- Fishberg Department of Neuroscience, Friedman Brain Institute, Mount Sinai School of Medicine, New York, NY, USA
The striatum plays a key role in mediating the acute and chronic effects of addictive drugs, with drugs of abuse causing long-lasting molecular and cellular alterations in both dorsal striatum and nucleus accumbens (ventral striatum). Despite the wealth of research on the biological actions of abused drugs in striatum, until recently, the distinct roles of the striatum’s two major subtypes of medium spiny neurons (MSNs) in drug addiction remained elusive. Recent advances in cell-type-specific technologies, including fluorescent reporter mice, transgenic, or knockout mice, and viral-mediated gene transfer, have advanced the field toward a more comprehensive understanding of the two MSN subtypes in the long-term actions of drugs of abuse. Here we review progress in defining the distinct molecular and functional contributions of the two MSN subtypes in mediating addiction.
Introduction
Drugs of abuse exert potent molecular and cellular alterations in both dorsal striatum (dStr) and ventral striatum (nucleus accumbens, NAc), and many of these changes occur in medium spiny neurons (MSNs), the principal projection neurons in dStr and NAc, which account for 90–95% of all neurons in these regions. However, researchers have until recently been unable to clearly define the differential role of the two MSN subtypes in addiction-related phenomena. The two MSN subtypes are differentiated by their enrichment of dopamine receptor 1 (D1) or dopamine receptor 2 (D2) as well as several other genes (Gerfen and Young, 1988; Gerfen et al., 1990; Le Moine et al., 1990, 1991; Bernard et al., 1992; Ince et al., 1997; Lobo et al., 2006, 2007; Heiman et al., 2008; gensat.org) and by their distinct projections through the cortico-basal ganglia pathway (the direct vs. indirect pathways; Gerfen, 1984, 1992). Early work suggested that drugs of abuse exert most influence on the D1+ MSNs, with the use of numerous dopamine receptor agonists and antagonists providing important insight into the functional and molecular roles of each MSN in drug reward behaviors (Self, 2010). However, current cell-type-specific methodologies, including fluorescent reporter mice that express GFP under D1 or D2 bacterial artificial chromosomes (BACs; Gong et al., 2003; Valjent et al., 2009; gensat.org), conditional mouse models such as the use of tetracycline-regulated inducible transgenic mice (Chen et al., 1998; Kelz et al., 1999), and transgenic mice expressing Cre-recombinase using D1 or D2 BACs, yeast artificial chromosomes (YACs), or knock-in mice (Gong et al., 2007; Lemberger et al., 2007; Heusner et al., 2008; Parkitna et al., 2009; Valjent et al., 2009; Bateup et al., 2010; Lobo et al., 2010; gensat.org) as well as cell-type-specific viral-mediated gene transfer (Cardin et al., 2010; Hikida et al., 2010; Lobo et al., 2010; Ferguson et al., 2011), have provided profound new insight into the precise molecular underpinnings of each MSN subtype and their regulation by drugs of abuse (Table 1).
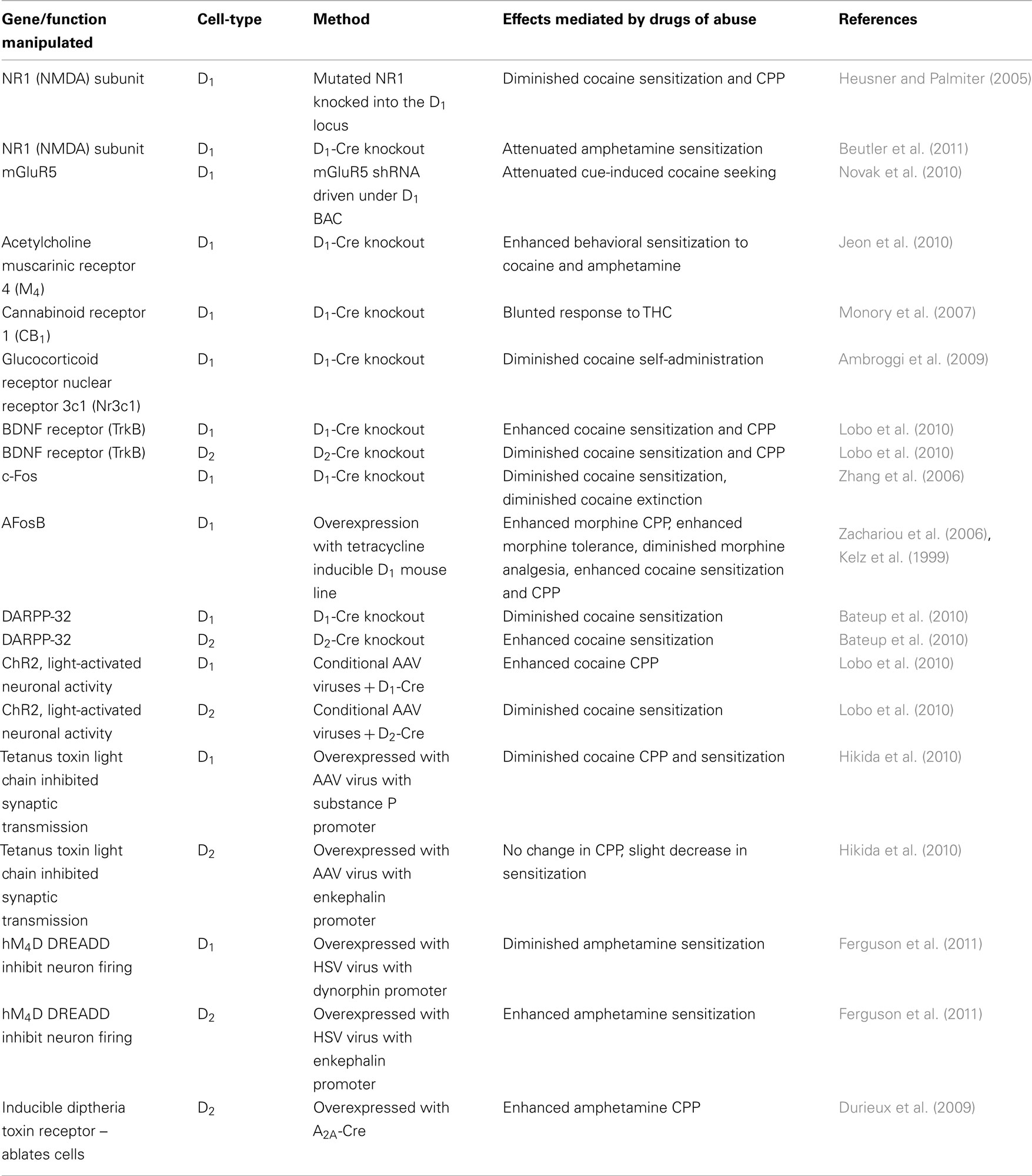
Table 1. Effects of cell-type-specific genetic manipulation in D1+ and D2+ MSNs in drug addiction models.
Recent findings support the conclusion of a more predominate role for D1+ MSNs in producing the reinforcing and sensitizing effect of drugs of abuse, with most robust molecular changes occurring in these MSNs. For instance, acute exposure to psychostimulants potently induces numerous signaling molecules including FosB, ERK, c-Fos, and Zif268 in the D1+ MSNs, while repeated cocaine preferentially induces ΔFosB and alters GABA receptor and other ion channel subunits in this cell-type as well (Robertson et al., 1991; Young et al., 1991; Berretta et al., 1992; Cenci et al., 1992; Moratalla et al., 1992; Hope et al., 1994; Bertran-Gonzalez et al., 2008; Heiman et al., 2008). Furthermore, disrupting or over-expressing specific molecules, such as ΔFosB, DARPP-32, or Nr3c1 (the glucocorticoid receptor), in D1+ MSNs typically mimics the drug-related behaviors observed when these alterations are made in a non-cell-type-specific manner, while disrupting such genes in D2+ MSNs often causes an opposite response (Fienberg et al., 1998; Kelz et al., 1999; Deroche-Gamonet et al., 2003; Zachariou et al., 2006; Ambroggi et al., 2009; Bateup et al., 2010). Nonetheless, we cannot rule out an important contribution of the D2+ MSNs in adaptations to drugs of abuse, because cocaine exposure alters gene expression in both MSN subtypes (Heiman et al., 2008) and D2-receptor agonists and antagonists exert potent effects in behavioral assays (Self, 2010). Indeed, recent findings show that molecular signaling adaptations in D2+ MSNs potently modify an animal’s behavioral response to drugs of abuse (Lobo et al., 2010). The latter findings showed that loss of TrkB (the receptor for BDNF) in D2+ MSNs results in similar behavioral responses to cocaine as total TrkB knockout from the NAc, showing for the first time a selective dominant role for a molecular pathway in D2+ MSNs in mediating the effects of drugs of abuse.
Finally, recent literature reveals that the two MSNs exert antagonistic effects in drug-related behaviors, where activation of D1+ MSNs or inhibition of D2+ MSNs enhances an animal’s sensitivity to a drug of abuse (Hikida et al., 2010; Lobo et al., 2010; Ferguson et al., 2011). These findings are consistent with opposing roles of the two MSNs and their direct vs. indirect pathways in the basal ganglia in motor behaviors (Alexander et al., 1986; Albin et al., 1989; Graybiel, 2000; Kravitz et al., 2010). This recent literature is in accord with the general idea that dopaminergic neurotransmission, which is activated by all drugs of abuse, facilitates glutamatergic activation of D1+ MSNs while inhibiting glutamatergic activation of D2+ MSNs through its actions on D1 vs. D2 dopamine receptors (Figure 1). In this review, we address the current knowledge of the distinct molecular signaling exhibited by these two MSN subtypes in relation to their functional roles and responses to drugs of abuse.
