- 1Department of Orthopaedics, China-Japan Union Hospital of Jilin University, Changchun, China
- 2Department of Laboratory Medicine Center, China-Japan Union Hospital of Jilin University, Changchun, China
- 3Radiotherapy Department, The First Bethune Hospital of Jilin University, Changchun, China
- 4School of Laboratory Medicine, Beihua University, Jilin, China
Spinal cord injury (SCI) induced catastrophic neurological disability is currently incurable, especially in elderly patients. Due to the limited axon regeneration capacity and hostile microenvironment in the lesion site, essential neural network reconstruction remains challenging. Owing to the blood–spinal cord barrier (BSCB) created immune cells and cytokines isolation, the immune elements were incorrectly recognized as innocent bystanders during the SCI pathological process traditionally. Emerging evidence demonstrated that the central nervous system (CNS) is an “immunological quiescent” rather than “immune privileged” area, and the CNS-associated immune response played mixed roles which dedicate beneficial and detrimental contributions throughout the SCI process. Consequently, coordinating double-edged immunomodulation is vital to promote tissue repair and neurological recovery post-SCI. The comprehensive exploration and understanding of the immune landscape post-SCI are essential in establishing new avenues for further basic and clinical studies. In this context, this review summarizes the recent significant breakthroughs in key aspects of SCI-related immunomodulation, including innate and adaptive immune response, immune organ changes, and holistic immune status modification. Moreover, the currently existing immune-oriented therapies for SCI will be outlined.
Introduction
Spinal cord injury (SCI) is a catastrophic clinical syndrome that results in permanent neurological disability and fatal complications, especially among elderly patients. The SCI occurrence rate has increased in recent years as an adverse effect of economic and industrial development. In 2016, the estimated global prevalence rate of SCI was 368 cases per 100,000 population, and the primary causes included falls and traffic injuries (Feigin et al., 2019). The annual SCI medical expenditure in the United States was up to US $1.7 billion. Notably, the indirect costs of each patient were more than US $70,000 (Lo et al., 2021). Unfortunately, SCI-triggered devastating neurological impairment currently has no cure.
Pathophysiologically, the poor functional restoration of SCI can be generally attributed to various internal and external elements (Zhang B. Y. et al., 2020). Internally, the restrained capacity of the adult axon regeneration and sheath remyelination after SCI hamper the neural circuit reconnection. Externally, the glial activation and immune response onset make the microenvironment intractable, thereby suppressing tissue repair and axon regrowth (Bo-Yin et al., 2021). Neurological recovery demands the valid organization of internal and external factors. Previous studies on axon regeneration or inhibitory glial reaction have identified a spectrum of potential regeneration-related candidates (Liu et al., 2021). However, an efficacious strategy to rescue neurological disability remains to be developed.
Among these elements, the roles that immune regulation plays in the SCI repair process are somewhat blind spots that have attracted massive attention. Traditionally, the central nervous system (CNS), including the spinal cord, was recognized as an “immune-privileged area” owing to the blood–spinal cord barrier (BSCB) created by immune cells and cytokines isolation. However, recent studies have demonstrated that the CNS is an “immunologically quiescent” area rather than “immune privileged,” as it is equipped with quiescent immune cells capable of triggering comprehensive immune responses when required (Louveau et al., 2015). Under the SCI scenario, the quiescent immune system is provoked spontaneously, and multiple immune responses can be activated and amplified via various mechanisms (Al Mamun et al., 2021). During this process, various immune cell types, including innate glial cells, peripheral immune cells, and lymphocytes, played versatile roles in the SCI repair process. First, the spinal cord resident glial cells and infiltrated immune cells innately exert immediate immunomodulation after SCI, like the innate immune response against pathogens, which directly maneuvers the inflammation-associated cytokines and chemokines released in the early stage of SCI (Table 1). Moreover, the peripheral and infiltrated lymphocytes modify the adaptive immune response and play essential roles in neurological function repair. Additionally, as the development and storage sites of immune cells, immune organs are indispensable for immune function maintenance. SCI-induced immune reactions in immune organs, such as the spleen and gut, alter the holistic immune response status, especially in the SCI chronic stage. Consequently, by orchestrating the SCI-related critical pathophysiological changes, such as inflammatory infiltration, glial response, and axon regeneration, SCI-related immune response dedicates beneficial and detrimental contributions depending on the specific spatiotemporal environment. Although the regulatory mechanism is still not fully understood, the way to coordinate the double-edged immunomodulation is of great importance to post-SCI tissue repair and neurological recovery.
Thus, this review summarizes the recent significant progress in CNS immunomodulation studies. It describes the SCI-associated immune cells and organ-related responses and summarizes the currently existing immune-oriented therapies for treating SCI.
The immune roles of spinal cord glial cells—Far beyond the glia
The immune roles of innate glial cells in the spinal cord
The spinal cord is primarily composed of two categories of cells: glial cells and neurons. Glial cells, including astrocytes, microglia, and oligodendrocytes, account for 90% of the total intraspinal cellular amount. The well-known functions of glial cells include nerve conduction activity maintenance and neuronal survival protection. Findings of recent studies indicate that glial cells also act as resident immune cells and play critical roles in the CNS's innate immune responses, especially in the spinal cord (Xu et al., 2020). Glial cells tend to exert immunomodulation innately, analogous to the innate immune response against pathogens. To illustrate, they directly regulate the inflammatory-related cytokine and chemokine expression without immune memory (besides microglia cells) and modulate the subsequent inflammatory cell infiltration and secondary injury inflammation cascade amplification (Figure 1).
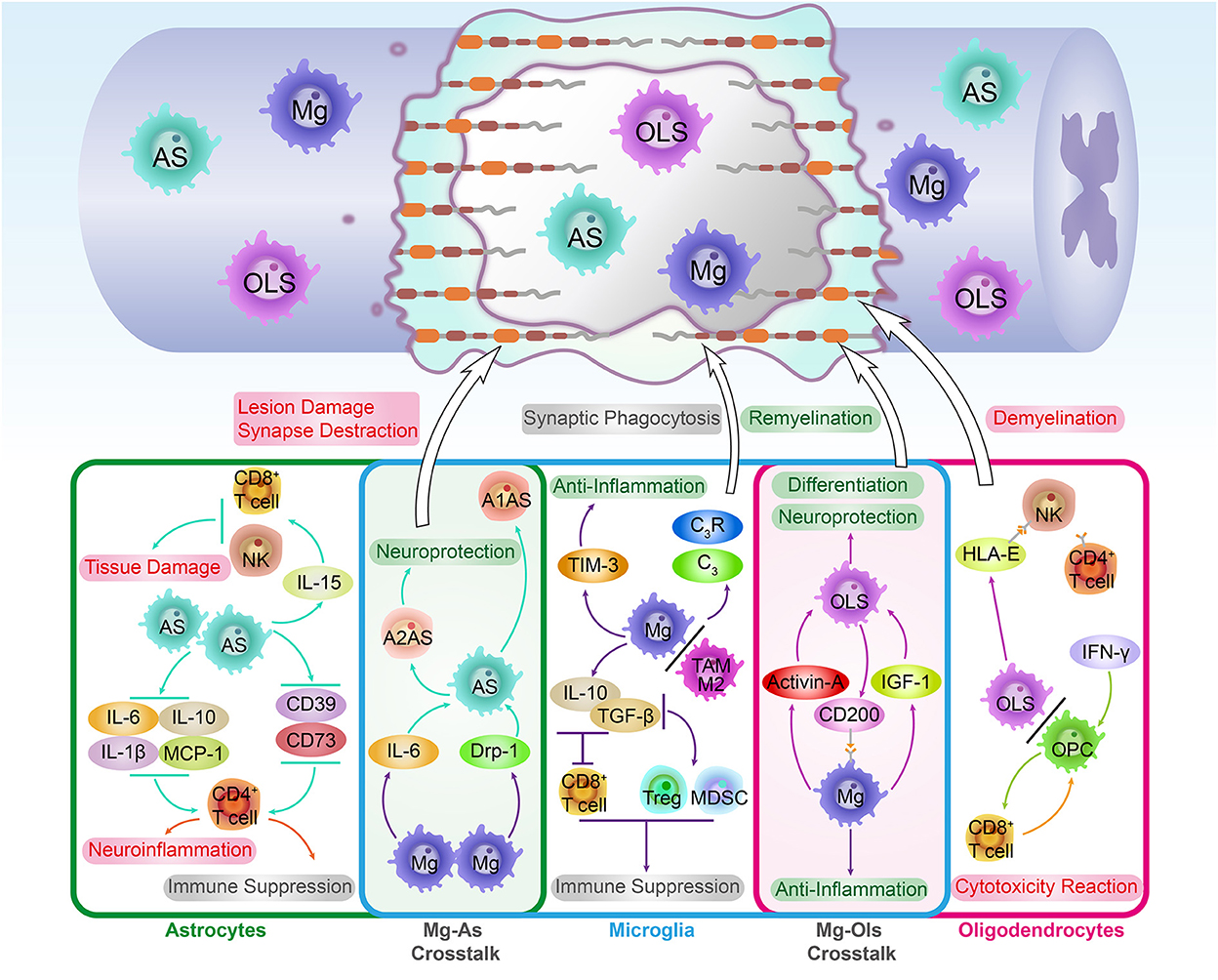
Figure 1. The immune roles and cross-talk of innate glial cells in the spinal cord. As the co-founder of CNS, the spinal cord's innate glial cells, including astrocytes, microglia, and oligodendrocytes, are essential for immune landscape establishment during SCI pathological repair process. From the SCI recovery point of view, these glial-related immune responses played mixed roles in the key steps of SCI pathological repair. To illustrate, by expressing pro-inflammatory and anti-inflammatory cytokines, glial cells modulate lymphocyte-induced neuroimmune functions. These functions could be beneficial and neuroprotective, which promote remyelination, axon regeneration, and glial differentiation. Instead, they also could be detrimental to SCI recovery, which aggravates tissue damage, cytotoxicity reaction, and demyelination. In addition, glial cells are still able to orchestrate the complement system activation and lymphocyte phenotypes transformation. Remarkably, the crosstalk among innate glial cells not only strengthens the communication between the CNS and immune system but supports the exploration of novel targets for SCI treatment. An important example of crosstalk is microglia-mediated astrocyte phenotype transformation, which induces the neuroprotective A2 phenotype astrocyte formation.
The neuroimmune roles of astrocytes
Naïve astrocytes were found to transform into the reaction pool and scar pool in response to SCI. Shortly after SCI, the reactive astrocytes provided trophic factors and elements, which are beneficial for synapse formation and axon regeneration. Afterward, the reactive astrocytes formed glial scars, restraining the lesion site's inflammation during the earlier stage of the SCI repair process. However, with the accumulation of mature glial scars, the spinal lesion microenvironment irreversibly turned hostile, which inhibited axon regrowth (Zhang B. Y. et al., 2020). Astrocytes produced soluble inflammatory mediators, which were found to modulate immune cell recruitment and activation as neurotrophic cells. Therefore, besides the glial functions, astrocytes play considerable roles in CNS immune regulation.
