- Department of Basic Neurosciences, University of Geneva Medical School, Geneva, Switzerland
Synaptic plasticity mechanisms are usually discussed in terms of changes in synaptic strength. The capacity of excitatory synapses to rapidly modify the membrane expression of glutamate receptors in an activity-dependent manner plays a critical role in learning and memory processes by re-distributing activity within neuronal networks. Recent work has however also shown that functional plasticity properties are associated with a rewiring of synaptic connections and a selective stabilization of activated synapses. These structural aspects of plasticity have the potential to continuously modify the organization of synaptic networks and thereby introduce specificity in the wiring diagram of cortical circuits. Recent work has started to unravel some of the molecular mechanisms that underlie these properties of structural plasticity, highlighting an important role of signaling pathways that are also major candidates for contributing to developmental psychiatric disorders. We review here some of these recent advances and discuss the hypothesis that alterations of structural plasticity could represent a common mechanism contributing to the cognitive and functional defects observed in diseases such as intellectual disability, autism spectrum disorders and schizophrenia.
Introduction
Dendritic spines are the major site for excitatory transmission in the brain. They are usually contacted by en passant presynaptic terminals and most often surrounded by astrocytic processes, forming complex structures that dysplay a high degree of functional and structural plasticity. While most research attention has usually focused on the functional aspects of synaptic plasticity and their key contribution to learning and memory mechanisms, work in the last decade has clearly demonstrated the importance of the associated structural rearrangements. These consist of different types of morphological changes (enlargement, growth, pruning, stabilization), affecting different partners (spines, terminals, astrocytic processes) and taking place on different time scales (minutes to days), making them sometimes difficult to relate to the functional changes. These structural rearrangements are also tightly controlled by activity, they are usually NMDA receptor dependent, and have the potential to significantly affect the development and organization of local synaptic networks. Recent advances have started to unravel some of complex molecular mechanisms and signaling systems regulating these synaptic rearrangements, notably at the postsynaptic level. We will therefore mainly focus on these aspects in this review and highlight the multiplicity of mechanisms that may affect structural plasticity and the development of synaptic networks and thereby contribute to cognitive disorders.
Morphological Variability of Excitatory Synapses
A particular characteristic of dendritic spines is the high variability of their morphological organization. They display major variations in volume, with large spines being several hundred times larger than small spines, but also in length, shape and content in organelles such as ribosomes, endosomal systems or spine apparatus. This high morphological variability is believed to reflect different functional properties of excitatory synapses linked to the size of the postsynaptic density, the number of postsynaptic receptors inserted in the postsynaptic density, the strength of the synapse, its developmental stage or even its stability over time. It is thus often considered that spine morphology correlates with functional parameters (Bourne and Harris, 2008). Large spines usually referred to as mushroom type of spines, or sometimes also as memory spines, are associated with mature, stable synapses that have been strengthened through a process of activity- or plasticity-mediated enlargement. In contrast, thin, elongated spines with small heads, sometimes called learning spines, are interpreted as representing young, newly formed synaptic structures that are more likely to be eliminated over time (Bourne and Harris, 2007). Several in vitro and in vivo studies have shown a high correlation between the size of the spine head, the size of the postsynaptic density, the size of glutamate-evoked responses and the stability of the spine (Kasai et al., 2003; Matsuzaki et al., 2004). In addition to thin spines, another type of protrusion often analyzed separately are filopodia, usually characterized by the absence of enlargement at the tip. Filopodia are believed to represent precursors of dendritic spines (Ziv and Smith, 1996; Petrak et al., 2005; Toni et al., 2007; Kayser et al., 2008) and they are mainly seen during early stages of development, where they can represent up to 20% of all protrusions. In adolescent and adult tissue however, they constitute only a few percent of all protrusions depending upon criteria used to identify them and their function remains largely unclear as a majority of filopodia just appear and disappear without transforming into spine synapses (Zuo et al., 2005; De Roo et al., 2008a). Overall, based on our current understanding of spine properties, it seems likely that the high morphological variability of dendritic spines reflects the different stages of maturation of excitatory synapses and their individual history. Accordingly, alterations of spine morphology or spine density, as seen in some developmental psychiatric disorders, are often interpreted as indicating defects in spine morphogenesis, stability or plasticity. A more in depth analysis of the dynamic properties of dendritic spines could however provide a better understanding of the underlying defects.
Mechanism Contributing to Structural Plasticity
A major breakthrough made possible by the development of in vivo confocal imaging approaches has been the demonstration that dendritic spines are highly plastic structures that not only continuously change in shape over time (Matus, 2000; Yasumatsu et al., 2008), but can also be formed and eliminated throughout life in an activity-dependent manner (Lendvai et al., 2000; Holtmaat et al., 2005; Caroni et al., 2012). These observations support the concept of a dynamic regulation of excitatory synaptic networks by activity and plasticity mechanisms. A striking aspect of this dynamic regulation of connectivity is its magnitude during periods of intense development such as critical periods and the fact that it then decreases with age (Zuo et al., 2005; Holtmaat and Svoboda, 2009). This is consistent with the notion that the control of spine dynamics by activity plays a central role in shaping the organization of local synaptic networks during development, whereas the reduced structural plasticity still present in older animals maintains possibilities of adaptation while minimizing the possible disruption of existing circuits.
These synaptic rearrangements involve multiple mechanisms to control the formation, maintenance and elimination of excitatory synapses. It is very likely that distinct molecular pathways regulate these different mechanisms independently, as specific manipulations can selectively affect formation, stabilization or elimination of protrusions (Mendez et al., 2010b; Koleske, 2013; Kehoe et al., 2014). Additionally there probably exists homeostatic regulations that coordinate these processes and can compensate for alterations of one or the other mechanism. For example, we have observed that transfection of pyramidal neurons with a dominant-negative mutant of N-cadherin, that strongly reduces spine stability, also leads to a compensatory increase in spine formation (Mendez et al., 2010a). Finally, these different mechanisms are finely tuned by activity and plasticity induction, adding another complexity to their regulation.
In the context of learning related paradigms (LTP), there appear to be two major structural types of changes that have been reported both in in vitro systems such as the hippocampal organotypic slice culture or under in vivo conditions in anesthetized mice (De Roo et al., 2008b; Hübener and Bonhoeffer, 2010). In slice cultures, induction of long-term potentiation results in two major changes: an increase in spine turnover, characterized by an increase in spine growth but also in spine elimination (Engert and Bonhoeffer, 1999; Nägerl et al., 2004; De Roo et al., 2008b), and a selective stabilization of activated synapses (De Roo et al., 2008b; Hill and Zito, 2013). In living mice, similar changes have also been observed in the visual or motor cortices (Keck et al., 2008; Xu et al., 2009). Notably, in mice trained for a dexterity motor task, spine turnover increases and correlates with the ability to learn the task, while there is also a specific stabilization of newly formed spines that correlates with the memory or retention of the task (Xu et al., 2009; Yang et al., 2009). Based on this type of results, one can thus propose that changes in spine turnover and changes in spine stability represent two key mechanisms associated with memory that could account for some of its antinomic properties: the capacity to learn, which requires adaptation of existing networks, and the capacity to retain information, which requires to maintain important functional circuits (Caroni et al., 2012; see Figure 1).
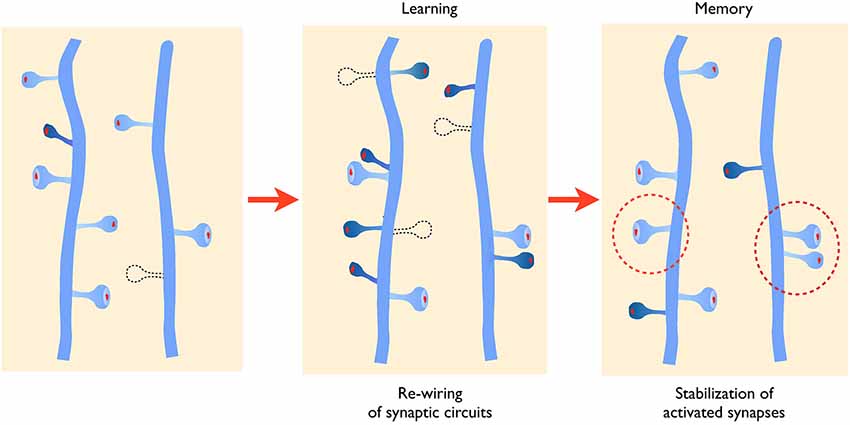
Figure 1. Activity-mediated structural plasticity. Left panel: synaptic networks are characterized by a continuous process of growth (dark blue spine) and elimination (dotted line) of dendritic spines that is developmentally regulated. Middle panel: during learning or learning related activity, baseline turnover is significantly enhanced leading to an increased formation and elimination of synaptic contacts, thus allowing remodeling and adaptation of connectivity. New spines also tend to grow in the proximity of activated synapses favoring the formation of spine clusters. Right panel: newly formed and activated synapses are preferentially stabilized (dotted circles) allowing to maintain important functional connections.