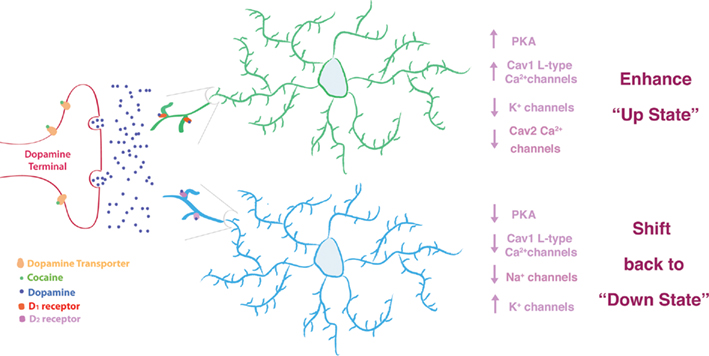
Figure 1. All drugs of abuse increase dopamine signaling in striatum, which can differentially modulate glutamatergic activity in the two MSN subtypes. In particular, cocaine binds to the dopamine transporter preventing dopamine reuptake into the terminals of VTA dopamine neurons. Activation of Gs/olf coupled D1 receptors enhances PKA activity and alters Ca2+ and K+ conductances to enhance the glutamate mediated "up-state" in these MSNs. In contrast, activation of Gi/Go D2-receptors diminishes PKA activity and alters Ca2+, Na+, and K+ conductances to diminish the glutamate mediated “up-state.” This shifts these MSNs back to their resting “down-state.”
Dopamine Receptor Signaling in D1 vs. D2 MSNs
As already noted, all drugs of abuse activate dopaminergic input to the NAc and related limbic brain regions (Volkow et al., 2004; Wise, 2004; Nestler, 2005). For instance, psychostimulants such as cocaine or amphetamine act directly on the dopaminergic reward pathway by interfering with the dopamine transporter: cocaine blocks the transporter and amphetamine reverses the transporter, both actions resulting in a build up of dopamine in the synapse which can activate downstream dopamine receptors on target neurons (Figure 1). The two MSNs are most notably differentiated by their enrichment of D1 vs. D2-receptors although single-cell RT-PCR studies reveal that D1+ MSNs express low levels of the D2-like receptor, D3 and D2+ MSNs express low levels of the D1-like receptor, D5 (Surmeier et al., 1996). The two MSNs require glutamatergic innervation to drive neural activity; dopamine oppositely modulates these functional responses via stimulation of distinct dopamine receptor subtypes: by positively modulating excitatory glutamatergic input through D1 receptor signaling via Gs or Golf, which stimulates adenylyl cyclase leading to increased PKA activity, whereas dopamine negatively modulates this input through D2-receptor signaling via Gi and Go which inhibit adenylyl cyclase causing decreased PKA activity (Surmeier et al., 2007; Gerfen and Surmeier, 2011). In reality, each receptor exerts complex effects on many additional downstream signaling pathways. At rest, the two MSN subtypes are generally inhibited, they are in what researchers have termed the down-state. Excitatory glutamatergic synaptic activity can release the MSNs from this down-state and shift them into a more depolarized state (the up-state). Dopamine oppositely modulates the excitatory glutamatergic shift to the up-state. D1 activation of PKA enhances Cav1 L-type Ca2+ channel activity, decreases somatic K+ channel activity, and downregulates Cav2 Ca2+ channels that control activation of Ca2+ dependent, small-conductance K+ (SK) channels, resulting in increased spiking in these MSNs (Surmeier et al., 2007; Gerfen and Surmeier, 2011). In contrast, D2 signaling inhibits the up-state transition, thereby preventing increased spiking, via reduction of Cav1 L-type Ca2+ channel activity and Nav1 Na+ channel activity while increasing K+ channel currents (Surmeier et al., 2007; Gerfen and Surmeier, 2011; Figure 1). Such opposite alterations in the two MSNs suggest that increased dopamine signaling elicited by drugs of abuse should enhance glutamatergic activation of D1+ MSNs and reduce glutamatergic activation of D2+ MSNs. In reality, such responses are far more varied and complex for reasons that remain poorly understood. This topic will be addressed further below.
The role of dopamine receptors in drug abuse is complex and often elusive (Self, 2010). There is an abundance of literature on the role of D1 and D2-receptor agonists and antagonists in modulating rewarding properties and self-administration of drugs of abuse, however, the results differ depending on the type of agonist/antagonist used, the type of delivery (systemic vs. brain region-specific), and the timing of the treatment (Self, 2010). Such results are further confounded by non-striatal specific effects, such as the contribution of pre-synaptic D2-receptors from the VTA or presence of D1 receptors in many other limbic regions, and the lack of specificity of the agonists/antagonists utilized as well as the expression of D1-like and D2-like receptors in both MSN subtypes as noted earlier. In general, it is thought that D1 receptors play a more predominant role in the primary rewarding properties of drugs of abuse, whereas D2-receptors play a role in drug seeking mechanisms (Self et al., 1996; Self, 2010). Studies with D1 receptor and D2-receptor knockout mice provide some insight into the role of these receptors in the two MSNs. D1 knockout mice show a blunted induction of immediate early genes (IEGs) c-Fos and Zif268 in response to cocaine, a diminished response to psychostimulant-induced locomotor activity but with no alterations in cocaine-conditioned place preference (CPP) – an indirect measure of drug reward, and diminished cocaine self-administration and ethanol consumption (Miner et al., 1995; Drago et al., 1996; Crawford et al., 1997; El-Ghundi et al., 1998; Caine et al., 2007). D2 knockout mice display diminished rewarding effects to opiates and cocaine as well as decreased ethanol consumption but no reduction in cocaine taking (Maldonado et al., 1997; Cunningham et al., 2000; Risinger et al., 2000; Caine et al., 2002; Chausmer et al., 2002; Elmer et al., 2002; Welter et al., 2007). Such data support important roles for D1 and D2-receptors in the two MSNs in multiple aspects of drug abuse, however, the knockouts lack striatal specificity and occur early in development, thus one cannot rule out other brain regions and cell-types and developmental factors in mediating these behaviors. Finally, decreased levels of D2/D3 receptors in striatum, as visualized by brain imaging, has become a common marker of addiction in human patients especially during periods of withdrawal (Volkow et al., 2009). Rodents receiving viral-mediated gene transfer of D2-receptors to the NAc display attenuated cocaine self-administration and ethanol consumption (Thanos et al., 2004, 2008). These studies were not performed in a cell-type-specific manner, so we cannot rule out the possible effect of D2-receptor overexpression influencing D1+ MSNs. This collection of data emphasizes the need to move to more selective approaches, including cell-type-specific, region-specific, and even temporally specific manipulations of the dopamine receptors to better elucidate their functional roles in the two MSN subtypes in drug addiction.
Finally, it has been reported recently that D2-GFP homozygote BAC transgenic mice display increased expression levels of the D2-receptor in striatum and enhanced behavioral sensitivity and dopamine signaling to D2 agonists. Moreover, both homozygotes and hemizygotes exhibit blunted behavioral responses to cocaine (Kramer et al., 2011). This study highlights the need to perform thorough characterization of D1 and D2 fluorescent reporter and Cre driver lines. However, the majority of the data collected in this study used homozygotes, which is not the ideal experimental genotype since 5–10% of transgene integrations result in insertional mutations (Meisler, 1992); therefore, the hemizygote genotype is the more reliable experimental genotype. Additionally, this study did not use littermate wildtype controls but used controls on a similar background (Swiss Webster) obtained from Taconic, while their transgenic lines were obtained from GENSAT and MMRRC. Finally, another group has shown normal cocaine locomotor behavioral responses in D2-GFP hemizygotes (Kim et al., 2011). Thus, future studies using proper controls and proper genotypes must be performed to fully characterize the various cell-type-specific transgenic lines available.
Glutamate and GABA Signaling in D1 vs. D2 MSNs
Medium spiny neurons receive glutamatergic input from multiple brain regions including prefrontal cortex, amygdala, and hippocampus, and GABAergic input from local interneurons and perhaps collateral inputs from other MSNs. Net excitatory and inhibitory regulation of MSNs is no doubt crucial in regulating the drug-addicted state, and there is now a growing literature on the complex ways in which drugs of abuse alter glutamatergic neurotransmission in particular in the NAc (Pierce et al., 1996; Thomas et al., 2001; Beurrier and Malenka, 2002; Kourrich et al., 2007; Bachtell and Self, 2008; Bachtell et al., 2008; Conrad et al., 2008; Kalivas, 2009; Wolf, 2010). Although MSNs are thought to primarily exist in an inhibited down-state under basal conditions with glutamate driving activity of both cell-types, there remains limited information with respect to distinct regulation occurring in D1 vs. D2 MSNs.
ΔFosB overexpression in D1+ MSNs (see below for more details) enhances the rewarding effects of cocaine and increases levels of the Ca2+-impermeable glutamate receptor subunit, GluR2, in NAc. Furthermore, viral-mediated gene transfer of GluR2 to the NAc similarly enhances the rewarding effects of cocaine (Kelz et al., 1999). However, it is not known whether the induction of GluR2 seen in response to ΔFosB overexpression in D1+ MSNs is also specific to these neurons, and the viral overexpression of GluR2 is not cell-type-specific, therefore we cannot infer direct conclusions about GluR2 function in these two MSNs in drug reward. Heusner and Palmiter (2005) assessed the role of NMDA glutamatergic conductance in cocaine behaviors by expressing an NR1 subunit, which contains a mutation in the pore that reduces calcium flux, selectively in D1+ MSNs. This group showed that lack of NMDA conductance in D1+ MSNs prevents cocaine-induced CPP and cocaine locomotor sensitization, highlighting the necessity for NMDA signaling in D1+ MSNs for the rewarding and sensitizing effects of cocaine (Heusner and Palmiter, 2005). Furthermore, recently it was found that knocking out the NR1 subunit in D1+ MSNs attenuates amphetamine sensitization and this phenotype was rescued by resupplying the NR1 subunit to D1+ MSNs specifically in the NAc (Beutler et al., 2011). Finally, knockdown of the mGluR5 subunit, using RNA interference, in D1+ MSNs has no effect on the initial rewarding properties of cocaine but diminishes the cue-induced reinstatement of cocaine seeking (Novak et al., 2010). While these data reveal compelling roles for glutamatergic signaling in D1+ MSNs, future work is needed to study glutamatergic systems in D2+ MSNs. Future research should also evaluate how modulation of these glutamate receptor subunits in the two MSN subtypes affects the structural synaptic changes observed in NAc after drugs of abuse (Dietz et al., 2009; Russo et al., 2010), particularly the dendritic alterations observed after cocaine exposure selectively in the D1+ MSNs (Lee et al., 2006; Kim et al., 2011) which may be associated with the increase in miniature excitatory postsynaptic currents observed in D1+ MSNs (Kim et al., 2011). Interestingly, ΔFosB induction in D1+ MSNs has been related directly to such dendritic adaptations after chronic cocaine (Maze et al., 2010).