Recent studies have demonstrated that astrocytes can mediate the T cell's polarization after CNS injury. The miR-409-3p and miR-1869 in astrocytes promote cytokine expression, such as IL-1β, IL-6, IL-10, and MCP-1, via the SOCS3/STAT3 pathway. Under the CNS injury condition, the astrocyte-oriented cytokine enrichment in the lesion induces the astrocyte-associated CD4+ T cells migration and polarization, which further results in neuroinflammation aggravation (Liu et al., 2019). Moreover, astrocytes also promote the plasma membrane ectonucleotidase expression (e.g., CD39, CD73) in activated CD4+ T cells, which promotes the T cells polarization to a Th17-like immunosuppressive phenotype during the neuroinflammation progression (Filipello et al., 2016). Additionally, under the CNS ischemic condition, astrocytes produced pro-inflammatory cytokine IL-15 up-regulation in astrocytes, which exacerbated the CD8+ T cells, and NK cells induced the immune-related cerebral damage. Instead, the deletion of astrocyte-derived IL-15 or IL-15 receptor knockdown alleviated the size of the lesion site and neurological dysfunction (Li et al., 2017).
In the CNS, the adventitial cuff (AC) structure is the perivascular terminal structure that hosts several kinds of immune cells. The integrity of AC expends the immune cell infiltration interfaces of immune organs when suffering from diseases such as infection and allergy (Dahlgren and Molofsky, 2019). Astrocytes around AC borders were demonstrated to serve as immune barriers against CNS inflammation invasion. The local astrocyte aggregation induced tight junction protein CLDN1 and CLDN4 upregulation, which encircled immune cells into AC borders when inflammatory infiltration occurred. Moreover, Sam et al. found that in vivo astrocyte-specific CLDN4 inactivation disrupted the astrocyte-mediated immune cell infiltration and deteriorated the lesion damage in a multiple sclerosis (MS) model (Horng et al., 2017).
The neuroimmune roles of microglia
Microglia are the first activated inflammatory responder in the lesion site after SCI. As the innate phagocytes in the CNS, microglia eliminate cellular debris and intracellular protein particles that may hinder remyelination. In addition, microglia also produce pro-inflammatory factors and neurotrophic factors that regulate the neuroinflammatory reaction. The SCI stress triggers the release of damage-associated molecular patterns (DAMPs) in the cytoplasm around the lesion site. Afterward, the DAMP signaling was recognized and captured by the pattern recognition receptors (PRRs) on microglia, and the DAMP/PRRs complex formed simultaneously. As a result, the complex stimulated microglia proliferation and triggered the microglia into the activated condition (Kigerl et al., 2014).
Activated microglia can be classified into two phenotypes, the immune-activated type (M1, pro-inflammatory) and the protective type (M2, anti-inflammatory). By releasing cytokines and inflammatory factors, the M1-type microglia trigger a neuroinflammatory cascade that turns the injury site into a hostile milieu that represses axon regeneration and neural survival. The SCI secondary injury turns microglia into the M1 type, which exaggerates the inflammatory response in the lesion site (Zhang B. Y. et al., 2020). In contrast, the branching morphology is the hallmark of the M2-type microglia, and the M2 microglia play beneficial roles after SCI by means of elevated phagocytic activity and anti-inflammatory cytokines expression (Bellver-Landete et al., 2019). To illustrate, by moderating the immune response of resident microglia, neuregulin-1 (Nrg-1) plays a neuroprotective role in SCI recovery. The systemic administration of Nrg-1 after SCI promotes the M2 phenotype formation at the SCI acute stage and increases the M1 phenotype formation during the SCI chronic stage (Alizadeh et al., 2018). Thus, the innate immune function of microglia after SCI was determined by the balance between the two phenotypes. In the CNS malignant tumor lesion, the microglia are involved in the tumor-associated microglia and macrophages (TAMs), which regulate tumor growth and immune response. Similar to CNS naive microglia, the M2 TAMs subtype cells have pro-tumorigenic and anti-inflammatory functions (Andersen et al., 2021). By secreting anti-inflammatory cytokines, such as TGF-β and IL-10, the M2-type TAM cells repressed the cytotoxic T cells and activated the regulatory T (Treg) cells and myeloid-derived suppressor cells. Consequently, the CNS tumor microenvironment turned into an immunosuppressive status that facilitated tumor growth and progression (Hambardzumyan et al., 2016).
Besides the innate immune response function and inflammatory roles, microglia also proved to possess immune memory in CNS inflammatory events. After experiencing the priming challenge of ligands, such as lipopolysaccharide (LPS) and β-glucan, microglia can be induced into immune training or immune tolerance status. Therefore, the immune response of primed microglia toward secondary stimulation could be activated or suppressed (Zhang et al., 2022). Yang et al. (2017) demonstrated that the distinct preconditioning protocols result in microglia's immune memory-related response, and the results provide promising treatment targets for microglia-associated diseases. For instance, the 2-day interval challenge protocol resulted in microglia immune training. However, the 7-day interval challenge protocol reversed the immune training into the immune tolerance status (Heng et al., 2021). Mechanically, the immune memory phenomenon of microglia results from the epigenetic remodeling navigated functional phenotype transformation. For instance, the LPS-induced gene expression was found to correlate with the enrichment of the permissive epigenetic markers in promoter regions, such as H3K4me3 and H3K27Ac (Zhang et al., 2022).
In addition to the inflammatory factors and cytokine secretion, microglia are competent in expressing special neuroimmune components. T-cell immunoglobulin and mucin domain protein-3 (Tim-3) are critical microglia-derived immune checkpoints closely related to CNS tissue damage and inflammation. It can serve as a therapeutic target for CNS disorders. In the brain, pathogens such as infectious disease or hypoxia–ischemia induced the Tim-3 up-regulation, which was recognized as an adverse factor in neurological recovery (Koh et al., 2015). A study found that the phagocytosis and secretion of microglia were modulated by Tim-3 activity. More particularly, Tim-3 activation promoted the microglia expression of inflammatory cytokines, such as TNF-α, IL-1β, and TGF-β. On the contrary, the Tim-3 blockade decreased the phagocytic activity of microglia (Wang et al., 2015). In addition, the Tim-3 blockade significantly reduced inflammatory cell infiltration and lesion volume in the cerebral hypoxia–ischemia model and improved neurological function (Koh et al., 2015). Furthermore, microglia still interact with complement proteins to regulate CNS function via immunological strategies (Cowan and Petri, 2018). For instance, microglia regulate synaptic refinement and nerve circuit projection by modulating the complement system-mediated synapse phagocytic effect. The effect was revealed to play a critical role in CNS diseases such as Alzheimer's disease and cerebral hypoperfusion (Zhang L. Y. et al., 2020). In this regard, the redundant synaptic cytoskeleton can be eliminated by microglia-expressed complement protein 3 (C3) and its receptor C3R (Hong et al., 2016).
The neuroimmune roles of oligodendrocytes
Oligodendrocytes are the CNS myelinating cells involved in both physiological and pathological processes. Previous studies primarily focused on the role of oligodendrocytes in myelination, and the immune function of oligodendrocytes is still underexplored (Dulamea, 2017).
Recently, the immunological profiles of oligodendrocytes were explored and recognized extensively. Fatma et al. reported that HLA-E, a subtype of non-classical MHC-1 factors, can be released by oligodendrocytes. By binding to the NKG2 receptor on NK cells and CD4+ T cells, HLA-E mediates the CNS immune responses toward oligodendrocytes. In the tissues of a patient with MS, the interaction between NKG2C+/CD4+ T cells and HLA-E+ oligodendrocytes induces the oligodendrocyte's death and demyelination modification (Zaguia et al., 2013). The differentiation of oligodendrocyte precursor cells (OPCs) rescues the oligodendrocyte loss following CNS injury or disease. Interestingly, OPCs also act as antigen-presenting cells (APCs) under neuroinflammatory conditions. When exposed to IFN-γ in the lesion, the MHC class-I presentation pathway of OPC was activated, and the antigen was cross-presented to CD8+ T cells. Therefore, the CD8+ T cell-mediated cytotoxicity reaction recognized OPCs as target cells and resulted in the OPC's sacrifice and extensive demyelination in the lesion site (Kirby et al., 2019).
Crosstalk among glial cells
Recent studies discovered various types of crosstalk among different types of glial cells. The communication between the CNS and the immune system is strengthened via crosstalk. In addition, with the coordination of the glial cells, the phenotype transformation is carried out after nerve injury spontaneously, which modulates the immune response and inflammatory reaction in the lesion site and supports the establishment of novel discovery targets.
Microglia–astrocyte interaction
One of the well-known crosstalk is the microglia-mediated astrocyte phenotype transformation. The reactive astrogliosis and scar formation effect were recognized as typical characteristics of the neuroprotective reaction of the A2-type astrocytes. In CNS traumatic injury, the exposure of microglia-derived pro-inflammatory cytokines, such as IL-6 and TNF-α, promotes the A2 phenotype astrocyte transformation, which accelerates the protective astrogliosis in the lesion epicenter. In addition, microglia can still modulate the astrocyte-associated glial reaction and phenotype transformation by regulating the astrocyte-specific P2Y1 receptor expression (Shinozaki et al., 2017). After the CNS injury, the microglia are activated earlier than the astrocytes. Therefore, microglia-released mitochondria debris and neurotoxic proteins, such as dynamin-related protein1 (Drp-1), accumulated in the extracellular milieu. The enrichment of Drp-1 promoted the astrocytes transformed into A1 neurotoxic phenotype, which was characterized by pro-inflammatory factor secretion, synapse destruction, and lesion damage, and eventually directly propagated neuronal death in the CNS (Joshi et al., 2019).
Microglia–oligodendrocyte crosstalk
The bi-directional crosstalk between microglia and oligodendrocytes exist in both CNS development and pathological condition. On the one hand, microglia orchestrated the oligodendrocytes' differentiation, remyelination, and survival process. On the other hand, oligodendrocyte-expressed proteins regulated the microglial activity. Studies found that the microglia are necessary to regulate postnatal oligodendrocyte linage homeostasis and maturation, and the in vivo microglia depletion is detrimental to the quality and quantity of oligodendrocytes (Hagemeyer et al., 2017). In addition, activated microglia-secreted growth factors modulated the oligodendrocyte survival and lineage selection during the remyelination process. For instance, microglia produced IGF-1 exerted the neuronal protective function, and it rescued the cuprizone-induced oligodendrocytes apoptosis and promoted remyelination after CNS disease attack (Sierra et al., 2010).
Moreover, Miron et al. reported that polarized M2-type microglia secreted activin-A, promoting oligodendrocyte lineage differentiation and axon remyelination. In this context, the finding is probably one of the potential mechanisms of M2 phenotype microglia-induced neuroprotective properties during the CNS remyelination process (Miron et al., 2013).
