- 1Institute for Regenerative Medicine, University of Zurich, Zurich, Switzerland
- 2Changes Technology Corporation Ltd., Shanghai, China
- 3PET Center, Huashan Hospital, Fudan University, Shanghai, China
- 4Department of Radiopharmacy and Molecular Imaging, School of Pharmacy, Fudan University, Shanghai, China
- 5Guangdong Robotics Association, Guangzhou, China
- 6Institute for Biomedical Engineering, ETH Zurich and University of Zurich, Zurich, Switzerland
The microtubule-associated protein tau (MAPT) plays an important role in Alzheimer’s disease and primary tauopathy diseases. The abnormal accumulation of tau contributes to the development of neurotoxicity, inflammation, neurodegeneration, and cognitive deficits in tauopathy diseases. Tau synergically interacts with amyloid-beta in Alzheimer’s disease leading to detrimental consequence. Thus, tau has been an important target for therapeutics development for Alzheimer’s disease and primary tauopathy diseases. Tauopathy animal models recapitulating the tauopathy such as transgenic, knock-in mouse and rat models have been developed and greatly facilitated the understanding of disease mechanisms. The advance in PET and imaging tracers have enabled non-invasive detection of the accumulation and spread of tau, the associated microglia activation, metabolic, and neurotransmitter receptor alterations in disease animal models. In vivo microPET studies on mouse or rat models of tauopathy have provided significant insights into the phenotypes and time course of pathophysiology of these models and allowed the monitoring of treatment targeting at tau. In this study, we discuss the utilities of PET and recently developed tracers for evaluating the pathophysiology in tauopathy animal models. We point out the outstanding challenges and propose future outlook in visualizing tau-related pathophysiological changes in brain of tauopathy disease animal models.
Introduction
The microtubule-associated protein tau (MAPT) locates intracellularly and is composed of six isoforms, classified into 4-repeat (4R) and 3-repeat (3R) species (Lee et al., 2001; Spillantini and Goedert, 2013). Tauopathy diseases include Alzheimer’s disease (AD), primary tauopathy such as progressive supranuclear palsy (PSP), corticobasal degeneration (CBD), and frontotemporal dementia (FTD) with Parkinsonism linked to chromosome 17 and Pick’s disease. In AD, amyloid-beta (Aβ) and tau interact synergically in the brain and trigger a complex cascade of biochemical and cellular processes, resulting in neurodegeneration (Busche and Hyman, 2020; Chang et al., 2021b). Tau has been an important target in therapeutics development for AD and primary tauopathies. Immunotherapies semorinemab, gosuranemab (Boxer et al., 2019; Ayalon et al., 2021; Grossman, 2021; Mullard, 2021; Novak et al., 2021), antisense oligonucleotides (DeVos et al., 2017), and aggregation inhibitors are at different stages of clinical trials (Congdon and Sigurdsson, 2018). Transgenic animal models recapitulating human tauopathy have enabled understanding of disease mechanisms and facilitated the development of treatment strategies (Albert et al., 2019; Roberts et al., 2020; Ayalon et al., 2021). Varieties of transgenic mouse lines with mutationson MAPT gene including P301S (PS19), P301L (JNPL3, rTg4510, pR5) (Lewis et al., 2000; Ramsden et al., 2005; Santacruz et al., 2005; Yoshiyama et al., 2007; de Calignon et al., 2012), knock-out hTau (Andorfer et al., 2003), and knock-in (Hashimoto et al., 2019; Saito et al., 2019) mouse models as well as transgenic rat models (Filipcik et al., 2012) have been developed. In addition, 3 × Tg mice and TgF344-AD rats harbor both the Aβ and tauopathy have been widely used (Oddo et al., 2003; Cohen et al., 2013). Recent advances in molecular imaging using PET and MRI have provided valuable insights into the time course of disease pathophysiology in tau animal models, including tau, neuroinflammation, and structural and functional alterations (Ishikawa et al., 2018; Ni et al., 2018; Tagai et al., 2020), thus providing a blueprint for tauopathy disease clinical study.
Tau Imaging
Different types of tau inclusions in AD and primary tauopathies have been observed. Neuropil thread, neurofibrillary tangles are observed in AD; Oligodendroglial coiled bodies and argyrophilic threads are common in PSP and CBD. For the glial tau inclusions, tufted astrocytes in PSP, and astrocytic plaques in CBD are observed (Lee et al., 2001). The cerebral tau load assessed by PET using various tau imaging tracers associates with brain regional atrophy assessed by using structural MRI and cognitive impairment in patients with AD (La Joie et al., 2020; Ossenkoppele et al., 2020, 2021; Vogel et al., 2021), CBD, and PSP (Robinson et al., 2020; Tagai et al., 2020; Whitwell et al., 2020). Tau spreading and misfolding follow a disease-specific brain region-dependent pattern first in the entorhinal cortex, hippocampus in human (Wegmann et al., 2019), and in transgenic mouse brain (Clavaguera et al., 2009). The onset and severity of the pathology and brain regions of atrophy vary among the different strains. The rTg4510 line develops tauopathy at a young age (4–5 months) and showed atrophy in both the cortex and hippocampus. In contrast, hTau mice mainly show atrophy in the cortex and PS19 mice demonstrate pathology mainly in the brain stem and spinal cord (Lewis et al., 2000; Andorfer et al., 2003; Ramsden et al., 2005; Santacruz et al., 2005; Yoshiyama et al., 2007; de Calignon et al., 2012). MicroPET imaging of tau in tauopathy rodent models has contributed to the development of novel PET tracers, understanding of disease mechanism, and monitoring of treatment effect. Several tau tracers have been tested in tau mouse models including 2,6-disubstituted naphthalene derivative [18F]FDDNP (Teng et al., 2011), pyridinyl-butadienyl-benzothiazole 3 derivatives [18F]PM-PBB3 (APN-1607), [11C]PBB3, [11C]mPBB5 (Maruyama et al., 2013; Ishikawa et al., 2018; Ni et al., 2018; Barron et al., 2020; Tagai et al., 2020), arylquinoline derivatives [18F]THK523 (Fodero-Tavoletti et al., 2011), [18F]THK5351 (Moreno-Gonzalez et al., 2021), [18F]THK5317 (Filip et al., 2021), [18F]THK5117 (Brendel et al., 2016; Chaney et al., 2021), pyridoindole derivative [18F]flortaucipir (Brendel et al., 2018), and lansoprazole derivative [18F]NML (Shao et al., 2012; Fawaz et al., 2014; Table 1). Using [18F]THK523, Brendel et al. (2018) showed significantly higher tracer retentions in brains of 6-month-old rTg4510 mice compared with non-transgenic mice or PS1/APP mice with Aβ pathology, indicating specific detection of tau. Brendel et al. (2016) and Chaney et al. (2021) demonstrated that PET using (S)-[18F]THK5117 showed higher tracer uptakes in PS19 biGT mice [glycogen synthase kinase-3β (GSK-3β) × P301L tau] and TgF344 rats compared to non-transgenic littermates, respectively (Figures 1F,G). However, Eckenweber et al. (2020) reported that in PS19 mice at 6 month-of-age, (S)-[18F]THK5117 was not able to detect the tau accumulation, although presence of tau accumulation was validated by using ex vivo immunohistochemical staining. Moreno-Gonzalez et al. (2021) recently showed an elevated regional [18F]THK5351 PET signaling in brain of PS19 tau mice, correlating with histological levels of tau. However, both the binding assays as well as in silico experiment showed that [18F]THK5351 had the limitation of off-target binding to monoamine oxidase B (Ng et al., 2017; Murugan et al., 2019). The most widely used first-generation tau tracer [18F]flortaucipir was reported to showed greater difference compared to [18F]THK5117 in tracer retention in APPswe × P301L vs. non-transgenic mice (Brendel et al., 2018; Figures 1D,E). However, ex vivo binding and autoradiography from two other studies showed lack of detection using [18F] flortaucipir in rTg4510 mice (Marquié et al., 2015; Ni et al., 2018). PET using [11C]PBB3 imaging for tau has been demonstrated in PS19 (Chang et al., 2021b) and rTg4510 mice (Ishikawa et al., 2018; Ni et al., 2018; Takuwa et al., 2020; Figures 1A–C). PS19 mice showed a mainly brainstem and spinal cord tracer retention, while in rTg4510 mice the retention was observed in the cortex and hippocampus in line with the ex vivo validation (Maruyama et al., 2013). An age-dependent increase in [11C]PBB3 signal was observed in 7–11-month-old rTg4510 mice, consistent with neuropathological observations (Ni et al., 2018). In addition, the tau load inversely correlated with neocortical volumes assessed by T2 structural MRI, indicating an association between tau and neurodegeneration (Ishikawa et al., 2018; Ni et al., 2018). The disadvantages of [11C]PBB3 include non-negligible binding to Aβ plaques in patients with AD and the short half-life. Thus, the second-generation [18F]PM-PBB3 with improved binding properties was developed to overcome the limitations. Similar observations were reported by Tagai et al. (2020) and Weng et al. (2020) using [18F]PM-PBB3 in rTg4510 mouse models with increased tracer retention in the cortical and hippocampal regions. Among the other second-generation tau tracers, [18F]JNJ-64349311 (Declercq et al., 2017) and [18F]PI-6240 (Kroth et al., 2019) have so far been reported in wild-type mice for brain uptake and biodistribution assessment. In addition, several new tau probes are underdevelopment such as [18F]IBIPF1 (Kaide et al., 2019), [18F]PI-2014 (Gabellieri et al., 2020), [18F]PPQ (Lerdsirisuk et al., 2021), [11C]LM229 (McMurray et al., 2021), [18F]2-phenylquinoxaline derivatives (Zhou K. et al., 2021), antibody-based imaging (Krishnaswamy et al., 2014), and 4R-tau specific tracer [18F]CBD-2115 (Lindberg et al., 2021).
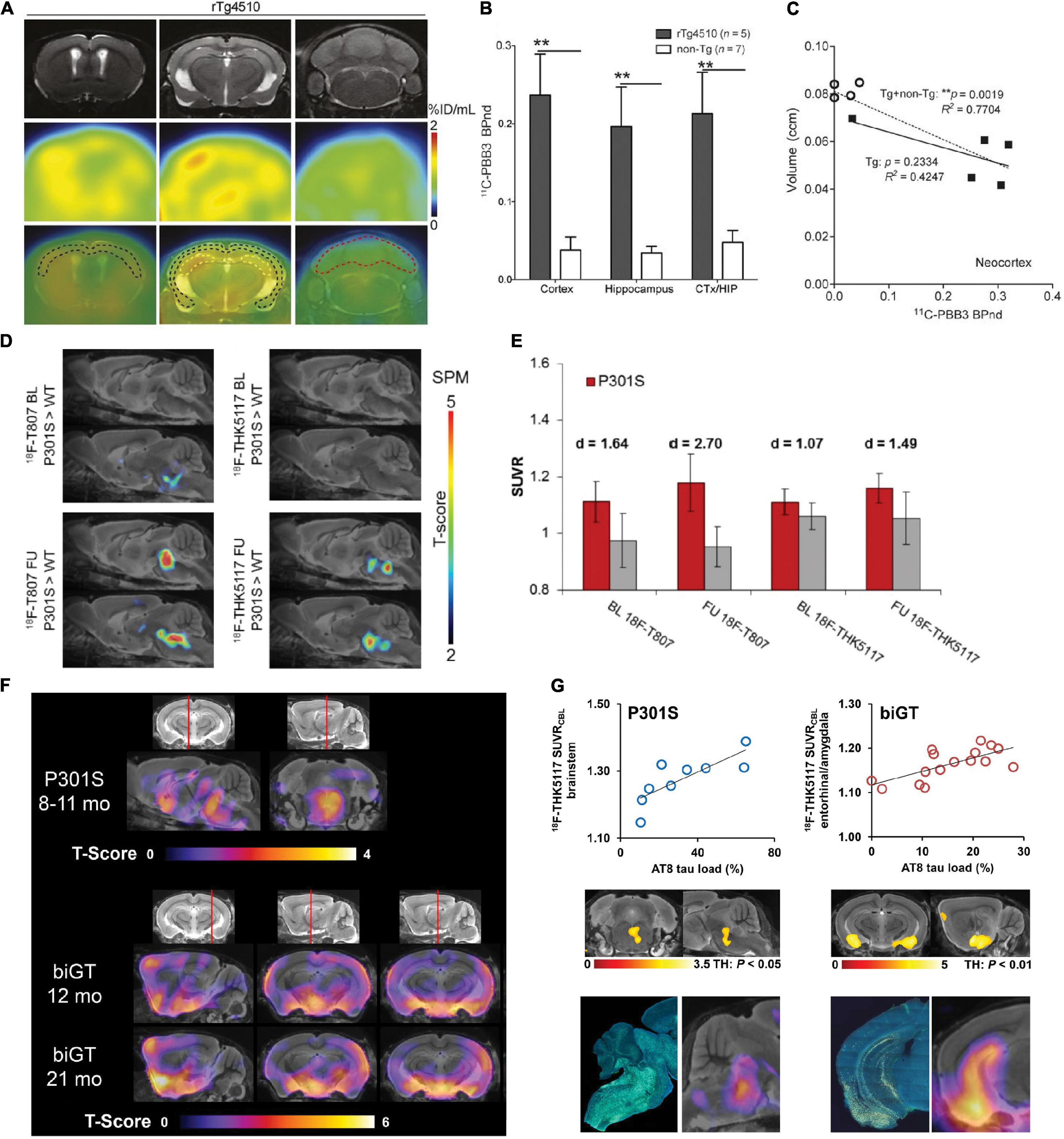
Figure 1. In vivo microPET tau imaging in mice (A) Representative T2-weighted MR, PET using [11C]PBB3 and PET/MR images of coronal brain sections of 9-month-old rTg4510 mice showing neocortical, hippocampal, and cerebellar VOIs (black, yellow, and red outlines, respectively). PET images were generated from averaged dynamic data at 30–60 min after injection of [11C]PBB3. (B) [11C]PBB3 binding potential in each VOI calculated by simplified reference tissue model with cerebellum as reference tissue and brain volume measured using structural MRI data including calculation of non-displaceable binding potential for neocortex and hippocampus (CTX/HIP). **p < 0.01, rTg4510 vs. non-transgenic mice. (C) Correlation between [11C]PBB3 non-displaceable binding potential and volume of neocortex and hippocampus in transgenic (∙) and non-transgenic (°) mice. **p < 0.01, for correlations in the transgenic plus non-transgenic group (Tg + non-Tg, dotted lines) and the transgenic group only (Tg, solid lines). Reproduced from Ni et al. (2018) with permission Society of Nuclear Medicine and Molecular Imaging. (D) Statistical parametric mapping (SPM) are depicted upon a MRI mouse atlas and extracerebral voxels are masked. The t-score threshold of 2 complies a significance threshold of 0.01 uncorrected. (E) Bar graphs show group mean relative standard uptake value (SUVR) of P301S (red) and WT (gray) mice for baseline and follow-up PET measurements of [18F]T807 and [18F]THK5117. Error bars indicate SD and effect sizes are given by Cohen’s d. Sagittal slices (median and 0.6 mm paramedian) show voxel-wise SPM between transgenic P301S and WT mice at baseline (BL) and follow-up (FU) for [18F]T807 and [18F]THK5117. Reproduced from Brendel et al. (2018) with permission from Frontiers SA. (F) Mean voxel-wise z score maps in sagittal and coronal planes of [18F]THK5117 binding for groups of aged P301S vs. pooled WT mice and biGT mice vs. pooled WT mice. Results of 2-sample t-test are expressed as z score maps projected on MRI mouse atlas (gray scale). (G) Validation of [18F]THK5117 small-animal PET results by immunohistochemical AT8 staining in vitro for P301S and biGT mice. Top row shows correlation plots of tau load (%) in corresponding AT8-stained areas with [18F]THK5117 SUVR. Middle row depicts linear regression between tau load (%) and small-animal PET SUVR images projected on MRI mouse atlas. Bottom row illustrates AT8-stained sections from single mice along with their individual SPM-derived z score maps (projected on MRI mouse atlas). Reproduced from Brendel et al. (2016) with permission Society of Nuclear Medicine and Molecular Imaging.
