- 1Vascular Cognitive Impairment and Neurodegeneration Program, Reynolds Oklahoma Center on Aging/Center for Geroscience and Healthy Brain Aging, Department of Neurosurgery, University of Oklahoma Health Sciences Center, Oklahoma City, OK, United States
- 2Stephenson Cancer Center, University of Oklahoma Health Sciences Center, Oklahoma City, OK, United States
- 3International Training Program in Geroscience, Doctoral School of Basic and Translational Medicine/Department of Public Health, Semmelweis University, Budapest, Hungary
- 4Department of Health Promotion Sciences, College of Public Health, University of Oklahoma Health Sciences Center, Oklahoma City, OK, United States
Transient receptor potential (TRP) proteins are part of a superfamily of polymodal cation channels that can be activated by mechanical, physical, and chemical stimuli. In the vascular endothelium, TRP channels regulate two fundamental parameters: the membrane potential and the intracellular Ca2+ concentration [(Ca2+)i]. TRP channels are widely expressed in the cerebrovascular endothelium, and are emerging as important mediators of several brain microvascular functions (e.g., neurovascular coupling, endothelial function, and blood–brain barrier permeability), which become impaired with aging. Aging is the most significant risk factor for vascular cognitive impairment (VCI), and the number of individuals affected by VCI is expected to exponentially increase in the coming decades. Yet, there are currently no preventative or therapeutic treatments available against the development and progression of VCI. In this review, we discuss the involvement of endothelial TRP channels in diverse physiological processes in the brain as well as in the pathogenesis of age-related VCI to explore future potential neuroprotective strategies.
1. Introduction
Age-related cognitive decline is a growing world health problem, which preferentially affects elderly people greater than the age of 60 years old. This unprecedented increase is caused by the continuous increase of life expectancy, and the consequent survival of a growing number of elderly individuals worldwide. Cognitive decline impairs memory, comprehension, learning capacity, and judgment, and has been demonstrated to be closely associated with several types of cerebrovascular pathologies, such as stroke and atherosclerosis. According to the World Health Organization (WHO), dementia is the seventh leading cause of death worldwide. It affected 55 million people in 2021, and is expected to reach 78 million in 2030 and 139 million in 2050 (World Health Organization, 2022). Notably, Alzheimer’s disease (AD) and vascular dementia (VaD) are, respectively, the first and second most common forms of dementia (Iadecola, 2013). In 1993, the term vascular cognitive impairment (VCI) was introduced to encompass any type of cognitive disease associated with cerebrovascular disorders, without considering the underlying mechanism; this terminology helped to emphasize the increasingly understood contribution of cerebrovascular function to cognitive health (Dichgans and Leys, 2017; Balasubramanian et al., 2020). Since then, it has been well accepted that cerebral microvascular dysfunction characterizes cognitive decline in neurodegenerative disorders, particularly in AD and VaD (Dichgans and Leys, 2017; Balasubramanian et al., 2020).
Brain endothelial cells (BECs) have lately emerged as important players in age-related neurogenesis, neuroinflammation and cognitive function (Chen et al., 2020). For instance, several chemical [e.g., neurotransmitters and reactive oxygen species (ROS)] and mechanical (e.g., shear stress) stimuli induce intracellular Ca2+ signals in BECs (Guerra et al., 2018; Zuccolo et al., 2018; Berra-Romani et al., 2019a,b; Negri et al., 2019). The subsequent increase in intracellular Ca2+ concentration [(Ca2+)i] leads to the production of multiple Ca2+-dependent vasoactive mediators, such as nitric oxide (NO), prostacyclin, prostaglandin H2, and thromboxane A2 (Guerra et al., 2018). Moreover, the increase in [Ca2+]i may activate intermediate and small-conductance Ca2+-dependent K+ channels (IK and SK, respectively), by inducing a membrane hyperpolarization that is conveyed to vascular smooth muscle cells through myoendothelial junctions (MEGJs), causing vasodilatation (Behringer, 2017; Garland and Dora, 2017). Notably, BECs, especially capillary BECs, present wide transcriptional and functional alterations in normal aging, by increasing innate immunity and oxidative stress response pathways. Moreover, it is well known that BECs’ Ca2+ machinery undergoes major changes following cerebrovascular damage in several cardiovascular disorders (e.g., AD and VaD; Iadecola, 2004, 2013; Cascella and Cecchi, 2021). Finally, by being the key constituent elements of the blood brain barrier (BBB), BECs are exposed to a wide range of circulatory proteins, mechanical stimuli, and chemical molecules from different compartments of the organism (Chen et al., 2020).
In this view, organisms have developed several strategies to sense environmental changes: one of which is represented by the superfamily of the transient receptor potential (TRP) channels. They are polymodal cation channels that are activated by mechanical, physical, and chemical stimuli, and regulate two fundamental endothelial parameters: the membrane potential and the [Ca2+]i (Samanta et al., 2018). The first TRP channel was discovered in a blind mutant of Drosophila in 1969 (Cosens and Manning, 1969), where the deletion of the trp gene caused impairment in the fly’s visual capacity and an alteration in its electrical response to light. Indeed, prolonged light exposure induced only a transient retinal depolarization in mutant drosophila photoreceptors, far from the normal steady-state depolarization recorded in wild-type flies (Earley and Brayden, 2015; Moran, 2018). The identity of the trp gene was unearthed several years later by Craig Montell and Gerald Rubin (Montell et al., 1985; Montell and Rubin, 1989). Six years later, three independent groups found TRP channel expression in vertebrates, and in particular, in mouse brains, xenopus oocytes (Petersen et al., 1995), and humans (Wes et al., 1995; Zhu et al., 1995). The first cloned mammalian TRP channel was denominated TRP Canonical 1 (TRPC1), due to its resemblance to the Drosophila TRP channel (Wes et al., 1995). Not surprisingly, the discovery of human TRPC1 started a 10 year long scientific race to identify other TRP channels. Nowadays, 28 members have been discovered, and are continuously studied in physiological and pathophysiological conditions as a promising therapeutic target for several neurological and cardiovascular disorders. TRP channels have emerged as important regulators of neurovascular functions, and their proper function is fundamental to maintain normal brain activity (Kuppusamy et al., 2021). In the last few decades, growing evidence highlights the importance of healthy lifestyle habits to prevent cardiovascular and neurovascular disorders typical of aging (Csipo et al., 2019; Balasubramanian et al., 2020). In this context, TRP channels have been tightly linked to some risk factors (e.g., obesity and diabetes) involved in the onset of these disorders (Moraes et al., 2021). It has been demonstrated that hyperglycemia and diabetes cause impaired endothelial-dependent vasodilatation(Makimattila et al., 1996) and endothelial dysfunction(Xu et al., 2003; Ren et al., 2017), which correlates with modifications in the expression and function of several TRP channels (Moraes et al., 2021). Moreover, it has long been known that the clearance of high levels of glucose and lipids in older adults is robustly decreased (Donato et al., 2015). Pathological increases in glucose and lipids cause oxidative stress and neuroinflammation, thereby leading to endothelial dysfunction typical of aging brains (Donato et al., 2015). This brings us to the last important issue: the role of dietary regimens (e.g., time restricted feeding and caloric restriction) in the prevention of VCI and cognitive decline (Csiszar et al., 2014; Balasubramanian et al., 2020). An appropriate fasting time may indeed resolve the acute metabolic insults (Donato et al., 2015), and similarly, specific food-derived compounds may ameliorate the aging-dependent vascular defects by targeting different TRP channels (Zhang et al., 2019). In this review, our focus will be on exploring the role of TRP channels in cerebrovascular mechanisms from various perspectives, with the aim of elucidating how targeted interventions could potentially enhance brain health and improve overall quality of life. Unlike traditional aging research which focused primarily on extending lifespan, modern aging research places a greater emphasis on improving the quality of life for individuals as they age.
2. TRP channels
2.1. Brief introduction
The TRP channel superfamily comprises 28 members subdivided in six subfamilies based on their sequence homology: TRP Canonical (TRPC1-7), TRP Mucolipin (TRPML1-3), TRP Ankyrin (TRPA1), TRP Melastatin (TRPM1-8), TRP Vanilloid (TRPV1-6), and TRP Polycystin (TRPP2, TRPP3, and TRPP5; Gees et al., 2010; Earley and Brayden, 2015; Smani et al., 2018). The TRP subunits consists of 553 to 2022 amino acids polypeptides (⁓ 64–230 kDa) organized in six transmembrane (TM1-6) α-helices with cytosolic NH2- and COOH-termini and a re-entrant loop between TM5 and TM6 (Negri et al., 2019). In particular, NH2- and COOH-extremities demonstrate very high variability in length and functions between the different subfamilies (Gaudet, 2008). For example: (i) TRPA, TRP, and TRPV NH2-terminals present a variable number of ankyrin repeats, which is important in sensing and gating (Gaudet, 2008); (ii) TRPC, TRPM, and TRPV present a 25-amino acid conserved element named TRP domain located distal to S6 and characterized by two highly conserved TRP-boxes, which delimit a more variable central region (Earley and Brayden, 2015); (iii) TRPM6 and TRPM7 COOH-terminals show an α-kinase domain that modify channel function (Zhang et al., 2014); (iv) TRPM2 COOH-extremity features an ADPR hydrolase domain (Nudix-like domain) (Scharenberg, 2005). Finally, (v) TRP channels may interact with multiple intracellular kinases and Ca2+-dependent sensors [i.e., Calmodulin (CaM) and Stromal Interacting Molecule 1 (STIM1)] due to the presence of CaM-inositol 1,4,5-trisphosphate receptors (InsP3R)-binding (CIRB) sites and Ca2+-binding EF hands domains at the COOH-extremity (Gaudet, 2008; Gees et al., 2010). Overall, the TRP channel’s structure is very similar to that of voltage-gated K+ channels, but TRP channels lack the series of charged amino acids in TM4, which explains their lower sensitivity to voltage changes. Moreover, the activation voltage-range is maintained at a non-physiological interval that is not usually experienced by the cell (Zheng, 2013). Notably, the TRP channel’s voltage response may be affected by other chemical and physical stimuli (e.g., pH variation, temperature, and agonists), which reconducts the voltage sensitivity to a physiological range (Voets et al., 2004). For instance, TRPV1 half-activation potential is +100 mV at room temperature; conversely, at 42°C, it decays to −50 mV, which is reachable by a sensory neuron (Voets et al., 2004). An analogous mechanism has also been characterized in TRPM8 (Voets et al., 2007) and TRPM4 and TRPM5 (Hofmann et al., 2003; Talavera et al., 2005).
The functional channel is composed of four subunits, which may aggregate into homomeric or heteromeric structures around a central pore delimitated by TM5, TM6, and the connecting re-entrant loop (Gaudet, 2008). TRP channels preferentially assemble into homomeric complexes; however, recent evidence demonstrates the presence of a large number of heteromeric channels in naive cells, including vascular endothelial cells (ECs; Zheng, 2013). Notably, heteromeric channels may be constituted by subunits from the same subfamily [e.g., TRPC1 aggregates with TRPC3, TRPC4 or TRPC5 (Smani et al., 2018)] or from different subfamilies [e.g., TRPC1/TRPP2 (Berrout et al., 2012) or TRPC1/TRPV4 complexes (Ma et al., 2011)].
2.2. Gating mechanisms and biophysical properties
Transient receptor potential channels are polymodal cation receptors that may be activated by multiple chemical and physical stimuli, including temperature variation, mechanical perturbation (e.g., laminar shear stress, and membrane stretch), exogenous dietary agonists (e.g., menthol, allyl isothiocyanate and capsaicin), hydrogen peroxide (H2O2), arachidonic acid (AA), intracellular ions (e.g., H+ and Ca2+), and finally, some TRP channels may be activated by the depletion of the endoplasmic reticulum (ER) Ca2+ store through interaction with STIM1 and Orai1, which are the main proteins involved in the activation of store-operated Ca2+ entry (SOCE)(Venkatachalam and Montell, 2007; Earley and Brayden, 2015; Ambudkar et al., 2017; Negri et al., 2019; Thakore and Earley, 2019). SOCE is mediated mainly by the Ca2+ release-activated Ca2+ (CRAC) channels, which comprises Orai1, and by the less selective store-operated Ca2+ (SOC) channels. After a long debate, TRPC1 is now considered a component of the SOC channels since it is involved in assembling the ternary complex with Orai1 and STIM1 (Jardin et al., 2008; Ambudkar et al., 2017).
