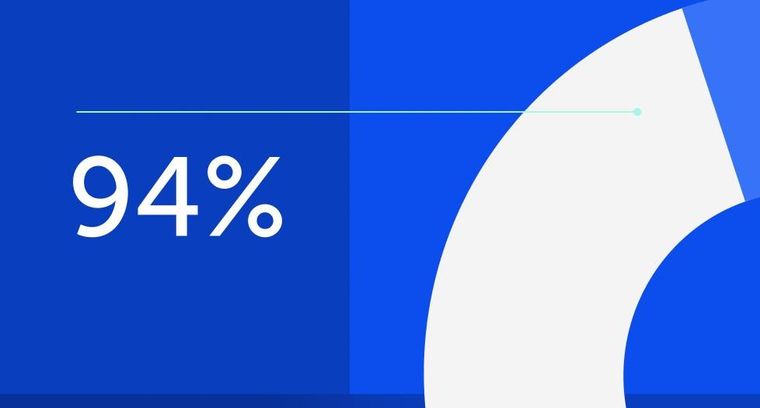
94% of researchers rate our articles as excellent or good
Learn more about the work of our research integrity team to safeguard the quality of each article we publish.
Find out more
REVIEW article
Front. Aging Neurosci., 24 June 2022
Sec. Parkinson’s Disease and Aging-related Movement Disorders
Volume 14 - 2022 | https://doi.org/10.3389/fnagi.2022.861035
This article is part of the Research TopicProtein Aggregation and Propagation in Neurodegenerative DiseasesView all 11 articles
Parkinson's disease (PD) is the second most common chronic progressive neurodegenerative disease. The main pathological features are progressive degeneration of neurons and abnormal accumulation of α-synuclein. At present, the pathogenesis of PD is not completely clear, and many changes in the intestinal tract may be the early pathogenic factors of PD. These changes affect the central nervous system (CNS) through both nervous and humoral pathways. α-Synuclein deposited in the intestinal nerve migrates upward along the vagus nerve to the brain. Inflammation and immune regulation mediated by intestinal immune cells may be involved, affecting the CNS through local blood circulation. In addition, microorganisms and their metabolites may also affect the progression of PD. Therefore, paying attention to the multiple changes in the intestinal tract may provide new insight for the early diagnosis and treatment of PD.
Parkinson's disease (PD) is the second most common neurodegenerative disease (Grosso Jasutkar et al., 2022). PD is characterized mainly by motor disorders such as tremor, muscle stiffness, motor retardation and gait impairment (Nalls et al., 2014). These motor symptoms appear mainly in the middle and late stages of the disease (Machado et al., 2016; Wiratman et al., 2019). In addition, PD also shows non-motor symptoms (NMS) in the prodromal stage (Hussein et al., 2021), such as gastrointestinal motility disorder, decreased sense of smell, and rapid-eye-movement (REM) sleep behavior disorder. NMS are also a major cause of disability during the clinical stages of PD and progress all through PD (Poewe et al., 2017). Gastrointestinal dysfunction is the main non-motor symptom in patients with PD. The clinical manifestations are dysphagia, delayed gastric emptying and constipation, among which constipation is the most common symptom (Knudsen et al., 2017; Ahn et al., 2021). Clinical reports have shown that nearly 30% of patients with PD develop constipation 20 years before motor symptoms appear (Savica et al., 2009; Schapira et al., 2017; Manfredsson et al., 2018). About 70–80% of PD patients suffer from constipation, which is four times higher than normal people of the same age and sex (Hurt et al., 2019). When motor symptoms occur in PD patients, the degenerative changes are accompanied by a loss of 40–60% of the nigral dopamine neurons (D'Andrea et al., 2019), and an 80% reduction in striatal dopamine (Rabiei et al., 2019). At this time, PD is at an irreparable stage (Savica et al., 2018). Therefore, detecting the early NMS of the disease and providing intervention as early as possible, would be an effective means of treating or delaying the development of the disease.
Several lines of evidence suggest a close relationship between the intestinal nerve and the central nervous system (CNS) during the pathogenesis of PD. Braak and collaborators initially hypothesized that the gut-brain axis is involved in PD (Braak et al., 2006). Pathogens in the environment could pass through the intestinal epithelium and induce misfolding and accumulation of α-synuclein in specific neurons of the intestinal nerve, and this agent may mediate the propagation of pathology. But this connection still remains a hypothesis and it will be very difficult to prove in humans or will remain controversial. Recently, it was discovered that patients who had undergone a total vagotomy due to peptic ulcers had a lower incidence of PD than people who had not undergone this procedure or who had a selective partial vagotomy (Svensson et al., 2015). However, another study discussed clinical evidence that the case is not very clear (Tysnes et al., 2015). In addition, experimental evidence in rodents showed that the injection of the aggregated form of α-synuclein into the intestinal wall could promote the accumulation of endogenous α-synuclein (Kim et al., 2019; Van Den Berge et al., 2019). These evidence indicated that α-synuclein aggregates contributed to the accumulation of aggregated α-synuclein in various brain regions through vagus nerve transmission. This transmission was time-specific and region-dependent (Kim et al., 2019). The gastrointestinal tract is innervated by parasympathetic vagal and sympathetic non-vagal pathway. Structures along these pathways also show α-synuclein pathology (Braak et al., 2007). This means that the propagation of α-synuclein pathology via sympathetic nerves, mediated by the intermediolateral nucleus of the spinal cord, provides an additional potential route to the brain (Van Den Berge et al., 2021). These studies have confirmed that colonic lesions triggers the CNS lesions. However, a seminal work provided detailed studies in postmortem tissue that the “body-first” hypothesis is not the ultimate disease mechanism for PD (Beach et al., 2021), indicating that the relationship between the CNS and the enteric nervous system (ENS) might be bidirectional (Garrido-Gil et al., 2018). The nervous systems of the brain and the intestine are interlinked and this gut-brain axis can play a critical role pathogenesis and progression of PD.
The intestinal tract is the largest and longest immune organ in mammals (Liu et al., 2018). Therefore, the mucosal immune barrier and abundant immune cells in the intestinal tract may play an important regulatory role in gastrointestinal inflammation in PD. Studies have confirmed that intestinal nerve-derived IL-18 signals can control intestinal immunity and have a far-reaching impact on the mucosal barrier (Jarret et al., 2020). Gastrointestinal infections are associated with an increased risk of PD (Nerius et al., 2020). A recent study showed that α-synuclein is required for normal immune function, such as the development of a normal inflammatory response to bacterial peptidoglycan introduced into the peritoneal cavity as well as antigen-specific and T cell responses following intraperitoneal immunization (Alam et al., 2022). These indicate the presence of information exchange between the ENS and the immune system. Enteric glial cells (EGC) around intestinal neurons have closely interaction with intestinal neurons (Clairembault et al., 2015b). Intestinal neurons and glial cells may be targets for the treatment of intestinal inflammatory diseases, such as inflammatory bowel disease (IBD), via regulating the barrier function or immune response (Puzan et al., 2018; Li et al., 2020). Some studies have confirmed that the expression of pro-inflammatory cytokines and glial markers was increased in colon biopsies of patients with PD (Devos et al., 2013).
Intestinal innate immunity is also involved in the follow-up process of the T cell immune response. The antigen-presenting cells, such as dendritic cells (DCs), may take up previous inclusion bodies and then present the major histocompatibility complex (MHC) peptides derived from this process. The MHC peptides can be recognized by specific T-cell receptors (TCRs) on T cells. This triggers activation of the adaptive immune cells in the intestine, such as T cells, causing chronic inflammation (Campos-Acuña et al., 2019). At the same time, regulatory T (Treg) cells or other immune cells can also be activated to play an anti-inflammatory role through the dopamine pathway (Levite, 2016; Xue et al., 2018; Campos-Acuña et al., 2019) and short-chain fatty acid pathway (Zeng and Chi, 2015; Yuan et al., 2021). A variety of immune cells acquire immunophenotype in the intestinal tract and are transported to the CNS through abundant local blood flow, which affects the central immune response (Korn and Kallies, 2017). T cells are a “double-edged sword” and these cells undoubtedly add a new possibility to the pathogenic and therapeutic mechanisms of PD.
The intestinal tract has close contact with the external environment. Changes in the microflora in the intestinal cavity may be closely related to immune inflammation and the aggregation of pathological α-synuclein (Sampson et al., 2016). Changes in the intestinal flora can also affect the integrity of the blood-brain barrier (Rutsch et al., 2020). Short-chain fatty acids (SCFAs), the metabolites of intestinal flora, have multiple protective effects. Changes in the intestinal flora and its metabolites are indispensable factors affecting the occurrence and development of PD (Cholan et al., 2020).
While there is also the hypothesis of “brain-first” for PD and the evidence for that is similar and based on neuropathology in humans, this review will focus on the “body-first” hypothesis with an emphasis on the role of the microbiome and immune pathways.
There are various gastrointestinal symptoms (GIS) in patients with PD, including dry mouth, drooling, dysphagia, constipation, and defecation dysfunction (Travagli et al., 2020). Clinical studies have confirmed that the symptoms of gastroparesis in patients with PD precede motor symptoms, although the prevalence rate between PD and the control group was not significantly different (Edwards et al., 1991; Cersosimo et al., 2013). Constipation and defecation dysfunction are the main pre-motor GIS of PD.
According to different diagnostic criteria of chronic constipation, the prevalence rate of constipation ranges from 24.6 to 63% (Stocchi and Torti, 2017). Constipation is one of the main and crippling NMS of PD. In this review, we will first discuss the pathophysiological mechanism of PD-related constipation.
α-Synuclein is the major component of the intraneuronal Lewy bodies (LBs) (Grochowska et al., 2021) and Lewy neurites (LNs) (Ehgoetz Martens and Lewis, 2017), the pathological hallmarks of PD (Koprich et al., 2010). Pathological aggregation of α-synuclein in gastric and colonic neurons has been detected in autopsies from patients with advanced PD (Wang et al., 2012). These neuroanatomical changes observed in patients with PD suggest that there is an abnormal accumulation of α-synuclein in the gastrointestinal system, which may play a role in the development of the gastrointestinal pathology in PD.
α-Synuclein is widely expressed in the brain and is thought to have a variety of functions, including regulating the release of neurotransmitters and vesicular circulation of central synapses (Burré et al., 2018). However, little is known about the physiological and pathological functions of α-synuclein in the peripheral nervous system. Studies on the gut of humans and guinea pigs have found that α-synuclein is expressed in the cell bodies of some intestinal neurons, especially in the varicosities and terminals of cholinergic neurons, and has an immune response to vesicular acetylcholine transporter (Vacht) (Sharrad et al., 2013). Some studies have confirmed that α-synuclein can regulate the development of cholinergic neurons (Swaminathan et al., 2019). It is not clear how α-synuclein affects and regulates intestinal function.
