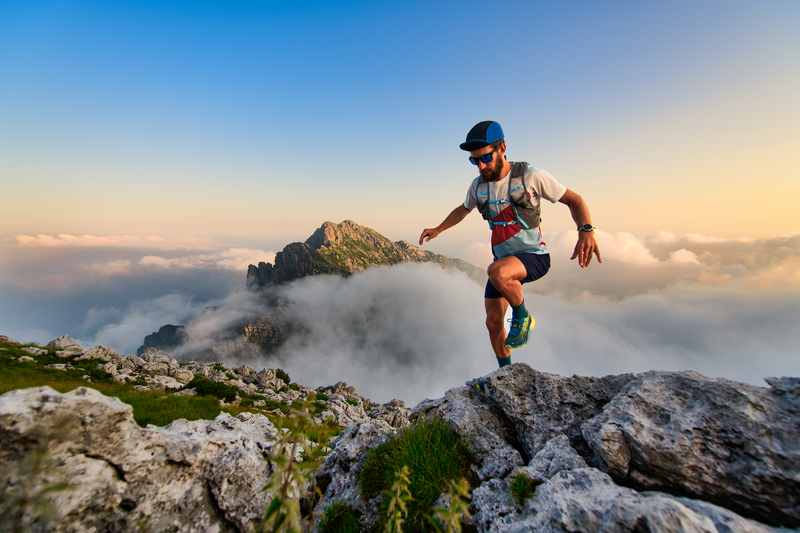
95% of researchers rate our articles as excellent or good
Learn more about the work of our research integrity team to safeguard the quality of each article we publish.
Find out more
ORIGINAL RESEARCH article
Front. Aging Neurosci. , 27 September 2021
Sec. Neuroinflammation and Neuropathy
Volume 13 - 2021 | https://doi.org/10.3389/fnagi.2021.717644
This article is part of the Research Topic Central Nervous System: From Aging to Repair and Regeneration View all 10 articles
Enriched environment (EE) is a complex containing social, cognitive, and motor stimuli. Exposure to EE can promote functional recovery after ischemia/reperfusion (I/R) injury. However, the underlying mechanisms remained unclear. Pyroptosis has recently been identified and demonstrated a significant role in ischemic stroke. The purpose of this study was to explore the effect of EE on neuronal pyroptosis after cerebral I/R injury. In the current study, middle cerebral artery occlusion/reperfusion (MCAO/R) was applied to establish the cerebral I/R injury model. Behavior tests including the modified Neurological Severity Scores (mNSS) and the Morris Water Maze (MWM) were performed. The infarct volume was evaluated by Nissl staining. To evaluate the levels of pyroptosis-related proteins, the levels of GSDMD-N and nod-like receptor protein 1/3 (NLRP1/3) inflammasome-related proteins were examined. The mRNA levels of IL-1β and IL-18 were detected by Quantitative Real-Time PCR (qPCR). The secretion levels of IL-1β and IL-18 were analyzed by ELISA. Also, the expression of p65 and p-p65 were detected. The results showed that EE treatment improved functional recovery, reduced infarct volume, attenuated neuronal pyroptosis after cerebral I/R injury. EE treatment also suppressed the activities of NLRP1/NLRP3 inflammasomes. These may be affected by inhabiting the NF-κB p65 signaling pathway. Our findings suggested that neuronal pyroptosis was probably the neuroprotective mechanism that EE treatment rescued neurological deficits after I/R injury.
Stroke is a disease with the highest mortality and disability rates in the world. Ischemic stroke is responsible for the majority of strokes (Campbell et al., 2019; Stinear et al., 2020). Despite the increasing improvement in the treatment of stroke, many survivors remain with residual functional deficits (Winstein et al., 2016). Therefore, the need for effective stroke rehabilitation is essential for the patients to deal with life challenges after stroke.
Enriched environment (EE) is a complex containing social, cognitive, and motor stimuli (Kempermann, 2019). EE provides laboratory animals with greater living space, further sensory stimulation, more possibilities for social interaction, and increasing opportunities for learning than the standard environment (Dahlqvist et al., 2003). The benefits of EE in neurological diseases have been extensive studied (Begenisic et al., 2015; Jungling et al., 2017; Song et al., 2017; Shin et al., 2018). In the EE, the ability of learning and memory has been significantly improved while the anxiety behavior was reduced (Benaroya–Milshtein et al., 2004). Meanwhile, exposure to EE enhanced the experience-dependent plasticity of the brain and promoted the recovery of cognitive and motor functions after ischemia/reperfusion (I/R) injury (Livingston-Thomas et al., 2016). EE improved cognitive function via neurogenesis and angiogenesis by regulating the activation of PI3K/AKT/GSK-3/β-catenin signaling pathways and intrinsic axon guidance molecules following I/R (Zhan et al., 2020). Moreover, EE mediated neurogenesis by inhibiting the production and secretion of IL-17A from astrocyte via NF-κB/p65 after I/R injury (Zhang et al., 2018). EE also facilitated cognitive recovery through remodeling bilateral synaptic after ischemic stroke (Wang C. et al., 2019). However, there has been little research on the relationship between EE-mediated ischemic stroke recovery and cell death. Evidence has shown that EE reduced spontaneous apoptotic cell death in the rat hippocampus (Young et al., 1999). A recent study has demonstrated that enriched environment-induced neuronal autophagy boosted the post-stroke recovery of neurological function (Deng et al., 2021). Our previous studies have illustrated that EE reduced neuronal apoptosis conducting to the superior recovery after I/R injury (Chen et al., 2017). However, the mechanisms by which EE attenuated cell death following stroke remained unclear.
Pyroptosis is a type of lytic cell death that features cell swelling, rapid rupture of the plasma membrane, and release of proinflammatory intracellular contents as a result of cleaving pore-forming proteins gasdermin D (GSDMD) following by activation of inflammasomes (Shi et al., 2017). Inflammasomes are large multimolecular complexes formed of a cytosolic sensor (nucleotide-binding domain and leucine-rich-repeat-containing [NLR] Pyrin domain containing NLRP1 and NLRP3), an adaptor protein (apoptosis-associated speck-like protein containing a CARD [ASC]), and an effector caspase pro-caspase-1 (Rathinam and Fitzgerald, 2016). After ischemic stroke attacks, the expression of inflammasomes is abundant in the brain (Abulafia et al., 2009). Pro-caspase-1 is activated through NLRP1 and NLRP3 signal cleaving GSDMD into the N-terminal gasdermin-N domain and the C-terminal gasdermin-C domain (Shi et al., 2015; Wang et al., 2020b). Then the pore-forming GSDMD-N domain causes membrane lysis inducing pyroptosis (Ding et al., 2016; Sborgi et al., 2016). Also, activated caspase-1 mediates the maturation of Interleukin-1β (IL-1β) and Interleukin-18 (IL-18) which are released into the extracellular environment subsequently (Brough and Rothwell, 2007). In recent years, increasing evidence indicated that inflammasome-mediated pyroptosis following ischemic stroke performed a crucial role in the course of functional recovery (Xu et al., 2019; Li et al., 2020). In addition, the activation of inflammasome was considered an essential step for neuroinflammation in subsequent brain injury (Walsh et al., 2014). Increasing expression of NLRP1 and NLRP3 inflammasome has been confirmed in neurons, microglia, and astrocytes (Barrington et al., 2017). Particularly, NLRP1 and NLRP3 inflammasome-mediated neuronal pyroptosis performed an increasingly crucial role in the course of ischemic stroke (Yang-Wei Fann et al., 2013; Ito et al., 2015). As a widely studied inflammation-associated transcriptional element, NF-κB regulated numerous genes and signaling pathways associated with inflammation (Afonina et al., 2017). Furthermore, emerging evidence suggested that the elevated expression of NLRP1 and NLRP3 inflammasome proteins could be modulated by the NF-κB signaling pathway in ischemic stroke (Gross et al., 2011; Fann et al., 2018). Although the accumulated evidence has shown that pyroptosis was involved in ischemic stroke injury, the relationship between neuronal pyroptosis and EE-mediated functional recovery following ischemic stroke was still unknown.
Since EE was neuroprotective and pyroptosis was involved in the progress of ischemic stroke. We formulated the hypothesis that post-stroke neurological outcomes could be improved by EE treatment to attenuate neuronal pyroptosis. In the current study, we investigated pyroptosis-related protein expression levels in the penumbra in a rat I/R injury model. Additionally, EE decreased the expression levels of NLRP1, NLRP3, and GSDMD-N. We firstly demonstrated that EE rescued neurological deficits after I/R injury involving the suppressing of neuronal pyroptosis. Generally, our findings indicated EE as a promising therapeutic method for ischemic stroke-mediated inflammasome activity.
