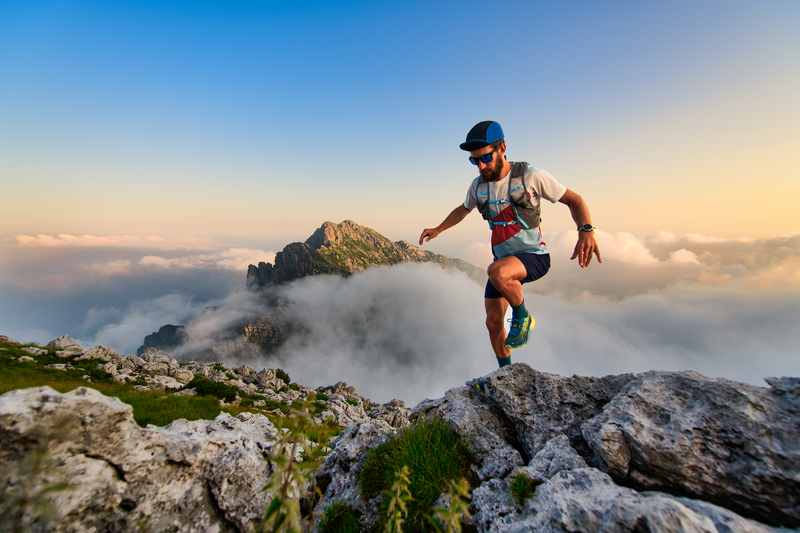
95% of researchers rate our articles as excellent or good
Learn more about the work of our research integrity team to safeguard the quality of each article we publish.
Find out more
REVIEW article
Front. Aging Neurosci. , 02 November 2022
Sec. Parkinson’s Disease and Aging-related Movement Disorders
Volume 14 - 2022 | https://doi.org/10.3389/fnagi.2022.976316
This article is part of the Research Topic Reproducibility and Replicability in Parkinson's Disease and Age-Related Movement Disorders View all 10 articles
Non-motor symptoms (NMS) of Parkinson's disease (PD), such as constipation, sleep disorders, and olfactory deficits, may emerge up to 20 years earlier than motor symptoms. A series of evidence indicates that the pathology of PD may occur from the gastrointestinal tract to the brain. Numerous studies support that the gut microbiota communicates with the brain through the immune system, special amino acid metabolism, and the nervous system in PD. Recently, there is growing recognition that the gut microbiota plays a vital role in the modulation of multiple neurochemical pathways via the “gut microbiota-brain axis” (GMBA). Many gut microbiota metabolites, such as fatty acids, amino acids, and bile acids, convey signaling functions as they mediate the crosstalk between gut microbiota and host physiology. Amino acids' abundance and species alteration, including glutamate and tryptophan, may disturb the signaling transmission between nerve cells and disrupt the normal basal ganglia function in PD. Specific amino acids and their receptors are considered new potential targets for ameliorating PD. The present study aimed to systematically summarize all available evidence on the gut microbiota-derived amino acid metabolism alterations associated with PD.
Parkinson's disease (PD) is the second most common neurodegenerative disease and affects an estimated 3 million people worldwide. The typical feature of PD is the accumulation of intracellular aggregates called Lewy bodies, composed largely of the presynaptic protein alpha-synuclein (Collier et al., 2017). In clinical practice, individuals with PD benefit from drugs developed to act on a single molecular target (Figure 1; Sarkar et al., 2016; Tambasco et al., 2018). Levodopa (L-DOPA) is still the gold standard therapy for patients with PD. However, long-term L-DOPA treatment is associated with motor fluctuations and dyskinesias. As the disease progresses, amino acid decarboxylase inhibitors (AADC inhibitors), catechol-O-methyl transferase inhibitors (COMT inhibitors), monoamine oxidase B inhibitors (MAO-B inhibitors), and dopamine receptor agonists are also used. The surgical treatment of deep brain stimulation can be used for patients with PD who have severe limitations in functioning despite optimal pharmacological treatment (Weiner, 2002; Malek, 2019). However, there is not currently a medication available to cure or halt the progression of the disease.
Figure 1. Drugs utilized in the treatment of Parkinson's disease. MAO-B, Monoamine oxidase-B inhibitors; COMT, catechol-O-methyl transferase inhibitors; L-DOPA, L-3,4-dihydroxyphenylalanine; AADC, amino acid decarboxylase (AADC, Dopa decarboxylase); DOPAC, 3,4-dihydroxyphenylacetic acid; 3-OMD, 3-O-methyl-dopa; 3-MT, 3-methoxytyramine.
The intestine is a complex and dynamic ecosystem caused by various microbial communities known as the gut microbiota (Zhao and Liu, 2020). The human intestinal tract contains more than 10-100 trillion microorganisms (Duttaroy, 2021; Zhang J. et al., 2021), which contribute to food digestion, nutrient provision, and combat pathogen invasion. Numerous studies have demonstrated that the gut microbiota plays a vital role in maintaining human health and adjusting numerous physiological functions, such as the nervous system, cardiovascular system, and immune system (Izco et al., 2021). Considering the importance of host-microbiota interactions for human health, access to yet unexplored resources will lead to the discovery of new actors regulating this crosstalk and therefore new potential therapeutic targets (Alam and Neish, 2018; Chang and Kao, 2019). Although genetic, societal, environmental, and other influencing factors are closely involved in disease etiology and progression, the host-microbiota interaction is increasingly recognized for its influence in a variety of diseases (Duttaroy, 2021), such as obesity (Arnoriaga-Rodríguez et al., 2020), inflammatory bowel disease (Sankarasubramanian et al., 2020), non-alcoholic fatty liver disease (Rom et al., 2020), and Parkinson's disease (Vascellari et al., 2020). More importantly, a better understanding of the host-microbiota interaction will improve an understanding of the relationship between gut microbiota and health. Interestingly, the change in microbial composition, intestinal dysfunction, bacterial metabolites, and endocrine function in the intestine is associated with PD (Sun et al., 2017). In addition, the abundance of Prevotellaceae presented a declining trend in PD patients compared with healthy individuals. Simultaneously, the abundance of Enterobacteriaceae has been found to be associated with the severity of postural instability and gait difficulty of PD patients (Scheperjans et al., 2015). Hence, the interaction between the gut microbiota and the occurrence of PD will provide a bright future for intervention, especially for the diagnosis and therapy of PD. Growing evidence suggests that the modulation of gut microbiota could be a novel therapeutic target in PD patients.
Amino acids are fundamental elements for protein and peptide synthesis. More importantly, a series of evidence indicates that amino acids are also important bioactive molecules that play key roles in signaling pathways and metabolic regulation (Sankarasubramanian et al., 2020). The gut microbiota composition related to protein metabolism in the intestinal tract is similar to that in feces and important in amino acid homeostasis and health (Bishu, 2016; Lin et al., 2017; Zhao et al., 2019). The result showed that the primary bacteria, such as Klebsiella spp., Escherichia coli, and Anaerovibrio lipolytica, are associated with protein metabolism. In addition, amino acids could be directly metabolized by some of these bacteria which own the ability to excrete numerous proteases and peptidases (Fan et al., 2017). Evidence shows that amino acids are exchanged between gut microbiota and the host (Metges, 2000). Thus, there is no doubt that a profound grasp of the significance of gut microbiota-derived amino acid metabolism has become imperative. Notwithstanding, the effects of amino acid metabolism on PD pathogenesis have not been comprehensively reviewed at present. In this review, detailed descriptions of the relationship between gut microbiota-derived amino acid metabolism and PD are summarized, and dysbiosis of microbial metabolite and microbiota-targeted interventions in PD disease are also discussed (Figure 2).
Figure 2. Microbiota-gut-brain axis communication pathway linking gut microbial dysbiosis with brain function in PD.
Few researchers investigated the pathogenesis of PD prior to 1980. The mechanism remained elusive until the description of Lewy bodies first appeared. The main hypotheses involved in the pathogenesis of PD were as follows: oxidative stress (Trist et al., 2019), mitochondrial dysfunction (Navarro and Boveris, 2009), excitotoxicity (Ambrosi et al., 2014), inflammation, neurotrophic factor-deficiency (Lindahl et al., 2020), and the theory of gut origin (Postuma, 2015). Among them, oxidative stress and PD originating from the gut have attracted more attention. Accumulating evidence illustrated that oxidative stress may be the cause of many neurodegenerative diseases, including PD (Trist et al., 2019). Low levels of oxidative stress could promote mitochondria elimination and protect their biological function, while opposite effects appear in high-level oxidative stress beyond the cellular capacity by affecting the mitochondrial membrane's potential and protein synthesis in cytoplasm (Lee and Wei, 2005; Barodia et al., 2017). Previous studies mainly focused on the abnormal deposition of α-syn in the central nervous system in PD pathogenesis. Recently, multitudes of studies have elucidated that gut microbiota homeostasis and metabolites are correlated with the pathogenesis of PD. Simultaneously, Yang et al. (2019) revealed the existence of a bidirectional network called GMBA. The GMBA could affect human behavior and brain neurochemistry by regulating neurotransmitters and their receptors in PD patients. Taken altogether, the gut origin hypothesis implicates the gut as a potential origin of PD pathogenesis, offering fresh insights into the mechanisms underlying PD.
Relevant preclinical and epidemiological studies illustrated that the occurrence of common intestinal disorders, such as colorectal cancer (CRC), constipation, irritable bowel syndrome (IBS), inflammatory bowel disease (IBD), and small intestinal bacterial overgrowth syndrome (SIBO), were associated with PD by affecting the central nervous system (Barboza et al., 2015). Interestingly, a bidirectional link between the brain and gut plays an essential role in the association between intestinal dysfunction and PD. The association between PD and intestinal disorders is described in Table 1.
PD and CRC are different diseases with diverse pathogenic mechanisms. Gut microbiota diversity (Xie et al., 2017), melatonin (Schernhammer et al., 2006), dopamine (Sarkar et al., 2015), and smoking (Hernán et al., 2002) may account for the bidirectional link between PD and CRC. The disorders of the ubiquitin-proteasome system may lead to the formation and accumulation of Lewy bodies including α-syn (Sherman and Goldberg, 2001). More importantly, the level of ubiquitin proteasome system is usually up-regulated in CRC patients (Manasanch and Orlowski, 2017). Notwithstanding, there is a clear need for more prospective studies to validate these hypotheses. Interestingly, phosphatidylinositol-3-kinase/protein kinase B/mammalian target of rapamycin (PI3K/Akt/mTOR) are over-expressed in CRCs (Bahrami et al., 2018), while the PI3K/Akt/mTOR activation could prevent PD via reducing the dopaminergic neuron apoptosis (Leikas et al., 2017).
Constipation, the second most common non-motor symptom of PD after anosmia, is characterized by infrequent stools, difficult stool passage, or both. Previous studies indicated that the main cause of constipation was slowed colonic transit. Furthermore, constipation is one of the indicators and occurs more than 20 years earlier than the diagnosis of PD (Frazzitta et al., 2019). Simultaneously, gastrointestinal dysfunction is a common feature of PD and could lead to the impaired absorption of L-DOPA, which also causes the motor fluctuations of PD (Svensson et al., 2016). Despite copious research, the exact mechanism of IBS remains unclear. Genetic variation, altered gut microbiota, increased gut permeability, and low-grade inflammation are risk factors for IBS (Ohman and Simrén, 2010). Compared with those without IBS, an individual with IBS is at a 48% increased risk of PD based on the meta-analysis and systematic review methods (Zhang J. et al., 2021). The risk factors of IBS can make the gastrointestinal tract more vulnerable to bacterial endotoxin and pathogens which may cause an increase in α-syn expression and aggregation (Mertsalmi et al., 2021). Furthermore, the previous research about the correlation between the gut microbiota and the occurrence and development of IBS has been observed and the gut-brain axis plays a vital role, which was consistent with the hypothesis of “PD originates in the gut” (Braak et al., 2006; Raskov et al., 2016; Canakis et al., 2020). The altered gut microbiota and increased gut permeability can activate systemic inflammation and enteric neuroglial cells, thereby initiating the development of α-syn pathology (Klingelhoefer and Reichmann, 2015). An increasing number of clinical studies revealed that IBD could cause neuroinflammation through the gut-brain axis which was consistent with the elevated stool calprotectin levels in PD patients (Schwiertz et al., 2018). Intestinal inflammation could accelerate the aggregation of α-syn in the gut and then spread to the brain, finally leading to PD (Stokholm et al., 2016).
