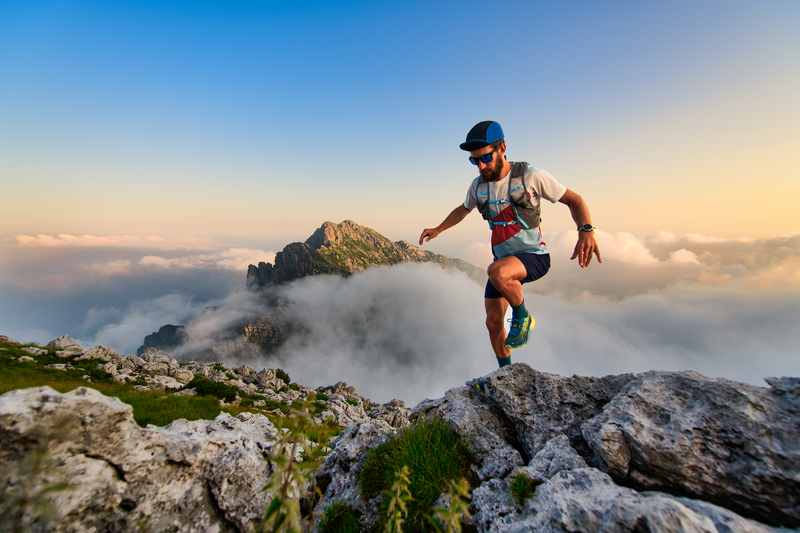
95% of researchers rate our articles as excellent or good
Learn more about the work of our research integrity team to safeguard the quality of each article we publish.
Find out more
REVIEW article
Front. Aging Neurosci. , 04 October 2022
Sec. Cellular and Molecular Mechanisms of Brain-aging
Volume 14 - 2022 | https://doi.org/10.3389/fnagi.2022.1020092
This article is part of the Research Topic Trends in Biomarkers for Neurodegenerative Diseases: Current Research and Future Perspectives View all 18 articles
COVID-19 is renowned as a multi-organ disease having subacute and long-term effects with a broad spectrum of clinical manifestations. The evolving scientific and clinical evidence demonstrates that the frequency of cognitive impairment after COVID-19 is high and it is crucial to explore more clinical research and implement proper diagnostic and treatment strategies. Several central nervous system complications have been reported as comorbidities of COVID-19. The changes in cognitive function associated with neurodegenerative diseases develop slowly over time and are only diagnosed at an already advanced stage of molecular pathology. Hence, understanding the common links between COVID-19 and neurodegenerative diseases will broaden our knowledge and help in strategizing prognostic and therapeutic approaches. The present review focuses on the diverse neurodegenerative changes associated with COVID-19 and will highlight the importance of major circulating biomarkers and microRNAs (miRNAs) associated with the disease progression and severity. The literature analysis showed that major proteins associated with central nervous system function, such as Glial fibrillary acidic protein, neurofilament light chain, p-tau 181, Ubiquitin C-terminal hydrolase L1, S100 calcium-binding protein B, Neuron-specific enolase and various inflammatory cytokines, were significantly altered in COVID-19 patients. Furthermore, among various miRNAs that are having pivotal roles in various neurodegenerative diseases, miR-146a, miR-155, Let-7b, miR-31, miR-16 and miR-21 have shown significant dysregulation in COVID-19 patients. Thus the review consolidates the important findings from the numerous studies to unravel the underlying mechanism of neurological sequelae in COVID-19 and the possible association of circulatory biomarkers, which may serve as prognostic predictors and therapeutic targets in future research.
Coronavirus disease 2019 (COVID-19), the disease caused by severe acute respiratory syndrome coronavirus 2 (SARS-CoV-2), has created morbidity and mortality at an unprecedented scale globally and was declared a pandemic by the World Health Organization (WHO) in March 2020 (Nalbandian et al., 2021). It was initially detected in Wuhan, China, which triggered a severe acute respiratory syndrome, contaminating more than 175 million people after one year and leading to the death of 3.8 million people worldwide (Huang et al., 2020; Hui et al., 2020; Wu et al., 2020a; Lopez-Leon et al., 2021). SARS-CoV-2 is a betacoronavirus, a member of the subfamily Coronavirinae, having a single-stranded positive-sense RNA genome (Wang et al., 2020b). SARS-CoV-2 is made up of at least 29 proteins, four of which are structural proteins, and the others are non-structural proteins (Yao et al., 2020). SARS-CoV-2 is prone to genetic evolution through mutations over time in human hosts. This leads to the generation of mutant variants having diverse characteristics than their ancestral strains. Several variants of SARS-CoV-2 have been described during the course of this pandemic and WHO has classified them based on their impact on public health. Currently, there are five SARS-CoV-2 variants of concern (VOC), Alpha, Beta, Gamma, Delta, and Omicron, and two SARS-CoV-2 variants of interest (VOI), Lambda and Mu (WHO SARS-CoV-2 Variants) (Liu et al., 2022). The emergence of these new SARS-CoV-2 variants are posing threats to vaccine development and other therapeutic options.
Several scientific and clinical studies have shown that subacute and long-term effects of COVID-19 can affect multiple organ systems (Gupta et al., 2020a). It is reported that the mechanism of infection and replication of SARS-CoV-2 is similar to that of SARS-CoV and MERS-CoV. The angiotensin-converting enzyme-2 (ACE-2) receptors are the primary binding receptors for the viral particle and are found highly expressed in alveolar epithelial cells of lungs, vascular endothelial cells, and enterocytes but can also be found in other organs, such as kidney, liver, and gastrointestinal tract (Azer, 2020; Bhavana et al., 2020; Harrison et al., 2020; Parasher, 2021). The internalization of the virus in host cells results in different inflammatory changes such as edema, necrosis, and tissue dysfunction. These changes can cause a cytokine storm, promoting changes in the immune response that cause excessive damage to the lung, gastrointestinal, neurological, and cardiopulmonary systems (Azer, 2020; Bhavana et al., 2020; Harrison et al., 2020; Rizzo et al., 2020; Xu et al., 2020; Anka et al., 2021).
As SARS-CoV-2 has the ability to affect different organs, recent clinical studies have demonstrated that there is an increased risk of long-term health problems in patients who have survived infection with SARS-CoV-2 (Seyedalinaghi et al., 2021). The most recurrent long-term complication is respiratory problems that may further develop pulmonary fibrosis, arterial complications, venous thrombo-embolic late complications associated with a hyperinflammatory and hypercoagulable state (Lodigiani et al., 2020; Puntmann et al., 2020). Likewise, cardiac dysfunction can be caused due to structural damage to the myocardium, pericardium, and conduction system, triggering arrhythmias in a large proportion of patients (Lindner et al., 2020; Liu et al., 2020). Renal lesions have also been reported in approximately 20-31% of patients who developed the severe form of COVID-19. The reduced glomerular filtration was related to extensive acute tubular necrosis observed in renal biopsies (Kudose et al., 2020). Diabetic ketoacidosis, liver dysfunction, joint pain, muscle weekness and dermatologic manifestations were also observed in post-covid patients (Freeman et al., 2020; Suwanwongse and Shabarek, 2021). In addition, late complications are reported in the central and peripheral nervous system, promoting decreased awareness and absorption, difficulties with concentration, disturbed memory, difficulty in communication, anxiety, depression, sleep problems, and olfactory and taste losses (Paybast et al., 2020; Varatharaj et al., 2020; Huang et al., 2021a). Figure 1 demonstrates the various complications associated with COVID-19.
Figure 1. Schematic representation showing the potential complications of COVID-19 causing wide range of complications in various organ systems.
The present review aims to elucidate the underlying mechanism that links COVID-19 with neurodegenerative changes that lead to cognitive dysfunction. As patients infected with SARS-CoV-2 are stratified according to their clinical manifestations, such as symptoms, oxygen saturation, and blood pressure (Malik et al., 2021), these manifestations are apparent at the late stages of infection, especially for neurological complications. Identifying factors that can lead to complications during the disease early is extremely important since it can significantly influence the quality of care and adequate treatment. It would be valuable to reveal the alterations of plasma biomarkers in various cognitive impairment stages since the cognitive manifestations are one of the most concerned post covid complications. Hence, based on recent literature, we will provide clinicians with updated and practical information on the role of circulating biomarkers and miRNAs in COVID-19-associated cognitive dysfunction that may act as possible therapeutic targets and prognostic predictors in future studies.
The purpose of this review is to highlight the importance of cognitive dysfunction and neurodegenerative changes associated with COVID-19 and the dysregulation of circulating biomarkers and miRNAs in the clinical condition being studied. A systematic search of relevant research articles was performed using the databases, namely, PubMed, ProQuest, Science Direct, and Google Scholar. The electronic search was conducted using a combination of search terms related to the following keywords: “COVID-19” OR “SARS-CoV-2” OR “Post COVID-19 complications” OR “Cognitive dysfunction” OR “Neurodegeneration” OR “Circulating Biomarkers” OR “miRNAs”. The articles retrieved from our search were further distinguished for relevancy. The inclusion and exclusion criteria were set to only evaluate articles published from 2000 to 2022 in order to limit this search. Moreover, articles that contain only abstracts without their full text and published in languages other than English were excluded.
As the population of patients recovering from COVID-19 grows, it is important to establish an understanding of the multi-organ dysfunction associated with post- COVID-19 complications. Of note, neurological manifestations in COVID-19 patients have been reported, showing a close correlation between COVID-19 and future development of neurodegenerative diseases (Wu et al., 2020b; Taquet et al., 2021; Frontera et al., 2022; Li et al., 2022a). It has also been established that the likelihood of developing COVID-19-associated cognitive impairment and the severity of these deficits is associated with the severity of the SARS-CoV-2 infection and the subsequent increases in specific circulating inflammatory mediators and biomarkers (Becker et al., 2021; Miskowiak et al., 2021; Zeng et al., 2022). Alternatively, some studies have demonstrated that even non-hospitalized COVID-19 patients have developed cognitive-associated post-COVID-19 symptoms, suggesting that regardless of illness severity, cognitive dysfunction can arise (Graham et al., 2021; Hadad et al., 2022; Van Kessel et al., 2022). Report shows that COVID-19-associated cognitive impairment can arise during post-acute COVID-19 infection 3 weeks following diagnosis, and 31.2% of participants experience cognitive dysfunction within the first week of symptoms (Davis et al., 2021). Another study found that 22% of individuals infected with SARS-CoV-2 developed symptoms of cognitive impairment, which remained after 12 weeks following their diagnosis (Ceban et al., 2022). These deficits can last for a prolonged period of time and clinically relevant cognitive impairments in verbal learning and executive function were found in 48% of patients 1 year following the onset of symptoms (Miskowiak et al., 2022).
Likewise, another report identified abnormalities in executive function, attention, and phonemic fluency in post-COVID-19 patients (Hadad et al., 2022). The results of a systemic review showed a high frequency of cognitive impairment after COVID-19 infection with defects in processing speed, inattention, or executive dysfunction (Tavares-Junior et al., 2022). A post-COVID-19 community clinic compared Montreal Cognitive Assessment (MoCA) index scores of participants who reported cognitive symptoms and found that index scores were significantly worse in language, executive function, and attention (Crivelli et al., 2022). With increasing severity of infection with SARS-CoV-2, there are corresponding increases in both the likelihood of developing cognitive dysfunction as well as the severity of the cognitive dysfunction in those who develop these sequelae (Wang et al., 2021). For these reasons, it is imperative to understand the underlying mechanisms by which covid-associated cognitive dysfunction and neurodegeneration occur.
The neurological complications of COVID-19 include damage to the central and the peripheral nervous system that consists of neuronal damage, neuroinflammation, rupture of the blood brain barrier, microvasculitis and hypoxia (Ellul et al., 2020; Boldrini et al., 2021). ACE2 receptors within the central nervous system (CNS) are most highly concentrated within the substantia nigra, ventricles, middle temporal gyrus, posterior cingulate cortex, olfactory bulb, motor cortex, and brainstem (Iodice et al., 2021). The disruption of the normal physiological functions of these areas due to infection with SARS-CoV-2 has been postulated to be a potential explanation for many of the reported symptoms associated with the long-Covid syndrome. This was validated by an observational study that examined cortical metabolism in the subacute and chronic (>6 months) stages of illness and found that hypometabolism in the frontoparietal and temporal cortex was associated with cognitive impairment (Hosp et al., 2021). Long- COVID-19 brain fog in patients has presented with abnormal FDG-PET scan results, with hypometabolic regions localized mostly to the anterior and posterior cingulate cortices (Hugon et al., 2022). The cingulate cortex plays a role in a variety of neurological functions including memory, emotions, and decisive action taking. The abundance of ACE2 receptors in this area could additionally provide some insight into their experience of brain fog (Hugon et al., 2022).
There are numerous potential mechanisms by which SARS-CoV-2 could access the CNS to elicit these pathologies, which can be broadly classified into direct invasion and indirect hematological entry following inflammatory mediated neurodegeneration of the blood-brain-barrier (BBB) (Iodice et al., 2021). Direct neurological invasion is hypothesized to occur through the olfactory epithelium and the cribriform plate into the olfactory bulbs following nasal inhalation of aerosolized droplets containing the infectious viral load. Additionally, it has been suggested that SARS-CoV-2 penetration into the CNS can result in heightened neuroinflammation which may cause neurodegeneration or exacerbate existing neuroinflammation from preexisting comorbidities such as Alzheimer’s dementia and Parkinson’s disease, leading to the development of neuropsychological symptoms (Iodice et al., 2021).
Hematological spread is another proposed mechanism by which SARS-CoV-2 can gain access to the CNS. One possible mechanism involves the attachment of SARS-CoV-2 to ACE2 expressed on BBB endothelial cells and the induction of neuroinflammation, which weakens the protective barrier of the brain and thus provides access for the virus. It is also hypothesized that it is possible that the virus may circumvent BBB altogether by infecting CNS-infiltrating macrophages and monocytes (Zhou et al., 2020b). Furthermore, astrocytes, a vital component of the BBB, can receive signals from circulating pro-inflammatory cytokines, generated in response to viral infection in the lungs, which in turn cause SARS-CoV-2 to enter the CNS and induce neuroinflammation (Murta et al., 2020). Moreover, SARS-CoV-2 can elicit the pro-inflammatory phenotype of microglia, the native immune cells in the CNS, which up-regulate the expression of genes involved in neuroinflammation (Amruta et al., 2021). In addition, it has been demonstrated that SARS-CoV-2 infection can facilitate neuronal injury, encephalitis, fibrosis, thrombosis and axonal damage (Bradley et al., 2020; Turski et al., 2020; Wang et al., 2020a). As, COVID-19-associated pathological changes are characterized by distinct changes in the levels of specific circulating biomarkers, the expression profile of these biomarkers may demonstrate a link between various disease states. The Schematic representation of various neurodegenerative changes associated with COVID-19 is shown in Figure 2. Understanding the changes in expression of these circulating biomarkers may provide insights into the holistic understanding of the underlying process by which COVID-19 long-haulers experience their cognitive dysfunction.
Figure 2. Schematic representation showing the neuronal infection of SARS-CoV-2 virus and CNS damage caused by the infection that leads to cognitive dysfunction and neurodegenerative diseases. Angiotensin-converting enzyme-2 (ACE-2), Alzheimer’s disease (AD), Parkinson’s disease (PD), Multiple sclerosis (MS), Amyotrophic lateral sclerosis (ALS), Multiple system atrophy (MSA), Ischemia stroke (IS).
Circulating biomarkers present a promising approach in the research and clinical practice of various diseases including neurodegenerative diseases as they are minimally invasive, highly cost-effective and provide high specificity (Solfrizzi et al., 2006; Pillai et al., 2020; Lakhani et al., 2021; Kivisakk et al., 2022). The prognostic utility of plasma biomarkers in neuroinflammation, vascular injury, and cognitive dysfunction may aid in the management of clinical care and treatment strategies. Hence in this section, we are highlighting the importance of some key biomarkers of cognitive decline and neurodegeneration that are altered in COVID-19 for their future application in research on therapeutic targets and prognostic deliberations of COVID-19- associated cognitive dysfunction. A summary of the findings are illustrated in Table 1.