Although microglia-derived factors, such as lipolytic enzymes, reactive oxygen, and excitotoxins, are necessary for pathogen clearance in the immune defense process, oligodendrocytes are highly sensitive to these factors. Oligodendrocytes in a hostile environment produce poor-quality myelin, which impedes the injured axon remyelination. Additionally, these microglia-released factors block OPC proliferation and induce OPC sacrifice (Peferoen et al., 2014).
CD200 is one of the membrane glycoproteins that contribute to the CNS immune suppressive milieu. Nathalie et al. found that the oligodendrocyte-expressed CD200 regulated the activity of microglia. By binding to its ligand CD200R located on the microglia surface, CD200 exerted the inhibitory regulation of microglia activity. On the contrary, the CD200 deletion resulted in the hyperactivity of microglia and CNS neuroinflammation (Koning et al., 2009).
Glial cell–myelin crosstalk
Myelin debris ubiquitously distributes in the lesion environment after SCI, which drives the ongoing neuroimmune activities. Microglia-mediated myelin debris clearance in the lesion environment facilitates axon regeneration and oligodendrocyte differentiation. As a receptor of damaged myelin lipid components, the TREM2 is expressed on the surface of microglia and is required for the myelin debris phagocytosis after SCI. A study reported that the mutations or deficiencies of TREM2 in the microglia inhibit the uptake and the subsequent phagocytosis of myelin fragments post-SCI (Poliani et al., 2015). Complement–myelin interaction was identified as another type of immune crosstalk post-SCI that contributed to the intraspinal inflammation regulation after injury. The binding of myelin fragments released oligodendrocyte myelin glycoprotein, and the complement initiated the complement activation and the complement-mediated opsonization. Once activated, complement C5a recruited the immune cells, such as macrophages and neutrophils, from the periphery blood to the injury site, which promoted myelin clearance and inflammatory process in the lesion (Brennan et al., 2015).
The immune roles of peripheral immune cells—The unfavorable guys?
BSCB disruption post-SCI
The blood–spinal cord barrier is a crucial barrier structure between the CNS and circulatory system, and it is composed of endotheliocytes, pericytes, astrocytic processes, intercellular connections, and membrane structures (Jin et al., 2021). Among these components, the endotheliocytes and the adherens junction proteins constitute a cellular barrier, which protects the spinal cord from harmful molecules. In addition, pericytes in spinal cord capillaries regulate the permeability of the BSCB by adjusting the blood flow status of the neurovascular system and modulating extracellular matrix (ECM) and growth factors. Previous studies demonstrated the sieve-like roles of the BSCB and considered it the key interface for molecular and neurotransmitter exchange of the spinal cord microenvironment (Cardoso et al., 2010). Of note, accumulating evidence suggests that the BSCB contributes to intraspinal immune homeostasis and CNS immune surveillance function.
After an SCI attack, both the histological structure and immune homeostasis of the BSCB are affected dramatically. First, the irreversible histological structure disruption permits the exogenous immune cells to recruit and infiltrate into the lesion site through the compromised BSCB. For instance, peripheral inflammatory cell infiltration, such as neutrophils and macrophages, leads to neuroinflammatory and demyelination. Lymphocytic infiltration may trigger necrosis of neural tissue and activation of glial cells (Aubé et al., 2014). Moreover, SCI-induced “peripheral guys” infiltration disrupts the BSCB-built immune homeostasis microenvironment in the spinal cord. Under this circumstance, the intraspinal molecular and glycoprotein are liable to be recognized as exogenous antigens by infiltrated peripheral immune cells. Although these peripheral infiltrated cells were traditionally considered “unfavorable guys,” emerging evidence demonstrates that these peripheral partners play complex immune roles during the SCI injury recovery (Figure 2). Consequently, a comprehensive understanding of the peripheral infiltration-induced immune response and BSCB morphological changes post-SCI might be indispensable for developing novel medical strategies for SCI treatment.
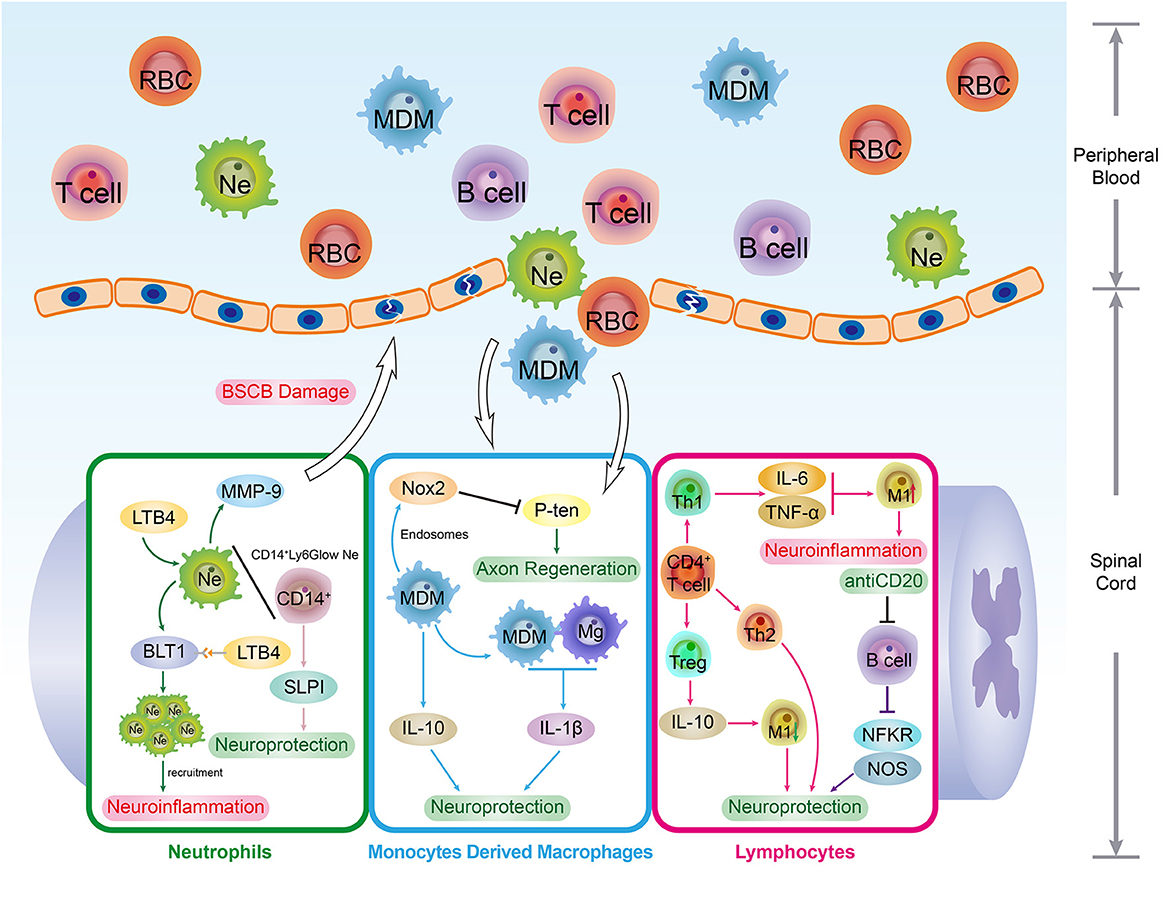
Figure 2. The immune roles of peripheral immune cells. The SCI events not only interrupt the BSCB integrity but break the intraspinal immune homeostasis. With the permeability elevation, peripheral immune cells such as neutrophils (Ne), monocytes derived macrophages (MDM) and lymphocytes are infiltrated in spinal cord lesions. In spinal cord lesions, these peripheral phagocytes. As the typical peripheral phagocytes, Ne and MDM cells also work as immune regulators in CNS. By interacting with immune factors in the spinal cord microenvironment, Ne and MDM played both positive and negative roles in regulating SCI recovery. In addition, the infiltration of lymphocytes enables the adaptive immune response to occur in the spinal cord, which provides significant immunomodulation for SCI pathological repair process. Interestingly, lymphocytes are competent in regulating the phenotype transformation of macrophages. To illustrate, the Th1 cells expressed cytokines such as TNF-α and IL-6 induce the transformation and activation of M1-type macrophages which results in extensive demyelination and neural tissue necrosis.
Peripheral neutrophils
Among these peripheral infiltrated cells, neutrophils are the first visitors that arrive at the spinal cord lesion within minutes post-injury (Wang, 2018). The typical role of the neutrophils is the phagocytic effect, which is essential for cellular and myelin debris clearance post-SCI (Zivkovic et al., 2021). Additionally, by secreting proteases, cytokines, and enzymes, neutrophils play versatile immune roles during the SCI. Studies found that inhibiting the recruitment of peripheral neutrophils and monocytes could not only reduce the oxidative stress associated with non-heme iron accumulation in the lesion but also downregulate the MMP-9 expression significantly. Under this circumstance, the long-term (28–42 days) neurological functional recovery after SCI improved markedly. In addition, a research group noted that the recruitment inhibition of neutrophils was conducive to protect the BSCB from MMP-9-induced disruption (Lee et al., 2011). Leukotriene B4 is one of the arachidonic acid metabolism productions. By interacting with neutrophils' leukotriene B4 receptor (BLT1), leukotriene B4 induces the recruitment of neutrophils. Studies found that the immunosuppressive effect of BLT1 deletion inhibited the post-injury neutrophil infiltration. Consequently, the inflammatory reaction and neuron apoptosis were limited to BLT1 deletion, thus promoting the recovery after SCI (Saiwai et al., 2010).
Although the neutrophil infiltration could exacerbate the SCI damage, recent evidence suggests that recruitment also exerts beneficial roles in the spinal cord lesion. Sas et al. discovered a novel subset of immature neutrophils, CD14+Ly6Glow, which possess neuroprotective and axon regenerative properties. The transplantation of the Ly6Glow promotes neuron survival and axon regeneration after CNS injury (Sas et al., 2020). The secretory leukocyte protease inhibitor (SLPI) is a neutrophil-produced serine protease inhibitor.
In the early stage of SCI, the SLPI's upregulation was detected in lesions, confirming the anti-inflammatory properties for neurological recovery (Zivkovic et al., 2021). In addition, by over-expressing the SLPI, Tang's group detected the neuroprotective role of SLPI post-SCI. These results suggest that the neutrophil-derived SLPI played a positive role in promoting the functional recovery of SCI (Tang et al., 2020).
Peripheral monocyte-derived macrophages
As the most prevalent glial cells in the spinal cord, the macrophages in the lesion generally originate from two sources: the spinal cord resident microglia (the innate group) and infiltrated peripheral blood monocyte-derived macrophages (the exotic group, MDM). Peripheral blood monocytes were recruited to the spinal cord lesion spontaneously post-injury and peaked at 7 days post-injury. Afterward, the differentiated descendants of infiltrated monocytes, the exotic group MDM, started to accumulate and activate onsite. Studies found that the exotic group macrophage activation is of great importance to SCI recovery. For instance, by secreting anti-inflammatory cytokines, such as IL-10, infiltrated macrophages not only essentially promoted the neurological behavior performance post-SCI but also limited the inflammation-associated nerve damage (Shechter et al., 2009).
