of phosphorylated tau and maintain their spines number during torpor. No reduction of synaptic proteins was observed. However, hibernation did impair the recall performance in a two-odor discrimination task. We conclude that hibernation is associated with a specific olfactory memory deficit, which might not be attributed to the formation of PHF-like phosphorylated tau within the olfactory bulb. We discuss a possible involvement of modulatory input provided by cholinergic neurons in the basal forebrain, which are affected by hibernation.
Introduction
The microtubule-associated protein tau promotes polymerization (Weingarten et al., 1975), stabilizes existing microtubules (Drechsel et al., 1992), organizes them into bundles (Elie et al., 2015) and mediates interaction of microtubules with filamentous actin (Biswas and Kalil, 2017; Cabrales Fontela et al., 2017). Phosphorylation of serine and threonine residues modulates these function and leads to a detachment of tau from microtubules. Whereas dephosphorylated tau is present in the axon, its phosphorylation results in its accumulation in the somatodendritic and subsynaptic compartment (Lindwall and Cole, 1984; Biernat et al., 1993; Buée et al., 2000; Morris et al., 2011). In neurodegenerative diseases commonly referred to as “tauopathies” the accumulation of hyper-phosphorylated tau leads to the formation of paired helical filaments (PHF), the structural subunits of neurofibrillary tangles and other aggregates (Avila, 2000; Shahani and Brandt, 2002; Brandt et al., 2005; Avila et al., 2008; Goedert and Spillantini, 2011). Long before the formation of PHFs and subsequent neuronal degeneration, the presence of hyperphosphorylated tau in the post-synaptic compartments leads to the deterioration of synaptic plasticity and cognitive decline (Yoshiyama et al., 2007; Hoover et al., 2010; Ittner et al., 2010; Morris et al., 2011). This is supported by the presence of tau aggregates in dendritic spines (Blazquez-Llorca et al., 2011) at very early stages of Alzheimer’s disease.
Paired helical filaments-like hyperphosphorylation of tau is not restricted to neurodegeneration, but occurs under physiologically controlled conditions such as hibernation (Arendt et al., 2003; Härtig et al., 2007; Stieler et al., 2009, 2011; Avila et al., 2012; Bullmann et al., 2016). Subsequent anatomical and biochemical studies on different hibernating species revealed a profound synaptic regression in cortex, hippocampus and thalamus (Popov and Bocharova, 1992; Arendt et al., 2003; von der Ohe et al., 2006, 2007). The likely consequence of synaptic changes is an impairment of cognitive functions, such as learning and memory, that has been studied using different learning paradigms in various species (McNamara and Riedesel, 1973; Millesi et al., 2001; Zhao et al., 2004; Clemens et al., 2009; Bullmann et al., 2016); for a review see (Arendt and Bullmann, 2013). However, the outcome of these studies revealed a dependency on species-specific preparedness (Bullmann et al., 2016). Under laboratory conditions, we observed a preservation of spatial memory following hibernation in golden hamsters (Bullmann et al., 2016), while an impairment was observed in European ground squirrels (Millesi et al., 2001). In their natural habitat, however, this two species employ different overwintering strategies and favor specific modalities to be preserved. The Golden hamster is strictly solitary and digs rather complex burrows to store the horded food for the hibernation period (Gattermann et al., 2001). In contrast, groups of European ground squirrel cuddle in a single nest, relying on body fat instead of stockpiled food as energy resource (Hut and Scharff, 1998).
Therefore, European ground squirrels must recognize conspecifics while the impairment of spatial memory (Millesi et al., 2001) does not impact survival. Contrary, the Golden hamster needs to preserve spatial memory but not necessarily social recognition. For regulating social interactions hamsters almost exclusively use olfactory cues in Johnston (1990), Petrulis (2009). Both main olfactory system (MOS) and the accessory or vomeronasal system (AOS) process social cues (Meredith, 1991). Both systems have separate sensory neurons and segregated representations in the olfactory bulbs. The sensory neurons of the MOS detect primarily volatile chemicals and make synapses with dendrites of mitral/tufted cell within the glomeruli of main olfactory bulb. Processing of olfactory information from the periphery to central brain structures occurs along a neuronal network, consisting of several well defined relays (Barkai and Saar, 2001; Wilson et al., 2006). This includes layer II pyramidal cells in the piriform cortex (Knafo et al., 2001) as well as hippocampal CA3 pyramidal cells (Hess et al., 1995), CA1 pyramidal cells (Knafo et al., 2004) and the prefrontal cortex (Alvarez and Eichenbaum, 2002). Already within the olfactory bulb, the lateral inhibition (Lledo and Lagier, 2006) by the reciprocal connections between mitral and granule cells increase discrimination of odors (Mori et al., 1999). Rodents have a highly effective olfactory modality and show rapid acquisition and sustained recall of odor discrimination (Slotnick and Katz, 1974; Schellinck et al., 2001; Friedrich, 2006).
Therefore, we combined behavioral, biochemical and morphological analyses to test whether hibernation induced phosphorylation of tau in the main olfactory bulb is associated with synapse regression and affects odor discrimination and recognition memory. Previously, we presented preliminary results in abstract form at a conference (Bullmann et al., 2009).
Materials and Methods
Animals
Male and female Golden hamsters (Syrian hamsters, Mesocricetus auratus) purchased from Harlan Winkelmann GmbH (Borchen, Germany) were bred and housed at the Medizinisch Technisches Zentrum of the Medical Faculty of the University of Leipzig. Animals had free access to food and water and were maintained on an artificial 12:12 h light-dark cycle under conditions of constant temperature (22°C) and humidity. All experimental procedures on animals were carried out in accordance with the European Council Directive of 24 November 1986 (86/609/EEC) and had been approved by the local authorities. All efforts were made to minimize the number of animals used and their suffering.
A total of 76 animals were subjected to hibernation conditions (Ueda and Ibuka, 1995) as described before (Bullmann et al., 2016). Briefly, they were maintained in an animal incubator (8:16 h light-dark cycle; 23–26°C) for four to 8 weeks and then in the cold room (4:20 h light-dark cycle; 5–7°C). General locomotor activity was monitored with custom build infrared detectors mounted on top of each cage allowing the discrimination between euthermic phases and torpor. Hibernating animals show torpor phases (over 24 h of inactivity) whereas non-hibernating euthermic animals did not. For sampling hamsters were allocated to one of five groups depending on their time of inactivity after an arousal episode as torpor early (TE; 8 h of inactivity) or torpor late (TL; 36–48 h of inactivity), according to the time after induction of arousal as arousal early (AE; 2.5 h) and arousal late (AL, 24–36 h), and those did not show torpor as euthermic (EU).
Sample preparation was performed as previously described (Bullmann et al., 2016): Animals were killed by CO2, followed by transcardial perfusion with physiological saline followed by 4% paraformaldehyde and 0.1% glutaraldehyde in phosphate buffered saline (PBS). After immersion for 2 days in 30% sucrose in PBS, brains where cut into 30 μm thick coronal sections and stored at 4°C in PBS with 0.01% sodium azide added as a preservative. For western blotting brains were dissected, samples were shock frozen in liquid nitrogen, and stored at -80°C.
Human Cases
For each case (Table 1), one olfactory bulb and a block containing the hippocampus and entorhinal cortex were fixed for 2 weeks in neutral formalin and immersed in 30% sucrose in phosphate buffered saline (PBS) with sodium azide added as a preservative. Afterward 30 μm thick frozen sections were cut and stored in PBS with sodium azide at 4°C.
All cases were neuropathologically assessed for neurofibrillary tangle stage (Braak and Braak, 1991; Braak et al., 2006), for amyloid beta/amyloid plaque score (Thal et al., 2002) and for neuritic plaque score according to CERAD (Gearing et al., 1995).
The diagnosis of Alzheimer’s disease (AD) was made on the basis of both clinical and neuropathological evidence according to the criteria of the International Working Group (IWG) for New Research Criteria for the diagnosis of AD in the revision of 2014 (IWG-2) (Dubois et al., 2014), the NIA-AA diagnostic criteria in the revision of 2011 (Albert et al., 2011) and the NIA-AA guidelines for the neuropathological assessment of AD (Mirra et al., 1991).
Immunohistochemistry
Immunohistochemical processing was performed as described before (Arendt et al., 2003; Bullmann et al., 2007, 2010, 2016; Härtig et al., 2007). Briefly, free-floating sections were rinsed in PBS, and incubated 30 min in 0.3% hydrogen peroxide in PBS (phosphate buffered saline). As from now TBS (Tris buffered saline: 100 mM NaCl, 50 mM Tris/HCl pH 7.4) was used with Triton X-100 added at 0.01% for rinsing and 0.1% for antibody incubation. Sections were rinsed, incubated 60 min in 5% normal donkey serum (Amersham), and primary antibodies where applied in 1% normal donkey serum overnight (see Table 2). For immunofluorescent labeling, sections were rinsed, incubated for 2 h with appropriate carbocyanine 2 (Cy2) and carbocyanine 3 (Cy3) conjugated secondary antibodies. The selected donkey antibodies recognizing goat, rabbit or mouse IgG, 1:1000 (Jackson Immuno Research, West Grove, PA, United States) were affinity-purified and showed minimal cross-reactivity. Sections were then rinsed, mounted, dehydrated, cleared, and coverslipped with Entellan. For immunoperoxidase labeling, sections were rinsed, incubated for 2 h with the appropriate biotin-conjugated secondary antibody. The selected donkey antibodies recognizing rabbit or mouse IgG, 1:1000 (Jackson Immuno Research, West Grove, PA, United States) were affinity-purified and showed minimal cross-reactivity. Afterward, sections were rinsed, incubated for 45 min with ExtrAvidin (1:1,000; Sigma), rinsed, and developed with nickel-enhanced diaminobenzidine, mounted, dehydrated, cleared, and coverslipped with Entellan.
Western Blots
Western blots were performed as previously described (Arendt et al., 2003; Härtig et al., 2005; Stieler et al., 2011; Bullmann et al., 2016). Briefly, tissue samples were homogenized in tenfold volume of ice cold buffer A (20 mM Tris–HCL, pH 7.2; 150 mM NaCl; 2 mM MgCl2; 2 mM EDTA; 2 mM EGTA; 5 mM NaF; 1 mM Na3VO4; 5 % glycerol) supplemented with protease inhibitors (1 mM PMSF; 1 μg/ml leupeptin; Complete protease inhibitor cocktail from Roche). Homogenates were centrifuged (50,000 × g, 30 min, 4°C), and supernatants (“cytosolic fraction”) were transferred into new tubes. Pellets were suspended in buffer B (buffer A + 0.5% Triton X-100) supplemented with protease inhibitors (see above), centrifuged again and supernatants (“membrane fraction”) were transferred into new tubes.
Protein samples in Laemmli buffer of 20 μg protein per lane were separated on a 10% polyacrylamide gel by SDS-PAGE and transferred to a PVDF membrane. After rinsing with TBS-Tw (100 mM NaCl, 50 mM Tris/HCl pH 7.4, 0.05% Tween 20) and blocking (1% w/v bovine serum albumin in TBS-Tw) they were incubated overnight with primary antibodies (Table 3) in blocking buffer. After rinsing they were incubated 2 h with the appropriate horseradish peroxidase conjugated secondary antibodies (GE Healthcare; 1:10,000 sheep-anti-Mouse IgG, NA931V; 1:10,000 donkey-anti-Rabbit IgG, NA934V) and rinsed. Immunoreactivity was determined by enhanced chemoluminescence (0.23 mg/ml Luminol; 0.1 mg/ml p-coumaric acid and 0.6 mg/ml sodium perborate in 0.1 M Tris–HCL, pH 8.6), acquired with a Kodak Image Station 2000R, and quantified by densitometric analysis using TINA (version 2.09 g, 1993, raytest Isotopenmeßgeräte GmbH). The expression level was set to 1 for the euthermic group, by dividing the arbitrary fluorescence units with the median of the respective euthermic group. Simple measurements were shown as dots and a line connects the median for each group (see Figure 2). For statistic testing these expression levels were log10-transformed in order to obtain normality of their distribution. In case of the six phosphorylation-dependent antibodies a MANOVA was performed before assessing the results for each individual antibody by separate ANOVA and Tukey’s HSD test. Preliminary experiments had shown that the blot and detection efficiency decreases toward the edges of the blot. In order to prevent a bias, we therefore applied the protein samples cyclically according to their state (EU>TE>TL>AE>AL) from the left to the right. Furthermore, the spatial difference in the blot and detection efficiency was then controlled in ANOVA by using the position on the blot (the cycle number WBCYCLE) as a blocking factor. If ANOVA showed significant influence of the physiological state, the fit was used for the post hoc Tukey’s HSD test for comparison between the different states.