Male Sprague–Dawley rats (6–7 weeks old, 200–220 g) from Beijing Vital River Laboratory Animal Technology Company were kept in individually ventilated cages (temperature: 20 ± 1°C, relative humidity: 55 ± 5%, lighting period: 8:00 ∼ 20:00) with free access to water and rat feed. Upon arrival, all rats had a 3-day acclimation before receiving the operation. Following acclimation, the animals were numbered and randomly divided into various groups: the sham + standard condition group (SSC), the sham + enriched environment group (SEE), the ischemia/reperfusion + standard condition group (ISC), and the ischemia/reperfusion + enriched environment group (IEE). The schematic representation of the experimental timeline and the setting of EE were shown in Figure 1. All animal experimental procedures were approved according to the Animal Care and Use Committee of Wuhan University. All efforts were made to minimize the mortality of animals and their suffering.
Figure 1. Flow chart of the experimental protocol and enrichment settings. (A) Timeline of behavioral testing after stroke. Modified Neurological Severity Scores (mNSS) were tested 3, 7, 14, and 21 days post-stroke (arrows) and compared with baseline performances to evaluate sensorimotor deficits. Morris Water Maze (MWM) test was performed from day 21 to day 26 post-stroke to evaluate spatial learning and memory. (B) The setting of an enriched environment.
Following adapting, male rats were subjected to transient Middle Cerebral Artery Occlusion and Reperfusion (MCAO/R) injury as previously described (Longa et al., 1989). All experimental animals were anesthetized by isoflurane through a face mask (inducing concentration: 4%, maintaining concentration: 2%, respectively, in 2:1 N2O:O2). An approximately 2 cm incision was made in the middle of the neck. The common carotid artery (CCA), internal carotid artery (ICA), and external carotid artery (ECA) were meticulously separated. And a 5–0 silk thread was used to ligate the left ECA. Then ligating the CCA with 5-0 silk thread, and clamping it at the bifurcation of the ICA with a blood vessel clip. The CCA was cut, and a monofilament nylon filament (Cinontech) was gently inserted into the ICA to approximately 18–20 mm distal to the carotid artery bifurcation. Then the left MCA was occluded. After 90 min, the filament was carefully removed to initiate reperfusion. All surgery procedures except insertion of the nylon filament were performed on rats in the sham-operation group. After recovering from anesthesia, all rats were assessed by a five-point neurological deficit score in a blinded fashion (Longa et al., 1989). Rats with scores of 1–3 points were included in this study, while the rats with scores of 0 or 4 were excluded from the study. N = 18/group in this study. All the experimental procedures in vivo were approved by The Animal Care and Use Committee of Wuhan University.
Twenty four hour after MCAO/R, the rats were returned to their respective housing conditions. The rats of the SSC, ISC groups were kept in the standard conditions (SC) while the rats in the SEE, IEE groups were kept in the enriched environment. The details of SC and EE were as follows:
The rats were kept in individually ventilated cages (length: 44 cm, width: 32 cm, height: 20 cm) with bedding for animals inside. Three rats were kept in one cage.
The animals are placed in a stainless-steel net cage (length: 75 cm, width: 90 cm, height: 50 cm) which contained ladders, platforms, swings, colorful balls, different-shaped wooden blocks, plastic tunnels, and a running wheel for sensorimotor stimulations. And 6–10 rats were grouping housed in the EE for social stimulations. The type and location of the items in the cage were changed three times a week to ensure novelty and exploration (Figure 1B).
Modified Neurological Severity Scores (mNSS) (Chen et al., 2001), an 18-point scoring system compositing of motor, sensory, reflex, and balance tests, was utilized 1 day before surgery and on day 3, 7, 14, and 21 post-stroke to evaluate sensorimotor deficits (n = 12/group).
For spatial learning and memory testing, Morris Water Maze (MWM) test was performed on days 21–26 following I/R in a blinded situation (Morris, 1984). A round black platform (9 cm diameter, 30 cm height) was concealed in a pool (150 cm diameter, 60 cm deep, water temperature: 20 ± 1°C). On day 1–5, rats were dropped into the water from four different quadrants in turn while the position of the platform was fixed. The mean of escaping tendency to the platform in the four trials was recorded. The rat was required to stay on the platform for 15 s when reaching the platform within 60 s. The rat was guided to the platform for 15 s when reaching the platform exceeding 60 s. On day 6, the rats underwent the probe trial that allowed them to swim freely for 60 s without the platform. Swimming trajectories and the average times to reach the submerged platform were captured using an Animal Video Tracking Analysis System (n = 12/group) (Anilab Scientific Instruments Co., Ltd., China).
After being fixed with 4% paraformaldehyde, tissues were embedded in paraffin cut into seriatim 4-μm-thick coronal sections with adjacent sections separated by 400 μm. The sections were placed in xylene, xylene, xylene, 100, 95, and 80% ethanol for 5 min each and rinsed under running water for 5 min. Then the sections were treated with Cresyl Violet Solution (Servicebio, China) for 3min. After washing in running water and drying thoroughly, the sections were coverslipped with neutral resin. The stained sections were scanned and measured with the ImageJ software. The total infarct volume was calculated by the formula as previously described (n = 6/group) (Chen et al., 2017).
Protein samples were harvested from penumbra. Tissues were ground separately in RIPA buffer comprising protease and phosphatase inhibitors (cocktails and PMSF from Aspen) for 30 min at 4°C. A BCA kit (Aspen) was used to detect the total protein concentration of each sample. Proteins were processed by SDS-PAGE (10–12.5%) and electro-blotted onto a PVDF membrane. And the membrane was then incubated in blocking buffer (5% skim milk) for 1 h at room temperature and incubated with primary antibodies including GSDMD (Abclonal), NLRP1, NLRP3, Caspase-1 (Novus), IL-1β,IL-18 (R&D), total p-65 (Proteintech),phosphorylated p-65 (Abclonal), GAPDH (Proteintech) overnight at 4°C. After washing three times, the membrane was incubated in secondary antibody for 1 h at 24°C. The proteins were scanned with a Bio-Rad system. ImageJ software was used to quantify protein levels which were normalized to GAPDH (n = 6/group).
Brain paraffin sections (4 μm) were hydrated and Tris/EDTA buffer performed heat-mediated antigen retrieval for 20 min. The sections blocked with 5% BSA for 1 h were incubated with Neun (Proteintech) along with primary antibodies Caspase-1 (Novus) overnight at 4°C and subsequently incubated in fluorescent secondary antibodies (Proteintech) for 1 h at 24°C. DAPI (Antgene) was used for nuclei staining. Images were taken with an Olympus BX53 microscope (Olympus). Positive cells were counted using ImageJ software (n = 6/group).
Brain paraffin sections (4 μm) were hydrated and Tris/EDTA buffer performed heat-mediated antigen retrieval for 20 min. The sections were then processed with 3% H2O2 for 10 min. The sections blocked with 5% BSA for 1 h were incubated with primary antibodies GSDMD (Abclonal), phosphorylated p65 (Abclonal) overnight at 4°C, and then incubated in HRP-labeled secondary antibodies (Proteintech). DAB (Servicebio) was utilized for dyeing while hematoxylin was used for nuclei staining. Images were acquired using the Olympus BX53 microscope (Olympus). The distribution and intensity of GSDMD and p-p65 staining was described by a semiquantitative score in a blinded fashion (0-negative, 1-weak, 2-moderate, 3-strong, and 4-strong and widely distributed) (n = 6/group) (Xu et al., 2020).
Rat (n = 3/group) penumbra tissues were separated and homogenized with PBS. After being centrifuged at 5000 rpm for 10 min at 4°C, the supernatants were collected. The secretion levels of inflammatory cytokines (IL-1β and IL-18) were analyzed by ELISA (Elabscience). Following the instructions on the ELISA kit, the optical density (OD) at 450 nm was measured by an enzyme-labeled instrument (PerkinElmer Singapore Pte. Ltd).