Neuroinflammation Imaging
Neuroinflammation play an important role in patient with AD and other primary tauopathy diseases as well as in animal models of tauopathy (Heneka et al., 2015; Ising et al., 2019; Wu et al., 2019; Linnerbauer et al., 2020; Richetin et al., 2020; Gaikwad et al., 2021; McAlpine et al., 2021; Palleis et al., 2021), featured by disease associated with microglia activation, reactive astrocytes, and activated cytokines such as complement C3 and interleukin-3. Immunohistochemical staining showed that synapse loss and microglial activation precede the appearance of tangles in PS19 mice (Yoshiyama et al., 2007). NLR family pyrin domain containing 3 (NLRP3) inflammasome activation was reported to drive tau pathology (Ising et al., 2019). A marked reduction of homeostatic microglial genes was found by single-cell sequencing of microglia isolated from rTg4510 mice, correlating with the degree of neuronal loss (Sobue et al., 2021). Shi et al. (2021a) and Wang C. et al. (2021) showed that microglia promoted apolipoprotein E-dependent neurodegeneration in tau mice (Shi et al., 2019) and that selective removal of astrocytic apolipoprotein E can protect against tau-mediated neurodegeneration and decrease synaptic phagocytosis by microglia. Microglia activation has been shown to occur preceding the tangle formation in tau mice (Yoshiyama et al., 2007). In addition, microglia was activated to engulf neuron containing tau aggregates, turned hypofunctional after phagocytosis, released the seed component of tau aggregates, and, thus, facilitated the spreading of tau in PS19 mice (Bellucci et al., 2004; Brelstaff et al., 2021). Thus, imaging of neuroinflammation in tauopathy mice offers crucial dynamic pathophysiological information and potential diagnostic parameter (Leng and Edison, 2021).
Translocator Protein
The most widely used neuroinflammation probes are those targeting at 18 kDa Translocator Protein (TSPO), locates on the outer mitochondrial membrane, and overexpressed by microglia during activation (Van Camp et al., 2021; Zhou R. et al., 2021). TSPO PET tracers that have been applied in tau animal models include first generation (R)-[11C]PK11195, second generation [18F]FEBMP, [18F]DPA-714, [11C]AC-5216, (125I) CLINDE, [11C]PBR28, [18F]FEDAA1106, and [11C]DAA1106 (Ji et al., 2008, 2021; Maeda et al., 2011; Jacobs and Tavitian, 2012; Mirzaei et al., 2016; Ishikawa et al., 2018; Tournier et al., 2019, 2020; Barron et al., 2020; Takuwa et al., 2020; Chaney et al., 2021; Fairley et al., 2021; Leng and Edison, 2021; Zhou X. et al., 2021), and third generation [18F]GE-180, etc. (Eckenweber et al., 2020; Chaney et al., 2021; Palleis et al., 2021). However, the first generation [11C]PK-11195 has several disadvantages such as high non-specific plasma binding, low signal-to-noise ratio as well as relatively low entrance into the brain and difficulty in quantitative analysis. The second-generation TSPO tracers improved the limitation of [11C]PK-11195 and demonstrated favorable binding properties. However, the binding of second-generation TSPO tracers differs greatly in humans depending on the rs6971 polymorphism in the TSPO gene and can be categorized into high-, mixed-, and low-affinity binders (Owen et al., 2012). This polymorphism adds complexity and introduces high variability among subjects. Therefore, the third-generation TSPO tracers are being developed to overcome this limitation. In preclinical TSPO imaging studies, no evidence with respect to rs6971 polymorphisms has been reported. Microglia activation assessed by using [11C]PK11195 PET was reported to co-localize with tau and can predict disease progression and tau accumulation assessed by [18F]flortaucipir in patients with PSP (Malpetti et al., 2020, 2021). Takuwa et al. (2020) demonstrated higher [11C]PBB3 uptake (tau accumulation) and [18F]AC-5216 (microglia activation) in rTg4510 mice at 6 month-of-age compared to wild-type littermates. The regional [18F]AC-5216 retention correlated with both the tau level and brain atrophy assessed by T2 structural MRI (Takuwa et al., 2020). Eckenweber et al. (2020) reported that [18F]GE-180 measures of microglia activation was accompanied by [18F]fluorodeoxyglucose (FDG) reduction with increasing age in PS19 mice at 2–6 months. This microglia activation also predicted the increased tau accumulation assessed by immunohistochemistry and deteriorated spatial learning in the Morris water maze (Eckenweber et al., 2020; Figures 2A,B). The specific detection of [18F]GE-180 was demonstrated by serial PET during pharmacological depletion of microglia in PS19 mice (Palleis et al., 2021). Barron et al. (2020), Fairley et al. (2021), and Ji et al. (2021) have assessed microglia activation by using [18F]FEBMP, which showed higher specificity to microglial TSPO and demonstrated increased microglia activation along with increased uptakes of [11C]PBB3 for tau deposits in rTg4510 and PS19 mice.
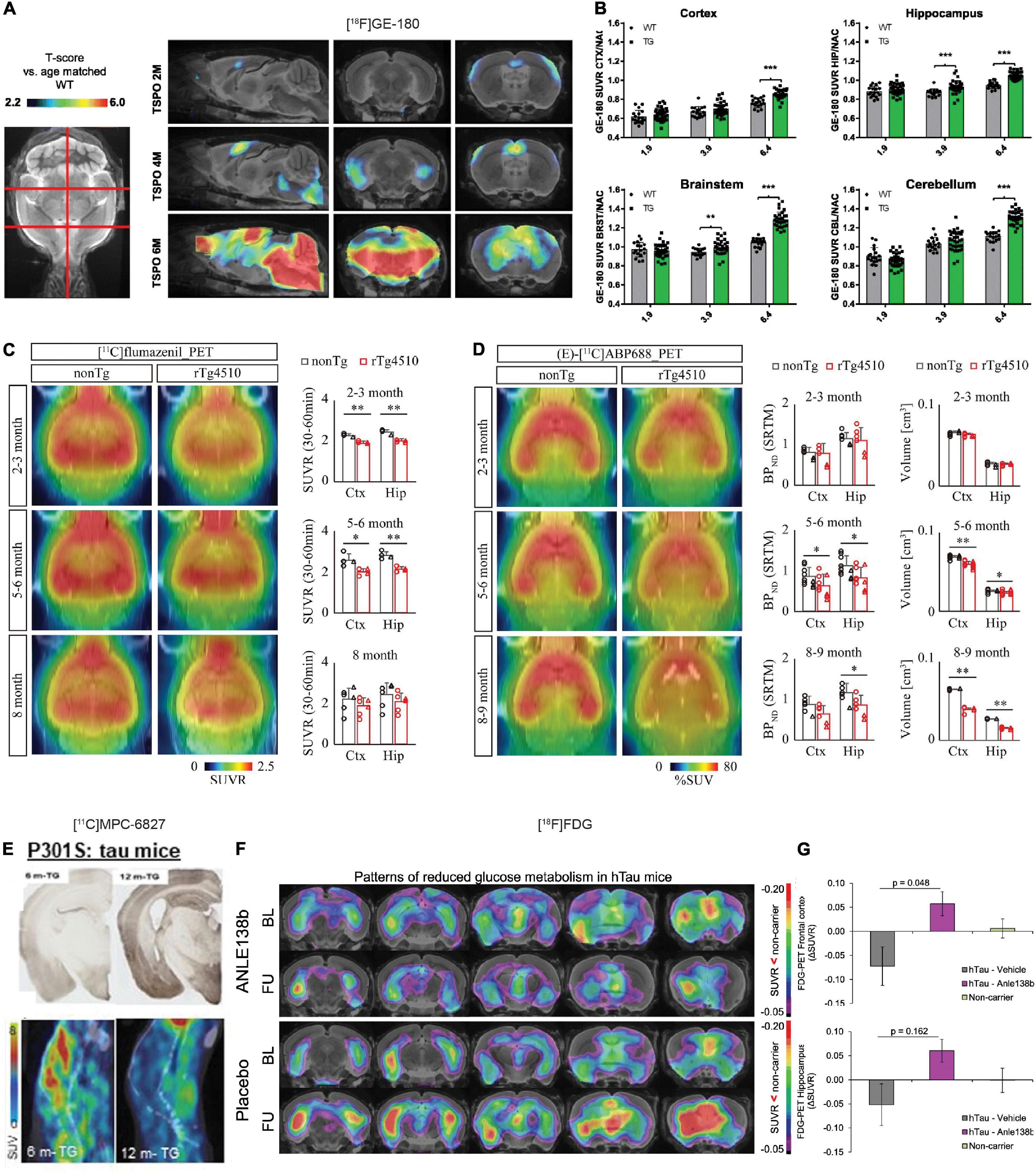
Figure 2. In vivo microPET translocator protein (TSPO), synaptic, and metabolic alterations imaging in tauopathy mice. (A) Age-dependent exponential increase of 18kDa TSPO expression in different target regions of the brain of P301S tau model mice. n(P301S/WT) = 1.9M, 33/18; 3.9M, 32/17; 6.4M, 29/17. (B) Voxel-wise SPM analysis of TSPO expression in the contrast of P301S vs. wild-type mice at different ages. T-score maps are projected upon an MRI template in sagittal and coronal slices. ***p < 0.001. Reproduced from Eckenweber et al. (2020) with permission Society of Nuclear Medicine and Molecular Imaging. (C) PET assessment of inhibitory synapse with [11C]flumazenil in non-Tg and rTg4510 mice at age 2–3, 5–6, and 8 months after peripheral bolus administration of [11C]flumazenil. Representative PET images generated by averaging dynamic scan data at 30–60 min are shown. Brainstem was set as reference region. *p < 0.05; **p < 0.01; Student’s t-test. (D) PET assessment of excitatory synapse with (E)-[11C]ABP688 in non-Tg and rTg4510 mice at age 2–3, 5–6, and 8–9 months after peripheral bolus administration of (E)-[11C]ABP688. Representative PET images generated by averaging dynamic scan data at 0–90 min are shown. (E)-[11C]ABP688 PET was analyzed in cortex (Ctx) and hippocampus (Hip) by simple reference tissue model with cerebellum as reference region. *p < 0.05 (Mann–Whitney U test). Reproduced from Shimojo et al. (2020) with permission from Biomed Central Ltd. (Springer Nature). (E) Immunohistochemical tau staining in 6-month- and 12-month-old transgenic P301S tau mice with their corresponding dynamic 0–45 min microPET/CT images with [11C]MPC-6827 injection. Decrease in [11C]MPC-6827 radioactive uptake with increase in tau loads. Reproduced from Sai et al. (2020) with permission from John Wiley and Sons. (F) Serial [18F]fluorodeoxyglucose (FDG)-PET imaging of relative cerebral metabolism at follow-up (FU) imaging 3 months after late-stage novel aggregation-inhibiting oligomer modulator Anle138b treatment in hTau mice, with reduced baseline compared to control mice. (G) Quantification of longitudinal changes in relative FDG uptake (ΔSUVR) indicates normalization of cerebral metabolism in the hTau-treated group, while ongoing decrease in hTau-vehicle group. Baseline (BL) at 14.5 months of age and FU at 17.5 months. Reproduced from Brendel et al. (2019) with permission from Springer Nature AG.
Beyond Translocator Protein
The probes utilized so far for microglia activation imaging are not specific for a specific activation status (proinflammatory M1 or anti-inflammatory M2) (Jain et al., 2020). TSPO tracers have several limitations such as diverse cellular sources on both the astrocytes and microglia as well as high non-specific binding level (Nutma et al., 2021). Different targets have been pursued for neuroinflammation imaging toward a clearer activation status and have been evaluated in tauopathy animal models. These include tracers for purinergic P2Y12 receptor [[11C]AZD1283], for organic anion-transporting polypeptide 1c1 [[18F]2B-SRF101], and for neutrophil infiltration [(68Ga) PEG-cFLFLFK] (Kreimerman et al., 2019; Kong et al., 2020; Maeda et al., 2021). P2X7 deficiency has been shown to improve plasticity and cognitive abilities in THY-Tau22 mice (Carvalho et al., 2021). Maeda et al. (2021) showed a distinct response of P2Y12 receptor to tau deposits using [11C]AZD1283 by ex vivo autoradiography and immunohistochemical staining in rTg4510 and PS19 tau mice. The levels of P2Y12 receptor declined in tau-laden region with increased total level of microglia (Maeda et al., 2021). However, low brain uptake was observed for this tracer, which hinders further in vivo usage. Kreimerman et al. (2019) recently reported N-[3-[18F]fluoropropyl] sulfonamide [[18F]2B-SRF101] as a potential astrocytosis tracer in 3 × Tg mice: a higher [18F]2B-SRF101 uptake was observed in the cortex and hippocampus of 3 × Tg compared to control mice, while the N-(11C-methyl)-L-deuterodeprenyl (DED) showed a different uptake pattern.