Table 1. Summary of circulating biomarkers associated with neurological dysfunction showing potential dysregulation in COVID-19.
Astrocytes are the most abundant cell type throughout the CNS and have many roles including but not limited to maintenance of BBB, neurotransmitter homeostasis, synaptogenesis, neurogenesis, ion and water homeostasis, and neuronal cholesterol synthesis (Cabezas et al., 2014; Liu et al., 2018). When neurological insult occurs, astrocytes become activated through reactive gliosis that involves the upregulation of glial fibrillary acidic protein (GFAP), a widely known biomarker of brain injury (Pekny and Pekna, 2014). As a structural protein that is unique to astrocytes, GFAP provides stability to astrocytes, thereby influencing their shape and movement (Eng et al., 2000; Hol and Capetanaki, 2017). Therefore, GFAP has been regarded as a biomarker of reactive astrocytes in a variety of neuropathological conditions (Eng et al., 2000). Elevated circulating levels of GFAP have been linked to a number of such neurological conditions including traumatic brain injury (TBI), spinal cord injury, multiple sclerosis (MS), Alzheimer’s Disease (AD), Alexander disease, Parkinson’s disease (PD), and neuromyelitis optica spectrum disorder (Elahi et al., 2020; Abdelhak et al., 2022; Heimfarth et al., 2022; Kim et al., 2022; Newcombe et al., 2022). It is evident that GFAP has been and continues to be explored as a potential biomarker of neurological injury across several neurodegenerative and inflammatory CNS diseases.
Recent studies have found elevated levels of GFAP in serum and/or plasma of COVID-19 patients (Kanberg et al., 2020, 2021; Frontera et al., 2022; Hanson et al., 2022; Sahin et al., 2022). This supports that GFAP correlates with disease severity, as it was found at significantly higher levels in COVID-19 patients who died during hospitalization when compared to those who survived (Frontera et al., 2022). Other results have supported elevated GFAP levels with disease severity but did not find a significant correlation with the presence of neurological symptoms (Sahin et al., 2022). One study found that GFAP levels normalized in all COVID-19 patients despite disease severity and the persistence of reported cognitive symptoms suggesting that the symptoms of COVID-19-associated cognitive impairment linger without the presence of active CNS injury (Kanberg et al., 2021). These findings may also further support the proposed mechanism that reactive gliosis following SARS-CoV-2 infection causes COVID-19-associated cognitive dysfunction by spreading hematogenously, infecting endothelial cells, and disrupting the BBB (Johansson et al., 2021; Mohamed et al., 2022). Taken together, these findings suggest that additional studies with larger sample sizes and standardized protocols should be explored to determine exactly how useful plasma or serum GFAP can be as a biomarker for COVID-19-associated cognitive impairment.
Neurofilaments (Nfs) are classified as type IV intermediate filaments that provide cytoskeletal stability and allow for radial growth of neurons (Yuan et al., 2017; Gaetani et al., 2019). Under normal conditions, axons release neurofilament light chain (NfL), the most abundant subunit of Nfs, into the blood at low levels, and this has been found to increase with age (Yuan et al., 2017; Gaetani et al., 2019; Zanella et al., 2022). Moreover, during neurological degeneration or injury, these levels increase significantly indicating its potential use as a biomarker of neuroaxonal injury (Zanella et al., 2022). Elevated levels of NfL in serum have been associated with various neuropathologies such as cognitive decline, TBI, AD, PD, MS, Lewy body dementia (LBD), frontotemporal dementia (FTD), Amyotrophic Lateral Sclerosis (ALS), and Huntington Disease (HD) (Palermo et al., 2020; Rajan et al., 2020; Verde et al., 2021; Chouliaras et al., 2022; Ebenau et al., 2022; Newcombe et al., 2022; Thijssen et al., 2022).
Studies examining various COVID-19 patient populations have also found elevated plasma and or serum levels of NfL (Ameres et al., 2020; Kanberg et al., 2020, 2021; Aamodt et al., 2021; De Lorenzo et al., 2021; Prudencio et al., 2021; Frontera et al., 2022; Hanson et al., 2022; Verde et al., 2022). Similarly to GFAP, NfL was elevated in hospitalized patients who had COVID-19 encephalopathy (Hanson et al., 2022). NfL also correlates with disease severity as elevated plasma levels were found in COVID-19 patients that died during hospitalization (Aamodt et al., 2021; Frontera et al., 2022). When compared to the control group consisting of non-COVID-19 AD patients, the COVID-19 patients exhibited higher levels of NfL (Frontera et al., 2022). COVID-19 patients who did not have obvious signs or symptoms of cognitive dysfunction also had elevated levels of serum NfL (Prudencio et al., 2021; Verde et al., 2022). In another group of intensive care unit (ICU) COVID-19 patients, those who did not survive the infection had higher levels of NfL when compared to those who survived (Aamodt et al., 2021). Report shows that after 30-70 days, plasma NfL levels increased persistently and then normalized after six months in COVID-19 patients who continued to report the presence of neurological symptoms (Kanberg et al., 2021). Also plasma NfL levels were found to be increased from the first follow-up to the last in the severe group (Kanberg et al., 2020). Both studies support their shared hypothesis of delayed axonal injury occurring in severe COVID-19 patients, while astrocyte activation occurs earlier and is not limited to the severe COVID-19 patient population (Kanberg et al., 2020, 2021). The elevated concentrations of plasma NfL found in these COVID-19 patient populations warrants further investigation to explore its neuropathological mechanism causing neuroaxonal injury to determine whether it can be a contributing factor to the cognitive sequelae that arises in post-COVID-19 infections.
The soluble tau proteins are important for maintaining neuronal microtubule integrity by providing stability and encouraging assembly (Wang and Mandelkow, 2016). The post-translational phosphorylation of tau is necessary for the protein to change its confirmation to operate under physiological conditions (Wang and Mandelkow, 2016; Kent et al., 2020). However, when phosphorylated excessively, the tau proteins dissociate from microtubules and aggregate with one another becoming insoluble and ultimately leading to extensive networks of neurofibrillary tangles (NFTs), which are characteristic of AD pathology (Metaxas and Kempf, 2016). The neurogenerative diseases distinctively express the hyperphosphorylation of Tau and subsequent aggregation are altogether known as tauopathies (Wang and Mandelkow, 2016). The role of p-tau 181 has been explored extensively in AD pathology and has been found to predict cognitive decline and AD several years before diagnosis and/or death (Guo et al., 2017; Lantero Rodriguez et al., 2020; Moscoso et al., 2021; Smirnov et al., 2022). These findings support p-tau 181 as a promising blood biomarker for AD and the potential for its application in other tauopathies.
Elevated levels of serum and/or plasma p-tau 181 have been found in COVID-19 patients (Sun et al., 2021; Frontera et al., 2022). COVID-19 patients who did not survive the infection and those who developed COVID-encephalopathy had elevated levels of p-tau 181, along with NfL and GFAP as mentioned previously. The study also found that hospitalized COVID-19 patients who experienced new cognitively related symptoms also had elevated levels p-tau 181 when compared to patients who did not experience additional cognitive sequelae (Frontera et al., 2022). In a study that examined the contents of neuronal-enriched extracellular vesicles (nEVs) in the plasma of COVID-19 patients, elevated levels of p-tau 181 were found and these levels also had a significant correlation with NfL in patients who reported neurological sequelae (Sun et al., 2021). There is some evidence suggesting that COVID-19 worsens pathology that has been implicated in AD such as tau, β-amyloid aggregation, neuroinflammation cerebral ischemia, and disruption of the BBB (Miners et al., 2020; Shen et al., 2022). Therefore, these findings suggest that p-tau 181 is yet another neurodegenerative biomarker correlated with COVID-19 disease severity during and/or following SARS-CoV-2 infection.
Ubiquitin is a regulatory protein that is widely known for its role in the ubiquitin-proteasome system (UPS), which is involved in cellular processes including protein degradation, DNA repair and cell trafficking (Bishop et al., 2016; Guo and Tadi, 2022). In neurons, ubiquitination is involved in regulating the function of various synapses influencing their maintenance, transmission, and plasticity by altering the quantity of proteins at each synapse (Mabb and Ehlers, 2010). Deubiquinating enzymes known as deubiquitinases (DUBs) are responsible for removing ubiquitin from their target proteins (Bishop et al., 2016). Ubiquitin C-terminal hydrolase L1 (UCH-L1) is a specific member of this group that is highly expressed in neurons (Bishop et al., 2016). Higher levels of plasma UCH-L1 have been associated with PD (Ng et al., 2020), AD (Bogdan et al., 2022), Friedreich’s Ataxia (Zeitlberger et al., 2018), TBI (Wang et al., 2018), and general cognitive capabilities (Zhang et al., 2022a). The loss of UCH-L1 has resulted in the loss of neurons and instability of axons, while in some cases oxidatively modified UCH-L1 may aggregate (Bishop et al., 2016). These findings support that UCH-L1 is utilized as a general marker of neurodegeneration and other CNS-related complications.
Various COVID-19 patient populations have also experienced elevated levels of plasma UCH-L1 (Cooper et al., 2020; Frontera et al., 2022). It was found to correlate with COVID-19 disease severity as it was significantly higher in COVID-19 patients with encephalopathy (Frontera et al., 2022). As mentioned previously, hospitalized COVID-19 patients who experienced new neurological symptoms during admission had elevated plasma levels of UCH-L1, p-tau 181, and NfL, when compared to the control group consisting of non-COVID AD patients (Frontera et al., 2022). A group of ICU COVID-19 patients were also found to have higher levels of UCH-L1 that was also associated with delirium (Cooper et al., 2020). In another group of COVID-19 patients, UCH-L1 and NfL yielded predictive values on whether patients required a transfer to the ICU (De Lorenzo et al., 2021). Hence, it remains a possibility that UCH-L1 may be used as a prognostic biomarker when combined with others in providing potential clinical outcomes in COVID-19 patients (De Lorenzo et al., 2021).
The S100 protein family consists of proteins that principally bind Ca2+ and are named S100 because they dissolve in a neutral pH solution consisting of 100% saturated ammonium sulfate (Sedaghat and Notopoulos, 2008). A frequently investigated member of this protein family, S100 calcium-binding protein B (S100B), is used to describe levels of both the heterodimer S100AB and homodimer S100BB (Thelin et al., 2017). Serum levels of S100B may indicate astrocyte damage or injury, though with less accuracy than GFAP due to its more extensive distribution throughout different cell types of the CNS (Steiner et al., 2007). The function of S100B also differs depending on the concentration, having cytotoxic effects when increased (micromolar) and neuroprotective effects when at lower levels (nanomolar) in serum (Lam et al., 2013). S100B has been implicated in several neurological disorders including TBI (Thelin et al., 2017; Mondello et al., 2021), AD (Cristovao and Gomes, 2019), PD (Angelopoulou et al., 2021), ALS (Serrano et al., 2017), and MS (Barateiro et al., 2016). Micromolar levels of S100B is reported to cause such disorders by activating astrocytes and microglia, inducing nitric oxide (NO) release, increasing reactive oxygen species (ROS) and ultimately leading to neuroinflammation and loss of neurons (Michetti et al., 2019). These findings support that S100B is continuing to be explored as a potential blood biomarker across neurological disorders.
Elevated levels of circulating S100B has also been found in different groups of COVID-19 patients (Aceti et al., 2020; Mete et al., 2021; Savarraj et al., 2021; Sahin et al., 2022). As mentioned with previous brain injury biomarkers, serum S100B has also been associated with disease severity in COVID-19 patients (Aceti et al., 2020; Mete et al., 2021). Report show that levels of serum S100B were not correlated with neurological symptoms overall in acute phase COVID-19 patients, but did find marginally elevated levels of S100B in patients with more than one neurological symptom (Sahin et al., 2022). This may suggest that the elevated levels of S100B represents CNS injury to some extent during the acute phase of COVID-19, but it is unclear what implications this may have both clinically and in long-COVID patients. S100B has also been described as a pro-inflammatory ligand by binding to the Receptor for Advanced Glycation Endproducts (RAGE), which itself has been associated with neuroinflammation following neurological insult (Michetti et al., 2019). These findings may partly explain the association of elevated levels S100B with both disease severity and cognitive sequelae in COVID-19 infection. The exact role of S100B in COVID-associated cognitive dysfunction remains to be discovered to confirm whether it is directly contributing to neuropathological damage and/or it is a reaction of downstream inflammatory processes.
Neuron specific enolase (NSE) is the gamma isozyme named due to its specificity for neuronal and neuroendocrine cells (Haque et al., 2018). NSE itself can be expressed as two different isozymes in neurons as either γγ or αγ (Polcyn et al., 2017). In astrocytes, NSE is expressed as γγ and in oligodendrocytes and microglia it is found as the αγ subunits (Polcyn et al., 2017). NSE has therefore been linked to both neurological and non-neurological pathologies due to its tissue specificity and upregulation following axonal injury (Polcyn et al., 2017). Similar to S100B, NSE can be both neuroprotective or neuroinflammatory depending on surrounding conditions such as the typical homeostatic environment, disease state, or presence of injury (Polcyn et al., 2017). The neuropathological conditions that have been associated with altered levels of plasma or serum NSE include AD (Chaves et al., 2010), HD (Ciancarelli et al., 2014), postoperative cognitive dysfunction (POCD) (Wan et al., 2021), and beta-propeller protein-associated neurodegeneration (BPAN) (Takano et al., 2017).
Neuron specific enolase levels have also been found at higher concentrations in the serum or plasma of COVID-19 patients (Wei et al., 2020; Cione et al., 2021; Savarraj et al., 2021). Increased levels of serum NSE has also been associated with disease severity as mentioned previously with other biomarkers specifically in a cohort of COVID-19 patients who developed dyspnea (Cione et al., 2021), in critical cases of COVID-19 patients (Wei et al., 2020), and in patients immediately following hospital admission for COVID-19 infection (Savarraj et al., 2021). A unique observation found was that serum NSE was found at significantly higher levels in men when compared to women, which may support increased susceptibility to COVID-associated cognitive dysfunction in men (Savarraj et al., 2021). NSE may be elevated in COVID-19 due to the potential presence of axonal injury (Polcyn et al., 2017), lung injury (Cione et al., 2021), neuroinflammation (Haque et al., 2018), or a combination of all. There is not enough data at this time to concretely validate the use of serum NSE as a biomarker of only neurodegeneration and/or prognosis in COVID-19 infection. Further investigation are required to support these findings and uncover the exact role of NSE in COVID-29 infection.
Pro-inflammatory cytokines, such as interleukin (IL)-6, IL-1β, and tumor necrosis factor-α (TNF-α) are well established as major contributing factors to neuropathological diseases (Wang et al., 2015; Gupta et al., 2020b; Xin et al., 2021; Lu et al., 2022). Anti-inflammatory cytokines such as IL-10 also play crucial roles in brain injury and neuroinflammation due to its ability to suppress inflammatory responses (Garcia et al., 2017; Burmeister and Marriott, 2018; Lu et al., 2022; Sanchez-Molina et al., 2022). C-reactive protein (CRP), a non-specific marker of systemic inflammation, is induced by pro-inflammatory cytokines such as IL-6, and has been associated with chronic inflammation, and various degrees of cognitive dysfunction (Watanabe et al., 2016; Luan and Yao, 2018; Vintimilla et al., 2019; Gupta et al., 2020b; Xin et al., 2021). A cascade of events, referred to as “cytokine storm” or “cytokine release syndrome (CRS),” has been implicated in neurotoxicity, disruption of the integrity of BBB, neuroglial cells activation, and ultimately neuroinflammation (Gupta et al., 2020b; Zhang et al., 2022b).
