- 1Brain Behavior Laboratory, School of Kinesiology, University of Michigan, Ann Arbor, MI, United States
- 2Motor Control Laboratory, School of Kinesiology, University of Michigan, Ann Arbor, MI, United States
Age-related changes in cortico-cortical connectivity in the human motor network in older adults are associated with declines in hand dexterity. Posterior parietal cortex (PPC) is strongly interconnected with motor areas and plays a critical role in many aspects of motor planning. Functional connectivity measures derived from dual-site transcranial magnetic stimulation (dsTMS) studies have found facilitatory inputs from PPC to ipsilateral primary motor cortex (M1) in younger adults. In this study, we investigated whether facilitatory inputs from PPC to M1 are altered by age. We used dsTMS in a conditioning-test paradigm to characterize patterns of functional connectivity between the left PPC and ipsilateral M1 and a standard pegboard test to assess skilled hand motor function in 13 young and 13 older adults. We found a PPC-M1 facilitation in young adults but not older adults. Older adults also showed a decline in motor performance compared to young adults. We conclude that the reduced PPC-M1 facilitation in older adults may be an early marker of age-related decline in the neural control of movement.
Introduction
Age-related decline in cognitive and sensorimotor functions in older adults has been linked with changes in the brain's structural and functional connectivity patterns (Seidler et al., 2010; Damoiseaux, 2017). These age-related differences in functional connectivity that mediate information flow across the brain have been attributed in part to the decline in white matter integrity in older adults (Wu and Hallett, 2005; Zahr et al., 2009; Sullivan et al., 2010; Bruijn, 2014). Additionally, mounting evidence from neuroimaging suggests age-related changes in cortico-cortical connectivity in the motor network of healthy older adults contribute to age-related declines in sensorimotor functions. Functional cortico-cortical connectivity measures derived from dual-site transcranial magnetic stimulation (dsTMS) in healthy older adults also have shown reduced facilitatory and inhibitory inputs from secondary motor areas, including the supplementary motor area (SMA) (Green et al., 2018) and dorsal premotor cortex (PMd) (Ni et al., 2014), to primary motor cortex (M1). Posterior parietal cortex (PPC), a region involved in transforming sensory information into motor commands (Crawford et al., 2003, 2004; Andersen and Cui, 2009), is strongly interconnected with motor areas through white-matter tracts of the superior longitudinal fasciculus (Makris, 2004). These reciprocal glutamatergic parietal-frontal circuits are likely excitatory (Tokuno and Nambu, 2000; Dum and Strick, 2002; Matsumoto et al., 2006) and underlie control processes for skilled voluntary movements such as dexterous finger movements required during the manipulation of objects (Filimon, 2010; Davare et al., 2011; Vesia and Crawford, 2012; Turella and Lingnau, 2014; Gallivan and Culham, 2015).
Human neuroimaging studies have implicated parieto-frontal brain regions in sensorimotor control of human hand behavior (Gallivan et al., 2011, 2013; Fabbri et al., 2014; Monaco et al., 2020; Turella et al., 2020). Anatomical findings in non-human primates have shown direct monosynaptic inputs to M1 from PPC in the control of hand movements (Strick and Kim, 1978; Rozzi et al., 2005; Bruni, 2018). A similar direct functional and anatomical parieto-motor pathway has been seen in human imaging (Koch et al., 2010). A number of dsTMS findings also have shown direct facilitatory parieto-motor connectivity in both the resting and active brain for hand actions in young adults (Koch et al., 2007, 2008; Ziluk et al., 2010; Cattaneo and Barchiesi, 2011; Karabanov et al., 2013; Vesia et al., 2013, 2017). Similarly, recent findings from intraoperative dual cortical stimulation in humans have provided direct evidence that the inferior parietal lobule exerts short-latency excitatory effects on cortical motor output (Cattaneo et al., 2020). Importantly, a recent neuroimaging study points to reduced coupling of parietal and premotor areas as a possible mechanism for the decreased perceptual motor speed observed in older adults (Michely et al., 2018). A question that remains, however, is whether the well-established age-related decline in sensorimotor performance relates to age-related differences in parieto-motor connectivity in older adults. We used dsTMS to characterize patterns of functional PPC-M1 connectivity and a standard pegboard test to estimate skilled motor performance in young and older adults. We hypothesized that facilitatory connectivity between PPC and M1 is reduced in older adults.
Methods
Participants
Thirteen young adults (YA, 8 females, 19.9 ± 1.3 years) and thirteen older adults (OA, 5 females, 72.2 ± 5.5 years) provided written consent to participate in the study. All participants were right-handed as assessed by the Edinburgh Handedness Inventory (Oldfield, 1971). All participants were screened for any contraindications to TMS (Keel et al., 2001; Rossi et al., 2011) and had no history of neurological disorders. To assess weekly frequency and duration of various physical activities undertaken by older adults, we administered the Community Health Activities Model Program for Seniors self-report questionnaire (CHAMPS), which revealed that all were very physically active (total caloric expenditure per week: 3,799.5 ± 869.6; (Stewart et al., 2001). Cognitive function was assessed in the older adults using the Montreal Cognitive Assessment (MoCA score, ≥26) (Nasreddine et al., 2005) and Mini-Mental State Exam (MMSE score ≥27) (Folstein et al., 1975). Those who took CNS-active medications within 48 h of the study were excluded. All procedures were approved by the University of Michigan Institutional Review Board (HUM00155459) in accordance with the Declaration of Helsinki.
