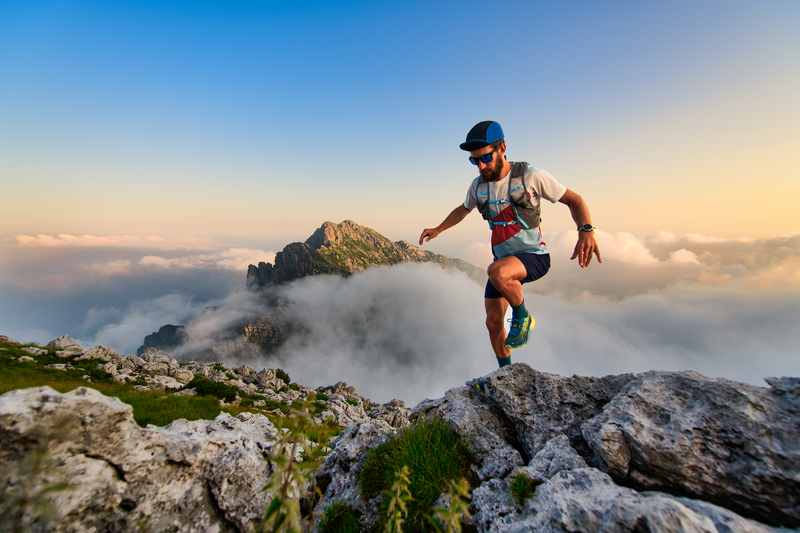
95% of researchers rate our articles as excellent or good
Learn more about the work of our research integrity team to safeguard the quality of each article we publish.
Find out more
REVIEW article
Front. Aging Neurosci. , 05 August 2021
Sec. Neuroinflammation and Neuropathy
Volume 13 - 2021 | https://doi.org/10.3389/fnagi.2021.717745
This article is part of the Research Topic Potential Neurotoxic Effects of Perioperative Factors View all 25 articles
Sevoflurane is one of the most commonly used inhaled anesthetics due to its low blood gas coefficient, fast onset, low airway irritation, and aromatic smell. However, recent studies have reported that sevoflurane exposure may have deleterious effects on cognitive function. Although neuroinflammation was most widely mentioned among the established mechanisms of sevoflurane-induced cognitive dysfunction, its upstream mechanisms have yet to be illustrated. Thus, we reviewed the relevant literature and discussed the most mentioned mechanisms, including the modulation of the microglial function, blood–brain barrier (BBB) breakdown, changes in gut microbiota, and ease of cholinergic neurotransmission to help us understand the properties of sevoflurane, providing us new perspectives for the prevention of sevoflurane-induced cognitive impairment.
Postoperative cognitive dysfunction, which has been included in the new conception of perioperative neurocognitive disorders, is a complication that occurs following surgery, especially in elderly people (>60 years old). It is worth noting that the trend for using anesthesia with surgery in elderly people is increasing (Miller et al., 2018). Among the anesthetics, sevoflurane has been widely used as an inhaled general anesthetic for anesthesia induction and maintenance in a variety of surgical treatments due to its low blood gas coefficient, fast onset, low airway irritation, and aromatic smell (Al Tmimi et al., 2015; Liu et al., 2015; Ye et al., 2016). In recent years, although sevoflurane was considered safe with a rapid clearance upon maintenance cessation, many studies have reported its capability to cause cognitive dysfunction (Fang et al., 2012; Lv et al., 2017; Zhu G. et al., 2017; Guo et al., 2018; Zhang et al., 2019).
To date, despite having various mechanisms of sevoflurane-induced cognitive dysfunction being mentioned by researchers, the exact pathophysiological changes remain unclear. Neuroinflammation, which was found to be associated with neurodegenerative diseases (Herranz et al., 2016; Melah et al., 2016), was found to be the most frequently mentioned mechanism. Several studies have shown that dysregulated neuroinflammation plays a key role in cognitive dysfunction (Cibelli et al., 2010; Terrando et al., 2011; Vacas et al., 2013; Riedel et al., 2014) through a variety of downstream mechanisms, including detrimental connectivity of brain network secondary to the dysfunction of synaptic transmission (Xiong et al., 2003), Tau phosphorylation, plaque formation, and dystrophic neurite growth (Streit et al., 2004). Due to this, sevoflurane might be one of the upstream causes of neuroinflammation (Zhang et al., 2013; Zheng et al., 2017; Cui et al., 2018), which can be exacerbated by other interferences such as sleep deprivation (Hou et al., 2019) and early-life adversity (Zhu Y. et al., 2017). However, the exact mechanisms underlying sevoflurane-induced neuroinflammation have yet to be elucidated.
Although the microglia were first recognized as immune surveillance cells of the central nervous system (CNS), recent studies have found them as the multifunctional cells that might interact with other CNS cells, which are associated with neurodegenerative diseases, such as Alzheimer’s disease (AD), Parkinson’s disease (PD), schizophrenia, autism, and multiple sclerosis (MS) (Prinz et al., 2019). Notably, one of the key features shared by these neurodegenerative diseases was microglia-mediated neuroinflammation. Concerning this, sevoflurane might induce the classical activation of microglia (M1), which is responsible for the production of proinflammatory cytokines, thereby reducing the alternative activation and acquired deactivation (M2), which are associated with anti-inflammation, reconstruction of extracellular matrix, and tissue repair (Tang and Le, 2016).
Aside from the inflammation initiated in the CNS, sevoflurane might also mediate the permeability of the blood–brain barrier (BBB) (Sun et al., 2019) and affect peripheral factors, such as peripheral immune cells and gut microbiota (Han et al., 2021), which have been widely explored in recent years (Figure 1). Another factor, the cholinergic synaptic transmission, has also been found to be involved in the inflammation modulation (Kalb et al., 2013), in which sevoflurane might play a proinflammatory role via the suppression of cholinergic neurotransmission (Yin et al., 2019; Figure 2).
Figure 1. Sevoflurane modulating microglia and peripheral factors involved in neuroinflammation. Sevoflurane induced Tau transfer from the neurons to the microglia, thereby activating it to produce neurotoxic inflammatory molecules and cytokines, such as IL-1β and TNF-α; later, Tau can be phagocytized and secreted extracellularly and transmitted into the neuron again; sevoflurane induced microglia polarized into M1 activation via the NF-κB signaling pathway and inhibited its M2 activation by mediating SOCS1, SOCS3, and STAT6; sevoflurane induced macrophage inflammation, as shown by IL-1α, IL-1β, MIP-2, IFN-γ, and TNF-α increases; sevoflurane flattened the endothelial cell luminal surface and enlarged its perivascular spaces; and cytokines (IL-1β, IL-1, TNF-α, and IL-6) induced depression and redistribution of BBB adherens junction proteins (ZO-1, occludin, and vinculin); sevoflurane increased RAGE and decreased IRP-1 expressions; sevoflurane decreased intestinal microbiome abundance and diversity.
Figure 2. Sevoflurane decreased cholinergic neurotransmission. Sevoflurane partially decreased presynaptic acetylcholine (ACh) by inhibiting calcium currents, sevoflurane decreased postsynaptic ACh by increasing acetylcholinesterase (AChE), and sevoflurane reduced ACh binding with ACh receptors (AChRs) on the immune cells to inhibit the release of proinflammatory cytokines.
In consideration of the clinical significance of sevoflurane, this study attempts to synthesize and discuss the mechanisms of sevoflurane-induced neuroinflammation, as all these mechanisms might be promising therapeutic targets that could be used to prevent, either partly or in total, neuroinflammation and consequent cognitive dysfunction.
