- 1Department of Traditional Chinese Medicine, Xuanwu Hospital, Capital Medical University, Beijing, China
- 2Beijing Geriatric Institute of Integrated Traditional and Western Medicine, Beijing, China
- 3Department of General Diseases, Wangjing Hospital, China Academy of Chinese Medical Sciences, Beijing, China
- 4Department of Biomedical Sciences, Institute of Medical Biochemistry, University of Veterinary Medicine Vienna, Vienna, Austria
Iron plays a crucial role in many physiological processes of the human body, but iron is continuously deposited in the brain as we age. Early studies found iron overload is directly proportional to cognitive decline in Alzheimer’s disease (AD). Amyloid precursor protein (APP) and tau protein, both of which are related to the AD pathogenesis, are associated with brain iron metabolism. A variety of iron metabolism-related proteins have been found to be abnormally expressed in the brains of AD patients and mouse models, resulting in iron deposition and promoting AD progression. Amyloid β (Aβ) and hyperphosphorylated tau, two pathological hallmarks of AD, can also promote iron deposition in the brain, forming a vicious cycle of AD development-iron deposition. Iron deposition and the subsequent ferroptosis has been found to be a potential mechanism underlying neuronal loss in many neurodegenerative diseases. Iron chelators, antioxidants and hepcidin were found useful for treating AD, which represents an important direction for AD treatment research and drug development in the future. The review explored the deep connection between iron dysregulation and AD pathogenesis, discussed the potential of new hypothesis related to iron dyshomeostasis and ferroptosis, and summarized the therapeutics capable of targeting iron, with the expectation to draw more attention of iron dysregulation and corresponding drug development.
Introduction
Iron is the most abundant transition element on Earth (Coby et al., 2011) and one of the most important minerals in the body. It plays an indispensable role in many physiological and pathological processes of the body. Iron homeostasis is even more crucial in the brain to maintain its normal function. Iron dyshomeostasis within the brain can cause oxidative stress and inflammatory responses, leading to cell damage and finally neurological diseases. Ferroptosis, a programmed cell death process associated with iron dysregulation, has been supposed to be linked to neurological diseases, especially neurodegenerative diseases (Ren et al., 2020). Alzheimer’s disease (AD) is the most common type of dementia, and there is no effective clinical drug yet that can delay its progression, posing a serious burden on patients’ families and the whole society. β-amyloid (Aβ) plaque and tau tangles are the pathological hallmarks of AD, accompanied by neuronal loss and damage to brain tissue (Braak and Braak, 1991). Growing evidence have proved that Aβ triggers the chain events downstream in the pathological course of AD, including tau pathology. However, other processes such as neural inflammation (Leng and Edison, 2021), oxidative stress (Song et al., 2021), apoptosis (Sharma et al., 2021), autophagy (Zhang et al., 2021) and metal dysregulation (Wang et al., 2020; Aaseth et al., 2021) are also found in AD pathology. Some of these processes are even involved in the deposition of Aβ. Up to date, it is impossible to explain AD with a single pathological path. Currently, metal dyshomeostasis in AD has been extensively studied (Wang et al., 2020). Studies have found intracellular iron deposition even before the formation of senile plaques (Cheignon et al., 2018) and neurofibrillary tangles (NFT) (Wan et al., 2019) and ferroptosis is proposed to be one of key causes of neuronal loss in AD patients (Ashraf et al., 2020; Park et al., 2021). This review aimed to discuss the associations of AD pathogenesis with iron dyshomeostasis and ferroptosis, in order to highlight the hypothesis regarding iron-induced AD and the possibility of targeting iron and ferroptosis to treat AD.
Iron Metabolism in Healthy and Alzheimer’s Disease Brain
About 48% of the iron in the body is bound to hemoglobin and is involved in oxygen transport in the body (Andreini et al., 2018). About 17% of the iron is found as the cofactor in proteins (Andreini et al., 2018) to carry out functions in several crucial biological processes such as the tricarboxylic acid cycle, oxidative phosphorylation, DNA synthesis and repair, and iron homeostasis (Sheftel et al., 2012; Andreini et al., 2018). In the brain, iron is involved in myelination, neurotransmitter synthesis, and antioxidant enzyme function, and its entry and exit are tightly regulated by a variety of molecules. Aging (Loeffler et al., 1995), inflammation (Yoshida et al., 1998; Urrutia et al., 2013), and oxidative stress, that disturb the functions of molecules involved in iron metabolism, present as the main contributors to iron dyshomeostasis.
Iron Transport Across Blood-Brain Barrier
In the brain, transferrin receptor 1 (TfR1), responsible for the strict control of the level of iron transported into the brain (Pardridge et al., 1987; Connor et al., 2011), is expressed on the luminal side of the brain microvascular endothelial cells (BMECs) and the blood-cerebrospinal fluid barrier. After circulating iron forming a complex (holo-Tf) with transferrin (Tf), it binds to TfR1 on the surface of the BMECs, followed by entry into the BMECs via clathrin-mediated endocytosis (Pardridge et al., 1987; McCarthy and Kosman, 2012). Fe3+ detaches from Tf in the acidic environment of the endosome and is reduced to Fe2+ by six-transmembrane epithelial antigen of prostrate 3 (STEAP3) (Ohgami et al., 2005) or duodenal cytochrome b (DCYTB) (Tulpule et al., 2010), both of which are metalloreductases. It then enters the cytoplasm via divalent metal transporter 1 (DMT1) (De Domenico et al., 2008). Fe2+ in BMECs can then enter the brain by the secretion of ferroportin 1 (FPN1) followed by the oxidation by extracellular ceruloplasmin (Cp) or hephaestin (McCarthy and Kosman, 2013; Burkhart et al., 2016). There is also a hypothesis that iron could be transported to the brain interstitium through transcytosis in the form of holo-Tf based on the observation of lack of DMT1 in BMECs (Moos et al., 2007), which is still controversial. Not transferrin-bond iron (NTBI) can cross the Blood-Brain Barrier (BBB) via receptor-mediated transcytosis after binding to heavy-chain ferritin (H-ferritin; Hulet et al., 1999a,b) or lactoferrin (Lf) (Fillebeen et al., 1999; Fisher et al., 2007). It was also reported that Lf increased in the brains of aged individuals and those with AD (Kawamata et al., 1993), allowing large amounts of non-Tf-bound iron to enter the brain.
Iron Transport and Storage Within the Brain
Neuronal Iron Metabolism
TfR1 is highly expressed on the surface of neurons (Giometto et al., 1990) and, similar to BMECs, iron enter neurons via clathrin-mediated endocytosis of holo-Tf/TfR1 and exit the endosomes in the form of reduced Fe2+ via DMT1 (Burdo et al., 2001). NTBI can also enter neurons in a DMT1-dependent manner independent of Tf. Cellular prion protein (PrPC) is abundantly expressed on the surface of neuronal membranes. It functions as a ferrireductase partner for DMT1, mediating Fe2+ uptake in the plasma membrane in the form of complex PrPc/DMT1 (Singh, 2014; Tripathi et al., 2015). PrPC knockout in mice can lead to iron deficiency in brain and uptake increase of holo-Tf (Singh et al., 2013). By comparing the brain tissues of juvenile, adult, and aged rats that had the pathological features of AD, Lu et al. (2017) found that DMT1 abnormally increased with age. They supposed that DMT1 may be one of the main reason why the iron concentration in the brain gradually increases with age (Lu et al., 2017).
Some Fe2+ undergoes normal metabolism in the cytoplasm of neurons, while some is stored in ferritin in the form of non-toxic Fe3+ (Nikseresht et al., 2019); when neurons are low in iron, ferritin can be degraded by lysosomes to release the stored iron to meet the physiological needs of the neurons (Mancias et al., 2014; Quiles Del Rey and Mancias, 2019). Ferritin is positively correlated with iron overload and is found deposited in senile plaques in the AD brain (Connor et al., 1992; Raha et al., 2022). It had been shown that there was an age-dependent increase in ferritin in the brain (Belaidi et al., 2018), probably a contributor to the iron overload in aged and AD brains. Autopsy studies of AD patients have revealed that mitochondrial ferritin is upregulated (Wang et al., 2011). Ferritin in the cerebrospinal fluid (CSF) of AD patients has been shown significantly increased, which is negatively correlated with cognitive decline and hippocampal atrophy in AD (Diouf et al., 2019). Additionally, iron can enter mitochondria to form iron sulfur cluster and participate in participate in the process of aerobic respiration (Peng et al., 2021).
Regarding the transport of excess iron out of neurons, FPN1 is the only known iron exporter (Ganz, 2005) to date. Both Cp and hephaestin (Heph) can oxidize Fe2+ and facilitate FPN1to export iron (Qian et al., 2007), so the FPN1/Cp and FPN1/Heph are the main iron efflux pathways (Dlouhy et al., 2019; Qian and Ke, 2019). Decrease of any of these three export proteins can induce iron retention and consequently the memory impairment (Jiang et al., 2015; Zheng et al., 2018; Bao et al., 2021). It was reported that FPN1 was downregulated in the brains of AD patients and triple-transgenic AD mouse models (Raha et al., 2013), thus excessive iron could not be excreted normally, initiating intracellular iron deposition. Since Cp is a crucial partner of FPN1 to oxidize Fe2+ before it is excreted by FPN1, the dysfunction of Cp serves as an upstream event of iron retention, which has been found in AD (Connor et al., 1993; Kristinsson et al., 2012; Zhao et al., 2018).
Noteworthy, both of amyloid precursor protein (APP) and tau, which are the substrates of the AD hallmarks in pathological condition, are crucial for neuronal iron efflux (McCarthy et al., 2014; Belaidi et al., 2018). APP is defined as a metalloprotein involved in iron homeostasis (Duce et al., 2010). With the assistance of soluble tau protein, APP is transported to the cell membrane (Lei et al., 2012) where it stabilizes FPN1 (Wong et al., 2014b) and facilitates the efflux of iron. APP or tau knockdown can lead to abnormal FPN1 function and the inability of neuronal iron to flow out normally, resulting in neuronal iron overload (Lei et al., 2012; Belaidi et al., 2018; Tsatsanis et al., 2020). APP with the pathogenic Italian mutation A673V is more prone to be cleaved by β-secretase to produce Aβ1–42, impeding its support of FPN1 and thus increasing iron retention (Tsatsanis et al., 2020). Because of the continuous cleavage of APP and hyperphosphorylation of tau in AD brain, the iron efflux was hindered in neurons (Tuo et al., 2017; Tsatsanis et al., 2020).
Glial Support for Neuronal Iron Metabolism
Glial cells help to maintain the iron availability at a safe level in neurons. Astrocytes and microglia respond during iron overload or deficiency in order to maintain neuronal iron homeostasis. As a buffer pool, astrocytes express abundant TfR1 and DMT1 (Pelizzoni et al., 2013; Zarruk et al., 2015), which facilitates taking up both of holo-Tf and NTBI from the abluminal side of BMECs and the brain interstitium, precisely regulating the iron concentration in neurons (Tulpule et al., 2010; Hohnholt and Dringen, 2013; Knutson, 2019). Microglia also express TfR1 and reduce iron toxicity by promoting the influx of excess iron (for storage in ferritin) via the TfR1/DMT1 pathway (Qian and Ke, 2019). Microglia and astrocytes are capable of releasing ferritin carrying Fe3+ to supplement the iron deficiency or to support oligodendrocytes for myelination or remyelination (Cheli et al., 2021). Iron is essential for myelination in oligodendrocytes, which are the most iron-rich cell type in the brain. TfR1 is absent in oligodendrocytes (Zhang et al., 2006), while H-ferritin is the main source of iron for oligodendrocyte by interaction with T-cell immunoglobulin mucin domain 2 (TIM2) (Todorich et al., 2009, 2011). Noteworthy, when iron is overloaded, oligodendrocytes provide an antioxidant defense for neurons by secreting H-ferritin, scavenging extracellular extra iron (Mukherjee et al., 2020).
Regulators of Iron Homeostasis Proteins in the Brain
Transcription Factors and Iron Regulatory Element/Iron Regulatory Protein Interaction
At the transcriptional level, many of the intracellular iron homeostasis-related genes described above can be regulated by hypoxia-inducible factorα (HIFα). Most of the mRNAs related to the intracerebral iron metabolism have a hairpin-like structure known as iron regulatory element (IRE) (Camaschella, 2019). The iron regulatory protein 1/2 (IRP1/2) is the iron sensor, which can bind to the IRE, post-transcriptionally regulating those iron homeostasis-related proteins. In a low iron or hypoxic state in cells, HIFα is activated and upregulates IRP. IRP then binds to the IRE at the 3′-untranslated region (UTR) end of TfR1 and DMT1 mRNAs, to promote their translation, and to the IRE at the 5′-UTR end of ferritin, FPN1, and APP mRNAs, to inhibit their translation (Rogers et al., 2002; Anderson and Frazer, 2017). As a result, iron intake is increased, stored iron is released, and iron efflux is reduced. However, under iron overload, HIFα is degraded, and the IRP-IRE interactions lead to iron storage in neurons and increased iron efflux (Meyron-Holtz et al., 2004). Nuclear factor E2-related factor 2 (Nrf2) is an important regulator of intracellular oxidative stress and is also involved in the regulation of iron metabolism-related proteins. Nrf2 is able to upregulate FPN1 (Chen et al., 2020), while Nrf2 knockout reduces FPN1 levels in BMECs and prevents iron entry into the brain (Han et al., 2021). Decrease of Nrf2 could be one of the means for defense against iron overload in the brain (Han et al., 2021). Nevertheless, most of the target genes of Nrf2 have antioxidative property (Hayes and Dinkova-Kostova, 2014), thereby endowing it as a potent inhibitor of lipid oxidation and ferroptosis (Sun et al., 2016). This will be discussed in the part of “Ferroptosis and AD.”
