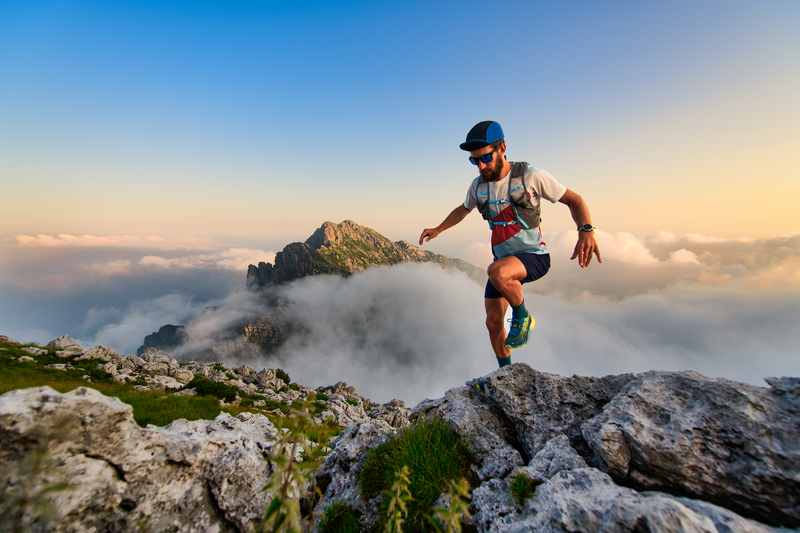
95% of researchers rate our articles as excellent or good
Learn more about the work of our research integrity team to safeguard the quality of each article we publish.
Find out more
REVIEW article
Front. Aging Neurosci. , 13 April 2021
Sec. Neuroinflammation and Neuropathy
Volume 13 - 2021 | https://doi.org/10.3389/fnagi.2021.632054
This article is part of the Research Topic Accurate Treatment of Cerebrovascular Diseases: Frontiers in Pathology, Diagnostic Methods and Treatment Targets View all 26 articles
Intracerebral hemorrhage (ICH) is the most fatal subtype of stroke with high disability and high mortality rates, and there is no effective treatment. The predilection site of ICH is in the area of the basal ganglia and internal capsule (IC), where exist abundant white matter (WM) fiber tracts, such as the corticospinal tract (CST) in the IC. Proximal or distal white matter injury (WMI) caused by intracerebral parenchymal hemorrhage is closely associated with poor prognosis after ICH, especially motor and sensory dysfunction. The pathophysiological mechanisms involved in WMI are quite complex and still far from clear. In recent years, the neuroprotection and repairment capacity of mesenchymal stem cells (MSCs) has been widely investigated after ICH. MSCs exert many unique biological effects, including self-recovery by producing growth factors and cytokines, regenerative repair, immunomodulation, and neuroprotection against oxidative stress, providing a promising cellular therapeutic approach for the treatment of WMI. Taken together, our goal is to discuss the characteristics of WMI following ICH, including the mechanism and potential promising therapeutic targets of MSCs, aiming at providing new clues for future therapeutic strategies.
Intracerebral hemorrhage (ICH) is triggered by the spontaneous rupture of blood vessels, wherein the blood constituents penetrate the brain parenchyma following a path of least resistance and thus destroy both gray and white matter (GM and WM) structures (Qureshi et al., 2009). ICH is the most lethal form of stroke characterized by high morbidity, high disability, and high mortality with no effective treatment. It was demonstrated that more than 77% of ICH patients suffered WM injury (WMI), and a better understanding of WMI and remyelination may shed new light on the treatment of ICH (Smith et al., 2004).
Damage to WM fibers which establish connections among different regions impairs brain connectivity, leading to functional deficits. Dysfunction following cerebral parenchymal hemorrhage derives not only from neuron or synapse losses, but also from primary damage to the WM axons. The area of the basal ganglia where abundant WM fiber tracts cross is the predominant location of hematoma in human ICH. The internal capsule (IC), which lies between the putamen (embraced in the basal ganglia) and the thalamus, contains both descending and ascending fiber bundles conducting sensory, motor, and visual information. Once bleeding occurs in this region, the physiological structure and function of the WM are interrupted to varying degrees, leading to various complications and dysfunctions, such as hemiplegia, hemianopia, hemidysesthesia, and aphasia (Kusano et al., 2009; Balami and Buchan, 2012; Keep et al., 2012; Wu et al., 2017). The corticospinal tracts (CSTs) and corticonuclear tracts’ damage can cause contralateral hemiplegia. Destruction of the optic radiation following deep basal ganglia ICH leads to hemianopia, while hemidysesthesia is caused due to lesions of the central thalamic radiations. It is worth mentioning that IC injury can present all these three clinical manifestations resulting in serious sensorimotor dysfunction in human ICH. WMI is considered the major cause of motor-sensory disorders commonly seen in ICH patients. Therefore, reducing WMI or repairing WM after ICH is critical for reducing long-term neurological deficits, especially for motor function recovery.
In the past few decades, stem cell therapy has been actively explored for the treatment of stroke. Mesenchymal stem cells (MSCs) are pivotal to tissue homeostasis, repair, and regeneration for their self-renewing and multipotent characteristics, which are emerging as the most promising means of allogeneic cell therapy. Basic and clinical research clarify that MSCs are not antigen-presenting cells and would not cause activation of the host’s immune system (Tse et al., 2003). These cells can be harvested and expanded from a variety of adult and perinatal tissues, such as bone marrow, adipose tissue, peripheral blood, fetal tissues, umbilical cord tissues, and placental tissues. The neuroprotection and repairment capacity of MSCs has been widely investigated after ICH. MSCs exert many unique biological effects in tissue replacement, neurotrophy, neurogenesis, angiogenesis, anti-apoptosis, and immunomodulation (Caplan and Dennis, 2006; Mine et al., 2013; Galipeau and Sensebe, 2018; Song et al., 2020), which provides a promising therapeutic strategy in the treatment of WMI. MSCs participate in both innate immunity and adaptive immunity. Their immunomodulatory functions are exerted mainly via the release of bioactive factors and interactions with immune cells, which then change the damaged microenvironment by shifting the balance from toxic to protective regenerative events.
Herein, this review is primarily focused on the pathophysiology and onset mechanism of post-ICH WMI and potential therapy methods of MSCs, in hopes that it can benefit clinical treatment.
White matter comprises over 40% of the total volume of adult brain tissue and takes an indispensable part in distributed neural networks that are responsible for neurobehavioral management (Bedell and Narayana, 1998; Herndon et al., 1998). The two main parts- myelinated axon tracts and supporting glial cells including oligodendrocytes (OLs), astrocytes, and microglia- are embraced in WM. The axons (nerve fibers) are surrounded by multiple dense myelin membranes produced by mature OLs (Kang and Yao, 2019), the molecular structure integrity of which makes axons insulated from each other, thus promising the quick and efficient conduction of electrical nerve impulses (transmitting the action potentials) and protecting the nerve fibers from injury (Roncagliolo et al., 2006).
Specifically, three parts constitute WM fiber bundles: projection tracts, commissural tracts, and association tracts (Gerrish et al., 2014). Projection tracts transmit nerve signals from the cortex to other regions of the central nervous system (CNS) (Ge et al., 2002). For instance, CST is the dominant pathway responsible for conveying descending information from the cerebral cortex to the spinal cord. The commissural tracts allow the communication between the left and right cerebral hemispheres (Ge et al., 2002). The association tracts build up connections among cortical lobes within the ipsilateral hemisphere. All these tracts form networks between different regions and serve diverse functions (Ge et al., 2002). Up to now, a large amount of research has clarified the brain functions associated with WM, such as cognitive function, motor function, reading, and practicing abilities (Schmahmann et al., 2008; Carreiras et al., 2009).
The pathophysiology change of WMI after ICH is mainly characterized by demyelination, axonal injury, and death of OLs. OLs are the sole source of myelin in the adult CNS, and generally form insulating myelin sheaths marked by myelin basic protein (MBP), enhancing the propagation of action potentials and supporting neuronal and axonal integrity through metabolic coupling under physical circumstances (Bacmeister et al., 2020). OLs contain a high level of iron and are especially sensitive to iron overload, which makes them quite vulnerable to hemorrhagic insults; meanwhile, missed OLs leave axons susceptible to degeneration (Windrem et al., 2020). With the degradation of hemoglobin released from dead erythrocytes, there is a sharp increase in intracellular Fe2+ destroying OLs, and iron chelator can inhibit this oxidative toxicity in OLs. Both apoptosis and necrosis are involved in the mechanism of OLs loss. With the onset of ICH, OLs’ expression of caspase-3 in the lesion increases and most of the damaged cells undergo necrosis. Mitochondrial dysfunction is also attributed to OLs apoptosis. Fortunately, OLs maintain the capacity of regeneration and repair after damage to the CNS. OLs death is accompanied by oligodendrocyte progenitor cells (OPCs) proliferation during the acute period in the perihematomal WMI, which is responsible for generating new OLs to remyelinate denuded axons and reconstruct neuronal function.
Dramatical demyelination and axonal damage were first observed at the perihematomal site within three days after ICH, and the axonal damage gradually extended to the adjacent parenchyma over time (Wasserman and Schlichter, 2008). These pathologic changes are highly related to brain edema and neurologic dysfunction, indicating that WMI plays a critical role in neurologic impairment. Hijioka et al. (2016) has investigated the exact relationship between axon pathology and impaired sensorimotor functions; the former mainly referred to axonal transport deficits and structural destruction. The axonal dysfunction in the IC was confirmed to be strongly linked with early motion disturbance after ICH in mice (Hijioka et al., 2016). Remyelination and axonal regeneration are considered valid repair forms in WMI (Joseph et al., 2016). Remyelination is defined as adult OPCs differentiating into new myelin-forming OLs in a regenerative process after CNS demyelination (Gensert and Goldman, 1997; Fancy et al., 2004; Kang and Yao, 2019). To successfully regenerate, damaged axons must reseal denuded stumps, rebuild the cytoskeleton, colligate and transport building substrates, package axon modules, and form growth cones, which is a highly energy-demanding process (Bradke et al., 2012; Lu et al., 2014; He and Jin, 2016). Based on this, Han et al. (2020) raised the “energy deficit” definition and proved that enhancing mitochondrial transport and energetic metabolism can dramatically stimulate axonal regeneration, promoting functional restoration in a spinal cord injury (SCI) animal model. Ultrastructural features of collagenase-induced ICH have been systematically examined in mice over time (Li et al., 2018). Obvious axonal demyelination and degeneration, and the presence of dystrophic neurites in the axons, were demonstrated but OLs proliferation was also observed, which is responsible for the myelination of axons (Li et al., 2018). They first showed a robust inflammatory response of erythrophagocytosis by microglia and macrophages after ICH by transmission electronic microscope (TEM) (Li et al., 2018). Myelination could also be regulated by the growth factor neuregulin (NRG) through binding to the transmembrane tyrosine kinase receptors (ErbB) on unsheathing OLs. Collectively, severe changes of myelin destruction and swelling axons are clear in the ICH animal models, but the exact mechanisms contributing to the loss of motor function are uncertain.