Prior to the use of the term “neuroinflammation,” immune responses have already been found to play a critical role in neurodegenerative diseases such as AD (McGeer et al., 1987; Griffin et al., 1989). Neurodegeneration is more aptly referred to the innate immune system in the brain rather than the adaptive immunity because leukocyte infiltration is limited. However, the intrinsic immune cells of the brain, especially microglia, are prominently activated in these situations (Streit et al., 2004). As early as 1932, the microglia were first mentioned by Pio del Río-Hortega to function as the macrophages of the CNS, but there have been few investigations followed up in the next 50 years using the limited methods that can distinguish microglia from other cells. Until 1995, microglia were known to be the vital components of the brain immune system (Streit and Kincaid-Colton, 1995). Specifically, they can be activated by adverse factors and are the first cells that appear to cause a neuroinflammatory response (Dheen et al., 2007). In fact, microglial activation is a hallmark of neuroinflammatory and neurodegenerative diseases, such as AD, PD, Huntington’s disease (HD), and amyotrophic lateral sclerosis (ALS) (Prinz et al., 2019).
Microglia can be polarized into two different activated states, namely, the classical activation (M1) that is characterized by proinflammation and production of reactive oxygen species, which is harmful to neurons, and the alternative activation (M2), which is typified by its opposite anti-inflammatory function and is helpful for tissue repair and wound healing (Boche et al., 2013). Sevoflurane can accelerate microglial migration, promote their activation, enhance their phagocytic efficiency, and promote their proliferation (Dang et al., 2018). In particular, (Zhang et al., 2013), reported that 4% sevoflurane for 6 h led to an increase of interleukin-6 (IL-6) via the nuclear factor-kappa B (NF-κB) pathway in isolated microglia (Zhang et al., 2013). The NF-κB signaling pathway has been well explored and is known to be associated with inflammation and cognitive impairment (Kaushal and Schlichter, 2008; Wang et al., 2014; Yang and Yuan, 2018), one of which is the microglial M1 activation (Kobayashi et al., 2013). Additionally, the activation of NF-κB signaling pathway can be induced by Ca2+ elevation, in which even the local Ca2+ transients may cause apparent NF-κB capacity (Meffert et al., 2003). Sevoflurane also increases the cytosolic calcium via the activation of inositol 1,4,5-trisphosphate receptors, leading to abnormal calcium release from the endoplasmic reticulum (Yang et al., 2008).
In contrast, sevoflurane not only enhances M1 polarization but also simultaneously suppresses M2 activation (Pei et al., 2017). Pei et al. (2017) found that in primary microglial cells isolated from the cerebral cortices of mice, the pretreatment of sevoflurane (2 or 4%) for 12 h before IL-4 (10 ng/ml) can suppress IL-4-induced increases of characteristic M2 marker genes Arg1, Ym1, and IL-10. The protein levels of the aforementioned genes were also inhibited by sevoflurane. Furthermore, other factors involved in M2 polarization, such as suppressor of cytokine signaling one upregulation, SOCS3 suppression, and signal transducer and activator of transcription 6 (STAT6) phosphorylation were found to be regulated by sevoflurane in a dose-dependent manner. In the same experiment, a similar conclusion was drawn in another model of human umbilical cord mesenchymal stromal cells–induced M2 polarization, in which sevoflurane attenuated Arg1, Ym1, and IL-10 protein and mRNA levels.
Moreover, both Tau and amyloid-beta (Aβ) peptides, as mentioned below, are canonical features of AD. Tau peptides can transfer between cells in a prion-like fashion, in which this seeding activity has been associated with AD progression (Laurent et al., 2018). Due to this, the relationship between microglia and the speed of Tau activity might be involved in a vicious cycle described as the following: first, Tau is transferred from the neurons to the microglia, thereby activating it to produce neurotoxic inflammatory molecules and cytokines, such as IL-1β and tumor necrosis factor-α (TNF-α); afterward, Tau can be phagocytized and secreted extracellularly, consequently increasing the progressive spread of tauopathy. Microglial phagocytosis further aggregates the spreading of Tau, given that the secreted Tau was easily transmissible to the neurons (Furman et al., 2017). Most recently, Dong et al. (2021) demonstrated that sevoflurane also induced Tau migration from the neurons to the microglia, leading to IL-6 generation and cognitive impairment. Therefore, the influence of sevoflurane on Tau migration might further worsen the neuroinflammation.
Previously, peripheral immune cells were thought to have less influence on the CNS due to the presence of the BBB; however, contradictory results have been found in models of patients with systemic inflammation and PD, in which monocytes are trafficked into the brain, influencing inflammatory CNS signaling (Zhou et al., 2009; Grozdanov et al., 2014). One of the specific mechanisms may be that proinflammatory factors break the BBB (reviewed in the section “Permeability Changes of Blood–Brain Barrier”).
The BBB, formed with a monolayer of brain vascular endothelial cells (BVECs) and supported by astrocytes, pericytes, and a basement membrane, is essential for the regulation of the neural environment and brain homeostasis (Kniesel and Wolburg, 2000; Begley and Brightman, 2003; Abbott et al., 2010). The core element of the BBB is the formation of the blood vessel by the BVECs, acting as a physical barrier to control the microenvironment of cerebral cells. Unlike the peripheral tissue endothelium, BVECs in the brain are unique, as they are connected by numerous tight junctions that severely restrict ionic penetration and fluid movements (Abbott et al., 2006). The dysfunction of BBB is associated with a wide range of neurodegenerative diseases like AD, PD (Desai et al., 2007), and multiple system atrophy (Song et al., 2011).
Previous studies have focused on the protective role of sevoflurane in the BBB, but these articles were often in the precondition of adverse factors, such as surgery (Gao et al., 2019) or cerebral ischemia and reperfusion (Yu Q. et al., 2019). For example, Gao et al. (2019) reported that a short-time (i.e., 2 h) exposure to sevoflurane did not change cognitive impairment in rats; on the contrary, the Aβ, one of the main factors of postoperative cognitive dysfunction (Xu et al., 2014), was significantly eliminated by sevoflurane treatment via upregulating the expression of Aquaporin-4, a water channel expressed in astrocytic end feet. Additionally, Yu Q. et al. (2019) reported that sevoflurane improved the integrity of BBB after ischemia and reperfusion in rats, and they found that 1.5% of sevoflurane preconditioning induced migration of astrocyte toward infarct areas to form scaffolds facilitating neuroblast migration, and then subsequently promoted the formation of neural networks, which closely resemble those of the normal brain.
However, with regards to the aim of this article, there are also studies on sevoflurane-induced BBB disruption, which have mainly focused on BVECs. In particular, Acharya et al. (2015) found in an in vivo study of rats that exposure to 1–3% sevoflurane for 3 h resulted in the compromised BBB integrity and increased permeability, as shown by immunoglobulin G augmentation that leaked into the brain. Additionally, the structural changes in BBB-associated BVECs were observed in this study, resulting in a general flattening of the BVEC luminal surface and subsequent death. Similarly, Sun et al. (2019) observed enlarged perivascular spaces after the sevoflurane exposure in a duration-dependent manner, in which 78% of the capillaries were destroyed after 6 h of 2% sevoflurane treatment.
Although the astrocytes play a vital role in the maintenance of BBB integrity, there have been few studies attributing the disruption of sevoflurane-induced BBB to these astrocytes. It has been reported that the lengthy sevoflurane exposure disrupted the maturation of “tripartite synapse” by altering astrocyte morphogenesis and causing consequential behavioral deficits (Zhou et al., 2019). Despite the apparent adverse impact of sevoflurane on astrocytes, no researchers have linked this impact to direct BBB breakdown. More research is needed to clarify whether sevoflurane can damage BBB by regulating the function of astrocytes.