Rat (n = 3/group) penumbra tissues were separated and homogenized with Trizol reagent (Invitrogen, United States). The PrimeScript RT Reagent Kit (RR047A, Takara, Japan) was used for the reverse transcription of RNA. According to the manufacturers’ protocol, we performed qPCR to detect the mRNA levels using SYBR Premix Ex Taq II (RR820A, Takara) in a 2.1 Real-Time PCR System (Bio-Rad, United States). The relative Ct method was adopted to compare the data and GAPDH was set as internal control. The primer sequences were listed as follows:
IL-1β (F): TGACTTCACCATGGAACCCG
IL-1β (R): TCCTGGGGAAGGCATTAGGA
IL-18 (F): TGACAAAAGAAAGCCGCCTG
IL-18 (R): ATAGGGTCACAGCCAGTCCT
GAPDH (F): CGCTAACATCAAATGGGGTG
GAPDH (R): TTGCTGACAATCTTGAGGGAG
SPSS 23.0 software and GraphPad Prism 8.0 were used for data analysis. Analysis of mNSS was implemented by a non-parametric Kruskal–Wallis test. Analysis of escape latency in the MWM test was implemented by two-way repeated-measures ANOVA followed by Tukey’s post hoc test. And differences between groups were compared by two-tailed Student’s t-test and one-way ANOVA followed by Tukey’s post hoc test. All experimental data are expressed as mean ± standard deviation (SD). Statistical significance was determined as p < 0.05.
Ischemia/reperfusion injury caused marked Behavioral dysfunction (Yirmiya and Goshen, 2011). To determine whether EE treatment improved functional recovery after I/R injury, a series of behavioral tests were performed. To evaluate the neurological function, mNSS was assessed on day 3, 7, 14, 21 after I/R injury. Rats had persistent sensorimotor defects after MCAO/R operation and EE treatment could effectively reverse the defects (Figure 2A; p < 0.001). To assess long-term spatial learning and memory functions, MWM was assessed on day 21–26 after I/R injury. In the spatial learning phase, the escape latency of rats in all groups decreased as the training days progressed. And MCAO/R rats spent more time reaching the platform compared with sham-operated rats on days 1–5 of training. However, rats housed in EE demonstrated the superior performance of shorter escape latency than rats housed in standard conditions following I/R injury (Figure 2B; p < 0.001). Probe trials proceeded 24 h after the final spatial learning trial. Rats of the IEE group spent more time in the correct quadrant and revealed more crossovers compared to rats of the ISC group (Figures 2C–E; p < 0.001 and p < 0.01). To sum up, EE treatment improved long-term neurobehavioral function after I/R injury.
Figure 2. Enriched environment improved long-term neurobehavioral function of MCAO/R rats. (A) The mNSS of ISC and IEE group. Rats were tested before MCAO surgery. (B) The escape latency in the spatial learning phase. (C,D) Time in the correct quadrant and the crossovers in the target quadrant was recorded and analyzed. (E) Representative swimming trajectories of SSC, SEE, ISC, and IEE group in the probe trials. n = 12. Data are expressed as mean ± SD. *p < 0.05 vs. SSC group; ##p < 0.01, ###p < 0.001 vs. ISC group.
As the improvement in functional outcome could be attributed to a reduction in brain damage, Nissl staining was performed to confirm the effects of EE on infarct volume after I/R injury. The schematic diagram of the ischemic border was shown in Figure 3A. EE treatment significantly reduced infarct volume in comparison with the ISC group (Figures 3B,D; p < 0.001). No lesion was found in SSC and SEE groups. Reduced post-stroke ischemic infarction has been reported to be associated with inhibition of pyroptosis (Ye et al., 2020). Next, we explored whether EE could rescue ischemia-induced pyroptosis. GSDMD was downstream of pyroptosis and GSDMD-N fragments transferred to the plasma membrane to form pores that caused lytic cell death and the secretion of mature IL-1β and mature IL-18 (Kovacs and Miao, 2017). The expression levels of GSDMD-N from the ischemic border were detected. Western blot results demonstrated enhanced expression levels of GSDMD-N in the ISC group. And the expression levels of GSDMD-N were apparently reduced in the IEE group in comparison with the ISC group (Figures 3C,E; p < 0.001).
Figure 3. Enriched environment decreased ischemic infarction and inhibited pyroptosis after I/R injury. (A) Schematic brain with a highlight of the ischemic border. (B,D) Representative cresyl violet stained brain slices and quantification of cerebral infarct volume percentage. Scale bars, 5000 μm. n = 6. (C,E) Protein levels of GSDMD-N in peri-infarct tissues. n = 6. (G,F) Representative IHC staining images for GSDMD in the penumbra and IHC score of GSDMD in the penumbra. Scale bars, 50 μm. n = 6. Data are expressed as mean ± SD. *p < 0.05, ***p < 0.001 vs. SSC group; ##p < 0.01, ###p < 0.001 vs. ISC group.
Furthermore, we performed immunohistochemistry GSDMD and found that the IEE group expressed an obviously lower level of GSDMD in comparison with the ISC group (Figures 3F,G; p < 0.01).
Collectively, all of these data suggested that ischemic infarction and pyroptosis after I/R injury were negatively regulated by EE treatment.
To investigate how EE influenced neuronal pyroptosis after ischemic stroke, the activation of inflammasomes which was regarded as the upstream signal in the early stage of pyroptosis was detected. As neuronal pyroptosis might be mediated by the activation of NLRP1 and NLRP3 inflammasomes in the course of ischemic stroke, western blot was performed to explore whether EE inhibited pyroptosis by suppressing the activities of NLRP1/NLRP3 inflammasomes. The expression levels of NLRP1 and NLRP3 inflammasome proteins, mature IL-1β and mature IL-18 in the ischemic border of MCAO/R rats were measured. It was obvious that the expression levels of NLRP1 and NLRP3 were increased following I/R in comparison with sham controls while EE treatment decreased the expression levels of NLRP1 and NLRP3 in comparison with the ISC group (Figures 4A–C; p < 0.05 and p < 0.01). The elevated levels of cleaved caspases-1, mature IL-1β, and mature IL-18 indicated the activation of NLRP1/NLRP3 inflammasomes. I/R increased the expression levels of cleaved caspases-1, mature IL-1β, and mature IL-18 in comparison with sham controls while rats in the IEE group expressed a lower level (Figures 4D–G; p < 0.01, p < 0.001, and p < 0.001). To compare the expression levels of inflammatory factors, ELISAs were performed to detect inflammatory cytokines IL-1β and IL-18. We found that the expression levels of IL-1β and IL-18 were significantly increased following I/R in comparison with sham controls while EE treatment decreased the expression levels of IL-1β and IL-18 in comparison with the ISC group (Figures 5A,B; p < 0.01 and p < 0.01). The IL-1β and IL-18 mRNA expression levels were further examined by q-PCR. The mRNA levels of IL-1β and IL-18 were significantly reduced following I/R when the rats were housed in EE (Figures 5C,D; p < 0.01 and p < 0.05).
Figure 4. Enriched environment suppressed the activities of NLRP1/NLRP3 inflammasomes of MCAO/R rats. (A–G) Western blots and quantification of NLRP1/NLRP3 inflammasomes related proteins including NLRP1, NLRP3, cleaved caspase-1, mature IL-1β, and IL-18 in peri-infarct tissues. n = 6. (H,I) Double immunostaining of Neun and Caspase-1 revealed a good co-localization of these two makers. Statistical analysis of the positive rate is shown. Treatment with EE reduced Caspase-1 positive neurons in the ischemic penumbra. Scale bars, 50 μm. n = 6. Data are expressed as mean ± SD. **p < 0.01, ***p < 0.001 vs. SSC group; #p < 0.05, ##p < 0.01, ###p < 0.001 vs. ISC group.
Figure 5. Enriched environment suppressed the expression levels of inflammatory cytokines in the penumbra. (A,B) Effects of EE treatment on IL-1β and IL-18 expression in penumbra according to ELISA. n = 3. (C,D) Quantitative analysis of IL-1β and IL-18 mRNA levels in penumbra. n = 3. Data are expressed as mean ± SD. *p < 0.05, **p < 0.01, ***p < 0.001 vs. SSC group; #p < 0.05, ##p < 0.01, ###p < 0.001 vs. ISC group.
In addition, immunofluorescence analysis in the penumbra from the IEE group demonstrated a lower level of caspase-1compared with the ISC group (Figures 4H,I, p < 0.001). Notably, caspase-1 was highly colocalized with Neun + neurons, suggesting the inflammasome activity in neurons. These results demonstrated EE inhibited neuronal pyroptosis by suppressing the activities of NLRP1/NLRP3 inflammasomes.
