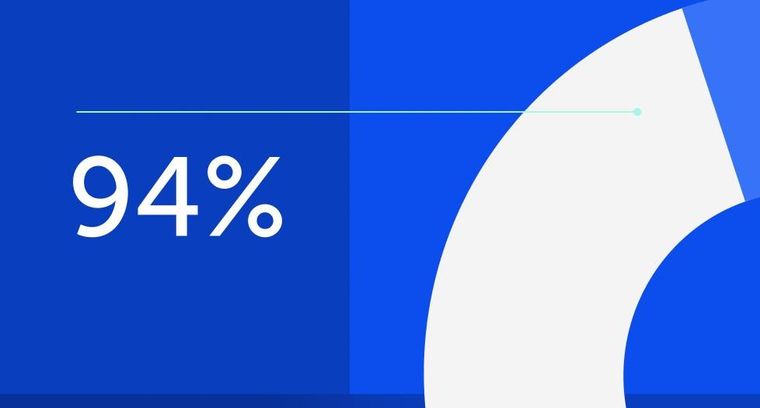
94% of researchers rate our articles as excellent or good
Learn more about the work of our research integrity team to safeguard the quality of each article we publish.
Find out more
REVIEW article
Front. Aging Neurosci., 19 December 2023
Sec. Alzheimer's Disease and Related Dementias
Volume 15 - 2023 | https://doi.org/10.3389/fnagi.2023.1300405
This article is part of the Research TopicNeurotoxins in Alzheimer's Disease and Other DementiasView all 8 articles
Copper (Cu) plays a crucial role as a trace element in various physiological processes in humans. Nonetheless, free copper ions accumulate in the brain over time, resulting in a range of pathological changes. Compelling evidence indicates that excessive free copper deposition contributes to cognitive decline in individuals with Alzheimer’s disease (AD). Free copper levels in the serum and brain of AD patients are notably elevated, leading to reduced antioxidant defenses and mitochondrial dysfunction. Moreover, free copper accumulation triggers a specific form of cell death, namely copper-dependent cell death (cuproptosis). This article aimed to review the correlation between copper dysregulation and the pathogenesis of AD, along with the primary pathways regulating copper homoeostasis and copper-induced death in AD. Additionally, the efficacy and safety of natural and synthetic agents, including copper chelators, lipid peroxidation inhibitors, and antioxidants, were examined. These treatments can restore copper equilibrium and prevent copper-induced cell death in AD cases. Another aim of this review was to highlight the significance of copper dysregulation and promote the development of pharmaceutical interventions to address it.
Alzheimer’s disease (AD) is a neurodegenerative condition characterized by cognitive memory impairment and is the primary form of senile dementia, representing 60–70% of all dementia cases (Gao et al., 2022; Huang, 2023). As the population ages, the incidence of Alzheimer’s has steadily increased, resulting in substantial societal and financial burdens (Huang, 2023). Earlier studies estimate that around 152 million individuals worldwide will develop AD by 2050, emphasizing the urgent need for effective drug therapies (Zhang et al., 2021). Currently, drugs can only alleviate the symptoms of AD and do not cease or alter its progression. Therefore, it is critical to discover efficient remedies for this condition that pose a notable obstacle for humanity.
Alzheimer’s disease (AD) is characterized by the accumulation of amyloid-beta (Aβ) plaques and tau neuronal tangles in the brain (van der Kant et al., 2020; Gao et al., 2022). However, the etiology and pathogenesis of AD have remained elusive due to the intricate pathological changes (Kaur D. et al., 2021). Two clinical trials targeting Aβ plaques as a therapeutic intervention for AD have not yielded positive outcomes, suggesting that Aβ plaques may not be the optimal target (Salloway et al., 2014). Therefore, the current research is focused on identifying additional pathomechanisms relevant to AD.
In recent decades, an increasing number of studies have linked copper (Cu) dyshomeostasis to the pathogenesis of AD (Squitti et al., 2014; Talwar et al., 2017; Pal et al., 2021a). Cu is a redox-active metal that participates in several metabolic processes in the brain under physiological conditions (Lei et al., 2021). It is either present as protein-bound Cu or non-protein-bound Cu (free Cu) in human tissues, and exerts its effects in the free form (Zhang et al., 2016). Construction of a premature aging model (CuSO4-SIPS) using copper sulfate (CuSO4) demonstrates the role of Cu in age-related functional decline and advancement in age-related diseases (Matos et al., 2015, 2017). Furthermore, higher levels of free Cu have been observed in the serum of patients with AD than in non-AD subjects, with a greater proportion of exchangeable Cu in the brains of patients with AD (Desai and Kaler, 2008; James et al., 2012; Squitti et al., 2014). Furthermore, high consumption of copper sulfate pentahydrate by mice is causally linked to cognitive impairment, suggesting a potential correlation between Cu exposure and AD (Noda et al., 2013; Chen et al., 2022; Zhang et al., 2023).
Cuproptosis is a recent term coined to refer to cell death caused by the aggregation of lipoylated proteins and proteotoxic stress from excessive accumulation of Cu (Tsvetkov et al., 2022). The literature reports a potential correlation between a higher proportion of labile Cu levels and oxidative damage in the brains of patients with AD (James et al., 2012), which is intricately associated with cuproptosis. Therefore, this review focuses on the impact of free Cu and cuproptosis on cognitive functions, as well as potential therapeutic interventions. We believe that a better understanding of the mechanism by which Cu and cuproptosis promote AD development will help in delineating the pathogenesis of the disease and offering insights into effective treatment strategies.
Copper is known to participate in the physiological functioning of the human body (Jomova et al., 2022; Tsvetkov et al., 2022). However, excessive accumulation of free Cu is linked to several pathological conditions, including neurodegenerative disorders, cancer, and cardiovascular diseases (Chen et al., 2022). In Chan et al. (1978) reported that a healthy fibroblast medium containing Cu concentrations above 30 μg/mL induced cell death. Although Cu concentrations in human serum are considerably lower (Barceloux, 1999), Chan et al. (1978) reported that Cu dysregulation contributes to the underlying pathology.
Tsvetkov et al. (2022) reported that excessive Cu2+ induces a new form of cell death, which is distinct from all other known apoptotic mechanisms, referred to as cuproptosis. Cuproptosis is mediated by protein lipoylation, and FDX1 serves as the upstream regulator. Thus, both ferredoxin 1 (FDX1) and protein lipoylation mediate cuproptosis. Under physiological conditions, protein lipoylation participates in the tricarboxylic acid (TCA) cycle (Solmonson and DeBerardinis, 2018). Previous studies have shown a correlation between mitochondrial metabolism and susceptibility to cuproptosis. Specifically, cells with an active TCA cycle exhibit increased protein lipoylation, and the lipoyl moiety acts as a Cu binder, further promoting protein lipoylation aggregation, Fe–S cluster protein loss, and heat shock protein (HSP)70 induction, ultimately causing acute protein toxic stress (Tsvetkov et al., 2022). Cuproptosis has been associated with several pathological conditions, including neurodegenerative diseases, Wilson’s disease, cancer, and cardiovascular disease (Chen et al., 2022).
Copper has been implicated in the maintenance of normal brain functions, and deviations from appropriate levels, whether insufficient or excessive, can cause several disorders (Madsen and Gitlin, 2007). Excessive Cu accumulation can result in Cu toxicity, leading to apoptosis, astrocytosis, and damage to the hippocampus (Kalita et al., 2018). Therefore, maintaining normal Cu levels is essential for optimal memory and learning. A significant amount of free Cu is present in the serum of patients with cognitive impairment and AD (Squitti et al., 2005, 2017; Rozzini et al., 2018). Moreover, a meta-analysis revealed a positive correlation between serum Cu levels and the risk of AD (Li et al., 2017). Excessive accumulation of Cu could contribute to the development of AD by mediating cuproptosis, oxidative stress, synaptic damage, Aβ plaque deposition, and neuronal death (Figure 1).
Figure 1. Alzheimer’s disease (AD) and copper ions. Copper is transported into brain cells through membrane copper transporter protein CTR1 and exits through ATP7A. The effect of copper on the development of AD encompasses a varied and intricate array of outcomes. (1) Copper regulates the expression of amyloid precursor protein (APP) and the aggregation of tau, both of which contribute to oxidative damage. (2) Accumulation of copper can result in cuproptosis and reduced activity in the cAMP-response element-binding (CREB) pathway, potentially leading to impaired synaptic plasticity and cognitive abilities. (3) Copper is closely linked to synaptic plasticity via the regulation of long-term potentiation (LTP) and neurotransmission. (4) The excessive accumulation of copper impacts microglial phagocytosis of Aβ and the release of pro-inflammatory cytokines. (5) IFN-γ boosts copper uptake by increasing copper-dependent translocation of ATP7A from Golgi to cytoplasmic vesicles as well as by upregulating the expression level of the copper importer, CTR1. This leads to a significant alteration in copper homeostasis.
The central nervous system (CNS) is greatly affected by Cu exposure (Rossi-George and Guo, 2016; Barca et al., 2019). A recent study demonstrated that Cu inhibits the expression of enzymatic antioxidants, namely superoxide dismutase (SOD) and glutathione peroxidase (GPX), in the brain tissue of mice (Zhang et al., 2023), indicating the induction of oxidative damage. In addition, Cu increased the levels of malondialdehyde (MDA), a lipid peroxide, in the hippocampus of rats with cognitive impairment, suggesting lipid damage (Zhang et al., 2023). In another study, Cu exposure elevated the levels of hippocampal nitric oxide and oxidative stress (OS), increased hippocampal tissue lipid peroxidation (LPO) levels, and decreased SOD and catalase (CAT) activities (Lamtai et al., 2020). Furthermore, Cu toxicity induces neuronal death and astrocyte proliferation in the hippocampus through glutamate and oxidative stress pathways, causing impaired memory and learning ability (Kalita et al., 2018). Thus, Cu-induced cognitive impairment is intricately associated with the induction of oxidative damage in the hippocampus (Kalita et al., 2018; Lu et al., 2022).
