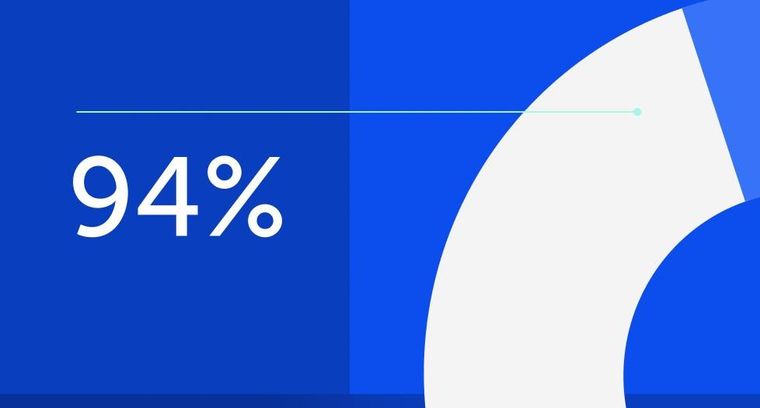
94% of researchers rate our articles as excellent or good
Learn more about the work of our research integrity team to safeguard the quality of each article we publish.
Find out more
REVIEW article
Front. Aging Neurosci., 10 November 2023
Sec. Alzheimer's Disease and Related Dementias
Volume 15 - 2023 | https://doi.org/10.3389/fnagi.2023.1251075
This article is part of the Research TopicEmotional Affective Disorders in Aging and Alzheimer’s Disease and Their Impact on Higher Order CognitionView all 6 articles
Dementia remains one of the leading causes of morbidity and mortality in older adults. Alzheimer’s disease (AD) is the most common type of dementia, affecting over 55 million people worldwide. AD is characterized by distinct neurobiological changes, including amyloid-beta protein deposits and tau neurofibrillary tangles, which cause cognitive decline and subsequent behavioral changes, such as distress, insomnia, depression, and anxiety. Recent literature suggests a strong connection between stress systems and AD progression. This presents a promising direction for future AD research. In this review, two systems involved in regulating stress and AD pathogenesis will be highlighted: serotonin (5-HT) and corticotropin releasing factor (CRF). Throughout the review, we summarize critical findings in the field while discussing common limitations with two animal models (3xTg-AD and TgF344-AD), novel pharmacotherapies, and potential early-intervention treatment options. We conclude by highlighting promising future pharmacotherapies and translational animal models of AD and anxiety.
Currently over 55 million people worldwide are diagnosed with dementia, with 60–70% of dementia cases caused by Alzheimer’s disease (AD; World Health Organization, 2023). In the United States, over 6 million people are currently diagnosed with AD, with this number projected to rise due to the large aging population (Zhao, 2020; Alzheimer’s Association, 2023). There are distinct neurobiological changes that occur in AD patients, including deposition of amyloid-beta (Aβ) plaques, neurofibrillary tangles of tau, neuroinflammation, and neuronal loss (Duyckaerts et al., 2009; Zhao, 2020). This neuropathological burden of Aβ and tangles in the brain leads to neurodegeneration, with key areas such as the hippocampus, frontal cortex, and raphe nucleus exhibiting evidence of synaptic dysfunction, severe neuronal loss, and tissue atrophy (Goedert and Spillantini, 2006; Duyckaerts et al., 2009). Importantly, it has been reported that neurobiological changes begin years before initial AD diagnosis, even in the absence of cognitive changes or symptoms (Müller-Spahn, 2003; Sperling et al., 2011). Given the extent of irreversible neurobiological changes that occur in AD, it is critical that the field strive to promote preventative and early-stage AD treatments.
AD patients are diagnosed on a continuum consisting of Preclinical AD, Mild Cognitive Impairment (MCI), and Mild, Moderate or Severe Dementia due to AD (Sperling et al., 2011; Zhao, 2020; Alzheimer’s Association, 2023). There are several risk factors for developing AD, including age, genetics, and family history (Zhao, 2020; Alzheimer’s Association, 2023). Recent research has suggested a set of modifiable risk factors for developing AD, including smoking, cardiovascular health, low socioeconomic status (SES), fewer years of formal education, and poor sleep quality (Alzheimer’s Association, 2023). Among the modifiable risk factors, perhaps the most intriguing is oxidative and psychological stress. Research has shown that early AD pathogenesis may result in subtle neuropsychological changes, including increased anxiety, depression, or agitation (Schmid et al., 2013; Escher et al., 2019). The hypothalamic–pituitary–adrenal (HPA) axis is a critical system responsible for the body’s response to a perceived or actual threat (i.e., stressor), as well as the return to homeostasis following stress exposure. Homeostasis is achieved through neurological coordination between endocrine, autonomic, and behavioral systems (Aguilera, 2011). Specifically, the HPA axis is activated when there is a perceived stressor leading to an increase in the release of corticotropin-releasing factor (CRF) and subsequent cortisol (corticosterone in rodents) release (Cook, 2004). Excessive exposure to cortisol can result in psychiatric, reproductive, cardiovascular, immune, and metabolic disorders (Aguilera, 2011; Justice, 2018). Importantly, overexposure to cortisol can result in oxidative stress, which has been linked to onset of early-AD neurobiological changes such as mitochondrial dysfunction and synaptic loss (Spiers et al., 2015; Tchekalarova and Tzoneva, 2023). Further, AD patients with posttraumatic stress disorder (PTSD) or generalized anxiety disorder (GAD) are more likely to experience hastened progression and worsening of AD symptoms compared to non-anxious patients (Justice, 2018; Escher et al., 2019; Tchekalarova and Tzoneva, 2023). One meta-analysis reports that anxiety symptoms are associated with a 29% increase in the risk for dementia (Santabárbara et al., 2019, 2020), while a recent meta-analysis reports no significant association between anxiety and AD neuropathology (Demnitz-King et al., 2023). Additionally, it remains unclear if increased anxiety precedes AD, is a consequence of neurodegeneration caused by AD, or is some combination of both possibilities. One study reports that high cerebrospinal fluid (CSF) markers of hyperphosphorylated tau and total tau but low CSF markers of Aβ(42) were associated with neuropsychological burden in cognitively normal adults (Krell-Roesch et al., 2022). Another study reports that anxiety symptomatology predicts Aβ accumulation in non-AD, cognitively normal adults (Johansson et al., 2020). Thus, the involvement of the HPA axis, anxiety, and AD symptomatology warrants further investigation.
There are two systems of interest that impact the HPA axis and AD symptomatology and have potential protective factors against developing AD: the CRF and serotonergic (5-HT) systems (Hajszan et al., 2005; Canet et al., 2019). It is important to note that other systems, such as the noradrenergic system, contribute to stress and AD; however, for the purpose of this specific review this will not be covered (see Poe et al., 2020 for a review). Critically, transgenic (Tg) animals treated with CRF type 1 receptor (CRFR1) antagonists exhibit slowed progression of AD symptomatology. Additionally, the raphe nucleus, which is responsible for 5-HT production in the brain, has significant neuronal loss and dense collections of neurofibrillary tangles in AD patients (Duyckaerts et al., 2009; Zhang C. et al., 2016). Recent research continues to point to the impact of stress on cortisol in AD. Clinical epidemiological studies report that individuals who experience chronic stress see increased incidents of AD neuropathology later in life (Machado et al., 2014; Justice, 2018). Further, AD pathogenesis in early-onset AD animal models consistently report accelerated deposition of Aβ and worse cognitive performance compared to control animals (Dong et al., 2004; Kang et al., 2007; Srivareerat et al., 2009; Devi et al., 2010; Huang et al., 2023). Given the intertwined nature of AD, CRF, and 5-HT, it is likely that there is a treatment capable of targeting these key systems. Future cognitive and pharmacotherapies may aim to regulate the HPA axis to prevent and treat AD symptoms, including anxiety. Importantly, neurodegeneration due to AD is estimated to start 20–30 years before the first signs of clinical symptoms. Identifying patients diagnosed with anxiety disorders such as PTSD and GAD could lead to the implementation of early-intervention treatments for AD. In this review, treatments targeting the HPA axis and anxiety, specifically CRF and 5-HT, will be described, with AD animal models evaluated on translational ability.
AD is a unique form of dementia due to the extended preclinical and prodromal phases of the disease. Typically, subclinical cognitive and neuropsychiatric symptoms are present two decades before a formal diagnosis (Masters et al., 2015). Currently, those who are diagnosed at the preclinical stage of AD have a confirmatory diagnosis of Aβ accumulation through positron emission tomography (PET) or CSF analysis (Dubois et al., 2014; Masters et al., 2015). It is possible that screening for neuropsychiatric symptoms may help improve rates of early diagnosis, especially because most AD patients present with anxiety and/or depression, and have trouble sleeping years prior to their AD diagnosis (Belleville et al., 2014; Burke et al., 2018; Eratne et al., 2018). This increased comprehensive screening is critical because people who receive an earlier diagnosis have improved outcomes and slowed progression of the disease (Rasmussen and Langerman, 2019). The current FDA-approved treatments available can only be prescribed, at the earliest, in the MCI stage (Zhao, 2020). FDA-approved drugs Aducanumab and Lecanemab are both Aβ monoclonal antibody medications that break down Aβ plaques in the brain; however, these medications have severe side effects, including brain hemorrhaging and swelling (Zhao, 2020; Alzheimer’s Association, 2023). Given the improved methodology for diagnosing AD patients in the preclinical stage and lack of early-intervention pharmacotherapies, there is a critical need for improved early-intervention treatments. Due to the increased rates of anxiety in patients in the prodromal and preclinical stages of AD, drugs targeting anxiety symptoms and circuitry, such as the HPA axis, present a potential path forward.
