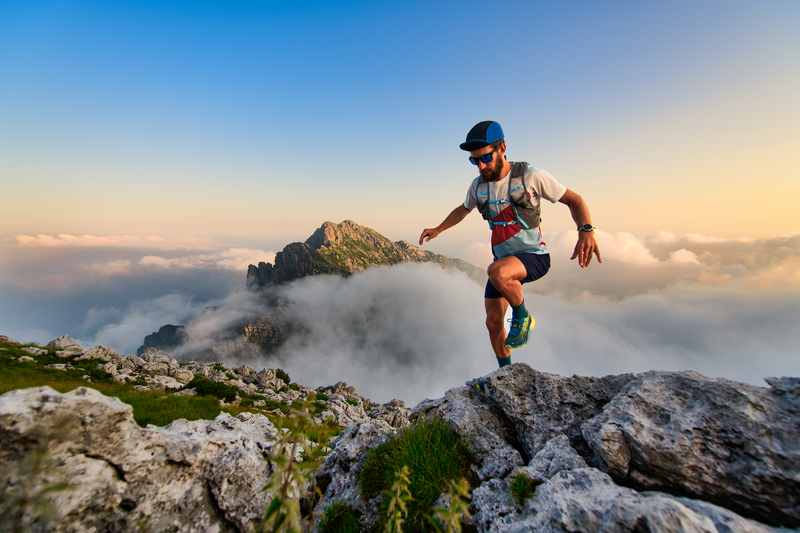
95% of researchers rate our articles as excellent or good
Learn more about the work of our research integrity team to safeguard the quality of each article we publish.
Find out more
ORIGINAL RESEARCH article
Front. Neuroanat. , 06 May 2014
Volume 8 - 2014 | https://doi.org/10.3389/fnana.2014.00026
This article is part of the Research Topic Neuroanatomy and transgenic technologies View all 12 articles
Over 90 years ago, Kolmer and Agduhr identified spinal cerebrospinal fluid-contacting neurons (CSF-cNs) based on their morphology and location within the spinal cord. In more than 200 vertebrate species, they observed ciliated neurons around the central canal that extended a brush of microvilli into the cerebrospinal fluid (CSF). Although their morphology is suggestive of a primitive sensory cell, their function within the vertebrate spinal cord remains unknown. The identification of specific molecular markers for these neurons in vertebrates would benefit the investigation of their physiological roles. PKD2L1, a transient receptor potential channel that could play a role as a sensory receptor, has been found in cells contacting the central canal in mouse. In this study, we demonstrate that PKD2L1 is a specific marker for CSF-cNs in the spinal cord of mouse (Mus musculus), macaque (Macaca fascicularis) and zebrafish (Danio rerio). In these species, the somata of spinal PKD2L1+ CSF-cNs were located below or within the ependymal layer and extended an apical bulbous extension into the central canal. We found GABAergic PKD2L1-expressing CSF-cNs in all three species. We took advantage of the zebrafish embryo for its transparency and rapid development to identify the progenitor domains from which pkd2l1+ CSF-cNs originate. pkd2l1+ CSF-cNs were all GABAergic and organized in two rows—one ventral and one dorsal to the central canal. Their location and marker expression is consistent with previously described Kolmer–Agduhr cells. Accordingly, pkd2l1+ CSF-cNs were derived from the progenitor domains p3 and pMN defined by the expression of nkx2.2a and olig2 transcription factors, respectively. Altogether our results suggest that a system of CSF-cNs expressing the PKD2L1 channel is conserved in the spinal cord across bony vertebrate species.
Spinal cerebrospinal fluid-contacting neurons (CSF-cNs) were identified and described by Kolmer (1921, 1931) and Agduhr (1922) in over 200 species based on cellular morphology, location, and Nissl staining. They independently noted that these cells exhibit an apical bulbous extension in the central canal and send basal axonal projections to other cells. Their observations suggested that CSF-cNs could constitute a sensory organ (referred to as the sagittal organ by Kolmer) interfacing the cerebrospinal fluid (CSF) with the nervous system at the level of the spinal cord.
Since the discovery of CSF-cNs, electron microscopy studies have shown that these cells exhibit a sensory tuft, previously referred to as a “brush border” (Dale et al., 1987b), a “central body” (Vigh and Vigh-Teichmann, 1973) or a “bud” (Stoeckel et al., 2003). The apical bulbous extension projects from the perikarya of CSF-cNs toward the central canal and ends with a terminal bud in contact with its lumen. This apical extension is characterized by the dendritic marker microtubule-associated protein 2 (MAP2) (Orts-Del'Immagine et al., 2014).
Immunohistochemistry (IHC) studies showed evidence for GABAergic CSF-cNs in many species such as rat (Barber et al., 1982; Stoeckel et al., 2003), turtle (Reali et al., 2011), African clawed frog (Dale et al., 1987a; Binor and Heathcote, 2001), zebrafish (Bernhardt et al., 1992; Martin et al., 1998; Higashijima et al., 2004a,b; Wyart et al., 2009; Yang et al., 2010), eel and trout (Roberts et al., 1995), dogfish (Sueiro et al., 2004), and lampreys (Brodin et al., 1990; Christenson et al., 1991a,b; Melendez-Ferro et al., 2003; Robertson et al., 2007; Rodicio et al., 2008; Villar-Cervino et al., 2008). In African clawed frog and zebrafish, these GABAergic CSF-cNs were named Kolmer–Agduhr cells (KAs) to distinguish them from ciliated ependymal cells (Dale et al., 1987a; Bernhardt et al., 1992). In these species CSF-cNs project an ascending axon ventrally in the spinal cord (Dale et al., 1987a; Bernhardt et al., 1992; Higashijima et al., 2004a; Wyart et al., 2009).
Despite the anatomical studies of CSF-cNs and their implication in modulating locomotion (Wyart et al., 2009), their physiological role in vertebrates remains unknown. One obstacle to answering this question is the lack of a specific genetic marker to identify these cells. Recently the calcium-permeable polycystic kidney disease 2-like 1 (PKD2L1) channel (Huang et al., 2006; Ishimaru et al., 2006), first identified in kidney, retina, and heart (Basora et al., 2002) was found to be expressed in cells contacting the CSF at the level of the brainstem and spinal cord in mouse (Huang et al., 2006; Orts-Del'Immagine et al., 2012). This channel belongs to the family of Transient Receptor Potential (TRP) channels. These are known to be chemo-, thermo- or mechano-sensitive (Delmas, 2004; Delmas et al., 2004). Expression of this channel and location of these cells at the interface with the CSF are consistent with the hypothesis that CSF-cNs have a proprioceptive sensory function.
In this study, we investigated whether PKD2L1 was a specific marker for CSF-cNs across vertebrates, by examining mouse, macaque and zebrafish. In mouse and macaque PKD2L1 was enriched in the sensory tuft of CSF-cNs in cervical, thoracic and lumbar spinal cord. The soma of PDK2L1+ cells was located in the ependymal layer or just underneath. In both species, a significant proportion of PKD2L1+ CSF-cNs was distinctly identified as GABAergic. We took advantage of the zebrafish model organism to thoroughly characterize the properties and lineage of pkd2l1+ cells in the spinal cord. In this model, all pkd2l1+ cells contacting the central canal were confirmed as GABAergic neurons. Reciprocally, all KAs, defined by their location and their GABAergic phenotype expressed pkd2l1. Using specific transgenic lines, we confirmed that these cells derive from two progenitor domains. The dorsal pkd2l1+ KAs originated from pMN and were labeled by the Enhanced Green Fluorescent Protein (EGFP) in the Tg(olig2:EGFP) while the ventral pkd2l1+ KAs originated from p3 and were labeled by Tg(nkx2.2a:mEGFP). Altogether our data show that PKD2L1 is a specific marker of CSF-cNs shared between multiple vertebrate species. We found evidence for PKD2L1-expressing GABAergic CSF-cNs in all examined species. Our results on the developmental origin of CSF-cNs in zebrafish open new paths of investigation in mammals.
Wild type (WT) mice (Mus musculus, OF1 strain) were kept on a 12 h light/dark cycle with free access to food and water. Experiments were performed at embryonic (E), postnatal (P) and adult stages: four WT adult mice, one WT newborn, and three WT embryos were used in the present study. One adult male and two E16.5 GAD67-GFP knock-in embryos (Tamamaki et al., 2003), resulting of the insertion of the cDNA encoding EGFP into the locus encoding GAD67, have been used. After mating, the day of detection of the vaginal plug was considered as embryonic day E0.5. Pregnant females were sacrificed by decapitation at E14.5, E16.5, or E18.5. Uterine horns were removed from the mother and embryos were excised from their individual bag, soaked in cold 0.1 M Phosphate Buffer (PB) for 10 min and fixed by immersion in cold 2% paraformaldehyde (PFA) solution in 0.1 M PB for 3 h at 4°C. Newborn mice (P1) were deeply anesthetized by an exposure to low temperature and the spinal cord was immediately removed and fixed with 2% PFA for 4 h. Adult mice were deeply anesthetized with intraperitoneal injection of Nembutal® (150 mg·kg−1) and transcardially perfused sequentially with NaCl 0.9% (20 ml) and PFA (2%, 30 ml for WT adults and 4%, 30 ml for the GAD67-GFP mouse). After fixation, spinal cords were dissected out and post-fixed for 24 h in the fixative solution (2% PFA for WT mice and 4% for the GAD67-GFP mouse) at 4°C. Following fixation (and post-fixation if appropriate), tissues were cryo-protected for 24–48 h in 30% sucrose in 0.1 M PB at 4°C and then stored at -20°C for later use. Experiments were performed in accordance with the European Communities Council (EEC) Directive of 22 September 2010 (2010/63/EU) and French law (87/848).
