- 1Neuroscience and Mental Health Institute, University of Alberta, Edmonton, AB, Canada
- 2Neuroscience and Mental Health Institute, University of Alberta, Edmonton, AB, Canada
- 3Department of Medicine, Division of Neurology, Faculty of Medicine and Dentistry, University of Alberta, Edmonton, AB, Canada
Stroke is among the leading causes of death and disability worldwide. Restoring blood flow through recanalization is currently the only acute treatment for cerebral ischemia. Unfortunately, many patients that achieve a complete recanalization fail to regain functional independence. Recent studies indicate that activation of peripheral immune cells, particularly neutrophils, may contribute to microcirculatory failure and futile recanalization. Stroke primarily affects the elderly population, and mortality after endovascular therapies is associated with advanced age. Previous analyses of differential gene expression across injury status and age identify ischemic stroke as a complex age-related disease. It also suggests robust interactions between stroke injury, aging, and inflammation on a cellular and molecular level. Understanding such interactions is crucial in developing effective protective treatments. The global stroke burden will continue to increase with a rapidly aging human population. Unfortunately, the mechanisms of age-dependent vulnerability are poorly defined. In this review, we will discuss how neutrophil-specific gene expression patterns may contribute to poor treatment responses in stroke patients. We will also discuss age-related transcriptional changes that may contribute to poor clinical outcomes and greater susceptibility to cerebrovascular diseases.
Cerebral ischemia and the ischemic cascade
Stroke is one of the leading causes of death and long-term disability globally (Sturm et al., 2004; Chen et al., 2010; Benjamin et al., 2019) and leads to significant healthcare and economic burden (Saka et al., 2009). A stroke can be characterized as ischemic or hemorrhagic (Donkor, 2018). Hemorrhagic stroke results from a ruptured blood vessel that bleeds into the surrounding area. Cerebral ischemia, or ischemic stroke (IS), is caused by a blockage of the artery supplying an area of the brain (Formisano et al., 2020). Over half of all IS occurs in the middle cerebral artery (MCA), one of the main blood supplies to the brain (Ng et al., 2007). The MCA derives directly from the internal carotid artery (ICA) and routes along the lateral sulcus before branching into the basal ganglia, frontal, parietal, and temporal lobes (Llovera et al., 2014). The MCA connects the anterior cerebral artery (ACA) and the posterior cerebral artery (PCA). Along with the ICA and PCA, these form the circle of Willis. There are four main branches in the MCA, termed M1, M2, M3, and M4. With respect to IS, the location of MCA occlusion determines the extent of cerebral injury. Proximal middle cerebral occlusion (MCAO) is an occlusion nearer to the origin of MCA and results in damage to a large cortical and subcortical region (Llovera et al., 2014). Distal MCAO is an occlusion more distally that damages a smaller area, often a purely cortical injury with a less severe clinical presentation (Bouet and Freret, 2012; Navarro-Orozco and Sánchez-Manso, 2021).
The pathophysiology of IS is complex. Focal IS begins with an occlusion of a cerebral artery (most commonly the MCA), leading to focal hypoperfusion in downstream territories. The lack of oxygen and reduced delivery of glucose in the ischemic region disrupts normal cellular ATP synthesis. This prompts cells to switch to anaerobic metabolism, promoting lactic acidosis and electrochemical gradient loss. Impaired ATP production prevents the normal function of ATP-reliant ion transport pumps, leading to cellular depolarization and intracellular Ca2+ elevation, which triggers free radical release, excitotoxicity, and cytotoxic edema (Dirnagl et al., 1999; Endres et al., 2008; Xing et al., 2012). Severe ischemia leads to rapid cell lysis due to anoxic depolarization, while less severe cellular injury triggers apoptosis. As the cell membrane and the mitochondria are damaged, there is an increase in intracellular toxins and apoptotic factors that initiates a caspase-dependent apoptotic cascade, causing cells to undergo cell death and further exacerbating local inflammation (Dirnagl et al., 1999; Allencherril et al., 2019). Without immediate restoration of blood flow, the ischemic cascade results in permanent cerebral damage (Hossmann, 2006; Durukan and Tatlisumak, 2007; Lakhan et al., 2009).
The rate and reversibility of IS vary with the degree of preserved blood flow and tissue viability at the time of treatment (Kalogeris et al., 2012). The current gold standard treatment for IS is recanalization through endovascular therapy (EVT), involving a catheter-based mechanical thrombectomy (MT), and adjunctive systemic intravenous (IV) thrombolysis with recombinant tissue plasminogen activator (r-tPA; Rothwell, 2018; Campbell et al., 2019). Both interventions aim to recanalize the affected blood vessels and reperfuse ischemic tissue to restore oxygen and nutrient delivery (del Zoppo et al., 1992; Panni et al., 2019). Studies have demonstrated the benefits of IV r-tPA for treating IS (Phan et al., 2017); however, its efficacy has been suboptimal, and many patients treated within its therapeutic window exhibited limited benefit (Allencherril et al., 2019). EVT is highly effective at opening large vessel occlusions and produces superior outcomes to IV thrombolysis (Goyal et al., 2016). Nevertheless, good outcomes are not always achieved with EVT, even when the vessel is fully recanalized. Clinical trials MR CLEAN, EXTEND-IA, ESCAPE, SWIFT PRIME, and REVASCAT showed the rate of “futile recanalization” after endovascular treatment to be 54%, even among patients with optimal conditions of recanalization timing and efficiency (Goyal et al., 2016; Leischner et al., 2019; Flottmann et al., 2021). These studies and trials suggest that tissue viability prior to recanalization is vital and that successfully reopening the vessels does not necessarily equate to improved tissue-level perfusion. This latter phenomenon of microcirculatory failure is known as “no-reflow.”
Collateral circulation and stroke outcome
In the late 1970s, Lynsay Symon demonstrated that in reversible MCAO, there is the presence of the ischemic penumbra and oligaemia, in addition to an ischemic core where irreversible neuronal death occurs within minutes after the occlusion (Astrup et al., 1981; Baron et al., 2020). In this core/penumbra model, Symon showed that there are three parts in the ischemic area, (1) the ischemic core with established irreversible cell death and the cerebral blood flow below 10 ml/100 g/min (as to a normal 50 ml/100 g/min flow); (2) the ischemic penumbra outside of the core with reduced perfusion rate but viable and salvageable if blood flow is restored; and (3) the oligaemia that is mildly hypoperfused and neuronal functions are preserved (Astrup et al., 1981; Dirnagl et al., 1999; Jackman and Iadecola, 2015; Baron et al., 2020). Unless reperfusion occurs quickly, irreversible cell death in the core expands; hence, the famous mantra “time is brain.”
Collateral circulation refers to auxiliary vascular pathways that allow for partial perfusion of ischemic tissue after the primary vascular routes are blocked (Liebeskind, 2003, 2012; Shuaib et al., 2011; Winship, 2015). Collateral circulation consists of the primary and secondary arterial collaterals and venous collaterals. Primary arterial collaterals are the arterial segments in the circle of Willis that carry blood between the areas of the ICA and vertebrobasilar system or between cerebral hemispheres. The secondary collaterals include leptomeningeal arteries at the pial surface of the cortex, which connect the distal branches of the ACA, PCA, and MCA (Liebeskind, 2003; Shuaib et al., 2011; Winship et al., 2014; Winship, 2015). Under normal conditions, leptomeningeal collaterals are dormant, but are recruited when blood flow in major arteries is arrested. During MCAO, the change in pressure gradient between the areas supplied by the ACA or PCA permits blood to flow through leptomeningeal collateral anastomoses from intact areas into the ischemic region. Thus, the extent of collateral flow defines the viability of the penumbral region (Winship, 2015). Blood flow through these collaterals drops once the pressure gradient is restored after successful recanalization (Iwasawa et al., 2016).
Cerebral collaterals are associated with IS pathophysiology (Liebeskind, 2003), penumbral volume (Jung et al., 2013), and response to treatment (Becker, 1998). Collateral circulation deterioration after IS is also correlated with continued infarct growth in patients without recanalization (Campbell et al., 2013). Adequate collateral circulation, measured by collateral status, can temporarily maintain tissue viability in the absence of recanalization. Collateral status can be determined by using computed tomographic angiography and a variety of scales including the binary modified Tan collateral scale, in which “good collaterals” fill more than 50% of the area distal to MCAO region and “poor collaterals” can only achieve <50% (Tan et al., 2009; Yeo et al., 2015; Gensicke et al., 2022). In both IV thrombolysis and EVT trials, a shorter time to treatment correlated with better odds for positive outcomes (Lees et al., 2010; Khatri et al., 2014; Prabhakaran et al., 2015). However, many patients with good collateral flow and prolonged time from symptom onset to treatment of up to 24 h could still benefit from EVT (Albers et al., 2018; Nogueira et al., 2018). This shows that there is a patient-to-patient variation in collateral circulation, and that collaterals extend the time window to treatment and predict good clinical responses (Ribo et al., 2011; Hwang et al., 2015). Furthermore, patients with better angiographically assessed collateral scores have shown smaller infarct volumes, a lower degree of dependence and disability, and better functional recovery from IS (Becker, 1998; Christoforidis et al., 2005).
It has been shown that the prognosis of stroke patients is influenced by collateral circulation, especially leptomeningeal anastomoses. Collaterals sustain tissue viability until reperfusion (Tariq and Khatri, 2008). In recent years, evidence of leptomeningeal anastomoses contributing to ischemic tissue viability has been documented using computerized tomography (CT) angiography, triphasic perfusion CT, Xenon CT, and magnetic resonance imaging (Wildermuth et al., 1998; Brozici et al., 2003; Tariq and Khatri, 2008). In the PROACT II clinical trial, researchers showed that leptomeningeal collaterals are associated with better clinical outcomes (evaluated by NIHSS score), lower infarct size (shown in 24-h CT scan), and more rapid recanalization (Roberts et al., 2002). Similarly, other studies using CT showed that collaterals are correlated with lower infarct volume post-recanalization and better discharge mRS scores (Ringelstein et al., 1992; Christoforidis et al., 2005; Mohammad et al., 2008). Lee and their colleagues demonstrated using triphasic CT that slow or few meningeal collaterals corresponded to severe perfusion deficits and hemorrhagic transformation (Lee et al., 2000). In 2017, a retrospective study of 135 patients with ischemia and good collateral scores demonstrated that these scores significantly predicted successful recanalization, as determined by 90-day mRS scores (Tong et al., 2017). These findings provide concrete evidence of the correlation between good collaterals and improved recanalization outcomes post-stroke.
Futile recanalization and the “no-reflow” phenomenon
The “no-reflow” phenomenon is defined as inadequate perfusion through a given segment of circulation without any angiographic evidence of mechanical vessel obstruction (Kloner et al., 2018; Allencherril et al., 2019; El Amki et al., 2020; Ter Schiphorst et al., 2021). This could be due to microcirculatory flow failure in small arterioles and capillaries despite a complete recanalization of the large, occluded artery (Soares et al., 2009; Pan et al., 2021). Although there are different processes involved in the ischemic cascade and IS pathogenesis, increasing evidence shows that inflammation is a primary driver of no-reflow (Muir et al., 2007; Jaffe et al., 2008; Kloner et al., 2018; Jayaraj et al., 2019).
The no-reflow phenomenon has been suggested to contribute to futile recanalization (El Amki and Wegener, 2017). Futile recanalization refers to the failure to improve neurological outcomes despite a successful recanalization, as indicated by the modified Rankin Scale (mRS) scores of 4–6 (Pan et al., 2021). The mRS measures the degree of disability or dependence in daily activities and is scaled from 0 (no symptoms) to 5 (severe disability) and 6 (death). Patients with near-complete or complete recanalization are divided into two groups, according to their functional outcomes at 3 months. Scores 0–2 are defined as “meaningful recanalization,” while scores 3–6 are “futile recanalization” (Patel et al., 2012; Saver et al., 2021). There are several factors that may account for futile recanalization, but neutrophil adhesion to the microvasculature has recently gained attention and may play a key role in no-reflow (Liebeskind, 2003; El Amki et al., 2020). Understanding the molecular changes in the hypoperfused tissues is paramount to resolving reperfusion injury, microcirculatory dysfunctions, no-reflow, and futile recanalization, as well as finding novel predictors of clinical deterioration and prevention strategies (Cowled and Fitridge, 2011). Notably, good collateral status is associated with reduced frequency of futile recanalization, as sufficient collateral flow may maintain tissue viability prior to restored flow in the occluded vessel (Bang et al., 2015). Moreover, good collaterals may also reduce the activation of neutrophils by cerebral ischemia, thereby reducing no-reflow/microcirculatory failure and improving tissue reperfusion after successful recanalization (Bang et al., 2015; Kloner et al., 2018).
Post-ischemic inflammation exacerbates stroke outcomes
Depending on the injury severity, acute inflammation can initiate within minutes and resolve after days (Chung et al., 2019; Endres et al., 2022). In IS, acute inflammation manifests immediately after vessel occlusion (Iadecola and Anrather, 2011; De Meyer et al., 2016).
Following IS, the ensuing hypoxia, reduced sheer stress on the post-capillary venule walls, and reactive oxygen species (ROS) production trigger leukocytes recruitment, including neutrophils, lymphocytes, and monocytes, to the injury site (Dereski et al., 1993; del Zoppo, 1994; Iadecola and Anrather, 2011; Perez-de-Puig et al., 2015). These cells have long been documented in the peripheral blood samples of patients with IS (Huang et al., 2006; Whiteley et al., 2009; Kim et al., 2012; Petrovic-Djergovic et al., 2016; Xue et al., 2017). Cell recruitment prompts a systemic inflammatory reaction characterized by an activation of peripheral immune cells and the release of pro-inflammatory mediators from the ischemic endothelium and brain parenchyma (Iadecola and Anrather, 2011; Jickling et al., 2015; Planas, 2018). Inflammatory cells release several cytotoxic agents, such as matrix metalloproteinases (MMPs) and nitric oxide (NO). These mediators intensify cellular damage and disrupt the extracellular matrix and blood–brain barrier (BBB), and can lead to hemorrhagic transformation (Danton and Dietrich, 2003; Prass et al., 2003). Within 24 h after onset, cell damage, ATP, fibrinogen, and ROS activate microglia, the primary immune cells of the CNS (Weinstein et al., 2010; Anttila et al., 2017). Microglia produce inflammatory cytokines (e.g., IL-1β, IL-6, and TNF-α) and chemokines (e.g., MIP-1, MIP-2, and MCP-1) in the brain parenchyma (Raghavendra Rao et al., 2002), which stimulates NF-κB expression and the upregulation of adhesion molecules (E-selectin, L-selectin, P-selectin, ICAM-1, and integrins) on the endothelial cell surface (Morioka et al., 1993; Becker, 1998). Cytokine release also facilitates progressive cell death (Zhang et al., 1994) and oxidative cellular injury (Nagayama et al., 2000), exacerbating the ischemic cascade.
Adhesion molecules permit leukocyte adhesion to the vascular endothelium and their infiltration into the brain parenchyma (Hallenbeck, 1996; Suzuki et al., 1997; Sughrue et al., 2004), which is a hallmark of acute inflammation (Perry and Granger, 1991; Bienvenu et al., 1992). Selectins generally slow down circulating leukocytes by attracting them to the endothelial surface. Upon activation, L-selectin recruits leukocytes to the injury site, while P-selectin and E-selectin bind to leukocytes on the endothelium (Becker, 1998), facilitating leukocyte rolling and adhesion (De Meyer et al., 2016; Petrovic-Djergovic et al., 2016; Figure 1). P-selection upregulation initiates as early as 15 min after ischemic onset while E-selection expression begins within 2 h (Yilmaz and Granger, 2008). Normally, leukocytes flow smoothly across capillaries without rotating or marginating. However, in IS, leukocytes, and specifically neutrophils, may clog the capillaries and disrupt blood flow (Doerschuk et al., 1993). Impeding inflammatory responses and, recently, reducing these neutrophil stalls have been shown to ameliorate injury in animal stroke models (Cuartero et al., 2013; Drieu et al., 2018; Kelly et al., 2018; El Amki et al., 2020; Erdener et al., 2021). For instance, inhibiting CXCR1 and CXCR2 in rat MCAO model, which are among the biomarkers of inflammation, improves behavioral measures, reduces ischemic brain damage, and decreases IL-1β level in the brain (Villa et al., 2007). IL1-receptor antagonist has also been found to decrease immune cell invasion in the brain, including one of neutrophils, as well as reducing infarct size and advancing behavioral outcomes in rodents (Rothwell, 2003). P-selectin expression is also associated with a reduced cerebral blood flow post-reperfusion, and animals that lack P-selectin have smaller infarct size and better survival (Connolly et al., 1997). ICAM-1 and integrins control leukocyte adhesion and movement across endothelial cells, or diapedesis, and eventual migration to the parenchyma (Blann et al., 2002). This can be observed as early as 30 min after onset and prolongs to the 48-h mark (Yilmaz and Granger, 2008). When leukocytes attached to adhesion molecules are activated, they produce and release ROS, proteases (e.g., elastase, collagenase, and gelatinase), and cationic proteins (e.g., defensins) that can proteolyze the endothelial membrane and interstitial matrix (Weiss, 1989; Granger, 1999). Blocking ICAM-1, thus reducing leukocyte adhesion, has been shown to reduce IS damage (Zhang et al., 1995).
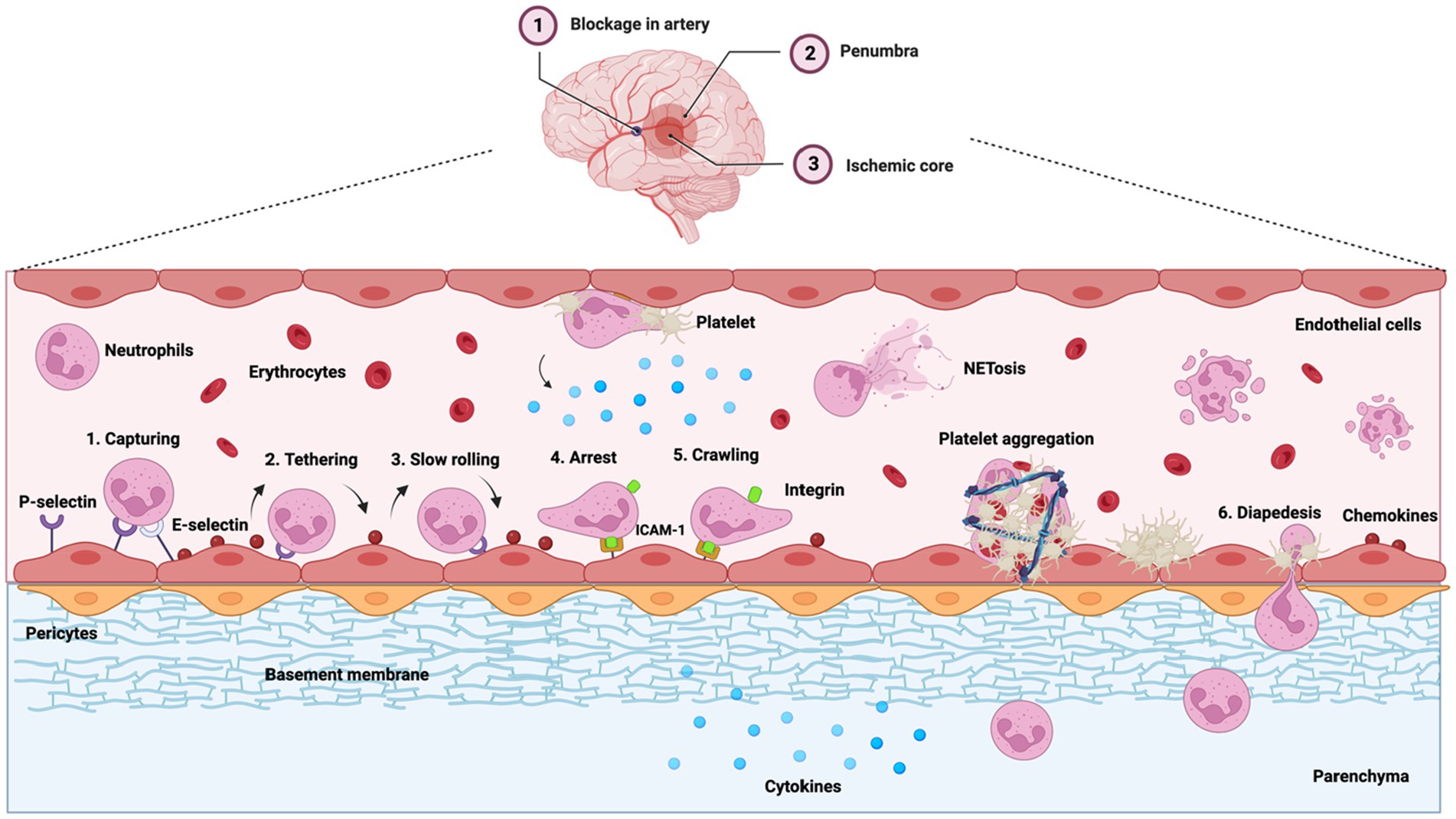
Figure 1. Leukocyte (neutrophil) extravasation and infiltration during inflammation are major contributors to poor ischemia outcomes. Middle cerebral artery occlusion is illustrated. During inflammation, cytokines (IL-1β, IL-6, and TNF-α) and the chemokines (MIP-1, MIP-2, and MCP-1) are upregulated, which stimulate the expression of selectins and ICAM-1 on the endothelial surface near the ischemic area. Chemokines (blue dots) are transcytosed through or deposited on the endothelium. E-selectin and P-selectin capture neutrophils from the blood flow, pulling the tethers at the rear of neutrophils and initiating their rolling motion. This activates integrins on neutrophils that firmly attach to ICAM-1, causing neutrophil adhesion. Neutrophils also recruit platelets, causing platelet aggregation and blocking the flow of red blood cells (erythrocytes). Neutrophils then infiltrate into the perivascular space through diapedesis while continuing to release pro-inflammatory factors into the ischemic area, causing neuronal damage.
Days and weeks after the initial insult, neutrophils and other inflammatory cells gradually undergo efferocytosis (a programmed cell death that is similar to apoptosis but engages a different signaling cascade), in which they are phagocytosed by macrophages to resolve inflammation (Schwartz and Baruch, 2014; Zhu et al., 2019; Cai et al., 2020; Sendama, 2020). Efferocytosis removes apoptotic neutrophils that could otherwise undergo necrosis and prevents further neutrophil recruitment (Huynh et al., 2002; Rydell-Törmänen et al., 2006). This dual role of inflammation in both injury and repair poses many unwanted side effects to anti-inflammatory treatments for IS, which is important to address, especially with current challenges in clinical translation despite promising preclinical results.
In recent years, multiple clinical trials have been conducted to determine whether anti-inflammatory strategies are beneficial to IS, including studies on anti-integrin (HALT stroke study, ASTIN), anti-ICAM-1 (Enlimomab Acute Stroke Trial), and minocycline (MINOS, MINOS-sub analysis, NeuMAST; Jiang et al., 1995; Becker, 1998; Caimi et al., 2001; Gendron et al., 2002; Lampl et al., 2007; Martin et al., 2008; Perez-de-Puig et al., 2015). Despite promising preclinical data, these trials failed to show benefits in patients with stroke. The rationale for these failures is multifactorial and includes challenges in both the preclinical evaluation and the clinical trials conducted (Jiang et al., 1995; Becker, 1998; DeGraba, 1998; Yenari et al., 1998; Perez-de-Puig et al., 2015; Lambertsen et al., 2019). For instance, some trials failed to account for species differences (Schneider et al., 1998; Perez-de-Puig et al., 2015) with regard to treatment responses (Kim et al., 2014; Jickling et al., 2015) and cerebrovascular collateralization (Del Zoppo, 1995; Liebeskind, 2003). The true goal of anti-inflammatory IS treatments should be inhibiting persistent detrimental inflammatory processes and restoring tissue homeostasis, rather than blocking all immunoregulatory functions (Lawrence and Gilroy, 2007).
Neutrophil stalls in capillaries aggravate inflammation and hypoperfusion
During IS, the stroke core develops severe focal hypoperfusion and irreversible injury and becomes a nidus for inflammation. The penumbra, while being more salvageable, is still at risk from ongoing ischemia (Shekhar et al., 2018; Semerano et al., 2019). These injuries can be aggravated by secondary damage occurring days or weeks after the initial insult (Chamorro et al., 2016). In addition to poor collaterals and collateral failure, which contribute to viability before recanalization (Hill et al., 1999; Boutin et al., 2001; Iadecola and Alexander, 2001), secondary microcirculatory failure (e.g., neutrophil stalls) in ischemic and penumbral regions (Jickling et al., 2015; Anrather and Iadecola, 2016) contributes to reperfusion failure and futile recanalization (Dalkara and Arsava, 2012; Iwasawa et al., 2016; Sheth et al., 2016; Erdener et al., 2021).
Neutrophils as an inflammatory modulator
Neutrophils, also known as polymorphonuclear leukocytes, are the most abundant white blood cell in humans, representing 50%–70% of leukocytes (Furze and Rankin, 2008; Perez-de-Puig et al., 2015; Nah et al., 2018). These cells are continually produced in the bone marrow through granulopoiesis and released into circulation, constituting the first line of defense in the innate immune response under homeostatic conditions (Furze and Rankin, 2008; Mayadas et al., 2014; Christoffersson and Phillipson, 2018). Neutrophils are short-lived, with an estimated cellular lifespan of ~10–18 h once released into the bloodstream, although they can survive longer (Pillay et al., 2010; Lahoz-Beneytez et al., 2016; Ballesteros et al., 2020).
Neutrophils are complex, transcriptionally active cells that perform their immune functions via phagocytosis, granules production, degranulation, neutrophil extracellular traps (NETs) release, and reactive species generation (Borregaard, 2010; Pillay et al., 2010; Kolaczkowska and Kubes, 2013; Lahoz-Beneytez et al., 2016; Sollberger et al., 2018; Ballesteros et al., 2020). During cellular differentiation in the bone marrow, neutrophils are densely packed with secretory and antimicrobial granules to be released upon encountering pathogens (Gullberg et al., 1997; Mitsios et al., 2007; Ericson et al., 2014). Neutrophils generate web-like structures known as NETs, which are extracellular fibers composed of DNA-histone complexes and antimicrobial granule proteins (Lacy, 2006; Masucci et al., 2020). They are highly effective in trapping and killing pathogens by releasing enzymes, histones, and DNA out of the cells before dying through a process called NETosis (Becker, 1998; Brinkmann and Zychlinsky, 2007). NETs-forming neutrophils are found throughout ischemic brain tissue (Laridan et al., 2017; Denorme et al., 2022).
As neutrophils circulate, they undergo “neutrophil aging,” a natural process that involves phenotypic and functional alterations that are distinct from organismal aging and follows a rigid diurnal regime (Martin et al., 2003; Casanova-Acebes et al., 2013; Zhang et al., 2015). This diurnal program of neutrophils is coordinated by CXCR2, which drives neutrophil aging, and CXCR4, which antagonizes it (Adrover et al., 2019). After their production, immature neutrophils are retained in the bone marrow through the ligation of CXCR4 receptor expressed on their surface and CXCL12. Immature neutrophils can be differentiated from the mature ones by their low-density fractions (Martin et al., 2003; Leliefeld et al., 2016; Figure 2). The upregulation of CXCR2 (ligands IL-8/CXCL1 and 2), along with G-CSF cleaving CXCR4-CXCL12 ligation via Cathepsin G and neutrophil elastase, release mature neutrophils from the bone marrow into circulation. However, these stimulators can also release immature neutrophils into circulation during significant inflammatory events (Lévesque et al., 2003; Grieshaber-Bouyer and Nigrovic, 2019). Mature neutrophils are morphologically smaller and can migrate to the injury site faster than the immature ones with enhanced phagocytic activities (Zhang et al., 2015; Uhl et al., 2016; Liu et al., 2021). As neutrophils circulate, they have increased CXCR4 and decreased CD62L expression, which triggers clearance by macrophages via efferocytosis to return to the bone marrow (Martin et al., 2003; Stark et al., 2005; Furze and Rankin, 2008; Casanova-Acebes et al., 2013; Figure 2). Thus, aged neutrophils have a shorter lifespan and faster apoptosis (Van Eeden et al., 1997; Simon, 2003; Milot and Filep, 2011; Casanova-Acebes et al., 2013; Drifte et al., 2013). These indicate that during inflammation, there are subsets of neutrophils in circulation with different functional properties (Perez-de-Puig et al., 2015).
