- 1Shenzhen Bao’an District Hospital of Traditional Chinese Medicine, Shenzhen, Guangdong, China
- 2Shenzhen Bao’an Traditional Chinese Medicine Hospital, Guangzhou University of Chinese Medicine, Shenzhen, Guangdong, China
Soluble Triggering Receptor Expressed on Myeloid Cells 2 (sTREM2) plays a crucial role in the pathogenesis of Alzheimer’s disease (AD). This review comprehensively examines sTREM2’s involvement in AD, focusing on its regulatory functions in microglial responses, neuroinflammation, and interactions with key pathological processes. We discuss the dynamic changes in sTREM2 levels in cerebrospinal fluid and plasma throughout AD progression, highlighting its potential as a therapeutic target. Furthermore, we explore the impact of genetic variants on sTREM2 expression and its interplay with other AD risk genes. The evidence presented in this review suggests that modulating sTREM2 activity could influence AD trajectory, making it a promising avenue for future research and drug development. By providing a holistic understanding of sTREM2’s multifaceted role in AD, this review aims to guide future studies and inspire novel therapeutic strategies.
1 Introduction: unveiling the role of sTREM2 in Alzheimer’s disease
Alzheimer’s disease (AD), as the predominant cause of dementia globally, is delineated by the progressive deposition of amyloid-beta (Aβ) plaques and neurofibrillary tangles composed of hyperphosphorylated tau protein within the cerebral cortex (Holtzman et al., 2011). Despite considerable scientific endeavors, the multifaceted etiology and pathogenesis of AD continue to be inadequately elucidated, thus stymying the advancement of efficacious therapeutic modalities. Recent discourse has increasingly recognized the integral role of neuroinflammation and innate immune responses in the progression of AD, with particular focus on microglia—the CNS’s inherent immune constituents—as pivotal in the disease’s initiation and development (Hansen et al., 2018; Wang and Colonna, 2019). Microglia, constituting 5–10% of total brain cells, are essential in sustaining CNS homeostasis by monitoring brain parenchyma, phagocytizing cellular debris and apoptotic cells, and modulating synaptic plasticity (Deczkowska et al., 2020). In AD, microglia accumulate around Aβ plaques and demonstrate diverse activation phenotypes, ranging from pro-inflammatory states that potentially aggravate neuronal degeneration to protective states facilitating Aβ clearance and tissue restitution (Keren-Shaul et al., 2017; Deczkowska et al., 2020). The regulation of these heterogeneous microglial responses is partly governed by various cell surface receptors, including the triggering receptor expressed on myeloid cells 2 (TREM2).
TREM2, an innate immune receptor predominantly expressed on microglia within the CNS, mediates its signaling through the adaptor protein DAP12 (Klesney-Tait et al., 2006; Ulland et al., 2017). Interaction with ligands such as anionic lipids, lipoproteins, and Aβ instigates intracellular signaling cascades that enhance microglial survival, proliferation, chemotaxis, and phagocytic activity (Keren-Shaul et al., 2017; Zhao et al., 2018; Deczkowska et al., 2020). The importance of TREM2 in AD pathology is underscored by the discovery of heterozygous TREM2 mutations, such as R47H, which significantly increase the risk of developing late-onset AD (Guerreiro et al., 2013; Jonsson et al., 2013). These mutations are posited to diminish the receptor’s protective functions, especially concerning Aβ plaque management (Condello et al., 2015; Yuan et al., 2016). Notably, a soluble variant of TREM2 (sTREM2) detected in cerebrospinal fluid (CSF) and plasma exhibits dynamic concentration changes through the AD continuum, with initial elevations during early symptomatic phases followed by reductions in later stages (Piccio et al., 2008, 2016; Heslegrave et al., 2016; Suárez-Calvet et al., 2016a,b; Zhou W. et al., 2023; Yin T. et al., 2024). Elevated CSF sTREM2 levels correlate with attenuated cognitive decline and reduced cerebral atrophy in AD subjects (Ewers et al., 2019), proposing sTREM2 as a potential biomarker for microglial activation and a moderator of disease trajectory (Gu et al., 2023). Recent findings further suggest an association between minimal depressive symptoms in prodromal AD and amyloid pathology, mediated in part by alterations in CSF sTREM2 levels (Wang et al., 2022b). The accruing evidence underscores the potential of sTREM2 as both a biomarker and a therapeutic target in AD (La Rosa et al., 2023). This review aims to furnish a comprehensive understanding of sTREM2 within the AD framework, exploring its biogenesis, functional roles, and clinical implications, thus underlining the imperative for additional research to delineate its precise function in AD pathogenesis.
2 Biogenesis of sTREM2: mechanisms and variants
sTREM2 is produced through two primary mechanisms (Figure 1): proteolytic cleavage of the TREM2 ectodomain and alternative splicing of the TREM2 gene. Metalloproteases ADAM10 and ADAM17 facilitate the cleavage at the H157-S158 bond, releasing sTREM2 into the extracellular space (Schlepckow et al., 2017; Thornton et al., 2017). Meprin β is a zinc metalloproteinase that belongs to the astacin family of proteases. It is known to cleave a wide range of substrates, including extracellular matrix proteins, cytokines, and cell surface proteins. In the context of sTREM2 biogenesis, meprin β has been shown to cleave TREM2 at a distinct site (R136-D137) compared to the cleavage site of ADAM10 and ADAM17 (H157-S158). This alternative cleavage by meprin β contributes to the generation of sTREM2 in macrophages (Berner et al., 2020). Alternatively, splicing variations produce mRNA lacking the transmembrane domain, which translates into sTREM2 and is subsequently released (Ma et al., 2016; Del-Aguila et al., 2019). Around 25% of the brain’s total sTREM2 is produced through this alternative splicing pathway (Del-Aguila et al., 2019). A recent genome-wide association study (GWAS) identified four loci correlating with CSF sTREM2 levels, including the well-known MS4A gene cluster on chromosome 11 and three novel loci: TREM2 on chromosome 6, TGFBR2/RBMS3 on chromosome 3, and NECTIN2/APOE on chromosome 19 (Wang et al., 2024).
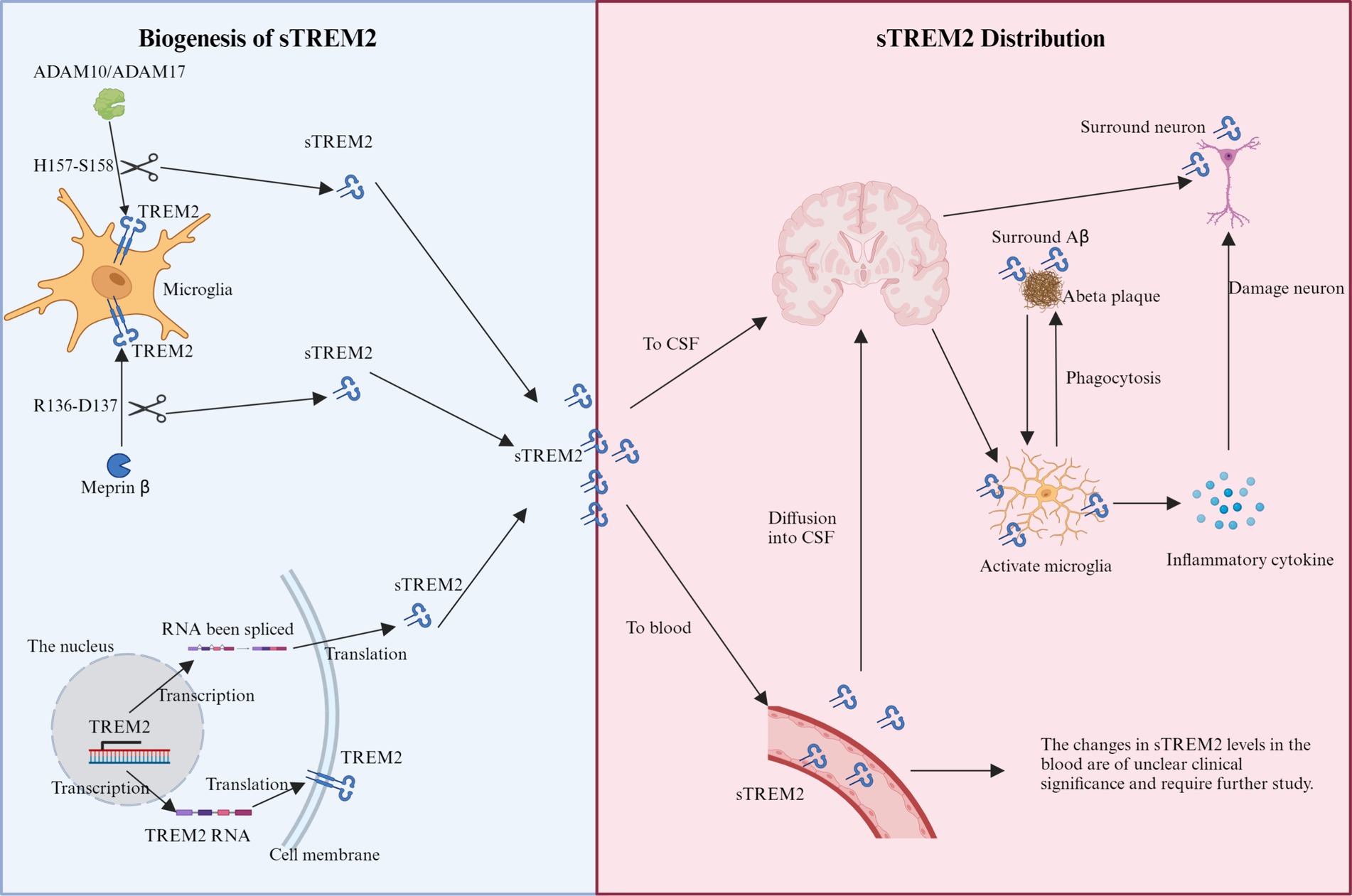
Figure 1. Schematic representation of the production and distribution of sTREM2. Biogenesis of sTREM2: the diagram illustrates two primary mechanisms of sTREM2 production within microglia. The proteolytic cleavage pathway involves the cleavage of the TREM2 ectodomain by metalloproteases ADAM10 and ADAM17 at the H157-S158 bond. Additionally, meprin β cleaves at the R136-D137 site. The splicing pathway shows mRNA variants being processed to produce sTREM2 lacking the transmembrane domain, which is then released into the extracellular space. sTREM2 distribution: sTREM2 is depicted as being distributed into two main compartments: the brain and the bloodstream. In the brain, sTREM2 penetrates the blood–brain barrier and accumulates around damaged neurons and amyloid-beta plaques, potentially exacerbating inflammatory responses. In the bloodstream, sTREM2 is shown being released by monocytes, illustrating its presence across different extracellular compartments.
Four principal TREM2 mRNA variants have been identified: ENST00000373113, ENST00000373122, ENST00000338469, and TREM2Δe2 (Jin et al., 2014; Kiianitsa et al., 2021). The canonical ENST00000373113 encodes the full-length TREM2 protein, comprising 230 amino acids across 5 exons. ENST00000373122, lacking exon 5, diverges in its sequence, initiating from an alternate start within exon 4, yielding a 222 amino acid variant. ENST00000338469, lacking the critical exon 4, likely results in a secretory form of TREM2 of 219 amino acids. TREM2Δe2, devoid of exon 2 which encodes the ligand-binding domain, might produce a non-functional receptor variant tethered to the membrane (Kiianitsa et al., 2021). Mutations within the TREM2 gene notably impact sTREM2 production, with certain variants leading to protein misfolding and retention in the endoplasmic reticulum, thereby reducing sTREM2 secretion (Kleinberger et al., 2014; Park et al., 2015; Song et al., 2017). Specific mutations in the receptor’s Ig-like domain, such as p.T66M and p.Y38C, lead to misfolding or retention, thus decreasing its cell surface expression and subsequent cleavage into sTREM2 and C-terminal fragments in vitro (Kleinberger et al., 2014; Park et al., 2015; Song et al., 2017). Conversely, variants like p.R47H do not affect intracellular trafficking (Kleinberger et al., 2014; Wang et al., 2015). Additionally, certain low-frequency genetic alterations in the TREM2 gene could alter splicing regulatory elements, potentially increasing the probability of exon 2 omission during splicing (Han et al., 2021). Thus, a spectrum of genetic variations influences both the cleavage and splicing of TREM2, affecting the observed levels of sTREM2.
