- 1Wallace H. Coulter Department of Biomedical Engineering, Emory University and Georgia Tech, Atlanta, GA, United States
- 2Division of Physical Therapy, Department of Rehabilitation Medicine, Emory University, Atlanta, GA, United States
- 3Department of Biomedical Informatics, Emory University School of Medicine, Atlanta, GA, United States
- 4Jean and Paul Amos PD and Movement Disorders Program, Department of Neurology, Emory University School of Medicine, Atlanta, GA, United States
The mechanisms underlying associations between cognitive set shifting impairments and balance dysfunction are unclear. Cognitive set shifting refers to the ability to flexibly adjust behavior to changes in task rules or contexts, which could be involved in flexibly adjusting balance recovery behavior to different contexts, such as the direction the body is falling. Prior studies found associations between cognitive set shifting impairments and severe balance dysfunction in populations experiencing frequent falls. The objective of this study was to test whether cognitive set shifting ability is expressed in successful balance recovery behavior in older adults with high clinical balance ability (N = 19, 71 ± 7 years, 6 female). We measured cognitive set shifting ability using the Trail Making Test and clinical balance ability using the miniBESTest. For most participants, cognitive set shifting performance (Trail Making Test B-A = 37 ± 20 s) was faster than normative averages (46 s for comparable age and education levels), and balance ability scores (miniBESTest = 25 ± 2/28) were above the threshold for fall risk (23 for people between 70 and 80 years). Reactive balance recovery in response to support-surface translations in anterior and posterior directions was assessed in terms of body motion, muscle activity, and brain activity. Across participants, lower cognitive set shifting ability was associated with smaller peak center of mass displacement during balance recovery, lower directional specificity of late phase balance-correcting muscle activity (i.e., greater antagonist muscle activity 200–300 ms after perturbation onset), and larger cortical N1 responses (100–200 ms). None of these measures were associated with clinical balance ability. Our results suggest that cognitive set shifting ability is expressed in balance recovery behavior even in the absence of profound clinical balance disability. Specifically, our results suggest that lower flexibility in cognitive task performance is associated with lower ability to incorporate the directional context into the cortically mediated later phase of the motor response. The resulting antagonist activity and stiffer balance behavior may help explain associations between cognitive set shifting impairments and frequent falls.
Introduction
Cognitive impairment is associated with balance dysfunction, but it is unclear whether or how cognitive ability relates to balance recovery behavior in relatively high-functioning preclinical populations. Subtle cognitive impairments in executive function (Muir et al., 2012), attention, and memory are associated with clinical balance impairments (Tangen et al., 2014) and predict the first (Herman et al., 2010) and recurring falls in older adults (Gleason et al., 2009; Mirelman et al., 2012). However, it is unclear whether subtle differences in cognitive ability in the absence of clinically detectable balance dysfunction are associated with changes in balance control. Associations between cognitive function and balance control could provide mechanistic insight into findings that cognitive engagement in balance control increases with age (Rankin et al., 2000), fall history (Shumway-Cook et al., 1997), and fall risk (Lundin-Olsson et al., 1997). Here, we focus on individual differences in cognitive set shifting ability (i.e., the ability to flexibly adjust a behavior to changes in task rules or contexts), which have previously been associated with clinical balance dysfunction (Tangen et al., 2014), fall history (McKay et al., 2018), and fall risk (Herman et al., 2010). We investigate balance recovery behavior in terms of body motion, muscle activity, and brain activity evoked by a sudden balance disturbance, in contrast to prior studies that used clinical instruments and falls tracking, which are less applicable to preclinical populations. Identifying associations between cognitive ability and balance recovery behavior in preclinical populations could provide insight into underlying mechanisms for balance impairments that could serve as therapeutic targets for rehabilitation prior to occurrence of a fall.
Cognitive set shifting is an executive function that pertains to the ability to flexibly adjust behavior to changes in task rules or contexts, but its potential role in balance behavior is unclear. The Trail Making Test is a common pen and paper assessment of cognitive set shifting, consisting of two parts. In Part A, a participant must rapidly draw lines to connect dots in numerical order, relying on sustained attention, working memory, visuomotor search, and dexterity (Sanchez-Cubillo et al., 2009). In Part B, the task is altered to incorporate switching between numbers and letters (1-A-2-B-3-C…), thereby adding in a component of cognitive set shifting (Sanchez-Cubillo et al., 2009). Scoring the difference in time to complete Part B – Part A accounts for the overlapping motor and cognitive aspects, leaving a relatively pure measure of cognitive set shifting (Sanchez-Cubillo et al., 2009). However, the construct of set shifting inherently includes an increased working memory load to maintain and switch between two rule sets, as well as response selection and inhibition to select the response according to the current rule set while suppressing the response to the previous rule set (Koch et al., 2010). Because the Trail Making Test is so far removed from standing balance behavior, it is unclear why it has been repeatedly associated with advanced balance impairments (Herman et al., 2010; Tangen et al., 2014; McKay et al., 2018). However, if the neural mechanisms for cognitive set shifting assessed by the Trail Making Test are involved in balance control, then variation in cognitive set shifting ability should be expressed in successful balance recovery behavior before people begin experiencing frequent falls.
Similar to effective cognitive control, successful balance recovery behavior requires quick and flexible execution of a contextually appropriate behavior. Support-surface translational perturbations rapidly displace the base of support (i.e., the feet) relative to the body’s center of mass, requiring a rapid neural and mechanical reaction to prevent a fall. Effective balance recovery behavior involves directionally specific motor responses, with muscles showing preferential activation in response to perturbation directions in which they can generate torque to counteract center of mass displacement (Henry et al., 1998; Torres-Oviedo and Ting, 2007). This type of directional specificity is reduced in people with balance impairments (Lang et al., 2019), resulting in simultaneous agonist-antagonist cocontraction, which increases joint stiffness, but ultimately limits joint torques as the actions of the agonist and antagonist muscles partially resist one another (Damiano, 1993). Although cocontraction is common when learning new or complex motor skills and can be beneficial in some contexts (Damiano, 1993), its association to balance impairments suggests it is not an ideal strategy for balance recovery behavior (Lang et al., 2019). It has been suggested that cognitive flexibility, a broader construct containing cognitive set shifting, may be needed to quickly adjust behavior to unpredictable demands, including the use of feedback from the body or environment to appropriately react to a sudden displacement of the body’s center of mass (Pieruccini-Faria et al., 2019). Here, we test whether cognitive set shifting ability is associated with the ability to modulate muscle activity between balance perturbations that displace the body’s center of mass in opposite directions.
Testing different phases of the motor response for associations to cognitive ability could provide insight into different mechanisms by which cognitive function may overlap with balance recovery behavior. A sudden balance perturbation evokes a relatively stereotyped brainstem-mediated balance-correcting motor response at ∼100 ms via integrated sensory inputs reflecting the task-level goal of upright posture, and not the local stretch of individual muscles (Nashner, 1979; Horak and Nashner, 1986; Dietz et al., 1987; Safavynia and Ting, 2013). While this early response is subcortically mediated, it can be influenced by pre-perturbation cognitive state, including arousal (Carpenter et al., 2004), expectations (Horak et al., 1989), and intentions (McIlroy and Maki, 1993; Burleigh et al., 1994; Burleigh and Horak, 1996; Weerdesteyn et al., 2008) in ways that may depend on descending cortical influence in anticipation of an upcoming balance disturbance. More variable motor responses occur at longer latencies (> 150 ms) that can incorporate cortically mediated motor responses to the balance disturbance (Jacobs and Horak, 2007). Cognitive dual task interference is limited to this later phase of the motor response, suggesting only the later phase depends on online cognitive processing (Rankin et al., 2000). If cognitive set shifting is associated with directional specificity in the early phase of the response, this would implicate cognitive set shifting in the maintenance of “central set,” which refers to the ability of the central nervous system to preselect the gain of stimulus-evoked behaviors in consideration of arousal, expectations, and intentions (Prochazka, 1989). If cognitive set shifting is associated with directional specificity only in the later phase, this would implicate cognitive set shifting in cortically mediated reactions to the balance perturbation, which can incorporate incoming sensory information into decisions about how to react.
Balance perturbations also evoke a cortical response that is associated with balance ability and cognitive processing, but it is unknown whether this cortical response reflects individual differences in cognitive ability. A cortical response, termed the “N1” for the first negative peak in the evoked electroencephalography signal, occurs in the supplementary motor area ∼150 ms after a balance disturbance (Marlin et al., 2014; Mierau et al., 2015). We have previously suggested that the cortical N1 may reflect compensatory cortical engagement in balance recovery because it is enhanced in young adults with lower balance ability (Payne and Ting, 2020b) and on trials in which compensatory steps are taken (Payne and Ting, 2020a). The cortical N1 is also influenced by cognitive processes including attention (Quant et al., 2004; Little and Woollacott, 2015), perceived threat (Adkin et al., 2008; Mochizuki et al., 2010), and predictability (Adkin et al., 2006, 2008; Mochizuki et al., 2008, 2010) and may therefore reflect cognitive-motor interactions. The possibility that the N1 reflects cognitive-motor interactions is further supported by its localization to the supplementary motor area (Marlin et al., 2014; Mierau et al., 2015), which is thought to mediate interactions between cognitive and motor processes by mediating interactions between neighboring prefrontal and motor cortical areas (Goldberg, 1985). Although investigations of the cortical N1 in older populations have been limited (Duckrow et al., 1999; Ozdemir et al., 2018), the N1 may be ideally suited for investigating relationships between cognitive and motor impairments with aging. Here, we test whether the cortical N1 is associated with individual differences in cognitive set shifting ability.
We investigated whether individual differences in cognitive set shifting ability were associated with perturbation-evoked balance recovery behavior and cortical activity in an older population with relatively high balance function to gain insight into possible mechanisms linking balance and cognitive function. We assessed clinical balance ability with the mini Balance Evaluation Systems Test (miniBESTest) (Magnani et al., 2020) and cognitive set shifting ability with the Trail Making Test (Tombaugh, 2004; Sanchez-Cubillo et al., 2009). We tested these ability measures for association with perturbation-evoked balance recovery behavior, including whole body stiffness, directional specificity of ankle muscle activity in early and late phases of the motor response, and the evoked cortical N1 response. We hypothesized that cognitive set shifting shares underlying mechanisms with balance control. We therefore predicted that inter-individual variation in cognitive set shifting ability would be associated with balance recovery behavior prior to the development of clinically significant balance disorders.
Materials and Methods
Participants
Nineteen older adults (age 71 ± 6, 6 female) participated in this study. Written consent was obtained from all participants after a detailed explanation of the protocol according to procedures approved by the Emory University Institutional Review Board.