Studies on α-synuclein pathology have shown that α-synuclein pathology, induced in the α-synuclein virus overexpression model and prefabricated fibril (PFF) model, leads to abnormal gastrointestinal motility in the ENS of rats and non-human primates (Manfredsson et al., 2018). Human A53T α-synuclein transgenic mice have gastrointestinal disorders in the early stage (<6 months), insufficient intestinal peristalsis and decreased motor response of the longitudinal and circular muscle layer of the colon (Rota et al., 2019). Verification on a variety of animal models showed a correlation between intestinal α-synuclein pathology, reduced cholinergic function and prolonged gastric transit time in PD (Van Den Berge et al., 2021; Van Den Berge and Ulusoy, 2022). While it has been inferred that the possible mechanism of constipation might be the weakening of cholinergic transport in the ENS by α-synuclein pathology, this remains to be further studied.
In PD, constipation is due to slower colonic transit or outlet dysfunction, or both (Stocchi and Torti, 2017). In either case, it may be related to an imbalance in the control of defecation by the intestinal nerve. The early involvement of intestinal neurons may explain the occurrence of constipation in early PD.
Previous studies have confirmed the loss of vasoactive intestinal peptide (VIP) neurons in the colon of patients with PD (Wakabayashi et al., 1993) and the loss of excitatory dopaminergic neurons in the colon of the 1-methyl-4-phenyl-1,2,3,6-tetrahydropyridine (MPTP) model (Anderson et al., 2007). Both showed a deficiency in the relaxation function of the colonic smooth muscle, which may be closely related to the occurrence of constipation. Some studies have also confirmed a large loss in the number of dopamine neurons in the colonic myenteric plexus in patients with PD (Singaram et al., 1995). In contrast, recent studies have detected Lewy pathology in the colonic submucosal biopsies from PD patients (Shannon et al., 2012). However, no significant difference was found in the intermuscular neuron density between PD patients and the control group (Annerino et al., 2012). This may mean that the decrease in the intermuscular neurons may be the result of the gastrointestinal symptoms rather than the cause. This study also suggested that the neuropathology of the dorsal motor nucleus of the vagus nerve (DMV) and/or submucosal plexus is more likely than myenteric plexus injury to be the cause of the gastrointestinal motility disturbance associated with PD.
Some studies have found that Lewy body dementia (DLB), another disease with the same Lewy body pathology as PD, also produces non-motor disorders (Fereshtehnejad et al., 2019). Literature statistics have shown that in DLB, α-synuclein aggregation appears earlier in the peripheral nerves than in the brain, such as the vagus nerve (86.7%), myenteric nerve plexus (86.7%), and cardiac sympathetic nerve (100%) (Gelpi et al., 2014). Constipation associated with Lewy bodies is called Lewy body constipation. Constipation in DLB may be more common than in PD (Sakakibara et al., 2019). Therefore, the current research on non-motor disorders in patients with PD should not be limited to PD but should include Lewy body disease and all neurodegenerative diseases.
What we know so far is that constipation occurs in the early stage of PD and there is a variety of pathological changes associated with it. So, is there a relationship between early intestinal changes and the occurrence and development of CNS lesions in the later stage? Furthermore, does PD originate from the intestinal tract and spread to the CNS through a particular mode of transmission to cause pathological changes? Next, we will discuss this part of the content (Figure 1).
Figure 1. Parkinson's disease pathology spreads along the brain-gut axis through the vagus nerve. This figure reflects the “gut-first” hypothesis as well as the “brain-first” hypothesis was omitted. The environmental changes in the intestinal lumen may pass through the gastrointestinal wall and enter the myenteric neurons, triggering the formation of α-synuclein inclusions in the enteric nervous system (ENS). Parkinson's disease (PD) pathology was first found in the ENS, and this process may cause gastrointestinal dysfunction in the early stage of PD. The change in the lumen environment may lead to pathological changes in α-synuclein in enteric endocrine cells (EEC). Through the structure named “neuropods,” this signal is transmitted to the ENS, and induces the pathological changes in α-synuclein in the ENS. A variety of immune cells in the lamina propria may participate in this process through a variety of immune responses. Subsequently, α-synuclein ascends retrograde through the vagus nerve to the neurons in the dorsal motor nucleus of the vagus (DMV) in the brainstem, and finally reaches the substantia nigra pars compacta (SNpc), causing dopaminergic neuronal degeneration. At this time patients show typical motor symptoms of PD. NTS, nucleus tractus solitarius; α-syn, α-synuclein; Tc, T cells; EGC, enteric glial cells.
Neurodegenerative diseases have always been regarded as central system diseases. Therefore, most studies have focused on the CNS. However, peripheral pathology is also closely related to the occurrence and development of CNS diseases. Braak et al. demonstrated that LBs are present in the neurons of the Meissner plexus of the stomach of patients with early stage PD, further supporting the hypothesis that the periphery, especially the ENS, is the origin of PD. The pathology of PD may start in the gastrointestinal tract and then spread to the brain through the vagus nerve. The findings by Braak and colleagues suggest that in the pathogenesis of PD, pathological alterations appear in the DMV and the ENS before they develop in the substantia nigra (Braak et al., 2006).
At present, this view is supported by pathophysiological evidence. It has been reported that α-synuclein inclusions appear in the ENS, glossopharyngeal nerve, and vagus nerve in the early stage of PD (Burré et al., 2018). Immunohistochemical detection in PD transgenic mice showed that a few months before the loss of striatal dopaminergic neurons, age-dependent α-synuclein-GFP was progressively expressed and accumulated in the Meissner and Auerbach plexus of the colon (Chen et al., 2018a). Some studies have shown that different forms of α-synuclein can be transmitted to the brain through the vagus nerve, which may be the mechanism of prion-like transmission of α-synuclein in PD and related diseases (Zhong et al., 2017; Kim et al., 2019; Liu et al., 2021a). This evidence all shows that the abnormal intestinal α-synuclein deposition is earlier than the occurrence of degenerative diseases of the CNS (Hilton et al., 2014).
The view that α-synuclein deposition in the intestine is earlier than in the CNS, and that Lewy pathology begins in the DMV has been supported. Moreover, the gastrointestinal system and the brain are anatomically connected through the vagus nerve. Although supported by the above theories, no studies have fully confirmed this process. The theory that PD begins in the intestinal tract and spreads by the vagus nerve is still widely debated (Gershanik, 2018).
The ENS is one of the earliest structures showing PD pathology, but the experimental results have not been confirmed in a large autopsy cohort study. It has also been shown that some patients show early pathology in the intermediolateral nucleus of the spinal cord (IML) and autonomic ganglia (data from large patient cohorts), also indicating a peripheral start of disease (Borghammer et al., 2021). α-Synuclein injected into the stomach of rats was retrogradely transported from the intestine to the brain through the vagus nerve (Kurnik et al., 2015). Another recent study confirmed that exogenous venereal α-synuclein injection could induce the accumulation of endogenous α-synuclein in the gastrointestinal tract and transmit it to the brain along the vagus nerve, causing corresponding pathological changes in various brain regions that finally lead to motor and cognitive impairment in the mice (Kim et al., 2019). A recent study indicated that propagation of α-synuclein pathology from the gut to the brain is more efficient in old vs. young wild-type rats, upon gastrointestinal injection of aggregated α-synuclein (Van Den Berge et al., 2021). However, overexpression of α-synuclein has also been proven to be transmitted from the brain to the ENS as a bidirectional pathway (Santos et al., 2019), which can not mean that the pathological changes in the brain must come from the intestinal tract. A study from Borghammer and Van Den Berge formulated two hypothesized PD-subtypes, a “body-first” subtype where pathogenic α-synuclein arises in the body and spreads to the brain, and a “brain-first” subtype where pathogenic α-synuclein arises in the brain and spreads to the body. Recent studies report new evidence in support of this hypothesis from newly diagnosed PD patients (Horsager et al., 2020; Borghammer et al., 2021; Knudsen et al., 2021) and animal models (Van Den Berge and Ulusoy, 2022). Moreover, the toxic changes of endogenous α-synuclein in the intestinal tract have not been solved. Therefore, the scientific question of whether and why the pathology of PD begins in the intestinal tract remains to be explored.
Enteric endocrine cells (EECs) are cells that sense the content of substances in the lumen, produce and release hormone/signal molecules, regulate a variety of physiological functions in the intestine and maintain homeostasis (Liddle, 2018). In recent years, the role of EECs in gut-brain/brain-gut communication has sparked people's interest (Ye et al., 2021). It was found that the cytoplasmic processes of cholecystokinin (CCK) and peptide tyrosine-tyrosine (PYY) cells are similar to axons, and their synaptic ends have been named “neuropods.” They contain a large number of secretory vesicles, many of which are distributed at the tip, indicating that they may guide the process of hormone secretion (Bohórquez et al., 2015). “Neuropods” contain intermediate filaments, are surrounded by glial cells, and appear to connect directly to nerves (including sensory nerve endings) (Latorre et al., 2016).
Intestinal nerves are not exposed to the intestinal lumen, so they are not directly affected by substances in the lumen. However, EECs have many neuron-like characteristics, which provide a possible way for intestinal luminal substances and intestinal nerves to communicate with each other. Rabies virus and mad cow disease prion can infect EECs and transfer to the intestinal nerve (Liddle, 2018). It has been confirmed that EECs express α-synuclein (Chandra et al., 2017), and that misfolded α-synuclein has the ability to transfer from nerve to nerve in a prion-like manner (Angot et al., 2012). Therefore, EECs may be involved in the neural transmission pathway in PD. α-Synuclein can misfold in EECs due to change in the intestinal environment and other factors. Through the prion-like characteristics and neuron-like properties of EECs, the misfolded α-synuclein may spread to the adjacent ENS and eventually spread to the brain through the vagus nerve pathway.
The transfer of gastrointestinal Lewy pathology to the CNS along the vagus nerve pathway in the initial stage of the disease leads to Lewy pathology and inflammation in the brain. However, the development of the disease may also be related to the peripheral intestinal tract. Because the intestine is the largest and longest immune organ of the body, and the intestinal cavity is in close contact with the external microenvironment, there are abundant immune cells and a variety of immune responses. Changes in intestinal permeability and continuous contact with a variety of antigens may trigger innate and acquired immune responses in the intestinal immune system, leading to gastrointestinal inflammation. Under normal circumstances, there is also an anti-inflammatory mechanism in the body. This immune balance is disrupted in the disease state. The activated immune cells are much more likely to affect the CNS through the humoral pathway and aggravate the level of pathology and inflammation in the brain. Therefore, the inflammatory changes caused by intestinal microecology and the regulation of immune cells involved in it provide new ideas for the potential risk factors and possible pathogenesis of PD (Figure 2, Table 1).