Antibodies
For summary see Tables 1, 2. Tau phosphorylation was studied by phosphorylation-dependent tau antibodies in hibernating animals including European ground squirrels [Spermophilus parryii: (Arendt et al., 2003)], Golden hamster [Mesocricetus auratus: (Härtig et al., 2005; Stieler et al., 2011; Bullmann et al., 2016)], arctic ground squirrel [Spermophilus citellus: (Stieler et al., 2011)] and black bear [Ursus americanus: (Stieler et al., 2011)]. Again, these antibodies will be used to determine the phosphorylation of Golden hamster tau. Microtubule stability was assessed by immunohistochemical detection of α-tubulin and its acetylation, because it is known that tubulin monomers with this modification are enriched in stable microtubules (Janke and Montagnac, 2017). Furthermore, transfection with microtubule-associated proteins MAP1B, MAP2 or tau increase both microtubule stability and tubulin acetylation (Takemura et al., 1992). Synaptic proteins were measured by antibodies directed against synaptophysin (present in all pre-synapses) (Calhoun et al., 1996; Tarsa and Goda, 2002; Strijkstra et al., 2003), gephyrin (present in GABAergic post-synapses) (Peden et al., 2008) as well as VGluT1 (glutamatergic pre-synapses) (Härtig et al., 2003; Graziano et al., 2008) and PSD95 (glutamatergic post-synapses) (Mondragón-Rodríguez et al., 2012; Nair et al., 2013).
Golgi Impregnation and Spine Measurements
Golgi impregnation of neurons was performed according to the Golgi-Bubenaite method (Bubenaite, 1929) as previously described (Bullmann et al., 2016). Briefly, brains were fixed by transcardial perfusion with 4% paraformaldehyde and 0.1% glutaraldehyde in phosphate buffered saline (PBS), olfactory bulb samples were immersed for one day in 2.5% potassium dichromate, one day in 3.5% potassium dichromate at 37°C, washed in 2% silver nitrate and incubated in the dark for 2 days in 2% silver nitrate at 37°C. Afterward samples were dehydrated and embedded in celloidin. Sections of 100 μm thickness were cut, mounted onto glass slides and coverslipped with Canada balsam.
In the main olfactory bulb, only GABAergic granule cells possess spines, which are part of the bidirectional dendrodendritic synapses with the Glutamatergic mitral cells. Blind measurements were performed on the spine density of single granule cell dendrites within the external plexiform layer of the olfactory bulb; short straight sections within one focal plane selected and traced using the NeurolucidaTM system (MicroBrightField, Inc.) at highest magnification (100×, immersion lens). The spine density (spines/μm) was log10-transformed in order to obtain normality of their distribution. The influence of physiological state on spine density was assessed by a nested ANOVA, where the single measurements were nested within each animal.
Olfactory Discrimination Task
Olfactory discrimination task was performed similar as described for mice (Schellinck, 2001). The test apparatus was constructed from a large housing cage divided into three equal compartments by two pieces of acrylic that had openings of 6 cm diameter that could be closed by sliding doors made of acrylic. Therefore, animals could be placed in the middle compartment and after opening of the doors, they could freely move from one compartment to another. Phenyl acetate (Sigma Aldrich, “rose”) and Linalool (Sigma Aldrich, “lemon”) where diluted to a concentration of 15% in mineral oil (Sigma Aldrich). The odors were the same as in the original procedure (Schellinck et al., 2001). Linalool is a naturally occurring terpene alcohol found in many flowers and spice plants, whereas phenyl acetate is an aromatic fatty acid metabolite of phenylalanine naturally occurring in mammals. They have been selected for having a different chemical structure, possess distinct smells and bind to different subsets of olfactory receptors and activate olfactory receptor neurons of the clusters B and C, respectively (Mori et al., 2006). A volume of 50 μl of either lemon or rose odor were pipetted onto filter paper, placed in petri dishes with holes in the top, and buried with pine chips each in one of the side chambers. The animal was placed in the middle chamber and the sliding doors were opened after 5 min of habituation. The behavior was then recorded for 30 min by a small IR-camera module (191710-62, Conrad Electronics, Germany) connected to a recorder and analyzed off-line. During testing, the three chamber apparatus was covered by a mesh top and was placed under a fume hood.
One week prior training animals were habituated to the test procedure. During the initial habituation we decided to not present any of the two odors, because after such initial presentation, the animal recognizes both them as an unrewarded cue and the subsequent training will elicit a weaker conditioned response. This is sometimes referred to as negative priming (Wagner, 1981). Animals were placed in new cages with fresh beddings at lights on, and 2 h after lights off habituation to the test procedure started as described above, but without odors in the petri dishes. Later animals were placed back into their home cages with free access to food and water.
After 2 months under short day conditions, 2 weeks before transfer to the cold room, animals were trained. One half of the hamsters received rose paired with food reward (three sunflower seeds) and lemon alone; the other half received lemon paired with food reward and rose alone. The choice of sunflower over the original sugar reward (Schellinck et al., 2001)seed was motivated by a study on suitable food rewards in learning and memory test in hamsters (Shettleworth, 1978) and we have used them successfully in a previous behavioral study of memory in hibernating hamsters (Bullmann et al., 2016). Animals were placed in cages without food at lights on, training started at 2 h after lights off and an hour after training animals were allowed free access to food. In two subsequent weeks, subjects were trained on four consecutive days and received four trials per day, which consisted of alternating trials of rose and lemon, resulting in a total of 16 trials for each CS+ and CS-. As described in the original procedure, in each trial only one odor was presented to the animal (see Supplementary Figure S2).
After training animals were transfer to the cold room and continuously monitored for hibernation behavior (see section 2.1). After half of the animals had entered hibernation, all animals were removed from the cold room and maintained under short day conditions with 25°C room temperature and free access to food and water for 2 days before testing. A total of 35 hamsters were tested, 17 hibernating and 18 non-hibernating. After final testing, all animals were transferred to the cold room, and approximately 90% entered hibernation afterward.
The behavior in the test chamber was measured using the timestamp from the recorded video. The preference for CS+ over CS- (conditioned stimulus with and without food reward, respectively) odor was measured by converting the time spend in the side-chambers to a preference index P. P was calculated (Kaut et al., 2003) according to the following formula: , where x is the amount of time spent in the CS+ chamber, and y is the amount of time spent in the CS- chamber. An index of P = +1 indicates a perfect preference for CS+, an index of P = -1 indicates a perfect preference for CS- whereas an index of 0 reflect chance performance. This preference index was used for the box-whisker plot in Figure 1C.
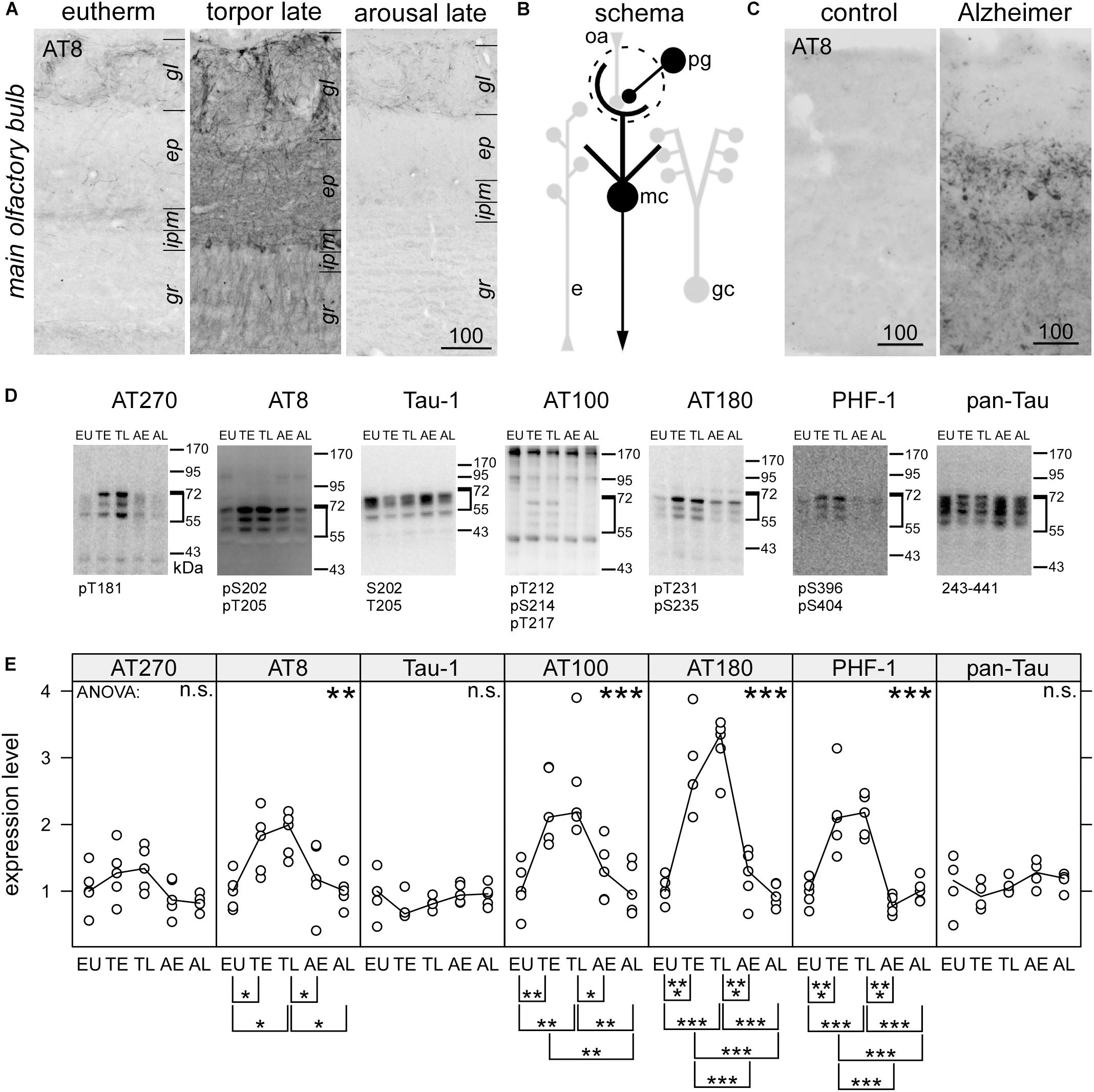
Figure 1. Immunohistochemical detection of phospho-tau by the monoclonal antibody AT8in the olfactory bulb of hamsters during hibernation (A) and humans (C). For the human cases, higher magnification images can be found in the Supplementary Figure S1. Legend: gl, glomerular layer; ep, external plexiform layer; m, mitral cell layer; gr, granule cell; lm, stratum lacunosum moleculare; r, stratum radiatum; p, pyramidal cell layer; o, stratum oriens. Scale bars in μm. A schematic description of the distribution of phosphorylated tau is shown for olfactory bulb (B). Structures containing phosphorylated tau are drawn black whereas those devoid of phosphorylated tau are depicted in light gray. Within the olfactory bulb, olfactory receptor cell axons (oa) are forming synapses to the apical dendritic tuft of mitral cells, which project outside the olfactory bulb. Activity of periglomerular cells (pg), lateral inhibition by granule cells (gc) and efferent modulatory input (e) influence this transmission. Phosphorylation of tau protein in the olfactory bulb was compared in euthermic animals (EU), early torpor (ET), late torpor (TL), and after early arousal (AE) and late arousal (AL). Immunoblots (D) were probed for the presence of specific PHF-like tau-phospho-epitopes (AT 270; AT8; AT 100; AT180; PHF-1), for tau, unphosphorylated at Ser198, Ser199, and Ser202 (Tau-1) and total tau (pan-tau). ANOVA showed significant differences after quantification (E)for AT8 (p = 0.017), AT100 (p = 0.00032), AT180 (p = 2.5e-08), and PHF-1 (p = 6.5e-08), but not for AT270 (p = 0.096), Tau-1 (p = 0.34), and pan-Tau (p = 0.21). The antibody epitope is indicated below each blot. Inside the diagrams the following significance code for p-values for ANOVA are shown: 0 < “∗∗∗” < 0.001 < “∗∗” < 0.01 < “∗” 0.05 < “n.s.”. The significance codes for p-values for the post hoc Tukey’s HSD test are shown below the diagrams.