Hepcidin
Hepcidin is a polypeptide that plays a major role in regulating peripheral iron homeostasis, and it has also been shown to be expressed in cortical neurons, intracerebral BMECs, and glial cells (Zechel et al., 2006; Raha et al., 2013; Raha-Chowdhury et al., 2015), playing an important role in the regulation of iron homeostasis in the brain. It binds to FPN1 in the cell membrane of BMECs, and then the hepcidin/FPN1 complex is internalized and degraded (Drakesmith et al., 2015) so that iron cannot cross the BBB into the brain. From this perspective, hepcidin is beneficial in preventing iron overload in neuron and maintaining iron homeostasis in the brain (Du et al., 2015; Vela, 2018). Studies have found hepcidin decreased in AD affected neurons (Raha et al., 2013), while exogenous hepcidin supplement normalized the levels of iron transport proteins, thereby reducing iron level in the brain (Du et al., 2015). The AD symptoms and Aβ burden can be ameliorated by astrocyte hepcidin (Xu et al., 2020; Zhang et al., 2020). Therefore, it is partially accepted that hepcidin is protective as an iron homeostasis regulator in the brain. However, it was also reported that hepcidin mRNA and protein increased in the brain with aging (Wang et al., 2010); it can be up-regulated by inflammation (Urrutia et al., 2013), especially by interleukin-6, with the result of FPN1 decrease and iron retention in AD brains (Chaudhary et al., 2021), exerting the detrimental impact on the brain. Up to date, studies on hepcidin in brain iron regulation remain controversial. How hepcidin allowing the normal iron efflux from neurons while causing FPN1 degradation remains an important issue to be studied.
Apolipoprotein E
Apolipoprotein E (apoE) is a protein responsible for the cholesterol transport to neurons, and also regulates Aβ transport and deposition. The allele ε4 of APOE (APOE4) is the strongest genetic risk factor for AD. CSF ferritin level is positively correlated with apoE4 expression, and it can be enhanced in APOE4 carriers (Ayton et al., 2015, 2017a). In old-aged individuals, cortical iron burden was found more severe by quantitative susceptibility mapping in APOE4 carriers (Kagerer et al., 2020). Therefore, brain iron deposition caused by APOE4 may be one of the reasons why APOE4 allele predisposes individuals to AD (Wood, 2015). Interestingly, increased extracellular iron levels can upregulate APOE4 expression in the neurons, but reduce the secretion of it, thereby reducing its ability to clear extracellular Aβ and aggravating Aβ deposition (Xu et al., 2016). From recent studies, iron could be the link between APOE4 and AD.
Impact of Iron Overload on Alzheimer’s Disease Pathology
Alzheimer’s disease is characterized by senile plaque and NFT pathologically. Senile plaque derives from the accumulation of insoluble Aβ, which is cleaved from APP by β-secretase. Hyperphosphorylation of tau is proposed to be the downstream event of Aβ formation. The pathological mechanism of AD is too complex to explain it merely with Aβ and tau accumulation. Scientists have found that many pathological processes, for instance, neural inflammation (Leng and Edison, 2021), oxidative stress (Song et al., 2021) and metal dysregulation (Wang et al., 2020; Aaseth et al., 2021), involved in Aβ deposition and tau hyperphosphorylation, driving the insidious progression before AD diagnosis. Currently, the involvement of iron in the early pathology of AD has been well accepted since the discovery of the link between dysregulation of brain iron homeostasis and AD pathogenesis in 1953 (Goodman, 1953).
In the preclinical stage of AD, there is significant abnormal iron elevation in cortical, hippocampal, and cerebellar neurons (Ding et al., 2009; Smith et al., 2010), while much severer in the cortex and hippocampus, the main brain areas affected by AD (Smith et al., 1997; Belaidi and Bush, 2016). The iron overload in the brain is corresponding to the severity of AD lesions and the rate of cognitive decline (van Duijn et al., 2017; Ayton et al., 2020). It is also proposed that hippocampal iron deposition could be the predictor of the rate of cognitive decline caused by Aβ (Ayton et al., 2017b). Iron overload drives a series of events, including glial activation, formation of Aβ plaque and tau tangles, and even neuronal loss (Singh et al., 2014; Gleason and Bush, 2021), pushing the progress of the disease and accelerating cognitive decline.
Iron Interaction With Aβ Plaques and Neurofibrillary Tangles
Iron accumulation was demonstrated to accelerate senile plaque deposition and the production of neurofibrillary tangles (Becerril-Ortega et al., 2014; Kim et al., 2018). Autopsy evidence and magnetic resonance imaging analysis provide evidence that there are a large amount of iron deposition not only in and around senile plaques (James et al., 2017), but also in the sites of cortical tau accumulation (Spotorno et al., 2020), indicating the potential crosstalk of iron with both of senile plaques and neurofibrillary tangles.
Perturbations in iron homeostasis is one of key players in Aβ deposition. High intracellular iron concentration enhances the interaction of IRP/IRE, inducing APP upregulation. Furthermore, the enzymes that cleave APP named α- and β-secretase are tightly balanced and modulated by furin (Silvestri and Camaschella, 2008; Guillemot et al., 2013). More β-secretase is activated when α- secretase is suppressed by furin impairment in the condition of excessive iron (Silvestri and Camaschella, 2008). Upregulated APP is cleaved by more β-secretase to Aβ40/42, accelerating the Aβ deposition. Meanwhile, APP can no longer assist FPN1, resulting in impaired iron efflux and aggravated iron deposition (Ward et al., 2014; Greenough, 2016). Some researchers have even proposed that Aβ is non-toxic in the absence of redox metals and that aggregation of Aβ requires the involvement of metals (Li et al., 2013; Belaidi and Bush, 2016). Soluble Aβ binds to Fe3+ when extracellular iron increases, so as to remove excess iron, but it is difficult to dissociate them after they interact; Aβ can promote the reduction of Fe3+ to Fe2+, and the reactive oxygen species (ROS) released during this process allows Aβ to be deposited more easily and rapidly, forming more senile plaques (Ha et al., 2007). The interactions of iron with APP and Aβ greatly increase the formation rate and degree of senile plaques (Rottkamp et al., 2001; Ha et al., 2007). Therefore, some researchers believe that iron deposition should be included in the “Aβ cascade hypothesis” of AD (Peters et al., 2015; Gleason and Bush, 2021).
Iron can also interact with tau. Reduced soluble tau in the brain of AD patients increased brain iron deposition by suppressing FPN1 activity (Lei et al., 2012). On the contrary, a diet high in iron can lead to cognitive decline in mice, increased abnormal tau phosphorylation in neurons, and abnormal expression of insulin pathway-related proteins. Insulin supplementation can reduce iron-induced phosphorylation of tau (Wan et al., 2019), indicating that iron deposition may lead to tau hyperphosphorylation by interfering insulin signaling. In vivo research has found that iron can be involved in tau hyperphosphorylation by activating the cyclin-dependent kinase 5 (CDK5)/P25 complex and glycogen synthase kinase-3β (GSK-3β) (Guo et al., 2013a). Excessive intracellular Fe2+-induced production of oxygen free radicals can also promote tau hyperphosphorylation by activating the extracellular signal-regulated kinase 1/2 (Erk1/2) or mitogen-activated protein kinase (MAPK) signaling pathways (Chan and Shea, 2006; Munoz et al., 2006).
Iron Overload and Glial Activation
Glial activation and neuroinflammation has been demonstrated to be a prominent characteristic of AD pathology (Newcombe et al., 2018; Leng and Edison, 2021). Microglial are highly reactive cells responding to increased iron levels in the brain. When iron level increases in brain, microglia become activated (Meng et al., 2017), with soma volume increased and process length decreased (Rathnasamy et al., 2013; Donley et al., 2021). Iron may activate microglia through proinflammatory cytokines mediated by the nuclear factor-κB (NF-κB) (Meng et al., 2017). After activated, they express more ferritin to scavenge the extracellular iron (Streit et al., 2021), resulting in intracellular iron retention (Kenkhuis et al., 2021), increased TNFα expression (Holland et al., 2018), and finally infiltrated with Aβ-plaques (Peters et al., 2018; Kenkhuis et al., 2021). Activated microglia also secret Lf, which can interact with APP, promoting the Aβ formation (Tsatsanis et al., 2021). Conversely, formation of Aβ induces more IL-1β expression in microglia in the environment of elevated iron, exacerbating the proinflammatory effects (Nnah et al., 2020).
Astrocytes are highly resistant to metal-induced toxicity within the brain (Kress et al., 2002) as the critical cell type in maintaining a balanced extracellular environment and supporting the normal functioning of neurons (Abbott et al., 2006). In the environment of high iron, astrocytes respond with a significant increase in glutathione, catalase and manganese superoxide dismutase levels to resist the oxidative stress (Iwata-Ichikawa et al., 1999; Shih et al., 2003). They show less impairment by iron than neurons and oligodendrocytes (Kress et al., 2002). But later, the astrocytes were found activated with increased glial fibrillary acidic protein (GFAP) (Kress et al., 2002). Activated astrocytes release inflammatory mediators and induce oxidative stress, which facilitate the formation of Aβ and tau tangles and hinder Aβ clearance (Cai et al., 2017).
Iron Overload Induces Oxidative Stress and Neuronal Loss
Iron toxicity is largely based on Fenton chemistry. As a redox-active transition metal, iron is a key player during the process of oxidative stress. Elevated iron promotes the production of ROS, which further depletes the cellular antioxidant GSH and promotes lipid peroxidation (Maher, 2018), finally triggering ferroptosis and neuronal loss. This will be discussed in the next part.
Ferroptosis and Alzheimer’s Disease
Ferroptosis in Brain
Ferroptosis is a programmed cell death process discovered by Dixon et al. (2012) that is distinct from other modalities of cell death such as apoptosis and necrosis. Ferroptosis is characterized by iron overload and triggered by iron-driven pile-up of the lipid peroxides to a lethal level (Dixon et al., 2012). The morphological changes of cells undergoing ferroptosis include cell swelling, reduced or absent mitochondrial cristae, decreased mitochondrial membrane density, nuclear chromatin condensation, and finally mitochondrial and cellular membrane rupture (Xie et al., 2016). It can be halted by iron chelators, antioxidants, and ferroptosis blockers.
With age, iron is gradually deposited in the body and iron deposition in the brain is more pronounced than in other organs. In addition, brain contains the highest level of polyunsaturated fatty acids (PUFAs), which are the preferential substrate for lipid peroxidation in the process of ferroptosis. So it is not surprising that brain is more susceptible to undergo ferroptosis. So far, ferroptosis has been found in neurodegenerative diseases (Masaldan et al., 2019), cerebrovascular diseases (Guan et al., 2019; Abdul et al., 2021), and other central nervous system diseases (Weiland et al., 2019).
It remains elusive of the precise role of iron even though it is indispensable in the execution of ferroptosis. Fe2+ itself has oxidation ability; it can produce a large amount of ROS via the Fenton reaction when it accumulates in excess (Wong et al., 2014a). This directly induces peroxidation of PUFA and leads to the lethal accumulation of the hydride PUFA-OOH (Angeli et al., 2017). Additionally, iron accumulation can activate iron-dependent lipoxygenases (LOXs) (Yang et al., 2016), thus excessively catalyzing the enzymatic oxidation of PUFA. Neuronal ferroptosis can be caused by both mechanisms.
Glutathione peroxidase 4 (GPX4) is the primary enzyme that negatively regulate ferroptosis. It physiologically reduces PUFA-OOH to non-toxic PUFA-OH (Ashraf et al., 2020), thus playing a detoxifying role and preventing ferroptosis. The intracellular level of glutathione (GSH; comprising cysteine, glycine, and glutamate), which is used by GPX4, is affected by the intracellular cysteine level. When the glutamate/cystine antiporter (xCT) encoded by SLC7A11 is inhibited under the condition of inflammation, aging, and other factors, the level of intracellular cysteine is reduced, resulting in insufficient GSH for the biosynthesis of GPX4 (Dixon et al., 2014). Excessive PUFA-OOH cannot be detoxified and accumulates to a lethal level, triggering ferroptosis (Weiland et al., 2019). Therefore, the dysfunction of xCT or GPX4 represents an upstream factor in neural ferroptosis (Ashraf et al., 2020). Restoration the functions of GPX4, GSH, or xCT has been demonstrated to be one of the strategies to inhibit ferroptosis (Seibt et al., 2019).
If ferritin, the major iron storage protein in cells, binds to its cargo receptor, nuclear receptor coactivator 4 (NCOA4) under the condition of inflammation, it is transported to lysosomes for autophagic degradation (Gao et al., 2016; Quiles Del Rey and Mancias, 2019), followed by the entry of iron into the cytoplasm. This is an important trigger for ferroptosis in cells. It has been found that multiple autophagy genes were associated with ferroptosis, while blocking or knocking down these genes reduced ferroptosis (Park and Chung, 2019). Thus, ferroptosis is considered to be an autophagic process.