In contrast to glutamate, there is a lack of research on GABA function in the two MSNs in addiction models, which is surprising considering both ethanol and benzodiazepines enhance the effects of GABA and the two MSNs receive dense GABAergic inputs as stated above. There is also considerable evidence pointing to enhanced inhibition in the NAc at least after chronic cocaine exposure (White et al., 1995; Peoples et al., 1998; Zhang et al., 1998; Thomas et al., 2001; Beurrier and Malenka, 2002). Heiman et al. (2008) performed high throughput genetic screening in the two MSNs after chronic cocaine exposure and, interestingly, the most altered biological process in the D1+ MSNs was GABA signaling. In particular, there was potent upregulation of GABAA receptor subunits Gabra1 and Gabra4 as well as the GABAB receptor subunit Gabrb3, and this group found that chronic cocaine increases the frequency of small-amplitude GABAergic mini inhibitory postsynaptic currents (mIPSCs) in D1+ MSNs (Heiman et al., 2008). On the other hand, another group recently showed that chronic cocaine results in an opposite response with decreased frequency and amplitude of mIPSCs in the D1+ MSNs (Kim et al., 2011). However, the latter group did show diminished membrane excitability in the D1+ MSNs after chronic cocaine, which could be a reflection of enhanced GABA tone and is consistent with the field’s assessment of enhanced inhibition in the NAc after exposure to chronic cocaine. Furthermore, such differences between the two groups could simply be due to the timing of cocaine exposure and withdrawal. In general, there is a need to study glutamatergic and GABAergic function in the two MSNs in response to drugs of abuse and the field is now equipped with the resources that make such a cell-type- and region-specific study possible.
Other Receptor Signaling in D1 vs. D2 MSN Subtypes
The two MSNs are differentially enriched in other G-protein-coupled receptors in addition to dopamine receptors. D1+ MSNs express higher levels of the acetylcholine muscarinic receptor 4 (M4; Bernard et al., 1992; Ince et al., 1997) and D2+ MSNs are enriched in both adenosine receptor 2A (A2A; Schiffmann et al., 1991; Schiffmann and Vanderhaeghen, 1993) and G-protein-coupled receptor 6 (Gpr6; Lobo et al., 2007; gensat.org). M4 is coupled to Gi/o, which would produce an opposite response, compared to D1 receptors, in D1+ MSNs by inhibiting cAMP/PKA activity. Indeed, a D1+ MSN selective M4 knockout displayed enhanced behavioral sensitization to cocaine and amphetamine (Jeon et al., 2010). Furthermore, recent studies using a designer receptor exclusively activated by a synthetic drug (DREADDs) showed that activation of the DREADD Gi/o-coupled human M4 receptor (hM4D) in D1+ MSNs diminished behavioral sensitization to amphetamine, with the opposite response seen in D2+ MSNs (Ferguson et al., 2011). Such data reveal the antagonizing role of M4 receptors in D1+ MSNs in drug abuse. As well, since the hM4D receptor potently inhibits these MSNs, the data provide insight into the effect of altered activity of these two MSNs in drug abuse, which will be discussed further below.
Both A2A and Gpr6 are positively coupled to Gs/Golf proteins, implicating their role in antagonizing the D2-receptor in D2+ MSNs. Indeed, stimulation of A2A receptors has been shown to reduce both the development and expression of cocaine sensitization (Filip et al., 2006), impair the initiation of cocaine self-administration (Knapp et al., 2001), and antagonize the reinstatement of cocaine seeking elicited by cocaine, D2-receptor stimulation, or cocaine-conditioned cues (Bachtell and Self, 2009). As Gpr6 is also enriched in D2+ MSNs (Lobo et al., 2007), its role in behavioral functions of the striatum should be evaluated. To date, it has been shown to influence instrumental learning (Lobo et al., 2007) but its role in drug abuse models is yet unknown.
The cannabinoid receptor 1 (CB1) is expressed ubiquitously throughout the central nervous system (Mackie, 2008), hence it is difficult to dissect the precise role of specific brain regions and cell-types in mediating Δ9-tetrahydrocannabinol (THC) addiction. Recently, deletion of CB1 from D1+ MSNs was found to modestly affect behavioral responses to THC, including blunted effects in THC-induced hypolocomotion, hypothermia, and analgesia (Monory et al., 2007). It would be interesting to evaluate cannabinoid receptor function in D2+ MSNs since these MSNs express endocannabinoid-mediated long-term depression (eCB-LTD), which requires dopamine D2-receptor activation (Kreitzer and Malenka, 2007).
The glucocorticoid receptor, Nr3c1, is also broadly expressed in the CNS and periphery. Stress-induced glucocorticoid secretion can potentiate maladaptive behaviors including drug addiction (Frank et al., 2011). In particular, disrupting glucocorticoid signaling in D1+ MSNs by deleting Nr3c1 diminished the motivation these mice display to self-administer cocaine, and this is consistent with previous data where Nr3c1 was deleted from the entire brain (Ambroggi et al., 2009). These data are consistent with other findings described in this review, showing a predominant role for D1+ MSNs in mediating many of the effects of drugs of abuse.
Finally, we recently disrupted BDNF signaling in the two MSNs by deleting its TrkB receptor selectively from each MSN subtype. We observed opposite effects on cocaine-elicited behaviors: cocaine-induced locomotor activity and the induction of cocaine CPP were enhanced after TrkB deletion from D1+ MSNs, but attenuated after deletion from D2+ MSNs (Lobo et al., 2010). Interestingly, the deletion of TrkB from D2+ MSNs mimics the effects of total deletion of TrkB from the NAc as well as disruption of BDNF signaling from the VTA (Horger et al., 1999; Graham et al., 2007, 2009; Bahi et al., 2008; Crooks et al., 2010). These findings thus show for the first time a predominant role of a signaling cascade in D2+ MSNs in mediating the effects of a drug of abuse. The predominant role of D2+ MSNs in mediating BDNF’s effects on cocaine-elicited behaviors is not surprising considering both TrkB mRNA and protein are enriched in D2+ MSNs (Lobo et al., 2010; Baydyuk et al., 2011). The behavioral changes observed in these mice were accompanied by enhanced neuronal activity in the D2+ MSNs upon a selective knockout of TrkB. These findings prompted us to use optogenetic technology to selectively manipulate MSN activity in cocaine reward (see below).
Transcription Factors in D1 vs. D2 MSNs
The most compelling evidence for the more robust role of D1+ MSNs in drug abuse comes from literature evaluating induction of intracellular signaling molecules. As stated above, acute doses of psychostimulants induce IEG expression, including c-Fos, Zif268 (Egr1), and FosB primarily in D1+ MSNs in NAc and dStr (Robertson et al., 1991; Young et al., 1991; Berretta et al., 1992; Cenci et al., 1992; Moratalla et al., 1992; Bertran-Gonzalez et al., 2008). This induction requires activation of D1 receptors, and the cell-type-specificity of the IEG induction in response to acute cocaine was recently confirmed using D1-GFP and D2-GFP reporter mice (Bertran-Gonzalez et al., 2008). Interestingly, the confirmation of cocaine’s induction of c-Fos primarily in D1-GFP throughout striatum with a small induction in D2-GFP MSNs only in dStr was confirmed using a context-dependent paradigm (mice were injected in a novel environment outside of their home cage). Furthermore, a previous study using in situ hybridization in mice also showed induction of c-Fos in D1+ and D2+ MSNs in dStr, although in this study representative bar graphs show greater number of D1+ c-Fos positive neurons (Ferguson et al., 2006). Interestingly, this study reveals significantly enhanced c-Fos induction in D2+ MSNs in the dStr after loss of ERK1, which parallels our findings of enhanced c-Fos induction in D2+ MSNs specifically in the NAc shell after disruption of BDNF signaling which is known to enhance ERK activity (Lobo et al., 2010). However, opposite behavioral responses to cocaine were observed in each study, which may reflect induction of c-Fos in D2+ MSNs in dStr vs. NAc shell. Finally, previous literature using in situ hybridization/immunohistochemistry in rats has shown acute psychostimulants can induce c-Fos equally in both MSNs when the drug is given in a novel environment (Badiani et al., 1999; Uslaner et al., 2001a,b; Ferguson and Robinson, 2004) and chronic administration of amphetamine is reported to selectively induce c-Fos in D2+ MSNs (Mattson et al., 2007). These different results could be a reflection of the experimental procedures used (in situ hybridization vs. GFP reporter mice) or even be due to the animal species used as the latter experiments used rats.