The influx of blood from vessels into the brain destroys GM and WM. Increasing studies have focused on the implicated pathophysiology of WMI after ICH (Wasserman and Schlichter, 2008; Gerrish et al., 2014; Chen W. et al., 2019; Chen Z. et al., 2019). It is demonstrated that the mass effect and barotrauma during hematoma formation present immediate compression of adjacent brain tissue the moment of ICH onset, which is defined as the primary brain injury. Soon afterward, secondary brain injury- excitotoxicity, oxidative stress, and neuroinflammation- aggravate the WMI leading to neurological deterioration (Aronowski and Zhao, 2011; Babu et al., 2012; Duan et al., 2016; Lan et al., 2017b), accompanied by a succession of pathologic changes which contain hemodynamic changes resulted from ischemia, increased cerebral edema, disruption of the blood–brain barrier (BBB) function, effects of erythrocytes decomposition products, and apoptosis (Aronowski and Zhao, 2011; Keep et al., 2012; Zhou et al., 2014; Chen S. et al., 2015). And it was shown that intracerebral hematoma extended via perivascular spaces and the perineurium (Yin et al., 2013). All these pathologic changes make the nerve fibers within the hematoma lesion distend, distort, and finally disrupt to a point at which they cannot be rescued (Figure 1). Those located in the perihematomal, meanwhile, exert varying degrees of impairment, exactly where researchers continuously focus on and try to intervene.
Figure 1. The mechanisms and signal pathways of white matter injury after intracerebral hemorrhage. WMI, white matter injury; ICH, intracerebral hemorrhage; ICP, intracranial pressure; DAMPs, damage-associated molecular pattern molecules; HO, hemeoxygenase; BBB, blood-brain barrier; NF-κB, nuclear factor-κB; ROS, reactive oxygen species.
Complex immune and inflammatory cascades characterized by the mobilization and activation of inflammatory molecules are triggered at the moment the blood composition is released into the substance, which plays a pivotal role in ICH-induced brain injury (Lan et al., 2017b), especially during the secondary WMI. The primary and secondary damage usually mutually affect the whole developing process. The leakage of BBB after ICH is mainly derived from endothelial cell activation and vascular ONOO– formation, leading to the reduction of key tight junction proteins which generally promise the BBB permeability such as claudin-5, occludin, and zonula occludens (ZO)-1 (Abbott et al., 2010). There is increasing evidence that an inflammatory response can promote the formation of edema by increasing the BBB permeability near the hematoma, thereby aggravating the mass effect, enhancing the process of cell death through secondary ischemia, and further causing inflammatory damage to the surrounding brain tissue (Chu et al., 2014; Wang T. et al., 2015). Many inflammatory cells have been demonstrated to be involved and work in concert to modulate the inflammatory response both in ischemic stroke and ICH. Herein, we introduce several immune cells involved in inflammation in detail as follows.
Microglia, the resident myeloid phagocytes in the brain parenchyma, constantly and rapidly monitor the brain microenvironment for threats and damage and play a critical role in maintaining homeostasis in the brain by removing pathogens and injured brain tissue debris, and reconstructing the extracellular matrix and synapses, just like a “cop.” When encountered with ICH, they can be activated within minutes and migrate to the lesion together with peripheral macrophages (Lan et al., 2017b), and become highly phagocytic to respond to factors secreted by necrotic neurons or astrocytes, like adenosine triphosphate (ATP), galactin-3, or high mobility group box 1 (HMGB1), which are all embraced in damage-associated molecular pattern molecules (DAMPs, also known as alarmins) (Kong and Le, 2011; Ohnishi et al., 2011; Fang et al., 2013; Zhou et al., 2014; Xiao et al., 2020). Meanwhile, microglia cells secrete multiple cytokines and chemokines associated with the axon-glial injury via the transcription factor nuclear factor-κB (NF-κB), and show enhanced phagocytosis, thereby aggravating structural WMI. DAMPs can initiate the activation of microglia via several toll-like receptors (TLRs) in the neuroinflammatory processes after ICH. The TLR4 on microglia cell surfaces generally interacts with TRIF and MyD88, and then the information is passed through downstream NF-κB, like phosphorylated P65, and several other secreted proinflammatory factors, causing inflammation (Zhou et al., 2014; Lan et al., 2017a). It was proven that traumatic brain injury (TBI) could induce a rapid and persistent up-regulation of Myd88, NF-κB, and proinflammatory cytokines (Ling et al., 2013). Microglial activation can also be induced by thrombin and complement factors via protease-activated receptors (PARs). A newly found adenosine diphosphate (ADP)/ATP-responsive G-protein coupled receptor, P2Y12, helps microglia migrate to the site of injury (Suzuki et al., 2020). Subsequently, microglia cells in an activated state and the toxic molecules secreted further destroy the BBB, leading to peripheral immune cells’ infiltration, thus propagating inflammatory damage.
According to the surface markers and intracellular cytokines expressed, microglia can be polarized into classically activated (M1, pro-inflammatory, and neurotoxic) and alternatively activated (M2, anti-inflammatory, and neuroprotective) phenotypes (Zhang et al., 2017). That the microglia can dynamically and temporally change their phenotypes in response to acute brain injury is exactly the pointcut of brain damage intervention researchers pay attention to. M1 microglia could release high levels of proinflammatory cytokines, that, in turn, hinder axonal regeneration and OLs maturation (Lampron et al., 2015; Chen et al., 2017; Qin et al., 2017). In contrast, polarized M2 microglia typically secrete restorative cytokines and growth factors, remove tissue debris through phagocytosis, and promote remyelination (Olah et al., 2012; Zhao et al., 2015). It was proven that activated microglia act as a double-edged sword and the transfer of M1 to M2 can dramatically alleviate the brain injury caused by inflammation (Zhao et al., 2019).
Astrocytes are recognized as active elements of the brain circuitry, and play key roles in maintaining homeostasis of the extracellular environment, including neurotrophic and structural supporting functions, stabilization of cell–cell communications, and anti-oxidative stress functions (Ransom and Ransom, 2012). Activated astrocytes usually respond to CNS disorders through reactive astrogliosis which represent a series of successive processes including changes of gene and protein expression, proliferation and migration of cells, cellular hypertrophy, and formation of glial scars. Some research demonstrated that reactive astrocytes generate neurotrophic factors (NTFs), isolate injured sites, and prevent harmful inflammation. Whereas it was shown that astrocytes could restrain axonal regeneration and hamper other repair processes in the brain by expressing a wide range of molecules as demonstrated in an earlier study (McKeon et al., 1991). Moreover, axonal regeneration and the reconnection among neurons are further inhibited by the resulting glial scar, inhibiting the formation of which does not always alleviate tissue damage in animal experiments (Wanner et al., 2013; Cregg et al., 2014).
Lymphocytes play a vital role in immune surveillance and homeostasis maintenance in the peripheral system, among which T cells mainly participate in adaptive cellular immunity. CD4+ T cells dominate the lymphocyte population whether in autoblood- or collagenase-induced ICH models, while CD8+ T cells constitute an extremely small infiltrating leukocyte population. Undisputedly, FoxP3+CD4+ regulatory T cells (Tregs) are the major immunosuppressive lineage of the CD4+ T cell compartment. It is generally supposed that T cells rarely enter the brain parenchyma except when pathologic changes occur, and only when the stroke onset, T cells would migrate largely and infiltrate into the lesion site following the activation of microglia (Ito et al., 2019).
Recent research demonstrated that it was the regulatory T cells inside the brain that exerted robust neuronal protection in ischemic stroke by suppressing neurotoxic astrogliosis through producing epidermal growth factor receptor (EGFR) ligand (Ito et al., 2019). This finding suggests that, in the repair of the ICH process, the function of brain T cells should also be highly focused on. Apart from sustaining immune tolerance (Sakaguchi et al., 2010; Lowther and Hafler, 2012), Tregs perform specialized functions in tissue homeostasis and remodeling mainly through restraining the activation and release of cytokines (Liesz et al., 2009; Ito et al., 2019). IL-10 originating from Tregs can trigger hemoglobin-activated microglia/macrophages toward the M2 phenotype. Similarly, it was indicated that Tregs protected against ICH-induced inflammatory injury by modulating microglia/macrophages polarization through the IL-10/GSK3β/PTEN axis which might exert importance in Treg-induced microglia polarization (Zhou et al., 2017). When co-cultured in vitro, Tregs also changed the polarization of microglia, decreased the expression of MHC-II, IL-6, and TNF-α, and increased expression of CD206.
CCR5 participates in the regulation of T cells and monocytes/macrophage lines’ migration, mainly expressed on the T cells at rest, monocytes, and immature dendritic cells (Joy et al., 2019). Also, accumulating evidence highlighted a central role for mTOR as a fundamental determinant of cell fate in antigen-activated CD4+ T cells. It was shown that suppression of activity in the Akt/mTOR pathway impaired Th17 and Th1 differentiation and promoted the development of Tregs. Fingolimod is recognized as an inhibitor of peripheral immune cell infiltration and can reduce inflammatory injury after ICH (Wei et al., 2011; Fu et al., 2014). These results demonstrated that the number of protective Tregs in the CNS was reduced when the infiltrating inflammatory cells were non-specifically inhibited. It was proven that the transfer of protective Tregs attenuated neurological deficit after ICH.
Oxidative stress initiated by the blood breakdown component or factors of the plasma plays a central role in the pathogenesis of WMI. Hemolysis after ICH is not that rapid. Rather, the main component of hematoma-erythrocytes starts to lyse 1 day after ICH and continues over days to weeks, followed by the release of decomposition products, including hemoglobin (Hb), heme, and iron, which exert neurotoxic effects on the lesion site and perihematomal regions (Cao et al., 2016). These metabolites, on the one hand, cause consecutive oxidative reactions; on the other hand, they can trigger inflammatory reaction via TLRs. Iron is derived from the collapse of heme by hemeoxygenase (HO)-1 and HO-2. Iron mediates neuronal ferroptosis, generating reactive oxygen species (ROS) and turning Fe2+ into Fe3+ via the Fenton reaction (Hu et al., 2016). ROS also mediates the inflammatory cascade, giving rise to cell death and perihematomal swelling, which finally leads to WMI.
In addition to increased ROS and oxidative stress, the levels of extracellular glutamate can also be augmented after ICH. It was confirmed followed by an increase of oxidative stress leading to sustained neuronal loss that ICH-induced striatal lesion produces distinct changes of EAAT1 and EAAT2 glutamate transporters expression and glutamate uptake activity. Besides, the level of perihematomal glutamate is highly related to the outcome in clinical ICH patients (Wu et al., 2013). It was demonstrated that in hemiplegic patients with basal ganglia bleeding affecting the IC, the earlier mini surgery was conducted, the less glutamate existing in peri-hematoma and the better prognosis, which means the level of glutamate is highly correlated with the outcome of ICH (Wu et al., 2013). Several pieces of research showed blood glutamate grabbing was effective at lessening the excitotoxicity of extracellular glutamate released during ischemic brain injury, although this did seemingly occur in ICH (Castillo et al., 2016; da Silva-Candal et al., 2018). It was indicated that blood glutamate grabbing cannot reduce the hematoma but still can serve as a safe excitotoxic treatment modality following ICH.
Ongoing research efforts are confirming our acknowledgment of the robust potential applications in regenerative medicine of stem cells (Chen et al., 2008), the therapeutic effectiveness of which has been reported in numerous previous experiments conducted in animal models of ICH. Among various stem cells, the multipotency and self-renewal capacity make MSCs a promising candidate for WMI treatment (Ramos-Cabrer et al., 2010; Huang et al., 2013; Mine et al., 2013), which can be expatiated as many unique biological effects including self-recovery by producing growth factors and cytokines, regenerative repair, inherent immunomodulation, and neuroprotection against oxidative stress, and can be further engineered to enhance immunomodulatory functions (Caplan and Dennis, 2006; Lee et al., 2014; Galipeau and Sensebe, 2018; Song et al., 2020), etc.