“Ferroptosis Hypothesis” of Alzheimer’s Disease
After the discovery of ferroptosis in 2012, research on ferroptosis continued, and the involvement of ferroptosis in neurodegenerative diseases has now been widely accepted. Ferroptosis may be a potential mechanism of neuronal loss in many neurodegenerative diseases (Hou et al., 2019; Ayton et al., 2021; Bao et al., 2021; Tian et al., 2021). Ayton et al. (2021) proposed the “ferroptosis hypothesis” in AD, which could be independent of the “Aβ hypothesis” and “tau protein hypothesis,” based on large cohort studies. Thus, iron and ferroptosis are supposed to be the promising therapeutic targets for treating neurodegenerative diseases.
Raha et al. (2013) found reduced hippocampal FPN1 expression in AD patients and AD transgenic mice, but the association with ferroptosis was not examined. Bao et al. (2021) found that FPN1 was decreased in the brains of APP/presenilin-1(PS1) mice and AD patients; moreover, genetic FPN1 deletion in the neurons of neocortex and hippocampus in NEX-Cre mice led to AD-like atrophy in the hippocampus and cognitive impairment. The characteristic neuronal morphology of ferroptosis was observed; the biomarkers of ferroptosis named acyl-CoA synthetase family member 2 (ACSF2) and iron response element binding protein 2 (IREB2) were both upregulated; GPX4 was downregulated in FPN1 deletion mice as well as in APP/PS1 mice (Bao et al., 2021). FPN1 restoration or ferroptosis inhibitors named liproxstatin-1 and ferrostatin-1 improved memory impairment in both types of the mice (Bao et al., 2021). This not only indicated that abnormal expression of FPN1 solely is capable of inducing AD, but also implicated the role of ferroptosis in the pathogenesis of AD.
The mRNA GPX4 and its protein level have been both found to be aberrantly expressed in the brains of AD patients and mice (Yoo et al., 2010; da Rocha et al., 2018). In glial cells, a mild hypoxic state is capable of decreasing the level of GSH, which is used for the biosynthesis of GPX4 (Makarov et al., 2006). In AD mouse models, GSH expression is reduced in the cortex and is positively correlated with cognitive decline (Karelson et al., 2001). Some researchers have proposed that GSH levels in the frontal lobe and hippocampus may serve as a biomarker to predict AD and mild cognitive impairment (Mandal et al., 2015; Ayton et al., 2020). The xCT activity determines GSH availability and, subsequently GPX4 activity in the brain (Ashraf et al., 2020). Furthermore, studies have found that most of the proteins that involved in ferroptosis can be regulated by Nrf2 (Habib et al., 2015; Lane et al., 2021). The target genes include FPN1, GSH as well as SLC7A11 that encode xCT. Nrf2 level in the brain decreases with age, and even worse in the brains of AD (Osama et al., 2020), representing a possible upstream that leads to ferroptosis (Habib et al., 2015). GPX4 expression was reported to be reduced in the brains of both AD mouse models and AD patients (Ansari and Scheff, 2010; Yoo et al., 2010). GPX4-knockout mice were proved to have significant hippocampal neuronal loss and cognitive impairment (Yoo et al., 2012; Hambright et al., 2017); the diet deficient in vitamin E, an antioxidant with anti-ferroptosis activity, deteriorated hippocampal neurodegeneration and behavior dysfunction; ferroptosis inhibitors liproxstatin 1 improved the cognitive function and ameliorated the neurodegeneration in these mice (Hambright et al., 2017).
In addition to findings in animal models, autopsy findings revealed that GPX4 was downregulated and arachidonate 12/15-lipoxygenase (ALOX15) was upregulated, and the enhanced lipid peroxidation was observed, evidenced by elevated 4-hydroxynonenal (4-HNE) in the brains of AD patients (Yoo et al., 2010). 4-HNE has the potential to modify the proteins that involved in the antioxidant and energy metabolism, promoting Aβ deposition and fibril formation (Siegel et al., 2007). Thus, it is evident that ferroptosis plays a key role in AD, causing neuronal loss and cognitive decline (the roles of iron dyshomeostasis and ferroptosis are shown in Figure 1). Therefore, regulating brain iron metabolism and reducing neuronal ferroptosis may be a promising treatment for AD.
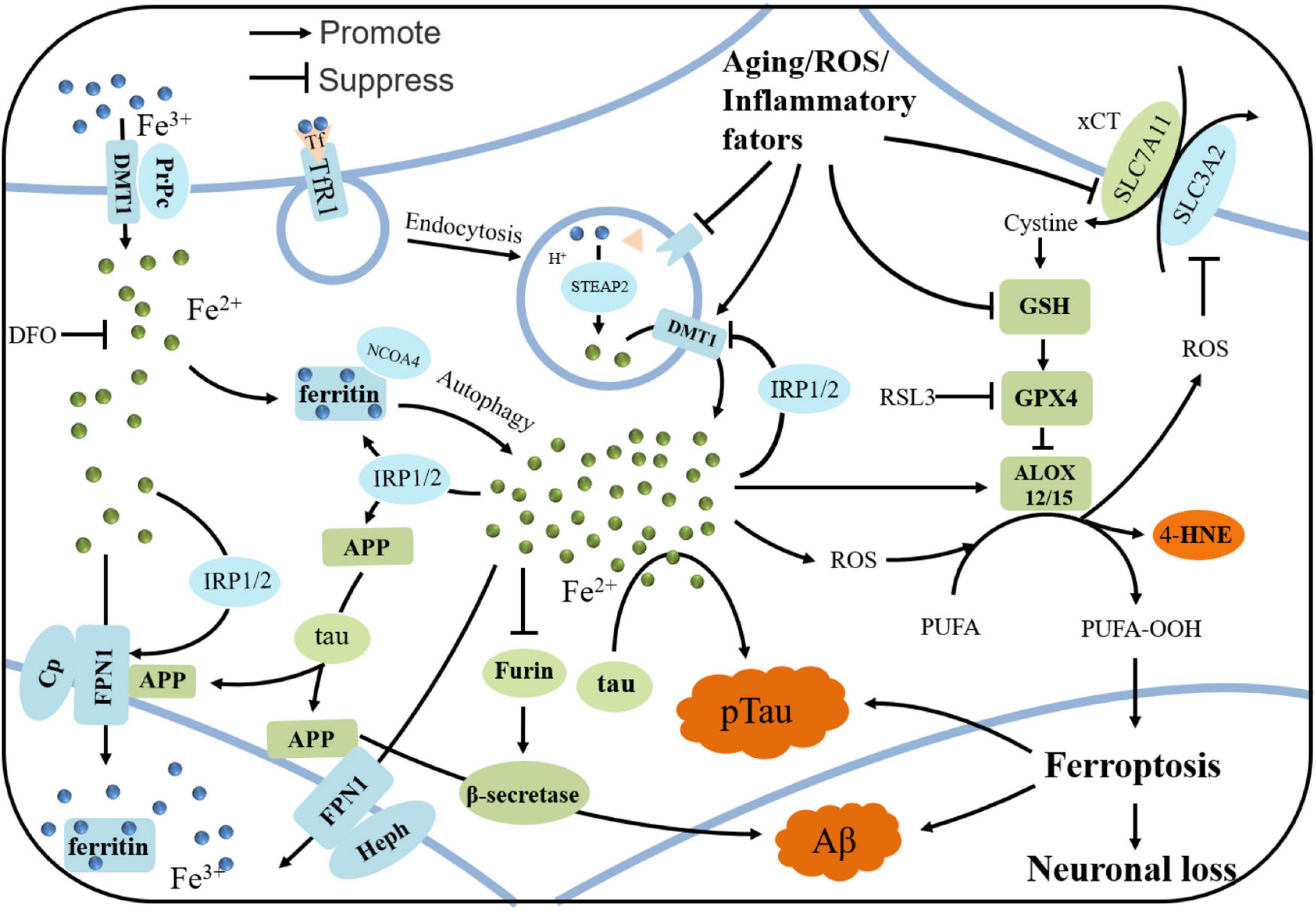
Figure 1. The iron regulation in neuron and the mechanism of ferroptosis in AD. Iron is uptaken by neurons via holo-Tf/TfR1 complex or DMT1/PrPC-dependent manners. PrPC reduce Fe3+ to Fe2+ and DMT1 transport Fe2+ into neurons. Tf carrying Fe3+ forms a complex with TfR1, and enters neurons via clathrin-mediated endocytosis. Fe3+ detaches from Tf and then is reduced by STEAP3. Fe2+ is pumped to cytoplasm by DMT1, and is stored in ferritin in the form of Fe3+ when overload. In some conditions, ferritin undergoes autophagy by binding with NCOA4, releasing iron and subsequently leading to lethal iron levels and ferroptosis. Elevated irons could be excreted by FPN1/Cp or FPN1/Heph, with the assistance of APP, which is transported to stabilize FPN1 by soluble tau protein. Aging, inflammation, and oxidative stress could dysregulate the iron transport proteins and cause iron retention. When overload, iron could upregulates the expressions of ferritin, FPN1, and APP by IRP-IRE interactions, while suppresses the normal function of furin, leading to the upregulation of β-secretase and thus accelerating Aβ deposition. When xCT or GSH decreases in the neurons under some states, the decreased GPX4 cannot exert the function of anti-lipid peroxidation. After Fenton reaction or ALOX-catalyzed process, PUFA-OOH can accumulate to a lethal level to trigger ferroptosis, which could be responsible for the tau hyperphosphorylation, Aβ formation and neuronal loss.
Targeting Iron Dyshomeostasis and Ferroptosis to Treat Alzheimer’s Disease
Iron Chelators
Deferoxamine and Deferiprone
Deferoxamine (DFO; also known as desferrioxamin) is an iron chelator that is commonly used in clinical practice. It may be an effective treatment for AD, and animal experiments and clinical trials have been conducted on DFO. Crapper et al. (1991) conducted a single-blind trial of intramuscular DFO in 48 AD patients for 24 months, and they found that DFO reduced the rate of clinical progression of AD by up to 50% compared to the control group. However, DFO also caused weight and appetite loss (Crapper et al., 1991).
Animal experiments involving the systemic administration of DFO to treat AD are rare, and whether DFO can cross the BBB has not yet been conclusively established. However, some studies have found that DFO crosses the BBB less efficiently than other iron chelators (Ward et al., 1995; Shachar et al., 2004), so nasal administration may be a better choice. Nasal administration of DFO has been found to reduce Aβ deposition and cognitive dysfunction in AD models (Fine et al., 2012, 2015; Guo et al., 2013b). However, nasal administration can cause gastrointestinal deposition of DFO, and there is inadequate penetration through the nasal mucosa thus low bioavailability (Lochhead and Thorne, 2012). Nasal administration of DFO nanoparticles may be a safer and effective way to treat AD (Rassu et al., 2015). This is currently being studied and may be used for treating AD in the near future.
Deferiprone (DFP) is a chelator that could be absorbed orally and cross BBB (Hider and Hoffbrand, 2018). In a randomized controlled trial, DFP improved neurological scores and iron-related neurological symptoms (Abbruzzese et al., 2011; Klopstock et al., 2019). DFP is currently being investigated in a randomized, double-blinded, placebo controlled, and multi-central phase II trial involving patients with mild cognitive impairment, prodromal AD or mild AD, who should have sedimentary Aβ confirmed by PET scan (NCT03234686). Giving the safety and lower systemic toxicities, DFP is a viable strategy to treat AD against iron dyshomeostasis and ferroptosis.
Clioquinol and Its Derivatives
Clioquinol and its derivatives, which chelate iron, zinc, and copper, have also been shown to improve cognitive ability, reduce Aβ deposition, and/or promote Aβ degradation in AD animal models (Grossi et al., 2009; Crouch et al., 2011). This may be related to their capture of iron from Aβ and prevention of Aβ aggregation (Opazo et al., 2006). It has also been found that clioquinol downregulates β- and γ-secretase and APP expression in the brain (Wang et al., 2012). In vitro experiment demonstrated that clioquinol was able to degrade oligomerized tau and reduce tau tangles (Lin et al., 2021). The effect of clioquinol in AD was also validated in a randomized controlled trial involving patients with moderate-to-severe AD, as oral clioquinol compared to placebo led to slower cognitive decline and reduced CSF Aβ42 (Ritchie et al., 2003). There is a derivative of clioquinol that have been studied extensively named PBT2 [5,7-dichloro-2-((dimethylamino)methyl) 8-quinolinol]. PBT2 has the effect to promote Aβ degradation (Crouch et al., 2011). A phase IIa, double-blind, randomized, placebo-controlled trial showed satisfactory safety and tolerability of PBT2 in AD, with the significant reduction in CSF Aβ42 concentration and improvement in cognition (Lannfelt et al., 2008; Faux et al., 2010). Because of the potential of clioquinol in treating AD, some derivatives or hybrids were synthesized to treat AD, and were proved effective both in vitro and in vivo (Perez et al., 2019). Yang et al. (2020) tested a series of novel flurbiprofen-clioquinol hybrids synthesized for Alzheimer’s disease therapy in vitro, and found they could prevent the accumulation of Aβ with antioxidant and anti-neuroinflammatory activity.
Clioquinol and its derivatives or hybrids were demonstrated to treat AD in the above studies ascribed to their chelation properties for zinc and copper. Other studies indicated clioquinol was also capable of preventing the loss of substantia nigra cells in the Parkinsonian transgenic mouse model because of its ability to chelate iron (Billings et al., 2016). Further studies on clioquinol to chelate iron in the treatment of AD need to be conducted in the future. Giving the roles of the three metals in AD, clioquinol chelating three of them should performed better in the treatment of AD. Because of the comparably less side-effects, clioquinol seems more promising than DFO for treating AD.
