- 1Department of Neurosurgery, Western Theater General Hospital, Chengdu, Sichuan, China
- 2Department of Neurosurgery, The Affiliated Hospital of Southwest Medical University, Luzhou, Sichuan, China
- 3Department of Pediatrics, Western Theater General Hospital, Chengdu, Sichuan, China
- 4Department of Neurosurgery, Meishan City People's Hospital, Meishan, Sichuan, China
- 5Department of Burn and Plastic, Western Theater General Hospital, Chengdu, Sichuan, China
The blood–brain barrier (BBB) is pivotal in maintaining neuronal physiology within the brain. This review delves into the alterations of the BBB specifically in the context of geriatric epilepsy. We examine how age-related changes in the BBB contribute to the pathogenesis of epilepsy in the elderly and present significant challenges in pharmacotherapy. Subsequently, we evaluate recent advancements in drug delivery methods targeting the BBB, as well as alternative approaches that could bypass the BBB’s restrictive nature. We particularly highlight the use of neurotropic viruses and various synthetic nanoparticles that have been investigated for delivering a range of antiepileptic drugs. Additionally, the advantage and limitation of these diverse delivery methods are discussed. Finally, we analyze the potential efficacy of different drug delivery approaches in the treatment of geriatric epilepsy, aiming to provide insights into more effective management of this condition in the elderly population.
1 Introduction
Geriatric epilepsy, characterized by an onset at or beyond the age of 65 years (Cloyd et al., 2006), presents a distinct epidemiological pattern. Epidemiological studies have delineated a bimodal distribution of epilepsy incidence, observing heightened rates in both young children and the elderly. Notably, the incidence of epilepsy escalates progressively post-50 years of age, reaching its zenith in individuals aged 75 and above (Hauser et al., 1993; Collaborators, 2019). In elderly patients, seizures are typically localized to specific brain regions, with convulsive symptoms being comparatively infrequent (Godfrey, 1989). This often leads to misdiagnoses of seizures as fainting or transient loss of consciousness in older adults (Ramsay et al., 2004). Such patients are at an increased risk of cognitive decline and psychiatric complications, factors that augment the likelihood of status epilepticus and related mortality (DeLorenzo et al., 1996; Martin et al., 2005).
Furthermore, geriatric populations exhibit heightened sensitivity to antiepileptic drugs, necessitating lower initial dosages and a more meticulous approach to medication regimen (Werhahn et al., 2011). Cerebrovascular disease is identified as the predominant cause of epilepsy in the elderly (Hauser et al., 1993), while blood–brain barrier (BBB) dysfunction emerges as a common pathological feature in this cohort (van Vliet and Marchi, 2022). Alterations in BBB functionality significantly influence drug delivery to the brain (Movahedpour et al., 2023), underscoring the need for a nuanced understanding of BBB dynamics in the context of elderly epilepsy treatment.
This review aims to elucidate the structure and function of the BBB and its role in the pathogenesis of geriatric epilepsy. It will also examine the impact of BBB on pharmacotherapy in elderly epileptic patients. Finally, the paper will explore innovative approaches to antiepileptic therapy, particularly strategies aimed at mitigating or circumventing BBB challenges, thereby offering fresh perspectives and strategies for treating geriatric epilepsy.
2 The structure and function of BBB
Comprehensive understanding of the BBB’s structure and the regulatory mechanisms of its permeability is paramount for developing diagnostic tools to assess BBB integrity and therapeutic interventions aimed at preserving or restoring normal BBB function in CNS diseases. The BBB is a critical selective barrier (Figure 1), constituted by brain endothelial cells, which regulates molecular exchanges between the bloodstream and neural tissue (Abbott et al., 2006). Its functionality hinges on the integrity of the neurovascular unit, comprising endothelial cells, pericytes, astrocytes, neurons, and the basement membrane (Sá-Pereira et al., 2012; Sweeney et al., 2016; Wang et al., 2021). Structurally, the BBB primarily consists of brain microvascular endothelial cells (BMECs) interconnected by tight junctions, adherens junctions, and gap junctions (Beard Jr et al., 2011; Spéder and Brand, 2014; Li et al., 2015). These intercellular junctional complexes significantly restrict the paracellular diffusion of solutes (Petty and Lo, 2002). Moreover, brain pericytes, astrocytic end-feet, neurons, and the extracellular matrix coalesce to form the neurovascular unit alongside BMECs, playing a pivotal role in inducing BBB properties during development and maintaining its integrity in adulthood (Kadry et al., 2020).
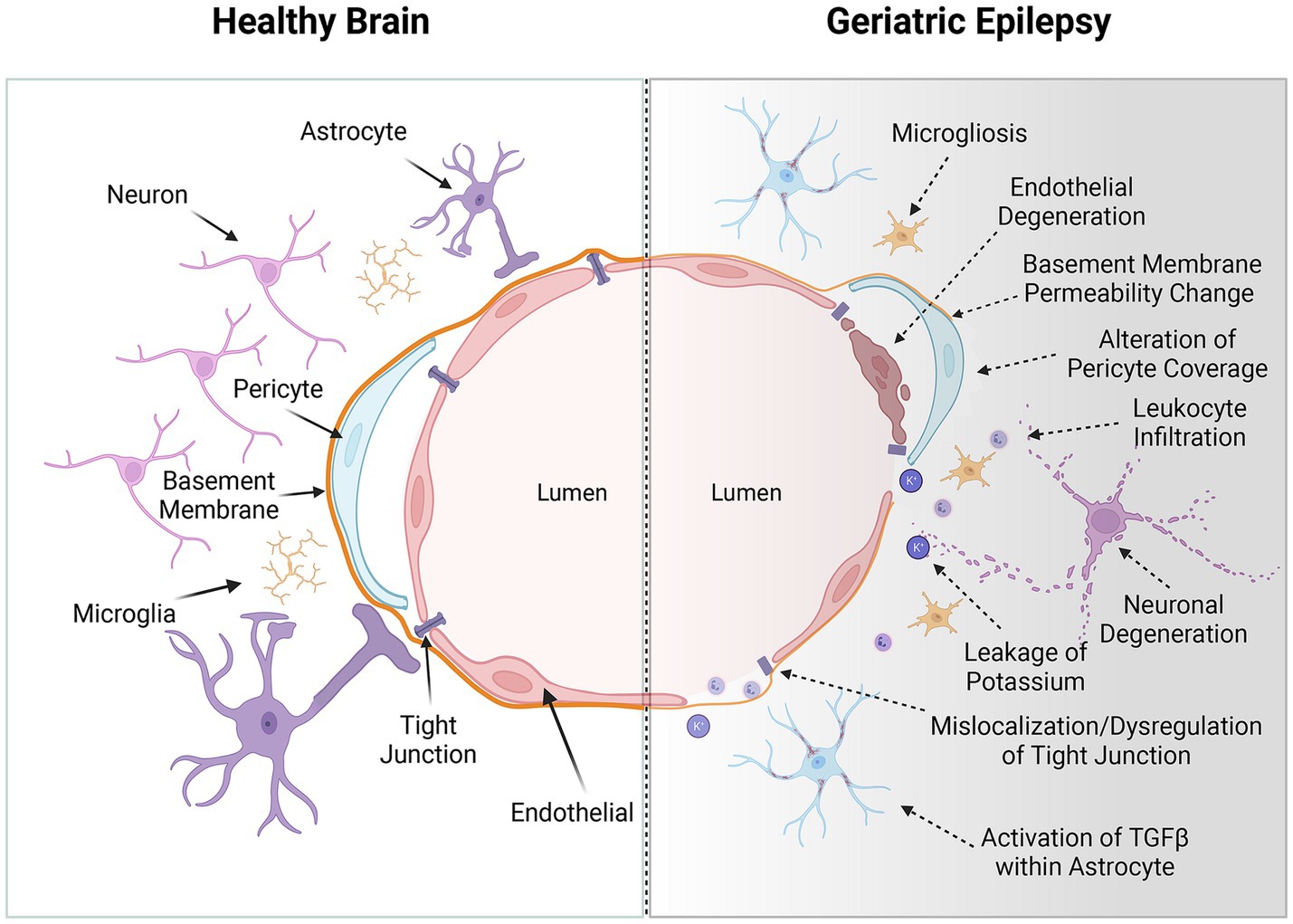
Figure 1. The blood–brain barrier (BBB) in the healthy brain and geriatric epilepsy patients’ brain. Left: In a healthy brain, the BBB consists of endothelial cells that are tightly connected through tight junction proteins, forming a primary physical barrier along the brain’s blood vessels. These cells are intricately surrounded by pericytes and perivascular macrophages embedded within the basement membrane. Additionally, the basement membrane is enveloped by the end feet of astrocytes. Furthermore, this unit contains inactive microglia and functional neurons, essential for the brain’s normal activation. Right: With advancing age, a convergence of adverse physiological processes can intersect with the pathogenesis of epilepsy. Age-related alterations include an increase in BBB permeability, leukocyte infiltration, pericellular shedding, mislocalization of tight junction, leakage of ions and activation of special signal pathway. Additionally, perivascular inflammation, along with the accumulation of amyloid and tau proteins, contributes to this complex scenario. These neuropathological changes, occurring concurrently, may collectively precipitate neurological disorders such as epilepsy and cognitive decline. The interplay of these factors underscores the multifaceted nature of age-associated neurological deterioration and highlights the need for targeted therapeutic strategies in the aging population. These alterations critically disrupt the central nervous system’s (CNS) delicate ‘excitation-inhibition’ balance, thereby potentially initiating or exacerbating the symptoms of epilepsy. Created with BioRender.com.
According to Yu et al. (2015), BMECs are distinguished from peripheral endothelial cells by their markedly low pinocytic activity and absence of fenestrations. The transcellular pathway across the BBB is intricately regulated by an array of membrane transporters and metabolic enzymes expressed in BMECs (Pottiez et al., 2009). Key efflux transporters such as P-glycoprotein (P-gp) and breast cancer resistance protein actively expel xenobiotics back into the bloodstream, whereas solute carrier transporters facilitate the influx of nutrients and ions into the brain (Kodaira et al., 2011). Additional membrane proteins and enzymes play crucial roles in controlling transcellular permeability (Raleigh et al., 2010; Del Vecchio et al., 2012; Sweeney et al., 2016). BMECs, in conjunction with the neurovascular unit, dynamically regulate BBB permeability to balance the metabolic demands of the central nervous system and protect against blood-borne toxins and pathogens (Daneman and Prat, 2015; Yan et al., 2021).
