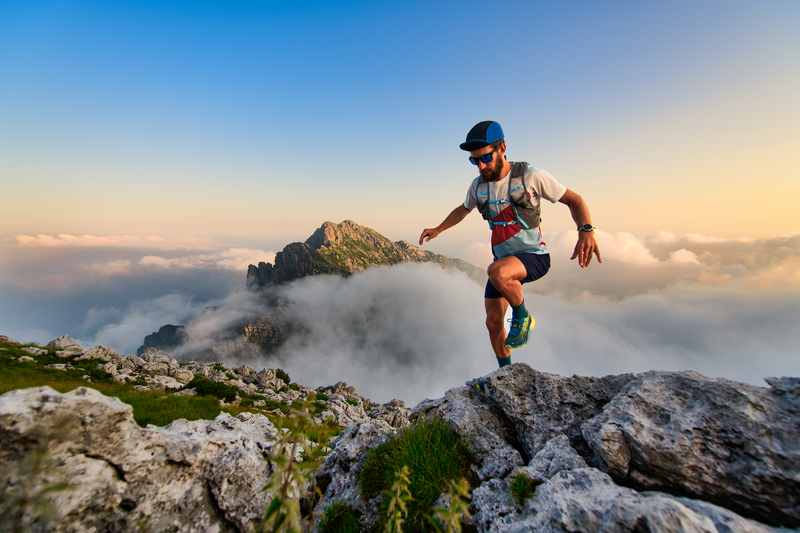
95% of researchers rate our articles as excellent or good
Learn more about the work of our research integrity team to safeguard the quality of each article we publish.
Find out more
MINI REVIEW article
Front. Neuroanat. , 02 October 2014
Volume 8 - 2014 | https://doi.org/10.3389/fnana.2014.00110
This article is part of the Research Topic Dendritic Spines: From Shape to Function View all 21 articles
Units of dendritic branches called dendritic spines represent more than simply decorative appendages of the neuron and actively participate in integrative functions of “spinous” nerve cells thereby contributing to the general phenomenon of synaptic plasticity. In animal models of drug addiction, spines are profoundly affected by treatments with drugs of abuse and represent important sub cellular markers which interfere deeply into the physiology of the neuron thereby providing an example of the burgeoning and rapidly increasing interest in “structural plasticity”. Medium Spiny Neurons (MSNs) of the Nucleus Accumbens (Nacc) show a reduced number of dendritic spines and a decrease in TH-positive terminals upon withdrawal from opiates, cannabinoids and alcohol. The reduction is localized “strictly” to second order dendritic branches where dopamine (DA)-containing terminals, impinging upon spines, make synaptic contacts. In addition, long-thin spines seems preferentially affected raising the possibility that cellular learning of these neurons may be selectively hampered. These findings suggest that dendritic spines are affected by drugs widely abused by humans and provide yet another example of drug-induced aberrant neural plasticity with marked reflections on the physiology of synapses, system structural organization, and neuronal circuitry remodeling.
Dendritic spines have been recognized, described and named, for the first time by Ramón y Cajal on the surface of Purkinje cells using the Golgi staining method (Cajal, 1888, 1891). While other investigators and even Golgi himself, disregarded spines as artifacts, Gray (1959) unambiguously showed that spines were sites of synaptic contact. It is now clear that dendritic spines are the main postsynaptic compartments of excitatory synapses in the brain with peculiar and distinctive morphological features.
Dendritic spines are heterogeneous in size and shape but, mostly mature ones, consist of a bulbous head and a thinner neck that connects the spine to the dendritic shaft (Wilson et al., 1983; Svoboda et al., 1996). This morphological configuration is particularly important for synaptic efficacy. In particular, dimensions of the spine head (Kirov and Harris, 1999; Holtmaat and Svoboda, 2009), rather than the neck, realistically reflect the observed differences in synaptic strength (Harris and Stevens, 1988). The neck constriction might serve to isolate metabolic events in the vicinity of activated synapses without significantly influencing the transfer of synaptic charge to the parent dendrite (Harris and Stevens, 1988) and thus favoring “local” changes in the number and shape of spines during synaptic plasticity (Engert and Bonhoeffer, 1999). Indeed, individual spines may represent partially autonomous compartments with a cytoskeleton composed mostly of F-actin, and may hold numerous specialized organelle such as the smooth endoplasmic reticulum, which in the largest spines forms the “spine apparatus” (Gray, 1959) with polyribosomes, near the base of the spine (Steward and Levy, 1982) offering the possibility of local protein synthesis.
At the ultrastructural level, the spine head is characterized by an electron-dense matrix of receptors and supporting proteins collectively known as the postsynaptic density (PSD; Yamauchi, 2002). This complex assembly, made of hundreds of distinct proteins (Moon et al., 1994), dynamically changes its structure and composition during development and in response to synaptic activity. The PSD contains signaling molecules including the subunits of the N-methyl-D-aspartate (NMDA) glutamate receptors, the a-amino-3-hydroxy-5-methyl-4-isoxazolepropionic-acid (AMPA), the subunits of Ca21/calmodulin-dependent protein kinase II (CaMKII; Kennedy et al., 1983) and synGAP, a ras GTPase-activating protein phosphorylated by CaMKII and dopamine (DA) receptors (Zhang et al., 2007). Other prominent PSD proteins are scaffold molecules, including the PSD-95 family (Cho et al., 1992), that link receptors to signaling proteins or to the cytoskeleton, thus helping organize the structure of PSDs (Kornau et al., 1995).
Spine development is a dynamic process which includes transition from small dendritic formations to large spines and vice versa, through a series of sophisticated structural refinements (Calabrese et al., 2006). The continuous and rapid change in shape of dendritic spines is essential for short and long term plasticity (Kasai et al., 2003, 2010) and different shapes may reflect dynamically different functions (Hering and Sheng, 2001).
A pioneering classification was proposed by Peters and Kaiserman-Abramof (1970), where they distinguished three categories: stubby, thin, and mushroom spines. However, it was necessary to introduce the dendritic filopodia in this classification. In some cases, following establishment of contact with an afferent fiber, these transient structures can become a spine (Ziv and Smith, 1996; Fiala et al., 1998; Sorra and Harris, 2000). On the other hand, some author prefers to distinguish mature spines into two broad categories: large and small considering the head size (Kasai et al., 2003, 2010) emphasizing spine function.
The confocal microscope is able to detect sufficient details of the Golgi-Cox-stained neurons. In this case is possible to extract numerical information from 3D recontruction and to establish an unambiguous criterion of classification (Figure 1) which was recently introduced (Spiga et al., 2014).
Figure 1. Representative Golgi-Cox stained MSN with various spines types. Inset shows details of different morphologies. Image is color-coded. Reconstructed with filament tracer algorithm (Imaris 7.4). Note relative abundance of blu (long thin) spines which amount for 52% of all spines (see Spiga et al., 2014 for further details).