As NF-κB was reported to mediate pyroptosis by regulating the transcription of NLRP and then regulated its downstream substrates (Fann et al., 2018), we next explored how EE treatment inhabited pyroptosis through evaluating the NF-κB signaling pathway proteins. First, a western blot was used for the detection of p-65 and p-p65 expression levels in the penumbra following I/R injury. The results suggested that p65 levels were not influenced by EE treatment (p > 0.05). However, p-p65 was reduced when the rats after I/R injury were housed in EE (Figures 6A–C, p < 0.01). Furthermore, we used immunohistochemistry to explore the expression levels of p-p65 in the penumbra and found that rats of the IEE group significantly down-regulated p-p65 expression in comparison with rats of the ISC group (Figures 6D,E, p < 0.05). As is well known, p65 phosphorylation indicates the activation of the p65 NF-κB signal (Pradère et al., 2016); thus, these results illustrate that EE can inhibit the p65 NF-κB signal activation after cerebral I/R injury.
Figure 6. Enriched environment inhibited p65 phosphorylation after I/R injury. (A–C) Western blots and quantification illustrating increases in the activation of NF-κB (p-p65) in the penumbra. (D) Representative IHC staining images for p-p65 in the penumbra. Scale bars, 50 μm. (E) IHC score of p-p65 in penumbra. n = 6. Data are expressed as mean ± SD. **p < 0.01, ***p < 0.001 vs. SSC group; #p < 0.05, ##p < 0.01, ###p < 0.001 vs. ISC group.
In general, these data revealed that EE treatment inhibited neuronal pyroptosis by attenuating the expression of NLRP1/NLRP3 inflammasomes following cerebral I/R injury. These may be affected by inhabiting the NF-κB p65 signaling pathway.
Despite the high rate of disability associated with stroke worldwide, valid therapeutic methods were restricted (Virani et al., 2020). Residual dysfunction of stroke greatly affected the life quality of the survivors (Stinear et al., 2020). It should not be overlooked to search methods for the recovery of the functional deficit caused by stroke. Abundant evidence showed that EE could effectively promote functional recovery after ischemic stroke (Chen J.Y. et al., 2017; Kubota et al., 2018; Lin et al., 2021, p. 1). However, due to the complexity of transforming EE into clinical practice, EE remained mainly a laboratory stage (Lang et al., 2015). Therefore, exploring the potential mechanism underlying the role of EE in promoting functional recovery may provide precise targets for the recovery of ischemic stroke and expedite its clinical application. Our previous study demonstrated the connections between neuroprotective effects of EE and neuronal cell death (Chen et al., 2017). A variety of pathological stimuli such as heart attacks, obesity, or cancer could trigger pyroptosis (Bergsbaken et al., 2009). Moreover, pyroptosis was closely related to central nervous system diseases (Fricker et al., 2018). In models of multiple sclerosis, pyroptosis inhibition preserved axons in the spinal cord lesions (McKenzie et al., 2018). In models of Alzheimer’s disease, pyroptosis relived the behavioral ability (Han et al., 2020). Previous research showed that neuronal pyroptosis affected the prognosis after ischemic stroke, which suggested that anti-pyroptosis was an effective treatment for all functional recovery following I/R injury (Lu et al., 2021). But there remained insufficient evidence that whether pyroptosis was essential for EE-mediated ischemic stroke recovery, and if so, how EE influenced neuronal pyroptosis after the pathological process. A key finding of our research was that EE attenuated pyroptosis and improved functional recovery after cerebral ischemia/reperfusion injury. The schematic mechanism was shown in Figure 7.
Figure 7. Schematic mechanism of EE treatment regulates post-ischemic pyroptosis. The NF-κB signaling pathway is activated following I/R injury, which stimulates the nucleus to induce transcription of NLRP1 and NLRP3 proteins to form the NLRP1 and NLRP3 inflammasome. Pro-caspase-1 is activated through NLRP1 and NLRP3 signal cleaving GSDMD into GSDMD-N and GSDMD-C. Then the pore-forming GSDMD-N domain causes membrane lysis inducing pyroptosis. Also, cleaved caspase-1 mediates the maturation of IL-1β and IL-18 which are released into the extracellular environment. EE attenuates pyroptosis resulting in ischemic stroke outcomes amelioration.
Being a novel type of cell death, pyroptosis mainly featured plasma-membrane pores formation, rapid plasma membrane rupture, and the release of intracellular inflammatory substances (Kuang et al., 2017). In the present study, we showed compelling evidence that the expression levels of GSDMD-N, the major pore-forming executive in pyroptosis, increased in the MCAO/R group versus the sham-operated group, and this alteration was counteracted in the EE treatment group. Then, to investigate how EE influenced neuronal pyroptosis after ischemic stroke, we detected the activation of inflammasomes which was regarded as the upstream signal in the early stage of pyroptosis. Inflammasomes were a group of the multimolecular complex that identified multiple inflammation-induced stimuli and mediate the maturation of critical proinflammatory cytokines in the process of pyroptosis (Strowig et al., 2012; Xue et al., 2019). It was worth noting that NLRP1 and NLRP3 inflammasomes were reported to be involved in ischemic stroke (Fann et al., 2014; Yang et al., 2014). However, it is not known whether EE treatment worked in the modulation of NLRP1/NLRP3 inflammasomes activation. The present research provided compelling evidence that EE treatment significantly modulated the activation of NLRP1/NLRP3 inflammasomes. The expression levels of NLRP1, NLRP3, the cleaved caspase-1, and the inflammatory cytokines mature IL-1β, and mature IL-18 were downregulated by EE treatment compared with standard conditions after I/R injury. And we found that the related proteins mainly expressed in the neurons through immunofluorescence double staining to locate its position. In summary, we found that EE treatment inhibited neuronal pyroptosis by affecting the activation of inflammasomes and thereby improved the functional recovery after I/R injury.
Next, we investigated the potential molecular mechanism of EE-reduced NLRP1 and NLRP3 inflammasome expression and activation in neurons. The activation of NLRP1 and NLRP3 inflammasome in the brain following I/R injury may be induced by pattern recognition receptors (PRRs) including toll-like receptors (TLRs), the receptor for advanced glycation end products (RAGE), and the IL-1 receptor 1 (IL-1R1) (Gelderblom et al., 2015). PRRs identified different pathological stimuli including endogenous damage-associated molecular patterns (DAMPs) released from damaged cells in the stroke core such as high mobility group box 1 protein (HMGB1), heat shock proteins, and peroxiredoxin family proteins (Tang et al., 2013; Wang et al., 2020a). DAMPs-activated PRRs further activated the intracellular NF−κB signaling pathway resulting in pyroptosis and the release of inflammatory factors (Schroder and Tschopp, 2010). As a transcription factor, NF-κB played a crucial role in cell death and inflammation (Kondylis et al., 2017; Liu et al., 2017). As a cytosolic sensor, the NF−κB signal activated and facilitated its nuclear translocation and DNA binding (Liu et al., 2017). Phosphorylation of p65 indicated the activation and functional status of the NF-κB signaling pathway (Pradère et al., 2016). Previous studies have indicated that activation of the NF-κB signaling pathway which could be activated by reactive oxygen species (ROS), hypoxia, and several inflammatory mediators occurred in neurons following I/R injury (Ridder and Schwaninger, 2009; Liu et al., 2019; He et al., 2020). The role of the NF-κB signaling pathway in regulating pyroptosis has been extensively studied in various diseases. Evidence has confirmed that NF-κB could regulate the transcription of NLRP by binding to their promoter region and then regulated its downstream substrates (Liu et al., 2017; Matias et al., 2019). The activation of the NF-κB signaling pathway was essential for the up-regulation of the protein synthesis of NLRP3 (Afonina et al., 2017). It has been demonstrated that the elevated expression level of IL-1β was induced by the activation of the NF-κB signaling pathway in ischemic damage (Zhou et al., 2012). Additionally, studies have confirmed that EE treatment was beneficial for the recovery of central nervous system diseases by inhibiting the NF-κB signaling pathway (Wu et al., 2016; Li et al., 2018). Moreover, Zhang et al. (2018) reported that EE mediated neurogenesis and functional recovery by inhibiting the NF-κB/IL-17A signaling pathway in astrocytes after ischemic stroke. In the present study, EE decreased the phosphorylation of p65 and the expression of NLRP1 and NLRP3 inflammasome proteins induced by I/R injury. This was supported by the study that NF-κB signaling promotes NLRP1 and NLRP3 inflammasome activation in neurons following I/R injury (Fann et al., 2018). In brief, our findings suggested that the anti-pyroptosis effect of EE after ischemic stroke was associated with the inhibition of the NF-κB p-65 signaling pathway and the reduced expression levels of NLRP1 and NLRP3 inflammasome-related proteins.