Interestingly, proinflammatory cytokines, such as IL-1 and TNF-α, can also induce BBB disruption, and their synergistic effects have also been reported (Quagliarello et al., 1991). As those tight junction proteins such as occludin play pivotal roles in maintaining the BBB integrity (Zehendner et al., 2011; Lv et al., 2018), TNF-α decreases the occludin in astrocytes through its specific effect on the TNF type-1 receptor via NF-κB activation (Wachtel et al., 2001). All these effects of TNF-α, such as the decreased levels of tight junction protein and the elevation of BBB permeability, have been attributed to the production of microvascular endothelial IL-6 (Rochfort et al., 2016). Similarly, IL-1β was found to induce neutrophil adhesion to the endothelial cells, decreasing the levels of occludin and zonula occludens protein 1 (ZO-1) and redistributing vinculin, another adherens junction protein, to enhance BBB permeability (Bolton et al., 1998). Thus, the breakdown of BBB may also still be the downstream mechanism of neuroinflammation, in turn, to further aggravate neuroinflammation.
Except for the structural breakdown of BBB, the receptors of the barrier, including receptors for advanced glycation end products (RAGE) and receptors for low-density lipoprotein receptor–related protein 1, play a vital role in the brain Aβ exchanges (Shibata et al., 2000; Deane et al., 2003; Herz and Marschang, 2003). More specifically, the expression of microglial RAGE enhanced the infiltration of astrocyte and microglial and increased the production of IL-1 and TNF-α (Fang et al., 2010). In particular, the study by Liu et al. (2013) proved that exposure to 2% sevoflurane for 2 h will lead to the increased RAGE and decreased IRP-1 in rats, possibly attributing that sevoflurane might induce neuroinflammation by regulating the expressions of RAGE and IRP-1, ultimately leading to Aβ and cytokine accumulation in the brain.
The dysregulation of gut microbe might also influence the brain through immune molecules, hormones, and relevant metabolites (Mayer et al., 2015; Smith, 2015), such as impairing BBB function by regulating tight junction proteins (Braniste et al., 2014), modulating brain development and behavior by altering canonical signaling pathways, expressing synaptic plasticity–related proteins and allowing neurotransmitter turnover (Diaz Heijtz et al., 2011). The correlations between gut microbes and human neurological diseases, such as MS, PD, AD, HD, ALS, and neuromyelitis optica spectrum disorders, have been well illustrated in previous studies (Tremlett et al., 2017). The gastrointestinal microbiota were further proven to be a key neuroinflammation modulator, possibly by recruiting monocytes into the brain and/or modulating the stress response via the regulation of the activity of the hypothalamic–pituitary–adrenal axis (Rea et al., 2016). Given that gut microbes have been well explored in human neurological diseases and Rea et al. (2016) have reviewed the relationship between gut microbiome and neuroinflammation, the role of gut microbes in regulating neuroinflammation may be compelling.
Frohlich et al. (2016) performed an in vivo study in rats that the short-term antibiotic use (mixed antibiotics of ampicillin, bacitracin, meropenem, neomycin, and vancomycin at 8–11 weeks of age for 11 days) significantly disrupted the gut bacterial community and impaired the cognitive performance. Although researchers have discussed the antibacterial activity of the halogenated anesthetic decades ago, these were always studied at very high concentrations (Wardley-Smith and Nunn, 1971; Molliex et al., 1998), which carries no actual clinical significance. Until recently, a new study performed by Han et al. (2021) finally gave us a certain answer, showing that a clinical concentration of sevoflurane did change the gut microbiota. More specifically, 1.3 minimum alveolar concentration (MAC) of sevoflurane gas (i.e., 2.21%) for 4 h was found to have decreased the diversity and abundance of the intestinal microbiome. Similarly, a previous study conducted on isoflurane proved that one MAC isoflurane for 4 h in juvenile rats (postnatal Day 7) significantly changed their gut microbiota in the long term (i.e., 42 days), in which such changes may be the potential mechanism of isoflurane-induced cognitive dysfunction (Wang et al., 2019).
It is worth noting that Chamberlain et al. (2017) provided a new perspective on the influence of sevoflurane on bacterial behavior, revealing that sevoflurane at clinically relevant concentrations (i.e., 1.5% and 3%) reduced Escherichia coli and Pseudomonas aeruginosa swimming, and conversely enhanced the biofilm formation of Staphylococcus aureus and Enterococcus faecalis, which are the important behaviors in bacteria for liquid mobility and consequent antibiotic resistance. This study also provides a new orientation to evaluate the potential impact of sevoflurane on gut bacteria, rather than focusing on the abundance and diversity of the intestinal microbiome. Unfortunately, neither sevoflurane nor isoflurane experiments have been further extended to the regulation of neuroinflammation after the disturbance of the intestinal microbiome. This should be one of the key directions for future research on neuroinflammation caused by the inhaled anesthetic.
Although there are few direct studies on the regulation of the intestinal microbiome by sevoflurane, in line with the results of the isoflurane study, the effect of sevoflurane on reducing the diversity and abundance of the intestinal microbiome may be convincing. According to the existing studies, when exploring the mechanism of sevoflurane-induced neuroinflammation, it may be safe to put forward the influence of the gut microbiota–brain axis, though more follow-up experiments are needed to prove these findings further.
The selected cholinergic neuron loss in the canonical neuroinflammation-related disease, AD, has been observed decades ago (Davies and Maloney, 1976). The principal neurotransmitter of the vagus nerve is acetylcholine (ACh), which is associated with cognition and learning ability (Conner et al., 2003; Gotti et al., 2006; Lima et al., 2014). There are two types of ACh receptors (AChRs), namely, the muscarinic receptor (mAChR) and nicotinic receptor (nAChR), which are distributed in both the CNS and periphery. Although the ACh anti-inflammatory function was mostly found with the nAChRs, or more specifically, the subdivided α7 nicotinic acetylcholine receptors (α7nAChRs) (Pavlov and Tracey, 2005), the contribution of mAChR has also been reported (Fujii et al., 2017). The core of the cholinergic anti-inflammatory pathway is the α7nAChRs, which are expressed on the surfaces of the immune cell and are known to be required for the cholinergic inhibition of TNF and other proinflammatory cytokines, such as IL-1β and high-mobility group protein B1 (Wang et al., 2003, 2004). Peripheral immune cells such as macrocytes and cerebral immune cells, such as microglia and astrocytes, all have α7nAChRs, in which ACh binding can reduce the production of proinflammatory cytokine.
In addition, it is clear that anti-inflammation and pro-resolution are not equivalent processes. Inflammation resolution, involving pro-resolving mediators, is also vital for the tissues affected by inflammation to regain function (Serhan and Savill, 2005). Meanwhile, Mirakaj et al. (2014) reported that the resolution and pro-resolving mediators of inflammation were controlled by the vagus nerve. Although the vagus nerve induced the anti-inflammatory pathway and pro-resolution function might be vital for neuroinflammation prevention, ACh transmission might also be inhibited by sevoflurane, and the potential mechanisms are reviewed as follows.
It has been reported that the clinical concentration of sevoflurane can inhibit ACh release in a dose-dependent manner (Shichino et al., 1998). Furthermore, an experiment by Chen et al. (2015) in Drosophila melanogaster showed that 1, 2, and 3% sevoflurane for 24 h reduced presynaptic cholinergic neurotransmission, partially by inhibiting calcium current. Interestingly, the in vitro study by Naruo et al. (2005) demonstrated that a clinically relevant sevoflurane concentration (i.e., 0.5–3%) inhibited the postsynaptic cholinergic neurotransmitters without the influence of presynaptic exocytosis or endocytosis, which instead functioned by nAChR blocking on the postsynaptic neuron. Despite these findings, the exact mechanism by which ACh moves to the α7nAChRs on immune cells remains to be elucidated, and it has been postulated that the ACh diffuses to α7nAChRs following its release from the vagus nerve axon terminals. Thus, supposing that this postulate is true, nAChR blockage on the postsynaptic neuron via sevoflurane induction increases ACh diffusion to the immune cells. However, this effect might be insignificant because aside from decreasing presynaptic and postsynaptic cholinergic neurotransmitters, sevoflurane might also increase acetylcholinesterase (AChE) activity and expression (Yin et al., 2019). In consideration of the rapid AChE catabolism, we assumed that ACh redundancy caused by the nAChR blockage on the postsynaptic neuron might not have influenced the diffused quantity, although more studies are needed to verify this hypothesis.
