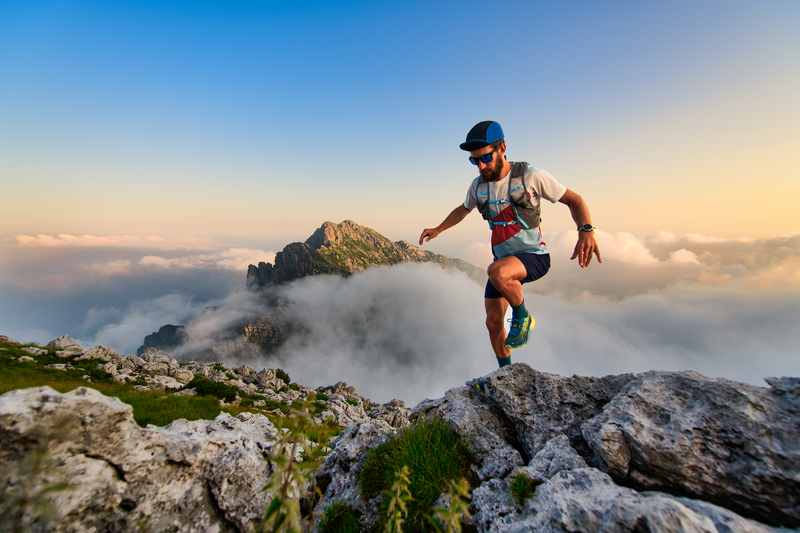
95% of researchers rate our articles as excellent or good
Learn more about the work of our research integrity team to safeguard the quality of each article we publish.
Find out more
REVIEW article
Front. Aging Neurosci. , 29 November 2021
Sec. Parkinson’s Disease and Aging-related Movement Disorders
Volume 13 - 2021 | https://doi.org/10.3389/fnagi.2021.745438
This article is part of the Research Topic Behavioral Models of Human Cognitive Diseases with Focus on Validity, Reproducibility, and Statistics View all 7 articles
A correction has been applied to this article in:
Corrigendum: Experimental Models of Cognitive Impairment for Use in Parkinson's Disease Research: The Distance Between Reality and Ideal
Parkinson’s disease (PD) is the second most common neurodegenerative disease. Cognitive impairment is one of the key non-motor symptoms of PD, affecting both mortality and quality of life. However, there are few experimental studies on the pathology and treatments of PD with mild cognitive impairment (PD-MCI) and PD dementia (PDD) due to the lack of representative models. To identify new strategies for developing representative models, we systematically summarized previous studies on PD-MCI and PDD and compared differences between existing models and diseases. Our initial search identified 5432 articles, of which 738 were duplicates. A total of 227 articles met our inclusion criteria and were included in the analysis. Models fell into three categories based on model design: neurotoxin-induced, transgenic, and combined. Although the neurotoxin-induced experimental model was the most common type that was used during every time period, transgenic and combined experimental models have gained significant recent attention. Unfortunately, there remains a big gap between ideal and actual experimental models. While each model has its own disadvantages, there have been tremendous advances in the development of PD models of cognitive impairment, and almost every model can verify a hypothesis about PD-MCI or PDD. Finally, our proposed strategies for developing novel models are as follows: a set of plans that integrate symptoms, biochemistry, neuroimaging, and other objective indicators to judge and identify that the novel model plays a key role in new strategies for developing representative models; novel models should simulate different clinical features of PD-MCI or PDD; inducible α-Syn overexpression and SH-SY5Y-A53T cellular models are good candidate models of PD-MCI or PDD.
Cognitive impairment, one of the key non-motor comorbid symptoms of PD, is not only a risk factor for early mortality but also has a greater impact on quality of life than motor symptoms (Oosterveld et al., 2015; Saredakis et al., 2019). Cognitive impairment in PD can be divided based on severity into mild cognitive impairment (PD-MCI) and dementia (PDD). PD-MCI, an intermediate cognitive stage on the continuum between normal cognitive function and dementia, can be identified in 15%–40% of PD patients at the time of diagnosis (Aarsland, 2016; Monastero et al., 2018). The prevalence of PD-MCI and PDD in the PD population is 40% and 30%, respectively (Aarsland and Kurz, 2010; Baiano et al., 2020). PDD occurs in 75%–90% of patients who have had PD for 10 years or more (Gratwicke et al., 2015). Understanding the pathophysiology of PD-MCI and PDD is critical to the development of disease-modifying treatments. Although only 5%–15% of PD cases are attributed to clear genetic factors, several studies have shown that genetic factors such as α-synuclein (α-Syn) variants play an important role in cognitive impairment (Ball et al., 2019; Chen et al., 2020). The 85%–95% remaining idiopathic cases of PD can be linked to environmental factors, such as heavy metals, pesticides, and illicit drugs, whose important role cannot be overlooked when studying cognitive impairment (Ball et al., 2019; Cariccio et al., 2019; Agnihotri and Aruoma, 2020). Systemic medical factors are also associated with PD cognitive impairment, including lower uric acid levels and the presence of an orthostatic heart rate or orthostatic hypotension (McDonald et al., 2016; Veselý et al., 2019; Tanaka et al., 2020). Gene–environment–medical history interactions may play a vital role in the pathogenesis of PD-MCI and PDD. Because the development of cognitive impairment in patients with PD is a multi-factor interaction, the heterogeneous pathophysiologic mechanisms underlying PD-MCI and PDD remain unclear. Recent studies have shown that inhibitors, amino acids, and actively targeted gold nanoparticle composites could improve the cognitive flexibility of experimental models. There are very few therapeutic options for PD patients with cognitive impairment due to the limited efficacy and significant side effects of therapeutic methods (Hsu et al., 2018; Byers et al., 2020; Liu L. et al., 2020; Wang et al., 2021). In order to identify risk factors, mechanisms, and treatment strategies for PD-MCI and PDD, research using appropriate experimental models is invaluable.
Experimental models, an important part of basic science research that emulates human physiology or pathology, are crucial to improving our understanding of PD-MCI and PDD pathophysiology and to the preclinical testing of novel therapeutics. Prior use of multitudinous PD experimental models has assessed the different symptoms and stages of PD and includes classical neurotoxin-induced, transgenic, and combined models (Kin et al., 2019). Some experimental PD models replicate PD cognitive impairment, which is very useful for studies on PD-MCI and PDD. These models include genetic and toxin-based exposure to a variety of animals (Prediger et al., 2010; Ba et al., 2019; Singh et al., 2019). However, each model exhibited varying degrees of motor and cognitive dysfunction symptoms or only produced mild symptoms of cognitive dysfunction alone because of the tangle modeling methods that were used. These experimental models, which were assessed with uneven behavioral tests such as the NOR, MWM, Y-maze test, T-maze test, and Barnes maze test demonstrated short-term or long-term spatial memory deficits, episodic-like memory deficits and recognition memory deficits (Huang et al., 2015; Singh et al., 2019; Ding et al., 2020). Regrettably, an ideal and representative experimental model that replicates all of the phenotypic and pathologic features of PD-MCI and PDD is still unavailable. However, each existing model has its own benefits and drawbacks that are important to consider when selecting an appropriate model based on the specific objectives of the study (Grandi et al., 2018). It is therefore vital to have a comprehensive understanding of the neuropathology of each model, permitting an accurate explanation of research results and a reasonable estimate of how well the model will correlate with human PD-MCI and PDD. In this review, we analyze trends in PD cognitive impairment modeling and discuss the features and limitations of current models based on the clinical features of PD-MCI and PDD.
The two cardinal symptoms of PD-MCI and PDD are motor deficits (i.e., asymmetric resting tremor, bradykinesia, postural instability, and rigidity) and one or more cognitive deficits in attention, working memory, executive function, language, memory, visuo-constructive ability, and visuospatial function (Dubois et al., 2007; Litvan et al., 2012; Hayes, 2019). Unlike PD-MCI patients, whose symptoms are insufficient to interfere with functional independence, PDD is characterized by the impairment of two or more of the four cognitive domains and leads to more severe cognitive deficits that impair daily life based on the proposed criteria of the Movement Disorder Society Task Force (Table 1) (Dubois et al., 2007; Litvan et al., 2012). Representative experimental models of PD-MCI or PDD therefore, need to develop both motor and cognitive deficiency symptoms. Moreover, the cognitive dysfunction shown by the ideal model of PDD should be distinct from that of PD-MCI. We need to evaluate more than four cognitive domains through a variety of behavioral studies to clarify the degree of cognitive impairment of the ideal model and prove whether this ideal model represents PD-MCI or PDD.
The representative pathologic substrate of both PD-MCI and PDD mainly includes LBs and AD pathology. A longitudinal clinical study found that the representative types of pathologies that contribute to PD-MCI includes α-synucleinopathy in the locus coeruleus, amygdala, cingulate gyrus, and middle temporal gyrus, argyrophilic grains, a single neurofibrillary tangle in the hippocampal CA1 region, white matter rarefaction in the frontal lobe, amyloid plaques and neurofibrillary tangles in the transentorhinal area, and thorn-shaped astrocytes characteristic of aging-related tau astrogliopathy in the subpial region of the amygdala (Knox et al., 2020). However, there was significant heterogeneity in the clinical presentation of PD-MCI due to the differing impact of the individual disease pathology on each cognitive domain (Halliday et al., 2014; Knox et al., 2020).
The most persuasive evidence to date suggests that Lewy-type α-synucleinopathy plays a paramount role in PDD. α-synucleinopathy was found in the pigmented neurons of the SN, amygdala, cortex, and hippocampal CA2 region (Halliday et al., 2014; Liu et al., 2019). Recent studies found that a progressively decreased α-Syn pathologic load between the anterior periallocortical agranular toward the intermediate dysgranular and posterior isocortical granular insular subregions and an increase in activated microglia in the amygdala were significantly related to the extent of α-Syn pathology (Fathy et al., 2019; Kouli et al., 2020). A systematic review found that tau and amyloid-β pathologies independently contributed to the development of PDD (Smith et al., 2019). Moreover, the intricate causal relationship between PD and cerebral amyloid angiopathy, hippocampal sclerosis, small vessel disease, and other pathological factors remains unclear and therefore requires further research (Halliday et al., 2014; Hase et al., 2020).
The relationships between PD cognitive dysfunction and biomarkers in biofluids [i.e., cerebrospinal fluid (CSF), serum/plasma] have been studied in great detail (Johar et al., 2017). Biomarkers in the CSF could represent cognitive decline in PD due to their proximity to the central nervous system. Multiple studies have focused on the AD markers amyloid-β 42 (Aβ42), total tau (t-tau), phosphorylated tau (p-tau), and α-Syn. Recent studies have shown that low CSF Aβ42 levels were related not only to cognitive impairment in PD but also to rapid cognitive decline (Bäckström et al., 2015; Stav et al., 2015). T-tau, p-tau, and α-Syn levels at each disease stage are inconsistent. CSF t-tau, p-tau, and total α-Syn levels may increase with disease stage, and high levels of these factors have been associated with cognitive impairment in patients with more advanced disease, but not those at an early disease stage (Hall et al., 2016). The above results suggest that CSF Aβ42, t-tau, p-tau, and total α-Syn can be used as objective indicators of cognitive function in PD experimental models.
The diagnosis of most diseases mainly depends on the detection of biomarkers in the serum or plasma. Glucosylceramidase (GBA), leucine-rich repeat serine/threonine-protein kinase 2 (LRRK2), and α-Syn mutations have been associated with PD-MCI and PDD (Aarsland et al., 2017). Recent studies have shown that high levels of serum/plasma α-Syn and low levels of serum glial cell line-derived neurotrophic factor (GDNF), miRNA-29s (miR-29s), vitamin D, and 25-hydroxyvitamin D may be involved in the cognitive impairment of PD-MCI and PDD patients (Bougea et al., 2020; Han L. et al., 2020; Liu Y. et al., 2020; Santangelo et al., 2020). Importantly, low levels of epidermal growth factor (EGF) may be predictive of cognitive decline (Pellecchia et al., 2013). However, the current body of literature on the association between serum/plasma biomarkers and cognitive impairment lacks long-term randomized controlled trials with multi-center large samples. Furthermore, the combination of CSF and serum markers was significantly better than serum alone at distinguishing between PDD and PD (Bougea et al., 2020). Although additional novel biomarkers have been found to be related to cognitive impairment in PD, Aβ42, t-tau, p-tau, and α-Syn are relatively reliable indicators of cognitive function.
Over the past decade, our understanding of the association between morphologic changes and cognitive impairment in PD has been characterized using structural and molecular neuroimaging techniques, such as MRI and PET. Although PD-MCI might show evidence of cortical volume loss, PDD was associated with extensive cortical and subcortical atrophy that can be quantified with a structural MRI (Duncan et al., 2013). Patients with PD-MCI have been found to have cortical volume loss in the parietal, posterior, and frontal cortices and atrophy in the hippocampus (Mak et al., 2015; Pereira et al., 2015; Yildiz et al., 2015). In contrast, PDD has more severe cortical thinning in the parietal, occipital, temporal, and frontal cortices and substantial atrophy of the hippocampus, including the parahippocampus, insula, and cingulate gyrus (Aarsland et al., 2017).