Activity-Dependent Changes in Spine Morphology and Stability
Identification of stimulated synapses within a network has been and still is one of the important limitations for understanding how activity and plasticity regulate synapse properties. This has been overcome in only a few studies using either 2-photon uncaging of glutamate to achieve local stimulation of identified synapses or calcium imaging coupled to electrical stimulation to reveal functional synapses. Although previous electron microscopic (EM) analyses had already suggested that LTP could be associated with morphological changes (Fifková and Anderson, 1981; Geinisman, 1993; Buchs and Muller, 1996), the early, elegant studies by Kasai group (Matsuzaki et al., 2004) were the first to provide a direct demonstration that induction of plasticity was indeed associated with a rapid increase in size of stimulated synapses and an increased sensitivity to glutamate. Other experiments further showed that this enlargement can last several hours (Zito et al., 2009) and that it is correlated with a reorganization of the actin cytoskeleton (Honkura et al., 2008). These observations were thus perfectly in line with accumulating evidence suggesting an implication of the actin cytoskeleton and various actin-regulatory proteins in both LTP maintenance and spine morphology (Fukazawa et al., 2003; Lisman, 2003; Chen et al., 2007; Bramham, 2008). As previously mentioned and revelaed by the studies in hippocampal slice cultures, the structural changes affecting stimulated synapses are also correlated with a major increase in their long-term stability (De Roo et al., 2008b; Hill and Zito, 2013). Very few stimulated synapses disappeared within the days that followed their stimulation, while in contrast non stimulated synapses located on the same dendrite tended to be eliminated at a faster rate. Whether the change in spine stability is directly related to the activity-dependent enlargement remains however unclear. Spine size and spine stability show indeed a high degree of correlation. Repetitive imaging experiments indicate that the size of spine heads continuously fluctuates over a time scale of days (Holtmaat et al., 2005; Yasumatsu et al., 2008). Furthermore, the fact that the activity-dependent spine enlargement seems to be only transient and to reverse within 24 h, while the improved stability can be measured over days suggests that they are regulated by different mechanisms (De Roo et al., 2008b).
The molecular nature of the mechanisms confering a high stability to activated spines remains also an open issue. Evidence from different studies suggest that the structural organization of the actin cytoskeleton within the spine head could contribute to modify spine stability (Fukazawa et al., 2003; Chen et al., 2007). Phosphorylation of the cytoskeleton-stabilizing protein beta-adducin appears to be required for spine stabilization (Bednarek and Caroni, 2011). Also, several molecules regulating actin polymerization and notably upstream and downstream regulators of Rho GTPases affect the capacity of spines to enlarge and their stability (Rex et al., 2009; Murakoshi et al., 2011; Dubos et al., 2012). Proteins that could also be important to confer stability are adhesion molecules expressed at excitatory synapses. Neuroligins, integrins, ephrins and N-cadherins have all been proposed to contribute to the persistence of spines (Wang et al., 2008; Matter et al., 2009; Bozdagi et al., 2010; McGeachie et al., 2011; Koleske, 2013), although a direct demonstration of their role in long-term activity-dependent spine stability is still often missing. In studies that we carried out to analyze the role of N-cadherin in structural plasticity, we found that an extracellular mutant of N-cadherin strongly affects the life time of dendritic spines and that it prevents activity-mediated spine stabilization (Mendez et al., 2010a). We also showed that N-cadherin is not expressed in all spines and that the spines in which it is expressed show an increased stability. Furthermore N-cadherin expression could be increased through activity patterns that induce LTP, and following stimulation we found that the adhesion molecule selectively accumulates in spines that had been activated. These results thus suggest that modifications in the adhesion profile of activated synapses contribute to regulate the stability and life time of spine synapses (Mendez et al., 2010a). It seems likely that this effect is mediated by increasing trans-synaptic interactions between pre- and postsynaptic structures as molecular complexes showing a periodic organization compatible with the existence of N-cadherin meshwork has been identified in the synaptic cleft of excitatory synapses (Zuber et al., 2005). Adhesion could also be reinforced between synaptic structures and the third partner of the tripartite synapse: the astroglial component. EM studies (Lushnikova et al., 2009) as well as work by Bernardinelli et al. (2014) suggest that synaptic activity and LTP induction can drive a reorganization of astrocytic processes around activated synapses. This glial structural remodeling is mediated by direct activation of glial metabotropic receptors by the released transmitter and calcium influx in the astrocytic process. This transient increase in motility of the astrocytic process leads to a better coverage of the synapse and an enhanced long-term stability of the synaptic contact. This effect has been observed both under in vitro and in vivo conditions in the somatosensory cortex and is summarized in Figure 2. These new results thus suggest a new role of astrocytic processes in the activity-dependent structural organization of the synapse and a contribution of the astrocyte to the generation of life-long, persistent synapses. In line with these observations, recent work has also demonstrated a critical role of astrocytes in LTP mechanisms and long-term memory in mice (Suzuki et al., 2011). Conversely, there are multiple examples showing that molecular interference with the mechanisms of spine stabilization described above significantly affect cognitive functions (Allen et al., 1998; Sudhof, 2008; Redies et al., 2012; Vukojevic et al., 2012; Ba et al., 2013).
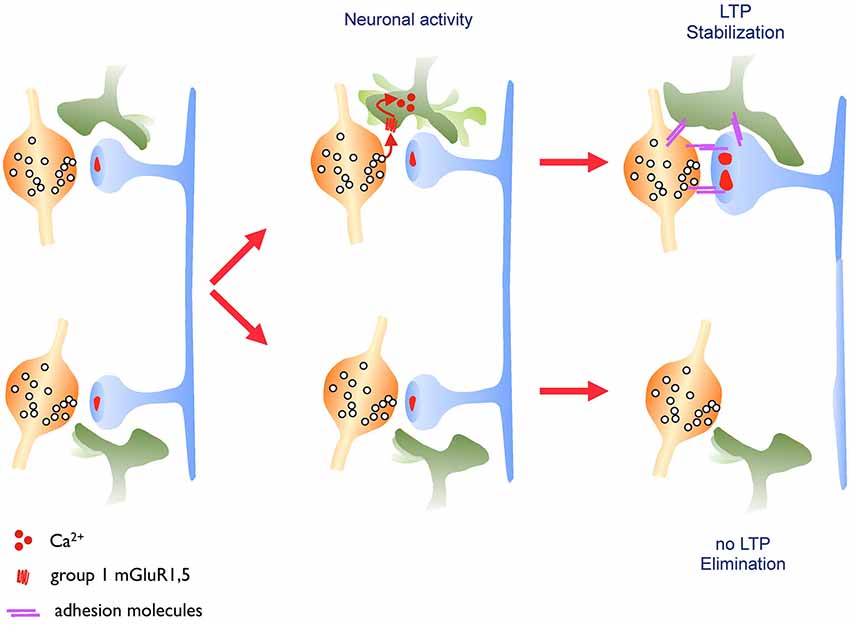
Figure 2. Astrocytic structural plasticity contributes to the maintenance of activated synapses. Left panel: excitatory synapses are surrounded by astrocytic processes that show a high level of motility. Middle panel: this astrocytic motility is regulated by the released neurotransmitter, which activates glutamate metabotropic receptors on the astrocytic process leading to an increased calcium flux and motility of the astrocytic process. Right panel: when driven by learning related paradigms (LTP, upper synapse), this enhanced motility leads to an increased and more stable coverage of the synapse by the astrocytic process resulting in a long-lasting stabilization of the synapse. This mechanism might involve, among other possibilities, a contribution of adhesion molecules.
Mechanisms of Activity-Dependent Spine Turnover
The now classical experiments made by Engert and Bonhoeffer (1999) provided the first evidence that induction of plasticity could result in the growth of new dendritic spines. Further studies by several groups confirmed that these new spines display synapses with all the characteristics of morphologically mature and functional contacts (Toni et al., 1999; Nägerl et al., 2007). In vivo studies then showed that spine formation and elimination occurred continuously in a developmentally regulated manner and independently of activity (Lendvai et al., 2000; Holtmaat et al., 2005; Zuo et al., 2005). These mechanisms however are strongly enhanced by sensory or motor activity (Holtmaat et al., 2006; Xu et al., 2009). Consistently theta activity or induction of plasticity in hippocampal slices also strongly promotes spine turnover, enhancing both formation and elimination of dendritic spines, leading to a significant activity-dependent rewiring of the hippocampal circuit (De Roo et al., 2008b).