Additionally, we assessed the motivational state by measuring the exploratory behavior. From the total time spend in the side-chambers during the 30 min test period, an exploratory index E was calculated by the following formula: , where z is the amount of time spent in the middle chamber. An index of 0 reflects that the hamster did not leave the starting chamber, whereas an higher index indicates more exploratory behavior. The preference index and exploratory index were used for the box-whisker plots in Figure 4.
Before the analysis of variance (ANOVA), both indices were Logit-transformed: and . This transformation then yields normally distributed data LP and LE for the relative quantities P and E, which presumably show a binomial distribution. We assumed that the animals might show preference not only for the conditioned over the unconditioned stimulus, but also for one of the odors or one of the side chambers. Furthermore, the performance might also depend on subtle differences during the training of the four cohorts. In this case a randomized block design should be used (Festing and Altman, 2002). Therefore, the ANOVA for LP included the factor HIBERNATION and the three blocking factors ODOR, SIDE, and COHORT. HIBERNATION encoded whether the animal showed at least one torpor episode (yes or no). ODOR and SIDE indicated the odor (rose or lemon) used as the CS+ and the side chamber, which contained the CS+ during the test (left or right). COHORT was a categorical variable with four levels. For the LE the P-values for the difference were taken from the ANOVA with COHORT as the only blocking factor. The raw data used for the statistical analysis of the two odor discrimination task can be found in Supplementary Table S1.
Software for Data, Statistics, Diagrams, Figures
Data were organized and stored using Excel and OpenOffice. R1 (R Development Core Team, 2011) was used for statistics and with the R package lattice2 (Sarkar, 2008) also for diagrams. Final figures were arranged using Canvas X (Deneba Systems). The following significance code for p-values were used: 0 < “∗∗∗” < 0.001 < “∗∗” < 0.01 < “∗”0.05 < “n.s.”.
Results
Phosphorylation of Tau
Both the olfactory bulb and hippocampus have been implicated in the formation and consolidation of olfactory memory as “peripheral” and “central” relays along the pathway for processing olfactory information (Hess et al., 1995; Lledo and Lagier, 2006). While phosphorylation of tau in the hippocampus of hibernating hamsters was documented recently (Stieler et al., 2011; Bullmann et al., 2016), it has not been assessed in the olfactory bulb before.
Using Western blots and immunocytochemistry we observed a profound tau phosphorylation in the olfactory bulb (Figures 1, 2). Western blots of olfactory bulb samples were probed with the following antibodies recognizing different epitopes consisting of phosphorylated serine (pS) and threonine (pT) residues or a combination of several epitopes (Figure 1D): AT270 (pT181), AT8 (pS202+pT205), AT180 (pT231+pS235), PHF-1 (pS396+pS404), and AT100 (pT212+pS214+pT217). The majority of antibodies showed a significant increase in phosphorylation at their respective epitopes during torpor, which was fully reversible after arousal (Figure 1E). Accordingly, a slightly retarded gel migration was observed during torpor using the pan-tau antibody (Figure 1D, rightmost panel). Conversely, dephosphorylation of tau protein after arousal was revealed by monoclonal antibody tau-1, which binds if both S202 and T205 are unphosphorylated.
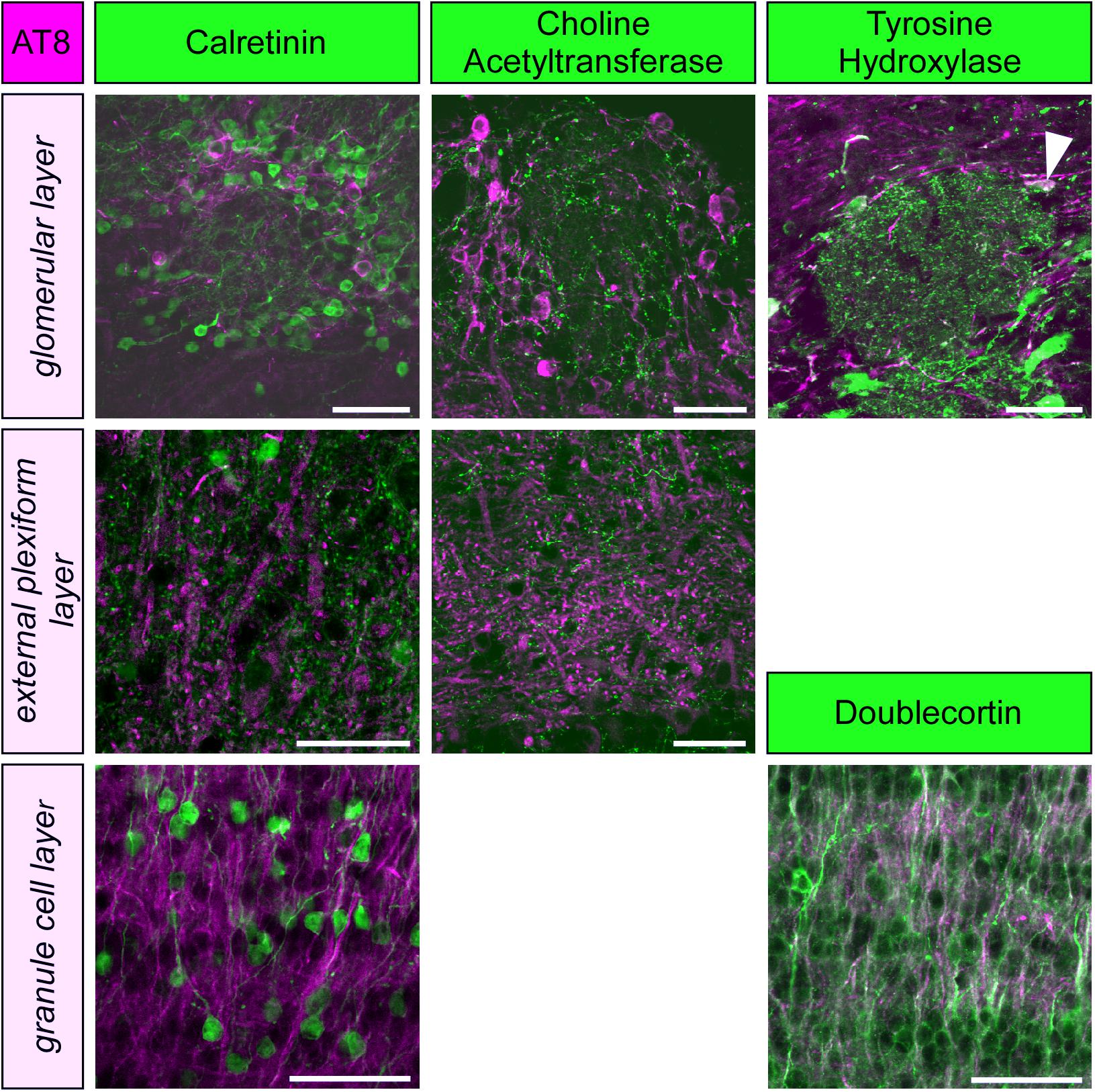
Figure 2. Phospho-tau, detected by the monoclonal antibody AT8 is not co-localized with markers for periglomerular neurons (calretinin, choline acetyltransferase, tyrosine hydroxylase) or granule cells (calretinin, doublecortin) within the olfactory bulb, except for a few tyrosine hydroxylase containing periglomerular neurons (arrowhead). Scale bars: 50 μm.
The epitope pS202+pT205, recognized by the monoclonal antibody AT8, is also found at very early, pre-tangle stages of Alzheimer’s disease (Braak and Braak, 1991). When we mapped the distribution of PHF-like hyperphosphorylated tau by immunohistochemistry using the AT8 antibody we observed an intense staining in the human olfactory bulb (Figure 1C) in cases with Alzheimer’s disease (N = 3) but not in control the case (N = 1).
Increased immunoreactivity for AT8 during torpor has previously also been found in both obligatory and facultative hibernators (Arendt et al., 2003; Härtig et al., 2007; Bullmann et al., 2016; Stieler et al., 2009, 2011). Within the olfactory bulb of torpid hamsters, a particularly strong labeling was seen in periglomerular cells and the neurites within the glomeruli (Figure 1A). Somewhat weaker reactivity was present in cell bodies of the mitral cell layer and in neurites within the external plexiform layer and the granule cell layer (summarized in Figure 1B). After arousal, immunoreactivity for AT8 vanished rapidly and returned to levels seen in euthermic animals.
To further specify the cellular identity of cells containing PHF-like phosphorylated tau, we performed double labeling with molecular markers of granule cells, periglomerular neurons and cholinergic efferent projections. Phosphorylated tau did not co-localize with any of these markers, and only a small fraction of dopaminergic periglomerular neurons contained phosphorylated tau (Figure 2). Therefore, it can be concluded that phosphorylated tau is mainly present in cell bodies, dendrites and axons of mitral cells.
Spines Density and Synaptic Proteins
Previously, apical dendrites hippocampal CA3 pyramidal neurons were affected much stronger by the accumulation of phosphorylated tau than basal dendrites, and this was correlated with a larger spine regression (Bullmann et al., 2016). Therefore, we analyzed the spine density on the dendrites of granule cells, which do not show accumulation of phosphorylated tau. Olfactory bulb granule cell dendrites were identified by their morphology and localization within the external plexiform layer (Figure 3A). Reconstructions were made from branches containing at least ten large spines (Figure 3B). Consistent with the absence of phosphorylated tau in granule cell dendrites, their spine density remained unaffected during hibernation (Figure 3C, left panel).
