- 1Department of Neurology, Weill Cornell Medical College, New York, NY, United States
- 2Department of Radiology, Weill Cornell Medical College, New York, NY, United States
- 3Department of Pharmacology, University of Arizona, Tucson, AZ, United States
- 4Department of Neurology, University of Arizona, Tucson, AZ, United States
Ovarian hormones, particularly 17β-estradiol, are involved in numerous neurophysiological and neurochemical processes, including those subserving cognitive function. Estradiol plays a key role in the neurobiology of aging, in part due to extensive interconnectivity of the neural and endocrine system. This aspect of aging is fundamental for women’s brains as all women experience a drop in circulating estradiol levels in midlife, after menopause. Given the importance of estradiol for brain function, it is not surprising that up to 80% of peri-menopausal and post-menopausal women report neurological symptoms including changes in thermoregulation (vasomotor symptoms), mood, sleep, and cognitive performance. Preclinical evidence for neuroprotective effects of 17β-estradiol also indicate associations between menopause, cognitive aging, and Alzheimer’s disease (AD), the most common cause of dementia affecting nearly twice more women than men. Brain imaging studies demonstrated that middle-aged women exhibit increased indicators of AD endophenotype as compared to men of the same age, with onset in perimenopause. Herein, we take a translational approach to illustrate the contribution of ovarian hormones in maintaining cognition in women, with evidence implicating menopause-related declines in 17β-estradiol in cognitive aging and AD risk. We will review research focused on the role of endogenous and exogenous estrogen exposure as a key underlying mechanism to neuropathological aging in women, with a focus on whether brain structure, function and neurochemistry respond to hormone treatment. While still in development, this research area offers a new sex-based perspective on brain aging and risk of AD, while also highlighting an urgent need for better integration between neurology, psychiatry, and women’s health practices.
Introduction
Sex is a genetic modifier of brain aging and risk of neurodegenerative disease
Sex differences in disease prevalence, manifestation, and response to treatment are rooted in genetic and hormonal differences between men and women. The effects of sex on neural aging phenotypes are often as large as, if not larger than the effects of other important variables (Cahill, 2006). In fact, female sex is the second greatest risk factor for late-onset Alzheimer’s disease (AD), second only to the aging process itself (Farrer et al., 1997). Moreover, susceptibility to aging-related neurodegenerative diseases and mental health conditions is greater in women than men, whereas men exhibit higher rates neuropsychiatric and learning disorders with developmental origins (Jazin and Cahill, 2010; McCarthy, 2016; Mauvais-Jarvis et al., 2020).
For decades, the general mindset was that sex differences in brain structure and function were controlled by a unitary program: genetic sex as the determinant of gonadal sex, and gonadal hormones as the determinants of brain sexual differentiation and subsequent neurophysiological and behavioral outcomes (McCarthy, 2016). Evidence has accumulated that numerous sex-specific factors, including hormonal, but also genetic and environment-driven epigenetic mechanisms, act in concert to provoke or eliminate sex differences in brain (McCarthy et al., 2009; Giatti et al., 2019). The combination of all these genetic and hormonal variables generates two different neurobiological systems in men and women. Starting at puberty, cells with androgen or estrogen receptors will be affected differently in men and women (McEwen, 2002), eliciting differences in disease predisposition, manifestation, and response to treatment. Overall, genetic sex is an important modifier of neurophysiology and neuropathology via genetic, epigenetic, and hormonal regulations (Cahill, 2006).
The value of understanding sex differences in brain aging and neurodegenerative disease is as self-evident as it is underappreciated. Historically, for multiple reasons, including the purported safety of women and their offspring, women of childbearing age were excluded from clinical trials (Clayton, 2016). As a result, for several decades, evidence-based medicine was defined by male physiology. In 1993, the US National Institutes of Health (NIH) mandated the inclusion of women in NIH-funded clinical trials, but many investigators did not follow this mandate (Schiebinger et al., 2016). This was followed by a 2014 mandate to consider sex as a biological variable in basic research (Mazure and Jones, 2015). However, based on arguments that ovarian hormone fluctuations made female animals too volatile to assess, preclinical research and drug development studies have also predominantly used male animal models (McCarthy et al., 2017). Today, even though women are included in biomedical research, the data from both clinical trials and research studies is rarely broken down by sex.
The field of cognitive aging and AD is no exception as sex and gender are more likely to be used as confounders than predictors. As of 2022, of all clinical trials of AD, none has set out to examine sex differences in efficacy or outcomes (Ferretti et al., 2018). Recent research, however, has elucidated the important neuroprotective role of ovarian steroid hormones and their receptors for cognitive aging and AD (Morrison et al., 2006; Brinton et al., 2015).
Focus of this review
This review explores the role of ovarian steroid hormones, especially 17β-estradiol, as contributors of brain aging and risk of AD or dementia. Endogenous exposures to ovarian hormones include chiefly pubertal timing, the menstrual cycle, pregnancy, and menopause. Exogenous hormonal exposures include chiefly use of hormonal therapy such as oral contraceptives and menopause hormone therapy (MHT). Throughout the review, the emphasis is on studies that used brain biomarkers of AD, primarily brain imaging, conducted in cisgender women with sound methodology.
Search strategy and selection criteria
We conducted a systematic review of neuroimaging studies of the menstrual cycle, oral contraceptives, menopausal status, and randomized clinical trials of MHT, as well as of imaging studies of pubertal timing and pregnancy as related to cognitive aging and AD risk. We also provide a narrative review of psychometric studies of all exposures. We searched PubMed and the Web of Science for papers published in English between 1998 and 2022, using “estrogen,” “sex steroids,” “ovarian hormones,” or “sex hormones,” all exposures and outcomes as search terms. Although we tried to cite seminal studies as necessary, because of space limitation, representative reviews were also selected. We also provide general information on the actions of ovarian steroid hormones in brain to provide context for the research findings linking these hormones to brain aging and AD risk.
Action of ovarian steroid hormones in brain
The brain is a target for ovarian steroid hormones
The primary hormones secreted by the ovaries are 17β-estradiol (estradiol, E2), the most prevalent form of estrogen produced before menopause, and progesterone, a type of progestagen. Both hormones pass through the blood–brain barrier and have receptors throughout the brain (McEwen, 2002). As reviewed below, estradiol receptors (chiefly ERα and ERβ) are present throughout areas of the brain involved in both reproductive and cognitive functions (McEwen et al., 2001). However, there is controversy regarding estrogen receptor expression across species, especially ERβ, due to limited ERβ antibody specificity (Maioli et al., 2021). Validated techniques have confirmed ERβ in rodent but not human brain (Maioli et al., 2021). Development of ERβ antibodies with higher binding specificity is needed to resolve this inconsistency, as discussed elsewhere (Andersson et al., 2017). Moreover, despite animal research demonstrating the presence of progesterone receptors (PRA and PRB) in brain regions involved in cognition, little is known about their location or function in the human brain (Brinton et al., 2008). As such, here we focus primarily on the action of estradiol on brain structure and function.
Estradiol is a steroid hormone synthesized in a series of enzymatic steps beginning with the conversion of cholesterol into pregnenolone in the mitochondria (McEwen, 2002). The final enzymatic step, the conversion of testosterone into estradiol, is catalyzed by the enzyme aromatase, or estrogen synthase (McEwen, 2002). In neurons and astrocytes, depending on tissue and time period, estradiol can also be synthesized from androstenedione and estrone (E1) (Cui et al., 2013). Starting at puberty, and for the duration of a woman’s reproductive life, estradiol is mainly produced in the ovary. Its levels in plasma change during development, fluctuate cyclically during the menstrual cycle, increase dramatically during pregnancy, drop during lactation, and eventually decline after menopause (McEwen, 2002).
Estradiol is also locally synthesized in different tissues, including brain. Recent research demonstrates that the brain is a steroidogenic organ (Arevalo et al., 2015) expressing the molecules and enzymes necessary for the conversion of endogenous cholesterol into local estradiol. As a result, the brain is a target for the action of both peripheral estradiol and neuroestradiol, e.g., estradiol synthesized in neural cells (Arevalo et al., 2015). There is emerging evidence that both types of estradiol, from ovaries and brain, control various neurobiological processes, including sexual behavior, but also neurological functions such as regulation of body temperature and blood pressure, response to stress, some aspects of mood and of cognition (Lupien et al., 2009). Importantly, brain steroidogenesis is regulated independently of peripheral steroidogenesis, and brain steroid levels do not correlate with plasma steroid levels in animals (Caruso et al., 2013).
Moreover, there is some evidence that the brain upregulates synthesis of neurosteroids in response to the drop in estrogen following oophorectomy as a compensatory adaptive reaction (Caruso et al., 2010). This suggests that similar mechanisms might be in place in response to naturally occurring declines in ovarian hormones following spontaneous menopause, though this remains to be confirmed.
Estradiol: The “master regulator” of the female brain
Estradiol has been called the “master regulator of the female brain” (Rettberg et al., 2014) due to its wide range effects on neuronal structure and function. Its neuroprotective role is of particular relevance for cognitive aging and AD. In mouse models of AD, decreasing estradiol levels in plasma following oophorectomy exacerbate brain damage under neurodegenerative conditions (Azcoitia et al., 1999), trigger decrease cerebral glucose metabolism (CRMglc) (Ding et al., 2013), and increase amyloid-β fibrillization (Yue et al., 2005).
Estrogen therapy reduces such damage (Azcoitia et al., 1999), normalizing CMRglc and reducing Aβ oligomers in oophorectomized mice (Yue et al., 2005). Estradiol’s neuroprotective action may be related to its role in maintaining metabolic homeostasis in body and brain (Frank et al., 2014; Rettberg et al., 2014). In brain, estradiol regulates glucose metabolism, glycolysis, oxidative phosphorylation and subsequent ATP generation in neurons (Rettberg et al., 2014). Substantial evidence indicates that metabolic alterations play a role in neurodegenerative diseases including AD (Lin and Beal, 2006).
Additionally, genetic studies have identified variants of the gene encoding for the aromatase enzyme that are associated with an increased risk for AD (Iivonen et al., 2004; Huang and Poduslo, 2006; Medway et al., 2014) These genetic variants may result in decreased estradiol synthesis in brain, which, together with decreased serum estradiol levels in post-menopausal women may increase the risk for AD (Huang and Poduslo, 2006). Aromatase expression is indeed increased in prefrontal cortex of patients with severe AD, a phenomenon that has been interpreted to be part of a “rescue program” (Luchetti et al., 2011).
Estrogen receptors (ERs) also coordinate multiple neuroprotective signaling cascades, either via direct activation or by the interaction of ERs with the receptors for other neuroprotective factors. Estradiol action in brain can be both delayed in onset and prolonged in duration (“genomic”) or rapid in onset and short in duration (“non-genomic”) (McEwen and Milner, 2017). Both ERα and ERβ are expressed in regions including hippocampus, amygdala, and hypothalamus, their distribution density differs. ERα shows greater distribution in hypothalamic nuclei associated with sexual behavior, whereas ERβ is expressed more in areas associated with cognition such as basal forebrain, prefrontal cortex, temporal and parietal regions, and posterior cingulate (Foster, 2012). Additionally, while both ERα and ERβ participate in the overall neuroprotective action of the estradiol, ERα is more closely involved in neuroprotection, as demonstrated by animal models of focal ischemia (Dubal et al., 2001), whereas ERβ has been shown to be involved in cognition, thought to promote learning and memory, neural plasticity, and regulating neurotrophic factors (Zhao et al., 2015). The G-protein coupled estrogen receptor (GPER1) shows widespread brain distribution, with heavy concentration in key brain regions including hippocampus and amygdala (Hadjimarkou and Vasudevan, 2018) and play a key role in mediating the rapid action of estradiol.
ERα and ERβ are also implicated in modulating the immune system. Both receptors are expressed on microglia and astrocytes, both involved in neuroinflammation and implicated in Alzheimer’s disease (Mishra and Brinton, 2018). Activation of ERα and ERβ via estradiol treatment has been reported to decrease inflammatory responses such as phagocytosis and cytokine secretion, ultimately having an anti-inflammatory and neuroprotective effect (Mishra and Brinton, 2018). Activation of ERα has also been reported to shorten the inflammatory response to infection in preclinical studies (Villa et al., 2015). There is increasing evidence that chronic inflammatory processes are activated during midlife chronological and endocrine aging, which ultimately limit the clearance capacity of microglia and lead to immune senescence (Mishra and Brinton, 2018). The inflammatory immune response is a possible unifying factor that bridges across the three major risk factors for AD in women: aging, menopause, and ApoE epsilon 4 (ApoE4) genotype (Mishra and Brinton, 2018).
Influence of sex hormones across a woman’s life
Ovarian hormones affect the nervous system in ways that extend beyond their essential actions of regulating gonadotropin secretion and modulating sexual behavior. As reviewed below, at a neurological level, estrogens are involved in regulation of thermoregulation, mood, sleep, and cognitive abilities, among other factors (McEwen et al., 1997). From a cognitive aging perspective, both estradiol and progesterone influence verbal memory, fluency, performance on spatial tasks, and fine motor skills (Maki and Henderson, 2016). Declines in these hormones with menopause have been associated with an increased risk of cognitive impairment, affective disorders, and AD pathology (Rahman et al., 2019; Jett et al., 2022).
In what follows, we review research elucidating the role of ovarian steroid hormones in cognitive aging and AD risk across the female lifespan, including studies of puberty, menstrual cycle, hormonal contraceptive use, the menopause transition, and hormone therapy for menopausal symptoms.
Pubertal timing and menstrual cycle
Puberty is characterized by surges in the production of sex hormones, which in turn prompt dramatic organizational changes in the brain, followed by transformative changes in cognition and behavior (Sisk and Foster, 2004). For girls, the maturation of the ovaries with the subsequent production of estradiol and progesterone typically occurs around age 11–12 years, ranging from 10 to 18 years (Anderson et al., 2003). This results in the development of secondary sexual characteristics and of menarche, or the first menstrual bleeding.
There is ample evidence that ovarian sex hormones influence brain development and cognition during adolescence. While reviewing these findings is beyond the scope of this review, we recommend prior reviews on the topic (Giedd et al., 1999; Sisk and Foster, 2004; Blakemore, 2008). Herein, we focus on links between early hormonal exposures and cognitive aging in midlife and older age. Of all the factors involved in puberty and adolescence, two have been consistently examined as possible predictors of future cognitive impairment and AD or dementia: pubertal timing and the menstrual cycle.
Pubertal timing and age at menarche
A recent hypothesis posits that the brain has declining sensitivity to sex hormones throughout adolescence, such that females who mature early have greater effective ovarian hormone exposure than those who mature late (Schulz et al., 2009). The age at which a woman enters menarche has gained attention for a possible relationship with cognition in later life due to longer estrogen exposure when menarche occurs at a younger age (Bernstein et al., 1991). While research on this topic is scant, some studies indicate associations between an early age at menarche and greater white matter integrity in frontal cortex in adolescence (Chahal et al., 2018). Thus, pubertal timing may facilitate brain maturation due to longer exposure to ovarian sex hormones, which may in turn confer greater brain reserve later in life.
Nonetheless, the majority of studies so far indicate null associations between age at menarche and cognitive impairment or AD risk (Geerlings et al., 2001; Henderson et al., 2003; Colucci et al., 2006; Fox et al., 2013; Prince et al., 2018; Najar et al., 2020; Song X. et al., 2020). On the other hand, in some studies, a younger age at menarche correlated with better visual memory performance on Benton’s visual retention test and psychomotor speed on a trail making task (task A) (Ryan et al., 2009), and with a reduced risk of dementia or AD in later life (Rasgon et al., 2005a; Gilsanz et al., 2019). Additionally, the Gothenburg H70 Birth Cohort study reported associations between a younger age at menarche and lower CSF Aβ42/40 ratio and higher hyperphosphorylated tau levels among older post-menopausal women free of dementia (Najar et al., 2021). More studies of pubertal timing, ideally spanning puberty and young adulthood to midlife and beyond, and including the use of AD biomarkers, are needed to clarify the strength and reproducibility of these associations.
Menstrual cycle
The typical menstrual cycle is 28 days long, with normal variation ranging from 22 to 35 days (Reed and Carr, 2000; Grieger and Norman, 2020). Menstruation is generally considered the beginning of the cycle, which is divided into two phases, follicular and luteal. The follicular phase begins after the first day of menstruation and is characterized by initial low levels of both estradiol and progesterone followed by rising estradiol. Estradiol levels peak before ovulation (∼day 14), triggering the release of luteinizing hormone (LH). The luteal phase begins after ovulation and is characterized by a decrease in estradiol that settles at moderate levels, while progesterone begins to rise. If the egg is not fertilized, estradiol and progesterone decline during the second half of the phase (i.e., premenstrual phase), triggering menstruation and a new cycle. As these phases are relatively easy to pinpoint, studies of the menstrual cycle offer a unique opportunity to clarify the influence of ovarian hormones on neuronal circuits implicated in the regulation of cognitive and emotional processing.
Seminal animal studies from the early 1990’s demonstrated that estradiol levels regulate synaptogenesis and synapse density on excitatory spines in hippocampal CA1 pyramidal neurons in female rats (Woolley and McEwen, 1992), which have been since replicated by many investigators (for example, Hara et al., 2015; McEwen and Milner, 2017; Sheppard et al., 2019). Fluctuations in synaptogenesis occur throughout the estrous cycle, with increases in synapses on dendritic spines after estrogen treatment, along with decreases in spine synapse density that occurs between the days of proestrus and estrus (Woolley and McEwen, 1992). Consistent with these observations, neuroimaging and cognitive studies provide evidence for changes in brain structure, function, and cognitive performance across the menstrual cycle or as a function of ovarian hormones.
The long-held view is that verbal memory and implicit memory are enhanced in the late follicular and midluteal phase, when estradiol is high (Hampson, 1990; Maki et al., 2002; Pletzer et al., 2011), whereas spatial and numerical abilities are enhanced in the early follicular phase, when estradiol is low (Hausmann et al., 2000; Courvoisier et al., 2013). Nonetheless, results are generally inconsistent (Sacher et al., 2013; Sundström Poromaa and Gingnell, 2014). Specifically for brain aging and AD, only one study to date has investigated possible associations of menstrual cycles and AD risk (Fox et al., 2013). In a cohort of 89 elderly British women, Fox et al. (2013) reported a marginally significant association between the number of menstrual cycles, defined as the number of months between menarche and menopause, free from oral contraceptive use, pregnancy, breastfeeding, and post-partum anovulation, and a lower risk of AD. Each additional month of having a menstrual cycle corresponded to a 0.3% reduction in risk of AD.
Neuroimaging studies of the menstrual cycle are summarized in Table 1. Several structural MRI studies report changes in the volume of several cortical and subcortical regions across the menstrual cycle (Figure 1). Most studies indicate increased hippocampal or amygdala volumes during the late follicular phase, when estradiol levels are rising and progesterone is low (Protopopescu et al., 2008; De Bondt et al., 2013a; Lisofsky et al., 2015; Pletzer et al., 2018), with some exceptions (Ossewaarde et al., 2013). Two studies also demonstrate a direct association between higher estradiol levels and larger hippocampal volume (Barth et al., 2016; Pletzer et al., 2018), while another study found a positive association between estradiol levels and the volume of another limbic structure, the parahippocampal gyrus (Lisofsky et al., 2015). Insular volume has also been reported, being positively associated with estradiol levels and higher during the follicular phase (De Bondt et al., 2016). Prefrontal cortex volume and thickness also appear to be positively associated with estradiol levels (Dubol et al., 2021).
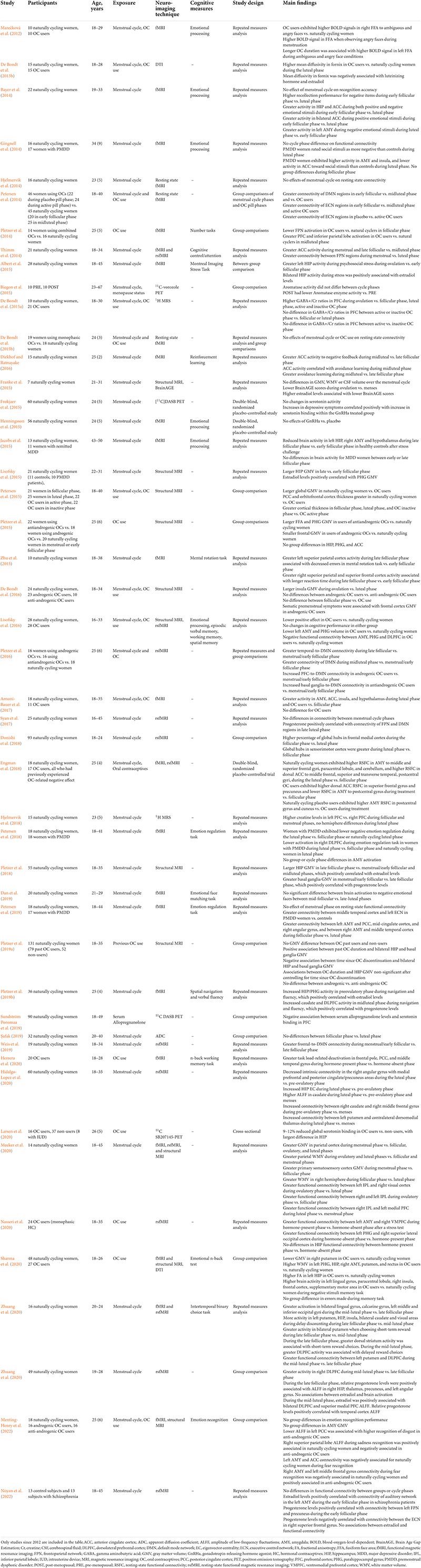
Table 1. Summary of studies investigating the effects of the menstrual cycle and of use of hormonal contraceptives on neuroimaging outcomes.