Usually, TRP channels are defined as non-selective cation channels by driving both monovalent (i.e., Na+ and K+) and divalent (i.e., Ca2+ and Mg2+) cation fluxes, but their relative selectivity (PCa/PNa) ranges from TRPM4 and TRPM5, which are substantially impermeable to Ca2+ (PCa/PNa < 0.01), to TRPV5 and TRPV6, which show higher Ca2+ permeability (PCa/PNa > 100) (Smani et al., 2018). The remaining fraction of TRP channels feature an intermediate selectivity, and some of them present a peculiar permeability to Mg2+ (e.g., TRPM6 and TRPM7), H+ (e.g., TRPV1, TRPML1 and TRPP3), and metal ions (e.g., manganese, zinc, barium, strontium nickel and cobalt; Gees et al., 2010; Smani et al., 2018).
3. Age-related cerebrovascular changes
Aging has been demonstrated to alter brain vascular structure and morphology (Fulop et al., 2019). Fortunately, several vascular mechanisms involved in cognitive decline are partially reversible and serve as potential therapeutic targets in aging-related cognitive disorders (Fulop et al., 2019). The structural and functional alterations of cerebral vasculature may cause ischemia, blood–brain barrier disruption, cerebral blood flow (CBF) modification, rarefaction of vasculature, increased neuroinflammation, and impaired neurovascular coupling, which, in turn, have a robust impact on the proper function of the brain (Tarantini et al., 2017; Csipo et al., 2019; Fulop et al., 2019). Notably, age-related changes in brain vasculature may derive from endothelial dysfunction (Donato et al., 2015), which are characterized by impaired endothelial-dependent dilatation (Lesniewski et al., 2009), angiogenesis (Zhuo et al., 2010), permeability (Pelegri et al., 2007), and fibrinolysis (Yamamoto et al., 2002). In addition, growing evidence recognizes the importance of oxidative stress and inflammation in endothelial functions, such as bioenergetics and mitochondrial functions (Donato et al., 2015). Finally, as mentioned above, the clearance of elevated levels of glucose and lipids is reduced in aging and is associated with acute endothelial dysfunction (Marchesi et al., 2000; Rudolph et al., 2007). It has long been known that Ca2+ homeostasis is crucial in the physiological functioning of cerebral microvascular endothelial cells (Stoica et al., 2021). For instance, an alteration in normal Ca2+ trafficking may cause mitochondrial dysfunction followed by ROS production and ATP depletion (Hong et al., 2020). In this context, TRP channels are characterized by a double role: they mediate extracellular Ca2+ entry and some isoforms are sensitive to ROS (Negri et al., 2019). For example, the accumulation of Aβ deposits, typically seen in AD, close to BECs may induce TRPM2-mediated intracellular Ca2+ signals in BECs, thereby triggering oxidative stress and modification of BBB permeability (Park et al., 2014; Mantzavinos and Alexiou, 2017). Similarly, TRP channel expression and TRP-mediated Ca2+ signals in BBB are modified in traumatic brain injury or stroke (Yang et al., 2019).
For all these reasons, TRP channels are important mediators of several microvascular functions and may be important tools in the prevention of aging-related cognitive deficits (Kuppusamy et al., 2021). Herein, we provide an overview of the TRP channels-dependent molecular mechanisms involved in aging and specifically in the modification of endothelial cells’ activity, highlighting their possible therapeutic role.
4. TRP channels in endothelial cells
4.1. TRP channels expression in BECs
The endothelium is an active cell layer found along all the vascular compartments. Similar to any other tissue, it can become dysfunctional, which may be caused by intrinsic and extrinsic factors (Kwan et al., 2007). Several vascular functions are regulated by endothelial cells, and specifically, by ROS-induced and Ca2+-dependent mechanisms (e.g., EDH, vascular permeability, and angiogenesis; Negri et al., 2021).
Transient receptor potential channels are widely express in the brain, where they are key players in the integration of several chemical and physical stimuli (Wang et al., 2020). In particular, a major fraction of TRP channels have been found in ECs (i.e., TRPC1-7; TRPV1-4; TRPA1; TRPP1-2, and TRPM1-4/6–8), but only 11 of them have been demonstrated to have a functional role in the vasculature (Thakore and Earley, 2019). Not surprisingly, TRP channel expression varies throughout the vascular tree and between different species. Furthermore, conflicting works reported that the same endothelial cell type presented different TRP expression patterns in vitro, supporting the hypothesis of a reorganization of ion channel and receptor expression depending on culture conditions, which is worsened by increasing passages of the cultured cells (Earley and Brayden, 2015; Negri et al., 2019). However, the TRP expression in ECs has been demonstrated in vivo, both in homomeric (e.g., TRPC1-6) and heteromeric (e.g., TRPC1-TRPC4; TRPV1-TRPV4; TRPC1-TRPP2-TRPV4) assembly (Negri et al., 2019).
Specifically, TRPA1 (Sullivan et al., 2015; Pires and Earley, 2018; Thakore et al., 2021), TRPM2 (Park et al., 2014), TRPP2 (Berrout et al., 2012), and TRPC3 (Kochukov et al., 2014) are expressed and have a functional role in mouse BECs. Furthermore, Luo and collaborators investigated TRPV1-4 expression in brain ECs from humans and rats and found significant differences. For instance, both human primary cultured brain microvascular cerebral ECs (BMECs) and brain microvascular endothelial cell line hCMEC/D3 (which models the human BBB, can be easily grown, and is amenable to cellular and molecular studies on cerebrovascular pathology), express more TRPV2 compared to the other isoforms. Conversely, rat cells mainly feature the TRPV4 isoform, followed by TRPV2, TRPV3, and TRPV1 in decreasing frequency (Luo et al., 2020). Herein, we focus our attention on TRP channels in BECs by highlighting their role in aging-related disorders.
4.2. Cerebral endothelial cell functions mediated by TRP channels
Endothelial TRP channels regulate EC functions by mediating extracellular Ca2+ influx and membrane potential variations in response to several chemical and physical stimuli, as reported in previous paragraphs (Sundivakkam et al., 2013). The increase in [Ca2+]i activates multiple signaling pathways and cellular functions. For example, TRPV4 (Zhang et al., 2009), TRPP2 (Berrout et al., 2012), and TRPM2(Park et al., 2014) channels are involved in the modulation of the vascular tone, thereby mediating endothelial nitric oxide synthase (eNOS) activation and the subsequent NO release in mice BECs.
On the other hand, TRP-mediated cation influx (e.g., Na+ and Ca2+) results in membrane depolarization or in activation of K+ channels, inducing membrane hyperpolarization (Earley and Brayden, 2015; Smani et al., 2018; Negri et al., 2019). For instance, it has been long known that TRPC3/6 and TRPM4 have a role in vascular smooth muscular cell depolarization. Conversely, TRPV4-driven Ca2+ entry activates Ca2+-dependent K+ channels that cause the hyperpolarization of vascular smooth muscle cells (Earley et al., 2005; Smani et al., 2018; Negri et al., 2019). Recently, it has been demonstrated by different groups that the interplay between TRP channels and the Ca2+-sensitive K+ channels is also present in ECs, and is called the endothelium-dependent hyperpolarization (EDH) mechanism (Feletou and Vanhoutte, 2006a; Longden et al., 2017). In this context, TRPA1 induces endothelium-dependent vasodilatation and smooth muscular cell membrane hyperpolarization in mouse cerebral arteries (Earley et al., 2009), by initiating the molecular mechanism in the deepest region of the capillary network (Thakore et al., 2021). Likewise, TRPV4 (Liu et al., 2011; Zhang et al., 2013; Harraz et al., 2018), TRPC3 (Marrelli et al., 2003), and TRPV3 (Earley, 2011; Pires et al., 2015) channels interact with endothelial Ca2+-sensitive K+ channels and inward-rectifying K+ (KIR) channels, thereby causing the onset of the EDH mechanism.
In addition, TRP channels are important in vascular permeability modulation (e.g., TRPV4, TRPV1, TRPC1, TRPC4, TRPC6, and TRPM2) by regulating cell–cell adhesions and endothelial shape variations driven by the cytoskeleton recently reviewed by Genova and collaborators (Genova et al., 2020). It is particularly important if we consider the central role of the BBB in the physical brain, serving as protection from systemic inflammation (Duarte-Delgado et al., 2019). Finally, TRP channels are involved in neuroinflammation, which is closely related to vascular permeability. In this context, TRPV1, TRPV4, TRPA1, and TRPM2 show anti-inflammatory properties, while TRPC1, TRPC3, and TRPC6 have pro-inflammatory activities (Thakore and Earley, 2019).
5. TRP channels in cerebrovascular diseases
5.1. Endothelial dysfunction and oxidative stress
In paragraph 4.2, we have already mentioned the role of BEC TRP channels in vascular smooth muscle contraction/dilatation and NO availability. An alteration in this fragile equilibrium is the source of neurovascular pathologies, such as hypertension and atherosclerosis (Goligorsky, 2000; Feletou and Vanhoutte, 2006b). Notably, aging-induced cerebral endothelium dysfunction causes disorders in cerebral blood supply that is worsened by the typical increase in ROS production present in VCI (Iadecola, 2013; Balasubramanian et al., 2020). It has long been known that some TRP channels (e.g., TRPC3, TRPC4, TRPM2, TRPM7, and TRPA1) may be activated by oxidative stress (Hecquet et al., 2008; Wong and Yao, 2011). Regarding brain endothelial dysfunction, the extracellular accumulation of Aβ typically seen in AD induces oxidative stress in BECs, which activates the DNA repair enzyme poly-ADPR polymerase in cultured mouse brain endothelial cells (Park et al., 2014). The production of poly-ADPR activates TRPM2 channel, thereby inducing an endothelial Ca2+ overload, endothelial dysfunction, and neurovascular impairment (Park et al., 2014). Furthermore, endothelial TRPA1 channels play a crucial role in CBF regulation at the artery, arteriole, and capillary level (Kuppusamy et al., 2021). For instance, TRPA1 is activated by superoxide anions generated by the NADPH oxidase isoform 2 (NOX2) enzyme. Interestingly, TRPA1 and NOX2 colocalize only in cerebral endothelium. Moreover, endothelial TRPA1 has been shown to be activated by ROS and 4-hydroxy-2-nonenal (4-HNE), which may be produced by lipid peroxidation or released by astrocytes and neurons during neuronal activity (Earley et al., 2009; Sullivan et al., 2015). Herein, TRPA1 mediates vasodilation by activating IK and SK channels, and inwardly rectifying K+ (KIR) channels and by inducing the EDH mechanism. Thereafter, cerebral endothelial TRPA1 channels may be activated by mitochondrial ROS in mouse pial arteries and parenchymal arterioles under hypoxic conditions (Pires and Earley, 2018). These data show that TRPA1 is a valuable candidate for oxidative stress sensing, and similarly to TRPM,2 an alteration in TRPA1 functioning may be a source of Ca2+ overloading in BECs, causing consequent brain endothelial dysfunction.
