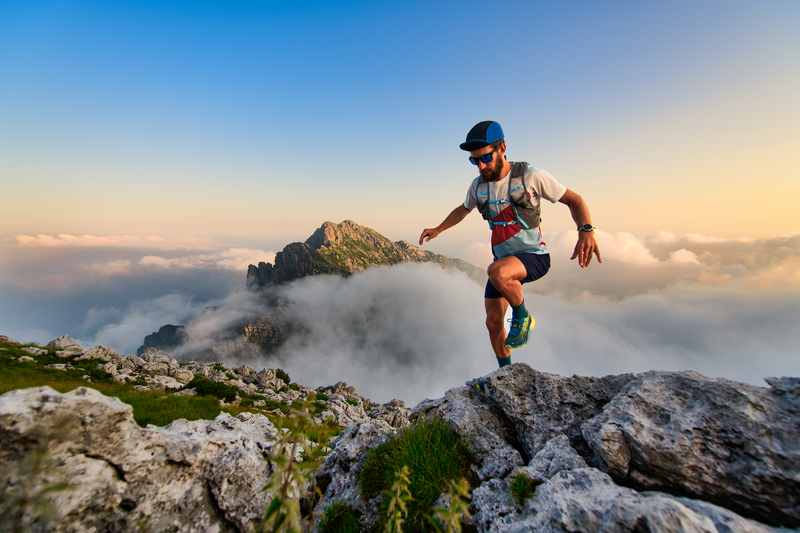
95% of researchers rate our articles as excellent or good
Learn more about the work of our research integrity team to safeguard the quality of each article we publish.
Find out more
REVIEW article
Front. Aging Neurosci. , 06 July 2023
Sec. Neuroinflammation and Neuropathy
Volume 15 - 2023 | https://doi.org/10.3389/fnagi.2023.1173987
Vagus nerve stimulation (VNS) is a technology that provides electrical stimulation to the cervical vagus nerve and can be applied in the treatment of a wide variety of neuropsychiatric and systemic diseases. VNS exerts its effect by stimulating vagal afferent and efferent fibers, which project upward to the brainstem nuclei and the relayed circuits and downward to the internal organs to influence the autonomic, neuroendocrine, and neuroimmunology systems. The neuroimmunomodulation effect of VNS is mediated through the cholinergic anti-inflammatory pathway that regulates immune cells and decreases pro-inflammatory cytokines. Traditional and non-invasive VNS have Food and Drug Administration (FDA)-approved indications for patients with drug-refractory epilepsy, treatment-refractory major depressive disorders, and headaches. The number of clinical trials and translational studies that explore the therapeutic potentials and mechanisms of VNS is increasing. In this review, we first introduced the anatomical and physiological bases of the vagus nerve and the immunomodulating functions of VNS. We covered studies that investigated the mechanisms of VNS and its therapeutic implications for a spectrum of brain disorders and systemic diseases in the context of neuroimmunomodulation.
Vagus nerve stimulation (VNS) is a modern technique that delivers electrical stimulation to the vagus nerve. Traditional VNS is the earliest model of VNS in which the stimulation electrode cuff is invasively implanted at the left cervical vagus nerve, and the electrical generator is embedded in the subcutaneous space of the left anterior chest (Table 1). Traditional VNS is Food and Drug Administration (FDA) approved as an adjunctive therapy for patients with drug-resistant epilepsy (DRE) and treatment-resistant depression (Ben-Menachem, 2002; Young et al., 2020). Non-invasive VNS (nVNS) has been developed to extracorporeally deliver electrical stimulation to the vagus nerve and includes transcutaneous or percutaneous delivery to the cervical or auricular vagus nerve branches, which has been applied in human and animal studies for the treatment of a variety of diseases (Butt et al., 2020; Hilz, 2022) (Table 1). The FDA has approved transcutaneous cervical VNS (tcVNS) for refractory migraine and cluster headache (CH) (Silberstein et al., 2016a). Currently, VNS is an emerging technique being investigated in clinical trials and experimental studies to explore its underlying mechanisms and therapeutic effects for a broad spectrum of general medical disorders beyond neuropsychiatric disorders (Downes et al., 2023). The mechanism of action of VNS is not fully understood (Henry, 2002; Wang Y. et al., 2021). It is believed to be mediated through the activation of vagal afferents and efferents projecting upward to the brain and downward to internal organs. The vagal afferents signal the activation of brainstem nuclei and the relayed cortical projections, leading to multiple effects on brain activities, neurotransmitters, and hypothalamic–pituitary–adrenal (HPA) axis-related endocrine functions. The vagal efferent fibers are widely distributed in internal organs, and transmit the communication of the nervous system with the immune system to modulate the neuroendocrine and neuroimmunology, mainly through the cholinergic anti-inflammatory pathway (CAP) (Manta et al., 2012; Thrivikraman et al., 2013; Tarn et al., 2019). CAP-mediated neuroimmunomodulation underlies the therapeutic effects of VNS. The modulations of neural circuit, neuroendocrine, and neuroimmune responses are thought to be underpinning the VNS therapeutic effects (Kelly et al., 2022). In this review, we introduced the anatomical and physiological bases of vagus nerve associated with immune systems, the immunomodulation by VNS, and its therapeutic implications.
The vagus nerve is the longest cranial nerve and is the 10th cranial nerve. It functions in bidirectional communication between the brain and internal organs and is involved in regulating the homeostasis of autonomic systems. The vagus nerve primarily comprises unmyelinated sensory afferent fibers, accounting for 80–90% of the nerve fiber, with the remaining 10–20% being myelinated efferent fibers (Foley and Dubois, 1937; Asala and Bower, 1986; Dolphin et al., 2022; Prescott and Liberles, 2022). Most known actions of the vagus nerve depend on the vagal efferent innervations that are distributed from the neck to the heart, lung, spleen, and gastrointestinal (GI) organs (Wang and Powley, 2007). The vagus efferent fibers originate from the nucleus ambiguous (NAm) and dorsal motor nucleus of the vagus nerve (DMV), both located in the medulla. Vagal somatic motor fibers arise from the NAm and terminate at the laryngeal and pharyngeal muscles that control the soft palate and function in speech and swallowing. Meanwhile, the NAm contributes to preganglionic parasympathetic fibers of the vagal cardiac branches that innervate the postganglionic parasympathetic neurons to decrease the heart rate (Machado and Brody, 1988). The DMV gives rise to the preganglionic parasympathetic fibers that are distributed throughout the lung and GI tract. These fibers primarily innervate the postganglionic neurons located in the myenteric plexuses of the muscularis propria and, to a lesser extent, the submucosal plexus and function in muscular contraction and glandular secretion (Berthoud et al., 1990). The majority of the vagus nerve fibers are sensory afferent fibers that transmit sensory information from internal organs to the central nervous system. The vagal afferent signals arise from the tension receptors of the stomach and esophagus, chemoreceptors and mechanoreceptors from the GI mucosa (Cirillo et al., 2022). And sensory inputs from the liver and pancreas project to the nucleus tractus solitarii (NTS) via the vagal nodose ganglion, with secondary projections to the raphe nucleus, locus coeruleus (LC), thalamus, hypothalamus, amygdala, and cerebral cortex (Nemeroff et al., 2006; Decarie-Spain et al., 2023). The LC is a crucial structure for norepinephrine release (Berger et al., 2021) and the raphe nucleus for widespread serotonergic innervations (Hornung, 2003). Therefore, the projections of the vagus nerve to the LC and raphe nucleus regulate the neurotransmitters norepinephrine and serotonin, respectively. The ascending bilateral vago-solitario-parabrachial pathways involve the central autonomic, reticular activating, and limbic systems. The ascending unilateral vago-trigemino-thalamocortical pathways project to the somatosensory system (Henry, 2002). Therefore, the vagal afferent input can activate several brainstem nuclei and circuits to affect multiple neuropsychological functions. The vagal afferent and efferent fibers function as the parasympathetic autonomic nervous system and bridge the communication between the central, cardiopulmonary, and enteric nervous systems. The vagus nerve allows bidirectional modulations between the brain and autonomic systems to influence cognition, emotion, enteroendocrine, and immunomodulation functions (Carabotti et al., 2015).
The vagal efferent route gives rise to the CAP, mediated through the release of acetylcholine (ACh) (Figure 1). ACh binding to the α-7-nicotinic ACh receptors (α7nAChRs) on macrophages inhibits tumor necrosis factor (TNF)-α synthesis and secretion and suppresses inflammation (Bonaz et al., 2016). The vagal efferent route synapses with the celiac ganglion, which relays the signal to T cells. As these T cells enter the spleen, they release ACh, which binds on the α7nAChR on the splenic sympathetic terminal nerves to release noradrenaline signaling to splenic macrophages to decrease the release of TNF-α (Martelli et al., 2014). Macrophages also respond to ACh when it binds to the α7nAChR, where ACh inhibits the macrophage TNF synthesis and reduces the levels of the inflammatory cytokine (Wang et al., 2003). The GI tract receives various antigens via food and liquid ingestion. The vagus nerve functions as the bridge for the brain–gut axis and has the primary role of immunomodulation in the GI tract. The vagal CAP synapses directly on the enteric neurons and modulates macrophages to reduce TNF-α synthesis (Berthoud and Neuhuber, 2019). The immunomodulation by the vagal afferent route is mediated through the HPA axis (Figure 1). Environmental stress and systemic pro-inflammatory cytokines can trigger the release of corticotropin-releasing factor (CRF) from the hypothalamus. CRF further stimulates the release of adrenocorticotropic hormone (ACTH) from the pituitary. After stimulation with ACTH, the adrenal medulla exerts the immunomodulating function by secreting cortisol, which acts on multiple systems, including the brain, bones, muscles, and body fat, as a response to stress (Mayer et al., 2014).
Figure 1. Proposed neuroimmunomodulation by vagus nerve stimulation. VNS can activate vagal afferent fibers projecting to the NTS, which relays to the PVN in the hypothalamus and DMV. The DMV transmits downward signals via vagal efferent fibers. The PVN activates the HPA axis, releasing glucocorticoids from the adrenal glands to exert an anti-inflammatory effect (Berthoud and Neuhuber, 2019). The sympathetic preganglionic neurons project to the splenic nerve. The splenic nerve sympathetic terminals can be modulated by ACh via the vagus-to-spleen circuit. In addition, the activation of ACh-synthesizing T cells can stimulate the sympathetic terminal of the splenic nerve to release NA and suppress macrophages to produce TNF-α (Martelli et al., 2014). Vagal efferent fibers in the gut innervate the postganglionic enteric neurons and suppress macrophage TNF-α production, via alpha-7 nicotinic receptors (Bonaz et al., 2017). The red arrow line indicates the vagus nerve pathway and regulation, whereas the blue line indicates the regulation by the sympathetic pathway. A, adrenaline; ACh, acetylcholine; ACTH, adrenocorticotrophic hormone; CRH, corticotrophin-releasing hormone; DMV, dorsal motor nucleus of the vagus; GR, glucocorticoid receptor; GI, gastrointestinal; HPA, hypothalamic–pituitary–adrenal; NA, noradrenaline; NTS, nucleus tractus solitarius; PVN, paraventricular nucleus at the hypothalamus; TNF-α, tumor necrosis factor-α; VNS, vagus nerve stimulation; α7nAChR, α-7-nicotinic ACh receptors; αAR, alpha-adrenergic receptor; βAR, beta-adrenergic receptor.