SIBO is characterized by the excessive levels of bacteria colonized in the small intestine causing inflammation and malabsorption. The pathogenesis, clinical manifestation, and progression of sporadic PD might be affected by SIBO (Dobbs et al., 2012; DiBaise et al., 2018; Manole et al., 2021). Several studies found that H2-predominant vs. methane-predominant SIBO shows various effects on PD progression. In addition, because of different drug bioavailability and absorption, SIBO has conflicting effects on intestinal symptoms of PD with different pharmacological interventions (Gibson and Barrett, 2010; Maini Rekdal et al., 2019; van Kessel et al., 2019). Among the pathogenesis of PD, SIBO may cause local and systemic reactions that would further destroy the integrity of the intestinal barrier by influencing tight junctions and intestinal permeability. Many studies illustrated that the intestinal disorder may serve as a warning sign for PD.
The association pathogenesis of PD and gastrointestinal disorders was mainly focused on genetic factors, diet, environmental toxins, and gut microbiota, etc. Interestingly, PD is characterized by severe motor and NMS that can result in debilitating gastrointestinal (GI) symptoms. Specifically, the association between intestinal disorders and PD pathogenesis attracting many researchers' attention may be due to the existence of the gut-brain axis. Overall, the PD risk was higher in IBS patients than others, indicating that the intestinal disorder may serve as a warning sign for PD. Further research is warranted to explore the underlying mechanisms of this correlation.
The key pathological characteristics of PD are the accumulation of α-syn and cell death in the brain's basal ganglia, affecting an estimated three million people (more than 60 years of age) (Wolters and Braak, 2006; Sulzer, 2007). This damage to dopaminergic neurons is responsible for the distinctive movement disorder and vagal nerve dysfunction associated with PD. Until recently, the NMS including constipation, dysphagia, disrupted sleep architecture, impaired olfaction, and depression were presented in PD patients (Raval et al., 2020). A great quantity of studies has shown that constipation is an early manifestation of the neurodegenerative process underlying PD. More importantly, the NMS of GI dysfunction (i.e., constipation) often appears much earlier in PD patients, predating the onset of motor symptoms by as many as 20 years (Abbott et al., 2001; Savica et al., 2009). Many studies found that gut microbiota composition alters in various PD-related NMS, possibly pre-dating motor symptoms (Heintz-Buschart et al., 2018). Interestingly, current research studies have illustrated that gut microbial disturbance can damage the intestinal barrier and raise chronic inflammation both in the gut (de La Serre et al., 2010; de Theije et al., 2014) and brain (Sampson and Mazmanian, 2015; Sampson et al., 2016).
The role of the intestinal barrier as an interface between the external and internal environments of the host is an obvious focus. The intestinal barrier facilitates the absorption of nutrients and prevents the entry of harmful intraluminal components into the blood circulation, such as endotoxins, and deleterious rhizobacteria (Furness et al., 1999; Sun and Shen, 2018). The tight junction complexes in epithelial cells, the mucosal surface, and the immune system are vital for the intact intestinal barrier and function, of which the first line of defense is the intestinal lumen itself (Figure 3; Schoultz and Keita, 2020; Gharib-Naseri et al., 2021).
Figure 3. A schematic overview of the main cell types and physical defense mechanisms as targets related to intestinal barrier function for therapeutic strategies in PD.
Commensal luminal bacteria inhibit the colonization of pathogens by, for example, the production of bacteriocins, pH modification of the luminal content, and competition for nutrients required for the growth of pathogens (Bharadia et al., 2020). Otherwise, the commensal microbiota resides in the intestinal epithelium enhanced physiological paracellular permeability, and the mucus layer (Schoultz and Keita, 2019).
The single cell layer of epithelium consists of plentiful cell types and could divide the body and the outside luminal milieu. The tight barrier of the intestinal luminal milieu is established by a large quantity of cells and their functions (Schoultz and Keita, 2019). It is shown that antimicrobial peptides could be produced by Paneth cells by eliminating pathogenic bacteria (Wang et al., 2018). Simultaneously, innate and adaptive immune cells, such as neutrophils, T cells, monocytes, and natural killer cells, respond to the intrusion of xenobiotics and suppress the inflammation. Neutrophils, a kind of immunological cells, are among the first cells to reach inflamed areas and eliminate microorganisms through phagocytosis (Rosales et al., 2016). T-regulatory cells, a specific subpopulation of lymphocytes, are critical in the maintenance of immune homeostasis in suppressing the activation of various immune cells related to inflammation (Corthay, 2009). The crosstalk established between the gut microbiota and the intestinal epithelium is key to maintaining homeostasis in the gut. More importantly, the bi-directional communications between the innate immune system and pattern recognition receptors, such as Toll-like receptors (TLRs), scavenger receptors, and nucleotide-binding oligomerization domain (NOD)-like receptors (NLRs), are crucial to the intestinal barrier homeostasis in some conditions. The intestinal epithelial and immune cells are constantly in contact with foreign material and then respond appropriately to their intrusions, protecting the host (Wells et al., 2011). This interactivity ability makes the identification of external ingredients possible based on the antigen presenting cells. Even more interesting is that these cells can migrate to the peripheral site and transmit antigens to T-cells, leading to increased secretion of pro-inflammatory cytokines, IFN-γ for example, to improve the intestinal barrier (Wallace et al., 2014). Simultaneously, the enzyme of indoleamine 2,3-dooxygenase (Bessede et al., 2014), owning the effect of regulating the inflammatory reaction and the ability of protective effect against IBD, is responsible for the transformation of tryptophan to kynurenine (Arsenescu et al., 2011). TLRs are pro-inflammatory and conserved transmembrane receptors that can identify conserved molecular structures to detect microorganisms (Kubinak and Round, 2012). Pathogen triggered TLRs are generally understood to cause an inflammatory immune response, supporting the subsequent clearance of pathogenic microorganisms (Mayne et al., 2020). da Silva et al. (2016) found that these receptors were regulated by peripheral leukocyte responses in PD patients.
Intestinal permeability (between intestinal epithelial cells) is mainly characterized by medium-sized hydrophilic molecules transporting along their concentration gradient in a system without the assistance of a carrier system (Schoultz and Keita, 2020). A vital function of the intestinal epithelium is the maintenance of normal intestinal barrier function, which allows the selective permeability of essential nutrients, water, and ions without the entry of bacterial toxins and pathogens (Tabler et al., 2020). The increase in intestinal permeability represents intestinal barrier function disorders. A number of studies have found that increased permeability is correlated with the development of several diseases. Higher intestinal permeability induces the translocation of gut bacteria and microbial components that initiate inflammation and oxidative stress in the enteric nervous system, resulting in enteric α-synucleinopathy in PD (Bjarnason et al., 2004). Simultaneously, these findings also have important clinical applications. Barrier integrity in IBD patients, whose endoscopy findings are normal, may be detected using confocal laser endomicroscopy, which may predict the relapses (Galipeau and Verdu, 2016). The development of reliable and sensitive methods to measure intestinal permeability could contribute to identifying patients who are at high risk of suffering from PD and assist in the development of preventative therapies (Galipeau and Verdu, 2016). PD is characterized by an altered gut microbiota composition, the intestinal barrier, and the enteric neuroimmune system, therefore it is possible that intestinal barrier dysfunction may play a key role in PD development and/or progression. Meanwhile, the significant role of intestinal permeability has been highlighted in PD, which lays the groundwork for novel PD therapy targeting the restoration of the intestinal barrier.
The maturation and function of microglia are affected by the altered cell proportions, while immature phenotypes are based on the circulating levels of pro-inflammatory and anti-inflammatory cytokines contributing to host homeostasis (Erny et al., 2015). The astrocytes and microglia can produce pro-inflammatory cytokines via TLRs activation (Lucas and Maes, 2013). TLRs can recognize microbial-associated molecular patterns (MAMPs), such as lipopolysaccharides (LPS), and are activated by changes in the composition of gut microbiota and gut permeability (Bowman et al., 2003). LPS can activate immune cells (such as macrophages, neutrophils, and dendritic) and promote the inflammation and permeability of the gut (Rhee, 2014). In particular, the activated immune cells can release various pro-inflammatory cytokines, binding with receptors expressed in neurons and glial cells, and then spread to the brain via the blood-brain-barrier and can result in the neuroinflammation and death of neurons in the brain (Dantzer et al., 2000). The new research illustrated that gut leakiness and endotoxemia owing to the alteration of microbiota led to the co-occurrence of intestinal inflammation and neuroinflammation via the activation of the TLR4 (Alhasson et al., 2017).
In the gastrointestinal tract, enterocyte peptidases further hydrolyze resultant peptide products into amino acids, dipeptides, and tripeptides for enterocyte transport into the portal venous system. At the same time, amino acids serve as substrates for protein synthesis that are utilized for energy production under extreme conditions, such as disease for example. Amino acids are classified as essential amino acids, non-essential amino acids, and conditionally essential amino acids based on the species, age, disease, and condition dependence (Thalacker-Mercer et al., 2020). Essential amino acids, including tryptophan, valine, lysine, leucine, isoleucine, phenylalanine, threonine, and methionine, must be provided by dietary protein (Church et al., 2020; Verzola et al., 2020) and non-essential amino acids can be synthesized through other pathways.
Recently, amino acids, including total amino acid and free amino acid, have been found to exist in mushrooms, corn grain, peanut, soybean, and white rice, etc. Research has found that the average total free amino acid content of the mushroom was 4.35% (Sun et al., 2017). The relative amount of total amino acid is 8.76% in coffee silverskin (Machado et al., 2020), 10.67% in corn grain, 29.29% in peanut, 9.08% potato, and 8.49% in white rice (Hou et al., 2019). It should be noted that amino acids are substrates in polyamine synthesis by numerous enzymes in humans and microbes (Krzystek-Korpacka et al., 2020).
Amino acids perform several crucial functions in the human body, either directly or indirectly. They are the main constituents of protein in bioactive substances and adjust the balance of energy and immunity in organisms. In addition, amino acids can be catalyzed through oxidized, reduced, fissioned, or coupled pathways (Davila et al., 2013). Several reactions including transamination, deamination, and decarboxylation are required steps in this process. Lots of work demonstrated that glycine, proline, and arginine usually act as the acceptors of hydrogen, and alanine, leucine, isoleucine, and histidine are hydrogen donors in the metabolic pathways (Rist et al., 2013). The metabolisms of relevant amino acids are summarized in Figure 4. The microorganisms are mixed with endogenous proteins, such as mucous proteins, chemicals secreted by the pancreas and gastric gland in the alimentary canal (Hayashi et al., 2005). The nitrogen-containing compounds are broken down into amino acids and peptides and related enzymes are excreted via the exocrine pancreas. The amino acids and peptides are absorbed by bacteria or spread from the intestinal lumen to the portal vein.
Amino acids are absorbed and digested in the intestinal epithelium (Bos et al., 2005; Hu and Guo, 2021). During the process, a tremendous amount of nitrogen-containing compounds passes by the ileocecal junction and gets into the posterior part of the intestine (Blachier et al., 2007). However, some proteins are still difficult to absorb and digest completely in the small intestinal. The remaining nitrogen-containing compounds without digestion are transported to the large intestine. Microorganisms play a vital role in protein hydrolysis to provide free amino acids for both host and microbial metabolism functions. Furthermore, most amino acids, synthesized by microorganisms, can be absorbed by the intestinal epithelial cells of the small and large intestines to implement a sequence of functions, like synthesis and catabolism (Laparra and Sanzm, 2010; Ma and Ma, 2019). The proteolysis and recycling of nitrogen-containing compounds are performed by proteolytic bacteria residing in the gastrointestinal tract (Macfarlane and Macfarlane, 2007). A large amount of amino acid maintains the immense and widespread metabolism via the function of microbiota. This diversity of amino acid metabolism in microorganisms may impart either active or passive effects on the host. Hence, adjusting protein or amino acid absorption and metabolization in the intestine may provide a strategy for shaping the amino acid fermenting bacteria and their metabolic pathways, thereby potentially affecting host metabolism.