In COVID-19 patients, elevated serum levels of IL-6, IL-1β, TNF- α, IL-10, and CRP have been measured and discussed (Chen et al., 2020; Han et al., 2020; Islam et al., 2021a; Lavillegrand et al., 2021; Lu et al., 2021; Acosta-Ampudia et al., 2022; Ferrando et al., 2022; Hirzel et al., 2022; Leretter et al., 2022; Montazersaheb et al., 2022; Peluso et al., 2022; PHOSP-COVID Collaborative Group, 2022; Schultheiss et al., 2022). IL-6, CRP, and IL-10 and have also been associated with disease severity amongst COVID-19 patients, correlating with severe clinical outcomes and fatality (Han et al., 2020; Lavillegrand et al., 2021; Alshammary et al., 2022; Ashktorab et al., 2022; Azaiz et al., 2022; Galliera et al., 2022; Jafrin et al., 2022; Mainous et al., 2022). CRP was found specifically to be positively correlated with two parts of a Continuous Performance Test (CPT) conducted in patients who had resolved COVID-19 infections (Zhou et al., 2020a) and was found at significantly elevated concentration in COVID-19 patients with moderate to severe cognitive dysfunction (Arica-Polat et al., 2022). Even though vast amount of evidence continues to validate the role of hyperinflammation in SARS-CoV-2 infection, there is a clear need for additional studies to explore the role of these inflammation in the CNS in COVID-19 patient populations.
Growing evidence suggests that as post-transcriptional regulators of gene expression, miRNAs are involved in physiological and pathological processes and their dysregulation in function is synonymous with a multiplicity of diseases (Condrat et al., 2020; Carini et al., 2021). The prominent role of non-coding microRNAs in CNS and their signature in the circulation has been well established in various neurodegenerative diseases (Thounaojam et al., 2013; Bahlakeh et al., 2021; Islam et al., 2021c; Perdoncin et al., 2021; Blount et al., 2022). Hence, miRNAs as possible therapeutic targets and disease markers for early diagnosis have strongly been advocated because of their stability and convenience in extraction from biological fluids (Szelenberger et al., 2019). In this section of the review, we are showcasing the importance of some dysregulated miRNAs in COVID-19 and their possible correlation with CNS to further explore the mechanism of COVID-19- associated cognitive dysfunction. A summary of the findings are illustrated in Table 2.
Table 2. Summary of circulating miRNAs associated with neurological dysfunction showing potential dysregulation in COVID-19.
miR-146a is produced by bone marrow mesenchymal stem cells and released in exosome granules, then it is taken up by activated astrocytes, particularly in the hippocampal region, indicating it may serve a neuroprotective role in seizure-related cognitive dysfunction (Kong et al., 2015). miR-146a exerts an anti-inflammatory effect by inactivating NF-κB activity through a reduction of interleukin-1 receptor-associated kinase 1 (IRAK1) and tumor necrosis factor associated factor 6 (TRAF6) (Nakano et al., 2020a,b). This will further lead to the supression of NF-κB’s target genes such as the interleukins IL-6, IL-8, IL-1β, and TNF alpha (TNF-α) (Saba et al., 2014; Fan et al., 2020). Increased expression of miR-146a in brain endothelial cells alters cytokine signaling and reduces NF-κB activity by reducing its nuclear translocation and thus decreasing the number of expressed T-cell adhesion molecules and limiting their entry into the CNS in the development of neuroinflammation (Wu et al., 2015). Circulating miR-146a is reduced in the blood of AD (Kumar and Reddy, 2016; Swarbrick et al., 2019), reducing the capability to deal with neurodegenerative inflammation. Overexpression of miR146a in microglia has been shown to reduce cognitive deficits in learning and memory, attenuate neuroinflammation, reduce beta-amyloid levels, and alleviate plaque-associated neuritic pathologies. miR-146a also influences microglial phenotype switching, allowing for the reduction of pro-inflammatory cytokine production and improved phagocytic functions in the clearance of beta-amyloid and tau (Liang et al., 2021). Thus, serum miR-146a levels are being considered for clinical use as a biomarker for neurodegenerative diseases (Kumar and Reddy, 2016; Mai et al., 2019; Swarbrick et al., 2019; Sabbatinelli et al., 2021).
Cumulative evidences suggest that decreased expression of miR-146a is associated with SARS-CoV-2 infection (Keikha and Jebali, 2021; Roganovic, 2021; Sabbatinelli et al., 2021). Furthermore, individuals with pre-existing inflammatory conditions which cause a decreased expression of miR-146a are thus predisposed to COVID-19 infection, and at risk for more serious progression of the illness (Roganovic, 2021; Sudhakar et al., 2022). Interestingly, COVID-19 patients showed a down-regulation of this anti-neuroinflammatory miRNA, which in turn causes an increase in expression of IL-6, IL-8, IL-17, and other inflammatory cytokines (Arghiani et al., 2021; Roganovic, 2021). Increased levels of the inflammatory cytokine IL-6 thus reduce the effectiveness of many drugs being currently tested for use against COVID, such as tocilizumab, because they act as antibodies against these inflammatory cytokines (Arghiani et al., 2021; Sabbatinelli et al., 2021). Conversely, some studies have shown that the expression of miR-146a is increased in COVID-19 patients when compared to healthy controls (Donyavi et al., 2021; Pinacchio et al., 2022). miR-146a is therefore uniquely can be positioned as one of a handful of micro-RNAs suited for use as a biomarker for cognitive dysfunction and SARS-CoV-2 infection and as a potential therapeutic for the treatment of those disease states. Given the altered expression of miR-146a in neurodegenerative diseases as well as in SARS-CoV-2 infection, and its role as an anti-neuroinflammatory microRNA, future studies with large populations may help to elucidate the actual mechanism of action of miR-146a in COVID-19 mediated cognitive decline and its importance as a circulating prognostic marker.
CNS upregulation of miR-155 has been associated cognitive dysfunction and is the most expressed chromosome 21 miRNA in Down’s Syndrome dementia, as it is co-expressed with hyperphosphorylated tau protein (Tili et al., 2018). miR-155 act as a prevalent CNS pro-inflammatory mediator and microglia activator by regulating inflammatory cytokines such as (IFN)-λ and IFN-β (Thounaojam et al., 2013). In the CNS, the action of miR-155 is mediated in microglia and macrophages through NF-κB following stimulation of the appropriate TLR and interferon-gamma release. It also causes a reduction in the anti-inflammatory response by targeting anti-inflammatory regulators such as Suppressor of Cytokine Signaling 1 (SOCS1), SH2 Domain-Containing Inositol 5’-Phosphatase1 (SHIP1), activator protein 1, Signal Transducers and Activators of Transcription 5 (STAT5) and IL-13 Receptor Alpha 1 (IL13Ra1), further increasing inflammation (Song and Lee, 2015; Zingale et al., 2021). miR-155 increases BBB permeability by targeting cell–cell complex molecules including claudin-1 and annexin-2 and focal adhesion components such as syntenin-1 and dedicator of cytokinesis 1 (DOCK-1) (Lopez-Ramirez et al., 2014). miR-155 is also associated with promotion of CNS T cell responses and the subsequent development of cognitive dysfunction symptomology. Through regulation of interferon-gamma signaling, miR-155 can influence CD8 + T cell activation, T cell development, various cell to cell interactions, and macrophage activation. T cell activation and IFN-y production, followed by infiltration into the CNS, results in the deposition of beta-amyloid and thus cognitive dysfunction (Song and Lee, 2015). The pro-neuroinflammatory role of miR-155 was affirmed through knockout studies which show reduced neuroinflammation, reduced cognitive impairment and better recovery in traumatic brain injury mouse models (Henry et al., 2019). Moreover, miR-155 overexpression is implicated in CCR2/CXCL2 translation disorders, causing impaired cell migration and clearance of beta-amyloid by blood-derived monocytes (BDMs) and monocyte-derived macrophages (MDMs) in AD (Guedes et al., 2016). The pro-inflammatory function of miR-155 also carries over into auto-immune conditions such as MS-associated cognitive dysfunction by promoting inflammatory damage to the neurovascular units of the blood-brain-barrier via down regulation of junctional proteins (Maciak et al., 2021). miR-155 contributes to CNS demyelination via microglia activation and subsequent production of TNF-a, IL-1, IL-6, interferon-inducible protein 10 (IP-10), macrophage inflammatory protein-1a (MIP-1a), monocyte chemoattractant protein-1 (MCP-1), and nitric oxide (NO) (Miller, 2012). It is reported that miR-155 mediated impairment to the BBB, damage of myelin and axons, synaptic dysfunction, and dysregulated neurotransmitter production due to acute inflammation can lead to brain atrophy and progressive cognitive impairment (Varma-Doyle et al., 2021).
Serum miR-155 levels are significantly increased during the acute and post-acute phases of SARS-CoV-2 infection (Bautista-Becerril et al., 2021; Abbasi-Kolli et al., 2022). The study which showed significantly increased expression of miR-155 in the peripheral blood mononuclear cell (PBMC) samples of patients with acute COVID-19 infection suggest it as a diagnostic marker for COVID-19 (Abbasi-Kolli et al., 2022). Similarly, another study demonstrates miR-155 as a biomarker to distinguish acute from post-acute phase of COVID-19 disease (Donyavi et al., 2021). Additionally, plasma miR-155 levels appear to be significantly correlated with chest CT findings, CRP and ferritin levels, mortality, d-dimer, WBC count and neutrophil percentage. miR-155 levels are 90% sensitive and 100% specific when used as a biomarker for the detection of COVID-19 and are 76% sensitive and specific for detection of severity of COVID-19 disease (Haroun et al., 2022). Overexpression of miR-155 in SARS-CoV-2 infection thus may partially explain the enhanced immune response that leads to CNS damage in the context of covid-associated cognitive dysfunction.
Let-7b a multifunctional miRNA that is differentially expressed in issues of cognitive dysfunction in comparison to healthy individuals (Rahman et al., 2020; Yuen et al., 2021). Levels of Let-7b appear to be increased in diseases of cognitive dysfunction such as MCI (Kenny et al., 2019), AD (Leidinger et al., 2013), and PD (Huang et al., 2021b). Overexpression of Let-7b in AD models was found to significantly reduce cell viability, inhibit autophagy and increase apoptosis through increased cleavage of caspase 3 and through increased expression levels of PI3K, p-AKT, and p-mTOR in upstream signaling pathways (Pang et al., 2022). Let-7b also appears to be involved in neurodegeneration through interaction with toll-like receptor 7 (TLR7) (Lehmann et al., 2012). TLR7 mediated pathway of Let-7b action is additionally seen in the postmortem hippocampal formations of alcoholics, where TLR7 and Let-7b expression was increased, leading to neuroinflammation and neurodegeneration (Coleman et al., 2017). Additional studies have also shown the role of Let-7b and TLR7 mediated mechanism of alcohol-associated cognitive dysfunction (Qin et al., 2021). Let-7b has been shown to regulate the function of high mobility group AT-hook 2 (HMGA2) protein in PD, causing a dysregulation of chromatin structure and transcription which leads to decreased self-renewal of neuronal stem cells, leading to neurodegeneration (Huang et al., 2021b).
Let-7b levels are elevated in peripheral blood samples in both the acute and post-acute stages of SARS-CoV-2 infection compared to healthy individuals (Donyavi et al., 2021; Nain et al., 2021), suggesting potential use as a clinical biomarker COVID-19 infection. Let-7b targets ACE2 causing dysregulation of ACE2 and potentially increasing susceptibility to SARS-CoV-2 infection, making it a potential target for therapeutic treatment of SARS-CoV-2 infection (Bellae Papannarao et al., 2022). Let-7b, in the context of SARS-CoV-2 infection, increases apoptosis through a reduction of BCL-2, an anti-apoptotic protein, and through modulation of immune responses, establishing a potential link between chronic inflammatory illness such as type 2 diabetes and COVID-19 (Islam et al., 2021b), which may have effect on cognitive dysfunction. Reports shows showcase Let-7b as a marker of lung disease which is highly prevalent in COVID-19 (Islam et al., 2021b; Nain et al., 2021). These reported upregulation of Let-7b in SARS-CoV-2 infection suggest its possible link with cognitive dysfunction that warrants future studies.
miR-31 is decreased in the serum of AD individuals compared to healthy controls (Dong et al., 2015; Klyucherev et al., 2022) and can be used as part of a panel in conjunction with miR-93 and miR-146a to differentiate Alzheimer’s Disease from Vascular Dementia (Dong et al., 2015). Expression of miR-31 was also decreased in the serum of PD and Multiple System Atrophy (MSA) individuals (Yan et al., 2020). Letiviral delivery of miR-31 was able to significantly ameliorate AD neuropathology by reducing Aβ deposition in both the hippocampus and subiculum of transgenic mice models (Barros-Viegas et al., 2020). The study shows that miRNA-31 targets amyloid precursor protein (APP) and β-secretase (BACE1), which further abolishes the pathogical aletrations in AD. The results showed improvements in memory deficits, reduced anxiety, and reduced cognitive inflexibility, suggesting future possibilities for miR-31 to be used as a therapeutic in the treatment of AD (Barros-Viegas et al., 2020). RhoA has been reported to modulate synaptic plasticity and inhibition of the RhoA pathway reduces cognitive impairment and deficits in synapses and dendritic spines (Pearn et al., 2018). miR-31 has been reported as a regulator of RhoA and decrease in miR-31 plays a role in the development of cognitive dysfunction in learning, memory, behavior, etc., patterns which are similarly seen in neurodegenerative disease (Qian et al., 2021). miRNA target prediction analysis have shown some PD- and MSA-related genes such as parkin E3 ubiquitin-protein ligase (PARK2), GRB10-interacting GYF protein 2 (GIGYF2) as potential target of miR-31 (Yan et al., 2020).
miR-31 is multifunctional in the context of COVID-19, particularly when discussing hypoxia and potential resultant neurodegenerative effects. miR-31 serum expression levels have been demonstrated to be decreased in COVID-19 infected patients (Bautista-Becerril et al., 2021). Expression levels of miR-31 appear to decrease with worsening severity of illness with COVID-19 infection also, making it a worthwhile candidate for use as a biomarker of COVID-19 infection and severity (Keikha et al., 2021). Decreased expression may play a role in the neurodegenerative pathologies seen with enhanced micro coagulation in SARS-CoV-2 infected individuals. Thus, decreased levels of miR-31 appear to have a multifactorial effect in cognitive dysfunction and in SARS-CoV-2 infection and presents as a potential link between the two disease processes. Conversely, microRNA expression profile analysis of another set of COVID-19 patients showed up-regulation of miR-31 expression (Farr et al., 2021). Hence, future studies with more population size and more detailed mechanistic approach may clarify the exact role of miR-31 in COVID-19-associated cognitive decline.
miR-16 is differentially expressed in various neurodegenerative diseases, with its levels being significantly decreased in the serum of AD patients (Denk et al., 2018; Mckeever et al., 2018). miR-16 regulates cell death in AD by targeting APP (Liu et al., 2012; Zhang et al., 2015; Turk et al., 2021). Downregulation of miR-16 in hippocampal neurons has been associated with increases in APP eventual processing to beta-amyloid followed by deposition into neurons within the brain (Zhang et al., 2015; Grinan-Ferre et al., 2018). In cell culture studies, miR16 has been shown to regulate Aβ production, and Tau phosphorylation (Hebert et al., 2012). Similarly report shows a reduction of the expression of a number of genes related to AD including APP, BACE1, tau, inflammation and oxidative stress through the delivery of miR-16 mimics directly to mouse brain (Parsi et al., 2015). The role of miR16 in targeting genes involved in neurite extension and branching in hippocampal neurons during presymptomatic prion disease has also been reported (Burak et al., 2018). miR-16 appears to have potential for future drug development because it simultaneously targets various endogenous targets of AD biomarkers. These findings suggest that further research is needed into the role of miR-16 in other forms of neurodegenerative diseases to evaluate its impact more completely on cognitive function.
