- 1Department of Medical Elementology and Toxicology, School of Chemical and Life Sciences, Jamia Hamdard, New Delhi, India
- 2Department of Pharmacology, School of Pharmaceutical Education and Research, Jamia Hamdard, New Delhi, India
- 3Department of Pharmacology and Toxicology, College of Pharmacy, Prince Sattam Bin Abdulaziz University, Al-Kharj, Saudi Arabia
A progressive degradation of the brain’s structure and function, which results in a reduction in cognitive and motor skills, characterizes neurodegenerative diseases (NDs) such as Alzheimer’s disease (AD), Parkinson’s disease (PD), Amyotrophic lateral sclerosis (ALS), and Huntington’s disease (HD). The morbidity linked to NDs is growing, which poses a severe threat to human being’s mental and physical ability to live well. The gut-brain axis (GBA) is now known to have a crucial role in the emergence of NDs. The gut microbiota is a conduit for the GBA, a two-way communication system between the gut and the brain. The myriad microorganisms that make up the gut microbiota can affect brain physiology by transmitting numerous microbial chemicals from the gut to the brain via the GBA or neurological system. The synthesis of neurotransmitters, the immunological response, and the metabolism of lipids and glucose have all been demonstrated to be impacted by alterations in the gut microbiota, such as an imbalance of helpful and harmful bacteria. In order to develop innovative interventions and clinical therapies for NDs, it is crucial to comprehend the participation of the gut microbiota in these conditions. In addition to using antibiotics and other drugs to target particular bacterial species that may be a factor in NDs, this also includes using probiotics and other fecal microbiota transplantation to maintain a healthy gut microbiota. In conclusion, the examination of the GBA can aid in understanding the etiology and development of NDs, which may benefit the improvement of clinical treatments for these disorders and ND interventions. This review indicates existing knowledge about the involvement of microbiota present in the gut in NDs and potential treatment options.
1. Introduction
The progressive loss of function and death of neurons in the brain and other areas of the nervous system characterizes neurodegenerative diseases (NDs). NDs have been associated with various factors, including genetics, environmental toxins, and abnormal protein aggregation in the brain. Some specific examples of neurotoxic chemicals that have been implicated in the development of NDs include beta-amyloid (Aβ) and tau proteins in Alzheimer’s disease (AD), α-synuclein (α-syn) in Parkinson’s disease (PD), and Huntington protein in Huntington’s disease (HD). The expansion of human life expectancy is attributable to the advancements in diet and healthcare brought by the growth of the economy and technology. With the advancement of age, the prevalence of these diseases rises to place heavier and heavier obligations on society. Unfortunately, despite of numerous current clinical trials, there is no effective treatment for these NDs due to their uncertain origin and the intricacy of the central nervous system (CNS).
The gut microbiota (GM) is a community that is a permanent resident of gastrointestinal tract (GIT). The GM are crucial for maintaining the gut homeostasis and overall well-being. The GM comprises a diverse group of microorganisms, including bacteria, viruses, fungi, and protozoa, which together functions to digest food, produce nutrients, and support the immune system (Arumugam et al., 2011). The GM significantly influences the physiology of the host in many ways, such as neuronal growth, maintaining immunological functions, produce an anti-infection effect, and enhancing nutritional efficiency (Sommer and Bäckhed, 2013; Geva-Zatorsky et al., 2017). There are multiple challenges faced by GM such as changes in food habits like more consumption of fast and processed food, rapid urbanization, and medical technologies, which makes GM more susceptible than in previous years (Sonnenburg and Sonnenburg, 2019).
The gut-brain axis (GBA) is a complex communication system that links the gut and the brain. This connection allows bidirectional communication between the gut and brain signals can be sent from the gut to the brain, and vice versa. The GBA plays a significant role in maintaining a human’s general health and wellbeing. It encompasses several pathways, including neural, hormonal, and immune, that work together to regulate various physiology, such as digestion, immune function, and mood regulation (O’Mahony et al., 2015; Dinan and Cryan, 2017). The GM produces a variety of by-products that eventually affect brain’s function through the GBA. These metabolites include short-chain fatty acids (SCFAs), like butyrate, acetate, and propionate. These secreted metabolites exert various functions like modulation of immune function, and influence secretion of neurotransmitters (NTs) like gamma-aminobutyric acid (GABA) and serotonin (5-HT), which participates in regulation of mood, cognition, and anxiety. For example, Kynurenines are synthesized by GM that plays role in modulation of immune response and affecting production of NTs such as glutamate and dopamine. In addition, other NTs like norepinephrine and histamine are also regulated under the influence of GM through the GBA. These metabolites exerts various physiological activities in the brain, including cognitive function, mood, and appetite (Kennedy et al., 2017; Lin and Zhang, 2017; Rowland et al., 2018). A condition known as “dysbiosis,” which is caused by the imbalance in the composition and overall diversity of GM, thus leading to disruption of the normal homeostasis of the gut through the GBA. This causes severe effects on the normal brain activities and affect wellbeing of an individual. The imbalanced state of GM is also responsible for releasing pro-inflammatory cytokines, establishing low-grade inflammatory state in the body, and increasing oxidative stress in the body which leads to cell damage and contributes to aging and disease pathologies. The other detrimental effects caused by the dysbiosis are disruption in the energy metabolism, increased cell death, and modulation of immune response, which contributes to the advancement of various diseases (Noble et al., 2017), adding to the pathogenic mechanisms of several disorders, including neurodegeneration (Mulak and Bonaz, 2015). Dysbiosis has been linked to several other diseases, including: (a) Inflammatory Bowel Disease (IBD): dysbiosis in the GM gut microbiome is associated with IBD, and other gut related disorder like Crohn’s disease and ulcerative colitis (Di Martino et al., 2023); (b) Irritable Bowel Syndrome (IBS): it is associated with dysbiosis, which is characterized by abdominal pain and altered bowel habits (Körner and Lorentz, 2023); (c) Obesity: GM is associated with obesity and has been linked with the dysbiosis due to increased body weight and fat accumulation (Zhang et al., 2023); (d) Type 2 diabetes (T2DM): various studies have demonstrated correlation of T2DM with dysbiosis and stated that may dysbiosis is a contributing factor to T2DM (He et al., 2023); (e) Allergies and Asthma: dysbiosis in the gut and respiratory microbiome has been associated with an increased risk of allergies and asthma (Kleniewska and Pawliczak, 2023); (f) Mental Health disorders: dysbiosis in the gut microbiome has been linked to mental health disorders, including depression, anxiety, and autism (Młynarska et al., 2022); (g) Autoimmune Diseases: dysbiosis in the GM is correlated with autoimmune diseases, including rheumatoid arthritis (RA) and multiple sclerosis (MS; Ordoñez-Rodriguez et al., 2023). In addition, variation in the diversity of GM is associated with aging and aging gut demonstrated a decrease in the microbial diversity (Cheng et al., 2013). Interestingly, elderly individuals with NDs demonstrated a steep change in GM (Claesson et al., 2011). Reportedly, changes in the diversity and composition of GM and dysbiosis is associated with development of brain pathologies like NDs through various mechanisms like promoting inflammation, increased oxidative stress, or disrupted energy metabolism. Few studies suggest that certain gut bacteria can impact proteins, like Aβ, and tau, which are associated with the pathogenesis of NDs. The illustration (Figure 1) represents the dysregulation of GM leading to the development of NDs. Further research is warranted to elucidate the relationship between the GM and NDs. Herein, we have highlighted influence of GM on several NDs and discussed their associated mechanisms, pertinent clinical consequences, and future applications. We have also discussed potential adjuvant therapeutic strategies like probiotics and fecal microbiota transplantation (FMT) for the treatment of NDs.
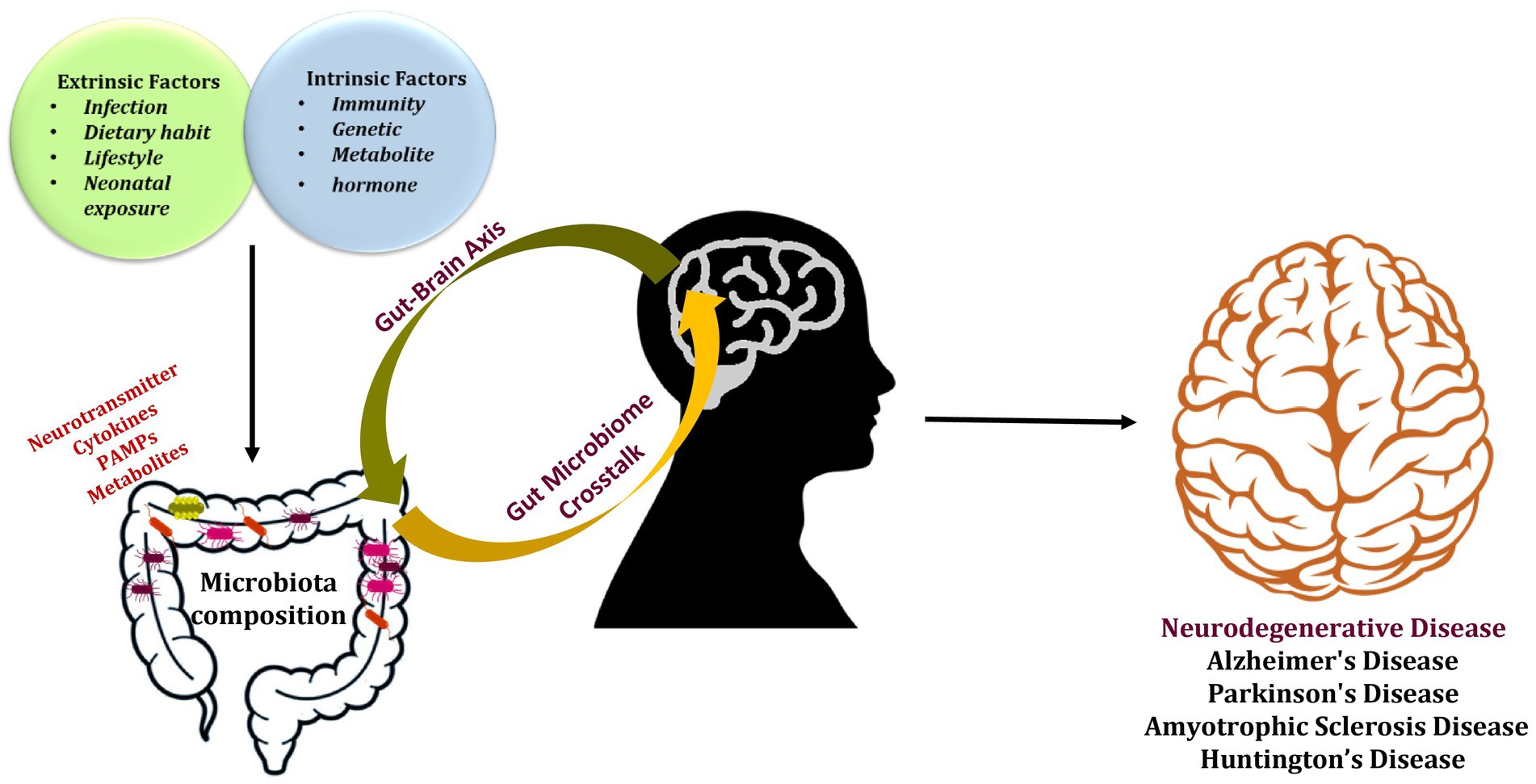
Figure 1. The gut microbiota’s composition is altered by external and internal factors, which also have a role in developing neurodegenerative disorders.
2. Gut microbiota
The gut microorganisms and the host possess a symbiotic relationship, which means they rely on each other for survival. The GM is responsible for various activities like aiding digestion, producing vitamins and other nutrients, and supporting the immune system. In turn, the host offers the GM an appropriate environment and nutrients to survive. This relationship is dynamic and can be influenced by factors such as diet, antibiotics, and stress (Lozupone et al., 2012; Geva-Zatorsky et al., 2017).
Various factors directly influence the diversity and individual variations in the GM. Interestingly, the acidic pH of the gut significantly impacts the different types of microorganisms that survive and thrive in the gut. Moreover, the immune system plays a crucial role in sculpting the curvature of the GM. Factors like genetics and environmental exposure can significantly influence the ability of the immune system to regulate a healthy balance of GM. Interestingly, the digestive enzyme also has a role in shaping the GM. At the strain level, the dynamics of GM is also influenced by other factors like genetics, medical conditions and history, diet and supplements, physical activity, and stress. All these factors contribute to the diversification of the GM and also cause individual variations in the GM (Yatsunenko et al., 2012). Each individual poses a unique microbial community that contributes to developing stable and resilient conditions (Lozupone et al., 2012). Firmicutes and Bacteroidetes are predominantly present in the gut mucosa of an adult, whereas Actinobacteria, Verrucomicrobia, and Proteobacteria are less common (Eckburg et al., 2005). By secreting microbial substances, including lipids, vitamins, and vital amino acids, the GM has the potential to impact human health directly (Diaz Heijtz et al., 2011; Ma et al., 2019; Needham et al., 2020). These elements affect the normal physiological processes of an individual, such as the gastrointestinal barrier, nutrition breakdown, immunological reaction, and aging-related neurological progress through neuronal, endocrine, and immune pathways (Gonzalez et al., 2011; Kau et al., 2011; O’Toole and Jeffery, 2015; Zhou et al., 2021).
3. Mechanisms underlying the effect of gut microbiota on neurodegeneration
3.1. BBB dysfunction
The blood–brain barrier (BBB) is a complex, multi-layered structure that separates the brain and spinal cord from the circulating blood. BBB comprises three cellular elements: endothelial cells, pericytes, and astrocytes (Ballabh et al., 2004; Daneman and Prat, 2015). Many essential elements needed for CNS function must flow through the BBB, and it also secretes elements into the brain and blood that are essential for preserving CNS homeostasis. Additionally, the BBB might restrict the entry of chemicals from the gut to the brain (Banks, 2019). Aging increases the vulnerability of BBB, which can cause CNS disorders and inflammation of the cerebrovascular system (Obermeier et al., 2013; Banks et al., 2021; Finger et al., 2022). Microorganism-associated molecular patterns (MAMPs) are crucial for regulating the structural integrity and vital cellular processes of microorganisms (Sellge and Kufer, 2015). Upregulation and downregulation of MAMPs are related to either acute or chronic inflammation, which is related to the etiology of a wide spectrum of neurological illnesses (Skaper et al., 2018). BBB permeability is linked to various microbial compounds, like trimethylamines (TMAs), SCFAs, lipopolysaccharides (LPS), and vitamins (Braniste et al., 2014; Harrington, 2015; Aho et al., 2021). The endocrine and immune systems stimulate by these molecules to protect against inflammatory reactions or neurodegeneration or might directly influence neurons by targeting BBB. The outer membrane of gram-negative bacteria comprises LPS, one of the most researched elements of bacteria that is required to induce immune system activation. The excessive amount of LPS can induce systemic inflammatory response and sepsis (Miller et al., 2005). It was reported that LPS administration in germ-free mice reduced the permeability of BBB (Braniste et al., 2014). Reportedly, via microglia activation, LPS induced neuroinflammation in germ-free mice (Wendeln et al., 2018). Propionate, acetate, and butyrate are examples of SCFAs produced when resistant starch and indigestible food fibers are digested by the GM, along with other metabolites like methane and hydrogen (Bach Knudsen, 2015). By influencing cellular processes such as G-protein coupled receptor activation and histone deacetylase activation, SCFAs influence psychological functioning and reduce inflammation. These processes also impact host epithelium integrity, BBB integrity, and neural function (Chen et al., 2018; Dalile et al., 2020). Trimethylamine-n-oxide (TMAO), a gut-derived TMA, is secreted by microbiomes including Anaerococcus, Desulfovibrio, Clostridium, and Providencia (Romano et al., 2015). The ability of TMAO to traverse the BBB was demonstrated by its presence in cerebrospinal fluid (CSF; Del Rio et al., 2017). The clinical investigation has reported an increased level of TMAO in the CSF of cognitively impaired AD patients. This clinical investigation might help researchers to develop a therapy for conditions like AD, which is characterized by protein misfolding (Vogt et al., 2018). This can be attributed to neurodegeneration linked to complex mechanisms, including aberrant signal transduction, excitation/inhibition imbalance, synaptic dysfunction, oxidative stress, and protein misfolding. These processes are allied with dramatic alterations in the structure and activity of the BBB (Salmina et al., 2021).
