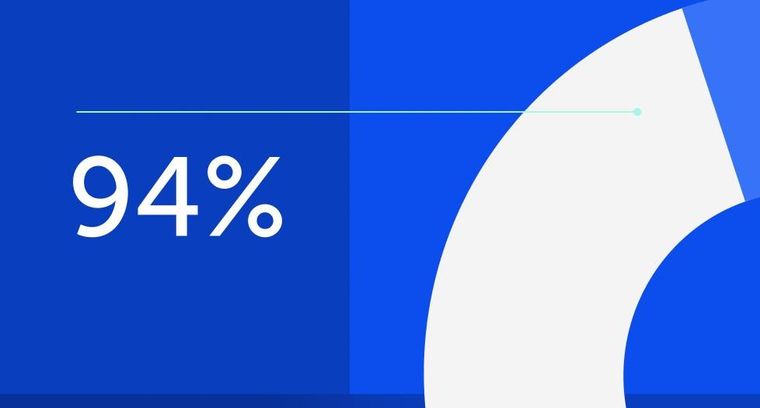
94% of researchers rate our articles as excellent or good
Learn more about the work of our research integrity team to safeguard the quality of each article we publish.
Find out more
REVIEW article
Front. Aging Neurosci., 16 August 2022
Sec. Neuroinflammation and Neuropathy
Volume 14 - 2022 | https://doi.org/10.3389/fnagi.2022.937999
Diabetic retinopathy (DR) is the most common complication of diabetes and has been historically regarded as a microangiopathic disease. Now, the paradigm is shifting toward a more comprehensive view of diabetic retinal disease (DRD) as a tissue-specific neurovascular complication, in which persistently high glycemia causes not only microvascular damage and ischemia but also intraretinal inflammation and neuronal degeneration. Despite the increasing knowledge on the pathogenic pathways involved in DR, currently approved treatments are focused only on its late-stage vasculopathic complications, and a single molecular target, vascular endothelial growth factor (VEGF), has been extensively studied, leading to drug development and approval. In this review, we discuss the state of the art of research on neuroinflammation and neurodegeneration in diabetes, with a focus on pathophysiological studies on human subjects, in vivo imaging biomarkers, and clinical trials on novel therapeutic options.
Diabetic retinopathy (DR) is the most common complication of diabetes mellitus (DM) and the leading cause of preventable blindness in developed countries. In the past, DR was regarded as a microangiopathy because its clinically detectable lesions are mainly vascular: even nowadays, its diagnosis and staging are still based on vascular abnormalities observed by fundoscopy. However, the American Diabetes Association has recently defined DR as a highly tissue-specific neurovascular complication of DM involving progressive disruption of the interdependence between multiple cell types in the retina (Solomon et al., 2017). Indeed, the retinal neurovascular unit (NVU) is composed of neurons, glial cells, and the intraretinal vascular network (Hawkins and Davis, 2005; Antonetti et al., 2012). Recent evidence suggests that DR is the result of a global dysfunction of the NVU: the activation of glial cells (astrocytes, Müller cells, and microglia) and degeneration of neural elements (ganglion, bipolar, horizontal, and amacrine cells) are distinct pathogenic events (and therefore therapeutic targets) that interplay with microvascular phenomena (Gardner and Davila, 2017; Nian et al., 2021), leading to the development of diabetic retinal disease (DRD).
Current treatments for DR are available only for its vision-threatening complications, such as diabetic macular edema (DME) and proliferative diabetic retinopathy (PDR). A better understanding of pathologic retinal changes in diabetes is crucial for the identification of novel pharmacological targets in order to prevent the development of late-stage complications, improve functional and anatomical outcomes and maybe slow the progression of irreversible retinal neurodegeneration. In this review, we discuss the state of the art of research on the role of neuroinflammation and neurodegeneration in DR, with a focus on pathophysiological studies on human subjects, in vivo imaging biomarkers, and clinical trials on novel therapeutic options.
We searched all English language and human subject scientific literature on PubMed library using the following keywords: DRD, DR, DME, inflammation, neurodegeneration, glia, multimodal imaging, biomarker. Additional articles were identified through the references of publications selected in the first instance. The website https://clinicaltrials.gov/ was used to find all clinical trials on DR and DME targeting neuroinflammation and neuroprotection.
Inflammation is a complex biological response of tissues and cells to pathogens and damaged cells that involves leucocytes, blood vessels, and many molecular mediators. Acute inflammation typically has beneficial effects in the acute setting, while becomes detrimental if persisting chronically. Initial suggestions for possible involvement of inflammation in DR came from reports of a lower incidence of DR in arthritic patients taking salicylates (Powell and Field, 1964). Experimental evidence for the presence of chronic-low grade inflammation from the early moments of DR pathogenesis began accumulating since Schröder et al. (1991) demonstrated in a murine model that leukostasis, a phenomenon that may cause retinal microvasculature dropout by monocytes and granulocytes, is present even in NPDR. Biochemical, molecular, and cellular mechanisms of neuroinflammation in DR significantly contributed to current knowledge of DR pathogenesis, are better studied in animal models, and are extensively reviewed elsewhere (Tang and Kern, 2011; Forrester et al., 2020; Pan et al., 2021). Before delving into the relationship between neuroinflammation and DR through the analysis of current evidence on clinical research in human subjects, a short overview is provided.
Neuroretinal inflammation is mediated by the retinal glia, which senses stress signals in the neural tissue (such as high glucose levels or glycated compounds, oxidative stress, and damaged cells) and secretes pro-inflammatory mediators. Retinal glia is composed of three cytotypes: astrocytes, Müller cells, and microglia. Astrocytes are not strictly of neuroepithelial origin but enter the developing retina from the optic nerve and present several fibrous processes radiating from the cell body that cover blood vessels in the superficial capillary plexus, contributing to inner retinal blood barrier (iBRB) formation (Stone and Dreher, 1987). Microglial cells are not of neuroglial origin, despite their name, but enter the retina with the mesenchymal precursors of retinal blood vessels and are believed to represent innate immune cells of the neural tissue, with a macrophage-like function (Rathnasamy et al., 2019). Müller cells are sustentacular cells that span from the external to the internal limiting membrane, connecting neurons, and vascular cells (Vecino et al., 2016).
Müller cells gliosis (activation) is a hallmark of DR, as demonstrated by a significant increase in aqueous biomarkers of glial activation (glial fibrillary acidic protein, aquaporin 1, and aquaporin 4) in aqueous humor (Vujosevic et al., 2015). Chronic hyperglycemia could induce gliosis through the formation of advanced glycation end products (AGEs) (Sorrentino et al., 2016; Xu et al., 2018), which are macromolecules that become abnormally glycated after exposure to chronically elevated blood glucose concentrations. The factors associated with AGEs formation include normal aging, degree of hyperglycemia, and glycated protein turnover (Sharma et al., 2012). Accumulated AGEs, both within retinal walls and serum, are capable of inducing pro-inflammatory responses by receptor (RAGE) dependent or independent pathways: AGEs can induce the upregulation of adhesion molecules (such as ICAM-1) on endothelial cells and directly activate leukocytes (Moore et al., 2003), while the activation of RAGE results in glial activation and inflammatory cytokines secretion. The formation of AGEs is also influenced by oxidizing conditions and reactive oxygen species (ROS) formation concentrations, thereby creating a positive feedback loop between retinal neuroinflammation and AGEs accumulation (Baynes and Thorpe, 2000).
Gliosis of Müller cells results in the secretion of pro-inflammatory cytokines, chemokines, and vascular endothelial growth factor (VEGF) (Bringmann and Wiedemann, 2012). While elevated VEGF levels directly cause iBRB instability and neovascularization development (Ozaki et al., 1997; Qaum et al., 2001), chemokines and cytokines attract and activate leukocytes. The adhesion of leukocytes to the endothelium for subsequent diapedesis is mediated by leukocyte integrins and endothelial cell adhesion molecules. Indeed, an elevated concentration of E-selectin in the plasma of diabetic subjects may play a role in the development of DR (Kasza et al., 2017). At this point, leucocytes recruited in the retinal capillaries appear to activate the Fas (CD95)/Fas-ligand pathway, eventually leading to endothelial cell apoptosis and further iBRB impairment (Joussen et al., 2003; Vincent and Mohr, 2007).
Several studies tried to determine the inflammatory profile of DR and DME in vivo by measuring cytokine concentrations on intraocular fluids (Sivalingam et al., 1990; Yuuki et al., 2001; Funatsu et al., 2002, 2009; Suzuki et al., 2011; Jonas et al., 2012; Lee et al., 2012; Schoenberger et al., 2012; Bromberg-White et al., 2013; Mao and Yan, 2014; Noma et al., 2014, 2017; Dong et al., 2015; Kim et al., 2015; Takeuchi et al., 2015; Ghodasra et al., 2016; Vujosevic et al., 2016b; Boss et al., 2017; Wu et al., 2017; Song et al., 2020). Table 1 lists all the inflammatory mediators investigated on intraocular fluids of human subjects with DR and DME. Among the many identified, the most promising is IL-6, according to multiple independent studies. Indeed, IL-6 and IL-8 are increased in the vitreous of eyes with PDR (Yuuki et al., 2001) and have an independent influence on macular volume and DME severity (Dong et al., 2015; Kim et al., 2015). However, conflicting data on the role of IL-8 can be found in literature (Ghodasra et al., 2016; Vujosevic et al., 2016b). Other studies suggest that glial activation biomarkers (MIP-1β, GM-CSF, RANTES, and sTNF-RII) in the aqueous humor of patients with DR (Vujosevic et al., 2016b), which are presumably secreted under the inflammatory drive of IL-6 and IL-8 from Müller cells (Boss et al., 2017), could be associated with the concentration of neurotrophic mediators (such as Nerve Growth Factor, Brain-Derived Neurotrophic Factor, and Glial cell–Derived Neurotrophic Factor).
Table 1. Summary of inflammation-related cytokines, chemokines, and growth factors investigated in the aqueous humor (A) or vitreous (V) of human patients with non-proliferative diabetic retinopathy (NPDR), proliferative diabetic retinopathy (PDR), and diabetic macular edema (DME).
The other promising molecule that appears to have a role in the development of DME is ICAM-1, a ligand for LFA-1 integrin receptor that mediates leukocytes’ adhesion to endothelial cells. Its concentration in the vitreous fluid is significantly higher in eyes with DME than in diabetic eyes without retinopathy and is correlated with the degree of fluorescein leakage and macular thickness (Funatsu et al., 2009). Also, Jonas and associates found that ICAM-1 concentration in the aqueous humor was the most associated with macular thickness (Jonas et al., 2012).