Participants were recruited from Emory University and the surrounding community. Adults over 55 years of age were screened for the following inclusion criteria: vision can be corrected to 20/40 or better with corrective lenses, no history of stroke or other neurologic condition, no musculoskeletal conditions or procedures that cause pain or limit mobility of the legs, ability to stand unassisted for at least 15 min, and cognitive ability to provide informed consent. Potential participants were excluded for prior experience on the perturbation platform. Study data were collected and managed using a Research Electronic Data Capture (REDCap) database hosted at Emory University (Harris et al., 2009, 2019).
Balance Ability
The miniBESTest1 was used as a measure of balance ability (Magnani et al., 2020) which assesses anticipatory postural control, reactive postural control, sensory orientation, and dynamic gait.
Set Shifting Ability
The set shifting ability score was measured as the difference in time to complete Part B minus Part A of the Trail Making Test (Sanchez-Cubillo et al., 2009; McKay et al., 2018). Part A requires participants to quickly connect sequentially numbered dots (1-2-3, etc.). Part B is similarly formatted but requires participants to shift between numbers and letters (1-A-2-B, etc.). A greater difference in time to complete Part B compared to Part A indicates slower cognitive set shifting and therefore lower cognitive set shifting ability.
Overall Cognitive Ability
The Montreal Cognitive Assessment (MoCA2) was given as a rapid assessment of overall cognitive ability that assesses cognitive domains including executive function, attention, and memory (Nasreddine et al., 2005). Participants also self-reported the number of years of education. These data were collected as potential covariates so we could test whether overall cognitive ability confounds more specific associations to cognitive set shifting and were not considered for exclusion, as a range of cognitive abilities was necessary to achieve the goals of this study.
Perturbations
Participants were exposed to 48 translational support-surface perturbations of unpredictable timing, direction, and magnitude using a custom perturbation platform (Payne et al., 2019a). Perturbations were evenly divided between forward and backward directions, and three perturbation magnitudes, which will be referred to as small, medium, and large. The small perturbation (0.15 g, 11.1 cm/s, 5.1 cm) was identical across participants. Medium (0.21–0.22 g, 15.2–16.1 cm/s, 7.0–7.4 cm) and large (0.26–0.29 g, 19.1–21.0 cm/s, 8.9–9.8 cm) perturbations were linearly scaled down from reference magnitudes (medium: 0.22 g, 16.7 cm/s, 7.7 cm; large: 0.30 g, 21.8 cm/s, 10.2 cm) by multiplying perturbation acceleration, velocity, and displacement characteristics by a scaling factor linearly related to the participant’s height [Eq. (1)] to account for the effect of participant height and deliver perturbations that are mechanically similar across body sizes (Payne et al., 2019a). The 48 perturbation series was divided into 8 blocks, each containing one replicate of each unique perturbation. Three different block-randomized perturbation orders were used across participants to randomize any possible effects of trial order. The perturbations for an example participant are shown in Figure 1.
Participants were instructed to cross their arms across their chest, focus their vision on a fixed location at eye-level approximately 4.5 meters away, and to try to recover balance without taking a step. The experimenter monitored continuous electromyography (EMG) and electroencephalography (EEG) activity to ensure activity had returned to quiet baseline levels prior to the onset of the next perturbation. Seated rest breaks were taken after 15 min of perturbations, or more frequently at the request of the participant or if the participant displayed signs of fatigue or loss of concentration. Excluding rest breaks, inter-trial-intervals, from perturbation onset to perturbation onset were 23 ± 11 s. Trials in which participants took steps (8% of all trials) were excluded from analysis.
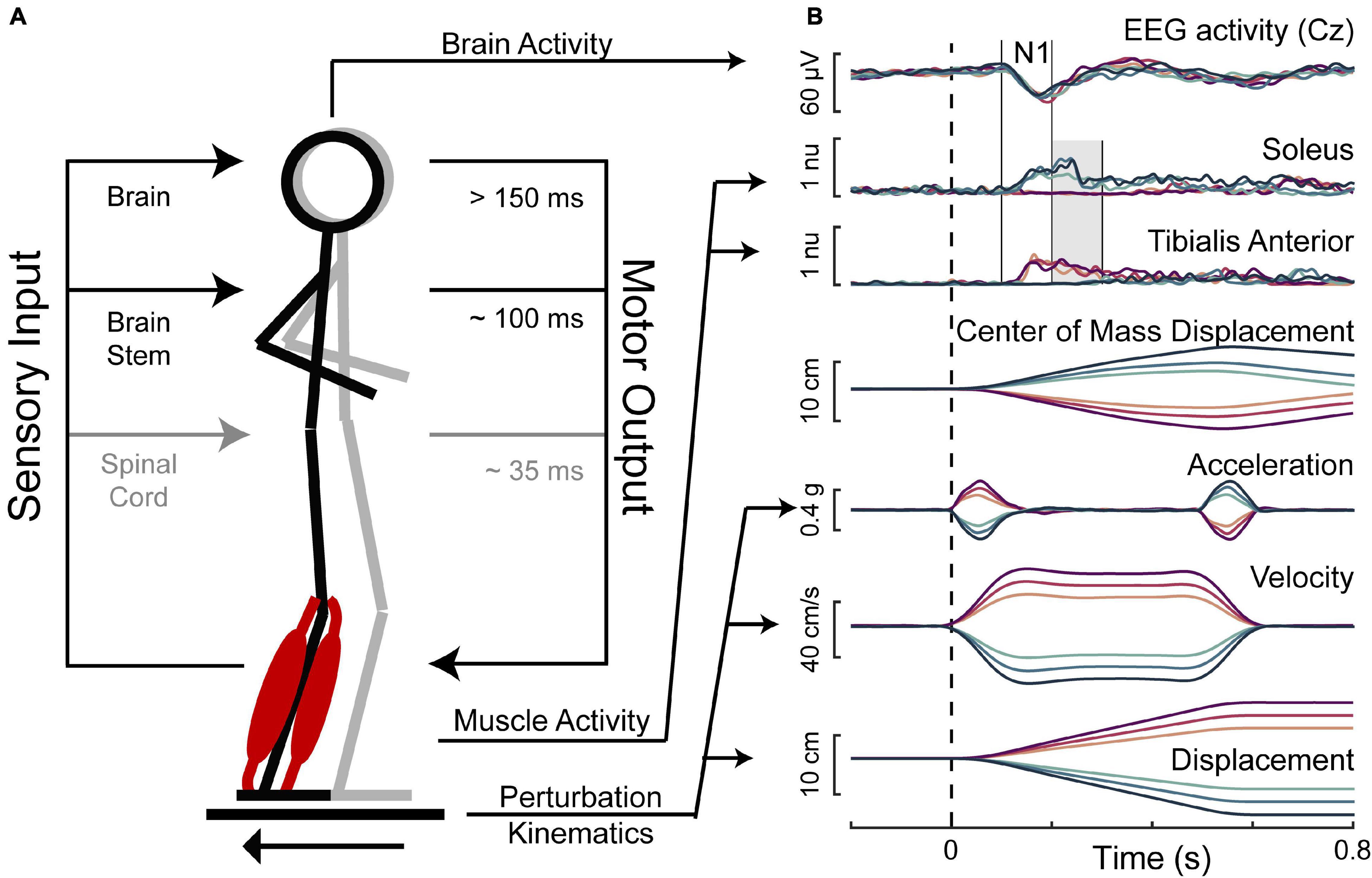
Figure 1. Balance perturbations. (A) The translational support-surface balance perturbation is depicted along with a schematic displaying hierarchical levels of control of the perturbation-evoked muscle activity. (B) Perturbation kinematics and the measured response variables are shown for an example participant with forward movements of the floor represented in magentas and backward movements of the floor represented in blues, with darker colors for larger perturbations. Perturbation onset is indicated with the dashed vertical line. Solid vertical lines indicate the time window of 100–200 ms, in which the cortical N1 and the early phase of muscle activity were assessed. The shaded gray area indicates the time window of 200–300 ms, in which the late phase of muscle activity was assessed.
Body Motion
Motion of the body’s center of mass was tracked using a 10-camera Vicon Nexus 3D motion analysis system. Reflective markers placed on areas of the body including the head, neck, hips, knees, ankles, and feet were used to create a model of the body, and Vicon’s plug-in-gait model was used to estimate the body’s center of mass. Body motion was referenced to motion of the support-surface to assess whole body stiffness in terms of the deviation of the center of mass from the base of support, where stiffer individuals will move less in response to the same perturbation. Body motion was then quantified for each participant in each trial type as the peak deviation of the center of mass from the base of support along the axis of perturbation motion in data averaged across perturbations for each direction and magnitude.
Muscle Activity
Surface EMGs (Motion Lab Systems, Baton Rouge, LA) were collected from tibialis anterior and soleus muscles, which are an agonist-antagonist pair of ankle muscles activated in forward and backward support-surface perturbations. EMG activity was collected using silver silver-chloride bipolar electrodes with 2 cm interelectrode spacing (Norotrode 20, Myotronics, Inc.) and sampled at 1000 Hz after an online analog 500 Hz low-pass filter. Skin was scrubbed with alcohol swabs and shaved if necessary prior to electrode placement.
Muscle activity was epoched into 2.4 s intervals, beginning 400 ms before perturbation onset. Epochs were 35 Hz high-pass filtered with a third-order zero-lag Butterworth filter, mean-subtracted, half-wave rectified, and then 40 Hz low-pass filtered. To avoid issues with normalization that could occur when averaging muscle activity across the left and right legs, only the muscle activity for the left leg was analyzed. As only non-stepping behaviors were analyzed in forward and backward perturbations, muscle activity is expected to be similar across left and right legs.
Muscle activity was assessed in two time bins of interest. This included an early (100–200 ms) time bin, which is expected to primarily contain involuntary brainstem-mediated activity, and a late (200–300 ms) time bin, in which cortical contributions to muscle activation can appear (Figure 1).
Antagonist muscle activation was quantified relative to agonist muscle activity (Lang et al., 2019) within each perturbation magnitude as a measure of directional specificity, or motor set shifting, in terms of how the same muscle is activated differently across perturbation directions. EMG activity for each muscle was averaged within each time bin for each of the perturbation magnitudes and directions. Then, EMG activity was quantified according to Equation 2, which takes the absolute value of the difference in EMG activity between forward and backward perturbation directions (i.e., agonist activity – antagonist activity) and divides it by the larger of the two values (i.e., agonist activity). This results in a value between 0 and 1, where values near 1 indicate nearly exclusive agonist activity, or high directional specificity, and values near zero indicate nearly identical agonist and antagonist activity, or low directional specificity.
Cortical Activity
Electroencephalography (EEG) data were collected during the perturbation series using thirty-two active electrodes (ActiCAP, Brain Products, Germany) placed according to the international 10–20 system, with the exception of two electrodes placed on the skin over the mastoid bones behind the ears for offline re-referencing. The electrodes were prepared with conductive electrode gel (SuperVisc 100 gr. HighViscosity Electrolyte-Gel for active electrodes, Brain Products) using a blunt-tipped needle, which was also used to rub the scalp to improve signal quality. Impedances for the Cz and mastoid electrodes were generally below 10 kOhm before the start of data collection.