In various central nervous system disorders, including stroke (Bernardo-Castro et al., 2020), seizures (Uzüm et al., 2006), multiple sclerosis (Cramer et al., 2014), Alzheimer’s disease (Rosenberg, 2014), and brain tumors (Gerstner and Fine, 2007), BBB disruption is implicated, leading to increased permeability. This disruption facilitates the influx of potentially neurotoxic plasma components into the brain parenchyma (Keaney and Campbell, 2015). Diseases common in the elderly population, such as aging, hypertension, and diabetes, can further impair the integrity and functionality of the BBB (Zlokovic, 2008; Popescu et al., 2009; Prasad et al., 2014; Katsi et al., 2020).
3 Impact of aging on BBB alterations associated with the onset of geriatric epilepsy
Neuroimaging studies of the living human brain have revealed that disruptions in the BBB within the hippocampus are strongly linked to aging, a correlation that is particularly pronounced in patients with mild cognitive impairment (van de Haar et al., 2016; Sweeney et al., 2018; Nation et al., 2019). This phenomenon is corroborated by animal model studies, which have shown that the downregulation of tight junction proteins such as claudin-5 and occludin occurs with age, resulting in increased paracellular permeability of the BBB (Keaney and Campbell, 2015; Bors et al., 2018; Knox et al., 2022).
As part of the aging process, the BBB undergoes considerable structural and functional degradation (Figure 1). This degradation becomes evident when the BBB is compromised, manifesting in extensive alterations in comparison to a healthy state (Persidsky, 1999; Knox et al., 2022). Notable age-related changes in the BBB include significant modifications in cell-to-cell interactions, resulting in endothelial cell degeneration or atrophy and the reduced expression or mislocalization of tight junction proteins (Obermeier et al., 2013; Sweeney et al., 2019b). These alterations critically affect paracellular transport mechanisms (Erdő et al., 2017). Pathological conditions further lead to the upregulation of leukocyte adhesion molecules in endothelial cells, promoting leukocyte infiltration into the brain parenchyma and exacerbating oxidative stress through the release of reactive oxygen species, cytokines, and other mediators (Brochard et al., 2009; Gerwien et al., 2016; Profaci et al., 2020; Iannucci and Grammas, 2023; Figure 2). The heightened Reactive oxygen species consumption within the brain amplifies its vulnerability to oxidative stress (Segarra et al., 2021), with deleterious effects including oxidative damage to cellular components, activation of matrix metalloproteinases, and dysregulation of tight junction proteins and inflammatory mediators (Pun et al., 2009; Olmez and Ozyurt, 2012; Obermeier et al., 2013). These disruptions impair key cellular functions, including transport, energy production, and ion homeostasis (Olmez and Ozyurt, 2012). Additionally, the efficiency of P-gp in the BBB diminishes from middle age onwards, exacerbating the BBB’s transport dysfunction in conjunction with neuropathological burdens (Chiu et al., 2015; Banks et al., 2021).
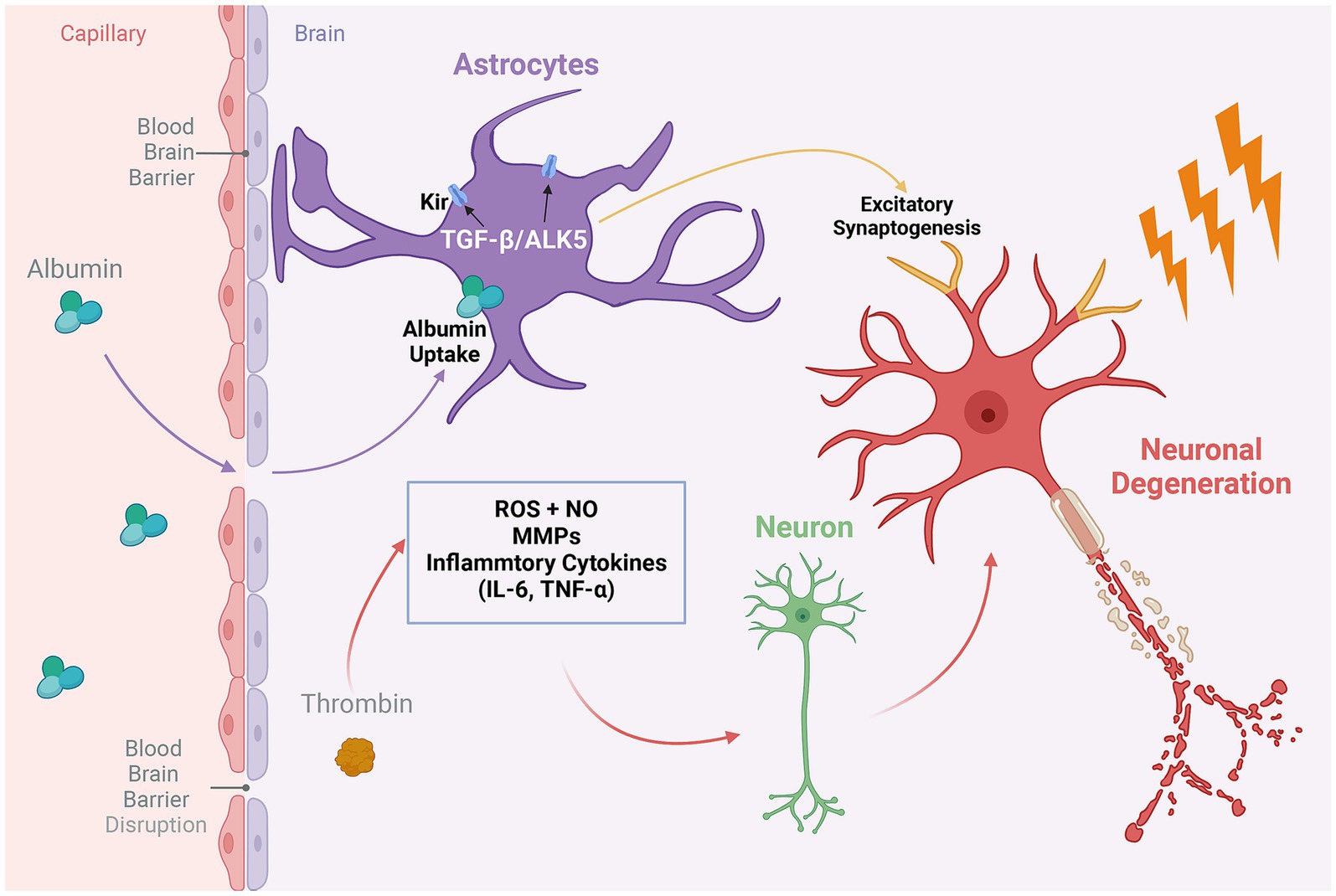
Figure 2. The role of proinflammatory proteins in the geriatric epilepsy brain. Thrombin, a key regulatory factor produced by endothelial cells during neuroinflammatory processes in the brain, impacts a variety of inflammatory mediators. These include inflammatory cytokines, matrix metalloproteinases (MMPs), reactive oxygen species (ROS), and nitric oxide (NO). The activity of these mediators can lead to the destruction of healthy neurons and precipitate neuronal degeneration, thereby exacerbating the neurological consequences of BBB disruption. On the other hand, compromised BBB integrity results in the leakage of serum albumin into the brain, subsequently triggering the activation of the TGFβ/ALK5 signaling pathway in astrocytes. This activation exerts a dual effect: it modulates the expression of potassium ion channels in astrocytes, affecting extracellular ion homeostasis, and concurrently promotes the formation of excitatory synapses in neurons. Together, these processes enhance neuronal excitability, potentially leading to epileptic discharges. Created with BioRender.com.
At the cellular level, the process of aging is correlated with a notable reduction in the density and coverage of BMECs and pericytes. This reduction is particularly pronounced in brain regions that are more susceptible to age-related degeneration, such as the hippocampus (Montagne et al., 2015; Verheggen et al., 2020). Importantly, this decline associated with aging encompasses not just cellular density but also includes significant changes in the heterogeneity of astrocytes, oligodendrocytes, and microglia. These alterations collectively exert a profound impact on brain clearance mechanisms, myelination processes, and immune surveillance within the central nervous system (Kettenmann et al., 2011; Rodríguez-Arellano et al., 2016). Furthermore, changes in pericyte behavior, alongside alterations in glial cell function, are known to adversely affect BBB permeability and are implicated in the exacerbation of brain inflammation, further complicating the pathophysiological landscape (Rustenhoven et al., 2017; Sweeney et al., 2019a).
Consequently, this decline in BBB integrity and functionality has profound implications for neuronal health. BBB damage can precipitate epileptic seizures through various interconnected mechanisms. The compromised barrier allows for the leakage of substances such as potassium, albumin, or immune cells, which are intrinsically associated with abnormal neuronal firing (Seiffert et al., 2004; David et al., 2009; Fabene et al., 2010). Furthermore, BBB deterioration alters the balance of adenosine and glutamate transporters and catalytic enzymes, characterized by a decrease in adenosine and a elevation in glutamate levels, contributing to an increase in neuronal excitability (Grant et al., 2003; Parkinson et al., 2003). Additionally, BBB disruption can lead to a decrease in pH, negatively impacting the coupling of cerebral blood flow to neuronal activity and resulting in enhanced neuronal firing (Aaslid, 2006). These changes disrupt the central nervous system’s ‘excitation-inhibition’ balance, potentially initiating or intensifying epileptic symptoms.
Among the various pathways implicated in this disruption, the role of transforming growth factor beta (TGFβ)-associated pathway stands out as particularly significant in the progression of epilepsy, especially among the elderly (Figure 2). Research utilizing rodent models to emulate BBB leakage has elucidated that the infusion of serum albumin precipitates the activation of TGFβ signaling within astrocytes. This activation is implicated in engendering age-related neurological dysfunctions, brain aging phenotypes, heightened susceptibility to epilepsy, and cognitive impairments, as evidenced by several studies (Cacheaux et al., 2009; Milikovsky et al., 2019; Senatorov Jr et al., 2019). Itai et al. have highlighted the crucial role of the astrocyte ALK5/TGF-β pathway in mediating excitatory synaptogenesis post-BBB disruption, leading to seizures (Weissberg et al., 2015). Furthermore, the presence of albumin in the brain mediates the downregulation of potassium channels in astrocytes through TGFβ receptors, impacting potassium buffering and leading to increased extracellular potassium levels and neuronal overexcitability, thereby inducing epileptic discharges. The blockade of TGFβ receptors has been shown to significantly reduce the incidence of albumin-induced epilepsy (Ivens et al., 2007). Subsequent research revealed that serum-derived albumin preferentially activates the TGF-β receptor I activin receptor-like kinase 5 pathways in astrocytes. Notably, the TGF-β signaling inhibitor losartan effectively inhibits this pathway, preventing delayed recurrent spontaneous seizures, with effects lasting for weeks after drug discontinuation, highlighting its therapeutic potential (Bar-Klein et al., 2014).