The roles of gut microbiota on amino acid metabolism have been well investigated (Zhao et al., 2019). The great majority of digestive products, such as small peptides, amino acids, and tripeptides, are utilized by certain microbes or transported into systemic circulation by the small intestine. The functions of amino acids between the gut microbiota and host are bi-directional (Zhao et al., 2019). The gut microbiota plays an essential role in dietary protein and nitrogen recycling. In addition, the microbial activity induced by indigestible amino acids promotes the production of metabolic end products including short-chain fatty acids (SCFA; acetate, butyrate, propionate), branched-chain fatty acids, ammines, indoles, and ammonia, etc. (Macfarlane et al., 1988). Previous work revealed that bacterial metabolites appear to have beneficial or opposite effects on the host depending on their concentrations. As the major nutrients in the diet, amino acids are the basic components of proteins and peptides, and are essential for the emergence of organism-active molecules involved in the signaling pathways and metabolism modulation (Dai et al., 2015). Additionally, the gut microbiota is crucial for the metabolism and recycling of amino acids. The amino acids, deriving from the diet or the host, are utilized as the fundamental element by gut microbiota for the synthesis of proteins or produce a large number of metabolites by the nutritional metabolism, such as polyamines, phenolic, nitric oxide (NO), and hydrogen sulfide (H2S) (Dai et al., 2015). In addition, the “essential” amino acids, as a regulator for amino acid homeostasis in humans, can be synthesized by intestinal bacteria. For instance, research has illustrated that a large number of bacteria, like Selenomonas ruminantium, perform de novo synthesis of amino acids in the existence of peptides using experiments in vitro (Atasoglu et al., 1998; Neis et al., 2015). These results suggest that amino acid metabolism provides nutrition for gut microbiota and supports physiological action for the host (Dai et al., 2011; Collins et al., 2012). The intestinal bacterial communities are influenced by constituents of dietary protein, especially protein sources and concentrations. Proteins derived from plants are generally utilized in humans and animals. Nevertheless, crude proteins are characterized by low digestibility because of the anti-nutritional elements (He et al., 2015). By contrast, proteins originating from animals are characterized by high digestibility by aerobes in the large intestine. The homeostasis of gut microbiota (mainly in the large intestine) can be disorganized, leading to intestinal disorders (Fan et al., 2015). Consequently, it is essential to explore the connection between gut microbiota and amino acids (which are necessary for protein metabolism). In addition, comprehending the functions of different constituents of dietary protein on gut microbiota is crucial.
An enormous number of metabolic pathways, such as glucose, lipid, and amino acid metabolism, are regulated by gut microbiota. The composition of gut microbiota-derived metabolites in the intestinal tract is very complex. The nutrition can be provided by amino acids acquired in the diet or synthesized by the host for the gut microbiota for protein production. Amino acids can be integrated into bacterial cells as amino acid residues in proteins, and further incorporated into the metabolic pathway. Amino acids and peptides are obtained by the hydrolysis of proteins through proteases and peptidases secreted by gut microbiota. Ultimately, amino acids and peptides could be absorbed by microbiota through specific transporters. However, the small bioactive molecules will encounter various consequences under different physiological conditions of humans (Davila et al., 2013). Transamination or deamination is the first stage of amino acid catabolism, followed by oxidation or reduction. Biogenic amines are alkaline organic compounds mainly produced by decarboxylation reactions of free amino acids present by the enzyme decarboxylase (Wu et al., 2021).
Recent studies demonstrated convincingly that a large number of amino acid-fermenting bacteria are inhabited the intestinal tract (up to 1,011 per gram of dry feces) (Atasoglu et al., 1998). The “essential” amino acid of lysine, produced by bacteria, is absorbed and integrated as a whole host protein in vivo studies (Metges et al., 1996, 1999). The research illustrated that the 15N-lysine originated from microbial lysine with the comparison of the integration of 15N from 15NH4Cl into the inside body system (Torrallardona et al., 1996; Gill et al., 2006). Additionally, gut microbiota-derived lysine and threonine were shown to be crucial for free lysine and threonine in a study on nitrogen adequate diets in humans (Metges et al., 1999). Furthermore, this research found that the abundance of gut microbiota was associated with the precursors generated by humans related to “essential” amino acids (Metges et al., 1999).
A genome-wide analysis illustrates that a number of clostridium species, Clostridium acetobutylicum for example, have comprehensive genes for the biosynthesis of amino acids, whereas Clostridium perfringens lack the genes for amino acid biosynthesis such as lysine, serine, glutamic acid, and aromatic amino acids (Kevin et al., 2016). Hence, in order to elucidate the functions of amino acids from the perspective of bacterial species, it is necessary to understand the biosynthesis of amino acids in microorganisms. However, nitrogen and amino acids, required for the growth and fermentation of glycolytic bacteria, could be provided by the residual dietary proteins in the large intestine (Mafra et al., 2013). Various Lactobacillus and Bifidobacterium strains can produce γ-aminobutyric acid which is a neural active substance (Barrett et al., 2012). It is acknowledged that imidazole propionate can be transformed into histidine-derived metabolite by gut microbiota (Koh et al., 2018). Moreover, gut microbiota can produce tryptophan owing to the existing of tryptophan decarboxylase in the intestinal tract (van de Wouw et al., 2017). Given the above, amino acids can be utilized for the synthesis of bacterial cell components and catabolized. Major amino acid-fermenting bacteria in the intestinal tract are concluded in Table 2.
The literature reports that short-chain fatty acids (SCFAs) constitute a major class of fermentation products. The microbiota-derived carbohydrates of SCFAs are cardinal examples of beneficial metabolites (Sonnenburg and Sonnenburg, 2014). Acetate (accounts for ~50–70%), propionate (produced by the species of Phylum Firmicutes), and butyrate (produced by a small quantity of Phylum Firmicutes, such as Faecalibacterium prausnitzii and Roseburia hominis) (Sokol et al., 2008; Machiels et al., 2014) are SCFAs. These SCFAs supply key metabolic signaling that underlies homeostasis and the function of colonic Tregs by different molecular mechanisms (Mu et al., 2017; Yang, 2022). In faith, a wide variety of amino acids generated from microbial protein fermentation in the large intestine can be presented as precursors for short-chain fatty acid synthesis (Ciarlo et al., 2016). The great majority of amino acids such as glutamate, ornithine, and glycine can be metabolized by anaerobic bacteria and utilized for the synthesis of butyrate (Neis et al., 2015). Zou et al. (2021) reported that phenylalanine, tyrosine, and tryptophan could be metabolized to indolic and phenolic compounds via the gut microbiota. However, indolic and phenolic compounds can be utilized to mitigate inflammatory responses. Concurrently, it plays a significant role to promote the comprehension of the pathophysiology of diseases.
SCFAs have many ameliorative effects on gut health. First, SCFAs can maintain the integrity of the intestinal barrier and exert therapeutic potential for intestinal inflammation (Lewis et al., 2010). For instance, butyrate can activate adenosine monophosphate kinase (AMPK) or down-regulate the expression of claudin 2 to regulate the expression of tight junction proteins, eventually enhancing intestinal barrier function (Daly and Shirazi-Beechey, 2006; Peng et al., 2009). Second, SCFAs contribute to the mucous production in the gastrointestinal tract (Dalile et al., 2019). The research (Gaudier et al., 2009) illustrated that butyrate regulated MUC2 gene expression to regulate the production of mucin. Additionally, SCFAs can affect gastrointestinal motility by inhibiting histone deacetylases (Cherbut et al., 1998). Ultimately, SCFAs can interact with vagal afferents by influencing inflammation and hormonal regulation (Dalile et al., 2019).
To summarize, amino acids can alter the gut microbiota composition by forming an intestinal microenvironment favorable to the survival and proliferation of certain microbes (Libao-Mercado et al., 2009). Conversely, gut microbiota delivers energy and reduces co-factors via providing amino acids to meet the needs of the host (Metges, 2000). Gut microbiota owns the substitutable role (both the host nutrition and physiology) in amino acid metabolism, and the phenomenon shows the bidirectional process between amino acids and gut microbiota (Macfarlane and Macfarlane, 2012). Briefly, SCFAs play numerous biological roles: (1) constitute an energy source for muscles; (2) implicate in the transportation and metabolism of epithelial cells; and (3) influence epithelial cell growth and differentiation.
It is widely known that several amino acids are likely absorbed in the large intestine. The significance of the balance between whole-body proteins and amino acids is still unclear, especially in humans. The amino acids and peptide transporters in the intestinal tract contribute to amino acid absorption and metabolism in the large intestine (Hendriks et al., 2012).
A tremendous amount of solute carrier (SLC) transporters, which contain a large number of families of distinct transporters, are adopted to transport amino acids and peptides (including di- and tripeptides). Many above-mentioned transporters are expressed in the large intestine. There is evidence for transporter expression at different levels, such as the protein level and the gene expression level. Peptide transporter 1 (PEPT1), encoded by SLC family 15 member 1 (SLC15A1), is involved in the intestinal uptake of oligopeptides and peptide-mimetic drugs (Liu et al., 2019). As we have seen previously, SLC15A1 is the unique transporter that has been verified at the protein level in the human large intestine. The host relies on the system L transporters to obtain essential amino acids, such as SLC7A5, also known as L-type amino acid transporter (LAT)1, SLC7A8, also known as LAT2, SLC43A1, also known as LAT3, and SLC43A2, also known as LAT4. Both SLC7A5 and SLC7A82 are amino acid exchangers, while SLC43A1 and SLC43A2 facilitate amino acid proliferation (Wang and Holst, 2015). Some of the amino acids, such as tryptophan, tyrosine, isoleucine, leucine, valine, and phenylalanine, can be transported into cells via LAT1 and LAT2. Meanwhile, the other amino acids, such as Leucine, isoleucine, valine, phenylalanine, and methionine can be transported into cells by LAT3 and LAT4 (Wang and Holst, 2015). Furthermore, SLC6A14, coupled with a Na+ gradient, a Cl− gradient, and membrane potential, shows higher expression in the colon than in the ileum and presents special characteristics with much a better sense of amino acids than the other three transporters (Anderson et al., 2009). Simultaneously, SLC36A1, also called proton-assisted amino acid transporter 1 (PAT1), shows a similar ability to SLC6A14. In addition, other transporter genes expressed in the large intestine discussed in current research also attract our attention. Furthermore, the SLC transporter functions of amino acids and peptides in the large intestine require further study.
The elementary mechanism of intestinal homeostasis relies on complex molecular crosstalk between host and gut microbiota. It is demonstrated that the catabolites of tryptophan by bacteria are various, such as tryptamine, skatole, indoleacrylic acid, indoleacetic acid, indolelactic acid, indolepropionic acid, and indolealdehyde, which are the ligands of the aryl hydrocarbon receptor. A recent study indicated that the catabolite of indole could improve intestinal epithelial barrier functions by regulating certain gene expressions (Roager and Licht, 2018). Simultaneously, the catabolite of indolepropionic acid acted as a ligand of the pregnane X receptor and showed the effect of adjusting the intestinal barrier function at the existence of indoles (Venkatesh et al., 2014). In addition, catabolite indoleacrylic acid was found to promote goblet cell differentiation and mucus production to regulate intestinal barrier function. In summary, the catabolites of tryptophan have the effect on improving intestinal barrier function by regulating the pregnane X receptor and aryl hydrocarbon receptor. The study demonstrated that glutamine improved IL-13-induced barrier dysfunction by up-regulating the expression of the tight junction protein claudin-1, through preventing the PI3K-Akt signaling pathway (Li et al., 2021), and consequently damaged the intestinal barrier function and increased intestinal permeability. At the same time, glutamate can up-regulate the expression of tight junction proteins and protect the diquat-induced oxidative stress to promote intestinal epithelial cell growth and health based on the new biochemical.
There is increasing evidence that dopamine dysfunction plays an important role in the pathogenesis of PD. There is also increasing evidence to suggest that the other amino acids, such as glutamate, γ-aminobutyric acid, homocysteine, and large neutral amino acids in the brain were involved in the pathogenesis of PD (Yuan et al., 2013; Figura et al., 2018). According to the research, dopamine treatment is associated with elevated homocysteine in Parkinson's disease (Kuhn et al., 1998). The three amino acids of glutamate, cysteine, and glycine are involved in the synthesis of glutathione. The ratio of the two forms of glutathione (reduced and oxidized forms) can affect the cellular redox status. Mitochondrial complex I has been considered central to the pathogenesis of PD. However, low glutathione concentrations may inhibit mitochondrial complex I (Müller et al., 2016). Meanwhile, the finding suggests that branched-chain amino acids (leucine, isoleucine, and valine) can serve as nitrogen donors to maintain the balance of glutamate-glutamine in astrocytes and neurons (Zhang et al., 2022). Branched-chain amino acids could promote the catabolism of glutamate which may exert beneficial effects on PD. Interestingly, tyrosine and phenylalanine are the key substrates for the production of dopamine in PD. The elevation of phenylacetyl-L-glutamine was positively correlated with firmer stool and constipation severity among PD patients. Simultaneously, gut microbiota-derived tryptophan catabolites could adjust the inflammatory response via affecting pro-inflammatory cytokines and lipogenesis in macrophages and hepatocytes (Shao et al., 2021). To compound the issue, glutamic acid and γ-aminobutyric acid are related to the clinical heterogeneity of PD, the symptoms of Parkinson's disease, and especially to the parkinsonian tremor (Yuan et al., 2013). Promisingly, the detection of amino acid neurotransmitter levels may offer new insight into the pathogenesis and early diagnosis of Parkinson's disease. Therefore, more stringent studies with a larger sample size are needed to explore the changes in amino acid neurotransmitter levels during Parkinson's disease progression.