3 sTREM2 distribution: cellular sources and pathophysiological implications
sTREM2 exhibits a varied distribution within the extracellular domain, significantly influenced by its cellular origin. It is secreted into the brain’s parenchyma by microglia (Henjum et al., 2016; Tan et al., 2017) and released into the bloodstream by monocytes (Feuerbach et al., 2017; Figure 1), illustrating its presence across different extracellular spaces. This variability in secretion highlights sTREM2’s involvement in a spectrum of functions, particularly evident during the early stages of AD, where its accumulation around damaged neurons and protein plaques suggests a role in initiating innate immune responses and potentially exacerbating the disease state (Kober and Brett, 2017; Song et al., 2018). Unregulated proteolytic cleavage, which results in increased sTREM2 levels, can have significant consequences, such as the potential disruption of the blood–brain barrier and the diffusion of sTREM2 into the CSF (Raha-Chowdhury et al., 2019; Tanaka et al., 2019). Additionally, minor quantities of sTREM2 found in the plasma may infiltrate through compromised vascular structures into the brain parenchyma (Pugazhenthi et al., 2017), offering a partial explanation for the increased sTREM2 concentrations observed in AD alongside other neurodegenerative diseases like multiple sclerosis (MS), frontal temporal dementia (FTD), and Lewy body dementia (LBD) (Piccio et al., 2008; Cooper-Knock et al., 2017; Zhou et al., 2019).
The dynamic progression of sTREM2 levels in the CSF throughout the AD continuum, particularly their elevation during early disease stages, is crucial for both diagnostic and staging purposes (Song et al., 2018; Rauchmann et al., 2019). Certain studies have reported a rise in sTREM2 levels in patients up to 5 years before the clinical onset of AD (Suárez-Calvet et al., 2016a,b), where these elevated levels also correlate with established neurodegenerative markers such as t-tau/p-tau and Aβ42 (Henjum et al., 2018). The presence of sTREM2 in the CSF is associated with microglial activation, which supports the hypothesis that neuroinflammation plays a central role in the early stages of AD. This is further reinforced by the observation that sTREM2 concentrations increase following amyloid deposition and subsequent neuronal damage (Gispert et al., 2016; Suárez-Calvet et al., 2016a). However, it’s noteworthy that sTREM2 levels in the CSF do not consistently remain elevated across all AD stages.
Currently, the clinical significance of elevated serum sTREM2 levels in AD patients remains uncertain (Tanaka et al., 2019). While several studies have documented higher sTREM2 levels in the peripheral blood of AD patients compared to healthy counterparts (Hu et al., 2014; Casati et al., 2018), others have found no substantial differences (Piccio et al., 2008; Liu et al., 2018). This discrepancy could be attributed to various factors, including differences in patient demographics like gender, age, and disease stage. The origin of serum sTREM2 is also under investigation (Fahrenhold et al., 2018), with sources such as monocyte-derived dendritic cells expressing high levels of membrane-bound TREM2, which are then cleaved by sheddases (Song et al., 2017). The potential contribution of other cells, such as osteoclasts, to the circulating sTREM2 pool complicates the understanding of its impact on AD development, necessitating further study.
4 Regulatory mechanisms of sTREM2 shedding and metabolic degradation
The release of sTREM2 from its membrane-bound precursor is influenced by various stimuli, including LPS, interleukin-1β (IL-1β), oligomeric Aβ, Vascular Endothelial Growth Factor (VEGF), viral infections like HIV, and cytokines such as IL-13 and IL-4, which promote sTREM2 secretion (Wu et al., 2015; Gisslén et al., 2019; Zhong et al., 2019; Vilalta et al., 2021). Notably, LPS stimulation has been shown to decrease TREM2 surface expression and increase sTREM2 release in RAW264.7 cells, highlighting its role in TREM2 shedding and sTREM2 generation (Bandow et al., 2022). Additionally, BRI2 modulates sTREM2 functionality by inhibiting α-secretase-mediated cleavage of TREM2, affecting microglial phagocytic capabilities under pathological conditions (Yin T. et al., 2024). VEGF is recognized as a pivotal regulator of microglial phagocytosis in AD, facilitating the cleavage and release of sTREM2, thus enhancing the clearance of Aβ oligomers (de Gea et al., 2023). Studies report an age-related increase in CSF sTREM2 levels, suggesting rapid, demand-driven conversion of TREM2 to sTREM2, reflecting a regulatory need or a functional necessity for sTREM2, with the full-length protein serving as its precursor (Henjum et al., 2016).
The degradation pathways and body elimination processes for sTREM2 are not fully defined. Macrophages efficiently ingest sTREM2 (Wu et al., 2015), and sTREM2 introduced into the mouse brain is cleared within 3 days (Zhong et al., 2019). The role of meprin β in sTREM2 metabolism in the brain is under investigation, particularly its capacity to degrade sTREM2 following ADAM10/17-mediated inflammation which releases soluble meprin β (Berner et al., 2020). Moreover, sTREM2 variants from TREM2 gene splicing, particularly isoforms ENST00000373122 and ENST00000338469, add complexity to the sTREM2 pool, necessitating advanced analytical techniques to differentiate these isoforms in CSF (Piccio et al., 2016; Rauchmann et al., 2020). This underscores the importance of developing isoform-specific antibodies to better understand their roles in disease mechanisms. Ongoing research is essential to elucidate the functionalities of these distinct C-terminal sequences.
In conclusion, sTREM2 production results from both proteolytic cleavage and alternative splicing of TREM2. It actively participates in innate immune responses within the extracellular space. Genetic variations in TREM2 influence sTREM2 levels, leading to considerable differences in sTREM2 concentrations in various conditions, such as AD. The mechanisms for sTREM2 breakdown and removal are still elusive, emphasizing the need for further investigation into the specific roles and implications of various sTREM2 forms in neurodegenerative diseases.
5 Modulators of sTREM2 levels: genetic, demographic, and environmental impacts
Research underscores the critical role of specific genetic loci and demographic variables in modulating levels of sTREM2 within the CSF. Key findings highlight a crucial genomic region on chromosome 11, adjacent to the MS4A gene cluster, significantly affecting CSF sTREM2 concentrations. Variants such as rs1582763, MS4A6A (p.A112T), and MS4A4A (p.M178V) have been found to elevate sTREM2 levels (Wang et al., 2024) whereas rs6591561 diminishes them (Winfree et al., 2024), and is also linked to an increased risk of AD (Piccio et al., 2016; Deming et al., 2019; Ferkingstad et al., 2021). This locus is not only expressed in microglia but also interacts functionally with TREM2, influencing microglial activation and predisposition to AD. Although this locus is pivotal, it shows no significant associations with other well-known AD risk factors like APOE and CD33 (Kamboh et al., 2012; Deming et al., 2019; Griciuc and Tanzi, 2021). Further research reveals that the rs2062323T allele in the TREM1 gene is associated with increased CSF sTREM2 levels (Wang et al., 2023).
Demographic factors—particularly age, sex, and ethnicity—also significantly influence sTREM2 dynamics. Aging is consistently linked to increased CSF sTREM2 levels, suggesting its potential as a biomarker for age-related microglial activation and neuroinflammatory responses (Henjum et al., 2016; Piccio et al., 2016; Suárez-Calvet et al., 2016a,b, 2019; Gisslén et al., 2019; Knapskog et al., 2020; Moore et al., 2021). This elevation correlates with other markers of astrocyte activation and synaptic injury, highlighting the intricate interplay between microglial activity and neurodegenerative processes during aging (Moore et al., 2021). A recent study demonstrated elevated sTREM2 levels in the serum of long-COVID patients with cognitive impairment compared to healthy controls (Besteher et al., 2024), suggesting a shift in microglial reactivity in response to neuronal degeneration. While some studies report higher sTREM2 levels in males compared to females, these findings are not consistent across all cohorts (Piccio et al., 2016; Suárez-Calvet et al., 2016a,b; Knapskog et al., 2020; Wilson et al., 2020). Additionally, African American populations exhibit lower sTREM2 levels than Non-Hispanic Whites, paralleling lower levels of other AD biomarkers, which may reflect different genetic predispositions toward sTREM2 dynamics (Schindler et al., 2021). Lifestyle factors like physical exercise may influence sTREM2 levels and microglial function, though more research is needed in this area (Schindler et al., 2021). Furthermore, while CSF sTREM2 concentrations are thought to primarily reflect intrathecal production without correlation to peripheral blood levels or blood–brain barrier integrity, some findings suggest a negative correlation between CSF and plasma sTREM2 levels, associating higher plasma levels with aging (Piccio et al., 2008, 2016; Park et al., 2015). Epidemiological evidence also links prolonged exposure to fine particulate matter (PM2.5) with reduced CSF sTREM2 levels, suggesting impaired microglial function and increased neuroinflammation, potentially exacerbating AD pathology (Li et al., 2022). Additionally, high ferritin levels, indicative of increased brain iron, have been associated with elevated sTREM2 levels, particularly in individuals with higher baseline ferritin (Shi et al., 2022).
This body of evidence collectively illuminates the complex web of genetic, demographic, and potentially modifiable factors shaping sTREM2 levels, offering valuable insights into AD’s pathophysiology and highlighting the need for tailored approaches in studying sTREM2 across different neurological conditions.
6 sTREM2 dynamics and Alzheimer’s disease progression
Research into sTREM2 levels in the blood and CSF of individuals with AD presents a diverse range of findings. While the majority of cross-sectional studies observed a moderate increase in CSF sTREM2 levels among AD patients compared to cognitively normal controls (Heslegrave et al., 2016; Piccio et al., 2016; Ewers et al., 2019), there are reports of reduced (Kleinberger et al., 2014) or unchanged levels (Henjum et al., 2016; Knapskog et al., 2020). This variability might stem from the differing stages of disease among participants, including the inclusion of individuals with preclinical AD in control groups (Figure 2). CSF sTREM2 has shown promise as an indicator of cognitive decline in Parkinson’s disease (PD) (Qin et al., 2022), especially in PD with mild cognitive impairment (PD-MCI), where baseline CSF levels were significantly higher in patients who exhibited cognitive deterioration over a two-year period compared to those who remained cognitively stable (Paolini Paoletti et al., 2023). Remarkably, elevated CSF sTREM2 levels can be detected 12 to 14 years before the estimated onset of symptoms, marking its potential as an early indicator of neuroinflammatory changes (Li et al., 2024). CSF sTREM2 concentrations exhibit a stage-dependent increase in AD (Figure 2), peaking during early symptomatic phases, which aligns with heightened microglial activation in response to neuronal damage (Hok-A-Hin et al., 2023). Insights from the Dominantly Inherited Alzheimer Network (DIAN) have shown that CSF sTREM2 levels are elevated in familial AD mutation carriers up to 5 years before and after symptom onset but not beyond this period (Suárez-Calvet et al., 2016a). This finding is corroborated by recent studies demonstrating significantly higher CSF sTREM2 levels in individuals with mild cognitive impairment due to AD, particularly in those testing positive for CSF AD biomarkers (Giannisis et al., 2023). These elevated levels of sTREM2 not only correlate with the severity of neurofibrillary degeneration and cognitive decline but also reflect increased inflammasome activation (Španić Popovački et al., 2023). Another study highlighted that both CSF and plasma sTREM2 levels serve as significant predictive biomarkers for progression from MCI to AD, with lower CSF TREM2 levels indicating an elevated risk of disease progression (Zhou W. et al., 2023). Lower CSF sTREM2 levels are also associated with increased depressive symptoms and enhanced amyloid deposition in the early stages of AD, especially in individuals lacking the APOE ε4 allele (Wang et al., 2022b). Interestingly, higher sTREM2 concentrations were noted in cognitively normal individuals suspected of non-AD pathology (tau positive, amyloid negative) (Wang et al., 2022a), suggesting that sTREM2 elevation might more accurately indicate neuronal injury associated with tau rather than amyloid pathology.