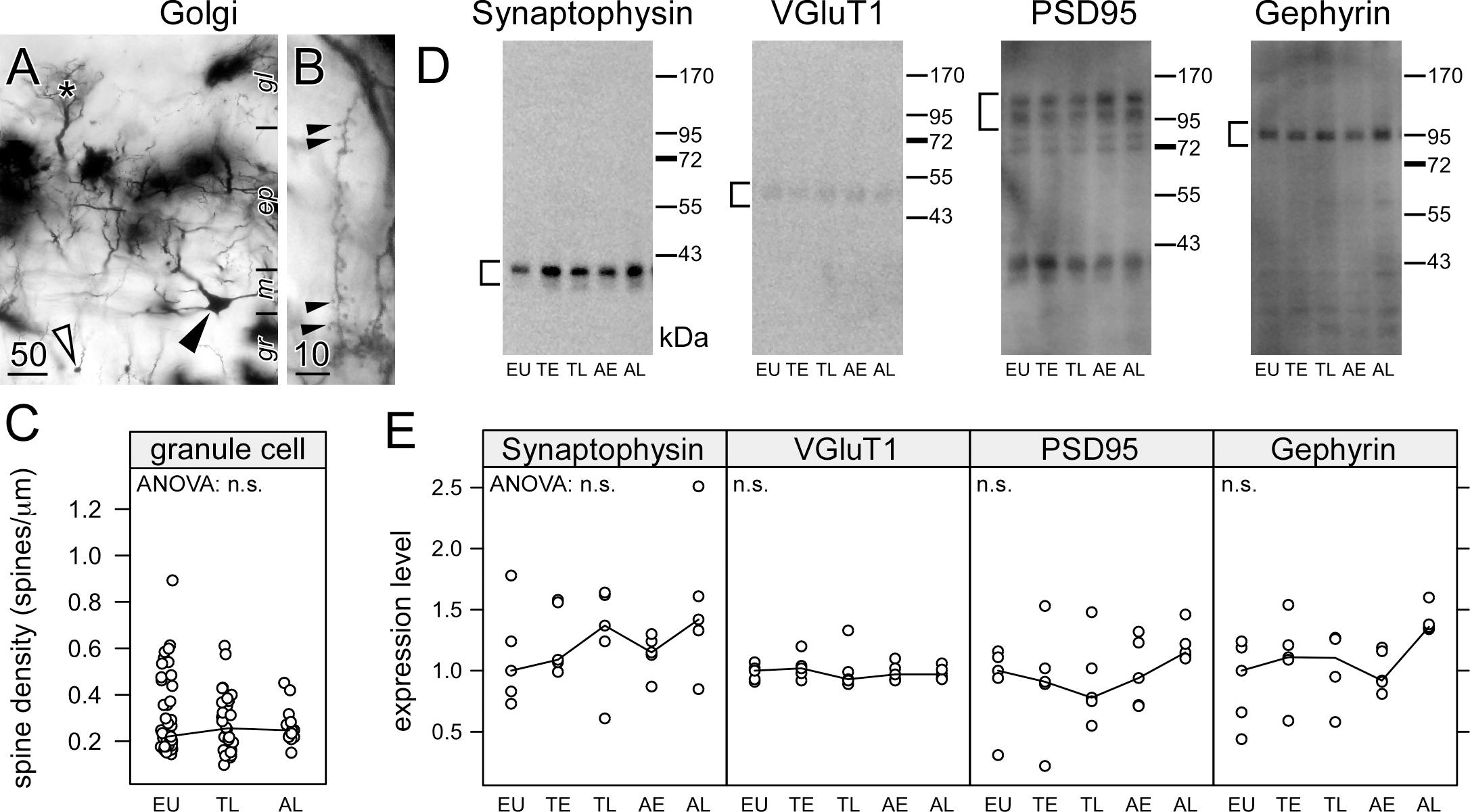
Figure 3. Hibernation does not lead to synaptic regression in the dendrites of granule cells in the olfactory bulb. Hemi brains were impregnated by the Golgi-Bubenaite method, embedded in celloidin and sectioned at 100 μm. In low magnification, granule cell bodies (open arrow) as well as cell bodies (closed arrow) and dendritic tufts of mitral cells within glomeruli (star) (A) are visible. Single granule cell dendrites in the external plexiform layer of the olfactory bulb were identified by their morphology and short segments showing numerous spines (B) were traced. Spine density (C) remained unchanged on granule cell dendrites (ANOVA: p = 0.61). Synaptic proteins in the olfactory bulb were compared in euthermic animals (EU), early torpor (ET), late torpor (TL), and after early arousal (AE) and late arousal (AL). Immunoblots (D) were reacted for the presence of synaptophysin, VGluT1, PSD95 and gephyrin. ANOVA showed no significant (p > 0.05, n.s.) variation after quantification (E) for each of them. Scale bars in μm.
Granule cell spines are associated with glutamatergic synapses from mitral to granule cells, but also with reciprocal GABAergic synapses from granule to mitral cells. Theses dendrodendritic synapses are the majority of synapses within the olfactory bulb. Synaptic proteins present in GABAergic synapses (gephyrin), glutamatergic synapses (VGluT1, PSD95) or synapses in general (synaptophysin) were quantified using western blots (Figure 3D). Consistent with the spine measurements, no changes were observed in the amount of synaptic proteins during the hibernation cycle (Figure 3E).
Olfactory Memory and Motivation
First, we analyzed whether hibernation affects memory in Golden hamsters using an olfactory discrimination task, which allows to reliably assess olfactory learning and memory (Figure 4). During the training phase, one odor (CS+) was paired with a food reward while another odor (CS-) remained unrewarded. After several weeks in the cold, animals were retested for retention. We removed any systematic bias of odor preference by randomizing the odor cue paired with the food reward and during testing half of the animals were presented with the rewarded odor on the left side, for the other half it was presented at the right side. Furthermore, we removed any confounding effect of any group preference for any odor by using it as a blocking factor in the ANOVA. The results show no preference for rose or lemon in hamsters (Table 4).
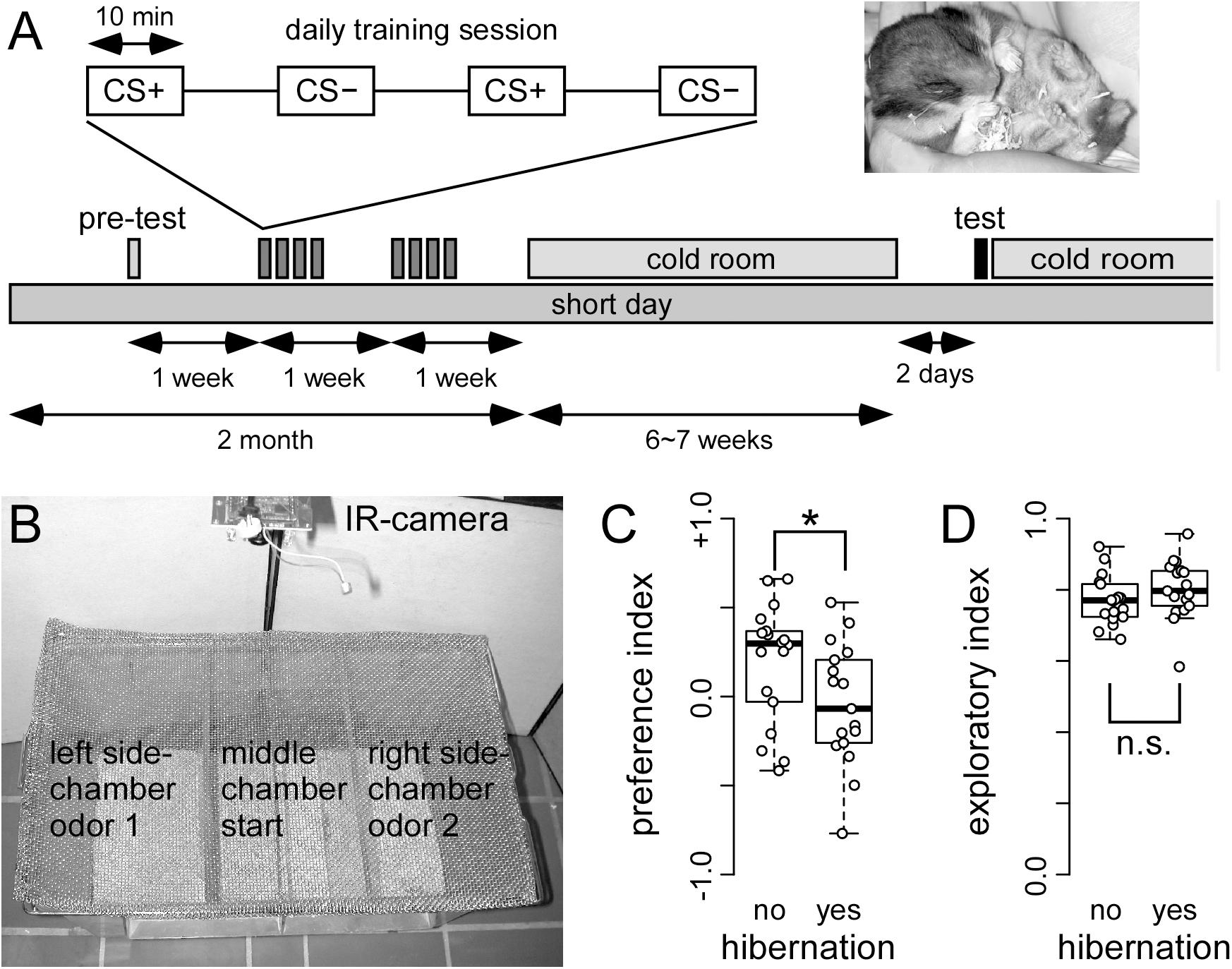
Figure 4. Hibernating hamster fail in a two odor discrimination task (A) On each training day, food deprived hamster received trials in which one odor (CS+) was paired with food reward (sunflower seed) and another odor (CS–) was paired with no reward. After a total of 16 of each CS+ and CS– pairings, hamsters were transferred to the cold room. When 50% of the cohort were hibernating (see photo insert), they were removed from the cold room, and tested the other day. A total of 17 and 18 hibernating and non-hibernating hamsters were tested, respectively. The test chamber (B) was placed under a fume hood. After being placed in the middle chamber, the hamster could choose to walk and explore the side chambers. These side-chambers were equipped with odor pots used during the training of CS+ and CS–. The behavioral response was recorded using a IR camera. The preference index (C) showed that non-hibernating hamster spend more time. This difference was significant (∗) as indicated by ANOVA (p = 0.027424) in the side-chamber with the CS+ odor. This preference was not observed in hamsters that did hibernate. In contrast, hibernation did not affect the exploration index (D) (ANOVA: p = 0.2190). The entire hamster did not stay in the middle chamber, but spend most of their time in the side-chambers.
Non-hibernating animals showed significantly higher preference for the rewarded (CS+) than for the unrewarded (CS-) odor (Figure 4C; p = 0.0274), while hibernating hamsters showed no preference. This indicates that non-hibernating animals remembered the odor, which was associated with food reward while animals gone through hibernation did not.
In order to test whether these behavioral changes might potentially be attributed to changes in motivation, we assessed the exploratory behavior of both hibernating and non-hibernating animals. To this end, the time was measured that animals spend in the side chambers of the test apparatus instead of remaining in the middle chamber. No differences were obtained between hibernating and non-hibernating animals (Figure 4D, p = 0.2190). This indicates that motivation is not different between non-hibernating animals and animals previously gone through hibernation.
Stability of Microtubules
Next, we analyzed the effects of hibernation on microtubule stabilization, the canonical function of tau protein. During the course of hibernation, there were no obvious changes in the acetylated Tubulin monomers (AcTub) compared to the total amount of alpha-Tubulin (Tub; see Figure 5). This indicates, that microtubule stability might largely remain unaffected during torpor.