Standard immunofluorescence procedures were used to localize antigens of interest (Voituron et al., 2011). At the cervical level, 10 μm-thick coronal sections of the spinal cord were obtained using a cryostat (Leica CM 3050S), mounted on silanized slides and stored at −20°C for later IHC using a rabbit anti-Polycystin-L (anti-human PKD2L1, 1:500 dilution; Millipore, Billerica, MA, USA). This PKD2L1 antibody was raised against the synthetic peptide from the N-terminal of human Polycystin-L. For PKD2L1 IHC at E14.5 and E16.5, we used an Antigen Unmasking Solution (Vector Laboratories). Briefly, the sections were incubated in 1% Bovine serum albumin (BSA) and 0.1% Triton X-100 for 20 min at room temperature (RT). After rinsing with 0.1 M phosphate buffered saline (PBS), the sections were incubated for 30 min at RT with DAPI (1.25 μg/ml; Life Technologies) and with Alexa-conjugated anti-rabbit secondary antibodies (1:500 dilution; Life Technologies). For GFP and PKD2L1 double IHC, 30 μm-thick coronal floating sections obtained using a cryostat (Leica CM 1850S) were immunostained by co-incubating two primary antibodies, a chicken anti-GFP (1:1000 dilution; Abcam, Cambridge, UK) with the rabbit anti-PKD2L1 (1:500 dilution) overnight at 4°C, and then the specific secondary antibodies Alexa Fluor 488 donkey anti-chicken IgG (1:500 dilution, Life Technologies) and Alexa 555-conjugated goat anti rabbit for 2 h at RT. In all IHC experiments, control sections were processed in parallel by omitting primary antibodies. Finally, sections were washed with PBS before being mounted onto glass slides with fluorescent mounting medium (AquaPolyMount, Biovalley, Marne La Vallée, France). Sections were observed under an Olympus FV1000 confocal microscope.
On 10 μm-thick sections, we performed Z-stacks with a step size of 1–1.5 μm in a region of interest (ROI) centered on the central canal (approximately 150 × 220 μm width, n = 13 sections for E16.5 GAD67-GFP and 115 × 140 μm width, n = 7 sections for adult GAD67-GFP sections). For each analyzed section, cells were identified by the DAPI staining in order to avoid counting multiple times the same cell. Within the ROI, all PKD2L1+ cells were counted manually and probed for GFP in the GAD67-GFP line.
Tissue from two adult macaques (Macaca fascicularis, a 25-year old female and an 8-year old male) was used for this study. These animals were sacrificed for other purposes and spinal cord tissue samples were subsequently collected and devoted to the present study. Spinal cord collection was performed after the sacrifice of animals, in strict accordance with the recommendations of the Weatherall Report regarding good animal practice. All surgical procedures and experimental protocols were carried out in strict accordance with the National Institutes of Health guidelines (2013) and the recommendations of the EEC (2010/63/EU).
Macaques were deeply anesthetized with intramuscular injection of ketamine and xylazine at 50 and 5 mg/kg, respectively. Following the anesthesia, each animal was exsanguinated and perfused with 0.9% NaCl before their tissues were fixed by an intracardial perfusion containing 4% PFA in 0.1 M PBS. Spinal cord was extracted and meninges were removed using fine forceps. Tissue was embedded in 5% agarose and sectioned at 50 μm using a vibratome (Leica VT1000S). Additionally, frozen tissue blocks were obtained by passing tissue through a series of cryoprotection solutions (20, 30% sucrose in PBS) and freezing in isopentane; frozen sections were cut at 20 μm (HM 650V Microtome, Thermo Scientific). All sections were stored in 0.4% Sodium Azide in 0.1 M PBS at 4°C until IHC.
Free-floating sections from cervical, thoracic, and lumbar spinal cord tissue were used for IHC. Sections were first incubated in a blocking solution composed of 1% BSA, 0.5% Triton X-100 and 2% normal goat serum in PBS to reduce non-specific labeling. Sections were then incubated in primary antibodies overnight at 4°C. After multiple washes, sections were incubated in secondary antibodies for 4 h at 4°C. Primary antibodies used were rabbit anti-Polycystin-L (anti-human PKD2L1, 1:1,000 dilution; Millipore, Billerica, MA, USA), guinea pig anti-vesicular GABA Transporter (anti-VGAT, 1:500; Synaptic Systems, Germany) and rabbit anti-GAD65/67 (1:500; Abcam, MA, USA). Secondary antibodies were Alexa Fluor 488- or Alexa Fluor 568-conjugated anti-rabbit (1:500; Life Technologies) and Alexa Fluor 488-anti-guinea pig (1:500; Life Technologies). The same blocking solution as previously was used to dilute the antibodies. PBS was used for washing sections between each step. Sections were mounted on glass slides, cover slipped with Prolong® Gold Antifade Reagent (Life Technologies) mounting medium and then imaged on a Leica SP2 AOBS AOTF inverted confocal laser scanning microscope. Single optical slice images of individual labeled cells were taken using a 63× oil objective.
All zebrafish (Danio rerio) lines were maintained and raised on a 14/10 h light cycle and water was regulated at 28.5°C, conductivity at 500 μS and pH at 7.4 (Westerfield, 2000). WT AB and Tüpfel long fin (TL) embryos were used for whole mount in situ hybridization (ISH). Tg(nkx2.2a:mEGFP) (Ng et al., 2005; Kirby et al., 2006) and Tg(olig2:EGFP) (Shin et al., 2003) transgenic lines were kindly provided by Prof. Bruce Appel, University of Colorado, Denver, USA. Embryos were dechorionated and staged according to number of somites as described (Kimmel et al., 1995): the 30-somite stage corresponds to Prim-5 or 24 h post fertilization when raised at 28.5°C. Adult fish were anesthetized in 0.02% MS 222 (Sandoz, Levallois-Perret, France) and killed by decapitation. All procedures were approved by the Institutional Ethics Committee at the Institut du Cerveau et de la Moelle épinière (ICM), Paris, France, the Ethical Committee Charles Darwin and received subsequent approval from the EEC (2010/63/EU).
To generate the pkd2l1 ISH probe, the coding fragment for pkd2l1 was amplified from zebrafish embryo total cDNA using the following primers (5′ to 3′): pkd2l1_For: TAGTGGTGATACTGCTTGCTGTGGTGG (from the end of the exon 6 of the pkd2l1 gene and the beginning of exon 7) and pkd2l1_Rev: TGGTTCCACACTGTTCTCGAGGTCACG (from the end of exon 13). The PCR fragment was cloned into the pCRII-TOPO vector (Life Technologies, Carlsbad, CA, USA). The resulting plasmid was linearized with NotI. The gad67 plasmid, kindly provided by Dr. Uwe Strähle, Karlsruhe Institute of Technology, Germany, was linearized with NcoI. Digoxigenin (DIG)- and fluorescein (Fluo)-labeled probes were synthesized using SP6 RNA polymerase with the RNA Labeling Kit (Roche Applied Science, Basel, Switzerland) to generate both pkd2l1 and gad67 antisense probes. To generate pkd2l1 sense probes, the plasmid was linearized with KpnI and transcription was carried out using T7 RNA polymerase. All probes were purified using the mini Quick Spin RNA Column (Roche, Basel, Switzerland).
Whole-mount ISH were performed as previously described (Parmentier et al., 2011; Alunni et al., 2013) on embryos or dissected adult spinal cords fixed in 4% PFA in PBS overnight at 4°C. To reveal pkd2l1 expression, probes were detected with anti-DIG or anti-Fluo antibodies conjugated to alkaline phosphatase followed by a chromogenic reaction using a solution of NBT/BCIP as substrate (Roche Diagnostics, France). To quantify cell density or ascertain transcript colocalization, probes were detected by the antibodies conjugated to horseradish peroxidase and were revealed by Tyramide Signal Amplification using Tyramide-FITC or Tyramide-TAMRA as substrates. The specificity of the pkd2l1 probe was verified using a pkd2l1 sense probe as negative control (data not shown). Double fluorescent in situ hybridization (FISH) for pkd2l1 and gad67 were performed on adult zebrafish spinal cords and coupled with respectively DIG and Fluo.
The following primary antibodies were used for IHC: rabbit anti-GABA (1:2000, Sigma-Aldrich, St. Louis, MO, USA), rabbit anti-GAD65/67 and chicken anti-GFP (both used at 1:500 dilution, Abcam, Cambridge, UK). Immunostaining specificity was established by omitting the primary specific antibodies, no immunoreactive signal was observed. Agarose sections were collected as described above. In the zebrafish embryo, we performed either 50 μm-thick sagittal (for anti-GAD65/67 IHC) or transverse (for anti-GFP IHC) sections. In the adult, we performed 50 μm-thick sagittal and frontal sections of previously FISH stained adult spinal cords.
pkd2l1 FISH was performed before IHC against GFP or GAD65/67: embryos were washed and immunostained with the chicken anti-GFP antibody or the rabbit anti-GAD65/67 antibody overnight at 4°C, and then incubated with the corresponding Alexa conjugated secondary antibodies IgG (1:500, Life Technologies) combined with DAPI (2.5 μg/ml, Life Technologies).
Whole-mount embryos stained by NBT/BCIP were mounted in 80% glycerol. Sections of adult spinal cord were mounted in a solution of Mowiol. Embryos were imaged using a Nikon AZ100M macroscope and a Leica DM5000 B Upright microscope. Adult spinal cord sections were imaged using a Nikon AZ100M macroscope. To quantify pkd2l1+ and GABA+ cells, stained embryos were imaged using the Fixed Stage microscope AxioExaminer Z1 equipped with a 20× water immersion objective and a Yokogawa CSU-X1 spinning disk unit (n = 13 embryos for pkd2l1 and n = 10 for GABA). To quantify the overlap of GFP with pkd2l1 FISH in the Tg(nkx2.2a:mEGFP) and the Tg(olig2:EGFP) transgenic embryos, 50 μm-thick sections were analyzed on an Olympus FV1000 confocal microscope equipped with a 40× water immersion objective using 405, 473, and 543 nm laser lines. Images were processed using Fiji (Schindelin et al., 2012) and Adobe Illustrator (Adobe Systems, Mountain View, CA, USA) software.
