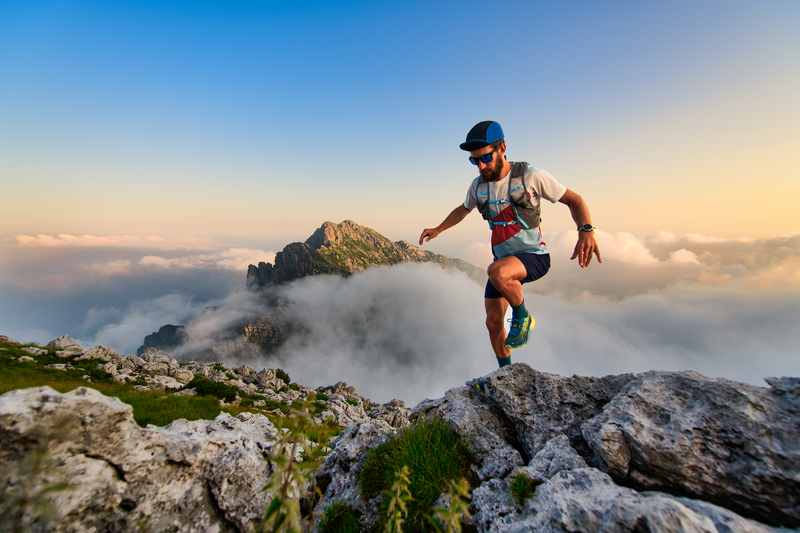
95% of researchers rate our articles as excellent or good
Learn more about the work of our research integrity team to safeguard the quality of each article we publish.
Find out more
REVIEW article
Front. Aging Neurosci. , 09 August 2022
Sec. Cellular and Molecular Mechanisms of Brain-aging
Volume 14 - 2022 | https://doi.org/10.3389/fnagi.2022.975248
This article is part of the Research Topic Molecular Biomarkers in the Prediction, Diagnosis, and Prognosis of Neurodegenerative Diseases View all 22 articles
Parkinson’s disease is the second most common neurodegenerative disease after Alzheimer’s disease, which imposes an ever-increasing burden on society. Many studies have indicated that oxidative stress may play an important role in Parkinson’s disease through multiple processes related to dysfunction or loss of neurons. Besides, several subtypes of non-coding RNAs are found to be involved in this neurodegenerative disorder. However, the interplay between oxidative stress and regulatory non-coding RNAs in Parkinson’s disease remains to be clarified. In this article, we comprehensively survey and overview the role of regulatory ncRNAs in combination with oxidative stress in Parkinson’s disease. The interaction between them is also summarized. We aim to provide readers with a relatively novel insight into the pathogenesis of Parkinson’s disease, which would contribute to the development of pre-clinical diagnosis and treatment.
Parkinson’s disease (PD) is a common neurologic disease, which affected about 6.1 million people around the world in 2016 (Bloem et al., 2021). The elderly are more likely to suffer from this disease, with most cases occurring after the age of 50 (Opara et al., 2017). PD is mainly characterized by the loss of dopaminergic neurons and the presence of Lewy bodies in surviving neurons, while its exact cause is unknown (Antony et al., 2013; Raza et al., 2019; Li W. et al., 2020). The disease can be intolerable because it is a progressive disease and can severely damage the somatic motor system, making it difficult for PD patients to use their hands or walk normally (Schneider et al., 2017; Cerri et al., 2019; Hayes, 2019). They may also exhibit multiple non-motor symptoms, including cognitive decline, depression, anxiety, and sleep disorders (Reich and Savitt, 2019). A number of studies illustrated that many types of RNA have been deeply involved in the whole disease progression even after onset, including but not limited to long non-coding RNAs (lncRNAs), microRNAs (miRNAs), and circularRNAs (circRNAs; Choi et al., 2018, Liu N. et al., 2020; Liu et al., 2021; Wu and Kuo, 2020; Wang W. et al., 2021). All of the three most well-known and most commonly studied RNAs belong to the regulatory non-coding RNAs (ncRNAs), a category of ncRNAs that are transcribed from DNA and not involved in coding proteins (Szymański and Barciszewski, 2002).
lncRNAs are a collective term for a group of highly heterogeneous regulatory ncRNAs (Hombach and Kretz, 2016). The common characteristics of lncRNA mainly include transcripts longer than 200 and a lack of ability to encode proteins (Yang et al., 2021). Given their wide variety, their biological roles can be quite diverse. An important function of lncRNA is to regulate gene expression at the transcriptional, post-transcriptional, and epigenetic levels (Panni et al., 2020). lncRNAs can bind certain RNAs and proteins to prevent them from interacting with other molecules (Wang, 2018). They can also affect epigenetic modifications of genes and histones. In addition, several lncRNAs have been found to have a role in the maintenance of chromosome stability and the regulation of the cell cycle (Lee et al., 2016; Munschauer et al., 2018; Wang R. et al., 2018; Zhang et al., 2018).
miRNAs, small RNA molecules with an average length of 22 nt, must be the most extensively studied and best understood small non-coding RNAs (sncRNAs; Zhang P. et al., 2019). The major function of miRNAs is to control mRNA translation, which is usually based on the complementary base pairing between seed regions of miRNAs and 3’ untranslated regions of mRNAs (Wei J. W. et al., 2017). The miRNA first incorporates Argonaute proteins to form an RNA-induced silencing complex (RISC; Scott and Ono, 2011). Then, if miRNAs show imperfect complementarity to their target mRNAs, which is usually the case in animals, deadenylation of the mRNA will occur, leading to translation inhibition (Carvalho Barbosa et al., 2020). Furthermore, a small number of miRNAs can upregulate gene expression, though this process is fairly rare (Gerin et al., 2010).
circRNAs, a unique group of regulatory ncRNAs, are characterized by their covalently closed structures (Zhou W. Y. et al., 2020). Due to a lack of capping and polyadenylation, circRNAs are resistant to RNA exonucleases and more stable than linear RNAs (Huang et al., 2020). The size of circRNA range from 100 nt to over 4 kb, so it might belong to lncRNA and sncRNA at the same time (Zhang P. et al., 2019). Increasing evidence has demonstrated that a subset of circRNAs exerts their functions by functioning as competing endogenous RNA (ceRNA) or miRNA sponges (Cao et al., 2020; Jiang Q. et al., 2020; Li L. et al., 2020; Peng et al., 2021). miRNAs captured by circRNAsare unable to regulate gene expression (Jin et al., 2020). In addition, several circRNAs can act as protein decoys. Through the interaction with these circRNAs, the biological functions of a variety of proteins can be changed (Altesha et al., 2019).
In addition to the aforementioned regulatory ncRNAs, oxidative stress is believed to have a notable effect on the pathological progression of PD (Wang X. et al., 2020). It results from an imbalance between oxidant production and antioxidative defenses (Forman and Zhang, 2021). The oxidant refers to reactive oxygen species (ROS) and reactive nitrogen species (RNS), which are mainly produced in mitochondria (Turrens, 2003). ROS includes superoxide (O•–2), hydrogen peroxide (H2O2), hydroxyl radical (•OH), ozone, and singlet oxygen. These small molecules are all derived from the reaction of oxygen with electrons (Brieger et al., 2012). The primary intermediate in the biosynthesis of RNS is nitric oxide (NO; Lushchak and Lushchak, 2021). NO reacts with ROS, thereby giving rise to other forms of RNS such as peroxynitrite and peroxynitrous acid (ONOOH; Tharmalingam et al., 2017). Disruption of the ATP production function of mitochondria as well as inflammation may cause an increase in oxidant production (Brand and Nicholls, 2011; Islam, 2017). In response to ROS and RON, organisms have evolved antioxidant defense systems. Such antioxidant defenses are largely provided by antioxidant enzymes, including superoxide dismutase (SOD), catalase (CAT), and glutathione peroxidase (GPx; Prasad et al., 2017). By catalyzing specific reactions, these enzymes can scavenge oxidants and repair oxidative damage. Excessive oxidant production combined with decreased expression and activity of antioxidant enzymes will induce oxidative stress, which can greatly impair cell viability (Nunomura et al., 2012; Chen and Zhong, 2014).
It is undeniable that a lot of research has been conducted on the role of regulatory ncRNAs or oxidative stress in PD (Lu et al., 2017, 2019; Pavlou and Outeiro, 2017; Cao et al., 2019; Nies et al., 2021; Zhu et al., 2021). However, the interaction between the minPD has not been well studied. It is worth noting that both regulatory ncRNAs and oxidative stress are closely involved in neurodegenerative disorders (Nunomura and Perry, 2020). On the one hand, oxidative stress can cause damage to nucleic acids and affect the expression levels of varieties of regulatory ncRNAs (Radi et al., 2014). On the other hand, several regulatory ncRNAs have the potential to regulate oxidative stress-related pathways, which represent promising therapeutic targets (Geng et al., 2017; Song et al., 2019; Li Y. et al., 2020; Li et al., 2021). Hence, further research on this molecular mechanism in PD may be beneficial to our understanding of the neurodegenerative disorder in conjunction with the development of novel strategies for pre-clinical diagnosis and therapeutic intervention.
Once it was thought that ncRNAs did not have any biological function. Only recently has it been discovered that the roles of RNAs are not limited to bridging genes and proteins (Carvalho Barbosa et al., 2020). The last decade has witnessed the discovery and annotation of thousands of both housekeeping and regulatory ncRNAs, which are emerging as key regulators of gene expression at the transcriptional or post-transcriptional level (Li et al., 2015; Merry et al., 2015; Yildirim et al., 2020; Rezaei et al., 2021). Several subtypes of regulatory ncRNAs, including miRNAs, lncRNAs, and circRNAs, are involved in the pathogenesis of PD (Majidinia et al., 2016). The PD models used and the regulatory ncRNAs identified are shown in Table 1.
PD is characterized by the loss of dopaminergic neurons (DAs) in the substantia nigra (Goh et al., 2019). Convincing evidence indicated that neuroinflammation was involved in DA death (Gordon et al., 2018; Rodriguez-Perez et al., 2018). Activated microglia may initiate the inflammatory process in the central nervous system (CNS). The upregulation of microRNA-132-3p (miR-132-3p) and microRNA-873 (miR-873) in PD led to the deficiency of ATP-binding cassette transporter A1 (ABCA1) and GLRX, which may give rise to the activation of microglial cells and subsequent neuronal death (Wu et al., 2020; Gong et al., 2022). microRNA-29b2/c (miR-29b2/c) and microRNA-124 (miR-124) played contrasting roles in microglia activation. Knockout of miR-29b2/c would inhibit microglia activation, whereas decreased expression of miR-124 correlated with the progression of microglia activation (Yao et al., 2018; Bai et al., 2021). Feng et al. found that activated microglia can be transformed into two different phenotypes, i.e., M1 polarization and M2 polarization. M1 microglia could produce proinflammatory cytokines to maintain the homeostasis of the central nervous system. However, this process may be prolonged by microRNA-330 (miR-330), which in turn caused inflammatory damage to neuronal cells (Feng et al., 2021). TNF-α was a proinflammatory factor released by M1 polarization, whose production was downregulated by micro-RNA-7116-5p (miR-7116-5p; He et al., 2017). Nod-like receptor protein 3 (Nlrp3) functioned in regulating proinflammatory cytokines. Both microRNA-30e (miR-30e) and microRNA-190 (miR-190) targeted the 3’ UTR of Nlrp3 mRNA and thus inhibited neuroinflammation (Li et al., 2018; Sun et al., 2019). Furthermore, Specific protein-1 (SP1), nuclear factor of activated T cells 5 (NFAT5), Rho-associated kinase 1 (ROCK1), and transcriptional activator 3 (STAT3) participated in regulating neuroinflammation. By suppressing the expression of SP1, NFAT5, ROCK1, and STAT3, microRNA-29c (miR-29c), microRNA-195 (miR-195), microRNA-93 (miR-93), and microRNA-let-7a (miR-let-7a) may reduce neuronal damage caused by inflammatory responses (Ren et al., 2019; Zhang et al., 2019; Wang et al., 2020a, b; Wang et al., 2021).
Another hallmark of PD is the formation of Lewy bodies in neurons (Singh and Sen, 2017). The main component of Lewy bodies was α-synuclein fibrils (α-syn) toxic to DA neurons. Apoptosis was induced when the formation of α-syn aggregates exceeded the limit of what the cell can tolerate, suggesting α-syn is an essential player in the PD neurodegenerative process (Rocha et al., 2018). miRNA-7 (miR-7) was found to not only inhibit α-syn expression by interacting with its messenger RNA (mRNA) but also help remove α-syn by promoting autophagy (Choi et al., 2018). As McMillan et al. stated in their study, miR-7 may promote autophagy by suppressing the expression of epidermal growth factor receptor (EGFR), since the activated EGFR was able to inhibit autophagy. Then, α-syn can be degraded by autophagy (McMillan et al., 2017). In addition to promoting α-syn degradation, researchers found that two RNAs affected the toxicity of α-syn. Both phosphorylation and acetylation were essential mechanisms regulating the neurotoxicity of α-syn. Su et al. discovered that miRNA-26a (miR-26a) directly downregulated the expression level of death-associated protein kinase 1 (DAPK1), and DAPK1 was capable of promoting the phosphorylation of α-syn (Su et al., 2019). Also, sirtuin 2 (SIRT2) was identified to catalyze the deacetylation reaction of α-syn, and miRNA-486-3p (miR-486-3p) may affect the toxicity of α-syn by regulating the expression of SIRT2 (Wang et al., 2018).
Accumulating evidence suggested that autophagy participated in the pathogenesis of PD. Autophagy dysregulation can impair many subcellular functions, including α-syn degradation (Lu et al., 2020). Based on the findings of Zhao et al., UNC51-like kinase (ULK1), a serine/threonine kinase responsible for promoting cell autophagy, was positively regulated by miRNA-132-5p (miR-132-5p; Zhao et al., 2020a). In addition, both miRNA-181b and miRNA-199a were able to mediate autophagy by targeting the PTEN/Akt/mTOR pathway. The downregulation of miRNA-181b in PD inhibited the Akt/mTOR signaling pathway, thereby improving autophagy (Li et al., 2018). Similarly, the downregulation of miRNA-199a in PD enhanced autophagy by regulating the activity of mTOR (Ba et al., 2020). Mitophagy is a form of autophagy in which cells remove damaged mitochondria to maintain cellular homeostasis. It has been reported that the accumulation of dysfunctional mitochondria would lead to the death of DA neurons (John et al., 2020). According to the research conducted by Zhou et al., miRNA-103a-3p (miR-103a-3p) was able to regulate mitophagy by mediating Parkin at the post-transcriptional level. Since Parkin is an E3 ubiquitin ligase that function in promoting the removal of damaged mitochondria via mitophagy, the upregulated level of miR-103a-3p in PD may harm the nervous system (Zhou J. et al., 2020).