Active electrodes are specifically designed to minimize movement artifacts by pre-amplifying the signal at the electrode before the signal passes through any cables. Movement artifacts were further minimized by the nature of the balance perturbations acting at the participants’ feet, evoking a cortical N1 response prior to significant head movement (Payne et al., 2019a), and ensuring that perturbations were only delivered during a stable EEG baseline as described above.
However, it was necessary to address spontaneous blinks and eye movement, which can occur during the evoked response and affect the measured outcomes. To subtract vertical eye movement and blink artifacts, electrooculography (EOG) data were collected with bipolar passive electrodes (E220x, Brain Products) vertically bisecting the right pupil and referenced to the forehead. Prior to placement, the EOG electrodes were prepared with high-chloride abrasive gel (ABRALYT HiCl 250 gr., High-chloride-10% abrasive electrolyte gel, Brain Products) and the skin was scrubbed with an alcohol swab. EEG and EOG data were sampled at 1000 Hz on an ActiCHamp amplifier (Brain Products) with a 24-bit A/D converter and an online 20 kHz anti-aliasing low-pass filter.
Electroencephalography (EEG) data were 1 Hz high-pass filtered offline with a third-order zero-lag Butterworth filter, mean-subtracted within channels, and then low-pass filtered at 25 Hz. Data at the Cz electrode were re-referenced to the average of the two mastoid electrodes and epoched into 2.4 s segments beginning 400 ms before perturbation onset. Electrooculography data were similarly filtered and segmented without re-referencing. The Gratton et al. (1983) algorithm was applied as described in Payne et al. (2019a) to remove blinks and eye movement artifacts through a serial regression-subtraction approach. Cz epochs were then averaged across trials within each trial type (7 ± 1 trials per subject per condition) and baseline subtracted using a baseline period of 50–150 ms before perturbation onset. Cortical N1 response amplitudes were then quantified as the negative of the most negative amplitude (μV, such that higher values indicate a larger component peak) at the Cz electrode between 100 and 200 ms after perturbation onset (Figure 1).
The decision to analyze a single electrode where the cortical N1 response is the largest, rather than a more complex analysis, such as independent components analysis, was based on the goal of identifying a clinically feasible and reproducible biomarker of brain engagement during balance recovery. However, as the cortical N1 may arise due to synchronization of multiple component sources (Peterson and Ferris, 2018, 2019; Varghese et al., 2019), this single electrode approach cannot clarify the phenomena underlying the cortical N1 response.
Statistical Analyses
Simple linear regressions were used to test for associations between pairs of study variables. Specifically, linear regressions were used to test set shifting ability scores as a predictor of: N1 amplitudes, peak center of mass displacements, and directional specificity of soleus and tibialis anterior muscle activity. Additional linear regressions were used to test each of these variables as a predictor of MiniBESTest scores. Variables that were not normally distributed as determined by Shapiro-Wilk test p-values < 0.05 were transformed to a normal distribution prior to regression using boxcox.m in MATLAB. Parameter estimates for the regression slopes were compared against the hypothesized value 0 with two-sided t-tests using PROC GLM in SAS. Figures display untransformed data with p-values and R2 values obtained from the adjusted variables. For significant associations, we also report Cohen’s F2 (Cohen, 1992) as a measure of effect size.
All tests for association with N1 amplitudes and peak center of mass motion were performed separately across the two perturbation directions and three perturbation magnitudes, and tests for association with EMG activity were performed separately across the three perturbation magnitudes. Associations were examined for consistency across testing conditions via visual inspection of regression plots and tabulated regression coefficients. Simple linear regressions that yielded significant associations were further tested for robustness to potential confounding variables, including age, sex, height, weight, overall cognition scores, years of education, and balance ability scores. Specifically, each significant regression was retested in a multivariate regression with each of the potential confounding variables added, one at a time, as an additional predictor in the model to confirm that the associations were insensitive to adjustment by the potential confound.
Results
Demographic characteristics of the participants are shown in Table 1. Overall, participants had high clinical balance ability and cognitive set shifting ability. On the clinical balance test, most participants scored above the fall-risk threshold of 23 for adults between 70 and 80 years old (Magnani et al., 2020) (Figure 2). Similarly, most participants performed better on the Trail Making Test (B-A) than the average of 46 s that would be expected for adults between 70 and 80 years old with 12 + years of education (Tombaugh, 2004).
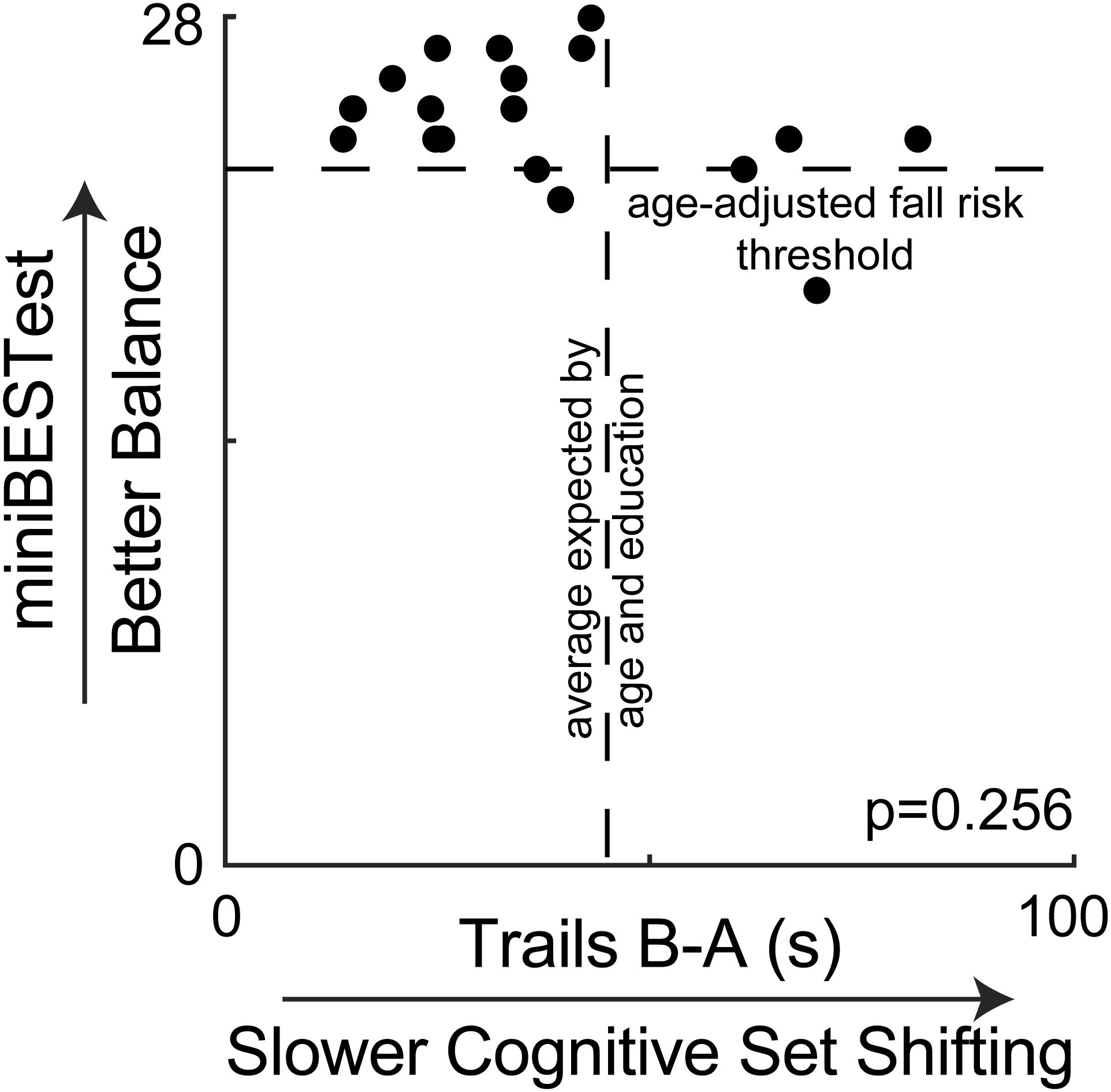
Figure 2. Clinical balance scores are plotted against cognitive set shifting performance, showing no association. Most participants scored above the suggested fall risk cutoff score of 23 for people between 70 and 80 years old on the miniBESTest (Magnani et al., 2020). Most participants also completed the Trail Making Test (B-A) faster than the average of 46 s that would be expected by their age and education from normative data (Tombaugh, 2004).
Clinical balance ability scores were not associated with any other study variable. Specifically, miniBESTest scores were not associated with cognitive set shifting (p = 0.256, Figure 2), the peak amplitude of center of mass displacement (p > = 0.123 across all perturbation magnitudes and directions, Figure 3), antagonist activity of the soleus (all p > = 0.078, Supplementary Figures) or tibialis anterior (all p > = 0.500, Supplementary Figures), or the peak amplitude of the cortical N1 response (all p > = 0.566, Supplementary Figures).