To quantify the total number of pkd2l1+ cells revealed by FISH per embryo, Z-stacks of the entire embryos from somite 1 to 30 were acquired. Total numbers of cells were counted per somite labeled on both sides of the midline. The boundaries for each somite were established with transmitted light. The three subtypes of pkd2l1 expressing cells were defined according to their position relative to the central canal. We distinguished: (i) the row of cells that was ventral and in proximity to the central canal, (ii) the row that was dorsal and in proximity to the central canal and (iii) the sparse cells that were dorsal and distant from the central canal. The proportion of pkd2l1+ cells double-labeled for GAD65/67 in 30-somite (Prim-5) embryos was quantified based on 50 μm-thick sagittal sections performed after the FISH and before GAD65/67 IHC. The proportion of pkd2l1+ cells double-labeled for GFP in Tg(nkx2.2a:mEGFP) and Tg(olig2:EGFP) in 30-somite embryos was quantified based on 50 μm-thick transverse sections performed after the FISH and IHC. The total number of cells is given as the mean ± Standard Error of the Mean (SEM). The same method was applied for counting of GABA+ KAs. There are four GABAergic interneuron types in the zebrafish embryo (Bernhardt et al., 1992; Higashijima et al., 2004a); KAs were identified as the only GABAergic ascending neurons with a soma located just below (KA”) or above (KA') the central canal.
PKD2L1 was originally identified in CSF-cNs at postnatal stages P1–P4 in the mouse spinal cord (Huang et al., 2006). We investigated PKD2L1 expression in the spinal cord at the embryonic, postnatal and adult stages. PKD2L1 expression could not be detected before E14.5 in the spinal cord at the cervical, thoracic and lumbar levels. At E14.5, few PKD2L1+ cells could be identified in the cervical spinal cord (Figure 1A). Starting at E16.5, PKD2L1+ cells localized around the central canal exhibited the typical morphology of spinal CSF-cNs (Figures 1B–E, arrows). At E18.5, the soma of PKD2L1+ cells was usually located under the layer of ependymal cells (91 out of 102, while 11 out of 102 were located within the ependyma, Table 1). PKD2L1+ cells sent an apical bulbous extension toward the central canal ending in the lumen with a bud enriched in PKD2L1 (Figures 1B–E\, arrows). In the adult, the soma of PKD2L1+ CSF-cNs was located in lamina X and always under the ependyma (95 out of 95 PKD2L1+ cells) (Figure 1E, Table 1). We also observed PKD2L1+ cells with similar morphology localized away from the central canal, though these cells lacked a visible apical extension to the central canal (Figures 1A–C\, arrowheads). Our data identify PKD2L1 as a general marker of CSF-cNs.
Figure 1. PKD2L1 expression in CSF-cNs is present from embryonic stage E14.5 to adulthood in mouse. (A–E) Immunostaining for PKD2L1 at E14.5 (A), E16.5 (B), E18.5 (C), P1 (D), and in the adult (E) performed on coronal sections of the mouse cervical spinal cord. At E14.5, few PKD2L1+ cells are detected (arrowhead). From E16.5 (B) to adult (E), multiple PKD2L1+ CSF-cNs (arrows) surround the central canal where they project an apical bulbous dendritic extension contacting its lumen. Note that PKD2L1+ cells exhibiting a similar morphology but not distinctly contacting the central canal (arrowheads) can also be observed (A–C). Dorsal is up. White dashed line delineates the central canal. DAPI staining appears in blue. Scale bars = 20 μm.
Table 1. Distribution of PKD2L1+ cells based on the location of their soma relative to the ependyma in the mouse spinal cord.
As mentioned before, cells contacting the CSF had been described as GABAergic in many species, but the neurotransmitter phenotype has not been thoroughly established yet in mouse. We tested whether CSF-cNs were GABAergic in the embryonic and adult mouse spinal cord (Figure 2). We took advantage of the well-characterized GAD67-GFP knock-in mouse where the enhanced GFP was targeted to the locus encoding GAD67 using homologous recombination (Tamamaki et al., 2003). In these animals, we performed IHC for GFP to amplify the endogenous GFP signals (Figure 2). In GAD67-GFP knock-in embryos, double IHC for GFP and PKD2L1 on coronal sections of E16.5 mice spinal cords (Figure 2A) revealed that most of PKD2L1+ cells were GFP+ (arrows) indicating their GABAergic nature (n = 2 embryos, 107 out of 162 PKD2L1+ cells). In one adult, we observed the same findings and found 47 out of 73 cells labeled for both PKD2L1 and GFP (Figure 2B, arrows). Note that both in the embryos and in the adult, we observed PKD2L1+ cells that were not distinctly GFP+ (Figures 2A,B, double arrowhead). Notably, we never observed GFP+ CSF-cNs that were not PKD2L1+. Altogether we show evidence for PKD2L1+ CSF-cNs that are GABAergic in the mouse spinal cord.
Figure 2. Some PKD2L1+ cells surrounding the central canal of cervical spinal cord of adult mouse are immunoreactive for GFP in the knock-in GAD67-GFP mouse. (A) 10 μm-thick coronal sections of GAD67-GFP E16.5 mouse spinal cord immunostained for GFP (A1,A3,A4) and PKD2L1 (A2–A4). Most PKD2L1+ cells are GFP+ (arrows) but not all (double arrowheads). (B) 30 μm-thick coronal sections of a GAD67-GFP adult mouse spinal cord immunostained for GFP (B1,B3,B4) and PKD2L1 (B2–B4). While many PKD2L1+cells are distinctly GFP+ (arrows), some cells are not (double arrowheads). Dorsal is up. White dashed line delineates the central canal. DAPI staining appears in blue. Scale bars = 25 μm (A), 15 μm (B).
We next asked whether PKD2L1 labels CSF-cNs in the spinal cord of primates (Figure 3). In transverse sections from cervical, thoracic and lumbar spinal cord, we observed the distribution of PKD2L1 immunofluorescence around the central canal in lamina X (Figures 3A–C\). PKD2L1+ cells were localized around the central canal and exhibited the typical morphology of spinal CSF-cNs with an apical extension toward the central canal ending with a bud in the lumen. PKD2L1 was enriched in the terminal bud of cerebrospinal fluid-contacting cells (Figures 3A–C\) and faintly expressed (Figures 3B,C) or absent (Figure 3G) in the cell soma and in the rest of the apical extensions. PKD2L1+CSF-cNs closely surrounded the central canal; they had a round nucleus and were located under (Figures 3B–E\) or within the ependyma (Figures 3F,G). In order to test whether PKD2L1+ CSF-cNs were GABAergic, we used IHC for the enzymes GAD65/67 and the VGAT transporter. Positive immunostaining for GAD65/67 and VGAT was found in cells surrounding the central canal at the level of their apical bulbous extension, soma and putative axon (Figures 3D–F\, arrowheads, arrows, and asterisks, respectively) but buds were labeled by GABAergic markers less frequently than they were by PKD2L1. Double immunofluorescence for PKD2L1 and VGAT showed evidence for CSF-cNs double-labeled at the level of the soma and the intraluminal bud (Figure 3G). Nonetheless VGAT could not be detected in all PKD2L1+ buds (Figure 3H, double arrowheads). Cells double-labeled for PKD2L1 and VGAT were observed at the cervical, thoracic and lumbar level in the spinal cord. Taken together, our data show for the first time the presence of immunoreactive CSF-cNs containing PKD2L1 and GABAergic markers (GAD65/67 or VGAT) in the macaque lamina X. These findings demonstrate that a population of GABAergic CSF-cNs expressing PKD2L1 is present as well in the spinal cord of adult primates. Our results also support the idea that PKD2L1 is a specific marker of CSF-cNs in primates and labels more of these cells than GABAergic markers do in our experimental conditions.
Figure 3. PKD2L1+ CSF-cNs can be found as GABAergic neurons surrounding the central canal in the spinal cord of the adult macaque. (A–C) PKD2L1 immunostaining labels intraluminal buds (A–C) and rarely subependymal or ependymal cell bodies (B,C). (D,E) GAD65/67 immunoreactive cells are numerous in the vicinity of the central canal: proximal cells show a clear apical extension contacting the lumen while cells located away do not. (F) CSF-cNs surrounding the central canal are immunoreactive for the vesicular GABA transporter VGAT. (G,H) Double immunostaining for VGAT and PKD2L1 shows that some but not all PKD2L1+ CSF-cNs are clearly GABAergic. The intraluminal buds can be found double-labeled for PKD2L1 and VGAT (arrowheads) but not systematically (double arrowheads), while apical extensions, cell body and putative axon are usually only labeled by VGAT. All panels are coronal sections of the macaque adult spinal cord taken at the lumbar level for (A,G), at the cervical level for (B–F) and at the thoracic level for (H). In all panels, arrows point to the somata, asterisks to the putative axons and arrowheads to the intraluminal buds of CSF-cNs. White dashed line delineates the central canal. DAPI staining appears in blue. Scale bars = 100 μm (A) and 10 μm (B–H).
The developmental origin of CSF-cNs has not been established yet in mammals. To tackle the question of the developmental origin of pkd2l1+ cells, we took advantage of the zebrafish embryo for its transparency and the rapidity of development of the spinal cord.