Copper exists in different oxidation states (Cu2+ and Cu+) during the Cu cycle, resulting in the dysregulation of Cu steady-states, which is hypothesized to be one of the key mechanisms underlying brain injury (Zhang et al., 2023). Copper can generate reactive free radicals through the Fenton reaction and directly interact with Aβ plaques and amyloid precursor protein (APP), thereby promoting the synthesis and aggregation of Aβ plaques (Kitazawa et al., 2016). Furthermore, Cu can bind to Aβ plaques, thereby directly contributing to the generation of reactive oxygen species (ROS) (Cheignon et al., 2018). The overproduction of ROS can trigger oxidative damage in several biological macromolecules, including the proteome, lipids, and DNA (Wu and Cederbaum, 2003; Tomanek, 2015; Pal et al., 2021b). In contrast, sequestering Cu from Aβ peptides can hinder its accumulation and maximize Aβ degradation (Cherny et al., 2001; Behbehani et al., 2012), inhibit hydroxyl radical (OH) production and oxidative damage, and ultimately decrease cell death (Chen et al., 2022).
The effects of Cu on memory function and oxidative stress in rats are gender-dependent, with marginally greater effects observed in female rats (Lamtai et al., 2020). This could be because estrogen enhances Cu retention, rendering females more susceptible to its neurotoxic effects (Amtage et al., 2014). However, further investigation is warranted to elucidate potential gender differences in the neurocognitive consequences of Cu.
Numerous studies have suggested that Cu-induced oxidative stress and lipid peroxide production could be used as an intervention target to prevent AD.
Copper can interact with several pathogenic factors, including Aβ plaques and tau (Chen et al., 2022). In addition, it promotes the toxic accumulation of Aβ plaques in the brain (Kitazawa et al., 2016), thereby increasing the risk of AD.
The formation and accumulation of Aβ are central to AD pathogenesis. These originate from Aβ precursor protein (AβPP), which deposits in neuronal plaques, wherein AβPP regulates Aβ synthesis (Selkoe, 2001; An et al., 2022). Cu binds to the Aβ protein to form a stable complex (Atrián-Blasco et al., 2019) that generates ROS (Atrián-Blasco et al., 2018) and exacerbates neuronal damage (An et al., 2022). Thus, Cu-promoted Aβ neurotoxicity could be responsible for the pathogenesis of AD.
A study reported that Cu governs the conversion of AβPP into Aβ (Becaria et al., 2006), thereby contributing to the neurodegeneration in AD. In addition, Kitazawa et al. (2016) stated that the Cu–Aβ complex downregulates the expression of lipoprotein receptor-related protein 1 (LRP1), eventually limiting the clearance of neurotoxic Aβ plaques and causing their accumulation in the brain. In contrast, Cu chelators upregulate the expression of ADAM 10 through the melatonin receptor (MT1/2) and its associated downstream signaling pathways, causing marked improvements in cognitive performance in AβPP/PS1 Tg mice (Wang et al., 2018c). Moreover, Cu can phosphorylate tau proteins, causing their aggregation and enhancing the formation of plaques and pathological tangles in the brain (Kitazawa et al., 2009; Du et al., 2014; Voss et al., 2014). Cognitive impairment in AD is attributed to synaptic damage caused by p-tau, both structurally and functionally (Polydoro et al., 2009; Tai et al., 2012).
The accumulation of Cu in the brain is known to trigger cuproptosis and reduce the phosphorylation of cAMP-response element-binding protein (CREB), as well as downregulate the expression of brain-derived neurotrophic factor (BDNF) and its receptor, tropomyosin receptor kinase B (TrkB) (Zhang et al., 2023). In turn, these changes lead to both structural and functional alterations in neurons, which subsequently impairs synaptic plasticity and cognitive abilities.
Furthermore, Cu ions can directly bind to fatty acylated proteins in the mitochondrial tricarboxylic acid (TCA) cycle, thereby stimulating abnormal oligomerization of fatty acylated proteins. This results in a loss of Fe-S cluster proteins, causing proteotoxic stress and, eventually, cuproptosis in neuronal cells. Several studies have demonstrated that neuronal loss can cause cognitive impairment (Hwang et al., 2006; Do Val-da Silva et al., 2016; Esquerda-Canals et al., 2019). The key pathological processes leading to cuproptosis are FDX1-mediated protein lipoacylation and Cu reduction reactions.
Copper accumulation-induced oxidative damage can inhibit CREB phosphorylation and impair CREB-mediated neuronal excitability, which is vital for memory formation (Lisman et al., 2018; Sharma and Singh, 2020; Lu et al., 2022). Phosphorylation of CREB promotes neuronal survival and neutrophin-induced differentiation (Bonni et al., 1995). BDNF is a neurotrophic factor widely distributed throughout the brain, which largely regulates synaptic maturation at morphological, molecular, and functional levels (Nieto et al., 2013). Intracellular signaling, stimulated by both BDNF and its receptor TrkB, contributes to neuronal survival, morphogenesis, and plasticity (Numakawa et al., 2010). In addition, CREB is known to mediate the neurotrophic and neuroprotective impacts of BDNF (Fujino et al., 2009; Wang et al., 2018a), BDNF promotes the phosphorylation of CREB by activating TrkB (Pizzorusso et al., 2000), and CREB phosphorylation promotes the transcription of the BDNF gene (Wang et al., 2018a). This sequence of events is critical for the initiation and preservation of synaptic functions. In addition, increasing the expression of CREB is known to prevent cuproptosis (Zhang et al., 2023).
Because excessive accumulation of Cu in the hippocampus can severely impair cognitive function through CREB in AD, it is essential to maintain Cu homeostasis in the hippocampus. Similarly, activating the CREB pathway and inhibiting neuronal cell death in the AD brain tissue could potentially serve as novel strategies to develop drugs against AD or intervene in it either by delaying or halting its progression.
Several studies have identified that pro-inflammatory pathways in microglia are associated with abnormal Cu homeostasis and AD (Zheng et al., 2010; Wang et al., 2018b). Although microglia can phagocytize Aβ or Aβ-antibody complexes (Das et al., 2003; Ennerfelt et al., 2022; Jäntti et al., 2022), an excessive accumulation of Cu ions could hinder phagocytosis. This could be attributed to the effect of Cu on the LDL receptor-related protein 1 (LRP1), which is responsible for transporting Aβ protein (Singh et al., 2013). Increased expression of LRP has been demonstrated to control Aβ accumulation and neuroinflammation in AD (Deane et al., 2008). Therefore, reduced microglial phagocytosis of Aβ and a decrease in LRP1 expression increase the risk of AD following Cu exposure.
Interferon (IFN)-γ is the only cytokine in the IFN family (Conlon et al., 2019) that elicits and facilitates inflammatory responses (Langer et al., 2019; Karki et al., 2021; Yang et al., 2022) and induces cellular death (Kano et al., 1997; Tirotta et al., 2012). IFN-γ transcription and production are significantly increased in reactive microglia and astrocytes surrounding the Aβ deposits in the cerebral cortical region of the transgenic AD mouse model (Abbas et al., 2002). Thus, IFN-γ could exert an inflammatory effect on the formation of amyloid plaques and activation of microglia and astrocytes. IFN-γ induces significant changes in copper homeostasis by increasing copper-dependent transport of ATP7A from the Golgi apparatus to cytosolic vesicles, enhancing copper uptake and raising expression levels of the CTR1 copper importer (Zheng et al., 2010). The finding infer that pro-inflammatory conditions associated with AD substantially alter microglial copper transport, which may account for the fluctuations in copper homeostasis in AD patients (Zheng et al., 2010).
The activation of nuclear factor (NF)-κB in microglia is associated with oxidative stress, inflammatory response, and apoptosis (Liu et al., 2020, 2021; Tastan et al., 2021). Copper facilitates the activation of microglia and ensues neurotoxicity via the phosphorylation and translocation of NF-κB p65. Paired helical filament (PHF) tau and advanced glycation end products in AD generate oxygen radicals that activate transcription via NF-κB, increase Aβ protein precursors, and release Aβ peptides (Yan et al., 1995).
The promoter activity of apolipoprotein E (APOE), which is intricately linked to the development of AD, is dependent on NF-κB (Du et al., 2005). APOE impedes microglial response, blocks Aβ clearance, hastens Aβ aggregation, affects tau pathology and tau-mediated neurodegeneration, and compromises synaptic integrity and plasticity, thereby contributing to the development of AD (Yamazaki et al., 2019).
Synaptic damage is the primary cause of cognitive impairment in AD (Polydoro et al., 2009; Tai et al., 2012). A study reported that Cu, an essential element, could exert a biphasic impact on the initiation of neurotransmission to uphold sufficient synaptic function (Opazo et al., 2014). Physiologically, Cu is essential for normal synaptic functions by improving neurotransmission via modification of the arrangement of neuronal proteins, such as by facilitating the accumulation of postsynaptic density protein (PSD) 95 protein (Opazo et al., 2014). Excessive Cu exposure is known to impact both pre- and post-synaptic regulatory mechanisms (Zhang et al., 2023). Copper sulfate (5 mg/kg) has been reported to substantially enhance the population spike (PS) amplitude elicited by hippocampal Schaffer collateral fiber stimulation and repress long-term potentiation (LTP) and paired-pulse index (PPI) in the hippocampal CA1 zone. Conversely, higher concentrations (10 mg/kg and 15 mg/kg) of copper sulfate fail to exert any significant effects on PS amplitude, LTP, or PPI inception (Jand et al., 2018). Another study reported that Cu administration caused a concentration-dependent increase in the total Cu content in the hippocampus. However, hippocampal-free Cu only increased after the administration of lower concentrations (0.2 mg/Kg) of Cu(OAc)2 and decreased after the administration of higher concentrations (2 mg/Kg and 20 mg/Kg) (Zhang et al., 2016). This could be attributed to higher concentrations of Cu that stimulate responses in Cu-binding proteins (Zhang et al., 2016).