In vivo studies on cytokines in intraocular fluids paved the way for the identification of an enormous number of novel molecular targets. However, few anti-inflammatory targeted therapies have been developed by pharmaceutical companies, even fewer are in late-phase clinical trials, and only old molecules acting more broadly on the inflammatory cascade, such as corticosteroids, have been approved for intravitreal use (see also “anti-inflammatory compounds” section in “novel treatment strategies” chapter). The reason could be linked to the lack of robust studies with adequate samples on cytokine levels in eyes at different stages of DR, leading to contradictory pieces of information due to the enormous number of small studies on the topic (Table 1). Moreover, many of these research indifferently sampled cytokines from the aqueous humor rather than the vitreous. Despite one study suggested that aqueous humor concentration of some inflammatory cytokines may be correlated with that in the vitreous chamber (Funatsu et al., 2005), subsequent works demonstrated the fallacy of assuming that the concentration of proteins in the aqueous correlate with their counterparts in the vitreous (Noma et al., 2010; Ecker et al., 2011). Therefore, caution should be taken when comparing studies on cytokine levels in different intraocular fluids and further research in this area should investigate which cytokine, in which compartment may be used as a biomarker of retinal inflammation in DR.
In conclusion, retinal inflammation can be considered a key pathogenic factor in DR and especially in the progression to PDR and development of DME. Further pharmacological research is needed to bring novel therapeutic options into clinical practice.
There is a growing scientific interest in the possibility of identifying retinal inflammation in vivo, by means of multimodal imaging, which includes optical coherence tomography (OCT), OCT-angiography (OCTA), fluorescein angiography (FA), indocyanine-green angiography (ICGA), and confocal MultiColor imaging among the others.
Retinal hyperreflective foci (HF) are small (<30 μm), punctiform lesions with reflectivity similar to that of NFL, scattered throughout the neuroretina, and visible on OCT B-scans. HF can be distinguished from hard intraretinal exudates and microaneurysms and have been proposed to represent aggregates of microglial cells (Vujosevic et al., 2017a; Pilotto et al., 2020). In diabetic eyes without clinical retinopathy, HF can be identified in the inner retina, where most microglial cells are present, while DR is associated with their outer retinal migration (Vujosevic et al., 2013). HF number increases with DR and DME severity (Schreur et al., 2020).
The inflammatory origin of HF is debated but supported by a histopathologic study that investigated microglial activation in human DR and found an increased number of hypertrophic microglial cells scattered in the inner retinal layers, which were also present in outer retinal layers in later stages of the disease (Zeng et al., 2008). However, it must be noted that immunolabeled microglial cells in retinal sections did not clearly resemble HF and were often located around retinal vasculature, microaneurysms, intraretinal hemorrhages, cotton-wool spots, and neovascularizations, which have not been described for HF, although no OCT-based investigation specifically addressed this area of uncertainty (Zeng et al., 2008). Another research supporting this hypothesis reported an association between aqueous humor concentration of soluble CD14, a molecule involved in the cellular recognition of inflammatory signals (such as lipopolysaccharide), and HF number in diabetics with DME (Lee et al., 2018).
Other works specifically investigated the functional, prognostic and predictive role of HF. In DME, HF number shows an inverse correlation with macular sensitivity, possibly linking microglial inflammatory response to functional neuroretinal impairment (Vujosevic et al., 2016a), but predicts a higher increase in sensitivity thresholds after intravitreal dexamethasone treatment (Vujosevic et al., 2017c). Also, the presence of numerous HF at baseline predicts a worse visual acuity at the end of follow-up in DME treated with observation (Busch et al., 2020). However, a recent systematic review found that it is still unclear whether HF presence in DME can predict treatment outcomes, even though their number decreases after treatment (Huang et al., 2022).
Vitreous HF are another potential biomarker of neuroinflammation. The resolution of spectral-domain OCT is in the range of 5–7 μm and, thus, has the potential of imaging leukocytes which can be up to 10–30 μm in size. These leukocytes would appear as vitreous HF, larger and brighter than the usual background speckle and about 20 μm in diameter (Saito et al., 2013). A study by Mizukami et al. (2017) found a correlation between the average number of vitreous HF and the severity of DR. Similarly, macrophage-like cells (MLCs) at the vitreoretinal interface can be seen using en-face OCT. Their signal corresponds to a ramified morphology and a recent study found that MLC density is higher in PDR compared with controls, diabetics without retinopathy, and NPDR (Ong et al., 2021). Interestingly, MLCs are more likely to localize on blood vessels and in perivascular areas than in ischemic areas (Ong et al., 2021).
Also, the OCT pattern of DME may become a key feature to guide treatment and predict outcomes. Current treatments for DME include intravitreal therapies that target different aspects of its pathophysiology: anti-VEGF for vasogenic edema and corticosteroids for inflammatory edema (Romero-Aroca et al., 2016; Schmidt-Erfurth et al., 2017). DME can present with various patterns on OCT, including subretinal fluid (SRF) accumulation, which is visible on OCT as a hyporeflective area under the neuroretina (Otani et al., 1999). This pattern is associated with higher concentrations of CXCL10, IL-6, IL-8, and PDGF-AA but not VEGF (Sonoda et al., 2014; Kim et al., 2015) and by a higher number of HF (Vujosevic et al., 2017b), suggesting that inflammation plays a pivotal role in the development of at least some cases of DME. Indeed, dexamethasone treatment of SRF-associated DME is associated with a greater improvement of CMT (central macular thickness), retinal HF, and disorganization of inner retinal layers and cysts area with respect to ranibizumab treatment (Vujosevic et al., 2020).
Choroidal OCT biomarkers of inflammation have been proposed to monitor the response of DME to intravitreal corticosteroids: choroidal HF, which again are thought to represent inflammatory aggregates (Lee et al., 2018; Schreur et al., 2018), and choroidal vascularity index (CVI), measured as the ratio of choroid occupied by vessels and providing information on choroidal congestion (Kim et al., 2018; Iovino et al., 2020). A recent prospective study from our research group evaluated the relationship between OCT biomarkers of inflammation in DME and the response to treatment with fluocinolone acetonide (FAc) 0.19 mg intravitreal implant over 1 year of follow-up. Good responders tend to show higher choroidal HF and lower CVI than poor responders and both metrics do not change over the follow-up in poor responders to FAc implant (Arrigo et al., 2020). A subsequent investigation on non-naïve DME eyes treated with anti-VEGF and/or dexamethasone or FAc implant found that foveal eversion is a negative biomarker associated with a higher prevalence of persistent DME (Arrigo et al., 2021). Since DME with foveal eversion is associated with a cytokine profile similar to that observed in inflammatory diseases such as uveitis or Irvine-Gass syndrome (Kiire et al., 2014), it could represent a sign of Müller cells impairment due to a chronic inflammatory milieu in the retinal tissue (Arrigo et al., 2021). These facts underline the compelling need for a better understanding of DME physiopathology and for validated OCT biomarkers that would guide the therapeutic strategy in accordance with the chorioretinal inflammatory profile.
Recently, it has been proposed that macular perfusion in DME eyes increases after intravitreal FAc administration, owing to a reduced leukostasis (Brambati et al., 2022). DME eyes have a decreased vessel density (VD), mainly at the deep capillary plexus (DCP) (Lee et al., 2016; AttaAllah et al., 2019), but OCTA has limited reliability in DME because cystic cavities interfere with flow detection from deeper retinal layers and with correct anatomical segmentation of retinal layers and vascular plexuses (Spaide, 2015; de Carlo et al., 2016; Tey et al., 2019). Previous studies reported conflicting data on VD change after intravitreal treatment for DME (Toto et al., 2017; Mastropasqua et al., 2018). However, Vujosevic et al. (2020) performed all quantitative OCTA analyses after image compensation for artifacts determined by intraretinal cysts and found a decrease in VD at DCP after dexamethasone but not ranibizumab treatment. The authors excluded an ischemic effect of dexamethasone because the VD decrease was not accompanied by a difference in vessel length or caliber between the two treatments (Vujosevic et al., 2020). Therefore, further studies on larger cohorts and novel methodological approaches are needed to clarify the relationship between macular perfusion and anti-inflammatory treatments for DME.
Retinal neurodegeneration is a process that features reactive gliosis, diminished neuronal function, and neuronal loss and it has long been known that in the diabetic retina the development of microangiopathic findings is preceded by neuronal functional changes and apoptosis (Simonsen, 1980; Barber et al., 1998; Carrasco et al., 2007). Intrinsic retinal microvasculature lacks autonomic innervation and vessel dilation is guaranteed by glia-mediated autoregulatory signals from neurons in case of high metabolic stress (functional hyperemia) (Metea and Newman, 2007; Newman, 2013). A number of studies demonstrated that this mechanism of neurovascular coupling is impaired even in the early stages of DR (Bek, 2017) and the term “DRD” has been proposed to express all neurovascular pathologic changes in diabetes (Figure 1; Abramoff et al., 2018; Levine et al., 2022). However, photographic imaging of clinically evident vascular lesions has limited the study and understanding of diabetic retinal neuropathy.
Figure 1. Schematic overview of pathogenic mechanisms and phenotypes in Diabetic Retinal Disease (DRD). Retinal complications of diabetes have historically been considered microangiopathic in nature. Now, the paradigm is shifting toward a novel view of DRD as a tissue-specific neurovascular complication due to blood-retinal barrier (BRB) breakdown, retinal and optic nerve head ischemia, and neuroinflammation.
Direct evidence for photoreceptor dysfunction early in the course of DRD was demonstrated in the 80′, with two studies showing a decreased contrast and color sensitivity in diabetics without retinopathy (Roy et al., 1986; Trick et al., 1988). Subsequently, photoreceptors’ transduction abnormalities in patients with early DR were detected by means of full-field electroretinogram (ERG) (Holopigian et al., 1997). Recently, multifocal ERG confirmed the presence of functional alterations even from the preclinical stage of DR, though in presence of an altered superficial capillary plexus (Reis et al., 2014). However, it must be noted that a significant proportion of diabetic patients with early microvascular disease have no detectable neuronal dysfunction on multifocal ERG (Santos et al., 2017).
On the other hand, an accurate in vivo assessment of the inner retinal status in diabetic eyes became possible only with the advent of OCT, despite diabetes-associated damage to nerve fiber layer (NFL) had already been demonstrated (Özdek et al., 2002; Takahashi et al., 2006). Macular NFL and ganglion cell/inner plexiform layer (GCIPL) thickness are decreased in diabetic eyes, even in absence of vascular signs (van Dijk et al., 2009; Vujosevic and Midena, 2013; Chhablani et al., 2015; Picconi et al., 2017; Scarinci et al., 2017). Sohn et al. (2016) found that this form of retinal diabetic neuropathy is progressive: diabetics without retinopathy display a loss of 0.25 μm/year in macular NFL and 0.29 μm/year in GCIPL over 4 years of follow-up. These neuroretinal changes may be related to poor metabolic control (Lonneville et al., 2003; Picconi et al., 2017).
However, the relationship between diabetic neuropathy and vasculopathy is still debated: neurodegeneration could be due to subclinical changes in intraretinal microvasculature that can be detected and quantified with the aid of OCTA. Indeed, recent work by Marques et al. (2022) demonstrated that the progression of neuroretinal thinning in patients with NPDR is associated with a decrease in VD at the DCP. Several investigations tried to clarify the pathogenetic sequence by studying the optic nerve head in diabetics, because of the intimate relationship between neural and vascular elements in the peripapillary area. Two investigations on DR (Rodrigues et al., 2019; Frizziero et al., 2021) found a reduction of both radial peripapillary capillary plexus (RPC) vascular density and peripapillary NFL thickness, but only one (Rodrigues et al., 2019) was able to demonstrate a direct correlation between the two parameters. Similarly, two studies on optic nerve head neurovascular tissue in diabetics without retinopathy were published in 2019: one reported a reduction of peripapillary VD in absence of a NFL loss (Li et al., 2019), confirming previous results (Takahashi and Chihara, 2008; Vujosevic and Midena, 2013), while the other suggested that both peripapillary VD and NFL thickness are decreased with respect to controls, albeit the former could be more prominent than the latter (Cao et al., 2019).