Procedures
Transcranial magnetic stimulation in a conditioning-test approach with two coils (Lafleur et al., 2016; Hallett et al., 2017; Goldenkoff et al., 2020) was used to measure connectivity between left PPC and left M1 (Figure 1A). A test stimulus (TS) was delivered to M1 with a figure-8 coil (D702, 7 cm diameter) connected to a Magstim 2002 stimulator (Magstim, Whitland, UK) with a monophasic waveform. The TS coil was held tangential to the skull at 45° from the mid-sagittal line, inducing a current in the posterior-anterior direction in the underlying cortical tissue. TS intensity was set to produce a motor evoked potential (MEP) of ~1 mV in the first dorsal interosseous muscle or the abductor pollicis brevis muscle in the right hand (Rossini et al., 2015). Left M1 was defined as the optimal scalp position for coil placement where stimulation evoked the largest MEP from the quiescent right-hand target muscle. PPC stimulation was applied to the P3 electrode position of the international 10-20 electroencephalogram (EEG) coordinate system using commercially available EEG head caps in each participant. The site is situated over angular gyrus (BA 39) of the inferior parietal lobule (Herwig et al., 2004; Okamoto et al., 2004) and corresponds with activation foci for hand actions identified by neuroimaging (Vesia and Crawford, 2012). A conditioning stimulus (CS) was delivered to PPC with another figure-8 coil (D50 Alpha B.I., 5 cm diameter) connected to a Magstim 2002 stimulator (Magstim, Whitland, UK) with a monophasic waveform and a posterior-anterior current direction. The CS coil was held tangential to the skull at 90° from the mid-sagittal line. CS preceded TS by an inter-stimulus interval (ISI) of 4, 6, or 8 ms. PPC stimulation intensity was applied at 70, 90, 110, and 130% of resting motor threshold (RMT), similar to previous work (Koch et al., 2007). RMT was defined as the lowest intensity that evoked MEPs of at least 50 μV in peak-to-peak amplitude in three of five consecutive trials with the PPC coil from the right-hand muscle (Rossini et al., 1994). Each stimulus-response curve was repeated for each ISI. Twelve single-pulse stimuli (TS alone) to M1 and paired-pulse stimuli (CS-TS) at each PPC stimulation intensity were delivered in random order within an experimental block (60 trials) with both hands at rest. Stimuli were applied every 5 s. The order of the ISI block for each stimulus-response curve was counterbalanced across participants. A frameless stereotactic neuronavigation system (Brainsight; Rogue Research, Montreal, Canada) was used to ensure consistency in the TMS coil position throughout the stimulation session. After the electrophysiological measurements were completed, motor skill performance was examined by a test of hand dexterity, the Grooved Pegboard Test (GPT, Lafayette Instrument # 32025) using standard procedures (Wang et al., 2011).
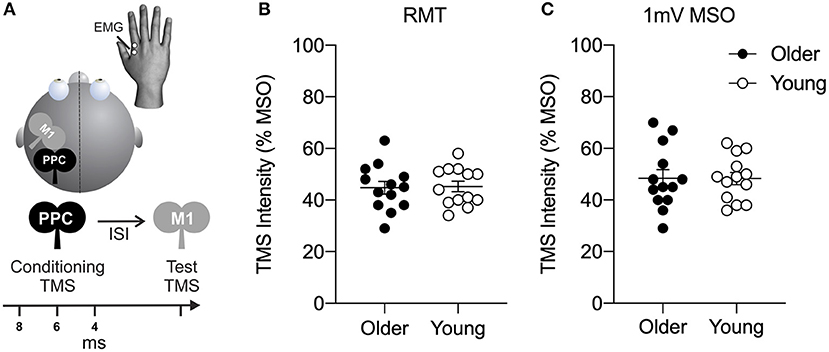
Figure 1. (A) Dual-site transcranial magnetic stimulation in a conditioning-test approach was used to probe connectivity between left posterior parietal cortex (PPC) and ipsilateral primary motor cortex (M1). The conditioning stimulus (CS) was delivered at an intensity of 70, 90, 110, or 130% of resting motor threshold (RMT) to the PPC. CS was delivered 4, 6, or 8 ms prior to a test stimulus (TS) delivered to primary motor cortex (M1). Resulting motor evoked potential (MEP) amplitudes were recorded using electromyography (EMG) in the right-hand target muscle at rest. (B) Motor excitability plots showing transcranial magnetic stimulation (TMS) intensity (expressed as a percentage of the maximum stimulator output, MSO) of resting motor threshold (RMT) and (C) TMS intensity to elicit a motor evoked potential (MEP) of 1 mV for older (filled circles) and young (open circles) adults. Mean and SE are presented.
Data Analysis
Electromyography (EMG) signals were recorded from the right-hand target muscle using bipolar surface electrodes (Model 2024F, Intronix Technologies Corporation), filtered (band-pass, 20 Hz to 2.5 kHz), and digitized at 5 kHz (Micro 1401 Cambridge Electronics Design). The peak-to-peak amplitude of the MEPs (mV) occurring between 15 and 100 ms after the TS were measured for each trial. Trials in which test pulses coincided with motor activity or failed to elicit reliable MEPs (i.e., value exceeded 1.5 times the interquartile range for the participant) were removed from the analysis (~2% of trials). The mean MEP amplitude for paired-pulse stimulation (CS-TS) was normalized by calculating the ratio of the amplitude relative to the mean single-pulse TS alone to M1 for each participant. Separate split-plot analysis of variances (ANOVAs) were carried out on the normalized MEP amplitudes for each PPC stimulus-response curve at each PPC stimulation intensity using Age (two levels: young or older adults) as a between-subjects factor and ISI (three levels: 4, 6, or 8 ms) as a within-subjects factor. The Bonferroni method was used for post-hoc t-test comparisons. The Greenhouse-Geiser method was used to correct for sphericity. Independent sample t-test was used to compare motor excitability and behavioral measures between groups. Paired t-tests also were conducted on the absolute amplitudes of the test MEP and conditioned MEP for the PPC stimulation intensity of 90% RMT at 6 ms ISI to evaluate facilitation and inhibition within the older and young adults. Correlations between neurophysiological and behavioral data were tested with Pearson's coefficient. Statistical analysis was performed using IBM-SPSS Statistics Version 26. A significance threshold was set at p < 0.05. Partial η squared () values were computed as a measure of effect size. Cutoffs for effect sizes are considered small (≥0.01), medium (≥0.06), and large (≥0.14) (Cohen, 1992). Mean and standard error values are reported.
