- 1Key Laboratory of Acupuncture and Massage for Treatment of Encephalopathy, College of Acupuncture, Tuina and Rehabilitation, Yunnan University of Traditional Chinese Medicine, Kunming, China
- 2Library, Kunming Medical University, Kunming, China
- 3School of Continuing Education, Yunnan University of Traditional Chinese Medicine, Kunming, China
- 4Department of Teaching Affairs and Administration, Kunming Medical University, Kunming, China
- 5School of Public Health, Kunming Medical University, Kunming, China
- 6Department of Pulmonary and Critical Care Medicine, The Sixth Affiliated Hospital of Kunming Medical University, Yuxi, China
Brain injury poses a heavy disease burden in the world, resulting in chronic deficits. Therapies for brain injuries have been focused on pharmacologic, small molecule, endocrine and cell-based therapies. Endogenous neural stem cells (eNSCs) are a group of stem cells which can be activated in vivo by damage, neurotrophic factors, physical factor stimulation, and physical exercise. The activated eNSCs can proliferate, migrate and differentiate into neuron, oligodendrocyte and astrocyte, and play an important role in brain injury repair and neural plasticity. The roles of eNSCs in the repair of brain injury include but are not limited to ameliorating cognitive function, improving learning and memory function, and promoting functional gait behaviors. The activation and mobilization of eNSCs is important to the repair of injured brain. In this review we describe the current knowledge of the common character of brain injury, the roles and mechanism of eNSCs in brain injury. And then we discuss the current mobilization strategy of eNSCs following brain injury. We hope that a comprehensive awareness of the roles and mobilization strategy of eNSCs in the repair of cerebral ischemia may help to find some new therapeutic targets and strategy for treatment of stroke.
Introduction
The brain is the center of the nervous system and controls all of our functions, including voluntary and involuntary movement, the secretion of hormones, study and memory formation, and so on. The damage and impairment of the brain directly brings about dysfunction in the body. The neural networks composed with neurons and their microenvironment is the functional foundation of the brain. And the destruction of neural networks is the common character of brain injury, such as stroke, trauma, cerebral palsy, neurodegenerative disorder, et al. (Larivière et al., 2019). Thus the reconstruction of the injured neural network is the ultimate purpose of the treatment for brain injury.
The key components in the reconstruction and repairmen of the neural network are neurogenesis and synaptogenesis (Ceanga et al., 2021; Puderbaugh and Emmady, 2022). The neurogenesis is the progress that new neurons generate and replace the injured neural cells in the adult brain after brain injury. And the synaptogenesis is the progress that neurites branch from the newborn neuron or injured neurons (with surviving bodies and dead axons or synapses), and repair the impaired neural networks. This progress needs a lot of newborn neurons derived from neural stem cells. Due to advances in brain biology, we already know that new neurons are generated from endogenous neural stem cells (eNSCs) throughout adulthood in the adult brain (Ottoboni et al., 2020). The eNSCs is a group of stem cells which have two essential properties: self-renewal and the potency of differentiation into neural cells. The most important neural stem cell pool in the adult brain is the subventricular zone (SVZ) and the dentate gyrus (DG) of hippocampus (Jinnou, 2021). These neural stem cells originated from SVZ and DG can proliferate, migrate and differentiate into neuron, oligodendrocyte and astrocyte. The newborn neurons can incorporate into network circuitry, the new oligodendrocytes can repair myelin sheaths, the new astrocytes can support, protect and nourish neural networks in the progress of reconstruction (Hamblin and Lee, 2021). Although the value and meaning of eNSCs in theory, the application of eNSCs in clinical setting limited by complex factors such as apoptosis, uncontrollable differentiation, and adverse microenvironment.
Thus a thorough clarification of the molecular mechanisms is essential for the application of eNSCs biology to promote repair strategies for brain injury. In this review, we firstly describe the current knowledge of the common character of brain injury, the roles and mechanism of eNSCs in brain injury. And then we discuss the current mobilization strategy of eNSCs following brain injury.
The common character of brain injury: The destruction of neural networks
Brain injury refers to injuries to the cerebral hemispheres, cerebellum, and brain stem. According to the tree structure of brain injuries in Medical Subject Headings (MeSH) in National Library of Medicine (NLM), the major categories of brain injuries include brain trauma (traumatic brain hemorrhage, diffuse brain injuries, traumatic brain injuries, chronic brain injury, post-traumatic epilepsy), cerebral ischemia/hemorrhagic injury, degenerative injury.
Ischemic stroke
Ischemic stroke caused by a disruption of blood flow to brain tissue leads to a lot of neuron loss and dysfunction, such as necrosis, apoptosis, autophagic cell death and synapse loss. The irreversible cerebral infarction is the primary lesion of ischemic stroke, which occurs within minutes in infarct core area, and gradually extends to the surrounding area of the lesion within a few hours (Feske, 2021). Ischemia leads to the malfunction of electron transport chain in the mitochondrial membrane, the calcium influx and calciumdependent excitotoxicity, the generation of reactive oxygen species, and ultimately leads to neural cells death. Meanwhile, ischemia triggers inflammatory response that leads to secondary brain injury and expand the infarction. These pathophysiology progress lead to the loss of the neuron and the synapse, and ultimately leads to the destruction of neural networks and neurological symptom (Ghelani et al., 2021; Wang et al., 2021). Neurological complications after ischemic stroke include brain edema, hemorrhagic transformation, seizures and epilepsy and delirium. Furthermore, deaths were one of the consequences of ischemic stroke within the first few days (Balami et al., 2011). During the acute phase of ischemic stroke, of all patients with the neurological worsening 33.6% of those encountered progressive stroke, 27.3% had brain swelling, 11.3% experienced a recurrent ischemic stroke, 10.5% suffered from parenchymal hemorrhage, and 17.3% had manifestations such as fever, hyperglycemia, and hypertension (Weimar et al., 2005).
Cerebral hemorrhage
Non-traumatic intracerebral hemorrhage (ICH) caused by rupture of blood vessel in brain and extravasation of blood into the brain parenchyma. ICH is the subtype of stroke with the greatest mortality (Poon et al., 2015). According to the site of hemorrhage, the ICH divide into subarachnoid hemorrhage, subdural hemorrhage, lobar intracerebral hemorrhage and deep intracerebral hemorrhage (the basal ganglia, periventricular white matter, internal capsule, brainstem, and so on (Carpenter et al., 2016; Ziai and Carhuapoma, 2018). The hematoma and hydrocephalus cased by ICH not only destroy the neural cells in brain parenchyma and increases intracranial pressure, but also result in the ischemic brain injury in the areas far from the bleeding site, and exacerbate the brain injury (Bhattacharjee et al., 2021; Magid-Bernstein et al., 2022).
Traumatic brain injury
Traumatic brain injury (TBI) is one of the most serious acute injuries with high incidence, high disability rate, and high mortality rate (Diaz-Arrastia, 2015). The TBI is usually caused by a violent bump, blow, or jolt to the head, or penetrating trauma, and the direct consequence of TBI is the damage of brain tissue and the destruction of neural networks induced by bruising, torn tissues, bleeding, neuroinflammation and apoptosis (Centers for Disease Control and Prevention (CDC), and National Center for Injury Prevention and Control., 2003; Peterson and Daugherty, 2019). In addition to acute injury, the chronic effects of TBI including impairment of motor, attention, memory, executive dysfunction and social deficits, can persist for months or years, and greatly reduce daily quality of life, and increase the burden for the patient and their family. The biological mechanisms involved in chronic TBI include neural apoptosis, neuroinflammatory reaction, demyelination, white matter pathology, which consistently damage the neural network and even lead to dementia (Fann et al., 2018; Haarbauer-Krupa et al., 2021).
Neurodegenerative disease
Differing from the TBI and cerebral stroke, the neurodegenerative disease without significant loss of brain tissue is not caused by a sudden injury or cerebrovascular accident. The typical neurodegenerative diseases are Alzheimer’s and Parkinson’s disease. Alzheimer’s disease (AD) is a devastating neurodegenerative disorder that accounts for 70–80% of dementia (Reitz and Mayeux, 2011; Prince et al., 2013). The symptoms of AD include memory loss and cognitive decline. As far as we know now, the etiology of AD is the production, aggregation, and deposition of amyloid β (Aβ) peptides in the brain. The amyloidogenesis and deposition of Aβ generates excessive reactive oxygen species (ROS) and nitrogen species (RNS) which break the membranes and mitochondria of the neuron (Dobson, 2003; Rajasekhar and Govindaraju, 2015, 2018). Also, the Aβ initiates neuro-inflammation by activating glia and deteriorates the microenvironment of neural networks (Wyss-Coray, 2006). Ultimately, the neural networks are broken by oxidative stress, inflammation, and mitochondrial dysfunction. Parkinson’s disease (PD) is a severe movement disorder with muscle rigidity, tremors, and changes in speech and gait. The PD is caused by the dysfunction and death of neurons that produce dopamine in substantia nigra (SN) in the brain. Although the injury occurs in the local region in the brain, the related neural networks are badly affected by PD. The supplement and rescue of the neurons in SN can repair the impaired neural networks and help relieves symptoms (MedicinePlus, 2022).
Recently, frontotemporal lobe degeneration (FTD) has been attracted great attention. FTD present with a spectrum of syndromes characterized by neuronal degeneration in the frontal and anterior temporal lobes. FTD often leads to motor, language and memory disorders, it is the third most common cause of dementia. Some neurologists think that Parkinsonism is a manifestation of FTD, but some FTD patients are negative in the presynaptic dopaminergic deficiency when they are detected by dopamine transporter-single photon emission computed tomography (DaTSPECT). These finding implied that FTD is a neurodegenerative disease differentiated to the PD, although both of them lead to dementia (Menéndez-González et al., 2018). The underlying pathologic mechanism of FTD is intracellular deposition of abnormal aggregates of transactive DNA-binding protein TDP-43 in the frontal and temporal lobes, which lead to the degeneration of neurons and astrocytosis, and ultimately lead to frontotemporal lobar degeneration (Miki et al., 2020; Khan and De Jesus, 2022).
