- 1Guangdong Provincial Key Laboratory of Orthopaedics and Traumatology, Orthopaedic Research Institute/Department of Spine Surgery, The First Affiliated Hospital of Sun Yat-sen University, Guangzhou, China
- 2Department of Neurology, The First Affiliated Hospital of Guangzhou Medical University, Guangzhou, China
Osteoporosis and neurodegenerative diseases are two kinds of common disorders of the elderly, which often co-occur. Previous studies have shown the skeletal and central nervous systems are closely related to pathophysiology. As the main structural scaffold of the body, the bone is also a reservoir for stem cells, a primary lymphoid organ, and an important endocrine organ. It can interact with the brain through various bone-derived cells, mostly the mesenchymal and hematopoietic stem cells (HSCs). The bone marrow is also a place for generating immune cells, which could greatly influence brain functions. Finally, the proteins secreted by bones (osteokines) also play important roles in the growth and function of the brain. This article reviews the latest research studying the impact of bone-derived cells, bone-controlled immune system, and bone-secreted proteins on the brain, and evaluates how these factors are implicated in the progress of neurodegenerative diseases and their potential use in the diagnosis and treatment of these diseases.
Introduction
Neurodegenerative diseases are characterized by the gradual loss of structure and function of selectively vulnerable neurons in different regions of the brain, affecting millions of people worldwide. Neurodegenerative disorders can be clinically identified by their representative features, mostly exhibiting extrapyramidal and/or pyramidal movement disorders, cognitive or behavioral disorders. Most patients suffer multiple clinical symptoms rather than pure phenotype (Dugger and Dickson, 2017). The related mechanism of neurodegenerative diseases has been studied. For example, the most common kind of dementia worldwide, Alzheimer’s disease (AD), is pathologically characterized by the extracellular amyloid-β (Aβ) peptides accumulation in senile plaques and the formation of intracytoplasmic neurofibrillary tangles in the brain (Querfurth and LaFerla, 2010; Livingston et al., 2017). And Parkinson’s disease (PD) is characterized by loss of dopaminergic neurons and insufficient synthesis of dopamine in the substantia nigra (SN) area (Dauer and Przedborski, 2003; Maiti et al., 2017), and the accumulation of intracytoplasmic α-synuclein polymers (Lewy bodies) in the brain (Goldman et al., 1983). Multiple sclerosis (MS) which shows both neuroinflammatory and neurodegenerative characteristics had been found it results from chronic demyelinating of the central nervous system (CNS; Compston and Coles, 2008). For the majority of MS patients (85–90%), tissue damage is more caused by inflammation rather than neuronal degeneration in the first 5–12 years of onset, whereas the balance between inflammatory response and neuronal degeneration shifts to the latter at the secondary stage of progression. However, for a minor proportion of MS patients (10–15%), neuronal degeneration plays a major role throughout the disease (Steinman, 2009).
With the increasing trend of social aging, neurodegenerative diseases and osteoporosis have become severe social issues. The bone-related effect in neurodegenerative diseases has so far gained an increased awareness of several epidemiological findings. A meta-analytic study showed that compared with healthy controls, AD patients have a lower hip bone mineral density and are more likely to suffer from hip fractures (Zhao Y. et al., 2012). Also, osteoporosis is regarded as a risk factor for AD, since osteoporosis correlates with the cognitive deficit and its severity in adults older than 50 (Zhou et al., 2011; Kang et al., 2018). Many PD patients have compulsory trunk scoliosis, known as Pisa syndrome, which causes severe late complications of disability (Poewe et al., 2017). In a more common scenario, AD or PD patients suffer from bone fracture, osteoarthritis, restricted movement, bed ulcer, and even death caused by severe osteoporosis. Osteoporosis and an abnormal amount of bone-derived factors in turn promote the progression of AD and PD, especially in women over 60 years old (Yuan et al., 2019).
Bone is a multifaceted, dynamic tissue, which participated in movement facilitation, mineral metabolism, immune cell generation, and mesenchymal stem cells (MSCs) or hematopoietic stem cells (HSCs) breading. Since the bone marrow (BM) contains enormous amounts of MSCs and serves as the primary lymphoid organ, both of which have already been proved to have effects on the brain by many studies, the significance of bone in the regulation of CNS homeostasis is un-doubtable. Moreover, some hormones or “osteokines” from bone cells such as osteocalcin (OCN), osteopontin (OPN), and fibroblast growth factor (FGF) 23 have endocrine functions (Vervloet et al., 2014; Han Y. et al., 2018). These proteins can act on the brain directly or indirectly by regulating phosphate homeostasis or systemic energy metabolism (Han Y. et al., 2018; Yuan et al., 2019). This review aims to clarify the inter-relationship between the bone and brain and to discuss its potential therapeutic implications for neurodegenerative diseases.
BM-Derived Cells
The bone organ consists of bone and Bone marrow(BM), both of which function as a single unit (Compston, 2002). BM is a soft and viscous tissue enclosed within the bone cortex, containing HSCs and MSCs. HSCs are responsible for producing blood cells, including leukocytes, monocytes, erythrocytes, and platelets, while MSCs are contributed to osteoclasts and osteoblasts’ origination. These stem cells are tightly involved in the neurodegenerative progressions. Transplantation of young BM can rejuvenate the hematopoietic system and preserve cognitive function in old recipient mice, suggesting the beneficial effects of BM to the brain (Castellano et al., 2015; Das et al., 2019). Several pathways are contributed to the beneficial effects such as these BM-derived cells migrating directly into the brain to exert their toxicant clearance, immuno-modulative and neuro-generative functions, or via secreting exosomes to enter the brain and execute their biological functions (Soulet and Rivest, 2008; Dennie et al., 2016; Nakano et al., 2016; Han K. H. et al., 2018; Figure 1).
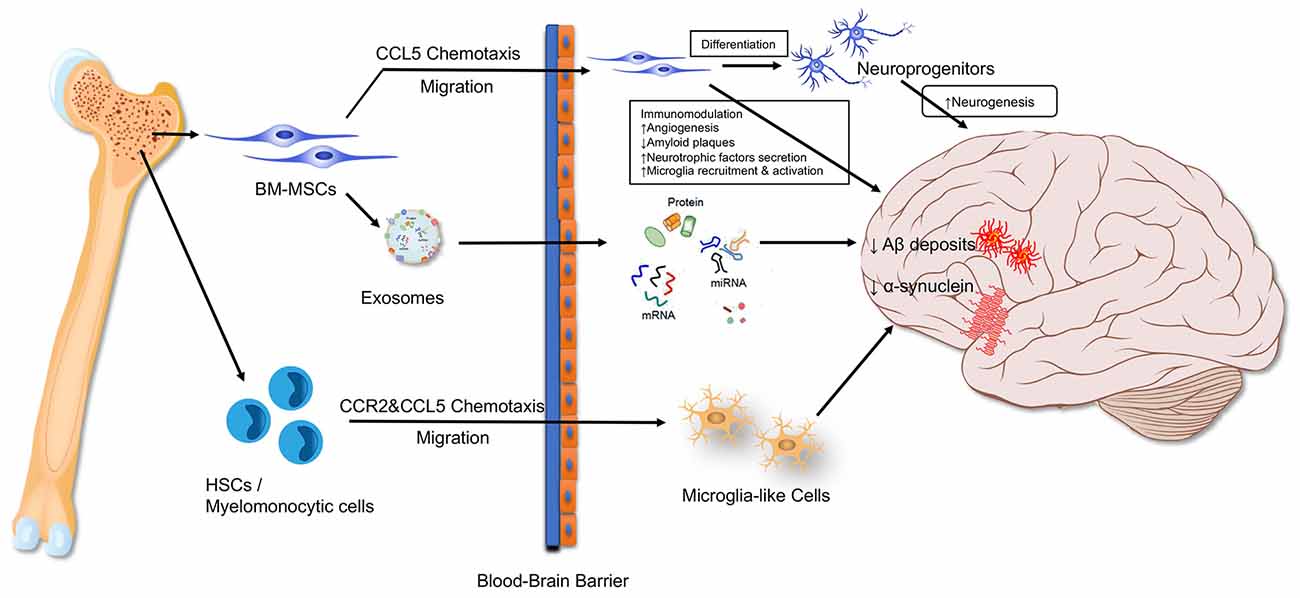
Figure 1. Bone marrow-derived cells influence the brain. BM-MSC, bone marrow mesenchymal stem cell; HSC, hematopoietic stem cell.