There are various sources of MSCs including adult tissues (e.g., bone marrow, adipose tissue, inner organs, and peripheral blood) and neonatal tissues (e.g., umbilical cord, placenta, amniotic fluid, and amniotic membrane). MSCs can be administered either via intracerebral injection into a specific brain region or by intravenous/intraarterial injection (Table 1). In an earlier study on the rat ICH model, intracerebral administration of MSCs enhanced motor coordination and balance, which was attributed to nerve fiber remyelination and axonal regeneration (Liu et al., 2010). Allogeneic and syngeneic BMSCs treatment after stroke in rats improved neurological recovery and enhanced reactive oligodendrocyte and astrocyte-related axonal remodeling with no indication of immunologic sensitization in the adult rat brain (Li et al., 2006). Furthermore, the safety and efficiency of MSCs therapy have also been proven in many clinical trials for stroke, and some of these trials proved the significant neuroprotective effects of MSCs (Table 2). These stem cells are mostly derived from umbilical cord blood, bone marrow, and adipose tissues. Human umbilical cord-derived MSCs (UCMSCs) have been used in clinical trials as a treatment for some neurological diseases since 2011. While the clinical evidence showing the regenerative and immunomodulatory potential of the MSCs on ischemic stroke continues to expand rapidly, the clinical studies on ICH are still scarce. Besides, the proliferation and functions of MSCs are known to decline during the process of senescence. The immunomodulatory functions of MSCs can be compromised due to increased reactive oxygen species and oxidative stress in aged cells. Therefore, early passage MSCs or strategies to prevent senescence must be considered to yield better therapeutic function (Li et al., 2017; Fafián-Labora et al., 2019). And freshly thawed MSCs seem to have an impaired immunomodulatory capacity compared to continuously cultured MSCs (Moll et al., 2016).
The organism itself possesses a few endogenous mechanisms, such as migration of endogenous stem cells and hematoma clearance, which benefit the repair of injured WM structures. Transplanting exogenous stem cells also exerts significant neuroprotection on ICH although the difficulty in obtainment limits their clinical applications. Other sources of stem cells are required for replacement therapy and MSCs rise in response to the proper time and conditions. MSCs can secrete many trophic molecules when transferred into the body generally by two approaches-orthotopic transplantation and caudal vein transplantation (Smith and Gavins, 2012; Shichinohe et al., 2015)- thus promoting endogenous repair mechanism, which eventually accelerates functional recovery after stroke.
Mesenchymal stem cells transplantation is promising in terms of angiogenesis. Pfeiffer et al. (2019) found human amnion–derived MSCs (hAMSCs), well-known for their favorable angiogenic potential, enhanced human placental endothelial cells (hPEC) viability, and network formation of endothelial cells by paracrine factors in vitro (Konig et al., 2015) and promoted angiogenesis in vivo (Kinzer et al., 2014; Tuca et al., 2016; Ertl et al., 2018). Brain-derived neurotrophic factor (BDNF), as the most abundant neurotrophin in the CNS, can promote neurogenesis, oligodendrocyte genesis, myelination, and synaptic plasticity through interaction with protein tropomyosin receptor kinase B (TrkB), which is also a receptor of neurotrophin-4 (NT4) (Tolar et al., 2010; Lim et al., 2011). Recently, given the fact that TrkB usually can’t be expressed by the MSCs in an undifferentiated state and the capacity of EA in promoting neurofunctional recovery through specific NTFs, such as VEGF, BDNF, and NT4 (Ahn et al., 2016), investigators combined the electroacupuncture (EA) with genetically modified TrkB gene-transfected MSCs (TrkB-MSCs) in a mouse model of ischemic stroke (Ahn et al., 2019). Consistent with the original assumption, the results showed the combination facilitated further neural survival and differentiation via stimulating the BDNF/NT4-TrkB signaling pathway rather than simply the administration of MSCs (Ahn et al., 2019). EA could directly stimulate the proliferation and differentiation of endogenous neural NSC in a rat model of ischemic stroke (Tan et al., 2018). BDNF can also exert neurotrophic effects on neuronal survival and neurite outgrowth, particularly the CST axons (Gupta et al., 2009). This procedure can be mediated by growth-associated protein-43 (GAP-43) which is highly distributed in the presynaptic membrane associated with neurite extension and long-term synaptic enhancement (Ramakers et al., 2000; Gupta et al., 2009; Morita and Miyata, 2013). Cui et al. (2017) elaborated that MSC graft increased GAP-43 expression via the ERK1/2 and pro-survival phosphatidylinositol 3-kinase (PI3K)/Akt signaling pathways after transplanting BMSC into a rat model of autologous blood injection. It means that MSC transplantation alleviated axonal damage and enhanced synaptic plasticity to some extent. GAP-43 may be considered a potential therapeutic target for MSCs in the treatment of axonal injury following ICH.
The CST is the only descending conduction pathway, in which some axons directly take shape synapses with spinal motoneurons, evolved in the major system for skilled voluntary movement in human and motor functions in rodents. Motor deficits in a stroke critically lie in an interruption of CST integrity, i.e., the motor fibers descending from the cortex to the spinal cord (Hijioka et al., 2016; Jang et al., 2018; Chen W. et al., 2019). Our previous research detected WM degeneration which lasted for at least 5 weeks after ICH, i.e., even during the chronic phase following stroke (Ng et al., 2020). And the longitudinal pathological alternations of the CST in the cervical portion of the spinal cord after unilateral striatal hemorrhage in adult mice were first illustrated, implying that the structural integrity of the CST was compromised extensively after ICH (Ng et al., 2020). In general, the establishment of compensatory re-innervation in the bilateral hemispheres after brain injury is mainly achieved through axonal sprouting of surviving neurons, new synapse formation, and factors produced by the brain. MSCs have been reported to promote neurogenesis and to alleviate side effects in injured brain regions, where both differentiation and secretion of MSCs involve axonal plasticity. In addition to the neuroprotective and neurotrophic effects, Choi et al. (2018) proved that MSCs may also prevent hematoma expansion in the hyperacute stage of ICH by enhancing endothelial integrity of cerebral vasculature by uplifting the expression of tight junction proteins (ZO-1, occludin).
The scientific fact that neuroinflammation makes a principal contribution to the progress of ICH-induced brain damage is well acknowledged. Thus, modulating the immune response could help improve brain injury outcomes following ICH. The highly anti-inflammatory and immunomodulatory properties make MSCs suitable therapeutic candidates in inflammatory diseases, through regulating infiltration of microglia and neutrophils and increasing anti-inflammatory cytokines levels, while also downregulating the expression of proinflammatory cytokines.
Emerging knowledge in targeting neuroinflammation argues MSCs are effective modifiers of microglial phenotype by maintaining a resting, pro-regenerative microglial phenotype, or by controlling the microglial activation following stroke (Wei et al., 2012; Yan et al., 2013). Neurological deficits of collagenase-induced ICH-bearing mice during the subacute phase were improved by human adipose-derived stem cells (hADSCs) which suppressed the acute inflammation mediated by CD11+CD45+ cells subpopulations (Kuramoto et al., 2019). In a middle cerebral artery occlusion (MCAO) model of rats, microglia activation and inflammatory signaling were dramatically reduced by transplanted MSCs activated by interferon (INF)-γ, along with oligodendrogenesis and the minimization of the infarct and penumbra (Tobin et al., 2020). The activation of microglia is largely determined by CX3CR1, and MSCs are known to shift activated inflammatory M1 macrophages to an M2 macrophage-like phenotype through prostaglandin E2 (PGE2). In a global cerebral ischemia (GCI) mice model conducted by Du et al. (2020), CX3CR1 downregulation markedly reduced activation of microglia and inflammatory responses and promoted the generation of mature OLs from OPCs, and thus protected myelin from ischemia-induced damage. Similarly, Hamzei Taj et al. (2018) transplanted the BMSCs line which was genetically engineered to express the anti-inflammatory cytokine IL-13 (further named as IL13-MSCs) to CX3CR1eGFP/+ CCR2RFP/+ knock-in fluorescent protein reporter mice to distinguish brain-resident microglia from infiltrated macrophages after ischemic stroke. They found the transplantation of IL13-MSCs shifted microglia and macrophages toward an anti-inflammatory, neuroprotective phenotype at 14 days after ischemia (Hamzei Taj et al., 2018). Compared with MSCs, IL13-MSCs were proven to better limit oligodendrocyte loss and demyelination in a model for neuroinflammation and demyelination of cuprizone-treated mice, and promote histopathological and functional recovery in SCI of mice (Hamzei Taj et al., 2018). Engineered MSCs were also applied in malignant glioma tumor models (Sun et al., 2011).
As one of the main components of glial cells, astrocytes’ regulation is also a promising target in WMI treatment (Zhang et al., 2006), although there is not adequate research recorded. Donega et al. (2014) reported that intranasal administration of human-MSC successfully reduced the expression of GFAP (a biomarker of astrocytes) and the formation of glial scars. Interestingly, in experiments conducted by Chen et al. (2020), transplanting BMSCs led to an elevation of GFAP level of expression; this difference might lie in the inherent double-edged features of activated astrocytes. Fortunately, the transplantation of MSCs into the CNS of ICH mice significantly improved cognitive and motor function and decreased hemorrhagic volume, which is consistent with previous research (Bedini et al., 2018). The key challenge in the treatment of ICH is to therefore understand how to magnify the advantages and minify the disadvantage of reactive astrocytes. Another factor that presents a double-edged sword function due to producing both pro-inflammatory and anti-inflammatory cytokines is IL-33; it was proven that IL-33 improved wound healing through enhanced M2 macrophage polarization in diabetic mice (He et al., 2017). It is a member of the IL-1 family mainly expressed in astrocytes, microglia, and OLs in CNS (Schmitz et al., 2005). Besides, a late research voted for IL-33 as a neuroprotective target which shifted microglia polarization from M1 to M2 and thus promoted OLs differentiation and WM repair banding with its ligand ST2 after ICH (Chen Z. et al., 2019).
The immunomodulatory functions of MSCs can also be exerted by secreting multifunctional paracrine signaling factors (Zhang, 2013; Zhao et al., 2013; Liu et al., 2014; Zhou et al., 2019), including cytokines, growth factors, and chemokines, which combine to regulate the immune cells’ function. Systemically delivered MSCs can pass through the BBB while very few of these cells are detected homing to and survive in the lesion site of the brain (Chen et al., 2001). Functional activities are still improved by transplantation therapy. It has been commonly accepted that the functional benefits of MSCs’ transplantation are due to increased trophic support from these cells that reduce overall inflammation, thereby eliminating the potentially toxic environment (Caplan and Dennis, 2006; Hess and Borlongan, 2008). Some studies described the bystander mechanism of MSCs that MSCs is related to some soluble factors such as IL-10, indoleamine 2,3-dioxygenase (IDO), PGE2, transforming growth factor-β1 (TGF-β1), tumor necrosis factor-α (TNF-α), and TNF-α stimulated gene/protein 6 (TSG-6) (Nemeth et al., 2009). These molecules are encapsulated in cell-secreted extracellular vesicles (EVs), which are usually divided into exosomes, microvesicles (MVs), and apoptotic bodies according to the size and cell of origin. TSG-6 is an anti-inflammatory factor that can suppress neutrophil migration into the inflammation region, interact through the CD44 receptor on resident macrophages, and inhibit the NF-κB signaling pathway (Chen M. et al., 2015). The BMSCs, injected from the jugular vein of ICH-bearing rats, attenuated the inflammatory response and decreased BBB disruption by secreting TSG-6 after being trapped in the lung (Chen M. et al., 2015). Most recently, researchers have verified the MSC-derived EVs (MSC-EVs), which mediate cell-to-cell inflammation and trophic signaling, to be feasible therapeutic targets for functional recovery after cortical injury in a monkey model (Medalla et al., 2020). Besides, MSC-EVs can also trigger macrophage polarization by increasing the formation of anti-inflammatory M2 phenotype over M1-like pro-inflammatory phenotype via downregulation of level of IL-23 and IL-22.