Prior works have also focused on the association between cognitive impairment and PET on dopaminergic (DAergic) molecular, glucose metabolism, and cholinergic molecular interactions. Several studies have suggested that PD-MCI has DAergic deficits in the striatum and the insula, while such defects in the PDD are in the striatum, anterior cingulate, and midbrain (Chou et al., 2014; Aarsland et al., 2017; Politis et al., 2017; Han et al., 2021). It was reported that [C-11]-PE2I (N-(3-iodoprop-2E-enyl)-2b-carbomethoxy-3b-(4-methyl-phenyl)nortropane) (PE2I), a PET radiotracer targeting neuronal dopamine (DA) transporters with high specificity, avidity in the caudate, putamen, and pallidum was indicative of more severe cognitive function deficits (Ivanidze et al., 2020). Moreover, PET imaging of glucose metabolism has shown lower levels of metabolic activity in the parietal, temporal, cingulate, and frontal cortices of patients with PD-MCI and PDD (González-Redondo et al., 2014; Ruppert et al., 2021). The cholinergic system, which is responsible for cognitive processing and is associated with the clinical manifestations of PDD, plays an important role in the structural and functional remodeling of the cortical circuits (Aarsland et al., 2017). PDD has been associated with the loss of cholinergic function in the temporal, frontal, and medial occipital cortices and the thalamus (Kotagal et al., 2012; Aarsland et al., 2017). However, most studies only identified a relationship between PET and cognitive impairment in patients with PDD, not PD-MCI.
To clarify trends in experimental modeling of PD cognitive impairment, we systematically searched PubMed using the following search terms: (“Parkinson’s disease [Title/Abstract]” AND “cognitive function [Title/Abstract]”) OR (“Parkinson’s disease [Title/Abstract]” AND “cognitive impairment [Title/Abstract]”) OR (“Parkinson’s disease mild cognitive impairment” AND “model”) OR (“Parkinson’s disease dementia” AND “model”). The publication range was from the start of the database to 30 May 2021. Two investigators (YF and JH) reviewed each topic and abstract independently to select references that met the inclusion criteria. Inconsistencies encountered by the reviewers would be resolved after consultation with a third reviewer (DC). The selection process is shown in Figure 1. We identified 5432 articles, of which 738 were duplicates. A total of 227 articles met our inclusion criteria and were included in the analysis.
Information on the experimental models used in each work was extracted. Models were divided into three categories based on model generation: neurotoxin-induced experimental model, transgenic experimental model, and combined experimental model. Models using any kind of neurotoxic substance were defined as a neurotoxin-induced experimental model. The transgenic experimental model group included models that involved gene manipulation. Models that included two or more methods were assigned to the combined experimental model group. Figure 2 demonstrates an increased focus on cognitive impairment in PD over time. Although neurotoxin-induced experimental models were the most prevalent during every time period, transgenic experimental models and combined experimental models have received increased recent attention.
1-Methyl-4-phenyl-1,2,3,6-tetrahydropyridine has been commonly used in experimental models of PD. MPTP is a highly lipophilic prodrug that crosses the blood–brain barrier (BBB) into the brain, where it is metabolized into the potent neurotoxin 1-methyl-4-phenylpyridinium (MPP+). MPP+ released from astrocytes into the extracellular space is taken up by neighboring DAergic neurons. It consequently becomes highly concentrated in the microglia and induces oxidative stress and decreased adenosine triphosphate (ATP) generation by inhibiting mitochondrial complex I of the mitochondrial electron transport chain (El-Gamal et al., 2021). This leads to apoptosis and necrosis of DAergic neurons due to the rapid decrease in the concentration of ATP in the SN and striatum.
A total of 73 articles showed that MPTP therapy alone can induce both the loss of DAergic neurons in the SN and clinical cognitive deficits (Table 2). An initial MPTP-treated PD model with cognitive deficits in macaque nemistrina monkeys was reported in 1990 (Schneider and Kovelowski, 1990). Based on different experimental conditions and requirements, scholars have created 16 modeling methods for MPTP-induced PD cognitive impairment using seven different methods of drug administration: oral (p.o.), intravenous (i.v.), intramuscular (i.m.), subcutaneous (s.c.), intraperitoneal (i.p.), stereotaxical, and intranasal (pr.nar.) (Table 2). Rodent and non-human primate (NHP) models have primarily been used to model PD with cognitive impairment. This suggests that MPTP is easily absorbed by animals and that experimenters require personal protection.
From 1990 to 2010, the majority of MPTP-treated experimental models used NHP to study cognitive impairment, including Cynomolgus macaque, Macaca fasciculari, Macaca mulatta, and eight other kinds of monkeys, on account of the specific sensitive and reproducible toxic effects of MPTP on the DAergic nigrostriatal pathway of NHPs (Taylor et al., 1990; Schneider and Pope-Coleman, 1995; Decamp and Schneider, 2009; Schneider et al., 2013). VDR, CPT, modified delayed response task (MDR), spatial delayed response task (SDR), attentional set-shifting test (ASST), and nine other kinds of tasks are commonly used to monitor the cognitive deficits of primates (Fernández-Ruiz et al., 1999; Millan et al., 2010; Schneider et al., 2013; Ko et al., 2016). Previous studies demonstrated that MPTP-treated NHPs developed motor dysfunction similar to that seen in PD, including tremors, bradykinesia, abnormal posture, decreased activity, low frequency (4.3 ± 1.7 Hz) tremor of the head at rest, and a high motor symptom score (Zhang et al., 2013; Choudhury and Daadi, 2018). NHP models also demonstrated evidence of cognitive impairments, such as spatial working memory deficits, attention deficits, learning deficits, executive function deficits, or deficits in the modulation of PFC-mediated cognitive performance (Schneider et al., 2002, 2013; Zhang et al., 2014; Ko et al., 2016; Phillips et al., 2017). A slowly progressing dose of MPTP administered to monkeys (ranging from an initial 0.05 mg/kg to 0.075 mg/kg, 2 to 3 times per week for up to 24 weeks) resulted in the development of cognitive deficits before motor deficits (Schneider and Pope-Coleman, 1995), which suggested that an MPTP-treated monkey model can yield significant individual differences in motor and cognitive impairment. There are several drawbacks to NHP models that limit their use in the study of mechanisms of cognitive impairment. First, the Unified Parkinson’s Disease Rating Scale, which assesses the severity of the disease phenotype, has not been validated in NHPs (Imbert et al., 2000). Secondly, a long experimental period, high costs, a complicated genetic manipulation process, and ethical considerations limit the employment of NHP in research (Chia et al., 2020).
As NHP studies require a high experimental threshold, rodents are valuable experimental subjects when evaluating cognitive impairment in PD. The motor symptoms of PD can also be induced in rodent models by MPTP, including tremors, muscle rigidity, hypotonia, hypokinesia, hollow back, catalepsy, dysfunctional dynamic gait, transitory hypolocomotion, loss of balance, and poor motor coordination. Cognitive impairment phenotypes in rodents can be quantified using a series of tests, such as the MWM, NOR, passive avoidance test (PAT), Barnes maze test, T-maze test, and Y-maze test. However, one report noted that acute (4 mg/kg/day, 4 days, i.p.) and chronic (22 mg/kg/day, 28 days, i.p.) MPTP-treated models did not develop long-term changes in locomotive and cognitive function (Fifel et al., 2013). Most studies have suggested that an MPTP-treated rodent model induces specific types of cognitive impairment, which include deficits in short-term spatial learning, working memory, memory acquisition and retention, short-term social and object recognition memory, short-term inhibitory avoidance memory, contextual memory, visuospatial attention, and episodic-like memory (Monaghan et al., 2010; Wang et al., 2010; Castro et al., 2012; Das et al., 2014; Ho et al., 2014; Yabuki et al., 2014; Zhang et al., 2018; Yang et al., 2020).
The above studies suggest that cognitive deficits in rodents are linked to degeneration of the nigrostriatal DAergic neurons, striatal DA depletions, and neuroinflammation (Perry et al., 2004; Wang et al., 2009; Hsieh et al., 2012a). In addition, α-Syn and phosphorylated-α-synuclein (p-α-Syn), which are significant contributors to the neuropathology of PD-MCI and PDD, accumulated abnormally in the striatum and SN of MPTP-treated mice (Han N. R. et al., 2020). Moreover, microglial activation in the SN, striatum, and hippocampus and cell loss in the hippocampal CA1 and dentate gyrus (DG) areas are related to deficits in the cognitive function of MPTP-treated rodents (Ho et al., 2011; Hsieh et al., 2012b). Due to differences in protocol and animal strains, reports to this end have presented inconsistent findings. However, these experimental models have been able to reproduce the partial cognitive impairment observed in parkinsonian patients. Several limitations of MPTP-treated experimental models cannot be ignored, which include the high mortality rate of treated rodents, neurologic damage that tends to heal spontaneously, and environmental exposure effects.
Treatment using 6-OHDA, a selective catecholaminergic neurotoxin that cannot cross the BBB, leads to a consistent behavioral phenotype in experimental models and predictable degeneration of DAergic neurons. In the 62 articles that utilized a 6-OHDA-treated experimental model, multiple different types of cognitive impairment in addition to motor symptoms were induced (Table 3). Except for zebrafish, which were exposed to 6-OHDA by dissolving it into the culture medium, rodent and gray treefrog models received stereotactic injections of 6-OHDA into different anatomic regions (Endepols et al., 2004; Ramos-Moreno et al., 2012; Choi et al., 2019; Abidar et al., 2020) such as the SN, the MFB, the striatum, PFC, VTA, hippocampus, and the third ventricle (Gasbarri et al., 1996; Gu et al., 2012; Kadowaki Horita et al., 2013; Razavinasab et al., 2013; Zhou et al., 2017; Bayo-Olugbami et al., 2020; Schneider et al., 2021). Of these, the target proportions for unilateral stereotactic 6-OHDA injection were more common in the MFB and the SN, whereas the target proportions for bilateral injection were more common in the striatum. Previous studies showed that the injection of 6-OHDA into the SN or the MFB led to rapid and enormous DA cell body degeneration, which involves the nigrostriatal pathway in an anterograde progression. Moreover, the administration of 6-OHDA into the striatum affected DA terminals and disrupted the nigrostriatal pathway in retrograde succession (More et al., 2016). Although there was a wide total dose range of 6-OHDA used in the literature (3–200 μg), 8–24 μg was most commonly used (Hsueh et al., 2018; Wang et al., 2019).
The common motor symptoms seen in a 6-OHDA-treated rodent model include hypolocomotion, a shuffling gait similar to that seen in PD, limb rigidity, forepaw akinesia in the limb-use asymmetry test, and impaired balance and coordination in the rotarod test (Hadadianpour et al., 2017; Hsueh et al., 2018; Bayo-Olugbami et al., 2020). This model had a specific stereotyped rotation after subcutaneous injection of apomorphine if the 6-OHDA is injected unilaterally. These models also showed various levels of cognitive impairment as assessed with the MWM, NOR, five-choice serial reaction time task (5-CSRT), and the spatial and egocentric task (S&E task). These generally cover long-term spatial learning acquisition and memory retention deficits (but not short-term), spatial and object location memory deficits, long-term object recognition deficits, visuo-spatial memory deficits, deficits in long- and short-term working memory, and provisionally on additional executive processes, cognitive flexibility performance deficits, attention deficits, episodic-like memory deficits, and short-term social memory deficits (Tadaiesky et al., 2010; Hsieh et al., 2012b; Razavinasab et al., 2013; Eagle et al., 2015; Betancourt et al., 2017; Haghparast et al., 2018; Marshall et al., 2019). However, in cognitive studies, bilateral injection of a low dose of 6-OHDA (4–6 μg/side) is preferred as it produces a balanced loss of DAergic neurons and mimics the early pathologic changes of PD with cognitive impairment (Ferro et al., 2005).
In general, 6-OHDA induces rapid non-enzymatic auto-oxidation in the cytosol to produce toxic species, such as hydrogen peroxide, quinones, superoxide radicals, and hydroxyl radicals. These species can not only induce oxidative stress via the generation of ROS but also cause mitochondrial dysfunction by impairing mitochondrial complex I and inhibiting the activity of the electron transport chain (More et al., 2016). Previous studies have found that oxidative stress, neuroinflammation, and DAergic neural apoptosis in the SN, VTA, PFC, striatum, and hippocampus are related to the cognitive impairment of 6-OHDA-treated models, as the DAergic neurons present in the VTA, striatum and SN project their axons into the hippocampus and PFC (Bitu Pinto et al., 2015; Haghparast et al., 2018; Singh et al., 2018). Mitochondrial dysfunction and glial activation have also been shown to be involved in the cognitive impairment seen in 6-OHDA-treated models (Smith et al., 2017; Real et al., 2019). Two factors play an important role in 6-OHDA-treated models: reduced DA, 3,4-dihydroxyphenylacetic acid (DOPAC), homovanillic acid (HVA), dopamine D3 receptor (D3Rs) and tyrosine hydroxylase (TH) levels in the striatum, and reduced DA metabolism and transport (Goes et al., 2014; Betancourt et al., 2017). 6-OHDA injection increased the expression of Aβ1–42 and NOX4 in the DG of the hippocampus by activating the MKK7-ERK-Fos-APP axis in the PD-MCI model (Ba et al., 2019; Choi et al., 2019). Although 6-OHDA-treated experimental models serve as a useful tool for exploring the potential mechanism and therapies of PD-MCI and PDD, several significant disadvantages of this model need to be noted. Firstly, 6-OHDA fails to produce LBs, a key part of PD-MCI and PDD pathology. Further, bilateral lesions are associated with a high rate of mortality. Due to its inability to penetrate the BBB, stereotactic injection of 6-OHDA damages the structure of the brain. Moreover, several articles suggest that 6-OHDA-treated models generate inconspicuous movement disorders and unstable cognitive dysfunction (Tadaiesky et al., 2008; Carvalho et al., 2013; Oliveira et al., 2020; Schneider et al., 2021). Finally, 6-OHDA has to be injected together with noradrenaline and serotonin transport inhibitors because it is taken up by catecholaminergic nerve terminals.