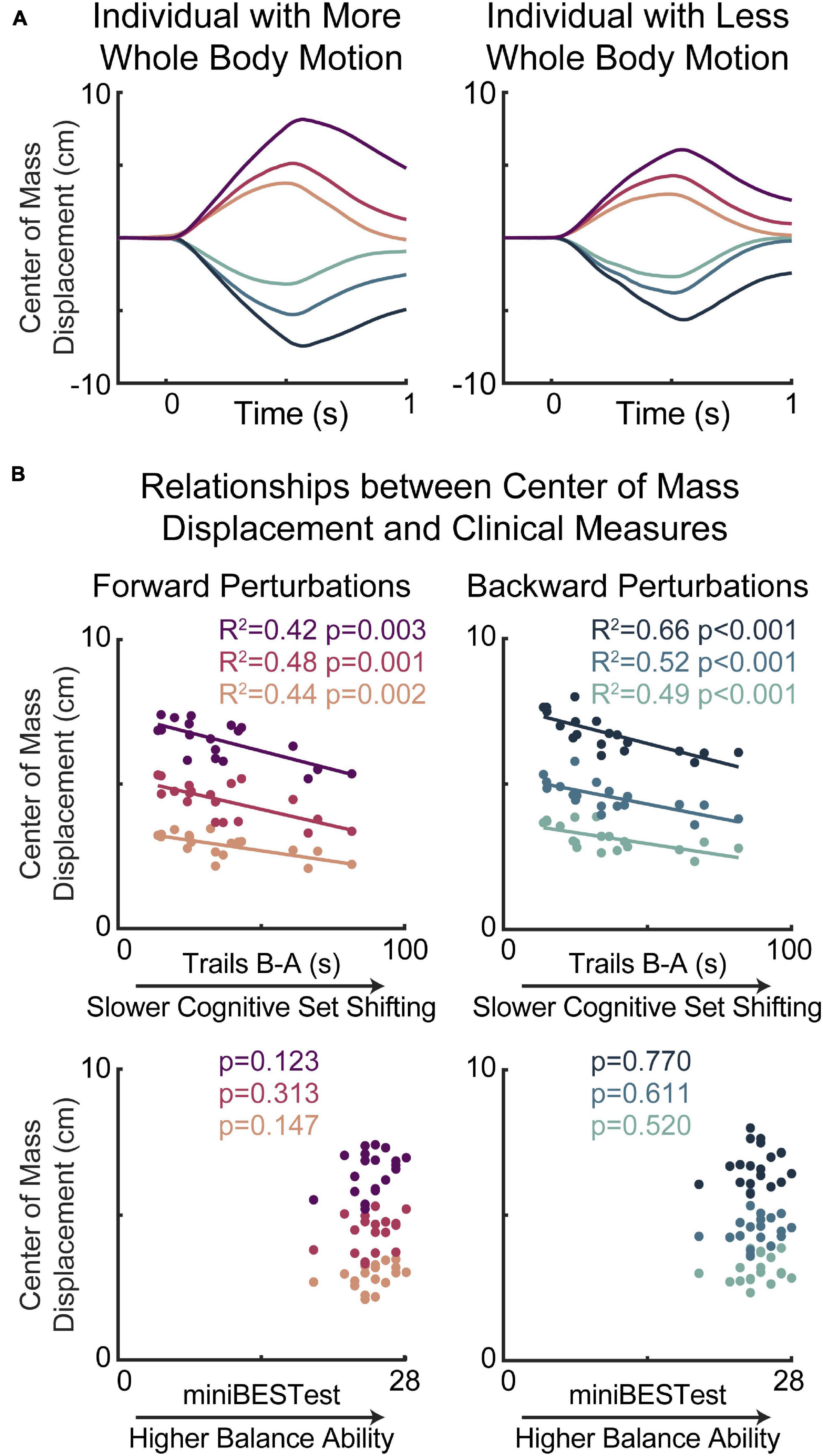
Figure 3. Slower cognitive set shifting was associated with stiffer behavioral responses to perturbation. (A) Center of mass displacements are shown for two individuals with more (left) or less (right) whole body motion for each of the perturbation types, with forward movements of the floor (resulting in backward leaning posture) represented in magentas and backward movements of the floor represented in blues, with darker colors for larger perturbations. (B) Peak center of mass displacements are plotted against cognitive set shifting scores (middle) and clinical balance scores (bottom) for forward (left) and backward (right) perturbations for each perturbation magnitude.
Lower cognitive set shifting ability was associated with stiffer responses to perturbations (Figure 3). Specifically, individuals who took longer to complete the cognitive set shifting task had smaller peak amplitudes of center of mass displacement with respect to the base of support in all perturbation magnitudes in both perturbation directions (forward perturbations: small p = 0.002 R2 = 0.44 Cohen’s F2 = 0.77, medium p = 0.001 R2 = 0.48 F2 = 0.92, large p = 0.003 R2 = 0.42 F2 = 0.72; backward perturbations: small p < 0.001 R2 = 0.49 F2 = 0.96, medium p < 0.001 R2 = 0.52 F2 = 1.07, large p < 0.001 R2 = 0.66 F2 = 1.98). Set shifting ability scores remained a significant predictor of center of mass displacement in all perturbation magnitudes and directions when potential confounding variables of age, sex, height, weight, overall cognition, education, and balance ability scores were included in the models (Supplementary Material).
Lower cognitive set shifting ability was associated with more antagonist activity in the late phase (200–300 ms) of soleus muscle activation (Figure 4). Specifically, individuals who took longer to complete the cognitive set shifting task displayed less directional specificity of late phase (200–300 ms) soleus activity (small perturbation p = 0.010, R2 = 0.33 F2 = 0.50, medium p = 0.007, R2 = 0.35 F2 = 0.55, large p = 0.004 R2 = 0.39 F2 = 0.64). Set shifting ability scores remained a significant predictor of directional specificity of late phase soleus activity in all perturbation magnitudes when potential confounding variables of age, sex, height, weight, overall cognition, education, and balance ability scores were included in the models (Supplementary Material). In contrast, set shifting ability was not associated with directional specificity of the early automatic phase (100–200 ms) of soleus muscle activation (small perturbation p = 0.121, medium p = 0.409, large p = 0.156).
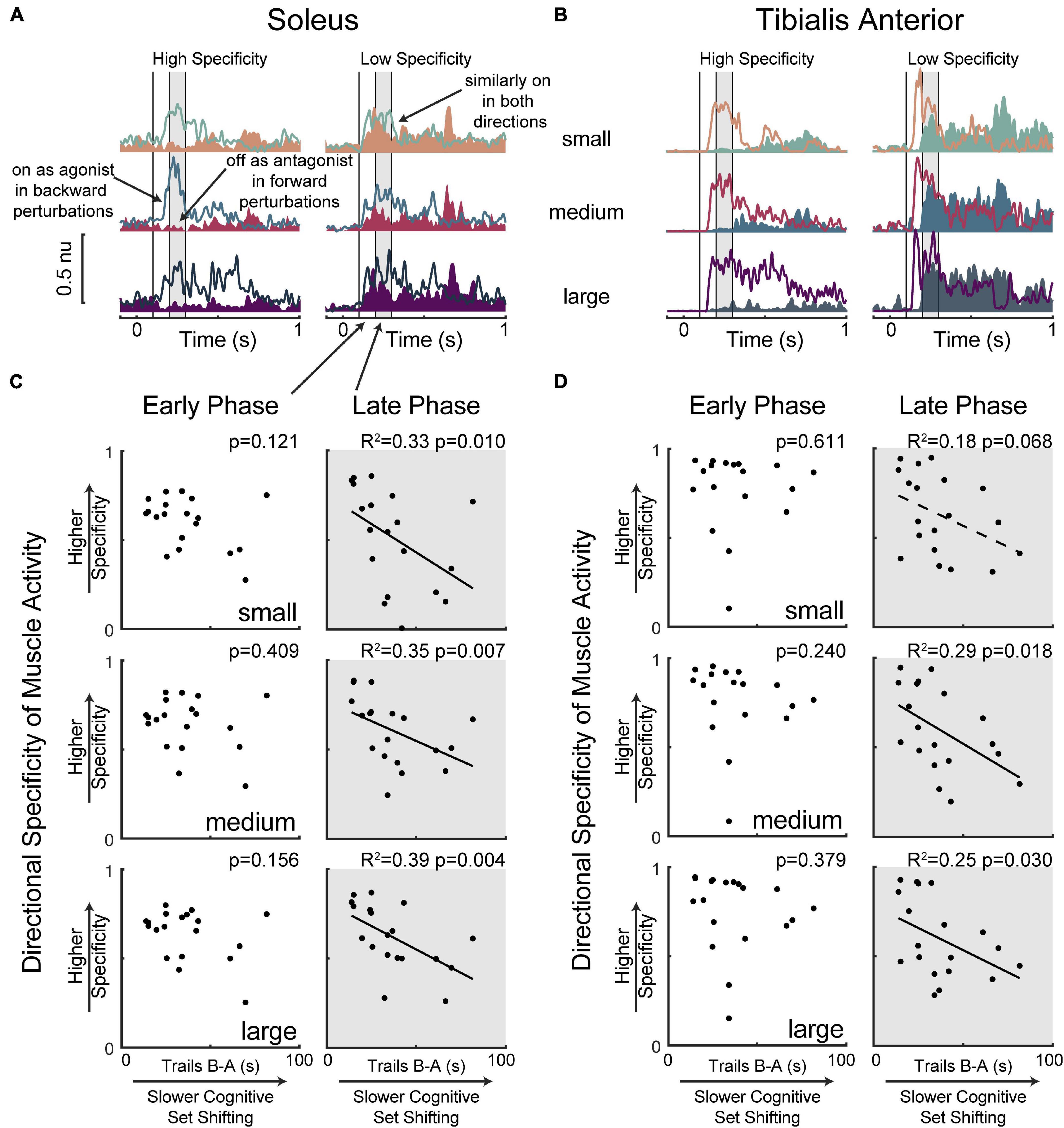
Figure 4. Slower cognitive set shifting was associated with lower directional specificity in the late phase of muscle activation. Condition-averaged muscle activity is shown for (A) soleus and (B) tibialis anterior muscles for example participants with higher or lower directional specificity scores. Muscle activity evoked by forward movements of the floor (resulting in backward leaning posture) is represented in magentas and activity evoked by backward movements of the floor is represented in blues, with darker colors for larger perturbations. Antagonist activity (i.e., soleus activity in forward perturbations and tibialis anterior activity in backward perturbations) is shaded for clarity. Vertical lines at 100 ms, 200 ms, and 300 ms mark the bounds of the time bins of interest. The later (200–300 ms) time bin is shaded in all panels. Directional specificity is plotted against cognitive set shifting scores for the (C) soleus muscle and (D) tibialis anterior muscle in each perturbation magnitude for early (100–200 ms) and late (200–300 ms) time bins.
Limited associations between cognitive set shifting ability and tibialis anterior muscle activity (Figure 4) were not robust to the inclusion of potential confounding variables. Specifically, individuals who took longer to complete the cognitive set shifting task displayed lower directional specificity of late phase (200–300 ms) tibialis anterior muscle activation in medium (p = 0.018 R2 = 0.29 F2 = 0.40) and large (p = 0.030 R2 = 0.25 F2 = 0.33) perturbations, but only a trend was observed in small (p = 0.068 R2 = 0.18 F2 = 0.22) perturbations. However, these associations were not robust to the inclusion of potential confounding variables (Supplementary Material). Specifically, set shifting scores were no longer a significant predictor of directional specificity of late phase tibialis anterior muscle activation in the medium perturbation magnitude upon the inclusion of sex (p = 0.053) or overall cognition (p = 0.096) into the model, and significance was similarly lost in the large perturbation magnitude upon the inclusion of height (p = 0.058), sex (p = 0.110), or overall cognition (p = 0.077) into the model. Set shifting ability scores were not significant predictors of directional specificity of the early automatic phase (100–200 ms) of tibialis anterior muscle activation (small perturbation p = 0.611, medium p = 0.240, large p = 0.379).