BM-Derived Microglia-Like Cells
Microglia are the primary immune effector cells in the CNS. These cells are monocytes that originated from mesodermal that invade the developing CNS in the embryonic period when the blood-brain barrier (BBB) is not fully formed. In addition to the primary resident microglia, another type of microglia that originates from precursors cells in the BM has been identified in the brain (Soulet and Rivest, 2008). To distinguish them from the originally brain-resident microglia cells, microglial cells originating from the outside of the brain are referred to as “microglia-like cells” (Han K. H. et al., 2018). Although BM-derived microglia-like cells can enter the CNS under normal physiological conditions, they are preferentially presented in regions suffering from neurodegeneration or exogenous insults (Soulet and Rivest, 2008). In the case of ischemic stroke, peripheral monocytes can migrate into the brain at the injury site and differentiate into ramified microglial-like cells (Priller et al., 2001a,b; Soulet and Rivest, 2008). This is even true for facial nerve axotomy and hypoglossal nerve axotomy models, in both cases the mouse models’ BBBs are not damaged (Priller et al., 2001a,b; Soulet and Rivest, 2008). In vitro studies showed Aβ-eliminating microglia-like cells could be differentiated from the BM-derived HSCs (Kuroda et al., 2020b) monocyte (Simard et al., 2006) and CD11b- or CD115-positive cells (Lebson et al., 2010; Koronyo et al., 2015). Further mechanism study showed that cells from the human and mouse BM can become microglia-like cells under the stimulation of colony-stimulating factor-1 (Kuroda et al., 2020a). An in vivo study showed that BM-derived microglia-like cells can move through the BBB and accumulate inside the brain in a CCR2 chemokine-dependent manner (El Khoury and Luster, 2008). The decreased CCR2 expression can reduce microglia accumulation and increase the level of Aβ in the brain, suggesting that early microglial accumulation can promote Aβ clearance in AD mouse models (El Khoury and Luster, 2008).
We reviewed the BM-derived microglia-like cells’ contribution to microgliosis in neurodegenerative diseases. Researchers transferred GFP-labeled myeloid cells into the BM of irradiated AD mice, and these GFP-labeled cells were later detected in the senile plaques in the brains (Simard et al., 2006). Similarly, early and rapid recruitment of BM-derived microglia to the brain also occurs in prion disease, bacterial meningitis, and PD mouse models (Kokovay and Cunningham, 2005; Djukic et al., 2006). Intracerebral injection of externally differentiated BM-derived microglia-like cells can also decrease Aβ deposits and improved cognitive function in AD mouse models (Kawanishi et al., 2018). These injected microglia-like cells can automatically migrate toward Aβ plaques, and reduce the number and area of these plaques (Lampron et al., 2011). Another study used a novel transgenic AD mouse to demonstrate that it is the peripheral blood-originated microglia, rather than their resident counterparts, that are capable of phagocytosing Aβ deposits (Simard et al., 2006). Besides, BM-derived microglia-like cells could also stimulate the phagocytic functions of brain-resident microglia in vitro and in vivo, via secreting large sums of transforming growth factor-β (TGF-β; Kuroda et al., 2020a). All these results suggest that BM-derived microglia-like cells have potential cell-based disease-modifying therapy against neurodegenerative diseases, especially AD.
BM-MSCs
Bone marrow MSCs (BM-MSCs) are pluripotent stem cells inside the BM. It has been proved that BM-MSCs can move into the brain and develop neuronal markers such as nestin, doublecortin, and NeuN under physiological conditions (Dennie et al., 2016). The CCR5 plays a critical role in regulating the BM-MSCs’ migration into the brain, both in physiological conditions and in response to injury (Dennie et al., 2016). Thus, BM-derived progenitors can migrate to the brain and become neurons at least in part, by firstly differentiating into neuronal precursor cells (Dennie et al., 2016). Apart from that, BM-MSCs can migrate to the brain and exert strong neuroprotective effects via regulating neurogenesis, apoptosis, angiogenesis, immunomodulation, and eliminating Aβ plaques in the brain (Naaldijk et al., 2017; Qin et al., 2020).
Transplanted BM-MSCs could significantly diminish the hippocampal Aβ plaques by activating several Aβ-degrading enzymes (Jha et al., 2015). Since vascular damage is also a pathogenic factor of AD, BM-MSCs can promote angiogenesis in the AD brain by secreting vascular endothelial growth factor (VEGF), epidermal growth factor (EGF), FGF-2, and Ang-1 (Gallina et al., 2015), and thus favor the cognitive and behavioral recovery (Garcia et al., 2014). The MSCs can also exert neuroprotective effects by secreting neurotrophic factors such as neurotrophin-3 (NT-3), hepatocyte growth factor (HGF), and brain-derived neurotrophic factor (BDNF; Wang et al., 2010). These factors stimulate endogenous regeneration and contribute to neurobehavioral function recovery. Animal studies demonstrate that the immunomodulatory effect of BM-MSCs plays a vital role in AD treatment as well (Salem et al., 2014; Zhang et al., 2020). Transplanted BM-MSCs can attract microglia-like cells by secreting CCL5 both in vitro and in the AD brain (Lee J. K. et al., 2012). Despite this, the activation levels of microglia and astrocyte were decreased in AD mice brains after BM-MSCs transplantation, manifested as the cerebral Iba-1 levels were down-regulated (Yokokawa et al., 2019). Our previous study showed that the transplanted BM-MSCs could inactivate microglia in the peri-infarct area via the CD200-CD200R1 signaling (Li et al., 2019). Also, BM-MSCs could lower expressional levels of pro-inflammatory genes, cytokines, and enzymes in astrocytes (Schäfer et al., 2012; Naaldijk et al., 2017). However, other studies report that the BM-MSCs could accelerate the microglia activation and thus the Aβ clearance in the AD brain (Lee et al., 2009). The BM-MSCs could also significantly increase the number of ChAT-positive cells and the intensity of ChAT spots in AD brains, which is an indicator for neurogenesis, neuronal differentiation, and integration (Mezey and Chandross, 2000; Mezey et al., 2000; Sanchez-Ramos et al., 2000). Nestin in neural cells, a neural precursor biomarker, is also up-regulated in the brain following the BM-MSC transplantation (Sanchez-Ramos et al., 2000). In fact, cells in different neurogenic stages, including proliferation, differentiation, migration, targeting, and integration, could be found in the hippocampus after the BM-MSC treatment (Perry et al., 2012).
Furthermore, BM-MSCs can alleviate cognitive defects related to various neurological disorders including traumatic brain injury (TBI), PD, and stroke by secreting microvesicles (MV) or exosomes (Xiong et al., 2017; Yang et al., 2017). It has been hypothesized that vesicles from BM-MSCs facilitate the transference of various functional factors, including regulatory non-coding RNAs, lipids, and proteins (Reza-Zaldivar et al., 2018). These regulatory factors within the MSC-derived exosomes are reported to act on critical cellular pathophysiological processes, such as energy metabolism, inflammation, and migration (Nakano et al., 2016; Borger et al., 2017). Li et al. (2017) reported that MSC-oriented exosomes shift the M1 microglia polarization toward an M2 phenotype, which ameliorates neuroinflammation and promotes functional recovery in a TBI model. Nakano et al. (2016) reported that BM-MSC-derived exosomes can alleviate the learning and cognitive damage in Streptozotocin (STZ)-induced diabetic mice by alleviating oxidative stress and promoting synaptogenesis. Functional miRNAs in exosomes might be an important mediator to inhibit neuronal apoptosis, promote synaptic plasticity and remodeling, and accelerate functional recovery (Xin et al., 2013, 2017; Cheng et al., 2018). For example, Xin et al. (2012, 2013) reported that MSC-released exosomes can transfer miR-133b into astrocytes and neurons in stroke rat models, and thereby promote the neurite outgrowth in the ischemic area. The authors further demonstrated the miR-133b exerts their neuroprotective function via decreasing the Ras homolog gene family member A (RhoA) and connective tissue growth factor (CTGF) expression. Besides, a study based on miRNA profiling and qPCR demonstrated the upregulation of miR-146a-5p in senescent MSCs and MSC-MVs (Lei et al., 2017). MiR-146a were also significantly upregulated in peripheral blood and cerebral spinal fluid (CSF) during the progression of AD, amyotrophic lateral sclerosis (ALS), and MS (Viswambharan et al., 2017). The miR-146a transferred by BM-MSCs-secreted exosomes is a key regulator in suppressing the astrocytic inflammation and protecting diabetic rats from cognitive decline (Kubota et al., 2018). Other neuroprotective mechanisms of MiR-146a-5p include inhibiting the NF-κB signaling (Iyer et al., 2012) and promoting remyelination (Zhang et al., 2017).