Approximately 40 million adults in the U.S. live with a diagnosed anxiety disorder (Merikangas et al., 2010). Additionally, 40% of AD patients report experiencing anxiety symptoms (Mendez, 2021). Early-life adversity and exposure to stress correlates strongly with later diagnosis of major depression disorder or anxiety disorders (Liu, 2017). The modifiable risk factors of AD and early-life adversity overlap significantly, with low SES/economic hardship, poor sleep quality, and psychological stress present in both measures. Neurobiologically, early-life adversity is linked to increased CRF type-1 receptor (CRF1) expression in the hypothalamus, amygdala, and prefrontal cortex (Wang et al., 2022). Those exposed to early-life adversity showed smaller hippocampal volume and HPA axis hypoactivity (Dahmen et al., 2018), while other individuals who experienced early-life adversity demonstrated HPA axis hyperactivity (Farrell et al., 2018). Additionally, abnormal HPA axis activity has been observed in those diagnosed with GAD and AD, with some studies reporting hypoactivity/reduced cortisol levels and others reporting hyperactivity/increased cortisol levels (Du and Pang, 2015; Juruena et al., 2020). This supports a hypothesis posited by Miller et al. that there are both HPA axis responders and non-responders to psychosocial stressors (Miller et al., 2013). More commonly, AD patients present with increased cortisol levels measured through saliva or plasma (Csernansky et al., 2006; Bangasser et al., 2017). CRF pathway activation is critical to increased cortisol levels due to its signaling and activation of the downstream Gs, cAMP, PKA pathway, ultimately leading to pro-AD signaling and resulting in a hastened progression of the disease (Yan et al., 2018). This CRF system signaling was first characterized extensively by Vale et al., who described the ability of high potency CRF1 to stimulate adrenocorticotropic hormone and glucocorticoid secretion through the paraventricular nucleus of the hypothalamus (Vale et al., 1981). SSRIs affect the same pathway through upregulation of 5-HT1A and subsequent inhibition of the Gs, cAMP, PKA pathway. An example of this is the SSRI escitalopram, which mediates HPA axis activity via CRF inhibition (Tafet and Nemeroff, 2020). Interestingly, clinical populations show a reduction of the 5-HT1A autoreceptor in the raphe nucleus after SSRI treatment (Gray et al., 2013). This finding has been consistent in the literature, with other studies reporting 5-HT1A autoreceptors having increased desensitization compared to 5-HT1A heteroreceptors. This is important to highlight when considering treatment with SSRIs for AD, as 5-HT1A receptors are critical to hippocampal signaling, activation of growth factor-regulated signaling pathways, and neuronal myelination processes (Gray et al., 2013; Kroeze et al., 2015; Albert and Vahid-Ansari, 2019). Given the extensive involvement of both the CRF and 5-HT systems in HPA axis regulation and downstream AD pathogenesis, early-intervention treatments should consider prioritizing these two systems.
Clinical AD populations treated with SSRIs report enhanced memory and cognition (Mowla et al., 2007; Bartels et al., 2018; Elsworthy and Aldred, 2019; Lenze et al., 2023). Critically, AD patients with behavioral and cognitive deficits who receive treatment with SSRIs show significant improvements and hippocampal neurogenesis (Santarelli et al., 2003; Correia et al., 2021). However, the rodent models of AD yield mixed results. A more recent study found no differences in amyloid burden or cognitive performance in AD patients treated with an SSRI and cognitively normal controls (Bouter and Bouter, 2022). Further, one meta-analysis reported no significant differences between AD patients treated with SSRIs and AD patients not treated with SSRIs (Jones et al., 2016), while another meta-analysis reported an increased risk of dementia in people prescribed SSRIs, monoamine oxidase inhibitors, and tricyclics (Caballero et al., 2006; Popovic et al., 2015; Wang et al., 2018). Ultimately, there are few randomized control trials examining the effects of SSRIs on dementia patients. Despite the mixed findings in clinical populations, preclinical data suggest that SSRIs may reduce AD pathogenesis while improving behavioral and cognitive outcomes (Elsworthy and Aldred, 2019; Lenze et al., 2023). It is possible that a more targeted pharmacotherapy, such as a CRF1 antagonist, with or without a standard antidepressant, may produce more robust effects in clinical populations due to their potency and specificity compared to antidepressants.
Currently, there are 214 animal models of early-onset AD (EOAD) available, with 197 AD mouse models and 17 AD rat models (Götz et al., 2018). Mouse models of AD are generally preferred for Tg experiments due to low breeding and husbandry costs and ease of gene manipulation. In contrast, rat models are generally preferred for behavioral tasks due to their increased sociality and increased translational relevance (Bryda, 2013). Regardless of species, there are five genes commonly targeted in AD Tg models: amyloid-precursor protein (APP), presenilin 1 (PS1), presenilin 2 (PS2), microtubule-associated protein tau (MAPT), and apolipoprotein E (APOE; Remy et al., 2014; Alzheimer’s Association, 2023). The APP, PS1, and PS2 genes are implicated in EOAD, while the APOE gene is implicated in late-onset AD (LOAD). MAPT is typically integrated into animal models that do not express robust tauopathy with other AD genetic manipulations alone. This could be a potential benefit of rat models, due to their natural expression of tau isoforms compared to mouse models that rely on integration of human mutant MAPT genes. Genes of animals can be manipulated in two ways, either through Tg technology or genome editing recombination. In contrast, genome editing recombination, such as homology directed recombination, knocks out or replaces the targeted AD genes, guaranteeing AD pathology expression in the selected rodents (Goedert and Spillantini, 2006; Filali et al., 2012; Götz et al., 2018; Alzheimer’s Association, 2023). Due to the abundance of AD animal models available, two AD models will be prioritized in the current review: the 3xTg-AD mouse model (see Figure 1) and the TgF344-AD rat model (see Figure 2). These models were chosen due to their common use in the literature and robust AD neuropathology expressed, including AB plaques and tau tangles.
It is important to highlight the potential differences in AD strains since their original development. Due to TgF344-AD rodents being developed and characterized more recently, there is less concern regarding genetic drift and consistency between Tg colonies, with multiple papers replicating the original phenotype findings reported by Cohen et al. (2013), Anckaerts et al. (2019), and Fowler et al. (2022). However, 3xTg mice were developed 20 years ago (Oddo et al., 2003) and there is evidence of genetic drift impacting both behavioral and neural AD pathology. A comparative study looking at the drift was conducted by the LaFerla Lab, which originally developed the 3xTg-AD model. The authors report that compared to their original study in 2003, 3xTg-AD mice develop age-dependent Aβ and tangles, but this development occurs significantly later in the model with AD pathology previously present at 12 mo. and now present at 18 mo. (Javonillo et al., 2022). According to several studies, Aβ plaque development occurs in female 3xTg-AD mice from 12 to 18 months, with males showing significantly less Aβ and hyperphosphorylated tau deposition at 18 months (Carroll and Pike, 2008; Creighton et al., 2019; Javonillo et al., 2022). Additionally, female 18-month-old 3xTg-AD mice present with increased microglial density compared to males (Javonillo et al., 2022; see Figure 3). Although genetic drift is present in the 3xTg mouse model, it is important to consider that there is no ideal animal model for EOAD. Scientists should remain vigilant for potential genetic drift and subsequent behavioral changes that may occur. In-depth phenotype characterization is necessary for each model in order to properly assess and quantify AD pathological and behavioral changes in Tg models (Javonillo et al., 2022). The present review aims to investigate two EOAD animal models that expressed robust AD pathology while prioritizing research that manipulated or analyzed the serotonergic and CRF systems. Given these parameters, the models most frequently observed in the literature were the 3xTg-AD mouse and TgF344-AD rat models. However, it is important to note that the effects of stress, anxiety, cortisol, and AD pathogenesis should be investigated in all AD Tg models currently available.
Figure 1. Timeline of AD pathogenesis in 3xTg-AD model (original characterization; Oddo et al., 2003). Created with BioRender.com.
This triple Tg model expresses the APP, PS1, and MAPT genes with Aβ plaques present at 6 months and tau/tangles present at 12 months. Additionally, 3xTg mice show synaptic dysfunction, including long-term potentiation deficits (Oddo et al., 2003; Blázquez et al., 2014). Considering the genetic drift of the 3xTg-AD mice, deposition of Aβ and tau, as well as LTP deficits, occur around 18 months, with more pronounced deposition reported in female 3xTg-AD mice (Javonillo et al., 2022). Increased anxiety, defined as the behavioral and/or physiological response to perceived threat, is typically measured in animal models through testing in elevated plus-maze (EPM) or the light–dark test (LDT; for a review of animal models see Pentkowski et al., 2021). In the EPM, rodents classified as exhibiting enhanced anxiety-like behavior spend more time in the closed arms compared to the open arms. Similarly, for the LDT, rodents exhibiting heightened anxiety-like behavior tend to spend more time in the dark compartment compared to the light compartment. Both tests capitalize on rodents’ tendency to seek shelter from potential predators by staying in dark, enclosed spaces. Further, entries into the light, exposed environments are recorded to assess for exploratory behavior (see Figure 4). Rodents that explore more are presumed to be less anxious (McCormick and Green, 2013). Extremely conflicting results have been reported in anxiety measures in the 3xTg-AD model. Prior to AD pathology being expressed, 3xTg-AD mice at 3 and 4 months do not exhibit enhanced anxiety-like behavior on the EPM, with Tg female mice spending increased time in the open arms compared to wild-type mice (WT; Pairojana et al., 2021; Várkonyi et al., 2022). Anxiety-like behaviors in LDT continue to be inconsistent at this age, with 4-month-old WT mice spending more time in the dark compartment compared to Tg mice (Várkonyi et al., 2022). At 6 months, 3xTg-AD behavior in the EPM is varied, with one study reporting that Tg mice spent more time in the closed arms and made fewer entries into the open arms (Zhang Y. L. et al., 2016), while other studies observed no differences between Tg and WT mice (Hebda-Bauer et al., 2013; Pairojana et al., 2021). For the LDT, 6-month-old 3xTg-AD mice spent more time in the dark compartment, consistent with elevated anxiety-like behavior (Zhang C. et al., 2016; refer to Table 1). Older 3x-Tg mice (7.5–12 months old) did not differ in amount of time spent in the open arms of the EPM or the amount of locomotor activity exhibited compared to controls. This observation suggests that the mice are not exhibiting enhanced anxiety at this time point, despite the progression of positive lesions and cognitive deficits (Sterniczuk et al., 2010; Pairojana et al., 2021). In the LDT, 8–12-month-old 3xTg mice spent significantly more time in the dark compartment and exhibited decreased locomotion, which is consistent with increased anxiety-like behavior (Blázquez et al., 2014; Várkonyi et al., 2022; refer to Table 2).
Figure 2. Timeline of AD pathogenesis in the TgF344-AD model (Cohen et al., 2013). Created with BioRender.com.
