- 1Ocular Molecular Biology and Mechanisms of Disease Group, Institute of Life Course and Medical Sciences, University of Liverpool, Liverpool, United Kingdom
- 2Faculty of Medicine, Siriraj Hospital, Mahidol University, Bangkok, Thailand
- 3Integrative Genomics of Ageing Group, Institute of Life Course and Medical Sciences, University of Liverpool, Liverpool, United Kingdom
The retinal pigment epithelium (RPE) and the choroid are ocular tissues with fundamental roles in supporting neuroretinal function. The pathogenesis of age-related macular degeneration (AMD), a leading cause of irreversible blindness for which aging is the highest risk factor is closely linked with progressive impairment of various functions of these tissues. Cellular senescence, marked by cell cycle arrest and secretion of proinflammatory factors, is known to be associated with aging and has been proposed as a potential driver of AMD. Here, we investigated the role played by intercellular communication in the RPE/choroid within the context of aging, senescence and AMD. We inferred cell–cell interactions in the RPE/choroid by applying CellChat and scDiffCom on a publicly available scRNA-seq dataset from three human donors with and without AMD. We identified age-regulated ligand and receptor genes by using limma on a separate publicly available bulk microarray dataset providing RPE/choroid samples at multiple time points. Cellular senescence was investigated by assigning a score to each cell and each sample of these scRNA-seq and microarray datasets, respectively, based on the expression of key signature genes determined by a previous senescence meta-analysis. We identified VEGF-, BMP-and tenascin-mediated pathways supporting some of the strongest cell–cell interactions between RPE cells, fibroblasts and choroidal endothelial cells and as strong intercellular communication pathways related to both aging and senescence. Their signaling strength was enhanced between subpopulations of cells having high senescence scores. Predominant ligands of these pathways were upregulated with age whereas predominant receptors were downregulated. Globally, we also observed that cells from AMD samples presented slightly bigger senescence scores than normal cells and that the senescence score positively correlated with age in bulk samples (R = 0.26, value of p < 0.01). Hence, our analysis provides novel information on RPE/choroid intercellular communication that gives insights into the connection between aging, senescence and AMD.
Introduction
Age-related macular degeneration (AMD) is the leading cause of irreversible blindness in adults in developed countries (Wong et al., 2014), which has been linked to numerous genetical and environmental risk factors and most importantly age (Group et al., 2012; Wong et al., 2014). However, the molecular mechanisms by which aging contributes to AMD pathogenesis are far from being fully characterized. One hypothesis suggests cellular senescence of the retinal pigment epithelium (RPE), the cell monolayer with essential role in supporting the function of the neuroretina, as a key process promoting the development of AMD (Kozlowski, 2012). Cellular senescence impairs the ability of self-renewal – a biological process that is particularly important for the mitotically inactive RPE – and contributes to an increased inflammatory phenotype, mostly through the secretion of inflammatory cytokines and proteases, collectively defined as the senescence-associated secretory phenotype (SASP) (Kozlowski, 2012; Wang et al., 2019; Basisty et al., 2020). Age-related factors like increased oxidative stress, altered proteostasis and DNA damage are thought to promote senescence in several age-related neurodegenerative diseases such as Alzheimer’s disease and Parkinson’s disease (Kritsilis et al., 2018; Martinez-Cue and Rueda, 2020). An increase in senescent cells in aged RPE and choroid tissues has also been reported (Chaum et al., 2015; Cabrera et al., 2016) consistent with altered cell signaling known to promote chronic inflammation and RPE cellular dysfunction (Cao et al., 2013). Senescent RPE cells likely influence neighboring cells, potentially contributing to the development of AMD characteristics such as increased choroidal endothelial stiffness and membrane attack complex deposition (Cabrera et al., 2016; Lee et al., 2021). Although an ongoing effort is trying to connect aging, cellular senescence and the pathogenesis of AMD, the molecular mechanisms underlying their relationship are still not well established (Blasiak et al., 2017; Sreekumar et al., 2020).
The RPE and the choroid form a system that plays crucial roles in the normal function of the neuroretina as well as in the pathology of AMD (Strauss, 2005). It supports the neuroretinal metabolism by supplying nutrients and facilitating the removal of waste. The junctional complex of the RPE is critical for the blood–brain barrier while the tissue itself acts as a secretory machinery to support the communication between the choroid and the retina (Paraoan et al., 2020). During AMD, lipoproteinaceous and other extracellular debris accumulate between the RPE and the choroid, leading to the formation of drusen (Ardeljan and Chan, 2013). Previous research investigating how this system ages focused on the RPE or the choroid alone (Paraoan et al., 2000; Kay et al., 2013, 2014; Porter et al., 2019; Sharif et al., 2019; Voigt et al., 2019, 2020; Dhirachaikulpanich et al., 2020; Butler et al., 2021; Saptarshi et al., 2021). However, given that the RPE/choroid system is a complex microenvironment composed of many cell types functioning together, including RPE cells, endothelial cells, fibroblasts and immune cells (Voigt et al., 2021, 2022), the communication between the cellular components of the RPE/choroid needs also to be characterized.
The advent of single-cell RNA sequencing (scRNA-seq) and the development of cell–cell communication software now enables the investigation of the interactions between different cell types in a tissue (Armingol et al., 2021). In this context, intercellular communication (ICC) is defined as a set of signaling interactions involving secreted proteins (ligands) from one cell type and membrane-bound proteins (receptors) from another (or the same) cell type (Jin et al., 2021). Inferring such intercellular communication patterns, including secreted protein crosstalk and extracellular matrix receptor interactions, could prove useful to identify key signaling pathways in normal and disease conditions (Armingol et al., 2021; Jin et al., 2021). This type of analysis has been applied to a variety of diseases, including COVID-19 (He et al., 2021; Yang et al., 2021), wound healing (Hu et al., 2021), inflammatory bowel disease (Corridoni et al., 2020). However, no study has yet investigated intercellular communication of the RPE/choroid in the context of aging, senescence and AMD. For this purpose here we leveraged publicly available microarray and scRNA-seq datasets of the RPE/choroid to investigate cell–cell signaling altered by aging and senescence in normal and AMD tissues. Our analysis predicts three specific age-related pathways (VEGF, BMP and tenascin) that are enriched in interactions taking place between a set of fibroblasts, RPE cells and endothelial cells characterized by high expression of senescence signature genes. Although the scarcity of the currently available data does not allow to firmly establish how these pathways differ between AMD and normal patients, we independently observe that AMD samples have a higher expression of senescence signature genes compared to normal samples. Together, our results support the notion that cellular senescence plays an important role in the aging of the RPE/choroid microenvironment and AMD pathogenesis.
Materials and methods
Single-cell RNA-seq data acquisition and processing
We retrieved publicly available scRNA-seq RPE/choroid data (GSE135922) containing samples from three human donors, including two normal eyes and one uncharacterized neovascular AMD eye (Voigt et al., 2019). This dataset was downloaded as a Seurat object through the human cell atlas galaxy portal (Moreno et al., 2021; Papatheodorou et al., 2020). The subsequent analysis was done in R (version 4.0.2). Cells were filtered out if they had unique gene counts lower than 300 or more than 7,000. Log normalization was performed with a scale factor of 10,000 using Seurat (version 4.0.1). The Seurat functions FindNeighbors and FindClusters were used to cluster the cells based on the first 10 principal components returned by the function RunPCA. For visualization purposes, UMAP dimensional reduction was performed with the function RunUMAP using the same 10 principal components (Hao et al., 2021). The gene markers’ identifier of each cell cluster was done with the function FindAllMarkers. These markers were used to annotate each cluster by cell types similarly to Voigt et al. (2019).
Intercellular communication analysis
Intercellular communication (ICC) analysis was performed on the pre-processed Seurat object described above with two R packages relying on different detection methods: CellChat (Jin et al., 2021) and scDiffCom (Lagger et al., 2021). Both algorithms combine the cell type-specific expression of known ligand and receptor genes with permutation tests to assess the biological relevance of potential cell–cell interactions (CCIs). They differ in the way they quantify the strength of these interactions (respectively referred to as “communication probability” and “CCI score”) and in their internal statistical implementations. scDiffCom was also specifically designed to perform differential ICC analysis between biological conditions, but this functionality was not used in the current study because of the low sample size of the aforementioned scRNA-seq dataset. Default settings of CellChat (version 1.0.0) were used as recommended (Jin et al., 2021). scDiffCom (version 0.2.3) was used in “detection-mode-only” with default parameters, except for the number of permutations that was set to 10,000 (instead of 1,000) and the “quantile expression threshold” that was set to 0 (instead of 0.2). The latter parameter is used by scDiffCom to filter out statistically significant but lowly expressed CCIs. As such filtering is not performed by CellChat by default, we disabled it in this analysis to obtain more similar results. Detection of CCIs by both packages crucially depends on the prior database of curated ligand-receptor interactions (LRI) they rely on. Such LRIs can be pairs of genes (e.g., AMD: CALCR) or include additional factors to describe heteromeric complexes (e.g., ANGPT2:ITGA1-ITGB1). CellChat manually created a curated list of 1,939 human LRIs principally based on knowledge extracted from the KEGG database (Kanehisa et al., 2016). However, scDiffCom relies on a larger collection of 4,785 LRIs obtained by combining seven LRI databases that had been manually curated by previous studies, including CellChat itself. As scDiffCom has therefore the potential to detect more CCIs than CellChat, we took this factor into account when comparing their results.