While MPTP and 6-OHDA are classic experimental inducers of PD in pre-clinical models, several pesticides, pharmacologic compounds and oligomers have been used to induce PD-like conditions that include DA depletion and cognitive deficits. A total of 26 articles describe 12 of these toxins (Table 4).
Because pesticide exposure is a well-known risk factor of PD, rotenone and permethrin are useful tools for simulating environmental exposure. Rotenone, an inhibitor of mitochondrial complex I by penetrating the BBB in a manner similar to MPTP, has been shown to induce PD-like motor symptoms and learning and memory deficits in rodents and drosophila (Dhanraj et al., 2018; Perez-Pardo et al., 2018; Bandookwala et al., 2019). Interestingly, rapid eye movement sleep deprivation, which is a way to damage cognitive function, improved memory impairment in a rotenone-treated rat model due to moderate compensatory striatal DA release (Dos Santos et al., 2013). A previous study found that LBs can be formed in the surviving DAergic neurons in a rotenone model; however, this has not been observed in models of cognitive impairment (Inden et al., 2011). Rats exposed to permethrin early in life can also develop a phenotype of PD that is characterized by poor motor coordination, gait changes, and working memory deficits due to oxidative stress, LB formation, mitochondrial complex I defects, and DA loss (Nasuti et al., 2013, 2017). While cognitive impairment observed in both rotenone- and permethrin-treated models appears to be related to DAergic neuron degeneration, the exact mechanism that causes this effect remains unclear.
Several pharmacological compounds, including reserpine, ibotenic acid, chlorpromazine, and haloperidol, have been used to induce reversible DA depletion (Camacho-Abrego et al., 2014; Ogundele et al., 2015; Campêlo et al., 2017; Naeem et al., 2017). Reserpine exposure decreases brain monoamine storage capacity and total volume by inhibiting the vesicular monoamine transporter VMAT2, which models early-stage PD that progresses to PDD (Ikram and Haleem, 2019; Leal et al., 2019). Reserpine-treated rodent models showed not only progressive catalepsy behavior and periods of oral twitching and reduced motor coordination but also developed impaired long- and short-term memory and learning (Campêlo et al., 2017; Leal et al., 2019). Stereotactic injection of ibotenic acid into the subthalamic nucleus (STN) and pedunculopontine tegmental nucleus (PPT) can affect cholinergic neurons and the cholinergic system, thereby disturbing DAergic neurotransmission, which is associated with learning and memory (Targa et al., 2018). Although chlorpromazine and haloperidol may trigger PD cognitive impairment, this has not been well demonstrated and requires further validation (Ogundele et al., 2015; Naeem et al., 2017).
The novel toxins human α-synuclein oligomers (H-αSynO) and recombinant α-synuclein fibrils can induce PD memory deficits by depleting early mitochondria in SN neurons, which damages nigrostriatal DAergic neurons and inhibits cortical and amygdala function (Boi et al., 2020; Stoyka et al., 2020). These toxins can also induce p-α-Syn deposition in the SN neurons and microglia and spread to the striatum, which is a similar pathology to that of PD-MCI and PDD (Boi et al., 2020).
Lipopolysaccharide (LPS), an endotoxin, has been used to simulate PD neuroinflammation, which can induce spatial learning and memory deficits and recognition memory deficits (Delattre et al., 2013; Saini et al., 2020). Although other novel toxins, including MnCl2, β-sitosterol β-d-glucoside (BSSG), β-N-methylamino-L-alanine (BMAA), have been found to trigger PD with cognitive deficits, their reliability still requires further verification (Peres et al., 2015; Van Kampen et al., 2015; Scott and Downing, 2017).
Only 5%–15% of PD cases are attributed to clear genetic factors; however, transgenic experimental models play a significant role in the study of idiopathic PD-MCI and PDD. In addition to common familial PD-linked genes, which include α-Syn, parkin, PTEN-induced putative (PINK1), protein deglycase (DJ-1), and LRRK2, 11 other transgenic models were used in a total of 38 articles to replicate PD-MCI or PDD (Table 5). The genes that these models interfered with included Pitx3, dopamine transporter (DAT), mitochondrial transcription factor A (TFAM), β-synuclein (β-Syn), A2A receptors, Atp13a2, B4galnt, Ifnb, midkine (Mdk), Ndufs4, tau, and Washc4 (Prediger et al., 2011; Lei et al., 2012; Sekiyama et al., 2012; Li et al., 2013; Moon et al., 2013; Schultheis et al., 2013; Ejlerskov et al., 2015; Choi et al., 2017; Hwang et al., 2019; Wu et al., 2020; Courtland et al., 2021). Rodent, NHP, and drosophila animal models based on α-Syn are currently available. Rodents and drosophila have been used in LRRK2 transgenic models. However, other rodent transgenic models have also been established.
α-Synuclein, a small neuronal protein present at presynaptic terminals, regulates membrane and vesicular dynamics (Kin et al., 2019). Transgenic models of α-Syn, such as those with mutations (A53T and A30P) or gene knockout, have developed cognitive deficits similar to PD-MCI or PDD (Freichel et al., 2007; Kokhan et al., 2012; Singh et al., 2019). As tau oligomers are elevated by α-Syn in the hippocampus, pons, and cerebellum, A53T mice showed not only freezing behavior and loss of motor coordination but also long- and short-term spatial learning and memory deficits at 12 months (but not 6 months) of age (Singh et al., 2019). Several mechanisms are related to the motor and cognitive deficits seen in α-Syn transgenic models, such as reduced dendritic spine density of the hippocampal and caudate putamen medium spiny neurons, lower synaptophysin and synapsin I levels in the frontal cortex and hippocampus, and impaired microglial response (Table 5). Moreover, α-Syn transgenic NHP models of PD develop more replicable and stable phenotypes and pathologic changes compared with those of toxin-treated NHP models of PD (Niu et al., 2015). Interestingly, α-Syn knockout mice also developed long-term learning and spatial memory deficits, which suggests that appropriate α-Syn is necessary for cognitive function of the hippocampus and limbic systems (Kokhan et al., 2012). Therefore, human α-Syn A53T and A30P appear to play a key role in the mechanisms of PD-MCI and PDD.
R1441C/G and G2019S are the two most common LRRK2 mutations that are related to the pathologic mechanisms of the autosomal dominant inheritance of familial PD. LRRK2 mutation models may be most useful for studying early compensation in the nigrostriatal DA system because they demonstrate loss of motor coordination and spatial recognition memory deficits consistent with late-stage DA transmission deficits in the dorsal striatum and low expression of PSD-95 in the hippocampus (Sloan et al., 2016; Adeosun et al., 2017).
Gene knockout (KO) models, including parkin, PINK1, DJ-1, Pitx3, Mdk, and Ndufs4, are useful models of the earliest abnormalities in the nigrostriatal DA system during PD pathogenesis. MitoPark (DAT+/cre-TfamloxP/loxP) mice and TGR (NSEhA2A) rats may play an important role in studies that involve the DA transporter. Transgenic models that more closely resemble human PD physiology and progression are valuable in the development of preventative treatments. However, it is not clear if results that are obtained from transgenic models can be applied directly to humans because most of the splicing events between humans and rodents are conserved (Kin et al., 2019). On the other hand, some types of transgenic models can serve as novel tools for generating different phenotypes of cognitive deficits and unstable motor symptoms of PD, although the repeatability and stability of these phenotypes require further verification. Thus, we should objectively analyze the results of transgenic models in future work, such as by observing the relationship between cognitive impairment and changes in different regions of the brain during transgenic animal development and aging using advanced imaging.
In order to imitate the complex pathogen and neuropathology of PD-MCI and PDD, combined experimental models have become increasingly popular. The number of articles that utilized models has gradually increased. A total of 24 kinds of combined experimental models were reported in 30 articles and can be divided into three categories: dual/multiple toxin-induced models, models combining toxins and transgenes, and models combining toxins and surgery (Tables 6–8).
There are currently six kinds of dual/multiple toxin-induced models of PD with cognitive deficits (Table 6). 6-OHDA- and LPS-combined lesions may provide a new model for verifying associations between memory process vulnerabilities and the stress reactivity of the temporal brain in PD (Hritcu et al., 2011). The hypocretin/orexin (HO) system plays a central role in memory processes. The model induced by 6-OHDA and saporin anti-orexin-B showed deficits in long-term and spatial memory, but not short-term and working memory, which suggests that the HO system is functionally involved in memory processes and consolidation in PD (Oliveira et al., 2020). A PD-MCI model induced by paraquat and maneb administered twice a week for a total of 12 times was characterized by deficits in recognition memory, spatial learning, and memory by damaging synapses in the hippocampus and DA neurons in the SN (Ding et al., 2020). It is a relatively mature combined model that has been widely used in pre-clinical studies of potential therapies for PD-MCI (Wang et al., 2021). On this basis, N-(2-chloroethyl)-N-ethyl-2-bromobenzylamine (DSP-4), which damages locus coeruleus/norepinephrine neurons and depletes norepinephrine, combined with paraquat and maneb, could exacerbate spatial learning and memory via ferroptosis and microglia-mediated neuroinflammation (Hou et al., 2019). Moreover, the model induced by DSP-4 and 6-OHDA developed a similar phenotype (Rampersaud et al., 2012). An LPS and recombinant monomeric human α-Syn co-induced model that has the pathologic features of LB-formation and neuroinflammation could potentiate the effects of each vector and may be a high potential model for studying PD-MCI and PDD (La Vitola et al., 2021).
There are currently 12 kinds of models that combine toxins and transgenes (Table 7). Because both genetic makeup and environmental factors have a crucial role in the early onset of PD, these combined rodent models are useful tools for assessing the effects of environmental factors on genetically susceptible individuals. The combination of one kind of toxin, such as 6-OHDA, MPTP, reserpine, α-Syn oligomers, human α-Syn preformed fibrils, and LPS, and one kind of transgenic mouse, including APPswe/PS1△E9 mice, CHTHET mice, mice expressing the human E4 isoform, hTau mice, TKO mice, Nestin-GFP mice, hD1 mice, Prnp0/0 mice, TLR4–/– mice, 5xFAD Tg mice, and A53T mice resulted in memory, attention, and motor defects (Zurkovsky et al., 2013; Melief et al., 2015; Klein et al., 2016; Bruns et al., 2018; La Vitola et al., 2018, 2019, 2021; Gratuze et al., 2019; Bassil et al., 2020; Torres et al., 2020). These models, which are more closely related to the pathogenesis of PD-MCI and PDD, may be more suitable for assessing the efficacy of a therapeutic agent. However, as generating models that combine a toxin and transgene is difficult, cumbersome, and cost-consuming, significant additional research and discussions are required.
Six kinds of models combined a toxin and surgery to understand the impact of the relationship between risk factors and cognitive deficits in PD, yielding a total of 10 articles (Table 8). For instance, the model combining a toxin (6-OHDA or MPTP) and a bilateral ovariectomy replicated PD-MCI and PDD phenotypes, indicating that parkinsonism aggravates during the premenstrual period in the setting of the lowest levels of estrogen and progesterone (Arbabi et al., 2016; Yadav et al., 2017). Maternal separation, a method that imitates a state of stress, worsens cognitive function in a PD model (Dallé et al., 2017). A model that combined MPTP and narrowing of the bilateral common carotid arteries explored the relationship between orthostatic hypotension and cognitive impairment in PD, reporting that hypotension aggravates cognitive impairment in PD by exacerbating hippocampal damage and white matter lesions. Finally, a model combining rotenone and sleep deprivation reported that the combination of pesticide exposure and insomnia is a key risk factor for cognitive deficits in PD (Gao et al., 2019; Kmita et al., 2019; Lv et al., 2019).
Various experimental models for PD-MCI or PDD have been generated using multiple methods and diverse animal species. Unfortunately, there is no perfect experimental model for studying PD-MCI or PDD, and five pivotal problems that are summarized in the proceeding paragraphs require further consideration and resolution.