Researchers have found that amino acids provide powerful support for human health. In recent years, the gut microbiota has received great attention, putting it in the spotlight of biomedical research. The balance of intestinal microbes and the host play a vital role in several disorders, including the impairment of the nervous system. Notably, gut microbiota, a major determinant of bidirectional communication between the gut and brain, could be regulated by a number of mechanisms, and it is illustrated that the microbiota and its produced biochemical messengers are major facets of maintaining a balanced intestinal microenvironment. Microbiota-derived amino acid molecules contribute to host health through modulating immune function, gastrointestinal physiology function, and microbiota composition. Striatal dopamine depletion in PD contributes to the hyperactivity of subthalamic nucleus output pathways, resulting in an abnormal increase in specific microbiota-derived amino acid molecules (glutamate for example), which are involved in the degeneration of the nigrostriatal system based on the excitotoxicity mechanisms. All the studies have shown that GMBA may be significantly correlated with PD pathogenesis.
Although microbiota-host interaction has drawn much attention both from clinicians and researchers in recent years, it still calls for a deeper understanding of the complex GMBA communication. Furthermore, microbiota-derived amino acid metabolism is a crucial factor suggesting major perturbations of the microbiome. These studies illustrated that amino acids metabolized by gut microbiota might affect the degeneration of the nigrostriatal system in PD patients. Gut microbiota and amino acids may be potential therapeutic targets for PD.
GZ and YZ contributed to the conceptualization. WW and GZ contributed to the investigation and funding. WW, GZ, SJ, CX, YL, and LT contributed to the writing and final approval. All authors contributed to the article and approved the submitted version.
This work was funded by the National Natural Science Foundation of China (82204375 and 82003933), the Science and Technology Development Planning Project of Traditional Chinese Medicine of Jiangsu Province of China (QN202103), the Natural Science Foundation of Nanjing University of Chinese Medicine (XZR2021046), the Nanjing Medical Science and Technology Development Project (YKK20167), and Nanjing Youth Talent Training Plan of TCM (ZYQ20006).
The authors declare that the research was conducted in the absence of any commercial or financial relationships that could be construed as a potential conflict of interest.
All claims expressed in this article are solely those of the authors and do not necessarily represent those of their affiliated organizations, or those of the publisher, the editors and the reviewers. Any product that may be evaluated in this article, or claim that may be made by its manufacturer, is not guaranteed or endorsed by the publisher.
Abbott, R. D., Petrovitch, H., White, L. R., Masaki, K. H., Tanner, C. M., Curb, J. D., et al. (2001). Frequency of bowel movements and the future risk of Parkinson's disease. Neurology 57, 456–462. doi: 10.1212/wnl.57.3.456
Adams-Carr, K. L., Bestwick, J. P., Shribman, S., Lees, A., Schrag, A., and Noyce, A. J. (2016). Constipation preceding Parkinson's disease: a systematic review and meta-analysis. J. Neurol. Neurosurg. Psychiatry 87, 710–716. doi: 10.1136/jnnp-2015-311680
Alam, A., and Neish, A. (2018). Role of gut microbiota in intestinal wound healing and barrier function. Tissue Barriers 6, 1539595. doi: 10.1212/WNL.57.3.456
Alhasson, F., Das, S., Seth, R., Dattaroy, D., Chandrashekaran, V., Ryan, C. N., et al. (2017). Altered gut microbiome in a mouse model of Gulf War Illness causes neuroinflammation and intestinal injury via leaky gut and TLR4 activation. PLoS ONE 12, e0172914. doi: 10.1371/journal.pone.0172914
Amano, G., Matsuzaki, S., Mori, Y., Miyoshi, K., Han, S., and Shikada, S. (2020). SCYL1 arginine methylation by PRMT1 is essential for neurite outgrowth via Golgi morphogenesis. Mol. Biol. Cell 31, 1963–1973. doi: 10.1091/mbc.E20-02-0100
Ambrosi, G., Cerri, S., and Blandini, F. (2014). A further update on the role of excitotoxicity in the pathogenesis of Parkinson's disease. J. Neural Transm. (Vienna) 121, 849–859. doi: 10.1007/s00702-013-1149-z
Anderson, C. M., Howard, A., Walters, J. R., Ganapathy, V., and Thwaites, D. T. (2009). Taurine uptake across the human intestinal brush-border membrane is via two transporters: H+-coupled PAT1 (SLC36A1) and Na+- and Cl(–)-dependent TauT (SLC6A6). J. Physiol. 587, 731–744. doi: 10.1113/jphysiol.2008.164228
Arnoriaga-Rodríguez, M., Mayneris-Perxachs, J., Burokas, A., Contreras-Rodríguez, O., Blasco, G., Coll, C., et al. (2020). Obesity impairs short-term and working memory through gut microbial metabolism of aromatic amino acids. Cell Metab. 32, 548–560.e7. doi: 10.1016/j.cmet.2020.09.002
Arsenescu, R., Arsenescu, V., Zhong, J., Nasser, M., Melinte, R., Dingle, R. W., et al. (2011). Role of the xenobiotic receptor in inflammatory bowel disease. Inflamm Bowel Dis. 17, 1149–1162. doi: 10.1002/ibd.21463
Atasoglu, C., Valdés, C., Walker, N. D., Newbold, C. J., and Wallace, R. J. (1998). De novo synthesis of amino acids by the ruminal bacteria Prevotella bryantii B14, Selenomonas ruminantium HD4, and Streptococcus bovis ES1. Appl Environ Microbiol. 64, 2836–2843. doi: 10.1128/AEM.64.8.2836-2843.1998
Attwood, G. T., Klieve, A. V., Ouwerkerk, D., and Patel, B. K. (1998). Ammonia-hyperproducing bacteria from New Zealand ruminants. Appl. Environ. Microbiol. 64, 1796–1804. doi: 10.1128/AEM.64.5.1796-1804.1998
Bahrami, A., Khazaei, M., Hasanzadeh, M., ShahidSales, S., Joudi Mashhad, M., Farazestanian, M., et al. (2018). Therapeutic potential of targeting PI3K/AKT pathway in treatment of colorectal cancer: rational and progress. J. Cell Biochem. 119, 2460–2469. doi: 10.1002/jcb.25950
Barboza, J. L., Okun, M. S., and Moshiree, B. (2015). The treatment of gastroparesis, constipation and small intestinal bacterial overgrowth syndrome in patients with Parkinson's disease. Exp. Opin. Pharmacother. 16, 2449–2464. doi: 10.1517/14656566.2015.1086747
Barodia, S. K., Creed, R. B., and Goldberg, M. S. (2017). Parkin and PINK1 functions in oxidative stress and neurodegeneration. Brain Res. Bull. 133, 51–59. doi: 10.1016/j.brainresbull.2016.12.004
Barrett, E., Ross, R. P., O'Toole, P. W., Fitzgerald, G. F., and Stanton, C. (2012). γ-Aminobutyric acid production by culturable bacteria from the human intestine. J. Appl. Microbiol. 113, 411–417. doi: 10.1111/j.1365-2672.2012.05344.x
Bessede, A., Gargaro, M., Pallotta, M.T., Matino, D., Servillo, G., Brunacci, C., et al. (2014). Aryl hydrocarbon receptor control of a disease tolerance defence pathway. Nature. 511, 184–190. doi: 10.1038/nature13323
Bharadia, L., Agrawal, N., and Joshi, N. (2020). Development and functions of the infant gut microflora: Western vs. Indian infants. Int. J. Pediatr. 2020, 7586264. doi: 10.1155/2020/7586264
Bishu, S. (2016). Sensing of nutrients and microbes in the gut. Curr. Opin. Gastroenterol. 32, 86–95. doi: 10.1097/MOG.0000000000000246
Bjarnason, I., Takeuchi, K., Bjarnason, A., Adler, S. N., and Teahon, K. (2004). The G.U.T. of gut. Scand. J. Gastroenterol. 39, 807–815. doi: 10.1080/00365520410003326
Blachier, F., Mariotti, F., Huneau, J. F., and Tomé, D. (2007). Effects of amino acid-derived luminal metabolites on the colonic epithelium and physiopathological consequences. Amino Acids 33, 547–562. doi: 10.1007/s00726-006-0477-9
Bos, C., Juillet, B., Fouilletm, H., Turlanm, L., Daré, S., Luengo, C., et al. (2005). Postprandial metabolic utilization of wheat protein in humans. Am. J. Clin. Nutr. 81, 87–94. doi: 10.1093/ajcn/81.1.87
Bowman, C. C., Rasley, A., Tranguch, S. L., and Marriott, I. (2003). Cultured astrocytes express toll-like receptors for bacterial products. Glia 43, 281–291. doi: 10.1002/glia.10256
Braak, H., de Vos, RA., Bohl, J., and Del Tredici, K. (2006). Gastric alpha-synuclein immunoreactive inclusions in Meissner's and Auerbach's plexuses in cases staged for Parkinson's disease-related brain pathology. Neurosci Lett. 396, 67–72. doi: 10.1016/j.neulet.2005.11.012
Camacho, M., Macleod, A. D., Maple-Grødem, J., Evans, J. R., Breen, D. P., Cummins, G., et al. (2021). Early constipation predicts faster dementia onset in Parkinson's disease. NPJ Parkinson's Dis. 7, 45. doi: 10.1038/s41531-021-00191-w
Campbell, H. A., Mashburn, L. T., Boyse, E. A., and Old, L. J. (1967). Two L-asparaginases from Escherichia coli B. Their separation, purification, and antitumor activity. Biochemistry 6, 721–730. doi: 10.1021/bi00855a011
Canakis, A., Haroon, M., and Weber, H. C. (2020). Irritable bowel syndrome and gut microbiota. Curr. Opin. Endocrinol. Diab. Obes. 27, 28–35. doi: 10.1097/MED.0000000000000523
Chang, C. S., and Kao, C. Y. (2019). Current understanding of the gut microbiota shaping mechanisms. J. Biomed. Sci. 26, 59. doi: 10.1186/s12929-019-0554-5
Cherbut, C., Ferrier, L., Rozé, C., Anini, Y., Blottière, H., Lecannu, G., et al. (1998). Short-chain fatty acids modify colonic motility through nerves and polypeptide YY release in the rat. Am. J. Physiol. 275, G1415–G1422. doi: 10.1152/ajpgi.1998.275.6.G1415
Church, D. D., Hirsch, K. R., Park, S., Kim, I. Y., Gwin, J. A., Pasiakos, S. M., et al. (2020). Essential amino acids and protein synthesis: insights into maximizing the muscle and whole-body response to feeding. Nutrients. 12, 3717. doi: 10.3390/nu12123717
Ciarlo, E., Heinonen, T., Herderscheem, J, Fenwickm, C, Mombelli, M., Le Roy, D., et al. (2016). Impact of the microbial derived short chain fatty acid propionate on host susceptibility to bacterial and fungal infections in vivo. Sci. Rep. 6, 37944. doi: 10.1038/srep37944
Collier, T. J., Kanaan, N. M., and Kordower, J. H. (2017). Aging and Parkinson's disease: different sides of the same coin? Mov. Disord. 32, 983–990. doi: 10.1002/mds.27037
Collins, S. M., Surette, M., and Bercik, P. (2012). The interplay between the intestinal microbiota and the brain. Nat. Rev. Microbiol. 10, 735–742. doi: 10.1038/nrmicro2876
Corthay, A. (2009). How do regulatory T cells work? Scand. J. Immunol. 70, 326–336. doi: 10.1111/j.1365-3083.2009.02308.x
da Silva, D. J., Borges, A. F., Souza, P. O., de Souza, P. R., Cardoso, C. R., Dorta, M. L., et al. (2016). Decreased toll-like receptor 2 and toll-like receptor 7/8-induced cytokines in Parkinson's disease patients. Neuroimmunomodulation 23, 58–66. doi: 10.1159/000443238