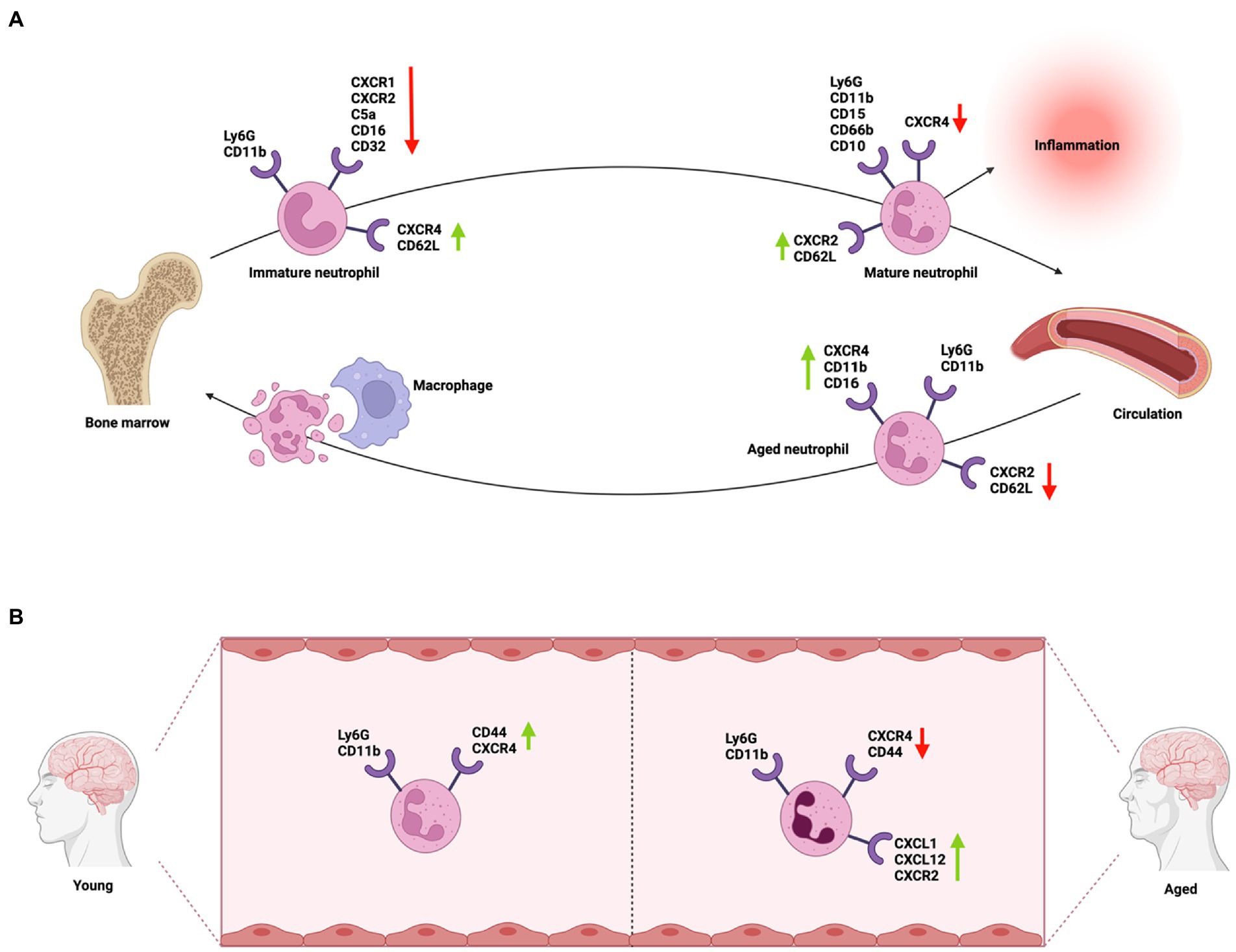
Figure 2. Neutrophil phenotypic differentiation during circulation and neutrophil phenotypic heterogeneity in aging. (A) Immature neutrophils are produced in the bone marrow, where they express either CXCR4 and CD62L on the cell surface to remain in the bone marrow or CXCR2 to be released into circulation as mature neutrophils. Aged neutrophils, after completing their immune functions in the circulation, have an increased expression of CXCR4 and a decreased expression of CXCR2 and CD62L to prompt their return to the bone marrow. (B) During organismal aging, circulating neutrophils demonstrated phenotypic changes compared to those in young individuals. Circulating neutrophils in aged individuals are hyperactive, having decreased CXCR4 and CD44 expression that diminish their efferocytosis and clearance. They also express high levels of CXCR1, CXCL1, and CXCL12 to help them remain in circulation, thus, creating a pro-inflammatory microenvironment. This condition of inflammaging makes aged individuals more susceptible to inflammatory stimuli.
A major explanation of neutrophil aging is to allow for temporal separation between subsequent neutrophil-mediated inflammatory responses, thus, honing their protective effects without damaging the vasculature (Adrover et al., 2019). Neutrophil aging has been correlated with various immunological and cardiovascular pathologies, including IS, atherosclerosis, sickle cell disease, rheumatoid arthritis, and asthma (Elliott, 1998; Coller, 2005; Scheiermann et al., 2013; Al-Ahwal et al., 2019; Esposito et al., 2020). In both humans and mice, neutrophil aging peaks in the morning, even though these two species have opposite circadian activities (Adrover et al., 2019). Consistent with neutrophils’ oscillatory signals and diurnal phenotypic changes, there is also a circadian variation in IS onset and progress (Aroca-Crevillén et al., 2020). Compared to hemorrhagic stroke and transient ischemic attack, IS has the highest risk between 6 a.m. and 12 p.m. but the lowest risk at night time between 12 a.m. and 6 a.m. (Elliott, 1998; Butt et al., 2009; Al-Ahwal et al., 2019). This may be partially explained by the rhythmic increase in immune activity and neutrophil infiltration (Scheiermann et al., 2013). Ischemic events that happen during sleep were found to have the worst prognosis and worst functional outcomes (measured by mRS), compared to those that occur during daytime (Jiménez-Conde et al., 2007; Al-Ahwal et al., 2019). In rodent models of stroke, it was shown that neuroprotective treatments can reduce infarct volume during their daytime onset (inactive phase), which corresponds to night time onset in humans, but not at the animals’ night onset (active phase; Esposito et al., 2020). Although inactive phase strokes are less frequent, they are severe with more cell death and infarct growth (Castellanos et al., 2008; Simonsen et al., 2016; Esposito et al., 2020). These patterns suggest an important pathological link between inflammation, neutrophils, and IS.
Neutrophil functional dynamics post-ischemic stroke
In IS, the same functions that make neutrophils an effective defense against pathogens also cause a significant amount of bystander tissue damage (Furze and Rankin, 2008; Mayadas et al., 2014), as documented in human patients (Jickling et al., 2015; Perez-de-Puig et al., 2015). Neutrophils are among the first responders to the ischemic brain (Gelderblom et al., 2009; Wu et al., 2015). Their increased cell counts in circulation are observed as early as 4–6 h after IS (Wang et al., 2007), and their migration into the brain parenchyma begins after 6–8 h (Jickling et al., 2015). NETs are detected 2–3 days after stroke (Cai et al., 2020). Neutrophils continue to circulate as post-ischemic injury mounts, peaking at 1–3 days (Xu and Jiang, 2014) with expression still being detected after 7–15 days, then declining afterwards (Becker, 1998).
Neutrophil influx post-ischemia could lead to microcirculatory failure due to microvascular plugging, elevated blood viscosity, and increased vascular resistance (Engler et al., 1983; del Zoppo et al., 1991; Pham and Bendszus, 2016). While cytokines and vasoactive factors released by neutrophils might contribute to collateral failure, the consequences of these factors or a direct effect of neutrophil plugging on collateral flow have not been demonstrated. As previously discussed, during IS, endothelial adhesion molecules are rapidly upregulated, expressing integrins (e.g., α2β1, α4β1, and α6β1), recognition molecules (e.g., chemokine receptors CCR1, CCR2, CCR3, CCR5, CXCR3, and CXCR4), and proteases (Nourshargh and Marelli-Berg, 2005; Hartl et al., 2008). These molecules recruit neutrophils via the chemotaxis thrombin (Reglero-Real et al., 2016). Thrombin can prolong neutrophil-adhesion molecules interactions by activating protease-activated receptors and NF-κB. It can also directly upregulate both C3 and C5 components of the complement system to disrupt endothelial barrier functions (Becker, 1998; Xue et al., 2009). These promote neutrophil occlusion in the capillary bed in mostly the stroke core and the penumbra (Jiang et al., 2014). Neutrophil occlusion is a prime contributor to tissue loss and unfavorable outcomes in patients receiving thrombolysis (Malhotra et al., 2018). Neutrophil adhesion to the endothelium could also lead to accumulated stalls and block erythrocyte movement through the microvasculature, resulting in infarct expansion (Rolfes et al., 2021). Higher neutrophil counts and infiltration are associated with larger infarct volumes post-IS and elevated neutrophil counts at admission are also associated with poor clinical outcomes (Price et al., 2004; Semerano et al., 2019). Thus, neutrophil depletion can reduce overall tissue damage, including infarct volume, edema formation, and hemorrhagic transformation in various animal stroke models, including MCAO mice and rats (Harris et al., 2005; Villa et al., 2007; Kenne et al., 2012; Cuartero et al., 2013; Herz et al., 2015; Wang et al., 2019; El Amki et al., 2020; Kang et al., 2020; Erdener et al., 2021). Blocking neutrophil entry into the brain can also drastically improve neurological outcomes in both MCAO rats and mice, evident in improved neurodeficit scores (e.g., forelimb strength, weight bearing, barrel rolling), rotarod, tight rope, corner turn, hanging wire, adhesive removal tests, T maze, foot-fault, water maze, and Garcia tests (Doycheva et al., 2014; Neumann et al., 2015; Zhao X. et al., 2017; Wang et al., 2019; El Amki et al., 2020; Roy-O’Reilly et al., 2020).
Aggregated neutrophils in capillaries have extended circulating time and increased cytotoxicity (Hartl et al., 2008; Allen et al., 2012; Dixon et al., 2012). Activated neutrophils at the surface of the endothelium release pro-inflammatory factors in and around the penumbra such as MMPs, ROS, and NOS. These destabilize the BBB, destroy the surrounding vasculature, and inflict secondary tissue damages (Garcia et al., 1994; Becker, 1998; Forster et al., 1999; Rosell et al., 2008; Yilmaz and Granger, 2008; Ludewig et al., 2013; Garcia-Bonilla et al., 2014; Perez-de-Puig et al., 2015; Figure 1). Neutrophil overproduction of MMPs can also lead to hemorrhagic transformation (Perez-de-Puig et al., 2015). Phospholipase activation in neutrophils produces prostaglandins and platelet-activating factors (Garcia et al., 1994), causing platelet aggregation, vasoconstriction, and flow stagnation (del Zoppo et al., 1991; Dawson et al., 1996; Ramiro et al., 2018). Neutrophils can then infiltrate the parenchyma and accumulate, inducing further neutrophil recruitment by continually releasing additional factors (e.g., ROS, cytokines, and proteases; Lorant et al., 1995; Wang et al., 2008; Tokgoz et al., 2013; Figure 1).
In addition to neutrophil stalls, NETs play a significant role in the no-reflow phenomenon. NETs close the time window for thrombolytic therapy and cause tPA resistance by promoting secondary thrombosis (Perez-de-Puig et al., 2015). NETs can also facilitate fibrin deposition, form scaffolds enclosing platelets, and activate the intrinsic coagulation pathway (Fuchs et al., 2010; Ionita et al., 2010; Massberg et al., 2010; Perez-de-Puig et al., 2015; Sørensen and Borregaard, 2016). NETs increase inflammatory cytokine levels and trigger the pro-inflammatory microglia subtype (Perez-de-Puig et al., 2015; Hanhai et al., 2021). The frequency and intensity of NETosis are significantly greater after permanent MCAO, and NETs marker levels are linked to stroke severity in patients, as evaluated by NIHSS and mRS scores (Vallés et al., 2017).
Neutrophils differ in their expression of surface markers and nucleus density (Perez-de-Puig et al., 2015). This heterogeneity impacts their immune functions (Perez-de-Puig et al., 2015). Ischemic environments and neutrophil interaction with endothelial adhesion molecules shift neutrophil phenotype from the protective N2 to the injurious N1 phenotype (Nourshargh and Marelli-Berg, 2005; Perez-de-Puig et al., 2015). N1 neutrophils have hyper-segmented nuclei that are seen in mature neutrophils while N2 neutrophils have banded or ring-shaped nuclei exhibited in immature neutrophils (Eruslanov et al., 2017). N1 neutrophils secrete inflammatory molecules, such as cytokines and effector molecules, including IL-1β, IL-12, TNFα, IFNγ, NO, CXCL13, CCL3, CC6, CXCL10, and hydrogen peroxide (Andzinski et al., 2016; Sionov, 2021). N1 neutrophils are also characterized by the surface expression of Ly6G, CD11b, CD54, and CD86 (Wang et al., 2018; Li et al., 2019; Figure 3). These cells are short-lived, highly cytotoxic and can worsen inflammatory damage. In contrast, N2 neutrophils are long-lived and anti-inflammatory. These cells express Ly6G, CD11b, CD206, MMP9, ARG1, and YM-1; and emerge at later stages (days 5–7; Rao et al., 2014; Perez-de-Puig et al., 2015; Ma et al., 2016; Figure 3). N2 cells produce anti-inflammatory cytokines, including TGFβ, IL-10, CCL2, CCL5, CCL17, CXCL4, and VEGF, which confer neuroprotection, tissue remodelling, and wound healing (Shirasuna et al., 2013; Perez-de-Puig et al., 2015; Sionov, 2021). It is notable here that VEGF upregulation confers biphasic roles in IS, with both deleterious impacts such as BBB disintegration and hemorrhagic transformation within 24 h and angiogenic functions 48 h post-ischemic onset (Zhang et al., 2000; Suzuki et al., 2016; Hu et al., 2022). N2 neutrophils facilitate neutrophil clearance by efferocytosis and are less harmful to ischemic neurons (Cuartero et al., 2013; Christoffersson and Phillipson, 2018; Cai et al., 2020). Skewing neutrophils toward the N2 phenotype with TGFβ treatment before IS has been shown to significantly reduce infarct volumes in MCAO mice (Cai et al., 2020). The binary classification of N1 and N2 neutrophils, in recent years, has been suggested to be oversimplified, as neutrophil phenotypes can exist as a continuum of activation states instead of extreme dichotomy (Eruslanov et al., 2017; Jaillon et al., 2020). One example of this is the recently discovered pro-angiogenic neutrophil subtype, which constitutes ~3%–5% of circulating neutrophils in both humans and mice. They have angiogenic properties (Tsuda et al., 2004; Fridlender et al., 2009; Christoffersson and Phillipson, 2018) and may contribute to collateral growth post-IS by releasing growth factors and pro-angiogenic factors (Jayaraj et al., 2019; Phillipson and Kubes, 2019). This type of neutrophils is prone to hypoxic stimulus and is recruited by increased VEGF-A expression, a potent inducer of endothelial cell chemotaxis (Barkefors et al., 2008; Piccard et al., 2012). They express high levels of CD11b, CXCR4, and VEGFR1 (Christoffersson et al., 2010, 2012; Massena et al., 2015) and overly express MMP-9, an angiogenic effector protein (Figure 3). MMP-9 can degrade the extracellular matrix to stimulate revascularization and neoformation of immature vessels at the ischemic site (Christoffersson et al., 2012; Massena et al., 2015). Pro-angiogenic neutrophils also engage different adhesion molecules, such as integrin VLA-4, in which its inhibition reduces new vessel growth and pro-angiogenic neutrophil presence at hypoxic site (Massena et al., 2015). Thus, these neutrophils increase the number of new vessels formed, including collateral conduits that can enhance blood supply during recovery (Weisenburger-Lile et al., 2019). Moreover, because collaterals reduce acute ischemia and maintain tissue viability, they might also reduce the activation of neutrophils via this reduced ischemia. Since distinct subpopulations of neutrophils perform opposing roles, targeting all neutrophils may not be ideal in stroke. A complete neutrophil removal, although showed positive effects in animal studies (Cuartero et al., 2013; El Amki et al., 2020; Erdener et al., 2021), is an impractical treatment clinically, as it requires an extensive amount of time and needs to be done before the stroke (Erdener et al., 2021). Complete inhibition of neutrophils could also increase risk of infection, as seen in patients with leukocyte adhesion deficiency (Perez-de-Puig et al., 2015). While the mechanism for therapeutic strategies for IS gearing against neutrophil adhesion is unclear and requires further investigation, it remains a promising avenue of research.
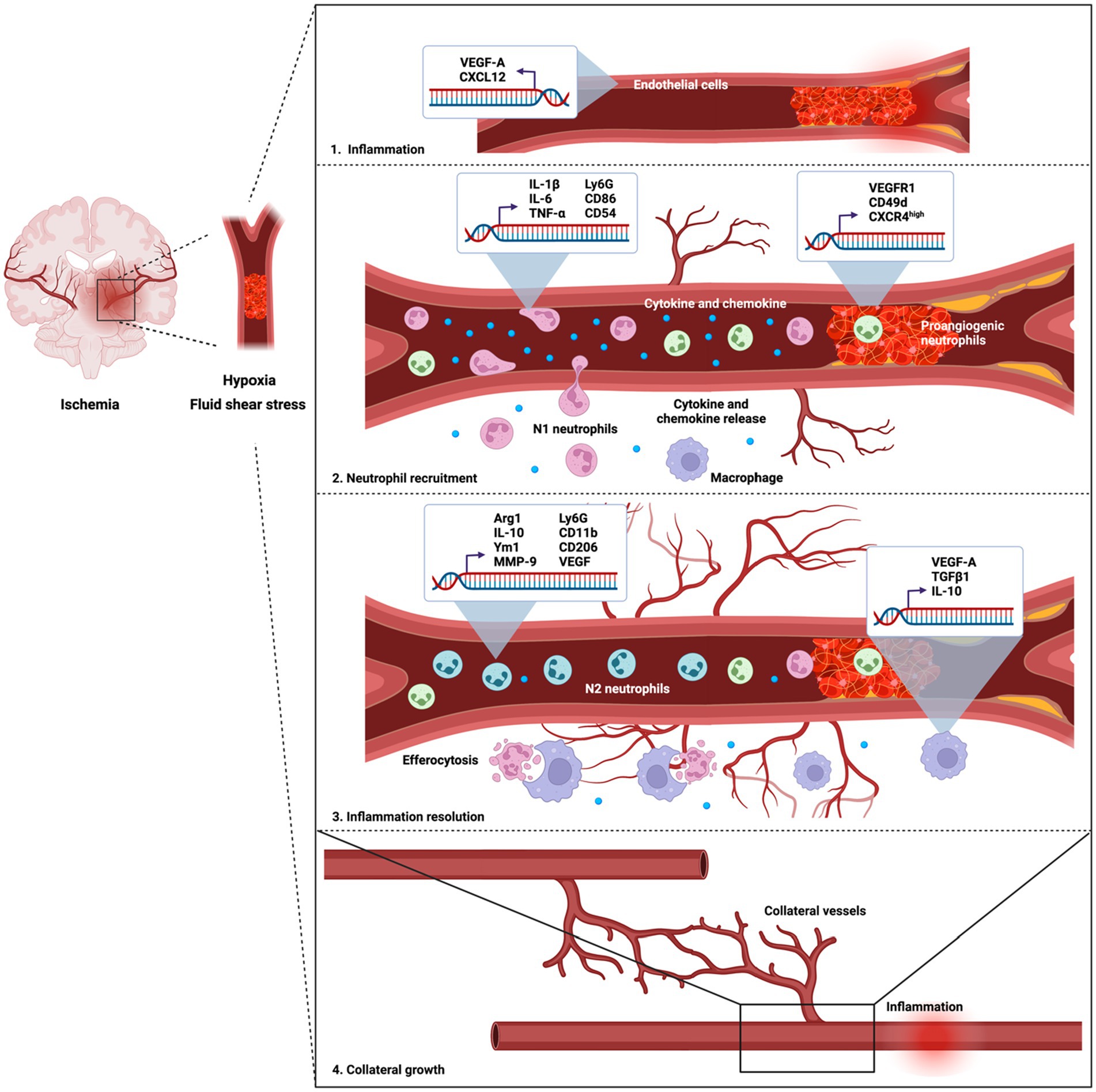
Figure 3. Different subpopulations of neutrophils have distinct effects on collateral growth and angiogenesis post-ischemia. Angiogenesis post-ischemia stimulates the growth of new collateral vessel conduits, which helps partially perfuse the tissues and sustain tissue viability until recanalization and reperfusion. Middle cerebral artery occlusion is depicted with collateral vessel growth post-ischemia. Three subtypes of neutrophils, pro-inflammatory N1, protective N2, and pro-angiogenic neutrophils are recruited to the inflammatory site post-IS in response to hypoxic stimuli and fluid shear stress. During ischemic injury, hypoxic genes, including VEGF-A, CXCL12, and DAMPs are upregulated. VEGF-A is overly expressed in endothelial cells, which recruit N1 neutrophils and pro-angiogenic neutrophils into the circulation. The later wave of recruitment consists of both pro-angiogenic and N2 neutrophils that initiate collateral development via MMP-9 release. This prompts collaterals recruitment from adjacent vascular arteries. Resolution of inflammation is completed by efferocytosis of neutrophils by macrophages.
Neutrophils, collaterals, and microvascular circulation
In addition to the adverse effects of neutrophil stalls post-ischemia, neutrophil counts and density in blood at admission are linked to hemorrhagic complications in patients who received MT, long-term outcomes, and collateral growth (Easton, 2013; Tokgoz et al., 2013, 2014; Xue et al., 2017). This is because continuous basal hyperactivation of circulating neutrophils can offset the equilibrium between apoptotic and necrotic immune cells, which may aggravate ischemic inflammation later (Perez-de-Puig et al., 2015). The extent of collateral circulation and the degree of reperfusion strengthen this association by modulating neutrophils’ capacity to reach the injury site (Semerano et al., 2019).
Neutrophil/lymphocyte ratio (NLR) has been proposed as a prognostic marker for inflammatory responses and clinical outcomes independent of age and recanalization (Brooks et al., 2014; Perez-de-Puig et al., 2015; Shantsila and Lip, 2015; Uysal et al., 2015; Albers et al., 2018; Giede-Jeppe et al., 2019; Li et al., 2021; Sharma et al., 2021). Prior to IS, neutrophils create a pro-inflammatory environment that can increase the severity of initial IS and further poor long-term outcomes (Kim et al., 2012; Zhang et al., 2020) while lymphocytes could induce neuroprotection (Wheeler et al., 2004; Gillum et al., 2005; Horne et al., 2005; Macrez et al., 2011). Elevated admission NLR was connected to hemorrhagic complications in patients receiving MT (Easton, 2013; Tokgoz et al., 2013, 2014; Perez-de-Puig et al., 2015; Guo et al., 2016; Xue et al., 2017) and can predict 3-month mortality (Goyal et al., 2018). The NLR value is also significantly higher in severe stroke cases compared to mild and moderate strokes (Ying et al., 2021).
With their different subpopulations, N1, N2, and proangiogenic neutrophils could exert both deleterious and protective effects on collaterals recruitment post-ischemia. During IS, peripheral neutrophils infiltration into the ischemic territory via circulation is severely reduced and their access is provided mainly through collateral blood vessels, peripheral brain regions, or when the ischemic areas are reperfused (Zhang et al., 1995; Prestigiacomo et al., 1999; Cuartero et al., 2013; Chu et al., 2014; Perez-de-Puig et al., 2015; Otxoa-de-Amezaga et al., 2019). Without reperfusion, the main route for neutrophil infiltration and extravasation is via retrograde collateral pathways, moving from leptomeningeal vessels to perivascular space and then parenchyma (Perez-de-Puig et al., 2015; Otxoa-de-Amezaga et al., 2019). Through this route, neutrophils and their NETs impair revascularization and tissue integrity (Yipp and Kubes, 2013) and patients with NETs-poor thrombi have better collateral flow and milder clinical symptoms with reperfusion (Kang et al., 2020; Chen et al., 2022). This suggests that treatments targeting NETs in thrombi might be beneficial for early neurological protection (Kang et al., 2020). Neutrophil influx and high local neutrophil density in circulation (counts/μL) are also correlated with poor retrograde collateral flow and greater infarct extent (Strinitz et al., 2021). Even in the subgroup with successful reperfusion and good collaterals, high neutrophils and low lymphocytes (thus, a higher NLR) are indicative of intracerebral hemorrhage (Guo et al., 2016; Gill et al., 2018; Semerano et al., 2019), collectively due to the destructive effects of neutrophils and the lack of vessel protecting effects from lymphocytes (Salas-Perdomo et al., 2018; Sharma et al., 2021, 2022).
There is significant evidence suggesting that ischemia, more specifically ischemic hypoxia and fluid shear stress, stimulates collateral circulation development in the peri-infarct regions after injury, as a result of angiogenesis and arteriogenesis (Wei et al., 2001; Heil et al., 2006; Faber et al., 2014; Phillipson and Kubes, 2019). Both angiogenesis and arteriogenesis presence after stroke have been observed in various animal models (Kanazawa et al., 2019). In rodents and non-human primates, angiogenesis can be detected as early as 4 days after ischemic onset in the peri-infarct region (Ijichi et al., 1995; Abumiya et al., 1999; Zhang et al., 2000; Tagaya et al., 2001; Hayashi et al., 2003; Wang et al., 2009; Li et al., 2012). An argument in favor of this is the upregulation of VEGF-A and EPO, which are central to collateral sprouting, at hypoxic sites (Semenza, 1999; Matsunaga et al., 2000; Resnick et al., 2003; Toyota et al., 2004; Kanazawa et al., 2019). VEGF recruits proangiogenic neutrophils selectively (Eltzschig and Carmeliet, 2011; Massena et al., 2015), which then release MMP-9 that breaks down the extracellular matrix, allowing for vessel growth by 4–7 days after focal ischemia (Grunewald et al., 2006; Nozawa et al., 2006; Christoffersson et al., 2012; Sun et al., 2017; Figure 3). VEGF-A has been implicated in collateral development (elevated pial collateral count), reduced infarct expansion after MCAO, and increased recanalization of occluded arteries (Clayton et al., 2008; Greenberg and Jin, 2013; Perez-de-Puig et al., 2015). Additionally, upon VEGF administration, both rat and mouse models have been shown to have higher new vessel density and better functional recovery (Zhang et al., 2000; Hayashi et al., 2003).
Another stimulator of post-ischemic collateral development is the fluid shear stress. The change in vascular tone and pressure during IS upregulates adhesion molecules expression, such as selectins, I-CAM, and V-CAM along the lumenal surface of collateral vessels (Scholz et al., 2000; Lee et al., 2004). Adhesion molecules recruit neutrophils to the site to activate collateral remodelling (Hoefer et al., 2004) and their inflammatory effects sustain vessel growth (Becker, 1998; Okyere et al., 2020). Removing these adhesion molecules has been shown to attenuate arteriogenesis (Hoefer et al., 2004).
Neutrophils, baseline collateral status, collateral growth post-stroke, and stroke outcomes are inextricably connected. Neutrophil infiltration and the expression of their deleterious subset in collateral vessels during IS can exacerbate stroke pathogenicity despite good collateral status at admission. On the other hand, the expression of pro-angiogenic and N2 neutrophils post-ischemia can enhance collateral growth during recovery. Although factors that determine stroke outcomes such as collateral status and circulating neutrophil levels at admission are fixed, many can still be done to improve functional recovery via post-ischemic neutrophil dynamics and collateral development manipulation (Wufuer et al., 2018; Wang et al., 2021). In patients with poor existing collateral networks, it may be possible to provide bone marrow-derived cell therapy (e.g., leukocytes and progenitor cells) to prompt endothelial functions and stimulate collateral growth and remodelling (Benest et al., 2008; Kinnaird et al., 2008). Upregulating pro-arteriogenic genes, such as VEGF, FGF, BDNF, and PDGF, after IS could also facilitate collateral development and functional recovery (Deindl et al., 2003; Clayton et al., 2008; Tomanek et al., 2008; Ergul et al., 2012; Chapouly et al., 2019).
Neutrophil-specific transcriptional changes post-ischemic stroke
Altered genomic patterns have been observed in human blood samples as early as 3 h after an ischemic onset (Tang et al., 2006). In human brain tissue, many differentially expressed genes were found 2–6 days and 9–20 days after IS, with the majority of differentially expressed genes being upregulated. The number of upregulated genes in the rat brain tissues increases steadily after MCAO at 1 h, peaking at 3 days and persisting for several days, implying that active mechanisms are initiated during the acute phase of IS. Downregulated genes are detected 24 h after MCAO and their number declines after 26–37 days, indicating that gene expression is the most dynamic and robust during the first few weeks following stroke (Rusanen et al., 2015; Nannoni et al., 2019; Tarkanyi et al., 2020).
Transcription factors that lead to inflammatory responses, necrosis in the anoxic core, and delayed apoptosis in the penumbra have been documented (Zhan et al., 2011; Drifte et al., 2013). For instance, although has been recognized as a passive process lacking genetic regulation, a recent study in Drosophila and MCAO rats showed that necrosis is regulated by MSK1/2 and JIL-1 through H3S28ph phosphorylation (Liu et al., 2014). Numerous studies were done on apoptosis gene expression in the penumbra, highlighting the overexpression of Smac/DIABLO, AIF, XAF1, HtrA2/Omi, CASP3, etc. (Uzdensky, 2019). Changes in gene expression of circulating peripheral immune cells have also been recorded, including neutrophils, platelets, and cytokines (Lee et al., 2004; Meisner and Price, 2010; Zbinden et al., 2010). However, relatively few studies have been conducted on the transcriptional regulatory effects of IS on neutrophils alone (Konstantinov et al., 2004). Using microarray analysis, Tang et al., 2006 identified a total of 29 probe sets with 18 signature genes that distinguish IS from healthy human controls, with most highly expressed by neutrophils, suggesting that they are among the molecular and genomic signatures of stroke (Tang et al., 2006). Similar results were obtained by Carmona-Mora et al. using RNA-seq analyses of human blood samples. They found that the majority of the differentially expressed genes (197/248, 79%) were upregulated in IS neutrophils (Carmona-Mora et al., 2021). This reinforces the concept that post-stroke transcriptional changes occur predominantly in neutrophils (Moore et al., 2005). Some signature neutrophil genomic markers include FPR1 and PGLYRP1, which represent the initial steps leading to neutrophil activation and granulocyte accumulation at the inflammation site (Le et al., 2002; Tang et al., 2006); S100P/A8/A9/A12 for binding specifically to endothelial cells and induce inflammatory response; and NCF-4 that produces free radicals in neutrophils (Tang et al., 2006; Perez-de-Puig et al., 2015). Immediately after IS, mRNA of neutrophil chemoattractant (e.g., CXCL1, CXCL2, CXCL3, CCL5) are expressed, which peaks after 12 h and returns to the sham level at 48 h. After a day, IL-12, MMP3, TIMP1, MPO, and NE expression associated with neutrophil extravasation is increased. The surge in the expression of MMP10, NLRP3, and BAFF is not detectable until after 2 days, while IL-23, IFNγ, MMP2, MMP8, MMP9, MMP13, PAD4, TLR7, and TLR9 not until the third day. In the protective N2 neutrophils only, CD206 is upregulated after 7 days post-MCAO (Cai et al., 2020).
Neutrophils activate many canonical pathways implicated in IS. Very few neutrophil-specific pathways are suppressed post-ischemic stroke (Carmona-Mora et al., 2021). Significant and well-studied neutrophil-specific pathways post-ischemia include mTOR (Maiese, 2014), ERK5 (Becker, 1998), integrin (Bouchon et al., 2000), thrombopoietin (Yang et al., 2019), TGF-β, BMP, GM-CSF, IL-3, TRK, STAT3, and calpain protease signaling pathways (Carmona-Mora et al., 2021).
To exert its pro-death effects, neutrophils activate oxidative phosphorylation via cytokine signaling, calpain protease (Carmona-Mora et al., 2021), and thrombopoietin pathways (Bretón and Rodríguez, 2012). mTOR is activated via Akt and mediates pro-inflammatory gene transcription, autophagy, and apoptosis (Maiese, 2014; Hadley et al., 2019). PDGF, along with integrin signaling, stimulates chemotaxis and membrane ruffling before neutrophil extravasation (Perez-de-Puig et al., 2015; Lee and Li, 2018; Edwards and Bix, 2019). Integrins interact with extracellular matrix components to disrupt BBB permeability after stroke (Edwards and Bix, 2019). Calpain proteases further dysregulate synapses (Curcio et al., 2016) and cause BBB breakdown (Perez-de-Puig et al., 2015).