One principal challenge is that the difference between PD-MCI and PDD has not been standardized in an experimental model, although there are analogous phenotypic, behavioral, biochemical, and anatomical impairments that represent both PD-MCI and PDD. A clear distinction between PD-MCI and PDD will help transform the results of experimental studies into clinical applications more easily. Based on the diagnostic criteria of PD-MCI and PDD, the severity of cognitive impairment is a key distinction between these pathologies (Dubois et al., 2007; Litvan et al., 2012). A PD model could be used as a PD-MCI model if any one of the following cognitive impairments appears: attention, executive function, memory, or visuospatial function as assessed using different kinds of behavioral tests. When the model has two or more of the above cognitive deficits, it can be considered a model of PDD. Importantly, researchers need to understand the various testing methods that are used to assess PD symptoms, such as dyskinesia and cognitive impairment, from different perspectives. In assessing dyskinesia, the foot-printing test, the rotarod test, the pole test, and the balance beam are commonly used and authoritative methods in rodents. Among these, the balance beam, a simple and direct balance and motor coordination test, is superior to the rotarod test for testing fine movement defects. For NHP, we can assess dyskinesia based on the Unified Parkinson’s Disease Rating Scale. Moreover, we can evaluate the motor function of the drosophila or zebrafish by analyzing their movements paths. For single-sided injections, such as 6-OHDA, specific stereotyped movements after injection of apomorphine is an important test for measuring dyskinesias (Smith et al., 2017; Real et al., 2019). As the DA content in the striatum of the injured side decreases, the number and sensitivity of DA D2 receptors increase significantly. Apomorphine, a DA receptor agonist, can make the excitatory effect of the injured side dominate, thereby inducing the spontaneous rotational movement of models. In addition, VDR, CPT, MDR, SDR, ASST, MWM, NOR, PAT, Barnes maze test, T-maze test, and Y-maze test are the authoritative and representative methods for measuring cognitive impairment. Among these, MWM, a common test to investigate spatial learning and memory in rodents, can be used to assess lesions in distinct brain regions, such as the hippocampus, striatum, cerebral cortex, basal forebrain, and cerebellum (D’Hooge and De Deyn, 2001). In addition to detecting spatial learning and memory functions, the Y maze can detect the ability of animals to recognize spatial position and orientation, the ability to avoid conditioned reflexes, spatial working memory, and fragmented memory. The Barnes maze test is used to evaluate spatial memory and non-spatial memory of experimental animals and can distinguish reference memory from working memory. NOR, a fine and sensitive behavioral method, uses rodents’ instincts to approach and explore novel objects to detect recognition and memory. Thus, we should select the corresponding method based on the different experimental requirements, conditions, and objects. This also requires the evaluation of motor and cognitive function in novel models using different types of behavioral experiments. Forming a set of plans that integrate symptoms, biochemistry, neuroimaging, and other objective indicators to judge and identify the success of a proposed novel model is therefore needed.
An additional challenge is that most novel models simply develop a cognitive deficit that is measurable by a single or analogous behavioral test in the setting of changes in pathology. No model has been integrated to evaluate phenotypes, such as motor deficits, cognitive impairment, and biomarkers in biofluids, and neuroimaging. It is therefore difficult to determine whether a model represents PD-MCI or PDD. The lack of a comprehensive evaluation tool is a principal obstacle to the mutual transformation of research results from basic experiments to clinical trials, especially with respect to clinical examination and radiology. For example, the CSF of rodents, drosophila, and zebrafish is difficult to obtain. MRI and PET instruments for animals are rare and expensive. However, these problems will be solved in the future with the development of technology. Thirdly, molding rate and mortality, which are significant indicators of the repeatability and stability of a novel model, are rarely mentioned. One example is a work that identified a 60%–80% molding rate in 6-OHDA-induced models (Marshall et al., 2019). Some MPTP-induced models also appeared to have normal cognitive function (Gao et al., 2019). However, the combination of MPTP and pesticides resulted in a high mortality rate in rodents. Mortality would be expected to increase in combined experimental models, and the molding rate and mortality of such models should be calculated in future studies.
Fourth, NHP, the closest species to humans, developed cognitive deficits in MPTP-treated and α-Syn transgenic models. However, the mechanism behind the cognitive impairment that results from these models is unclear (Schneider and Kovelowski, 1990; Vezoli et al., 2011; Niu et al., 2015). Moreover, handling and breeding NHP processes are difficult, time-consuming, and expensive. Thus, how to effectively use NHP for PD research remains a significant issue. We could observe the dynamic transition process from PD-MCI to PDD in NHPs, such as symptoms, biomarkers in biofluids, and neuroimaging, and provide new objective evidence for distinguishing PD-MCI from PDD. Furthermore, in addition to the striatum, SN, and hippocampus, researchers need to pay attention to lesions of the PFC, amygdala, anterior commissure, locus coeruleus, and putamen in the NHP model. Importantly, we need to use a neural network perspective to study the pathological mechanisms of PD-MCI and PDD in the NHP model, given their complex pathogenesis (Gratwicke et al., 2015). Finally, cellular models are necessary for mechanism research but have not been used in studies on PD-MCI or PDD. Abnormal aggregation of α-Syn and human α-Syn oligomers were positively correlated with the severity of cognitive deficits in PD (Finkelstein et al., 2016; Liu L. et al., 2020). Inducible α-Syn overexpression and SH-SY5Y-A53T cellular models are good candidate models of PD-MCI or PDD (Zhang et al., 2020; Zhu et al., 2021). However, it is currently difficult to distinguish PD-MCI from PDD at the cellular level.
While each of the models discussed in the present work has their own disadvantages, there have been a tremendous number of advances in PD cognitive impairment models. Almost every model can verify a hypothesis about PD-MCI or PDD. Based on previous research, we identified a set of plans that integrate symptom, biochemistry, neuroimaging, and other objective indicators to judge and identify that the novel model plays a key role in new strategies for developing representative models. Moreover, we may develop representative models based on the different clinical features of PD-MCI and PDD. Simultaneously, inducible α-Syn overexpression and SH-SY5Y-A53T cellular models and good candidate models of PD-MCI or PDD are important directions for the development of novel models. With the development of improved technology and in-depth research methods, novel models will become increasingly in line with the complex neurodegenerative symptoms and mechanisms of PD-MCI and PDD.
YF and MZ designed the research. YF and JH analyzed the data. YF and LZ wrote and reviewed the manuscript. ZH, XH, and YJ reviewed the manuscript. XH, CW, and PW edited figures and tables. DC and MZ supervised the research and reviewed the manuscript. All authors contributed to the article and approved the submitted version.
This present study was supported by the Traditional Chinese Medicine Bureau of Guangdong Province, China (20203010), the Natural Science Foundation of Guangdong Province (2020A151501325), the Shenzhen Science and Technology Innovation Committee subject (JCYJ20190807112405520 and JCYJ20210324123614040), and Bao’an TCM Development Foundation (2020KJCX-KTYJ-131).
The authors declare that the research was conducted in the absence of any commercial or financial relationships that could be construed as a potential conflict of interest.
All claims expressed in this article are solely those of the authors and do not necessarily represent those of their affiliated organizations, or those of the publisher, the editors and the reviewers. Any product that may be evaluated in this article, or claim that may be made by its manufacturer, is not guaranteed or endorsed by the publisher.
PD, Parkinson’s disease; PD-MCI, Parkinson’s disease mild cognitive impairment; PDD, Parkinson’s disease’s disease dementia; α-Syn, α-synuclein; LBs, Lewy bodies; AD, Alzheimer’s disease; SN, substantia nigra; LRRK2, leucine-rich repeat serine/threonine-protein kinase 2; MRI, magnetic resonance imaging; PET, positron emission tomography; DAergic, dopaminergic; MPTP, 1-methyl-4-phenyl-1,2,3,6-tetrahydropyridine; 6-OHDA, 6-hydroxy dopamine; MFB, medial forebrain bundle; PFC, prefrontal cortex; VTA, ventral tegmental area; NHP, non-human primates; VDR, variable delayed response task; CPT, continuous performance task; NOR, novel object recognition test; MWM, Morris water maze task.
Aarsland, D. (2016). Cognitive impairment in Parkinson’s disease and dementia with Lewy bodies. Parkinsonism Relat. Disord. 22(Suppl. 1), S144–S148. doi: 10.1016/j.parkreldis.2015.09.034
Aarsland, D., Creese, B., Politis, M., Chaudhuri, K. R., Ffytche, D. H., Weintraub, D., et al. (2017). Cognitive decline in Parkinson disease. Nat. Rev. Neurol. 13, 217–231. doi: 10.1038/nrneurol.2017.27
Aarsland, D., and Kurz, M. W. (2010). The epidemiology of dementia associated with Parkinson disease. J. Neurol. Sci. 289, 18–22. doi: 10.1016/j.jns.2009.08.034
Abidar, S., Boiangiu, R. S., Dumitru, G., Todirascu-Ciornea, E., Amakran, A., Cioanca, O., et al. (2020). The aqueous extract from Ceratonia siliqua leaves protects against 6-hydroxydopamine in zebrafish: understanding the underlying mechanism. Antioxidants (Basel) 9:304. doi: 10.3390/antiox9040304
Adeosun, S. O., Hou, X., Zheng, B., Melrose, H. L., Mosley, T., and Wang, J. M. (2017). Human LRRK2 G2019S mutation represses post-synaptic protein PSD95 and causes cognitive impairment in transgenic mice. Neurobiol. Learn. Mem. 142(Pt B), 182–189. doi: 10.1016/j.nlm.2017.05.001
Agnihotri, A., and Aruoma, O. I. (2020). Alzheimer’s disease and Parkinson’s disease: a nutritional toxicology perspective of the impact of oxidative stress, mitochondrial dysfunction, nutrigenomics and environmental chemicals. J. Am. Coll. Nutr. 39, 16–27. doi: 10.1080/07315724.2019.1683379
Arbabi, E., Hamidi, G., Talaei, S. A., and Salami, M. (2016). Estrogen agonist genistein differentially influences the cognitive and motor disorders in an ovariectomized animal model of Parkinsonism. Iran. J. Basic Med. Sci. 19, 1285–1290. doi: 10.22038/ijbms.2016.7911
Ba, F., Zhou, Y., Zhou, J., and Chen, X. (2019). Repetitive transcranial magnetic stimulation protects mice against 6-OHDA-induced Parkinson’s disease symptoms by regulating brain amyloid β(1-42) level. Mol. Cell. Biochem. 458, 71–78. doi: 10.1007/s11010-019-03531-w
Bäckström, D. C., Eriksson Domellöf, M., Linder, J., Olsson, B., Öhrfelt, A., Trupp, M., et al. (2015). Cerebrospinal fluid patterns and the risk of future dementia in early, incident Parkinson disease. JAMA Neurol. 72, 1175–1182. doi: 10.1001/jamaneurol.2015.1449
Baiano, C., Barone, P., Trojano, L., and Santangelo, G. (2020). Prevalence and clinical aspects of mild cognitive impairment in Parkinson’s disease: a meta-analysis. Mov. Disord. 35, 45–54. doi: 10.1002/mds.27902
Ball, N., Teo, W. P., Chandra, S., and Chapman, J. (2019). Parkinson’s disease and the environment. Front. Neurol. 10:218. doi: 10.3389/fneur.2019.00218
Bandookwala, M., Sahu, A. K., Thakkar, D., Sharma, M., Khairnar, A., and Sengupta, P. (2019). Edaravone-caffeine combination for the effective management of rotenone induced Parkinson’s disease in rats: an evidence based affirmative from a comparative analysis of behavior and biomarker expression. Neurosci. Lett. 711:134438. doi: 10.1016/j.neulet.2019.134438
Bassil, F., Brown, H. J., Pattabhiraman, S., Iwasyk, J. E., Maghames, C. M., Meymand, E. S., et al. (2020). Amyloid-Beta (Aβ) plaques promote seeding and spreading of alpha-synuclein and tau in a mouse model of lewy body disorders with Aβ pathology. Neuron 105, 260–275.e6. doi: 10.1016/j.neuron.2019.10.010
Bayo-Olugbami, A., Nafiu, A. B., Amin, A., Ogundele, O. M., Lee, C. C., and Owoyele, B. V. (2020). Vitamin D attenuated 6-OHDA-induced behavioural deficits, dopamine dysmetabolism, oxidative stress, and neuro-inflammation in mice. Nutr. Neurosci. 23, 1–12. doi: 10.1080/1028415x.2020.1815331