Antioxidants
Vitamin E
Antioxidants have been widely used to treat AD, and many of them can inhibit lipid peroxidation and thus ferroptosis. Vitamin E has been found to reduce lipid peroxidation in the brains of GPX4-knockout mice, mitigate the ferroptosic morphology of neurons, and improve the cognitive function of the mice. However, vitamin E didn’t show any benefit in patients with AD or mild cognitive impairment in a randomized clinical trial (Farina et al., 2017). Moreover, another clinical trial found that vitamin E treatment accelerated cognitive decline compared to placebo (Lloret et al., 2009). Therefore, the use of vitamin E in AD remains questionable and more clinical trials are needed to ascertain its effect.
Alpha-Lipoic Acid
Alpha-lipoic acid (α-LA) is an antioxidant with benefits for AD. In a clinical study of 129 patients with possible AD, α-LA slowed cognitive decline and reduced cognitive impairment (Fava et al., 2013). α-LA relieved tau hyperphosphorylation in P301S tau transgenic mice, and it also blocked tau-induced iron overload, lipid peroxidation, and inflammation (Zhang et al., 2018), thus exerting the effect to ameliorate ferroptosis in the brain. As no serious side effects of α-LA have been observed so far, α-LA may be useful for developing treatments for AD.
Selenium
Selenium (Se) is present in a variety of proteins in the body, such as GPX4, and it has antioxidant activity. Research have found that decreased Se in the brain of AD patients may be associated with AD progression (Cardoso et al., 2017; Varikasuvu et al., 2019). Primary neuron culture indicated that Se might reduce Aβ production by reducing 4-HNE-induced β-secretase transcription, thereby preventing Aβ-mediated toxicity (Gwon et al., 2010). Se -containing compounds may inhibit ferroptosis by upregulating GPX4. A clinical trial of 40 AD patients found that, on average, oral sodium selenate effectively supplemented brain Se levels without significant side effects, and among those who responded (i.e., had improved brain selenium levels), the Mini-Mental State Examination (MMSE) score did not significantly deteriorate over time (Cardoso et al., 2019). However, Kryscio et al. (2017) studied the effects of Se and vitamin E on AD progression in male patients in the PREADViSE trial and found that each of them had deleterious effects. In summary, the current knowledge provides no valid evidence for a beneficial role of Se in the treatment of AD, and clinical trials are still needed to provide definitive answers.
Ferrostatin-1
Ferrostatin-1 (Fer-1), a radical scavenger, is a common ferroptosis inhibitor much more efficiently than phenolic antioxidants (Miotto et al., 2020). It has been demonstrated to inhibit oxidative lipid damage and cell death in diverse disease models, including Huntington’s disease, periventricular leukomalacia, kidney dysfunction, intracerebral hemorrhage, and cardiomyopathy (Skouta et al., 2014; Li et al., 2017; Fang et al., 2019). Fer-1 was able to alleviate angiotensin II -induced inflammation and ferroptosis in astrocytes by suppressing the ROS levels and downregulating Nrf2 and GPX4 (Li et al., 2021). In the treatment of AD, Fer-1 was evidenced to ameliorate neuronal death and memory impairment both in vitro and in vivo (Bao et al., 2021). After traumatic brain injury, the mice administrated Fer-1 was shown significantly reduced iron deposition and neuronal degeneration in the brains as well as improved long-term motor and cognitive function (Xie et al., 2019). Although in vivo and in vitro studies have proved the pronounced effect of Fer-1 in ameliorating oxidative stress and preventing ferroptosis, there is still no clinical trials as yet. Its exact efficacy in AD needs to be further excavated as well as its side-effects.
Hepcidin
As hepcidin can reduce the transport of iron across the BBB and prevent iron overload in the brain, some researchers have proposed that it can treat AD, which has been confirmed in cell-based and animal experiments. In cultured microvascular endothelial cells, hepcidin significantly inhibited FPN1, and consequently low expression of TfR1 and DMT1, as well as reduced iron uptake and iron release in neurons (Du et al., 2015). In APP/PS1 mice, overexpressing hepcidin in astrocytes reduced neuronal iron level in cortex and hippocampus, where the formation of Aβ plaque were also alleviated; the mice cognitive function was elevated (Xu et al., 2020). Consistently, another study found that treatment with recombinant adenovirus carrying the hepcidin gene reduced iron retention and oxidative stress in the brain (Gong et al., 2016). These studies demonstrated the excellent potential of hepcidin in AD treatment, but hepcidin is currently only being investigated in preclinical studies. How to deliver hepcidin to brain and its side-effects yet need to be further studied and solid evidenced.
Conclusion and Future Prospects
As the most common type of dementia, AD has become a major challenge for society and health systems because the globe population is rapidly aging. Most clinical studies of treatments based on the mainstream hypotheses regarding AD pathogenesis have not been highly fruitful. The gradual understanding of the roles of iron dyshomeostasis and ferroptosis in the pathogenesis of AD may offer a promising direction to establish a new hypothesis, which will be of great significance and worthy to be studied deeper. In the future, the upstream and downstream roles of iron in AD pathogenesis are yet to be elucidated, and iron quantification methods will definitely be improved with technological advances to assist the scientists better understand the iron dyshomeostasis in different brain regions. Even though a various of molecules have been found to have the potential to alleviate iron dyshomeostasis and prevent ferroptosis, finding a way to allow these potentially useful drugs, such as iron chelators and antioxidants, to effectively cross the BBB into the brain in order to mediate their effects while avoiding systemic side effects will be the greatest challenge for future pharmacological research on AD treatment based on the potential “ferroptosis hypothesis.”
Author Contributions
XH: conceptualization. FW, JW, and YS: methodology and investigation. JW, HL, W-DR, and XH: validation. FW: writing – original draft preparation. FW, HL, W-DR, and XH: writing – review and editing. YS and XH: supervision. YS: project administration. FW, YS, and XH: funding acquisition. All authors: contributed to the article and approved the submitted version.
Funding
This work was supported by Beijing Municipal Natural Science Foundation (Grant No. 71720947), Capital Medical University Scientific Research Cultivation Project (Grant No. PYZ20128), and Shihezi Science and Technology Project (Grant No. 2019ZH10).
Conflict of Interest
The authors declare that the research was conducted in the absence of any commercial or financial relationships that could be construed as a potential conflict of interest.
Publisher’s Note
All claims expressed in this article are solely those of the authors and do not necessarily represent those of their affiliated organizations, or those of the publisher, the editors and the reviewers. Any product that may be evaluated in this article, or claim that may be made by its manufacturer, is not guaranteed or endorsed by the publisher.
References
Aaseth, J., Skalny, A. V., Roos, P. M., Alexander, J., Aschner, M., and Tinkov, A. A. (2021). Copper, Iron, Selenium and Lipo-Glycemic Dysmetabolism in Alzheimer’s Disease. Int. J. Mol. Sci. 22:ijms22179461. doi: 10.3390/ijms22179461
Abbott, N. J., Ronnback, L., and Hansson, E. (2006). Astrocyte-endothelial interactions at the blood-brain barrier. Nat. Rev. Neurosci. 7, 41–53. doi: 10.1038/nrn1824
Abbruzzese, G., Cossu, G., Balocco, M., Marchese, R., Murgia, D., Melis, M., et al. (2011). A pilot trial of deferiprone for neurodegeneration with brain iron accumulation. Haematologica 96, 1708–1711. doi: 10.3324/haematol.2011.043018
Abdul, Y., Li, W., Ward, R., Abdelsaid, M., Hafez, S., Dong, G., et al. (2021). Deferoxamine Treatment Prevents Post-Stroke Vasoregression and Neurovascular Unit Remodeling Leading to Improved Functional Outcomes in Type 2 Male Diabetic Rats: Role of Endothelial Ferroptosis. Transl. Stroke Res. 12, 615–630. doi: 10.1007/s12975-020-00844-7
Anderson, G. J., and Frazer, D. M. (2017). Current understanding of iron homeostasis. Am. J. Clin. Nutr. 106(Suppl. 6), 1559S–1566S. doi: 10.3945/ajcn.117.155804
Andreini, C., Putignano, V., Rosato, A., and Banci, L. (2018). The human iron-proteome. Metallomics 10, 1223–1231. doi: 10.1039/c8mt00146d
Angeli, J. P. F., Shah, R., Pratt, D. A., and Conrad, M. (2017). Ferroptosis Inhibition: Mechanisms and Opportunities. Trends Pharmacol. Sci. 38, 489–498. doi: 10.1016/j.tips.2017.02.005
Ansari, M. A., and Scheff, S. W. (2010). Oxidative stress in the progression of Alzheimer disease in the frontal cortex. J. Neuropathol. Exp. Neurol. 69, 155–167. doi: 10.1097/NEN.0b013e3181cb5af4
Ashraf, A., Jeandriens, J., Parkes, H. G., and So, P. W. (2020). Iron dyshomeostasis, lipid peroxidation and perturbed expression of cystine/glutamate antiporter in Alzheimer’s disease: Evidence of ferroptosis. Redox Biol. 32:101494. doi: 10.1016/j.redox.2020.101494
Ayton, S., Faux, N. G., and Bush, A. I. (2017a). Association of Cerebrospinal Fluid Ferritin Level With Preclinical Cognitive Decline in APOE-epsilon4 Carriers. JAMA Neurol. 74, 122–125. doi: 10.1001/jamaneurol.2016.4406
Ayton, S., Fazlollahi, A., Bourgeat, P., Raniga, P., Ng, A., Lim, Y. Y., et al. (2017b). Cerebral quantitative susceptibility mapping predicts amyloid-beta-related cognitive decline. Brain 140, 2112–2119. doi: 10.1093/brain/awx137
Ayton, S., Faux, N. G., Bush, A. I., and Alzheimer’s Disease Neuroimaging (2015). Ferritin levels in the cerebrospinal fluid predict Alzheimer’s disease outcomes and are regulated by APOE. Nat. Commun. 6:6760. doi: 10.1038/ncomms7760
Ayton, S., Portbury, S., Kalinowski, P., Agarwal, P., Diouf, I., Schneider, J. A., et al. (2021). Regional brain iron associated with deterioration in Alzheimer’s disease: A large cohort study and theoretical significance. Alzheimers Dement. 17, 1244–1256. doi: 10.1002/alz.12282
Ayton, S., Wang, Y., Diouf, I., Schneider, J. A., Brockman, J., Morris, M. C., et al. (2020). Brain iron is associated with accelerated cognitive decline in people with Alzheimer pathology. Mol. Psychiatry 25, 2932–2941. doi: 10.1038/s41380-019-0375-7
Bao, W. D., Pang, P., Zhou, X. T., Hu, F., Xiong, W., Chen, K., et al. (2021). Loss of ferroportin induces memory impairment by promoting ferroptosis in Alzheimer’s disease. Cell Death Differ. 28, 1548–1562. doi: 10.1038/s41418-020-00685-9