In addition to autophagy dysregulation, dysregulated apoptosis had a crucial role in the pathogenesis of PD (Li D. et al., 2020). Although apoptosis was necessary for building neural networks, excessive apoptosis would accelerate the progression of PD (Liu et al., 2019). Three miRNAs were found to downregulate neuroprotective factors, including miRNA-183 (miR-183), miRNA-384-5p (miR-384-5p), and miRNA-421 (miR-421; Gao et al., 2019; Tao et al., 2020; Dong et al., 2021). Conversely, several miRNAs were able to inhibit neuronal apoptosis and therefore played a protective role in PD. Increasing evidence has shown that SNCA, Bcl-2-like protein 11 (BCL2L11), Ras-related C3 botulinum toxin substrate 1 (RAC1), Bax, caspase-3, and p53 were apoptotic activators. Their overexpression can be attenuated by microRNA-30b (miR-30b), microRNA-181c (miR-181c), microRNA-133a (miR-133a), microRNA-216a (miR-216a), let-7d, and microRNA-132 (miR-132), respectively (Li et al., 2017; Wei M. et al., 2017; Lu W. et al., 2020; Shen et al., 2020; Yang et al., 2020; Qazi et al., 2021). However, this effect may be diminished due to the downregulation of these six miRNAs in PD. p38 MAPK pathway has been proven to be important in regulating cell apoptosis. microRNA-599 (miR-599) and microRNA-181a (miR-181a) functioned in inactivating the p38 MAPK pathway, thereby protecting neurons (Liu Y. et al., 2017; Wu Q. et al., 2019). In addition, the activation of ERK1/2 and AMPK/mTOR pathways were essential to cell proliferation, which could be suppressed by microRNA-133b (miR-133b) and microRNA-185 (miR-185; Wen et al., 2018; Dong et al., 2020). miR-7 and microRNA-128 (miR-128) regulated the Wnt/beta-catenin signaling pathway differently. miR-7 suppressed the proliferation of DAs by inhibiting Wnt/beta-catenin pathway, whereas microRNA-128 (miR-128) alleviated the inhibiting effect of axis inhibition protein 1 (AXIN1) on this pathway and blocked DA apoptosis (Zhou et al., 2018; Adusumilli et al., 2020). microRNA-221 (miR-221) and miR-124 could extend cell lifespan via indirect regulation of PI3K/Akt and Hedgehog pathways (Li L. et al., 2018; Wang J. et al., 2019).
In recent years, the neuropathological consequences of dysregulated glutamate homeostasis have been recognized, and glutamate excitotoxicity has been associated with PD. Glutamate is an essential neurotransmitter in the mammalian central nervous system, responsible for transmitting excitatory signals between neurons. The amount of glutamate at the synapse above the physiological range was toxic and could have a detrimental effect on neuron cells, which was termed glutamate excitotoxicity (Iovino et al., 2020). Glutamate transporter-1 (GLT-1) was responsible for clearing excess glutamate from the synaptic gap to maintain glutamate homeostasis. It was found that GLT-1 mRNA was directly targeted by miRNA-543-3p (miR-543-3p). The overexpression of miR-543-3p in PD was able to suppress the expression and function of GLT-1 protein (Wu X. et al., 2019). miRNA-30a-5p (miR-30a-5p) was also upregulated in a mouse model of PD, while the regulatory mechanism of PKCα by miR-30a-5p needs further research. Meng et al. revealed that once PKCα was activated, it could induce ubiquitination and subsequent degradation of GLT-1 (Meng X. et al., 2021). Hence, both miR-543-3p and miR-30a-5p contributed to the pathology of PD by reducing the level of GLT-1, making them promising targets for treatment.
Briefly, numerous studies have validated those miRNAs were capable of regulating recognized causative factors of PD such as neuronal cell damage, microglia activation, and α-syn production, and chemical modifications by repressing the expression of target genes. The lncRNA can also influence the progress of this disease, however, it does not do so in the same way as the miRNA.
The progression of PD is normally accompanied by apoptosis and inflammation of nerve cells (Bhattacharyya et al., 2021). Two lncRNAs, namely nuclear-enriched abundant transcript 1 (NEAT1) and SNHG gene 14 (SNHG14), were identified to accelerate this progression (Zhou S. et al., 2020; Liu et al., 2021). The main hallmark of PD is the loss of dopaminergic neurons (DAs) in the substantia nigra, which correlates with typical PD symptoms including resting tremors and bradykinesia (Xin and Liu, 2021). SNHG14 reduced the number of DAsby sponging miR-133b, whereas H19 exerted a protective role against DAneuron damage (Zhang L. M. et al., 2019; Jiang J. et al., 2020). This protection was based on the overexpression of hypoxanthine-guanine phosphoribosyltransferase (HPRT), which was prompted by H19 sponging microRNA-301b-3p (miR-301b-3p; Jiang J. et al., 2020).
In PD, the main form of DA neuron death is apoptosis. Abnormalities in apoptosis are a sign of the loss of DAs in the substantia nigra, which have a notable effect on the development of PD. Zhang et al. found that microRNA-583-3p (miR-583-3p) downregulated the expression of PIK3R3. lncRNA H19 could attenuate the apoptosis of neurons by interacting with miR-583-3p (Zhang Y. et al., 2020). lncRNA myocardial infarction-associated transcript (MIAT) also exerted a neuroprotective role in PD. Shen et al. revealed that MIAT regulated synaptotagmin-1 (SYT1) by binding to microRNA-34-5p (miR-34-5p), which enhanced cell viability and inhibited apoptosis (Shen et al., 2021a). In contrast, growth arrest-specific 5 (GAS5) may cause loss of neuronal cells. The underlying mechanism was that GAS5 negatively regulated microRNA-150 (miR-150) and positively regulated fos-like antigen-1 (Fosl1), which resulted in cell apoptosis (Ma et al., 2022). In addition, a number of evidence indicated that LINC00943, long intergenic noncoding RNA-p21 (lincRNA-p21), NEAT1, and small molecule RNA host gene 1 (SNHG1) acted as molecular sponges of microRNA-338-3p (miR-338-3p), microRNA-1277-5p (miR-1277-5p), miR-124, microRNA-1301-3p (miR-1301-3p), and microRNA-216-3p (miR-216-3p), thereby inhibiting cell viability (Xu et al., 2018, Liu J. et al., 2020; Meng C. et al., 2021; Wang et al., 2021b). LRRK2 gene has been acknowledged to be associated with PD. Researchers found that Hox transcript antisense intergenic RNA (HOTAIR), X-inactive specific transcript (XIST), and metastasis-associated lung adenocarcinoma transcript 1 (MALAT1) indirectly enhanced the expression of LRRK2, which would increase the rate of apoptosis (Wang et al., 2017; Chen Q. et al., 2018; Zhou Q. et al., 2021). HOTAIR may also promote apoptosis of neuronal cells through microRNA-126-5p (miR-126-5p)/RAB3A-interacting protein (RAB3IP) axis (Lin et al., 2019). Furthermore, MALAT1 contributed to the apoptosis of DAs by combining with miR-124 and microRNA-135-5p (miR-135-5p; Liu W. et al., 2017; Lv et al., 2021).
Neuroinflammation is believed to play important role in the development of PD. Multiple proinflammatory cytokines released by activated microglia are involved in neuroinflammatory responses, which would eventually induce apoptosis of DA neurons (Xin and Liu, 2021). Cao et al. revealed that lncRNA HOXA11-AS activated microglia and promoted neuroinflammation via regulating miR-124-3p/NF-κB axis (Cao et al., 2021). Besides, lncRNA nuclear enriched abundant transcript 1 (NEAT1) acted as sponges of miR-124, microRNA-212-5p (miR-212-5p), and microRNA-519a-3p, thereby increasing the expression of phosphodiesterase 4B (PDE4B), RAB3A-interacting protein (RAB3IP), and specific protein 1 (SP1; Xie S. P. et al., 2019; Liu R. et al., 2020; Chen M. Y. et al., 2021). Since PDE4B, RAB3IP, and SP1were shown to facilitate cell inflammation, the knockdown of NEAT1 could be a potential strategy for treating PD patients. Based on the study of Sun et al., LINC00943 also positively regulated SP1 expression, making it a possible therapeutic target in PD (Sun et al., 2022). Small molecule RNA host gene 1 (SNHG1) was found to prevent microRNA-181a-5p (miR-181a-5p) from suppressing C-X-C motif chemokine 12 (CXCL12). This may trigger inflammatory responses (Wang et al., 2021a). In addition, SNHG1 as well as growth arrest-specific 5 (GAS5) had a promotion effect on the expression of an inflammasome named nod-like receptor protein 3 (NLRP3), which would stimulate the secretion of inflammatory factors (Cao et al., 2018; Xu et al., 2020).
Abnormal aggregation of α-syn also causes damage to DA neurons. Liu et al. discovered that the expression of nuclear-enriched abundant transcript (NEAT) was positively correlated with the α-syn expression, suggesting that the knockdown of NEAT may protect neurons (Liu and Lu, 2018). Both UCA1 and small nucleolar RNA host gene 1 (SNHG1) were able to promote the accumulation of α-syn. Based on the study of Lu et al., UCA1 could upregulate the expression of SCNA (Lu et al., 2018). In addition, Chen et al. found that the inhibitory effect of microRNA-15b-5p (miR-15b-5p) on the expression of seven in absentia homolog 1 (SIAH1) could be reversed by SNHG1. The overexpression of SIAH1 would induce the aggregation of α-syn and elevate its toxicity (Chen Y. et al., 2018).
Autophagy is responsible for the degradation of α-syn. The dysregulation of this process is an important contributor to the development of PD (Xin and Liu, 2021). Yan et al. revealed that lncRNA nuclear paraspeckle assembly transcript 1 (NEAT1) might promote autophagy in PD by stabilizing PTEN-induced kinase 1 (PINK1; Yan et al., 2018). Brain-derived neurotrophic factor anti-sense (BDNF-AS) was also able to promote autophagy. According to the study by Fan et al., BDNF-AS enhanced the number of autophagosomes by regulating microRNA-125b-5p (miR-125b-5p) negatively (Fan Y. et al., 2020). In contrast, SNHG1 was found to increase the expression level of p27 via sponging microRNA-221/222 (miR-221/222). Since p27 was believed to have a role in inhibiting autophagy, the downregulation of SNHG1 may promote autophagic activation (Qian et al., 2019). PLK2 was associated with a pathway that inducedα-syn degradation via autophagy. microRNA-126 (miR-126) suppressed the expression of p27, which could be blocked by Opa interacting protein five antisense RNA 1 (OIP5-AS1). Hence, OIP5-AS1 exerted a protective role in PD by accelerating the clearance of α-syn (Song and Xie, 2021). Based on the findings of Zhao et al., OIP5-AS1 also had a role in promoting mitochondrial autophagy, a process that selectively removes unwanted or damaged mitochondria. Specifically, the expression of NIX was down-regulated by microRNA-137 (miR-137), which was reversed by OIP5-AS1. Overexpression of NIX was proved to promote mitochondrial autophagy, which would prevent neuronal death (Zhao et al., 2022). For further review on targeting α-syn as a therapy for PD, please refer to Taylor et al. (2002), Wong and Cuervo (2010), Vidal et al. (2014), Martire et al. (2015), Pickrell and Youle (2015), Dunn et al. (2019), and Fields et al. (2019).
To sum up, lncRNAs have been shown to affect apoptosis and autophagy of neurons, the accumulation and degradation of α-syn, and neuronal inflammation. The regulatory roles they play in PD are largely accomplished by sponging miRNAs.
The in vitro and in vivo experiments have indicated the feasibility of treating PD by targeting miRNAs and lncRNAs. These regulatory ncRNAs may serve as targets in PD treatment, while the effectiveness and safety of this therapy have yet to be tested in human trials. Other regulatory ncRNAs, such as circRNAs, may sponge specific miRNAs, thus contributing to the development of PD (Lu et al., 2019; Feng et al., 2020; Wang W. et al., 2021). Further research is needed to unravel the role of a wider class of regulatory ncRNAs in PD progression, which would not only further our understanding but also lead to the development of novel and effective therapeutic strategies (Acharya et al., 2020).
ROS generated in the body perform physiologic functions such as stimulating growth factors, promoting inflammatory responses, and regulating cell production. However, when their levels far exceed that of antioxidants, human cells will be subjected to devastating effects (Surendran and Rajasankar, 2010; Zuo and Motherwell, 2013; Hemmati-Dinarvand et al., 2019). Neurons are likely to be attacked by oxidative stress since they consume large amounts of oxygen and possess relatively modest levels of antioxidant enzymes. Accumulating evidence has demonstrated that oxidative stress is an important factor in the etiology and progression of PD (Figure 1; Percario et al., 2020).
Mitochondrial dysfunction was found in the substantia nigra (SN) in some patients with PD. Although the mechanism by which mitochondrial depletion causes oxidative stress and bioenergetic deficiency is not fully understood, researchers have correlated the impairment or inhibition of mitochondrial complex I with elevated levels of ROS (Schapira et al., 1990a, b, c). Complex I (NADH-ubiquinone oxidoreductase) is a major component of the oxidative phosphorylation system responsible for converting molecular oxygen into water and driving energy synthesis (Hauser and Hastings, 2013). Reduced activity of complex I may lead to disruption of electron transfer, which would result in excessive ROS production (Figure 2; Sarkar et al., 2016).
Mitochondrial-related energy failure may also disrupt the vesicular storage of DA (Puspita et al., 2017). Under normal circumstances, dopamine was preserved in synaptic vesicles, which were an acidic and stable environment that protected dopamine from oxidation (Jin et al., 2014). In the SN of PD patients, however, a rise in the amount of free dopamine in the cytoplasm has been observed (Sackner-Bernstein, 2021). Monoamine oxidases (MAO) functioned in catalyzing the transition from cytosolic dopamine to H2O2and 3,4-dihydroxyphenylacetaldehyde (DOPAL; Raza et al., 2019; Zaman et al., 2021). In addition, dopamine may undergo auto-oxidation to form DA quinones (Janda et al., 2012; Smeyne and Smeyne, 2013). H2O2 was a by-product of both of these reactions, which could be further transformed into •OH via the Fenton reaction (Vallee et al., 2020). This reaction was largely dependent on the presence of iron, which was also identified to be elevated in the PD SN (Vallée et al., 2021a). Therefore, enhanced dopamine metabolism in combination with iron accumulation may contribute to cellular ROS in dopaminergic neurons.
In addition to the alternations occurring within neuronal cells, microglia activation has been regarded as an essential contributor to ROS production (Hassanzadeh and Rahimmi, 2018). Usually, microglia cells, the main immune cells in the brain, remained quiescent. Once activated, microglia released ROS and RNS such as H2O2, O•–2, and NO (Onyango, 2008). The reaction of the latter two free radicals produced peroxynitrite (ONOO−), a highly reactive molecule that may induce apoptosis of DA neurons (Varcin et al., 2012). Furthermore, the capacity of activated microglia to produce ROS and RNS was enhanced due to the increased release of inducible nitric oxide synthase (iNOS) and NADPH oxidase. These two enzymes promoted the generation of O•–2 and NO, respectively (Drechsel and Patel, 2008; Koppula et al., 2012). Excessive intracellular and intercellular free radicals would lead to oxidative stress (Hald and Lotharius, 2005).