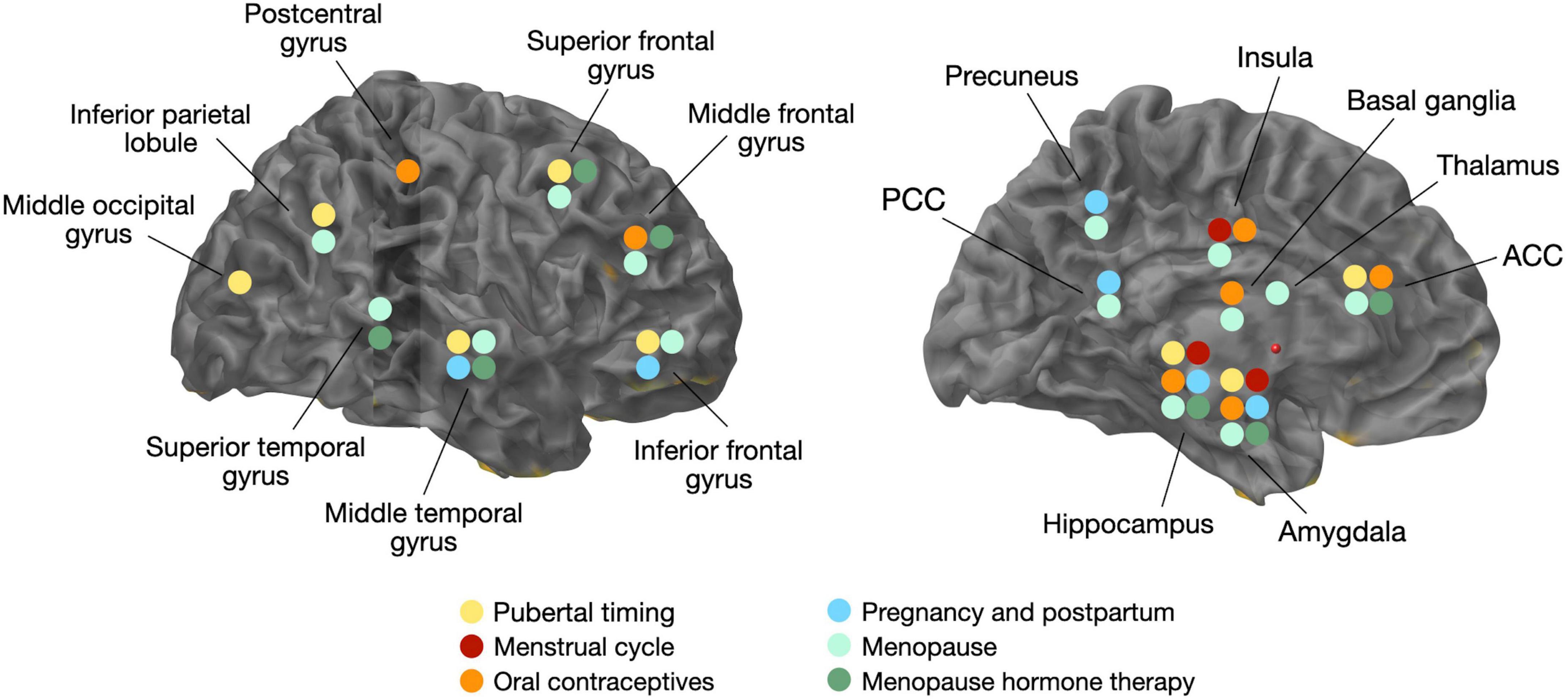
Figure 1. Overview of volumetric gray matter changes related to changes in estradiol levels and/or hormonal transitions. ACC, anterior cingulate cortex; PCC, posterior cingulate cortex.
On the contrary, the volume of the basal ganglia and anterior cingulate cortex (ACC) is reduced during the late follicular phase, opposite the pattern observed for the hippocampus (Protopopescu et al., 2008; De Bondt et al., 2013a). In the mid-luteal phase, when estradiol levels are moderate and progesterone levels are high, ACC volume increased. The increase in ACC volume was inversely correlated with estradiol levels, and positively correlated with progesterone levels (De Bondt et al., 2013a; Pletzer et al., 2018). Other regions, including fusiform gyrus, insula, and some parts of the temporal and frontal cortices, also change in size across the menstrual cycle (Pletzer et al., 2018).
Functional MRI studies also provide evidence of differential activation patterns during the menstrual cycle. A recent systematic review of neuroimaging studies indicates increased prefrontal cortical activity during cognitive tasks during the mid-luteal phase (Dubol et al., 2021). There is mixed evidence for preferential ACC activation exhibits greater activation and functional connectivity during the early follicular (menstrual) phase and late follicular phase compared with the midluteal phase (Thimm et al., 2014), or in the midluteal compared to the late follicular phase (Diekhof and Ratnayake, 2016). Activity in hippocampus (Pletzer et al., 2019b) and insular cortex (Dubol et al., 2021) during cognitive activities tend to be greater during the follicular phase.
Additionally, resting state fMRI studies indicate that some regions within the Default Mode Network (DMN) are more connected in the early follicular phase, when estradiol and progesterone levels are low (Petersen et al., 2014; Weis et al., 2019). Instead, another study reported no impact of menstrual cycle phase on DMN connectivity but increased connectivity between basal ganglia and frontoparietal attention network in midluteal phase, when both progesterone and estradiol are high (Pletzer et al., 2016). Some studies showed higher functional connectivity between amygdala and cingulate cortex, and amygdala with middle frontal gyrus (Petersen et al., 2019), and between ACC and the executive control network during the follicular phase as compared to the luteal phase, whereas dorsolateral prefrontal cortex and sensorimotor cortex are more connected with hippocampus (Arélin et al., 2015), resulting in greater activity in response to stimuli (Dubol et al., 2021), during the luteal phase compared to the follicular phase. Another study has reported that the hippocampus has greater whole brain functional connectivity at rest during the mid-luteal phase (Hidalgo-Lopez et al., 2020). Finally, a study comparing all three phases of the menstrual cycle showed higher hippocampal activation during the pre-ovulatory phase (e.g., higher estradiol) and higher fronto-striatal activation during the luteal cycle phase (e.g., higher progesterone) (Pletzer et al., 2019b). However, other studies comparing the three phases of the menstrual cycle did not confirm these associations (Hjelmervik et al., 2014; De Bondt et al., 2015b). Additionally, a study comparing the early follicular and mid-luteal phases found increased connectivity between angular gyrus and DMN, and between ACC with executive control network (ECN), during the follicular phase as compared to the mid-luteal phase (Petersen et al., 2014). A smaller study comparing mid-follicular and late luteal phases found no functional connectivity differences between menstrual phases (Syan et al., 2017). However, progesterone levels were positively correlated with connectivity of frontoparietal network (FPN) and DMN regions during the late luteal phase.
Although PET studies of the menstrual cycle are scarce and limited by small sample sizes, they did provide evidence for bioenergetic changes over the menstrual cycle, and limited to no effects on neurotransmitter activity. On [18F]fluorodeoxyglucose (FDG) PET, cerebral glucose metabolism (CMRglc) was higher in thalamic, prefrontal, temporoparietal, and inferior temporal regions in the mid-follicular as compared to the luteal phase, whereas CMRglc in superior temporal, occipital, cerebellar, cingulate and anterior insular regions was higher in the luteal as compared to the follicular phase (Reiman et al., 1996). There were also no differences in overall brain glucose metabolic activity between the follicular and luteal phases as measured via FDG-PET (Rapkin et al., 2011). There is no evidence for changes in D2 dopamine receptor density during different phases of the menstrual cycle on [11C]raclopride PET (Nordström et al., 1998), or for differences in serotonin binding between men and women in the follicular phase on [11C]WAY-100635 PET (Stein et al., 2008). One study of [11C]vorozole PET found no differences in aromatase activity between midcycle and late luteal phases (Biegon et al., 2015). A double-blind, randomized, placebo-controlled study investigating the effects of a gonadotropin-releasing hormone agonist (GnRHa) used [11C]DASB PET to image serotonin transporter (SERT) binding during the follicular phase in naturally cycling women (Frokjaer et al., 2015). The researchers found that increased SERT binding in neocortex and lower estradiol levels in the GnRHa group was associated with depressive symptoms as compared to placebo (Frokjaer et al., 2015). Another study using [11C]DASB PET reported that lower serum levels of allopregnanolone, which typically occurs during the follicular phase, was associated with greater SERT binding in prefrontal cortex (PFC) (Sundström Poromaa et al., 2019). However, several studies did not perform follow-up scans during different menstrual cycle phases (Frokjaer et al., 2015; Sundström Poromaa et al., 2019), thus additional work is needed to elucidate the relationship between menstrual cycle effects on PET brain imaging.
Altogether, neuroimaging results indicate that hormonal changes during the menstrual cycle may impact widespread networks involved in memory, learning, attention, and emotion. It is possible that, as effects of ovarian hormones on synaptic activity are generally subtle, neuroimaging might be more sensitive to detecting these changes than cognitive tests. Since most fMRI studies show no links to cognitive performance despite detecting activational changes during the menstrual cycle, it’s been hypothesized that not all effects of ovarian hormones might immediately translate to changes in cognition (Pletzer et al., 2019b). It is also possible that the brain compensates for cycling variations in ovarian hormone levels, leaving cognitive performance broadly unchanged throughout the menstrual cycle. Further, recent reviews suggest that menstrual cycle-related changes in cognition may be smaller than those in affective function and mood (Sacher et al., 2013). It is well established that the risk of depression becomes higher in women than in men starting at puberty (McGuire et al., 2019), and midlife depression is a risk factor for AD in turn (Livingston et al., 2020). Whether links between menstruation and mood are predictors of cognitive vulnerability later in life is under investigation.
Oral contraceptives
Hormonal contraceptives consist either of a synthetic progesterone (i.e., progestin), or a progestin and a synthetic estrogen (e.g., combined formulation). These exogenous hormones control ovulation by inhibition of follicular development, and suppression of the production of endogenous estradiol and progesterone (Taylor et al., 2021). Hormonal contraceptives have various routes of administration, including oral, transdermal, intrauterine, and transvaginal. The most common form of birth control is by means of oral contraceptives (OC), which are used by over 85% of women in the United States (Taylor et al., 2021). Most OC formulations contain 21 active pills followed by seven placebo pills, which do not halt menstruation. Placebo pills are placeholders meant to help you stay on track by taking one pill every day until the next month starts. Some formulations have longer or shorter pill phases. Other formulations contain 28 active (monophasic) pills, which halt menstruation. Most OC contain ethinylestradiol, a potent form of estradiol, and synthetic progestins with different hormone derivatives. As a result, pills can either be androgenic or anti-androgenic (Pletzer et al., 2019a; Taylor et al., 2021).
Given the effects of ovarian hormones on brain structure and function, examination of the effects of OCs on cognitive aging and AD risk provides important information for preventative efforts. Nonetheless, few studies have investigated whether OC use influences cognition. Most of these studies were conducted on young adult women, while a handful examined associations between OC use in young adulthood and midlife, and future risk of cognitive decline in older age. While some studies report no differences in cognitive performance between young adult women with natural cycles and OC users (Lisofsky et al., 2016), others suggest that OC therapy supports verbal memory (Warren et al., 2014; Beltz et al., 2015) but not verbal fluency (Griksiene et al., 2018). Users of pills with androgenic progestins may also show increased spatial ability (Griksiene et al., 2018). For the long-term, some studies report higher performance on cognitive testing (Egan and Gleason, 2012; Karim et al., 2016) or a reduced risk of cognitive impairment (Li et al., 2016; Song X. et al., 2020) in midlife women taking OC. One study reported an almost 50% lower risk of cognitive impairment in women aged 60 or older who had used birth control as compared to never-users (Li et al., 2016). The remaining studies report no associations between OC use and cognitive performance (Ryan et al., 2009; Tierney et al., 2013), cognitive decline (McLay et al., 2003), or dementia incidence (Najar et al., 2020). Inconsistent findings may be a result of discrepancies in several factors including the age of initiation, OC formulations, dosage and duration of use (Taylor et al., 2021).
Neuroimaging studies of OC use are summarized in Table 1. Generally, structural MRI studies of young adult women indicate that OC users have larger regional gray matter (GM) volumes than natural cycling women, chiefly in frontal, temporal and anterior cingulate cortices, as well as hippocampus, parahippocampal gyrus, and cerebellum (Pletzer et al., 2010, 2015; De Bondt et al., 2013a; Figure 1). Limited data from longitudinal studies suggest that frontal and ACC volumes may be larger during the active phase compared with the placebo phase, during which no hormones are given (Pletzer et al., 2010; De Bondt et al., 2013a). Another study observed larger hippocampal volume with longer duration of OC treatment in young adult women, although the associations were mild (Pletzer et al., 2019a). In a recent MRI study of midlife women at risk for AD, OC users exhibited greater GM volume in medial temporal lobe, precuneus, fusiform gyrus, parietal and frontal cortex as compared to never-users (Schelbaum et al., 2021), which is in line with findings in younger women (Pletzer and Kerschbaum, 2014). However, other studies reported reduced GM volume of amygdala, parahippocampal gyrus, hypothalamus, pituitary gland, posterior cingulate cortex and orbitofrontal cortex of OC users compared to non-users (Petersen et al., 2015; Lisofsky et al., 2016; Chen et al., 2021). When comparing the follicular phase of naturally cycling women with the inactive phase of androgenic progestins or antiandrogenic pills, OC users had lower GM volume in cingulate gyrus and bilateral culmen, although these effects did not survive correction for multiple comparisons (De Bondt et al., 2016). The OC formulation also seems to matter, as women taking pills with androgenic progestins demonstrated smaller frontal volume and lower face recognition performance as compared to non-users, whereas those taking antiandrogenic pills had larger parahippocampal and fusiform volumes and better cognitive scores (Pletzer et al., 2015).
Most fMRI studies report an overall lack of performance differences between OC users and naturally cycling women during processing tasks (Brønnick et al., 2020; Taylor et al., 2021), although some studies indicate reduced frontoparietal activation in OC users compared with non-users in the follicular phase, and greater medial PFC and inferior parietal activation in OC users compared with non-users in the midluteal phase (Pletzer et al., 2014). Resting state fMRI studies have also produced mixed results, as some studies report no differences between women using OC and naturally cycling women (De Bondt et al., 2015b), whereas others report mixed effects (Brønnick et al., 2020; Taylor et al., 2021). On Diffusion Tensor Imaging (DTI), young OC users exhibited higher mean diffusivity (MD) when compared to naturally cycling women in the luteal phase (De Bondt et al., 2013b). Another study of 45–80 year old women reported reductions in fractional anisotropy (FA) with duration and age at onset of OC use (Nabulsi et al., 2020), while a separate study reported higher FA in younger OC users compared to naturally cycling women (Sharma et al., 2020).
Overall, research concerning OC effects on cognitive aging is just emerging. Although samples are small and differences between OC formulations were not reported in most studies, there is some indication that exogenous hormones influence brain volumes among young adult OC users, and may play a role in verbal functions, consistent with research on the menstrual cycle. Future systematic work is needed to better elucidate androgenic vs. anti-androgenic OC effects on cognitive health, and to probe between OC use pre-menopause and cognition post-menopause. Given the widespread use of OC, this work carries significant implications.
Pregnancy
Pregnancy induces significant changes in endogenous estrogen levels, with reported effects on brain structure and function (de Lange et al., 2020). High levels of estradiol observed during pregnancy may lend neuroprotective support due to cumulative estrogen exposure (Deems and Leuner, 2020). However, the neurological impact of pregnancy is multifaceted and the biological mechanisms impacting cognitive aging remain to be elucidated. On one hand, compared to women who have never been pregnant, the levels of circulating estrogen are lower in women who have experienced pregnancy, a difference which extends into menopause (Bernstein et al., 1985). On the other hand, brain sensitivity to estrogen is increased in pre-clinical models of pregnancy, as evidenced by increased numbers of ERα positive cells in parous rats compared to nulliparous rats (Byrnes et al., 2009). Reports also suggest these effects may be evident in the human brain, as parity has been associated with increased responsiveness to estrogen in older aged women (de Lange et al., 2019).
Nonetheless, the vast majority of studies have focused on the short-term effects of pregnancy and postpartum on brain structure, function, and cognition, with the longest follow-ups conducted at 2–6 years postpartum (Brunton and Russell, 2008; Barth and de Lange, 2020). Studies investigating the long-term effects of pregnancy and childbearing on cognitive aging and AD risk are scant, as summarized below.
There is some evidence for a positive effect of pregnancy on cognitive aging. Several studies have reported that midlife women who had experienced pregnancy exhibited better cognitive performance in verbal and visual memory performance (Henderson et al., 2003; Ning et al., 2020), and another reported lower AD risk in later life (Fox et al., 2018). Studies examining gravidity (total number of pregnancies including stillbirth, miscarriage, and/or abortion) have reported a reduced risk of AD in elderly women who had spent more cumulative months pregnant and breastfeeding throughout their life (Fox et al., 2013, 2018). Another study supported these findings in reporting protection against AD dementia with longer breastfeeding duration (Heys et al., 2011). During lactation, estrogen levels are lower, and thus there are likely other factors contributing to these associations.
However, other studies report detrimental effects of pregnancy on cognitive aging. Compared to nulliparous women, parous women had greater cognitive decline on Mini-Mental State Examination (MMSE) scores (McLay et al., 2003), increased AD risk (Colucci et al., 2006) and AD onset at a younger age (Ptok et al., 2002), which may be limited to non-carriers of the ApoE4 gene (Corbo et al., 2007). A post-mortem study reported no clear associations between cognition and parity, though parity was associated with higher levels of AD-related neuropathology (Beeri et al., 2009).
Other studies have reported null associations between parity and cognitive performance or dementia risk (Ptok et al., 2002; Corbo et al., 2007; Ryan et al., 2009; Bae et al., 2020). In the Rancho Bernardo Study, 1,025 women between the ages of 44–99 who were followed over time showed no long-term effect on cognitive performance in relation to their prior pregnancies (Ilango et al., 2019).
Discrepancies may be in part due to how studies define parity. Studies defining parity as the number of childbirths or time spent pregnant more commonly report associations with cognition as compared to studies defining parity as parous vs. nulliparous. The number of children may play an important role, studies report having 1–4 children provides neuroprotection in women (Heys et al., 2011; Ning et al., 2020; Song X. et al., 2020), having 5 or more children, or grand multiparity, has been linked to negative effects as measured by cognitive performance or dementia risk (Rasgon et al., 2005a; Bae et al., 2020; Song X. et al., 2020).
While neuroimaging results are also mixed, MRI studies generally report positive effects of pregnancy and parity on structural brain aging (Figure 1). Two large studies reported that in comparison to nulliparous women, parous women, especially with a higher number of childbirths, exhibited less apparent brain aging as predicted via MRI-based machine learning models (de Lange et al., 2020; Ning et al., 2020). A recent volumetric MRI study of cognitively normal midlife individuals at risk for AD reported positive associations between number of children (between 2 and 5) and larger GM volume in frontal and temporal regions in women, whereas no associations were observed among men (Schelbaum et al., 2021). While there was no direct association between cognitive performance and number of children, there was a positive association between temporal cortex GMV with memory and global cognition performance, which suggests a mediation effect of pregnancy on cognition (Schelbaum et al., 2021).
Overall, studies investigating the associations between pregnancy and later life cognition are limited by small samples, heterogeneity of cognitive assessments and diagnostic criteria, possible inclusion of non-biological children, and different exposure variables. Pregnancy-related factors, including age at first birth, breastfeeding, or complications such as gestational diabetes or pre-eclampsia, have rarely been considered yet may have significant contributions. Later life cognitive testing or dementia diagnosis may also contribute to contrasting results, as the effects of pregnancy are likely more apparent closer to the time of childbirth than many years later after cumulative experiences have affected the brain.
The menopause transition
Menopause represents the permanent cessation of ovulation and menstrual cycles, which is defined retrospectively, after 12 months of amenorrhea without obvious pathologic cause (Harlow et al., 2012). Hormonally, menopause is characterized by drastic reductions in estradiol and progesterone levels and elevated levels of gonadotropins follicle-stimulating hormone (FSH) and luteinizing hormone (LH) (Santoro, 2005). Menopause occurs either as the result of a natural midlife aging process (spontaneous menopause) or iatrogenically, via surgical or pharmacological intervention (induced menopause). In most cases, induced menopause results from bilateral oophorectomy or salpingo-oophorectomy, which lead to an abrupt cessation of ovarian estrogen production. Hysterectomy without oophorectomy can reduce ovarian estrogen production by disturbing blood flow to the ovaries, thus indirectly influencing the onset of menopause (Jett et al., 2022). Endocrine therapy for cancer and radiation therapies can also damage the ovaries and precipitate menopause (Jett et al., 2022). The reduction in ovarian hormones, particularly estradiol, is thought to elicit vasomotor (e.g., hot flashes) and urogenital (e.g., vaginal dryness) symptoms, while also increasing risk for cardiovascular disease and osteoporosis (Harlow et al., 2012), as well as neurological and psychiatric disorders including depression, anxiety, and dementia (Monteleone et al., 2018).