Figure 2. Gastrointestinal immune inflammatory response participates in the progression of α-synuclein. α-Synuclein may activate T cells to trigger downstream inflammatory responses through APCs (①) and EGCs (③), resulting in traumatic changes in intestinal structure and function. The inflammatory reaction further aggravates the aggregation and toxicity of α-synuclein. The excessive accumulation of α-synuclein in intestinal neurons can inhibit synaptic vesicle transport and reduce dopamine production, then inhibit the transformation of T cells to protective regulatory T (Treg) cells, and aggravate inflammation (②). Dopamine in this process participates in T cell protective regulation through the dopamine receptor. The pro-inflammatory or anti-inflammatory reaction caused by the above processes further aggravate the aggregation and toxicity of α-synuclein. Subsequently, these changes affect the central nervous system (CNS) through the dual pathways of nerve (vagal and non-vagal circuits) and blood circulation. α-syn, α-synuclein; APC, antigen presenting cell; EGC, enteric glial cells; ENS, enteric nervous system.
Table 1. Types of gastrointestinal immune cells associated with PD and their pro-/anti-inflammatory effects.
One of the important functions of the gastrointestinal tract is to act as a semi-permeable barrier, regulating the absorption of nutrients, ions, and water, and regulating host contact with a large number of dietary antigens and bacteria (Ménard et al., 2010). Intestinal permeability can be defined as a facility in which intestinal epithelial cells allow molecules to spread through passive diffusion, and it is an important index for evaluating the integrity of the mucosal barrier (Luissint et al., 2016). Several chronic autoimmune intestinal diseases, such as IBD, are associated with increased intestinal permeability (Chang et al., 2017). Case studies with patients with PD have confirmed that there is a significant increase in intestinal permeability, and increased exposure to intestinal bacteria and bacterial endotoxins in patients with early diagnosed PD (Karunaratne et al., 2020). The increased α-synuclein in intestinal biopsies was associated with high intestinal permeability (Oreja-Guevara et al., 2011; Schwiertz et al., 2018). Some literatures have detected changes in the distribution of the tight junction proteins ZO-1 and occludin in colonic mucosa samples of PD patients and decreased amounts of occludin (Clairembault et al., 2015a), which is related to changes in colonic permeability. The LPS mouse model has shown different intestinal permeability changes. That is, the increased intestinal permeability occurred mainly in the colon, while phosphorylated α-synuclein serine 129 was detected in the intermuscular neurons of the colon (Kelly et al., 2014).
Prolonged intestinal permeability dysfunction can lead to the translocation of bacteria (such as Escherichia coli) and bacterial products (such as lipopolysaccharide), which creates a pro-inflammatory environment and increases the burden of oxidative stress on the ENS. Various factors contribute to the appearance of pathological α-synuclein in the gastrointestinal tract and make the immune cells acquire an antigenic phenotype. Therefore, intestinal leakage in patients with a genetic susceptibility to PD may be a key early step in promoting the pro-inflammatory/oxidative environment. Combined with the transmission theory of PD, this change in the microenvironment would contribute to the initiation and/or progression of PD.
Crohn's disease (CD) and ulcerative colitis (UC) are two IBD. UC involves the colon and rectum, while CD involves the small intestine and colon (Chang, 2020). Coincidentally, IBD and PD have some common characteristics, including several common risk genes, such as LRRK2 (Hui et al., 2018). In recent years, several studies have reported the causal relationship between IBD and PD (Lin et al., 2016; Peter et al., 2018; Brudek, 2019; Kishimoto et al., 2019; Zhu et al., 2019; Rolli-Derkinderen et al., 2020; Wan et al., 2020; Lee et al., 2021). Therefore, the two diseases are often compared.
It has been confirmed that initially α-synuclein aggregation and subsequently Lewy body generation occur in neurons such as the olfactory bulb and gastrointestinal tract that are exposed to adverse environmental factors (Del Tredici and Braak, 2016). A study using endoscopic biopsy from children with intestinal inflammation found a significant correlation between the level of α-synuclein accumulation in the ENS and the degree of intestinal inflammation (Stolzenberg et al., 2017). Recent literature has determined the capacity of the appendix to modify PD risk and influence pathogenesis (Gray et al., 2014; Killinger et al., 2018; Gordevicius et al., 2021). Lewy bodies stimulated Toll-like receptor 2 (TLR2) and Toll-like receptor 4 (TLR4) on local glial cells to induce NF-κB activation through Toll-like receptor (TLR) signaling pathway, thus inducing initial inflammation in the microenvironment (González et al., 2015). The EGC population is species specific and as complex as CNS glia (Grundmann et al., 2019). Some literature has hypothesized that the inflammatory mediators produced by EGC may damage the surrounding tissue, and induce and aggravate the misfolding and accumulation of α-synuclein in the intestinal nerve (Fellner and Stefanova, 2013).
At present, there is sufficient evidence that innate immune cells are involved in PD-related gastrointestinal inflammation. Through the analysis of colon biopsies from patients with PD, it has been determined that pro-inflammatory cytokines (TNF-α, IF-γ, IL-6, and IL-1β) and glial cell markers GFAP and SOX-10 are significantly increased in patients with PD (Devos et al., 2013). Recent findings demonstrated that chronic colitis promotes parkinsonism in genetically susceptible mice, and TNF-α plays a detrimental role in the gut-brain axis of PD (Lin et al., 2021).
The interaction between intestinal inflammation and intestinal nerves has also been reported. It has been found that partial knockout of M1 monocytes has a neuroprotective effect on the myenteric plexus in the MPTP model, but has no protective effect on basal ganglia (Côté et al., 2015). Moreover, the ENS provides a key link in the innate immune response, which is not only important for coordinating mucosal barrier homeostasis, but also for combating invasive bacterial infections. Therefore, intestinal nerve damage caused by inflammation may further aggravate local inflammation (Jarret et al., 2020).
How local gastrointestinal inflammation in PD affects the follow-up progress of the disease in the CNS will be summarized in 4.4.
Innate immunity may play an initiating role in gastrointestinal inflammation. In addition to innate immune inflammation, innate immunity can also participate in subsequently acquired immune inflammation. Acquired immunity plays the role of inflammatory cascade amplification and anti-inflammatory response, resulting in follow-up regulation of inflammation. Acquired immunity plays an important role in both neurodegenerative diseases and IBD (Table 2). Early studies showed that while B cells were not significantly increased, T cells were significantly increased in the postmortem brains of patients with PD and brains of the MPTP model. A decrease in dopaminergic cell death induced by MPTP was observed in CD4-deficient mice (Brochard et al., 2009). CD4+ T cells contribute to neurodegeneration in Lewy body dementia (Gate et al., 2021). This suggests that T cells, especially CD4+ T cells, may be involved in the pathogenesis of PD (Chen et al., 2019) or other neurodegenerative disease.
α-Synuclein can be an antigenic substance. It has been reported that the peptides of its two regions (Tyr39 and phosphorylated Ser129 region) can be used as antigenic epitopes to stimulate infiltrating antigen-presenting cells (APCs) (that is, monocytes/macrophages and DC). These cells capture and receive stimulation through the TLR signaling pathway, then process Lewy bodies into suitable small peptides, before forming Lewy body-specific MHC-II antigens (Sulzer et al., 2017). Then, APCs present MHC-II antigen to naive CD4+ T cells (González et al., 2015; Sulzer et al., 2017). CD4+ T cells activate, proliferate, and differentiate into Th1 and Th17 cells, infiltrate into the lamina propria of the colon, and release IFN-γ, IL-17, and other inflammatory mediators that recruit and stimulate neutrophils and macrophages, thereby inducing chronic inflammation in the intestinal mucosa (Dardalhon et al., 2008). Reactive oxygen species (ROS) induced by Th1 and Th17 immunization in local phagocytes promotes the further accumulation of α-synuclein in ENS neurons (González et al., 2015). This mechanism creates a cycle of increased toxicity of α-synuclein. Moreover, the intestinal inflammation of IBD is driven mainly by Th1 and Th17 cells of the CD4+ T cell subset (Granlund et al., 2013). A study defined that a compromised immune system increases the accumulation of pathological α-synuclein in the brain (George et al., 2021). This suggests that the pro-inflammatory responses of CD4+ Th1 and Th17 play an important role in gastrointestinal inflammation in both IBD and PD.
Regulatory T (Treg) cells are an inhibitory subtype of lymphocytes and play an important role in maintaining intestinal homeostasis (Chen et al., 2021). Treg cells can inhibit the inflammation induced by effector T cells (Th1 and Th17) in chronic UC models induced by T cells transferred to lymphocyte knockout mice. One of the main inhibitory mechanisms depends on the secretion of IL-10 by Treg cells (Powrie and Mason, 1990). IL-22 produced by Treg cells induces the expression of tight junction proteins (claudin1 and ZO-1) in epithelial cells, thereby reducing intestinal infiltration, increasing the integrity of the intestinal mucosal barrier and protecting it from intestinal inflammation (Fang et al., 2018). In the presence of chronic neuroinflammation in the CNS, there is evidence that peripheral immune cells can infiltrate the CNS through the damaged blood-brain barrier. It has been confirmed that the adoptive transfer of CD4+CD25+ Tregs into the MPTP model could reduce neuroinflammation and protect dopaminergic neurons in the substantia nigra compacta of the model mice (Huang et al., 2020). Dopamine can enhance the protective effect of CD4+ T cells or reduce the inflammatory damage of CD4+ T cells so as to inhibit inflammation (Contreras et al., 2016; Ahlers-Dannen et al., 2020).
To sum up, Lewy body-derived antigens may be an important target for T cell-mediated immunity. Inflammation induced by CD4+ T cells promotes the further accumulation of α-synuclein. The pro-inflammatory response mediated by Th cells and the anti-inflammatory response driven by Treg cells show the subsequent regulation of acquired immunity on the inflammatory process and represent the central process of PD and IBD, and dopamine may regulate this process.
It has been reported that a variety of neurodegenerative diseases (Sweeney et al., 2018), including PD, exist in the presence of blood-brain barrier damage (Varatharaj and Galea, 2017). Similarly, a variety of animal models of PD, such as the rotenone model (Ravenstijn et al., 2012), MPTP model (Liu et al., 2017), and LPS model (Sweeney et al., 2018), also show different degrees of damage to the blood-brain barrier. This damage to the blood-brain barrier may be attributed to the ascending transmission of Lewy bodies along the vagus pathway. Lewy bodies accumulate in the brain to produce pro-inflammatory mediators, such as IL-1β, which activate microglia and related inflammatory cytokines to cause damage to the blood-brain barrier (Varatharaj and Galea, 2017; Gordon et al., 2018). Peripheral inflammatory factors can also cause damage to the blood-brain barrier. There is abundant blood flow in the intestinal tract. Innate immune cells or antigen-activated T cells and corresponding inflammatory factors obtained in the gastrointestinal tract can enter the blood circulation, pass through the damaged blood-brain barrier, and migrate to the brain parenchyma, leading to neuroinflammation and neurodegeneration (Chen et al., 2018b). This mechanism represents a vicious cycle: Inflammation may continue to aggravate the production of Lewy bodies and damage the blood-brain barrier in the brain, which in turn leads to the continuous aggravation of inflammation and progression of disease.