Results
As shown in Figure 1, no significant age difference was found in measures of motor excitability (all t24 < 0.71, all p > 0.49). The RMT of maximum stimulator output (MSO) was 45.2 ± 2.0% MSO for young adults and 44.8 ± 2.5% MSO for older adults (Figure 1B). The intensity to elicit a MEP amplitude of 1 mV in the right-hand target muscle was 48.3 ± 2.4% MSO for young adults and 48.4 ± 3.7% MSO for older adults (Figure 1C).
Figure 2A shows PPC-M1 connectivity in young and older adults. Facilitation seen in the MEP amplitude ratio in young adults was reduced in older adults with PPC stimulation at 90% RMT for the ISI of 6 ms (significant Age and ISI interaction: F(1.96,47.0) = 3.4, p = 0.043, = 0.12; no main effect of Age: F(1,24)1.17, p = 0.29, = 0.05; no main effect of ISI: F(1.96,47.0) = 0.34, p = 0.71, = 0.01). Specifically, post hoc tests confirmed that the ratio of the MEP amplitude was significantly different between the age groups for the PPC stimulus-response curve at 90% RMT at the 6 ms ISI (Bonferroni's t-test: t22.9 = 2.84, p = 0.028). The results show that the facilitation between left PPC and ipsilateral M1 connections in young adults is reduced in older adults. Closer inspection of the individual normalized data confirmed that left PPC stimulation intensity of 90% RMT at 6 ms ISI caused inhibition of corticospinal excitability in ipsilateral M1 in about 69% of the older adults (Figure 2B). A similar pattern emerged at this timing and intensity when comparing the absolute amplitude of the conditioned and test MEP (Figure 2C). Paired t-tests revealed a trend toward significance in the facilitation of the conditioned MEP compared to TS alone in young adults (t12 = 2.04, p = 0.06), while the analysis within older adults did not reach significance (t12 = 1.38, p = 0.19). It is worth noting that single-pulse TS MEPs for the older adults were more variable than young adults, possibly influencing the normalized MEP amplitude ratio.
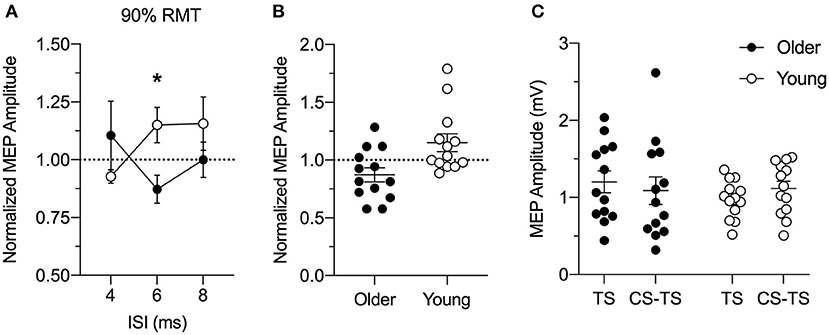
Figure 2. (A) Group analysis of the effects of left posterior parietal cortex (PPC) stimulation at 90% resting motor threshold (RMT) intensity on motor evoked potential (MEP) amplitude induced by left M1 stimulation for older (n = 13; closed circles) and young (n = 13; open circles) adults at rest. The conditioning stimulus (CS) to PPC preceded a test stimulus (TS) to the primary motor cortex (M1) by an inter-stimulus interval (ISI) of 4, 6, or 8 ms. MEP amplitude was normalized as a ratio of the MEP amplitude evoked by paired-pulse stimulation (CS-TS) to that evoked by single-pulse stimulation (TS) to M1 alone (dashed line). Y = 1 indicates no effect of TMS to PPC on M1 excitability, whereas ratios higher than 1 indicate increased and ratios lower than 1 indicate decreased M1 excitability because of PPC stimulation. Facilitation in the ratio of MEP amplitude in young adults was reduced in older adults with PPC stimulation delivered at an intensity of 90% RMT at ISI of 6 ms. (B) Individual conditioned MEP amplitudes for the stimulus-response curve at 90% of RMT at the 6 ms ISI for older (n = 13, filled circles) and young (n = 13, open circles) adults normalized to TS alone (dashed line). (C) Individual absolute values of MEP amplitudes (mV) for the single-pulse TS to M1 and paired-pulse CS-TS to PPC at an intensity of 90% RMT at the 6 ms ISI for older (filled circles) and young (open circles) adults. Mean and SE are presented. *p < 0.05.
In a series of control experiments, we also verified whether the PPC-M1 connectivity at rest would differ with different PPC stimulation intensities. In each case, no significant age difference was found in the PPC stimulus-response curve at intensities of 70, 110, or 130% RMT (Figure 3). At 70% RMT (Figure 3A), a split-plot ANOVA showed that there was no significant effect of Age [F(1,24) = 1.42, p = 0.25, = 0.06], ISI (F(1.44,34.6) = 0.40, p = 0.60, = 0.02), nor an interaction effect [F(1.44,34.6) = 2.52, p = 0.11, = 0.10]. At 110% RMT (Figure 3B), a split-plot ANOVA showed that there was no significant effect of Age [F(1,24) = 0.28, p = 0.60, = 0.01], ISI [F(1.73,41.5) = 0.11, p = 0.90, = 0.005], nor an interaction effect [F(1.73,41.5) = 1.05, p = 0.35, = 0.04]. Similarly, at 130% RMT (Figure 3C), a split-plot ANOVA showed that there was no significant effect of Age [F(1,24) = 1.32, p = 0.26, = 0.05], ISI [F(1.93,46.29) = 0.64, p = 0.53, = 0.03], nor an interaction effect [F(1.93,46.29) = 0.61, p = 0.54, = 0.03].