The average age at spontaneous menopause in industrialized countries is 49–51 years (Monteleone et al., 2018). Therefore, women live at least a third of their lives in a hypogonadal state, and that number increases to up to half for women with induced menopause (Monteleone et al., 2018). Recent evidence that AD starts in midlife (Sperling et al., 2013), thus proximate to the menopause transition, has highlighted a previously overlooked connection between menopause and AD risk. Currently, menopause is the most widely investigated female-specific risk factor for AD (Rahman et al., 2020). Estrogen withdrawal during menopause has been linked to accelerated brain cellular aging, possibly increasing risk of neurodegenerative events and AD later in life (Wang et al., 2020b; Mosconi et al., 2021).
Spontaneous menopause is a normal physiological event without long-term adverse effects for the majority of women (Monteleone et al., 2018). However, as high as 80% of women are vulnerable to the neurological shifts that can occur during this transition (Brinton et al., 2015), experiencing not only vasomotor symptoms such as hot flashes, but also “brain fog” and cognitive complaints. While the term “brain fog” is not a medically accepted entity, it reflects the common self-reported awareness of a decline in memory, attention and concentration during the menopause transition (Gold et al., 2000). While statistics on this vary, over 60% of women report changes in their ability to think clearly, concentrate, remember, or make use of new information during the menopause transition (Greendale et al., 2020). Most women experience a 15–20% increase in forgetfulness during perimenopause relative to pre-menopausal levels (Gold et al., 2000).
Nonetheless, whether menopause-related cognitive complaints can be confirmed objectively is a topic of debate (Mitchell and Woods, 2011; Weber et al., 2012). The first evidence for associations between menopause and memory decline stemmed from studies of oophorectomy, which reported an almost doubled long-term risk of dementia in oophorectomized women (Rocca et al., 2007, 2014; Phung et al., 2010; Bove et al., 2014). Dementia risk is generally highest following bilateral oophorectomy, intermediate with unilateral oophorectomy, and lowest but significant following hysterectomy without oophorectomy (Yaffe et al., 1998; Hogervorst et al., 2000; LeBlanc et al., 2001; Rocca et al., 2007; Phung et al., 2010; Bove et al., 2014; Gilsanz et al., 2019). For example, The Mayo Clinic Cohort Study of Oophorectomy (MCSO) observed an 84% higher risk of dementia for women who underwent unilateral oophorectomy with or without hysterectomy before age 42 years, and a 70% to double higher risk in women who underwent bilateral oophorectomy before the onset of natural menopause (Rocca et al., 2012). Phung et al. (2010) reported a 38% higher risk of dementia before the age of 50 for hysterectomy alone [RR = 1.38, 95% confidence interval (CI) = 1.07–1.78], and over double the risk with unilateral oophorectomy (RR = 2.10, 95% CI = 1.28–3.45) and bilateral oophorectomy (RR = 2.33, 95% CI = 1.44–3.77) (Phung et al., 2010). Dementia risk increases with younger age at the time of surgery (Rocca et al., 2008; Phung et al., 2010), which has also been associated with an increased burden of AD neuropathology at post-mortem (Bove et al., 2014; Agca et al., 2020). Surgical menopause may also have more severe consequences on cognitive function, including lower performance in verbal learning, visual memory (Rocca et al., 2007), and delayed word recall tasks (Zhou et al., 2011). Decline in short-term verbal memory was more severe in women who had greater than 50% decline in serum estradiol levels following surgery (Nappi et al., 1999; Farrag et al., 2002).
Overall, studies including surgical and spontaneous menopause cases indicate measurable, yet modest declines in verbal episodic memory on delayed recall tests, or lack of improvement in verbal memory and processing speed with repeated testing (Fuh et al., 2006; Greendale et al., 2009, 2011; Bromberger et al., 2010; Berent-Spillson et al., 2012; Epperson et al., 2013; Weber et al., 2013). In some studies, peri-menopausal women exhibited declines in working memory and complex attention rather than verbal episodic learning or memory (Weber et al., 2012), suggesting that operations demanding higher cognitive effort contribute to women’s perception of cognitive difficulties.
Some studies indicate that cognitive changes are possibly transient, as evidenced by longitudinal reports suggesting that they are mostly present at the peri-menopausal and early post-menopausal stages, with a rebound to almost pre-menopausal levels after menopause (Greendale et al., 2009; Weber et al., 2013). In the Study of Women Across the Nation (SWAN), over 2,300 midlife women followed for 4 years showed a decrease in verbal memory and processing speed in perimenopause compared to their pre-menopausal scores (Greendale et al., 2009). These declines resolved post-menopause, when cognitive performance returned to pre-menopausal levels, or closer to baseline (Greendale et al., 2009). In the Rochester Investigation of Cognition Across Menopause, peri-menopausal and early post-menopausal women had lower verbal memory, attention, and working memory scores, which improved in late postmenopause (Weber et al., 2013). However, other studies report conflicting results of reduced memory still in postmenopause (Epperson et al., 2013). While cognitive effects are for the most part independent of non-cognitive menopausal symptoms such as anxiety and disturbed sleep (Greendale et al., 2010), frequent hot flashes and a negative mood have been linked with more severe cognitive disturbances (Maki et al., 2008; Drogos et al., 2013).
Importantly, memory declines during perimenopause and early postmenopause ranged from subtle to moderate, and remained within normal limits for age and education in most studies (Maki and Henderson, 2016). Moreover, women maintain an advantage in verbal memory as compared to age-controlled men regardless of menopausal status (Rentz et al., 2017), which strongly argue for development of gender-specific tests that also take account women’s reproductive stage. Generally, cognitive complaints during menopause are unlikely to result in objectively measured impairments, thus often falling under the diagnostic category of subjective cognitive decline (SCD). Current evidence suggests that people ages 65 and older experiencing SCD may be at higher risk for MCI and dementia (Jessen et al., 2014), especially women (Pérès et al., 2011).
Although neuroimaging research of menopause is scant, and the majority of studies has been carried out in women who had already transitioned through the menopause, recent translational neuroimaging studies corroborate animal findings by showing associations between menopause and biomarker indicators of AD risk in midlife women (Rahman et al., 2019; Jett et al., 2022). Neuroimaging studies of menopause status are summarized in Table 2.
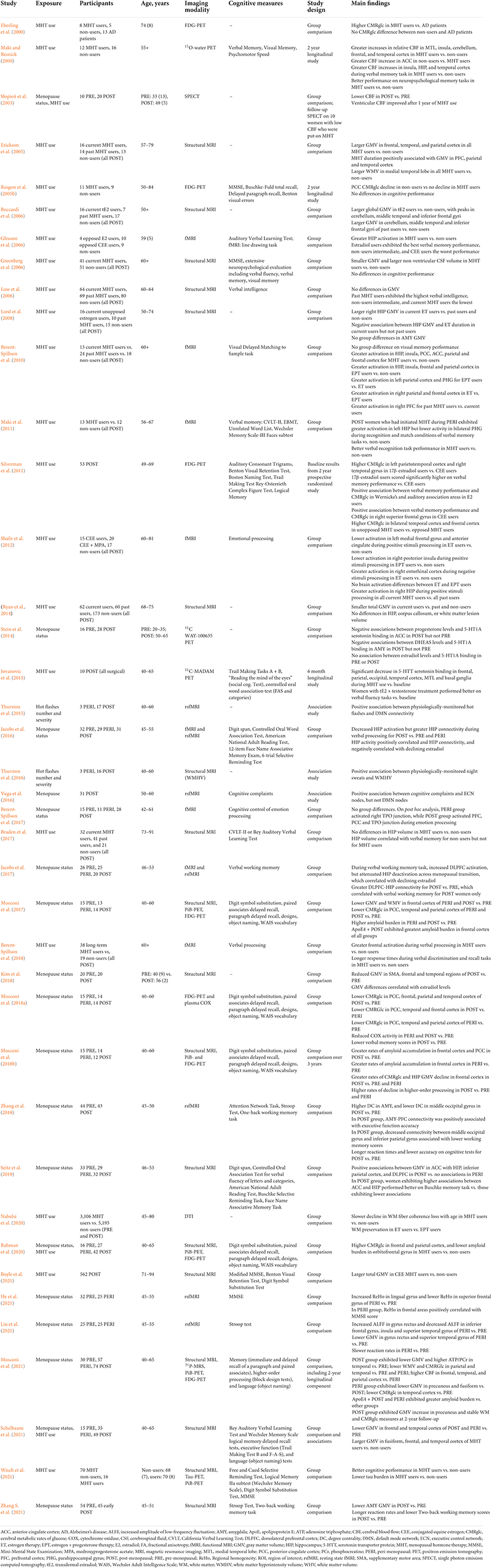
Table 2. Observational studies of menopause status and menopausal hormone therapy (MHT) use on neuroimaging outcomes.
Recent multi-modality neuroimaging investigations targeting women at different menopausal stages (pre-menopausal, peri-menopausal, and post-menopausal), all carrying risk factors for AD, such as ApoE4 genotype and a family history of late-onset AD, demonstrate emergence of AD endophenotypes in women of peri-menopausal age (Mosconi et al., 2017, 2018a,b, 2021; Rahman et al., 2020). AD endophenotypes included higher Aβ load, lower CMRglc, and lower GM and WM volume in brain regions vulnerable to AD, chiefly posterior cingulate, precuneus, medial temporal, parieto-temporal, and frontal cortices as compared to pre-menopausal women and to age-controlled men, independent of age and midlife health indicators (Mosconi et al., 2017, 2018a,b, 2021; Rahman et al., 2020; Figure 1). Biomarker abnormalities increased post-menopause (Mosconi et al., 2017, 2018a,b, 2021; Rahman et al., 2020). Additionally, peri-menopausal and post-menopausal women positive for ApoE4 genotype exhibited the highest Aβ burden (Mosconi et al., 2017, 2021), supporting the notion that ApoE4 genotype exacerbates AD-related brain changes in women with onset in the perimenopause (Riedel et al., 2016). While menopause effects on Aβ deposition were overall mild, the earlier onset and longer exposure to Aβ pathology could help account for the higher prevalence of AD in women.
Longitudinal evaluations showed progressive AD biomarker abnormalities in the menopause transition, including chiefly declines in hippocampal and temporal lobe GM volumes, CMRglc declines in temporal regions and PCC, and increased Aβ deposition in frontal cortex (Mosconi et al., 2018b, 2021). Figure 2 provides an overview of menopause effects on Aβ deposition among midlife women.
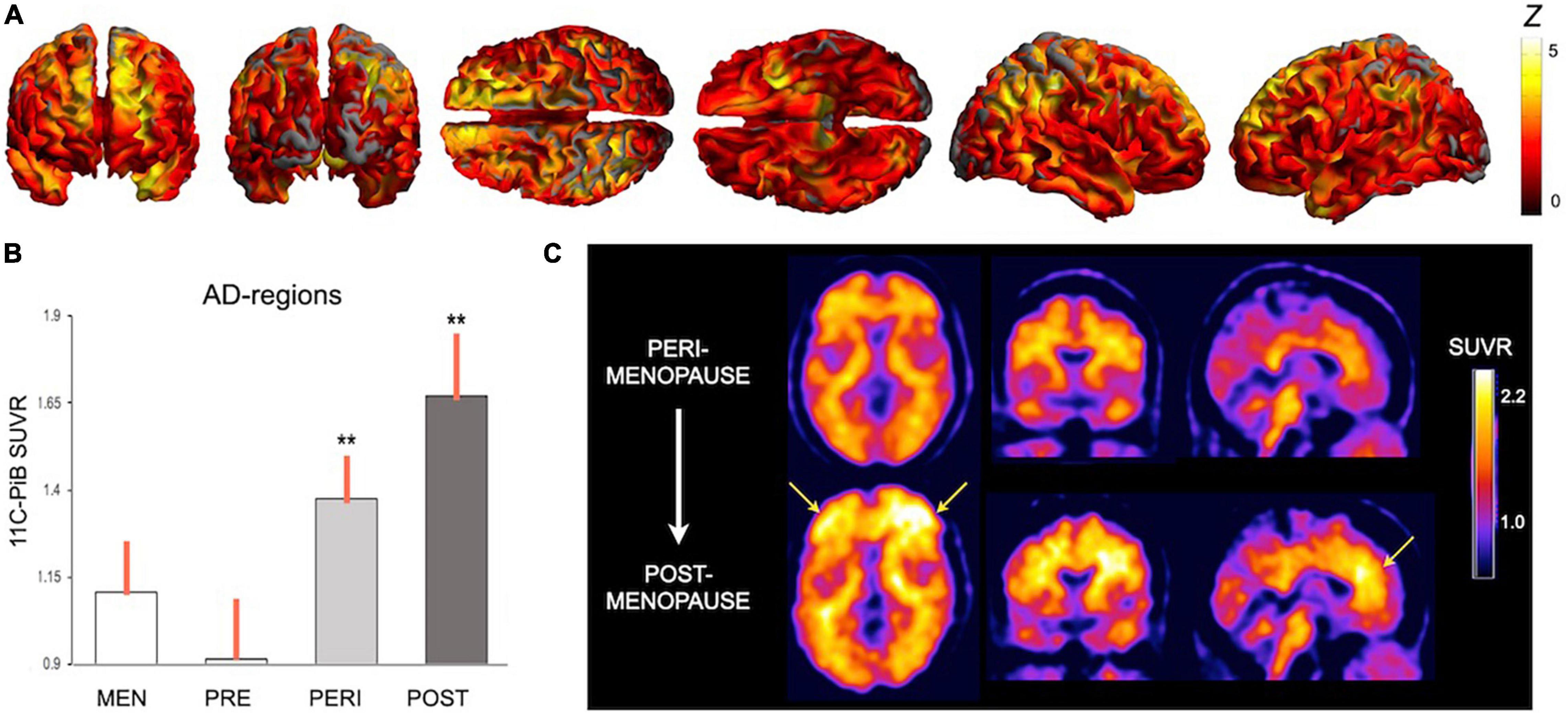
Figure 2. Effects of menopause on brain amyloid-beta deposition. Summary of Pittsburgh compound B PET (PiB-PET) studies showing menopause status effects on Aβ deposition: (A) Statistical parametric maps showing higher PiB uptake, a marker of Aβ load, in key brain regions for AD in a group of post-menopausal and peri-menopausal women vs. age-controlled men (Z scores > 2 correspond to p < 0.001). (B) In these regions, Aβ load was associated with menopausal status, e.g., was highest post-menopause, intermediate in peri-menopause, and lowest pre-menopause (**different from men at p < 0.001). (C) Aβ deposition is progressive during the menopause transition, as evidenced in a representative case who underwent PiB-PET at baseline, when she was peri-menopausal, and 3 years later, when she was post-menopausal. Images are adapted from data presented in (A) Mosconi et al. (2021), (B) Mosconi et al. (2017), and (C) Mosconi et al. (2018b). PiB, Pittsburgh compound B; SUVR, standardized uptake value ratio.
Nonetheless, a follow-up study provided preliminary evidence for biomarker stabilization or recovery in late post-menopause (Mosconi et al., 2021). For example, GM volume declined during peri-menopausal and early post-menopausal stages (Mosconi et al., 2018b), but plateaued in temporal cortex, and showed a rebound in precuneus in late post-menopause (Mosconi et al., 2021). WM declines in major WM tracts and CMRglc in parieto-temporal areas also appeared to plateau in late post-menopause (Mosconi et al., 2021). Additionally, cerebral blood flow (CBF) measured by means of Arterial Spin Labeling (ASL) was higher in the post-menopausal group as compared to pre-menopausal controls and to age-controlled men, and so was the ratio of adenosine triphosphate (ATP) to phosphocreatine (PCr) levels measured by means of 31Phosphorus Magnetic Resonance Spectroscopy (31P-MRS), reflecting higher ATP synthesis (Mosconi et al., 2021). Importantly, cognitive performance was intact post-menopause, which correlated with GM volume and ATP levels (Mosconi et al., 2021). Biomarker “recovery” was, however, attenuated in peri-menopausal and post-menopausal ApoE4 carriers (Mosconi et al., 2021). Overall, while these findings need to be replicated in larger samples, in keeping with preclinical work (Wang et al., 2020c), they suggest presence of compensatory mechanisms that allow brain adaptation to the hypo-estrogenic post-menopausal state, at least in some women. Brain adaptation may also account for cognitive preservation and for the easing of menopausal symptoms observed in the late post-menopausal stage (Monteleone et al., 2018).
Other natural history studies indicate lower GM volume in post-menopausal as compared with pre-menopausal women in frontal and temporal regions, which positively corelated with estradiol levels (Kim et al., 2018). The type of menopause also seems to have an impact, as induced menopausal cases exhibited smaller medial temporal lobe volume as compared to spontaneous post-menopausal cases (Zeydan et al., 2019). Moreover, physiologically-monitored night sweats correlated with estrogen levels and white matter hyperintensities (Thurston et al., 2016). Albeit limited by the small samples and by the fact that cognition was not studied (Thurston et al., 2016), this study suggests a link between vasomotor symptoms and cerebral small vessel disease, a risk factor for later stroke and dementia (Debette and Markus, 2010).
fMRI studies also provide emerging evidence for menopause-related changes in brain activation during verbal tasks and emotion processing. In some studies, post-menopausal women showed the least hippocampal activation, in spite of increased hippocampal connectivity, during verbal processing (Jacobs et al., 2016, 2017). Post-menopausal women also exhibited increased dorsolateral prefrontal cortex activation during verbal working memory (Jacobs et al., 2017). There is also evidence that post-menopausal women exhibited increased activation of regions involved in cognitive control during emotion decision making, such as the PFC, posterior cingulate, and temporoparietal junction, but not in limbic system (Berent-Spillson et al., 2017). Finally, presence of subjective cognitive complaints was associated with increased connectivity of the prefrontal cortex (Vega et al., 2016) while physiologically-monitored hot flashes were linked to increased DMN connectivity (Thurston et al., 2015).
Overall, a growing literature indicates that ovarian steroid hormones, particularly declines in estradiol, reshape the landscape of the female brain during the menopause transition. Some aspects of memory, such as verbal memory, are negatively impacted by menopause, along with more variable declines in processing speed, attention, and verbal fluency. These effects are, however, mild and tend to resolve in the late post-menopausal stage, e.g., approximately 6 years after the last menstrual period. Novel neuroimaging data also suggest that negative effects of menopause on neurophysiology may be transient, with the exception of women at risk for AD, who exhibit preclinical AD endophenotypes already during perimenopause. However, given that all women experience menopause but only a fraction will develop dementia, more work is warranted to elucidate which protective mechanisms may offset the effects of menopause on AD risk. Population-based studies indicate that over 30% of all AD cases could potentially be prevented by addressing modifiable medical and lifestyle factors such as smoking, depression, obesity, diabetes, and lack of physical activity (Livingston et al., 2020). Many of these factors also impact the age of onset and severity of menopause (Monteleone et al., 2018). More studies are needed to examine the effects of lifestyle and medical comorbidities on the brain changes occurring during menopause in association with future AD risk.
It also remains unclear whether altered brain biomarkers and memory fluctuations during perimenopause are predictive of dementia in later life. There are also preliminary findings of links between white matter hyperintensities and vasomotor symptoms, and of menopause-related changes in cognitive processing during emotion identification and in resting state networks, which need further clarification. While available findings need to be replicated in larger samples with longitudinal follow-ups and with the use of AD biomarkers, the evidence so far indicates that window of opportunity for support of estrogen-based neuroplasticity is early in the endocrine aging process.
Menopausal hormone therapy
Menopause hormonal therapy (MHT) includes oral and transdermal preparations thought to have systemic effects, and localized administrations (e.g., vaginal creams) that do not have systemic effects. Herein, we focus on systemic MHT. The treatment of choice for women with a uterus consists of combined (opposed) estrogens and progestins. The treatment of choice for women without a uterus is unopposed, or estrogen-only therapy. Estrogens can be estradiol or conjugated equine estrogens (CEE). Progestins vary in their hormone derivatives, as in hormonal contraceptives. The most commonly used are medroxyprogesterone acetate (MPA) and micronized progesterone.
There is a relatively large literature on MHT effects on brain and cognitive aging. In spite of this, results of whether MHT is viable for support of cognitive function and AD risk reduction are mixed. The hypothesis that MHT might protect against AD arose in large part from early observational studies and small clinical trials demonstrating a protective effect of MHT on cognitive function and AD risk among MHT users compared with never-users (Kawas et al., 1997; Zandi et al., 2002; Paganini-Hill et al., 2006; Sherwin, 2006; Whitmer et al., 2011), especially among younger, 50–59 year-old women (LeBlanc et al., 2001). Positive effects were particularly consistent with estrogen-only, or unopposed MHT for hysterectomized women (Sherwin and Phillips, 1990; Henderson et al., 2005; Rocca et al., 2007, 2010; Whitmer et al., 2011; Shao et al., 2012). Recent observational studies continue to provide conflicting results. For example, an analysis of health insurance claims from nearly 400,000 women reported a protective effect against AD and other neurodegenerative diseases with use of MHT (Kim et al., 2021). Compared with non-users, MHT users exhibited a 57% reduced risk of AD (Kim et al., 2021), with the greatest risk reduction for long-term MHT users (Kim et al., 2021). On the contrary, a population study in Finland of nearly 170,000 women reported that MHT use was associated with a 9–17% increased risk of AD, with higher risk for opposed MHT (Savolainen-Peltonen et al., 2019). In women younger than 60 at hormone therapy initiation, the increase in AD risk was mostly associated with MHT exposure over 10 years (Savolainen-Peltonen et al., 2019). However, ApoE4 status was not evaluated in this study. As Finland has a higher rate of ApoE4 carriers than most countries, with nearly 20% frequency, this is an important confounder as the effectiveness of MHT may be impacted by ApoE4 status (Depypere et al., 2016). Additionally, there is some evidence that oophorectomy before the natural age of menopause, but not after, is associated with an increased risk of AD (Rocca et al., 2007), which is mitigated by post-operative MHT (Sherwin and Phillips, 1990; Henderson et al., 2005; Rocca et al., 2007, 2010; Whitmer et al., 2011; Shao et al., 2012).