The vagus nerve has immunomodulatory functions through vagal efferent fibers mediated by the CAP and vagal afferent circuits mediated by the HPA axis. Therefore, stimulating the vagus nerve using VNS enhances vagus nerve-mediated anti-inflammatory effects and is applied in the treatment of multiple diseases with underlying inflammatory etiologies (Kelly et al., 2022). Typically, VNS exerts its immunomodulating effects via the CAP to reduce pro-inflammatory cytokines, activate microglia and macrophages, and alter the consequences of neuroinflammation (Figure 1). The activated vagus nerve by VNS increases the release of ACh, which binds to the α7nAChR in cytokine-producing immune cells and decreases the production of inflammatory cytokines. Wang et al. found that TNF synthesis was inhibited in wild-type mice treated with VNS; however, this inhibition was diminished in α7-deficient mice, suggesting that the anti-inflammatory action of VNS is mediated via ACh and the α7nAChR (Wang et al., 2003). In an ovine fetus with umbilical cord occlusion-induced ischemic injury, heart rate variability (HRV), which reflects vagus nerve activity, was positively correlated with plasma interleukin (IL)-1β levels and negatively correlated with white matter microglia cell counts. High-mobility group box protein 1 (HMGB1) is a DNA-binding protein, and its translocation functions to promote pro-inflammatory cytokines and induce microglial activation. The hypoxic insult increases HMGB1 translocation in α7nAChR+ microglia in a brain region-dependent manner. Higher HRV values are correlated with lower HMGB1 translocation and higher α7nAChR intensity in a brain region-specific manner, suggesting the CAP-mediated anti-inflammatory role of the vagus nerve in early perinatal hypoxic brain injury (Frasch et al., 2016). Stimulating the vagus nerve using VNS significantly reduces the levels of pro-inflammatory cytokines (IL-1β, IL-6, and TNF-α) and the percentage of CD11b+CD45low microglia and CD11b+/CD45high macrophages in the brain. In mice with lipopolysaccharide-induced inflammation treated with VNS, a significantly reduced level of ionized calcium-binding adapter molecule 1 (Iba-1) expression, implying a reduction in activated microglia, was observed following VNS in the brain under an inflammatory context (Meneses et al., 2016). In an endotoxemic rat model, Mihaylova et al. demonstrated that VNS decreased the plasma levels of interleukin (IL)-6, tumor necrosis factor (TNF)-α, interferon (IFN)-γ, IL-10, and cerebral hypoxia-inducible factor (HIF)-2α expression following sepsis and stabilized hemodynamic responses in the animals with sepsis (Mihaylova et al., 2012). Caravaca et al. reported the effect of VNS on lipid metabolomes during inflammation. In mice with iatrogenic peritonitis, the duration of inflammation resolution was significantly reduced, and efferocytosis was significantly increased in the mice treated with VNS. In supernatants of peritoneal exudates, IL-6 and IL-1β levels were significantly lower in VNS-treated mice. Mice treated with VNS had higher levels of specialized pro-resolving mediators (SPMs), particularly from the omega-3 docosahexaenoic (DHA) and n-3 docosapentaenoic acid (DPA) metabolomes, in the peritoneal exudates. VNS also shifted the ratio between pro-inflammatory and pro-resolving lipid mediators toward a pro-resolving profile. This effect was absent in mice deficient in 12/15-lipoxygenase (Alox15), a key enzyme in SPM biosynthesis. The significant VNS-mediated reduction of neutrophil numbers in peritoneal exudates was diminished in mice deficient in α7nAChR. These findings support the notion that the anti-inflammatory effect induced by VNS in peritonitis is likely mediated via α7nAChR and involves Alox15 (Caravaca et al., 2022). The HPA axis can be affected by VNS via vagal afferent pathways (Hosoi et al., 2000; Thrivikraman et al., 2013; Bonaz et al., 2021). A few in vivo evidence suggest the causal relationship between HPA axis regulation and VNS neuromodulation. The CRF-induced ACTH serum concentrations were decreased in normal rats treated with VNS (Chen et al., 2021). Similarly, a reduction of the over-elevated ACTH responses under CRF challenge tests was reported in patients with chronic depression following VNS (O'keane et al., 2005). Increased serum corticosterone levels, neuronal activation measured by c-Fos at NTS, and reduced food intake with body weight loss were observed in rats fed with high-fat diet following VNS treatment (De Herdt et al., 2009; Thrivikraman et al., 2013). The changes in CRF-induced ACTH responses and serum corticosterone support the notion that VNS modulates the neuroendocrine functions via the HPA axis.
VNS was applied in variable animal models of neuropsychiatric disorders to examine whether it can ameliorate the disorders through its immunomodulatory effects (Table 2).
Farrand et al. demonstrated that VNS significantly improved locomotion and increased the expression of tyrosine hydroxylase (TH) in the striatum, substantia nigra (SN), and LC in an animal model of Parkinson's disease induced by bilateral intrastriatal injection of dopaminergic neurotoxin 6-hydroxydopamine (6-OHDA). VNS decreased α-synuclein expression in the SN and glial markers in the SN and LC of lesioned rats. Furthermore, higher LC TH and lower SN Iba-1 were observed following VNS. Furthermore, they discovered that higher VNS frequencies, specifically microburst VNS, provided greater improvements in motor function, attenuation of TH-positive cell loss in the SN and LC, and norepinephrine concentration in the prefrontal cortex. Additionally, higher VNS frequencies resulted in lower intrastromal α-synuclein accumulation and glial density in the SN (Farrand et al., 2017, 2020).
Tang et al. showed that VNS inhibited pyroptosis following a cerebral ischemic/reperfusion injury through the CAP, mediated by α7nAChR. The VNS-treated group showed decreased expressions of the nucleotide-binding domain, leucine-rich-containing family, pyrin domain-containing-3 (NLRP3), and its inflammasome, cleaved caspase-1, and N-terminal fragment of gasdermin D (GSDMD-N), which would cause cell membrane rupture and release of inflammatory cytokines. The apoptosis-associated speck-like protein containing a caspase-recruitment domain (ASC), the number of pyroptotic cells, and membrane pores were also decreased in VNS-treated animals. Furthermore, VNS downregulated IL-1β and IL-18 in brain tissues. Administration of an α7nAChR-agonist mimicked the VNS effects in terms of the improvement of neurological deficits, reduction of infarct volumes, and inhibition of neuronal pyroptosis after cerebral ischemia/reperfusion injury. The neuroprotection provided by VNS could be reversed by the administration of an α7nAChR antagonist (Tang et al., 2022).
Cerebral microinfarction (CMI) was aggravated by colitis in mice with CMI treated with dextran sodium sulfate (DSS). In mice with CMI and colitis, VNS significantly reversed the increases in blood–brain barrier permeability, infarct volume, activation of Iba-1+ microglia and GFAP+ astrocytes, and TNF-α level in mice with colitis and CMI. Brain lesions in mice with colitis and CMI were significantly ameliorated when VNS was performed during acute colitis (Chen et al., 2018). VNS attenuated the systemic inflammatory response to endotoxins and intestinal inflammation in the animal model of inflammatory bowel disease (IBD). In animals with chronic colitis and IBD, VNS resulted in a reduction of inflammatory markers and an improvement in colitis symptoms (Sinniger et al., 2016; Breit et al., 2018; Bonaz et al., 2021).
In rats with chronic stress, the levels of TNF-α, IL-1β, and IL-6 in the gastric and hippocampal tissues significantly decreased by VNS. After VNS treatment, Iba-1-labeled microglial cells in the hippocampus also showed changes in morphological features from activated inflammatory cells to normal shapes. VNS increased hippocampal expressions of α7nAChR, and pharmacological blockade of α7nAChR increased the production of TNF-α, IL-1β, and IL-6 and suppressed cholinergic anti-inflammatory activities mediated by VNS. Chronic VNS downregulated hippocampal expression of the active form of caspase 3 and 5-HT1A receptors and decreased the levels of TNF-α, IL-1β, and IL-6 in the gastric and hippocampal tissues. Furthermore, pain sensitivity and depressive-like behaviors were improved by chronic VNS (Namgung et al., 2022).
The Gulf War illness comprises multiple symptoms, such as cognitive, behavioral, and emotional deficits, all of which were often reported by the troops in the Gulf War. A chemical-induced animal model analogous to the Gulf War illness showed an impaired lower nociceptive threshold. VNS restored the impaired nociceptive threshold, relieved anxiety, and improved cognitive deficits in tasks involving object location and pattern separation. VNS significantly reduced neuroinflammation and astrocyte number in the hippocampal CA1, CA3, and dentate gyrus hilus. Meanwhile, VNS improved neurogenesis, with increased doublecortin-expressing cells in the hippocampal dentate gyrus compared with the control group (Nizamutdinov et al., 2018; Venkatasamy et al., 2021; Iannucci et al., 2022).
Recent human studies have shown inconclusive results on the immunomodulatory effects of VNS in patients with DRE. In a pilot study of five pediatric patients with DRE implanted VNS, the variations of IgA, IgE, IgG, CD19, and pentraxin-3 (PTX-3) displayed a tendency toward a positive correlation between pre-surgery and 6 weeks after VNS. IL-1β and PTX-3 levels tended to decrease more in patients with >80–100% seizure reduction, whereas TNF-α levels decreased in patients with 60–79% seizure reduction and slightly increased in patients with >80–100% seizure reduction at 6 weeks after VNS. However, these results did not reach statistical significance, which may require a larger sample size (Baro et al., 2022). Another postmortem study recruited four patients with VNS and four without VNS as controls. Three of the four VNS-treated patients and all four control patients without VNS died from a sudden unexpected death in epilepsy (SUDEP). There was no laterality difference in the expressions of neuronal nuclear protein, glial fibrillary acidic protein, human leukocyte antigen (HLA)-DR isotype, and Iba-1 in the LC and NTS of VNS-treated patients. Similarly, there was no difference in the rostral pontine group of the raphe nuclei, LC, and NTS between the VNS-treated and non–VNS-treated groups (Ding et al., 2021). Kaur et al. analyzed the gene expression in peripheral blood mononuclear cells in patients with DRE treated with VNS compared with the non–VNS-treated groups. They reported that genes related to stress, inflammatory response, and immunity were downregulated in VNS-treated patients, suggesting an anti-inflammatory effect of VNS for patients with epilepsy (Kaur et al., 2023).
In a rat model of spinal cord injury (SCI), the VNS-treated group exhibited better functional recovery, with reduced glial and fibrotic scar formation and tissue damage. The beneficial effects of VNS were diminished after α7nAChR blockade. VNS inhibited the pro-inflammatory cytokines TNF-α, IL-1β, and IL-6 and increased the expression of IL-10 in the injured spinal cord. In Iba-1-stained microglia, several protein markers can recognize its pro-inflammatory M1 and anti-inflammatory M2 polarization phenotypes, e.g., CD16, CD32, CD40, and CD86 for M1 whereas CD206 and CD163 for M2 (Jurga et al., 2020). VNS promoted the shift of M1-polarized Iba-1+/CD86+ microglia to M2-polarized Iba-1+/CD206+ microglia via upregulating α7nAChR to alleviate the neuroinflammation after SCI (Chen et al., 2022).