4 Impact of BBB alterations on pharmacological challenges in geriatric epilepsy
In the management of epilepsy among elderly patients, antiepileptic drugs (AEDs) play a crucial role (Vu et al., 2018). Compared to their younger counterparts, older adults may derive greater benefits from AEDs but are simultaneously at an elevated risk for potential side effects (Hernández-Ronquillo et al., 2018). Consequently, in geriatric epilepsy treatment, the initial dosing and dosage increments of AEDs are typically reduced to half of those prescribed for younger individuals to enhance drug tolerance. It is often observed that elderly patients require only half the standard dose recommended for patients under 65 years of age (Sen et al., 2020). Moreover, the selection of appropriate AEDs for older adults is constrained by the increased likelihood of side effects and drug–drug interactions. For instance, traditional AEDs such as carbamazepine and phenytoin are often eschewed due to their adverse impacts on bone health, lipid metabolism, balance, and their propensity for enzyme induction (Sen et al., 2020). A meta-analysis has indicated that lamotrigine is better tolerated than carbamazepine in the elderly with epilepsy. Additionally, levetiracetam demonstrated a higher probability of seizure control compared to lamotrigine, with no significant difference in tolerability. Furthermore, no notable differences in efficacy and tolerability were observed between carbamazepine and levetiracetam (Lezaic et al., 2019). Another meta-analysis posited that lacosamide, lamotrigine, and levetiracetam are the most effective in terms of achieving seizure remission for old patients (Lattanzi et al., 2019). Patients with drug-resistant epilepsy, particularly older adults, may require an increased number of medications to control seizures and are more vulnerable to the neurotoxic effects of specific drugs (Trinka, 2003). This situation highlights the ongoing need for research into various drug delivery methods, including those involving the BBB.
The BBB defined by its intricate tight junctions and sophisticated efflux transport systems, is a critical physiological structure. Its role extends beyond the mere obstruction of drug delivery to the brain; it also regulates the distribution of therapeutic agents within brain tissues, presenting a significant challenge in treating central nervous system disorders (Cardoso et al., 2010; Li et al., 2011). Contemporary research has identified three protective mechanisms that drugs targeting the central nervous system encounter when interacting with the BBB (Angelow and Yu, 2007; Pardridge, 2007). The first is the enzymatic barrier, which restricts the entry of drugs and organic substances into capillary endothelial cells. The second is the cellular barrier, composed of contacts between BBB cells, where endothelial cells limit the passage of water-soluble substances and regulate vesicular transport and endocytosis. The third mechanism involves the ATP-binding cassette protein transport system, actively extruding certain drugs from the endothelial cells of brain blood vessels.
The BBB’s significance is particularly notable in epilepsy treatment, as it impedes the penetration of biotechnological drugs into the brain, thereby diminishing the effectiveness of antiepileptic medications (Zhao et al., 2020). In elderly epilepsy patients, a disrupted BBB structural integrity has been observed, which introduces complexities in traditional antiepileptic drug therapies. While it might seem intuitive that a compromised BBB would allow greater drug access to the brain, the reality is more nuanced. In fact, BBB leakage enables the entry of serum albumin into the brain, which adversely affects the brain distribution of many antiepileptic drugs that bind to plasma proteins, leading to suboptimal treatment outcomes (Marchi et al., 2009; Salar et al., 2014). Moreover, damage to the BBB can result in an increased expression of various drug transporters. This phenomenon contributes to pharmacoresistance in epilepsy, particularly due to the overexpression of ATP-binding cassette efflux transporters like P-gp and breast cancer resistance protein (van Vliet et al., 2014; Gonçalves et al., 2021). The excessive presence of these transporters restricts the entry of antiepileptic drugs into the brain, thereby playing a crucial role in the development of resistance to these drugs. Furthermore, oxidative stress, whether due to disease progression or AED side effects, may alter the expression of these BBB transport proteins (Grewal et al., 2017). Additionally, genetic variations and the increased expression of efflux transporter genes could be potential factors in pharmacoresistance. These transporters collaboratively act to inhibit antiepileptic drugs from delivering their therapeutic impact on the central nervous system (Soares et al., 2016).
Traditionally, one potential strategy to counteract the reduction in drug concentration within the central nervous system, caused by BBB disruption in elderly epilepsy patients, has been to increase the drug dosage. However, it is important to acknowledge that while most antiepileptic drugs can penetrate brain tissue, they also distribute to other organs, potentially leading to significant systemic toxicity (Perucca, 2002; Li and Ma, 2006). Such chronic toxicity, encompassing hematological disorders, hepatotoxicity, and other adverse effects, considerably constrains the clinical application of antiepileptic drugs (Perucca and Gilliam, 2012; Schmidt and Schachter, 2014). Consequently, developing targeted drug delivery systems specifically designed for the central nervous system may offer a promising approach to enhance drug efficacy in the brain while minimizing peripheral side effects. This strategy could prove invaluable in optimizing therapeutic outcomes in the treatment of epilepsy, particularly in the elderly.
5 Overcoming the BBB: advancements in targeted drug delivery for brain
Contemporary approaches to drug administration across the BBB have been extensively reviewed in prior literature (van Vliet and Marchi, 2022), and thus, will not be reiterated here. In summary, the current therapeutic strategies for managing epilepsy in elderly patients predominantly encompass four mechanistic pathways: the modulation of pro- and anti-inflammatory balance (Fabene et al., 2008), the PDGF/TGF signaling pathways (Shen et al., 2019), pathways addressing oxidative stress (Luo et al., 2018), and the manipulation of matrix metalloproteinases (Broekaart et al., 2021). While these methodologies have shown potential in restoring cerebral vascular integrity and broadly controlling brain inflammation, their efficacy in epilepsy management and the efficiency of drug delivery via these mechanisms warrant further investigation. Given the unique physiological attributes of the BBB, there is a critical need for research focused on enhancing drug delivery efficiency through the BBB. Alternatively, exploring methods to directly bypass the BBB for the targeted delivery of antiepileptic drugs to the central nervous system is equally imperative. Here, we explore recent advancements in strategies designed to bypass the BBB for effective drug delivery to the central nervous system (Figure 3).
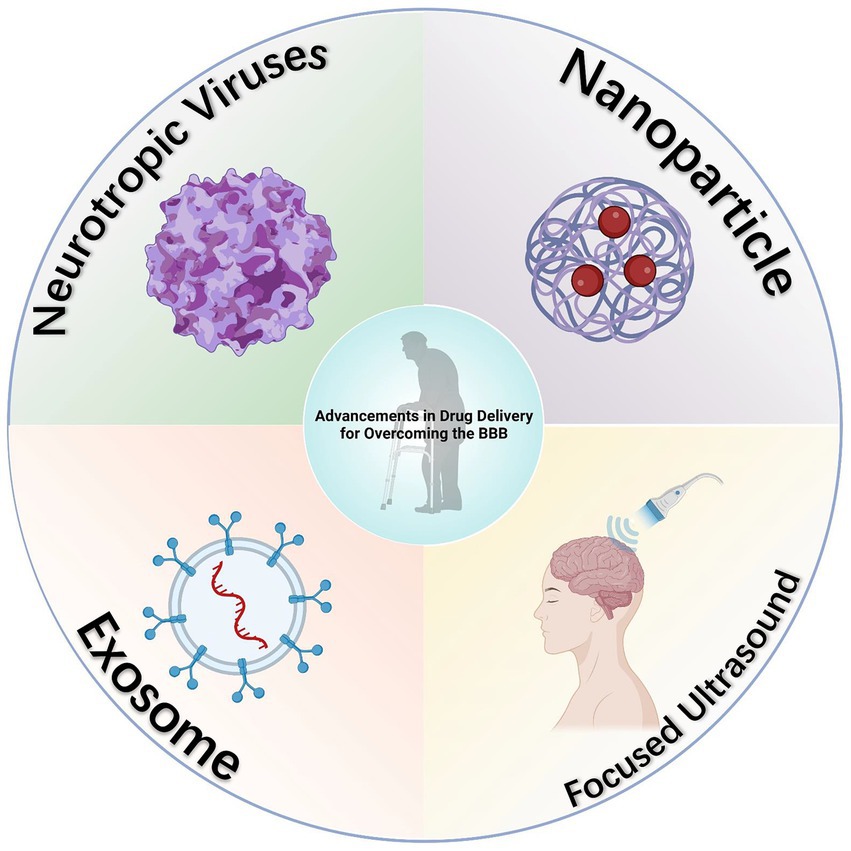
Figure 3. Diagram of advancements in drug delivery for overcoming the BBB. Several innovative methods for bypassing the BBB have been developed, and while not yet directly applied in the delivery of antiepileptic drugs, these approaches have demonstrated considerable potential in this area, as evidenced by existing research. Created with BioRender.com.
5.1 Neurotropic viruses
Neurotropic viruses exhibit a unique capacity to infiltrate the central nervous system via diverse mechanisms. These viruses inherently target the central nervous system, achieving entry by either directly crossing the BBB (Dahm et al., 2016) or utilizing immune cells as transport vehicles (Salinas et al., 2010). This attribute is critical for facilitating the delivery of therapeutic genes to brain cells (Körbelin et al., 2016; Powell et al., 2016), positioning neurotropic viruses as potential vectors for central nervous system targeted drug delivery.
In research focused on chronic temporal lobe epilepsy, the overexpression of neuropeptide Y (NPY) induced by recombinant adeno-associated virus (rAAV) vectors in epileptogenic regions has been shown to slow the progression of epileptic discharges. This approach has also been correlated with a notable reduction in the frequency of seizures (Noè et al., 2008; Noe et al., 2010). Moreover, the use of human foamy virus (HFV) as a gene transfer vector demonstrates efficiency in the expression of human glutamate decarboxylase (GAD) cDNA in hippocampal neurons. This expression leads to increased synthesis and release of gamma-aminobutyric acid (GABA) upon stimulation, thereby elevating the neuronal excitability threshold and contributing to an antiepileptic effect (Liu et al., 2005).