3.2. Metabolites
3.2.1. Tryptophan metabolites
Tryptophan metabolism by gut bacteria plays a crucial role in regulating host immune responses, GM, and brain functions. Several gut bacteria can produce tryptophan metabolites, including (i) Lactobacillus: This is a common genus of lactic acid bacteria in the human gut. Lactobacillus can produce several tryptophan metabolites, including indole-3-lactic acid, tryptamine, and 5-HT. (ii) Bacteroides: This is a genus of Gram-negative bacteria that are dominant members of the human GM. Bacteroides can produce several tryptophan metabolites, including indole-3-propionic acid (IPA), indole-3-acetic acid (IAA), and indole-3-aldehyde. (iii) Clostridium: This is a genus of Gram-positive bacteria, also a member of the human GM. Clostridium can produce several tryptophan metabolites, including IPA, IAA, and tryptamine. (iv) Escherichia coli: This is a species of Gram-negative bacteria that are commonly found in the human gut. Escherichia coli can produce several tryptophan metabolites, including IAA, indole-3-aldehyde, and indole-3-ethanol.
Tryptophan-kynurenine (TRP-KYN) pathway and its by-products were found to be crucial in the development of neuroinflammation (Tanaka et al., 2021a,b). Tryptophan (TRP), a member of the eight amino acids required by humans, is the sole amino acid with an indole structure and is only supplied by protein in the diet. Food consumption and the activation of three tryptophan metabolic processes affect the amount of free TRP in the body. In contrast to the 5-HT and kynurenine channels, which are indirectly controlled by the microbiota of the gut, the indole pathway is controlled directly. Overall, only a small portion of free TRP is used for protein synthesis and synthesizing neurotransmitters like 5-HT and neuromodulators like tryptamine. In contrast, more than 95% of free TRP is the substrate for the TRP-KYN pathway’s degradation (Le Floc’h et al., 2011; Stone et al., 2013), where a variety of bioactive molecules are produced, including immunomodulators, neuroprotective antioxidants, neuroprotectants, and neurotoxins (Tanaka et al., 2021a). Kynurenine uric acid (KA) and Quinolinic acid (QA) are noteworthy examples of the TRP-KYN pathway’s important intrinsic metabolites. Through NMDA-mediated excitotoxicity, QA can cause neurodegeneration (Braidy et al., 2009), while KA, an innate NMDA receptor antagonist, has neuroprotective properties and can control QA’s neurotoxic effects (Vamos et al., 2009). Numerous studies have shown that the TRP-KYN pathway is essential for both: (i) neurodegeneration and (ii) severe brain damage (Schwarcz et al., 2012). Tryptophan 2,3-dioxygenase (TDO) and indoleamine 2,3-dioxygenase (IDO) are the route’s rate-limiting enzymes, which also trigger the TRP-KYN system and produce neuroactive molecules like QA and KA. In addition, the kynurenine per tryptophan quotient (KYN/TRP) ratio is a marker of IDO/TDO activity. Reportedly, the severity of the cognitive function impairment is directly correlated with the rise in the KYN/TRP proportion (Widner et al., 2000). Furthermore, there is proof that the GM can affect how important enzymes in the TRP-KYN pathway function directly. It is important to note that although the activity of IDO is reduced in the intestine of germ-free mice, it can be returned to normal by populating the intestinal system with microorganisms as soon as the animals are weaned (Wikoff et al., 2009; Clarke et al., 2013). A growing body of research has revealed that in AD animal models, the serum levels of KYN and 3-hydroxykynurenine (3-HK), intermediates of the TRP-KYN pathway are significantly increased; in contrast, the concentration levels of TRP and KA are reduced, which was directly connected to impairment of cognitive performance (Ting et al., 2007). In PD, 3-HK concentrations dramatically rose, whereas KA concentrations fell in the substantia nigra putamen and frontal cortex (Ogawa et al., 1992). However, the pathological progression of HD was also thought to be connected to the TRP-KYN pathway metabolites. Furthermore, compared with the control group, HD patients and transgenic mice had higher amounts of 3-HK and QA in their cortex and neostriatum (Guidetti et al., 2004). However, it is interesting that probiotic therapy modifies KYN levels (Desbonnet et al., 2008). Furthermore, several investigations have demonstrated that 5-HT, an important neurotransmitter, can control cognitive performance and lessen Aβ plaque formation (Cirrito et al., 2011). Noteworthy, the digestive system’s chromaffin cells are responsible for secreting 90% of 5-HT. In addition, the common intestinal bacteria Enterococcus and Escherichia coli can secrete 5-HT (Cryan and Dinan, 2012). Therefore, by regulating 5-HT synthesis, the GM may have an impact on how the CNS regulates.
3.2.2. Short chain fatty acids
A critical metabolic by-product of the GM is SCFAs. Some of the bacterial genera commonly associated with SCFA production in the gut include Bacteroides, Prevotella, Clostridium, Ruminococcus, and Fecalibacterium. Propionate, butyrate, and acetate, formed from incompletely digested dietary proteins and carbohydrates, comprises most SCFAs (Hamer et al., 2008). SCFAs formed by the GM mainly depend on the relative abundance of the microbiota subgroups or microbiota makeup. For instance, Bifidobacteria species mainly synthesize lactate and acetate while Firmicutes microbes primarily create butyrate (Tagliabue and Elli, 2013). Concerning the roles of SCFAs, these tiny molecules take part in the physiological signaling of the intestinal epithelium through FFAR2 (G-protein-coupled free fatty acid receptors 2) and FFAR3. However, SCFAs can also reach the systemic circulation either actively or passively, having a wide variety of physiological consequences (den Besten et al., 2013), involving contribution in the breakdown of lipids and glucose (Todesco et al., 1991; Fushimi et al., 2006; Gao et al., 2009). The CNS’s functionality and growth are both impacted by SCFA. For example, it has been demonstrated that SCFA worsens the motor function in sterile PD mouse (Sampson et al., 2016), nevertheless, they enhanced experimental stroke mouse recovery (Sadler et al., 2020). It has been demonstrated that acetate can cross the BBB and inhibit mice’s urge to eat (Wyss et al., 2011; Frost et al., 2014). Butyric acid is a versatile chemical with beneficial neuroprotective properties that enhances brain health. Butyric acid is a crucial energy-producing substrate that can also boost ATP and mitochondrial respiration rates, inhibit histone deacetylase, and influence the activity of various genes and cellular proteins (Bourassa et al., 2016). Additionally, via binding to homologous receptors like GPR43, GPR41, peptide YY (PYY), and Glucagon-like peptide 1 (GLP-1), which have a particular function in intestinal endocrine signaling, SCFAs can promote the release of neuropeptides. Once produced, these peptides impact the control of energy balance by stimulating the vagal and intestinal primary afferent pathways (Kuwahara, 2014).
3.2.3. Neurometabolites
Due to the activation of microbiota, a significant number of neurometabolites are directly produced by gut microbes or mucosal epithelial cells. These neurometabolites encompass NTs that directly affect the CNS, signaling cascades, and other signal transduction pathways, all of which have an impact on the CNS’s normal function either directly or indirectly (Forsythe et al., 2012; Lyte, 2014). For instance, when a sufficient substrate is accessible, Lactobacillus and Bifidobacterium strains can create significant levels of GABA (Barrett et al., 2012). Neurometabolites from the gut interact with the CNS by activating nearby afferent vagal fibers or exerting a distant endocrine effect. Behavioral changes due to differences in NT levels, such as the enhanced activity of spontaneous motor neurons brought on by increased monoamines, 5-HT, and dopamine concentration in the striatum (Barrett et al., 2012). Furthermore, the management of NDs is affected by this phenomenon since the production of NTs is frequently dysregulated and ultimately causes the onset of the disease.
3.3. Modification of the nervous system
The bidirectional communication network comprises the CNS, autonomic nervous system (ANS), enteric nervous system (ENS), and the hypothalamic–pituitary–adrenal (HPA) axis. The vagus nerve may be the most direct link between the GM and the brain because it is connected to enteroendocrine cells (Latorre et al., 2016). The solitary tract’s nucleus receives sensory vagal inputs, which are widely distributed to the cerebral cortex and medulla oblongata (Liu and Forsythe, 2021). 20% of vagus fibers transmit signals from the CNS to periphery organs via efferent fibers, and 80% of vagus fibers transmit signals to the CNS via afferent pathways (Bonaz et al., 2018). The former is associated with anti-inflammatory reflexes, gastric secretions, and gut motility. Vagotomy, a surgical procedure that involves removing a portion of the nerve, is a reliable tool used in the field to examine gut-brain communication (Liu and Forsythe, 2021). Using vagotomy in animal models showed that particular bacterial strains communicate with the brain via the vagus nerve, changing behavior performance (Bravo et al., 2011; Buffington et al., 2016). Through vagus nerve pathways, Lactobacillus rhamnosus JB1 ameliorates anxiety-related and depressive-like behavior in mice by modulating the GABAergic system in the brain (Bravo et al., 2011). Vagotomised germ-free animals showed no unique neurochemical or behavioral consequences, indicating that the vagal pathway is a crucial channel for gut and brain communication (Forsythe et al., 2014). Direct neurochemical signals from the GM to the brain and vice versa are produced by the ENS in order to communicate with the CNS via the vagus nerve (Heiss and Olofsson, 2019). The limbic system’s HPA axis, which controls memory and emotional reactions, comprises the hypothalamus, hippocampus, and amygdala. Stress or inflammatory cytokines like interleukin (IL)-6 raise levels of corticotropin-releasing factor (CRF) released from the hypothalamus and adrenocorticotropic hormone (ACTH) produced from the pituitary gland, which causes the adrenal gland to produce cortisol that is harmful to the brain (Frankiensztajn et al., 2020). Consequently, the CNS can affect the activity and functions of intestinal cells owing to the interaction between neurological and hormonal communications (Sharon et al., 2016; Kaelberer et al., 2018). Additionally, through controlling gut cells and preserving intestinal metabolic and immunological homeostasis, the GM impacts host health (Smith et al., 2013; Sun et al., 2015; Honda and Littman, 2016). For instance, immune-related intestinal and extraintestinal illnesses are thought to include features such as microbial intestinal dysbiosis and increased intestinal permeability linked to Clostridium expansion (Zheng et al., 2020). It is interesting to note that the microbiota also affects the production of NTs and hormones that act on the CNS or ENS directly via the vagus nerve or indirectly by entering the circulation, such as dopamine, adrenaline, noradrenaline, 5-HT, glucagon-like peptide-1 (GLP-1), GABA, and peptide YY or their precursor chemicals (Strandwitz, 2018; Huang and Wu, 2021).
3.4. Modification of the host immune system
Gastrointestinal homeostasis and the development of the host’s immune system depend on GM health (Sommer and Bäckhed, 2013). Immune signaling is induced when the connection between the immune system and the microbiota is dysfunctional, suggesting consequences for NDs (Fung et al., 2017). Numerous immunological signaling pathways, including the inflammasome signaling pathway, type I interferon signaling pathway (IFN-I), and nuclear factor (NF)-κB signaling system, are regulated by the gut bacteria, according to studies (Ma et al., 2019; Oswal et al., 2021). In a study, caspase-1-, the ASC-, and IL-18 deletion mice models displayed altered microbiota compared to the wild-type mouse model, which is characterized by the presence of Prevotellaceae species (Gagliani et al., 2014). Additionally, research suggests that major depressive disorder is linked to an active inflammasome and an increase in pro-inflammatory cytokines, such as IL-6, IL-1β, and IL-18 proteins (Young et al., 2014). IFN can prevent inflammasome signaling in multiple sclerosis (Inoue and Shinohara, 2013). IFN-I is linked to the development of dendritic cells (DCs), the expansion of cytotoxic T cells, and the bidirectional communication between the GM and the host (Giles and Stagg, 2017). However, toll-like receptor (TLR) 3-mediated IFN-I production of intestinal DCs can be induced by symbiotic lactic acid bacteria (Kawashima et al., 2013). Additionally, the signs of amnesia and colitis were lessened after the restoration of GM in a colitis model. The crucial transcription factor contributes to immune response; the elevated NF-κB level with the collaborative expression of TNF-α was identified in the intestinal and hippocampal areas that are connected with amnesia (Jang et al., 2018).
4. Involvement of gut microbiota in major neurodegenerative diseases
In the coming sections, we explain the mechanisms underpinning how the GM affects the causes of neurodegenerative illnesses (Table 1; Figure 2).
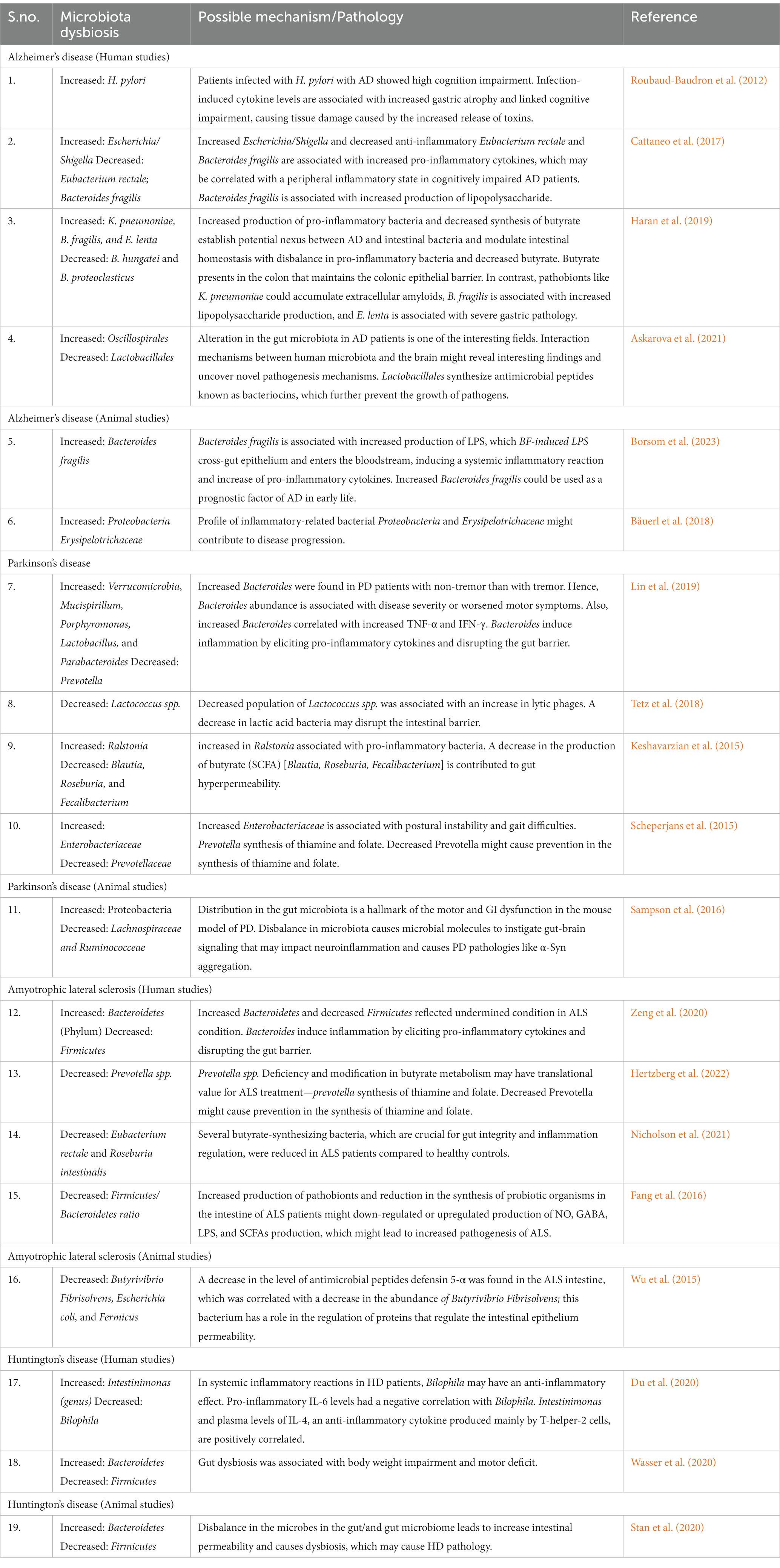
Table 1. Involvement of the gut microbiome in neurodegenerative diseases and their GM related plausible mechanisms.