5.2. TRP channels in neurovascular coupling
Neurovascular coupling is the mechanism whereby an increase in neuronal activity leads to an increase in CBF to ensure local supply of oxygen and nutrients to the activated areas (Iadecola, 2017). In this context, during the first Stroke Progress Review Group meeting of the National Institute of Neurological Disorders and Stroke of the NIH (2001), the concept of the neurovascular unit (NVU), or rather the functional and anatomical complex composed by ECs, basal lamina, pericytes, smooth muscular cells and neuronal cells (i.e., astrocytes, neurons, and interneurons), emerged (Iadecola, 2017; Guerra et al., 2018). NVC impairment in age-related neurodegenerative disorders has been demonstrated again and again in pre-clinical (Tarantini et al., 2021a,b) and clinical studies (Toth et al., 2022; Zhang et al., 2022) in AD and VaD (Iadecola, 2004; Iadecola, 2013). Notably, NVC is mediated by both vasoactive molecules (e.g., NO and prostacyclin) and by EDH (Iadecola, 2017), and as reported in the previous paragraphs, endothelial TRP channels have a fundamental role in these mechanisms. For instance, TRPV4 activation by arachidonic acid metabolites [e.g., 5,6 epoxyeicosatrienoic acids (5,6-EET), 8,9-EET and 11,12-EET] mediates an increase in [Ca2+]i that activates phospholipase A2 and induces EDH-driven vasodilatation in rat middle cerebral arteries (You et al., 2002; Marrelli et al., 2007). Moreover, TRPV4-dependet Ca2+ signals trigger IK and SK-mediated EDH mechanism in rodent cortical arterioles in response to ATP (You et al., 2002; Liu et al., 2011) and in mouse posterior cerebral arteries in response to acetylcholine (Zhang et al., 2013). Notably, TRPV4-mediated dilation in mouse pial arteries is impaired in the mouse model of AD (Zhang et al., 2013); likewise, TRPV4 is shown to be fundamental in parenchymal arteriolar dilation and cognitive function in hypertension (Diaz-Otero et al., 2018, 2019). Furthermore, a recent investigation by Nelson’s group demonstrated that TRPV4-mediated Ca2+ influx, enhanced by intracellular Ca2+ release from the endoplasmic reticulum through InsP3Rs, causes a robust NO release in the arteriolar-capillary transitional zone, thereby stimulating pericyte relaxation. This mechanism results in focal dilation limited to the branch region with Ca2+ signals and thus in close proximity to the neuronal activity site (Longden et al., 2021). The TRPV4 channel is also expressed in capillary BECs (Longden et al., 2017; Figure 1), where it mediates Ca2+-dependent eNOS activation and the consequent NO release in human brain microvascular endothelial cell line (hCMEC/D3; Berra-Romani et al., 2019a). Interestingly, capillary ECs lack SK and IK channels (Longden et al., 2017), and indeed, TRPV4 causes endothelial depolarization by conducting a Ca2+ influx. However, recently, endothelial KIR2.1 channels have emerged as important players in CBF regulation since, unlike IK and SK channels, they are expressed in capillary BECs. For instance, Harraz et al. have reported that Gq protein-coupled receptor (GqPCR)-mediated phosphatidylinositol 4,5-bisphosphate (PIP2) hydrolysis activated TRPV4 channels and simultaneously inhibited KIR2.1 channel activity in mouse capillary BECs (Harraz et al., 2018). Several GqPCR agonists (e.g., acetylcholine, ATP, and PGE2) are released by astrocytes and neurons during NA (Harraz et al., 2018), and they may be fundamental in endothelial membrane repolarization after the onset of the hyperpolarizing signal. Indeed, they act by inhibiting KIR channels, and consequently, reduce the retrograde propagation of the electric signal by activating TRPV4-dependent endothelial depolarization (Harraz et al., 2018).
Another important TRP channel involved in NVC is TRPA1 channel, expressed in rat cerebral arteries-MEGJs, where it co-localizes with IK and SK channels and participates to the onset of the EDH phenomenon (Sullivan et al., 2015). For instance, the dietary compound allyl isothiocyanate (AITC) selectively activates TRPA1, and the consequent IK opening that induces vasodilation in rat cerebral arteries (Earley et al., 2009; Qian et al., 2013). Furthermore, endothelial TRPA1 is responsible for neuronal activity sensing and NVC initiation in deep brain capillaries through a ROS-dependent mechanism (Thakore et al., 2021). Herein, 4-HNE-induced TRPA1 activation elicits an increase in [Ca2+]i, which is propagated to the upstream arterioles through the Ca2+-dependent ATP release via pannexin 1 (Panx1; Thakore et al., 2021). ATP, in turn, binds to P2X receptors in adjacent cells, thereby spreading the Ca2+ wave until it reaches the arterioles. Herein, the chemical signal is converted into the hyperpolarizing electric signal by IK and SK channels (Pires and Earley, 2018; Alvarado et al., 2021). Likewise, EDH generation may be induced by TRPC3 and TRPV3 in cerebral arteries (Earley and Brayden, 2015). TRPC3 is stimulated by the phospholipase C product Diacylglycerol (DAG; Negri et al., 2019), and is responsible for the ATP-induced hyperpolarization and consequent vasodilatation in mouse middle cerebral arteries and posterior cerebral arteries through a TRPA1-like mechanism. Specifically, IK channels drive the initial phase of endothelial hyperpolarization, and conversely, SK channels sustain the following delayed hyperpolarization phase (Kochukov et al., 2014). Likewise, TRPV3 elicits IK and SK channels opening and triggers EDH in rat isolated posterior cerebral and superior cerebellar arteries, but, unlike TRPA1, it is mainly localized on the endothelial membrane and not in MEGJs (Earley, 2011; Pires et al., 2015). Of note, TRPV3-dependent Ca2+ signals were significantly higher compared to other TRP channels (e.g., three times bigger than TRPV4-induced and 1.5 times bigger than TRPA1-induced Ca2+ sparklets). Finally, Berrout and collaborators showed that stretch-induced Ca2+ signals and NO production in mouse BMECs were significantly reduced by TRPC1 and TRPP2 pharmacological and genetic manipulation (Berrout et al., 2012), suggesting an additional activation mechanism of TRP channels involved in endothelial NO-dependent vasodilation.
5.3. BBB disruption and neuroinflammation
The BBB is a specialized vasculature that separates the brain from peripheral circulation. The BBB presents a limited permeability to macromolecules and peripheral immune cells by exerting a protective effect against neuroinflammation (Chen et al., 2020). The NVU is responsible for the formation and maintenance of the BBB10, of which malfunction and disruption have been shown to be involved in age-related neurodegeneration(Iadecola, 2013; Kerkhofs et al., 2021; Towner et al., 2021; Montagne et al., 2022) in several neuropsychiatric, vascular, metabolic, and immunologic diseases(Luo et al., 2020). Notably, BBB integrity is regulated by BECs calcium signals, which may be largely mediated by TRP channels (Negri et al., 2019). For instance, TRPV channels, and especially TRPV2 in humans and TRPV4 in rodents, have been demonstrated to have a major role in BBB integrity (Luo et al., 2020). Indeed, the potent TRPV2 antagonist cannabidiol (CBD) prevents the BBB disruption induced by oxygen glucose deprivation (OGD) in a human in vitro model of BBB, consisting of human BMECs and human astrocytes coculture (Hind et al., 2016). A subsequent work showed that CBD-induced TRPV2 activation modulates human BBB permeability defined by transendothelial electrical resistance (TEER; Luo et al., 2019). On the other hand, TRPV4 influences mouse BBB permeability by sensing the osmotic changes and BMECs volume alteration (Brown et al., 2008). In this context, TRPV4 activation may assume a role in driving Ca2+ entry in ECs and regulating BBB functions (Cig et al., 2021). For instance, Friese’s group has shown an amelioration of BBB transendothelial resistance after TRPV4 pharmacological inhibition in mouse BMECs (Rosenkranz et al., 2020). This effect is abrogated by the TRPV4 downregulation with interferon-γ and tumor necrosis factor-α. Moreover, in vivo treatment did not prevent BBB dysfunction in a TRPV4 knock-out mouse model of multiple sclerosis or in MCAO/R-induced brain damage (Rosenkranz et al., 2020). Accordingly, Jie and collaborators have demonstrated that the injection of the potent TRPV4 agonist, GSK1016790A, caused BBB disruption in mice. Notably, the effect of GSK1016790 is prevented by co-injecting the TRPV4 antagonist HC-067047(Jie et al., 2015). Taken together, these data demonstrate that TRPV4 regulates BBB integrity upon different non-physiological conditions (Rosenkranz et al., 2020). Regarding TRPV1, it has been demonstrated to be scarcely expressed in BECs; however, the co-culture of human primary BMECs with primary astrocytes from the same patient increased TRPV1 expression by 4.8-fold (Luo et al., 2020), which confirms the importance of the cellular interplay within the NVU. Early work from Zygmunt and collaborators have shown that anandamide-dependent TRPV1 activation reduced human BBB permeability, thereby stimulating the release of the vasodilator neuropeptide, calcitonin gene-related peptide (CGRP; Zygmunt et al., 1999; Hind et al., 2016). However, subsequent investigations have demonstrated the opposite role of TRPV1 in BBB permeability regulation (Hu et al., 2005; Yang et al., 2019). An earlier work has shown that the potent TRPV1 agonist, capsaicin, induced an increase in BBB permeability in an ex vivo rat model (Hu et al., 2005). Therefore, recently, Yang and collaborators have provided the evidence that TRPV1 inhibition with the specific blocker capsazepine, avoids BBB disruption in an in vivo mouse model of TBI (Yang et al., 2019).
Transient receptor potential conical channels have also been correlated to BBB function maintenance (Li and Ehrich, 2013; Ryu et al., 2013; Kuppusamy et al., 2021). Multiple studies have put forward TRPC6 as a regulator of endothelial permeability. For instance, DAG-induced TRPC6-mediated Ca2+ entry stimulates the Ras homolog family member A (RhoA), the following myosin light chain-dependent EC shape variation, and the raising gap formation between adjacent cells (Singh et al., 2007; Figure 1). Furthermore, the TRPC3 channel is overexpressed in the rat brain’s piriform cortex ECs in status epilepticus. TRPC3 channel upregulation reduces the expression of SMI-71, a rat endothelial BBB antigen correlated with BBB disruption and neuronal damage (Ryu et al., 2013). Conversely, the insecticide Chlorpyrifos reduces TRPC4 channel expression by inducing BBB disruption in Sprague–Dawley rats (Li and Ehrich, 2013). Moreover, there is an emerging theory regarding the protein interaction between TRP channels and scaffold proteins. In this view, TRP channels may interact with caveolin-1, which is overexpressed after an ischemic insult, thereby leading a major BBB permeability. Caveolin-1 is an important structural protein of the endothelial caveolae, which are essential elements in neurovascular coupling (Chow et al., 2020). It has been shown to anchor eNOS, which, in turn, is regulated by TRP channels activation (Goligorsky et al., 2002; Daneva et al., 2021). However, further investigations are needed to understand the molecular interaction and interdependence of these three proteins in the context of neurovascular coupling and cerebral blood flow regulation. In summary, these data demonstrate that TRP channels are important modulators of BBB permeability.
5.4. TRP channels in ischemic brain damage
Elderly individuals are particularly at risk for stroke; in the United States alone, it is estimated that strokes kill one person every 4 min (Shekhar et al., 2021; Kaucsar et al., 2022). Recently, Zhu’s group reviewed the role of TRPC channel during ischemic episodes (Jeon et al., 2020). The major studies are conducted on middle cerebral artery occlusion/reperfusion (MCAO/R) in an in vivo rat model of ischemic stroke with middle carotid artery occlusion followed by reperfusion. Likewise, multiple groups have performed oxygen glucose deprivation/re-oxygenation (OGD/R) assays on in vitro models of ischemia/reperfusion, which mimic neuronal death induced by oxygen and glucose deprivation followed by re-oxygenation. These investigations are then combined with additional in vivo evaluations of TRPC knock-out mice or with the in vitro pharmacological and genetic manipulation of TRP channels. Of note, TRPC3, TRPC4, TRPC6, and TRPC7 have been shown to play a key role in ischemic brain damage (Jeon et al., 2020). Although, a controversial result from Xu et al. have demonstrated that genetic deletion of TRPC1 ameliorates OGD/R-induced neuronal death (Xu et al., 2018), it seems that TRPC1 has a protective role in ischemic damage by reducing the production of ROS. Conversely, ROS-dependent TRPA1 activation results in the limitation of ischemic brain damage (Pires and Earley, 2018; Alvarado et al., 2021). For instance, hypoxia promotes mitochondrial ROS production followed by lipid peroxidation and TRPA1-mediated vasodilation in cerebral pial arteries and intraparenchymal arterioles (Pires and Earley, 2018). This could result from the angiogenic function of TRPC5, which dampens injury-induced inflammation. Indeed, TRPC5 has been reported to promote endothelial cell sprouting, angiogenesis, and blood perfusion in ischemic tissues through activation of nuclear factor of activated T cell (NFAT) isoform c3 and angiopoietin 1(Zhu et al., 2019; Figure 1).