However, the clinical application of these findings faces significant challenges. Primary challenges involve addressing the immunogenicity (Rapti et al., 2012; Rabinowitz et al., 2019) and the inconsistencies in intracranial transduction efficiency (Foust et al., 2009; Gray et al., 2013) associated with these vectors. Additionally, the potential for inflammatory responses (Armangue et al., 2014; Ji et al., 2016) and toxicity underscores the need for rigorous evaluation and cautious application. Therefore, the optimization of viral vector designs is crucial. This entails minimizing immunogenicity and toxicity risks while maximizing targeting specificity to brain tissue. Such advancements are vital for ensuring the safe and effective utilization of neurotropic viral vectors in central nervous system drug delivery.
5.2 Nanoparticle
Nanoparticle (NP)-mediated drug delivery has emerged as a viable solution to the challenge of enhancing drug permeability across the BBB. Nanoparticles, defined as colloidal systems ranging from 1 to 1,000 nm in size, are adept at encapsulating therapeutic agents (Choradiya and Patil, 2021). This encapsulation not only improves the passage of drugs through the BBB but also enables precise targeting of specific brain regions affected by neurodegenerative and ischemic conditions (Solans et al., 2005). A diverse array of nanoparticles has demonstrated efficacy in brain delivery therapies. The effectiveness of these NPs is notably enhanced when their surfaces are modified with targeted moieties, thereby improving their specificity and efficiency in drug delivery (Farhoudi et al., 2022). In our investigation into the potential of nanoparticles to circumvent the BBB, we found their distinct advantages. These include their capacity to encapsulate diverse drug types, encompassing both hydrophilic and lipophilic compounds (Fonseca-Santos et al., 2015; Kamaly et al., 2016; DeMarino et al., 2017), and the potential for enhanced tissue-specific targeting through surface modifications (Saraiva et al., 2016). Furthermore, evidence suggests that nanoparticles can significantly increase the efficiency of drug delivery to the brain (Song et al., 2014; Maussang et al., 2016; Vilella et al., 2018), with exosome-based nanoparticles demonstrating enhanced stability in the bloodstream due to their resistance to phagocytic clearance (Yildiz et al., 2012; Hovlid et al., 2014).
Biodegradable nanoparticles have demonstrated considerable promise in augmenting the efficacy of antiepileptic drug therapies. Notably, surface functionalization of these nanoparticles has markedly improved their ability to permeate the BBB (Bonilla et al., 2022). For instance, polyssorbate-80 coated albumin nanoparticles have been effectively utilized to target the delivery of levetiracetam, a widely used antiepileptic drug, enhancing its brain-specific delivery and thus optimizing the treatment of epilepsy (Wilson et al., 2020). Additionally, the overexpression of P-gp is recognized as a pivotal contributor to the development of antiepileptic drug resistance (Löscher and Potschka, 2002; Löscher and Potschka, 2005; Luna-Tortós et al., 2008). To counteract this challenge, researchers have investigated co-embedding P-gp inhibitors and antiepileptic drugs in Pluronic P85-modified nanocarriers. This strategy has shown effectiveness in surmounting P-gp mediated drug resistance, particularly in the delivery of phenytoin (Fang et al., 2016). Furthermore, the innovative polypyrrole-poly-dopamine-phenytoin-Angiopep (PPY-PDA-PHT-ANG) nanoparticle system developed by Wu et al. represents a significant advancement in the field. This system employing copolymerization and surface functionalization techniques, not only achieves efficient drug loading and electro responsive release but also ensures precise brain-targeted delivery. This system distinguishes itself from traditional delivery methods through its capacity to respond to abnormal brain electrical activity, potentially contributing valuable insights to antiepileptic treatment strategies (Wu et al., 2022).
While conventional research continues to explore ways to exploit the physiological properties of the BBB for efficient brain drug delivery, some researchers have pursued an alternative approach through intranasal administration. This method directly bypasses the BBB, circumventing digestive tract absorption and first-pass liver metabolism, thereby enhancing drug bioavailability (Illum, 2003). Intranasal delivery can also target brain tissue directly, allowing for reduced dosages to achieve therapeutic effectiveness while minimizing systemic side effects (Pardeshi and Belgamwar, 2013). Its non-invasive nature and rapid efficacy make it particularly suitable for acute seizure management (Grassin-Delyle et al., 2012). Moreover, the potential for controlled release formulations extends its utility in chronic treatment, improving adherence, especially in patient groups with special needs, such as the elderly, where oral administration or intravenous injection may pose challenges (Illum, 2012).
In pre-clinical investigations, nanoparticles synthesized from polymers such as polylactic-polyethylene glycol copolymer and chitosan have demonstrated substantial promise for encapsulating antiepileptic drugs (Musumeci et al., 2018). These nanoparticle-based drug carriers have been notably effective in enhancing the transnasal cerebral delivery of drugs like carbamazepine and diazepam, significantly increasing their brain uptake (Sharma et al., 2015; Musumeci et al., 2018). Additionally, the incorporation of oxcarbazepine into nanoemulsions facilitated its transport through the nasal mucosa, resulting in an extended drug release profile and prolonged residence time in the brain (El-Zaafarany et al., 2016). In animal model trials for epilepsy treatment, these nanoparticle-encapsulated drugs exhibited superior antiepileptic efficacy compared to equivalent doses of the drugs in free form (Sharma et al., 2014; Musumeci et al., 2018). While these pre-clinical findings offer robust support for the utilization of nanocarriers in epilepsy treatment via the naso-brain route, comprehensive clinical studies are essential to validate their therapeutic effectiveness (Kapoor et al., 2016). Overall, the deployment of polymer-based nanoparticles for drug delivery through the naso-brain pathway emerges as a highly promising approach in the management of epilepsy.
Despite these promising attributes, there are notable limitations associated with nanoparticle technology. Their susceptibility to phagocytic engulfment and subsequent clearance from the circulatory system poses a significant hurdle (Hare et al., 2017). The intrinsic factors that influence drug release and targeting specificity further complicate their application. The necessity for brain-targeting ligands to enhance intracranial drug concentration introduces increased complexity and production costs (Saraiva et al., 2016). Moreover, the long-term stability of nanoparticles and potential issues of drug leakage warrant careful consideration (Dai et al., 2018). Future research endeavors should concentrate on optimizing critical attributes of nanoparticles, such as their stability, targeting accuracy, and drug loading capacity (Hare et al., 2017). The development of a safe, effective nanocarrier platform, and elucidating its interaction with disease mechanisms, are paramount for the successful integration of this technology in medical applications.
5.3 Beyond conventional methods
Numerous methods currently exist for circumventing the BBB, while not yet directly applied to the delivery of antiepileptic drugs, have shown significant potential in this domain according to existing studies. A notable example is the application of magnetic resonance guided low-intensity focused ultrasound (FUS). This technique has been evidenced to reversibly open the BBB, thereby enhancing the targeted delivery of drugs for brain therapies. In this field, Ali et al. have reported the findings of a preliminary clinical trial. This trial assessed the safety, feasibility, and reversibility of employing FUS techniques to breach the BBB in the hippocampus and entorhinal cortex areas (Rezai et al., 2020). Considering that these regions are focal points in epilepsy treatment, the deployment of FUS technology for the precise delivery of high-dose antiepileptic drugs offers substantial promise (Wiest and Beisteiner, 2019).
Moreover, exosomes represent another area of potential breakthrough. Owing to their endogenous nature, reduced toxicity, enhanced resistance to macrophage clearance, and extended half-life in the bloodstream, they are garnering significant interest. Research indicates that extracellular vesicles secreted by intranasally injected human bone marrow mesenchymal stem cells manifest robust anti-inflammatory properties in epileptic conditions. These exosomes are capable of reaching the hippocampal area within 6 h post-administration, effectively mitigating the loss of glutamatergic and GABAergic neurons associated with the epileptic state, and consequently, substantially diminishing hippocampal inflammation (Long et al., 2017). Despite the absence of studies on exosome-mediated antiepileptic drug delivery and the limitations of exosomes in drug payload capacity and efficiency, their advantages in safety, targeting specificity, and in vivo circulation duration position them as promising contenders to synthetic nanoparticles in the realm of antiepileptic drug delivery (de la Torre et al., 2020; Fu et al., 2020).
6 Conclusion and perspective
In summary, this review underscores the paramount importance of comprehending the dynamics of the BBB in managing epilepsy in the elderly. Although various innovative anti-epileptic strategies targeting the BBB have been discussed, their efficacy in elderly patients with epilepsy necessitates further exploration. With the rising prevalence of epilepsy in an aging population, unique pharmacological challenges emerge due to age-related alterations in the BBB. Future research endeavors should be directed towards understanding how these novel treatment strategies can be optimized in the context of such age-associated pathological changes. Given the intricacy of the BBB, advancements in targeted therapy and non-invasive drug delivery methods hold promise for enhancing treatment efficacy and, consequently, the quality of life for the elderly. The integration of precision medicine and emerging technologies in this field aims not only to ameliorate health outcomes but also to enrich our understanding of the aging nervous system. Adopting this approach will facilitate more personalized and compassionate care for our aging society, ultimately contributing to improved health management and enhanced quality of life for individuals with geriatric epilepsy.
Author contributions
XC: Writing – original draft, Writing – review & editing. JL: Writing – review & editing, Investigation. MS: Writing – review & editing. LP: Writing – review & editing. ZQ: Data curation, Software, Writing – review & editing. BH: Conceptualization, Software, Writing – review & editing. SY: Writing – review & editing. HS: Writing – review & editing.
Funding
The author(s) declare financial support was received for the research, authorship, and/or publication of this article. This work was supported by Joint key project [grant number 2019LH01]; Department of Science and Technology of Sichuan Province [grant number 22CXRC0178]; Medical Innovation Project [grant number 21WQ040]; Hospital Management Project of Western Command General Hospital [grant number 2021-XZYG-B22]; Hospital Management Project of Western Command General Hospital [grant number 2021-XZYG-B21].
Conflict of interest
The authors declare that the research was conducted in the absence of any commercial or financial relationships that could be construed as a potential conflict of interest.
Publisher’s note
All claims expressed in this article are solely those of the authors and do not necessarily represent those of their affiliated organizations, or those of the publisher, the editors and the reviewers. Any product that may be evaluated in this article, or claim that may be made by its manufacturer, is not guaranteed or endorsed by the publisher.