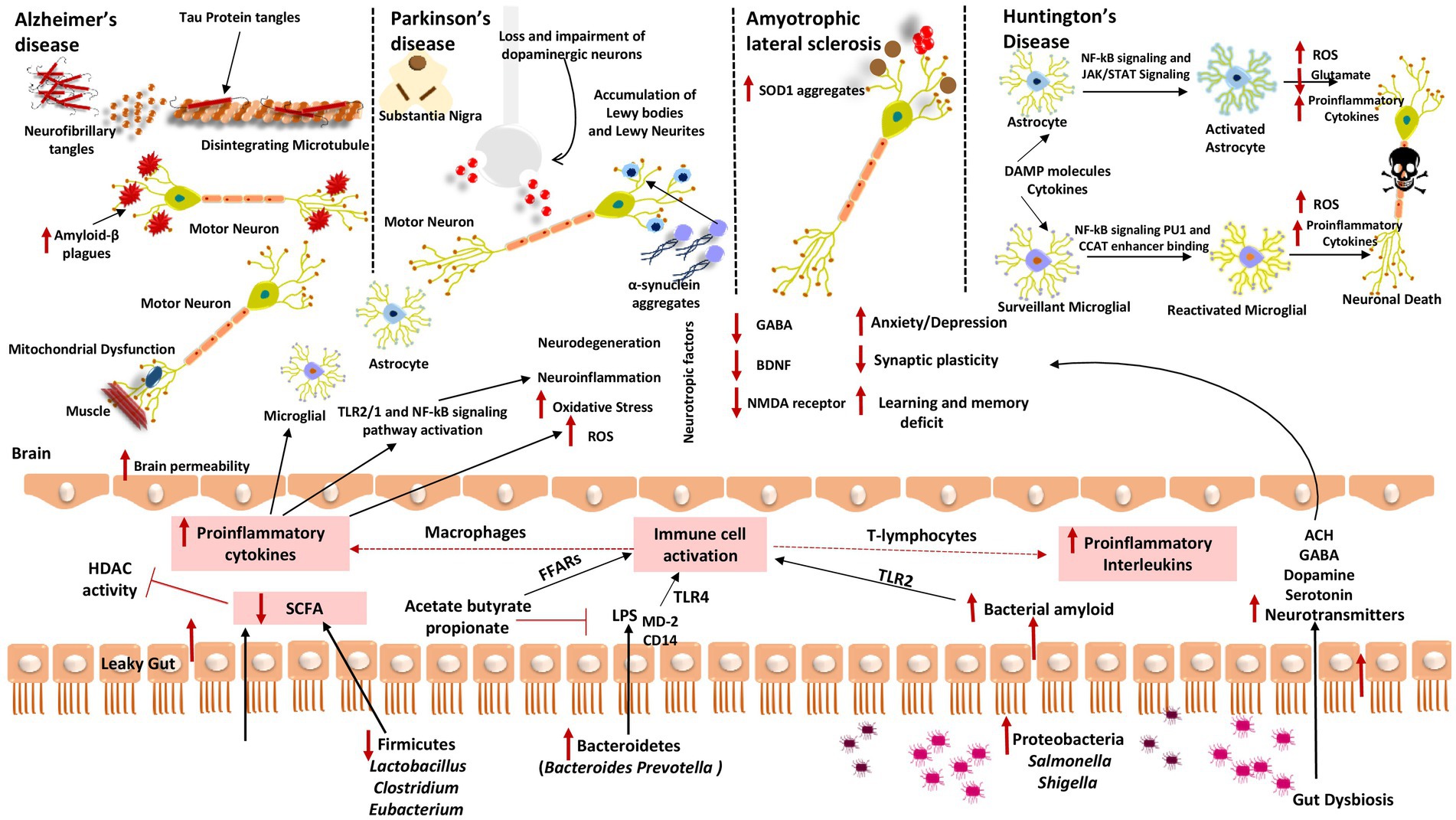
Figure 2. Gut dysbiosis mechanisms underlying several neurodegenerative diseases (A) Alzheimer’s disease; (B) Parkinson’s disease; (C) Amyotrophic lateral sclerosis; and (D) Huntington’s disease. When fewer healthy microbes are around to create SCFAs like Firmicutes and Actinobacteria, HDAC activity and LPS-induced inflammation cannot be inhibited. Contrarily, gut microorganisms like Bacteroidetes have a high excretion rate of LPS, which stimulates the TLR4 receptor by interacting with CD14 and MD-2 proteins and results in an inflammatory process. Additionally, proteobacteria produce a lot of bacterial amyloids like curli peptide, which binds to TLR2 to activate macrophages and cause them to release pro-inflammatory cytokines like TNF-α, IL-6, and IL-1β. Additionally, T-lymphocyte activation causes Th17 cells to produce pro-inflammatory interleukins like IL-17A and IL-22. These cytokines can cross the BBB, boost ROS production, and encourage oxidative stress, which results in neuroinflammation and neurodegeneration. These cytokines also stimulate the transcription of pro-inflammatory miRNAs, activate neuroinflammatory mediators, and inhibit microglial cell phagocytosis, which promotes the progression of neurodegenerative diseases. These cytokines also initiate TLR2/1 and NF-kB signal transduction pathways in microglia and astrocytes.
4.1. The gut microbiota on Aging
Some significant studies on the GM and normal aging preceded interest in the microbiota and NDs linked to aging. Increased intestinal permeability, inflammation, lowered digestion, and altered nutrient absorption are all frequently associated with aging, and each of these conditions interacts in both directions with the gut flora (An et al., 2018). According to most sequencing research, the makeup of the GM changes from adulthood to old age, which is comparable with these linkages (Langille et al., 2014; O’Toole and Jeffery, 2015). However, there is limited agreement over the specific taxa linked to aging discovered in this research. For instance, some research linked a rise in Bacteroidetes with aging (Claesson et al., 2011; Odamaki et al., 2016); in contrast, another study observed increases in Bifidobacterium, Akkermansia, and Christensenellaceae and losses in Lachnospiraceae, Bacteroidaceae, and Ruminococcaceae (Biagi et al., 2016). Several confounding factors likely cause the lack of study consensus, in addition to variations in study design and technical methodologies. For instance, medication intake was linked favorably with Bradyrhizobium, Coprobacter, Helicobacter, and Prevotella and negatively with Massilia and Lachnospiraceae in a study of hospitalized elderly patients and healthy controls (Ticinesi et al., 2017). In a 60-year longitudinal research, married people had more microbial diversity and richness than people who lived alone (Dill-McFarland et al., 2019), which may be connected to findings about how social interaction affects the microbiota in the stomach. Animal models have been beneficial for proving proof of concept for microbial effects on aging due to the technological difficulties of doing mechanistic aging-related research in humans. Changing the GM can alter the lifespan in various fish, flies, worms, and rodents (Gordon et al., 1966; Smith et al., 2017; Obata et al., 2018). Age-related changes in the GM of Drosophila melanogaster indicated and preceded age-related intestinal barrier failure and subsequent deterioration (Clark et al., 2015). After exposure to oxidants as children, specific Acetobacter depletion from the gut increased longevity (Obata et al., 2018). In contrast, excessive Lactobacillus plantarum growth increases reactive oxygen species, which in turn shortens lifespan (Iatsenko et al., 2018). Extending the longevity of African turquoise killifish by transplanting their middle-aged recipients’ microbiota from young donors coincided with the transfection of Exiguobacterium, Psychrobacter, Planococcus, and Propionigenium (Smith et al., 2017). In line with age-related decreases in Akkermansia muciniphila in mice and humans (Fransen et al., 2017; Sovran et al., 2019), A. muciniphila treatment considerably increased lifespan in progerian mice (Bárcena et al., 2019). Many studies indicate that microbial modulation of immunological homeostasis may be implicated, even though little is known about the precise cellular and molecular mechanisms underpinning these numerous events. Germ-free mice infected with the GM of conventional mice that were old but not young showed heightened production of cytokines in the serum and increased intestinal permeability, indicating that aging-associated alterations in the GM increase inflammatory processes (Thevaranjan et al., 2017). In line with this, transplanting young mice’s fecal microbiota restored deficient B cell proliferation in the Peyer’s patches of old mice (Stebegg et al., 2019), demonstrating defenses against immunological dysregulation brought on by aging in young mice’s microbiome. This pioneering research on the microbiome’s functions throughout healthy aging reveals changes in the microbiome that affect lifespan and health, perhaps due to immunological and stress responses. These findings further make way for active research in microbial influences on aging-related neurological conditions, such as AD, PD, ALS, and HD given that aging is a significant risk factor for many NDs.
4.2. Alzheimer’s disease
Alzheimer’s disease (AD), the most prevalent degenerative illness, which is characterized by a loss in cognitive abilities, including memory, speech, and problem-solving (Alzheimer’s Association, 2016). Neuroinflammation, Aβ plaque build-up, and neurofibrillary tau tangles are pathological features of AD. The amyloid precursor protein (APP) cleaved form is Aβ (Aβ40 or Aβ42). By self-aggregation, the Aβ polymerizes form fibrils in the CNS, where it further causes inflammatory reactions and neurotoxicity. Recent research has shown that microbial dysfunction and infection play a significant role in the etiology of AD, particularly in the stimulation of neuroinflammation and the production of amyloids. Patients with AD have been found to have elevated levels of Tumor necrosis factor-α (TNF-α), IL-1β, and other neuroinflammation-related variables (Bagyinszky et al., 2017; Wang et al., 2018). Gareau et al. (2011) demonstrated that infection under acute stress may worsen memory loss and dysfunction. Inflammation and amyloid fibrillogenesis are sparked by releasing LPS in the brain (Asti and Gioglio, 2014). The microglia can identify and remove amyloid molecules by using TLR Pathway (TLR4 and TLR2). Noteworthy, TNF-α and NF-κB are activated by signaling of myeloid differentiation primary response 88 (MyD88) from microglial TLR2, which likewise causes the synthesis of Aβ by increasing α- and β-secretase, respectively (Allen, 2016). Anything insoluble, prone to aggregation, and rich in lipoprotein deposit that matches carbohydrate starches is referred to be amyloid (Schwartz and Boles, 2013). Recently, it has been demonstrated that bacterial metabolites and by-products aggravate AD. Curli, tau, Aβ, α-Syn, and prion, bacteria-derived amyloids, can catalyze cross-section and assemble host amyloids in AD patients (Zhou et al., 2021). Despite having different sequences, amyloids like CsgA and Aβ42 can cause brain deposition and set off a series of AD-related pathogenic events (Hill and Lukiw, 2015). According to reports, persistent H. pylori infection could cause AD patients to generate inflammatory cytokines and amyloids (Roubaud-Baudron et al., 2012; Bu et al., 2015). The cell model has demonstrated that hyperphosphorylation of tau might be induced by H. pylori filtrate (Wang X. L. et al., 2015). Curli fibers, a significant CsgA subunit, are extracellular amyloids found to be produced by E. coli. Other amyloids generated by microorganisms include phenol-soluble Modulins by Staphylococcus aureus, TasA by Bacillus subtilis, Chaplins by Streptomyces coelicolor, FapC by Pseudomonas fluorescens, MccE492 by Klebsiella pneumonia, and CsgA by Salmonella spp. (Schwartz et al., 2012; Schwartz and Boles, 2013; Friedland and Chapman, 2017). In fecal specimens from an APP-transgenic mouse, Harach and the group have identified a striking change in the GM and concluded that the microbiota contributed to the formation of this degenerative brain disease. Comparing controls and intestinal germ-free APP transgenic mice, it was discovered that the later had less cerebral amyloid pathology (Harach et al., 2017). More recently, it has been shown that Chlamydia pneumoniae invasion in astrocytes contributes to the production of Aβ, which encourages AD (Al-Atrache et al., 2019). It was suggested that the use and implementation of probiotic is helpful in AD. In a mouse model where Aβ was administered, Athari Nik Azm et al. (2018) showed that Lactobacilli and Bifidobacteria were successful in improving learning and memory deficits. Wang and Liang and colleagues have demonstrated the implementation of Lactobacillus fermentum NS9 and Lactobacillus helveticus NS8 have improved spatial memory under persistent restraint stress and reduced the symptoms of ampicillin-induced impairment (Liang et al., 2015; Wang T. et al., 2015). Akbari et al. (2016) demonstrated that mini-mental state examination (MMSE) scores significantly improved after 12 weeks of supplementation of Lactobacillus and Bifidobacterium species through fermented milk in a randomized clinical study of probiotic treatment in 60 AD patients. In addition, glucose and lipid metabolism modifications were noted, which were believed to improve the cognitive evaluation score (Akbari et al., 2016). There were improvements in cognition measures seen in AD patients when H. pylori is eradicated with triple treatment (Kountouras et al., 2009). The established studies demonstrated that using probiotics and antibiotics could open a new window in treating AD. More recently, it was demonstrated that probiotics supplementation and exercise could improve cognition function, reduce the amount of Aβ plaques in the hippocampal region, and eventually reduce disease progression in the AD mice model (Abraham et al., 2019).
4.3. Parkinson’s disease
More than 1% of the adult population > 65 years of age has PD, which is a common neurodegenerative illness (Reeve et al., 2014). It is hypothesized that PD results from the interaction of environmental and genetic factors. Motor impairments and non-motor symptoms (NMS) are the hallmarks of this neuropathology, which ultimately impacts life quality (Felice et al., 2016). The GM attained more interest by the scientific society because it has a potential connection with the development of PD (Felice et al., 2016). PD is accompanied by several enteral dysfunctions, including malnutrition, infection due to H. pylori infection, and constipation (Fasano et al., 2015). A greater incidence of α-syn presence is identified in patients compared with controls from various studies regarding the function of GI tract disease in PD (Chiang and Lin, 2019). Animal studies have established that vagus nerve excision can prevent α-syn from traveling from the gut to the CNS (Ulusoy et al., 2017). Additionally, bowel inflammation can lead to neuroinflammation, which encourages dopaminergic neuron death in the substantia nigra region of rodents (Villarán et al., 2010). Forsyth and co-workers discovered that PD progression is impacted by “leaky intestines” and intestinal inflammation (Forsythe et al., 2014). Increasing E. coli and α-syn deposition in PD patients’ sigmoid was observed to be linked with increased colonic permeability (Salat-Foix et al., 2012). While the butyrate-producing and anti-inflammatory bacterial genera Blautia, Coprococcus, and Roseburia are markedly deficient in PD patients, the numbers of the LPS-producing genera Bacteroides and Oscillospira are noticeably higher (Keshavarzian et al., 2015). Ralstonia, a pro-inflammatory genus, is much more prevalent in the mucosa of PD patients, suggesting that the inflammatory gut barrier is harmful. Scheperjans et al. (2015) demonstrated that PD could be diagnosed using the changed gut flora and the associated motor phenotype. Prevotellaceae’s relative abundance is markedly decreased in PD, which has been proven to be a particularly profound biomarker for PD diagnosis. 90.3% specificity in diagnosing PD was demonstrated by a model based on the presence of constipation and several bacterial families. PD problems like constipation have been shown to be improved by consuming fermented milk for 4 weeks (Barichella et al., 2016). According to two studies, immunosuppressants and anti-TNF medication lower the risk of PD (Camacho-Soto et al., 2018; Peter et al., 2018). However, there is a severe lack of clinical evidence supporting the probiotics use in treating PD, and more research is required.