Metabolism Imaging
Cerebral Glucose Metabolism
[18F]FDG is commonly used in assisting the early and differential diagnosis of AD, FTD, and Parkinson’s disease, of which regional cerebral glucose hypometabolism is present. Most studies report global cerebral glucose hypometabolism in PS19, tauVLW mice as well as in 3 × Tg, 5 × FAD mice with both the amyloid and tau pathologies (Sancheti et al., 2013; Macdonald et al., 2014; DeBay et al., 2017; Hara et al., 2017; Son et al., 2018; Adlimoghaddam et al., 2019; Brendel et al., 2019; Eckenweber et al., 2020; Franke et al., 2020). However, Rojas et al. (2013) reported an increased [18F]FDG uptake resulted from glial activation in 5 × FAD mice compared to wild-type mice. Hara et al. (2017) showed cerebral glucose hypometabolism first in the medial septum measured by using [18F]FDG PET and spread to the hippocampal dentate gyrus in aged Tg601 tau mice. Brendel et al. (2019) demonstrated a reduced [18F]FDG uptake in brain of hTau mice compared to wild-type mice and that treatment using novel aggregation-inhibiting oligomer modulator Anle138b can rescue this reduction in [18F]FDG measures at follow-up scan after treatment (Figures 2F,G). Recent study by Xiang et al. (2021) showed that [18F]FDG uptake reflected metabolism derived from microglia and that the increased [18F]FDG might instead due to microglia activation.
Mitochondria
Mitochondrial ATP production is crucial in brain bioenergetics and is associated with brain homeostasis, functions, synaptic plasticity, and neurotransmitter processes (Du et al., 2008). The mitochondrial complex 1 (MC-1) plays an important role in the ATP production process, maintains calcium homeostasis, and regulates the apoptosis pathways. Altered MC1 function has been associated with neuronal toxicity, which contributes to the development of various neurodegenerative diseases including AD, FTD, and Parkinson’s disease. Thus, MC-1 has been an attractive target for imaging biomarker indicative of neuronal damage and metabolic changes. As MC-1 locates inside neuron and not microglia or astrocytes, the probe will detect specifically the neuronal metabolism. Several tracers such as [18F]BCPP-EF, [18F]BCPP-BF, [18F]BCPP-EM, and [18F]BMS-747158-02 have been developed (Harada et al., 2013; Tsukada et al., 2014). And among these tracers, [18F]BCPP-EF shows the sufficient brain uptake and a reversible binding pattern. Terada et al. (2021) recently demonstrated reduced uptake of brain [18F]BCPP-EF, associating with [11C]PBB3 measures of tauopathy and cognitive performance in patients with mild AD. In addition, the reduction of [18F]BCPP-EF in the parahippocampus of early-stage AD may precede cerebral glucose hypometabolism assessed by using [18F]FDG (Terada et al., 2020). Barron et al. (2020) and Fairley et al. (2021) showed a reduced [18F]BCPP-EF uptake in the forebrain and hippocampus in rTg4510 mice compared to wild-type mice. Moreover, the [18F]BCPP-EF signal co-localized with tau accumulation assessed by using [11C]PBB3, regional atrophy assayed by structural T2 MRI, and negatively associated with microglia activation assessed by using [18F]FEBMP in rTg4510 mice (Barron et al., 2020; Fairley et al., 2021).
Synaptic Neurotransmitter Receptors
Aberrant accumulation of tau, especially the toxic oligomeric type, has been shown to be associated with altered synaptic protein expression, impairment in axonal transport, neurotransmitter deficits (e.g., cholinergic and glutamatergic), and leading to synaptic loss (Lasagna-Reeves et al., 2011; Spires-Jones and Hyman, 2014; Ait-Bouziad et al., 2017; Lo et al., 2019; Chang et al., 2021b). Several receptors have been shown to be involved in the tau-induced neurotoxicity: Tau modulates the N-methyl-D-aspartate (NMDA) receptor-dependent excitotoxicity and depotentiation in mouse models (Miyamoto et al., 2017). M1 and M3 muscarinic receptors have been shown to mediating the tau-induced intracellular calcium increase and alter calcium ion homeostasis (Gómez-Ramos et al., 2008). Oligomeric forms of tau have been shown to impair synaptic function and recognition memory function in mice (Lasagna-Reeves et al., 2011). Neuronal activity, in turn, enhances tau propagation and pathology (Wu et al., 2016). Chang et al. (2021a,b) recently showed that tau reduction reduced the excitation-inhibition ratio and decreased network hypersynchrony. The abnormal accumulation of tau deposits is directly associated with neuronal loss in animal models (Gómez-Isla et al., 1997; Park et al., 2020), gray matter atrophy, and cognitive deficit in AD (Clavaguera et al., 2009; Murray et al., 2011; Nelson et al., 2012; Bejanin et al., 2017; Ossenkoppele et al., 2021). Recent studies have showed a close correlation between postmortem tauopathy and atrophy assessed by structural MRI as well as functional connectivity in patients with CBD and PSP (Spina et al., 2019). Neuronal hyperactivity has been reported to enhance tau spread in vivo in tau mice (Wu et al., 2016). Selective disruption of inhibitory synapses led to neuronal hyperexcitability at an early stage of tau pathogenesis in PS19 mice (Shimojo et al., 2020).
Nicotinic Acetylcholine Receptors
The cholinergic system is important for memory and cognitive function. Impairment in cholinergic signaling, cholinesterase, decreased levels of nicotinic acetylcholine receptors (nAChRs) that was found at an early stage of AD and primary tauopathy diseases (Ni et al., 2013; Murley and Rowe, 2018). Several new α7 nAChR tracers have been developed including [11C]NS14492 (Ettrup et al., 2011), [18F]DBT-10 (Hillmer et al., 2016), [18F]YLF-DW (Wang D. et al., 2021), and [18F]ASEM (Horti et al., 2014). In animal models, Chaney et al. (2021) showed using an age-dependent increase in [18F]ASEM uptake in the striatum and nucleus basalis of Meynert in the wild-type rats and was higher compared to that in the TgF344 rats at 18 month-of-age. [18F]ASEM has also been evaluated in Parkinson’s disease animal model with striatal injection of 6-hydroxydopamine, in which a transient increase in the level of α7 nAChR was detected (Vetel et al., 2020). A comparative PET study comparing [18F]ASEM and [18F]DBT-10 indicated that [18F]ASEM harbored better brain uptake and kinetic behavior in non-human primates (Hillmer et al., 2017). [18F]ASEM imaging was reported in patients with mild cognitive impairment (Coughlin et al., 2020), psychosis (Coughlin J. et al., 2018), schizophrenia (Wong et al., 2018), and in healthy aging (Coughlin J. M. et al., 2018). Coughlin et al. (2020) showed an increased cerebral [18F]ASEM measure of α7 nAChR in patients with mild cognitive impairment compared to healthy controls.
Metabotropic Glutamate Receptors
Metabotropic glutamate receptors (mGluRs) play important roles in memory and learning in regulating neuronal cell death and survival (Murley and Rowe, 2018). Among the mGluRs, the mGluR5 subtype has been most implicated in AD and FTD (Lee et al., 2004), with several tracers been developed and evaluated in animal and in human, e.g., [18F]FPEB (Mecca et al., 2020), (E)-[11C]ABP688 (Treyer et al., 2007), and [18F]PSS232 (Sephton et al., 2015; Warnock et al., 2018). Lee et al. (2019) reported a 35% decrease in the cortical and subcortical [18F]FPEB binding in 5 × FAD mice at 9 month-of-age compared to 3 month-of-age. Shimojo et al. (2020) demonstrated a reduced level of inhibitory synapse by using [11C]flumazenil at 2–3 month-of-age and a reduced level of excitatory synapse by using (E)-[11C]ABP688 at 5–6 month-of-age in rTg4510 mice compared to wild-type littermates, preceding tau accumulation and brain regional atrophy (Figures 2C,D). Similarly, Treyer et al. (2020) demonstrated a reduced (E)-[11C]ABP688 uptake in the hippocampus and amygdala in patients with AD compared to healthy controls. Mecca et al. (2020) showed an age-dependent reduction of [18F]FPEB-PET level in health control cases probably due to tissue loss. However, conflicting result was reported, where a higher level of [18F]PSS232 binding was detected in autoradiography using postmortem brain slices from AD compared with healthy controls (Müller Herde et al., 2019).
Discussion
Recent advances in PET imaging tracers have enabled in vivo visualization of the time course of central pathologies in patients with AD and primary tauopathy diseases as well as in disease animal models (Jacobs and Tavitian, 2012). The multiplex molecular, structural, and functional imaging readouts have provided important etiological insights, facilitating the understanding of disease mechanism (Hoenig et al., 2018; Jacobs et al., 2018; Franzmeier et al., 2019; Hanseeuw et al., 2019; La Joie et al., 2020; Vogel et al., 2020). In this study, we summarize several considerations in further PET studies in tauopathy animal models.
1. Improvement in animal models: Recent studies have indicated that genomic disruption in addition to mutant tau led to neuronal loss in the widely used rTg4510 mouse model (Ramsden et al., 2005; Santacruz et al., 2005). Moreover, the C57BL/6 strain background also impacts on the development of tauopathy in the rTg4510 model (Bailey et al., 2014). Tau propagates more quickly when human tau is knocked into the mouse locus (Gamache et al., 2019). New models such as the knock-in mouse model (Hashimoto et al., 2019; Saito et al., 2019) or non-human primate model (Beckman et al., 2021) that better recapitulate the human tauopathy diseases are essential for evaluation of imaging tracers and for understanding the tau-related pathophysiological alterations.
2. Difference in the structure of tauopathy between animal models and human: The mostly widely used P301L and P301S transgenic mouse models, which overexpress human 4R tau, show accumulation of pretangle, hyperphosphorylated tau, and neurofibrillary tangles in the brain parenchymal. However, the tau fibril structure is different in animal models compared to that in human with 4R tauopathy diseases, partly due to difference in seeding potency, posttranslational modification, cell-type specificity, as well as a much shorter disease development period (Narasimhan et al., 2017). Recent cryogenic electron microscopy study further demonstrated the complexity and structural differences in the folding of tau filaments among AD (3R/4R), primary tauopathy such as CBD (4R), PSP (4R), argyrophilic grain disease (4R), and Pick’s disease (3R) (Shi et al., 2021b).
3. Quantification and reference brain region: Different observations across different PET imaging in tauopathy animal models were observed, partly due to the use of different strains and age groups, as the pathophysiology spatial distribution and time course differ among different models. For the quantification of PET tracer uptake, standard uptake value (SUV), percent injected dose per gram (ID%/g), and more advanced reference brain region modeling have been utilized (Kuntner and Stout, 2014). For quantifying tau tracer uptake in animal models, relative SUV (SUVR) was calculated using cerebellum as reference brain region. Difficulty in identifying a suitable reference brain region and high non-specific binding of tracer (such as TSPO tracer) hinder the accuracy of advanced analysis.
4. Emerging synaptic targets: Synaptic loss assessed by PET using synaptic vesicle proteins 2A (SV2A) tracer [11C]UCB-J was recently reported in patients with primary tauopathies (Holland et al., 2020) and in AD (Coomans et al., 2021) where a link between tau [assessed by [18F]flortaucipir] and SV2A was detected. No study has so far been reported using SV2A PET in tauopathy animal models. In addition, other emerging targets such as o-GlcNAcase (Paul et al., 2019; Wang et al., 2020) and GSK-3β (Liang et al., 2016; Hu et al., 2017; Prabhakaran et al., 2017; Bernard-Gauthier et al., 2019; Varlow et al., 2021) that associated with tauopathy remain to be explored in tauopathy models. Pilot study results from microtubule imaging using novel tracer [11C]MPC-6827 in PS19 mice and cynomolgus monkeys showed promising results (Damuka et al., 2020; Sai et al., 2020), where a decrease level of [11C]MPC-6827 uptake accompanied by an increased level of tau was detected (Figure 2E).
5. Imaging microglia activation and astrocytosis: There is a lack of imaging tracers for detecting the dynamic phenotypes of microglia, especially the disease-associated microglia with more specific cellular location and activation status (proinflammatory/anti-inflammatory). Several promising targets are currently under investigation such as purinergic P2X7 receptors, P2Y12 receptors (Beaino et al., 2017, 2020; Van Weehaeghe et al., 2020), cyclooxygenase-1 and cyclooxygenase-2 (Ohnishi et al., 2014, 2016; Shukuri et al., 2016), macrophage colony-stimulating factor 1 receptor (Horti et al., 2019; Zhou X. et al., 2021), cannabinoid receptor type 2 (Savonenko et al., 2015; Ni et al., 2019, 2021a), imidazoline-2 binding sites, and receptor for advanced glycation end products (Cary et al., 2016; Luzi et al., 2020). Recent studies highlighted the role of astrocyte in tau pathology and the associated neurodegeneration (Bussian et al., 2018; Ferrer et al., 2018; Richetin et al., 2020; Maté de Gérando et al., 2021; Wang C. et al., 2021; Wang and Ye, 2021). Tau oligomer exposure was shown to trigger the senescence and toxic subpopulation of astrocytes (Gaikwad et al., 2021; Maté de Gérando et al., 2021). For astrocytosis, imaging several tracers targeting at monoamine oxidase-B [e.g., [11C]deuterium-L-deprenyl and [11C]SMBT-1] (Marutle et al., 2013; Rodriguez-Vieitez et al., 2015, 2016; Schöll et al., 2015; Carter et al., 2019; Bellaver et al., 2021; Ni et al., 2021b) and at imidazoline binding site (I2-BS) [e.g., [11C]BU99008] have shown promising results (Calsolaro et al., 2021). How the astrocytosis evolves longitudinally in tauopathy animal models remains to be demonstrated.