The gastrointestinal tract of healthy people is inhabited by a wide variety of microorganisms called intestinal flora. Bacteria in the human gastrointestinal tract can form a large and complex ecosystem. These microbes are involved in almost all intestinal functions, affecting host metabolism, and behavior, neural circuits, hormone secretion, and immune response (Cox and Weiner, 2018). In recent years, with the introduction of the concept of microorganism-intestine-brain axis, it was shown that there is a two-way interaction between intestinal flora and the brain (Cryan et al., 2019). This may play an important role in neurological diseases, including anxiety disorder, depression (Foster and McVey Neufeld, 2013), autism, multiple sclerosis, PD (Elfil et al., 2020; Liu et al., 2021b), and Alzheimer's disease (Quigley, 2017; Srivastav and Mohideen, 2021).
The gastrointestinal microbiome is altered in PD and likely plays a key role in its pathophysiology. A study showed that compared with healthy controls, the abundance of Prevotella in the feces of patients with PD is decreased (Unger et al., 2016). This results in decreased intestinal mucus secretion, increased intestinal permeability, and increased local and systemic susceptibility to bacterial antigens and endotoxins, as well as a large amount of α-synuclein expression and misfolding. However, changes in intestinal flora can also affect the occurrence of PD. Intestinal gram-negative bacterial infection in mice can induce a decrease in dopaminergic neurons in the substantia nigra of PINK1–/– mice and produce PD-like behavioral changes, such as dyskinesia (Matheoud et al., 2019). In the intestinal tissue of germ-free mice, the activation of microglia is decreased, the content of pathological α-synuclein is also significantly decreased (Sampson et al., 2016). There are obvious changes in the intestinal flora in patients with PD. Therefore, gut dysbiosis has a significant potential as a therapeutic target in PD.
Some studies have found that the intestinal flora can change the permeability of the blood-brain barrier in germ-free mice, which indicates that changes in the intestinal flora will affect the defense function of the blood-brain barrier (Rutsch et al., 2020). The expression of different types of TLR in brain endothelial cells can respond to bacterial cell wall components such as lipopolysaccharide (LPS) of gram-negative bacteria and lipoteichoic acid (LTA) of gram-positive bacteria, and directly affect the function of the blood-brain barrier (Tang et al., 2017). LPS can also induce other cell types to produce and release pro-inflammatory mediators, and thus regulates the function of the blood-brain barrier (Nagyoszi et al., 2010).
SCFAs, such as butyrate, acetate and propionate, are produced by the fermentation of dietary fiber by intestinal microflora. After reaching the CNS through the blood circulation, SCFAs enhance the blood-brain barrier function by up-regulating the expression of tight junction protein in the blood-brain barrier. SCFAs also have neurotrophic and anti-inflammatory effects (Al-Asmakh and Hedin, 2015; Cholan et al., 2020). Reductions in fecal SCFAs but increased plasma SCFAs were observed in PD patients (Aho et al., 2021; Chen et al., 2022). SCFAs can also improve the dysfunctional blood-brain barrier in germ-free mice (Braniste et al., 2014), and it is also beneficial to the intestinal mucosal barrier (Chen et al., 2017).
SCFAs can up-regulate brain-derived nerve growth factor and glial cell line-derived neurotrophic factor. They can also protect dopaminergic neurons by activating the expression of G protein coupled receptors and inhibiting histone deacetylase (Abdel-Haq et al., 2019). In animal experiments, microglia in the brain of germ-free mice have shown immaturity and had almost no response to inflammatory stimuli (Erny et al., 2015). After supplementing with SCFAs, immature microglia routinely matured and could be activated to respond to inflammation and stimulation, suggesting that SCFAs have a neuroprotective effect (Scott et al., 2017).
SCFAs can also regulate immunity through a variety of mechanisms (Yao et al., 2022). G protein-coupled receptor (GPR)-mediated SCFA signaling can stimulate the differentiation of Treg cells and inhibit intestinal inflammation, or regulate the differentiation of Treg cells through epigenetic modification, which is helpful for the dynamic balance of immunity in the colon. At the same time, SCFAs promote the production of IL-10 by microbiota antigen-specific Th1 cells to limit the induction of colitis (Sun et al., 2018).
PD is the second most common neurodegenerative disease. The main clinical manifestations are dyskinesia and non-motor symptoms. Gastrointestinal dysfunction is the main non-motor symptom in patients with PD, among which constipation is the most common. Constipation symptoms may appear 20 years earlier than exercise symptoms, suggesting that PD may originate from the intestinal tract.
The occurrence of constipation may be closely related to the loss of intestinal neurons and the pathology of α-synuclein. At present, although evidence supports the view that α-synuclein deposition occurs earlier in the intestine than in the CNS, whether PD pathology originates from the periphery and then affects the CNS, or whether PD begins in the intestinal tract, is still widely debated. In recent years, studies on the pathogenesis of the microorganism-gut-brain axis in PD suggest that early changes in the intestinal flora and α-synuclein expression in intestinal nerves cause toxic and aggregation-like changes under inflammation. These changes affect the CNS through the dual pathways of nerve (vagal and non-vagal circuits) and blood circulation. This causes CNS damage and promotes disease progression. In this process, the anti-inflammatory responses of Treg cells, the T cell anti-inflammatory pathway mediated by dopamine, and the multiple protective effects of SCFAs, produce certain anti-inflammatory effects. These results enrich the theory of the intestinal origin of PD and provide theoretical support for the discovery of new therapeutic targets for PD.
In the prodromal stage of PD, the changes in neural or immune molecules in the gastrointestinal tract and peripheral blood may become biomarkers of PD and provide the basis for early diagnosis. Based on the microorganism-gut-brain axis hypothesis in PD, each part of it may become a potential therapeutic target. Early application of drugs and antibodies against gastrointestinal α-synuclein or immune cells may reduce the transmission of α-synuclein. Dietary or pharmacological interventions aimed at modifying the gut microbiota composition and enhancing the intestinal epithelial barrier integrity in PD patients or subjects at higher risk for the disease may delay disease progression. Cellular therapies using Treg cells are currently undergoing clinical trials for the treatment of autoimmune diseases, transplant rejection, and Treg cells have also been shown to have neuroprotective effects in mouse models of Alzheimer's disease.
In this review, we first focused on the possible mechanism of constipation in PD. Then, by analyzing the effects of intestinal changes on the CNS, we analyzed the involvement of the vagus nerve in the transmission of α-synuclein to the brain, as well as the pro-inflammatory and anti-inflammatory responses of congenital and adaptive immune cells. We also considered the anti-inflammatory and protective functions of dopamine. Finally, we analyzed how the flora and its metabolites participate in this process. Combining these findings suggest that intestinal lesions may be the origin of PD. Intestinal pathological changes may spread to the CNS through the vagus nerve, causing pathological changes in the brain and inflammation. This leads to damage of the blood-brain barrier, which occurs at the initial stage of the disease. However, the immune response activated by the intestinal tract is key to the subsequent vicious cycle: Intestinal immune response aggravates intestinal α-synuclein deposition; activated immune cells pass through the damaged blood-brain barrier through blood circulation. Both may cause CNS inflammation and irreversible neurodegeneration.
RY performed the literature review, drafted and reviewed the manuscript, and designed the figures. GG reviewed the manuscript. HY made critical revisions to the manuscript and provided study supervision. All authors contributed to the article and approved the submitted version.
This work was supported by grant of National Natural Science Foundation of China (81870994).
The authors declare that the research was conducted in the absence of any commercial or financial relationships that could be construed as a potential conflict of interest.
All claims expressed in this article are solely those of the authors and do not necessarily represent those of their affiliated organizations, or those of the publisher, the editors and the reviewers. Any product that may be evaluated in this article, or claim that may be made by its manufacturer, is not guaranteed or endorsed by the publisher.
Abdel-Haq, R., Schlachetzki, J. C. M., Glass, C. K., and Mazmanian, S. K. (2019). Microbiome-microglia connections via the gut-brain axis. J. Exp. Med. 216, 41–59. doi: 10.1084/jem.20180794
Ahlers-Dannen, K. E., Spicer, M. M., and Fisher, R. A. (2020). RGS Proteins as critical regulators of motor function and their implications in Parkinson's disease. Mol. Pharmacol. 98, 730–738. doi: 10.1124/mol.119.118836