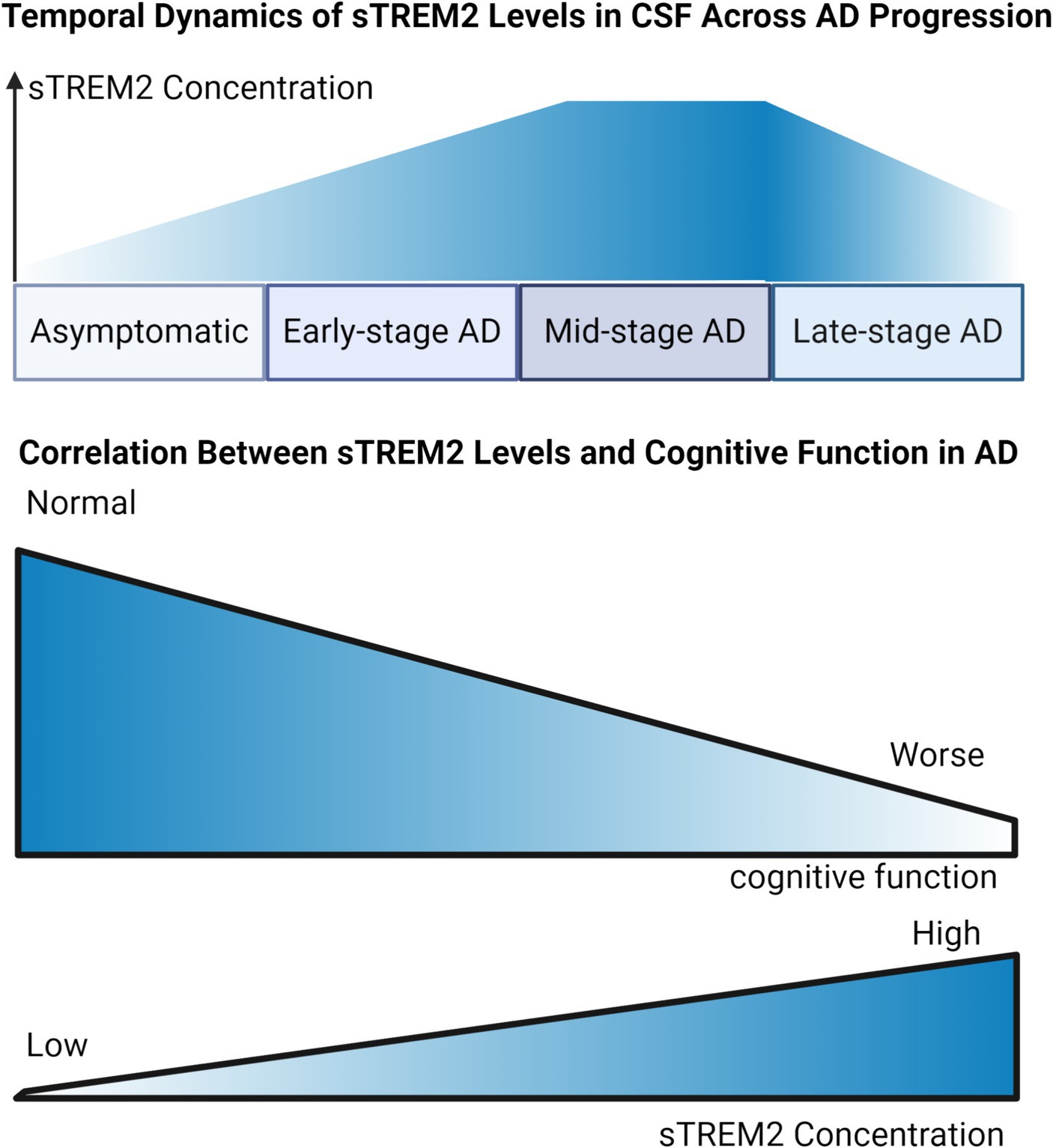
Figure 2. Dynamic Trends of sTREM2 Levels in Alzheimer’s Disease Progression. Temporal Dynamic of sTREM2 Levels in CSF Across AD Progression: Illustrating the trend of sTREM2 levels, the graph depicts an ascending trajectory against the stages of AD from asymptomatic to late-stage. It suggests an initial increase in sTREM2 concentration, which peaks during the early symptomatic phases, corresponding to heightened microglial activation, followed by a plateau or potential decrease in the late stages of AD. Correlation Between sTREM2 Levels and Cognitive Function in AD: Serving as a focused examination of the sTREM2-MMSE correlation, this graph presents a direct association between increased sTREM2 levels and changes in MMSE scores. It highlights how elevated sTREM2 levels are potentially linked with cognitive stability or decline, thereby reflecting their significance as a biomarker within the AD diagnostic spectrum.
In addition to its potential as a diagnostic marker, sTREM2 levels may offer prognostic insights. A study found that higher baseline CSF sTREM2 levels were linked to less severe declines in memory and cognitive functions over an 11-year span in individuals with AD, implying a protective role of microglial activation in the early stages of the disease (Ewers et al., 2019). Moreover, a higher CSF sTREM2 to p-tau181 ratio predicted slower progression from MCI to AD dementia or to more advanced symptomatic stages. Recent findings also revealed increased sTREM2 levels in the serum of long-COVID patients with cognitive impairment when compared to healthy controls (Besteher et al., 2024), suggesting a corresponding shift in microglial reactivity in response to neuronal degeneration. In patients whose diabetes control deteriorated, those with higher baseline levels of sTREM2 experienced a more pronounced decline in Mini-Mental State Examination (MMSE) scores over a 2-year period, which suggests a potential link between higher sTREM2 levels and the acceleration of cognitive impairment under conditions of poor glycemic control (Tanaka et al., 2022). This aligns with previous studies demonstrating an association between sTREM2 levels and neuronal injury markers in the early stages of AD.
Collectively, these findings enhance our understanding of sTREM2’s involvement in AD and MCI, highlighting its potential both as a biomarker of disease stage and as an indicator of microglial neuroprotective effects in the early stages of Alzheimer’s pathology.
7 Functional insights into sTREM2 in Alzheimer’s disease
7.1 The impact of sTREM2 shedding on TREM2 receptor signaling
The precise mechanisms through which sTREM2 shedding influences TREM2 receptor signaling and the inherent functions of sTREM2 remain somewhat enigmatic. It has been observed that stabilizing TREM2 on the cellular membrane with antibodies to hinder its proteolytic shedding by sTREM2 enhances not only downstream TREM2 signaling but also critical functions of the receptor, such as the phagocytic activity of microglial cells (Schlepckow et al., 2020; Szykowska et al., 2021). This observation suggests that the detachment of TREM2 from the membrane, facilitated by ADAM metalloprotease activity leading to sTREM2 production, might play a regulatory role in attenuating TREM2 receptor signaling. Genetic variant rs7922621 within a microglia-specific cis-regulatory element significantly impacts sTREM2 levels by modulating tetraspanin 14 expression, thereby influencing ADAM10 activity and the inflammatory response in AD (Yang et al., 2023), further supporting the idea that sTREM2 shedding may serve to modulate receptor activity. In line with this concept, an increase in both TREM2 protein levels and sTREM2 concentrations in the CSF has been associated with heightened microglial activation, as observed in inflammatory neurological conditions (Piccio et al., 2008; Diaz-Lucena et al., 2021), indicating elevated receptor signaling. It is posited that sTREM2 shedding may naturally follow ligand engagement, serving to regulate receptor activity by intercepting downstream signaling pathways. Consequently, the presence of sTREM2 in CSF or plasma could serve as an indicator of the extent of TREM2’s interaction with its ligand, including the dynamics of its shedding and the turnover of newly synthesized TREM2 within the cell, which is necessary for sustained receptor function. However, the precise dynamics between ligand engagement and the shedding process require further elucidation.
7.2 Ligand interactions with TREM2 and sTREM2: binding dynamics and functional consequences
The complexity of TREM2 ligands and their interactions with the receptor adds layers to our understanding of sTREM2’s functionality. TREM2 is recognized for its versatility, capable of binding a wide array of molecules with polyanionic traits, including bacterial membranes, myelin, lipoproteins such as ApoE, Aβ, DNA, and others (Poliani et al., 2015; Wang et al., 2015; Yeh et al., 2016; Cignarella et al., 2020), suggesting that TREM2’s activation is highly context-dependent. Notably, TREM2’s ability to bind to membrane-associated ligands in-trans on macrophages (Hamerman et al., 2006) and astrocytes (Piccio et al., 2007), with emerging evidence of sTREM2 interactions with neuronal surfaces (Song et al., 2018; Zhong et al., 2019), points to a multifaceted role in cellular communication and immune response. However, the extent to which sTREM2, like TREM2, can engage in-trans ligands present on the surface of neighboring cells remains a question that warrants further investigation.
TREM2’s pivotal role in Aβ phagocytosis within the brain is underscored by the specific interaction between the TREM2 ectodomain and Aβ fibrils, facilitated by the V-type immunoglobulin-like domain (Zhong et al., 2018). Modifications such as glycosylation and sulfidation further refine TREM2’s ligand affinity (Kober et al., 2016), enhancing its functional capacity. Among TREM2’s ligands, lipoprotein particles and apolipoproteins, notably Low-Density Lipoprotein (LDL), Clusterin (CLU)/APOJ, and APOE, have been thoroughly investigated (Daws et al., 2003; Yeh et al., 2016; Nugent et al., 2020). Studies reveal that APOE, particularly its ε4 allele—a known risk factor for late-onset AD (Nicolas, 2024)-acts as an agonist in this interaction, affecting AD pathology through the TREM2-APOE complex (Bailey et al., 2015; Jendresen et al., 2017). This interaction is significant, with specific amino acid sequences in APOE identified as critical for binding to TREM2’s ectodomain (Yeh et al., 2016; Zhao et al., 2018), promoting the clearance of apoptotic neurons and misfolded proteins via the TREM2 pathway (Atagi et al., 2015). Furthermore, Aβ’s interaction with APOE’s lipid-binding region activates TREM2, contributing to the amyloid plaque-associated TREM2 activation. This evidence collectively suggests a synergistic relationship between TREM2 and APOE in modulating AD progression, highlighting their collaborative role in Aβ uptake and clearance in the context of the disease. In a pivotal study exploring the role of sTREM2 in post-stroke recovery, sTREM2 was identified as a negative regulator of erythrophagocytosis through CD36 receptor recycling, suggesting that modulation of sTREM2 activity could enhance hematoma resolution and neurorehabilitation outcomes following intracerebral hemorrhage (Zhou H. et al., 2023). These findings underscore the multifaceted nature of sTREM2’s interactions and its potential as a therapeutic target in various neurological conditions.
7.3 sTREM2 and Aβ: modulating amyloid aggregation and neurotoxicity
sTREM2 exhibits specificity in binding to oligomeric forms of Aβ, with significantly less affinity for monomeric or fibrillar structures (Lessard et al., 2018; Zhao et al., 2018; Zhong et al., 2018; Vilalta et al., 2021). Research reveals that the TREM2 ectodomain’s affinity varies across different amyloid structures, with Aβ oligomers demonstrating the highest binding affinity, regardless of whether they are Aβ42 monomers, Aβ42 fibrils, or Aβ40 monomers (Lessard et al., 2018). Notably, the interaction between sTREM2 and Aβ oligomers is characterized by a slow dissociation rate, effectively hindering Aβ oligomers from binding to other potential ligands. Further investigation uncovered that sTREM2 inhibits both the oligomerization and fibrillization of Aβ at specific molar ratios (1 sTREM2 to 100 Aβ), and higher concentrations of sTREM2 can disaggregate Aβ oligomers and fibrils, suggesting sTREM2’s role as an extracellular chaperone (Vilalta et al., 2021). This function allows sTREM2 to prevent Aβ from forming neurotoxic aggregates and even revert such aggregates back to less harmful forms, thus attenuating Aβ’s neurotoxic effects (Kober et al., 2020; Vilalta et al., 2021). Conversely, the R47H variant of sTREM2 demonstrates diminished binding to Aβ oligomers, paradoxically enhancing the aggregation of Aβ into protofibrils and exacerbating neuronal loss in vitro (Vilalta et al., 2021). This suggests that the R47H mutation might not only diminish sTREM2’s neuroprotective abilities but could also contribute to Aβ’s neurotoxicity by promoting its aggregation into more harmful structures. Administering recombinant sTREM2 has been shown to reduce Aβ accumulation and ameliorate behavioral impairments in the 5 × FAD mouse model of AD (Zhong et al., 2019), underscoring sTREM2’s potential in mitigating Aβ-driven pathology in AD. These findings collectively highlight sTREM2’s therapeutic value in targeting neurodegenerative disorders, offering insights into its ability to modulate Aβ aggregation and attenuate its neurotoxic effects. As research continues to unravel the intricacies of sTREM2’s interactions with Aβ, it is becoming increasingly evident that harnessing sTREM2’s unique properties could pave the way for novel therapeutic strategies in the fight against AD and other neurodegenerative diseases.
7.4 Evaluating sTREM2: effects on amyloid pathology in transgenic animal models
Injecting sTREM2 into the brains of mice engineered to express amyloid precursor protein (APP) led to a notable reduction in amyloid plaque burden (Zhong et al., 2019). Moreover, employing viral vectors to express sTREM2 in these APP mice diminished plaque loads and significantly reversed spatial memory deficits and impairments in long-term potentiation (LTP) (Moutinho et al., 2023). These observations underscore sTREM2’s role in guarding against amyloid pathology, potentially through mechanisms that involve altering Aβ aggregation dynamics or enhancing microglial activity to engulf plaques. Interestingly, specific sTREM2 segments (amino acids 51–81) activated microglia without binding Aβ or tangibly affecting amyloid pathology, whereas a slightly larger fragment (amino acids 41–81) demonstrated the capability to both bind Aβ and significantly reduce amyloid deposition, outperforming the full-length protein (Sheng et al., 2021). Further, sTREM2 influences microglial morphology and sustains activation by delivering Fc-TREM2 to the hippocampus in both wild-type and Trem2 knockout mice (Zhong et al., 2017). This indicates a primary protective mechanism of sTREM2 against amyloid pathology through its interaction with Aβ.