In consideration of the clinical importance of sevoflurane, understanding its properties and figuring out its potential noxious effects are significant. Although numerous studies have explored the mechanism of sevoflurane-induced cognitive dysfunction and have brought up multiple mechanisms to support these hypotheses, neuroinflammation was the most mentioned in recent years. Meanwhile, our understanding of the upstream mechanisms of how sevoflurane induces neuroinflammation was enriched with time. Aside from cerebral tissue–initiated inflammation, BBB breakdown, modulation of cholinergic anti-inflammatory pathway, the influence of sevoflurane on peripheral factors and gut microbiota was also investigated. Although the mechanisms of sevoflurane induced-neuroinflammation might not limit to the aforementioned mechanisms, they should still be promising orientations for researchers to understand the impact of sevoflurane on cognitive function well.
In fact, the effects of sevoflurane on neuroinflammation remain controversial. In addition to its proinflammatory function, this anesthetic was also shown to reduce neuroinflammation caused by adverse effects, such as brain ischemic diseases, which was its most evidenced benefit. For instance, the study by Hwang et al. (2017) showed that sevoflurane postconditioning decreased the production of multiple proinflammatory cytokines such as TNF-α, IL-6, and IL-1β, via the Toll-like receptor-4/NF-κB pathway, which was activated by cerebral ischemia/reperfusion injury. Similarly, Xue et al. (2020) reported that sevoflurane postconditioning significantly attenuated the activation of hypoxic–ischemic brain injury–induced microglia/macrophage and impairment of the cognitive function.
In line with this, sevoflurane might also play a dual role in microglial activation, in which Xie et al., found that sevoflurane treatment increased expressions of NF-κB and cytokine IL-6 in isolated neuroglioma cells (Zhang et al., 2013); however, Yu X. et al. (2019) observed different results, in which sevoflurane reduced NF-κB expression and production of proinflammatory cytokines following the lipopolysaccharide (LPS) treatment. These contradictory results might be caused by the concentration and/or duration differences between the studies. In the study by Xie et al., 4.1% sevoflurane for 6 h was used, whereas 3.3% sevoflurane for l h in the study by Yu et al., was only used. Similarly, TNF-α decrease was found in resting isolated microglia after 2% sevoflurane treatment, but a significant increase was observed after 4% sevoflurane. Another similar result was also found in LPS-activated microglia, in which 4% sevoflurane increased IL-1β and IL-6, but this was not observed on using 2% sevoflurane (Ye et al., 2013). This concentration-dependent and/or duration-dependent phenomenon was also observed in the effect of sevoflurane on peripheral immune cells. It is apparent to see that the suppressive effect of sevoflurane on peripheral immune cells was found at relatively low concentrations, which, in most cases, was less than 3% (Mitsuhata et al., 1995; Wada et al., 2007; Kong et al., 2010). In contrast, its stimulative function is always observed in relatively high concentrations, such as two MAC in rats (Morisaki et al., 1997; Kotani et al., 1999) and 5% in humans (Morisaki et al., 1998).
The gut microbes were well explored in human neurological diseases, such as MS, PD, AD, HD, and ALS (Tremlett et al., 2017). Furthermore, the Rea et al. (2016) reviewed the relationship between gut microbiome and neuroinflammation. The gut microbiome–neuroinflammation process might partly be bridged by the breakdown of BBB. For example, the study by Braniste et al. (2014) showed that the lack of gut microbiota was associated with lower expression of the tight junction proteins (e.g., occludin, ZO-1, and claudin-5) and increased the permeability of BBB subsequently. As we reviewed in the section “Permeability Changes of Blood–Brain Barrier,” abundant studies confirmed that sevoflurane induced the breakdown of BBB to cause neuroinflammation. Unfortunately, to date, there is only one study published this year (Han et al., 2021) which reported that sevoflurane might decrease the diversity and abundance of the intestinal microbiome, and this study only focused on the effect of sevoflurane on intestinal bacteria yet did not extend to downstream neuroinflammation. Even so, in line with the similar conclusion drawn by a previous isoflurane study (Wang et al., 2019), the regulative function of sevoflurane on gut bacteria seems convincing. Although the evidence is not particularly strong at present, sevoflurane may be a promising area of research considering the regulation of neuroinflammation by intestinal flora, and further studies are needed to fill in this gap.
It is worth noting that the resulting increase in inflammatory cytokines can increase the permeability of BBB and in turn aggravate neuroinflammation (Quagliarello et al., 1991). Similarly, more research is needed to confirm whether the decrease in the intestinal flora caused by sevoflurane was mediated by neuroinflammation. After all, the brain–gut axis was known as a bidirectional axis (Dinan and Cryan, 2017). This means that the breakdown of BBB and intestinal flora disorder may also be the result of the occurrence of neuroinflammation, which in turn further aggravates neuroinflammation.
Although the inhibitory effect of sevoflurane on the cholinergic nerve is well described in multiple studies, different findings can still be seen. Silva et al. (2005) conversely reported that sevoflurane increased the ACh release, and his opinion was enforced by similar findings in other volatile anesthetics, such as halothane (Gomez et al., 1999; Adachi et al., 2002) and isoflurane (Gomez et al., 2000). This discrepancy might be explained by the differences in the models and drug administration, which was observed in an in vivo study with 0.5 MAC (i.e., 1.1%) to 1.5 MAC (i.e., 3.3%) of sevoflurane gas (Shichino et al., 1998); however, the study by Silva et al. (2005) and relevant isoflurane studies were performed in vitro. As previously mentioned, sevoflurane partially reduced cholinergic neurotransmissions by blockage of the postsynaptic nAChRs, but the question remains on whether the anesthetic also directly blocks the α7nAChRs on the immune cells. This is an interesting question to explore in future studies.
Sevoflurane might induce neuroinflammation through complicated and interacting mechanisms, in which its proinflammatory function is dependent on concentration and duration under certain pathological models. However, the neuroprotective function of sevoflurane should also not be ignored. Therefore, future studies with uniform models should find the thresholds of both the proinflammatory and anti-inflammatory functions of sevoflurane, which will be more instructive for its clinical use.
XH and DY reviewed the literature and drafted the manuscript. FH, JY, and PF participated in the design and coordination of the review. XW, BZ, and LZ participated in the preparation of the manuscript. YF, WY, XL, and QZ participated in the review of the literature. All authors read and approved the final manuscript.
This work was supported by grants from the National Natural Science Foundation of China (81760261 and 82060219), Provincial Science Foundation of Jiangxi (20192BCB23024 and 20202BABL206016), and Youth Team Project of the Second Affiliated Hospital of Nanchang University (2019YNTD12003).
The authors declare that the research was conducted in the absence of any commercial or financial relationships that could be construed as a potential conflict of interest.
All claims expressed in this article are solely those of the authors and do not necessarily represent those of their affiliated organizations, or those of the publisher, the editors and the reviewers. Any product that may be evaluated in this article, or claim that may be made by its manufacturer, is not guaranteed or endorsed by the publisher.
We would like to thank Editage (www.editage.com) for English language editing.
Abbott, N. J., Patabendige, A. A., Dolman, D. E., Yusof, S. R., and Begley, D. J. (2010). Structure and function of the blood-brain barrier. Neurobiol. Dis. 37, 13–25.