Ahn, E. H., Kang, S. S., Liu, X., Cao, X., Choi, S. Y., Musazzi, L., et al. (2021). BDNF and Netrin-1 repression by C/EBPβ in the gut triggers Parkinson's disease pathologies, associated with constipation and motor dysfunctions. Prog. Neurobiol. 198, 101905. doi: 10.1016/j.pneurobio.2020.101905
Aho, V. T. E., Houser, M. C., Pereira, P. A. B., Chang, J., Rudi, K., Paulin, L., et al. (2021). Relationships of gut microbiota, short-chain fatty acids, inflammation, and the gut barrier in Parkinson's disease. Mol. Neurodegener. 16, 6. doi: 10.1186/s13024-021-00427-6
Alam, M. M., Yang, D., Li, X. Q., Liu, J., Back, T. C., Trivett, A., et al. (2022). Alpha synuclein, the culprit in Parkinson disease, is required for normal immune function. Cell Rep. 38, 110090. doi: 10.1016/j.celrep.2021.110090
Al-Asmakh, M., and Hedin, L. (2015). Microbiota and the control of blood-tissue barriers. Tissue Barriers 3, e1039691. doi: 10.1080/21688370.2015.1039691
Anderson, G., Noorian, A. R., Taylor, G., Anitha, M., Bernhard, D., Srinivasan, S., et al. (2007). Loss of enteric dopaminergic neurons and associated changes in colon motility in an MPTP mouse model of Parkinson's disease. Exp. Neurol. 207, 4–12. doi: 10.1016/j.expneurol.2007.05.010
Angot, E., Steiner, J. A., Lema Tomé, C. M., Ekström, P., Mattsson, B., Björklund, A., et al. (2012). Alpha-synuclein cell-to-cell transfer and seeding in grafted dopaminergic neurons in vivo. PLoS ONE 7:e39465. doi: 10.1371/journal.pone.0039465
Annerino, D. M., Arshad, S., Taylor, G. M., Adler, C. H., Beach, T. G., and Greene, J. G. (2012). Parkinson's disease is not associated with gastrointestinal myenteric ganglion neuron loss. Acta Neuropathol. 124, 665–680. doi: 10.1007/s00401-012-1040-2
Baird, J. K., Bourdette, D., Meshul, C. K., and Quinn, J. F. (2019). The key role of T cells in Parkinson's disease pathogenesis and therapy. Parkinson. Relat. Disord. 60, 25–31. doi: 10.1016/j.parkreldis.2018.10.029
Baruch, K., Rosenzweig, N., Kertser, A., Deczkowska, A., Sharif, A. M., Spinrad, A., et al. (2015). Breaking immune tolerance by targeting Foxp3(+) regulatory T cells mitigates Alzheimer's disease pathology. Nat. Commun. 6, 7967. doi: 10.1038/ncomms8967
Beach, T. G., Adler, C. H., Sue, L. I., Shill, H. A., Driver-Dunckley, E., Mehta, S. H., et al. (2021). Vagus nerve and stomach synucleinopathy in parkinson's disease, incidental lewy body disease, and normal elderly subjects: evidence against the “Body-First” hypothesis. J. Parkinsons. Dis. 11, 1833–1843. doi: 10.3233/jpd-212733
Bohórquez, D. V., Shahid, R. A., Erdmann, A., Kreger, A. M., Wang, Y., Calakos, N., et al. (2015). Neuroepithelial circuit formed by innervation of sensory enteroendocrine cells. J. Clin. Invest. 125, 782–786. doi: 10.1172/jci78361
Borghammer, P., Horsager, J., Andersen, K., Van Den Berge, N., Raunio, A., Murayama, S., et al. (2021). Neuropathological evidence of body-first vs. brain-first Lewy body disease. Neurobiol. Dis. 161, 105557. doi: 10.1016/j.nbd.2021.105557
Braak, H., De Vos, R. A., Bohl, J., and Del Tredici, K. (2006). Gastric alpha-synuclein immunoreactive inclusions in Meissner's and Auerbach's plexuses in cases staged for Parkinson's disease-related brain pathology. Neurosci. Lett. 396, 67–72. doi: 10.1016/j.neulet.2005.11.012
Braak, H., Sastre, M., Bohl, J. R., De Vos, R. A., and Del Tredici, K. (2007). Parkinson's disease: lesions in dorsal horn layer I, involvement of parasympathetic and sympathetic pre- and postganglionic neurons. Acta Neuropathol. 113, 421–429. doi: 10.1007/s00401-007-0193-x
Braniste, V., Al-Asmakh, M., Kowal, C., Anuar, F., Abbaspour, A., Tóth, M., et al. (2014). The gut microbiota influences blood-brain barrier permeability in mice. Sci. Transl. Med. 6, 263ra158. doi: 10.1126/scitranslmed.3009759
Brochard, V., Combadière, B., Prigent, A., Laouar, Y., Perrin, A., Beray-Berthat, V., et al. (2009). Infiltration of CD4+ lymphocytes into the brain contributes to neurodegeneration in a mouse model of Parkinson disease. J. Clin. Invest. 119, 182–192. doi: 10.1172/JCI36470
Brudek, T. (2019). Inflammatory bowel diseases and Parkinson's disease. J. Parkinsons. Dis. 9, S331–s344. doi: 10.3233/jpd-191729
Burré, J., Sharma, M., and Südhof, T. C. (2018). Cell biology and pathophysiology of α-synuclein. Cold Spring Harb. Perspect. Med. 8, a024091. doi: 10.1101/cshperspect.a024091
Campos-Acuña, J., Elgueta, D., and Pacheco, R. (2019). T-cell-driven inflammation as a mediator of the gut-brain axis involved in Parkinson's disease. Front. Immunol. 10:239. doi: 10.3389/fimmu.2019.00239
Cersosimo, M. G., Raina, G. B., Pecci, C., Pellene, A., Calandra, C. R., Gutierrez, C., et al. (2013). Gastrointestinal manifestations in Parkinson's disease: prevalence and occurrence before motor symptoms. J. Neurol. 260, 1332–1338. doi: 10.1007/s00415-012-6801-2
Chandra, R., Hiniker, A., Kuo, Y. M., Nussbaum, R. L., and Liddle, R. A. (2017). α-Synuclein in gut endocrine cells and its implications for Parkinson's disease. JCI Insight 2, e92295. doi: 10.1172/jci.insight.92295
Chang, J., Leong, R. W., Wasinger, V. C., Ip, M., Yang, M., and Phan, T. G. (2017). Impaired intestinal permeability contributes to ongoing bowel symptoms in patients with inflammatory bowel disease and mucosal healing. Gastroenterology 153, 723–731.e721. doi: 10.1053/j.gastro.2017.05.056
Chang, J. T. (2020). Pathophysiology of inflammatory bowel diseases. N. Engl. J. Med. 383, 2652–2664. doi: 10.1056/NEJMra2002697
Chen, Q. Q., Haikal, C., Li, W., and Li, J. Y. (2019). Gut inflammation in association with pathogenesis of Parkinson's disease. Front. Mol. Neurosci. 12:218. doi: 10.3389/fnmol.2019.00218
Chen, Q. Q., Haikal, C., Li, W., Li, M. T., Wang, Z. Y., and Li, J. Y. (2018a). Age-dependent alpha-synuclein accumulation and aggregation in the colon of a transgenic mouse model of Parkinson's disease. Transl. Neurodegener. 7, 13. doi: 10.1186/s40035-018-0118-8
Chen, S. J., Chen, C. C., Liao, H. Y., Lin, Y. T., Wu, Y. W., Liou, J. M., et al. (2022). Association of fecal and plasma levels of short-chain fatty acids with gut microbiota and clinical severity in Parkinson disease patients. Neurology 98, e848–e858. doi: 10.1212/wnl.0000000000013225
Chen, T., Kim, C. Y., Kaur, A., Lamothe, L., Shaikh, M., Keshavarzian, A., et al. (2017). Dietary fibre-based SCFA mixtures promote both protection and repair of intestinal epithelial barrier function in a Caco-2 cell model. Food Funct. 8, 1166–1173. doi: 10.1039/c6fo01532h
Chen, X., Berin, M. C., Gillespie, V. L., Sampson, H. A., and Dunkin, D. (2021). Treatment of intestinal inflammation with epicutaneous immunotherapy requires TGF-β and IL-10 but not Foxp3(+) tregs. Front. Immunol. 12:637630. doi: 10.3389/fimmu.2021.637630
Chen, Z., Chen, S., and Liu, J. (2018b). The role of T cells in the pathogenesis of Parkinson's disease. Prog. Neurobiol. 169, 1–23. doi: 10.1016/j.pneurobio.2018.08.002
Cholan, P. M., Han, A., Woodie, B. R., Watchon, M., Kurz, A. R., Laird, A. S., et al. (2020). Conserved anti-inflammatory effects and sensing of butyrate in zebrafish. Gut Microbes 12, 1–11. doi: 10.1080/19490976.2020.1824563
Clairembault, T., Leclair-Visonneau, L., Coron, E., Bourreille, A., Le Dily, S., Vavasseur, F., et al. (2015a). Structural alterations of the intestinal epithelial barrier in Parkinson's disease. Acta Neuropathol. Commun. 3, 12. doi: 10.1186/s40478-015-0196-0
Clairembault, T., Leclair-Visonneau, L., Neunlist, M., and Derkinderen, P. (2015b). Enteric glial cells: new players in Parkinson's disease? Mov. Disord. 30, 494–498. doi: 10.1002/mds.25979
Contreras, F., Prado, C., González, H., Franz, D., Osorio-Barrios, F., Osorio, F., et al. (2016). Dopamine receptor D3 signaling on CD4+ T cells favors Th1- and Th17-mediated immunity. J. Immunol. 196, 4143–4149. doi: 10.4049/jimmunol.1502420
Côté, M., Drouin-Ouellet, J., Cicchetti, F., and Soulet, D. (2011). The critical role of the MyD88-dependent pathway in non-CNS MPTP-mediated toxicity. Brain Behav. Immun. 25, 1143–1152. doi: 10.1016/j.bbi.2011.02.017
Côté, M., Poirier, A. A., Aubé, B., Jobin, C., Lacroix, S., and Soulet, D. (2015). Partial depletion of the proinflammatory monocyte population is neuroprotective in the myenteric plexus but not in the basal ganglia in a MPTP mouse model of Parkinson's disease. Brain Behav. Immun. 46, 154–167. doi: 10.1016/j.bbi.2015.01.009
Cox, L. M., and Weiner, H. L. (2018). Microbiota signaling pathways that influence neurologic disease. Neurotherapeuticss 15, 135–145. doi: 10.1007/s13311-017-0598-8
Cryan, J. F., O'riordan, K. J., Cowan, C. S. M., Sandhu, K. V., Bastiaanssen, T. F. S., Boehme, M., et al. (2019). The microbiota-gut-brain axis. Physiol. Rev. 99, 1877–2013. doi: 10.1152/physrev.00018.2018
D'Andrea, G., Pizzolato, G., Gucciardi, A., Stocchero, M., Giordano, G., Baraldi, E., et al. (2019). Different circulating trace amine profiles in de novo and treated Parkinson's disease patients. Sci. Rep. 9, 6151. doi: 10.1038/s41598-019-42535-w
Dardalhon, V., Korn, T., Kuchroo, V. K., and Anderson, A. C. (2008). Role of Th1 and Th17 cells in organ-specific autoimmunity. J. Autoimmun. 31, 252–256. doi: 10.1016/j.jaut.2008.04.017
Del Tredici, K., and Braak, H. (2016). Review: sporadic Parkinson's disease: development and distribution of α-synuclein pathology. Neuropathol. Appl. Neurobiol. 42, 33–50. doi: 10.1111/nan.12298
Devos, D., Lebouvier, T., Lardeux, B., Biraud, M., Rouaud, T., Pouclet, H., et al. (2013). Colonic inflammation in Parkinson's disease. Neurobiol. Dis. 50, 42–48. doi: 10.1016/j.nbd.2012.09.007