Recent studies demonstrated that macrophage-released endosomes, such as NOX2, can be recognized and transported retrogradely to soma post-DRG conditioning lesions. Then, the NOX2 enrichment inactivated the PTEN signaling pathway via intracellular oxidation, thereby stimulating axon regeneration (Hervera et al., 2018). Additionally, Hong et al. found that the macrophage-mediated ECM remodeling capacity was promoted by polymer hydrogel (imidazole-poly-organophosphazenes) microinjection. In a study, the exogenous hydrogel stimulated the macrophage's histamine receptor-mediated MMP-9 upregulation in the lesion site, which induced the fibronectin-rich ECM formation and bridged the physical barrier at the epicenter. Consequently, the post-traumatic cystic cavities were eliminated dramatically, and the axonal reinnervation and neurological function were largely improved (Hong et al., 2017). Interestingly, the interactions between the exotic and innate groups of macrophages also play beneficial roles post-SCI. The innate microglia-mediated pathological reactions post-SCI, such as phagocytic and inflammatory response, can be limited by exotic macrophage infiltration. Moreover, the lesion MDM and microglia co-culture decreased the microglial-associated inflammatory cytokine expression, such as IL-1β, which further promoted lesion site healing and neurological functional recovery (Greenhalgh et al., 2018).
The roles of lymphocytes—Friends or foes?
The adaptive immune system constructs a crucial defense barrier against pathogen invasion via a lymphocyte-mediated immune response. Under normal conditions of the CNS, sole adaptive immune components are present in the meningeal lymphatic system (including T, B, and dendritic cells), which provide immune surveillance and protection for neurons (Louveau et al., 2015). After an SCI, the BSCB damage leads to lymphocyte infiltration, and these peripheral immune cells are spontaneously transported into the lesion microenvironment. Although a previous study found that the overall inactivation of T and B cells promoted axon regeneration and neurological functional recovery post-SCI, recent studies suggest that T and B cell-mediated adaptive immune responses exert complex effects during the repair process (Wu et al., 2012).
B cells
Mechanically, B cells exert the immune response in the CNS by mediating the antibody-associated complement activation and inflammatory cell recruitment. Pathophysiologically, the peripheral B cell populations underwent reduction owing to the surplus of glucocorticoids and norepinephrine induced by hypothalamic–pituitary–adrenal axis dysregulation post-SCI. However, due to the BSCB disruption-induced influx, the B cell elevation was detected in the spinal cord lesion site (Oropallo et al., 2014).
Giovanna et al. demonstrated that the glycoengineered anti-muCD20 antibody-mediated B cell blockade is conducive to SCI recovery. First, the inhibited pro-inflammatory factors and cytokine expression significantly decreased the neuron casualties and histological damage post-SCI, namely, NFκB, GFAP, and nitric oxide synthase. Moreover, the B cell inactivation modulated the immune response condition of other immune cells, such as T cells, NK cells, and microglial, which create the neuroprotective immune microenvironment in a lesion (Casili et al., 2016). Likewise, the B-cell knockout was demonstrated to exert neuroprotective effects against SCI, including improved locomotor recovery, decreased lesion volume, and immunoglobulin secretion. A possible explanation for neuroprotection is B cell inactivation-induced maturing of plasma cells and antibody deficiency (e.g., IgM, IgG) that are beneficial for SCI recovery (Jones, 2014).
T cells
Soon after SCI, heterogeneity of T cell influx is involved in immune microenvironment modification, such as T-helper (Th) cells, including Th1, Th2, Th17, and Treg cells (Roy and Awasthi, 2019). Catarina et al. showed that Th1 cells are indispensable for the anti-inflammatory milieu reconstruction and tissue repair post-SCI. Th1 cell deletion not only disrupts the balance between anti- and pro-inflammatory responses in the spinal cord microenvironment but cumbers the functional recovery post-SCI (Raposo et al., 2014). In addition to the beneficial functions, Th1 cell activation leads to detrimental results. Under the chronic spinal cord compression condition, Th1-expressed cytokines, such as TNF-α and IL-6, induce the transformation and activation of the M1-type macrophages. As a consequence, the detrimental inflammatory cascade in the lesion site was exacerbated by the accumulation of M1 macrophages, which gradually resulted in extensive demyelination and neural tissue necrosis (Hirai et al., 2013). Compared with Th1 cells, which have a mixed contribution, Th2 cells are conductive to SCI recovery and neuroplasticity in the SCI repair process. The myelin basic protein activated T cells (MBP-T) transition is a crucial CNS trauma-induced autoimmunity regulation, which is regarded as a protective autoimmunity mechanism during SCI. Activated MBP-T cells can be transformed into either Th1 (immunity and phagocyte activation type) or Th2 (immunity and phagocyte inactivation type) subtype under certain circumstances. He et al. found that the Th2-dominant condition in the CNS facilitates neuron survival and axon remyelination post-SCI. Moreover, promoting the MBP-T transformation from the Th1 to Th2 subtype and raising the Th2 quantity is beneficial for SCI recovery (Hu et al., 2016).
Recent studies have proven that Treg cells act as an immune orchestrator in the hostile lesion microenvironment, which can suppress the overactive immune responses in the CNS. For instance, the Nrg-1 infusion post-SCI influences the T cell phenotype transformation, which exerts immunomodulatory roles. In the Nrg-1-administrated SCI model, the Treg cell population increased remarkably in both spinal cord lesion tissue and peripheral blood (Alizadeh et al., 2018). Liesz et al. found that in CNS ischemia models, the HDAC inhibition-induced Treg cell formation and activation not only ameliorated the neuroinflammation but also promoted neurological recovery. During this process, Treg cell-expressed IL-10 suppressed the M1 microglia transformation and leukocyte activation and inhibited the pro-inflammatory cascades in the brain ischemia lesion site (Liesz et al., 2013). Interestingly, the absence of Treg cells at the subacute or chronic stage inhibited the neurological recovery following SCI. In contrast, an earlier depletion of Treg cells before the injury was beneficial for SCI repair (Raposo et al., 2014). Consequently, the Treg cell-mediated neuroprotection is still subject to the constraints of time and space during the SCI pathological process.
The immune depression post-SCI—The great recession
Cellular vitality and metabolic status are crucial for neurological recovery post-SCI. Unfortunately, during senescence, the regenerative and inflammatory response properties tend to reduce. A clinical cross-section study discovered that the immune function frailty (immunosenescence status) onset prematurely in patients with SCI. As a typical characteristic of immunosenescence, the T cells phenotype transformation from naive to memory was detected in younger patients with SCI. Under this circumstance, the secretion and proliferation capacity of T cells declined remarkably, which induced SCI-related immune deficiency (Pavlicek et al., 2017). Moreover, the cytokine expression and immune response activity of T and NK cells declined post-SCI (Schwartz and Raposo, 2014). SCI-induced immune depression syndrome (SCI-IDS) is another type of systemic maladaptive immune dysfunction post-SCI. Unlike immunosenescence, SCI-IDS is a neurogenic immune paralysis scenario. Owing to the SCI-induced immune system-associated dysautonomia, the immune response was found to be profoundly depressed. As a consequence, the SCI-IDS onset not only conjugated with leukopenia and leukocyte dysfunction but also facilitated the life-threatening infection spreading and tissue damage (Kopp et al., 2013). As the largest immune organ, the spleen is the first victim of the SCI-IDS condition, which is caused by sympathetic over-activation. Moreover, the SCI-caused spleen dysfunction deteriorated immunosuppression. For instance, the supraphysiological levels of norepinephrine in the splenic microenvironment post-SCI impaired the immune activity and cytokine secretion capacity of T cells, which accelerated the T cell exhaustion status (Zha et al., 2014). Of note, the splenic sympathectomy was shown to accomplish reversing the immune depression status post-SCI (Schwab et al., 2014).
The immune organs and SCI recovery—The familiar strangers
The spinal cord injury triggers systemic and extensive inflammatory and immune responses. Nevertheless, most studies have only paid attention to nerve regeneration and its regulation mechanisms due to the CNS centralized conception, and the rest of organs and tissues dysfunction has long been overlooked. As the development and storage places of immune cells, immune organs are indispensable for the effective operation of the immune system. Recent studies have focused on SCI-induced immune organ pathological modifications and immune function influences (Figure 3).
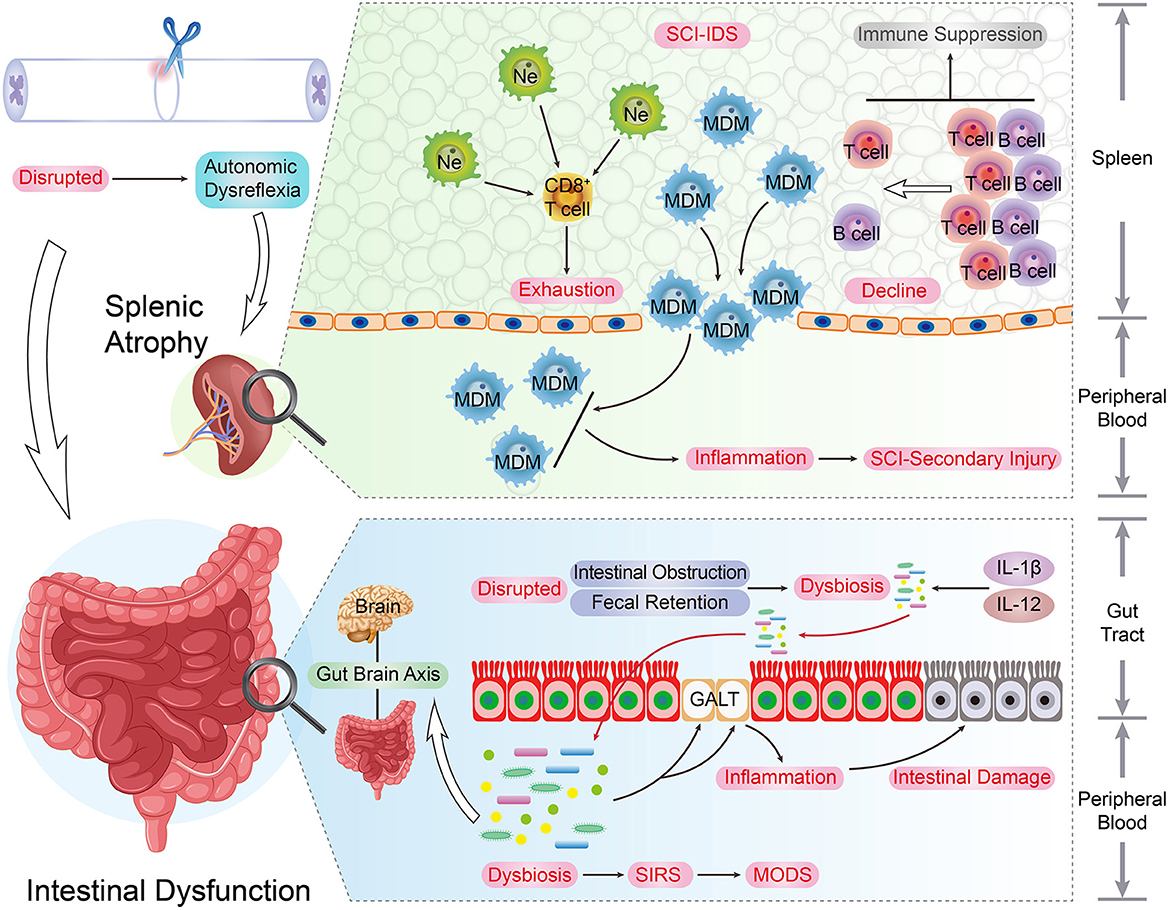
Figure 3. The immune organs and SCI recovery. As the development and storage places of immune cells, immune organs are indispensable for the immune system's smooth and effective operation. The upper spinal cord segment injury led to the autonomic dysreflexia status. Under this circumstance, the excessive norepinephrine and activated β-AR signaling result in adverse spleen dysfunction, such as splenic atrophy and immune cells secretion inhibition, and the quantity of peripheral and splenic CD4+ and CD8+ T cells underwent a decrease post-SCI. In addition, due to the surplus norepinephrine stimulation post-SCI, the immune activity and cytokine secretion capacity of splenic CD8+ T cells were impaired. These changes can be regarded as the typical hallmarks of SCI-IDS. Post SCI, the stress-induced intrinsic enteric nervous system interruption results in intestinal complications such as intestinal obstruction, fecal retention, and infections. As consequence, this situation raises the incidence of dysbiosis and induces immunity dysfunction, systemic Inflammatory response syndrome (SIRS), and multiple organ dysfunction syndromes (MODS). Moreover, the SCI-induced pro-inflammatory cytokines up-regulation (IL-1β and IL-12) takes place in the gut, and the intestinal immune factors disorder condition is parallel with beneficial bacteria reduction extent.