In summary, although the causes of these brain injuries are different in pathology and etiology, all of these brain injuries involves the loss of neurons and nerve tissue. This loss eventually leads to the dysfunction and destruction of neural networks. Thus the treatment of brain injury is not only to protect the neuron and its network in the early stage but also to repair the injured neural networks in the late stage.
The roles and mechanism of endogenous neural stem cells in brain injury
Currently, the therapies for brain injuries have been focused on pharmacologic, rehabilitative training and cell-based therapies (Aertker and Cox, 2016; Szelenberger et al., 2020). Unfortunately, to date, we still lack effective pharmacologic in the treatment of brain injury because of the poor clinical effect and adverse side effects. Thus the cell-based therapies will become increasingly important in the treatment of brain injury. The cells used for neural repair can derive from activated eNSCs and cultured neural stem cells in vitro (Pereira and Rylander Ottosson, 2019). Numerous studies have confirmed that neural stem cell grafts promoted neural repair in brain injury. However, the great difficulty in cell source, transplantation methods, complex microenvironment and directed differentiation limited the application of stem cell transplantation. eNSCs have more advantages in the repair of the brain. Firstly, eNSCs do not initiate immune rejection and inflammatory response. Secondly, eNSCs are not affected by in vitro culture conditions and transplant operation, and the proliferation, generation and repair of eNSCs are sustainable. Thirdly, eNSCs will migrate to the site of injury under the action of chemokines. At last, eNSCs can migrate and differentiate in a more appropriate microenvironment. To date, extensive studies have shown that eNSCs have shown a beneficial effect in improving the functional recovery of the injured brain (Levison, 2018; Fang et al., 2019).
Factually, brain injury, such as stroke, trauma and neurodegeneration, activate the eNSCs (Liu et al., 1998; Wu, 1999; Gu and Wester, 2000; Nakagawa et al., 2000). Following stroke, in addition to the eNSCs derived from SVZ and DG, the local eNSCs in ischemic core and peri-infarct area, and microvascular pericytes residing near blood vessels can be activated and differentiate into neurosphere-like cell clusters expressing nestin (Nakagomi et al., 2009, 2011; Shimada et al., 2010; Takagi et al., 2017). In a photothrombotic stroke model, Vandeputte et al. (2014) found that 36% of eNSCs differentiate into astrocytes in the peri-infarct area and 21% into mature neurons at 90 days post stroke. In neonatal brain injury, the eNSCs derived from the ventricular-subventricular zone migrate to the damaged area and differentiate into neurons and oligodendrocytes, and improve functional gait behaviors (Felling et al., 2006; Jinnou, 2021). In an intraventricular hemorrhage model of rats, the proliferation and differentiation of eNSCs were enhanced by brain injury and the combination treatment with granulocyte colony stimulating factor (G-CSF) and lithium chloride, and attenuate the development of hydrocephalus and neuronal apoptosis (Yuan et al., 2016).
Similarly, TBI triggered the proliferation, differentiation of the eNSCs and migrated to the injured side (Sui and Jiang, 2016). The results from the group of Sun D showed that the proliferation of eNSCs in the DG had been activated by TBI in juvenile and adult rats. These eNSCs differentiated into mature neurons and integrated into the existing neuronal circuitry, and finally, promoted the cognitive recovery in the TBI model (Sun et al., 2005, 2007).
In a degenerative model of corticothalamic projection neurons in layer VI of the anterior cortex of mice, Magavi and Macklis (2000) and Macklis (2001) confirmed that the injury promoted neurogenesis and these newly generated eNSCs differentiated into mature neurons and formed long-distance corticothalamic connections. In animals with Huntington’s disease, the neurodegenerative process triggered immediately intensive cell proliferation and differentiation which led to the characteristic enlargement of the subependymal zone (SEZ) of lateral brain ventricles, which showed higher activation next to the degenerated striatum compared to the rostral part of the SEZ (R-SEZ). In the activated L-SEZ, niche cells with immature astrocytic phenotype ensheathed clusters of neural progenitors (Mazurová et al., 2006). Scharff et al. (2000) observed that the injured neurons caused by photolysis in the high vocal center (HVC) of adult songbirds were replaced and restore the impaired behavior. The neurogenesis was the potential therapeutic approaches of neurodegenerative disease including AD and PD (Lamm et al., 2014; Sung et al., 2020). Thus the restoration of neurogenesis and the replacement of injured neurons will be beneficial to the recovery of function from neurodegenerative disease (Yoneyama et al., 2011; van den Berge and Hol, 2013).
In summary, the injured brain retains the repair and regenerative potential to restore damaged neural networks through eNSCs (Table 1). And the mechanism of eNSCs involve a variety of mechanisms (Baker and West, 2019). The first is the neuronal replacement. Under an appropriate stimulus, the eNSCs primarily derived from SVA and DG can migrate to injured brain regions, differentiate into neurons, integrate into preexisting neural networks, and eventually replace the damaged neuron (Romanko et al., 2004). The second is remyelination. The eNSCs can differentiate into oligodendroglia cells and participate in myelination in the axons from newly generated neurons and the injured neurons (surviving bodies and dead axons), and play an important role in the reconstruction of the injured neural networks in brain injury (Kaneko and Sawamoto, 2013; Jinnou, 2021). The third is the secretion of trophic function. The neural stem cells can release neurotrophic factors such as nerve growth factor (NGF), brain derived neurotrophic factor (BDNF), and glial-derived neurotrophic factor (GDNF), and so on. These neurotrophic factors can improve the microenvironment of neural networks and promote nerve repair (Lu et al., 2003; Lladó et al., 2004). At last, the epigenetic mechanisms such as DNA methylation, histone acetylation and some microRNAs have been involved in the neurogenesis induced by brain injury (Liu et al., 2013, 2020).
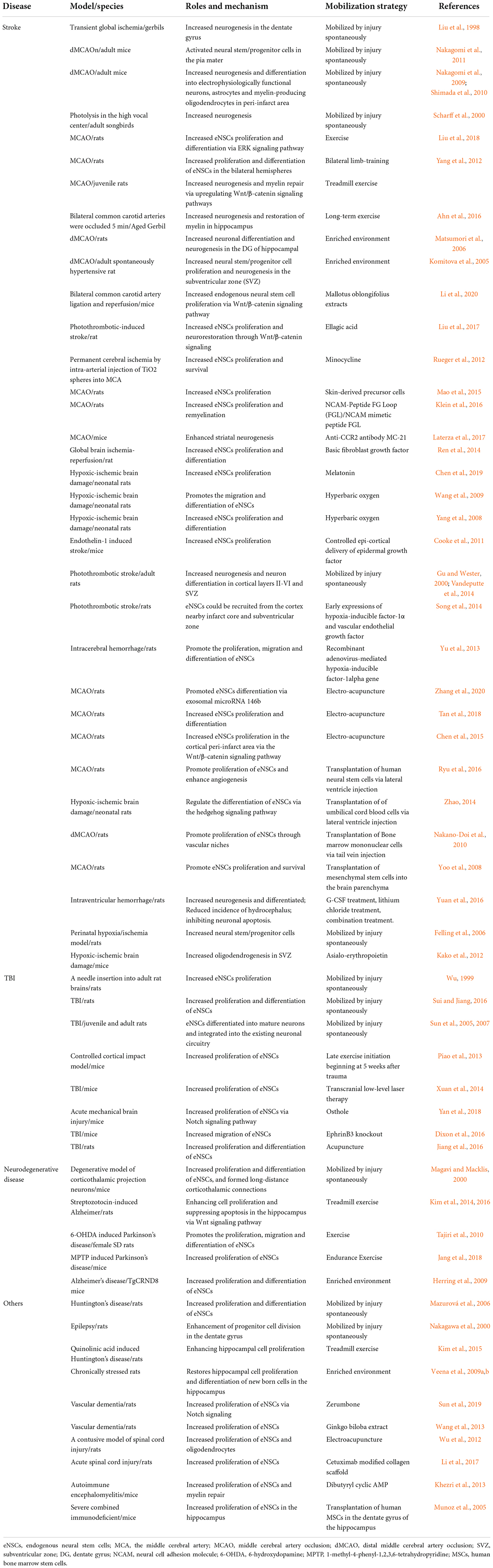
Table 1. The roles, mechanism, and mobilization strategy of endogenous neural stem cells in brain injury.
Mobilization strategies and mechanisms of endogenous neural stem cells for the repair of brain injury
Physical exercise
Physical exercise is the therapeutic schedule commonly used for the treatment of brain injury (Liang et al., 2021). In cerebral ischemia, physical exercise was reported to enhance the proliferation and differentiation of eNSCs in the hippocampus via the ERK signaling pathway in a rat model of cerebral infarction (Liu et al., 2018). In a Wistar rat model induced by middle cerebral artery occlusion, bilateral limb-training was observed to improve the proliferation and differentiation of eNSCs in the bilateral hemispheres and accelerate the recovery of neurologic function (Yang et al., 2012). The findings from the group of Ji Hyeon Ahn showed that long-term exercise improved memory impairment through enhanced neurogenesis, restoration of myelin and microvessel damage, and decreased synaptic adhesion molecule induced by a stroke in the aged gerbil hippocampus (Ahn et al., 2016, 2018). The undergoing mechanism of neurogenesis and myelin repair involved activated Wnt/β-catenin signaling pathways and upregulated BDNF (Alcantara et al., 2018; Cheng et al., 2020). In the injury of TBI, physical exercise enhanced the generation of new neurons by activating the eNSCs in the hippocampus and promoted cognitive recovery (Piao et al., 2013). Similarly, physical exercise ameliorated the learning and memory impairment induced by Alzheimer’s disease through increasing cell proliferation and suppressing apoptosis in the DG (Azimi et al., 2018; Jahangiri and Hosseini, 2019). In Parkinson’s Disease, physical exercise induced neurogenesis and increased the TH-immunopositive neurons in SN, and attenuated the loss of dopaminergic neurons (Tajiri et al., 2010; Petzinger et al., 2013; Jang et al., 2018; Palasz et al., 2019). Using streptozotocin-induced Alzheimer model, Kim et al. (2014, 2016) found that postnatal treadmill exercise enhanced cell proliferation and suppressed apoptosis in the hippocampus through Wnt signaling pathway. In addition, physical therapy, such as low-level laser therapy and deep brain stimulation (DBS), provided the neuroprotective effects through enhancing neurogenesis in brain injury (Xuan et al., 2014; Tsai et al., 2019).