However, the neuroprotective functions of MSCs may be restricted with age, since both the number and function of MSCs are decreased (Lee et al., 2010; Bang et al., 2016). The senescent MSCs commonly present enlarged and more granular morphology, limited proliferation and differentiation capacity, and a distinct secretory phenotype referred to as “senescence-associated secretory phenotype” (SASP; Watanabe et al., 2017). The senescence of MSCs is believed to be the underlying cause of osteoporosis (Román et al., 2017), and neurodegenerative diseases including AD and PD (Lei et al., 2017). Furthermore, MSCs in the senescent late period release higher levels but smaller-sized MSC-MVs than the early passage of MSCs (Lei et al., 2017), which correlated with the phenomenon that the levels of myeloid MVs in plasma and cerebrospinal fluid were positively associated with neurodegenerative disease (Joshi et al., 2014).
In conclusion, BM-MSCs can migrate into the brain to differentiate toward neurons themselves, or recruit microglia cells for toxicant clearance. BM-MSCs can also promote neuronal repair and functional recovery by secreting exosomes. However, BM-MSC’s curative effects decreased greatly in the aging process.
BM-Controlled Immune System
The BM is the primary organ for producing common lymphoid progenitors (CLPs), which are responsible for generating innate and adaptive immune cells (Zhao E. et al., 2012). This is why the BM is called the primary lymphoid organ. The function of the BM and CNS is closely interconnected. There are norepinephrine (NE)-releasing sympathetic nerve fibers in BM, which are essential for preserving the HSC niche, promoting the HSC mobility, and regulating their differentiation into immune cells (Maryanovich et al., 2018). At the same time, the changes in the immune system originated from the BM will also affect the functions of the CNS by various mechanisms, including promoting inflammatory cytokines secretion, microglial activation, neuronal apoptosis, demyelination, and tissue damage (Liang et al., 2017). With age, the hematopoietic tissue is gradually replaced by fat tissue, and the proliferative and developmental capacity of HSCs, as well as the lymphogenic capacity decrease (Geiger et al., 2013). Studies have shown that the pathogenesis of AD and PD may be related to systemic immune dysfunction, especially to the T cell subtypes (Pellicano et al., 2012). Compared with the age-matched controls, the difference of immunity in AD patients was greater than that caused by age. The immune alterations mainly include less naïve T cells, more terminated-differentiated cells, and more dysfunctional Treg cells in AD patients. In PD patients, CD4+ and CD8+ T lymphocytes are found aggregated around the SN compacta (Brochard et al., 2009). These CD4+ cells are highly pro-inflammatory, which can promote the microglia activation and the degeneration of dopaminergic neurons. These studies suggest that immune alterations can contribute to the progression of neurodegenerative diseases (Figure 2).
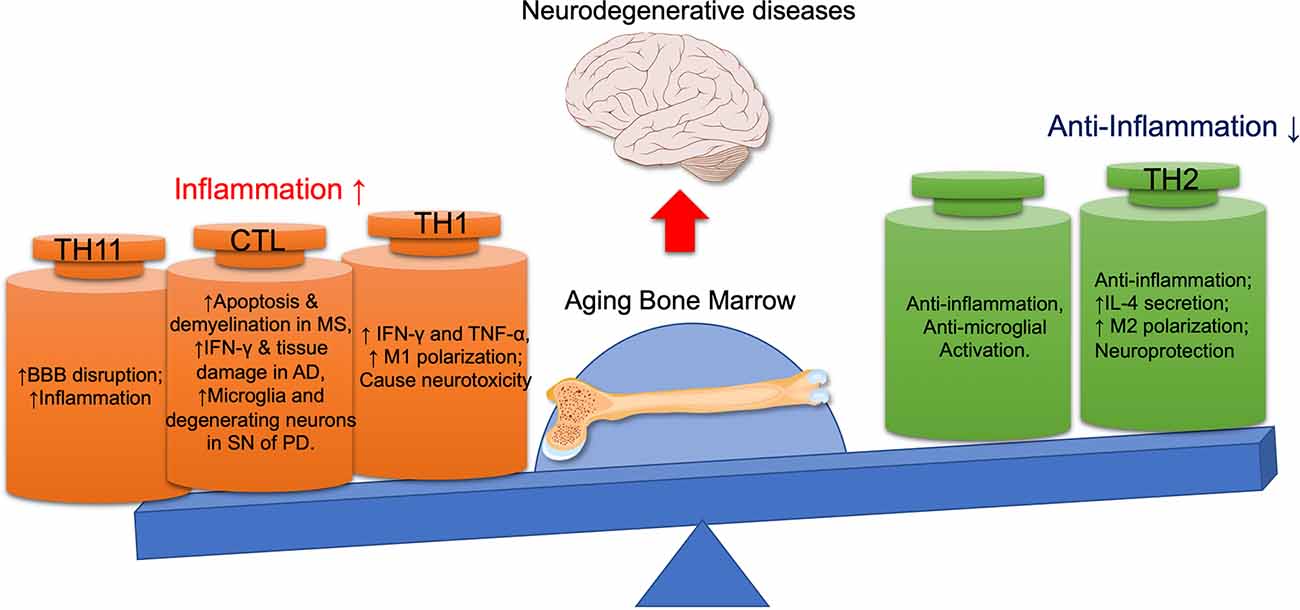
Figure 2. The detrimental and beneficial roles of different immune cells in neurodegenerative diseases. With the aging bone marrow, pro-inflammatory lymphocytes (CTL, Th1, Th17) are activated, while immunomodulatory lymphocytes (Th2, Tregs) are suppressed, thus promoting the progression of neurodegenerative diseases. AD, Alzheimer’s disease; BBB, blood-brain barrier; CTL, cytotoxic T lymphocyte; IFN-γ, interferon-γ; MS, multiple sclerosis; PD, Parkinson disease; SN, substantia nigra; TNF-α, tumor necrosis factor-α.
CD8+ T Cells
CD8+ T cells also referred to as cytotoxic T lymphocytes (CTL), play vital roles in the immune system to defend against intracellular pathogens by secreting pro-inflammatory cytokines, primarily the TNF-α and IFN-γ, or directly killing target cells via Fas/FasL interactions or cytotoxic granules containing perforin and granzymes (Harty et al., 2000). CD8+ T cells are also related to the pathogenesis of neurodegenerative diseases. Studies indicated that the CD8+ T cells outnumber the CD4+ T cells in MS lesions (Goverman et al., 2005). Moreover, the injection of CD8+ myelin-specific T cells rather than myelin-specific CD4+ T cells into wild-type mice can induce a demyelinating disease similar to MS, suggesting CD8+ T cells’ unique roles in the pathogenesis of MS (Huseby et al., 2001; Goverman, 2009). Furthermore, in the brains of both postmortem human PD specimens and PD mouse models, CD8+ T cells are often located next to the activated microglia and degenerating neurons in the SN area, which indicates that these cells might be implicated in PD neuronal loss (Brochard et al., 2009). Additionally, CD8+ T cells mediated inflammation and IFN-γ levels are associated with microstructural tissue damage and neurological deficits in AD patients (Baglio et al., 2013; Lueg et al., 2015).
Th1 Cells
CD4+ Th1 cells referred to as type 1 T helper cells are characterized by T-bet expression and Type 1 cytokines (mainly IFN-γ, TNF-α, and IL-2) secretion (Romagnani, 2014). CD4+ Th1 infiltration in the brain is seen in various neurodegenerative diseases (Liang et al., 2017). Browne et al. (2013) showed that Th cells infiltrated in the brains of APP/PS1 mouse models secrete abundant IFN-γ and IL-17, suggesting these Th cells are mainly the Th1 and Th17 subsets. They further demonstrated that it is the Th1 cells, but not Th2 nor Th17 cells, that cause microglial activation, Aβ plaque burden increase, and impaired cognitive function in APP/PS1 mouse models (Browne et al., 2013). Much of the Th1 cell-mediated inflammatory damage in the context of neurodegenerative diseases can be attributed to the release of pro-inflammatory cytokines and the M1 polarization of macrophages and microglia. These cellular processes could significantly aggravate neurodegenerative diseases by promoting neurotoxicity and tissue damage in the brain (Sanchez-Guajardo et al., 2013). Administrating anti-IFN-γ antibody and immuno-modulative TGF-β in AD mouse models could effectively alleviate the Th1 cell-mediated neuroinflammation and cognitive decline (Browne et al., 2013; Chen et al., 2015). However, some studies show the beneficial roles of Th1 cells in AD pathology. Intracerebroventricular (ICV) injection of Aβ-specific Th1 cells lowers the Aβ levels and enhances neurogenesis, while exerts no impact on apoptosis in AD mouse models (Fisher et al., 2014). Thus, it seems that different routes of Th cells migration into the brain might play different roles in neurodegeneration.