Betancourt, E., Wachtel, J., Michaelos, M., Haggerty, M., Conforti, J., and Kritzer, M. F. (2017). The impact of biological sex and sex hormones on cognition in a rat model of early, pre-motor Parkinson’s disease. Neuroscience 345, 297–314. doi: 10.1016/j.neuroscience.2016.05.041
Bitu Pinto, N., da Silva Alexandre, B., Neves, K. R., Silva, A. H., Leal, L. K., and Viana, G. S. (2015). Neuroprotective properties of the standardized extract from Camellia sinensis (Green Tea) and its main bioactive components, epicatechin and epigallocatechin gallate, in the 6-OHDA model of Parkinson’s disease. Evid. Based Complement. Alternat. Med. 2015:161092. doi: 10.1155/2015/161092
Boi, L., Pisanu, A., Palmas, M. F., Fusco, G., Carboni, E., Casu, M. A., et al. (2020). Modeling Parkinson’s disease neuropathology and symptoms by intranigral inoculation of preformed human α-synuclein oligomers. Int. J. Mol. Sci. 21:8535. doi: 10.3390/ijms21228535
Bougea, A., Stefanis, L., Emmanouilidou, E., Vekrelis, K., and Kapaki, E. (2020). High discriminatory ability of peripheral and CFSF biomarkers in Lewy body diseases. J. Neural Transm. (Vienna) 127, 311–322. doi: 10.1007/s00702-019-02137-2
Bruns, R. F., Mitchell, S. N., Wafford, K. A., Harper, A. J., Shanks, E. A., Carter, G., et al. (2018). Preclinical profile of a dopamine D1 potentiator suggests therapeutic utility in neurological and psychiatric disorders. Neuropharmacology 128, 351–365. doi: 10.1016/j.neuropharm.2017.10.032
Byers, S., Buchler, I. P., DePasquale, M., Rowley, H. L., Kulkarni, R. S., Pinder, L., et al. (2020). Novel, non-nitrocatechol catechol-O-methyltransferase inhibitors modulate dopamine neurotransmission in the frontal cortex and improve cognitive flexibility. Psychopharmacology (Berl.) 237, 2695–2707. doi: 10.1007/s00213-020-05566-0
Camacho-Abrego, I., Tellez-Merlo, G., Melo, A. I., Rodríguez-Moreno, A., Garcés, L., De La Cruz, F., et al. (2014). Rearrangement of the dendritic morphology of the neurons from prefrontal cortex and hippocampus after subthalamic lesion in Sprague-Dawley rats. Synapse 68, 114–126. doi: 10.1002/syn.21722
Campêlo, C. L. C., Santos, J. R., Silva, A. F., Dierschnabel, A. L., Pontes, A., Cavalcante, J. S., et al. (2017). Exposure to an enriched environment facilitates motor recovery and prevents short-term memory impairment and reduction of striatal BDNF in a progressive pharmacological model of parkinsonism in mice. Behav. Brain Res. 328, 138–148. doi: 10.1016/j.bbr.2017.04.028
Cariccio, V. L., Samà, A., Bramanti, P., and Mazzon, E. (2019). Mercury involvement in neuronal damage and in neurodegenerative diseases. Biol. Trace Elem. Res. 187, 341–356. doi: 10.1007/s12011-018-1380-4
Carvalho, M. M., Campos, F. L., Coimbra, B., Pêgo, J. M., Rodrigues, C., Lima, R., et al. (2013). Behavioral characterization of the 6-hydroxidopamine model of Parkinson’s disease and pharmacological rescuing of non-motor deficits. Mol. Neurodegener. 8:14. doi: 10.1186/1750-1326-8-14
Castro, A. A., Ghisoni, K., Latini, A., Quevedo, J., Tasca, C. I., and Prediger, R. D. (2012). Lithium and valproate prevent olfactory discrimination and short-term memory impairments in the intranasal 1-methyl-4-phenyl-1,2,3,6-tetrahydropyridine (MPTP) rat model of Parkinson’s disease. Behav. Brain. Res. 229, 208–215. doi: 10.1016/j.bbr.2012.01.016
Chen, Y., Gu, X., Ou, R., Zhang, L., Hou, Y., Liu, K., et al. (2020). Evaluating the Role of SNCA, LRRK2, and GBA in Chinese patients with Early-Onset Parkinson’s disease. Mov. Disord. 35, 2046–2055. doi: 10.1002/mds.28191
Chia, S. J., Tan, E. K., and Chao, Y. X. (2020). Historical perspective: models of Parkinson’s disease. Int. J. Mol. Sci. 21:2464. doi: 10.3390/ijms21072464
Choi, D. H., Choi, I. A., Lee, C. S., Yun, J. H., and Lee, J. (2019). The Role of NOX4 in Parkinson’s disease with dementia. Int. J. Mol. Sci. 20:696. doi: 10.3390/ijms20030696
Choi, W. S., Kim, H. W., Tronche, F., Palmiter, R. D., Storm, D. R., and Xia, Z. (2017). Conditional deletion of Ndufs4 in dopaminergic neurons promotes Parkinson’s disease-like non-motor symptoms without loss of dopamine neurons. Sci. Rep. 7:44989. doi: 10.1038/srep44989
Chou, K. L., Lenhart, A., Koeppe, R. A., and Bohnen, N. I. (2014). Abnormal MoCA and normal range MMSE scores in Parkinson disease without dementia: cognitive and neurochemical correlates. Parkinsonism Relat. Disord. 20, 1076–1080. doi: 10.1016/j.parkreldis.2014.07.008
Choudhury, G. R., and Daadi, M. M. (2018). Charting the onset of Parkinson-like motor and non-motor symptoms in nonhuman primate model of Parkinson’s disease. PLoS One 13:e0202770. doi: 10.1371/journal.pone.0202770
Courtland, J. L., Bradshaw, T. W., Waitt, G., Soderblom, E. J., Ho, T., Rajab, A., et al. (2021). Genetic disruption of WASHC4 drives endo-lysosomal dysfunction and cognitive-movement impairments in mice and humans. Elife 10:e61590. doi: 10.7554/eLife.61590
Dallé, E., Daniels, W. M. U., and Mabandla, M. V. (2017). Fluvoxamine maleate effects on dopamine signaling in the prefrontal cortex of stressed Parkinsonian rats: implications for learning and memory. Brain Res. Bull. 132, 75–81. doi: 10.1016/j.brainresbull.2017.05.014
Das, N. R., Gangwal, R. P., Damre, M. V., Sangamwar, A. T., and Sharma, S. S. (2014). A PPAR-β/δ agonist is neuroprotective and decreases cognitive impairment in a rodent model of Parkinson’s disease. Curr. Neurovasc. Res. 11, 114–124. doi: 10.2174/1567202611666140318114037
Decamp, E., and Schneider, J. S. (2009). Interaction between nicotinic and dopaminergic therapies on cognition in a chronic Parkinson model. Brain Res. 1262, 109–114. doi: 10.1016/j.brainres.2009.01.028
Delattre, A. M., Carabelli, B., Mori, M. A., Pudell, C., da Silva, D. R., Menezes, I., et al. (2013). Multiple intranigral unilateral LPS infusion protocol generates a persistent cognitive impairment without cumulative dopaminergic impairment. CNS Neurol. Disord. Drug Targets 12, 1002–1010. doi: 10.2174/18715273113129990074
Dhanraj, V., Karuppaiah, J., Balakrishnan, R., and Elangovan, N. (2018). Myricetin attenuates neurodegeneration and cognitive impairment in Parkinsonism. Front. Biosci. (Elite Ed.) 10, 481–494. doi: 10.2741/E835
D’Hooge, R., and De Deyn, P. P. (2001). Applications of the Morris water maze in the study of learning and memory. Brain Res. Brain Res. Rev. 36, 60–90. doi: 10.1016/s0165-0173(01)00067-4
Ding, W., Lin, H., Hong, X., Ji, D., and Wu, F. (2020). Poloxamer 188-mediated anti-inflammatory effect rescues cognitive deficits in paraquat and maneb-induced mouse model of Parkinson’s disease. Toxicology 436:152437. doi: 10.1016/j.tox.2020.152437
Dos Santos, A. C., Castro, M. A., Jose, E. A., Delattre, A. M., Dombrowski, P. A., Da Cunha, C., et al. (2013). REM sleep deprivation generates cognitive and neurochemical disruptions in the intranigral rotenone model of Parkinson’s disease. J. Neurosci. Res. 91, 1508–1516. doi: 10.1002/jnr.23258
Dubois, B., Burn, D., Goetz, C., Aarsland, D., Brown, R. G., Broe, G. A., et al. (2007). Diagnostic procedures for Parkinson’s disease dementia: recommendations from the movement disorder society task force. Mov. Disord. 22, 2314–2324. doi: 10.1002/mds.21844
Duncan, G. W., Firbank, M. J., O’Brien, J. T., and Burn, D. J. (2013). Magnetic resonance imaging: a biomarker for cognitive impairment in Parkinson’s disease? Mov. Disord. 28, 425–438. doi: 10.1002/mds.25352
Eagle, A. L., Olumolade, O. O., and Otani, H. (2015). Partial dopaminergic denervation-induced impairment in stimulus discrimination acquisition in parkinsonian rats: a model for early Parkinson’s disease. Neurosci. Res. 92, 71–79. doi: 10.1016/j.neures.2014.11.002
Ejlerskov, P., Hultberg, J. G., Wang, J., Carlsson, R., Ambjørn, M., Kuss, M., et al. (2015). Lack of neuronal IFN-β-IFNAR causes Lewy body- and Parkinson’s disease-like dementia. Cell 163, 324–339. doi: 10.1016/j.cell.2015.08.069
El-Gamal, M., Salama, M., Collins-Praino, L. E., Baetu, I., Fathalla, A. M., Soliman, A. M., et al. (2021). Neurotoxin-induced rodent models of Parkinson’s disease: benefits and drawbacks. Neurotox Res. 39, 897–923. doi: 10.1007/s12640-021-00356-8
Endepols, H., Schul, J., Gerhardt, H. C., and Walkowiak, W. (2004). 6-hydroxydopamine lesions in anuran amphibians: a new model system for Parkinson’s disease? J. Neurobiol. 60, 395–410. doi: 10.1002/neu.20047
Fathy, Y. Y., Jonker, A. J., Oudejans, E., de Jong, F. J. J., van Dam, A. W., Rozemuller, A. J. M., et al. (2019). Differential insular cortex subregional vulnerability to α-synuclein pathology in Parkinson’s disease and dementia with Lewy bodies. Neuropathol. Appl. Neurobiol. 45, 262–277. doi: 10.1111/nan.12501
Fernández-Ruiz, J., Doudet, D., and Aigner, T. G. (1999). Spatial memory improvement by levodopa in parkinsonian MPTP-treated monkeys. Psychopharmacology (Berl.) 147, 104–107. doi: 10.1007/s002130051148
Ferro, M. M., Bellissimo, M. I., Anselmo-Franci, J. A., Angellucci, M. E., Canteras, N. S., and Da Cunha, C. (2005). Comparison of bilaterally 6-OHDA- and MPTP-lesioned rats as models of the early phase of Parkinson’s disease: histological, neurochemical, motor and memory alterations. J. Neurosci. Methods 148, 78–87. doi: 10.1016/j.jneumeth.2005.04.005
Fifel, K., Dkhissi-Benyahya, O., and Cooper, H. M. (2013). Lack of long-term changes in circadian, locomotor, and cognitive functions in acute and chronic MPTP (1-methyl-4-phenyl-1,2,3,6-tetrahydropyridine) mouse models of Parkinson’s disease. Chronobiol. Int. 30, 741–755. doi: 10.3109/07420528.2012.762011
Finkelstein, D. I., Hare, D. J., Billings, J. L., Sedjahtera, A., Nurjono, M., Arthofer, E., et al. (2016). Clioquinol improves cognitive, motor function, and microanatomy of the alpha-synuclein hA53T transgenic mice. ACS Chem. Neurosci. 7, 119–129. doi: 10.1021/acschemneuro.5b00253
Freichel, C., Neumann, M., Ballard, T., Müller, V., Woolley, M., Ozmen, L., et al. (2007). Age-dependent cognitive decline and amygdala pathology in alpha-synuclein transgenic mice. Neurobiol. Aging 28, 1421–1435. doi: 10.1016/j.neurobiolaging.2006.06.013
Gao, Y., Tang, H., Nie, K., Zhu, R., Gao, L., Feng, S., et al. (2019). Hippocampal damage and white matter lesions contribute to cognitive impairment in MPTP-lesioned mice with chronic cerebral hypoperfusion. Behav. Brain Res. 368:111885. doi: 10.1016/j.bbr.2019.03.054
Gasbarri, A., Sulli, A., Innocenzi, R., Pacitti, C., and Brioni, J. D. (1996). Spatial memory impairment induced by lesion of the mesohippocampal dopaminergic system in the rat. Neuroscience 74, 1037–1044. doi: 10.1016/0306-4522(96)00202-3
Goes, A. T., Souza, L. C., Filho, C. B., Del Fabbro, L., De Gomes, M. G., Boeira, S. P., et al. (2014). Neuroprotective effects of swimming training in a mouse model of Parkinson’s disease induced by 6-hydroxydopamine. Neuroscience 256, 61–71. doi: 10.1016/j.neuroscience.2013.09.042
González-Redondo, R., García-García, D., Clavero, P., Gasca-Salas, C., García-Eulate, R., Zubieta, J. L., et al. (2014). Grey matter hypometabolism and atrophy in Parkinson’s disease with cognitive impairment: a two-step process. Brain 137(Pt 8), 2356–2367. doi: 10.1093/brain/awu159
Grandi, L. C., Di Giovanni, G., and Galati, S. (2018). Animal models of early-stage Parkinson’s disease and acute dopamine deficiency to study compensatory neurodegenerative mechanisms. J. Neurosci. Methods 308, 205–218. doi: 10.1016/j.jneumeth.2018.08.012
Gratuze, M., Josset, N., Petry, F. R., Pflieger, M., Eyoum Jong, L., Truchetti, G., et al. (2019). The toxin MPTP generates similar cognitive and locomotor deficits in hTau and tau knock-out mice. Brain Res. 1711, 106–114. doi: 10.1016/j.brainres.2019.01.016
Gratwicke, J., Jahanshahi, M., and Foltynie, T. (2015). Parkinson’s disease dementia: a neural networks perspective. Brain 138(Pt 6), 1454–1476. doi: 10.1093/brain/awv104
Gu, P., Zhang, Z., Cui, D., Wang, Y., Ma, L., Geng, Y., et al. (2012). Intracerebroventricular transplanted bone marrow stem cells survive and migrate into the brain of rats with Parkinson’s disease. Neural Regen. Res. 7, 978–984. doi: 10.3969/j.issn.1673-5374.2012.13.003
Hadadianpour, Z., Fatehi, F., Ayoobi, F., Kaeidi, A., Shamsizadeh, A., and Fatemi, I. (2017). The effect of orexin-A on motor and cognitive functions in a rat model of Parkinson’s disease. Neurol. Res. 39, 845–851. doi: 10.1080/01616412.2017.1352185
Haghparast, E., Esmaeili-Mahani, S., Abbasnejad, M., and Sheibani, V. (2018). Apelin-13 ameliorates cognitive impairments in 6-hydroxydopamine-induced substantia nigra lesion in rats. Neuropeptides 68, 28–35. doi: 10.1016/j.npep.2018.01.001
Hall, S., Surova, Y., Öhrfelt, A., Blennow, K., Zetterberg, H., and Hansson, O. (2016). Longitudinal measurements of cerebrospinal fluid biomarkers in Parkinson’s disease. Mov. Disord. 31, 898–905. doi: 10.1002/mds.26578