One interesting issue raised by these observations is whether mechanisms of spine growth and elimination are independently regulated. Although activity appears to stimulate both processes simultaneously, several recent results suggest that these two mechanisms are regulated independently of each other. For example, treatment with estrogens results in a significant increase in spine density both in in vitro and in vivo experiments (Yankova et al., 2001; Sakamoto et al., 2003; Mendez et al., 2010b). Turnover analyses revealed that this effect resulted from a significant increase in spine growth mechanisms, but without changes in spine elimination, thereby accounting for the changes in density. Similarly, specific effects on spine growth mechanisms without alterations of spine elimination have been observed following interference with P21 activated kinase (PAK3; Dubos et al., 2012), by direct activation of the cytoskeletal regulatory protein vasodilator-stimulated phosphoprotein (VASP; Lin et al., 2007; Nikonenko et al., 2013) and following application of BDNF or the phosphatase and tensin homolog (PTEN) inhibitor BpV (Tanaka et al., 2008; Boda et al., 2014). This suggests therefore that mechanisms that promote actin polymerization can directly stimulate spine growth and that signaling pathways implicated in the regulation of protein synthesis such as the PI3K-AKT-mTor pathway participate in the control of this growth process. Conversely, there are also mechanisms that selectively affect spine elimination without altering spine growth processes. This might be the case of long-term depression that has been shown in several recent studies to result in spine shrinkage and disappearance (Becker et al., 2008; Oh et al., 2013; Wiegert and Oertner, 2013). The molecular mechanisms implicated in this effect remain unclear but are likely to require activation of both NMDA and glutamate metabotropic receptors (Oh et al., 2013). Consistent with this interpretation, we recently identified a specific role of the inclusion of the GluN3A subunit in NMDA receptors in the regulation of spine pruning (Kehoe et al., 2013). The GluN3A subunit is highly expressed during critical periods of development and its expression is associated with a decrease in spine density (Roberts et al., 2009). By manipulating the expression of this subunit using gain or loss of function experiments, we found that a central mechanism accounting for these effects is a selective increase in spine pruning associated with expression of the GluN3A subunit (Kehoe et al., 2014). These results thus clearly indicate that spine density can be differentially regulated by interfering with either spine growth or elimination mechanisms.
Whether and how these mechanisms of spine turnover contribute to cognitive functions remains still unclear. However alterations of spine turnover have been reported for cases of intellectual disability or autism spectrum disorders (Cruz-Martín et al., 2010; Pan et al., 2010; Dubos et al., 2012) and mechanisms that regulate spine growth and turnover are also associated with cognitive defects and autism spectrum disorders (Sharma et al., 2010; Cao et al., 2013; Boda et al., 2014).
Mechanisms Underlying Spine Clustering
An interesting observation related to activity-dependent spine growth mechanisms is that the formation of the new spines occurs in a non-random fashion but is more readily observed in close proximity to activated synapses leading sometimes to the formation of spine clusters (De Roo et al., 2008b; Dubos et al., 2012). This phenomenon, initially detected in hippocampal slice cultures, was then also observed under in vivo conditions following a motor learning task (Fu et al., 2012). An intriguing question relates to the mechanism that could promote the growth of new spines in clusters or in regions of activity. Several hypotheses can be considered. First, there is evidence from in vitro work that application of glutamate or uncaging of glutamate on a dendrite can rapidly trigger the growth of a new spine (Richards et al., 2005; Kwon and Sabatini, 2011). The phenomenon was shown to depend upon the activity of protein kinases in the postsynaptic dendrite. One could thus consider that intense presynaptic activity and glutamate release could trigger the growth of new spines in small brain areas. One major difficulty with this interpretation is the timing of spine formation. Spine growth was observed to occur within minutes after glutamate uncaging, whereas activity-dependent spine growth is a much slower process that can only be visualized hours after stimulation, long after glutamate release has taken place (Engert and Bonhoeffer, 1999; De Roo et al., 2008b). Although this does not exclude a role for glutamate release, it certainly indicates that activity-induced spine growth is not equivalent to the effects of glutamate uncaging. Another interpretation has recently been proposed based on experiments analyzing the role of nitric oxide (NO) on synapse development (Nikonenko et al., 2013). Nitric oxide can be generated at excitatory synapses in response to NMDA receptor activation of NO synthase, which is closely associated with the postsynaptic density (Burette et al., 2002; Ishii et al., 2006; d’Anglemont de Tassigny et al., 2007). Interventions that blocked NO production or guanilate kinase, the main downstream effector of NO, strongly reduced spine growth mechanisms including the enhancement produced by induction of plasticity (Nikonenko et al., 2013). Conversely, application of cGMP enhanced spine formation, occluding the effects of synaptic activity. Interestingly, these effects could be traced to the phosphorylation of the actin regulatory protein VASP by cGMP kinase. Expression of VASP phospho-mutants that either mimicked or prevented phosphorylation by cGMP kinase reproduced or blocked the effects of activity on spine growth mechanisms (Nikonenko et al., 2013). Moreover in mice deficient in NO synthase, the development of spine synapses was considerably affected and exposure of these mice to an enriched environment failed to promote an increase in synapse density. Analysis of the distribution of spine synapses also revealed a lack of clustering of synapses, a phenomenon readily observed in wild type mice (Nikonenko et al., 2013). These findings thus suggest that one mechanism that may account for the activity-dependent clustering of spines around active sites could involve the production of NO by activated synapses, which in turn stimulates protrusion growth through the phosphorylation of the regulatory protein VASP, promoting in this way the formation of synapses in areas where active partners are found (summary in Figure 3).
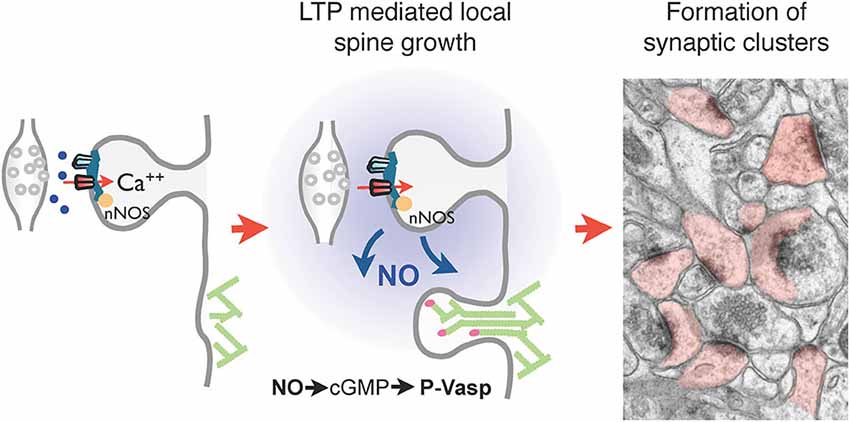
Figure 3. Regulation of local spine growth by nitric oxide (NO). Left panel: neuronal NO synthase (nNOS), present in the postsynaptic density and tightly associated with PSD-95 and NMDA receptors, generates NO upon NMDA receptor activation and calcium influx. Middle panel: NO generated at activated synapses by LTP diffuses locally and triggers the activation of a cGMP-dependent cascade leading to the phosphorylation of the cytoskeleton regulatory protein VASP by cGMP-dependent kinase. This in turn promotes actin filament elongation and spine formation. Right panel: this mechanisms allows the formation of clusters of spine synapses around activated contacts, a phenomenon absent in nNOS deficient mice. The electron microscopy image illustrates clusters of dendritic spines (filled in rose) in the hippocampus of wild type mouse raised in an enriched environment.
While the functional importance of this clustering phenomenon remains still unclear, it is interesting that interference with NO production or signaling is associated with impaired cognitive performance and social dysfunction (Kirchner et al., 2004; Kelley et al., 2009; Tanda et al., 2009; Wass et al., 2009) and variants of the NO synthase 1 gene have been linked to schizophrenia (Shinkai et al., 2002; Bernstein et al., 2011).
Synaptic Defects in Developmental Psychiatric Disorders
All these data highlight the complexity of the mechanisms regulating the dynamics of excitatory synapses during development and strongly suggest that any interference with these structural plasticity properties may have a significant impact on the organization and functional properties of excitatory networks. Changes in spine stability or spine turnover can be expected to affect the number, but also the specificity of excitatory synapses resulting in the formation of synaptic circuits displaying various abnormal features such as hyper- or hypo-connectivity and hyper- or hypo-dynamic responses to activity (Mukai et al., 2008; Pan et al., 2010; Qiu et al., 2011). All these different alterations, by affecting the development and functional properties of synaptic networks, might decrease the reliability of information processing and lead to the formation of maladaptative brain circuits (Sigurdsson et al., 2010). Depending on the molecular mechanism involved, the alterations could affect differentially various brain areas or have a greater impact during different phases of development, thereby accounting for the variability in clinical or behavioral phenotypes. One would also anticipate that alterations of structural plasticity with a strong impact during critical periods of development might have more damaging consequences that could be difficult to reverse and would require very early interventions in order to compensate for the defects. Such early approaches are now tested in many situations related to autism spectrum disorders (Canitano, 2014).