Transient receptor potential vanilloid channels may also interfere with ischemic episodes through a controversial TRPV1 role in this process. On the one hand, pre-ischemia treatment with capsaicin, a selective TRPV1 channel agonist, protects the Mongolian gerbil’s brain from global ischemia (Pegorini et al., 2005). Accordingly, Khatibi and collaborators have confirmed the neuroprotective effect of TRPV1 channel in another rat model of brain ischemia. Herein, capsaicin pre-treatment reduced the infarcted area and normalized vessel reactivity (Khatibi et al., 2011). On the other hand, more recent investigation demonstrates that post-ischemia TRPV1 inhibition limited neuronal damage by decreasing toll-like receptor 2 (TLR2) and TLR4 (Hakimizadeh et al., 2017), which are usually upregulated after brain ischemia and modulate inflammation and neuronal death (Lehnardt et al., 2007). Accordingly, TRPV1 inhibition has been demonstrated to have a neuroprotective role during brain ischemia in mice, and its expression is enhanced post-stroke (Luo et al., 2020). In addition, CBD elicited a protective effect in human BMECs upon OGD, but the investigation did not demonstrate the direct activation of TRPV2 in BMECs (Hind et al., 2016). Likewise, the pharmacological inhibition of TRPV4 with HC-067047 ameliorates BBB disruption in rats with focal cerebral ischemia and reperfusion (Xie and Lu, 2018). TRPV4 inhibition or genetic deletion is also responsible for reduced BBB disruption after intracerebral hemorrhage in rats, which is reputed to be a fatal stroke subtype (Zhao et al., 2018). Finally, TRPM4 is found to be upregulated in vascular endothelium in the penumbra region of a rat model with permanent middle cerebral artery occlusion (Loh et al., 2014). In addition, the genetic silencing of TRPM4 increases angiogenesis and capillary integrity in the same animal model (Loh et al., 2014). Based on the reported data, further work is needed to clarify some roles of TRP channels (e.g., TRPC1 and TRPV1) in ischemic episodes. Nevertheless, they are likely to be promising therapeutic targets to treat stroke-induced brain damage.
6. TRP channels as promising targets in the treatment in age-related cerebrovascular disorders
Previous paragraphs underlined the important role of endothelial TRP channels in the onset of microvasculature defects linked to cognitive decline (summarized in Table 1). As already mentioned, with these conditions, the pathological environment is characterized by the presence of higher levels of ROS and dysregulated Ca2+ homeostasis (Hong et al., 2020). The major studies done to understand the involvement of TRP channels in neurodegenerative disorders have been done on TRP-KO mice and by using TRP channels agonists and antagonists. In this view, we can delineate the role of TRP channels in several neurological diseases, but we cannot rule out their specific involvement in the malfunction of different cell types that are impaired in the disorders (Hong et al., 2020; Koivisto et al., 2022). For instance, Jang et al. have demonstrated that genetic elimination and pharmacological inhibition of TRPA1 in mice brain had a neuroprotective role on stroke-derived hypoxia and reduced myelin damage (Hamilton et al., 2016). On the contrary, TRPV1-dependent hypothermia has been shown to reduce stroke volume by 50% and increase the post-ischemia recovery in mice (Cao et al., 2017). Regarding cognitive decline, Borbély and collaborators have revealed that aging Trpa1−/− mice exhibited an improved memory (Borbely et al., 2019). In addition, TRPA1 antagonism may be a potential therapeutic target for AD-associated seizures (Payrits et al., 2020). Similarly, triple-transgenic AD mouse model KO for Trpv1 showed an ameliorated memory function (Kim et al., 2020). Finally, a recent work by Thapak and collaborators showed that Tranilast, a powerful TRPV2 inhibitor, ameliorates cognitive impairment in a rat model of AD, delineating TRPV2 as a potential therapeutic target for AD137.
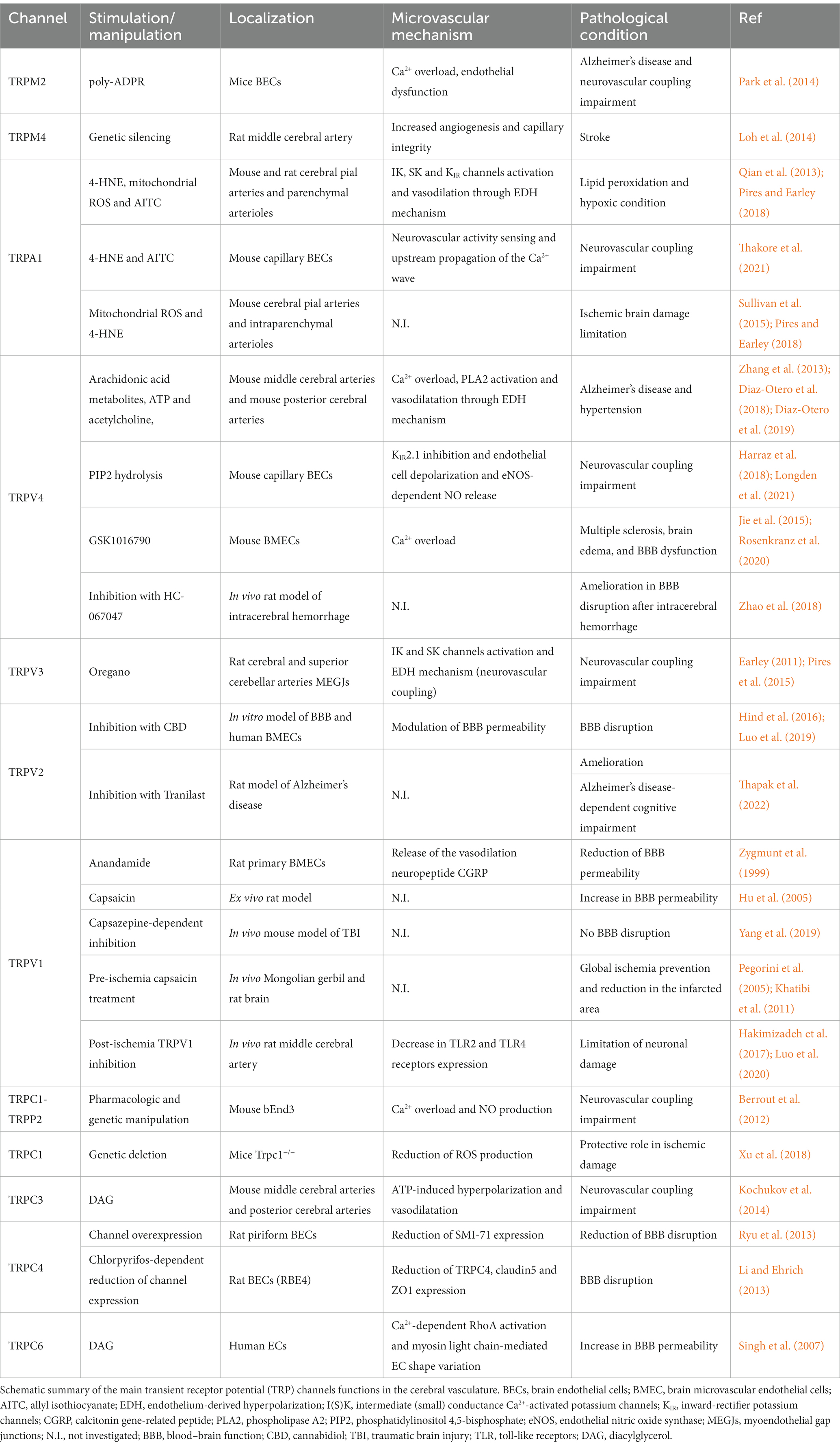
Table 1. Endothelial transient receptor potential (TRP) channels involved in cerebrovascular functions.
In this review, we have clearly shown the importance of TRP channels in microvasculature mechanisms. Nevertheless, there are not specific therapies targeting endothelial TRP channels currently. As mentioned above, the main problem is to selectively silence or activate TRP channels on endothelial cells without affecting other cell types. An elegant example of TRP stimulation characterized by temporal and spatial precision is represented by the optical stimulation of endothelial colony forming cells (ECFCs) plated on a light-sensitive organic semiconductor (poly(3-hexylthiophene-2,5-diyl), P3HT)(Lodola et al., 2019; Negri et al., 2022). Intriguingly, the authors have demonstrated that the optical stimulation of P3HT thin films can induce the Ca2+-dependent increase of ECFCs proliferation and tubulogenesis in vitro through the ROS-dependent TRPV1 activation (Lodola et al., 2019; Negri et al., 2022). In addition, the two investigations have shown that the role of ROS in the phototransduction mechanism is important if we consider the fact that several ROS mechanisms(?) may be activated by nontoxic ROS levels (Negri et al., 2019). Notably, organic semiconductors thin films may be used as implantable patches to stimulate the region of interest, or conversely, they may be engineered as nanoparticles and function to selectively target endothelial cells, avoiding the previously mentioned problem of non-specificity.
Finally, several works highlight the importance of different nutrition regimens (e.g., caloric restriction and time restricted feeding) in the improvement of aging-related neurovascular disorders (Csiszar et al., 2014; Balasubramanian et al., 2020; Oikawa et al., 2021; Dobreva et al., 2022; Maroto-Rodriguez et al., 2022). In this context, TRP channels are intriguing molecular elements, since they may be activated by several dietary compounds (Negri et al., 2019). Several food-derived molecules stimulate TRPV channels, such as: (i) capsaicin and piperine from pepper(Kobata et al., 1999), eugenol from cloves(Yang et al., 2003) and gingerol from ginger(Kim et al., 2016) activate TRPV1; (ii) eugenol and carvacrol from oregano stimulate TRPV3(Xu et al., 2006); and (iii) apigenin (Ma et al., 2012) and bisandrographolide A target TRPV4(Akbar, 2011). Apigenin is a plant-derived flavone that stimulates endothelial TRPV4 to ameliorate hypertension (Ma et al., 2012). Additionally, TRPV4 activity is modulated by dietary ω-3 polyunsaturated fatty acids intake, ω-3 eicosanoid epoxide derivatives are required for TRPV4 function in worm neurons, and eicosapentaenoic acid enhances TRPV4 activity in human endothelial cells (Caires et al., 2017). Besides TRPV channels, TRPA1 may also be bound by different reactive electrophiles, including allyl isothiocyanate that is present in mustard oil and pungent vegetables (e.g., cauliflower, radish, wasabi, and cabbage; Appendino et al., 2008); aldehyde cinnamaldehyde from cinnamon (Bandell et al., 2004); and polygodial that is obtained from different varieties of pepper (Escalera et al., 2008). Finally, also TRPC channels activated by DAG (e.g., TRPC3, TRPC6, and TRPC7) may be influenced by a lipid diet, which introduces many edible fats and lipids (e.g., Olive oil and nuts) rich in DAG (Trebak et al., 2007). Although there are several pieces of evidence about TRP channels and food-derived molecules, the field remains controversial, requiring a lot more work to understand the effective role of these relationships in several cardio- and neurovascular disease.