Therefore, to present knowledge, retinal neurodegeneration can only be considered as a different pathologic feature of DRD, at least partly independent from microvascular alterations. This new view of DR underpins the need for better phenotyping and stratification of DRD in order to identify the subset of patients with chronic neurodysfunction and validate biomarkers to measure therapy outcomes, considering the emergent development of neuroprotective drugs.
Currently, a robust amount of research is conducted on developing new pharmacological agents for DR and DME. At the moment the only strategy to prevent or halt the progression of DR is intensive blood glucose control (Diabetes Control and Complications Trial Research Group, 1995) while effective therapies are available to treat only late-stage complications related to ischemia and DME, even though the incidence of persistent DME after 1 or 2 years of anti-VEGF treatment is still very high (Dugel et al., 2016; Bressler et al., 2018). Several alternative strategies have been tested in the pre-clinical setting and here we summarize the advancement in research on novel therapeutics targeting inflammation (Table 2) and neurodegeneration (Table 3) in DR and DME, with a focus on those under investigation in clinical trials.
Despite an early clinical trial (NCT00367133) on intravitreal triamcinolone demonstrated its inferiority to focal/grid laser photocoagulation (Diabetic Retinopathy Clinical Research Network, 2008), intravitreal corticosteroids are the only category of pure anti-inflammatory drugs to be approved for clinical use in DR complicated by DME: FAc 0.2 mg implant (Iluvien; NCT00367133) (Campochiaro et al., 2011) and dexamethasone 0.7 mg (Ozurdex; NCT00168337, NCT00168389) (Boyer et al., 2014). Their ability to reduce intraocular inflammation has been extensively demonstrated. A study on diabetic rats demonstrated that dexamethasone reduces retinal leukostasis and vascular permeability (Wang et al., 2008), while in vivo effects of intravitreal corticosteroids in human subjects have been investigated by measuring the concentration of proinflammatory cytokines in intraocular fluids of eyes with DME. Indeed, triamcinolone decreases the aqueous levels of IL-6 and MCP-1 (Sohn et al., 2011), and FAc those of IL-6, IP-10, MCP-1, and ICAM-1 in the vitreous (Deuchler et al., 2022). Since the pathogenesis of DME is not only vasogenic but also inflammatory and around 40% of patients treated show suboptimal visual acuity improvements when treated with anti-VEGF (Gonzalez et al., 2016), corticosteroids use has become increasingly important, especially in chronic DME (Cunha-Vaz et al., 2014) and in DME displaying biomarkers of retinal inflammation (Arrigo et al., 2020). However, 20% of eyes receiving FAc experience persistent or recurrent DME in the first year after injection and require additional anti-VEGF treatments (Cicinelli et al., 2020). Therefore, new drugs targeting other pathways of retinal inflammation are needed.
NSAIDs mechanism of action pertains the inhibition of cyclooxygenases, which are enzymes involved in the synthesis of prostaglandins, one of the key biological mediators of inflammation. Prostaglandin E2 (PGE2) signaling seems to be involved in the pathogenesis of DR (Schoenberger et al., 2012; Wang et al., 2019) but clinical investigations on the role of topical NSAIDs in DME suggest that these medications have scant therapeutic potential. Indeed, topical nepafenac showed no effect on visual function in DRCR Network Protocol R (NCT01331005), just like bromfenac in a smaller pilot study (Friedman et al., 2015; Pinna et al., 2017). The poor efficacy may be due to the topical administration route, but a small, randomized trial on intravitreal diclofenac confirmed no beneficial effect on visual function, despite a reduction of DME (Elbendary and Shahin, 2011).
IL-6 is a key molecule that leads to retinal inflammation in DR and DME since it has been found elevated in vitreous in several studies, as described previously (Koleva-Georgieva et al., 2011; Sonoda et al., 2014). Two registered clinical trials investigated the blockage of the IL-6 pathway with monoclonal antibodies in DME: both NCT02842541 for EBI-031 and NCT02511067 for tocilizumab are withdrawn at the present moment.
TNF-α is another inflammatory cytokine secreted by activated macrophages and monocytes. Its serum concentrations correlate with the presence and severity of DR (Koleva-Georgieva et al., 2011) and a phase III trial (NCT00505947) demonstrated that intravenous anti-TNF-α monoclonal antibody infliximab significantly improved visual acuity over placebo in patients with DME (Sfikakis et al., 2010). Interestingly, a subsequent retrospective study from Wu et al. (2011) found that intravitreal infliximab (and adalimumab) does not improve visual acuity or CMT in refractory DME.
Integrin receptors are transmembrane adhesion proteins that mediate cell-to-cell and cell-to-extracellular matrix attachment and intracellular signal transduction, playing an essential role in leukocytes’ adhesion to the endothelium and extravasation. Most of their ligands are proteins containing arginine-glycine-aspartate (RGD) or leucine-aspartate-valine (LDV) motifs (van Hove et al., 2021). Risuteganib (Luminate) is an intravitreal antagonist of RGD integrin receptors and a phase IIb trial in DME (NCT02348918) showed its non-inferiority to bevacizumab with respect to visual acuity improvement at 24 weeks of follow-up (Quiroz-Mercado et al., 2018). THR-687 is another RGD integrin receptors antagonist that demonstrated an excellent safety profile with no dose-limiting toxicities along with a rapid gain in visual acuity that lasted about 3 months in a phase I trial (NCT03666923) (Khanani et al., 2021). Also OTT-166 (formerly SF0166) is an antagonist that demonstrated biological effects after topical administration in an early clinical trial (NCT02914613) (Edwards et al., 2018), while AXT107 is currently under investigation in a phase I/IIa trial (NCT04697758).
Vascular adhesion protein-1 (VAP-1) is an endothelial and soluble enzyme with amine oxidase activity found elevated in the serum of diabetic patients and vitreous of eyes with PDR (Murata et al., 2012; Luo et al., 2013) and involved in leukocyte diapedesis and vessel leakage (Noda et al., 2009). Its oral inhibitor ASP8232 had no effect alone or in combination with ranibizumab in a phase II trial on DME (NCT02302079) (Nguyen et al., 2019).
Chemokine receptor type 2 and type 5 (CCR2 and CCR5) are expressed by monocytes and play a key role in homing inflammatory cells to retinal tissue (Rangasamy et al., 2014). Since the concentration of CCR2 and CCR5 ligands (monocyte chemoattractant protein-1 and RANTES, respectively) is elevated in the vitreous and aqueous humor of patients with DR and DME (Funatsu et al., 2009; Roh et al., 2009) and CCR2 has been implicated in VEGF production and vascular leakage (Krause et al., 2014), a phase II trial on CCR2/5 dual antagonist in DME has been performed (NCT01994291). Treatment with the CCR2/5 inhibitor was associated with a modest improvement in visual acuity, though inferior to intravitreal ranibizumab, despite the high level of CCR2 antagonism observed in the study (Gale et al., 2018).
Bradykinin (BK) plays a role in vascular inflammation, ischemic vasogenic edema, and angioedema. BK is cleaved from high-molecular-weight kininogen by the proteolytically active plasma kallikrein (PKal) and stimulates two receptors, B1R and B2R: the former is constitutively expressed whereas the latter is regulated by inflammatory mediators such as IL-1β or TNF-α (Abdulaal et al., 2016). PKal concentration is increased in the vitreous of DME eyes and BK-induced retinal thickening in mice is not affected by the blockade of VEGF receptor (Kita et al., 2015). Indeed, the inhibition of B1R has been shown to inhibit retinal inflammation in the animal model (Pouliot et al., 2012) and intravitreal KVD001, which act upstream in the pathway as a PKal inhibitor, showed promising results in a phase Ib (NCT02193113) and II (NCT03466099) trial on DME (Sun et al., 2019). Also THR-149, a novel bicyclic peptide inhibitor of PKal, demonstrated clinical potential in a phase I trial (NCT03511898) (Dugel et al., 2021).
No pharmacologic compound to halt neurodegeneration is approved for clinical use, yet some agents are under investigation.
The EUROCONDOR phase II–III clinical trial (NCT01726075) investigated the neuroprotective potential of topically administered brimonidine (α2-adrenergic agonist) and somatostatin (endogenous neuroprotective hormone) in patients with type 2 diabetes and early stage or no DR (Simó et al., 2019). Indeed, animal studies have demonstrated that topical brimonidine enhances neuronal function and reduces apoptosis (Saylor et al., 2009); similarly, topical somatostatin prevents ERG abnormalities, glial activation, and neuronal apoptosis (Hernández et al., 2013). Results from the trial have shown that somatostatin and brimonidine do not prevent neurodysfunction in diabetics; however, in the subset of patients displaying abnormal mfERG at baseline, these two compounds arrested the progression of neural dysfunction (Simó et al., 2019).
Citicoline is a precursor in the synthesis of phosphatidylcholine, a component of the neuronal plasma membrane. Since GC dysfunction seems an early event in DR (Parisi and Uccioli, 2001; Vujosevic and Midena, 2013; Sohn et al., 2016; Scarinci et al., 2017) and the topical administration of citicoline improves GC function (Parisi et al., 2018), a recent double-blind, randomized, placebo-controlled trial (NCT04009980) investigated the role of citicoline plus vitamin B12 eyedrops in mild DR and found a reduction of functional, structural and vascular progression over 36-months of follow-up (Parravano et al., 2020).
Cardiolipin is a phospholipid of the inner mitochondrial membrane which has an active role in mitochondrial-dependent apoptosis (Birk et al., 2014). Elamipretide (formerly MTP-131) is a soluble tetrapeptide that selectively stabilizes mitochondrial cardiolipin and promotes efficient electron transfer and reversed the visual decline without improving glycemic control or reducing body weight in mouse models of diabetes (Alam et al., 2015). A phase I/II clinical trial has been carried out to determine the effects of topical ocular administration of elamipretide in DME (NCT02314299).
Erythropoietin (EPO) is produced by RPE and can inhibit apoptosis of retinal neurons (Becerra and Amaral, 2002; Shen et al., 2010). EPO overexpression is an early event in the natural history of DR (Garciìa-Ramiìrez et al., 2008) and high levels of this growth factor can be found in the vitreous of DME eyes (Hernaìndez et al., 2006). However, the exogenous administration of EPO could exacerbate retinal neoangiogenesis and thrombosis (Chen et al., 2009, 2021). Cibinetide (formerly known as ARA290 and helix B surface peptide) is a non-erythrogenic EPO-derived peptide that retains tissue-protective properties (Brines et al., 2008). Treatment with an EPO-derived peptide in diabetic rats prevents glial dysfunction, microglial activation, and neuronal damage without altering hematocrit or exacerbating neovascularization (McVicar et al., 2011). A phase II clinical trial in DME (EudraCT 2015-001940-12) demonstrated safety but no improvements in BCVA, retinal sensitivity, or CMT (Lois et al., 2020).