Serum miR-16 levels are likewise reduced in COVID-19 infected patients suggesting a potential link between differential miR-16 expression and covid-associated cognitive dysfunction. miR-16 levels were established to be inversely correlated with length of ICU stay in COVID-19 infected patients as well, opening the possibility for its use as a biomarker of disease and severity (De Gonzalo-Calvo et al., 2021). Also, miR-16 has already been used as a biomarker for other viral respiratory illnesses such as community acquired pneumonia (Galvan-Roman et al., 2020). miR-16 is capable of high affinity binding to the SARS-CoV-2 genome, opening future avenues of potential drug research (Kim et al., 2020; Nersisyan et al., 2020). A single-cell RNA-sequencing based study identified miR-16 as a potential virus targeting miRNAs across multiple cell types from bronchoalveolar lavage fuid samples (Li et al., 2022b). Down regulation of miR-16 levels in individuals with SARS-CoV-2 suggests miR-16 may play a role in covid-associated cognitive dysfunction due to its previously defined role in other forms of neurodegenerative cognitive dysfunction.
miR-21 is extensively involved in processes governing apoptosis and neuroinflammation in neurodegenerative diseases and thus in cognitive dysfunction (Bai and Bian, 2022). Serum and CSF levels of miR-21 are decreased in patients with AD compared to individuals with Lewy Body Dementia and healthy controls (Gamez-Valero et al., 2019). miR-21 acts as an anti-inflammatory microRNA by acting as a negative feedback regulator on NF-κB in response to pro-inflammatory signaling (Ma et al., 2011). miR-21 has been shown to ameliorate cognitive impairments associated with brain injury from subarachnoid hemorrhaging by modulation of the PTEN/AKT pathway and reducing apoptosis in the hippocampus and prefrontal cortex (Gao et al., 2020). Use of miR-21 mimics in cell culture studies of AD has shown that miR21 is capable of inhibiting beta-amyloid induced apoptosis by increasing expression of PI3K, AKT, and GSK-3B (Feng et al., 2018). Overexpression of miR-21 was demonstrated to protect neurons of the hippocampus in epileptic rat studies by inhibiting STAT3 (Bai and Bian, 2022). Microglial miR-21 has been reported to protect neurons from cell death under hypoxic conditions (Zhang et al., 2012). miR-21 has been shown to restore neurogenesis and reverse cellular senescence via inhibition of the mTOR1 pathway in models of vascular dementia, making a candidate as a potential therapeutic in the treatment of vascular dementia and its associated cognitive impairment (Blount et al., 2022).
Serum miR-21 levels have been reported to be decreased in COVID-19 infected individuals (Li et al., 2020; Sabbatinelli et al., 2021). The down-regulation in the relative expression of miR-21 in COVID-19 patients was concomitant with up-regulation of its target proinflammatory genes (Keikha and Jebali, 2021). The study also demonstrate miR-21 as an anti- neuroinflammatory miRNA, for correlating the disease grade from asymptomatic to critical illness in COVID-19. Down regulation of miR-21 in COVID-19 patients exacerbates systemic inflammation through hyperactive immune response, loss of T cell function, and immune dysregulation (Tang et al., 2020). Increased systemic inflammation can weaken the blood-brain-barrier, causing heightened neuroinflammation and resulting in neurodegeneration. Thus, decreases in expression of miR-21 could directly and indirectly contribute to the development and progression of covid-associated cognitive dysfunction, or worsening of pre-existing cognitive dysfunction after infection with SARS-CoV-2.
The different sequelae presented by post-COVID-19 patients are being increasingly studied from the improvement of clinical and laboratory experience. Cumulative evidences suggest that surviving patients of COVID-19 have a high risk of developing some neuropsychiatric impairment, which can occur in different forms, such as cases of depression, anxiety, and severe mental illness. The sequelae related to cognitive decline and neurodegeneration are diverse and there is a need for detailed assessments to identify new neurological conditions. An extremely relevant factor to be considered in the fight against COVID-19 is the use of biomarkers in the early recognition of patients susceptible to developing the severe form of the disease. The discovery of potential markers could be used to provide essential information that will assist in stratifying these patients, improving primary care, and developing optimal individualized therapy according to the patient’s response to cognitive damage. The review summarizes the neuropathological changes associated with COVID-19 and signifies the importance of circulating biomarkers and miRNAs in these neurodegenerative changes (Figure 3). Thus, the clinical use of the markers reported in this review will significantly improve the development of new policies to prevent, address and manage the neurological conditions caused by SARS-CoV-2 infection and may aid in future research exploring the mechanistic aspects of COVID-19 associated neurodegeneration and cognitive dysfunction.
Figure 3. Schematic representation of the main mechanisms involved in the neurophysiology caused by SARS-CoV2 virus and the role of biomarkers and miRNAs in neuronal damage. The SARS-CoV2 virus has the ability to infect brain tissue by mechanisms involving the olfactory and hematological pathway, which will lead to an oxidative and inflammatory state in nervous tissue, due to the activation of neutrophils, astrocytes, and microglial cells releasing excessive ROS and pro-inflammatory molecules. The imbalance in brain homeostasis leads to a dysfunction in the expression pattern of different biomarkers and miRNAs, potentiating neuroinflammatory mechanisms, responsible for brain damage and the consequent progression of cognitive dysfunction and neurodegenerative disorders. GFAP, Glial fibrillary acid protein; NFL, Neurofilament light chain; P-tau-181, Phosphorylated tau at threonine-181; UCH-L1, Ubiquitin Carboxy-Terminal Hydrolase L1; S100B, S100 calcium-binding protein B; NSE, Neuron Specific Enolase.
SP: conceptualization, project administration, and writing—review and editing. KS: conceptualization and project administration. MA, ET, BG, DP, RP, and NF: writing—original draft preparation. All authors contributed to the article and approved the submitted version.
The authors declare that the research was conducted in the absence of any commercial or financial relationships that could be construed as a potential conflict of interest.
All claims expressed in this article are solely those of the authors and do not necessarily represent those of their affiliated organizations, or those of the publisher, the editors and the reviewers. Any product that may be evaluated in this article, or claim that may be made by its manufacturer, is not guaranteed or endorsed by the publisher.
Aamodt, A. H., Hogestol, E. A., Popperud, T. H., Holter, J. C., Dyrhol-Riise, A. M., Tonby, K., et al. (2021). Blood neurofilament light concentration at admittance: A potential prognostic marker in COVID-19. J. Neurol. 268, 3574–3583. doi: 10.1007/s00415-021-10517-6
Abbasi-Kolli, M., Sadri Nahand, J., Kiani, S. J., Khanaliha, K., Khatami, A., Taghizadieh, M., et al. (2022). The expression patterns of MALAT-1, NEAT-1, THRIL, and miR-155-5p in the acute to the post-acute phase of COVID-19 disease. Braz. J. Infect. Dis. 26:102354. doi: 10.1016/j.bjid.2022.102354
Abdelhak, A., Foschi, M., Abu-Rumeileh, S., Yue, J. K., D’anna, L., Huss, A., et al. (2022). Blood GFAP as an emerging biomarker in brain and spinal cord disorders. Nat. Rev. Neurol. 18, 158–172. doi: 10.1038/s41582-021-00616-3
Aceti, A., Margarucci, L. M., Scaramucci, E., Orsini, M., Salerno, G., Di Sante, G., et al. (2020). Serum S100B protein as a marker of severity in Covid-19 patients. Sci. Rep. 10:18665. doi: 10.1038/s41598-020-75618-0
Acosta-Ampudia, Y., Monsalve, D. M., Rojas, M., Rodriguez, Y., Zapata, E., Ramirez-Santana, C., et al. (2022). Persistent autoimmune activation and proinflammatory state in post-coronavirus disease 2019 syndrome. J. Infect. Dis. 225, 2155–2162. doi: 10.1093/infdis/jiac017
Alshammary, A. F., Alsughayyir, J. M., Alharbi, K. K., Al-Sulaiman, A. M., Alshammary, H. F., and Alshammary, H. F. (2022). T-Cell subsets and interleukin-10 levels are predictors of severity and mortality in COVID-19: A systematic review and meta-analysis. Front. Med. (Lausanne) 9:852749. doi: 10.3389/fmed.2022.852749
Ameres, M., Brandstetter, S., Toncheva, A. A., Kabesch, M., Leppert, D., Kuhle, J., et al. (2020). Association of neuronal injury blood marker neurofilament light chain with mild-to-moderate COVID-19. J. Neurol. 267, 3476–3478. doi: 10.1007/s00415-020-10050-y
Amruta, N., Chastain, W. H., Paz, M., Solch, R. J., Murray-Brown, I. C., Befeler, J. B., et al. (2021). SARS-CoV-2 mediated neuroinflammation and the impact of COVID-19 in neurological disorders. Cytokine Growth Factor Rev. 58, 1–15. doi: 10.1016/j.cytogfr.2021.02.002
Angelopoulou, E., Paudel, Y. N., and Piperi, C. (2021). Emerging role of S100B protein implication in Parkinson’s disease pathogenesis. Cell Mol. Life Sci. 78, 1445–1453. doi: 10.1007/s00018-020-03673-x
Anka, A. U., Tahir, M. I., Abubakar, S. D., Alsabbagh, M., Zian, Z., Hamedifar, H., et al. (2021). Coronavirus disease 2019 (COVID-19): An overview of the immunopathology, serological diagnosis and management. Scand. J. Immunol. 93:e12998. doi: 10.1111/sji.12998
Arghiani, N., Nissan, T., and Matin, M. M. (2021). Role of microRNAs in COVID-19 with implications for therapeutics. Biomed. Pharmacother. 144:112247. doi: 10.1016/j.biopha.2021.112247
Arica-Polat, B. S., Gundogdu, A. A., Cinar, N., Uncu, G., Ayas, Z. O., Iseri, P., et al. (2022). Evaluation of cognitive deficits in patients infected with COVID-19. Eur. Rev. Med. Pharmacol. Sci. 26, 678–685.
Ashktorab, H., Pizuorno, A., Adeleye, F., Laiyemo, A., Dalivand, M. M., Aduli, F., et al. (2022). Symptomatic, clinical and biomarker associations for mortality in hospitalized COVID-19 patients enriched for African Americans. BMC Infect. Dis. 22:552. doi: 10.1186/s12879-022-07520-1
Azaiz, M. B., Jemaa, A. B., Sellami, W., Romdhani, C., Ouslati, R., Gharsallah, H., et al. (2022). Deciphering the balance of IL-6/IL-10 cytokines in severe to critical COVID-19 patients. Immunobiology 227:152236. doi: 10.1016/j.imbio.2022.152236
Azer, S. A. (2020). COVID-19: Pathophysiology, diagnosis, complications and investigational therapeutics. New Microbes New Infect. 37:100738. doi: 10.1016/j.nmni.2020.100738
Bahlakeh, G., Gorji, A., Soltani, H., and Ghadiri, T. (2021). MicroRNA alterations in neuropathologic cognitive disorders with an emphasis on dementia: Lessons from animal models. J. Cell Physiol. 236, 806–823. doi: 10.1002/jcp.29908
Bai, X., and Bian, Z. (2022). MicroRNA-21 is a versatile regulator and potential treatment target in central nervous system disorders. Front. Mol. Neurosci. 15:842288. doi: 10.3389/fnmol.2022.842288
Barateiro, A., Afonso, V., Santos, G., Cerqueira, J. J., Brites, D., Van Horssen, J., et al. (2016). S100B as a potential biomarker and therapeutic target in multiple sclerosis. Mol. Neurobiol. 53, 3976–3991. doi: 10.1007/s12035-015-9336-6
Barros-Viegas, A. T., Carmona, V., Ferreiro, E., Guedes, J., Cardoso, A. M., Cunha, P., et al. (2020). miRNA-31 improves cognition and abolishes amyloid-beta pathology by targeting APP and BACE1 in an animal model of Alzheimer’s disease. Mol. Ther. Nucleic Acids 19, 1219–1236. doi: 10.1016/j.omtn.2020.01.010
Bautista-Becerril, B., Perez-Dimas, G., Sommerhalder-Nava, P. C., Hanono, A., Martinez-Cisneros, J. A., Zarate-Maldonado, B., et al. (2021). miRNAs, from evolutionary junk to possible prognostic markers and therapeutic targets in COVID-19. Viruses 14:41. doi: 10.3390/v14010041
Becker, J. H., Lin, J. J., Doernberg, M., Stone, K., Navis, A., Festa, J. R., et al. (2021). Assessment of cognitive function in patients after COVID-19 infection. JAMA Netw. Open 4:e2130645. doi: 10.1001/jamanetworkopen.2021.30645
Bellae Papannarao, J., Schwenke, D. O., Manning, P., and Katare, R. (2022). Upregulated miR-200c is associated with downregulation of the functional receptor for severe acute respiratory syndrome coronavirus 2 ACE2 in individuals with obesity. Int. J. Obes. (Lond) 46, 238–241. doi: 10.1038/s41366-021-00984-2
Bhavana, V., Thakor, P., Singh, S. B., and Mehra, N. K. (2020). COVID-19: Pathophysiology, treatment options, nanotechnology approaches, and research agenda to combating the SARS-CoV2 pandemic. Life Sci. 261:118336. doi: 10.1016/j.lfs.2020.118336
Bishop, P., Rocca, D., and Henley, J. M. (2016). Ubiquitin C-terminal hydrolase L1 (UCH-L1): Structure, distribution and roles in brain function and dysfunction. Biochem. J. 473, 2453–2462. doi: 10.1042/BCJ20160082
Blount, G. S., Coursey, L., and Kocerha, J. (2022). MicroRNA networks in cognition and dementia. Cells 11:1882. doi: 10.3390/cells11121882
Bogdan, S., Puscion-Jakubik, A., Klimiuk, K., Socha, K., Kochanowicz, J., and Gorodkiewicz, E. (2022). UCHL1 and proteasome in blood serum in relation to dietary habits, concentration of selected antioxidant minerals and total antioxidant status among patients with Alzheimer’s disease. J. Clin. Med. 11:412. doi: 10.3390/jcm11020412
Boldrini, M., Canoll, P. D., and Klein, R. S. (2021). How COVID-19 Affects the Brain. JAMA Psychiatry 78, 682–683. doi: 10.1001/jamapsychiatry.2021.0500
Bradley, B. T., Maioli, H., Johnston, R., Chaudhry, I., Fink, S. L., Xu, H., et al. (2020). Histopathology and ultrastructural findings of fatal COVID-19 infections in Washington State: A case series. Lancet 396, 320–332. doi: 10.1016/S0140-6736(20)31305-2
Burak, K., Lamoureux, L., Boese, A., Majer, A., Saba, R., Niu, Y., et al. (2018). MicroRNA-16 targets mRNA involved in neurite extension and branching in hippocampal neurons during presymptomatic prion disease. Neurobiol. Dis. 112, 1–13. doi: 10.1016/j.nbd.2017.12.011
Burmeister, A. R., and Marriott, I. (2018). The interleukin-10 family of cytokines and their role in the CNS. Front. Cell Neurosci. 12:458. doi: 10.3389/fncel.2018.00458
Cabezas, R., Avila, M., Gonzalez, J., El-Bacha, R. S., Baez, E., Garcia-Segura, L. M., et al. (2014). Astrocytic modulation of blood brain barrier: Perspectives on Parkinson’s disease. Front. Cell Neurosci. 8:211. doi: 10.3389/fncel.2014.00211
Carini, G., Musazzi, L., Bolzetta, F., Cester, A., Fiorentini, C., Ieraci, A., et al. (2021). The potential role of miRNAs in cognitive frailty. Front. Aging Neurosci. 13:763110. doi: 10.3389/fnagi.2021.763110
Ceban, F., Ling, S., Lui, L. M. W., Lee, Y., Gill, H., Teopiz, K. M., et al. (2022). Fatigue and cognitive impairment in Post-COVID-19 Syndrome: A systematic review and meta-analysis. Brain Behav. Immun. 101, 93–135. doi: 10.1016/j.bbi.2021.12.020
Chaves, M. L., Camozzato, A. L., Ferreira, E. D., Piazenski, I., Kochhann, R., Dall’igna, O., et al. (2010). Serum levels of S100B and NSE proteins in Alzheimer’s disease patients. J. Neuroinflamm. 7:6. doi: 10.1186/1742-2094-7-6
Chen, X., Zhao, B., Qu, Y., Chen, Y., Xiong, J., Feng, Y., et al. (2020). Detectable serum severe acute respiratory syndrome coronavirus 2 viral load (RNAemia) is closely correlated with drastically elevated interleukin 6 level in critically ill patients with coronavirus disease 2019. Clin. Infect. Dis. 71, 1937–1942. doi: 10.1093/cid/ciaa449