7. Conclusion
Recent evidence in both animal models and humans, which has been highlighted in this review, suggest that TRPs in the brain cerebrovasculature are one of the key cellular mechanisms that mediate age-related endothelial dysfunction, leading to poor cognitive outcomes in elderly individuals. Endothelial TRP channels are not only key players in physiological and pathological vascular functions thanks to their ability to sense a wide spectrum of chemical and physical stimuli (Negri et al., 2019), but also are emerging as fundamental regulators of endothelial-dependent dilation, vascular permeability, and neuroinflammation. This is particularly interesting in age-related microvascular disorders, such as VCI, and related neurodegenerative diseases, such as dementia, which are characterized by alterations in cerebral vascular functions (Balasubramanian et al., 2020). There is evidence that TRP channels in the cerebrovascular system can be modulated by both endogenous and exogenous agents. This suggests that targeting TRP channel activity through dietary and pharmacological interventions may be a viable approach for restoring cerebrovascular function. Lifestyle interventions, such as dietary regimens, dietary compounds, and exercise, have been shown to improve health span, and could be beneficial for targeting TRP channels in the treatment of age-related VCI and related dementias. Specifically, endothelial TRP channels may be promising targets for dietary and pharmacological studies. Further research is needed to investigate the effects of dietary compounds, such as oregano, pepper, and green tea, on brain endothelial TRP channels.
Author contributions
SN, MS, and ST contributed substantially to the conception or design of the manuscript. SN, MS, and ST contributed to the generating, drafting, and revising the intellectual content and provided approval for publication of the content. HS contibuted in generating, drafting, and revising the intellectual content and provided approval for publication of the content All authors contributed to the article and approved the submitted version.
Funding
This work was supported by grants from the National Institute on Aging (NIA R03AG070479 and NIA K01AG073614 to ST), the American Heart Association AHA CDA941290 to ST, the NIA-supported Geroscience Training Program in Oklahoma (T32AG052363), the NIA-supported Oklahoma Nathan Shock Center, the NIGMS supported Center of Biomedical Research Excellence (CoBRE) (1P20GM125528-01A1), and the NCI Cancer Center Support Grant (P30 CA225520) and the Oklahoma Tobacco Settlement Endowment Trust.
Conflict of interest
The authors declare that the research was conducted in the absence of any commercial or financial relationships that could be construed as a potential conflict of interest.
Publisher’s note
All claims expressed in this article are solely those of the authors and do not necessarily represent those of their affiliated organizations, or those of the publisher, the editors and the reviewers. Any product that may be evaluated in this article, or claim that may be made by its manufacturer, is not guaranteed or endorsed by the publisher.
References
Akbar, S. (2011). Andrographis paniculata: a review of pharmacological activities and clinical effects. Altern. Med. Rev. 16, 66–77.
Alvarado, M. G., Thakore, P., and Earley, S. (2021). Transient receptor potential channel Ankyrin 1: a unique regulator of vascular function. Cells 10:1167. doi: 10.3390/cells10051167
Ambudkar, I. S., de Souza, L. B., and Ong, H. L. (2017). TRPC1, Orai1, and STIM1 in SOCE: friends in tight spaces. Cell Calcium 63, 33–39. doi: 10.1016/j.ceca.2016.12.009
Appendino, G., Minassi, A., Pagani, A., and Ech-Chahad, A. (2008). The role of natural products in the ligand deorphanization of TRP channels. Curr. Pharm. Des. 14, 2–17. doi: 10.2174/138161208783330781
Balasubramanian, P., DelFavero, J., Ungvari, A., Papp, M., Tarantini, A., Price, N., et al. (2020). Time-restricted feeding (TRF) for prevention of age-related vascular cognitive impairment and dementia. Ageing Res. Rev. 64:101189. doi: 10.1016/j.arr.2020.101189
Bandell, M., Story, G. M., Hwang, S. W., Viswanath, V., Eid, S. R., Petrus, M. J., et al. (2004). Noxious cold ion channel TRPA1 is activated by pungent compounds and bradykinin. Neuron 41, 849–857. doi: 10.1016/S0896-6273(04)00150-3
Behringer, E. J. (2017). Calcium and electrical signaling in arterial endothelial tubes: new insights into cellular physiology and cardiovascular function. Microcirculation 24:e12328. doi: 10.1111/micc.12328
Berra-Romani, R., Faris, P., Negri, S., Botta, L., Genova, T., and Moccia, F. (2019a). Arachidonic acid evokes an increase in intracellular ca(2+) concentration and nitric oxide production in endothelial cells from human brain microcirculation. Cells 8:689. doi: 10.3390/cells8070689
Berra-Romani, R., Faris, P., Pellavio, G., Orgiu, M., Negri, S., Forcaia, G., et al. (2019b). Histamine induces intracellular ca(2+) oscillations and nitric oxide release in endothelial cells from brain microvascular circulation. J. Cell. Physiol. 235, 1515–1530. doi: 10.1002/jcp.29071
Berrout, J., Jin, M., and O'Neil, R. G. (2012). Critical role of TRPP2 and TRPC1 channels in stretch-induced injury of blood-brain barrier endothelial cells. Brain Res. 1436, 1–12. doi: 10.1016/j.brainres.2011.11.044
Borbely, E., Payrits, M., Hunyady, A., Mezo, G., and Pinter, E. (2019). Important regulatory function of transient receptor potential ankyrin 1 receptors in age-related learning and memory alterations of mice. Geroscience 41, 643–654. doi: 10.1007/s11357-019-00083-1
Brown, R. C., Wu, L., Hicks, K., and O'Neil, R. G. (2008). Regulation of blood-brain barrier permeability by transient receptor potential type C and type v calcium-permeable channels. Microcirculation 15, 359–371. doi: 10.1080/10739680701762656
Caires, R., Sierra-Valdez, F. J., Millet, J. R. M., Herwig, J. D., Roan, E., Vasquez, V., et al. (2017). Omega-3 fatty acids modulate TRPV4 function through plasma membrane remodeling. Cell Rep. 21, 246–258. doi: 10.1016/j.celrep.2017.09.029
Cao, Z., Balasubramanian, A., Pedersen, S. E., Romero, J., Pautler, R. G., and Marrelli, S. P. (2017). TRPV1-mediated pharmacological hypothermia promotes improved functional recovery following ischemic stroke. Sci. Rep. 7:17685. doi: 10.1038/s41598-017-17548-y
Cascella, R., and Cecchi, C. (2021). Calcium Dyshomeostasis in Alzheimer's disease pathogenesis. Int. J. Mol. Sci. 22:4914. doi: 10.3390/ijms22094914
Chen, M. B., Yang, A. C., Yousef, H., Lee, D., Chen, W., Schaum, N., et al. (2020). Brain endothelial cells are exquisite sensors of age-related circulatory cues. Cell Rep. 30, 4418–4432.e4. doi: 10.1016/j.celrep.2020.03.012
Chow, B. W., Nunez, V., Kaplan, L., Granger, A. J., Bistrong, K., Zucker, H. L., et al. (2020). Caveolae in CNS arterioles mediate neurovascular coupling. Nature 579, 106–110. doi: 10.1038/s41586-020-2026-1
Cig, B., Derouiche, S., and Jiang, L. H. (2021). Editorial: emerging roles of TRP channels in brain pathology. Front. Cell Dev. Biol. 9:705196. doi: 10.3389/fcell.2021.705196
Cosens, D. J., and Manning, A. (1969). Abnormal electroretinogram from a drosophila mutant. Nature 224, 285–287. doi: 10.1038/224285a0
Csipo, T., Lipecz, A., Fulop, G. A., Hand, R. A., Ngo, B. N., Dzialendzik, M., et al. (2019). Age-related decline in peripheral vascular health predicts cognitive impairment. Geroscience 41, 125–136. doi: 10.1007/s11357-019-00063-5
Csiszar, A., Gautam, T., Sosnowska, D., Tarantini, S., Banki, E., Tucsek, Z., et al. (2014). Caloric restriction confers persistent anti-oxidative, pro-angiogenic, and anti-inflammatory effects and promotes anti-aging miRNA expression profile in cerebromicrovascular endothelial cells of aged rats. Am. J. Physiol. Heart Circ. Physiol. 307, H292–H306. doi: 10.1152/ajpheart.00307.2014
Daneva, Z., Marziano, C., Ottolini, M., Chen, Y. L., Baker, T. M., Kuppusamy, M., et al. (2021). Caveolar peroxynitrite formation impairs endothelial TRPV4 channels and elevates pulmonary arterial pressure in pulmonary hypertension. Proc. Natl. Acad. Sci. U. S. A. 118:e2023130118. doi: 10.1073/pnas.2023130118
Diaz-Otero, J. M., Yen, T. C., Ahmad, A., Laimon-Thomson, E., Abolibdeh, B., Kelly, K., et al. (2019). Transient receptor potential vanilloid 4 channels are important regulators of parenchymal arteriole dilation and cognitive function. Microcirculation 26:12535. doi: 10.1111/micc.12535
Diaz-Otero, J. M., Yen, T. C., Fisher, C., Bota, D., Jackson, W. F., and Dorrance, A. M. (2018). Mineralocorticoid receptor antagonism improves parenchymal arteriole dilation via a TRPV4-dependent mechanism and prevents cognitive dysfunction in hypertension. Am. J. Physiol. Heart Circ. Physiol. 315, 1304–1315.