Halliday, G. M., Leverenz, J. B., Schneider, J. S., and Adler, C. H. (2014). The neurobiological basis of cognitive impairment in Parkinson’s disease. Mov. Disord. 29, 634–650. doi: 10.1002/mds.25857
Han, L., Lu, J., Tang, Y., Fan, Y., Chen, Q., Li, L., et al. (2021). Dopaminergic and metabolic correlations with cognitive domains in non-demented Parkinson’s disease. Front. Aging Neurosci. 13:627356. doi: 10.3389/fnagi.2021.627356
Han, L., Tang, Y., Bai, X., Liang, X., Fan, Y., Shen, Y., et al. (2020). Association of the serum microRNA-29 family with cognitive impairment in Parkinson’s disease. Aging (Albany N. Y.) 12, 13518–13528. doi: 10.18632/aging.103458
Han, N. R., Kim, Y. K., Ahn, S., Hwang, T. Y., Lee, H., and Park, H. J. (2020). A comprehensive phenotype of non-motor impairments and distribution of alpha-synuclein deposition in Parkinsonism-induced mice by a combination injection of MPTP and probenecid. Front. Aging Neurosci. 12:599045. doi: 10.3389/fnagi.2020.599045
Hase, Y., Polvikoski, T. M., Firbank, M. J., Craggs, L. J. L., Hawthorne, E., Platten, C., et al. (2020). Small vessel disease pathological changes in neurodegenerative and vascular dementias concomitant with autonomic dysfunction. Brain Pathol. 30, 191–202. doi: 10.1111/bpa.12769
Hayes, M. T. (2019). Parkinson’s disease and parkinsonism. Am. J. Med. 132, 802–807. doi: 10.1016/j.amjmed.2019.03.001
Ho, S. C., Hsu, C. C., Yu, C. H., Huang, W. N., Tikhonova, M. A., Ho, M. C., et al. (2014). Measuring attention in a Parkinson’s disease rat model using the 5-arm maze test. Physiol. Behav. 130, 176–181. doi: 10.1016/j.physbeh.2014.03.017
Ho, Y. J., Ho, S. C., Pawlak, C. R., and Yeh, K. Y. (2011). Effects of D-cycloserine on MPTP-induced behavioral and neurological changes: potential for treatment of Parkinson’s disease dementia. Behav. Brain Res. 219, 280–290. doi: 10.1016/j.bbr.2011.01.028
Hou, L., Sun, F., Sun, W., Zhang, L., and Wang, Q. (2019). Lesion of the locus coeruleus damages learning and memory performance in paraquat and maneb-induced mouse Parkinson’s disease model. Neuroscience 419, 129–140. doi: 10.1016/j.neuroscience.2019.09.006
Hritcu, L., Ciobica, A., Stefan, M., Mihasan, M., Palamiuc, L., and Nabeshima, T. (2011). Spatial memory deficits and oxidative stress damage following exposure to lipopolysaccharide in a rodent model of Parkinson’s disease. Neurosci. Res. 71, 35–43. doi: 10.1016/j.neures.2011.05.016
Hsieh, M. H., Gu, S. L., Ho, S. C., Pawlak, C. R., Lin, C. L., Ho, Y. J., et al. (2012a). Effects of MK-801 on recognition and neurodegeneration in an MPTP-induced Parkinson’s rat model. Behav. Brain Res. 229, 41–47. doi: 10.1016/j.bbr.2011.12.035
Hsieh, M. H., Ho, S. C., Yeh, K. Y., Pawlak, C. R., Chang, H. M., Ho, Y. J., et al. (2012b). Blockade of metabotropic glutamate receptors inhibits cognition and neurodegeneration in an MPTP-induced Parkinson’s disease rat model. Pharmacol. Biochem. Behav. 102, 64–71. doi: 10.1016/j.pbb.2012.03.022
Hsu, W. Y., Lane, H. Y., and Lin, C. H. (2018). Medications used for cognitive enhancement in patients with Schizophrenia, Bipolar disorder, Alzheimer’s disease, and Parkinson’s disease. Front. Psychiatry 9:91. doi: 10.3389/fpsyt.2018.00091
Hsueh, S. C., Chen, K. Y., Lai, J. H., Wu, C. C., Yu, Y. W., Luo, Y., et al. (2018). Voluntary physical exercise improves subsequent motor and cognitive impairments in a rat model of Parkinson’s disease. Int. J. Mol. Sci. 19:508. doi: 10.3390/ijms19020508
Huang, C. K., Chang, Y. T., Amstislavskaya, T. G., Tikhonova, M. A., Lin, C. L., Hung, C. S., et al. (2015). Synergistic effects of ceftriaxone and erythropoietin on neuronal and behavioral deficits in an MPTP-induced animal model of Parkinson’s disease dementia. Behav. Brain Res. 294, 198–207. doi: 10.1016/j.bbr.2015.08.011
Hwang, J., Estick, C. M., Ikonne, U. S., Butler, D., Pait, M. C., Elliott, L. H., et al. (2019). The role of lysosomes in a broad disease-modifying approach evaluated across transgenic mouse models of Alzheimer’s disease and Parkinson’s disease and models of mild cognitive impairment. Int. J. Mol. Sci. 20:4432. doi: 10.3390/ijms20184432
Ikram, H., and Haleem, D. J. (2019). Repeated treatment with a low dose of reserpine as a progressive model of Parkinson’s dementia. Pak. J. Pharm. Sci. 32, 555–562.
Imbert, C., Bezard, E., Guitraud, S., Boraud, T., and Gross, C. E. (2000). Comparison of eight clinical rating scales used for the assessment of MPTP-induced parkinsonism in the Macaque monkey. J. Neurosci. Methods 96, 71–76. doi: 10.1016/s0165-0270(99)00184-3
Inden, M., Kitamura, Y., Abe, M., Tamaki, A., Takata, K., and Taniguchi, T. (2011). Parkinsonian rotenone mouse model: reevaluation of long-term administration of rotenone in C57BL/6 mice. Biol. Pharm. Bull. 34, 92–96. doi: 10.1248/bpb.34.92
Ivanidze, J., Skafida, M., Pandya, S., Patel, D., Osborne, J. R., Raj, A., et al. (2020). Molecular imaging of striatal dopaminergic neuronal loss and the neurovascular unit in Parkinson disease. Front. Neurosci. 14:528809. doi: 10.3389/fnins.2020.528809
Johar, I., Mollenhauer, B., and Aarsland, D. (2017). Cerebrospinal fluid biomarkers of cognitive decline in Parkinson’s disease. Int. Rev. Neurobiol. 132, 275–294. doi: 10.1016/bs.irn.2016.12.001
Kadowaki Horita, T., Kobayashi, M., Mori, A., Jenner, P., and Kanda, T. (2013). Effects of the adenosine A2A antagonist istradefylline on cognitive performance in rats with a 6-OHDA lesion in prefrontal cortex. Psychopharmacology (Berl.) 230, 345–352. doi: 10.1007/s00213-013-3158-x
Kin, K., Yasuhara, T., Kameda, M., and Date, I. (2019). Animal models for Parkinson’s disease research: trends in the 2000s. Int. J. Mol. Sci. 20:5402. doi: 10.3390/ijms20215402
Klein, C., Rasińska, J., Empl, L., Sparenberg, M., Poshtiban, A., Hain, E. G., et al. (2016). Physical exercise counteracts MPTP-induced changes in neural precursor cell proliferation in the hippocampus and restores spatial learning but not memory performance in the water maze. Behav. Brain Res. 307, 227–238. doi: 10.1016/j.bbr.2016.02.040
Kmita, L. C., Ilkiw, J. L., Rodrigues, L. S., Targa, A. D., Noseda, A. C. D., Dos-Santos, P., et al. (2019). Absence of a synergic nigral proapoptotic effect triggered by REM sleep deprivation in the rotenone model of Parkinson’s disease. Sleep Sci. 12, 196–202. doi: 10.5935/1984-0063.20190078
Knox, M. G., Adler, C. H., Shill, H. A., Driver-Dunckley, E., Mehta, S. A., Belden, C., et al. (2020). Neuropathological findings in Parkinson’s disease with mild cognitive impairment. Mov. Disord. 35, 845–850. doi: 10.1002/mds.27991
Ko, W. K. D., Camus, S. M., Li, Q., Yang, J., McGuire, S., Pioli, E. Y., et al. (2016). An evaluation of istradefylline treatment on Parkinsonian motor and cognitive deficits in 1-methyl-4-phenyl-1,2,3,6-tetrahydropyridine (MPTP)-treated macaque models. Neuropharmacology 110(Pt A), 48–58. doi: 10.1016/j.neuropharm.2016.07.012
Kokhan, V. S., Afanasyeva, M. A., and Van’kin, G. I. (2012). α-Synuclein knockout mice have cognitive impairments. Behav. Brain Res. 231, 226–230. doi: 10.1016/j.bbr.2012.03.026
Kotagal, V., Müller, M. L., Kaufer, D. I., Koeppe, R. A., and Bohnen, N. I. (2012). Thalamic cholinergic innervation is spared in Alzheimer disease compared to parkinsonian disorders. Neurosci. Lett. 514, 169–172. doi: 10.1016/j.neulet.2012.02.083
Kouli, A., Camacho, M., Allinson, K., and Williams-Gray, C. H. (2020). Neuroinflammation and protein pathology in Parkinson’s disease dementia. Acta Neuropathol. Commun. 8:211. doi: 10.1186/s40478-020-01083-5
La Vitola, P., Balducci, C., Baroni, M., Artioli, L., Santamaria, G., Castiglioni, M., et al. (2021). Peripheral inflammation exacerbates α-synuclein toxicity and neuropathology in Parkinson’s models. Neuropathol. Appl. Neurobiol. 47, 43–60. doi: 10.1111/nan.12644
La Vitola, P., Balducci, C., Cerovic, M., Santamaria, G., Brandi, E., Grandi, F., et al. (2018). Alpha-synuclein oligomers impair memory through glial cell activation and via Toll-like receptor 2. Brain Behav. Immun. 69, 591–602. doi: 10.1016/j.bbi.2018.02.012
La Vitola, P., Beeg, M., Balducci, C., Santamaria, G., Restelli, E., Colombo, L., et al. (2019). Cellular prion protein neither binds to alpha-synuclein oligomers nor mediates their detrimental effects. Brain 142, 249–254. doi: 10.1093/brain/awy318
Leal, P. C., Bispo, J. M. M., Lins, L., Souza, M. F., Gois, A. M., Moore, C., et al. (2019). Cognitive and anxiety-like impairments accompanied by serotonergic ultrastructural and immunohistochemical alterations in early stages of parkinsonism. Brain Res. Bull. 146, 213–223. doi: 10.1016/j.brainresbull.2019.01.009
Lei, P., Ayton, S., Finkelstein, D. I., Spoerri, L., Ciccotosto, G. D., Wright, D. K., et al. (2012). Tau deficiency induces parkinsonism with dementia by impairing APP-mediated iron export. Nat. Med. 18, 291–295. doi: 10.1038/nm.2613
Li, X., Redus, L., Chen, C., Martinez, P. A., Strong, R., Li, S., et al. (2013). Cognitive dysfunction precedes the onset of motor symptoms in the MitoPark mouse model of Parkinson’s disease. PLoS One 8:e71341. doi: 10.1371/journal.pone.0071341
Litvan, I., Goldman, J. G., Tröster, A. I., Schmand, B. A., Weintraub, D., Petersen, R. C., et al. (2012). Diagnostic criteria for mild cognitive impairment in Parkinson’s disease: movement disorder society task force guidelines. Mov. Disord. 27, 349–356. doi: 10.1002/mds.24893
Liu, A. K. L., Chau, T. W., Lim, E. J., Ahmed, I., Chang, R. C., Kalaitzakis, M. E., et al. (2019). Hippocampal CA2 Lewy pathology is associated with cholinergic degeneration in Parkinson’s disease with cognitive decline. Acta Neuropathol. Commun. 7:61. doi: 10.1186/s40478-019-0717-3
Liu, L., Li, M., Xu, M., Wang, Z., Zeng, Z., Li, Y., et al. (2020). Actively targeted gold nanoparticle composites improve behavior and cognitive impairment in Parkinson’s disease mice. Mater. Sci. Eng. C Mater. Biol. Appl. 114:111028. doi: 10.1016/j.msec.2020.111028
Liu, Y., Tong, S., Ding, L., Liu, N., and Gao, D. (2020). Serum levels of glial cell line-derived neurotrophic factor and multiple neurotransmitters: in relation to cognitive performance in Parkinson’s disease with mild cognitive impairment. Int. J. Geriatr. Psychiatry 35, 153–162. doi: 10.1002/gps.5222
Lv, D. J., Li, L. X., Chen, J., Wei, S. Z., Wang, F., Hu, H., et al. (2019). Sleep deprivation caused a memory defects and emotional changes in a rotenone-based zebrafish model of Parkinson’s disease. Behav. Brain Res. 372:112031. doi: 10.1016/j.bbr.2019.112031
Mak, E., Su, L., Williams, G. B., Firbank, M. J., Lawson, R. A., Yarnall, A. J., et al. (2015). Baseline and longitudinal grey matter changes in newly diagnosed Parkinson’s disease: ICICLE-PD study. Brain 138(Pt 10), 2974–2986. doi: 10.1093/brain/awv211
Marshall, C. A., King, K. M., and Kortagere, S. (2019). Limitations of the rat medial forebrain lesion model to study prefrontal cortex mediated cognitive tasks in Parkinson’s disease. Brain Res. 1702, 105–113. doi: 10.1016/j.brainres.2018.03.035
McDonald, C., Newton, J. L., and Burn, D. J. (2016). Orthostatic hypotension and cognitive impairment in Parkinson’s disease: causation or association? Mov. Disord. 31, 937–946. doi: 10.1002/mds.26632
Melief, E. J., Cudaback, E., Jorstad, N. L., Sherfield, E., Postupna, N., Wilson, A., et al. (2015). Partial depletion of striatal dopamine enhances penetrance of cognitive deficits in a transgenic mouse model of Alzheimer’s disease. J. Neurosci. Res. 93, 1413–1422. doi: 10.1002/jnr.23592
Millan, M. J., Buccafusco, J. J., Loiseau, F., Watson, D. J., Decamp, E., Fone, K. C., et al. (2010). The dopamine D3 receptor antagonist, S33138, counters cognitive impairment in a range of rodent and primate procedures. Int. J. Neuropsychopharmacol. 13, 1035–1051. doi: 10.1017/s1461145710000775
Monaghan, M. M., Leddy, L., Sung, M. L., Albinson, K., Kubek, K., Pangalos, M. N., et al. (2010). Social odor recognition: a novel behavioral model for cognitive dysfunction in Parkinson’s disease. Neurodegener. Dis. 7, 153–159. doi: 10.1159/000289227
Monastero, R., Cicero, C. E., Baschi, R., Davì, M., Luca, A., Restivo, V., et al. (2018). Mild cognitive impairment in Parkinson’s disease: the Parkinson’s disease cognitive study (PACOS). J. Neurol. 265, 1050–1058. doi: 10.1007/s00415-018-8800-4
Moon, J., Lee, H. S., Kang, J. M., Park, J., Leung, A., Hong, S., et al. (2013). Stem cell grafting improves both motor and cognitive impairments in a genetic model of Parkinson’s disease, the aphakia (ak) mouse. Cell Transplant. 22, 1263–1279. doi: 10.3727/096368912x657242
More, S. V., Kumar, H., Cho, D. Y., Yun, Y. S., and Choi, D. K. (2016). Toxin-induced experimental models of learning and memory impairment. Int. J. Mol. Sci. 17:1447. doi: 10.3390/ijms17091447
Naeem, S., Ikram, R., Khan, S. S., and Rao, S. S. (2017). NSAIDs ameliorate cognitive and motor impairment in a model of parkinsonism induced by chlorpromazine. Pak. J. Pharm. Sci. 30, 801–808.