Randomized, placebo-controlled clinical trials of MHT for AD prevention have also provided conflicting results. The first large trial to test MHT for dementia prevention was the Women’s Health Initiative (WHI). The WHI included two studies, the WHI Estrogen-plus-Progestin Study, in which women with a uterus were randomly assigned to receive either combined MHT (Prempro) or a placebo; and the WHI Estrogen-Alone Study, in which women without a uterus were randomly assigned to receive either estrogen-alone therapy (Premarin) or a placebo. Cumulatively, the WHI showed some benefits related to use of MHT, including one-third fewer hip and vertebral fractures, and one-third lower risk of colorectal cancer relative to placebo (Rossouw et al., 2002; LaCroix et al., 2011). However, the trials were stopped prematurely as both MHT types were associated with an increased risk of coronary artery disease, stroke and blood clots (Rossouw et al., 2002; Anderson et al., 2004). Additionally, the Estrogen-plus-Progestin arm of the study initially showed an increased risk of cancer (Rossouw et al., 2002; Anderson et al., 2004), although subsequent analysis found no increase in risk (Anderson et al., 2012; Lobo, 2017).
The WHI included an additional arm, the WHI Memory Study (WHIMS), which examined the impact of MHT for dementia prevention among women ages 65 and older, thus in late post-menopause. These studies focused on oral CEEs in women with prior hysterectomy, and CEE/MPA in naturally post-menopausal women (Shumaker et al., 2003). Although AD was the a priori primary outcome of interest, all-cause dementia became the default primary outcome because of the lack of a sufficient number of AD cases at follow-up. In a sample of 2,947 post-menopausal women with prior hysterectomy, there was no evidence that CEE lowered the risk of all-cause dementia (Espeland et al., 2004; Shumaker et al., 2004). However, in a sample of 4,532 spontaneous post-menopausal women, CEE/MPA doubled the risk for all-cause dementia (Shumaker et al., 2003). Thus, the WHIMS study demonstrated no protective effects of unopposed MHT, and a substantial increase in dementia risk with opposed MHT among late post-menopausal women.
In terms of MHT effects on cognition, the WHIMS trial found that both opposed and unopposed therapy were associated with slightly worse mean scores in global cognitive function compared to placebo (Rapp et al., 2003; Espeland et al., 2004). These effects were observed within the first 3–4 years of the trial follow-up and remained fairly constant several years thereafter. The subsequent Women’s Health Initiative Study of Cognitive Aging (WHISCA) study examined whether MHT influenced domain-specific cognitive function at initial assessment, an average of 3 years after randomization to MHT or placebo, and after an additional ∼3 year of on-trial follow-up. Among the 2,304 participants, only small mean differences in cognitive test scores changes were noted (Resnick et al., 2006, 2009). Together, these findings suggest that if MHT use produces an initial decrement in at least some aspects of cognitive function, this decrement does not markedly widen or diminish thereafter. Notably, all the above studies involved post-menopausal women above age 65, thus possibly already harboring pre-existing cardiovascular or neurodegenerative conditions. As such, it may have been too late for MHT to prevent those conditions. These considerations, together with evidence from observational studies, has led to the understanding that the efficacy of MHT depends on the timing of initiation and the use of progestogens (LeBlanc et al., 2001; Manson et al., 2006; Maki, 2013; Bove et al., 2014).
However, the newer Early versus Late Intervention Trial with Estradiol (ELITE)-cog and Kronos Early Estrogen Prevention Study (KEEPS) trials have reported no beneficial or adverse effects of MHT on cognition among recently post-menopausal women within 6 years of the menopause diagnosis (Gleason et al., 2015; Henderson et al., 2016; Miller et al., 2019). Nonetheless, MHT reduced the progression of subclinical atherosclerosis when therapy was initiated soon after menopause (Hodis et al., 2016), which has been linked to a 30% reduced number of heart attacks and cardiac deaths (Salpeter et al., 2009).
To date, eight meta-analysis have examined the neuroprotective effects of MHT on AD risk (Yaffe et al., 1998; Hogervorst et al., 2000; LeBlanc et al., 2001; Lethaby et al., 2008; O’Brien et al., 2014; Song Y. J. et al., 2020; Zhang G. Q. et al., 2021). Early meta-analyses were based almost entirely on observational studies, and indicated a 29–35% reduced risk of AD in MHT users (Yaffe et al., 1998; LeBlanc et al., 2001). However, the large majority of women in those studies had started MHT before they experienced natural or surgical menopause, generally used estrogen-only therapy (typically CEEs), and stopped using MHT after age 60. As such, the hypothesis that MHT protects against AD was developed based on studies of estrogen-only therapy beginning in early post-menopause (or prior) and stopping a few years post-menopause. In fact, MHT initiated more than 10 years after menopause did not protect against AD (Zandi et al., 2002). Rather, women who initiated MHT between ages 61 and 68 had about double the risk of developing AD as compared to those who had begun MHT at younger ages (Zandi et al., 2002). Today, although results are still mixed, MHT use remains more consistently associated with reduced risk of AD or all-cause dementia as compared to placebo and/or lack of use, especially for estrogen-alone therapy, although all reports indicated substantial heterogeneity and large variability (Hogervorst et al., 2000; Lethaby et al., 2008; O’Brien et al., 2014; Song Y. J. et al., 2020; Zhang G. Q. et al., 2021). As possible biases and lack of control for potential confounders limit interpretation of these studies, more work is warranted to better clarify the role of MHT for AD prevention and preservation of cognitive function.
There is some evidence that MHT may facilitate maintenance of some aspects of cognition when initiated in early post-menopause or prior. Verbal memory is consistently seen to be maintained or sometimes enhanced with estrogen-alone treatment. A review of randomized, placebo-controlled trials of MHT and verbal memory indicate a beneficial effect of estrogen alone therapy in women younger than age 65, especially surgically post-menopausal cases (Maki and Sundermann, 2009). Additionally, different forms of progestogen may have different effects, with negative effects of CEE/MPA on verbal memory in younger women (Maki et al., 2007). There is also indication of positive, yet mild effects of MHT on learning and processing speed (Maki and Sundermann, 2009). Effects vary, however, with MHT type and timing, and there are individual differences, in particular related to time since menopause, type of menopause, and overall neurocognitive health prior to menopause.
Clinical trials using brain scans as endpoints lend support to the hypothesis that both age at treatment initiation and type of MHT are important factors to consider. As summarized in Table 3, the first generation of neuroimaging studies of MHT indicated a generally stimulating or preserving effects of MHT on CBF and CRMglc (Eberling et al., 2000; Maki and Resnick, 2000; Słopień et al., 2003; Rasgon et al., 2005b, 2014; Silverman et al., 2011). Among for women at risk for AD, PET studies provided evidence of differential changes in CMRglc as related to MHT use (Rasgon et al., 2005b, 2014; Silverman et al., 2011). A 2-year longitudinal study showed that non-users exhibited significant CMRglc declines in PCC, whereas MHT users did not exhibit significant CMRglc changes (Rasgon et al., 2005b). Two subsequent prospective, randomized clinical trials investigated post-menopausal women who were taking estrogen-alone MHT for at least 1 year prior to enrollment in the study, and were then randomized to continue or discontinue therapy. Over a 2-year period, women randomized to continue MHT exhibited a relative preservation of frontal and parietal CMRglc as compared with those randomized to discontinue MHT (Silverman et al., 2011; Rasgon et al., 2014). In addition, those continuing unopposed estradiol-based MHT showed additional preservation of CMRglc in PCC and precuneus (Rasgon et al., 2014). Additionally, unopposed MHT use was associated with higher CMRglc in frontal and temporal cortices, as well as better cognitive performance, as compared to opposed MHT, suggesting regionally specific neuroprotective effects (Eberling et al., 2000; Maki and Resnick, 2000; Silverman et al., 2011).
Structural MRI studies reported less consistent evidence of protective effects of MHT. Some report greater GM volumes in MHT users versus non-users (Erickson et al., 2005; Boccardi et al., 2006; Lord et al., 2008) or versus placebo (Eberling et al., 2003; Albert et al., 2017), mostly localized in frontal and temporal cortices, and hippocampus. In some studies, hippocampal volume was positively linked to verbal memory in treated post-menopausal women (Zhang et al., 2016; Braden et al., 2017). However, there are just as many contradictory reports showing decreased frontal GM volume in MHT users versus non-users (Coker et al., 2014; Ryan et al., 2014; Zhang et al., 2016), and decreased hippocampal volume in MHT users versus non-users (Greenberg et al., 2006; Low et al., 2006; Resnick et al., 2009) and in MHT users versus placebo, although there was no further decline from 1–3 to 6–7 years post-treatment (Coker et al., 2014). Notably, reports of positive effects of MHT focused on post-menopausal women in their 60s, whereas negative reports included mostly women of advanced age (71–89 years), sometimes with scanning conducted years after MHT ended. Additionally, two MRI studies showed no differences comparing current or past MHT users to non-users (Ryan et al., 2014; Braden et al., 2017). However, these studies were based on longitudinal WHIMS data collected several years after MHT cessation, and grouped users of estrogen-only and combined therapies, therefore not taking into account possible effects of MHT formulation (Ryan et al., 2014; Braden et al., 2017).
There are also reports of increased white matter hyperintensities with MHT use (Kantarci et al., 2016b) although results on this are mixed (Coker et al., 2014; Zhang et al., 2016) suggesting that effects of MHT on WMH are either small or moderated by confounders, such as age and overall cardiovascular health before treatment.
In addition, randomized controlled trials that incorporated fMRI indicated a higher activation of fronto-cingulate regions and hippocampus during verbal, non-verbal and spatial working memory tasks, although results are not always consistent (Shaywitz et al., 1999; Joffe et al., 2006; Smith et al., 2006; Dumas et al., 2010; Davison et al., 2013; Thomas et al., 2014; Berent-Spillson et al., 2015; Girard et al., 2017). Since these studies reported MHT-related effects in absence of differences in cognitive performance, it remains unclear whether higher activation during task performance reflects a beneficial response or a less efficient use of neuronal resources (Shaywitz et al., 1999; Thomas et al., 2014; Girard et al., 2017).
Overall, brain imaging studies of MHT suggest a putative positive role of estrogen against regional cerebral atrophy and metabolic decline, with an advantage of unopposed over combined MHT (Silverman et al., 2011; Rasgon et al., 2014), and of transdermal estradiol over oral CEE (Resnick et al., 2009; Zhang et al., 2016; Kantarci et al., 2018). However, brain imaging data suffers from several limitations (Comasco et al., 2014). Most studies are statistically under-powered due to relatively small samples and high heterogeneity, including differences in study design (controlled randomization vs. cross-sectional trials, parallel vs. cross-over design, baseline vs. placebo control state), different duration of MHT use, different routes of administration and posology/dose, and different type of therapy (unopposed vs. combined MHT), differences in the timing of initiation with respect to age and/or the menopausal transition, as well as use of different neuroimaging techniques, different neuropsychological paradigms in activation studies, and different processing and analysis pipelines.
In conclusion, active debate remains on whether MHT has value for neuroprotection. Natural history studies and some clinical trials suggest that MHT may support cognition and brain function in peri-menopausal and recently post-menopausal women. However, most studies demonstrating benefits are based on observational studies, or studies of younger women which may have better captured the critical window for MHT action vs. larger clinical trials of older post-menopausal women. Observational studies are subject to bias as women who choose to use MHT have in general higher education, and tend to have healthier lifestyles and better overall health before and after taking MHT than women who do not (Matthews et al., 1996). Taking MHT may therefore be associated with a healthier lifestyle which in turn might be driving cognitive function. In addition, despite estrogen’s biologically plausible mechanisms for supporting brain aging, most reviews have concluded that many observational studies and clinical trials are limited by methodological problems such as small size and short duration, and display substantial heterogeneity.
Conclusion
Understanding sex-driven effects of ovarian hormones on dementia risk is a crucial step toward development of precision medicine strategies for AD prevention. In recent years, significant progress has been made in discovering how ovarian steroid hormones influence cognitive aging, prompted in part by advancements in the research on sex differences in AD. Across the female lifespan, there is compelling evidence that estradiol levels influence brain structure, function, and biochemistry in many regions affected by AD. There are also increasing indications of complex interactions of estradiol with other sex hormones, chiefly progesterone and androgens.
In this review we examined the effects of puberty, the menstrual cycle, hormonal contraceptives, menopause, and MHT on cognitive aging and neuroimaging biomarkers of AD. While this field is still in its infancy, there is increasing evidence for associations between indicators of estrogen exposure, such as pubertal timing, menstrual cycle frequency, number of pregnancies, and OC use, and cognitive function over the course of a woman’s life (Egan and Gleason, 2012; Li et al., 2016).
More work has been done to investigate changes in cognition and AD biomarkers during the transition to menopause, and more so as due to MHT use. Clinical studies indicate a dip in cognitive performance, mostly verbal memory, during peri-menopause, possibly followed by a rebound post-menopause. Significant heterogeneity has been noted as related to age, menopause status, use of MHT, and genetic risk factors. Hardly any clinical studies analyzed data in relation to women’s existing genetic predisposition to AD or other neurological conditions. On the other hand, neuroimaging studies of midlife women at genetic risk for AD have provided robust evidence for emergence of AD endophenotypes with onset in peri-menopause among natural cyclers (Mosconi et al., 2017, 2018a,b, 2021; Rahman et al., 2020). Surgically induced menopause is also associated with a higher risk of AD, especially in presence of an earlier age at oophorectomy (Bove et al., 2014). Across studies, the risk of AD is over 30% higher following hysterectomy alone, and over two times higher in presence of oophorectomy relative to spontaneous menopause. For contrast, women’s risk of AD is increased 4- and 12–15-fold with one or two ApoE4 alleles, respectively (Riedel et al., 2016). More work is needed to examine the combined effects of ApoE4 and hysterectomy/oophorectomy status prior to menopause on AD biomarkers, and whether the associations are modified by MHT use.
Menopause hormone therapy use has been heavily scrutinized due to the disparity between basic science, observational studies, and large randomized clinical trials. Overall, MHT action on brain is dependent on multiple factors, including chronological age, stage of reproductive aging, duration of hypogonadism, and presence of symptoms, as well as the formulation of MHT, route of administration, and the health status of the brain. Currently, MHT is not indicated to alleviate cognitive complaints or for AD prevention. However, some argue that MHT given to healthy peri-menopausal and early post-menopausal women under age 60 for about 5 years may be recommended for support of cognitive function with careful consideration of other risks (Stuenkel et al., 2015; Baber et al., 2016). There is mounting evidence that MHT use during early menopause, and in presence of symptoms, may help sustain neurological health and reduce the risk of AD (Brinton, 2008), whereas MHT initiated >5 years after menopause may be less beneficial if not detrimental as in the case of combined therapy (Shumaker et al., 2003). Personalized physician advice which takes into consideration key factors including age, menopausal stage, symptoms, and comorbidities, may offer a greater look at how MHT impacts AD risk as compared to the one-size-fits-all approach of randomized clinical trials, and argues for a precision medicine approach to MHT use (Kim and Brinton, 2021; Kim et al., 2021). More research is warranted to further understand this critical window of estrogen sensitivity.
Previous work has shown that, in vitro and in vivo, ApoE expression can be differentially regulated either by 17-beta-estradiol or specific agonists, depending on activation of ER subtypes (Wang et al., 2006). These data suggest that use of ER-selective ligands might provide therapeutic benefit to reduce AD risk by decreasing ApoE expression in ApoE4 allele carriers. Moreover, because ERβ promotes estrogen-mediated neuronal plasticity and memory function, a formula that selectively targets ERβ may be a novel and plausible solution for menopause-related vasomotor symptoms and cognitive impairment. In 2022, we obtained NIH funds to carry out a Phase IIb randomized, placebo-controlled clinical trial testing the efficacy of PhytoSERM, a selective estrogen receptor beta (ERβ) modulator comprised of three phytoestrogens: genistein, daidzein, and S-equol (Zhao et al., 2009), for AD prevention in midlife women. The PhytoSERM formulation has been shown to promote estrogenic action in brain while remaining largely inactive or inhibitory in reproductive tissue (Zhao et al., 2009). The initial phase Ib/IIa clinical trial (ClinicalTrial.gov ID: NCT01723917) demonstrated safety and established the pharmacokinetics profile of PhytoSERM (Wang et al., 2020a). Results of the ongoing Phase IIb trial will become available by 2026.
In conclusion, ovarian steroid hormones are long overlooked but critical contributors to brain aging and AD risk. While the neurobiological consequences of hormonal activity have only begun to be understood, converging evidence supports a role for cumulative estrogen exposure in reducing risk of developing AD later in life. This strongly argues for continued examination of sex hormones and reproductive history factors in AD prevention strategies for women. There is an urgent need for prospective epidemiological, clinical and biomarkers studies with data taken at several time-points starting at midlife that examine the associations between lifetime estrogen exposure and neurological function in later life. Understanding the dynamic interplay between sex, chronological aging, endocrine aging, and additional AD risk factors is crucial to inform and justify primary precision-medicine strategies for AD prevention.
Author contributions
LM and SJ discussed the concepts and wrote the manuscript. ES, GJ, CB, JD, SP, and RD reviewed the literature and provided critical revision of the manuscript for important intellectual content. All authors contributed to the article and approved the submitted version.
Funding
This study was supported by grants from NIH/NIA (PO1AG026572, RO1AG05793, and RO1AG0755122), NIH/NCATS UL1TR002384, the Cure Alzheimer’s Fund, Maria Shriver’s Women’s Alzheimer’s Movement; and philanthropic support to the Weill Cornell Alzheimer’s Prevention Program.
Conflict of interest
The authors declare that the research was conducted in the absence of any commercial or financial relationships that could be construed as a potential conflict of interest.
Publisher’s note
All claims expressed in this article are solely those of the authors and do not necessarily represent those of their affiliated organizations, or those of the publisher, the editors and the reviewers. Any product that may be evaluated in this article, or claim that may be made by its manufacturer, is not guaranteed or endorsed by the publisher.