Based on these observations, we propose that a possibly common feature of various developmental psychiatric disorders could be the existence of alterations in the dynamic properties of excitatory synapses. This would be consistent with the strong developmental component of these disorders and the multitude of synaptic proteins possibly implicated. This could also account for the fact that regions showing a high degree of plasticity, such as the prefrontal cortex and the hippocampus, are very often showing functional defects. Finally, in line with this hypothesis, signaling pathways that play an important role in regulating spine dynamics are major candidates for contributing to psychiatric disorders. Examples include Rho GTPases signaling and their regulation of the actin cytoskeleton and the signaling cascades implicated in the control of protein synthesis (Sawicka and Zukin, 2012; Ba et al., 2013). These two signaling systems are particularly important at excitatory synapses to control spine morphology and plasticity, and several genetic alterations associated with psychiatric disorders affect molecules implicated in these two pathways (Boda et al., 2010; Fromer et al., 2014).
The Rho GTPases signaling pathway comprises several molecules that have been associated with intellectual disability, autism spectrum disorders or schizophrenia. Examples include membrane receptors and adhesion molecules able to activate Rho GTPases (cadherins, ephrins), regulators of Rho GTPases (oligophrenin1, GDI1, ARHGEF6, EPAC2, CNK2 (connector enhancer of KSR-2), srGAP3, Disc1, SynGAP), effectors of Rho GTPases (PAK3), as well as cytoskeletal regulatory proteins (Endris et al., 2002; Govek et al., 2004; Nodé-Langlois et al., 2006; Woolfrey et al., 2009; Hayashi-Takagi et al., 2010; Clement et al., 2012; Lim et al., 2014). In many of these cases, alterations of synapse morphology or function have been reported, although analyses of spine dynamics are still often missing. There is one example that we have studied in more details and that concerns the intellectual disability protein PAK3. Several mutations of PAK3 are associated with intellectual disability in humans. Expression of the mutant PAK3 in hippocampal neurons or genetic and pharmacological suppression of PAK3 were all found to result in alterations of spine morphology and spine dynamics (Kreis et al., 2007; Dubos et al., 2012). The two main features observed following interference with PAK3 function were an increase in spontaneous spine growth mechanisms and a block of activity-dependent spine stabilization. Consequently, this resulted in an excessively dynamic synaptic network in which activity failed to selectively stabilize activated synapses (Dubos et al., 2012). This was also reflected by the presence of a high proportion of thin, immature spines and a reduced number of large mushroom type spines (Boda et al., 2004). It can be assumed therefore that these defects resulted in a failure to maintain important connections and to develop specificity in the wiring organization.
The second important pathway strongly implicated in psychiatric disorders involves a number of molecules regulating local protein synthesis. This includes membrane receptors (BDNF and TrkB, Insulin receptor, Ephrins) or adhesion and scaffold molecules (integrins, neuroligin/neurexin complex, shank, SynGAP), intracellular mediators implicated in mTOR signaling (PI3K, Akt, PTEN, TSC2) and regulators of protein synthesis (FMRP, CYFIP1) (Kumar et al., 2005; Lai and Ip, 2009; Mendez et al., 2010a; Sharma et al., 2010; Cuesto et al., 2011; Clement et al., 2012; Boda et al., 2014). This pathway is also critically implicated in the regulation of synaptic strength and in most cases alterations of spine morphology or density have been reported, suggesting impairments of structural plasticity. One of the most studied examples is certainly Fragile X syndrome, associated to multiple CGG repeats in the fmr1 gene (Bhakar et al., 2012). Mice deficient in FMRP protein show alterations of spine morphology with an increased proportion of thin, elongated spines (Comery et al., 1997, but see also Wijetunge et al., 2014). Recent work using Fmr1 knocout mice provided evidence that these spines are hyper-dynamic, do not respond to sensory stimulation (Pan et al., 2010) and lack stability (Cruz-Martín et al., 2010). In slice culture experiments, we also found that spine turnover was excessively sensitive to activity and more specifically that induction of plasticity failed to induce a differential stabilization of activated spines. Interestingly, in these experiments, we were able to reverse this deficit through application of a PTEN inhibitor, which enhances the PI3K-Akt-mTOR signaling pathway (Boda et al., 2014), while interfering with metabotropic glutamate receptors was only partly effective (Cruz-Martín et al., 2010; Boda et al., 2014). This PTEN inhibitor also improved an associated deficit in LTP mechanisms and when injected in FMR1 deficient mice restored reversal learning in the Morris water maze task (Boda et al., 2014). While much remains to be understood regarding the mechanisms through which local protein synthesis could affect structural plasticity, these data suggest that this system may be an interesting target for modulating properties of spine dynamics and eventually counterbalance some of the deficits observed in the associated disorders.
Conclusion
Functional synaptic plasticity properties, by quickly changing synaptic strength, allow fast adaptations of network activity which are critical for information processing. However, on a longer time scale, structural plasticity properties may allow a more significant and stable rewiring of synaptic networks through both the formation of new connections and the stabilization of specific contacts. These properties of structural plasticity are particularly important during development where they contribute to shape the structural organization of brain circuits through activity. Molecular analyses of these structural properties started to identify key signaling pathways implicated in these synaptic reorganizations, which also appear to be strong candidates for contributing to cognitive and psychiatric disorders. Hence a common denominator of developmental disorders could involve alterations in spine dynamics that would affect the connectivity and specificity of brain circuits. More systematic analyses of these properties and their functional consequences should allow a better understanding of how they affect information processing and this could eventually lead to new possibilities of treatment of these disorders.
Conflict of Interest Statement
The authors declare that the research was conducted in the absence of any commercial or financial relationships that could be construed as a potential conflict of interest.
Acknowledgments
This work was supported by the Swiss Science Foundation (grant 310030B_144080) and NCCR Synapsy.
References
Allen, K. M., Gleeson, J. G., Bagrodia, S., Partington, M. W., MacMillan, J. C., Cerione, R. A., et al. (1998). PAK3 mutation in nonsyndromic X-linked mental retardation. Nat. Genet. 20, 25–30. doi: 10.1038/1675
Pubmed Abstract | Pubmed Full Text | CrossRef Full Text | Google Scholar
Ba, W., van der Raadt, J., and Nadif Kasri, N. (2013). Rho GTPase signaling at the synapse: implications for intellectual disability. Exp. Cell Res. 319, 2368–2374. doi: 10.1016/j.yexcr.2013.05.033
Pubmed Abstract | Pubmed Full Text | CrossRef Full Text | Google Scholar
Becker, N., Wierenga, C. J., Fonseca, R., Bonhoeffer, T., and Nägerl, U. V. (2008). LTD induction causes morphological changes of presynaptic boutons and reduces their contacts with spines. Neuron 60, 590–597. doi: 10.1016/j.neuron.2008.09.018
Pubmed Abstract | Pubmed Full Text | CrossRef Full Text | Google Scholar
Bednarek, E., and Caroni, P. (2011). β-Adducin is required for stable assembly of new synapses and improved memory upon environmental enrichment. Neuron 69, 1132–1146. doi: 10.1016/j.neuron.2011.02.034
Pubmed Abstract | Pubmed Full Text | CrossRef Full Text | Google Scholar
Bernardinelli, Y., Randall, J., Janett, E., Nikonenko, I., Konig, S., Jones, E. V., et al. (2014). Activity-dependent structural plasticity of perisynaptic astrocytic domains promotes excitatory synapse stability. Curr. Biol. 24, 1679–1688. doi: 10.1016/j.cub.2014.06.025
Pubmed Abstract | Pubmed Full Text | CrossRef Full Text | Google Scholar
Bernstein, H. G., Keilhoff, G., Steiner, J., Dobrowolny, H., and Bogerts, B. (2011). Nitric oxide and schizophrenia: present knowledge and emerging concepts of therapy. CNS Neurol. Disord. Drug Targets 10, 792–807. doi: 10.2174/187152711798072392
Pubmed Abstract | Pubmed Full Text | CrossRef Full Text | Google Scholar
Bhakar, A. L., Dölen, G., and Bear, M. F. (2012). The pathophysiology of fragile X (and what it teaches us about synapses). Annu. Rev. Neurosci. 35, 417–443. doi: 10.1146/annurev-neuro-060909-153138
Pubmed Abstract | Pubmed Full Text | CrossRef Full Text | Google Scholar
Boda, B., Alberi, S., Nikonenko, I., Node-Langlois, R., Jourdain, P., Moosmayer, M., et al. (2004). The mental retardation protein PAK3 contributes to synapse formation and plasticity in hippocampus. J. Neurosci. 24, 10816–10825. doi: 10.1523/jneurosci.2931-04.2004
Pubmed Abstract | Pubmed Full Text | CrossRef Full Text | Google Scholar
Boda, B., Dubos, A., and Muller, D. (2010). Signaling mechanisms regulating synapse formation and function in mental retardation. Curr. Opin. Neurobiol. 20, 519–527. doi: 10.1016/j.conb.2010.03.012
Pubmed Abstract | Pubmed Full Text | CrossRef Full Text | Google Scholar
Boda, B., Mendez, P., Boury-Jamot, B., Magara, F., and Muller, D. (2014). Reversal of activity-mediated spine dynamics and learning impairment in a mouse model of Fragile X syndrome. Eur. J. Neurosci. 39, 1130–1137. doi: 10.1111/ejn.12488
Pubmed Abstract | Pubmed Full Text | CrossRef Full Text | Google Scholar
Bourne, J., and Harris, K. M. (2007). Do thin spines learn to be mushroom spines that remember? Curr. Opin. Neurobiol. 17, 381–3886. doi: 10.1016/j.conb.2007.04.009
Pubmed Abstract | Pubmed Full Text | CrossRef Full Text | Google Scholar
Bourne, J. N., and Harris, K. M. (2008). Balancing structure and function at hippocampal dendritic spines. Annu. Rev. Neurosci. 31, 47–67. doi: 10.1146/annurev.neuro.31.060407.125646
Pubmed Abstract | Pubmed Full Text | CrossRef Full Text | Google Scholar
Bozdagi, O., Wang, X. B., Nikitczuk, J. S., Anderson, T. R., Bloss, E. B., Radice, G. L., et al. (2010). Persistence of coordinated long-term potentiation and dendritic spine enlargement at mature hippocampal CA1 synapses requires N-cadherin. J. Neurosci. 30, 9984–9989. doi: 10.1523/jneurosci.1223-10.2010
Pubmed Abstract | Pubmed Full Text | CrossRef Full Text | Google Scholar
Bramham, C. R. (2008). Local protein synthesis, actin dynamics and LTP consolidation. Curr. Opin. Neurobiol. 18, 524–531. doi: 10.1016/j.conb.2008.09.013
Pubmed Abstract | Pubmed Full Text | CrossRef Full Text | Google Scholar
Buchs, P. A., and Muller, D. (1996). Induction of long-term potentiation is associated with major ultrastructural changes of activated synapses. Proc. Natl. Acad. Sci. U S A 93, 8040–8045. doi: 10.1073/pnas.93.15.8040
Pubmed Abstract | Pubmed Full Text | CrossRef Full Text | Google Scholar
Burette, A., Zabel, U., Weinberg, R. J., Schmidt, H. H., and Valtschanoff, J. G. (2002). Synaptic localization of nitric oxide synthase and soluble guanylyl cyclase in the hippocampus. J. Neurosci. 22, 8961–8970.