Other behaviors measured in AD animal models include deficits in locomotion, and spatial learning and navigation. The Morris Water Task (MWT) measures spatial learning ability by training rodents to swim to a platform over a period of days, while the open-field task (OFT) measures locomotor activity and exploratory behavior organized around salient locations termed a “home base” (Whishaw and Tomie, 1996; Seibenhener and Wooten, 2015; Thompson et al., 2018; Donaldson et al., 2019; see Figure 2). Importantly, the OFT can also be used to assess anxiety-like behaviors due to thigmotaxic behaviors present in animals and the proclivity of animals to spend long periods of time at home base locations (Whishaw et al., 2006). Due to the increase in cognitive and neurological deficits as AD progresses, it should be expected that mice perform progressively worse on the MWT and have a reduction in locomotion. Few studies observed 3xTg-AD mice prior to development of AD pathology; however, one study reports that 4-month-old 3xTg-AD mice show no deficits in spatial learning compared to WT mice (Várkonyi et al., 2022). Further, 3–4-month-old 3xTg-AD mice exhibit decreased locomotion and increased grooming behaviors compared to WT controls (Pairojana et al., 2021; Várkonyi et al., 2022). At the onset of AD pathogenesis, 8-month-old 3xTg mice spent more time locating the platform in the MWT on the final of training compared to their WT counterparts (Várkonyi et al., 2022; refer to Table 3). 6–8-month-old 3xTg mice have inconsistent locomotor behaviors in the OFT, with some mice exhibiting less active movement and others not differing from WT controls (Sterniczuk et al., 2010; Hebda-Bauer et al., 2013; Zhang Y. L. et al., 2016; Pairojana et al., 2021; Várkonyi et al., 2022). Older 3xTg-AD mice (12–15 months old) perform consistently worse on the MWT compared to WT controls, showing severe deficits in spatial memory. However, it is important to note that these effects were captured on the first days of training. The differences between Tg and WT 3xTg-AD mice were not seen on the final days of MWT testing when performance was at asymptote (Blázquez et al., 2014; Torres-Lista et al., 2015). Most studies report a significant reduction in locomotion in the OFT in older 3xTg mice, but one study found no differences in locomotor behavior compared to WT controls (Sterniczuk et al., 2010; Blázquez et al., 2014; Torres-Lista et al., 2015; Pairojana et al., 2021). Overall, behavior measured in the MWT and OFT varied between labs and studies (refer to Table 4).
Although neurobiological changes reported in the 3xTg-AD mouse model remain consistent with development of Aβ plaques (6 months/18 months) and tau/tangles (12 months/18 months; see Figures 1 and 3), behavioral results are more inconsistent (Oddo et al., 2003; Zhang C. et al., 2016). For the anxiety measures using the EPM and LDT, LDT findings were more consistent, with 3xTg-AD mice consistently exhibiting elevated anxiety-like behavior, with increased time spent in the dark compartment and fewer entries into the light compartment compared to controls. The EPM findings varied heavily, with some Tg mice spending increased time in the open arms and others more time in the closed arms. In the MWT, 3xTg-AD mice generally performed worse than their WT counterparts; however, OFT findings were more varied. At all ages, 3xTg-AD mice expressed differing behavior, with some Tg mice expressing decreased locomotion and speed while other Tg mice did not differ significantly from WT controls. Although the behavioral results were more varied, the 3xTg-AD model is still an ideal model for examining anxiety-like behaviors and the impact on AD. This is due to the robust AD pathology expressed, as well as enhanced anxiety-like behavior and locomotor deficits present from 6–12 months of age. Despite genetic drift reported in the 3xTg-AD model, there is still consistent behavioral and AD pathology reports at 18 months. The 3xTg-AD model is heavily cited and widely used, allowing for robust meta-analyses and comparisons of colonies. Additionally, the 3xTg-AD model may be more translational to a clinical population compared to other mouse AD models due to the gradual onset of AD symptoms and pathology (Liu et al., 2021). However, the implantation of the tau gene, MAPT, may cause this model to be slightly less translationally valid compared to AD models that do not rely on genetic manipulation for expression of tau. Future studies should verify the presence of microglia, Aβ, and tau, to ensure standardization across various colonies, labs, and studies.
Figure 3. Updated characterization of AD pathogenesis in the 3xTg-AD model (Javonillo et al., 2022). Created with BioRender.com.
This Tg rat model expresses both the mutant human amyloid precursor protein (APPswe) and presenilin 1 (PS1ΔE9) genes. The model consistently shows age-dependent cerebral Aβ plaque and tau deposition, neuronal loss, and cognitive disturbance (Cohen et al., 2013). Typically, TgF344-AD rats begin to express Aβ plaque deposition at 5–6 months, prior to marked cognitive deficits being observed. This is followed by robust tauopathy at 12–16 months and neuronal loss and neuroinflammation present around 16 months (Cohen et al., 2013; Wu et al., 2020) For rat studies, primarily the EPM and OFT were used to measure anxiety-like behavior, with measures examining exploratory behavior via time spent and entries into the open arms (EPM), and time spent in the center of the area (OFT; see Figure 4). Younger TgF344-AD rats yielded some mixed behavioral results. 2- and 6-month-old TgF344-AD rats did not exhibit altered anxiety-like behavior in the EPM or OFT according to some studies (Wu et al., 2020; Kelberman et al., 2022), while other studies reported increased anxiety-like behavior demonstrated by reduced open arm time in the EPM and reduced locomotion in the OFT in TgF344-AD rats aged 4.5–6.5 months (Pentkowski et al., 2018, 2022; Saré et al., 2020). Most of the variability in the anxiety behavioral measures occurs in TgF344-AD rats aged 6 months, with studies finding no differences between TgF344-AD and WT in the OFT, with TgF344-AD rats spending less time in the center of the OFT compared to WT rats, or TgF344-AD hypoactivity (Cohen et al., 2013; Saré et al., 2020; Kelberman et al., 2022). At 9 months, findings are more consistent, with two studies finding increased anxiety-like behaviors in Tg rats. Specifically, compared to WT rats, Tg rats spent less time in the open arms of the EPM and less time in the center of the open field (Tournier et al., 2021; Srivastava et al., 2023). After rats were aged 12 months or older, anxiety-like behavior was more consistently demonstrated in both the EPM and OFT (Cohen et al., 2013; Saré et al., 2020; Wu et al., 2020; Kelberman et al., 2023). However, some studies still report no differences between older TgF344-AD and WT rats during testing in the EPM and OFT (Saré et al., 2020; Srivastava et al., 2023; refer to Tables 5, 6).
To assess cognitive deficits in spatial learning and navigation, studies utilized the MWT (see Figure 4). One study examined cognition and spatial learning in TgF344-AD rats prior to AD pathogenesis and found no significant differences in spatial learning at 4.5–6.5 months (Pentkowski et al., 2018). After 6 months, TgF344-AD rats show deficits in initial acquisition and take longer paths to the platform (Bernaud et al., 2022). However, another study reported no differences in path length between Tg and WT rats at 7–8 months (Berkowitz et al., 2018). Studies continue to report Tg rats taking longer paths at 9–10 months (Berkowitz et al., 2018; Bernaud et al., 2022), yet one study reports no differences in locating the platform during the probe trial in 9-month-old Tg and WT animals (Srivastava et al., 2023). TgF344-AD rats aged 12 months or older continue to execute longer paths and less direct trajectories and have more trouble locating the platform (Bernaud et al., 2022; Bac et al., 2023; Srivastava et al., 2023), with only one study reporting no differences between TgF344-AD and WT rats at 12 months (Kelberman et al., 2023; refer to Table 7).
Neurobiological changes in TgF344-AD rats remain consistent throughout studies, with this model expressing robust AD neuropathology not expressed in many other animal models of AD (Saré et al., 2020; Wu et al., 2020; See Figure 2). A distinct difference between the TgF344-AD and the 3xTg-AD models is the expression of neuronal loss, gliosis, neuroinflammation, and tau expression in TgF344-AD rats that is not reliant on implanted mutated human tau. Given this robust and complete AD pathology expression, this rat model may generate more translational results than mouse models, including the 3xTg-AD model. Further, the TgF344-AD rat model yielded more consistent cognitive and behavioral results, with TgF344-AD rats aged more than 6 months typically exhibiting more anxiety-like behavior and deficits in spatial navigation and learning. However, this model was developed a decade after the 3xTg-AD model and needs more extensive research into the consistency of behavioral and cognitive results reported in the present review (Oddo et al., 2003; Cohen et al., 2013).
Figure 4. Examples of the behavioral test examined in the review including: elevated plus maze (EPM), light-dark test (LDT), open field test (OFT), and Morris water task (MWT). Created with BioRender.com.
Dysregulation of the HPA axis and CRF1 are critical to AD pathogenesis, and investigation of these systems could lead to novel treatments for both EOAD and LOAD due to the modifiable environmental risk factors implicated in the onset of both EOAD and LOAD (Cañete et al., 2015). Indeed, chronic stressor-induced HPA axis dysregulation leads to increased activation, synthesis, and release of glucocorticoids, which can have deleterious consequences on brain morphology and function (De Bellis et al., 1999), as well as the diminished ability to suppress cortisol secretion, which is seen in AD patients and rodents in the preclinical/prodromal stage (Csernansky et al., 2006; Rothman et al., 2012; Nguyen et al., 2020). Critical to HPA axis regulation are glucocorticoid receptors (GR) and CRF1. In rodents, often females have more central CRF1 expression compared to males (Donner and Lowry, 2013; Bangasser et al., 2017), which may represent one mechanism underlying higher rates of AD in females. Further, in AD rodent models, increased cortisol levels are thought to result from increased GR and CRF1 expression in key areas such as the hippocampus and cortex (Dong et al., 2008; Rissman et al., 2012; see Mohammadi et al., 2022 for a review). The impacts of HPA dysfunction, GR and CRF1 expression, and novel pharmacotherapies targeting these systems will be presented below.