References
Agca, C., Klakotskaia, D., Stopa, E. G., Schachtman, T. R., and Agca, Y. (2020). Ovariectomy influences cognition and markers of Alzheimer’s disease. J. Alzheimers Dis. 73, 529–541. doi: 10.3233/jad-190935
Albert, K., Hiscox, J., Boyd, B., Dumas, J., Taylor, W., and Newhouse, P. (2017). Estrogen enhances hippocampal gray-matter volume in young and older postmenopausal women: a prospective dose-response study. Neurobiol. Aging 56, 1–6. doi: 10.1016/j.neurobiolaging.2017.03.033
Albert, K., Pruessner, J., and Newhouse, P. (2015). Estradiol levels modulate brain activity and negative responses to psychosocial stress across the menstrual cycle. Psychoneuroendocrinology 59, 14–24. doi: 10.1016/j.psyneuen.2015.04.022
Anderson, G. L., Chlebowski, R. T., Aragaki, A. K., Kuller, L. H., Manson, J. E., Gass, M., et al. (2012). Conjugated equine oestrogen and breast cancer incidence and mortality in postmenopausal women with hysterectomy: extended follow-up of the Women’s Health Initiative randomised placebo-controlled trial. Lancet Oncol. 13, 476–486. doi: 10.1016/S1470-2045(12)70075-X
Anderson, G. L., Limacher, M., Assaf, A. R., Bassford, T., Beresford, S. A., Black, H., et al. (2004). Effects of conjugated equine estrogen in postmenopausal women with hysterectomy: the Women’s Health Initiative randomized controlled trial. JAMA 291, 1701–1712. doi: 10.1001/jama.291.14.1701
Anderson, S. E., Dallal, G. E., and Must, A. (2003). Relative weight and race influence average age at menarche: results from two nationally representative surveys of Us girls studied 25 years apart. Pediatrics 111, 844–850. doi: 10.1542/peds.111.4.844
Andersson, S., Sundberg, M., Pristovsek, N., Ibrahim, A., Jonsson, P., Katona, B., et al. (2017). Insufficient antibody validation challenges oestrogen receptor beta research. Nat. Commun. 8:15840. doi: 10.1038/ncomms15840
Arélin, K., Mueller, K., Barth, C., Rekkas, P. V., Kratzsch, J., Burmann, I., et al. (2015). Progesterone mediates brain functional connectivity changes during the menstrual cycle-a pilot resting state MRI study. Front. Neurosci. 9:44. doi: 10.3389/fnins.2015.00044
Arevalo, M. A., Azcoitia, I., and Garcia-Segura, L. M. (2015). The neuroprotective actions of oestradiol and oestrogen receptors. Nat. Rev. Neurosci. 16, 17–29. doi: 10.1038/nrn3856
Arnoni-Bauer, Y., Bick, A., Raz, N., Imbar, T., Amos, S., Agmon, O., et al. (2017). Is it me or my hormones? Neuroendocrine activation profiles to visual food stimuli across the menstrual cycle. J. Clin. Endocrinol. Metab. 102, 3406–3414. doi: 10.1210/jc.2016-3921
Azcoitia, I., Fernandez-Galaz, C., Sierra, A., and Garcia-Segura, L. M. (1999). Gonadal hormones affect neuronal vulnerability to excitotoxin-induced degeneration. J. Neurocytol. 28, 699–710. doi: 10.1023/a:1007025219044
Baber, R. J., Panay, N., and Fenton, A. (2016). 2016 IMS Recommendations on women’s midlife health and menopause hormone therapy. Climacteric 19, 109–150. doi: 10.3109/13697137.2015.1129166
Bae, J. B., Lipnicki, D. M., Han, J. W., Sachdev, P. S., Kim, T. H., Kwak, K. P., et al. (2020). Parity and the risk of incident dementia: a COSMIC study. Epidemiol. Psychiatr. Sci. 29:e176. doi: 10.1017/s2045796020000876
Barth, C., and de Lange, A. G. (2020). Towards an understanding of women’s brain aging: the immunology of pregnancy and menopause. Front. Neuroendocrinol. 58:100850. doi: 10.1016/j.yfrne.2020.100850
Barth, C., Steele, C. J., Mueller, K., Rekkas, V. P., Arélin, K., Pampel, A., et al. (2016). In-vivo dynamics of the human hippocampus across the menstrual cycle. Sci. Rep. 6:32833. doi: 10.1038/srep32833
Bayer, J., Schultz, H., Gamer, M., and Sommer, T. (2014). Menstrual-cycle dependent fluctuations in ovarian hormones affect emotional memory. Neurobiol. Learn. Mem. 110, 55–63. doi: 10.1016/j.nlm.2014.01.017
Beeri, M. S., Rapp, M., Schmeidler, J., Reichenberg, A., Purohit, D. P., Perl, D. P., et al. (2009). Number of children is associated with neuropathology of Alzheimer’s disease in women. Neurobiol. Aging 30, 1184–1191. doi: 10.1016/j.neurobiolaging.2007.11.011
Beltz, A. M., Hampson, E., and Berenbaum, S. A. (2015). Oral contraceptives and cognition: a role for ethinyl estradiol. Horm Behav. 74, 209–217. doi: 10.1016/j.yhbeh.2015.06.012
Berent-Spillson, A., Briceno, E., Pinsky, A., Simmen, A., Persad, C. C., Zubieta, J. K., et al. (2015). Distinct cognitive effects of estrogen and progesterone in menopausal women. Psychoneuroendocrinology 59, 25–36. doi: 10.1016/j.psyneuen.2015.04.020
Berent-Spillson, A., Kelley, A. S., Persad, C. C., Love, T., Frey, K. A., Reame, N. E., et al. (2018). Postmenopausal hormone treatment alters neural pathways but does not improve verbal cognitive function. Menopause 25, 1424–1431. doi: 10.1097/gme.0000000000001157
Berent-Spillson, A., Marsh, C., Persad, C., Randolph, J., Zubieta, J. K., and Smith, Y. (2017). Metabolic and hormone influences on emotion processing during menopause. Psychoneuroendocrinology 76, 218–225. doi: 10.1016/j.psyneuen.2016.08.026
Berent-Spillson, A., Persad, C. C., Love, T., Sowers, M., Randolph, J. F., Zubieta, J. K., et al. (2012). Hormonal environment affects cognition independent of age during the menopause transition. J. Clin. Endocrinol. Metab. 97, E1686–E1694. doi: 10.1210/jc.2012-1365
Berent-Spillson, A., Persad, C. C., Love, T., Tkaczyk, A., Wang, H., Reame, N. K., et al. (2010). Early menopausal hormone use influences brain regions used for visual working memory. Menopause 17, 692–699. doi: 10.1097/gme.0b013e3181cc49e9
Bernstein, L., Pike, M. C., Ross, R. K., and Henderson, B. E. (1991). Age at menarche and estrogen concentrations of adult women. Cancer Causes Control 2, 221–225. doi: 10.1007/bf00052137
Bernstein, L., Pike, M. C., Ross, R. K., Judd, H. L., Brown, J. B., and Henderson, B. E. (1985). Estrogen and sex hormone-binding globulin levels in nulliparous and parous women. J. Natl. Cancer Inst. 74, 741–745.
Biegon, A., Alexoff, D. L., Kim, S. W., Logan, J., Pareto, D., Schlyer, D., et al. (2015). Aromatase imaging with [N-methyl-11C]vorozole PET in healthy men and women. J. Nucl. Med. 56, 580–585. doi: 10.2967/jnumed.114.150383
Blakemore, S. J. (2008). The social brain in adolescence. Nat. Rev. Neurosci. 9, 267–277. doi: 10.1038/nrn2353
Boccardi, M., Ghidoni, R., Govoni, S., Testa, C., Benussi, L., Bonetti, M., et al. (2006). Effects of hormone therapy on brain morphology of healthy postmenopausal women: a voxel-based morphometry study. Menopause 13, 584–591. doi: 10.1097/01.gme.0000196811.88505.10
Bove, R., Secor, E., Chibnik, L. B., Barnes, L. L., Schneider, J. A., Bennett, D. A., et al. (2014). Age at surgical menopause influences cognitive decline and Alzheimer pathology in older women. Neurology 82, 222–229. doi: 10.1212/wnl.0000000000000033
Boyle, C. P., Raji, C. A., Erickson, K. I., Lopez, O. L., Becker, J. T., Gach, H. M., et al. (2021). Estrogen, brain structure, and cognition in postmenopausal women. Hum. Brain Mapp. 42, 24–35. doi: 10.1002/hbm.25200
Braden, B. B., Dassel, K. B., Bimonte-Nelson, H. A., O’Rourke, H. P., Connor, D. J., Moorhous, S., et al. (2017). Sex and post-menopause hormone therapy effects on hippocampal volume and verbal memory. Neuropsychol. Dev. Cogn. B Aging Neuropsychol. Cogn. 24, 227–246. doi: 10.1080/13825585.2016.1182962
Brinton, R. D. (2008). The healthy cell bias of estrogen action: mitochondrial bioenergetics and neurological implications. Trends Neurosci. 31, 529–537. doi: 10.1016/j.tins.2008.07.003
Brinton, R. D., Thompson, R. F., Foy, M. R., Baudry, M., Wang, J., Finch, C. E., et al. (2008). Progesterone receptors: form and function in brain. Front. Neuroendocrinol. 29:313–339. doi: 10.1016/j.yfrne.2008.02.001
Brinton, R. D., Yao, J., Yin, F., Mack, W. J., and Cadenas, E. (2015). Perimenopause as a neurological transition state. Nat. Rev. Endocrinol. 11, 393–405. doi: 10.1038/nrendo.2015.82
Bromberger, J. T., Schott, L. L., Kravitz, H. M., Sowers, M., Avis, N. E., Gold, E. B., et al. (2010). Longitudinal change in reproductive hormones and depressive symptoms across the menopausal transition: results from the Study of Women’s Health Across the Nation (SWAN). Arch. Gen. Psychiatry 67, 598–607. doi: 10.1001/archgenpsychiatry.2010.55
Brønnick, M. K., Økland, I., Graugaard, C., and Brønnick, K. K. (2020). The effects of hormonal contraceptives on the brain: a systematic review of neuroimaging studies. Front. Psychol. 11:556577. doi: 10.3389/fpsyg.2020.556577
Brunton, P. J., and Russell, J. A. (2008). The expectant brain: adapting for motherhood. Nat. Rev. Neurosci. 9, 11–25. doi: 10.1038/nrn2280
Byrnes, E. M., Babb, J. A., and Bridges, R. S. (2009). Differential expression of oestrogen receptor alpha following reproductive experience in young and middle-aged female rats. J. Neuroendocrinol. 21, 550–557. doi: 10.1111/j.1365-2826.2009.01874.x
Cahill, L. (2006). Why sex matters for neuroscience. Nat. Rev. Neurosci. 7, 477–484. doi: 10.1038/nrn1909
Caruso, D., Pesaresi, M., Abbiati, F., Calabrese, D., Giatti, S., Garcia-Segura, L. M., et al. (2013). Comparison of plasma and cerebrospinal fluid levels of neuroactive steroids with their brain, spinal cord and peripheral nerve levels in male and female rats. Psychoneuroendocrinology 38, 2278–2290. doi: 10.1016/j.psyneuen.2013.04.016
Caruso, D., Pesaresi, M., Maschi, O., Giatti, S., Garcia-Segura, L. M., and Melcangi, R. C. (2010). Effect of short-and long-term gonadectomy on neuroactive steroid levels in the central and peripheral nervous system of male and female rats. J. Neuroendocrinol. 22, 1137–1147. doi: 10.1111/j.1365-2826.2010.02064.x
Chahal, R., Vilgis, V., Grimm, K. J., Hipwell, A. E., Forbes, E. E., Keenan, K., et al. (2018). Girls’ pubertal development is associated with white matter microstructure in late adolescence. Neuroimage 181, 659–669. doi: 10.1016/j.neuroimage.2018.07.050
Chen, K. X., Worley, S., Foster, H., Edasery, D., Roknsharifi, S., Ifrah, C., et al. (2021). Oral contraceptive use is associated with smaller hypothalamic and pituitary gland volumes in healthy women: a structural MRI study. PLoS One 16:e0249482. doi: 10.1371/journal.pone.0249482
Clayton, J. A. (2016). Studying both sexes: a guiding principle for biomedicine. FASEB J. 30, 519–524. doi: 10.1096/fj.15-279554
Coker, L. H., Espeland, M. A., Hogan, P. E., Resnick, S. M., Bryan, R. N., Robinson, J. G., et al. (2014). Change in brain and lesion volumes after CEE therapies: the WHIMS-MRI studies. Neurology 82, 427–434. doi: 10.1212/wnl.0000000000000079
Coker, L. H., Hogan, P. E., Bryan, N. R., Kuller, L. H., Margolis, K. L., Bettermann, K., et al. (2009). Postmenopausal hormone therapy and subclinical cerebrovascular disease: the WHIMS-MRI Study. Neurology 72, 125–134. doi: 10.1212/01.wnl.0000339036.88842.9e
Colucci, M., Cammarata, S., Assini, A., Croce, R., Clerici, F., Novello, C., et al. (2006). The number of pregnancies is a risk factor for Alzheimer’s disease. Eur. J. Neurol. 13, 1374–1377. doi: 10.1111/j.1468-1331.2006.01520.x
Comasco, E., Frokjaer, V. G., and Sundström-Poromaa, I. (2014). Functional and molecular neuroimaging of menopause and hormone replacement therapy. Front. Neurosci. 8:388. doi: 10.3389/fnins.2014.00388
Corbo, R. M., Gambina, G., Ulizzi, L., Monini, P., Broggio, E., Rosano, A., et al. (2007). Combined effect of apolipoprotein e genotype and past fertility on age at onset of Alzheimer’s disease in women. Dement. Geriatr. Cogn. Disord. 24, 82–85. doi: 10.1159/000103866
Courvoisier, D. S., Renaud, O., Geiser, C., Paschke, K., Gaudy, K., and Jordan, K. (2013). Sex hormones and mental rotation: an intensive longitudinal investigation. Horm Behav. 63, 345–351. doi: 10.1016/j.yhbeh.2012.12.007
Cui, J., Shen, Y., and Li, R. (2013). Estrogen synthesis and signaling pathways during aging: from periphery to brain. Trends Mol. Med. 19, 197–209. doi: 10.1016/j.molmed.2012.12.007
Dan, R., Canetti, L., Keadan, T., Segman, R., Weinstock, M., Bonne, O., et al. (2019). Sex differences during emotion processing are dependent on the menstrual cycle phase. Psychoneuroendocrinology 100, 85–95. doi: 10.1016/j.psyneuen.2018.09.032
Davison, S. L., Bell, R. J., Robinson, P. J., Jane, F., Leech, J., Maruff, P., et al. (2013). Continuous-combined oral estradiol/drospirenone has no detrimental effect on cognitive performance and improves estrogen deficiency symptoms in early postmenopausal women: a randomized placebo-controlled trial. Menopause 20, 1020–1026. doi: 10.1097/GME.0b013e318287474f
De Bondt, T., De Belder, F., Vanhevel, F., Jacquemyn, Y., and Parizel, P. M. (2015a). Prefrontal GABA concentration changes in women—Influence of menstrual cycle phase, hormonal contraceptive use, and correlation with premenstrual symptoms. Brain Res. 1597, 129–138. doi: 10.1016/j.brainres.2014.11.051
De Bondt, T., Jacquemyn, Y., Van Hecke, W., Sijbers, J., Sunaert, S., and Parizel, P. M. (2013a). Regional gray matter volume differences and sex-hormone correlations as a function of menstrual cycle phase and hormonal contraceptives use. Brain Res. 1530, 22–31. doi: 10.1016/j.brainres.2013.07.034
De Bondt, T., Pullens, P., Van Hecke, W., Jacquemyn, Y., and Parizel, P. M. (2016). Reproducibility of hormone-driven regional grey matter volume changes in women using SPM8 and SPM12. Brain Struct. Funct. 221, 4631–4641. doi: 10.1007/s00429-016-1193-1
De Bondt, T., Smeets, D., Pullens, P., Van Hecke, W., Jacquemyn, Y., and Parizel, P. M. (2015b). Stability of resting state networks in the female brain during hormonal changes and their relation to premenstrual symptoms. Brain Res. 1624, 275–285. doi: 10.1016/j.brainres.2015.07.045
De Bondt, T., Van Hecke, W., Veraart, J., Leemans, A., Sijbers, J., Sunaert, S., et al. (2013b). Does the use of hormonal contraceptives cause microstructural changes in cerebral white matter? Preliminary results of a DTI and tractography study. Eur. Radiol. 23, 57–64. doi: 10.1007/s00330-012-2572-5
de Lange, A. G., Barth, C., Kaufmann, T., Anatürk, M., Suri, S., Ebmeier, K. P., et al. (2020). The maternal brain: region-specific patterns of brain aging are traceable decades after childbirth. Hum. Brain Mapp. 41, 4718–4729. doi: 10.1002/hbm.25152
de Lange, A.-M. G., Kaufmann, T., van der Meer, D., Maglanoc, L. A., Alnćs, D., Moberget, T., et al. (2019). Population-based neuroimaging reveals traces of childbirth in the maternal brain. Proc. Natl. Acad. Sci. U.S.A. 116, 22341–22346. doi: 10.1073/pnas.1910666116
Debette, S., and Markus, H. S. (2010). The clinical importance of white matter hyperintensities on brain magnetic resonance imaging: systematic review and meta-analysis. BMJ 341:c3666. doi: 10.1136/bmj.c3666
Deems, N. P., and Leuner, B. (2020). Pregnancy, postpartum and parity: resilience and vulnerability in brain health and disease. Front. Neuroendocrinol. 57:100820. doi: 10.1016/j.yfrne.2020.100820
Depypere, H., Vierin, A., Weyers, S., and Sieben, A. (2016). Alzheimer’s disease, apolipoprotein E and hormone replacement therapy. Maturitas 94, 98–105. doi: 10.1016/j.maturitas.2016.09.009
Diekhof, E. K., and Ratnayake, M. (2016). Menstrual cycle phase modulates reward sensitivity and performance monitoring in young women: preliminary fMRI evidence. Neuropsychologia 84, 70–80. doi: 10.1016/j.neuropsychologia.2015.10.016
Ding, F., Yao, J., Rettberg, J. R., Chen, S., and Brinton, R. D. (2013). Early decline in glucose transport and metabolism precedes shift to ketogenic system in female aging and Alzheimer’s mouse brain: implication for bioenergetic intervention. PLoS One 8:e79977. doi: 10.1371/journal.pone.0079977
Donishi, T., Terada, M., and Kaneoke, Y. (2018). Effects of gender, digit ratio, and menstrual cycle on intrinsic brain functional connectivity: a whole-brain, voxel-wise exploratory study using simultaneous local and global functional connectivity mapping. Brain Behav. 8:e00890. doi: 10.1002/brb3.890
Drogos, L. L., Rubin, L. H., Geller, S. E., Banuvar, S., Shulman, L. P., and Maki, P. M. (2013). Objective cognitive performance is related to subjective memory complaints in midlife women with moderate to severe vasomotor symptoms. Menopause 20, 1236–1242. doi: 10.1097/GME.0b013e318291f5a6
Dubal, D. B., Zhu, H., Yu, J., Rau, S. W., Shughrue, P. J., Merchenthaler, I., et al. (2001). Estrogen receptor alpha, not beta, is a critical link in estradiol-mediated protection against brain injury. Proc. Natl. Acad. Sci. U.S.A. 98, 1952–1957. doi: 10.1073/pnas.041483198
Dubol, M., Epperson, C. N., Sacher, J., Pletzer, B., Derntl, B., Lanzenberger, R., et al. (2021). Neuroimaging the menstrual cycle: a multimodal systematic review. Front. Neuroendocrinol. 60:100878. doi: 10.1016/j.yfrne.2020.100878
Dumas, J. A., Kutz, A. M., Naylor, M. R., Johnson, J. V., and Newhouse, P. A. (2010). Increased memory load-related frontal activation after estradiol treatment in postmenopausal women. Horm Behav. 58, 929–935. doi: 10.1016/j.yhbeh.2010.09.003
Eberling, J. L., Reed, B. R., Coleman, J. E., and Jagust, W. J. (2000). Effect of estrogen on cerebral glucose metabolism in postmenopausal women. Neurology 55, 875–877. doi: 10.1212/wnl.55.6.875
Eberling, J. L., Wu, C., Haan, M. N., Mungas, D., Buonocore, M., and Jagust, W. J. (2003). Preliminary evidence that estrogen protects against age-related hippocampal atrophy. Neurobiol. Aging 24, 725–732. doi: 10.1016/s0197-4580(02)00056-8
Egan, K. R., and Gleason, C. E. (2012). Longer duration of hormonal contraceptive use predicts better cognitive outcomes later in life. J. Womens Health 21, 1259–1266. doi: 10.1089/jwh.2012.3522
Engman, J., Sundström Poromaa, I., Moby, L., Wikström, J., Fredrikson, M., and Gingnell, M. (2018). Hormonal cycle and contraceptive effects on amygdala and salience resting-state networks in women with previous affective side effects on the pill. Neuropsychopharmacology 43, 555–563. doi: 10.1038/npp.2017.157
Epperson, C. N., Sammel, M. D., and Freeman, E. W. (2013). Menopause effects on verbal memory: findings from a longitudinal community cohort. J. Clin. Endocrinol. Metab. 98, 3829–3838. doi: 10.1210/jc.2013-1808
Erickson, K. I., Colcombe, S. J., Raz, N., Korol, D. L., Scalf, P., Webb, A., et al. (2005). Selective sparing of brain tissue in postmenopausal women receiving hormone replacement therapy. Neurobiol. Aging 26, 1205–1213. doi: 10.1016/j.neurobiolaging.2004.11.009
Espeland, M. A., Rapp, S. R., Shumaker, S. A., Brunner, R., Manson, J. E., Sherwin, B. B., et al. (2004). Conjugated equine estrogens and global cognitive function in postmenopausal women: women’s health initiative memory study. JAMA 291, 2959–2968. doi: 10.1001/jama.291.24.2959
Farrag, A. K., Khedr, E. M., Abdel-Aleem, H., and Rageh, T. A. (2002). Effect of surgical menopause on cognitive functions. Dement. Geriatr. Cogn. Disord. 13, 193–198. doi: 10.1159/000048652
Farrer, L. A., Cupples, L. A., Haines, J. L., Hyman, B., Kukull, W. A., Mayeux, R., et al. (1997). Effects of age, sex, and ethnicity on the association between apolipoprotein E genotype and Alzheimer disease. a meta-analysis. APOE and Alzheimer Disease Meta Analysis Consortium. JAMA 278, 1349–1356.