Canitano, R. (2014). New experimental treatments for core social domain in autism spectrum disorders. Front. Pediatr. 2:61. doi: 10.3389/fped.2014.00061
Pubmed Abstract | Pubmed Full Text | CrossRef Full Text | Google Scholar
Cao, C., Rioult-Pedotti, M. S., Migani, P., Yu, C. J., Tiwari, R., Parang, K., et al. (2013). Impairment of TrkB-PSD-95 signaling in Angelman syndrome. PLoS Biol. 11:e1001478. doi: 10.1371/journal.pbio.1001848
Pubmed Abstract | Pubmed Full Text | CrossRef Full Text | Google Scholar
Caroni, P., Donato, F., and Muller, D. (2012). Structural plasticity upon learning: regulation and functions. Nat. Rev. Neurosci. 13, 478–490. doi: 10.1038/nrn3258
Pubmed Abstract | Pubmed Full Text | CrossRef Full Text | Google Scholar
Chen, L. Y., Rex, C. S., Casale, M. S., Gall, C. M., and Lynch, G. (2007). Changes in synaptic morphology accompany actin signaling during LTP. J. Neurosci. 27, 5363–5372. doi: 10.1523/jneurosci.0164-07.2007
Pubmed Abstract | Pubmed Full Text | CrossRef Full Text | Google Scholar
Clement, J. P., Aceti, M., Creson, T. K., Ozkan, E. D., Shi, Y., Reish, N. J., et al. (2012). Pathogenic SYNGAP1 mutations impair cognitive development by disrupting maturation of dendritic spine synapses. Cell 151, 709–723. doi: 10.1016/j.cell.2012.08.045
Pubmed Abstract | Pubmed Full Text | CrossRef Full Text | Google Scholar
Comery, T. A., Harris, J. B., Willems, P. J., Oostra, B. A., Irwin, S. A., Weiler, I. J., et al. (1997). Abnormal dendritic spines in fragile X knockout mice: maturation and pruning deficits. Proc. Natl. Acad. Sci. U S A 94, 5401–5404. doi: 10.1073/pnas.94.10.5401
Pubmed Abstract | Pubmed Full Text | CrossRef Full Text | Google Scholar
Cruz-Martín, A., Crespo, M., and Portera-Cailliau, C. (2010). Delayed stabilization of dendritic spines in fragile X mice. J. Neurosci. 30, 7793–7803. doi: 10.1523/JNEUROSCI.0577-10.2010
Pubmed Abstract | Pubmed Full Text | CrossRef Full Text | Google Scholar
Cuesto, G., Enriquez-Barreto, L., Caramés, C., Cantarero, M., Gasull, X., Sandi, C., et al. (2011). Phosphoinositide-3-kinase activation controls synaptogenesis and spinogenesis in hippocampal neurons. J. Neurosci. 31, 2721–2733. doi: 10.1523/JNEUROSCI.4477-10.2011
Pubmed Abstract | Pubmed Full Text | CrossRef Full Text | Google Scholar
d’Anglemont de Tassigny, X., Campagne, C., Dehouck, B., Leroy, D., Holstein, G. R., Beauvillain, J. C., et al. (2007). Coupling of neuronal nitric oxide synthase to NMDA receptors via postsynaptic density-95 depends on estrogen and contributes to the central control of adult female reproduction. J. Neurosci. 27, 6103–6114. doi: 10.1523/jneurosci.5595-06.2007
Pubmed Abstract | Pubmed Full Text | CrossRef Full Text | Google Scholar
De Roo, M., Klauser, P., Mendez, P., Poglia, L., and Muller, D. (2008a). Activity-dependent PSD formation and stabilization of newly formed spines in hippocampal slice cultures. Cereb. Cortex 18, 151–161. doi: 10.1093/cercor/bhm041
Pubmed Abstract | Pubmed Full Text | CrossRef Full Text | Google Scholar
De Roo, M., Klauser, P., and Muller, D. (2008b). LTP promotes a selective long-term stabilization and clustering of dendritic spines. PLoS Biol. 6:e219. doi: 10.3410/f.1124276.581429
Pubmed Abstract | Pubmed Full Text | CrossRef Full Text | Google Scholar
Dubos, A., Combeau, G., Bernardinelli, Y., Barnier, J. V., Hartley, O., Gaertner, H., et al. (2012). Alteration of synaptic network dynamics by the intellectual disability protein PAK3. J. Neurosci. 32, 519–527. doi: 10.1523/JNEUROSCI.3252-11.2012
Pubmed Abstract | Pubmed Full Text | CrossRef Full Text | Google Scholar
Endris, V., Wogatzky, B., Leimer, U., Bartsch, D., Zatyka, M., Latif, F., et al. (2002). The novel Rho-GTPase activating gene MEGAP/ srGAP3 has a putative role in severe mental retardation. Proc. Natl. Acad. Sci. U S A 99, 11754–11759. doi: 10.1073/pnas.162241099
Pubmed Abstract | Pubmed Full Text | CrossRef Full Text | Google Scholar
Engert, F., and Bonhoeffer, T. (1999). Dendritic spine changes associated with hippocampal long-term synaptic plasticity. Nature 399, 66–70. doi: 10.1038/19978
Pubmed Abstract | Pubmed Full Text | CrossRef Full Text | Google Scholar
Fifková, E., and Anderson, C. L. (1981). Stimulation-induced changes in dimensions of stalks of dendritic spines in the dentate molecular layer. Exp. Neurol. 74, 621–627. doi: 10.1016/0014-4886(81)90197-7
Pubmed Abstract | Pubmed Full Text | CrossRef Full Text | Google Scholar
Fromer, M., Pocklington, A. J., Kavanagh, D. H., Williams, H. J., Dwyer, S., Gormley, P., et al. (2014). De novo mutations in schizophrenia implicate synaptic networks. Nature 506, 179–184. doi: 10.1038/nature12929
Pubmed Abstract | Pubmed Full Text | CrossRef Full Text | Google Scholar
Fu, M., Yu, X., Lu, J., and Zuo, Y. (2012). Repetitive motor learning induces coordinated formation of clustered dendritic spines in vivo. Nature 483, 92–95. doi: 10.1038/nature10844
Pubmed Abstract | Pubmed Full Text | CrossRef Full Text | Google Scholar
Fukazawa, Y., Saitoh, Y., Ozawa, F., Ohta, Y., Mizuno, K., and Inokuchi, K. (2003). Hippocampal LTP is accompanied by enhanced F-actin content within the dendritic spine that is essential for late LTP maintenance in vivo. Neuron 38, 447–460. doi: 10.1016/s0896-6273(03)00206-x
Pubmed Abstract | Pubmed Full Text | CrossRef Full Text | Google Scholar
Geinisman, Y. (1993). Perforated axospinous synapses with multiple, completely partitioned transmission zones: probable structural intermediates in synaptic plasticity. Hippocampus 3, 417–433. doi: 10.1002/hipo.450030404
Pubmed Abstract | Pubmed Full Text | CrossRef Full Text | Google Scholar
Govek, E. E., Newey, S. E., Akerman, C. J., Cross, J. R., Van der Veken, L., and Van Aelst, L. (2004). The X-linked mental retardation protein oligophrenin-1 is required for dendritic spine morphogenesis. Nat. Neurosci. 7, 364–372. doi: 10.1038/nn1210
Pubmed Abstract | Pubmed Full Text | CrossRef Full Text | Google Scholar
Hayashi-Takagi, A., Takaki, M., Graziane, N., Seshadri, S., Murdoch, H., Dunlop, A. J., et al. (2010). Disrupted-in-Schizophrenia 1 (DISC1) regulates spines of the glutamate synapse via Rac1. Nat. Neurosci. 13, 327–332. doi: 10.1038/nn.2487
Pubmed Abstract | Pubmed Full Text | CrossRef Full Text | Google Scholar
Hill, T. C., and Zito, K. (2013). LTP-induced long-term stabilization of individual nascent dendritic spines. J. Neurosci. 33, 678–686. doi: 10.1523/JNEUROSCI.1404-12.2013
Pubmed Abstract | Pubmed Full Text | CrossRef Full Text | Google Scholar
Holtmaat, A., and Svoboda, K. (2009). Experience-dependent structural synaptic plasticity in the mammalian brain. Nat. Rev. Neurosci. 10, 647–658. doi: 10.1038/nrn2699
Pubmed Abstract | Pubmed Full Text | CrossRef Full Text | Google Scholar