References
Aaslid, R. (2006). Cerebral autoregulation and vasomotor reactivity. Front. Neurol. Neurosci. 21, 216–228. doi: 10.1159/000092434
Abbott, N. J., Rönnbäck, L., and Hansson, E. (2006). Astrocyte-endothelial interactions at the blood-brain barrier. Nat. Rev. Neurosci. 7, 41–53. doi: 10.1038/nrn1824
Angelow, S., and Yu, A. S. (2007). Claudins and paracellular transport: an update. Curr. Opin. Nephrol. Hypertens. 16, 459–464. doi: 10.1097/MNH.0b013e32820ac97d
Armangue, T., Leypoldt, F., Málaga, I., Raspall-Chaure, M., Marti, I., Nichter, C., et al. (2014). Herpes simplex virus encephalitis is a trigger of brain autoimmunity. Ann. Neurol. 75, 317–323. doi: 10.1002/ana.24083
Banks, W. A., Reed, M. J., Logsdon, A. F., Rhea, E. M., and Erickson, M. A. (2021). Healthy aging and the blood-brain barrier. Nat Aging 1, 243–254. doi: 10.1038/s43587-021-00043-5
Bar-Klein, G., Cacheaux, L. P., Kamintsky, L., Prager, O., Weissberg, I., Schoknecht, K., et al. (2014). Losartan prevents acquired epilepsy via TGF-β signaling suppression. Ann. Neurol. 75, 864–875. doi: 10.1002/ana.24147
Beard, R. S. Jr., Reynolds, J. J., and Bearden, S. E. (2011). Hyperhomocysteinemia increases permeability of the blood-brain barrier by NMDA receptor-dependent regulation of adherens and tight junctions. Blood 118, 2007–2014. doi: 10.1182/blood-2011-02-338269
Bernardo-Castro, S., Sousa, J. A., Brás, A., Cecília, C., Rodrigues, B., Almendra, L., et al. (2020). Pathophysiology of blood-brain barrier permeability throughout the different stages of ischemic stroke and its implication on hemorrhagic transformation and recovery. Front. Neurol. 11:594672. doi: 10.3389/fneur.2020.594672
Bonilla, L., Esteruelas, G., Ettcheto, M., Espina, M., García, M. L., Camins, A., et al. (2022). Biodegradable nanoparticles for the treatment of epilepsy: from current advances to future challenges. Epilepsia Open 7, S121–s132. doi: 10.1002/epi4.12567
Bors, L., Tóth, K., Tóth, E. Z., Bajza, Á., Csorba, A., Szigeti, K., et al. (2018). Age-dependent changes at the blood-brain barrier. A comparative structural and functional study in young adult and middle aged rats. Brain Res. Bull. 139, 269–277. doi: 10.1016/j.brainresbull.2018.03.001
Brochard, V., Combadière, B., Prigent, A., Laouar, Y., Perrin, A., Beray-Berthat, V., et al. (2009). Infiltration of CD4+ lymphocytes into the brain contributes to neurodegeneration in a mouse model of Parkinson disease. J. Clin. Invest. 119, 182–192. doi: 10.1172/jci36470
Broekaart, D. W., Bertran, A., Jia, S., Korotkov, A., Senkov, O., Bongaarts, A., et al. (2021). The matrix metalloproteinase inhibitor IPR-179 has antiseizure and antiepileptogenic effects. J. Clin. Invest. 131:e138332. doi: 10.1172/jci138332
Cacheaux, L. P., Ivens, S., David, Y., Lakhter, A. J., Bar-Klein, G., Shapira, M., et al. (2009). Transcriptome profiling reveals TGF-beta signaling involvement in epileptogenesis. J. Neurosci. 29, 8927–8935. doi: 10.1523/jneurosci.0430-09.2009
Cardoso, F. L., Brites, D., and Brito, M. A. (2010). Looking at the blood-brain barrier: molecular anatomy and possible investigation approaches. Brain Res. Rev. 64, 328–363. doi: 10.1016/j.brainresrev.2010.05.003
Chiu, C., Miller, M. C., Monahan, R., Osgood, D. P., Stopa, E. G., and Silverberg, G. D. (2015). P-glycoprotein expression and amyloid accumulation in human aging and Alzheimer's disease: preliminary observations. Neurobiol. Aging 36, 2475–2482. doi: 10.1016/j.neurobiolaging.2015.05.020
Choradiya, B. R., and Patil, S. B. (2021). A comprehensive review on nanoemulsion as an ophthalmic drug delivery system. J. Mol. Liq. 339:116751. doi: 10.1016/j.molliq.2021.116751
Cloyd, J., Hauser, W., Towne, A., Ramsay, R., Mattson, R., Gilliam, F., et al. (2006). Epidemiological and medical aspects of epilepsy in the elderly. Epilepsy Res. 68, 39–48. doi: 10.1016/j.eplepsyres.2005.07.016
Collaborators, G. E. (2019). Global, regional, and national burden of epilepsy, 1990-2016: a systematic analysis for the global burden of disease study 2016. Lancet Neurol. 18, 357–375. doi: 10.1016/s1474-4422(18)30454-x
Cramer, S. P., Simonsen, H., Frederiksen, J. L., Rostrup, E., and Larsson, H. B. (2014). Abnormal blood-brain barrier permeability in normal appearing white matter in multiple sclerosis investigated by MRI. Neuroimage Clin 4, 182–189. doi: 10.1016/j.nicl.2013.12.001
Dahm, T., Rudolph, H., Schwerk, C., Schroten, H., and Tenenbaum, T. (2016). Neuroinvasion and inflammation in viral central nervous system infections. Mediat. Inflamm. 2016, 8562805–8562816. doi: 10.1155/2016/8562805
Dai, T., Jiang, K., and Lu, W. (2018). Liposomes and lipid disks traverse the BBB and BBTB as intact forms as revealed by two-step Förster resonance energy transfer imaging. Acta Pharm. Sin. B 8, 261–271. doi: 10.1016/j.apsb.2018.01.004
Daneman, R., and Prat, A. (2015). The blood-brain barrier. Cold Spring Harb. Perspect. Biol. 7:a020412. doi: 10.1101/cshperspect.a020412
David, Y., Cacheaux, L. P., Ivens, S., Lapilover, E., Heinemann, U., Kaufer, D., et al. (2009). Astrocytic dysfunction in epileptogenesis: consequence of altered potassium and glutamate homeostasis? J. Neurosci. 29, 10588–10599. doi: 10.1523/jneurosci.2323-09.2009
de la Torre, P., Pérez-Lorenzo, M. J., Alcázar-Garrido, Á., and Flores, A. I. (2020). Cell-based nanoparticles delivery systems for targeted cancer therapy: lessons from anti-angiogenesis treatments. Molecules 25:715. doi: 10.3390/molecules25030715
Del Vecchio, G., Tscheik, C., Tenz, K., Helms, H. C., Winkler, L., Blasig, R., et al. (2012). Sodium caprate transiently opens claudin-5-containing barriers at tight junctions of epithelial and endothelial cells. Mol. Pharm. 9, 2523–2533. doi: 10.1021/mp3001414
DeLorenzo, R. J., Hauser, W. A., Towne, A. R., Boggs, J. G., Pellock, J. M., Penberthy, L., et al. (1996). A prospective, population-based epidemiologic study of status epilepticus in Richmond, Virginia. Neurology 46, 1029–1035. doi: 10.1212/wnl.46.4.1029
DeMarino, C., Schwab, A., Pleet, M., Mathiesen, A., Friedman, J., El-Hage, N., et al. (2017). Biodegradable nanoparticles for delivery of therapeutics in CNS infection. J. Neuroimmune Pharmacol. 12, 31–50. doi: 10.1007/s11481-016-9692-7
El-Zaafarany, G. M., Soliman, M. E., Mansour, S., and Awad, G. A. (2016). Identifying lipidic emulsomes for improved oxcarbazepine brain targeting: in vitro and rat in vivo studies. Int. J. Pharm. 503, 127–140. doi: 10.1016/j.ijpharm.2016.02.038
Erdő, F., Denes, L., and de Lange, E. (2017). Age-associated physiological and pathological changes at the blood-brain barrier: a review. J. Cereb. Blood Flow Metab. 37, 4–24. doi: 10.1177/0271678x16679420
Fabene, P. F., Bramanti, P., and Constantin, G. (2010). The emerging role for chemokines in epilepsy. J. Neuroimmunol. 224, 22–27. doi: 10.1016/j.jneuroim.2010.05.016
Fabene, P. F., Navarro Mora, G., Martinello, M., Rossi, B., Merigo, F., Ottoboni, L., et al. (2008). A role for leukocyte-endothelial adhesion mechanisms in epilepsy. Nat. Med. 14, 1377–1383. doi: 10.1038/nm.1878
Fang, Z., Chen, S., Qin, J., Chen, B., Ni, G., Chen, Z., et al. (2016). Pluronic P85-coated poly(butylcyanoacrylate) nanoparticles overcome phenytoin resistance in P-glycoprotein overexpressing rats with lithium-pilocarpine-induced chronic temporal lobe epilepsy. Biomaterials 97, 110–121. doi: 10.1016/j.biomaterials.2016.04.021
Farhoudi, M., Sadigh-Eteghad, S., Mahmoudi, J., Farjami, A., Mahmoudian, M., and Salatin, S. (2022). The therapeutic benefits of intravenously administrated nanoparticles in stroke and age-related neurodegenerative diseases. Curr. Pharm. Des. 28, 1985–2000. doi: 10.2174/1381612828666220608093639
Fonseca-Santos, B., Gremião, M. P., and Chorilli, M. (2015). Nanotechnology-based drug delivery systems for the treatment of Alzheimer's disease. Int. J. Nanomedicine 10, 4981–5003. doi: 10.2147/ijn.S87148
Foust, K. D., Nurre, E., Montgomery, C. L., Hernandez, A., Chan, C. M., and Kaspar, B. K. (2009). Intravascular AAV9 preferentially targets neonatal neurons and adult astrocytes. Nat. Biotechnol. 27, 59–65. doi: 10.1038/nbt.1515
Fu, S., Wang, Y., Xia, X., and Zheng, J. C. (2020). Exosome engineering: current progress in cargo loading and targeted delivery. NanoImpact 20:100261. doi: 10.1016/j.impact.2020.100261