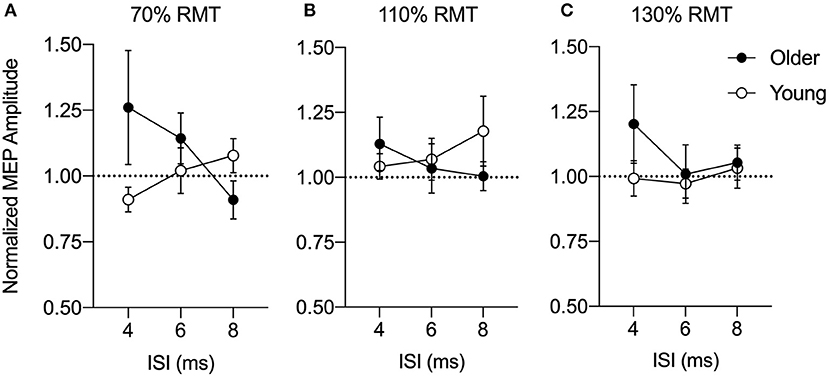
Figure 3. Group-averaged conditioned motor evoked potential (MEP) amplitudes for a posterior parietal cortex (PPC) stimulus-response curve at 70% (A), 110% (B), and 130% (C) of resting motor threshold (RMT) at an inter-stimulus interval (ISI) of 4, 6, or 8 ms normalized to a test stimulus (TS) alone (dashed line). MEP amplitudes are expressed as a ratio of the amplitude relative to the mean single-pulse TS alone to the primary motor cortex (M1) for older (n = 13, filled circles) and young adults (n = 13, open circles). Mean and SE are presented.
As shown in Figure 4A, young adults were significantly faster than older adults at completing the GPT (young adults = 68.0 ± 8.6 s vs. older adults = 90.7 ± 22.2 s, t24 = 3.44, p = 0.003). Figure 4B shows associations between the PPC-M1 connectivity at rest and motor skill performance in young and older adults. A correlation analysis for each age group showed that the normalized MEP amplitude for the PPC stimulation intensity of 90% RMT at the 6 ms ISI did not correlate with GPT completion time in both young (r = 0.130; p = 0.23) and older adults (r = 0.015; p = 0.69).
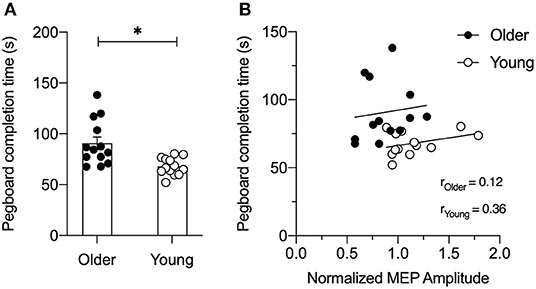
Figure 4. (A) Bar graph for group averaged completion times for the Grooved Pegboard Test in seconds (s). Individual data are presented for older (filled circles) and young (open circles) adults. As expected, young adults performed the task faster compared to older adults. Error bars represent SE. *p < 0.05. (B) Correlation between normalized motor evoked potential (MEP) at the 6 ms inter-stimulus interval (ISI) for PPC stimulus-response curve at 90% resting motor threshold (RMT) and Grooved Pegboard Test completion time for each older (filled circles) and young adult (open circles). A simple linear regression line is superimposed over the individual data points for each group. Normalized MEP amplitude did not correlate with the Grooved Pegboard Test performance in both young and older adults (all p's > 0.2).
Discussion
A reduction of facilitation in a parieto-motor connection responsible for skilled hand movements was demonstrated in older adults compared to young adults. Using a dsTMS approach, we found that a conditioning stimulus to PPC with a 90% RMT intensity delivered 6 ms prior to a test stimulus to M1 resulted in a facilitation of the MEP amplitude in young but not older adults. In fact, the PPC-M1 interaction in the older group changed from facilitation to inhibition. The present findings are in line with prior dsTMS work in young adults showing short-latency PPC-M1 functional interactions are selectively facilitated at rest when applying CS to PPC at an intensity of 90% RMT (Koch et al., 2007; Karabanov et al., 2013). In the current study, the reduced PPC-M1 facilitation in older adults may be related to impairments of high-level movement planning signals in PPC as demonstrated by their slowed completion time of the GPT (Andersen and Cui, 2009). However, future work will need to better characterize the relationship between functional PPC-M1 connectivity and skilled motor performance. For instance, previous dsTMS studies have shown that functional PPC-M1 connectivity is modulated early in the motor plan for different types of hand actions at a similar ISI and conditioning stimulation intensity in young adults (Koch et al., 2008; Vesia et al., 2013, 2017). Yet, no work has investigated whether there is a relationship between functional PPC-M1 interactions during grasp preparation and manual dexterity in older adults. Future investigation is needed to determine whether similar age-related differences occur during these action-associated processes. It also should be noted that it remains unclear whether the non-significant association between reduced facilitation of parieto-motor functional connections and manual dexterity in older adults is present in a larger sample size given the moderate sample size in the current study. One possible explanation of the current results is that widespread motor plans involving multiple, parallel parieto-premotor-motor circuits could modulate corticospinal output associated with sensorimotor hand control (Koch and Rothwell, 2009; Davare et al., 2010, 2011; Vesia and Davare, 2011; Turella and Lingnau, 2014; Vesia et al., 2018). It also is possible that the relationship between cortico-cortical connections in the motor system and skilled hand behavior likely encompasses a much broader range of brain regions within frontal, parietal, and temporal cortices to support the flexibility of human hand behavior (Grafton, 2010; Gallivan and Culham, 2015; Monaco et al., 2020; Turella et al., 2020).