Edwards, L. L., Pfeiffer, R. F., Quigley, E. M., Hofman, R., and Balluff, M. (1991). Gastrointestinal symptoms in Parkinson's disease. Mov. Disord. 6, 151–156. doi: 10.1002/mds.870060211
Ehgoetz Martens, K. A., and Lewis, S. J. (2017). Pathology of behavior in PD: what is known and what is not? J. Neurol. Sci. 374, 9–16. doi: 10.1016/j.jns.2016.12.062
Elfil, M., Kamel, S., Kandil, M., Koo, B. B., and Schaefer, S. M. (2020). Implications of the gut microbiome in Parkinson's disease. Mov. Disord. 35, 921–933. doi: 10.1002/mds.28004
Erny, D., Hrabě De Angelis, A. L., Jaitin, D., Wieghofer, P., Staszewski, O., David, E., et al. (2015). Host microbiota constantly control maturation and function of microglia in the CNS. Nat. Neurosci. 18, 965–977. doi: 10.1038/nn.4030
Fang, L., Pang, Z., Shu, W., Wu, W., Sun, M., Cong, Y., et al. (2018). Anti-TNF therapy induces CD4+ T-cell production of IL-22 and promotes epithelial repairs in patients with Crohn's disease. Inflamm. Bowel Dis. 24, 1733–1744. doi: 10.1093/ibd/izy126
Fellner, L., and Stefanova, N. (2013). The role of glia in α-synucleinopathies. Mol. Neurobiol. 47, 575–586. doi: 10.1007/s12035-012-8340-3
Fereshtehnejad, S.-M., Yao, C., Pelletier, A., Montplaisir, J. Y., Gagnon, J.-F., and Postuma, R. B. (2019). Evolution of prodromal Parkinson's disease and dementia with Lewy bodies: a prospective study. Brain 142, 2051–2067. doi: 10.1093/brain/awz111
Foster, J. A., and McVey Neufeld, K.-A. (2013). Gut-brain axis: how the microbiome influences anxiety and depression. Trends Neurosci. 36, 305–312. doi: 10.1016/j.tins.2013.01.005
Garrido-Gil, P., Rodriguez-Perez, A. I., Dominguez-Meijide, A., Guerra, M. J., and Labandeira-Garcia, J. L. (2018). Bidirectional neural interaction between central dopaminergic and gut lesions in Parkinson's disease models. Mol. Neurobiol. 55, 7297–7316. doi: 10.1007/s12035-018-0937-8
Gate, D., Tapp, E., Leventhal, O., Shahid, M., Nonninger, T. J., Yang, A. C., et al. (2021). CD4(+) T cells contribute to neurodegeneration in Lewy body dementia. Science 374, 868–874. doi: 10.1126/science.abf7266
Gelpi, E., Navarro-Otano, J., Tolosa, E., Gaig, C., Compta, Y., Rey, M. J., et al. (2014). Multiple organ involvement by alpha-synuclein pathology in Lewy body disorders. Mov. Disord. 29, 1010–1018. doi: 10.1002/mds.25776
George, S., Tyson, T., Rey, N. L., Sheridan, R., Peelaerts, W., Becker, K., et al. (2021). T cells limit accumulation of aggregate pathology following intrastriatal injection of α-synuclein fibrils. J. Parkinsons. Dis. 11, 585–603. doi: 10.3233/jpd-202351
Gershanik, O. S. (2018). Does Parkinson's disease start in the gut? Arq. Neuropsiquiatr. 76, 67–70. doi: 10.1590/0004-282X20170188
González, H., Contreras, F., and Pacheco, R. (2015). Regulation of the neurodegenerative process associated to Parkinson's disease by CD4+ T-cells. J. Neuroimmune Pharmacol. 10, 561–575. doi: 10.1007/s11481-015-9618-9
Gordevicius, J., Li, P., Marshall, L. L., Killinger, B. A., Lang, S., Ensink, E., et al. (2021). Epigenetic inactivation of the autophagy-lysosomal system in appendix in Parkinson's disease. Nat. Commun. 12, 5134. doi: 10.1038/s41467-021-25474-x
Gordon, R., Albornoz, E. A., Christie, D. C., Langley, M. R., Kumar, V., Mantovani, S., et al. (2018). Inflammasome inhibition prevents α-synuclein pathology and dopaminergic neurodegeneration in mice. Sci. Transl. Med. 10, eaah4066. doi: 10.1126/scitranslmed.aah4066
Granlund, A. V. B., Flatberg, A., Østvik, A. E., Drozdov, I., Gustafsson, B. I., Kidd, M., et al. (2013). Whole genome gene expression meta-analysis of inflammatory bowel disease colon mucosa demonstrates lack of major differences between Crohn's disease and ulcerative colitis. PLoS ONE 8:e56818. doi: 10.1371/journal.pone.0056818
Gray, M. T., Munoz, D. G., Gray, D. A., Schlossmacher, M. G., and Woulfe, J. M. (2014). Alpha-synuclein in the appendiceal mucosa of neurologically intact subjects. Mov. Disord. 29, 991–998. doi: 10.1002/mds.25779
Grochowska, M. M., Carreras Mascaro, A., Boumeester, V., Natale, D., Breedveld, G. J., Geut, H., et al. (2021). LRP10 interacts with SORL1 in the intracellular vesicle trafficking pathway in non-neuronal brain cells and localises to Lewy bodies in Parkinson's disease and dementia with Lewy bodies. Acta Neuropathol. 142, 117–137. doi: 10.1007/s00401-021-02313-3
Grosso Jasutkar, H., Oh, S. E., and Mouradian, M. M. (2022). Therapeutics in the pipeline targeting α-synuclein for Parkinson's disease. Pharmacol. Rev. 74, 207–237. doi: 10.1124/pharmrev.120.000133
Grundmann, D., Loris, E., Maas-Omlor, S., Huang, W., Scheller, A., Kirchhoff, F., et al. (2019). Enteric glia: S100, GFAP, and beyond. Anat. Rec. 302, 1333–1344. doi: 10.1002/ar.24128
Hilton, D., Stephens, M., Kirk, L., Edwards, P., Potter, R., Zajicek, J., et al. (2014). Accumulation of alpha-synuclein in the bowel of patients in the pre-clinical phase of Parkinson's disease. Acta Neuropathol. 127, 235–241. doi: 10.1007/s00401-013-1214-6
Horsager, J., Andersen, K. B., Knudsen, K., Skjærbæk, C., Fedorova, T. D., Okkels, N., et al. (2020). Brain-first versus body-first Parkinson's disease: a multimodal imaging case-control study. Brain 143, 3077–3088. doi: 10.1093/brain/awaa238
Huang, Y., Liu, Z., Cao, B. B., Qiu, Y. H., and Peng, Y. P. (2017). Treg cells protect dopaminergic neurons against MPP+ neurotoxicity via CD47-SIRPA interaction. Cell Physiol. Biochem. 41, 1240–1254. doi: 10.1159/000464388
Huang, Y., Liu, Z., Cao, B. B., Qiu, Y. H., and Peng, Y. P. (2020). Treg cells attenuate neuroinflammation and protect neurons in a mouse model of Parkinson's disease. J. Neuroimmune Pharmacol. 15, 224–237. doi: 10.1007/s11481-019-09888-5
Hui, K. Y., Fernandez-Hernandez, H., Hu, J., Schaffner, A., Pankratz, N., Hsu, N.-Y., et al. (2018). Functional variants in the gene confer shared effects on risk for Crohn's disease and Parkinson's disease. Sci. Transl. Med. 10, eaai7795. doi: 10.1126/scitranslmed.aai7795
Hurt, C. S., Rixon, L., Chaudhuri, K. R., Moss-Morris, R., Samuel, M., and Brown, R. G. (2019). Barriers to reporting non-motor symptoms to health-care providers in people with Parkinson's. Parkinson. Relat. Disord. 64, 220–225. doi: 10.1016/j.parkreldis.2019.04.014
Hussein, A., Guevara, C. A., Del Valle, P., Gupta, S., Benson, D. L., and Huntley, G. W. (2021). Non-motor symptoms of Parkinson's disease: the neurobiology of early psychiatric and cognitive dysfunction. Neuroscientist doi: 10.1177/10738584211011979. [Epub ahead of print].
Jarret, A., Jackson, R., Duizer, C., Healy, M. E., Zhao, J., Rone, J. M., et al. (2020). Enteric nervous system-derived IL-18 orchestrates mucosal barrier immunity. Cell 180, 50–63 e12. doi: 10.1016/j.cell.2019.12.016
Kaiko, G. E., Horvat, J. C., Beagley, K. W., and Hansbro, P. M. (2008). Immunological decision-making: how does the immune system decide to mount a helper T-cell response? Immunology 123, 326–338. doi: 10.1111/j.1365-2567.2007.02719.x
Karunaratne, T. B., Okereke, C., Seamon, M., Purohit, S., Wakade, C., and Sharma, A. (2020). Niacin and butyrate: nutraceuticals targeting dysbiosis and intestinal permeability in Parkinson's disease. Nutrients 13, 28. doi: 10.3390/nu13010028
Kelly, L. P., Carvey, P. M., Keshavarzian, A., Shannon, K. M., Shaikh, M., Bakay, R. A., et al. (2014). Progression of intestinal permeability changes and alpha-synuclein expression in a mouse model of Parkinson's disease. Mov. Disord. 29, 999–1009. doi: 10.1002/mds.25736
Killinger, B. A., Madaj, Z., Sikora, J. W., Rey, N., Haas, A. J., Vepa, Y., et al. (2018). The vermiform appendix impacts the risk of developing Parkinson's disease. Sci. Transl. Med. 10, eaar5280. doi: 10.1126/scitranslmed.aar5280
Kim, S., Kwon, S. H., Kam, T. I., Panicker, N., Karuppagounder, S. S., Lee, S., et al. (2019). Transneuronal propagation of pathologic alpha-synuclein from the gut to the brain models Parkinson's disease. Neuron 103, 627–641.e7. doi: 10.1016/j.neuron.2019.05.035
Kishimoto, Y., Zhu, W., Hosoda, W., Sen, J. M., and Mattson, M. P. (2019). Chronic mild gut inflammation accelerates brain neuropathology and motor dysfunction in α-synuclein mutant mice. Neuromol. Med. 21, 239–249. doi: 10.1007/s12017-019-08539-5
Knudsen, K., Fedorova, T. D., Horsager, J., Andersen, K. B., Skjærbæk, C., Berg, D., et al. (2021). Asymmetric dopaminergic dysfunction in brain-first versus body-first Parkinson's disease subtypes. J. Parkinsons. Dis. 11, 1677–1687. doi: 10.3233/jpd-212761
Knudsen, K., Krogh, K., Østergaard, K., and Borghammer, P. (2017). Constipation in parkinson's disease: subjective symptoms, objective markers, and new perspectives. Mov. Disord. 32, 94–105. doi: 10.1002/mds.26866
Koprich, J. B., Johnston, T. H., Reyes, M. G., Sun, X., and Brotchie, J. M. (2010). Expression of human A53T alpha-synuclein in the rat substantia nigra using a novel AAV1/2 vector produces a rapidly evolving pathology with protein aggregation, dystrophic neurite architecture and nigrostriatal degeneration with potential to model the pathology of Parkinson's disease. Mol. Neurodegener. 5, 43. doi: 10.1186/1750-1326-5-43
Korn, T., and Kallies, A. (2017). T cell responses in the central nervous system. Nat. Rev. Immunol. 17, 179–194. doi: 10.1038/nri.2016.144
Kredel, L. I., Jödicke, L. J., Scheffold, A., Gröne, J., Glauben, R., Erben, U., et al. (2019). T-cell composition in ileal and colonic creeping fat – separating ileal from colonic Crohn's disease. J. Crohns Colitis 13, 79–91. doi: 10.1093/ecco-jcc/jjy146