To assess whether pkd2l1 was expressed in CSF-cNs in zebrafish, we performed ISH using the antisense probe for pkd2l1 (Figure 4). We observed the expression of pkd2l1 in CSF-cNs along the central canal in sagittal sections of the adult spinal cord (Figure 4A). pkd2l1+ cells contacting the CSF were located ventrally and dorsally to the central canal (Figures 4A1,A2). In the zebrafish embryo, we investigated the 10-, 14-, 18-, 20-, and 30-somite stages (n > 6 embryos per condition). A weak signal in the rostral spinal cord (black arrow) could be detected at the 18-somite stage (Figure 4B). Gradually, the staining appeared rostro-caudally within the spinal cord (Figures 4C,D). Cells with dense labeling were located in two parallel rows along the ventral margin of the spinal cord (Figures 4E,F). Dorsal views of the spinal cord showed that these cells were located on either side of the midline (Figure 4G). At the larval stages, we observed that pkd2l1+ CSF-cNs covered the entire length of the spinal cord and the organization in two parallel rows was not distinct anymore (data not shown). In zebrafish, CSF-cNs (named KAs) have been shown to derive from two progenitor domains, p3 and pMN (Park et al., 2004; Schafer et al., 2007; Shin et al., 2007; Yeo and Chitnis, 2007; Yang et al., 2010; England et al., 2011; Huang et al., 2012). KAs were therefore divided into two subpopulations on this basis, dorsal KA' and ventral KA” (Park et al., 2004). To identify the nature of pkd2l1 expressing cells, we performed FISH in 30-somite stage zebrafish embryos (i.e., Prim-5 stage or 24 h post fertilization, Figure 5). This staining confirmed that expression of pkd2l1 mRNA was localized in the ventral spinal cord and mainly distributed in two rows of cells along the rostro-caudal axis (Figures 5A–C\). At higher magnification, we observed that these cells were lining the central canal either ventrally (arrowhead) or dorsally (arrow) (Figures 5B–D\). Transverse sections confirmed that the somata of these cells directly surround the central canal (Figure 5D). While most pkd2l1+ cells lined the central canal, we observed faint pkd2l1 expressing cells away from the central canal in the dorsal spinal cord (Figures 5C,D, double arrowhead). We quantified the number of cells for each subpopulation based on lateral views originating from 13 embryos at the 30-somite stage (Figure 5E). pkd2l1+ cells localized mainly in the rostral two thirds of the spinal cord in 30-somite stage zebrafish embryos with 3–4 cells per somite for both ventral KA” and dorsal KA' between somites 5 and 15. At this stage, cell density decreased from somite 16 to 21 after which expression ceased (Figure 5E). We quantified the total number of pkd2l1+ cells for each subpopulation per embryo (Figure 5F). We found that pkd2l1+ cells from the ventral row reached 45.4 ± 2.4 cells (n = 13 embryos), similar to counts performed previously on KA” at the same stage (Huang et al., 2012). These observations on cell body location and number suggest that the ventral pkd2l1+ cells are KA” and dorsal ones are KA'.
Figure 4. pkd2l1 is expressed from the embryonic 18-somite stage to adulthood in the zebrafish spinal cord. (A) Adult; (B–G) embryonic stages: 18-somite (B), 20-somite (C) and 30-somite stages (D–G). (A) In sagittal sections from the adult spinal cord, pkd2l1+ CSF-contacting cells are located ventral and dorsal to the central canal. White dash line: central canal, dark gray line: ventral limit of the spinal cord. pkd2l1 expression appears in the rostral spinal cord (B,C, arrows) and is distributed in two rows of cells along the rostro-caudal axis (E,F) and on each side of the midline (G). (E) is a close-up of the black box from (D). Rostral is to the left for all panels. Lateral views with dorsal up for (A–F) and dorsal view for (G). DAPI staining appears in blue. Scale bars = 40 μm (A), 100 μm for (B–D), 50 μm for (E–G).
Figure 5. pkd2l1 is expressed in cells contacting the CSF and surrounding the central canal in the spinal cord of the zebrafish embryo. (A–D) FISH of pkd2l1 mRNA at the 30-somite stage. Lateral views for (A–C) and transverse section for (D). Two subpopulations of pkd2l1 bright expressing cells surround the central canal ventrally (arrowhead) and dorsally (arrow). Note the existence of dorsal pkd2l1 weak expressing cells away from the central canal (double arrowhead). The white dashed line, dark gray solid line and the solid white lines indicate respectively the central canal, the ventral limit of the spinal cord, and the somite boundaries. DAPI staining appears in blue. Scale bars = 50 μm for (A), 30 μm for (B,C), and 20 μm for (D). (E) Mean number of pkd2l1+ cells per somite along the rostro-caudal axis at the 30-somite stage. (F) Total cell counts per embryo (n = 13 embryos). We counted 45.4 ± 2.4 ventral pkd2l1+ cells, 47.1 ± 3.4 dorsal pkd2l1+ cells and 7.6 ± 2.3 dorsal away from the central canal pkd2l1+ cells per embryo. (E,F) Mean values are given ± s.e.m.
To demonstrate that zebrafish pkd2l1+ CSF-cNs are GABAergic, we investigated the expression of GABA and of GAD65/67 (Figure 6). An immunostaining for GABA at the 30-somite stage revealed multiple GABAergic cell types (Bernhardt et al., 1992; Higashijima et al., 2004a) including ventral and dorsal cells surrounding the central canal (Figure 6A, arrowhead and arrow, respectively). A short ascending axon originating from the soma of these cells can be observed (Figure 6A, asterisks). Based on morphology and location (see Materials and Methods), we estimated the number of GABAergic ventral (34.6 ± 2.3) and dorsal (49.9 ± 1.8) CSF-cNs per embryo (n = 10 embryos). We performed pkd2l1 FISH followed by IHC for GAD65/67 to determine whether pkd2l1+ CSF-cNs are also GABAergic. All pkd2l1+ cells (n = 160) along the central canal were GAD65/67 immunoreactive (Figure 6B) (92 ventral pkd2l1+ cells, 68 dorsal pkd2l1+ cells). Based on their location, density and GABAergic phenotype, these pkd2l1+ CSF-cNs correspond to KA” and KA'. To test whether zebrafish CSF-cNs persist as GABAergic neurons until adulthood, we performed pkd2l1 and gad67 double FISH on whole adult spinal cord indicating that pkd2l1+ CSF-cNs express gad67 throughout development (Figure 6C). Contrary to what we observed in mouse and macaque, these results demonstrate that in zebrafish all pkd2l1+ CSF-cNs are clearly GABAergic.
Figure 6. pkd2l1+ CSF-cNs are GABAergic neurons expressing GABA and GAD in zebrafish. (A) GABA IHC on WT 30-somite embryos shows ventral (arrowhead) and dorsal (arrow) KAs and their ascending axon (asterisks). (B1–B4) Ventral and dorsal pkd2l1+ KAs are GAD65/67 immunoreactive in 30-somite embryos as shown by FISH for pkd2l1 (green) coupled to a GAD65/67 IHC (red). (C1–C4) In the adult, pkd2l1+ KAs are gad67+ as shown by FISH for gad67 (C1,C3,C4) and pkd2l1 (C2–C4) on sections of WT spinal cord. The white dash line indicates the central canal, the dark line the ventral limit of the spinal cord. (A) is a projection from the lateral view of a whole-mount embryo immunostained for GABA while (B1–B4) correspond to sagittal sections and (C1–C4) to frontal sections. DAPI staining appears in blue. Scale bars = 30 μm.
To confirm the developmental origins of pkd2l1+ cells, we took advantage of the zebrafish model in which pkd2l1 is expressed in the 30-somite stage embryo when progenitor domains are well-defined. Previous studies have shown that dorsal KA' cells are derived from the pMN domain and express the transcription factor olig2 (Park et al., 2004). Ventral KA” cells originate from the p3 progenitor domain (Schafer et al., 2007) and express the transcription factor nkx2.2a (Yang et al., 2010; Huang et al., 2012). We therefore performed pkd2l1 FISH combined with an anti-GFP immunostaining in the Tg(nkx2.2a:mEGFP) and the Tg(olig2:EGFP) transgenic lines (Shin et al., 2003; Ng et al., 2005) (Figures 7, 8). In the Tg(nkx2.2a:mEGFP) transgenic embryos, the p3 domain was labeled by GFP staining as expected (Figure 7A). Ventral pkd2l1+ cells contacting the central canal were labeled for GFP while dorsal cells were not (Figure 7B). In the Tg(olig2:EGFP) transgenic embryos (Figure 8), the progenitor domain pMN was labeled by GFP (Figure 8A). In these animals, only dorsal pkd2l1+ cells contacting the central canal were GFP+ (Figure 8B). Transverse sections allowed to estimate the number of pkd2l1+ cells contacting the central canal that were positive for GFP in the Tg(nkx2.2a:mEGFP) and in the Tg(olig2:EGFP) transgenic embryos (Table 2). While only ventral pkd2l1+ cells expressed GFP in the Tg(nkx2.2a:mEGFP) line (38 out of 38 cells), dorsal cells solely expressed GFP in the Tg(olig2:EGFP) line (93 out of 93 cells) (Table 2). Our data indicate that pkd2l1+ cells lining the central canal in zebrafish are KAs originating from p3 (for KA”) and from pMN (for KA') depending on their dorso-ventral location relative to the central canal.
Figure 7. Ventral pkd2l1+ cells express nkx2.2a and derive from the p3 progenitor domain in the zebrafish embryo. (A,B) pkd2l1 FISH in Tg(nkx2.2a: mEGFP) embryos immunostained for GFP at the 30-somite stage. IHC for GFP reveals the p3 domain. (A) Lateral view showing that the most ventral pkd2l1+ cells are GFP+. (B) A typical transverse section shows a ventral pkd2l1+ cell contacting the central canal and expressing GFP (arrowhead) while a dorsal pkd2l1+ cell contacting the central canal does not express GFP (arrow) in the Tg(nkx2.2a: mEGFP) transgenic embryo. White dashed line delineates the central canal, dark line the ventral limit of the spinal cord. DAPI staining appears in blue. Scale bars = 20 μm.
Figure 8. Dorsal pkd2l1+ CSF-cNs express olig2 and derive from the pMN progenitor domain in the embryonic spinal cord of zebrafish. (A,B) pkd2l1 FISH in Tg(olig2:EGFP) embryos immunostained for GFP at the 30-somite stage. IHC for GFP reveals the pMN domain. (A) Lateral view showing dorsal pkd2l1+ cells express GFP. (B) Transverse sections showing dorsal pkd2l1+ cells contacting the central canal express GFP (arrow) while a ventral pkd2l1+ cell contacting the central canal (arrowhead) does not express GFP in the Tg(olig2:EGFP) transgenic line. White dashed line delineates the central canal, dark line the ventral limit of the spinal cord. DAPI staining appears in blue. Scale bars = 20 μm.