Recently, researchers genetically profiled the cocaine context-dependent, c-Fos activated neurons in rats using immunolabeled fluorescence activated cell sorting (FACS) and showed that the c-Fos+ neurons are enriched in a D1+ MSN gene, prodynorphin (Pdyn), but have lower levels of D2 and A2A, both D2+ MSN genes (Guez-Barber et al., 2011), suggesting that the c-Fos+ activated neurons consist primarily of D1+ MSNs. Furthermore, this group previously showed that c-Fos expressing MSNs are important for this context-dependent sensitization, as ablation of these neurons abolishes this behavioral phenotype (Koya et al., 2009). Although previous data showed that the cocaine context-dependent induction of c-Fos occurs in both D1+ and D2+ MSNs in rats, the more recent results correspond to findings in which deletion of c-Fos selectively from D1+ MSNs blunts cocaine-induced locomotor sensitization in mice (Zhang et al., 2006). Furthermore, this group found that deletion of c-Fos in D1+ MSNs blunts the dendritic spine changes normally induced by cocaine in the NAc, indicating a role for c-Fos in mediating these synaptic plasticity changes. Finally, the group observed no change in the induction of cocaine CPP, but found that loss of c-Fos in D1+ MSNs prevented extinction of cocaine CPP. Such data illustrate a dynamic role for c-Fos induction in D1+ MSNs, however, one cannot rule out the differential effects at the behavioral level as being mediated by any of several other limbic brain regions that express the D1 receptor.
Another IEG that has been extensively studied in the two MSN subtypes is FosB. Acute exposure to cocaine induces FosB in D1+ MSNs (Berretta et al., 1992), whereas chronic exposure induces ΔFosB, a stable product of the FosB gene generated by alternative splicing (Hope et al., 1994; Nestler et al., 2001; Nestler, 2008), in D1+ MSNs (Nye et al., 1995; Moratalla et al., 1996; Lee et al., 2006). Similar findings are observed with many other drugs of abuse as well as with natural rewards such as food, sex, and wheel running. For example, chronic wheel running, which is a natural reward (Iversen, 1993; Belke, 1997; Lett et al., 2000), induces ΔFosB in D1+ MSNs but not D2+ MSNs (Werme et al., 2002). To gain functional insight into the role of ΔFosB in the two MSNs, our group generated NSE-tTa lines, termed 11A and 11B, which direct transgene expression to either D1+ or D2+ MSNs, respectively (Chen et al., 1998; Kelz et al., 1999; Werme et al., 2002). Line 11A mice crossed with a Tet-Op ΔFosB line show increased responses to the rewarding and locomotor effects of cocaine (Kelz et al., 1999), which is consistent with ΔFosB induction in D1+ MSNs (Nye et al., 1995; Moratalla et al., 1996). Furthermore, these same mice display increased morphine reward (evaluated by CPP) as well as diminished morphine analgesia and enhanced morphine tolerance, while the 11B Tet-Op ΔFosB mice show no change in morphine reward. Overexpression of a dominant negative antagonist of ΔFosB exerts effects opposite to those seen with ΔFosB, although this mouse model does not distinguish D1 vs. D2 MSNs (Peakman et al., 2003). Together, these data further supports the role of ΔFosB induction in D1+ MSNs as an important molecular player in the rewarding properties of drugs of abuse (Zachariou et al., 2006). This phenomenon is also observed in other reward behaviors, in particular, wheel running: 11A Tet-Op ΔFosB mice display increased wheel running behavior, whereas 11B Tet-Op ΔFosB mice display diminished wheel running (Werme et al., 2002). The finding that ΔFosB induction in D1 MSNs promotes reward is consistent with recent findings that such cell-type-selective induction also promotes resilience responses to chronic stress (Vialou et al., 2010). Finally, chronic cocaine induction of ΔFosB in D1+ MSNs was shown to be accompanied by robust long-lasting increases in dendritic spine densities (Lee et al., 2006) and recently ΔFosB in the NAc was shown to be both necessary and sufficient in mediating the increased density of dendritic spines in this brain region (Maze et al., 2010). Such data support a role for ΔFosB in D1+ MSNs in mediating the rewarding aspects of drugs of abuse and natural rewards as well as the accompanying structural plasticity changes. The data also suggest that induction of ΔFosB in D2+ MSNs confers negative consequences to rewarding stimuli. Since ΔFosB induction in D2+ MSNs is seen in response to chronic stress and antipsychotic drug exposure (Hiroi and Graybiel, 1996; Perrotti et al., 2004), further studies of the latter actions are needed.
Other Intracellular Signaling Molecules in D1 vs. D2 MSNs
One signaling molecule that has been well studied in the two MSNs in the context of drug abuse is the protein kinase, ERK (extracellular signal related kinase). Acute or chronic exposure to cocaine induces phosphorylated ERK (pERK), the activated form of the protein, in the NAc and dStr in D1+ MSNs using D1-GFP and D2-GFP BAC transgenic reporter mice (Bertran-Gonzalez et al., 2008) and this response is mediated through D1 receptors (Valjent et al., 2000; Lu et al., 2006). This group also showed that pMSK-1 (phospho-MAP and stress activated kinase-1) and histone H3, both targets of pERK signaling, are robustly induced in pERK containing D1+ MSNs after acute cocaine exposure and modestly increased after chronic cocaine (Bertran-Gonzalez et al., 2008). pERK is also induced is response to chronic morphine, in particular, pERK is robustly induced in D1+ MSNs and modestly induced in D2+ MSNs in the NAc shell after withdrawal in response to the context-specific association with morphine (Borgkvist et al., 2008). The precise functional role of pERK in drug addiction remains to be determined. Pharmacological treatment with ERK inhibitors has been shown to decrease cocaine reward, however, a knockout of ERK1 potentiates cocaine reward, suggesting that ERK inhibitors may preferentially be affecting ERK2. Recently, we showed that optogenetic activation of D1+ MSNs in the NAc, which increases an animal’s rewarding responses to cocaine, potently reduces both pERK1 and pERK2. Future studies manipulating ERK expression in a cell-type-specific manner are necessary to fully address the functional role of ERK signaling in the two MSNs in drug abuse.
DARPP-32 is another signaling molecule that has been extensively studied in response to drugs of abuse. It is well known that acute psychostimulants lead to PKA phosphorylation of DARPP-32 at threonine 34 (T34), causing it to become a potent inhibitor of protein phosphatase 1 (PP-1), which regulates the phosphorylation state of many effector proteins, including transcription factors, ionotropic receptors, and ion channels (Greengard et al., 1999). However, until recently, it was unclear which MSN subtype mediates this biochemical change. Greengard et al. (1999) generated BAC transgenic mouse models that enable the evaluation of DARPP-32 phosphorylation in D1+ or D2+ MSNs by expressing tagged versions of DARPP-32 using D1 or D2 BACs allowing for immunoprecipitation of DARPP-32 from each MSN subtype. These studies demonstrated that acute cocaine treatment increases T34 phosphorylation in D1+ MSNs and induces phosphorylation of threonine 75 (T75) by Cdk5, which inhibits PKA signaling, selectively in D2+ MSNs (Bateup et al., 2008). Finally this group showed that deletion of DARPP-32 from each MSN subtype using D1-Cre and D2-Cre BAC transgenic mice results in opposite regulation of cocaine-induced locomotor activity (Bateup et al., 2010). Loss of DARPP-32 from D1+ MSNs diminished the locomotor effects of cocaine, which mimics previous data evaluating a total DARPP-32 knockout (Fienberg et al., 1998), whereas loss of DARPP-32 from D2+ MSNs enhanced cocaine locomotor responses. Such data provide concrete evidence for differential roles of DARPP-32 in the two MSNs in response to drugs of abuse and illustrate the importance of cell-type-specific methods to fully understand the contribution of these two neuronal types in drug addiction.
Modulating Activity of D1 or D2 MSNs
Directly modulating the activity of the two MSN subtypes has recently provided novel insight into the molecular and functional role of D1 and D2 MSNs in addiction. We used optogenetic tools combined with a conditional (i.e., Cre-dependent) adeno-associated viral (AAV) vector expressing the blue light-activated cation channel, channelrhodopsin-2 (ChR2). We injected the vector, or a control, into the NAc of D1-Cre or D2-Cre BAC transgenic mice and then stimulated the injected region with blue light to selectively activate D1+ vs. D2+ MSNs in the context of cocaine CPP. We found that activation of D1+ MSNs potentiates induction of cocaine CPP, whereas activation of D2+ MSNs inhibits this induction (Lobo et al., 2010). As noted previously, we observed the same behavioral effects when TrkB was deleted selectively from these MSN subtypes: enhanced cocaine CPP and locomotor activity after TrkB deletion from D1+ MSNs, and reduced cocaine CPP and locomotor activity after TrkB deletion from D2+ MSNs. The likely common action of TrkB knockout and optogenetic stimulation in D2+ MSNs is their increased activity, since deletion of TrkB from these cells increases their electrical excitability. As mentioned earlier, we also found a robust reduction of pERK after TrkB deletion from D1+ MSNs. pERK is a known downstream target of BDNF signaling, therefore, the shared behavioral effects observed after TrkB deletion from D1+ MSNs and from optogenetic activation of these cells might be due to converging effects on pERK activity. However, future work is needed to determine the precise, shared molecular underpinnings that govern the behavioral effects seen after disruption of BDNF signaling and optogenetic control of these two neuronal subtypes.
Other groups have used different tools to modulate activity of the two MSNs in drug abuse models. Hikida et al. (2010) used AAV vectors to express tetracycline-repressive transcription factor (tTa) using the substance P (a D1+ MSN gene) or enkephalin (a D2+ MSN gene) promoters. These vectors were injected into the NAc of mice, in which tetanus toxin light chain (TN) – a bacterial toxin that cleaves the synaptic vesicle-associated protein, VAMP2 – was controlled by the tetracycline-responsive element, to selectively abolish synaptic transmission in each MSN subtype. Consistent with our optogenetic approach, these data showed a role of D1+ MSN activity in enhancing cocaine CPP as well as cocaine-induced locomotor activity, since abolishing synaptic transmission in D1+ MSNs diminished both behavioral effects. In contrast to the optogenetic studies, the authors found no alterations in cocaine CPP after abolishing synaptic transmission in D2+ MSNs, but did observe reduced cocaine-induced locomotor activity in response to the first two cocaine exposures. Interestingly, this group showed that inactivation of the D2+ MSNs played a more profound role in mediating aversive behaviors.