In parallel with pro-apoptotic pathways, neutrophils activate various neuroprotective pathways. ERK5 signaling, a MAP kinase family member, induces PPARδ, which is protective against stroke-induce brain injuries and its agonists are suggested as potential stroke treatments (Woo et al., 2006; Yin et al., 2011; Jin et al., 2013; Su et al., 2014). Neutrophin/TRK signaling activates hematopoietic cell survival and neurite outgrowth and differentiation (Reichardt, 2006). GM-CSF signaling, enriched at 48 h after stroke, initiates cell survival and differentiation (Cox et al., 1992; Becker, 1998; Lanfranconi et al., 2011; Carmona-Mora et al., 2021, 2022). Activation of TGF-β signaling, an N2 marker, exerts both deleterious and neuroprotective post-stroke, including upregulating the anti-inflammatory and anti-apoptosis IL-10 and IL-2 pathways (Boey et al., 1989, 1; Perez-de-Puig et al., 2015; Carmona-Mora et al., 2022). BMP signaling mediates glial scar formation after stroke (Shin et al., 2012) and TGFB3 signaling regulates N1 to N2 neutrophil polarization involved in inflammation resolution (Cuartero et al., 2013; Liao et al., 2014; Perez-de-Puig et al., 2015). These signaling pathways, once again, highlight the dual role of neutrophils during IS.
“Inflammaging”
Organismal aging is characterized by a systemic dysfunction of the immune system and failed somatic maintenance, which involves mechanisms that maintain tissue integrity and promoted lifespan expansion. This leads to a chronic, sustaining inflammatory state (Kirkwood, 2005; Shaw et al., 2013; Childs et al., 2015; Perez-de-Puig et al., 2015; Boe et al., 2017; Rozhok and DeGregori, 2019). This phenomenon is referred to as “inflammaging” (Lencel and Magne, 2011; Franceschi and Campisi, 2014; Franceschi et al., 2018; Lu et al., 2022). 90% of the differentially expressed genes in human aged hippocampal brain tissue are linked to inflammation (Nikas, 2013). In a microarray study, aged hippocampal tissues showed an overexpression of pro-inflammatory genes, including (1) IL1B, IL6, IL10, and TNF that express cytokines, (2) IRAK3 and SOCS3 that modulate cytokine signaling, and (3) MYD88, TLR2, TLR4, and TLR7 that control TLR signaling (Cribbs et al., 2012). Inflammaging can diminish the immune system’s ability to fight and clear pathogens, causing greater susceptibility to infections, mortality, and age-related pathologies (Solana et al., 2006; Dorshkind et al., 2009; Lencel and Magne, 2011; Shaw et al., 2013; Pawelec et al., 2014; Akbar and Gilroy, 2020). The overall decline in immunity state is termed “immunosenescence” and is attributed to telomere erosion, defective protein catabolism, autophagy, and mitophagy (Perez-de-Puig et al., 2015).
With age, the mitochondria slowly become less efficient (Sun et al., 2013; West et al., 2015), which compromises energy production, causing a redox imbalance (Harman, 1956). This promotes an overproduction of ROS, DAMPs (e.g., HMGN1 that regulates neutrophil activity), cardiolipin, and mitDNA that induces oxidative stress and inflammasome activation (Nakahira et al., 2015; Gkikas et al., 2018). Aging is also associated with an overall decline in the body’s anti-oxidative defense system, including efferocytosis (Sendama, 2020), which is supposed to remove cellular debris, senescent cells, (Xuan et al., 2011; Mohan et al., 2016; Wiggins and Clarke, 2019), and misfolded molecules, such as DNA, proteins, and lipids (Harman, 1956; Sohal and Orr, 2012). These molecules increase microglial pro-inflammatory cytokines, such as IL-6, TNF-α, IL-β, and microglial exposure to the anti-inflammatory TGFβ (Flanary et al., 2007; Perry and Holmes, 2014; Heneka et al., 2015; Figure 4). Chronic exposure of microglia to TGFβ impairs their capacity to secrete anti-inflammatory cytokines (Doyle et al., 2010; Cohen et al., 2014), producing more pro-inflammatory blood-borne factors and inflammasome (Villeda et al., 2011; Pishel et al., 2012; Perry and Holmes, 2014; Smith et al., 2015) and leading to chronic inflammation (Becker, 1998; Bruunsgaard et al., 2003; Ferrucci et al., 2005; Pinti et al., 2014). In many studies, manipulation of the immune system was shown to attenuate the negative effects of inflammaging. For instance, surgical parabiosis of young and old mice can partially reverse deficits in neurogenesis, remyelination, and muscle regeneration, as mice share youthful plasma factors via the circulation (Ruckh et al., 2012; Katsimpardi et al., 2014; Sinha et al., 2014; Villeda et al., 2014). Similar effects were seen in bone marrow transplantation, which ameliorated age-related frailty and reduced hemorrhagic transformation after stroke (Heidt et al., 2014; Ritzel et al., 2018).
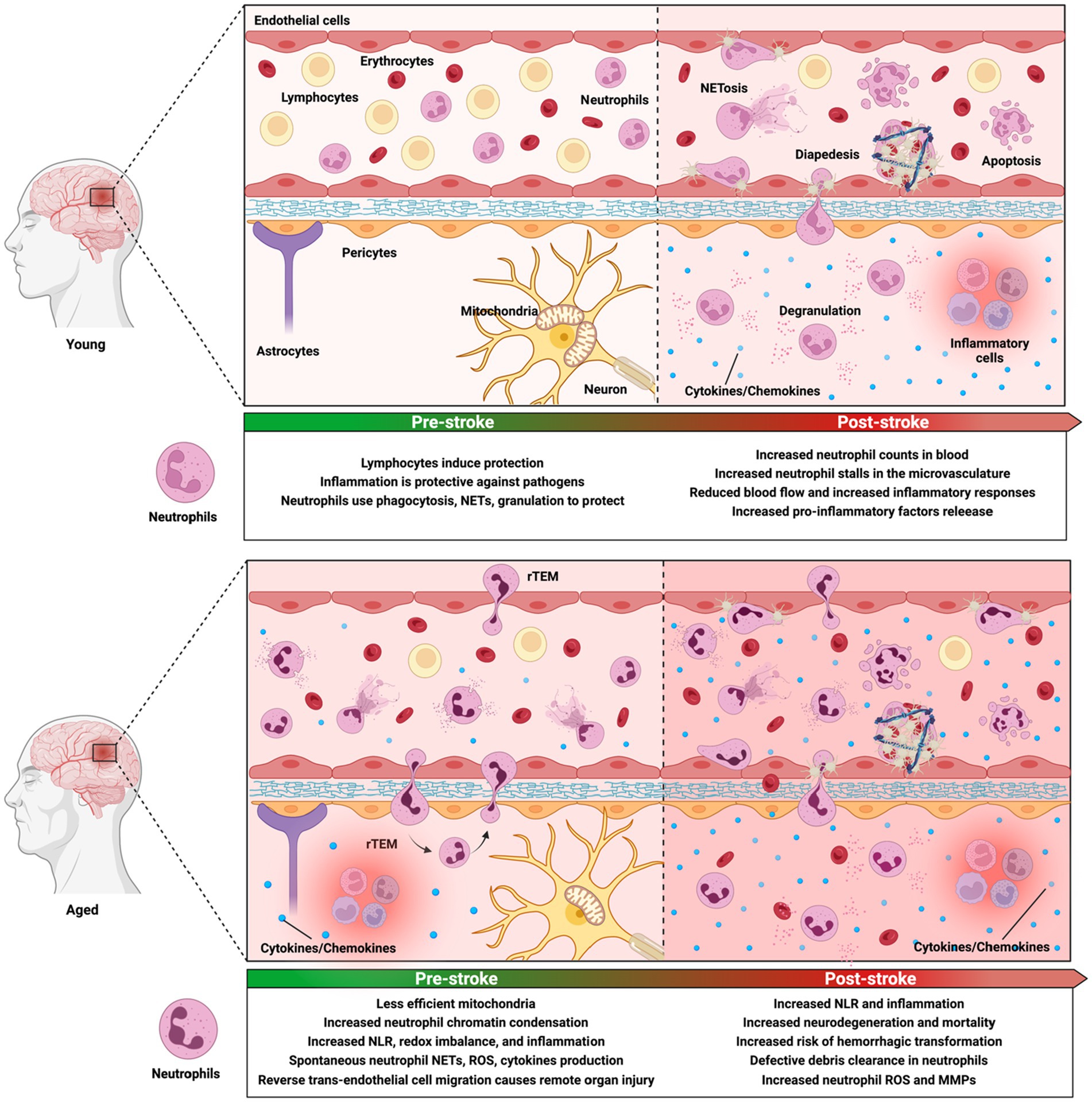
Figure 4. Aging alters the immune microenvironment as well as neutrophil phenotype and functions both before and after ischemic stroke. In normal condition, inflammation serves as a mechanism that protects the body against pathogens. Upon encountering pathogens, neutrophils undergo immune responses by producing granules, releasing neutrophil extracellular traps (NETs) and reactive species, and performing phagocytosis. Ischemic injury induces significant changes in the microvasculature, including increased neutrophil count, neutrophil stalls, and aberrant neutrophil activities that are correlated with neurological deficits and poor outcomes. During aging, the brain is primed with a basal elevated neuroinflammatory environment, which is evident in less efficient mitochondria, chemical gradient loss, and increased basal neutrophil counts and pro-inflammatory activities. With aging, due to increased levels of CXCL1 production, a higher level of neutrophil reverse transendothelial migration (rTEM) is seen. After neutrophils undergo diapesis, they can re-enter the vascular lumen and return to the circulation. They can stay in downstream organs, causing multiorgan failure. Aged individuals are significantly more susceptible to ischemic injuries than young individuals. They have increased risk of hemorrhagic transformation and worse neurological deficits. Their system has defective neutrophil clearance and heightened reactive species and MMP-9, causing sustained inflammatory responses. These further ischemic injuries and cause significantly more irreversible damage in aged subjects.
Inflammaging leads to epigenetic changes in cells via DNA methylation (De Martinis et al., 2005; Perez-de-Puig et al., 2015; Chen et al., 2016). In general, these genes are neurodegenerative and linked to exacerbated immune responses and cellular oxidative stress, which are also commonly observed in neurodegenerative disorders including Alzheimer’s disease (Lee et al., 2000; Blalock et al., 2003; Verbitsky et al., 2004; Fraser et al., 2005; Rowe et al., 2007; de Magalhães et al., 2009; Lipinski et al., 2010; Zeier et al., 2011; Yuan et al., 2012). Some inflammatory signatures include (1) differentially overexpressed inflammatory genes C1QA, C3, C1QB, CTSS, FCGR2B, IGJ, LYZ, MGST1, GSTA1, S100A4, S100A6, ANXA3, ANXA5, IL18, IL18BP LILRB3, IGJ, RAB27A, RAB32, PTPRC, SWAP70, SLC11AL, ST14, TEP1, TREM2, and TYROBP; and (2) underexpressed mitochondrial genes (ATP5G3, NDUFB11, UQCRQ, and UQCRFS1; de Magalhães et al., 2009; Wang et al., 2012; Ianov et al., 2016). Other pro-inflammatory biological processes (e.g., autophagy, oxidative stress) are elevated with age, including the overexpression of LYZ, CLU, MGST1, GSTA1, S100A4, S100A6, ANXA3, and ANXA5 and the underexpression of mitochondrial genes ATP5G3, NDUFB11, UQCRQ, and UQCRFS1 (de Magalhães et al., 2009). Additionally, the complement system genes, including C1QA, C1QB, C1QC, C1S, C3, C3AR1, C4α, C4β, C5, and C5AR1, are significantly upregulated with age (Cribbs et al., 2012). Additionally, with age, angiogenesis and neurogenesis that may contribute to recovery are diminished, as pro-angiogenic genes ANGPT2 and VEGFA are significantly downregulated (Guo et al., 2021). Genes related to synaptic transmission, axonal projection, dendrite growth, and neuroplasticity show age-related downregulation (Blalock et al., 2003; Lu et al., 2004; Verbitsky et al., 2004; Burger et al., 2007; Aenlle and Foster, 2010; Zeier et al., 2011; Berchtold et al., 2019; Androvic et al., 2020). These include genes related to axon functions (ANKS1B, CLSTN3, CBLN1, CHRM2, CHRNA5, CYP19A1, DOC2A, DNM3, GABRA4, GAD1, GRIP1, GRID1, GRID2, GRIK3, GRM7, GRM8, GLRB, LIN7B, MAGREE1, SCAMP1, PRKACA, SLC2A3, SV2B, SYT6, YWHAZ) and neurite growth (BACE1, DCC, DPYSL2, DPYSL5, DCTN2, GOT1, KLHL1, MAP2K4, KCNJ12, PGR, PTPRN2, and TACR3; Ianov et al., 2016). Neurons in the peri-infarct exhibit axonal sprouting associated with recovery after stroke and increase pro-inflammatory genes IGF1 and SPP1 activation (Li et al., 2010). Consistently, Berchtold et al. showed in the aged human hippocampus that anti-aging genes are highly enriched in neuron projection, cell junction, and axon (Berchtold et al., 2019).
Microglial are the principal cells driving the innate immune response in the brain and are also impacted by inflammaging. Differentially expressed genes include C4B, CCL4, CCL8, CLEC7A, CST7, CYBB, LGALS3, MMP12, and SPP1 (Holtman et al., 2015; Grabert et al., 2016; Androvic et al., 2020). These genes are in line with the transcriptional profile of microglia in neurodegenerative diseases (Mathys et al., 2017; Friedman et al., 2018; Hammond et al., 2019). For instance, in an Alzheimer’s disease mouse model, microglia significantly overexpressed complement components (C3, C4B, and CFB), chemokines (CCL12, CCL3, CCL4, and CXCL16), and immune-related genes (H2-D1, AXL, APOE, and LGALS3B; Mathys et al., 2017); APOE, SPP1, and LPL are specifically upregulated in microglia surrounding amyloid plaques (Keren-Shaul et al., 2017). CCL3 and CCL4 are upregulated in humans with multiple sclerosis (Omari et al., 2005; Szczuciński and Losy, 2007; Hammond et al., 2019). Many upregulated inflammaging genes are also the signatures of other immune cells, such as astrocytes (ANLN, C4B, GFAP, LYZ2, NEAT1, PCDHB6, PLIN4, and SERPINA3N; Boisvert et al., 2018; Clarke et al., 2018) and oligodendrocyte precursor cells (RAB37 and TNFAIP2; Spitzer et al., 2019; Androvic et al., 2020).
Transcriptional changes in peripheral blood neutrophils from aged Beagle dogs, mice, and humans are overwhelmingly related to chromatin and cell cycle (Zhang et al., 2004; Hall et al., 2010; Grassi et al., 2018; Tabula Muris Consortium et al., 2018; Kalucka et al., 2020; Lu et al., 2021). Chromatin compaction is essential to cellular functions as it represses genome transcription (Janssen et al., 2018). Lu et al. showed that 18 histone-encoding genes are significantly downregulated with aging in mouse bone marrow cells. The same study showed that neutrophils experience age-dependent changes in chromatin organization (Lu et al., 2021), with increased median neutrophil nucleosomal occupancy (Brinkmann et al., 2004; Papayannopoulos, 2018) and chromatin condensation (Lu et al., 2021; Figure 4). Since chromatin decompaction is a limiting step of NETosis, this suggests that aging can directly influence NETosis (Neubert et al., 2018; Sollberger et al., 2018). Transcriptional changes with aging, specifically in neutrophils, are complex due to exogenous and endogenous confounding factors, such as the increased number of medications taken by older individuals (Perez-de-Puig et al., 2015). Thus, the genomic link between inflammaging and neutrophils and strategies to reverse neutrophil immunosenescence in the elderly population requires further investigation (Simmons et al., 2021).
Despite changes in receptor expression with aging (decreased CXCR4 expression as described below), circulating neutrophil counts remain stable in individuals without co-morbidities (Chatta et al., 1994; Van Avondt et al., 2022). Their production is not diminished (Corberand et al., 1986; Perez-de-Puig et al., 2015), rather, their functions and signaling pathways are impaired (Hazeldine et al., 2014; Sapey et al., 2014; Tseng and Liu, 2014; Simmons et al., 2021). Neutrophil mobilization from bone marrow and proliferative response to G-CSF are significantly reduced with aging (Chatta et al., 1993, 1994). Neutrophils from older individuals release more neutrophil elastase and proteolytic enzymes, spontaneously produce NETs, ROS, and cytokines (e.g., TNF-α; Tseng et al., 2012; Hazeldine et al., 2014; Zhang et al., 2015), express high levels of extracellular matrix-degrading enzymes (e.g., MMP-9), and increase degranulation (Carter et al., 2013; Figure 4). Several autophagy-related pathways are upregulated in neutrophils during aging, consistent with the age-dependent proteostasis loss (López-Otín et al., 2013). Since control of autophagy is critical for neutrophil differentiation (Riffelmacher et al., 2017), regulation of NET formation (Park et al., 2017), and degranulation (Bhattacharya et al., 2015), this can not only damage the vasculature but also blunt local immune response (McLaughlin et al., 1986; Chua and Laurent, 2006; Gomez et al., 2007; Eskan et al., 2012; Nomellini et al., 2012; Wulfert et al., 2012).
During organismal aging, neutrophils have an increase in basal levels of activation of MAPK pathways and PI3K signaling (Fortin et al., 2006; De Maeyer et al., 2020). Excessive PI3K signaling impairs neutrophil chemotaxis and migration toward the insult (Fulop et al., 2004; Hinojosa et al., 2009; Chen et al., 2014; Hazeldine et al., 2014). Since PI3K directs PIP3 phosphorylation and accumulation to the edge of neutrophil, inducing the molecular cascade that recruits elements needed for neutrophil environmental sampling and propulsion, inhibiting PI3K can improve neutrophil mobilization in aged individuals. Furthermore, increased PI3K activity could impair PI3K/SHIP1/PTEN balance and causes neutrophil to be insensitive to inflammatory signals (Sapey et al., 2014). Serum levels of CXCL1/IL-8 are significantly higher in the circulation of aged patients and animals, causing increased respiratory burst, altered neutrophil trafficking, and degranulation (Bréchard et al., 2005; Wright et al., 2014; Figure 2). CCL5/RANTES concentrations significantly increase in old mice after stroke (Desai et al., 2010; Sieber et al., 2011, 2014). This is associated with neutrophil migratory defects, diminished neutrophil cytokine production (Kirkwood, 2005; Dalboni et al., 2013; Qian et al., 2014), reduced host phagocytic ability to control pathogen burdens (Butcher et al., 2001; Weiskopf et al., 2009; Domon et al., 2016, 2018; Bou Ghanem et al., 2017), and poor stroke outcome (Terao et al., 2008; Dénes et al., 2010; Dhungana et al., 2013).
Neutrophil apoptosis (Tortorella et al., 2006; Gasparoto et al., 2012) and clearance to bone marrow (Martin et al., 2003; Buckley et al., 2006; Casanova-Acebes et al., 2013) are also reduced with aging via GM-CSF, LPS, and IL-2 stimulation and activation (Fülöp et al., 1997). This leads to a persistent release of inflammatory signals that recruit more immune cells (Sendama, 2020), such as IFN-I signaling (Androvic et al., 2020), causing a continuous influx of neutrophils in blood (Danton and Dietrich, 2003). Aged animals also under-express CXCR4, CXCL12, and CD44 with increased CXCR2 expression, reducing neutrophil clearance via efferocytosis and enhancing their circulation in blood (Hart et al., 1997; Furze and Rankin, 2008; Figure 2). CXCR4 is known to be a CXCR2 antagonist, as CXCR2 induces natural aging phenotypic changes in mice (Martin et al., 2003; Eash et al., 2010; Adrover et al., 2019; Yan et al., 2021; Figure 2). This difference in neutrophil phenotype might explain why neutrophil depletion using anti-Ly6G rescue behavior deficits in MCAO aged animals but not young animals (Perez-de-Puig et al., 2015; Roy-O’Reilly et al., 2020). When given anti-Ly6G treatment after a 60-min MCAO, young mice (3 months) showed no functional benefits in corner testing and hang-wire testing; while aged animals (21–22 months) showed significantly improved recovery without changes in long-term mortality (Roy-O’Reilly et al., 2020). This is because anti-Ly6G treatment is less efficient for CXCR2− neutrophils in all mice spleen, blood and lungs (Pollenus et al., 2019). Moreover, these unique patterns in behavioral rescues may be independent of the improvement in gross tissue damage observed with anti-Ly6G in previous studies, which has no correlation with age (Perez-de-Puig et al., 2015; El Amki et al., 2020; Erdener et al., 2021).
Neutrophil endothelial adhesion (Niwa et al., 1989; MacGregor and Shalit, 1990; Fortin et al., 2006, 200; Nogueira et al., 2018) and transepithelial migration (Bou Ghanem et al., 2017) are preserved with age. Neutrophils that have undergone diapedesis into inflamed tissues can exhibit retrograde motility and re-enter the vascular lumen. This is called reverse trans-endothelial cell migration (rTEM) and is mediated by elevated CXCL1 production by tissue-resident vascular and perivascular cells (Buckley et al., 2006; Mathias et al., 2006; Woodfin et al., 2011; Perez-de-Puig et al., 2015; Owen-Woods et al., 2020; Figure 4). Neutrophils that undergo rTEM have distinct surface phenotypes as to circulating and tissue-resident neutrophils which drive inflammation (Buckley et al., 2006; Woodfin et al., 2011). rTEM neutrophil express elevated ICAM-1 and CD54 expression and reduced CXCR1 expression (Buckley et al., 2006; Woodfin et al., 2011; Weisenburger-Lile et al., 2019). In aged tissues, a high level of CXCL1 expression is sustained by increased expression of ACKR1 at endothelial cell junctions, which facilitates CXCR2 downregulation. Since CXCR2 is responsible for transmigrating neutrophils, this causes impaired neutrophil motility and directs them back into circulation (Marki and Ley, 2020; Barkaway et al., 2021). Neutrophil excessive production of elastase in aged mice also cleaves the endothelial junctional adhesion molecule JAM-C and triggers rTEM (Woodfin et al., 2011; Colom et al., 2015; Girbl et al., 2018; Owen-Woods et al., 2020; Barkaway et al., 2021). Increased neutrophil elastase and decreased JAM-C have been shown to induce rTEM in mice (Jin et al., 2019; Kim Y. R. et al., 2020). rTEM neutrophils have longer survival, lower apoptosis rate, slower passage through the microvasculature, and higher ROS release (Buckley et al., 2006; Perez-de-Puig et al., 2015), suggesting that they may inflict systemic damage if reinfiltrated into remote organs (Ellett et al., 2015). rTEM neutrophils also have elevated integrins and high ICAM-1 phenotype, which may support rTEM neutrophil aggregation, degranulation, and ROS release within small blood vessels (Owen-Woods et al., 2020). With aging, CXCL1 and ACKR1 upregulation can bring rTEM neutrophils back into the circulation and entrap them in downstream organs, leading to multiorgan failure (Ellett et al., 2015). rTEM process has been reported recently in ischemia/reperfusion model mouse, rabbit models, and human patients (Clark et al., 1991; Colom et al., 2015; Cho et al., 2017; Weisenburger-Lile et al., 2019).
Aging exacerbates neutrophil pathogenicity in ischemia
IS is a well-recognized disease of aging, with 75%–89% of strokes occurring in people over 65 years old, and the incidence of stroke doubles each decade after the age of 55 years (Chen et al., 2010). Along with a higher incidence, aged patients have higher mortality, suffer more severe deficits (as measured by the NIH and mRS scales), and recover more slowly (Kammersgaard et al., 2004; Marini et al., 2004; Perez-de-Puig et al., 2015; Scapini et al., 2016; Benjamin et al., 2018). Thus, understanding age-related molecular mechanisms of ischemic injury is crucial to translate preclinical findings to clinical interventions (Chen et al., 2010). In the past few decades, despite significant breakthroughs made by preclinical studies in animal stroke models, there still is a substantial translational roadblock for stroke treatments. This is because unlike patients in clinical trials, most preclinical studies used young animals with a homogeneous genetic background (Chen et al., 2010; Roy-O’Reilly and McCullough, 2018). Biological variables such as age, sex, and comorbidities that profoundly affect the clinical outcome are often unaccounted for (Bosetti et al., 2017; McBride and Zhang, 2017). The complex factors underlying worsened outcomes in older individuals remain poorly defined, particularly in females, who are often underrepresented in research studies (van der Worp et al., 2005; Sohrabji et al., 2017; Tsivgoulis et al., 2017; Carcel et al., 2019). Older animals also have higher mortality and more severe neurological deficits, making them more challenging in research studies (Rosen et al., 2005; DiNapoli et al., 2008).
An explanation for increased risk and worse stroke outcomes with aging is the coupling of chronic inflammation in the aged brain and ischemic injury, which amplifies neurodegeneration and tissue loss (Li et al., 2018; Tsai et al., 2019). Aged mice showed increased risks of hemorrhagic transformation and higher neurological deficits with stroke (Liu et al., 2009, 2010, 2012; Manwani et al., 2013; Ritzel et al., 2018; Roy-O’Reilly et al., 2020). Reports on age-related infarct size in the literature are mixed. Many showed that aged mice have significantly smaller infarct sizes (Liu et al., 2009; Manwani et al., 2014; Zhao S. et al., 2017; Korf et al., 2022), while the opposite result has also been recorded (Shen et al., 2019; Liberale et al., 2021). Recent data suggests that this difference might be because inflammatory insults that compromise BBB are independent of infarct sizes (Dénes et al., 2011). Furthermore, ischemic functional deficits seen in behavior testing might also happen independently from histological damage (shown in infarct size, edema formation, and hemorrhagic transformation; Liu et al., 2009). This partially explains why anti-Ly6G treatment reduces tissue damage and infarct volume in MCAO animals regardless of age (Harris et al., 2005; Villa et al., 2007; Kenne et al., 2012; Cuartero et al., 2013; Herz et al., 2015; Wang et al., 2019; El Amki et al., 2020; Kang et al., 2020; Erdener et al., 2021), while it only rescued behavior deficits in aged animals (Roy-O’Reilly et al., 2020). When aged mice received young bone marrow, they showed improved motor responses and fewer brain-infiltrating neutrophils post-ischemia. On the contrary, young mice that received aged bone marrow had worse behavioral outcomes and mortality. Removal of peripheral immune cells via splenectomy also decreases stroke-induced inflammation and injury in aged mice, thus, improving cognitive recovery (Chauhan et al., 2018; Tsai et al., 2019). Aged mice given a fecal transfer of young mice’s microbiome, a modulator of immune activation, produced improved outcomes (Zhang et al., 2015).
Age is a critical factor in studying neutrophil dynamics in stroke, since aging alters neutrophil functions and older brains are more susceptible to IS injury (Schulte-Herbrüggen et al., 2006; Manwani et al., 2013; Chen et al., 2014; Venna et al., 2014; Grønhøj et al., 2017; Ritzel et al., 2018). In preclinical studies, aged animals have a higher basal neutrophil proportion in the bone marrow and brain and greater admission NLR, which is associated with increased hemorrhagic risk after IS (Jickling et al., 2015; Maestrini et al., 2015; Perez-de-Puig et al., 2015). Aged brain-infiltrating neutrophils have heightened elevated ROS and MMP-9 production that degrades the extracellular matrix and BBB and has been connected to enlarged infarct volume (Montaner et al., 2003; Tang et al., 2006; Perez-de-Puig et al., 2015). Aged neutrophils in the ischemic brain also have defective debris clearance, leading to more risk of tissue death (Chen and Sun, 2007; Arumugam et al., 2010). Old animals have higher levels of circulating CXCL12 (increased CXCR4 expression) in the sham group but significantly lower levels of CXCL12 than young animals after IS, implying defects in neutrophil clearance (Roy-O’Reilly et al., 2020). Following IS, aged mice were also shown to have elevated neutrophil-activating IL-6 and CXCL1 compared to young animals (Roy-O’Reilly et al., 2020). Depletion of neutrophils via a specific monoclonal antibody after IS led to long-term benefits in functional outcomes only in aged animals. These results demonstrate that aging is tightly linked to neutrophil pathogenicity in IS, and that neutrophil-targeted therapies may confer greater benefit in aged subjects than the young ones (Perez-de-Puig et al., 2015; Figure 3).
In clinical studies, it has been shown that circulating neutrophils in IS patients are hyperactive and can produce significantly more ROS both in non-inflammatory and inflammatory conditions than the controls. These neutrophils bear a lower CD62L expression and higher CD11b expression, suggesting that they are mature neutrophils and can readily and rapidly respond to inflammatory stimuli. Patients with IS also have lower levels of circulating NETosis products than the controls, as well as higher circulating levels of JAM. Serum neutrophil elastase levels are also higher in IS patients (Weisenburger-Lile et al., 2019).