Dai, Z., Wu, Z., Hang, S., Zhu, W., and Wu, G. (2015). Amino acid metabolism in intestinal bacteria and its potential implications for mammalian reproduction. Mol. Hum. Reprod. 21, 389–409. doi: 10.1093/molehr/gav003
Dai, Z. L., Wu, G., and Zhu, W. Y. (2011). Amino acid metabolism in intestinal bacteria: links between gut ecology and host health. Front. Biosci. (Landmark Ed). 16, 1768–1786. doi: 10.2741/3820
Dalile, B., Van Oudenhove, L., Vervliet, B., and Verbeke, K. (2019). The role of short-chain fatty acids in microbiota-gut-brain communication. Nat. Rev. Gastroenterol. Hepatol. 16, 461–478. doi: 10.1038/s41575-019-0157-3
Daly, K., and Shirazi-Beechey, S. P. (2006). Microarray analysis of butyrate regulated genes in colonic epithelial cells. DNA Cell Biol. 25, 49–62. doi: 10.1089/dna.2006.25.49
Dǎnǎu, A., Dumitrescu, L., Lefter, A., Tulbă, D., and Popescu, B. O. (2021). Small intestinal bacterial overgrowth as potential therapeutic target in Parkinson's disease. Int. J. Mol. Sci. 22, 11663. doi: 10.3390/ijms222111663
Dantzer, R., Konsman, J. P., Bluthé, R. M., and Kelley, K. W. (2000). Neural and humoral pathways of communication from the immune system to the brain: parallel or convergent? Auton. Neurosci. 85, 60–65. doi: 10.1016/S1566-0702(00)00220-4
Davila, A. M., Blachier, F., Gotteland, M., Andriamihaja, M., Benetti, P. H., Sanz, Y., et al. (2013). Intestinal luminal nitrogen metabolism: role of the gut microbiota and consequences for the host. Pharmacol. Res. 68, 95–107. doi: 10.1016/j.phrs.2012.11.005
de La Serre, C. B., Ellis, C. L., Lee, J., Hartman, A. L., Rutledge, J. C., and Raybould, H. E. (2010). Propensity to high-fat diet-induced obesity in rats is associated with changes in the gut microbiota and gut inflammation. Am. J. Physiol. Gastrointest. Liver Physiol. 299, G440–G448. doi: 10.1152/ajpgi.00098.2010
de Theije, C. G., Wopereis, H., Ramadan, M., van Eijndthoven, T., Lambert, J., Knol, J., et al. (2014). Altered gut microbiota and activity in a murine model of autism spectrum disorders. Brain Behav. Immun. 37, 197–206. doi: 10.1016/j.bbi.2013.12.005
Dehhaghi, M., Kazemi Shariat Panahi, H., and Guillemin, G. J. (2019). Microorganisms, tryptophan metabolism, and kynurenine pathway: a complex interconnected loop influencing human health status. Int. J. Tryptoph. Res. IJTR 12, 1178646919852996. doi: 10.1177/1178646919852996
DiBaise, J. K., Crowell, M. D., Driver-Dunckley, E., Mehta, S. H., Hoffman-Snyder, C., Lin, T., et al. (2018). Weight loss in Parkinson's disease: no evidence for role of small intestinal bacterial overgrowth. J Parkinsons Dis. 8, 571–581. doi: 10.3233/JPD-181386
Dobbs, R. J., Charlett, A., Dobbs, S. M., Weller, C. A., Ibrahim, M. A., Iguodala, O., et al. (2012). Leukocyte-subset counts in idiopathic parkinsonism provide clues to a pathogenic pathway involving small intestinal bacterial overgrowth. A surveillance study. Gut Pathog. 4, 12. doi: 10.1186/1757-4749-4-12
Dodd, D., Spitzer, M. H., Van Treuren, W., Merrill, B. D., Hryckowian, A. J., Higginbottom, S. K., et al. (2017). A gut bacterial pathway metabolizes aromatic amino acids into nine circulating metabolites. Nature 551, 648–652. doi: 10.1038/nature24661
Donatti, A., Canto, A. M., Godoi, A. B., da Rosa, D. C., and Lopes-Cendes, I. (2020). Circulating metabolites as potential biomarkers for neurological disorders-metabolites in neurological disorders. Metabolites 10, 389. doi: 10.3390/metabo10100389
Duttaroy, A. K. (2021). Role of gut microbiota and their metabolites on atherosclerosis, hypertension and human blood platelet function: a review. Nutrients. 13, 144. doi: 10.3390/nu13010144
Erny, D., Hrabě de Angelis, A. L., Jaitin, D., Wieghofer, P., Staszewski, O., David, E., et al. (2015). Host microbiota constantly control maturation and function of microglia in the CNS. Nat. Neurosci. 18, 965–977. doi: 10.1038/nn.4030
Fan, P., Li, L., Rezaei, A., Eslamfam, S., Che, D., and Ma, X. (2015). Metabolites of dietary protein and peptides by intestinal microbes and their impacts on gut. Curr. Protein Pept. Sci. 16, 646–654. doi: 10.2174/1389203716666150630133657
Fan, P., Liu, P., Song, P., Chen, X., and Ma, X. (2017). Moderate dietary protein restriction alters the composition of gut microbiota and improves ileal barrier function in adult pig model. Sci. Rep. 7, 43412. doi: 10.1038/srep43412
Fang, H., Du, Y., Pan, S., Zhong, M., and Tang, J. (2021). Patients with Parkinson's disease predict a lower incidence of colorectal cancer. BMC Geriatr. 21, 564. doi: 10.1186/s12877-021-02497-z
Figura, M., Kuśmierska, K., Bucior, E., Szlufik, S., Koziorowski, D., Jamrozik, Z., et al. (2018). Serum amino acid profile in patients with Parkinson's disease. PLoS One 13, e0191670. doi: 10.1371/journal.pone.0191670
Frazzitta, G., Ferrazzoli, D., Folini, A., Palamara, G., and Maestri, R. (2019). Severe constipation in Parkinson's disease and in parkinsonisms: prevalence and affecting factors. Front. Neurol. 10, 621. doi: 10.3389/fneur.2019.00621
Fu, P., Gao, M., and Yung, K. (2020). Association of intestinal disorders with parkinson's disease and alzheimer's disease: a systematic review and meta-analysis. ACS Chem. Neurosci. 11, 395–405. doi: 10.1021/acschemneuro.9b00607
Furness, J. B., Kunze, W. A., and Clerc, N. (1999). Nutrient tasting and signaling mechanisms in the gut. II. The intestine as a sensory organ: neural, endocrine, and immune responses. Am. J. Physiol. 277, G922–G928. doi: 10.1152/ajpgi.1999.277.5.G922
Galipeau, H. J., and Verdu, E. F. (2016). The complex task of measuring intestinal permeability in basic and clinical science. Neurogastroenterol. Motil. 28, 957-65. doi: 10.1111/nmo.12871
Gan, J., Wan, Y., Shi, J., Zhou, M., Lou, Z., and Liu, Z. (2018). A survey of subjective constipation in Parkinson's disease patients in shanghai and literature review. BMC Neurol. 18, 29. doi: 10.1186/s12883-018-1034-3
Gaudier, E., Rival, M., Buisine, M. P., Robineau, I., and Hoebler, C. (2009). Butyrate enemas upregulate Muc genes expression but decrease adherent mucus thickness in mice colon. Physiol. Res. 58, 111–119. doi: 10.33549/physiolres.931271
Gharib-Naseri, K., Kheravii, S., Keerqin, C., Swick, R. A., Choct, M., and Wu, S. B. (2021). Differential expression of intestinal genes in necrotic enteritis challenged broiler chickens with 2 different Clostridium perfringens strains. Poult. Sci. 100, 100886. doi: 10.1016/j.psj.2020.11.063
Gibson, P. R., and Barrett, J. S. (2010). The concept of small intestinal bacterial overgrowth in relation to functional gastrointestinal disorders. Nutrition 26, 1038–1043. doi: 10.1016/j.nut.2010.01.005
Gill, S. R., Pop, M., Deboy, R. T., Eckburg, P. B., Turnbaugh, P. J., Samuel, B. S., et al. (2006). Metagenomic analysis of the human distal gut microbiome. Science 312, 1355–1359. doi: 10.1126/science.1124234
Guccione, E., Leon-Kempis, M., Pearson, B. M., Hitchin, E., Mulholland, F., van Diemen, P. M., et al. (2008). Amino acid-dependent growth of Campylobacter jejuni: key roles for aspartase (AspA) under microaerobic and oxygen-limited conditions and identification of AspB (Cj0762), essential for growth on glutamate. Mol. Microbiol. 69, 77–93. doi: 10.1111/j.1365-2958.2008.06263.x
Hatano, T., Saiki, S., Okuzumi, A., Mohney, R. P., and Hattori, N. (2016). Identification of novel biomarkers for Parkinson's disease by metabolomic technologies. J. Neurol. Neurosurg. Psychiatry 87, 295–301. doi: 10.1136/jnnp-2014-309676
Hayashi, H., Takahashi, R., Nishi, T., Sakamoto, M., and Benno, Y. (2005). Molecular analysis of jejunal, ileal, caecal and recto-sigut microbiotaoidal human colonic microbiota using 16S rRNA gene libraries and terminal restriction fragut microbiotaent length polymorphism. J. Med. Microbiol. 54, 1093–1101. doi: 10.1099/jmm.0.45935-0
He, L., Han, M., Qiao, S., He, P., Li, D., Li, N., et al. (2015). Soybean antigen proteins and their intestinal sensitization activities. Curr. Protein Pept. Sci. 16, 613–621. doi: 10.2174/1389203716666150630134602
Heintz-Buschart, A., Pandey, U., Wicke, T., Sixel-Döring, F., Janzen, A., Sittig-Wiegand, E. et al. (2018). The nasal and gut microbiome in Parkinson's disease and idiopathic rapid eye movement sleep behavior disorder. Mov. Disord. 33, 88–98. doi: 10.1002/mds.27105
Hendriks, W. H., van Baal, J., and Bosch, G. (2012). Ileal and faecal protein digestibility measurement in humans and other non-ruminants—a comparative species view. Br. J. Nutr. 108, S247–S257. doi: 10.1017/S0007114512002395
Hernán, M. A., Takkouche, B., Caamaño-Isorna, F., and Gestal-Otero, J. J. (2002). A meta-analysis of coffee drinking, cigarette smoking, and the risk of Parkinson's disease. Ann Neurol. 52, 276–284. doi: 10.1002/ana.10277
Herrick, M. K., and Tansey, M. G. (2021). Is LRRK2 the missing link between inflammatory bowel disease and Parkinson's disease? NPJ Parkinson's Dis. 7, 26. doi: 10.1038/s41531-021-00170-1
Hirayama, M., Tsunoda, M., Yamamoto, M., Tsuda, T., and Ohno, K. (2016). Serum tyrosine-to-phenylalanine ratio is low in Parkinson's disease. J. Parkinson's Dis. 6, 423–431. doi: 10.3233/JPD-150736
Hou, Y., He, W., Hu, S., and Wu, G. (2019). Composition of polyamines and amino acids in plant-source foods for human consumption. Amino Acids. 51, 1153–1165. doi: 10.1007/s00726-019-02751-0
Hu, X., and Guo, F. (2021). Amino acid sensing in metabolic homeostasis and health. Endocr Rev. 42, 56-76. doi: 10.1210/endrev/bnaa026
Izco, M., Vettorazzi, A., de Toro, M., Sáenz, Y., and Alvarez-Ervitim, L. (2021). Oral sub-chronic ochratoxin A exposure induces gut microbiota alterations in mice. Toxins (Basel). 13, 106. doi: 10.3390/toxins13020106
Jiménez-Jiménez, F. J., Alonso-Navarro, H., García-Martín, E., and Agúndez, J. (2020). Cerebrospinal and blood levels of amino acids as potential biomarkers for Parkinson's disease: review and meta-analysis. Eur. J. Neurol. 27, 2336–2347. doi: 10.1111/ene.14470
Kang, S. H., Lee, J., and Koh, S. B. (2022). Constipation is associated with mild cognitive impairment in patients with de novo Parkinson's disease. J. Mov. Disord. 15, 38–42. doi: 10.14802/jmd.21074
Karkache, I. Y., Damodaran, J. R., Molstad, D., and Bradley, E. W. (2021). Serine/threonine phosphatases in osteoclastogenesis and bone resorption. Gene 771, 145362. doi: 10.1016/j.gene.2020.145362
Kaye, J., Gage, H., Kimber, A., Storey, L., and Trend, P. (2006). Excess burden of constipation in Parkinson's disease: a pilot study. Mov. Disord. 21, 1270–1273. doi: 10.1002/mds.20942