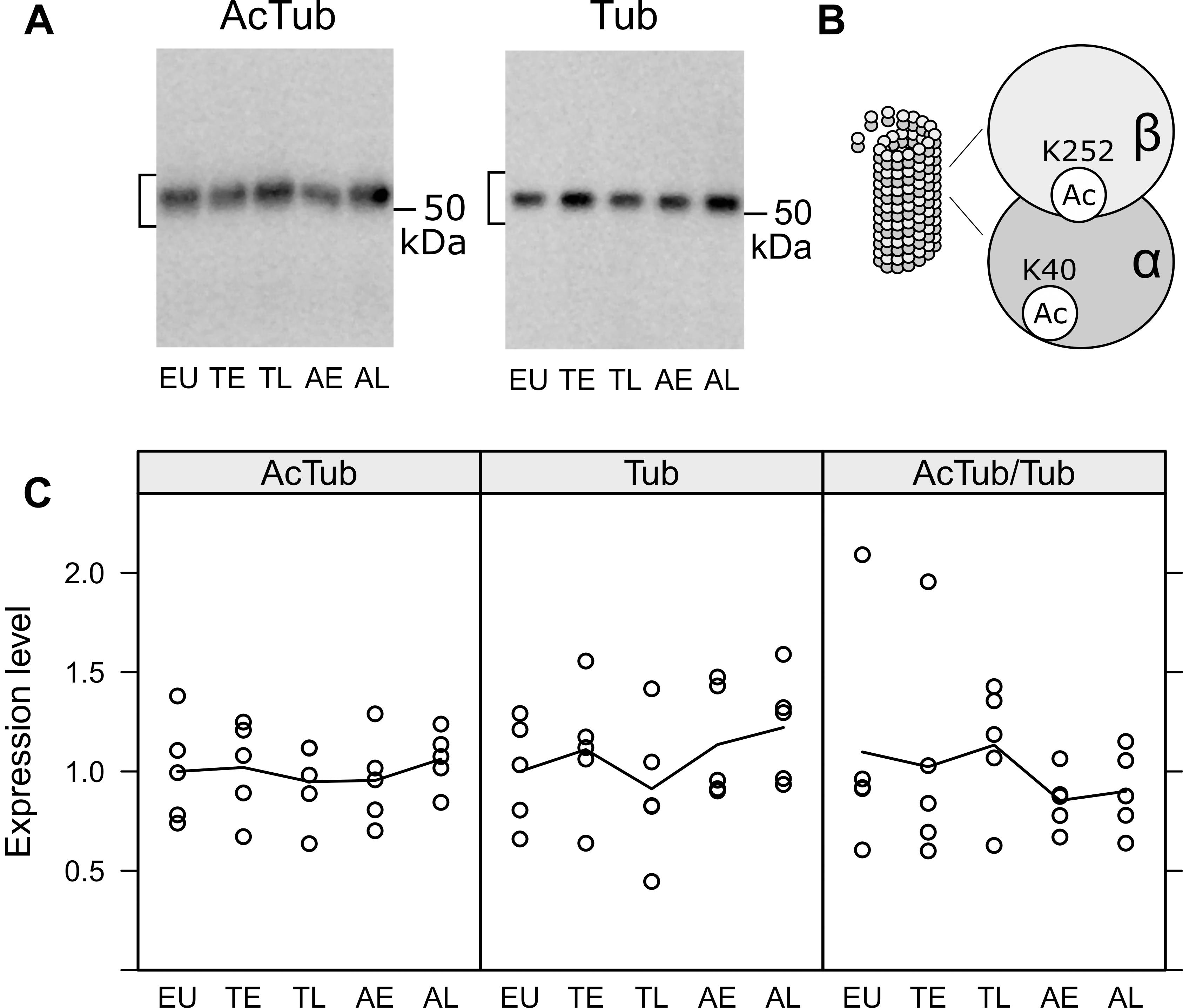
Figure 5. Acetylation of tubulin in the olfactory bulb was compared in euthermic animals (EU), early torpor (ET), late torpor (TL), and after early arousal (AE) and late arousal (AL). Immunoblots (A) were probed for acetylated tubulin (AcTub) and alpha tubulin (Tub). Stable microtubules are enriched in acetylated tubulin monomers (B). The densitometric quantification of both as well as the ratio of AcTub/Tub are shown in the diagrams below (C). ANOVA showed no significant differences (p = 0.915, 0.548 and 0.759 for AcTub, Tub, and AcTub/Tub), indicating no change in microtubule stability during the course of hibernation.
Discussion
In the present study, we combined behavioral, biochemical and morphological analyses to investigate whether hibernation induced phosphorylation of tau in the main olfactory bulb is associated with synaptic regression and a loss of long-term memory for discrimination of odors.
Phosphorylated Tau in the Olfactory Bulb
Paired helical filaments-like hyperphosphorylation of tau is a typical feature of AD, however, it also occurs under physiologically controlled conditions such as hibernation (Arendt et al., 2003; Härtig et al., 2007; Stieler et al., 2009, 2011; Avila et al., 2012; Bullmann et al., 2016). Previous reports have shown, that both temperature dependent mechanisms (Planel et al., 2004) as well as the hibernation-state dependent activity of tau kinases (Stieler et al., 2011) are responsible for the reversible tau phosphorylation that occurs during hibernation. Compared to other brain areas of the golden hamster the pattern and time course of tau phosphorylation in the olfactory bulb resembles closely the midbrain region as reported previously (Stieler et al., 2011). The only exception is the almost twofold expression of S396/S404 in the olfactory bulb, whereas in the other regions show only an increase up to 1.5 fold. Similar to basal forebrain and diagonal band (Härtig et al., 2007), phosphorylated tau protein was present in only some cell types while it was absent in others. In the periglomerular neurons of the olfactory bulb phosphorylated tau is present in some dopaminergic neurons, but is absent in GABAergic and acetylcholinergic neurons. There is no colocalization of hyperphosphorylated tau in cholinergic fibers and buttons in the external plexiform layer. Immature and mature granule cells, as identified by expression of doublecortin and calretinin, respectively, are devoid of phosphorylated tau. Phosphorylated tau is present in a few cell bodies located in the mitral cell layer and therefore we assume that the expression in the external plexiform layer belongs to the apical dendrites of mitral (and perhaps tufted cells). However, fluorescence double labeling using a suitable marker of mitral cells in adult Golden hamsters is necessary.
Synaptic Plasticity
Spine density in Golgi-impregnated specimens (Popov and Bocharova, 1992; Popov et al., 1992, 2007; Magariños et al., 2006; Bullmann et al., 2016) and expression of synaptic proteins can be used to quantify the plastic changes in hibernation (Arendt et al., 2003; Strijkstra et al., 2003; von der Ohe et al., 2007; Bullmann et al., 2016). There is a single report (von der Ohe et al., 2007) using immunohistochemistry to show that plastic synaptic changes in hibernation are associated with a dissociation of proteins from the post-synaptic density rather than a degradation of these proteins. However, western blots that measure the total amount of proteins have shown changes in the expression of synaptic proteins during hibernation (Mirra et al., 1991; Härtig et al., 2007; Hoover et al., 2010) parallel to the spines regression (Popov and Bocharova, 1992; Magariños et al., 2006; Popov et al., 2007; Bullmann et al., 2016). Synaptic protein expression remained unchanged during hibernation as well as the spine density on olfactory bulb granule cells. This sparing of granule cell spines during hibernation was consistent with the absence of phosphorylated tau in olfactory bulb granule cells.
Stability of Microtubules
Dendritic spines contain neurotransmitter receptors organized by specific scaffolding proteins, extensive actin cytoskeleton, which together with microtubules (Jaworski et al., 2009) regulates spine plasticity (Hotulainen and Hoogenraad, 2010). The microtubule binding protein tau stabilizes microtubules (Weingarten et al., 1975; Drechsel et al., 1992; Goode and Feinstein, 1994; Fryer et al., 1996; Prezel et al., 2018) and co-organizes dynamic microtubules and actin networks (Elie et al., 2015). Such cross-linking of microtubules and actin filaments depends on the phosphorylation of tau (Elie et al., 2015). Probably, tau phosphorylation during torpor uncouples microtubule in the spine neck and actin cytoskeleton in the spine head thus leading to spine retraction (Popov and Bocharova, 1992; Arendt et al., 2003; von der Ohe et al., 2006, 2007). Furthermore, its presence in post-synaptic spines is activity dependent (Frandemiche et al., 2014) and mediates the targeting of the src family kinase fyn to glutamatergic NMDA receptors (Ittner et al., 2010) and a reduction of tau expression results in synapse reduction (Chen et al., 2011). However, consistent with the constant synaptic protein expression and spine density in the olfactory bulb during hibernation we did not detect major changes of tubulin stability: This is also in agreement with previous reports (Yu et al., 1994; Planel et al., 2008) which indicated that tau does not markedly contribute to cold stability of microtubules. Tau phosphorylation and its subsequent detachment from microtubules during hibernation apparently have, thus, no influence on microtubule stability.
Hibernation Might Impair Olfactory Memory
The effect of hibernation on olfactory discrimination was tested using an established two-odor discrimination task (Schellinck et al., 2001). After multiple exposures to a rewarded and a non-rewarded odor, animals were housed in a cold environment. When half of the animals went into hibernation, all animals were removed from the cold environment and tested for their odor preference. Hibernating animals did not show a significant preference for the odor that was previously presented with a food reward. On the other hand, non-hibernating animals showed a clear preference, which implies that they remembered the rewarded odor. Our study shows that this behavioral task originally described for mice can efficiently be used to assess olfactory learning and memory in hamsters. In the control group, olfactory memory traces last for almost 2 months, whereas the recall was impaired in the hibernation group (where animals experienced at least one torpor phase).
The observed impairment after hibernation could be explained in terms of sensation (reception), perception, memory and motivation. The odor sensation is the binding of the odor molecules to the receptor in the olfactory epithelium and the increased activity of a specific subtype of olfactory receptor neurons. Although we did not test for the sensation of odors, e.g., by calcium imaging of olfactory receptor responses (Ma and Shepherd, 2000) in hibernating animals, hematoxylin and eosin staining of the olfactory epithelium does not show any obvious damage after hibernation (not shown). The perception of odors takes place in the olfactory bulb; each type of receptor neurons projects to a different glomerulus and the discrimination between odor features is enhanced by lateral inhibition (Lledo and Lagier, 2006) of mitral cells mediated by the granule cells increase discrimination of odors (Mori et al., 1999). Mitral cells then project to principal cells in the piriform cortex (Knafo et al., 2001), which relay this olfactory information to the hippocampus (Hess et al., 1995; Knafo et al., 2004) as well as the prefrontal cortex (Alvarez and Eichenbaum, 2002). In turn granule and mitral cells are modulated by efferent inputs originating in central brain areas. It has been argued (Wilson et al., 2004), that peripheral olfactory system is more involved in implicit memory (e.g., perceptual learning) (Fletcher and Wilson, 2003) whereas the central olfactory system is encodes explicit memory (e.g., olfactory discrimination learning) (Eichenbaum et al., 1989; Alvarez and Eichenbaum, 2002). However, in the last 15 years it has become clear that adult born granule cells play a large role in olfactory discrimination learning. Olfactory discrimination learning specifically increases proximal spine density in adult born granule cells, their activity promotes odor-reward association while their immediate post-training ablation impair olfactory memory. Interestingly, ablation 28 days after training did not impair memory, indicating that long-term memory is independent of adult born granule cells (Arruda-Carvalho et al., 2014).
Previously we did not observed memory loss in a hippocampus-dependent task (Bullmann et al., 2016). Here we used the same reward (sunflower seeds) and the same hibernation paradigm. Therefore we do not expect that the impairment of odor discrimination is due to differences in motivation, as it has been argued before (McNamara and Riedesel, 1973; Alloway, 2008). We did not observe any difference in exploratory activity, further suggesting that the memory recall test was not affected by a change of motivation. As we summarized previously (Bullmann et al., 2016), the effect of hibernation on memory (McNamara and Riedesel, 1973; Millesi et al., 2001; Zhao et al., 2004; Clemens et al., 2009; Arendt and Bullmann, 2013; Bullmann et al., 2016) varies from species to species and depends on the testing paradigm. Therefore, we argue for species-specific differences in memory consolidation as required for specific behavior in the natural habitat. Previously, we hypothesized that the golden hamster must preserve spatial memory, but not social recognition. The Golden hamsters almost exclusively use olfactory cues processed by both main olfactory system (MOS) and the accessory or vomeronasal system (AOS) (Meredith, 1991) for regulating social interactions (Johnston, 1990; Petrulis, 2009). The impairment observed in the two-odor discrimination task is consistent with our hypothesis that during hibernation non-essential memory content may prone to erasure. Further behavioral experiments are needed to prove that this extends to impaired social kin recognition in Golden hamsters after arousal.
Cholinergic Hypothesis of Odor Discrimination Impairment — Implications for Alzheimer’s Disease
Aggregated tau is present in the olfactory system in all definite Alzheimer’s disease cases and shows highly significant correlation with Braak staging in the brain (Attems et al., 2014). All layers are affected by the tau pathology and there are conflicting reports whether the dramatic loss of mitral cells is preceded by development of neurofibrillary tangles (Struble and Clark, 1992; Kovács et al., 1999). It has been argued that consequence of either tau pathology or mitral cell degeneration that leads to a dramatic loss of smell in Alzheimer’s disease might be a useful screening tool (Wesson et al., 2011; Attems et al., 2014). However, olfactory deficit may not be caused by tau phosphorylation, aggregation and cell death in the MOB, but by the diminished modulatory input, most important from acetylcholinergic neurons in the basal forebrain (Chaudhury et al., 2009; Gottfried, 2010; Ma and Luo, 2012; Devore et al., 2014; Rothermel et al., 2014). Cholinergic projection neurons in the basal forebrain can be classified according to their projection targets as Ch1–Ch4 regions (Mesulam et al., 1983). According to that classification, the lateral portion of the horizontal limb nucleus of the diagonal band (Ch3) provides the major cholinergic innervation to the olfactory bulb. Previously, we showed (Härtig et al., 2007) in the basal forebrain projection system of hibernating hamsters that cholinergic neurons are selectively affected by PHF-like phosphorylated tau, while γ-aminobutyric acid (GABA)ergic neurons are largely spared, similar to what has been observed in AD (Davies and Maloney, 1976; Whitehouse et al., 1981; Arendt et al., 1983; Härtig et al., 2002). Cognitive dysfunction in patients with AD correlates with both neuronal loss and tangle load in the basal forebrain (Holzer et al., 1994; Samuel et al., 1994; Iraizoz et al., 1999). It is possible that phosphorylation of tau in torpor is associated with a cholinergic deficit during the arousal phase. Such disruption cholinergic homoeostasis in nucleus basalis of Meynert and frontal cortex has been observed by activation of a major tau kinase, GSK3β (Wang et al., 2017). This might explain the observed impairment of olfactory discrimination in hibernation and perhaps in the very early, preclinical stages of Alzheimer’s disease (Attems and Jellinger, 2006; Attems et al., 2014; Roberts et al., 2016).