Rats receiving chemotherapy with paclitaxel exhibited a decrease in heat and mechanical pain thresholds. The pain threshold in the chemotherapy-treated rats significantly increased on day 1 post-VNS but not on day 7 post-VNS. IL-10 level was elevated in the dorsal root ganglia of rats treated with VNS compared with those treated with sham treatment, whereas no apparent changes in nuclear factor kappa B (NF-κB) or TNF-α levels were observed (Zhang et al., 2020).
Neuroinflammation-related cognitive decline: VNS can be delivered using a percutaneous echo-guided needle inserted into the carotid sheath, termed percutaneous cervical VNS. Percutaneous cervical VNS decreased the heart rate and increased c-Fos and choline acetyltransferase expression in the brainstem nuclei. Percutaneous cervical VNS significantly reduced plasma levels of TNF-α at 3 h post-lipopolysaccharide (LPS) injection. Furthermore, it decreased Iba-1+ microglia numbers in the hippocampus, altered the morphology of microglia, and restored cognitive dysfunction in animals with LPS-induced neuroinflammation (Huffman et al., 2019).
Parkinson's disease: In a study by Jiang et al., percutaneous auricular VNS treatment delivered by acupuncture needle significantly improved motor deficits, increased the expressions of TH in the SN and α7nAChR in the ventral midbrain, and reduced the levels of inflammatory cytokines TNF-α and IL-1β. Additionally, percutaneous auricular VNS increased the number of regulatory T cells while decreasing the number of T helper 17 cells in an animal model of 6-OHDA-induced Parkinson's disease (Jiang et al., 2018). Post-operative cognitive decline: Post-operative memory impairment after exploratory laparotomy surgery was alleviated in animals treated with percutaneous auricular VNS. The animals treated with VNS showed shorter swimming latency and distance in Morris water maze tests. Treatment with percutaneous auricular VNS decreased the levels of IL-1β and TNF-α and the nuclear protein expression of NF-κB. Neurodegenerative pathology: Neurodegenerative pathology included tau phosphorylation and Aβ40 and Aβ42 expressions in the hippocampus in aged rats (Cai et al., 2019). Percutaneous auricular VNS delivered by an acupuncture needle significantly reversed the abovementioned phenomena; however, improvements in α7nAChR (-/-) rats and rats receiving α7nAChR antagonists were diminished. These findings are consistent with those of the previous studies on the vital role of α7nAChR in the VNS-mediated CAP (Wang J. Y. et al., 2021).
Migraine: In a rat model of migraine, Liu et al. demonstrated that non-invasive nVNS suppressed the susceptibility of cortical spreading depression (CSD) in a stimulation intensity-dependent manner. Low-intensity VNS was ineffective, whereas medium-intensity VNS had an efficacy comparable to that of high-intensity VNS. Two 2-min nVNS spaced 5 min apart showed the highest efficacy on the electrical CSD threshold and frequency of KCl-evoked CSD. The optimal nVNS also attenuated CSD-induced upregulation of cortical cyclooxygenase-2, calcitonin gene-related peptide in trigeminal ganglia, and c-Fos expression in the trigeminal nucleus caudalis (Liu et al., 2022). In a double-blinded, sham-controlled study that enrolled 48 patients with migraine, 2 months of adjunctive cervical nVNS significantly reduced the number of severe headaches per month. Pro-inflammatory IL-1β plasma levels (interictal) were higher in the sham-treated patients compared with the nVNS-treated group. However, pro- (IL-6, HMGB1, TNF-a, and leptin) and anti-inflammatory (IL-10, adiponectin, and ghrelin) mediators did not significantly differ between the nVNS and sham groups (Chaudhry et al., 2019).
Most of the commercial devices of taVNS are applied at the concha of the external ear, but it is not exclusively stimulating the vagus nerve for complicated nerve distributions. The anterior and superior parts are innervated by auriculotemporal nerve, a sensory branch of the mandibular nerve. The external acoustic meatus is innervated by the facial nerve and auricular branch of the vagus nerve. The inferior and posterior parts of the external ear are innervated by the greater auricular and lesser occipital nerves, which are superficial branches of the cervical plexus with fibers from the C2 and C3 spinal nerves. The precise cutaneous map remains unclear because the cutaneous distribution of a particular nerve varies in some cases, and the boundaries between particular dermatomes often overlap. Moreover, some nerve fibers may cross-communicate with others along their intracranial course (Butt et al., 2020). Depression: Wang et al. demonstrated that depression-like behavior and hippocampal neuroinflammation developed in rats with chronic, unpredictable, and mild stress-induced depression. Downregulated expression of α7nAChR, upregulated expression of NF-κB, p65, and IL-1β, and microglial morphology changes in amoebic-like activated states were observed in the depressed rats (Wang J. Y. et al., 2021). Guo et al. provided a novel approach to delivering transcutaneous auricular VNS (taVNS) by a self-adsorptive magnet fixed in the bilateral cavities of the auricular concha. The levels of plasma TNF-α and the expression of TNF-α in the prefrontal cortex, hippocampus, amygdala, and hypothalamus increased in depressed animals. taVNS showed a trend of decreased weight gain, improved sucrose preference, increased mechanical withdrawal threshold, and suppression of elevated TNF-α levels in rats with depression and chronic somatic pain (Guo et al., 2020). These studies indicate that taVNS exerts antidepressive effects via an α7nAChR-dependent mechanism to modulate TNF-α and microglia-mediated neuroinflammation.
We introduced the FDA-approved indications and the extended clinical trials of VNS based on the discussed diseases. We summarized the modalities, diseases, study sample sizes, and indications with FDA approval or under clinical trial investigation in an integrated figure to provide an overview of the clinical implications of VNS (Figure 2).
Figure 2. Clinical implications of VNS by different modalities and diseases. Traditional VNS is applied in the treatment of refractory epilepsy and depression and stroke, with FDA-approved indications. VNS is undergoing clinical trials for the treatment of epilepsy, tinnitus, generalized anxiety disorders, fibromyalgia, and rheumatic arthritis. Transcutaneous cervical VNS is FDA approved for cluster headache and migraine and is under clinical trials of COVID-19-related inflammation, stroke, atrial fibrillation, post-traumatic stress disorder, opioid use disorder, and painful chronic pancreatitis. Transcutaneous auricular VNS was applied in a broad spectrum of clinical studies. Most of the VNS studies have achieved the primary outcome, with significant effects, except for references 26, 29, and 33. VNS, vagus nerve stimulation; FDA, Food and Drug Administration; COVID-19, coronavirus disease 2019; ns, non-significant effect on study measure outcome. Reference number, 1 (Addorisio et al., 2019), 2 (Arsava et al., 2022), 3 (Badran et al., 2020), 4 (Chang et al., 2021), 5 (Courties et al., 2022), 6 (Dawson et al., 2021), 7 (De Ridder et al., 2015), 8 (Diener et al., 2019), 9 (Fisher et al., 2016), 10 (Garcia et al., 2021), 11 (Gaul et al., 2016), 12 (Gazi et al., 2022), 13 (Genovese et al., 2020), 14 (George et al., 2005), 15 (George et al., 2008), 16 (Goadsby et al., 2018), 17 (Gomolka et al., 2018), 18 (Grazzi et al., 2017), 19 (Kawai et al., 2017), 20 (Kozorosky et al., 2022), 21 (Kreuzer et al., 2014), 22 (Labar et al., 1999), 23 (Lange et al., 2011), 24 (Manning et al., 2019), 25 (Merchant et al., 2022), 26 (Mertens et al., 2022), 27 (Morris et al., 2013), 28 (Morris and Mueller, 1999), 29 (Muthulingam et al., 2021), 30 (Najib et al., 2022), 31 (Paccione et al., 2022), 32 (Redgrave et al., 2018), 33 (Rush et al., 2005a), 34 (Rush et al., 2005b), 35 (Salama et al., 2020), 36 (Shi et al., 2021), 37 (Silberstein et al., 2016a), 38 (Silberstein et al., 2016b), 39 (Stavrakis et al., 2020), 40 (Tassorelli et al., 2018), 41 (Tirado et al., 2022), 42 (Tornero et al., 2022), 43 (Tyler et al., 2017), 44 (Verrier et al., 2016), and 45 (Wittbrodt et al., 2021).
The FDA has approved the use of traditional VNS as adjunctive therapy for patients with DRE and treatment-resistant depression and paired use with post-stroke rehabilitation. nVNS has been approved for the acute treatment of episodic CH and episodic migraines and the prevention of chronic CH and episodic or chronic migraines (Table 3).
VNS was first approved as an adjunctive therapy in adults and adolescents aged 12–17 years with DRE in 1997, based on the results of the clinical trials E-01, E-02, E-03, E-04, and E-05 by Morris and Mueller (1999). A total of 454 patients were implanted with VNS, among whom 440 had assessable data. A ≥50% seizure reduction post-implantation was observed in 36.8% of the patients at 1 year, 43.2% of the patients at 2 years, and 42.7% of the patients at 3 years. Median seizure reductions compared with baseline were 35% at 1 year, 44.3% at 2 years, and 44.1% at 3 years. Continuation with VNS rates were 96.7% at 1 year, 84.7% at 2 years, and 72.1% at 3 years. The device was safe and well-tolerated. In 2017, the FDA approved VNS for patients aged 4–11 years with DRE. In the subgroup analysis of the E-04 trial by Labar et al., 24 patients aged > 2 years with drug-resistant generalized epilepsy were prospectively enrolled. The median seizure rate reduction was 46%. Sixteen out of the 24 patients had a > 30% reduction in seizure rate, and 11 had a > 50% reduction. In this cohort, patients with higher baseline seizure rates and later-onset age of epilepsy had the best response to VNS. Furthermore, VNS was an effective treatment for DRE even in patients as young as 4 years old (Labar et al., 1999). According to the evidence-based guideline published by Morris et al. (2013). VNS was possibly effective in achieving >50% seizure frequency reduction, based on the data from 14 Class III studies. In a pooled analysis of data from 481 children, the responder rate was 55%. A Japanese post-approval study published by Kawai et al. prospectively enrolled 78 patients aged <12 years treated with VNS. The median decreases in all the seizures after 3, 6, 12, 24, and 36 months following VNS therapy were 9.0%, 40.2%, 50.0%, 50.0%, and 60.0%, respectively (Kawai et al., 2017).
In 2005, the FDA approved the use of VNS for treatment-resistant depression, based on the randomized, double-blind D-02 trial. In the acute phase of the trial, there were no significant differences in the 24-item Hamilton Rating Scale for Depression (HRSD-24) and Inventory of Depressive Symptomatology Self-Report (IDS-SR30) after 10 weeks of VNS stimulation compared with the sham group (Rush et al., 2005a). Twelve months after VNS implantation, there was a significant reduction in HRSD-24. The response rate measured by the HRSD-24 was 27.2%, and the remission rate was 15.8%. Similar results were obtained with the Montgomery Asberg Depression Rating Scale (28.2% response rate) and Clinical Global Impression-Improvement scale (34.0% response rate) after 12 months of VNS. Voice alteration, dyspnea, and neck pain were the most frequently reported adverse events (Rush et al., 2005b). Furthermore, compared with the treatment-as-usual (TAU) group, the VNS + TAU group was associated with greater improvement per month in the IDS-SR30. The response rates of the HRSD-24 were 27% for the VNS + TAU group and 13% for the TAU group (George et al., 2005).