As stated earlier, Ferguson et al. (2011) used herpes simplex virus (HSV) vectors to express an engineered GPCR (a Gi/o-coupled human muscarinic M4 designer receptor exclusively activated by a designer drug, hM4D) that is activated by an otherwise pharmacologically inert ligand using enkephalin and dynorphin promoters to selectively silence D1+ or D2+ MSNs in the dStr. The authors showed that transiently disrupting D2+ MSN activity in dStr facilitated amphetamine sensitization, whereas decreasing excitability of D1+ MSNs impaired the persistence of amphetamine-induced sensitization. Finally, abolishing D2+ MSNs in the NAc at adult ages using diptheria toxin receptor enhances the rewarding effect of amphetamine (Durieux et al., 2009). Such data are in accordance with our optogenetic findings, and together implicate opposite roles of D1+ vs. D2+ MSNs in drug addiction, with D1+ MSNs promoting both reward and sensitizing responses to psychostimulants and D2+ MSNs dampening these behaviors.
Future Directions
The field has made tremendous advances toward understanding the selective role of the D1+ and D2+ MSN subtypes in NAc and dStr in mediating the effects of drugs of abuse. In particular, recently developed tools that enable the selective manipulation of these cell-types have played a predominant role in obtaining the majority of this information. What are the next steps? Since the underlying molecular adaptations in drug addiction models are not static, but very dynamic, it is crucial to develop the capability to selectively manipulate signaling molecules of interest in D1+ vs. D2+ MSNs in a temporally precise way. DREADDs and optogenetic tools can help with this time scale manipulation. DREADD ligands can be administered at different time courses throughout drug behavioral paradigms to parcel out the selective role of signaling receptors in the two MSNs in drug models. Optogenetic tools in particular provide an extremely powerful means to temporally regulate not only neuronal activity but G-protein-coupled receptor signaling using OptoXRs (Airan et al., 2009), glutamatergic signaling(Volgraf et al., 2006; Numano et al., 2009), GABAergic signaling, and even certain intracellular signaling molecules (Wu et al., 2009; Hahn and Kuhlman, 2010). Ultimately, it may be possible to extend these capabilities to optogenetic regulation of transcriptional activity. Likewise, optogenetic tools are making it possible for the first time to study the influence of specific inputs to striatum and to determine whether such inputs impinge in selective ways on D1+ vs. D2+ MSNs (Higley and Sabatini, 2010). The ability to control such signaling and molecular properties with great temporal resolution will allow major steps to be made toward a more comprehensive understanding of the two MSN subtypes, and other cell subtypes in NAc and dStr, in mediating the time course and different phases of drug addiction.
Conflict of Interest Statement
The authors declare that the research was conducted in the absence of any commercial or financial relationships that could be construed as a potential conflict of interest.
References
Airan, R. D., Thompson, K. R., Fenno, L. E., Bernstein, H., and Deisseroth, K. (2009). Temporally precise in vivo control of intracellular signalling. Nature 458, 1025–1029.
Albin, R. L., Young, A. B., and Penney, J. B. (1989). The functional anatomy of basal ganglia disorders. Trends Neurosci. 12, 366–375.
Alexander, G. E., Delong, M. R., and Strick, P. L. (1986). Parallel organization of functionally segregated circuits linking basal ganglia and cortex. Annu. Rev. Neurosci. 9, 357–381.
Ambroggi, F., Turiault, M., Milet, A., Deroche-Gamonet, V., Parnaudeau, S., Balado, E., Barik, J., Van Der Veen, R., Maroteaux, G., Lemberger, T., Schutz, G., Lazar, M., Marinelli, M., Piazza, P. V., and Tronche, F. (2009). Stress and addiction: glucocorticoid receptor in dopaminoceptive neurons facilitates cocaine seeking. Nat. Neurosci. 12, 247–249.
Bachtell, R. K., Choi, K. H., Simmons, D. L., Falcon, E., Monteggia, L. M., Neve, R. L., and Self, D. W. (2008). Role of GluR1 expression in nucleus accumbens neurons in cocaine sensitization and cocaine-seeking behavior. Eur. J. Neurosci. 27, 2229–2240.
Bachtell, R. K., and Self, D. W. (2008). Renewed cocaine exposure produces transient alterations in nucleus accumbens AMPA receptor-mediated behavior. J. Neurosci. 28, 12808–12814.
Bachtell, R. K., and Self, D. W. (2009). Effects of adenosine A2A receptor stimulation on cocaine-seeking behavior in rats. Psychopharmacology (Berl.) 206, 469–478.
Badiani, A., Oates, M. M., Day, H. E., Watson, S. J., Akil, H., and Robinson, T. E. (1999). Environmental modulation of amphetamine-induced c-fos expression in D1 versus D2 striatal neurons. Behav. Brain Res. 103, 203–209.
Bahi, A., Boyer, F., Chandrasekar, V., and Dreyer, J. L. (2008). Role of accumbens BDNF and TrkB in cocaine-induced psychomotor sensitization, conditioned-place preference, and reinstatement in rats. Psychopharmacology (Berl.) 199, 169–182.
Bateup, H. S., Santini, E., Shen, W., Birnbaum, S., Valjent, E., Surmeier, D. J., Fisone, G., Nestler, E. J., and Greengard, P. (2010). Distinct subclasses of medium spiny neurons differentially regulate striatal motor behaviors. Proc. Natl. Acad. Sci. U.S.A. 107, 14845–14850.
Bateup, H. S., Svenningsson, P., Kuroiwa, M., Gong, S., Nishi, A., Heintz, N., and Greengard, P. (2008). Cell type-specific regulation of DARPP-32 phosphorylation by psychostimulant and antipsychotic drugs. Nat. Neurosci. 11, 932–939.
Baydyuk, M., Nguyen, M. T., and Xu, B. (2011). Chronic deprivation of TrkB signaling leads to selective late-onset nigrostriatal dopaminergic degeneration. Exp. Neurol. 228, 118–125.
Belke, T. W. (1997). Running and responding reinforced by the opportunity to run: effect of reinforcer duration. J. Exp. Anal. Behav. 67, 337–351.
Bernard, V., Normand, E., and Bloch, B. (1992). Phenotypical characterization of the rat striatal neurons expressing muscarinic receptor genes. J. Neurosci. 12, 3591–3600.
Berretta, S., Robertson, H. A., and Graybiel, A. M. (1992). Dopamine and glutamate agonists stimulate neuron-specific expression of Fos-like protein in the striatum. J. Neurophysiol. 68, 767–777.
Bertran-Gonzalez, J., Bosch, C., Maroteaux, M., Matamales, M., Herve, D., Valjent, E., and Girault, J. A. (2008). Opposing patterns of signaling activation in dopamine D1 and D2 receptor-expressing striatal neurons in response to cocaine and haloperidol. J. Neurosci. 28, 5671–5685.
Beurrier, C., and Malenka, R. C. (2002). Enhanced inhibition of synaptic transmission by dopamine in the nucleus accumbens during behavioral sensitization to cocaine. J. Neurosci. 22, 5817–5822.
Beutler, L. R., Wanat, M. J., Quintana, A., Sanz, E., Bamford, N. S., Zweifel, L. S., and Palmiter, R. D. (2011). Balanced NMDA receptor activity in dopamine D1 receptor (D1R)- and D2R-expressing medium spiny neurons is required for amphetamine sensitization. Proc. Natl. Acad. Sci. U.S.A. 108, 4206–4211.
Borgkvist, A., Valjent, E., Santini, E., Herve, D., Girault, J. A., and Fisone, G. (2008). Delayed, context- and dopamine D1 receptor-dependent activation of ERK in morphine-sensitized mice. Neuropharmacology 55, 230–237.
Caine, S. B., Negus, S. S., Mello, N. K., Patel, S., Bristow, L., Kulagowski, J., Vallone, D., Saiardi, A., and Borrelli, E. (2002). Role of dopamine D2-like receptors in cocaine self-administration: studies with D2 receptor mutant mice and novel D2 receptor antagonists. J. Neurosci. 22, 2977–2988.
Caine, S. B., Thomsen, M., Gabriel, K. I., Berkowitz, J. S., Gold, L. H., Koob, G. F., Tonegawa, S., Zhang, J., and Xu, M. (2007). Lack of self-administration of cocaine in dopamine D1 receptor knock-out mice. J. Neurosci. 27, 13140–13150.
Cardin, J. A., Carlen, M., Meletis, K., Knoblich, U., Zhang, F., Deisseroth, K., Tsai, L. H., and Moore, C. I. (2010). Targeted optogenetic stimulation and recording of neurons in vivo using cell-type-specific expression of channelrhodopsin-2. Nat. Protoc. 5, 247–254.
Cenci, M. A., Campbell, K., Wictorin, K., and Bjorklund, A. (1992). Striatal c-fos induction by cocaine or apomorphine occurs preferentially in output neurons projecting to the substantia nigra in the rat. Eur. J. Neurosci. 4, 376–380.
Chausmer, A. L., Elmer, G. I., Rubinstein, M., Low, M. J., Grandy, D. K., and Katz, J. L. (2002). Cocaine-induced locomotor activity and cocaine discrimination in dopamine D2 receptor mutant mice. Psychopharmacology (Berl.) 163, 54–61.
Chen, J., Kelz, M. B., Zeng, G., Sakai, N., Steffen, C., Shockett, P. E., Picciotto, M. R., Duman, R. S., and Nestler, E. J. (1998). Transgenic animals with inducible, targeted gene expression in brain. Mol. Pharmacol. 54, 495–503.
Conrad, K. L., Tseng, K. Y., Uejima, J. L., Reimers, J. M., Heng, L. J., Shaham, Y., Marinelli, M., and Wolf, M. E. (2008). Formation of accumbens GluR2-lacking AMPA receptors mediates incubation of cocaine craving. Nature 454, 118–121.