4.4. Amyotrophic lateral sclerosis
Amyotrophic lateral sclerosis is a progressive ND that results in the death of motor neurons, spinal cord cells, and brain cells. Muscle twitching, cramping, stiffness, coordination issues, and muscle weakness are among the symptoms. This eventually makes it harder to breathe, speak, and swallow. ALS exists in two forms: sporadic ALS, which is the most prevalent kind with an unclear etiology, and familial ALS, which is brought on by gene alterations (Toepfer et al., 1997). The G93A SOD1 (superoxide dismutase) transgenic ALS mouse model is utilized to study the function of GM in the illness. In a preclinical model, three stages can be distinguished in the ALS progression: pre-onset syndrome lasts 60–70 days, the onset lasts 90–100 days, and progressive lasts 120–130 days (Zhou et al., 2015). The transgenic mice exhibit higher intestinal permeability and impaired BBB during the pre-onset period. It has been documented that lower levels of E-cadherin and zonula occludens (ZO)-1 cause gut wall damage. Reduced quantities of butyrate-producing bacteria, such as Peptostreptococcus and Butyrivibrio fibrosolvens were found in the feces of SOD1G93A mice, which led to an upsurge in serum and intestine inflammatory cytokine, IL-17 (Wu et al., 2015; Fang, 2016). Zhang and co-workers demonstrated that 2% supplementation of butyrate in their drinking water to SOD1 transgenic mice prevented the progression of ALS. The prevention in ALS progression is possible because of a significant increase in the abundance of B. fibrosolvens, improving the function of the intestinal barrier (Zhang et al., 2017). Interestingly, fecal sample of ALS patients showed less butyrate-producing bacteria such Anaerostipes, Oscillibacter, Lachnospira, and more Dorea species. Dorea may produce ethanol as a by-product of glucose metabolism, so it is deemed toxic (Fang et al., 2016). It has also been found that ALS patients’ plasma levels of LPS and β-Methylamino-L-alanine differ. Circulation of this chemical in the bloodstream may enter the CNS and contributes to ALS development by causing brain inflammation and disrupting BBB function (Bengmark, 2013; Catanzaro et al., 2015). BBB disruption is widespread in ALS patients, including humans and animals (Garbuzova-Davis and Sanberg, 2014). Probiotics’ therapeutic potential for treating ALS-like diseases is still being investigated.
4.5. Huntington’s disease
Huntington’s disease is an autosomal dominant, uncommon ND with an estimated global prevalence of 2.7 cases per 100,000 people with an onset age between 35 and 44 years (Erkkinen et al., 2018; Gatto et al., 2020). Cognitive impairment, mental problems, and motor symptoms are the three classical pathological signatures of HD. Dysphagia develops from motor disruption, causes loss of weight and aspiration issues, and is lethal (Rosas et al., 2008; Erkkinen et al., 2018).
The prospect of incorporating GM into HD diagnosis and treatment arises from recent research that links GM with neurological health (Wasser et al., 2020). Alterations in gut microbiome diversity or abundance may indicate HD, and these changes may involve variances in biological sex (Du et al., 2020). A study has reported that the microbiota in the gut of HD mouse models compared to wild-type mice found that male HD mouse models had higher abundances of Bacteroidales and Lactobacillales and lower levels of Clostridiales. Female HD mice, on the other hand, had higher levels of Coriobacteriales, Erysipelotrichales, Bacteroidales, and Burkholderiale and a lower abundance of Clostridiales. In addition, compared to the female and wild-type mice, male HD mice displayed greater microbial diversity (Kong et al., 2020). Subsequently, a study found that microbiota-deficient animals had lower numbers of mature oligodendrocytes and myelin-related proteins in the prefrontal cortex, which inhibited the plasticity of white matter and callosal myelination (Radulescu et al., 2020). This study demonstrated that depletion of the microbiome could exacerbate HD symptoms. In context to the GM association with HD, some SCFAs and bioactive elements secreted by the GM are observed in HD development and progression. These substances primarily affect the GBA’s biological functions (Konjevod et al., 2021). Tyrosine, IPA, 2-hydroxyphenylacetic acid, 3-hydroxyphenylacetic acid, and 4-hydroxyphenylacetic acid can all result in dietary and bioactive compounds dysbiosis in GBA, while 5-HT, tyrosine, these acids, and hydroxyphenylacetic acids can all cause intestinal permeability (Rosas et al., 2015). The intricate communication between the GM and HD will be better understood with more research on these compounds from the GM. This research will also aid with early detection and HD therapeutics.
5. Microbiota-based treatments
Probiotics therapy and fecal microbiota transplantation (FMT) are the two main types of microbiota-associated therapy that is being used as adjuvants nowadays to treat neurological illnesses (Abuaish et al., 2021; Table 2).
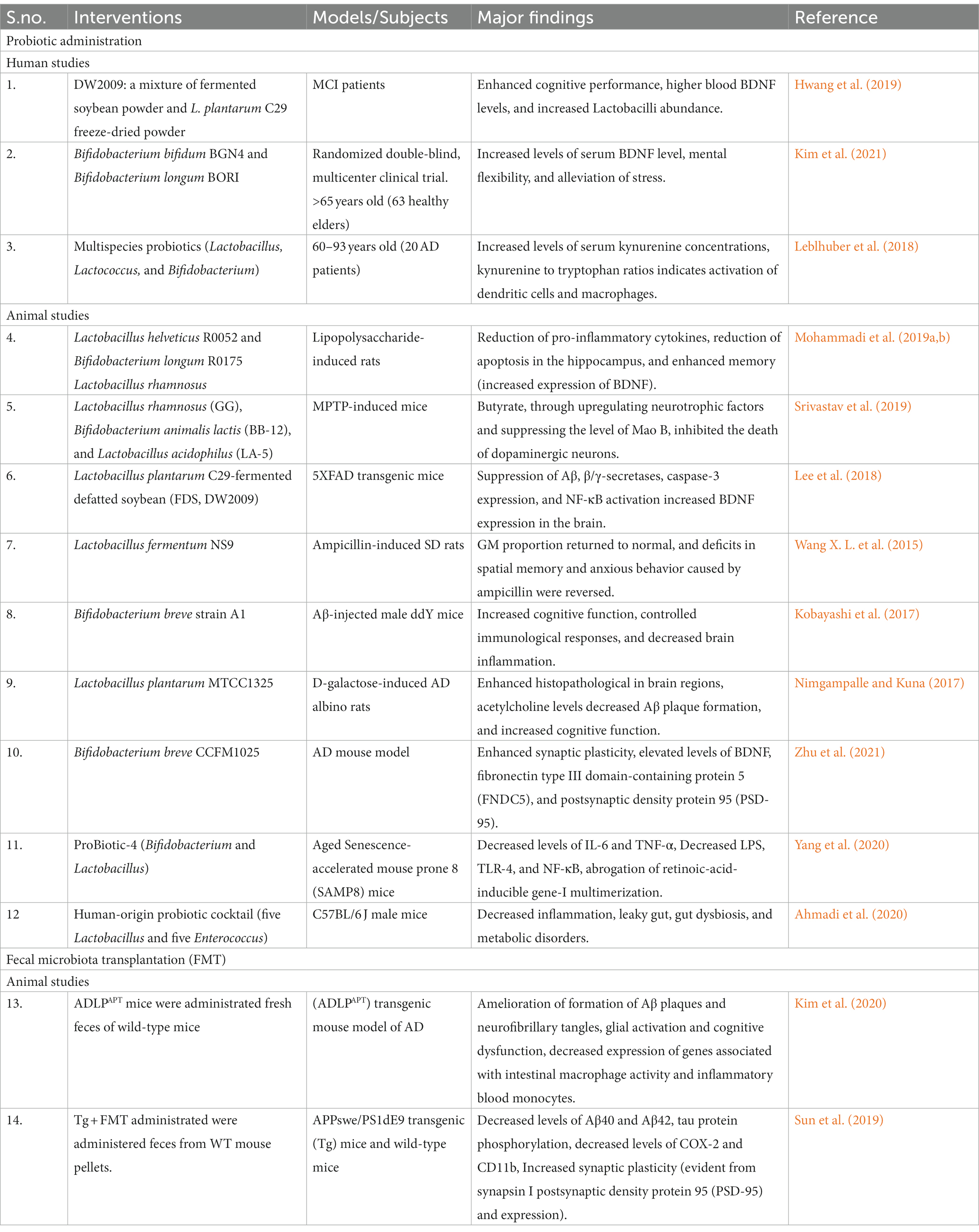
Table 2. Modification of the gut microbiome in preclinical and clinical investigations using various microbiota therapies.
5.1. Probiotics
Numerous studies have demonstrated that implementing beneficial bacteria and probiotics effectively prevents and treats NDs. Oral delivery of probiotics as a cocktail of strains or with a single strain has demonstrated promising results as effective adjuvant therapy. In Probiotic-4, for instance, Lactobacillus casei, Bifidobacterium lactis, Lactobacillus acidophilus, and Bifidobacterium bifidum are all present. By suppressing the NF-κB signaling cascade and the inflammation, which RIG-I and TLR4 regulate, it acts to considerably inhibit Pseudomonas, Proteobacteria, and the Lachnospiraceae NK4A136 group; also, it decreases the proportion of Firmicutes to Bacteroidetes and enhances cognitive performance in a significant manner in SAMP8 mice (aged Yang et al., 2020). In another study, rats were given (receiving Aβ injections for 8 weeks), a probiotic mixture of Lactobacillus fermentum, Lactobacillus acidophilus, Bifidobacterium longum, and Bifidobacterium lactis. The altered elements of the GIT microbiota were found to be accompanied by enhanced spatial memory, learning disabilities, and a decrease in reactive oxygen species, according to the research (Athari Nik Azm et al., 2018). The microbiome of 3xTG AD mice was recently shown to be modulated by SLAB51, a preparation of Bifidobacterium and Lactobacillus, boosting the number of Bifidobacterium spp. while decreasing the Campylobacter population. The investigational study concluded that these changes in the composition of the microbiota, along with the large quantities of SCFAs in the intestine and the raised amount of the intestinal neuroprotective peptide hormone, may lessen cognitive dysfunction by reduction of Aβ aggregates and, consequently, neuron injury (Bonfili et al., 2017). By expressing BDNF proteins, Bifidobacterium longum R0175 and Lactobacillus helveticus R0052 were able to reduce the inflammatory mediators levels in a significant manner that were brought on by LPS in the serum and hippocampus, lowering the apoptotic cell death of hippocampus, and lessening the detrimental effects of LPS on memory (Mohammadi et al., 2019a,b). In a different study, Bifidobacterium breve A1 was administered to mice who had received an injection of Aβ; this treatment enhanced the mice’s behavior and cognition while suppressing the immune reaction and expression of genes responsible for inflammation in the hippocampal region (Kobayashi et al., 2017). According to a different study, 60 days of therapy with Lactobacillus plantarum MTCC1325 in a rat model of D-galactose consumption reduced cognitive impairment and returned acetylcholine levels and histopathological characteristics to normal levels (Nimgampalle and Kuna, 2017). According to studies, Lactobacillus helveticus NS8 and Lactobacillus fermentum NS9 improved spatial memory under persistent restraint stress and reduced the symptoms of ampicillin-induced impairment (Liang et al., 2015; Wang T. et al., 2015). Recently, a study also demonstrated that a probiotic combination and physical activity could lessen Aβ plaque deposition in the hippocampal region, enhance memory function, and eventually delay AD onset in an APP/PS1 model of mice (Abraham et al., 2019). According to another study, G93A mice’s intestinal epithelial barrier integrity and GM balance were restored following a 2% butyrate treatment, a naturally occurring product from bacteria that aids in restoring the homeostasis of the gut microbiome. In addition, G93A mice showed extended survival and decreased body weight in addition to alleviating the disease’s peripheral and central symptoms (Zhang et al., 2017). Bifidobacterium and Lactobacillus are frequently employed in probiotic formulations as these bacteria are frequently used to boost people’s condition and are classified as GRAS (generally regulated as safe) for the consumption of humans (Fijan, 2014). In another study, AD patients received 200 mL/D of Lactobacillus acidophilus, Lactobacillus fermentum, Bifidobacterium bifidum, and Lactobacillus casei for 12 weeks. Probiotic implementation resulted in positive impact on cognitive performance of AD patient and insulin metabolism but no discernible impact on inflammation, oxidative damage, fasting blood glucose, or lipid distribution compared to the control group (Akbari et al., 2016). Nevertheless, according to Agahi et al. (2018), the serum inflammatory molecules: IL-6, TNF-α, and IL-10, oxidative stress markers: glutathione (GSH), nitric oxide (NO), and malondialdehyde (MDA), did not significantly change after patients with AD received Lactobacillus plantarum, Lactobacillus fermentum, Bifidobacterium lactis, or Lactobacillus acidophilus, Bifidum, and Longum for 12 weeks. As a result, the dosage and formulation of the administered probiotics are only one factor in determining how effective probiotic intervention will be for AD patients. The severity of the disease itself has a significant impact. The results of a Repeatable Battery for the Assessment of Neuropsychological Status (RBANS) and Mini Mental State Examination (MMSE) showed significant changes after 12 weeks of administration of Bifidobacterium breve A1 capsule, while shifts in the serum lipid levels, inflammatory mediators, and oxidative stress were insignificant. This suggests that Bifidobacterium breve A1 is secure and can enhance reduced cognitive abilities (Kobayashi et al., 2019). Additionally, it has been demonstrated that consuming fermented milk for 4 weeks can reduce the severity of PD problems, especially the symptoms of constipation (Barichella et al., 2016). Numerous research showing the crucial functions endogenous bacteria play in our health, particularly concerning neurodegeneration, provided compelling evidence for the safe use of probiotics (Table 2). These investigations have demonstrated that using particular microbial strains as probiotics could provide favorable outcomes.
5.2. Fecal microbiota transplantation
Fecal microbiota transplantation (FMT) is the method of transferring GM from the healthy donors’ from their feces into dysbacteriosis patients’ intestines through enema, nasogastric tube, nasointestinal tube, or endoscopic methods to return the gut microbiome to its healthy biodiversity and functioning (Staley et al., 2017; Parker et al., 2020). This approach is thought to be a successful remedy for Clostridium difficile infections that come back several times (Gulati et al., 2020). FMT is regarded as a viable adjuvant therapeutic for some extraintestinal disorders, particularly NDs, due to the GBA’ bidirectional signal connection. For instance, fecal microbiome from APP/PS1-21 male mice was transplanted into age-matched, antibiotic-treated APP/PS1-21 mice, and Dodiya et al. (2019) discovered that this restored the normal GM and partly reversed Aβ disease and microglia architecture. Another investigation discovered that mice transplanted with feces from AD patients had worsened cognitive abilities and less fecal metabolites linked to the neurological system than healthy control mice (Fujii et al., 2019). Clearly, there are not many studies on how FMT affects neurological disorders in people right now, and safety is still a big problem for applying FMT research to human trials. Fecal samples and donors from FMT are tested for potential pathogenic viruses, bacteria, and various other microorganism just like in past or present human investigations (Woodworth et al., 2017). However, the precise and ideal microbial makeup of the transplantable samples is still being studied, which, in terms of human trials, creates possible safety questions and makes it difficult to evaluate results.
6. Conclusion
The GM has role in sustaining brain physiology and intestinal flora and particular field received attention by medical fraternity lately. However, there are various challenges that are associated with the current research on GM. The GM controls neurotransmission and vascular barriers via the immune function and microbial metabolites, influencing host cognitive function and cerebral vascular physiology. The plausible identified treatment is currently under investigational and developmental phase. The experimental design, individuals, models, analytical technique, pipelines, and quality assurance protocols employed in metabolomics investigations continue to present difficulties. Evidence has shown correlations, not causative links, between the host’s physiology and the microbiota. More sample-size research is required for metagenomics biomarker screening because human feces experiments have many confounding factors. When exposure, demography, food, and socioeconomic conditions are considered, such research are more trustworthy. Although the outcome variables in population-based metagenomics investigations are substantially affected by confounding and irreducible variables, the microbiota composition may be described by a relatively limited number of variables (Falony et al., 2016; Rothschild et al., 2018). Another challenge is the moderate and long-term impacts on cognitive or psychological function identified in contemporary microflora translational medicine. Microbiota-based treatments may take months or years to impact neuropsychiatric illnesses, but their impact on host coagulation can be seen much more quickly. Animal models, alongside human cohort and cross-sectional research, offer crucial data that help to determine how particular microorganism impact the host. In order to verify the roles of specific microbial species, preclinical experiments frequently use animal models, particularly rodents. The initial animal studies aimed to identify relationships between host physiology and the impact of the microbiome in germ-free species (Jonsson and Bäckhed, 2017). The specific characteristics and actions of the microbiota are mostly understood through probiotics, specialized bacteria that colonize germ-free animals, and FMT techniques. Antibiotic-treated animal models are alternatives to evaluating the microbial depletion effects on mature immune wild-type animals (Knight et al., 2018). Mice have a significantly small intestine and larger cecum than humans have in terms of intestinal structure. The surface of mouse mucosa is smoother, in contrast to the human small intestine, which has more circular folds that increase its surface area (Scholtens et al., 2012; Hugenholtz and de Vos, 2018). As animals and humans have dissimilar life histories, the routine of physical activity, circadian rhythm, social constraints, and food composition, life experiences come up during data processing. However, in human research, quantitative cohort research has provided approval than retrospective questionnaires, and sometimes, lower sample numbers are sufficient to test an idea (Zhu et al., 2017). Additional significant problems are the choice of analysis of microbiome methodologies and the associated technical stability (Costea et al., 2017). The microbiota-based therapeutics is a probable strategy to be applied in treatments for brain related diseases in the near future, although being far from perfection.