Chouliaras, L., Thomas, A., Malpetti, M., Donaghy, P., Kane, J., Mak, E., et al. (2022). Differential levels of plasma biomarkers of neurodegeneration in Lewy body dementia. Alzheimer’s disease, frontotemporal dementia and progressive supranuclear palsy. J. Neurol. Neurosurg. Psychiatry 93, 651–658. doi: 10.1136/jnnp-2021-327788
Ciancarelli, I., De Amicis, D., Di Massimo, C., Di Scanno, C., Pistarini, C., D’orazio, N., et al. (2014). Peripheral biomarkers of oxidative stress and their limited potential in evaluation of clinical features of Huntington’s patients. Biomarkers 19, 452–456. doi: 10.3109/1354750X.2014.935955
Cione, E., Siniscalchi, A., Gangemi, P., Cosco, L., Colosimo, M., Longhini, F., et al. (2021). Neuron-specific enolase serum levels in COVID-19 are related to the severity of lung injury. PLoS One 16:e0251819. doi: 10.1371/journal.pone.0251819
Coleman, L. G. Jr., Zou, J., and Crews, F. T. (2017). Microglial-derived miRNA let-7 and HMGB1 contribute to ethanol-induced neurotoxicity via TLR7. J Neuroinflamm. 14:22. doi: 10.1186/s12974-017-0799-4
Condrat, C. E., Thompson, D. C., Barbu, M. G., Bugnar, O. L., Boboc, A., Cretoiu, D., et al. (2020). miRNAs as biomarkers in disease: Latest findings regarding their role in diagnosis and prognosis. Cells 9:276. doi: 10.3390/cells9020276
Cooper, J., Stukas, S., Hoiland, R. L., Fergusson, N. A., Thiara, S., Foster, D., et al. (2020). Quantification of neurological blood-based biomarkers in critically Ill patients with coronavirus disease 2019. Crit. Care Explor. 2:e0238. doi: 10.1097/CCE.0000000000000238
Cristovao, J. S., and Gomes, C. M. (2019). S100 proteins in Alzheimer’s disease. Front. Neurosci. 13:463. doi: 10.3389/fnins.2019.00463
Crivelli, L., Palmer, K., Calandri, I., Guekht, A., Beghi, E., Carroll, W., et al. (2022). Changes in cognitive functioning after COVID-19: A systematic review and meta-analysis. Alzheimers Dement. 18, 1047–1066. doi: 10.1002/alz.12644
Davis, H. E., Assaf, G. S., Mccorkell, L., Wei, H., Low, R. J., Re’em, Y., et al. (2021). Characterizing long COVID in an international cohort: 7 months of symptoms and their impact. E Clin.Med. 38:101019. doi: 10.1016/j.eclinm.2021.101019
De Gonzalo-Calvo, D., Benitez, I. D., Pinilla, L., Carratala, A., Moncusi-Moix, A., Gort-Paniello, C., et al. (2021). Circulating microRNA profiles predict the severity of COVID-19 in hospitalized patients. Transl. Res. 236, 147–159. doi: 10.1016/j.trsl.2021.05.004
De Lorenzo, R., Lore, N. I., Finardi, A., Mandelli, A., Cirillo, D. M., Tresoldi, C., et al. (2021). Blood neurofilament light chain and total tau levels at admission predict death in COVID-19 patients. J. Neurol. 268, 4436–4442. doi: 10.1007/s00415-021-10595-6
Denk, J., Oberhauser, F., Kornhuber, J., Wiltfang, J., Fassbender, K., Schroeter, M. L., et al. (2018). Specific serum and CSF microRNA profiles distinguish sporadic behavioural variant of frontotemporal dementia compared with Alzheimer patients and cognitively healthy controls. PLoS One 13:e0197329. doi: 10.1371/journal.pone.0197329
Dong, H., Li, J., Huang, L., Chen, X., Li, D., Wang, T., et al. (2015). Serum MicroRNA profiles serve as novel biomarkers for the diagnosis of Alzheimer’s disease. Dis. Markers 2015:625659. doi: 10.1155/2015/625659
Donyavi, T., Bokharaei-Salim, F., Baghi, H. B., Khanaliha, K., Alaei Janat-Makan, M., Karimi, B., et al. (2021). Acute and post-acute phase of COVID-19: Analyzing expression patterns of miRNA-29a-3p, 146a-3p, 155-5p, and let-7b-3p in PBMC. Int. Immunopharmacol. 97:107641. doi: 10.1016/j.intimp.2021.107641
Ebenau, J. L., Pelkmans, W., Verberk, I. M. W., Verfaillie, S. C. J., Van Den Bosch, K. A., Van Leeuwenstijn, M., et al. (2022). Association of CSF, plasma, and imaging markers of neurodegeneration with clinical progression in people with subjective cognitive decline. Neurology 98, e1315–e1326. doi: 10.1212/WNL.0000000000200035
Elahi, F. M., Casaletto, K. B., La Joie, R., Walters, S. M., Harvey, D., Wolf, A., et al. (2020). Plasma biomarkers of astrocytic and neuronal dysfunction in early- and late-onset Alzheimer’s disease. Alzheimers Dement. 16, 681–695. doi: 10.1016/j.jalz.2019.09.004
Ellul, M. A., Benjamin, L., Singh, B., Lant, S., Michael, B. D., Easton, A., et al. (2020). Neurological associations of COVID-19. Lancet Neurol. 19, 767–783. doi: 10.1016/S1474-4422(20)30221-0
Eng, L. F., Ghirnikar, R. S., and Lee, Y. L. (2000). Glial fibrillary acidic protein: GFAP-thirty-one years (1969-2000). Neurochem. Res. 25, 1439–1451. doi: 10.1023/A:1007677003387
Fan, W., Liang, C., Ou, M., Zou, T., Sun, F., Zhou, H., et al. (2020). MicroRNA-146a is a wide-reaching neuroinflammatory regulator and potential treatment target in neurological diseases. Front. Mol. Neurosci. 13:90. doi: 10.3389/fnmol.2020.00090
Farr, R. J., Rootes, C. L., Rowntree, L. C., Nguyen, T. H. O., Hensen, L., Kedzierski, L., et al. (2021). Altered microRNA expression in COVID-19 patients enables identification of SARS-CoV-2 infection. PLoS Pathog. 17:e1009759. doi: 10.1371/journal.ppat.1009759
Feng, M. G., Liu, C. F., Chen, L., Feng, W. B., Liu, M., Hai, H., et al. (2018). MiR-21 attenuates apoptosis-triggered by amyloid-beta via modulating PDCD4/PI3K/AKT/GSK-3beta pathway in SH-SY5Y cells. Biomed. Pharmacother. 101, 1003–1007. doi: 10.1016/j.biopha.2018.02.043
Ferrando, S. J., Dornbush, R., Lynch, S., Shahar, S., Klepacz, L., Karmen, C. L., et al. (2022). Neuropsychological, medical, and psychiatric findings after recovery from acute COVID-19: A cross-sectional study. J. Acad. Consult Liaison Psychiatry 63, 474–484. doi: 10.1016/j.jaclp.2022.01.003
Freeman, E. E., Mcmahon, D. E., Lipoff, J. B., Rosenbach, M., Kovarik, C., Desai, S. R., et al. (2020). The spectrum of COVID-19-associated dermatologic manifestations: An international registry of 716 patients from 31 countries. J. Am. Acad. Dermatol. 83, 1118–1129. doi: 10.1016/j.jaad.2020.06.1016
Frontera, J. A., Boutajangout, A., Masurkar, A. V., Betensky, R. A., Ge, Y., Vedvyas, A., et al. (2022). Comparison of serum neurodegenerative biomarkers among hospitalized COVID-19 patients versus non-COVID subjects with normal cognition, mild cognitive impairment, or Alzheimer’s dementia. Alzheimers Dement. 18, 899–910. doi: 10.1002/alz.12556
Gaetani, L., Blennow, K., Calabresi, P., Di Filippo, M., Parnetti, L., and Zetterberg, H. (2019). Neurofilament light chain as a biomarker in neurological disorders. J. Neurol. Neurosurg. Psychiatry 90, 870–881. doi: 10.1136/jnnp-2018-320106
Galliera, E., Massaccesi, L., Yu, L., He, J., Ranucci, M., and Corsi Romanelli, M. M. (2022). SCD14-ST and new generation inflammatory biomarkers in the prediction of COVID-19 outcome. Biomolecules 12:826. doi: 10.3390/biom12060826
Galvan-Roman, J. M., Lancho-Sanchez, A., Luquero-Bueno, S., Vega-Piris, L., Curbelo, J., Manzaneque-Pradales, M., et al. (2020). Usefulness of circulating microRNAs miR-146a and miR-16-5p as prognostic biomarkers in community-acquired pneumonia. PLoS One 15:e0240926. doi: 10.1371/journal.pone.0240926
Gamez-Valero, A., Campdelacreu, J., Vilas, D., Ispierto, L., Rene, R., Alvarez, R., et al. (2019). Exploratory study on microRNA profiles from plasma-derived extracellular vesicles in Alzheimer’s disease and dementia with Lewy bodies. Transl. Neurodegener. 8:31. doi: 10.1186/s40035-019-0169-5
Gao, X., Xiong, Y., Li, Q., Han, M., Shan, D., Yang, G., et al. (2020). Extracellular vesicle-mediated transfer of miR-21-5p from mesenchymal stromal cells to neurons alleviates early brain injury to improve cognitive function via the PTEN/Akt pathway after subarachnoid hemorrhage. Cell Death Dis. 11:363. doi: 10.1038/s41419-020-2530-0
Garcia, J. M., Stillings, S. A., Leclerc, J. L., Phillips, H., Edwards, N. J., Robicsek, S. A., et al. (2017). Role of interleukin-10 in acute brain injuries. Front. Neurol. 8:244. doi: 10.3389/fneur.2017.00244
Graham, E. L., Clark, J. R., Orban, Z. S., Lim, P. H., Szymanski, A. L., Taylor, C., et al. (2021). Persistent neurologic symptoms and cognitive dysfunction in non-hospitalized Covid-19 “long haulers”. Ann. Clin. Transl. Neurol. 8, 1073–1085. doi: 10.1002/acn3.51350
Grinan-Ferre, C., Corpas, R., Puigoriol-Illamola, D., Palomera-Avalos, V., Sanfeliu, C., and Pallas, M. (2018). Understanding epigenetics in the neurodegeneration of Alzheimer’s disease: SAMP8 mouse model. J. Alzheimers Dis. 62, 943–963. doi: 10.3233/JAD-170664
Guedes, J. R., Santana, I., Cunha, C., Duro, D., Almeida, M. R., Cardoso, A. M., et al. (2016). MicroRNA deregulation and chemotaxis and phagocytosis impairment in Alzheimer’s disease. Alzheimers Dement. (Amst) 3, 7–17. doi: 10.1016/j.dadm.2015.11.004
Guo, T., Noble, W., and Hanger, D. P. (2017). Roles of tau protein in health and disease. Acta Neuropathol. 133, 665–704. doi: 10.1007/s00401-017-1707-9
Gupta, K. K., Khan, M. A., and Singh, S. K. (2020b). Constitutive inflammatory cytokine storm: A major threat to human health. J. Interferon Cytokine Res. 40, 19–23. doi: 10.1089/jir.2019.0085
Gupta, A., Madhavan, M. V., Sehgal, K., Nair, N., Mahajan, S., Sehrawat, T. S., et al. (2020a). Extrapulmonary manifestations of COVID-19. Nat. Med. 26, 1017–1032. doi: 10.1038/s41591-020-0968-3
Hadad, R., Khoury, J., Stanger, C., Fisher, T., Schneer, S., Ben-Hayun, R., et al. (2022). Cognitive dysfunction following COVID-19 infection. J. Neurovirol. 28, 430–437. doi: 10.1007/s13365-022-01079-y
Han, H., Ma, Q., Li, C., Liu, R., Zhao, L., Wang, W., et al. (2020). Profiling serum cytokines in COVID-19 patients reveals IL-6 and IL-10 are disease severity predictors. Emerg. Microbes Infect. 9, 1123–1130. doi: 10.1080/22221751.2020.1770129
Hanson, B. A., Visvabharathy, L., Ali, S. T., Kang, A. K., Patel, T. R., Clark, J. R., et al. (2022). Plasma biomarkers of neuropathogenesis in hospitalized patients with COVID-19 and those with postacute sequelae of SARS-CoV-2 infection. Neurol. Neuroimmunol. Neuroinflamm. 9:e1151. doi: 10.1212/NXI.0000000000001151
Haque, A., Polcyn, R., Matzelle, D., and Banik, N. L. (2018). New insights into the role of neuron-specific enolase in neuro-inflammation, neurodegeneration, and neuroprotection. Brain Sci. 8:33. doi: 10.3390/brainsci8020033
Haroun, R. A., Osman, W. H., Amin, R. E., Hassan, A. K., Abo-Shanab, W. S., and Eessa, A. M. (2022). Circulating plasma miR-155 is a potential biomarker for the detection of SARS-CoV-2 infection. Pathology 54, 104–110. doi: 10.1016/j.pathol.2021.09.006
Harrison, A. G., Lin, T., and Wang, P. (2020). Mechanisms of SARS-CoV-2 transmission and pathogenesis. Trends Immunol. 41, 1100–1115. doi: 10.1016/j.it.2020.10.004
Hebert, S. S., Sergeant, N., and Buee, L. (2012). MicroRNAs and the regulation of tau metabolism. Int. J. Alzheimers Dis. 2012:406561. doi: 10.1155/2012/406561
Heimfarth, L., Passos, F. R. S., Monteiro, B. S., Araujo, A.A.S, Quintans Junior, L. J., and Quintans, J. S. S. (2022). Serum glial fibrillary acidic protein is a body fluid biomarker: A valuable prognostic for neurological disease–A systematic review. Int. Immunopharmacol. 107:108624. doi: 10.1016/j.intimp.2022.108624
Henry, R. J., Doran, S. J., Barrett, J. P., Meadows, V. E., Sabirzhanov, B., Stoica, B. A., et al. (2019). Inhibition of miR-155 limits neuroinflammation and improves functional recovery after experimental traumatic brain injury in mice. Neurotherapeutics 16, 216–230. doi: 10.1007/s13311-018-0665-9
Hirzel, C., Grandgirard, D., Surial, B., Wider, M. F., Leppert, D., Kuhle, J., et al. (2022). Neuro-axonal injury in COVID-19: The role of systemic inflammation and SARS-CoV-2 specific immune response. Ther. Adv. Neurol. Disord. 15:17562864221080528. doi: 10.1177/17562864221080528
Hol, E. M., and Capetanaki, Y. (2017). Type III intermediate filaments desmin, glial fibrillary acidic protein (GFAP), vimentin, and peripherin. Cold Spring Harb. Perspect. Biol. 9:a021642. doi: 10.1101/cshperspect.a021642
Hosp, J. A., Dressing, A., Blazhenets, G., Bormann, T., Rau, A., Schwabenland, M., et al. (2021). Cognitive impairment and altered cerebral glucose metabolism in the subacute stage of COVID-19. Brain 144, 1263–1276. doi: 10.1093/brain/awab009
Huang, Y., Liu, Y., Huang, J., Gao, L., Wu, Z., Wang, L., et al. (2021b). Let7b5p promotes cell apoptosis in Parkinson’s disease by targeting HMGA2. Mol. Med. Rep. 24:820. doi: 10.3892/mmr.2021.12461
Huang, C., Huang, L., Wang, Y., Li, X., Ren, L., Gu, X., et al. (2021a). 6-month consequences of COVID-19 in patients discharged from hospital: A cohort study. Lancet 397, 220–232. doi: 10.1016/S0140-6736(20)32656-8
Huang, C., Wang, Y., Li, X., Ren, L., Zhao, J., Hu, Y., et al. (2020). Clinical features of patients infected with 2019 novel coronavirus in Wuhan. China Lancet 395, 497–506. doi: 10.1016/S0140-6736(20)30183-5
Hugon, J., Msika, E. F., Queneau, M., Farid, K., and Paquet, C. (2022). Long COVID: Cognitive complaints (brain fog) and dysfunction of the cingulate cortex. J. Neurol. 269, 44–46. doi: 10.1007/s00415-021-10655-x
Hui, D. S., I Azhar, E., Madani, T. A., Ntoumi, F., Kock, R., Dar, O., et al. (2020). The continuing 2019-nCoV epidemic threat of novel coronaviruses to global health–The latest 2019 novel coronavirus outbreak in Wuhan, China. Int. J. Infect. Dis. 91, 264–266. doi: 10.1016/j.ijid.2020.01.009
Iodice, F., Cassano, V., and Rossini, P. M. (2021). Direct and indirect neurological, cognitive, and behavioral effects of COVID-19 on the healthy elderly, mild-cognitive-impairment, and Alzheimer’s disease populations. Neurol. Sci. 42, 455–465. doi: 10.1007/s10072-020-04902-8
Islam, H., Chamberlain, T. C., Mui, A. L., and Little, J. P. (2021a). Elevated interleukin-10 Levels in COVID-19: Potentiation of pro-inflammatory responses or impaired anti-inflammatory action? Front. Immunol. 12:677008. doi: 10.3389/fimmu.2021.677008
Islam, M. B., Chowdhury, U. N., Nain, Z., Uddin, S., Ahmed, M. B., and Moni, M. A. (2021b). Identifying molecular insight of synergistic complexities for SARS-CoV-2 infection with pre-existing type 2 diabetes. Comput. Biol. Med. 136:104668. doi: 10.1016/j.compbiomed.2021.104668
Islam, M. R., Kaurani, L., Berulava, T., Heilbronner, U., Budde, M., Centeno, T. P., et al. (2021c). A microRNA signature that correlates with cognition and is a target against cognitive decline. EMBO Mol. Med. 13:e13659. doi: 10.15252/emmm.202013659
Jafrin, S., Aziz, M. A., and Islam, M. S. (2022). Elevated levels of pleiotropic interleukin-6 (IL-6) and interleukin-10 (IL-10) are critically involved with the severity and mortality of COVID-19: An updated longitudinal meta-analysis and systematic review on 147 studies. Biomark. Insights 17:11772719221106600. doi: 10.1177/11772719221106600
Johansson, A., Mohamed, M. S., Moulin, T. C., and Schioth, H. B. (2021). Neurological manifestations of COVID-19: A comprehensive literature review and discussion of mechanisms. J. Neuroimmunol. 358:577658. doi: 10.1016/j.jneuroim.2021.577658
Kanberg, N., Ashton, N. J., Andersson, L. M., Yilmaz, A., Lindh, M., Nilsson, S., et al. (2020). Neurochemical evidence of astrocytic and neuronal injury commonly found in COVID-19. Neurology 95, e1754–e1759. doi: 10.1212/WNL.0000000000010111
Kanberg, N., Simren, J., Eden, A., Andersson, L. M., Nilsson, S., Ashton, N. J., et al. (2021). Neurochemical signs of astrocytic and neuronal injury in acute COVID-19 normalizes during long-term follow-up. EBioMedicine 70:103512. doi: 10.1016/j.ebiom.2021.103512
Keikha, R., and Jebali, A. (2021). [The miRNA neuroinflammatory biomarkers in COVID-19 patients with different severity of illness]. Neurologia (Engl Ed). doi: 10.1016/j.nrl.2021.06.005 [Epub ahead of print].