The spleen and SCI
The spleen is the biggest lymphatic and immune organ. Physiologically, the sympathetic nervous system manipulates the immune defense function of the spleen, such as peripheral blood leukocyte filtration. The upper spinal cord segment injury disrupts the neural connection between the upper-level inhibitory signal and post-ganglionic circuits, which leads to autonomic dysreflexia status. Under this circumstance, the excessive norepinephrine and over-activated β-AR signaling resulted in adverse spleen dysfunction, such as splenic atrophy and immune cells secretion inhibition, and these changes were recognized as the typical hallmarks of SCI-IDS (Noble et al., 2018). This was illustrated by the decline of peripheral and splenic CD4+ and CD8+ T cell quantity after SCI (Zhang et al., 2013).
The spleen is the primary storage and refinery organ of MDMs. Despite the immune cell secretion inhibition, SCI triggers the release of MDMs into the peripheral circulation. These peripheral MDMs are further infiltrated into the SCI lesion and deteriorate the inflammatory response of the SCI secondary injury. Blomster et al. noted that the SCI-induced MDM infiltration and accumulation were strongly suppressed by splenectomy, promoting neurological recovery and alleviating the immunosuppression condition (Blomster et al., 2013).
Under physiological conditions, the splenic lymphocytes are tolerant to CNS endogenous antigens. However, immune tolerance can be compromised owing to SCI-induced BSCB disruption and CNS antigen exposure. When these endogenous molecules were captured by splenic lymphocytes, the autoimmune reactions were elicited, and the roles they played depended on the characteristics of target antigens. For example, by producing neurotrophic factors and antithrombin, myelin-reactive T cells enabled the protective autoreactive immune response post-SCI (Schwartz and Raposo, 2014). In contrast, due to the norepinephrine stimulation post-SCI, the immune activity and cytokine secretion capacity of splenic CD8+ T cells were impaired (Zha et al., 2014).
The gut and SCI
The gut microbial system contains trillions of gastrointestinal resident microbes and was recently recognized as an independent organ for its self-reliant metabolism and absorption regulation functions (Fung et al., 2017). Moreover, as the key component of the gut–brain axis networks, gut microbiota exerts critical influences on CNS physiological or pathological modulation by means of neuroactive metabolites and neurotransmitters, for example, short-chain fatty acids, GABA, and dopamine. For instance, under the autoimmune encephalomyelitis condition, the transplantation of Bacteroides fragilis alleviates inflammation reaction and enhances the activity of the Treg cells (Jogia and Ruitenberg, 2020).
Post SCI, the stress-induced intrinsic enteric nervous system interruption may result in intestinal dysfunction, such as intestinal obstruction, fecal retention, and infections. Consequently, this situation raises the incidence of dysbiosis and induces holistic complications, such as immunity dysfunction, systemic inflammatory response syndrome, and multiple organ dysfunction syndromes. Remarkably, the SCI and dysbiosis form a vicious circle, and the dysbiosis triggers the mucosal immune cell activation in gut-associated lymphoid tissues (GALT), which eventually deteriorates the lesion inflammatory size and neurological impairment (Kigerl et al., 2016). In patients with traumatic SCI, although the gut microbiota diversity has reduced tremendously, bacterial abundance augment has been detected, such as the proteobacteria, bacteroides, and verrucomicrobia phyla. Interestingly, these bacterial spectrum modifications are related to the extent of neurological dysfunction of SCI (Zhang et al., 2018). Moreover, the SCI-induced pro-inflammatory cytokine upregulation (IL-1β and IL-12) takes place in the gut, and the intestinal immune factors disorder condition is parallel with the extent of reduction of beneficial bacteria (Gungor et al., 2016).
Therapeutic perspective—A silver lining
The spinal cord injury-induced catastrophic neurological disability is currently incurable. Recent studies demonstrated that comprehensive decoding and manipulation of SCI-induced immune microenvironment modification are crucial to the spinal cord's neurological recovery. Essentially, these breakthroughs propose promising immune-associated candidates for further SCI studies and clinical treatment. Generally, based on the immune checkpoints and pathological mechanisms of SCI, the immunotherapy principles should be targetable, for instance, the inflammation regulation-oriented (innate glia or WBCs) and immunomodulation-oriented (adaptive immune cells or organs) treatments.
Minocycline, as a semi-synthetic second-generation tetracycline, has been used as an antibiotic for decades. Recently, the neuroprotective characteristics of minocycline were extensively explored, which are distinct from its well-known antibiotic identity. More specifically, by inhibiting the canonical inflammatory pathway, minocycline prevents microglia activation, and thus, the secretion of inflammatory mediators and cytokines was found to be restricted (Bowes and Yip, 2014). In a randomized clinical study involving 52 patients with SCI, the neurological treatment effect and safety of minocycline were affirmed (Casha et al., 2012). Remarkably, the minocycline SCI treatment dosage was optimized to 90 mg/kg on the first-day post-injury, followed by half dosage per day (Chio et al., 2021).
At present, the roles of immune checkpoints in CNS traumatic injury-related neuroimmune and neuroinflammatory responses are finely discovered. The programmed cell death protein 1 (PD-1) is a crucial T cell-related immune checkpoint for anti-tumor immunotherapy. Recent studies found that PD-1 can be expressed by multiple SCI inflammation participants, such as Treg cells and glial cells. For instance, the M1/M2 phenotypes transformation in microglia post-SCI was closely related to PD-1 signaling activation. The PD-1 upregulation promotes the anti-inflammatory cytokine expression and drives the microglia M2 polarization. Meanwhile, the PD-1 signaling suppresses the M1 phenotype microglia formation. As a consequence, the PD-1-induced M2 microglia polarization inhibits the neuroinflammation ignition post-SCI, which is beneficial to SCI function recovery (Zhao et al., 2021); PMID: 33877518). Likewise, the programmed death-ligand 1 (PD-L1) is a vital inhibitory immune checkpoint, which was shown to regulate the neuroimmune responses in the CNS. The de novo expression of PD-L1-induced positive reactive astrocyte enrichment occurred around the lesion site of the CNS traumatic injury. With the accumulation of these reactive astrocytes, the inflammatory cell recruitment and activation were repressed, which retarded the tissue damage and neurological disability. In contrast, the PD-L1 signaling inactivation promoted inflammatory cell infiltration and deteriorated the functional outcomes of traumatic brain injury (Gao et al., 2022).
The stimulator of the interferon gene (STING) is a critical immune sensor that recognizes the foreign DNA for anti-tumor immunity (Hu et al., 2022). By regulating inflammatory factor expression, such as interferon I (IFN-I), the STING signaling pathway promotes the clearance of neoplastic and pathogens (Donnelly et al., 2021). Besides, STING facilitates the neuroimmune and inflammatory response in CNS pathophysiology disorders, which provides a novel potential strategy for SCI treatment. In an SCI lesion, STING upregulation can be detected, which is parallel with the inflammatory cytokine expression in the lesion. Wang et al. found that STING can be captured and phosphorylated by TANK-binding kinase 1 (TBK1). Thus, the downstream inflammatory pathway targets of STING, such as NF-kB and MAPK, were activated to trigger the inflammation progress (Wang et al., 2019). Instead, the STING ablation relieved the spinal cord inflammation and promoted SCI recovery via the TBK1/STING pathway.
Human immunoglobulin G (hIgG) is an effective FDA-approved immunomodulator that is widely applied in clinical CNS immunotherapy. A recent study indicated that the hIgG treatment conferred neuroprotective and immunomodulatory activities in both a dose- and time-dependent manner. Notably, studies optimized the effective dosage of hIgG (2 g/kg) to execute the immunomodulatory effects, such as inflammatory infiltration attenuation, immune cell inactivation, and vascular integrity improvement (Chio et al., 2019).
Notwithstanding these promising immunotherapy breakthroughs, owing to the BSCB isolation, effective drug penetration and spinal targeting approach remain to be adequately explored. With developments in nanodrug loading technologies, effective immunotherapy drugs can be delivered to the lesion site, which would endow SCI clinical treatment with favorable weapons (e.g., the novel synthesized lesion targeting nanoparticles named triblock copolymer-MMPs targeting peptide [PPP-ACPP]). In a study, the etanercept (a well-established TNF-α antibody, ET) was released and accumulated into the lesion site via the ET- PPP-ACPP loading manner. Thus, the SCI epicenter ET enrichment not only decreased the M1-type macrophage quantity but also alleviated the secondary injury-associated inflammation cascade (Shen et al., 2021).
Recently, a novel subset of neutrophils (the N2 cells) with neuroprotective capacities was identified, providing a promising candidate for neurorestorative immunotherapies. Unlike the traditional neutrophils, these novel N2 neutrophils and CD14+ cells are unable to produce pro-inflammatory cytokines and chemokines. Conversely, N2 cells induce a robust axon regeneration post-SCI via a dependent manner, such as NGF and IGF1 (Sas et al., 2020).