Author contributions
SK, NK, SR, and GB carried out the literature review, and drafted the manuscript. SK and NK conceptualized, edited, and provided necessary suggestions, provided critical revisions, contributed to the final manuscript, and also designed the figures. All authors contributed to the article and approved the submitted version.
Conflict of interest
The authors declare that the research was conducted in the absence of any commercial or financial relationships that could be construed as a potential conflict of interest.
Publisher’s note
All claims expressed in this article are solely those of the authors and do not necessarily represent those of their affiliated organizations, or those of the publisher, the editors and the reviewers. Any product that may be evaluated in this article, or claim that may be made by its manufacturer, is not guaranteed or endorsed by the publisher.
Abbreviations
3-HK, 3-Hydroxykynurenine; 5-HT, Serotonin; ACTH, Adrenocorticotropic hormone; AD, Alzheimer’s disease; ALS, Amyotrophic lateral sclerosis; ANS, Autonomic nervous system; APP, Amyloid precursor protein; Aβ, Amyloid beta; BBB, Blood brain barrier; BDNF, Brain derived neurotrophic factor; CNS, Central nervous system; CRF, Corticotropin-releasing factor; CSF, cerebrospinal fluid; DCs, Dendritic cells; ENS, Enteric nervous system; FFAR2, G-protein-coupled free fatty acid receptors 2; FMT, Fecal microbiota transplantation; GABA, Gamma-aminobutyric acid; GBA, Gut-brain axis; GIT, Gastrointestinal tract; GLP-1, Glucagon-like peptide; GM, Gut microbiota; GRAS, Generally regarded as safe; GSH, Glutathione; HD, Huntington’s disease; HPA, Hypothalamic–pituitary–adrenal axis; IAA, Indole-3-acetic acid; IBD, Inflammatory bowel disease; IBS, Irritable bowel syndrome; IDO, Indoleamine 2,3-dioxygenase; IFN-1, Type I interferon; IL, Interleukin; IPA, Indole-3-propionic acid; KA, Kynurenine uric acid; LPS, Lipopolysaccharides; MAMPs, Microorganism-associated molecular patterns; MDA, Malondialdehyde; MMSE, Mini-mental state examination; MyD88, Myeloid differentiation primary response 88; NDs, Neurodegenerative diseases; NF-κB, Nuclear factor-κB; NMS, Non-motor symptoms; NO, Nitric oxide; NTs, Neurotransmitters; PD, Parkinson’s disease; PYY, peptide YY; QA, Quinolinic acid; RBANS, Repeatable battery for the assessment of neuropsychological status; SCFAs, Short-chain fatty acids; SOD, Superoxide dismutase; T2DM, Type 2 diabetes mellitus; TDO, Tryptophan 2,3-dioxygenase; TLR, Toll-like receptor; TMAO, Trimethylamine-n-oxide; TMAs, Trimethylamines; TNF-α, Tumor necrosis factor-α; TRP, Tryptophan; TRP-KYN, Tryptophan-kynurenine; α-syn, α-Synuclein.
References
Abraham, D., Feher, J., Scuderi, G. L., Szabo, D., Dobolyi, A., Cservenak, M., et al. (2019). Exercise and probiotics attenuate the development of Alzheimer’s disease in transgenic mice: role of microbiome. Exp. Gerontol. 115, 122–131. doi: 10.1016/j.exger.2018.12.005
Abuaish, S., Al-Otaibi, N. M., Abujamel, T. S., Alzahrani, S. A., Alotaibi, S. M., AlShawakir, Y. A., et al. (2021). Fecal transplant and Bifidobacterium treatments modulate gut Clostridium Bacteria and rescue social impairment and hippocampal BDNF expression in a rodent model of autism. Brain Sci. 11:1038. doi: 10.3390/brainsci11081038
Agahi, A., Hamidi, G. A., Daneshvar, R., Hamdieh, M., Soheili, M., Alinaghipour, A., et al. (2018). Does severity of Alzheimer’s disease contribute to its responsiveness to modifying gut microbiota? A double blind clinical trial. Front. Neurol. 9:662. doi: 10.3389/fneur.2018.00662
Ahmadi, S., Wang, S., Nagpal, R., Wang, B., Jain, S., Razazan, A., et al. (2020). A human-origin probiotic cocktail ameliorates aging-related leaky gut and inflammation via modulating the microbiota/taurine/tight junction axis. JCI Insight 5:e132055. doi: 10.1172/jci.insight.132055
Aho, V. T. E., Houser, M. C., Pereira, P. A. B., Chang, J., Rudi, K., Paulin, L., et al. (2021). Relationships of gut microbiota, short-chain fatty acids, inflammation, and the gut barrier in Parkinson’s disease. Mol. Neurodegener. 16:6. doi: 10.1186/s13024-021-00427-6
Akbari, E., Asemi, Z., Daneshvar Kakhaki, R., Bahmani, F., Kouchaki, E., Tamtaji, O. R., et al. (2016). Effect of probiotic supplementation on cognitive function and metabolic status in Alzheimer’s disease: a randomized, double-blind and controlled trial. Front. Aging Neurosci. 8:256. doi: 10.3389/fnagi.2016.00256
Al-Atrache, Z., Lopez, D. B., Hingley, S. T., and Appelt, D. M. (2019). Astrocytes infected with Chlamydia pneumoniae demonstrate altered expression and activity of secretases involved in the generation of β-amyloid found in Alzheimer disease. BMC Neurosci. 20:6. doi: 10.1186/s12868-019-0489-5
Allen, H. B. (2016). Alzheimer’s disease: assessing the role of spirochetes, biofilms, the immune system, and amyloid-β with regard to potential treatment and prevention. J. Alzheimers Dis. 53, 1271–1276. doi: 10.3233/JAD-160388
Alzheimer’s Association (2016). 2016 Alzheimer’s disease facts and figures. Alzheimers Dement. J. Alzheimers Assoc. 12, 459–509. doi: 10.1016/j.jalz.2016.03.001
An, R., Wilms, E., Masclee, A. A. M., Smidt, H., Zoetendal, E. G., and Jonkers, D. (2018). Age-dependent changes in GI physiology and microbiota: time to reconsider? Gut 67, 2213–2222. doi: 10.1136/gutjnl-2017-315542
Arumugam, M., Raes, J., Pelletier, E., Le Paslier, D., Yamada, T., Mende, D. R., et al. (2011). Enterotypes of the human gut microbiome. Nature 473, 174–180. doi: 10.1038/nature09944
Askarova, S., Kaiyrlykyzy, A., Kozhakhmetov, S., Babenko, D., Olzhayev, F., and Kushugulova, A. (2021). Gut microbiome alterations in Alzheimer’s patients from Kazakhstan. Alzheimers Dement. 17. doi: 10.1002/alz.052741
Asti, A., and Gioglio, L. (2014). Can a bacterial endotoxin be a key factor in the kinetics of amyloid fibril formation? J. Alzheimers Dis. 39, 169–179. doi: 10.3233/JAD-131394
Athari Nik Azm, S., Djazayeri, A., Safa, M., Azami, K., Ahmadvand, B., Sabbaghziarani, F., et al. (2018). Lactobacilli and bifidobacteria ameliorate memory and learning deficits and oxidative stress in β-amyloid (1-42) injected rats. Appl. Physiol. Nutr. Metab. Physiol. Appl. Nutr. Metab. 43, 718–726. doi: 10.1139/apnm-2017-0648
Bach Knudsen, K. E. (2015). Microbial degradation of whole-grain complex carbohydrates and impact on short-chain fatty acids and health. Adv. Nutr. 6, 206–213. doi: 10.3945/an.114.007450
Bagyinszky, E., Giau, V. V., Shim, K., Suk, K., An, S. S. A., and Kim, S. (2017). Role of inflammatory molecules in the Alzheimer’s disease progression and diagnosis. J. Neurol. Sci. 376, 242–254. doi: 10.1016/j.jns.2017.03.031
Ballabh, P., Braun, A., and Nedergaard, M. (2004). The blood-brain barrier: an overview: structure, regulation, and clinical implications. Neurobiol. Dis. 16, 1–13. doi: 10.1016/j.nbd.2003.12.016
Banks, W. A. (2019). The blood-brain barrier as an endocrine tissue. Nat. Rev. Endocrinol. 15, 444–455. doi: 10.1038/s41574-019-0213-7
Banks, W. A., Reed, M. J., Logsdon, A. F., Rhea, E. M., and Erickson, M. A. (2021). Healthy aging and the blood-brain barrier. Nat. Aging 1, 243–254. doi: 10.1038/s43587-021-00043-5
Bárcena, C., Valdés-Mas, R., Mayoral, P., Garabaya, C., Durand, S., Rodríguez, F., et al. (2019). Healthspan and lifespan extension by fecal microbiota transplantation into progeroid mice. Nat. Med. 25, 1234–1242. doi: 10.1038/s41591-019-0504-5
Barichella, M., Pacchetti, C., Bolliri, C., Cassani, E., Iorio, L., Pusani, C., et al. (2016). Probiotics and prebiotic fiber for constipation associated with Parkinson disease: An RCT. Neurology 87, 1274–1280. doi: 10.1212/WNL.0000000000003127
Barrett, E., Ross, R. P., O’Toole, P. W., Fitzgerald, G. F., and Stanton, C. (2012). γ-Aminobutyric acid production by culturable bacteria from the human intestine. J. Appl. Microbiol. 113, 411–417. doi: 10.1111/j.1365-2672.2012.05344.x
Bäuerl, C., Collado, M. C., Diaz Cuevas, A., Viña, J., and Pérez Martínez, G. (2018). Shifts in gut microbiota composition in an APP/PSS1 transgenic mouse model of Alzheimer’s disease during lifespan. Lett. Appl. Microbiol. 66, 464–471. doi: 10.1111/lam.12882
Bengmark, S. (2013). Gut microbiota, immune development and function. Pharmacol. Res. 69, 87–113. doi: 10.1016/j.phrs.2012.09.002
Biagi, E., Franceschi, C., Rampelli, S., Severgnini, M., Ostan, R., Turroni, S., et al. (2016). Gut microbiota and extreme longevity. Curr. Biol. 26, 1480–1485. doi: 10.1016/j.cub.2016.04.016
Bonaz, B., Bazin, T., and Pellissier, S. (2018). The Vagus nerve at the Interface of the microbiota-gut-brain Axis. Front. Neurosci. 12:49. doi: 10.3389/fnins.2018.00049
Bonfili, L., Cecarini, V., Berardi, S., Scarpona, S., Suchodolski, J. S., Nasuti, C., et al. (2017). Microbiota modulation counteracts Alzheimer’s disease progression influencing neuronal proteolysis and gut hormones plasma levels. Sci. Rep. 7:2426. doi: 10.1038/s41598-017-02587-2
Borsom, E. M., Conn, K., Keefe, C. R., Herman, C., Orsini, G. M., Hirsch, A. H., et al. (2023). Predicting neurodegenerative disease using Prepathology gut microbiota composition: a longitudinal study in mice modeling Alzheimer’s disease pathologies. Microbiol. Spectr., 6, 11–e0345822. doi: 10.1128/spectrum.03458-22
Bourassa, M. W., Alim, I., Bultman, S. J., and Ratan, R. R. (2016). Butyrate, neuroepigenetics and the gut microbiome: can a high fiber diet improve brain health? Neurosci. Lett. 625, 56–63. doi: 10.1016/j.neulet.2016.02.009
Braidy, N., Grant, R., Adams, S., Brew, B. J., and Guillemin, G. J. (2009). Mechanism for quinolinic acid cytotoxicity in human astrocytes and neurons. Neurotox. Res. 16, 77–86. doi: 10.1007/s12640-009-9051-z
Braniste, V., Al-Asmakh, M., Kowal, C., Anuar, F., Abbaspour, A., Tóth, M., et al. (2014). The gut microbiota influences blood-brain barrier permeability in mice. Sci. Transl. Med. 6:263ra158. doi: 10.1126/scitranslmed.3009759
Bravo, J. A., Forsythe, P., Chew, M. V., Escaravage, E., Savignac, H. M., Dinan, T. G., et al. (2011). Ingestion of Lactobacillus strain regulates emotional behavior and central GABA receptor expression in a mouse via the vagus nerve. Proc. Natl. Acad. Sci. U. S. A. 108, 16050–16055. doi: 10.1073/pnas.1102999108
Bu, X.-L., Yao, X.-Q., Jiao, S.-S., Zeng, F., Liu, Y.-H., Xiang, Y., et al. (2015). A study on the association between infectious burden and Alzheimer’s disease. Eur. J. Neurol. 22, 1519–1525. doi: 10.1111/ene.12477
Buffington, S. A., Di Prisco, G. V., Auchtung, T. A., Ajami, N. J., Petrosino, J. F., and Costa-Mattioli, M. (2016). Microbial reconstitution reverses maternal diet-induced social and synaptic deficits in offspring. Cells 165, 1762–1775. doi: 10.1016/j.cell.2016.06.001
Camacho-Soto, A., Gross, A., Searles Nielsen, S., Dey, N., and Racette, B. A. (2018). Inflammatory bowel disease and risk of Parkinson’s disease in Medicare beneficiaries. Parkinsonism Relat. Disord. 50, 23–28. doi: 10.1016/j.parkreldis.2018.02.008
Catanzaro, R., Anzalone, M., Calabrese, F., Milazzo, M., Capuana, M., Italia, A., et al. (2015). The gut microbiota and its correlations with the central nervous system disorders. Panminerva Med. 57, 127–143.