An in vitro experiment was performed which preclinically investigated the potential effect of MSCs on Treg and showed that MSCs induced the generation of Treg via epigenetic conversion of human conventional CD4 T cells, possibly partly through TGF-β and/or PD-1/PD-L1 pathway (Azevedo et al., 2020). PD-L1 was found to result in generating more Th2 and Treg cells but fewer Th1 and Th17 cells from naive CD4+ T cells via inhibiting the mTOR pathway in vitro experiment. Administrating PD-L1 could promote the development of Treg cells and inhibit the differentiation of Th17 cells and thus significantly alleviate symptoms and suppress disease progression in several murine models (Fujiwara et al., 2014; Ding et al., 2016; Han et al., 2017). Besides, PD-L1 usually promotes the phosphorylation of immune-receptor tyrosine and conveys negative modulation signals, leading to cell inactivation via STAT or Janus kinase (JAK). When the STAT1 phosphorylation was hampered, overexpressing PD-1/PD-L1 could decrease the M1 microglia, indicating a potential approach of transferring to the anti-inflammatory phenotype via inhibiting STAT1. The findings mentioned above may attract researchers to conduct further investigation on the specific mechanism and therapeutic effect of MSCs transplantation based on PD-1/PD-L1 after ICH.
The antioxidative stress properties of MSCs have been validated in many previous studies (Shalaby et al., 2014), as diminishing ROS via the Nrf2 signaling pathway and protecting the body from oxidative stress was proven in many diseases such as acute lung injury (ALI), acute respiratory distress syndrome (ARDS), acute myocardial infarction (AMI), and acute liver failure (ALF). Various preconditioning strategies have been used to enhance the therapeutic efficacy of MSCs. Hypoxia preconditioning is thought to enhance MSCs’ survival and the expression of various trophic factors of MSCs, and even to strengthen the engraftment and paracrine properties (Hu and Li, 2018).
For the past two decades, great progress in understanding the mechanisms of ICH-induced brain injury has been made (Balami and Buchan, 2012; Fang et al., 2013; Chen S. et al., 2015; Duan et al., 2016; Bobinger et al., 2018). But not until recently has the importance of WM damage in ICH, which exerts a high correlation with functional outcomes, been acknowledged. The WM is involved in the transmission of motor and sensory information between the cerebral cortex and spinal cord. Therefore, whether in hemorrhagic or ischemic stroke, WMI can cause serious cognitive dysfunction, emotional disorders, and motor disturbance. Without the parallel protection of WM, true lasting neurorestoration cannot be achieved.
Mesenchymal stem cells have proven to be an extremely promising therapy for WMI due to their multipotency and self-renewal capacity. Furthermore, they exert reduced immunogenicity because of a low MHC class I expression and the absence of MHC class II molecules and co-stimulatory factors. Most importantly, MSCs can produce many immunomodulatory, neurotrophic, and angiogenic factors and have a potential immunomodulatory effect on immune cells (Caplan and Dennis, 2006; Chen et al., 2008; Bedini et al., 2018; Galipeau and Sensebe, 2018). And the neuroprotective effects have been well recognized in numerous pieces of research. To enhance the therapeutic effects of MSC transplantation by boosting the immunomodulatory properties of MSCs, investigators can also make some improvements to the MSC and the results show enhanced therapeutic effect, especially for the inflammatory modulation.
It is true that numerous signaling molecular pathways are involved in inflammatory responses and further exacerbate secondary brain damage (Zhou et al., 2014; Zhu et al., 2019). Although the modulation of immunological response after ICH showed promising results in a small proof-of-concept study, larger trials need to be done to further verify this. There are still several questions that remain to be addressed. First, studies in WMI are insufficient, whether effective drug targets of MSCs in the diseases mentioned above are equally effective in WM damage after ICH requires further verification. Second, human ICH pathomechanisms cannot be entirely mimicked by experimental models. The proportion of WM in rodent animals, especially in rats and mice, is much smaller than that of humans. It is essential to apply animals whose brain structures fit better with humans in future studies. Third, a majority of studies on post-ICH WMI after ICH concentrate on single−factor intervention; agents with multiple targets or combined drug therapy strategies remain to be designed and tested in further research. Last but not least, cell resources, invasive extraction procedures, and cell quantity make future research of this therapy challenging. An improvement on MSCs calls for further investigation so that it can be better applied in ICH treatment.
The work presented here was carried out in collaboration with all authors. HS conceived and designed the review. JL wrote the manuscript. LX, DH, and YL helped with literature searching and summarizing. All authors read, commented on, and approved this manuscript.
This research was funded by the National Natural Science Foundation of China (81701243), Guangdong Basic and Applied Basic Research Foundation (2020A1515010038), the Pearl River S&T Nova Program of Guangzhou (201710010047), and the Presidential Foundation of Zhujiang Hospital of Southern Medical University (No. yzjj2018rc03).
The authors declare that the research was conducted in the absence of any commercial or financial relationships that could be construed as a potential conflict of interest.
The support from the National Natural Science Foundation of China is gratefully acknowledged.
Abbott, N. J., Patabendige, A. A., Dolman, D. E., Yusof, S. R., and Begley, D. J. (2010). Structure and function of the blood-brain barrier. Neurobiol. Dis. 37, 13–25. doi: 10.1016/j.nbd.2009.07.030
Ahn, S. M., Kim, Y. R., Kim, H. N., Shin, Y. I., Shin, H. K., and Choi, B. T. (2016). Electroacupuncture ameliorates memory impairments by enhancing oligodendrocyte regeneration in a mouse model of prolonged cerebral hypoperfusion. Sci. Rep. 6:28646. doi: 10.1038/srep28646
Ahn, S. M., Kim, Y. R., Shin, Y. I., Ha, K. T., Lee, S. Y., Shin, H. K., et al. (2019). Therapeutic potential of a combination of electroacupuncture and TrkB-Expressing mesenchymal stem cells for ischemic stroke. Mol. Neurobiol. 56, 157–173. doi: 10.1007/s12035-018-1067-z
Ahn, S. Y., Chang, Y. S., Sung, S. I., and Park, W. S. (2018). Mesenchymal stem cells for severe intraventricular hemorrhage in preterm infants: phase I dose-escalation clinical trial. Stem Cells Transl. Med. 7, 847–856. doi: 10.1002/sctm.17-0219
Aronowski, J., and Zhao, X. (2011). Molecular pathophysiology of cerebral hemorrhage: secondary brain injury. Stroke 42, 1781–1786. doi: 10.1161/strokeaha.110.596718
Azevedo, R. I., Minskaia, E., Fernandes-Platzgummer, A., Vieira, A. I. S., da Silva, C. L., Cabral, J. M. S., et al. (2020). Mesenchymal stromal cells induce regulatory T cells via epigenetic conversion of human conventional CD4 T cells in vitro. Stem Cells 38, 1007–1019. doi: 10.1002/stem.3185
Babu, R., Bagley, J. H., Di, C., Friedman, A. H., and Adamson, C. (2012). Thrombin and hemin as central factors in the mechanisms of intracerebral hemorrhage-induced secondary brain injury and as potential targets for intervention. Neurosurg. Focus 32:E8. doi: 10.3171/2012.1.Focus11366
Bacmeister, C. M., Barr, H. J., McClain, C. R., Thornton, M. A., Nettles, D., Welle, C. G., et al. (2020). Motor learning promotes remyelination via new and surviving oligodendrocytes. Nat. Neurosci. 23, 819–831. doi: 10.1038/s41593-020-0637-3
Balami, J. S., and Buchan, A. M. (2012). Complications of intracerebral haemorrhage. Lancet Neurol. 11, 101–118. doi: 10.1016/s1474-4422(11)70264-2
Bao, X. J., Liu, F. Y., Lu, S., Han, Q., Feng, M., Wei, J. J., et al. (2013). Transplantation of Flk-1+ human bone marrow-derived mesenchymal stem cells promotes behavioral recovery and anti-inflammatory and angiogenesis effects in an intracerebral hemorrhage rat model. Int. J, Mol. Med. 31, 1087–1096. doi: 10.3892/ijmm.2013.1290
Bedell, B. J., and Narayana, P. A. (1998). Volumetric analysis of white matter, gray matter, and CSF using fractional volume analysis. Magn. Reson. Med. 39, 961–969. doi: 10.1002/mrm.1910390614
Bedini, G., Bersano, A., Zanier, E. R., Pischiutta, F., and Parati, E. A. (2018). Mesenchymal stem cell therapy in intracerebral haemorrhagic stroke. Curr. Med. Chem. 25, 2176–2197. doi: 10.2174/0929867325666180111101410
Bhasin, A., Srivastava, M. V., Kumaran, S. S., Mohanty, S., Bhatia, R., Bose, S., et al. (2011). Autologous mesenchymal stem cells in chronic stroke. Cerebrovasc. Dis. Extra 1, 93–104. doi: 10.1159/000333381
Bhasin, A., Srivastava, M. V., Mohanty, S., Bhatia, R., Kumaran, S. S., and Bose, S. (2013). Stem cell therapy: a clinical trial of stroke. Clin. Neurol. Neurosurg. 115, 1003–1008. doi: 10.1016/j.clineuro.2012.10.015