Network analysis to compute centrality scores of outgoing, incoming or mediator communication pathways was performed with CellChat built-in function netAnalysis_computeCentrality. The determination of the dominant and most relevant pathways was performed by exploring and comparing network results manually and by using either visualization tools provided by CellChat (e.g., netAnalysis_signalingRole_network) or custom scripts for scDiffCom results.
Differential analysis of ligand/receptor genes in aging RPE/choroid microarray datasets
Transcriptomic microarray data were retrieved from GSE29801 (Newman et al., 2012). This dataset contained 96 post-mortem RPE/choroid samples with no previous ocular disease. The donors’ age ranged from 9 to 93 years old. We identified genes differentially expressed with age using the R package limma (Ritchie et al., 2015) with the linear model indicated below,
In this regression model, Yij is the expression level of gene j in sample i; Agei denotes the age of sample i; Sexi denotes the sex of sample i and Macular/non-Maculari denotes the anatomical location of RPE/choroid of sample i.
The genes were filtered to include only factors relevant to intercellular communication. As a reference, we used the genes from the LRI database of scDiffCom (that also includes those from CellChat, as explained above). Age-associated differential expression was considered significant for adjusted p-values (Benjamini-Hochberg) <0.1 and absolute log2 fold change bigger than log2(1.5)/70 years.
Senescence gene expression and intercellular communication analysis
We scored each cell from the scRNA-seq dataset and each sample from the microarray dataset in relation to the level of expressed senescence signature genes. Such signatures were obtained from a recent meta-analysis that identified genes up-and down-regulated with senescence across 20 microarray datasets (Chatsirisupachai et al., 2019). This dataset provided 1,232 senescence signature genes. As recommended in the GSEA user guide to limit gene set within 500 genes (Hanzelmann et al., 2013), we selected only genes with a q-value from the meta-analysis <0.001, and obtained 135 upregulated and 271 downregulated genes (Supplementary Tables 1, 2). The senescence score was defined as follows:
where each term corresponds to a gene set enrichment analysis (GSEA) score computed either on each cell or sample (ssGSEA) using the upregulated, respectively downregulated, senescence signatures as gene sets. ssGSEA scores were computed from the scRNA-seq Seurat object described above by using the function enrichIt from the R package escape (version 1.1.1; Borcherding et al., 2021). ssGSEA scores for microarray samples were computed using the R package gsva (version 1.38.2; Hanzelmann et al., 2013).
Results
Inferring intercellular communication in the RPE/choroid
To initiate the analysis of the intercellular communication occurring at the level of the RPE and choroid, we used RPE/choroid scRNA-seq data from the publicly available dataset GSE135922 (Voigt et al., 2019) that had been obtained from post-mortem tissues of 3 donors, including one patient with neovascular (wet) AMD and two non-AMD patients (Figure 1A). Following standard pre-processing (see Materials and methods), we annotated 4,766 cells based on previously reported cell-type-specific marker genes as follows: fibroblasts (APOD), melanocytes (PMEL), lymphocytes (CXCR4), Schwann cells (PLP1), RPE (RPE65), macrophages (IGKC), endothelial cells (VWF) and mast cells (TPSB2; Figures 1B–E). Our annotation is similar to the original work from Voigt et al. (2019), although they have described more specific clusters such as subpopulations of Schwann cells and lymphocytes that are not relevant to our current study. We next applied the CellChat algorithm to the healthy and AMD samples separately and detected 3,600 and 3,430 cell-type to cell-type interactions (CCIs), respectively (Supplementary Tables 3, 4). As it has been recently pointed out that different intercellular communication algorithms might be prone to returning different results (Dimitrov et al., 2021, 2022), we also used a second package, scDiffCom, to further confirm CellChat findings. When using the same prior LRI database as CellChat, scDiffCom returned 2,597 and 2,081 CCIs in normal and AMD samples, respectively. Out of those, 2,274 and 1827 CCIs, respectively, were commonly detected by both packages (Supplementary Figures 1A–C), indicating that the scDiffCom algorithm is generally more conservative. As expected, when using its extended curated database including more LRIs than those present in CellChat, scDiffCom returned significantly more CCIs, namely 8,629 and 7,176, respectively, (Supplementary Tables 5, 6). The detected interactions provide a global atlas of intercellular communication in the human RPE/choroid. Although we considered normal and AMD tissues separately, we note that a sample size of only three patients was not large enough to perform a complete differential ICC analysis.
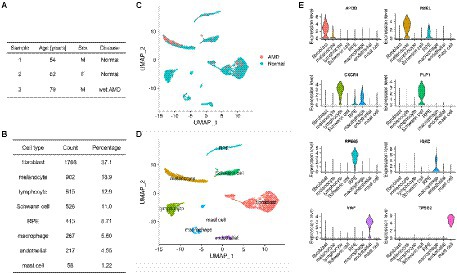
Figure 1. Summary of the RPE/choroid scRNA-seq dataset used for cell–cell communication analysis. (A) Demographic data including disease status of 3 RPE/choroid donors from GSE135922. (B) Count and percentage of each specific cell type detected from the 4,766 cells of the scRNA-seq data. (C,D) Dimensionality reduction (UMAP) of the scRNA-seq data showing eight cell types in the RPE/choroid. (C) Cells are colored by disease status (normal or AMD). (D) Cells are colored by cell-type annotations. (E) Violin plots showing the expression of marker genes used to annotate cell clusters.
Secreted proteins-mediated signaling and extracellular matrix interactions
As the RPE plays an important role in the homeostasis of surrounding tissues, we were particularly interested in secreted proteins-mediated signaling pathways engaging RPE and/or other cells. Network centrality analysis on CellChat data revealed that vascular endothelial growth factor (VEGF), bone morphogenic proteins (BMP), pleiotrophin (PTN), growth differentiation factors (GDF), granulin (GRN) and hepatocyte growth factor (HGF) were among the top such pathways between RPE cells and cells from the choroid (Figure 2A; Supplementary Figures 2A–F). The CellChat cell-type-specific networks of VEGF and BMP (Figures 2B,C) showed that RPE cells are the prominent source of secretion of effectors for these two pathways whereas endothelial cells and, respectively, fibroblast/endothelial cells are the major targets. Results derived from scDiffCom also confirmed these findings (Supplementary Figures 3A,B). VEGF denotes a group of signal proteins that promote blood vessel formation or angiogenesis (Senger et al., 1983; Ferrara et al., 2003; Gogat et al., 2004) that have been studied in various diseases (Ellis and Hicklin, 2008) as their main inhibitor (anti-VEGF) could reduce the severity of many pathological conditions, including wet AMD (Schmidt-Erfurth et al., 2014). BMP are cytokines related to tissue regeneration and development (Sieber et al., 2009). BMP has been associated with the development of the neural retina and RPE (Hocking and McFarlane, 2007), RPE migration and maintenance of RPE barrier integrity (Ibrahim et al., 2020). Here, our cell–cell communication analysis highlighted VEGF and BMP as dominant signaling pathways from the RPE to the choroid.
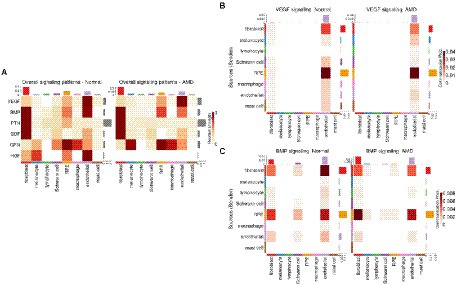
Figure 2. Secreted proteins-mediated signaling involving RPE cells derived from CellChat results. (A) Heatmap showing the relative strength of selected signaling pathways across cell types in both normal and AMD tissues in the scRNA-seq dataset from Voigt et al. (2019). Pathways were selected as those being the most relevant to communication patterns involving RPE cells. (B) Cell-type to cell-type heatmap of CellChat communication probabilities aggregated from each VEGF LRI. VEGF signaling was predominantly targeting endothelial cells and was especially elevated when originating from RPE cells. (C) Cell-type to cell-type heatmap of CellChat communication probabilities aggregated from each BMP LRI. BMP signaling was predominantly targeting fibroblasts and endothelial cells and was especially elevated when originating from RPE cells and fibroblasts.
As alterations of the extracellular matrix have been associated with dysfunction of the RPE/choroid and with AMD, especially in relation to migration/wound healing and angiogenesis (Pouw et al., 2021), we then extracted all CCIs detected by CellChat and scDiffCom involving ECM-receptor interactions. Prominent ECM-related pathways included collagen, laminin, fibronectin 1 (FN1), thrombospondin (THBS), tenascin and vitronectin (VTN; Figure 3A). CellChat cell-type-specific networks showed that fibroblasts, Schwann cells and endothelial cells were the major sources of effectors for these pathways (Figures 3B–D). Again, the same patterns were revealed by scDiffCom (Supplementary Figures 4A–C).