Crawford, C. A., Drago, J., Watson, J. B., and Levine, M. S. (1997). Effects of repeated amphetamine treatment on the locomotor activity of the dopamine D1A-deficient mouse. Neuroreport 8, 2523–2527.
Crooks, K. R., Kleven, D. T., Rodriguiz, R. M., Wetsel, W. C., and Mcnamara, J. O. (2010). TrkB signaling is required for behavioral sensitization and conditioned place preference induced by a single injection of cocaine. Neuropharmacology 58, 1067–1077.
Cunningham, C. L., Howard, M. A., Gill, S. J., Rubinstein, M., Low, M. J., and Grandy, D. K. (2000). Ethanol-conditioned place preference is reduced in dopamine D2 receptor-deficient mice. Pharmacol. Biochem. Behav. 67, 693–699.
Deroche-Gamonet, V., Sillaber, I., Aouizerate, B., Izawa, R., Jaber, M., Ghozland, S., Kellendonk, C., Le Moal, M., Spanagel, R., Schutz, G., Tronche, F., and Piazza, P. V. (2003). The glucocorticoid receptor as a potential target to reduce cocaine abuse. J. Neurosci. 23, 4785–4790.
Dietz, D. M., Dietz, K. C., Nestler, E. J., and Russo, S. J. (2009). Molecular mechanisms of psychostimulant-induced structural plasticity. Pharmacopsychiatry 42(Suppl. 1), S69–S78.
Drago, J., Gerfen, C. R., Westphal, H., and Steiner, H. (1996). D1 dopamine receptor-deficient mouse: cocaine-induced regulation of immediate-early gene and substance P expression in the striatum. Neuroscience 74, 813–823.
Durieux, P. F., Bearzatto, B., Guiducci, S., Buch, T., Waisman, A., Zoli, M., Schiffmann, S. N., and De Kerchove D’Exaerde, A. (2009). D2R striatopallidal neurons inhibit both locomotor and drug reward processes. Nat. Neurosci. 12, 393–395.
El-Ghundi, M., George, S. R., Drago, J., Fletcher, P. J., Fan, T., Nguyen, T., Liu, C., Sibley, D. R., Westphal, H., and O’Dowd, B. F. (1998). Disruption of dopamine D1 receptor gene expression attenuates alcohol-seeking behavior. Eur. J. Pharmacol. 353, 149–158.
Elmer, G. I., Pieper, J. O., Rubinstein, M., Low, M. J., Grandy, D. K., and Wise, R. A. (2002). Failure of intravenous morphine to serve as an effective instrumental reinforcer in dopamine D2 receptor knock-out mice. J. Neurosci. 22, RC224.
Ferguson, S. M., Eskenazi, D., Ishikawa, M., Wanat, M. J., Phillips, P. E., Dong, Y., Roth, B. L., and Neumaier, J. F. (2011). Transient neuronal inhibition reveals opposing roles of indirect and direct pathways in sensitization. Nat. Neurosci. 14, 22–24.
Ferguson, S. M., Fasano, S., Yang, P., Brambilla, R., and Robinson, T. E. (2006). Knockout of ERK1 enhances cocaine-evoked immediate early gene expression and behavioral plasticity. Neuropsychopharmacology 31, 2660–2668.
Ferguson, S. M., and Robinson, T. E. (2004). Amphetamine-evoked gene expression in striatopallidal neurons: regulation by corticostriatal afferents and the ERK/MAPK signaling cascade. J. Neurochem. 91, 337–348.
Fienberg, A. A., Hiroi, N., Mermelstein, P. G., Song, W., Snyder, G. L., Nishi, A., Cheramy, A., O’Callaghan, J. P., Miller, D. B., Cole, D. G., Corbett, R., Haile, C. N., Cooper, D. C., Onn, S. P., Grace, A. A., Ouimet, C. C., White, F. J., Hyman, S. E., Surmeier, D. J., Girault, J., Nestler, E. J., and Greengard, P. (1998). DARPP-32: regulator of the efficacy of dopaminergic neurotransmission. Science 281, 838–842.
Filip, M., Frankowska, M., Zaniewska, M., Przegalinski, E., Muller, C. E., Agnati, L., Franco, R., Roberts, D. C., and Fuxe, K. (2006). Involvement of adenosine A2A and dopamine receptors in the locomotor and sensitizing effects of cocaine. Brain Res. 1077, 67–80.
Frank, M. G., Watkins, L. R., and Maier, S. F. (2011). Stress- and glucocorticoid-induced priming of neuroinflammatory responses: potential mechanisms of stress-induced vulnerability to drugs of abuse. Brain Behav. Immun. 25, S21–S28.
Gerfen, C. R. (1984). The neostriatal mosaic: compartmentalization of corticostriatal input and striatonigral output systems. Nature 311, 461–464.
Gerfen, C. R. (1992). The neostriatal mosaic: multiple levels of compartmental organization in the basal ganglia. Annu. Rev. Neurosci. 15, 285–320.
Gerfen, C. R., Engber, T. M., Mahan, L. C., Susel, Z., Chase, T. N., Monsma, F. J. Jr., and Sibley, D. R. (1990). D1 and D2 dopamine receptor-regulated gene expression of striatonigral and striatopallidal neurons. Science 250, 1429–1432.
Gerfen, C. R., and Surmeier, D. J. (2011). Modulation of striatal projection systems by dopamine. Annu. Rev. Neurosci. 34, 441–466.
Gerfen, C. R., and Young, W. S. III. (1988). Distribution of striatonigral and striatopallidal peptidergic neurons in both patch and matrix compartments: an in situ hybridization histochemistry and fluorescent retrograde tracing study. Brain Res. 460, 161–167.
Gong, S., Doughty, M., Harbaugh, C. R., Cummins, A., Hatten, M. E., Heintz, N., and Gerfen, C. R. (2007). Targeting Cre recombinase to specific neuron populations with bacterial artificial chromosome constructs. J. Neurosci. 27, 9817–9823.
Gong, S., Zheng, C., Doughty, M. L., Losos, K., Didkovsky, N., Schambra, U. B., Nowak, N. J., Joyner, A., Leblanc, G., Hatten, M. E., and Heintz, N. (2003). A gene expression atlas of the central nervous system based on bacterial artificial chromosomes. Nature 425, 917–925.
Graham, D. L., Edwards, S., Bachtell, R. K., Dileone, R. J., Rios, M., and Self, D. W. (2007). Dynamic BDNF activity in nucleus accumbens with cocaine use increases self-administration and relapse. Nat. Neurosci. 10, 1029–1037.
Graham, D. L., Krishnan, V., Larson, E. B., Graham, A., Edwards, S., Bachtell, R. K., Simmons, D., Gent, L. M., Berton, O., Bolanos, C. A., Dileone, R. J., Parada, L. F., Nestler, E. J., and Self, D. W. (2009). Tropomyosin-related kinase B in the mesolimbic dopamine system: region-specific effects on cocaine reward. Biol. Psychiatry 65, 696–701.
Greengard, P., Allen, P. B., and Nairn, A. C. (1999). Beyond the dopamine receptor: the DARPP-32/protein phosphatase-1 cascade. Neuron 23, 435–447.
Guez-Barber, D., Fanous, S., Golden, S. A., Schrama, R., Koya, E., Stern, A. L., Bossert, J. M., Harvey, B. K., Picciotto, M. R., and Hope, B. T. (2011). FACS identifies unique cocaine-induced gene regulation in selectively activated adult striatal neurons. J. Neurosci. 31, 4251–4259.
Heiman, M., Schaefer, A., Gong, S., Peterson, J. D., Day, M., Ramsey, K. E., Suarez-Farinas, M., Schwarz, C., Stephan, D. A., Surmeier, D. J., Greengard, P., and Heintz, N. (2008). A translational profiling approach for the molecular characterization of CNS cell types. Cell 135, 738–748.
Heusner, C. L., Beutler, L. R., Houser, C. R., and Palmiter, R. D. (2008). Deletion of GAD67 in dopamine receptor-1 expressing cells causes specific motor deficits. Genesis 46, 357–367.
Heusner, C. L., and Palmiter, R. D. (2005). Expression of mutant NMDA receptors in dopamine D1 receptor-containing cells prevents cocaine sensitization. J. Neurosci. 25, 6651–6657.
Higley, M. J., and Sabatini, B. L. (2010). Competitive regulation of synaptic Ca2+ influx by D2 dopamine and A2A adenosine receptors. Nat. Neurosci. 13, 958–966.
Hikida, T., Kimura, K., Wada, N., Funabiki, K., and Nakanishi, S. (2010). Distinct roles of synaptic transmission in direct and indirect striatal pathways to reward and aversive behavior. Neuron 66, 896–907.
Hiroi, N., and Graybiel, A. M. (1996). Atypical and typical neuroleptic treatments induce distinct programs of transcription factor expression in the striatum. J. Comp. Neurol. 374, 70–83.
Hope, B. T., Nye, H. E., Kelz, M. B., Self, D. W., Iadarola, M. J., Nakabeppu, Y., Duman, R. S., and Nestler, E. J. (1994). Induction of a long-lasting AP-1 complex composed of altered Fos-like proteins in brain by chronic cocaine and other chronic treatments. Neuron 13, 1235–1244.
Horger, B. A., Iyasere, C. A., Berhow, M. T., Messer, C. J., Nestler, E. J., and Taylor, J. R. (1999). Enhancement of locomotor activity and conditioned reward to cocaine by brain-derived neurotrophic factor. J. Neurosci. 19, 4110–4122.
Ince, E., Ciliax, B. J., and Levey, A. I. (1997). Differential expression of D1 and D2 dopamine and m4 muscarinic acetylcholine receptor proteins in identified striatonigral neurons. Synapse 27, 357–366.
Iversen, I. H. (1993). Techniques for establishing schedules with wheel running as reinforcement in rats. J. Exp. Anal. Behav. 60, 219–238.