In contrast, TREM2-deficient mice, crossed with APP mice, exhibited plaques that were more fibrous and less compact (Condello et al., 2015; Wang et al., 2016; Yuan et al., 2016; Song et al., 2018), a phenomenon previously attributed to diminished microglial plaque phagocytosis due to the absence of full-length TREM2. Alternatively, this could result from an impaired ability of sTREM2 to hinder Aβ aggregation or its reduced capacity to stimulate microglial plaque clearance. Upon the formation of the TREM2-Aβ complex, microglia are spurred into action, embarking on targeted phagocytosis to clear aggregated proteins while concurrently preventing the spread of plaques to adjacent healthy tissue. This is complemented by the activation of microglia around plaques to release inflammatory mediators and kick-start anti-plaque signaling mechanisms. Further, TREM2-deficient models show an increase in Aβ seeding (Parhizkar et al., 2019), potentially due to a decrease in microglial phagocytosis of Aβ seeds, a process facilitated by full-length TREM2, or possibly due to a lack of Aβ aggregation blockade by sTREM2. In models expressing human wild-type TREM2, sTREM2 has been observed in close association with amyloid plaques (Song et al., 2018), reinforcing its regulatory role in plaque management. Notably, both sTREM2 and its full-length counterpart share the ability to bind Aβ oligomers, though the comparative efficacy of these interactions in plaque regulation remains to be explored (Vilalta et al., 2021). Furthermore, individuals and mice harboring the R47H TREM2 variant exhibit plaques with increased fibrousness and neuritic pathology (Yuan et al., 2016), which could be explained by either the variant sTREM2 fostering Aβ fibrillation or a diminished microglial response to plaque phagocytosis. This nuanced understanding of sTREM2’s interplay with amyloid pathology not only elucidates its protective mechanisms but also opens avenues for therapeutic interventions targeting AD.
7.5 TREM2 and sTREM2: central players in neuroinflammation and tau pathology
AD is widely recognized not merely as a disorder of abnormal protein accumulation but also as one deeply characterized by extensive neuroinflammation (Liu et al., 2023; Cohen et al., 2024). Fundamental to understanding AD’s complexity is the role of TREM2, a key regulator in the immune response associated with this disease. Genetic studies have robustly demonstrated that TREM2 is instrumental in managing neuroinflammatory processes (Nicolas, 2024), and its activity is crucial even before the deposition of Aβ (Shen et al., 2024). The critical function of TREM2 begins with its ectodomain binding to a specific ligand, which triggers a sequence of events starting with proteolytic cleavage (Wunderlich et al., 2013). This cleavage allows the intracellular fragment of TREM2 to interact with DAP12, subsequently activating the Syk–PI3K/MAPK signaling pathway. This activation is essential as it promotes the release of IP3−gated Ca2+ stores that are vital for phagocytosis (Sasaki et al., 2015; Glebov et al., 2016). sTREM2 emerges as a significant modulator in this process. It plays a crucial role in microglial activation and significantly influences cytokine expression in THP-1 monocytes and macrophages through the MAPK–JNK signaling pathway. This modulation helps initiate pro-inflammatory responses at the early stages of AD and fosters anti-inflammatory activities in the later stages (Rauchmann et al., 2020; Pascoal et al., 2021; Arsenault et al., 2024). Moreover, alterations in peripheral sTREM2-related inflammatory markers such as Fibroblast Growth Factor 2 (FGF-2), Granulocyte-Macrophage Colony-Stimulating Factor (GM-CSF), and IL-1β significantly correlate with stages of AD, reflecting sTREM2 levels (Weber et al., 2022). In primary microglia from both wild-type and Trem2-knockout mice models, sTREM2 stimulates the production of inflammatory cytokines through the NF-κB pathway and promotes microglial survival via the Akt/GSK3β/β-catenin signaling pathway (Zhong et al., 2017, 2019). The diversity in microglial responses further complicates the immune landscape in AD. Microglia exhibit heterogeneity with M1 and M2 phenotypes, where M1 microglia are pro-inflammatory and cytotoxic, while M2 microglia provide anti-inflammatory and neuroprotective functions (Li and Zhang, 2018). Notably, overexpressing TREM2 in P301S tau transgenic mice has been shown to activate M2 microglia, which leads to a reduction in the release of pro-inflammatory cytokines, demonstrating TREM2’s therapeutic potential (Neumann and Takahashi, 2007; Jiang et al., 2016). Furthermore, the levels of sTREM2 in CSF serve as a dynamic biomarker for AD progression, reflecting microglial states and their engagement in clearing amyloid plaques and regulating inflammation (Kuchenbecker et al., 2024; Yin S. et al., 2024). This is particularly notable during the early symptomatic stages of AD. Additionally, studies have identified a close link between sTREM2 levels and specific CSF metabolites such as stearoyl sphingomyelin and palmitoyl sphingomyelin, which are involved in cellular signaling and potentially influence microglial activation (Dong et al., 2023). However, the role of TREM2 in AD presents a complex paradox. While TREM2’s activation generally supports anti-inflammatory processes, its deficiency or loss of function has been found to reduce neurodegeneration and mitigate tau pathology in some models, suggesting that its exact role may vary significantly depending on the pathological context (Leyns et al., 2017; Jiang et al., 2018; Sayed et al., 2018). Furthermore, research has shown that elevated sTREM2 levels correlate with increased phosphorylated tau, enhanced glucose metabolism, and decreased tau propagation in brain networks, highlighting sTREM2’s impact on both tau pathology and neuronal connectivity (Biel et al., 2023; Nabizadeh, 2023). Complex interactions continue to be explored, including how TREM2 and sTREM2 correlate with tau and phosphorylated tau (p-tau) levels in both CSF samples and post-mortem AD brain tissues, unaffected by the presence of Aβ (Cruchaga et al., 2013; Suárez-Calvet et al., 2019; Vogels et al., 2019). These findings suggest that TREM2 and sTREM2’s roles extend beyond simple inflammatory modulation to significant implications in tauopathy and overall disease progression. The roles of TREM2 and sTREM2 are further highlighted in other contexts such as long-COVID, where cognitive impairments associated with elevated IFNγ and IL-10 levels suggest a sustained inflammatory state that may perpetuate tissue damage and symptom severity (Besteher et al., 2024). Additionally, interactions with neuronal transgelin-2 (TG2) indicate novel pathways for mitigating tau phosphorylation and cognitive deficits, presenting new therapeutic possibilities for addressing neurodegeneration (Zhang et al., 2023b).
7.6 Synaptic roles of TREM2 and sTREM2: pruning and connectivity in the brain
TREM2 and sTREM2 are hypothesized to play a crucial role in synaptic functions within the brain. It’s suggested that TREM2’s ectodomain, or sTREM2, acts as an extracellular signal that binds to synaptic surface receptors, thereby guiding microglia during the synaptic pruning necessary for proper brain connectivity (Filipello et al., 2018). This involvement is pivotal in maintaining inter-neuronal signal transmission. However, the precise mechanisms by which TREM2 influences human synapses remain to be fully elucidated. Studies in AD mouse models have linked TREM2 deficiency with impairments in synaptic structure and cognitive decline, indicating that proper synaptic pruning, which is essential for normal cognition, is compromised under TREM2 deficiency. This leads to synaptic and axonal dystrophy, and a reduction in hippocampal microglia, which are crucial for synaptic pruning (Kajiwara et al., 2016; Bohlen et al., 2019; Jay et al., 2019; Sheng et al., 2019). Furthermore, TREM2 interacts with complement proteins such as C1q and C3, playing a significant role in the elimination of inappropriate synaptic connections during the maturation of neural circuits (Schafer et al., 2012; Stephan et al., 2012). Research by Woo et al. demonstrated that sTREM2 mediates synaptic loss independently before tau accumulation becomes evident. However, in later stages of the disease, when tau accumulation is visible, astrogliosis also contributes to synaptic damage (Woo et al., 2023). Moreover, elevated levels of sTREM2 in the CSF have been independently associated with presynaptic loss, as evidenced by increased Growth Associated Protein 43 (GAP-43) levels, suggesting that sTREM2-mediated microglial reactivity might drive synaptic dysfunction independently of β-amyloid and tau pathologies (Lan et al., 2024). Additionally, concentrations of various forms of sTREM2, reported in the CSF of AD patients, have been found to inhibit LTP independently of amyloid plaque presence and related pathology. Intriguingly, blocking Gamma-Aminobutyric Acid (GABA) receptors prevented the LTP-impairing actions of sTREM2, suggesting a dependency on the activation of these receptors, or that the removal of tonic GABA transmission could counteract the inhibitory impact of sTREM2 on neuronal excitability (Moutinho et al., 2023).
8 Concluding perspectives and future directions in TREM2 and sTREM2 research
The extensive evidence presented in this review underscores the pivotal roles of TREM2 and sTREM2 in the complex pathogenesis of AD. Their involvement in microglial function, neuroinflammation, and the modulation of amyloid and tau pathologies highlights their potential as therapeutic targets and biomarkers for disease progression. However, despite the significant progress made in understanding their functions, several key aspects of TREM2 and sTREM2 biology remain to be elucidated. In this concluding section, we summarize the main findings, discuss the challenges and opportunities, and outline the critical directions for future research in this field.
8.1 Overview of findings
TREM2, through its extracellular domain, binds to a variety of ligands, orchestrating a myriad of functions within the brain. These interactions not only sequester harmful substances but also activate the DAP12–Syk signaling pathway, potentially leading to an inflammatory response. Additionally, sTREM2 has the capacity to directly engage with pathogenic entities like Aβ or indirectly modulate inflammatory processes (Song et al., 2018; Karanfilian et al., 2020), even independently causes synaptic cleavage before tau accumulation appears. These insights underscore the pivotal roles of TREM2’s extracellular domain and sTREM2 in the evolution of AD, positioning them as promising targets for therapeutic intervention. However, the interplay between protein aggregation and neuroinflammation presents conflicting data (Mosley, 2015; Labzin et al., 2018), with some studies suggesting that misfolded Aβ fibrils disrupt brain homeostasis, while others argue that protein accumulation triggers inflammation. The initiating factor of AD—be it protein deposition or disturbed homeostasis—remains uncertain, though the involvement of the innate immune system is clear (Castro-Gomez and Heneka, 2024).
Elevated serum levels of sTREM2 may also serve as a promising biomarker for identifying mild cognitive impairment in patients with obstructive sleep apnea, correlating with lower cognitive performance as assessed by the Montreal Cognitive Assessment (Jiahuan et al., 2022). These findings advocate for sTREM2’s role in mediating microglial-associated inflammatory responses and suggest its potential as a protective agent in AD. Nonetheless, the regulatory effects of TREM2 on immune responses remain contentious, with some studies indicating that TREM2 may incite microglia to release neurotoxic pro-inflammatory cytokines, such as IL-1β, TNFα, and IL-6 (Nordengen et al., 2019). To reconcile these divergent results, an understanding of AD’s progression and TREM2’s impact on resident microglia is essential (Zhang et al., 2023a). At early and mid-stages of AD, TREM2 appears protective, but this role may pivot to a detrimental one by activating the adaptive immune system in the disease’s later phases (Suárez-Calvet et al., 2016b; Jay et al., 2017), reflecting the dynamic activation states of microglia throughout AD. sTREM2, acting as a decoy receptor, can induce the production of inflammatory cytokines, prompting microglial transformation and immune responses to counteract early-stage AD threats (Karanfilian et al., 2020).
In summary, TREM2 and sTREM2’s roles in AD pathogenesis are complex, involving ligand interactions, microglial function regulation, neuroinflammation modulation, and direct impacts on amyloid and tau pathologies. While evidence predominantly supports TREM2/sTREM2’s neuroprotective function in AD’s early stages, conflicting observations and unanswered questions about their actions, especially in later disease stages, persist. Future research is essential to clarify TREM2/sTREM2’s nuanced roles across AD’s spectrum and to verify their therapeutic viability.
8.2 Progress in the development of TREM2 and sTREM2-related drugs
Several companies had identified antibodies targeting the ectodomain of TREM2, which likely stimulate TREM2 signaling via crosslinking (Cheng et al., 2018; Cignarella et al., 2020; Price et al., 2020). These antibodies, such as AL002a/c developed by Alector, bind to the extracellular domain of TREM2 and activate downstream signaling pathways, including phosphorylation of DAP12 and Syk. In preclinical studies, these agonistic antibodies have been shown to enhance microglial survival, proliferation, and phagocytosis of myelin debris and Aβ.