Dichgans, M., and Leys, D. (2017). Vascular Cognitive Impairment. Circ. Res. 120, 573–591. doi: 10.1161/CIRCRESAHA.116.308426
Dobreva, I., Marston, L., and Mukadam, N. (2022). Which components of the Mediterranean diet are associated with dementia? A UK biobank cohort study. Geroscience 44, 2541–2554. doi: 10.1007/s11357-022-00615-2
Donato, A. J., Morgan, R. G., Walker, A. E., and Lesniewski, L. A. (2015). Cellular and molecular biology of aging endothelial cells. J. Mol. Cell. Cardiol. 89, 122–135. doi: 10.1016/j.yjmcc.2015.01.021
Duarte-Delgado, N. P., Vasquez, G., and Ortiz-Reyes, B. L. (2019). Blood-brain barrier disruption and neuroinflammation as pathophysiological mechanisms of the diffuse manifestations of neuropsychiatric systemic lupus erythematosus. Autoimmun. Rev. 18, 426–432. doi: 10.1016/j.autrev.2018.12.004
Earley, S. (2011). Endothelium-dependent cerebral artery dilation mediated by transient receptor potential and Ca2+−activated K+ channels. J. Cardiovasc. Pharmacol. 57, 148–153. doi: 10.1097/FJC.0b013e3181f580d9
Earley, S., and Brayden, J. E. (2015). Transient receptor potential channels in the vasculature. Physiol. Rev. 95, 645–690. doi: 10.1152/physrev.00026.2014
Earley, S., Gonzales, A. L., and Crnich, R. (2009). Endothelium-dependent cerebral artery dilation mediated by TRPA1 and Ca2+-activated K+ channels. Circ. Res. 104, 987–994. doi: 10.1161/CIRCRESAHA.108.189530
Earley, S., Heppner, T. J., Nelson, M. T., and Brayden, J. E. (2005). TRPV4 forms a novel Ca2+ signaling complex with ryanodine receptors and BKCa channels. Circ. Res. 97, 1270–1279. doi: 10.1161/01.RES.0000194321.60300.d6
Escalera, J., von Hehn, C. A., Bessac, B. F., Sivula, M., and Jordt, S. E. (2008). TRPA1 mediates the noxious effects of natural sesquiterpene deterrents. J. Biol. Chem. 283, 24136–24144. doi: 10.1074/jbc.M710280200
Feletou, M., and Vanhoutte, P. M. (2006a). Endothelium-derived hyperpolarizing factor: where are we now? Arterioscler. Thromb. Vasc. Biol. 26, 1215–1225. doi: 10.1161/01.ATV.0000217611.81085.c5
Feletou, M., and Vanhoutte, P. M. (2006b). Endothelial dysfunction: a multifaceted disorder (the Wiggers award lecture). Am. J. Physiol. Heart Circ. Physiol. 291, H985–H1002. doi: 10.1152/ajpheart.00292.2006
Fulop, G. A., Tarantini, S., Yabluchanskiy, A., Molnar, A., Prodan, C. I., Kiss, T., et al. (2019). Role of age-related alterations of the cerebral venous circulation in the pathogenesis of vascular cognitive impairment. Am J Physiol-Heart C. 316, H1124–H1140. doi: 10.1152/ajpheart.00776.2018
Garland, C. J., and Dora, K. A. (2017). EDH: endothelium-dependent hyperpolarization and microvascular signalling. Acta Physiol (Oxford) 219, 152–161. doi: 10.1111/apha.12649
Gaudet, R. (2008). TRP channels entering the structural era. J. Physiol. 586, 3565–3575. doi: 10.1113/jphysiol.2008.155812
Gees, M., Colsoul, B., and Nilius, B. (2010). The role of transient receptor potential cation channels in Ca2+ signaling. Cold Spring Harb. Perspect. Biol. 2:a003962. doi: 10.1101/cshperspect.a003962
Genova, T., Gaglioti, D., and Munaron, L. (2020). Regulation of vessel permeability by TRP channels. Front. Physiol. 11:421. doi: 10.3389/fphys.2020.00421
Goligorsky, M. S. (2000). Endothelial cell dysfunction and nitric oxide synthase. Kidney Int. 58, 1360–1376. doi: 10.1046/j.1523-1755.2000.00292.x
Goligorsky, M. S., Li, H., Brodsky, S., and Chen, J. (2002). Relationships between caveolae and eNOS: everything in proximity and the proximity of everything. Am. J. Physiol. Ren. Physiol. 283, F1–F10. doi: 10.1152/ajprenal.00377.2001
Guerra, G., Lucariello, A., Perna, A., Botta, L., De Luca, A., and Moccia, F. (2018). The role of endothelial ca(2+) signaling in neurovascular coupling: a view from the lumen. Int. J. Mol. Sci. 19:938. doi: 10.3390/ijms19040938
Hakimizadeh, E., Shamsizadeh, A., Roohbakhsh, A., Arababadi, M. K., Hajizadeh, M. R., Shariati, M., et al. (2017). TRPV1 receptor-mediated expression of toll-like receptors 2 and 4 following permanent middle cerebral artery occlusion in rats. Iran. J. Basic Med. Sci. 20, 863–869. doi: 10.22038/IJBMS.2017.9107
Hamilton, N. B., Kolodziejczyk, K., Kougioumtzidou, E., and Attwell, D. (2016). Proton-gated ca(2+)-permeable TRP channels damage myelin in conditions mimicking ischaemia. Nature 529, 523–527. doi: 10.1038/nature16519
Harraz, O. F., Longden, T. A., Dabertrand, F., Hill-Eubanks, D., and Nelson, M. T. (2018). Endothelial GqPCR activity controls capillary electrical signaling and brain blood flow through PIP2 depletion. Proc. Natl. Acad. Sci. U. S. A. 115, 3569–3577. doi: 10.1073/pnas.1800201115
Harraz, O. F., Longden, T. A., Hill-Eubanks, D., and Nelson, M. T. (2018). PIP2 depletion promotes TRPV4 channel activity in mouse brain capillary endothelial cells. elife 7:e38689. doi: 10.7554/eLife.38689
Hecquet, C. M., Ahmmed, G. U., Vogel, S. M., and Malik, A. B. (2008). Role of TRPM2 channel in mediating H2O2-induced Ca2+ entry and endothelial hyperpermeability. Circ. Res. 102, 347–355. doi: 10.1161/CIRCRESAHA.107.160176
Hind, W. H., England, T. J., and O'Sullivan, S. E. (2016). Cannabidiol protects an in vitro model of the blood-brain barrier from oxygen-glucose deprivation via PPARgamma and 5-HT1A receptors. Br. J. Pharmacol. 173, 815–825. doi: 10.1111/bph.13368
Hofmann, T., Chubanov, V., Gudermann, T., and Montell, C. (2003). TRPM5 is a voltage-modulated and ca(2+)-activated monovalent selective cation channel. Curr. Biol. 13, 1153–1158. doi: 10.1016/S0960-9822(03)00431-7
Hong, C., Jeong, B., Park, H. J., Chung, J. Y., Lee, J. E., Kim, J., et al. (2020). TRP channels as emerging therapeutic targets for neurodegenerative diseases. Front. Physiol. 11:238. doi: 10.3389/fphys.2020.00238
Hu, D. E., Easton, A. S., and Fraser, P. A. (2005). TRPV1 activation results in disruption of the blood-brain barrier in the rat. Br. J. Pharmacol. 146, 576–584. doi: 10.1038/sj.bjp.0706350
Iadecola, C. (2004). Neurovascular regulation in the normal brain and in Alzheimer's disease. Nat. Rev. Neurosci. 5, 347–360. doi: 10.1038/nrn1387
Iadecola, C. (2013). The pathobiology of vascular dementia. Neuron 80, 844–866. doi: 10.1016/j.neuron.2013.10.008
Iadecola, C. (2017). The neurovascular unit coming of age: a journey through neurovascular coupling in health and disease. Neuron 96, 17–42. doi: 10.1016/j.neuron.2017.07.030
Jardin, I., Lopez, J. J., Salido, G. M., and Rosado, J. A. (2008). Orai1 mediates the interaction between STIM1 and hTRPC1 and regulates the mode of activation of hTRPC1-forming Ca2+ channels. J. Biol. Chem. 283, 25296–25304. doi: 10.1074/jbc.M802904200
Jeon, J., Bu, F., Sun, G., Tian, J. B., Ting, S. M., Li, J., et al. (2020). Contribution of TRPC channels in neuronal excitotoxicity associated with neurodegenerative disease and ischemic stroke. Front. Cell Dev. Biol. 8:618663. doi: 10.3389/fcell.2020.618663
Jie, P., Tian, Y., Hong, Z., Li, L., Zhou, L., Chen, L., et al. (2015). Blockage of transient receptor potential vanilloid 4 inhibits brain edema in middle cerebral artery occlusion mice. Front. Cell. Neurosci. 9:141. doi: 10.3389/fncel.2015.00141
Kaucsar, T., Roka, B., Tod, P., Do, P. T., Hegedus, Z., Szenasi, G., et al. (2022). Divergent regulation of lncRNA expression by ischemia in adult and aging mice. Geroscience 44, 429–445. doi: 10.1007/s11357-021-00460-9
Kerkhofs, D., Wong, S. M., Zhang, E., Uiterwijk, R., Hoff, E. I., Jansen, J. F. A., et al. (2021). Blood-brain barrier leakage at baseline and cognitive decline in cerebral small vessel disease: a 2-year follow-up study. Geroscience 43, 1643–1652. doi: 10.1007/s11357-021-00399-x
Khatibi, N. H., Jadhav, V., Charles, S., Chiu, J., Buchholz, J., Tang, J., et al. (2011). Capsaicin pre-treatment provides neurovascular protection against neonatal hypoxic-ischemic brain injury in rats. Acta Neurochir. Suppl. 111, 225–230. doi: 10.1007/978-3-7091-0693-8_38
Kim, Y. S., Hong, C. S., Lee, S. W., Nam, J. H., and Kim, B. J. (2016). Effects of ginger and its pungent constituents on transient receptor potential channels. Int. J. Mol. Med. 38, 1905–1914. doi: 10.3892/ijmm.2016.2791
Kim, J., Lee, S., Kim, J., Ham, S., Park, J. H. Y., Han, S., et al. (2020). Ca2+−permeable TRPV1 pain receptor knockout rescues memory deficits and reduces amyloid-beta and tau in a mouse model of Alzheimer's disease. Hum. Mol. Genet. 29, 228–237. doi: 10.1093/hmg/ddz276
Kobata, K., Sutoh, K., Todo, T., Yazawa, S., Iwai, K., and Watanabe, T. (1999). Nordihydrocapsiate, a new capsinoid from the fruits of a nonpungent pepper, capsicum annuum. J. Nat. Prod. 62, 335–336. doi: 10.1021/np9803373
Kochukov, M. Y., Balasubramanian, A., Abramowitz, J., Birnbaumer, L., and Marrelli, S. P. (2014). Activation of endothelial transient receptor potential C3 channel is required for small conductance calcium-activated potassium channel activation and sustained endothelial hyperpolarization and vasodilation of cerebral artery. J. Am. Heart Assoc. 3:e000913. doi: 10.1161/JAHA.114.000913
Koivisto, A. P., Belvisi, M. G., Gaudet, R., and Szallasi, A. (2022). Advances in TRP channel drug discovery: from target validation to clinical studies. Nat. Rev. Drug Discov. 21, 41–59. doi: 10.1038/s41573-021-00268-4
Kuppusamy, M., Ottolini, M., and Sonkusare, S. K. (2021). Role of TRP ion channels in cerebral circulation and neurovascular communication. Neurosci. Lett. 765:136258. doi: 10.1016/j.neulet.2021.136258
Kwan, H. Y., Huang, Y., and Yao, X. (2007). TRP channels in endothelial function and dysfunction. Biochim. Biophys. Acta 1772, 907–914. doi: 10.1016/j.bbadis.2007.02.013
Lehnardt, S., Lehmann, S., Kaul, D., Tschimmel, K., Hoffmann, O., Cho, S., et al. (2007). Toll-like receptor 2 mediates CNS injury in focal cerebral ischemia. J. Neuroimmunol. 190, 28–33. doi: 10.1016/j.jneuroim.2007.07.023
Lesniewski, L. A., Connell, M. L., Durrant, J. R., Folian, B. J., Anderson, M. C., Donato, A. J., et al. (2009). B6D2F1 mice are a suitable model of oxidative stress-mediated impaired endothelium-dependent dilation with aging. J. Gerontol. A Biol. Sci. Med. Sci. 64, 9–20. doi: 10.1093/gerona/gln049
Li, W., and Ehrich, M. (2013). Transient alterations of the blood-brain barrier tight junction and receptor potential channel gene expression by chlorpyrifos. J. Appl. Toxicol. 33, 1187–1191. doi: 10.1002/jat.2762