Several studies have reported that Cu impedes long-term potentiation and reduces synaptic plasticity (Doreulee et al., 1997; Leiva et al., 2003; Goldschmith et al., 2005). Goldschmith administered high doses of copper (dissolved in water) (8–10 mg/day) to rats and observed inhibition of LTP onset, with a significant reduction in synapse sensitivity and facilitation (Goldschmith et al., 2005). Similarly, Leiva et al. (2003, 2009) investigated the effect of long-term copper sulfate administration (1 mg/kg) on hippocampal LTP, and the findings align with the previously mentioned results. Overall, these findings indicate that copper exerts an effect on synaptic function (Gaier et al., 2013).
In addition, Cu-induced dysregulation of acetylcholine (ACh), glutamate, γ-aminobutyric acid (GABA), and other synaptic transmitters, is strongly associated with cognitive abnormalities and behavioral changes in AD (Pal et al., 2013; Pavandi et al., 2014; Zhang et al., 2016; Kaur S. et al., 2021). Copper is released into the synaptic cleft, where it inhibits excitatory neurotransmission by blocking glutamate receptors (Opazo et al., 2014). However, its excessive accumulation significantly decreases the levels of dopamine, 5-hydroxytryptamine, and GABA and increases those of glutamate (Kaur S. et al., 2021). Glutamate contributes to neurological disorders by binding with glutamate receptors (Traynelis et al., 2010). An excessive amount of glutamate has been demonstrated to induce oxidative stress and activate caspase-3 and glial fibrillary acidic protein (GFAP) (Kalita et al., 2018). The overabundance of extracellular Glu and subsequent excessive activation of ionic Glu receptors eventually cause neuronal cell death (Lamtai et al., 2020). In addition, excessive accumulation of Cu decreases the serum acetylcholinesterase activity and causes neuronal degeneration (Pal et al., 2013). A recent study concluded that exposure to copper downregulates the expression of serotonin (5-HT), dopamine (DA), and GABA in the hippocampus of mice (Zhang et al., 2023).
Heavy metals are known to initiate free radical-mediated chain reactions, causing oxidative deterioration of biomolecules, lipid peroxidation, protein oxidation, and oxidation of nucleic acids (Flora et al., 2013). Free metals significantly contribute to the pathomechanisms of AD. Because patients with AD often experience disruptions in metal homeostasis and OS within their brains (Sestito et al., 2019), metal chelators could be used as a potential therapeutic strategy to prevent excessive free Cu from contributing to redox reactions. Metal chelators have been demonstrated to significantly reduce the generation of ROS and hydroxyl radicals (Csire et al., 2020).
Clioquinol (CQ) is a mild metal chelating agent in the body for iron, Cu and zinc (Cahoon, 2009). For example, a 9-week CQ treatment inhibited and could have reversed the accumulation of Aβ deposits in APP 2,576 transgenic animals. Furthermore in vitro experiments demonstrated that CQ blocked the interaction of Cu2+ and Zn2+ within brain Aβ plaques, thus reversing metal ion-induced aggregation (Cherny et al., 2001). However, several studies have reported that CQ does not exhibit a significant advantage over alternative chelators in effectively treating AD (Adlard et al., 2008; Sun et al., 2022). Furthermore, clinical trials of CQ for AD have been unsuccessful (Ho et al., 2022).
5,7-Dichloro-2-(dimethylamino)methyl-8-hydroxyquinoline (PBT2) is a second-generation derivative of 8-OH quinoline (Lannfelt et al., 2008) that has been developed as an ion carrier (Bush, 2008; Cahoon, 2009). It has demonstrated satisfactory efficacy in phase IIa clinical trials (Bush, 2008). However, the results of existing clinical trials are questionable including phase IIa trials. For example, there exists a bias toward reporting outcomes of clinical trials of therapeutic Cu chelators as positive and beneficial for patients (Drew, 2017). In addition, a recent study demonstrated that PBT2 could not chelate Cu(II) from Aβ(1-42) than CQ and B2 Q. Therefore, it can be hypothesized that PBT2 has a poor anti-AD effect (Summers et al., 2022). Further studies are required to completely and accurately assess the protective properties of PBT2 in AD.
Tetrathiomolybdate (TTM) is a kind of chelating agent that can inhibit Cu absorption, has a rapid onset of action, and does not cause neurological deterioration (Ejaz et al., 2020). Although TTM is toxic following injection into the hippocampus, the formation of metal ion/TTM complexes with Cu2+ reduces the toxicity (Armstrong et al., 2001). TTM can form a high-affinity triple complex with Cu and albumin, chelating Cu in the bloodstream (Yu et al., 2006). However, ammonium preparations have been considered highly unstable for routine use (Ejaz et al., 2020). Moreover, TTM is not effective when used as a single agent (Yu et al., 2006) and its clinical applicability remains limited (Weiss et al., 2017).
Bis-choline TTM (WTX101) is an orally available Cu protein-binding molecule that targets Cu in hepatocytes and reduces plasma non-ceruloplasmin-bound copper (NCC) by forming a triple complex with albumin and enhances biliary Cu excretion, and is more stable than TTM (Weiss et al., 2017).
Weiss et al. (2017) conducted a Phase II study to evaluate the efficacy and safety of WTX101 in the initial or early treatment of patients with Wilson’s disease (WD). The results suggested that low-dose WTX101 could be a promising novel treatment for WD (Weiss et al., 2017). Molybdenum accumulates in the major organs of Sprague–Dawley rats following repeated oral administration of bis-choline TTM. Therefore, it is hypothesized that prolonged exposure could result in adverse pathophysiological cellular functions (Foster et al., 2022).
Sun et al. (2022) designed specific Cu chelators [tetradentate monoquinolines (TDMQs)] and investigated changes in protein profiles expressed in 5xFAD mice following oral treatment with TDMQ20. The results implied that TDMQ20, a Cu chelator, functions on the cholinergic system and mediates Cu homeostasis in the brains of patients with AD and inhibits the deleterious oxidative stress catalyzed by Cu–Aβ complexes, thereby improving cognitive and behavioral performance in AD rats (Sun et al., 2022). In another study, Zhao et al. (2021) demonstrated that TDMQ20 reduced memory impairment in a mouse model of AD. However, there exists a dearth of extensive clinical trials assessing the effects of TDMQ20 in human subjects.
In addition to potential side effects and instability, numerous objective reasons restrict the use of Cu chelators (Table 1). Certain chelators for Cu are hydrophilic and require small hydrophobic molecules to cross the blood–brain barrier (BBB) (Kenche and Barnham, 2011). However, nanoparticle delivery systems could be promising and intriguing methods for metal chelation in AD treatment (Bonda et al., 2012). This novel technology has been used in multiple studies to treat different illnesses (Hernando et al., 2016; Xiao et al., 2022; Guadarrama-Escobar et al., 2023). Furthermore, Cu is known to maintain proper physiological functions (Opazo et al., 2014; Jomova et al., 2022; Tsvetkov et al., 2022). However, chelating agents involve the risk of depleting the body’s essential Cu levels (Andersen, 2004), adding to the limitation on chelator usage. Thus, it is imperative to explore safer Cu chelators.
The generation of ROS and accumulation of lipid peroxides have been known to contribute to cuproptosis. It could be feasible to employ antioxidants to impede cuproptosis to treat AD.
In Hall (1992) discovered a range of efficient lipid peroxidation inhibitors known as “lazaroids,” which can alleviate such peroxidation in the brain tissue and impede the degeneration of motor nerve fibers, contributing to the treatment of AD. However, the inability of the Lazaroid compound to sustain cytoprotective effects during the advanced stages of cellular injury could be ascribed to its restricted clinical effectiveness (Huang et al., 2001). Another study demonstrated that a combination of multi-antioxidant nutritional supplements improved the memory and cognitive functions of adults living in the community (Chan et al., 2010).
Natural polyphenols, including resveratrol, epigallocatechin gallate, and curcumin, are potential therapeutic agents in treating oxidative stress, reducing extracellular amyloid deposition, and managing AD (Jayasena et al., 2013).
Curcumin is extracted from turmeric and possesses therapeutic properties, including anti-inflammatory, neuroprotective, neurotoxic metal chelating, anti-amyloidogenic, and antioxidative effects on mitochondria and DNA (Lakey-Beitia et al., 2017; Khayatan et al., 2023; Maghool et al., 2023). This natural extract also modifies microglia activity and inhibits acetylcholinesterase, making it an effective treatment for AD (Tang and Taghibiglou, 2017). However, its potential therapeutic value may be limited by its low bioavailability (Tang and Taghibiglou, 2017). There may be hope for curcumin-based treatments for AD in the future, provided that the issue of poor bioavailability can be effectively addressed (Tang and Taghibiglou, 2017).
A study demonstrated that green tea extract, epigallocatechin-3-gallate (EGCG) can reduce the toxicity of metal-free Aβ and metal-Aβ plaques and exhibit a distinctive anti-amyloidogenic reaction to metal-aβ plaques (Hyung et al., 2013). However, several studies have demonstrated that high concentrations of EGCG can cause liver toxicity (Wang et al., 2015a,b).