Another approach involves preventing the shedding of the TREM2 extracellular domain by ADAM proteases using antibodies that bind to the stalk region near the cleavage site, such as clone 4D9 (Schlepckow et al., 2020). However, more work is required to identify a “therapeutic molecular signature” and functional outcomes stimulated by TREM2 agonistic antibodies. Additionally, it remains to be demonstrated whether CSF concentrations of sTREM2 can be used as a therapeutic biomarker, as recently proposed (Wang et al., 2020).
Several TREM2 agonists were in clinical. These included Denali’s DNL919 and Alector’s AL002 antibodies, which were being evaluated in Phase 1 and Phase 2 trials, respectively, though neither reported results at the AD/PD conference that year. Vigil Neuroscience, a company based in Watertown, Massachusetts, was evaluating its TREM2 agonist antibody, VGL101, in people with ALSP, a rare genetic disorder caused by a mutation in the CSF-1R gene. However, for AD—a much larger indication that requires chronic dosing—Vigil took a small-molecule approach. The company had originally acquired its TREM2 agonist antibody and a suite of TREM2 small-molecule agonists from Amgen in 2020, after Amgen discontinued its neuroscience programs. At the 2023 AD/PD conference, Vigil’s Christian Mirescu presented preclinical data on the company’s lead TREM2 agonists, which had been selected and optimized for brain permeability, oral availability, and potency. Mirescu claimed they worked like “molecular glue, “promoting the clustering of TREM2 receptors on the microglial cell surface. In cultured human microglia, this clustering boosted TREM2 signaling and reduced shedding. Vigil aimed to file an IND application for its lead TREM2 agonist. Before testing the agonists in the broader AD population, the company planned to evaluate them in people who carry loss-of-function mutations in TREM2 or other microglial genes.
8.3 Directions for future research
1. Development of Advanced Imaging Tools: There is a critical need for non-invasive imaging technologies capable of monitoring TREM2 and sTREM2 in living patients. Techniques such as PET imaging tagged to specific TREM2/sTREM2 antibodies could allow real-time observation of their distribution and activity, facilitating a better understanding of their roles throughout the progression of AD.
2. Longitudinal Cohort Studies: Implementing longitudinal studies that track TREM2 and sTREM2 levels alongside cognitive decline and other biomarkers of AD could clarify their roles as potential predictive or diagnostic tools. Such studies should aim to include a diverse participant pool to assess the impact of genetic backgrounds and environmental exposures on TREM2/sTREM2 dynamics.
3. Integrated Systems Biology Approaches: Utilizing systems biology to integrate data from genomics, proteomics, and metabolomics could help elucidate the complex interactions and regulatory networks involving TREM2 and sTREM2. This approach would also aid in identifying potential therapeutic targets within these pathways.
4. Clinical Trials Targeting TREM2/sTREM2 Pathways: Despite the theoretical benefits of modulating TREM2 and sTREM2 activity, fundamental research based on clinical studies and non-interventional approaches is necessary to evaluate the potential efficacy and safety of targeting TREM2/sTREM2 pathways. These studies should be designed to not only assess potential therapeutic outcomes but also to monitor any unintended effects on immune function within the brain and the circulatory system, given the roles of TREM2 and sTREM2 in neuroinflammation and systemic immune responses.
Author contributions
CL: Writing – original draft. YK: Writing – original draft. QC: Writing – original draft. JZ: Writing – original draft. XP: Writing – review & editing. JM: Writing – review & editing.
Funding
The author(s) declare that no financial support was received for the research, authorship, and/or publication of this article.
Acknowledgments
Figures were created with BioRender.com.
Conflict of interest
The authors declare that the research was conducted in the absence of any commercial or financial relationships that could be construed as a potential conflict of interest.
Publisher’s note
All claims expressed in this article are solely those of the authors and do not necessarily represent those of their affiliated organizations, or those of the publisher, the editors and the reviewers. Any product that may be evaluated in this article, or claim that may be made by its manufacturer, is not guaranteed or endorsed by the publisher.
References
Arsenault, R., Marshall, S., Salois, P., Li, Q., and Zhang, W. (2024). sTREM2 differentially affects cytokine expression in myeloid-derived cell models via MAPK–JNK signaling pathway. Biology (Basel). 13:87. doi: 10.3390/biology13020087
Atagi, Y., Liu, C.-C., Painter, M. M., Chen, X.-F., Verbeeck, C., Zheng, H., et al. (2015). Apolipoprotein E is a ligand for triggering receptor expressed on myeloid cells 2 (TREM2). J. Biol. Chem. 290, 26043–26050. doi: 10.1074/jbc.M115.679043
Bailey, C. C., DeVaux, L. B., and Farzan, M. (2015). The triggering receptor expressed on myeloid cells 2 binds apolipoprotein E. J. Biol. Chem. 290, 26033–26042. doi: 10.1074/jbc.M115.677286
Bandow, K., Smith, A., and Garlick, J. (2022). Soluble triggering receptor expressed on myeloid cells 2 (sTREM2) positively regulates lipopolysaccharide-induced expression of CXC chemokine ligand 10 and 11 in mouse macrophages. Biochem. Biophys. Res. Commun. 635, 227–235. doi: 10.1016/j.bbrc.2022.10.048
Berner, D. K., Wessolowski, L., Armbrust, F., Schneppenheim, J., Schlepckow, K., Koudelka, T., et al. (2020). Meprin β cleaves TREM2 and controls its phagocytic activity on macrophages. FASEB. J. Off. Publ. Fed. Am. Soc. Exp. Biol. 34, 6675–6687. doi: 10.1096/fj.201902183R
Besteher, B., Rocktäschel, T., Garza, A. P., Machnik, M., Ballez, J., Helbing, D. L., et al. (2024). Cortical thickness alterations and systemic inflammation define long-COVID patients with cognitive impairment. Brain Behav. Immun. 116, 175–184. doi: 10.1016/j.bbi.2023.11.028
Biel, D., Suárez-Calvet, M., Hager, P., Rubinski, A., Dewenter, A., Steward, A., et al. (2023). sTREM2 is associated with amyloid-related p-tau increases and glucose hypermetabolism in Alzheimer’s disease. EMBO Mol. Med. 15, e16987–e16913. doi: 10.15252/emmm.202216987
Bohlen, C. J., Friedman, B. A., Dejanovic, B., and Sheng, M. (2019). Microglia in brain development, homeostasis, and neurodegeneration. Annu. Rev. Genet. 53, 263–288. doi: 10.1146/annurev-genet-112618-043515
Casati, M., Ferri, E., Gussago, C., Mazzola, P., Abbate, C., Bellelli, G., et al. (2018). Increased expression of TREM2 in peripheral cells from mild cognitive impairment patients who progress into Alzheimer’s disease. Eur. J. Neurol. 25, 805–810. doi: 10.1111/ene.13583
Castro-Gomez, S., and Heneka, M. T. (2024). Innate immune activation in neurodegenerative diseases. Immunity 57, 790–814. doi: 10.1016/j.immuni.2024.03.010
Cheng, Q., Danao, J., Talreja, S., Wen, P., Yin, J., Sun, N., et al. (2018). TREM2-activating antibodies abrogate the negative pleiotropic effects of the Alzheimer's disease variant Trem2(R47H) on murine myeloid cell function. J. Biol. Chem. 293, 12620–12633. doi: 10.1074/jbc.RA118.001848
Cignarella, F., Filipello, F., Bollman, B., Cantoni, C., Locca, A., Mikesell, R., et al. (2020). TREM2 activation on microglia promotes myelin debris clearance and remyelination in a model of multiple sclerosis. Acta Neuropathol. 140, 513–534. doi: 10.1007/s00401-020-02193-z
Cohen, J., Mathew, A., Dourvetakis, K. D., Sanchez-Guerrero, E., Pangeni, R. P., Gurusamy, N., et al. (2024). Recent research trends in Neuroinflammatory and neurodegenerative disorders. Cells 13:511. doi: 10.3390/cells13060511
Condello, C., Yuan, P., Schain, A., and Grutzendler, J. (2015). Microglia constitute a barrier that prevents neurotoxic protofibrillar Aβ42 hotspots around plaques. Nat. Commun. 6:6176. doi: 10.1038/ncomms7176
Cooper-Knock, J., Green, C., Altschuler, G., Wei, W., Bury, J. J., Heath, P. R., et al. (2017). A data-driven approach links microglia to pathology and prognosis in amyotrophic lateral sclerosis. Acta Neuropathol. Commun. 5:23. doi: 10.1186/s40478-017-0424-x
Cruchaga, C., Kauwe, J. S. K., Harari, O., Jin, S. C., Cai, Y., Karch, C. M., et al. (2013). GWAS of cerebrospinal fluid tau levels identifies risk variants for Alzheimer’s disease. Neuron 78, 256–268. doi: 10.1016/j.neuron.2013.02.026
Daws, M. R., Sullam, P. M., Niemi, E. C., Chen, T. T., Tchao, N. K., and Seaman, W. E. (2003). Pattern recognition by TREM-2: binding of anionic ligands. J. Immunol. 171, 594–599. doi: 10.4049/jimmunol.171.2.594
de Gea, P., Benkeder, S., Bouvet, P., Aimard, M., Chounlamountri, N., Honnorat, J., et al. (2023). VEGF controls microglial phagocytic response to amyloid-β. Front. Cell. Neurosci. 17, 1–13. doi: 10.3389/fncel.2023.1264402
Deczkowska, A., Weiner, A., and Amit, I. (2020). The physiology, pathology, and potential therapeutic applications of the TREM2 signaling pathway. Cell 181, 1207–1217. doi: 10.1016/j.cell.2020.05.003
Del-Aguila, J. L., Benitez, B. A., Li, Z., Dube, U., Mihindukulasuriya, K. A., Budde, J. P., et al. (2019). TREM2 brain transcript-specific studies in AD and TREM2 mutation carriers. Mol. Neurodegener. 14:18. doi: 10.1186/s13024-019-0319-3
Deming, Y., Filipello, F., Cignarella, F., Cantoni, C., Hsu, S., Mikesell, R., et al. (2019). The MS4A gene cluster is a key modulator of soluble TREM2 and Alzheimer’s disease risk. Sci. Transl. Med. 11:eaau2291. doi: 10.1126/scitranslmed.aau2291
Diaz-Lucena, D., Kruse, N., Thüne, K., Schmitz, M., Villar-Piqué, A., da Cunha, J. E. G., et al. (2021). TREM2 expression in the brain and biological fluids in prion diseases. Acta Neuropathol. 141, 841–859. doi: 10.1007/s00401-021-02296-1
Dong, R., Lu, Q., Kang, H., Suridjan, I., Kollmorgen, G., Wild, N., et al. (2023). CSF metabolites associated with biomarkers of Alzheimer’s disease pathology. Front. Aging Neurosci. 15:1214932. doi: 10.3389/fnagi.2023.1214932
Ewers, M., Franzmeier, N., Suárez-Calvet, M., Morenas-Rodriguez, E., Caballero, M. A. A., Kleinberger, G., et al. (2019). Increased soluble TREM2 in cerebrospinal fluid is associated with reduced cognitive and clinical decline in Alzheimer’s disease. Sci. Transl. Med. 11:eaav6221. doi: 10.1126/scitranslmed.aav6221