6. Sex difference: In human, sex modifies APOE ε4 dose effect on brain tau deposition in cognitively impaired individuals. Sex differences in cerebrospinal fluid tau levels and a mediating effect of testosterone were reported (Sundermann et al., 2020). In amyloidosis animal model, higher load of cerebral Aβ level in female compared to male mice and difference in immune system and metabolism have been reported (Eede et al., 2020; Ni, 2021). In tau animal models, the gender influence on the pathophysiology has been less well documented. A recent study showed increased olfactory, motor deficits, and tau pathology in Tau-P301L male mice compared to female mice (Camargo et al., 2021). Imaging of GSK-3β also indicated a sex difference in P301L mouse model with difference in tracer uptake only between male P301L and wild-type mice (Knight et al., 2021). In other studies, no behavioral, structural, and metabolic difference was observed in the P301S (Criver.com, 2021) and P301L mice (Ni et al., 2020; Massalimova et al., 2021). Several histology and behavior studies in 3 × Tg mice have also indicated a higher amyloid load in female mice compared to male mice, while no difference in tau level between groups (Hirata-Fukae et al., 2008; Carroll et al., 2010; Yang et al., 2018). The recent platform such as MODEL-AD provides an excellent platform for comparative studies and further understanding of the phenotype- and sex-related behavioral and pathological differences in the animal models (Oblak et al., 2020; Sukoff Rizzo et al., 2020; Vitek et al., 2020).
In conclusion, PET has provided a systematic non-invasive approaches to probe the spreading of tauopathy and the related neuroinflammatory, metabolic, and synaptic alterations as well as monitoring of treatment effect in tauopathy animal models.
Author Contributions
LC, YK, and RN wrote the manuscript draft. All authors contributed to the manuscript and approved the final version of the manuscript.
Funding
RN received funding from Helmut Horten Stiftung and Vontobel Stiftung, University of Zurich (reference no. MEDEF-20-021).
Conflict of Interest
LC was employed by Changes Technology Corporation Ltd.
The remaining authors declare that the research was conducted in the absence of any commercial or financial relationships that could be construed as a potential conflict of interest.
Publisher’s Note
All claims expressed in this article are solely those of the authors and do not necessarily represent those of their affiliated organizations, or those of the publisher, the editors and the reviewers. Any product that may be evaluated in this article, or claim that may be made by its manufacturer, is not guaranteed or endorsed by the publisher.
References
Adlimoghaddam, A., Snow, W. M., Stortz, G., Perez, C., Djordjevic, J., Goertzen, A. L., et al. (2019). Regional hypometabolism in the 3xTg mouse model of Alzheimer’s disease. Neurobiol. Dis. 127, 264–277. doi: 10.1016/j.nbd.2019.03.008
Ait-Bouziad, N., Lv, G., Mahul-Mellier, A. L., Xiao, S., Zorludemir, G., Eliezer, D., et al. (2017). Discovery and characterization of stable and toxic Tau/phospholipid oligomeric complexes. Nat. Commun. 8:1678. doi: 10.1038/s41467-017-01575-4
Albert, M., Mairet-Coello, G., Danis, C., Lieger, S., Caillierez, R., Carrier, S., et al. (2019). Prevention of tau seeding and propagation by immunotherapy with a central tau epitope antibody. Brain 142, 1736–1750. doi: 10.1093/brain/awz100
Andorfer, C., Kress, Y., Espinoza, M., de Silva, R., Tucker, K. L., Barde, Y. A., et al. (2003). Hyperphosphorylation and aggregation of tau in mice expressing normal human tau isoforms. J. Neurochem. 86, 582–590. doi: 10.1046/j.1471-4159.2003.01879.x
Ayalon, G., Lee, S. H., Adolfsson, O., Foo-Atkins, C., Atwal, J. K., Blendstrup, M., et al. (2021). Antibody semorinemab reduces tau pathology in a transgenic mouse model and engages tau in patients with Alzheimer’s disease. Sci. Transl. Med. 13:abb2639. doi: 10.1126/scitranslmed.abb2639
Bailey, R. M., Howard, J., Knight, J., Sahara, N., Dickson, D. W., and Lewis, J. (2014). Effects of the C57BL/6 strain background on tauopathy progression in the rTg4510 mouse model. Mol. Neurodegener. 9:8. doi: 10.1186/1750-1326-9-8
Barron, A. M., Ji, B., Fujinaga, M., Zhang, M. R., Suhara, T., Sahara, N., et al. (2020). In vivo positron emission tomography imaging of mitochondrial abnormalities in a mouse model of tauopathy. Neurobiol. Aging 94, 140–148. doi: 10.1016/j.neurobiolaging.2020.05.003
Beaino, W., Janssen, B., Kooij, G., van der Pol, S. M. A., van Het Hof, B., van Horssen, J., et al. (2017). Purinergic receptors P2Y12R and P2X7R: potential targets for PET imaging of microglia phenotypes in multiple sclerosis. J. Neuroinflamm. 14:259. doi: 10.1186/s12974-017-1034-z
Beaino, W., Janssen, B., Kooijman, E., Vos, R., Schuit, R. C., O’Brien-Brown, J., et al. (2020). PET imaging of P2X7R in the experimental autoimmune encephalomyelitis model of multiple sclerosis using [11C]SMW139. J. Neuroinflamm. 17:300. doi: 10.1186/s12974-020-01962-7
Beckman, D., Chakrabarty, P., Ott, S., Dao, A., Zhou, E., Janssen, W. G., et al. (2021). A novel tau-based rhesus monkey model of Alzheimer’s pathogenesis. Alzheimers Dement. 17, 933–945. doi: 10.1002/alz.12318
Bejanin, A., Schonhaut, D. R., La Joie, R., Kramer, J. H., Baker, S. L., Sosa, N., et al. (2017). Tau pathology and neurodegeneration contribute to cognitive impairment in Alzheimer’s disease. Brain 140, 3286–3300. doi: 10.1093/brain/awx243
Bellaver, B., Ferrari-Souza, J. P., Uglione da Ros, L., Carter, S. F., Rodriguez-Vieitez, E., Nordberg, A., et al. (2021). Astrocyte Biomarkers in Alzheimer Disease: A Systematic Review and Meta-analysis. Neurology [Preprint]. doi: 10.1212/wnl.0000000000012109
Bellucci, A., Westwood, A. J., Ingram, E., Casamenti, F., Goedert, M., and Spillantini, M. G. (2004). Induction of inflammatory mediators and microglial activation in mice transgenic for mutant human P301S tau protein. Am. J. Pathol. 165, 1643–1652. doi: 10.1016/s0002-9440(10)63421-9
Bernard-Gauthier, V., Mossine, A. V., Knight, A., Patnaik, D., Zhao, W. N., Cheng, C., et al. (2019). Structural Basis for Achieving GSK-3β Inhibition with High Potency, Selectivity, and Brain Exposure for Positron Emission Tomography Imaging and Drug Discovery. J. Med. Chem. 62, 9600–9617. doi: 10.1021/acs.jmedchem.9b01030
Boxer, A. L., Qureshi, I., Ahlijanian, M., Grundman, M., Golbe, L. I., Litvan, I., et al. (2019). Dam: Safety of the tau-directed monoclonal antibody BIIB092 in progressive supranuclear palsy: a randomised, placebo-controlled, multiple ascending dose phase 1b trial. Lancet Neurol. 18, 549–558. doi: 10.1016/s1474-4422(19)30139-5
Brelstaff, J. H., Mason, M., Katsinelos, T., McEwan, W. A., Ghetti, B., Tolkovsky, A. M., et al. (2021). Microglia become hypofunctional and release metalloproteases and tau seeds when phagocytosing live neurons with P301S tau aggregates. Sci. Adv. 7:eabg4980. doi: 10.1126/sciadv.abg4980
Brendel, M., Deussing, M., Blume, T., Kaiser, L., Probst, F., Overhoff, F., et al. (2019). Late-stage Anle138b treatment ameliorates tau pathology and metabolic decline in a mouse model of human Alzheimer’s disease tau. Alzheimers Res. Ther. 11:67. doi: 10.1186/s13195-019-0522-z
Brendel, M., Jaworska, A., Probst, F., Overhoff, F., Korzhova, V., Lindner, S., et al. (2016). Small-Animal PET Imaging of Tau Pathology with [18F]-THK5117 in 2 Transgenic Mouse Models. J. Nucl. Med. 57, 792–798. doi: 10.2967/jnumed.115.163493
Brendel, M., Yousefi, B. H., Blume, T., Herz, M., Focke, C., Deussing, M., et al. (2018). Comparison of [18F]-T807 and [18F]-THK5117 PET in a Mouse Model of Tau Pathology. Front. Aging Neurosci. 10:174. doi: 10.3389/fnagi.2018.00174
Busche, M. A., and Hyman, B. T. (2020). Synergy between amyloid-β and tau in Alzheimer’s disease. Nat. Neurosci. 23, 1183–1193. doi: 10.1038/s41593-020-0687-6
Bussian, T. J., Aziz, A., Meyer, C. F., Swenson, B. L., van Deursen, J. M., and Baker, D. J. (2018). Clearance of senescent glial cells prevents tau-dependent pathology and cognitive decline. Nature 562, 578–582. doi: 10.1038/s41586-018-0543-y
Calsolaro, V., Matthews, P. M., Donat, C. K., Livingston, N. R., Femminella, G. D., Guedes, S. S., et al. (2021). Astrocyte reactivity with late-onset cognitive impairment assessed in vivo using 11C-BU99008 PET and its relationship with amyloid load. Mol. Psychiatry [Preprint]. doi: 10.1038/s41380-021-01193-z
Camargo, L. C., Honold, D., Bauer, R., Shah, N. J., Langen, K. J., Willbold, D., et al. (2021). Sex-Related Motor Deficits in the Tau-P301L Mouse Model. Biomedicines 9:biomedicines9091160. doi: 10.3390/biomedicines9091160
Carroll, J. C., Rosario, E. R., Kreimer, S., Villamagna, A., Gentzschein, E., Stanczyk, F. Z., et al. (2010). Sex differences in β-amyloid accumulation in 3xTg-AD mice: role of neonatal sex steroid hormone exposure. Brain Res. 1366, 233–245. doi: 10.1016/j.brainres.2010.10.009
Carter, S. F., Chiotis, K., Nordberg, A., and Rodriguez-Vieitez, E. (2019). Longitudinal association between astrocyte function and glucose metabolism in autosomal dominant Alzheimer’s disease. Eur. J. Nucl. Med. Mol. Imaging 46, 348–356. doi: 10.1007/s00259-018-4217-7
Carvalho, K., Martin, E., Ces, A., Sarrazin, N., Lagouge-Roussey, P., Nous, C., et al. (2021). P2X7-deficiency improves plasticity and cognitive abilities in a mouse model of Tauopathy. Prog. Neurobiol. 2021:102139. doi: 10.1016/j.pneurobio.2021.102139
Cary, B. P., Brooks, A. F., Fawaz, M. V., Drake, L. R., Desmond, T. J., Sherman, P., et al. (2016). Synthesis and Evaluation of [18F]RAGER: A First Generation Small-Molecule PET Radioligand Targeting the Receptor for Advanced Glycation Endproducts. ACS Chem. Neurosci. 7, 391–398. doi: 10.1021/acschemneuro.5b00319
Chaney, A. M., Lopez-Picon, F. R., Serrière, S., Wang, R., Bochicchio, D., Webb, S. D., et al. (2021). Prodromal neuroinflammatory, cholinergic and metabolite dysfunction detected by PET and MRS in the TgF344-AD transgenic rat model of AD: a collaborative multi-modal study. Theranostics 11, 6644–6667. doi: 10.7150/thno.56059
Chang, C. W., Shao, E., and Mucke, L. (2021b). Tau: Enabler of diverse brain disorders and target of rapidly evolving therapeutic strategies. Science 371:abb8255. doi: 10.1126/science.abb8255
Chang, C. W., Evans, M. D., Yu, X., Yu, G. Q., and Mucke, L. (2021a). Tau reduction affects excitatory and inhibitory neurons differently, reduces excitation/inhibition ratios, and counteracts network hypersynchrony. Cell Rep. 37:109855. doi: 10.1016/j.celrep.2021.109855
Chiquita, S., Ribeiro, M., Castelhano, J., Oliveira, F., Sereno, J., Batista, M., et al. (2019). A longitudinal multimodal in vivo molecular imaging study of the 3xTg-AD mouse model shows progressive early hippocampal and taurine loss. Hum. Mol. Genet. 28, 2174–2188. doi: 10.1093/hmg/ddz045
Clavaguera, F., Bolmont, T., Crowther, R. A., Abramowski, D., Frank, S., Probst, A., et al. (2009). Transmission and spreading of tauopathy in transgenic mouse brain. Nat. Cell Biol. 11, 909–913. doi: 10.1038/ncb1901
Cohen, R. M., Rezai-Zadeh, K., Weitz, T. M., Rentsendorj, A., Gate, D., Spivak, I., et al. (2013). A transgenic Alzheimer rat with plaques, tau pathology, behavioral impairment, oligomeric aβ, and frank neuronal loss. J. Neurosci. 33, 6245–6256. doi: 10.1523/jneurosci.3672-12.2013
Congdon, E. E., and Sigurdsson, E. M. (2018). Tau-targeting therapies for Alzheimer disease. Nat. Rev. Neurol. 14, 399–415. doi: 10.1038/s41582-018-0013-z
Coomans, E. M., Schoonhoven, D. N., Tuncel, H., Verfaillie, S. C. J., Wolters, E. E., Boellaard, R., et al. (2021). In vivo tau pathology is associated with synaptic loss and altered synaptic function. Alzheimers Res. Ther. 13:35. doi: 10.1186/s13195-021-00772-0