Keikha, R., Hashemi-Shahri, S. M., and Jebali, A. (2021). The relative expression of miR-31, miR-29, miR-126, and miR-17 and their mRNA targets in the serum of COVID-19 patients with different grades during hospitalization. Eur. J. Med. Res. 26:75. doi: 10.1186/s40001-021-00544-4
Kenny, A., Mcardle, H., Calero, M., Rabano, A., Madden, S. F., Adamson, K., et al. (2019). Elevated plasma microRNA-206 levels predict cognitive decline and progression to dementia from mild cognitive impairment. Biomolecules 9:734. doi: 10.3390/biom9110734
Kent, S. A., Spires-Jones, T. L., and Durrant, C. S. (2020). The physiological roles of tau and Abeta: Implications for Alzheimer’s disease pathology and therapeutics. Acta Neuropathol. 140, 417–447. doi: 10.1007/s00401-020-02196-w
Kim, H., Lee, E. J., Lim, Y. M., and Kim, K. K. (2022). Glial fibrillary acidic protein in blood as a disease biomarker of neuromyelitis optica spectrum disorders. Front. Neurol. 13:865730. doi: 10.3389/fneur.2022.865730
Kim, W. R., Park, E. G., Kang, K. W., Lee, S. M., Kim, B., and Kim, H. S. (2020). Expression analyses of MicroRNAs in hamster lung tissues infected by SARS-CoV-2. Mol. Cells 43, 953–963. doi: 10.14348/molcells.2020.0177
Kivisakk, P., Magdamo, C., Trombetta, B. A., Noori, A., Kuo, Y. K. E., Chibnik, L. B., et al. (2022). Plasma biomarkers for prognosis of cognitive decline in patients with mild cognitive impairment. Brain Commun. 4:fcac155. doi: 10.1093/braincomms/fcac155
Klyucherev, T. O., Olszewski, P., Shalimova, A. A., Chubarev, V. N., Tarasov, V. V., Attwood, M. M., et al. (2022). Advances in the development of new biomarkers for Alzheimer’s disease. Transl. Neurodegener. 11:25. doi: 10.1186/s40035-022-00296-z
Kong, H., Yin, F., He, F., Omran, A., Li, L., Wu, T., et al. (2015). The effect of miR-132, miR-146a, and miR-155 on MRP8/TLR4-induced astrocyte-related inflammation. J Mol Neurosci 57, 28–37. doi: 10.1007/s12031-015-0574-x
Kudose, S., Batal, I., Santoriello, D., Xu, K., Barasch, J., Peleg, Y., et al. (2020). Kidney biopsy findings in patients with COVID-19. J. Am. Soc. Nephrol. 31, 1959–1968. doi: 10.1681/ASN.2020060802
Kumar, S., and Reddy, P. H. (2016). Are circulating microRNAs peripheral biomarkers for Alzheimer’s disease? Biochim. Biophys. Acta 1862, 1617–1627. doi: 10.1016/j.bbadis.2016.06.001
Lakhani, H. V., Pillai, S. S., Zehra, M., Dao, B., Tirona, M. T., Thompson, E., et al. (2021). Detecting early onset of anthracyclines-induced cardiotoxicity using a novel panel of biomarkers in West-Virginian population with breast cancer. Sci. Rep. 11:7954. doi: 10.1038/s41598-021-87209-8
Lam, V., Albrecht, M. A., Takechi, R., Giles, C., James, A. P., Foster, J. K., et al. (2013). The serum concentration of the calcium binding protein S100B is positively associated with cognitive performance in older adults. Front. Aging Neurosci. 5:61. doi: 10.3389/fnagi.2013.00061
Lantero Rodriguez, J., Karikari, T. K., Suarez-Calvet, M., Troakes, C., King, A., Emersic, A., et al. (2020). Plasma p-tau181 accurately predicts Alzheimer’s disease pathology at least 8 years prior to post-mortem and improves the clinical characterisation of cognitive decline. Acta Neuropathol. 140, 267–278. doi: 10.1007/s00401-020-02195-x
Lavillegrand, J. R., Garnier, M., Spaeth, A., Mario, N., Hariri, G., Pilon, A., et al. (2021). Elevated plasma IL-6 and CRP levels are associated with adverse clinical outcomes and death in critically ill SARS-CoV-2 patients: Inflammatory response of SARS-CoV-2 patients. Ann. Intensive Care 11:9. doi: 10.1186/s13613-020-00798-x
Lehmann, S. M., Kruger, C., Park, B., Derkow, K., Rosenberger, K., Baumgart, J., et al. (2012). An unconventional role for miRNA: Let-7 activates Toll-like receptor 7 and causes neurodegeneration. Nat. Neurosci. 15, 827–835. doi: 10.1038/nn.3113
Leidinger, P., Backes, C., Deutscher, S., Schmitt, K., Mueller, S. C., Frese, K., et al. (2013). A blood based 12-miRNA signature of Alzheimer disease patients. Genome Biol. 14:R78. doi: 10.1186/gb-2013-14-7-r78
Leretter, M. T., Vulcanescu, D. D., Horhat, F. G., Matichescu, A., Rivis, M., Rusu, L. C., et al. (2022). COVID-19: Main findings after a year and half of unease and the proper scientific progress (Review). Exp. Ther. Med. 23:424. doi: 10.3892/etm.2022.11350
Li, C., Hu, X., Li, L., and Li, J. H. (2020). Differential microRNA expression in the peripheral blood from human patients with COVID-19. J. Clin. Lab. Anal. 34:e23590. doi: 10.1002/jcla.23590
Li, C., Wang, R., Wu, A., Yuan, T., Song, K., Bai, Y., et al. (2022b). SARS-COV-2 as potential microRNA sponge in COVID-19 patients. BMC Med. Genomics 15:94. doi: 10.1186/s12920-022-01243-7
Li, C., Liu, J., Lin, J., and Shang, H. (2022a). COVID-19 and risk of neurodegenerative disorders: A mendelian randomization study. Transl. Psychiatry 12:283. doi: 10.1038/s41398-022-02052-3
Liang, C., Zou, T., Zhang, M., Fan, W., Zhang, T., Jiang, Y., et al. (2021). MicroRNA-146a switches microglial phenotypes to resist the pathological processes and cognitive degradation of Alzheimer’s disease. Theranostics 11, 4103–4121. doi: 10.7150/thno.53418
Lindner, D., Fitzek, A., Brauninger, H., Aleshcheva, G., Edler, C., Meissner, K., et al. (2020). Association of cardiac infection with SARS-CoV-2 in confirmed COVID-19 autopsy cases. JAMA Cardiol. 5, 1281–1285. doi: 10.1001/jamacardio.2020.3551
Liu, C. Y., Yang, Y., Ju, W. N., Wang, X., and Zhang, H. L. (2018). Emerging roles of astrocytes in neuro-vascular unit and the tripartite synapse with emphasis on reactive gliosis in the context of Alzheimer’s disease. Front. Cell Neurosci. 12:193. doi: 10.3389/fncel.2018.00193
Liu, H., Wei, P., Kappler, J. W., Marrack, P., and Zhang, G. (2022). SARS-CoV-2 variants of concern and variants of interest receptor binding domain mutations and virus infectivity. F. Immunol. 13:825256. doi: 10.3389/fimmu.2022.825256
Liu, P. P., Blet, A., Smyth, D., and Li, H. (2020). The science underlying COVID-19: Implications for the cardiovascular system. Circulation 142, 68–78. doi: 10.1161/CIRCULATIONAHA.120.047549
Liu, W., Liu, C., Zhu, J., Shu, P., Yin, B., Gong, Y., et al. (2012). MicroRNA-16 targets amyloid precursor protein to potentially modulate Alzheimer’s-associated pathogenesis in SAMP8 mice. Neurobiol. Aging 33, 522–534. doi: 10.1016/j.neurobiolaging.2010.04.034
Lodigiani, C., Iapichino, G., Carenzo, L., Cecconi, M., Ferrazzi, P., Sebastian, T., et al. (2020). Venous and arterial thromboembolic complications in COVID-19 patients admitted to an academic hospital in Milan. Italy. Thromb. Res. 191, 9–14. doi: 10.1016/j.thromres.2020.04.024
Lopez-Leon, S., Wegman-Ostrosky, T., Perelman, C., Sepulveda, R., Rebolledo, P. A., Cuapio, A., et al. (2021). More than 50 Long-term effects of COVID-19: A systematic review and meta-analysis. medRxiv [Preprint]. doi: 10.1101/2021.01.27.21250617
Lopez-Ramirez, M. A., Wu, D., Pryce, G., Simpson, J. E., Reijerkerk, A., King-Robson, J., et al. (2014). MicroRNA-155 negatively affects blood-brain barrier function during neuroinflammation. FASEB J. 28, 2551–2565. doi: 10.1096/fj.13-248880
Lu, Q., Zhu, Z., Tan, C., Zhou, H., Hu, Y., Shen, G., et al. (2021). Changes of serum IL-10. IL-1beta, IL-6, MCP-1, TNF-alpha, IP-10 and IL-4 in COVID-19 patients. Int. J. Clin. Pract. 75:e14462. doi: 10.1111/ijcp.14462
Lu, Y., Li, J., and Hu, T. (2022). Analysis of Correlation between Serum Inflammatory Factors and Cognitive Function. Language, and Memory in Alzheimer’s Disease and Its Clinical Significance. Comput. Math. Methods Med. 2022:2701748. doi: 10.1155/2022/2701748
Luan, Y. Y., and Yao, Y. M. (2018). The clinical significance and potential role of c-reactive protein in chronic inflammatory and neurodegenerative diseases. Front. Immunol. 9:1302. doi: 10.3389/fimmu.2018.01302
Ma, X., Becker Buscaglia, L. E., Barker, J. R., and Li, Y. (2011). MicroRNAs in NF-kappaB signaling. J. Mol. Cell Biol. 3, 159–166. doi: 10.1093/jmcb/mjr007
Mabb, A. M., and Ehlers, M. D. (2010). Ubiquitination in postsynaptic function and plasticity. Annu. Rev. Cell Dev. Biol. 26, 179–210. doi: 10.1146/annurev-cellbio-100109-104129
Maciak, K., Dziedzic, A., Miller, E., and Saluk-Bijak, J. (2021). miR-155 as an Important regulator of multiple sclerosis pathogenesis. A review. Int. J. Mol. Sci. 22:4332. doi: 10.3390/ijms22094332
Mai, H., Fan, W., Wang, Y., Cai, Y., Li, X., Chen, F., et al. (2019). Intranasal administration of miR-146a agomir rescued the pathological process and cognitive impairment in an AD mouse model. Mol. Ther. Nucleic Acids 18, 681–695. doi: 10.1016/j.omtn.2019.10.002
Mainous, A. G. III, Rooks, B. J., and Orlando, F. A. (2022). The impact of initial COVID-19 episode inflammation among adults on mortality within 12 months post-hospital discharge. Front. Med. (Lausanne) 9:891375. doi: 10.3389/fmed.2022.891375
Malik, P., Patel, U., Mehta, D., Patel, N., Kelkar, R., Akrmah, M., et al. (2021). Biomarkers and outcomes of COVID-19 hospitalisations: Systematic review and meta-analysis. BMJ Evid. Based Med. 26, 107–108. doi: 10.1136/bmjebm-2020-111536
Mckeever, P. M., Schneider, R., Taghdiri, F., Weichert, A., Multani, N., Brown, R. A., et al. (2018). MicroRNA expression levels are altered in the cerebrospinal fluid of patients with young-onset Alzheimer’s Disease. Mol. Neurobiol. 55, 8826–8841. doi: 10.1007/s12035-018-1032-x
Metaxas, A., and Kempf, S. J. (2016). Neurofibrillary tangles in Alzheimer’s disease: Elucidation of the molecular mechanism by immunohistochemistry and tau protein phospho-proteomics. Neural Regen. Res. 11, 1579–1581. doi: 10.4103/1673-5374.193234
Mete, E., Sabirli, R., Goren, T., Turkcuer, I., Kurt, O., and Koseler, A. (2021). Association Between S100b Levels and COVID-19 Pneumonia: A Case Control Study. In. Vivo 35, 2923–2928. doi: 10.21873/invivo.12583
Michetti, F., D’ambrosi, N., Toesca, A., Puglisi, M. A., Serrano, A., Marchese, E., et al. (2019). The S100B story: From biomarker to active factor in neural injury. J. Neurochem. 148, 168–187. doi: 10.1111/jnc.14574
Miller, E. (2012). Multiple sclerosis. Adv. Exp. Med. Biol. 724, 222–238. doi: 10.1007/978-1-4614-0653-2_17
Miners, S., Kehoe, P. G., and Love, S. (2020). Cognitive impact of COVID-19: Looking beyond the short term. Alzheimers Res. Ther. 12:170. doi: 10.1186/s13195-020-00744-w
Miskowiak, K. W., Fugledalen, L., Jespersen, A. E., Sattler, S. M., Podlekareva, D., Rungby, J., et al. (2022). Trajectory of cognitive impairments over 1 year after COVID-19 hospitalisation: Pattern, severity, and functional implications. Eur. Neuropsychopharmacol. 59, 82–92. doi: 10.1016/j.euroneuro.2022.04.004