Kevin, J., Portune, M. B., Anne-Marie, D., Daniel, T., François, B., and Yolanda, S. (2016). Gut microbiota role in dietary protein metabolism and health-related outcomes: the two sides of the coin. Trends Food Sci. Technol. 57, 213–232. doi: 10.1016/j.tifs.2016.08.011
Killinger, B. A., Madaj, Z., Sikora, J. W., Rey, N., Haas, A. J., and Vepa, Y. (2018). The vermiform appendix impacts the risk of developing Parkinson's disease. Sci. Transl. Med. 10, eaar5280. doi: 10.1126/scitranslmed.aar5280
Kim, G. H., Lee, Y. C., Kim, T. J., Kim, E. R., Hong, S. N., Chang, D. K., et al. (2022). Risk of neurodegenerative diseases in patients with inflammatory bowel disease: a nationwide population-based cohort study. J. Crohn's Colit. 16, 436–443. doi: 10.1093/ecco-jcc/jjab162
Klingelhoefer, L., and Reichmann, H. (2015). Pathogenesis of Parkinson disease—the gut-brain axis and environmental factors. Nat. Rev. Neurol. 11, 625–636. doi: 10.1038/nrneurol.2015.197
Koh, A., Molinaro, A., Ståhlman, M., Khan, M. T., Schmidt, C., Mannerås-Holm, L., et al. (2018). Microbially produced imidazole propionate impairs insulin signaling through mTORC1. Cell 175, 947–961.e17. doi: 10.1016/j.cell.2018.09.055
Krzystek-Korpacka, M., Fleszar, M. G., Bednarz-Misa, I., Lewandowski, Ł., Szczuka, I., Kempiński, R., et al. (2020). Transcriptional and metabolomic analysis of L-arginine/nitric oxide pathway in inflammatory bowel disease and its association with local inflammatory and angiogenic response: preliminary findings. Int. J. Mol. Sci. 21, 1641. doi: 10.3390/ijms21051641
Kubinak, J. L., and Round, J. L. (2012). Toll-like receptors promote mutually beneficial commensal-host interactions. PLoS Pathog. 8, e1002785. doi: 10.1371/journal.ppat.1002785
Kuhn, W., Roebroek, R., Blom, H., van Oppenraaij, D., Przuntek, H., Kretschmer, A., et al. (1998). Elevated plasma levels of homocysteine in Parkinson's disease. Eur. Neurol. 40, 225–227. doi: 10.1159/000007984
Kumari, S., Kumaran, S. S., Goyal, V., Sharma, R. K., Sinha, N., Dwivedi, S. N., et al. (2020). Identification of potential urine biomarkers in idiopathic parkinson's disease using NMR. Clin. Chim. Acta 510, 442–449. doi: 10.1016/j.cca.2020.08.005
Lai, S. W., Liao, K. F., Lin, C. L., and Sung, F. C. (2014). Irritable bowel syndrome correlates with increased risk of Parkinson's disease in Taiwan. Eur. J. Epidemiol. 29, 57–62. doi: 10.1007/s10654-014-9878-3
Laparra, J. M., and Sanzm, Y. (2010). Interactions of gut microbiota with functional food components and nutraceuticals. Pharmacol. Res. 61, 219–225. doi: 10.1016/j.phrs.2009.11.001
Lee, H. C., and Wei, Y. H. (2005). Mitochondrial biogenesis and mitochondrial DNA maintenance of mammalian cells under oxidative stress. Int. J. Biochem. Cell Biol. 37, 822–834. doi: 10.1016/j.biocel.2004.09.010
Leikas, J. V., Kohtala, S., Theilmann, W., Jalkanen, A. J., Forsberg, M. M., and Rantamäki, T. (2017). Brief isoflurane anesthesia regulates striatal AKT-GSK3β signaling and ameliorates motor deficits in a rat model of early-stage Parkinson's disease. J. Neurochem. 142, 456–463. doi: 10.1111/jnc.14066
Lewis, K., Lutgendorff, F., Phan, V., Söderholm, J. D., Sherman, P. M., and McKay, D. M. (2010). Enhanced translocation of bacteria across metabolically stressed epithelia is reduced by butyrate. Inflamm. Bowel Dis. 16, 1138–1148. doi: 10.1002/ibd.21177
Li, M., Oshima, T., Ito, C., Yamada, M., Tomita, T., Fukui, H., et al. (2021). Glutamine blocks interleukin-13-induced intestinal epithelial barrier dysfunction. Digestion 102, 170–179. doi: 10.1159/000502953
Libao-Mercado, A. J., Zhu, C. L., Cant, J. P., Lapierre, H., Thibault, J. N., Sève, B., et al. (2009). Dietary and endogenous amino acids are the main contributors to microbial protein in the upper gut of normally nourished pigs. J. Nutr. 139, 1088–1094. doi: 10.3945/jn.108.103267
Lin, J. C., Lin, C. S., Hsu, C. W., Lin, C. L., and Kao, C. H. (2016). Association between Parkinson's disease and inflammatory bowel disease: a nationwide Taiwanese retrospective cohort study. Inflamm. Bowel Dis. 22, 1049–1055. doi: 10.1097/MIB.0000000000000735
Lin, R., Liu, W., Piao, M., and Zhu, H. (2017). A review of the relationship between the gut microbiota and amino acid metabolism. Amino Acids 49, 2083–2090. doi: 10.1007/s00726-017-2493-3
Lindahl, M., Chalazonitis, A., Palm, E., Pakarinen, E., Danilova, T., Pham, T. D., et al. (2020). Cerebral dopamine neurotrophic factor-deficiency leads to degeneration of enteric neurons and altered brain dopamine neuronal function in mice. Neurobiol. Dis. 134, 104696. doi: 10.1016/j.nbd.2019.104696
Liu, B., Sjölander, A., Pedersen, N. L., Ludvigsson, J. F., Chen, H., Fang, F., et al. (2021). Irritable bowel syndrome and Parkinson's disease risk: register-based studies. NPJ Parkinson's Dis. 7, 5. doi: 10.1038/s41531-020-00145-8
Liu, J. R., Miao, H., Deng, D. Q., Vaziri, N. D., Li, P., and Zhao, Y. Y. (2021). Gut microbiota-derived tryptophan metabolism mediates renal fibrosis by aryl hydrocarbon receptor signaling activation. Cell. Mol. Life Sci. CMLS 78, 909–922. doi: 10.1007/s00018-020-03645-1
Liu, S., Wang, C., Chen, Y., Peng, S., Chen, X., and Tan, Z. (2019). Association of SLC15A1 polymorphisms with susceptibility to dyslipidaemia in a Chinese Han population. J. Clin. Pharm. Therap. 44, 868–874. doi: 10.1111/jcpt.13016
Lu, S., Jiang, H. Y., and Shi, Y. D. (2022). Association between irritable bowel syndrome and Parkinson's disease: a systematic review and meta-analysis. Acta Neurol. Scand. 145, 442–448. doi: 10.1111/ane.13570
Luan, H., Liu, L. F., Tang, Z., Zhang, M., Chua, K. K., Song, J. X., et al. (2015). Comprehensive urinary metabolomic profiling and identification of potential noninvasive marker for idiopathic Parkinson's disease. Sci. Rep. 5, 13888. doi: 10.1038/srep13888
Lucas, K., and Maes, M. (2013). Role of the toll like receptor (TLR) radical cycle in chronic inflammation: possible treatments targeting the TLR4 pathway. Mol. Neurobiol. 48, 190–204. doi: 10.1007/s12035-013-8425-7
Ma, N., and Ma, X. (2019). Dietary amino acids and the gut-microbiome-immune axis: physiological metabolism and therapeutic prospects. Comprehens. Rev. Food Sci. Food Saf. 18, 221–242. doi: 10.1111/1541-4337.12401
Ma, X., Ma, L., Wang, Z., Liu, Y., Long, L., Ma, X., et al. (2020). Clinical features and gut microbial alterations in anti-leucine-rich glioma-inactivated 1 encephalitis—a pilot study. Front. Neurol. 11, 585977. doi: 10.3389/fneur.2020.585977
Macfarlane, G. T., Allison, C., Gibson, S. A., and Cummings, J. H. (1988). Contribution of the microflora to proteolysis in the human large intestine. J. Appl. Bacteriol. 64, 37–46. doi: 10.1111/j.1365-2672.1988.tb02427.x
Macfarlane, G. T., and Macfarlane, S. (2007). Models for intestinal fermentation: association between food components, delivery systems, bioavailability and functional interactions in the gut. Curr. Opin Biotechnol. 18, 156–162. doi: 10.1016/j.copbio.2007.01.011
Macfarlane, G. T., and Macfarlane, S. (2012). Bacteria, colonic fermentation, and gastrointestinal health. J. AOAC Int. 95, 50–60. doi: 10.5740/jaoacint.SGE_Macfarlane
Machado, S., Costa, A., Pimentel, B, F., Oliveira, M., and Alves, R. C. (2020). A study on the protein fraction of coffee silverskin: protein/non-protein nitrogen and free and total amino acid profiles. Food Chem. 326, 126940. doi: 10.1016/j.foodchem.2020.126940
Machiels, K., Joossens, M., Sabino, J., De Preter, V., Arijs, I., Eeckhaut, V., et al. (2014). A decrease of the butyrate-producing species Roseburia hominis and Faecalibacterium prausnitzii defines dysbiosis in patients with ulcerative colitis. Gut 63, 1275–1283. doi: 10.1136/gutjnl-2013-304833
Mafra, D., Barros, A. F., and Fouque, D. (2013). Dietary protein metabolism by gut microbiota and its consequences for chronic kidney disease patients. Fut. Microbiol. 8, 1317–1323. doi: 10.2217/fmb.13.103
Maini Rekdal, V., Bess, E. N., Bisanz, J. E., Turnbaugh, P. J., and Balskus, E. P. (2019). Discovery and inhibition of an interspecies gut bacterial pathway for Levodopa metabolism. Science (New York, N.Y.) 364, eaau6323. doi: 10.1126/science.aau6323
Malek, N. (2019). Deep brain stimulation in Parkinson's disease. Neurol. India 67, 968–978. doi: 10.4103/0028-3886.266268
Manasanch, E. E., and Orlowski, R. Z. (2017). Proteasome inhibitors in cancer therapy. Nat. Rev. Clin. Oncol. 14, 417–433. doi: 10.1038/nrclinonc.2016.206
Manole, E., Dumitrescu, L., Niculite, C., Popescu, B. O., and Ceafalan, L. C. (2021). Potential roles of functional bacterial amyloid proteins, bacterial biosurfactants and other putative gut microbiota products in the etiopathogeny of Parkinson's disease. Biocell 45, 1–16. doi: 10.32604/biocell.2021.013452
Marrinan, S., Emmanuel, A. V., and Burn, D. J. (2014). Delayed gastric emptying in Parkinson's disease. Mov. Disord. 29, 23–32. doi: 10.1002/mds.25708
Martí I Líndez, A. A., and Reith, W. (2021). Arginine-dependent immune responses. Cell. Mol. Life Sci. CMLS 78, 5303–5324. doi: 10.1007/s00018-021-03828-4
Mayne, K., White, J. A., McMurran, C. E., Rivera, F. J., and de la Fuente, A. G. (2020). Aging and neurodegenerative disease: is the adaptive immune system a friend or foe? Front. Aging Neurosci. 12, 572090. doi: 10.3389/fnagi.2020.572090
Mertsalmi, T. H., Aho, V., Pereira, P., Paulin, L., Pekkonen, E., Auvinen, P., et al. (2017). More than constipation—bowel symptoms in Parkinson's disease and their connection to gut microbiota. Eur. J. Neurol. 24, 1375–1383. doi: 10.1111/ene.13398
Mertsalmi, T. H., But, A., Pekkonen, E., and Scheperjans, F. (2021). Irritable bowel syndrome and risk of Parkinson's disease in finland: a nationwide registry-based cohort study. J. Parkinson's Dis. 11, 641–651. doi: 10.3233/JPD-202330
Metges, C. C. (2000). Contribution of microbial amino acids to amino acid homeostasis of the host. J Nutr. 130, 1857S−1864S. doi: 10.1093/jn/130.7.1857S
Metges, C. C., El-Khoury, A. E., Henneman, L., Petzke, K. J., Grant, I., Bedri, S., et al. (1999). Availability of intestinal microbial lysine for whole body lysine homeostasis in human subjects. Am. J. Physiol. 277, E597–E607. doi: 10.1152/ajpendo.1999.277.4.E597
Metges, C. C., Petzke, K. J., and Hennig, U. (1996). Gas chromatography/combustion/isotope ratio mass spectrometric comparison of N-acetyl- and N-pivaloyl amino acid esters to measure 15N isotopic abundances in physiological samples: a pilot study on amino acid synthesis in the upper gastro-intestinal tract of minipigs. J. Mass Spectr. JMS 31, 367–376. doi: 10.1002/(SICI)1096-9888(199604)31:4<367::AID-JMS310>3.0.CO;2-V