Enriching environments
Enriching environments (EE) that consists of physical, social and sensory stimuli are effective treatment measures for the normal and injured brain through promoting neurogenesis and plasticity (Hannan, 2014). There is plenty of evidence that EE improved spatial learning ability in neurological disease (Olson et al., 2006; Gelfo et al., 2011; Kempermann, 2019). EE induces various molecular and cellular changes in the hippocampus, including upregulated the expression of neurotrophic factors, enhanced neurogenesis, and synaptic plasticity (Pham et al., 2002; Bekinschtein et al., 2011). The disturbed neurogenesis induced by cerebral ischemia has been restored by EE, and the density of NeuN positive cells has been enhanced (Matsumori et al., 2006). In rats with stroke, EE promoted neural stem/progenitor cell proliferation in the SVZ and ameliorated the impairment in spatial learning and memory, and passive avoidance memory and long-term potentiation (LTP) (Komitova et al., 2005; Ahmadalipour et al., 2017; Wang et al., 2019). EE increased the number of newborn mature hippocampal neurons and upregulated the expression of multiple plasticity associated molecules compared to the standard housed control mice in transgenic mice with Alzheimer-like pathology (Herring et al., 2009). The results from David and Lisa group respectively showed that EE improved cognitive impairment in AD transgenic mice through a dual mechanism in both amyloid dependence and independence (Costa et al., 2007; Robison et al., 2020). Exposure to an enriched environment ameliorated depressive symptoms by promoting the survival and differentiation of eNSCs in the hippocampus in chronically stressed rats (Veena et al., 2009a,b). Despite EE has been demonstrated the efficacy in the treatment of brain injury through enhancing neurogenesis and plasticity, there was still a long way to apply to the clinical setting because of the lack of standards (McDonald et al., 2018).
Small molecule drugs and herbal extract
There are many exogenous small molecule drugs reported to be capable of triggering the proliferation of eNSCs (Wu et al., 2017). Zerumbone is a sesquiterpenoid and cyclic ketone, which is obtained by steam distillation from Zingiber zerumbet Smith, a type of edible ginger found in southeast Asia particularly (Pubchem, 2022). 50 mg/kg and 100 mg/kg intraperitoneally Zerumbone was reported to stimulate the proliferation of neural stem cells in rats by regulating the Notch signaling (Sun et al., 2019). Osthole was found to promote eNSC proliferation and improve neurological function via the Notch signaling pathway in mice with acute mechanical brain injury (Yan et al., 2018). In a mouse model of ischemic injuries induced by bilateral common carotid artery ligation, mallotus oblongifolius was identified to improve the morphology of neurons, resist the loss of neurons, and enhance the content of the nestin protein in the cerebral cortex and subgranular zone area (Li et al., 2020). In vitro, mallotus oblongifolius was observed to activate the Wnt/β-catenin signaling pathway, increase the proliferation of neural stem cells, and enhance the protein expression levels of β-catenin and CyclinD1 in the oxygen-glucose deprivation and reperfusion (OGD/R) treated cell model. EGb761, one of the ginkgo biloba extracts, enhanced the proliferation of neural stem cells in the subventricular zone and DG in a rat model of vascular dementia (Wang and Wang, 2013). In a photothrombotic-induced rate model of brain injury, administration of polyphenol ellagic acid could improve brain injury outcomes and increase the proliferation of neural stem cells through the Wnt/β-catenin signaling pathway (Liu et al., 2017). Cetuximab is an EGFR signaling antagonist, which was found to significantly promote neurogenesis in the lesion site of a rat model of severe spinal cord injury (SCI). On the other hand, it was also indicated that implanted cetuximab modified linear ordered collagen scaffolds (LOCS) into SCI lesion sites in dogs leading to neuronal regeneration including neuronal differentiation, maturation, myelination, and synapse formation (Li et al., 2017). Aromatic-turmerone derived from Curcuma longa, which is an herb of the Zingiberaceae family, was reported to promote the mobilization of endogenous stem cells resident in the nervous system (Androutsellis-Theotokis, 2014). Minocycline was observed to increase eNSCs in both the SVZ as well as the hippocampus in a rat stroke model. In addition, minocycline showed positive effects on eNSC survival (Rueger et al., 2012). Hyperbaric oxygen (HBO) showed the capacity to improve the proliferation of neural stem cells in the SVZ and DG, promote the eNSCs to migrate to the cortex and differentiate into mature neurocytes in neonatal rats with hypoxic-ischemic brain damage (HIBD) (Yang et al., 2008; Wang et al., 2009), which was correlated with the activation of Wnt signaling (Wang et al., 2007).
Cytokines
Some cytokines are also reported to promote the proliferation of eNSCs. The repressor element-1 silencing transcription factor (REST), polycomb group (PcG) proteins and other chromatin remodeling factors play important roles in neural stem and progenitor cell biology, and can be applied to potentially promote eNSCs- mediated brain repair (Mehler, 2011). The anti-CCR2 antibody MC-21 was used to deplete circulating monocytes during the first week after stroke, which enhanced striatal neurogenesis 1 week later, probably by means of improving the short-term survival of the newly formed neuroblasts in the subventricular zone and close to the striatum in a mouse model (Laterza et al., 2017). After subcutaneously injected on cellular compartments affected by degeneration and regeneration after stroke, the FG loop (FGL) peptide profoundly promoted the mobilization of eNSCs in the neurogenic niches and triggered remyelination (Klein et al., 2016). The chitosan-neurotrophic factor 3 was applied to bridge the transected spinal cord and release neurotrophic factor 3 slowly to provide a favorable microenvironment for neural stem cell activation and migration (De Filippis and Pang, 2015). It was indicated that the slow release of neurotrophic factor 3 by chitosan promoted physiological repair processes in SCI in an animal model, implying NT3 contributed to the generation of neurons. Skin-derived precursor cells could secrete basic fibroblast growth factor (bFGF) and vascular endothelial growth factor (VEGF) in the ischemic region and further significantly promote the proliferation of endogenous nestin (+) and βIII-tubulin (+) neural stem cells in cerebral ischemia rats (Mao et al., 2015). Increased expression of epidermal growth factor (EGF) and bFGF after human cerebral infarction probably led to endogenous reparation and it correlated with the proliferation of eNSCs in humans (Duan et al., 2008). In a rat model of the cerebral cortex with global brain ischemia-reperfusion, basic fibroblast growth factor (bFGF) could be used to promote and extend the proliferation of eNSCs in situ, as well as to promote the differentiation of eNSCs into neurons (Ren et al., 2014). Controlled epi-cortical delivery of EGF was used to stimulate eNSCs proliferation in a mouse model of stroke (Cooke et al., 2011). The non-erythropoietic derivative (asialo-erythropoietin) had been confirmed to promote the maturation of SVZ-derived oligodendrocyte progenitor cells and improve neurological function in a Hypoxic-ischemic brain damage mice (Kako et al., 2012). In the rats with hypoxic-ischemic brain damage, single-dose immediate melatonin treatment and 7-day continuous melatonin treatment could improved the proliferation of eNSCs and ameliorated long-term histological injury in the brain of neonatal (Chen et al., 2019). In fetal Sprague-Dawley rats, neural stem cells derived from hippocampi were treated with ciliary neurotrophic factor for 7 days, which showed an increased number of microtubule associated protein-2-positive cells and decreased number of glial fibrillary acidic protein-positive cells (Zhang et al., 2016). The administration of intraventricular growth factors promoted eNSCs mobilization (Nakatomi and Saito, 2016). Using an ephrinB3 knockout mice model, Dixon et al. (2016) found that ephrinB3 expression in tissues surrounding neurogenic regions functioned to restrict neuroblast migration outside the rostral migratory stream (RMS) by limiting chain migration. The early expressions of hypoxia-inducible factor-1α (HIF-1α) and VEGF were observed to promote the proliferation, migration and differentiation of eNSCs in a rat stroke and ICH model (Yu et al., 2013; Song et al., 2014). Dibutyryl cyclic AMP (dbcAMP) might serve as a potential treatment option for inducing myelin repair in the context of demyelinating diseases like multiple sclerosis partially because of the eNSCs and their increased recruitment (Khezri et al., 2013).