Becerril-Ortega, J., Bordji, K., Freret, T., Rush, T., and Buisson, A. (2014). Iron overload accelerates neuronal amyloid-beta production and cognitive impairment in transgenic mice model of Alzheimer’s disease. Neurobiol. Aging 35, 2288–2301. doi: 10.1016/j.neurobiolaging.2014.04.019
Belaidi, A. A., and Bush, A. I. (2016). Iron neurochemistry in Alzheimer’s disease and Parkinson’s disease: targets for therapeutics. J. Neurochem. 139(Suppl. 1), 179–197. doi: 10.1111/jnc.13425
Belaidi, A. A., Gunn, A. P., Wong, B. X., Ayton, S., Appukuttan, A. T., Roberts, B. R., et al. (2018). Marked Age-Related Changes in Brain Iron Homeostasis in Amyloid Protein Precursor Knockout Mice. Neurotherapeutics 15, 1055–1062. doi: 10.1007/s13311-018-0656-x
Billings, J. L., Hare, D. J., Nurjono, M., Volitakis, I., Cherny, R. A., Bush, A. I., et al. (2016). Effects of Neonatal Iron Feeding and Chronic Clioquinol Administration on the Parkinsonian Human A53T Transgenic Mouse. ACS Chem. Neurosci. 7, 360–366. doi: 10.1021/acschemneuro.5b00305
Braak, H., and Braak, E. (1991). Neuropathological stageing of Alzheimer-related changes. Acta Neuropathol. 82, 239–259. doi: 10.1007/BF00308809
Burdo, J. R., Menzies, S. L., Simpson, I. A., Garrick, L. M., Garrick, M. D., Dolan, K. G., et al. (2001). Distribution of divalent metal transporter 1 and metal transport protein 1 in the normal and Belgrade rat. J. Neurosci. Res. 66, 1198–1207. doi: 10.1002/jnr.1256
Burkhart, A., Skjorringe, T., Johnsen, K. B., Siupka, P., Thomsen, L. B., Nielsen, M. S., et al. (2016). Expression of Iron-Related Proteins at the Neurovascular Unit Supports Reduction and Reoxidation of Iron for Transport Through the Blood-Brain Barrier. Mol. Neurobiol. 53, 7237–7253. doi: 10.1007/s12035-015-9582-7
Cai, Z., Wan, C. Q., and Liu, Z. (2017). Astrocyte and Alzheimer’s disease. J. Neurol. 264, 2068–2074. doi: 10.1007/s00415-017-8593-x
Cardoso, B. R., Hare, D. J., Lind, M., McLean, C. A., Volitakis, I., Laws, S. M., et al. (2017). The APOE epsilon4 Allele Is Associated with Lower Selenium Levels in the Brain: Implications for Alzheimer’s Disease. ACS Chem. Neurosci. 8, 1459–1464. doi: 10.1021/acschemneuro.7b00014
Cardoso, B. R., Roberts, B. R., Malpas, C. B., Vivash, L., Genc, S., Saling, M. M., et al. (2019). Supranutritional Sodium Selenate Supplementation Delivers Selenium to the Central Nervous System: Results from a Randomized Controlled Pilot Trial in Alzheimer’s Disease. Neurotherapeutics 16, 192–202. doi: 10.1007/s13311-018-0662-z
Chan, A., and Shea, T. B. (2006). Dietary and genetically-induced oxidative stress alter tau phosphorylation: influence of folate and apolipoprotein E deficiency. J. Alzheimers Dis. 9, 399–405. doi: 10.3233/jad-2006-9405
Chaudhary, S., Ashok, A., McDonald, D., Wise, A. S., Kritikos, A. E., Rana, N. A., et al. (2021). Upregulation of Local Hepcidin Contributes to Iron Accumulation in Alzheimer’s Disease Brains. J. Alzheimers Dis. 82, 1487–1497. doi: 10.3233/JAD-210221
Cheignon, C., Tomas, M., Bonnefont-Rousselot, D., Faller, P., Hureau, C., and Collin, F. (2018). Oxidative stress and the amyloid beta peptide in Alzheimer’s disease. Redox Biol. 14, 450–464. doi: 10.1016/j.redox.2017.10.014
Cheli, V. T., Santiago Gonzalez, D. A., Wan, Q., Denaroso, G., Wan, R., Rosenblum, S. L., et al. (2021). H-ferritin expression in astrocytes is necessary for proper oligodendrocyte development and myelination. Glia 69, 2981–2998. doi: 10.1002/glia.24083
Chen, X., Yu, C., Kang, R., and Tang, D. (2020). Iron Metabolism in Ferroptosis. Front. Cell Dev. Biol. 8:590226. doi: 10.3389/fcell.2020.590226
Coby, A. J., Picardal, F., Shelobolina, E., Xu, H., and Roden, E. E. (2011). Repeated anaerobic microbial redox cycling of iron. Appl. Environ. Microbiol. 77, 6036–6042. doi: 10.1128/AEM.00276-11
Connor, J. R., Menzies, S. L., St Martin, S. M., and Mufson, E. J. (1992). A histochemical study of iron, transferrin, and ferritin in Alzheimer’s diseased brains. J. Neurosci. Res. 31, 75–83. doi: 10.1002/jnr.490310111
Connor, J. R., Ponnuru, P., Wang, X. S., Patton, S. M., Allen, R. P., and Earley, C. J. (2011). Profile of altered brain iron acquisition in restless legs syndrome. Brain 134(Pt 4), 959–968. doi: 10.1093/brain/awr012
Connor, J. R., Tucker, P., Johnson, M., and Snyder, B. (1993). Ceruloplasmin levels in the human superior temporal gyrus in aging and Alzheimer’s disease. Neurosci. Lett. 159, 88–90. doi: 10.1016/0304-3940(93)90805-u
Crapper McLachlan, D. R., Dalton, A. J., Kruck, T. P., Bell, M. Y., Smith, W. L., et al. (1991). Intramuscular desferrioxamine in patients with Alzheimer’s disease. Lancet 337, 1304–1308. doi: 10.1016/0140-6736(91)92978-b
Crouch, P. J., Savva, M. S., Hung, L. W., Donnelly, P. S., Mot, A. I., Parker, S. J., et al. (2011). The Alzheimer’s therapeutic PBT2 promotes amyloid-beta degradation and GSK3 phosphorylation via a metal chaperone activity. J. Neurochem. 119, 220–230. doi: 10.1111/j.1471-4159.2011.07402.x
da Rocha, T. J., Silva Alves, M., Guisso, C. C., de Andrade, F. M., Camozzato, A., de Oliveira, A. A., et al. (2018). Association of GPX1 and GPX4 polymorphisms with episodic memory and Alzheimer’s disease. Neurosci. Lett. 666, 32–37. doi: 10.1016/j.neulet.2017.12.026
De Domenico, I., McVey Ward, D., and Kaplan, J. (2008). Regulation of iron acquisition and storage: consequences for iron-linked disorders. Nat. Rev. Mol. Cell Biol. 9, 72–81. doi: 10.1038/nrm2295
Ding, B., Chen, K. M., Ling, H. W., Sun, F., Li, X., Wan, T., et al. (2009). Correlation of iron in the hippocampus with MMSE in patients with Alzheimer’s disease. J. Magn. Reson. Imaging 29, 793–798. doi: 10.1002/jmri.21730
Diouf, I., Fazlollahi, A., Bush, A. I., Ayton, S., and Alzheimer’s disease Neuroimaging, I. (2019). Cerebrospinal fluid ferritin levels predict brain hypometabolism in people with underlying beta-amyloid pathology. Neurobiol. Dis. 124, 335–339. doi: 10.1016/j.nbd.2018.12.010
Dixon, S. J., Lemberg, K. M., Lamprecht, M. R., Skouta, R., Zaitsev, E. M., Gleason, C. E., et al. (2012). Ferroptosis: an iron-dependent form of nonapoptotic cell death. Cell 149, 1060–1072. doi: 10.1016/j.cell.2012.03.042
Dixon, S. J., Patel, D. N., Welsch, M., Skouta, R., Lee, E. D., Hayano, M., et al. (2014). Pharmacological inhibition of cystine-glutamate exchange induces endoplasmic reticulum stress and ferroptosis. Elife 3:e02523. doi: 10.7554/eLife.02523
Dlouhy, A. C., Bailey, D. K., Steimle, B. L., Parker, H. V., and Kosman, D. J. (2019). Fluorescence resonance energy transfer links membrane ferroportin, hephaestin but not ferroportin, amyloid precursor protein complex with iron efflux. J. Biol. Chem. 294, 4202–4214. doi: 10.1074/jbc.RA118.005142
Donley, D. W., Realing, M., Gigley, J. P., and Fox, J. H. (2021). Iron activates microglia and directly stimulates indoleamine-2,3-dioxygenase activity in the N171-82Q mouse model of Huntington’s disease. PLoS One 16:e0250606. doi: 10.1371/journal.pone.0250606
Drakesmith, H., Nemeth, E., and Ganz, T. (2015). Ironing out Ferroportin. Cell Metab. 22, 777–787. doi: 10.1016/j.cmet.2015.09.006
Du, F., Qian, Z. M., Luo, Q., Yung, W. H., and Ke, Y. (2015). Hepcidin Suppresses Brain Iron Accumulation by Downregulating Iron Transport Proteins in Iron-Overloaded Rats. Mol. Neurobiol. 52, 101–114. doi: 10.1007/s12035-014-8847-x
Duce, J. A., Tsatsanis, A., Cater, M. A., James, S. A., Robb, E., Wikhe, K., et al. (2010). Iron-export ferroxidase activity of beta-amyloid precursor protein is inhibited by zinc in Alzheimer’s disease. Cell 142, 857–867. doi: 10.1016/j.cell.2010.08.014
Fang, X., Wang, H., Han, D., Xie, E., Yang, X., Wei, J., et al. (2019). Ferroptosis as a target for protection against cardiomyopathy. Proc. Natl. Acad. Sci. U S A. 116, 2672–2680. doi: 10.1073/pnas.1821022116
Farina, N., Llewellyn, D., Isaac, M. G., and Tabet, N. (2017). Vitamin E for Alzheimer’s dementia and mild cognitive impairment. Cochrane Database Syst. Rev. 1:CD002854. doi: 10.1002/14651858.CD002854.pub4
Faux, N. G., Ritchie, C. W., Gunn, A., Rembach, A., Tsatsanis, A., Bedo, J., et al. (2010). PBT2 rapidly improves cognition in Alzheimer’s Disease: additional phase II analyses. J. Alzheimers Dis. 20, 509–516. doi: 10.3233/JAD-2010-1390
Fava, A., Pirritano, D., Plastino, M., Cristiano, D., Puccio, G., Colica, C., et al. (2013). The Effect of Lipoic Acid Therapy on Cognitive Functioning in Patients with Alzheimer’s Disease. J. Neurodegener. Dis. 2013:454253. doi: 10.1155/2013/454253
Fillebeen, C., Descamps, L., Dehouck, M. P., Fenart, L., Benaissa, M., Spik, G., et al. (1999). Receptor-mediated transcytosis of lactoferrin through the blood-brain barrier. J. Biol. Chem. 274, 7011–7017. doi: 10.1074/jbc.274.11.7011
Fine, J. M., Baillargeon, A. M., Renner, D. B., Hoerster, N. S., Tokarev, J., Colton, S., et al. (2012). Intranasal deferoxamine improves performance in radial arm water maze, stabilizes HIF-1alpha, and phosphorylates GSK3beta in P301L tau transgenic mice. Exp. Brain Res. 219, 381–390. doi: 10.1007/s00221-012-3101-0
Fine, J. M., Renner, D. B., Forsberg, A. C., Cameron, R. A., Galick, B. T., Le, C., et al. (2015). Intranasal deferoxamine engages multiple pathways to decrease memory loss in the APP/PS1 model of amyloid accumulation. Neurosci. Lett. 584, 362–367. doi: 10.1016/j.neulet.2014.11.013
Fisher, J., Devraj, K., Ingram, J., Slagle-Webb, B., Madhankumar, A. B., Liu, X., et al. (2007). Ferritin: a novel mechanism for delivery of iron to the brain and other organs. Am. J. Physiol. Cell Physiol. 293, C641–C649. doi: 10.1152/ajpcell.00599.2006
Ganz, T. (2005). Cellular iron: ferroportin is the only way out. Cell Metab. 1, 155–157. doi: 10.1016/j.cmet.2005.02.005
Gao, M., Monian, P., Pan, Q., Zhang, W., Xiang, J., and Jiang, X. (2016). Ferroptosis is an autophagic cell death process. Cell Res. 26, 1021–1032. doi: 10.1038/cr.2016.95
Giometto, B., Bozza, F., Argentiero, V., Gallo, P., Pagni, S., Piccinno, M. G., et al. (1990). Transferrin receptors in rat central nervous system. An immunocytochemical study. J. Neurol. Sci. 98, 81–90. doi: 10.1016/0022-510x(90)90183-n
Gleason, A., and Bush, A. I. (2021). Iron and Ferroptosis as Therapeutic Targets in Alzheimer’s Disease. Neurotherapeutics 18, 252–264. doi: 10.1007/s13311-020-00954-y
Gong, J., Du, F., Qian, Z. M., Luo, Q. Q., Sheng, Y., Yung, W. H., et al. (2016). Pre-treatment of rats with ad-hepcidin prevents iron-induced oxidative stress in the brain. Free Radic. Biol. Med. 90, 126–132. doi: 10.1016/j.freeradbiomed.2015.11.016
Goodman, L. (1953). Alzheimer’s disease; a clinico-pathologic analysis of twenty-three cases with a theory on pathogenesis. J. Nerv. Ment. Dis. 118, 97–130.