Coughlin, J. M., Du, Y., Rosenthal, H. B., Slania, S., Min Koo, S., Park, A., et al. (2018). The distribution of the alpha7 nicotinic acetylcholine receptor in healthy aging: An in vivo positron emission tomography study with [18F]ASEM. Neuroimage 165, 118–124. doi: 10.1016/j.neuroimage.2017.10.009
Coughlin, J. M., Rubin, L. H., Du, Y., Rowe, S. P., Crawford, J. L., Rosenthal, H. B., et al. (2020). High Availability of the α7-Nicotinic Acetylcholine Receptor in Brains of Individuals with Mild Cognitive Impairment: A Pilot Study Using (18)F-ASEM PET. J. Nucl. Med. 61, 423–426. doi: 10.2967/jnumed.119.230979
Coughlin, J., Du, Y., Crawford, J. L., Rubin, L. H., Behnam Azad, B., Lesniak, W. G., et al. (2018). The availability of the α7 nicotinic acetylcholine receptor in recent-onset psychosis: a study using (18)F-ASEM PET. J. Nucl. Med. 2018:213686. doi: 10.2967/jnumed.118.213686
Criver.com (2021). Characterization of Tau Expressing P301S Mouse Model for Tauopathz Longitudinal Brain Structural and Metabolic Profile. https://www.criver.com/sites/default/files/resource-files/SP-SFN-17-characterization_tau-expressing-p301s.pdf (accessed November 16, 2021)
Damuka, N., Czoty, P. W., Davis, A. T., Nader, M. A., Nader, S. H., Craft, S., et al. (2020). PET Imaging of [11C]MPC-6827, a Microtubule-Based Radiotracer in Non-Human Primate Brains. Molecules 25:molecules25102289. doi: 10.3390/molecules25102289
de Calignon, A., Polydoro, M., Suárez-Calvet, M., William, C., Adamowicz, D. H., Kopeikina, K. J., et al. (2012). Propagation of tau pathology in a model of early Alzheimer’s disease. Neuron 73, 685–697. doi: 10.1016/j.neuron.2011.11.033
de Cristóbal, J., García-García, L., Delgado, M., Pérez, M., Pozo, M. A., and Medina, M. (2014). Longitudinal assessment of a transgenic animal model of tauopathy by FDG-PET imaging. J. Alzheimers Dis. 40, (Suppl. 1), S79–S89. doi: 10.3233/jad-132276
DeBay, D. R., Reid, G. A., Macdonald, I. R., Mawko, G., Burrell, S., Martin, E., et al. (2017). Butyrylcholinesterase-knockout reduces fibrillar β-amyloid and conserves 18FDG retention in 5XFAD mouse model of Alzheimer’s disease. Brain Res. 1671, 102–110. doi: 10.1016/j.brainres.2017.07.009
Declercq, L., Rombouts, F., Koole, M., Fierens, K., Mariën, J., Langlois, X., et al. (2017). Preclinical evaluation of [18F]-JNJ64349311, a novel PET tracer for tau imaging. J. Nuclear Med. 58:185199. doi: 10.2967/jnumed.116.185199
DeVos, S. L., Miller, R. L., Schoch, K. M., Holmes, B. B., Kebodeaux, C. S., Wegener, A. J., et al. (2017). Tau reduction prevents neuronal loss and reverses pathological tau deposition and seeding in mice with tauopathy. Sci. Translat. Med. 9:eaag0481. doi: 10.1126/scitranslmed.aag0481
Du, F., Zhu, X. H., Zhang, Y., Friedman, M., Zhang, N., Ugurbil, K., et al. (2008). Tightly coupled brain activity and cerebral ATP metabolic rate. Proc. Natl. Acad. Sci. U S A. 105, 6409–6414. doi: 10.1073/pnas.0710766105
Eckenweber, F., Medina-Luque, J., Blume, T., Sacher, C., Biechele, G., Wind, K., et al. (2020). Longitudinal TSPO expression in tau transgenic P301S mice predicts increased tau accumulation and deteriorated spatial learning. J. Neuroinflamm. 17:208. doi: 10.1186/s12974-020-01883-5
Eede, P., Obst, J., Benke, E., Yvon-Durocher, G., Richard, B. C., Gimber, N., et al. (2020). Interleukin-12/23 deficiency differentially affects pathology in male and female Alzheimer’s disease-like mice. EMBO Rep. 21:e48530. doi: 10.15252/embr.201948530
Ettrup, A., Mikkelsen, J. D., Lehel, S., Madsen, J., Nielsen, E., Palner, M., et al. (2011). [11C]-NS14492 as a novel PET radioligand for imaging cerebral alpha7 nicotinic acetylcholine receptors: in vivo evaluation and drug occupancy measurements. J. Nucl. Med. 52, 1449–1456. doi: 10.2967/jnumed.111.088815
Fairley, L. H., Sahara, N., Aoki, I., Ji, B., Suhara, T., Higuchi, M., et al. (2021). Neuroprotective effect of mitochondrial translocator protein ligand in a mouse model of tauopathy. J. Neuroinflamm. 18:76. doi: 10.1186/s12974-021-02122-1
Fawaz, M. V., Brooks, A. F., Rodnick, M. E., Carpenter, G. M., Shao, X., Desmond, T. J., et al. (2014). High Affinity Radiopharmaceuticals Based Upon Lansoprazole for PET Imaging of Aggregated Tau in Alzheimer’s Disease and Progressive Supranuclear Palsy: Synthesis, Preclinical Evaluation, and Lead Selection. ACS Chem. Neurosci. 5, 718–730. doi: 10.1021/cn500103u
Ferrer, I., García, M. A., González, I. L., Lucena, D. D., Villalonga, A. R., Tech, M. C., et al. (2018). Aging-related tau astrogliopathy (ARTAG): not only tau phosphorylation in astrocytes. Brain Pathol. 28, 965–985. doi: 10.1111/bpa.12593
Filip, T., Mairinger, S., Neddens, J., Sauberer, M., Flunkert, S., Stanek, J., et al. (2021). Characterization of an APP/tau rat model of Alzheimer’s disease by positron emission tomography and immunofluorescent labeling. Alzheimer’s Res. Therapy 13:175. doi: 10.1186/s13195-021-00916-2
Filipcik, P., Zilka, N., Bugos, O., Kucerak, J., Koson, P., Novak, P., et al. (2012). First transgenic rat model developing progressive cortical neurofibrillary tangles. Neurobiol. Aging 33, 1448–1456. doi: 10.1016/j.neurobiolaging.2010.10.015
Fodero-Tavoletti, M. T., Okamura, N., Furumoto, S., Mulligan, R. S., Connor, A. R., McLean, C. A., et al. (2011). [18F]-THK523: a novel in vivo tau imaging ligand for Alzheimer’s disease. Brain 134(Pt 4), 1089–1100. doi: 10.1093/brain/awr038
Franke, T. N., Irwin, C., Bayer, T. A., Brenner, W., Beindorff, N., Bouter, C., et al. (2020). In vivo Imaging With [18F]-FDG- and [18F]-Florbetaben-PET/MRI Detects Pathological Changes in the Brain of the Commonly Used 5XFAD Mouse Model of Alzheimer’s Disease. Front. Med. 7:529. doi: 10.3389/fmed.2020.00529
Franzmeier, N., Rubinski, A., Neitzel, J., Kim, Y., Damm, A., Na, D. L., et al. (2019). Functional connectivity associated with tau levels in ageing, Alzheimer’s, and small vessel disease. Brain 142, 1093–1107. doi: 10.1093/brain/awz026
Gabellieri, E., Capotosti, F., Molette, J., Sreenivasachary, N., Mueller, A., Berndt, M., et al. (2020). Discovery of 2-(4-(2-fluoroethoxy)piperidin-1-yl)-9-methyl-9H-pyrrolo[2,3-b:4,5-c’]dipyridine ([18F]PI-2014) as PET tracer for the detection of pathological aggregated tau in Alzheimer’s disease and other tauopathies. Eur. J. Med. Chem. 204:112615. doi: 10.1016/j.ejmech.2020.112615
Gaikwad, S., Puangmalai, N., Bittar, A., Montalbano, M., Garcia, S., McAllen, S., et al. (2021). Tau oligomer induced HMGB1 release contributes to cellular senescence and neuropathology linked to Alzheimer’s disease and frontotemporal dementia. Cell Rep. 36:109419. doi: 10.1016/j.celrep.2021.109419
Gamache, J., Benzow, K., Forster, C., Kemper, L., Hlynialuk, C., Furrow, E., et al. (2019). Factors other than hTau overexpression that contribute to tauopathy-like phenotype in rTg4510 mice. Nat. Commun. 10, 2479–2479. doi: 10.1038/s41467-019-10428-1
Gómez-Isla, T., Hollister, R., West, H., Mui, S., Growdon, J. H., Petersen, R. C., et al. (1997). Neuronal loss correlates with but exceeds neurofibrillary tangles in Alzheimer’s disease. Ann. Neurol. 41, 17–24. doi: 10.1002/ana.410410106
Gómez-Ramos, A., Díaz-Hernández, M., Rubio, A., Miras-Portugal, M. T., and Avila, J. (2008). Extracellular tau promotes intracellular calcium increase through M1 and M3 muscarinic receptors in neuronal cells. Mol. Cell Neurosci. 37, 673–681. doi: 10.1016/j.mcn.2007.12.010
Grossman, M. (2021). Lessons learned from a progressive supranuclear palsy trial. Lancet Neurol. 20, 162–163. doi: 10.1016/s1474-4422(21)00035-1
Hanseeuw, B. J., Betensky, R. A., Jacobs, H. I. L., Schultz, A. P., Sepulcre, J., Becker, J. A., et al. (2019). Association of Amyloid and Tau With Cognition in Preclinical Alzheimer Disease: A Longitudinal Study. JAMA Neurol. 76, 915–924. doi: 10.1001/jamaneurol.2019.1424
Hara, Y., Motoi, Y., Hikishima, K., Mizuma, H., Onoe, H., Matsumoto, S. E., et al. (2017). Involvement of the Septo-Hippocampal Cholinergic Pathway in Association with Septal Acetylcholinesterase Upregulation in a Mouse Model of Tauopathy. Curr. Alzheimer Res. 14, 94–103. doi: 10.2174/1567205013666160602235800
Harada, N., Nishiyama, S., Kanazawa, M., and Tsukada, H. (2013). Development of novel PET probes, [18F]BCPP-EF, [18F]BCPP-BF, and [11C]BCPP-EM for mitochondrial complex 1 imaging in the living brain. J. Labelled Comp. Radiopharm. 56, 553–561. doi: 10.1002/jlcr.3056
Hashimoto, S., Matsuba, Y., Kamano, N., Mihira, N., Sahara, N., Takano, J., et al. (2019). Tau binding protein CAPON induces tau aggregation and neurodegeneration. Nat. Commun. 10:2394. doi: 10.1038/s41467-019-10278-x
Heneka, M. T., Carson, M. J., El Khoury, J., Landreth, G. E., Brosseron, F., Feinstein, D. L., et al. (2015). Neuroinflammation in Alzheimer’s disease. Lancet Neurol. 14, 388–405. doi: 10.1016/s1474-4422(15)70016-5
Hillmer, A. T., Li, S., Zheng, M. Q., Scheunemann, M., Lin, S. F., Nabulsi, N., et al. (2017). PET imaging of α(7) nicotinic acetylcholine receptors: a comparative study of [18F]ASEM and [18F]DBT-10 in nonhuman primates, and further evaluation of [18F]ASEM in humans. Eur. J. Nucl. Med. Mol. Imaging 44, 1042–1050. doi: 10.1007/s00259-017-3621-8
Hillmer, A. T., Zheng, M. Q., Li, S., Scheunemann, M., Lin, S. F., Holden, D., et al. (2016). PET imaging evaluation of [18F]DBT-10, a novel radioligand specific to α7 nicotinic acetylcholine receptors, in nonhuman primates. Eur. J. Nucl. Med. Mol. Imaging 43, 537–547. doi: 10.1007/s00259-015-3209-0
Hirata-Fukae, C., Li, H. F., Hoe, H. S., Gray, A. J., Minami, S. S., Hamada, K., et al. (2008). Females exhibit more extensive amyloid, but not tau, pathology in an Alzheimer transgenic model. Brain Res. 1216, 92–103. doi: 10.1016/j.brainres.2008.03.079
Hoenig, M. C., Bischof, G. N., Seemiller, J., Hammes, J., Kukolja, J., Onur, A., et al. (2018). Networks of tau distribution in Alzheimer’s disease. Brain 141, 568–581. doi: 10.1093/brain/awx353
Holland, N., Jones, P. S., Savulich, G., Wiggins, J. K., Hong, Y. T., Fryer, T. D., et al. (2020). Synaptic Loss in Primary Tauopathies Revealed by [(11) C]UCB-J Positron Emission Tomography. Mov. Disord. 35, 1834–1842. doi: 10.1002/mds.28188
Horti, A. G., Gao, Y., Kuwabara, H., Wang, Y., Abazyan, S., Yasuda, R. P., et al. (2014). [18F]-ASEM, a radiolabeled antagonist for imaging the α7-nicotinic acetylcholine receptor with PET. J. Nucl. Med. 55, 672–677. doi: 10.2967/jnumed.113.132068
Horti, A. G., Naik, R., Foss, C. A., Minn, I., Misheneva, V., Du, Y., et al. (2019). PET imaging of microglia by targeting macrophage colony-stimulating factor 1 receptor (CSF1R). Proc. Natl. Acad. Sci. U S A. 116, 1686–1691. doi: 10.1073/pnas.1812155116
Hu, K., Patnaik, D., Collier, T. L., Lee, K. N., Gao, H., Swoyer, M. R., et al. (2017). Development of [18F]Maleimide-Based Glycogen Synthase Kinase-3β Ligands for Positron Emission Tomography Imaging. ACS Med. Chem. Lett. 8, 287–292. doi: 10.1021/acsmedchemlett.6b00405
Ishikawa, A., Tokunaga, M., Maeda, J., Minamihisamatsu, T., Shimojo, M., Takuwa, H., et al. (2018). In Vivo Visualization of Tau Accumulation, Microglial Activation, and Brain Atrophy in a Mouse Model of Tauopathy rTg4510. J. Alzheimers Dis. 61, 1037–1052. doi: 10.3233/jad-170509
Ising, C., Venegas, C., Zhang, S., Scheiblich, H., Schmidt, S. V., Vieira-Saecker, A., et al. (2019). NLRP3 inflammasome activation drives tau pathology. Nature 575, 669–673. doi: 10.1038/s41586-019-1769-z
Jacobs, A. H., and Tavitian, B. (2012). Noninvasive molecular imaging of neuroinflammation. J. Cereb. Blood Flow Metab. 32, 1393–1415. doi: 10.1038/jcbfm.2012.53