Data Availability
The datasets generated for this study are available on request to the corresponding author.
Ethics Statement
All experimental procedures on animals were carried out in accordance with the European Council Directive 86/609/EEC and 2010/63/EU to improve the welfare of animals used in scientific procedures and had been approved by the local authorities (T74/05, Regierungspräsidium Leipzig). Case recruitment, autopsy, and data handling have been performed in accordance with the ethical standards as laid down in the 1964 Declaration of Helsinki and its later amendments as well as with the convention of the Council of Europe on Human Rights and Biomedicine and had been approved by the responsible Ethics Committee of the Leipzig University.
Author Contributions
TB designed the study, built the equipment, performed the experiments, analyzed the data, assembled the figures, interpreted the results, and prepared and revised the manuscript. EF performed the behavioral experiments, immunohistochemical staining, and spine measurements. TK performed the Western blotting and histochemical staining. VO performed the sampling of human olfactory bulb. MH and TA interpreted the results, and prepared and revised the manuscript.
Funding
This work was supported by a grant of the Medical Faculty of the University of Leipzig awarded to TB (Grant No. 984000-102) and a grant by the DFG awarded to MH (HO2368-4/1).
Conflict of Interest Statement
The authors declare that the research was conducted in the absence of any commercial or financial relationships that could be construed as a potential conflict of interest.
Acknowledgments
The authors would like to thank Ute Bauer and Hildegard Gruschka for their expert technical assistance with animal perfusion and Golgi staining and sectioning, Jens T. Stieler for advice on sample preparation and helpful discussions, and Ulrich Bullmann and Thomas Müller for help in building activity monitoring and behavioral testing equipment. The authors acknowledge support from the German Research Foundation (DFG) and the Universität Leipzig within the program of Open Access Publishing.
Supplementary Material
The Supplementary Material for this article can be found online at: https://www.frontiersin.org/articles/10.3389/fnana.2019.00069/full#supplementary-material
FIGURE S1 | High magnification images of the human olfactory bulb, showing the distribution of phosphorylated tau by AT8 immunohistochemistry and the distribution of tangles by standard Gallyas/Nissl stainind.
FIGURE S2 | Habituation: The hamsters are initially placed in the middle of the three-chamber-apparatus for accomodation to the test procedure. No odors are present and they can freely explore the apparatus. Training: The hamsters are presented with an alternation of two odors, one of them paired with reward (sunflower seeds), the other without. They are given 4 trials each day, 4 times a week, for two weeks. They experience 16 CS+ and 16 CS- pairings in total. Testing: The hamsters are again placed in the middle of the three-chamber-apparatus, this time with both CS+ and CS- odors presented in the side chamber. This time, no food reward is given with CS+ odor.
TABLE S1 | Raw data for the two odor discrimination task.
Footnotes
References
Albert, M. S., DeKosky, S. T., Dickson, D., Dubois, B., Feldman, H. H., Fox, N. C., et al. (2011). The diagnosis of mild cognitive impairment due to Alzheimer’s disease: recommendations from the National Institute on Aging-Alzheimer’s Association workgroups on diagnostic guidelines for Alzheimer’s disease. Alzheimer’s Dement 7, 270–279.
Alloway, K. D. (2008). Information processing streams in rodent barrel cortex: the differential functions of barrel and septal circuits. Cereb. Cortex 18, 979–989. doi: 10.1093/cercor/bhm138
Alvarez, P., and Eichenbaum, H. (2002). Representations of odors in the rat orbitofrontal cortex change during and after learning. Behav. Neurosci. 116, 421–433. doi: 10.1037//0735-7044.116.3.421
Arendt, T., Bigl, V., Arendt, A., and Tennstedt, A. (1983). Loss of neurons in the nucleus basalis of Meynert in Alzheimer’s disease, paralysis agitans and Korsakoff’s disease. Acta Neuropathol. 61, 101–108. doi: 10.1007/bf00697388
Arendt, T., and Bullmann, T. (2013). Neuronal plasticity in hibernation and the proposed role of the microtubule-associated protein tau as a ‘master switch’ regulating synaptic gain in neuronal networks. Am. J. Physiol. Regul. Integr. Comp. Physiol. 305, R478–R489.
Arendt, T., Stieler, J., Strijkstra, A. M., Hut, R. A., Rüdiger, J., Van der Zee, E. A., et al. (2003). Reversible paired helical filament-like phosphorylation of tau is an adaptive process associated with neuronal plasticity in hibernating animals. J. Neurosci. 23, 6972–6981. doi: 10.1523/jneurosci.23-18-06972.2003
Arruda-Carvalho, M., Akers, K. G., Guskjolen, A., Sakaguchi, M., Josselyn, S. A., and Frankland, P. W. (2014). Posttraining ablation of adult-generated olfactory granule cells degrades odor–reward memories. J. Neurosci. 34, 15793–15803. doi: 10.1523/jneurosci.2336-13.2014
Attems, J., and Jellinger, K. A. (2006). Olfactory tau pathology in Alzheimer disease and mild cognitive impairment. Clin. Neuropathol. 25, 265–271.
Attems, J., Walker, L., and Jellinger, K. A. (2014). Olfactory bulb involvement in neurodegenerative diseases. Acta Neuropathol. 127, 459–475. doi: 10.1007/s00401-014-1261-7
Avila, J. (2000). Tau aggregation into fibrillar polymers: taupathies. FEBS Lett. 476, 89–92. doi: 10.1016/s0014-5793(00)01676-8
Avila, J., León-Espinosa, G., García, E., García-Escudero, V., Hernández, F., and Defelipe, J. (2012). Tau phosphorylation by GSK3 in different conditions. Int. J. Alzheimers Dis. 2012:578373. doi: 10.1155/2012/578373
Avila, J., Soares, H., Fanarraga, M. L., and Zabala, J. C. (2008). Isolation of microtubules and microtubule proteins. Curr. Protoc. Cell Biol. 39, 3.29.1–3.29.28.
Barkai, E., and Saar, D. (2001). Cellular correlates of olfactory learning in the rat piriform cortex. Rev. Neurosci. 12, 111–120.
Biernat, J., Gustke, N., Drewes, G., Mandelkow, E. M., and Mandelkow, E. (1993). Phosphorylation of Ser262 strongly reduces binding of tau to microtubules: distinction between PHF-like immunoreactivity and microtubule binding. Neuron 11, 153–163. doi: 10.1016/0896-6273(93)90279-z
Biswas, S., and Kalil, K. (2017). The microtubule-associated protein tau mediates the organization of microtubules and their dynamic exploration of actin-rich lamellipodia and filopodia of cortical growth cones. J. Neurosci. 38, 291–307. doi: 10.1523/JNEUROSCI.2281-17.2017
Blazquez-Llorca, L., Garcia-Marin, V., Merino-Serrais, P., Ávila, J., and DeFelipe, J. (2011). Abnormal tau phosphorylation in the thorny excrescences of CA3 hippocampal neurons in patients with Alzheimer’s disease. J. Alzheimer’s Dis. 26, 683–698. doi: 10.3233/JAD-2011-110659
Braak, H., Alafuzoff, I., Arzberger, T., Kretzschmar, H., and Tredici, K. (2006). Staging of Alzheimer disease-associated neurofibrillary pathology using paraffin sections and immunocytochemistry. Acta Neuropathol. 112, 389–404. doi: 10.1007/s00401-006-0127-z
Braak, H., and Braak, E. (1991). Neuropathological stageing of Alzheimer-related changes. Acta Neuropathol. 82, 239–259. doi: 10.1007/bf00308809
Brandt, R., Hundelt, M., and Shahani, N. (2005). Tau alteration and neuronal degeneration in tauopathies: mechanisms and models. Biochim. Biophys. Acta 1739, 331–354. doi: 10.1016/j.bbadis.2004.06.018
Bubenaite, J. (1929). Über einige erfahrungen mit der golgi-methode. Zeitschrift Für Wissenschaftliche Mikroskopie 46, 359–360.
Buée, L., Bussière, T., Buée-Scherrer, V., Delacourte, A., and Hof, P. R. (2000). Tau protein isoforms, phosphorylation and role in neurodegenerative disorders. Brain Res. Rev. 33, 95–130. doi: 10.1016/s0165-0173(00)00019-9
Bullmann, T., De Silva, R., Holzer, M., Mori, H., and Arendt, T. (2007). Expression of embryonic tau protein isoforms persist during adult neurogenesis in the hippocampus. Hippocampus 17, 98–102. doi: 10.1002/hipo.20255
Bullmann, T., Feneberg, E., Treutlein, T., Alpar, A., Ogunlade, V., Holzer, M., et al. (2009). Olfactory memory, tau phosphorylation and synaptic plasticity in hibernating hamsters. J. Neurochem. 110:47.
Bullmann, T., Härtig, W., Holzer, M., and Arendt, T. (2010). Expression of the embryonal isoform (0n/3R) of the microtubule-associated protein tau in the adult rat central nervous system. J. Comp. Neurol. 518, 2538–2553. doi: 10.1002/cne.22351
Bullmann, T., Seeger, G., Stieler, J., Hanics, J., Reimann, K., Kretzschmann, T. P., et al. (2016). Tau phosphorylation-associated spine regression does not impair hippocampal-dependent memory in hibernating golden hamsters. Hippocampus 26, 301–318. doi: 10.1002/hipo.22522
Cabrales Fontela, Y., Kadavath, H., Biernat, J., Riedel, D., Mandelkow, E., and Zweckstetter, M. (2017). Multivalent cross-linking of actin filaments and microtubules through the microtubule-associated protein Tau. Nat. Commun. 8:1981. doi: 10.1038/s41467-017-02230-8
Calhoun, M. E., Jucker, M., Martin, L. J., Thinakaran, G., Price, D. L., and Mouton, P. R. (1996). Comparative evaluation of synaptophysin-based methods for quantification of synapses. J. Neurocytol. 25, 821–828. doi: 10.1007/bf02284844
Chaudhury, D., Escanilla, O., and Linster, C. (2009). Bulbar acetylcholine enhances neural and perceptual odor discrimination. J. Neurosci. 29, 52–60. doi: 10.1523/JNEUROSCI.4036-08.2009
Chen, Q., Zhou, Z., Zhang, L., Wang, Y., Zhang, Y. W., Zhong, M., et al. (2011). Tau protein is involved in morphological plasticity in hippocampal neurons in response to BDNF. Neurochem. Int. 60, 233–242. doi: 10.1016/j.neuint.2011.12.013
Clemens, L. E., Heldmaier, G., and Exner, C. (2009). Keep cool: memory is retained during hibernation in alpine marmots. Physiol. Behav. 98, 78–84. doi: 10.1016/j.physbeh.2009.04.013
Davies, P., and Maloney, A. J. F. (1976). Selective loss of central cholinergic neurons in Alzheimer’s disease. Lancet 2:1403. doi: 10.1016/s0140-6736(76)91936-x
Devore, S., de Almeida, L., and Linster, C. (2014). Distinct roles of bulbar muscarinic and nicotinic receptors in olfactory discrimination learning. J. Neurosci. 34, 11244–11260. doi: 10.1523/JNEUROSCI.1499-14.2014
Drechsel, D. N., Hyman, A. A., Cobb, M. H., and Kirschner, M. W. (1992). Modulation of the dynamic instability of tubulin assembly by the microtubule-associated protein tau. Mol. Biol. Cell 3, 1141–1154. doi: 10.1091/mbc.3.10.1141
Dubois, B., Feldman, H. H., Jacova, C., Hampel, H., Molinuevo, J. L., Blennow, K., et al. (2014). Advancing research diagnostic criteria for Alzheimer’s disease: the IWG-2 criteria. Lancet Neurol. 13, 614–629.