Acupuncture and moxibustion
In addition, electro-acupuncture was identified to promote the differentiation of eNSCs. Electro-acupuncture was reported to promote the differentiation of eNSCs via exosomal microRNA 146b after ischemic stroke in rats (Zhang et al., 2020). Electro-acupuncture also promoted the proliferation and differentiation of eNSCs probably via modulating PRG5/RhoA signaling (Tan et al., 2018). In a rat model of SCI at spinal segment T8-9, the electroacupuncture of acupoints Huantiao (GB30) and Huatuojiaji (Ex-B05) significantly increased the numbers of BrdU(+)/NG2(+) cells at spinal cord tissue 15 mm away from the injury center in the rostral and caudal directions (Wu et al., 2012). Acupuncture was reported to induce the proliferation and differentiation of eNSCs, therefore neural repair was promoted in traumatic brain injury rats (Jiang et al., 2016). In a raumatic brain model established by Feeney’s free fall epidural impact method, the acupuncture at acupoints (Baihui, Shuigou, Fengfu, Yamen, and bilateral Hegu) increased significantly the number of nestin-expressing cells (Nestin+), bromodeoxyuridine/glial fibrillary acidic protein (BrdU+/GFAP+), BrdU/S100 calcium-binding protein B, BrdU/microtubule-associated protein 2- and BrdU/galactocerebrosidase-positive cells (Jiang et al., 2016). The results from Chen Bin et al. indicated that electro-acupuncture significantly improved neurological deficits, reduced the infarct volume and enhanced NPC proliferation through the Wnt β-catenin signaling pathway (Chen et al., 2015).
Transplantation of exogenous cells
Transplantation of exogenous cells was found to improve the proliferation of eNSCs. After transplanted into the subventricular zone, human neural stem cells in the brain of a rat model of focal cerebral ischemia triggered the proliferation of eNSCs and their differentiation into mature neural-like cells, and improved angiogenesis (Ryu et al., 2016). Umbilical cord blood mononuclear cells (UCBMC) were reported to probably promote eNSCs proliferation, ameliorate brain injury, and reduce glial differentiation in hypoxia-ischemia neonatal rats through the Sonic hedgehog (Shh) signaling pathway (Zhao, 2014). Administration of bone marrow mononuclear cells (BMMCs) could contribute to the proliferation of endogenous ischemia-induced neural stem/progenitor cells through vascular niche regulation in a mouse model of cortical infarction, which includes regulation of endothelial proliferation (Nakano-Doi et al., 2010). Bone marrow-derived mesenchymal stem cells (MSCs) were transplanted into the brain parenchyma 3 days after induction of stroke by occluding the middle cerebral artery for 2 h, which promoted the proliferation of eNSCs and suppressed the cell death of newly generated cells. The newborn cells were observed to migrate toward ischemic territory and differentiate in ischemic boundaries into doublecortin + neuroblasts at higher rates in animals with mesenchymal stem cells (MSCs) compared to the control group, partly because MSCs had properties of enhancing endogenous neurogenesis and protecting newborn cells from the deleterious environment (Yoo et al., 2008). The implanted human MSCs significantly promoted the proliferation of eNSCs which expressed the stem cell marker Sox2 (Munoz et al., 2005) (see Table 1 in detail).
Negative factors in endogenous neural stem cells mobilization
There are also some factors reported to curb the proliferation of eNSCs. Gli1 is a gene that encodes a member of the Kruppel family of zinc finger proteins. The encoded transcription factor is activated by the sonic hedgehog signal transduction cascade and regulates stem cell proliferation (NCBI, 2021). eNSCs can be mobilized for the repair of demyelinated lesions by inhibiting Gli1 (Samanta et al., 2015). Methylprednisolone was found to inhibit the proliferation of eNSCs in cynomolgus monkeys with SCI (Ye et al., 2018). Chronic alcohol consumption have a negative impact on the survival of neural stem cells in the subventricular zone and altered neural stem cell differentiation in the subventricular zone, subgranular zone, and tanycyte layer in an inducible transgenic mouse model (McGrath et al., 2017). The cytosine arabinoside inhibited the activation of eNSCs (Zhang et al., 2016). eNSCs were observed the proliferation and differentiation in a mild SCI in rats, while the ability to differentiate into neurons is limited partly because of the high expression of inhibitory Notch1 and Hes1 genes after injury (Liu et al., 2015).
Summary and outlook for endogenous neural stem cells in the repair of brain injury
Neural stem cells have proven strikingly valuable to the repair in brain injury. Although exogenous neural stem cells are a potential strategy for repairing brain injuries, it is not popular because of tumorigenic, immunological, and ethical problems. As a result, eNSCs appear to be more practical in brain repair. However, there are still lots of unsolved difficulties which limit the maximum therapeutic effect of eNSCs. Firstly, although the brain injury and some exogenous medicines can trigger neurogenesis in SVZ and hippocampus DG, even some of them can migrate to the site of ischemic area, most of them die during the next few weeks after stroke. Thus we need to explore some strategies to enhance the number of surviving neurons, increased cell survival rate. Secondly, in addition to the role of nutrient factor secretion, the differentiation of eNSCs into mature neurons is more important for repair of brain injury. The new-formed neurons can replace the injured neurons and repair the disrupted neural network. However, at present the mechanisms that affected differentiation of eNSCs is not understood clearly and the effective promotion strategies is still lack. Finally, a single treatment strategy may not be enough to maximize the effect of eNSCs. Some strategies are better at promoting eNSCs proliferation, while others are better at promoting eNSCs migration or differentiation. The comprehensive treatment program that integrated small drugs, neurotrophic factor and growth factor, rehabilitation, electro-acupuncture, and so on may lead to a better outcomes. Thus we need to further investigate the optimal combination of treatment options and the optimum application time window of every option.
Author contributions
PZ and KY developed the idea and revised the manuscript. QH, WL, YY, YJ, and DW reviewed the literature and prepared the tables. HL and TW prepared and revised the manuscript. All authors have read and approved the final manuscript.
Funding
This study was supported by the National Natural Science Foundation of China (81960731 and 81860878), Yunnan Province biological medicine major special project (202102AA100016), Joint Special Project of Traditional Chinese Medicine in Science and Technology Department of Yunnan Province [2019FF002(-008) and 202001AZ070001-002 and 030], and Yunnan Province University Innovation Team Projects (Acupuncture prevents Mental disease: 2019YGC04).
Conflict of interest
The authors declare that the research was conducted in the absence of any commercial or financial relationships that could be construed as a potential conflict of interest.
Publisher’s note
All claims expressed in this article are solely those of the authors and do not necessarily represent those of their affiliated organizations, or those of the publisher, the editors and the reviewers. Any product that may be evaluated in this article, or claim that may be made by its manufacturer, is not guaranteed or endorsed by the publisher.
References
Aertker, B. M., and Cox, C. S. Jr. (2016). Strategies for CNS repair following TBI. Exp. Neurol. 275, 411–426. doi: 10.1016/j.expneurol.2015.01.008
Ahmadalipour, A., Sadeghzadeh, J., and Rashidy-Pour, A. (2017). Protective Effects of Enriched Environment Against Transient Cerebral Ischemia-Induced Impairment of Passive Avoidance Memory and Long-Term Potentiation in Rats. Basic Clin. Neurosci. 8, 443–452. doi: 10.29252/nirp.bcn.8.6.443
Ahn, J. H., Choi, J. H., Park, J. H., Kim, I. H., Cho, J. H., Lee, J. C., et al. (2016). Long-Term Exercise Improves Memory Deficits via Restoration of Myelin and Microvessel Damage, and Enhancement of Neurogenesis in the Aged Gerbil Hippocampus After Ischemic Stroke. Neurorehabil. Neural Repair 30, 894–905. doi: 10.1177/1545968316638444
Ahn, J. H., Park, J. H., Park, J., Shin, M. C., Cho, J. H., Kim, I. H., et al. (2018). Long-term treadmill exercise improves memory impairment through restoration of decreased synaptic adhesion molecule 1/2/3 induced by transient cerebral ischemia in the aged gerbil hippocampus. Exp. Gerontol. 103, 124–131. doi: 10.1016/j.exger.2018.01.015
Alcantara, C. C., García-Salazar, L. F., Silva-Couto, M. A., Santos, G. L., and Russo, T. L. (2018). Post-stroke BDNF Concentration Changes Following Physical Exercise: A Systematic Review. Front. Neurol. 9:637. doi: 10.3389/fneur.2018.00637
Androutsellis-Theotokis, A. (2014). Spicing up endogenous neural stem cells: aromatic-turmerone offers new possibilities for tackling neurodegeneration. Stem Cell Res. Ther. 5:127. doi: 10.1186/scrt517
Azimi, M., Gharakhanlou, R., Naghdi, N., and Heysieattalab, S. (2018). Moderate treadmill exercise ameliorates amyloid-β-induced learning and memory impairment, possibly via increasing AMPK activity and up-regulation of the PGC-1α/FNDC5/BDNF pathway. Peptides 102, 78–88. doi: 10.1016/j.peptides.2017.12.027
Baker, E. W., and West, F. D. (2019). Neural stem cell therapy for stroke: A multimechanistic approach to restoring neurological function. Brain Behav. 9:e01214. doi: 10.1002/brb3.1214
Balami, J. S., Chen, R. L., and Buchan, A. M. (2011). Neurological complications of acute ischaemic stroke. Lancet Neurol. 10, 357–371. doi: 10.1016/S1474-4422(10)70313-6
Bekinschtein, P., Oomen, C. A., and Bussey, T. J. (2011). Effects of environmental enrichment and voluntary exercise on neurogenesis, learning and memory, and pattern separation: BDNF as a critical variable? Semin. Cell Dev. Biol. 22, 536–542. doi: 10.1016/j.semcdb.2011.07.002
Bhattacharjee, S., Rakesh, D., Ramnadha, R., and Manas, P. (2021). Subarachnoid Hemorrhage and Hydrocephalus. Neurol. Ind. 69, S429–S433. doi: 10.4103/0028-3886.332266
Carpenter, A. M., Singh, I. P., Gandhi, C. D., and Prestigiacomo, C. J. (2016). Genetic risk factors for spontaneous intracerebral haemorrhage. Nat. Rev. Neurol. 12, 40–49. doi: 10.1038/nrneurol.2015.226
Ceanga, M., Dahab, M., Witte, O. W., and Keiner, S. (2021). Adult Neurogenesis and Stroke: A Tale of Two Neurogenic Niches. Front. Neurosci. 15:700297. doi: 10.3389/fnins.2021.700297
Centers for Disease Control and Prevention (CDC), and National Center for Injury Prevention and Control. (2003). Report to Congress on Mild Traumatic Brain Injury in the United States; Steps to Prevent a Serious Public Health Problem. Available online at https://stacks.cdc.gov/view/cdc/6544.(accessed on Apr 7 2022).