Lower cognitive set shifting ability was associated with larger perturbation-evoked cortical N1 responses (Figure 5). Specifically, individuals who took longer to complete the cognitive set shifting task had larger cortical N1 peak amplitudes in response to all perturbation magnitudes in both perturbation directions (forward perturbations: small p = 0.004 R2 = 0.40 F2 = 0.65, medium p = 0.002 R2 = 0.44 F2 = 0.80, large p = 0.003 R2 = 0.41 F2 = 0.69; backward perturbations: small p < 0.001 R2 = 0.60 F2 = 1.48, medium p = 0.004 R2 = 0.39 F2 = 0.64, large p = 0.016 R2 = 0.30 F2 = 0.42). Set shifting ability scores remained a significant predictor of N1 peak amplitudes in all perturbation magnitudes and directions when potential confounding variables of age, sex, height, weight, overall cognition, education, and balance ability scores were included in the models, with one exception (Supplementary Material). This exception was in large backward perturbations, where set shifting ability fell below significance as a predictor of N1 amplitudes upon inclusion of sex into the model (p = 0.055).
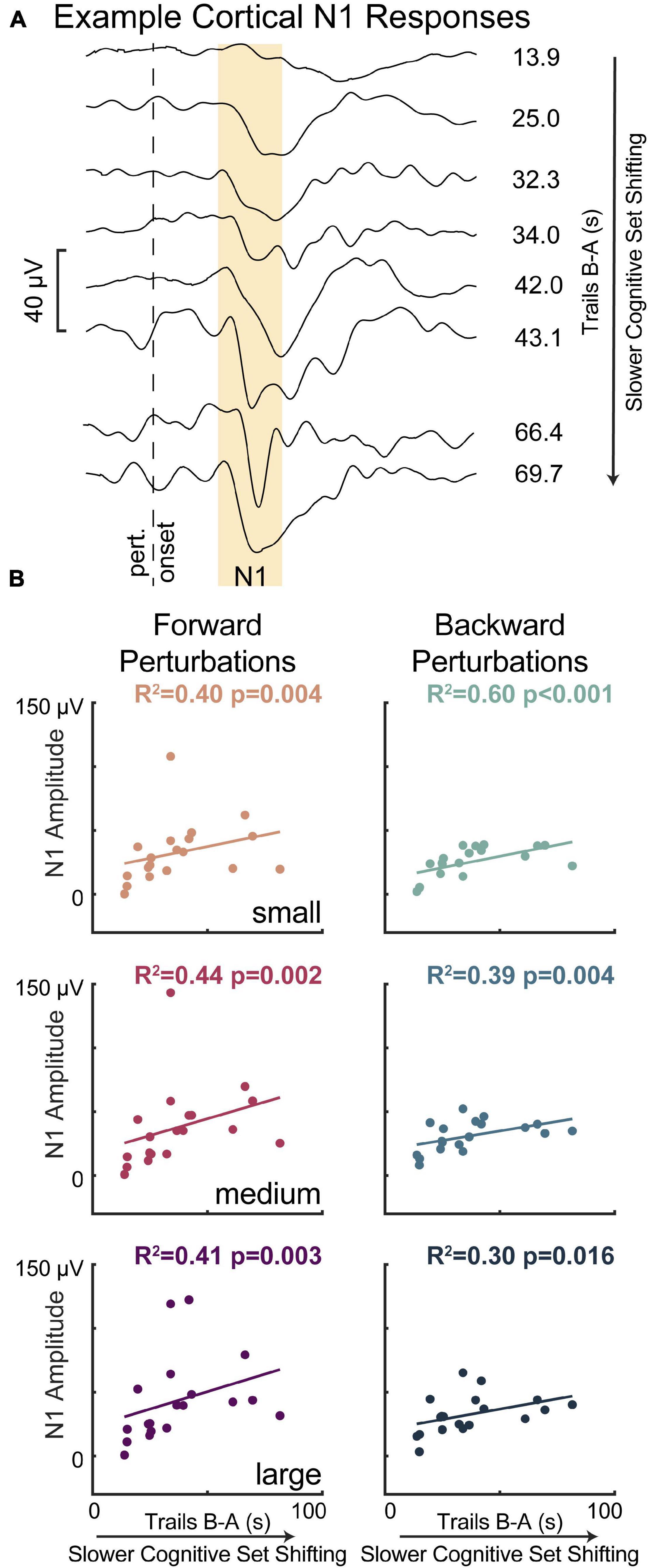
Figure 5. Slower cognitive set shifting was associated with larger perturbation-evoked cortical N1 responses. (A) Cortical responses are shown at the Cz electrode for eight different participants (averages across all trials) as examples along with their set shifting scores. The gold box highlights the window of 100–200 ms, in which the N1 amplitude was quantified. (B) Cortical N1 response amplitudes are plotted against cognitive set shifting scores in each of the perturbation conditions. Forward movements of the floor (resulting in backward leaning posture) are represented in magentas and backward movements of the floor are represented in blues, with darker colors for larger perturbations.
Discussion
Our results suggest that cortically mediated reactions to a balance disturbance may share common mechanisms with cognitive set shifting ability in older adults with relatively high balance ability. While prior studies have linked set shifting impairments to severe balance dysfunction and frequent falls (Herman et al., 2010; Tangen et al., 2014; McKay et al., 2018), our results demonstrate that set shifting ability is expressed in successful balance recovery behavior even in the absence of profound clinical balance disability. Individuals with lower cognitive set shifting ability had stiffer whole-body behavior in terms of lower center of mass displacement after balance perturbations, which may be caused by excessive agonist-antagonist coactivation related to difficulty incorporating the directional context into the cortically mediated phase of the motor response. The associations between cognitive set shifting and the late phase of the motor response as well as the larger cortical N1 responses both suggest that cognitive set shifting shares common mechanisms with the cortically mediated reaction to the perturbation, rather than cortical preparatory activity, which would have been observed in the early phase of the motor response. The associations between balance and cognitive set shifting cannot be explained by age, as all associations remained significant when accounting for age. Our data suggest that cognitive set shifting ability may indicate variation in balance control that is not yet detectable in the clinical balance test, but it remains to be tested whether cognitive set shifting ability could serve as an earlier predictor of falls in preclinical populations. Earlier detection of changes in balance control could enable treatment when these changes are more amenable to adaptation (Zaback et al., 2019). These findings warrant further investigation of cortical engagement in balance recovery behavior guided by cognitive set shifting ability rather than just clinical balance ability, which may help explain why balance rehabilitation can be enhanced by cognitive training (Hagovska and Olekszyova, 2016).
These findings suggest that cognitive set shifting ability is expressed in balance recovery behavior earlier than previously suggested. The clinical balance test (Balance Evaluation Systems Test, or BESTest, later shortened to the miniBESTest) was designed to distinguish between levels of balance function and fall risk in people seeking treatment for balance-related disability (Horak et al., 2009; Franchignoni et al., 2010). The miniBESTest was not designed to measure subtle differences in balance recovery behavior in preclinical balance function, which may explain why we did not find associations between clinical balance ability and any other variable in the present study. By assessing more precise neuromechanical metrics of balance control in response to balance destabilization, we found that cognitive set shifting ability was associated with stiffer balance recovery mechanics and antagonist muscle activity that were not observed in the clinical balance test. While the majority of our participants had a high level of balance function, we chose not to exclude people with mild cognitive impairment to allow for variation in cognitive function to relate to balance recovery behavior. Although our cognitive set shifting scores were typical given the age and relatively high level of education of our participant cohort (Giovagnoli et al., 1996; Tombaugh, 2004; Steinberg et al., 2005), this should not be misinterpreted as an indication that our participants are free of the cognitive decline that would be expected for their ages. Indeed, roughly a quarter of our participants (5 of 19) scored below the cutoff value for mild cognitive impairment on the Montreal Cognitive Assessment (Nasreddine et al., 2005). In any case, most of the observed associations between cognitive set shifting and perturbation-evoked balance recovery behavior remained significant when accounting for age, education, overall cognition, and most of the other potentially confounding variables considered, indicating that our associations are specific to cognitive set shifting and not better explained by these other factors.
Difficulty shifting cognitive sets may extend to a related difficulty shifting motor sets in the cortically mediated phase of balance recovery behavior. People with lower cognitive set shifting ability had stiffer whole-body mechanics as evidenced by less center of mass motion in response to balance perturbations. While greater resistance to a balance disturbance would seem to suggest greater stability (Horak et al., 2005), the accompanying increase in antagonist activity, which is associated with clinical balance impairments (Lang et al., 2019), suggests this biomechanical stiffness does not reflect better balance control. Our directional specificity measure of antagonist activity was originally developed as a proxy measure for simultaneous cocontraction of agonist-antagonist muscle pairs that overcomes issues of comparing activity levels between muscles by instead comparing the activity of an individual muscle between agonist and antagonist contexts (Lang et al., 2019). However, because this measure compares the shift in activity of individual muscles between agonist and antagonist contexts, it could also be considered as a measure of motor set shifting. Directional specificity of the late phase of the soleus muscle was robustly associated with cognitive set shifting ability across perturbation magnitudes and robust to all potential confounds, but associations with the late phase of the tibialis anterior muscle were limited to larger perturbations and may be explained by lower overall cognition or female sex. The majority of our findings displayed a large effect size (i.e., Cohen’s F2 > 0.35), as smaller effects would require a larger sample size to observe. While these muscles are a small fraction of the muscle activity contributing to the overall balance recovery behavior, our data provide evidence that difficulty shifting cognitive sets may extend to a related difficulty shifting motor sets between perturbation directions in the cortically mediated phase of the motor response. A relationship between cognitive and motor set shifting is further supported by a recent finding that older adults with difficulty shifting cognitive sets had a related difficulty shifting between locomotor patterns on a split belt treadmill (Sombric and Torres-Oviedo, 2021). Because the motor set shifting association was not observed in the early phase of the perturbation-evoked motor response, it is unlikely that the influence of pre-perturbation cognitive state, such as anticipation, readiness, or arousal, on the brainstem-mediated response has overlapping mechanisms with cognitive set shifting. The association of motor set shifting during the later cortically mediated phase of the motor response suggests that instead the cortically mediated reaction of the perturbation may have overlapping mechanisms with cognitive set shifting, which is further supported by the enhanced cortical N1 responses.