This interpretation is in line with prior dsTMS studies demonstrating age-related decline between M1 and frontal areas in the motor cortical network such as dorsolateral prefrontal cortex (Fujiyama et al., 2016), SMA (Green et al., 2018), and PMd (Ni et al., 2014). Together, these findings suggest an age-related reduction in functional connectivity from action-associated cortical areas to M1 in older adults. It is possible that the degraded facilitatory inputs from PPC to M1 in older adults likely represent an early marker of age-related decline for functional connectivity underlying complex motor skills. This view is in line with theoretical suggestions that older adults recruit frontal cortical areas to compensate for bottom-up sensory processing in posterior cortical areas when performing more cognitive-demanding motor tasks (Davis et al., 2008). Indeed, a large body of research provides complementary evidence linking these age-related differences in functional activation and connectivity patterns with cognitive and motor performance (see reviews Seidler et al., 2010; Damoiseaux, 2017). Functional neuroimaging studies have consistently demonstrated enhanced prefrontal influences on the motor system in response to increased task demands in older adults (Heuninckx et al., 2005; Wu and Hallett, 2005; Cabeza et al., 2018). One such study found that prefrontal areas compensated for decreased parietal influences on premotor areas associated with a decline in perceptual motor speed with advancing age (Michely et al., 2018). This is also consistent with evidence linking age-related decline in cognitively demanding motor tasks with structural changes in white matter tracts that connect sensorimotor, frontal, and parietal regions in older adults (Stewart et al., 2014). The reduced efficacy of this connection is also supported by findings in individuals with Parkinson's disease (Palomar et al., 2013) and stroke (Schulz et al., 2015) that show a more favorable motor outcome related to higher levels of communication between frontal and parietal areas in the motor system. Additionally, dsTMS evidence has shown that this selective age-related decrease in PPC-M1 facilitation in healthy older adults is exacerbated at early clinical stages of Alzheimer's disease (Bonnı̀ et al., 2012). This decreased functional connectivity precedes disease-related changes in cognitive-related frontal areas and could in part represent a key driver of cognitive decline in Alzheimer's disease (Koch et al., 2019).
It is worth noting that neurobiological aging is a complex process involving interactions between local cortical and brain network plasticity (Freitas et al., 2013). In the case of aging, compensatory processes likely depend on the neural reorganization and recruitment of alternative circuits to attenuate cognitive and motor declines that occur with age (Cabeza et al., 2018). Future longitudinal studies delineating the specific contribution of PPC on motor related frontal circuits combining TMS with neuroimaging approaches will be needed to gain insight into the mechanisms of system-level plasticity across the lifespan. We recognize that age-related neural decline such as brain atrophy, synaptic loss, and white matter degradation could account for the observed MEP amplitude differences between young and older adults (Giorgio et al., 2010; Farokhian et al., 2017). However, we believe a global effect on mechanisms underlying aging is unlikely to account for the highly specific reduction in PPC-M1 facilitation in the current study. A methodological limitation of the study is that we did not selectively localize PPC sites for hand actions at the individual level using fMRI or task-based dsTMS. Prior dsTMS work has shown anatomical and functional differences in connectivity between PPC regions and M1 (Karabanov et al., 2013). This raises the possibility that the effects at other ISIs and conditioning stimulation intensities in our study could be dependent on different neural substrates. Further studies will need to examine the effect of nearby parietal regions on motor excitability to account for the individual differences in functional connectivity among older adults. Finally, we recognize that the older adults tested in the current study were high-functioning and in relatively good health based on self-reports. Therefore, future work is needed with a more heterogenous subset of older participants to clarify the effects of physical activity and other environmental factors on age-related cognitive and motor deficits.
We conclude that the reduced PPC-M1 facilitation in older adults may be an early marker of age-related decline in the neural control of movement. Our findings could have implications for understanding functional parieto-frontal connectivity affected by advancing age in both healthy and clinical populations. Importantly, dsTMS methods could be used to develop better diagnostic tools and treatment approaches (Fox et al., 2012, Hallett et al., 2017; Goldenkoff et al., 2020). We propose that prospective strengthening of PPC-M1 circuitry in healthy adults might be a fruitful therapeutic path to counteract the gradual age-related breakdown in functional connectivity within the motor-related network associated with motor impairments. It is possible that the preservation of these neural substrates could enhance resilience of the intact circuitry and minimize compensatory shifts in brain networks that maintain optimal cognitive and motor performance.
Data Availability Statement
The raw data supporting the conclusions of this article will be made available by the authors, without undue reservation.
Ethics Statement
The studies involving human participants were reviewed and approved by University of Michigan Institutional Review Board. The patients/participants provided their written informed consent to participate in this study.
Author Contributions
EG: methodology, formal analysis, investigation, writing—original draft, writing—review & editing, visualization, and project administration. RL: methodology, investigation, project administration, formal analysis, and writing—review & editing. SB: methodology, resources, writing—review & editing, and supervision. MV: conceptualization, methodology, resources, writing—review & editing, supervision, and funding acquisition. All authors contributed to the article and approved the submitted version.
Funding
The work was supported by the University of Michigan School of Kinesiology.
Conflict of Interest
The authors declare that the research was conducted in the absence of any commercial or financial relationships that could be construed as a potential conflict of interest.