The Medium Spiny Neuron (MSN) of the Nucleus Accumbens (Nacc) plays a central role in the integration of cortical, thalamic and mesencephalic afferents and MSNs (accounting for 90–95% of the total striatal complex) are involved in various behavioral sequelae including movement control (Björklund and Dunnett, 2007; Pissadaki and Bolam, 2013), motivation (Ostlund et al., 2014) and addiction (Diana, 2011). Terminals of DA containing neurons from the ventral tegmentum (VTA) are jumbled in a dense network of connections in many forebrain regions. Although the number of these neurons is relatively small, the projections from individual neurons are very extensive having a total axonal length (including collaterals) of roughly 74 cm with 500,000 terminals (Björklund and Dunnett, 2007; Pissadaki and Bolam, 2013) forming, in the striatum, approximately 20% of all synapses (Zhou et al., 2002, 2003). Basically, in this area every MSN is innervated by a conspicuous number of DAergic axons (Yao et al., 2008). MSNs also receive glutamate inputs from the PFC, thalamus, hippocampus (Harris and Stevens, 1989), and amygdala (Bredt and Nicoll, 2003). Accordingly, the Nacc plays a central role in the integration of cortical and mesencephalic afferent systems. Cell body and different portions of dendrites of MSNs, are targeted by various inputs. Mainly the soma and most proximal dendrites receive recurrent collaterals from other MSN (Groves, 1983), while cortical and DAergic afferents synapse onto spines located more distally on the dendrite. On distal dendrites a significant subpopulation of spines shows a particular synaptic architecture, called “striatal microcircuit” or “synaptic triad” (Freund et al., 1984), that involves both DAergic and glutamatergic axons (Figure 2). Similar innervation architecture is also observed in pyramidal neurons in the cortex (Sesack and Pickel, 1992), hippocampus (Totterdell and Smith, 1989) and magnocellualar neurons of basolateral amygdala (Johnson et al., 1994). In this configuration, DAergic terminals make a symmetric synapse with the neck whereas cortical terminals form an asymmetric contact in the spine head (Bouyer et al., 1984; Freund et al., 1984; Smith et al., 1994). In other words, DArgic and prefrontal cortical terminals in the MSNs dually synapse on a common dendritic spine (Sesack and Pickel, 1992; Moss and Bolam, 2008). The significance of this heterosynaptic formation is not very clear but it seems to suggest that DA (Pascoli et al., 2011) is able to modulate the influence of cortical glutamatergic axons (see Spiga et al., 2014 for discussion on this point). This aspect is particularly important because, despite their distinct targets, all addictive drugs commonly abused by humans evoke variations on DA concentrations within the Nacc (Di Chiara and Imperato, 1988) and it may have a role in spine density, morphology and synaptic strength. Because of this particular synaptic configuration, even modest changes in the number of dendritic spines, can have major effects on the entire neuronal pathway. Accordingly, conditions of lowered DA tone such as morphine withdrawal has been associated with spine loss (Spiga et al., 2005). Similarly, cannabis-dependent subjects undergo spine pruning in the shell of the Nacc (Spiga et al., 2010) with a reduced MSN intrinsic excitability (Spiga et al., 2010) and alcohol-dependent rats show a DA-dependent selective loss of long thin spines associated with a lack of long term depression (Spiga et al., 2014).
Figure 2. Synaptic triad in the Nucleus Accumbens. Tyrosine Hydroxylase-positive terminals (green) are forming a putative contact with the neck of a spine on a second order dendritic trunk (red), while the head of the same spine is reached by a Golgi-Cox impregnated fiber (blue) from an adjacent neuron.
The number and shape of dendritic spines, during pathological events, are extremely variable. A broad variety of psychiatric diseases and neurological disorders are accompanied by patterns of spine disruption (Huttenlocher, 1970; Fiala et al., 2002) and changes in morphology (Irwin et al., 2000; Kaufmann and Moser, 2000). Schizophrenia, for example, is commonly associated with fewer spines and synapses in many brain areas and neuronal types (Garey et al., 1998; Glantz and Lewis, 2000; Lewis and Levitt, 2002). Further, neurodegenerative disorders such as Parkinson’s disease are characterized by a loss of dendritic spines in striatal neurons (Villalba et al., 2009). Likewise, neural events related to chronic drug intake are linked to long-lasting drug-induced whole cell plasticity (Miller et al., 2012; Diana, 2013) and abnormal spine structure and density in critical brain areas (Robinson and Kolb, 2004; Russo et al., 2010). Four functionally connected structures of the brain: medial PFC, Nacc, lateral hypothalamus and the mesencephalic VTA, represent the neuroanatomical substrate of the so-called reward pathway (Koob, 1992; Melis et al., 2005). This fundamental system of regulation of complex behavior, influences rudimentary functions like food intake (Wise, 2006), sexual behavior (Robbins and Everitt, 1996), sensory perception (Berridge and Robinson, 1998), emotions (LeDoux, 2000), intellectual evaluations and processes of memory and learning (Robbins and Everitt, 2002; Hyman et al., 2006). Drugs of abuse “illegally” occupy this circuit over-stimulating the reward mechanism, causing cumulative impacts on neurotransmission. Addictive drugs, for example, can release 2–10 times the amount of DA (Di Chiara and Imperato, 1988) that natural rewards do and they do it more quickly and more reliably. Accordingly, addiction can be considered an example of experience-dependent plasticity (Robinson and Kolb, 2004).
Drug-induced structural plasticity of dendritic spines was first described by Kunz et al. (1976) and by Riley and Halkar (1978) in hippocampal pyramidal neurons following long-term alcohol consumption and is now an emerging field of investigation (Chen et al., 2010). While chronic administration of ethanol (Zhou et al., 2007) and morphine is accompanied by a decrease in the density of dendritic spines and dendritic branching of NAcc MSNs and mPFC pyramidal neurons (Robinson and Kolb, 1999b; Robinson et al., 2002), administration (or self administration) of amphetamine (Robinson and Kolb, 1997, 1999a; Heijtz et al., 2003; Kolb et al., 2003; Li et al., 2003; Crombag et al., 2005), cocaine (Robinson and Kolb, 1999a; Robinson et al., 2001; Li et al., 2003; Norrholm et al., 2003) and nicotine (Brown and Kolb, 2001; Gonzalez et al., 2005) increases spine density and dendritic branching on NAcc MSNs and pyramidal cells in the mPFC (Kolb et al., 2003). Indeed, a direct comparison among different substances is not easy because researchers use a wide variety of doses and ways of drug administration, producing, very often, divergent results on neuron morphology, during different phases of treatment with the same substance. In particular, the withdrawal syndrome after chronic drug administration seems to be a crucial point of the addictive process that is manifested by the induction of rapid changes in dendritic spine density and morphology and is thus experimentally appealing to gain insights when the drug is not on-board, to avoid possible confounds. Accordingly, we observed radical changes on spine density in accumbal MSNs during the early phases of abstinence of various drugs of abuse (Spiga et al., 2005, 2010). In fact, spontaneous and naloxone-induced morphine withdrawal, after 14 days of escalating chronic morphine administration, selectively alters spine density in the MSN second order dendrites of the NAcc shell (Spiga et al., 2005; Diana et al., 2006). Similar results we found when rats were subjected to a chronic treatment with two different cannabinoid agonists (Delta(9)-tetrahydrocannabinol and CP 55 940) and withdrawn spontaneously and pharmacologically with the CB1 antagonist SR141716A. Confocal analysis of Golgi-Cox-stained MSNs of the NAcc revealed a decrease in spine density in the shell, but not in the core only during withdrawal (both spontaneous and pharmacologically-precipitated) (Spiga et al., 2010). In contrast, no changes in the number of spines were observed during chronic morphine, cannabis and ethanol treatment, thereby suggesting that as long as the drug is “on-board” it supports spine persistence and function, whereas abrupt withdrawal discloses spine pruning and synaptic dysfunction. Interestingly, 3 weeks of daily cocaine administration did not seem to alter spine density in the core subregion of the Nacc (Shen et al., 2009) whereas other studies showed an increased spine density in the shell (Ren et al., 2010) 1–2 days after interruption of consecutive cocaine injections in mice. Further, increases were seen in the whole Nacc (Lee et al., 2006) and the core (Kim et al., 2009). However, there are no clear indications how and whether (and if) these additional spines participate in the network activity (but see Heck et al., 2014). These experiments cast doubt and urge caution on the notion that chronic cocaine or morphine treatments are unequivocally accompanied by an increase or a decrease of dendritic spines density in the NAcc, but suggest that the withdrawal itself might be the time-window in which to observe unequivocally the reported functional and morphological changes. Indeed, chronic treatment (per se) without exact dosing, regimen, degree of tolerance etc., cannot offer clear-cut results. On the other hand, it should be considered that withdrawal, after (not during) chronic drug intake, is one of the most powerful factor (negative reinforcement) driving dependence (Koob and Volkow, 2010). Accordingly, it is during this phase, to expect major changes at the neural level which in turn, will elicit behavioral changes and is considered the “driving force” in the transition from chronic drug intake to “addiction” (George et al., 2014). On the contrary, repeated exposure of drugs of abuse (drug on-board) likely alters the brain, but adaptive mechanisms intervened over the course of treatment may hide objective observations, potentially misleading judgement and spoiling conclusions (Kosten and George, 2002) because, mainly due to the wide variety of drugs, diverse treatment regimens, ample dosing, different pharmacokinetic properties, and various degrees of adaptive mechanisms such as tolerance, sensitization and others.