Fahrenhold, M., Rakic, S., Classey, J., Brayne, C., Ince, P. G., Nicoll, J. A. R., et al. (2018). TREM2 expression in the human brain: a marker of monocyte recruitment? Brain Pathol. 28, 595–602. doi: 10.1111/bpa.12564
Ferkingstad, E., Sulem, P., Atlason, B. A., Sveinbjornsson, G., Magnusson, M. I., Styrmisdottir, E. L., et al. (2021). Large-scale integration of the plasma proteome with genetics and disease. Nat. Genet. 53, 1712–1721. doi: 10.1038/s41588-021-00978-w
Feuerbach, D., Schindler, P., Barske, C., Joller, S., Beng-Louka, E., Worringer, K. A., et al. (2017). ADAM17 is the main sheddase for the generation of human triggering receptor expressed in myeloid cells (hTREM2) ectodomain and cleaves TREM2 after histidine 157. Neurosci. Lett. 660, 109–114. doi: 10.1016/j.neulet.2017.09.034
Filipello, F., Morini, R., Corradini, I., Zerbi, V., Canzi, A., Michalski, B., et al. (2018). The microglial innate immune receptor TREM2 is required for synapse elimination and Normal brain connectivity. Immunity 48, 979–991.e8. doi: 10.1016/j.immuni.2018.04.016
Giannisis, A., Al-Grety, A., Carlsson, H., Howell, J. C., Hu, W. T., Kultima, K., et al. (2023). Plasma apolipoprotein E levels, isoform composition, and dimer profile in relation to plasma lipids in racially diverse patients with Alzheimer’s disease and mild cognitive impairment. Alzheimers Res. Ther. 15:119. doi: 10.1186/s13195-023-01262-1
Gispert, J. D., Suárez-Calvet, M., Monté, G. C., Tucholka, A., Falcon, C., Rojas, S., et al. (2016). Cerebrospinal fluid sTREM2 levels are associated with gray matter volume increases and reduced diffusivity in early Alzheimer’s disease. Alzheimers Dement. 12, 1259–1272. doi: 10.1016/j.jalz.2016.06.005
Gisslén, M., Heslegrave, A., Veleva, E., Yilmaz, A., Andersson, L.-M., Hagberg, L., et al. (2019). CSF concentrations of soluble TREM2 as a marker of microglial activation in HIV-1 infection. Neurol. Neuroimmunol. Neuroinflam. 6:e512. doi: 10.1212/NXI.0000000000000512
Glebov, K., Wunderlich, P., Karaca, I., and Walter, J. (2016). Functional involvement of γ-secretase in signaling of the triggering receptor expressed on myeloid cells-2 (TREM2). J. Neuroinflammation 13:17. doi: 10.1186/s12974-016-0479-9
Griciuc, A., and Tanzi, R. E. (2021). The role of innate immune genes in Alzheimer’s disease. Curr. Opin. Neurol. 34, 228–236. doi: 10.1097/WCO.0000000000000911
Gu, L., Shu, H., and Wang, Y. (2023). Soluble TREM2 in body fluid in Alzheimer’s disease and Parkinson’s disease. Neurol. Sci. 44, 2743–2751. doi: 10.1007/s10072-023-06729-5
Guerreiro, R., Wojtas, A., Bras, J., Carrasquillo, M., Rogaeva, E., Majounie, E., et al. (2013). TREM2 variants in Alzheimer’s disease. N. Engl. J. Med. 368, 117–127. doi: 10.1056/NEJMoa1211851
Hamerman, J. A., Jarjoura, J. R., Humphrey, M. B., Nakamura, M. C., Seaman, W. E., and Lanier, L. L. (2006). Cutting edge: inhibition of TLR and FcR responses in macrophages by triggering receptor expressed on myeloid cells (TREM)-2 and DAP12. J. Immunol. 177, 2051–2055. doi: 10.4049/jimmunol.177.4.2051
Han, S., Na, Y., Koh, I., Nho, K., and Lee, Y. (2021). Alternative splicing regulation of low-frequency genetic variants in exon 2 of TREM2 in Alzheimer’s disease by splicing-based aggregation. Int. J. Mol. Sci. 22:9865. doi: 10.3390/ijms22189865
Hansen, D. V., Hanson, J. E., and Sheng, M. (2018). Microglia in Alzheimer’s disease. J. Cell Biol. 217, 459–472. doi: 10.1083/jcb.201709069
Henjum, K., Almdahl, I. S., Årskog, V., Minthon, L., Hansson, O., Fladby, T., et al. (2016). Cerebrospinal fluid soluble TREM2 in aging and Alzheimer’s disease. Alzheimers Res. Ther. 8:17. doi: 10.1186/s13195-016-0182-1
Henjum, K., Quist-Paulsen, E., Zetterberg, H., Blennow, K., Nilsson, L. N. G., and Watne, L. O. (2018). CSF sTREM2 in delirium-relation to Alzheimer’s disease CSF biomarkers Aβ42, t-tau and p-tau. J. Neuroinflammation 15:304. doi: 10.1186/s12974-018-1331-1
Heslegrave, A., Heywood, W., Paterson, R., Magdalinou, N., Svensson, J., Johansson, P., et al. (2016). Increased cerebrospinal fluid soluble TREM2 concentration in Alzheimer’s disease. Mol. Neurodegener. 11:3. doi: 10.1186/s13024-016-0071-x
Hok-A-Hin, Y. S., del Campo, M., Boiten, W. A., Stoops, E., Vanhooren, M., Lemstra, A. W., et al. (2023). Neuroinflammatory CSF biomarkers MIF, sTREM1, and sTREM2 show dynamic expression profiles in Alzheimer’s disease. J. Neuroinflammation 20, 107–110. doi: 10.1186/s12974-023-02796-9
Holtzman, D. M., Morris, J. C., and Goate, A. M. (2011). Alzheimer’s disease: the challenge of the second century. Sci. Transl. Med. 3:77sr1. doi: 10.1126/scitranslmed.3002369
Hu, N., Tan, M.-S., Yu, J.-T., Sun, L., Tan, L., Wang, Y.-L., et al. (2014). Increased expression of TREM2 in peripheral blood of Alzheimer’s disease patients. J. Alzheimers Dis. 38, 497–501. doi: 10.3233/JAD-130854
Jay, T. R., Hirsch, A. M., Broihier, M. L., Miller, C. M., Neilson, L. E., Ransohoff, R. M., et al. (2017). Disease progression-dependent effects of TREM2 deficiency in a mouse model of Alzheimer’s disease. J. Neurosci. 37, 637–647. doi: 10.1523/JNEUROSCI.2110-16.2016
Jay, T. R., von Saucken, V. E., Muñoz, B., Codocedo, J. F., Atwood, B. K., Lamb, B. T., et al. (2019). TREM2 is required for microglial instruction of astrocytic synaptic engulfment in neurodevelopment. Glia 67, 1873–1892. doi: 10.1002/glia.23664
Jendresen, C., Årskog, V., Daws, M. R., and Nilsson, L. N. G. (2017). The Alzheimer’s disease risk factors apolipoprotein E and TREM2 are linked in a receptor signaling pathway. J. Neuroinflammation 14:59. doi: 10.1186/s12974-017-0835-4
Jiahuan, X., Ying, Z., Hongyu, J., Zhijing, W., Shibo, G., Chengyue, D., et al. (2022). Serum sTREM2: A potential biomarker for mild cognitive impairment in patients with obstructive sleep apnea. Front. Aging Neurosci. 14:843828. doi: 10.3389/fnagi.2022.843828
Jiang, T., Zhang, Y.-D., Chen, Q., Gao, Q., Zhu, X.-C., Zhou, J.-S., et al. (2016). TREM2 modifies microglial phenotype and provides neuroprotection in P301S tau transgenic mice. Neuropharmacology 105, 196–206. doi: 10.1016/j.neuropharm.2016.01.028
Jiang, T., Zhang, Y.-D., Gao, Q., Ou, Z., Gong, P.-Y., Shi, J.-Q., et al. (2018). TREM2 ameliorates neuronal tau pathology through suppression of microglial inflammatory response. Inflammation 41, 811–823. doi: 10.1007/s10753-018-0735-5
Jin, S. C., Benitez, B. A., Karch, C. M., Cooper, B., Skorupa, T., Carrell, D., et al. (2014). Coding variants in TREM2 increase risk for Alzheimer’s disease. Hum. Mol. Genet. 23, 5838–5846. doi: 10.1093/hmg/ddu277
Jonsson, T., Stefansson, H., Steinberg, S., Jonsdottir, I., Jonsson, P. V., Snaedal, J., et al. (2013). Variant of TREM2 associated with the risk of Alzheimer’s disease. N. Engl. J. Med. 368, 107–116. doi: 10.1056/NEJMoa1211103
Kajiwara, Y., McKenzie, A., Dorr, N., Gama Sosa, M. A., Elder, G., Schmeidler, J., et al. (2016). The human-specific CASP4 gene product contributes to Alzheimer-related synaptic and behavioural deficits. Hum. Mol. Genet. 25, 4315–4327. doi: 10.1093/hmg/ddw265
Kamboh, M. I., Demirci, F. Y., Wang, X., Minster, R. L., Carrasquillo, M. M., Pankratz, V. S., et al. (2012). Genome-wide association study of Alzheimer’s disease. Transl. Psychiatry 2:e117. doi: 10.1038/tp.2012.45
Karanfilian, L., Tosto, M. G., and Malki, K. (2020). The role of TREM2 in Alzheimer’s disease; evidence from transgenic mouse models. Neurobiol. Aging 86, 39–53. doi: 10.1016/j.neurobiolaging.2019.09.004
Keren-Shaul, H., Spinrad, A., Weiner, A., Matcovitch-Natan, O., Dvir-Szternfeld, R., Ulland, T. K., et al. (2017). A unique microglia type associated with restricting development of Alzheimer’s disease. Cell 169, 1276–1290.e17. doi: 10.1016/j.cell.2017.05.018
Kiianitsa, K., Kurtz, I., Beeman, N., Matsushita, M., Chien, W.-M., Raskind, W. H., et al. (2021). Novel TREM2 splicing isoform that lacks the V-set immunoglobulin domain is abundant in the human brain. J. Leukoc. Biol. 110, 829–837. doi: 10.1002/JLB.2HI0720-463RR
Kleinberger, G., Yamanishi, Y., Suárez-Calvet, M., Czirr, E., Lohmann, E., Cuyvers, E., et al. (2014). TREM2 mutations implicated in neurodegeneration impair cell surface transport and phagocytosis. Sci. Transl. Med. 6:243ra86. doi: 10.1126/scitranslmed.3009093
Klesney-Tait, J., Turnbull, I. R., and Colonna, M. (2006). The TREM receptor family and signal integration. Nat. Immunol. 7, 1266–1273. doi: 10.1038/ni1411
Knapskog, A.-B., Henjum, K., Idland, A.-V., Eldholm, R. S., Persson, K., Saltvedt, I., et al. (2020). Cerebrospinal fluid sTREM2 in Alzheimer’s disease: comparisons between clinical presentation and AT classification. Sci. Rep. 10:15886. doi: 10.1038/s41598-020-72878-8
Kober, D. L., Alexander-Brett, J. M., Karch, C. M., Cruchaga, C., Colonna, M., Holtzman, M. J., et al. (2016). Neurodegenerative disease mutations in TREM2 reveal a functional surface and distinct loss-of-function mechanisms. eLife 5:e20391. doi: 10.7554/eLife.20391
Kober, D. L., and Brett, T. J. (2017). TREM2-ligand interactions in health and disease. J. Mol. Biol. 429, 1607–1629. doi: 10.1016/j.jmb.2017.04.004
Kober, D. L., Stuchell-Brereton, M. D., Kluender, C. E., Dean, H. B., Strickland, M. R., Steinberg, D. F., et al. (2020). Functional insights from biophysical study of TREM2 interactions with apoE and Aβ(1-42). Alzheimers Dement. doi: 10.1002/alz.12194
Kuchenbecker, L. A., Tipton, P. W., Martens, Y., Brier, M. R., Satyadev, N., Dunham, S. R., et al. (2024). Diagnostic utility of cerebrospinal fluid biomarkers in patients with rapidly progressive dementia. Ann. Neurol. 95, 299–313. doi: 10.1002/ana.26822
La Rosa, F., Agostini, S., Piancone, F., Marventano, I., Hernis, A., Fenoglio, C., et al. (2023). TREM2 expression and amyloid-Beta phagocytosis in Alzheimer’s disease. Int. J. Mol. Sci. 24:8626. doi: 10.3390/ijms24108626
Labzin, L. I., Heneka, M. T., and Latz, E. (2018). Innate immunity and neurodegeneration. Annu. Rev. Med. 69, 437–449. doi: 10.1146/annurev-med-050715-104343
Lan, G., Chen, X., Yang, J., Sun, P., Cai, Y., Li, A., et al. (2024). Microglial reactivity correlates with presynaptic loss independent of β-amyloid and tau. Ann. Neurol. 95, 917–928. doi: 10.1002/ana.26885
Lessard, C. B., Malnik, S. L., Zhou, Y., Ladd, T. B., Cruz, P. E., Ran, Y., et al. (2018). High-affinity interactions and signal transduction between Aβ oligomers and TREM2. EMBO Mol. Med. 10:e9027. doi: 10.15252/emmm.201809027
Leyns, C. E. G., Ulrich, J. D., Finn, M. B., Stewart, F. R., Koscal, L. J., Remolina Serrano, J., et al. (2017). TREM2 deficiency attenuates neuroinflammation and protects against neurodegeneration in a mouse model of tauopathy. Proc. Natl. Acad. Sci. USA 114, 11524–11529. doi: 10.1073/pnas.1710311114