Alpha-lipoic acid is a pro-energetic and antioxidant compound that seems to be a valuable neuroprotective option for Alzheimer’s disease (Hager et al., 2007; Fava et al., 2013). Moreover, alpha-lipoic acid treatment reduced VEGF levels and preserved ganglion cells, inner and outer layers in diabetic mouse retinas (Kan et al., 2017). A randomized trial on diabetic subjects receiving 300 mg of ALA orally once daily for 3 months demonstrated that contrast sensitivity remained stable in patients with type 1 DM and improved in those with type 2 while contrast sensitivity declined in diabetics without ALA supplementation; however, visual acuity and eye fundus image was stable in all studied subjects (Gêbka et al., 2014).
Lutein, zeaxanthin, and meso-zeaxanthin are exogenous retinal antioxidants. The neuroprotective properties of lutein have been demonstrated in several preclinical studies (Muriach et al., 2006; Li et al., 2009). A retrospective study in which patients received a carotenoid supplement containing lutein 10 mg, zeaxanthin 2 mg, and meso-zeaxanthin 10 mg once a day observed an increase in CMT and mfERG responses after 2 years of treatment (Moschos et al., 2017).
DR is characterized by extremely complex pathogenesis, in which microvascular damage holds a pivotal role. However, neuroinflammation and neuronal degeneration are common phenomena occurring in all DR stages, entangled with the effects of vascular exudation and retinal ischemia but at least partially independent, possibly explaining why some patients experience poor outcomes despite optimal treatment. The wide range of molecular targets prompts the development of new drugs, some of which are already under evaluation in clinical trials, in order to provide more bullets in the management of DR while in vivo multimodal imaging biomarkers promise a patient-tailored therapeutic strategy according to the vascular, inflammatory and neurodegenerative DRD phenotype.
AAr and LB: study design, data collection, and manuscript draft. AAn, AS, EA, and AB: data collection and critical revision of the text. FB and MB: manuscript revision and study supervision. All authors contributed to the article and approved the submitted version.
The cartoon in Figure 1 is adapted from “Diabetic Retinopathy Hallmarks” template by BioRender (2022).
FB consultant for: Alcon (Fort Worth, Texas, United States), Alimera Sciences (Alpharetta, Georgia, United States), Allergan Inc. (Irvine, California, United States), Farmila-Thea (Clermont-Ferrand, France), Bayer Shering-Pharma (Berlin, Germany), Bausch And Lomb (Rochester, New York, United States), Genentech (San Francisco, California, United States), Hoffmann-La-Roche (Basel, Switzerland), NovagaliPharma (Évry, France), Novartis (Basel, Switzerland), Sanofi-Aventis (Paris, France), Thrombogenics (Heverlee, Belgium), and Zeiss (Dublin, United States).
The remaining authors declare that the research was conducted in the absence of any commercial or financial relationships that could be construed as a potential conflict of interest.
All claims expressed in this article are solely those of the authors and do not necessarily represent those of their affiliated organizations, or those of the publisher, the editors and the reviewers. Any product that may be evaluated in this article, or claim that may be made by its manufacturer, is not guaranteed or endorsed by the publisher.
Abdulaal, M., Haddad, N. M. N., Sun, J. K., and Silva, P. S. (2016). The role of plasma kallikrein–kinin pathway in the development of diabetic retinopathy: Pathophysiology and therapeutic approaches. Semin. Ophthalmol. 31, 19–24. doi: 10.3109/08820538.2015.1114829
Abramoff, M. D., Fort, P. E., Han, I. C., Jayasundera, K. T., Sohn, E. H., and Gardner, T. W. (2018). Approach for a clinically useful comprehensive classification of vascular and neural aspects of diabetic retinal disease. Investig. Opthalmol. Vis. Sci. 59, 519–527. doi: 10.1167/iovs.17-21873
Alam, N. M., Mills, W. C., Wong, A. A., Douglas, R. M., Szeto, H. H., and Prusky, G. T. (2015). A mitochondrial therapeutic reverses visual decline in mouse models of diabetes. Dis. Models Mech. 8, 701–710. doi: 10.1242/dmm.020248
Antonetti, D. A., Klein, R., and Gardner, T. W. (2012). Diabetic retinopathy. N. Engl. J. Med. 366, 1227–1239. doi: 10.1056/NEJMra1005073
Arrigo, A., Aragona, E., Capone, L., Lattanzio, R., Zollet, P., and Bandello, F. (2021). Foveal eversion: A possible biomarker of persistent diabetic macular edema. Ophthalmol. Ther. 10, 115–126. doi: 10.1007/s40123-020-00324-z
Arrigo, A., Capone, L., Lattanzio, R., Aragona, E., Zollet, P., and Bandello, F. (2020). Optical coherence tomography biomarkers of inflammation in diabetic macular edema treated by fluocinolone acetonide intravitreal drug-delivery system implant. Ophthalmol. Ther. 9, 971–980. doi: 10.1007/s40123-020-00297-z
AttaAllah, H. R., Mohamed, A. A. M., and Ali, M. A. (2019). Macular vessels density in diabetic retinopathy: Quantitative assessment using optical coherence tomography angiography. Int. Ophthalmol. 39, 1845–1859. doi: 10.1007/s10792-018-1013-0
Barber, A. J., Lieth, E., Khin, S. A., Antonetti, D. A., Buchanan, A. G., and Gardner, T. W. (1998). Neural apoptosis in the retina during experimental and human diabetes. Early onset and effect of insulin. J. Clin. Invest. 102, 783–791. doi: 10.1172/JCI2425
Baynes, J. W., and Thorpe, S. R. (2000). Glycoxidation and lipoxidation in atherogenesis. Free Radic. Biol. Med. 28, 1708–1716. doi: 10.1016/S0891-5849(00)00228-8
Becerra, S. P., and Amaral, J. (2002). Erythropoietin — an endogenous retinal survival factor. N. Engl. J. Med. 347, 1968–1970. doi: 10.1056/NEJMcibr022629
Bek, T. (2017). Diameter changes of retinal vessels in diabetic retinopathy. Curr. Diabetes Rep. 17:82. doi: 10.1007/s11892-017-0909-9
Birk, A. V., Chao, W. M., Bracken, C., Warren, J. D., and Szeto, H. H. (2014). Targeting mitochondrial cardiolipin and the cytochrome c /cardiolipin complex to promote electron transport and optimize mitochondrial ATP synthesis. Br. J. Pharmacol. 171, 2017–2028. doi: 10.1111/bph.12468
Boss, J. D., Singh, P. K., Pandya, H. K., Tosi, J., Kim, C., Tewari, A., et al. (2017). Assessment of neurotrophins and inflammatory mediators in vitreous of patients with diabetic retinopathy. Invest. Opthalmol. Vis. Sci. 58, 5594. doi: 10.1167/iovs.17-21973
Boyer, D. S., Yoon, Y. H., Belfort, R., Bandello, F., Maturi, R. K., Augustin, A. J., et al. (2014). Three-year, randomized, sham-controlled trial of dexamethasone intravitreal implant in patients with diabetic macular edema. Ophthalmology 121, 1904–1914. doi: 10.1016/j.ophtha.2014.04.024
Brambati, M., Borrelli, E., Capone, L., Querques, L., Sacconi, R., Battista, M., et al. (2022). Changes in macular perfusion after ILUVIEN§intravitreal implant for diabetic macular edema: An OCTA study. Ophthalmol. Ther. 11, 653–660. doi: 10.1007/s40123-022-00455-5
Bressler, N. M., Beaulieu, W. T., Glassman, A. R., Blinder, K. J., Bressler, S. B., Jampol, L. M., et al. (2018). Persistent macular thickening following intravitreous aflibercept, bevacizumab, or ranibizumab for central-involved diabetic macular edema with vision impairment. JAMA Ophthalmol. 136, 257–269. doi: 10.1001/jamaophthalmol.2017.6565
Brines, M., Patel, N. S. A., Villa, P., Brines, C., Mennini, T., de Paola, M., et al. (2008). Nonerythropoietic, tissue-protective peptides derived from the tertiary structure of erythropoietin. Proc. Natl. Acad. Sci. U.S.A. 105, 10925–10930. doi: 10.1073/pnas.0805594105
Bringmann, A., and Wiedemann, P. (2012). Müller glial cells in retinal disease. Ophthalmologica 227, 1–19. doi: 10.1159/000328979
Bromberg-White, J. L., Glazer, L., Downer, R., Furge, K., Boguslawski, E., and Duesbery, N. S. (2013). Identification of VEGF-independent cytokines in proliferative diabetic retinopathy vitreous. Invest. Opthalmol. Vis. Sci. 54, 6472–6480. doi: 10.1167/iovs.13-12518
Busch, C., Okada, M., Zur, D., Fraser-Bell, S., Rodríguez-Valdés, P. J., Cebeci, Z., et al. (2020). Baseline predictors for visual acuity loss during observation in diabetic macular oedema with good baseline visual acuity. Acta Ophthalmol. 98, e801–e806. doi: 10.1111/aos.14390
Campochiaro, P. A., Brown, D. M., Pearson, A., Ciulla, T., Boyer, D., Holz, F. G., et al. (2011). Long-term benefit of sustained-delivery fluocinolone acetonide vitreous inserts for diabetic macular edema. Ophthalmology 118, 626–635.e2. doi: 10.1016/j.ophtha.2010.12.028
Cao, D., Yang, D., Yu, H., Xie, J., Zeng, Y., Wang, J., et al. (2019). Optic nerve head perfusion changes preceding peripapillary retinal nerve fibre layer thinning in preclinical diabetic retinopathy. Clin. Exp. Ophthalmol. 47, 219–225. doi: 10.1111/ceo.13390
Carrasco, E., Hernaìndez, C., Miralles, A., Huguet, P., Farreìs, J., and Simoì, R. (2007). Lower somatostatin expression is an early event in diabetic retinopathy and is associated with retinal neurodegeneration. Diabetes Care 30, 2902–2908. doi: 10.2337/dc07-0332
Chen, J., Connor, K. M., Aderman, C. M., Willett, K. L., Aspegren, O. P., and Smith, L. E. H. (2009). Suppression of retinal neovascularization by erythropoietin siRNA in a mouse model of proliferative retinopathy. Invest. Opthalmol. Vis. Sci. 50, 1329–1335. doi: 10.1167/iovs.08-2521
Chen, L., Feng, J., Shi, Y., Luan, F., Ma, F., Wang, Y., et al. (2021). Reduced expression of erythropoietin after intravitreal ranibizumab in proliferative diabetic retinopathy patients—retrospective interventional study. Front. Med. 8:710079. doi: 10.3389/fmed.2021.710079
Chhablani, J., Sharma, A., Goud, A., Peguda, H. K., Rao, H. L., Begum, V. U., et al. (2015). Neurodegeneration in type 2 diabetes: Evidence from spectral-domain optical coherence tomography. Invest. Opthalmol. Vis. Sci. 56, 6333–6338. doi: 10.1167/iovs.15-17334
Cicinelli, M. V., Rabiolo, A., Zollet, P., Capone, L., Lattanzio, R., and Bandello, F. (2020). Persistent or recurrent diabetic macular edema after fluocinolone acetonide 0.19 mg implant: Risk factors and management. Am. J. Ophthalmol. 215, 14–24. doi: 10.1016/j.ajo.2020.03.016
Cunha-Vaz, J., Ashton, P., Iezzi, R., Campochiaro, P., Dugel, P. U., Holz, F. G., et al. (2014). Sustained delivery fluocinolone acetonide vitreous implants: Long-term benefit in patients with chronic diabetic macular edema. Ophthalmology 121, 1892–1903.e3. doi: 10.1016/j.ophtha.2014.04.019
de Carlo, T. E., Chin, A. T., Joseph, T., Baumal, C. R., Witkin, A. J., Duker, J. S., et al. (2016). Distinguishing diabetic macular edema from capillary nonperfusion using optical coherence tomography angiography. Ophthalmic Surg. Lasers Imaging Retina 47, 108–114. doi: 10.3928/23258160-20160126-02
Deuchler, S. K., Schubert, R., Singh, P., Chedid, A., Kenikstul, N., Scott, J., et al. (2022). Vitreous cytokine levels following the administration of a single 0.19 mg fluocinolone acetonide (ILUVIEN§) implant in patients with refractory diabetic macular edema (DME)—results from the ILUVIT study. Graefes Arch. Clin. Exp. Ophthalmol. 260, 2537–2547. doi: 10.1007/s00417-022-05564-2
Diabetes Control and Complications Trial Research Group (1995). Progression of retinopathy with intensive versus conventional treatment in the diabetes control and complications trial. Ophthalmology 102, 647–661. doi: 10.1016/S0161-6420(95)30973-6
Diabetic Retinopathy Clinical Research Network (2008). A randomized trial comparing intravitreal triamcinolone acetonide and focal/grid photocoagulation for diabetic macular edema. Ophthalmology 115, 1447–1459. doi: 10.1016/j.ophtha.2008.06.015
Dong, N., Xu, B., Chu, L., and Tang, X. (2015). Study of 27 aqueous humor cytokines in type 2 diabetic patients with or without macular edema. PLoS One 10:e0125329. doi: 10.1371/journal.pone.0125329
Dugel, P. U., Khanani, A. M., Berger, B. B., Patel, S., Fineman, M., Jaffe, G. J., et al. (2021). Phase 1 dose-escalation study of plasma kallikrein inhibitor THR-149 for the treatment of diabetic macular edema. Transl. Vis. Sci. Technol. 10:28. doi: 10.1167/tvst.10.14.28
Dugel, P. U., Layton, A., and Varma, R. (2016). Diabetic macular edema diagnosis and treatment in the real world: An analysis of medicare claims data (2008 to 2010). Ophthalmic Surg. Lasers Imaging Retina 47, 258–267. doi: 10.3928/23258160-20160229-09
Ecker, S. M., Hines, J. C., Pfahler, S. M., and Glaser, B. M. (2011). Aqueous cytokine and growth factor levels do not reliably reflect those levels found in the vitreous. Mol. Vis. 17, 2856–2863.