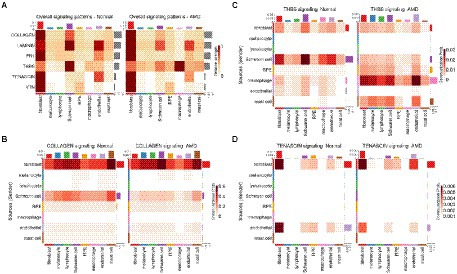
Figure 3. ECM-related signaling in the RPE/choroid derived from CellChat results. (A) Heatmap showing the relative strength of highly expressed ECM-related pathways across cell types in both normal and AMD tissues in the scRNA-seq dataset from Voigt et al. (2019). (B) Cell-type to cell-type heatmap of CellChat communication probabilities aggregated from each COLLAGEN LRI. COLLAGEN signaling was mostly originating from fibroblast and Schwann cells targeting all cell types similarly. (C) Cell-type to cell-type heatmap of CellChat communication probabilities aggregated from each thrombospondin LRI. Thrombospondin signaling was mostly originating from fibroblast and Schwann cells and targeting various cell types. (D) Cell-type to cell-type heatmap of CellChat communication probabilities aggregated from each tenascin LRI. Tenascin signaling was mostly originating from fibroblast and endothelial cells targeting predominantly themselves as well as RPE and Schwann cells to a lesser extent.
Age-associated ligands and receptors in the RPE/choroid
As there is currently no scRNA-seq dataset of the RPE/choroid across the human lifespan, we relied on the bulk microarray data from (Newman et al., 2012) to investigate potential age-related expression changes in ligand and receptor genes. We performed a linear regression across age and accounted for potential confounding factors such as retinal location (macular versus non-macular). Out of the 1,854 unique ligand/receptor genes present in the scDiffCom LRI database, we found that 60 of them were upregulated and, respectively, 103 of them were downregulated, with age in the bulk data (BH adjusted p-value < 0.1 and|log2 (fold change)| > log2(1.5)/70 years; Supplementary Table 7). To focus on the communication between the RPE and the cell types of the choroid, we then selected the genes that were explicitly taking part in a least one cell–cell interaction detected by scDiffCom either originating from or targeting the RPE. We obtained 46 and 43 ligand/receptor genes upregulated and, respectively, downregulated with age (Table 1).
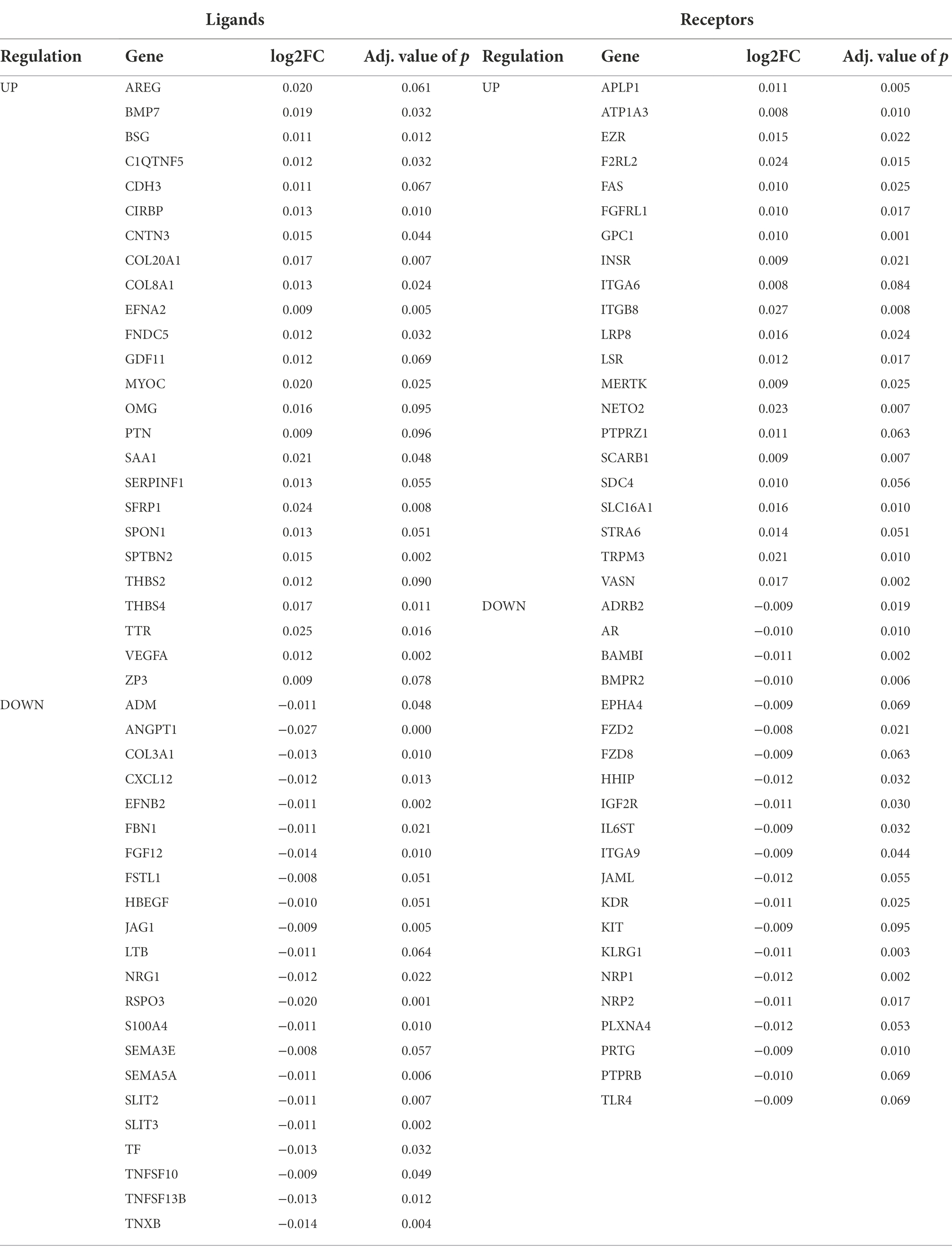
Table 1. Ligands and receptors that are differentially expressed with age in RPE/choroid microarray samples and taking part in cell–cell interactions (detected by scDiffCom in scRNA-seq data) specifically from or to the RPE cell type.
Several of the genes differentially expressed with age were part of the top secreted pathways detected by CellChat/scDiffCom from or to RPE cells, including VEGFA, KDR, BMP7, BMPR2, PTN, SDC4 and GDF11 (Table 1). Regarding VEGF pathway, the ligand gene VEGFA was upregulated with age in RPE/choroid bulk data (Figure 4A) and mainly expressed by RPE cells (Figure 4A) in scRNA-seq data. KDR, a receptor of the VEGF pathway, was downregulated with age in RPE/choroid bulk data (Figure 4A) and predominantly expressed by endothelial cells in scRNA-seq data (Figure 4B). Regarding the BMP pathway, the ligand gene BMP7 was upregulated in aging RPE/choroid bulk data (Figure 4C) and mainly expressed by RPE cells (Figure 4D) in scRNA-seq data. BMPR2, a receptor of the BMP pathway, was downregulated with age in RPE/choroid bulk data (Figure 4C) and mainly expressed by endothelial cells (Figure 4D). Collectively, these results support the idea that aging RPE cells tend to increase the secretion of VEGF and BMP factors toward the choroid, whereas endothelial cells tend to decrease the expression of the corresponding receptors, suggesting a potential compensatory mechanism, which may offer some protection against initial pathological changes.
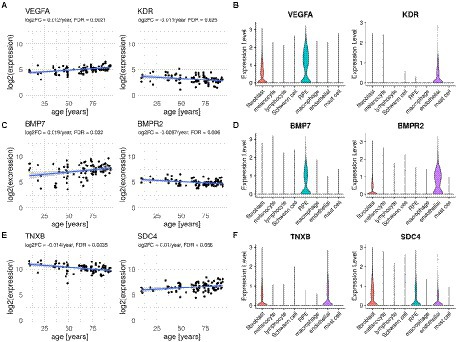
Figure 4. Ligand and receptor genes differentially expressed with age in the RPE/choroid with their typical cell type-specific expression. (A,C,E) Scatter plots of selected ligands and receptors showing expression changes with age in human RPE/choroid microarray samples from Newman et al. (2012). Displayed Log2FC and FDR are those computed with Limma according to the linear model described in Methods. (B,D,F) Violin plots showing expression levels of corresponding genes in RPE/choroid cell types from scRNA-seq samples of Voigt et al. (2019).
Several genes belonging to the top ECM-related pathways detected by CellChat from the scRNA-seq data were also differentially expressed with age in the bulk dataset, including collagen and integrin genes, THBS2, THBS4, SDC4 and TNXB (Table 1). Among these, the ligand-receptor pair TNXB/SDC4 was particularly noteworthy. TNXB was expressed mostly by fibroblasts and endothelial cells in the scRNA-seq data (Figure 4F) and downregulated with age in the bulk data (Figure 4E). SDC4 was expressed by RPE cells, fibroblasts and endothelial cells in the scRNA-seq data (Figure 4F) and upregulated with age in the bulk data (Figure 4E). Changes in tenascin expression have been previously related to development and aging (Choi et al., 2020; Matsumoto and Aoki, 2020) as well as to pathologic conditions such as cancer, inflammation and fibrosis (Albacete-Albacete et al., 2021; Tajiri et al., 2021). Disruption of the tenascin pathway also results in promoting inflammatory processes and angiogenesis (Kobayashi et al., 2016). Tenascin pathway genes were also reported as being enriched among differentially expressed genes in the RPE/choroid in AMD, including Tenascin-C (Dhirachaikulpanich et al., 2020) and TNXB (Porter et al., 2019).