Bobinger, T., Burkardt, P., Huttner, H. B., and Manaenko, A. (2018). programmed cell death after intracerebral hemorrhage. Curr. Neuropharmacol. 16, 1267–1281. doi: 10.2174/1570159X15666170602112851
Bradke, F., Fawcett, J. W., and Spira, M. E. (2012). Assembly of a new growth cone after axotomy: the precursor to axon regeneration. Nat. Rev. Neurosci. 13, 183–193. doi: 10.1038/nrn3176
Cao, S., Zheng, M., Hua, Y., Chen, G., Keep, R. F., and Xi, G. (2016). Hematoma changes during clot resolution after experimental intracerebral hemorrhage. Stroke 47, 1626–1631. doi: 10.1161/strokeaha.116.013146
Caplan, A. I., and Dennis, J. E. (2006). Mesenchymal stem cells as trophic mediators. J. Cell Biochem. 98, 1076–1084. doi: 10.1002/jcb.20886
Carreiras, M., Seghier, M. L., Baquero, S., Estévez, A., Lozano, A., Devlin, J. T., et al. (2009). An anatomical signature for literacy. Nature 461, 983–986. doi: 10.1038/nature08461
Castillo, J., Loza, M. I., Mirelman, D., Brea, J., Blanco, M., Sobrino, T., et al. (2016). A novel mechanism of neuroprotection: blood glutamate grabber. J. Cereb. Blood Flow Metab. 36, 292–301. doi: 10.1177/0271678x15606721
Chen, J., Li, Y., Wang, L., Zhang, Z., Lu, D., Lu, M., et al. (2001). Therapeutic benefit of intravenous administration of bone marrow stromal cells after cerebral ischemia in rats. Stroke 32, 1005–1011. doi: 10.1161/01.str.32.4.1005
Chen, L., Xi, H., Huang, H., Zhang, F., Liu, Y., Chen, D., et al. (2013). Multiple cell transplantation based on an intraparenchymal approach for patients with chronic phase stroke. Cell Transplant. 22(Suppl. 1), S83–S91. doi: 10.3727/096368913x672154
Chen, M., Li, X., Zhang, X., He, X., Lai, L., Liu, Y., et al. (2015). The inhibitory effect of mesenchymal stem cell on blood-brain barrier disruption following intracerebral hemorrhage in rats: contribution of TSG-6. J. Neuroinflammation 12:61. doi: 10.1186/s12974-015-0284-x
Chen, S., Yang, Q., Chen, G., and Zhang, J. H. (2015). An update on inflammation in the acute phase of intracerebral hemorrhage. Transl. Stroke Res. 6, 4–8. doi: 10.1007/s12975-014-0384-4
Chen, W., Xia, M., Guo, C., Jia, Z., Wang, J., Li, C., et al. (2019). Modified behavioural tests to detect white matter injury- induced motor deficits after intracerebral haemorrhage in mice. Sci. Rep. 9:16958. doi: 10.1038/s41598-019-53263-6
Chen, X., Liang, H., Xi, Z., Yang, Y., Shan, H., Wang, B., et al. (2020). BM-MSC transplantation alleviates intracerebral hemorrhage-induced brain injury, promotes astrocytes vimentin expression, and enhances astrocytes antioxidation via the Cx43/Nrf2/HO-1 Axis. Front. Cell Dev. Biol. 8:302. doi: 10.3389/fcell.2020.00302
Chen, Y., Shao, J.-Z., Xiang, L.-X., Dong, X.-J., and Zhang, G.-R. (2008). Mesenchymal stem cells: a promising candidate in regenerative medicine. Int. J. Biochem. Cell Biol. 40, 815–820. doi: 10.1016/j.biocel.2008.01.007
Chen, Y., Tian, H., Yao, E., Tian, Y., Zhang, H., Xu, L., et al. (2017). Soluble epoxide hydrolase inhibition promotes white matter integrity and long-term functional recovery after chronic hypoperfusion in mice. Sci. Rep. 7:7758. doi: 10.1038/s41598-017-08227-z
Chen, Z., Xu, N., Dai, X., Zhao, C., Wu, X., Shankar, S., et al. (2019). Interleukin-33 reduces neuronal damage and white matter injury via selective microglia M2 polarization after intracerebral hemorrhage in rats. Brain Res. Bull. 150, 127–135. doi: 10.1016/j.brainresbull.2019.05.016
Choi, B. Y., Kim, O. J., Min, S. H., Jeong, J. H., Suh, S. W., and Chung, T. N. (2018). Human placenta-derived mesenchymal stem cells reduce mortality and hematoma size in a rat intracerebral hemorrhage model in an acute phase. Stem Cells Int. 2018:1658195. doi: 10.1155/2018/1658195
Chu, H., Ding, H., Tang, Y., and Dong, Q. (2014). Erythropoietin protects against hemorrhagic blood-brain barrier disruption through the effects of aquaporin-4. Lab Invest. 94, 1042–1053. doi: 10.1038/labinvest.2014.84
Cregg, J. M., DePaul, M. A., Filous, A. R., Lang, B. T., Tran, A., and Silver, J. (2014). Functional regeneration beyond the glial scar. Exp. Neurol. 253, 197–207. doi: 10.1016/j.expneurol.2013.12.024
Cui, J., Cui, C., Cui, Y., Li, R., Sheng, H., Jiang, X., et al. (2017). Bone marrow mesenchymal stem cell transplantation increases GAP-43 Expression via ERK1/2 and PI3K/Akt pathways in intracerebral hemorrhage. Cell Physiol. Biochem. 42, 137–144. doi: 10.1159/000477122
da Silva-Candal, A., Pérez-Díaz, A., Santamaría, M., Correa-Paz, C., Rodríguez-Yáñez, M., Ardá, A., et al. (2018). Clinical validation of blood/brain glutamate grabbing in acute ischemic stroke. Ann. Neurol. 84, 260–273. doi: 10.1002/ana.25286
Deng, L., Peng, Q., Wang, H., Pan, J., Zhou, Y., Pan, K., et al. (2019). Intrathecal injection of allogenic bone marrow-derived mesenchymal stromal cells in treatment of patients with severe ischemic stroke: study protocol for a randomized controlled observer-blinded trial. Transl Stroke Res. 10, 170–177. doi: 10.1007/s12975-018-0634-y
Díez-Tejedor, E., Gutiérrez-Fernández, M., Martínez-Sánchez, P., Rodríguez-Frutos, B., Ruiz-Ares, G., Lara, M. L., et al. (2014). Reparative therapy for acute ischemic stroke with allogeneic mesenchymal stem cells from adipose tissue: a safety assessment: a phase II randomized, double-blind, placebo-controlled, single-center, pilot clinical trial. J. Stroke Cerebrovasc. Dis. 23, 2694–2700. doi: 10.1016/j.jstrokecerebrovasdis.2014.06.011
Ding, Y., Han, R., Jiang, W., Xiao, J., Liu, H., Chen, X., et al. (2016). Programmed death ligand 1 plays a neuroprotective role in experimental autoimmune neuritis by controlling peripheral nervous system inflammation of rats. J. Immunol. 197, 3831–3840. doi: 10.4049/jimmunol.1601083
Donega, V., Nijboer, C. H., Braccioli, L., Slaper-Cortenbach, I., Kavelaars, A., van Bel, F., et al. (2014). Intranasal administration of human MSC for ischemic brain injury in the mouse: in vitro and in vivo neuroregenerative functions. PLoS One 9:e112339. doi: 10.1371/journal.pone.0112339
Du, B., Liang, M., Zheng, H., Fan, C., Zhang, H., Lu, X., et al. (2020). Anti-mouse CX3CR1 antibody alleviates cognitive impairment, neuronal loss and myelin deficits in an animal model of brain ischemia. Neuroscience 438, 169–181. doi: 10.1016/j.neuroscience.2020.05.011
Duan, X., Wen, Z., Shen, H., Shen, M., and Chen, G. (2016). Intracerebral hemorrhage, oxidative stress, and antioxidant therapy. Oxid. Med. Cell Longev. 2016:1203285. doi: 10.1155/2016/1203285
Ertl, J., Pichlsberger, M., Tuca, A. C., Wurzer, P., Fuchs, J., Geyer, S. H., et al. (2018). Comparative study of regenerative effects of mesenchymal stem cells derived from placental amnion, chorion and umbilical cord on dermal wounds. Placenta 65, 37–46. doi: 10.1016/j.placenta.2018.04.004
Fafián-Labora, J. A., Morente-López, M., and Arufe, M. C. (2019). Effect of aging on behaviour of mesenchymal stem cells. World J. Stem Cells 11, 337–346. doi: 10.4252/wjsc.v11.i6.337
Fancy, S. P., Zhao, C., and Franklin, R. J. (2004). Increased expression of Nkx2.2 and Olig2 identifies reactive oligodendrocyte progenitor cells responding to demyelination in the adult CNS. Mol. Cell Neurosci. 27, 247–254. doi: 10.1016/j.mcn.2004.06.015
Fang, H., Wang, P. F., Zhou, Y., Wang, Y. C., and Yang, Q. W. (2013). Toll-like receptor 4 signaling in intracerebral hemorrhage-induced inflammation and injury. J. Neuroinflammation 10:27. doi: 10.1186/1742-2094-10-27
Fatar, M., Stroick, M., Griebe, M., Marwedel, I., Kern, S., Bieback, K., et al. (2008). Lipoaspirate-derived adult mesenchymal stem cells improve functional outcome during intracerebral hemorrhage by proliferation of endogenous progenitor cells stem cells in intracerebral hemorrhages. Neurosci. Lett. 443, 174–178. doi: 10.1016/j.neulet.2008.07.077
Feng, M., Zhu, H., Zhu, Z., Wei, J., Lu, S., Li, Q., et al. (2010). Serial 18F-FDG PET demonstrates benefit of human mesenchymal stem cells in treatment of intracerebral hematoma: a translational study in a primate model. J. Nucl. Med. 52, 90–97. doi: 10.2967/jnumed.110.080325
Fu, Y., Hao, J., Zhang, N., Ren, L., Sun, N., Li, Y. J., et al. (2014). Fingolimod for the treatment of intracerebral hemorrhage: a 2-arm proof-of-concept study. JAMA Neurol. 71, 1092–1101. doi: 10.1001/jamaneurol.2014.1065
Fujiwara, H., Maeda, Y., Kobayashi, K., Nishimori, H., Matsuoka, K., Fujii, N., et al. (2014). Programmed death-1 pathway in host tissues ameliorates Th17/Th1-mediated experimental chronic graft-versus-host disease. J. Immunol. 193, 2565–2573. doi: 10.4049/jimmunol.1400954
Galipeau, J., and Sensebe, L. (2018). Mesenchymal stromal cells: clinical challenges and therapeutic opportunities. Cell Stem Cell 22, 824–833. doi: 10.1016/j.stem.2018.05.004
Ge, Y., Grossman, R. I., Babb, J. S., Rabin, M. L., Mannon, L. J., and Kolson, D. L. (2002). Age-related total gray matter and white matter changes in normal adult brain. Part II: quantitative magnetization transfer ratio histogram analysis. AJNR Am. J. Neuroradiol. 23, 1334–1341.