Ferretti, M. T., Iulita, M. F., Cavedo, E., Chiesa, P. A., Schumacher Dimech, A., Santuccione Chadha, A., et al. (2018). Sex differences in Alzheimer disease - the gateway to precision medicine. Nat. Rev. Neurol. 14, 457–469. doi: 10.1038/s41582-018-0032-9
Foster, T. C. (2012). Role of estrogen receptor alpha and beta expression and signaling on cognitive function during aging. Hippocampus 22, 656–669. doi: 10.1002/hipo.20935
Fox, M., Berzuini, C., and Knapp, L. A. (2013). Cumulative estrogen exposure, number of menstrual cycles, and Alzheimer’s risk in a cohort of British women. Psychoneuroendocrinology 38, 2973–2982. doi: 10.1016/j.psyneuen.2013.08.005
Fox, M., Berzuini, C., Knapp, L. A., and Glynn, L. M. (2018). Women’s pregnancy life history and alzheimer’s risk: can immunoregulation explain the link? Am. J. Alzheimers Dis. Other Demen. 33, 516–526. doi: 10.1177/1533317518786447
Frank, A., Brown, L. M., and Clegg, D. J. (2014). The role of hypothalamic estrogen receptors in metabolic regulation. Front. Neuroendocrinol. 35:550–557. doi: 10.1016/j.yfrne.2014.05.002
Franke, K., Hagemann, G., Schleussner, E., and Gaser, C. (2015). Changes of individual BRAINAGE during the course of the menstrual cycle. Neuroimage 115, 1–6. doi: 10.1016/j.neuroimage.2015.04.036
Frokjaer, V. G., Pinborg, A., Holst, K. K., Overgaard, A., Henningsson, S., Heede, M., et al. (2015). Role of serotonin transporter changes in depressive responses to sex-steroid hormone manipulation: a positron emission tomography study. Biol. Psychiatry 78, 534–543. doi: 10.1016/j.biopsych.2015.04.015
Fuh, J. L., Wang, S. J., Lee, S. J., Lu, S. R., and Juang, K. D. (2006). A longitudinal study of cognition change during early menopausal transition in a rural community. Maturitas 53, 447–453. doi: 10.1016/j.maturitas.2005.07.009
Geerlings, M. I., Ruitenberg, A., Witteman, J. C., van Swieten, J. C., Hofman, A., van Duijn, C. M., et al. (2001). Reproductive period and risk of dementia in postmenopausal women. JAMA 285, 1475–1481. doi: 10.1001/jama.285.11.1475
Giatti, S., Garcia-Segura, L. M., Barreto, G. E., and Melcangi, R. C. (2019). Neuroactive steroids, neurosteroidogenesis and sex. Progr. Neurobiol. 176, 1–17. doi: 10.1016/j.pneurobio.2018.06.007
Giedd, J. N., Blumenthal, J., Jeffries, N. O., Castellanos, F. X., Liu, H., Zijdenbos, A., et al. (1999). Brain development during childhood and adolescence: a longitudinal MRI study. Nat. Neurosci. 2, 861–863. doi: 10.1038/13158
Gilsanz, P., Lee, C., Corrada, M. M., Kawas, C. H., Quesenberry, C. P. Jr., and Whitmer, R. A. (2019). Reproductive period and risk of dementia in a diverse cohort of health care members. Neurology 92, e2005–e2014. doi: 10.1212/wnl.0000000000007326
Gingnell, M., Ahlstedt, V., Bannbers, E., Wikström, J., Sundström-Poromaa, I., and Fredrikson, M. (2014). Social stimulation and corticolimbic reactivity in premenstrual dysphoric disorder: a preliminary study. Biol. Mood Anxiety Disord. 4:3. doi: 10.1186/2045-5380-4-3
Girard, R., Météreau, E., Thomas, J., Pugeat, M., Qu, C., and Dreher, J. C. (2017). Hormone therapy at early post-menopause increases cognitive control-related prefrontal activity. Sci. Rep. 7:44917. doi: 10.1038/srep44917
Gleason, C. E., Dowling, N. M., Wharton, W., Manson, J. E., Miller, V. M., Atwood, C. S., et al. (2015). Effects of hormone therapy on cognition and mood in recently postmenopausal women: findings from the randomized, controlled KEEPS-cognitive and affective study. PLoS Med. 12:e1001833;discussione1001833. doi: 10.1371/journal.pmed.1001833
Gleason, C. E., Schmitz, T. W., Hess, T., Koscik, R. L., Trivedi, M. A., Ries, M. L., et al. (2006). Hormone effects on fMRI and cognitive measures of encoding: importance of hormone preparation. Neurology 67, 2039–2041. doi: 10.1212/01.wnl.0000247277.81400.43
Gold, E. B., Sternfeld, B., Kelsey, J. L., Brown, C., Mouton, C., Reame, N., et al. (2000). Relation of demographic and lifestyle factors to symptoms in a multi-racial/ethnic population of women 40-55 years of age. Am. J. Epidemiol. 152, 463–473. doi: 10.1093/aje/152.5.463
Greenberg, D. L., Payne, M. E., MacFall, J. R., Provenzale, J. M., Steffens, D. C., and Krishnan, R. R. (2006). Differences in brain volumes among males and female hormone-therapy users and nonusers. Psychiatry Res. 147, 127–134. doi: 10.1016/j.pscychresns.2006.01.001
Greendale, G. A., Derby, C. A., and Maki, P. M. (2011). Perimenopause and cognition. Obstet. Gynecol. Clin. North Am. 38, 519–535. doi: 10.1016/j.ogc.2011.05.007
Greendale, G. A., Huang, M. H., Wight, R. G., Seeman, T., Luetters, C., Avis, N. E., et al. (2009). Effects of the menopause transition and hormone use on cognitive performance in midlife women. Neurology 72, 1850–1857. doi: 10.1212/WNL.0b013e3181a71193
Greendale, G. A., Karlamangla, A. S., and Maki, P. M. (2020). The menopause transition and cognition. JAMA 323, 1495–1496. doi: 10.1001/jama.2020.1757
Greendale, G. A., Wight, R. G., Huang, M. H., Avis, N., Gold, E. B., Joffe, H., et al. (2010). Menopause-associated symptoms and cognitive performance: results from the study of women’s health across the nation. Am. J. Epidemiol. 171, 1214–1224. doi: 10.1093/aje/kwq067
Grieger, J. A., and Norman, R. J. (2020). Menstrual cycle length and patterns in a global cohort of women using a mobile phone app: retrospective cohort study. J. Med. Int. Res. 22:e17109. doi: 10.2196/17109
Griksiene, R., Monciunskaite, R., Arnatkeviciute, A., and Ruksenas, O. (2018). Does the use of hormonal contraceptives affect the mental rotation performance? Horm Behav. 100, 29–38. doi: 10.1016/j.yhbeh.2018.03.004
Hadjimarkou, M. M., and Vasudevan, N. (2018). GPER1/GPR30 in the brain: crosstalk with classical estrogen receptors and implications for behavior. J. Steroid Biochem. Mol. Biol. 176, 57–64. doi: 10.1016/j.jsbmb.2017.04.012
Hampson, E. (1990). Variations in sex-related cognitive abilities across the menstrual cycle. Brain Cogn. 14, 26–43. doi: 10.1016/0278-2626(90)90058-v
Hara, Y., Waters, E. M., McEwen, B. S., and Morrison, J. H. (2015). Estrogen effects on cognitive and synaptic health over the lifecourse. Physiol. Rev. 95, 785–807. doi: 10.1152/physrev.00036.2014
Harlow, S. D., Gass, M., Hall, J. E., Lobo, R., Maki, P., Rebar, R. W., et al. (2012). Executive summary of the stages of reproductive aging workshop + 10: addressing the unfinished agenda of staging reproductive aging. J. Clin. Endocrinol. Metab. 97, 1159–1168. doi: 10.1210/jc.2011-3362
Hausmann, M., Slabbekoorn, D., Van Goozen, S. H., Cohen-Kettenis, P. T., and Güntürkün, O. (2000). Sex hormones affect spatial abilities during the menstrual cycle. Behav. Neurosci. 114, 1245–1250. doi: 10.1037//0735-7044.114.6.1245
He, L., Guo, W., Qiu, J., An, X., and Lu, W. (2021). Altered spontaneous brain activity in women during menopause transition and its association with cognitive function and serum estradiol level. Front. Endocrinol. 12:652512. doi: 10.3389/fendo.2021.652512
Henderson, V. W., Benke, K. S., Green, R. C., Cupples, L. A., and Farrer, L. A. (2005). Postmenopausal hormone therapy and Alzheimer’s disease risk: interaction with age. J. Neurol. Neurosurg. Psychiatry 76, 103–105. doi: 10.1136/jnnp.2003.024927
Henderson, V. W., Guthrie, J. R., Dudley, E. C., Burger, H. G., and Dennerstein, L. (2003). Estrogen exposures and memory at midlife: a population-based study of women. Neurology 60, 1369–1371. doi: 10.1212/01.wnl.0000059413.75888.be
Henderson, V. W., St John, J. A., Hodis, H. N., McCleary, C. A., Stanczyk, F. Z., Shoupe, D., et al. (2016). Cognitive effects of estradiol after menopause: a randomized trial of the timing hypothesis. Neurology 87, 699–708. doi: 10.1212/wnl.0000000000002980
Henningsson, S., Madsen, K. H., Pinborg, A., Heede, M., Knudsen, G. M., Siebner, H. R., et al. (2015). Role of emotional processing in depressive responses to sex-hormone manipulation: a pharmacological fMRI study. Transl. Psychiatry 5:e688. doi: 10.1038/tp.2015.184
Herrera, A. Y., Velasco, R., Faude, S., White, J. D., Opitz, P. C., Huang, R., et al. (2020). Brain activity during a post-stress working memory task differs between the hormone-present and hormone-absent phase of hormonal contraception. Neurobiol. Stress 13:100248. doi: 10.1016/j.ynstr.2020.100248
Heys, M., Jiang, C., Cheng, K. K., Zhang, W., Yeung, S. L. A., Lam, T. H., et al. (2011). Life long endogenous estrogen exposure and later adulthood cognitive function in a population of naturally postmenopausal women from Southern China: the Guangzhou Biobank Cohort Study. Psychoneuroendocrinology 36, 864–873. doi: 10.1016/j.psyneuen.2010.11.009
Hidalgo-Lopez, E., Mueller, K., Harris, T., Aichhorn, M., Sacher, J., and Pletzer, B. (2020). Human menstrual cycle variation in subcortical functional brain connectivity: a multimodal analysis approach. Brain Struct. Funct. 225, 591–605. doi: 10.1007/s00429-019-02019-z
Hjelmervik, H., Hausmann, M., Craven, A. R., Hirnstein, M., Hugdahl, K., and Specht, K. (2018). Sex- and sex hormone-related variations in energy-metabolic frontal brain asymmetries: a magnetic resonance spectroscopy study. Neuroimage 172, 817–825. doi: 10.1016/j.neuroimage.2018.01.043
Hjelmervik, H., Hausmann, M., Osnes, B., Westerhausen, R., and Specht, K. (2014). Resting states are resting traits–an FMRI study of sex differences and menstrual cycle effects in resting state cognitive control networks. PLoS One 9:e103492. doi: 10.1371/journal.pone.0103492
Hodis, H. N., Mack, W. J., Henderson, V. W., Shoupe, D., Budoff, M. J., Hwang-Levine, J., et al. (2016). Vascular effects of early versus late postmenopausal treatment with estradiol. N. Engl. J. Med. 374, 1221–1231. doi: 10.1056/NEJMoa1505241
Hogervorst, E., Williams, J., Budge, M., Riedel, W., and Jolles, J. (2000). The nature of the effect of female gonadal hormone replacement therapy on cognitive function in post-menopausal women: a meta-analysis. Neuroscience 101, 485–512. doi: 10.1016/s0306-4522(00)00410-3
Huang, R., and Poduslo, S. E. (2006). CYP19 haplotypes increase risk for Alzheimer’s disease. J. Med. Genet. 43:e42. doi: 10.1136/jmg.2005.039461
Iivonen, S., Corder, E., Lehtovirta, M., Helisalmi, S., Mannermaa, A., Vepsäläinen, S., et al. (2004). Polymorphisms in the CYP19 gene confer increased risk for Alzheimer disease. Neurology 62, 1170–1176. doi: 10.1212/01.wnl.0000118208.16939.60
Ilango, S. D., McEvoy, L. K., Laughlin, G. A., Bergstrom, J., Barrett-Connor, E., and Kritz-Silverstein, D. (2019). Pregnancy history and cognitive aging among older women: the Rancho Bernardo Study. Menopause 26, 750–757. doi: 10.1097/GME.0000000000001318
Jacobs, E. G., Holsen, L. M., Lancaster, K., Makris, N., Whitfield-Gabrieli, S., Remington, A., et al. (2015). 17β-Estradiol differentially regulates stress circuitry activity in healthy and depressed women. Neuropsychopharmacology 40, 566–576. doi: 10.1038/npp.2014.203
Jacobs, E. G., Weiss, B. K., Makris, N., Whitfield-Gabrieli, S., Buka, S. L., Klibanski, A., et al. (2016). Impact of sex and menopausal status on episodic memory circuitry in early midlife. J. Neurosci. 36, 10163–10173. doi: 10.1523/jneurosci.0951-16.2016
Jacobs, E. G., Weiss, B., Makris, N., Whitfield-Gabrieli, S., Buka, S. L., Klibanski, A., et al. (2017). Reorganization of functional networks in verbal working memory circuitry in early midlife: the impact of sex and menopausal status. Cereb. Cortex 27, 2857–2870. doi: 10.1093/cercor/bhw127
Jayachandran, M., Lahr, B. D., Bailey, K. R., Miller, V. M., and Kantarci, K. (2020). Menopausal hormone therapy, blood thrombogenicity, and development of white matter hyperintensities in women of the kronos early estrogen prevention study. Menopause 27, 305–310. doi: 10.1097/gme.0000000000001465
Jazin, E., and Cahill, L. (2010). Sex differences in molecular neuroscience: from fruit flies to humans. Nat. Rev. Neurosci. 11, 9–17. doi: 10.1038/nrn2754
Jessen, F., Amariglio, R. E., van Boxtel, M., Breteler, M., Ceccaldi, M., Chételat, G., et al. (2014). A conceptual framework for research on subjective cognitive decline in preclinical Alzheimer’s disease. Alzheimers Dement. 10, 844–852. doi: 10.1016/j.jalz.2014.01.001
Jett, S., Malviya, N., Schelbaum, E., Jang, G., Jahan, E., Clancy, K., et al. (2022). Endogenous and exogenous estrogen exposures: how women’s reproductive health can drive brain aging and inform Alzheimer’s prevention. Front. Aging Neurosci. 14:831807. doi: 10.3389/fnagi.2022.831807
Joffe, H., Hall, J. E., Gruber, S., Sarmiento, I. A., Cohen, L. S., Yurgelun-Todd, D., et al. (2006). Estrogen therapy selectively enhances prefrontal cognitive processes: a randomized, double-blind, placebo-controlled study with functional magnetic resonance imaging in perimenopausal and recently postmenopausal women. Menopause 13, 411–422. doi: 10.1097/01.gme.0000189618.48774.7b
Jovanovic, H., Kocoska-Maras, L., Rådestad, A. F., Halldin, C., Borg, J., Hirschberg, A. L., et al. (2015). Effects of estrogen and testosterone treatment on serotonin transporter binding in the brain of surgically postmenopausal women – a PET study. Neuroimage 106, 47–54. doi: 10.1016/j.neuroimage.2014.11.003
Kantarci, K., Lowe, V. J., Lesnick, T. G., Tosakulwong, N., Bailey, K. R., Fields, J. A., et al. (2016a). Early postmenopausal transdermal 17β-estradiol therapy and Amyloid-β deposition. J. Alzheimers Dis. 53, 547–556. doi: 10.3233/jad-160258
Kantarci, K., Tosakulwong, N., Lesnick, T. G., Zuk, S. M., Gunter, J. L., Gleason, C. E., et al. (2016b). Effects of hormone therapy on brain structure: a randomized controlled trial. Neurology 87, 887–896. doi: 10.1212/wnl.0000000000002970
Kantarci, K., Tosakulwong, N., Lesnick, T. G., Zuk, S. M., Lowe, V. J., Fields, J. A., et al. (2018). Brain structure and cognition 3 years after the end of an early menopausal hormone therapy trial. Neurology 90, e1404–e1412. doi: 10.1212/wnl.0000000000005325
Karim, R., Dang, H., Henderson, V. W., Hodis, H. N., St John, J., Brinton, R. D., et al. (2016). Effect of reproductive history and exogenous hormone use on cognitive function in mid- and late life. J. Am. Geriatr. Soc. 64, 2448–2456. doi: 10.1111/jgs.14658
Kawas, C., Resnick, S., Morrison, A., Brookmeyer, R., Corrada, M., Zonderman, A., et al. (1997). A prospective study of estrogen replacement therapy and the risk of developing Alzheimer’s disease: the baltimore longitudinal study of aging. Neurology 48, 1517–1521. doi: 10.1212/wnl.48.6.1517
Kim, G. W., Park, K., and Jeong, G. W. (2018). Effects of sex hormones and age on brain volume in post-menopausal women. J. Sex Med. 15, 662–670. doi: 10.1016/j.jsxm.2018.03.006
Kim, Y. J., and Brinton, R. D. (2021). Precision hormone therapy: identification of positive responders. Climacteric 24, 350–358. doi: 10.1080/13697137.2021.1882418
Kim, Y. J., Soto, M., Branigan, G. L., Rodgers, K., and Brinton, R. D. (2021). Association between menopausal hormone therapy and risk of neurodegenerative diseases: implications for precision hormone therapy. Alzheimers Dement. 7:12174. doi: 10.1002/trc2.12174
Kling, J. M., Miller, V. M., Tosakulwong, N., Lesnick, T., and Kantarci, K. (2020). Associations of pituitary-ovarian hormones and white matter hyperintensities in recently menopausal women using hormone therapy. Menopause 27, 872–878. doi: 10.1097/gme.0000000000001557
Kranz, G. S., Rami-Mark, C., Kaufmann, U., Baldinger, P., Hahn, A., Höflich, A., et al. (2014). Effects of hormone replacement therapy on cerebral serotonin-1A receptor binding in postmenopausal women examined with [carbonyl-11C]WAY-100635. Psychoneuroendocrinology 45, 1–10. doi: 10.1016/j.psyneuen.2014.03.004
LaCroix, A. Z., Chlebowski, R. T., Manson, J. E., Aragaki, A. K., Johnson, K. C., Martin, L., et al. (2011). Health outcomes after stopping conjugated equine estrogens among postmenopausal women with prior hysterectomy: a randomized controlled trial. JAMA 305, 1305–1314. doi: 10.1001/jama.2011.382
Larsen, S. V., Köhler-Forsberg, K., Dam, V. H., Poulsen, A. S., Svarer, C., Jensen, P. S., et al. (2020). Oral contraceptives and the serotonin 4 receptor: a molecular brain imaging study in healthy women. Acta Psychiatr. Scand. 142, 294–306. doi: 10.1111/acps.13211
LeBlanc, E. S., Janowsky, J., Chan, B. K., and Nelson, H. D. (2001). Hormone replacement therapy and cognition: systematic review and meta-analysis. JAMA 285, 1489–1499. doi: 10.1001/jama.285.11.1489
Lethaby, A., Hogervorst, E., Richards, M., Yesufu, A., and Yaffe, K. (2008). Hormone replacement therapy for cognitive function in postmenopausal women. Cochrane Database Syst. Rev. 2008:CD003122. doi: 10.1002/14651858.CD003122.pub2
Li, F. D., He, F., Chen, T. R., Xiao, Y. Y., Lin, S. T., Shen, W., et al. (2016). Reproductive history and risk of cognitive impairment in elderly women: a cross-sectional study in eastern China. J. Alzheimers Dis. 49, 139–147. doi: 10.3233/jad-150444
Lin, M. T., and Beal, M. F. (2006). Mitochondrial dysfunction and oxidative stress in neurodegenerative diseases. Nature 443, 787–795. doi: 10.1038/nature05292
Lisofsky, N., Mårtensson, J., Eckert, A., Lindenberger, U., Gallinat, J., and Kühn, S. (2015). Hippocampal volume and functional connectivity changes during the female menstrual cycle. Neuroimage 118, 154–162. doi: 10.1016/j.neuroimage.2015.06.012
Lisofsky, N., Riediger, M., Gallinat, J., Lindenberger, U., and Kühn, S. (2016). Hormonal contraceptive use is associated with neural and affective changes in healthy young women. Neuroimage 134, 597–606. doi: 10.1016/j.neuroimage.2016.04.042
Liu, N., Zhang, Y., Liu, S., Zhang, X., and Liu, H. (2021). Brain functional changes in perimenopausal women: an amplitude of low-frequency fluctuation study. Menopause 28, 384–390. doi: 10.1097/gme.0000000000001720
Livingston, G., Huntley, J., Sommerlad, A., Ames, D., Ballard, C., Banerjee, S., et al. (2020). Dementia prevention, intervention, and care: 2020 report of the lancet Commission. Lancet 396, 413–446. doi: 10.1016/S0140-6736(20)30367-6
Lobo, R. A. (2017). Hormone-replacement therapy: current thinking. Nat. Rev. Endocrinol. 13, 220–231. doi: 10.1038/nrendo.2016.164
Lord, C., Buss, C., Lupien, S. J., and Pruessner, J. C. (2008). Hippocampal volumes are larger in postmenopausal women using estrogen therapy compared to past users, never users and men: a possible window of opportunity effect. Neurobiol. Aging 29, 95–101. doi: 10.1016/j.neurobiolaging.2006.09.001
Love, T., Smith, Y. R., Persad, C. C., Tkaczyk, A., and Zubieta, J. K. (2010). Short-term hormone treatment modulates emotion response circuitry in postmenopausal women. Fertil. Steril. 93, 1929–1937. doi: 10.1016/j.fertnstert.2008.12.056
Low, L. F., Anstey, K. J., Maller, J., Kumar, R., Wen, W., Lux, O., et al. (2006). Hormone replacement therapy, brain volumes and white matter in postmenopausal women aged 60-64 years. Neuroreport 17, 101–104. doi: 10.1097/01.wnr.0000194385.10622.8e
Luchetti, S., Bossers, K., Van de Bilt, S., Agrapart, V., Morales, R. R., Frajese, G. V., et al. (2011). Neurosteroid biosynthetic pathways changes in prefrontal cortex in Alzheimer’s disease. Neurobiol. Aging 32, 1964–1976. doi: 10.1016/j.neurobiolaging.2009.12.014
Lupien, S. J., McEwen, B. S., Gunnar, M. R., and Heim, C. (2009). Effects of stress throughout the lifespan on the brain, behaviour and cognition. Nat. Rev. Neurosci. 10, 434–445. doi: 10.1038/nrn2639
Maioli, S., Leander, K., Nilsson, P., and Nalvarte, I. (2021). Estrogen receptors and the aging brain. Essays Biochem. 65, 913–925. doi: 10.1042/ebc20200162
Maki, P. M. (2013). Critical window hypothesis of hormone therapy and cognition: a scientific update on clinical studies. Menopause 20, 695–709. doi: 10.1097/GME.0b013e3182960cf8
Maki, P. M., and Henderson, V. W. (2016). Cognition and the menopause transition. Menopause 23, 803–805. doi: 10.1097/gme.0000000000000681
Maki, P. M., and Resnick, S. M. (2000). Longitudinal effects of estrogen replacement therapy on PET cerebral blood flow and cognition. Neurobiol. Aging 21, 373–383. doi: 10.1016/s0197-4580(00)00123-8
Maki, P. M., and Sundermann, E. (2009). Hormone therapy and cognitive function. Hum. Reprod. Update 15, 667–681. doi: 10.1093/humupd/dmp022
Maki, P. M., Dennerstein, L., Clark, M., Guthrie, J., LaMontagne, P., Fornelli, D., et al. (2011). Perimenopausal use of hormone therapy is associated with enhanced memory and hippocampal function later in life. Brain Res. 1379, 232–243. doi: 10.1016/j.brainres.2010.11.030
Maki, P. M., Drogos, L. L., Rubin, L. H., Banuvar, S., Shulman, L. P., and Geller, S. E. (2008). Objective hot flashes are negatively related to verbal memory performance in midlife women. Menopause 15, 848–856. doi: 10.1097/gme.0b013e31816d815e
Maki, P. M., Gast, M. J., Vieweg, A. J., Burriss, S. W., and Yaffe, K. (2007). Hormone therapy in menopausal women with cognitive complaints: a randomized, double-blind trial. Neurology 69, 1322–1330. doi: 10.1212/01.wnl.0000277275.42504.93
Maki, P. M., Rich, J. B., and Rosenbaum, R. S. (2002). Implicit memory varies across the menstrual cycle: estrogen effects in young women. Neuropsychologia 40, 518–529. doi: 10.1016/s0028-3932(01)00126-9
Manson, J. E., Bassuk, S. S., Harman, S. M., Brinton, E. A., Cedars, M. I., Lobo, R., et al. (2006). Postmenopausal hormone therapy: new questions and the case for new clinical trials. Menopause 13, 139–147. doi: 10.1097/01.gme.0000177906.94515.ff
Marečková, K., Perrin, J. S., Nawaz Khan, I., Lawrence, C., Dickie, E., McQuiggan, D. A., et al. (2012). Hormonal contraceptives, menstrual cycle and brain response to faces. Soc. Cogn. Affect. Neurosci. 9, 191–200. doi: 10.1093/scan/nss128
Matthews, K. A., Kuller, L. H., Wing, R. R., Meilahn, E. N., and Plantinga, P. (1996). Prior to use of estrogen replacement therapy, are users healthier than nonusers? Am. J. Epidemiol. 143, 971–978. doi: 10.1093/oxfordjournals.aje.a008678
Mauvais-Jarvis, F., Bairey Merz, N., Barnes, P. J., Brinton, R. D., Carrero, J. J., DeMeo, D. L., et al. (2020). Sex and gender: modifiers of health, disease, and medicine. Lancet 396, 565–582. doi: 10.1016/S0140-6736(20)31561-0
Mazure, C. M., and Jones, D. P. (2015). Twenty years and still counting: including women as participants and studying sex and gender in biomedical research. BMC Womens Health 15:94. doi: 10.1186/s12905-015-0251-9
McCarthy, M. M. (2016). Sex differences in the developing brain as a source of inherent risk. Dialogues Clin. Neurosci. 18, 361–372.