Generally, 3xTg mice aged 3–4 months do not exhibit differing corticosterone (CORT) levels compared to controls; however, some 3xTg males did exhibit increased GR mRNA expression in the hippocampus and paraventricular nucleus, suggesting a possible progression toward increased CORT in this AD model (Hebda-Bauer et al., 2013; Nguyen et al., 2020). In the early stages of AD progression, CORT measurements vary. Additionally, dexamethasone non-suppression was found in 4-month-old 3xTg mice (Várkonyi et al., 2022), suggesting reduced levels of GR. Higher CORT levels were reported in 6-month-old 3xTg-AD males and females, while another study reported no differences between 3xTg-AD and WT mice (Hebda-Bauer et al., 2013; Baeta-Corral et al., 2023). At 9 months, 3xTg-AD males have higher basal CORT levels compared to 3xTg-AD females and WT (Clinton et al., 2007; Nguyen et al., 2020). 3xTg-AD animals aged beyond 12 months consistently have increased CORT levels compared to controls, which aligns with full expression of AD pathology in this model (Rothman et al., 2012; Muntsant and Giménez-Llort, 2021). Overall, this remains consistent with the findings of age-dependent increases in cortisol serum levels (Green et al., 2006). This steady increase in GR and subsequent CORT measurements suggests that the HPA axis is severely dysregulated in the 3xTg-AD model. Thus, novel pharmacotherapies could target GR and CRF1 receptors to reduce downstream Gs, cAMP, PKA activation and increased cortisol release. Indeed, chronic administration of the GR antagonist (RU486) in 10-month-old mice reversed Aβ deposition and reduced tau/NFT (Baglietto-Vargas et al., 2013). This trend is well supported in similar mouse models of AD, with Tg mice treated with CRF1 antagonists (Antalarmin, R121919) demonstrating lower levels of Aβ, tau, and CORT (Dong et al., 2014; Campbell et al., 2015; Zhang Y. L. et al., 2016; Zhang and Rissman, 2017). Research utilizing AD rat models is more limited because AD rat models were developed more recently. However, some data from studies indicate increased CORT levels when Aβ is present (Brureau et al., 2013; refer to Table 8). Additionally, current trends in the literature suggest that due to the well-established behavioral phenotype of TgF344-AD rats, future research should implement novel pharmacotherapies aimed at HPA axis regulation (Justice, 2018; Pentkowski et al., 2022).
The monoaminergic system in general, and the serotonergic system specifically, have been implicated in HPA axis activation, AD pathogenesis, and cognition. The serotonergic system is vital and heavily implicated in the experience of anxiety and depressive symptoms in animal and human models (Yohn et al., 2017). Additionally, risk factors such as early-life stress can alter 5-HT transmission. For instance, rats that experience early-life stress show significantly altered 5-HT transmission (Van Riel et al., 2004). Specifically, 5-HT1A receptor density has been reduced in AD, which could account for some anxiety and depression symptoms experienced in AD patients (Chakraborty et al., 2019). In rodent models of AD, reports indicate that one of the areas severely impacted by Aβ and tau burden is the raphe nucleus, which may account for the disruption in 5-HT system signaling and receptor density. Restoring the effectiveness of this system could promote psychological well-being (e.g., reduced anxiety and/or depression) and possibly delay cognitive impairments in AD patients.
SSRIs are often used often in scientific literature due to their widespread availability and history of treating neuropsychiatric disorders. Research has examined the effects of SSRIs in Tg AD animals, with some studies reporting improved cognition and behavioral performance (Wang et al., 2014; Jin et al., 2017; Ma et al., 2017; Marwari and Dawe, 2018; Torrisi et al., 2019; Zhou et al., 2019). Specifically, several studies in AD mouse models (APP/PS1, Aβ-injected C57BL/6 mice) report efficacy of SSRIs (e.g., fluoxetine) in restoring cognition and cell density in the hippocampus (Cirrito et al., 2011; Ma et al., 2017; Caruso et al., 2021). Additional studies report that treatments with SSRIs or 5-HT receptor agonists increase brain derived neurotropic factor production and stimulate neurogenesis (Santarelli et al., 2003). Multiple studies report reduced Aβ deposition in APP/PSI mice and APP treated cells that received short-term and long-term treatment with SSRIs (Fisher et al., 2016; Reddy et al., 2021). Specifically, in 3xTg models, administration of risperidone attenuates anxiety-like behaviors and cognitive deficits in the OFT and MWT, respectively (Nelson et al., 2007; Torres-Lista et al., 2015). However, there are issues in both consistency and translational ability of SSRIs’ effectiveness in reducing AD pathogenesis and improving cognitive and behavioral deficits. In the APP/PS1 model, studies report no change in Aβ deposition, nor cognitive or behavioral measures (Severino et al., 2018; Sivasaravanaparan et al., 2022; see Mdawar et al., 2020; for a review; refer to Table 9). Although treatments with SSRIs varied in AD rodent models, given that treatment with SSRIs worked in a subsection of AD animal models, both in reducing AD pathological burden and reducing cognitive and behavioral disturbances, more targeted 5-HT treatment may be more effective. Further, with the lack of behavioral measure consistency in some AD animal models, it is possible that a more specific 5-HT treatment could produce more consistent and translational outcomes.
Overall, continuing improvements in AD animal models are crucial; more translational animal models will improve treatment efficacy, leading to better outcomes for AD patients. Additionally, early intervention is critical due to improved patient outcomes; however, diagnosing patients in the Preclinical/Prodromal Stage of AD presents challenges and concerns. Due to high sensitivity in more recent diagnostic measures, a false positive is more likely (Sperling et al., 2011). However, a focus on reducing psychological stress and anxiety, as well as improving HPA axis regulation, could still benefit individuals who receive a false positive (Plotsky et al., 1998). Animal models are important in pharmacotherapy development, but translational ability is perhaps the most important factor for researchers to consider. In this review, models of anxiety and locomotion were explored, including the OFT, LDT, EPM, and MWT. Of note, both 3xTg-AD and TgF344-AD had inconsistencies in behavioral and cognitive outcomes measured. In the 3xTg mouse model, the EPM was an unreliable measure of anxiety-like behavior, but the LDT findings remained consistent. Although many of these studies suggest that the EPM should not be used in this model, it is important to elucidate the reasons why the EPM is not reliable in these animals. Contrastingly, the EPM results were more consistent in the TgF344-AD rat model, suggesting that the EPM can be used for some AD animal models. Further, it is important to investigate mouse capabilities in wet tasks such as the MWT. Mice have been found to perform unreliably, anxiously, and slowly in the MWT; however, rats perform extraordinarily well in both wet and dry tasks, perhaps making them the preferred subjects for AD models (Whishaw and Tomie, 1996; Cohen et al., 2013). It is important to consider the age of the WT control rats as well, due to a steady decline in MWT performance (Lindner, 1997). Although this may explain some inconsistencies outlined in the present paper, the Tg animals should still demonstrate increased impairment compared to WT animals provided that control performance is not at the “floor.”
It is also important to consider potential differences in methodology, breeding, and housing. Although the same behavioral tests (EPM, MWT LDT, OFT) were conducted in each study, findings could be inconsistent due to variables such as housing, handling, breeding, and colony genetics. In the 3xTg-AD model, this may account for some variance observed in Tables 2–6 since the mice were all tested prior to 18 months, which is when Aβ, tau, microglia, and LTP deficits are seen in the more contemporary 3xTg-AD colonies (Javonillo et al., 2022). Although genetic drift has not yet been observed or reported in TgF344-AD rats, other factors, such as breeding and/or the amount of handling, could contribute to the varying behavioral results observed. Additionally, the behavioral testing apparatuses’, such as an EPM or open field, may differ between labs, which could also contribute to variance between studies. In order to increase standardization of research methods, scientists should include detailed methods sections allowing for more seamless replication of studies across laboratories.
Although inconsistencies were present in behavioral and cognitive measures in the AD models, both the 3xTg-AD and TgF344-AD animals consistently expressed robust AD pathology (Oddo et al., 2003; Cohen et al., 2013). Despite the extensive literature available on the 3xTg mouse model, the TgF344 rat model may be preferred in the future due to its more consistent behavioral findings and expression of neuroinflammation and neuronal loss, which remains lacking in mouse AD models. Additionally, mouse models rely on implantation of human tau while rat models rely on the naturally occurring rat tau gene (Cohen et al., 2013). Regardless of species chosen in the AD model, treatments with CRF or 5-HT ligands present a promising path forward. Due to the elevated cortisol and HPA axis activity consistently reported in animal AD models, there is opportunity to model stress and discover novel treatments (Ahmad et al., 2019). Targeting 5-HT could be an easy preventative measure to implement due to its widespread access and the high percentage of US adults having taken an antidepressant in their lifetime (Brody and Gu, 2020). Side effects of SSRIs can lead to noncompliance; however, more specific manipulations to both the 5-HT and CRF systems could lead to fewer side effects. Targeting CRF1 may improve AD outcomes in patients based on animal literature citing a reduction in AD pathology and studies showing anxiolytic responses to CRF1 antagonists. The specificity of medications, such as CRF1 antagonists, may limit side effects further while still improving clinical outcomes in AD patients.
NR, NP, and BC produced original conceptualization of the current manuscript topic. NR performed literature searches, selected relevant papers, and wrote the manuscript. All figures made by NR using BioRender.com. DH provided table formatting and effect size calculation assistance. BC, DH, and NP were involved in critically reading and editing the manuscript. All authors contributed to the article and approved the submitted version.
The current work was supported by the New Mexico Alzheimer’s Disease Research Center (P20-AG068077, PI: Gary Rosenberg) with pilot funding awarded to NR and by the UNM Center for Brain Recovery and Repair (P20 GM109089). The authors thank the Quad-L Foundation at UNM for supporting publication cost.
The authors declare that the research was conducted in the absence of any commercial or financial relationships that could be construed as a potential conflict of interest.
All claims expressed in this article are solely those of the authors and do not necessarily represent those of their affiliated organizations, or those of the publisher, the editors and the reviewers. Any product that may be evaluated in this article, or claim that may be made by its manufacturer, is not guaranteed or endorsed by the publisher.