We know that SCI-induced intestinal dysfunction deteriorates immune disorders. Unfortunately, SCI-caused sensory deficiency makes these gut complications more undetectable, which aggravates gut microbiota dysfunction and dysbiosis. Consequently, timely gut management is essential for gut barrier strengthening and complication reduction post-SCI. A recent study reported that an earlier oral administration of a probiotic cocktail (named VSL#3, eight probiotic strains enriched mixture) not only benefits the gut lactic acid–producing bacteria abundance elevation but also promotes neurological recovery (Kigerl et al., 2016). Another study in rodents indicates that timely oral feeding of diluted fecal matter from healthy counterparts may rescue SCI-induced dysbiosis, intestinal permeability disorder, and neurological disability via a short-chain fatty acids anti-inflammation manner (Jing et al., 2021). Consequently, compared with sophisticated surgery operations and complicated immunotherapy, these gut-associated breakthroughs provide promising SCI treatment that is more feasible for patient daily care scenarios, for example, the oral administration agents of gut microbiota.
Credible biomarkers to predict clinical outcomes are crucial and desirable. Unfortunately, owing to the complicated pathological changes of SCI, establishing a reliable prognosis system is a challenging task. As the early infiltrated immune cells, the neutrophils are essential for the immunomodulation and inflammation reaction of SCI. Recently, a retrospective study involving 163 patients found that acute neutrophilia (circulating neutrophil > 7.7–8.0 × 109 cells/L) post-SCI was positively correlated with the severity of neurological impairment. Moreover, the ratios of peripheral blood neutrophils and lymphocytes were demonstrated to be closely related to patient respiratory system infection (Jogia et al., 2021).
Conclusion
Traditionally, owing to the BSCB seemingly creating an “immune privileged” area, immune elements tend to be treated as “hidden players” in SCI, which garnered less attention in previous studies. However, recent studies present the panoramic immune landscape of SCI pathophysiological changes, providing a deep understanding of how immune components modulate the SCI recovery process (Figure 4). Around the spinal cord epicenter, the SCI-associated immune response can be immediately aroused in either an innate or adaptive manner.
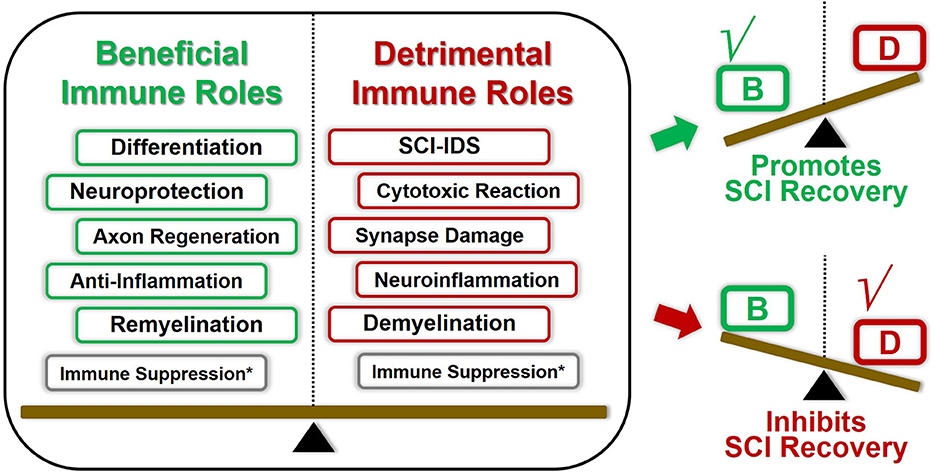
Figure 4. Double-edged immune factors modulate the overall immune landscape SCI recovery. Most of these aforementioned immune factors played both beneficial and harmful roles in regulating SCI pathological repair process. The overall outcomes of SCI pathological repair are the superimposed results of multiple immune elements. For instance, once the beneficial immune roles overcome these detrimental counterparts under certain conditions, the overall immunomodulation tent to promote SCI repair, and vice versa. Consequently, coordinating the panoramic immune landscape of SCI pathophysiological changes will provide us deepening understanding of how immune factors modulate the SCI recovery process. *The immunosuppression effects could be both beneficial and detrimental toward SCI recovery, which presents various characteristics due to the spatiotemporal difference.
These activated immune cell-produced immune molecules and antibodies modulated the immune organ's function and even induced immunosuppression conditions. Remarkably, the aforementioned neuroimmune regulation mechanisms are likely to play both beneficial and harmful roles in governing the SCI pathological repair process (Table 2). First, the same categories of immune cells tend to present various characteristics owing to the spatiotemporal difference. In addition, the overall outcomes of SCI pathological repair are the superimposed results of multiple aspects of immunomodulation. Consequently, favorable orchestration of these pros and cons aspects of neuroimmune factors is prone to turn the injured spinal cord into a regenerative competent state, eventually promoting SCI recovery. Even so, specific immune mechanisms underlying SCI remain to be illustrated.
Moreover, some technical challenges are expected to be solved, for instance, the stable and safe loading-delivery system and the specific cellular or organ of targeting technique. Based on these favorable breakthroughs, it is reasonable to believe that these uncertainties will be solved gradually by deepening the understanding of SCI-associated neuroimmune mechanisms. Altogether, further exploring immune-based mechanisms and treatment targets will broaden SCI clinical therapy perspectives.
Author contributions
LZ-G: drafting figure and writing section–The immune roles of spinal cord glial cells–Far beyond the glia. YF: writing sections–The immune roles of peripheral immune cells–The unfavorable guys? and The roles of lymphocytes–Friends or foes?. CP: writing section–The immune depression post post-SCI–The great recession and revising the manuscript. ZB-Y: conceptualization, writing sections–The immune organs and SCI recovery–The familiar strangers, Therapeutic perspective–A silver lining, and conclusion and revising the manuscript. All authors contributed to the article and approved the submitted version.
Funding
This work was supported by Jilin Province Health and Health Young Scientist Cultivation Program (2020Q020, ZB-Y), Jilin Province Science and Technology Department Grant (20200201436JC, ZB-Y), Jilin Province Health and Health Young Scientist Special Program (JLSWSRCZX 2020-0093, CP), Jilin Provincial Department of Education Science and Technology Research Planning Project (JJKH20211181KJ, SJ), and National Natural Science Foundation of China (NSFC, 81874254, CP).
Conflict of interest
The authors declare that the research was conducted in the absence of any commercial or financial relationships that could be construed as a potential conflict of interest.
Publisher's note
All claims expressed in this article are solely those of the authors and do not necessarily represent those of their affiliated organizations, or those of the publisher, the editors and the reviewers. Any product that may be evaluated in this article, or claim that may be made by its manufacturer, is not guaranteed or endorsed by the publisher.
References
Al Mamun, A., Monalisa, I., Tul Kubra, K., Akter, A., Akter, J., Sarker, T., et al. (2021). Advances in immunotherapy for the treatment of spinal cord injury. Immunobiology 226, 152033. doi: 10.1016/j.imbio.2020.152033
Alizadeh, A., Santhosh, K. T., Kataria, H., Gounni, A. S., and Karimi-Abdolrezaee, S. (2018). Neuregulin-1 elicits a regulatory immune response following traumatic spinal cord injury. J. Neuroinflammation 15, 53. doi: 10.1186/s12974-018-1093-9
Andersen, R. S., Anand, A., Harwood, D. S. L., and Kristensen, B. W. (2021). Tumor-associated microglia and macrophages in the glioblastoma microenvironment and their implications for therapy. Cancers 13:4255. doi: 10.3390/cancers13174255
Aubé, B., Lévesque, S. A., Paré, A., Chamma, É., Kébir, H., Gorina, R., et al. (2014). Neutrophils mediate blood-spinal cord barrier disruption in demyelinating neuroinflammatory diseases. J. Immunol. 193, 2438–2454. doi: 10.4049/jimmunol.1400401
Bellver-Landete, V., Bretheau, F., Mailhot, B., Vallières, N., Lessard, M., Janelle, M. E., et al. (2019). Microglia are an essential component of the neuroprotective scar that forms after spinal cord injury. Nat. Commun. 10, 518. doi: 10.1038/s41467-019-08446-0
Blomster, L. V., Brennan, F. H., Lao, H. W., Harle, D. W., Harvey, A. R., and Ruitenberg, M. J. (2013). Mobilisation of the splenic monocyte reservoir and peripheral CX3CR1 deficiency adversely affects recovery from spinal cord injury. Exp. Neurol. 247, 226–240. doi: 10.1016/j.expneurol.2013.05.002
Bowes, A. L., and Yip, P. K. (2014). Modulating inflammatory cell responses to spinal cord injury: all in good time. J. Neurotrauma 31, 1753–1766. doi: 10.1089/neu.2014.3429
Bo-Yin, Z., Qingsan, Z., Yihang, M., Fan, Y., Yuhang, Z., and Pengyu, C. (2021). Unlocking the recovery potential: JMJD3 inhibition-mediated SAPK/JNK signaling inactivation supports endogenous oligodendrocyte-lineage commitment post mammalian spinal cord injury. Neurochem. Res. 46, 792–803. doi: 10.1007/s11064-020-03210-z
Brennan, F. H., Gordon, R., Lao, H. W., Biggins, P. J., Taylor, S. M., Franklin, R. J., et al. (2015). The complement receptor C5aR controls acute inflammation and astrogliosis following spinal cord injury. J. Neurosci. 35, 6517–6531. doi: 10.1523/jneurosci.5218-14.2015
Cardoso, F. L., Brites, D., and Brito, M. A. (2010). Looking at the blood-brain barrier: molecular anatomy and possible investigation approaches. Brain Res. Rev. 64, 328–363. doi: 10.1016/j.brainresrev.2010.05.003
Casha, S., Zygun, D., McGowan, M. D., Bains, I., Yong, V. W., and Hurlbert, R. J. (2012). Results of a phase II placebo-controlled randomized trial of minocycline in acute spinal cord injury. Brain 135(Pt 4), 1224–1236. doi: 10.1093/brain/aws072