Gerstner, E. R., and Fine, R. L. (2007). Increased permeability of the blood-brain barrier to chemotherapy in metastatic brain tumors: establishing a treatment paradigm. J. Clin. Oncol. 25, 2306–2312. doi: 10.1200/jco.2006.10.0677
Gerwien, H., Hermann, S., Zhang, X., Korpos, E., Song, J., Kopka, K., et al. (2016). Imaging matrix metalloproteinase activity in multiple sclerosis as a specific marker of leukocyte penetration of the blood-brain barrier. Sci. Transl. Med. 8:364ra152. doi: 10.1126/scitranslmed.aaf8020
Godfrey, J. B. (1989). Misleading presentation of epilepsy in elderly people. Age Ageing 18, 17–20. doi: 10.1093/ageing/18.1.17
Gonçalves, J., Silva, S., Gouveia, F., Bicker, J., Falcão, A., Alves, G., et al. (2021). A combo-strategy to improve brain delivery of antiepileptic drugs: focus on BCRP and intranasal administration. Int. J. Pharm. 593:120161. doi: 10.1016/j.ijpharm.2020.120161
Grant, G. A., Meno, J. R., Nguyen, T. S., Stanness, K. A., Janigro, D., and Winn, R. H. (2003). Adenosine-induced modulation of excitatory amino acid transport across isolated brain arterioles. J. Neurosurg. 98, 554–560. doi: 10.3171/jns.2003.98.3.0554
Grassin-Delyle, S., Buenestado, A., Naline, E., Faisy, C., Blouquit-Laye, S., Couderc, L. J., et al. (2012). Intranasal drug delivery: an efficient and non-invasive route for systemic administration: focus on opioids. Pharmacol. Ther. 134, 366–379. doi: 10.1016/j.pharmthera.2012.03.003
Gray, S. J., Nagabhushan Kalburgi, S., McCown, T. J., and Jude Samulski, R. (2013). Global CNS gene delivery and evasion of anti-AAV-neutralizing antibodies by intrathecal AAV administration in non-human primates. Gene Ther. 20, 450–459. doi: 10.1038/gt.2012.101
Grewal, G. K., Kukal, S., Kanojia, N., Saso, L., Kukreti, S., and Kukreti, R. (2017). Effect of oxidative stress on ABC transporters: contribution to epilepsy pharmacoresistance. Molecules 22:365. doi: 10.3390/molecules22030365
Hare, J. I., Lammers, T., Ashford, M. B., Puri, S., Storm, G., and Barry, S. T. (2017). Challenges and strategies in anti-cancer nanomedicine development: an industry perspective. Adv. Drug Deliv. Rev. 108, 25–38. doi: 10.1016/j.addr.2016.04.025
Hauser, W. A., Annegers, J. F., and Kurland, L. T. (1993). Incidence of epilepsy and unprovoked seizures in Rochester, Minnesota: 1935-1984. Epilepsia 34, 453–458. doi: 10.1111/j.1528-1157.1993.tb02586.x
Hernández-Ronquillo, L., Adams, S., Ballendine, S., and Téllez-Zenteno, J. F. (2018). Epilepsy in an elderly population: classification, etiology and drug resistance. Epilepsy Res. 140, 90–94. doi: 10.1016/j.eplepsyres.2017.12.016
Hovlid, M. L., Lau, J. L., Breitenkamp, K., Higginson, C. J., Laufer, B., Manchester, M., et al. (2014). Encapsidated atom-transfer radical polymerization in Qβ virus-like nanoparticles. ACS Nano 8, 8003–8014. doi: 10.1021/nn502043d
Iannucci, J., and Grammas, P. (2023). Thrombin, a key driver of pathological inflammation in the brain. Cell 12:1222. doi: 10.3390/cells12091222
Illum, L. (2003). Nasal drug delivery--possibilities, problems and solutions. J. Control. Release 87, 187–198. doi: 10.1016/s0168-3659(02)00363-2
Illum, L. (2012). Nasal drug delivery - recent developments and future prospects. J. Control. Release 161, 254–263. doi: 10.1016/j.jconrel.2012.01.024
Ivens, S., Kaufer, D., Flores, L. P., Bechmann, I., Zumsteg, D., Tomkins, O., et al. (2007). TGF-beta receptor-mediated albumin uptake into astrocytes is involved in neocortical epileptogenesis. Brain 130, 535–547. doi: 10.1093/brain/awl317
Ji, N., Weng, D., Liu, C., Gu, Z., Chen, S., Guo, Y., et al. (2016). Adenovirus-mediated delivery of herpes simplex virus thymidine kinase administration improves outcome of recurrent high-grade glioma. Oncotarget 7, 4369–4378. doi: 10.18632/oncotarget.6737
Kadry, H., Noorani, B., and Cucullo, L. (2020). A blood-brain barrier overview on structure, function, impairment, and biomarkers of integrity. Fluids Barriers CNS 17:69. doi: 10.1186/s12987-020-00230-3
Kamaly, N., Yameen, B., Wu, J., and Farokhzad, O. C. (2016). Degradable controlled-release polymers and polymeric nanoparticles: mechanisms of controlling drug release. Chem. Rev. 116, 2602–2663. doi: 10.1021/acs.chemrev.5b00346
Kapoor, M., Cloyd, J. C., and Siegel, R. A. (2016). A review of intranasal formulations for the treatment of seizure emergencies. J. Control. Release 237, 147–159. doi: 10.1016/j.jconrel.2016.07.001
Katsi, V., Marketou, M., Maragkoudakis, S., Didagelos, M., Charalambous, G., Parthenakis, F., et al. (2020). Blood-brain barrier dysfunction: the undervalued frontier of hypertension. J. Hum. Hypertens. 34, 682–691. doi: 10.1038/s41371-020-0352-2
Keaney, J., and Campbell, M. (2015). The dynamic blood-brain barrier. FEBS J. 282, 4067–4079. doi: 10.1111/febs.13412
Kettenmann, H., Hanisch, U. K., Noda, M., and Verkhratsky, A. (2011). Physiology of microglia. Physiol. Rev. 91, 461–553. doi: 10.1152/physrev.00011.2010
Knox, E. G., Aburto, M. R., Clarke, G., Cryan, J. F., and O'Driscoll, C. M. (2022). The blood-brain barrier in aging and neurodegeneration. Mol. Psychiatry 27, 2659–2673. doi: 10.1038/s41380-022-01511-z
Kodaira, H., Kusuhara, H., Fujita, T., Ushiki, J., Fuse, E., and Sugiyama, Y. (2011). Quantitative evaluation of the impact of active efflux by p-glycoprotein and breast cancer resistance protein at the blood-brain barrier on the predictability of the unbound concentrations of drugs in the brain using cerebrospinal fluid concentration as a surrogate. J. Pharmacol. Exp. Ther. 339, 935–944. doi: 10.1124/jpet.111.180398
Körbelin, J., Dogbevia, G., Michelfelder, S., Ridder, D. A., Hunger, A., Wenzel, J., et al. (2016). A brain microvasculature endothelial cell-specific viral vector with the potential to treat neurovascular and neurological diseases. EMBO Mol. Med. 8, 609–625. doi: 10.15252/emmm.201506078
Lattanzi, S., Trinka, E., Del Giovane, C., Nardone, R., Silvestrini, M., and Brigo, F. (2019). Antiepileptic drug monotherapy for epilepsy in the elderly: a systematic review and network meta-analysis. Epilepsia 60, 2245–2254. doi: 10.1111/epi.16366
Lezaic, N., Gore, G., Josephson, C. B., Wiebe, S., Jetté, N., and Keezer, M. R. (2019). The medical treatment of epilepsy in the elderly: a systematic review and meta-analysis. Epilepsia 60, 1325–1340. doi: 10.1111/epi.16068
Li, J., Feng, L., Fan, L., Zha, Y., Guo, L., Zhang, Q., et al. (2011). Targeting the brain with PEG-PLGA nanoparticles modified with phage-displayed peptides. Biomaterials 32, 4943–4950. doi: 10.1016/j.biomaterials.2011.03.031
Li, L. F., and Ma, C. (2006). Epidemiological study of severe cutaneous adverse drug reactions in a city district of China. Clin. Exp. Dermatol. 31, 642–647. doi: 10.1111/j.1365-2230.2006.02185.x
Li, C. H., Shyu, M. K., Jhan, C., Cheng, Y. W., Tsai, C. H., Liu, C. W., et al. (2015). Gold nanoparticles increase endothelial Paracellular permeability by altering components of endothelial tight junctions, and increase blood-brain barrier permeability in mice. Toxicol. Sci. 148, 192–203. doi: 10.1093/toxsci/kfv176
Liu, W., He, X., Cao, Z., Sheng, J., Liu, H., Li, Z., et al. (2005). Efficient therapeutic gene expression in cultured rat hippocampal neurons mediated by human foamy virus vectors: a potential for the treatment of neurological diseases. Intervirology 48, 329–335. doi: 10.1159/000085102
Long, Q., Upadhya, D., Hattiangady, B., Kim, D. K., An, S. Y., Shuai, B., et al. (2017). Intranasal MSC-derived A1-exosomes ease inflammation, and prevent abnormal neurogenesis and memory dysfunction after status epilepticus. Proc. Natl. Acad. Sci. USA 114, E3536–e3545. doi: 10.1073/pnas.1703920114
Löscher, W., and Potschka, H. (2002). Role of multidrug transporters in pharmacoresistance to antiepileptic drugs. J. Pharmacol. Exp. Ther. 301, 7–14. doi: 10.1124/jpet.301.1.7
Löscher, W., and Potschka, H. (2005). Blood-brain barrier active efflux transporters: ATP-binding cassette gene family. NeuroRx 2, 86–98. doi: 10.1602/neurorx.2.1.86
Luna-Tortós, C., Fedrowitz, M., and Löscher, W. (2008). Several major antiepileptic drugs are substrates for human P-glycoprotein. Neuropharmacology 55, 1364–1375. doi: 10.1016/j.neuropharm.2008.08.032
Luo, W. D., Min, J. W., Huang, W. X., Wang, X., Peng, Y. Y., Han, S., et al. (2018). Vitexin reduces epilepsy after hypoxic ischemia in the neonatal brain via inhibition of NKCC1. J. Neuroinflammation 15:186. doi: 10.1186/s12974-018-1221-6