Chen, B., Tao, J., Lin, Y., Lin, R., Liu, W., and Chen, L. (2015). Electro-acupuncture exerts beneficial effects against cerebral ischemia and promotes the proliferation of neural progenitor cells in the cortical peri-infarct area through the Wnt/β-catenin signaling pathway. Int. J. Mol. Med. 36, 1215–1222. doi: 10.3892/ijmm.2015.2334
Chen, W., Chen, L. F., Zhang, M. B., Xia, Y. P., Zhao, Y. H., Li, G. Z., et al. (2019). Effects of different melatonin treatment regimens on the proliferation of endogenous neural stem cells in neonatal rats with hypoxic-ischemic brain damage. Zhongguo Dang Dai Er Ke Za Zhi 21, 830–835.
Cheng, J., Shen, W., Jin, L., Pan, J., Zhou, Y., Pan, G., et al. (2020). Treadmill exercise promotes neurogenesis and myelin repair via upregulating Wnt/β−catenin signaling pathways in the juvenile brain following focal cerebral ischemia/reperfusion. Int. J. Mol. Med. 45, 1447–1463. doi: 10.3892/ijmm.2020.4515
Cooke, M. J., Wang, Y., and Shoichet, M. S. (2011). Controlled epi-cortical delivery of epidermal growth factor for the stimulation of endogenous neural stem cell proliferation in stroke-injured brain. Biomaterials 32, 5688–5697. doi: 10.1016/j.biomaterials.2011.04.032
Costa, D. A., Cracchiolo, J. R., Bachstetter, A. D., Hughes, T. F., Bales, K. R., Paul, S. M., et al. (2007). Enrichment improves cognition in AD mice by amyloid-related and unrelated mechanisms. Neurobiol. Aging 28, 831–844. doi: 10.1016/j.neurobiolaging.2006.04.009
De Filippis, L., and Pang, Z. P. (2015). Harness the power of endogenous neural stem cells by biomaterials to treat spinal cord injury. Sci. Chin. Life Sci. 58, 1167–1168. doi: 10.1007/s11427-015-4943-z
Diaz-Arrastia, R. R. (2015). Diagnosis, prognosis, and clinical management of mild traumatic brain injury. Lancet Neurol. 14, 506–517. doi: 10.1016/S1474-4422(15)00002-2
Dixon, K. J., Mier, J., Gajavelli, S., Turbic, A., Bullock, R., Turnley, A. M., et al. (2016). EphrinB3 restricts endogenous neural stem cell migration after traumatic brain injury. Stem Cell Res. 17, 504–513. doi: 10.1016/j.scr.2016.09.029
Duan, S. R., Wang, H. H., Qi, J. P., Teng, W. L., Xu, R., Wang, D. S., et al. (2008). Influences of bFGF and EGF on the proliferation and differentiation of endogenous neural stem cells after cerebral infarction in human. Zhonghua Yi Xue Za Zhi 88, 3337–3341.
Fang, C. Y., Wu, X. S., Zhang, H., Gu, Y. L., Wang, S. B., Ren, H. W., et al. (2019). Research advances in endogenous neural stem cells promoting neural repair after ischemic stroke. Sheng Li Xue Bao 71, 454–462.
Fann, J. R., Ribe, A. R., Pedersen, H. S., Fenger-Grøn, M., Christensen, J., Benros, M. E., et al. (2018). Long-term risk of dementia among people with traumatic brain injury in Denmark: a population-based observational cohort study. Lancet Psychiatry 5, 424–431. doi: 10.1016/S2215-0366(18)30065-8
Felling, R. J., Snyder, M. J., Romanko, M. J., Rothstein, R. P., Ziegler, A. N., Yang, Z., et al. (2006). Neural stem/progenitor cells participate in the regenerative response to perinatal hypoxia/ischemia. J. Neurosci. 26, 4359–4369. doi: 10.1523/JNEUROSCI.1898-05.2006
Gelfo, F., Cutuli, D., Foti, F., Laricchiuta, D., De Bartolo, P., Caltagirone, C., et al. (2011). Enriched environment improves motor function and increases neurotrophins in hemicerebellar lesioned rats. Neurorehabil. Neural Repair 25, 243–252. doi: 10.1177/1545968310380926
Ghelani, D. P., Kim, H. A., Zhang, S. R., Drummond, G. R., Sobey, C. G., and De Silva, T. M. (2021). Ischemic stroke and infection: A brief update on mechanisms and potential therapies. Biochem. Pharmacol. 193:114768. doi: 10.1016/j.bcp.2021.114768
Gu, W., and Wester, P. (2000). Cortical neurogenesis in adult rats after reversible photothrombotic stroke. J. Cereb. Blood Flow Metab. 20, 1166–1173. doi: 10.1097/00004647-200008000-00002
Haarbauer-Krupa, J., Pugh, M. J., Prager, E. M., Harmon, N., Wolfe, J., and Yaffe, K. (2021). Epidemiology of Chronic Effects of Traumatic Brain Injury. J. Neurotrauma 38, 3235–3247. doi: 10.1089/neu.2021.0062
Hamblin, M. H., and Lee, J. P. (2021). Neural Stem Cells for Early Ischemic Stroke. Int. J. Mol. Sci. 22:7703. doi: 10.3390/ijms22147703
Hannan, A. J. (2014). Environmental enrichment and brain repair: harnessing the therapeutic effects of cognitive stimulation and physical activity to enhance experience-dependent plasticity. Neuropathol. Appl. Neurobiol. 40, 13–25. doi: 10.1111/nan.12102
Herring, A., Ambrée, O., Tomm, M., Habermann, H., Sachser, N., Paulus, W., et al. (2009). Environmental enrichment enhances cellular plasticity in transgenic mice with Alzheimer-like pathology. Exp. Neurol. 216, 184–192. doi: 10.1016/j.expneurol.2008.11.027
Jahangiri, Z., and Hosseini, M. (2019). Neuroprotective effects of exercise in rodent models of memory deficit and Alzheimer’s. Metab. Brain Dis. 34, 21–37. doi: 10.1007/s11011-018-0343-y
Jang, Y., Kwon, I., Song, W., and Lee, Y. (2018). Endurance Exercise Mediates Neuroprotection Against MPTP-mediated Parkinson’s Disease via Enhanced Neurogenesis. Antioxidant Capacity, and Autophagy. Neuroscience 379, 292–301. doi: 10.1016/j.neuroscience.2018.03.015
Jiang, S., Chen, W., Zhang, Y., Chen, A., Dai, Q., Lin, S., et al. (2016). Acupuncture Induces the Proliferation and Differentiation of Endogenous Neural Stem Cells in Rats with Traumatic Brain Injury. Evid. Based Complement Alternat. Med. 2016:2047412. doi: 10.1155/2016/2047412
Jinnou, H. (2021). Regeneration using endogenous neural stem cells following neonatal brain injury. Pediatr. Int. 63, 13–21. doi: 10.1111/ped.14368
Kako, E., Kaneko, N., Aoyama, M., Hida, H., Takebayashi, H., et al. (2012). Subventricular zone-derived oligodendrogenesis in injured neonatal white matter in mice enhanced by a nonerythropoietic erythropoietin derivative. Stem cells 30, 2234–2247. doi: 10.1002/stem.1202
Kaneko, N., and Sawamoto, K. (2013). Enhancement of ventricular-subventricular zone-derived neurogenesis and oligodendrogenesis by erythropoietin and its derivatives. Front. Cell Neurosci. 7:235. doi: 10.3389/fncel.2013.00235
Kempermann, G. (2019). Environmental enrichment, new neurons and the neurobiology of individuality. Nat. Rev. Neurosci. 20, 235–245. doi: 10.1038/s41583-019-0120-x
Khezri, S., Javan, M., Goudarzvand, M., and Baharvand, H. (2013). Dibutyryl cyclic AMP inhibits the progression of experimental autoimmune encephalomyelitis and potentiates recruitment of endogenous neural stem cells. J. Mol. Neurosci. 51, 298–306. doi: 10.1007/s12031-013-9959-x
Kim, D. Y., Jung, S. Y., Kim, K., and Kim, C. J. (2016). Treadmill exercise ameliorates Alzheimer disease-associated memory loss through the Wnt signaling pathway in the streptozotocin-induced diabetic rats. J. Exerc. Rehabil. 12, 276–283. doi: 10.12965/jer.1632678.339
Kim, Y. H., Sung, Y. H., Lee, H. H., Ko, I. G., Kim, S. E., Shin, M. S., et al. (2014). Postnatal treadmill exercise alleviates short-term memory impairment by enhancing cell proliferation and suppressing apoptosis in the hippocampus of rat pups born to diabetic rats. J. Exerc. Rehabil. 10, 209–217. doi: 10.12965/jer.140145
Kim, Y. M., Ji, E. S., Kim, S. H., Kim, T. W., Ko, I. G., Jin, J. J., et al. (2015). Treadmill exercise improves short-term memory by enhancing hippocampal cell proliferation in quinolinic acid-induced Huntington’s disease rats. J. Exerc. Rehabil. 11, 5–11. doi: 10.12965/jer.150182
Klein, R., Mahlberg, N., Ohren, M., Ladwig, A., Neumaier, B., Graf, R., et al. (2016). The Neural Cell Adhesion Molecule-Derived (NCAM)-Peptide FG Loop (FGL) Mobilizes Endogenous Neural Stem Cells and Promotes Endogenous Regenerative Capacity after Stroke. J. Neuroimmun. Pharmacol. 11, 708–720. doi: 10.1007/s11481-016-9694-5
Komitova, M., Mattsson, B., and Eriksson, P. S. (2005). Enriched environment increases neural stem/progenitor cell proliferation and neurogenesis in the subventricular zone of stroke-lesioned adult rats. Stroke 36, 1278–1282. doi: 10.1161/01.STR.0000166197.94147.59