References
Andersen, R. A., and Cui, H. (2009). Intention, action planning, and decision making in parietal-frontal circuits. Neuron 63, 568–583. doi: 10.1016/j.neuron.2009.08.028
Bonnì, S., Lupo, F., Gerfo, E., Martorana, A., Perri, R., Caltagirone, C., et al. (2012). Altered parietal-motor connections in Alzheimer's disease patients. JAD 33, 525–533. doi: 10.3233/JAD-2012-121144
Bruijn, S. M. (2014). White matter microstructural organization and gait stability in older adults. Front Aging Neurosci. 6:104. doi: 10.3389/fnagi.2014.00104/abstract
Bruni, S. (2018). Cortical and subcortical connections of parietal and premotor nodes of the monkey hand mirror neuron network. Brain Struct. Funct. 223, 1713–1729. doi: 10.1007/s00429-017-1582-0
Cabeza, R., Albert, M., Belleville, S., Craik, F. I. M., Duarte, A., Grady, C. L., et al. (2018). Maintenance, reserve and compensation: the cognitive neuroscience of healthy ageing. Nat. Rev. Neurosci. 19, 701–710. doi: 10.1038/s41583-018-0068-2
Cattaneo, L., and Barchiesi, G. (2011). Transcranial magnetic mapping of the short-latency modulations of corticospinal activity from the ipsilateral hemisphere during rest. Front. Neural Circuits 5:14. doi: 10.3389/fncir.2011.00014
Cattaneo, L., Giampiccolo, D., Pietro, M., Tramontano, V., and Sala, F. (2020). Cortico-cortical connectivity between the superior and inferior parietal lobules and the motor cortex assessed by intraoperative dual cortical stimulation. Brain Stimul. 13, 819–831. doi: 10.1016/j.brs.2020.02.023
Crawford, J. D., Martinez-Trujillo, J. C., and Klier, E. M. (2003). Neural control of three-dimensional eye and head movements. Curr. Opin. Neurobiol. 13, 655–662. doi: 10.1016/j.conb.2003.10.009
Crawford, J. D., Medendorp, W. P., and Marotta, J. J. (2004). Spatial transformations for eye-hand coordination. J. Neurophysiol. 92, 10–19. doi: 10.1152/jn.00117.2004
Damoiseaux, J. S. (2017). Effects of aging on functional and structural brain connectivity. Neuroimage 160, 32–40. doi: 10.1016/j.neuroimage.2017.01.077
Davare, M., Kraskov, A., Rothwell, J. C., and Lemon, R. N. (2011). Interactions between areas of the cortical grasping network. Curr. Opin. Neurobiol. 21, 565–570. doi: 10.1016/j.conb.2011.05.021
Davare, M., Rothwell, J. C., and Lemon, R. N. (2010). Causal connectivity between the human anterior intraparietal area and premotor cortex during grasp. Curr. Biol. 20, 176–181. doi: 10.1016/j.cub.2009.11.063
Davis, S. W., Dennis, N. A., Daselaar, S. M., Fleck, M. S., and Cabeza, R. (2008). Que PASA? The posterior-anterior shift in aging. Cereb. Cortex 18, 1201–1209. doi: 10.1093/cercor/bhm155
Dum, R. P., and Strick, P. L. (2002). Motor areas in the frontal lobe of the primate. Physiol. Behav. 77, 677–682. doi: 10.1016/s0031-9384(02)00929-0
Fabbri, S., Strnad, L., Caramazza, A., and Lingnau, A. (2014). Overlapping representations for grip type and reach direction. Neuroimage 94, 138–146. doi: 10.1016/j.neuroimage.2014.03.017
Farokhian, F., Yang, C., Beheshti, I., Matsuda, H., and Wu, S. (2017). Age-related gray and white matter changes in normal adult brains. A&D 8, 899–811. doi: 10.14336/AD.2017.0502
Filimon, F. (2010). Human cortical control of hand movements: parietofrontal networks for reaching, grasping, and pointing. Neuroscientist 16, 388–407. doi: 10.1177/1073858410375468
Folstein, M. F., Folstein, S. E., and McHugh, P. R. (1975). “Mini-mental state.” A practical method for grading the cognitive state of patients for the clinician. J. Psychiatr. Res. 12, 189–198. doi: 10.1016/0022-3956(75)90026-6
Fox, M. D., Halko, M. A., Eldaief, M. C., and Pascual-Leone, A. (2012). Measuring and manipulating brain connectivity with resting state functional connectivity magnetic resonance imaging (fcMRI) and transcranial magnetic stimulation (TMS). Neuroimage 62, 2232–2243. doi: 10.1016/j.neuroimage.2012.03.035
Freitas, C., Farzan, F., and Pascual-Leone, A. (2013). Assessing brain plasticity across the lifespan with transcranial magnetic stimulation: why, how, and what is the ultimate goal? Front. Neurosci. 7:42. doi: 10.3389/fnins.2013.00042
Fujiyama, H., Van Soom, J., Rens, G., Gooijers, J., Leunissen, I., Levin, O., et al. (2016). Age-related changes in frontal network structural and functional connectivity in relation to bimanual movement control. J. Neurosci. 36, 1808–1822. doi: 10.1523/JNEUROSCI.3355-15.2016
Gallivan, J. P., and Culham, J. C. (2015). Neural coding within human brain areas involved in actions. Curr. Opin. Neurobiol. 33, 141–149. doi: 10.1016/j.conb.2015.03.012
Gallivan, J. P., McLean, D. A., Flanagan, J. R., and Culham, J. C. (2013). Where one hand meets the other: limb-specific and action-dependent movement plans decoded from preparatory signals in single human frontoparietal brain areas. J. Neurosci. 33, 1991–2008. doi: 10.1523/JNEUROSCI.0541-12.2013
Gallivan, J. P., McLean, D. A., Valyear, K. F., Pettypiece, C. E., and Culham, J. C. (2011). Decoding action intentions from preparatory brain activity in human parieto-frontal networks. J. Neurosci. 31, 9599–9610. doi: 10.1523/JNEUROSCI.0080-11.2011
Giorgio, A., Santelli, L., Tomassini, V., Bosnell, R., Smith, S., De Stefano, N., et al. (2010). Age-related changes in grey and white matter structure throughout adulthood. Neuroimage 51, 943–951. doi: 10.1016/j.neuroimage.2010.03.004
Goldenkoff, E. R., Mashni, A., Michon, K. J., Lavis, H., and Vesia, M. (2020). Measuring and manipulating functionally specific neural pathways in the human motor system with transcranial magnetic stimulation. JoVE 23:106. doi: 10.3791/60706