Miskowiak, K. W., Johnsen, S., Sattler, S. M., Nielsen, S., Kunalan, K., Rungby, J., et al. (2021). Cognitive impairments four months after COVID-19 hospital discharge: Pattern, severity and association with illness variables. Eur. Neuropsychopharmacol. 46, 39–48. doi: 10.1016/j.euroneuro.2021.03.019
Mohamed, M. S., Johansson, A., Jonsson, J., and Schioth, H. B. (2022). Dissecting the molecular mechanisms surrounding post-COVID-19 syndrome and neurological features. Int. J. Mol. Sci. 23:4275. doi: 10.3390/ijms23084275
Mondello, S., Sorinola, A., Czeiter, E., Vamos, Z., Amrein, K., Synnot, A., et al. (2021). Blood-based protein biomarkers for the management of traumatic brain injuries in adults presenting to emergency departments with mild brain injury: A living systematic review and meta-analysis. J. Neurotrauma. 38, 1086–1106. doi: 10.1089/neu.2017.5182
Montazersaheb, S., Hosseiniyan Khatibi, S. M., Hejazi, M. S., Tarhriz, V., Farjami, A., Ghasemian Sorbeni, F., et al. (2022). COVID-19 infection: An overview on cytokine storm and related interventions. Virol. J. 19:92. doi: 10.1186/s12985-022-01814-1
Moscoso, A., Grothe, M. J., Ashton, N. J., Karikari, T. K., Rodriguez, J. L., Snellman, A., et al. (2021). Time course of phosphorylated-tau181 in blood across the Alzheimer’s disease spectrum. Brain 144, 325–339. doi: 10.1093/brain/awaa399
Murta, V., Villarreal, A., and Ramos, A. J. (2020). Severe acute respiratory syndrome coronavirus 2 impact on the central nervous system: Are astrocytes and microglia main players or merely bystanders? ASN Neuro 12:1759091420954960. doi: 10.1177/1759091420954960
Nain, Z., Rana, H. K., Lio, P., Islam, S. M. S., Summers, M. A., and Moni, M. A. (2021). Pathogenetic profiling of COVID-19 and SARS-like viruses. Brief. Bioinform. 22, 1175–1196. doi: 10.1093/bib/bbaa173
Nakano, M., Kubota, K., Kobayashi, E., Chikenji, T. S., Saito, Y., Konari, N., et al. (2020b). Bone marrow-derived mesenchymal stem cells improve cognitive impairment in an Alzheimer’s disease model by increasing the expression of microRNA-146a in hippocampus. Sci. Rep. 10:10772. doi: 10.1038/s41598-020-67460-1
Nakano, M., Kubota, K., Hashizume, S., Kobayashi, E., Chikenji, T. S., Saito, Y., et al. (2020a). An enriched environment prevents cognitive impairment in an Alzheimer’s disease model by enhancing the secretion of exosomal microRNA-146a from the choroid plexus. Brain Behav. Immun. Health 9:100149. doi: 10.1016/j.bbih.2020.100149
Nalbandian, A., Sehgal, K., Gupta, A., Madhavan, M. V., Mcgroder, C., Stevens, J. S., et al. (2021). Post-acute COVID-19 syndrome. Nat. Med. 27, 601–615. doi: 10.1038/s41591-021-01283-z
Nersisyan, S., Engibaryan, N., Gorbonos, A., Kirdey, K., Makhonin, A., and Tonevitsky, A. (2020). Potential role of cellular miRNAs in coronavirus-host interplay. PeerJ 8:e9994. doi: 10.7717/peerj.9994
Newcombe, V. F. J., Ashton, N. J., Posti, J. P., Glocker, B., Manktelow, A., Chatfield, D. A., et al. (2022). Post-acute blood biomarkers and disease progression in traumatic brain injury. Brain 145, 2064–2076. doi: 10.1093/brain/awac126
Ng, A. S. L., Tan, Y. J., Lu, Z., Ng, E. Y., Ng, S. Y. E., Chia, N. S. Y., et al. (2020). Plasma ubiquitin C-terminal hydrolase L1 levels reflect disease stage and motor severity in Parkinson’s disease. Aging (Albany NY) 12, 1488–1495. doi: 10.18632/aging.102695
Palermo, G., Mazzucchi, S., Della Vecchia, A., Siciliano, G., Bonuccelli, U., Azuar, C., et al. (2020). Different clinical contexts of use of blood neurofilament light chain protein in the spectrum of neurodegenerative diseases. Mol. Neurobiol. 57, 4667–4691. doi: 10.1007/s12035-020-02035-9
Pang, Y., Lin, W., Zhan, L., Zhang, J., Zhang, S., Jin, H., et al. (2022). Inhibiting autophagy pathway of PI3K/AKT/mTOR promotes apoptosis in SK-N-SH cell model of Alzheimer’s Disease. J. Healthc. Eng. 2022:6069682. doi: 10.1155/2022/6069682
Parasher, A. (2021). COVID-19: Current understanding of its Pathophysiology. Clinical presentation and Treatment. Postgrad, Med. J. 97, 312–320. doi: 10.1136/postgradmedj-2020-138577
Parsi, S., Smith, P. Y., Goupil, C., Dorval, V., and Hebert, S. S. (2015). Preclinical evaluation of miR-15/107 family members as multifactorial drug targets for Alzheimer’s disease. Mol. Ther. Nucleic Acids 4:e256. doi: 10.1038/mtna.2015.33
Paybast, S., Emami, A., Koosha, M., and Baghalha, F. (2020). Novel coronavirus disease (COVID-19) and central nervous system complications: What neurologist need to know. Acta Neurol. Taiwan. 29, 24–31.
Pearn, M. L., Schilling, J. M., Jian, M., Egawa, J., Wu, C., Mandyam, C. D., et al. (2018). Inhibition of RhoA reduces propofol-mediated growth cone collapse, axonal transport impairment, loss of synaptic connectivity, and behavioural deficits. Br. J. Anaesth. 120, 745–760. doi: 10.1016/j.bja.2017.12.033
Pekny, M., and Pekna, M. (2014). Astrocyte reactivity and reactive astrogliosis: Costs and benefits. Physiol. Rev. 94, 1077–1098. doi: 10.1152/physrev.00041.2013
Peluso, M. J., Sans, H. M., Forman, C. A., Nylander, A. N., Ho, H. E., Lu, S., et al. (2022). Plasma markers of neurologic injury and inflammation in people with self-reported neurologic postacute sequelae of SARS-CoV-2 infection. Neurol. Neuroimmunol. Neuroinflamm. 9:e200003. doi: 10.1212/NXI.0000000000200003
Perdoncin, M., Konrad, A., Wyner, J. R., Lohana, S., Pillai, S. S., Pereira, D. G., et al. (2021). A review of miRNAs as biomarkers and effect of dietary modulation in obesity associated cognitive decline and neurodegenerative disorders. Front. Mol. Neurosci. 14:756499. doi: 10.3389/fnmol.2021.756499
PHOSP-COVID Collaborative Group (2022). Clinical characteristics with inflammation profiling of long COVID and association with 1-year recovery following hospitalisation in the UK: A prospective observational study. Lancet Respir. Med. 10, 761–775.
Pillai, S. S., Lakhani, H. V., Zehra, M., Wang, J., Dilip, A., Puri, N., et al. (2020). Predicting nonalcoholic fatty liver disease through a panel of plasma biomarkers and MicroRNAs in female west virginia population. Int. J. Mol. Sci. 21:6698. doi: 10.3390/ijms21186698
Pinacchio, C., Scordio, M., Santinelli, L., Frasca, F., Sorrentino, L., Bitossi, C., et al. (2022). Analysis of serum microRNAs and rs2910164 GC single-nucleotide polymorphism of miRNA-146a in COVID-19 patients. J. Immunoassay Immunochem. 43, 347–364. doi: 10.1080/15321819.2022.2035394
Polcyn, R., Capone, M., Hossain, A., Matzelle, D., Banik, N. L., and Haque, A. (2017). Neuron specific enolase is a potential target for regulating neuronal cell survival and death: Implications in neurodegeneration and regeneration. Neuroimmunol. Neuroinflamm. 4, 254–257. doi: 10.20517/2347-8659.2017.59
Prudencio, M., Erben, Y., Marquez, C. P., Jansen-West, K. R., Franco-Mesa, C., Heckman, M. G., et al. (2021). Serum neurofilament light protein correlates with unfavorable clinical outcomes in hospitalized patients with COVID-19. Sci. Transl. Med. 13:eabi7643. doi: 10.1126/scitranslmed.abi7643
Puntmann, V. O., Carerj, M. L., Wieters, I., Fahim, M., Arendt, C., Hoffmann, J., et al. (2020). Outcomes of cardiovascular magnetic resonance imaging in patients recently recovered from coronavirus disease 2019 (COVID-19). JAMA Cardiol. 5, 1265–1273. doi: 10.1001/jamacardio.2020.3557
Qian, H., Shang, Q., Liang, M., Gao, B., Xiao, J., Wang, J., et al. (2021). MicroRNA-31-3p/RhoA signaling in the dorsal hippocampus modulates methamphetamine-induced conditioned place preference in mice. Psychopharmacology (Berl) 238, 3207–3219. doi: 10.1007/s00213-021-05936-2
Qin, L., Zou, J., Barnett, A., Vetreno, R. P., Crews, F. T., and Coleman, L. G. Jr. (2021). TRAIL mediates neuronal death in AUD: A link between neuroinflammation and neurodegeneration. Int. J. Mol. Sci. 22:2547. doi: 10.3390/ijms22052547
Rahman, M. R., Islam, T., Zaman, T., Shahjaman, M., Karim, M. R., Huq, F., et al. (2020). Identification of molecular signatures and pathways to identify novel therapeutic targets in Alzheimer’s disease: Insights from a systems biomedicine perspective. Genomics 112, 1290–1299. doi: 10.1016/j.ygeno.2019.07.018
Rajan, K. B., Aggarwal, N. T., Mcaninch, E. A., Weuve, J., Barnes, L. L., Wilson, R. S., et al. (2020). Remote blood biomarkers of longitudinal cognitive outcomes in a population study. Ann. Neurol. 88, 1065–1076. doi: 10.1002/ana.25874
Rizzo, P., Vieceli Dalla Sega, F., Fortini, F., Marracino, L., Rapezzi, C., and Ferrari, R. (2020). COVID-19 in the heart and the lungs: Could we “Notch” the inflammatory storm? Basic Res. Cardiol. 115:31. doi: 10.1007/s00395-020-0791-5
Roganovic, J. (2021). Downregulation of microRNA-146a in diabetes, obesity and hypertension may contribute to severe COVID-19. Med. Hypotheses 146:110448. doi: 10.1016/j.mehy.2020.110448
Saba, R., Sorensen, D. L., and Booth, S. A. (2014). MicroRNA-146a: A Dominant. Negative regulator of the innate immune response. Front. Immunol. 5:578. doi: 10.3389/fimmu.2014.00578
Sabbatinelli, J., Giuliani, A., Matacchione, G., Latini, S., Laprovitera, N., Pomponio, G., et al. (2021). Decreased serum levels of the inflammaging marker miR-146a are associated with clinical non-response to tocilizumab in COVID-19 patients. Mech. Ageing Dev. 193:111413. doi: 10.1016/j.mad.2020.111413
Sahin, B. E., Celikbilek, A., Kocak, Y., Saltoglu, G. T., Konar, N. M., and Hizmali, L. (2022). Plasma biomarkers of brain injury in COVID-19 patients with neurological symptoms. J. Neurol. Sci. 439:120324. doi: 10.1016/j.jns.2022.120324
Sanchez-Molina, P., Almolda, B., Gimenez-Llort, L., Gonzalez, B., and Castellano, B. (2022). Chronic IL-10 overproduction disrupts microglia-neuron dialogue similar to aging, resulting in impaired hippocampal neurogenesis and spatial memory. Brain Behav. Immun. 101, 231–245. doi: 10.1016/j.bbi.2021.12.026
Savarraj, J., Park, E. S., Colpo, G. D., Hinds, S. N., Morales, D., Ahnstedt, H., et al. (2021). Brain injury, endothelial injury and inflammatory markers are elevated and express sex-specific alterations after COVID-19. J. Neuroinflamm. 18:277. doi: 10.1186/s12974-021-02323-8
Schultheiss, C., Willscher, E., Paschold, L., Gottschick, C., Klee, B., Henkes, S. S., et al. (2022). The IL-1beta. IL-6, and TNF cytokine triad is associated with post-acute sequelae of COVID-19. Cell Rep. Med. 3:100663. doi: 10.1016/j.xcrm.2022.100663
Sedaghat, F., and Notopoulos, A. (2008). S100 protein family and its application in clinical practice. Hippokratia 12, 198–204.