The FDA has approved nVNS for the acute treatment of episodic CHs, based on the ACT1 and ACT2 trials. The ACT1 trial by Silberstein et al. included 133 patients with CH randomized into an nVNS-treated group (60 patients: episodic CH, n = 38; chronic CH, n = 22) and a sham-treated group (73 patients: episodic CH, n = 47; cluster CH, n = 26). Response rates were significantly higher with nVNS than with a sham treatment in the episodic CH cohort (nVNS, 34.2%; sham, 10.6%) but not in the chronic CH cohort (nVNS, 13.6%; sham, 23.1%). Sustained response rates were significantly higher with nVNS for the episodic CH cohort and total population (Silberstein et al., 2016b). The ACT2 trial by Goadsby et al. included 48 nVNS-treated (14 episodic CH and 34 chronic CH) and 44 sham-treated patients (13 episodic CH, 31 chronic CH). Regarding the proportion of all treated attacks that achieved a pain-free status within 15 min after treatment initiation, nVNS (14%) and sham (12%) treatments were not significantly different for the total cohort. However, nVNS (48%) was superior to sham treatment (6%, p < 0.01) in the episodic CH subgroup. No significant differences between the nVNS (5%) and sham (13%) treatments were observed in the chronic CH subgroup (Goadsby et al., 2018).
In 2018, nVNS was approved for the acute treatment of episodic migraine, based on the double-blind, randomized PRESTO study. A total of 248 participants with episodic migraine with/without aura were randomized to receive nVNS or sham stimulation within 20 min of pain onset. Results showed that nVNS (n = 120) was superior to sham treatment (n = 123) for pain freedom at 30 min (12.7% vs. 4.2%, p = 0.012) and 60 min (21.0% vs. 10.0%, p = 0.023) but not at 120 min after the first treated attack. A post hoc repeated-measures test showed the therapeutic benefit of nVNS at 30, 60, and 120 min (odds ratio, 2.3; 95% confidence interval, 1.2–4.4; p = 0.012). nVNS demonstrated benefits across other endpoints, including pain relief at 120 min, and was safe and well-tolerated (Tassorelli et al., 2018). In 2021, the FDA approved nVNS for the acute treatment of episodic migraine in adolescents, based on the results of the study of Grazzi et al., which enrolled 47 adolescent patients with migraine without aura. Of the treated migraine attacks, 46.8% were considered successfully treated and did not require any rescue medication. No device-related adverse events were recorded (Grazzi et al., 2017).
In 2018, the FDA approved nVNS for the prevention of chronic CHs, based on the results of the PREVA trial. The PREVA trial was an open-label randomized study conducted by Gaul et al. Patients with chronic CHs were randomized into the adjunctive prophylactic nVNS (n = 48) or standard of care (SoC) alone (control, n = 49) group. During the randomized phase, the SoC + nVNS group had a significantly greater reduction in the number of attacks per week compared with the controls, with a mean therapeutic gain of 3.9 fewer attacks per week. A greater percentage of ≥50% response rates was also observed in the SoC + nVNS (40%) group compared with the control group (8.3%) (Gaul et al., 2016).
In 2021, the FDA approved nVNS for adults and adolescents aged 12–17 years for the prevention of episodic and chronic migraines, based on the result of the EVENT, PREMIUM I, and PREMIUM II trials. In the EVENT trial, 59 adults with chronic migraines were enrolled. The mean changes in the number of headache days were −1.4 with nVNS and −0.2 with sham treatment, without significant statistical differences. Among the 27 participants who completed the open-label phase, 15 were initially assigned to nVNS, and their mean change from baseline in headache days after 8 months of treatment was −7.9 (95% confidence interval, −11.9 to −3.8; p < 0.01) (Silberstein et al., 2016a). In the PREMIUM trial, 477 adults with episodic migraines were enrolled. Mean reductions in migraine days per month were not significantly different from those in the sham group. Post hoc analysis of patients with ≥ 67% adherence per month demonstrated a significant difference between nVNS and sham for the reduction in migraine days (2.27 vs. 1.53, p = 0.043). Patients with migraine with aura responded better than those with migraine without aura (Diener et al., 2019). In the PREMIUM II trial, 336 adults with episodic or chronic migraines were enrolled. There was a significant mean reduction in monthly migraine days in the nVNS group compared with the sham group (3.12, 2.29, difference, −0.83, p = 0.2329). The responder rate was greater in the nVNS group (44.87%) than in the sham group (26.81%, p = 0.0481) (Najib et al., 2022).
The VNS-REHAB trial was a pivotal, randomized, triple-blinded, and sham-controlled study. This trial enrolled 108 adults at least 9 months after an ischemic stroke with moderate-to-severe arm weakness. The patients were assigned to the rehabilitation paired with active VNS (VNS group, n = 53) or rehabilitation paired with sham stimulation (sham group, n = 55) groups. Among the 106 patients who completed the study, there was a significant improvement in the mean Fugl–Meyer Assessment-Upper Extremity (FMA-UE) score in the VNS group compared with the control group. A clinically meaningful response in the FMA-UE score was achieved in 23 (47%) out of 53 patients in the VNS group vs. 13 (24%) out of 55 patients in the control group 90 days after in-clinic therapy, which was significantly greater in the VNS group compared with the control group (between-group difference, 24%; p = 0.0098) (Dawson et al., 2021).
Currently, there are several ongoing studies on the clinical utility of VNS. We reviewed recently completed clinical trials with available published data to provide a scope for current practice, off-label use, and unmet needs (Table 4).
Some trials were conducted on healthy participants to understand the mechanism of action of VNS and its effect on the immune response. In healthy participants, transvenous VNS was insufficient to modulate the innate immune response during endotoxemia (Kox et al., 2015). Short-term non-invasive auricular VNS did not significantly affect the motor performance of young healthy individuals in the cycle ergometer test (Hatik et al., 2022). Short trains of taVNS decreased the heart rate in a stimulation parameter-dependent manner (Badran et al., 2018). taVNS showed a trend in improving the global sleep score and significantly improving gastric motility (Steidel et al., 2021; Jackowska et al., 2022). Transcutaneous VNS was applied to test its protective effect on stress-induced intestinal barrier dysfunction. A reduction in paracellular permeability of the small intestine was observed in the VNS-treated group measured by intestinal fatty acid-binding protein release, although it did not entirely mitigate intestinal barrier dysfunction (Mogilevski et al., 2022).
In patients with epilepsy, traditional VNS with an open-loop system has been shown with safety, tolerability, and a significant seizure reduction rate (Batson et al., 2022). The closed-loop device of VNS equipped with seizure detection and automatic stimulation mode upon sensing tachycardia also showed beneficial effects in patients with epilepsy (Fisher et al., 2016). Furthermore, VNS may reduce the interictal cardiac electrical instability and T-wave alternans in patients with DRE and may further provide better prevention of SUDEP (Verrier et al., 2016). Mertens et al. used traditional or taVNS in patients with DRE to investigate the effects of VNS on verbal memory. There was improved verbal memory performance after 6 weeks of traditional VNS treatment. Acute VNS and taVNS did not improve verbal memory performance (Mertens et al., 2022). Currently, the efficacy of tcVNS and taVNS on DRE remained to be elucidated. Several compelling studies have suggested that stimulating large-diameter afferent myelinated fibers by traditional VNS can alter the activation and connectivity of the neural network through the vagal–NTS–parabrachial nucleus–hypothalamus–thalamus–limbic system to the cortex and induce catecholamine releases, whereby VNS effectively reduces seizures in patients with DRE (Carron et al., 2023).
Regarding tinnitus, variable results of efficacy were reported due to the small number of patients and heterogeneity of the study design. One investigation of taVNS for the control of tinnitus showed no improvement after 6 months of stimulation (Kreuzer et al., 2014). In another case report by De Ridder et al. (2015) a patient had improvement of tinnitus after VNS (paired with tones) and had a recurrence of tinnitus after sham stimulation. In a small, double-blind, control pilot trial, Tyler et al. found improvement in tinnitus in patients receiving VNS paired with tone but not in the VNS-only-treated group, particularly with tonal and non–blast-induced tinnitus (De Ridder et al., 2015; Tyler et al., 2017).
Regarding stroke, VNS is thought to enhance the task-related circuit plasticity, thus facilitating post-stroke rehabilitation to improve motor, speech, and swallowing functions (Morrison et al., 2021). Redgrave et al. demonstrated the feasibility and safety of taVNS with upper limb repetitive movement for post-stroke rehabilitation (Redgrave et al., 2018). taVNS with upper limb robotic training reduced the spasticity and variability of the biceps brachii surface electromyography peak amplitude in extension movements (Chang et al., 2021). Furthermore, one pilot study by Arsava et al. (2022) showed feasibility, safety, and a reduction of stroke size via tcVNS in the setting of acute ischemic and hemorrhagic stroke. Badran et al. (2020) found that 57% of enrolled premature infants or infants with hypoxic–ischemic encephalopathy and oromotor dysfunction who received taVNS-paired feeding rehabilitation achieved adequate feeding volumes without the need for G-tube insertion at discharge, with a significant increase in feeding volume compared with pre-stimulation.
taVNS was found to lower postprandial plasma ghrelin levels in healthy participants receiving a high-calorie beverage, which posed its probable therapeutic application for reducing oral intake in patients with diabetes mellitus (Kozorosky et al., 2022). Gomolka et al. (2018) demonstrated that the Higuchi fractal dimension method on HRV could readily differentiate patients with diabetes mellitus from normal controls and could provide stimulation feedback for taVNS. VNS exerts a variety of influences on heart rate and HRV among different study populations with variable stimulation modalities, sites, and parameters (Dolphin et al., 2022). It may have therapeutic implications for patients with cardiovascular diseases, such as heart failure and arrhythmia (Kharbanda et al., 2022; Konstam et al., 2022; Verrier et al., 2022). tcVNS reduced the burden of atrial fibrillation after 6 months of daily treatment (Stavrakis et al., 2020).
Chronic stress may aggravate pre-existing psychiatric diseases, and VNS provides an opportunity to treat such diseases by the augmentation of the HPA axis. In patients with generalized anxiety disorder, a pilot study by George et al. showed the tolerability of VNS treatment with the evidence of acute and long-term improvement in some patients (George et al., 2008). In patients with fibromyalgia, Lange et al. demonstrated the feasibility and tolerability of VNS (Lange et al., 2011). taVNS improved overall fibromyalgia severity by clinical evaluation. However, there were no significant differences in the visual analog scale rated by patients or HRV compared with the sham group (Paccione et al., 2022). Wittbrodt et al. (2021) recruited healthy participants with traumatic experiences and found that tcVNS increased anterior cingulate and hippocampal activity when exposed to trauma scripts. Transcutaneous VNS was effective in reducing temper outbursts in Prader–Willi syndrome (Manning et al., 2019). In contrast to traditional VNS with FDA-approved indication for the treatment of patients with refractory depression, the effects of taVNS have been explored in patients with depression (Austelle et al., 2022). Recently, a novel device with respiratory-gated auricular vagal afferent nerve stimulation (RAVANS) effectively modulated the brain circuitries that regulate the response to negative stress. RAVANS was associated with a significant acute reduction in symptoms of depression and anxiety in women with recurrent major depressive disorder (Garcia et al., 2021). Regarding opioid withdrawal syndrome, studies have also demonstrated that tcVNS may decrease subjective opioid withdrawal, reduce distress, and lower the heart rate during acute opioid withdrawal (Gazi et al., 2022; Tirado et al., 2022). Based on the rationale that VNS can activate the LC and catecholamines in the hippocampus and cortex, VNS undergoes some clinical trials to improve cognitive functions in patients with Alzheimer's disease, but these trials did not achieve a conclusive significance (Vargas-Caballero et al., 2022).