Li, M., Ma, Y. H., Fu, Y., Liu, J. Y., Hu, H. Y., Zhao, Y. L., et al. (2022). Association between air pollution and CSF sTREM2 in cognitively normal older adults: the CABLE study. Ann. Clin. Transl. Neurol. 9, 1752–1763. doi: 10.1002/acn3.51671
Li, Y., Yen, D., Hendrix, R. D., Gordon, B. A., Dlamini, S., Barthélemy, N. R., et al. (2024). Timing of biomarker changes in sporadic Alzheimer’s disease in estimated years from symptom onset. Ann. Neurol. 95, 951–965. doi: 10.1002/ana.26891
Li, J.-T., and Zhang, Y. (2018). TREM2 regulates innate immunity in Alzheimer’s disease. J. Neuroinflammation 15:107. doi: 10.1186/s12974-018-1148-y
Liu, D., Cao, B., Zhao, Y., Huang, H., McIntyre, R. S., Rosenblat, J. D., et al. (2018). Soluble TREM2 changes during the clinical course of Alzheimer’s disease: a meta-analysis. Neurosci. Lett. 686, 10–16. doi: 10.1016/j.neulet.2018.08.038
Liu, G., Zhang, L., Fan, Y., and Ji, W. (2023). The pathogenesis in Alzheimer’s disease: TREM2 as a potential target. J. Integr. Neurosci. 22:150. doi: 10.31083/j.jin2206150
Ma, L., Allen, M., Sakae, N., Ertekin-Taner, N., Graff-Radford, N. R., Dickson, D. W., et al. (2016). Expression and processing analyses of wild type and p.R47H TREM2 variant in Alzheimer’s disease brains. Mol. Neurodegener. 11:72. doi: 10.1186/s13024-016-0137-9
Moore, E. E., Liu, D., Li, J., Schimmel, S. J., Cambronero, F. E., Terry, J. G., et al. (2021). Association of Aortic Stiffness with Biomarkers of Neuroinflammation, synaptic dysfunction, and neurodegeneration. Neurology 97, e329–e340. doi: 10.1212/WNL.0000000000012257
Mosley, R. L. (2015). Adaptive immunity in neurodegenerative and neuropsychological disorders. J. Neuroimmune Pharmacol. 10, 522–527. doi: 10.1007/s11481-015-9640-y
Moutinho, M., Coronel, I., Tsai, A. P., Di Prisco, G. V., Pennington, T., Atwood, B. K., et al. (2023). TREM2 splice isoforms generate soluble TREM2 species that disrupt long-term potentiation. Genome Med. 15, 11–16. doi: 10.1186/s13073-023-01160-z
Nabizadeh, F. (2023). sTREM2 is associated with attenuated tau aggregate accumulation in the presence of amyloid-β pathology. Brain Commun. 5, 1–13. doi: 10.1093/braincomms/fcad286
Neumann, H., and Takahashi, K. (2007). Essential role of the microglial triggering receptor expressed on myeloid cells-2 (TREM2) for central nervous tissue immune homeostasis. J. Neuroimmunol. 184, 92–99. doi: 10.1016/j.jneuroim.2006.11.032
Nicolas, G. (2024). Lessons from genetic studies in Alzheimer disease. Rev. Neurol. (Paris) 180, 368–377. doi: 10.1016/j.neurol.2023.12.006
Nordengen, K., Kirsebom, B.-E., Henjum, K., Selnes, P., Gísladóttir, B., Wettergreen, M., et al. (2019). Glial activation and inflammation along the Alzheimer’s disease continuum. J. Neuroinflammation 16:46. doi: 10.1186/s12974-019-1399-2
Nugent, A. A., Lin, K., van Lengerich, B., Lianoglou, S., Przybyla, L., Davis, S. S., et al. (2020). TREM2 regulates microglial cholesterol metabolism upon chronic phagocytic challenge. Neuron 105, 837–854.e9. doi: 10.1016/j.neuron.2019.12.007
Paolini Paoletti, F., Gaetani, L., Bellomo, G., Chipi, E., Salvadori, N., Montanucci, C., et al. (2023). CSF neurochemical profile and cognitive changes in Parkinson’s disease with mild cognitive impairment. NPJ Park. Dis. 9:68. doi: 10.1038/s41531-023-00509-w
Parhizkar, S., Arzberger, T., Brendel, M., Kleinberger, G., Deussing, M., Focke, C., et al. (2019). Loss of TREM2 function increases amyloid seeding but reduces plaque-associated ApoE. Nat. Neurosci. 22, 191–204. doi: 10.1038/s41593-018-0296-9
Park, J.-S., Ji, I. J., An, H. J., Kang, M.-J., Kang, S.-W., Kim, D.-H., et al. (2015). Disease-associated mutations of TREM2 Alter the processing of N-linked oligosaccharides in the Golgi apparatus. Traffic 16, 510–518. doi: 10.1111/tra.12264
Pascoal, T. A., Benedet, A. L., Ashton, N. J., Kang, M. S., Therriault, J., Chamoun, M., et al. (2021). Microglial activation and tau propagate jointly across Braak stages. Nat. Med. 27, 1592–1599. doi: 10.1038/s41591-021-01456-w
Piccio, L., Buonsanti, C., Cella, M., Tassi, I., Schmidt, R. E., Fenoglio, C., et al. (2008). Identification of soluble TREM-2 in the cerebrospinal fluid and its association with multiple sclerosis and CNS inflammation. Brain 131, 3081–3091. doi: 10.1093/brain/awn217
Piccio, L., Buonsanti, C., Mariani, M., Cella, M., Gilfillan, S. C., Cross, A. . H., et al. (2007). Blockade of TREM-2 exacerbates experimental autoimmune encephalomyelitis. Eur. J. Immunol. 37, 1290–1301. doi: 10.1002/eji.200636837
Piccio, L., Deming, Y., Del-Águila, J. L., Ghezzi, L., Holtzman, D. M., Fagan, A. M., et al. (2016). Cerebrospinal fluid soluble TREM2 is higher in Alzheimer disease and associated with mutation status. Acta Neuropathol. 131, 925–933. doi: 10.1007/s00401-016-1533-5
Poliani, P., Wang, Y., Fontana, E., Robinette, M. L., Yamanishi, Y., Gilfillan, S., et al. (2015). TREM2 sustains microglial expansion during aging and response to demyelination. J. Clin. Invest. 125, 2161–2170. doi: 10.1172/JCI77983
Price, B. R., Sudduth, T. L., Weekman, E. M., Johnson, S., Hawthorne, D., Woolums, A., et al. (2020). Therapeutic Trem2 activation ameliorates amyloid-beta deposition and improves cognition in the 5XFAD model of amyloid deposition. J. Neuroinflammation 17:238. doi: 10.1186/s12974-020-01915-0
Pugazhenthi, S., Qin, L., and Reddy, P. H. (2017). Common neurodegenerative pathways in obesity, diabetes, and Alzheimer’s disease. Biochim. Biophys. Acta Mol. basis Dis. 1863, 1037–1045. doi: 10.1016/j.bbadis.2016.04.017
Qin, Q., Wan, H., Wang, D., Li, J., Qu, Y., Zhao, J., et al. (2022). The association of CSF sTREM2 with cognitive decline and its dynamic change in Parkinson’s disease: analysis of the PPMI cohort. Front. Aging Neurosci. 14, 1–11. doi: 10.3389/fnagi.2022.892493
Raha-Chowdhury, R., Henderson, J. W., Raha, A. A., Vuono, R., Bickerton, A., Jones, E., et al. (2019). Choroid plexus acts as gatekeeper for TREM2, abnormal accumulation of ApoE, and fibrillary tau in Alzheimer’s disease and in down syndrome dementia. J. Alzheimers Dis. 69, 91–109. doi: 10.3233/JAD-181179
Rauchmann, B.-S., Sadlon, A., and Perneczky, R. (2020). Soluble TREM2 and inflammatory proteins in Alzheimer’s disease cerebrospinal fluid. J. Alzheimers Dis. 73, 1615–1626. doi: 10.3233/JAD-191120
Rauchmann, B.-S., Schneider-Axmann, T., Alexopoulos, P., and Perneczky, R. (2019). CSF soluble TREM2 as a measure of immune response along the Alzheimer’s disease continuum. Neurobiol. Aging 74, 182–190. doi: 10.1016/j.neurobiolaging.2018.10.022
Sasaki, A., Kakita, A., Yoshida, K., Konno, T., Ikeuchi, T., Hayashi, S., et al. (2015). Variable expression of microglial DAP12 and TREM2 genes in Nasu-Hakola disease. Neurogenetics 16, 265–276. doi: 10.1007/s10048-015-0451-3
Sayed, F. A., Telpoukhovskaia, M., Kodama, L., Li, Y., Zhou, Y., Le, D., et al. (2018). Differential effects of partial and complete loss of TREM2 on microglial injury response and tauopathy. Proc. Natl. Acad. Sci. USA 115, 10172–10177. doi: 10.1073/pnas.1811411115
Schafer, D. P., Lehrman, E. K., Kautzman, A. G., Koyama, R., Mardinly, A. R., Yamasaki, R., et al. (2012). Microglia sculpt postnatal neural circuits in an activity and complement-dependent manner. Neuron 74, 691–705. doi: 10.1016/j.neuron.2012.03.026
Schindler, S. E., Cruchaga, C., Joseph, A., McCue, L., Farias, F. H. G., Wilkins, C. H., et al. (2021). African Americans have differences in CSF soluble TREM2 and associated genetic variants. Neurol. Genet. 7:e571. doi: 10.1212/NXG.0000000000000571
Schlepckow, K., Kleinberger, G., Fukumori, A., Feederle, R., Lichtenthaler, S. F., Steiner, H., et al. (2017). An Alzheimer-associated TREM2 variant occurs at the ADAM cleavage site and affects shedding and phagocytic function. EMBO Mol. Med. 9, 1356–1365. doi: 10.15252/emmm.201707672
Schlepckow, K., Monroe, K. M., Kleinberger, G., Cantuti-Castelvetri, L., Parhizkar, S., Xia, D., et al. (2020). Enhancing protective microglial activities with a dual function TREM2 antibody to the stalk region. EMBO Mol. Med. 12:e11227. doi: 10.15252/emmm.201911227
Shen, Z., Yang, X., Lan, Y., and Chen, G. (2024). The neuro-inflammatory microenvironment: An important regulator of stem cell survival in Alzheimer’s disease. J. Alzheimers Dis. 98, 741–754. doi: 10.3233/JAD-231159
Sheng, L., Chen, M., Cai, K., Song, Y., Yu, D., Zhang, H., et al. (2019). Microglial Trem2 induces synaptic impairment at early stage and prevents amyloidosis at late stage in APP/PS1 mice. FASEB J. Off. Publ. Fed. Am. Soc. Exp. Biol. 33, 10425–10442. doi: 10.1096/fj.201900527R
Sheng, X., Yao, Y., Huang, R., Xu, Y., Zhu, Y., Chen, L., et al. (2021). Identification of the minimal active soluble TREM2 sequence for modulating microglial phenotypes and amyloid pathology. J. Neuroinflammation 18:286. doi: 10.1186/s12974-021-02340-7
Shi, X., Zhong, X., Zhou, H., Zhou, N., Hu, Y., and Ning, Y. (2022). The association between cerebrospinal ferritin and soluble triggering receptor expressed on myeloid cells 2 along Alzheimer’s continuum. Front. Neurol. 13:961842. doi: 10.3389/fneur.2022.961842
Song, W., Hooli, B., Mullin, K., Jin, S. C., Cella, M., Ulland, T. K., et al. (2017). Alzheimer’s disease-associated TREM2 variants exhibit either decreased or increased ligand-dependent activation. Alzheimers Dement. 13, 381–387. doi: 10.1016/j.jalz.2016.07.004
Song, W. M., Joshita, S., Zhou, Y., Ulland, T. K., Gilfillan, S., and Colonna, M. (2018). Humanized TREM2 mice reveal microglia-intrinsic and -extrinsic effects of R47H polymorphism. J. Exp. Med. 215, 745–760. doi: 10.1084/jem.20171529
Španić Popovački, E., Babić Leko, M., Langer Horvat, L., Brgić, K., Vogrinc, Ž., Boban, M., et al. (2023). Soluble TREM2 concentrations in the cerebrospinal fluid correlate with the severity of neurofibrillary degeneration, cognitive impairment, and inflammasome activation in Alzheimer’s disease. Neurol. Int. 15, 842–856. doi: 10.3390/neurolint15030053
Stephan, A. H., Barres, B. A., and Stevens, B. (2012). The complement system: an unexpected role in synaptic pruning during development and disease. Annu. Rev. Neurosci. 35, 369–389. doi: 10.1146/annurev-neuro-061010-113810
Suárez-Calvet, M., Araque Caballero, M. Á., Kleinberger, G., Bateman, R. J., Fagan, A. M., Morris, J. C., et al. (2016a). Early changes in CSF sTREM2 in dominantly inherited Alzheimer’s disease occur after amyloid deposition and neuronal injury. Sci. Transl. Med. 8:369ra178. doi: 10.1126/scitranslmed.aag1767