Previous gene expression studies of experimental stroke using microarrays (Jin et al., 2001; Schmidt-Kastner et al., 2002; Kim et al., 2004; Ford et al., 2006; Mitsios et al., 2007; Jeyaseelan et al., 2008; Hori et al., 2012) and more recently RNA sequencing (Dergunova et al., 2018; Androvic et al., 2020) have provided useful insights into the pathophysiology of IS. However, few studies included aged animals or focused on neutrophils. Androvic et al. found in aged post-ischemic mice an upregulation of more than 400 genes, many involved in the inflammatory cascade, and accompanied by a great influx of neutrophils (Androvic et al., 2020). Gene ontology terms related to inflammatory responses (e.g., IFN-1 signaling) and cellular organization are overexpressed in aged MCAO mice. Consistent with findings on inflammaging transcriptional changes, synaptic communication and plasticity-related genes are downregulated more in the aged MCAO group (Blalock et al., 2003; Lu et al., 2004; Verbitsky et al., 2004; Burger et al., 2007; Aenlle and Foster, 2010; Zeier et al., 2011; Berchtold et al., 2019; Androvic et al., 2020). These include CADPS, PNKD, LIN7A, BRAF, DNM1, RIMS1, CPLX1, SYT2, and NRXN3, indicative of impaired synaptic transmission (Androvic et al., 2020). Strikingly, chemotaxis and leukocyte migration are among the most enriched gene categories in these animals, which aligns with reports on neutrophil infiltration and poor stroke outcomes in the aged (Engler et al., 1983; del Zoppo et al., 1991; Pham and Bendszus, 2016; Androvic et al., 2020; El Amki et al., 2020; Table 1). Pathways associated with cytokine secretion, oxidative phosphorylation, and cell cycle regulation (e.g., ERK/MAPK signaling, cAMP/cGMP-mediated signaling) are significantly enriched, suggesting an inflated neuroinflammatory environment contributing to secondary damage in aged MCAO mice (Androvic et al., 2020). The persistent upregulation of pro-inflammatory (CXCL12, MMP8, MMP12, MMP14, MPEG1, TNFRSF1a, and TNFRSF1B) and genes causing fibrotic scar buildup (CTHRC1, IL6RA, IL13AR1, IL18, MMP2, RASSF4, TGFB1, TGFB2, and TIMP-1) diminish angiogenesis response in aged MCAO rats. The upregulation of pro-angiogenic genes (ANGPT2, ANGPTL4, CIB1, COL8A1, NRP1, PECAM1, LEF1, PTTG1IP, RAC2, RUNX1, TNP4, and WNT4) are delayed in aged MCAO rats, as well as and basal lamina/extracellular matrix reconstruction genes (COL4A2, FN1, LAMC1, NID2, PLOD3), compared to the young ones (Buga et al., 2014; Table 1). Preclinical studies of aged individuals are essential as the behavior of neutrophils in young and aged individuals may not be the same, and as almost all stroke patients are old, it is essential to study neutrophil dynamic changes with aging.
Potential therapeutic targets and future research directions
Numerous studies over the years have shown that aging exacerbates stroke pathology in both human patients and experimental models (Li et al., 2005, 2005; Ritzel et al., 2016). Therefore, investigating the molecular mechanisms that underlie the effects of age on stroke, especially in light of the aging population, is important (Camici and Liberale, 2017). Inflammaging, or more specifically, age-related changes in neutrophils, could constitute a novel therapeutic target. Many studies have shown that blocking neutrophil-associated pathways, such as reactive species production, can reduce infarct volume (Jickling et al., 2015). However, clinical translation of these results still faces many challenges (Mitsios et al., 2007; Warner et al., 2014; Yang and Paschen, 2017). Experiments in aged animals are needed for better translation to clinical trials (Manwani et al., 2013).
Aging and IS involve similar pathophysiology, particularly heightened inflammation (Spescha et al., 2013, 2015; Camici et al., 2015; Diaz-Cañestro et al., 2019; Liberale et al., 2020). To achieve the maximum protective effect, further experiments must be conducted to determine whether promising anti-inflammatory treatments targeting neutrophils should be administered well before the initiation of recanalization therapy (Kollikowski et al., 2022). It is necessary to include aged animals in both mechanistic and therapeutic studies examining neutrophil contributions to acute IS since previous anti-neutrophil therapies have only been tested on young animals (Benjamin et al., 2019).
Author contributions
TAB researched and wrote the manuscript and created the figures. IW and GJ co-wrote and edited the manuscript and figures. All authors contributed to the article and approved the submitted version.
Funding
This work was supported by CIHR (180224) and HSFC (G-19-0026316) funding to IW.
Conflict of interest
The authors declare that the research was conducted in the absence of any commercial or financial relationships that could be construed as a potential conflict of interest.
Publisher’s note
All claims expressed in this article are solely those of the authors and do not necessarily represent those of their affiliated organizations, or those of the publisher, the editors and the reviewers. Any product that may be evaluated in this article, or claim that may be made by its manufacturer, is not guaranteed or endorsed by the publisher.
References
Abumiya, T., Lucero, J., Heo, J. H., Tagaya, M., Koziol, J. A., Copeland, B. R., et al. (1999). Activated microvessels express vascular endothelial growth factor and integrin alpha (v)beta 3 during focal cerebral ischemia. J. Cereb. Blood Flow Metab. 19, 1038–1050. doi: 10.1097/00004647-199909000-00012
Adrover, J. M., del Fresno, C., Crainiciuc, G., Cuartero, M. I., Casanova-Acebes, M., Weiss, L. A., et al. (2019). A neutrophil timer coordinates immune defense and vascular protection. Immunity 50, 390.e10–402.e10. doi: 10.1016/j.immuni.2019.01.002
Aenlle, K. K., and Foster, T. C. (2010). Aging alters the expression of genes for neuroprotection and synaptic function following acute estradiol treatment. Hippocampus 20, 1047–1060. doi: 10.1002/hipo.20703
Akbar, A. N., and Gilroy, D. W. (2020). Aging immunity may exacerbate COVID-19. Science 369, 256–257. doi: 10.1126/science.abb0762
Al-Ahwal, S. A., Ragab, O. A., Abo Elsafa, A. A., and Ghali, A. A. (2019). Circadian and circannual patterns of stroke. Egypt. J. Neurol. Psychiatry Neurosurg. 55:5. doi: 10.1186/s41983-019-0051-5
Albers, G. W., Marks, M. P., Kemp, S., Christensen, S., Tsai, J. P., Ortega-Gutierrez, S., et al. (2018). Thrombectomy for stroke at 6 to 16 hours with selection by perfusion imaging. N. Engl. J. Med. 378, 708–718. doi: 10.1056/NEJMoa1713973
Allen, C., Thornton, P., Denes, A., McColl, B. W., Pierozynski, A., Monestier, M., et al. (2012). Neutrophil cerebrovascular transmigration triggers rapid neurotoxicity through release of proteases associated with decondensed DNA. J. Immunol. 189, 381–392. doi: 10.4049/jimmunol.1200409
Allencherril, J., Jneid, H., Atar, D., Alam, M., Levine, G., Kloner, R. A., et al. (2019). Pathophysiology, diagnosis, and management of the no-Reflow Phenomenon. Cardiovasc. Drugs Ther. 33, 589–597. doi: 10.1007/s10557-019-06901-0
Androvic, P., Kirdajova, D., Tureckova, J., Zucha, D., Rohlova, E., Abaffy, P., et al. (2020). Decoding the transcriptional response to ischemic stroke in Young and aged mouse brain. Cell Rep. 31:107777. doi: 10.1016/j.celrep.2020.107777
Andzinski, L., Kasnitz, N., Stahnke, S., Wu, C.-F., Gereke, M., von Köckritz-Blickwede, M., et al. (2016). Type I IFNs induce anti-tumor polarization of tumor associated neutrophils in mice and human. Int. J. Cancer 138, 1982–1993. doi: 10.1002/ijc.29945
Anrather, J., and Iadecola, C. (2016). Inflammation and stroke: an overview. Neurotherapeutics 13, 661–670. doi: 10.1007/s13311-016-0483-x
Anttila, J. E., Whitaker, K. W., Wires, E. S., Harvey, B. K., and Airavaara, M. (2017). Role of microglia in ischemic focal stroke and recovery: focus on toll-like receptors. Prog. Neuro-Psychopharmacol. Biol. Psychiatry 79, 3–14. doi: 10.1016/j.pnpbp.2016.07.003
Aroca-Crevillén, A., Adrover, J. M., and Hidalgo, A. (2020). Circadian features of neutrophil biology. Front. Immunol. 11:576. doi: 10.3389/fimmu.2020.00576
Arumugam, T. V., Phillips, T. M., Cheng, A., Morrell, C. H., Mattson, M. P., and Wan, R. (2010). Age and energy intake interact to modify cell stress pathways and stroke outcome. Ann. Neurol. 67, 41–52. doi: 10.1002/ana.21798
Astrup, J., Siesjö, B. K., and Symon, L. (1981). Thresholds in cerebral ischemia – the ischemic penumbra. Stroke 12, 723–725. doi: 10.1161/01.STR.12.6.723
Ballesteros, I., Rubio-Ponce, A., Genua, M., Lusito, E., Kwok, I., Fernández-Calvo, G., et al. (2020). Co-option of neutrophil fates by tissue environments. Cells 183, 1282.e18–1297.e18. doi: 10.1016/j.cell.2020.10.003
Bang, O. Y., Goyal, M., and Liebeskind, D. S. (2015). Collateral circulation in ischemic stroke: assessment tools and therapeutic strategies. Stroke 46, 3302–3309. doi: 10.1161/STROKEAHA.115.010508
Barkaway, A., Rolas, L., Joulia, R., Bodkin, J., Lenn, T., Owen-Woods, C., et al. (2021). Age-related changes in the local milieu of inflamed tissues cause aberrant neutrophil trafficking and subsequent remote organ damage. Immunity 54, 1494.e7–1510.e7. doi: 10.1016/j.immuni.2021.04.025
Barkefors, I., Jan, S. L., Jakobsson, L., Hejll, E., Carlson, G., Johansson, H., et al. (2008). Endothelial cell migration in stable gradients of vascular endothelial growth factor a and fibroblast growth factor 2: EFFECTS ON CHEMOTAXIS AND CHEMOKINESIS *. J. Biol. Chem. 283, 13905–13912. doi: 10.1074/jbc.M704917200
Baron, J.-C., Markus, H. S., Pickard, J. D., Davis, S. M., and Donnan, G. A. (2020). Lindsay Symon: a giant of stroke. Int. J. Stroke 15, 356–360. doi: 10.1177/1747493020913088
Becker, K. J. (1998). Inflammation and acute stroke. Curr. Opin. Neurol. 11, 45–49. doi: 10.1097/00019052-199802000-00008
Benest, A. V., Stone, O. A., Miller, W. H., Glover, C. P., Uney, J. B., Baker, A. H., et al. (2008). Arteriolar genesis and angiogenesis induced by endothelial nitric oxide synthase overexpression results in a mature vasculature. Arterioscler. Thromb. Vasc. Biol. 28, 1462–1468. doi: 10.1161/ATVBAHA.108.169375
Benjamin, E. J., Muntner, P., Alonso, A., Bittencourt, M. S., Callaway, C. W., Carson, A. P., et al. (2019). Heart disease and stroke Statistics-2019 update: a report from the American Heart Association. Circulation 139, e56–e528. doi: 10.1161/CIR.0000000000000659
Benjamin, E. J., Virani, S. S., Callaway, C. W., Chamberlain, A. M., Chang, A. R., Cheng, S., et al. (2018). Heart disease and stroke Statistics-2018 update: a report from the American Heart Association. Circulation 137, e67–e492. doi: 10.1161/CIR.0000000000000558
Berchtold, N. C., Prieto, G. A., Phelan, M., Gillen, D. L., Baldi, P., Bennett, D. A., et al. (2019). Hippocampal gene expression patterns linked to late-life physical activity oppose age and AD-related transcriptional decline. Neurobiol. Aging 78, 142–154. doi: 10.1016/j.neurobiolaging.2019.02.012
Bhattacharya, A., Wei, Q., Shin, J. N., Abdel Fattah, E., Bonilla, D. L., Xiang, Q., et al. (2015). Autophagy is required for neutrophil-mediated inflammation. Cell Rep. 12, 1731–1739. doi: 10.1016/j.celrep.2015.08.019
Bienvenu, K., Russell, J., and Granger, D. N. (1992). Leukotriene B4 mediates shear rate-dependent leukocyte adhesion in mesenteric venules. Circ. Res. 71, 906–911. doi: 10.1161/01.RES.71.4.906
Blalock, E. M., Chen, K.-C., Sharrow, K., Herman, J. P., Porter, N. M., Foster, T. C., et al. (2003). Gene microarrays in hippocampal aging: statistical profiling identifies novel processes correlated with cognitive impairment. J. Neurosci. 23, 3807–3819. doi: 10.1523/JNEUROSCI.23-09-03807.2003
Blann, A. D., Ridker, P. M., and Lip, G. Y. H. (2002). Inflammation, cell adhesion molecules, and stroke: tools in pathophysiology and epidemiology? Stroke 33, 2141–2143. doi: 10.1161/01.STR.0000029008.00497.D3
Boe, D. M., Boule, L. A., and Kovacs, E. J. (2017). Innate immune responses in the ageing lung. Clin. Exp. Immunol. 187, 16–25. doi: 10.1111/cei.12881
Boey, H., Rosenbaum, R., Castracane, J., and Borish, L. (1989). Interleukin-4 is a neutrophil activator. J. Allergy Clin. Immunol. 83, 978–984. doi: 10.1016/0091-6749(89)90115-2
Boisvert, M. M., Erikson, G. A., Shokhirev, M. N., and Allen, N. J. (2018). The aging astrocyte Transcriptome from multiple regions of the mouse brain. Cell Rep. 22, 269–285. doi: 10.1016/j.celrep.2017.12.039
Borregaard, N. (2010). Neutrophils, from marrow to microbes. Immunity 33, 657–670. doi: 10.1016/j.immuni.2010.11.011
Bosetti, F., Koenig, J. I., Ayata, C., Back, S. A., Becker, K., Broderick, J. P., et al. (2017). Translational stroke research: vision and opportunities. Stroke 48, 2632–2637. doi: 10.1161/STROKEAHA.117.017112
Bou Ghanem, E. N., Lee, J. N., Joma, B. H., Meydani, S. N., Leong, J. M., and Panda, A. (2017). The alpha-Tocopherol form of vitamin E boosts Elastase activity of human PMNs and their ability to kill Streptococcus pneumoniae. Front. Cell. Infect. Microbiol. 7:161. doi: 10.3389/fcimb.2017.00161
Bouchon, A., Dietrich, J., and Colonna, M. (2000). Cutting edge: inflammatory responses can be triggered by TREM-1, a novel receptor expressed on neutrophils and monocytes. J. Immunol. 164, 4991–4995. doi: 10.4049/jimmunol.164.10.4991
Bouet, V., and Freret, T. (2012). “A master key to assess stroke consequences across species: the adhesive removal test,” in Advances in the Preclinical Study of Ischemic Stroke. ed. M. Balestrino (London, United Kingdom: InTech).
Boutin, H., LeFeuvre, R. A., Horai, R., Asano, M., Iwakura, Y., and Rothwell, N. J. (2001). Role of IL-1alpha and IL-1beta in ischemic brain damage. J. Neurosci. 21, 5528–5534. doi: 10.1523/JNEUROSCI.21-15-05528.2001
Bréchard, S., Bueb, J.-L., and Tschirhart, E. J. (2005). Interleukin-8 primes oxidative burst in neutrophil-like HL-60 through changes in cytosolic calcium. Cell Calcium 37, 531–540. doi: 10.1016/j.ceca.2005.01.019
Bretón, R. R., and Rodríguez, J. C. G. (2012). Excitotoxicity and oxidative stress in acute ischemic stroke. Acute Ischemic Stroke 200, 29–50. doi: 10.5772/28300
Brinkmann, V., Reichard, U., Goosmann, C., Fauler, B., Uhlemann, Y., Weiss, D. S., et al. (2004). Neutrophil extracellular traps kill bacteria. Science 303, 1532–1535. doi: 10.1126/science.1092385
Brinkmann, V., and Zychlinsky, A. (2007). Beneficial suicide: why neutrophils die to make NETs. Nat. Rev. Microbiol. 5, 577–582. doi: 10.1038/nrmicro1710
Brooks, S. D., Spears, C., Cummings, C., Van Gilder, R. L., Stinehart, K. R., Gutmann, L., et al. (2014). Admission neutrophil-lymphocyte ratio predicts 90 day outcome after endovascular stroke therapy. J. Neurointerv. Surg. 6, 578–583. doi: 10.1136/neurintsurg-2013-010780
Brozici, M., van der Zwan, A., and Hillen, B. (2003). Anatomy and functionality of leptomeningeal anastomoses: a review. Stroke 34, 2750–2762. doi: 10.1161/01.STR.0000095791.85737.65
Bruunsgaard, H., Ladelund, S., Pedersen, A. N., Schroll, M., Jørgensen, T., and Pedersen, B. K. (2003). Predicting death from tumour necrosis factor-alpha and interleukin-6 in 80-year-old people. Clin. Exp. Immunol. 132, 24–31. doi: 10.1046/j.1365-2249.2003.02137.x
Buckley, C. D., Ross, E. A., McGettrick, H. M., Osborne, C. E., Haworth, O., Schmutz, C., et al. (2006). Identification of a phenotypically and functionally distinct population of long-lived neutrophils in a model of reverse endothelial migration. J. Leukoc. Biol. 79, 303–311. doi: 10.1189/jlb.0905496
Buga, A. M., Margaritescu, C., Scholz, C. J., Radu, E., Zelenak, C., and Popa-Wagner, A. (2014). Transcriptomics of post-stroke angiogenesis in the aged brain. Front. Aging Neurosci. 6:44. doi: 10.3389/fnagi.2014.00044
Burger, C., López, M. C., Feller, J. A., Baker, H. V., Muzyczka, N., and Mandel, R. J. (2007). Changes in transcription within the CA1 field of the hippocampus are associated with age-related spatial learning impairments. Neurobiol. Learn. Mem. 87, 21–41. doi: 10.1016/j.nlm.2006.05.003
Butcher, S. K., Chahal, H., Nayak, L., Sinclair, A., Henriquez, N. V., Sapey, E., et al. (2001). Senescence in innate immune responses: reduced neutrophil phagocytic capacity and CD16 expression in elderly humans. J. Leukoc. Biol. 70, 881–886. doi: 10.1189/jlb.70.6.881
Butt, M.-U.-R. A., Zakaria, M., and Hussain, H. M. (2009). Circadian pattern of onset of ischaemic and haemorrhagic strokes, and their relation to sleep/wake cycle. J. Pak. Med. Assoc. 59, 129–132.
Cai, W., Liu, S., Hu, M., Huang, F., Zhu, Q., Qiu, W., et al. (2020). Functional dynamics of neutrophils after ischemic stroke. Transl. Stroke Res. 11, 108–121. doi: 10.1007/s12975-019-00694-y
Caimi, G., Canino, B., Ferrara, F., Montana, M., Musso, M., Porretto, F., et al. (2001). Granulocyte integrins before and after activation in acute ischaemic stroke. J. Neurol. Sci. 186, 23–26. doi: 10.1016/s0022-510x(01)00495-6
Camici, G. G., and Liberale, L. (2017). Aging: the next cardiovascular disease? Eur. Heart J. 38, 1621–1623. doi: 10.1093/eurheartj/ehx239
Camici, G. G., Savarese, G., Akhmedov, A., and Lüscher, T. F. (2015). Molecular mechanism of endothelial and vascular aging: implications for cardiovascular disease. Eur. Heart J. 36, 3392–3403. doi: 10.1093/eurheartj/ehv587
Campbell, B. C., Christensen, S., Tress, B. M., Churilov, L., Desmond, P. M., Parsons, M. W., et al. (2013). Failure of collateral blood flow is associated with infarct growth in ischemic stroke. J. Cereb. Blood Flow Metab. 33, 1168–1172. doi: 10.1038/jcbfm.2013.77
Campbell, B. C. V., Ma, H., Ringleb, P. A., Parsons, M. W., Churilov, L., Bendszus, M., et al. (2019). Extending thrombolysis to 4·5-9 h and wake-up stroke using perfusion imaging: a systematic review and meta-analysis of individual patient data. Lancet 394, 139–147. doi: 10.1016/S0140-6736(19)31053-0
Carcel, C., Woodward, M., Balicki, G., Koroneos, G. L., de Sousa, D. A., Cordonnier, C., et al. (2019). Trends in recruitment of women and reporting of sex differences in large-scale published randomized controlled trials in stroke. Int. J. Stroke 14, 931–938. doi: 10.1177/1747493019851292
Carmona-Mora, P., Ander, B. P., Jickling, G. C., Dykstra-Aiello, C., Zhan, X., Ferino, E., et al. (2021). Distinct peripheral blood monocyte and neutrophil transcriptional programs following intracerebral hemorrhage and different etiologies of ischemic stroke. J. Cereb. Blood Flow Metab. 41, 1398–1416. doi: 10.1177/0271678X20953912
Carmona-Mora, P., Knepp, B., Jickling, G. C., Zhan, X., Hakoupian, M., Hull, H., et al. (2022). Monocyte, neutrophil and whole blood Transcriptome dynamics following ischemic stroke. medRxiv 2022.03.03.22271866. doi: 10.1101/2022.03.03.22271866
Carter, R. I., Ungurs, M. J., Mumford, R. A., and Stockley, R. A. (2013). Aα-Val 360: a marker of neutrophil elastase and COPD disease activity. Eur. Respir. J. 41, 31–38. doi: 10.1183/09031936.00197411
Casanova-Acebes, M., Pitaval, C., Weiss, L. A., Nombela-Arrieta, C., Chèvre, R., A-González, N., et al. (2013). Rhythmic modulation of the hematopoietic niche through neutrophil clearance. Cells 153, 1025–1035. doi: 10.1016/j.cell.2013.04.040
Castellanos, M., Sobrino, T., Pedraza, S., Moldes, O., Pumar, J. M., Silva, Y., et al. (2008). High plasma glutamate concentrations are associated with infarct growth in acute ischemic stroke. Neurology 71, 1862–1868. doi: 10.1212/01.wnl.0000326064.42186.7e
Chamorro, Á., Dirnagl, U., Urra, X., and Planas, A. M. (2016). Neuroprotection in acute stroke: targeting excitotoxicity, oxidative and nitrosative stress, and inflammation. Lancet Neurol. 15, 869–881. doi: 10.1016/S1474-4422(16)00114-9
Chapouly, C., Guimbal, S., Hollier, P.-L., and Renault, M.-A. (2019). Role of hedgehog signaling in vasculature development, differentiation, and maintenance. Int. J. Mol. Sci. 20:3076. doi: 10.3390/ijms20123076
Chatta, G. S., Andrews, R. G., Rodger, E., Schrag, M, Hammond, W. P., and Dale, D. C. (1993). Hematopoietic progenitors and aging: alterations in granulocytic precursors and responsiveness to recombinant human G-CSF, GM-CSF, and IL-3. J Gerontol. 48, M207–12. doi: 10.1093/geronj/48.5.m207
Chatta, G. S., Price, T. H., Stratton, J. R., and Dale, D. C. (1994). Aging and marrow neutrophil reserves. J. Am. Geriatr. Soc. 42, 77–81. doi: 10.1111/j.1532-5415.1994.tb06077.x
Chauhan, A., Al Mamun, A., Spiegel, G., Harris, N., Zhu, L., and McCullough, L. D. (2018). Splenectomy protects aged mice from injury after experimental stroke. Neurobiol. Aging 61, 102–111. doi: 10.1016/j.neurobiolaging.2017.09.022
Chen, R.-L., Balami, J. S., Esiri, M. M., Chen, L.-K., and Buchan, A. M. (2010). Ischemic stroke in the elderly: an overview of evidence. Nat. Rev. Neurol. 6, 256–265. doi: 10.1038/nrneurol.2010.36
Chen, L., Ge, B., Casale, F. P., Vasquez, L., Kwan, T., Garrido-Martín, D., et al. (2016). Genetic drivers of epigenetic and transcriptional variation in human immune cells. Cells 167, 1398.e24–1414.e24. doi: 10.1016/j.cell.2016.10.026
Chen, M. M., Palmer, J. L., Plackett, T. P., Deburghgraeve, C. R., and Kovacs, E. J. (2014). Age-related differences in the neutrophil response to pulmonary pseudomonas infection. Exp. Gerontol. 54, 42–46. doi: 10.1016/j.exger.2013.12.010
Chen, Y., and Sun, F.-Y. (2007). Age-related decrease of striatal neurogenesis is associated with apoptosis of neural precursors and newborn neurons in rat brain after ischemia. Brain Res. 1166, 9–19. doi: 10.1016/j.brainres.2007.06.043
Chen, X., Wang, L., Jiang, M., Lin, L., Ba, Z., Tian, H., et al. (2022). Leukocytes in cerebral thrombus respond to large-vessel occlusion in a time-dependent manner and the association of NETs with collateral flow. Front. Immunol. 13:834562. doi: 10.3389/fimmu.2022.834562
Childs, B. G., Durik, M., Baker, D. J., and van Deursen, J. M. (2015). Cellular senescence in aging and age-related disease: from mechanisms to therapy. Nat. Med. 21, 1424–1435. doi: 10.1038/nm.4000
Cho, W., Song, J.-Y., Oh, S. W., Kim, M. G., Ko, Y. S., Lee, H. Y., et al. (2017). Fate of neutrophils during the recovery phase of ischemia/reperfusion induced acute kidney injury. J. Korean Med. Sci. 32, 1616–1625. doi: 10.3346/jkms.2017.32.10.1616
Christoffersson, G., Henriksnäs, J., Johansson, L., Rolny, C., Ahlström, H., Caballero-Corbalan, J., et al. (2010). Clinical and experimental pancreatic islet transplantation to striated muscle: establishment of a vascular system similar to that in native islets. Diabetes 59, 2569–2578. doi: 10.2337/db10-0205
Christoffersson, G., and Phillipson, M. (2018). The neutrophil: one cell on many missions or many cells with different agendas? Cell Tissue Res. 371, 415–423. doi: 10.1007/s00441-017-2780-z
Christoffersson, G., Vågesjö, E., Vandooren, J., Lidén, M., Massena, S., Reinert, R. B., et al. (2012). VEGF-A recruits a proangiogenic MMP-9-delivering neutrophil subset that induces angiogenesis in transplanted hypoxic tissue. Blood 120, 4653–4662. doi: 10.1182/blood-2012-04-421040
Christoforidis, G. A., Mohammad, Y., Kehagias, D., Avutu, B., and Slivka, A. P. (2005). Angiographic assessment of pial collaterals as a prognostic indicator following intra-arterial thrombolysis for acute ischemic stroke. AJNR Am. J. Neuroradiol. 26, 1789–1797.
Chu, H. X., Kim, H. A., Lee, S., Moore, J. P., Chan, C. T., Vinh, A., et al. (2014). Immune cell infiltration in malignant middle cerebral artery infarction: comparison with transient cerebral ischemia. J. Cereb. Blood Flow Metab. 34, 450–459. doi: 10.1038/jcbfm.2013.217
Chua, F., and Laurent, G. J. (2006). Neutrophil elastase: mediator of extracellular matrix destruction and accumulation. Proc. Am. Thorac. Soc. 3, 424–427. doi: 10.1513/pats.200603-078AW
Chung, H. Y., Kim, D. H., Lee, E. K., Chung, K. W., Chung, S., Lee, B., et al. (2019). Redefining chronic inflammation in aging and age-related diseases: proposal of the Senoinflammation concept. Aging Dis. 10, 367–382. doi: 10.14336/AD.2018.0324
Clark, W. M., Madden, K. P., Rothlein, R., and Zivin, J. A. (1991). Reduction of central nervous system ischemic injury by monoclonal antibody to intercellular adhesion molecule. J. Neurosurg. 75, 623–627. doi: 10.3171/jns.1991.75.4.0623
Clarke, L. E., Liddelow, S. A., Chakraborty, C., Münch, A. E., Heiman, M., and Barres, B. A. (2018). Normal aging induces A1-like astrocyte reactivity. Proc. Natl. Acad. Sci. U. S. A. 115, E1896–E 1905. doi: 10.1073/pnas.1800165115
Clayton, J. A., Chalothorn, D., and Faber, J. E. (2008). Vascular endothelial growth factor-a specifies formation of native collaterals and regulates collateral growth in ischemia. Circ. Res. 103, 1027–1036. doi: 10.1161/CIRCRESAHA.108.181115
Cohen, M., Matcovitch, O., David, E., Barnett-Itzhaki, Z., Keren-Shaul, H., Blecher-Gonen, R., et al. (2014). Chronic exposure to TGFβ1 regulates myeloid cell inflammatory response in an IRF7-dependent manner. EMBO J. 33, 2906–2921. doi: 10.15252/embj.201489293
Coller, B. S. (2005). Leukocytosis and ischemic vascular disease morbidity and mortality: is it time to intervene? Arterioscler. Thromb. Vasc. Biol. 25, 658–670. doi: 10.1161/01.ATV.0000156877.94472.a5
Colom, B., Bodkin, J. V., Beyrau, M., Woodfin, A., Ody, C., Rourke, C., et al. (2015). Leukotriene B4-neutrophil Elastase Axis drives neutrophil reverse Transendothelial cell migration in vivo. Immunity 42, 1075–1086. doi: 10.1016/j.immuni.2015.05.010
Connolly, E. S., Winfree, C. J., Prestigiacomo, C. J., Kim, S. C., Choudhri, T. F., Hoh, B. L., et al. (1997). Exacerbation of cerebral injury in mice that express the P-Selectin gene: identification of P-Selectin blockade as a new target for the treatment of stroke. Circ. Res. 81, 304–310. doi: 10.1161/01.RES.81.3.304
Corberand, J. X., Laharrague, P. F., and Fillola, G. (1986). Neutrophils of healthy aged humans are normal. Mech. Ageing Dev. 36, 57–63. doi: 10.1016/0047-6374(86)90138-7
Cowled, P., and Fitridge, R. (2011). “Pathophysiology of reperfusion injury,” in Mechanisms of Vascular Disease: A Reference Book for Vascular Specialists, eds. R. Fitridge and M. Thompson (Adelaide, AU: University of Adelaide Press). Available at: http://www.ncbi.nlm.nih.gov/books/NBK534267/ (Accessed June 28, 2022).