Abbott, N. J., Rönnbäck, L., and Hansson, E. (2006). Astrocyte-endothelial interactions at the blood-brain barrier. Nat. Rev. Neurosci. 7, 41–53. doi: 10.1038/nrn1824
Acharya, N. K., Goldwaser, E. L., Forsberg, M. M., Godsey, G. A., Johnson, C. A., Sarkar, A., et al. (2015). Sevoflurane and Isoflurane induce structural changes in brain vascular endothelial cells and increase blood-brain barrier permeability: possible link to postoperative delirium and cognitive decline. Brain Res. 1620, 29–41. doi: 10.1016/j.brainres.2015.04.054
Adachi, Y. U., Watanabe, K., Higuchi, H., Satoh, T., and Zsilla, G. (2002). Halothane enhances acetylcholine release by decreasing dopaminergic activity in rat striatal slices. Neurochem. Int. 40, 189–193. doi: 10.1016/s0197-0186(01)00092-4
Al Tmimi, L., Van de Velde, M., Herijgers, P., Meyns, B., Meyfroidt, G., Milisen, K., et al. (2015). Xenon for the prevention of postoperative delirium in cardiac surgery: study protocol for a randomized controlled clinical trial. Trials 16:449.
Begley, D. J., and Brightman, M. W. (2003). Structural and functional aspects of the blood-brain barrier. Prog. Drug Res. 61, 39–78. doi: 10.1007/978-3-0348-8049-7_2
Boche, D., Perry, V. H., and Nicoll, J. A. (2013). Review: activation patterns of microglia and their identification in the human brain. Neuropathol. Appl. Neurobiol. 39, 3–18. doi: 10.1111/nan.12011
Bolton, S. J., Anthony, D. C., and Perry, V. H. (1998). Loss of the tight junction proteins occludin and zonula occludens-1 from cerebral vascular endothelium during neutrophil-induced blood-brain barrier breakdown in vivo. Neuroscience 86, 1245–1257. doi: 10.1016/s0306-4522(98)00058-x
Braniste, V., Al-Asmakh, M., Kowal, C., Anuar, F., Abbaspour, A., Tóth, M., et al. (2014). The gut microbiota influences blood-brain barrier permeability in mice. Sci. Transl. Med. 6:263ra158.
Chamberlain, M., Koutsogiannaki, S., Schaefers, M., Babazada, H., Liu, R., and Yuki, K. (2017). The Differential Effects of Anesthetics on Bacterial Behaviors. PLoS One 12:e0170089. doi: 10.1371/journal.pone.0170089
Chen, R., Zhang, T., Kuang, L., Chen, Z., Ran, D., Niu, Y., et al. (2015). Cholinergic synaptic transmissions were altered after single sevoflurane exposure in Drosophila pupa. BioMed Res. Int. 2015:485709.
Cibelli, M., Fidalgo, A. R., Terrando, N., Ma, D., Monaco, C., Feldmann, M., et al. (2010). Role of interleukin-1beta in postoperative cognitive dysfunction. Ann. Neurol. 68, 360–368.
Conner, J. M., Culberson, A., Packowski, C., Chiba, A. A., and Tuszynski, M. H. (2003). Lesions of the Basal forebrain cholinergic system impair task acquisition and abolish cortical plasticity associated with motor skill learning. Neuron 38, 819–829. doi: 10.1016/s0896-6273(03)00288-5
Cui, R. S., Wang, K., and Wang, Z. L. (2018). Sevoflurane anesthesia alters cognitive function by activating inflammation and cell death in rats. Exp. Ther. Med. 15, 4127–4130.
Dang, D.-D., Saiyin, H., Yu, Q., and Liang, W.-M. (2018). Effects of sevoflurane preconditioning on microglia/macrophage dynamics and phagocytosis profile against cerebral ischemia in rats. CNS Neurosci. Ther. 24, 564–571. doi: 10.1111/cns.12823
Davies, P., and Maloney, A. J. (1976). Selective loss of central cholinergic neurons in Alzheimer’s disease. Lancet 2:1403. doi: 10.1016/s0140-6736(76)91936-x
Deane, R., Du Yan, S., Submamaryan, R. K., LaRue, B., Jovanovic, S., Hogg, E., et al. (2003). RAGE mediates amyloid-beta peptide transport across the blood-brain barrier and accumulation in brain. Nat. Med. 9, 907–913. doi: 10.1038/nm890
Desai, B. S., Monahan, A. J., Carvey, P. M., and Hendey, B. (2007). Blood-brain barrier pathology in Alzheimer’s and Parkinson’s disease: implications for drug therapy. Cell Transplant. 16, 285–299. doi: 10.3727/000000007783464731
Dheen, S. T., Kaur, C., and Ling, E. A. (2007). Microglial activation and its implications in the brain diseases. Curr. Med. Chem. 14, 1189–1197. doi: 10.2174/092986707780597961
Diaz Heijtz, R., Wang, S., Anuar, F., Qian, Y., Bjorkholm, B., Samuelsson, A., et al. (2011). Normal gut microbiota modulates brain development and behavior. Proc. Natl. Acad Sci. U. S. A. 108, 3047–3052. doi: 10.1073/pnas.1010529108
Dinan, T. G., and Cryan, J. F. (2017). Brain-Gut-Microbiota Axis and Mental Health. Psychosom. Med. 79, 920–926. doi: 10.1097/psy.0000000000000519
Dong, Y., Liang, F., Huang, L., Fang, F., Yang, G., Tanzi, R. E., et al. (2021). The anesthetic sevoflurane induces tau trafficking from neurons to microglia. Commun. Biol. 4:560.
Fang, F., Lue, L. F., Yan, S., Xu, H., Luddy, J. S., Chen, D., et al. (2010). RAGE-dependent signaling in microglia contributes to neuroinflammation, Abeta accumulation, and impaired learning/memory in a mouse model of Alzheimer’s disease. FASEB J. 24, 1043–1055. doi: 10.1096/fj.09-139634
Fang, F., Xue, Z., and Cang, J. (2012). Sevoflurane exposure in 7-day-old rats affects neurogenesis, neurodegeneration and neurocognitive function. Neurosci. Bull. 28, 499–508. doi: 10.1007/s12264-012-1260-4
Frohlich, E. E., Farzi, A., Mayerhofer, R., Reichmann, F., Jacan, A., Wagner, B., et al. (2016). Cognitive impairment by antibiotic-induced gut dysbiosis: analysis of gut microbiota-brain communication. Brain Behav. Immun. 56, 140–155. doi: 10.1016/j.bbi.2016.02.020
Fujii, T., Mashimo, M., Moriwaki, Y., Misawa, H., Ono, S., Horiguchi, K., et al. (2017). Expression and Function of the Cholinergic System in Immune Cells. Front. Immunol. 8:1085. doi: 10.3389/fimmu.2017.01085
Furman, J. L., Vaquer-Alicea, J., White, C. L., Cairns, N. J., Nelson, P. T., and Diamond, M. I. (2017). Widespread tau seeding activity at early Braak stages. Acta Neuropathol. 133, 91–100. doi: 10.1007/s00401-016-1644-z
Gao, X., Ming, J., Liu, S., Lai, B., Fang, F., and Cang, J. (2019). Sevoflurane enhanced the clearance of Aβ1-40 in hippocampus under surgery via up-regulating AQP-4 expression in astrocyte. Life Sci. 221, 143–151. doi: 10.1016/j.lfs.2019.02.024
Gomez, R. S., Gomez, M. V., and Prado, M. A. (2000). The effect of isoflurane on the release of [(3)H]-acetylcholine from rat brain cortical slices. Brain Res. Bull. 52, 263–267. doi: 10.1016/s0361-9230(00)00259-8