Mu, C., Yang, Y., Yu, K., Yu, M., Zhang, C., Su, Y., et al. (2017). Alteration of metabolomic markers of amino-acid metabolism in piglets with in-feed antibiotics. Amino Acids 49, 771–781. doi: 10.1007/s00726-017-2379-4
Müller, T., Trommer, I., Muhlack, S., and Mueller, B. K. (2016). Levodopa increases oxidative stress and repulsive guidance molecule A levels: a pilot study in patients with Parkinson's disease. J. Neural Transm. 123, 401–406. doi: 10.1007/s00702-016-1519-4
Nagaraja, S., Cai, M. W., Sun, J., Varet, H., Sarid, L., Trebicz-Geffen, M., et al. (2021). Queuine is a nutritional regulator of Entamoeba histolytica response to oxidative stress and a virulence attenuator. MBio. 12, e03549–e03520. doi: 10.1128/mBio.03549-20
Navarro, A., and Boveris, A. (2009). Brain mitochondrial dysfunction and oxidative damage in Parkinson's disease. J. Bioenerg. Biomembr. 41, 517–521. doi: 10.1007/s10863-009-9250-6
Neis, E. P., Dejong, C. H., and Rensen, S. S. (2015). The role of microbial amino acid metabolism in host metabolism. Nutrients 7, 2930–2946. doi: 10.3390/nu7042930
Nielsen, P. M., Mariager, C. Ø., Mølmer, M., Sparding, N., Genovese, F., Karsdal, M. A., et al. (2020). Hyperpolarized [1-13 C] alanine production: a novel imaging biomarker of renal fibrosis. Magn. Reson. Med. 84, 2063–2073. doi: 10.1002/mrm.28326
Niu, X. L., Liu, L., Song, Z. X., Li, Q., Wang, Z. H., Zhang, J. L., et al. (2016). Prevalence of small intestinal bacterial overgrowth in Chinese patients with Parkinson's disease. J. Neural Transm. (Vienna, Austria: 1996) 123, 1381–1386. doi: 10.1007/s00702-016-1612-8
Noh, H., Jang, J., Kwon, S., Cho, S. Y., Jung, W. S., Moon, S. K., et al. (2020). The impact of korean medicine treatment on the incidence of Parkinson's disease in patients with inflammatory bowel disease: a nationwide population-based cohort study in South Korea. J. Clin. Med. 9, 2422. doi: 10.3390/jcm9082422
Ohman, L., and Simrén, M. (2010). Pathogenesis of IBS: role of inflammation, immunity and neuroimmune interactions. Nat. Rev. Gastroenterol. Hepatol. 7, 163–173. doi: 10.1038/nrgastro.2010.4
Park, S., Kim, J., Chun, J., Han, K., Soh, H., Kang, E. A., et al. (2019). Patients with inflammatory bowel disease are at an increased risk of Parkinson's disease: a South Korean nationwide population-based study. J. Clin. Med. 8, 1191. doi: 10.3390/jcm8081191
Peng, L., Li, Z. R., Green, R. S., Holzman, I. R., and Lin, J. (2009). Butyrate enhances the intestinal barrier by facilitating tight junction assembly via activation of AMP-activated protein kinase in Caco-2 cell monolayers. J. Nutr. 139, 1619–1625. doi: 10.3945/jn.109.104638
Peter, I., Dubinsky, M., Bressman, S., Park, A., Lu, C., and Chen, N. (2018). Anti-tumor necrosis factor therapy and incidence of Parkinson disease among patients with inflammatory bowel disease. JAMA Neurol. 75, 939–946. doi: 10.1001/jamaneurol.2018.0605
Postuma, R. B. (2015). Can Parkinson's disease come from the gut? Mov. Disord. 30, 1325. doi: 10.1002/mds.26337
Potrykus, J., White, R. L., and Bearne, S. L. (2008). Proteomic investigation of amino acid catabolism in the indigenous gut anaerobe Fusobacterium varium. Proteomics 8, 2691–2703. doi: 10.1002/pmic.200700437
Raskov, H., Burcharth, J., Pommergaard, H. C., and Rosenberg, J. (2016). Irritable bowel syndrome, the microbiota and the gut-brain axis. Gut Microbes 7, 365–383. doi: 10.1080/19490976.2016.1218585
Raval, U., Harary, J. M., Zeng, E., and Pasinetti, G. M. (2020). The dichotomous role of the gut microbiome in exacerbating and ameliorating neurodegenerative disorders. Expert Rev. Neurother. 20, 673–686. doi: 10.1080/14737175.2020.1775585
Rhee, S. H. (2014). Lipopolysaccharide: basic biochemistry, intracellular signaling, and physiological impacts in the gut. Intest Res. 12, 90–95. doi: 10.5217/ir.2014.12.2.90
Rist, V. T., Weiss, E., Eklund, M., and Mosenthin, R. (2013). Impact of dietary protein on microbiota composition and activity in the gastrointestinal tract of piglets in relation to gut health: a review. Animal 7, 1067–1078. doi: 10.1017/S1751731113000062
Roager, H. M., and Licht, T. R. (2018). Microbial tryptophan catabolites in health and disease. Nat. Commun. 9, 3294. doi: 10.1038/s41467-018-05470-4
Rom, O., Liu, Y., Liu, Z., Zhao, Y., Wu, J., Ghrayeb, A., et al. (2020). Glycine-based treatment ameliorates NAFLD by modulating fatty acid oxidation, glutathione synthesis, and the gut microbiome. Sci. Transl. Med. 12, eaaz2841. doi: 10.1126/scitranslmed.aaz2841
Rosales, C., Demaurex, N., Lowell, C. A., and Uribe-Querol, E. (2016). Neutrophils: their role in innate and adaptive immunity. J. Immunol. Res. 2016, 1469780. doi: 10.1155/2016/1469780
Rychlik, J. L., and Russell, J. B. (2002). The adaptation and resistance of Clostridium aminophilum F to the butyrivibriocin-like substance of Butyrivibrio fibrisolvens JL5 and monensin. FEMS Microbiol. Lett. 209, 93–98. doi: 10.1111/j.1574-6968.2002.tb11115.x
Sampson, T. R., Debelius, J. W., Thron, T., Janssen, S., Shastri, G. G., and Ilhan, Z. E. (2016). Gut microbiota regulate motor deficits and neuroinflammation in a model of Parkinson's disease. Cell 167, 1469–1480.e12. doi: 10.1016/j.cell.2016.11.018
Sampson, T. R., and Mazmanian, S. K. (2015). Control of brain development, function, and behavior by the microbiome. Cell Host Microbe 17, 565–576. doi: 10.1016/j.chom.2015.04.011
Sánchez-Andrea, I., Guedes, I. A., Hornung, B., Boeren, S., Lawson, C. E., Sousa, D. Z., et al. (2020). The reductive glycine pathway allows autotrophic growth of Desulfovibrio desulfuricans. Nat. Commun. 11, 5090. doi: 10.1038/s41467-020-18906-7
Sankarasubramanian, J., Ahmad, R., Avuthu, N., Singh, A. B., and Guda, C. (2020). Gut microbiota and metabolic specificity in ulcerative colitis and Crohn's disease. Front. Med. 7, 606298. doi: 10.3389/fmed.2020.606298
Santos García, D., García Roca, L., de Deus Fonticoba, T., Cores Bartolomé, C., Naya Ríos, L., Canfield, H., et al. (2022). Constipation predicts cognitive decline in Parkinson's disease: results from the COPPADIS cohort at 2-year follow-up and comparison with a control group. J. Parkinson's Dis. 12, 315–331. doi: 10.3233/JPD-212868
Sarkar, S., Gough, B., Raymick, J., Beaudoin, M. A., Ali, S. F., Virmani, A., et al. (2015). Histopathological and electrophysiological indices of rotenone-evoked dopaminergic toxicity: Neuroprotective effects of acetyl-L-carnitine. Neurosci. Lett. 606, 53–59. doi: 10.1016/j.neulet.2015.08.044
Sarkar, S., Raymick, J., and Imam, S. (2016). Neuroprotective and therapeutic strategies against parkinson's disease: recent perspectives. Int. J. Mol. Sci. 17, 904. doi: 10.3390/ijms17060904
Savica, R., Carlin, J. M., Grossardt, B. R., Bower, J. H., Ahlskog, J. E., Maraganore, D. M., et al. (2009). Medical records documentation of constipation preceding Parkinson disease: a case-control study. Neurology 73, 1752–1758. doi: 10.1212/WNL.0b013e3181c34af5
Scheperjans, F., Aho, V., Pereira, P. A., Koskinen, K., Paulin, L., Pekkonen, E., et al. (2015). Gut microbiota are related to Parkinson's disease and clinical phenotype. Mov. Disord. 30,350–358. doi: 10.1002/mds.26069
Schernhammer, E., Chen, H., and Ritz, B. (2006). Circulating melatonin levels: possible link between Parkinson's disease and cancer risk? Cancer Causes Control 17, 577–582. doi: 10.1007/s10552-005-9002-9
Schierack, P., Walk, N., Reiter, K., Weyrauch, K. D., and Wieler, L. H. (2007). Composition of intestinal Enterobacteriaceae populations of healthy domestic pigs. Microbiology(Reading, England) 153, 3830–3837. doi: 10.1099/mic.0.2007/010173-0
Schoultz, I., and Keita, Å. V. (2019). The intestinal barrier and current techniques for the assessment of gut permeability. Cells 8, 193. doi: 10.3390/cells8020193
Schoultz, I., and Keita, Å. V. (2020). The intestinal barrier and current techniques for the assessment of gut permeability. Cells 9, 1909. doi: 10.3390/cells9081909
Schwiertz, A., Spiegel, J., Dillmann, U., Grundmann, D., Bürmann, J., Faßbender, K., et al. (2018). Fecal markers of intestinal inflammation and intestinal permeability are elevated in Parkinson's disease. Parkinsonism Relat. Disord. 50, 104–107. doi: 10.1016/j.parkreldis.2018.02.022
Shao, Y., Li, T., Liu, Z., Wang, X., Xu, X., Li, S., et al. (2021). Comprehensive metabolic profiling of Parkinson's disease by liquid chromatography-mass spectrometry. Mol. Neurodegener. 16, 4. doi: 10.1186/s13024-021-00425-8
Shen, G., Wu, J., Ye, B. C., and Qi, N. (2021). Gut microbiota-derived metabolites in the development of diseases. Can. J. Infect. Dis. Med. Microbiol. 2021, 6658674. doi: 10.1155/2021/6658674
Sherman, M. Y., and Goldberg, A. L. (2001). Cellular defenses against unfolded proteins: a cell biologist thinks about neurodegenerative diseases. Neuron 29, 15–32. doi: 10.1016/S0896-6273(01)00177-5
Sokol, H., Pigneur, B., Watterlot, L., Lakhdari, O., Bermúdez-Humarán, L. G., Gratadoux, J. J., et al. (2008). Faecalibacterium prausnitzii is an anti-inflammatory commensal bacterium identified by gut microbiota analysis of Crohn disease patients. Proc. Natl. Acad. Sci. USA. 105, 16731–16736. doi: 10.1073/pnas.0804812105
Sonnenburg, E. D., and Sonnenburg, J. L. (2014). Starving our microbial self: the deleterious consequences of a diet deficient in microbiota-accessible carbohydrates. Cell Metab. 20, 779–786. doi: 10.1016/j.cmet.2014.07.003
Stokholm, M. G., Danielsen, E. H., Hamilton-Dutoit, S. J., and Borghammer, P. (2016). Pathological α-synuclein in gastrointestinal tissues from prodromal Parkinson disease patients. Ann. Neurol. 79, 940–949. doi: 10.1002/ana.24648
Sugihara, P. T., Sutter, V. L., Attebery, H. R., Bricknell, K. S., and Finegold, S. M. (1974). Isolation of Acidaminococcus fermentans and Megasphaera elsdenii from normal human feces. Appl. Microbiol. 27, 274–275. doi: 10.1128/am.27.1.274-275.1974
Sulzer, D. (2007). Multiple hit hypotheses for dopamine neuron loss in Parkinson's disease. Trends Neurosci. 30, 244–250. doi: 10.1016/j.tins.2007.03.009
Sun, L., Liu, Q., Bao, C., and Fan, J. (2017). Comparison of free total amino acid compositions and their functional classifications in 13 wild edible mushrooms. Molecules (Basel, Switzerland) 22, 350. doi: 10.3390/molecules22030350
Sun, M. F., and Shen, Y. Q. (2018). Dysbiosis of gut microbiota and microbial metabolites in Parkinson's Disease. Ageing Res. Rev. 45, 53–61. doi: 10.1016/j.arr.2018.04.004
Svensson, E., Henderson, V. W., Borghammer, P., Horváth-Puhó, E., and Sørensen, H. T. (2016). Constipation and risk of Parkinson's disease: a Danish population-based cohort study. Parkinsonism Relat Disord. 28, 18–22. doi: 10.1016/j.parkreldis.2016.05.016