Holtmaat, A. J., Trachtenberg, J. T., Wilbrecht, L., Shepherd, G. M., Zhang, X., Knott, G. W., et al. (2005). Transient and persistent dendritic spines in the neocortex in vivo. Neuron 45, 279–291. doi: 10.1016/j.neuron.2005.01.003
Pubmed Abstract | Pubmed Full Text | CrossRef Full Text | Google Scholar
Holtmaat, A., Wilbrecht, L., Knott, G. W., Welker, E., and Svoboda, K. (2006). Experience-dependent and cell-type-specific spine growth in the neocortex. Nature 441, 979–983. doi: 10.1038/nature04783
Pubmed Abstract | Pubmed Full Text | CrossRef Full Text | Google Scholar
Honkura, N., Matsuzaki, M., Noguchi, J., Ellis-Davies, G. C., and Kasai, H. (2008). The subspine organization of actin fibers regulates the structure and plasticity of dendritic spines. Neuron 57, 719–729. doi: 10.1016/j.neuron.2008.01.013
Pubmed Abstract | Pubmed Full Text | CrossRef Full Text | Google Scholar
Hübener, M., and Bonhoeffer, T. (2010). Searching for engrams. Neuron 67, 363–371. doi: 10.1016/j.neuron.2010.06.033
Pubmed Abstract | Pubmed Full Text | CrossRef Full Text | Google Scholar
Ishii, H., Shibuya, K., Ohta, Y., Mukai, H., Uchino, S., Takata, N., et al. (2006). Enhancement of nitric oxide production by association of nitric oxide synthase with N-methyl-D-aspartate receptors via postsynaptic density 95 in genetically engineered Chinese hamster ovary cells: real-time fluorescence imaging using nitric oxide sensitive dye. J. Neurochem. 96, 1531–1539. doi: 10.1111/j.1471-4159.2006.03656.x
Pubmed Abstract | Pubmed Full Text | CrossRef Full Text | Google Scholar
Kasai, H., Matsuzaki, M., Noguchi, J., Yasumatsu, N., and Nakahara, H. (2003). Structure-stability-function relationships of dendritic spines. Trends Neurosci. 26, 360–368. doi: 10.1016/s0166-2236(03)00162-0
Pubmed Abstract | Pubmed Full Text | CrossRef Full Text | Google Scholar
Kayser, M. S., Nolt, M. J., and Dalva, M. B. (2008). EphB receptors couple dendritic filopodia motility to synapse formation. Neuron 59, 56–69. doi: 10.1016/j.neuron.2008.05.007
Pubmed Abstract | Pubmed Full Text | CrossRef Full Text | Google Scholar
Keck, T., Mrsic-Flogel, T. D., Vaz Afonso, M., Eysel, U. T., Bonhoeffer, T., and Hübener, M. (2008). Massive restructuring of neuronal circuits during functional reorganization of adult visual cortex. Nat. Neurosci. 11, 1162–1167. doi: 10.1038/nn.2181
Pubmed Abstract | Pubmed Full Text | CrossRef Full Text | Google Scholar
Kehoe, L. A., Bellone, C., De Roo, M., Zandueta, A., Dey, P. N., Pérez-Otaño, I., et al. (2014). GluN3A promotes dendritic spine pruning and destabilization during postnatal development. J. Neurosci. 34, 9213–9221. doi: 10.1523/JNEUROSCI.5183-13.2014
Pubmed Abstract | Pubmed Full Text | CrossRef Full Text | Google Scholar
Kehoe, L. A., Bernardinelli, Y., and Muller, D. (2013). GluN3A: an NMDA receptor subunit with exquisite properties and functions. Neural Plast. 2013:145387. doi: 10.1155/2013/145387
Pubmed Abstract | Pubmed Full Text | CrossRef Full Text | Google Scholar
Kelley, J. B., Balda, M. A., Anderson, K. L., and Itzhak, Y. (2009). Impairments in fear conditioning in mice lacking the nNOS gene. Learn. Mem. 16, 371–378. doi: 10.1101/lm.1329209
Pubmed Abstract | Pubmed Full Text | CrossRef Full Text | Google Scholar
Kirchner, L., Weitzdoerfer, R., Hoeger, H., Url, A., Schmidt, P., Engelmann, M., et al. (2004). Impaired cognitive performance in neuronal nitric oxide synthase knockout mice is associated with hippocampal protein derangements. Nitric Oxide 11, 316–330. doi: 10.1016/j.niox.2004.10.005
Pubmed Abstract | Pubmed Full Text | CrossRef Full Text | Google Scholar
Koleske, A. J. (2013). Molecular mechanisms of dendrite stability. Nat. Rev. Neurosci. 14, 536–550. doi: 10.1038/nrn3486
Pubmed Abstract | Pubmed Full Text | CrossRef Full Text | Google Scholar
Kreis, P., Thévenot, E., Rousseau, V., Boda, B., Muller, D., and Barnier, J. V. (2007). The p21-activated kinase 3 implicated in mental retardation regulates spine morphogenesis through a Cdc42-dependent pathway. J. Biol. Chem. 282, 21497–21506. doi: 10.1074/jbc.m703298200
Pubmed Abstract | Pubmed Full Text | CrossRef Full Text | Google Scholar
Kumar, V., Zhang, M. X., Swank, M. W., Kunz, J., and Wu, G. Y. (2005). Regulation of dendritic morphogenesis by Ras-PI3K-Akt-mTOR and Ras-MAPK signaling pathways. J. Neurosci. 25, 11288–11299. doi: 10.1523/jneurosci.2284-05.2005
Pubmed Abstract | Pubmed Full Text | CrossRef Full Text | Google Scholar
Kwon, H. B., and Sabatini, B. L. (2011). Glutamate induces de novo growth of functional spines in developing cortex. Nature 474, 100–104. doi: 10.1038/nature09986
Pubmed Abstract | Pubmed Full Text | CrossRef Full Text | Google Scholar
Lai, K. O., and Ip, N. Y. (2009). Synapse development and plasticity: roles of ephrin/Eph receptor signaling. Curr. Opin. Neurobiol. 19, 275–283. doi: 10.1016/j.conb.2009.04.009
Pubmed Abstract | Pubmed Full Text | CrossRef Full Text | Google Scholar
Lendvai, B., Stern, E. A., Chen, B., and Svoboda, K. (2000). Experience-dependent plasticity of dendritic spines in the developing rat barrel cortex in vivo. Nature 404, 876–881. doi: 10.1038/35009107
Pubmed Abstract | Pubmed Full Text | CrossRef Full Text | Google Scholar
Lim, J., Ritt, D. A., Zhou, M., and Morrison, D. K. (2014). The CNK2 scaffold interacts with vilse and modulates Rac cycling during spine morphogenesis in hippocampal neurons. Curr. Biol. 24, 786–792. doi: 10.1016/j.cub.2014.02.036
Pubmed Abstract | Pubmed Full Text | CrossRef Full Text | Google Scholar
Lin, Y. L., Lei, Y. T., Hong, C. J., and Hsueh, Y. P. (2007). Syndecan-2 induces filopodia and dendritic spine formation via the neurofibromin-PKA-Ena/VASP pathway. J. Cell Biol. 177, 829–841. doi: 10.1083/jcb.200608121
Pubmed Abstract | Pubmed Full Text | CrossRef Full Text | Google Scholar
Lisman, J. (2003). Actin’s actions in LTP-induced synapse growth. Neuron 38, 361–362. doi: 10.1016/s0896-6273(03)00257-5
Pubmed Abstract | Pubmed Full Text | CrossRef Full Text | Google Scholar
Lushnikova, I., Skibo, G., Muller, D., and Nikonenko, I. (2009). Synaptic potentiation induces increased glial coverage of excitatory synapses in CA1 hippocampus. Hippocampus 19, 753–762. doi: 10.1002/hipo.20551
Pubmed Abstract | Pubmed Full Text | CrossRef Full Text | Google Scholar
Matsuzaki, M., Honkura, N., Ellis-Davies, G. C., and Kasai, H. (2004). Structural basis of long-term potentiation in single dendritic spines. Nature 429, 761–766. doi: 10.1038/nature02617
Pubmed Abstract | Pubmed Full Text | CrossRef Full Text | Google Scholar
Matter, C., Pribadi, M., Liu, X., and Trachtenberg, J. T. (2009). Delta-catenin is required for the maintenance of neural structure and function in mature cortex in vivo. Neuron 64, 320–327. doi: 10.1016/j.neuron.2009.09.026
Pubmed Abstract | Pubmed Full Text | CrossRef Full Text | Google Scholar
Matus, A. (2000). Actin-based plasticity in dendritic spines. Science 290, 754–758. doi: 10.1126/science.290.5492.754