Gomez, R. S., Prado, M. A., Carazza, F., and Gomez, M. V. (1999). Halothane enhances exocytosis of [3H]-acetylcholine without increasing calcium influx in rat brain cortical slices. Br. J. Pharmacol. 127, 679–684. doi: 10.1038/sj.bjp.0702603
Gotti, C., Zoli, M., and Clementi, F. (2006). Brain nicotinic acetylcholine receptors: native subtypes and their relevance. Trends Pharmacol. Sci. 27, 482–491. doi: 10.1016/j.tips.2006.07.004
Griffin, W. S., Stanley, L. C., Ling, C., White, L., MacLeod, V., Perrot, L. J., et al. (1989). Brain interleukin 1 and S-100 immunoreactivity are elevated in Down syndrome and Alzheimer disease. Proc. Natl. Acad. Sci. U. S. A. 86, 7611–7615. doi: 10.1073/pnas.86.19.7611
Grozdanov, V., Bliederhaeuser, C., Ruf, W. P., Roth, V., Fundel-Clemens, K., Zondler, L., et al. (2014). Inflammatory dysregulation of blood monocytes in Parkinson’s disease patients. Acta Neuropathol. 128, 651–663. doi: 10.1007/s00401-014-1345-4
Guo, S., Liu, L., Wang, C., Jiang, Q., Dong, Y., and Tian, Y. (2018). Repeated exposure to sevoflurane impairs the learning and memory of older male rats. Life Sci. 192, 75–83. doi: 10.1016/j.lfs.2017.11.025
Han, C., Zhang, Z., Guo, N., Li, X., Yang, M., Peng, Y., et al. (2021). Effects of Sevoflurane Inhalation Anesthesia on the Intestinal Microbiome in Mice. Front. Cell Infect. Microbiol. 11:633527. doi: 10.3389/fcimb.2021.633527
Herranz, E., Gianni, C., Louapre, C., Treaba, C. A., Govindarajan, S. T., Ouellette, R., et al. (2016). Neuroinflammatory component of gray matter pathology in multiple sclerosis. Ann. Neurol. 80, 776–790. doi: 10.1002/ana.24791
Herz, J., and Marschang, P. (2003). Coaxing the LDL receptor family into the fold. Cell 112, 289–292. doi: 10.1016/s0092-8674(03)00073-4
Hou, J., Shen, Q., Wan, X., Zhao, B., Wu, Y., and Xia, Z. (2019). REM sleep deprivation-induced circadian clock gene abnormalities participate in hippocampal-dependent memory impairment by enhancing inflammation in rats undergoing sevoflurane inhalation. Behav. Brain Res. 364, 167–176. doi: 10.1016/j.bbr.2019.01.038
Hwang, J.-W., Jeon, Y.-T., Lim, Y.-J., and Park, H.-P. (2017). Sevoflurane Postconditioning-Induced Anti-Inflammation via Inhibition of the Toll-Like Receptor-4/Nuclear Factor Kappa B Pathway Contributes to Neuroprotection against Transient Global Cerebral Ischemia in Rats. Int. J. Mol. Sci. 18:2347. doi: 10.3390/ijms18112347
Kalb, A., von Haefen, C., Sifringer, M., Tegethoff, A., Paeschke, N., Kostova, M., et al. (2013). Acetylcholinesterase inhibitors reduce neuroinflammation and -degeneration in the cortex and hippocampus of a surgery stress rat model. PLoS One 8:e62679. doi: 10.1371/journal.pone.0062679
Kaushal, V., and Schlichter, L. C. (2008). Mechanisms of microglia-mediated neurotoxicity in a new model of the stroke penumbra. J. Neurosci. 28, 2221–2230. doi: 10.1523/jneurosci.5643-07.2008
Kniesel, U., and Wolburg, H. (2000). Tight junctions of the blood-brain barrier. Cell. Mol. Neurobiol. 20, 57–76.
Kobayashi, K., Imagama, S., Ohgomori, T., Hirano, K., Uchimura, K., Sakamoto, K., et al. (2013). Minocycline selectively inhibits M1 polarization of microglia. Cell Death Dis. 4:e525. doi: 10.1038/cddis.2013.54
Kong, H. Y., Zhu, S. M., Wang, L. Q., He, Y., Xie, H. Y., and Zheng, S. S. (2010). Sevoflurane protects against acute kidney injury in a small-size liver transplantation model. Am. J. Nephrol. 32, 347–355. doi: 10.1159/000319623
Kotani, N., Takahashi, S., Sessler, D. I., Hashiba, E., Kubota, T., Hashimoto, H., et al. (1999). Volatile anesthetics augment expression of proinflammatory cytokines in rat alveolar macrophages during mechanical ventilation. Anesthesiology 91, 187–197. doi: 10.1097/00000542-199907000-00027
Laurent, C., Buée, L., and Blum, D. (2018). Tau and neuroinflammation: what impact for Alzheimer’s Disease and Tauopathies? Biomed J. 41, 21–33. doi: 10.1016/j.bj.2018.01.003
Lima, A. P., Silva, K., Padovan, C. M., Almeida, S. S., and Fukuda, M. T. (2014). Memory, learning, and participation of the cholinergic system in young rats exposed to environmental enrichment. Behav. Brain Res. 259, 247–252. doi: 10.1016/j.bbr.2013.10.046
Liu, J., Zhang, X., Zhang, W., Gu, G., and Wang, P. (2015). Effects of Sevoflurane on Young Male Adult C57BL/6 Mice Spatial Cognition. PLoS One 10:e0134217. doi: 10.1371/journal.pone.0134217
Liu, Y., Gao, M., Ma, L., Zhang, L., and Pan, N. (2013). Sevoflurane alters the expression of receptors and enzymes involved in Abeta clearance in rats. Acta Anaesthesiol. Scand. 57, 903–910. doi: 10.1111/aas.12098
Lv, J., Hu, W., Yang, Z., Li, T., Jiang, S., Ma, Z., et al. (2018). Focusing on claudin-5: a promising candidate in the regulation of BBB to treat ischemic stroke. Prog. Neurobiol. 161, 79–96. doi: 10.1016/j.pneurobio.2017.12.001
Lv, X., Yan, J., Jiang, J., Zhou, X., Lu, Y., and Jiang, H. (2017). MicroRNA-27a-3p suppression of peroxisome proliferator-activated receptor-gamma contributes to cognitive impairments resulting from sevoflurane treatment. J. Neurochem. 143, 306–319. doi: 10.1111/jnc.14208
Mayer, E. A., Tillisch, K., and Gupta, A. (2015). Gut/brain axis and the microbiota. J. Clin. Investig. 125, 926–938.
McGeer, P. L., Itagaki, S., Tago, H., and McGeer, E. G. (1987). Reactive microglia in patients with senile dementia of the Alzheimer type are positive for the histocompatibility glycoprotein HLA-DR. Neurosci. Lett. 79, 195–200. doi: 10.1016/0304-3940(87)90696-3
Meffert, M. K., Chang, J. M., Wiltgen, B. J., Fanselow, M. S., and Baltimore, D. (2003). NF-kappa B functions in synaptic signaling and behavior. Nat. Neurosci. 6, 1072–1078. doi: 10.1038/nn1110
Melah, K. E., Lu, S. Y., Hoscheidt, S. M., Alexander, A. L., Adluru, N., Destiche, D. J., et al. (2016). Cerebrospinal Fluid Markers of Alzheimer’s Disease Pathology and Microglial Activation are Associated with Altered White Matter Microstructure in Asymptomatic Adults at Risk for Alzheimer’s Disease. J. Alzheimers Dis. 50, 873–886. doi: 10.3233/jad-150897
Miller, D., Lewis, S. R., Pritchard, M. W., Schofield-Robinson, O. J., Shelton, C. L., Alderson, P., et al. (2018). Intravenous versus inhalational maintenance of anaesthesia for postoperative cognitive outcomes in elderly people undergoing non-cardiac surgery. Cochrane Database Syst. Rev. 8:CD012317.