Edwards, D., Boyer, D. S., Kaiser, P. K., Heier, J. S., and Askew, B. (2018). First-in human study of SF0166 topical ophthalmic solution in patients with diabetic macular edema. Invest. Opthalmol. Vis. Sci. 59:1961.
Elbendary, A. M., and Shahin, M. M. (2011). Intravitreal diclofenac versus intravitreal triamcinolone acetonide in the treatment of diabetic macular edema. Retina 31, 2058–2064. doi: 10.1097/IAE.0b013e31822a042a
Fava, A., Pirritano, D., Plastino, M., Cristiano, D., Puccio, G., Colica, C., et al. (2013). The effect of lipoic acid therapy on cognitive functioning in patients with Alzheimer’s disease. J. Neurodegener. Dis. 2013:454253. doi: 10.1155/2013/454253
Forrester, J. V., Kuffova, L., and Delibegovic, M. (2020). The role of inflammation in diabetic retinopathy. Front. Immunol. 11:583687. doi: 10.3389/fimmu.2020.583687
Friedman, S. M., Almukhtar, T. H., Baker, C. W., Glassman, A. R., Elman, M. J., Bressler, N. M., et al. (2015). Topical nepafenec in eyes with noncentral diabetic macular edema. Retina 35, 944–956. doi: 10.1097/IAE.0000000000000403
Frizziero, L., Parrozzani, R., Londei, D., Pilotto, E., and Midena, E. (2021). Quantification of vascular and neuronal changes in the peripapillary retinal area secondary to diabetic retinopathy. Br. J. Ophthalmol. 105, 1577–1583. doi: 10.1136/bjophthalmol-2020-316468
Funatsu, H., Noma, H., Mimura, T., Eguchi, S., and Hori, S. (2009). Association of vitreous inflammatory factors with diabetic macular edema. Ophthalmology 116, 73–79. doi: 10.1016/j.ophtha.2008.09.037
Funatsu, H., Yamashita, H., Noma, H., Mimura, T., Nakamura, S., Sakata, K., et al. (2005). Aqueous humor levels of cytokines are related to vitreous levels and progression of diabetic retinopathy in diabetic patients. Graefes Arch. Clin. Exp. Ophthalmol. 243, 3–8. doi: 10.1007/s00417-004-0950-7
Funatsu, H., Yamashita, H., Noma, H., Mimura, T., Yamashita, T., and Hori, S. (2002). Increased levels of vascular endothelial growth factor and interleukin-6 in the aqueous humor of diabetics with macular edema. Am. J. Ophthalmol. 133, 70–77. doi: 10.1016/S0002-9394(01)01269-7
Gale, J. D., Berger, B., Gilbert, S., Popa, S., Sultan, M. B., Schachar, R. A., et al. (2018). A CCR2/5 inhibitor, PF-04634817, is inferior to monthly ranibizumab in the treatment of diabetic macular edema. Invest. Opthalmol. Vis. Sci. 59, 2659–2669. doi: 10.1167/iovs.17-22731
Garciìa-Ramiìrez, M., Hernaìndez, C., and Simoì, R. (2008). Expression of erythropoietin and its receptor in the human retina. Diabetes Care 31, 1189–1194. doi: 10.2337/dc07-2075
Gardner, T. W., and Davila, J. R. (2017). The neurovascular unit and the pathophysiologic basis of diabetic retinopathy. Graefes Arch. Clin. Exp. Ophthalmol. 255, 1–6. doi: 10.1007/s00417-016-3548-y
Gêbka, A., Serkies-Minuth, E., and Raczyñska, D. (2014). Effect of the administration of alpha-lipoic acid on contrast sensitivity in patients with type 1 and type 2 diabetes. Mediat. Inflamm. 2014:131538. doi: 10.1155/2014/131538
Ghodasra, D. H., Fante, R., Gardner, T. W., Langue, M., Niziol, L. M., Besirli, C., et al. (2016). Safety and feasibility of quantitative multiplexed cytokine analysis from office-based vitreous aspiration. Invest. Opthalmol. Vis. Sci. 57, 3017–3023. doi: 10.1167/iovs.15-18721
Gonzalez, V. H., Campbell, J., Holekamp, N. M., Kiss, S., Loewenstein, A., Augustin, A. J., et al. (2016). Early and long-term responses to anti–vascular endothelial growth factor therapy in diabetic macular edema: Analysis of protocol I data. Am. J. Ophthalmol. 172, 72–79. doi: 10.1016/j.ajo.2016.09.012
Hager, K., Kenklies, M., McAfoose, J., Engel, J., and Münch, G. (2007). “α-Lipoic acid as a new treatment option for Alzheimer’s disease — a 48 months follow-up analysis,” in Neuropsychiatric disorders an integrative approach, eds M. Gerlach, J. Deckert, K. Double, and E. Koutsilieri (Vienna: Springer), 189–193. doi: 10.1007/978-3-211-73574-9_24
Hawkins, B. T., and Davis, T. P. (2005). The blood-brain barrier/neurovascular unit in health and disease. Pharmacol. Rev. 57, 173–185. doi: 10.1124/pr.57.2.4
Hernández, C., García-Ramírez, M., Corraliza, L., Fernández-Carneado, J., Farrera-Sinfreu, J., Ponsati, B., et al. (2013). Topical administration of somatostatin prevents retinal neurodegeneration in experimental diabetes. Diabetes 62, 2569–2578. doi: 10.2337/db12-0926
Hernaìndez, C., Fonollosa, A., Garciìa-Ramiìrez, M., Higuera, M., Catalaìn, R., Miralles, A., et al. (2006). Erythropoietin is expressed in the human retina and it is highly elevated in the vitreous fluid of patients with diabetic macular edema. Diabetes Care 29, 2028–2033. doi: 10.2337/dc06-0556
Holopigian, K., Greenstein, V. C., Seiple, W., Hood, D. C., and Carr, R. E. (1997). Evidence for photoreceptor changes in patients with diabetic retinopathy. Invest. Opthalmol. Vis. Sci. 38, 2355–2365.
Huang, H., Jansonius, N. M., Chen, H., and Los, L. I. (2022). Hyperreflective dots on OCT as a predictor of treatment outcome in diabetic macular edema. Ophthalmol. Retina. doi: 10.1016/j.oret.2022.03.020 [Epub ahead of print].
Iovino, C., Pellegrini, M., Bernabei, F., Borrelli, E., Sacconi, R., Govetto, A., et al. (2020). Choroidal vascularity index: An in-depth analysis of this novel optical coherence tomography parameter. J. Clin. Med. 9:595. doi: 10.3390/jcm9020595
Jonas, J. B., Jonas, R. A., Neumaier, M., and Findeisen, P. (2012). Cytokine concentration in aqueous humor of eyes with diabetic macular edema. Retina 32, 2150–2157. doi: 10.1097/IAE.0b013e3182576d07
Joussen, A. M., Poulaki, V., Mitsiades, N., Cai, W., Suzuma, I., Pak, J., et al. (2003). Suppression of Fas-FasL-induced endothelial cell apoptosis prevents diabetic blood-retinal barrier breakdown in a model of streptozotocin-induced diabetes. FASEB J. 17, 76–78. doi: 10.1096/fj.02-0157fje
Kan, E., Alici, Ö., Kan, E. K., and Ayar, A. (2017). Effects of alpha-lipoic acid on retinal ganglion cells, retinal thicknesses, and VEGF production in an experimental model of diabetes. Int. Ophthalmol. 37, 1269–1278. doi: 10.1007/s10792-016-0396-z
Kasza, M., Meleg, J., Vardai, J., Nagy, B., Szalai, E., Damjanovich, J., et al. (2017). Plasma E-selectin levels can play a role in the development of diabetic retinopathy. Graefes Arch. Clin. Exp. Ophthalmol. 255, 25–30. doi: 10.1007/s00417-016-3411-1
Khanani, A. M., Patel, S. S., Gonzalez, V. H., Moon, S. J., Jaffe, G. J., Wells, J. A., et al. (2021). Phase 1 study of THR-687, a novel, highly potent integrin antagonist for the treatment of diabetic macular edema. Ophthalmol. Sci. 1:100040. doi: 10.1016/j.xops.2021.100040
Kiire, C., Broadgate, S., Halford, S., and Chong, V. (2014). Diabetic macular edema with foveal eversion shows a distinct cytokine profile to other forms of diabetic macular edema in patients with type 2 diabetes. Invest. Ophthalmol. Vis. Sci. 55:4408.