Jeon, J., Dencker, D., Wortwein, G., Woldbye, D. P., Cui, Y., Davis, A. A., Levey, A. I., Schutz, G., Sager, T. N., Mork, A., Li, C., Deng, C. X., Fink-Jensen, A., and Wess, J. (2010). A subpopulation of neuronal M4 muscarinic acetylcholine receptors plays a critical role in modulating dopamine-dependent behaviors. J. Neurosci. 30, 2396–2405.
Kalivas, P. W. (2009). The glutamate homeostasis hypothesis of addiction. Nat. Rev. Neurosci. 10, 561–572.
Kelz, M. B., Chen, J., Carlezon, W. A. Jr., Whisler, K., Gilden, L., Beckmann, A. M., Steffen, C., Zhang, Y. J., Marotti, L., Self, D. W., Tkatch, T., Baranauskas, G., Surmeier, D. J., Neve, R. L., Duman, R. S., Picciotto, M. R., and Nestler, E. J. (1999). Expression of the transcription factor deltaFosB in the brain controls sensitivity to cocaine. Nature 401, 272–276.
Kim, J., Park, B. H., Lee, J. H., Park, S. K., and Kim, J. H. (2011). Cell type-specific alterations in the nucleus accumbens by repeated exposures to cocaine. Biol. Psychiatry 69, 1026–1034.
Knapp, C. M., Foye, M. M., Cottam, N., Ciraulo, D. A., and Kornetsky, C. (2001). Adenosine agonists CGS 21680 and NECA inhibit the initiation of cocaine self-administration. Pharmacol. Biochem. Behav. 68, 797–803.
Kourrich, S., Rothwell, P. E., Klug, J. R., and Thomas, M. J. (2007). Cocaine experience controls bidirectional synaptic plasticity in the nucleus accumbens. J. Neurosci. 27, 7921–7928.
Koya, E., Golden, S. A., Harvey, B. K., Guez-Barber, D. H., Berkow, A., Simmons, D. E., Bossert, J. M., Nair, S. G., Uejima, J. L., Marin, M. T., Mitchell, T. B., Farquhar, D., Ghosh, S. C., Mattson, B. J., and Hope, B. T. (2009). Targeted disruption of cocaine-activated nucleus accumbens neurons prevents context-specific sensitization. Nat. Neurosci. 12, 1069–1073.
Kramer, P. F., Christensen, C. H., Hazelwood, L. H., Dobi, A., Bock, R., Sibley, D. R., Mateo, Y., and Alvarez, V. A. (2011). Dopamine D2 receptor overexpression alters behavior and physiology in Drd2-EGFP mice. J. Neurosci. 31, 126–132.
Kravitz, A. V., Freeze, B. S., Parker, P. R., Kay, K., Thwin, M. T., Deisseroth, K., and Kreitzer, A. C. (2010). Regulation of parkinsonian motor behaviours by optogenetic control of basal ganglia circuitry. Nature 466, 622–626.
Kreitzer, A. C., and Malenka, R. C. (2007). Endocannabinoid-mediated rescue of striatal LTD and motor deficits in Parkinson’s disease models. Nature 445, 643–647.
Le Moine, C., Normand, E., and Bloch, B. (1991). Phenotypical characterization of the rat striatal neurons expressing the D1 dopamine receptor gene. Proc. Natl. Acad. Sci. U.S.A. 88, 4205–4209.
Le Moine, C., Normand, E., Guitteny, A. F., Fouque, B., Teoule, R., and Bloch, B. (1990). Dopamine receptor gene expression by enkephalin neurons in rat forebrain. Proc. Natl. Acad. Sci. U.S.A. 87, 230–234.
Lee, K. W., Kim, Y., Kim, A. M., Helmin, K., Nairn, A. C., and Greengard, P. (2006). Cocaine-induced dendritic spine formation in D1 and D2 dopamine receptor-containing medium spiny neurons in nucleus accumbens. Proc. Natl. Acad. Sci. U.S.A. 103, 3399–3404.
Lemberger, T., Parlato, R., Dassesse, D., Westphal, M., Casanova, E., Turiault, M., Tronche, F., Schiffmann, S. N., and Schutz, G. (2007). Expression of Cre recombinase in dopaminoceptive neurons. BMC Neurosci. 8, 4. doi: 10.1186/1471-2202-8-4
Lett, B. T., Grant, V. L., Byrne, M. J., and Koh, M. T. (2000). Pairings of a distinctive chamber with the aftereffect of wheel running produce conditioned place preference. Appetite 34, 87–94.
Lobo, M. K., Covington, H. E. III, Chaudhury, D., Friedman, A. K., Sun, H., Damez-Werno, D., Dietz, D. M., Zaman, S., Koo, J. W., Kennedy, P. J., Mouzon, E., Mogri, M., Neve, R. L., Deisseroth, K., Han, M. H., and Nestler, E. J. (2010). Cell type-specific loss of BDNF signaling mimics optogenetic control of cocaine reward. Science 330, 385–390.
Lobo, M. K., Cui, Y., Ostlund, S. B., Balleine, B. W., and Yang, X. W. (2007). Genetic control of instrumental conditioning by striatopallidal neuron-specific S1P receptor Gpr6. Nat. Neurosci. 10, 1395–1397.
Lobo, M. K., Karsten, S. L., Gray, M., Geschwind, D. H., and Yang, X. W. (2006). FACS-array profiling of striatal projection neuron subtypes in juvenile and adult mouse brains. Nat. Neurosci. 9, 443–452.
Lu, L., Koya, E., Zhai, H., Hope, B. T., and Shaham, Y. (2006). Role of ERK in cocaine addiction. Trends Neurosci. 29, 695–703.
Mackie, K. (2008). Cannabinoid receptors: where they are and what they do. J. Neuroendocrinol. 20(Suppl. 1), 10–14.
Maldonado, R., Saiardi, A., Valverde, O., Samad, T. A., Roques, B. P., and Borrelli, E. (1997). Absence of opiate rewarding effects in mice lacking dopamine D2 receptors. Nature 388, 586–589.
Mattson, B. J., Crombag, H. S., Mitchell, T., Simmons, D. E., Kreuter, J. D., Morales, M., and Hope, B. T. (2007). Repeated amphetamine administration outside the home cage enhances drug-induced Fos expression in rat nucleus accumbens. Behav. Brain Res. 185, 88–98.
Maze, I., Covington, H. E. III, Dietz, D. M., Laplant, Q., Renthal, W., Russo, S. J., Mechanic, M., Mouzon, E., Neve, R. L., Haggarty, S. J., Ren, Y., Sampath, S. C., Hurd, Y. L., Greengard, P., Tarakhovsky, A., Schaefer, A., and Nestler, E. J. (2010). Essential role of the histone methyltransferase G9a in cocaine-induced plasticity. Science 327, 213–216.
Meisler, M. H. (1992). Insertional mutation of “classical” and novel genes in transgenic mice. Trends Genet. 8, 341–344.
Miner, L. L., Drago, J., Chamberlain, P. M., Donovan, D., and Uhl, G. R. (1995). Retained cocaine conditioned place preference in D1 receptor deficient mice. Neuroreport 6, 2314–2316.
Monory, K., Blaudzun, H., Massa, F., Kaiser, N., Lemberger, T., Schutz, G., Wotjak, C. T., Lutz, B., and Marsicano, G. (2007). Genetic dissection of behavioural and autonomic effects of Delta(9)-tetrahydrocannabinol in mice. PLoS Biol. 5, e269. doi: 10.1371/journal.pbio.0050269
Moratalla, R., Robertson, H. A., and Graybiel, A. M. (1992). Dynamic regulation of NGFI-A (zif268, egr1) gene expression in the striatum. J. Neurosci. 12, 2609–2622.
Moratalla, R., Vallejo, M., Elibol, B., and Graybiel, A. M. (1996). D1-class dopamine receptors influence cocaine-induced persistent expression of Fos-related proteins in striatum. Neuroreport 8, 1–5.
Nestler, E. J. (2005). Is there a common molecular pathway for addiction? Nat. Neurosci. 8, 1445–1449.
Nestler, E. J. (2008). Review. Transcriptional mechanisms of addiction: role of DeltaFosB. Philos. Trans. R. Soc. Lond. B Biol. Sci. 363, 3245–3255.
Nestler, E. J., Barrot, M., and Self, D. W. (2001). DeltaFosB: a sustained molecular switch for addiction. Proc. Natl. Acad. Sci. U.S.A. 98, 11042–11046.
Novak, M., Halbout, B., O’Connor, E. C., Rodriguez Parkitna, J., Su, T., Chai, M., Crombag, H. S., Bilbao, A., Spanagel, R., Stephens, D. N., Schutz, G., and Engblom, D. (2010). Incentive learning underlying cocaine-seeking requires mGluR5 receptors located on dopamine D1 receptor-expressing neurons. J. Neurosci. 30, 11973–11982.
Numano, R., Szobota, S., Lau, A. Y., Gorostiza, P., Volgraf, M., Roux, B., Trauner, D., and Isacoff, E. Y. (2009). Nanosculpting reversed wavelength sensitivity into a photoswitchable iGluR. Proc. Natl. Acad. Sci. U.S.A. 106, 6814–6819.
Nye, H. E., Hope, B. T., Kelz, M. B., Iadarola, M., and Nestler, E. J. (1995). Pharmacological studies of the regulation of chronic FOS-related antigen induction by cocaine in the striatum and nucleus accumbens. J. Pharmacol. Exp. Ther. 275, 1671–1680.
Parkitna, J. R., Engblom, D., and Schutz, G. (2009). Generation of Cre recombinase-expressing transgenic mice using bacterial artificial chromosomes. Methods Mol. Biol. 530, 325–342.