Quercetin belongs to the flavonol class of flavonoids; it is one of the most effective plant antioxidants (Brüll et al., 2015). Quercetin protects neural cells by attenuating neuroinflammation (Bournival et al., 2012) and inhibiting Aβ aggregation and tau phosphorylation (Khan et al., 2019), anti-oxidative, anti-lipid peroxidative, and acetylcholinesterase inhibitory activities (Rishitha and Muthuraman, 2018). However, its limited penetration via the BBB restricts its efficacy against neurodegenerative diseases (Khan et al., 2019).
Resveratrol (RES) is an effective antioxidant, scavenger of ROS, and a metal chelator (Olas and Wachowicz, 2005). A previous study demonstrated that RES treatment protected high-fat diet (HFD)-induced insulin resistance (IR) rats from diet-induced IR and elevated the expression of sirtuin 1 (SIRT1) and sirtuin 3 (SIRT3), mitochondrial DNA, and mitochondrial biogenesis (Haohao et al., 2015). In addition, RES enhances mitochondrial antioxidant enzyme activity, thereby reducing oxidative stress (Haohao et al., 2015).
Naturally occurring polyphenols possess considerable therapeutic potential due to their intrinsic antioxidative and chelating properties. In addition, certain polyphenols can potentially cross the BBB through chemical modification of their structure (Grabska-Kobyłecka et al., 2023). However, potential interactions between antioxidants and other medications should be considered as patients with AD could have additional underlying conditions requiring long-term treatment (Table 1). For instance, resveratrol can influence the effectiveness of other medications by inhibiting intestinal enzymes such as CYP3A4 or P-glycoprotein, especially in drugs with higher first-pass effects, such as specific calcium channel blockers, sildenafil, midazolam, and nefazodone (Detampel et al., 2012). In addition, quercetin and EGCG have been reported to display a similar effect (Choi and Burm, 2009; Choi et al., 2011; Tang et al., 2023). Curcumin can cause changes in pharmacokinetics during its simultaneous use with pharmacological agents such as cardiovascular drugs, antidepressants, anticoagulants, antibiotics, chemotherapeutic agents, and antihistamines (Bahramsoltani et al., 2017).
It has been established that Cu can activate the NF-κB signaling pathway, causing increased production of several inflammatory factors (Liu et al., 2020, 2021; Tastan et al., 2021) and APOE activity activation (Du et al., 2005), ultimately driving AD progression. Therefore, the application of NF-κB inhibitors could hinder the activity of NF-κB-induced APOE, consequently obstructing the progression of AD.
Reports have stated that p53 can inhibit glucose uptake and glycolysis, promoting a metabolic shift in the TCA cycle and oxidative phosphorylation and contributing to Cu toxicity (Li et al., 2023; Xiong et al., 2023). In addition, p53 regulates the biogenesis of Fe-S clusters and glutathione (GSH) (Ho et al., 2022; Xiong et al., 2023), thereby mitigating or enhancing Cu toxicity (Xiong et al., 2023)—two processes crucial for cuproptosis. Thus, p53-related therapeutic targets could be associated with cuproptosis, although its detailed mechanism of regulating Cu toxicity requires further research.
Alternatively, because Cu significantly contributes to the complex pathological mechanisms underlying AD, treating patients with multiple heterozygous compounds acting simultaneously on different biological targets could be a promising approach (Dias and Viegas, 2014). For instance, a clinical trial demonstrated that administering a nutritional formulation containing folic acid, α-tocopherol, B12, S-adenosylmethionine, N-acetylcysteine, and acetyl-L-carnitine to patients with AD for over a year effectively delayed AD progression (Remington et al., 2016).
The majority of clinical studies based on the mainstream hypothesis of AD pathogenesis have not yielded significantly positive outcomes. Therefore, it is essential to unravel the complex pathophysiological mechanisms underlying AD and discover and develop novel AD therapeutic targets and drugs. Despite the potential of Cu-chelating agents, lipid peroxidation inhibitors, antioxidants, and other molecules to prevent homeostatic disorders and cuproptosis, we need to identify drugs that can effectively cross the BBB with minimal systemic side effects. In this regard, nanomedicine delivery systems present a promising avenue for AD therapy. However, it is crucial to emphasize the possibility of drug–drug interactions in patients simultaneously taking multiple medications.
Major challenges for forthcoming studies entail comprehensively identifying the roles of copper in AD pathogenesis and potential druggable targets. Our team has been dedicated to investigating methods to improve cognitive capabilities for an extended period. Our next goal is to develop a copper-targeted therapy for the treatment of AD. We aimed to provide a scientific basis for the clinical development of novel treatment approaches for AD, which is a promising and challenging process.
In conclusion, our review of the mechanisms of oxidative stress, cuproptosis death, amyloid deposition, neuronal death, and synaptic damage in AD caused by Cu dysregulation demonstrated that Cu and cuproptosis are promising therapeutic avenues for the future treatment of AD through the development of safe and effective Cu-chelating drugs.
XL: Investigation, Writing – original draft. XC: Investigation, Writing – review & editing. XG: Formal analysis, Supervision, Writing – review & editing.
The authors declare that no financial support was received for the research, authorship, and/or publication of this article.
We thank Bullet Edits Limited for the linguistic editing and proofreading of the manuscript. We also thank Jing Wen, Ph.D. for linguistically editing the original version.
The authors declare that the research was conducted in the absence of any commercial or financial relationships that could be construed as a potential conflict of interest.
All claims expressed in this article are solely those of the authors and do not necessarily represent those of their affiliated organizations, or those of the publisher, the editors and the reviewers. Any product that may be evaluated in this article, or claim that may be made by its manufacturer, is not guaranteed or endorsed by the publisher.
Abbas, N., Bednar, I., Mix, E., Marie, S., Paterson, D., Ljungberg, A., et al. (2002). Up-regulation of the inflammatory cytokines IFN-gamma and IL-12 and down-regulation of IL-4 in cerebral cortex regions of APP(SWE) transgenic mice. J. Neuroimmunol. 126, 50–57. doi: 10.1016/s0165-5728(02)00050-4
Adlard, P., Cherny, R., Finkelstein, D., Gautier, E., Robb, E., Cortes, M., et al. (2008). Rapid restoration of cognition in Alzheimer’s transgenic mice with 8-hydroxy quinoline analogs is associated with decreased interstitial Abeta. Neuron 59, 43–55. doi: 10.1016/j.neuron.2008.06.018
Amtage, F., Birnbaum, D., Reinhard, T., Niesen, W., Weiller, C., Mader, I., et al. (2014). Estrogen intake and copper depositions: implications for Alzheimer’s disease? Case Rep. Neurol. 6, 181–187. doi: 10.1159/000363688
An, Y., Li, S., Huang, X., Chen, X., Shan, H., and Zhang, M. (2022). The role of copper homeostasis in brain disease. Int. J. Mol. Sci. 23:13850. doi: 10.3390/ijms232213850
Andersen, O. (2004). Chemical and biological considerations in the treatment of metal intoxications by chelating agents. Mini. Rev. Med. Chem. 4, 11–21. doi: 10.2174/1389557043487583
Armstrong, C., Leong, W., and Lees, G. (2001). Comparative effects of metal chelating agents on the neuronal cytotoxicity induced by copper (Cu+2), iron (Fe+3) and zinc in the hippocampus. Brain Res. 892, 51–62. doi: 10.1016/s0006-8993(00)03195-4
Atrián-Blasco, E., Cerrada, E., Faller, P., Laguna, M., and Hureau, C. (2019). Role of PTA in the prevention of Cu(amyloid-β) induced ROS formation and amyloid-β oligomerisation in the presence of Zn. Metallomics 11, 1154–1161. doi: 10.1039/c9mt00011a
Atrián-Blasco, E., Del Barrio, M., Faller, P., and Hureau, C. (2018). Ascorbate Oxidation by Cu(Amyloid-β) Complexes: Determination of the Intrinsic Rate as a Function of Alterations in the Peptide Sequence Revealing Key Residues for Reactive Oxygen Species Production. Anal. Chem. 90, 5909–5915. doi: 10.1021/acs.analchem.8b00740