One possible explanation for these conflicting results, is provided by the particular nature of dendritic spines, relationships with afferents and their dynamic nature in changing size, shape and function (Kasai et al., 2010). For example, in the striatum the loss of DA terminals, in animal models of Parkinson disease (Schintu et al., 2009) and/or aging (Darbin, 2012), on the spine neck removes a modulatory influence that determines if cortically derived signals invade the dendritic shaft (Garcia et al., 2010). Conversely, a decrease in activity results in elongation of spines and a collapse of their heads (Segal, 2010) or a loss altogether (Nägerl et al., 2004). Remodeling in size and morphology of dendritic spines seems to be important at least as much as their changes in density on behavioral plasticity (Grutzendler et al., 2002; Trachtenberg et al., 2002). In drug addiction (Dumitriu et al., 2012) and schizophrenia (Faludi and Mirnics, 2011), in some brain areas, spines are approximately 30% smaller than controls (Roberts et al., 1996). Two spine types seem to be particularly involved in excitatory synaptic activity: long thin and mushroom. Mushroom are large and more stable spines that can persist for months (Bourne and Harris, 2007), whereas long thin seem to be “designed” for rapid responses to changes imposed by salient stimuli (Matsuzaki et al., 2004). Although long thin spines can change their volume even independently from synaptic activity, reflecting a native instability of these structures (Yasumatsu et al., 2008), the stimulation of a single spine cause a nearly immediate expansion of the spine head volume by 3–4-fold (Matsuzaki et al., 2004). During the course of cocaine treatment, spines shift from small to large (Shen et al., 2009) as a consequence of changes in synaptic strength (Bourne and Harris, 2007). On the contrary, thin spines shift toward smaller size in response to cocaine withdrawal with the addition of new thin spines (Dumitriu et al., 2012), perhaps immature, and silent synapses (Huang et al., 2009), that contain NMDA but few or no AMPA receptors (Russo et al., 2010). These newly formed spines appear to be highly “plastic”, being able to retract or consolidate into larger spines (Shen et al., 2009). Therefore, the stabilization of heads enlargement of potentiated spines is associated with recruitment of additional AMPA-type glutamate receptors (Nusser et al., 1998; Kharazia and Weinberg, 1999) and an increase of protein synthesis as well as actin remodeling (Matsuzaki et al., 2004; Okamoto et al., 2004; Bramham, 2008; Honkura et al., 2008). In line with an active remodeling theory, by the introduction of a new staining method combining Golgi-Cox impregnation with immunofluorescence (Spiga et al., 2011), we recently found that the reduction in spine density in ethanol abstinent rats could be attributed almost entirely to long thin spines (while “mushroom” remains relatively unaffected) (Spiga et al., 2014). At the same time, PSD-95 and tyrosine hydroxilase (but not DA transporters) immunoreactivity were similarly reduced in association with ethanol withdrawal. These results show a close relationship between morphology and function of spines and reiterate on the trophic role of DA on spines in addictive states (Melis et al., 2005; Diana, 2011) and further support the “hypodopaminergic state” as a key element in animal models of addiction. On the other hand, long thin spines, in MSNs, could be strategically used as elements highly modifiable to support important modulatory roles in synaptic transmission (Jones, 2011).
It seems clear that even a single neuron respond differently as a result of exposure to different drugs and different modality of intake of the same drug in a sort of learned addictive behavior or “memory of addiction” (Mello, 1972; Kalant, 1973; Boening, 2001; Nestler, 2013; Dong and Nestler, 2014). This kind of “memory” may be similar to the long-term learning model supported by excitatory synapses located on dendritic spines (Kasai et al., 2010) of neurons in the dopaminoceptive areas such as PFC and hippocampus. This raises the possibility that long lasting changes in synapse formation and synaptic organization induced by drugs of abuse, may interact and hinder those produced by experience in the reward pathway. These drug-paired memories and the drug withdrawal-associated aversive feeling have been suggested to contribute to the high rate of relapse among addicts (Nestler, 2001; Hyman et al., 2006; Robbins et al., 2008). This wrong (aberrant) learning mechanism should be strongly related to synapse formation, changes in efficacy of synaptic transmission and morphology, modulated by DA tone in different cell types and brain regions. The resulting changes in neuronal connectivity are likely to contribute to hamper cognitive functions such as decision making and emotional rigidity typical of addicts.
The authors declare that the research was conducted in the absence of any commercial or financial relationships that could be construed as a potential conflict of interest.
This work was supported, in part, by funds from Regione Autonoma della Sardegna (RAS), Progetti di ricerca di base—Bando 2008, to Marco Diana.
Berridge, K. C., and Robinson, T. E. (1998). What is the role of dopamine in reward: hedonic impact, reward learning, or incentive salience? Brain Res. Brain Res. Rev. 28, 309–369. doi: 10.1016/s0165-0173(98)00019-8
Pubmed Abstract | Pubmed Full Text | CrossRef Full Text | Google Scholar
Björklund, A., and Dunnett, S. B. (2007). Dopamine neuron systems in the brain: an update. Trends Neurosci. 5, 194–202. doi: 10.1016/j.tins.2007.03.006
Pubmed Abstract | Pubmed Full Text | CrossRef Full Text | Google Scholar
Boening, J. A. (2001). Neurobiology of an addiction memory. J. Neural Transm. 108, 755–765. doi: 10.1007/s007020170050
Pubmed Abstract | Pubmed Full Text | CrossRef Full Text | Google Scholar
Bourne, J., and Harris, K. M. (2007). Do thin spines learn to be mushroom spines that remember? Curr. Opin. Neurobiol. 17, 381–386. doi: 10.1016/j.conb.2007.04.009
Pubmed Abstract | Pubmed Full Text | CrossRef Full Text | Google Scholar
Bouyer, J. J., Park, D. H., Joh, T. H., and Pickel, V. M. (1984). Chemical and structural analysis of the relation between cortical inputs and tyrosine hydroxylase-containing terminals in rat neostriatum. Brain Res. 302, 267–275. doi: 10.1016/0006-8993(84)90239-7
Pubmed Abstract | Pubmed Full Text | CrossRef Full Text | Google Scholar
Bramham, C. R. (2008). Local protein synthesis, actin dynamics and LTP consolidation. Curr. Opin. Neurobiol. 18, 524–531. doi: 10.1016/j.conb.2008.09.013
Pubmed Abstract | Pubmed Full Text | CrossRef Full Text | Google Scholar
Bredt, D. S., and Nicoll, R. A. (2003). AMPA receptor trafficking at excitatory synapses. Neuron 40, 361–379. doi: 10.1016/s0896-6273(03)00640-8
Pubmed Abstract | Pubmed Full Text | CrossRef Full Text | Google Scholar
Brown, R. W., and Kolb, B. (2001). Nicotine sensitization increases dendritic length and spine density in the nucleus accumbens and cingulate cortex. Brain Res. 899, 94–100. doi: 10.1016/s0006-8993(01)02201-6
Pubmed Abstract | Pubmed Full Text | CrossRef Full Text | Google Scholar
Cajal, S. (1888). Estructura de los centros nerviosos de las aves. Rev. Trim. Histol. Norm. Patol. 1, 1–10.