Less perfection was that the upstream regulator of p65 phosphorylation remained to be explored in this study. As the activation of NLRP1 and NLRP3 inflammasome may be induced by PRRs which identified DAMPs released from dying neural cells and stimulated NF-κB translocation during the I/R process, the sources of danger signals that promoted inflammatory response remained to be further investigated (Dong et al., 2018). Downregulation of the HMGB1/TLR4/NF-κB pathway was associated with inhibition of pyroptosis (Sun et al., 2020). HMGB1 activated the NLRP3 inflammasome via the NF-κB signaling pathway in acute glaucoma (Chi et al., 2015). The activation of the TLR4/NF-κB signaling pathway could modulate NLRP3 inflammasome activation in inflammatory bowel disease and induce GSDMD-mediated pyroptosis in tubular cells in diabetic kidney disease (Chen et al., 2019; Wang Y. et al., 2019). Moreover, SYK expression which was downregulated by the activation of miRNA-27a could stimulate the NF-κB signaling pathway and facilitate NLRP3-mediated pyroptosis (Li et al., 2021). The previous study has shown that EE could regulate the expression of HMGB1 and mediate post-stroke angiogenesis (Chen J.Y. et al., 2017). EE was also associated with growth factors (epithelial growth factor, hepatocyte growth factor) and signaling pathways (STAT3, JNK, EKR1/2, NF-κB) expressed in the gastrocnemius muscle (Le Guennec et al., 2020). However, it remained to be explored whether EE regulated the NF-κB signaling pathway by regulating the expression of these growth factors or DAMPs. It was essential to figure out the upstream signaling pathway to reveal the underlying mechanism of the EE-mediated effect on the NF-κB activation. In our future research, we would focus on solving this problem. In another hand, to confirm the exact effect of EE on the NF-κB signaling pathway, the agonist of NF-κB should be included. Our future work would dwell on this. Moreover, NF-κB pathway activation resulted in pyroptosis and the release of inflammatory factors. In turn, these inflammatory factors may act by activating the NF-κB signaling which would keep the cells in an activated cyclic state (Vallabhapurapu and Karin, 2009).
There was increasing evidence that many cell death pathways including apoptosis, autophagy and pyroptosis were simultaneously present in the ischemic core and penumbral area, which were fine-tuned and had either beneficial, deleterious or dual roles in the progression of post-stroke brain damage (Şekerdağ et al., 2018). However, the relevant research of EE on different types of cell death following ischemic stroke was extremely limited. In our previous studies, EE performed beneficial effects by inhibiting apoptosis of neurons following I/R (Chen et al., 2017). EE treatment increased the levels of anti-apoptotic protein Bcl-2 while decreased the levels of pro-apoptotic protein Bax, cytochrome c, caspase-3 in the penumbra after cerebral I/R injury. Caspases, the main drivers of apoptosis, were also involved in the crosstalk between apoptosis and autophagy. Activated caspases could inhibit autophagy by degrading autophagy proteins such as beclin-1, Atg5 and Atg7 (Wu et al., 2014). A recent study also demonstrated that EE promoted autophagy by increasing the expression of beclin-1 and enhancing the lysosomal activities of lysosomal-associated membrane protein 1, cathepsin B, and cathepsin D, which eventually boosted neurological function recovery following ischemic stroke (Deng et al., 2021). This kind of crosstalk in EE regulated cell death pathways after stroke could also be found in autophagy and pyroptosis. The evidence for the role of NLRP1/3 inflammasomes and pyroptosis in stroke pathology was well defined in the previous studies (Yang-Wei Fann et al., 2013; Barrington et al., 2017). Recently, autophagy has been linked to the regulation of inflammatory response. In Beclin1 +/- cells, the levels of NLRP3 and cleaved caspase1 were increased and the number of cells with inflammasome were elevated, indicating that autophagy inhibited inflammasome activation through NLRP3 degradation (Houtman et al., 2019, p. 1). Meanwhile, NLRs have been shown to increase the synthesis of autophagy-related proteins and assist in the localization of autophagy proteins (Deretic, 2012). In the present study, we found that EE inhabited neuronal pyroptosis by suppressing the activities of NLRP1/NLRP3 inflammasomes after I/R injury. The cell death pathways after I/R injury were overlapping each other in several steps of the cascades and shared common features. Keeping on exploring the effect of EE on different cell death types and how these mechanisms worked individually or correlatively might bring us a step closer to a promising approach for stroke treatment. What’s more, whether EE affected pyroptosis and the cell types experiencing pyroptosis after I/R injury remained unclear. Evidence showed that pyroptosis occurring in neurons, astrocytes, and microglia was involved in the pathological process in diseases other than ischemic stroke (Jamilloux et al., 2013; Alfonso-Loeches et al., 2014; Tan et al., 2014). In this study, we proved the effect of EE on neuronal pyroptosis after I/R injury. However, it was of great importance to notice that EE might not only influence neuronal pyroptosis but also affect pyroptosis or inflammatory reaction of additional cell types including astrocytes and microglia. Our future work would dwell on revealing the pleiotropic roles of EE-inhibited pyroptosis in other cell types.
Our finding showed that EE treatment presented a prospective cerebral-protective effect against I/R injury. EE treatment promoted functional recovery after I/R injury, involving inhibition of pyroptosis by suppressing the activities of NLRP1/NLRP3 inflammasomes. The beneficial effect of EE may result from the inhibition of NF-κB p65 phosphorylation. As a result, the therapeutic application of EE after I/R injury, including several potential therapeutic targets was probably a promising strategy for stroke recovery.
The original contributions presented in the study are included in the article/supplementary material, further inquiries can be directed to the corresponding author/s.
The animal study was reviewed and approved by the experimental animal Ethics Committee of Wuhan University (WP2020-08052).
JL performed the research. XZ, YX, and JL were responsible for experimental design. WC, JW, and BW contributed essential reagents and tools. LZ and JZ helped in collecting and analyzing the data. JL and JZ wrote the manuscript. XZ and WL reviewed and edited the manuscript. All the authors contributed to the article and approved the submitted version.
This work was supported by grants from the National Natural Science Foundation of China (No. 81902304 to XZ).
The authors declare that the research was conducted in the absence of any commercial or financial relationships that could be construed as a potential conflict of interest.
All claims expressed in this article are solely those of the authors and do not necessarily represent those of their affiliated organizations, or those of the publisher, the editors and the reviewers. Any product that may be evaluated in this article, or claim that may be made by its manufacturer, is not guaranteed or endorsed by the publisher.
The authors thank the personnel in the department of rehabilitation medicine at the Zhongnan Hospital of Wuhan University. In particular, JL wants to thank YX for his care, companionship and encouragement over the years. I love you!