Cox, G., Gauldie, J., and Jordana, M. (1992). Bronchial epithelial cell-derived cytokines (G-CSF and GM-CSF) promote the survival of peripheral blood neutrophils in vitro. Am. J. Respir. Cell Mol. Biol. 7, 507–513. doi: 10.1165/ajrcmb/7.5.507
Cribbs, D. H., Berchtold, N. C., Perreau, V., Coleman, P. D., Rogers, J., Tenner, A. J., et al. (2012). Extensive innate immune gene activation accompanies brain aging, increasing vulnerability to cognitive decline and neurodegeneration: a microarray study. J. Neuroinflammation 9:179. doi: 10.1186/1742-2094-9-179
Cuartero, M. I., Ballesteros, I., Moraga, A., Nombela, F., Vivancos, J., Hamilton, J. A., et al. (2013). N2 neutrophils, novel players in brain inflammation after stroke: modulation by the PPARγ agonist rosiglitazone. Stroke 44, 3498–3508. doi: 10.1161/STROKEAHA.113.002470
Curcio, M., Salazar, I. L., Mele, M., Canzoniero, L. M. T., and Duarte, C. B. (2016). Calpains and neuronal damage in the ischemic brain: the swiss knife in synaptic injury. Prog. Neurobiol. 143, 1–35. doi: 10.1016/j.pneurobio.2016.06.001
Dalboni, T. M., Abe, A. E., de Oliveira, C. E., Lara, V. S., Campanelli, A. P., Gasparoto, C. T., et al. (2013). Activation profile of CXCL8-stimulated neutrophils and aging. Cytokine 61, 716–719. doi: 10.1016/j.cyto.2013.01.016
Dalkara, T., and Arsava, E. M. (2012). Can restoring incomplete microcirculatory reperfusion improve stroke outcome after thrombolysis? J. Cereb. Blood Flow Metab. 32, 2091–2099. doi: 10.1038/jcbfm.2012.139
Danton, G. H., and Dietrich, W. D. (2003). Inflammatory mechanisms after ischemia and stroke. J. Neuropathol. Exp. Neurol. 62, 127–136. doi: 10.1093/jnen/62.2.127
Dawson, D. A., Ruetzler, C. A., Carlos, T. M., Kochanek, P. M., and Hallenbeck, J. M. (1996). Polymorphonuclear leukocytes and microcirculatory perfusion in acute stroke in the SHR. Keio J. Med. 45, 248–252. doi: 10.2302/kjm.45.248
De Maeyer, R. P. H., van de Merwe, R. C., Louie, R., Bracken, O. V., Devine, O. P., Goldstein, D. R., et al. (2020). Blocking elevated p 38 MAPK restores efferocytosis and inflammatory resolution in the elderly. Nat. Immunol. 21, 615–625. doi: 10.1038/s41590-020-0646-0
de Magalhães, J. P., Curado, J., and Church, G. M. (2009). Meta-analysis of age-related gene expression profiles identifies common signatures of aging. Bioinformatics 25, 875–881. doi: 10.1093/bioinformatics/btp073
De Martinis, M., Franceschi, C., Monti, D., and Ginaldi, L. (2005). Inflamm-ageing and lifelong antigenic load as major determinants of ageing rate and longevity. FEBS Lett. 579, 2035–2039. doi: 10.1016/j.febslet.2005.02.055
De Meyer, S. F., Denorme, F., Langhauser, F., Geuss, E., Fluri, F., and Kleinschnitz, C. (2016). Thromboinflammation in stroke brain damage. Stroke 47, 1165–1172. doi: 10.1161/STROKEAHA.115.011238
DeGraba, T. J. (1998). The role of inflammation after acute stroke: utility of pursuing anti-adhesion molecule therapy. Neurology 51, S62–S68. doi: 10.1212/WNL.51.3_Suppl_3.S62
Deindl, E., Hoefer, I. E., Fernandez, B., Barancik, M., Heil, M., Strniskova, M., et al. (2003). Involvement of the fibroblast growth factor system in adaptive and chemokine-induced Arteriogenesis. Circ. Res. 92, 561–568. doi: 10.1161/01.RES.0000061181.80065.7D
del Zoppo, G. J. (1994). Microvascular changes during cerebral ischemia and reperfusion. Cerebrovasc. Brain Metab. Rev. 6, 47–96.
Del Zoppo, G. J. (1995). Why do all drugs work in animals but none in stroke patients? 1. Drugs promoting cerebral blood flow. J. Intern. Med. 237, 79–88. doi: 10.1111/j.1365-2796.1995.tb01144.x
del Zoppo, G. J., Poeck, K., Pessin, M. S., Wolpert, S. M., Furlan, A. J., Ferbert, A., et al. (1992). Recombinant tissue plasminogen activator in acute thrombotic and embolic stroke. Ann. Neurol. 32, 78–86. doi: 10.1002/ana.410320113
del Zoppo, G. J., Schmid-Schönbein, G. W., Mori, E., Copeland, B. R., and Chang, C. M. (1991). Polymorphonuclear leukocytes occlude capillaries following middle cerebral artery occlusion and reperfusion in baboons. Stroke 22, 1276–1283. doi: 10.1161/01.STR.22.10.1276
Dénes, A., Ferenczi, S., and Kovács, K. J. (2011). Systemic inflammatory challenges compromise survival after experimental stroke via augmenting brain inflammation, blood-brain barrier damage and brain oedema independently of infarct size. J. Neuroinflammation 8:164. doi: 10.1186/1742-2094-8-164
Dénes, A., Humphreys, N., Lane, T. E., Grencis, R., and Rothwell, N. (2010). Chronic systemic infection exacerbates ischemic brain damage via a CCL5 (regulated on activation, normal T-cell expressed and secreted)-mediated proinflammatory response in mice. J. Neurosci. 30, 10086–10095. doi: 10.1523/JNEUROSCI.1227-10.2010
Denorme, F., Portier, I., Rustad, J. L., Cody, M. J., de Araujo, C. V., Hoki, C., et al. (2022). Neutrophil extracellular traps regulate ischemic stroke brain injury. J. Clin. Invest. 132:e154225. doi: 10.1172/JCI154225
Dereski, M. O., Chopp, M., Knight, R. A., Rodolosi, L. C., and Garcia, J. H. (1993). The heterogeneous temporal evolution of focal ischemic neuronal damage in the rat. Acta Neuropathol. 85, 327–333. doi: 10.1007/BF00227730
Dergunova, L. V., Filippenkov, I. B., Stavchansky, V. V., Denisova, A. E., Yuzhakov, V. V., Mozerov, S. A., et al. (2018). Genome-wide transcriptome analysis using RNA-Seq reveals a large number of differentially expressed genes in a transient MCAO rat model. BMC Genomics 19:655. doi: 10.1186/s12864-018-5039-5
Desai, A., Grolleau-Julius, A., and Yung, R. (2010). Leukocyte function in the aging immune system. J. Leukoc. Biol. 87, 1001–1009. doi: 10.1189/jlb.0809542
Dhungana, H., Malm, T., Denes, A., Valonen, P., Wojciechowski, S., Magga, J., et al. (2013). Aging aggravates ischemic stroke-induced brain damage in mice with chronic peripheral infection. Aging Cell 12, 842–850. doi: 10.1111/acel.12106
Diaz-Cañestro, C., Reiner, M. F., Bonetti, N. R., Liberale, L., Merlini, M., Wüst, P., et al. (2019). AP-1 (activated Protein-1) transcription factor Jun D regulates ischemia/reperfusion brain damage via IL-1β (interleukin-1β). Stroke 50, 469–477. doi: 10.1161/STROKEAHA.118.023739
DiNapoli, V. A., Huber, J. D., Houser, K., Li, X., and Rosen, C. L. (2008). Early disruptions of the blood-brain barrier may contribute to exacerbated neuronal damage and prolonged functional recovery following stroke in aged rats. Neurobiol. Aging 29, 753–764. doi: 10.1016/j.neurobiolaging.2006.12.007
Dirnagl, U., Iadecola, C., and Moskowitz, M. A. (1999). Pathobiology of ischaemic stroke: an integrated view. Trends Neurosci. 22, 391–397. doi: 10.1016/S0166-2236(99)01401-0
Dixon, G., Elks, P. M., Loynes, C. A., Whyte, M. K. B., and Renshaw, S. A. (2012). A method for the in vivo measurement of zebrafish tissue neutrophil lifespan. ISRN Hematol 2012:915868. doi: 10.5402/2012/915868
Doerschuk, C. M., Beyers, N., Coxson, H. O., Wiggs, B., and Hogg, J. C. (1993). Comparison of neutrophil and capillary diameters and their relation to neutrophil sequestration in the lung. J. Appl. Physiol. 1985, 3040–3045. doi: 10.1152/jappl.1993.74.6.3040
Domon, H., Nagai, K., Maekawa, T., Oda, M., Yonezawa, D., Takeda, W., et al. (2018). Neutrophil Elastase subverts the immune response by cleaving toll-like receptors and cytokines in pneumococcal pneumonia. Front. Immunol. 9:732. doi: 10.3389/fimmu.2018.00732
Domon, H., Oda, M., Maekawa, T., Nagai, K., Takeda, W., and Terao, Y. (2016). Streptococcus pneumoniae disrupts pulmonary immune defence via elastase release following pneumolysin-dependent neutrophil lysis. Sci. Rep. 6:38013. doi: 10.1038/srep38013
Donkor, E. S. (2018). Stroke in the 21st century: a snapshot of the burden, epidemiology, and quality of life. Stroke Res. Treat. 2018:3238165. doi: 10.1155/2018/3238165
Dorshkind, K., Montecino-Rodriguez, E., and Signer, R. A. J. (2009). The ageing immune system: is it ever too old to become young again? Nat. Rev. Immunol. 9, 57–62. doi: 10.1038/nri2471
Doycheva, D. M., Hadley, T., Li, L., Applegate, R. L., Zhang, J. H., and Tang, J. (2014). Anti-neutrophil antibody enhances the neuroprotective effects of G-CSF by decreasing number of neutrophils in hypoxic ischemic neonatal rat model. Neurobiol. Dis. 69, 192–199. doi: 10.1016/j.nbd.2014.05.024
Doyle, K. P., Cekanaviciute, E., Mamer, L. E., and Buckwalter, M. S. (2010). TGFβ signaling in the brain increases with aging and signals to astrocytes and innate immune cells in the weeks after stroke. J. Neuroinflammation 7:62. doi: 10.1186/1742-2094-7-62
Drieu, A., Levard, D., Vivien, D., and Rubio, M. (2018). Anti-inflammatory treatments for stroke: from bench to bedside. Ther. Adv. Neurol. Disord. 11:1756286418789854. doi: 10.1177/1756286418789854
Drifte, G., Dunn-Siegrist, I., Tissières, P., and Pugin, J. (2013). Innate immune functions of immature neutrophils in patients with sepsis and severe systemic inflammatory response syndrome. Crit. Care Med. 41, 820–832. doi: 10.1097/CCM.0b013e318274647d
Durukan, A., and Tatlisumak, T. (2007). Acute ischemic stroke: overview of major experimental rodent models, pathophysiology, and therapy of focal cerebral ischemia. Pharmacol. Biochem. Behav. 87, 179–197. doi: 10.1016/j.pbb.2007.04.015
Eash, K. J., Greenbaum, A. M., Gopalan, P. K., and Link, D. C. (2010). CXCR2 and CXCR4 antagonistically regulate neutrophil trafficking from murine bone marrow. J. Clin. Invest. 120, 2423–2431. doi: 10.1172/JCI41649
Easton, A. S. (2013). Neutrophils and stroke – can neutrophils mitigate disease in the central nervous system? Int. Immunopharmacol. 17, 1218–1225. doi: 10.1016/j.intimp.2013.06.015
Edwards, D. N., and Bix, G. J. (2019). Roles of blood-brain barrier integrins and extracellular matrix in stroke. Am. J. Physiol. Cell Physiol. 316, C252–C263. doi: 10.1152/ajpcell.00151.2018
El Amki, M., Glück, C., Binder, N., Middleham, W., Wyss, M. T., Weiss, T., et al. (2020). Neutrophils obstructing brain capillaries are a major cause of no-reflow in ischemic stroke. Cell Rep. 33:108260. doi: 10.1016/j.celrep.2020.108260
El Amki, M., and Wegener, S. (2017). Improving cerebral blood flow after arterial recanalization: a novel therapeutic strategy in stroke. Int. J. Mol. Sci. 18:E 2669. doi: 10.3390/ijms18122669
Ellett, F., Elks, P. M., Robertson, A. L., Ogryzko, N. V., and Renshaw, S. A. (2015). Defining the phenotype of neutrophils following reverse migration in zebrafish. J. Leukoc. Biol. 98, 975–981. doi: 10.1189/jlb.3MA0315-105R
Elliott, W. J. (1998). Circadian variation in the timing of stroke onset. Stroke 29, 992–996. doi: 10.1161/01.STR.29.5.992
Eltzschig, H. K., and Carmeliet, P. (2011). Hypoxia and inflammation. N. Engl. J. Med. 364, 656–665. doi: 10.1056/NEJMra0910283
Endres, M., Dirnagl, U., and Moskowitz, M. A. (2008). “Chapter 2 the ischemic cascade and mediators of ischemic injury,” in Handbook of Clinical Neurology (Amsterdam, Netherlands: Elsevier), 31–41.
Endres, M., Moro, M. A., Nolte, C. H., Dames, C., Buckwalter, M. S., and Meisel, A. (2022). Immune pathways in etiology, acute phase, and chronic Sequelae of ischemic stroke. Circ. Res. 130, 1167–1186. doi: 10.1161/CIRCRESAHA.121.319994
Engler, R. L., Schmid-Schönbein, G. W., and Pavelec, R. S. (1983). Leukocyte capillary plugging in myocardial ischemia and reperfusion in the dog. Am. J. Pathol. 111, 98–111.
Erdener, Ş. E., Tang, J., Kılıç, K., Postnov, D., Giblin, J. T., Kura, S., et al. (2021). Dynamic capillary stalls in reperfused ischemic penumbra contribute to injury: a hyperacute role for neutrophils in persistent traffic jams. J. Cereb. Blood Flow Metab. 41, 236–252. doi: 10.1177/0271678X20914179
Ergul, A., Alhusban, A., and Fagan, S. C. (2012). Angiogenesis: a harmonized target for recovery after stroke. Stroke 43, 2270–2274. doi: 10.1161/STROKEAHA.111.642710
Ericson, J. A., Duffau, P., Yasuda, K., Ortiz-Lopez, A., Rothamel, K., Rifkin, I. R., et al. (2014). Gene expression during the generation and activation of mouse neutrophils: implication of novel functional and regulatory pathways. PLoS One 9:e108553. doi: 10.1371/journal.pone.0108553
Eruslanov, E. B., Singhal, S., and Albelda, S. M. (2017). Mouse versus human neutrophils in cancer–a major knowledge gap. Trends Cancer 3, 149–160. doi: 10.1016/j.trecan.2016.12.006
Eskan, M. A., Jotwani, R., Abe, T., Chmelar, J., Lim, J.-H., Liang, S., et al. (2012). The leukocyte integrin antagonist Del-1 inhibits IL-17-mediated inflammatory bone loss. Nat. Immunol. 13, 465–473. doi: 10.1038/ni.2260
Esposito, E., Li, W., Mandeville, E., Park, J. H., Şencan, I., Guo, S., et al. (2020). Potential circadian effects on translational failure for neuroprotection. Nature 582, 395–398. doi: 10.1038/s41586-020-2348-z
Faber, J. E., Chilian, W. M., Deindl, E., van Royen, N., and Simons, M. (2014). A brief etymology of the collateral circulation. Arterioscler. Thromb. Vasc. Biol. 34, 1854–1859. doi: 10.1161/ATVBAHA.114.303929
Ferrucci, L., Corsi, A., Lauretani, F., Bandinelli, S., Bartali, B., Taub, D. D., et al. (2005). The origins of age-related proinflammatory state. Blood 105, 2294–2299. doi: 10.1182/blood-2004-07-2599
Flanary, B. E., Sammons, N. W., Nguyen, C., Walker, D., and Streit, W. J. (2007). Evidence that aging and amyloid promote microglial cell senescence. Rejuvenation Res. 10, 61–74. doi: 10.1089/rej.2006.9096
Flottmann, F., Brekenfeld, C., Broocks, G., Leischner, H., McDonough, R., Faizy, T. D., et al. (2021). Good clinical outcome decreases with number of retrieval attempts in stroke thrombectomy. Stroke 52, 482–490. doi: 10.1161/STROKEAHA.120.029830
Ford, G., Xu, Z., Gates, A., Jiang, J., and Ford, B. D. (2006). Expression analysis systematic explorer (EASE) analysis reveals differential gene expression in permanent and transient focal stroke rat models. Brain Res. 1071, 226–236. doi: 10.1016/j.brainres.2005.11.090
Formisano, L., Guida, N., Mascolo, L., Serani, A., Laudati, G., Pizzorusso, V., et al. (2020). Transcriptional and epigenetic regulation of ncx 1 and ncx 3 in the brain. Cell Calcium 87:102194. doi: 10.1016/j.ceca.2020.102194
Forster, C., Clark, H. B., Ross, M. E., and Iadecola, C. (1999). Inducible nitric oxide synthase expression in human cerebral infarcts. Acta Neuropathol. 97, 215–220. doi: 10.1007/s004010050977
Fortin, C. F., Larbi, A., Lesur, O., Douziech, N., and Fulop, T. (2006). Impairment of SHP-1 down-regulation in the lipid rafts of human neutrophils under GM-CSF stimulation contributes to their age-related, altered functions. J. Leukoc. Biol. 79, 1061–1072. doi: 10.1189/jlb.0805481
Franceschi, C., and Campisi, J. (2014). Chronic inflammation (inflammaging) and its potential contribution to age-associated diseases. J. Gerontol. A Biol. Sci. Med. Sci. 69, S4–S9. doi: 10.1093/gerona/glu057
Franceschi, C., Garagnani, P., Parini, P., Giuliani, C., and Santoro, A. (2018). Inflammaging: a new immune-metabolic viewpoint for age-related diseases. Nat. Rev. Endocrinol. 14, 576–590. doi: 10.1038/s41574-018-0059-4
Fraser, H. B., Khaitovich, P., Plotkin, J. B., Pääbo, S., and Eisen, M. B. (2005). Aging and gene expression in the primate brain. PLoS Biol. 3:e274. doi: 10.1371/journal.pbio.0030274
Fridlender, Z. G., Sun, J., Kim, S., Kapoor, V., Cheng, G., Ling, L., et al. (2009). Polarization of tumor-associated neutrophil phenotype by TGF-beta: “N1” versus “N2” TAN. Cancer Cell 16, 183–194. doi: 10.1016/j.ccr.2009.06.017
Friedman, B. A., Srinivasan, K., Ayalon, G., Meilandt, W. J., Lin, H., Huntley, M. A., et al. (2018). Diverse brain myeloid expression profiles reveal distinct microglial activation states and aspects of Alzheimer’s disease not evident in mouse models. Cell Rep. 22, 832–847. doi: 10.1016/j.celrep.2017.12.066
Fuchs, T. A., Brill, A., Duerschmied, D., Schatzberg, D., Monestier, M., Myers, D. D., et al. (2010). Extracellular DNA traps promote thrombosis. Proc. Natl. Acad. Sci. U. S. A. 107, 15880–15885. doi: 10.1073/pnas.1005743107
Fülöp, T., Fouquet, C., Allaire, P., Perrin, N., Lacombe, G., Stankova, J., et al. (1997). Changes in apoptosis of human polymorphonuclear granulocytes with aging. Mech. Ageing Dev. 96, 15–34. doi: 10.1016/S0047-6374(96)01881-7
Fulop, T., Larbi, A., Douziech, N., Fortin, C., Guérard, K.-P., Lesur, O., et al. (2004). Signal transduction and functional changes in neutrophils with aging. Aging Cell 3, 217–226. doi: 10.1111/j.1474-9728.2004.00110.x
Furze, R. C., and Rankin, S. M. (2008). Neutrophil mobilization and clearance in the bone marrow. Immunology 125, 281–288. doi: 10.1111/j.1365-2567.2008.02950.x
Garcia, J. H., Liu, K. F., Yoshida, Y., Lian, J., Chen, S., and del Zoppo, G. J. (1994). Influx of leukocytes and platelets in an evolving brain infarct (Wistar rat). Am. J. Pathol. 144, 188–199.
Garcia-Bonilla, L., Moore, J. M., Racchumi, G., Zhou, P., Butler, J. M., Iadecola, C., et al. (2014). Inducible nitric oxide synthase in neutrophils and endothelium contributes to ischemic brain injury in mice. J. Immunol. 193, 2531–2537. doi: 10.4049/jimmunol.1400918
Gasparoto, T. H., de Oliveira, C. E., Vieira, N. A., Porto, V. C., Cunha, F. Q., Garlet, G. P., et al. (2012). Activation pattern of neutrophils from blood of elderly individuals with Candida-related denture stomatitis. Eur. J. Clin. Microbiol. Infect. Dis. 31, 1271–1277. doi: 10.1007/s10096-011-1439-z
Gelderblom, M., Leypoldt, F., Steinbach, K., Behrens, D., Choe, C.-U., Siler, D. A., et al. (2009). Temporal and spatial dynamics of cerebral immune cell accumulation in stroke. Stroke 40, 1849–1857. doi: 10.1161/STROKEAHA.108.534503
Gendron, A., Teitelbaum, J., Cossette, C., Nuara, S., Dumont, M., Geadah, D., et al. (2002). Temporal effects of left versus right middle cerebral artery occlusion on spleen lymphocyte subsets and mitogenic response in Wistar rats. Brain Res. 955, 85–97. doi: 10.1016/S0006-8993(02)03368-1
Gensicke, H., Al-Ajlan, F., Fladt, J., Campbell, B. C. V., Majoie, C. B. L. M., Bracard, S., et al. (2022). Comparison of three scores of collateral status for their association with clinical outcome: the HERMES collaboration. Stroke. 53, 3548–3556. doi: 10.1161/STROKEAHA.122.039717
Giede-Jeppe, A., Reichl, J., Sprügel, M. I., Lücking, H., Hoelter, P., Eyüpoglu, I. Y., et al. (2019). Neutrophil-to-lymphocyte ratio as an independent predictor for unfavorable functional outcome in aneurysmal subarachnoid hemorrhage. J. Neurosurg. 132, 400–407. doi: 10.3171/2018.9.JNS181975
Gill, D., Sivakumaran, P., Aravind, A., Tank, A., Dosh, R., and Veltkamp, R. (2018). Temporal trends in the levels of peripherally circulating leukocyte subtypes in the hours after ischemic stroke. J. Stroke Cerebrovasc. Dis. 27, 198–202. doi: 10.1016/j.jstrokecerebrovasdis.2017.08.023
Gillum, R. F., Mussolino, M. E., and Madans, J. H. (2005). Counts of neutrophils, lymphocytes, and monocytes, cause-specific mortality and coronary heart disease: the NHANES-I epidemiologic follow-up study. Ann. Epidemiol. 15, 266–271. doi: 10.1016/j.annepidem.2004.08.009
Girbl, T., Lenn, T., Perez, L., Rolas, L., Barkaway, A., Thiriot, A., et al. (2018). Distinct compartmentalization of the chemokines CXCL1 and CXCL2 and the atypical receptor ACKR1 determine discrete stages of neutrophil diapedesis. Immunity 49, 1062.e6–1076.e6. doi: 10.1016/j.immuni.2018.09.018
Gkikas, I., Palikaras, K., and Tavernarakis, N. (2018). The role of mitophagy in innate immunity. Front. Immunol. 9:1283. doi: 10.3389/fimmu.2018.01283
Gomez, C. R., Hirano, S., Cutro, B. T., Birjandi, S., Baila, H., Nomellini, V., et al. (2007). Advanced age exacerbates the pulmonary inflammatory response after lipopolysaccharide exposure. Crit. Care Med. 35, 246–251. doi: 10.1097/01.CCM.0000251639.05135.E0
Goyal, M., Menon, B. K., van Zwam, W. H., Dippel, D. W. J., Mitchell, P. J., Demchuk, A. M., et al. (2016). Endovascular thrombectomy after large-vessel ischaemic stroke: a meta-analysis of individual patient data from five randomised trials. Lancet 387, 1723–1731. doi: 10.1016/S0140-6736(16)00163-X
Goyal, N., Tsivgoulis, G., Chang, J. J., Malhotra, K., Pandhi, A., Ishfaq, M. F., et al. (2018). Admission neutrophil-to-lymphocyte ratio as a prognostic biomarker of outcomes in large vessel occlusion strokes. Stroke 49, 1985–1987. doi: 10.1161/STROKEAHA.118.021477
Grabert, K., Michoel, T., Karavolos, M. H., Clohisey, S., Baillie, J. K., Stevens, M. P., et al. (2016). Microglial brain region-dependent diversity and selective regional sensitivities to aging. Nat. Neurosci. 19, 504–516. doi: 10.1038/nn.4222
Granger, D. N. (1999). Ischemia-reperfusion: mechanisms of microvascular dysfunction and the influence of risk factors for cardiovascular disease. Microcirculation 6, 167–178. doi: 10.1111/j.1549-8719.1999.tb00099.x
Grassi, L., Pourfarzad, F., Ullrich, S., Merkel, A., Were, F., Carrillo-de-Santa-Pau, E., et al. (2018). Dynamics of transcription regulation in human bone marrow myeloid differentiation to mature blood neutrophils. Cell Rep. 24, 2784–2794. doi: 10.1016/j.celrep.2018.08.018
Greenberg, D. A., and Jin, K. (2013). Vascular endothelial growth factors (VEGFs) and stroke. Cell. Mol. Life Sci. 70, 1753–1761. doi: 10.1007/s00018-013-1282-8
Grieshaber-Bouyer, R., and Nigrovic, P. A. (2019). Neutrophil heterogeneity as therapeutic opportunity in immune-mediated disease. Front. Immunol. 10:346. doi: 10.3389/fimmu.2019.00346
Grønhøj, M. H., Clausen, B. H., Fenger, C. D., Lambertsen, K. L., and Finsen, B. (2017). Beneficial potential of intravenously administered IL-6 in improving outcome after murine experimental stroke. Brain Behav. Immun. 65, 296–311. doi: 10.1016/j.bbi.2017.05.019
Grunewald, M., Avraham, I., Dor, Y., Bachar-Lustig, E., Itin, A., Jung, S., et al. (2006). VEGF-induced adult neovascularization: recruitment, retention, and role of accessory cells. Cells 124, 175–189. doi: 10.1016/j.cell.2005.10.036
Gullberg, U., Andersson, E., Garwicz, D., Lindmark, A., and Olsson, I. (1997). Biosynthesis, processing and sorting of neutrophil proteins: insight into neutrophil granule development. Eur. J. Haematol. 58, 137–153. doi: 10.1111/j.1600-0609.1997.tb00940.x
Guo, Z., Yu, S., Xiao, L., Chen, X., Ye, R., Zheng, P., et al. (2016). Dynamic change of neutrophil to lymphocyte ratio and hemorrhagic transformation after thrombolysis in stroke. J. Neuroinflammation 13:199. doi: 10.1186/s12974-016-0680-x
Guo, Y., Zhou, J., Li, X., Xiao, Y., Zhang, J., Yang, Y., et al. (2021). The Association of Suppressed Hypoxia-Inducible Factor-1 transactivation of angiogenesis with defective recovery from cerebral ischemic injury in aged rats. Front. Aging Neurosci. 13:648115. doi: 10.3389/fnagi.2021.648115
Hadley, G., Beard, D. J., Couch, Y., Neuhaus, A. A., Adriaanse, B. A., DeLuca, G. C., et al. (2019). Rapamycin in ischemic stroke: old drug, new tricks? J. Cereb. Blood Flow Metab. 39, 20–35. doi: 10.1177/0271678X18807309
Hall, J. A., Chinn, R. M., Vorachek, W. R., Gorman, M. E., and Jewell, D. E. (2010). Aged beagle dogs have decreased neutrophil phagocytosis and neutrophil-related gene expression compared to younger dogs. Vet. Immunol. Immunopathol. 137, 130–135. doi: 10.1016/j.vetimm.2010.05.002
Hallenbeck, J. M. (1996). Significance of the inflammatory response in brain ischemia. Acta Neurochir. Suppl. 66, 27–31. doi: 10.1007/978-3-7091-9465-2_5
Hammond, T. R., Dufort, C., Dissing-Olesen, L., Giera, S., Young, A., Wysoker, A., et al. (2019). Single-cell RNA sequencing of microglia throughout the mouse lifespan and in the injured brain reveals complex cell-state changes. Immunity 50, 253.e6–271.e6. doi: 10.1016/j.immuni.2018.11.004
Hanhai, Z., Bin, Q., Shengjun, Z., Jingbo, L., Yinghan, G., Lingxin, C., et al. (2021). Neutrophil extracellular traps, released from neutrophil, promote microglia inflammation and contribute to poor outcome in subarachnoid hemorrhage. Aging (Albany NY) 13, 13108–13123. doi: 10.18632/aging.202993
Harman, D. (1956). Aging: a theory based on free radical and radiation chemistry. J. Gerontol. 11, 298–300. doi: 10.1093/geronj/11.3.298
Harris, A. K., Ergul, A., Kozak, A., Machado, L. S., Johnson, M. H., and Fagan, S. C. (2005). Effect of neutrophil depletion on gelatinase expression, edema formation and hemorrhagic transformation after focal ischemic stroke. BMC Neurosci. 6:49. doi: 10.1186/1471-2202-6-49
Hart, S. P., Dougherty, G. J., Haslett, C., and Dransfield, I. (1997). CD44 regulates phagocytosis of apoptotic neutrophil granulocytes, but not apoptotic lymphocytes, by human macrophages. J. Immunol. 159, 919–925.