Cattaneo, A., Cattane, N., Galluzzi, S., Provasi, S., Lopizzo, N., Festari, C., et al. (2017). Association of brain amyloidosis with pro-inflammatory gut bacterial taxa and peripheral inflammation markers in cognitively impaired elderly. Neurobiol. Aging 49, 60–68. doi: 10.1016/j.neurobiolaging.2016.08.019
Chen, G., Huang, B., Fu, S., Li, B., Ran, X., He, D., et al. (2018). G protein-coupled receptor 109A and host microbiota modulate intestinal epithelial integrity during Sepsis. Front. Immunol. 9:2079. doi: 10.3389/fimmu.2018.02079
Cheng, J., Palva, A. M., de Vos, W. M., and Satokari, R. (2013). Contribution of the intestinal microbiota to human health: from birth to 100 years of age. Curr. Top. Microbiol. Immunol. 358, 323–346. doi: 10.1007/82_2011_189
Chiang, H.-L., and Lin, C.-H. (2019). Altered gut microbiome and intestinal pathology in Parkinson’s disease. J. Mov. Disord. 12, 67–83. doi: 10.14802/jmd.18067
Cirrito, J. R., Disabato, B. M., Restivo, J. L., Verges, D. K., Goebel, W. D., Sathyan, A., et al. (2011). Serotonin signaling is associated with lower amyloid-β levels and plaques in transgenic mice and humans. Proc. Natl. Acad. Sci. U. S. A. 108, 14968–14973. doi: 10.1073/pnas.1107411108
Claesson, M. J., Cusack, S., O’Sullivan, O., Greene-Diniz, R., de Weerd, H., Flannery, E., et al. (2011). Composition, variability, and temporal stability of the intestinal microbiota of the elderly. Proc. Natl. Acad. Sci. U. S. A. 108, 4586–4591. doi: 10.1073/pnas.1000097107
Clark, R. I., Salazar, A., Yamada, R., Fitz-Gibbon, S., Morselli, M., Alcaraz, J., et al. (2015). Distinct shifts in microbiota composition during Drosophila aging impair intestinal function and drive mortality. Cell Rep. 12, 1656–1667. doi: 10.1016/j.celrep.2015.08.004
Clarke, G., Grenham, S., Scully, P., Fitzgerald, P., Moloney, R. D., Shanahan, F., et al. (2013). The microbiome-gut-brain axis during early life regulates the hippocampal serotonergic system in a sex-dependent manner. Mol. Psychiatry 18, 666–673. doi: 10.1038/mp.2012.77
Costea, P. I., Zeller, G., Sunagawa, S., Pelletier, E., Alberti, A., Levenez, F., et al. (2017). Towards standards for human fecal sample processing in metagenomic studies. Nat. Biotechnol. 35, 1069–1076. doi: 10.1038/nbt.3960
Cryan, J. F., and Dinan, T. G. (2012). Mind-altering microorganisms: the impact of the gut microbiota on brain and behaviour. Nat. Rev. Neurosci. 13, 701–712. doi: 10.1038/nrn3346
Dalile, B., Vervliet, B., Bergonzelli, G., Verbeke, K., and Van Oudenhove, L. (2020). Colon-delivered short-chain fatty acids attenuate the cortisol response to psychosocial stress in healthy men: a randomized, placebo-controlled trial. Neuropsychopharmacol. Off. Publ. Am. Coll. Neuropsychopharmacol. 45, 2257–2266. doi: 10.1038/s41386-020-0732-x
Daneman, R., and Prat, A. (2015). The blood-brain barrier. Cold Spring Harb. Perspect. Biol. 7:a020412. doi: 10.1101/cshperspect.a020412
Del Rio, D., Zimetti, F., Caffarra, P., Tassotti, M., Bernini, F., Brighenti, F., et al. (2017). The gut microbial metabolite trimethylamine-N-oxide is present in human cerebrospinal fluid. Nutrients 9:1053. doi: 10.3390/nu9101053
den Besten, G., van Eunen, K., Groen, A. K., Venema, K., Reijngoud, D.-J., and Bakker, B. M. (2013). The role of short-chain fatty acids in the interplay between diet, gut microbiota, and host energy metabolism. J. Lipid Res. 54, 2325–2340. doi: 10.1194/jlr.R036012
Desbonnet, L., Garrett, L., Clarke, G., Bienenstock, J., and Dinan, T. G. (2008). The probiotic Bifidobacteria infantis: An assessment of potential antidepressant properties in the rat. J. Psychiatr. Res. 43, 164–174. doi: 10.1016/j.jpsychires.2008.03.009
Di Martino, L., Osme, A., Ghannoum, M., and Cominelli, F. (2023). A novel probiotic combination ameliorates Crohn’s disease-like ileitis by increasing short-chain fatty acid production and modulating essential adaptive immune pathways. Inflamm. Bowel Dis. :izac284. doi: 10.1093/ibd/izac284
Diaz Heijtz, R., Wang, S., Anuar, F., Qian, Y., Björkholm, B., Samuelsson, A., et al. (2011). Normal gut microbiota modulates brain development and behavior. Proc. Natl. Acad. Sci. U. S. A. 108, 3047–3052. doi: 10.1073/pnas.1010529108
Dill-McFarland, K. A., Tang, Z.-Z., Kemis, J. H., Kerby, R. L., Chen, G., Palloni, A., et al. (2019). Close social relationships correlate with human gut microbiota composition. Sci. Rep. 9:703. doi: 10.1038/s41598-018-37298-9
Dinan, T. G., and Cryan, J. F. (2017). Gut instincts: microbiota as a key regulator of brain development, ageing and neurodegeneration. J. Physiol. 595, 489–503. doi: 10.1113/JP273106
Dodiya, H. B., Kuntz, T., Shaik, S. M., Baufeld, C., Leibowitz, J., Zhang, X., et al. (2019). Sex-specific effects of microbiome perturbations on cerebral Aβ amyloidosis and microglia phenotypes. J. Exp. Med. 216, 1542–1560. doi: 10.1084/jem.20182386
Du, G., Dong, W., Yang, Q., Yu, X., Ma, J., Gu, W., et al. (2020). Altered gut microbiota related to inflammatory responses in patients with Huntington’s disease. Front. Immunol. 11:603594. doi: 10.3389/fimmu.2020.603594
Eckburg, P. B., Bik, E. M., Bernstein, C. N., Purdom, E., Dethlefsen, L., Sargent, M., et al. (2005). Diversity of the human intestinal microbial flora. Science 308, 1635–1638. doi: 10.1126/science.1110591
Erkkinen, M. G., Kim, M.-O., and Geschwind, M. D. (2018). Clinical neurology and epidemiology of the major neurodegenerative diseases. Cold Spring Harb. Perspect. Biol. 10:a033118. doi: 10.1101/cshperspect.a033118
Falony, G., Joossens, M., Vieira-Silva, S., Wang, J., Darzi, Y., Faust, K., et al. (2016). Population-level analysis of gut microbiome variation. Science 352, 560–564. doi: 10.1126/science.aad3503
Fang, X. (2016). Potential role of gut microbiota and tissue barriers in Parkinson’s disease and amyotrophic lateral sclerosis. Int. J. Neurosci. 126, 771–776. doi: 10.3109/00207454.2015.1096271
Fang, X., Wang, X., Yang, S., Meng, F., Wang, X., Wei, H., et al. (2016). Evaluation of the microbial diversity in amyotrophic lateral sclerosis using high-throughput sequencing. Front. Microbiol. 7:1479. doi: 10.3389/fmicb.2016.01479
Fasano, A., Visanji, N. P., Liu, L. W. C., Lang, A. E., and Pfeiffer, R. F. (2015). Gastrointestinal dysfunction in Parkinson’s disease. Lancet Neurol. 14, 625–639. doi: 10.1016/S1474-4422(15)00007-1
Felice, V. D., Quigley, E. M., Sullivan, A. M., O’Keeffe, G. W., and O’Mahony, S. M. (2016). Microbiota-gut-brain signalling in Parkinson’s disease: implications for non-motor symptoms. Parkinsonism Relat. Disord. 27, 1–8. doi: 10.1016/j.parkreldis.2016.03.012
Fijan, S. (2014). Microorganisms with claimed probiotic properties: an overview of recent literature. Int. J. Environ. Res. Public Health 11, 4745–4767. doi: 10.3390/ijerph110504745
Finger, C. E., Moreno-Gonzalez, I., Gutierrez, A., Moruno-Manchon, J. F., and McCullough, L. D. (2022). Age-related immune alterations and cerebrovascular inflammation. Mol. Psychiatry 27, 803–818. doi: 10.1038/s41380-021-01361-1
Forsythe, P., Bienenstock, J., and Kunze, W. A. (2014). Vagal pathways for microbiome-brain-gut axis communication. Adv. Exp. Med. Biol. 817, 115–133. doi: 10.1007/978-1-4939-0897-4_5
Forsythe, P., Kunze, W. A., and Bienenstock, J. (2012). On communication between gut microbes and the brain. Curr. Opin. Gastroenterol. 28, 557–562. doi: 10.1097/MOG.0b013e3283572ffa
Frankiensztajn, L. M., Elliott, E., and Koren, O. (2020). The microbiota and the hypothalamus-pituitary-adrenocortical (HPA) axis, implications for anxiety and stress disorders. Curr. Opin. Neurobiol. 62, 76–82. doi: 10.1016/j.conb.2019.12.003
Fransen, F., van Beek, A. A., Borghuis, T., Aidy, S. E., Hugenholtz, F., et al. (2017). Aged gut microbiota contributes to Systemical Inflammaging after transfer to germ-free mice. Front. Immunol. 8::1385. doi: 10.3389/fimmu.2017.01385
Friedland, R. P., and Chapman, M. R. (2017). The role of microbial amyloid in neurodegeneration. PLoS Pathog. 13:e1006654. doi: 10.1371/journal.ppat.1006654
Frost, G., Sleeth, M. L., Sahuri-Arisoylu, M., Lizarbe, B., Cerdan, S., Brody, L., et al. (2014). The short-chain fatty acid acetate reduces appetite via a central homeostatic mechanism. Nat. Commun. 5:3611. doi: 10.1038/ncomms4611
Fujii, Y., Nguyen, T. T. T., Fujimura, Y., Kameya, N., Nakamura, S., Arakawa, K., et al. (2019). Fecal metabolite of a gnotobiotic mouse transplanted with gut microbiota from a patient with Alzheimer’s disease. Biosci. Biotechnol. Biochem. 83, 2144–2152. doi: 10.1080/09168451.2019.1644149
Fung, T. C., Olson, C. A., and Hsiao, E. Y. (2017). Interactions between the microbiota, immune and nervous systems in health and disease. Nat. Neurosci. 20, 145–155. doi: 10.1038/nn.4476
Fushimi, T., Suruga, K., Oshima, Y., Fukiharu, M., Tsukamoto, Y., and Goda, T. (2006). Dietary acetic acid reduces serum cholesterol and triacylglycerols in rats fed a cholesterol-rich diet. Br. J. Nutr. 95, 916–924. doi: 10.1079/bjn20061740
Gagliani, N., Palm, N. W., de Zoete, M. R., and Flavell, R. A. (2014). Inflammasomes and intestinal homeostasis: regulating and connecting infection, inflammation and the microbiota. Int. Immunol. 26, 495–499. doi: 10.1093/intimm/dxu066
Gao, Z., Yin, J., Zhang, J., Ward, R. E., Martin, R. J., Lefevre, M., et al. (2009). Butyrate improves insulin sensitivity and increases energy expenditure in mice. Diabetes 58, 1509–1517. doi: 10.2337/db08-1637
Garbuzova-Davis, S., and Sanberg, P. R. (2014). Blood-CNS barrier impairment in ALS patients versus an animal model. Front. Cell. Neurosci. 8:21. doi: 10.3389/fncel.2014.00021
Gareau, M. G., Wine, E., Rodrigues, D. M., Cho, J. H., Whary, M. T., Philpott, D. J., et al. (2011). Bacterial infection causes stress-induced memory dysfunction in mice. Gut 60, 307–317. doi: 10.1136/gut.2009.202515
Gatto, E. M., Rojas, N. G., Persi, G., Etcheverry, J. L., Cesarini, M. E., and Perandones, C. (2020). Huntington disease: advances in the understanding of its mechanisms. Clin. Park. Relat. Disord. 3:100056. doi: 10.1016/j.prdoa.2020.100056
Geva-Zatorsky, N., Sefik, E., Kua, L., Pasman, L., Tan, T. G., Ortiz-Lopez, A., et al. (2017). Mining the human gut microbiota for immunomodulatory organisms. Cells 168, 928–943.e11. doi: 10.1016/j.cell.2017.01.022
Giles, E. M., and Stagg, A. J. (2017). Type 1 interferon in the human intestine-a co-ordinator of the immune response to the microbiota. Inflamm. Bowel Dis. 23, 524–533. doi: 10.1097/MIB.0000000000001078
Gonzalez, A., Stombaugh, J., Lozupone, C., Turnbaugh, P. J., Gordon, J. I., and Knight, R. (2011). The mind-body-microbial continuum. Dialogues. Clin. Neurosci. 13, 55–62. doi: 10.31887/DCNS.2011.13.1/agonzalez
Gordon, H. A., Bruckner-kardoss, E., and Wostmann, B. S. (1966). Aging in germ-free mice: life tables and lesions observed at natural death. J. Gerontol. 21, 380–387. doi: 10.1093/geronj/21.3.380
Guidetti, P., Luthi-Carter, R. E., Augood, S. J., and Schwarcz, R. (2004). Neostriatal and cortical quinolinate levels are increased in early grade Huntington’s disease. Neurobiol. Dis. 17, 455–461. doi: 10.1016/j.nbd.2004.07.006
Gulati, M., Singh, S. K., Corrie, L., Kaur, I. P., and Chandwani, L. (2020). Delivery routes for fecal microbiota transplants: available, anticipated and aspired. Pharmacol. Res. 159:104954. doi: 10.1016/j.phrs.2020.104954
Hamer, H. M., Jonkers, D., Venema, K., Vanhoutvin, S., Troost, F. J., and Brummer, R.-J. (2008). Review article: the role of butyrate on colonic function. Aliment. Pharmacol. Ther. 27, 104–119. doi: 10.1111/j.1365-2036.2007.03562.x
Harach, T., Marungruang, N., Duthilleul, N., Cheatham, V., Mc Coy, K. D., Frisoni, G., et al. (2017). Reduction of Abeta amyloid pathology in APPPS1 transgenic mice in the absence of gut microbiota. Sci. Rep. 7:41802. doi: 10.1038/srep41802
Haran, J. P., Bhattarai, S. K., Foley, S. E., Dutta, P., Ward, D. V., Bucci, V., et al. (2019). Alzheimer’s disease microbiome is associated with dysregulation of the anti-inflammatory P-glycoprotein pathway. MBio 10:e00632. doi: 10.1128/mBio.00632-19
Harrington, M. (2015). For lack of gut microbes, the blood-brain barrier “leaks.”. Lab. Anim. 44:6. doi: 10.1038/laban.682
He, Q.-L., Wang, H.-C., Ma, Y.-K., Yang, R.-L., Dai, Z.-F., Yang, J.-N., et al. (2023). Changes in the microbiota and their roles in patients with type 2 diabetes mellitus. Curr. Microbiol. 80:132. doi: 10.1007/s00284-023-03219-x
Heiss, C. N., and Olofsson, L. E. (2019). The role of the gut microbiota in development, function and disorders of the central nervous system and the enteric nervous system. J. Neuroendocrinol. 31:e12684. doi: 10.1111/jne.12684
Hertzberg, V. S., Singh, H., Fournier, C. N., Moustafa, A., Polak, M., Kuelbs, C. A., et al. (2022). Gut microbiome differences between amyotrophic lateral sclerosis patients and spouse controls. Amyotroph. Lat. Scler. Front. Degener. 23, 91–99. doi: 10.1080/21678421.2021.1904994
Hill, J. M., and Lukiw, W. J. (2015). Microbial-generated amyloids and Alzheimer’s disease (AD). Front. Aging Neurosci. 7:9. doi: 10.3389/fnagi.2015.00009
Honda, K., and Littman, D. R. (2016). The microbiota in adaptive immune homeostasis and disease. Nature 535, 75–84. doi: 10.1038/nature18848
Huang, F., and Wu, X. (2021). Brain neurotransmitter modulation by gut microbiota in anxiety and depression. Front. Cell Dev. Biol. 9:649103. doi: 10.3389/fcell.2021.649103
Hugenholtz, F., and de Vos, W. M. (2018). Mouse models for human intestinal microbiota research: a critical evaluation. Cell. Mol. Life Sci. 75, 149–160. doi: 10.1007/s00018-017-2693-8
Hwang, Y.-H., Park, S., Paik, J.-W., Chae, S.-W., Kim, D.-H., Jeong, D.-G., et al. (2019). Efficacy and safety of Lactobacillus Plantarum C29-fermented soybean (DW2009) in individuals with mild cognitive impairment: a 12-week, multi-center, randomized, double-blind. Nutrients 11:305. doi: 10.3390/nu11020305