Table 2. Distribution of ventral and dorsal pkd2l1+ cells expressing GFP in Tg(nkx2.2a:mEGFP) and Tg(olig2:EGFP) zebrafish transgenic lines at the 30-somite stage.
PKD2L1+ neurons projecting their apical bulbous dendritic extension into the central canal of the spinal cord had been described only in mouse at the P1–P4 stages (Huang et al., 2006) and in the adult (Orts-Del'Immagine et al., 2012, 2014). We hypothesized that the channel PKD2L1 could be a specific marker of spinal CSF-cNs shared among vertebrates. We demonstrate here that the TRP channel PKD2L1 is a marker of CSF-cNs in three vertebrate species: Mus musculus, Macaca fascicularis, and Danio rerio. For the first time, we show the expression of this channel in primate and zebrafish CSF-cNs. In the adult macaque spinal cord, CSF-cNs express PKD2L1 at the cervical, thoracic and lumbar levels. In the zebrafish embryo, pkd2l1+ CSF-cNs originally differentiate in two rows along rostro-caudal gradient.
Taken together, our results demonstrate the shared expression of PKD2L1 in spinal CSF-cNs across bony vertebrate species. GABA has been previously reported to label some CSF-cNs (Barber et al., 1982; Dale et al., 1987a,b; Brodin et al., 1990; Christenson et al., 1991a,b; Bernhardt et al., 1992; Martin et al., 1998; Binor and Heathcote, 2001; Stoeckel et al., 2003; Robertson et al., 2007; Rodicio et al., 2008; Villar-Cervino et al., 2008; Reali et al., 2011). In mouse and macaque, PKD2L1 appeared always highly enriched in the intraluminal buds of CSF-cNs. On the contrary, GABAergic markers (GAD65/67, VGAT) rarely labeled in these species the soma or the apical bulbous extension of CSF-cNs. Notably we never observed CSF-cNs labeled by GABAergic markers and not by PKD2L1. In mammals, PKD2L1 seems therefore to label more CSF-contacting cells than GABAergic markers do. Nonetheless, with the approach we developed here, we cannot assess the existence of CSF-cNs that would not express PKD2L1.
In mouse and zebrafish, we detected the expression of the channel at early embryonic stages of development (E14.5 stage for the mouse and 18-somite stage for the zebrafish). In mouse, PKD2L1 expression could be found in few spinal cells contacting the central canal at E14.5 but became more evenly expressed from E16.5 to adulthood. This observation is consistent with a recent report showing that CSF-cNs emerged at E14 in the rat spinal cord (Kutna et al., 2013). It suggests that PKD2L1 expression may start soon after the cell differentiates into a CSF-cN.
We demonstrate here that within the spinal cord of the zebrafish embryo pkd2l1 mRNA is enriched in CSF-cNs that are mainly arranged in two rows, one ventral and one dorsal to the central canal. Previous studies have shown that KA cells can be subdivided into two populations of CSF-cNs: the ventral KA” and the dorsal KA' (Park et al., 2004; Schafer et al., 2007; Shin et al., 2007; Yeo and Chitnis, 2007; Yang et al., 2010; England et al., 2011; Huang et al., 2012). We showed that pkd2l1 can be detected in both KA” and KA'.
In the three species studied here, we observed PKD2L1+ perikarya away from the central canal and for which we could not observe an apical bulbous extension reaching the lumen. In the zebrafish embryonic spinal cord, pkd2l1 expression was weak in cells that were localized dorsally and away from the central canal. These cells were less than 8% (7.6% ± 2.3) of the pkd2l1+ cells in our FISH experiments (Figure 5). Some of them expressed GFP in the Tg(olig2:EGFP) line (Table 2) suggesting that they could be either CSF-cNs originating from pMN, motoneurons or ventral longitudinal descending neurons (VeLDs) (Bernhardt et al., 1990; Park et al., 2004; Warp et al., 2012). A more extensive characterization would be necessary to identify the nature of these marginal dorsal cells. In cross sections of mouse, macaque and zebrafish spinal cords, those distant cells did not distinctly contact the central canal. However, as shown in rats (Lu et al., 2008), they could extend a long apical bulbous extension reaching the central canal that would be difficult to capture in thin sections.
At the embryonic stage, the neural tube is subdivided into molecularly defined neural progenitor domains generating distinct neuronal subtypes in vertebrates (Ericson et al., 1997; Briscoe et al., 1999; Jessell, 2000; Novitch et al., 2001; Goulding, 2009). In zebrafish, CSF-cNs referred to as KA cells derive from the two most ventral domains of the spinal cord; the ventral to the central canal p3 domain labeled by nkx2.2a and the more dorsal pMN marked by olig2 (Park et al., 2004; Schafer et al., 2007; Shin et al., 2007; Yeo and Chitnis, 2007; Yang et al., 2010; England et al., 2011; Huang et al., 2012). By analyzing transverse sections of stable transgenic lines where GFP reports the expression of these transcription factors, we found that pkd2l1+ CSF-cNs were derived from the nkx2.2a and from the olig2 expression domains. This observation confirms the double developmental origin of CSF-cNs in zebrafish.
In the embryonic mouse spinal cord, the progenitor domains p3 and pMN form well-defined bands labeled by NKX2.2 and OLIG2, respectively, between E9.5 and E12.5 (Briscoe et al., 1999; Jessell, 2000; Novitch et al., 2001). At these stages, PKD2L1 is not yet expressed (data not shown). Therefore, by IHC for these transcription factors and PKD2L1, we could not test whether PKD2L1+CSF-cNs originate as well from p3 and pMN (data not shown). To reveal the developmental origin of these cells in mouse, lineage tracing based on the use of inducible transgenic lines for p3 and pMN markers will be necessary. Previous results relying on a tamoxifen-inducible Cre-recombinase inserted into the Olig2 locus indicate that a subpopulation of cells originating from pMN between E9.5 and E14.5 are located at the ependymal border circling the central canal (Srinivas et al., 2001; Masahira et al., 2006). This observation suggests that some CSF-cNs could originate from pMN in mouse, although a thorough investigation would be necessary to address this question in this model organism.
GABAergic CSF-cNs have been described in the spinal cord of various vertebrate species (Barber et al., 1982; Dale et al., 1987a,b; Brodin et al., 1990; Christenson et al., 1991a,b; Bernhardt et al., 1992; Martin et al., 1998; Binor and Heathcote, 2001; Stoeckel et al., 2003; Robertson et al., 2007; Rodicio et al., 2008; Villar-Cervino et al., 2008; Reali et al., 2011). Here we used different GABAergic markers, GABA itself, the synthesis enzyme GAD or the GABA transporter VGAT, to test whether PKD2L1+ CSF-cNs were GABAergic. In zebrafish, we demonstrate that all pkd2l1+ cells are GABAergic in spinal CSF-cNs. In mouse and macaque, we could not demonstrate a complete co-localization of PKD2L1 with GABAergic markers (GAD67-GFP or VGAT) in CSF-cNs. In macaque, a minority of PKD2L1+ intraluminal buds was clearly double-labeled with VGAT. In mouse, the observation of GABAergic PKD2L1+ CSF-cNs was confirmed in the GAD67-GFP transgenic line by the co-expression of PKD2L1 and GFP in only some cells. The lack of systematic colocalization between PKD2L1 and GABAergic markers in CSF-cNs could be due to the expression of other isoforms of GAD (such as GAD65) or to other neurotransmitters expressed in PKD2L1+ CSF-cNs. Although we could not demonstrate that GABA is expressed in all CSF-cNs in mammals, our results show evidence for GABAergic PKD2L1+ CSF-cNs in the spinal cord of the three species studied here.
Previous studies characterized a diversity of markers potentially expressed in CSF-cNs in multiple species. Peptides such as the vasoactive intestinal polypeptide (VIP), somatostatin or urotensin II-related peptide (URP2) have been found in CSF-cNs (Buchanan et al., 1987; Yulis and Lederis, 1988a,b; Christenson et al., 1991a; Lamotte and Shapiro, 1991; Lopez et al., 2007; Wyart et al., 2009; Parmentier et al., 2011; Jalalvand et al., 2014). Ventral CSF-cNs were found dopaminergic in tetrapods such as birds (Acerbo et al., 2003) and amphibians (Gonzalez and Smeets, 1991, 1993; Gonzalez et al., 1993), as well as in some teleosts such as the eel and the trout (Roberts et al., 1995), in dogfish (Sueiro et al., 2004) and lampreys (Schotland et al., 1996; Rodicio et al., 2008). It would be interesting to determine in these species whether the dopaminergic and non-dopaminergic CSF-cNs derive as well from two different progenitor domains. However, the dopaminergic phenotype does not seem to be conserved in all vertebrate species as shown in some teleosts and in mammals (Nagatsu et al., 1988; McLean and Fetcho, 2004).
Other markers such as the subunit P2X2 of the purinergic receptor have been found in adult CSF-cNs together with early neuronal markers such as the polysialylated neural cell adhesion molecule (PSA-NCAM) in rats (Stoeckel et al., 2003) and HuC in turtles (Reali et al., 2011) and dogfish (Sueiro et al., 2004).
All markers listed above are not highly specific for CSF-cNs. However, in the three species studies here, PKD2L1 appears as a specific marker of CSF-cNs that is highly expressed and broadly targets CSF-cNs within the spinal cord. Despite existing variations in expression of peptides, neuromodulators and receptors, our results in zebrafish, mouse and macaque suggest the existence of a conserved system of spinal CSF-cNs defined by their morphology, their location—ependymally or subependymally—and their enriched expression of PKD2L1.