Peakman, M. C., Colby, C., Perrotti, L. I., Tekumalla, P., Carle, T., Ulery, P., Chao, J., Duman, C., Steffen, C., Monteggia, L., Allen, M. R., Stock, J. L., Duman, R. S., Mcneish, J. D., Barrot, M., Self, D. W., Nestler, E. J., and Schaeffer, E. (2003). Inducible, brain region-specific expression of a dominant negative mutant of c-Jun in transgenic mice decreases sensitivity to cocaine. Brain Res. 970, 73–86.
Peoples, L. L., Uzwiak, A. J., Guyette, F. X., and West, M. O. (1998). Tonic inhibition of single nucleus accumbens neurons in the rat: a predominant but not exclusive firing pattern induced by cocaine self-administration sessions. Neuroscience 86, 13–22.
Perrotti, L. I., Hadeishi, Y., Ulery, P. G., Barrot, M., Monteggia, L., Duman, R. S., and Nestler, E. J. (2004). Induction of deltaFosB in reward-related brain structures after chronic stress. J. Neurosci. 24, 10594–10602.
Pierce, R. C., Bell, K., Duffy, P., and Kalivas, P. W. (1996). Repeated cocaine augments excitatory amino acid transmission in the nucleus accumbens only in rats having developed behavioral sensitization. J. Neurosci. 16, 1550–1560.
Risinger, F. O., Freeman, P. A., Rubinstein, M., Low, M. J., and Grandy, D. K. (2000). Lack of operant ethanol self-administration in dopamine D2 receptor knockout mice. Psychopharmacology (Berl.) 152, 343–350.
Robertson, H. A., Paul, M. L., Moratalla, R., and Graybiel, A. M. (1991). Expression of the immediate early gene c-fos in basal ganglia: induction by dopaminergic drugs. Can. J. Neurol. Sci. 18, 380–383.
Russo, S. J., Dietz, D. M., Dumitriu, D., Morrison, J. H., Malenka, R. C., and Nestler, E. J. (2010). The addicted synapse: mechanisms of synaptic and structural plasticity in nucleus accumbens. Trends Neurosci. 33, 267–276.
Schiffmann, S. N., Libert, F., Vassart, G., and Vanderhaeghen, J. J. (1991). Distribution of adenosine A2 receptor mRNA in the human brain. Neurosci. Lett. 130, 177–181.
Schiffmann, S. N., and Vanderhaeghen, J. J. (1993). Adenosine A2 receptors regulate the gene expression of striatopallidal and striatonigral neurons. J. Neurosci. 13, 1080–1087.
Self, D. W. (2010). “Dopamine receptor subtypes in reward and relapse,” in The Dopamine Receptors, ed. K. A. Neve (New York, NY: Humana Press), 479–523.
Self, D. W., Barnhart, W. J., Lehman, D. A., and Nestler, E. J. (1996). Opposite modulation of cocaine-seeking behavior by D1- and D2-like dopamine receptor agonists. Science 271, 1586–1589.
Surmeier, D. J., Ding, J., Day, M., Wang, Z., and Shen, W. (2007). D1 and D2 dopamine-receptor modulation of striatal glutamatergic signaling in striatal medium spiny neurons. Trends Neurosci. 30, 228–235.
Surmeier, D. J., Song, W. J., and Yan, Z. (1996). Coordinated expression of dopamine receptors in neostriatal medium spiny neurons. J. Neurosci. 16, 6579–6591.
Thanos, P. K., Michaelides, M., Umegaki, H., and Volkow, N. D. (2008). D2R DNA transfer into the nucleus accumbens attenuates cocaine self-administration in rats. Synapse 62, 481–486.
Thanos, P. K., Taintor, N. B., Rivera, S. N., Umegaki, H., Ikari, H., Roth, G., Ingram, D. K., Hitzemann, R., Fowler, J. S., Gatley, S. J., Wang, G. J., and Volkow, N. D. (2004). DRD2 gene transfer into the nucleus accumbens core of the alcohol preferring and nonpreferring rats attenuates alcohol drinking. Alcohol. Clin. Exp. Res. 28, 720–728.
Thomas, M. J., Beurrier, C., Bonci, A., and Malenka, R. C. (2001). Long-term depression in the nucleus accumbens: a neural correlate of behavioral sensitization to cocaine. Nat. Neurosci. 4, 1217–1223.
Uslaner, J., Badiani, A., Day, H. E., Watson, S. J., Akil, H., and Robinson, T. E. (2001a). Environmental context modulates the ability of cocaine and amphetamine to induce c-fos mRNA expression in the neocortex, caudate nucleus, and nucleus accumbens. Brain Res. 920, 106–116.
Uslaner, J., Badiani, A., Norton, C. S., Day, H. E., Watson, S. J., Akil, H., and Robinson, T. E. (2001b). Amphetamine and cocaine induce different patterns of c-fos mRNA expression in the striatum and subthalamic nucleus depending on environmental context. Eur. J. Neurosci. 13, 1977–1983.
Valjent, E., Bertran-Gonzalez, J., Herve, D., Fisone, G., and Girault, J. A. (2009). Looking BAC at striatal signaling: cell-specific analysis in new transgenic mice. Trends Neurosci. 32, 538–547.
Valjent, E., Corvol, J. C., Pages, C., Besson, M. J., Maldonado, R., and Caboche, J. (2000). Involvement of the extracellular signal-regulated kinase cascade for cocaine-rewarding properties. J. Neurosci. 20, 8701–8709.
Vialou, V., Robison, A. J., Laplant, Q. C., Covington, H. E. III, Dietz, D. M., Ohnishi, Y. N., Mouzon, E., Rush, A. J. III, Watts, E. L., Wallace, D. L., Iniguez, S. D., Ohnishi, Y. H., Steiner, M. A., Warren, B. L., Krishnan, V., Bolanos, C. A., Neve, R. L., Ghose, S., Berton, O., Tamminga, C. A., and Nestler, E. J. (2010). DeltaFosB in brain reward circuits mediates resilience to stress and antidepressant responses. Nat. Neurosci. 13, 745–752.
Volgraf, M., Gorostiza, P., Numano, R., Kramer, R. H., Isacoff, E. Y., and Trauner, D. (2006). Allosteric control of an ionotropic glutamate receptor with an optical switch. Nat. Chem. Biol. 2, 47–52.
Volkow, N. D., Fowler, J. S., Wang, G. J., Baler, R., and Telang, F. (2009). Imaging dopamine’s role in drug abuse and addiction. Neuropharmacology 56(Suppl. 1), 3–8.
Volkow, N. D., Fowler, J. S., Wang, G. J., and Swanson, J. M. (2004). Dopamine in drug abuse and addiction: results from imaging studies and treatment implications. Mol. Psychiatry 9, 557–569.
Welter, M., Vallone, D., Samad, T. A., Meziane, H., Usiello, A., and Borrelli, E. (2007). Absence of dopamine D2 receptors unmasks an inhibitory control over the brain circuitries activated by cocaine. Proc. Natl. Acad. Sci. U.S.A. 104, 6840–6845.
Werme, M., Messer, C., Olson, L., Gilden, L., Thoren, P., Nestler, E. J., and Brene, S. (2002). Delta FosB regulates wheel running. J. Neurosci. 22, 8133–8138.
White, F. J., Hu, X. T., Zhang, X. F., and Wolf, M. E. (1995). Repeated administration of cocaine or amphetamine alters neuronal responses to glutamate in the mesoaccumbens dopamine system. J. Pharmacol. Exp. Ther. 273, 445–454.
Wolf, M. E. (2010). Regulation of AMPA receptor trafficking in the nucleus accumbens by dopamine and cocaine. Neurotox. Res. 18, 393–409.
Wu, Y. I., Frey, D., Lungu, O. I., Jaehrig, A., Schlichting, I., Kuhlman, B., and Hahn, K. M. (2009). A genetically encoded photoactivatable Rac controls the motility of living cells. Nature 461, 104–108.
Young, S. T., Porrino, L. J., and Iadarola, M. J. (1991). Cocaine induces striatal c-fos-immunoreactive proteins via dopaminergic D1 receptors. Proc. Natl. Acad. Sci. U.S.A. 88, 1291–1295.
Zachariou, V., Bolanos, C. A., Selley, D. E., Theobald, D., Cassidy, M. P., Kelz, M. B., Shaw-Lutchman, T., Berton, O., Sim-Selley, L. J., Dileone, R. J., Kumar, A., and Nestler, E. J. (2006). An essential role for DeltaFosB in the nucleus accumbens in morphine action. Nat. Neurosci. 9, 205–211.
Zhang, J., Zhang, L., Jiao, H., Zhang, Q., Zhang, D., Lou, D., Katz, J. L., and Xu, M. (2006). c-Fos facilitates the acquisition and extinction of cocaine-induced persistent changes. J. Neurosci. 26, 13287–13296.
Keywords: medium spiny neurons, addiction, nucleus accumbens, cell-type-specific, D1+ MSNs, D2+ MSNs, cocaine, dopamine
Citation: Lobo MK and Nestler EJ (2011) The striatal balancing act in drug addiction: distinct roles of direct and indirect pathway medium spiny neurons. Front. Neuroanat. 5:41. doi: 10.3389/fnana.2011.00041
Received: 12 May 2011; Paper pending published: 31 May 2011;
Accepted: 05 July 2011; Published online: 18 July 2011.
Edited by:
Emmanuel Valjent, Université Montpellier 1 & 2, FranceReviewed by:
Bruce Thomas Hope, National Institute on Drug Abuse, USAJohn Neumaier, University of Washington, USA
Copyright: © 2011 Lobo and Nestler. This is an open-access article subject to a non-exclusive license between the authors and Frontiers Media SA, which permits use, distribution and reproduction in other forums, provided the original authors and source are credited and other Frontiers conditions are complied with.
*Correspondence: Eric J. Nestler, Department of Neuroscience, Friedman Brain Institute, Mount Sinai School of Medicine, One Gustave L. Levy Place, Box 1065, New York, NY 10029-6574, USA. e-mail:ZXJpYy5uZXN0bGVyQG1zc20uZWR1