Liu, X., Li, C., Gebremedhin, D., Hwang, S. H., Hammock, B. D., Falck, J. R., et al. (2011). Epoxyeicosatrienoic acid-dependent cerebral vasodilation evoked by metabotropic glutamate receptor activation in vivo. Am. J. Physiol. Heart Circ. Physiol. 301, H373–H381. doi: 10.1152/ajpheart.00745.2010
Lodola, F., Rosti, V., Tullii, G., Desii, A., Tapella, L., Catarsi, P., et al. (2019). Conjugated polymers optically regulate the fate of endothelial colony-forming cells. Sci. Adv. 5:9. doi: 10.1126/sciadv.aav4620
Loh, K. P., Ng, G., Yu, C. Y., Fhu, C. K., Yu, D., Vennekens, R., et al. (2014). TRPM4 inhibition promotes angiogenesis after ischemic stroke. Pflugers Arch. 466, 563–576. doi: 10.1007/s00424-013-1347-4
Longden, T. A., Dabertrand, F., Koide, M., Gonzales, A. L., Tykocki, N. R., Brayden, J. E., et al. (2017). Capillary K(+)-sensing initiates retrograde hyperpolarization to increase local cerebral blood flow. Nat. Neurosci. 20, 717–726. doi: 10.1038/nn.4533
Longden, T. A., Mughal, A., Hennig, G. W., Harraz, O. F., Shui, B., Lee, F. K., et al. (2021). Local IP3 receptor-mediated ca(2+) signals compound to direct blood flow in brain capillaries. Sci. Adv. 7:eabh0101. doi: 10.1126/sciadv.abh0101
Luo, H., Rossi, E., Saubamea, B., Chasseigneaux, S., Cochois, V., Choublier, N., et al. (2019). Cannabidiol increases proliferation, migration, Tubulogenesis, and integrity of human brain endothelial cells through TRPV2 activation. Mol. Pharm. 16, 1312–1326. doi: 10.1021/acs.molpharmaceut.8b01252
Luo, H., Saubamea, B., Chasseigneaux, S., Cochois, V., Smirnova, M., Glacial, F., et al. (2020). Molecular and functional study of transient receptor potential Vanilloid 1-4 at the rat and human blood-brain barrier reveals interspecies differences. Front. Cell Dev. Biol. 8:578514. doi: 10.3389/fcell.2020.578514
Ma, X., Cheng, K. T., Wong, C. O., O'Neil, R. G., Birnbaumer, L., Ambudkar, I. S., et al. (2011). Heteromeric TRPV4-C1 channels contribute to store-operated ca(2+) entry in vascular endothelial cells. Cell Calcium 50, 502–509. doi: 10.1016/j.ceca.2011.08.006
Ma, X., He, D., Ru, X., Chen, Y., Cai, Y., Bruce, I. C., et al. (2012). Apigenin, a plant-derived flavone, activates transient receptor potential vanilloid 4 cation channel. Br. J. Pharmacol. 166, 349–358. doi: 10.1111/j.1476-5381.2011.01767.x
Makimattila, S., Virkamaki, A., Groop, P. H., Cockcroft, J., Utriainen, T., Fagerudd, J., et al. (1996). Chronic hyperglycemia impairs endothelial function and insulin sensitivity via different mechanisms in insulin-dependent diabetes mellitus. Circulation 94, 1276–1282. doi: 10.1161/01.CIR.94.6.1276
Mantzavinos, V., and Alexiou, A. (2017). Biomarkers for Alzheimer's disease diagnosis. Curr. Alzheimer Res. 14, 1149–1154. doi: 10.2174/1567205014666170203125942
Marchesi, S., Lupattelli, G., Schillaci, G., Pirro, M., Siepi, D., Roscini, A. R., et al. (2000). Impaired flow-mediated vasoactivity during post-prandial phase in young healthy men. Atherosclerosis 153, 397–402. doi: 10.1016/S0021-9150(00)00415-9
Maroto-Rodriguez, J., Delgado-Velandia, M., Ortola, R., Carballo-Casla, A., Garcia-Esquinas, E., Rodriguez-Artalejo, F., et al. (2022). Plant-based diets and risk of frailty in community-dwelling older adults: the seniors-ENRICA-1 cohort. Geroscience 45, 221–232. doi: 10.1007/s11357-022-00614-3
Marrelli, S. P., Eckmann, M. S., and Hunte, M. S. (2003). Role of endothelial intermediate conductance KCa channels in cerebral EDHF-mediated dilations. Am. J. Physiol. Heart Circ. Physiol. 285, H1590–H1599. doi: 10.1152/ajpheart.00376.2003
Marrelli, S. P., O'Neil, R. G., Brown, R. C., and Bryan, R. M. Jr. (2007). PLA2 and TRPV4 channels regulate endothelial calcium in cerebral arteries. Am. J. Physiol. Heart Circ. Physiol. 292, H1390–H1397. doi: 10.1152/ajpheart.01006.2006
Montagne, A., Barnes, S. R., Nation, D. A., Kisler, K., Toga, A. W., and Zlokovic, B. V. (2022). Imaging subtle leaks in the blood-brain barrier in the aging human brain: potential pitfalls, challenges, and possible solutions. Geroscience 44, 1339–1351. doi: 10.1007/s11357-022-00571-x
Montell, C., Jones, K., Hafen, E., and Rubin, G. (1985). Rescue of the Drosophila phototransduction mutation trp by germline transformation. Science 230, 1040–1043. doi: 10.1126/science.3933112
Montell, C., and Rubin, G. M. (1989). Molecular characterization of the drosophila trp locus: a putative integral membrane protein required for phototransduction. Neuron 2, 1313–1323. doi: 10.1016/0896-6273(89)90069-X
Moraes, R. D. A., Webb, R. C., and Silva, D. F. (2021). Vascular dysfunction in diabetes and obesity: focus on TRP channels. Front. Physiol. 12:645109. doi: 10.3389/fphys.2021.645109
Moran, M. M. (2018). TRP channels as potential drug targets. Annu. Rev. Pharmacol. Toxicol. 58, 309–330. doi: 10.1146/annurev-pharmtox-010617-052832
Negri, S., Faris, P., Berra-Romani, R., Guerra, G., and Moccia, F. (2019). Endothelial transient receptor potential channels and vascular remodeling: extracellular ca(2 +) entry for angiogenesis, Arteriogenesis and Vasculogenesis. Front. Physiol. 10:1618. doi: 10.3389/fphys.2019.01618
Negri, S., Faris, P., and Moccia, F. (2021). Reactive oxygen species and endothelial ca(2+) signaling: brothers in arms or Partners in Crime? Int. J. Mol. Sci. 22:9821. doi: 10.3390/ijms22189821
Negri, S., Faris, P., Pellavio, G., Botta, L., Orgiu, M., Forcaia, G., et al. (2019). Group 1 metabotropic glutamate receptors trigger glutamate-induced intracellular ca(2+) signals and nitric oxide release in human brain microvascular endothelial cells. Cell. Mol. Life Sci. 77, 2235–2253. doi: 10.1007/s00018-019-03284-1
Negri, S., Faris, P., Tullii, G., Vismara, M., Pellegata, A. F., Lodola, F., et al. (2022). Conjugated polymers mediate intracellular Ca2+ signals in circulating endothelial colony forming cells through the reactive oxygen species-dependent activation of transient receptor potential Vanilloid 1 (TRPV1). Cell Calcium 101:102502. doi: 10.1016/j.ceca.2021.102502
Oikawa, S. Y., Brisbois, T. D., van Loon, L. J. C., and Rollo, I. (2021). Eat like an athlete: insights of sports nutrition science to support active aging in healthy older adults. Geroscience 43, 2485–2495. doi: 10.1007/s11357-021-00419-w
Park, L., Wang, G., Moore, J., Girouard, H., Zhou, P., Anrather, J., et al. (2014). The key role of transient receptor potential melastatin-2 channels in amyloid-beta-induced neurovascular dysfunction. Nat. Commun. 5:5318. doi: 10.1038/ncomms6318
Payrits, M., Borbely, E., Godo, S., Ernszt, D., Kemeny, A., Kardos, J., et al. (2020). Genetic deletion of TRPA1 receptor attenuates amyloid beta-1-42 (a beta(1-42))-induced neurotoxicity in the mouse basal forebrain in vivo. Mech. Ageing Dev. 189:111268. doi: 10.1016/j.mad.2020.111268
Pegorini, S., Braida, D., Verzoni, C., Guerini-Rocco, C., Consalez, G. G., Croci, L., et al. (2005). Capsaicin exhibits neuroprotective effects in a model of transient global cerebral ischemia in Mongolian gerbils. Br. J. Pharmacol. 144, 727–735. doi: 10.1038/sj.bjp.0706115
Pelegri, C., Canudas, A. M., del Valle, J., Casadesus, G., Smith, M. A., Camins, A., et al. (2007). Increased permeability of blood-brain barrier on the hippocampus of a murine model of senescence. Mech. Ageing Dev. 128, 522–528. doi: 10.1016/j.mad.2007.07.002
Petersen, C. C., Berridge, M. J., Borgese, M. F., and Bennett, D. L. (1995). Putative capacitative calcium entry channels: expression of drosophila trp and evidence for the existence of vertebrate homologues. Biochem. J. 311, 41–44. doi: 10.1042/bj3110041
Pires, P. W., and Earley, S. (2018). Neuroprotective effects of TRPA1 channels in the cerebral endothelium following ischemic stroke. elife 7:e35316. doi: 10.7554/eLife.35316
Pires, P. W., Sullivan, M. N., Pritchard, H. A., Robinson, J. J., and Earley, S. (2015). Unitary TRPV3 channel Ca2+ influx events elicit endothelium-dependent dilation of cerebral parenchymal arterioles. Am. J. Physiol. Heart Circ. Physiol. 309, H2031–H2041. doi: 10.1152/ajpheart.00140.2015
Qian, X., Francis, M., Solodushko, V., Earley, S., and Taylor, M. S. (2013). Recruitment of dynamic endothelial Ca2+ signals by the TRPA1 channel activator AITC in rat cerebral arteries. Microcirculation 20, 138–148. doi: 10.1111/micc.12004
Ren, X., Ren, L., Wei, Q., Shao, H., Chen, L., and Liu, N. (2017). Advanced glycation end-products decreases expression of endothelial nitric oxide synthase through oxidative stress in human coronary artery endothelial cells. Cardiovasc. Diabetol. 16:52. doi: 10.1186/s12933-017-0531-9
Rosenkranz, S. C., Shaposhnykov, A., Schnapauff, O., Epping, L., Vieira, V., Heidermann, K., et al. (2020). TRPV4-mediated regulation of the blood brain barrier is abolished during inflammation. Front. Cell Dev. Biol. 8:849. doi: 10.3389/fcell.2020.00849
Rudolph, T. K., Ruempler, K., Schwedhelm, E., Tan-Andresen, J., Riederer, U., Boger, R. H., et al. (2007). Acute effects of various fast-food meals on vascular function and cardiovascular disease risk markers: the Hamburg burger trial. Am. J. Clin. Nutr. 86, 334–340. doi: 10.1093/ajcn/86.2.334
Ryu, H. J., Kim, J. E., Kim, Y. J., Kim, J. Y., Kim, W. I., Choi, S. Y., et al. (2013). Endothelial transient receptor potential conical channel (TRPC)-3 activation induces vasogenic edema formation in the rat piriform cortex following status epilepticus. Cell. Mol. Neurobiol. 33, 575–585. doi: 10.1007/s10571-013-9931-x
Samanta, A., Hughes, T. E. T., and Moiseenkova-Bell, V. Y. (2018). Transient receptor potential (TRP) channels. Subcell. Biochem. 87, 141–165. doi: 10.1007/978-981-10-7757-9_6
Scharenberg, A. M. (2005). TRPM2 and TRPM7: channel/enzyme fusions to generate novel intracellular sensors. Pflugers Arch. 451, 220–227. doi: 10.1007/s00424-005-1444-0
Shekhar, S., Liu, Y., Wang, S., Zhang, H., Fang, X., Zhang, J., et al. (2021). Novel mechanistic insights and potential therapeutic impact of TRPC6 in neurovascular coupling and ischemic stroke. Int. J. Mol. Sci. 22:2074. doi: 10.3390/ijms22042074
Singh, I., Knezevic, N., Ahmmed, G. U., Kini, V., Malik, A. B., and Mehta, D. (2007). Galphaq-TRPC6-mediated Ca2+ entry induces RhoA activation and resultant endothelial cell shape change in response to thrombin. J. Biol. Chem. 282, 7833–7843. doi: 10.1074/jbc.M608288200
Smani, T., Gomez, L. J., Regodon, S., Woodard, G. E., Siegfried, G., Khatib, A. M., et al. (2018). TRP channels in angiogenesis and other endothelial functions. Front. Physiol. 9:1731. doi: 10.3389/fphys.2018.01731