Hartl, D., Krauss-Etschmann, S., Koller, B., Hordijk, P. L., Kuijpers, T. W., Hoffmann, F., et al. (2008). Infiltrated neutrophils acquire novel chemokine receptor expression and chemokine responsiveness in chronic inflammatory lung diseases. J. Immunol. 181, 8053–8067. doi: 10.4049/jimmunol.181.11.8053
Hayashi, T., Noshita, N., Sugawara, T., and Chan, P. H. (2003). Temporal profile of angiogenesis and expression of related genes in the brain after ischemia. J. Cereb. Blood Flow Metab. 23, 166–180. doi: 10.1097/01.WCB.0000041283.53351.CB
Hazeldine, J., Harris, P., Chapple, I. L., Grant, M., Greenwood, H., Livesey, A., et al. (2014). Impaired neutrophil extracellular trap formation: a novel defect in the innate immune system of aged individuals. Aging Cell 13, 690–698. doi: 10.1111/acel.12222
Heidt, T., Sager, H. B., Courties, G., Dutta, P., Iwamoto, Y., Zaltsman, A., et al. (2014). Chronic variable stress activates hematopoietic stem cells. Nat. Med. 20, 754–758. doi: 10.1038/nm.3589
Heil, M., Eitenmüller, I., Schmitz-Rixen, T., and Schaper, W. (2006). Arteriogenesis versus angiogenesis: similarities and differences. J. Cell. Mol. Med. 10, 45–55. doi: 10.1111/j.1582-4934.2006.tb00290.x
Heneka, M. T., Carson, M. J., El Khoury, J., Landreth, G. E., Brosseron, F., Feinstein, D. L., et al. (2015). Neuroinflammation in Alzheimer’s disease. Lancet Neurol. 14, 388–405. doi: 10.1016/S1474-4422(15)70016-5
Herz, J., Sabellek, P., Lane, T. E., Gunzer, M., Hermann, D. M., and Doeppner, T. R. (2015). Role of neutrophils in exacerbation of brain injury after focal cerebral ischemia in hyperlipidemic mice. Stroke 46, 2916–2925. doi: 10.1161/STROKEAHA.115.010620
Hill, J. K., Gunion-Rinker, L., Kulhanek, D., Lessov, N., Kim, S., Clark, W. M., et al. (1999). Temporal modulation of cytokine expression following focal cerebral ischemia in mice. Brain Res. 820, 45–54. doi: 10.1016/S0006-8993(98)01140-8
Hinojosa, E., Boyd, A. R., and Orihuela, C. J. (2009). Age-associated inflammation and toll-like receptor dysfunction prime the lungs for pneumococcal pneumonia. J. Infect. Dis. 200, 546–554. doi: 10.1086/600870
Hoefer, I. E., van Royen, N., Rectenwald, J. E., Deindl, E., Hua, J., Jost, M., et al. (2004). Arteriogenesis proceeds via ICAM-1/Mac-1-mediated mechanisms. Circ. Res. 94, 1179–1185. doi: 10.1161/01.RES.0000126922.18222.F0
Holtman, I. R., Raj, D. D., Miller, J. A., Schaafsma, W., Yin, Z., Brouwer, N., et al. (2015). Induction of a common microglia gene expression signature by aging and neurodegenerative conditions: a co-expression meta-analysis. Acta Neuropathol. Commun. 3:31. doi: 10.1186/s40478-015-0203-5
Hori, M., Nakamachi, T., Rakwal, R., Shibato, J., Nakamura, K., Wada, Y., et al. (2012). Unraveling the ischemic brain transcriptome in a permanent middle cerebral artery occlusion mouse model by DNA microarray analysis. Dis. Model. Mech. 5, 270–283. doi: 10.1242/dmm.008276
Horne, B. D., Anderson, J. L., John, J. M., Weaver, A., Bair, T. L., Jensen, K. R., et al. (2005). Which white blood cell subtypes predict increased cardiovascular risk? J. Am. Coll. Cardiol. 45, 1638–1643. doi: 10.1016/j.jacc.2005.02.054
Hossmann, K.-A. (2006). Pathophysiology and therapy of experimental stroke. Cell. Mol. Neurobiol. 26, 1055–1081. doi: 10.1007/s10571-006-9008-1
Hu, Y., Zheng, Y., Wang, T., Jiao, L., and Luo, Y. (2022). VEGF, a key factor for blood brain barrier injury after cerebral ischemic stroke. Aging Dis. 13, 647–654. doi: 10.14336/AD.2021.1121
Huang, J., Upadhyay, U. M., and Tamargo, R. J. (2006). Inflammation in stroke and focal cerebral ischemia. Surg. Neurol. 66, 232–245. doi: 10.1016/j.surneu.2005.12.028
Huynh, M.-L. N., Fadok, V. A., and Henson, P. M. (2002). Phosphatidylserine-dependent ingestion of apoptotic cells promotes TGF-beta 1 secretion and the resolution of inflammation. J. Clin. Invest. 109, 41–50. doi: 10.1172/JCI0211638
Hwang, Y.-H., Kang, D.-H., Kim, Y.-W., Kim, Y.-S., Park, S.-P., and Liebeskind, D. S. (2015). Impact of time-to-reperfusion on outcome in patients with poor collaterals. AJNR Am. J. Neuroradiol. 36, 495–500. doi: 10.3174/ajnr.A4151
Iadecola, C., and Alexander, M. (2001). Cerebral ischemia and inflammation. Curr. Opin. Neurol. 14, 89–94. doi: 10.1097/00019052-200102000-00014
Iadecola, C., and Anrather, J. (2011). The immunology of stroke: from mechanisms to translation. Nat. Med. 17, 796–808. doi: 10.1038/nm.2399
Ianov, L., Rani, A., Beas, B. S., Kumar, A., and Foster, T. C. (2016). Transcription profile of aging and cognition-related genes in the medial prefrontal cortex. Front. Aging Neurosci. 8:113. doi: 10.3389/fnagi.2016.00113
Ijichi, A., Sakuma, S., and Tofilon, P. J. (1995). Hypoxia-induced vascular endothelial growth factor expression in normal rat astrocyte cultures. Glia 14, 87–93. doi: 10.1002/glia.440140203
Ionita, M. G., van den Borne, P., Catanzariti, L. M., Moll, F. L., de Vries, J.-P. P. M., Pasterkamp, G., et al. (2010). High neutrophil numbers in human carotid atherosclerotic plaques are associated with characteristics of rupture-prone lesions. Arterioscler. Thromb. Vasc. Biol. 30, 1842–1848. doi: 10.1161/ATVBAHA.110.209296
Iwasawa, E., Ichijo, M., Ishibashi, S., and Yokota, T. (2016). Acute development of collateral circulation and therapeutic prospects in ischemic stroke. Neural Regen. Res. 11, 368–371. doi: 10.4103/1673-5374.179033
Jackman, K., and Iadecola, C. (2015). Neurovascular regulation in the ischemic brain. Antioxid. Redox Signal. 22, 149–160. doi: 10.1089/ars.2013.5669
Jaffe, R., Charron, T., Puley, G., Dick, A., and Strauss, B. H. (2008). Microvascular obstruction and the no-reflow phenomenon after percutaneous coronary intervention. Circulation 117, 3152–3156. doi: 10.1161/CIRCULATIONAHA.107.742312
Jaillon, S., Ponzetta, A., Di Mitri, D., Santoni, A., Bonecchi, R., and Mantovani, A. (2020). Neutrophil diversity and plasticity in tumour progression and therapy. Nat. Rev. Cancer 20, 485–503. doi: 10.1038/s41568-020-0281-y
Janssen, A., Colmenares, S. U., and Karpen, G. H. (2018). Heterochromatin: Guardian of the genome. Annu. Rev. Cell Dev. Biol. 34, 265–288. doi: 10.1146/annurev-cellbio-100617-062653
Jayaraj, R. L., Azimullah, S., Beiram, R., Jalal, F. Y., and Rosenberg, G. A. (2019). Neuroinflammation: friend and foe for ischemic stroke. J. Neuroinflammation 16:142. doi: 10.1186/s12974-019-1516-2
Jeyaseelan, K., Lim, K. Y., and Armugam, A. (2008). Micro RNA expression in the blood and brain of rats subjected to transient focal ischemia by middle cerebral artery occlusion. Stroke 39, 959–966. doi: 10.1161/STROKEAHA.107.500736
Jiang, R., Hu, M.-B., Zhang, H. M., Gao, Z.-Y., Jia, B., Wu, Q.-S., et al. (2014). Traffic experiment reveals the nature of car-following. PLoS One 9:e94351. doi: 10.1371/journal.pone.0094351
Jiang, N., Moyle, M., Soule, H. R., Rote, W. E., and Chopp, M. (1995). Neutrophil inhibitory factor is neuroprotective after focal ischemia in rats. Ann. Neurol. 38, 935–942. doi: 10.1002/ana.410380615
Jickling, G. C., Liu, D., Ander, B. P., Stamova, B., Zhan, X., and Sharp, F. R. (2015). Targeting neutrophils in ischemic stroke: translational insights from experimental studies. J. Cereb. Blood Flow Metab. 35, 888–901. doi: 10.1038/jcbfm.2015.45
Jiménez-Conde, J., Ois, A., Rodríguez-Campello, A., Gomis, M., and Roquer, J. (2007). Does sleep protect against ischemic stroke? Less frequent ischemic strokes but more severe ones. J. Neurol. 254, 782–788. doi: 10.1007/s00415-006-0438-y
Jin, H., Aziz, M., Ode, Y., and Wang, P. (2019). CIRP induces neutrophil reverse transendothelial migration in sepsis. Shock 51, 548–556. doi: 10.1097/SHK.0000000000001257
Jin, R., Liu, L., Zhang, S., Nanda, A., and Li, G. (2013). Role of inflammation and its mediators in acute ischemic stroke. J. Cardiovasc. Transl. Res. 6, 834–851. doi: 10.1007/s12265-013-9508-6
Jin, K., Mao, X. O., Eshoo, M. W., Nagayama, T., Minami, M., Simon, R. P., et al. (2001). Microarray analysis of hippocampal gene expression in global cerebral ischemia. Ann. Neurol. 50, 93–103. doi: 10.1002/ana.1073
Jung, S., Gilgen, M., Slotboom, J., El-Koussy, M., Zubler, C., Kiefer, C., et al. (2013). Factors that determine penumbral tissue loss in acute ischaemic stroke. Brain 136, 3554–3560. doi: 10.1093/brain/awt246
Kalogeris, T., Baines, C. P., Krenz, M., and Korthuis, R. J. (2012). Cell biology of ischemia/reperfusion injury. Int. Rev. Cell Mol. Biol. 298, 229–317. doi: 10.1016/B978-0-12-394309-5.00006-7
Kalucka, J., de Rooij, L. P. M. H., Goveia, J., Rohlenova, K., Dumas, S. J., Meta, E., et al. (2020). Single-cell transcriptome atlas of murine endothelial cells. Cells 180, 764.e20–779.e20. doi: 10.1016/j.cell.2020.01.015
Kammersgaard, L. P., Jørgensen, H. S., Reith, J., Nakayama, H., Pedersen, P. M., and Olsen, T. S. (2004). Short- and long-term prognosis for very old stroke patients. The Copenhagen stroke study. Age Ageing 33, 149–154. doi: 10.1093/ageing/afh052
Kanazawa, M., Takahashi, T., Ishikawa, M., Onodera, O., Shimohata, T., and del Zoppo, G. J. (2019). Angiogenesis in the ischemic core: a potential treatment target? J. Cereb. Blood Flow Metab. 39, 753–769. doi: 10.1177/0271678X19834158
Kang, L., Yu, H., Yang, X., Zhu, Y., Bai, X., Wang, R., et al. (2020). Neutrophil extracellular traps released by neutrophils impair revascularization and vascular remodeling after stroke. Nat. Commun. 11:2488. doi: 10.1038/s41467-020-16191-y
Katsimpardi, L., Litterman, N. K., Schein, P. A., Miller, C. M., Loffredo, F. S., Wojtkiewicz, G. R., et al. (2014). Vascular and neurogenic rejuvenation of the aging mouse brain by young systemic factors. Science 344, 630–634. doi: 10.1126/science.1251141
Kelly, P. J., Murphy, S., Coveney, S., Purroy, F., Lemmens, R., Tsivgoulis, G., et al. (2018). Anti-inflammatory approaches to ischaemic stroke prevention. J. Neurol. Neurosurg. Psychiatry 89, 211–218. doi: 10.1136/jnnp-2016-314817
Kenne, E., Erlandsson, A., Lindbom, L., Hillered, L., and Clausen, F. (2012). Neutrophil depletion reduces edema formation and tissue loss following traumatic brain injury in mice. J. Neuroinflammation 9:17. doi: 10.1186/1742-2094-9-17
Keren-Shaul, H., Spinrad, A., Weiner, A., Matcovitch-Natan, O., Dvir-Szternfeld, R., Ulland, T. K., et al. (2017). A unique microglia type associated with restricting development of Alzheimer’s disease. Cells 169, 1276.e17–1290.e17. doi: 10.1016/j.cell.2017.05.018
Khatri, P., Yeatts, S. D., Mazighi, M., Broderick, J. P., Liebeskind, D. S., Demchuk, A. M., et al. (2014). Time to angiographic reperfusion and clinical outcome after acute ischaemic stroke: an analysis of data from the interventional Management of Stroke (IMS III) phase 3 trial. Lancet Neurol. 13, 567–574. doi: 10.1016/S1474-4422(14)70066-3
Kim, J. Y., Kawabori, M., and Yenari, M. A. (2014). Innate inflammatory responses in stroke: mechanisms and potential therapeutic targets. Curr. Med. Chem. 21, 2076–2097. doi: 10.2174/0929867321666131228205146
Kim, Y. R., Kim, Y. M., Lee, J., Park, J., Lee, J. E., and Hyun, Y.-M. (2020). Neutrophils return to bloodstream through the brain blood vessel after crosstalk with microglia during LPS-induced Neuroinflammation. Front. Cell Dev. Biol. 8:613733. doi: 10.3389/fcell.2020.613733
Kim, J., Krichevsky, A., Grad, Y., Hayes, G. D., Kosik, K. S., Church, G. M., et al. (2004). Identification of many micro RNAs that copurify with polyribosomes in mammalian neurons. Proc. Natl. Acad. Sci. U. S. A. 101, 360–365. doi: 10.1073/pnas.2333854100
Kim, H., Seo, J. S., Lee, S.-Y., Ha, K.-T., Choi, B. T., Shin, Y.-I., et al. (2020). AIM2 inflammasome contributes to brain injury and chronic post-stroke cognitive impairment in mice. Brain Behav. Immun. 87, 765–776. doi: 10.1016/j.bbi.2020.03.011
Kim, J., Song, T.-J., Park, J. H., Lee, H. S., Nam, C. M., Nam, H. S., et al. (2012). Different prognostic value of white blood cell subtypes in patients with acute cerebral infarction. Atherosclerosis 222, 464–467. doi: 10.1016/j.atherosclerosis.2012.02.042
Kinnaird, T., Stabile, E., Zbinden, S., Burnett, M.-S., and Epstein, S. E. (2008). Cardiovascular risk factors impair native collateral development and may impair efficacy of therapeutic interventions. Cardiovasc. Res. 78, 257–264. doi: 10.1093/cvr/cvm116
Kirkwood, T. B. L. (2005). Understanding the odd science of aging. Cells 120, 437–447. doi: 10.1016/j.cell.2005.01.027
Kloner, R. A., King, K. S., and Harrington, M. G. (2018). No-reflow phenomenon in the heart and brain. Am. J. Physiol. Heart Circ. Physiol. 315, H550–H562. doi: 10.1152/ajpheart.00183.2018
Kolaczkowska, E., and Kubes, P. (2013). Neutrophil recruitment and function in health and inflammation. Nat. Rev. Immunol. 13, 159–175. doi: 10.1038/nri3399
Kollikowski, A. M., Cattus, F., Haag, J., Feick, J., März, A. G., Weidner, F., et al. (2022). Progression of cerebral infarction before and after thrombectomy is modified by prehospital pathways. J. Neurointerv. Surg. 14:neurintsurg-2020-017155. doi: 10.1136/neurintsurg-2020-017155
Konstantinov, I. E., Arab, S., Kharbanda, R. K., Li, J., Cheung, M. M. H., Cherepanov, V., et al. (2004). The remote ischemic preconditioning stimulus modifies inflammatory gene expression in humans. Physiol. Genomics 19, 143–150. doi: 10.1152/physiolgenomics.00046.2004
Korf, J. M., Honarpisheh, P., Mohan, E. C., Banerjee, A., Blasco-Conesa, M. P., Honarpisheh, P., et al. (2022). CD11bhigh B cells increase after stroke and regulate microglia. J. Immunol. 209, 288–300. doi: 10.4049/jimmunol.2100884
Lacy, P. (2006). Mechanisms of degranulation in neutrophils. Allergy Asthma Clin. Immunol. 2, 98–108. doi: 10.1186/1710-1492-2-3-98
Lahoz-Beneytez, J., Elemans, M., Zhang, Y., Ahmed, R., Salam, A., Block, M., et al. (2016). Human neutrophil kinetics: modeling of stable isotope labeling data supports short blood neutrophil half-lives. Blood 127, 3431–3438. doi: 10.1182/blood-2016-03-700336
Lakhan, S. E., Kirchgessner, A., and Hofer, M. (2009). Inflammatory mechanisms in ischemic stroke: therapeutic approaches. J. Transl. Med. 7:97. doi: 10.1186/1479-5876-7-97
Lambertsen, K. L., Finsen, B., and Clausen, B. H. (2019). Post-stroke inflammation—target or tool for therapy? Acta Neuropathol. 137, 693–714. doi: 10.1007/s00401-018-1930-z
Lampl, Y., Boaz, M., Gilad, R., Lorberboym, M., Dabby, R., Rapoport, A., et al. (2007). Minocycline treatment in acute stroke: an open-label, evaluator-blinded study. Neurology 69, 1404–1410. doi: 10.1212/01.wnl.0000277487.04281.db
Lanfranconi, S., Locatelli, F., Corti, S., Candelise, L., Comi, G. P., Baron, P. L., et al. (2011). Growth factors in ischemic stroke. J. Cell. Mol. Med. 15, 1645–1687. doi: 10.1111/j.1582-4934.2009.00987.x
Laridan, E., Denorme, F., Desender, L., François, O., Andersson, T., Deckmyn, H., et al. (2017). Neutrophil extracellular traps in ischemic stroke thrombi. Ann. Neurol. 82, 223–232. doi: 10.1002/ana.24993
Lawrence, T., and Gilroy, D. W. (2007). Chronic inflammation: a failure of resolution? Int. J. Exp. Pathol. 88, 85–94. doi: 10.1111/j.1365-2613.2006.00507.x
Le, Y., Murphy, P. M., and Wang, J. M. (2002). Formyl-peptide receptors revisited. Trends Immunol. 23, 541–548. doi: 10.1016/S1471-4906(02)02316-5
Lee, C., and Li, X. (2018). Platelet-derived growth factor-C and -D in the cardiovascular system and diseases. Mol. Asp. Med. 62, 12–21. doi: 10.1016/j.mam.2017.09.005
Lee, C. W., Stabile, E., Kinnaird, T., Shou, M., Devaney, J. M., Epstein, S. E., et al. (2004). Temporal patterns of gene expression after acute hindlimb ischemia in mice: insights into the genomic program for collateral vessel development. J. Am. Coll. Cardiol. 43, 474–482. doi: 10.1016/j.jacc.2003.09.033
Lee, C. K., Weindruch, R., and Prolla, T. A. (2000). Gene-expression profile of the ageing brain in mice. Nat. Genet. 25, 294–297. doi: 10.1038/77046
Lees, K. R., Bluhmki, E., von Kummer, R., Brott, T. G., Toni, D., Grotta, J. C., et al. (2010). Time to treatment with intravenous alteplase and outcome in stroke: an updated pooled analysis of ECASS, ATLANTIS, NINDS, and EPITHET trials. Lancet 375, 1695–1703. doi: 10.1016/S0140-6736(10)60491-6
Leischner, H., Flottmann, F., Hanning, U., Broocks, G., Faizy, T. D., Deb-Chatterji, M., et al. (2019). Reasons for failed endovascular recanalization attempts in stroke patients. J. Neurointerv. Surg. 11, 439–442. doi: 10.1136/neurintsurg-2018-014060
Leliefeld, P. H. C., Wessels, C. M., Leenen, L. P. H., Koenderman, L., and Pillay, J. (2016). The role of neutrophils in immune dysfunction during severe inflammation. Crit. Care 20:73. doi: 10.1186/s13054-016-1250-4
Lencel, P., and Magne, D. (2011). Inflammaging: the driving force in osteoporosis? Med. Hypotheses 76, 317–321. doi: 10.1016/j.mehy.2010.09.023
Lévesque, J.-P., Hendy, J., Takamatsu, Y., Simmons, P. J., and Bendall, L. J. (2003). Disruption of the CXCR4/CXCL12 chemotactic interaction during hematopoietic stem cell mobilization induced by GCSF or cyclophosphamide. J. Clin. Invest. 111, 187–196. doi: 10.1172/JCI15994
Li, W., Hou, M., Ding, Z., Liu, X., Shao, Y., and Li, X. (2021). Prognostic value of neutrophil-to-lymphocyte ratio in stroke: a systematic review and meta-analysis. Front. Neurol. 12:786353. doi: 10.3389/fneur.2021.786353
Li, L., Liu, F., Welser-Alves, J. V., McCullough, L. D., and Milner, R. (2012). Upregulation of Fibronectin and the α5β1 and αvβ 3 Integrins on blood vessels within the cerebral ischemic penumbra. Exp. Neurol. 233, 283–291. doi: 10.1016/j.expneurol.2011.10.017
Li, S., Overman, J. J., Katsman, D., Kozlov, S. V., Donnelly, C. J., Twiss, J. L., et al. (2010). An age-related sprouting transcriptome provides molecular control of axonal sprouting after stroke. Nat. Neurosci. 13, 1496–1504. doi: 10.1038/nn.2674
Li, P., Stetler, R. A., Leak, R. K., Shi, Y., Li, Y., Yu, W., et al. (2018). Oxidative stress and DNA damage after cerebral ischemia: potential therapeutic targets to repair the genome and improve stroke recovery. Neuropharmacology 134, 208–217. doi: 10.1016/j.neuropharm.2017.11.011
Li, Y., Wang, W., Yang, F., Xu, Y., Feng, C., and Zhao, Y. (2019). The regulatory roles of neutrophils in adaptive immunity. Cell Commun. Signal 17:147. doi: 10.1186/s12964-019-0471-y
Li, S., Zheng, J., and Carmichael, S. T. (2005). Increased oxidative protein and DNA damage but decreased stress response in the aged brain following experimental stroke. Neurobiol. Dis. 18, 432–440. doi: 10.1016/j.nbd.2004.12.014
Liao, Y., Smyth, G. K., and Shi, W. (2014). Feature counts: an efficient general purpose program for assigning sequence reads to genomic features. Bioinformatics 30, 923–930. doi: 10.1093/bioinformatics/btt656
Liberale, L., Bonetti, N. R., Puspitasari, Y. M., Vukolic, A., Akhmedov, A., Diaz-Cañestro, C., et al. (2021). TNF-α antagonism rescues the effect of ageing on stroke: perspectives for targeting inflamm-ageing. Eur. J. Clin. Investig. 51:e13600. doi: 10.1111/eci.13600
Liberale, L., Gaul, D. S., Akhmedov, A., Bonetti, N. R., Nageswaran, V., Costantino, S., et al. (2020). Endothelial SIRT6 blunts stroke size and neurological deficit by preserving blood-brain barrier integrity: a translational study. Eur. Heart J. 41, 1575–1587. doi: 10.1093/eurheartj/ehz712
Liebeskind, D. S. (2003). Collateral circulation. Stroke 34, 2279–2284. doi: 10.1161/01.STR.0000086465.41263.06
Liebeskind, D. S. (2012). Collateral perfusion: time for novel paradigms in cerebral ischemia. Int. J. Stroke 7, 309–310. doi: 10.1111/j.1747-4949.2012.00818.x
Lipinski, M. M., Zheng, B., Lu, T., Yan, Z., Py, B. F., Ng, A., et al. (2010). Genome-wide analysis reveals mechanisms modulating autophagy in normal brain aging and in Alzheimer’s disease. Proc. Natl. Acad. Sci. U. S. A. 107, 14164–14169. doi: 10.1073/pnas.1009485107
Liu, F., Akella, P., Benashski, S. E., Xu, Y., and McCullough, L. D. (2010). Expression of Na-K-cl cotransporter and edema formation are age dependent after ischemic stroke. Exp. Neurol. 224, 356–361. doi: 10.1016/j.expneurol.2010.04.010
Liu, F., Benashski, S. E., Persky, R., Xu, Y., Li, J., and McCullough, L. D. (2012). Age-related changes in AMP-activated protein kinase after stroke. Age (Dordr.) 34, 157–168. doi: 10.1007/s11357-011-9214-8
Liu, K., Ding, L., Li, Y., Yang, H., Zhao, C., Lei, Y., et al. (2014). Neuronal necrosis is regulated by a conserved chromatin-modifying cascade. Proc. Natl. Acad. Sci. U. S. A. 111, 13960–13965. doi: 10.1073/pnas.1413644111
Liu, K., Huang, H.-H., Yang, T., Jiao, Y.-M., Zhang, C., Song, J.-W., et al. (2021). Increased neutrophil aging contributes to T cell immune suppression by PD-L1 and Arginase-1 in HIV-1 treatment Naïve patients. Front. Immunol. 12:773896. doi: 10.3389/fimmu.2021.773896
Liu, F., Yuan, R., Benashski, S. E., and McCullough, L. D. (2009). Changes in experimental stroke outcome across the life span. J. Cereb. Blood Flow Metab. 29, 792–802. doi: 10.1038/jcbfm.2009.5
Llovera, G., Roth, S., Plesnila, N., Veltkamp, R., and Liesz, A. (2014). Modeling stroke in mice: permanent coagulation of the distal middle cerebral artery. J. Vis. Exp. 51729:e51729. doi: 10.3791/51729
López-Otín, C., Blasco, M. A., Partridge, L., Serrano, M., and Kroemer, G. (2013). The hallmarks of aging. Cells 153, 1194–1217. doi: 10.1016/j.cell.2013.05.039
Lorant, D. E., McEver, R. P., McIntyre, T. M., Moore, K. L., Prescott, S. M., and Zimmerman, G. A. (1995). Activation of polymorphonuclear leukocytes reduces their adhesion to P-selectin and causes redistribution of ligands for P-selectin on their surfaces. J. Clin. Invest. 96, 171–182. doi: 10.1172/JCI118018
Lu, T., Pan, Y., Kao, S.-Y., Li, C., Kohane, I., Chan, J., et al. (2004). Gene regulation and DNA damage in the ageing human brain. Nature 429, 883–891. doi: 10.1038/nature02661
Lu, R. J., Taylor, S., Contrepois, K., Kim, M., Bravo, J. I., Ellenberger, M., et al. (2021). Multi-omic profiling of primary mouse neutrophils predicts a pattern of sex and age-related functional regulation. Nat. Aging 1, 715–733. doi: 10.1038/s43587-021-00086-8
Lu, R. J., Wang, E. K., and Benayoun, B. A. (2022). Functional genomics of inflamm-aging and immunosenescence. Brief. Funct. Genomics 21, 43–55. doi: 10.1093/bfgp/elab009
Ludewig, P., Sedlacik, J., Gelderblom, M., Bernreuther, C., Korkusuz, Y., Wagener, C., et al. (2013). Carcinoembryonic antigen-related cell adhesion molecule 1 inhibits MMP-9-mediated blood-brain-barrier breakdown in a mouse model for ischemic stroke. Circ. Res. 113, 1013–1022. doi: 10.1161/CIRCRESAHA.113.301207
Ma, Y., Yabluchanskiy, A., Iyer, R. P., Cannon, P. L., Flynn, E. R., Jung, M., et al. (2016). Temporal neutrophil polarization following myocardial infarction. Cardiovasc. Res. 110, 51–61. doi: 10.1093/cvr/cvw024
MacGregor, R. R., and Shalit, M. (1990). Neutrophil function in healthy elderly subjects. J. Gerontol. 45, M55–M60. doi: 10.1093/geronj/45.2.M55
Macrez, R., Ali, C., Toutirais, O., Le Mauff, B., Defer, G., Dirnagl, U., et al. (2011). Stroke and the immune system: from pathophysiology to new therapeutic strategies. Lancet Neurol. 10, 471–480. doi: 10.1016/S1474-4422(11)70066-7
Maestrini, I., Strbian, D., Gautier, S., Haapaniemi, E., Moulin, S., Sairanen, T., et al. (2015). Higher neutrophil counts before thrombolysis for cerebral ischemia predict worse outcomes. Neurology 85, 1408–1416. doi: 10.1212/WNL.0000000000002029
Maiese, K. (2014). Cutting through the complexities of mTOR for the treatment of stroke. Curr. Neurovasc. Res. 11:177. doi: 10.2174/1567202611666140408104831
Malhotra, K., Goyal, N., Chang, J. J., Broce, M., Pandhi, A., Kerro, A., et al. (2018). Differential leukocyte counts on admission predict outcomes in patients with acute ischaemic stroke treated with intravenous thrombolysis. Eur. J. Neurol. 25, 1417–1424. doi: 10.1111/ene.13741
Manwani, B., Friedler, B., Verma, R., Venna, V. R., McCullough, L. D., and Liu, F. (2014). Perfusion of ischemic brain in Young and aged animals. Stroke 45, 571–578. doi: 10.1161/STROKEAHA.113.002944
Manwani, B., Liu, F., Scranton, V., Hammond, M. D., Sansing, L. H., and McCullough, L. D. (2013). Differential effects of aging and sex on stroke induced inflammation across the lifespan. Exp. Neurol. 249, 120–131. doi: 10.1016/j.expneurol.2013.08.011
Marini, C., Baldassarre, M., Russo, T., De Santis, F., Sacco, S., Ciancarelli, I., et al. (2004). Burden of first-ever ischemic stroke in the oldest old: evidence from a population-based study. Neurology 62, 77–81. doi: 10.1212/01.WNL.0000101461.61501.65
Marki, A., and Ley, K. (2020). Leaking chemokines confuse neutrophils. J. Clin. Invest. 130, 2177–2179. doi: 10.1172/JCI136259
Martin, A., Aguirre, J., Sarasa-Renedo, A., Tsoukatou, D., Garofalakis, A., Meyer, H., et al. (2008). Imaging changes in lymphoid organs in vivo after brain ischemia with three-dimensional fluorescence molecular tomography in transgenic mice expressing green fluorescent protein in T lymphocytes. Mol. Imaging 7, 157–167.