Our findings suggest the cortical N1 has overlapping mechanisms with cognitive set shifting, and prior work linking the N1 to attention and perceived threat may help explain the subsequent antagonist activity. While prior studies have shown within-subjects changes in the cortical N1 with cognitive processes including attention (Quant et al., 2004; Little and Woollacott, 2015), perceived threat (Adkin et al., 2008; Mochizuki et al., 2010), and predictability (Adkin et al., 2006, 2008; Mochizuki et al., 2008, 2010), we believe this is the first study to demonstrate an association to between-subjects differences in cognitive ability. Given that set shifting ability is reflected in the cortical activity (100–200 ms) prior to the muscle activity (200–300 ms), it is possible that the N1 reflects cortical activity contributing to the subsequent antagonist activity, which could be tested in future studies by modulating cortical activity through therapeutic non-invasive brain stimulation (Taube et al., 2006; Jacobs et al., 2009). For example, after the perturbation, the participant may perceive a high threat or need for attention, which is reflected in the cortical N1 (Quant et al., 2004; Adkin et al., 2008; Mochizuki et al., 2010; Little and Woollacott, 2015), and subsequently engage a non-specific cocontraction strategy that does not incorporate the directional context into the behavior. Indeed, people report paying more attention to balance under more threatening conditions (Huffman et al., 2009) and display greater stiffness of postural sway (Carpenter et al., 2001, 2004, 2006). Greater perceived threat or fear of falling is also associated with agonist-antagonist cocontraction in both younger and older adults (Okada et al., 2001; Carpenter et al., 2006). Further, habituation of agonist-antagonist cocontraction with practice in high threat conditions is associated with habituation of the emotional response (Zaback et al., 2019), which may be easier to modify than the abnormal involuntary behavior observed at more severe stages of balance impairment (McKay et al., 2021). We previously suggested that the cortical N1 could reflect compensatory cortical control based on larger amplitudes in young adults with lower balance ability (Payne and Ting, 2020b) and on trials with compensatory steps (Payne and Ting, 2020a). This non-specific cocontraction could be another way in which compensatory cortical control is engaged. Although the supplementary motor area has direct connections to motor neurons (Goldberg, 1985), potential connections between the cortical N1 and subsequent muscle activation also include indirect routes, such as through projections from the supplementary motor area to the motor cortex or basal ganglia (Goldberg, 1985). However, any causal links between the cortical N1 and balance recovery behavior remain speculative until tested by further studies, particularly through methods that would disrupt the cortical activity, such as non-invasive brain stimulation, or dual task interference, which reduces the N1 amplitude (Quant et al., 2004; Little and Woollacott, 2015) and the late phase of the muscle activity (Rankin et al., 2000). However, the cortical N1 could serve as a potential biomarker for the development of a balance disorder without requiring a direct relationship to the muscle activity on a within-trial timescale. The cortical N1 has been frequently compared to the error-related negativity (Payne et al., 2019b), which occurs in response to mistakes in cognitive tasks. The error-related negativity, measured in 6-year-olds, can predict who will develop an anxiety disorder by 9 years old (Meyer et al., 2015), using a reduced electrode setup and measurement at a single electrode. As the N1 is in the early stages of validation as a between-subjects measure, much more work is needed to determine whether the N1 could predict the development of disorders on longitudinal timescales.
Well-established changes in prefrontal cortical activation in older adults may explain links between motor and cognitive behavior and have been previously demonstrated as a potential target for rehabilitation. As cognitive set shifting and the cortical N1 response have previously been localized to distinct brain areas, we can only speculate as to why they would be associated in the present findings. Cognitive set shifting depends on the dorsolateral prefrontal cortex (Zgaljardic et al., 2006; Ko et al., 2008a,b; Leite et al., 2011, 2013, 2020; Luthi et al., 2014; Gerrits et al., 2015; Tayeb and Lavidor, 2016; Imburgio and Orr, 2018), and the cortical N1 has been localized to the supplementary motor area (Marlin et al., 2014; Mierau et al., 2015), but there are several potential explanations as to why cognitive set shifting would be associated with the cortical N1 response despite their distinct brain regions. First, older adults recruit prefrontal cortical areas to a greater extent and more broadly than young adults for the same tasks (Reuter-Lorenz and Cappell, 2008), and lose functional segregation between different cortical areas (Chen et al., 2011; Damoiseaux, 2017; Chong et al., 2019; Cassady et al., 2020), which may result in coupled activation between cognitive and motor cortical areas. Accordingly, older adults tend to recruit prefrontal cortical areas broadly for balance and walking tasks (Stuart et al., 2018; Nobrega-Sousa et al., 2020; St George et al., 2021). However, the cortical N1 may not arise exclusively from the supplementary motor area, as there is evidence to suggest that multiple cortical sources synchronize in the theta frequency band to contribute to the cortical N1 response even in young adults (Peterson and Ferris, 2018, 2019). We speculate that increased synchronization between prefrontal and motor cortical areas during balance recovery with aging may explain associations between cognitive function and balance control in older adults. For example, we recently showed that functional connectivity in the beta frequency band between motor and prefrontal cortical areas during balance recovery in older adults is associated with cognitive dual task interference in walking (Palmer et al., 2021). A better understanding of the mechanisms linking balance and cognitive function in aging could reveal new therapeutic targets for rehabilitation and enable a more targeted exploration of the effects of cognitive training on balance rehabilitation (Smith-Ray et al., 2015; Hagovska and Olekszyova, 2016). For instance, it is well established that non-invasive stimulation of the dorsolateral prefrontal cortex can affect cognitive set shifting performance (Ko et al., 2008a,b; Leite et al., 2011, 2013, 2020; Luthi et al., 2014; Gerrits et al., 2015; Tayeb and Lavidor, 2016; Imburgio and Orr, 2018), but similar stimulation protocols are rarely applied to impact balance function despite links between cognitive set shifting and balance function dysfunction (Herman et al., 2010; Tangen et al., 2014; McKay et al., 2018) and evidence that such stimulation can reduce cognitive dual task interference on balance and walking behaviors (Manor et al., 2018).
Data Availability Statement
The raw data supporting the conclusions of this article will be made available by the authors, without undue reservation.
Ethics Statement
The studies involving human participants were reviewed and approved by Institutional Review Board of Emory University. The patients/participants provided their written informed consent to participate in this study.
Author Contributions
AP and LT conceived and designed the experiment. AP collected the data, performed all analyses, and drafted and revised the manuscript and figures. JP and JM contributed to the data analysis. LT and JP contributed to the interpretation of results and manuscript revision. All authors approved of the final manuscript.
Funding
This work was supported by the National Institutes of Health (Eunice Kennedy Shriver National Institute of Child Health and Human: R01 HD46922, F32 HD096816; National Institute of Neurological Disorders and Stroke: P50 NS 098685; National Center for Advancing Translational Sciences: UL1 TR000424), the Fulton County Elder Health Scholarship (2015–2017), and the Zebrowitz Award (2018).
Author Disclaimer
The content is solely the responsibility of the authors and does not necessarily represent the official views of the National Institutes of Health or other funding agencies.
Conflict of Interest
The authors declare that the research was conducted in the absence of any commercial or financial relationships that could be construed as a potential conflict of interest.
Publisher’s Note
All claims expressed in this article are solely those of the authors and do not necessarily represent those of their affiliated organizations, or those of the publisher, the editors and the reviewers. Any product that may be evaluated in this article, or claim that may be made by its manufacturer, is not guaranteed or endorsed by the publisher.
Supplementary Material
The Supplementary Material for this article can be found online at: https://www.frontiersin.org/articles/10.3389/fnagi.2021.742243/full#supplementary-material
Supplementary Figure 1 | No significant associations were observed between clinical balance ability and directional specificity of muscle activity. Condition-averaged muscle activity is shown for (A) soleus and (B) tibialis anterior muscles for example participants with higher or lower directional specificity scores. Muscle activity evoked by forward movements of the floor (resulting in backward leaning posture) is represented in magentas and activity evoked by backward movements of the floor is represented in blues, with darker colors for larger perturbations. Antagonist activity (i.e., soleus activity in forward perturbations and tibialis anterior activity in backward perturbations) is shaded for clarity. Vertical lines at 100 ms, 200 ms, and 300 ms mark the bounds of the time bins of interest. The later (200–300 ms) time bin is shaded in all panels. Directional specificity is plotted against miniBESTest scores for the (C) soleus muscle and (D) tibialis anterior muscle in each perturbation magnitude for early (100–200 ms) and late (200–300 ms) time bins.
Supplementary Figure 2 | Perturbation-evoked cortical N1 responses were not associated with clinical balance ability. Cortical N1 response amplitudes are plotted against miniBESTest scores in each of the perturbation conditions. Forward movements of the floor (resulting in backward leaning posture) are represented in magentas and backward movements of the floor are represented in blues, with darker colors for larger perturbations.
Footnotes
References
Adkin, A. L., Campbell, A. D., Chua, R., and Carpenter, M. G. (2008). The influence of postural threat on the cortical response to unpredictable and predictable postural perturbations. Neurosci. Lett. 435, 120–125. doi: 10.1016/j.neulet.2008.02.018
Adkin, A. L., Quant, S., Maki, B. E., and McIlroy, W. E. (2006). Cortical responses associated with predictable and unpredictable compensatory balance reactions. Exp. Brain Res. 172, 85–93. doi: 10.1007/s00221-005-0310-319
Burleigh, A., and Horak, F. (1996). Influence of instruction, prediction, and afferent sensory information on the postural organization of step initiation. J. Neurophysiol. 75, 1619–1628. doi: 10.1152/jn.1996.75.4.1619
Burleigh, A. L., Horak, F. B., and Malouin, F. (1994). Modification of postural responses and step initiation: evidence for goal-directed postural interactions. J. Neurophysiol. 72, 2892–2902. doi: 10.1152/jn.1994.72.6.2892
Carpenter, M. G., Adkin, A. L., Brawley, L. R., and Frank, J. S. (2006). Postural, physiological and psychological reactions to challenging balance: does age make a difference? Age Ageing 35, 298–303. doi: 10.1093/ageing/afl002
Carpenter, M. G., Frank, J. S., Adkin, A. L., Paton, A., and Allum, J. H. (2004). Influence of postural anxiety on postural reactions to multi-directional surface rotations. J. Neurophysiol. 92, 3255–3265. doi: 10.1152/jn.01139.2003
Carpenter, M. G., Frank, J. S., Silcher, C. P., and Peysar, G. W. (2001). The influence of postural threat on the control of upright stance. Exp. Brain Res. 138, 210–218. doi: 10.1007/s002210100681
Cassady, K., Ruitenberg, M. F. L., Reuter-Lorenz, P. A., Tommerdahl, M., and Seidler, R. D. (2020). Neural dedifferentiation across the lifespan in the motor and somatosensory systems. Cereb. Cortex 30, 3704–3716. doi: 10.1093/cercor/bhz336
Chen, Z. J., He, Y., Rosa-Neto, P., Gong, G., and Evans, A. C. (2011). Age-related alterations in the modular organization of structural cortical network by using cortical thickness from MRI. Neuroimage 56, 235–245. doi: 10.1016/j.neuroimage.2011.01.010
Chong, J. S. X., Ng, K. K., Tandi, J., Wang, C., Poh, J. H., Lo, J. C., et al. (2019). Longitudinal changes in the cerebral cortex functional organization of healthy elderly. J. Neurosci. 39, 5534–5550. doi: 10.1523/JNEUROSCI.1451-18.2019
Damiano, D. L. (1993). Reviewing muscle cocontraction. Phys. Occup. Ther. Pediatr. 12, 3–20. doi: 10.1080/J006v12n04_02
Damoiseaux, J. S. (2017). Effects of aging on functional and structural brain connectivity. Neuroimage 160, 32–40. doi: 10.1016/j.neuroimage.2017.01.077
Dietz, V., Quintern, J., and Sillem, M. (1987). Stumbling reactions in man: significance of proprioceptive and pre-programmed mechanisms. J. Physiol. 386, 149–163. doi: 10.1113/jphysiol.1987.sp016527
Duckrow, R. B., Abu-Hasaballah, K., Whipple, R., and Wolfson, L. (1999). Stance perturbation-evoked potentials in old people with poor gait and balance. Clin. Neurophysiol. 110, 2026–2032. doi: 10.1016/S1388-245700195-199
Franchignoni, F., Horak, F., Godi, M., Nardone, A., and Giordano, A. (2010). Using psychometric techniques to improve the balance evaluation systems test: the mini-BESTest. J. Rehabil. Med. 42, 323–331. doi: 10.2340/16501977-16501537
Gerrits, N. J. H. M., van den Heuvel, O. A., and van der Werf, Y. D. (2015). Decreased neural activity and neural connectivity while performing a set-shifting task after inhibiting repetitive transcranial magnetic stimulation on the left dorsal prefrontal cortex. BMC Neurosci. 16:45. doi: 10.1186/s12868-015-0181-183
Giovagnoli, A. R., Del Pesce, M., Mascheroni, S., Simoncelli, M., Laiacona, M., and Capitani, E. (1996). Trail making test: normative values from 287 normal adult controls. Ital. J. Neurol. Sci. 17, 305–309. doi: 10.1007/BF01997792
Gleason, C. E., Gangnon, R. E., Fischer, B. L., and Mahoney, J. E. (2009). Increased risk for falling associated with subtle cognitive impairment: secondary analysis of a randomized clinical trial. Dement. Geriatr. Cogn. Disord 27, 557–563. doi: 10.1159/000228257
Goldberg, G. (1985). Supplementary motor area structure and function: review and hypotheses. Behav. Brain Sci. 8, 567–616.