Grafton, S. T. (2010). The cognitive neuroscience of prehension: recent developments. Exp. Brain Res. 204, 475–491. doi: 10.1007/s00221-010-2315-2
Green, P. E., Ridding, M. C., Hill, K. D., Semmler, J. G., Drummond, P. D., and Vallence, A.-M. (2018). Supplementary motor area-primary motor cortex facilitation in younger but not older adults. Neurobiol. Aging 64, 85–91. doi: 10.1016/j.neurobiolaging.2017.12.016
Hallett, M., Di Iorio, R., Rossini, P. M., Park, J. E., Chen, R., Celnik, P., et al. (2017). Contribution of transcranial magnetic stimulation to assessment of brain connectivity and networks. Clin. Neurophysiol. 128, 2125–2139. doi: 10.1016/j.clinph.2017.08.007
Herwig, U., Satrapi, P., and Schönfeldt-Lecuona, C. (2004). Using the international 10-20 EEG system for positioning of transcranial magnetic stimulation. Brain Topogr. 16, 95–99. doi: 10.1023/b:brat.0000006333.93597.9d
Heuninckx, S., Wenderoth, N., Debaere, F., Peeters, R., and Swinnen, S. P. (2005). Neural basis of aging: the penetration of cognition into action control. J. Neurosci. 25, 6787–6796. doi: 10.1523/JNEUROSCI.1263-05.2005
Karabanov, A. N., Chao, C.-C., Paine, R., and Hallett, M. (2013). Mapping different intra-hemispheric parietal-motor networks using twin coil TMS. Brain Stimul. 6, 384–389. doi: 10.1016/j.brs.2012.08.002
Keel, J. C., Smith, M. J., and Wassermann, E. M. (2001). A safety screening questionnaire for transcranial magnetic stimulation. Clin. Neurophysiol. 112:720. doi: 10.1016/s1388-2457(00)00518-6
Koch, G., Cercignani, M., Pecchioli, C., Versace, V., Oliveri, M., Caltagirone, C., et al. (2010). In vivo definition of parieto-motor connections involved in planning of grasping movements. Neuroimage 51, 300–312. doi: 10.1016/j.neuroimage.2010.02.022
Koch, G., Fernandez Del Olmo, M., Cheeran, B., Ruge, D., Schippling, S., Caltagirone, C., et al. (2007). Focal stimulation of the posterior parietal cortex increases the excitability of the ipsilateral motor cortex. J. Neurosci. 27, 6815–6822. doi: 10.1523/JNEUROSCI.0598-07.2007
Koch, G., Fernandez Del Olmo, M., Del Olmo, M. F., Cheeran, B., Schippling, S., Caltagirone, C., et al. (2008). Functional interplay between posterior parietal and ipsilateral motor cortex revealed by twin-coil transcranial magnetic stimulation during reach planning toward contralateral space. J. Neurosci. 28, 5944–5953. doi: 10.1523/JNEUROSCI.0957-08.2008
Koch, G., Martorana, A., and Caltagirone, C. (2019). Transcranial magnetic stimulation_ emerging biomarkers and novel therapeutics in Alzheimer's disease. Neurosci. Lett. 719:134355. doi: 10.1016/j.neulet.2019.134355
Koch, G., and Rothwell, J. C. (2009). TMS investigations into the task-dependent functional interplay between human posterior parietal and motor cortex. Behav. Brain Res. 202, 147–152. doi: 10.1016/j.bbr.2009.03.023
Lafleur, L.-P., Tremblay, S., Whittingstall, K., and Lepage, J.-F. (2016). Assessment of effective connectivity and plasticity with dual-coil transcranial magnetic stimulation. Brain Stimul. 9, 347–355. doi: 10.1016/j.brs.2016.02.010
Makris, N. (2004). Segmentation of subcomponents within the superior longitudinal fascicle in humans: a quantitative, in vivo, DT-MRI study. Cerebral Cortex 15, 854–869. doi: 10.1093/cercor/bhh186
Matsumoto, R., Nair, D. R., LaPresto, E., Bingaman, W., Shibasaki, H., and Luders, H. O. (2006). Functional connectivity in human cortical motor system: a cortico-cortical evoked potential study. Brain 130, 181–197. doi: 10.1093/brain/awl257
Michely, J., Volz, L. J., Hoffstaedter, F., Tittgemeyer, M., Eickhoff, S. B., Fink, G. R., et al. (2018). Network connectivity of motor control in the ageing brain. YNICL 18, 443–455. doi: 10.1016/j.nicl.2018.02.001
Monaco, S., Malfatti, G., Culham, J. C., Cattaneo, L., and Turella, L. (2020). Decoding motor imagery and action planning in the early visual cortex: overlapping but distinct neural mechanisms. Neuroimage 218, 116981–116915. doi: 10.1016/j.neuroimage.2020.116981
Nasreddine, Z. S., Phillips, N. A., Bédirian, V., Charbonneau, S., Whitehead, V., Collin, I., et al. (2005). The montreal cognitive assessment, MoCA: a brief screening tool for mild cognitive impairment. J. Am. Geriatr. Soc. 53, 695–699. doi: 10.1111/j.1532-5415.2005.53221.x
Ni, Z., Isayama, R., Castillo, G., Gunraj, C., Saha, U., and Chen, R. (2014). Reduced dorsal premotor cortex and primary motor cortex connectivity in older adults. Neurobiol. Aging 36, 301–303. doi: 10.1016/j.neurobiolaging.2014.08.017
Okamoto, M., Dan, H., Sakamoto, K., Takeo, K., Shimizu, K., Kohno, S., et al. (2004). Three-dimensional probabilistic anatomical cranio-cerebral correlation via the international 10-20 system oriented for transcranial functional brain mapping. Neuroimage 21, 99–111. doi: 10.1016/j.neuroimage.2003.08.026
Oldfield, R. C. (1971). The assessment and analysis of handedness: the Edinburgh inventory. Neuropsychologia 9, 97–113.
Palomar, F. J., Conde, V., Carrillo, F., Fernandez Del Olmo, M., Koch, G., and Mir, P. (2013). Parieto-motor functional connectivity is impaired in Parkinson's disease. Brain Stimul. 6, 147–154. doi: 10.1016/j.brs.2012.03.017
Rossi, S., Hallett, M., Rossini, P. M., and Pascual-Leone, A. (2011). Screening questionnaire before TMS: an update. Clin. Neurophysiol. 122:1686. doi: 10.1016/j.clinph.2010.12.037
Rossini, P. M., Barker, A. T., Berardelli, A., Caramia, M. D., Caruso, G., Cracco, R. Q., et al. (1994). Non-invasive electrical and magnetic stimulation of the brain, spinal cord and roots: basic principles and procedures for routine clinical application. Report of an IFCN committee. Electroencephalogr. Clin. Neurophysiol. 91, 79–92.