Eichenbaum, H., Mathews, P., and Cohen, N. J. (1989). Further studies of hippocampal representation during odor discrimination learning. Behav. Neurosci. 103, 1207–1216. doi: 10.1037//0735-7044.103.6.1207
Elie, A., Prezel, E., Guérin, C., Denarier, E., Ramirez-Rios, S., Serre, L., et al. (2015). Tau co-organizes dynamic microtubule and actin networks. Sci. Rep. 5:9964. doi: 10.1038/srep09964
Festing, M. F. W., and Altman, D. G. (2002). Guidelines for the design and statistical analysis of experiments using laboratory animals. ILAR J. 43, 244–258. doi: 10.1093/ilar.43.4.244
Fletcher, M. L., and Wilson, D. A. (2003). Olfactory bulb mitral-tufted cell plasticity: odorant-specific tuning reflects previous odorant exposure. J. Neurosci. 23, 6946–6955. doi: 10.1523/jneurosci.23-17-06946.2003
Frandemiche, M. L., De Seranno, S., Rush, T., Borel, E., Elie, A., Arnal, I., et al. (2014). Activity-dependent tau protein translocation to excitatory synapse is disrupted by exposure to amyloid-beta oligomers. J. Neurosci. 34, 6084–6097. doi: 10.1523/JNEUROSCI.4261-13.2014
Friedrich, R. W. (2006). Mechanisms of odor discrimination: neurophysiological and behavioral approaches. Trends Neurosci. 29, 40–47. doi: 10.1016/j.tins.2005.10.004
Fryer, R. H., Kaplan, D. R., Feinstein, S. C., Radeke, M. J., Grayson, D. R., and Kromer, L. F. (1996). Developmental and mature expression of full-length and truncated TrkB receptors in the rat forebrain. J. Comp. Neurol. 374, 21–40. doi: 10.1002/(sici)1096-9861(19961007)374:1<21::aid-cne2>3.0.co;2-p
Gattermann, R., Johnston, R. E., Yigit, N., Fritzsche, P. A., Larimer, S., Ozkurt, S. O., et al. (2001). Notes on the current distribution and the ecology of wild golden hamsters (Mesocricetus auratus). J. Zool. 254, 359–365. doi: 10.1017/s0952836901000851
Gearing, M., Mirra, S. S., Hedreen, J. C., Sumi, S. M., Hansen, L. A., and Heyman, A. (1995). The consortium to establish a registry for Alzheimer’s disease (CERAD). Part X. neuropathology confirmation of the clinical diagnosis of alzheimer’s disease. Neurology 45, 461–466. doi: 10.1212/wnl.45.3.461
Goedert, M., and Spillantini, M. G. (2011). Pathogenesis of the Tauopathies. J. Mol. Neurosci. 45, 425–431. doi: 10.1007/s12031-011-9593-4
Goode, B. L., and Feinstein, S. C. (1994). Identification of a novel microtubule binding and assembly domain in the developmentally regulated inter-repeat region of tau. J. Cell Biol. 124, 769–781.
Gottfried, J. A. (2010). Central mechanisms of odour object perception. Nat. Rev. Neurosci. 11, 628–641. doi: 10.1038/nrn2883
Graziano, A., Liu, X.-B., Murray, K. D., and Jones, E. G. (2008). Vesicular glutamate transporters define two sets of glutamatergic afferents to the somatosensory thalamus and two thalamocortical projections in the mouse. J. Comp. Neurol. 507, 1258–1276. doi: 10.1002/cne.21592
Härtig, W., Bauer, A., Brauer, K., Grosche, J., Hortobágyi, T., Penke, B., et al. (2002). Functional recovery of cholinergic basal forebrain neurons under disease conditions: Old problems, new solutions? Rev. Neurosci. 13, 95–165.
Härtig, W., Oklejewicz, M., Strijkstra, A. M., Boerema, A. S., Stieler, J., and Arendt, T. (2005). Phosphorylation of the tau protein sequence 199-205 in the hippocampal CA3 region of Syrian hamsters in adulthood and during aging. Brain Res. 1056, 100–104. doi: 10.1016/j.brainres.2005.07.017
Härtig, W., Riedel, A., Grosche, J., Edwards, R. H., Fremeau, R. T. Jr., Harkany, T., et al. (2003). Complementary distribution of vesicular glutamate transporters 1 and 2 in the nucleus accumbens of rat: Relationship to calretinin-containing extrinsic innervation and calbindin-immunoreactive neurons. J. Comp. Neurol. 465, 1–10. doi: 10.1002/cne.10789
Härtig, W., Stieler, J., Boerema, A. S., Wolf, J., Schmidt, U., Weissfuss, J., et al. (2007). Hibernation model of tau phosphorylation in hamsters: Selective vulnerability of cholinergic basal forebrain neurons - Implications for Alzheimer’s disease. Eur. J. Neurosci. 25, 69–80. doi: 10.1111/j.1460-9568.2006.05250.x
Hess, U. S., Lynch, G., and Gall, C. M. (1995). Regional patterns of c-fos mRNA expression in rat hippocampus following exploration of a novel environment versus performance of a well-learned discrimination. J. Neurosci. 15, 7796–7809. doi: 10.1523/jneurosci.15-12-07796.1995
Holzer, M., Holzapfel, H. P., Zedlick, D., Brückner, M. K., and Arendt, T. (1994). Abnormally phosphorylated tau protein in Alzheimer’s disease: Heterogeneity of individual regional distribution and relationship to clinical severity. Neuroscience 63, 499–516. doi: 10.1016/0306-4522(94)90546-0
Hoover, B. R., Reed, M. N., Su, J., Penrod, R. D., Kotilinek, L. A., Grant, M. K., et al. (2010). Tau mislocalization to dendritic spines mediates synaptic dysfunction independently of neurodegeneration. Neuron 68, 1067–1081. doi: 10.1016/j.neuron.2010.11.030
Hotulainen, P., and Hoogenraad, C. C. (2010). Actin in dendritic spines: connecting dynamics to function. J. Cell Biol. 189, 619–629. doi: 10.1083/jcb.201003008
Hut, R. A., and Scharff, A. (1998). Endoscopic observations on tunnel blocking behaviour in the European ground squirrel (Spermophilus citellus). Int. J. Mamm. Biol. 63, 377–380.
Iraizoz, I., Guijarro, J. L., Gonzalo, L. M., and De Lacalle, S. (1999). Neuropathological changes in the nucleus basalis correlate with clinical measures of dementia. Acta Neuropathol. 98, 186–196. doi: 10.1007/s004010051068
Ittner, L. M., Ke, Y. D., Delerue, F., Bi, M., Gladbach, A., van Eersel, J., et al. (2010). Dendritic function of tau mediates amyloid-beta toxicity in Alzheimer’s disease mouse models. Cell 142, 387–397. doi: 10.1016/j.cell.2010.06.036
Janke, C., and Montagnac, G. (2017). Causes and consequences of microtubule acetylation. Curr. Biol. 27, R1287–R1292. doi: 10.1016/j.cub.2017.10.044
Jaworski, J., Kapitein, L. C., Gouveia, S. M., Dortland, B. R., Wulf, P. S., Grigoriev, I., et al. (2009). Dynamic microtubules regulate dendritic spine morphology and synaptic plasticity. Neuron 61, 85–100. doi: 10.1016/j.neuron.2008.11.013
Johnston, R. E. (1990). “Chemical communication in golden hamsters: From behavior to molecules and neural mechanisms,” in Contemporary Issues in Comparative Psychology, ed. D. A. Dewsbury (Sunderland, MA: Sinauer Associates), 381–409. doi: 10.1037/11525-017
Kaut, K. P., Bunsey, M. D., and Riccio, D. C. (2003). Olfactory learning and memory impairments following lesions to the hippocampus and perirhinal-entorhinal cortex. Behav. Neurosci. 117, 304–319. doi: 10.1037/0735-7044.117.2.304
Knafo, S., Ariav, G., Barkai, E., and Libersat, F. (2004). Olfactory learning-induced increase in spine density along the apical dendrites of CA1 hippocampal neurons. Hippocampus 14, 819–825. doi: 10.1002/hipo.10219
Knafo, S., Grossman, Y., Barkai, E., and Benshalom, G. (2001). Olfactory learning is associated with increased spine density along apical dendrites of pyramidal neurons in the rat piriform cortex. Eur. J. Neurosci. 13, 633–638. doi: 10.1046/j.1460-9568.2001.01422.x
Kovács, T., Cairns, N. J., and Lantos, P. L. (1999). beta-amyloid deposition and neurofibrillary tangle formation in the olfactory bulb in ageing and Alzheimer’s disease. Neuropathol. Appl. Neurobiol. 25, 481–491. doi: 10.1046/j.1365-2990.1999.00208.x
Lindwall, G., and Cole, R. D. (1984). Phosphorylation affects the ability of tau protein to promote microtubule assembly. J. Biol. Chem. 259, 5301–5305.
Lledo, P. M., and Lagier, S. (2006). Adjusting neurophysiological computations in the adult olfactory bulb. Semin. Cell Dev. Biol. 17, 443–453. doi: 10.1016/j.semcdb.2006.04.011
Ma, M., and Luo, M. (2012). Optogenetic activation of basal forebrain cholinergic neurons modulates neuronal excitability and sensory responses in the main olfactory bulb. J. Neurosci. 32, 10105–10116. doi: 10.1523/JNEUROSCI.0058-12.2012
Ma, M., and Shepherd, G. M. (2000). Functional mosaic organization of mouse olfactory receptor neurons. Proc. Natl. Acad. Sci. U.S.A. 97, 12869–12874. doi: 10.1073/pnas.220301797
Magariños, A. M., McEwen, B. S., Saboureau, M., and Pevet, P. (2006). Rapid and reversible changes in intrahippocampal connectivity during the course of hibernation in European hamsters. Proc. Natl. Acad. Sci. U.S.A. 103, 18775–18780. doi: 10.1073/pnas.0608785103
McNamara, M. C., and Riedesel, M. L. (1973). Memory and hibernation in Citellus lateralis. Science 179, 92–94. doi: 10.1126/science.179.4068.92
Meredith, M. (1991). Sensory processing in the main and accessory olfactory systems: comparisons and contrasts. J. Steroid Biochem. Mol. Biol. 39, 601–614. doi: 10.1016/0960-0760(91)90258-7
Mesulam, M. M., Mufson, E. J., Wainer, B. H., and Levey, A. I. (1983). Central cholinergic pathways in the rat: an overview based on an alternative nomenclature (Ch1-Ch6). Neuroscience 10, 1185–1201. doi: 10.1016/0306-4522(83)90108-2
Millesi, E., Prossinger, H., Dittami, J. P., and Fieder, M. (2001). Hibernation effects on memory in European ground squirrels (Spermophilus citellus). J. Biol. Rhythms 16, 264–271.