Jacobs, H. I. L., Hedden, T., Schultz, A. P., Sepulcre, J., Perea, R. D., Amariglio, R. E., et al. (2018). Structural tract alterations predict downstream tau accumulation in amyloid-positive older individuals. Nat. Neurosci. 21, 424–431. doi: 10.1038/s41593-018-0070-z
Jain, P., Chaney, A. M., Carlson, M. L., Jackson, I. M., Rao, A., and James, M. L. (2020). Neuroinflammation PET Imaging: Current Opinion and Future Directions. J. Nucl. Med. 61, 1107–1112. doi: 10.2967/jnumed.119.229443
Ji, B., Maeda, J., Sawada, M., Ono, M., Okauchi, T., Inaji, M., et al. (2008). Imaging of peripheral benzodiazepine receptor expression as biomarkers of detrimental versus beneficial glial responses in mouse models of Alzheimer’s and other CNS pathologies. J. Neurosci. 28, 12255–12267. doi: 10.1523/jneurosci.2312-08.2008
Ji, B., Ono, M., Yamasaki, T., Fujinaga, M., Zhang, M. R., Seki, C., et al. (2021). Detection of Alzheimer’s disease-related neuroinflammation by a PET ligand selective for glial versus vascular translocator protein. J. Cereb. Blood. Flow Metab. 2021:21992457. doi: 10.1177/0271678x21992457
Kaide, S., Watanabe, H., Shimizu, Y., Tatsumi, H., Iikuni, S., Nakamoto, Y., et al. (2019). (18)F-labeled benzimidazopyridine derivatives for PET imaging of tau pathology in Alzheimer’s disease. Bioorg. Med. Chem. 27, 3587–3594. doi: 10.1016/j.bmc.2019.06.039
Knight, A. C., Varlow, C., Tong, J., and Vasdev, N. (2021). In Vitro and In Vivo Evaluation of GSK-3 Radioligands in Alzheimer’s Disease: Preliminary Evidence of Sex Differences. ACS Pharmacol. Transl. Sci. 4, 1287–1294. doi: 10.1021/acsptsci.1c00132
Kong, Y., Liu, K., Hua, T., Zhang, C., Sun, B., and Guan, Y. (2020). PET Imaging of Neutrophils Infiltration in Alzheimer’s Disease Transgenic Mice. Front. Neurol. 11:523798. doi: 10.3389/fneur.2020.523798
Kreimerman, I., Reyes, A. L., Paolino, A., Pardo, T., Porcal, W., Ibarra, M., et al. (2019). Biological Assessment of a [18F]-Labeled Sulforhodamine 101 in a Mouse Model of Alzheimer’s Disease as a Potential Astrocytosis Marker. Front. Neurosci. 13:734. doi: 10.3389/fnins.2019.00734
Krishnaswamy, S., Lin, Y., Rajamohamedsait, W. J., Rajamohamedsait, H. B., Krishnamurthy, P., and Sigurdsson, E. M. (2014). Antibody-derived in vivo imaging of tau pathology. J. Neurosci. 34, 16835–16850. doi: 10.1523/jneurosci.2755-14.2014
Kroth, H., Oden, F., Molette, J., Schieferstein, H., Capotosti, F., Mueller, A., et al. (2019). Discovery and preclinical characterization of [18F]PI-2620, a next-generation tau PET tracer for the assessment of tau pathology in Alzheimer’s disease and other tauopathies. Eur. J. Nucl. Med. Mol. Imaging 46, 2178–2189. doi: 10.1007/s00259-019-04397-2
Kuntner, C., and Stout, D. (2014). Quantitative preclinical PET imaging: opportunities and challenges. Front. Phys. 2:12. doi: 10.3389/fphy.2014.00012
La Joie, R., Visani, A. V., Baker, S. L., Brown, J. A., Bourakova, V., Cha, J., et al. (2020). Prospective longitudinal atrophy in Alzheimer’s disease correlates with the intensity and topography of baseline tau-PET. Sci. Translat. Med. 12:eaau5732. doi: 10.1126/scitranslmed.aau5732
Lasagna-Reeves, C. A., Castillo-Carranza, D. L., Sengupta, U., Clos, A. L., Jackson, G. R., and Kayed, R. (2011). Tau oligomers impair memory and induce synaptic and mitochondrial dysfunction in wild-type mice. Mol. Neurodegenerat. 6:39. doi: 10.1186/1750-1326-6-39
Lee, H. G., Ogawa, O., Zhu, X., O’Neill, M. J., Petersen, R. B., Castellani, R. J., et al. (2004). Aberrant expression of metabotropic glutamate receptor 2 in the vulnerable neurons of Alzheimer’s disease. Acta Neuropathol. 107, 365–371. doi: 10.1007/s00401-004-0820-8
Lee, M., Lee, H. J., Jeong, Y. J., Oh, S. J., Kang, K. J., Han, S. J., et al. (2019). Age dependency of mGluR5 availability in 5xFAD mice measured by PET. Neurobiol. Aging 84, 208–216. doi: 10.1016/j.neurobiolaging.2019.08.006
Lee, V. M., Goedert, M., and Trojanowski, J. Q. (2001). Neurodegenerative tauopathies. Annu. Rev. Neurosci. 24, 1121–1159. doi: 10.1146/annurev.neuro.24.1.1121
Leng, F., and Edison, P. (2021). Neuroinflammation and microglial activation in Alzheimer disease: where do we go from here? Nat. Rev. Neurol. 17, 157–172. doi: 10.1038/s41582-020-00435-y
Lerdsirisuk, P., Harada, R., Hayakawa, Y., Shimizu, Y., Ishikawa, Y., Iwata, R., et al. (2021). Synthesis and evaluation of 2-pyrrolopyridinylquinoline derivatives as selective tau PET tracers for the diagnosis of Alzheimer’s disease. Nucl. Med. Biol. 93, 11–18. doi: 10.1016/j.nucmedbio.2020.10.002
Lewis, J., McGowan, E., Rockwood, J., Melrose, H., Nacharaju, P., Van Slegtenhorst, M., et al. (2000). Neurofibrillary tangles, amyotrophy and progressive motor disturbance in mice expressing mutant (P301L) tau protein. Nat. Genet. 25, 402–405. doi: 10.1038/78078
Liang, S. H., Chen, J. M., Normandin, M. D., Chang, J. S., Chang, G. C., Taylor, C. K., et al. (2016). Discovery of a Highly Selective Glycogen Synthase Kinase-3 Inhibitor (PF-04802367) That Modulates Tau Phosphorylation in the Brain: Translation for PET Neuroimaging. Angew Chem. Int. Ed. Engl. 55, 9601–9605. doi: 10.1002/anie.201603797
Lindberg, A., Knight, A. C., Sohn, D., Rakos, L., Tong, J., Radelet, A., et al. (2021). Radiosynthesis, In Vitro and In Vivo Evaluation of [18F]CBD-2115 as a First-in-Class Radiotracer for Imaging 4R-Tauopathies. ACS Chem. Neurosci. 12, 596–602. doi: 10.1021/acschemneuro.0c00801
Linnerbauer, M., Wheeler, M. A., and Quintana, F. J. (2020). Astrocyte Crosstalk in CNS Inflammation. Neuron 108, 608–622. doi: 10.1016/j.neuron.2020.08.012
Lo, C. H., Lim, C. K., Ding, Z., Wickramasinghe, S. P., Braun, A. R., Ashe, K. H., et al. (2019). Targeting the ensemble of heterogeneous tau oligomers in cells: A novel small molecule screening platform for tauopathies. Alzheimers Dement. 15, 1489–1502. doi: 10.1016/j.jalz.2019.06.4954
Luzi, F., Savickas, V., Taddei, C., Hader, S., Singh, N., Gee, A. D., et al. (2020). Radiolabeling of [(11)C]FPS-ZM1, a receptor for advanced glycation end products-targeting positron emission tomography radiotracer, using a [(11)C]CO(2)-to-[(11)C]CO chemical conversion. Fut. Med. Chem. 12, 511–521. doi: 10.4155/fmc-2019-0329
Macdonald, I. R., DeBay, D. R., Reid, G. A., O’Leary, T. P., Jollymore, C. T., Mawko, G., et al. (2014). Early detection of cerebral glucose uptake changes in the 5XFAD mouse. Curr. Alzheimer. Res. 11, 450–460. doi: 10.2174/1567205011666140505111354
Maeda, J., Minamihisamatsu, T., Shimojo, M., Zhou, X., Ono, M., Matsuba, Y., et al. (2021). Distinct microglial response against Alzheimer’s amyloid and tau pathologies characterized by P2Y12 receptor. Brain Commun. 3:fcab011. doi: 10.1093/braincomms/fcab011
Maeda, J., Zhang, M. R., Okauchi, T., Ji, B., Ono, M., Hattori, S., et al. (2011). In vivo positron emission tomographic imaging of glial responses to amyloid-beta and tau pathologies in mouse models of Alzheimer’s disease and related disorders. J. Neurosci. 31, 4720–4730. doi: 10.1523/jneurosci.3076-10.2011
Malpetti, M., Passamonti, L., Jones, P. S., Street, D., Rittman, T., Fryer, T. D., et al. (2021). Neuroinflammation predicts disease progression in progressive supranuclear palsy. J. Neurol. Neurosurg. Psychiatry 92, 769–775. doi: 10.1136/jnnp-2020-325549
Malpetti, M., Passamonti, L., Rittman, T., Jones, P. S., Vázquez Rodríguez, P., Bevan-Jones, W. R., et al. (2020). Neuroinflammation and Tau Colocalize in vivo in Progressive Supranuclear Palsy. Ann. Neurol. 88, 1194–1204. doi: 10.1002/ana.25911
Marquié, M., Normandin, M. D., Vanderburg, C. R., Costantino, I. M., Bien, E. A., Rycyna, L. G., et al. (2015). Validating novel tau positron emission tomography tracer [F-18]-AV-1451 (T807) on postmortem brain tissue. Ann. Neurol. 78, 787–800. doi: 10.1002/ana.24517
Marutle, A., Gillberg, P. G., Bergfors, A., Yu, W. F., Ni, R. Q., Nennesmo, I., et al. (2013). H-3-Deprenyl and H-3-PIB autoradiography show different laminar distributions of astroglia and fibrillar beta-amyloid in Alzheimer brain. J. Neuroinflamm. 10:90. doi: 10.1186/1742-2094-10-90
Maruyama, M., Shimada, H., Suhara, T., Shinotoh, H., Ji, B., Maeda, J., et al. (2013). Imaging of tau pathology in a tauopathy mouse model and in Alzheimer patients compared to normal controls. Neuron 79, 1094–1108. doi: 10.1016/j.neuron.2013.07.037
Massalimova, A., Ni, R., Nitsch, R. M., Reisert, M., von Elverfeldt, D., and Klohs, J. (2021). Diffusion Tensor Imaging Reveals Whole-Brain Microstructural Changes in the P301L Mouse Model of Tauopathy. Neurodegener. Dis. 2021, 1–12. doi: 10.1159/000515754
Maté de Gérando, A., d’Orange, M., Augustin, E., Joséphine, C., Aurégan, G., Gaudin-Guérif, M., et al. (2021). Neuronal tau species transfer to astrocytes and induce their loss according to tau aggregation state. Brain 144, 1167–1182. doi: 10.1093/brain/awab011
McAlpine, C. S., Park, J., Griciuc, A., Kim, E., Choi, S. H., Iwamoto, Y., et al. (2021). Astrocytic interleukin-3 programs microglia and limits Alzheimer’s disease. Nature [Preprint]. doi: 10.1038/s41586-021-03734-6
McMurray, L., Macdonald, J. A., Ramakrishnan, N. K., Zhao, Y., Williamson, D. W., Tietz, O., et al. (2021). Synthesis and Assessment of Novel Probes for Imaging Tau Pathology in Transgenic Mouse and Rat Models. ACS Chem. Neurosci. [Preprint]. doi: 10.1021/acschemneuro.0c00790
Mecca, A. P., McDonald, J. W., Michalak, H. R., Godek, T. A., Harris, J. E., Pugh, E. A., et al. (2020). PET imaging of mGluR5 in Alzheimer’s disease. Alzheimers Res. Ther. 12:15. doi: 10.1186/s13195-020-0582-0
Mirzaei, N., Tang, S. P., Ashworth, S., Coello, C., Plisson, C., Passchier, J., et al. (2016). In vivo imaging of microglial activation by positron emission tomography with [(11)C]PBR28 in the 5XFAD model of Alzheimer’s disease. Glia 64, 993–1006. doi: 10.1002/glia.22978
Miyamoto, T., Stein, L., Thomas, R., Djukic, B., Taneja, P., Knox, J., et al. (2017). Phosphorylation of tau at Y18, but not tau-fyn binding, is required for tau to modulate NMDA receptor-dependent excitotoxicity in primary neuronal culture. Mol. Neurodegenerat. 12:41. doi: 10.1186/s13024-017-0176-x
Moreno-Gonzalez, I., Edwards, G. A. III, Hasan, O., Gamez, N., Schulz, J. E., Fernandez-Valenzuela, J. J., et al. (2021). Longitudinal Assessment of Tau-Associated Pathology by (18)F-THK5351 PET Imaging: A Histological, Biochemical, and Behavioral Study. Diagnostics 11:11101874. doi: 10.3390/diagnostics11101874
Mullard, A. (2021). Failure of first anti-tau antibody in Alzheimer disease highlights risks of history repeating. Nat. Rev. Drug Discov. 2021:7. doi: 10.1038/d41573-020-00217-7
Müller Herde, A., Schibli, R., Weber, M., and Ametamey, S. M. (2019). Metabotropic glutamate receptor subtype 5 is altered in LPS-induced murine neuroinflammation model and in the brains of AD and ALS patients. Eur. J. Nucl. Med. Mol. Imaging 46, 407–420. doi: 10.1007/s00259-018-4179-9
Murley, A. G., and Rowe, J. B. (2018). Neurotransmitter deficits from frontotemporal lobar degeneration. Brain 141, 1263–1285. doi: 10.1093/brain/awx327
Murray, M. E., Graff-Radford, N. R., Ross, O. A., Petersen, R. C., Duara, R., and Dickson, D. W. (2011). Neuropathologically defined subtypes of Alzheimer’s disease with distinct clinical characteristics: a retrospective study. Lancet Neurol. 10, 785–796. doi: 10.1016/s1474-4422(11)70156-9
Murugan, N. A., Chiotis, K., Rodriguez-Vieitez, E., Lemoine, L., Ågren, H., and Nordberg, A. (2019). Cross-interaction of tau PET tracers with monoamine oxidase B: evidence from in silico modelling and in vivo imaging. Eur. J. Nucl. Med. Mol. Imaging 46, 1369–1382. doi: 10.1007/s00259-019-04305-8