Bahramsoltani, R., Rahimi, R., and Farzaei, M. (2017). Pharmacokinetic interactions of curcuminoids with conventional drugs: A review. J. Ethnopharmacol. 209, 1–12. doi: 10.1016/j.jep.2017.07.022
Barca, A., Ippati, S., Urso, E., Vetrugno, C., Storelli, C., Maffia, M., et al. (2019). Carnosine modulates the Sp1-Slc31a1/Ctr1 copper-sensing system and influences copper homeostasis in murine CNS-derived cells. Am. J. Physiol. Cell Physiol. 316, C235–C245. doi: 10.1152/ajpcell.00106.2018
Becaria, A., Lahiri, D., Bondy, S., Chen, D., Hamadeh, A., Li, H., et al. (2006). Aluminum and copper in drinking water enhance inflammatory or oxidative events specifically in the brain. J. Neuroimmunol. 176, 16–23. doi: 10.1016/j.jneuroim.2006.03.025
Behbehani, G., Barzegar, L., Mohebbian, M., and Saboury, A. A. A. (2012). Comparative Interaction between Copper Ions with Alzheimer’s β Amyloid Peptide and Human Serum Albumin. Bioinorg. Chem. Appl. 2012:208641. doi: 10.1155/2012/208641
Bonda, D., Liu, G., Men, P., Perry, G., Smith, M., and Zhu, X. (2012). Nanoparticle delivery of transition-metal chelators to the brain: Oxidative stress will never see it coming! CNS Neurol. Disord. Drug Targets 11, 81–85. doi: 10.2174/187152712799960709
Bonni, A., Ginty, D., Dudek, H., and Greenberg, M. (1995). Serine 133-phosphorylated CREB induces transcription via a cooperative mechanism that may confer specificity to neurotrophin signals. Mol. Cell Neurosci. 6, 168–183. doi: 10.1006/mcne.1995.1015
Bournival, J., Plouffe, M., Renaud, J., Provencher, C., and Martinoli, M. (2012). Quercetin and sesamin protect dopaminergic cells from MPP+-induced neuroinflammation in a microglial (N9)-neuronal (PC12) coculture system. Oxid. Med. Cell Longev. 2012:921941. doi: 10.1155/2012/921941
Brüll, V., Burak, C., Stoffel-Wagner, B., Wolffram, S., Nickenig, G., Müller, C., et al. (2015). Effects of a quercetin-rich onion skin extract on 24 h ambulatory blood pressure and endothelial function in overweight-to-obese patients with (pre-)hypertension: a randomised double-blinded placebo-controlled cross-over trial. Br. J. Nutr. 114, 1263–1277. doi: 10.1017/S0007114515002950
Bush, A. (2008). Drug development based on the metals hypothesis of Alzheimer’s disease. J. Alzheimers Dis. 15, 223–240. doi: 10.3233/jad-2008-15208
Chan, A., Remington, R., Kotyla, E., Lepore, A., Zemianek, J., and Shea, T. B. (2010). A vitamin/nutriceutical formulation improves memory and cognitive performance in community-dwelling adults without dementia. J. Nutr. Health Aging 14, 224–230. doi: 10.1007/s12603-010-0054-5
Chan, W., Garnica, A., and Rennert, O. (1978). Cell culture studies of Menkes kinky hair disease. Clin. Chim. Acta 88, 495–507. doi: 10.1016/0009-8981(78)90284-x
Cheignon, C., Tomas, M., Bonnefont-Rousselot, D., Faller, P., Hureau, C., and Collin, F. (2018). Oxidative stress and the amyloid beta peptide in Alzheimer’s disease. Redox Biol. 14, 450–464. doi: 10.1016/j.redox.2017.10.014
Chen, L., Min, J., and Wang, F. (2022). Copper homeostasis and cuproptosis in health and disease. Signal Transduct. Target Ther. 7:378. doi: 10.1038/s41392-022-01229-y
Cherny, R., Atwood, C., Xilinas, M., Gray, D., Jones, W., McLean, C., et al. (2001). Treatment with a copper-zinc chelator markedly and rapidly inhibits beta-amyloid accumulation in Alzheimer’s disease transgenic mice. Neuron 30, 665–676. doi: 10.1016/s0896-6273(01)00317-8
Choi, J., and Burm, J. (2009). Effects of oral epigallocatechin gallate on the pharmacokinetics of nicardipine in rats. Arch. Pharm. Res. 32, 1721–1725. doi: 10.1007/s12272-009-2209-7
Choi, J., Piao, Y., and Kang, K. (2011). Effects of quercetin on the bioavailability of doxorubicin in rats: role of CYP3A4 and P-gp inhibition by quercetin. Arch. Pharm. Res. 34, 607–613. doi: 10.1007/s12272-011-0411-x
Conlon, K., Miljkovic, M., and Waldmann, T. (2019). Cytokines in the Treatment of Cancer. J. Interferon Cytokine Res. 39, 6–21. doi: 10.1089/jir.2018.0019
Csire, G., Canabady-Rochelle, L., Averlant-Petit, M., Selmeczi, K., and Stefan, L. (2020). Both metal-chelating and free radical-scavenging synthetic pentapeptides as efficient inhibitors of reactive oxygen species generation. Metallomics 12, 1220–1229. doi: 10.1039/d0mt00103a
Das, P., Howard, V., Loosbrock, N., Dickson, D., Murphy, M., and Golde, T. (2003). Amyloid-beta immunization effectively reduces amyloid deposition in FcRgamma-/- knock-out mice. J. Neurosci. 23, 8532–8538. doi: 10.1523/JNEUROSCI.23-24-08532.2003
Deane, R., Sagare, A., and Zlokovic, B. (2008). The role of the cell surface LRP and soluble LRP in blood-brain barrier Abeta clearance in Alzheimer’s disease. Curr. Pharm. Des. 14, 1601–1605. doi: 10.2174/138161208784705487
Desai, V., and Kaler, S. (2008). Role of copper in human neurological disorders. Am. J. Clin. Nutr. 88, 855S–858S. doi: 10.1093/ajcn/88.3.855S
Detampel, P., Beck, M., Krähenbühl, S., and Huwyler, J. (2012). Drug interaction potential of resveratrol. Drug Metab. Rev. 44, 253–265. doi: 10.3109/03602532.2012.700715
Dias, K., and Viegas, C. (2014). Multi-Target Directed Drugs: A Modern Approach for Design of New Drugs for the treatment of Alzheimer’s Disease. Curr. Neuropharmacol. 12, 239–255. doi: 10.2174/1570159X1203140511153200
Do Val-da Silva, R., Peixoto-Santos, J., Scandiuzzi, R., Balista, P., Bassi, M., Glass, M., et al. (2016). Decreased neuron loss and memory dysfunction in pilocarpine-treated rats pre-exposed to hypoxia. Neuroscience 332, 88–100. doi: 10.1016/j.neuroscience.2016.06.047
Doreulee, N., Yanovsky, Y., and Haas, H. (1997). Suppression of long-term potentiation in hippocampal slices by copper. Hippocampus 7, 666–669.
Drew, S. (2017). The Case for Abandoning Therapeutic Chelation of Copper Ions in Alzheimer’s Disease. Front. Neurosci. 11:317. doi: 10.3389/fnins.2017.00317
Du, X., Zheng, Y., Wang, Z., Chen, Y., Zhou, R., Song, G., et al. (2014). Inhibitory act of selenoprotein P on Cu(+)/Cu(2+)-induced tau aggregation and neurotoxicity. Inorg. Chem. 53, 11221–11230. doi: 10.1021/ic501788v
Du, Y., Chen, X., Wei, X., Bales, K., Berg, D., Paul, S., et al. (2005). NF-(kappa)B mediates amyloid beta peptide-stimulated activity of the human apolipoprotein E gene promoter in human astroglial cells. Brain Res. Mol. Brain Res. 136, 177–188. doi: 10.1016/j.molbrainres.2005.02.001
Ejaz, H., Wang, W., and Lang, M. (2020). Copper toxicity links to pathogenesis of Alzheimer’s disease and therapeutics approaches. Int. J. Mol. Sci. 21:7660. doi: 10.3390/ijms21207660
Ennerfelt, H., Frost, E., Shapiro, D., Holliday, C., Zengeler, K., Voithofer, G., et al. (2022). SYK coordinates neuroprotective microglial responses in neurodegenerative disease. Cell 185, 4135–4152. doi: 10.1016/j.cell.2022.09.030
Esquerda-Canals, G., Roda, A., Martí-Clúa, J., Montoliu-Gaya, L., Rivera-Hernández, G., and Villegas, S. (2019). Treatment with scFv-h3D6 prevented neuronal loss and improved spatial memory in young 3xTg-AD Mice by Reducing the Intracellular Amyloid-β Burden. J. Alzheimers Dis. 70, 1069–1091. doi: 10.3233/JAD-190484
Flora, S., Shrivastava, R., and Mittal, M. (2013). Chemistry and pharmacological properties of some natural and synthetic antioxidants for heavy metal toxicity. Curr. Med. Chem. 20, 4540–4574. doi: 10.2174/09298673113209990146
Foster, J., Billimoria, K., Del Castillo Busto, M., Strekopytov, S., Goenaga-Infante, H., and Morley, T. (2022). Accumulation of molybdenum in major organs following repeated oral administration of bis-choline tetrathiomolybdate in the Sprague Dawley rat. J. Appl. Toxicol. 42, 1807–1821. doi: 10.1002/jat.4358
Fujino, H., Kitaoka, Y., Hayashi, Y., Munemasa, Y., Takeda, H., Kumai, T., et al. (2009). Axonal protection by brain-derived neurotrophic factor associated with CREB phosphorylation in tumor necrosis factor-alpha-induced optic nerve degeneration. Acta Neuropathol. 117, 75–84. doi: 10.1007/s00401-008-0440-9
Gaier, E. D., Eipper, B., and Mains, R. (2013). Copper signaling in the mammalian nervous system: synaptic effects. J. Neurosci. Res. 91, 2–19. doi: 10.1002/jnr.23143