Th2 Cells
CD4+ Th2 cells are T helper cells expressing the GATA3 and producing Type-2 cytokines (mainly IL-4, IL-5, IL-9, and IL-13; Walker and McKenzie, 2018). Th2 cells mainly exhibit an anti-inflammatory function, such as suppressing the Th1-related IFN-γ-driven immune response and inducing macrophage to polarize into an M2-like phenotype (Walker and McKenzie, 2018). In the Th1/Th2 balance paradigm, the Th1-secreted IFN-γ and Th2-derived IL-10 inhibit the proliferation of each other’s cells. CD4+ Th2 cells mainly exhibit neuroprotective properties in the context of neurodegenerative diseases. For example, myelin basic protein (MBP)-primed Th2 cells can enter the brain and restrict the AD and PD neurotoxicant induced microglial inflammation (Roy and Pahan, 2013). In MS models, Th2 cells can inhibit the activation of lipopolysaccharide (LPS)-stimulated microglia via direct cell contact, and ultimately inhibit its IL-1b and nitric oxide production (Roy and Pahan, 2013). Additionally, MBP-primed Th2 cells can even induce the neurotrophic molecules (including BDNF and NT-3) expression in microglia and astroglia via direct cell contact (Roy et al., 2007).
Th17 Cells
Th17 cells are CD4+ Th cells expressing the RORγτ transcriptional factors and secreting characteristic cytokines including IL-17 and IL-22 (Annunziato et al., 2013). They are involved in the progression of neurodegenerative diseases, mainly by secreting IL-17 to recruit inflammatory neutrophils and IL-22 to stimulate epithelial cells generating antimicrobial peptides (Saresella et al., 2011). Saresella et al. (2011) analyzed the function of various T lymphocytes in AD patients and compared the data with those of mild cognitive impairment participants or aged-matched healthy people. They found that the Th17 lymphocytes are significantly increased in AD. In MS or experimental autoimmune encephalomyelitis (EAE), Th17 cells secrete cytokines to act on IL-17 and IL-22 receptors on the BBB endothelium, causing BBB damage and subsequent neural inflammation (Kebir et al., 2007).
Interestingly, Th17 is tightly associated with bone destruction. In postmenopausal osteoporosis patients, serum IL-17A is significantly higher, while the IFN-γ and IL-4 are significantly lower, suggesting osteoporosis may be more associated with the Th17 cells rather than the Th1 or Th2 cells (Zhang et al., 2015). The further study demonstrates IL-17A is pro-osteoclastogenic at the cellular level (Le Goff et al., 2019). In vivo study shows that although IL-17A is not required for normal bone homeostasis, it plays a vital role in bone loss of ovariectomized osteoporosis mouse models. Furthermore, preliminary data from clinical trials show that anti-IL-17A antibodies stabilize bone density in inflammatory arthritis (Le Goff et al., 2019). These results suggest an inter-relationship between osteoporosis and neurodegenerative diseases.
Treg Cells
Regulatory T cells (Tregs) are T cells expressing the CD4+CD25+Foxp3+ surface markers. They inhibit immune response and thus to maintain homeostasis and self-tolerance. Treg cells play a substantial role in slowing down the progression of ALS by lowering pro-inflammatory cytokine expressions and reducing microglial activation in mouse models (Beers et al., 2011). The mechanisms behind are associated with Tregs’ secretion of immuno-modulative cytokines (TGF-β, IL-10, and IL-4) which inhibits the microglia and pro-inflammatory T cells activation (Beers et al., 2011; Xie et al., 2015). However, functional Tregs are usually deficient in patients with neurodegenerative diseases for several reasons. Under normal physiological conditions, 30% of CD4+ T cells are functional Treg cells in BM (Zou et al., 2004), and over 15% of CD4+ T cells in rat cerebrum are Treg cells (Xie et al., 2015). However, the absolute lymphocyte numbers and the percentage of Treg subsets were both decreased in the BM and peripheral blood of the aged participants, indicating the Treg production in BM is reduced (Freitas et al., 2019). Moreover, a decrease of functional Treg seems to be closely related to MS, since the relapsed MS patients have fewer Tregs and significantly reduced Treg/Th17 ratio in the peripheral blood compared with healthy individuals (Jamshidian et al., 2013). Furthermore, Tregs are extremely rare or even undetectable in MS brain lesions, which might be due to Treg’s impaired migration across the BBB (Fritzsching et al., 2011). In vitro studies show healthy Tregs can cross the human brain endothelium easily, whereas the migration ability of Tregs in MS patients is severely impaired (Schneider-Hohendorf et al., 2010). Moreover, their neural protective functionality might also be hindered in neurodegenerative disease because of the Th17 subset’s inhibitory effect on Tregs. Treg cells rely on TGF-β, which is competitively bound by the abundant Th17 cells in a neurodegenerative context, for differentiation and maintenance (Afzali et al., 2010). However, Tregs can be transformed into pro-inflammatory Th17 cells themselves in some circumstances (Afzali et al., 2010). These multiple factors result in low Treg levels in neurodegenerative patients.
Bone Secretory Proteins
Previous evidence showed that several bone cell-secreted hormones or “osteokines” have endocrine functions, such as OCN, OPN, FGF23, Lipocalin 2(LCN2), osteoprotegerin (OPG), sclerostin (SOST), and Dickkopf-1 (DKK1; Vervloet et al., 2014; Han Y. et al., 2018). Most of these proteins are produced by osteoblasts and osteocytes and have roles in regulating phosphate and systemic energy metabolism (Han Y. et al., 2018). Osteoblasts-secreted OCN regulates energy metabolism, reproduction, and cognition (Zoch et al., 2016; Mizokami et al., 2017; Obri et al., 2018). OPN in the osseous tissue is released from osteoblasts and osteoclasts and is associated with bone destruction and suppression of ectopic calcification (Lund et al., 2009; Uede, 2011). It mainly regulates phosphate homeostasis (Huang et al., 2013). LCN2 secreted by osteoblasts can act on the brain to suppress appetite (Mosialou et al., 2017). OPG is synthesized by osteoblasts and its main function is to antagonize the effects of receptor activator of nuclear factor-kappa-B ligand (RANKL; Bonnet, 2017; Rochette et al., 2019). Both SOST and DKK1 (Ke et al., 2012) are mainly secreted by osteocytes and function through antagonizing the canonical Wnt pathway. These bone secretory proteins are reported to play diverse roles in neurodegenerative diseases (Table 1).
OCN
OCN is uniquely secreted by osteoblasts (Hauschka et al., 1975; Price et al., 1976) and is thus a specific biomarker for bone formation (Ducy et al., 1996; Ducy, 2011). OCN is also an important regulator of energy metabolism, as it can enhance insulin secretion, decrease insulin resistance, improve glucose tolerance and blood lipid profile, and regulate brown adipose tissue differentiation (Karsenty and Ferron, 2012; Wei et al., 2014).
OCN, uncarboxylated in most circumstances, can pass through the BBB and bind specifically with neurons in the brainstem, thalamus, and hypothalamus (Oury et al., 2013; Shan et al., 2019). Upon binding, OCN can influence the signals that regulate neurotransmitter syntheses, such as decreasing the synthesis of glutamate decarboxylase 1 (Gad1), an enzyme involved in GABA biosynthesis, and increasing the tyrosine hydroxylase (Th) and tryptophan hydroxylase 2 (Tph2) synthesis, which are the key enzymes involved in the serotonin, dopamine, and norepinephrine generation in the brainstem and midbrain explants (Oury et al., 2013). In the hippocampus, OCN mainly combines with neurons’ Gpr158/Gaq receptors, and functions in part by activating IP3 and promoting the secretion of BDNF (Khrimian et al., 2017), a molecule well known to promote hippocampal-dependent memory (Hall et al., 2000; Dean et al., 2009). Adult mice lacking OCN displayed a substantial increase in anxiety-like behavior and had a major deficit in memory and learning (Nakazawa et al., 2002; Oury et al., 2013). Anatomically, the brains of OCN–/– mice are consistently smaller, mainly in the hippocampal region, and often lost the corpus callosum compared with wild-type littermates (Nakazawa et al., 2002; Oury et al., 2013). OCN–/– mice injected with OCN showed ameliorated anxiety and depression, and improved memory and learning abilities (Mera et al., 2016). OCN also has neuroprotective effects in the context of PD. As mentioned above, OCN could enhance dopamine synthesis in neurons, thereby reducing the Th loss and relieving the PD symptoms in PD rat models (Guo et al., 2018; Obri et al., 2018). Also, OCN could modulate neuroinflammation in the SN of PD rats by inhibiting astrocyte and microglia proliferation, together with partially decreased levels of TNF-α and IL-1β (Guo et al., 2018).