VNS is possibly therapeutic in the field of systemic inflammatory response, including sepsis and autoimmune diseases. In patients with multidrug-refractory rheumatoid arthritis, traditional VNS showed effectiveness in reducing signs and symptoms (Genovese et al., 2020). taVNS also showed efficacy in the inhibition of peripheral blood production of TNF, IL-1β, and IL-6 in healthy participants and attenuated systemic inflammatory responses in patients with rheumatoid arthritis (Addorisio et al., 2019). A recent randomized control trial enrolled patients with coronavirus disease 2019 (COVID) and found that tcVNS significantly reduced the levels of inflammatory markers, specifically C-reactive protein (CRP) and procalcitonin (Czura et al., 2022; Tornero et al., 2022). Post-operative taVNS after lung lobectomy attenuated the acute postsurgical inflammatory response by regulating IL-6 and IL-10, resulting in reduced incidence of Post-operative pneumonia and hospitalization time (Salama et al., 2020). In a pilot study of erosive hand osteoarthritis, taVNS significantly reduced the hand pain in the visual analog scale score and functional index for hand arthropathy score (Courties et al., 2022). In a small trial that included patients with idiopathic nephrotic syndrome, taVNS was associated with clinical remission in frequently relapsing nephrotic syndrome and reduced proteinuria in non-congenital steroid-resistant nephrotic syndrome (Merchant et al., 2022). In constipation-predominant irritable bowel syndrome, 4 weeks of taVNS improved both constipation and abdominal pain (Shi et al., 2021). However, the pain-relieving effect was not observed in patients with painful chronic pancreatitis receiving tcVNS (Muthulingam et al., 2021).
The neuroimmunomodulation effect of VNS is mediated through the CAP, which is an α7nAChR-dependent pathway that reduces pro-inflammatory cytokines. Studies on the mechanisms of VNS included in vivo or in vitro experiments, and the therapeutic effects were verified in animal studies with a broad spectrum of diseases, including systemic diseases with inflammatory pathophysiology and neuropsychiatric diseases. However, whether the immunomodulating effects shown in animal studies can be reproduced in humans and particularly patients requires further evidence. nVNS is currently being explored in device developments and clinical applications to examine whether the immunomodulation in traditional VNS can be repeated using nVNS as the stimulation modalities and protocols are different from those in traditional VNS. Large prospective randomized controlled trials are required to elucidate the optimal stimulation parameters. Moreover, the acute and long-lasting effects of VNS should be carefully monitored as chronic stimulation would change neural plasticity and excitability. Furthermore, the vagus nerve connects the brain and internal organs. This opens up a unique opportunity to investigate the bidirectional interactions of cognition, mood, and systemic diseases with VNS modulation, e.g., the emerging topics in the gut–vagus–brain axis in inflammatory and neuropsychiatric diseases. VNS activates both vagal afferent and efferent fibers. However, most of the knowledge on the neuroimmunomodulatory effects of VNS involves the vagal efferent pathway and its related peripheral mechanisms. Little is known about how the brain is activated by the vagal afferent pathway and its relationship to the modulation of neuroendocrine and immune systems. Therefore, further studies are warranted to elucidate the neural substrates and neurophysiological mechanisms of VNS via the vagal afferent pathway to the brain and their contributions to regulating the inflammatory process that underlies the therapeutic effects of VNS.
Y-TF, Y-TL, W-LT, G-LH, PT, Y-JC, and Y-JW wrote the manuscript. Y-JW organized the manuscript. PT and Y-JW edited the manuscript. Y-TL and W-LT drew the figures. Y-TF generated the tables. All authors contributed to the article and approved the submitted version.
We appreciate the research grants to Y-JW from the National Cheng Kung University Hospital (NCKUH-11202033) and the National Science and Technology Council, Taiwan (NSTC 111-2314-B-006-100).
The authors thank Dr. Kuei-Sen Hsu and Ms. Hsin-Ling Shih from the Department of Pharmacology, College of Medicine, National Cheng Kung University for Dr. Hsu's thoughtful comments on the article and for Ms. Shih's assistance in the graphic editing.
The authors declare that the research was conducted in the absence of any commercial or financial relationships that could be construed as a potential conflict of interest.
All claims expressed in this article are solely those of the authors and do not necessarily represent those of their affiliated organizations, or those of the publisher, the editors and the reviewers. Any product that may be evaluated in this article, or claim that may be made by its manufacturer, is not guaranteed or endorsed by the publisher.
Addorisio, M. E., Imperato, G. H., Vos, De., Forti, A. F., Goldstein, S., Pavlov, R. S., et al. (2019). Investigational treatment of rheumatoid arthritis with a vibrotactile device applied to the external ear. Bioelectron. Med. 5, 4. doi: 10.1186/s42234-019-0020-4
Arsava, E. M., Topcuoglu, M. A., Ay, I., Ozdemir, A. O., Gungor, I. L., Togay Isikay, C., et al. (2022). Assessment of safety and feasibility of non-invasive vagus nerve stimulation for treatment of acute stroke. Brain Stimul. 15, 1467–1474. doi: 10.1016/j.brs.2022.10.012
Asala, S. A., and Bower, A. J. (1986). An electron microscope study of vagus nerve composition in the ferret. Anat. Embryol. 175, 247–253. doi: 10.1007/BF00389602
Austelle, C. W. O'leary, G.H., Thompson, S., Gruber, E., Kahn, A., Manett, A.J., Short, B., et al. (2022). A comprehensive review of vagus nerve stimulation for depression. Neuromodulation 25, 309–315. doi: 10.1111/ner.13528
Badran, B. W., Jenkins, D. D., Cook, D., Thompson, S., Dancy, M., Devries, W. H., et al. (2020). Transcutaneous auricular vagus nerve stimulation-paired rehabilitation for oromotor feeding problems in newborns: an open-label pilot study. Front. Hum. Neurosci. 14, 77. doi: 10.3389/fnhum.2020.00077
Badran, B. W., Mithoefer, O. J., Summer, C. E., Labate, N. T., Glusman, C. E., Badran, A. W., et al. (2018). Short trains of transcutaneous auricular vagus nerve stimulation (taVNS) have parameter-specific effects on heart rate. Brain Stimul. 11, 699–708. doi: 10.1016/j.brs.2018.04.004
Baro, V., Bonavina, M. V., Saettini, F. D'amico, G., Trezza, A., Denaro, L., Grioni, D., et al. (2022). Effect of vagus nerve stimulation on blood inflammatory markers in children with drug-resistant epilepsy: a pilot study. Children. 9, 1133. doi: 10.3390/children9081133
Batson, S., Shankar, R., Conry, J., Boggs, J., Radtke, R., Mitchell, S., et al. (2022). Efficacy and safety of VNS therapy or continued medication management for treatment of adults with drug-resistant epilepsy: systematic review and meta-analysis. J. Neurol. 269, 2874–2891. doi: 10.1007/s00415-022-10967-6
Ben-Menachem, E. (2002). Vagus-nerve stimulation for the treatment of epilepsy. Lancet Neurol. 1, 477–482. doi: 10.1016/S1474-4422(02)00220-X
Berger, A., Vespa, S., Dricot, L., Dumoulin, M., Iachim, E., Doguet, P., et al. (2021). How is the norepinephrine system involved in the antiepileptic effects of vagus nerve stimulation? Front. Neurosci. 15, 790943. doi: 10.3389/fnins.2021.790943
Berthoud, H. R., Jedrzejewska, A., and Powley, T. L. (1990). Simultaneous labeling of vagal innervation of the gut and afferent projections from the visceral forebrain with dil injected into the dorsal vagal complex in the rat. J. Comp. Neurol. 301, 65–79. doi: 10.1002/cne.903010107
Berthoud, H. R., and Neuhuber, W. L. (2019). Vagal mechanisms as neuromodulatory targets for the treatment of metabolic disease. Ann. N. Y. Acad. Sci. 1454, 42–55. doi: 10.1111/nyas.14182
Bonaz, B., Sinniger, V., and Pellissier, S. (2016). Anti-inflammatory properties of the vagus nerve: potential therapeutic implications of vagus nerve stimulation. J. Physiol. 594, 5781–5790. doi: 10.1113/JP271539
Bonaz, B., Sinniger, V., and Pellissier, S. (2017). Vagus nerve stimulation: a new promising therapeutic tool in inflammatory bowel disease. J. Intern. Med. 282, 46–63. doi: 10.1111/joim.12611
Bonaz, B., Sinniger, V., and Pellissier, S. (2021). Therapeutic potential of vagus nerve stimulation for inflammatory bowel diseases. Front. Neurosci. 15, 650971. doi: 10.3389/fnins.2021.650971
Breit, S., Kupferberg, A., Rogler, G., and Hasler, G. (2018). Vagus nerve as modulator of the brain-gut axis in psychiatric and inflammatory disorders. Front. Psychiatry. 9, 44. doi: 10.3389/fpsyt.2018.00044
Butt, M. F., Albusoda, A., Farmer, A. D., and Aziz, Q. (2020). The anatomical basis for transcutaneous auricular vagus nerve stimulation. J. Anat. 236, 588–611. doi: 10.1111/joa.13122
Cai, L., Lu, K., Chen, X., Huang, J. Y., Zhang, B. P., Zhang, H., et al. (2019). Auricular vagus nerve stimulation protects against postoperative cognitive dysfunction by attenuating neuroinflammation and neurodegeneration in aged rats. Neurosci. Lett. 703, 104–110. doi: 10.1016/j.neulet.2019.03.034
Carabotti, M., Scirocco, A., Maselli, M. A., and Severi, C. (2015). The gut-brain axis: interactions between enteric microbiota, central and enteric nervous systems. Ann. Gastroenterol. 28, 203–209.