PKD2L1 has been involved in multiple functions from sour taste (Huang et al., 2006; Ishimaru et al., 2006; Inada et al., 2008; Ishii et al., 2009; Shimizu et al., 2009; Chang et al., 2010; Shimizu et al., 2011; Horio et al., 2011) to primary cilium signaling (Decaen et al., 2013; Delling et al., 2013). The peculiar location of CSF-cNs in contact with the CSF strongly suggests a role for PKD2L1 as a sensor of CSF composition, pH and/or osmolarity (Huang et al., 2006; Orts-Del'Immagine et al., 2012) since the channel is activated upon acidification (Ishimaru et al., 2006; Inada et al., 2008; Orts-Del'Immagine et al., 2012), alkalinization (Shimizu et al., 2011; Orts-Del'Immagine et al., 2012) or hypo-osmotic variations (Shimizu et al., 2009; Orts-Del'Immagine et al., 2012).
The role(s) of CSF-cNs in the vertebrate spinal cord is (are) poorly understood. They could be proprioceptors sensitive to the CSF composition (Huang et al., 2006; Orts-Del'Immagine et al., 2012) and modulating locomotion (Wyart et al., 2009), or enabling the differentiation of progenitors in the ependymal neurogenic niche via GABA release (Reali et al., 2011). The investigation of PKD2L1 functions in CSF-cNs across multiple species should reveal whether its physiological role in the spinal cord is conserved in vertebrates.
Lydia Djenoune and Claire Wyart conceived, designed, and supervised all experiments. Lydia Djenoune performed the experiments on zebrafish embryos helped by Céline Burcklé at the early stages of the project. Hanen Khabou performed all experiments on the macaque spinal cord. Fanny Joubert and Laurence Bodineau performed experiments on the mouse spinal cord. Feng B. Quan performed FISH experiments on the zebrafish adult spinal cord. Sophie Nunes Figueiredo performed GABA immunohistochemistry on zebrafish embryos. Filippo Del Bene designed the pkd2l1 in situ probe. Lydia Djenoune analyzed the data under supervision of Claire Wyart and Hervé Tostivint. Lydia Djenoune and Claire Wyart wrote the manuscript. All authors discussed the results and implications and commented on the manuscript.
The authors declare that the research was conducted in the absence of any commercial or financial relationships that could be construed as a potential conflict of interest.
We thank Prof. Bruce Appel, University of Colorado in Denver, USA for sharing the Tg(nkx2.2a:mEGFP) and Tg(olig2:EGFP) fish transgenic lines and Prof. Yuchio Yanagawa, Gunma University Graduate School of Medicine in Maebashi, Japan for sharing the GAD67-GFP knock-in mice used in this study. We thank Dr. Uwe Strähle, Karlsruhe Institute of Technology, Germany for sharing the gad67 plasmid. We thank Jean Simonnet, Dr. Desdemona Fricker, and Dr. Richard Miles for help with the GAD67-GFP knock-in mouse. We thank Dr. Vanessa Ribes for helpful advices regarding experiments on the embryonic mouse spinal cord. We thank Dr. Carlos Parras, Sowmya Sekizar, Dr. Mariana Graciarena, Melissa Fauveau, and Dr. Brahim Nait-Oumesmar who gave valuable insights for IHC in the mouse embryonic spinal cord. We thank Dr. Pierre Pouget and Prof. Marie Vidailhet for providing access to the tissue of adult macaque spinal cord and Virgile Brochard and Dominique Tandé for precious advices. We thank Prof. Herwig Baier, Max Planck Institute of Neurobiology in Martinsried, Germany, the team of the “Plateforme d'Imagerie Cellulaire Pitié Salpêtrière” and Prof. Thomas Similowski for their support. We thank Urs Lucas Böhm, Kevin Fidelin, Jenna Sternberg, Dr. Pierre-Luc Bardet, Dr. Andrew E. Prendergast, Dr. Jean Paul Rio, and Dr. Kristen Severi for critical reading of the manuscript. This work received financial support from the Institut du Cerveau et de la Moelle épinière (ICM with the French program “Investissements d'avenir” ANR-10-IAIHU-06), the network Ecole des Neurosciences de Paris (ENP), the Fondation Bettencourt Schueller (FBS), Mr Pierre Belle, the City of Paris Emergence program, the Atip/Avenir junior program from Institut National de la Santé et de la Recherche Médicale and Centre National de la Recherche Scientifique, the Fyssen foundation, the International Reintegration Grant from Marie Curie Actions Framework Program 6, and the European Research Council (ERC) starter grant “OptoLoco.”
cc, Central canal; CSF, Cerebrospinal fluid; CSF-cNs, Cerebrospinal fluid-contacting neurons; dpf, days post fertilization; FISH, Fluorescent in situ hybridization; GAD, Glutamic Acid Decarboxylase; GABA, γ-aminobutyric acid; GFP, Green Fluorescent Protein; GFP+, GFP positive; hpf, hours post fertilization; IHC, Immunohistochemistry; KAs, Kolmer–Agduhr cells; MAP2, microtubule-associated protein 2; PB, Phosphate Buffer; PBS, Phosphate Buffered Saline; PFA, paraformaldehyde; pkd2l1, mRNA form of polycystic kidney disease 2-like 1 in zebrafish; pkd2l1+, pkd2l1 positive; PKD2L1, polycystic kidney disease 2-like 1 protein in mouse and macaque; PKD2L1+, PKD2L1 positive; TRP, Transient Receptor Potential; VGAT, vesicular GABA transporter.
Acerbo, M. J., Hellmann, B., and Gunturkun, O. (2003). Catecholaminergic and dopamine-containing neurons in the spinal cord of pigeons: an immunohistochemical study. J. Chem. Neuroanat. 25, 19–27. doi: 10.1016/S0891-0618(02)00072-8
Agduhr, E. (1922). Über ein Zentrales Sinnesorgan (?) bei den Vertebraten. Z. Anat. Entwicklungs. 66, 223–360. doi: 10.1007/BF02593586
Alunni, A., Krecsmarik, M., Bosco, A., Galant, S., Pan, L., Moens, C. B., et al. (2013). Notch3 signaling gates cell cycle entry and limits neural stem cell amplification in the adult pallium. Development 140, 3335–3347. doi: 10.1242/dev.095018
Barber, R. P., Vaughn, J. E., and Roberts, E. (1982). The cytoarchitecture of GABAergic neurons in rat spinal cord. Brain Res. 238, 305–328. doi: 10.1016/0006-8993(82)90107-X
Basora, N., Nomura, H., Berger, U. V., Stayner, C., Guo, L., Shen, X., et al. (2002). Tissue and cellular localization of a novel polycystic kidney disease-like gene product, polycystin-L. J. Am. Soc. Nephrol. 13, 293–301.
Bernhardt, R. R., Chitnis, A. B., Lindamer, L., and Kuwada, J. Y. (1990). Identification of spinal neurons in the embryonic and larval zebrafish. J. Comp. Neurol. 302, 603–616. doi: 10.1002/cne.903020315
Bernhardt, R. R., Patel, C. K., Wilson, S. W., and Kuwada, J. Y. (1992). Axonal trajectories and distribution of GABAergic spinal neurons in wildtype and mutant zebrafish lacking floor plate cells. J. Comp. Neurol. 326, 263–272. doi: 10.1002/cne.903260208
Binor, E., and Heathcote, R. D. (2001). Development of GABA-immunoreactive neuron patterning in the spinal cord. J. Comp. Neurol. 438, 1–11. doi: 10.1002/cne.1298
Briscoe, J., Sussel, L., Serup, P., Hartigan-O'Connor, D., Jessell, T. M., Rubenstein, J. L., et al. (1999). Homeobox gene Nkx2.2 and specification of neuronal identity by graded Sonic hedgehog signalling. Nature 398, 622–627. doi: 10.1038/19315
Brodin, L., Dale, N., Christenson, J., Storm-Mathisen, J., Hokfelt, T., and Grillner, S. (1990). Three types of GABA-immunoreactive cells in the lamprey spinal cord. Brain Res. 508, 172–175. doi: 10.1016/0006-8993(90)91134-3
Buchanan, J. T., Brodin, L., Hokfelt, T., Van Dongen, P. A., and Grillner, S. (1987). Survey of neuropeptide-like immunoreactivity in the lamprey spinal cord. Brain Res. 408, 299–302. doi: 10.1016/0006-8993(87)90392-1
Chang, R. B., Waters, H., and Liman, E. R. (2010). A proton current drives action potentials in genetically identified sour taste cells. Proc. Natl. Acad. Sci. U.S.A. 107, 22320–22325. doi: 10.1073/pnas.1013664107
Christenson, J., Alford, S., Grillner, S., and Hokfelt, T. (1991a). Co-localized GABA and somatostatin use different ionic mechanisms to hyperpolarize target neurons in the lamprey spinal cord. Neurosci. Lett. 134, 93–97. doi: 10.1016/0304-3940(91)90516-V
Christenson, J., Bongianni, F., Grillner, S., and Hokfelt, T. (1991b). Putative GABAergic input to axons of spinal interneurons and primary sensory neurons in the lamprey spinal cord as shown by intracellular Lucifer yellow and GABA immunohistochemistry. Brain Res. 538, 313–318. doi: 10.1016/0006-8993(91)90446-3
Dale, N., Roberts, A., Ottersen, O. P., and Storm-Mathisen, J. (1987a). The development of a population of spinal cord neurons and their axonal projections revealed by GABA immunocytochemistry in frog embryos. Proc. R. Soc. Lond. B Biol. Sci. 232, 205–215. doi: 10.1098/rspb.1987.0069