Cajal, S. (1891). Significación fisiológica de las expansiones protoplásmicas y nerviosas de las células de la sustancia gris. Rev. Cienc. Méd. Barc. 22, 23–37.
Calabrese, B., Wilson, M. S., and Halpain, S. (2006). Development and regulation of dendritic spine synapses. Physiology (Bethesda) 21, 38–47. doi: 10.1152/physiol.00042.2005
Pubmed Abstract | Pubmed Full Text | CrossRef Full Text | Google Scholar
Chen, B. T., Hopf, F. W., and Bonci, A. (2010). Synaptic plasticity in the mesolimbic system: therapeutic implications for substance abuse. Ann. N Y Acad. Sci. 1187, 129–139. doi: 10.1111/j.1749-6632.2009.05154.x
Pubmed Abstract | Pubmed Full Text | CrossRef Full Text | Google Scholar
Cho, K.-O., Hunt, C. A., and Kennedy, M. B. (1992). The rat brain postsynaptic density fraction contains a homolog of the Drosophila discs-large tumor suppressor protein. Neuron 9, 929–942. doi: 10.1016/0896-6273(92)90245-9
Pubmed Abstract | Pubmed Full Text | CrossRef Full Text | Google Scholar
Crombag, H. S., Gorny, G., Li, Y., Kolb, B., and Robinson, T. E. (2005). Opposite effects of amphetamine self-administration experience on dendritic spines in the medial and orbital prefrontal cortex. Cereb. Cortex 3, 341–348. doi: 10.1093/cercor/bhh136
Pubmed Abstract | Pubmed Full Text | CrossRef Full Text | Google Scholar
Darbin, O. (2012). The aging striatal dopamine function. Parkinsonism Relat. Disord. 18, 426–432. doi: 10.1016/j.parkreldis.2011.11.025
Pubmed Abstract | Pubmed Full Text | CrossRef Full Text | Google Scholar
Diana, M. (2011). The dopamine hypothesis of drug addiction and its potential therapeutic value. Front. Psychiatry 2:64. doi: 10.3389/fpsyt.2011.00064
Pubmed Abstract | Pubmed Full Text | CrossRef Full Text | Google Scholar
Diana, M. (2013). The addicted brain. Front. Psychiatry 4:40. doi: 10.3389/fpsyt.2013.00040
Pubmed Abstract | Pubmed Full Text | CrossRef Full Text | Google Scholar
Diana, M., Spiga, S., and Acquas, E. (2006). Persistent and reversible morphine withdrawal-induced morphological changes in the nucleus accumbens. Ann. N Y Acad. Sci. 1074, 446–457. doi: 10.1196/annals.1369.045
Pubmed Abstract | Pubmed Full Text | CrossRef Full Text | Google Scholar
Di Chiara, G., and Imperato, A. (1988). Drugs abused by humans preferentially increase synaptic dopamine concentrations in the mesolimbic system of freely moving rats. Proc. Natl. Acad. Sci. U S A 85, 5274–5278. doi: 10.1073/pnas.85.14.5274
Pubmed Abstract | Pubmed Full Text | CrossRef Full Text | Google Scholar
Dong, Y., and Nestler, E. J. (2014). The neural rejuvenation hypothesis of cocaine addiction. Trends Pharmacol. Sci. 35, 374–383. doi: 10.1016/j.tips.2014.05.005
Pubmed Abstract | Pubmed Full Text | CrossRef Full Text | Google Scholar
Dumitriu, D., LaPlant, Q., Grossman, Y. S., Dias, C., Janssen, W. G., Russo, S. J., et al. (2012). Subregional, dendritic compartment and spine subtype specificity in cocaine-regulation of dendritic spines in the nucleus accumbens. J. Neurosci. 20, 6957–6966. doi: 10.1523/JNEUROSCI.5718-11.2012
Pubmed Abstract | Pubmed Full Text | CrossRef Full Text | Google Scholar
Engert, F., and Bonhoeffer, T. (1999). Dendritic spine changes associated with hippocampal long-term synaptic plasticity. Nature 399, 66–70. doi: 10.1038/19978
Pubmed Abstract | Pubmed Full Text | CrossRef Full Text | Google Scholar
Faludi, G., and Mirnics, K. (2011). Synaptic changes in the brain of subjects with schizophrenia. Int. J. Dev. Neurosci. 29, 305–309. doi: 10.1016/j.ijdevneu.2011.02.013
Pubmed Abstract | Pubmed Full Text | CrossRef Full Text | Google Scholar
Fiala, J. C., Feinberg, M., Popov, V., and Harris, K. M. (1998). Synaptogenesis via dendritic filopodia in developing hippocampal area CA1. J. Neurosci. 18, 8900–8911.
Fiala, J. C., Spacek, J., and Harris, K. M. (2002). Dendritic spine pathology: cause or consequence of neurological disorders? Brain Res. Brain Res. Rev. 39, 29–54. doi: 10.1016/s0165-0173(02)00158-3
Pubmed Abstract | Pubmed Full Text | CrossRef Full Text | Google Scholar
Freund, T. F., Powell, J. F., and Smith, A. D. (1984). Tyrosine hydroxylase-immunoreactive boutons in synaptic contact with identified striatonigral neurons, with particular reference to dendritic spines. Neuroscience 13, 1189–1215. doi: 10.1016/0306-4522(84)90294-x
Pubmed Abstract | Pubmed Full Text | CrossRef Full Text | Google Scholar
Garcia, B. G., Neely, M. D., and Deutch, A. Y. (2010). Cortical regulation of striatal medium spiny neuron dendritic remodeling in parkinsonism: modulation of glutamate release reverses dopamine depletion-induced dendritic spine loss. Cereb. Cortex 20, 2423–2432. doi: 10.1093/cercor/bhp317
Pubmed Abstract | Pubmed Full Text | CrossRef Full Text | Google Scholar
Garey, L. J., Ong, W. Y., Patel, T. S., Kanani, M., Davis, A., Mortimer, A. M., et al. (1998). Reduced dendritic spine density on cerebral cortical pyramidal neurons in schizophrenia. J. Neurol. Neurosurg. Psychiatry 65, 446–453. doi: 10.1136/jnnp.65.4.446
Pubmed Abstract | Pubmed Full Text | CrossRef Full Text | Google Scholar
George, O., Koob, G. F., and Vendruscolo, L. F. (2014). Negative reinforcement via motivational withdrawal is the driving force behind the transition to addiction. Psychopharmacology (Berl) 231, 3911–3917. doi: 10.1007/s00213-014-3623-1
Pubmed Abstract | Pubmed Full Text | CrossRef Full Text | Google Scholar
Glantz, L. A., and Lewis, D. A. (2000). Decreased dendritic spine density on prefrontal cortical pyramidal neurons in schizophrenia. Arch. Gen. Psychiatry 57, 65–73. doi: 10.1001/archpsyc.57.1.65
Pubmed Abstract | Pubmed Full Text | CrossRef Full Text | Google Scholar
Gonzalez, C. L. R., Gharbawie, O. A., Whishaw, I. Q., and Kolb, B. (2005). Nicotine stimulates dendritic arborization in motor cortex and improves concurrent motor skill but impairs subsequent motor learning. Synapse 55, 183–191. doi: 10.1002/syn.20106
Pubmed Abstract | Pubmed Full Text | CrossRef Full Text | Google Scholar
Gray, E. G. (1959). Electron microscopy of synaptic contacts on dendritic spines of the cerebral cortex. Nature 183, 1592–1593. doi: 10.1038/1831592a0
Groves, P. M. (1983). A theory of the functional organization of the neostriatum and the neostriatal control of voluntary movement. Brain Res. 286, 109–132. doi: 10.1016/0165-0173(83)90011-5
Pubmed Abstract | Pubmed Full Text | CrossRef Full Text | Google Scholar
Grutzendler, J., Kasthuri, N., and Gan, W. B. (2002). Long-term dendritic spine stability in the adult cortex. Nature 420, 812–816. doi: 10.1038/nature01276
Pubmed Abstract | Pubmed Full Text | CrossRef Full Text | Google Scholar
Harris, K. M., and Stevens, J. K. (1988). Dendritic spines of rat cerebellar Purkinje cells: serial electron microscopy with reference to their biophysical characteristics. J. Neurosci. 8, 4455–4469.