Gensert, J. M., and Goldman, J. E. (1997). Endogenous progenitors remyelinate demyelinated axons in the adult CNS. Neuron 19, 197–203. doi: 10.1016/s0896-6273(00)80359-1
Gerrish, A. C., Thomas, A. G., and Dineen, R. A. (2014). Brain white matter tracts: functional anatomy and clinical relevance. Semin. Ultrasound. CT MR 35, 432–444. doi: 10.1053/j.sult.2014.06.003
Gupta, S. K., Mishra, R., Kusum, S., Spedding, M., Meiri, K. F., Gressens, P., et al. (2009). GAP-43 is essential for the neurotrophic effects of BDNF and positive AMPA receptor modulator S18986. Cell Death Differ. 16, 624–637. doi: 10.1038/cdd.2008.188
Hamzei Taj, S., Le Blon, D., Hoornaert, C., Daans, J., Quarta, A., Praet, J., et al. (2018). Targeted intracerebral delivery of the anti-inflammatory cytokine IL13 promotes alternative activation of both microglia and macrophages after stroke. J. Neuroinflammation 15:174. doi: 10.1186/s12974-018-1212-7
Han, Q., Xie, Y., Ordaz, J. D., Huh, A. J., Huang, N., Wu, W., et al. (2020). Restoring cellular energetics promotes axonal regeneration and functional recovery after spinal cord injury. Cell Metab. 31, 623.e8–641.e8. doi: 10.1016/j.cmet.2020.02.002
Han, R., Luo, J., Shi, Y., Yao, Y., and Hao, J. (2017). PD-L1 (Programmed Death Ligand 1) protects against experimental intracerebral hemorrhage-induced brain injury. Stroke 48, 2255–2262. doi: 10.1161/strokeaha.117.016705
He, R., Yin, H., Yuan, B., Liu, T., Luo, L., Huang, P., et al. (2017). IL-33 improves wound healing through enhanced M2 macrophage polarization in diabetic mice. Mol. Immunol. 90, 42–49. doi: 10.1016/j.molimm.2017.06.249
He, Z., and Jin, Y. (2016). Intrinsic control of axon regeneration. Neuron 90, 437–451. doi: 10.1016/j.neuron.2016.04.022
Herndon, R. C., Lancaster, J. L., Giedd, J. N., and Fox, P. T. (1998). Quantification of white matter and gray matter volumes from three-dimensional magnetic resonance volume studies using fuzzy classifiers. J. Magn. Reson. Imaging 8, 1097–1105. doi: 10.1002/jmri.1880080515
Hess, D. C., and Borlongan, C. V. (2008). Stem cells and neurological diseases. Cell Prolif. 41(Suppl. 1), 94–114. doi: 10.1111/j.1365-2184.2008.00486.x
Hijioka, M., Anan, J., Matsushita, H., Ishibashi, H., Kurauchi, Y., Hisatsune, A., et al. (2016). Axonal dysfunction in internal capsule is closely associated with early motor deficits after intracerebral hemorrhage in mice. Neurosci. Res. 106, 38–46. doi: 10.1016/j.neures.2015.10.006
Honmou, O., Houkin, K., Matsunaga, T., Niitsu, Y., Ishiai, S., Onodera, R., et al. (2011). Intravenous administration of auto serum-expanded autologous mesenchymal stem cells in stroke. Brain 134(Pt 6), 1790–1807. doi: 10.1093/brain/awr063
Hu, C., and Li, L. (2018). Preconditioning influences mesenchymal stem cell properties in vitro and in vivo. J. Cell Mol. Med. 22, 1428–1442. doi: 10.1111/jcmm.13492
Hu, X., Tao, C., Gan, Q., Zheng, J., Li, H., and You, C. (2016). Oxidative stress in intracerebral hemorrhage: sources. mechanisms, and therapeutic targets. Oxid. Med. Cell Longev. 2016:3215391. doi: 10.1155/2016/3215391
Huang, W., Mo, X., Qin, C., Zheng, J., Liang, Z., and Zhang, C. (2013). Transplantation of differentiated bone marrow stromal cells promotes motor functional recovery in rats with stroke. Neurol. Res. 35, 320–328. doi: 10.1179/1743132812y.0000000151
Ito, M., Komai, K., Mise-Omata, S., Iizuka-Koga, M., Noguchi, Y., Kondo, T., et al. (2019). Brain regulatory T cells suppress astrogliosis and potentiate neurological recovery. Nature 565, 246–250. doi: 10.1038/s41586-018-0824-5
Jaillard, A., Hommel, M., Moisan, A., Zeffiro, T. A., Favre-Wiki, I. M., Barbieux-Guillot, M., et al. (2020). Autologous mesenchymal stem cells improve motor recovery in subacute ischemic stroke: a randomized clinical trial. Transl. Stroke Res. 11, 910–923. doi: 10.1007/s12975-020-00787-z
Jang, S., Kwon, Y., and Kwak, S. (2018). Change of an injured corticospinal tract during 3 weeks’ rehabilitation after putaminal hemorrhage. Am. J. Phys. Med. Rehabil. 97, e29–e30. doi: 10.1097/phm.0000000000000787
Jiang, Y., Zhu, W., Zhu, J., Wu, L., Xu, G., and Liu, X. (2013). Feasibility of delivering mesenchymal stem cells via catheter to the proximal end of the lesion artery in patients with stroke in the territory of the middle cerebral artery. Cell Transplant 22, 2291–2298. doi: 10.3727/096368912x658818
Joseph, M. J. E., Caliaperumal, J., and Schlichter, L. C. (2016). After intracerebral hemorrhage, oligodendrocyte precursors proliferate and differentiate inside white-matter tracts in the rat striatum. Transl. Stroke Res. 7, 192–208. doi: 10.1007/s12975-015-0445-3
Joy, M. T., Ben Assayag, E., Shabashov-Stone, D., Liraz-Zaltsman, S., Mazzitelli, J., Arenas, M., et al. (2019). CCR5 is a therapeutic target for recovery after stroke and traumatic brain injury. Cell 176, 1143.e13–1157.e13. doi: 10.1016/j.cell.2019.01.044
Kang, M., and Yao, Y. (2019). Oligodendrocytes in intracerebral hemorrhage. CNS Neurosc. Ther. 25, 1075–1084. doi: 10.1111/cns.13193
Keep, R. F., Hua, Y., and Xi, G. (2012). Intracerebral haemorrhage: mechanisms of injury and therapeutic targets. Lancet Neurol. 11, 720–731. doi: 10.1016/s1474-4422(12)70104-7
Kim, K., Park, H. W., Moon, H. E., Kim, J. W., Bae, S., Chang, J. W., et al. (2015). The effect of human umbilical cord blood-derived mesenchymal stem cells in a collagenase-induced intracerebral hemorrhage rat model. Exp. Neurobiol. 24, 146–155. doi: 10.5607/en.2015.24.2.146
Kim, S. J., Moon, G. J., Chang, W. H., Kim, Y. H., and Bang, O. Y. (2013). Intravenous transplantation of mesenchymal stem cells preconditioned with early phase stroke serum: current evidence and study protocol for a randomized trial. Trials 14:317. doi: 10.1186/1745-6215-14-317
Kinzer, M., Hingerl, K., Konig, J., Reinisch, A., Strunk, D., Huppertz, B., et al. (2014). Mesenchymal stromal cells from the human placenta promote neovascularization in a mouse model in vivo. Placenta 35, 517–519. doi: 10.1016/j.placenta.2014.04.004
Kong, Y., and Le, Y. (2011). Toll-like receptors in inflammation of the central nervous system. Int/Immunopharmacol. 11, 1407–1414. doi: 10.1016/j.intimp.2011.04.025
Konig, J., Weiss, G., Rossi, D., Wankhammer, K., Reinisch, A., Kinzer, M., et al. (2015). Placental mesenchymal stromal cells derived from blood vessels or avascular tissues: what is the better choice to support endothelial cell function? Stem Cells Dev. 24, 115–131. doi: 10.1089/scd.2014.0115
Kuramoto, Y., Takagi, T., Tatebayashi, K., Beppu, M., Doe, N., Fujita, M., et al. (2019). Intravenous administration of human adipose-derived stem cells ameliorates motor and cognitive function for intracerebral hemorrhage mouse model. Brain Res. 1711, 58–67. doi: 10.1016/j.brainres.2018.12.042
Kusano, Y., Seguchi, T., Horiuchi, T., Kakizawa, Y., Kobayashi, T., Tanaka, Y., et al. (2009). Prediction of functional outcome in acute cerebral hemorrhage using diffusion tensor imaging at 3T: a prospective study. AJNR Am. J. Neuroradiol. 30, 1561–1565. doi: 10.3174/ajnr.A1639
Lampron, A., Larochelle, A., Laflamme, N., Préfontaine, P., Plante, M. M., Sánchez, M. G., et al. (2015). Inefficient clearance of myelin debris by microglia impairs remyelinating processes. J. Exp. Med. 212, 481–495. doi: 10.1084/jem.20141656
Lan, X., Han, X., Li, Q., Li, Q., Gao, Y., Cheng, T., et al. (2017a). Pinocembrin protects hemorrhagic brain primarily by inhibiting toll-like receptor 4 and reducing M1 phenotype microglia. Brain Behav. Immun. 61, 326–339. doi: 10.1016/j.bbi.2016.12.012
Lan, X., Han, X., Li, Q., Yang, Q. W., and Wang, J. (2017b). Modulators of microglial activation and polarization after intracerebral haemorrhage. Nat. Rev. Neurol. 13, 420–433. doi: 10.1038/nrneurol.2017.69
Lee, H. K., Finniss, S., Cazacu, S., Xiang, C., and Brodie, C. (2014). Mesenchymal stem cells deliver exogenous miRNAs to neural cells and induce their differentiation and glutamate transporter expression. Stem Cells Dev. 23, 2851–2861. doi: 10.1089/scd.2014.0146
Lee, H. S., Kim, K. S., Lim, H. S., Choi, M., Kim, H. K., Ahn, H. Y., et al. (2015). Priming Wharton’s jelly-derived mesenchymal stromal/stem cells with ROCK inhibitor improves recovery in an intracerebral hemorrhage model. J. Cell Biochem. 116, 310–319. doi: 10.1002/jcb.24969
Lee, J. S., Hong, J. M., Moon, G. J., Lee, P. H., Ahn, Y. H., and Bang, O. Y. (2010). A long-term follow-up study of intravenous autologous mesenchymal stem cell transplantation in patients with ischemic stroke. Stem Cells 28, 1099–1106. doi: 10.1002/stem.430
Levy, M. L., Crawford, J. R., Dib, N., Verkh, L., Tankovich, N., and Cramer, S. C. (2019). Phase I/II study of safety and preliminary efficacy of intravenous allogeneic mesenchymal stem cells in chronic stroke. Stroke 50, 2835–2841. doi: 10.1161/strokeaha.119.026318
Li, Q., Weiland, A., Chen, X., Lan, X., Han, X., Durham, F., et al. (2018). Ultrastructural characteristics of neuronal death and white matter injury in mouse brain tissues after intracerebral hemorrhage: coexistence of ferroptosis. Autophagy, and Necrosis. Front. Neurol. 9:581. doi: 10.3389/fneur.2018.00581
Li, Y., McIntosh, K., Chen, J., Zhang, C., Gao, Q., Borneman, J., et al. (2006). Allogeneic bone marrow stromal cells promote glial-axonal remodeling without immunologic sensitization after stroke in rats. Exp. Neurol. 198, 313–325. doi: 10.1016/j.expneurol.2005.11.029
Li, Y., Wu, Q., Wang, Y., Li, L., Bu, H., and Bao, J. (2017). Senescence of mesenchymal stem cells (Review). Int. J. Mol. Med. 39, 775–782. doi: 10.3892/ijmm.2017.2912
Li, Z. M., Zhang, Z. T., Guo, C. J., Geng, F. Y., Qiang, F., and Wang, L. X. (2013). Autologous bone marrow mononuclear cell implantation for intracerebral hemorrhage-a prospective clinical observation. Clin. Neurol. Neurosurg. 115, 72–76. doi: 10.1016/j.clineuro.2012.04.030
Liao, W., Zhong, J., Yu, J., Xie, J., Liu, Y., Du, L., et al. (2009). Therapeutic benefit of human umbilical cord derived mesenchymal stromal cells in intracerebral hemorrhage rat: implications of anti-inflammation and angiogenesis. Cell Physiol. Biochem. 24, 307–316. doi: 10.1159/000233255
Liesz, A., Suri-Payer, E., Veltkamp, C., Doerr, H., Sommer, C., Rivest, S., et al. (2009). Regulatory T cells are key cerebroprotective immunomodulators in acute experimental stroke. Nat. Med. 15, 192–199. doi: 10.1038/nm.1927
Lim, J. Y., Park, S. I., Kim, S. M., Jun, J. A., Oh, J. H., Ryu, C. H., et al. (2011). Neural differentiation of brain-derived neurotrophic factor-expressing human umbilical cord blood-derived mesenchymal stem cells in culture via TrkB-mediated ERK and β-catenin phosphorylation and following transplantation into the developing brain. Cell Transplant 20, 1855–1866. doi: 10.3727/096368910x557236
Ling, H. P., Li, W., Zhou, M. L., Tang, Y., Chen, Z. R., and Hang, C. H. (2013). Expression of intestinal myeloid differentiation primary response protein 88 (Myd88) following experimental traumatic brain injury in a mouse model. J. Surg. Res. 179, e227–e234. doi: 10.1016/j.jss.2012.03.030
Liu, A. M., Lu, G., Tsang, K. S., Li, G., Wu, Y., Huang, Z. S., et al. (2010). Umbilical cord-derived mesenchymal stem cells with forced expression of hepatocyte growth factor enhance remyelination and functional recovery in a rat intracerebral hemorrhage model. Neurosurgery 67, 357–365. doi: 10.1227/01.NEU.0000371983.06278.B3
CrossRef Full Text | discussion 365-6,Google Scholar