Gao, D., Li, P., Gao, F., Feng, Y., Li, X., Li, D., et al. (2022). Preparation and Multitarget Anti-AD Activity Study of Chondroitin Sulfate Lithium in AD Mice Induced by Combination of D-Gal/AlCl3. Oxid. Med. Cell Longev. 2022:9466166. doi: 10.1155/2022/9466166
Goldschmith, A., Infante, C., Leiva, J., Motles, E., and Palestini, M. (2005). Interference of chronically ingested copper in long-term potentiation (LTP) of rat hippocampus. Brain Res. 1056, 176–182. doi: 10.1016/j.brainres.2005.07.030
Grabska-Kobyłecka, I., Szpakowski, P., Król, A., Książek-Winiarek, D., Kobyłecki, A., Głąbiński, A., et al. (2023). Polyphenols and Their Impact on the Prevention of Neurodegenerative Diseases and Development. Nutrients 15:3454. doi: 10.3390/nu15153454
Guadarrama-Escobar, O., Serrano-Castañeda, P., Anguiano-Almazán, E., Vázquez-Durán, A., Peña-Juárez, M., Vera-Graziano, R., et al. (2023). Chitosan Nanoparticles as Oral Drug Carriers. Int. J. Mol. Sci. 24:4289. doi: 10.3390/ijms24054289
Hall, E. (1992). Novel inhibitors of iron-dependent lipid peroxidation for neurodegenerative disorders. Ann. Neurol. 32, S137–S142. doi: 10.1002/ana.410320724
Haohao, Z., Guijun, Q., Juan, Z., Wen, K., and Lulu, C. (2015). Resveratrol improves high-fat diet induced insulin resistance by rebalancing subsarcolemmal mitochondrial oxidation and antioxidantion. J. Physiol. Biochem. 71, 121–131. doi: 10.1007/s13105-015-0392-1
Hernando, S., Gartziandia, O., Herran, E., Pedraz, J., Igartua, M., and Hernandez, R. (2016). Advances in nanomedicine for the treatment of Alzheimer’s and Parkinson’s diseases. Nanomedicine 11, 1267–1285. doi: 10.2217/nnm-2016-0019
Ho, T., Ahmadi, S., and Kerman, K. (2022). Do glutathione and copper interact to modify Alzheimer’s disease pathogenesis? Free Radic. Biol. Med. 181, 180–196. doi: 10.1016/j.freeradbiomed.2022.01.025
Huang, H., Patel, P., and Salahudeen, A. (2001). Lazaroid compounds prevent early but not late stages of oxidant-induced cell injury: potential explanation for the lack of efficacy of lazaroids in clinical trials. Pharmacol. Res. 43, 55–61. doi: 10.1006/phrs.2000.0740
Huang, X. A. (2023). Concise review on oxidative stress-mediated ferroptosis and cuproptosis in Alzheimer’s disease. Cells 12:1369. doi: 10.3390/cells12101369
Hwang, I., Yoo, K., Jung, B., Cho, J., Kim, D., Kang, T., et al. (2006). Correlations between neuronal loss, decrease of memory, and decrease expression of brain-derived neurotrophic factor in the gerbil hippocampus during normal aging. Exp. Neurol. 201, 75–83. doi: 10.1016/j.expneurol.2006.02.129
Hyung, S., DeToma, A., Brender, J., Lee, S., Vivekanandan, S., Kochi, A., et al. (2013). Insights into antiamyloidogenic properties of the green tea extract (-)-epigallocatechin-3-gallate toward metal-associated amyloid-β species. Proc. Natl. Acad. Sci. U. S. A. 110, 3743–3748. doi: 10.1073/pnas.1220326110
James, S., Volitakis, I., Adlard, P., Duce, J., Masters, C., Cherny, R., et al. (2012). Elevated labile Cu is associated with oxidative pathology in Alzheimer disease. Free Radic. Biol. Med. 52, 298–302. doi: 10.1016/j.freeradbiomed.2011.10.446
Jand, A., Taheri-Nejad, M., Mosleh, M., and Palizvan, M. (2018). Low, but Not High, Doses of Copper Sulfate Impair Synaptic Plasticity in the Hippocampal CA1 Region In Vivo. Biol. Trace Elem. Res. 185, 143–147. doi: 10.1007/s12011-017-1234-5
Jäntti, H., Sitnikova, V., Ishchenko, Y., Shakirzyanova, A., Giudice, L., Ugidos, I., et al. (2022). Microglial amyloid beta clearance is driven by PIEZO1 channels. J. Neuroinflammation 19:147. doi: 10.1186/s12974-022-02486-y
Jayasena, T., Poljak, A., Smythe, G., Braidy, N., Münch, G., and Sachdev, P. (2013). The role of polyphenols in the modulation of sirtuins and other pathways involved in Alzheimer’s disease. Ageing Res. Rev. 12, 867–883. doi: 10.1016/j.arr.2013.06.003
Jomova, K., Makova, M., Alomar, S., Alwasel, S., Nepovimova, E., Kuca, K., et al. (2022). Essential metals in health and disease. Chem. Biol. Interact. 367:110173. doi: 10.1016/j.cbi.2022.110173
Kalita, J., Kumar, V., Misra, U., and Bora, H. (2018). Memory and Learning Dysfunction Following Copper Toxicity: Biochemical and Immunohistochemical Basis. Mol. Neurobiol. 55, 3800–3811. doi: 10.1007/s12035-017-0619-y
Kano, A., Watanabe, Y., Takeda, N., Aizawa, S., and Akaike, T. (1997). Analysis of IFN-gamma-induced cell cycle arrest and cell death in hepatocytes. J. Biochem. 121, 677–683. doi: 10.1093/oxfordjournals.jbchem.a021639
Karki, R., Sharma, B., Tuladhar, S., Williams, E., Zalduondo, L., Samir, P., et al. (2021). Synergism of TNF-α and IFN-γ triggers inflammatory cell death, tissue damage, and mortality in SARS-CoV-2 infection and cytokine shock syndromes. Cell 184, 149–168. doi: 10.1016/j.cell.2020.11.025
Kaur, D., Behl, T., Sehgal, A., Singh, S., Sharma, N., and Bungau, S. (2021). Multifaceted Alzheimer’s disease: Building a roadmap for advancement of novel therapies. Neurochem. Res. 46, 2832–2851. doi: 10.1007/s11064-021-03415-w
Kaur, S., Raj, K., Gupta, Y., and Singh, S. (2021). Allicin ameliorates aluminium- and copper-induced cognitive dysfunction in Wistar rats: relevance to neuro-inflammation, neurotransmitters and Aβ(1-42) analysis. J. Biol. Inorg. Chem. 26, 495–510. doi: 10.1007/s00775-021-01866-8
Kenche, V., and Barnham, K. (2011). Alzheimer’s disease & metals: therapeutic opportunities. Br. J. Pharmacol. 163, 211–219. doi: 10.1111/j.1476-5381.2011.01221.x
Khan, H., Ullah, H., Aschner, M., Cheang, W., and Akkol, E. (2019). Neuroprotective Effects of Quercetin in Alzheimer’s Disease. Biomolecules 10:59. doi: 10.3390/biom10010059
Khayatan, D., Razavi, S., Arab, Z., Hosseini, Y., Niknejad, A., Momtaz, S., et al. (2023). Superoxide dismutase: a key target for the neuroprotective effects of curcumin. Mol. Cell Biochem. doi: 10.1007/s11010-023-04757-5 [Epub ahead of print].
Kitazawa, M., Cheng, D., and Laferla, F. (2009). Chronic copper exposure exacerbates both amyloid and tau pathology and selectively dysregulates cdk5 in a mouse model of AD. J. Neurochem. 108, 1550–1560. doi: 10.1111/j.1471-4159.2009.05901.x
Kitazawa, M., Hsu, H., and Medeiros, R. (2016). Copper Exposure Perturbs Brain Inflammatory Responses and Impairs Clearance of Amyloid-Beta. Toxicol. Sci. 152, 194–204. doi: 10.1093/toxsci/kfw081
Lakey-Beitia, J., González, Y., Doens, D., Stephens, D., Santamaría, R., Murillo, E., et al. (2017). Assessment of Novel Curcumin Derivatives as Potent Inhibitors of Inflammation and Amyloid-β Aggregation in Alzheimer’s Disease. J. Alzheimers Dis. 60, S59–S68. doi: 10.3233/JAD-170071
Lamtai, M., Zghari, O., Ouakki, S., Marmouzi, I., Mesfioui, A., El Hessni, A., et al. (2020). Chronic copper exposure leads to hippocampus oxidative stress and impaired learning and memory in male and female rats. Toxicol. Res. 36, 359–366. doi: 10.1007/s43188-020-00043-4
Langer, V., Vivi, E., Regensburger, D., Winkler, T., Waldner, M., Rath, T., et al. (2019). IFN-γ drives inflammatory bowel disease pathogenesis through VE-cadherin-directed vascular barrier disruption. J. Clin. Invest. 129, 4691–4707. doi: 10.1172/JCI124884
Lannfelt, L., Blennow, K., Zetterberg, H., Batsman, S., Ames, D., Harrison, J., et al. (2008). Safety, efficacy, and biomarker findings of PBT2 in targeting Abeta as a modifying therapy for Alzheimer’s disease: a phase IIa, double-blind, randomised, placebo-controlled trial. Lancet Neurol. 7, 779–786. doi: 10.1016/S1474-4422(08)70167-4
Lei, P., Ayton, S., and Bush, A. (2021). The essential elements of Alzheimer’s disease. J. Biol. Chem. 296:100105. doi: 10.1074/jbc.REV120.008207
Leiva, J., Gaete, P., and Palestini, M. (2003). Copper interaction on the long-term potentiation. Arch. Ital. Biol. 141, 149–155.
Leiva, J., Palestini, M., Infante, C., Goldschmidt, A., and Motles, E. (2009). Copper suppresses hippocampus LTP in the rat, but does not alter learning or memory in the morris water maze. Brain Res. 1256, 69–75. doi: 10.1016/j.brainres.2008.12.041
Li, D., Zhang, W., Wang, Z., and Zhao, P. (2017). Serum Copper, Zinc, and Iron Levels in Patients with Alzheimer’s Disease: A Meta-Analysis of Case-Control Studies. Front. Aging Neurosci. 9:300. doi: 10.3389/fnagi.2017.00300
Li, Q., Wang, T., Zhou, Y., and Shi, J. (2023). Cuproptosis in lung cancer: mechanisms and therapeutic potential. Mol. Cell Biochem. doi: 10.1007/s11010-023-04815-y [Epub ahead of print].