Marchi, N., Betto, G., Fazio, V., Fan, Q., Ghosh, C., Machado, A., et al. (2009). Blood-brain barrier damage and brain penetration of antiepileptic drugs: role of serum proteins and brain edema. Epilepsia 50, 664–677. doi: 10.1111/j.1528-1167.2008.01989.x
Martin, R. C., Griffith, H. R., Faught, E., Gilliam, F., Mackey, M., and Vogtle, L. (2005). Cognitive functioning in community dwelling older adults with chronic partial epilepsy. Epilepsia 46, 298–303. doi: 10.1111/j.0013-9580.2005.02104.x
Maussang, D., Rip, J., van Kregten, J., van den Heuvel, A., van der Pol, S., van der Boom, B., et al. (2016). Glutathione conjugation dose-dependently increases brain-specific liposomal drug delivery in vitro and in vivo. Drug Discov. Today Technol. 20, 59–69. doi: 10.1016/j.ddtec.2016.09.003
Milikovsky, D. Z., Ofer, J., Senatorov, V. V. Jr., Friedman, A. R., Prager, O., Sheintuch, L., et al. (2019). Paroxysmal slow cortical activity in Alzheimer's disease and epilepsy is associated with blood-brain barrier dysfunction. Sci. Transl. Med. 11:eaaw8954. doi: 10.1126/scitranslmed.aaw8954
Montagne, A., Barnes, S. R., Sweeney, M. D., Halliday, M. R., Sagare, A. P., Zhao, Z., et al. (2015). Blood-brain barrier breakdown in the aging human hippocampus. Neuron 85, 296–302. doi: 10.1016/j.neuron.2014.12.032
Movahedpour, A., Taghvaeefar, R., Asadi-Pooya, A. A., Karami, Y., Tavasolian, R., Khatami, S. H., et al. (2023). Nano-delivery systems as a promising therapeutic potential for epilepsy: current status and future perspectives. CNS Neurosci. Ther. 29, 3150–3159. doi: 10.1111/cns.14355
Musumeci, T., Serapide, M. F., Pellitteri, R., Dalpiaz, A., Ferraro, L., Dal Magro, R., et al. (2018). Oxcarbazepine free or loaded PLGA nanoparticles as effective intranasal approach to control epileptic seizures in rodents. Eur. J. Pharm. Biopharm. 133, 309–320. doi: 10.1016/j.ejpb.2018.11.002
Nation, D. A., Sweeney, M. D., Montagne, A., Sagare, A. P., D'Orazio, L. M., Pachicano, M., et al. (2019). Blood-brain barrier breakdown is an early biomarker of human cognitive dysfunction. Nat. Med. 25, 270–276. doi: 10.1038/s41591-018-0297-y
Noè, F., Pool, A. H., Nissinen, J., Gobbi, M., Bland, R., Rizzi, M., et al. (2008). Neuropeptide Y gene therapy decreases chronic spontaneous seizures in a rat model of temporal lobe epilepsy. Brain 131, 1506–1515. doi: 10.1093/brain/awn079
Noe, F., Vaghi, V., Balducci, C., Fitzsimons, H., Bland, R., Zardoni, D., et al. (2010). Anticonvulsant effects and behavioural outcomes of rAAV serotype 1 vector-mediated neuropeptide Y overexpression in rat hippocampus. Gene Ther. 17, 643–652. doi: 10.1038/gt.2010.23
Obermeier, B., Daneman, R., and Ransohoff, R. M. (2013). Development, maintenance and disruption of the blood-brain barrier. Nat. Med. 19, 1584–1596. doi: 10.1038/nm.3407
Olmez, I., and Ozyurt, H. (2012). Reactive oxygen species and ischemic cerebrovascular disease. Neurochem. Int. 60, 208–212. doi: 10.1016/j.neuint.2011.11.009
Pardeshi, C. V., and Belgamwar, V. S. (2013). Direct nose to brain drug delivery via integrated nerve pathways bypassing the blood-brain barrier: an excellent platform for brain targeting. Expert Opin. Drug Deliv. 10, 957–972. doi: 10.1517/17425247.2013.790887
Pardridge, W. M. (2007). Blood-brain barrier delivery. Drug Discov. Today 12, 54–61. doi: 10.1016/j.drudis.2006.10.013
Parkinson, F. E., Friesen, J., Krizanac-Bengez, L., and Janigro, D. (2003). Use of a three-dimensional in vitro model of the rat blood-brain barrier to assay nucleoside efflux from brain. Brain Res. 980, 233–241. doi: 10.1016/s0006-8993(03)02980-9
Persidsky, Y. (1999). Model systems for studies of leukocyte migration across the blood–brain barrier. J. Neurovirol. 5, 579–590. doi: 10.3109/13550289909021287
Perucca, E. (2002). Overtreatment in epilepsy: adverse consequences and mechanisms. Epilepsy Res. 52, 25–33. doi: 10.1016/s0920-1211(02)00182-1
Perucca, P., and Gilliam, F. G. (2012). Adverse effects of antiepileptic drugs. Lancet Neurol. 11, 792–802. doi: 10.1016/s1474-4422(12)70153-9
Petty, M. A., and Lo, E. H. (2002). Junctional complexes of the blood-brain barrier: permeability changes in neuroinflammation. Prog. Neurobiol. 68, 311–323. doi: 10.1016/s0301-0082(02)00128-4
Popescu, B. O., Toescu, E. C., Popescu, L. M., Bajenaru, O., Muresanu, D. F., Schultzberg, M., et al. (2009). Blood-brain barrier alterations in ageing and dementia. J. Neurol. Sci. 283, 99–106. doi: 10.1016/j.jns.2009.02.321
Pottiez, G., Flahaut, C., Cecchelli, R., and Karamanos, Y. (2009). Understanding the blood-brain barrier using gene and protein expression profiling technologies. Brain Res. Rev. 62, 83–98. doi: 10.1016/j.brainresrev.2009.09.004
Powell, S. K., Khan, N., Parker, C. L., Samulski, R. J., Matsushima, G., Gray, S. J., et al. (2016). Characterization of a novel adeno-associated viral vector with preferential oligodendrocyte tropism. Gene Ther. 23, 807–814. doi: 10.1038/gt.2016.62
Prasad, S., Sajja, R. K., Naik, P., and Cucullo, L. (2014). Diabetes mellitus and blood-brain barrier dysfunction: An overview. J Pharmacovigil 2:125. doi: 10.4172/2329-6887.1000125
Profaci, C. P., Munji, R. N., Pulido, R. S., and Daneman, R. (2020). The blood-brain barrier in health and disease: important unanswered questions. J. Exp. Med. 217:e20190062. doi: 10.1084/jem.20190062
Pun, P. B., Lu, J., and Moochhala, S. (2009). Involvement of ROS in BBB dysfunction. Free Radic. Res. 43, 348–364. doi: 10.1080/10715760902751902
Rabinowitz, J., Chan, Y. K., and Samulski, R. J. (2019). Adeno-associated virus (AAV) versus immune response. Viruses 11:102. doi: 10.3390/v11020102
Raleigh, D. R., Marchiando, A. M., Zhang, Y., Shen, L., Sasaki, H., Wang, Y., et al. (2010). Tight junction-associated MARVEL proteins marveld3, tricellulin, and occludin have distinct but overlapping functions. Mol. Biol. Cell 21, 1200–1213. doi: 10.1091/mbc.e09-08-0734
Ramsay, R. E., Rowan, A. J., and Pryor, F. M. (2004). Special considerations in treating the elderly patient with epilepsy. Neurology 62, S24–S29. doi: 10.1212/wnl.62.5_suppl_2.s24
Rapti, K., Louis-Jeune, V., Kohlbrenner, E., Ishikawa, K., Ladage, D., Zolotukhin, S., et al. (2012). Neutralizing antibodies against AAV serotypes 1, 2, 6, and 9 in sera of commonly used animal models. Mol. Ther. 20, 73–83. doi: 10.1038/mt.2011.177
Rezai, A. R., Ranjan, M., D'Haese, P. F., Haut, M. W., Carpenter, J., Najib, U., et al. (2020). Noninvasive hippocampal blood-brain barrier opening in Alzheimer's disease with focused ultrasound. Proc. Natl. Acad. Sci. USA 117, 9180–9182. doi: 10.1073/pnas.2002571117
Rodríguez-Arellano, J. J., Parpura, V., Zorec, R., and Verkhratsky, A. (2016). Astrocytes in physiological aging and Alzheimer's disease. Neuroscience 323, 170–182. doi: 10.1016/j.neuroscience.2015.01.007
Rosenberg, G. A. (2014). Blood-brain barrier permeability in aging and Alzheimer's disease. J. Prev Alzheimers Dis. 1, 138–139. doi: 10.14283/jpad.2014.25
Rustenhoven, J., Jansson, D., Smyth, L. C., and Dragunow, M. (2017). Brain Pericytes as mediators of Neuroinflammation. Trends Pharmacol. Sci. 38, 291–304. doi: 10.1016/j.tips.2016.12.001
Salar, S., Maslarova, A., Lippmann, K., Nichtweiss, J., Weissberg, I., Sheintuch, L., et al. (2014). Blood-brain barrier dysfunction can contribute to pharmacoresistance of seizures. Epilepsia 55, 1255–1263. doi: 10.1111/epi.12713
Salinas, S., Schiavo, G., and Kremer, E. J. (2010). A hitchhiker's guide to the nervous system: the complex journey of viruses and toxins. Nat. Rev. Microbiol. 8, 645–655. doi: 10.1038/nrmicro2395
Sá-Pereira, I., Brites, D., and Brito, M. A. (2012). Neurovascular unit: a focus on pericytes. Mol. Neurobiol. 45, 327–347. doi: 10.1007/s12035-012-8244-2
Saraiva, C., Praça, C., Ferreira, R., Santos, T., Ferreira, L., and Bernardino, L. (2016). Nanoparticle-mediated brain drug delivery: overcoming blood-brain barrier to treat neurodegenerative diseases. J. Control. Release 235, 34–47. doi: 10.1016/j.jconrel.2016.05.044
Schmidt, D., and Schachter, S. C. (2014). Drug treatment of epilepsy in adults. BMJ 348:g254. doi: 10.1136/bmj.g254
Segarra, M., Aburto, M. R., and Acker-Palmer, A. (2021). Blood-brain barrier dynamics to maintain brain homeostasis. Trends Neurosci. 44, 393–405. doi: 10.1016/j.tins.2020.12.002