Casili, G., Impellizzeri, D., Cordaro, M., Esposito, E., and Cuzzocrea, S. (2016). B-cell depletion with CD20 antibodies as new approach in the treatment of inflammatory and immunological events associated with spinal cord injury. Neurotherapeutics 13, 880–894. doi: 10.1007/s13311-016-0446-2
Chio, J. C. T., Wang, J., Badner, A., Hong, J., Surendran, V., and Fehlings, M. G. (2019). The effects of human immunoglobulin G on enhancing tissue protection and neurobehavioral recovery after traumatic cervical spinal cord injury are mediated through the neurovascular unit. J. Neuroinflammation 16, 141. doi: 10.1186/s12974-019-1518-0
Chio, J. C. T., Xu, K. J., Popovich, P., David, S., and Fehlings, M. G. (2021). Neuroimmunological therapies for treating spinal cord injury: evidence and future perspectives. Exp. Neurol. 341, 113704. doi: 10.1016/j.expneurol.2021.113704
Cowan, M., and Petri, W. A. Jr. (2018). Microglia: immune regulators of neurodevelopment. Front. Immunol. 9, 2576. doi: 10.3389/fimmu.2018.02576
Dahlgren, M. W., and Molofsky, A. B. (2019). Adventitial cuffs: regional hubs for tissue immunity. Trends Immunol. 40, 877–887. doi: 10.1016/j.it.2019.08.002
Donnelly, C. R., Jiang, C., Andriessen, A. S., Wang, K., Wang, Z., Ding, H., et al. (2021). STING controls nociception via type I interferon signalling in sensory neurons. Nature 591, 275–280. doi: 10.1038/s41586-020-03151-1
Dulamea, A. O. (2017). Role of oligodendrocyte dysfunction in demyelination, remyelination and neurodegeneration in multiple sclerosis. Adv. Exp. Med. Biol. 958, 91–127. doi: 10.1007/978-3-319-47861-6_7
Feigin, V. L., Nichols, E., Alam, T., Bannick, M. S., Beghi, E., Blake, N., et al. (2019). Global, regional, and national burden of neurological disorders, 1990–2016: a systematic analysis for the Global Burden of Disease Study 2016. Lancet Neurol. 18, 459–480. doi: 10.1016/s1474-4422(18)30499-x
Filipello, F., Pozzi, D., Proietti, M., Romagnani, A., Mazzitelli, S., Matteoli, M., et al. (2016). Ectonucleotidase activity and immunosuppression in astrocyte-CD4 T cell bidirectional signaling. Oncotarget 7, 5143–5156. doi: 10.18632/oncotarget.6914
Fung, T. C., Olson, C. A., and Hsiao, E. Y. (2017). Interactions between the microbiota, immune and nervous systems in health and disease. Nat. Neurosci. 20, 145–155. doi: 10.1038/nn.4476
Gao, X., Li, W., Syed, F., Yuan, F., Li, P., and Yu, Q. (2022). PD-L1 signaling in reactive astrocytes counteracts neuroinflammation and ameliorates neuronal damage after traumatic brain injury. J. Neuroinflammation 19, 43. doi: 10.1186/s12974-022-02398-x
Greenhalgh, A. D., Zarruk, J. G., Healy, L. M., Baskar Jesudasan, S. J., Jhelum, P., Salmon, C. K., et al. (2018). Peripherally derived macrophages modulate microglial function to reduce inflammation after CNS injury. PLoS Biol. 16, e2005264. doi: 10.1371/journal.pbio.2005264
Gungor, B., Adiguzel, E., Gursel, I., Yilmaz, B., and Gursel, M. (2016). Intestinal microbiota in patients with spinal cord injury. PLoS ONE 11, e0145878. doi: 10.1371/journal.pone.0145878
Hagemeyer, N., Hanft, K. M., Akriditou, M. A., Unger, N., Park, E. S., Stanley, E. R., et al. (2017). Microglia contribute to normal myelinogenesis and to oligodendrocyte progenitor maintenance during adulthood. Acta Neuropathol. 134, 441–458. doi: 10.1007/s00401-017-1747-1
Hambardzumyan, D., Gutmann, D. H., and Kettenmann, H. (2016). The role of microglia and macrophages in glioma maintenance and progression. Nat. Neurosci. 19, 20–27. doi: 10.1038/nn.4185
Heng, Y., Zhang, X., Borggrewe, M., van Weering, H. R. J., Brummer, M. L., Nijboer, T. W., et al. (2021). Systemic administration of β-glucan induces immune training in microglia. J. Neuroinflammation 18, 57. doi: 10.1186/s12974-021-02103-4
Hervera, A., De Virgiliis, F., Palmisano, I., Zhou, L., Tantardini, E., Kong, G., et al. (2018). Reactive oxygen species regulate axonal regeneration through the release of exosomal NADPH oxidase 2 complexes into injured axons. Nat. Cell Biol. 20, 307–319. doi: 10.1038/s41556-018-0039-x
Hirai, T., Uchida, K., Nakajima, H., Guerrero, A. R., Takeura, N., Watanabe, S., et al. (2013). The prevalence and phenotype of activated microglia/macrophages within the spinal cord of the hyperostotic mouse (twy/twy) changes in response to chronic progressive spinal cord compression: implications for human cervical compressive myelopathy. PLoS ONE 8, e64528. doi: 10.1371/journal.pone.0064528
Hong, L. T. A., Kim, Y. M., Park, H. H., Hwang, D. H., Cui, Y., Lee, E. M., et al. (2017). An injectable hydrogel enhances tissue repair after spinal cord injury by promoting extracellular matrix remodeling. Nat. Commun. 8, 533. doi: 10.1038/s41467-017-00583-8
Hong, S., Beja-Glasser, V. F., Nfonoyim, B. M., Frouin, A., Li, S., Ramakrishnan, S., et al. (2016). Complement and microglia mediate early synapse loss in Alzheimer mouse models. Science 352, 712–716. doi: 10.1126/science.aad8373
Horng, S., Therattil, A., Moyon, S., Gordon, A., Kim, K., Argaw, A. T., et al. (2017). Astrocytic tight junctions control inflammatory CNS lesion pathogenesis. J. Clin. Invest. 127, 3136–3151. doi: 10.1172/jci91301
Hu, J. G., Shi, L. L., Chen, Y. J., Xie, X. M., Zhang, N., Zhu, A. Y., et al. (2016). Differential effects of myelin basic protein-activated Th1 and Th2 cells on the local immune microenvironment of injured spinal cord. Exp. Neurol. 277, 190–201. doi: 10.1016/j.expneurol.2016.01.002
Hu, X., Zhang, H., Zhang, Q., Yao, X., Ni, W., and Zhou, K. (2022). Emerging role of STING signalling in CNS injury: inflammation, autophagy, necroptosis, ferroptosis and pyroptosis. J. Neuroinflammation 19, 242. doi: 10.1186/s12974-022-02602-y
Jin, L. Y., Li, J., Wang, K. F., Xia, W. W., Zhu, Z. Q., Wang, C. R., et al. (2021). Blood-spinal cord barrier in spinal cord injury: a review. J. Neurotrauma 38, 1203–1224. doi: 10.1089/neu.2020.7413
Jing, Y., Yu, Y., Bai, F., Wang, L., Yang, D., Zhang, C., et al. (2021). Effect of fecal microbiota transplantation on neurological restoration in a spinal cord injury mouse model: involvement of brain-gut axis. Microbiome 9, 59. doi: 10.1186/s40168-021-01007-y
Jogia, T., Lübstorf, T., Jacobson, E., Scriven, E., Atresh, S., Nguyen, Q. H., et al. (2021). Prognostic value of early leukocyte fluctuations for recovery from traumatic spinal cord injury. Clin. Transl. Med. 11, e272. doi: 10.1002/ctm2.272
Jogia, T., and Ruitenberg, M. J. (2020). Traumatic spinal cord injury and the gut microbiota: current insights and future challenges. Front. Immunol. 11, 704. doi: 10.3389/fimmu.2020.00704
Jones, T. B. (2014). Lymphocytes and autoimmunity after spinal cord injury. Exp. Neurol. 258, 78–90. doi: 10.1016/j.expneurol.2014.03.003
Joshi, A. U., Minhas, P. S., Liddelow, S. A., Haileselassie, B., Andreasson, K. I., Dorn, G. W. II, et al. (2019). Fragmented mitochondria released from microglia trigger A1 astrocytic response and propagate inflammatory neurodegeneration. Nat. Neurosci. 22, 1635–1648. doi: 10.1038/s41593-019-0486-0
Kigerl, K. A., de Rivero Vaccari, J. P., Dietrich, W. D., Popovich, P. G., and Keane, R. W. (2014). Pattern recognition receptors and central nervous system repair. Exp. Neurol. 258, 5–16. doi: 10.1016/j.expneurol.2014.01.001
Kigerl, K. A., Hall, J. C., Wang, L., Mo, X., Yu, Z., and Popovich, P. G. (2016). Gut dysbiosis impairs recovery after spinal cord injury. J. Exp. Med. 213, 2603–2620. doi: 10.1084/jem.20151345
Kirby, L., Jin, J., Cardona, J. G., Smith, M. D., Martin, K. A., Wang, J., et al. (2019). Oligodendrocyte precursor cells present antigen and are cytotoxic targets in inflammatory demyelination. Nat. Commun. 10, 3887. doi: 10.1038/s41467-019-11638-3
Koh, H. S., Chang, C. Y., Jeon, S. B., Yoon, H. J., Ahn, Y. H., Kim, H. S., et al. (2015). The HIF-1/glial TIM-3 axis controls inflammation-associated brain damage under hypoxia. Nat. Commun. 6, 6340. doi: 10.1038/ncomms7340
Koning, N., Swaab, D. F., Hoek, R. M., and Huitinga, I. (2009). Distribution of the immune inhibitory molecules CD200 and CD200R in the normal central nervous system and multiple sclerosis lesions suggests neuron-glia and glia-glia interactions. J. Neuropathol. Exp. Neurol. 68, 159–167. doi: 10.1097/NEN.0b013e3181964113
Kopp, M. A., Druschel, C., Meisel, C., Liebscher, T., Prilipp, E., Watzlawick, R., et al. (2013). The SCIentinel study–prospective multicenter study to define the spinal cord injury-induced immune depression syndrome (SCI-IDS)–study protocol and interim feasibility data. BMC Neurol. 13, 168. doi: 10.1186/1471-2377-13-168
Lee, S. M., Rosen, S., Weinstein, P., van Rooijen, N., and Noble-Haeusslein, L. J. (2011). Prevention of both neutrophil and monocyte recruitment promotes recovery after spinal cord injury. J. Neurotrauma 28, 1893–1907. doi: 10.1089/neu.2011.1860
Li, M., Li, Z., Yao, Y., Jin, W. N., Wood, K., Liu, Q., et al. (2017). Astrocyte-derived interleukin-15 exacerbates ischemic brain injury via propagation of cellular immunity. Proc. Natl. Acad. Sci. U.S.A. 114, E396–e405. doi: 10.1073/pnas.1612930114
Liesz, A., Zhou, W., Na, S. Y., Hämmerling, G. J., Garbi, N., Karcher, S., et al. (2013). Boosting regulatory T cells limits neuroinflammation in permanent cortical stroke. J. Neurosci. 33, 17350–17362. doi: 10.1523/jneurosci.4901-12.2013