Sylte, M. J., Shippy, D. C., Bearson, B. L., and Bearson, S. (2020). Detection of Campylobacter jejuni liver dissemination in experimentally colonized turkey poults. Poult. Sci. 99, 4028–4033. doi: 10.1016/j.psj.2020.03.042
Tabler, T. W., Greene, E. S., Orlowski, S. K., Hiltz, J. Z., Anthony, N. B., and Dridi, S. (2020). Intestinal barrier integrity in heat-stressed modern broilers and their ancestor wild jungle fowl. Front. Vet. Sci. 7, 249. doi: 10.3389/fvets.2020.00249
Tambasco, N., Romoli, M., and Calabresi, P. (2018). Levodopa in Parkinson's disease: current status and future developments. Curr. Neuropharmacol. 16, 1239–1252. doi: 10.2174/1570159X15666170510143821
Tan, A. H., Mahadeva, S., Thalha, A. M., Gibson, P. R., Kiew, C. K., Yeat, C. M., et al. (2014). Small intestinal bacterial overgrowth in Parkinson's disease. Parkinsonism Relat. Disord. 20, 535–540. doi: 10.1016/j.parkreldis.2014.02.019
Thalacker-Mercer, A., Riddle, E., and Barre, L. (2020). Protein and amino acids for skeletal muscle health in aging. Adv. Food Nutr. Res. 91, 29–64. doi: 10.1016/bs.afnr.2019.08.002
Toczylowska, B., Zieminska, E., Michałowska, M., Chalimoniuk, M., and Fiszer, U. (2020). Changes in the metabolic profiles of the serum and putamen in Parkinson's disease patients—in vitro and in vivo NMR spectroscopy studies. Brain Res. 1748, 147118. doi: 10.1016/j.brainres.2020.147118
Torrallardona, D., Harris, C. I., Coates, M. E., and Fuller, M. F. (1996). Microbial amino acid synthesis and utilization in rats: incorporation of 15N from 15NH4Cl into lysine in the tissues of germ-free and conventional rats. Br. J. Nutr. 76, 689–700. doi: 10.1079/BJN19960076
Trist, B. G., Hare, D. J., and Double, K. L. (2019). Oxidative stress in the aging substantia nigra and the etiology of Parkinson's disease. Aging Cell 18, e13031. doi: 10.1111/acel.13031
van de Wouw, M., Schellekens, H., Dinan, T. G., and Cryan, J. F. (2017). Microbiota-gut-brain axis: modulator of host metabolism and appetite. J. Nutr. 147, 727–745. doi: 10.3945/jn.116.240481
van Kessel, S. P., Frye, A. K., El-Gendy, A. O., Castejon, M., Keshavarzian, A., van Dijk, G., et al. (2019). Gut bacterial tyrosine decarboxylases restrict levels of levodopa in the treatment of Parkinson's disease. Nat. Commun. 10, 310. doi: 10.1038/s41467-019-08294-y
Vascellari, S., Palmas, V., Melis, M., Pisanu, S., Cusano, R., Uva, P., et al. (2020). Gut microbiota and metabolome alterations associated with Parkinson's disease. mSystems 5, e00561–e00520. doi: 10.1128/mSystems.00561-20
Venkatesh, M., Mukherjee, S., Wang, H., Li, H., Sun, K., Benechet, A. P., et al. (2014). Symbiotic bacterial metabolites regulate gastrointestinal barrier function via the xenobiotic sensor PXR and toll-like receptor 4. Immunity 41, 296–310. doi: 10.1016/j.immuni.2014.06.014
Verzola, D., Picciotto, D., Saio, M., Aimasso, F., Bruzzone, F., Sukkar, S. G., et al. (2020). Low protein diets and plant-based low protein diets: do they meet protein requirements of patients with chronic kidney disease? Nutrients 13, 83. doi: 10.3390/nu13010083
Villumsen, M., Aznar, S., Pakkenberg, B., Jess, T., and Brudek, T. (2019). Inflammatory bowel disease increases the risk of Parkinson's disease: a Danish nationwide cohort study 1977-2014. Gut 68, 18–24. doi: 10.1136/gutjnl-2017-315666
Wallace, K. L., Zheng, L. B., Kanazawa, Y., and Shih, D. Q. (2014). Immunopathology of inflammatory bowel disease. World J. Gastroenterol. 20, 6–21. doi: 10.3748/wjg.v20.i1.6
Wan, J., Liu, H., Chu, J., and Zhang, H. (2019). Functions and mechanisms of lysine crotonylation. J. Cell. Mol. Med. 23, 7163–7169. doi: 10.1111/jcmm.14650
Wang, Q., and Holst, J. (2015). L-type amino acid transport and cancer: targeting the mTORC1 pathway to inhibit neoplasia. Am. J. Cancer Res. 5, 1281–1294.
Wang, S. L., Shao, B. Z., Zhao, S. B., Fang, J., Gu, L., Miao, C. Y., et al. (2018). Impact of paneth cell autophagy on inflammatory bowel disease. Front. Immunol. 9, 693. doi: 10.3389/fimmu.2018.00693
Weiner, WJ. (2002). An algorithm (decision tree) for the management of Parkinson's disease (2001): treatment guidelines. Neurology 58, 156. doi: 10.1212/WNL.58.1.156
Wells, J. M., Rossi, O., Meijerink, M., and van Baarlen, P. (2011). Epithelial crosstalk at the microbiota-mucosal interface. Proc. Natl. Acad. Sci. USA. 108 (Suppl 1), 4607–4614. doi: 10.1073/pnas.1000092107
Whitehead, T. R., and Cotta, M. A. (2004). Isolation and identification of hyper-ammonia producing bacteria from swine manure storage pits. Curr. Microbiol. 48, 20–26. doi: 10.1007/s00284-003-4084-7
Wolters, E., and Braak, H. (2006). Parkinson's disease: premotor clinico-pathological correlations. J. Neural Transm. Supplementum (70), 309–319. doi: 10.1007/978-3-211-45295-0_47
Wu, L., and Deng, H. (2020). Defluorination of 4-fluorothreonine by threonine deaminase. Organ. Biomol. Chem. 18, 6236–6240. doi: 10.1039/D0OB01358G
Wu, L., Tang, Z., Chen, H., Ren, Z., Ding, Q., Liang, K., et al. (2021). Mutual interaction between gut microbiota and protein/amino acid metabolism for host mucosal immunity and health. Anim. Nutr. 7, 11–16. doi: 10.1016/j.aninu.2020.11.003
Xie, X., Luo, X., and Xie, M. (2017). Association between Parkinson's disease and risk of colorectal cancer. Parkinsonism Relat. Disord. 35, 42–47. doi: 10.1016/j.parkreldis.2016.11.011
Yang, D., Zhao, D., Ali Shah, S. Z., Wu, W., Lai, M., Zhang, X., et al. (2019). The role of the gut microbiota in the pathogenesis of Parkinson's disease. Front. Neurol. 10, 1155. doi: 10.3389/fneur.2019.01155
Yang, K. (2022). Regulation of treg cell metabolism and function in non-lymphoid tissues. Front. Immunol. 13, 909705. doi: 10.3389/fimmu.2022.909705
Yoon, S. Y., Shin, J., Heo, S. J., Chang, J. S., Sunwoo, M. K., and Kim, Y. W. (2022). Irritable bowel syndrome and subsequent risk of Parkinson's disease: a nationwide population-based matched-cohort study. J. Neurol. 269, 1404–1412. doi: 10.1007/s00415-021-10688-2
Yuan, Y. S., Zhou, X. J., Tong, Q., Zhang, L., Zhang, L., Qi, Z. Q., et al. (2013). Change in plasma levels of amino acid neurotransmitters and its correlation with clinical heterogeneity in early Parkinson's disease patients. CNS Neurosci. Therap. 19, 889–896. doi: 10.1111/cns.12165
Zhang, J., Zhu, S., Ma, N., Johnston, L. J., Wu, C., and Ma, X. (2021). Metabolites of microbiota response to tryptophan and intestinal mucosal immunity: A therapeutic target to control intestinal inflammation. Med. Res. Rev. 41, 1061–1088. doi: 10.1002/med.21752
Zhang, X., Svn, Z., Liv, M., Yang, Y., Zeng, R., Huang, Q., et al. (2021). Association between irritable bowel syndrome and risk of Parkinson's disease: a systematic review and meta-analysis. Front. Neurol. 12, 720958. doi: 10.3389/fneur.2021.720958
Zhang, Y., He, X., Qian, Y., Xu, S., Mo, C., Yan, Z., et al. (2022). Plasma branched-chain and aromatic amino acids correlate with the gut microbiota and severity of Parkinson's disease. NPJ Parkinson's Dis. 8, 48. doi: 10.1038/s41531-022-00312-z
Zhao, J., Zhang, X., Liu, H., Brown, M. A., and Qiao, S. (2019). Dietary protein and gut microbiota composition and function. Curr. Protein Peptide Sci. 20, 145–154. doi: 10.2174/1389203719666180514145437
Zhao, Z., and Liu, W. (2020). Pancreatic cancer: a review of risk factors, diagnosis, and treatment. Technol. Cancer Res. Treatm. 19, 1533033820962117. doi: 10.1177/1533033820962117
Zheng, J. H., Sun, W. H., Ma, J. J., Wang, Z. D., Chang, Q. Q., Dong, L. R., et al. (2022). Resting-state functional magnetic resonance imaging in patients with Parkinson's disease with and without constipation: a prospective study. Clin. Auton. Res. 32, 51–58. doi: 10.1007/s10286-022-00851-8
Zhou, Y., Su, Y., Xu, W., Wang, W., and Yao, S. (2019). Constipation increases disability and decreases dopamine levels in the nigrostriatal system through gastric inflammatory factors in Parkinson's disease. Curr. Neurovasc. Res. 16, 241–249. doi: 10.2174/1567202616666190618170103
Zhu, F., Li, C., Gong, J., Zhu, W., Gu, L., and Li, N. (2019). The risk of Parkinson's disease in inflammatory bowel disease: a systematic review and meta-analysis. Dig. Liver Dis. 51, 38–42. doi: 10.1016/j.dld.2018.09.017
Zhu, Y., Yuan, M., Liu, Y., Yang, F., Chen, W. Z., Xu, Z. Z., et al. (2022). Association between inflammatory bowel diseases and Parkinson's disease: systematic review and meta-analysis. Neural Regener. Res. 17, 344–353. doi: 10.4103/1673-5374.317981
Zou, B., Sun, Y., Xu, Z., Chen, Y., Li, L., Lin, L., et al. (2021). Rapid simultaneous determination of gut microbial phenylalanine, tyrosine, and tryptophan metabolites in rat serum, urine, and faeces using LC-MS/MS and its application to a type 2 diabetes mellitus study. Biomed. Chromatogr. BMC 35, e4985. doi: 10.1002/bmc.4985
Keywords: Parkinson's disease, gut microbiota, amino acid metabolism, microbiota-host interaction, gut microbiota-brain axis
Citation: Wang W, Jiang S, Xu C, Tang L, Liang Y, Zhao Y and Zhu G (2022) Interactions between gut microbiota and Parkinson's disease: The role of microbiota-derived amino acid metabolism. Front. Aging Neurosci. 14:976316. doi: 10.3389/fnagi.2022.976316
Received: 23 June 2022; Accepted: 29 August 2022;
Published: 02 November 2022.
Edited by:
Weidong Le, Dalian Medical University, ChinaReviewed by:
Heinz Reichmann, University Hospital Carl Gustav Carus, GermanyCopyright © 2022 Wang, Jiang, Xu, Tang, Liang, Zhao and Zhu. This is an open-access article distributed under the terms of the Creative Commons Attribution License (CC BY). The use, distribution or reproduction in other forums is permitted, provided the original author(s) and the copyright owner(s) are credited and that the original publication in this journal is cited, in accordance with accepted academic practice. No use, distribution or reproduction is permitted which does not comply with these terms.
*Correspondence: Yang Zhao, eWFuZ3poYW90Y21Abmp1Y20uZWR1LmNu; Guoxue Zhu, emd4dWUwMTIyQG5qdWNtLmVkdS5jbg==
Disclaimer: All claims expressed in this article are solely those of the authors and do not necessarily represent those of their affiliated organizations, or those of the publisher, the editors and the reviewers. Any product that may be evaluated in this article or claim that may be made by its manufacturer is not guaranteed or endorsed by the publisher.
Research integrity at Frontiers
Learn more about the work of our research integrity team to safeguard the quality of each article we publish.