Greenough, M. A. (2016). The Role of Presenilin in Protein Trafficking and Degradation-Implications for Metal Homeostasis. J. Mol. Neurosci. 60, 289–297. doi: 10.1007/s12031-016-0826-4
Grossi, C., Francese, S., Casini, A., Rosi, M. C., Luccarini, I., Fiorentini, A., et al. (2009). Clioquinol decreases amyloid-beta burden and reduces working memory impairment in a transgenic mouse model of Alzheimer’s disease. J. Alzheimers Dis. 17, 423–440. doi: 10.3233/JAD-2009-1063
Guan, X., Li, X., Yang, X., Yan, J., Shi, P., Ba, L., et al. (2019). The neuroprotective effects of carvacrol on ischemia/reperfusion-induced hippocampal neuronal impairment by ferroptosis mitigation. Life Sci. 235:116795. doi: 10.1016/j.lfs.2019.116795
Guillemot, J., Canuel, M., Essalmani, R., Prat, A., and Seidah, N. G. (2013). Implication of the proprotein convertases in iron homeostasis: proprotein convertase 7 sheds human transferrin receptor 1 and furin activates hepcidin. Hepatology 57, 2514–2524. doi: 10.1002/hep.26297
Guo, C., Wang, P., Zhong, M. L., Wang, T., Huang, X. S., Li, J. Y., et al. (2013a). Deferoxamine inhibits iron induced hippocampal tau phosphorylation in the Alzheimer transgenic mouse brain. Neurochem. Int. 62, 165–172. doi: 10.1016/j.neuint.2012.12.005
Guo, C., Wang, T., Zheng, W., Shan, Z. Y., Teng, W. P., and Wang, Z. Y. (2013b). Intranasal deferoxamine reverses iron-induced memory deficits and inhibits amyloidogenic APP processing in a transgenic mouse model of Alzheimer’s disease. Neurobiol. Aging 34, 562–575. doi: 10.1016/j.neurobiolaging.2012.05.009
Gwon, A. R., Park, J. S., Park, J. H., Baik, S. H., Jeong, H. Y., Hyun, D. H., et al. (2010). Selenium attenuates A beta production and A beta-induced neuronal death. Neurosci. Lett. 469, 391–395. doi: 10.1016/j.neulet.2009.12.035
Ha, C., Ryu, J., and Park, C. B. (2007). Metal ions differentially influence the aggregation and deposition of Alzheimer’s beta-amyloid on a solid template. Biochemistry 46, 6118–6125. doi: 10.1021/bi7000032
Habib, E., Linher-Melville, K., Lin, H. X., and Singh, G. (2015). Expression of xCT and activity of system xc(-) are regulated by NRF2 in human breast cancer cells in response to oxidative stress. Redox. Biol. 5, 33–42. doi: 10.1016/j.redox.2015.03.003
Hambright, W. S., Fonseca, R. S., Chen, L., Na, R., and Ran, Q. (2017). Ablation of ferroptosis regulator glutathione peroxidase 4 in forebrain neurons promotes cognitive impairment and neurodegeneration. Redox. Biol. 12, 8–17. doi: 10.1016/j.redox.2017.01.021
Han, K., Jin, X., Guo, X., Cao, G., Tian, S., Song, Y., et al. (2021). Nrf2 knockout altered brain iron deposition and mitigated age-related motor dysfunction in aging mice. Free Radic. Biol. Med. 162, 592–602. doi: 10.1016/j.freeradbiomed.2020.11.019
Hayes, J. D., and Dinkova-Kostova, A. T. (2014). The Nrf2 regulatory network provides an interface between redox and intermediary metabolism. Trends Biochem. Sci. 39, 199–218. doi: 10.1016/j.tibs.2014.02.002
Hider, R. C., and Hoffbrand, A. V. (2018). The Role of Deferiprone in Iron Chelation. N. Engl. J. Med. 379, 2140–2150. doi: 10.1056/NEJMra1800219
Hohnholt, M. C., and Dringen, R. (2013). Uptake and metabolism of iron and iron oxide nanoparticles in brain astrocytes. Biochem. Soc. Trans. 41, 1588–1592. doi: 10.1042/BST20130114
Holland, R., McIntosh, A. L., Finucane, O. M., Mela, V., Rubio-Araiz, A., Timmons, G., et al. (2018). Inflammatory microglia are glycolytic and iron retentive and typify the microglia in APP/PS1 mice. Brain Behav. Immun. 68, 183–196. doi: 10.1016/j.bbi.2017.10.017
Hou, L., Huang, R., Sun, F., Zhang, L., and Wang, Q. (2019). NADPH oxidase regulates paraquat and maneb-induced dopaminergic neurodegeneration through ferroptosis. Toxicology 417, 64–73. doi: 10.1016/j.tox.2019.02.011
Hulet, S. W., Hess, E. J., Debinski, W., Arosio, P., Bruce, K., Powers, S., et al. (1999a). Characterization and distribution of ferritin binding sites in the adult mouse brain. J. Neurochem. 72, 868–874. doi: 10.1046/j.1471-4159.1999.720868.x
Hulet, S. W., Powers, S., and Connor, J. R. (1999b). Distribution of transferrin and ferritin binding in normal and multiple sclerotic human brains. J. Neurol. Sci. 165, 48–55. doi: 10.1016/s0022-510x(99)00077-5
Iwata-Ichikawa, E., Kondo, Y., Miyazaki, I., Asanuma, M., and Ogawa, N. (1999). Glial cells protect neurons against oxidative stress via transcriptional up-regulation of the glutathione synthesis. J. Neurochem. 72, 2334–2344. doi: 10.1046/j.1471-4159.1999.0722334.x
James, S. A., Churches, Q. I., de Jonge, M. D., Birchall, I. E., Streltsov, V., McColl, G., et al. (2017). Iron, Copper, and Zinc Concentration in Abeta Plaques in the APP/PS1 Mouse Model of Alzheimer’s Disease Correlates with Metal Levels in the Surrounding Neuropil. ACS Chem. Neurosci. 8, 629–637. doi: 10.1021/acschemneuro.6b00362
Jiang, R., Hua, C., Wan, Y., Jiang, B., Hu, H., Zheng, J., et al. (2015). Hephaestin and ceruloplasmin play distinct but interrelated roles in iron homeostasis in mouse brain. J. Nutr. 145, 1003–1009. doi: 10.3945/jn.114.207316
Kagerer, S. M., van Bergen, J. M. G., Li, X., Quevenco, F. C., Gietl, A. F., Studer, S., et al. (2020). APOE4 moderates effects of cortical iron on synchronized default mode network activity in cognitively healthy old-aged adults. Alzheimers Dement. 12:e12002. doi: 10.1002/dad2.12002
Karelson, E., Bogdanovic, N., Garlind, A., Winblad, B., Zilmer, K., Kullisaar, T., et al. (2001). The cerebrocortical areas in normal brain aging and in Alzheimer’s disease: noticeable differences in the lipid peroxidation level and in antioxidant defense. Neurochem. Res. 26, 353–361. doi: 10.1023/a:1010942929678
Kawamata, T., Tooyama, I., Yamada, T., Walker, D. G., and McGeer, P. L. (1993). Lactotransferrin immunocytochemistry in Alzheimer and normal human brain. Am. J. Pathol. 142, 1574–1585.
Kenkhuis, B., Somarakis, A., de Haan, L., Dzyubachyk, O., Me, I. J., de Miranda, N., et al. (2021). Iron loading is a prominent feature of activated microglia in Alzheimer’s disease patients. Acta Neuropathol. Commun. 9:27. doi: 10.1186/s40478-021-01126-5
Kim, A. C., Lim, S., and Kim, Y. K. (2018). Metal Ion Effects on Abeta and Tau Aggregation. Int. J. Mol. Sci. 19:ijms19010128. doi: 10.3390/ijms19010128
Klopstock, T., Tricta, F., Neumayr, L., Karin, I., Zorzi, G., Fradette, C., et al. (2019). Safety and efficacy of deferiprone for pantothenate kinase-associated neurodegeneration: a randomised, double-blind, controlled trial and an open-label extension study. Lancet Neurol. 18, 631–642. doi: 10.1016/S1474-4422(19)30142-5
Knutson, M. D. (2019). Non-transferrin-bound iron transporters. Free Radic. Biol. Med. 133, 101–111. doi: 10.1016/j.freeradbiomed.2018.10.413
Kress, G. J., Dineley, K. E., and Reynolds, I. J. (2002). The relationship between intracellular free iron and cell injury in cultured neurons, astrocytes, and oligodendrocytes. J. Neurosci. 22, 5848–5855. doi: 10.1523/JNEUROSCI.22-14-05848.2002
Kristinsson, J., Snaedal, J., Torsdottir, G., and Johannesson, T. (2012). Ceruloplasmin and iron in Alzheimer’s disease and Parkinson’s disease: a synopsis of recent studies. Neuropsychiatr. Dis. Treat. 8, 515–521. doi: 10.2147/NDT.S34729
Kryscio, R. J., Abner, E. L., Caban-Holt, A., Lovell, M., Goodman, P., Darke, A. K., et al. (2017). Association of Antioxidant Supplement Use and Dementia in the Prevention of Alzheimer’s Disease by Vitamin E and Selenium Trial (PREADViSE). JAMA Neurol. 74, 567–573. doi: 10.1001/jamaneurol.2016.5778
Lane, D. J. R., Metselaar, B., Greenough, M., Bush, A. I., and Ayton, S. J. (2021). Ferroptosis and NRF2: an emerging battlefield in the neurodegeneration of Alzheimer’s disease. Essays Biochem. 65, 925–940. doi: 10.1042/EBC20210017
Lannfelt, L., Blennow, K., Zetterberg, H., Batsman, S., Ames, D., Harrison, J., et al. (2008). Safety, efficacy, and biomarker findings of PBT2 in targeting Abeta as a modifying therapy for Alzheimer’s disease: a phase IIa, double-blind, randomised, placebo-controlled trial. Lancet Neurol. 7, 779–786. doi: 10.1016/S1474-4422(08)70167-4
Lei, P., Ayton, S., Finkelstein, D. I., Spoerri, L., Ciccotosto, G. D., Wright, D. K., et al. (2012). Tau deficiency induces parkinsonism with dementia by impairing APP-mediated iron export. Nat. Med. 18, 291–295. doi: 10.1038/nm.2613
Leng, F., and Edison, P. (2021). Neuroinflammation and microglial activation in Alzheimer disease: where do we go from here? Nat. Rev. Neurol. 17, 157–172. doi: 10.1038/s41582-020-00435-y
Li, J., Li, W., Jiang, Z. G., and Ghanbari, H. A. (2013). Oxidative stress and neurodegenerative disorders. Int. J. Mol. Sci. 14, 24438–24475. doi: 10.3390/ijms141224438
Li, Q., Han, X., Lan, X., Gao, Y., Wan, J., Durham, F., et al. (2017). Inhibition of neuronal ferroptosis protects hemorrhagic brain. JCI Insight 2:e90777. doi: 10.1172/jci.insight.90777
Li, S., Zhou, C., Zhu, Y., Chao, Z., Sheng, Z., Zhang, Y., et al. (2021). Ferrostatin-1 alleviates angiotensin II (Ang II)- induced inflammation and ferroptosis in astrocytes. Int. Immunopharmacol. 90:107179. doi: 10.1016/j.intimp.2020.107179
Lin, G., Zhu, F., Kanaan, N. M., Asano, R., Shirafuji, N., Sasaki, H., et al. (2021). Clioquinol Decreases Levels of Phosphorylated, Truncated, and Oligomerized Tau Protein. Int. J. Mol. Sci. 22:ijms222112063. doi: 10.3390/ijms222112063
Lloret, A., Badia, M. C., Mora, N. J., Pallardo, F. V., Alonso, M. D., and Vina, J. (2009). Vitamin E paradox in Alzheimer’s disease: it does not prevent loss of cognition and may even be detrimental. J. Alzheimers Dis. 17, 143–149. doi: 10.3233/JAD-2009-1033
Lochhead, J. J., and Thorne, R. G. (2012). Intranasal delivery of biologics to the central nervous system. Adv. Drug Deliv. Rev. 64, 614–628. doi: 10.1016/j.addr.2011.11.002
Loeffler, D. A., Connor, J. R., Juneau, P. L., Snyder, B. S., Kanaley, L., DeMaggio, A. J., et al. (1995). Transferrin and iron in normal, Alzheimer’s disease, and Parkinson’s disease brain regions. J. Neurochem. 65, 710–724. doi: 10.1046/j.1471-4159.1995.65020710.x
Lu, L. N., Qian, Z. M., Wu, K. C., Yung, W. H., and Ke, Y. (2017). Expression of Iron Transporters and Pathological Hallmarks of Parkinson’s and Alzheimer’s Diseases in the Brain of Young, Adult, and Aged Rats. Mol. Neurobiol. 54, 5213–5224. doi: 10.1007/s12035-016-0067-0
Maher, P. (2018). Potentiation of glutathione loss and nerve cell death by the transition metals iron and copper: Implications for age-related neurodegenerative diseases. Free Radic. Biol. Med. 115, 92–104. doi: 10.1016/j.freeradbiomed.2017.11.015
Makarov, P., Kropf, S., Wiswedel, I., Augustin, W., and Schild, L. (2006). Consumption of redox energy by glutathione metabolism contributes to hypoxia/reoxygenation-induced injury in astrocytes. Mol. Cell Biochem. 286, 95–101. doi: 10.1007/s11010-005-9098-y
Mancias, J. D., Wang, X., Gygi, S. P., Harper, J. W., and Kimmelman, A. C. (2014). Quantitative proteomics identifies NCOA4 as the cargo receptor mediating ferritinophagy. Nature 509, 105–109. doi: 10.1038/nature13148
Mandal, P. K., Saharan, S., Tripathi, M., and Murari, G. (2015). Brain glutathione levels–a novel biomarker for mild cognitive impairment and Alzheimer’s disease. Biol. Psychiatry 78, 702–710. doi: 10.1016/j.biopsych.2015.04.005