Liu, C., Liu, J., Liu, C., Zhou, Q., Zhou, Y., Zhang, B., et al. (2021). The intrinsic axon regenerative properties of mature neurons after injury. Acta Biochim. Biophys. Sin. 53, 1–9. doi: 10.1093/abbs/gmaa148
Liu, X., Zhou, F., Yang, Y., Wang, W., Niu, L., Zuo, D., et al. (2019). MiR-409-3p and MiR-1896 co-operatively participate in IL-17-induced inflammatory cytokine production in astrocytes and pathogenesis of EAE mice via targeting SOCS3/STAT3 signaling. Glia 67, 101–112. doi: 10.1002/glia.23530
Lo, J., Chan, L., and Flynn, S. (2021). A systematic review of the incidence, prevalence, costs, and activity and work limitations of amputation, osteoarthritis, rheumatoid arthritis, back pain, multiple sclerosis, spinal cord injury, stroke, and traumatic brain injury in the United States: a 2019 update. Arch. Phys. Med. Rehabil. 102, 115–131. doi: 10.1016/j.apmr.2020.04.001
Louveau, A., Harris, T. H., and Kipnis, J. (2015). Revisiting the mechanisms of CNS immune privilege. Trends Immunol. 36, 569–577. doi: 10.1016/j.it.2015.08.006
Miron, V. E., Boyd, A., Zhao, J. W., Yuen, T. J., Ruckh, J. M., Shadrach, J. L., et al. (2013). M2 microglia and macrophages drive oligodendrocyte differentiation during CNS remyelination. Nat. Neurosci. 16, 1211–1218. doi: 10.1038/nn.3469
Noble, B. T., Brennan, F. H., and Popovich, P. G. (2018). The spleen as a neuroimmune interface after spinal cord injury. J. Neuroimmunol. 321, 1–11. doi: 10.1016/j.jneuroim.2018.05.007
Oropallo, M. A., Goenka, R., and Cancro, M. P. (2014). Spinal cord injury impacts B cell production, homeostasis, and activation. Semin. Immunol. 26, 421–427. doi: 10.1016/j.smim.2014.09.014
Pavlicek, D., Krebs, J., Capossela, S., Bertolo, A., Engelhardt, B., Pannek, J., et al. (2017). Immunosenescence in persons with spinal cord injury in relation to urinary tract infections -a cross-sectional study. Immun. Ageing 14, 22. doi: 10.1186/s12979-017-0103-6
Peferoen, L., Kipp, M., van der Valk, P., van Noort, J. M., and Amor, S. (2014). Oligodendrocyte-microglia cross-talk in the central nervous system. Immunology 141, 302–313. doi: 10.1111/imm.12163
Poliani, P. L., Wang, Y., Fontana, E., Robinette, M. L., Yamanishi, Y., Gilfillan, S., et al. (2015). TREM2 sustains microglial expansion during aging and response to demyelination. J. Clin. Invest. 125, 2161–2170. doi: 10.1172/jci77983
Raposo, C., Graubardt, N., Cohen, M., Eitan, C., London, A., Berkutzki, T., et al. (2014). CNS repair requires both effector and regulatory T cells with distinct temporal and spatial profiles. J. Neurosci. 34, 10141–10155. doi: 10.1523/jneurosci.0076-14.2014
Roy, S., and Awasthi, A. (2019). Emerging roles of noncoding RNAs in T cell differentiation and functions in autoimmune diseases. Int. Rev. Immunol. 38, 232–245. doi: 10.1080/08830185.2019.1648454
Saiwai, H., Ohkawa, Y., Yamada, H., Kumamaru, H., Harada, A., Okano, H., et al. (2010). The LTB4-BLT1 axis mediates neutrophil infiltration and secondary injury in experimental spinal cord injury. Am. J. Pathol. 176, 2352–2366. doi: 10.2353/ajpath.2010.090839
Sas, A. R., Carbajal, K. S., Jerome, A. D., Menon, R., Yoon, C., Kalinski, A. L., et al. (2020). A new neutrophil subset promotes CNS neuron survival and axon regeneration. Nat. Immunol. 21, 1496–1505. doi: 10.1038/s41590-020-00813-0
Schwab, J. M., Zhang, Y., Kopp, M. A., Brommer, B., and Popovich, P. G. (2014). The paradox of chronic neuroinflammation, systemic immune suppression, autoimmunity after traumatic chronic spinal cord injury. Exp. Neurol. 258, 121–129. doi: 10.1016/j.expneurol.2014.04.023
Schwartz, M., and Raposo, C. (2014). Protective autoimmunity: a unifying model for the immune network involved in CNS repair. Neuroscientist 20, 343–358. doi: 10.1177/1073858413516799
Shechter, R., London, A., Varol, C., Raposo, C., Cusimano, M., Yovel, G., et al. (2009). Infiltrating blood-derived macrophages are vital cells playing an anti-inflammatory role in recovery from spinal cord injury in mice. PLoS Med. 6, e1000113. doi: 10.1371/journal.pmed.1000113
Shen, K., Sun, G., Chan, L., He, L., Li, X., Yang, S., et al. (2021). Anti-inflammatory nanotherapeutics by targeting matrix metalloproteinases for immunotherapy of spinal cord injury. Small 17, e2102102. doi: 10.1002/smll.202102102
Shinozaki, Y., Shibata, K., Yoshida, K., Shigetomi, E., Gachet, C., Ikenaka, K., et al. (2017). Transformation of astrocytes to a neuroprotective phenotype by microglia via P2Y(1) receptor downregulation. Cell Rep. 19, 1151–1164. doi: 10.1016/j.celrep.2017.04.047
Sierra, A., Encinas, J. M., Deudero, J. J., Chancey, J. H., Enikolopov, G., Overstreet-Wadiche, L. S., et al. (2010). Microglia shape adult hippocampal neurogenesis through apoptosis-coupled phagocytosis. Cell Stem Cell 7, 483–495. doi: 10.1016/j.stem.2010.08.014
Tang, R., Botchway, B. O. A., Meng, Y., Zhang, Y., Zhou, C., Jiang, J., et al. (2020). The inhibition of inflammatory signaling pathway by secretory leukocyte protease inhibitor can improve spinal cord injury. Cell Mol. Neurobiol. 40, 1067–1073. doi: 10.1007/s10571-020-00799-1
Wang, H. W., Zhu, X. L., Qin, L. M., Qian, H. J., and Wang, Y. (2015). Microglia activity modulated by T cell Ig and mucin domain protein 3 (Tim-3). Cell Immunol. 293, 49–58. doi: 10.1016/j.cellimm.2014.12.005
Wang, J. (2018). Neutrophils in tissue injury and repair. Cell Tissue Res 371, 531–539. doi: 10.1007/s00441-017-2785-7
Wang, Y. Y., Shen, D., Zhao, L. J., Zeng, N., and Hu, T. H. (2019). Sting is a critical regulator of spinal cord injury by regulating microglial inflammation via interacting with TBK1 in mice. Biochem. Biophys. Res. Commun. 517, 741–748. doi: 10.1016/j.bbrc.2019.07.125
Wu, B., Matic, D., Djogo, N., Szpotowicz, E., Schachner, M., and Jakovcevski, I. (2012). Improved regeneration after spinal cord injury in mice lacking functional T- and B-lymphocytes. Exp. Neurol. 237, 274–285. doi: 10.1016/j.expneurol.2012.07.016
Xu, S., Lu, J., Shao, A., Zhang, J. H., and Zhang, J. (2020). Glial cells: role of the immune response in ischemic stroke. Front. Immunol. 11, 294. doi: 10.3389/fimmu.2020.00294
Yang, Y, Liu, H, Zhang, H, Ye, Q, Wang, J, and Yang, B. (2017). ST2/IL-33-Dependent microglial response limits acute ischemic brain injury. J. Neurosci. 37:4692–704. doi: 10.1523/JNEUROSCI.3233-16.2017
Zaguia, F., Saikali, P., Ludwin, S., Newcombe, J., Beauseigle, D., McCrea, E., et al. (2013). Cytotoxic NKG2C+ CD4 T cells target oligodendrocytes in multiple sclerosis. J. Immunol. 190, 2510–2518. doi: 10.4049/jimmunol.1202725
Zha, J., Smith, A., Andreansky, S., Bracchi-Ricard, V., and Bethea, J. R. (2014). Chronic thoracic spinal cord injury impairs CD8+ T-cell function by up-regulating programmed cell death-1 expression. J. Neuroinflammation 11, 65. doi: 10.1186/1742-2094-11-65
Zhang, B. J., Men, X. J., Lu, Z. Q., Li, H. Y., Qiu, W., and Hu, X. Q. (2013). Splenectomy protects experimental rats from cerebral damage after stroke due to anti-inflammatory effects. Chin. Med. J. 126, 2354–2360. doi: 10.3760/cma.j.issn.0366-6999.20122483
Zhang, B. Y., Chang, P. Y., Zhu, Q. S., and Zhu, Y. H. (2020). Decoding epigenetic codes: new frontiers in exploring recovery from spinal cord injury. Neural Regen. Res. 15, 1613–1622. doi: 10.4103/1673-5374.276323
Zhang, C., Zhang, W., Zhang, J., Jing, Y., Yang, M., Du, L., et al. (2018). Gut microbiota dysbiosis in male patients with chronic traumatic complete spinal cord injury. J. Transl. Med. 16, 353. doi: 10.1186/s12967-018-1735-9
Zhang, L. Y., Pan, J., Mamtilahun, M., Zhu, Y., Wang, L., Venkatesh, A., et al. (2020). Microglia exacerbate white matter injury via complement C3/C3aR pathway after hypoperfusion. Theranostics 10, 74–90. doi: 10.7150/thno.35841
Zhang, X., Kracht, L., Lerario, A. M., Dubbelaar, M. L., Brouwer, N., Wesseling, E. M., et al. (2022). Epigenetic regulation of innate immune memory in microglia. J. Neuroinflammation 19, 111. doi: 10.1186/s12974-022-02463-5
Zhao, J., Roberts, A., Wang, Z., Savage, J., and Ji, R. R. (2021). Emerging role of PD-1 in the central nervous system and brain diseases. Neurosci. Bull. 37, 1188–1202. doi: 10.1007/s12264-021-00683-y
Keywords: spinal cord injury, central nervous system, immune cells, glial cells, immunotherapy
Citation: Zhen-Gang L, Fan Y, Jingwei S, Pengyu C, Shengman Y and Bo-Yin Z (2022) Revisiting the immune landscape post spinal cord injury: More than black and white. Front. Aging Neurosci. 14:963539. doi: 10.3389/fnagi.2022.963539
Received: 07 June 2022; Accepted: 10 November 2022;
Published: 07 December 2022.
Edited by:
Ning Song, Qingdao University, ChinaCopyright © 2022 Zhen-Gang, Fan, Jingwei, Pengyu, Shengman and Bo-Yin. This is an open-access article distributed under the terms of the Creative Commons Attribution License (CC BY). The use, distribution or reproduction in other forums is permitted, provided the original author(s) and the copyright owner(s) are credited and that the original publication in this journal is cited, in accordance with accepted academic practice. No use, distribution or reproduction is permitted which does not comply with these terms.
*Correspondence: Zhang Bo-Yin, ZHJib3lpbkBqbHUuZWR1LmNu
†These authors have contributed equally to this work and share first authorship