Kim, M., Ha, M. J., Choi, S. Y., and Park, Y.-H. (2018). Choroidal vascularity index in type-2 diabetes analyzed by swept-source optical coherence tomography. Sci. Rep. 8:70. doi: 10.1038/s41598-017-18511-7
Kim, M., Kim, Y., and Lee, S.-J. (2015). Comparison of aqueous concentrations of angiogenic and inflammatory cytokines based on optical coherence tomography patterns of diabetic macular edema. Indian J. Ophthalmol. 63, 312–317. doi: 10.4103/0301-4738.158069
Kita, T., Clermont, A. C., Murugesan, N., Zhou, Q., Fujisawa, K., Ishibashi, T., et al. (2015). Plasma kallikrein-kinin system as a VEGF-independent mediator of diabetic macular edema. Diabetes 64, 3588–3599. doi: 10.2337/db15-0317
Koleva-Georgieva, D. N., Sivkova, N. P., and Terzieva, D. (2011). Serum inflammatory cytokines IL-1β, IL-6, TNF-α and VEGF have influence on the development of diabetic retinopathy. Folia Med. 53, 44–50. doi: 10.2478/v10153-010-0036-8
Krause, T. A., Alex, A. F., Engel, D. R., Kurts, C., and Eter, N. (2014). VEGF-production by CCR2-dependent macrophages contributes to laser-induced choroidal neovascularization. PLoS One 9:e94313. doi: 10.1371/journal.pone.0094313
Lee, H., Jang, H., Choi, Y. A., Kim, H. C., and Chung, H. (2018). Association between soluble CD14 in the aqueous humor and hyperreflective foci on optical coherence tomography in patients with diabetic macular edema. Invest. Opthalmol. Vis. Sci. 59, 715–721. doi: 10.1167/iovs.17-23042
Lee, J., Moon, B. G., Cho, A. R., and Yoon, Y. H. (2016). Optical coherence tomography angiography of DME and its association with anti-VEGF treatment response. Ophthalmology 123, 2368–2375. doi: 10.1016/j.ophtha.2016.07.010
Lee, W. J., Kang, M. H., Seong, M., and Cho, H. Y. (2012). Comparison of aqueous concentrations of angiogenic and inflammatory cytokines in diabetic macular oedema and macular oedema due to branch retinal vein occlusion. Br. J. Ophthalmol. 96, 1426–1430. doi: 10.1136/bjophthalmol-2012-301913
Levine, S. R., Sapieha, P., Dutta, S., Sun, J. K., and Gardner, T. W. (2022). It is time for a moonshot to find “Cures” for diabetic retinal disease. Prog. Retinal Eye Res. 101051. doi: 10.1016/j.preteyeres.2022.101051 [Epub ahead of printf].
Li, S.-Y., Fu, Z.-J., Ma, H., Jang, W.-C., So, K.-F., Wong, D., et al. (2009). Effect of lutein on retinal neurons and oxidative stress in a model of acute retinal ischemia/reperfusion. Invest. Opthalmol. Vis. Sci. 50, 836–843. doi: 10.1167/iovs.08-2310
Li, Z., Wen, X., Zeng, P., Liao, Y., Fan, S., Zhang, Y., et al. (2019). Do microvascular changes occur preceding neural impairment in early-stage diabetic retinopathy? Evidence based on the optic nerve head using optical coherence tomography angiography. Acta Diabetol. 56, 531–539. doi: 10.1007/s00592-019-01288-8
Lois, N., Gardner, E., McFarland, M., Armstrong, D., McNally, C., Lavery, N. J., et al. (2020). A phase 2 clinical trial on the use of cibinetide for the treatment of diabetic macular edema. J. Clin. Med. 9:2225. doi: 10.3390/jcm9072225
Lonneville, Y. H., Özdek, Ş. C., Önol, M., Yetkin, Ý., Gürelik, G., and Hasanreisoğlu, B. (2003). The effect of blood glucose regulation on retinal nerve fiber layer thickness in diabetic patients. Ophthalmologica 217, 347–350. doi: 10.1159/000071350
Luo, W., Xie, F., Zhang, Z., and Sun, D. (2013). Vascular adhesion protein 1 in the eye. J. Ophthalmol. 2013:925267. doi: 10.1155/2013/925267
Mao, C., and Yan, H. (2014). Roles of elevated intravitreal IL-1β and IL-10 levels in proliferative diabetic retinopathy. Indian J. Ophthalmol. 62, 699–701. doi: 10.4103/0301-4738.136220
Marques, I. P., Ferreira, S., Santos, T., Madeira, M. H., Santos, A. R., Mendes, L., et al. (2022). Association between neurodegeneration and macular perfusion in the progression of diabetic retinopathy. A 3-year longitudinal study. Ophthalmologica 245, 335–341. doi: 10.1159/000522527
Mastropasqua, R., D’Aloisio, R., di Nicola, M., di Martino, G., Lamolinara, A., di Antonio, L., et al. (2018). Relationship between aqueous humor cytokine level changes and retinal vascular changes after intravitreal aflibercept for diabetic macular edema. Sci. Rep. 8:16548. doi: 10.1038/s41598-018-35036-9
McVicar, C. M., Hamilton, R., Colhoun, L. M., Gardiner, T. A., Brines, M., Cerami, A., et al. (2011). Intervention with an erythropoietin-derived peptide protects against neuroglial and vascular degeneration during diabetic retinopathy. Diabetes 60, 2995–3005. doi: 10.2337/db11-0026
Metea, M. R., and Newman, E. A. (2007). Signalling within the neurovascular unit in the mammalian retina. Exp. Physiol. 92, 635–640. doi: 10.1113/expphysiol.2006.036376
Mizukami, T., Hotta, Y., and Katai, N. (2017). Higher numbers of hyperreflective foci seen in the vitreous on spectral-domain optical coherence tomographic images in eyes with more severe diabetic retinopathy. Ophthalmologica 238, 74–80. doi: 10.1159/000473886
Moore, T. C. B., Moore, J. E., Kaji, Y., Frizzell, N., Usui, T., Poulaki, V., et al. (2003). The role of advanced glycation end products in retinal microvascular leukostasis. Invest. Opthalmol. Vis. Sci. 44, 4457–4464. doi: 10.1167/iovs.02-1063
Moschos, M. M., Dettoraki, M., Tsatsos, M., Kitsos, G., and Kalogeropoulos, C. (2017). Effect of carotenoids dietary supplementation on macular function in diabetic patients. Eye Vis. 4:23. doi: 10.1186/s40662-017-0088-4
Murata, M., Noda, K., Fukuhara, J., Kanda, A., Kase, S., Saito, W., et al. (2012). Soluble vascular adhesion protein-1 accumulates in proliferative diabetic retinopathy. Invest. Opthalmol. Vis. Sci. 53, 4055–4062. doi: 10.1167/iovs.12-9857
Muriach, M., Bosch-Morell, F., Alexander, G., Blomhoff, R., Barcia, J., Arnal, E., et al. (2006). Lutein effect on retina and hippocampus of diabetic mice. Free Radic. Biol. Med. 41, 979–984. doi: 10.1016/j.freeradbiomed.2006.06.023
Newman, E. A. (2013). Functional hyperemia and mechanisms of neurovascular coupling in the retinal vasculature. J. Cereb. Blood Flow Metab. 33, 1685–1695. doi: 10.1038/jcbfm.2013.145
Nguyen, Q. D., Sepah, Y. J., Berger, B., Brown, D., Do, D. V., Garcia-Hernandez, A., et al. (2019). Primary outcomes of the VIDI study: Phase 2, double-masked, randomized, active-controlled study of ASP8232 for diabetic macular edema. Int. J. Retina Vitr. 5:28. doi: 10.1186/s40942-019-0178-7
Nian, S., Lo, A. C. Y., Mi, Y., Ren, K., and Yang, D. (2021). Neurovascular unit in diabetic retinopathy: Pathophysiological roles and potential therapeutical targets. Eye Vis. 8:15. doi: 10.1186/s40662-021-00239-1
Noda, K., Nakao, S., Zandi, S., Engelstädter, V., Mashima, Y., and Hafezi-Moghadam, A. (2009). Vascular adhesion protein-1 regulates leukocyte transmigration rate in the retina during diabetes. Exp. Eye Res. 89, 774–781. doi: 10.1016/j.exer.2009.07.010
Noma, H., Funatsu, H., Mimura, T., Harino, S., and Hori, S. (2010). Aqueous humor levels of vasoactive molecules correlate with vitreous levels and macular edema in central retinal vein occlusion. Eur. J. Ophthalmol. 20, 402–409. doi: 10.1177/112067211002000222
Noma, H., Mimura, T., Yasuda, K., Motohashi, R., Kotake, O., and Shimura, M. (2017). Aqueous humor levels of soluble vascular endothelial growth factor receptor and inflammatory factors in diabetic macular edema. Ophthalmologica 238, 81–88. doi: 10.1159/000475603
Noma, H., Mimura, T., Yasuda, K., and Shimura, M. (2014). Role of inflammation in diabetic macular edema. Ophthalmologica 232, 127–135. doi: 10.1159/000364955
Ong, J. X., Nesper, P. L., Fawzi, A. A., Wang, J. M., and Lavine, J. A. (2021). Macrophage-like cell density is increased in proliferative diabetic retinopathy characterized by optical coherence tomography angiography. Invest. Opthalmol. Vis. Sci. 62:2. doi: 10.1167/iovs.62.10.2
Otani, T., Kishi, S., and Maruyama, Y. (1999). Patterns of diabetic macular edema with optical coherence tomography. Am. J. Ophthalmol. 127, 688–693. doi: 10.1016/S0002-9394(99)00033-1
Ozaki, H., Hayashi, H., Vinores, S. A., Moromizato, Y., Campochiaro, P. A., and Oshima, K. (1997). Intravitreal sustained release of VEGF causes retinal neovascularization in rabbits and breakdown of the blood– retinal barrier in rabbits and primates. Exp. Eye Res. 64, 505–517. doi: 10.1006/exer.1996.0239
Özdek, S., Lonneville, Y. H., Önol, M., Yetkin, I., and Hasanreisoðlu, B. B. (2002). Assessment of nerve fiber layer in diabetic patients with scanning laser polarimetry. Eye 16, 761–765. doi: 10.1038/sj.eye.6700207
Pan, W. W., Lin, F., and Fort, P. E. (2021). The innate immune system in diabetic retinopathy. Prog. Retin. Eye Res. 84:100940. doi: 10.1016/j.preteyeres.2021.100940
Parisi, V., Oddone, F., Ziccardi, L., Roberti, G., Coppola, G., and Manni, G. (2018). Citicoline and retinal ganglion cells: Effects on morphology and function. Curr. Neuropharmacol. 16, 919–932. doi: 10.2174/1570159X15666170703111729
Parisi, V., and Uccioli, L. (2001). Visual electrophysiological responses in persons with type 1 diabetes. Diabetes Metab. Res. Rev. 17, 12–18. doi: 10.1002/dmrr.177
Parravano, M., Scarinci, F., Parisi, V., Giorno, P., Giannini, D., Oddone, F., et al. (2020). Citicoline and vitamin B12 eye drops in type 1 diabetes: Results of a 3-year pilot study evaluating morpho-functional retinal changes. Adv. Ther. 37, 1646–1663. doi: 10.1007/s12325-020-01284-3
Picconi, F., Parravano, M., Ylli, D., Pasqualetti, P., Coluzzi, S., Giordani, I., et al. (2017). Retinal neurodegeneration in patients with type 1 diabetes mellitus: The role of glycemic variability. Acta Diabetol. 54, 489–497. doi: 10.1007/s00592-017-0971-4
Pilotto, E., Miante, S., Torresin, T., Puthenparampil, M., Frizziero, L., Federle, L., et al. (2020). Hyperreflective foci in the retina of active relapse-onset multiple sclerosis. Ophthalmology 127, 1774–1776. doi: 10.1016/j.ophtha.2020.03.024
Pinna, A., Blasetti, F., Ricci, G. D., and Boscia, F. (2017). Bromfenac eyedrops in the treatment of diabetic macular edema: A pilot study. Eur. J. Ophthalmol. 27, 326–330. doi: 10.5301/ejo.5000888
Pouliot, M., Talbot, S., Sénécal, J., Dotigny, F., Vaucher, E., and Couture, R. (2012). Ocular application of the kinin B1 receptor antagonist LF22-0542 inhibits retinal inflammation and oxidative stress in streptozotocin-diabetic rats. PLoS One 7:e33864. doi: 10.1371/journal.pone.0033864
Powell, E. D., and Field, R. A. (1964). Diabetic retinopathy and rheumatoid arthritis. Lancet 2, 17–18. doi: 10.1016/s0140-6736(64)90008-x
Qaum, T., Xu, Q., Joussen, A. M., Clemens, M. W., Qin, W., Miyamoto, K., et al. (2001). VEGF-initiated blood-retinal barrier breakdown in early diabetes. Invest. Opthalmol. Vis. Sci. 42, 2408–2413.