Aguilera, G. (2011). HPA axis responsiveness to stress: implications for healthy aging. Exp. Gerontol. 46, 90–95. doi: 10.1016/j.exger.2010.08.023
Ahmad, M. H., Fatima, M., and Mondal, A. C. (2019). Role of hypothalamic-pituitary-adrenal axis, hypothalamic-pituitary-gonadal axis and insulin signaling in the pathophysiology of Alzheimer’s disease. Neuropsychobiology 77, 197–205. doi: 10.1159/000495521
Albert, P. R., and Vahid-Ansari, F. (2019). The 5-HT1A receptor: signaling to behavior. Biochimie 161, 34–45. doi: 10.1016/j.biochi.2018.10.015
Alzheimer’s Association (2023). Alzheimer’s disease facts and figures. Alzheimers Dement. 19, 1598–1695. doi: 10.1002/alz.13016
Anckaerts, C., Blockx, I., Summer, P., Michael, J., Hamaide, J., Kreutzer, C., et al. (2019). Early functional connectivity deficits and progressive microstructural alterations in the TgF344-AD rat model of Alzheimer’s disease: a longitudinal MRI study. Neurobiol. Dis. 124, 93–107. doi: 10.1016/j.nbd.2018.11.010
Bac, B., Hicheri, C., Weiss, C., Buell, A., Vilcek, N., Spaeni, C., et al. (2023). The TgF344-AD rat: behavioral and proteomic changes associated with aging and protein expression in a transgenic rat model of Alzheimer's disease. Neurobiol. Aging 123, 98–110. doi: 10.1016/j.neurobiolaging.2022.12.015
Baeta-Corral, R., De la Fuente, M., and Giménez-Llort, L. (2023). Sex-dependent worsening of NMDA-induced responses, anxiety, hypercortisolemia, and organometry of early peripheral immunoendocrine impairment in adult 3xTg-AD mice and their long-lasting ontogenic modulation by neonatal handling. Behav. Brain Res. 438:114189. doi: 10.1016/j.bbr.2022.114189
Baglietto-Vargas, D., Medeiros, R., Martinez-Coria, H., LaFerla, F. M., and Green, K. N. (2013). Mifepristone alters amyloid precursor protein processing to preclude amyloid beta and also reduces tau pathology. Biol. Psychiatry 74, 357–366. doi: 10.1016/j.biopsych.2012.12.003
Bangasser, D. A., Dong, H., Carroll, J., Plona, Z., Ding, H., Rodriguez, L., et al. (2017). Corticotropin-releasing factor overexpression gives rise to sex differences in Alzheimer’s disease-related signaling. Mol. Psychiatry 22, 1126–1133. doi: 10.1038/mp.2016.185
Bartels, C., Wagner, M., Wolfsgruber, S., Ehrenreich, H., and Schneider, A., Alzheimer’s Disease Neuroimaging Initiative (2018). Impact of SSRI therapy on risk of conversion from mild cognitive impairment to Alzheimer’s dementia in individuals with previous depression. Am. J. Psychiatr. 175, 232–241. doi: 10.1176/appi.ajp.2017.17040404
Belleville, S., Fouquet, C., Duchesne, S., Collins, D. L., and Hudon, C. (2014). Detecting early preclinical Alzheimer's disease via cognition, neuropsychiatry, and neuroimaging: qualitative review and recommendations for testing. J. Alzheimers Dis. 42, S375–S382. doi: 10.3233/JAD-141470
Berkowitz, L. E., Harvey, R. E., Drake, E., Thompson, S. M., and Clark, B. J. (2018). Progressive impairment of directional and spatially precise trajectories by TgF344-Alzheimer’s disease rats in the Morris water task. Sci. Rep. 8, 16153–16114. doi: 10.1038/s41598-018-34368-w
Bernaud, V. E., Bulen, H. L., Peña, V. L., Koebele, S. V., Northup-Smith, S. N., Manzo, A. A., et al. (2022). Task-dependent learning and memory deficits in the TgF344-AD rat model of Alzheimer’s disease: three key timepoints through middle-age in females. Sci. Rep. 12:14596. doi: 10.1038/s41598-022-18415-1
Blázquez, G., Cañete, T., Tobeña, A., Giménez-Llort, L., and Fernández-Teruel, A. (2014). Cognitive and emotional profiles of aged Alzheimer’s disease (3×TgAD) mice: effects of environmental enrichment and sexual dimorphism. Behav. Brain Res. 268, 185–201. doi: 10.1016/j.bbr.2014.04.008
Bouter, Y., and Bouter, C. (2022). Selective serotonin reuptake inhibitor-treatment does not show beneficial effects on cognition or amyloid burden in cognitively impaired and cognitively Normal subjects. Front. Aging Neurosci. 14:883256. doi: 10.3389/fnagi.2022.883256
Brody, DJ, and Gu, Q. (2020). Antidepressant use among adults: United States, 2015–2018. NCHS Data Brief, no 377. Hyattsville, MD: National Center for Health Statistics.
Brureau, A., Zussy, C., Delair, B., Ogier, C., Ixart, G., Maurice, T., et al. (2013). Deregulation of hypothalamic-pituitary-adrenal axis functions in an Alzheimer's disease rat model. Neurobiol. Aging 34, 1426–1439. doi: 10.1016/j.neurobiolaging.2012.11.015
Bryda, E. C. (2013). The mighty mouse: the impact of rodents on advances in biomedical research. Mo. Med. 110, 207–211.
Burke, S. L., Cadet, T., Alcide, A., O’Driscoll, J., and Maramaldi, P. (2018). Psychosocial risk factors and Alzheimer’s disease: the associative effect of depression, sleep disturbance, and anxiety. Aging and mental health, 22, 1577–1584. doi: 10.1080/13607863.2017.1387760
Caballero, J., Hitchcock, M., Beversdorf, D., Scharre, D., and Nahata, M. (2006). Long‐term effects of antidepressants on cognition in patients with Alzheimer’s disease. Journal of clinical pharmacy and therapeutics, 31, 593–598. doi: 10.1111/j.1365-2710.2006.00778.x
Campbell, S. N., Zhang, C., Roe, A. D., Lee, N., Lao, K. U., Monte, L., et al. (2015). Impact of CRFR1 ablation on amyloid-β production and accumulation in a mouse model of Alzheimer's disease. J. Alzheimers Dis. 45, 1175–1184. doi: 10.3233/JAD-142844
Canet, G., Hernandez, C., Zussy, C., Chevallier, N., Desrumaux, C., and Givalois, L. (2019). Is AD a stress-related disorder? Focus on the HPA Axis and its promising therapeutic targets. Front. Aging Neurosci. 11:269. doi: 10.3389/fnagi.2019.00269
Cañete, T., Blázquez, G., Tobeña, A., Giménez-Llort, L., and Fernández-Teruel, A. (2015). Cognitive and emotional alterations in young Alzheimer's disease (3xTgAD) mice: effects of neonatal handling stimulation and sexual dimorphism. Behav. Brain Res. 281, 156–171. doi: 10.1016/j.bbr.2014.11.004
Carroll, J. C., and Pike, C. J. (2008). Selective estrogen receptor modulators differentially regulate Alzheimer-like changes in female 3xTg-AD mice. Endocrinology 149, 2607–2611. doi: 10.1210/en.2007-1346
Caruso, G., Grasso, M., Fidilio, A., Torrisi, S. A., Musso, N., Geraci, F., et al. (2021). Antioxidant activity of fluoxetine and vortioxetine in a non-transgenic animal model of alzheimer’s disease. Front. Pharmacol. 12:809541. doi: 10.3389/fphar.2021.809541
Chakraborty, S., Lennon, J. C., Malkaram, S. A., Zeng, Y., Fisher, D. W., and Dong, H. (2019). Serotonergic system, cognition, and BPSD in Alzheimer’s disease. Neurosci. Lett. 704, 36–44. doi: 10.1016/j.neulet.2019.03.050
Cirrito, J. R., Disabato, B. M., Restivo, J. L., Verges, D. K., Goebel, W. D., Sathyan, A., et al. (2011). Serotonin signaling is associated with lower amyloid-β levels and plaques in transgenic mice and humans. Proc. Natl. Acad. Sci. 108, 14968–14973. doi: 10.1073/pnas.1107411108
Clinton, L. K., Billings, L. M., Green, K. N., Caccamo, A., Ngo, J., Oddo, S., et al. (2007). Age-dependent sexual dimorphism in cognition and stress response in the 3xTg-AD mice. Neurobiol. Dis. 28, 76–82. doi: 10.1016/j.nbd.2007.06.013
Cohen, R. M., Rezai-Zadeh, K., Weitz, T. M., Rentsendorj, A., Gate, D., Spivak, I., et al. (2013). A transgenic Alzheimer rat with plaques, tau pathology, behavioral impairment, oligomeric aβ, and frank neuronal loss. J. Neurosci. 33, 6245–6256. doi: 10.1523/JNEUROSCI.3672-12.2013
Cook, C. J. (2004). Stress induces CRF release in the paraventricular nucleus, and both CRF and GABA release in the amygdala. Physiol. Behav. 82, 751–762. doi: 10.1016/j.physbeh.2004.06.013
Correia, A. S., Cardoso, A., and Vale, N. (2021). Highlighting immune system and stress in major depressive disorder, Parkinson’s, and Alzheimer’s diseases, with a connection with serotonin. Int. J. Mol. Sci. 22:8525. doi: 10.3390/ijms22168525
Creighton, S. D., Mendell, A. L., Palmer, D., Kalisch, B. E., MacLusky, N. J., Prado, V. F., et al. (2019). Dissociable cognitive impairments in two strains of transgenic Alzheimer’s disease mice revealed by a battery of object-based tests. Sci. Rep. 9:57. doi: 10.1038/s41598-018-37312-0
Csernansky, J. G., Dong, H., Fagan, A. M., Wang, L., Xiong, C., Holtzman, D. M., et al. (2006). Plasma cortisol and progression of dementia in subjects with Alzheimer-type dementia. Am. J. Psychiatr. 163, 2164–2169. doi: 10.1176/ajp.2006.163.12.2164
Dahmen, B., Puetz, V. B., Scharke, W., von Polier, G. G., Herpertz-Dahlmann, B., and Konrad, K. (2018). Effects of early-life adversity on hippocampal structures and associated HPA axis functions. Dev. Neurosci. 40, 13–22. doi: 10.1159/000484238