McCarthy, M. M., Auger, A. P., Bale, T. L., De Vries, G. J., Dunn, G. A., Forger, N. G., et al. (2009). The epigenetics of sex differences in the brain. J. Neurosci. 29, 12815–12823. doi: 10.1523/JNEUROSCI.3331-09.2009
McCarthy, M. M., Woolley, C. S., and Arnold, A. P. (2017). Incorporating sex as a biological variable in neuroscience: what do we gain? Nat. Rev. Neurosci. 18, 707–708. doi: 10.1038/nrn.2017.137
McEwen, B. (2002). Estrogen actions throughout the brain. Recent Prog. Horm. Res. 57, 357–384. doi: 10.1210/rp.57.1.357
McEwen, B. S., Alves, S. E., Bulloch, K., and Weiland, N. G. (1997). Ovarian steroids and the brain: implications for cognition and aging. Neurology 48(5 Suppl. 7), 8S–15S.
McEwen, B. S., and Milner, T. A. (2017). Understanding the broad influence of sex hormones and sex differences in the brain. J. Neurosci. Res. 95, 24–39. doi: 10.1002/jnr.23809
McEwen, B., Akama, K., Alves, S., Brake, W. G., Bulloch, K., Lee, S., et al. (2001). Tracking the estrogen receptor in neurons: implications for estrogen-induced synapse formation. Proc. Natl. Acad. Sci. U.S.A. 98, 7093–7100. doi: 10.1073/pnas.121146898
McGuire, T. C., McCormick, K. C., Koch, M. K., and Mendle, J. (2019). Pubertal maturation and trajectories of depression during early adolescence. Front. Psychol. 10:1362. doi: 10.3389/fpsyg.2019.01362
McLay, R. N., Maki, P. M., and Lyketsos, C. G. (2003). Nulliparity and late menopause are associated with decreased cognitive decline. J. Neuropsychiatry Clin. Neurosci. 15, 161–167. doi: 10.1176/jnp.15.2.161
Medway, C., Combarros, O., Cortina-Borja, M., Butler, H. T., Ibrahim-Verbaas, C. A., de Bruijn, R. F., et al. (2014). The sex-specific associations of the aromatase gene with Alzheimer’s disease and its interaction with IL10 in the Epistasis Project. Eur. J. Hum. Genet. 22, 216–220. doi: 10.1038/ejhg.2013.116
Meeker, T. J., Veldhuijzen, D. S., Keaser, M. L., Gullapalli, R. P., and Greenspan, J. D. (2020). Menstrual cycle variations in gray matter volume, white matter volume and functional connectivity: critical impact on parietal lobe. Front. Neurosci. 14:594588. doi: 10.3389/fnins.2020.594588
Menting-Henry, S., Hidalgo-Lopez, E., Aichhorn, M., Kronbichler, M., Kerschbaum, H., and Pletzer, B. (2022). Oral contraceptives modulate the relationship between resting brain activity, amygdala connectivity and emotion recognition – a resting state fMRI study. Front. Behav. Neurosci. 16:775796. doi: 10.3389/fnbeh.2022.775796
Miller, V. M., Naftolin, F., Asthana, S., Black, D. M., Brinton, E. A., Budoff, M. J., et al. (2019). The Kronos Early Estrogen Prevention Study (KEEPS): what have we learned? Menopause 26, 1071–1084. doi: 10.1097/gme.0000000000001326
Mishra, A., and Brinton, R. D. (2018). Inflammation: bridging age, menopause and APOEε4 genotype to Alzheimer’s disease. Front. Aging Neurosci. 10:312. doi: 10.3389/fnagi.2018.00312
Mitchell, E. S., and Woods, N. F. (2011). Cognitive symptoms during the menopausal transition and early postmenopause. Climacteric 14, 252–261. doi: 10.3109/13697137.2010.516848
Monteleone, P., Mascagni, G., Giannini, A., Genazzani, A. R., and Simoncini, T. (2018). Symptoms of menopause - global prevalence, physiology and implications. Nat. Rev. Endocrinol. 14, 199–215. doi: 10.1038/nrendo.2017.180
Morrison, J. H., Brinton, R. D., Schmidt, P. J., and Gore, A. C. (2006). Estrogen, menopause, and the aging brain: how basic neuroscience can inform hormone therapy in women. J. Neurosci. 26, 10332–10348. doi: 10.1523/JNEUROSCI.3369-06.2006
Mosconi, L., Berti, V., Dyke, J., Schelbaum, E., Jett, S., Loughlin, L., et al. (2021). Menopause impacts human brain structure, connectivity, energy metabolism, and amyloid-beta deposition. Sci. Rep. 11:10867. doi: 10.1038/s41598-021-90084-y
Mosconi, L., Berti, V., Quinn, C., McHugh, P., Petrongolo, G., Osorio, R. S., et al. (2018a). Correction: perimenopause and emergence of an Alzheimer’s bioenergetic phenotype in brain and periphery. PLoS One 13:e0193314. doi: 10.1371/journal.pone.0193314
Mosconi, L., Berti, V., Quinn, C., McHugh, P., Petrongolo, G., Varsavsky, I., et al. (2017). Sex differences in Alzheimer risk: brain imaging of endocrine vs chronologic aging. Neurology 89, 1382–1390. doi: 10.1212/wnl.0000000000004425
Mosconi, L., Rahman, A., Diaz, I., Wu, X., Scheyer, O., Hristov, H. W., et al. (2018b). Increased Alzheimer’s risk during the menopause transition: a 3-year longitudinal brain imaging study. PLoS One 13:e0207885. doi: 10.1371/journal.pone.0207885
Nabulsi, L., Lawrence, K., Santhalingam, V., Abaryan, Z., Boyle, C., Villalon-Reina, J., et al. (2020). Exogenous Sex Hormone Effects on Brain Microstructure in Women: A Diffusion MRI Study in the UK Biobank. Bellingham, WA: SPIE.
Najar, J., Hällström, T., Zettergren, A., Johansson, L., Joas, E., Fässberg, M. M., et al. (2021). Reproductive period and preclinical cerebrospinal fluid markers for Alzheimer disease: a 25-year study. Menopause 28, 1099–1107. doi: 10.1097/gme.0000000000001816
Najar, J., Ostling, S., Waern, M., Zettergren, A., Kern, S., Wetterberg, H., et al. (2020). Reproductive period and dementia: a 44-year longitudinal population study of Swedish women. Alzheimers Dement. 16, 1153–1163. doi: 10.1002/alz.12118
Nappi, R. E., Sinforiani, E., Mauri, M., Bono, G., Polatti, F., and Nappi, G. (1999). Memory functioning at menopause: impact of age in ovariectomized women. Gynecol. Obstet. Invest. 47, 29–36. doi: 10.1159/000010058
Nasseri, P., Herrera, A. Y., Gillette, K., Faude, S., White, J. D., Velasco, R., et al. (2020). Hormonal contraceptive phases matter: resting-state functional connectivity of emotion-processing regions under stress. Neurobiol. Stress 13:100276. doi: 10.1016/j.ynstr.2020.100276
Ning, K., Zhao, L., Franklin, M., Matloff, W., Batta, I., Arzouni, N., et al. (2020). Parity is associated with cognitive function and brain age in both females and males. Sci. Rep. 10:6100. doi: 10.1038/s41598-020-63014-7
Nordström, A. L., Olsson, H., and Halldin, C. (1998). A PET study of D2 dopamine receptor density at different phases of the menstrual cycle. Psychiatry Res. 83, 1–6. doi: 10.1016/s0925-4927(98)00021-3
Noyan, H., Hamamci, A., Firat, Z., Sarsilmaz, A., and Ucok, A. (2022). Menstrual cycle-related changes in women with schizophrenia: a resting-state fMRI study. Neuropsychobiology Online ahead of print, doi: 10.1159/000522002
O’Brien, J., Jackson, J. W., Grodstein, F., Blacker, D., and Weuve, J. (2014). Postmenopausal hormone therapy is not associated with risk of all-cause dementia and Alzheimer’s disease. Epidemiol. Rev. 36, 83–103. doi: 10.1093/epirev/mxt008
Ossewaarde, L., van Wingen, G. A., Rijpkema, M., Bäckström, T., Hermans, E. J., and Fernández, G. (2013). Menstrual cycle-related changes in amygdala morphology are associated with changes in stress sensitivity. Hum. Brain Mapp. 34, 1187–1193. doi: 10.1002/hbm.21502
Paganini-Hill, A., Corrada, M. M., and Kawas, C. H. (2006). Increased longevity in older users of postmenopausal estrogen therapy: the leisure world cohort study. Menopause 13, 12–18. doi: 10.1097/01.gme.0000172880.40831.3b
Pérès, K., Helmer, C., Amieva, H., Matharan, F., Carcaillon, L., Jacqmin-Gadda, H., et al. (2011). Gender differences in the prodromal signs of dementia: memory complaint and IADL-restriction. a prospective population-based cohort. J. Alzheimers Dis. 27, 39–47. doi: 10.3233/jad-2011-110428
Persad, C. C., Zubieta, J. K., Love, T., Wang, H., Tkaczyk, A., and Smith, Y. R. (2009). Enhanced neuroactivation during verbal memory processing in postmenopausal women receiving short-term hormone therapy. Fertil. Steril. 92, 197–204. doi: 10.1016/j.fertnstert.2008.04.040
Petersen, N., Ghahremani, D. G., Rapkin, A. J., Berman, S. M., Liang, L., and London, E. D. (2018). Brain activation during emotion regulation in women with premenstrual dysphoric disorder. Psychol. Med. 48, 1795–1802. doi: 10.1017/S0033291717003270
Petersen, N., Ghahremani, D. G., Rapkin, A. J., Berman, S. M., Wijker, N., Liang, L., et al. (2019). Resting-state functional connectivity in women with PMDD. Transl. Psychiatry 9:339. doi: 10.1038/s41398-019-0670-8
Petersen, N., Kilpatrick, L. A., Goharzad, A., and Cahill, L. (2014). Oral contraceptive pill use and menstrual cycle phase are associated with altered resting state functional connectivity. Neuroimage 90, 24–32. doi: 10.1016/j.neuroimage.2013.12.016
Petersen, N., Touroutoglou, A., Andreano, J. M., and Cahill, L. (2015). Oral contraceptive pill use is associated with localized decreases in cortical thickness. Hum. Brain Mapp. 36, 2644–2654. doi: 10.1002/hbm.22797
Phung, T. K., Waltoft, B. L., Laursen, T. M., Settnes, A., Kessing, L. V., Mortensen, P. B., et al. (2010). Hysterectomy, oophorectomy and risk of dementia: a nationwide historical cohort study. Dement. Geriatr. Cogn. Disord. 30, 43–50. doi: 10.1159/000314681
Pletzer, B. A., and Kerschbaum, H. H. (2014). 50 years of hormonal contraception-time to find out, what it does to our brain. Front. Neurosci. 8:256. doi: 10.3389/fnins.2014.00256
Pletzer, B. A., Kronbichler, M., Nuerk, H. C., and Kerschbaum, H. (2014). Hormonal contraceptives masculinize brain activation patterns in the absence of behavioral changes in two numerical tasks. Brain Res. 1543, 128–142. doi: 10.1016/j.brainres.2013.11.007
Pletzer, B., Crone, J. S., Kronbichler, M., and Kerschbaum, H. (2016). Menstrual cycle and hormonal contraceptive-dependent changes in intrinsic connectivity of resting-state brain networks correspond to behavioral changes due to hormonal status. Brain Connect. 6, 572–585. doi: 10.1089/brain.2015.0407
Pletzer, B., Harris, T. A., Scheuringer, A., and Hidalgo-Lopez, E. (2019b). The cycling brain: menstrual cycle related fluctuations in hippocampal and fronto-striatal activation and connectivity during cognitive tasks. Neuropsychopharmacology 44, 1867–1875. doi: 10.1038/s41386-019-0435-3
Pletzer, B., Harris, T., and Hidalgo-Lopez, E. (2018). Subcortical structural changes along the menstrual cycle: beyond the hippocampus. Sci. Rep. 8:16042. doi: 10.1038/s41598-018-34247-4
Pletzer, B., Harris, T., and Hidalgo-Lopez, E. (2019a). Previous contraceptive treatment relates to grey matter volumes in the hippocampus and basal ganglia. Sci. Rep. 9:11003. doi: 10.1038/s41598-019-47446-4
Pletzer, B., Kronbichler, M., Aichhorn, M., Bergmann, J., Ladurner, G., and Kerschbaum, H. H. (2010). Menstrual cycle and hormonal contraceptive use modulate human brain structure. Brain Res. 1348, 55–62. doi: 10.1016/j.brainres.2010.06.019
Pletzer, B., Kronbichler, M., and Kerschbaum, H. (2015). Differential effects of androgenic and anti-androgenic progestins on fusiform and frontal gray matter volume and face recognition performance. Brain Res. 1596, 108–115. doi: 10.1016/j.brainres.2014.11.025
Pletzer, B., Kronbichler, M., Ladurner, G., Nuerk, H. C., and Kerschbaum, H. (2011). Menstrual cycle variations in the BOLD-response to a number bisection task: implications for research on sex differences. Brain Res. 1420, 37–47. doi: 10.1016/j.brainres.2011.08.058
Prince, M. J., Acosta, D., Guerra, M., Huang, Y., Jimenez-Velazquez, I. Z., Llibre Rodriguez, J. J., et al. (2018). Reproductive period, endogenous estrogen exposure and dementia incidence among women in Latin America and China; A 10/66 population-based cohort study. PLoS One 13:e0192889. doi: 10.1371/journal.pone.0192889
Protopopescu, X., Butler, T., Pan, H., Root, J., Altemus, M., Polanecsky, M., et al. (2008). Hippocampal structural changes across the menstrual cycle. Hippocampus 18, 985–988. doi: 10.1002/hipo.20468
Ptok, U., Barkow, K., and Heun, R. (2002). Fertility and number of children in patients with Alzheimer’s disease. Arch. Womens Ment. Health 5, 83–86. doi: 10.1007/s00737-002-0142-6
Rahman, A., Jackson, H., Hristov, H., Isaacson, R. S., Saif, N., Shetty, T., et al. (2019). Sex and gender driven modifiers of alzheimer’s: the role for estrogenic control across age, race, medical and lifestyle risks. Front. Aging Neurosci. 11:315. doi: 10.3389/fnagi.2019.00315
Rahman, A., Schelbaum, E., Hoffman, K., Diaz, I., Hristov, H., Andrews, R., et al. (2020). Sex-driven modifiers of Alzheimer risk: a multimodality brain imaging study. Neurology 95, e166–e178. doi: 10.1212/wnl.0000000000009781
Rapkin, A. J., Berman, S. M., Mandelkern, M. A., Silverman, D. H. S., Morgan, M., and London, E. D. (2011). Neuroimaging evidence of cerebellar involvement in premenstrual dysphoric disorder. Biol. Psychiatry 69, 374–380. doi: 10.1016/j.biopsych.2010.09.029
Rapp, S. R., Espeland, M. A., Shumaker, S. A., Henderson, V. W., Brunner, R. L., Manson, J. E., et al. (2003). Effect of estrogen plus progestin on global cognitive function in postmenopausal women: the Women’s Health Initiative Memory Study: a randomized controlled trial. JAMA 289, 2663–2672. doi: 10.1001/jama.289.20.2663
Rasgon, N. L., Geist, C. L., Kenna, H. A., Wroolie, T. E., Williams, K. E., and Silverman, D. H. (2014). Prospective randomized trial to assess effects of continuing hormone therapy on cerebral function in postmenopausal women at risk for dementia. PLoS One 9:e89095. doi: 10.1371/journal.pone.0089095
Rasgon, N. L., Magnusson, C., Johansson, A. L. V., Pedersen, N. L., Elman, S., and Gatz, M. (2005a). Endogenous and exogenous hormone exposure and risk of cognitive impairment in Swedish twins: a preliminary study. Psychoneuroendocrinology 30, 558–567. doi: 10.1016/j.psyneuen.2005.01.004
Rasgon, N. L., Silverman, D., Siddarth, P., Miller, K., Ercoli, L. M., Elman, S., et al. (2005b). Estrogen use and brain metabolic change in postmenopausal women. Neurobiol. Aging 26, 229–235. doi: 10.1016/j.neurobiolaging.2004.03.003
Reed, B. G., and Carr, B. R. (2000). “The normal menstrual cycle and the control of ovulation,” in Endotext, eds K. R. Feingold, B. Anawalt, A. Boyce, G. Chrousos, W. W. de Herder, K. Dhatariya, et al. (South Dartmouth, MA: MDText.com, Inc).
Reiman, E. M., Armstrong, S. M., Matt, K. S., and Mattox, J. H. (1996). The application of positron emission tomography to the study of the normal menstrual cycle. Hum. Reprod. 11, 2799–2805. doi: 10.1093/oxfordjournals.humrep.a019214
Rentz, D. M., Weiss, B. K., Jacobs, E. G., Cherkerzian, S., Klibanski, A., Remington, A., et al. (2017). Sex differences in episodic memory in early midlife: impact of reproductive aging. Menopause 24, 400–408.
Resnick, S. M., Espeland, M. A., Jaramillo, S. A., Hirsch, C., Stefanick, M. L., Murray, A. M., et al. (2009). Postmenopausal hormone therapy and regional brain volumes: the WHIMS-MRI Study. Neurology 72, 135–142. doi: 10.1212/01.wnl.0000339037.76336.cf
Resnick, S. M., Maki, P. M., Rapp, S. R., Espeland, M. A., Brunner, R., Coker, L. H., et al. (2006). Effects of combination estrogen plus progestin hormone treatment on cognition and affect. J. Clin. Endocrinol. Metab. 91, 1802–1810. doi: 10.1210/jc.2005-2097
Rettberg, J. R., Yao, J., and Brinton, R. D. (2014). Estrogen: a master regulator of bioenergetic systems in the brain and body. Front. Neuroendocrinol. 35:8–30. doi: 10.1016/j.yfrne.2013.08.001
Riedel, B. C., Thompson, P. M., and Brinton, R. D. (2016). Age, APOE and sex: triad of risk of Alzheimer’s disease. J. Steroid Biochem. Mol. Biol. 160, 134–147. doi: 10.1016/j.jsbmb.2016.03.012
Rocca, W. A., Bower, J. H., Maraganore, D. M., Ahlskog, J. E., Grossardt, B. R., de Andrade, M., et al. (2007). Increased risk of cognitive impairment or dementia in women who underwent oophorectomy before menopause. Neurology 69, 1074–1083. doi: 10.1212/01.wnl.0000276984.19542.e6
Rocca, W. A., Grossardt, B. R., and Maraganore, D. M. (2008). The long-term effects of oophorectomy on cognitive and motor aging are age dependent. Neurodegener. Dis. 5, 257–260. doi: 10.1159/000113718
Rocca, W. A., Grossardt, B. R., and Shuster, L. T. (2010). Oophorectomy, menopause, estrogen, and cognitive aging: the timing hypothesis. Neurodegener. Dis. 7, 163–166.