To deal with high contents of ROS and protect themselves from oxidative damage, neuronal cells utilized an antioxidant system consisting of antioxidant enzymes in conjunction with low molecular compounds (Manoharan et al., 2016). The enzymes included catalase (CAT), superoxide dismutase (SOD), and glutathione peroxidase (GPx), whereas glutathione (GSH) was a major non-enzymatic antioxidant (Redensek et al., 2019; Robea et al., 2020). CAT and GPx were believed to be responsible for scavenging H2O2 (Foley and Riederer, 2000). In the substantia nigra pars compacta (SNc) of PD patients, however, a dramatic decrease in the activity of antioxidative enzymes and the levels of non-enzymatic antioxidants has been observed (Damier et al., 1993; Mythri et al., 2011). Such an imbalance may induce oxidative stress and accelerate PD progression (Hauser and Hastings, 2013).
Oxidative stress correlates with increased oxidation of macromolecules, including lipid, nucleic acid, and protein (Vallée et al., 2021a). Lipids are components of cell membranes, maintaining membrane fluidity and permeability. Hence, lipid oxidation would directly cause structural damage to cell membranes, which may lead to neuronal damage or even death (Dalle and Mabandla, 2018). Elevated oxidative damage to nucleic acids was also revealed in the PD SN (Hegde et al., 2006). The conformation and stability of DNA were altered because of oxidative stress, which could result in cell death (Guo et al., 2018). Protein oxidation is believed to be a feature of oxidative damage in the PD SN (Taylor et al., 2013). Oxidative stress caused nitration or carbonylation of proteins, which may lead to loss of function or aggregation (Maguire-Zeiss et al., 2005). The cellular systems responsible for the removal of misfolded or aggregated proteins were impaired by oxidative stress, which also contributed to the formation of protein aggregates (Hassanzadeh and Rahimmi, 2018).
Mitochondria are the main source of ROS in cells and are highly vulnerable to oxidative damage (Yuan et al., 2007). A dramatic outbreak of free radicals impaired the capability of the ETC to transfer electrons, which would result in a steady decline in mitochondrial activity and increased ROS production (Subramaniam and Chesselet, 2013). Significant elevation of ROS levels in neurons was found to be responsible for GSH leakage, mitochondrial DNA (mtDNA) mutation, and DA oxidation, which further promoted the production of free radicals (Janda et al., 2012; Yan et al., 2013; Vallée et al., 2021a, b). The damage to neurons caused by the positive feedback loop consisting of these PD elements will eventually lead to apoptosis (Trist et al., 2019).
Oxidative stress was also found to induce the formation of α-syn aggregates. Under normal physiological conditions, the α-syn existed as monomers or tetramers (Figure 2). Due to sensitivity to the excessive accumulation of ROS, the α-syn was induced to misfold by oxidative stress (Tsang and Chung, 2009). Misfolded α-syn proteins formed oligomers or fibrils and eventually insoluble aggregates (Jiang et al., 2016). The degradation of α-syn aggregates within Das is dependent mainly on the ubiquitin-proteasomal system (UPS) or chaperone-mediated autophagy (CMA). However, in a highly oxidized environment, these two pathways became ineffective in mediating the degradation of α-syn aggregates (Ganguly et al., 2017). This is because oxidative stress along with α-syn proteins subjected to oxidative modifications impaired the UPS and CMA (Jimenez-Moreno and Lane, 2020). α-syn accumulation and aggregation were found to inhibit the synthesis of ATP by mitochondria and induce microglia activation, which led to chronic effects of oxidative stress on the SN (Maguire-Zeiss et al., 2005). Hence, the interaction between oxidative stress and α-syn proteins can be regarded as a positive feedback loop that drives pathological conditions, which ultimately leads to the development of PD (Puspita et al., 2017).
PD remains an incurable neurodegenerative disease, and the etiologies of it is not completely understood (Jiang et al., 2016; Raza et al., 2019). However, since researchers have found that oxidative stress can trigger PD or accelerate its progression, there is a consensus that combating oxidative stress is a promising medicinal strategy (Janda et al., 2012). Many molecules and natural compounds exert antioxidant properties, including carvacrol, coenzyme Q10 (CoQ10), creatine, curcumin, melatonin, lipoic acid (LA), lycopene, N-acetyl-cysteine (NAC), vitamin B3, vitamin C, vitamin D3, and urate (Chen et al., 2012; Crotty et al., 2017; Ciulla et al., 2019; Figure 3). These antioxidants may serve as neuroprotective agents, while they need to be proven safe and effective in clinical trials (Henchcliffe and Beal, 2008; Hassanzadeh and Rahimmi, 2018). In addition, regular physical exercise has been identified to have a positive impact on PD via reducing oxidative stress (Fan B. et al., 2020; Robea et al., 2020).
As mentioned above, both regulatory non-coding RNAs, as well as oxidative stress, are closely associated with PD. Furthermore, oxidative stress can cause oxidative damage to RNA. In contrast, regulatory ncRNAs such as miRNAs and lncRNAs play a role in regulating ROS production (Figure 2). Their interactions have been confirmed to be involved in the pathophysiology of PD (Konovalova et al., 2019).
RNA is susceptible to oxidative stress due to its single-stranded structure and dense distribution near the mitochondria, where most intracellular ROS are generated (Nunomura et al., 2009; Liu Z. et al., 2020). Excessive amounts of ROS may lead to RNA strand breaks and chemical modification and excision of RNA bases (Song et al., 2011; Zhao et al., 2017). Due to the lack of advanced repair mechanisms, oxidatively damaged RNA would accumulate in cells, resulting in reduced protein synthesis, erroneous protein generation, and eventual cell death (Zhang et al., 1999; Nunomura et al., 2006). RNA oxidation is not only a common feature of PD but also an early event in the progression of this disease (Nunomura et al., 2007; Cervinkova et al., 2017). Among oxidative marks on RNA, 8-oxo-7, 8-dihydroguanosine (8-oxoG) might be the most abundant and most extensively studied one (Gonzalez-Rivera et al., 2020). This base adduct can be produced by the exposure of guanine to free radicals and may cause incorrect base pairing (Zhang and Li, 2020). Researchers have found that 8-OHG levels in cerebrospinal fluid (CSF) and serum are significantly higher in PD patients than in healthy controls, indicating that 8-OHG may serve as a biomarker for PD (Alam et al., 1997; Kikuchi et al., 2002; Abe et al., 2003).
Non-coding RNAs, which are not responsible for encoding proteins, make up the majority of RNAs in human cells (Moreira et al., 2008). As a category of ncRNAs, regulatory ncRNAs, including miRNAs, lncRNAs, and circRNAs, are involved in the regulation of gene expression (Kong and Lin, 2010). Compared to mRNAs, these regulatory ncRNAs live relatively longer. Hence, oxidative damage that impairs their function would have a detrimental effect on cellular homeostasis (Yan and Zaher, 2019). For example, miRNAs attacked by free radicals may fail to correctly recognize their target mRNAs, which may lead to increased expression of certain proteins (Nunomura and Perry, 2020). In the experiment conducted by Je and Kim, miR-7 and miR-153 were identified to suppress the expression of α-SYN. Their mediated translational inhibition was abolished by oxidative stress, resulting in increased α-SYN levels and subsequent development of PD (Je and Kim, 2017). Furthermore, the study of Chen et al. showed that oxidative stress induced N6-methyladenosine (m6A) modification of circRNAs. m6Amodified circRNAs influenced the expression of stress response genes, which could be a potential mechanism for oxidative stress-induced neurodegenerative diseases (Chen N. et al., 2021).
α-syn is responsible for inducing oxidative stress. Both microRNA-141-3p (miR-141-3p) and microRNA-9-5p (miR-9-5p) were found to target the 3’ UTR of SIRT1 mRNA. Since SIRT1 inhibited the formation of α-syn aggregates, knockdown of miR-141-3p and miR-9-5p may alleviate oxidative stress and boost the viability of Das (Wang Z. et al., 2019; Zheng et al., 2020). In addition, microglia are thought to have a role in the pathophysiology of PD, since cytotoxic substances released from activated microglia can exacerbate oxidative stress. miR-124 inhibited microglia activation, thereby representing a neuroprotective factor (Lushchak and Lushchak, 2021). Recently, researchers found that dysregulation of Fe2+ homeostasis may lead to the accumulation of ROS in cells. This homeostasis was maintained by ferritin heavy chain 1 (FTH1) since FTH1 converted Fe2+ ions into soluble, non-toxic Fe3+ ions. microRNA-335 (miR-335) suppressed the expression of FTH1, thereby promoting the release of Fe2+ ions and the generation of free radicals (Li et al., 2021). Furthermore, downregulation of microRNA-410 (miR-410) expression in PD was identified to be associated with elevated ROS production, although the underlying mechanism by which miR-410 exerted its neuroprotective role needs further study (Ge et al., 2019).
SOD, CAT, and GPx are responsible for detoxifying oxidants and repairing oxidative damage. Based on some research, microRNA-375 (miR-375), microRNA-218-5p (miR-218-5p), and miR-185 attenuated oxidative stress, as evidenced by elevated SOD and GPx activity in PD rats treated with these miRNAs (Cai et al., 2020; Ma et al., 2021b; Qin et al., 2021). In contrast, miR-137 and microRNA-494-3p (miR-494-3p) aggravated oxidative stress by reducing the level of SOD (Geng et al., 2018; Jiang et al., 2019). The activity of SOD was down-regulated by microRNA-155-5p (miR-155-5p; Lv et al., 2020).
Mitochondrial dysfunction, a common feature of PD, is directly related to the excessive production of ROS. This process can be inhibited by upregulated NORAD (Song et al., 2019). In addition, the formation of α-syn aggregates is capable of exacerbating oxidative stress via downregulating complex I activity or activating microglia. Li et al. found that the upregulation of lncRNA beta-amyloid cleaving enzyme-antisense (BACE1-AS) in PD wasassociatedwithrisingα-syn levels (Li Y. et al., 2020). Besides, Zhang et al. discovered that the lncRNA miR-17-92a-1 cluster host gene (MIR17HG) played a role in promoting the expression of α-syn. MIR17HG sponged microRNA-153-5p (miR-153-5p), thereby preventing miR-153-5p from downregulating α-syn expression (Zhang et al., 2022). GSK3β was revealed to promote α-syn accumulation via inhibiting autophagy. The expression of GSK3β was suppressed by miR-15b-5p, which was reversed by SNHG1 binding to miR-15b-5p (Xie N. et al., 2019).
Among the 17 newly studied lncRNAs, 13 were identified to aggravate oxidative stress and inflammatory responses in neurons, namely AL049437, HOTAIR, LINC00943, lncRNA-p21 (lnc-p21), MIAT, NEAT1, rhabdomyosarcoma 2-associate transcript (RMST), SNHG1, SNHG7, SOS1 intronic transcript 1 (SOS1-IT1), SRY-box transcription factor 2 overlapping transcript (SOX2-OT), taurine upregulated gene 1 (TUG1), and UCA1 (Cai et al., 2019; Ding et al., 2019; Zhai et al., 2020; Zhang L. et al., 2020; Zhao et al., 2020b; Guo et al., 2021; Lian et al., 2021; Ma et al., 2021a; Zhang et al., 2021; Zhou S. et al., 2021; Fan et al., 2022; Lang et al., 2022). The other four lncRNAs, namely JHDM1D antisense 1 (JHDM1D-AS1), myocardial infarction associated transcript 2 (Mirt2), small nucleolar RNA host gene 12 (SNHG12), and PART1, exhibited anti-oxidant and anti-inflammatory roles in models of PD, as evidenced by the decline in proinflammatory cytokines and increase in SOD contents (Han et al., 2019; Shen et al., 2021b; Wang C. et al., 2021; Yan et al., 2021). Nevertheless, the precise mechanism by which these 17 RNAs affect oxidative stress and thereby regulate PD progression remains unclear.
At present, we are in the early stage of investigating the cellular consequences of oxidatively damaged RNA and the mechanism by which regulatory ncRNAs affect oxidative stress (Kong et al., 2008; Xu et al., 2021). Further investigations are needed to explore the association between regulatory ncRNA oxidation and PD, which is beneficial to the development of early diagnosis and treatment for this disease (Nunomura et al., 2017).
We described the role of regulatory ncRNAs, oxidative stress, and their interactions in the regulation of PD. In recent years, the intrinsic correlation between regulatory ncRNAs and oxidative stress in PD has been increasingly studied. It is worth pointing out that some regulatory ncRNAs have been found to influence the progression of PD via regulating oxidative stress, which makes them potential therapeutic targets. However, there is fairly limited research that uncovers the precise mechanisms. In addition, because of the differences between the brains of laboratory animals and the human brain in combination with the inability of experimental models to accurately recapitulate the various features of PD, translating the results from PD models to humans may face considerable difficulties (Chia et al., 2020). There are still many hurdles to be overcome in the study of the interplay between regulatory ncRNAs and oxidative stress in PD. With the continuous innovation of experimental methods and techniques, the application of safe and effective targeted drugs for PD treatment is foreseeable.
The outline of this review was conceived by MT and XG. The draft manuscript was accomplished by HZ and polished by XL and GL. The tables were provided by JL and the figures were constructed by YL. All authors made a direct and intellectual contribution to this topic. All authors contributed to the article and approved the submitted version.
This work was financially supported by grants from the National Natural Science Foundation of China (32002235).
The authors declare that the research was conducted in the absence of any commercial or financial relationships that could be construed as a potential conflict of interest.
All claims expressed in this article are solely those of the authors and do not necessarily represent those of their affiliated organizations, or those of the publisher, the editors and the reviewers. Any product that may be evaluated in this article, or claim that may be made by its manufacturer, is not guaranteed or endorsed by the publisher.
We thank all the individuals who have helped us in this study. We acknowledge the valuable work of the many investigators whose published articles we were unable to cite owing to space limitations.