Mirra, S. S., Heyman, A., McKeel, D., Sumi, S. M., Crain, B. J., Brownlee, L. M., et al. (1991). The consortium to establish a registry for Alzheimer’s Disease (CERAD): Part II. standardization of the neuropathologic assessment of Alzheimer’s disease. Neurology 41, 479–479. doi: 10.1212/wnl.41.4.479
Mondragón-Rodríguez, S., Trillaud-Doppia, E., Dudilot, A., Bourgeois, C., Lauzon, M., Leclerc, N., et al. (2012). Interaction of endogenous tau protein with synaptic proteins is regulated by N-methyl-D-aspartate receptor-dependent tau phosphorylation. J. Biol. Chem. 287, 32040–32053. doi: 10.1074/jbc.M112.401240
Mori, K., Nagao, H., and Yoshihara, Y. (1999). The olfactory bulb: coding and processing of odor molecule information. Science 286, 711–715. doi: 10.1126/science.286.5440.711
Mori, K., Takahashi, Y. K., Igarashi, K. M., and Yamaguchi, M. (2006). Maps of odorant molecular features in the mammalian olfactory bulb. Physiol. Rev. 86, 409–433. doi: 10.1152/physrev.00021.2005
Morris, M., Maeda, S., Vossel, K., and Mucke, L. (2011). The many faces of tau. Neuron 70, 410–426. doi: 10.1016/j.neuron.2011.04.009
Nair, D., Hosy, E., Petersen, J. D., Constals, A., Giannone, G., Choquet, D., et al. (2013). Super-resolution imaging reveals that AMPA receptors inside synapses are dynamically organized in nanodomains regulated by PSD95. J. Neurosci. 33, 13204–13224. doi: 10.1523/JNEUROSCI.2381-12.2013
Peden, D. R., Petitjean, C. M., Herd, M. B., Durakoglugil, M. S., Rosahl, T. W., Wafford, K., et al. (2008). Developmental maturation of synaptic and extrasynaptic GABAA receptors in mouse thalamic ventrobasal neurones. J. Physiol. 586, 965–987. doi: 10.1113/jphysiol.2007.145375
Petrulis, A. (2009). Neural mechanisms of individual and sexual recognition in Syrian hamsters (Mesocricetus auratus). Behav. Brain Res. 200, 260–267. doi: 10.1016/j.bbr.2008.10.027
Planel, E., Krishnamurthy, P., Miyasaka, T., Liu, L., Herman, M., Kumar, A., et al. (2008). Anesthesia-induced hyperphosphorylation detaches 3-repeat tau from microtubules without affecting their stability in vivo. J. Neurosci. 28, 12798–12807. doi: 10.1523/JNEUROSCI.4101-08.2008
Planel, E., Miyasaka, T., Launey, T., Chui, D. H., Tanemura, K., Sato, S., et al. (2004). Alterations in glucose metabolism induce hypothermia leading to tau hyperphosphorylation through differential inhibition of kinase and phosphatase activities: implications for Alzheimer’s disease. J. Neurosci. 24, 2401–2411. doi: 10.1523/jneurosci.5561-03.2004
Popov, V. I., and Bocharova, L. S. (1992). Hibernation-induced structural changes in synaptic contacts between mossy fibres and hippocampal pyramidal neurons. Neuroscience 48, 53–62. doi: 10.1016/0306-4522(92)90337-2
Popov, V. I., Bocharova, L. S., and Bragin, A. G. (1992). Repeated changes of dendritic morphology in the hippocampus of ground squirrels in the course of hibernation. Neuroscience 48, 45–51. doi: 10.1016/0306-4522(92)90336-z
Popov, V. I., Medvedev, N. I., Patrushev, I. V., Ignat’ev, D. A., Morenkov, E. D., and Stewart, M. G. (2007). Reversible reduction in dendritic spines in CA1 of rat and ground squirrel subjected to hypothermia-normothermia in vivo: a three-dimensional electron microscope study. Neuroscience 149, 549–560. doi: 10.1016/j.neuroscience.2007.07.059
Prezel, E., Elie, A., Delaroche, J., Stoppin-Mellet, V., Bosc, C., Serre, L., et al. (2018). Tau can switch microtubule network organizations: from random networks to dynamic and stable bundles. Mol. Biol. Cell 29, 154–165. doi: 10.1091/mbc.E17-06-0429
R Development Core Team (2011). R: A Language and Environment For Statistical Computing. Vienna: R Foundation for Statistical Computing. Available at: http://www.R-project.org
Roberts, R. O., Christianson, T. J., Kremers, W. K., Mielke, M. M., Machulda, M. M., Vassilaki, M., et al. (2016). Association between olfactory dysfunction and amnestic mild cognitive impairment and Alzheimer disease dementia. JAMA Neurol. 73, 93–101. doi: 10.1001/jamaneurol.2015.2952
Rothermel, M., Carey, R. M., Puche, A., Shipley, M. T., and Wachowiak, M. (2014). Cholinergic inputs from basal forebrain add an excitatory bias to odor coding in the olfactory bulb. J. Neurosci. 34, 4654–4664. doi: 10.1523/JNEUROSCI.5026-13.2014
Samuel, W., Terry, R. D., Deteresa, R., Butters, N., and Masliah, E. (1994). Clinical correlates of cortical and nucleus basalis pathology in Alzheimer Dementia. Arch. Neurol. 51, 772–778. doi: 10.1001/archneur.1994.00540200048015
Schellinck, H. M. (2001). A simple and reliable test of olfactory learning and memory in mice. Chem. Senses 26, 663–672. doi: 10.1093/chemse/26.6.663
Schellinck, H. M., Forestell, C. A., and LoLordo, V. M. (2001). A simple and reliable test of olfactory learning and memory in mice. Chem. Senses 26, 663–672. doi: 10.1093/chemse/26.6.663
Shahani, N., and Brandt, R. (2002). Functions and malfunctions of the tau proteins. Cell. Mol. Life Sci. 59, 1668–1680. doi: 10.1007/pl00012495
Shettleworth, S. J. (1978). Reinforcement and the organization of behaviour in golden hamsters: sunflower seed and nest paper reinforcers. Anim. Learn. Behav. 6, 352–362. doi: 10.3758/bf03209626
Slotnick, B. M., and Katz, H. M. (1974). Olfactory learning-set formation in rats. Science 185, 796–798. doi: 10.1126/science.185.4153.796
Stieler, J. T., Bullmann, T., Kohl, F., Barnes, B. M., and Arendt, T. (2009). PHF-like tau phosphorylation in mammalian hibernation is not associated with p25-formation. J. Neural Transm. 116, 345–350. doi: 10.1007/s00702-008-0181-x
Stieler, J. T., Bullmann, T., Kohl, F., Tøien,Ø, Brückner, M. K., Härtig, W., et al. (2011). The physiological link between metabolic rate depression and tau phosphorylation in mammalian hibernation. PLoS One 6:e14530. doi: 10.1371/journal.pone.0014530
Strijkstra, A. M., Hut, R. A., de Wilde, M. C., Stieler, J., and Van der Zee, E. A. (2003). Hippocampal synaptophysin immunoreactivity is reduced during natural hypothermia in ground squirrels. Neurosci. Lett. 344, 29–32. doi: 10.1016/s0304-3940(03)00399-9
Struble, R. G., and Clark, H. B. (1992). Olfactory bulb lesions in alzheimer’s disease. Neurobiol. Aging 13, 469–473. doi: 10.1016/0197-4580(92)90074-8
Takemura, R., Okabe, S., Umeyama, T., Kanai, Y., Cowan, N. J., and Hirokawa, N. (1992). Increased microtubule stability and alpha tubulin acetylation in cells transfected with microtubule-associated proteins MAP1B, MAP2 or tau. J. Cell Sci. 103, 953–964.
Tarsa, L., and Goda, Y. (2002). Synaptophysin regulates activity-dependent synapse formation in cultured hippocampal neurons,”. Proc. Natl. Acad. Sci. U.S.A. 99, 1012–1016. doi: 10.1073/pnas.022575999
Thal, D. R., Rüb, U., Orantes, M., and Braak, H. (2002). Phases of Aβ-deposition in the human brain and its relevance for the development of AD. Neurology 58, 1791–1800. doi: 10.1212/wnl.58.12.1791
Ueda, S., and Ibuka, N. (1995). An analysis of factors that induce hibernation in Syrian hamsters. Physiol. Behav. 58, 653–657. doi: 10.1016/0031-9384(95)00095-z
von der Ohe, C. G., Darian-Smith, C., Garner, C. C., and Heller, H. C. (2006). Ubiquitous and temperature-dependent neural plasticity in hibernators. J. Neurosci. 26, 10590–10598. doi: 10.1523/jneurosci.2874-06.2006
von der Ohe, C. G., Garner, C. C., Darian-Smith, C., and Heller, H. C. (2007). Synaptic protein dynamics in hibernation. J. Neurosci. 27, 84–92. doi: 10.1523/jneurosci.4385-06.2007
Wagner, A. (1981). “SOP: A model of automatic memory processing in animal behavior,” in Information Processing in Animals, Memory Mechanisms, eds N. E. Spear and R. R. Miller (Hillsdale, NJ: Erlbaum), 5–47.
Wang, Y., Tian, Q., Liu, E. J., Zhao, L., Song, J., Liu, X. A., et al. (2017). Activation of GSK-3 disrupts cholinergic homoeostasis in nucleus basalis of Meynert and frontal cortex of rats. J. Cell. Mol. Med. 21, 3515–3528. doi: 10.1111/jcmm.13262
Weingarten, M. D., Lockwood, A. H., Hwo, S. Y., and Kirschner, M. W. (1975). A protein factor essential for microtubule assembly. Proc. Natl. Acad. Sci. U.S.A. 72, 1858–1862. doi: 10.1073/pnas.72.5.1858
Wesson, D. W., Borkowski, A. H., Landreth, G. E., Nixon, R. A., Levy, E., and Wilson, D. A. (2011). Sensory network dysfunction, behavioral impairments, and their reversibility in an Alzheimer’s β-amyloidosis mouse model. J. Neurosci. 31, 15962–15971. doi: 10.1523/jneurosci.2085-11.2011
Whitehouse, P. J., Price, D. L., Clark, A. W., Coyle, J. T., and DeLong, M. R. (1981). Alzheimer disease: evidence for selective loss of cholinergic neurons in the nucleus basalis. Ann. Neurol. 10, 122–126. doi: 10.1002/ana.410100203
Wilson, D. A., Best, A. R., and Sullivan, R. M. (2004). Plasticity in the olfactory system: lessons for the neurobiology of memory. Neuroscience 10, 513–524. doi: 10.1177/1073858404267048
Wilson, D. A., Kadohisa, M., and Fletcher, M. L. (2006). Cortical contributions to olfaction: plasticity and perception. Semin. Cell Dev. Biol. 17, 462–470. doi: 10.1016/j.semcdb.2006.04.008
Yoshiyama, Y., Higuchi, M., Zhang, B., Huang, S. M., Iwata, N., Saido, T. C., et al. (2007). Synapse loss and microglial activation precede tangles in a P301S tauopathy mouse model. Neuron 53, 337–351. doi: 10.1016/j.neuron.2007.01.010
Yu, W., Ahmad, F. J., and Baas, P. W. (1994). Microtubule fragmentation and partitioning in the axon during collateral branch formation. J. Neurosci. 14, 5872–5884. doi: 10.1523/jneurosci.14-10-05872.1994
Keywords: Alzheimer’s disease, microtubule associated protein tau, protein phosphorylation, spines, torpor, memory
Citation: Bullmann T, Feneberg E, Kretzschmann TP, Ogunlade V, Holzer M and Arendt T (2019) Hibernation Impairs Odor Discrimination – Implications for Alzheimer’s Disease. Front. Neuroanat. 13:69. doi: 10.3389/fnana.2019.00069
Received: 26 February 2019; Accepted: 21 June 2019;
Published: 16 July 2019.
Edited by:
Laurent Gautron, UT Southwestern Medical Center, United StatesReviewed by:
Nixon M. Abraham, Indian Institute of Science Education and Research, Pune, IndiaJacques Epelbaum, Institut National de la Santé et de la Recherche Médicale (INSERM), France William Israelsen
Copyright © 2019 Bullmann, Feneberg, Kretzschmann, Ogunlade, Holzer and Arendt. This is an open-access article distributed under the terms of the Creative Commons Attribution License (CC BY). The use, distribution or reproduction in other forums is permitted, provided the original author(s) and the copyright owner(s) are credited and that the original publication in this journal is cited, in accordance with accepted academic practice. No use, distribution or reproduction is permitted which does not comply with these terms.
*Correspondence: Thomas Arendt, VGhvbWFzLkFyZW5kdEBtZWRpemluLnVuaS1sZWlwemlnLmRl; Torsten Bullmann, YnVsbG1hbm4udG9yc3RlbkBnbWFpbC5jb20=
†Present address: Emily Feneberg, Nuffield Department of Clinical Neurosciences, University of Oxford, Oxford, United Kingdom