Masaldan, S., Bush, A. I., Devos, D., Rolland, A. S., and Moreau, C. (2019). Striking while the iron is hot: Iron metabolism and ferroptosis in neurodegeneration. Free Radic. Biol. Med. 133, 221–233. doi: 10.1016/j.freeradbiomed.2018.09.033
McCarthy, R. C., and Kosman, D. J. (2012). Mechanistic analysis of iron accumulation by endothelial cells of the BBB. Biometals 25, 665–675. doi: 10.1007/s10534-012-9538-6
McCarthy, R. C., and Kosman, D. J. (2013). Ferroportin and exocytoplasmic ferroxidase activity are required for brain microvascular endothelial cell iron efflux. J. Biol. Chem. 288, 17932–17940. doi: 10.1074/jbc.M113.455428
McCarthy, R. C., Park, Y. H., and Kosman, D. J. (2014). sAPP modulates iron efflux from brain microvascular endothelial cells by stabilizing the ferrous iron exporter ferroportin. EMBO Rep. 15, 809–815. doi: 10.15252/embr.201338064
Meng, F. X., Hou, J. M., and Sun, T. S. (2017). In vivo evaluation of microglia activation by intracranial iron overload in central pain after spinal cord injury. J. Orthop. Surg. Res. 12:75. doi: 10.1186/s13018-017-0578-z
Meyron-Holtz, E. G., Ghosh, M. C., Iwai, K., LaVaute, T., Brazzolotto, X., Berger, U. V., et al. (2004). Genetic ablations of iron regulatory proteins 1 and 2 reveal why iron regulatory protein 2 dominates iron homeostasis. EMBO J. 23, 386–395. doi: 10.1038/sj.emboj.7600041
Miotto, G., Rossetto, M., Di Paolo, M. L., Orian, L., Venerando, R., Roveri, A., et al. (2020). Insight into the mechanism of ferroptosis inhibition by ferrostatin-1. Redox. Biol. 28:101328. doi: 10.1016/j.redox.2019.101328
Moos, T., Rosengren Nielsen, T., Skjorringe, T., and Morgan, E. H. (2007). Iron trafficking inside the brain. J. Neurochem. 103, 1730–1740. doi: 10.1111/j.1471-4159.2007.04976.x
Mukherjee, C., Kling, T., Russo, B., Miebach, K., Kess, E., Schifferer, M., et al. (2020). Oligodendrocytes Provide Antioxidant Defense Function for Neurons by Secreting Ferritin Heavy Chain. Cell Metab. 32, 259–272e210. doi: 10.1016/j.cmet.2020.05.019
Munoz, P., Zavala, G., Castillo, K., Aguirre, P., Hidalgo, C., and Nunez, M. T. (2006). Effect of iron on the activation of the MAPK/ERK pathway in PC12 neuroblastoma cells. Biol. Res. 39, 189–190. doi: 10.4067/s0716-97602006000100021
Newcombe, E. A., Camats-Perna, J., Silva, M. L., Valmas, N., Huat, T. J., and Medeiros, R. (2018). Inflammation: the link between comorbidities, genetics, and Alzheimer’s disease. J. Neuroinflamm. 15:276. doi: 10.1186/s12974-018-1313-3
Nikseresht, S., Bush, A. I., and Ayton, S. (2019). Treating Alzheimer’s disease by targeting iron. Br. J. Pharmacol. 176, 3622–3635. doi: 10.1111/bph.14567
Nnah, I. C., Lee, C. H., and Wessling-Resnick, M. (2020). Iron potentiates microglial interleukin-1beta secretion induced by amyloid-beta. J. Neurochem. 154, 177–189. doi: 10.1111/jnc.14906
Ohgami, R. S., Campagna, D. R., Greer, E. L., Antiochos, B., McDonald, A., Chen, J., et al. (2005). Identification of a ferrireductase required for efficient transferrin-dependent iron uptake in erythroid cells. Nat. Genet. 37, 1264–1269. doi: 10.1038/ng1658
Opazo, C., Luza, S., Villemagne, V. L., Volitakis, I., Rowe, C., Barnham, K. J., et al. (2006). Radioiodinated clioquinol as a biomarker for beta-amyloid: Zn complexes in Alzheimer’s disease. Aging Cell 5, 69–79. doi: 10.1111/j.1474-9726.2006.00196.x
Osama, A., Zhang, J., Yao, J., Yao, X., and Fang, J. (2020). Nrf2: a dark horse in Alzheimer’s disease treatment. Ageing Res. Rev. 64:101206. doi: 10.1016/j.arr.2020.101206
Pardridge, W. M., Eisenberg, J., and Yang, J. (1987). Human blood-brain barrier transferrin receptor. Metabolism 36, 892–895. doi: 10.1016/0026-0495(87)90099-0
Park, E., and Chung, S. W. (2019). ROS-mediated autophagy increases intracellular iron levels and ferroptosis by ferritin and transferrin receptor regulation. Cell Death Dis. 10:822. doi: 10.1038/s41419-019-2064-5
Park, M. W., Cha, H. W., Kim, J., Kim, J. H., Yang, H., Yoon, S., et al. (2021). NOX4 promotes ferroptosis of astrocytes by oxidative stress-induced lipid peroxidation via the impairment of mitochondrial metabolism in Alzheimer’s diseases. Redox. Biol. 41:101947. doi: 10.1016/j.redox.2021.101947
Pelizzoni, I., Zacchetti, D., Campanella, A., Grohovaz, F., and Codazzi, F. (2013). Iron uptake in quiescent and inflammation-activated astrocytes: a potentially neuroprotective control of iron burden. Biochim. Biophys. Acta 1832, 1326–1333. doi: 10.1016/j.bbadis.2013.04.007
Peng, Y., Chang, X., and Lang, M. (2021). Iron Homeostasis Disorder and Alzheimer’s Disease. Int. J. Mol. Sci. 22:ijms222212442. doi: 10.3390/ijms222212442
Perez, D. R., Sklar, L. A., and Chigaev, A. (2019). Clioquinol: To harm or heal. Pharmacol. Ther. 199, 155–163. doi: 10.1016/j.pharmthera.2019.03.009
Peters, D. G., Connor, J. R., and Meadowcroft, M. D. (2015). The relationship between iron dyshomeostasis and amyloidogenesis in Alzheimer’s disease: Two sides of the same coin. Neurobiol. Dis. 81, 49–65. doi: 10.1016/j.nbd.2015.08.007
Peters, D. G., Pollack, A. N., Cheng, K. C., Sun, D., Saido, T., Haaf, M. P., et al. (2018). Dietary lipophilic iron alters amyloidogenesis and microglial morphology in Alzheimer’s disease knock-in APP mice. Metallomics 10, 426–443. doi: 10.1039/c8mt00004b
Qian, Z. M., and Ke, Y. (2019). Brain iron transport. Biol. Rev. Camb. Philos. Soc. 94, 1672–1684. doi: 10.1111/brv.12521
Qian, Z. M., Chang, Y. Z., Zhu, L., Yang, L., Du, J. R., Ho, K. P., et al. (2007). Development and iron-dependent expression of hephaestin in different brain regions of rats. J. Cell Biochem. 102, 1225–1233. doi: 10.1002/jcb.21352
Quiles Del Rey, M., and Mancias, J. D. (2019). NCOA4-Mediated Ferritinophagy: A Potential Link to Neurodegeneration. Front. Neurosci. 13:238. doi: 10.3389/fnins.2019.00238
Raha, A. A., Biswas, A., Henderson, J., Chakraborty, S., Holland, A., Friedland, R. P., et al. (2022). Interplay of Ferritin Accumulation and Ferroportin Loss in Ageing Brain: Implication for Protein Aggregation in Down Syndrome Dementia, Alzheimer’s, and Parkinson’s Diseases. Int. J. Mol. Sci. 23:ijms23031060. doi: 10.3390/ijms23031060
Raha, A. A., Vaishnav, R. A., Friedland, R. P., Bomford, A., and Raha-Chowdhury, R. (2013). The systemic iron-regulatory proteins hepcidin and ferroportin are reduced in the brain in Alzheimer’s disease. Acta Neuropathol. Commun. 1:55. doi: 10.1186/2051-5960-1-55
Raha-Chowdhury, R., Raha, A. A., Forostyak, S., Zhao, J. W., Stott, S. R., and Bomford, A. (2015). Expression and cellular localization of hepcidin mRNA and protein in normal rat brain. BMC Neurosci. 16:24. doi: 10.1186/s12868-015-0161-7
Rassu, G., Soddu, E., Cossu, M., Brundu, A., Cerri, G., Marchetti, N., et al. (2015). Solid microparticles based on chitosan or methyl-beta-cyclodextrin: a first formulative approach to increase the nose-to-brain transport of deferoxamine mesylate. J. Control Release 201, 68–77. doi: 10.1016/j.jconrel.2015.01.025
Rathnasamy, G., Ling, E. A., and Kaur, C. (2013). Consequences of iron accumulation in microglia and its implications in neuropathological conditions. CNS Neurol. Disord. Drug Targets 12, 785–798. doi: 10.2174/18715273113126660169
Ren, J. X., Sun, X., Yan, X. L., Guo, Z. N., and Yang, Y. (2020). Ferroptosis in Neurological Diseases. Front. Cell Neurosci. 14:218. doi: 10.3389/fncel.2020.00218
Ritchie, C. W., Bush, A. I., Mackinnon, A., Macfarlane, S., Mastwyk, M., MacGregor, L., et al. (2003). Metal-protein attenuation with iodochlorhydroxyquin (clioquinol) targeting Abeta amyloid deposition and toxicity in Alzheimer disease: a pilot phase 2 clinical trial. Arch. Neurol. 60, 1685–1691. doi: 10.1001/archneur.60.12.1685
Rogers, J. T., Randall, J. D., Cahill, C. M., Eder, P. S., Huang, X., Gunshin, H., et al. (2002). An iron-responsive element type II in the 5’-untranslated region of the Alzheimer’s amyloid precursor protein transcript. J. Biol. Chem. 277, 45518–45528. doi: 10.1074/jbc.M207435200
Rottkamp, C. A., Raina, A. K., Zhu, X., Gaier, E., Bush, A. I., Atwood, C. S., et al. (2001). Redox-active iron mediates amyloid-beta toxicity. Free Radic. Biol. Med. 30, 447–450. doi: 10.1016/s0891-5849(00)00494-9
Seibt, T. M., Proneth, B., and Conrad, M. (2019). Role of GPX4 in ferroptosis and its pharmacological implication. Free Radic. Biol. Med. 133, 144–152. doi: 10.1016/j.freeradbiomed.2018.09.014
Shachar, D. B., Kahana, N., Kampel, V., Warshawsky, A., and Youdim, M. B. (2004). Neuroprotection by a novel brain permeable iron chelator, VK-28, against 6-hydroxydopamine lession in rats. Neuropharmacology 46, 254–263. doi: 10.1016/j.neuropharm.2003.09.005
Sharma, V. K., Singh, T. G., Singh, S., Garg, N., and Dhiman, S. (2021). Apoptotic Pathways and Alzheimer’s Disease: Probing Therapeutic Potential. Neurochem. Res. 46, 3103–3122. doi: 10.1007/s11064-021-03418-7
Sheftel, A. D., Mason, A. B., and Ponka, P. (2012). The long history of iron in the Universe and in health and disease. Biochim. Biophys. Acta 1820, 161–187. doi: 10.1016/j.bbagen.2011.08.002
Shih, A. Y., Johnson, D. A., Wong, G., Kraft, A. D., Jiang, L., Erb, H., et al. (2003). Coordinate regulation of glutathione biosynthesis and release by Nrf2-expressing glia potently protects neurons from oxidative stress. J. Neurosci. 23, 3394–3406. doi: 10.1523/JNEUROSCI.23-08-03394.2003
Siegel, S. J., Bieschke, J., Powers, E. T., and Kelly, J. W. (2007). The oxidative stress metabolite 4-hydroxynonenal promotes Alzheimer protofibril formation. Biochemistry 46, 1503–1510. doi: 10.1021/bi061853s
Silvestri, L., and Camaschella, C. (2008). A potential pathogenetic role of iron in Alzheimer’s disease. J. Cell Mol. Med. 12, 1548–1550. doi: 10.1111/j.1582-4934.2008.00356.x
Singh, A., Haldar, S., Horback, K., Tom, C., Zhou, L., Meyerson, H., et al. (2013). Prion protein regulates iron transport by functioning as a ferrireductase. J. Alzheimers Dis. 35, 541–552. doi: 10.3233/JAD-130218
Singh, N. (2014). The role of iron in prion disease and other neurodegenerative diseases. PLoS Pathog. 10:e1004335. doi: 10.1371/journal.ppat.1004335
Singh, N., Haldar, S., Tripathi, A. K., Horback, K., Wong, J., Sharma, D., et al. (2014). Brain iron homeostasis: from molecular mechanisms to clinical significance and therapeutic opportunities. Antioxid. Redox. Signal. 20, 1324–1363. doi: 10.1089/ars.2012.4931
Skouta, R., Dixon, S. J., Wang, J., Dunn, D. E., Orman, M., Shimada, K., et al. (2014). Ferrostatins inhibit oxidative lipid damage and cell death in diverse disease models. J. Am. Chem. Soc. 136, 4551–4556. doi: 10.1021/ja411006a
Smith, M. A., Harris, P. L., Sayre, L. M., and Perry, G. (1997). Iron accumulation in Alzheimer disease is a source of redox-generated free radicals. Proc. Natl. Acad. Sci. U S A. 94, 9866–9868. doi: 10.1073/pnas.94.18.9866
Smith, M. A., Zhu, X., Tabaton, M., Liu, G., McKeel, D. W. Jr., Cohen, M. L., et al. (2010). Increased iron and free radical generation in preclinical Alzheimer disease and mild cognitive impairment. J. Alzheimers Dis. 19, 363–372. doi: 10.3233/JAD-2010-1239
Song, T., Song, X., Zhu, C., Patrick, R., Skurla, M., Santangelo, I., et al. (2021). Mitochondrial dysfunction, oxidative stress, neuroinflammation, and metabolic alterations in the progression of Alzheimer’s disease: A meta-analysis of in vivo magnetic resonance spectroscopy studies. Ageing Res. Rev. 72:101503. doi: 10.1016/j.arr.2021.101503