Abe, T., Isobe, C., Murata, T., Sato, C., and Tohgi, H. (2003). Alteration of 8-hydroxyguanosine concentrations in the cerebrospinal fluid and serum from patients with Parkinson’s disease. Neurosci. Lett. 336, 105–108. doi: 10.1016/s0304-3940(02)01259-4
Acharya, S., Salgado-Somoza, A., Stefanizzi, F. M., Lumley, A. I., Zhang, L., Glaab, E., et al. (2020). Non-coding RNAs in the brain-heart axis: the case of Parkinson’s disease. Int. J. Mol. Sci. 21:6513. doi: 10.3390/ijms21186513
Adusumilli, L., Facchinello, N., Teh, C., Busolin, G., Le, M. T., Yang, H., et al. (2020). miR-7 controls the dopaminergic/oligodendroglial fate through Wnt/β-catenin signaling regulation. Cells 9:711. doi: 10.3390/cells9030711
Alam, Z. I., Jenner, A., Daniel, S. E., Lees, A. J., Cairns, N., Marsden, C. D., et al. (1997). Oxidative DNA damage in the parkinsonian brain: an apparent selective increase in 8-hydroxyguanine levels in substantia nigra. J. Neurochem. 69, 1196–1203. doi: 10.1046/j.1471-4159.1997.69031196.x
Altesha, M. A., Ni, T., Khan, A., Liu, K., and Zheng, X. (2019). Circular RNA in cardiovascular disease. J. Cell Physiol. 234, 5588–5600. doi: 10.1002/jcp.27384
Antony, P. M., Diederich, N. J., Kruger, R., and Balling, R. (2013). The hallmarks of Parkinson’s disease. FEBS J. 280, 5981–5993. doi: 10.1111/febs.12335
Ba, R. Q., Liu, J., Fan, X. J., Jin, G. L., Huang, B. G., Liu, M. W., et al. (2020). Effects of miR-199a on autophagy by targeting glycogen synthase kinase 3β to activate PTEN/AKT/mTOR signaling in an MPP+ in vitro model of Parkinson’s disease. Neurol. Res. 42, 308–318. doi: 10.1080/01616412.2020.1726584
Bai, X., Zhang, X., Fang, R., Wang, J., Ma, Y., Liu, Z., et al. (2021). Deficiency of miR-29b2/c leads to accelerated aging and neuroprotection in MPTP-induced Parkinson’s disease mice. Aging (Albany NY) 13, 22390–22411. doi: 10.18632/aging.203545
Bhattacharyya, N., Pandey, V., Bhattacharyya, M., and Dey, A. (2021). Regulatory role of long non coding RNAs (lncRNAs) in neurological disorders: from novel biomarkers to promising therapeutic strategies. Asian J. Pharm. Sci. 16, 533–550. doi: 10.1016/j.ajps.2021.02.006
Bloem, B. R., Okun, M. S., and Klein, C. (2021). Parkinson’s disease. Lancet 397, 2284–2303. doi: 10.1016/S0140-6736(21)00218-X
Brand, M. D., and Nicholls, D. G. (2011). Assessing mitochondrial dysfunction in cells. Biochem. J. 435, 297–312. doi: 10.1042/BJ20110162
Brieger, K., Schiavone, S., Miller, F. J., Jr., and Krause, K. H. (2012). Reactive oxygen species: from health to disease. Swiss Med. Wkly 142:w13659. doi: 10.4414/smw.2012.13659
Cai, L., Tu, L., Li, T., Yang, X., Ren, Y., Gu, R., et al. (2019). Downregulation of lncRNA UCA1 ameliorates the damage of dopaminergic neurons, reduces oxidative stress and inflammation in Parkinson’s disease through the inhibition of the PI3K/Akt signaling pathway. Int. Immunopharmacol. 75:105734. doi: 10.1016/j.intimp.2019.105734
Cai, L. J., Tu, L., Li, T., Yang, X. L., Ren, Y. P., Gu, R., et al. (2020). Up-regulation of microRNA-375 ameliorates the damage of dopaminergic neurons, reduces oxidative stress and inflammation in Parkinson’s disease by inhibiting SP1. Aging (Albany NY) 12, 672–689. doi: 10.18632/aging.102649
Cao, Q., Guo, Z., Yan, Y., Wu, J., and Song, C. (2019). Exosomal long noncoding RNAs in aging and age-related diseases. IUBMB Life 71, 1846–1856. doi: 10.1002/iub.2141
Cao, H., Han, X., Jia, Y., and Zhang, B. (2021). Inhibition of long non-coding RNA HOXA11-AS against neuroinflammation in Parkinson’s disease model via targeting miR-124-3p mediated FSTL1/NF-κB axis. Aging (Albany NY) 13, 11455–11469. doi: 10.18632/aging.202837
Cao, L., Wang, M., Dong, Y., Xu, B., Chen, J., Ding, Y., et al. (2020). Circular RNA circRNF20 promotes breast cancer tumorigenesis and Warburg effect through miR-487a/HIF-1α/HK2. Cell Death Dis. 11:145. doi: 10.1038/s41419-020-2336-0
Cao, B., Wang, T., Qu, Q., Kang, T., and Yang, Q. (2018). Long noncoding RNA SNHG1 promotes neuroinflammation in Parkinson’s disease via regulating miR-7/NLRP3 pathway. Neuroscience 388, 118–127. doi: 10.1016/j.neuroscience.2018.07.019
Carvalho Barbosa, C., Calhoun, S. H., and Wieden, H. J. (2020). Non-coding RNAs: what are we missing. Biochem. Cell Biol. 98, 23–30. doi: 10.1139/bcb-2019-0037
Cerri, S., Mus, L., and Blandini, F. (2019). Parkinson’s disease in women and men: what’s the difference. J. Parkinsons Dis. 9, 501–515. doi: 10.3233/JPD-191683
Cervinkova, B., Krcmova, L. K., Sestakova, V., Solichova, D., and Solich, P. (2017). A fully validated bioanalytical method using an UHPLC-MS/MS system for quantification of DNA and RNA oxidative stress biomarkers. Anal. Bioanal. Chem. 409, 3611–3621. doi: 10.1007/s00216-017-0301-2
Chen, M. Y., Fan, K., Zhao, L. J., Wei, J. M., Gao, J. X., and Li, Z. F. (2021). Long non-coding RNA nuclear enriched abundant transcript 1 (NEAT1) sponges microRNA-124-3p to up-regulate phosphodiesterase 4B (PDE4B) to accelerate the progression of Parkinson’s disease. Bioengineered 12, 708–719. doi: 10.1080/21655979.2021.1883279
Chen, Q., Huang, X., and Li, R. (2018). lncRNA MALAT1/miR-205-5p axis regulates MPP+-induced cell apoptosis in MN9D cells by directly targeting LRRK2. Am. J. Transl. Res. 10, 563–572.
Chen, Y., Lian, Y. J., Ma, Y. Q., Wu, C. J., Zheng, Y. K., and Xie, N. C. (2018). LncRNA SNHG1 promotes alpha-synuclein aggregation and toxicity by targeting miR-15b-5p to activate SIAH1 in human neuroblastoma SH-SY5Y cells. Neurotoxicology 68, 212–221. doi: 10.1016/j.neuro.2017.12.001
Chen, N., Tang, J., Su, Q., Chou, W. C., Zheng, F., Guo, Z., et al. (2021). Paraquat-induced oxidative stress regulates N6-methyladenosine (m(6)A) modification of circular RNAs. Environ. Pollut. 290:117816. doi: 10.1016/j.envpol.2021.117816
Chen, X., Wu, G., and Schwarzschild, M. A. (2012). Urate in Parkinson’s disease: more than a biomarker. Curr. Neurol. Neurosci. Rep. 12, 367–375. doi: 10.1007/s11910-012-0282-7
Chen, Z., and Zhong, C. (2014). Oxidative stress in Alzheimer’s disease. Neurosci. Bull. 30, 271–281. doi: 10.1007/s12264-013-1423-y
Chia, S. J., Tan, E. K., and Chao, Y. X. (2020). Historical perspective: models of Parkinson’s disease. Int. J. Mol. Sci. 21:2464. doi: 10.3390/ijms21072464
Choi, D. C., Yoo, M., Kabaria, S., and Junn, E. (2018). MicroRNA-7 facilitates the degradation of alpha-synuclein and its aggregates by promoting autophagy. Neurosci. Lett. 678, 118–123. doi: 10.1016/j.neulet.2018.05.009
Ciulla, M., Marinelli, L., Cacciatore, I., and Stefano, A. D. (2019). Role of dietary supplements in the management of Parkinson’s disease. Biomolecules 9:271. doi: 10.3390/biom9070271
Crotty, G. F., Ascherio, A., and Schwarzschild, M. A. (2017). Targeting urate to reduce oxidative stress in Parkinson disease. Exp. Neurol. 298, 210–224. doi: 10.1016/j.expneurol.2017.06.017
Dalle, E., and Mabandla, M. V. (2018). Early life stress, depression and Parkinson’s disease: a new approach. Mol. Brain 11:18. doi: 10.1186/s13041-018-0356-9
Damier, P., Hirsch, E. C., Zhang, P., Agid, Y., and Javoy-Agid, F. (1993). Glutathione peroxidase, glial cells and Parkinson’s disease. Neuroscience 52, 1–6. doi: 10.1016/0306-4522(93)90175-f
Ding, X. M., Zhao, L. J., Qiao, H. Y., Wu, S. L., and Wang, X. H. (2019). Long non-coding RNA-p21 regulates MPP+-induced neuronal injury by targeting miR-625 and derepressing TRPM2 in SH-SY5Y cells. Chem. Biol. Interact. 307, 73–81. doi: 10.1016/j.cbi.2019.04.017
Dong, L. G., Lu, F. F., Zu, J., Zhang, W., Xu, C. Y., Jin, G. L., et al. (2020). MiR-133b inhibits MPP+-induced apoptosis in Parkinson’s disease model by inhibiting the ERK1/2 signaling pathway. Eur. Rev. Med. Pharmacol. Sci. 24, 11192–11198. doi: 10.26355/eurrev_202011_23607
Dong, Y., Xiong, J., Ji, L., and Xue, X. (2021). MiR-421 aggravates neurotoxicity and promotes cell death in Parkinson’s disease models by directly targeting MEF2D. Neurochem. Res. 46, 299–308. doi: 10.1007/s11064-020-03166-0
Drechsel, D. A., and Patel, M. (2008). Role of reactive oxygen species in the neurotoxicity of environmental agents implicated in Parkinson’s disease. Free Radic. Biol. Med. 44, 1873–1886. doi: 10.1016/j.freeradbiomed.2008.02.008
Dunn, A. R., O’connell, K. M. S., and Kaczorowski, C. C. (2019). Gene-by-environment interactions in Alzheimer’s disease and Parkinson’s disease. Neurosci. Biobehav. Rev. 103, 73–80. doi: 10.1016/j.neubiorev.2019.06.018
Fan, B., Jabeen, R., Bo, B., Guo, C., Han, M., Zhang, H., et al. (2020). What and how can physical activity prevention function on Parkinson’s disease. Oxid. Med. Cell Longev. 2020:4293071. doi: 10.1155/2020/4293071
Fan, J., Wu, D., Guo, Y., and Yang, Z. (2022). SOS1-IT1 silencing alleviates MPP+-induced neuronal cell injury through regulating the miR-124-3p/PTEN/AKT/mTOR pathway. J. Clin. Neurosci. 99, 137–146. doi: 10.1016/j.jocn.2022.01.003
Fan, Y., Zhao, X., Lu, K., and Cheng, G. (2020). LncRNA BDNF-AS promotes autophagy and apoptosis in MPTP-induced Parkinson’s disease via ablating microRNA-125b-5p. Brain Res. Bull. 157, 119–127. doi: 10.1016/j.brainresbull.2020.02.003
Feng, Y., Li, T., Xing, C., Wang, C., Duan, Y., Yuan, L., et al. (2021). Effective inhibition of miR-330/SHIP1/NF-κB signaling pathway via miR-330 sponge repolarizes microglia differentiation. Cell Biol. Int. 45, 785–794. doi: 10.1002/cbin.11523
Feng, Z., Zhang, L., Wang, S., and Hong, Q. (2020). Circular RNA circDLGAP4 exerts neuroprotective effects via modulating miR-134-5p/CREB pathway in Parkinson’s disease. Biochem. Biophys. Res. Commun. 522, 388–394. doi: 10.1016/j.bbrc.2019.11.102
Fields, C. R., Bengoa-Vergniory, N., and Wade-Martins, R. (2019). Targeting alpha-synuclein as a therapy for Parkinson’s disease. Front. Mol. Neurosci. 12:299. doi: 10.1016/j.bbrc.2019.11.102
Foley, P., and Riederer, P. (2000). Influence of neurotoxins and oxidative stress on the onset and progression of Parkinson’s disease. J. Neurol. 247, II82–II94. doi: 10.1007/pl00007766
Forman, H. J., and Zhang, H. (2021). Targeting oxidative stress in disease: promise and limitations of antioxidant therapy. Nat. Rev. Drug Discov. 20, 689–709. doi: 10.1038/s41573-021-00233-1
Ganguly, G., Chakrabarti, S., Chatterjee, U., and Saso, L. (2017). Proteinopathy, oxidative stress and mitochondrial dysfunction: cross talk in Alzheimer’s disease and Parkinson’s disease. Drug Des. Dev. Ther. 11, 797–810. doi: 10.2147/DDDT.S130514
Gao, J. X., Li, Y., Wang, S. N., Chen, X. C., Lin, L. L., and Zhang, H. (2019). Overexpression of microRNA-183 promotes apoptosis of substantia nigra neurons via the inhibition of OSMR in a mouse model of Parkinson’s disease. Int. J. Mol. Med. 43, 209–220. doi: 10.3892/ijmm.2018.3982
Ge, H., Yan, Z., Zhu, H., and Zhao, H. (2019). MiR-410 exerts neuroprotective effects in a cellular model of Parkinson’s disease induced by 6-hydroxydopamine via inhibiting the PTEN/AKT/mTOR signaling pathway. Exp. Mol. Pathol. 109, 16–24. doi: 10.1016/j.yexmp.2019.05.002
Geng, L., Liu, W., and Chen, Y. (2017). miR-124-3p attenuates MPP+-induced neuronal injury by targeting STAT3 in SH-SY5Y cells. Exp. Biol. Med. (Maywood) 242, 1757–1764. doi: 10.1177/1535370217734492
Geng, L., Zhang, T., Liu, W., and Chen, Y. (2018). miR-494-3p modulates the progression of in vitro and in vivo Parkinson’s disease models by targeting SIRT3. Neurosci. Lett. 675, 23–30. doi: 10.1128/JVI.00955-15
Gerin, I., Bommer, G. T., Mccoin, C. S., Sousa, K. M., Krishnan, V., and Macdougald, O. A. (2010). Roles for miRNA-378/378* in adipocyte gene expression and lipogenesis. Am. J. Physiol. Endocrinol. Metab. 299, E198–E206. doi: 10.1152/ajpendo.00179.2010
Goh, S. Y., Chao, Y. X., Dheen, S. T., Tan, E. K., and Tay, S. S. (2019). Role of MicroRNAs in Parkinson’s disease. Int. J. Mol. Sci. 20:5649. doi: 10.3390/ijms20225649
Gong, X., Huang, M., and Chen, L. (2022). Mechanism of miR-132-3p promoting neuroinflammation and dopaminergic neurodegeneration in Parkinson’s disease. eNeuro 9:ENEURO.0393-21.2021. doi: 10.1101/lm.039578.115
Gonzalez-Rivera, J. C., Sherman, M. W., Wang, D. S., Chuvalo-Abraham, J. C. L., Hildebrandt Ruiz, L., and Contreras, L. M. (2020). RNA oxidation in chromatin modification and DNA-damage response following exposure to formaldehyde. Sci. Rep. 10:16545. doi: 10.1038/s41598-020-73376-7
Gordon, R., Albornoz, E. A., Christie, D. C., Langley, M. R., Kumar, V., Mantovani, S., et al. (2018). Inflammasome inhibition prevents α-synuclein pathology and dopaminergic neurodegeneration in mice. Sci. Transl. Med. 10:eaah4066. doi: 10.1126/scitranslmed.aah4066