Nasuti, C., Brunori, G., Eusepi, P., Marinelli, L., Ciccocioppo, R., and Gabbianelli, R. (2017). Early life exposure to permethrin: a progressive animal model of Parkinson’s disease. J. Pharmacol. Toxicol. Methods 83, 80–86. doi: 10.1016/j.vascn.2016.10.003
Nasuti, C., Carloni, M., Fedeli, D., Gabbianelli, R., Di Stefano, A., Serafina, C. L., et al. (2013). Effects of early life permethrin exposure on spatial working memory and on monoamine levels in different brain areas of pre-senescent rats. Toxicology 303, 162–168. doi: 10.1016/j.tox.2012.09.016
Niu, Y., Guo, X., Chen, Y., Wang, C. E., Gao, J., Yang, W., et al. (2015). Early Parkinson’s disease symptoms in α-synuclein transgenic monkeys. Hum. Mol. Genet. 24, 2308–2317. doi: 10.1093/hmg/ddu748
Ogundele, O. M., Nanakumo, E. T., Ishola, A. O., Obende, O. M., Enye, L. A., Balogun, W. G., et al. (2015). -NMDA R/+VDR pharmacological phenotype as a novel therapeutic target in relieving motor-cognitive impairments in Parkinsonism. Drug Chem. Toxicol. 38, 415–427. doi: 10.3109/01480545.2014.975355
Oliveira, L. M., Henrique, E., Bustelli, I. B., Netto, N. F. C., Moreira, T. S., Takakura, A. C., et al. (2020). Depletion of hypothalamic hypocretin/orexin neurons correlates with impaired memory in a Parkinson’s disease animal model. Exp. Neurol. 323:113110. doi: 10.1016/j.expneurol.2019.113110
Oosterveld, L. P., Allen, J. C. Jr., Reinoso, G., Seah, S. H., Tay, K. Y., Au, W. L., et al. (2015). Prognostic factors for early mortality in Parkinson’s disease. Parkinsonism Relat. Disord. 21, 226–230. doi: 10.1016/j.parkreldis.2014.12.011
Pellecchia, M. T., Santangelo, G., Picillo, M., Pivonello, R., Longo, K., Pivonello, C., et al. (2013). Serum epidermal growth factor predicts cognitive functions in early, drug-naive Parkinson’s disease patients. J. Neurol. 260, 438–444. doi: 10.1007/s00415-012-6648-6
Pereira, J. B., Aarsland, D., Ginestet, C. E., Lebedev, A. V., Wahlund, L. O., Simmons, A., et al. (2015). Aberrant cerebral network topology and mild cognitive impairment in early Parkinson’s disease. Hum. Brain Mapp. 36, 2980–2995. doi: 10.1002/hbm.22822
Peres, T. V., Eyng, H., Lopes, S. C., Colle, D., Gonçalves, F. M., Venske, D. K., et al. (2015). Developmental exposure to manganese induces lasting motor and cognitive impairment in rats. Neurotoxicology 50, 28–37. doi: 10.1016/j.neuro.2015.07.005
Perez-Pardo, P., Broersen, L. M., Kliest, T., van Wijk, N., Attali, A., Garssen, J., et al. (2018). Additive effects of levodopa and a neurorestorative diet in a mouse model of Parkinson’s disease. Front. Aging Neurosci. 10:237. doi: 10.3389/fnagi.2018.00237
Perry, J. C., Da Cunha, C., Anselmo-Franci, J., Andreatini, R., Miyoshi, E., Tufik, S., et al. (2004). Behavioural and neurochemical effects of phosphatidylserine in MPTP lesion of the substantia nigra of rats. Eur. J. Pharmacol. 484, 225–233. doi: 10.1016/j.ejphar.2003.11.029
Phillips, K. A., Ross, C. N., Spross, J., Cheng, C. J., Izquierdo, A., Biju, K. C., et al. (2017). Behavioral phenotypes associated with MPTP induction of partial lesions in common marmosets (Callithrix jacchus). Behav. Brain Res. 325(Pt A), 51–62. doi: 10.1016/j.bbr.2017.02.010
Politis, M., Pagano, G., and Niccolini, F. (2017). Imaging in Parkinson’s disease. Int. Rev. Neurobiol. 132, 233–274. doi: 10.1016/bs.irn.2017.02.015
Prediger, R. D., Aguiar, A. S. Jr., Rojas-Mayorquin, A. E., Figueiredo, C. P., Matheus, F. C., Ginestet, L., et al. (2010). Single intranasal administration of 1-methyl-4-phenyl-1,2,3,6-tetrahydropyridine in C57BL/6 mice models early preclinical phase of Parkinson’s disease. Neurotox. Res. 17, 114–129. doi: 10.1007/s12640-009-9087-0
Prediger, R. D., Rojas-Mayorquin, A. E., Aguiar, A. S. Jr., Chevarin, C., Mongeau, R., Hamon, M., et al. (2011). Mice with genetic deletion of the heparin-binding growth factor midkine exhibit early preclinical features of Parkinson’s disease. J. Neural Transm. (Vienna) 118, 1215–1225. doi: 10.1007/s00702-010-0568-3
Ramos-Moreno, T., Castillo, C. G., and Martínez-Serrano, A. (2012). Long term behavioral effects of functional dopaminergic neurons generated from human neural stem cells in the rat 6-OH-DA Parkinson’s disease model. Effects of the forced expression of BCL-X(L). Behav. Brain Res. 232, 225–232. doi: 10.1016/j.bbr.2012.04.020
Rampersaud, N., Harkavyi, A., Giordano, G., Lever, R., Whitton, J., and Whitton, P. (2012). Exendin-4 reverts behavioural and neurochemical dysfunction in a pre-motor rodent model of Parkinson’s disease with noradrenergic deficit. Br. J. Pharmacol. 167, 1467–1479. doi: 10.1111/j.1476-5381.2012.02100.x
Razavinasab, M., Shamsizadeh, A., Shabani, M., Nazeri, M., Allahtavakoli, M., Asadi-Shekaari, M., et al. (2013). Pharmacological blockade of TRPV1 receptors modulates the effects of 6-OHDA on motor and cognitive functions in a rat model of Parkinson’s disease. Fundam. Clin. Pharmacol. 27, 632–640. doi: 10.1111/fcp.12015
Real, C. C., Doorduin, J., Kopschina Feltes, P., Vállez García, D., de Paula Faria, D., Britto, L. R., et al. (2019). Evaluation of exercise-induced modulation of glial activation and dopaminergic damage in a rat model of Parkinson’s disease using [(11)C]PBR28 and [(18)F]FDOPA PET. J. Cereb. Blood Flow Metab. 39, 989–1004. doi: 10.1177/0271678x17750351
Ruppert, M. C., Greuel, A., Freigang, J., Tahmasian, M., Maier, F., Hammes, J., et al. (2021). The default mode network and cognition in Parkinson’s disease: a multimodal resting-state network approach. Hum. Brain Mapp. 42, 2623–2641. doi: 10.1002/hbm.25393
Saini, N., Akhtar, A., Chauhan, M., Dhingra, N., and Pilkhwal Sah, S. (2020). Protective effect of Indole-3-carbinol, an NF-κB inhibitor in experimental paradigm of Parkinson’s disease: in silico and in vivo studies. Brain Behav. Immun. 90, 108–137. doi: 10.1016/j.bbi.2020.08.001
Santangelo, G., Raimo, S., Erro, R., Picillo, M., Amboni, M., Pellecchia, M. T., et al. (2020). Vitamin D as a possible biomarker of mild cognitive impairment in parkinsonians. Aging Ment. Health 25, 1998–2002. doi: 10.1080/13607863.2020.1839860
Saredakis, D., Collins-Praino, L. E., Gutteridge, D. S., Stephan, B. C. M., and Keage, H. A. D. (2019). Conversion to MCI and dementia in Parkinson’s disease: a systematic review and meta-analysis. Parkinsonism Relat. Disord. 65, 20–31. doi: 10.1016/j.parkreldis.2019.04.020
Schneider, J. S., Giardiniere, M., and Morain, P. (2002). Effects of the prolyl endopeptidase inhibitor S 17092 on cognitive deficits in chronic low dose MPTP-treated monkeys. Neuropsychopharmacology 26, 176–182. doi: 10.1016/s0893-133x(01)00307-4
Schneider, J. S., and Kovelowski, C. J. II (1990). Chronic exposure to low doses of MPTP. I. Cognitive deficits in motor asymptomatic monkeys. Brain Res. 519, 122–128. doi: 10.1016/0006-8993(90)90069-n
Schneider, J. S., Marshall, C. A., Keibel, L., Snyder, N. W., Hill, M. P., Brotchie, J. M., et al. (2021). A novel dopamine D3R agonist SK609 with norepinephrine transporter inhibition promotes improvement in cognitive task performance in rodent and non-human primate models of Parkinson’s disease. Exp. Neurol. 335:113514. doi: 10.1016/j.expneurol.2020.113514
Schneider, J. S., Pioli, E. Y., Jianzhong, Y., Li, Q., and Bezard, E. (2013). Levodopa improves motor deficits but can further disrupt cognition in a macaque Parkinson model. Mov. Disord. 28, 663–667. doi: 10.1002/mds.25258
Schneider, J. S., and Pope-Coleman, A. (1995). Cognitive deficits precede motor deficits in a slowly progressing model of parkinsonism in the monkey. Neurodegeneration 4, 245–255. doi: 10.1016/1055-8330(95)90014-4
Schultheis, P. J., Fleming, S. M., Clippinger, A. K., Lewis, J., Tsunemi, T., Giasson, B., et al. (2013). Atp13a2-deficient mice exhibit neuronal ceroid lipofuscinosis, limited α-synuclein accumulation and age-dependent sensorimotor deficits. Hum. Mol. Genet. 22, 2067–2082. doi: 10.1093/hmg/ddt057
Scott, L. L., and Downing, T. G. (2017). B -N-Methylamino-L-Alanine (BMAA) toxicity is gender and exposure-age dependent in rats. Toxins (Basel) 10:16. doi: 10.3390/toxins10010016
Sekiyama, K., Fujita, M., Sekigawa, A., Takamatsu, Y., Waragai, M., Takenouchi, T., et al. (2012). Ibuprofen ameliorates protein aggregation and astrocytic gliosis, but not cognitive dysfunction, in a transgenic mouse expressing dementia with Lewy bodies-linked P123H β-synuclein. Neurosci. Lett. 515, 97–101. doi: 10.1016/j.neulet.2012.03.037
Singh, B., Covelo, A., Martell-Martínez, H., Nanclares, C., Sherman, M. A., Okematti, E., et al. (2019). Tau is required for progressive synaptic and memory deficits in a transgenic mouse model of α-synucleinopathy. Acta Neuropathol. 138, 551–574. doi: 10.1007/s00401-019-02032-w
Singh, S., Mishra, A., Srivastava, N., Shukla, R., and Shukla, S. (2018). Acetyl-L-carnitine via upegulating dopamine D1 receptor and attenuating microglial activation prevents neuronal loss and improves memory functions in Parkinsonian rats. Mol. Neurobiol. 55, 583–602. doi: 10.1007/s12035-016-0293-5
Sloan, M., Alegre-Abarrategui, J., Potgieter, D., Kaufmann, A. K., Exley, R., Deltheil, T., et al. (2016). LRRK2 BAC transgenic rats develop progressive, L-DOPA-responsive motor impairment, and deficits in dopamine circuit function. Hum. Mol. Genet. 25, 951–963. doi: 10.1093/hmg/ddv628