Seiffert, E., Dreier, J. P., Ivens, S., Bechmann, I., Tomkins, O., Heinemann, U., et al. (2004). Lasting blood-brain barrier disruption induces epileptic focus in the rat somatosensory cortex. J. Neurosci. 24, 7829–7836. doi: 10.1523/jneurosci.1751-04.2004
Sen, A., Jette, N., Husain, M., and Sander, J. W. (2020). Epilepsy in older people. Lancet 395, 735–748. doi: 10.1016/s0140-6736(19)33064-8
Senatorov, V. V. Jr., Friedman, A. R., Milikovsky, D. Z., Ofer, J., Saar-Ashkenazy, R., Charbash, A., et al. (2019). Blood-brain barrier dysfunction in aging induces hyperactivation of TGFβ signaling and chronic yet reversible neural dysfunction. Sci. Transl. Med. 11:eaaw8283. doi: 10.1126/scitranslmed.aaw8283
Sharma, D., Maheshwari, D., Philip, G., Rana, R., Bhatia, S., Singh, M., et al. (2014). Formulation and optimization of polymeric nanoparticles for intranasal delivery of lorazepam using box-Behnken design: in vitro and in vivo evaluation. Biomed. Res. Int. 2014:156010. doi: 10.1155/2014/156010
Sharma, D., Sharma, R. K., Sharma, N., Gabrani, R., Sharma, S. K., Ali, J., et al. (2015). Nose-to-brain delivery of PLGA-diazepam nanoparticles. AAPS PharmSciTech 16, 1108–1121. doi: 10.1208/s12249-015-0294-0
Shen, J., Xu, G., Zhu, R., Yuan, J., Ishii, Y., Hamashima, T., et al. (2019). PDGFR-β restores blood-brain barrier functions in a mouse model of focal cerebral ischemia. J. Cereb. Blood Flow Metab. 39, 1501–1515. doi: 10.1177/0271678x18769515
Soares, R. V., Do, T. M., Mabondzo, A., Pons, G., and Chhun, S. (2016). Ontogeny of ABC and SLC transporters in the microvessels of developing rat brain. Fundam. Clin. Pharmacol. 30, 107–116. doi: 10.1111/fcp.12175
Solans, C., Izquierdo, P., Nolla, J., Azemar, N., and Garcia-Celma, M. J. (2005). Nano-emulsions. Curr. Opin. Colloid Interface Sci. 10, 102–110. doi: 10.1016/j.cocis.2005.06.004
Song, Q., Huang, M., Yao, L., Wang, X., Gu, X., Chen, J., et al. (2014). Lipoprotein-based nanoparticles rescue the memory loss of mice with Alzheimer's disease by accelerating the clearance of amyloid-beta. ACS Nano 8, 2345–2359. doi: 10.1021/nn4058215
Spéder, P., and Brand, A. H. (2014). Gap junction proteins in the blood-brain barrier control nutrient-dependent reactivation of Drosophila neural stem cells. Dev. Cell 30, 309–321. doi: 10.1016/j.devcel.2014.05.021
Sweeney, M. D., Ayyadurai, S., and Zlokovic, B. V. (2016). Pericytes of the neurovascular unit: key functions and signaling pathways. Nat. Neurosci. 19, 771–783. doi: 10.1038/nn.4288
Sweeney, M. D., Montagne, A., Sagare, A. P., Nation, D. A., Schneider, L. S., Chui, H. C., et al. (2019a). Vascular dysfunction-the disregarded partner of Alzheimer's disease. Alzheimers Dement. 15, 158–167. doi: 10.1016/j.jalz.2018.07.222
Sweeney, M. D., Sagare, A. P., and Zlokovic, B. V. (2018). Blood-brain barrier breakdown in Alzheimer disease and other neurodegenerative disorders. Nat. Rev. Neurol. 14, 133–150. doi: 10.1038/nrneurol.2017.188
Sweeney, M. D., Zhao, Z., Montagne, A., Nelson, A. R., and Zlokovic, B. V. (2019b). Blood-brain barrier: from physiology to disease and Back. Physiol. Rev. 99, 21–78. doi: 10.1152/physrev.00050.2017
Trinka, E. (2003). Epilepsy: comorbidity in the elderly. Acta Neurol. Scand. Suppl. 108, 33–36. doi: 10.1034/j.1600-0404.108.s180.5.x
Uzüm, G., Sarper Diler, A., Bahçekapili, N., and Ziya Ziylan, Y. (2006). Erythropoietin prevents the increase in blood-brain barrier permeability during pentylentetrazol induced seizures. Life Sci. 78, 2571–2576. doi: 10.1016/j.lfs.2005.10.027
van de Haar, H. J., Jansen, J. F. A., van Osch, M. J. P., van Buchem, M. A., Muller, M., Wong, S. M., et al. (2016). Neurovascular unit impairment in early Alzheimer's disease measured with magnetic resonance imaging. Neurobiol. Aging 45, 190–196. doi: 10.1016/j.neurobiolaging.2016.06.006
van Vliet, E. A., Aronica, E., and Gorter, J. A. (2014). Role of blood-brain barrier in temporal lobe epilepsy and pharmacoresistance. Neuroscience 277, 455–473. doi: 10.1016/j.neuroscience.2014.07.030
van Vliet, E. A., and Marchi, N. (2022). Neurovascular unit dysfunction as a mechanism of seizures and epilepsy during aging. Epilepsia 63, 1297–1313. doi: 10.1111/epi.17210
Verheggen, I. C. M., de Jong, J. J. A., van Boxtel, M. P. J., Gronenschild, E., Palm, W. M., Postma, A. A., et al. (2020). Increase in blood-brain barrier leakage in healthy, older adults. Geroscience 42, 1183–1193. doi: 10.1007/s11357-020-00211-2
Vilella, A., Belletti, D., Sauer, A. K., Hagmeyer, S., Sarowar, T., Masoni, M., et al. (2018). Reduced plaque size and inflammation in the APP23 mouse model for Alzheimer's disease after chronic application of polymeric nanoparticles for CNS targeted zinc delivery. J. Trace Elem. Med. Biol. 49, 210–221. doi: 10.1016/j.jtemb.2017.12.006
Vu, L. C., Piccenna, L., Kwan, P., and O'Brien, T. J. (2018). New-onset epilepsy in the elderly. Br. J. Clin. Pharmacol. 84, 2208–2217. doi: 10.1111/bcp.13653
Wang, L., Xiong, X., Zhang, L., and Shen, J. (2021). Neurovascular Unit: A critical role in ischemic stroke. CNS Neurosci. Ther. 27, 7–16. doi: 10.1111/cns.13561
Weissberg, I., Wood, L., Kamintsky, L., Vazquez, O., Milikovsky, D. Z., Alexander, A., et al. (2015). Albumin induces excitatory synaptogenesis through astrocytic TGF-β/ALK5 signaling in a model of acquired epilepsy following blood-brain barrier dysfunction. Neurobiol. Dis. 78, 115–125. doi: 10.1016/j.nbd.2015.02.029
Werhahn, K. J., Klimpe, S., Balkaya, S., Trinka, E., and Krämer, G. (2011). The safety and efficacy of add-on levetiracetam in elderly patients with focal epilepsy: a one-year observational study. Seizure 20, 305–311. doi: 10.1016/j.seizure.2010.12.015
Wiest, R., and Beisteiner, R. (2019). Recent developments in imaging of epilepsy. Curr. Opin. Neurol. 32, 530–538. doi: 10.1097/wco.0000000000000704
Wilson, B., Selvam, J., Mukundan, G. K., Premakumari, K. B., and Jenita, J. L. (2020). Albumin nanoparticles coated with polysorbate 80 for the targeted delivery of antiepileptic drug levetiracetam into the brain. Drug Deliv. Transl. Res. 10, 1853–1861. doi: 10.1007/s13346-020-00831-3
Wu, D., Fei, F., Zhang, Q., Wang, X., Gong, Y., Chen, X., et al. (2022). Nanoengineered on-demand drug delivery system improves efficacy of pharmacotherapy for epilepsy. Sci. Adv. 8:eabm3381. doi: 10.1126/sciadv.abm3381
Yan, L., Moriarty, R. A., and Stroka, K. M. (2021). Recent progress and new challenges in modeling of human pluripotent stem cell-derived blood-brain barrier. Theranostics 11, 10148–10170. doi: 10.7150/thno.63195
Yildiz, I., Tsvetkova, I., Wen, A. M., Shukla, S., Masarapu, M. H., Dragnea, B., et al. (2012). Engineering of brome mosaic virus for biomedical applications. RSC Adv. 2, 3670–3677. doi: 10.1039/c2ra01376b
Yu, Q. J., Tao, H., Wang, X., and Li, M. C. (2015). Targeting brain microvascular endothelial cells: a therapeutic approach to neuroprotection against stroke. Neural Regen. Res. 10, 1882–1891. doi: 10.4103/1673-5374.170324
Zhao, J., Ye, Z., Yang, J., Zhang, Q., Shan, W., Wang, X., et al. (2020). Nanocage encapsulation improves antiepileptic efficiency of phenytoin. Biomaterials 240:119849. doi: 10.1016/j.biomaterials.2020.119849
Keywords: blood–brain barrier, geriatric epilepsy, antiepileptic therapy, antiepileptic drug, nanoparticle
Citation: Chen X, Luo J, Song M, Pan L, Qu Z, Huang B, Yu S and Shu H (2024) Challenges and prospects in geriatric epilepsy treatment: the role of the blood–brain barrier in pharmacotherapy and drug delivery. Front. Aging Neurosci. 16:1342366. doi: 10.3389/fnagi.2024.1342366
Edited by:
Rubem C. A. Guedes, Federal University of Pernambuco, BrazilReviewed by:
Yanfang Li, Xiamen University, ChinaDan Z. Milikovsky, Tel Aviv Sourasky Medical Center, Israel
Copyright © 2024 Chen, Luo, Song, Pan, Qu, Huang, Yu and Shu. This is an open-access article distributed under the terms of the Creative Commons Attribution License (CC BY). The use, distribution or reproduction in other forums is permitted, provided the original author(s) and the copyright owner(s) are credited and that the original publication in this journal is cited, in accordance with accepted academic practice. No use, distribution or reproduction is permitted which does not comply with these terms.
*Correspondence: Sixun Yu, YmluZ3l1dGlhbjE5ODJAMTI2LmNvbQ==; Haifeng Shu, c2h1aGFpZmVuZ0Bzd2p0dS5lZHUuY24=