Caravaca, A. S., Gallina, A. L., Tarnawski, L., Shavva, V. S., Colas, R. A., Dalli, J., et al. (2022). Vagus nerve stimulation promotes resolution of inflammation by a mechanism that involves Alox15 and requires the alpha7nAChR subunit. Proc. Natl. Acad. Sci. U. S. A. 119, e2023285119. doi: 10.1073/pnas.2023285119
Carron, R., Roncon, P., Lagarde, S., Dibue, M., Zanello, M., Bartolomei, F., et al. (2023). Latest views on the mechanisms of action of surgically implanted cervical vagal nerve stimulation in epilepsy. Neuromodulation 26, 498–506. doi: 10.1016/j.neurom.2022.08.447
Chang, J. L., Coggins, A. N., Saul, M., Paget-Blanc, A., Straka, M., Wright, J., et al. (2021). Transcutaneous auricular vagus nerve stimulation (tAVNS) delivered during upper limb interactive robotic training demonstrates novel antagonist control for reaching movements following stroke. Front. Neurosci. 15, 767302. doi: 10.3389/fnins.2021.767302
Chaudhry, S. R., Lendvai, I. S., Muhammad, S., Westhofen, P., Kruppenbacher, J., Scheef, L., et al. (2019). Inter-ictal assay of peripheral circulating inflammatory mediators in migraine patients under adjunctive cervical non-invasive vagus nerve stimulation (nVNS): a proof-of-concept study. Brain Stimul. 12, 643–651. doi: 10.1016/j.brs.2019.01.008
Chen, H., Feng, Z., Min, L., Deng, W., Tan, M., Hong, J., et al. (2022). Vagus nerve stimulation reduces neuroinflammation through microglia polarization regulation to improve functional recovery after spinal cord injury. Front. Neurosci. 16, 813472. doi: 10.3389/fnins.2022.813472
Chen, X., He, X., Luo, S., Feng, Y., Liang, F., Shi, T., et al. (2018). Vagus nerve stimulation attenuates cerebral microinfarct and colitis-induced cerebral microinfarct aggravation in mice. Front. Neurol. 9, 798. doi: 10.3389/fneur.2018.00798
Chen, X., Liang, H., Hu, K., Sun, Q., Sun, B., Bian, L., et al. (2021). Vagus nerve stimulation suppresses corticotropin-releasing factor-induced adrenocorticotropic hormone release in rats. Neuroreport 32, 792–796. doi: 10.1097/WNR.0000000000001656
Cirillo, G., Negrete-Diaz, F., Yucuma, D., Virtuoso, A., Korai, S. A., De Luca, M., et al. (2022). Vagus nerve stimulation: a personalized therapeutic approach for Crohn's and other inflammatory bowel diseases. Cells 11, 4103. doi: 10.3390/cells11244103
Courties, A., Deprouw, C., Maheu, E., Gibert, E., Gottenberg, J. E., Champey, J., et al. (2022). Effect of transcutaneous vagus nerve stimulation in erosive hand osteoarthritis: results from a pilot trial. J. Clin. Med. 11, 1087. doi: 10.3390/jcm11041087
Czura, C. J., Bikson, M., Charvet, L., Chen, J. D. Z., Franke, M., Fudim, M., et al. (2022). Neuromodulation strategies to reduce inflammation and improve lung complications in COVID-19 patients. Front. Neurol. 13, 897124. doi: 10.3389/fneur.2022.897124
Dawson, J., Liu, C. Y., Francisco, G. E., Cramer, S. C., Wolf, S. L., Dixit, A. O'dell, M.W., et al. (2021). Vagus nerve stimulation paired with rehabilitation for upper limb motor function after ischaemic stroke (VNS-REHAB): a randomized, blinded, pivotal, device trial. Lancet 397, 1545–1553. doi: 10.1016/S0140-6736(21)00475-X
De Herdt Puimege, V., De Waele, L., Raedt, J., Wyckhuys, R., El Tahry, T. P., et al. (2009). Increased rat serum corticosterone suggests immunomodulation by stimulation of the vagal nerve. J. Neuroimmunol. 212, 102–105. doi: 10.1016/j.jneuroim.2009.04.013
De Ridder, Kilgard, D., Engineer, M. N., and Vanneste, S. (2015). Placebo-controlled vagus nerve stimulation paired with tones in a patient with refractory tinnitus: a case report. Otol. Neurotol. 36, 575–580. doi: 10.1097/MAO.0000000000000704
Decarie-Spain, L., Hayes, A. M. R., Lauer, L. T., and Kanoski, S. E. (2023). The gut-brain axis and cognitive control: a role for the vagus nerve. Semin. Cell Dev. Biol. 3, S1084-9521(23)00032–0. doi: 10.1016/j.semcdb.2023.02.004
Diener, H. C., Goadsby, P. J., Ashina, M., Al-Karagholi, M. A., Sinclair, A., Mitsikostas, D., et al. (2019). Non-invasive vagus nerve stimulation (nVNS) for the preventive treatment of episodic migraine: the multicentre, double-blind, randomized, sham-controlled PREMIUM trial. Cephalalgia 39, 1475–1487. doi: 10.1177/0333102419876920
Ding, J. J., Liu, P., Rebernig, H., Suller-Marti, A., Parrent, A. G., Burneo, J. G., et al. (2021). Vagus nerve stimulation does not alter brainstem nuclei morphology in patients with refractory epilepsy. Epilepsy Behav. 118, 107940. doi: 10.1016/j.yebeh.2021.107940
Dolphin, H., Dukelow, T., Finucane, C., Commins, S., Mcelwaine, P., Kennelly, S., et al. (2022). “The wandering nerve linking heart and mind” - the complementary role of transcutaneous vagus nerve stimulation in modulating neuro-cardiovascular and cognitive performance. Front. Neurosci. 16, 897303. doi: 10.3389/fnins.2022.897303
Downes, M. H., Kalagara, R., Chennareddy, S., Vasan, V., Reford, E., Schuldt, B. R., et al. (2023). Vagal nerve stimulation: a bibliometric analysis of current research trends. Neuromodulation 26, 529–537. doi: 10.1016/j.neurom.2022.07.001
Farrand, A. Q., Helke, K. L., Gregory, R. A., Gooz, M., Hinson, V. K., Boger, H. A., et al. (2017). Vagus nerve stimulation improves locomotion and neuronal populations in a model of Parkinson's disease. Brain Stimul. 10, 1045–1054. doi: 10.1016/j.brs.2017.08.008
Farrand, A. Q., Verner, R. S., Mcguire, R. M., Helke, K. L., Hinson, V. K., Boger, H. A., et al. (2020). Differential effects of vagus nerve stimulation paradigms guide clinical development for Parkinson's disease. Brain Stimul. 13, 1323–1332. doi: 10.1016/j.brs.2020.06.078
Fisher, R. S., Afra, P., Macken, M., Minecan, D. N., Bagic, A., Benbadis, S. R., et al. (2016). Automatic vagus nerve stimulation triggered by ictal tachycardia: clinical outcomes and device performance–the U.S. E-37 trial. Neuromodulation 19, 188–195. doi: 10.1111/ner.12376
Foley, J. O., and Dubois, F. S. (1937). Quantitative studies of the vagus nerve in the cat: I. The ratio of sensory to motor fibers. J. Nerv. Ment. Dis. 86, 587. doi: 10.1097/00005053-193711000-00019
Frasch, M. G., Szynkaruk, M., Prout, A. P., Nygard, K., Cao, M., Veldhuizen, R., et al. (2016). Decreased neuroinflammation correlates to higher vagus nerve activity fluctuations in near-term ovine fetuses: a case for the afferent cholinergic anti-inflammatory pathway? J. Neuroinflammation 13, 103. doi: 10.1186/s12974-016-0567-x
Garcia, R. G., Cohen, J. E., Stanford, A. D., Gabriel, A., Stowell, J., Aizley, H., et al. (2021). Respiratory-gated auricular vagal afferent nerve stimulation (RAVANS) modulates brain response to stress in major depression. J. Psychiatr. Res. 142, 188–197. doi: 10.1016/j.jpsychires.2021.07.048
Gaul, C., Diener, H. C., Silver, N., Magis, D., Reuter, U., Andersson, A., et al. (2016). Non-invasive vagus nerve stimulation for PREVention and Acute treatment of chronic cluster headache (PREVA): a randomised controlled study. Cephalalgia 36, 534–546. doi: 10.1177/0333102415607070
Gazi, A.H., Harrison, A.B., Lambert, T.P., Obideen, M., Alavi, P., Murrah, N., et al. (2022). Transcutaneous cervical vagus nerve stimulation reduces behavioral and physiological manifestations of withdrawal in patients with opioid use disorder: A double-blind, randomized, sham-controlled pilot study. Brain Stimul. 15, 1206–1214. doi: 10.1016/j.brs.2022.08.017
Genovese, M. C., Gaylis, N. B., Sikes, D., Kivitz, A., Horowitz, D. L., Peterfy, C., et al. (2020). Safety and efficacy of neurostimulation with a miniaturised vagus nerve stimulation device in patients with multidrug-refractory rheumatoid arthritis: a two-stage multicentre, randomised pilot study. Lancet Rheumatol. 2, e527–e538. doi: 10.1016/S2665-9913(20)30172-7
George, M. S., Rush, A. J., Marangell, L. B., Sackeim, H. A., Brannan, S. K., Davis, S. M., et al. (2005). A 1-year comparison of vagus nerve stimulation with treatment as usual for treatment-resistant depression. Biol. Psychiatry. 58, 364–373. doi: 10.1016/j.biopsych.2005.07.028
George, M. S., Ward, H. E. Jr., Ninan, P.T., Pollack, M., Nahas, Z., Anderson, B., et al. (2008). A pilot study of vagus nerve stimulation (VNS) for treatment-resistant anxiety disorders. Brain Stimul. 1, 112–121. doi: 10.1016/j.brs.2008.02.001
Goadsby, P. J., De Coo Silver, I. F., Tyagi, N., Ahmed, A., Gaul, F., et al. (2018). Non-invasive vagus nerve stimulation for the acute treatment of episodic and chronic cluster headache: a randomized, double-blind, sham-controlled ACT2 study. Cephalalgia 38, 959–969. doi: 10.1177/0333102417744362
Gomolka, R. S., Kampusch, S., Kaniusas, E., Thurk, F., Szeles, J. C., Klonowski, W., et al. (2018). Higuchi fractal dimension of heart rate variability during percutaneous auricular vagus nerve stimulation in healthy and diabetic subjects. Front. Physiol. 9, 1162. doi: 10.3389/fphys.2018.01162
Grazzi, L., Egeo, G., Liebler, E., Padovan, A. M., and Barbanti, P. (2017). Non-invasive vagus nerve stimulation (nVNS) as symptomatic treatment of migraine in young patients: a preliminary safety study. Neurol. Sci. 38, 197–199. doi: 10.1007/s10072-017-2942-5
Guo, X., Zhao, Y., Huang, F., Li, S., Luo, M., Wang, Y., et al. (2020). Effects of transcutaneous auricular vagus nerve stimulation on peripheral and central tumor necrosis factor alpha in rats with depression-chronic somatic pain comorbidity. Neural. Plast. 2020, 8885729. doi: 10.1155/2020/8885729
Hatik, S., Pehlivanoglu, B., Arslan, M., Taşkin, C., and Özden, A. (2022). Acute effect of auricular vagus nerve stimulation on cycle ergometer test and physiological parameters in healthy young individuals: a pilot study. Int. J. Sports Exerc. Med. 8, 211. doi: 10.23937/2469-5718/1510211