Effects of cellular senescence on the RPE/choroid intercellular communication
We next sought to characterize potential cellular senescence signatures in the RPE/choroid as well as their effects on intercellular communication. We first assigned a senescence score to each cell of the RPE/choroid scRNA-seq dataset from Voigt et al. (2019), using the ssGSEA approach (Barbie et al., 2009; Borcherding et al., 2021). As reference signatures, we used the results from a previous meta-analysis that inferred genes up-and down-regulated with senescence by comparing 20 bulk transcriptomics datasets (Chatsirisupachai et al., 2019; Supplementary Tables 1, 2). The senescence score was defined as the difference between the ssGSEA score of upregulated signature genes and the ssGSEA score of downregulated signature genes (Figure 5A). We noticed that cells from the patient with AMD had a slightly larger average senescence score (mean = 0.21, SD = 0.21) compared to cells from healthy samples (mean = 0.18, SD = 0.22; Wilcoxon Rank Sum Test, value of p = 1.37E-6; Figure 5B). However, more data with a larger sample size in terms of patients will be required in the future to better establish if AMD is consistently associated with an increased expression of senescence-related genes.
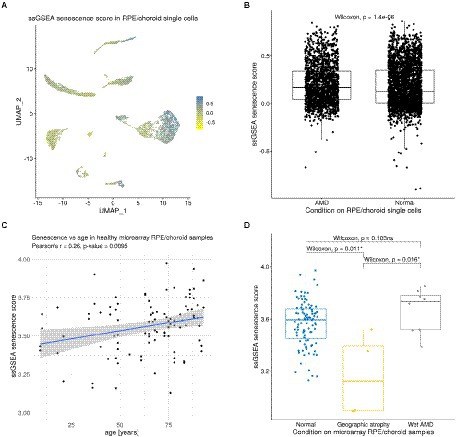
Figure 5. Expression of senescence signature genes in RPE/choroid scRNA-seq and microarray data. (A) Dimensionality reduction (UMAP) showing the ssGSEA senescence score of each cell in the scRNA-seq dataset from Voigt et al. (2019). (B) Boxplot showing a slight increase in the senescence score of AMD cells compared to normal cells from Voigt et al. (2019). (C) Positive correlation between senescence scores and age in the non-AMD microarray samples of Newman et al. (2012) (Pearson’s correlation R = 0.26, value of p = 0.0095). (D) Boxplot showing significant and non-significant differences in senescence scores between normal, wet AMD and geographic atrophy RPE/choroid samples from Newman et al. (2012).
We then used the senescence score to label cells as either “normal” or “senescent-like.” It is important to note that this method did not allow us to claim with certainty that cells with high scores were actually in a senescent state before being captured for sequencing. Nevertheless, it allowed us to extract cells that at least showed a senescence-like gene expression profile. In practice, we defined such cells as those having a score in the top 20% of all senescence scores across all cell types (Supplementary Figures 5A–C). We did not find any relevant differences in the distribution of the number of such senescent-like cells across cell types between the three donors. However, we confirmed that the expression of genes involved in typical senescence pathways, including TP53, CDKN1A, RB1, NFKB1 and NOTCH1, was generally higher in senescent-like cells compared to normal cells (Supplementary Figure 5D).
We performed a new intercellular communication analysis on the same scRNA-seq data as above by splitting each cell type into the two subpopulations of normal and senescent-like cells (and by merging the samples of all three donors together). As we did not consider groups with <11 cells, the analysis was performed on 12 subpopulations (Supplementary Figure 5C). When using the LRI database from Cellchat, CellChat and scDiffCom returned, respectively, 11,162 and 5,637 CCIs, out of which 5,044 were detected by both methods (Supplementary Figures 6A; Supplementary Tables 8, 9). When using its extended LRI database, scDiffCom returned 18,908 CCIs (Supplementary Table 9). Ranking pathways according to centrality measures with CellChat revealed that the pathways previously found to be associated with aging were among the top signaling patterns associated with senescent cell subpopulations. VEGF was predominantly expressed from senescent-like (sl-) RPE cells toward sl-endothelial cells (Figure 6A). Similarly, BMP showed stronger signaling from sl-RPE cells toward sl-endothelial cells, sl-Schwann cells and sl-fibroblasts compared to all other cell types (Figure 6B). Finally, tenascin-mediated signaling was also stronger between sl-fibroblasts and sl-endothelial cells and from these two cell populations toward sl-RPE cells (Figure 6C).
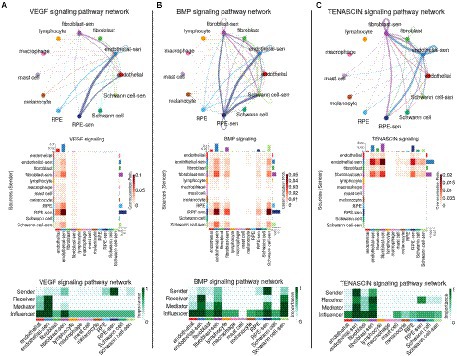
Figure 6. Network representation of the impact of senescence on VEGF, BMP and TENASCIN intercellular communication in the RPE/choroid. Signaling network summary between senescent-like and non-senescent cell types for (A) VEGF, (B) BMP and (C) TENASCIN pathways. Cell type to cell type networks (top panels) and heatmaps (middle panels) show the CellChat communication probability (aggregated over all LRIs from the given pathway) between each cell type pair. Centrality heatmaps (bottom panels) show the various CellChat centrality scores across each cell type for the selected pathways.
Results from scDiffCom generally recapitulated most of these findings from CellChat, with some differences to mention. First, scDiffCom did not predict as CellChat that sl-endothelial cells were more targeted by VEGF than endothelial cells. Instead, these two cell populations shared similar overall “receiver scores” (Supplementary Figure 6B). We still noted, in agreement with CellChat, that VEGF was secreted more by sl-RPE cells than by RPE or other cells. Second, scDiffCom did not predict a strong BMP signaling from sl-RPE cells toward sl-Schwann cells, but instead toward Schwann cells (Supplementary Figure 6C). Finally, although scDiffCom predicted as CellChat a strong tenascin signaling from sl-endothelial cells toward sl-fibroblasts, the opposite signaling from sl-fibroblasts toward sl-endothelial cells was much weaker than as predicted by CellChat (Supplementary Figure 6D). Also more generally, the overall decrease of tenascin signaling involving fibroblasts compared with sl-fibroblasts appeared less prominent when looking at scDiffCom compared to CellChat analysis.
Despite these differences, the results from both CellChat and scDiffCom analyses highlighted how senescence state might influence RPE/choroid tissue in both normal and AMD, specifically by enhancing ICC via VEGF, BMP and tenascin pathways.
Effects of age on cellular senescence scores in microarray RPE/choroid data
As we showed that senescence might influence ICC in the RPE/choroid, we further explored other potential factors that might be related to senescence in this tissue. To test the hypothesis that senescence might increase with age in the RPE/choroid, we assigned a senescence score to each sample of the bulk microarray dataset from Newman et al. (2012) and explored its evolution with time. We found that the senescence score was significantly correlated with age (Pearson’s correlation R = 0.26, value of p = 0.0095; Figure 5C). This is consistent with earlier observations reporting a similar accumulation of senescent cells in different other tissues (Chatsirisupachai et al., 2019). We also compared the senescence score between control (non-AMD) samples and different types of AMD, including neovascular AMD and geographic atrophy (an end-stage non-neovascular type AMD). The results showed that neovascular AMD samples (n = 8) had a slightly, but non-significantly, higher senescence score than non-AMD ones (n = 96; Wilcoxon Rank Sum Test, value of p = 0.103), while geographic atrophy samples (n = 4) had a significantly lower senescence score than non-AMD samples (n = 96; value of p = 0.011) and neovascular AMD samples (n = 8; value of p = 0.016; Figure 5D). Overall, these two analyses suggested that the senescence score in the RPE/choroid may be affected by both age and AMD types. However, the fact that when comparing non-AMD to wet AMD there is no significant difference in the bulk microarray dataset (Figure 5D) but a significant difference in the single-cell dataset (Figure 5B) shows that further studies leading to more data and further analyses are necessary. Nevertheless, the fact that the RPE/choroid cells with high senescence scores presented increased signaling via VEGF, BMP and tenascin pathways suggests a possible connection between senescence and AMD RPE/choroid.
Discussion
An increasing number of patients suffer from the main age-related degenerative eye disease involving retinal tissues, AMD, which may lead to irreversible blindness. Current standard treatments can only address a small subset of the disease by slowing down the disease progression (Wong et al., 2014; Mitchell et al., 2018), highlighting the importance and need for better understanding the biology of the RPE/choroid tissue in the context of aging and cellular senescence (Sreekumar et al., 2020; Lee et al., 2021). At the histological level, it is known that aging leads to abnormality of pigmentation with increase of soft drusen (a lipoproteinaceous deposit) between the RPE and the underlying choroid (Boulton et al., 2004; Curcio et al., 2009; Ardeljan and Chan, 2013; Curcio, 2018). At molecular level, a recent first RNA-seq global gene expression study of the aging human RPE reported upregulation of the visual cycle genes in the RPE with increasing age (Butler et al., 2021) and bulk and single-cell gene expression analyses of the aging human choriocapillaris reported an increase of the pro-inflammatory environment in the choroid (Voigt et al., 2020).