Spotorno, N., Acosta-Cabronero, J., Stomrud, E., Lampinen, B., Strandberg, O. T., van Westen, D., et al. (2020). Relationship between cortical iron and tau aggregation in Alzheimer’s disease. Brain 143, 1341–1349. doi: 10.1093/brain/awaa089
Streit, W. J., Rotter, J., Winter, K., Muller, W., Khoshbouei, H., and Bechmann, I. (2021). Droplet Degeneration of Hippocampal and Cortical Neurons Signifies the Beginning of Neuritic Plaque Formation. J. Alzheimers Dis. 2021:215334. doi: 10.3233/JAD-215334
Sun, X., Ou, Z., Chen, R., Niu, X., Chen, D., Kang, R., et al. (2016). Activation of the p62-Keap1-NRF2 pathway protects against ferroptosis in hepatocellular carcinoma cells. Hepatology 63, 173–184. doi: 10.1002/hep.28251
Tian, R., Abarientos, A., Hong, J., Hashemi, S. H., Yan, R., Drager, N., et al. (2021). Genome-wide CRISPRi/a screens in human neurons link lysosomal failure to ferroptosis. Nat. Neurosci. 2021:862. doi: 10.1038/s41593-021-00862-0
Todorich, B., Pasquini, J. M., Garcia, C. I., Paez, P. M., and Connor, J. R. (2009). Oligodendrocytes and myelination: the role of iron. Glia 57, 467–478. doi: 10.1002/glia.20784
Todorich, B., Zhang, X., and Connor, J. R. (2011). H-ferritin is the major source of iron for oligodendrocytes. Glia 59, 927–935. doi: 10.1002/glia.21164
Tripathi, A. K., Haldar, S., Qian, J., Beserra, A., Suda, S., Singh, A., et al. (2015). Prion protein functions as a ferrireductase partner for ZIP14 and DMT1. Free Radic. Biol. Med. 84, 322–330. doi: 10.1016/j.freeradbiomed.2015.03.037
Tsatsanis, A., McCorkindale, A. N., Wong, B. X., Patrick, E., Ryan, T. M., Evans, R. W., et al. (2021). The acute phase protein lactoferrin is a key feature of Alzheimer’s disease and predictor of Abeta burden through induction of APP amyloidogenic processing. Mol. Psychiatry 26, 5516–5531. doi: 10.1038/s41380-021-01248-1
Tsatsanis, A., Wong, B. X., Gunn, A. P., Ayton, S., Bush, A. I., Devos, D., et al. (2020). Amyloidogenic processing of Alzheimer’s disease beta-amyloid precursor protein induces cellular iron retention. Mol. Psychiat. 25, 1958–1966. doi: 10.1038/s41380-020-0762-0
Tulpule, K., Robinson, S. R., Bishop, G. M., and Dringen, R. (2010). Uptake of ferrous iron by cultured rat astrocytes. J. Neurosci. Res. 88, 563–571. doi: 10.1002/jnr.22217
Tuo, Q. Z., Lei, P., Jackman, K. A., Li, X. L., Xiong, H., Li, X. L., et al. (2017). Tau-mediated iron export prevents ferroptotic damage after ischemic stroke. Mol. Psychiatry 22, 1520–1530. doi: 10.1038/mp.2017.171
Urrutia, P., Aguirre, P., Esparza, A., Tapia, V., Mena, N. P., Arredondo, M., et al. (2013). Inflammation alters the expression of DMT1, FPN1 and hepcidin, and it causes iron accumulation in central nervous system cells. J. Neurochem. 126, 541–549. doi: 10.1111/jnc.12244
van Duijn, S., Bulk, M., van Duinen, S. G., Nabuurs, R. J. A., van Buchem, M. A., van der Weerd, L., et al. (2017). Cortical Iron Reflects Severity of Alzheimer’s Disease. J. Alzheimers Dis. 60, 1533–1545. doi: 10.3233/JAD-161143
Varikasuvu, S. R., Prasad, V. S., Kothapalli, J., and Manne, M. (2019). Brain Selenium in Alzheimer’s Disease (BRAIN SEAD Study): a Systematic Review and Meta-Analysis. Biol. Trace Elem. Res. 189, 361–369. doi: 10.1007/s12011-018-1492-x
Vela, D. (2018). Hepcidin, an emerging and important player in brain iron homeostasis. J. Transl. Med. 16:25. doi: 10.1186/s12967-018-1399-5
Wan, W., Cao, L., Kalionis, B., Murthi, P., Xia, S., and Guan, Y. (2019). Iron Deposition Leads to Hyperphosphorylation of Tau and Disruption of Insulin Signaling. Front. Neurol. 10:607. doi: 10.3389/fneur.2019.00607
Wang, L., Yang, H., Zhao, S., Sato, H., Konishi, Y., Beach, T. G., et al. (2011). Expression and localization of mitochondrial ferritin mRNA in Alzheimer’s disease cerebral cortex. PLoS One 6:e22325. doi: 10.1371/journal.pone.0022325
Wang, L., Yin, Y. L., Liu, X. Z., Shen, P., Zheng, Y. G., Lan, X. R., et al. (2020). Current understanding of metal ions in the pathogenesis of Alzheimer’s disease. Transl. Neurodegener. 9:10. doi: 10.1186/s40035-020-00189-z
Wang, S. M., Fu, L. J., Duan, X. L., Crooks, D. R., Yu, P., Qian, Z. M., et al. (2010). Role of hepcidin in murine brain iron metabolism. Cell Mol. Life Sci. 67, 123–133. doi: 10.1007/s00018-009-0167-3
Wang, T., Wang, C. Y., Shan, Z. Y., Teng, W. P., and Wang, Z. Y. (2012). Clioquinol reduces zinc accumulation in neuritic plaques and inhibits the amyloidogenic pathway in AbetaPP/PS1 transgenic mouse brain. J. Alzheimers Dis. 29, 549–559. doi: 10.3233/JAD-2011-111874
Ward, R. J., Dexter, D., Florence, A., Aouad, F., Hider, R., Jenner, P., et al. (1995). Brain iron in the ferrocene-loaded rat: its chelation and influence on dopamine metabolism. Biochem. Pharmacol. 49, 1821–1826. doi: 10.1016/0006-2952(94)00521-m
Ward, R. J., Zucca, F. A., Duyn, J. H., Crichton, R. R., and Zecca, L. (2014). The role of iron in brain ageing and neurodegenerative disorders. Lancet Neurol. 13, 1045–1060. doi: 10.1016/S1474-4422(14)70117-6
Weiland, A., Wang, Y., Wu, W., Lan, X., Han, X., Li, Q., et al. (2019). Ferroptosis and Its Role in Diverse Brain Diseases. Mol. Neurobiol. 56, 4880–4893. doi: 10.1007/s12035-018-1403-3
Wong, B. X., Tsatsanis, A., Lim, L. Q., Adlard, P. A., Bush, A. I., and Duce, J. A. (2014b). beta-Amyloid precursor protein does not possess ferroxidase activity but does stabilize the cell surface ferrous iron exporter ferroportin. PLoS One 9:e114174. doi: 10.1371/journal.pone.0114174
Wong, B. X., Ayton, S., Lam, L. Q., Lei, P., Adlard, P. A., Bush, A. I., et al. (2014a). A comparison of ceruloplasmin to biological polyanions in promoting the oxidation of Fe(2+) under physiologically relevant conditions. Biochim. Biophys. Acta 1840, 3299–3310. doi: 10.1016/j.bbagen.2014.08.006
Wood, H. (2015). Alzheimer disease: Iron–the missing link between ApoE and Alzheimer disease? Nat. Rev. Neurol. 11:369. doi: 10.1038/nrneurol.2015.96
Xie, B. S., Wang, Y. Q., Lin, Y., Mao, Q., Feng, J. F., Gao, G. Y., et al. (2019). Inhibition of ferroptosis attenuates tissue damage and improves long-term outcomes after traumatic brain injury in mice. CNS Neurosci. Ther. 25, 465–475. doi: 10.1111/cns.13069
Xie, Y., Hou, W., Song, X., Yu, Y., Huang, J., Sun, X., et al. (2016). Ferroptosis: process and function. Cell Death Differ. 23, 369–379. doi: 10.1038/cdd.2015.158
Xu, H., Perreau, V. M., Dent, K. A., Bush, A. I., Finkelstein, D. I., and Adlard, P. A. (2016). Iron Regulates Apolipoprotein E Expression and Secretion in Neurons and Astrocytes. J. Alzheimers Dis. 51, 471–487. doi: 10.3233/JAD-150797
Xu, Y., Zhang, Y., Zhang, J. H., Han, K., Zhang, X., Bai, X., et al. (2020). Astrocyte hepcidin ameliorates neuronal loss through attenuating brain iron deposition and oxidative stress in APP/PS1 mice. Free Radic. Biol. Med. 158, 84–95. doi: 10.1016/j.freeradbiomed.2020.07.012
Yang, W. S., Kim, K. J., Gaschler, M. M., Patel, M., Shchepinov, M. S., and Stockwell, B. R. (2016). Peroxidation of polyunsaturated fatty acids by lipoxygenases drives ferroptosis. Proc. Natl. Acad. Sci. U S A. 113, E4966–E4975. doi: 10.1073/pnas.1603244113
Yang, Z., Song, Q., Cao, Z., Yu, G., Liu, Z., Tan, Z., et al. (2020). Design, synthesis and evaluation of flurbiprofen-clioquinol hybrids as multitarget-directed ligands against Alzheimer’s disease. Bioorg. Med. Chem. 28:115374. doi: 10.1016/j.bmc.2020.115374
Yoo, M. H., Gu, X., Xu, X. M., Kim, J. Y., Carlson, B. A., Patterson, A. D., et al. (2010). Delineating the role of glutathione peroxidase 4 in protecting cells against lipid hydroperoxide damage and in Alzheimer’s disease. Antioxid. Redox. Signal. 12, 819–827. doi: 10.1089/ars.2009.2891
Yoo, S. E., Chen, L., Na, R., Liu, Y., Rios, C., Van Remmen, H., et al. (2012). Gpx4 ablation in adult mice results in a lethal phenotype accompanied by neuronal loss in brain. Free Radic. Biol. Med. 52, 1820–1827. doi: 10.1016/j.freeradbiomed.2012.02.043
Yoshida, T., Tanaka, M., Sotomatsu, A., Hirai, S., and Okamoto, K. (1998). Activated microglia cause iron-dependent lipid peroxidation in the presence of ferritin. Neuroreport 9, 1929–1933. doi: 10.1097/00001756-199806220-00003
Zarruk, J. G., Berard, J. L., Passos dos Santos, R., Kroner, A., Lee, J., Arosio, P., et al. (2015). Expression of iron homeostasis proteins in the spinal cord in experimental autoimmune encephalomyelitis and their implications for iron accumulation. Neurobiol. Dis. 81, 93–107. doi: 10.1016/j.nbd.2015.02.001
Zechel, S., Huber-Wittmer, K., and von Bohlen und Halbach, O. (2006). Distribution of the iron-regulating protein hepcidin in the murine central nervous system. J. Neurosci. Res. 84, 790–800. doi: 10.1002/jnr.20991
Zhang, X., Gou, Y. J., Zhang, Y., Li, J., Han, K., Xu, Y., et al. (2020). Hepcidin overexpression in astrocytes alters brain iron metabolism and protects against amyloid-beta induced brain damage in mice. Cell Death Discov. 6:113. doi: 10.1038/s41420-020-00346-3
Zhang, X., Surguladze, N., Slagle-Webb, B., Cozzi, A., and Connor, J. R. (2006). Cellular iron status influences the functional relationship between microglia and oligodendrocytes. Glia 54, 795–804. doi: 10.1002/glia.20416
Zhang, Y. H., Wang, D. W., Xu, S. F., Zhang, S., Fan, Y. G., Yang, Y. Y., et al. (2018). alpha-Lipoic acid improves abnormal behavior by mitigation of oxidative stress, inflammation, ferroptosis, and tauopathy in P301S Tau transgenic mice. Redox. Biol. 14, 535–548. doi: 10.1016/j.redox.2017.11.001
Zhang, Z., Yang, X., Song, Y. Q., and Tu, J. (2021). Autophagy in Alzheimer’s disease pathogenesis: Therapeutic potential and future perspectives. Ageing Res. Rev. 72:101464. doi: 10.1016/j.arr.2021.101464
Zhao, Y. S., Zhang, L. H., Yu, P. P., Gou, Y. J., Zhao, J., You, L. H., et al. (2018). Ceruloplasmin, a Potential Therapeutic Agent for Alzheimer’s Disease. Antioxid. Redox. Signal. 28, 1323–1337. doi: 10.1089/ars.2016.6883
Keywords: Alzheimer’s disease, iron, ferroptosis, FPN1, GPX4, lipid peroxidation, iron chelator, hepcidin
Citation: Wang F, Wang J, Shen Y, Li H, Rausch W-D and Huang X (2022) Iron Dyshomeostasis and Ferroptosis: A New Alzheimer’s Disease Hypothesis? Front. Aging Neurosci. 14:830569. doi: 10.3389/fnagi.2022.830569
Received: 07 December 2021; Accepted: 25 February 2022;
Published: 22 March 2022.
Edited by:
Xudong Huang, Massachusetts General Hospital and Harvard Medical School, United StatesReviewed by:
Scott Ayton, University of Melbourne, AustraliaJames Connor, Penn State Milton S. Hershey Medical Center, United States
Copyright © 2022 Wang, Wang, Shen, Li, Rausch and Huang. This is an open-access article distributed under the terms of the Creative Commons Attribution License (CC BY). The use, distribution or reproduction in other forums is permitted, provided the original author(s) and the copyright owner(s) are credited and that the original publication in this journal is cited, in accordance with accepted academic practice. No use, distribution or reproduction is permitted which does not comply with these terms.
*Correspondence: Xiaobo Huang, aHVhbmd4aWFvYm9AeHdoLmNjbXUuZWR1LmNu