Henry, T. R. (2002). Therapeutic mechanisms of vagus nerve stimulation. Neurology 59, S3–S14. doi: 10.1212/WNL.59.6_suppl_4.S3
Hilz, M. J. (2022). Transcutaneous vagus nerve stimulation—A brief introduction and overview. Auton. Neurosci. 243, 103038. doi: 10.1016/j.autneu.2022.103038
Hornung, J. P. (2003). The human raphe nuclei and the serotonergic system. J. Chem. Neuroanat. 26, 331–343. doi: 10.1016/j.jchemneu.2003.10.002
Hosoi, T., Okuma, Y., and Nomura, Y. (2000). Electrical stimulation of afferent vagus nerve induces IL-1beta expression in the brain and activates HPA axis. Am. J. Physiol. Regul. Integr. Comp. Physiol. 279, R141–R147. doi: 10.1152/ajpregu.2000.279.1.R141
Huffman, W. J., Subramaniyan, S., Rodriguiz, R. M., Wetsel, W. C., Grill, W. M., Terrando, N., et al. (2019). Modulation of neuroinflammation and memory dysfunction using percutaneous vagus nerve stimulation in mice. Brain Stimul. 12, 19–29. doi: 10.1016/j.brs.2018.10.005
Iannucci, J., Nizamutdinov, D., and Shapiro, L. A. (2022). Neurogenesis and chronic neurobehavioral outcomes are partially improved by vagus nerve stimulation in a mouse model of Gulf War illness. Neurotoxicology. 90, 205–215. doi: 10.1016/j.neuro.2022.04.001
Jackowska, M., Koenig, J., Vasendova, V., and Jandackova, V. K. (2022). A two-week course of transcutaneous vagal nerve stimulation improves global sleep: findings from a randomised trial in community-dwelling adults. Auton. Neurosci. 240, 102972. doi: 10.1016/j.autneu.2022.102972
Jiang, Y., Cao, Z., Ma, H., Wang, G., Wang, X., Wang, Z., et al. (2018). Auricular vagus nerve stimulation exerts antiinflammatory effects and immune regulatory function in a 6-OHDA model of Parkinson's disease. Neurochem. Res. 43, 2155–2164. doi: 10.1007/s11064-018-2639-z
Jurga, A. M., Paleczna, M., and Kuter, K. Z. (2020). Overview of general and discriminating markers of differential microglia phenotypes. Front. Cell Neurosci. 14, 198. doi: 10.3389/fncel.2020.00198
Kaur, S., Selden, N. R., and Aballay, A. (2023). Anti-inflammatory effects of vagus nerve stimulation in pediatric patients with epilepsy. Front. Immunol. 14, 403. doi: 10.3389/fimmu.2023.1093574
Kawai, K., Tanaka, T., Baba, H., Bunker, M., Ikeda, A., Inoue, Y., et al. (2017). Outcome of vagus nerve stimulation for drug-resistant epilepsy: the first 3 years of a prospective Japanese registry. Epileptic Disord. 19, 327–338. doi: 10.1684/epd.2017.0929
Kelly, M. J., Breathnach, C., Tracey, K. J., and Donnelly, S. C. (2022). Manipulation of the inflammatory reflex as a therapeutic strategy. Cell Rep. Med. 3, 100696. doi: 10.1016/j.xcrm.2022.100696
Kharbanda, R. K., Van Der Does, W. F. B., Van Staveren, L. N., Taverne, Y., Bogers, A., De Groot, et al. (2022). Vagus nerve stimulation and atrial fibrillation: revealing the paradox. Neuromodulation. 25, 356–365. doi: 10.1016/j.neurom.2022.01.008
Konstam, M. A., Mann, D. L., Udelson, J. J. E., Ardell, J. L., De Ferrari Cowie, G. M., et al. (2022). Advances in our clinical understanding of autonomic regulation therapy using vagal nerve stimulation in patients living with heart failure. Front. Physiol. 13, 857538. doi: 10.3389/fphys.2022.857538
Kox, M., Van Eijk, L. T., Verhaak, T., Frenzel, T., Kiers, H. D., Gerretsen, J., et al. (2015). Transvenous vagus nerve stimulation does not modulate the innate immune response during experimental human endotoxemia: a randomized controlled study. Arthritis Res. Ther. 17, 150. doi: 10.1186/s13075-015-0667-5
Kozorosky, E. M., Lee, C. H., Lee, J. G., Nunez Martinez, V., Padayachee, L. E., Stauss, H. M., et al. (2022). Transcutaneous auricular vagus nerve stimulation augments postprandial inhibition of ghrelin. Physiol. Rep. 10, e15253. doi: 10.14814/phy2.15253
Kreuzer, P. M., Landgrebe, M., Resch, M., Husser, O., Schecklmann, M., Geisreiter, F., et al. (2014). Feasibility, safety and efficacy of transcutaneous vagus nerve stimulation in chronic tinnitus: an open pilot study. Brain Stimul. 7, 740–747. doi: 10.1016/j.brs.2014.05.003
Labar, D., Murphy, J., and Tecoma, E. (1999). Vagus nerve stimulation for medication-resistant generalized epilepsy. E04 VNS study group. Neurology 52, 1510–1512. doi: 10.1212/WNL.52.7.1510
Lange, G., Janal, M. N., Maniker, A., Fitzgibbons, J., Fobler, M., Cook, D., et al. (2011). Safety and efficacy of vagus nerve stimulation in fibromyalgia: a phase I/II proof of concept trial. Pain Med. 12, 1406–1413. doi: 10.1111/j.1526-4637.2011.01203.x
Liu, T. T., Morais, A., Takizawa, T., Mulder, I., Simon, B. J., Chen, S. P., et al. (2022). Efficacy profile of non-invasive vagus nerve stimulation on cortical spreading depression susceptibility and the tissue response in a rat model. J. Headache Pain. 23, 12. doi: 10.1186/s10194-022-01384-1
Machado, B. H., and Brody, M. J. (1988). Role of the nucleus ambiguus in the regulation of heart rate and arterial pressure. Hypertension 11, 602–607. doi: 10.1161/01.HYP.11.6.602
Manning, K. E., Beresford-Webb, J. A., Aman, L. C. S., Ring, H. A., Watson, P. C., Porges, S. W., et al. (2019). Transcutaneous vagus nerve stimulation (t-VNS): a novel effective treatment for temper outbursts in adults with Prader-Willi syndrome indicated by results from a non-blind study. PLoS ONE. 14, e0223750. doi: 10.1371/journal.pone.0223750
Manta, S., El Mansari, M., and Blier, P. (2012). Novel attempts to optimize vagus nerve stimulation parameters on serotonin neuronal firing activity in the rat brain. Brain Stimul. 5, 422–429. doi: 10.1016/j.brs.2011.04.005
Martelli, D., Mckinley, M. J., and Mcallen, R. M. (2014). The cholinergic anti-inflammatory pathway: a critical review. Auton. Neurosci. 182, 65–69. doi: 10.1016/j.autneu.2013.12.007
Mayer, E. A., Savidge, T., and Shulman, R. J. (2014). Brain-gut microbiome interactions and functional bowel disorders. Gastroenterology 146, 1500–1512. doi: 10.1053/j.gastro.2014.02.037
Meneses, G., Bautista, M., Florentino, A., Diaz, G., Acero, G., Besedovsky, H., et al. (2016). Electric stimulation of the vagus nerve reduced mouse neuroinflammation induced by lipopolysaccharide. J. Inflamm. 13, 33. doi: 10.1186/s12950-016-0140-5
Merchant, K., Zanos, S., Datta-Chaudhuri, T., Deutschman, C. S., and Sethna, C. B. (2022). Transcutaneous auricular vagus nerve stimulation (taVNS) for the treatment of pediatric nephrotic syndrome: a pilot study. Bioelectron. Med. 8, 1. doi: 10.1186/s42234-021-00084-6
Mertens, A., Gadeyne, S., Lescrauwaet, E., Carrette, E., Meurs, A., De Herdt, P., et al. (2022). The potential of invasive and non-invasive vagus nerve stimulation to improve verbal memory performance in epilepsy patients. Sci. Rep. 12, 1984. doi: 10.1038/s41598-022-05842-3
Mihaylova, S., Killian, A., Mayer, K., Pullamsetti, S. S., Schermuly, R., Rosengarten, B., et al. (2012). Effects of anti-inflammatory vagus nerve stimulation on the cerebral microcirculation in endotoxinemic rats. J. Neuroinflammation. 9, 183. doi: 10.1186/1742-2094-9-183
Mogilevski, T., Rosella, S., Aziz, Q., and Gibson, P. R. (2022). Transcutaneous vagal nerve stimulation protects against stress-induced intestinal barrier dysfunction in healthy adults. Neurogastroenterol. Motil. 34, e14382. doi: 10.1111/nmo.14382
Morris, G. L. III., Gloss, D., Buchhalter, J., Mack, K. J., Nickels, K., and Harden, C. (2013). Evidence-based guideline update: vagus nerve stimulation for the treatment of epilepsy: report of the guideline development subcommittee of the American academy of neurology. Neurology 81, 1453–1459. doi: 10.1212/WNL.0b013e3182a393d1
Morris G. L. III. and Mueller W. M. (1999). Long-term treatment with vagus nerve stimulation in patients with refractory epilepsy. The vagus nerve stimulation study group E01-E05. Neurology 53, 1731–1735. doi: 10.1212/WNL.53.8.1731
Morrison, R. A., Hays, S. A., and Kilgard, M. P. (2021). Vagus nerve stimulation as a potential adjuvant to rehabilitation for post-stroke motor peech disorders. Front. Neurosci. 15, 15928. doi: 10.3389/fnins.2021.715928
Muthulingam, J. A., Olesen, S. S., Hansen, T. M., Brock, C., Drewes, A. M., Frokjaer, J. B., et al. (2021). Cervical transcutaneous vagal neuromodulation in chronic pancreatitis patients with chronic pain: a randomised sham controlled clinical trial. PLoS ONE 16, e0247653. doi: 10.1371/journal.pone.0247653
Najib, U., Smith, T., Hindiyeh, N., Saper, J., Nye, B., Ashina, S., et al. (2022). Non-invasive vagus nerve stimulation for prevention of migraine: the multicenter, randomized, double-blind, sham-controlled PREMIUM II trial. Cephalalgia 42, 560–569. doi: 10.1177/03331024211068813
Namgung, U., Kim, K. J., Jo, B. G., and Park, J. M. (2022). Vagus nerve stimulation modulates hippocampal inflammation caused by continuous stress in rats. J. Neuroinflammation. 19, 33. doi: 10.1186/s12974-022-02396-z
Nemeroff, C. B., Mayberg, H. S., Krahl, S. E., Mcnamara, J., Frazer, A., Henry, T. R., et al. (2006). VNS therapy in treatment-resistant depression: clinical evidence and putative neurobiological mechanisms. Neuropsychopharmacology 31, 1345–1355. doi: 10.1038/sj.npp.1301082
Nizamutdinov, D., Mukherjee, S., Deng, C., Stauss, H. M., and Shapiro, L. A. (2018). Gulf War agents pyridostigmine bromide and permethrin cause hypersensitive nociception that is restored after vagus nerve stimulation. Neurotoxicology 69, 93–96. doi: 10.1016/j.neuro.2018.09.007
O'keane, V., Dinan, T.G., Scott, L., and Corcoran, C. (2005). Changes in hypothalamic-pituitary-adrenal axis measures after vagus nerve stimulation therapy in chronic depression. Biol. Psychiatry. 58, 963–968. doi: 10.1016/j.biopsych.2005.04.049