Iatsenko, I., Boquete, J.-P., and Lemaitre, B. (2018). Microbiota-derived lactate activates production of reactive oxygen species by the intestinal NADPH oxidase Nox and shortens Drosophila lifespan. Immunity 49, 929–942.e5. doi: 10.1016/j.immuni.2018.09.017
Inoue, M., and Shinohara, M. L. (2013). The role of interferon-β in the treatment of multiple sclerosis and experimental autoimmune encephalomyelitis—in the perspective of inflammasomes. Immunology 139, 11–18. doi: 10.1111/imm.12081
Jang, S.-E., Lim, S.-M., Jeong, J.-J., Jang, H.-M., Lee, H.-J., Han, M. J., et al. (2018). Gastrointestinal inflammation by gut microbiota disturbance induces memory impairment in mice. Mucosal Immunol. 11, 369–379. doi: 10.1038/mi.2017.49
Jonsson, A. L., and Bäckhed, F. (2017). Role of gut microbiota in atherosclerosis. Nat. Rev. Cardiol. 14, 79–87. doi: 10.1038/nrcardio.2016.183
Kaelberer, M. M., Buchanan, K. L., Klein, M. E., Barth, B. B., Montoya, M. M., Shen, X., et al. (2018). A gut-brain neural circuit for nutrient sensory transduction. Science 361:eaat5236. doi: 10.1126/science.aat5236
Kau, A. L., Ahern, P. P., Griffin, N. W., Goodman, A. L., and Gordon, J. I. (2011). Human nutrition, the gut microbiome and the immune system. Nature 474, 327–336. doi: 10.1038/nature10213
Kawashima, T., Kosaka, A., Yan, H., Guo, Z., Uchiyama, R., Fukui, R., et al. (2013). Double-stranded RNA of intestinal commensal but not pathogenic bacteria triggers production of protective interferon-β. Immunity 38, 1187–1197. doi: 10.1016/j.immuni.2013.02.024
Kennedy, P. J., Cryan, J. F., Dinan, T. G., and Clarke, G. (2017). Kynurenine pathway metabolism and the microbiota-gut-brain axis. Neuropharmacology 112, 399–412. doi: 10.1016/j.neuropharm.2016.07.002
Keshavarzian, A., Green, S. J., Engen, P. A., Voigt, R. M., Naqib, A., Forsyth, C. B., et al. (2015). Colonic bacterial composition in Parkinson’s disease. Mov. Disord. Off. J. Mov. Disord. Soc. 30, 1351–1360. doi: 10.1002/mds.26307
Kim, C.-S., Cha, L., Sim, M., Jung, S., Chun, W. Y., Baik, H. W., et al. (2021). Probiotic supplementation improves cognitive function and mood with changes in gut microbiota in community-dwelling older adults: a randomized, double-blind, placebo-controlled, multicenter trial. J. Gerontol. A Biol. Sci. Med. Sci. 76, 32–40. doi: 10.1093/gerona/glaa090
Kim, M.-S., Kim, Y., Choi, H., Kim, W., Park, S., Lee, D., et al. (2020). Transfer of a healthy microbiota reduces amyloid and tau pathology in an Alzheimer’s disease animal model. Gut 69, 283–294. doi: 10.1136/gutjnl-2018-317431
Kleniewska, P., and Pawliczak, R. (2023). Does oxidative stress along with Dysbiosis participate in the pathogenesis of asthma in the obese? Cell Biochem. Biophysics 81, 117–126. doi: 10.1007/s12013-022-01114-z
Knight, R., Vrbanac, A., Taylor, B. C., Aksenov, A., Callewaert, C., Debelius, J., et al. (2018). Best practices for analysing microbiomes. Nat. Rev. Microbiol. 16, 410–422. doi: 10.1038/s41579-018-0029-9
Kobayashi, Y., Kuhara, T., Oki, M., and Xiao, J.-Z. (2019). Effects of Bifidobacterium breve A1 on the cognitive function of older adults with memory complaints: a randomised, double-blind, placebo-controlled trial. Benefic. Microbes 10, 511–520. doi: 10.3920/BM2018.0170
Kobayashi, Y., Sugahara, H., Shimada, K., Mitsuyama, E., Kuhara, T., Yasuoka, A., et al. (2017). Therapeutic potential of Bifidobacterium breve strain A1 for preventing cognitive impairment in Alzheimer’s disease. Sci. Rep. 7:13510. doi: 10.1038/s41598-017-13368-2
Kong, G., Cao, K.-A. L., Judd, L. M., Li, S., Renoir, T., and Hannan, A. J. (2020). Microbiome profiling reveals gut dysbiosis in a transgenic mouse model of Huntington’s disease. Neurobiol. Dis. 135:104268. doi: 10.1016/j.nbd.2018.09.001
Konjevod, M., Nikolac Perkovic, M., Sáiz, J., Svob Strac, D., Barbas, C., and Rojo, D. (2021). Metabolomics analysis of microbiota-gut-brain axis in neurodegenerative and psychiatric diseases. J. Pharm. Biomed. Anal. 194:113681. doi: 10.1016/j.jpba.2020.113681
Körner, E., and Lorentz, A. (2023). Fecal microbiota transplantation in patients with irritable bowel syndrome: an overview of current studies. J. Appl. Microbiol. lxad044. doi: 10.1093/jambio/lxad044
Kountouras, J., Boziki, M., Gavalas, E., Zavos, C., Grigoriadis, N., Deretzi, G., et al. (2009). Eradication of Helicobacter pylori may be beneficial in the management of Alzheimer’s disease. J. Neurol. 256, 758–767. doi: 10.1007/s00415-009-5011-z
Kuwahara, A. (2014). Contributions of colonic short-chain fatty acid receptors in energy homeostasis. Front. Endocrinol. 5:144. doi: 10.3389/fendo.2014.00144
Langille, M. G., Meehan, C. J., Koenig, J. E., Dhanani, A. S., Rose, R. A., Howlett, S. E., et al. (2014). Microbial shifts in the aging mouse gut. Microbiome 2:50. doi: 10.1186/s40168-014-0050-9
Latorre, R., Sternini, C., De Giorgio, R., and Greenwood-Van Meerveld, B. (2016). Enteroendocrine cells: a review of their role in brain-gut communication. Neurogastroenterol. Motil. Off. J. Eur. Gastrointest. Motil. Soc. 28, 620–630. doi: 10.1111/nmo.12754
Le Floc’h, N., Otten, W., and Merlot, E. (2011). Tryptophan metabolism, from nutrition to potential therapeutic applications. Amino Acids 41, 1195–1205. doi: 10.1007/s00726-010-0752-7
Leblhuber, F., Steiner, K., Schuetz, B., Fuchs, D., and Gostner, J. M. (2018). Probiotic supplementation in patients with Alzheimer’s dementia—An explorative intervention study. Curr. Alzheimer Res. 15, 1106–1113. doi: 10.2174/1389200219666180813144834
Lee, H.-J., Hwang, Y.-H., and Kim, D.-H. (2018). Lactobacillus plantarum C29-fermented soybean (DW2009) alleviates memory impairment in 5XFAD transgenic mice by regulating microglia activation and gut microbiota composition. Mol. Nutr. Food Res. 62:e1800359. doi: 10.1002/mnfr.201800359
Liang, S., Wang, T., Hu, X., Luo, J., Li, W., Wu, X., et al. (2015). Administration of Lactobacillus helveticus NS8 improves behavioral, cognitive, and biochemical aberrations caused by chronic restraint stress. Neuroscience 310, 561–577. doi: 10.1016/j.neuroscience.2015.09.033
Lin, C.-H., Chen, C.-C., Chiang, H.-L., Liou, J.-M., Chang, C.-M., Lu, T.-P., et al. (2019). Altered gut microbiota and inflammatory cytokine responses in patients with Parkinson’s disease. J. Neuroinflammation 16:129. doi: 10.1186/s12974-019-1528-y
Lin, L., and Zhang, J. (2017). Role of intestinal microbiota and metabolites on gut homeostasis and human diseases. BMC Immunol. 18:2. doi: 10.1186/s12865-016-0187-3
Liu, Y., and Forsythe, P. (2021). Vagotomy and insights into the microbiota-gut-brain axis. Neurosci. Res. 168, 20–27. doi: 10.1016/j.neures.2021.04.001
Lozupone, C. A., Stombaugh, J. I., Gordon, J. I., Jansson, J. K., and Knight, R. (2012). Diversity, stability and resilience of the human gut microbiota. Nature 489, 220–230. doi: 10.1038/nature11550
Lyte, M. (2014). Microbial endocrinology: host-microbiota neuroendocrine interactions influencing brain and behavior. Gut Microbes 5, 381–389. doi: 10.4161/gmic.28682
Ma, Q., Xing, C., Long, W., Wang, H. Y., Liu, Q., and Wang, R.-F. (2019). Impact of microbiota on central nervous system and neurological diseases: the gut-brain axis. J. Neuroinflammation 16:53. doi: 10.1186/s12974-019-1434-3
Miller, S. I., Ernst, R. K., and Bader, M. W. (2005). LPS, TLR4 and infectious disease diversity. Nat. Rev. Microbiol. 3, 36–46. doi: 10.1038/nrmicro1068
Młynarska, E., Gadzinowska, J., Tokarek, J., Forycka, J., Szuman, A., Franczyk, B., et al. (2022). The role of the microbiome-brain-gut Axis in the pathogenesis of depressive disorder. Nutrients 14:1921. doi: 10.3390/nu14091921
Mohammadi, G., Dargahi, L., Naserpour, T., Mirzanejad, Y., Alizadeh, S. A., Peymani, A., et al. (2019a). Probiotic mixture of Lactobacillus helveticus R0052 and Bifidobacterium longum R0175 attenuates hippocampal apoptosis induced by lipopolysaccharide in rats. Int. Microbiol. Off. J. Span. Soc. Microbiol. 22, 317–323. doi: 10.1007/s10123-018-00051-3
Mohammadi, G., Dargahi, L., Peymani, A., Mirzanejad, Y., Alizadeh, S. A., Naserpour, T., et al. (2019b). The effects of probiotic formulation pretreatment (Lactobacillus helveticus R0052 and Bifidobacterium longum R0175) on a lipopolysaccharide rat model. J. Am. Coll. Nutr. 38, 209–217. doi: 10.1080/07315724.2018.1487346
Mulak, A., and Bonaz, B. (2015). Brain-gut-microbiota axis in Parkinson’s disease. World J. Gastroenterol. 21, 10609–10620. doi: 10.3748/wjg.v21.i37.10609
Needham, B. D., Kaddurah-Daouk, R., and Mazmanian, S. K. (2020). Gut microbial molecules in behavioural and neurodegenerative conditions. Nat. Rev. Neurosci. 21, 717–731. doi: 10.1038/s41583-020-00381-0
Nicholson, K., Bjornevik, K., Abu-Ali, G., Chan, J., Cortese, M., Dedi, B., et al. (2021). The human gut microbiota in people with amyotrophic lateral sclerosis. Amyotroph. Lat. Scler. Front. Degener. 22, 186–194. doi: 10.1080/21678421.2020.1828475
Nimgampalle, M., and Kuna, Y. (2017). Anti-Alzheimer properties of probiotic, Lactobacillus plantarum MTCC 1325 in Alzheimer’s disease induced albino rats. J. Clin. Diagn. Res. 11, KC01–KC05. doi: 10.7860/JCDR/2017/26106.10428
Noble, E. E., Hsu, T. M., and Kanoski, S. E. (2017). Gut to brain Dysbiosis: mechanisms linking Western diet consumption, the microbiome, and cognitive impairment. Front. Behav. Neurosci. 11:9. doi: 10.3389/fnbeh.2017.00009
O’Mahony, S. M., Clarke, G., Borre, Y. E., Dinan, T. G., and Cryan, J. F. (2015). Serotonin, tryptophan metabolism and the brain-gut-microbiome axis. Behav. Brain Res. 277, 32–48. doi: 10.1016/j.bbr.2014.07.027
O’Toole, P. W., and Jeffery, I. B. (2015). Gut microbiota and aging. Science 350, 1214–1215. doi: 10.1126/science.aac8469
Obata, F., Fons, C. O., and Gould, A. P. (2018). Early-life exposure to low-dose oxidants can increase longevity via microbiome remodelling in Drosophila. Nat. Commun. 9:975. doi: 10.1038/s41467-018-03070-w
Obermeier, B., Daneman, R., and Ransohoff, R. M. (2013). Development, maintenance and disruption of the blood-brain barrier. Nat. Med. 19, 1584–1596. doi: 10.1038/nm.3407
Odamaki, T., Kato, K., Sugahara, H., Hashikura, N., Takahashi, S., Xiao, J., et al. (2016). Age-related changes in gut microbiota composition from newborn to centenarian: a cross-sectional study. BMC Microbiol. 16:90. doi: 10.1186/s12866-016-0708-5
Ogawa, T., Matson, W. R., Beal, M. F., Myers, R. H., Bird, E. D., Milbury, P., et al. (1992). Kynurenine pathway abnormalities in Parkinson’s disease. Neurology 42, 1702–1706. doi: 10.1212/wnl.42.9.1702
Ordoñez-Rodriguez, A., Roman, P., Rueda-Ruzafa, L., Campos-Rios, A., and Cardona, D. (2023). Changes in gut microbiota and multiple sclerosis: a systematic review. Int. J. Environ. Res. Public Health 20:4624. doi: 10.3390/ijerph20054624
Oswal, A., Cao, C., Yeh, C.-H., Neumann, W.-J., Gratwicke, J., Akram, H., et al. (2021). Neural signatures of hyperdirect pathway activity in Parkinson’s disease. Nat. Commun. 12:5185. doi: 10.1038/s41467-021-25366-0
Parker, A., Fonseca, S., and Carding, S. R. (2020). Gut microbes and metabolites as modulators of blood-brain barrier integrity and brain health. Gut Microbes 11, 135–157. doi: 10.1080/19490976.2019.1638722
Peter, I., Dubinsky, M., Bressman, S., Park, A., Lu, C., Chen, N., et al. (2018). Anti-tumor necrosis factor therapy and incidence of Parkinson disease among patients with inflammatory bowel disease. JAMA Neurol. 75, 939–946. doi: 10.1001/jamaneurol.2018.0605
Radulescu, C. I., Garcia-Miralles, M., Sidik, H., Bardile, C. F., Yusof, N. A. B. M., Lee, H. U., et al. (2020). Reprint of: manipulation of microbiota reveals altered callosal myelination and white matter plasticity in a model of Huntington disease. Neurobiol. Dis. 135:104744. doi: 10.1016/j.nbd.2020.104744
Reeve, A., Simcox, E., and Turnbull, D. (2014). Ageing and Parkinson’s disease: why is advancing age the biggest risk factor? Ageing Res. Rev. 14, 19–30. doi: 10.1016/j.arr.2014.01.004
Romano, K. A., Vivas, E. I., Amador-Noguez, D., and Rey, F. E. (2015). Intestinal microbiota composition modulates choline bioavailability from diet and accumulation of the proatherogenic metabolite trimethylamine-N-oxide. MBio 6:e02481. doi: 10.1128/mBio.02481-14
Rosas, H. D., Doros, G., Bhasin, S., Thomas, B., Gevorkian, S., Malarick, K., et al. (2015). A systems-level “misunderstanding”: the plasma metabolome in Huntington’s disease. Ann. Clin. Transl. Neurol. 2, 756–768. doi: 10.1002/acn3.214
Rosas, H. D., Salat, D. H., Lee, S. Y., Zaleta, A. K., Pappu, V., Fischl, B., et al. (2008). Cerebral cortex and the clinical expression of Huntington’s disease: complexity and heterogeneity. Brain J. Neurol. 131, 1057–1068. doi: 10.1093/brain/awn025
Rothschild, D., Weissbrod, O., Barkan, E., Kurilshikov, A., Korem, T., Zeevi, D., et al. (2018). Environment dominates over host genetics in shaping human gut microbiota. Nature 555, 210–215. doi: 10.1038/nature25973
Roubaud-Baudron, C., Krolak-Salmon, P., Quadrio, I., Mégraud, F., and Salles, N. (2012). Impact of chronic Helicobacter pylori infection on Alzheimer’s disease: preliminary results. Neurobiol. Aging 33, 1009.e11–1009.e19. doi: 10.1016/j.neurobiolaging.2011.10.021
Rowland, I., Gibson, G., Heinken, A., Scott, K., Swann, J., Thiele, I., et al. (2018). Gut microbiota functions: metabolism of nutrients and other food components. Eur. J. Nutr. 57, 1–24. doi: 10.1007/s00394-017-1445-8
Sadler, R., Cramer, J. V., Heindl, S., Kostidis, S., Betz, D., Zuurbier, K. R., et al. (2020). Short-chain fatty acids improve Poststroke recovery via immunological mechanisms. J. Neurosci. 40, 1162–1173. doi: 10.1523/JNEUROSCI.1359-19.2019