Stoica, R., Rusu, C. M., Staicu, C. E., Burlacu, A. E., Radu, M., and Radu, B. M. (2021). Ca(2+) homeostasis in brain microvascular endothelial cells. Int. Rev. Cell Mol. Biol. 362, 55–110. doi: 10.1016/bs.ircmb.2021.01.001
Sullivan, M. N., Gonzales, A. L., Pires, P. W., Bruhl, A., Leo, M. D., Li, W., et al. (2015). Localized TRPA1 channel Ca2+ signals stimulated by reactive oxygen species promote cerebral artery dilation. Sci. Signal. 8:2. doi: 10.1126/scisignal.2005659
Sundivakkam, P. C., Natarajan, V., Malik, A. B., and Tiruppathi, C. (2013). Store-operated Ca2+ entry (SOCE) induced by protease-activated receptor-1 mediates STIM1 protein phosphorylation to inhibit SOCE in endothelial cells through AMP-activated protein kinase and p38beta mitogen-activated protein kinase. J. Biol. Chem. 288, 17030–17041. doi: 10.1074/jbc.M112.411272
Talavera, K., Yasumatsu, K., Voets, T., Droogmans, G., Shigemura, N., Ninomiya, Y., et al. (2005). Heat activation of TRPM5 underlies thermal sensitivity of sweet taste. Nature 438, 1022–1025. doi: 10.1038/nature04248
Tarantini, S., Balasubramanian, P., Yabluchanskiy, A., Ashpole, N. M., Logan, S., Kiss, T., et al. (2021a). IGF1R signaling regulates astrocyte-mediated neurovascular coupling in mice: implications for brain aging. Geroscience 43, 901–911. doi: 10.1007/s11357-021-00350-0
Tarantini, S., Nyul-Toth, A., Yabluchanskiy, A., Csipo, T., Mukli, P., Balasubramanian, P., et al. (2021b). Endothelial deficiency of insulin-like growth factor-1 receptor (IGF1R) impairs neurovascular coupling responses in mice, mimicking aspects of the brain aging phenotype. Geroscience 43, 2387–2394. doi: 10.1007/s11357-021-00405-2
Tarantini, S., Tran, C. H. T., Gordon, G. R., Ungvari, Z., and Csiszar, A. (2017). Impaired neurovascular coupling in aging and Alzheimer's disease: contribution of astrocyte dysfunction and endothelial impairment to cognitive decline. Exp. Gerontol. 94, 52–58. doi: 10.1016/j.exger.2016.11.004
Thakore, P., Alvarado, M. G., Ali, S., Mughal, A., Pires, P. W., Yamasaki, E., et al. (2021). Brain endothelial cell TRPA1 channels initiate neurovascular coupling. elife. 10: e63040 doi: 10.7554/eLife.63040
Thakore, P., and Earley, S. (2019). Transient receptor potential channels and endothelial cell calcium signaling. Compr. Physiol. 9, 1249–1277. doi: 10.1002/cphy.c180034
Thapak, P., Bishnoi, M., and Sharma, S. S. (2022). Tranilast, a transient receptor potential Vanilloid 2 channel (TRPV2) inhibitor attenuates amyloid beta-induced cognitive impairment: possible mechanisms. NeuroMol. Med. 24, 183–194. doi: 10.1007/s12017-021-08675-x
Toth, L., Czigler, A., Hegedus, E., Komaromy, H., Amrein, K., Czeiter, E., et al. (2022). Age-related decline in circulating IGF-1 associates with impaired neurovascular coupling responses in older adults. Geroscience 44, 2771–2783. doi: 10.1007/s11357-022-00623-2
Towner, R. A., Gulej, R., Zalles, M., Saunders, D., Smith, N., Lerner, M., et al. (2021). Rapamycin restores brain vasculature, metabolism, and blood-brain barrier in an inflammaging model. Geroscience 43, 563–578. doi: 10.1007/s11357-021-00363-9
Trebak, M., Lemonnier, L., Smyth, J. T., Vazquez, G., and Putney, J. W. Jr. (2007). Phospholipase C-coupled receptors and activation of TRPC channels. Handb. Exp. Pharmacol. 179, 593–614. doi: 10.1007/978-3-540-34891-7_35
Venkatachalam, K., and Montell, C. (2007). TRP channels. Annu. Rev. Biochem. 76, 387–417. doi: 10.1146/annurev.biochem.75.103004.142819
Voets, T., Droogmans, G., Wissenbach, U., Janssens, A., Flockerzi, V., and Nilius, B. (2004). The principle of temperature-dependent gating in cold- and heat-sensitive TRP channels. Nature 430, 748–754. doi: 10.1038/nature02732
Voets, T., Owsianik, G., Janssens, A., Talavera, K., and Nilius, B. (2007). TRPM8 voltage sensor mutants reveal a mechanism for integrating thermal and chemical stimuli. Nat. Chem. Biol. 3, 174–182. doi: 10.1038/nchembio862
Wang, R., Tu, S., Zhang, J., and Shao, A. (2020). Roles of TRP channels in neurological diseases. Oxidative Med. Cell. Longev. 2020, 1–13. doi: 10.1155/2020/7289194
Wes, P. D., Chevesich, J., Jeromin, A., Rosenberg, C., Stetten, G., and Montell, C. (1995). TRPC1, a human homolog of a drosophila store-operated channel. Proc. Natl. Acad. Sci. U. S. A. 92, 9652–9656. doi: 10.1073/pnas.92.21.9652
Wong, C. O., and Yao, X. (2011). TRP channels in vascular endothelial cells. Adv. Exp. Med. Biol. 704, 759–780. doi: 10.1007/978-94-007-0265-3_40
World Health Organization. (2022). Dementia. https://www.who.int/news-room/fact-sheets/detail/dementia
Xie, H., and Lu, W. C. (2018). Inhibition of transient receptor potential vanilloid 4 decreases the expressions of caveolin-1 and caveolin-2 after focal cerebral ischemia and reperfusion in rats. Neuropathology 38, 337–346. doi: 10.1111/neup.12469
Xu, B., Chibber, R., Ruggiero, D., Kohner, E., Ritter, J., and Ferro, A. (2003). Impairment of vascular endothelial nitric oxide synthase activity by advanced glycation end products. FASEB J. 17, 1289–1291. doi: 10.1096/fj.02-0490fje
Xu, H., Delling, M., Jun, J. C., and Clapham, D. E. (2006). Oregano, thyme and clove-derived flavors and skin sensitizers activate specific TRP channels. Nat. Neurosci. 9, 628–635. doi: 10.1038/nn1692
Xu, N., Meng, H., Liu, T., Feng, Y., Qi, Y., and Wang, H. (2018). TRPC1 deficiency exacerbates cerebral ischemia/reperfusion-induced neurological injury by potentiating Nox4-derived reactive oxygen species generation. Cell. Physiol. Biochem. 51, 1723–1738. doi: 10.1159/000495676
Yamamoto, K., Takeshita, K., Shimokawa, T., Yi, H., Isobe, K., Loskutoff, D. J., et al. (2002). Plasminogen activator inhibitor-1 is a major stress-regulated gene: implications for stress-induced thrombosis in aged individuals. Proc. Natl. Acad. Sci. U. S. A. 99, 890–895. doi: 10.1073/pnas.022608799
Yang, D. X., Jing, Y., Liu, Y. L., Xu, Z. M., Yuan, F., Wang, M. L., et al. (2019). Inhibition of transient receptor potential Vanilloid 1 attenuates blood-brain barrier disruption after traumatic brain injury in mice. J. Neurotrauma 36, 1279–1290. doi: 10.1089/neu.2018.5942
Yang, B. H., Piao, Z. G., Kim, Y. B., Lee, C. H., Lee, J. K., Park, K., et al. (2003). Activation of vanilloid receptor 1 (VR1) by eugenol. J. Dent. Res. 82, 781–785. doi: 10.1177/154405910308201004
You, J., Marrelli, S. P., and Bryan, R. M. Jr. (2002). Role of cytoplasmic phospholipase A2 in endothelium-derived hyperpolarizing factor dilations of rat middle cerebral arteries. J. Cereb. Blood Flow Metab. 22, 1239–1247. doi: 10.1097/01.WCB.0000037996.34930.2E
Zhang, D. X., Mendoza, S. A., Bubolz, A. H., Mizuno, A., Ge, Z. D., Li, R., et al. (2009). Transient receptor potential vanilloid type 4-deficient mice exhibit impaired endothelium-dependent relaxation induced by acetylcholine in vitro and in vivo. Hypertension 53, 532–538. doi: 10.1161/HYPERTENSIONAHA.108.127100
Zhang, L., Papadopoulos, P., and Hamel, E. (2013). Endothelial TRPV4 channels mediate dilation of cerebral arteries: impairment and recovery in cerebrovascular pathologies related to Alzheimer's disease. Br. J. Pharmacol. 170, 661–670. doi: 10.1111/bph.12315
Zhang, H., Roman, R. J., and Fan, F. (2022). Hippocampus is more susceptible to hypoxic injury: has the Rosetta stone of regional variation in neurovascular coupling been deciphered? Geroscience 44, 127–130. doi: 10.1007/s11357-021-00449-4
Zhang, Z. M., Wu, X. L., Zhang, G. Y., Ma, X., and He, D. X. (2019). Functional food development: insights from TRP channels. J. Funct. Foods 56, 384–394. doi: 10.1016/j.jff.2019.03.023
Zhang, Z., Yu, H., Huang, J., Faouzi, M., Schmitz, C., Penner, R., et al. (2014). The TRPM6 kinase domain determines the mg.ATP sensitivity of TRPM7/M6 heteromeric ion channels. J. Biol. Chem. 289, 5217–5227. doi: 10.1074/jbc.M113.512285
Zhao, H., Zhang, K., Tang, R., Meng, H., Zou, Y., Wu, P., et al. (2018). TRPV4 blockade preserves the blood-brain barrier by inhibiting stress fiber formation in a rat model of intracerebral hemorrhage. Front. Mol. Neurosci. 11:97. doi: 10.3389/fnmol.2018.00097
Zheng, J. (2013). Molecular mechanism of TRP channels. Compr. Physiol. 3, 221–242. doi: 10.1002/cphy.c120001
Zhu, X., Chu, P. B., Peyton, M., and Birnbaumer, L. (1995). Molecular cloning of a widely expressed human homologue for the drosophila trp gene. FEBS Lett. 373, 193–198. doi: 10.1016/0014-5793(95)01038-G
Zhu, Y., Gao, M., Zhou, T., Xie, M., Mao, A., Feng, L., et al. (2019). The TRPC5 channel regulates angiogenesis and promotes recovery from ischemic injury in mice. J. Biol. Chem. 294, 28–37. doi: 10.1074/jbc.RA118.005392
Zhuo, Y., Li, S. H., Chen, M. S., Wu, J., Kinkaid, H. Y., Fazel, S., et al. (2010). Aging impairs the angiogenic response to ischemic injury and the activity of implanted cells: combined consequences for cell therapy in older recipients. J. Thorac. Cardiovasc. Surg. 139, 1286-94–1294 1-2. doi: 10.1016/j.jtcvs.2009.08.052
Zuccolo, E., Negri, S., Pellavio, G., Scarpellino, G., Laforenza, U., Sancini, G., et al. (2018). Acetylcholine induces Ca2+ signals and nitric oxide release from human brain microvascular endothelial cells. Vasc. Pharmacol. 103-105:65. doi: 10.1016/j.vph.2017.12.049
Keywords: aging, neurodegeneration, neurovascular coupling, Geroscience, cognitive dysfunction, dementia, lifestyle intervention
Citation: Negri S, Sanford M, Shi H and Tarantini S (2023) The role of endothelial TRP channels in age-related vascular cognitive impairment and dementia. Front. Aging Neurosci. 15:1149820. doi: 10.3389/fnagi.2023.1149820
Edited by:
Satoshi Saito, National Cerebral and Cardiovascular Center (Japan), JapanReviewed by:
Erik Josef Behringer, Loma Linda University, United StatesEwa Maria Koźniewska, Mossakowski Medical Research Institute, Polish Academy of Sciences, Poland
Copyright © 2023 Negri, Sanford, Shi and Tarantini. This is an open-access article distributed under the terms of the Creative Commons Attribution License (CC BY). The use, distribution or reproduction in other forums is permitted, provided the original author(s) and the copyright owner(s) are credited and that the original publication in this journal is cited, in accordance with accepted academic practice. No use, distribution or reproduction is permitted which does not comply with these terms.
*Correspondence: Stefano Tarantini, c3RlZmFuby10YXJhbnRpbmlAb3Voc2MuZWR1