Dale, N., Roberts, A., Ottersen, O. P., and Storm-Mathisen, J. (1987b). The morphology and distribution of ‘Kolmer-Agduhr cells,’ a class of cerebrospinal-fluid-contacting neurons revealed in the frog embryo spinal cord by GABA immunocytochemistry. Proc. R. Soc. Lond. B Biol. Sci. 232, 193–203. doi: 10.1098/rspb.1987.0068
Decaen, P. G., Delling, M., Vien, T. N., and Clapham, D. E. (2013). Direct recording and molecular identification of the calcium channel of primary cilia. Nature 504, 315–318. doi: 10.1038/nature12832
Delling, M., Decaen, P. G., Doerner, J. F., Febvay, S., and Clapham, D. E. (2013). Primary cilia are specialized calcium signalling organelles. Nature 504, 311–314. doi: 10.1038/nature12833
Delmas, P. (2004). Polycystins: from mechanosensation to gene regulation. Cell 118, 145–148. doi: 10.1016/j.cell.2004.07.007
Delmas, P., Padilla, F., Osorio, N., Coste, B., Raoux, M., and Crest, M. (2004). Polycystins, calcium signaling, and human diseases. Biochem. Biophys. Res. Commun. 322, 1374–1383. doi: 10.1016/j.bbrc.2004.08.044
England, S., Batista, M. F., Mich, J. K., Chen, J. K., and Lewis, K. E. (2011). Roles of Hedgehog pathway components and retinoic acid signalling in specifying zebrafish ventral spinal cord neurons. Development 138, 5121–5134. doi: 10.1242/dev.066159
Ericson, J., Rashbass, P., Schedl, A., Brenner-Morton, S., Kawakami, A., Van Heyningen, V., et al. (1997). Pax6 controls progenitor cell identity and neuronal fate in response to graded Shh signaling. Cell 90, 169–180. doi: 10.1016/S0092-8674(00)80323-2
Gonzalez, A., and Smeets, W. J. (1991). Comparative analysis of dopamine and tyrosine hydroxylase immunoreactivities in the brain of two amphibians, the anuran Rana ridibunda and the urodele Pleurodeles waltlii. J. Comp. Neurol. 303, 457–477. doi: 10.1002/cne.903030311
Gonzalez, A., and Smeets, W. J. (1993). Noradrenaline in the brain of the South African clawed frog Xenopus laevis: a study with antibodies against noradrenaline and dopamine-beta-hydroxylase. J. Comp. Neurol. 331, 363–374. doi: 10.1002/cne.903310306
Gonzalez, A., Tuinhof, R., and Smeets, W. J. (1993). Distribution of tyrosine hydroxylase and dopamine immunoreactivities in the brain of the South African clawed frog Xenopus laevis. Anat. Embryol. 187, 193–201. doi: 10.1007/BF00171750
Goulding, M. (2009). Circuits controlling vertebrate locomotion: moving in a new direction. Nat. Rev. Neurosci. 10, 507–518. doi: 10.1038/nrn2608
Higashijima, S., Mandel, G., and Fetcho, J. R. (2004a). Distribution of prospective glutamatergic, glycinergic, and GABAergic neurons in embryonic and larval zebrafish. J. Comp. Neurol. 480, 1–18. doi: 10.1002/cne.20278
Higashijima, S., Schaefer, M., and Fetcho, J. R. (2004b). Neurotransmitter properties of spinal interneurons in embryonic and larval zebrafish. J. Comp. Neurol. 480, 19–37. doi: 10.1002/cne.20279
Horio, N., Yoshida, R., Yasumatsu, K., Yanagawa, Y., Ishimaru, Y., Matsunami, H., et al. (2011). Sour taste responses in mice lacking PKD channels. PLoS ONE 6:e20007. doi: 10.1371/journal.pone.0020007
Huang, A. L., Chen, X., Hoon, M. A., Chandrashekar, J., Guo, W., Trankner, D., et al. (2006). The cells and logic for mammalian sour taste detection. Nature 442, 934–938. doi: 10.1038/nature05084
Huang, P., Xiong, F., Megason, S. G., and Schier, A. F. (2012). Attenuation of Notch and Hedgehog signaling is required for fate specification in the spinal cord. PLoS Genet. 8:e1002762. doi: 10.1371/journal.pgen.1002762
Inada, H., Kawabata, F., Ishimaru, Y., Fushiki, T., Matsunami, H., and Tominaga, M. (2008). Off-response property of an acid-activated cation channel complex PKD1L3-PKD2L1. EMBO Rep. 9, 690–697. doi: 10.1038/embor.2008.89
Ishii, S., Misaka, T., Kishi, M., Kaga, T., Ishimaru, Y., and Abe, K. (2009). Acetic acid activates PKD1L3-PKD2L1 channel–a candidate sour taste receptor. Biochem. Biophys. Res. Commun. 385, 346–350. doi: 10.1016/j.bbrc.2009.05.069
Ishimaru, Y., Inada, H., Kubota, M., Zhuang, H., Tominaga, M., and Matsunami, H. (2006). Transient receptor potential family members PKD1L3 and PKD2L1 form a candidate sour taste receptor. Proc. Natl. Acad. Sci. U.S.A. 103, 12569–12574. doi: 10.1073/pnas.0602702103
Jalalvand, E., Robertson, B., Wallen, P., Hill, R. H., and Grillner, S. (2014). Laterally projecting cerebrospinal fluid-contacting cells in the lamprey spinal cord are of two distinct types. J. Comp. Neurol. 522, 1753–1768. doi: 10.1002/cne.23542
Jessell, T. M. (2000). Neuronal specification in the spinal cord: inductive signals and transcriptional codes. Nat. Rev. Genet. 1, 20–29. doi: 10.1038/35049541
Kimmel, C. B., Ballard, W. W., Kimmel, S. R., Ullmann, B., and Schilling, T. F. (1995). Stages of embryonic development of the zebrafish. Dev. Dyn. 203, 253–310. doi: 10.1002/aja.1002030302
Kirby, B. B., Takada, N., Latimer, A. J., Shin, J., Carney, T. J., Kelsh, R. N., et al. (2006). In vivo time-lapse imaging shows dynamic oligodendrocyte progenitor behavior during zebrafish development. Nat. Neurosci. 9, 1506–1511. doi: 10.1038/nn1803
Kolmer, W. (1921). Das “Sagittalorgan” der Wirbeltiere. Z. Anat. Entwicklungs. 60, 652–717. doi: 10.1007/BF02593657
Kolmer, W. (1931). Über das Sagittalorgan, ein Zentrales Sinnesorgan der Wirbeltiere, Insbesondere Beim Affen. Z. Zellforsch. Mik. Ana. 13, 236–248. doi: 10.1007/BF00406356
Kutna, V., Sevc, J., Gombalova, Z., Matiasova, A., and Daxnerova, Z. (2013). Enigmatic cerebrospinal fluid-contacting neurons arise even after the termination of neurogenesis in the rat spinal cord during embryonic development and retain their immature-like characteristics until adulthood. Acta Histochem. 116, 278–285. doi: 10.1016/j.acthis.2013.08.004
Lamotte, C. C., and Shapiro, C. M. (1991). Ultrastructural localization of substance P, met-enkephalin, and somatostatin immunoreactivity in lamina X of the primate spinal cord. J. Comp. Neurol. 306, 290–306. doi: 10.1002/cne.903060206
Lopez, J. M., Moreno, N., Morona, R., Munoz, M., Dominguez, L., and Gonzalez, A. (2007). Distribution of somatostatin-like immunoreactivity in the brain of the caecilian Dermophis mexicanus (Amphibia: Gymnophiona): comparative aspects in amphibians. J. Comp. Neurol. 501, 413–430. doi: 10.1002/cne.21244
Lu, X., Geng, X., Zhang, L., and Zeng, Y. (2008). The methodology for labeling the distal cerebrospinal fluid-contacting neurons in rats. J. Neurosci. Methods 168, 98–103. doi: 10.1016/j.jneumeth.2007.09.033
Martin, S. C., Heinrich, G., and Sandell, J. H. (1998). Sequence and expression of glutamic acid decarboxylase isoforms in the developing zebrafish. J. Comp. Neurol. 396, 253–266. doi: 10.1002/(SICI)1096-9861(19980629)396:2<253::AID-CNE9>3.0.CO;2-#
Masahira, N., Takebayashi, H., Ono, K., Watanabe, K., Ding, L., Furusho, M., et al. (2006). Olig2-positive progenitors in the embryonic spinal cord give rise not only to motoneurons and oligodendrocytes, but also to a subset of astrocytes and ependymal cells. Dev. Biol. 293, 358–369. doi: 10.1016/j.ydbio.2006.02.029
McLean, D. L., and Fetcho, J. R. (2004). Ontogeny and innervation patterns of dopaminergic, noradrenergic, and serotonergic neurons in larval zebrafish. J. Comp. Neurol. 480, 38–56. doi: 10.1002/cne.20280
Melendez-Ferro, M., Perez-Costas, E., Villar-Cheda, B., Rodriguez-Munoz, R., Anadon, R., and Rodicio, M. C. (2003). Ontogeny of gamma-aminobutyric acid-immunoreactive neurons in the rhombencephalon and spinal cord of the sea lamprey. J. Comp. Neurol. 464, 17–35. doi: 10.1002/cne.10773
Nagatsu, I., Sakai, M., Yoshida, M., and Nagatsu, T. (1988). Aromatic L-amino acid decarboxylase-immunoreactive neurons in and around the cerebrospinal fluid-contacting neurons of the central canal do not contain dopamine or serotonin in the mouse and rat spinal cord. Brain Res. 475, 91–102. doi: 10.1016/0006-8993(88)90202-8
Ng, A. N., De Jong-Curtain, T. A., Mawdsley, D. J., White, S. J., Shin, J., Appel, B., et al. (2005). Formation of the digestive system in zebrafish: III. Intestinal epithelium morphogenesis. Dev. Biol. 286, 114–135. doi: 10.1016/j.ydbio.2005.07.013
Novitch, B. G., Chen, A. I., and Jessell, T. M. (2001). Coordinate regulation of motor neuron subtype identity and pan-neuronal properties by the bHLH repressor Olig2. Neuron 31, 773–789. doi: 10.1016/S0896-6273(01)00407-X
Orts-Del'Immagine, A., Kastner, A., Tillement, V., Tardivel, C., Trouslard, J., and Wanaverbecq, N. (2014). Morphology, distribution and phenotype of polycystin kidney disease 2-like 1-positive cerebrospinal fluid contacting neurons in the brainstem of adult mice. PLoS ONE 9:e87748. doi: 10.1371/journal.pone.0087748