De Bellis, M. D., Baum, A. S., Birmaher, B., Keshavan, M. S., Eccard, C. H., Boring, A. M., et al. (1999). Developmental traumatology part I: biological stress systems. Biol. Psychiatry 45, 1259–1270. doi: 10.1016/S0006-3223(99)00044-X
Demnitz-King, H., Saba, L., Lau, Y., Munns, L., Zabihi, S., Schlosser, M., et al. (2023). Association between anxiety symptoms and Alzheimer's disease biomarkers in cognitively healthy adults: a systematic review and meta-analysis. J. Psychosom. Res. 166:111159. doi: 10.1016/j.jpsychores.2023.111159
Devi, L., Alldred, M. J., Ginsberg, S. D., and Ohno, M. (2010). Sex-and brain region-specific acceleration of β-amyloidogenesis following behavioral stress in a mouse model of Alzheimer's disease. Mol. Brain 3, 1–11. doi: 10.1186/1756-6606-3-34
Donaldson, T. N., Jennings, K. T., Cherep, L. A., Blankenship, P. A., Blackwell, A. A., Yoder, R. M., et al. (2019). Progression and stop organization reveals conservation of movement organization during dark exploration across rats and mice. Behav. Process. 162, 29–38. doi: 10.1016/j.beproc.2019.01.003
Dong, H., Goico, B., Martin, M., Csernansky, C. A., Bertchume, A., and Csernansky, J. G. (2004). Modulation of hippocampal cell proliferation, memory, and amyloid plaque deposition in APPsw (Tg2576) mutant mice by isolation stress. Neuroscience 127, 601–609. doi: 10.1016/j.neuroscience.2004.05.040
Dong, H., Wang, S., Zeng, Z., Li, F., Montalvo-Ortiz, J., Tucker, C., et al. (2014). Effects of corticotrophin-releasing factor receptor 1 antagonists on amyloid-β and behavior in Tg2576 mice. Psychopharmacology 231, 4711–4722. doi: 10.1007/s00213-014-3629-8
Dong, H., Yuede, C. M., Yoo, H. S., Martin, M. V., Deal, C., Mace, A. G., et al. (2008). Corticosterone and related receptor expression are associated with increased β-amyloid plaques in isolated Tg2576 mice. Neuroscience 155, 154–163. doi: 10.1016/j.neuroscience.2008.05.017
Donner, N. C., and Lowry, C. A. (2013). Sex differences in anxiety and emotional behavior. Pflügers Archiv-Europ. J. Physiol. 465, 601–626. doi: 10.1007/s00424-013-1271-7
Du, X., and Pang, T. Y. (2015). Is dysregulation of the HPA-axis a core pathophysiology mediating co-morbid depression in neurodegenerative diseases? Front. Psychol. 6:32. doi: 10.3389/fpsyt.2015.00032
Dubois, B., Feldman, H. H., Jacova, C., Hampel, H., Molinuevo, J. L., Blennow, K., et al. (2014). Advancing research diagnostic criteria for Alzheimer's disease: the IWG-2 criteria. Lancet Neurol. 13, 614–629. doi: 10.1016/S1474-4422(14)70090-0
Duyckaerts, C., Delatour, B., and Potier, M.-C. (2009). Classification and basic pathology of Alzheimer disease. Acta Neuropathol. 118, 5–36. doi: 10.1007/s00401-009-0532-1
Elsworthy, R. J., and Aldred, S. (2019). Depression in Alzheimer’s disease: an alternative role for selective serotonin reuptake inhibitors? J. Alzheimers Dis. 69, 651–661. doi: 10.3233/JAD-180780
Eratne, D., Loi, S. M., Farrand, S., Kelso, W., Velakoulis, D., and Looi, J. C. (2018). Alzheimer’s disease: clinical update on epidemiology, pathophysiology and diagnosis. Australasian psychiatry, 26, 347–357. doi: 10.1177/1039856218762308
Escher, C. M., Sannemann, L., and Jessen, F. (2019). Stress and Alzheimer’s disease. J. Neural Transm. 126, 1155–1161. doi: 10.1007/s00702-019-01988-z
Farrell, C., Doolin, K., O’Leary, N., Jairaj, C., Roddy, D., Tozzi, L., et al. (2018). DNA methylation differences at the glucocorticoid receptor gene in depression are related to functional alterations in hypothalamic–pituitary–adrenal axis activity and to early life emotional abuse. Psychiatry Res. 265, 341–348. doi: 10.1016/j.psychres.2018.04.064
Filali, M., Lalonde, R., Theriault, P., Julien, C., Calon, F., and Planel, E. (2012). Cognitive and non-cognitive behaviors in the triple transgenic mouse model of Alzheimer’s disease expressing mutated APP, PS1, and Mapt (3xTg-AD). Behav. Brain Res. 234, 334–342. doi: 10.1016/j.bbr.2012.07.004
Fisher, J. R., Wallace, C. E., Tripoli, D. L., Sheline, Y. I., and Cirrito, J. R. (2016). Redundant Gs-coupled serotonin receptors regulate amyloid-β metabolism in vivo. Mol. Neurodegener. 11, 45–12. doi: 10.1186/s13024-016-0112-5
Fowler, C. F., Goerzen, D., Devenyi, G. A., Madularu, D., Chakravarty, M. M., and Near, J. (2022). Neurochemical and cognitive changes precede structural abnormalities in the TgF344-AD rat model. Brain Commun. 4:fcac072. doi: 10.1093/braincomms/fcac072
Goedert, M., and Spillantini, M. G. (2006). A century of Alzheimer’s disease. Science 314, 777–781. doi: 10.1126/science.1132814
Götz, J., Bodea, L.-G., and Goedert, M. (2018). Rodent models for Alzheimer disease. Nat. Rev. Neurosci. 19:10. doi: 10.1038/s41583-018-0054-8
Gray, N. A., Milak, M. S., DeLorenzo, C., Ogden, R. T., Huang, Y. Y., Mann, J. J., et al. (2013). Antidepressant treatment reduces serotonin-1A autoreceptor binding in major depressive disorder. Biol. Psychiatry 74, 26–31. doi: 10.1016/j.biopsych.2012.11.012
Green, K. N., Billings, L. M., Roozendaal, B., McGaugh, J. L., and LaFerla, F. M. (2006). Glucocorticoids increase amyloid-β and tau pathology in a mouse model of Alzheimer’s disease. J. Neurosci. 26, 9047–9056. doi: 10.1523/JNEUROSCI.2797-06.2006
Hajszan, T., MacLusky, N. J., and Leranth, C. (2005). Short-term treatment with the antidepressant fluoxetine triggers pyramidal dendritic spine synapse formation in rat hippocampus. Eur. J. Neurosci. 21, 1299–1303. doi: 10.1111/j.1460-9568.2005.03968.x
Hebda-Bauer, E. K., Simmons, T. A., Sugg, A., Ural, E., Stewart, J. A., Beals, J. L., et al. (2013). 3xTg-AD mice exhibit an activated central stress Axis during early-stage pathology. J. Alzheimer’s Dis. 33, 407–422. doi: 10.3233/JAD-2012-121438
Huang, Z., Jordan, J. D., and Zhang, Q. (2023). Early life adversity as a risk factor for cognitive impairment and Alzheimer’s disease. Transl. Neurodegen. 12, 25–18. doi: 10.1186/s40035-023-00355-z
Javonillo, D. I., Tran, K. M., Phan, J., Hingco, E., Kramár, E. A., da Cunha, C., et al. (2022). Systematic phenotyping and characterization of the 3xTg-AD mouse model of Alzheimer’s disease. Front. Neurosci. 15:785276. doi: 10.3389/fnins.2021.785276
Jin, L., Gao, L. F., Sun, D. S., Wu, H., Wang, Q., Ke, D., et al. (2017). Long-term ameliorative effects of the antidepressant fluoxetine exposure on cognitive deficits in 3× TgAD mice. Mol. Neurobiol. 54, 4160–4171. doi: 10.1007/s12035-016-9952-9
Johansson, M., Stomrud, E., Lindberg, O., Westman, E., Johansson, P. M., van Westen, D., et al. (2020). Apathy and anxiety are early markers of Alzheimer's disease. Neurobiol. Aging 85, 74–82. doi: 10.1016/j.neurobiolaging.2019.10.008
Jones, H. E., Joshi, A., Shenkin, S., and Mead, G. E. (2016). The effect of treatment with selective serotonin reuptake inhibitors in comparison to placebo in the progression of dementia: a systematic review and meta-analysis. Age Ageing 45, 448–456. doi: 10.1093/ageing/afw053
Juruena, M. F., Eror, F., Cleare, A. J., and Young, A. H. (2020). The role of early life stress in HPA axis and anxiety. Anxiety Disord. 1191, 141–153. doi: 10.1007/978-981-32-9705-0_9
Justice, N. J. (2018). The relationship between stress and Alzheimer’s disease. Neurobiol. Stress 8, 127–133. doi: 10.1016/j.ynstr.2018.04.002
Kang, J. E., Cirrito, J. R., Dong, H., Csernansky, J. G., and Holtzman, D. M. (2007). Acute stress increases interstitial fluid amyloid-β via corticotropin-releasing factor and neuronal activity. Proc. Natl. Acad. Sci. 104, 10673–10678. doi: 10.1073/pnas.0700148104
Kelberman, M. A., Anderson, C. R., Chlan, E., Rorabaugh, J. M., McCann, K. E., and Weinshenker, D. (2022). Consequences of hyperphosphorylated tau in the locus coeruleus on behavior and cognition in a rat model of Alzheimer’s disease. J. Alzheimers Dis. 86, 1037–1059. doi: 10.3233/JAD-215546
Kelberman, M. A., Rorabaugh, J. M., Anderson, C. R., Marriott, A., DePuy, S. D., Rasmussen, K., et al. (2023). Age-dependent dysregulation of locus coeruleus firing in a transgenic rat model of Alzheimer's disease. Neurobiol. Aging 125, 98–108. doi: 10.1016/j.neurobiolaging.2023.01.016
Kotrlik, J. W. K. J. W., and Williams, H. A. W. H. A. (2003). The incorporation of effect size in information technology, learning, information technology, learning, and performance research and performance research. Inf. Technol. Learn. Perform. J. 21:1.