Serrano, A., Donno, C., Giannetti, S., Peric, M., Andjus, P., D’ambrosi, N., et al. (2017). The Astrocytic S100B protein with its receptor rage is aberrantly expressed in SOD1(G93A) models, and its inhibition decreases the expression of proinflammatory genes. Mediators Inflamm. 2017:1626204. doi: 10.1155/2017/1626204
Seyedalinaghi, S., Afsahi, A. M., Mohssenipour, M., Behnezhad, F., Salehi, M. A., Barzegary, A., et al. (2021). Late complications of COVID-19; a systematic review of current evidence. Arch. Acad. Emerg. Med. 9:e14. doi: 10.5501/wjv.v9.i5.79
Shen, W. B., Logue, J., Yang, P., Baracco, L., Elahi, M., Reece, E. A., et al. (2022). SARS-CoV-2 invades cognitive centers of the brain and induces Alzheimer’s-like neuropathology. bioRxiv [Preprint]. doi: 10.1101/2022.01.31.478476
Smirnov, D. S., Ashton, N. J., Blennow, K., Zetterberg, H., Simren, J., Lantero-Rodriguez, J., et al. (2022). Plasma biomarkers for Alzheimer’s Disease in relation to neuropathology and cognitive change. Acta Neuropathol. 143, 487–503. doi: 10.1007/s00401-022-02408-5
Solfrizzi, V., D’introno, A., Colacicco, A. M., Capurso, C., Todarello, O., Pellicani, V., et al. (2006). Circulating biomarkers of cognitive decline and dementia. Clin. Chim. Acta 364, 91–112. doi: 10.1016/j.cca.2005.06.015
Song, J., and Lee, J. E. (2015). miR-155 is involved in Alzheimer’s disease by regulating T lymphocyte function. Front. Aging Neurosci. 7:61. doi: 10.3389/fnagi.2015.00061
Steiner, J., Bernstein, H. G., Bielau, H., Berndt, A., Brisch, R., Mawrin, C., et al. (2007). Evidence for a wide extra-astrocytic distribution of S100B in human brain. BMC Neurosci. 8:2. doi: 10.1186/1471-2202-8-2
Sudhakar, M., Winfred, S. B., Meiyazhagan, G., and Venkatachalam, D. P. (2022). Mechanisms contributing to adverse outcomes of COVID-19 in obesity. Mol. Cell Biochem. 477, 1155–1193. doi: 10.1007/s11010-022-04356-w
Sun, B., Tang, N., Peluso, M. J., Iyer, N. S., Torres, L., Donatelli, J. L., et al. (2021). Characterization and biomarker analyses of Post-COVID-19 complications and neurological manifestations. Cells 10:386. doi: 10.3390/cells10020386
Suwanwongse, K., and Shabarek, N. (2021). Newly diagnosed diabetes mellitus. DKA, and COVID-19: Causality or coincidence? A report of three cases. J. Med. Virol. 93, 1150–1153. doi: 10.1002/jmv.26339
Swarbrick, S., Wragg, N., Ghosh, S., and Stolzing, A. (2019). Systematic review of miRNA as biomarkers in Alzheimer’s Disease. Mol. Neurobiol. 56, 6156–6167. doi: 10.1007/s12035-019-1500-y
Szelenberger, R., Kacprzak, M., Saluk-Bijak, J., Zielinska, M., and Bijak, M. (2019). Plasma MicroRNA as a novel diagnostic. Clin. Chim. Acta 499, 98–107. doi: 10.1016/j.cca.2019.09.005
Takano, K., Goto, K., Motobayashi, M., Wakui, K., Kawamura, R., Yamaguchi, T., et al. (2017). Early manifestations of epileptic encephalopathy, brain atrophy, and elevation of serum neuron specific enolase in a boy with beta-propeller protein-associated neurodegeneration. Eur. J. Med. Genet. 60, 521–526. doi: 10.1016/j.ejmg.2017.07.008
Tang, H., Gao, Y., Li, Z., Miao, Y., Huang, Z., Liu, X., et al. (2020). The noncoding and coding transcriptional landscape of the peripheral immune response in patients with COVID-19. Clin. Transl. Med. 10:e200. doi: 10.1002/ctm2.200
Taquet, M., Geddes, J. R., Husain, M., Luciano, S., and Harrison, P. J. (2021). 6-month neurological and psychiatric outcomes in 236 379 survivors of COVID-19: A retrospective cohort study using electronic health records. Lancet Psychiatry 8, 416–427. doi: 10.1016/S2215-0366(21)00084-5
Tavares-Junior, J. W. L., De Souza, A. C. C., Borges, J. W. P., Oliveira, D. N., Siqueira-Neto, J. I., Sobreira-Neto, M. A., et al. (2022). COVID-19 associated cognitive impairment: A systematic review. Cortex 152, 77–97. doi: 10.1016/j.cortex.2022.04.006
Thelin, E. P., Nelson, D. W., and Bellander, B. M. (2017). A review of the clinical utility of serum S100B protein levels in the assessment of traumatic brain injury. Acta Neurochir. (Wien) 159, 209–225. doi: 10.1007/s00701-016-3046-3
Thijssen, E. H., Verberk, I. M. W., Kindermans, J., Abramian, A., Vanbrabant, J., Ball, A. J., et al. (2022). Differential diagnostic performance of a panel of plasma biomarkers for different types of dementia. Alzheimers Dement. (Amst) 14:e12285. doi: 10.1002/dad2.12285
Thounaojam, M. C., Kaushik, D. K., and Basu, A. (2013). MicroRNAs in the brain: It’s regulatory role in neuroinflammation. Mol. Neurobiol. 47, 1034–1044. doi: 10.1007/s12035-013-8400-3
Tili, E., Mezache, L., Michaille, J. J., Amann, V., Williams, J., Vandiver, P., et al. (2018). microRNA 155 up regulation in the CNS is strongly correlated to Down’s syndrome dementia. Ann. Diagn. Pathol. 34, 103–109. doi: 10.1016/j.anndiagpath.2018.03.006
Turk, A., Kunej, T., and Peterlin, B. (2021). MicroRNA-target interaction regulatory network in Alzheimer’s disease. J. Pers. Med. 11:1275. doi: 10.3390/jpm11121275
Turski, W. A., Wnorowski, A., Turski, G. N., Turski, C. A., and Turski, L. (2020). AhR and IDO1 in pathogenesis of Covid-19 and the “Systemic AhR Activation Syndrome:” a translational review and therapeutic perspectives. Restor. Neurol. Neurosci. 38, 343–354. doi: 10.3233/RNN-201042
Van Kessel, S. A. M, Olde Hartman, T. C., Lucassen, P., and Van Jaarsveld, C. H. M. (2022). Post-acute and long-COVID-19 symptoms in patients with mild diseases: A systematic review. Fam. Pract. 39, 159–167. doi: 10.1093/fampra/cmab076
Varatharaj, A., Thomas, N., Ellul, M. A., Davies, N. W. S., Pollak, T. A., Tenorio, E. L., et al. (2020). Neurological and neuropsychiatric complications of COVID-19 in 153 patients: A UK-wide surveillance study. Lancet Psychiatry 7, 875–882. doi: 10.1016/S2215-0366(20)30287-X
Varma-Doyle, A. V., Lukiw, W. J., Zhao, Y., Lovera, J., and Devier, D. (2021). A hypothesis-generating scoping review of miRs identified in both multiple sclerosis and dementia, their protein targets, and miR signaling pathways. J. Neurol. Sci. 420:117202. doi: 10.1016/j.jns.2020.117202
Verde, F., Milone, I., Bulgarelli, I., Peverelli, S., Colombrita, C., Maranzano, A., et al. (2022). Serum neurofilament light chain levels in Covid-19 patients without major neurological manifestations. J. Neurol. 1–11. doi: 10.1007/s00415-022-11233-5 [Epub ahead of print].
Verde, F., Otto, M., and Silani, V. (2021). Neurofilament light chain as biomarker for amyotrophic lateral sclerosis and frontotemporal dementia. Front. Neurosci. 15:679199. doi: 10.3389/fnins.2021.679199
Vintimilla, R., Hall, J., Johnson, L., and O’bryant, S. (2019). The relationship of CRP and cognition in cognitively normal older Mexican Americans: A cross-sectional study of the HABLE cohort. Medicine (Baltimore) 98:e15605. doi: 10.1097/MD.0000000000015605
Wan, Z., Li, Y., Ye, H., Zi, Y., Zhang, G., and Wang, X. (2021). Plasma S100beta and neuron-specific enolase, but not neuroglobin, are associated with early cognitive dysfunction after total arch replacement surgery: A pilot study. Medicine (Baltimore) 100:e25446. doi: 10.1097/MD.0000000000025446
Wang, M. Y., Zhao, R., Gao, L. J., Gao, X. F., Wang, D. P., and Cao, J. M. (2020b). SARS-CoV-2: Structure. Biology, and Structure-Based Therapeutics Development. Front. Cell Infect. Microbiol. 10:587269. doi: 10.3389/fcimb.2020.587269
Wang, F., Kream, R. M., and Stefano, G. B. (2020a). Long-term respiratory and neurological sequelae of COVID-19. Med. Sci. Monit. 26:e928996. doi: 10.12659/MSM.928996
Wang, K. K., Yang, Z., Zhu, T., Shi, Y., Rubenstein, R., Tyndall, J. A., et al. (2018). An update on diagnostic and prognostic biomarkers for traumatic brain injury. Expert. Rev. Mol. Diagn. 18, 165–180. doi: 10.1080/14737159.2018.1428089
Wang, Q., Davis, P. B., Gurney, M. E., and Xu, R. (2021). COVID-19 and dementia: Analyses of risk, disparity, and outcomes from electronic health records in the US. Alzheimers Dement. 17, 1297–1306. doi: 10.1002/alz.12296
Wang, W. Y., Tan, M. S., Yu, J. T., and Tan, L. (2015). Role of pro-inflammatory cytokines released from microglia in Alzheimer’s disease. Ann. Transl. Med. 3:136.
Wang, Y., and Mandelkow, E. (2016). Tau in physiology and pathology. Nat. Rev. Neurosci. 17, 5–21. doi: 10.1038/nrn.2015.1
Watanabe, Y., Kitamura, K., Nakamura, K., Sanpei, K., Wakasugi, M., Yokoseki, A., et al. (2016). Elevated C-reactive protein is associated with cognitive decline in outpatients of a general hospital: The project in sado for total health (PROST). Dement. Geriatr. Cogn. Dis. Extra 6, 10–19. doi: 10.1159/000442585
Wei, X., Su, J., Yang, K., Wei, J., Wan, H., Cao, X., et al. (2020). Elevations of serum cancer biomarkers correlate with severity of COVID-19. J. Med. Virol. 92, 2036–2041. doi: 10.1002/jmv.25957
Wu, D., Cerutti, C., Lopez-Ramirez, M. A., Pryce, G., King-Robson, J., Simpson, J. E., et al. (2015). Brain endothelial miR-146a negatively modulates T-cell adhesion through repressing multiple targets to inhibit NF-kappaB activation. J. Cereb. Blood Flow Metab. 35, 412–423. doi: 10.1038/jcbfm.2014.207
Wu, J. T., Leung, K., and Leung, G. M. (2020a). Nowcasting and forecasting the potential domestic and international spread of the 2019-nCoV outbreak originating in Wuhan, China: A modelling study. Lancet 395, 689–697. doi: 10.1016/S0140-6736(20)30260-9
Wu, Y., Xu, X., Chen, Z., Duan, J., Hashimoto, K., Yang, L., et al. (2020b). Nervous system involvement after infection with COVID-19 and other coronaviruses. Brain Behav. Immun. 87, 18–22. doi: 10.1016/j.bbi.2020.03.031
Xin, Y., Zhang, L., Hu, J., Gao, H., and Zhang, B. (2021). Correlation of early cognitive dysfunction with inflammatory factors and metabolic indicators in patients with Alzheimer’s disease. Am. J. Transl. Res. 13, 9208–9215. doi: 10.3389/fneur.2022.944205
Xu, Y., Li, X., Zhu, B., Liang, H., Fang, C., Gong, Y., et al. (2020). Characteristics of pediatric SARS-CoV-2 infection and potential evidence for persistent fecal viral shedding. Nat. Med. 26, 502–505. doi: 10.1038/s41591-020-0817-4
Yan, J. H., Hua, P., Chen, Y., Li, L. T., Yu, C. Y., Yan, L., et al. (2020). Identification of microRNAs for the early diagnosis of Parkinson’s disease and multiple system atrophy. J. Integr. Neurosci. 19, 429–436. doi: 10.31083/j.jin.2020.03.163
Yao, H., Song, Y., Chen, Y., Wu, N., Xu, J., Sun, C., et al. (2020). Molecular architecture of the SARS-CoV-2 Virus. Cell 183, 730–738e713. doi: 10.1016/j.cell.2020.09.018
Yuan, A., Rao, M. V., and Veeranna Nixon, R. A. (2017). Neurofilaments and neurofilament proteins in health and disease. Cold Spring Harb. Perspect. Biol. 9:a018309. doi: 10.1101/cshperspect.a018309
Yuen, S. C., Liang, X., Zhu, H., Jia, Y., and Leung, S. W. (2021). Prediction of differentially expressed microRNAs in blood as potential biomarkers for Alzheimer’s disease by meta-analysis and adaptive boosting ensemble learning. Alzheimers Res. Ther. 13:126. doi: 10.1186/s13195-021-00862-z
Zanella, I., Blasco, H., Filosto, M., and Biasiotto, G. (2022). Editorial: The impact of neurofilament light chain (NFL) quantification in serum and cerebrospinal fluid in neurodegenerative diseases. Front. Neurosci. 16:915115. doi: 10.3389/fnins.2022.915115
Zeitlberger, A. M., Thomas-Black, G., Garcia-Moreno, H., Foiani, M., Heslegrave, A. J., Zetterberg, H., et al. (2018). Plasma markers of neurodegeneration are raised in friedreich’s ataxia. Front. Cell Neurosci. 12:366. doi: 10.3389/fncel.2018.00366
Zeng, N., Zhao, Y. M., Yan, W., Li, C., Lu, Q. D., Liu, L., et al. (2022). A systematic review and meta-analysis of long term physical and mental sequelae of COVID-19 pandemic: Call for research priority and action. Mol. Psychiatry 1–11. doi: 10.1038/s41380-022-01614-7 [Epub ahead of print].
Zhang, B., Chen, C. F., Wang, A. H., and Lin, Q. F. (2015). MiR-16 regulates cell death in Alzheimer’s disease by targeting amyloid precursor protein. Eur. Rev. Med. Pharmacol. Sci. 19, 4020–4027.
Zhang, L., Dong, L. Y., Li, Y. J., Hong, Z., and Wei, W. S. (2012). miR-21 represses FasL in microglia and protects against microglia-mediated neuronal cell death following hypoxia/ischemia. Glia 60, 1888–1895. doi: 10.1002/glia.22404
Zhang, M., Chen, M. Y., Wang, S. L., Ding, X. M., Yang, R., Li, J., et al. (2022a). Association of Ubiquitin C-Terminal Hydrolase-L1 (Uch-L1) serum levels with cognition and brain energy metabolism. Eur. Rev. Med. Pharmacol. Sci. 26, 3656–3663.
Zhang, Y., Chen, X., Jia, L., and Zhang, Y. (2022b). Potential mechanism of SARS-CoV-2-associated central and peripheral nervous system impairment. Acta Neurol. Scand. 146, 225–236. doi: 10.1111/ane.13657
Zhou, Z., Kang, H., Li, S., and Zhao, X. (2020b). Understanding the neurotropic characteristics of SARS-CoV-2: From neurological manifestations of COVID-19 to potential neurotropic mechanisms. J. Neurol. 267, 2179–2184. doi: 10.1007/s00415-020-09929-7
Zhou, H., Lu, S., Chen, J., Wei, N., Wang, D., Lyu, H., et al. (2020a). The landscape of cognitive function in recovered COVID-19 patients. J. Psychiatr. Res. 129, 98–102. doi: 10.1016/j.jpsychires.2020.06.022
Keywords: COVID-19, cognitive impairment, neurodegenerative diseases, circulating biomarkers, microRNAs
Citation: Alvarez M, Trent E, Goncalves BDS, Pereira DG, Puri R, Frazier NA, Sodhi K and Pillai SS (2022) Cognitive dysfunction associated with COVID-19: Prognostic role of circulating biomarkers and microRNAs. Front. Aging Neurosci. 14:1020092. doi: 10.3389/fnagi.2022.1020092
Received: 15 August 2022; Accepted: 13 September 2022;
Published: 04 October 2022.
Edited by:
Suman Dutta, University of California, Los Angeles, United StatesReviewed by:
Seong Lin Teoh, National University of Malaysia, MalaysiaCopyright © 2022 Alvarez, Trent, Goncalves, Pereira, Puri, Frazier, Sodhi and Pillai. This is an open-access article distributed under the terms of the Creative Commons Attribution License (CC BY). The use, distribution or reproduction in other forums is permitted, provided the original author(s) and the copyright owner(s) are credited and that the original publication in this journal is cited, in accordance with accepted academic practice. No use, distribution or reproduction is permitted which does not comply with these terms.
*Correspondence: Sneha S. Pillai, cGlsbGFpc0BtYXJzaGFsbC5lZHU=
†These authors have contributed equally to this work
Disclaimer: All claims expressed in this article are solely those of the authors and do not necessarily represent those of their affiliated organizations, or those of the publisher, the editors and the reviewers. Any product that may be evaluated in this article or claim that may be made by its manufacturer is not guaranteed or endorsed by the publisher.
Research integrity at Frontiers
Learn more about the work of our research integrity team to safeguard the quality of each article we publish.