Rocca, W. A., Grossardt, B. R., and Shuster, L. T. (2014). Oophorectomy, estrogen, and dementia: a 2014 update. Mol. Cell Endocrinol. 389, 7–12. doi: 10.1016/j.mce.2014.01.020
Rocca, W. A., Grossardt, B. R., Shuster, L. T., and Stewart, E. A. (2012). Hysterectomy, oophorectomy, estrogen, and the risk of dementia. Neurodegener. Dis. 10, 175–178. doi: 10.1159/000334764
Rossouw, J. E., Anderson, G. L., Prentice, R. L., LaCroix, A. Z., Kooperberg, C., Stefanick, M. L., et al. (2002). Risks and benefits of estrogen plus progestin in healthy postmenopausal women: principal results From the Women’s Health Initiative randomized controlled trial. JAMA 288, 321–333. doi: 10.1001/jama.288.3.321
Ryan, J., Artero, S., Carrière, I., Scali, J., Maller, J. J., Meslin, C., et al. (2014). Brain volumes in late life: gender, hormone treatment, and estrogen receptor variants. Neurobiol. Aging 35, 645–654. doi: 10.1016/j.neurobiolaging.2013.09.026
Ryan, J., Carrière, I., Scali, J., Ritchie, K., and Ancelin, M.-L. (2009). Life-time estrogen exposure and cognitive functioning in later life. Psychoneuroendocrinology 34, 287–298. doi: 10.1016/j.psyneuen.2008.09.008
Sacher, J., Okon-Singer, H., and Villringer, A. (2013). Evidence from neuroimaging for the role of the menstrual cycle in the interplay of emotion and cognition. Front. Hum. Neurosci. 7:374. doi: 10.3389/fnhum.2013.00374
Şafak, K. Y. (2019). Variability of apparent diffusion coefficient in the brain in women during follicular and luteal phases of the menstrual cycle. Neuroradiol. J. 32, 127–131. doi: 10.1177/1971400918819088
Salpeter, S. R., Cheng, J., Thabane, L., Buckley, N. S., and Salpeter, E. E. (2009). Bayesian meta-analysis of hormone therapy and mortality in younger postmenopausal women. Am. J. Med. 122, 1016–1022.e1. doi: 10.1016/j.amjmed.2009.05.021
Santoro, N. (2005). The menopausal transition. Am. J. Med. 118 Suppl 12B, 8–13. doi: 10.1016/j.amjmed.2005.09.008
Savolainen-Peltonen, H., Rahkola-Soisalo, P., Hoti, F., Vattulainen, P., Gissler, M., Ylikorkala, O., et al. (2019). Use of postmenopausal hormone therapy and risk of Alzheimer’s disease in Finland: nationwide case-control study. BMJ 364:l665. doi: 10.1136/bmj.l665
Schelbaum, E., Loughlin, L., Jett, S., Zang, C., Jang, G., Malviya, N., et al. (2021). Association of reproductive history with brain MRI biomarkers of dementia risk in midlife. Neurology 97, e2328-e2339. doi: 10.1212/WNL.0000000000012941
Schiebinger, L., Leopold, S. S., and Miller, V. M. (2016). Editorial policies for sex and gender analysis. Lancet 388, 2841–2842. doi: 10.1016/S0140-6736(16)32392-3
Schulz, K. M., Molenda-Figueira, H. A., and Sisk, C. L. (2009). Back to the future: the organizational-activational hypothesis adapted to puberty and adolescence. Horm. Behav. 55, 597–604. doi: 10.1016/j.yhbeh.2009.03.010
Seitz, J., Kubicki, M., Jacobs, E. G., Cherkerzian, S., Weiss, B. K., Papadimitriou, G., et al. (2019). Impact of sex and reproductive status on memory circuitry structure and function in early midlife using structural covariance analysis. Hum. Brain Mapp. 40, 1221–1233. doi: 10.1002/hbm.24441
Shafir, T., Love, T., Berent-Spillson, A., Persad, C. C., Wang, H., Reame, N. K., et al. (2012). Postmenopausal hormone use impact on emotion processing circuitry. Behav. Brain Res. 226, 147–153. doi: 10.1016/j.bbr.2011.09.012
Shao, H., Breitner, J. C., Whitmer, R. A., Wang, J., Hayden, K., Wengreen, H., et al. (2012). Hormone therapy and Alzheimer disease dementia: new findings from the Cache County Study. Neurology 79, 1846–1852. doi: 10.1212/WNL.0b013e318271f823
Sharma, R., Smith, S. A., Boukina, N., Dordari, A., Mistry, A., Taylor, B. C., et al. (2020). Use of the birth control pill affects stress reactivity and brain structure and function. Horm. Behav. 124, 104783. doi: 10.1016/j.yhbeh.2020.104783
Shaywitz, S. E., Shaywitz, B. A., Pugh, K. R., Fulbright, R. K., Skudlarski, P., Mencl, W. E., et al. (1999). Effect of estrogen on brain activation patterns in postmenopausal women during working memory tasks. JAMA 281, 1197–1202. doi: 10.1001/jama.281.13.1197
Sheppard, P. A. S., Choleris, E., and Galea, L. A. M. (2019). Structural plasticity of the hippocampus in response to estrogens in female rodents. Mol. Brain 12:22. doi: 10.1186/s13041-019-0442-7
Sherwin, B. B. (2006). Estrogen and cognitive aging in women. Neuroscience 138, 1021–1026. doi: 10.1016/j.neuroscience.2005.07.051
Sherwin, B. B., and Phillips, S. (1990). Estrogen and cognitive functioning in surgically menopausal women. Ann. N. Y. Acad. Sci. 592, 474–475.
Shumaker, S. A., Legault, C., Kuller, L., Rapp, S. R., Thal, L., Lane, D. S., et al. (2004). Conjugated equine estrogens and incidence of probable dementia and mild cognitive impairment in postmenopausal women: women’s Health Initiative Memory Study. JAMA 291, 2947–2958. doi: 10.1001/jama.291.24.2947
Shumaker, S. A., Legault, C., Rapp, S. R., Thal, L., Wallace, R. B., Ockene, J. K., et al. (2003). Estrogen plus progestin and the incidence of dementia and mild cognitive impairment in postmenopausal women: the Women’s Health Initiative Memory Study: a randomized controlled trial. JAMA 289, 2651–2662. doi: 10.1001/jama.289.20.2651
Silverman, D. H., Geist, C. L., Kenna, H. A., Williams, K., Wroolie, T., Powers, B., et al. (2011). Differences in regional brain metabolism associated with specific formulations of hormone therapy in postmenopausal women at risk for AD. Psychoneuroendocrinology 36, 502–513. doi: 10.1016/j.psyneuen.2010.08.002
Sisk, C. L., and Foster, D. L. (2004). The neural basis of puberty and adolescence. Nat. Neurosci. 7, 1040–1047. doi: 10.1038/nn1326
Słopień, R., Junik, R., Meczekalski, B., Halerz-Nowakowska, B., Maciejewska, M., Warenik-Szymankiewicz, A., et al. (2003). Influence of hormonal replacement therapy on the regional cerebral blood flow in postmenopausal women. Maturitas 46, 255–262. doi: 10.1016/s0378-5122(03)00144-0
Smith, Y. R., Love, T., Persad, C. C., Tkaczyk, A., Nichols, T. E., and Zubieta, J. K. (2006). Impact of combined estradiol and norethindrone therapy on visuospatial working memory assessed by functional magnetic resonance imaging. J. Clin. Endocrinol. Metab. 91, 4476–4481. doi: 10.1210/jc.2006-0907
Song, X., Wu, J., Zhou, Y., Feng, L., Yuan, J.-M., Pan, A., et al. (2020). Reproductive and hormonal factors and risk of cognitive impairment among Singapore Chinese women. Am. J. Obstetr. Gynecol. 223, 410.e1-410.e23. doi: 10.1016/j.ajog.2020.02.032
Song, Y. J., Li, S. R., Li, X. W., Chen, X., Wei, Z. X., Liu, Q. S., et al. (2020). The effect of estrogen replacement therapy on Alzheimer’s disease and Parkinson’s disease in postmenopausal women: a meta-analysis. Front. Neurosci. 14:157. doi: 10.3389/fnins.2020.00157
Sperling, R. A., Karlawish, J., and Johnson, K. A. (2013). Preclinical Alzheimer disease-the challenges ahead. Nat. Rev. Neurol. 9, 54–58. doi: 10.1038/nrneurol.2012.241
Stein, P., Baldinger, P., Kaufmann, U., Christina, R. M., Hahn, A., Höflich, A., et al. (2014). Relation of progesterone and DHEAS serum levels to 5-HT1A receptor binding potential in pre- and postmenopausal women. Psychoneuroendocrinology 46, 52–63. doi: 10.1016/j.psyneuen.2014.04.008
Stein, P., Savli, M., Wadsak, W., Mitterhauser, M., Fink, M., Spindelegger, C., et al. (2008). The serotonin-1A receptor distribution in healthy men and women measured by PET and [carbonyl-11C]WAY-100635. Eur. J. Nucl. Med. Mol. Imaging 35, 2159–2168. doi: 10.1007/s00259-008-0850-x
Stuenkel, C. A., Davis, S. R., Gompel, A., Lumsden, M. A., Murad, M. H., Pinkerton, J. V., et al. (2015). Treatment of symptoms of the menopause: an endocrine society clinical practice guideline. J. Clin. Endocrinol. Metab. 100, 3975–4011. doi: 10.1210/jc.2015-2236
Sundström Poromaa, I., and Gingnell, M. (2014). Menstrual cycle influence on cognitive function and emotion processing-from a reproductive perspective. Front. Neurosci. 8:380. doi: 10.3389/fnins.2014.00380
Sundström Poromaa, I., Comasco, E., Bäckström, T., Bixo, M., Jensen, P., and Frokjaer, V. G. (2019). Negative association between allopregnanolone and cerebral serotonin transporter binding in healthy women of fertile age. Front. Psychol. 9:2767. doi: 10.3389/fpsyg.2018.02767
Syan, S. K., Minuzzi, L., Costescu, D., Smith, M., Allega, O. R., Coote, M., et al. (2017). Influence of endogenous estradiol, progesterone, allopregnanolone, and dehydroepiandrosterone sulfate on brain resting state functional connectivity across the menstrual cycle. Fertil. Steril. 107, 1246-1255.e4. doi: 10.1016/j.fertnstert.2017.03.021
Taylor, C. M., Pritschet, L., and Jacobs, E. G. (2021). The scientific body of knowledge – Whose body does it serve? A spotlight on oral contraceptives and women’s health factors in neuroimaging. Front. Neuroendocrinol. 60:100874. doi: 10.1016/j.yfrne.2020.100874
Thimm, M., Weis, S., Hausmann, M., and Sturm, W. (2014). Menstrual cycle effects on selective attention and its underlying cortical networks. Neuroscience 258, 307–317. doi: 10.1016/j.neuroscience.2013.11.010
Thomas, J., Météreau, E., Déchaud, H., Pugeat, M., and Dreher, J.-C. (2014). Hormonal treatment increases the response of the reward system at the menopause transition: a counterbalanced randomized placebo-controlled fMRI study. Psychoneuroendocrinology 50, 167–180. doi: 10.1016/j.psyneuen.2014.08.012
Thurston, R. C., Aizenstein, H. J., Derby, C. A., Sejdić, E., and Maki, P. M. (2016). Menopausal hot flashes and white matter hyperintensities. Menopause 23, 27–32. doi: 10.1097/gme.0000000000000481
Thurston, R. C., Maki, P. M., Derby, C. A., Sejdić, E., and Aizenstein, H. J. (2015). Menopausal hot flashes and the default mode network. Fertil. Steril. 103, 1572-8.e1. doi: 10.1016/j.fertnstert.2015.03.008
Tierney, M. C., Ryan, J., Ancelin, M.-L., Moineddin, R., Rankin, S., Yao, C., et al. (2013). Lifelong estrogen exposure and memory in older postmenopausal women. J. Alzheimers Dis. 34, 601–608. doi: 10.3233/JAD-122062
Vega, J. N., Zurkovsky, L., Albert, K., Melo, A., Boyd, B., Dumas, J., et al. (2016). Altered brain connectivity in early postmenopausal women with subjective cognitive impairment. Front. Neurosci. 10:433. doi: 10.3389/fnins.2016.00433
Villa, A., Rizzi, N., Vegeto, E., Ciana, P., and Maggi, A. (2015). Estrogen accelerates the resolution of inflammation in macrophagic cells. Sci. Rep. 5:15224. doi: 10.1038/srep15224
Wang, J. M., Irwin, R. W., and Brinton, R. D. (2006). Activation of estrogen receptor alpha increases and estrogen receptor beta decreases apolipoprotein E expression in hippocampus in vitro and in vivo. Proc. Natl. Acad. Sci. U.S.A. 103, 16983–16988. doi: 10.1073/pnas.0608128103
Wang, Y., Hernandez, G., Mack, W. J., Schneider, L. S., Yin, F., and Brinton, R. D. (2020a). Retrospective analysis of phytoSERM for management of menopause-associated vasomotor symptoms and cognitive decline: a pilot study on pharmacogenomic effects of mitochondrial haplogroup and APOE genotype on therapeutic efficacy. Menopause 27, 57–65. doi: 10.1097/gme.0000000000001418
Wang, Y., Mishra, A., and Brinton, R. D. (2020b). Transitions in metabolic and immune systems from pre-menopause to post-menopause: implications for age-associated neurodegenerative diseases. F1000Res 9, F1000 Faculty Rev-68. doi: 10.12688/f1000research.21599.1
Wang, Y., Shang, Y., Mishra, A., Bacon, E., Yin, F., and Brinton, R. (2020c). Midlife chronological and endocrinological transitions in brain metabolism: system biology basis for increased Alzheimer’s risk in female brain. Sci. Rep. 10:8528. doi: 10.1038/s41598-020-65402-5
Warren, A. M., Gurvich, C., Worsley, R., and Kulkarni, J. (2014). A systematic review of the impact of oral contraceptives on cognition. Contraception 90, 111–116. doi: 10.1016/j.contraception.2014.03.015
Weber, M. T., Mapstone, M., Staskiewicz, J., and Maki, P. M. (2012). Reconciling subjective memory complaints with objective memory performance in the menopausal transition. Menopause 19, 735–741. doi: 10.1097/gme.0b013e318241fd22
Weber, M. T., Rubin, L. H., and Maki, P. M. (2013). Cognition in perimenopause: the effect of transition stage. Menopause 20, 511–517. doi: 10.1097/gme.0b013e31827655e5
Weis, S., Hodgetts, S., and Hausmann, M. (2019). Sex differences and menstrual cycle effects in cognitive and sensory resting state networks. Brain Cogn. 131, 66–73. doi: 10.1016/j.bandc.2017.09.003
Whitmer, R. A., Quesenberry, C. P., Zhou, J., and Yaffe, K. (2011). Timing of hormone therapy and dementia: the critical window theory revisited. Ann. Neurol. 69, 163–169. doi: 10.1002/ana.22239
Wisch, J. K., Meeker, K. L., Gordon, B. A., Flores, S., Dincer, A., Grant, E. A., et al. (2021). Sex-related differences in tau Positron Emission Tomography (PET) and the effects of Hormone Therapy (HT). Alzheimer Dis. Assoc. Disord. 35, 164–168. doi: 10.1097/wad.0000000000000393
Woolley, C. S., and McEwen, B. S. (1992). Estradiol mediates fluctuation in hippocampal synapse density during the estrous cycle in the adult rat [published erratum appears in J Neurosci 1992 Oct; 12 (10): following table of contents]. J. Neurosci. 12, 2549–2554.
Yaffe, K., Sawaya, G., Lieberburg, I., and Grady, D. (1998). Estrogen therapy in postmenopausal women: effects on cognitive function and dementia. JAMA 279, 688–695. doi: 10.1001/jama.279.9.688
Yue, X., Lu, M., Lancaster, T., Cao, P., Honda, S., Staufenbiel, M., et al. (2005). Brain estrogen deficiency accelerates Abeta plaque formation in an Alzheimer’s disease animal model. Proc. Natl. Acad. Sci. U.S.A. 102, 19198–19203. doi: 10.1073/pnas.0505203102
Zandi, P. P., Carlson, M. C., Plassman, B. L., Welsh-Bohmer, K. A., Mayer, L. S., Steffens, D. C., et al. (2002). Hormone replacement therapy and incidence of Alzheimer disease in older women: the Cache County Study. JAMA 288, 2123–2129. doi: 10.1001/jama.288.17.2123
Zeydan, B., Tosakulwong, N., Schwarz, C. G., Senjem, M. L., Gunter, J. L., Reid, R. I., et al. (2019). Association of bilateral salpingo-oophorectomy before menopause onset with medial temporal lobe neurodegeneration. JAMA Neurol. 76, 95–100. doi: 10.1001/jamaneurol.2018.3057
Zhang, G. Q., Chen, J. L., Luo, Y., Mathur, M. B., Anagnostis, P., Nurmatov, U., et al. (2021). Menopausal hormone therapy and women’s health: an umbrella review. PLoS Med. 18:e1003731. doi: 10.1371/journal.pmed.1003731
Zhang, S., Fan, W., Hu, H., Wen, L., Gong, M., Liu, B., et al. (2021). Subcortical volume changes in early menopausal women and correlation with neuropsychological tests. Front. Aging Neurosci. 13:738679. doi: 10.3389/fnagi.2021.738679
Zhang, S., Hu, J., Fan, W., Liu, B., Wen, L., Wang, G., et al. (2018). Aberrant cerebral activity in early postmenopausal women: a resting-state functional magnetic resonance imaging study. Front. Cell Neurosci. 12:454. doi: 10.3389/fncel.2018.00454
Zhang, T., Casanova, R., Resnick, S. M., Manson, J. E., Baker, L. D., Padual, C. B., et al. (2016). Effects of hormone therapy on brain volumes changes of postmenopausal women revealed by optimally-discriminative voxel-based morphometry. PLoS One 11:e0150834. doi: 10.1371/journal.pone.0150834
Zhao, L., Mao, Z., and Brinton, R. D. (2009). A select combination of clinically relevant phytoestrogens enhances estrogen receptor beta-binding selectivity and neuroprotective activities in vitro and in vivo. Endocrinology 150, 770–783. doi: 10.1210/en.2008-0715
Zhao, L., Woody, S. K., and Chhibber, A. (2015). Estrogen receptor β in Alzheimer’s disease: from mechanisms to therapeutics. Ageing Res. Rev. 24(Pt. B), 178–190. doi: 10.1016/j.arr.2015.08.001
Zhou, G., Liu, J., Sun, F., Duan, L., Yan, B., and Peng, Q. (2011). Cognitive functioning in elderly women who underwent unilateral oophorectomy before menopause. Int. J. Neurosci. 121, 196–200. doi: 10.3109/00207454.2010.542842
Zhu, X., Kelly, T. H., Curry, T. E. Jr., Lal, C., and Joseph, J. E. (2015). Altered functional brain asymmetry for mental rotation: effect of estradiol changes across the menstrual cycle. Neuroreport 26, 814–819. doi: 10.1097/wnr.0000000000000429
Keywords: hormones, menopause, estrogen, neuroimaging, Alzheimer’s disease, hormone therapy, menstrual cycle, pregnancy
Citation: Jett S, Schelbaum E, Jang G, Boneu Yepez C, Dyke JP, Pahlajani S, Diaz Brinton R and Mosconi L (2022) Ovarian steroid hormones: A long overlooked but critical contributor to brain aging and Alzheimer’s disease. Front. Aging Neurosci. 14:948219. doi: 10.3389/fnagi.2022.948219
Received: 19 May 2022; Accepted: 28 June 2022;
Published: 19 July 2022.
Edited by:
Ana María Genaro, Consejo Nacional de Investigaciones Científicas y Técnicas (CONICET), ArgentinaReviewed by:
Alejandro O. Sodero, CONICET Institute for Biomedical Research (BIOMED), ArgentinaIvan Nalvarte, Karolinska Institutet (KI), Sweden
Amandine Grimm, University of Basel, Switzerland
Copyright © 2022 Jett, Schelbaum, Jang, Boneu Yepez, Dyke, Pahlajani, Diaz Brinton and Mosconi. This is an open-access article distributed under the terms of the Creative Commons Attribution License (CC BY). The use, distribution or reproduction in other forums is permitted, provided the original author(s) and the copyright owner(s) are credited and that the original publication in this journal is cited, in accordance with accepted academic practice. No use, distribution or reproduction is permitted which does not comply with these terms.
*Correspondence: Lisa Mosconi, bGltMjAzNUBtZWQuY29ybmVsbC5lZHU=