Guo, Y., Liu, Y., Wang, H., and Liu, P. (2021). Long noncoding RNA SRY-box transcription factor 2 overlapping transcript participates in Parkinson’s disease by regulating the microRNA-942-5p/nuclear apoptosis-inducing factor 1 axis. Bioengineered 12, 8570–8582. doi: 10.1080/21655979.2021.1987126
Guo, J. D., Zhao, X., Li, Y., Li, G. R., and Liu, X. L. (2018). Damage to dopaminergic neurons by oxidative stress in Parkinson’s disease (Review). Int. J. Mol. Med. 41, 1817–1825. doi: 10.3892/ijmm.2018.3406
Hald, A., and Lotharius, J. (2005). Oxidative stress and inflammation in Parkinson’s disease: is there a causal link. Exp. Neurol. 193, 279–290. doi: 10.1016/j.expneurol.2005.01.013
Han, Y., Kang, C., Kang, M., Quan, W., Gao, H., and Zhong, Z. (2019). Long non-coding RNA Mirt2 prevents TNF-alpha-triggered inflammation via the repression of microRNA-101. Int. Immunopharmacol. 76:105878. doi: 10.1016/j.intimp.2019.105878
Hassanzadeh, K., and Rahimmi, A. (2018). Oxidative stress and neuroinflammation in the story of Parkinson’s disease: could targeting these pathways write a good ending. J. Cell Physiol. 234, 23–32. doi: 10.1002/jcp.26865
Hauser, D. N., and Hastings, T. G. (2013). Mitochondrial dysfunction and oxidative stress in Parkinson’s disease and monogenic parkinsonism. Neurobiol. Dis. 51, 35–42. doi: 10.1016/j.nbd.2012.10.011
Hayes, M. T. (2019). Parkinson’s disease and Parkinsonism. Am. J. Med. 132, 802–807. doi: 10.1016/j.amjmed.2019.03.001
He, Q., Wang, Q., Yuan, C., and Wang, Y. (2017). Downregulation of miR-7116-5p in microglia by MPP+ sensitizes TNF-alpha production to induce dopaminergic neuron damage. Glia 65, 1251–1263. doi: 10.1002/glia.23153
Hegde, M. L., Gupta, V. B., Anitha, M., Harikrishna, T., Shankar, S. K., Muthane, U., et al. (2006). Studies on genomic DNA topology and stability in brain regions of Parkinson’s disease. Arch. Biochem. Biophys. 449, 143–156. doi: 10.1016/j.abb.2006.02.018
Hemmati-Dinarvand, M., Saedi, S., Valilo, M., Kalantary-Charvadeh, A., Alizadeh Sani, M., Kargar, R., et al. (2019). Oxidative stress and Parkinson’s disease: conflict of oxidant-antioxidant systems. Neurosci. Lett. 709:134296. doi: 10.1016/j.neulet.2019.134296
Henchcliffe, C., and Beal, M. F. (2008). Mitochondrial biology and oxidative stress in Parkinson disease pathogenesis. Nat. Clin. Pract. Neurol. 4, 600–609. doi: 10.1038/ncpneuro0924
Hombach, S., and Kretz, M. (2016). Non-coding RNAs: classification, biology and functioning. Adv. Exp. Med. Biol. 937, 3–17. doi: 10.1007/978-3-319-42059-2_1
Huang, A., Zheng, H., Wu, Z., Chen, M., and Huang, Y. (2020). Circular RNA-protein interactions: functions, mechanisms and identification. Theranostics 10, 3503–3517. doi: 10.7150/thno.42174
Iovino, L., Tremblay, M. E., and Civiero, L. (2020). Glutamate-induced excitotoxicity in Parkinson’s disease: the role of glial cells. J. Pharmacol. Sci. 144, 151–164. doi: 10.1016/j.jphs.2020.07.011
Islam, M. T. (2017). Oxidative stress and mitochondrial dysfunction-linked neurodegenerative disorders. Neurol. Res. 39, 73–82. doi: 10.1080/01616412.2016.1251711
Janda, E., Isidoro, C., Carresi, C., and Mollace, V. (2012). Defective autophagy in Parkinson’s disease: role of oxidative stress. Mol. Neurobiol. 46, 639–661. doi: 10.1007/s12035-012-8318-1
Je, G., and Kim, Y. S. (2017). Mitochondrial ROS-mediated post-transcriptional regulation of alpha-synuclein through miR-7 and miR-153. Neurosci. Lett. 661, 132–136. doi: 10.1016/j.neulet.2017.09.065
Jiang, Y., Liu, J., Chen, L., Jin, Y., Zhang, G., Lin, Z., et al. (2019). Serum secreted miR-137-containing exosomes affects oxidative stress of neurons by regulating OXR1 in Parkinson’s disease. Brain Res. 1722:146331. doi: 10.1016/j.brainres.2019.146331
Jiang, Q., Liu, C., Li, C. P., Xu, S. S., Yao, M. D., Ge, H. M., et al. (2020). Circular RNA-ZNF532 regulates diabetes-induced retinal pericyte degeneration and vascular dysfunction. J. Clin. Invest. 130, 3833–3847. doi: 10.1172/JCI123353
Jiang, J., Piao, X., Hu, S., Gao, J., and Bao, M. (2020). LncRNA H19 diminishes dopaminergic neuron loss by mediating microRNA-301b-3p in Parkinson’s disease via the HPRT1-mediated Wnt/β-catenin signaling pathway. Aging (Albany NY) 12, 8820–8836. doi: 10.18632/aging.102877
Jiang, T., Sun, Q., and Chen, S. (2016). Oxidative stress: a major pathogenesis and potential therapeutic target of antioxidative agents in Parkinson’s disease and Alzheimer’s disease. Prog. Neurobiol. 147, 1–19. doi: 10.1016/j.pneurobio.2016.07.005
Jimenez-Moreno, N., and Lane, J. D. (2020). Autophagy and redox homeostasis in Parkinson’s: a crucial balancing act. Oxid. Med. Cell Longev. 2020:8865611. doi: 10.1155/2020/8865611
Jin, H., Kanthasamy, A., Ghosh, A., Anantharam, V., Kalyanaraman, B., and Kanthasamy, A. G. (2014). Mitochondria-targeted antioxidants for treatment of Parkinson’s disease: preclinical and clinical outcomes. Biochim. Biophys. Acta 1842, 1282–1294. doi: 10.1016/j.bbadis.2013.09.007
Jin, J., Sun, H., Shi, C., Yang, H., Wu, Y., Li, W., et al. (2020). Circular RNA in renal diseases. J. Cell Mol. Med. 24, 6523–6533. doi: 10.1111/jcmm.15295
John, A., Kubosumi, A., and Reddy, P. H. (2020). Mitochondrial MicroRNAs in aging and neurodegenerative diseases. Cells 9:1345. doi: 10.3390/cells9061345
Kikuchi, A., Takeda, A., Onodera, H., Kimpara, T., Hisanaga, K., Sato, N., et al. (2002). Systemic increase of oxidative nucleic acid damage in Parkinson’s disease and multiple system atrophy. Neurobiol. Dis. 9, 244–248. doi: 10.1006/nbdi.2002.0466
Kong, Q., and Lin, C. L. (2010). Oxidative damage to RNA: mechanisms, consequences and diseases. Cell Mol. Life Sci. 67, 1817–1829. doi: 10.1007/s00018-010-0277-y
Kong, Q., Shan, X., Chang, Y., Tashiro, H., and Lin, C. L. (2008). RNA oxidation: a contributing factor or an epiphenomenon in the process of neurodegeneration. Free Radic Res. 42, 773–777. doi: 10.1080/10715760802311187
Konovalova, J., Gerasymchuk, D., Parkkinen, I., Chmielarz, P., and Domanskyi, A. (2019). Interplay between MicroRNAs and oxidative stress in neurodegenerative diseases. Int. J. Mol. Sci. 20:6055. doi: 10.3390/ijms20236055
Koppula, S., Kumar, H., Kim, I. S., and Choi, D. K. (2012). Reactive oxygen species and inhibitors of inflammatory enzymes, NADPH oxidase and iNOS in experimental models of Parkinson’s disease. Mediators Inflamm. 2012:823902. doi: 10.1155/2012/823902
Lang, Y., Zhang, H., Yu, H., Li, Y., Liu, X., and Li, M. (2022). Long non-coding RNA myocardial infarction-associated transcript promotes 1-Methyl-4-phenylpyridinium ion-induced neuronal inflammation and oxidative stress in Parkinson’s disease through regulating microRNA-221-3p/ transforming growth factor /nuclear factor E2-related factor 2 axis. Bioengineered 13, 930–940. doi: 10.1080/21655979.2021.2015527
Lee, S., Kopp, F., Chang, T. C., Sataluri, A., Chen, B., Sivakumar, S., et al. (2016). Noncoding RNA NORAD regulates genomic stability by sequestering PUMILIO proteins. Cell 164, 69–80. doi: 10.1016/j.cell.2015.12.017
Li, Y., Fang, J., Zhou, Z., Zhou, Q., Sun, S., Jin, Z., et al. (2020). Downregulation of lncRNA BACE1-AS improves dopamine-dependent oxidative stress in rats with Parkinson’s disease by upregulating microRNA-34b-5p and downregulating BACE1. Cell Cycle 19, 1158–1171. doi: 10.1080/15384101.2020.1749447
Li, L., Liu, H., Song, H., Qin, Y., Wang, Y., Xu, M., et al. (2017). Let-7d microRNA attenuates 6-OHDA-induced injury by targeting Caspase-3 in MN9D cells. J. Mol. Neurosci. 63, 403–411. doi: 10.1007/s12031-017-0994-x
Li, W., Jiang, Y., Wang, Y., Yang, S., Bi, X., Pan, X., et al. (2018). MiR-181b regulates autophagy in a model of Parkinson’s disease by targeting the PTEN/Akt/mTOR signaling pathway. Neurosci. Lett. 675, 83–88. doi: 10.1016/j.neulet.2018.03.041
Li, W., Kui, L., Demetrios, T., Gong, X., and Tang, M. (2020). A glimmer of hope: maintain mitochondrial homeostasis to mitigate Alzheimer’s disease. Aging Dis. 11, 1260–1275. doi: 10.14336/AD.2020.0105
Li, D., Mastaglia, F. L., Fletcher, S., and Wilton, S. D. (2020). Progress in the molecular pathogenesis and nucleic acid therapeutics for Parkinson’s disease in the precision medicine era. Med. Res. Rev. 40, 2650–2681. doi: 10.1002/med.21718
Li, X., Si, W., Li, Z., Tian, Y., Liu, X., Ye, S., et al. (2021). miR-335 promotes ferroptosis by targeting ferritin heavy chain 1 in in vivo and in vitro models of Parkinson’s disease. Int. J. Mol. Med. 47:61. doi: 10.3892/ijmm.2021.4894
Li, H., Xu, J. D., Fang, X. H., Zhu, J. N., Yang, J., Pan, R., et al. (2020). Circular RNA circRNA_000203 aggravates cardiac hypertrophy via suppressing miR-26b-5p and miR-140-3p binding to Gata4. Cardiovasc. Res. 116, 1323–1334. doi: 10.1093/cvr/cvz215
Li, L., Xu, J., Wu, M., and Hu, J. M. (2018). Protective role of microRNA-221 in Parkinson’s disease. Bratisl Lek Listy 119, 22–27. doi: 10.4149/BLL_2018_005
Li, D., Yang, H., Ma, J., Luo, S., Chen, S., and Gu, Q. (2018). MicroRNA-30e regulates neuroinflammation in MPTP model of Parkinson’s disease by targeting Nlrp3. Hum. Cell 31, 106-115. doi: 10.1007/s13577-017-0187-5
Li, J., Zhang, Y., Li, D., Liu, Y., Chu, D., Jiang, X., et al. (2015). Small non-coding RNAs transfer through mammalian placenta and directly regulate fetal gene expression. Protein Cell 6, 391–396. doi: 10.1007/s13238-015-0156-2
Lian, H., Wang, B., Lu, Q., Chen, B., and Yang, H. (2021). LINC00943 knockdown exerts neuroprotective effects in Parkinson’s disease through regulates CXCL12 expression by sponging miR-7-5p. Genes Genomics 43, 797–805. doi: 10.1007/s13258-021-01084-1
Lin, Q., Hou, S., Dai, Y., Jiang, N., and Lin, Y. (2019). LncRNA HOTAIR targets miR-126-5p to promote the progression of Parkinson’s disease through RAB3IP. Biol. Chem 400, 1217–1228. doi: 10.1515/hsz-2018-0431
Liu, Z., Chen, X., Li, Z., Ye, W., Ding, H., Li, P., et al. (2020). Role of RNA oxidation in neurodegenerative diseases. Int. J. Mol. Sci. 21:5022. doi: 10.3390/ijms21145022
Liu, R., Li, F., and Zhao, W. (2020). Long noncoding RNA NEAT1 knockdown inhibits MPP+-induced apoptosis, inflammation and cytotoxicity in SK-N-SH cells by regulating miR-212-5p/RAB3IP axis. Neurosci. Lett. 731:135060. doi: 10.1016/j.neulet.2020.135060
Liu, J., Liu, W., and Yang, H. (2019). Balancing apoptosis and autophagy for Parkinson’s disease therapy: targeting BCL-2. ACS Chem. Neurosci. 10, 792–802. doi: 10.1021/acschemneuro.8b00356
Liu, J., Liu, D., Zhao, B., Jia, C., Lv, Y., Liao, J., et al. (2020). Long non-coding RNA NEAT1 mediates MPTP/MPP+-induced apoptosis via regulating the miR-124/KLF4 axis in Parkinson’s disease. Open Life Sci. 15, 665–676. doi: 10.1515/biol-2020-0069
Liu, Y., and Lu, Z. (2018). Long non-coding RNA NEAT1 mediates the toxic of Parkinson’s disease induced by MPTP/MPP+ via regulation of gene expression. Clin. Exp. Pharmacol. Physiol. 45, 841–848. doi: 10.1111/1440-1681.12932
Liu, N., Wang, Z. Z., Zhao, M., Zhang, Y., and Chen, N. H. (2020). Role of non-coding RNA in the pathogenesis of depression. Gene 735:144276. doi: 10.1016/j.gene.2019.144276
Liu, T., Zhang, Y., Liu, W., and Zhao, J. (2021). LncRNA NEAT1 regulates the development of Parkinson’s disease by targeting AXIN1 via sponging miR-212-3p. Neurochem. Res. 46, 230–240. doi: 10.1007/s11064-020-03157-1
Liu, W., Zhang, Q., Zhang, J., Pan, W., Zhao, J., and Xu, Y. (2017). Long non-coding RNA MALAT1 contributes to cell apoptosis by sponging miR-124 in Parkinson disease. Cell Biosci. 7:19. doi: 10.1186/s13578-017-0147-5
Liu, Y., Song, Y., and Zhu, X. (2017). MicroRNA-181a regulates apoptosis and autophagy process in Parkinson’s disease by inhibiting p38 mitogen-activated protein kinase (MAPK)/c-Jun N-Terminal kinases (JNK) signaling pathways. Med. Sci. Monit. 23, 1597–1606. doi: 10.12659/msm.900218
Lu, W., Lin, J., Zheng, D., Hong, C., Ke, L., Wu, X., et al. (2020). Overexpression of MicroRNA-133a inhibits apoptosis and autophagy in a cell model of Parkinson’s disease by downregulating ras-related C3 botulinum toxin substrate 1 (RAC1). Med. Sci. Monit. 26:e922032. doi: 10.12659/MSM.922032
Lu, M., Sun, W. L., Shen, J., Wei, M., Chen, B., Qi, Y. J., et al. (2018). LncRNA-UCA1 promotes PD development by upregulating SNCA. Eur. Rev. Med. Pharmacol. Sci. 22, 7908–7915. doi: 10.26355/eurrev_201811_16417
Lu, J., Wu, M., and Yue, Z. (2020). Autophagy and Parkinson’s disease. Adv. Exp. Med. Biol. 1207, 21–51. doi: 10.1007/978-981-15-4272-5_2