Harris, K. M., and Stevens, J. K. (1989). Dendritic spines of CA 1 pyramidal cells in the rat hippocampus: serial electron microscopy with reference to their biophysical characteristics. J. Neurosci. 9, 2982–2997.
Heck, N., Dos Santos, M., Amairi, B., Salery, M., Besnard, A., Herzog, E., et al. (2014). A new automated 3D detection of synaptic contacts reveals the formation of cortico-striatal synapses upon cocaine treatment in vivo. Brain Struct. Funct. doi: 10.1007/s00429-014-0837-2. [Epub ahead of print].
Pubmed Abstract | Pubmed Full Text | CrossRef Full Text | Google Scholar
Heijtz, R. D., Kolb, B., and Forssberg, H. (2003). Can a therapeutic dose of amphetamine during pre-adolescence modify the pattern of synaptic organization in the brain? Eur. J. Neurosci. 18, 3394–3399. doi: 10.1046/j.0953-816x.2003.03067.x
Pubmed Abstract | Pubmed Full Text | CrossRef Full Text | Google Scholar
Hering, H., and Sheng, M. (2001). Dendritic spines: structure, dynamics and regulation. Nat. Rev. Neurosci. 2, 880–888. doi: 10.1038/35104061
Pubmed Abstract | Pubmed Full Text | CrossRef Full Text | Google Scholar
Holtmaat, A., and Svoboda, K. (2009). Experience-dependent structural synaptic plasticity in the mammalian brain. Nat. Rev. Neurosci. 10, 64–758. doi: 10.1038/nrn2699
Pubmed Abstract | Pubmed Full Text | CrossRef Full Text | Google Scholar
Honkura, N., Matsuzaki, M., Noguchi, J., Ellis-Davies, G. C., and Kasai, H. (2008). The subspine organization of actin fibers regulates the structure and plasticity of dendritic spines. Neuron 57, 719–729. doi: 10.1016/j.neuron.2008.01.013
Pubmed Abstract | Pubmed Full Text | CrossRef Full Text | Google Scholar
Huang, Y. H., Lin, Y., Mu, P., Lee, B. R., Brown, T. E., Wayman, G., et al. (2009). In vivo cocaine experience generates silent synapses. Neuron 1, 40–47. doi: 10.1016/j.neuron.2009.06.007
Pubmed Abstract | Pubmed Full Text | CrossRef Full Text | Google Scholar
Hyman, S. E., Malenka, R. C., and Nestler, E. J. (2006). Neural mechanisms of addiction: the role of reward-related learning and memory. Annu. Rev. Neurosci. 29, 565–598. doi: 10.1146/annurev.neuro.29.051605.113009
Pubmed Abstract | Pubmed Full Text | CrossRef Full Text | Google Scholar
Irwin, S. A., Galvez, R., and Greenough, W. T. (2000). Dendritic spine structural anomalies in fragile-X mental retardation syndrome. Cereb. Cortex 10, 1038–1044. doi: 10.1093/cercor/10.10.1038
Pubmed Abstract | Pubmed Full Text | CrossRef Full Text | Google Scholar
Johnson, L. R., Aylward, R. L. M., Hussain, Z., and Totterdell, S. (1994). Input from the amygdala to the rat nucleus accubens: its relationship with tyrosine hydroxylase immunoreactivity and identified neurons. Neuroscience 61, 851–865. doi: 10.1016/0306-4522(94)90408-1
Pubmed Abstract | Pubmed Full Text | CrossRef Full Text | Google Scholar
Jones, S. (2011). “Dopamine—glutamate interactions in the basal ganglia,” in Frontiers in Neuroscience Series, ed S. Jones (Switzerland: Taylor & Francis/CRC Press), 71–105.
Kalant, H. (1973). Relationship between psychological and physiological dependence and drug addiction. Vie Med. Can. Fr. 2, 766–774.
Kasai, H., Fukuda, M., Watanabe, S., Hayashi-Takagi, A., and Noguchi, J. (2010). Structural dynamics of dendritic spines in memory and cognition. Trends Neurosci. 33, 121–129. doi: 10.1016/j.tins.2010.01.001
Pubmed Abstract | Pubmed Full Text | CrossRef Full Text | Google Scholar
Kasai, H., Matsuzaki, M., Noguchi, J., Yasumatsu, N., and Nakahara, H. (2003). Structure-stability-function relationships of dendritic spines. Trends Neurosci. 26, 360–368. doi: 10.1016/s0166-2236(03)00162-0
Pubmed Abstract | Pubmed Full Text | CrossRef Full Text | Google Scholar
Kaufmann, W. E., and Moser, H. W. (2000). Dendritic anomalies in disorders associated with mental retardation. Cereb. Cortex 10, 981–991. doi: 10.1093/cercor/10.10.981
Pubmed Abstract | Pubmed Full Text | CrossRef Full Text | Google Scholar
Kennedy, M. B., McGuinness, T., and Greengard, P. (1983). A calcium/calmodulin-dependent protein kinase from mammalian brain that phosphorylates synapsin I: partial purification and characterization. J. Neurosci. 3, 818–831.