Suárez-Calvet, M., Kleinberger, G., Araque Caballero, M. Á., Brendel, M., Rominger, A., Alcolea, D., et al. (2016b). sTREM2 cerebrospinal fluid levels are a potential biomarker for microglia activity in early-stage Alzheimer’s disease and associate with neuronal injury markers. EMBO Mol. Med. 8, 466–476. doi: 10.15252/emmm.201506123
Suárez-Calvet, M., Morenas-Rodríguez, E., Kleinberger, G., Schlepckow, K., Araque Caballero, M. Á., Franzmeier, N., et al. (2019). Early increase of CSF sTREM2 in Alzheimer’s disease is associated with tau related-neurodegeneration but not with amyloid-β pathology. Mol. Neurodegener. 14:1. doi: 10.1186/s13024-018-0301-5
Szykowska, A., Chen, Y., Smith, T. B., Preger, C., Yang, J., Qian, D., et al. (2021). Selection and structural characterization of anti-TREM2 scFvs that reduce levels of shed ectodomain. Structure 29, 1241–1252.e5. doi: 10.1016/j.str.2021.06.010
Tan, Y. J., Ng, A. S. L., Vipin, A., Lim, J. K. W., Chander, R. J., Ji, F., et al. (2017). Higher peripheral TREM2 mRNA levels relate to cognitive deficits and hippocampal atrophy in Alzheimer’s disease and amnestic mild cognitive impairment. J. Alzheimers Dis. 58, 413–423. doi: 10.3233/JAD-161277
Tanaka, M., Yamakage, H., Masuda, S., Inoue, T., Ohue-Kitano, R., Araki, R., et al. (2019). Serum soluble TREM2 is a potential novel biomarker of cognitive impairment in Japanese non-obese patients with diabetes. Diabetes Metab. 45, 86–89. doi: 10.1016/j.diabet.2017.06.006
Tanaka, M., Yamakage, H., Muranaka, K., Yamada, T., Araki, R., Ogo, A., et al. (2022). Higher serum soluble TREM2 as a potential indicative biomarker for cognitive impairment in inadequately controlled type 2 diabetes without obesity: the DOR-KyotoJ-1. Front. Endocrinol. 13, 1–10. doi: 10.3389/fendo.2022.880148
Thornton, P., Sevalle, J., Deery, M. J., Fraser, G., Zhou, Y., Ståhl, S., et al. (2017). TREM2 shedding by cleavage at the H157-S158 bond is accelerated for the Alzheimer’s disease-associated H157Y variant. EMBO Mol. Med. 9, 1366–1378. doi: 10.15252/emmm.201707673
Ulland, T. K., Song, W. M., Huang, S. C.-C., Ulrich, J. D., Sergushichev, A., Beatty, W. L., et al. (2017). TREM2 maintains microglial metabolic fitness in Alzheimer’s disease. Cell 170, 649–663.e13. doi: 10.1016/j.cell.2017.07.023
Vilalta, A., Zhou, Y., Sevalle, J., Griffin, J. K., Satoh, K., Allendorf, D. H., et al. (2021). Wild-type sTREM2 blocks Aβ aggregation and neurotoxicity, but the Alzheimer’s R47H mutant increases Aβ aggregation. J. Biol. Chem. 296:100631. doi: 10.1016/j.jbc.2021.100631
Vogels, T., Murgoci, A.-N., and Hromádka, T. (2019). Intersection of pathological tau and microglia at the synapse. Acta Neuropathol. Commun. 7:109. doi: 10.1186/s40478-019-0754-y
Wang, Y., Cella, M., Mallinson, K., Ulrich, J. D., Young, K. L., Robinette, M. L., et al. (2015). TREM2 lipid sensing sustains the microglial response in an Alzheimer’s disease model. Cell 160, 1061–1071. doi: 10.1016/j.cell.2015.01.049
Wang, S., and Colonna, M. (2019). Microglia in Alzheimer’s disease: a target for immunotherapy. J. Leukoc. Biol. 106, 219–227. doi: 10.1002/JLB.MR0818-319R
Wang, Z. T., Fu, Y., Chen, S. D., Huang, Y. Y., Ma, Y. H., Wang, Y. J., et al. (2023). Association of rs2062323 in the TREM1 gene with Alzheimer’s disease and cerebrospinal fluid-soluble TREM2. CNS Neurosci. Ther. 29, 1657–1666. doi: 10.1111/cns.14129
Wang, Z. B., Ma, Y. H., Sun, Y., Tan, L., Wang, H. F., and Yu, J. T. (2022a). Interleukin-3 is associated with sTREM2 and mediates the correlation between amyloid-β and tau pathology in Alzheimer’s disease. J. Neuroinflammation 19, 316–311. doi: 10.1186/s12974-022-02679-5
Wang, S., Mustafa, M., Yuede, C. M., Salazar, S. V., Kong, P., Long, H., et al. (2020). Anti-human TREM2 induces microglia proliferation and reduces pathology in an Alzheimer’s disease model. J. Exp. Med. 217:e20200785. doi: 10.1084/jem.20200785
Wang, L., Nykänen, N. P., Western, D., Gorijala, P., Timsina, J., Li, F., et al. (2024). Proteo-genomics of soluble TREM2 in cerebrospinal fluid provides novel insights and identifies novel modulators for Alzheimer’s disease. Mol. Neurodegener. 19, 1–25. doi: 10.1186/s13024-023-00687-4
Wang, Z. B., Sun, Y., Ma, Y. H., Fu, Y., Hu, H., Xu, W., et al. (2022b). sTREM2 mediates the associations of minimal depressive symptoms with amyloid pathology in prodromal Alzheimer’s disease: the CABLE study. Transl. Psychiatry 12, 140–147. doi: 10.1038/s41398-022-01910-4
Wang, Y., Ulland, T. K., Ulrich, J. D., Song, W., Tzaferis, J. A., Hole, J. T., et al. (2016). TREM2-mediated early microglial response limits diffusion and toxicity of amyloid plaques. J. Exp. Med. 213, 667–675. doi: 10.1084/jem.20151948
Weber, G. E., Khrestian, M., Tuason, E. D., Shao, Y., Pillai, J., Rao, S., et al. (2022). Peripheral sTREM2-related inflammatory activity alterations in early-stage Alzheimer’s disease. J. Immunol. 208, 2283–2299. doi: 10.4049/jimmunol.2100771
Wilson, E. N., Swarovski, M. S., Linortner, P., Shahid, M., Zuckerman, A. J., Wang, Q., et al. (2020). Soluble TREM2 is elevated in Parkinson’s disease subgroups with increased CSF tau. Brain 143, 932–943. doi: 10.1093/brain/awaa021
Winfree, R. L., Nolan, E., Dumitrescu, L., Blennow, K., Zetterberg, H., Gifford, K. A., et al. (2024). Variants in the MS4A cluster interact with soluble TREM2 expression on biomarkers of neuropathology. Mol. Neurodegener. 19:41. doi: 10.1186/s13024-024-00727-7
Woo, M. S., Nilsson, J., Therriault, J., Rahmouni, N., Brinkmalm, A., Benedet, A. L., et al. (2023). 14-3-3 ζ/δ -reported early synaptic injury in Alzheimer’s disease is independently mediated by sTREM2. J. Neuroinflammation 20, 1–12. doi: 10.1186/s12974-023-02962-z
Wu, K., Byers, D. E., Jin, X., Agapov, E., Alexander-Brett, J., Patel, A. C., et al. (2015). TREM-2 promotes macrophage survival and lung disease after respiratory viral infection. J. Exp. Med. 212, 681–697. doi: 10.1084/jem.20141732
Wunderlich, P., Glebov, K., Kemmerling, N., Tien, N. T., Neumann, H., and Walter, J. (2013). Sequential proteolytic processing of the triggering receptor expressed on myeloid cells-2 (TREM2) protein by ectodomain shedding and γ-secretase-dependent intramembranous cleavage. J. Biol. Chem. 288, 33027–33036. doi: 10.1074/jbc.M113.517540
Yang, X., Wen, J., Yang, H., Jones, I. R., Zhu, X., Liu, W., et al. (2023). Functional characterization of Alzheimer’s disease genetic variants in microglia. Nat. Genet. 55, 1735–1744. doi: 10.1038/s41588-023-01506-8
Yeh, F. L., Wang, Y., Tom, I., Gonzalez, L. C., and Sheng, M. (2016). TREM2 binds to apolipoproteins, including APOE and CLU/APOJ, and thereby facilitates uptake of amyloid-Beta by microglia. Neuron 91, 328–340. doi: 10.1016/j.neuron.2016.06.015
Yin, S., Gao, P. Y., Ou, Y. N., Fu, Y., Liu, Y., Wang, Z. T., et al. (2024). ANU-ADRI scores, tau pathology, and cognition in non-demented adults: the CABLE study. Alzheimers Res. Ther. 16, 65–11. doi: 10.1186/s13195-024-01427-6
Yin, T., Yesiltepe, M., and D’Adamio, L. (2024). Functional BRI2-TREM2 interactions in microglia: implications for Alzheimer’s and related dementias. EMBO Rep. 25, 1326–1360. doi: 10.1038/s44319-024-00077-x
Yuan, P., Condello, C., Keene, C. D., Wang, Y., Bird, T. D., Paul, S. M., et al. (2016). TREM2 Haplodeficiency in mice and humans impairs the microglia barrier function leading to decreased amyloid compaction and severe axonal dystrophy. Neuron 90, 724–739. doi: 10.1016/j.neuron.2016.05.003
Zhang, X., Chen, X., Zhang, L., Sun, Y., Liang, Y., Li, H., et al. (2023a). Role of trigger receptor 2 expressed on myeloid cells in neuroinflammation-neglected multidimensional regulation of microglia. Neurochem. Int. 171:105639. doi: 10.1016/j.neuint.2023.105639
Zhang, X., Tang, L., Yang, J., Meng, L., Chen, J., Zhou, L., et al. (2023b). Soluble TREM2 ameliorates tau phosphorylation and cognitive deficits through activating transgelin-2 in Alzheimer’s disease. Nat. Commun. 14:6670. doi: 10.1038/s41467-023-42505-x
Zhao, Y., Wu, X., Li, X., Jiang, L.-L., Gui, X., Liu, Y., et al. (2018). TREM2 is a receptor for β-amyloid that mediates microglial function. Neuron 97, 1023–1031.e7. doi: 10.1016/j.neuron.2018.01.031
Zhong, L., Chen, X.-F., Wang, T., Wang, Z., Liao, C., Wang, Z., et al. (2017). Soluble TREM2 induces inflammatory responses and enhances microglial survival. J. Exp. Med. 214, 597–607. doi: 10.1084/jem.20160844
Zhong, L., Wang, Z., Wang, D., Wang, Z., Martens, Y. A., Wu, L., et al. (2018). Amyloid-beta modulates microglial responses by binding to the triggering receptor expressed on myeloid cells 2 (TREM2). Mol. Neurodegener. 13:15. doi: 10.1186/s13024-018-0247-7
Zhong, L., Xu, Y., Zhuo, R., Wang, T., Wang, K., Huang, R., et al. (2019). Soluble TREM2 ameliorates pathological phenotypes by modulating microglial functions in an Alzheimer’s disease model. Nat. Commun. 10, 1365–1316. doi: 10.1038/s41467-019-09118-9
Zhou, H., Li, J., Hu, L., Yu, J., Fu, X., Liang, F., et al. (2023). Soluble Trem2 is a negative regulator of erythrophagocytosis after intracerebral hemorrhage in a CD36 receptor recycling manner. J. Adv. Res. 44, 185–199. doi: 10.1016/j.jare.2022.03.011
Zhou, S.-L., Tan, C.-C., Hou, X.-H., Cao, X.-P., Tan, L., and Yu, J.-T. (2019). TREM2 variants and neurodegenerative diseases: A systematic review and Meta-analysis. J. Alzheimers Dis. 68, 1171–1184. doi: 10.3233/JAD-181038
Keywords: sTREM2, Alzheimer’s disease, neuroinflammation, microglial activation, TREM2
Citation: Lin C, Kong Y, Chen Q, Zeng J, Pan X and Miao J (2024) Decoding sTREM2: its impact on Alzheimer’s disease – a comprehensive review of mechanisms and implications. Front. Aging Neurosci. 16:1420731. doi: 10.3389/fnagi.2024.1420731
Edited by:
DuoYao Cao, Cedars-Sinai Medical Center, United StatesReviewed by:
Minhong Neenah Huang, Mayo Clinic, United StatesEnrica Caterina Pietronigro, University of Verona, Italy
Copyright © 2024 Lin, Kong, Chen, Zeng, Pan and Miao. This is an open-access article distributed under the terms of the Creative Commons Attribution License (CC BY). The use, distribution or reproduction in other forums is permitted, provided the original author(s) and the copyright owner(s) are credited and that the original publication in this journal is cited, in accordance with accepted academic practice. No use, distribution or reproduction is permitted which does not comply with these terms.
*Correspondence: Xiaojin Pan, cGFueGlhb2ppbkBzemJhenl5Lm5ldA==; Jifei Miao, MzU4NTkxOTUzQHFxLmNvbQ==