Pubmed Abstract | Pubmed Full Text | CrossRef Full Text | Google Scholar
McGeachie, A. B., Cingolani, L. A., and Goda, Y. (2011). A stabilising influence: integrins in regulation of synaptic plasticity. Neurosci. Res. 70, 24–29. doi: 10.1016/j.neures.2011.02.006
Pubmed Abstract | Pubmed Full Text | CrossRef Full Text | Google Scholar
Mendez, P., De Roo, M., Poglia, L., Klauser, P., and Muller, D. (2010a). N-cadherin mediates plasticity-induced long-term spine stabilization. J. Cell Biol. 189, 589–600. doi: 10.1083/jcb.201003007
Pubmed Abstract | Pubmed Full Text | CrossRef Full Text | Google Scholar
Mendez, P., Garcia-Segura, L. M., and Muller, D. (2010b). Estradiol promotes spine growth and synapse formation without affecting pre-established networks. Hippocampus 21, 1263–1267. doi: 10.1002/hipo.20875
Pubmed Abstract | Pubmed Full Text | CrossRef Full Text | Google Scholar
Mukai, J., Dhilla, A., Drew, L. J., Stark, K. L., Cao, L., MacDermott, A. B., et al. (2008). Palmitoylation-dependent neurodevelopmental deficits in a mouse model of 22q11 microdeletion. Nat. Neurosci. 11, 1302–1310. doi: 10.1038/nn.2204
Pubmed Abstract | Pubmed Full Text | CrossRef Full Text | Google Scholar
Murakoshi, H., Wang, H., and Yasuda, R. (2011). Local, persistent activation of Rho GTPases during plasticity of single dendritic spines. Nature 472, 100–104. doi: 10.1038/nature09823
Pubmed Abstract | Pubmed Full Text | CrossRef Full Text | Google Scholar
Nägerl, U. V., Eberhorn, N., Cambridge, S. B., and Bonhoeffer, T. (2004). Bidirectional activity-dependent morphological plasticity in hippocampal neurons. Neuron 44, 759–767. doi: 10.1016/j.neuron.2004.11.016
Pubmed Abstract | Pubmed Full Text | CrossRef Full Text | Google Scholar
Nägerl, U. V., Köstinger, G., Anderson, J. C., Martin, K. A., and Bonhoeffer, T. (2007). Protracted synaptogenesis after activity-dependent spinogenesis in hippocampal neurons. J. Neurosci. 27, 8149–8156. doi: 10.1523/JNEUROSCI.0511-07.2007
Pubmed Abstract | Pubmed Full Text | CrossRef Full Text | Google Scholar
Nikonenko, I., Nikonenko, A., Mendez, P., Michurina, T. V., Enikolopov, G., and Muller, D. (2013). Nitric oxide mediates local activity-dependent excitatory synapse development. Proc. Natl. Acad. Sci. U S A 110, E4142–E4151. doi: 10.1073/pnas.1311927110
Pubmed Abstract | Pubmed Full Text | CrossRef Full Text | Google Scholar
Nodé-Langlois, R., Muller, D., and Boda, B. (2006). Sequential implication of the mental retardation proteins ARHGEF6 and PAK3 in spine morphogenesis. J. Cell Sci. 119, 4986–4993. doi: 10.1242/jcs.03273
Pubmed Abstract | Pubmed Full Text | CrossRef Full Text | Google Scholar
Oh, W. C., Hill, T. C., and Zito, K. (2013). Synapse-specific and size-dependent mechanisms of spine structural plasticity accompanying synaptic weakening. Proc. Natl. Acad. Sci. U S A 110, E305–E312. doi: 10.1073/pnas.1214705110
Pubmed Abstract | Pubmed Full Text | CrossRef Full Text | Google Scholar
Pan, F., Aldridge, G. M., Greenough, W. T., and Gan, W. B. (2010). Dendritic spine instability and insensitivity to modulation by sensory experience in a mouse model of fragile X syndrome. Proc. Natl. Acad. Sci. U S A 107, 17768–17773. doi: 10.1073/pnas.1012496107
Pubmed Abstract | Pubmed Full Text | CrossRef Full Text | Google Scholar
Petrak, L. J., Harris, K. M., and Kirov, S. A. (2005). Synaptogenesis on mature hippocampal dendrites occurs via filopodia and immature spines during blocked synaptic transmission. J. Comp. Neurol. 484, 183–190. doi: 10.1002/cne.20468
Pubmed Abstract | Pubmed Full Text | CrossRef Full Text | Google Scholar
Qiu, S., Anderson, C. T., Levitt, P., and Shepherd, G. M. (2011). Circuit-specific intracortical hyperconnectivity in mice with deletion of the autism-associated Met receptor tyrosine kinase. J. Neurosci. 31, 5855–5864. doi: 10.1523/JNEUROSCI.6569-10.2011
Pubmed Abstract | Pubmed Full Text | CrossRef Full Text | Google Scholar
Redies, C., Hertel, N., and Hübner, C. A. (2012). Cadherins and neuropsychiatric disorders. Brain Res. 1470, 130–144. doi: 10.1016/j.brainres.2012.06.020
Pubmed Abstract | Pubmed Full Text | CrossRef Full Text | Google Scholar
Rex, C. S., Chen, L. Y., Sharma, A., Liu, J., Babayan, A. H., Gall, C. M., et al. (2009). Different Rho GTPase-dependent signaling pathways initiate sequential steps in the consolidation of long-term potentiation. J. Cell Biol. 186, 85–97. doi: 10.1083/jcb.200901084
Pubmed Abstract | Pubmed Full Text | CrossRef Full Text | Google Scholar
Richards, D. A., Mateos, J. M., Hugel, S., de Paola, V., Caroni, P., Gahwiler, B. H., et al. (2005). Glutamate induces the rapid formation of spine head protrusions in hippocampal slice cultures. Proc. Natl. Acad. Sci. U S A 102, 6166–6171. doi: 10.1073/pnas.0501881102
Pubmed Abstract | Pubmed Full Text | CrossRef Full Text | Google Scholar
Roberts, A. C., Díez-García, J., Rodriguiz, R. M., López, I. P., Luján, R., Martínez-Turrillas, R., et al. (2009). Downregulation of NR3A-containing NMDARs is required for synapse maturation and memory consolidation. Neuron 63, 342–356. doi: 10.1016/j.neuron.2009.06.016
Pubmed Abstract | Pubmed Full Text | CrossRef Full Text | Google Scholar
Sakamoto, H., Mezaki, Y., Shikimi, H., Ukena, K., and Tsutsui, K. (2003). Dendritic growth and spine formation in response to estrogen in the developing Purkinje cell. Endocrinology 144, 4466–4477. doi: 10.1210/en.2003-0307
Pubmed Abstract | Pubmed Full Text | CrossRef Full Text | Google Scholar
Sawicka, K., and Zukin, R. S. (2012). Dysregulation of mTOR signaling in neuropsychiatric disorders: therapeutic implications. Neuropsychopharmacology 37, 305–306. doi: 10.1038/npp.2011.210
Pubmed Abstract | Pubmed Full Text | CrossRef Full Text | Google Scholar
Sharma, A., Hoeffer, C. A., Takayasu, Y., Miyawaki, T., McBride, S. M., Klann, E., et al. (2010). Dysregulation of mTOR signaling in fragile X syndrome. J. Neurosci. 30, 694–702. doi: 10.1523/jneurosci.3696-09.2010
Pubmed Abstract | Pubmed Full Text | CrossRef Full Text | Google Scholar
Shinkai, T., Ohmori, O., Hori, H., and Nakamura, J. (2002). Allelic association of the neuronal nitric oxide synthase (NOS1) gene with schizophrenia. Mol. Psychiatry 7, 560–563. doi: 10.1038/sj.mp.4001041
Pubmed Abstract | Pubmed Full Text | CrossRef Full Text | Google Scholar
Sigurdsson, T., Stark, K. L., Karayiorgou, M., Gogos, J. A., and Gordon, J. A. (2010). Impaired hippocampal-prefrontal synchrony in a genetic mouse model of schizophrenia. Nature 464, 763–767. doi: 10.1038/nature08855
Pubmed Abstract | Pubmed Full Text | CrossRef Full Text | Google Scholar
Sudhof, T. C. (2008). Neuroligins and neurexins link synaptic function to cognitive disease. Nature 455, 903–911. doi: 10.1038/nature07456
Pubmed Abstract | Pubmed Full Text | CrossRef Full Text | Google Scholar