Abulafia, D. P., de Rivero Vaccari, J. P., Lozano, J. D., Lotocki, G., Keane, R. W., and Dietrich, W. D. (2009). Inhibition of the inflammasome complex reduces the inflammatory response after thromboembolic stroke in mice. J. Cereb. Blood Flow Metab. 29, 534–544. doi: 10.1038/jcbfm.2008.143
Afonina, I. S., Zhong, Z., Karin, M., and Beyaert, R. (2017). Limiting inflammation-the negative regulation of NF-κB and the NLRP3 inflammasome. Nat. Immunol. 18, 861–869. doi: 10.1038/ni.3772
Alfonso-Loeches, S., Ureña-Peralta, J. R., Morillo-Bargues, M. J., Oliver-De La Cruz, J., and Guerri, C. (2014). Role of mitochondria ROS generation in ethanol-induced NLRP3 inflammasome activation and cell death in astroglial cells. Front. Cell Neurosci. 8:216. doi: 10.3389/fncel.2014.00216
Barrington, J., Lemarchand, E., and Allan, S. M. (2017). A brain in flame; do inflammasomes and pyroptosis influence stroke pathology? Brain Pathol. 27, 205–212. doi: 10.1111/bpa.12476
Begenisic, T., Sansevero, G., Baroncelli, L., Cioni, G., and Sale, A. (2015). Early environmental therapy rescues brain development in a mouse model of Down syndrome. Neurobiol. Dis. 82, 409–419. doi: 10.1016/j.nbd.2015.07.014
Benaroya–Milshtein, N., Hollander, N., Apter, A., Kukulansky, T., Raz, N., Wilf, A., et al. (2004). Environmental enrichment in mice decreases anxiety, attenuates stress responses and enhances natural killer cell activity. Eur. J. Neurosci. 20, 1341–1347. doi: 10.1111/j.1460-9568.2004.03587.x
Bergsbaken, T., Fink, S. L., and Cookson, B. T. (2009). Pyroptosis: host cell death and inflammation. Nat. Rev. Microbiol. 7, 99–109. doi: 10.1038/nrmicro2070
Brough, D., and Rothwell, N. J. (2007). Caspase-1-dependent processing of pro-interleukin-1beta is cytosolic and precedes cell death. J. Cell Sci. 120, 772–781. doi: 10.1242/jcs.03377
Campbell, B. C. V., De Silva, D. A., Macleod, M. R., Coutts, S. B., Schwamm, L. H., Davis, S. M., et al. (2019). Ischaemic stroke. Nat. Rev. Dis. Primers 5:70. doi: 10.1038/s41572-019-0118-8
Chen, J., Li, Y., Wang, L., Zhang, Z., Lu, D., Lu, M., et al. (2001). Therapeutic benefit of intravenous administration of bone marrow stromal cells after cerebral ischemia in rats. Stroke 32, 1005–1011. doi: 10.1161/01.str.32.4.1005
Chen, J.-Y., Yu, Y., Yuan, Y., Zhang, Y.-J., Fan, X.-P., Yuan, S.-Y., et al. (2017). Enriched housing promotes post-stroke functional recovery through astrocytic HMGB1-IL-6-mediated angiogenesis. Cell Death Discov. 3:17054. doi: 10.1038/cddiscovery.2017.54
Chen, X., Zhang, X., Xue, L., Hao, C., Liao, W., and Wan, Q. (2017). Treatment with enriched environment reduces neuronal apoptosis in the periinfarct cortex after cerebral ischemia/reperfusion injury. Cell Physiol. Biochem. 41, 1445–1456. doi: 10.1159/000468368
Chen, X., Liu, G., Yuan, Y., Wu, G., Wang, S., and Yuan, L. (2019). NEK7 interacts with NLRP3 to modulate the pyroptosis in inflammatory bowel disease via NF-κB signaling. Cell Death Dis. 10:906. doi: 10.1038/s41419-019-2157-1
Chi, W., Chen, H., Li, F., Zhu, Y., Yin, W., and Zhuo, Y. (2015). HMGB1 promotes the activation of NLRP3 and caspase-8 inflammasomes via NF-κB pathway in acute glaucoma. J. Neuroinflamm. 12:137. doi: 10.1186/s12974-015-0360-2
Dahlqvist, P., Rönnbäck, A., Risedal, A., Nergårdh, R., Johansson, I.-M., Seckl, J. R., et al. (2003). Effects of postischemic environment on transcription factor and serotonin receptor expression after permanent focal cortical ischemia in rats. Neuroscience 119, 643–652. doi: 10.1016/S0306-4522(03)00195-7
Deng, Y.-H., Dong, L.-L., Zhang, Y.-J., Zhao, X.-M., and He, H.-Y. (2021). Enriched environment boosts the post-stroke recovery of neurological function by promoting autophagy. Neural Regen. Res. 16, 813–819. doi: 10.4103/1673-5374.297084
Deretic, V. (2012). Autophagy as an innate immunity paradigm: expanding the scope and repertoire of pattern recognition receptors. Curr. Opin. Immunol. 24, 21–31. doi: 10.1016/j.coi.2011.10.006
Ding, J., Wang, K., Liu, W., She, Y., Sun, Q., Shi, J., et al. (2016). Pore-forming activity and structural autoinhibition of the gasdermin family. Nature 535, 111–116. doi: 10.1038/nature18590
Dong, Z., Pan, K., Pan, J., Peng, Q., and Wang, Y. (2018). The possibility and molecular mechanisms of cell pyroptosis after cerebral ischemia. Neurosci. Bull. 34, 1131–1136. doi: 10.1007/s12264-018-0294-7
Fann, D. Y.-W., Lee, S.-Y., Manzanero, S., Chunduri, P., Sobey, C. G., and Arumugam, T. V. (2013). Pathogenesis of acute stroke and the role of inflammasomes. Ageing Res. Rev. 12, 941–966. doi: 10.1016/j.arr.2013.09.004
Fann, D. Y.-W., Lim, Y.-A., Cheng, Y.-L., Lok, K.-Z., Chunduri, P., Baik, S.-H., et al. (2018). Evidence that NF-κB and MAPK signaling promotes NLRP inflammasome activation in neurons following ischemic stroke. Mol. Neurobiol. 55, 1082–1096. doi: 10.1007/s12035-017-0394-9
Fann, D. Y.-W., Santro, T., Manzanero, S., Widiapradja, A., Cheng, Y.-L., Lee, S.-Y., et al. (2014). Intermittent fasting attenuates inflammasome activity in ischemic stroke. Exp. Neurol. 257, 114–119. doi: 10.1016/j.expneurol.2014.04.017
Fricker, M., Tolkovsky, A. M., Borutaite, V., Coleman, M., and Brown, G. C. (2018). Neuronal cell death. Physiol. Rev. 98, 813–880. doi: 10.1152/physrev.00011.2017
Gelderblom, M., Sobey, C. G., Kleinschnitz, C., and Magnus, T. (2015). Danger signals in stroke. Ageing Res. Rev. 24, 77–82. doi: 10.1016/j.arr.2015.07.004
Gross, O., Thomas, C. J., Guarda, G., and Tschopp, J. (2011). The inflammasome: an integrated view. Immunol. Rev. 243, 136–151. doi: 10.1111/j.1600-065X.2011.01046.x
Han, C., Yang, Y., Guan, Q., Zhang, X., Shen, H., Sheng, Y., et al. (2020). New mechanism of nerve injury in Alzheimer’s disease: β-amyloid-induced neuronal pyroptosis. J. Cell Mol. Med. 24, 8078–8090. doi: 10.1111/jcmm.15439
He, X., Zeng, Y., Li, G., Feng, Y., Wu, C., Liang, F., et al. (2020). Extracellular ASC exacerbated the recurrent ischemic stroke in an NLRP3-dependent manner. J. Cereb. Blood Flow Metab. 40, 1048–1060. doi: 10.1177/0271678X19856226
Houtman, J., Freitag, K., Gimber, N., Schmoranzer, J., Heppner, F. L., and Jendrach, M. (2019). Beclin1-driven autophagy modulates the inflammatory response of microglia via NLRP3. EMBO J. 38. doi: 10.15252/embj.201899430
Ito, M., Shichita, T., Okada, M., Komine, R., Noguchi, Y., Yoshimura, A., et al. (2015). Bruton’s tyrosine kinase is essential for NLRP3 inflammasome activation and contributes to ischaemic brain injury. Nat. Commun. 6:7360. doi: 10.1038/ncomms8360
Jamilloux, Y., Pierini, R., Querenet, M., Juruj, C., Fauchais, A.-L., Jauberteau, M.-O., et al. (2013). Inflammasome activation restricts Legionella pneumophila replication in primary microglial cells through flagellin detection. Glia 61, 539–549. doi: 10.1002/glia.22454
Jungling, A., Reglodi, D., Karadi, Z. N., Horvath, G., Farkas, J., Gaszner, B., et al. (2017). Effects of postnatal enriched environment in a model of Parkinson’s disease in adult rats. Int. J. Mol. Sci. 18:406. doi: 10.3390/ijms18020406