Lu, J., Xu, Y., Quan, Z., Chen, Z., Sun, Z., and Qing, H. (2017). Dysregulated microRNAs in neural system: implication in pathogenesis and biomarker development in Parkinson’s disease. Neuroscience 365, 70–82. doi: 10.1016/j.neuroscience.2017.09.033
Lu, S., Yang, X., Wang, C., Chen, S., Lu, S., Yan, W., et al. (2019). Current status and potential role of circular RNAs in neurological disorders. J. Neurochem. 150, 237–248. doi: 10.1111/jnc.14724
Lushchak, V. I., and Lushchak, O. (2021). Interplay between reactive oxygen and nitrogen species in living organisms. Chem. Biol. Interact. 349:109680. doi: 10.1016/j.cbi.2021.109680
Lv, R., Du, L., Zhou, F., Yuan, X., Liu, X., and Zhang, L. (2020). Rosmarinic acid alleviates inflammation, apoptosis and oxidative stress through regulating miR-155-5p in a mice model of Parkinson’s disease. ACS Chem. Neurosci. 11, 3259–3266. doi: 10.1021/acschemneuro.0c00375
Lv, K., Liu, Y., Zheng, Y., Dai, S., Yin, P., and Miao, H. (2021). Long non-coding RNA MALAT1 regulates cell proliferation and apoptosis via miR-135b-5p/GPNMB axis in Parkinson’s disease cell model. Biol. Res. 54:10. doi: 10.1186/s40659-021-00332-8
Ma, J., Sun, W., Chen, S., Wang, Z., Zheng, J., Shi, X., et al. (2022). The long noncoding RNA GAS5 potentiates neuronal injury in Parkinson’s disease by binding to microRNA-150 to regulate Fosl1 expression. Exp. Neurol. 347:113904. doi: 10.1016/j.expneurol.2021.113904
Ma, X., Wang, Y., Yin, H., Hua, L., Zhang, X., Xiao, J., et al. (2021a). Down-regulated long non-coding RNA RMST ameliorates dopaminergic neuron damage in Parkinson’s disease rats via regulation of TLR/NF-kappaB signaling pathway. Brain Res. Bull. 174, 22–30. doi: 10.1016/j.brainresbull.2021.04.026
Ma, X., Zhang, H., Yin, H., Geng, S., Liu, Y., Liu, C., et al. (2021b). Up-regulated microRNA-218-5p ameliorates the damage of dopaminergic neurons in rats with Parkinson’s disease via suppression of LASP1. Brain Res. Bull. 166, 92–101. doi: 10.1016/j.brainresbull.2020.10.019
Maguire-Zeiss, K. A., Short, D. W., and Federoff, H. J. (2005). Synuclein, dopamine and oxidative stress: co-conspirators in Parkinson’s disease. Brain Res. Mol. Brain Res. 134, 18–23. doi: 10.1016/j.molbrainres.2004.09.014
Majidinia, M., Mihanfar, A., Rahbarghazi, R., Nourazarian, A., Bagca, B., and Avci, C. B. (2016). The roles of non-coding RNAs in Parkinson’s disease. Mol. Biol. Rep. 43, 1193–1204. doi: 10.1007/s11033-016-4054-3
Manoharan, S., Guillemin, G. J., Abiramasundari, R. S., Essa, M. M., Akbar, M., and Akbar, M. D. (2016). The role of reactive oxygen species in the pathogenesis of Alzheimer’s disease, Parkinson’s disease and Huntington’s disease: a mini review. Oxid. Med. Cell Longev. 2016:8590578. doi: 10.1155/2016/8590578
Martire, S., Mosca, L., and D’erme, M. (2015). PARP-1 involvement in neurodegeneration: a focus on Alzheimer’s and Parkinson’s diseases. Mech. Ageing Dev. 146–148, 53–64. doi: 10.1016/j.mad.2015.04.001
McMillan, K. J., Murray, T. K., Bengoa-Vergniory, N., Cordero-Llana, O., Cooper, J., Buckley, A., et al. (2017). Loss of MicroRNA-7 regulation leads to alpha-synuclein accumulation and dopaminergic neuronal loss in vivo. Mol. Ther. 25, 2404–2414. doi: 10.1016/j.ymthe.2017.08.017
Meng, C., Gao, J., Ma, Q., Sun, Q., and Qiao, T. (2021). LINC00943 knockdown attenuates MPP+-induced neuronal damage via miR-15b-5p/RAB3IP axis in SK-N-SH cells. Neurol. Res. 43, 181–190. doi: 10.1080/01616412.2020.1834290
Meng, X., Zhong, J., Zeng, C., Yung, K. K. L., Zhang, X., Wu, X., et al. (2021). MiR-30a-5p regulates GLT-1 function via a PKCα-mediated ubiquitin degradation pathway in a mouse model of Parkinson’s disease. ACS Chem. Neurosci. 12, 1578–1592. doi: 10.1021/acschemneuro.1c00076
Merry, C. R., Forrest, M. E., Sabers, J. N., Beard, L., Gao, X. H., Hatzoglou, M., et al. (2015). DNMT1-associated long non-coding RNAs regulate global gene expression and DNA methylation in colon cancer. Hum. Mol. Genet. 24, 6240–6253. doi: 10.1093/hmg/ddv343
Moreira, P. I., Nunomura, A., Nakamura, M., Takeda, A., Shenk, J. C., Aliev, G., et al. (2008). Nucleic acid oxidation in Alzheimer disease. Free Radic. Biol. Med. 44, 1493–1505. doi: 10.1016/j.freeradbiomed.2008.01.002
Munschauer, M., Nguyen, C. T., Sirokman, K., Hartigan, C. R., Hogstrom, L., Engreitz, J. M., et al. (2018). The NORAD lncRNA assembles a topoisomerase complex critical for genome stability. Nature 561, 132–136. doi: 10.1038/s41586-018-0453-z
Mythri, R. B., Venkateshappa, C., Harish, G., Mahadevan, A., Muthane, U. B., Yasha, T. C., et al. (2011). Evaluation of markers of oxidative stress, antioxidant function and astrocytic proliferation in the striatum and frontal cortex of Parkinson’s disease brains. Neurochem. Res. 36, 1452–1463. doi: 10.1007/s11064-011-0471-9
Nies, Y. H., Mohamad Najib, N. H., Lim, W. L., Kamaruzzaman, M. A., Yahaya, M. F., and Teoh, S. L. (2021). MicroRNA dysregulation in Parkinson’s disease: a narrative review. Front. Neurosci. 15:660379. doi: 10.3389/fnins.2021.660379
Nunomura, A., Hofer, T., Moreira, P. I., Castellani, R. J., Smith, M. A., and Perry, G. (2009). RNA oxidation in Alzheimer disease and related neurodegenerative disorders. Acta Neuropathol. 118, 151–166. doi: 10.1007/s00401-009-0508-1
Nunomura, A., Honda, K., Takeda, A., Hirai, K., Zhu, X., Smith, M. A., et al. (2006). Oxidative damage to RNA in neurodegenerative diseases. J. Biomed. Biotechnol. 2006:82323. doi: 10.1155/JBB/2006/82323
Nunomura, A., Lee, H. G., Zhu, X., and Perry, G. (2017). Consequences of RNA oxidation on protein synthesis rate and fidelity: implications for the pathophysiology of neuropsychiatric disorders. Biochem. Soc. Trans. 45, 1053–1066. doi: 10.1042/BST20160433
Nunomura, A., Moreira, P. I., Castellani, R. J., Lee, H. G., Zhu, X., Smith, M. A., et al. (2012). Oxidative damage to RNA in aging and neurodegenerative disorders. Neurotox. Res. 22, 231–248. doi: 10.1007/s12640-012-9331-x
Nunomura, A., Moreira, P. I., Takeda, A., Smith, M. A., and Perry, G. (2007). Oxidative RNA damage and neurodegeneration. Curr. Med. Chem. 14, 2968–2975. doi: 10.2174/092986707782794078
Nunomura, A., and Perry, G. (2020). RNA and oxidative stress in Alzheimer’s disease: focus on microRNAs. Oxid. Med. Cell Longev. 2020:2638130. doi: 10.1155/2020/2638130
Onyango, I. G. (2008). Mitochondrial dysfunction and oxidative stress in Parkinson’s disease. Neurochem. Res. 33, 589–597. doi: 10.1007/s11064-007-9482-y
Opara, J., Malecki, A., Malecka, E., and Socha, T. (2017). Motor assessment in Parkinson’s disease. Ann. Agric. Environ. Med. 24, 411–415. doi: 10.5604/12321966.1232774
Panni, S., Lovering, R. C., Porras, P., and Orchard, S. (2020). Non-coding RNA regulatory networks. Biochim. Biophys. Acta Gene Regul. Mech. 1863:194417. doi: 10.1016/j.bbagrm.2019.194417
Pavlou, M. a. S., and Outeiro, T. F. (2017). Epigenetics in Parkinson’s disease. Adv. Exp. Med. Biol. 978, 363–390. doi: 10.1007/978-3-319-53889-1_19
Peng, F., Gong, W., Li, S., Yin, B., Zhao, C., Liu, W., et al. (2021). circRNA_010383 acts as a sponge for miR-135a and its downregulated expression contributes to renal fibrosis in diabetic nephropathy. Diabetes 70, 603–615. doi: 10.2337/db20-0203
Percario, S., Da Silva Barbosa, A., Varela, E. L. P., Gomes, A. R. Q., Ferreira, M. E. S., De Nazare Araujo Moreira, T., et al. (2020). Oxidative stress in Parkinson’s disease: potential benefits of antioxidant supplementation. Oxid. Med. Cell Longev. 2020:2360872. doi: 10.1155/2020/2360872
Pickrell, A. m., and Youle, R. j. (2015). The roles of PINK1, parkin and mitochondrial fidelity in Parkinson’s disease. Neuron 85, 257–273. doi: 10.1016/j.neuron.2014.12.007
Prasad, S., Gupta, S. C., and Tyagi, A. K. (2017). Reactive oxygen species (ROS) and cancer: role of antioxidative nutraceuticals. Cancer Lett. 387, 95–105. doi: 10.1016/j.canlet.2016.03.042
Puspita, L., Chung, S. Y., and Shim, J. W. (2017). Oxidative stress and cellular pathologies in Parkinson’s disease. Mol. Brain 10:53. doi: 10.1186/s13041-017-0340-9
Qazi, T. J., Lu, J., Duru, L., Zhao, J., and Qing, H. (2021). Upregulation of mir-132 induces dopaminergic neuronal death via activating SIRT1/P53 pathway. Neurosci. Lett. 740:135465. doi: 10.1016/j.neulet.2020.135465
Qian, C., Ye, Y., Mao, H., Yao, L., Sun, X., Wang, B., et al. (2019). Downregulated lncRNA-SNHG1 enhances autophagy and prevents cell death through the miR-221/222 /p27/mTOR pathway in Parkinson’s disease. Exp. Cell Res. 384:111614. doi: 10.1016/j.yexcr.2019.111614
Qin, X., Zhang, X., Li, P., Wang, M., Yan, L., Pan, P., et al. (2021). MicroRNA-185 activates PI3K/AKT signalling pathway to alleviate dopaminergic neuron damage via targeting IGF1 in Parkinson’s disease. J. Drug Target. 29, 875–883. doi: 10.1080/1061186X.2021.1886300
Radi, E., Formichi, P., Battisti, C., and Federico, A. (2014). Apoptosis and oxidative stress in neurodegenerative diseases. J. Alzheimers Dis. 42, S125–S152. doi: 10.3233/JAD-132738
Raza, C., Anjum, R., and Shakeel, N. U. A. (2019). Parkinson’s disease: mechanisms, translational models and management strategies. Life Sci. 226, 77–90. doi: 10.1016/j.lfs.2019.03.057
Redensek, S., Flisar, D., Kojovic, M., Kramberger, M. G., Georgiev, D., Pirtosek, Z., et al. (2019). Genetic variability of inflammation and oxidative stress genes does not play a major role in the occurrence of adverse events of dopaminergic treatment in Parkinson’s disease. J. Neuroinflammation 16:50. doi: 10.1186/s12974-019-1439-y
Reich, S. G., and Savitt, J. M. (2019). Parkinson’s disease. Med. Clin. North Am. 103, 337–350. doi: 10.1016/j.mcna.2018.10.014
Ren, Y., Li, H., Xie, W., Wei, N., and Liu, M. (2019). MicroRNA195 triggers neuroinflammation in Parkinson’s disease in a Rho-associated kinase 1-dependent manner. Mol. Med. Rep. 19, 5153–5161. doi: 10.3892/mmr.2019.10176
Rezaei, O., Nateghinia, S., Estiar, M. A., Taheri, M., and Ghafouri-Fard, S. (2021). Assessment of the role of non-coding RNAs in the pathophysiology of Parkinson’s disease. Eur. J. Pharmacol. 896:173914. doi: 10.1016/j.ejphar.2021.173914
Robea, M. A., Balmus, I. M., Ciobica, A., Strungaru, S., Plavan, G., Gorgan, L. D., et al. (2020). Parkinson’s disease-induced zebrafish models: focussing on oxidative stress implications and sleep processes. Oxid. Med. Cell. Longev. 2020:1370837. doi: 10.1155/2020/1370837
Rocha, E. M., De Miranda, B., and Sanders, L. H. (2018). Alpha-synuclein: pathology, mitochondrial dysfunction and neuroinflammation in Parkinson’s disease. Neurobiol. Dis. 109, 249–257. doi: 10.1016/j.nbd.2017.04.004
Rodriguez-Perez, A. I., Sucunza, D., Pedrosa, M. A., Garrido-Gil, P., Kulisevsky, J., Lanciego, J. L., et al. (2018). Angiotensin type 1 receptor antagonists protect against alpha-synuclein-induced neuroinflammation and dopaminergic neuron death. Neurotherapeutics 15, 1063–1081. doi: 10.1007/s13311-018-0646-z
Sackner-Bernstein, J. (2021). Estimates of intracellular dopamine in parkinson’s disease: a systematic review and meta-analysis. J. Parkinsons Dis. 11, 1011–1018. doi: 10.3233/JPD-212715
Sarkar, S., Raymick, J., and Imam, S. (2016). Neuroprotective and therapeutic strategies against Parkinson’s disease: recent perspectives. Int. J. Mol. Sci. 17:904. doi: 10.3390/ijms17060904
Schapira, A. H., Cooper, J. M., Dexter, D., Clark, J. B., Jenner, P., and Marsden, C. D. (1990a). Mitochondrial complex I deficiency in Parkinson’s disease. J. Neurochem. 54, 823–827. doi: 10.1111/j.1471-4159.1990.tb02325.x
Schapira, A. H., Holt, I. J., Sweeney, M., Harding, A. E., Jenner, P., and Marsden, C. D. (1990b). Mitochondrial DNA analysis in Parkinson’s disease. Mov. Disord. 5, 294–297. doi: 10.1002/mds.870050406
Schapira, A. H., Mann, V. M., Cooper, J. M., Dexter, D., Daniel, S. E., Jenner, P., et al. (1990c). Anatomic and disease specificity of NADH CoQ1 reductase (complex I) deficiency in Parkinson’s disease. J. Neurochem. 55, 2142–2145. doi: 10.1111/j.1471-4159.1990.tb05809.x
Schneider, R. B., Iourinets, J., and Richard, I. H. (2017). Parkinson’s disease psychosis: presentation, diagnosis and management. Neurodegener. Dis. Manage. 7, 365–376. doi: 10.2217/nmt-2017-0028
Scott, M. S., and Ono, M. (2011). From snoRNA to miRNA: dual function regulatory non-coding RNAs. Biochimie 93, 1987–1992. doi: 10.1016/j.biochi.2011.05.026
Shen, Y., Cui, X., Hu, Y., Zhang, Z., and Zhang, Z. (2021a). LncRNA-MIAT regulates the growth of SHSY5Y cells by regulating the miR-34-5p-SYT1 axis and exerts a neuroprotective effect in a mouse model of Parkinson’s disease. Am. J. Transl. Res. 13, 9993–10013.