OPN
OPN was first discovered as a protein to anchor osteoclast to the mineral surface of bones, thereby to facilitate the osteolytic process (Reinholt et al., 1990). Serum OPN levels have negative correlations with bone mineral density in postmenopausal women (Cho et al., 2013). OPN is mainly secreted by osteoblasts, osteoclasts, and BM-derived myelomonocytic cells (Lund et al., 2009; Uede, 2011). In the brain, OPN is a constituent of the normal extracellular matrix and is expressed mainly in the basal ganglia, especially in the substantia nigra (SN; Iczkiewicz et al., 2004). In neurodegenerative diseases, OPN is considered to play dual roles in neuroinflammation and neuroprotection (Carecchio and Comi, 2011; Yu et al., 2017).
Previous studies showed OPN’s detrimental role in MS. Abundant OPN transcript was found in plaques dissected from MS patients’ brains, while it was absent in the control group (Chabas et al., 2001). Further study demonstrated that OPN-deficient mice had a milder disease course than wild type animals, and displayed only a single relapse without subsequent exacerbations or progression (Chabas et al., 2001; Jansson et al., 2002). Administrating OPN-deficient EAE mice with recombinant OPN exacerbates the disease (Hur et al., 2007). Two mechanisms may be involved in OPN’s detrimental effects: by stimulating the pro-inflammatory mediators in MS lesions, and by inhibiting the apoptosis of autoreactive immune cells (Carecchio and Comi, 2011). In contrast, the OPN is mainly studied for its anti-inflammatory and anti-apoptotic properties in PD (Khan et al., 2002; Lund et al., 2009; Rittling and Singh, 2015). Iczkiewicz et al. (2010) showed that the arginine-glycine-aspartic acid (RGD)-binding domain of OPN protects dopaminergic cells against toxic insult induced by MPP+ and LPS. Also, the OPN’s effect decline with age, a major predisposing factor for PD, further reinforced the hypothesis (Hwang et al., 1994). However, Maetzler et al. (2007) showed that OPN knock-out PD mice displayed less nigral cell death and a lower glial response compared to wild-type PD mice. He also reported that PD patients’ serum and CSF OPN levels were higher, with CSF levels positively correlated with concomitant dementia and serum levels with more severe motor symptoms, suggesting OPN may promote the PD progression (Maetzler et al., 2007). In the context of AD, studies show OPN can promote the monocyte-macrophage’ recruitment into AD mouse brains, and their polarization towards an anti-inflammatory, highly phagocytic phenotype to facilitate Aβ clearance (Rentsendorj et al., 2018). The FDA-approved anti-AD drug glatiramer acetate increases the plasma levels of OPN, and therefore promotes a macrophage phenotype that is highly phagocytic of Aβ and anti-inflammatory (Rentsendorj et al., 2018). Also, OPN can bind to the CD44 receptor and exert its anti-apoptotic function (Lin and Yang-Yen, 2001). This may be another potential mechanism for OPN to protect neurons from injury in AD.
Therefore, we can conclude that the levels of OPN increase with age. OPN accelerates the progression of bone demineralization, while exerts different influence on various kinds of neurodegenerative disorders.
FGF-23
FGF-23 is mainly secreted by osteoblasts and osteocytes. It suppresses the phosphate resorption and 1, 25(OH)2D3 production in the kidney by binding to FGFR1 and its co-receptor Klotho (Urakawa et al., 2006). FGF-23 changes significantly in the aging process (Cardoso et al., 2018). Still, FGF-23 is an independent predictor for dementia and AD, after adjusting for age, sex, cardiovascular disease, diabetes mellitus, etc. (McGrath et al., 2019). In another study, researchers used high-resolution MRI to find out that increased FGF-23 was associated with axonal loss and white matter disruption in the frontal lobe only in patients with cardiovascular risk factors (Marebwa et al., 2018). FGF-23-deficient mice show ectopic calcifications in the brain, fewer immature neurons in the sub-granular zone (SGZ), and significant cognitive impairment compared with wild type controls (Kunert et al., 2017; Laszczyk et al., 2019). Mice overexpressing FGF-23 also showed impaired spatial learning and memory (Liu et al., 2011). However, these peripheral symptoms could be largely alleviated by dietary correction of phosphate levels (Morishita et al., 2001; Liu et al., 2011), suggesting that FGF-23 may indirectly affect brain health and cognition, but probably by affecting phosphate homeostasis which acts synergistically with renal or cardiovascular risk factors.
Another explanation is that too much FGF-23 may exacerbate neurodegenerative diseases by decreasing the level of vitamin D3. FGF-23 suppresses the vitamin D3 production by blocking the 1α-hydroxylase in the renal proximal and distal tubules (Karsenty and Olson, 2016). The detrimental effects of vitamin D deficiency on the brain and its roles in neurodegenerative diseases have already been elaborated on in other studies (Koduah et al., 2017; Lv et al., 2020).
In conclusion, an abnormal amount (too much or too little) of FGF-23 is sufficient to affect brain function and cognition, most likely through an indirect way by affecting ion (phosphate) and/or vitamin D homeostasis.
LCN2
LCN2 is an endocrine hormone secreted by osteoblasts and can suppress appetite by crossing the BBB to bind to the melanocortin-4 receptor (MC4R) in the brainstem (Mosialou et al., 2017). Besides, LCN2 can promote insulin resistance and cause hyperglycemia, diabetes, cardiovascular disease, and metabolic syndrome (Yan et al., 2007). LCN2 can also be secreted by neutrophils and glial cells, and play essential roles in inflammation, infection, and injury to cells (Pinyopornpanish et al., 2019).
LCN2 acts through its two receptors, 24p3R and megalin (Chakraborty et al., 2012), both of which can be found in the brain in basal and pathological conditions (Ip et al., 2011). LCN2 production is increased in progressive MS patients, and this effect could be relieved by the MS-treating drug natalizumab (Al Nimer et al., 2016). Further in vitro study shows that LCN2 plays detrimental roles by inhibiting remyelination in a dose-dependent manner (Al Nimer et al., 2016). LCN2 is also upregulated in the SN of PD patients and neurotoxin-induced PD animal models (Kim et al., 2016). The higher LCN2 levels could disrupt the SN dopaminergic projection and contribute to abnormal locomotor behaviors through neurotoxic iron accumulation and neuroinflammation, which were alleviated in LCN2-deficient mice (Kim et al., 2016). LCN2 is significantly decreased in CSF of AD patients and increased in brain regions related to AD pathology (Naudé et al., 2012). in vitro study demonstrates that LCN2 makes nerve cells susceptible to Aβ toxicity, and suppresses the neural protective tumor necrosis factor receptor 2 (TNFR2) signaling pathway in neurons (Naudé et al., 2012).
In animal experiments, ICV injection of recombinant mouse LCN2 protein can cause neuronal death in the hippocampal CA1 area and cognitive dysfunction (Kim et al., 2017). Moreover, LCN2 usually acts synergistically and exacerbates the neurotoxic effects of Aβ, TNF-α, or LPS (Mesquita et al., 2014; Yang et al., 2017). The expression of 24p3R increases after the administration of Aβ (Mesquita et al., 2014). An increase in cell death is reported when astrocytes or neurons are co-cultured with LCN2 and Aβ (Marebwa et al., 2018), and the depletion of LCN2 helps to protect astrocytes from Aβ toxicity (Mesquita et al., 2014). Also, LCN2 could undermine the neuroprotective effect of the TNFR2 signaling pathway induced by TNF-α (Hemmings and Restuccia, 2012; Naudé et al., 2012). Further in vitro studies demonstrate that LCN2 can directly induce neuronal apoptosis through the BCL2 mediated cell death signaling pathway in a time and dose-dependent manner (Lee S. et al., 2012; Bi et al., 2013).
In short, LCN2 could exert pro-apoptotic effects on brain cells and increase the susceptibility of neurons to toxic stimuli. LCN2 could also increase glial activity, increase inflammation, and inhibit remyelination.
OPG
OPG is a soluble glycoprotein that belongs to the TNF receptor superfamily. It is a decoy receptor for RANKL and TNF-related apoptosis-inducing ligand (TRAIL), and thus inhibits the association of RANKL and TRAIL with their receptors. It is secreted by osteoblast and prevents the RANKL from binding to its receptor on osteoclasts, thereby inhibiting the osteolysis (Bonnet, 2017; Rochette et al., 2019). Increased blood levels of OPG are associated with osteoporosis in postmenopausal women (Yano et al., 1999).