Kurnik, M., Gil, K., Gajda, M., Thor, P., and Bugajski, A. (2015). Neuropathic alterations of the myenteric plexus neurons following subacute intraperitoneal administration of salsolinol. Folia Histochem. Cytobiol. 53, 49–61. doi: 10.5603/FHC.a2015.0010
Kustrimovic, N., Comi, C., Magistrelli, L., Rasini, E., Legnaro, M., Bombelli, R., et al. (2018). Parkinson's disease patients have a complex phenotypic and functional Th1 bias: cross-sectional studies of CD4+ Th1/Th2/T17 and Treg in drug-naïve and drug-treated patients. J. Neuroinflammation 15, 205. doi: 10.1186/s12974-018-1248-8
Latorre, R., Sternini, C., De Giorgio, R., and Greenwood-Van Meerveld, B. (2016). Enteroendocrine cells: a review of their role in brain-gut communication. Neurogastroenterol. Motil. 28, 620–630. doi: 10.1111/nmo.12754
Lee, H. S., Lobbestael, E., Vermeire, S., Sabino, J., and Cleynen, I. (2021). Inflammatory bowel disease and Parkinson's disease: common pathophysiological links. Gut 70, 408–417. doi: 10.1136/gutjnl-2020-322429
Levite, M. (2016). Dopamine and T cells: dopamine receptors and potent effects on T cells, dopamine production in T cells, and abnormalities in the dopaminergic system in T cells in autoimmune, neurological and psychiatric diseases. Acta Physiol. 216, 42–89. doi: 10.1111/apha.12476
Li, H., Fan, C., Lu, H., Feng, C., He, P., Yang, X., et al. (2020). Protective role of berberine on ulcerative colitis through modulating enteric glial cells-intestinal epithelial cells-immune cells interactions. Acta Pharm. Sin. B 10, 447–461. doi: 10.1016/j.apsb.2019.08.006
Li, J., Ueno, A., Fort Gasia, M., Luider, J., Wang, T., Hirota, C., et al. (2016). Profiles of lamina propria T helper cell subsets discriminate between ulcerative colitis and Crohn's disease. Inflamm. Bowel Dis. 22, 1779–1792. doi: 10.1097/mib.0000000000000811
Liddle, R. A. (2018). Interactions of gut endocrine cells with epithelium and neurons. Compr. Physiol. 8, 1019–1030. doi: 10.1002/cphy.c170044
Lin, C. H., Lin, H. Y., Ho, E. P., Ke, Y. C., Cheng, M. F., Shiue, C. Y., et al. (2021). Mild chronic colitis triggers Parkinsonism in LRRK2 mutant mice through activating TNF-α pathway. Mov. Disord. 37, 745–757. doi: 10.1002/mds.28890
Lin, J. C., Lin, C. S., Hsu, C. W., Lin, C. L., and Kao, C. H. (2016). Association between Parkinson's disease and inflammatory bowel disease: a nationwide Taiwanese retrospective cohort study. Inflamm. Bowel Dis. 22, 1049–1055. doi: 10.1097/mib.0000000000000735
Liu, D., Guo, J. J., Su, J. H., Svanbergsson, A., Yuan, L., Haikal, C., et al. (2021a). Differential seeding and propagating efficiency of α-synuclein strains generated in different conditions. Transl. Neurodegener. 10, 20. doi: 10.1186/s40035-021-00242-5
Liu, X., Du, Z. R., Wang, X., Luk, K. H., Chan, C. H., Cao, X., et al. (2021b). Colonic dopaminergic neurons changed reversely with those in the midbrain via gut microbiota-mediated autophagy in a chronic Parkinson's disease mice model. Front. Aging Neurosci. 13:649627. doi: 10.3389/fnagi.2021.649627
Liu, Y.-H., Ding, Y., Gao, C.-C., Li, L.-S., Wang, Y.-X., and Xu, J.-D. (2018). Functional macrophages and gastrointestinal disorders. World J. Gastroenterol. 24, 1181–1195. doi: 10.3748/wjg.v24.i11.1181
Liu, Z., Huang, Y., Cao, B.-B., Qiu, Y.-H., and Peng, Y.-P. (2017). Th17 cells induce dopaminergic neuronal death via LFA-1/ICAM-1 interaction in a mouse model of Parkinson's disease. Mol. Neurobiol. 54, 7762–7776. doi: 10.1007/s12035-016-0249-9
Luissint, A. C., Parkos, C. A., and Nusrat, A. (2016). Inflammation and the intestinal barrier: leukocyte-epithelial cell interactions, cell junction remodeling, and mucosal repair. Gastroenterology 151, 616–632. doi: 10.1053/j.gastro.2016.07.008
Machado, A. R. P., Zaidan, H. C., Paixão, A. P. S., Cavalheiro, G. L., Oliveira, F. H. M., Júnior, J., et al. (2016). Feature visualization and classification for the discrimination between individuals with Parkinson's disease under levodopa and DBS treatments. Biomed. Eng. Online 15, 169. doi: 10.1186/s12938-016-0290-y
Manfredsson, F. P., Luk, K. C., Benskey, M. J., Gezer, A., Garcia, J., Kuhn, N. C., et al. (2018). Induction of alpha-synuclein pathology in the enteric nervous system of the rat and non-human primate results in gastrointestinal dysmotility and transient CNS pathology. Neurobiol. Dis. 112, 106–118. doi: 10.1016/j.nbd.2018.01.008
Matheoud, D., Cannon, T., Voisin, A., Penttinen, A.-M., Ramet, L., Fahmy, A. M., et al. (2019). Intestinal infection triggers Parkinson's disease-like symptoms in Pink1 mice. Nature 571, 565–569. doi: 10.1038/s41586-019-1405-y
Ménard, S., Cerf-Bensussan, N., and Heyman, M. (2010). Multiple facets of intestinal permeability and epithelial handling of dietary antigens. Mucosal Immunol. 3, 247–259. doi: 10.1038/mi.2010.5
Moehle, M. S., and West, A. B. (2015). M1 and M2 immune activation in Parkinson's disease: foe and ally? Neuroscience 302, 59–73. doi: 10.1016/j.neuroscience.2014.11.018
Nagyoszi, P., Wilhelm, I., Farkas, A. E., Fazakas, C., Dung, N. T. K., Haskó, J., et al. (2010). Expression and regulation of toll-like receptors in cerebral endothelial cells. Neurochem. Int. 57, 556–564. doi: 10.1016/j.neuint.2010.07.002
Nalls, M. A., Pankratz, N., Lill, C. M., Do, C. B., Hernandez, D. G., Saad, M., et al. (2014). Large-scale meta-analysis of genome-wide association data identifies six new risk loci for Parkinson's disease. Nat. Genet. 46, 989–993. doi: 10.1038/ng.3043
Nerius, M., Doblhammer, G., and Tamgüney, G. (2020). GI infections are associated with an increased risk of Parkinson's disease. Gut 69, 1154–1156. doi: 10.1136/gutjnl-2019-318822
Oreja-Guevara, C., Forsyth, C. B., Shannon, K. M., Kordower, J. H., Voigt, R. M., Shaikh, M., et al. (2011). Increased intestinal permeability correlates with sigmoid mucosa alpha-synuclein staining and endotoxin exposure markers in early Parkinson's disease. PLoS ONE 6:e28032. doi: 10.1371/journal.pone.0028032
Pacheco, R., Prado, C. E., Barrientos, M. J., and Bernales, S. (2009). Role of dopamine in the physiology of T-cells and dendritic cells. J. Neuroimmunol. 216, 8–19. doi: 10.1016/j.jneuroim.2009.07.018
Peter, I., Dubinsky, M., Bressman, S., Park, A., Lu, C., Chen, N., et al. (2018). Anti-tumor necrosis factor therapy and incidence of parkinson disease among patients with inflammatory bowel disease. JAMA Neurol. 75, 939–946. doi: 10.1001/jamaneurol.2018.0605
Poewe, W., Seppi, K., Tanner, C. M., Halliday, G. M., Brundin, P., Volkmann, J., et al. (2017). Parkinson disease. Nat. Rev. Dis. Primers 3, 17013. doi: 10.1038/nrdp.2017.13
Powrie, F., and Mason, D. (1990). OX-22high CD4+ T cells induce wasting disease with multiple organ pathology: prevention by the OX-22low subset. J. Exp. Med. 172, 1701–1708. doi: 10.1084/jem.172.6.1701
Puzan, M., Hosic, S., Ghio, C., and Koppes, A. (2018). Enteric nervous system regulation of intestinal stem cell differentiation and epithelial monolayer function. Sci. Rep. 8, 6313. doi: 10.1038/s41598-018-24768-3
Quigley, E. M. M. (2017). Microbiota-brain-gut axis and neurodegenerative diseases. Curr. Neurol. Neurosci. Rep. 17, 94. doi: 10.1007/s11910-017-0802-6
Quinn, J. L., and Axtell, R. C. (2018). Emerging role of follicular T helper cells in multiple sclerosis and experimental autoimmune encephalomyelitis. Int. J. Mol. Sci. 19. doi: 10.3390/ijms19103233
Rabiei, Z., Solati, K., and Amini-Khoei, H. (2019). Phytotherapy in treatment of Parkinson's disease: a review. Pharm. Biol. 57, 355–362. doi: 10.1080/13880209.2019.1618344
Ravenstijn, P. G., Drenth, H.-J., O'neill, M. J., Danhof, M., and De Lange, E. C. (2012). Evaluation of blood-brain barrier transport and CNS drug metabolism in diseased and control brain after intravenous L-DOPA in a unilateral rat model of Parkinson's disease. Fluids Barriers CNS 9, 4. doi: 10.1186/2045-8118-9-4
Rolli-Derkinderen, M., Leclair-Visonneau, L., Bourreille, A., Coron, E., Neunlist, M., and Derkinderen, P. (2020). Is Parkinson's disease a chronic low-grade inflammatory bowel disease? J. Neurol. 267, 2207–2213. doi: 10.1007/s00415-019-09321-0
Rota, L., Pellegrini, C., Benvenuti, L., Antonioli, L., Fornai, M., Blandizzi, C., et al. (2019). Constipation, deficit in colon contractions and alpha-synuclein inclusions within the colon precede motor abnormalities and neurodegeneration in the central nervous system in a mouse model of alpha-synucleinopathy. Transl. Neurodegener. 8, 5. doi: 10.1186/s40035-019-0146-z
Rutsch, A., Kantsjö, J. B., and Ronchi, F. (2020). The gut-brain axis: how microbiota and host inflammasome influence brain physiology and pathology. Front. Immunol. 11:604179. doi: 10.3389/fimmu.2020.604179
Sakakibara, R., Doi, H., and Fukudo, S. (2019). Lewy body constipation. J. Anus Rectum Colon 3, 10–17. doi: 10.23922/jarc.2018-022
Sampson, T. R., Debelius, J. W., Thron, T., Janssen, S., Shastri, G. G., Ilhan, Z. E., et al. (2016). Gut microbiota regulate motor deficits and neuroinflammation in a model of Parkinson's disease. Cell 167, 1469–1480.e12. doi: 10.1016/j.cell.2016.11.018
Santos, S. F., De Oliveira, H. L., Yamada, E. S., Neves, B. C., and Pereira, A. Jr. (2019). The gut and Parkinson's disease-a bidirectional pathway. Front. Neurol. 10:574. doi: 10.3389/fneur.2019.00574
Savica, R., Bradley, B. F., and Mielke, M. M. (2018). When do α-synucleinopathies start? An epidemiological timeline: a review. JAMA Neurol. 75, 503–509. doi: 10.1001/jamaneurol.2017.4243