Lamm, O., Ganz, J., and Offen, D. (2014). Harnessing neurogenesis for the possible treatment of Parkinson’s disease. J. Comp. Neurol. 522, 2817–2830. doi: 10.1002/cne.23607
Larivière, S., Vos, de Wael, R., Paquola, C., Hong, S. J., Mišić, B., et al. (2019). Microstructure-Informed Connectomics: Enriching Large-Scale Descriptions of Healthy and Diseased Brains. Brain Connect. 9, 113–127. doi: 10.1089/brain.2018.0587
Laterza, C., Wattananit, S., Uoshima, N., Ge, R., Pekny, R., Tornero, D., et al. (2017). Monocyte depletion early after stroke promotes neurogenesis from endogenous neural stem cells in adult brain. Exp. Neurol. 297, 129–137. doi: 10.1016/j.expneurol.2017.07.012
Levison, S. W. (2018). Pediatric brain repair from endogenous neural stem cells of the subventricular zone. Pediatr. Res. 83, 385–396. doi: 10.1038/pr.2017.261
Li, S. R., Song, Y. J., Deng, R., Li, X. W., Cheng, Y., Zhang, Z. Q., et al. (2020). Mallotus oblongifolius extracts ameliorate ischemic nerve damage by increasing endogenous neural stem cell proliferation through the Wnt/β-catenin signaling pathway. Food Funct. 11, 1027–1036. doi: 10.1039/C9FO01790A
Li, X., Zhao, Y., Cheng, S., Han, S., Shu, M., Chen, B., et al. (2017). Cetuximab modified collagen scaffold directs neurogenesis of injury-activated endogenous neural stem cells for acute spinal cord injury repair. Biomaterials 137, 73–86. doi: 10.1016/j.biomaterials.2017.05.027
Liang, J., Wang, H., Zeng, Y., Qu, Y., Liu, Q., Zhao, F., et al. (2021). Physical exercise promotes brain remodeling by regulating epigenetics, neuroplasticity and neurotrophins. Rev. Neurosci. 32, 615–629. doi: 10.1515/revneuro-2020-0099
Liu, J., Solway, K., and Sharp, F. R. (1998). Increased neurogenesis in the dentate gyrus after transient global ischemia in gerbils. J. Neurosci. 18, 7768–7778. doi: 10.1523/JNEUROSCI.18-19-07768.1998
Liu, Q. S., Li, S. R., Li, K., Li, X., and Pang, Z. (2017). Ellagic acid improves endogenous neural stem cells proliferation and neurorestoration through Wnt/β-catenin signaling in vivo and in vitro. Mol. Nutr. Food Res. 61:1600587. doi: 10.1002/mnfr.201600587
Liu, W., Wu, W., Lin, G., Cheng, J., and Shi, Y. (2018). Physical exercise promotes proliferation and differentiation of endogenous neural stem cells via ERK in rats with cerebral infarction. Mol. Med. Rep. 18, 1455–1464. doi: 10.3892/mmr.2018.9147
Liu, X., Fan, B., and Zhang, Z. (2020). Epigenetic Mechanisms Underlying Adult Post Stroke Neurogenesis. Int. J. Mol. Sci. 21:6179. doi: 10.3390/ijms21176179
Liu, X. S., Chopp, M., and Zhang, Z. G. (2013). MicroRNAs in cerebral ischemia-induced neurogenesis. J. Neuropathol. Exp. Neurol. 72, 718–722. doi: 10.1097/NEN.0b013e31829e4963
Liu, Y., Tan, B., Wang, L., Long, Z., Li, Y., Liao, W., et al. (2015). Endogenous neural stem cells in central canal of adult rats acquired limited ability to differentiate into neurons following mild spinal cord injury. Int. J. Clin. Exp. Pathol. 8, 3835–3842.
Lladó, J., Haenggeli, C., Maragakis, N. J., and Rothstein, J. D. (2004). Neural stem cells protect against glutamate-induced excitotoxicity and promote survival of injured motor neurons through the secretion of neurotrophic factors. Mol. Cell Neurosci. 27, 322–331. doi: 10.1016/j.mcn.2004.07.010
Lu, P., Jones, L. L., and Tuszynski, M. H. (2003). Neural stem cells constitutively secrete neurotrophic factors and promote extensive host axonal growth after spinal cord injury. Exp. Neurol. 181, 115–129. doi: 10.1016/S0014-4886(03)00037-2
Macklis, J. D. (2001). Manipulation of neural precursors in situ: induction of neurogenesis in the neocortex of adult mice. Neuropsychopharmacology 25, 816–835. doi: 10.1016/S0893-133X(01)00357-8
Magavi, S. S., and Macklis, J. D. (2000). Induction of neurogenesis in the neocortex of adult mice. Nature 405, 951–955. doi: 10.1038/35016083
Magid-Bernstein, J., Girard, R., Polster, S., Srinath, A., Romanos, S., Awad, I. A., et al. (2022). Cerebral Hemorrhage: Pathophysiology. Treatment, and Future Directions. Circ. Res. 130, 1204–1229. doi: 10.1161/CIRCRESAHA.121.319949
Mao, D., Yao, X., Feng, G., Yang, X., Mao, L., Wang, X., et al. (2015). Skin-derived precursor cells promote angiogenesis and stimulate proliferation of endogenous neural stem cells after cerebral infarction. Biomed. Res. Int. 2015:945846. doi: 10.1155/2015/945846
Matsumori, Y., Hong, S. M., Fan, Y., Kayama, T., Hsu, C. Y., Weinstein, P. R., et al. (2006). Enriched environment and spatial learning enhance hippocampal neurogenesis and salvages ischemic penumbra after focal cerebral ischemia. Neurobiol. Dis. 22, 187–198. doi: 10.1016/j.nbd.2005.10.015
Mazurová, Y., Rudolf, E., and Osterreicher, J. (2006). Proliferation and differentiation of adult endogenous neural stem cells in response to neurodegenerative process within the striatum. Neurodegener. Dis. 3, 12–18. doi: 10.1159/000092087
McDonald, M. W., Hayward, K. S., Rosbergen, I. C. M., and Corbett, D. (2018). Is Environmental Enrichment Ready for Clinical Application in Human Post-stroke Rehabilitation? Front. Behav. Neurosci. 12:135. doi: 10.3389/fnbeh.2018.00135
McGrath, E. L., Gao, J., Kuo, Y. F., Dunn, T. J., Ray, M. J., Dineley, K. T., et al. (2017). Spatial and Sex-Dependent Responses of Adult Endogenous Neural Stem Cells to Alcohol Consumption. Stem Cell Rep. 9, 1916–1930. doi: 10.1016/j.stemcr.2017.10.007
MedicinePlus. (2022). Parkinson’s Disease. Available online at https://medlineplus.gov/parkinsonsdisease.html.(accessed on Apr 14 2022).
Mehler, M. F. (2011). Chromatin-modifying agents for epigenetic reprogramming and endogenous neural stem cell-mediated repair in stroke. Transl. Stroke Res. 2, 7–16. doi: 10.1007/s12975-010-0051-3
Menéndez-González, M., Álvarez-Avellón, T., Salas-Pacheco, J. M., de Celis-Alonso, B., Wyman-Chick, K. A., and Arias-Carrión, O. (2018). Frontotemporal Lobe Degeneration as Origin of Scans Without Evidence of Dopaminergic Deficit. Front. Neurol. 9:335. doi: 10.3389/fneur.2018.00335
Miki, Y., Ling, H., Crampsie, S., Mummery, C. J., Rohrer, J. D., Jaunmuktane, Z., et al. (2020). Corticospinal tract degeneration and temporal lobe atrophy in frontotemporal lobar degeneration TDP-43 type C pathology. Neuropathol. Appl. Neurobiol. 46, 296–299. doi: 10.1111/nan.12582
Munoz, J. R., Stoutenger, B. R., Robinson, A. P., and Prockop, D. J. (2005). Human stem/progenitor cells from bone marrow promote neurogenesis of endogenous neural stem cells in the hippocampus of mice. Proc. Natl. Acad. Sci. U.S.A 102, 18171–18176. doi: 10.1073/pnas.0508945102
Nakagawa, E., Aimi, Y., Yasuhara, O., Tooyama, I., Shimada, M., McGeer, P. L., et al. (2000). Enhancement of progenitor cell division in the dentate gyrus triggered by initial limbic seizures in rat models of epilepsy. Epilepsia 41, 10–18. doi: 10.1111/j.1528-1157.2000.tb01498.x
Nakagomi, T., Molnár, Z., Nakano-Doi, A., Taguchi, A., Saino, O., Kubo, S., et al. (2011). Ischemia-induced neural stem/progenitor cells in the pia mater following cortical infarction. Stem Cells Dev. 20, 2037–2051. doi: 10.1089/scd.2011.0279
Nakagomi, T., Taguchi, A., Fujimori, Y., Saino, O., Nakano-Doi, A., Kubo, S., et al. (2009). Isolation and characterization of neural stem/progenitor cells from post-stroke cerebral cortex in mice. Eur. J. Neurosci. 29, 1842–1852. doi: 10.1111/j.1460-9568.2009.06732.x
Nakano-Doi, A., Nakagomi, T., Fujikawa, M., Nakagomi, N., Kubo, S., Lu, S., et al. (2010). Bone marrow mononuclear cells promote proliferation of endogenous neural stem cells through vascular niches after cerebral infarction. Stem Cells 28, 1292–1302. doi: 10.1002/stem.454
Nakatomi, H., and Saito, N. (2016). Endogenous neural stem cell mobilization with intraventricular growth factor administration. Nihon Rinsho 74, 655–660.