High OPG levels are also detected in the CSF, and as the OPG level in the CSF increases with age, it may play a role in inflammatory and degenerative disorders of the CNS (Hofbauer et al., 2004). A study demonstrates that after adjusting for age, sex, and APOE ε4 allele, OPG is an independent predictor of AD and vascular dementia (Emanuele et al., 2004). OPG might influence cognition by affecting the immune environment and the perfusion of the brain. For the former, studies have demonstrated that the RANKL/RANK signaling suppresses inflammation through a Toll-like receptor pathway in microglia. Increased OPG could inhibit the RANKL/RANK signaling and aggravate the post-ischemic inflammation (Shimamura et al., 2014). Whereas for the latter, the RANK/RANKL/OPG triad might play a critical role in vascular calcification (Rochette et al., 2019). Therefore, OPG can prevent vascular calcification as a RANKL inhibitor (Wu et al., 2013). Emanuele et al. (2004) measured plasma OPG levels in vascular dementia patients and compared them with OPG in AD and age-matched healthy individuals. They found that compared with the non-demented control group, OPG concentrations were significantly higher in both vascular dementia and AD patients, wherein the OPG level in vascular dementia was the highest. These results were interpreted to be that OPG could mirror atherosclerotic disease, most so in vascular dementia and that vascular factors may also play a role in the pathogenesis of AD (Emanuele et al., 2004). Increases of OPG in vascular dementia can be regarded as a compensatory defense mechanism to relieve the atherosclerotic burden (Schoppet et al., 2002).
DKK1
Both DKK1 and SOST are soluble Wnt inhibitors (Ke et al., 2012). Although these two molecules share some homologies in action, they have distinct biological effects and different expression patterns (Gifre et al., 2013). The DKK1 and SOST use a different receptor to inhibit the low-density lipoprotein receptor-related protein (LRP)5-LRP6 receptor complex formation (Monroe et al., 2012). The SOST protein seems to be a specific Wnt inhibitor because it is almost exclusively bone-derived, and is predominantly secreted by osteocytes and osteoclast precursors (Poole et al., 2005; Vervloet et al., 2014). Although DKK1 is also mainly expressed by osteocytes, osteoblasts, and BM-MSCs, it is not as highly selective as SOST (Han Y. et al., 2018). We mainly focus on the DKK1 in this review, since it is more deeply involved in brain pathologies, while the SOST is found unrelated to cognition change (Ross et al., 2018).
Wnt signaling has been proven to have strong neuroprotective effects, and can even regulate neo-neuronal generation in the adult brain (Inestrosa and Varela-Nallar, 2014). Wnt signaling activation facilitates synaptic remodeling and memory consolidation (Inestrosa and Varela-Nallar, 2014). Therefore, it is easy to understand that Dkk1, a Wnt signaling inhibitor, is associated with the severity of neurodegenerative diseases (Ross et al., 2018). The expression of DKK1 in the brain tissue, CSF, and plasma of AD patients and animal models increases significantly compared to healthy controls (Caricasole et al., 2004; Rosi et al., 2010). It has also been reported that at high concentrations, DKK1 can pass through the BBB, and thus accelerate the AD progression (Ren et al., 2019). Some studies show that the occurrence of familial/early-onset AD, sporadic/late-onset AD, and patient’s cognitive decline are related to DKK1 and Wnt/β-catenin signaling disruptions (Scott and Brann, 2013). The increased expression of DKK1 inhibits the Wnt signaling pathway, which further increases the tau phosphorylation, while the DKK1 knock-out inhibits the formation of neurofibrillary tangles, and reduces the neurotoxicity of Aβ (Caricasole et al., 2004). Further study shows that injecting different concentrations of DKK1 into the dorsal hippocampus can lead to object recognition memory loss, which is regulated by the canonical Wnt-dependent signaling pathway (Fortress and Frick, 2016). DKK1 could also aggravate the Aβ-induced neuronal apoptosis and synaptic loss by blocking the Wnt signaling pathway (Purro et al., 2012). Other related studies show that short-term exposure to Aβ in mouse brain slices increases the DKK1 expression and reduces the synaptic formation (Matrisciano et al., 2011), while injecting DKK1-specific antibodies could alleviate the Aβ-induced damage to neuronal synapses (Huang et al., 2018). Some scholars suggest that the endogenous Wnt ligands, which are inhibited by DKK1, are vital to maintaining the neuronal synapses (Purro et al., 2014). Also, the potential therapeutic effects of the DKK1 inhibitor may be associated with synaptic preservation and hippocampal circuit reconstruction (Marzo et al., 2016; Ortiz-Matamoros and Arias, 2018). Animal tests show that inhibiting DKK1 improves subjects’ spatial memory tasks (Marzo et al., 2016; Ortiz-Matamoros and Arias, 2018) and their electrophysiological findings (Marzo et al., 2016).
Potential Therapeutic Implications
BM-Derived Cells
After the transplantation of BM-MSCs, learning ability and spatial memory performance were significantly improved in AD animal models (Ohsawa et al., 2008; Kanamaru et al., 2015; Safar et al., 2016). It was revealed that the gene expression patterns are altered in AD brains, and the alterations are correlated with the severity of neuropathology (Qin et al., 2020). Transplantation of BM-MSCs could also alter some gene levels in the AD brains, such as genes related to pro-inflammatory cytokines, enzymes, receptors, and intermediate filaments. These differentially expressed representative genes are mostly responsible for neuropathological phenotypes in AD (Qin et al., 2020).
In terms of feasibility, the intravenous delivery of stem cells is convenient and sufficient to lower the levels of cerebral amyloidosis (Salem et al., 2014; Harach et al., 2017). The intravenous transplanted stem cells could be easily detected in the brain parenchyma, i.e., could be found in the hippocampus 1 h after administration (Harach et al., 2017), and expressed neuronal phenotypes in the brain for 1-6 months (Brazelton et al., 2000). The expression of male BM-MSCs’ sry gene in female AD model’s brain tissue proves that exogenous stem cells with intravenous delivery could successfully migrate to the brain injury site (Salem et al., 2014). As for the cell dose for intravenous administration, our previous data showed 5 × 105 BM-MSCs is an appropriate dose for treating ischemic stroke in rats (He et al., 2016). The optimal cell dose for AD and other neurodegenerative disease therapies are remained to be explored.
Bone-Derived Exosomes
Previous studies report positive correlations between MVs in CSF and neurodegenerative diseases and show that many exosomes are enriched with undigested lysosomal substrates, such as Aβ and APP (Malm et al., 2016). However, these MVs are mainly derived from inflammatory cells, microglia, or tumor (Rajendran et al., 2006; Saman et al., 2012, 2014; Joshi et al., 2014; Levy, 2017), and there is no evidence suggested that BM-derived exosomes can cause or exacerbate neurodegenerative diseases. Some studies indicate that using stem cell-secreted exosomes as an alternative therapeutic would be much safer compared with stem cell transplantation (Smith et al., 2020). Administrating exosomes rather than viable replicating cells can mitigate many complications and therefore be much safer (Smith et al., 2020). The transplanted stem cells may persist or be amplified even after treatment is terminated. The transplanted stem cells’ potential of differentiating into other cell types has also raised long-term safety concerns (Breitbach et al., 2007).
Apart from its innate therapeutic effect discussed above, exosomes from the BM can be modified into appropriate nanocarriers to transport siRNA. To strengthen exosomes’ targeting ability, researchers reshaped them by attaching neuron-specific Rabies Viral Glycoprotein (RVG) peptides onto their surface (Alvarez-Erviti et al., 2011). The remodeled RVG exosomes successfully transported the GAPDH siRNA into targeted neurons, oligodendrocytes, and microglia, and subsequently downregulated the BACE1 gene expression, which is crucial in the AD progression.
Bone Secretory Proteins
Several bone-secreted proteins were summarized above as regulators of CNS homeostasis and neurodegenerative diseases. Although the direct use of these bone-secreted proteins as drugs to treat neurodegenerative diseases are rare, a few studies indicated that these proteins might be indirectly involved in other therapeutics. For example, metformin could ameliorate spatial memory loss (Ahmed et al., 2017), reduce stress-induced behaviors (Mourya et al., 2018), and relieve the related symptoms in the PD mouse model (Katila et al., 2017). Metformin can affect OCN and related bone diseases. In an osteoporosis mouse model, metformin could ameliorate the bone loss with the increased OCN expression level (Liu et al., 2019). The drug could also improve the osteogenic functions of adipose-derived stromal cells by increasing the OCN expression and AMPK signaling (Smieszek et al., 2018). It should be noted that AMPK is also a regulator for brain energy metabolism (Garza-Lombó et al., 2018), which are related to cognition and motor coordination (Kobilo et al., 2014), and can inhibit NF-κB signaling and inflammation (Salminen et al., 2011). Moreover, metformin can behavior by increasing the BDNF secretion (Katila et al., 2017; Fatemi et al., 2019), which coordinates with OCN’s effect in age-related memory loss (Khrimian et al., 2017). These findings suggested that metformin therapy could influence brain functions, wherein the OCN may act as a mediator in promoting neurotrophic signal, energy metabolism, and immune modulation. A role for OCN may also be implicated in exercise-induced cognitive improvement through the IL-6/gp130/OCN axis (Shan et al., 2019). Moreover, the MS-treating drug natalizumab and the anti-AD drug glatiramer acetate mentioned above are another two examples to treat neurodegenerative diseases via the mechanisms related to bone secretory proteins. The two drugs reduce the LCN2 or increase the OPN expression, respectively.