Gratton, G., Coles, M. G., and Donchin, E. (1983). A new method for off-line removal of ocular artifact. Electroencephalogr. Clin. Neurophysiol. 55, 468–484. doi: 10.1016/0013-469490135-90139
Hagovska, M., and Olekszyova, Z. (2016). Impact of the combination of cognitive and balance training on gait, fear and risk of falling and quality of life in seniors with mild cognitive impairment. Geriatr. Gerontol. Int. 16, 1043–1050. doi: 10.1111/ggi.12593
Harris, P. A., Taylor, R., Minor, B. L., Elliott, V., Fernandez, M., O’Neal, L., et al. (2019). The REDCap consortium: building an international community of software platform partners. J. Biomed. Inform. 95:103208. doi: 10.1016/j.jbi.2019.103208
Harris, P. A., Taylor, R., Thielke, R., Payne, J., Gonzalez, N., and Conde, J. G. (2009). Research electronic data capture (REDCap)–a metadata-driven methodology and workflow process for providing translational research informatics support. J. Biomed. Inform. 42, 377–381. doi: 10.1016/j.jbi.2008.08.010
Henry, S. M., Fung, J., and Horak, F. B. (1998). EMG responses to maintain stance during multidirectional surface translations. J. Neurophysiol. 80, 1939–1950. doi: 10.1152/jn.1998.80.4.1939
Herman, T., Mirelman, A., Giladi, N., Schweiger, A., and Hausdorff, J. M. (2010). Executive control deficits as a prodrome to falls in healthy older adults: a prospective study linking thinking, walking, and falling. J. Gerontol. A Biol. Sci. Med. Sci. 65, 1086–1092. doi: 10.1093/gerona/glq077
Horak, F. B., Diener, H. C., and Nashner, L. M. (1989). Influence of central set on human postural responses. J. Neurophysiol. 62, 841–853. doi: 10.1152/jn.1989.62.4.841
Horak, F. B., Dimitrova, D., and Nutt, J. G. (2005). Direction-specific postural instability in subjects with Parkinson’s disease. Exp. Neurol. 193, 504–521. doi: 10.1016/j.expneurol.2004.12.008
Horak, F. B., and Nashner, L. M. (1986). Central programming of postural movements: adaptation to altered support-surface configurations. J. Neurophysiol. 55, 1369–1381. doi: 10.1152/jn.1986.55.6.1369
Horak, F. B., Wrisley, D. M., and Frank, J. (2009). The balance evaluation systems test (BESTest) to differentiate balance deficits. Phys. Ther. 89, 484–498. doi: 10.2522/ptj.20080071
Huffman, J. L., Horslen, B. C., Carpenter, M. G., and Adkin, A. L. (2009). Does increased postural threat lead to more conscious control of posture? Gait Posture 30, 528–532. doi: 10.1016/j.gaitpost.2009.08.001
Imburgio, M. J., and Orr, J. M. (2018). Effects of prefrontal tDCS on executive function: methodological considerations revealed by meta-analysis. Neuropsychologia 117, 156–166. doi: 10.1016/j.neuropsychologia.2018.04.022
Jacobs, J. V., and Horak, F. B. (2007). Cortical control of postural responses. J. Neural. Transm. 114, 1339–1348. doi: 10.1007/s00702-007-0657-650
Jacobs, J. V., Lou, J. S., Kraakevik, J. A., and Horak, F. B. (2009). The supplementary motor area contributes to the timing of the anticipatory postural adjustment during step initiation in participants with and without Parkinson’s disease. Neuroscience 164, 877–885. doi: 10.1016/j.neuroscience.2009.08.002
Ko, J. H., Monchi, O., Ptito, A., Bloomfield, P., Houle, S., and Strafella, A. P. (2008a). Theta burst stimulation-induced inhibition of dorsolateral prefrontal cortex reveals hemispheric asymmetry in striatal dopamine release during a set-shifting task: a TMS-[C]raclopride PET study. Eur. J. Neurosci. 28, 2147–2155. doi: 10.1111/j.1460-9568.2008.06501.x
Ko, J. H., Monchi, O., Ptito, A., Petrides, M., and Strafella, A. P. (2008b). Repetitive transcranial magnetic stimulation of dorsolateral prefrontal cortex affects performance of the wisconsin card sorting task during provision of feedback. Int. J. Biomed. Imaging 2008:143238. doi: 10.1155/2008/143238
Koch, I., Gade, M., Schuch, S., and Philipp, A. M. (2010). The role of inhibition in task switching: a review. Psychon. Bull. Rev. 17, 1–14. doi: 10.3758/PBR.17.1.1
Lang, K. C., Hackney, M. E., Ting, L. H., and McKay, J. L. (2019). Antagonist muscle activity during reactive balance responses is elevated in Parkinson’s disease and in balance impairment. PLoS One 14:e0211137. doi: 10.1371/journal.pone.0211137
Leite, J., Carvalho, S., Fregni, F., Boggio, P. S., and Goncalves, O. F. (2013). The effects of cross-hemispheric dorsolateral prefrontal cortex transcranial direct current stimulation (tDCS) on task switching. Brain Stimul. 6, 660–667. doi: 10.1016/j.brs.2012.10.006
Leite, J., Carvalho, S., Fregni, F., and Goncalves, O. F. (2011). Task-specific effects of tDCS-induced cortical excitability changes on cognitive and motor sequence set shifting performance. PLoS One 6:e24140. doi: 10.1371/journal.pone.0024140
Leite, J., Gonçalves, ÓF., and Carvalho, S. (2020). Task switching ability is compromised after cross-hemispheric tDCS over the parietal cortex. Principles Practice Clin. Res. J. 6, 42–47. doi: 10.21801/ppcrj.2020.63.5
Little, C. E., and Woollacott, M. (2015). EEG measures reveal dual-task interference in postural performance in young adults. Exp. Brain Res. 233, 27–37. doi: 10.1007/s00221-014-4111-x
Lundin-Olsson, L., Nyberg, L., and Gustafson, Y. (1997). “Stops walking when talking” as a predictor of falls in elderly people. Lancet 349:617.