Lisman, J., Cooper, K., Sehgal, M., and Silva, A. (2018). Memory formation depends on both synapse-specific modifications of synaptic strength and cell-specific increases in excitability. Nat. Neurosci. 21, 309–314. doi: 10.1038/s41593-018-0076-6
Liu, Z., Yao, X., Jiang, W., Li, W., Zhu, S., Liao, C., et al. (2020). Advanced oxidation protein products induce microglia-mediated neuroinflammation via MAPKs-NF-κB signaling pathway and pyroptosis after secondary spinal cord injury. J. Neuroinflammation 17:90. doi: 10.1186/s12974-020-01751-2
Liu, Z., Yao, X., Sun, B., Jiang, W., Liao, C., Dai, X., et al. (2021). Pretreatment with kaempferol attenuates microglia-mediate neuroinflammation by inhibiting MAPKs-NF-κB signaling pathway and pyroptosis after secondary spinal cord injury. Free Radic. Biol. Med. 168, 142–154. doi: 10.1016/j.freeradbiomed.2021.03.037
Lu, Q., Zhang, Y., Zhao, C., Zhang, H., Pu, Y., and Yin, L. (2022). Copper induces oxidative stress and apoptosis of hippocampal neuron via pCREB/BDNF/ and Nrf2/HO-1/NQO1 pathway. J. Appl. Toxicol. 42, 694–705. doi: 10.1002/jat.4252
Madsen, E., and Gitlin, J. (2007). Copper and iron disorders of the brain. Annu. Rev. Neurosci. 30, 317–337. doi: 10.1146/annurev.neuro.30.051606.094232
Maghool, F., Emami, M., Alipour, R., Mohammadzadeh, S., Sereshki, N., Dehkordi, S., et al. (2023). Rescue effect of curcumin against copper toxicity. J. Trace Elem. Med. Biol. 78:127153. doi: 10.1016/j.jtemb.2023.127153
Matos, L., Gouveia, A., and Almeida, H. (2017). Resveratrol Attenuates Copper-Induced Senescence by Improving Cellular Proteostasis. Oxid. Med. Cell Longev. 2017:3793817. doi: 10.1155/2017/3793817
Matos, L., Gouveia, A., and Almeida, H. E. R. (2015). Stress Response in Human Cellular Models of Senescence. J. Gerontol. A Biol. Sci. Med. Sci. 70, 924–935. doi: 10.1093/gerona/glu129
Nieto, R., Kukuljan, M., and Silva, H. (2013). BDNF and schizophrenia: from neurodevelopment to neuronal plasticity, learning, and memory. Front Psychiatry. 4:45. doi: 10.3389/fpsyt.2013.00045
Noda, Y., Asada, M., Kubota, M., Maesako, M., Watanabe, K., Uemura, M., et al. (2013). Copper enhances APP dimerization and promotes Aβ production. Neurosci. Lett. 547, 10–15. doi: 10.1016/j.neulet.2013.04.057
Numakawa, T., Suzuki, S., Kumamaru, E., Adachi, N., Richards, M., and Kunugi, H. (2010). BDNF function and intracellular signaling in neurons. Histol. Histopathol. 25, 237–258. doi: 10.14670/HH-25.237
Olas, B., and Wachowicz, B. (2005). Resveratrol, a phenolic antioxidant with effects on blood platelet functions. Platelets 16, 251–260. doi: 10.1080/09537100400020591
Opazo, C., Greenough, M., and Bush, A. (2014). Copper: from neurotransmission to neuroproteostasis. Front. Aging Neurosci. 6:143. doi: 10.3389/fnagi.2014.00143
Pal, A., Badyal, R., Vasishta, R., Attri, S., Thapa, B., and Prasad, R. (2013). Biochemical, histological, and memory impairment effects of chronic copper toxicity: a model for non-Wilsonian brain copper toxicosis in Wistar rat. Biol. Trace Elem. Res. 153, 257–268. doi: 10.1007/s12011-013-9665-0
Pal, A., Rani, I., Pawar, A., Picozza, M., Rongioletti, M., and Squitti, R. (2021a). Microglia and Astrocytes in Alzheimer’s Disease in the Context of the Aberrant Copper Homeostasis Hypothesis. Biomolecules 11:1598. doi: 10.3390/biom11111598
Pal, A., Sengupta, S., and Kundu, R. (2021b). Tiliacora racemosa leaves induce oxidative stress mediated DNA damage leading to G2/M phase arrest and apoptosis in cervical cancer cells SiHa. J. Ethnopharmacol. 269:113686. doi: 10.1016/j.jep.2020.113686
Pavandi, M., Messripour, M., and Moshtaghi, A. (2014). Effect of Aluminium and Copper on Dopamine Synthesis in Striatal Synaptosomes of Rat’s Brain. Bull. Env. Pharmacol. Life Sci. 3, 12–16.
Pizzorusso, T., Ratto, G., Putignano, E., and Maffei, L. (2000). Brain-derived neurotrophic factor causes cAMP response element-binding protein phosphorylation in absence of calcium increases in slices and cultured neurons from rat visual cortex. J. Neurosci. 20, 2809–2816. doi: 10.1523/JNEUROSCI.20-08-02809.2000
Polydoro, M., Acker, C., Duff, K., Castillo, P., and Davies, P. (2009). Age-dependent impairment of cognitive and synaptic function in the htau mouse model of tau pathology. J. Neurosci. 29, 10741–10749. doi: 10.1523/JNEUROSCI.1065-09.2009
Remington, R., Bechtel, C., Larsen, D., Samar, A., Page, R., Morrell, C., et al. (2016). Maintenance of Cognitive Performance and Mood for Individuals with Alzheimer’s Disease Following Consumption of a Nutraceutical Formulation: A one-year open-label study. J. Alzheimers Dis. 51, 991–995. doi: 10.3233/JAD-151098
Rishitha, N., and Muthuraman, A. (2018). Therapeutic evaluation of solid lipid nanoparticle of quercetin in pentylenetetrazole induced cognitive impairment of zebrafish. Life Sci. 199, 80–87. doi: 10.1016/j.lfs.2018.03.010
Rossi-George, A., and Guo, C. (2016). Copper disrupts S-nitrosothiol signaling in activated BV2 microglia. Neurochem. Int. 99, 1–8. doi: 10.1016/j.neuint.2016.05.011
Rozzini, L., Lanfranchi, F., Pilotto, A., Catalani, S., Gilberti, M., Paganelli, M., et al. (2018). Serum Non-Ceruloplasmin Non-Albumin Copper Elevation in Mild Cognitive Impairment and Dementia due to Alzheimer’s Disease: A Case Control Study. J. Alzheimers Dis. 61, 907–912. doi: 10.3233/JAD-170552
Salloway, S., Sperling, R., Fox, N., Blennow, K., Klunk, W., Raskind, M., et al. (2014). Two phase 3 trials of bapineuzumab in mild-to-moderate Alzheimer’s disease. N. Engl. J. Med. 370, 322–333. doi: 10.1056/NEJMoa1304839
Selkoe, D. (2001). Presenilins, β-amyloid precursor protein and the molecular basis of Alzheimer’s disease. Physiol. Rev. 81, 741–766.