The study presented herein focused on the communication occurring at the level and between these two tissues, and on the effect that the aging process has on this communication. By applying algorithms that have proved useful to study intercellular communication in various tissues from scRNA-seq data (Armingol et al., 2021; He et al., 2021; Hu et al., 2021; Jin et al., 2021; Lagger et al., 2021), we were able to extract important signaling patterns between the RPE and the choroid. Together with our aging and cellular senescence analyses, our analysis predicted three pathways (VEGF, BMP and tenascin) that support some of the strongest RPE/choroid cell–cell interactions, while being significantly affected by age and enhanced between subpopulations of cells showing a senescent-like gene expression profile.
The analysis of secreted molecules with roles in cell signaling revealed age-related changes in communication involving VEGF and BMP. VEGF signaling has been studied extensively in many retinal diseases, especially AMD and diabetic retinopathy (Marneros, 2016). Treatments targeting VEGF can slow down the progression of neovascular AMD, despite not reversing it (Schmidt-Erfurth et al., 2014). VEGFA is secreted basolaterally from the RPE and interacts with VEGF receptors such as KDR and VEGFR-2 (Blaauwgeers et al., 1999; Gogat et al., 2004; Hocking and McFarlane, 2007). By combining our aging analysis on bulk microarray samples with cell-type-specific gene expression knowledge extracted from scRNA-seq data, we suggested that aging correlated with increased VEGFA expression in RPE cells and with decreased expression of the KDR in choroidal endothelial cells. Similarly, we identified BMP as another signaling pathway of the RPE/choroid affected by aging. BMP signaling is involved in cell regulation processes, including cell proliferation, differentiation and eye morphogenesis (Solursh et al., 1996; Ibrahim et al., 2020). BMP7 plays a role in epithelial-mesenchymal transition, angiogenesis, and antifibrotic activity (Yang et al., 2020). A previous study showed that BMP7 could reduce proliferative vitreoretinopathy, a major complication of end-stage retinal detachment, by inhibiting RPE cells’ fibrosis in a rabbit model (Yao et al., 2019). Our findings indicated an increase in BMP7 expression in RPE cells and a decreased expression of the BMPR2 receptor in choroidal endothelial cells during human aging. This result underscores the importance of the BMP signaling pathway for the homeostasis of the RPE/choroid. Although it is unclear why the expression of the receptor and ligand genes change in opposite directions, we hypothesized that it could be a protective adaptation to maintain the overall activity level of these two signaling pathways during aging. Although available data do not provide information about the precise dynamics of molecular interactions between ligands and receptors, we suggest that a decrease in receptor expression might be a compensatory response to maintain a relatively stable level of signaling when facing an increase in ligand concentration. It has also been reported that choriocapillaris coverage of Bruch’s membrane decreases with age (Ramrattan et al., 1994; Lutty et al., 2020), while some reports point toward changes in the number of RPE cells during aging (Gao and Hollyfield, 1992; Harman et al., 1997; Del Priore et al., 2002; Ach et al., 2014). Whether compensatory gene expression occurs in aging in order to maintain an appropriate level of cellular signaling needs to be investigated further experimentally.
Age-associated changes in the extracellular matrix of the RPE/choroid have been observed in both fundus and post-mortem tissues (Ardeljan and Chan, 2013; Boulton, 2013; Sura et al., 2020). Typically, AMD is associated with a thickening of the RPE basal lamina-Bruch’s membrane complex driven by the accumulation of basal laminar deposit (Sura et al., 2020). Genes associated with ECM were also reported to be altered in AMD, including factors regulating wound healing and angiogenesis (Pouw et al., 2021). Here, our analysis suggested that the tenascin-mediated pathway might have an age-related significance to the RPE/choroid intercellular communication. The tenascin pathway contributes to ECM maintenance (Reinhard et al., 2017; Matsumoto and Aoki, 2020; Miller, 2020) and the absence of TNXB was reported to cause a reduction of collagen and of tissue strength (Mao et al., 2002). Tenascin has also a proangiogenic effect when interacting with the VEGF pathway (Ikuta et al., 2000). Our group previously identified TNXB as a methylation target that shows a decrease in methylation in its exon3 in RPE/choroid affected by AMD (Porter et al., 2019). Genome-wide association studies (GWAS) previously identified TNXB as a genetic variant associated with AMD (Cipriani et al., 2012; Fritsche et al., 2016). Our current analysis suggested that TNXB is expressed by choroidal fibroblasts and endothelial cells and is globally downregulated with age while its receptor SDC4 is expressed by fibroblasts, RPE cells and endothelial cells and is globally upregulated with age. This again suggested a potential compensatory mechanism. Together, our transcriptomic analysis and previous studies on DNA methylation and genetic variants emphasized the importance of TNXB and the tenascin pathway on the pathological mechanisms associated with aging and AMD, especially in relation to ECM interactions between the RPE and the choroid affected by AMD.
We acknowledge that this current analysis has several limitations. Firstly, the single-cell data we retrieved contains only provided 3 RPE/choroid samples: two from normal eyes and one from an eye with neovascular AMD (Voigt et al., 2019). To the best of our knowledge, there are currently no other public datasets thatcontain more samples suitable for our analysis. For example, although a recent scRNA-seq dataset from Voigt et al. (2022) contains more eye samples, it is not suitable for our intercellular communication study. Specifically, as cells have been sorted based on CD31 expression to enable the capture of endothelial cells, only a low number of RPE cells are present in the dataset and they are moreover confounded with photoreceptors (Voigt et al., 2019, 2022). Secondly, the bulk microarray dataset that we used for our aging-related analysis is missing clinical details regarding the post-mortem normal eyes (Newman et al., 2012). The only quality control that is provided is RNA integrity but not the detailed pathology of these eyes. Along the same line, both the scRNA-seq data (Voigt et al., 2019) and the bulk microarray data (Newman et al., 2012) are missing a clear nomenclature of neovascular AMD. The diagnosis of neovascular AMD was acquired from ophthalmic notes for the scRNA-seq dataset, while the diagnosis for the bulk microarray dataset was obtained from retina specialists and fundus photographs. However, the current clinical nomenclature (Jung et al., 2014; Spaide et al., 2020) indicates that specific subtypes of neovascularization, such as intraretinal neovascularization, might have different gene expression profiles. This emphasizes the importance of improving the clinical documentation of recovered samples in future experiments and data collections. Thirdly, a common limitation of both the single-cell and bulk datasets we analyzed is the absence of detailed spatial information. As samples originate from 8-mm tissue punches, details regarding regional differences such as between the fovea, parafovea and perifovea are lost. Indeed, several studies such as some based on imaging techniques have illustrated the importance of the topographic nature of AMD. For example, a higher concentration of melanolipofuscin is found in foveal RPE (Bermond et al., 2020). Other studies using optical coherence tomography suggest that hyperreflective foci (potentially associated with AMD progression) may be due to migrating RPE cells undergoing transdifferentiation (Ouyang et al., 2013; Cao et al., 2021), highlighting the three-dimensional nature of the disease.
Some recent studies indicate that senescent RPE cells accumulate during AMD in human donors, primates and RPE cell cultures (Wang et al., 2019; Blasiak, 2020; Lee et al., 2021), thus raising the interest in the role of cellular senescence in AMD. In addition to RPE, cellular senescence also may affect choroidal tissues and the neuronal retina (Ma et al., 2013; Cabrera et al., 2016). However, it is still unclear what are the causal role and function of the potential senescent cells in human pathology as most studies are limited to animal models and cell cultures (Cao et al., 2013; Chaum et al., 2015; Cabrera et al., 2016). Our results further highlight the importance of cellular senescence in the RPE/choroid tissues in relation to prominent cell–cell communication pathways mediated by VEGF, BMP and tenascin. These findings support potential alternative treatment approaches for AMD based on targeting senescent cells using senotherapies and senolytic drugs. The strategy to target senescent cells to prevent or slow down the progress of AMD has also been independently suggested by a recent review (Lee et al., 2021). A proposed approach is to inhibit pro-inflammatory signaling molecules and to regulate oxidative stress-induced senescence (Sreekumar et al., 2020). Notably, as our results suggested that different subtypes of AMD (geographic atrophy and neovascular AMD) may present differences in senescence status, such subtypes should be taken into consideration before applying senotherapies. To achieve this promising goal, further studies on the biological mechanisms of senescence in AMD and further clinical studies using senotherapies to treat AMD are needed (El-Nimri et al., 2020).
Data availability statement
The datasets presented in this study can be found in online repositories. The names of the repository/repositories and accession number(s) can be found at: https://github.com/CyrilLagger/amd_aging.
Author contributions
DD and KC conceptualized the study. DD, CL, and KC performed the analysis and wrote the first draft of the manuscript. LP conceived the overall project. LP and JM supervised the study and provided critical insights for the interpretation of results. All authors contributed to the article and approved the submitted version.
Acknowledgments
DD and KC were recipients of a Liverpool-Mahidol Partnership Scholarship. CL is grateful for the funding provided by the Human Frontier Science Program (fellowship LT000741/2019-C).
Conflict of interest
JM is an advisor/consultant for the Longevity Vision Fund, NOVOS, Insilico Medicine, YouthBio Therapeutics and the founder of Magellan Science Ltd, a company providing consulting services in longevity science.
The remaining authors declare that the research was conducted in the absence of any commercial or financial relationships that could be construed as a potential conflict of interest.
Publisher’s note
All claims expressed in this article are solely those of the authors and do not necessarily represent those of their affiliated organizations, or those of the publisher, the editors and the reviewers. Any product that may be evaluated in this article, or claim that may be made by its manufacturer, is not guaranteed or endorsed by the publisher.