Krell-Roesch, J., Rakusa, M., Syrjanen, J. A., van Harten, A. C., Lowe, V. J., Jack, C. R. Jr., et al. (2022). Association between CSF biomarkers of Alzheimer's disease and neuropsychiatric symptoms: Mayo Clinic study of aging. Alzheimers Dement. 19, 4498–4506. doi: 10.1002/alz.12557
Kroeze, Y., Peeters, D., Boulle, F., Van Den Hove, D. L. A., Van Bokhoven, H., Zhou, H., et al. (2015). Long-term consequences of chronic fluoxetine exposure on the expression of myelination-related genes in the rat hippocampus. Transl. Psychiatry 5:e642. doi: 10.1038/tp.2016.60
Lakens, D. (2013). Calculating and reporting effect sizes to facilitate cumulative science: a practical primer for t-tests and ANOVAs. Front. Psychol. 4:863. doi: 10.3389/fpsyg.2013.00863
Lenze, E. J., Reiersen, A. M., Zorumski, C. F., and Santosh, P. J. (2023). Beyond “psychotropic”: repurposing psychiatric drugs for COVID-19, Alzheimer’s disease, and Cancer. J. Clin. Psychiatry 84:46251. doi: 10.4088/JCP.22r14494
Lindner, M. D. (1997). Reliability, distribution, and validity of age-related cognitive deficits in the Morris water maze. Neurobiology of learning and memory, 68, 203–220. doi: 10.1006/nlme.1997.3782
Liu, R. T. (2017). Childhood adversities and depression in adulthood: current findings and future directions. Clin. Psychol. Sci. Pract. 24, 140–153. doi: 10.1111/cpsp.12190
Liu, Y., Yao, J., Song, Z., Guo, W., Sun, B., Wei, J., et al. (2021). Limiting RyR2 open time prevents Alzheimer's disease-related deficits in the 3xTG-AD mouse model. J. Neurosci. Res. 99, 2906–2921. doi: 10.1002/jnr.24936
Ma, J., Gao, Y., Jiang, L., Chao, F. L., Huang, W., Zhou, C. N., et al. (2017). Fluoxetine attenuates the impairment of spatial learning ability and prevents neuron loss in middle-aged APPswe/PSEN1dE9 double transgenic Alzheimer's disease mice. Oncotarget 8, 27676–27692. doi: 10.18632/oncotarget.15398
Machado, A., Herrera, A. J., de Pablos, R. M., Espinosa-Oliva, A. M., Sarmiento, M., Ayala, A., et al. (2014). Chronic stress as a risk factor for Alzheimer’s disease. Rev. Neurosci. 25, 785–804. doi: 10.1515/revneuro-2014-0035
Marwari, S., and Dawe, G. S. (2018). (R)-fluoxetine enhances cognitive flexibility and hippocampal cell proliferation in mice. J. Psychopharmacol. 32, 441–457. doi: 10.1177/0269881118754733
Masters, C. L., Bateman, R., Blennow, K., Rowe, C. C., Sperling, R. A., and Cummings, J. L. (2015). Alzheimer's disease. Nat. Rev. Dis. Primers 1, 1–18. doi: 10.1038/nrdp.2015.56
McCormick, C. M., and Green, M. R. (2013). From the stressed adolescent to the anxious and depressed adult: investigations in rodent models. Neuroscience 249, 242–257. doi: 10.1016/j.neuroscience.2012.08.063
Mdawar, B., Ghossoub, E., and Khoury, R. (2020). Selective serotonin reuptake inhibitors and Alzheimer’s disease. Neural Regen. Res. 15, 41–46. doi: 10.4103/1673-5374.264445
Mendez, M. F. (2021). The relationship between anxiety and Alzheimer’s disease. J. Alzheimer's Dis. Rep. 5, 171–177. doi: 10.3233/ADR-210294
Merikangas, K. R., He, J. P., Burstein, M., Swanson, S. A., Avenevoli, S., Cui, L., et al. (2010). Lifetime prevalence of mental disorders in U.S. adolescents: results from the National Comorbidity Survey Replication--Adolescent Supplement (NCS-A). J. Am. Acad. Child Adolesc. Psychiatry 49, 980–989. doi: 10.1016/j.jaac.2010.05.017
Miller, R., Plessow, F., Kirschbaum, C., and Stalder, T. (2013). Classification criteria for distinguishing cortisol responders from nonresponders to psychosocial stress: evaluation of salivary cortisol pulse detection in panel designs. Psychosom. Med. 75, 832–840. doi: 10.1097/PSY.0000000000000002
Mohammadi, S., Zandi, M., Dousti Kataj, P., and Karimi Zandi, L. (2022). Chronic stress and Alzheimer's disease. Biotechnol. Appl. Biochem. 69, 1451–1458. doi: 10.1002/bab.2216
Mowla, A., Mosavinasab, M., and Pani, A. (2007). Does fluoxetine have any effect on the cognition of patients with mild cognitive impairment?: a double-blind, placebo-controlled, clinical trial. J. Clin. Psychopharmacol. 27, 67–70. doi: 10.1097/JCP.0b013e31802e0002
Müller-Spahn, F. (2003). Behavioral disturbances in dementia. Dialogues Clin. Neurosci. 5, 49–59. doi: 10.31887/DCNS.2003.5.1/fmuellerspahn
Muntsant, A., and Giménez-Llort, L. (2021). Genotype Load modulates amyloid burden and anxiety-like patterns in male 3xTg-AD survivors despite similar neuro-Immunoendocrine, synaptic and cognitive impairments. Biomedicine 9:715. doi: 10.3390/biomedicines9070715
Nelson, R. L., Guo, Z., Halagappa, V. M., Pearson, M., Gray, A. J., Matsuoka, Y., et al. (2007). Prophylactic treatment with paroxetine ameliorates behavioral deficits and retards the development of amyloid and tau pathologies in 3xTgAD mice. Exp. Neurol. 205, 166–176. doi: 10.1016/j.expneurol.2007.01.037
Nguyen, E. T., Selmanovic, D., Maltry, M., Morano, R., Franco-Villanueva, A., Estrada, C. M., et al. (2020). Endocrine stress responsivity and social memory in 3xTg-AD female and male mice: a tale of two experiments. Horm. Behav. 126:104852. doi: 10.1016/j.yhbeh.2020.104852
Oddo, S., Caccamo, A., Shepherd, J. D., Murphy, M. P., Golde, T. E., Kayed, R., et al. (2003). Triple-transgenic model of Alzheimer’s disease with plaques and tangles: intracellular Aβ and synaptic dysfunction. Neuron 39, 409–421. doi: 10.1016/s0896-6273(03)00434-3
Pairojana, T., Phasuk, S., Suresh, P., Huang, S.-P., Pakaprot, N., Chompoopong, S., et al. (2021). Age and gender differences for the behavioral phenotypes of 3xTg alzheimer’s disease mice. Brain Res. 1762:147437. doi: 10.1016/j.brainres.2021.147437
Paterson, T. A., Harms, P. D., Steel, P., and Credé, M. (2016). An assessment of the magnitude of effect sizes: evidence from 30 years of meta-analysis in management. J. Leadersh. Org. Stud. 23, 66–81. doi: 10.1177/1548051815614321
Pentkowski, N. S., Berkowitz, L. E., Thompson, S. M., Drake, E. N., Olguin, C. R., and Clark, B. J. (2018). Anxiety-like behavior as an early endophenotype in the TgF344-AD rat model of Alzheimer's disease. Neurobiol. Aging 61, 169–176. doi: 10.1016/j.neurobiolaging.2017.09.024
Pentkowski, N. S., Bouquin, S. J., Maestas-Olguin, C. R., Villasenor, Z. M., and Clark, B. J. (2022). Differential effects of chronic stress on anxiety-like behavior and contextual fear conditioning in the TgF344-AD rat model of Alzheimer’s disease. Behav. Brain Res. 418:113661. doi: 10.1016/j.bbr.2021.113661
Pentkowski, N. S., Rogge-Obando, K. K., Donaldson, T. N., Bouquin, S. J., and Clark, B. J. (2021). Anxiety and Alzheimer’s disease: behavioral analysis and neural basis in rodent models of Alzheimer’s-related neuropathology. Neurosci. Biobehav. Rev. 127, 647–658. doi: 10.1016/j.neubiorev.2021.05.005
Plotsky, P. M., Owens, M. J., and Nemeroff, C. B. (1998). Psychoneuroendocrinology of depression: hypothalamic-pituitary-adrenal axis. Psychiatr. Clin. N. Am. 21, 293–307. doi: 10.1016/S0193-953X(05)70006-X
Poe, G. R., Foote, S., Eschenko, O., Johansen, J. P., Bouret, S., Aston-Jones, G., et al. (2020). Locus coeruleus: a new look at the blue spot. Nat. Rev. Neurosci. 21, 644–659. doi: 10.1038/s41583-020-0360-9
Popovic, D., Vieta, E., Fornaro, M., and Perugi, G. (2015). Cognitive tolerability following successful long term treatment of major depression and anxiety disorders with SSRi antidepressants. J. Affect. Disord. 173, 211–215. doi: 10.1016/j.jad.2014.11.008
Rasmussen, J., and Langerman, H. (2019). Alzheimer’s disease–why we need early diagnosis. Degen. Neurol. Neuromuscular Dis. 9, 123–130. doi: 10.2147/DNND.S228939
Reddy, A. P., Yin, X., Sawant, N., and Reddy, P. H. (2021). Protective effects of antidepressant citalopram against abnormal APP processing and amyloid beta-induced mitochondrial dynamics, biogenesis, mitophagy and synaptic toxicities in Alzheimer’s disease. Hum. Mol. Genet. 30, 847–864. doi: 10.1093/hmg/ddab054
Remy, S., Tesson, L., Menoret, S., Usal, C., De Cian, A., Thepenier, V., et al. (2014). Efficient gene targeting by homology-directed repair in rat zygotes using TALE nucleases. Genome Res. 24, 1371–1383. doi: 10.1101/gr.171538.113
Rissman, R. A., Staup, M. A., Lee, A. R., Justice, N. J., Rice, K. C., Vale, W., et al. (2012). Corticotropin-releasing factor receptor-dependent effects of repeated stress on tau phosphorylation, solubility, and aggregation. Proc. Natl. Acad. Sci. 109, 6277–6282. doi: 10.1073/pnas.1203140109
Rothman, S. M., Herdener, N., Camandola, S., Texel, S. J., Mughal, M. R., Cong, W. N., et al. (2012). 3xTgAD mice exhibit altered behavior and elevated Aβ after chronic mild social stress. Neurobiol. Aging 33, 830.e1–830.e12. doi: 10.1016/j.neurobiolaging.2011.07.005
Santabárbara, J., Lipnicki, D. M., Bueno-Notivol, J., Olaya-Guzmán, B., Villagrasa, B., and López-Antón, R. (2020). Updating the evidence for an association between anxiety and risk of Alzheimer's disease: a meta-analysis of prospective cohort studies. J. Affect. Disord. 262, 397–404. doi: 10.1016/j.jad.2019.11.065
Santabárbara, J., Lipnicki, D. M., Villagrasa, B., Lobo, E., and Lopez-Anton, R. (2019). Anxiety and risk of dementia: systematic review and meta-analysis of prospective cohort studies. Maturitas 119, 14–20. doi: 10.1016/j.maturitas.2018.10.014