Kempermann, G. (2019). Environmental enrichment, new neurons and the neurobiology of individuality. Nat. Rev. Neurosci. 20, 235–245. doi: 10.1038/s41583-019-0120-x
Kondylis, V., Kumari, S., Vlantis, K., and Pasparakis, M. (2017). The interplay of IKK, NF-κB and RIPK1 signaling in the regulation of cell death, tissue homeostasis and inflammation. Immunol. Rev. 277, 113–127. doi: 10.1111/imr.12550
Kovacs, S. B., and Miao, E. A. (2017). Gasdermins: effectors of pyroptosis. Trends Cell Biol. 27, 673–684. doi: 10.1016/j.tcb.2017.05.005
Kuang, S., Zheng, J., Yang, H., Li, S., Duan, S., Shen, Y., et al. (2017). Structure insight of GSDMD reveals the basis of GSDMD autoinhibition in cell pyroptosis. Proc. Natl. Acad. Sci. U.S.A. 114, 10642–10647. doi: 10.1073/pnas.1708194114
Kubota, K., Nakano, M., Kobayashi, E., Mizue, Y., Chikenji, T., Otani, M., et al. (2018). An enriched environment prevents diabetes-induced cognitive impairment in rats by enhancing exosomal miR-146a secretion from endogenous bone marrow-derived mesenchymal stem cells. PLoS One 13:e0204252. doi: 10.1371/journal.pone.0204252
Lang, C. E., Lohse, K. R., and Birkenmeier, R. L. (2015). Dose and timing in neurorehabilitation: prescribing motor therapy after stroke. Curr. Opin. Neurol. 28, 549–555. doi: 10.1097/WCO.0000000000000256
Le Guennec, D., Hatte, V., Farges, M.-C., Rougé, S., Goepp, M., Caldefie-Chezet, F., et al. (2020). Modulation of inter-organ signalling in obese mice by spontaneous physical activity during mammary cancer development. Sci. Rep. 10:8794. doi: 10.1038/s41598-020-65131-9
Li, B., Xu, P., Wu, S., Jiang, Z., Huang, Z., Li, Q., et al. (2018). Diosgenin attenuates lipopolysaccharide-induced Parkinson’s disease by inhibiting the TLR/NF- κB pathway. J. Alzheimer’s Dis. 64, 943–955. doi: 10.3233/JAD-180330
Li, Q., Cao, Y., Dang, C., Han, B., Han, R., Ma, H., et al. (2020). Inhibition of double-strand DNA-sensing cGAS ameliorates brain injury after ischemic stroke. EMBO Mol. Med. 12:e11002. doi: 10.15252/emmm.201911002
Li, Y., Song, W., Tong, Y., Zhang, X., Zhao, J., Gao, X., et al. (2021). Isoliquiritin ameliorates depression by suppressing NLRP3-mediated pyroptosis via miRNA-27a/SYK/NF-κB axis. J. Neuroinflamm. 18:1. doi: 10.1186/s12974-020-02040-8
Lin, Y., Yao, M., Wu, H., Wu, F., Cao, S., Ni, H., et al. (2021). Environmental enrichment implies GAT-1 as a potential therapeutic target for stroke recovery. Theranostics 11, 3760–3780. doi: 10.7150/thno.53316
Liu, H., Wu, X., Luo, J., Wang, X., Guo, H., Feng, D., et al. (2019). Pterostilbene attenuates astrocytic inflammation and neuronal oxidative injury after ischemia-reperfusion by inhibiting NF-κB phosphorylation. Front. Immunol. 10:2408. doi: 10.3389/fimmu.2019.02408
Liu, Z., Gan, L., Xu, Y., Luo, D., Ren, Q., Wu, S., et al. (2017). Melatonin alleviates inflammasome-induced pyroptosis through inhibiting NF-κB/GSDMD signal in mice adipose tissue. J. Pineal Res. 63:e12414. doi: 10.1111/jpi.12414
Livingston-Thomas, J., Nelson, P., Karthikeyan, S., Antonescu, S., Jeffers, M. S., Marzolini, S., et al. (2016). Exercise and environmental enrichment as enablers of task-specific neuroplasticity and stroke recovery. Neurotherapeutics 13, 395–402. doi: 10.1007/s13311-016-0423-9
Longa, E. Z., Weinstein, P. R., Carlson, S., and Cummins, R. (1989). Reversible middle cerebral artery occlusion without craniectomy in rats. Stroke 20, 84–91. doi: 10.1161/01.str.20.1.84
Lu, L.-Q., Tian, J., Luo, X.-J., and Peng, J. (2021). Targeting the pathways of regulated necrosis: a potential strategy for alleviation of cardio-cerebrovascular injury. Cell. Mol. Life Sci. 78, 63–78. doi: 10.1007/s00018-020-03587-8
Matias, M. L., Gomes, V. J., Romao-Veiga, M., Ribeiro, V. R., Nunes, P. R., Romagnoli, G. G., et al. (2019). Silibinin downregulates the NF-κB pathway and NLRP1/NLRP3 inflammasomes in monocytes from pregnant women with preeclampsia. Molecules 24:1548. doi: 10.3390/molecules24081548
McKenzie, B. A., Mamik, M. K., Saito, L. B., Boghozian, R., Monaco, M. C., Major, E. O., et al. (2018). Caspase-1 inhibition prevents glial inflammasome activation and pyroptosis in models of multiple sclerosis. Proc. Natl. Acad. Sci. U.S.A. 115, E6065–E6074. doi: 10.1073/pnas.1722041115
Morris, R. (1984). Developments of a water-maze procedure for studying spatial learning in the rat. J. Neurosci. Methods 11, 47–60. doi: 10.1016/0165-0270(84)90007-4
Pradère, J.-P., Hernandez, C., Koppe, C., Friedman, R. A., Luedde, T., and Schwabe, R. F. (2016). Negative regulation of NF-κB p65 activity by serine 536 phosphorylation. Sci. Signal. 9:ra85. doi: 10.1126/scisignal.aab2820
Rathinam, V. A. K., and Fitzgerald, K. A. (2016). Inflammasome complexes: emerging mechanisms and effector functions. Cell 165, 792–800. doi: 10.1016/j.cell.2016.03.046
Ridder, D. A., and Schwaninger, M. (2009). NF-kappaB signaling in cerebral ischemia. Neuroscience 158, 995–1006. doi: 10.1016/j.neuroscience.2008.07.007
Sborgi, L., Rühl, S., Mulvihill, E., Pipercevic, J., Heilig, R., Stahlberg, H., et al. (2016). GSDMD membrane pore formation constitutes the mechanism of pyroptotic cell death. EMBO J. 35, 1766–1778. doi: 10.15252/embj.201694696
Schroder, K., and Tschopp, J. (2010). The inflammasomes. Cell 140, 821–832. doi: 10.1016/j.cell.2010.01.040
Şekerdağ, E., Solaroğlu, I., and Gürsoy-Özdemir, Y. (2018). Cell Death Mechanisms in stroke and novel molecular and cellular treatment options. Curr. Neuropharmacol. 16:1396. doi: 10.2174/1570159X16666180302115544
Shi, J., Gao, W., and Shao, F. (2017). Pyroptosis: gasdermin-mediated programmed necrotic cell death. Trends Biochem. Sci. 42, 245–254. doi: 10.1016/j.tibs.2016.10.004
Shi, J., Zhao, Y., Wang, K., Shi, X., Wang, Y., Huang, H., et al. (2015). Cleavage of GSDMD by inflammatory caspases determines pyroptotic cell death. Nature 526, 660–665. doi: 10.1038/nature15514
Shin, S. S., Krishnan, V., Stokes, W., Robertson, C., Celnik, P., Chen, Y., et al. (2018). Transcranial magnetic stimulation and environmental enrichment enhances cortical excitability and functional outcomes after traumatic brain injury. Brain Stimul. 11, 1306–1313. doi: 10.1016/j.brs.2018.07.050
Song, Y., Gan, Y., Wang, Q., Meng, Z., Li, G., Shen, Y., et al. (2017). Enriching the housing environment for mice enhances their NK cell antitumor immunity via sympathetic nerve–dependent regulation of NKG2D and CCR5. Cancer Res. 77, 1611–1622. doi: 10.1158/0008-5472.CAN-16-2143
Stinear, C. M., Lang, C. E., Zeiler, S., and Byblow, W. D. (2020). Advances and challenges in stroke rehabilitation. Lancet Neurol. 19, 348–360. doi: 10.1016/S1474-4422(19)30415-6
Strowig, T., Henao-Mejia, J., Elinav, E., and Flavell, R. (2012). Inflammasomes in health and disease. Nature 481, 278–286. doi: 10.1038/nature10759
Sun, Z., Nyanzu, M., Yang, S., Zhu, X., Wang, K., Ru, J., et al. (2020). VX765 attenuates pyroptosis and HMGB1/TLR4/NF-κB pathways to improve functional outcomes in TBI mice. Oxid. Med. Cell Longev. 2020:7879629. doi: 10.1155/2020/7879629