Supplementary material
The Supplementary material for this article can be found online at: https://www.frontiersin.org/articles/10.3389/fnagi.2022.1016293/full#supplementary-material
References
Ach, T., Huisingh, C., Mcgwin, G. Jr., Messinger, J. D., Zhang, T., Bentley, M. J., et al. (2014). Quantitative autofluorescence and cell density maps of the human retinal pigment epithelium. Invest. Ophthalmol. Vis. Sci. 55, 4832–4841. doi: 10.1167/iovs.14-14802
Albacete-Albacete, L., Sanchez-Alvarez, M., and Del Pozo, M. A. (2021). Extracellular vesicles: an emerging mechanism governing the secretion and biological roles of tenascin-C. Front. Immunol. 12:671485. doi: 10.3389/fimmu.2021.671485
Ardeljan, D., and Chan, C. C. (2013). Aging is not a disease: distinguishing age-related macular degeneration from aging. Prog. Retin. Eye Res. 37, 68–89. doi: 10.1016/j.preteyeres.2013.07.003
Armingol, E., Officer, A., Harismendy, O., and Lewis, N. E. (2021). Deciphering cell-cell interactions and communication from gene expression. Nat. Rev. Genet. 22, 71–88. doi: 10.1038/s41576-020-00292-x
Barbie, D. A., Tamayo, P., Boehm, J. S., Kim, S. Y., Moody, S. E., Dunn, I. F., et al. (2009). Systematic RNA interference reveals that oncogenic KRAS-driven cancers require TBK1. Nature 462, 108–112. doi: 10.1038/nature08460
Basisty, N., Kale, A., Jeon, O. H., Kuehnemann, C., Payne, T., Rao, C., et al. (2020). A proteomic atlas of senescence-associated secretomes for aging biomarker development. PLoS Biol. 18:e3000599. doi: 10.1371/journal.pbio.3000599
Bermond, K., Wobbe, C., Tarau, I. S., Heintzmann, R., Hillenkamp, J., Curcio, C. A., et al. (2020). Autofluorescent granules of the human retinal pigment epithelium: phenotypes, intracellular distribution, and age-related topography. Invest. Ophthalmol. Vis. Sci. 61:35. doi: 10.1167/iovs.61.5.35
Blaauwgeers, H. G., Holtkamp, G. M., Rutten, H., Witmer, A. N., Koolwijk, P., Partanen, T. A., et al. (1999). Polarized vascular endothelial growth factor secretion by human retinal pigment epithelium and localization of vascular endothelial growth factor receptors on the inner choriocapillaris. Evidence for a trophic paracrine relation. Am. J. Pathol. 155, 421–428. doi: 10.1016/S0002-9440(10)65138-3
Blasiak, J. (2020). Senescence in the pathogenesis of age-related macular degeneration. Cell. Mol. Life Sci. 77, 789–805. doi: 10.1007/s00018-019-03420-x
Blasiak, J., Piechota, M., Pawlowska, E., Szatkowska, M., Sikora, E., and Kaarniranta, K. (2017). Cellular senescence in age-related macular degeneration: can autophagy and DNA damage response play a role? Oxidative Med. Cell. Longev. 2017, 1–15. doi: 10.1155/2017/5293258
Borcherding, N., Vishwakarma, A., Voigt, A. P., Bellizzi, A., Kaplan, J., Nepple, K., et al. (2021). Mapping the immune environment in clear cell renal carcinoma by single-cell genomics. Commun. Biol. 4:122. doi: 10.1038/s42003-020-01625-6
Boulton, M. E. (2013). “Ageing of the retina and retinal pigment epithelium,” in Age-Related Macular Degeneration eds. F. G. Holz, D. Pauleikhoff, R. F. Spaide, and A. C. Bird (Berlin Heidelberg: Springer), 45–63.
Boulton, M., Roanowska, M., and Wess, T. (2004). Ageing of the retinal pigment epithelium: implications for transplantation. Graefes Arch. Clin. Exp. Ophthalmol. 242, 76–84. doi: 10.1007/s00417-003-0812-8
Butler, J. M., Supharattanasitthi, W., Yang, Y. C., and Paraoan, L. (2021). RNA-seq analysis of ageing human retinal pigment epithelium: unexpected up-regulation of visual cycle gene transcription. J. Cell. Mol. Med. 25, 5572–5585. doi: 10.1111/jcmm.16569
Cabrera, A. P., Bhaskaran, A., Xu, J., Yang, X., Scott, H. A., Mohideen, U., et al. (2016). Senescence increases choroidal endothelial stiffness and susceptibility to complement injury: implications for Choriocapillaris loss in AMD. Invest. Ophthalmol. Vis. Sci. 57, 5910–5918. doi: 10.1167/iovs.16-19727
Cao, D., Leong, B., Messinger, J. D., Kar, D., Ach, T., Yannuzzi, L. A., et al. (2021). Hyperreflective foci, optical coherence tomography progression indicators in age-related macular degeneration, include transdifferentiated retinal pigment epithelium. Invest. Ophthalmol. Vis. Sci. 62:34. doi: 10.1167/iovs.62.10.34
Cao, L., Wang, H., Wang, F., Xu, D., Liu, F., and Liu, C. (2013). Abeta-induced senescent retinal pigment epithelial cells create a proinflammatory microenvironment in AMD. Invest. Ophthalmol. Vis. Sci. 54, 3738–3750. doi: 10.1167/iovs.13-11612
Chatsirisupachai, K., Palmer, D., Ferreira, S., and De Magalhaes, J. P. (2019). A human tissue-specific transcriptomic analysis reveals a complex relationship between aging, cancer, and cellular senescence. Aging Cell 18:e13041. doi: 10.1111/acel.13041
Chaum, E., Winborn, C. S., and Bhattacharya, S. (2015). Genomic regulation of senescence and innate immunity signaling in the retinal pigment epithelium. Mamm. Genome 26, 210–221. doi: 10.1007/s00335-015-9568-9
Choi, Y. E., Song, M. J., Hara, M., Imanaka-Yoshida, K., Lee, D. H., Chung, J. H., et al. (2020). Effects of tenascin C on the integrity of extracellular matrix and skin aging. Int. J. Mol. Sci. 21:8693. doi: 10.3390/ijms21228693
Cipriani, V., Leung, H. T., Plagnol, V., Bunce, C., Khan, J. C., Shahid, H., et al. (2012). Genome-wide association study of age-related macular degeneration identifies associated variants in the TNXB-FKBPL-NOTCH4 region of chromosome 6p21.3. Hum. Mol. Genet. 21, 4138–4150. doi: 10.1093/hmg/dds225
Corridoni, D., Antanaviciute, A., Gupta, T., Fawkner-Corbett, D., Aulicino, A., Jagielowicz, M., et al. (2020). Single-cell atlas of colonic CD8(+) T cells in ulcerative colitis. Nat. Med. 26, 1480–1490. doi: 10.1038/s41591-020-1003-4
Curcio, C. A. (2018). Soft Drusen in age-related macular degeneration: biology and targeting via the oil spill strategies. Invest. Ophthalmol. Vis. Sci. 59, AMD160–AMD181. doi: 10.1167/iovs.18-24882
Curcio, C. A., Johnson, M., Huang, J. D., and Rudolf, M. (2009). Aging, age-related macular degeneration, and the response-to-retention of apolipoprotein B-containing lipoproteins. Prog. Retin. Eye Res. 28, 393–422. doi: 10.1016/j.preteyeres.2009.08.001
Del Priore, L. V., Kuo, Y. H., and Tezel, T. H. (2002). Age-related changes in human RPE cell density and apoptosis proportion in situ. Invest. Ophthalmol. Vis. Sci. 43, 3312–3318.
Dhirachaikulpanich, D., Li, X., Porter, L. F., and Paraoan, L. (2020). Integrated microarray and RNAseq transcriptomic analysis of retinal pigment epithelium/choroid in age-related macular degeneration. Front. Cell Dev. Biol. 8:808. doi: 10.3389/fcell.2020.00808
Dimitrov, D., Türei, D., Boys, C., Nagai, J. S., Ramirez Flores, R. O., Kim, H., et al. (2021). Comparison of Resources and Methods to Infer Cell-Cell Communication From Single-Cell RNA Data Cold Spring Harbor Laboratory.
Dimitrov, D., Türei, D., Garrido-Rodriguez, M., Burmedi, P. L., Nagai, J. S., Boys, C., et al. (2022). Comparison of methods and resources for cell-cell communication inference from single-cell RNA-Seq data. Nat. Commun. 13:3224. doi: 10.1038/s41467-022-30755-0
Ellis, L. M., and Hicklin, D. J. (2008). VEGF-targeted therapy: mechanisms of anti-tumour activity. Nat. Rev. Cancer 8, 579–591. doi: 10.1038/nrc2403
El-Nimri, N. W., Moore, S. M., Zangwill, L. M., Proudfoot, J. A., Weinreb, R. N., Skowronska-Krawczyk, D., et al. (2020). Evaluating the neuroprotective impact of senolytic drugs on human vision. Sci. Rep. 10:21752. doi: 10.1038/s41598-020-78802-4
Ferrara, N., Gerber, H. P., and Lecouter, J. (2003). The biology of VEGF and its receptors. Nat. Med. 9, 669–676. doi: 10.1038/nm0603-669
Fritsche, L. G., Igl, W., Bailey, J. N., Grassmann, F., Sengupta, S., Bragg-Gresham, J. L., et al. (2016). A large genome-wide association study of age-related macular degeneration highlights contributions of rare and common variants. Nat. Genet. 48, 134–143. doi: 10.1038/ng.3448
Gao, H., and Hollyfield, J. G. (1992). Aging of the human retina. Differential loss of neurons and retinal pigment epithelial cells. Invest. Ophthalmol. Vis. Sci. 33, 1–17.