Smith, C., Malek, N., Grosset, K., Cullen, B., Gentleman, S., and Grosset, D. G. (2019). Neuropathology of dementia in patients with Parkinson’s disease: a systematic review of autopsy studies. J. Neurol. Neurosurg. Psychiatry 90, 1234–1243. doi: 10.1136/jnnp-2019-321111
Smith, E. S., Clark, M. E., Hardy, G. A., Kraan, D. J., Biondo, E., Gonzalez-Lima, F., et al. (2017). Daily consumption of methylene blue reduces attentional deficits and dopamine reduction in a 6-OHDA model of Parkinson’s disease. Neuroscience 359, 8–16. doi: 10.1016/j.neuroscience.2017.07.001
Stav, A. L., Aarsland, D., Johansen, K. K., Hessen, E., Auning, E., and Fladby, T. (2015). Amyloid-β and α-synuclein cerebrospinal fluid biomarkers and cognition in early Parkinson’s disease. Parkinsonism Relat. Disord. 21, 758–764. doi: 10.1016/j.parkreldis.2015.04.027
Stoyka, L. E., Arrant, A. E., Thrasher, D. R., Russell, D. L., Freire, J., Mahoney, C. L., et al. (2020). Behavioral defects associated with amygdala and cortical dysfunction in mice with seeded α-synuclein inclusions. Neurobiol. Dis. 134:104708. doi: 10.1016/j.nbd.2019.104708
Tadaiesky, M. T., Dombrowski, P. A., Da Cunha, C., and Takahashi, R. N. (2010). Effects of SR141716A on cognitive and depression-related behavior in an animal model of premotor Parkinson’s disease. Parkinsons Dis. 2010:238491. doi: 10.4061/2010/238491
Tadaiesky, M. T., Dombrowski, P. A., Figueiredo, C. P., Cargnin-Ferreira, E., Da Cunha, C., and Takahashi, R. N. (2008). Emotional, cognitive and neurochemical alterations in a premotor stage model of Parkinson’s disease. Neuroscience 156, 830–840. doi: 10.1016/j.neuroscience.2008.08.035
Tanaka, R., Yamashiro, K., Ogawa, T., Oyama, G., Nishioka, K., Umemura, A., et al. (2020). The absence of orthostatic heart rate increase is associated with cognitive impairment in Parkinson’s disease. PLoS One 15:e0240491. doi: 10.1371/journal.pone.0240491
Targa, A. D. S., Noseda, A. C. D., Rodrigues, L. S., Aurich, M. F., and Lima, M. M. S. (2018). REM sleep deprivation and dopaminergic D2 receptors modulation increase recognition memory in an animal model of Parkinson’s disease. Behav. Brain Res. 339, 239–248. doi: 10.1016/j.bbr.2017.11.008
Taylor, J. R., Roth, R. H., Sladek, J. R. Jr., and Redmond, D. E. Jr. (1990). Cognitive and motor deficits in the performance of an object retrieval task with a barrier-detour in monkeys (Cercopithecus aethiops sabaeus) treated with MPTP: long-term performance and effect of transparency of the barrier. Behav. Neurosci. 104, 564–576. doi: 10.1037//0735-7044.104.4.564
Torres, E. R. S., Weber Boutros, S., Meshul, C. K., and Raber, J. (2020). ApoE isoform-specific differences in behavior and cognition associated with subchronic MPTP exposure. Learn. Mem. 27, 372–379. doi: 10.1101/lm.052126.120
Van Kampen, J. M., Baranowski, D. C., Robertson, H. A., Shaw, C. A., and Kay, D. G. (2015). The progressive BSSG rat model of Parkinson’s: recapitulating multiple key features of the human disease. PLoS One 10:e0139694. doi: 10.1371/journal.pone.0139694
Veselý, B., Korit’áková, E., Bohnen, N. I., Viszlayová, D., Királová, S., Valkovič, P., et al. (2019). The contribution of cerebrovascular risk factors, metabolic and inflammatory changes to cognitive decline in Parkinson’s disease: preliminary observations. J. Neural Transm. (Vienna) 126, 1303–1312. doi: 10.1007/s00702-019-02043-7
Vezoli, J., Fifel, K., Leviel, V., Dehay, C., Kennedy, H., Cooper, H. M., et al. (2011). Early presymptomatic and long-term changes of rest activity cycles and cognitive behavior in a MPTP-monkey model of Parkinson’s disease. PLoS One 6:e23952. doi: 10.1371/journal.pone.0023952
Wang, A. L., Liou, Y. M., Pawlak, C. R., and Ho, Y. J. (2010). Involvement of NMDA receptors in both MPTP-induced neuroinflammation and deficits in episodic-like memory in Wistar rats. Behav. Brain Res. 208, 38–46. doi: 10.1016/j.bbr.2009.11.006
Wang, K., Shi, Y., Liu, W., Liu, S., and Sun, M. Z. (2021). Taurine improves neuron injuries and cognitive impairment in a mouse Parkinson’s disease model through inhibition of microglial activation. Neurotoxicology 83, 129–136. doi: 10.1016/j.neuro.2021.01.002
Wang, W. F., Wu, S. L., Liou, Y. M., Wang, A. L., Pawlak, C. R., and Ho, Y. J. (2009). MPTP lesion causes neuroinflammation and deficits in object recognition in Wistar rats. Behav. Neurosci. 123, 1261–1270. doi: 10.1037/a0017401
Wang, Z., Lin, Y., Liu, W., Kuang, P., Lao, W., Ji, Y., et al. (2019). Voltage-gated sodium channels are involved in cognitive impairments in Parkinson’s disease- like Rats. Neuroscience 418, 231–243. doi: 10.1016/j.neuroscience.2019.08.024
Wu, G., Lu, Z. H., Seo, J. H., Alselehdar, S. K., DeFrees, S., and Ledeen, R. W. (2020). Mice deficient in GM1 manifest both motor and non-motor symptoms of Parkinson’s disease; successful treatment with synthetic GM1 ganglioside. Exp. Neurol. 329:113284. doi: 10.1016/j.expneurol.2020.113284
Yabuki, Y., Ohizumi, Y., Yokosuka, A., Mimaki, Y., and Fukunaga, K. (2014). Nobiletin treatment improves motor and cognitive deficits seen in MPTP-induced Parkinson model mice. Neuroscience 259, 126–141. doi: 10.1016/j.neuroscience.2013.11.051
Yadav, S. K., Pandey, S., and Singh, B. (2017). Role of estrogen and levodopa in 1-methyl-4-pheny-l-1, 2, 3, 6-tetrahydropyridine (mptp)-induced cognitive deficit in Parkinsonian ovariectomized mice model: a comparative study. J. Chem. Neuroanat. 85, 50–59. doi: 10.1016/j.jchemneu.2017.07.002
Yang, Y., Kong, F., Ding, Q., Cai, Y., Hao, Y., and Tang, B. (2020). Bruceine D elevates Nrf2 activation to restrain Parkinson’s disease in mice through suppressing oxidative stress and inflammatory response. Biochem. Biophys. Res. Commun. 526, 1013–1020. doi: 10.1016/j.bbrc.2020.03.097
Yildiz, D., Erer, S., Zarifoǧlu, M., Hakyemez, B., Bakar, M., Karli, N., et al. (2015). Impaired cognitive performance and hippocampal atrophy in Parkinson disease. Turk. J. Med. Sci. 45, 1173–1177. doi: 10.3906/sag-1408-68
Zhang, D., Bordia, T., McGregor, M., McIntosh, J. M., Decker, M. W., and Quik, M. (2014). ABT-089 and ABT-894 reduce levodopa-induced dyskinesias in a monkey model of Parkinson’s disease. Mov. Disord. 29, 508–517. doi: 10.1002/mds.25817
Zhang, D., Mallela, A., Sohn, D., Carroll, F. I., Bencherif, M., Letchworth, S., et al. (2013). Nicotinic receptor agonists reduce L-DOPA-induced dyskinesias in a monkey model of Parkinson’s disease. J. Pharmacol. Exp. Ther. 347, 225–234. doi: 10.1124/jpet.113.207639
Zhang, Q., Gao, Y., Zhang, J., Li, Y., Chen, J., Huang, R., et al. (2020). L-Asparaginase exerts neuroprotective effects in an SH-SY5Y-A53T model of Parkinson’s disease by regulating glutamine metabolism. Front. Mol. Neurosci. 13:563054. doi: 10.3389/fnmol.2020.563054
Zhang, X., Bai, L., Zhang, S., Zhou, X., Li, Y., and Bai, J. (2018). Trx-1 ameliorates learning and memory deficits in MPTP-induced Parkinson’s disease model in mice. Free Radic. Biol. Med. 124, 380–387. doi: 10.1016/j.freeradbiomed.2018.06.029
Zhou, X., Doorduin, J., Elsinga, P. H., Dierckx, R., de Vries, E. F. J., and Casteels, C. (2017). Altered adenosine 2A and dopamine D2 receptor availability in the 6-hydroxydopamine-treated rats with and without levodopa-induced dyskinesia. Neuroimage 157, 209–218. doi: 10.1016/j.neuroimage.2017.05.066
Zhu, Q., Zhuang, X. X., Chen, J. Y., Yuan, N. N., Chen, Y., Cai, C. Z., et al. (2021). Lycorine, a natural alkaloid, promotes the degradation of alpha-synuclein via PKA-mediated UPS activation in transgenic Parkinson’s disease models. Phytomedicine 87:153578. doi: 10.1016/j.phymed.2021.153578
Keywords: Parkinson’s disease, cognitive impairment, experimental model, toxins, transgenic animal, limitations
Citation: Fan Y, Han J, Zhao L, Wu C, Wu P, Huang Z, Hao X, Ji Y, Chen D and Zhu M (2021) Experimental Models of Cognitive Impairment for Use in Parkinson’s Disease Research: The Distance Between Reality and Ideal. Front. Aging Neurosci. 13:745438. doi: 10.3389/fnagi.2021.745438
Received: 22 July 2021; Accepted: 01 November 2021;
Published: 29 November 2021.
Edited by:
Alena Savonenko, Johns Hopkins University, United StatesReviewed by:
Angeliki Maria Nikolakopoulou, University of Southern California, United StatesCopyright © 2021 Fan, Han, Zhao, Wu, Wu, Huang, Hao, Ji, Chen and Zhu. This is an open-access article distributed under the terms of the Creative Commons Attribution License (CC BY). The use, distribution or reproduction in other forums is permitted, provided the original author(s) and the copyright owner(s) are credited and that the original publication in this journal is cited, in accordance with accepted academic practice. No use, distribution or reproduction is permitted which does not comply with these terms.
*Correspondence: Dongfeng Chen, Y2RmMjcyMTJAMjFjbi5jb20=; Meiling Zhu, bWVpbGluZ3podTIwMjBAMTI2LmNvbQ==
†These authors share first authorship
Disclaimer: All claims expressed in this article are solely those of the authors and do not necessarily represent those of their affiliated organizations, or those of the publisher, the editors and the reviewers. Any product that may be evaluated in this article or claim that may be made by its manufacturer is not guaranteed or endorsed by the publisher.
Research integrity at Frontiers
Learn more about the work of our research integrity team to safeguard the quality of each article we publish.