Liu, L., Yu, Y., Hou, Y., Chai, J., Duan, H., Chu, W., et al. (2014). Human umbilical cord mesenchymal stem cells transplantation promotes cutaneous wound healing of severe burned rats. PLoS One 9:e88348. doi: 10.1371/journal.pone.0088348
Lowther, D. E., and Hafler, D. A. (2012). Regulatory T cells in the central nervous system. Immunol. Rev. 248, 156–169. doi: 10.1111/j.1600-065X.2012.01130.x
Lu, P., Woodruff, G., Wang, Y., Graham, L., Hunt, M., Wu, D., et al. (2014). Long-distance axonal growth from human induced pluripotent stem cells after spinal cord injury. Neuron 83, 789–796. doi: 10.1016/j.neuron.2014.07.014
McKeon, R. J., Schreiber, R. C., Rudge, J. S., and Silver, J. (1991). Reduction of neurite outgrowth in a model of glial scarring following CNS injury is correlated with the expression of inhibitory molecules on reactive astrocytes. J. Neurosci. 11, 3398–3411. doi: 10.1523/jneurosci.11-11-03398.1991
Medalla, M., Chang, W., Calderazzo, S. M., Go, V., Tsolias, A., Goodliffe, J. W., et al. (2020). Treatment with mesenchymal-derived extracellular vesicles reduces injury-related pathology in pyramidal neurons of monkey perilesional ventral premotor cortex. J. Neurosci. 40, 3385–3407. doi: 10.1523/JNEUROSCI.2226-19.2020
Mine, Y., Tatarishvili, J., Oki, K., Monni, E., Kokaia, Z., and Lindvall, O. (2013). Grafted human neural stem cells enhance several steps of endogenous neurogenesis and improve behavioral recovery after middle cerebral artery occlusion in rats. Neurobiol. Dis. 52, 191–203. doi: 10.1016/j.nbd.2012.12.006
Moll, G., Geißler, S., Catar, R., Ignatowicz, L., Hoogduijn, M. J., Strunk, D., et al. (2016). Cryopreserved or fresh mesenchymal stromal cells: only a matter of taste or key to unleash the full clinical potential of MSC therapy? Adv. Exp. Med. Biol. 951, 77–98. doi: 10.1007/978-3-319-45457-3_7
Morita, S., and Miyata, S. (2013). Synaptic localization of growth-associated protein 43 in cultured hippocampal neurons during synaptogenesis. Cell Biochem. Funct. 31, 400–411. doi: 10.1002/cbf.2914
Nagai, A., Kim, W. K., Lee, H. J., Jeong, H. S., Kim, K. S., Hong, S. H., et al. (2007). Multilineage potential of stable human mesenchymal stem cell line derived from fetal marrow. PLoS One 2:e1272. doi: 10.1371/journal.pone.0001272
Nemeth, K., Leelahavanichkul, A., Yuen, P. S., Mayer, B., Parmelee, A., Doi, K., et al. (2009). Bone marrow stromal cells attenuate sepsis via prostaglandin E(2)-dependent reprogramming of host macrophages to increase their interleukin-10 production. Nat. Med. 15, 42–49. doi: 10.1038/nm.1905
Ng, A. C. K., Yao, M., Cheng, S. Y., Li, J., Huang, J. D., Wu, W., et al. (2020). Protracted morphological changes in the corticospinal tract within the cervical spinal cord after intracerebral hemorrhage in the right striatum of mice. Front. Neurosci. 14:506. doi: 10.3389/fnins.2020.00506
Ohnishi, M., Katsuki, H., Fukutomi, C., Takahashi, M., Motomura, M., Fukunaga, M., et al. (2011). HMGB1 inhibitor glycyrrhizin attenuates intracerebral hemorrhage-induced injury in rats. Neuropharmacology 61, 975–980. doi: 10.1016/j.neuropharm.2011.06.026
Olah, M., Amor, S., Brouwer, N., Vinet, J., Eggen, B., Biber, K., et al. (2012). Identification of a microglia phenotype supportive of remyelination. Glia 60, 306–321. doi: 10.1002/glia.21266
Oppliger, B., Joerger-Messerli, M., Mueller, M., Reinhart, U., Schneider, P., Surbek, D. V., et al. (2016). Intranasal delivery of umbilical cord-derived mesenchymal stem cells preserves myelination in perinatal brain damage. Stem Cells Dev. 25, 1234–1242. doi: 10.1089/scd.2016.0027
Otero-Ortega, L., Gutierrez-Fernandez, M., Ramos-Cejudo, J., Rodriguez-Frutos, B., Fuentes, B., Sobrino, T., et al. (2015). White matter injury restoration after stem cell administration in subcortical ischemic stroke. Stem Cell Res. Ther. 6:121. doi: 10.1186/s13287-015-0111-4
Pfeiffer, D., Wankhammer, K., Stefanitsch, C., Hingerl, K., Huppertz, B., Dohr, G., et al. (2019). Amnion-derived mesenchymal stem cells improve viability of endothelial cells exposed to shear stress in ePTFE grafts. Int. J. Artif. Organs. 42, 80–87. doi: 10.1177/0391398818815470
Qiao, L. Y., Huang, F. J., Zhao, M., Xie, J. H., Shi, J., Wang, J., et al. (2014). A two-year follow-up study of cotransplantation with neural stem/progenitor cells and mesenchymal stromal cells in ischemic stroke patients. Cell Transplant 23(Suppl. 1), S65–S72. doi: 10.3727/096368914x684961
Qin, C., Fan, W. H., Liu, Q., Shang, K., Murugan, M., Wu, L. J., et al. (2017). Fingolimod protects against ischemic white matter damage by modulating microglia toward M2 polarization via STAT3 pathway. Stroke 48, 3336–3346. doi: 10.1161/strokeaha.117.018505
Qureshi, A. I., Mendelow, A. D., and Hanley, D. F. (2009). Intracerebral haemorrhage. Lancet 373, 1632–1644. doi: 10.1016/s0140-6736(09)60371-8
Ramakers, G. M., Heinen, K., Gispen, W. H., and de Graan, P. N. (2000). Long term depression in the CA1 field is associated with a transient decrease in pre- and postsynaptic PKC substrate phosphorylation. J. Biol. Chem. 275, 28682–28687. doi: 10.1074/jbc.M003068200
Ramos-Cabrer, P., Justicia, C., Wiedermann, D., and Hoehn, M. (2010). Stem cell mediation of functional recovery after stroke in the rat. PLoS One 5:e12779. doi: 10.1371/journal.pone.0012779
Ransom, B. R., and Ransom, C. B. (2012). Astrocytes: multitalented stars of the central nervous system. Methods Mol. Biol. 814, 3–7. doi: 10.1007/978-1-61779-452-0_1
Roncagliolo, M., Schlageter, C., León, C., Couve, E., Bonansco, C., and Eguibar, J. R. (2006). Developmental impairment of compound action potential in the optic nerve of myelin mutant taiep rats. Brain Res. 1067, 78–84. doi: 10.1016/j.brainres.2005.10.010
Sakaguchi, S., Miyara, M., Costantino, C. M., and Hafler, D. A. (2010). FOXP3+ regulatory T cells in the human immune system. Nat. Rev. Immunol. 10, 490–500. doi: 10.1038/nri2785
Schmahmann, J. D., Smith, E. E., Eichler, F. S., and Filley, C. M. (2008). Cerebral white matter: neuroanatomy, clinical neurology, and neurobehavioral correlates. Ann. N. Y. Acad. Sci. 1142, 266–309. doi: 10.1196/annals.1444.017
Schmitz, J., Owyang, A., Oldham, E., Song, Y., Murphy, E., McClanahan, T. K., et al. (2005). IL-33, an interleukin-1-like cytokine that signals via the IL-1 receptor-related protein ST2 and induces T helper type 2-associated cytokines. Immunity 23, 479–490. doi: 10.1016/j.immuni.2005.09.015
Seyfried, D. M., Han, Y., Yang, D., Ding, J., Savant-Bhonsale, S., Shukairy, M. S., et al. (2008). Mannitol enhances delivery of marrow stromal cells to the brain after experimental intracerebral hemorrhage. Brain Res. 1224, 12–19. doi: 10.1016/j.brainres.2008.05.080
Shalaby, S. M., El-Shal, A. S., Abd-Allah, S. H., Selim, A. O., Selim, S. A., Gouda, Z. A., et al. (2014). Mesenchymal stromal cell injection protects against oxidative stress in Escherichia coli-induced acute lung injury in mice. Cytotherapy 16, 764–775. doi: 10.1016/j.jcyt.2013.12.006
Shichinohe, H., Ishihara, T., Takahashi, K., Tanaka, Y., Miyamoto, M., Yamauchi, T., et al. (2015). Bone marrow stromal cells rescue ischemic brain by trophic effects and phenotypic change toward neural cells. Neurorehabil. Neural. Repair. 29, 80–89. doi: 10.1177/1545968314525856
Shichinohe, H., Kawabori, M., Iijima, H., Teramoto, T., Abumiya, T., Nakayama, N., et al. (2017). Research on advanced intervention using novel bone marrOW stem cell (RAINBOW): a study protocol for a phase I, open-label, uncontrolled, dose-response trial of autologous bone marrow stromal cell transplantation in patients with acute ischemic stroke. BMC Neurol. 17:179. doi: 10.1186/s12883-017-0955-6
Smith, E. E., Gurol, M. E., Eng, J. A., Engel, C. R., Nguyen, T. N., Rosand, J., et al. (2004). White matter lesions, cognition, and recurrent hemorrhage in lobar intracerebral hemorrhage. Neurology 63, 1606–1612. doi: 10.1212/01.wnl.0000142966.22886.20
Smith, H. K., and Gavins, F. N. (2012). The potential of stem cell therapy for stroke: is PISCES the sign? Faseb J. 26, 2239–2252. doi: 10.1096/fj.11-195719
Song, N., Scholtemeijer, M., and Shah, K. (2020). Mesenchymal stem cell immunomodulation: mechanisms and therapeutic potential. Trends Pharmacol. Sci. 41, 653–664. doi: 10.1016/j.tips.2020.06.009
Steinberg, G. K., Kondziolka, D., Wechsler, L. R., Lunsford, L. D., Coburn, M. L., Billigen, J. B., et al. (2016). Clinical outcomes of transplanted modified bone marrow-derived mesenchymal stem cells in stroke: a phase 1/2a study. Stroke 47, 1817–1824. doi: 10.1161/strokeaha.116.012995
Sun, J., Wei, Z. Z., Gu, X., Zhang, J. Y., Zhang, Y., Li, J., et al. (2015). Intranasal delivery of hypoxia-preconditioned bone marrow-derived mesenchymal stem cells enhanced regenerative effects after intracerebral hemorrhagic stroke in mice. Exp. Neurol. 272, 78–87. doi: 10.1016/j.expneurol.2015.03.011
Sun, X. L., Xu, Z. M., Ke, Y. Q., Hu, C. C., Wang, S. Y., Ling, G. Q., et al. (2011). Molecular targeting of malignant glioma cells with an EphA2-specific immunotoxin delivered by human bone marrow-derived mesenchymal stem cells. Cancer Lett. 312, 168–177. doi: 10.1016/j.canlet.2011.07.035
Suzuki, T., Kohyama, K., Moriyama, K., Ozaki, M., Hasegawa, S., Ueno, T., et al. (2020). Extracellular ADP augments microglial inflammasome and NF-κB activation via the P2Y12 receptor. Eur. J. Immunol. 50, 205–219. doi: 10.1002/eji.201848013
Tan, F., Wang, J., Liu, J. X., Wang, C., Li, M. D., and Gu, Y. (2018). Electroacupuncture stimulates the proliferation and differentiation of endogenous neural stem cells in a rat model of ischemic stroke. Exp. Ther. Med. 16, 4943–4950. doi: 10.3892/etm.2018.6848
Tobin, M. K., Stephen, T. K. L., Lopez, K. L., Pergande, M. R., Bartholomew, A. M., Cologna, S. M., et al. (2020). Activated mesenchymal stem cells induce recovery following stroke via regulation of inflammation and oligodendrogenesis. J. Am. Heart Assoc. 9:e013583. doi: 10.1161/jaha.119.013583
Tolar, J., Le Blanc, K., Keating, A., and Blazar, B. R. (2010). Concise review: hitting the right spot with mesenchymal stromal cells. Stem Cells 28, 1446–1455. doi: 10.1002/stem.459
Tse, W. T., Pendleton, J. D., Beyer, W. M., Egalka, M. C., and Guinan, E. C. (2003). Suppression of allogeneic T-cell proliferation by human marrow stromal cells: implications in transplantation. Transplantation 75, 389–397. doi: 10.1097/01.TP.0000045055.63901.A9
Tuca, A. C., Ertl, J., Hingerl, K., Pichlsberger, M., Fuchs, J., Wurzer, P., et al. (2016). Comparison of matrigel and matriderm as a carrier for human amnion-derived mesenchymal stem cells in wound healing. Placenta 48, 99–103. doi: 10.1016/j.placenta.2016.10.015
van Velthoven, C. T., Dzietko, M., Wendland, M. F., Derugin, N., Faustino, J., Heijnen, C. J., et al. (2017). Mesenchymal stem cells attenuate MRI-identifiable injury, protect white matter, and improve long-term functional outcomes after neonatal focal stroke in rats. J. Neurosci. Res. 95, 1225–1236. doi: 10.1002/jnr.23954
Vaquero, J., Otero, L., Bonilla, C., Aguayo, C., Rico, M. A., Rodriguez, A., et al. (2013). Cell therapy with bone marrow stromal cells after intracerebral hemorrhage: impact of platelet-rich plasma scaffolds. Cytotherapy 15, 33–43. doi: 10.1016/j.jcyt.2012.10.005
Wang, C., Fei, Y., Xu, C., Zhao, Y., and Pan, Y. (2015). Bone marrow mesenchymal stem cells ameliorate neurological deficits and blood-brain barrier dysfunction after intracerebral hemorrhage in spontaneously hypertensive rats. Int. J. Clin. Exp. Pathol. 8, 4715–4724.