Kharazia, V. N., and Weinberg, R. J. (1999). Immunogold localization of AMPA and NMDA receptors in somatic sensory cortex of albino rat. J. Comp. Neurol. 412, 292–302. doi: 10.1002/(sici)1096-9861(19990920)412:2<292::aid-cne8>3.0.co;2-g
Pubmed Abstract | Pubmed Full Text | CrossRef Full Text | Google Scholar
Kim, Y., Teylan, M. A., Baron, M., Sands, A., Nairn, A. C., and Greengard, P. (2009). Methylphenidate-induced dendritic spine formation and DeltaFosB expression in nucleus accumbens. Proc. Natl. Acad. Sci. U S A 106, 2915–2920. doi: 10.1073/pnas.0813179106
Pubmed Abstract | Pubmed Full Text | CrossRef Full Text | Google Scholar
Kirov, S. A., and Harris, K. M. (1999). Dendrites are more spiny on mature hippocampal neurons when synapses are inactivated. Nat. Neurosci. 2, 878–883. doi: 10.1038/13178
Pubmed Abstract | Pubmed Full Text | CrossRef Full Text | Google Scholar
Kolb, B., Gorny, G., Li, Y., Samaha, A. N., and Robinson, T. E. (2003). Amphetamine or cocaine limits the ability of later experience to promote structural plasticity in the neocortex and nucleus accumbens. Proc. Natl. Acad. Sci. U S A 100, 10523–10528. doi: 10.1073/pnas.1834271100
Pubmed Abstract | Pubmed Full Text | CrossRef Full Text | Google Scholar
Koob, G. F. (1992). Drugs of abuse: anatomy, pharmacology and function of reward pathways. Trends Pharmacol. Sci. 13, 177–184. doi: 10.1016/0165-6147(92)90060-j
Pubmed Abstract | Pubmed Full Text | CrossRef Full Text | Google Scholar
Koob, G. F., and Volkow, N. D. (2010). Neurocircuitry of addiction. Neuropsychopharmacology 35, 217–238. doi: 10.1038/npp.2009.110
Pubmed Abstract | Pubmed Full Text | CrossRef Full Text | Google Scholar
Kornau, H.-C., Schenker, L. T., Kennedy, M. B., and Seeburg, P. H. (1995). Domain interaction between NMDA receptor subunits and the postsynaptic density protein PSD-95. Science 269, 1737–1740. doi: 10.1126/science.7569905
Pubmed Abstract | Pubmed Full Text | CrossRef Full Text | Google Scholar
Kosten, T. R., and George, T. P. (2002). The neurobiology of opioid dependence: implications for treatment. Sci. Pract. Perspect. 1, 13–20. doi: 10.1151/spp021113
Pubmed Abstract | Pubmed Full Text | CrossRef Full Text | Google Scholar
Kunz, G., Englisch, H. J., and Wenzel, J. (1976). Spine-distribution of pyramidal neurons of the CAl-region of the rat hippocampus following long-term oral alcohol administration. J. Hirnforsch. 17, 351–363.
LeDoux, J. E. (2000). Emotion circuits in the brain. Annu. Rev. Neurosci. 23, 155–184. doi: 10.1146/annurev.neuro.23.1.155
Pubmed Abstract | Pubmed Full Text | CrossRef Full Text | Google Scholar
Lee, K. W., Kim, Y., Kim, A. M., Helmin, K., Nairn, A. C., and Greengard, P. (2006). Cocaine-induced dendritic spine formation in D1 and D2 dopamine receptor-containing medium spiny neurons in nucleus accumbens. Proc. Natl. Acad. Sci. U S A 103, 3399–3404. doi: 10.1073/pnas.0511244103
Pubmed Abstract | Pubmed Full Text | CrossRef Full Text | Google Scholar
Lewis, D. A., and Levitt, P. (2002). Schizophrenia as a disorder of neurodevelopment. Annu. Rev. Neurosci. 25, 409–432. doi: 10.1146/annurev.neuro.25.112701.142754
Pubmed Abstract | Pubmed Full Text | CrossRef Full Text | Google Scholar
Li, Y., Kolb, B., and Robinson, T. E. (2003). The location of persistent amphetamine-induced changes in the density of dendritic spines on medium spiny neurons in the nucleus accumbens and caudate putamen. Neuropsychopharmacology 238, 1082–1085. doi: 10.1038/sj.npp.1300115
Pubmed Abstract | Pubmed Full Text | CrossRef Full Text | Google Scholar
Matsuzaki, M., Honkura, N., Ellis-Davies, G. C., and Kasai, H. (2004). Structural basis of long-term potentiationin single dendritic spines. Nature 429, 761–766. doi: 10.1038/nature02617
Pubmed Abstract | Pubmed Full Text | CrossRef Full Text | Google Scholar
Melis, M., Spiga, S., and Diana, M. (2005). The dopamine hypothesis of drug addiction: hypodopaminergic state. Int. Rev. Neurobiol. 63, 101–154. doi: 10.1016/s0074-7742(05)63005-x
Pubmed Abstract | Pubmed Full Text | CrossRef Full Text | Google Scholar
Mello, N. K. (1972). “Behavioral studies of alcoholism,” in Biology of Alcoholism, eds B. Kissin and H. Begleiter (New York: Plenum Press), 210–219.
Miller, E. C., Zhang, L., Dummer, B. W., Cariveau, D. R., Loh, H., Law, P. Y., et al. (2012). Differential modulation of drug-induced structural and functional plasticity of dendritic spines. Mol. Pharmacol. 82, 333–343. doi: 10.1124/mol.112.078162
Pubmed Abstract | Pubmed Full Text | CrossRef Full Text | Google Scholar
Moon, I. S., Apperson, M. L., and Kennedy, M. B. (1994). The major tyrosine-phosphorylated protein in the postsynaptic density fraction is N-methyl-D-aspartate receptor subunit 2B. Proc. Natl. Acad. Sci. U S A 91, 3954–3958. doi: 10.1073/pnas.91.9.3954
Pubmed Abstract | Pubmed Full Text | CrossRef Full Text | Google Scholar
Moss, J., and Bolam, J. P. (2008). A dopaminergic axon lattice in the striatum and its relationship with cortical and thalamic terminals. J. Neurosci. 28, 11221–11230. doi: 10.1523/JNEUROSCI.2780-08.2008
Pubmed Abstract | Pubmed Full Text | CrossRef Full Text | Google Scholar
Nägerl, U. V., Eberhorn, N., Cambridge, S. B., and Bonhoeffer, T. (2004). Bidirectional activity-dependent morphological plasticity in hippocampal neurons. Neuron 44, 759–767. doi: 10.1016/j.neuron.2004.11.016
Pubmed Abstract | Pubmed Full Text | CrossRef Full Text | Google Scholar
Nestler, E. J. (2001). Neurobiology. Total recall-the memory of addiction. Science 292, 2266–2267. doi: 10.1126/science.1063024
Pubmed Abstract | Pubmed Full Text | CrossRef Full Text | Google Scholar
Nestler, E. J. (2013). Cellular basis of memory for addiction. Dialogues Clin. Neurosci. 4, 431–443.