Martin, C., Burdon, P. C. E., Bridger, G., Gutierrez-Ramos, J. C., Williams, T. J., and Rankin, S. M. (2003). Chemokines acting via CXCR2 and CXCR4 control the release of neutrophils from the bone marrow and their return following senescence. Immunity 19, 583–593. doi: 10.1016/S1074-7613(03)00263-2
Massberg, S., Grahl, L., von Bruehl, M.-L., Manukyan, D., Pfeiler, S., Goosmann, C., et al. (2010). Reciprocal coupling of coagulation and innate immunity via neutrophil serine proteases. Nat. Med. 16, 887–896. doi: 10.1038/nm.2184
Massena, S., Christoffersson, G., Vågesjö, E., Seignez, C., Gustafsson, K., Binet, F., et al. (2015). Identification and characterization of VEGF-A–responsive neutrophils expressing CD49d, VEGFR1, and CXCR4 in mice and humans. Blood 126, 2016–2026. doi: 10.1182/blood-2015-03-631572
Masucci, M. T., Minopoli, M., Del Vecchio, S., and Carriero, M. V. (2020). The emerging role of neutrophil extracellular traps (NETs) in tumor progression and metastasis. Front. Immunol. 11:1749. doi: 10.3389/fimmu.2020.01749
Mathias, J. R., Perrin, B. J., Liu, T.-X., Kanki, J., Look, A. T., and Huttenlocher, A. (2006). Resolution of inflammation by retrograde chemotaxis of neutrophils in transgenic zebrafish. J. Leukoc. Biol. 80, 1281–1288. doi: 10.1189/jlb.0506346
Mathys, H., Adaikkan, C., Gao, F., Young, J. Z., Manet, E., Hemberg, M., et al. (2017). Temporal tracking of microglia activation in Neurodegeneration at single-cell resolution. Cell Rep. 21, 366–380. doi: 10.1016/j.celrep.2017.09.039
Matsunaga, T., Warltier, D. C., Weihrauch, D. W., Moniz, M., Tessmer, J., and Chilian, W. M. (2000). Ischemia-induced coronary collateral growth is dependent on vascular endothelial growth factor and nitric oxide. Circulation 102, 3098–3103. doi: 10.1161/01.CIR.102.25.3098
Mayadas, T. N., Cullere, X., and Lowell, C. A. (2014). The multifaceted functions of neutrophils. Annu. Rev. Pathol. Mech. Dis. 9, 181–218. doi: 10.1146/annurev-pathol-020712-164023
McBride, D. W., and Zhang, J. H. (2017). Precision stroke animal models: the permanent MCAO model should be the primary model, not transient MCAO. Transl. Stroke Res. 8, 397–404. doi: 10.1007/s12975-017-0554-2
McLaughlin, M. E., Kao, R., Liener, I. E., and Hoidal, J. R. (1986). A quantitative in vitro assay of polymorphonuclear leukocyte migration through human amnion membrane utilizing 111in-oxine. J. Immunol. Methods 95, 89–98. doi: 10.1016/0022-1759(86)90321-2
Meisner, J. K., and Price, R. J. (2010). Spatial and temporal coordination of bone marrow-derived cell activity during arteriogenesis: regulation of the endogenous response and therapeutic implications. Microcirculation 17, 583–599. doi: 10.1111/j.1549-8719.2010.00051.x
Milot, E., and Filep, J. G. (2011). Regulation of neutrophil survival/apoptosis by Mcl-1. Sci. World J. 11, 1948–1962. doi: 10.1100/2011/131539
Mitsios, N., Saka, M., Krupinski, J., Pennucci, R., Sanfeliu, C., Wang, Q., et al. (2007). A microarray study of gene and protein regulation in human and rat brain following middle cerebral artery occlusion. BMC Neurosci. 8:93. doi: 10.1186/1471-2202-8-93
Mohammad, Y. M., Christoforidis, G. A., Bourekas, E. C., and Slivka, A. P. (2008). Qureshi grading scheme predicts subsequent volume of brain infarction following intra-arterial thrombolysis in patients with acute anterior circulation ischemic stroke. J. Neuroimaging 18, 262–267. doi: 10.1111/j.1552-6569.2007.00233.x
Mohan, A., Mather, K. A., Thalamuthu, A., Baune, B. T., and Sachdev, P. S. (2016). Gene expression in the aging human brain: an overview. Curr. Opin. Psychiatry 29, 159–167. doi: 10.1097/YCO.0000000000000238
Montaner, J., Rovira, A., Molina, C. A., Arenillas, J. F., Ribó, M., Chacón, P., et al. (2003). Plasmatic level of neuroinflammatory markers predict the extent of diffusion-weighted image lesions in hyperacute stroke. J. Cereb. Blood Flow Metab. 23, 1403–1407. doi: 10.1097/01.WCB.0000100044.07481.97
Moore, D. F., Li, H., Jeffries, N., Wright, V., Cooper, R. A., Elkahloun, A., et al. (2005). Using peripheral blood mononuclear cells to determine a gene expression profile of acute ischemic stroke: a pilot investigation. Circulation 111, 212–221. doi: 10.1161/01.CIR.0000152105.79665.C6
Morioka, T., Kalehua, A. N., and Streit, W. J. (1993). Characterization of microglial reaction after middle cerebral artery occlusion in rat brain. J. Comp. Neurol. 327, 123–132. doi: 10.1002/cne.903270110
Muir, K. W., Tyrrell, P., Sattar, N., and Warburton, E. (2007). Inflammation and ischaemic stroke. Curr. Opin. Neurol. 20, 334–342. doi: 10.1097/WCO.0b013e32813ba151
Nagayama, T., Lan, J., Henshall, D. C., Chen, D., O’Horo, C., Simon, R. P., et al. (2000). Induction of oxidative DNA damage in the peri-infarct region after permanent focal cerebral ischemia. J. Neurochem. 75, 1716–1728. doi: 10.1046/j.1471-4159.2000.0751716.x
Nah, E. H., Kim, S., Cho, S., and Cho, H. I. (2018). Complete blood count reference intervals and patterns of changes across pediatric, adult, and geriatric ages in Korea. Ann. Lab. Med. 38, 503–511. doi: 10.3343/alm.2018.38.6.503
Nakahira, K., Hisata, S., and Choi, A. M. K. (2015). The roles of mitochondrial damage-associated molecular patterns in diseases. Antioxid. Redox Signal. 23, 1329–1350. doi: 10.1089/ars.2015.6407
Nannoni, S., Cereda, C. W., Sirimarco, G., Lambrou, D., Strambo, D., Eskandari, A., et al. (2019). Collaterals are a major determinant of the core but not the penumbra volume in acute ischemic stroke. Neuroradiology 61, 971–978. doi: 10.1007/s00234-019-02224-x
Navarro-Orozco, D., and Sánchez-Manso, J. C. (2021). “Neuroanatomy, middle cerebral artery,” in Stat Pearls (Treasure Island, FL: Stat Pearls Publishing). Available at: http://www.ncbi.nlm.nih.gov/books/NBK526002/ (Accessed November 10, 2021).
Neubert, E., Meyer, D., Rocca, F., Günay, G., Kwaczala-Tessmann, A., Grandke, J., et al. (2018). Chromatin swelling drives neutrophil extracellular trap release. Nat. Commun. 9:3767. doi: 10.1038/s41467-018-06263-5
Neumann, J., Riek-Burchardt, M., Herz, J., Doeppner, T. R., König, R., Hütten, H., et al. (2015). Very-late-antigen-4 (VLA-4)-mediated brain invasion by neutrophils leads to interactions with microglia, increased ischemic injury and impaired behavior in experimental stroke. Acta Neuropathol. 129, 259–277. doi: 10.1007/s00401-014-1355-2
Ng, Y. S., Stein, J., Ning, M., and Black-Schaffer, R. M. (2007). Comparison of clinical characteristics and functional outcomes of ischemic stroke in different vascular territories. Stroke 38, 2309–2314. doi: 10.1161/STROKEAHA.106.475483
Nikas, J. B. (2013). Inflammation and immune system activation in aging: a mathematical approach. Sci. Rep. 3:3254. doi: 10.1038/srep03254
Niwa, Y., Kasama, T., Miyachi, Y., and Kanoh, T. (1989). Neutrophil chemotaxis, phagocytosis and parameters of reactive oxygen species in human aging: cross-sectional and longitudinal studies. Life Sci. 44, 1655–1664. doi: 10.1016/0024-3205(89)90482-7
Nogueira, R. G., Jadhav, A. P., Haussen, D. C., Bonafe, A., Budzik, R. F., Bhuva, P., et al. (2018). Thrombectomy 6 to 24 hours after stroke with a mismatch between deficit and infarct. N. Engl. J. Med. 378, 11–21. doi: 10.1056/NEJMoa1706442
Nomellini, V., Brubaker, A. L., Mahbub, S., Palmer, J. L., Gomez, C. R., and Kovacs, E. J. (2012). Dysregulation of neutrophil CXCR2 and pulmonary endothelial icam-1 promotes age-related pulmonary inflammation. Aging Dis. 3, 234–247.
Nourshargh, S., and Marelli-Berg, F. M. (2005). Transmigration through venular walls: a key regulator of leukocyte phenotype and function. Trends Immunol. 26, 157–165. doi: 10.1016/j.it.2005.01.006
Nozawa, H., Chiu, C., and Hanahan, D. (2006). Infiltrating neutrophils mediate the initial angiogenic switch in a mouse model of multistage carcinogenesis. Proc. Natl. Acad. Sci. U. S. A. 103, 12493–12498. doi: 10.1073/pnas.0601807103
Okyere, B., Mills, W. A., Wang, X., Chen, M., Chen, J., Hazy, A., et al. (2020). Eph A4/tie 2 crosstalk regulates leptomeningeal collateral remodeling following ischemic stroke. J. Clin. Invest. 130, 1024–1035. doi: 10.1172/JCI131493
Omari, K. M., John, G. R., Sealfon, S. C., and Raine, C. S. (2005). CXC chemokine receptors on human oligodendrocytes: implications for multiple sclerosis. Brain 128, 1003–1015. doi: 10.1093/brain/awh479
Otxoa-de-Amezaga, A., Gallizioli, M., Pedragosa, J., Justicia, C., Miró-Mur, F., Salas-Perdomo, A., et al. (2019). Location of neutrophils in different compartments of the damaged mouse brain after severe ischemia/reperfusion. Stroke 50, 1548–1557. doi: 10.1161/STROKEAHA.118.023837
Owen-Woods, C., Joulia, R., Barkaway, A., Rolas, L., Ma, B., Nottebaum, A. F., et al. (2020). Local microvascular leakage promotes trafficking of activated neutrophils to remote organs. J. Clin. Invest. 130, 2301–2318. doi: 10.1172/JCI133661
Pan, H., Lin, C., Chen, L., Qiao, Y., Huang, P., Liu, B., et al. (2021). Multiple-factor analyses of futile recanalization in acute ischemic stroke patients treated with mechanical Thrombectomy. Front. Neurol. 12:704088. doi: 10.3389/fneur.2021.704088
Panni, P., Gory, B., Xie, Y., Consoli, A., Desilles, J.-P., Mazighi, M., et al. (2019). Acute stroke with large ischemic core treated by Thrombectomy. Stroke 50, 1164–1171. doi: 10.1161/STROKEAHA.118.024295
Papayannopoulos, V. (2018). Neutrophil extracellular traps in immunity and disease. Nat. Rev. Immunol. 18, 134–147. doi: 10.1038/nri.2017.105
Park, S. Y., Shrestha, S., Youn, Y.-J., Kim, J.-K., Kim, S.-Y., Kim, H. J., et al. (2017). Autophagy primes neutrophils for neutrophil extracellular trap formation during sepsis. Am. J. Respir. Crit. Care Med. 196, 577–589. doi: 10.1164/rccm.201603-0596OC
Patel, N., Rao, V., Heilman-Espinoza, E., Lai, R., Quesada, R., and Flint, A. (2012). Simple and reliable determination of the modified Rankin scale score in neurosurgical and neurological patients: the mRS-9Q. Neurosurgery 71, 971–975. doi: 10.1227/NEU.0b013e31826a8a56
Pawelec, G., Goldeck, D., and Derhovanessian, E. (2014). Inflammation, ageing and chronic disease. Curr. Opin. Immunol. 29, 23–28. doi: 10.1016/j.coi.2014.03.007
Perez-de-Puig, I., Miró-Mur, F., Ferrer-Ferrer, M., Gelpi, E., Pedragosa, J., Justicia, C., et al. (2015). Neutrophil recruitment to the brain in mouse and human ischemic stroke. Acta Neuropathol. 129, 239–257. doi: 10.1007/s00401-014-1381-0
Perry, M. A., and Granger, D. N. (1991). Role of CD11/CD18 in shear rate-dependent leukocyte-endothelial cell interactions in cat mesenteric venules. J. Clin. Invest. 87, 1798–1804. doi: 10.1172/JCI115200
Perry, V. H., and Holmes, C. (2014). Microglial priming in neurodegenerative disease. Nat. Rev. Neurol. 10, 217–224. doi: 10.1038/nrneurol.2014.38
Petrovic-Djergovic, D., Goonewardena, S. N., and Pinsky, D. J. (2016). Inflammatory disequilibrium in stroke. Circ. Res. 119, 142–158. doi: 10.1161/CIRCRESAHA.116.308022
Pham, M., and Bendszus, M. (2016). Facing time in ischemic stroke: an alternative hypothesis for collateral failure. Clin. Neuroradiol. 26, 141–151. doi: 10.1007/s00062-016-0507-2
Phan, K., Dmytriw, A. A., Maingard, J., Asadi, H., Griessenauer, C. J., Ng, W., et al. (2017). Endovascular Thrombectomy alone versus combined with intravenous thrombolysis. World Neurosurg. 108, 850.e2–858.e2. doi: 10.1016/j.wneu.2017.08.040
Phillipson, M., and Kubes, P. (2019). The healing power of neutrophils. Trends Immunol. 40, 635–647. doi: 10.1016/j.it.2019.05.001
Piccard, H., Muschel, R. J., and Opdenakker, G. (2012). On the dual roles and polarized phenotypes of neutrophils in tumor development and progression. Crit. Rev. Oncol. Hematol. 82, 296–309. doi: 10.1016/j.critrevonc.2011.06.004
Pillay, J., den Braber, I., Vrisekoop, N., Kwast, L. M., de Boer, R. J., Borghans, J. A. M., et al. (2010). In vivo labeling with 2H2O reveals a human neutrophil lifespan of 5.4 days. Blood 116, 625–627. doi: 10.1182/blood-2010-01-259028
Pinti, M., Cevenini, E., Nasi, M., De Biasi, S., Salvioli, S., Monti, D., et al. (2014). Circulating mitochondrial DNA increases with age and is a familiar trait: implications for “inflamm-aging.”. Eur. J. Immunol. 44, 1552–1562. doi: 10.1002/eji.201343921
Pishel, I., Shytikov, D., Orlova, T., Peregudov, A., Artyuhov, I., and Butenko, G. (2012). Accelerated aging versus rejuvenation of the immune system in heterochronic parabiosis. Rejuvenation Res. 15, 239–248. doi: 10.1089/rej.2012.1331
Planas, A. M. (2018). Role of immune cells migrating to the ischemic brain. Stroke 49, 2261–2267. doi: 10.1161/STROKEAHA.118.021474
Pollenus, E., Malengier-Devlies, B., Vandermosten, L., Pham, T.-T., Mitera, T., Possemiers, H., et al. (2019). Limitations of neutrophil depletion by anti-Ly6G antibodies in two heterogenic immunological models. Immunol. Lett. 212, 30–36. doi: 10.1016/j.imlet.2019.06.006
Prabhakaran, S., Ruff, I., and Bernstein, R. A. (2015). Acute stroke intervention: a systematic review. JAMA 313, 1451–1462. doi: 10.1001/jama.2015.3058
Prass, K., Meisel, C., Höflich, C., Braun, J., Halle, E., Wolf, T., et al. (2003). Stroke-induced immunodeficiency promotes spontaneous bacterial infections and is mediated by sympathetic activation reversal by poststroke T helper cell type 1-like immunostimulation. J. Exp. Med. 198, 725–736. doi: 10.1084/jem.20021098
Prestigiacomo, C. J., Kim, S. C., Connolly, E. S., Liao, H., Yan, S. F., and Pinsky, D. J. (1999). CD18-mediated neutrophil recruitment contributes to the pathogenesis of reperfused but not nonreperfused stroke. Stroke 30, 1110–1117. doi: 10.1161/01.STR.30.5.1110
Price, C. J. S., Menon, D. K., Peters, A. M., Ballinger, J. R., Barber, R. W., Balan, K. K., et al. (2004). Cerebral neutrophil recruitment, histology, and outcome in acute ischemic stroke: an imaging-based study. Stroke 35, 1659–1664. doi: 10.1161/01.STR.0000130592.71028.92
Qian, F., Guo, X., Wang, X., Yuan, X., Chen, S., Malawista, S. E., et al. (2014). Reduced bioenergetics and toll-like receptor 1 function in human polymorphonuclear leukocytes in aging. Aging (Albany NY) 6, 131–139. doi: 10.18632/aging.100642
Raghavendra Rao, V. L., Bowen, K. K., Dhodda, V. K., Song, G., Franklin, J. L., Gavva, N. R., et al. (2002). Gene expression analysis of spontaneously hypertensive rat cerebral cortex following transient focal cerebral ischemia. J. Neurochem. 83, 1072–1086. doi: 10.1046/j.1471-4159.2002.01208.x
Ramiro, L., Simats, A., García-Berrocoso, T., and Montaner, J. (2018). Inflammatory molecules might become both biomarkers and therapeutic targets for stroke management. Ther. Adv. Neurol. Disord. 11:1756286418789340. doi: 10.1177/1756286418789340
Rao, X., Zhong, J., and Sun, Q. (2014). The heterogenic properties of monocytes/macrophages and neutrophils in inflammatory response in diabetes. Life Sci. 116, 59–66. doi: 10.1016/j.lfs.2014.09.015
Reglero-Real, N., Colom, B., Bodkin, J. V., and Nourshargh, S. (2016). Endothelial cell Junctional adhesion molecules: role and regulation of expression in inflammation. Arterioscler. Thromb. Vasc. Biol. 36, 2048–2057. doi: 10.1161/ATVBAHA.116.307610
Reichardt, L. F. (2006). Neurotrophin-regulated signalling pathways. Philos. Trans. R. Soc. Lond. Ser. B Biol. Sci. 361, 1545–1564. doi: 10.1098/rstb.2006.1894
Resnick, N., Yahav, H., Shay-Salit, A., Shushy, M., Schubert, S., Zilberman, L. C. M., et al. (2003). Fluid shear stress and the vascular endothelium: for better and for worse. Prog. Biophys. Mol. Biol. 81, 177–199. doi: 10.1016/S0079-6107(02)00052-4
Ribo, M., Flores, A., Rubiera, M., Pagola, J., Sargento-Freitas, J., Rodriguez-Luna, D., et al. (2011). Extending the time window for endovascular procedures according to collateral pial circulation. Stroke 42, 3465–3469. doi: 10.1161/STROKEAHA.111.623827
Riffelmacher, T., Clarke, A., Richter, F. C., Stranks, A., Pandey, S., Danielli, S., et al. (2017). Autophagy-dependent generation of free fatty acids is critical for Normal neutrophil differentiation. Immunity 47, 466.e5–480.e5. doi: 10.1016/j.immuni.2017.08.005
Ringelstein, E. B., Biniek, R., Weiller, C., Ammeling, B., Nolte, P. N., and Thron, A. (1992). Type and extent of hemispheric brain infarctions and clinical outcome in early and delayed middle cerebral artery recanalization. Neurology 42, 289–298. doi: 10.1212/WNL.42.2.289
Ritzel, R. M., Crapser, J., Patel, A. R., Verma, R., Grenier, J. M., Chauhan, A., et al. (2016). Age-associated resident memory CD8 T cells in the central nervous system are primed to potentiate inflammation after ischemic brain injury. J. Immunol. 196, 3318–3330. doi: 10.4049/jimmunol.1502021
Ritzel, R. M., Lai, Y.-J., Crapser, J. D., Patel, A. R., Schrecengost, A., Grenier, J. M., et al. (2018). Aging alters the immunological response to ischemic stroke. Acta Neuropathol. 136, 89–110. doi: 10.1007/s00401-018-1859-2
Roberts, H. C., Dillon, W. P., Furlan, A. J., Wechsler, L. R., Rowley, H. A., Fischbein, N. J., et al. (2002). Computed tomographic findings in patients undergoing intra-arterial thrombolysis for acute ischemic stroke due to middle cerebral artery occlusion: results from the PROACT II trial. Stroke 33, 1557–1565. doi: 10.1161/01.STR.0000018011.66817.41
Rolfes, L., Riek-Burchardt, M., Pawlitzki, M., Minnerup, J., Bock, S., Schmidt, M., et al. (2021). Neutrophil granulocytes promote flow stagnation due to dynamic capillary stalls following experimental stroke. Brain Behav. Immun. 93, 322–330. doi: 10.1016/j.bbi.2021.01.011
Rosell, A., Cuadrado, E., Ortega-Aznar, A., Hernández-Guillamon, M., Lo, E. H., and Montaner, J. (2008). MMP-9-positive neutrophil infiltration is associated to blood-brain barrier breakdown and basal lamina type IV collagen degradation during hemorrhagic transformation after human ischemic stroke. Stroke 39, 1121–1126. doi: 10.1161/STROKEAHA.107.500868
Rosen, H. J., Allison, S. C., Schauer, G. F., Gorno-Tempini, M. L., Weiner, M. W., and Miller, B. L. (2005). Neuroanatomical correlates of behavioural disorders in dementia. Brain 128, 2612–2625. doi: 10.1093/brain/awh628
Rothwell, N. (2003). Interleukin-1 and neuronal injury: mechanisms, modification, and therapeutic potential. Brain Behav. Immun. 17, 152–157. doi: 10.1016/S0889-1591(02)00098-3
Rothwell, P. M. (2018). Clinical innovation in stroke: getting the simple things right. Lancet Neurol. 17, 491–493. doi: 10.1016/S1474-4422(18)30113-3
Rowe, W. B., Blalock, E. M., Chen, K.-C., Kadish, I., Wang, D., Barrett, J. E., et al. (2007). Hippocampal expression analyses reveal selective association of immediate-early, neuroenergetic, and myelinogenic pathways with cognitive impairment in aged rats. J. Neurosci. 27, 3098–3110. doi: 10.1523/JNEUROSCI.4163-06.2007
Roy-O’Reilly, M. A., Ahnstedt, H., Spychala, M. S., Munshi, Y., Aronowski, J., Sansing, L. H., et al. (2020). Aging exacerbates neutrophil pathogenicity in ischemic stroke. Aging (Albany NY) 12, 436–461. doi: 10.18632/aging.102632
Roy-O’Reilly, M., and McCullough, L. D. (2018). Age and sex are critical factors in ischemic stroke pathology. Endocrinology 159, 3120–3131. doi: 10.1210/en.2018-00465
Rozhok, A., and DeGregori, J. (2019). Somatic maintenance impacts the evolution of mutation rate. BMC Evol. Biol. 19:172. doi: 10.1186/s12862-019-1496-y
Ruckh, J. M., Zhao, J.-W., Shadrach, J. L., van Wijngaarden, P., Rao, T. N., Wagers, A. J., et al. (2012). Rejuvenation of regeneration in the aging central nervous system. Cell Stem Cell 10, 96–103. doi: 10.1016/j.stem.2011.11.019
Rusanen, H., Saarinen, J. T., and Sillanpää, N. (2015). Collateral circulation predicts the size of the infarct Core and the proportion of salvageable penumbra in Hyperacute ischemic stroke patients treated with intravenous thrombolysis. Cerebrovasc. Dis. 40, 182–190. doi: 10.1159/000439064
Rydell-Törmänen, K., Uller, L., and Erjefält, J. S. (2006). Direct evidence of secondary necrosis of neutrophils during intense lung inflammation. Eur. Respir. J. 28, 268–274. doi: 10.1183/09031936.06.00126905
Saka, O., McGuire, A., and Wolfe, C. (2009). Cost of stroke in the United Kingdom. Age Ageing 38, 27–32. doi: 10.1093/ageing/afn281
Salas-Perdomo, A., Miró-Mur, F., Urra, X., Justicia, C., Gallizioli, M., Zhao, Y., et al. (2018). T cells prevent hemorrhagic transformation in ischemic stroke by P-Selectin binding. Arterioscler. Thromb. Vasc. Biol. 38, 1761–1771. doi: 10.1161/ATVBAHA.118.311284
Sapey, E., Greenwood, H., Walton, G., Mann, E., Love, A., Aaronson, N., et al. (2014). Phosphoinositide 3-kinase inhibition restores neutrophil accuracy in the elderly: toward targeted treatments for immunosenescence. Blood 123, 239–248. doi: 10.1182/blood-2013-08-519520
Saver, J. L., Chaisinanunkul, N., Campbell, B. C. V., Grotta, J. C., Hill, M. D., Khatri, P., et al. (2021). Standardized nomenclature for modified Rankin scale global disability outcomes: consensus recommendations from stroke therapy academic industry roundtable XI. Stroke 52, 3054–3062. doi: 10.1161/STROKEAHA.121.034480
Scapini, P., Marini, O., Tecchio, C., and Cassatella, M. A. (2016). Human neutrophils in the saga of cellular heterogeneity: insights and open questions. Immunol. Rev. 273, 48–60. doi: 10.1111/imr.12448
Scheiermann, C., Kunisaki, Y., and Frenette, P. S. (2013). Circadian control of the immune system. Nat. Rev. Immunol. 13, 190–198. doi: 10.1038/nri3386
Schmidt-Kastner, R., Zhang, B., Belayev, L., Khoutorova, L., Amin, R., Busto, R., et al. (2002). DNA microarray analysis of cortical gene expression during early recirculation after focal brain ischemia in rat. Brain Res. Mol. Brain Res. 108, 81–93. doi: 10.1016/S0169-328X(02)00516-8
Schneider, D., Berrouschot, J., Brandt, T., Hacke, W., Ferbert, A., Norris, S. H., et al. (1998). Safety, pharmacokinetics and biological activity of enlimomab (anti-ICAM-1 antibody): an open-label, dose escalation study in patients hospitalized for acute stroke. Eur. Neurol. 40, 78–83. doi: 10.1159/000007962
Scholz, D., Ito, W., Fleming, I., Deindl, E., Sauer, A., Wiesnet, M., et al. (2000). Ultrastructure and molecular histology of rabbit hind-limb collateral artery growth (arteriogenesis). Virchows Arch. 436, 257–270. doi: 10.1007/s004280050039
Schulte-Herbrüggen, O., Klehmet, J., Quarcoo, D., Meisel, C., and Meisel, A. (2006). Mouse strains differ in their susceptibility to poststroke infections. Neuroimmunomodulation 13, 13–18. doi: 10.1159/000092109
Schwartz, M., and Baruch, K. (2014). The resolution of neuroinflammation in neurodegeneration: leukocyte recruitment via the choroid plexus. EMBO J. 33, 7–22. doi: 10.1002/embj.201386609
Semenza, G. L. (1999). Regulation of mammalian O2 homeostasis by hypoxia-inducible factor 1. Annu. Rev. Cell Dev. Biol. 15, 551–578. doi: 10.1146/annurev.cellbio.15.1.551
Semerano, A., Laredo, C., Zhao, Y., Rudilosso, S., Renú, A., Llull, L., et al. (2019). Leukocytes, collateral circulation, and reperfusion in ischemic stroke patients treated with mechanical Thrombectomy. Stroke 50, 3456–3464. doi: 10.1161/STROKEAHA.119.026743
Sendama, W. (2020). The effect of ageing on the resolution of inflammation. Ageing Res. Rev. 57:101000. doi: 10.1016/j.arr.2019.101000
Shantsila, E., and Lip, G. Y. H. (2015). Stroke in atrial fibrillation and improving the identification of “high-risk” patients: the crossroads of immunity and thrombosis. J. Thromb. Haemost. 13, 1968–1970. doi: 10.1111/jth.13121
Sharma, D., Spring, K. J., and Bhaskar, S. M. M. (2021). Neutrophil-lymphocyte ratio in acute ischemic stroke: immunopathology, management, and prognosis. Acta Neurol. Scand. 144, 486–499. doi: 10.1111/ane.13493
Sharma, D., Spring, K. J., and Bhaskar, S. M. M. (2022). Role of neutrophil-lymphocyte ratio in the prognosis of acute Ischaemic stroke after reperfusion therapy: a systematic review and Meta-analysis. J. Cent. Nerv. Syst. Dis. 14:11795735221092518. doi: 10.1177/11795735221092518
Shaw, A. C., Goldstein, D. R., and Montgomery, R. R. (2013). Age-dependent dysregulation of innate immunity. Nat. Rev. Immunol. 13, 875–887. doi: 10.1038/nri3547
Shekhar, S., Cunningham, M. W., Pabbidi, M. R., Wang, S., Booz, G. W., and Fan, F. (2018). Targeting vascular inflammation in ischemic stroke: recent developments on novel immunomodulatory approaches. Eur. J. Pharmacol. 833, 531–544. doi: 10.1016/j.ejphar.2018.06.028
Shen, F., Jiang, L., Han, F., Degos, V., Chen, S., and Su, H. (2019). Increased inflammatory response in old mice is associated with more severe neuronal injury at the acute stage of ischemic stroke. Aging Dis. 10, 12–22. doi: 10.14336/AD.2018.0205
Sheth, S. A., Sanossian, N., Hao, Q., Starkman, S., Ali, L. K., Kim, D., et al. (2016). Collateral flow as causative of good outcomes in endovascular stroke therapy. J. Neurointerv. Surg. 8, 2–7. doi: 10.1136/neurintsurg-2014-011438
Shin, J. A., Kang, J. L., Lee, K.-E., and Park, E.-M. (2012). Different temporal patterns in the expressions of bone morphogenetic proteins and noggin during astroglial scar formation after ischemic stroke. Cell. Mol. Neurobiol. 32, 587–597. doi: 10.1007/s10571-012-9806-6
Shirasuna, K., Shimizu, T., Matsui, M., and Miyamoto, A. (2013). Emerging roles of immune cells in luteal angiogenesis. Reprod. Fertil. Dev. 25, 351–361. doi: 10.1071/RD12096
Shuaib, A., Butcher, K., Mohammad, A. A., Saqqur, M., and Liebeskind, D. S. (2011). Collateral blood vessels in acute ischaemic stroke: a potential therapeutic target. Lancet Neurol. 10, 909–921. doi: 10.1016/S1474-4422(11)70195-8
Sieber, M. W., Claus, R. A., Witte, O. W., and Frahm, C. (2011). Attenuated inflammatory response in aged mice brains following stroke. PLoS One 6:e26288. doi: 10.1371/journal.pone.0026288
Sieber, M. W., Guenther, M., Jaenisch, N., Albrecht-Eckardt, D., Kohl, M., Witte, O. W., et al. (2014). Age-specific transcriptional response to stroke. Neurobiol. Aging 35, 1744–1754. doi: 10.1016/j.neurobiolaging.2014.01.012
Simmons, S. R., Bhalla, M., Herring, S. E., Tchalla, E. Y. I., and Bou Ghanem, E. N. (2021). Older but not wiser: the age-driven changes in neutrophil responses during pulmonary infections. Infect. Immun. 89, e00653–e00620. doi: 10.1128/IAI.00653-20
Simon, H.-U. (2003). Neutrophil apoptosis pathways and their modifications in inflammation. Immunol. Rev. 193, 101–110. doi: 10.1034/j.1600-065X.2003.00038.x
Simonsen, C. Z., Schmitz, M. L., Madsen, M. H., Mikkelsen, I. K., Chandra, R. V., Leslie-Mazwi, T., et al. (2016). Early neurological deterioration after thrombolysis: clinical and imaging predictors. Int. J. Stroke 11, 776–782. doi: 10.1177/1747493016650454
Sinha, M., Jang, Y. C., Oh, J., Khong, D., Wu, E. Y., Manohar, R., et al. (2014). Restoring systemic GDF11 levels reverses age-related dysfunction in mouse skeletal muscle. Science 344, 649–652. doi: 10.1126/science.1251152
Sionov, R. V. (2021). Leveling up the controversial role of neutrophils in cancer: when the complexity becomes entangled. Cells 10:2486. doi: 10.3390/cells10092486
Smith, L. K., He, Y., Park, J.-S., Bieri, G., Snethlage, C. E., Lin, K., et al. (2015). β2-microglobulin is a systemic pro-aging factor that impairs cognitive function and neurogenesis. Nat. Med. 21, 932–937. doi: 10.1038/nm.3898
Soares, B. P., Chien, J. D., and Wintermark, M. (2009). MR and CT monitoring of recanalization, reperfusion, and penumbra salvage: everything that recanalizes does not necessarily reperfuse! Stroke 40, S24–S27. doi: 10.1161/STROKEAHA.108.526814
Sohal, R. S., and Orr, W. C. (2012). The redox stress hypothesis of aging. Free Radic. Biol. Med. 52, 539–555. doi: 10.1016/j.freeradbiomed.2011.10.445
Sohrabji, F., Park, M. J., and Mahnke, A. H. (2017). Sex differences in stroke therapies. J. Neurosci. Res. 95, 681–691. doi: 10.1002/jnr.23855
Solana, R., Pawelec, G., and Tarazona, R. (2006). Aging and innate immunity. Immunity 24, 491–494. doi: 10.1016/j.immuni.2006.05.003
Sollberger, G., Tilley, D. O., and Zychlinsky, A. (2018). Neutrophil extracellular traps: the biology of chromatin externalization. Dev. Cell 44, 542–553. doi: 10.1016/j.devcel.2018.01.019
Sørensen, O. E., and Borregaard, N. (2016). Neutrophil extracellular traps – the dark side of neutrophils. J. Clin. Invest. 126, 1612–1620. doi: 10.1172/JCI84538
Spescha, R. D., Klohs, J., Semerano, A., Giacalone, G., Derungs, R. S., Reiner, M. F., et al. (2015). Post-ischaemic silencing of p66Shc reduces ischaemia/reperfusion brain injury and its expression correlates to clinical outcome in stroke. Eur. Heart J. 36, 1590–1600. doi: 10.1093/eurheartj/ehv140
Spescha, R. D., Shi, Y., Wegener, S., Keller, S., Weber, B., Wyss, M. M., et al. (2013). Deletion of the ageing gene p 66 (Shc) reduces early stroke size following ischaemia/reperfusion brain injury. Eur. Heart J. 34, 96–103. doi: 10.1093/eurheartj/ehs331
Spitzer, S. O., Sitnikov, S., Kamen, Y., Evans, K. A., Kronenberg-Versteeg, D., Dietmann, S., et al. (2019). Oligodendrocyte progenitor cells become regionally diverse and heterogeneous with age. Neuron 101, 459–471.e5. doi: 10.1016/j.neuron.2018.12.020
Stark, M. A., Huo, Y., Burcin, T. L., Morris, M. A., Olson, T. S., and Ley, K. (2005). Phagocytosis of apoptotic neutrophils regulates granulopoiesis via IL-23 and IL-17. Immunity 22, 285–294. doi: 10.1016/j.immuni.2005.01.011
Strinitz, M., Pham, M., März, A. G., Feick, J., Weidner, F., Vogt, M. L., et al. (2021). Immune cells invade the collateral circulation during human stroke: prospective replication and extension. Int. J. Mol. Sci. 22:9161. doi: 10.3390/ijms22179161
Sturm, J. W., Donnan, G. A., Dewey, H. M., Macdonell, R. A. L., Gilligan, A. K., Srikanth, V., et al. (2004). Quality of life after stroke: the north East Melbourne stroke incidence study (NEMESIS). Stroke 35, 2340–2345. doi: 10.1161/01.STR.0000141977.18520.3b
Su, C., Sun, F., Cunningham, R. L., Rybalchenko, N., and Singh, M. (2014). ERK5/KLF4 signaling as a common mediator of the neuroprotective effects of both nerve growth factor and hydrogen peroxide preconditioning. Age (Dordr.) 36:9685. doi: 10.1007/s11357-014-9685-5
Sughrue, M. E., Mehra, A., Connolly, E. S., and D’Ambrosio, A. L. (2004). Anti-adhesion molecule strategies as potential neuroprotective agents in cerebral ischemia: a critical review of the literature. Inflamm. Res. 53, 497–508. doi: 10.1007/s00011-004-1282-0
Sun, L., Wu, J., Du, F., Chen, X., and Chen, Z. J. (2013). Cyclic GMP-AMP synthase is a cytosolic DNA sensor that activates the type I interferon pathway. Science 339, 786–791. doi: 10.1126/science.1232458
Sun, J., Yu, L., Huang, S., Lai, X., Milner, R., and Li, L. (2017). Vascular expression of angiopoietin 1, α5β1 integrin and tight junction proteins is tightly regulated during vascular remodeling in the post-ischemic brain. Neuroscience 362, 248–256. doi: 10.1016/j.neuroscience.2017.08.040
Suzuki, H., Abe, K., Tojo, S., Morooka, S., Kimura, K., Mizugaki, M., et al. (1997). Postischemic expression of P-selectin immunoreactivity in rat brain. Neurosci. Lett. 228, 151–154. doi: 10.1016/S0304-3940(97)00385-6
Suzuki, Y., Nagai, N., and Umemura, K. (2016). A review of the mechanisms of blood-brain barrier permeability by tissue-type plasminogen activator treatment for cerebral ischemia. Front. Cell. Neurosci. 10:2. doi: 10.3389/fncel.2016.00002
Szczuciński, A., and Losy, J. (2007). Chemokines and chemokine receptors in multiple sclerosis. Potential targets for new therapies. Acta Neurol. Scand. 115, 137–146. doi: 10.1111/j.1600-0404.2006.00749.x
Tabula Muris Consortium, Overall coordination, Logistical coordination, Organ collection and processing, Library preparation and sequencing, Computational data analysis, et al. (2018). Single-cell transcriptomics of 20 mouse organs creates a tabula Muris. Nature 562, 367–372. doi: 10.1038/s41586-018-0590-4
Tagaya, M., Haring, H.-P., Stuiver, I., Wagner, S., Abumiya, T., Lucero, J., et al. (2001). Rapid loss of microvascular integrin expression during focal brain ischemia reflects neuron injury. J. Cereb. Blood Flow Metab. 21, 835–846. doi: 10.1097/00004647-200107000-00009
Tan, I. Y. L., Demchuk, A. M., Hopyan, J., Zhang, L., Gladstone, D., Wong, K., et al. (2009). CT angiography clot burden score and collateral score: correlation with clinical and radiologic outcomes in acute middle cerebral artery infarct. AJNR. Am. J. Neuroradiol. 30:525. doi: 10.3174/ajnr.A1408
Tang, Y., Xu, H., Du, X. L., Lit, L., Walker, W., Lu, A., et al. (2006). Gene expression in blood changes rapidly in neutrophils and monocytes after ischemic stroke in humans: a microarray study. J. Cereb. Blood Flow Metab. 26, 1089–1102. doi: 10.1038/sj.jcbfm.9600264
Tariq, N., and Khatri, R. (2008). Leptomeningeal collaterals in acute ischemic stroke. J. Vasc. Interv. Neurol. 1, 91–95.