Quiroz-Mercado, H., Boyer, D., Campochiaro, P., Heier, J., Kaiser, P., Kornfield, J., et al. (2018). Randomized, prospective, double-masked, controlled phase 2b trial to evaluate the safety & efficacy of ALG-1001 (Luminate§) in diabetic macular edema. Invest. Ophthalmol. Vis. Sci. 59:1960. doi: 10.1016/j.ophtha.2018.03.059
Rangasamy, S., McGuire, P. G., Franco Nitta, C., Monickaraj, F., Oruganti, S. R., and Das, A. (2014). Chemokine mediated monocyte trafficking into the retina: Role of inflammation in alteration of the blood-retinal barrier in diabetic retinopathy. PLoS One 9:e108508. doi: 10.1371/journal.pone.0108508
Rathnasamy, G., Foulds, W. S., Ling, E.-A., and Kaur, C. (2019). Retinal microglia – a key player in healthy and diseased retina. Prog. Neurobiol. 173, 18–40. doi: 10.1016/j.pneurobio.2018.05.006
Reis, A., Mateus, C., Melo, P., Figueira, J., Cunha-Vaz, J., and Castelo-Branco, M. (2014). Neuroretinal dysfunction with intact blood-retinal barrier and absent vasculopathy in type 1 diabetes. Diabetes 63, 3926–3937. doi: 10.2337/db13-1673
Rodrigues, T. M., Marques, J. P., Soares, M., Dolan, M.-J., Melo, P., Simão, S., et al. (2019). Peripapillary neurovascular coupling in the early stages of diabetic retinopathy. Retina 39, 2292–2302. doi: 10.1097/IAE.0000000000002328
Roh, M. I., Kim, H. S., Song, J. H., Lim, J. B., and Kwon, O. W. (2009). Effect of intravitreal bevacizumab injection on aqueous humor cytokine levels in clinically significant macular edema. Ophthalmology 116, 80–86. doi: 10.1016/j.ophtha.2008.09.036
Romero-Aroca, P., Baget-Bernaldiz, M., Pareja-Rios, A., Lopez-Galvez, M., Navarro-Gil, R., and Verges, R. (2016). Diabetic macular edema pathophysiology: Vasogenic versus inflammatory. J. Diabetes Res. 2016:2156273. doi: 10.1155/2016/2156273
Roy, M. S., Gunkel, R. D., and Podgor, M. J. (1986). Color vision defects in early diabetic retinopathy. Arch. Ophthalmol. 104, 225–228. doi: 10.1001/archopht.1986.01050140079024
Saito, M., Barbazetto, I. A., and Spaide, R. F. (2013). Intravitreal cellular infiltrate imaged as punctate spots by spectral-domain optical coherence tomography in eyes with posterior segment inflammatory disease. Retina 33, 559–565. doi: 10.1097/IAE.0b013e31826710ea
Santos, A. R., Ribeiro, L., Bandello, F., Lattanzio, R., Egan, C., Frydkjaer-Olsen, U., et al. (2017). Functional and structural findings of neurodegeneration in early stages of diabetic retinopathy: Cross-sectional analyses of baseline data of the EUROCONDOR project. Diabetes 66, 2503–2510. doi: 10.2337/db16-1453
Saylor, M., McLoon, L., Harrison, A., and Lee, M. (2009). Experimental and clinical evidence for brimonidine as an optic nerve and retinal neuroprotective agent. Arch. Ophthalmol. 127, 402. doi: 10.1001/archophthalmol.2009.9
Scarinci, F., Picconi, F., Virgili, G., Giorno, P., di Renzo, A., Varano, M., et al. (2017). Single retinal layer evaluation in patients with type 1 diabetes with no or early signs of diabetic retinopathy: The first hint of neurovascular crosstalk damage between neurons and capillaries? Ophthalmologica 237, 223–231. doi: 10.1159/000453551
Schmidt-Erfurth, U., Garcia-Arumi, J., Bandello, F., Berg, K., Chakravarthy, U., Gerendas, B. S., et al. (2017). Guidelines for the management of diabetic macular edema by the European Society of Retina Specialists (EURETINA). Ophthalmologica 237, 185–222. doi: 10.1159/000458539
Schoenberger, S. D., Kim, S. J., Sheng, J., Rezaei, K. A., Lalezary, M., and Cherney, E. (2012). Increased prostaglandin E2 (PGE 2) levels in proliferative diabetic retinopathy, and correlation with VEGF and inflammatory cytokines. Invest. Opthalmol. Vis. Sci. 53, 5906–5911. doi: 10.1167/iovs.12-10410
Schreur, V., Altay, L., van Asten, F., Groenewoud, J. M. M., Fauser, S., Klevering, B. J., et al. (2018). Hyperreflective foci on optical coherence tomography associate with treatment outcome for anti-VEGF in patients with diabetic macular edema. PLoS One 13:e0206482. doi: 10.1371/journal.pone.0206482
Schreur, V., de Breuk, A., Venhuizen, F. G., Sánchez, C. I., Tack, C. J., Klevering, B. J., et al. (2020). Retinal hyperreflective foci in type 1 diabetes mellitus. Retina 40, 1565–1573. doi: 10.1097/IAE.0000000000002626
Schröder, S., Palinski, W., and Schmid-Schönbein, G. W. (1991). Activated monocytes and granulocytes, capillary nonperfusion, and neovascularization in diabetic retinopathy. Am. J. Pathol. 139, 81–100.