NCBI. (2021). GLI1 GLI family zinc finger 1. Available online at: https://www.ncbi.nlm.nih.gov/gtr/genes/2735/ (accessed July 26, 2022).
Olson, A. K., Eadie, B. D., and Christie, B. R. (2006). Environmental enrichment and voluntary exercise massively increase neurogenesis in the adult hippocampus via dissociable pathways. Hippocampus 16, 250–260. doi: 10.1002/hipo.20157
Ottoboni, L., von Wunster, B., and Martino, G. (2020). Therapeutic Plasticity of Neural Stem Cells. Front. Neurol. 11:148. doi: 10.3389/fneur.2020.00148
Palasz, E., Niewiadomski, W., Gasiorowska, A., Wysocka, A., and Niewiadomska, G. (2019). Exercise-Induced Neuroprotection and Recovery of Motor Function in Animal Models of Parkinson’s Disease. Front. Neurol. 10:1143. doi: 10.3389/fneur.2019.01143
Pereira, M., and Rylander Ottosson, D. (2019). Direct reprogramming into interneurons: potential for brain repair. Cell. Mol. Life Sci. 76, 3953–3967. doi: 10.1007/s00018-019-03193-3
Peterson, A. B., and Daugherty, J. (2019). Surveillance Report of Traumatic Brain Injury-Related Emergency Department Visits, Hospitalizations, and Deaths, United States, 2014. Available online at https://stacks.cdc.gov/view/cdc/78062.(accessed on Apr 7 2022).
Petzinger, G. M., Fisher, B. E., McEwen, S., Beeler, J. A., and Jakowec, M. W. (2013). Exercise-enhanced neuroplasticity targeting motor and cognitive circuitry in Parkinson’s disease. Lancet Neurol. 12, 716–726. doi: 10.1016/S1474-4422(13)70123-6
Pham, T. M., Winblad, B., and Mohammed, A. H. (2002). Environmental influences on brain neurotrophins in rats. Pharmacol. Biochem. Behav. 73, 167–175. doi: 10.1016/S0091-3057(02)00783-9
Piao, C. S., Stoica, B. A., Wu, J., Sabirzhanov, B., Zhao, Z., Cabatbat, R., et al. (2013). Late exercise reduces neuroinflammation and cognitive dysfunction after traumatic brain injury. Neurobiol. Dis. 54, 252–263. doi: 10.1016/j.nbd.2012.12.017
Poon, M. T., Bell, S. M., and Al-Shahi Salman, R. (2015). Epidemiology of Intracerebral Haemorrhage. Front. Neurol. Neurosci. 37, 1–12. doi: 10.1159/000437109
Prince, M., Bryce, R., Albanese, E., Wimo, A., and Ferri, C. P. (2013). The global prevalence of dementia: a systematic review and metaanalysis. Alzheimers Dement. 6:e62. doi: 10.1016/j.jalz.2012.11.007
Pubchem. (2022). Zerumbone. Available online at https://pubchem.ncbi.nlm.nih.gov/compound/Zerumbone (accessed July 26, 2022).
Rajasekhar, K., and Govindaraju, T. (2015). Function and toxicity of amyloid beta and recent therapeutic interventions targeting amyloid beta in Alzheimer’s disease. Chem. Commun. 51, 13434–13450. doi: 10.1039/C5CC05264E
Rajasekhar, K., and Govindaraju, T. (2018). Hybrid Multifunctional Modulators Inhibit Multifaceted Aβ Toxicity and Prevent Mitochondrial Damage. ACS Chem. Neurosci. 9, 1432–1440. doi: 10.1021/acschemneuro.8b00033
Reitz, C., and Mayeux, R. (2011). Epidemiology of Alzheimer disease. Nat. Rev. Neurol. 7, 137–152. doi: 10.1038/nrneurol.2011.2
Ren, M., Deng, X., Guo, Y., and Feng, Z. (2014). Effect of basic fibroblast growth factor on endogenous neural stem cell in rat cerebral cortex with global cerebral ischemia-reperfusion. Sheng Wu Yi Xue Gong Cheng Xue Za Zhi 31, 846–849.
Robison, L. S., Francis, N., Popescu, D. L., Anderson, M. E., Hatfield, J., Xu, F., et al. (2020). Environmental Enrichment: Disentangling the Influence of Novelty, Social, and Physical Activity on Cerebral Amyloid Angiopathy in a Transgenic Mouse Model. Int. J. Mol. Sci. 21:843. doi: 10.3390/ijms21030843
Romanko, M. J., Rola, R., Fike, J. R., Szele, F. G., Dizon, M. L., Felling, R. J., et al. (2004). Roles of the mammalian subventricular zone in cell replacement after brain injury. Prog. Neurobiol. 74, 77–99. doi: 10.1016/j.pneurobio.2004.07.001
Rueger, M. A., Muesken, S., Walberer, M., Jantzen, S. U., Schnakenburg, K., Backes, H., et al. (2012). Effects of minocycline on endogenous neural stem cells after experimental stroke. Neuroscience 215, 174–183. doi: 10.1016/j.neuroscience.2012.04.036
Ryu, S., Lee, S. H., and Yoon, B. W. (2016). Human neural stem cells promote proliferation of endogenous neural stem cells and enhance angiogenesis in ischemic rat brain. Neural Regen. Res. 11, 298–304. doi: 10.4103/1673-5374.177739
Samanta, J., Grund, E. M., Silva, H. M., Lafaille, J. J., and Salzer, J. L. (2015). Inhibition of Gli1 mobilizes endogenous neural stem cells for remyelination. Nature 526, 448–452. doi: 10.1038/nature14957
Scharff, C., Kirn, J. R., Grossman, M., and Nottebohm, F. (2000). Targeted neuronal death affects neuronal replacement and vocal behavior in adult songbirds. Neuron 25, 481–492. doi: 10.1016/S0896-6273(00)80910-1
Shimada, I. S., Peterson, B. M., and Spees, J. L. (2010). Isolation of locally derived stem/progenitor cells from the peri-infarct area that do not migrate from the lateral ventricle after cortical stroke. Stroke 41:e552–e560. doi: 10.1161/STROKEAHA.110.589010
Song, S., Park, J. T., Na, J. Y., Park, M. S., Lee, J. K., Lee, M. C., et al. (2014). Early expressions of hypoxia-inducible factor 1alpha and vascular endothelial growth factor increase the neuronal plasticity of activated endogenous neural stem cells after focal cerebral ischemia. Neural Regen. Res. 9, 912–918. doi: 10.4103/1673-5374.133136
Sui, L. S., and Jiang, X. D. (2016). [Proliferation and differentiation of endogenous neural stem cells in subventricular zone in rats after traumatic craniocerebral injury]. Nan Fang Yi Ke Da Xue Xue Bao 36, 1094–1099.
Sun, D., Colello, R. J., Daugherty, W. P., Kwon, T. H., McGinn, M. J., Harvey, H. B., et al. (2005). Cell proliferation and neuronal differentiation in the dentate gyrus in juvenile and adult rats following traumatic brain injury. J. Neurotrauma 22, 95–105. doi: 10.1089/neu.2005.22.95
Sun, D., McGinn, M. J., Zhou, Z., Harvey, H. B., and Colello, R. J. (2007). Anatomical integration of newly generated dentate granule neurons following traumatic brain injury in adult rats and its association to cognitive recovery. Exp. Neurol. 204, 264–272. doi: 10.1016/j.expneurol.2006.11.005
Sun, L., Li, M., and Li, X. (2019). Zerumbone promotes proliferation of endogenous neural stem cells in vascular dementia by regulating Notch signalling. Folia Neuropathol. 57, 277–284. doi: 10.5114/fn.2019.88458
Sung, P. S., Lin, P. Y., Liu, C. H., and Tsai, K. J. (2020). Neuroinflammation and Neurogenesis in Alzheimer’s Disease and Potential Therapeutic Approaches. Int. J. Mol. Sci. 21:701. doi: 10.3390/ijms21030701
Szelenberger, R., Kostka, J., and Miller, E. (2020). Pharmacological Interventions and Rehabilitation Approach for Enhancing Brain Self-repair and Stroke Recovery. Curr. Neuropharmacol. 18, 51–64. doi: 10.2174/1570159X17666190726104139
Tajiri, N., Yasuhara, T., Shingo, T., Kondo, A., Yuan, W., Kadota, T., et al. (2010). Exercise exerts neuroprotective effects on Parkinson’s disease model of rats. Brain Res. 1310, 200–207. doi: 10.1016/j.brainres.2009.10.075
Takagi, T., Yoshimura, S., Sakuma, R., Nakano-Doi, A., Matsuyama, T., and Nakagomi, T. (2017). Novel Regenerative Therapies Based on Regionally Induced Multipotent Stem Cells in Post-Stroke Brains: Their Origin. Characterization, and Perspective. Transl. Stroke Res. 8, 515–528. doi: 10.1007/s12975-017-0556-0
Tan, F., Wang, J., Liu, J. X., Wang, C., and Gu, Y. (2018). Electroacupuncture stimulates the proliferation and differentiation of endogenous neural stem cells in a rat model of ischemic stroke. Exp. Ther. Med. 16, 4943–4950. doi: 10.3892/etm.2018.6848
Tsai, S. T., Harn, H. J., Lin, S. Z., and Chen, S. Y. (2019). Deep Brain Stimulation for Amelioration of Cognitive Impairment in Neurological Disorders: Neurogenesis and Circuit Reanimation. Cell Transplant 28, 813–818. doi: 10.1177/0963689718804144
van den Berge, S. A., and Hol, E. M. (2013). Resident adult neural stem cells in Parkinson’s disease–the brain’s own repair system? Eur. J. Pharmacol. 719, 117–127. doi: 10.1016/j.ejphar.2013.04.058
Vandeputte, C., Reumers, V., Aelvoet, S. A., Thiry, I., De Swaef, S., Van den Haute, C., et al. (2014). Bioluminescence imaging of stroke-induced endogenous neural stem cell response. Neurobiol. Dis. 69, 144–155. doi: 10.1016/j.nbd.2014.05.014
Veena, J., Srikumar, B. N., Mahati, K., Bhagya, V., and Shankaranarayana Rao, B. S. (2009a). Enriched environment restores hippocampal cell proliferation and ameliorates cognitive deficits in chronically stressed rats. J. Neurosci. Res. 87, 831–843. doi: 10.1002/jnr.21907
Veena, J., Srikumar, B. N., and Shankaranarayana Rao, B. S. (2009b). Exposure to enriched environment restores the survival and differentiation of new born cells in the hippocampus and ameliorates depressive symptoms in chronically stressed rats. Neurosci. Lett. 455, 178–182. doi: 10.1016/j.neulet.2009.03.059
Wang, B., Chen, L., Liu, B., Liu, Z., Zhang, Z., Pan, Y., et al. (2012). Differentiation of endogenous neural stem cells in adult versus neonatal rats after brachial plexus root avulsion injury. Neural Regen. Res. 7, 1786–1790.