Mirakaj, V., Dalli, J., Granja, T., Rosenberger, P., and Serhan, C. N. (2014). Vagus nerve controls resolution and pro-resolving mediators of inflammation. J. Exp. Me0d. 211, 1037–1048. doi: 10.1084/jem.20132103
Mitsuhata, H., Shimizu, R., and Yokoyama, M. M. (1995). Suppressive effects of volatile anesthetics on cytokine release in human peripheral blood mononuclear cells. Int. J. Immunopharmacol. 17, 529–534. doi: 10.1016/0192-0561(95)00026-x
Molliex, S., Montravers, P., Dureuil, B., and Desmonts, J. M. (1998). Halogenated anesthetics inhibit Pseudomonas aeruginosa growth in culture conditions reproducing the alveolar environment. Anesth. Analg. 86, 1075–1078. doi: 10.1213/00000539-199805000-00033
Morisaki, H., Aoyama, Y., Shimada, M., Ochiai, R., and Takeda, J. (1998). Leucocyte distribution during sevoflurane anaesthesia. Br. J. Anaesth. 80, 502–503. doi: 10.1093/bja/80.4.502
Morisaki, H., Suematsu, M., Wakabayashi, Y., Moro-oka, S., Fukushima, K., Ishimura, Y., et al. (1997). Leukocyte-endothelium interaction in the rat mesenteric microcirculation during halothane or sevoflurane anesthesia. Anesthesiology 87, 591–598. doi: 10.1097/00000542-199709000-00020
Naruo, H., Onizuka, S., Prince, D., Takasaki, M., and Syed, N. I. (2005). Sevoflurane blocks cholinergic synaptic transmission postsynaptically but does not affect short-term potentiation. Anesthesiology 102, 920–928. doi: 10.1097/00000542-200505000-00010
Pavlov, V. A., and Tracey, K. J. (2005). The cholinergic anti-inflammatory pathway. Brain Behav. Immun. 19, 493–499.
Pei, Z., Wang, S., and Li, Q. (2017). Sevoflurane suppresses microglial M2 polarization. Neurosci. Lett. 655, 160–165. doi: 10.1016/j.neulet.2017.07.001
Prinz, M., Jung, S., and Priller, J. (2019). Microglia Biology: one Century of Evolving Concepts. Cell 179, 292–311. doi: 10.1016/j.cell.2019.08.053
Quagliarello, V. J., Wispelwey, B., Long, W. J. Jr., and Scheld, W. M. (1991). Recombinant human interleukin-1 induces meningitis and blood-brain barrier injury in the rat. Characterization and comparison with tumor necrosis factor. J. Clin. Investig. 87, 1360–1366. doi: 10.1172/jci115140
Rea, K., Dinan, T. G., and Cryan, J. F. (2016). The microbiome: a key regulator of stress and neuroinflammation. Neurobiol. Stress 4, 23–33. doi: 10.1016/j.ynstr.2016.03.001
Riedel, B., Browne, K., and Silbert, B. (2014). Cerebral protection: inflammation, endothelial dysfunction, and postoperative cognitive dysfunction. Curr. Opin. Anaesthesiol. 27, 89–97. doi: 10.1097/aco.0000000000000032
Rochfort, K. D., Collins, L. E., McLoughlin, A., and Cummins, P. M. (2016). Tumour necrosis factor-alpha-mediated disruption of cerebrovascular endothelial barrier integrity in vitro involves the production of proinflammatory interleukin-6. J. Neurochem. 136, 564–572.
Serhan, C. N., and Savill, J. (2005). Resolution of inflammation: the beginning programs the end. Nat. Immunol. 6, 1191–1197. doi: 10.1038/ni1276
Shibata, M., Yamada, S., Kumar, S. R., Calero, M., Bading, J., Frangione, B., et al. (2000). Clearance of Alzheimer’s amyloid-ss(1-40) peptide from brain by LDL receptor-related protein-1 at the blood-brain barrier. J. Clin. Investig. 106, 1489–1499. doi: 10.1172/jci10498
Shichino, T., Murakawa, M., Adachi, T., Arai, T., Miyazaki, Y., and Mori, K. (1998). Effects of inhalation anaesthetics on the release of acetylcholine in the rat cerebral cortex in vivo. Br. J. Anaesth. 80, 365–370. doi: 10.1093/bja/80.3.365
Silva, J. H., Gomez, R. S., Pinheiro, A. C., Gomez, M. V., and Guatimosim, C. (2005). Acetylcholine release induced by the volatile anesthetic sevoflurane in rat brain cortical slices. Cell. Mol. Neurobiol. 25, 807–818. doi: 10.1007/s10571-005-4934-x
Smith, P. A. (2015). The tantalizing links between gut microbes and the brain. Nature 526, 312–314. doi: 10.1038/526312a
Song, S. K., Lee, S.-K., Lee, J. J., Lee, J. E., Choi, H. S., Sohn, Y. H., et al. (2011). Blood-brain barrier impairment is functionally correlated with clinical severity in patients of multiple system atrophy. Neurobiol. Aging 32, 2183–2189. doi: 10.1016/j.neurobiolaging.2009.12.017
Streit, W. J., Mrak, R. E., and Griffin, W. S. (2004). Microglia and neuroinflammation: a pathological perspective. J. Neuroinflammation 1:14.
Sun, Z., Satomoto, M., Adachi, Y. U., and Makita, K. (2019). Blood-brain barrier disruption caused by neonatal sevoflurane-induced depends on exposure time and is reversible in mice. Korean J. Anesthesiol. 72, 389–391. doi: 10.4097/kja.d.19.00029
Tang, Y., and Le, W. (2016). Differential Roles of M1 and M2 Microglia in Neurodegenerative Diseases. Mol. Neurobiol. 53, 1181–1194. doi: 10.1007/s12035-014-9070-5
Terrando, N., Eriksson, L. I., Ryu, J. K., Yang, T., Monaco, C., Feldmann, M., et al. (2011). Resolving postoperative neuroinflammation and cognitive decline. Ann. Neurol. 70, 986–995. doi: 10.1002/ana.22664
Tremlett, H., Bauer, K. C., Appel-Cresswell, S., Finlay, B. B., and Waubant, E. (2017). The gut microbiome in human neurological disease: a review. Ann. Neurol. 81, 369–382. doi: 10.1002/ana.24901
Vacas, S., Degos, V., Feng, X., and Maze, M. (2013). The neuroinflammatory response of postoperative cognitive decline. Br. Med. Bull. 106, 161–178. doi: 10.1093/bmb/ldt006
Wachtel, M., Bolliger, M. F., Ishihara, H., Frei, K., Bluethmann, H., and Gloor, S. M. (2001). Down-regulation of occludin expression in astrocytes by tumour necrosis factor (TNF) is mediated via TNF type-1 receptor and nuclear factor-kappaB activation. J. Neurochem. 78, 155–162. doi: 10.1046/j.1471-4159.2001.00399.x
Wada, H., Seki, S., Takahashi, T., Kawarabayashi, N., Higuchi, H., Habu, Y., et al. (2007). Combined spinal and general anesthesia attenuates liver metastasis by preserving TH1/TH2 cytokine balance. Anesthesiology 106, 499–506. doi: 10.1097/00000542-200703000-00014
Wang, H., Liao, H., Ochani, M., Justiniani, M., Lin, X., Yang, L., et al. (2004). Cholinergic agonists inhibit HMGB1 release and improve survival in experimental sepsis. Nat. Med. 10, 1216–1221. doi: 10.1038/nm1124
Wang, H., Yu, M., Ochani, M., Amella, C. A., Tanovic, M., Susarla, S., et al. (2003). Nicotinic acetylcholine receptor alpha7 subunit is an essential regulator of inflammation. Nature 421, 384–388.