Norrholm, S. D., Bibb, J. A., Nestler, E. J., Ouimet, C. C., Taylor, J. R., and Greengard, P. (2003). Cocaine-induced proliferation of dendritic spines in nucleus accumbens is dependent on the activity of cyclin-dependent kinase-5. Neuroscience 116, 19–22. doi: 10.1016/s0306-4522(02)00560-2
Pubmed Abstract | Pubmed Full Text | CrossRef Full Text | Google Scholar
Nusser, Z., Lujan, R., Laube, G., Roberts, J. D., Molnar, E., and Somogyi, P. (1998). Cell type and pathway dependence of synaptic AMPA receptor number and variability in the hippocampus. Neuron 1, 545–559. doi: 10.1016/s0896-6273(00)80565-6
Pubmed Abstract | Pubmed Full Text | CrossRef Full Text | Google Scholar
Okamoto, K., Nagai, T., Miyawaki, A., and Hayashi, Y. (2004). Rapid and persistent modulation of actin dynamics regulates postsynaptic reorganization underlying bidirectional plasticity. Nat. Neurosci. 7, 1104–1112. doi: 10.1038/nn1311
Pubmed Abstract | Pubmed Full Text | CrossRef Full Text | Google Scholar
Ostlund, S. B., LeBlanc, K. H., Kosheleff, A. R., Wassum, K. M., and Maidment, N. T. (2014). Phasic mesolimbic dopamine signaling encodes the facilitation of incentive motivation produced by repeated cocaine exposure. Neuropsychopharmacology 39, 2441–2449. doi: 10.1038/npp.2014.96
Pubmed Abstract | Pubmed Full Text | CrossRef Full Text | Google Scholar
Pascoli, V., Turiault, M., and Lüscher, C. (2011). Reversal of cocaine-evoked synaptic potentiation resets drug-induced adaptive behaviour. Nature 481, 71–75. doi: 10.1038/nature10709
Pubmed Abstract | Pubmed Full Text | CrossRef Full Text | Google Scholar
Peters, A., and Kaiserman-Abramof, I. R. (1970). The small pyramidal neuron of the rat cerebral cortex. The perikaryon, dendrites and spines. Am. J. Anat. 127, 321–355. doi: 10.1002/aja.1001270402
Pubmed Abstract | Pubmed Full Text | CrossRef Full Text | Google Scholar
Pissadaki, E. K., and Bolam, J. P. (2013). The energy cost of action potential propagation in dopamine neurons: clues to susceptibility in Parkinson’s disease. Front. Comput. Neurosci. 7:13. doi: 10.3389/fncom.2013.00013
Pubmed Abstract | Pubmed Full Text | CrossRef Full Text | Google Scholar
Ren, Z., Sun, W. L., Jiao, H., Zhang, D., Kong, H., Wang, X., et al. (2010). Dopamine D1 and N-methyl-d-aspartate receptors and extracellular signal-regulated kinase mediate neuronal morphological changes induced by repeated cocaine administration. Neuroscience 168, 48–60. doi: 10.1016/j.neuroscience.2010.03.034
Pubmed Abstract | Pubmed Full Text | CrossRef Full Text | Google Scholar
Riley, J. N., and Halkar, D.-W. (1978). Morphological alterations in hippocampus after long-term alcohol consumption in mice. Science 210, 646–648. doi: 10.1126/science.566953
Pubmed Abstract | Pubmed Full Text | CrossRef Full Text | Google Scholar
Robbins, T. W., Ersche, K. D., and Everitt, B. J. (2008). Drug addiction and the memory systems of the brain. Ann. N Y Acad. Sci. 1141, 1–21. doi: 10.1196/annals.1441.020
Pubmed Abstract | Pubmed Full Text | CrossRef Full Text | Google Scholar
Robbins, T. W., and Everitt, B. J. (1996). Neurobehavioural mechanisms of reward and motivation. Curr. Opin. Neurobiol. 6, 228–236. doi: 10.1016/s0959-4388(96)80077-8
Pubmed Abstract | Pubmed Full Text | CrossRef Full Text | Google Scholar
Robbins, T. W., and Everitt, B. J. (2002). Limbic-striatal memory systems and drug addiction. Neurobiol. Learn. Mem. 78, 625–636. doi: 10.1006/nlme.2002.4103
Pubmed Abstract | Pubmed Full Text | CrossRef Full Text | Google Scholar
Roberts, R. C., Conley, R., Kung, L., Peretti, F. J., and Chute, D. J. (1996). Reduced striatal spine size in schizophrenia: a postmortem ultrastructural study. Neuroreport 7, 1214–1218. doi: 10.1097/00001756-199604260-00024
Pubmed Abstract | Pubmed Full Text | CrossRef Full Text | Google Scholar
Robinson, T. E., Gorny, G., Mitton, E., and Kolb, B. (2001). Cocaine self-administration alters the morphology of dendrites and dendritic spines in the nucleus accumbens and neocortex. Synapse 39, 257–266. doi: 10.1002/1098-2396(20010301)39:3<257::aid-syn1007>3.3.co;2-t
Pubmed Abstract | Pubmed Full Text | CrossRef Full Text | Google Scholar
Robinson, T. E., Gorny, G., Savage, V. R., and Kolb, B. (2002). Widespread but regionally specific effects of experimenter- versus self-administered morphine on dendritic spines in the nucleus accumbens, hippocampus and neocortex of adult rats. Synapse 46, 271–279. doi: 10.1002/syn.10146
Pubmed Abstract | Pubmed Full Text | CrossRef Full Text | Google Scholar
Robinson, T. E., and Kolb, B. (1997). Persistent structural modifications in nucleus accumbens and prefrontal cortex neurons produced by previous experience with amphetamine. J. Neurosci. 17, 8491–8497.
Robinson, T. E., and Kolb, B. (1999a). Alterations in the morphology of dendrites and dendritic spines in the nucleus accumbens and prefrontal cortex following repeated treatment with amphetamine or cocaine. Eur. J. Neurosci. 11, 1598–1604. doi: 10.1046/j.1460-9568.1999.00576.x
Pubmed Abstract | Pubmed Full Text | CrossRef Full Text | Google Scholar
Robinson, T. E., and Kolb, B. (1999b). Morphine alters the structure of neurons in the nucleus accumbens and neocortex of rats. Synapse 33, 160–162. doi: 10.1002/(sici)1098-2396(199908)33:2<160::aid-syn6>3.3.co;2-j
Pubmed Abstract | Pubmed Full Text | CrossRef Full Text | Google Scholar
Robinson, T. E., and Kolb, B. (2004). Structural plasticity associated with exposure to drugs of abuse. Neuropharmacology 47, 33–46. doi: 10.1016/j.neuropharm.2004.06.025
Pubmed Abstract | Pubmed Full Text | CrossRef Full Text | Google Scholar
Russo, S. J., Dietz, D. M., Dumitriu, D., Morrison, J. H., Malenka, R. C., and Nestler, E. J. (2010). The addicted synapse: mechanisms of synaptic and structural plasticity in nucleus accumbens. Trends Neurosci. 33, 267–276. doi: 10.1016/j.tins.2010.02.002
Pubmed Abstract | Pubmed Full Text | CrossRef Full Text | Google Scholar
Schintu, N., Frau, L., Ibba, M., Garau, A., Carboni, E., and Carta, A. R. (2009). Progressive dopaminergic degeneration in the chronic MPTPp mouse model of Parkinson’s disease. Neurotox. Res. 16, 127–139. doi: 10.1007/s12640-009-9061-x
Pubmed Abstract | Pubmed Full Text | CrossRef Full Text | Google Scholar
Segal, M. (2010). Dendritic spines, synaptic plasticity and neuronal survival: activity shapes dendritic spines toenhance neuronal viability. Eur. J. Neurosci. 31, 2178–2184. doi: 10.1111/j.1460-9568.2010.07270.x
Pubmed Abstract | Pubmed Full Text | CrossRef Full Text | Google Scholar
Sesack, S. R., and Pickel, V. M. (1992). Prefrontal cortical efferents in the rat synapse on unlabeled neuronal targets of catecholamine terminals in the nucleus accumbens septi and on dopamine neurons in the ventral tegmental area. J. Comp. Neurol. 320, 145–160. doi: 10.1002/cne.903200202
Pubmed Abstract | Pubmed Full Text | CrossRef Full Text | Google Scholar
Shen, H. W., Toda, S., Moussawi, K., Bouknight, A., Zahm, D. S., and Kalivas, P. W. (2009). Altered dendritic spine plasticity in cocaine-withdrawn rats. J. Neurosci. 29, 2876–2884. doi: 10.1523/JNEUROSCI.5638-08.2009
Pubmed Abstract | Pubmed Full Text | CrossRef Full Text | Google Scholar
Smith, Y., Bennett, B. D., Bolam, J. P., Parent, A., and Sadikot, A. F. (1994). Synaptic relationships between dopaminergic afferents and cortical or thalamic input in the sensorimotor territory of the striatum in monkey. J. Comp. Neurol. 344, 1–19. doi: 10.1002/cne.903440102
Pubmed Abstract | Pubmed Full Text | CrossRef Full Text | Google Scholar
Sorra, K. E., and Harris, K. M. (2000). Overview on the structure, composition, function, development and plasticity of hippocampal dendritic spines. Hippocampus 10, 501–511. doi: 10.1002/1098-1063(2000)10:5<501::aid-hipo1>3.3.co;2-k
Pubmed Abstract | Pubmed Full Text | CrossRef Full Text | Google Scholar
Spiga, S., Acquas, E., Puddu, M. C., Mulas, G., Lintas, A., Diana, M., et al. (2011). Simultaneous Golgi-Cox and immunofluorescence using confocal microscopy. Brain Struct. Funct. 216, 171–182. doi: 10.1007/s00429-011-0312-2
Pubmed Abstract | Pubmed Full Text | CrossRef Full Text | Google Scholar
Spiga, S., Lintas, A., Migliore, M., and Diana, M. (2010). Altered architecture and functional consequences of the mesolimbic dopamine system in cannabis dependence. Addict. Biol. 15, 266–276. doi: 10.1111/j.1369-1600.2010.00218.x
Pubmed Abstract | Pubmed Full Text | CrossRef Full Text | Google Scholar
Spiga, S., Puddu, M. C., Pisano, M., and Diana, M. (2005). Morphine withdrawal-induced morphological changes in the nucleus accumbens. Eur. J. Neurosci. 22, 2332–2340. doi: 10.1111/j.1460-9568.2005.04416.x
Pubmed Abstract | Pubmed Full Text | CrossRef Full Text | Google Scholar
Spiga, S., Talani, G., Mulas, G., Licheri, V., Fois, G. R., Muggironi, G., et al. (2014). Hampered LTD formation and thin spine loss in the nucleus accumbens of ethanol dependent rats. Proc. Natl. Acad. Sci. U S A 111, E3745–E3754. doi: 10.1073/pnas.1406768111
Pubmed Abstract | Pubmed Full Text | CrossRef Full Text | Google Scholar
Steward, O., and Levy, W. B. (1982). Preferential localization of polyribosomes under the base of dendritic spines in granule cells of the dentate gyrus. J. Neurosci. 2, 284–291.