Orts-Del'Immagine, A., Wanaverbecq, N., Tardivel, C., Tillement, V., Dallaporta, M., and Trouslard, J. (2012). Properties of subependymal cerebrospinal fluid contacting neurones in the dorsal vagal complex of the mouse brainstem. J. Physiol. 590, 3719–3741. doi: 10.1113/jphysiol.2012.227959
Park, H. C., Shin, J., and Appel, B. (2004). Spatial and temporal regulation of ventral spinal cord precursor specification by Hedgehog signaling. Development 131, 5959–5969. doi: 10.1242/dev.01456
Parmentier, C., Hameury, E., Dubessy, C., Quan, F. B., Habert, D., Calas, A., et al. (2011). Occurrence of two distinct urotensin II-related peptides in zebrafish provides new insight into the evolutionary history of the urotensin II gene family. Endocrinology 152, 2330–2341. doi: 10.1210/en.2010-1500
Reali, C., Fernandez, A., Radmilovich, M., Trujillo-Cenoz, O., and Russo, R. E. (2011). GABAergic signalling in a neurogenic niche of the turtle spinal cord. J. Physiol. 589, 5633–5647. doi: 10.1113/jphysiol.2011.214312
Roberts, B. L., Maslam, S., Scholten, G., and Smit, W. (1995). Dopaminergic and GABAergic cerebrospinal fluid-contacting neurons along the central canal of the spinal cord of the eel and trout. J. Comp. Neurol. 354, 423–437. doi: 10.1002/cne.903540310
Robertson, B., Auclair, F., Menard, A., Grillner, S., and Dubuc, R. (2007). GABA distribution in lamprey is phylogenetically conserved. J. Comp. Neurol. 503, 47–63. doi: 10.1002/cne.21348
Rodicio, M. C., Villar-Cervino, V., Barreiro-Iglesias, A., and Anadon, R. (2008). Colocalization of dopamine and GABA in spinal cord neurones in the sea lamprey. Brain Res. Bull. 76, 45–49. doi: 10.1016/j.brainresbull.2007.10.062
Schafer, M., Kinzel, D., and Winkler, C. (2007). Discontinuous organization and specification of the lateral floor plate in zebrafish. Dev. Biol. 301, 117–129. doi: 10.1016/j.ydbio.2006.09.018
Schindelin, J., Arganda-Carreras, I., Frise, E., Kaynig, V., Longair, M., Pietzsch, T., et al. (2012). Fiji: an open-source platform for biological-image analysis. Nat. Methods 9, 676–682. doi: 10.1038/nmeth.2019
Schotland, J. L., Shupliakov, O., Grillner, S., and Brodin, L. (1996). Synaptic and nonsynaptic monoaminergic neuron systems in the lamprey spinal cord. J. Comp. Neurol. 372, 229–244. doi: 10.1002/(SICI)1096-9861(19960819)372:2<229::AID-CNE6>3.0.CO;2-5
Shimizu, T., Higuchi, T., Fujii, T., Nilius, B., and Sakai, H. (2011). Bimodal effect of alkalization on the polycystin transient receptor potential channel, PKD2L1. Pflugers Arch. 461, 507–513. doi: 10.1007/s00424-011-0934-5
Shimizu, T., Janssens, A., Voets, T., and Nilius, B. (2009). Regulation of the murine TRPP3 channel by voltage, pH, and changes in cell volume. Pflugers Arch. 457, 795–807. doi: 10.1007/s00424-008-0558-6
Shin, J., Park, H. C., Topczewska, J. M., Mawdsley, D. J., and Appel, B. (2003). Neural cell fate analysis in zebrafish using olig2 BAC transgenics. Methods Cell Sci. 25, 7–14. doi: 10.1023/B:MICS.0000006847.09037.3a
Shin, J., Poling, J., Park, H. C., and Appel, B. (2007). Notch signaling regulates neural precursor allocation and binary neuronal fate decisions in zebrafish. Development 134, 191–120. doi: 10.1242/dev.001602
Srinivas, S., Watanabe, T., Lin, C. S., William, C. M., Tanabe, Y., Jessell, T. M., et al. (2001). Cre reporter strains produced by targeted insertion of EYFP and ECFP into the ROSA26 locus. BMC Dev. Biol. 1:4. doi: 10.1186/1471-213X-1-4
Stoeckel, M. E., Uhl-Bronner, S., Hugel, S., Veinante, P., Klein, M. J., Mutterer, J., et al. (2003). Cerebrospinal fluid-contacting neurons in the rat spinal cord, a gamma-aminobutyric acidergic system expressing the P2X2 subunit of purinergic receptors, PSA-NCAM, and GAP-43 immunoreactivities: light and electron microscopic study. J. Comp. Neurol. 457, 159–174. doi: 10.1002/cne.10565
Sueiro, C., Carrera, I., Molist, P., Rodriguez-Moldes, I., and Anadon, R. (2004). Distribution and development of glutamic acid decarboxylase immunoreactivity in the spinal cord of the dogfish Scyliorhinus canicula (elasmobranchs). J. Comp. Neurol. 478, 189–206. doi: 10.1002/cne.20285
Tamamaki, N., Yanagawa, Y., Tomioka, R., Miyazaki, J., Obata, K., and Kaneko, T. (2003). Green fluorescent protein expression and colocalization with calretinin, parvalbumin, and somatostatin in the GAD67-GFP knock-in mouse. J. Comp. Neurol. 467, 60–79. doi: 10.1002/cne.10905
Vigh, B., and Vigh-Teichmann, I. (1973). Comparative ultrastructure of the cerebrospinal fluid-contacting neurons. Int. Rev. Cytol. 35, 189–251. doi: 10.1016/S0074-7696(08)60355-1
Villar-Cervino, V., Holstein, G. R., Martinelli, G. P., Anadon, R., and Rodicio, M. C. (2008). Glycine-immunoreactive neurons in the developing spinal cord of the sea lamprey: comparison with the gamma-aminobutyric acidergic system. J. Comp. Neurol. 508, 112–130. doi: 10.1002/cne.21661
Voituron, N., Frugiere, A., Mc Kay, L. C., Romero-Granados, R., Dominguez-Del-Toro, E., Saadani-Makki, F., et al. (2011). The kreisler mutation leads to the loss of intrinsically hypoxia-activated spots in the region of the retrotrapezoid nucleus/parafacial respiratory group. Neuroscience 194, 95–111. doi: 10.1016/j.neuroscience.2011.07.062
Warp, E., Agarwal, G., Wyart, C., Friedmann, D., Oldfield, C. S., Conner, A., et al. (2012). Emergence of patterned activity in the developing zebrafish spinal cord. Curr. Biol. 22, 93–102. doi: 10.1016/j.cub.2011.12.002
Westerfield, M. (2000). The Zebrafish Book. A Guide for the Laboratory Use of Zebrafish (Danio rerio). Eugene, OR: University of Oregon Press.
Wyart, C., Del Bene, F., Warp, E., Scott, E. K., Trauner, D., Baier, H., et al. (2009). Optogenetic dissection of a behavioural module in the vertebrate spinal cord. Nature 461, 407–410. doi: 10.1038/nature08323
Yang, L., Rastegar, S., and Strahle, U. (2010). Regulatory interactions specifying Kolmer-Agduhr interneurons. Development 137, 2713–2722. doi: 10.1242/dev.048470
Yeo, S. Y., and Chitnis, A. B. (2007). Jagged-mediated Notch signaling maintains proliferating neural progenitors and regulates cell diversity in the ventral spinal cord. Proc. Natl. Acad. Sci. U.S.A. 104, 5913–5918. doi: 10.1073/pnas.0607062104
Yulis, C. R., and Lederis, K. (1988a). Occurrence of an anterior spinal, cerebrospinal fluid-contacting, urotensin II neuronal system in various fish species. Gen. Comp. Endocrinol. 70, 301–311. doi: 10.1016/0016-6480(88)90150-5
Keywords: cerebrospinal fluid-contacting neurons (CSF-cNs), PKD2L1, mouse, macaque, zebrafish, GABAergic neurons, spinal cord
Citation: Djenoune L, Khabou H, Joubert F, Quan FB, Nunes Figueiredo S, Bodineau L, Del Bene F, Burcklé C, Tostivint H and Wyart C (2014) Investigation of spinal cerebrospinal fluid-contacting neurons expressing PKD2L1: evidence for a conserved system from fish to primates. Front. Neuroanat. 8:26. doi: 10.3389/fnana.2014.00026
Received: 05 January 2014; Accepted: 10 April 2014;
Published online: 06 May 2014.
Edited by:
Laurent Gautron, The University of Texas Southwestern Medical Center, USAReviewed by:
Marina Bentivoglio, Université di Verona, ItalyCopyright © 2014 Djenoune, Khabou, Joubert, Quan, Nunes Figueiredo, Bodineau, Del Bene, Burcklé, Tostivint and Wyart. This is an open-access article distributed under the terms of the Creative Commons Attribution License (CC BY). The use, distribution or reproduction in other forums is permitted, provided the original author(s) or licensor are credited and that the original publication in this journal is cited, in accordance with accepted academic practice. No use, distribution or reproduction is permitted which does not comply with these terms.
*Correspondence: Claire Wyart, Institut du Cerveau et de la Moelle épinière, Hôpital de la Pitié-Salpêtrière, 83, bld de l'hôpital, 75013 Paris, France e-mail:Y2xhaXJlLnd5YXJ0QGluc2VybS5mcg==
†Present address: Céline Burcklé, Institut de Biologie du Développement de Marseille, Marseille, France
‡These authors have contributed equally to this work.
Disclaimer: All claims expressed in this article are solely those of the authors and do not necessarily represent those of their affiliated organizations, or those of the publisher, the editors and the reviewers. Any product that may be evaluated in this article or claim that may be made by its manufacturer is not guaranteed or endorsed by the publisher.
Research integrity at Frontiers
Learn more about the work of our research integrity team to safeguard the quality of each article we publish.