Suzuki, A., Stern, S. A., Bozdagi, O., Huntley, G. W., Walker, R. H., Magistretti, P. J., et al. (2011). Astrocyte-neuron lactate transport is required for long-term memory formation. Cell 144, 810–823. doi: 10.1016/j.cell.2011.02.018
Pubmed Abstract | Pubmed Full Text | CrossRef Full Text | Google Scholar
Tanaka, J., Horiike, Y., Matsuzaki, M., Miyazaki, T., Ellis-Davies, G. C., and Kasai, H. (2008). Protein synthesis and neurotrophin-dependent structural plasticity of single dendritic spines. Science 319, 1683–1687. doi: 10.1126/science.1152864
Pubmed Abstract | Pubmed Full Text | CrossRef Full Text | Google Scholar
Tanda, K., Nishi, A., Matsuo, N., Nakanishi, K., Yamasaki, N., Sugimoto, T., et al. (2009). Abnormal social behavior, hyperactivity, impaired remote spatial memory and increased D1-mediated dopaminergic signaling in neuronal nitric oxide synthase knockout mice. Mol. Brain 2:19. doi: 10.1186/1756-6606-2-19
Pubmed Abstract | Pubmed Full Text | CrossRef Full Text | Google Scholar
Toni, N., Buchs, P. A., Nikonenko, I., Bron, C. R., and Muller, D. (1999). LTP promotes formation of multiple spine synapses between a single axon terminal and a dendrite. Nature 402, 421–425. doi: 10.1038/46574
Pubmed Abstract | Pubmed Full Text | CrossRef Full Text | Google Scholar
Toni, N., Teng, E. M., Bushong, E. A., Aimone, J. B., Zhao, C., Consiglio, A., et al. (2007). Synapse formation on neurons born in the adult hippocampus. Nat. Neurosci. 10, 727–734. doi: 10.1038/nn1908
Pubmed Abstract | Pubmed Full Text | CrossRef Full Text | Google Scholar
Vukojevic, V., Gschwind, L., Vogler, C., Demougin, P., de Quervain, D. J., Papassotiropoulos, A., et al. (2012). A role for alpha-adducin (ADD-1) in nematode and human memory. EMBO J. 31, 1453–1466. doi: 10.1038/emboj.2012.14
Pubmed Abstract | Pubmed Full Text | CrossRef Full Text | Google Scholar
Wang, X. B., Bozdagi, O., Nikitczuk, J. S., Zhai, Z. W., Zhou, Q., and Huntley, G. W. (2008). Extracellular proteolysis by matrix metalloproteinase-9 drives dendritic spine enlargement and long-term potentiation coordinately. Proc. Natl. Acad. Sci. U S A 105, 19520–19525. doi: 10.1073/pnas.0807248105
Pubmed Abstract | Pubmed Full Text | CrossRef Full Text | Google Scholar
Wass, C., Klamer, D., Fejgin, K., and Palsson, E. (2009). The importance of nitric oxide in social dysfunction. Behav. Brain Res. 200, 113–116. doi: 10.1016/j.bbr.2009.01.002
Pubmed Abstract | Pubmed Full Text | CrossRef Full Text | Google Scholar
Wiegert, J. S., and Oertner, T. G. (2013). Long-term depression triggers the selective elimination of weakly integrated synapses. Proc. Natl. Acad. Sci. U S A 110, E4510–E4519. doi: 10.1073/pnas.1315926110
Pubmed Abstract | Pubmed Full Text | CrossRef Full Text | Google Scholar
Wijetunge, L. S., Angibaud, J., Frick, A., Kind, P. C., and Nägerl, U. V. (2014). Stimulated emission depletion (STED) microscopy reveals nanoscale defects in the developmental trajectory of dendritic spine morphogenesis in a mouse model of fragile X syndrome. J. Neurosci. 34, 6405–6412. doi: 10.1523/jneurosci.2974-14.2014
Pubmed Abstract | Pubmed Full Text | CrossRef Full Text | Google Scholar
Woolfrey, K. M., Srivastava, D. P., Photowala, H., Yamashita, M., Barbolina, M. V., Cahill, M. E., et al. (2009). Epac2 induces synapse remodeling and depression and its disease-associated forms alter spines. Nat. Neurosci. 12, 1275–1284. doi: 10.1038/nn.2386
Pubmed Abstract | Pubmed Full Text | CrossRef Full Text | Google Scholar
Xu, T., Yu, X., Perlik, A. J., Tobin, W. F., Zweig, J. A., Tennant, K., et al. (2009). Rapid formation and selective stabilization of synapses for enduring motor memories. Nature 462, 915–919. doi: 10.1038/nature08389
Pubmed Abstract | Pubmed Full Text | CrossRef Full Text | Google Scholar
Yang, G., Pan, F., and Gan, W. B. (2009). Stably maintained dendritic spines are associated with lifelong memories. Nature 462, 920–924. doi: 10.1038/nature08577
Pubmed Abstract | Pubmed Full Text | CrossRef Full Text | Google Scholar
Yankova, M., Hart, S. A., and Woolley, C. S. (2001). Estrogen increases synaptic connectivity between single presynaptic inputs and multiple postsynaptic CA1 pyramidal cells: a serial electron-microscopic study. Proc. Natl. Acad. Sci. U S A 98, 3525–3530. doi: 10.1073/pnas.051624598
Pubmed Abstract | Pubmed Full Text | CrossRef Full Text | Google Scholar
Yasumatsu, N., Matsuzaki, M., Miyazaki, T., Noguchi, J., and Kasai, H. (2008). Principles of long-term dynamics of dendritic spines. J. Neurosci. 28, 13592–13608. doi: 10.1523/jneurosci.0603-08.2008
Pubmed Abstract | Pubmed Full Text | CrossRef Full Text | Google Scholar
Zito, K., Scheuss, V., Knott, G., Hill, T., and Svoboda, K. (2009). Rapid functional maturation of nascent dendritic spines. Neuron 61, 247–258. doi: 10.1016/j.neuron.2008.10.054
Pubmed Abstract | Pubmed Full Text | CrossRef Full Text | Google Scholar
Ziv, N. E., and Smith, S. J. (1996). Evidence for a role of dendritic filopodia in synaptogenesis and spine formation. Neuron 17, 91–102. doi: 10.1016/s0896-6273(00)80283-4
Pubmed Abstract | Pubmed Full Text | CrossRef Full Text | Google Scholar
Zuber, B., Nikonenko, I., Klauser, P., Muller, D., and Dubochet, J. (2005). The mammalian central nervous synaptic cleft contains a high density of periodically organized complexes. Proc. Natl. Acad. Sci. U S A 102, 19192–19197. doi: 10.1073/pnas.0509527102
Pubmed Abstract | Pubmed Full Text | CrossRef Full Text | Google Scholar
Zuo, Y., Lin, A., Chang, P., and Gan, W. B. (2005). Development of long-term dendritic spine stability in diverse regions of cerebral cortex. Neuron 46, 181–189. doi: 10.1016/j.neuron.2005.04.001
Pubmed Abstract | Pubmed Full Text | CrossRef Full Text | Google Scholar
Keywords: dendritic spines, excitatory synapses, plasticity, morphology, astrocyte
Citation: Bernardinelli Y, Nikonenko I and Muller D (2014) Structural plasticity: mechanisms and contribution to developmental psychiatric disorders. Front. Neuroanat. 8:123. doi: 10.3389/fnana.2014.00123
Received: 23 July 2014; Accepted: 14 October 2014;
Published online: 03 November 2014.
Edited by:
Ruth Benavides-Piccione, Cajal Institute, SpainReviewed by:
Valentin Nägerl, Bordeaux Segalen University, FranceJean-Pierre Hornung, University of Lausanne, Switzerland
Copyright © 2014 Bernardinelli, Nikonenko and Muller. This is an open-access article distributed under the terms of the Creative Commons Attribution License (CC BY). The use, distribution and reproduction in other forums is permitted, provided the original author(s) or licensor are credited and that the original publication in this journal is cited, in accordance with accepted academic practice. No use, distribution or reproduction is permitted which does not comply with these terms.
*Correspondence: Dominique Muller, Department of Basic Neurosciences, University of Geneva Medical School, 1, M. Servet, 1211 Geneva 4, Switzerland e-mail: Dominique.Muller@unige.ch