Shen, Y., Cui, X., Xu, N., Hu, Y., and Zhang, Z. (2021b). lncRNA PART1 mitigates MPP+-induced neuronal injury in SH-SY5Y cells via micRNA-106b-5p/MCL1 axis. Am. J. Transl. Res. 13, 8897–8908.
Shen, Y. F., Zhu, Z. Y., Qian, S. X., Xu, C. Y., and Wang, Y. P. (2020). miR-30b protects nigrostriatal dopaminergic neurons from MPP+-induced neurotoxicity via SNCA. Brain Behav. 10:e01567. doi: 10.1002/brb3.1567
Singh, A., and Sen, D. (2017). MicroRNAs in Parkinson’s disease. Exp. Brain Res. 235, 2359–2374. doi: 10.1007/s00221-017-4989-1
Smeyne, M., and Smeyne, R. J. (2013). Glutathione metabolism and Parkinson’s disease. Free Radic. Biol. Med. 62, 13–25. doi: 10.1016/j.freeradbiomed.2013.05.001
Song, Q., Geng, Y., Li, Y., Wang, L., and Qin, J. (2019). Long noncoding RNA NORAD regulates MPP+-induced Parkinson’s disease model cells. J. Chem. Neuroanat. 101:101668. doi: 10.1016/j.jchemneu.2019.101668
Song, Z., and Xie, B. (2021). LncRNA OIP5-AS1 reduces alpha-synuclein aggregation and toxicity by targeting miR-126 to activate PLK2 in human neuroblastoma SH-SY5Y cells. Neurosci. Lett. 740:135482. doi: 10.1016/j.neulet.2020.135482
Song, X. N., Zhang, L. Q., Liu, D. G., Lin, J., Zheng, J. D., Dai, D. P., et al. (2011). Oxidative damage to RNA and expression patterns of MTH1 in the hippocampi of senescence-accelerated SAMP8 mice and Alzheimer’s disease patients. Neurochem. Res. 36, 1558–1565. doi: 10.1007/s11064-011-0484-4
Su, Y., Deng, M. F., Xiong, W., Xie, A. J., Guo, J., Liang, Z. H., et al. (2019). MicroRNA-26a/death-associated protein kinase 1 signaling induces synucleinopathy and dopaminergic neuron degeneration in Parkinson’s disease. Biol. Psychiatry 85, 769–781. doi: 10.1016/j.biopsych.2018.12.008
Subramaniam, S. R., and Chesselet, M. F. (2013). Mitochondrial dysfunction and oxidative stress in Parkinson’s disease. Prog. Neurobiol. 106–107, 17–32. doi: 10.1016/j.pneurobio.2013.04.004
Sun, Q., Wang, S., Chen, J., Cai, H., Huang, W., Zhang, Y., et al. (2019). MicroRNA-190 alleviates neuronal damage and inhibits neuroinflammation via Nlrp3 in MPTP-induced Parkinson’s disease mouse model. J. Cell. Physiol. 234, 23379–23387. doi: 10.1002/jcp.28907
Sun, X., Zhang, C., Tao, H., Yao, S., and Wu, X. (2022). LINC00943 acts as miR-338-3p sponge to promote MPP+-induced SK-N-SH cell injury by directly targeting SP1 in Parkinson’s disease. Brain Res. 1782:147814. doi: 10.1016/j.brainres.2022.147814
Surendran, S., and Rajasankar, S. (2010). Parkinson’s disease: oxidative stress and therapeutic approaches. Neurol. Sci. 31, 531–540. doi: 10.1007/s10072-010-0245-1
Szymański, M., and Barciszewski, J. (2002). Beyond the proteome: non-coding regulatory RNAs. Genome Biol. 3:reviews0005. doi: 10.1186/gb-2002-3-5-reviews0005
Tao, H., Liu, Y., and Hou, Y. (2020). miRNA-384-5p regulates the progression of Parkinson’s disease by targeting SIRT1 in mice and SH-SY5Y cell. Int. J. Mol. Med. 45, 441–450. doi: 10.3892/ijmm.2019.4426
Taylor, J. P., Hardy, J., and Fischbeck, K. H. (2002). Toxic proteins in neurodegenerative disease. Science 296, 1991–1995. doi: 10.1126/science.1067122
Taylor, J. M., Main, B. S., and Crack, P. J. (2013). Neuroinflammation and oxidative stress: co-conspirators in the pathology of Parkinson’s disease. Neurochem. Int. 62, 803–819. doi: 10.1016/j.neuint.2012.12.016
Tharmalingam, S., Alhasawi, A., Appanna, V. P., Lemire, J., and Appanna, V. D. (2017). Reactive nitrogen species (RNS)-resistant microbes: adaptation and medical implications. Biol. Chem. 398, 1193–1208. doi: 10.1515/hsz-2017-0152
Trist, B. G., Hare, D. J., and Double, K. L. (2019). Oxidative stress in the aging substantia nigra and the etiology of Parkinson’s disease. Aging Cell 18:e13031. doi: 10.1111/acel.13031
Tsang, A. H., and Chung, K. K. (2009). Oxidative and nitrosative stress in Parkinson’s disease. Biochim. Biophys. Acta 1792, 643–650. doi: 10.1016/j.bbadis.2008.12.006
Turrens, J. F. (2003). Mitochondrial formation of reactive oxygen species. J. Physiol. 552, 335–344. doi: 10.1113/jphysiol.2003.049478
Vallee, A., Lecarpentier, Y., Guillevin, R., and Vallee, J. N. (2020). Circadian rhythms, neuroinflammation and oxidative stress in the story of Parkinson’s disease. Cells 9:314. doi: 10.3390/cells9020314
Vallée, A., Vallée, J. N., and Lecarpentier, Y. (2021a). Parkinson’s disease: potential actions of lithium by targeting the WNT/β-catenin pathway, oxidative stress, inflammation and glutamatergic pathway. Cells 10:230. doi: 10.3390/cells10020230
Vallée, A., Vallée, J. N., and Lecarpentier, Y. (2021b). Potential role of cannabidiol in Parkinson’s disease by targeting the WNT/β-catenin pathway, oxidative stress and inflammation. Aging (Albany NY) 13, 10796–10813. doi: 10.18632/aging.202951
Varcin, M., Bentea, E., Michotte, Y., and Sarre, S. (2012). Oxidative stress in genetic mouse models of Parkinson’s disease. Oxid. Med. Cell. Longev. 2012:624925. doi: 10.1155/2012/624925
Vidal, R. L., Matus, S., Bargsted, L., and Hetz, C. (2014). Targeting autophagy in neurodegenerative diseases. Trends Pharmacol. Sci. 35, 583–591. doi: 10.1016/j.tips.2014.09.002
Wang, Z. (2018). Diverse roles of regulatory non-coding RNAs. J. Mol. Cell. Biol. 10, 91–92. doi: 10.1093/jmcb/mjy026
Wang, Y., Cai, Y., Huang, H., Chen, X., Chen, X., Chen, X., et al. (2018). miR-486-3p influences the neurotoxicity of a-synuclein by targeting the SIRT2 gene and the polymorphisms at target sites contributing to Parkinson’s disease. Cell. Physiol. Biochem. 51, 2732–2745. doi: 10.1159/000495963
Wang, R., Li, Q., He, Y., Yang, Y., Ma, Q., and Li, C. (2020a). miR-29c-3p inhibits microglial NLRP3 inflammasome activation by targeting NFAT5 in Parkinson’s disease. Genes Cells 25, 364–374. doi: 10.1111/gtc.12764
Wang, R., Yang, Y., Wang, H., He, Y., and Li, C. (2020b). MiR-29c protects against inflammation and apoptosis in Parkinson’s disease model in vivo and in vitro by targeting SP1. Clin. Exp. Pharmacol. Physiol. 47, 372–382. doi: 10.1111/1440-1681.13212
Wang, X., Liu, Z., and Wang, F. (2021). MicroRNA-93 blocks signal transducers and activator of transcription 3 to reduce neuronal damage in Parkinson’s disease. Neurochem. Res. 46, 1859–1868. doi: 10.1007/s11064-021-03333-x
Wang, W., Lv, R., Zhang, J., and Liu, Y. (2021). circSAMD4A participates in the apoptosis and autophagy of dopaminergic neurons via the miR-29c-3p-mediated AMPK/mTOR pathway in Parkinson’s disease. Mol. Med. Rep. 24:540. doi: 10.3892/mmr.2021.12179
Wang, R., Ma, Z., Feng, L., Yang, Y., Tan, C., Shi, Q., et al. (2018). LncRNA MIR31HG targets HIF1A and P21 to facilitate head and neck cancer cell proliferation and tumorigenesis by promoting cell-cycle progression. Mol. Cancer 17:162. doi: 10.1186/s12943-018-0916-8
Wang, Z., Sun, L., Jia, K., Wang, H., and Wang, X. (2019). miR-9-5p modulates the progression of Parkinson’s disease by targeting SIRT1. Neurosci. Lett. 701, 226–233. doi: 10.1016/j.neulet.2019.02.038
Wang, J., Wang, W., and Zhai, H. (2019). MicroRNA-124 enhances dopamine receptor expression and neuronal proliferation in mouse models of Parkinson’s disease via the hedgehog signaling pathway by targeting EDN2. Neuroimmunomodulation 26, 174–187. doi: 10.1159/000501339
Wang, H., Wang, X., Zhang, Y., and Zhao, J. (2021a). LncRNA SNHG1 promotes neuronal injury in Parkinson’s disease cell model by miR-181a-5p/CXCL12 axis. J. Mol. Histol. 52, 153–163. doi: 10.1007/s10735-020-09931-3
Wang, H., Zhang, M., Wei, T., Zhou, J., Zhang, Y., and Guo, D. (2021b). Long non-coding RNA SNHG1 mediates neuronal damage in Parkinson’s disease model cells by regulating miR-216a-3p/Bcl-2-associated X protein. Ann. Transl. Med. 9:851. doi: 10.21037/atm-21-1613
Wang, S., Zhang, X., Guo, Y., Rong, H., and Liu, T. (2017). The long noncoding RNA HOTAIR promotes Parkinson’s disease by upregulating LRRK2 expression. Oncotarget 8, 24449–24456. doi: 10.18632/oncotarget.15511
Wang, C., Zhang, H., and Li, J. (2021). LncRNA JHDM1D-AS1 suppresses MPP+-induced neuronal injury in Parkinson’s disease via miR-134-5p/PIK3R3 axis. Neurotox. Res. 39, 1771–1781. doi: 10.1007/s12640-021-00437-8
Wang, X., Zhou, Y., Gao, Q., Ping, D., Wang, Y., Wu, W., et al. (2020). The role of exosomal microRNAs and oxidative stress in neurodegenerative diseases. Oxid. Med. Cell. Longev. 2020:3232869. doi: 10.1155/2020/3232869
Wei, M., Cao, L. J., Zheng, J. L., Xue, L. J., Chen, B., Xiao, F., et al. (2017). MicroRNA-181c functions as a protective factor in a 1-methyl-4-phenylpyridinium iodide-induced cellular Parkinson’s disease model via BCL2L11. Eur. Rev. Med. Pharmacol. Sci. 21, 3296–3304.