Wang, S., Cheng, H., Dai, G., Wang, X., Hua, R., Liu, X., et al. (2013). Umbilical cord mesenchymal stem cell transplantation significantly improves neurological function in patients with sequelae of traumatic brain injury. Brain Res. 1532, 76–84. doi: 10.1016/j.brainres.2013.08.001
Wang, T., Chen, X., Wang, Z., Zhang, M., Meng, H., Gao, Y., et al. (2015). Poloxamer-188 can attenuate blood-brain barrier damage to exert neuroprotective effect in mice intracerebral hemorrhage model. J. Mol. Neurosci. 55, 240–250. doi: 10.1007/s12031-014-0313-8
Wanner, I. B., Anderson, M. A., Song, B., Levine, J., Fernandez, A., Gray-Thompson, Z., et al. (2013). Glial scar borders are formed by newly proliferated, elongated astrocytes that interact to corral inflammatory and fibrotic cells via STAT3-dependent mechanisms after spinal cord injury. J. Neurosci. 33, 12870–12886. doi: 10.1523/jneurosci.2121-13.2013
Wasserman, J. K., and Schlichter, L. C. (2008). White matter injury in young and aged rats after intracerebral hemorrhage. Exp. Neurol. 214, 266–275. doi: 10.1016/j.expneurol.2008.08.010
Wei, L., Fraser, J. L., Lu, Z. Y., Hu, X., and Yu, S. P. (2012). Transplantation of hypoxia preconditioned bone marrow mesenchymal stem cells enhances angiogenesis and neurogenesis after cerebral ischemia in rats. Neurobiol. Dis. 46, 635–645. doi: 10.1016/j.nbd.2012.03.002
Wei, Y., Yemisci, M., Kim, H. H., Yung, L. M., Shin, H. K., Hwang, S. K., et al. (2011). Fingolimod provides long-term protection in rodent models of cerebral ischemia. Ann. Neurol. 69, 119–129. doi: 10.1002/ana.22186
Windrem, M. S., Schanz, S. J., Zou, L., Chandler-Militello, D., Kuypers, N. J., and Nedergaard, M. (2020). Human glial progenitor cells effectively remyelinate the demyelinated adult brain. Cell Rep. 31:107658. doi: 10.1016/j.celrep.2020.107658
Wu, G., Li, S., Wang, L., and Mao, Y. (2013). The perihematomal glutamate level is associated with the outcome of patients with basal ganglia hematomas treated by minimally invasive procedures. Neurol. Res. 35, 829–836. doi: 10.1179/1743132813y.0000000220
Wu, W., Xiong, W., Zhang, P., Chen, L., Fang, J., Shields, C., et al. (2017). Increased threshold of short-latency motor evoked potentials in transgenic mice expressing Channelrhodopsin-2. PLoS One 12:e0178803. doi: 10.1371/journal.pone.0178803
Xiao, L., Zheng, H., Li, J., Wang, Q., and Sun, H. (2020). Neuroinflammation mediated by NLRP3 inflammasome after intracerebral hemorrhage and potential therapeutic targets. Mol. Neurobiol. 57, 5130–5149. doi: 10.1007/s12035-020-02082-2
Xie, J., Wang, B., Wang, L., Dong, F., Bai, G., and Liu, Y. (2016). Intracerebral and intravenous transplantation represents a favorable approach for application of human umbilical cord mesenchymal stromal cells in intracerebral hemorrhage rats. Med. Sci. Monit. 22, 3552–3561. doi: 10.12659/msm.900512
Yan, K., Zhang, R., Sun, C., Chen, L., Li, P., Liu, Y., et al. (2013). Bone marrow-derived mesenchymal stem cells maintain the resting phenotype of microglia and inhibit microglial activation. PLoS One 8:e84116. doi: 10.1371/journal.pone.0084116
Yang, C., Zhou, L., Gao, X., Chen, B., Tu, J., Sun, H., et al. (2011). Neuroprotective effects of bone marrow stem cells overexpressing glial cell line-derived neurotrophic factor on rats with intracerebral hemorrhage and neurons exposed to hypoxia/reoxygenation. Neurosurgery 68, 691–704. doi: 10.1227/NEU.0b013e3182098a8a
Yin, J., Lü, T. M., Qiu, G., Huang, R. Y., Fang, M., Wang, Y. Y., et al. (2013). Intracerebral hematoma extends via perivascular spaces and perineurium. Tohoku J. Exp. Med. 230, 133–139. doi: 10.1620/tjem.230.133
Yu, X., Wu, H., Zhao, Y., Guo, Y., Chen, Y., Dong, P., et al. (2018). Bone marrow mesenchymal stromal cells alleviate brain white matter injury via the enhanced proliferation of oligodendrocyte progenitor cells in focal cerebral ischemic rats. Brain Res. 1680, 127–136. doi: 10.1016/j.brainres.2017.12.019
Zhang, H., Huang, Z., Xu, Y., and Zhang, S. (2006). Differentiation and neurological benefit of the mesenchymal stem cells transplanted into the rat brain following intracerebral hemorrhage. Neurol,. Res. 28, 104–112. doi: 10.1179/016164106x91960
Zhang, R. (2013). Anti-inflammatory and immunomodulatory mechanisms of mesenchymal stem cell transplantation in experimental traumatic brain injury. J. Neuroinflammation 10:106.
Zhang, Z., Zhang, Z., Lu, H., Yang, Q., Wu, H., and Wang, J. (2017). Microglial polarization and inflammatory mediators after intracerebral hemorrhage. Mol. Neurobiol. 54, 1874–1886. doi: 10.1007/s12035-016-9785-6
Zhao, X., Li, J., and Sun, H. (2019). CD200-CD200R interaction: an important regulator after stroke. Front. Neurosci. 13:840. doi: 10.3389/fnins.2019.00840
Zhao, X., Wang, H., Sun, G., Zhang, J., Edwards, N. J., and Aronowski, J. (2015). Neuronal Interleukin-4 as a modulator of microglial pathways and ischemic brain damage. J. Neurosci. 35, 11281–11291. doi: 10.1523/jneurosci.1685-15.2015
Zhao, Y., Gao, J., and Lu, F. (2013). Human adipose-derived stem cell adipogenesis induces paracrine regulation of the invasive ability of MCF-7 human breast cancer cells in vitro. Exp. Ther. Med. 6, 937–942. doi: 10.3892/etm.2013.1237
Zhou, K., Zhong, Q., Wang, Y. C., Xiong, X. Y., Meng, Z. Y., Zhao, T., et al. (2017). Regulatory T cells ameliorate intracerebral hemorrhage-induced inflammatory injury by modulating microglia/macrophage polarization through the IL-10/GSK3beta/PTEN axis. J. Cereb. Blood Flow Metab. 37, 967–979. doi: 10.1177/0271678X16648712
Zhou, Y., Wang, Y., Wang, J., Anne Stetler, R., and Yang, Q. W. (2014). Inflammation in intracerebral hemorrhage: from mechanisms to clinical translation. Prog. Neurobiol. 115, 25–44. doi: 10.1016/j.pneurobio.2013.11.003
Zhou, Y., Yamamoto, Y., Xiao, Z., and Ochiya, T. (2019). The immunomodulatory functions of mesenchymal stromal/stem cells mediated via paracrine activity. J. Clin. Med. 8:1025. doi: 10.3390/jcm8071025
Keywords: intracerebral hemorrhage, white matter injury, mesenchymal stem cells, corticospinal tract, cell therapy
Citation: Li J, Xiao L, He D, Luo Y and Sun H (2021) Mechanism of White Matter Injury and Promising Therapeutic Strategies of MSCs After Intracerebral Hemorrhage. Front. Aging Neurosci. 13:632054. doi: 10.3389/fnagi.2021.632054
Received: 22 November 2020; Accepted: 18 March 2021;
Published: 13 April 2021.
Edited by:
Yuanli Zhao, Capital Medical University, ChinaReviewed by:
Gang Chen, First Affiliated Hospital of Soochow University, ChinaCopyright © 2021 Li, Xiao, He, Luo and Sun. This is an open-access article distributed under the terms of the Creative Commons Attribution License (CC BY). The use, distribution or reproduction in other forums is permitted, provided the original author(s) and the copyright owner(s) are credited and that the original publication in this journal is cited, in accordance with accepted academic practice. No use, distribution or reproduction is permitted which does not comply with these terms.
*Correspondence: Haitao Sun, MjAwOXNodEBzbXUuZWR1LmNu
Disclaimer: All claims expressed in this article are solely those of the authors and do not necessarily represent those of their affiliated organizations, or those of the publisher, the editors and the reviewers. Any product that may be evaluated in this article or claim that may be made by its manufacturer is not guaranteed or endorsed by the publisher.
Research integrity at Frontiers
Learn more about the work of our research integrity team to safeguard the quality of each article we publish.