Gogat, K., Le Gat, L., Van Den Berghe, L., Marchant, D., Kobetz, A., Gadin, S., et al. (2004). VEGF and KDR gene expression during human embryonic and fetal eye development. Invest. Ophthalmol. Vis. Sci. 45, 7–14. doi: 10.1167/iovs.02-1096
Group, A. R., Chew, E. Y., Clemons, T., Sangiovanni, J. P., Danis, R., Domalpally, A., et al. (2012). The age-related eye disease study 2 (AREDS2): study design and baseline characteristics (AREDS2 report number 1). Ophthalmology 119, 2282–2289. doi: 10.1016/j.ophtha.2012.05.027
Hanzelmann, S., Castelo, R., and Guinney, J. (2013). GSVA: gene set variation analysis for microarray and RNA-seq data. BMC Bioinf. 14:7. doi: 10.1186/1471-2105-14-7
Hao, Y., Hao, S., Andersen-Nissen, E., Mauck, W. M. 3rd, Zheng, S., Butler, A., et al. (2021). Integrated analysis of multimodal single-cell data. Cells 184, 3573–3587.e29. doi: 10.1016/j.cell.2021.04.048
Harman, A. M., Fleming, P. A., Hoskins, R. V., and Moore, S. R. (1997). Development and aging of cell topography in the human retinal pigment epithelium. Invest. Ophthalmol. Vis. Sci. 38, 2016–2026.
He, L., Zhang, Q., Zhang, Y., Fan, Y., Yuan, F., and Li, S. (2021). Single-cell analysis reveals cell communication triggered by macrophages associated with the reduction and exhaustion of CD8(+) T cells in COVID-19. Cell Commun. Signal 19:73. doi: 10.1186/s12964-021-00754-7
Hocking, J. C., and Mcfarlane, S. (2007). Expression of bmp ligands and receptors in the developing Xenopus retina. Int. J. Dev. Biol. 51, 161–165. doi: 10.1387/ijdb.062185jh
Hu, C., Chu, C., Liu, L., Wang, C., Jin, S., Yang, R., et al. (2021). Dissecting the microenvironment around biosynthetic scaffolds in murine skin wound healing. Sci. Adv. 7:eabf0787. doi: 10.1126/sciadv.abf0787
Ibrahim, A. S., Hussein, K., Wang, F., Wan, M., Saad, N., Essa, M., et al. (2020). Bone morphogenetic protein (BMP)4 but not BMP2 disrupts the barrier integrity of retinal pigment epithelia and induces their migration: a potential role in Neovascular age-related macular degeneration. J. Clin. Med. 9:2293. doi: 10.3390/jcm9072293
Ikuta, T., Ariga, H., and Matsumoto, K. (2000). Extracellular matrix tenascin-X in combination with vascular endothelial growth factor B enhances endothelial cell proliferation. Genes Cells 5, 913–927. doi: 10.1046/j.1365-2443.2000.00376.x
Jin, S., Guerrero-Juarez, C. F., Zhang, L., Chang, I., Ramos, R., Kuan, C. H., et al. (2021). Inference and analysis of cell-cell communication using CellChat. Nat. Commun. 12:1088. doi: 10.1038/s41467-021-21246-9
Jung, J. J., Chen, C. Y., Mrejen, S., Gallego-Pinazo, R., Xu, L., Marsiglia, M., et al. (2014). The incidence of neovascular subtypes in newly diagnosed neovascular age-related macular degeneration. Am J. Ophthalmol. 158:e762, 769–779.e2. doi: 10.1016/j.ajo.2014.07.006
Kanehisa, M., Sato, Y., Kawashima, M., Furumichi, M., and Tanabe, M. (2016). KEGG as a reference resource for gene and protein annotation. Nucleic Acids Res. 44, D457–D462. doi: 10.1093/nar/gkv1070
Kay, P., Yang, Y. C., Hiscott, P., Gray, D., Maminishkis, A., and Paraoan, L. (2014). Age-related changes of cystatin C expression and polarized secretion by retinal pigment epithelium: potential age-related macular degeneration links. Invest. Ophthalmol. Vis. Sci. 55, 926–934. doi: 10.1167/iovs.13-13239
Kay, P., Yang, Y. C., and Paraoan, L. (2013). Directional protein secretion by the retinal pigment epithelium: roles in retinal health and the development of age-related macular degeneration. J. Cell. Mol. Med. 17, 833–843. doi: 10.1111/jcmm.12070
Kobayashi, Y., Yoshida, S., Zhou, Y., Nakama, T., Ishikawa, K., Arima, M., et al. (2016). Tenascin-C promotes angiogenesis in fibrovascular membranes in eyes with proliferative diabetic retinopathy. Mol. Vis. 22, 436–445.
Kozlowski, M. R. (2012). RPE cell senescence: a key contributor to age-related macular degeneration. Med. Hypotheses 78, 505–510. doi: 10.1016/j.mehy.2012.01.018
Kritsilis, M. S. V. R., Koutsoudaki, P. N., Evangelou, K., Gorgoulis, V. G., and Papadopoulos, D. (2018). Ageing, cellular senescence and neurodegenerative disease. Int. J. Mol. Sci. 19:2937. doi: 10.3390/ijms19102937
Lagger, C., Ursu, E., Equey, A., Avelar, R. A., Pisco, A. O., Tacutu, R., et al. (2021). scAgeCom: A Murine Atlas of Age-Related Changes in Intercellular Communication Inferred with the Package scDiffCom. bioRxiv. 8:456238 doi: 10.1101/2021.08.13.456238
Lee, K. S., Lin, S., Copland, D. A., Dick, A. D., and Liu, J. (2021). Cellular senescence in the aging retina and developments of senotherapies for age-related macular degeneration. J. Neuroinflammation 18:32. doi: 10.1186/s12974-021-02088-0
Lutty, G. A., Mcleod, D. S., Bhutto, I. A., Edwards, M. M., and Seddon, J. M. (2020). Choriocapillaris dropout in early age-related macular degeneration. Exp. Eye Res. 192:107939. doi: 10.1016/j.exer.2020.107939
Ma, W., Cojocaru, R., Gotoh, N., Gieser, L., Villasmil, R., Cogliati, T., et al. (2013). Gene expression changes in aging retinal microglia: relationship to microglial support functions and regulation of activation. Neurobiol. Aging 34, 2310–2321. doi: 10.1016/j.neurobiolaging.2013.03.022
Mao, J. R., Taylor, G., Dean, W. B., Wagner, D. R., Afzal, V., Lotz, J. C., et al. (2002). Tenascin-X deficiency mimics Ehlers-Danlos syndrome in mice through alteration of collagen deposition. Nat. Genet. 30, 421–425. doi: 10.1038/ng850
Marneros, A. G. (2016). Increased VEGF-A promotes multiple distinct aging diseases of the eye through shared pathomechanisms. EMBO Mol. Med. 8, 208–231. doi: 10.15252/emmm.201505613
Martinez-Cue, C., and Rueda, N. (2020). Cellular senescence in neurodegenerative diseases. Front. Cell. Neurosci. 14:16. doi: 10.3389/fncel.2020.00016
Matsumoto, K. I., and Aoki, H. (2020). The roles of tenascins in cardiovascular, inflammatory, and heritable connective tissue diseases. Front. Immunol. 11:609752. doi: 10.3389/fimmu.2020.609752
Miller, W. L. (2020). Tenascin-X-discovery and early research. Front. Immunol. 11:612497. doi: 10.3389/fimmu.2020.612497
Mitchell, P., Liew, G., Gopinath, B., and Wong, T. Y. (2018). Age-related macular degeneration. Lancet 392, 1147–1159. doi: 10.1016/S0140-6736(18)31550-2
Moreno, P., Huang, N., Manning, J. R., Mohammed, S., Solovyev, A., Polanski, K., et al. (2021). User-friendly, scalable tools and workflows for single-cell RNA-seq analysis. Nat. Methods 18, 327–328. doi: 10.1038/s41592-021-01102-w
Newman, A. M., Gallo, N. B., Hancox, L. S., Miller, N. J., Radeke, C. M., Maloney, M. A., et al. (2012). Systems-level analysis of age-related macular degeneration reveals global biomarkers and phenotype-specific functional networks. Genome Med. 4:16. doi: 10.1186/gm315
Ouyang, Y., Heussen, F. M., Hariri, A., Keane, P. A., and Sadda, S. R. (2013). Optical coherence tomography-based observation of the natural history of drusenoid lesion in eyes with dry age-related macular degeneration. Ophthalmology 120, 2656–2665. doi: 10.1016/j.ophtha.2013.05.029
Papatheodorou, I., Moreno, P., Manning, J., Fuentes, A. M., George, N., Fexova, S., et al. (2020). Expression atlas update: from tissues to single cells. Nucleic Acids Res. 48, D77–D83. doi: 10.1093/nar/gkz947
Paraoan, L., Grierson, I., and Maden, B. E. (2000). Analysis of expressed sequence tags of retinal pigment epithelium: cystatin C is an abundant transcript. Int. J. Biochem. Cell Biol. 32, 417–426. doi: 10.1016/S1357-2725(99)00143-0
Paraoan, L., Sharif, U., Carlsson, E., Supharattanasitthi, W., Mahmud, N. M., Kamalden, T. A., et al. (2020). Secretory proteostasis of the retinal pigmented epithelium: impairment links to age-related macular degeneration. Prog. Retin. Eye Res. 79:100859. doi: 10.1016/j.preteyeres.2020.100859
Porter, L. F., Saptarshi, N., Fang, Y., Rathi, S., Den Hollander, A. I., De Jong, E. K., et al. (2019). Whole-genome methylation profiling of the retinal pigment epithelium of individuals with age-related macular degeneration reveals differential methylation of the SKI, GTF2H4, and TNXB genes. Clin. Epigenetics 11:6. doi: 10.1186/s13148-019-0608-2
Pouw, A. E., Greiner, M. A., Coussa, R. G., Jiao, C., Han, I. C., Skeie, J. M., et al. (2021). Cell-matrix interactions in the eye: from cornea to choroid. Cells 10:687. doi: 10.3390/cells10030687
Ramrattan, R. S., Van Der Schaft, T. L., Mooy, C. M., De Bruijn, W. C., Mulder, P. G., and De Jong, P. T. (1994). Morphometric analysis of Bruch's membrane, the choriocapillaris, and the choroid in aging. Invest. Ophthalmol. Vis. Sci. 35, 2857–2864.