Wei, J. W., Huang, K., Yang, C., and Kang, C. S. (2017). Non-coding RNAs as regulators in epigenetics (review). Oncol. Rep. 37, 3–9. doi: 10.3892/or.2016.5236
Wen, Z., Zhang, J., Tang, P., Tu, N., Wang, K., and Wu, G. (2018). Overexpression of miR185 inhibits autophagy and apoptosis of dopaminergic neurons by regulating the AMPK/mTOR signaling pathway in Parkinson’s disease. Mol. Med. Rep. 17, 131–137. doi: 10.3892/mmr.2017.7897
Wong, E., and Cuervo, A. M. (2010). Autophagy gone awry in neurodegenerative diseases. Nat. Neurosci. 13, 805–811. doi: 10.1038/nn.2575
Wu, Y. Y., and Kuo, H. C. (2020). Functional roles and networks of non-coding RNAs in the pathogenesis of neurodegenerative diseases. J. Biomed. Sci. 27:49. doi: 10.1186/s12929-020-00636-z
Wu, X., Meng, X., Tan, F., Jiao, Z., Zhang, X., Tong, H., et al. (2019). Regulatory mechanism of miR-543-3p on GLT-1 in a mouse model of Parkinson’s disease. ACS Chem. Neurosci. 10, 1791–1800. doi: 10.1021/acschemneuro.8b00683
Wu, Q., Xi, D. Z., and Wang, Y. H. (2019). MicroRNA-599 regulates the development of Parkinson’s disease through mediating LRRK2 expression. Eur. Rev. Med. Pharmacol. Sci. 23, 724–731. doi: 10.26355/eurrev_201901_16886
Wu, J., Yu, X., Xue, K., Wu, J., Wang, R., Xie, X., et al. (2020). The inhibition of miR-873 provides therapeutic benefit in a lipopolysaccharide-induced neuroinflammatory model of Parkinson’s disease. Oxid. Med. Cell. Longev. 2020:8735249. doi: 10.1155/2020/8735249
Xie, N., Qi, J., Li, S., Deng, J., Chen, Y., and Lian, Y. (2019). Upregulated lncRNA small nucleolar RNA host gene 1 promotes 1-methyl-4-phenylpyridinium ion-induced cytotoxicity and reactive oxygen species production through miR-15b-5p/GSK3beta axis in human dopaminergic SH-SY5Y cells. J. Cell. Biochem. 120, 5790–5801. doi: 10.1002/jcb.27865
Xie, S. P., Zhou, F., Li, J., and Duan, S. J. (2019). NEAT1 regulates MPP+-induced neuronal injury by targeting miR-124 in neuroblastoma cells. Neurosci. Lett. 708:134340. doi: 10.1016/j.neulet.2019.134340
Xin, C., and Liu, J. (2021). Long Non-coding RNAs in Parkinson’s disease. Neurochem. Res. 46, 1031–1042. doi: 10.1007/s11064-021-03230-3
Xu, Z., Huang, J., Gao, M., Guo, G., Zeng, S., Chen, X., et al. (2021). Current perspectives on the clinical implications of oxidative RNA damage in aging research: challenges and opportunities. Geroscience 43, 487–505. doi: 10.1007/s11357-020-00209-w
Xu, W., Zhang, L., Geng, Y., Liu, Y., and Zhang, N. (2020). Long noncoding RNA GAS5 promotes microglial inflammatory response in Parkinson’s disease by regulating NLRP3 pathway through sponging miR-223-3p. Int. Immunopharmacol. 85:106614. doi: 10.1016/j.intimp.2020.106614
Xu, X., Zhuang, C., Wu, Z., Qiu, H., Feng, H., and Wu, J. (2018). LincRNA-p21 inhibits cell viability and promotes cell apoptosis in Parkinson’s disease through activating alpha-synuclein expression. Biomed. Res. Int. 2018:8181374. doi: 10.1155/2018/8181374
Yan, W., Chen, Z. Y., Chen, J. Q., and Chen, H. M. (2018). LncRNA NEAT1 promotes autophagy in MPTP-induced Parkinson’s disease through stabilizing PINK1 protein. Biochem. Biophys. Res. Commun. 496, 1019–1024. doi: 10.1016/j.bbrc.2017.12.149
Yan, L., Li, L., and Lei, J. (2021). Long noncoding RNA small nucleolar RNA host gene 12/microRNA-138-5p/nuclear factor I/B regulates neuronal apoptosis, inflammatory response and oxidative stress in Parkinson’s disease. Bioengineered 12, 12867–12879. doi: 10.1080/21655979.2021.2005928
Yan, M. H., Wang, X., and Zhu, X. (2013). Mitochondrial defects and oxidative stress in Alzheimer disease and Parkinson disease. Free Radic. Biol. Med. 62, 90–101. doi: 10.1016/j.freeradbiomed.2012.11.014
Yan, L. L., and Zaher, H. S. (2019). How do cells cope with RNA damage and its consequences? J. Biol. Chem. 294, 15158–15171. doi: 10.1074/jbc.REV119.006513
Yang, S., Yang, H., Luo, Y., Deng, X., Zhou, Y., and Hu, B. (2021). Long non-coding RNAs in neurodegenerative diseases. Neurochem. Int. 148:105096. doi: 10.1016/j.neuint.2021.105096
Yang, X., Zhang, M., Wei, M., Wang, A., Deng, Y., and Cao, H. (2020). MicroRNA-216a inhibits neuronal apoptosis in a cellular Parkinson’s disease model by targeting Bax. Metab. Brain Dis. 35, 627–635. doi: 10.1007/s11011-020-00546-x
Yao, L., Ye, Y., Mao, H., Lu, F., He, X., Lu, G., et al. (2018). MicroRNA-124 regulates the expression of MEKK3 in the inflammatory pathogenesis of Parkinson’s disease. J. Neuroinflammation 15:13. doi: 10.1186/s12974-018-1053-4
Yildirim, O., Izgu, E. C., Damle, M., Chalei, V., Ji, F., Sadreyev, R. I., et al. (2020). S-phase enriched non-coding RNAs regulate gene expression and cell cycle progression. Cell Rep. 31:107629. doi: 10.1016/j.celrep.2020.107629
Yuan, H., Zheng, J. C., Liu, P., Zhang, S. F., Xu, J. Y., and Bai, L. M. (2007). Pathogenesis of Parkinson’s disease: oxidative stress, environmental impact factors and inflammatory processes. Neurosci. Bull. 23, 125–130. doi: 10.1007/s12264-007-0018-x
Zaman, V., Shields, D. C., Shams, R., Drasites, K. P., Matzelle, D., Haque, A., et al. (2021). Cellular and molecular pathophysiology in the progression of Parkinson’s disease. Metab. Brain Dis. 36, 815–827. doi: 10.1007/s11011-021-00689-5
Zhai, K., Liu, B., and Gao, L. (2020). Long-noncoding RNA TUG1 promotes Parkinson’s disease via modulating MiR-152-3p/PTEN pathway. Hum. Gene Ther. 31, 1274–1287. doi: 10.1089/hum.2020.106
Zhang, J., Dongwei, Z., Zhang, Z., Xinhui, Q., Kunwang, B., Guohui, L., et al. (2019). miR-let-7a suppresses alpha-Synuclein-induced microglia inflammation through targeting STAT3 in Parkinson’s disease. Biochem. Biophys. Res. Commun. 519, 740–746. doi: 10.1016/j.bbrc.2019.08.140
Zhang, X., and Li, L. (2020). The significance of 8-oxoGsn in aging-related diseases. Aging Dis. 11, 1329–1338. doi: 10.14336/AD.2019.1021
Zhang, L., Kang, W., Lu, X., Ma, S., Dong, L., and Zou, B. (2018). LncRNA CASC11 promoted gastric cancer cell proliferation, migration and invasion in vitro by regulating cell cycle pathway. Cell Cycle 17, 1886–1900. doi: 10.1080/15384101.2018.1502574
Zhang, J., Perry, G., Smith, M. A., Robertson, D., Olson, S. J., Graham, D. G., et al. (1999). Parkinson’s disease is associated with oxidative damage to cytoplasmic DNA and RNA in substantia nigra neurons. Am. J. Pathol. 154, 1423–1429. doi: 10.1016/S0002-9440(10)65396-5
Zhang, H., Wang, Z., Hu, K., and Liu, H. (2021). Downregulation of long noncoding RNA SNHG7 protects against inflammation and apoptosis in Parkinson’s disease model by targeting the miR-425-5p/TRAF5/NF-κB axis. J. Biochem. Mol. Toxicol. 35:e22867. doi: 10.1002/jbt.22867
Zhang, L., Wang, J., Liu, Q., Xiao, Z., and Dai, Q. (2020). Knockdown of long non-coding RNA AL049437 mitigates MPP+-induced neuronal injury in SH-SY5Y cells via the microRNA-205-5p/MAPK1 axis. Neurotoxicology 78, 29–35. doi: 10.1016/j.neuro.2020.02.004
Zhang, L. M., Wang, M. H., Yang, H. C., Tian, T., Sun, G. F., Ji, Y. F., et al. (2019). Dopaminergic neuron injury in Parkinson’s disease is mitigated by interfering lncRNA SNHG14 expression to regulate the miR-133b/ α-synuclein pathway. Aging (Albany NY) 11, 9264–9279. doi: 10.18632/aging.102330
Zhang, P., Wu, W., Chen, Q., and Chen, M. (2019). Non-coding RNAs and their integrated networks. J. Integr. Bioinform. 16:20190027. doi: 10.1515/jib-2019-0027
Zhang, Y., Xia, Q., and Lin, J. (2020). LncRNA H19 attenuates apoptosis in MPTP-induced Parkinson’s disease through regulating miR-585-3p/PIK3R3. Neurochem. Res. 45, 1700–1710. doi: 10.1007/s11064-020-03035-w
Zhang, J., Yang, Y., Zhou, C., Zhu, R., Xiao, X., Zhou, B., et al. (2022). LncRNA miR-17-92a-1 cluster host gene (MIR17HG) promotes neuronal damage and microglial activation by targeting the microRNA-153-3p/alpha-synuclein axis in Parkinson’s disease. Bioengineered 13, 4493–4516. doi: 10.1080/21655979.2022.2033409
Zhao, Y., Xie, Y., Yao, W. Y., Wang, Y. Y., and Song, N. (2022). Long non-coding RNA Opa interacting protein 5-antisense RNA 1 promotes mitochondrial autophagy and protects SH-SY5Y cells from 1-methyl-4-phenylpyridine-induced damage by binding to microRNA-137 and upregulating NIX. Kaohsiung J. Med. Sci. 38, 207–217. doi: 10.1002/kjm2.12485
Zhao, J., Yang, M., Li, Q., Pei, X., and Zhu, X. (2020a). miR-132-5p regulates apoptosis and autophagy in MPTP model of Parkinson’s disease by targeting ULK1. Neuroreport 31, 959–965. doi: 10.1097/WNR.0000000000001494
Zhao, J., Li, H., and Chang, N. (2020b). LncRNA HOTAIR promotes MPP+-induced neuronal injury in Parkinson’s disease by regulating the miR-874-5p/ATG10 axis. EXCLI J. 19, 1141–1153. doi: 10.17179/excli2020-2286
Zhao, J., Yu, S., Zheng, Y., Yang, H., and Zhang, J. (2017). Oxidative modification and its implications for the neurodegeneration of Parkinson’s disease. Mol. Neurobiol. 54, 1404–1418. doi: 10.1007/s12035-016-9743-3
Zheng, Y., Dong, L., Liu, N., Luo, X., and He, Z. (2020). Mir-141-3p regulates apoptosis and mitochondrial membrane potential via targeting sirtuin1 in a 1-methyl-4-phenylpyridinium in vitro model of Parkinson’s disease. Biomed. Res. Int. 2020:7239895. doi: 10.1002/kjm2.12485
Zhou, W. Y., Cai, Z. R., Liu, J., Wang, D. S., Ju, H. Q., and Xu, R. H. (2020). Circular RNA: metabolism, functions and interactions with proteins. Mol. Cancer 19:172. doi: 10.1186/s12943-020-01286-3
Zhou, L., Yang, L., Li, Y. J., Mei, R., Yu, H. L., Gong, Y., et al. (2018). MicroRNA-128 protects dopamine neurons from apoptosis and upregulates the expression of excitatory amino acid transporter 4 in Parkinson’s disease by binding to AXIN1. Cell. Physiol. Biochem. 51, 2275–2289. doi: 10.1159/000495872
Zhou, J., Zhao, Y., Li, Z., Zhu, M., Wang, Z., Li, Y., et al. (2020). miR-103a-3p regulates mitophagy in Parkinson’s disease through Parkin/Ambra1 signaling. Pharmacol. Res. 160:105197. doi: 10.1016/j.phrs.2020.105197
Zhou, S., Zhang, D., Guo, J., Chen, Z., Chen, Y., and Zhang, J. (2021). Deficiency of NEAT1 prevented MPP+-induced inflammatory response, oxidative stress and apoptosis in dopaminergic SK-N-SH neuroblastoma cells via miR-1277-5p/ARHGAP26 axis. Brain Res. 1750:147156. doi: 10.1016/j.brainres.2020.147156
Zhou, S., Zhang, D., Guo, J., Zhang, J., and Chen, Y. (2020). Knockdown of SNHG14 alleviates MPP+-induced injury in the cell model of Parkinson’s disease by targeting the miR-214-3p/KLF4 axis. Front. Neurosci. 14:930. doi: 10.3389/fnins.2020.00930
Zhou, Q., Zhang, M. M., Liu, M., Tan, Z. G., Qin, Q. L., and Jiang, Y. G. (2021). LncRNA XIST sponges miR-199a-3p to modulate the Sp1/LRRK2 signal pathway to accelerate Parkinson’s disease progression. Aging (Albany NY) 13, 4115–4137. doi: 10.18632/aging.202378
Zhu, W., Zhang, H., Gao, J., and Xu, Y. (2021). Silencing of miR-497-5p inhibits cell apoptosis and promotes autophagy in Parkinson’s disease by upregulation of FGF2. Environ. Toxicol. 36, 2302–2312. doi: 10.1002/tox.23344
Keywords: Parkinson’s disease, microRNAs, long non-coding RNAs, circular RNAs, oxidative stress
Citation: Zhang H, Liu X, Liu Y, Liu J, Gong X, Li G and Tang M (2022) Crosstalk between regulatory non-coding RNAs and oxidative stress in Parkinson’s disease. Front. Aging Neurosci. 14:975248. doi: 10.3389/fnagi.2022.975248
Received: 22 June 2021; Accepted: 09 July 2022;
Published: 09 August 2022.
Edited by:
Shenfeng Qiu, University of Arizona, United StatesReviewed by:
Weinan Zhou, University of Illinois at Urbana-Champaign, United StatesCopyright © 2022 Zhang, Liu, Liu, Liu, Gong, Li and Tang. This is an open-access article distributed under the terms of the Creative Commons Attribution License (CC BY). The use, distribution or reproduction in other forums is permitted, provided the original author(s) and the copyright owner(s) are credited and that the original publication in this journal is cited, in accordance with accepted academic practice. No use, distribution or reproduction is permitted which does not comply with these terms.
*Correspondence: Gang Li, bGlnYW5nMTExNjY2QDE2My5jb20=; Min Tang, bXQzMTM4QHVqcy5lZHUuY24=
Disclaimer: All claims expressed in this article are solely those of the authors and do not necessarily represent those of their affiliated organizations, or those of the publisher, the editors and the reviewers. Any product that may be evaluated in this article or claim that may be made by its manufacturer is not guaranteed or endorsed by the publisher.
Research integrity at Frontiers
Learn more about the work of our research integrity team to safeguard the quality of each article we publish.