Before clinical application, further studies on specific bone regulative processes and their effects on the brain are required. For example, even though BM-derived cells can enter the CNS and become neural progenitors or microglia-like cells, more details about how this process is controlled in vivo should be elucidated (Ajami et al., 2007; Mildner et al., 2007). Moreover, most studies examining the bone-derived cells or molecules’ roles in neurodegenerative diseases were performed in mouse models rather than in patients. These results should further be confirmed in human samples. Furthermore, except for OCN and DKK1, there is still a lack of direct evidence to show that these bone-secreted osteokines can cross the BBB and influence the neurodegenerative diseases’ course. Although these cytokines are mainly secreted from the bone, and show altered expression in the context of neurodegenerative diseases, most osteokines above are not bone-specific and some of them can be secreted within the brain. It remains to be determined how these osteokines are evolved in the process of bone-brain crosstalk and contribute to the progression of neurodegenerative diseases by using bone-defect models. Last but not least, although bone is a primary lymphoid organ, it does not sufficiently control the entire immune system, other factors are also needed for triggering the onset of neurodegenerative diseases. Although there are still many difficulties, the potential application of bone-based therapy in neurodegenerative diseases is worthy of further experimental and clinical studies.
Conclusions
Elderly people are prone to osteoporosis and neurodegenerative diseases including cognitive decline, PD, and MS. All these disorders have common soil, mainly the metabolic-related disorders and immune dysregulation, suggesting the bone and brain may be closely interacted. When anyone of the two sides breaks, the whole interconnected balanced system collapses. Finding out the interactive mechanisms and looking for early detection and intervention may be a promising way to prevent the disease progress. This review summarizes the roles of the bone-brain axis in the progression of neurodegenerative diseases. We focus on the effects of BM-derived cells, mainly the microglia-like cells and MSCs, the BM-controlled immune system, and the bone secreted proteins on the brain. Evidence from experimental studies is encouraging, wherein bone-derived cells and factors can influence brain development, neurotransmitter synthesis, ion homeostasis, neuroinflammation, and neurotoxicant clearance. Therefore, targeting and modulating bone physiological processes can be promising for developing novel therapeutic approaches for neurodegenerative diseases.
Author Contributions
ZY drafted the article. ZL and LL revised it critically for important intellectual content. JZ and XC contributed to the revision of the manuscript and figure illustrations. XZ and PX made substantial contributions to the conception and design of the review and gave final approval of the version to be published. All authors contributed to the article and approved the submitted version.
Funding
This work was supported in part by grants from National Key R&D Program of China (2016YFC1306601 and 2017YFC1310300), National Natural Science Foundation of China (32071341, 82071416, 31430030, 81901282, 81870992, and 81870856), Natural Science Foundation of Guangdong, China (2017A030308004), Science and Technology Program of Guangzhou, China (201804020011), and Technology project of Guangzhou (2018-1202-SF-0019 and 2019ZD09).
Conflict of Interest
The authors declare that the research was conducted in the absence of any commercial or financial relationships that could be construed as a potential conflict of interest.
References
Afzali, B., Mitchell, P., Lechler, R. I., John, S., and Lombardi, G. (2010). Translational mini-review series on Th17 cells: induction of interleukin-17 production by regulatory T cells. Clin. Exp. Immunol. 159, 120–130. doi: 10.1111/j.1365-2249.2009.04038.x
Ahmed, S., Mahmood, Z., Javed, A., Hashmi, S. N., Zerr, I., Zafar, S., et al. (2017). Effect of metformin on adult hippocampal neurogenesis: comparison with donepezil and links to cognition. J. Mol. Neurosci. 62, 88–98. doi: 10.1007/s12031-017-0915-z
Ajami, B., Bennett, J. L., Krieger, C., Tetzlaff, W., and Rossi, F. M. (2007). Local self-renewal can sustain CNS microglia maintenance and function throughout adult life. Nat. Neurosci. 10, 1538–1543. doi: 10.1038/nn2014
Al Nimer, F., Elliott, C., Bergman, J., Khademi, M., Dring, A. M., Aeinehband, S., et al. (2016). Lipocalin-2 is increased in progressive multiple sclerosis and inhibits remyelination. Neurol. Neuroimmunol. Neuroinflamm. 3:e191. doi: 10.1212/NXI.0000000000000191
Alvarez-Erviti, L., Seow, Y., Yin, H., Betts, C., Lakhal, S., and Wood, M. J. (2011). Delivery of siRNA to the mouse brain by systemic injection of targeted exosomes. Nat. Biotechnol. 29, 341–345. doi: 10.1038/nbt.1807
Annunziato, F., Cosmi, L., Liotta, F., Maggi, E., and Romagnani, S. (2013). Main features of human T helper 17 cells. Ann. N Y Acad. Sci. 1284, 66–70. doi: 10.1111/nyas.12075
Baglio, F., Saresella, M., Preti, M. G., Cabinio, M., Griffanti, L., Marventano, I., et al. (2013). Neuroinflammation and brain functional disconnection in Alzheimer’s disease. Front. Aging Neurosci. 5:81. doi: 10.3389/fnagi.2013.00081
Bang, O. Y., Kim, E. H., Cha, J. M., and Moon, G. J. (2016). Adult stem cell therapy for stroke: challenges and progress. J. Stroke 18, 256–266. doi: 10.5853/jos.2016.01263
Beers, D. R., Henkel, J. S., Zhao, W., Wang, J., Huang, A., Wen, S., et al. (2011). Endogenous regulatory T lymphocytes ameliorate amyotrophic lateral sclerosis in mice and correlate with disease progression in patients with amyotrophic lateral sclerosis. Brain 134, 1293–1314. doi: 10.1093/brain/awr074
Bi, F., Huang, C., Tong, J., Qiu, G., Huang, B., Wu, Q., et al. (2013). Reactive astrocytes secrete lcn2 to promote neuron death. Proc. Natl. Acad. Sci. U S A 110, 4069–4074. doi: 10.1073/pnas.1218497110
Bonnet, N. (2017). Bone-derived factors: a new gateway to regulate glycemia. Calcif. Tissue Int. 100, 174–183. doi: 10.1007/s00223-016-0210-y
Borger, V., Bremer, M., Ferrer-Tur, R., Gockeln, L., Stambouli, O., Becic, A., et al. (2017). Mesenchymal stem/stromal cell-derived extracellular vesicles and their potential as novel immunomodulatory therapeutic agents. Int. J. Mol. Sci. 18:1450. doi: 10.3390/ijms18071450
Brazelton, T. R., Rossi, F. M., Keshet, G. I., and Blau, H. M. (2000). From marrow to brain: expression of neuronal phenotypes in adult mice. Science 290, 1775–1779. doi: 10.1126/science.290.5497.1775
Breitbach, M., Bostani, T., Roell, W., Xia, Y., Dewald, O., Nygren, J. M., et al. (2007). Potential risks of bone marrow cell transplantation into infarcted hearts. Blood 110, 1362–1369. doi: 10.1182/blood-2006-12-063412
Brochard, V., Combadiere, B., Prigent, A., Laouar, Y., Perrin, A., Beray-Berthat, V., et al. (2009). Infiltration of CD4+ lymphocytes into the brain contributes to neurodegeneration in a mouse model of Parkinson disease. J. Clin. Invest. 119, 182–192. doi: 10.1172/JCI36470
Browne, T. C., McQuillan, K., McManus, R. M., O’Reilly, J. A., Mills, K. H., and Lynch, M. A. (2013). IFN-γ Production by amyloid β-specific Th1 cells promotes microglial activation and increases plaque burden in a mouse model of Alzheimer’s disease. J. Immunol. 190, 2241–2251. doi: 10.4049/jimmunol.1200947