Tarkanyi, G., Karadi, Z. N., Szabo, Z., Szegedi, I., Csiba, L., and Szapary, L. (2020). Relationship between leukocyte counts and large vessel occlusion in acute ischemic stroke. BMC Neurol. 20:440. doi: 10.1186/s12883-020-02017-3
Ter Schiphorst, A., Charron, S., Hassen, W. B., Provost, C., Naggara, O., Benzakoun, J., et al. (2021). Tissue no-reflow despite full recanalization following thrombectomy for anterior circulation stroke with proximal occlusion: a clinical study. J. Cereb. Blood Flow Metab. 41, 253–266. doi: 10.1177/0271678X20954929
Terao, S., Yilmaz, G., Stokes, K. Y., Russell, J., Ishikawa, M., Kawase, T., et al. (2008). Blood cell-derived RANTES mediates cerebral microvascular dysfunction, inflammation, and tissue injury after focal ischemia-reperfusion. Stroke 39, 2560–2570. doi: 10.1161/STROKEAHA.107.513150
Tokgoz, S., Kayrak, M., Akpinar, Z., Seyithanoğlu, A., Güney, F., and Yürüten, B. (2013). Neutrophil lymphocyte ratio as a predictor of stroke. J. Stroke Cerebrovasc. Dis. 22, 1169–1174. doi: 10.1016/j.jstrokecerebrovasdis.2013.01.011
Tokgoz, S., Keskin, S., Kayrak, M., Seyithanoglu, A., and Ogmegul, A. (2014). Is neutrophil/lymphocyte ratio predict to short-term mortality in acute cerebral infarct independently from infarct volume? J. Stroke Cerebrovasc. Dis. 23, 2163–2168. doi: 10.1016/j.jstrokecerebrovasdis.2014.04.007
Tomanek, R. J., Hansen, H. K., and Christensen, L. P. (2008). Temporally expressed PDGF and FGF-2 regulate embryonic coronary artery formation and growth. Arterioscler. Thromb. Vasc. Biol. 28, 1237–1243. doi: 10.1161/ATVBAHA.108.166454
Tong, E., Patrie, J., Tong, S., Evans, A., Michel, P., Eskandari, A., et al. (2017). Time-resolved CT assessment of collaterals as imaging biomarkers to predict clinical outcomes in acute ischemic stroke. Neuroradiology 59, 1101–1109. doi: 10.1007/s00234-017-1914-z
Tortorella, C., Simone, O., Piazzolla, G., Stella, I., Cappiello, V., and Antonaci, S. (2006). Role of phosphoinositide 3-kinase and extracellular signal-regulated kinase pathways in granulocyte macrophage-colony-stimulating factor failure to delay fas-induced neutrophil apoptosis in elderly humans. J. Gerontol. A Biol. Sci. Med. Sci. 61, 1111–1118. doi: 10.1093/gerona/61.11.1111
Toyota, E., Matsunaga, T., and Chilian, W. M. (2004). Myocardial angiogenesis. Mol. Cell. Biochem. 264, 35–44. doi: 10.1023/B:MCBI.0000044372.65864.18
Tsai, A. S., Berry, K., Beneyto, M. M., Gaudilliere, D., Ganio, E. A., Culos, A., et al. (2019). A year-long immune profile of the systemic response in acute stroke survivors. Brain 142, 978–991. doi: 10.1093/brain/awz022
Tseng, C. W., Kyme, P. A., Arruda, A., Ramanujan, V. K., Tawackoli, W., and Liu, G. Y. (2012). Innate immune dysfunctions in aged mice facilitate the systemic dissemination of methicillin-resistant S. aureus. PLoS One 7:e41454. doi: 10.1371/journal.pone.0041454
Tseng, C. W., and Liu, G. Y. (2014). Expanding roles of neutrophils in aging hosts. Curr. Opin. Immunol. 29, 43–48. doi: 10.1016/j.coi.2014.03.009
Tsivgoulis, G., Katsanos, A. H., and Caso, V. (2017). Under-representation of women in stroke randomized controlled trials: inadvertent selection bias leading to suboptimal conclusions. Ther. Adv. Neurol. Disord. 10, 241–244. doi: 10.1177/1756285617699588
Tsuda, Y., Takahashi, H., Kobayashi, M., Hanafusa, T., Herndon, D. N., and Suzuki, F. (2004). Three different neutrophil subsets exhibited in mice with different susceptibilities to infection by methicillin-resistant Staphylococcus aureus. Immunity 21, 215–226. doi: 10.1016/j.immuni.2004.07.006
Uhl, B., Vadlau, Y., Zuchtriegel, G., Nekolla, K., Sharaf, K., Gaertner, F., et al. (2016). Aged neutrophils contribute to the first line of defense in the acute inflammatory response. Blood 128, 2327–2337. doi: 10.1182/blood-2016-05-718999
Uysal, O. K., Turkoglu, C., Sahin, D. Y., Duran, M., Yıldırım, A., Elbasan, Z., et al. (2015). The relationship between neutrophil-to-lymphocyte ratio and coronary collateral circulation. Clin. Appl. Thromb. Hemost. 21, 329–333. doi: 10.1177/1076029613503399
Uzdensky, A. B. (2019). Apoptosis regulation in the penumbra after ischemic stroke: expression of pro- and antiapoptotic proteins. Apoptosis 24, 687–702. doi: 10.1007/s10495-019-01556-6
Vallés, J., Lago, A., Santos, M. T., Latorre, A. M., Tembl, J. I., Salom, J. B., et al. (2017). Neutrophil extracellular traps are increased in patients with acute ischemic stroke: prognostic significance. Thromb. Haemost. 117, 1919–1929. doi: 10.1160/TH17-02-0130
Van Avondt, K., Strecker, J.-K., Tulotta, C., Minnerup, J., Schulz, C., and Soehnlein, O. (2022). Neutrophils in aging and aging-related pathologies. Immunol. Rev. doi: 10.1111/imr.13153 [Epub ahead of print]
van der Worp, H. B., de Haan, P., Morrema, E., and Kalkman, C. J. (2005). Methodological quality of animal studies on neuroprotection in focal cerebral ischaemia. J. Neurol. 252, 1108–1114. doi: 10.1007/s00415-005-0802-3
Van Eeden, S. F., Bicknell, S., Walker, B. A., and Hogg, J. C. (1997). Polymorphonuclear leukocytes L-selectin expression decreases as they age in circulation. Am. J. Phys. Heart Circ. Phys. 272, H401–H408. doi: 10.1152/ajpheart.1997.272.1.H401
Venna, V. R., Xu, Y., Doran, S. J., Patrizz, A., and McCullough, L. D. (2014). Social interaction plays a critical role in neurogenesis and recovery after stroke. Transl. Psychiatry 4:e351. doi: 10.1038/tp.2013.128
Verbitsky, M., Yonan, A. L., Malleret, G., Kandel, E. R., Gilliam, T. C., and Pavlidis, P. (2004). Altered hippocampal transcript profile accompanies an age-related spatial memory deficit in mice. Learn. Mem. 11, 253–260. doi: 10.1101/lm.68204
Villa, P., Triulzi, S., Cavalieri, B., Di Bitondo, R., Bertini, R., Barbera, S., et al. (2007). The interleukin-8 (IL-8/CXCL8) receptor inhibitor reparixin improves neurological deficits and reduces long-term inflammation in permanent and transient cerebral ischemia in rats. Mol. Med. 13, 125–133. doi: 10.2119/2007-00008.Villa
Villeda, S. A., Luo, J., Mosher, K. I., Zou, B., Britschgi, M., Bieri, G., et al. (2011). The ageing systemic milieu negatively regulates neurogenesis and cognitive function. Nature 477, 90–94. doi: 10.1038/nature10357
Villeda, S. A., Plambeck, K. E., Middeldorp, J., Castellano, J. M., Mosher, K. I., Luo, J., et al. (2014). Young blood reverses age-related impairments in cognitive function and synaptic plasticity in mice. Nat. Med. 20, 659–663. doi: 10.1038/nm.3569
Wang, Y.-Q., Cui, H.-R., Yang, S.-Z., Sun, H.-P., Qiu, M.-H., Feng, X.-Y., et al. (2009). VEGF enhance cortical newborn neurons and their neurite development in adult rat brain after cerebral ischemia. Neurochem. Int. 55, 629–636. doi: 10.1016/j.neuint.2009.06.007
Wang, W. Z., Fang, X.-H., Stephenson, L. L., Khiabani, K. T., and Zamboni, W. A. (2008). Ischemia/reperfusion-induced necrosis and apoptosis in the cells isolated from rat skeletal muscle. J. Orthop. Res. 26, 351–356. doi: 10.1002/jor.20493
Wang, Y., Jin, H., Wang, W., Wang, F., and Zhao, H. (2019). Myosin 1f-mediated neutrophil migration contributes to acute neuroinflammation and brain injury after stroke in mice. J. Neuroinflammation 16:77. doi: 10.1186/s12974-019-1465-9
Wang, X., Qiu, L., Li, Z., Wang, X.-Y., and Yi, H. (2018). Understanding the multifaceted role of neutrophils in cancer and autoimmune diseases. Front. Immunol. 9:2456. doi: 10.3389/fimmu.2018.02456
Wang, Q., Tang, X. N., and Yenari, M. A. (2007). The inflammatory response in stroke. J. Neuroimmunol. 184, 53–68. doi: 10.1016/j.jneuroim.2006.11.014
Wang, C., Zhang, Q., Ji, M., Mang, J., and Xu, Z. (2021). Prognostic value of the neutrophil-to-lymphocyte ratio in acute ischemic stroke patients treated with intravenous thrombolysis: a systematic review and meta-analysis. BMC Neurol. 21:191. doi: 10.1186/s12883-021-02222-8
Wang, I.-M., Zhang, B., Yang, X., Zhu, J., Stepaniants, S., Zhang, C., et al. (2012). Systems analysis of eleven rodent disease models reveals an inflammatome signature and key drivers. Mol. Syst. Biol. 8:594. doi: 10.1038/msb.2012.24
Warner, D. S., James, M. L., Laskowitz, D. T., and Wijdicks, E. F. (2014). Translational research in acute central nervous system injury: lessons learned and the future. JAMA Neurol. 71, 1311–1318. doi: 10.1001/jamaneurol.2014.1238
Wei, L., Erinjeri, J. P., Rovainen, C. M., and Woolsey, T. A. (2001). Collateral growth and angiogenesis around cortical stroke. Stroke 32, 2179–2184. doi: 10.1161/hs0901.094282
Weinstein, J. R., Koerner, I. P., and Möller, T. (2010). Microglia in ischemic brain injury. Future Neurol. 5, 227–246. doi: 10.2217/fnl.10.1
Weisenburger-Lile, D., Dong, Y., Yger, M., Weisenburger, G., Polara, G. F., Chaigneau, T., et al. (2019). Harmful neutrophil subsets in patients with ischemic stroke. Neurol. Neuroimmunol. Neuroinflamm. 6:e571. doi: 10.1212/NXI.0000000000000571
Weiskopf, D., Weinberger, B., and Grubeck-Loebenstein, B. (2009). The aging of the immune system. Transpl. Int. 22, 1041–1050. doi: 10.1111/j.1432-2277.2009.00927.x
Weiss, S. J. (1989). Tissue destruction by neutrophils. N. Engl. J. Med. 320, 365–376. doi: 10.1056/NEJM198902093200606
West, A. P., Khoury-Hanold, W., Staron, M., Tal, M. C., Pineda, C. M., Lang, S. M., et al. (2015). Mitochondrial DNA stress primes the antiviral innate immune response. Nature 520:553. doi: 10.1038/nature14156
Wheeler, J. G., Mussolino, M. E., Gillum, R. F., and Danesh, J. (2004). Associations between differential leucocyte count and incident coronary heart disease: 1764 incident cases from seven prospective studies of 30, 374 individuals. Eur. Heart J. 25, 1287–1292. doi: 10.1016/j.ehj.2004.05.002
Whiteley, W., Jackson, C., Lewis, S., Lowe, G., Rumley, A., Sandercock, P., et al. (2009). Inflammatory markers and poor outcome after stroke: a prospective cohort study and systematic review of Interleukin-6. PLoS Med. 6:e1000145. doi: 10.1371/journal.pmed.1000145
Wiggins, K. A., and Clarke, M. C. (2019). Senescence utilises inflammatory caspases to drive SASP. Aging (Albany NY) 11, 3891–3892. doi: 10.18632/aging.102031
Wildermuth, S., Knauth, M., Brandt, T., Winter, R., Sartor, K., and Hacke, W. (1998). Role of CT angiography in patient selection for thrombolytic therapy in acute hemispheric stroke. Stroke 29, 935–938. doi: 10.1161/01.STR.29.5.935
Winship, I. R. (2015). Cerebral collaterals and collateral therapeutics for acute ischemic stroke. Microcirculation 22, 228–236. doi: 10.1111/micc.12177
Winship, I. R., Armitage, G. A., Ramakrishnan, G., Dong, B., Todd, K. G., and Shuaib, A. (2014). Augmenting collateral blood flow during ischemic stroke via transient aortic occlusion. J. Cereb. Blood Flow Metab. 34, 61–71. doi: 10.1038/jcbfm.2013.162
Woo, C.-H., Massett, M. P., Shishido, T., Itoh, S., Ding, B., McClain, C., et al. (2006). ERK5 activation inhibits inflammatory responses via peroxisome proliferator-activated receptor delta (PPARdelta) stimulation. J. Biol. Chem. 281, 32164–32174. doi: 10.1074/jbc.M602369200
Woodfin, A., Voisin, M.-B., Beyrau, M., Colom, B., Caille, D., Diapouli, F.-M., et al. (2011). The junctional adhesion molecule JAM-C regulates polarized transendothelial migration of neutrophils in vivo. Nat. Immunol. 12, 761–769. doi: 10.1038/ni.2062
Wright, H. L., Cross, A. L., Edwards, S. W., and Moots, R. J. (2014). Effects of IL-6 and IL-6 blockade on neutrophil function in vitro and in vivo. Rheumatology (Oxford) 53, 1321–1331. doi: 10.1093/rheumatology/keu035
Wu, L., Walas, S., Leung, W., Sykes, D. B., Wu, J., Lo, E. H., et al. (2015). Neuregulin 1-β decreases IL-1β-induced neutrophil adhesion to human brain microvascular endothelial cells. Transl. Stroke Res. 6, 116–124. doi: 10.1007/s12975-014-0347-9
Wufuer, A., Wubuli, A., Mijiti, P., Zhou, J., Tuerxun, S., Cai, J., et al. (2018). Impact of collateral circulation status on favorable outcomes in thrombolysis treatment: a systematic review and meta-analysis. Exp. Ther. Med. 15, 707–718. doi: 10.3892/etm.2017.5486
Wulfert, F. M., van Meurs, M., Kurniati, N. F., Jongman, R. M., Houwertjes, M. C., Heeringa, P., et al. (2012). Age-dependent role of microvascular endothelial and polymorphonuclear cells in lipopolysaccharide-induced acute kidney injury. Anesthesiology 117, 126–136. doi: 10.1097/ALN.0b013e31825b57c9
Xing, C., Arai, K., Lo, E. H., and Hommel, M. (2012). Pathophysiologic cascades in ischemic stroke. Int. J. Stroke 7, 378–385. doi: 10.1111/j.1747-4949.2012.00839.x
Xu, X., and Jiang, Y. (2014). The Yin and Yang of innate immunity in stroke. Biomed. Res. Int. 2014:807978. doi: 10.1155/2014/807978
Xuan, Q., Xu, S.-L., Lu, D.-H., Yu, S., Zhou, M., Uéda, K., et al. (2011). Increased expression of α-synuclein in aged human brain associated with neuromelanin accumulation. J. Neural Transm. (Vienna) 118, 1575–1583. doi: 10.1007/s00702-011-0636-3
Xue, J., Huang, W., Chen, X., Li, Q., Cai, Z., Yu, T., et al. (2017). Neutrophil-to-lymphocyte ratio is a prognostic marker in acute ischemic stroke. J. Stroke Cerebrovasc. Dis. 26, 650–657. doi: 10.1016/j.jstrokecerebrovasdis.2016.11.010
Xue, J., Thippegowda, P. B., Hu, G., Bachmaier, K., Christman, J. W., Malik, A. B., et al. (2009). NF-κB regulates thrombin-induced ICAM-1 gene expression in cooperation with NFAT by binding to the intronic NF-κB site in the ICAM-1 gene. Physiol. Genomics 38, 42–53. doi: 10.1152/physiolgenomics.00012.2009
Yan, S. L.-S., Hwang, I.-Y., Kamenyeva, O., Kabat, J., Kim, J. S., Park, C., et al. (2021). Unrestrained Gαi 2 signaling disrupts neutrophil trafficking, aging, and clearance. Front. Immunol. 12:679856. doi: 10.3389/fimmu.2021.679856
Yang, Q., Huang, Q., Hu, Z., and Tang, X. (2019). Potential Neuroprotective treatment of stroke: targeting Excitotoxicity, oxidative stress, and inflammation. Front. Neurosci. 13:1036. doi: 10.3389/fnins.2019.01036
Yang, W., and Paschen, W. (2017). Is age a key factor contributing to the disparity between success of neuroprotective strategies in young animals and limited success in elderly stroke patients? Focus on protein homeostasis. J. Cereb. Blood Flow Metab. 37, 3318–3324. doi: 10.1177/0271678X17723783
Yenari, M. A., Kunis, D., Sun, G. H., Onley, D., Watson, L., Turner, S., et al. (1998). Hu23F2G, an antibody recognizing the leukocyte CD11/CD18 integrin, reduces injury in a rabbit model of transient focal cerebral ischemia. Exp. Neurol. 153, 223–233. doi: 10.1006/exnr.1998.6876
Yeo, L. L. L., Paliwal, P., Teoh, H. L., Seet, R. C., Chan, B. P., Ting, E., et al. (2015). Assessment of intracranial collaterals on CT angiography in anterior circulation acute ischemic stroke. AJNR Am. J. Neuroradiol. 36, 289–294. doi: 10.3174/ajnr.A4117
Yilmaz, G., and Granger, D. N. (2008). Cell adhesion molecules and ischemic stroke. Neurol. Res. 30, 783–793. doi: 10.1179/174313208X341085
Yin, K.-J., Deng, Z., Hamblin, M., Zhang, J., and Chen, Y. E. (2011). Vascular PPARδ protects against stroke-induced brain injury. Arterioscler. Thromb. Vasc. Biol. 31, 574–581. doi: 10.1161/ATVBAHA.110.221267
Ying, Y., Yu, F., Luo, Y., Feng, X., Liao, D., Wei, M., et al. (2021). Neutrophil-to-lymphocyte ratio as a predictive biomarker for stroke severity and short-term prognosis in acute ischemic stroke with intracranial atherosclerotic stenosis. Front. Neurol. 12:705949. doi: 10.3389/fneur.2021.705949
Yipp, B. G., and Kubes, P. (2013). NETosis: how vital is it? Blood 122, 2784–2794. doi: 10.1182/blood-2013-04-457671
Yuan, Y., Chen, Y.-P. P., Boyd-Kirkup, J., Khaitovich, P., and Somel, M. (2012). Accelerated aging-related transcriptome changes in the female prefrontal cortex. Aging Cell 11, 894–901. doi: 10.1111/j.1474-9726.2012.00859.x
Zbinden, S., Wang, J., Adenika, R., Schmidt, M., Tilan, J. U., Najafi, A. H., et al. (2010). Metallothionein enhances angiogenesis and arteriogenesis by modulating smooth muscle cell and macrophage function. Arterioscler. Thromb. Vasc. Biol. 30, 477–482. doi: 10.1161/ATVBAHA.109.200949
Zeier, Z., Madorsky, I., Xu, Y., Ogle, W. O., Notterpek, L., and Foster, T. C. (2011). Gene expression in the hippocampus: regionally specific effects of aging and caloric restriction. Mech. Ageing Dev. 132, 8–19. doi: 10.1016/j.mad.2010.10.006
Zhan, X., Jickling, G. C., Tian, Y., Stamova, B., Xu, H., Ander, B. P., et al. (2011). Transient ischemic attacks characterized by RNA profiles in blood. Neurology 77, 1718–1724. doi: 10.1212/WNL.0b013e318236eee6
Zhang, D., Chen, G., Manwani, D., Mortha, A., Xu, C., Faith, J. J., et al. (2015). Neutrophil ageing is regulated by the microbiome. Nature 525, 528–532. doi: 10.1038/nature15367
Zhang, R. L., Chopp, M., Chen, H., and Garcia, J. H. (1994). Temporal profile of ischemic tissue damage, neutrophil response, and vascular plugging following permanent and transient (2H) middle cerebral artery occlusion in the rat. J. Neurol. Sci. 125, 3–10. doi: 10.1016/0022-510x(94)90234-8
Zhang, R. L., Chopp, M., Jiang, N., Tang, W. X., Prostak, J., Manning, A. M., et al. (1995). Anti-intercellular adhesion molecule-1 antibody reduces ischemic cell damage after transient but not permanent middle cerebral artery occlusion in the Wistar rat. Stroke 26, 1438–1442. doi: 10.1161/01.STR.26.8.1438
Zhang, X., Kluger, Y., Nakayama, Y., Poddar, R., Whitney, C., DeTora, A., et al. (2004). Gene expression in mature neutrophils: early responses to inflammatory stimuli. J. Leukoc. Biol. 75, 358–372. doi: 10.1189/jlb.0903412
Zhang, W.-B., Zeng, Y.-Y., Wang, F., Cheng, L., Tang, W.-J., and Wang, X.-Q. (2020). A high neutrophil-to-lymphocyte ratio predicts hemorrhagic transformation of large atherosclerotic infarction in patients with acute ischemic stroke. Aging (Albany NY) 12, 2428–2439. doi: 10.18632/aging.102752
Zhang, Z. G., Zhang, L., Jiang, Q., Zhang, R., Davies, K., Powers, C., et al. (2000). VEGF enhances angiogenesis and promotes blood-brain barrier leakage in the ischemic brain. J. Clin. Invest. 106, 829–838. doi: 10.1172/JCI9369
Zhao, X., Ting, S.-M., Liu, C.-H., Sun, G., Kruzel, M., Roy-O’Reilly, M., et al. (2017). Neutrophil polarization by IL-27 as a therapeutic target for intracerebral hemorrhage. Nat. Commun. 8:602. doi: 10.1038/s41467-017-00770-7
Zhao, S., Wang, C., Xu, H., Wu, W., Chu, Z., Ma, L., et al. (2017). Age-related differences in interferon regulatory factor-4 and -5 signaling in ischemic brains of mice. Acta Pharmacol. Sin. 38, 1425–1434. doi: 10.1038/aps.2017.122
Keywords: neutrophils, aging, inflammaging, stroke, ischemia, futile recanalization, collaterals, collateral circulation
Citation: Bui TA, Jickling GC and Winship IR (2022) Neutrophil dynamics and inflammaging in acute ischemic stroke: A transcriptomic review. Front. Aging Neurosci. 14:1041333. doi: 10.3389/fnagi.2022.1041333
Edited by:
Ying Xu, University at Buffalo, United StatesReviewed by:
Craig Edward Brown, University of Victoria, CanadaMohamad El Amki, University Hospital Zürich, Switzerland
Copyright © 2022 Bui, Jickling and Winship. This is an open-access article distributed under the terms of the Creative Commons Attribution License (CC BY). The use, distribution or reproduction in other forums is permitted, provided the original author(s) and the copyright owner(s) are credited and that the original publication in this journal is cited, in accordance with accepted academic practice. No use, distribution or reproduction is permitted which does not comply with these terms.
*Correspondence: Ian R. Winship, aXdpbnNoaXBAdWFsYmVydGEuY2E=