Santarelli, L., Saxe, M., Gross, C., Surget, A., Battaglia, F., Dulawa, S., et al. (2003). Requirement of hippocampal neurogenesis for the behavioral effects of antidepressants. Science 301, 805–809. doi: 10.1126/science.1083328
Saré, R. M., Cooke, S. K., Krych, L., Zerfas, P. M., Cohen, R. M., and Smith, C. B. (2020). Behavioral phenotype in the TgF344-AD rat model of Alzheimer’s disease. Front. Neurosci. 14:601. doi: 10.3389/fnins.2020.00601
Schmid, N. S., Taylor, K. I., Foldi, N. S., Berres, M., and Monsch, A. U. (2013). Neuropsychological signs of Alzheimer's disease 8 years prior to diagnosis. J. Alzheimers Dis. 34, 537–546. doi: 10.3233/JAD-121234
Seibenhener, M. L., and Wooten, M. C. (2015). Use of the open field maze to measure locomotor and anxiety-like behavior in mice. J. Visual. Exp. 96, 4–5. doi: 10.3791/52434
Severino, M., Sivasaravanaparan, M., Olesen, L. Ø., von Linstow, C. U., Metaxas, A., Bouzinova, E. V., et al. (2018). Established amyloid-β pathology is unaffected by chronic treatment with the selective serotonin reuptake inhibitor paroxetine. Alzheimer's Dement. 4, 215–223. doi: 10.1016/j.trci.2018.04.005
Sivasaravanaparan, M., Olesen, L. Ø., Severino, M., von Linstow, C. U., Lambertsen, K. L., Gramsbergen, J. B., et al. (2022). Efficacy of chronic paroxetine treatment in mitigating amyloid pathology and microgliosis in APP SWE/PS1 ΔE9 transgenic mice. J. Alzheimers Dis. 87, 685–699. doi: 10.3233/JAD-220019
Sperling, R. A., Aisen, P. S., Beckett, L. A., Bennett, D. A., Craft, S., Fagan, A. M., et al. (2011). Toward defining the preclinical stages of Alzheimer’s disease: recommendations from the National Institute on Aging-Alzheimer’s association workgroups on diagnostic guidelines for Alzheimer’s disease. Alzheimers Dement. 7, 280–292. doi: 10.1016/j.jalz.2011.03.003
Spiers, J. G., Chen, H. J. C., Sernia, C., and Lavidis, N. A. (2015). Activation of the hypothalamic-pituitary-adrenal stress axis induces cellular oxidative stress. Front. Neurosci. 8:456. doi: 10.3389/fnins.2014.00456
Srivareerat, M., Tran, T. T., Alzoubi, K. H., and Alkadhi, K. A. (2009). Chronic psychosocial stress exacerbates impairment of cognition and long-term potentiation in β-amyloid rat model of Alzheimer's disease. Biol. Psychiatry 65, 918–926. doi: 10.1016/j.biopsych.2008.08.021
Srivastava, H., Lasher, A. T., Nagarajan, A., and Sun, L. Y. (2023). Sexual dimorphism in the peripheral metabolic homeostasis and behavior in the TgF344-AD rat model of Alzheimer's disease. Aging Cell 22:e13854. doi: 10.1111/acel.13854
Sterniczuk, R., Antle, M. C., LaFerla, F. M., and Dyck, R. H. (2010). Characterization of the 3xTg-AD mouse model of Alzheimer’s disease: part 2. Behavioral and cognitive changes. Brain Res. 1348, 149–155. doi: 10.1016/j.brainres.2010.06.011
Tafet, G. E., and Nemeroff, C. B. (2020). Pharmacological treatment of anxiety disorders: the role of the HPA axis. Front. Psychol. 11:443. doi: 10.3389/fpsyt.2020.00443
Tchekalarova, J., and Tzoneva, R. (2023). Oxidative stress and aging as risk factors for Alzheimer’s disease and Parkinson’s disease: the role of the antioxidant melatonin. Int. J. Mol. Sci. 24, 1–9. doi: 10.3390/ijms24033022
Thompson, S. M., Berkowitz, L. E., and Clark, B. J. (2018). Behavioral and neural subsystems of rodent exploration. Learn. Motiv. 61, 3–15. doi: 10.1016/j.lmot.2017.03.009
Torres-Lista, V., López-Pousa, S., and Giménez-Llort, L. (2015). Marble-burying is enhanced in 3xTg-AD mice, can be reversed by risperidone and it is modulable by handling. Behav. Process. 116, 69–74. doi: 10.1016/j.beproc.2015.05.001
Torrisi, S. A., Geraci, F., Tropea, M. R., Grasso, M., Caruso, G., Fidilio, A., et al. (2019). Fluoxetine and vortioxetine reverse depressive-like phenotype and memory deficits induced by Aβ1-42 oligomers in mice: a key role of transforming growth factor-β1. Front. Pharmacol. 10:693. doi: 10.3389/fphar.2019.00693
Tournier, B. B., Barca, C., Fall, A. B., Gloria, Y., Meyer, L., Ceyzériat, K., et al. (2021). Spatial reference learning deficits in absence of dysfunctional working memory in the TgF344-AD rat model of Alzheimer's disease. Genes Brain Behav. 20:e12712. doi: 10.1111/gbb.12712
Vale, W., Spiess, J., Rivier, C., and Rivier, J. (1981). Characterization of a 41-residue ovine hypothalamic peptide that stimulates secretion of corticotropin and β-endorphin. Science 213, 1394–1397. doi: 10.1126/science.6267699
Van Riel, E., Van Gemert, N. G., Meijer, O. C., and Joëls, M. (2004). Effect of early life stress on serotonin responses in the hippocampus of young adult rats. Synapse 53, 11–19. doi: 10.1002/syn.20033
Várkonyi, D., Török, B., Sipos, E., Fazekas, C. L., Bánrévi, K., Correia, P., et al. (2022). Investigation of anxiety- and depressive-like symptoms in 4- and 8-month-old male triple transgenic mouse models of Alzheimer’s disease. Int. J. Mol. Sci. 23, 2–14. doi: 10.3390/ijms231810816
Wang, W., Liu, X., Huang, J., Zeng, S., Bao, J., Lu, Y., et al. (2022). The connection of early life adversity and post-traumatic stress disorder: an updated review. Discov. Med. 34, 25–32.
Wang, Y. C., Tai, P. A., Poly, T. N., Islam, M. M., Yang, H. C., Wu, C. C., et al. (2018). Increased risk of dementia in patients with antidepressants: a meta-analysis of observational studies. Behav. Neurol. 2018, 1–8. doi: 10.1155/2018/5315098
Wang, J., Zhang, Y., Xu, H., Zhu, S., Wang, H., He, J., et al. (2014). Fluoxetine improves behavioral performance by suppressing the production of soluble β-amyloid in APP/PS1 mice. Curr. Alzheimer Res. 11, 672–680. doi: 10.2174/1567205011666140812114715
Whishaw, I. Q., Gharbawie, O. A., Clark, B. J., and Lehmann, H. (2006). The exploratory behavior of rats in an open environment optimizes security. Behav. Brain Res. 171, 230–239. doi: 10.1016/j.bbr.2006.03.037
Whishaw, I. Q., and Tomie, J. A. (1996). Of mice and mazes: similarities between mice and rats on dry land but not water mazes. Physiol. Behav. 60, 1191–1197. doi: 10.1016/S0031-9384(96)00176-X
Wu, C., Yang, L., Li, Y., Dong, Y. A. N., Yang, B., Tucker, L. D., et al. (2020). Effects of exercise training on anxious–depressive-like behavior in Alzheimer rat. Med. Sci. Sports Exerc. 52, 1456–1469. doi: 10.1249/MSS.0000000000002294
Yan, Y., Dominguez, S., Fisher, D. W., and Dong, H. (2018). Sex differences in chronic stress responses and Alzheimer's disease. Neurobiol. Stress 8, 120–126. doi: 10.1016/j.ynstr.2018.03.002
Yohn, C. N., Gergues, M. M., and Samuels, B. A. (2017). The role of 5-HT receptors in depression. Mol. Brain 10, 28–12. doi: 10.1186/s13041-017-0306-y
Zhang, C., Kuo, C. C., Moghadam, S. H., Monte, L., Campbell, S. N., Rice, K. C., et al. (2016). Corticotropin-releasing factor receptor-1 antagonism mitigates beta amyloid pathology and cognitive and synaptic deficits in a mouse model of Alzheimer's disease. Alzheimers Dement. 12, 527–537. doi: 10.1016/j.jalz.2015.09.007
Zhang, C., and Rissman, R. A. (2017). Corticotropin-releasing factor receptor-1 modulates biomarkers of DNA oxidation in Alzheimer’s disease mice. PLoS One 12:e0181367. doi: 10.1371/journal.pone.0181367
Zhang, Y. L., Xing, R. Z., Luo, X. B., Xu, H., Chang, R. C., Zou, L. Y., et al. (2016). Anxiety-like behavior and dysregulation of miR-34a in triple transgenic mice of Alzheimer’s disease. Eur. Rev. Med. Pharmacol. Sci. 20, 2853–2862.
Zhao, L. H. (2020). Alzheimer’s disease facts and figures. Alzheimers Dement. 16, 391–460. doi: 10.1002/alz.12068
Keywords: Alzheimer’s disease, corticotropin releasing factor, serotonin, stress, HPA Axis
Citation: Reyna NC, Clark BJ, Hamilton DA and Pentkowski NS (2023) Anxiety and Alzheimer’s disease pathogenesis: focus on 5-HT and CRF systems in 3xTg-AD and TgF344-AD animal models. Front. Aging Neurosci. 15:1251075. doi: 10.3389/fnagi.2023.1251075
Received: 30 June 2023; Accepted: 25 October 2023;
Published: 10 November 2023.
Edited by:
Caesar Miguel Hernandez, University of Alabama at Birmingham, United StatesReviewed by:
Andrew Arrant, University of Alabama at Birmingham, United StatesCopyright © 2023 Reyna, Clark, Hamilton and Pentkowski. This is an open-access article distributed under the terms of the Creative Commons Attribution License (CC BY). The use, distribution or reproduction in other forums is permitted, provided the original author(s) and the copyright owner(s) are credited and that the original publication in this journal is cited, in accordance with accepted academic practice. No use, distribution or reproduction is permitted which does not comply with these terms.
*Correspondence: Nicole C. Reyna, bnJleW5hQHVubS5lZHU=
Disclaimer: All claims expressed in this article are solely those of the authors and do not necessarily represent those of their affiliated organizations, or those of the publisher, the editors and the reviewers. Any product that may be evaluated in this article or claim that may be made by its manufacturer is not guaranteed or endorsed by the publisher.
Research integrity at Frontiers
Learn more about the work of our research integrity team to safeguard the quality of each article we publish.