Tan, M.-S., Tan, L., Jiang, T., Zhu, X.-C., Wang, H.-F., Jia, C.-D., et al. (2014). Amyloid-β induces NLRP1-dependent neuronal pyroptosis in models of Alzheimer’s disease. Cell Death Dis. 5:e1382. doi: 10.1038/cddis.2014.348
Tang, S.-C., Wang, Y.-C., Li, Y.-I., Lin, H.-C., Manzanero, S., Hsieh, Y.-H., et al. (2013). Functional role of soluble receptor for advanced glycation end products in stroke. Arterioscler Thromb Vasc. Biol. 33, 585–594. doi: 10.1161/ATVBAHA.112.300523
Vallabhapurapu, S., and Karin, M. (2009). Regulation and function of NF-κB transcription factors in the immune system. Annu. Rev. Immunol. 27, 693–733. doi: 10.1146/annurev.immunol.021908.132641
Virani, S. S., Alonso, A., Benjamin, E. J., Bittencourt, M. S., Callaway, C. W., Carson, A. P., et al. (2020). Heart disease and stroke statistics-2020 update: a report from the American heart association. Circulation 141, e139–e596. doi: 10.1161/CIR.0000000000000757
Walsh, J. G., Muruve, D. A., and Power, C. (2014). Inflammasomes in the CNS. Nat. Rev. Neurosci. 15, 84–97. doi: 10.1038/nrn3638
Wang, C., Zhang, Q., Yu, K., Shen, X., Wu, Y., and Wu, J. (2019). Enriched environment promoted cognitive function via bilateral synaptic remodeling after cerebral ischemia. Front. Neurol. 10:1189. doi: 10.3389/fneur.2019.01189
Wang, Y., Zhu, X., Yuan, S., Wen, S., Liu, X., Wang, C., et al. (2019). TLR4/NF-κB signaling induces GSDMD-related pyroptosis in tubular cells in diabetic kidney disease. Front. Endocrinol. (Lausanne) 10:603. doi: 10.3389/fendo.2019.00603
Wang, K., Ru, J., Zhang, H., Chen, J., Lin, X., Lin, Z., et al. (2020a). Melatonin enhances the therapeutic effect of plasma exosomes against cerebral ischemia-induced pyroptosis through the TLR4/NF-κB pathway. Front. Neurosci. 14:848. doi: 10.3389/fnins.2020.00848
Wang, K., Sun, Q., Zhong, X., Zeng, M., Zeng, H., Shi, X., et al. (2020b). Structural mechanism for GSDMD targeting by autoprocessed caspases in pyroptosis. Cell 180, 941.e20–955.e20. doi: 10.1016/j.cell.2020.02.002
Winstein, C. J., Stein, J., Arena, R., Bates, B., Cherney, L. R., Cramer, S. C., et al. (2016). Guidelines for adult stroke rehabilitation and recovery: a guideline for healthcare professionals from the American Heart Association/American stroke association. Stroke 47, e98–e169. doi: 10.1161/STR.0000000000000098
Wu, H., Che, X., Zheng, Q., Wu, A., Pan, K., Shao, A., et al. (2014). Caspases: a molecular switch node in the crosstalk between autophagy and apoptosis. Int. J. Biol. Sci. 10, 1072–1083. doi: 10.7150/ijbs.9719
Wu, J., Bie, B., and Naguib, M. (2016). Epigenetic manipulation of brain-derived neurotrophic factor improves memory deficiency induced by neonatal anesthesia in rats. Anesthesiology 124, 624–640. doi: 10.1097/ALN.0000000000000981
Xu, P., Zhang, X., Liu, Q., Xie, Y., Shi, X., Chen, J., et al. (2019). Microglial TREM-1 receptor mediates neuroinflammatory injury via interaction with SYK in experimental ischemic stroke. Cell Death Dis. 10, 1–17. doi: 10.1038/s41419-019-1777-9
Xu, Y., Sun, Q., Yuan, F., Dong, H., Zhang, H., Geng, R., et al. (2020). RND2 attenuates apoptosis and autophagy in glioblastoma cells by targeting the p38 MAPK signalling pathway. J. Exp. Clin. Cancer Res. 39:174. doi: 10.1186/s13046-020-01671-2
Xue, Y., Enosi Tuipulotu, D., Tan, W. H., Kay, C., and Man, S. M. (2019). Emerging activators and regulators of inflammasomes and pyroptosis. Trends Immunol. 40, 1035–1052. doi: 10.1016/j.it.2019.09.005
Yang, F., Wang, Z., Wei, X., Han, H., Meng, X., Zhang, Y., et al. (2014). NLRP3 deficiency ameliorates neurovascular damage in experimental ischemic stroke. J. Cereb. Blood Flow. Metab. 34, 660–667. doi: 10.1038/jcbfm.2013.242
Yang-Wei Fann, D., Lee, S.-Y., Manzanero, S., Tang, S.-C., Gelderblom, M., Chunduri, P., et al. (2013). Intravenous immunoglobulin suppresses NLRP1 and NLRP3 inflammasome-mediated neuronal death in ischemic stroke. Cell Death Dis. 4:e790. doi: 10.1038/cddis.2013.326
Ye, A., Li, W., Zhou, L., Ao, L., Fang, W., and Li, Y. (2020). Targeting pyroptosis to regulate ischemic stroke injury: molecular mechanisms and preclinical evidences. Brain Res. Bull. 165, 146–160. doi: 10.1016/j.brainresbull.2020.10.009
Yirmiya, R., and Goshen, I. (2011). Immune modulation of learning, memory, neural plasticity and neurogenesis. Brain Behav. Immun. 25, 181–213. doi: 10.1016/j.bbi.2010.10.015
Young, D., Lawlor, P. A., Leone, P., Dragunow, M., and During, M. J. (1999). Environmental enrichment inhibits spontaneous apoptosis, prevents seizures and is neuroprotective. Nat. Med. 5, 448–453. doi: 10.1038/7449
Zhan, Y., Li, M.-Z., Yang, L., Feng, X.-F., Lei, J.-F., Zhang, N., et al. (2020). The three-phase enriched environment paradigm promotes neurovascular restorative and prevents learning impairment after ischemic stroke in rats. Neurobiol. Dis. 146:105091. doi: 10.1016/j.nbd.2020.105091
Zhang, Y., Xu, D., Qi, H., Yuan, Y., Liu, H., Yao, S., et al. (2018). Enriched environment promotes post-stroke neurogenesis through NF-κB-mediated secretion of IL-17A from astrocytes. Brain Res. 1687, 20–31. doi: 10.1016/j.brainres.2018.02.030
Keywords: cerebral ischemia/reperfusion, inflammasome, ischemic stroke, enriched environment, neuronal pyroptosis
Citation: Liu J, Zheng J, Xu Y, Cao W, Wang J, Wang B, Zhao L, Zhang X and Liao W (2021) Enriched Environment Attenuates Pyroptosis to Improve Functional Recovery After Cerebral Ischemia/Reperfusion Injury. Front. Aging Neurosci. 13:717644. doi: 10.3389/fnagi.2021.717644
Received: 31 May 2021; Accepted: 01 September 2021;
Published: 27 September 2021.
Edited by:
Homaira Nawabi, Institut National de la Santé et de la Recherche Médicale (INSERM), FranceReviewed by:
Yuanyuan Liu, National Institute of Dental and Craniofacial Research (NIDCR), United StatesCopyright © 2021 Liu, Zheng, Xu, Cao, Wang, Wang, Zhao, Zhang and Liao. This is an open-access article distributed under the terms of the Creative Commons Attribution License (CC BY). The use, distribution or reproduction in other forums is permitted, provided the original author(s) and the copyright owner(s) are credited and that the original publication in this journal is cited, in accordance with accepted academic practice. No use, distribution or reproduction is permitted which does not comply with these terms.
*Correspondence: Xin Zhang, emhhbmd4aW4wNDM5QDE2My5jb20=; Weijing Liao, d2VpamluZ2xpYW9yZWhhYkAxNjMuY29t
†These authors have contributed equally to this work
Disclaimer: All claims expressed in this article are solely those of the authors and do not necessarily represent those of their affiliated organizations, or those of the publisher, the editors and the reviewers. Any product that may be evaluated in this article or claim that may be made by its manufacturer is not guaranteed or endorsed by the publisher.
Research integrity at Frontiers
Learn more about the work of our research integrity team to safeguard the quality of each article we publish.