Wang, L., Yang, X., and Wu, H. (2019). Juvenile Rats Show Altered Gut Microbiota After Exposure to Isoflurane as Neonates. Neurochem. Res. 44, 776–786. doi: 10.1007/s11064-018-02707-y
Wang, Q., Zhao, Y., Sun, M., Liu, S., Li, B., Zhang, L., et al. (2014). 2-Deoxy-d-glucose attenuates sevoflurane-induced neuroinflammation through nuclear factor-kappa B pathway in vitro. Toxicol. In Vitro 28, 1183–1189. doi: 10.1016/j.tiv.2014.05.006
Wardley-Smith, B., and Nunn, J. F. (1971). The effect of halothane on bacterial growth rate. Br. J. Anaesth. 43, 919–925. doi: 10.1093/bja/43.10.919
Xiong, H., Boyle, J., Winkelbauer, M., Gorantla, S., Zheng, J., Ghorpade, A., et al. (2003). Inhibition of long-term potentiation by interleukin-8: implications for human immunodeficiency virus-1-associated dementia. J. Neurosci. Res. 71, 600–607. doi: 10.1002/jnr.10503
Xu, Z., Dong, Y., Wang, H., Culley, D. J., Marcantonio, E. R., Crosby, G., et al. (2014). Age-dependent postoperative cognitive impairment and Alzheimer-related neuropathology in mice. Sci. Rep. 4:3766.
Xue, H., Zhang, Y.-H., Gao, Q.-S., Wu, Z.-Y., Niu, J.-Y., Li, C., et al. (2020). Sevoflurane Post-Conditioning Ameliorates Neuronal Deficits and Axon Demyelination After Neonatal Hypoxic Ischemic Brain Injury: role of Microglia/Macrophage. Cell. Mol. Neurobiol. doi: 10.1007/s10571-020-00949-5 [Online ahead of print]
Yang, H., Liang, G., Hawkins, B. J., Madesh, M., Pierwola, A., and Wei, H. (2008). Inhalational anesthetics induce cell damage by disruption of intracellular calcium homeostasis with different potencies. Anesthesiology 109, 243–250. doi: 10.1097/aln.0b013e31817f5c47
Yang, Z. Y., and Yuan, C. X. (2018). IL-17A promotes the neuroinflammation and cognitive function in sevoflurane anesthetized aged rats via activation of NF-kappaB signaling pathway. BMC Anesthesiol. 18:147. doi: 10.1186/s12871-018-0607-4
Ye, J., Zhang, Z., Wang, Y., Chen, C., Xu, X., Yu, H., et al. (2016). Altered hippocampal microRNA expression profiles in neonatal rats caused by sevoflurane anesthesia: microRNA profiling and bioinformatics target analysis. Exp. Ther. Med. 12, 1299–1310. doi: 10.3892/etm.2016.3452
Ye, X., Lian, Q., Eckenhoff, M. F., Eckenhoff, R. G., and Pan, J. Z. (2013). Differential general anesthetic effects on microglial cytokine expression. PLoS One 8:e52887. doi: 10.1371/journal.pone.0052887
Yin, J., Zhao, X., Wang, L., Xie, X., Geng, H., Zhan, X., et al. (2019). Sevoflurane-induced inflammation development: involvement of cholinergic anti-inflammatory pathway. Behav. Pharmacol. 30, 730–737.
Yu, Q., Li, L., and Liang, W.-M. (2019). Effect of sevoflurane preconditioning on astrocytic dynamics and neural network formation after cerebral ischemia and reperfusion in rats. Neural Regen. Res. 14, 265–271. doi: 10.4103/1673-5374.244790
Yu, X., Zhang, F., and Shi, J. (2019). Effect of sevoflurane treatment on microglia activation, NF-kB and MAPK activities. Immunobiology 224, 638–644. doi: 10.1016/j.imbio.2019.07.004
Zehendner, C. M., Librizzi, L., de Curtis, M., Kuhlmann, C. R., and Luhmann, H. J. (2011). Caspase-3 contributes to ZO-1 and Cl-5 tight-junction disruption in rapid anoxic neurovascular unit damage. PLoS One 6:e16760. doi: 10.1371/journal.pone.0016760
Zhang, D., Xue, B., You, J., Zhang, B., and Chai, G. (2019). Suberoylanilide hydroxamic acid reversed cognitive and synaptic plasticity impairments induced by sevoflurane exposure in adult mice. Neuroreport 30, 274–279. doi: 10.1097/wnr.0000000000001196
Zhang, L., Zhang, J., Yang, L., Dong, Y., Zhang, Y., and Xie, Z. (2013). Isoflurane and sevoflurane increase interleukin-6 levels through the nuclear factor-kappa B pathway in neuroglioma cells. Br. J. Anaesth. 110, i82–i91.
Zheng, J. W., Meng, B., Li, X. Y., Lu, B., Wu, G. R., and Chen, J. P. (2017). NF-kappaB/P65 signaling pathway: a potential therapeutic target in postoperative cognitive dysfunction after sevoflurane anesthesia. Eur. Rev. Med. Pharmacol. Sci. 21, 394–407.
Zhou, B., Chen, L., Liao, P., Huang, L., Chen, Z., Liao, D., et al. (2019). Astroglial dysfunctions drive aberrant synaptogenesis and social behavioral deficits in mice with neonatal exposure to lengthy general anesthesia. PLoS Biol. 17:e3000086. doi: 10.1371/journal.pbio.3000086
Zhou, H., Andonegui, G., Wong, C. H., and Kubes, P. (2009). Role of endothelial TLR4 for neutrophil recruitment into central nervous system microvessels in systemic inflammation. J. Immunol. 183, 5244–5250. doi: 10.4049/jimmunol.0901309
Zhu, G., Tao, L., Wang, R., Xue, Y., Wang, X., Yang, S., et al. (2017). Endoplasmic reticulum stress mediates distinct impacts of sevoflurane on different subfields of immature hippocampus. J. Neurochem. 142, 272–285. doi: 10.1111/jnc.14057
Zhu, Y., Wang, Y., Yao, R., Hao, T., Cao, J., Huang, H., et al. (2017). Enhanced neuroinflammation mediated by DNA methylation of the glucocorticoid receptor triggers cognitive dysfunction after sevoflurane anesthesia in adult rats subjected to maternal separation during the neonatal period. J. Neuroinflammation 14:6.
Keywords: sevoflurane, neuroinflammation, microglia, blood–brain barrier, gut microbiota, cholinergic neurotransmission
Citation: Huang X, Ying J, Yang D, Fang P, Wang X, Zhou B, Zhang L, Fang Y, Yu W, Liu X, Zhen Q and Hua F (2021) The Mechanisms of Sevoflurane-Induced Neuroinflammation. Front. Aging Neurosci. 13:717745. doi: 10.3389/fnagi.2021.717745
Received: 31 May 2021; Accepted: 13 July 2021;
Published: 05 August 2021.
Edited by:
Zhongcong Xie, Massachusetts General Hospital and Harvard Medical School, United StatesReviewed by:
Mian Peng, Wuhan University, ChinaCopyright © 2021 Huang, Ying, Yang, Fang, Wang, Zhou, Zhang, Fang, Yu, Liu, Zhen and Hua. This is an open-access article distributed under the terms of the Creative Commons Attribution License (CC BY). The use, distribution or reproduction in other forums is permitted, provided the original author(s) and the copyright owner(s) are credited and that the original publication in this journal is cited, in accordance with accepted academic practice. No use, distribution or reproduction is permitted which does not comply with these terms.
*Correspondence: Fuzhou Hua, aHVhZnV6aG91QDEyNi5jb20=
Disclaimer: All claims expressed in this article are solely those of the authors and do not necessarily represent those of their affiliated organizations, or those of the publisher, the editors and the reviewers. Any product that may be evaluated in this article or claim that may be made by its manufacturer is not guaranteed or endorsed by the publisher.
Research integrity at Frontiers
Learn more about the work of our research integrity team to safeguard the quality of each article we publish.