Sestito, S., Wang, S., Chen, Q., Lu, J., Bertini, S., Pomelli, C., et al. (2019). Multi-targeted ChEI-copper chelating molecules as neuroprotective agents. Eur. J. Med. Chem. 174, 216–225. doi: 10.1016/j.ejmech.2019.04.060
Sharma, V., and Singh, T. (2020). CREB: A Multifaceted Target for Alzheimer’s Disease. Curr. Alzheimer Res. 17, 1280–1293. doi: 10.2174/1567205018666210218152253
Singh, I., Sagare, A., Coma, M., Perlmutter, D., Gelein, R., Bell, R., et al. (2013). Low levels of copper disrupt brain amyloid-β homeostasis by altering its production and clearance. Proc. Natl. Acad. Sci. U. S. A. 110, 14771–14776. doi: 10.1073/pnas.1302212110
Solmonson, A., and DeBerardinis, R. (2018). Lipoic acid metabolism and mitochondrial redox regulation. J. Biol. Chem. 293, 7522–7530. doi: 10.1074/jbc.TM117.000259
Squitti, R., Pasqualetti, P., Dal Forno, G., Moffa, F., Cassetta, E., Lupoi, D., et al. (2005). Excess of serum copper not related to ceruloplasmin in Alzheimer disease. Neurology 64, 1040–1046. doi: 10.1212/01.WNL.0000154531.79362.23
Squitti, R., Simonelli, I., Ventriglia, M., Siotto, M., Pasqualetti, P., Rembach, A., et al. (2014). Meta-analysis of serum non-ceruloplasmin copper in Alzheimer’s disease. J. Alzheimers Dis. 38, 809–822. doi: 10.3233/JAD-131247
Squitti, R., Siotto, M., Cassetta, E., El Idrissi, I., and Colabufo, N. (2017). Measurements of serum non-ceruloplasmin copper by a direct fluorescent method specific to Cu(II). Clin. Chem. Lab. Med. 55, 1360–1367. doi: 10.1515/cclm-2016-0843
Summers, K., Roseman, G., Schilling, K., Dolgova, N., Pushie, M., Sokaras, D., et al. (2022). Alzheimer’s Drug PBT2 Interacts with the Amyloid β 1-42 Peptide Differently than Other 8-Hydroxyquinoline Chelating Drugs. Inorg. Chem. 61, 14626–14640. doi: 10.1021/acs.inorgchem.2c01694
Sun, F., Zhao, J., Zhang, H., Shi, Q., Liu, Y., Robert, A., et al. (2022). Proteomics Evidence of the Role of TDMQ20 in the Cholinergic System and Synaptic Transmission in a Mouse Model of Alzheimer’s Disease. ACS Chem. Neurosci. 13, 3093–3107. doi: 10.1021/acschemneuro.2c00455
Tai, H., Serrano-Pozo, A., Hashimoto, T., Frosch, M., Spires-Jones, T., and Hyman, B. (2012). The synaptic accumulation of hyperphosphorylated tau oligomers in Alzheimer disease is associated with dysfunction of the ubiquitin-proteasome system. Am. J. Pathol. 181, 1426–1435. doi: 10.1016/j.ajpath.2012.06.033
Talwar, P., Grover, S., Sinha, J., Chandna, P., Agarwal, R., Kushwaha, S., et al. (2017). Multifactorial Analysis of a Biomarker Pool for Alzheimer Disease Risk in a North Indian Population. Dement. Geriatr. Cogn. Disord. 44, 25–34. doi: 10.1159/000477206
Tang, H., Kuang, Y., Wu, W., Peng, B., and Fu, Q. (2023). Quercetin inhibits the metabolism of arachidonic acid by inhibiting the activity of CYP3A4, thereby inhibiting the progression of breast cancer. Mol. Med. 29:127. doi: 10.1186/s10020-023-00720-8
Tang, M., and Taghibiglou, C. (2017). The mechanisms of action of curcumin in Alzheimer’s disease. J. Alzheimers Dis. 58, 1003–1016. doi: 10.3233/JAD-170188
Tastan, B., Arioz, B., Tufekci, K., Tarakcioglu, E., Gonul, C., Genc, K., et al. (2021). Dimethyl Fumarate Alleviates NLRP3 Inflammasome Activation in Microglia and Sickness Behavior in LPS-Challenged Mice. Front. Immunol. 12:737065. doi: 10.3389/fimmu.2021.737065
Tirotta, E., Kirby, L., Hatch, M., and Lane, T. E. (2012). IFN-γ-induced apoptosis of human embryonic stem cell derived oligodendrocyte progenitor cells is restricted by CXCR2 signaling. Stem Cell Res. 9, 208–217. doi: 10.1016/j.scr.2012.06.005
Tomanek, L. (2015). Proteomic responses to environmentally induced oxidative stress. J. Exp. Biol. 218, 1867–1879. doi: 10.1242/jeb.116475
Traynelis, S., Wollmuth, L., McBain, C., Menniti, F., Vance, K., Ogden, K., et al. (2010). Glutamate receptor ion channels: structure, regulation, and function. Pharmacol. Rev. 62, 405–496. doi: 10.1124/pr.109.002451
Tsvetkov, P., Coy, S., Petrova, B., Dreishpoon, M., Verma, A., Abdusamad, M., et al. (2022). Copper induces cell death by targeting lipoylated TCA cycle proteins. Science 375, 1254–1261. doi: 10.1126/science.abf0529
van der Kant, R., Goldstein, L., and Ossenkoppele, R. (2020). Amyloid-β-independent regulators of tau pathology in Alzheimer disease. Nat. Rev. Neurosci. 21, 21–35. doi: 10.1038/s41583-019-0240-3
Voss, K., Harris, C., Ralle, M., Duffy, M., Murchison, C., and Quinn, J. (2014). Modulation of tau phosphorylation by environmental copper. Transl. Neurodegener. 3:24. doi: 10.1186/2047-9158-3-24
Wang, D., Wang, Y., Wan, X., Yang, C., and Zhang, J. (2015a). Green tea polyphenol (-)-epigallocatechin-3-gallate triggered hepatotoxicity in mice: responses of major antioxidant enzymes and the Nrf2 rescue pathway. Toxicol. Appl. Pharmacol. 283, 65–74. doi: 10.1016/j.taap.2014.12.018
Wang, D., Wei, Y., Wang, T., Wan, X., Yang, C., Reiter, R., et al. (2015b). Melatonin attenuates (-)-epigallocatehin-3-gallate-triggered hepatotoxicity without compromising its downregulation of hepatic gluconeogenic and lipogenic genes in mice. J. Pineal Res. 59, 497–507. doi: 10.1111/jpi.12281
Wang, H., Xu, J., Lazarovici, P., Quirion, R., and Zheng, W. (2018a). cAMP response element-binding protein (CREB): A possible signaling molecule link in the pathophysiology of schizophrenia. Front. Mol. Neurosci. 11:255. doi: 10.3389/fnmol.2018.00255
Wang, Z., Zhang, Y., Guo, C., Gao, H., Zhong, M., Huang, T., et al. (2018b). Tetrathiomolybdate treatment leads to the suppression of inflammatory responses through the TRAF6/NFκB Pathway in LPS-stimulated BV-2 Microglia. Front. Aging Neurosci. 10:9. doi: 10.3389/fnagi.2018.00009
Wang, Z., Zhang, Y., Zhang, W., Gao, H., Zhong, M., Huang, T., et al. (2018c). Copper chelators promote nonamyloidogenic processing of AβPP via MT1/2 /CREB-dependent signaling pathways in AβPP/PS1 transgenic mice. J. Pineal Res. 65, e12502. doi: 10.1111/jpi.12502
Weiss, K., Askari, F., Czlonkowska, A., Ferenci, P., Bronstein, J., Bega, D., et al. (2017). Bis-choline tetrathiomolybdate in patients with Wilson’s disease: an open-label, multicentre, phase 2 study. Lancet Gastroenterol. Hepatol. 2, 869–876. doi: 10.1016/S2468-1253(17)30293-5
Wu, D., and Cederbaum, A. (2003). Alcohol, oxidative stress, and free radical damage. Alcohol Res. Health 27, 277–284.
Xiao, H., Qin, G., and Fang, B. (2022). Nanoparticle-mediated delivery system alleviates the formation of infection stones by activating TRPV5. Gen. Physiol. Biophys. 41, 465–471. doi: 10.4149/gpb_2022028
Xiong, C., Ling, H., Hao, Q., and Zhou, X. (2023). Cuproptosis: p53-regulated metabolic cell death? Cell Death Differ. 30, 876–884. doi: 10.1038/s41418-023-01125-0
Yamazaki, Y., Zhao, N., Caulfield, T., Liu, C., and Bu, G. (2019). Apolipoprotein E and Alzheimer disease: pathobiology and targeting strategies. Nat. Rev. Neurol. 15, 501–518. doi: 10.1038/s41582-019-0228-7
Yan, S., Yan, S., Chen, X., Fu, J., Chen, M., Kuppusamy, P., et al. (1995). Non-enzymatically glycated tau in Alzheimer’s disease induces neuronal oxidant stress resulting in cytokine gene expression and release of amyloid beta-peptide. Nat. Med. 1, 693–699. doi: 10.1038/nm0795-693
Yang, T., Ma, X., Wang, R., Liu, H., Wei, S., Jing, M., et al. (2022). Berberine inhibits IFN-γ signaling pathway in DSS-induced ulcerative colitis. Saudi Pharm. J. 30, 764–778. doi: 10.1016/j.jsps.2022.03.015
Yu, Y., Wong, J., Lovejoy, D., Kalinowski, D., and Richardson, D. (2006). Chelators at the cancer coalface: desferrioxamine to Triapine and beyond. Clin. Cancer Res. 12, 6876–6883. doi: 10.1158/1078-0432.CCR-06-1954
Zhang, X., Tian, Y., Wang, Z., Ma, Y., Tan, L., and Yu, J. (2021). The epidemiology of Alzheimer’s disease modifiable risk factors and prevention. J. Prev. Alzheimers Dis. 8, 313–321. doi: 10.14283/jpad.2021.15
Zhang, Y., Lu, W., Han, M., Li, H., Luo, H., Li, W., et al. (2016). Biphasic effects of copper on rat learning and memory in the morris water maze. Ann. Clin. Lab. Sci. 46, 346–352.
Zhang, Y., Zhou, Q., Lu, L., Su, Y., Shi, W., Zhang, H., et al. (2023). Copper Induces Cognitive Impairment in Mice via Modulation of Cuproptosis and CREB Signaling. Nutrients 15:972. doi: 10.3390/nu15040972
Zhao, J., Shi, Q., Tian, H., Li, Y., Liu, Y., Xu, Z., et al. (2021). TDMQ20, a specific copper chelator, reduces memory impairments in Alzheimer’s disease mouse models. ACS Chem. Neurosci. 12, 140–149. doi: 10.1021/acschemneuro.0c00621
Keywords: copper, cuproptosis, Alzheimer’s disease, long-term potentiation, chelators
Citation: Li X, Chen X and Gao X (2023) Copper and cuproptosis: new therapeutic approaches for Alzheimer’s disease. Front. Aging Neurosci. 15:1300405. doi: 10.3389/fnagi.2023.1300405
Received: 23 September 2023; Accepted: 13 November 2023;
Published: 19 December 2023.
Edited by:
Alexandre Henriques, Neuro-Sys, FranceReviewed by:
Bilal El Waly, Neuro-Sys, FranceCopyright © 2023 Li, Chen and Gao. This is an open-access article distributed under the terms of the Creative Commons Attribution License (CC BY). The use, distribution or reproduction in other forums is permitted, provided the original author(s) and the copyright owner(s) are credited and that the original publication in this journal is cited, in accordance with accepted academic practice. No use, distribution or reproduction is permitted which does not comply with these terms.
*Correspondence: Xiyan Gao, Z2FveGl5YW5AeWVhaC5uZXQ=
Disclaimer: All claims expressed in this article are solely those of the authors and do not necessarily represent those of their affiliated organizations, or those of the publisher, the editors and the reviewers. Any product that may be evaluated in this article or claim that may be made by its manufacturer is not guaranteed or endorsed by the publisher.
Research integrity at Frontiers
Learn more about the work of our research integrity team to safeguard the quality of each article we publish.