Sfikakis, P. P., Grigoropoulos, V., Emfietzoglou, I., Theodossiadis, G., Tentolouris, N., Delicha, E., et al. (2010). Infliximab for diabetic macular edema refractory to laser photocoagulation. Diabetes Care 33, 1523–1528. doi: 10.2337/dc09-2372
Sharma, Y., Saxena, S., Mishra, A., Saxena, A., and Natu, S. M. (2012). Advanced glycation end products and diabetic retinopathy. J. Ocul. Biol. Dis. Infor. 5, 63–69. doi: 10.1007/s12177-013-9104-7
Shen, J., Wu, Y., Xu, J.-Y., Zhang, J., Sinclair, S. H., Yanoff, M., et al. (2010). ERK- and Akt-dependent neuroprotection by erythropoietin (EPO) against glyoxal-AGEs via modulation of Bcl-xL, Bax, and BAD. Invest. Opthalmol. Vis. Sci. 51, 35–46. doi: 10.1167/iovs.09-3544
Simó, R., Hernández, C., Porta, M., Bandello, F., Grauslund, J., Harding, S. P., et al. (2019). Effects of topically administered neuroprotective drugs in early stages of diabetic retinopathy: Results of the EUROCONDOR clinical trial. Diabetes 68, 457–463. doi: 10.2337/db18-0682
Simonsen, S. E. (1980). The value of the oscillatory potential in selecting juvenile diabetics at risk of developing proliferative retinopathy. Acta Ophthalmol. 58, 865–878. doi: 10.1111/j.1755-3768.1980.tb08312.x
Sivalingam, A., Kenney, J., Brown, G. C., Benson, W. E., and Donoso, L. (1990). Basic fibroblast growth factor levels in the vitreous of patients with proliferative diabetic retinopathy. Arch. Ophthalmol. 108, 869–872. doi: 10.1001/archopht.1990.01070080113046
Sohn, E. H., van Dijk, H. W., Jiao, C., Kok, P. H. B., Jeong, W., Demirkaya, N., et al. (2016). Retinal neurodegeneration may precede microvascular changes characteristic of diabetic retinopathy in diabetes mellitus. Proc. Natl. Acad. Sci. U.S.A. 113, E2655–E2664. doi: 10.1073/pnas.1522014113
Sohn, H. J., Han, D. H., Kim, I. T., Oh, I. K., Kim, K. H., Lee, D. Y., et al. (2011). Changes in aqueous concentrations of various cytokines after intravitreal triamcinolone versus bevacizumab for diabetic macular edema. Am. J. Ophthalmol. 152, 686–694. doi: 10.1016/j.ajo.2011.03.033
Solomon, S. D., Chew, E., Duh, E. J., Sobrin, L., Sun, J. K., VanderBeek, B. L., et al. (2017). Diabetic retinopathy: A position statement by the American Diabetes Association. Diabetes Care 40, 412–418. doi: 10.2337/dc16-2641
Song, S., Yu, X., Zhang, P., and Dai, H. (2020). Increased levels of cytokines in the aqueous humor correlate with the severity of diabetic retinopathy. J. Diabetes Complications 34, 107641. doi: 10.1016/j.jdiacomp.2020.107641
Sonoda, S., Sakamoto, T., Yamashita, T., Shirasawa, M., Otsuka, H., and Sonoda, Y. (2014). Retinal morphologic changes and concentrations of cytokines in eyes with diabetic macular edema. Retina 34, 741–748. doi: 10.1097/IAE.0b013e3182a48917
Sorrentino, F. S., Allkabes, M., Salsini, G., Bonifazzi, C., and Perri, P. (2016). The importance of glial cells in the homeostasis of the retinal microenvironment and their pivotal role in the course of diabetic retinopathy. Life Sci. 162, 54–59. doi: 10.1016/j.lfs.2016.08.001
Spaide, R. F. (2015). Volume-rendered optical coherence tomography of diabetic retinopathy pilot study. Am. J. Ophthalmol. 160, 1200–1210. doi: 10.1016/j.ajo.2015.09.010
Stone, J., and Dreher, Z. (1987). Relationship between astrocytes, ganglion cells and vasculature of the retina. J. Comp. Neurol. 255, 35–49. doi: 10.1002/cne.902550104
Sun, J. K., Maturi, R. K., Boyer, D. S., Wells, J. A., Gonzalez, V. H., Tansley, R., et al. (2019). One-time intravitreal injection of KVD001, a plasma kallikrein inhibitor, in patients with central-involved diabetic macular edema and reduced vision. Ophthalmol. Retina 3, 1107–1109. doi: 10.1016/j.oret.2019.07.006
Suzuki, Y., Nakazawa, M., Suzuki, K., Yamazaki, H., and Miyagawa, Y. (2011). Expression profiles of cytokines and chemokines in vitreous fluid in diabetic retinopathy and central retinal vein occlusion. Jpn. J. Ophthalmol. 55, 256–263. doi: 10.1007/s10384-011-0004-8
Takahashi, H., and Chihara, E. (2008). Impact of diabetic retinopathy on quantitative retinal nerve fiber layer measurement and glaucoma screening. Invest. Opthalmol. Vis. Sci. 49, 687–692. doi: 10.1167/iovs.07-0655
Takahashi, H., Goto, T., Shoji, T., Tanito, M., Park, M., and Chihara, E. (2006). Diabetes-associated retinal nerve fiber damage evaluated with scanning laser polarimetry. Am. J. Ophthalmol. 142, 88–94. doi: 10.1016/j.ajo.2006.02.016
Takeuchi, M., Sato, T., Tanaka, A., Muraoka, T., Taguchi, M., Sakurai, Y., et al. (2015). Elevated levels of cytokines associated with Th2 and Th17 cells in vitreous fluid of proliferative diabetic retinopathy patients. PLoS One 10:e0137358. doi: 10.1371/journal.pone.0137358
Tang, J., and Kern, T. S. (2011). Inflammation in diabetic retinopathy. Prog. Retin. Eye Res. 30, 343–358. doi: 10.1016/j.preteyeres.2011.05.002
Tey, K. Y., Teo, K., Tan, A. C. S., Devarajan, K., Tan, B., Tan, J., et al. (2019). Optical coherence tomography angiography in diabetic retinopathy: A review of current applications. Eye Vis. 6:37. doi: 10.1186/s40662-019-0160-3
Toto, L., D’Aloisio, R., di Nicola, M., di Martino, G., di Staso, S., Ciancaglini, M., et al. (2017). Qualitative and quantitative assessment of vascular changes in diabetic macular edema after dexamethasone implant using optical coherence tomography angiography. Int. J. Mol. Sci. 18:1181. doi: 10.3390/ijms18061181
Trick, G. L., Burde, R. M., Cordon, M. O., Santiago, J. V., and Kilo, C. (1988). The relationship between hue discrimination and contrast sensitivity deficits in patients with diabetes mellitus. Ophthalmology 95, 693–698. doi: 10.1016/S0161-6420(88)33125-8
van Dijk, H. W., Kok, P. H. B., Garvin, M., Sonka, M., Devries, J. H., Michels, R. P. J., et al. (2009). Selective loss of inner retinal layer thickness in type 1 diabetic patients with minimal diabetic retinopathy. Invest. Opthalmol. Vis. Sci. 50, 3404–3409. doi: 10.1167/iovs.08-3143
van Hove, I., Hu, T.-T., Beets, K., van Bergen, T., Etienne, I., Stitt, A. W., et al. (2021). Targeting RGD-binding integrins as an integrative therapy for diabetic retinopathy and neovascular age-related macular degeneration. Prog. Retin. Eye Res. 85:100966. doi: 10.1016/j.preteyeres.2021.100966
Vecino, E., Rodriguez, F. D., Ruzafa, N., Pereiro, X., and Sharma, S. C. (2016). Glia–neuron interactions in the mammalian retina. Prog. Retin. Eye Res. 51, 1–40. doi: 10.1016/j.preteyeres.2015.06.003
Vincent, J. A., and Mohr, S. (2007). Inhibition of caspase-1/interleukin-1β signaling prevents degeneration of retinal capillaries in diabetes and galactosemia. Diabetes 56, 224–230. doi: 10.2337/db06-0427
Vujosevic, S., Bini, S., Midena, G., Berton, M., Pilotto, E., and Midena, E. (2013). Hyperreflective intraretinal spots in diabetics without and with nonproliferative diabetic retinopathy: An in vivo study using spectral domain OCT. J. Diabetes Res. 2013:491835. doi: 10.1155/2013/491835
Vujosevic, S., Micera, A., Bini, S., Berton, M., Esposito, G., and Midena, E. (2016b). Proteome analysis of retinal glia cells-related inflammatory cytokines in the aqueous humour of diabetic patients. Acta Ophthalmol. 94, 56–64. doi: 10.1111/aos.12812
Vujosevic, S., Berton, M., Bini, S., Casciano, M., Cavarzeran, F., and Midena, E. (2016a). Hyperreflective retinal spots and visual function after anti-vascular endothelial growth factor treatment in center-involving diabetic macular edema. Retina 36, 1298–1308. doi: 10.1097/IAE.0000000000000912
Vujosevic, S., Micera, A., Bini, S., Berton, M., Esposito, G., and Midena, E. (2015). Aqueous humor biomarkers of müller cell activation in diabetic eyes. Invest. Opthalmol. Vis. Sci. 56, 3913–3918. doi: 10.1167/iovs.15-16554
Vujosevic, S., and Midena, E. (2013). Retinal layers changes in human preclinical and early clinical diabetic retinopathy support early retinal neuronal and Müller cells alterations. J. Diabetes Res. 2013:905058. doi: 10.1155/2013/905058
Vujosevic, S., Toma, C., Villani, E., Muraca, A., Torti, E., Florimbi, G., et al. (2020). Diabetic macular edema with neuroretinal detachment: OCT and OCT-angiography biomarkers of treatment response to anti-VEGF and steroids. Acta Diabetol. 57, 287–296. doi: 10.1007/s00592-019-01424-4
Vujosevic, S., Bini, S., Torresin, T., Berton, M., Midena, G., Parrozzani, R., et al. (2017a). Hyperreflective retinal spots in normal and diabetic eyes. Retina 37, 1092–1103. doi: 10.1097/IAE.0000000000001304
Vujosevic, S., Torresin, T., Bini, S., Convento, E., Pilotto, E., Parrozzani, R., et al. (2017c). Imaging retinal inflammatory biomarkers after intravitreal steroid and anti-VEGF treatment in diabetic macular oedema. Acta Ophthalmol. 95, 464–471. doi: 10.1111/aos.13294
Vujosevic, S., Torresin, T., Berton, M., Bini, S., Convento, E., and Midena, E. (2017b). Diabetic macular edema with and without subfoveal neuroretinal detachment: Two different morphologic and functional entities. Am. J. Ophthalmol. 181, 149–155. doi: 10.1016/j.ajo.2017.06.026
Wang, K., Wang, Y., Gao, L., Li, X., Li, M., and Guo, J. (2008). Dexamethasone inhibits leukocyte accumulation and vascular permeability in retina of streptozotocin-induced diabetic rats via reducing vascular endothelial growth factor and intercellular adhesion molecule-1 expression. Biol. Pharm. Bull. 31, 1541–1546. doi: 10.1248/bpb.31.1541
Wang, M., Wang, Y., Xie, T., Zhan, P., Zou, J., Nie, X., et al. (2019). Prostaglandin E2/EP2 receptor signalling pathway promotes diabetic retinopathy in a rat model of diabetes. Diabetologia 62, 335–348. doi: 10.1007/s00125-018-4755-3
Wu, H., Hwang, D.-K., Song, X., and Tao, Y. (2017). Association between aqueous cytokines and diabetic retinopathy stage. J. Ophthalmol. 2017:9402198. doi: 10.1155/2017/9402198
Wu, L., Hernandez-Bogantes, E., Roca, J. A., Arevalo, J. F., Barraza, K., and Lasave, A. F. (2011). Intravitreal tumor necrosis factor inhibitors in the treatment of refractory diabetic macular edema. Retina 31, 298–303. doi: 10.1097/IAE.0b013e3181eac7a6
Xu, J., Chen, L.-J., Yu, J., Wang, H.-J., Zhang, F., Liu, Q., et al. (2018). Involvement of advanced glycation end products in the pathogenesis of diabetic retinopathy. Cell. Physiol. Biochem. 48, 705–717. doi: 10.1159/000491897
Yuuki, T., Kanda, T., Kimura, Y., Kotajima, N., Tamura, J., Kobayashi, I., et al. (2001). Inflammatory cytokines in vitreous fluid and serum of patients with diabetic vitreoretinopathy. J. Diabetes Complications 15, 257–259. doi: 10.1016/S1056-8727(01)00155-6
Keywords: diabetic retinopathy, neuroinflammation, diabetic retinal neuropathy, neurodegeneration, diabetes
Citation: Bianco L, Arrigo A, Aragona E, Antropoli A, Berni A, Saladino A, Battaglia Parodi M and Bandello F (2022) Neuroinflammation and neurodegeneration in diabetic retinopathy. Front. Aging Neurosci. 14:937999. doi: 10.3389/fnagi.2022.937999
Received: 06 May 2022; Accepted: 28 July 2022;
Published: 16 August 2022.
Edited by:
Yu-Min Kuo, National Cheng Kung University, TaiwanReviewed by:
Steven F. Abcouwer, Michigan Medicine, University of Michigan, United StatesCopyright © 2022 Bianco, Arrigo, Aragona, Antropoli, Berni, Saladino, Battaglia Parodi and Bandello. This is an open-access article distributed under the terms of the Creative Commons Attribution License (CC BY). The use, distribution or reproduction in other forums is permitted, provided the original author(s) and the copyright owner(s) are credited and that the original publication in this journal is cited, in accordance with accepted academic practice. No use, distribution or reproduction is permitted which does not comply with these terms.
*Correspondence: Alessandro Arrigo, YWxlc3NhbmRyby5hcnJpZ29AaG90bWFpbC5jb20=
Disclaimer: All claims expressed in this article are solely those of the authors and do not necessarily represent those of their affiliated organizations, or those of the publisher, the editors and the reviewers. Any product that may be evaluated in this article or claim that may be made by its manufacturer is not guaranteed or endorsed by the publisher.
Research integrity at Frontiers
Learn more about the work of our research integrity team to safeguard the quality of each article we publish.