Svoboda, K., Tank, D. W., and Denk, W. (1996). Direct measurement of coupling between dendritic spines and shafts. Science 272, 716–719. doi: 10.1126/science.272.5262.716
Pubmed Abstract | Pubmed Full Text | CrossRef Full Text | Google Scholar
Totterdell, S., and Smith, A. S. (1989). Convergence of hippocampal and DA-ergic input onto identified neurons in the nucleus accumbens of the rat. J. Chem. Neuroanat. 2, 285–298.
Trachtenberg, J. T., Chen, B. E., Knott, G. W., Feng, G., Sanes, J. R., Welker, E., et al. (2002). Long-term in vivo imaging of experience-dependent synaptic plasticity in adult cortex. Nature 420, 788–794. doi: 10.1038/nature01273
Pubmed Abstract | Pubmed Full Text | CrossRef Full Text | Google Scholar
Villalba, R. M., Lee, H., and Smith, Y. (2009). Dopaminergic denervation and spine loss in the striatum of MPTP-treated monkeys. Exp. Neurol. 215, 220–227. doi: 10.1016/j.expneurol.2008.09.025
Pubmed Abstract | Pubmed Full Text | CrossRef Full Text | Google Scholar
Wilson, C. J., Groves, P. M., Kitai, S. T., and Linder, J. C. (1983). Three-dimensional structure of dendritic spines in the rat neostriatum. J. Neurosci. 3, 383–398.
Wise, R. (2006). Role of brain dopamine in food reward and reinforcement. Philos. Trans. R. Soc. Lond. B Biol. Sci. 361, 1149–1158. doi: 10.1098/rstb.2006.1854
Pubmed Abstract | Pubmed Full Text | CrossRef Full Text | Google Scholar
Yamauchi, T. (2002). Molecular constituents and phosphorylation dependent regulation of the post-synaptic density. Mass Spectrom. Rev. 21, 266–286. doi: 10.1002/mas.10033
Pubmed Abstract | Pubmed Full Text | CrossRef Full Text | Google Scholar
Yao, W. D., Spealman, R. D., and Zhang, J. (2008). Dopaminergic signaling in dendritic spines. Biochem. Pharmacol. 75, 2055–2069. doi: 10.1016/j.bcp.2008.01.018
Pubmed Abstract | Pubmed Full Text | CrossRef Full Text | Google Scholar
Yasumatsu, N., Matsuzaki, M., Miyazaki, T., Noguchi, J., and Kasai, H. (2008). Principles of long-term dynamics of dendritic spines. J. Neurosci. 28, 13592–13608. doi: 10.1523/JNEUROSCI.0603-08.2008
Pubmed Abstract | Pubmed Full Text | CrossRef Full Text | Google Scholar
Zhang, J., Vinuela, A., Neely, M. H., Hallett, P. J., Grant, S. G., Miller, G. M., et al. (2007). Inhibition of the dopamine D1 receptor signaling by PSD-95. J. Biol. Chem. 282, 15778–15789. doi: 10.1074/jbc.m611485200
Pubmed Abstract | Pubmed Full Text | CrossRef Full Text | Google Scholar
Zhou, F. C., Anthony, B., Dunn, K. W., Lindquist, W. B., Xu, Z. C., and Deng, P. (2007). Chronic alcohol drinking alters neuronal dendritic spines in the brain reward center nucleus accumbens. Brain Res. 1134, 148–161. doi: 10.1016/j.brainres.2006.11.046
Pubmed Abstract | Pubmed Full Text | CrossRef Full Text | Google Scholar
Zhou, F. M., Wilson, C. J., and Dani, J. A. (2002). Cholinergic interneuron characteristics and nicotinic properties in the striatum. J. Neurobiol. 53, 590–605. doi: 10.1002/neu.10150
Pubmed Abstract | Pubmed Full Text | CrossRef Full Text | Google Scholar
Zhou, F. M., Wilson, C. J., and Dani, J. A. (2003). Muscarinic and nicotinic cholinergic mechanisms in the mesostriataldopamine systems. Neuroscientist 9, 23–36. doi: 10.1177/1073858402239588
Pubmed Abstract | Pubmed Full Text | CrossRef Full Text | Google Scholar
Ziv, N. E., and Smith, S. J. (1996). Evidence for a role of dendritic filopodia in synaptogenesis and spine formation. Neuron 17, 91–102. doi: 10.1016/s0896-6273(00)80283-4
Pubmed Abstract | Pubmed Full Text | CrossRef Full Text | Google Scholar
Keywords: spines, long thin, learning, dopamine, nucleus accumbens
Citation: Spiga S, Mulas G, Piras F and Diana M (2014) The “addicted” spine. Front. Neuroanat. 8:110. doi: 10.3389/fnana.2014.00110
Received: 04 August 2014; Accepted: 16 September 2014;
Published online: 02 October 2014.
Edited by:
Nicolas Heck, University Pierre and Marie Curie, FranceReviewed by:
Yan Dong, University of Pittsburgh, USACopyright © 2014 Spiga, Mulas, Piras and Diana. This is an open-access article distributed under the terms of the Creative Commons Attribution License (CC BY). The use, distribution and reproduction in other forums is permitted, provided the original author(s) or licensor are credited and that the original publication in this journal is cited, in accordance with accepted academic practice. No use, distribution or reproduction is permitted which does not comply with these terms.
*Correspondence: Marco Diana, “G.Minardi” Laboratory of Cognitive Neuroscience, Department of Chemistry and Pharmacy, University of Sassari, Via Muroni 23, 07100, Sassari, Italy e-mail:ZHNmZGlhbmFAdW5pc3MuaXQ=
Disclaimer: All claims expressed in this article are solely those of the authors and do not necessarily represent those of their affiliated organizations, or those of the publisher, the editors and the reviewers. Any product that may be evaluated in this article or claim that may be made by its manufacturer is not guaranteed or endorsed by the publisher.
Research integrity at Frontiers
Learn more about the work of our research integrity team to safeguard the quality of each article we publish.