Narasimhan, S., Guo, J. L., Changolkar, L., Stieber, A., McBride, J. D., Silva, L. V., et al. (2017). Pathological Tau Strains from Human Brains Recapitulate the Diversity of Tauopathies in Nontransgenic Mouse Brain. J. Neurosci. 37, 11406–11423. doi: 10.1523/JNEUROSCI.1230-17.2017
Nelson, P. T., Alafuzoff, I., Bigio, E. H., Bouras, C., Braak, H., Cairns, N. J., et al. (2012). Correlation of Alzheimer disease neuropathologic changes with cognitive status: a review of the literature. J. Neuropathol. Exp. Neurol. 71, 362–381. doi: 10.1097/NEN.0b013e31825018f7
Ng, K. P., Pascoal, T. A., Mathotaarachchi, S., Therriault, J., Kang, M. S., Shin, M., et al. (2017). Monoamine oxidase B inhibitor, selegiline, reduces (18)F-THK5351 uptake in the human brain. Alzheimer’s Res. Ther. 9, 25–25. doi: 10.1186/s13195-017-0253-y
Ni, R. (2021). Positron Emission Tomography in Animal Models of Alzheimer’s Disease Amyloidosis. [Preprint]. doi: 10.20944/PREPRINTS202110.0222.V1
Ni, R., Ji, B., Ono, M., Sahara, N., Zhang, M. R., Aoki, I., et al. (2018). Comparative in-vitro and in-vivo quantifications of pathological tau deposits and their association with neurodegeneration in tauopathy mouse models. J. Nucl. Med. 59, 960–966. doi: 10.2967/jnumed.117.201632
Ni, R., Marutle, A., and Nordberg, A. (2013). Modulation of α7 nicotinic acetylcholine receptor and fibrillar amyloid-β interactions in Alzheimer’s disease brain. J. Alzheimers Dis. 33, 841–851. doi: 10.3233/jad-2012-121447
Ni, R., Mu, L., and Ametamey, S. (2019). Positron emission tomography of type 2 cannabinoid receptors for detecting inflammation in the central nervous system. Acta Pharmacol. Sin. 40, 351–357. doi: 10.1038/s41401-018-0035-5
Ni, R., Müller Herde, A., Haider, A., Keller, C., Louloudis, G., Vaas, M., et al. (2021a). In vivo Imaging of Cannabinoid Type 2 Receptors: Functional and Structural Alterations in Mouse Model of Cerebral Ischemia by PET and MRI. Mol. Imaging Biol. 2021:4. doi: 10.1007/s11307-021-01655-4
Ni, R., Röjdner, J., Voytenko, L., Dyrks, T., Thiele, A., Marutle, A., et al. (2021b). In vitro Characterization of the Regional Binding Distribution of Amyloid PET Tracer Florbetaben and the Glia Tracers Deprenyl and PK11195 in Autopsy Alzheimer’s Brain Tissue. J. Alzheimers Dis. 80, 1723–1737. doi: 10.3233/jad-201344
Ni, R., Zarb, Y., Kuhn, G. A., Müller, R., Yundung, Y., Nitsch, R. M., et al. (2020). SWI and phase imaging reveal intracranial calcifications in the P301L mouse model of human tauopathy. Magma 33, 769–781. doi: 10.1007/s10334-020-00855-3
Novak, P., Kovacech, B., Katina, S., Schmidt, R., Scheltens, P., Kontsekova, E., et al. (2021). ADAMANT: a placebo-controlled randomized phase 2 study of AADvac1, an active immunotherapy against pathological tau in Alzheimer’s disease. Nat. Aging 1, 521–534. doi: 10.1038/s43587-021-00070-2
Nutma, E., Ceyzériat, K., Amor, S., Tsartsalis, S., Millet, P., Owen, D. R., et al. (2021). Cellular sources of TSPO expression in healthy and diseased brain. Eur. J. Nuclear Med. Mol. Imaging [Preprint]. doi: 10.1007/s00259-020-05166-2
Oblak, A. L., Forner, S., Territo, P. R., Sasner, M., Carter, G. W., Howell, G. R., et al. (2020). Model organism development and evaluation for late-onset Alzheimer’s disease: MODEL-AD. Alzheimers Dement. 6:e12110. doi: 10.1002/trc2.12110
Oddo, S., Caccamo, A., Shepherd, J. D., Murphy, M. P., Golde, T. E., Kayed, R., et al. (2003). Triple-transgenic model of Alzheimer’s disease with plaques and tangles: intracellular Abeta and synaptic dysfunction. Neuron 39, 409–421. doi: 10.1016/s0896-6273(03)00434-3
Ohnishi, A., Senda, M., Yamane, T., Mikami, T., Nishida, H., Nishio, T., et al. (2016). Exploratory human PET study of the effectiveness of (11)C-ketoprofen methyl ester, a potential biomarker of neuroinflammatory processes in Alzheimer’s disease. Nucl. Med. Biol. 43, 438–444. doi: 10.1016/j.nucmedbio.2016.04.005
Ohnishi, A., Senda, M., Yamane, T., Sasaki, M., Mikami, T., Nishio, T., et al. (2014). Human whole-body biodistribution and dosimetry of a new PET tracer, [(11)C]ketoprofen methyl ester, for imagings of neuroinflammation. Nucl. Med. Biol. 41, 594–599. doi: 10.1016/j.nucmedbio.2014.04.008
Ossenkoppele, R., Lyoo, C. H., Sudre, C. H., van Westen, D., Cho, H., Ryu, Y. H., et al. (2020). Distinct tau PET patterns in atrophy-defined subtypes of Alzheimer’s disease. Alzheimers Dement. 16, 335–344. doi: 10.1016/j.jalz.2019.08.201
Ossenkoppele, R., Smith, R., Mattsson-Carlgren, N., Groot, C., Leuzy, A., Strandberg, O., et al. (2021). Accuracy of Tau Positron Emission Tomography as a Prognostic Marker in Preclinical and Prodromal Alzheimer Disease: A Head-to-Head Comparison Against Amyloid Positron Emission Tomography and Magnetic Resonance Imaging. JAMA Neurol. [Preprint]. doi: 10.1001/jamaneurol.2021.1858
Owen, D. R., Yeo, A. J., Gunn, R. N., Song, K., Wadsworth, G., Lewis, A., et al. (2012). An 18-kDa translocator protein (TSPO) polymorphism explains differences in binding affinity of the PET radioligand PBR28. J. Cereb. Blood Flow Metabol. 32, 1–5. doi: 10.1038/jcbfm.2011.147
Palleis, C., Sauerbeck, J., Beyer, L., Harris, S., Schmitt, J., Morenas-Rodriguez, E., et al. (2021). In Vivo Assessment of Neuroinflammation in 4-Repeat Tauopathies. Mov. Disord. 36, 883–894. doi: 10.1002/mds.28395
Park, L., Hochrainer, K., Hattori, Y., Ahn, S. J., Anfray, A., Wang, G., et al. (2020). Tau induces PSD95-neuronal NOS uncoupling and neurovascular dysfunction independent of neurodegeneration. Nat. Neurosci. 23, 1079–1089. doi: 10.1038/s41593-020-0686-7
Paul, S., Haskali, M. B., Liow, J. S., Zoghbi, S. S., Barth, V. N., Kolodrubetz, M. C., et al. (2019). Evaluation of a PET Radioligand to Image O-GlcNAcase in Brain and Periphery of Rhesus Monkey and Knock-Out Mouse. J. Nucl. Med. 60, 129–134. doi: 10.2967/jnumed.118.213231
Prabhakaran, J., Zanderigo, F., Sai, K. K. S., Rubin-Falcone, H., Jorgensen, M. J., Kaplan, J. R., et al. (2017). Radiosynthesis and in Vivo Evaluation of [(11)C]A1070722, a High Affinity GSK-3 PET Tracer in Primate Brain. ACS Chem. Neurosci. 8, 1697–1703. doi: 10.1021/acschemneuro.6b00376
Ramsden, M., Kotilinek, L., Forster, C., Paulson, J., McGowan, E., SantaCruz, K., et al. (2005). Age-dependent neurofibrillary tangle formation, neuron loss, and memory impairment in a mouse model of human tauopathy (P301L). J. Neurosci. 25, 10637–10647. doi: 10.1523/jneurosci.3279-05.2005
Richetin, K., Steullet, P., Pachoud, M., Perbet, R., Parietti, E., Maheswaran, M., et al. (2020). Tau accumulation in astrocytes of the dentate gyrus induces neuronal dysfunction and memory deficits in Alzheimer’s disease. Nat. Neurosci. 23, 1567–1579. doi: 10.1038/s41593-020-00728-x
Roberts, M., Sevastou, I., Imaizumi, Y., Mistry, K., Talma, S., Dey, M., et al. (2020). Pre-clinical characterisation of E2814, a high-affinity antibody targeting the microtubule-binding repeat domain of tau for passive immunotherapy in Alzheimer’s disease. Acta Neuropathol. Commun. 8:13. doi: 10.1186/s40478-020-0884-2
Robinson, J. L., Yan, N., Caswell, C., Xie, S. X., Suh, E., Van Deerlin, V. M., et al. (2020). Primary Tau Pathology, Not Copathology, Correlates With Clinical Symptoms in PSP and CBD. J. Neuropathol. Exp. Neurol. 79, 296–304. doi: 10.1093/jnen/nlz141
Rodriguez-Vieitez, E., Ni, R., Gulyás, B., Tóth, M., Häggkvist, J., Halldin, C., et al. (2015). Astrocytosis precedes amyloid plaque deposition in Alzheimer APPswe transgenic mouse brain: a correlative positron emission tomography and in vitro imaging study. Eur. J. Nucl. Med. Mol. Imaging 42, 1119–1132. doi: 10.1007/s00259-015-3047-0
Rodriguez-Vieitez, E., Saint-Aubert, L., Carter, S. F., Almkvist, O., Farid, K., Schöll, M., et al. (2016). Diverging longitudinal changes in astrocytosis and amyloid PET in autosomal dominant Alzheimer’s disease. Brain 139(Pt 3), 922–936. doi: 10.1093/brain/awv404
Rojas, S., Herance, J. R., Gispert, J. D., Abad, S., Torrent, E., Jiménez, X., et al. (2013). Invivo evaluation of amyloid deposition and brain glucose metabolism of 5XFAD mice using positron emission tomography. Neurobiol. Aging 34, 1790–1798. doi: 10.1016/j.neurobiolaging.2012.12.027
Sai, K. S., Damuka, N., Mintz, A., Whitlow, C. T., Craft, S., and Macauley-Rambach, S. (2020). [11C]MPC-6827, a microtubule-based PET imaging tracer: A potential early imaging biomarker for AD and other ADRDs. Alzheimer’s Dement. 16:e037790. doi: 10.1002/alz.037790
Saito, T., Mihira, N., Matsuba, Y., Sasaguri, H., Hashimoto, S., Narasimhan, S., et al. (2019). Humanization of the entire murine Mapt gene provides a murine model of pathological human tau propagation. J. Biol. Chem. 294, 12754–12765. doi: 10.1074/jbc.RA119.009487
Sancheti, H., Akopian, G., Yin, F., Brinton, R. D., Walsh, J. P., and Cadenas, E. (2013). Age-dependent modulation of synaptic plasticity and insulin mimetic effect of lipoic acid on a mouse model of Alzheimer’s disease. PLoS One 8:e69830. doi: 10.1371/journal.pone.0069830
Santacruz, K., Lewis, J., Spires, T., Paulson, J., Kotilinek, L., Ingelsson, M., et al. (2005). Tau suppression in a neurodegenerative mouse model improves memory function. Science 309, 476–481. doi: 10.1126/science.1113694
Savonenko, A. V., Melnikova, T., Wang, Y., Ravert, H., Gao, Y., Koppel, J., et al. (2015). Cannabinoid CB2 Receptors in a Mouse Model of Aβ Amyloidosis: Immunohistochemical Analysis and Suitability as a PET Biomarker of Neuroinflammation. PLoS One 10:e0129618. doi: 10.1371/journal.pone.0129618
Schöll, M., Carter, S. F., Westman, E., Rodriguez-Vieitez, E., Almkvist, O., Thordardottir, S., et al. (2015). Early astrocytosis in autosomal dominant Alzheimer’s disease measured in vivo by multi-tracer positron emission tomography. Sci. Rep. 5:16404. doi: 10.1038/srep16404
Sephton, S. M., Herde, A. M., Mu, L., Keller, C., Rüdisühli, S., Auberson, Y., et al. (2015). Preclinical evaluation and test-retest studies of [18F]PSS232, a novel radioligand for targeting metabotropic glutamate receptor 5 (mGlu5). Eur. J. Nucl. Med. Mol. Imaging 42, 128–137. doi: 10.1007/s00259-014-2883-7
Shao, X., Carpenter, G. M., Desmond, T. J., Sherman, P., Quesada, C. A., Fawaz, M., et al. (2012). Evaluation of [(11)C]N-Methyl Lansoprazole as a Radiopharmaceutical for PET Imaging of Tau Neurofibrillary Tangles. ACS Med. Chem. Lett. 3, 936–941. doi: 10.1021/ml300216t
Shi, Y., Andhey, P. S., Ising, C., Wang, K., Snipes, L. L., Boyer, K., et al. (2021a). Overexpressing low-density lipoprotein receptor reduces tau-associated neurodegeneration in relation to apoE-linked mechanisms. Neuron [Preprint]. doi: 10.1016/j.neuron.2021.05.034
Shi, Y., Zhang, W., Yang, Y., Murzin, A. G., Falcon, B., Kotecha, A., et al. (2021b). Structure-based classification of tauopathies. Nature [Preprint]. doi: 10.1038/s41586-021-03911-7
Shi, Y., Manis, M., Long, J., Wang, K., Sullivan, P. M., Remolina Serrano, J., et al. (2019). Microglia drive APOE-dependent neurodegeneration in a tauopathy mouse model. J. Exp. Med. 216, 2546–2561. doi: 10.1084/jem.20190980
Shimojo, M., Takuwa, H., Takado, Y., Tokunaga, M., Tsukamoto, S., Minatohara, K., et al. (2020). Selective Disruption of Inhibitory Synapses Leading to Neuronal Hyperexcitability at an Early Stage of Tau Pathogenesis in a Mouse Model. J. Neurosci. 40, 3491–3501. doi: 10.1523/jneurosci.2880-19.2020
Shukuri, M., Mawatari, A., Ohno, M., Suzuki, M., Doi, H., Watanabe, Y., et al. (2016). Detection of Cyclooxygenase-1 in Activated Microglia During Amyloid Plaque Progression: PET Studies in Alzheimer’s Disease Model Mice. J. Nucl. Med. 57, 291–296. doi: 10.2967/jnumed.115.166116
Sobue, A., Komine, O., Hara, Y., Endo, F., Mizoguchi, H., Watanabe, S., et al. (2021). Microglial gene signature reveals loss of homeostatic microglia associated with neurodegeneration of Alzheimer’s disease. Acta Neuropathol. Commun. 9:1. doi: 10.1186/s40478-020-01099-x