Rossini, P. M., Burke, D., Chen, R., Cohen, L. G., Daskalakis, Z., Di Iorio, R., et al. (2015). Non-invasive electrical and magnetic stimulation of the brain, spinal cord, roots and peripheral nerves: basic principles and procedures for routine clinical and research application. An updated report from an I.F.C.N. Committee. Clin. Neurophysiol. 126, 1071–1107. doi: 10.1016/j.clinph.2015.02.001
Rozzi, S., Calzavara, R., Belmalih, A., Borra, E., Gregoriou, G. G., Matelli, M., et al. (2005). Cortical connections of the inferior parietal cortical convexity of the macaque monkey. Cereb. Cortex 16, 1389–1417. doi: 10.1093/cercor/bhj076
Schulz, R., Koch, P., Zimerman, M., Wessel, M., Bönstrup, M., Thomalla, G., et al. (2015). Parietofrontal motor pathways and their association with motor function after stroke. Brain 138, 1949–1960. doi: 10.1093/brain/awv100
Seidler, R. D., Bernard, J. A., Burutolu, T. B., Fling, B. W., Gordon, M. T., Gwin, J. T., et al. (2010). Motor control and aging: links to age-related brain structural, functional, and biochemical effects. Neurosci. Biobehav. Rev. 34, 721–733. doi: 10.1016/j.neubiorev.2009.10.005
Stewart, A. L., Mills, K. M., King, A. C., Haskell, W. L., Gillis, D., and Ritter, P. L. (2001). CHAMPS physical activity questionnaire for older adults: outcomes for interventions. Med. Sci. Sports Exercise 33, 1126–1141. doi: 10.1097/00005768-200107000-00010
Stewart, J. C., Tran, X., and Cramer, S. C. (2014). Age-related variability in performance of a motor action selection task is related to differences in brain function and structure among older adults. Neuroimage 86, 326–334. doi: 10.1016/j.neuroimage.2013.10.016
Strick, P. L., and Kim, C. C. (1978). Input to primate motor cortex from posterior parietal cortex (area 5). I. Demonstration by retrograde transport. Brain Res. 157, 325–330. doi: 10.1016/0006-8993(78)90035-5
Sullivan, E. V., Rohlfing, T., and Pfefferbaum, A. (2010). Quantitative fiber tracking of lateral and interhemispheric white matter systems in normal aging: relations to timed performance. Neurobiol. Aging 31, 464–481. doi: 10.1016/j.neurobiolaging.2008.04.007
Tokuno, H., and Nambu, A. (2000). Organization of nonprimary motor cortical inputs on pyramidal and nonpyramidal tract neurons of primary motor cortex: an electrophysiological study in the macaque monkey. Cereb. Cortex 10, 58–68. doi: 10.1093/cercor/10.1.58
Turella, L., and Lingnau, A. (2014). Neural correlates of grasping. Front. Hum. Neurosci. 8:686. doi: 10.3389/fnhum.2014.00686
Turella, L., Rumiati, R., and Lingnau, A. (2020). Hierarchical action encoding within the human brain. Cereb. Cortex 30, 2924–2938. doi: 10.1093/cercor/bhz284
Vesia, M., Barnett-Cowan, M., Elahi, B., Jegatheeswaran, G., Isayama, R., Neva, J. L., et al. (2017). Human dorsomedial parieto-motor circuit specifies grasp during the planning of goal-directed hand actions. Cortex 92, 175–186. doi: 10.1016/j.cortex.2017.04.007
Vesia, M., Bolton, D. A., Mochizuki, G., and Staines, W. R. (2013). Human parietal and primary motor cortical interactions are selectively modulated during the transport and grip formation of goal-directed hand actions. Neuropsychologia 51, 410–417. doi: 10.1016/j.neuropsychologia.2012.11.022
Vesia, M., and Crawford, J. D. (2012). Specialization of reach function in human posterior parietal cortex. Exp. Brain Res. 221, 1–18. doi: 10.1007/s00221-012-3158-9
Vesia, M., Culham, J. C., Jegatheeswaran, G., Isayama, R., Le, A., Davare, M., et al. (2018). Functional interaction between human dorsal premotor cortex and the ipsilateral primary motor cortex for grasp plans. Neuroreport 29, 1355–1359. doi: 10.1097/WNR.0000000000001117
Vesia, M., and Davare, M. (2011). Decoding action intentions in parietofrontal circuits. J. Neurosci. 31, 16491–16493. doi: 10.1523/JNEUROSCI.4408-11.2011
Wang, Y.-C., Magasi, S. R., Bohannon, R. W., Reuben, D. B., McCreath, H. E., Bubela, D. J., et al. (2011). Assessing dexterity function: a comparison of two alternatives for the NIH Toolbox. J. Hand Therap. 24, 313–321. doi: 10.1016/j.jht.2011.05.001
Wu, T., and Hallett, M. (2005). The influence of normal human ageing on automatic movements. J. Physiol. 562, 605–615. doi: 10.1113/jphysiol.2004.076042
Zahr, N. M., Rohlfing, T., Pfefferbaum, A., and Sullivan, E. V. (2009). Problem solving, working memory, and motor correlates of association and commissural fiber bundles in normal aging: a quantitative fiber tracking study. Neuroimage 44, 1050–1062. doi: 10.1016/j.neuroimage.2008.09.046
Keywords: aging, posterior parietal cortex (PPC), primary motor cortex (M1), motor evoked potential, transcrancial magnetic stimulation
Citation: Goldenkoff ER, Logue RN, Brown SH and Vesia M (2021) Reduced Facilitation of Parietal-Motor Functional Connections in Older Adults. Front. Aging Neurosci. 13:595288. doi: 10.3389/fnagi.2021.595288
Received: 15 August 2020; Accepted: 11 January 2021;
Published: 01 February 2021.
Edited by:
Ivan Aprahamian, Faculty of Medicine of Jundiaí, BrazilReviewed by:
Anke Ninija Karabanov, University of Copenhagen, DenmarkLuca Turella, University of Trento, Italy
Copyright © 2021 Goldenkoff, Logue, Brown and Vesia. This is an open-access article distributed under the terms of the Creative Commons Attribution License (CC BY). The use, distribution or reproduction in other forums is permitted, provided the original author(s) and the copyright owner(s) are credited and that the original publication in this journal is cited, in accordance with accepted academic practice. No use, distribution or reproduction is permitted which does not comply with these terms.
*Correspondence: Michael Vesia, bXZlc2lhQHVtaWNoLmVkdQ==