Luthi, M., Henke, K., Gutbrod, K., Nyffeler, T., Chaves, S., and Muri, R. M. (2014). In your eyes only: deficits in executive functioning after frontal TMS reflect in eye movements. Front. Behav. Neurosci. 8:7. doi: 10.3389/fnbeh.2014.00007
Magnani, P. E., Genovez, M. B., Porto, J. M., Zanellato, N. F. G., Alvarenga, I. C., Freire, R. C., et al. (2020). Use of the BESTest and the Mini-BESTest for fall risk prediction in community-dwelling older adults between 60 and 102 years of age. J. Geriatr. Phys. Ther. 43, 179–184. doi: 10.1519/JPT.0000000000000236
Manor, B., Zhou, J., Harrison, R., Lo, O. Y., Travison, T. G., Hausdorff, J. M., et al. (2018). Transcranial direct current stimulation may improve cognitive-motor function in functionally limited older adults. Neurorehabil. Neural Repair 32, 788–798. doi: 10.1177/1545968318792616
Marlin, A., Mochizuki, G., Staines, W. R., and McIlroy, W. E. (2014). Localizing evoked cortical activity associated with balance reactions: does the anterior cingulate play a role? J. Neurophysiol. 111, 2634–2643. doi: 10.1152/jn.00511.2013
McIlroy, W. E., and Maki, B. E. (1993). Changes in early ‘automatic’ postural responses associated with the prior-planning and execution of a compensatory step. Brain Res. 631, 203–211. doi: 10.1016/0006-899391536-91532
McKay, J. L., Lang, K. C., Bong, S. M., Hackney, M. E., Factor, S. A., and Ting, L. H. (2021). Abnormal center of mass feedback responses during balance: a potential biomarker of falls in Parkinson’s disease. PLoS One 16:e0252119. doi: 10.1371/journal.pone.0252119
McKay, J. L., Lang, K. C., Ting, L. H., and Hackney, M. E. (2018). Impaired set shifting is associated with previous falls in individuals with and without Parkinson’s disease. Gait Posture 62, 220–226. doi: 10.1016/j.gaitpost.2018.02.027
Meyer, A., Hajcak, G., Torpey-Newman, D. C., Kujawa, A., and Klein, D. N. (2015). Enhanced error-related brain activity in children predicts the onset of anxiety disorders between the ages of 6 and 9. J. Abnorm. Psychol. 124, 266–274. doi: 10.1037/abn0000044
Mierau, A., Hulsdunker, T., and Struder, H. K. (2015). Changes in cortical activity associated with adaptive behavior during repeated balance perturbation of unpredictable timing. Front. Behav. Neurosci. 9:272. doi: 10.3389/fnbeh.2015.00272
Mirelman, A., Herman, T., Brozgol, M., Dorfman, M., Sprecher, E., Schweiger, A., et al. (2012). Executive function and falls in older adults: new findings from a five-year prospective study link fall risk to cognition. PLoS One 7:e40297. doi: 10.1371/journal.pone.0040297
Mochizuki, G., Boe, S., Marlin, A., and McIlRoy, W. E. (2010). Perturbation-evoked cortical activity reflects both the context and consequence of postural instability. Neuroscience 170, 599–609. doi: 10.1016/j.neuroscience.2010.07.008
Mochizuki, G., Sibley, K. M., Esposito, J. G., Camilleri, J. M., and McIlroy, W. E. (2008). Cortical responses associated with the preparation and reaction to full-body perturbations to upright stability. Clin. Neurophysiol. 119, 1626–1637. doi: 10.1016/j.clinph.2008.03.020
Muir, S. W., Gopaul, K., and Montero Odasso, M. M. (2012). The role of cognitive impairment in fall risk among older adults: a systematic review and meta-analysis. Age. Ageing 41, 299–308. doi: 10.1093/ageing/afs012
Nashner, L. M. (1979). Organization and programming of motor activity during posture control. Prog. Brain Res. 50, 177–184. doi: 10.1016/S0079-612360818-60813
Nasreddine, Z. S., Phillips, N. A., Bedirian, V., Charbonneau, S., Whitehead, V., Collin, I., et al. (2005). The montreal cognitive assessment, MoCA: a brief screening tool for mild cognitive impairment. J. Am. Geriatr. Soc. 53, 695–699. doi: 10.1111/j.1532-5415.2005.53221.x
Nobrega-Sousa, P., Gobbi, L. T. B., Orcioli-Silva, D., Conceicao, N. R. D., Beretta, V. S., and Vitorio, R. (2020). Prefrontal cortex activity during walking: effects of aging and associations with gait and executive function. Neurorehabil. Neural Repair 34, 915–924. doi: 10.1177/1545968320953824
Okada, S., Hirakawa, K., Takada, Y., and Kinoshita, H. (2001). Relationship between fear of falling and balancing ability during abrupt deceleration in aged women having similar habitual physical activities. Eur. J. Appl. Physiol. 85, 501–506. doi: 10.1007/s004210100437
Ozdemir, R. A., Contreras-Vidal, J. L., and Paloski, W. H. (2018). Cortical control of upright stance in elderly. Mech. Ageing Dev. 169, 19–31. doi: 10.1016/j.mad.2017.12.004
Palmer, J. A., Payne, A. M., Ting, L. H., and Borich, M. R. (2021). Cortical engagement metrics during reactive balance are associated with distinct aspects of balance behavior in older adults. Front. Aging Neurosci. 13:684743. doi: 10.3389/fnagi.2021.684743
Payne, A. M., Hajcak, G., and Ting, L. H. (2019a). Dissociation of muscle and cortical response scaling to balance perturbation acceleration. J. Neurophysiol. 121, 867–880. doi: 10.1152/jn.00237.2018
Payne, A. M., Ting, L. H., and Hajcak, G. (2019b). Do sensorimotor perturbations to standing balance elicit an error-related negativity? Psychophysiology 56:e13359. doi: 10.1111/psyp.13359
Payne, A. M., and Ting, L. H. (2020a). Balance perturbation-evoked cortical N1 responses are larger when stepping and not influenced by motor planning. J. Neurophysiol. 124, 1875–1884. doi: 10.1152/jn.00341.2020
Payne, A. M., and Ting, L. H. (2020b). Worse balance is associated with larger perturbation-evoked cortical responses in healthy young adults. Gait Posture 80, 324–330. doi: 10.1016/j.gaitpost.2020.06.018
Peterson, S. M., and Ferris, D. P. (2018). Differentiation in theta and beta electrocortical activity between visual and physical perturbations to walking and standing balance. eNeuro 5:ENEURO.0207-18.2018. doi: 10.1523/ENEURO.0207-18.2018ENEURO0207182018.
Peterson, S. M., and Ferris, D. P. (2019). Group-level cortical and muscular connectivity during perturbations to walking and standing balance. Neuroimage 198, 93–103. doi: 10.1016/j.neuroimage.2019.05.038
Pieruccini-Faria, F., Lord, S. R., Toson, B., Kemmler, W., and Schoene, D. (2019). Mental flexibility influences the association between poor balance and falls in older people - a secondary analysis. Front. Aging Neurosci. 11:133. doi: 10.3389/fnagi.2019.00133
Prochazka, A. (1989). Sensorimotor gain control: a basic strategy of motor systems? Prog. Neurobiol. 33, 281–307.
Quant, S., Adkin, A. L., Staines, W. R., Maki, B. E., and McIlroy, W. E. (2004). The effect of a concurrent cognitive task on cortical potentials evoked by unpredictable balance perturbations. BMC Neurosci. 5:18. doi: 10.1186/1471-2202-5-18
Rankin, J. K., Woollacott, M. H., Shumway-Cook, A., and Brown, L. A. (2000). Cognitive influence on postural stability: a neuromuscular analysis in young and older adults. J. Gerontol. A Biol. Sci. Med. Sci. 55, M112–M119.
Reuter-Lorenz, P. A., and Cappell, K. A. (2008). Neurocognitive aging and the compensation hypothesis. Curr. Direct. Psychol. Sci. 17, 177–182. doi: 10.1111/j.1467-8721.2008.00570.x
Safavynia, S. A., and Ting, L. H. (2013). Long-latency muscle activity reflects continuous, delayed sensorimotor feedback of task-level and not joint-level error. J. Neurophysiol. 110, 1278–1290. doi: 10.1152/jn.00609.2012
Sanchez-Cubillo, I., Perianez, J. A., Adrover-Roig, D., Rodriguez-Sanchez, J. M., Rios-Lago, M., Tirapu, J., et al. (2009). Construct validity of the trail making test: role of task-switching, working memory, inhibition/interference control, and visuomotor abilities. J. Int. Neuropsychol. Soc. 15, 438–450. doi: 10.1017/S1355617709090626
Shumway-Cook, A., Woollacott, M., Kerns, K. A., and Baldwin, M. (1997). The effects of two types of cognitive tasks on postural stability in older adults with and without a history of falls. J. Gerontol. A Biol. Sci. Med. Sci. 52, M232–M240.
Smith-Ray, R. L., Hughes, S. L., Prohaska, T. R., Little, D. M., Jurivich, D. A., and Hedeker, D. (2015). Impact of cognitive training on balance and gait in older adults. J. Gerontol. B Psychol. Sci. Soc. Sci. 70, 357–366. doi: 10.1093/geronb/gbt097
Sombric, C. J., and Torres-Oviedo, G. (2021). Cognitive and motor perseveration are associated in older adults. Front. Aging Neurosci. 13:610359. doi: 10.3389/fnagi.2021.610359
St George, R. J., Hinder, M. R., Puri, R., Walker, E., and Callisaya, M. L. (2021). Functional near-infrared spectroscopy reveals the compensatory potential of pre-frontal cortical activity for standing balance in young and older adults. Neuroscience 452, 208–218. doi: 10.1016/j.neuroscience.2020.10.027
Steinberg, B. A., Bieliauskas, L. A., Smith, G. E., and Ivnik, R. J. (2005). Mayo’s older americans normative studies: age- and iq-adjusted norms for the trail-making test, the stroop test, and MAE controlled oral word association test. Clin. Neuropsychol. 19, 329–377. doi: 10.1080/13854040590945210
Stuart, S., Vitorio, R., Morris, R., Martini, D. N., Fino, P. C., and Mancini, M. (2018). Cortical activity during walking and balance tasks in older adults and in people with Parkinson’s disease: a structured review. Maturitas 113, 53–72. doi: 10.1016/j.maturitas.2018.04.011
Tangen, G. G., Engedal, K., Bergland, A., Moger, T. A., and Mengshoel, A. M. (2014). Relationships between balance and cognition in patients with subjective cognitive impairment, mild cognitive impairment, and Alzheimer disease. Phys. Ther. 94, 1123–1134. doi: 10.2522/ptj.20130298
Taube, W., Schubert, M., Gruber, M., Beck, S., Faist, M., and Gollhofer, A. (2006). Direct corticospinal pathways contribute to neuromuscular control of perturbed stance. J. Appl. Physiol. 101, 420–429. doi: 10.1152/japplphysiol.01447.2005
Tayeb, Y., and Lavidor, M. (2016). Enhancing switching abilities: improving practice effect by stimulating the dorsolateral pre frontal cortex. Neuroscience 313, 92–98. doi: 10.1016/j.neuroscience.2015.11.050
Tombaugh, T. (2004). Trail making test A and B: normative data stratified by age and education. Arch. Clin. Neuropsychol. 19, 203–214. doi: 10.1016/s0887-617700039-38
Torres-Oviedo, G., and Ting, L. H. (2007). Muscle synergies characterizing human postural responses. J. Neurophysiol. 98, 2144–2156. doi: 10.1152/jn.01360.2006
Varghese, J. P., Staines, W. R., and McIlroy, W. E. (2019). Activity in functional cortical networks temporally associated with postural instability. Neuroscience 401, 43–58. doi: 10.1016/j.neuroscience.2019.01.008
Weerdesteyn, V., Laing, A. C., and Robinovitch, S. N. (2008). Automated postural responses are modified in a functional manner by instruction. Exp. Brain Res. 186, 571–580. doi: 10.1007/s00221-007-1260-1261
Zaback, M., Adkin, A. L., and Carpenter, M. G. (2019). Adaptation of emotional state and standing balance parameters following repeated exposure to height-induced postural threat. Sci. Rep. 9:12449. doi: 10.1038/s41598-019-48722-z
Keywords: posture, aging, cortex, antagonist, cocontraction, EEG, motor
Citation: Payne AM, Palmer JA, McKay JL and Ting LH (2021) Lower Cognitive Set Shifting Ability Is Associated With Stiffer Balance Recovery Behavior and Larger Perturbation-Evoked Cortical Responses in Older Adults. Front. Aging Neurosci. 13:742243. doi: 10.3389/fnagi.2021.742243
Received: 15 July 2021; Accepted: 16 November 2021;
Published: 06 December 2021.
Edited by:
Simone Reppermund, University of New South Wales, AustraliaReviewed by:
Federico Gennaro, Children’s Hospital Los Angeles, United StatesIng-Shiou Hwang, National Cheng Kung University, Taiwan
Copyright © 2021 Payne, Palmer, McKay and Ting. This is an open-access article distributed under the terms of the Creative Commons Attribution License (CC BY). The use, distribution or reproduction in other forums is permitted, provided the original author(s) and the copyright owner(s) are credited and that the original publication in this journal is cited, in accordance with accepted academic practice. No use, distribution or reproduction is permitted which does not comply with these terms.
*Correspondence: Lena H. Ting, bHRpbmdAZW1vcnkuZWR1