Son, Y., Kim, J. S., Jeong, Y. J., Jeong, Y. K., Kwon, J. H., Choi, H.-D., et al. (2018). Long-term RF exposure on behavior and cerebral glucose metabolism in 5xFAD mice. Neurosci. Lett. 666, 64–69. doi: 10.1016/j.neulet.2017.12.042
Spillantini, M. G., and Goedert, M. (2013). Tau pathology and neurodegeneration. Lancet Neurol. 12, 609–622. doi: 10.1016/S1474-4422(13)70090-5
Spina, S., Brown, J. A., Deng, J., Gardner, R. C., Nana, A. L., Hwang, J.-H. L., et al. (2019). Neuropathological correlates of structural and functional imaging biomarkers in 4-repeat tauopathies. Brain 142, 2068–2081. doi: 10.1093/brain/awz122
Spires-Jones, T. L., and Hyman, B. T. (2014). The intersection of amyloid beta and tau at synapses in Alzheimer’s disease. Neuron 82, 756–771. doi: 10.1016/j.neuron.2014.05.004
Sukoff Rizzo, S. J., Masters, A., Onos, K. D., Quinney, S., Sasner, M., Oblak, A., et al. (2020). Improving preclinical to clinical translation in Alzheimer’s disease research. Alzheimer’s Dement. 6:e12038. doi: 10.1002/trc2.12038
Sundermann, E. E., Panizzon, M. S., Chen, X., Andrews, M., Galasko, D., and Banks, S. J. (2020). Sex differences in Alzheimer’s-related Tau biomarkers and a mediating effect of testosterone. Biol. Sex Differ. 11:33. doi: 10.1186/s13293-020-00310-x
Tagai, K., Ono, M., Kubota, M., Kitamura, S., Takahata, K., Seki, C., et al. (2020). High-Contrast InVivo Imaging of Tau Pathologies in Alzheimer’s and Non-Alzheimer’s Disease Tauopathies. Neuron 2020:042. doi: 10.1016/j.neuron.2020.09.042
Takuwa, H., Orihara, A., Takado, Y., Urushihata, T., Shimojo, M., Ishikawa, A., et al. (2020). Tracking tau fibrillogenesis and consequent primary phagocytosis of neurons mediated by microglia in a living tauopathy model. bioRxiv 2020:368977. doi: 10.1101/2020.11.04.368977
Teng, E., Kepe, V., Frautschy, S. A., Liu, J., Satyamurthy, N., Yang, F., et al. (2011). [F-18]FDDNP microPET imaging correlates with brain Aβ burden in a transgenic rat model of Alzheimer disease: effects of aging, in vivo blockade, and anti-Aβ antibody treatment. Neurobiol. Dis. 43, 565–575. doi: 10.1016/j.nbd.2011.05.003
Terada, T., Obi, T., Bunai, T., Matsudaira, T., Yoshikawa, E., Ando, I., et al. (2020). In vivo mitochondrial and glycolytic impairments in patients with Alzheimer disease. Neurology 94, e1592–e1604. doi: 10.1212/wnl.0000000000009249
Terada, T., Therriault, J., Kang, M. S. P., Savard, M., Pascoal, T. A., Lussier, F., et al. (2021). Mitochondrial complex I abnormalities is associated with tau and clinical symptoms in mild Alzheimer’s disease. Mol. Neurodegener. 16:28. doi: 10.1186/s13024-021-00448-1
Tournier, B. B., Tsartsalis, S., Ceyzériat, K., Fraser, B. H., Grégoire, M. C., Kövari, E., et al. (2020). Astrocytic TSPO Upregulation Appears Before Microglial TSPO in Alzheimer’s Disease. J. Alzheimers Dis. 77, 1043–1056. doi: 10.3233/jad-200136
Tournier, B. B., Tsartsalis, S., Rigaud, D., Fossey, C., Cailly, T., Fabis, F., et al. (2019). TSPO and amyloid deposits in sub-regions of the hippocampus in the 3xTgAD mouse model of Alzheimer’s disease. Neurobiol. Dis. 121, 95–105. doi: 10.1016/j.nbd.2018.09.022
Treyer, V., Gietl, A. F., Suliman, H., Gruber, E., Meyer, R., Buchmann, A., et al. (2020). Reduced uptake of [11C]-ABP688, a PET tracer for metabolic glutamate receptor 5 in hippocampus and amygdala in Alzheimer’s dementia. Brain Behav. 10:e01632. doi: 10.1002/brb3.1632
Treyer, V., Streffer, J., Wyss, M. T., Bettio, A., Ametamey, S. M., Fischer, U., et al. (2007). Evaluation of the metabotropic glutamate receptor subtype 5 using PET and [11C]-ABP688: assessment of methods. J. Nucl. Med. 48, 1207–1215. doi: 10.2967/jnumed.107.039578
Tsukada, H., Nishiyama, S., Fukumoto, D., Kanazawa, M., and Harada, N. (2014). Novel PET probes [18F]-BCPP-EF and [18F]-BCPP-BF for mitochondrial complex I: a PET study in comparison with [18F]-BMS-747158-02 in rat brain. J. Nucl. Med. 55, 473–480. doi: 10.2967/jnumed.113.125328
Van Camp, N., Lavisse, S., Roost, P., Gubinelli, F., Hillmer, A., and Boutin, H. (2021). TSPO imaging in animal models of brain diseases. Eur. J. Nucl. Med. Mol. Imaging [Preprint]. doi: 10.1007/s00259-021-05379-z
Van de Bittner, G. C., Riley, M. M., Cao, L., Ehses, J., Herrick, S. P., Ricq, E. L., et al. (2017). Nasal neuron PET imaging quantifies neuron generation and degeneration. J. Clin. Invest. 127, 681–694. doi: 10.1172/jci89162
Van Weehaeghe, D., Van Schoor, E., De Vocht, J., Koole, M., Attili, B., Celen, S., et al. (2020). TSPO Versus P2X7 as a Target for Neuroinflammation: An In Vitro and In Vivo Study. J. Nucl. Med. 61, 604–607. doi: 10.2967/jnumed.119.231985
Varlow, C., Mossine, A. V., Bernard-Gauthier, V., Scott, P. J. H., and Vasdev, N. (2021). Radiofluorination of oxazole-carboxamides for preclinical PET neuroimaging of GSK-3. J. Fluor Chem. 245:109760. doi: 10.1016/j.jfluchem.2021.109760
Vetel, S., Vercouillie, J., Buron, F., Vergote, J., Tauber, C., Busson, J., et al. (2020). Longitudinal PET Imaging of α7 Nicotinic Acetylcholine Receptors with [18F]ASEM in a Rat Model of Parkinson’s Disease. Mol. Imaging Biol. 22, 348–357. doi: 10.1007/s11307-019-01400-y
Vitek, M. P., Araujo, J. A., Fossel, M., Greenberg, B. D., Howell, G. R., Rizzo, S. J. S., et al. (2020). Translational animal models for Alzheimer’s disease: An Alzheimer’s Association Business Consortium Think Tank. Alzheimer’s Dement. Translat. Res. Clin. Intervent. 6:e12114. doi: 10.1002/trc2.12114
Vogel, J. W., Iturria-Medina, Y., Strandberg, O. T., Smith, R., Levitis, E., Evans, A. C., et al. (2020). Spread of pathological tau proteins through communicating neurons in human Alzheimer’s disease. Nat. Commun. 11:2612. doi: 10.1038/s41467-020-15701-2
Vogel, J. W., Young, A. L., Oxtoby, N. P., Smith, R., Ossenkoppele, R., Strandberg, O. T., et al. (2021). Four distinct trajectories of tau deposition identified in Alzheimer’s disease. Nat. Med. 27, 871–881. doi: 10.1038/s41591-021-01309-6
Wang, C., Xiong, M., Gratuze, M., Bao, X., Shi, Y., Andhey, P. S., et al. (2021). Selective removal of astrocytic APOE4 strongly protects against tau-mediated neurodegeneration and decreases synaptic phagocytosis by microglia. Neuron 109, 1657.e–1674.e. doi: 10.1016/j.neuron.2021.03.024
Wang, D., Yao, Y., Wang, S., Zhang, H., and He, Z.-X. (2021). The Availability of the α7-Nicotinic Acetylcholine Receptor in Early Identification of Vulnerable Atherosclerotic Plaques: A Study Using a Novel [18F]-Label Radioligand PET. Front. Bioengine. Biotechnol. 9:640037. doi: 10.3389/fbioe.2021.640037
Wang, P., and Ye, Y. (2021). Filamentous recombinant human Tau activates primary astrocytes via an integrin receptor complex. Nat. Commun. 12:95. doi: 10.1038/s41467-020-20322-w
Wang, X., Li, W., Marcus, J., Pearson, M., Song, L., Smith, K., et al. (2020). MK-8719, a Novel and Selective O-GlcNAcase Inhibitor That Reduces the Formation of Pathological Tau and Ameliorates Neurodegeneration in a Mouse Model of Tauopathy. J. Pharmacol. Exp. Ther. 374, 252–263. doi: 10.1124/jpet.120.266122
Warnock, G., Sommerauer, M., Mu, L., Pla Gonzalez, G., Geistlich, S., Treyer, V., et al. (2018). A first-in-man PET study of [18F]PSS232, a fluorinated ABP688 derivative for imaging metabotropic glutamate receptor subtype 5. Eur. J. Nucl. Med. Mol. Imaging 45, 1041–1051. doi: 10.1007/s00259-017-3879-x
Wegmann, S., Bennett, R. E., Delorme, L., Robbins, A. B., Hu, M., McKenzie, D., et al. (2019). Experimental evidence for the age dependence of tau protein spread in the brain. Sci. Adv. 5:eaaw6404. doi: 10.1126/sciadv.aaw6404
Weng, C. C., Hsiao, I. T., Yang, Q. F., Yao, C. H., Tai, C. Y., Wu, M. F., et al. (2020). Characterization of (18)F-PM-PBB3 ((18)F-APN-1607) Uptake in the rTg4510 Mouse Model of Tauopathy. Molecules 25:25071750. doi: 10.3390/molecules25071750
Whitwell, J. L., Tosakulwong, N., Botha, H., Ali, F., Clark, H. M., Duffy, J. R., et al. (2020). Brain volume and flortaucipir analysis of progressive supranuclear palsy clinical variants. NeuroImage Clin. 25:102152. doi: 10.1016/j.nicl.2019.102152
Wong, D. F., Kuwabara, H., Horti, A. G., Roberts, J. M., Nandi, A., Cascella, N., et al. (2018). Brain PET Imaging of α7-nAChR with [18F]ASEM: Reproducibility, Occupancy, Receptor Density, and Changes in Schizophrenia. Int. J. Neuropsychopharmacol. 21, 656–667. doi: 10.1093/ijnp/pyy021
Wu, J. W., Hussaini, S. A., Bastille, I. M., Rodriguez, G. A., Mrejeru, A., Rilett, K., et al. (2016). Neuronal activity enhances tau propagation and tau pathology in vivo. Nat. Neurosci. 19, 1085–1092. doi: 10.1038/nn.4328
Wu, T., Dejanovic, B., Gandham, V. D., Gogineni, A., Edmonds, R., Schauer, S., et al. (2019). Complement C3 Is Activated in Human AD Brain and Is Required for Neurodegeneration in Mouse Models of Amyloidosis and Tauopathy. Cell Rep. 28, 2111.e–2123.e. doi: 10.1016/j.celrep.2019.07.060
Xiang, X., Wind, K., Wiedemann, T., Blume, T., Shi, Y., Briel, N., et al. (2021). Microglial activation states drive glucose uptake and FDG-PET alterations in neurodegenerative diseases. Sci. Transl. Med. 13:eabe5640. doi: 10.1126/scitranslmed.abe5640
Yang, J.-T., Wang, Z.-J., Cai, H.-Y., Yuan, L., Hu, M.-M., Wu, M.-N., et al. (2018). Sex Differences in Neuropathology and Cognitive Behavior in APP/PS1/tau Triple-Transgenic Mouse Model of Alzheimer’s Disease. Neurosci. Bull. 34, 736–746. doi: 10.1007/s12264-018-0268-9
Yoshiyama, Y., Higuchi, M., Zhang, B., Huang, S. M., Iwata, N., Saido, T. C., et al. (2007). Synapse loss and microglial activation precede tangles in a P301S tauopathy mouse model. Neuron 53, 337–351. doi: 10.1016/j.neuron.2007.01.010
Zhou, K., Yang, F., Li, Y., Chen, Y., Zhang, X., Zhang, J., et al. (2021). Synthesis and Evaluation of Fluorine-18 Labeled 2-Phenylquinoxaline Derivatives as Potential Tau Imaging Agents. Mol. Pharm. 18, 1176–1195. doi: 10.1021/acs.molpharmaceut.0c01078
Zhou, R., Ji, B., Kong, Y., Qin, L., Ren, W., Guan, Y., et al. (2021). PET Imaging of Neuroinflammation in Alzheimer’s Disease. Front. Immunol. 12:3750. doi: 10.1007/s00702-017-1731-x
Zhou, X., Ji, B., Seki, C., Nagai, Y., Minamimoto, T., Fujinaga, M., et al. (2021). PET imaging of colony-stimulating factor 1 receptor: A head-to-head comparison of a novel radioligand, (11)C-GW2580, and (11)C-CPPC, in mouse models of acute and chronic neuroinflammation and a rhesus monkey. J. Cereb. Blood Flow Metab. 2021:211004146. doi: 10.1177/0271678x211004146
Keywords: Alzheimer’s disease, tau, animal model, positron emission tomography, FTD (fronto-temporal dementia), neurotransmitter, neuroinflammation
Citation: Cao L, Kong Y, Ji B, Ren Y, Guan Y and Ni R (2022) Positron Emission Tomography in Animal Models of Tauopathies. Front. Aging Neurosci. 13:761913. doi: 10.3389/fnagi.2021.761913
Received: 02 September 2021; Accepted: 30 November 2021;
Published: 10 January 2022.
Edited by:
Jiehui Jiang, Shanghai University, ChinaReviewed by:
Elena Rodriguez-Vieitez, Karolinska Institutet (KI), SwedenDaichi Sone, University College London, United Kingdom
Copyright © 2022 Cao, Kong, Ji, Ren, Guan and Ni. This is an open-access article distributed under the terms of the Creative Commons Attribution License (CC BY). The use, distribution or reproduction in other forums is permitted, provided the original author(s) and the copyright owner(s) are credited and that the original publication in this journal is cited, in accordance with accepted academic practice. No use, distribution or reproduction is permitted which does not comply with these terms.
*Correspondence: Ruiqing Ni, bmlAYmlvbWVkLmVlLmV0aHouY2g=
†These authors have contributed equally to this work