Paccione, C. E., Stubhaug, A., Diep, L. M., Rosseland, L. A., and Jacobsen, H. B. (2022). Meditative-based diaphragmatic breathing vs. vagus nerve stimulation in the treatment of fibromyalgia-a randomized controlled trial: body vs. machine. Front. Neurol. 13, 1030927. doi: 10.3389/fneur.2022.1030927
Prescott, S. L., and Liberles, S. D. (2022). Internal senses of the vagus nerve. Neuron 110, 579–599. doi: 10.1016/j.neuron.2021.12.020
Redgrave, J. N., Moore, L., Oyekunle, T., Ebrahim, M., Falidas, K., Snowdon, N., et al. (2018). Transcutaneous auricular vagus nerve stimulation with concurrent upper limb repetitive task practice for poststroke motor recovery: a pilot study. J. Stroke Cerebrovasc. Dis. 27, 1998–2005. doi: 10.1016/j.jstrokecerebrovasdis.2018.02.056
Rush, A. J., Marangell, L. B., Sackeim, H. A., George, M. S., Brannan, S. K., Davis, S. M., et al. (2005a). Vagus nerve stimulation for treatment-resistant depression: a randomized, controlled acute phase trial. Biol. Psychiatry. 58, 347–354. doi: 10.1016/j.biopsych.2005.05.025
Rush, A. J., Sackeim, H. A., Marangell, L. B., George, M. S., Brannan, S. K., Davis, S. M., et al. (2005b). Effects of 12 months of vagus nerve stimulation in treatment-resistant depression: a naturalistic study. Biol. Psychiatry. 58, 355–363. doi: 10.1016/j.biopsych.2005.05.024
Salama, M., Akan, A., and Mueller, M. R. (2020). Transcutaneous stimulation of auricular branch of the vagus nerve attenuates the acute inflammatory response after lung lobectomy. World J. Surg. 44, 3167–3174. doi: 10.1007/s00268-020-05543-w
Shi, X., Hu, Y., Zhang, B., Li, W., Chen, J. D., Liu, F., et al. (2021). Ameliorating effects and mechanisms of transcutaneous auricular vagal nerve stimulation on abdominal pain and constipation. JCI Insight. 6, e150052. doi: 10.1172/jci.insight.150052
Silberstein, S. D., Calhoun, A. H., Lipton, R. B., Grosberg, B. M., Cady, R. K., Dorlas, S., et al. (2016a). Chronic migraine headache prevention with non-invasive vagus nerve stimulation: the EVENT study. Neurology 87, 529–538. doi: 10.1212/WNL.0000000000002918
Silberstein, S. D., Mechtler, L. L., Kudrow, D. B., Calhoun, A. H., Mcclure, C., Saper, J. R., et al. (2016b). Non-invasive vagus nerve stimulation for the acute treatment of cluster headache: findings from the randomized, double-blind, sham-controlled ACT1 study. Headache 56, 1317–1332. doi: 10.1111/head.12896
Sinniger, V., Pellissier, S., Hoffmann, D., Mathieu, N., Trocme, C., Vercueil, L., et al. (2016). Electrical vagus nerve stimulation as an innovative treatment in inflammatory bowel diseases. Gastroenterology 150, S776–S776. doi: 10.1016/S0016-5085(16)32630-0
Stavrakis, S., Stoner, J. A., Humphrey, M. B., Morris, L., Filiberti, A., Reynolds, J. C., et al. (2020). TREAT AF (transcutaneous electrical vagus nerve stimulation to suppress atrial fibrillation): a randomized clinical trial. JACC Clin. Electrophysiol. 6, 282–291. doi: 10.1016/j.jacep.2019.11.008
Steidel, K., Krause, K., Menzler, K., Strzelczyk, A., Immisch, I., Fuest, S., et al. (2021). Transcutaneous auricular vagus nerve stimulation influences gastric motility: a randomized, double-blind trial in healthy individuals. Brain Stimul. 14, 1126–1132. doi: 10.1016/j.brs.2021.06.006
Tang, H., Li, J., Zhou, Q., Li, S., Xie, C., Niu, L., et al. (2022). Vagus nerve stimulation alleviated cerebral ischemia and reperfusion injury in rats by inhibiting pyroptosis via alpha7 nicotinic acetylcholine receptor. Cell Death Discov. 8, 54. doi: 10.1038/s41420-022-00852-6
Tarn, J., Legg, S., Mitchell, S., Simon, B., and Ng, W. F. (2019). The effects of non-invasive vagus nerve stimulation on fatigue and immune responses in patients with primary Sjogren's syndrome. Neuromodulation 22, 580–585. doi: 10.1111/ner.12879
Tassorelli, C., Grazzi, L., De Tommaso Pierangeli, M., Martelletti, G., Rainero, P. P., et al. (2018). Noninvasive vagus nerve stimulation as acute therapy for migraine: the randomized PRESTO study. Neurology 91, e364–e373. doi: 10.1212/WNL.0000000000005857
Thrivikraman, K. V., Zejnelovic, F., Bonsall, R. W., and Owens, M. J. (2013). Neuroendocrine homeostasis after vagus nerve stimulation in rats. Psychoneuroendocrinology 38, 1067–1077. doi: 10.1016/j.psyneuen.2012.10.015
Tirado, C. F., Washburn, S. N., Covalin, A., Hedenberg, C., Vanderpool, H., Benner, C., et al. (2022). Delivering transcutaneous auricular neurostimulation (tAN) to improve symptoms associated with opioid withdrawal: results from a prospective clinical trial. Bioelectron. Med. 8, 12. doi: 10.1186/s42234-022-00095-x
Tornero, C., Pastor, E., Garzando, M. D. M., Orduna, J., Forner, M. J., Bocigas, I., et al. (2022). Non-invasive vagus nerve stimulation for COVID-19: results from a randomized controlled trial (SAVIOR I). Front. Neurol. 13, 820864. doi: 10.3389/fneur.2022.820864
Tyler, R., Cacace, A., Stocking, C., Tarver, B., Engineer, N., Martin, J., et al. (2017). Vagus nerve stimulation paired with tones for the treatment of tinnitus: a prospective randomized double-blind controlled pilot study in humans. Sci. Rep. 7, 11960. doi: 10.1038/s41598-017-12178-w
Vargas-Caballero, M., Warming, H., Walker, R., Holmes, C., Cruickshank, G., Patel, B., et al. (2022). Vagus nerve stimulation as a potential therapy in early Alzheimer's disease: a review. Front. Hum. Neurosci. 16, 866434. doi: 10.3389/fnhum.2022.866434
Venkatasamy, L., Nizamutdinov, D., Jenkins, J., and Shapiro, L. A. (2021). Vagus nerve stimulation ameliorates cognitive impairment and increased hippocampal astrocytes in a mouse model of Gulf War illness. Neurosci. Insights. 16, 26331055211018456. doi: 10.1177/26331055211018456
Verrier, R. L., Libbus, I., Nearing, B. D., and Kenknight, B. H. (2022). Multifactorial benefits of chronic vagus nerve stimulation on autonomic function and cardiac electrical stability in heart failure patients with reduced ejection fraction. Front. Physiol. 13, 855756. doi: 10.3389/fphys.2022.855756
Verrier, R. L., Nearing, B. D., Olin, B., Boon, P., and Schachter, S. C. (2016). Baseline elevation and reduction in cardiac electrical instability assessed by quantitative T-wave alternans in patients with drug-resistant epilepsy treated with vagus nerve stimulation in the AspireSR E-36 trial. Epilepsy Behav. 62, 85–89. doi: 10.1016/j.yebeh.2016.06.016
Wang, F. B., and Powley, T. L. (2007). Vagal innervation of intestines: afferent pathways mapped with new en bloc horseradish peroxidase adaptation. Cell Tissue Res. 329, 221–230. doi: 10.1007/s00441-007-0413-7
Wang, H., Yu, M., Ochani, M., Amella, C. A., Tanovic, M., Susarla, S., et al. (2003). Nicotinic acetylcholine receptor alpha7 subunit is an essential regulator of inflammation. Nature 421, 384–388. doi: 10.1038/nature01339
Wang, J. Y., Zhang, Y., Chen, Y., Wang, Y., Li, S. Y., Wang, Y. F., et al. (2021). Mechanisms underlying antidepressant effect of transcutaneous auricular vagus nerve stimulation on CUMS model rats based on hippocampal alpha7nAchR/NF-kappaB signal pathway. J. Neuroinflammation. 18, 291. doi: 10.1186/s12974-021-02341-6
Wang, Y., Zhan, G., Cai, Z., Jiao, B., Zhao, Y., Li, S., et al. (2021). Vagus nerve stimulation in brain diseases: therapeutic applications and biological mechanisms. Neurosci. Biobehav. Rev. 127, 37–53. doi: 10.1016/j.neubiorev.2021.04.018
Wittbrodt, M. T., Gurel, N. Z., Nye, J. A., Shandhi, M. M. H., Gazi, A. H., Shah, A. J., et al. (2021). Noninvasive cervical vagal nerve stimulation alters brain activity during traumatic stress in individuals with posttraumatic stress disorder. Psychosom. Med. 83, 969–977. doi: 10.1097/PSY.0000000000000987
Young, A. H., Juruena, M. F., and De Zwaef Demyttenaere, R. (2020). Vagus nerve stimulation as adjunctive therapy in patients with difficult-to-treat depression (RESTORE-LIFE): study protocol design and rationale of a real-world post-market study. BMC Psychiatry 20, 471. doi: 10.1186/s12888-020-02869-6
Keywords: vagus nerve, vagus nerve stimulation, non-invasive VNS, neuroimmunomodulation, cholinergic anti-inflammatory pathway, inflammatory disease
Citation: Fang Y-T, Lin Y-T, Tseng W-L, Tseng P, Hua G-L, Chao Y-J and Wu Y-J (2023) Neuroimmunomodulation of vagus nerve stimulation and the therapeutic implications. Front. Aging Neurosci. 15:1173987. doi: 10.3389/fnagi.2023.1173987
Received: 25 February 2023; Accepted: 12 June 2023;
Published: 06 July 2023.
Edited by:
Vanessa Castelli, University of L'Aquila, ItalyReviewed by:
Stephanie Chee Yee Tjen-A-Looi, University of California, Irvine, United StatesCopyright © 2023 Fang, Lin, Tseng, Tseng, Hua, Chao and Wu. This is an open-access article distributed under the terms of the Creative Commons Attribution License (CC BY). The use, distribution or reproduction in other forums is permitted, provided the original author(s) and the copyright owner(s) are credited and that the original publication in this journal is cited, in accordance with accepted academic practice. No use, distribution or reproduction is permitted which does not comply with these terms.
*Correspondence: Yi-Jen Wu, d3V5akBtYWlsLm5ja3UuZWR1LnR3
†These authors have contributed equally to this work
Disclaimer: All claims expressed in this article are solely those of the authors and do not necessarily represent those of their affiliated organizations, or those of the publisher, the editors and the reviewers. Any product that may be evaluated in this article or claim that may be made by its manufacturer is not guaranteed or endorsed by the publisher.
Research integrity at Frontiers
Learn more about the work of our research integrity team to safeguard the quality of each article we publish.