Wang, C. J., Wu, Y., Zhang, Q., and Wang, Y. Y. (2019). An enriched environment promotes synaptic plasticity and cognitive recovery after permanent middle cerebral artery occlusion in mice. Neural Regen. Res. 14, 462–469. doi: 10.4103/1673-5374.245470
Wang, H., Wang, Z., Wu, Q., Yuan, Y., Cao, W., and Zhang, X. (2021). Regulatory T cells in ischemic stroke. CNS Neurosci. Ther. 27, 643–651. doi: 10.1111/cns.13611
Wang, J., and Wang, Y. (2013). A ginkgo biloba extract promotes proliferation of endogenous neural stem cells in vascular dementia rats. Neural Regen. Res. 8, 1655–1662. doi: 10.4103/1673-5374.121654
Wang, X. L., Yang, Y. J., Wang, Q. H., Xie, M., Yu, X. H., Liu, C. T., et al. (2007). Changes of Wnt-3 protein during the proliferation of endogenous neural stem cells in neonatal rats with hypoxic-ischemic brain damage after hyperbaric oxygen therapy. Zhongguo Dang Dai Er Ke Za Zhi 9, 241–246. doi: 10.1097/WNR.0b013e3282f0ec09
Wang, X. L., Yang, Y. J., Xie, M., and Wang, Q. H. (2009). Hyperbaric oxygen promotes the migration and differentiation of endogenous neural stem cells in neonatal rats with hypoxic-ischemic brain damage. Zhongguo Dang Dai Er Ke Za Zhi 11, 749–752.
Wang, X. L., Zhao, Y. S., Hu, M. Y., Sun, Y. Q., and Bi, X. H. (2013). Umbilical cord blood cells regulate endogenous neural stem cell proliferation via hedgehog signaling in hypoxic ischemic neonatal rats. Brain Res. 1518, 26–35. doi: 10.1016/j.brainres.2013.04.038
Weimar, C., Mieck, T., Buchthal, J., Ehrenfeld, C. E., and Diener, H. C. (2005). Neurologic worsening during the acute phase of ischemic stroke. Arch. Neurol. 62, 393–397. doi: 10.1001/archneur.62.3.393
Wu, H., Hu, M., Yuan, D., Wang, Y., Wang, J., Li, T., et al. (2012). Electroacupuncture promotes the proliferation of endogenous neural stem cells and oligodendrocytes in the injured spinal cord of adult rats. Neural Regen. Res. 7, 1138–1144.
Wu, J. P. (1999). Responses of microglia and neural progenitors to mechanical brain injury. Neuroreport 10, 2287–2292. doi: 10.1097/00001756-199908020-00012
Wu, K. J., Yu, S., Lee, J. Y., and Wang, Y. (2017). Improving Neurorepair in Stroke Brain Through Endogenous Neurogenesis-Enhancing Drugs. Cell Transplant 26, 1596–1600. doi: 10.1177/0963689717721230
Wyss-Coray, T. (2006). Inflammation in Alzheimer disease: driving force, bystander or beneficial response? Nat. Med. 12, 1005–1015.
Xuan, W., Vatansever, F., and Hamblin, M. R. (2014). Transcranial low-level laser therapy enhances learning, memory, and neuroprogenitor cells after traumatic brain injury in mice. J. Biomed. Opt. 19:108003. doi: 10.1117/1.JBO.19.10.108003
Yan, Y., Kong, L., Xia, Y., Liang, W., Wang, L., Song, J., et al. (2018). Osthole promotes endogenous neural stem cell proliferation and improved neurological function through Notch signaling pathway in mice acute mechanical brain injury. Brain Behav. Immun. 67, 118–129. doi: 10.1016/j.bbi.2017.08.011
Yang, X., Zhu, F., Zhang, X., and Cao, Y. (2012). Ipsilateral versus bilateral limb-training in promoting the proliferation and differentiation of endogenous neural stem cells following cerebral infarction in rats. Neural Regen. Res. 7, 2698–2704.
Yang, Y. J., Wang, X. L., Yu, X. H., Wang, X., and Liu, C. T. (2008). Hyperbaric oxygen induces endogenous neural stem cells to proliferate and differentiate in hypoxic-ischemic brain damage in neonatal rats. Undersea Hyperb. Med. 35, 113–129.
Ye, J., Qin, Y., Tang, Y., Ma, M., Wang, P., Huang, L., et al. (2018). Methylprednisolone inhibits the proliferation of endogenous neural stem cells in nonhuman primates with spinal cord injury. J. Neurosurg. Spine 29, 199–207. doi: 10.3171/2017.12.SPINE17669
Yoneyama, M., Shiba, T., and Ogita, K. (2011). Adult neurogenesis is regulated by endogenous factors produced during neurodegeneration. J. Pharmacol. Sci. 115, 425–432. doi: 10.1254/jphs.11R02CP
Yoo, S. W., Kim, S. S., Lee, S. Y., Lee, H. S., Kim, H. S., Lee, Y. D., et al. (2008). Mesenchymal stem cells promote proliferation of endogenous neural stem cells and survival of newborn cells in a rat stroke model. Exp. Mol. Med. 40, 387–397. doi: 10.3858/emm.2008.40.4.387
Yu, Z., Chen, L. F., and Hu, C. L. (2013). Effects of recombinant adenovirus-mediated hypoxia-inducible factor-1alpha gene on proliferation and differentiation of endogenous neural stem cells in rats following intracerebral hemorrhage. Asian Pac. J. Trop. Med. 6, 762–767. doi: 10.1016/S1995-7645(13)60134-0
Yuan, Q., Bu, X. Y., Yan, Z. Y., Liu, X. Z., Wei, Z. Y., Ma, C. X., et al. (2016). Combination of endogenous neural stem cell mobilization and lithium chloride treatment for hydrocephalus following intraventricular hemorrhage. Exp. Ther. Med. 12, 3275–3281. doi: 10.3892/etm.2016.3778
Zhang, L., Han, X., Cheng, X., Tan, X. F., and Zhang, X. H. (2016). Denervated hippocampus provides a favorable microenvironment for neuronal differentiation of endogenous neural stem cells. Neural Regen. Res. 11, 597–603. doi: 10.4103/1673-5374.180744
Zhang, S., Jin, T., Wang, L., Liu, W., Zhang, Y., Zheng, Y., et al. (2020). Electro-Acupuncture Promotes the Differentiation of Endogenous Neural Stem Cells via Exosomal microRNA 146b After Ischemic Stroke. Front. Cell Neurosci. 14:223. doi: 10.3389/fncel.2020.00223
Zhao, Y. (2014). Umbilical cord blood cells regulate the differentiation of endogenous neural stem cells in hypoxic ischemic neonatal rats via the hedgehog signaling pathway. Brain Res. 1560, 18–26. doi: 10.1016/j.brainres.2014.02.019
Keywords: brain injury, neuroregeneration, endogenous neural stem cell, therapeutic mechanism, mobilization strategy, therapeutic approaches
Citation: Liu H, Wei T, Huang Q, Liu W, Yang Y, Jin Y, Wu D, Yuan K and Zhang P (2022) The roles, mechanism, and mobilization strategy of endogenous neural stem cells in brain injury. Front. Aging Neurosci. 14:924262. doi: 10.3389/fnagi.2022.924262
Received: 20 April 2022; Accepted: 18 July 2022;
Published: 17 August 2022.
Edited by:
Jiawei Zhou, Wenzhou Medical University, ChinaReviewed by:
Hong Xu, University of Pennsylvania, United StatesTakayuki Nakagomi, Hyogo College of Medicine, Japan
Copyright © 2022 Liu, Wei, Huang, Liu, Yang, Jin, Wu, Yuan and Zhang. This is an open-access article distributed under the terms of the Creative Commons Attribution License (CC BY). The use, distribution or reproduction in other forums is permitted, provided the original author(s) and the copyright owner(s) are credited and that the original publication in this journal is cited, in accordance with accepted academic practice. No use, distribution or reproduction is permitted which does not comply with these terms.
*Correspondence: Kai Yuan, MTkwODc2MDcyQHFxLmNvbQ==; Pengyue Zhang, enB5MTk4MDIwMDBAMTYzLmNvbQ==
†These authors have contributed equally to this work and share first authorship