Reinhard, J., Roll, L., and Faissner, A. (2017). Tenascins in retinal and optic nerve neurodegeneration. Front. Integr. Neurosci. 11:30. doi: 10.3389/fnint.2017.00030
Ritchie, M. E., Phipson, B., Wu, D., Hu, Y., Law, C. W., Shi, W., et al. (2015). Limma powers differential expression analyses for RNA-sequencing and microarray studies. Nucleic Acids Res. 43:e47. doi: 10.1093/nar/gkv007
Saptarshi, N., Green, D., Cree, A., Lotery, A., Paraoan, L., and Porter, L. F. (2021). Epigenetic age acceleration is not associated with age-related macular degeneration. Int. J. Mol. Sci. 22:13457. doi: 10.3390/ijms222413457
Schmidt-Erfurth, U., Chong, V., Loewenstein, A., Larsen, M., Souied, E., Schlingemann, R., et al. (2014). Guidelines for the management of neovascular age-related macular degeneration by the European Society of Retina Specialists (EURETINA). Br. J. Ophthalmol. 98, 1144–1167. doi: 10.1136/bjophthalmol-2014-305702
Senger, D. R., Galli, S. J., Dvorak, A. M., Perruzzi, C. A., Harvey, V. S., and Dvorak, H. F. (1983). Tumor cells secrete a vascular permeability factor that promotes accumulation of ascites fluid. Science 219, 983–985. doi: 10.1126/science.6823562
Sharif, U., Mahmud, N. M., Kay, P., Yang, Y. C., Harding, S. P., Grierson, I., et al. (2019). Advanced glycation end products-related modulation of cathepsin L and NF-kappaB signalling effectors in retinal pigment epithelium lead to augmented response to TNFalpha. J. Cell. Mol. Med. 23, 405–416. doi: 10.1111/jcmm.13944
Sieber, C., Kopf, J., Hiepen, C., and Knaus, P. (2009). Recent advances in BMP receptor signaling. Cytokine Growth Factor Rev. 20, 343–355. doi: 10.1016/j.cytogfr.2009.10.007
Solursh, M., Langille, R. M., Wood, J., and Kuber Sampath, T. (1996). Osteogenic Protein-1 is required for mammalian eye development. Biochem. Biophys. Res. Commun. 218, 438–443. doi: 10.1006/bbrc.1996.0078
Spaide, R. F., Jaffe, G. J., Sarraf, D., Freund, K. B., Sadda, S. R., Staurenghi, G., et al. (2020). Consensus nomenclature for reporting Neovascular age-related macular degeneration data: consensus on Neovascular age-related macular degeneration nomenclature study Group. Ophthalmology 127, 616–636. doi: 10.1016/j.ophtha.2019.11.004
Sreekumar, P. G., Hinton, D. R., and Kannan, R. (2020). The emerging role of senescence in ocular disease. Oxidative Med. Cell. Longev. 2020, 1–19. doi: 10.1155/2020/2583601
Strauss, O. (2005). The retinal pigment epithelium in visual function. Physiol. Rev. 85, 845–881. doi: 10.1152/physrev.00021.2004
Sura, A. A., Chen, L., Messinger, J. D., Swain, T. A., Mcgwin, G. Jr., Freund, K. B., et al. (2020). Measuring the contributions of basal laminar deposit and Bruch's membrane in age-related macular degeneration. Invest. Ophthalmol. Vis. Sci. 61:19. doi: 10.1167/iovs.61.13.19
Tajiri, K., Yonebayashi, S., Li, S., and Ieda, M. (2021). Immunomodulatory role of tenascin-C in myocarditis and inflammatory cardiomyopathy. Front. Immunol. 12:624703. doi: 10.3389/fimmu.2021.624703
Voigt, A. P., Mulfaul, K., Mullin, N. K., Flamme-Wiese, M. J., Giacalone, J. C., Stone, E. M., et al. (2019). Single-cell transcriptomics of the human retinal pigment epithelium and choroid in health and macular degeneration. Proc. Natl. Acad. Sci. U. S. A. 116, 24100–24107. doi: 10.1073/pnas.1914143116
Voigt, A. P., Mullin, N. K., Mulfaul, K., Lozano, L. P., Wiley, L. A., Flamme-Wiese, M. J., et al. (2022). Choroidal endothelial and macrophage gene expression in atrophic and neovascular macular degeneration. Hum. Mol. Genet. 31, 2406–2423. doi: 10.1093/hmg/ddac043
Voigt, A. P., Mullin, N. K., Stone, E. M., Tucker, B. A., Scheetz, T. E., and Mullins, R. F. (2021). Single-cell RNA sequencing in vision research: insights into human retinal health and disease. Prog. Retin. Eye Res. 83:100934. doi: 10.1016/j.preteyeres.2020.100934
Voigt, A. P., Whitmore, S. S., Mulfaul, K., Chirco, K. R., Giacalone, J. C., Flamme-Wiese, M. J., et al. (2020). Bulk and single-cell gene expression analyses reveal aging human choriocapillaris has pro-inflammatory phenotype. Microvasc. Res. 131:104031. doi: 10.1016/j.mvr.2020.104031
Wang, S., Wang, X., Cheng, Y., Ouyang, W., Sang, X., Liu, J., et al. (2019). Autophagy dysfunction, cellular senescence, and abnormal immune-inflammatory responses in AMD: from mechanisms to therapeutic potential. Oxidative Med. Cell. Longev. 2019, 1–13. doi: 10.1155/2019/3632169
Wong, W. L., Su, X., Li, X., Cheung, C. M., Klein, R., Cheng, C. Y., et al. (2014). Global prevalence of age-related macular degeneration and disease burden projection for 2020 and 2040: a systematic review and meta-analysis. Lancet Glob. Health 2, e106–e116. doi: 10.1016/S2214-109X(13)70145-1
Yang, A. C., Kern, F., Losada, P. M., Agam, M. R., Maat, C. A., Schmartz, G. P., et al. (2021). Dysregulation of brain and choroid plexus cell types in severe COVID-19. Nature 595, 565–571. doi: 10.1038/s41586-021-03710-0
Yang, P., Troncone, L., Augur, Z. M., Kim, S. S. J., Mcneil, M. E., and Yu, P. B. (2020). The role of bone morphogenetic protein signaling in vascular calcification. Bone 141:115542. doi: 10.1016/j.bone.2020.115542
Keywords: RPE, choroid, aging, senescence, VEGF, BMP, tenascin, AMD
Citation: Dhirachaikulpanich D, Lagger C, Chatsirisupachai K, de Magalhães JP and Paraoan L (2022) Intercellular communication analysis of the human retinal pigment epithelial and choroidal cells predicts pathways associated with aging, cellular senescence and age-related macular degeneration. Front. Aging Neurosci. 14:1016293. doi: 10.3389/fnagi.2022.1016293
Edited by:
Christian Neri, Institut National de la Santé et de la Recherche Médicale, FranceReviewed by:
Robert Mullins, The University of Iowa, United StatesChristine A. Curcio, University of Alabama at Birmingham, United States
Copyright © 2022 Dhirachaikulpanich, Lagger, Chatsirisupachai, de Magãlhaes and Paraoan. This is an open-access article distributed under the terms of the Creative Commons Attribution License (CC BY). The use, distribution or reproduction in other forums is permitted, provided the original author(s) and the copyright owner(s) are credited and that the original publication in this journal is cited, in accordance with accepted academic practice. No use, distribution or reproduction is permitted which does not comply with these terms.
*Correspondence: João Pedro de Magalhães, anBAc2VuZXNjZW5jZS5pbmZv; Luminita Paraoan, THVtaW5pdGEuUGFyYW9hbkBlZGdlaGlsbC5hYy51aw==
†Present address: João Pedro de Magalhães, Institute of Inflammation and Ageing, University of Birmingham Queen Elizabeth Hospital, Mindelsohn Way, Birmingham, United Kingdom
Luminita Paraoan, Ocular Molecular Biology and Mechanisms of Disease Group, Department of Biology, Faculty of Arts and Sciences, Edge Hill University, Ormskirk, United Kingdom