Savica, R., Carlin, J. M., Grossardt, B. R., Bower, J. H., Ahlskog, J. E., Maraganore, D. M., et al. (2009). Medical records documentation of constipation preceding Parkinson disease: a case-control study. Neurology 73, 1752–1758. doi: 10.1212/WNL.0b013e3181c34af5
Schapira, A. H. V., Chaudhuri, K. R., and Jenner, P. (2017). Non-motor features of Parkinson disease. Nat. Rev. Neurosci. 18, 435–450. doi: 10.1038/nrn.2017.62
Schwartz, M., and Deczkowska, A. (2016). Neurological disease as a failure of brain-immune crosstalk: the multiple faces of neuroinflammation. Trends Immunol. 37, 668–679. doi: 10.1016/j.it.2016.08.001
Schwiertz, A., Spiegel, J., Dillmann, U., Grundmann, D., Burmann, J., Fassbender, K., et al. (2018). Fecal markers of intestinal inflammation and intestinal permeability are elevated in Parkinson's disease. Parkinson. Relat. Disord. 50, 104–107. doi: 10.1016/j.parkreldis.2018.02.022
Scott, K. A., Ida, M., Peterson, V. L., Prenderville, J. A., Moloney, G. M., Izumo, T., et al. (2017). Revisiting Metchnikoff: age-related alterations in microbiota-gut-brain axis in the mouse. Brain Behav. Immun. 65, 20–32. doi: 10.1016/j.bbi.2017.02.004
Shannon, K. M., Keshavarzian, A., Mutlu, E., Dodiya, H. B., Daian, D., Jaglin, J. A., et al. (2012). Alpha-synuclein in colonic submucosa in early untreated Parkinson's disease. Mov. Disord. 27, 709–715. doi: 10.1002/mds.23838
Sharrad, D. F., De Vries, E., and Brookes, S. J. H. (2013). Selective expression of α-synuclein-immunoreactivity in vesicular acetylcholine transporter-immunoreactive axons in the guinea pig rectum and human colon. J. Comp. Neurol. 521, 657–676. doi: 10.1002/cne.23198
Singaram, C., Ashraf, W., Gaumnitz, E. A., Torbey, C., Sengupta, A., Pfeiffer, R., et al. (1995). Dopaminergic defect of enteric nervous system in Parkinson's disease patients with chronic constipation. Lancet 346, 861–864. doi: 10.1016/s0140-6736(95)92707-7
Srivastav, A., and Mohideen, S. S. (2021). Intestinal microbial dysbiosis induced tau accumulation establishes a gut-brain correlation for pathology Alzheimer's disease in Drosophila melanogaster. Alzheimers Dement. 17(Suppl. 2), e058708. doi: 10.1002/alz.058708
Stocchi, F., and Torti, M. (2017). Constipation in Parkinson's disease. Int. Rev. Neurobiol. 134, 811–826. doi: 10.1016/bs.irn.2017.06.003
Stolzenberg, E., Berry, D., Yang, L.ee, E. Y., Kroemer, A., Kaufman, S., Wong, G. C. L., et al. (2017). A role for neuronal alpha-synuclein in gastrointestinal immunity. J. Innate Immun. 9, 456–463. doi: 10.1159/000477990
Sulzer, D., Alcalay, R. N., Garretti, F., Cote, L., Kanter, E., Agin-Liebes, J., et al. (2017). T cells from patients with Parkinson's disease recognize α-synuclein peptides. Nature 546, 656–661. doi: 10.1038/nature22815
Sun, M., Wu, W., Chen, L., Yang, W., Huang, X., Ma, C., et al. (2018). Microbiota-derived short-chain fatty acids promote Th1 cell IL-10 production to maintain intestinal homeostasis. Nat. Commun. 9, 3555. doi: 10.1038/s41467-018-05901-2
Svensson, E., Horvath-Puho, E., Thomsen, R. W., Djurhuus, J. C., Pedersen, L., Borghammer, P., et al. (2015). Vagotomy and subsequent risk of Parkinson's disease. Ann. Neurol. 78, 522–529. doi: 10.1002/ana.24448
Swaminathan, M., Fung, C., Finkelstein, D. I., Bornstein, J. C., and Foong, J. P. P. (2019). Alpha-synuclein regulates development and function of cholinergic enteric neurons in the mouse colon. Neuroscience 423, 76–85. doi: 10.1016/j.neuroscience.2019.10.029
Sweeney, M. D., Sagare, A. P., and Zlokovic, B. V. (2018). Blood-brain barrier breakdown in Alzheimer disease and other neurodegenerative disorders. Nat. Rev. Neurol. 14, 133–150. doi: 10.1038/nrneurol.2017.188
Tang, A. T., Choi, J. P., Kotzin, J. J., Yang, Y., Hong, C. C., Hobson, N., et al. (2017). Endothelial TLR4 and the microbiome drive cerebral cavernous malformations. Nature 545, 305–310. doi: 10.1038/nature22075
Travagli, R. A., Browning, K. N., and Camilleri, M. (2020). Parkinson disease and the gut: new insights into pathogenesis and clinical relevance. Nat. Rev. Gastroenterol. Hepatol. 17, 673–685. doi: 10.1038/s41575-020-0339-z
Tysnes, O. B., Kenborg, L., Herlofson, K., Steding-Jessen, M., Horn, A., Olsen, J. H., et al. (2015). Does vagotomy reduce the risk of Parkinson's disease? Ann. Neurol. 78, 1011–1012. doi: 10.1002/ana.24531
Unger, M. M., Spiegel, J., Dillmann, K. U., Grundmann, D., Philippeit, H., Burmann, J., et al. (2016). Short chain fatty acids and gut microbiota differ between patients with Parkinson's disease and age-matched controls. Parkinson. Relat. Disord. 32, 66–72. doi: 10.1016/j.parkreldis.2016.08.019
Van Den Berge, N., Ferreira, N., Gram, H., Mikkelsen, T. W., Alstrup, A. K. O., Casadei, N., et al. (2019). Evidence for bidirectional and trans-synaptic parasympathetic and sympathetic propagation of alpha-synuclein in rats. Acta Neuropathol. 138, 535–550. doi: 10.1007/s00401-019-02040-w
Van Den Berge, N., Ferreira, N., Mikkelsen, T. W., Alstrup, A. K. O., Tamgüney, G., Karlsson, P., et al. (2021). Ageing promotes pathological alpha-synuclein propagation and autonomic dysfunction in wild-type rats. Brain 144, 1853–1868. doi: 10.1093/brain/awab061
Van Den Berge, N., and Ulusoy, A. (2022). Animal models of brain-first and body-first Parkinson's disease. Neurobiol. Dis. 163, 105599. doi: 10.1016/j.nbd.2021.105599
Varatharaj, A., and Galea, I. (2017). The blood-brain barrier in systemic inflammation. Brain Behav. Immun. 60. doi: 10.1016/j.bbi.2016.03.010
Wakabayashi, K., Takahashi, H., Ohama, E., Takeda, S., and Ikuta, F. (1993). Lewy bodies in the visceral autonomic nervous system in Parkinson's disease. Adv. Neurol. 60, 609–612.
Wan, Q.-Y., Zhao, R., and Wu, X.-T. (2020). Older patients with IBD might have higher risk of Parkinson's disease. Gut 69, 193–194. doi: 10.1136/gutjnl-2018-317103
Wang, L., Magen, I., Yuan, P. Q., Subramaniam, S. R., Richter, F., Chesselet, M. F., et al. (2012). Mice overexpressing wild-type human alpha-synuclein display alterations in colonic myenteric ganglia and defecation. Neurogastroenterol. Motil. 24, e425–e436. doi: 10.1111/j.1365-2982.2012.01974.x
Wiratman, W., Kobayashi, S., Chang, F.-Y., Asano, K., and Ugawa, Y. (2019). Assessment of cognitive and motor skills in Parkinson's disease by a robotic object hitting game. Front. Neurol. 10:19. doi: 10.3389/fneur.2019.00019
Xue, R., Zhang, H., Pan, J., Du, Z., Zhou, W., Zhang, Z., et al. (2018). Peripheral dopamine controlled by gut microbes inhibits invariant natural killer T cell-mediated hepatitis. Front. Immunol. 9:2398. doi: 10.3389/fimmu.2018.02398
Yamasaki, R., Lu, H., Butovsky, O., Ohno, N., Rietsch, A. M., Cialic, R., et al. (2014). Differential roles of microglia and monocytes in the inflamed central nervous system. J. Exp. Med. 211, 1533–1549. doi: 10.1084/jem.20132477
Yao, Y., Cai, X., Fei, W., Ye, Y., Zhao, M., and Zheng, C. (2022). The role of short-chain fatty acids in immunity, inflammation and metabolism. Crit. Rev. Food Sci. Nutr. 62, 1–12. doi: 10.1080/10408398.2020.1854675
Ye, L., Bae, M., Cassilly, C. D., Jabba, S. V., Thorpe, D. W., Martin, A. M., et al. (2021). Enteroendocrine cells sense bacterial tryptophan catabolites to activate enteric and vagal neuronal pathways. Cell Host Microbe 29, 179–196.e179. doi: 10.1016/j.chom.2020.11.011
Yuan, X., Tang, H., Wu, R., Li, X., Jiang, H., Liu, Z., et al. (2021). Short-chain fatty acids calibrate RARα activity regulating food sensitization. Front. Immunol. 12:737658. doi: 10.3389/fimmu.2021.737658
Zeng, H., and Chi, H. (2015). Metabolic control of regulatory T cell development and function. Trends Immunol. 36, 3–12. doi: 10.1016/j.it.2014.08.003
Zhong, C. B., Chen, Q. Q., Haikal, C., Li, W., Svanbergsson, A., Diepenbroek, M., et al. (2017). Age-dependent alpha-synuclein accumulation and phosphorylation in the enteric nervous system in a transgenic mouse model of Parkinson's disease. Neurosci. Bull. 33, 483–492. doi: 10.1007/s12264-017-0179-1
Keywords: Parkinson's disease (PD), α-synuclein (α-syn), propagation, vagus nerve, immune inflammation, microbial-intestinal-brain axis
Citation: Yang R, Gao G and Yang H (2022) The Pathological Mechanism Between the Intestine and Brain in the Early Stage of Parkinson's Disease. Front. Aging Neurosci. 14:861035. doi: 10.3389/fnagi.2022.861035
Received: 24 January 2022; Accepted: 02 June 2022;
Published: 24 June 2022.
Edited by:
Robert Petersen, Central Michigan University, United StatesReviewed by:
Markus Britschgi, Roche, SwitzerlandCopyright © 2022 Yang, Gao and Yang. This is an open-access article distributed under the terms of the Creative Commons Attribution License (CC BY). The use, distribution or reproduction in other forums is permitted, provided the original author(s) and the copyright owner(s) are credited and that the original publication in this journal is cited, in accordance with accepted academic practice. No use, distribution or reproduction is permitted which does not comply with these terms.
*Correspondence: Hui Yang, aHVpeWFuZ0BjY3VtLmVkdS5jbg==
Disclaimer: All claims expressed in this article are solely those of the authors and do not necessarily represent those of their affiliated organizations, or those of the publisher, the editors and the reviewers. Any product that may be evaluated in this article or claim that may be made by its manufacturer is not guaranteed or endorsed by the publisher.
Research integrity at Frontiers
Learn more about the work of our research integrity team to safeguard the quality of each article we publish.