Salat-Foix, D., Tran, K., Ranawaya, R., Meddings, J., and Suchowersky, O. (2012). Increased intestinal permeability and Parkinson disease patients: chicken or egg? Can. J. Neurol. Sci. J. Can. Sci. Neurol. 39, 185–188. doi: 10.1017/s0317167100013202
Salmina, A. B., Kharitonova, E. V., Gorina, Y. V., Teplyashina, E. A., Malinovskaya, N. A., Khilazheva, E. D., et al. (2021). Blood-brain barrier and neurovascular unit in vitro models for studying mitochondria-driven molecular mechanisms of neurodegeneration. Int. J. Mol. Sci. 22:4661. doi: 10.3390/ijms22094661
Sampson, T. R., Debelius, J. W., Thron, T., Janssen, S., Shastri, G. G., Ilhan, Z. E., et al. (2016). Gut microbiota regulate motor deficits and Neuroinflammation in a model of Parkinson’s disease. Cells 167, 1469–1480.e12. doi: 10.1016/j.cell.2016.11.018
Scheperjans, F., Aho, V., Pereira, P. A. B., Koskinen, K., Paulin, L., Pekkonen, E., et al. (2015). Gut microbiota are related to Parkinson’s disease and clinical phenotype. Mov. Disord. Off. J. Mov. Disord. Soc. 30, 350–358. doi: 10.1002/mds.26069
Scholtens, P. A. M. J., Oozeer, R., Martin, R., Amor, K. B., and Knol, J. (2012). The early settlers: intestinal microbiology in early life. Annu. Rev. Food Sci. Technol. 3, 425–447. doi: 10.1146/annurev-food-022811-101120
Schwarcz, R., Bruno, J. P., Muchowski, P. J., and Wu, H.-Q. (2012). Kynurenines in the mammalian brain: when physiology meets pathology. Nat. Rev. Neurosci. 13, 465–477. doi: 10.1038/nrn3257
Schwartz, K., and Boles, B. R. (2013). Microbial amyloids--functions and interactions within the host. Curr. Opin. Microbiol. 16, 93–99. doi: 10.1016/j.mib.2012.12.001
Schwartz, K., Syed, A. K., Stephenson, R. E., Rickard, A. H., and Boles, B. R. (2012). Functional amyloids composed of phenol soluble modulins stabilize Staphylococcus aureus biofilms. PLoS Pathog. 8:e1002744. doi: 10.1371/journal.ppat.1002744
Sellge, G., and Kufer, T. A. (2015). PRR-signaling pathways: learning from microbial tactics. Semin. Immunol. 27, 75–84. doi: 10.1016/j.smim.2015.03.009
Sharon, G., Sampson, T. R., Geschwind, D. H., and Mazmanian, S. K. (2016). The central nervous system and the gut microbiome. Cells 167, 915–932. doi: 10.1016/j.cell.2016.10.027
Skaper, S. D., Facci, L., Zusso, M., and Giusti, P. (2018). An inflammation-centric view of neurological disease: beyond the neuron. Front. Cell. Neurosci. 12:72. doi: 10.3389/fncel.2018.00072
Smith, P. M., Howitt, M. R., Panikov, N., Michaud, M., Gallini, C. A., Bohlooly-Y, M., et al. (2013). The microbial metabolites, short-chain fatty acids, regulate colonic Treg cell homeostasis. Science 341, 569–573. doi: 10.1126/science.1241165
Smith, P., Willemsen, D., Popkes, M., Metge, F., Gandiwa, E., Reichard, M., et al. (2017). Regulation of life span by the gut microbiota in the short-lived African turquoise killifish. elife 6:e27014. doi: 10.7554/eLife.27014
Sommer, F., and Bäckhed, F. (2013). The gut microbiota--masters of host development and physiology. Nat. Rev. Microbiol. 11, 227–238. doi: 10.1038/nrmicro2974
Sonnenburg, J. L., and Sonnenburg, E. D. (2019). Vulnerability of the industrialized microbiota. Science 366:eaaw9255. doi: 10.1126/science.aaw9255
Sovran, B., Hugenholtz, F., Elderman, M., Van Beek, A. A., Graversen, K., Huijskes, M., et al. (2019). Age-associated impairment of the mucus barrier function is associated with profound changes in microbiota and immunity. Sci. Rep. 9:1437. doi: 10.1038/s41598-018-35228-3
Srivastav, S., Neupane, S., Bhurtel, S., Katila, N., Maharjan, S., Choi, H., et al. (2019). Probiotics mixture increases butyrate, and subsequently rescues the nigral dopaminergic neurons from MPTP and rotenone-induced neurotoxicity. J. Nutr. Biochem. 69, 73–86. doi: 10.1016/j.jnutbio.2019.03.021
Staley, C., Khoruts, A., and Sadowsky, M. J. (2017). Contemporary applications of fecal microbiota transplantation to treat intestinal diseases in humans. Arch. Med. Res. 48, 766–773. doi: 10.1016/j.arcmed.2017.11.006
Stan, T. L., Soylu-Kucharz, R., Burleigh, S., Prykhodko, O., Cao, L., Franke, N., et al. (2020). Increased intestinal permeability and gut dysbiosis in the R6/2 mouse model of Huntington’s disease. Sci. Rep. 10:18270. doi: 10.1038/s41598-020-75229-9
Stebegg, M., Silva-Cayetano, A., Innocentin, S., Jenkins, T. P., Cantacessi, C., Gilbert, C., et al. (2019). Heterochronic faecal transplantation boosts gut germinal centres in aged mice. Nat. Commun. 10:2443. doi: 10.1038/s41467-019-10430-7
Stone, T. W., Stoy, N., and Darlington, L. G. (2013). An expanding range of targets for kynurenine metabolites of tryptophan. Trends Pharmacol. Sci. 34, 136–143. doi: 10.1016/j.tips.2012.09.006
Strandwitz, P. (2018). Neurotransmitter modulation by the gut microbiota. Brain Res. 1693, 128–133. doi: 10.1016/j.brainres.2018.03.015
Sun, M., He, C., Cong, Y., and Liu, Z. (2015). Regulatory immune cells in regulation of intestinal inflammatory response to microbiota. Mucosal Immunol. 8, 969–978. doi: 10.1038/mi.2015.49
Sun, J., Xu, J., Ling, Y., Wang, F., Gong, T., Yang, C., et al. (2019). Fecal microbiota transplantation alleviated Alzheimer’s disease-like pathogenesis in APP/PS1 transgenic mice. Transl. Psychiatry 9:189. doi: 10.1038/s41398-019-0525-3
Tagliabue, A., and Elli, M. (2013). The role of gut microbiota in human obesity: recent findings and future perspectives. Nutr. Metab. Cardiovasc. Dis. 23, 160–168. doi: 10.1016/j.numecd.2012.09.002
Tanaka, M., Török, N., Tóth, F., Szabó, Á., and Vécsei, L. (2021a). Co-players in chronic pain: Neuroinflammation and the tryptophan-kynurenine metabolic pathway. Biomedicine 9:897. doi: 10.3390/biomedicines9080897
Tanaka, M., Tóth, F., Polyák, H., Szabó, Á., Mándi, Y., and Vécsei, L. (2021b). Immune influencers in action: metabolites and enzymes of the tryptophan-kynurenine metabolic pathway. Biomedicine 9:734. doi: 10.3390/biomedicines9070734
Tetz, G., Brown, S. M., Hao, Y., and Tetz, V. (2018). Parkinson’s disease and bacteriophages as its overlooked contributors. Sci. Rep. 8:10812. doi: 10.1038/s41598-018-29173-4
Thevaranjan, N., Puchta, A., Schulz, C., Naidoo, A., Szamosi, J. C., Verschoor, C. P., et al. (2017). Age-associated microbial Dysbiosis promotes intestinal permeability, systemic inflammation, and macrophage dysfunction. Cell Host Microbe 21, 455–466.e4. doi: 10.1016/j.chom.2017.03.002
Ticinesi, A., Milani, C., Lauretani, F., Nouvenne, A., Mancabelli, L., Lugli, G. A., et al. (2017). Gut microbiota composition is associated with polypharmacy in elderly hospitalized patients. Sci. Rep. 7:11102. doi: 10.1038/s41598-017-10734-y
Ting, K. K., Brew, B., and Guillemin, G. (2007). The involvement of astrocytes and kynurenine pathway in Alzheimer’s disease. Neurotox. Res. 12, 247–262. doi: 10.1007/BF03033908
Todesco, T., Rao, A. V., Bosello, O., and Jenkins, D. J. (1991). Propionate lowers blood glucose and alters lipid metabolism in healthy subjects. Am. J. Clin. Nutr. 54, 860–865. doi: 10.1093/ajcn/54.5.860
Toepfer, M., Schroeder, M., Klauser, A., Lochmüller, H., Hirschmann, M., Riepl, R. L., et al. (1997). Delayed colonic transit times in amyotrophic lateral sclerosis assessed with radio-opaque markers. Eur. J. Med. Res. 2, 473–476.
Ulusoy, A., Phillips, R. J., Helwig, M., Klinkenberg, M., Powley, T. L., and Di Monte, D. A. (2017). Brain-to-stomach transfer of α-synuclein via vagal preganglionic projections. Acta Neuropathol. 133, 381–393. doi: 10.1007/s00401-016-1661-y
Vamos, E., Pardutz, A., Klivenyi, P., Toldi, J., and Vecsei, L. (2009). The role of kynurenines in disorders of the central nervous system: possibilities for neuroprotection. J. Neurol. Sci. 283, 21–27. doi: 10.1016/j.jns.2009.02.326
Villarán, R. F., Espinosa-Oliva, A. M., Sarmiento, M., De Pablos, R. M., Argüelles, S., Delgado-Cortés, M. J., et al. (2010). Ulcerative colitis exacerbates lipopolysaccharide-induced damage to the nigral dopaminergic system: potential risk factor in Parkinson’s disease. J. Neurochem. 114, 1687–1700. doi: 10.1111/j.1471-4159.2010.06879.x
Vogt, N. M., Romano, K. A., Darst, B. F., Engelman, C. D., Johnson, S. C., Carlsson, C. M., et al. (2018). The gut microbiota-derived metabolite trimethylamine N-oxide is elevated in Alzheimer’s disease. Alzheimers Res. Ther. 10:124. doi: 10.1186/s13195-018-0451-2
Wang, T., Hu, X., Liang, S., Li, W., Wu, X., Wang, L., et al. (2015). Lactobacillus fermentum NS9 restores the antibiotic induced physiological and psychological abnormalities in rats. Benefic. Microbes 6, 707–717. doi: 10.3920/BM2014.0177
Wang, M.-M., Miao, D., Cao, X.-P., Tan, L., and Tan, L. (2018). Innate immune activation in Alzheimer’s disease. Ann. Transl. Med. 6:177. doi: 10.21037/atm.2018.04.20
Wang, X. L., Zeng, J., Yang, Y., Xiong, Y., Zhang, Z.-H., Qiu, M., et al. (2015). Helicobacter pylori filtrate induces Alzheimer-like tau hyperphosphorylation by activating glycogen synthase kinase-3β. J. Alzheimers Dis. 43, 153–165. doi: 10.3233/JAD-140198
Wasser, C. I., Mercieca, E.-C., Kong, G., Hannan, A. J., McKeown, S. J., Glikmann-Johnston, Y., et al. (2020). Gut dysbiosis in Huntington’s disease: associations among gut microbiota, cognitive performance and clinical outcomes. Brain Commun. 2:fcaa110. doi: 10.1093/braincomms/fcaa110
Wendeln, A.-C., Degenhardt, K., Kaurani, L., Gertig, M., Ulas, T., Jain, G., et al. (2018). Innate immune memory in the brain shapes neurological disease hallmarks. Nature 556, 332–338. doi: 10.1038/s41586-018-0023-4
Widner, B., Leblhuber, F., Walli, J., Tilz, G. P., Demel, U., and Fuchs, D. (2000). Tryptophan degradation and immune activation in Alzheimer’s disease. J. Neural Transm. 1996, 343–353. doi: 10.1007/s007020050029
Wikoff, W. R., Anfora, A. T., Liu, J., Schultz, P. G., Lesley, S. A., Peters, E. C., et al. (2009). Metabolomics analysis reveals large effects of gut microflora on mammalian blood metabolites. Proc. Natl. Acad. Sci. U. S. A. 106, 3698–3703. doi: 10.1073/pnas.0812874106
Woodworth, M. H., Carpentieri, C., Sitchenko, K. L., and Kraft, C. S. (2017). Challenges in fecal donor selection and screening for fecal microbiota transplantation: a review. Gut Microbes 8, 225–237. doi: 10.1080/19490976.2017.1286006
Wu, S., Yi, J., Zhang, Y.-G., Zhou, J., and Sun, J. (2015). Leaky intestine and impaired microbiome in an amyotrophic lateral sclerosis mouse model. Phys. Rep. 3:e12356. doi: 10.14814/phy2.12356
Wyss, M. T., Magistretti, P. J., Buck, A., and Weber, B. (2011). Labeled acetate as a marker of astrocytic metabolism. J. Cereb. Blood flow Metab. Off. J. Int. Soc. Cereb. Blood Flow Metab. 31, 1668–1674. doi: 10.1038/jcbfm.2011.84
Yang, X., Yu, D., Xue, L., Li, H., and Du, J. (2020). Probiotics modulate the microbiota-gut-brain axis and improve memory deficits in aged SAMP8 mice. Acta Pharm. Sin. B 10, 475–487. doi: 10.1016/j.apsb.2019.07.001
Yatsunenko, T., Rey, F. E., Manary, M. J., Trehan, I., Dominguez-Bello, M. G., Contreras, M., et al. (2012). Human gut microbiome viewed across age and geography. Nature 486, 222–227. doi: 10.1038/nature11053
Young, J. J., Bruno, D., and Pomara, N. (2014). A review of the relationship between proinflammatory cytokines and major depressive disorder. J. Affect. Disord. 169, 15–20. doi: 10.1016/j.jad.2014.07.032
Zeng, Q., Shen, J., Chen, K., Zhou, J., Liao, Q., Lu, K., et al. (2020). The alteration of gut microbiome and metabolism in amyotrophic lateral sclerosis patients. Sci. Rep. 10:12998. doi: 10.1038/s41598-020-69845-8
Zhang, Q., Bai, Y., Wang, W., Li, J., Zhang, L., Tang, Y., et al. (2023). Role of herbal medicine and gut microbiota in the prevention and treatment of obesity. J. Ethnopharmacol. 305:116127. doi: 10.1016/j.jep.2022.116127
Zhang, Y.-G., Wu, S., Yi, J., Xia, Y., Jin, D., Zhou, J., et al. (2017). Target intestinal microbiota to alleviate disease progression in amyotrophic lateral sclerosis. Clin. Ther. 39, 322–336. doi: 10.1016/j.clinthera.2016.12.014
Zheng, D., Liwinski, T., and Elinav, E. (2020). Interaction between microbiota and immunity in health and disease. Cell Res. 30, 492–506. doi: 10.1038/s41422-020-0332-7
Zhou, Y., Hu, G., and Wang, M. C. (2021). Host and microbiota metabolic signals in aging and longevity. Nat. Chem. Biol. 17, 1027–1036. doi: 10.1038/s41589-021-00837-z
Zhou, Y., Lu, Y., Fang, X., Zhang, J., Li, J., Li, S., et al. (2015). An astrocyte regenerative response from vimentin-containing cells in the spinal cord of amyotrophic lateral sclerosis’s disease-like transgenic (G93A SOD1) mice. Neurodegener. Dis. 15, 1–12. doi: 10.1159/000369466
Zhu, W., Wang, Z., Tang, W. H. W., and Hazen, S. L. (2017). Gut microbe-generated trimethylamine N-oxide from dietary choline is Prothrombotic in subjects. Circulation 135, 1671–1673. doi: 10.1161/CIRCULATIONAHA.116.025338
Keywords: neurodegenerative diseases, gut-brain axis, microbiota, Alzheimer’s disease, Parkinson’s disease, Huntington’s disease
Citation: Khatoon S, Kalam N, Rashid S and Bano G (2023) Effects of gut microbiota on neurodegenerative diseases. Front. Aging Neurosci. 15:1145241. doi: 10.3389/fnagi.2023.1145241
Edited by:
Shampa Ghosh, ICMR – National Institute of Nutrition (NIN), IndiaReviewed by:
Kathy R. Magnusson, Oregon State University, United StatesRakesh Bhaskar, Yeungnam University, Republic of Korea
Copyright © 2023 Khatoon, Kalam, Rashid and Bano. This is an open-access article distributed under the terms of the Creative Commons Attribution License (CC BY). The use, distribution or reproduction in other forums is permitted, provided the original author(s) and the copyright owner(s) are credited and that the original publication in this journal is cited, in accordance with accepted academic practice. No use, distribution or reproduction is permitted which does not comply with these terms.
*Correspondence: Saima Khatoon, c2FpbWEua2hhdG9vbjkwQHlhaG9vLmNvbQ==
†These authors have contributed equally to this work