Cardoso, A. L., Fernandes, A., Aguilar-Pimentel, J. A., de Angelis, M. H., Guedes, J. R., Brito, M. A., et al. (2018). Towards frailty biomarkers: candidates from genes and pathways regulated in aging and age-related diseases. Ageing Res. Rev. 47, 214–277. doi: 10.1016/j.arr.2018.07.004
Carecchio, M., and Comi, C. (2011). The role of osteopontin in neurodegenerative diseases. J. Alzheimers Dis. 25, 179–185. doi: 10.3233/JAD-2011-102151
Caricasole, A., Copani, A., Caraci, F., Aronica, E., Rozemuller, A. J., Caruso, A., et al. (2004). Induction of Dickkopf-1, a negative modulator of the Wnt pathway, is associated with neuronal degeneration in Alzheimer’s brain. J. Neurosci. 24, 6021–6027. doi: 10.1523/JNEUROSCI.1381-04.2004
Castellano, J. M., Kirby, E. D., and Wyss-Coray, T. (2015). Blood-borne revitalization of the aged brain. JAMA Neurol. 72, 1191–1194. doi: 10.1001/jamaneurol.2015.1616
Chabas, D., Baranzini, S. E., Mitchell, D., Bernard, C. C., Rittling, S. R., Denhardt, D. T., et al. (2001). The influence of the proinflammatory cytokine, osteopontin, on autoimmune demyelinating disease. Science 294, 1731–1735. doi: 10.1126/science.1062960
Chakraborty, S., Kaur, S., Guha, S., and Batra, S. K. (2012). The multifaceted roles of neutrophil gelatinase associated lipocalin (NGAL) in inflammation and cancer. Biochim. Biophys. Acta 1826, 129–169. doi: 10.1016/j.bbcan.2012.03.008
Chen, J.-H., Ke, K.-F., Lu, J.-H., Qiu, Y.-H., and Peng, Y.-P. (2015). Protection of TGF-β1 against neuroinflammation and neurodegeneration in Aβ1–42-induced Alzheimer’s disease model rats. PLoS One 10:e0116549. doi: 10.1371/journal.pone.0116549
Cheng, X., Zhang, G., Zhang, L., Hu, Y., Zhang, K., Sun, X., et al. (2018). Mesenchymal stem cells deliver exogenous miR-21 via exosomes to inhibit nucleus pulposus cell apoptosis and reduce intervertebral disc degeneration. J. Cell. Mol. Med. 22, 261–276. doi: 10.1111/jcmm.13316
Cho, E.-H., Cho, K.-H., Lee, H. A., and Kim, S.-W. (2013). High serum osteopontin levels are associated with low bone mineral density in postmenopausal women. J. Korean Med. Sci. 28, 1496–1499. doi: 10.3346/jkms.2013.28.10.1496
Compston, J. E. (2002). Bone marrow and bone: a functional unit. J. Endocrinol. 173, 387–394. doi: 10.1677/joe.0.1730387
Compston, A., and Coles, A. (2008). Multiple sclerosis. Lancet 372, 1502–1517. doi: 10.1016/S0140-6736(08)61620-7
Das, M. M., Godoy, M., Chen, S., Moser, V. A., Avalos, P., Roxas, K. M., et al. (2019). Young bone marrow transplantation preserves learning and memory in old mice. Commun. Biol. 2:73. doi: 10.1038/s42003-019-0298-5
Dauer, W., and Przedborski, S. (2003). Parkinson’s disease: mechanisms and models. Neuron 39, 889–909. doi: 10.1016/s0896-6273(03)00568-3
Dean, C., Liu, H., Dunning, F. M., Chang, P. Y., Jackson, M. B., and Chapman, E. R. (2009). Synaptotagmin-IV modulates synaptic function and long-term potentiation by regulating BDNF release. Nat. Neurosci. 12, 767–776. doi: 10.1038/nn.2315
Dennie, D., Louboutin, J. P., and Strayer, D. S. (2016). Migration of bone marrow progenitor cells in the adult brain of rats and rabbits. World J. Stem Cells 8, 136–157. doi: 10.4252/wjsc.v8.i4.136
Djukic, M., Mildner, A., Schmidt, H., Czesnik, D., Bruck, W., Priller, J., et al. (2006). Circulating monocytes engraft in the brain, differentiate into microglia and contribute to the pathology following meningitis in mice. Brain 129, 2394–2403. doi: 10.1093/brain/awl206
Ducy, P. (2011). The role of osteocalcin in the endocrine cross-talk between bone remodelling and energy metabolism. Diabetologia 54, 1291–1297. doi: 10.1007/s00125-011-2155-z
Ducy, P., Desbois, C., Boyce, B., Pinero, G., Story, B., Dunstan, C., et al. (1996). Increased bone formation in osteocalcin-deficient mice. Nature 382, 448–452. doi: 10.1038/382448a0
Dugger, B. N., and Dickson, D. W. (2017). Pathology of neurodegenerative diseases. Cold Spring Harb. Perspect. Biol. 9:a028035. doi: 10.1101/cshperspect.a028035
El Khoury, J., and Luster, A. D. (2008). Mechanisms of microglia accumulation in Alzheimer’s disease: therapeutic implications. Trends Pharmacol. Sci. 29, 626–632. doi: 10.1016/j.tips.2008.08.004
Emanuele, E., Peros, E., Scioli, G. A., D’Angelo, A., Olivieri, C., Montagna, L., et al. (2004). Plasma osteoprotegerin as a biochemical marker for vascular dementia and Alzheimer’s disease. Int. J. Mol. Med. 13, 849–853. doi: 10.3892/ijmm.13.6.849
Fatemi, I., Delrobaee, F., Bahmani, M., Shamsizadeh, A., and Allahtavakoli, M. (2019). The effect of the anti-diabetic drug metformin on behavioral manifestations associated with ovariectomy in mice. Neurosci. Lett. 690, 95–98. doi: 10.1016/j.neulet.2018.10.024
Fisher, Y., Strominger, I., Biton, S., Nemirovsky, A., Baron, R., and Monsonego, A. (2014). Th1 polarization of T cells injected into the cerebrospinal fluid induces brain immunosurveillance. J. Immunol. 192, 92–102. doi: 10.4049/jimmunol.1301707
Fortress, A. M., and Frick, K. M. (2016). Hippocampal Wnt signaling: memory regulation and hormone interactions. Neuroscientist 22, 278–294. doi: 10.1177/1073858415574728
Freitas, G. R. R., da Luz Fernandes, M., Agena, F., Jaluul, O., Silva, S. C., Lemos, F. B. C., et al. (2019). Aging and end stage renal disease cause a decrease in absolute circulating lymphocyte counts with a shift to a memory profile and diverge in treg population. Aging Dis. 10, 49–61. doi: 10.14336/AD.2018.0318
Fritzsching, B., Haas, J., Konig, F., Kunz, P., Fritzsching, E., Poschl, J., et al. (2011). Intracerebral human regulatory T cells: analysis of CD4+ CD25+ FOXP3+ T cells in brain lesions and cerebrospinal fluid of multiple sclerosis patients. PLoS One 6:e17988. doi: 10.1371/journal.pone.0017988
Gallina, C., Turinetto, V., and Giachino, C. (2015). A new paradigm in cardiac regeneration: the mesenchymal stem cell secretome. Stem Cells Int. 2015:765846. doi: 10.1155/2015/765846
Garcia, K. O., Ornellas, F. L., Martin, P. K., Patti, C. L., Mello, L. E., Frussa-Filho, R., et al. (2014). Therapeutic effects of the transplantation of VEGF overexpressing bone marrow mesenchymal stem cells in the hippocampus of murine model of Alzheimer’s disease. Front. Aging Neurosci. 6:30. doi: 10.3389/fnagi.2014.00030
Garza-Lombó, C., Schroder, A., Reyes-Reyes, E. M., and Franco, R. (2018). mTOR/AMPK signaling in the brain: cell metabolism, proteostasis and survival. Curr. Opin. Toxicol. 8, 102–110. doi: 10.1016/j.cotox.2018.05.002
Geiger, H., de Haan, G., and Florian, M. C. (2013). The ageing haematopoietic stem cell compartment. Nat. Rev. Immunol. 13, 376–389. doi: 10.1038/nri3433
Gifre, L., Ruiz-Gaspà, S., Monegal, A., Nomdedeu, B., Filella, X., Guañabens, N., et al. (2013). Effect of glucocorticoid treatment on Wnt signalling antagonists (sclerostin and Dkk-1) and their relationship with bone turnover. Bone 57, 272–276. doi: 10.1016/j.bone.2013.08.016
Goldman, J. E., Yen, S. H., Chiu, F. C., and Peress, N. S. (1983). Lewy bodies of Parkinson’s disease contain neurofilament antigens. Science 221, 1082–1084. doi: 10.1126/science.6308771
Goverman, J. (2009). Autoimmune T cell responses in the central nervous system. Nat. Rev. Immunol. 9, 393–407. doi: 10.1038/nri2550
Goverman, J., Perchellet, A., and Huseby, E. S. (2005). The role of CD8(+) T cells in multiple sclerosis and its animal models. Curr. Drug Targets Inflamm. Allergy 4, 239–245. doi: 10.2174/1568010053586264
Guo, X.-Z., Shan, C., Hou, Y.-F., Zhu, G., Tao, B., Sun, L. H., et al. (2018). Osteocalcin ameliorates motor dysfunction in a 6-hydroxydopamine-induced Parkinson’s disease rat model through AKT/GSK3β signaling. Front. Mol. Neurosci. 11:343. doi: 10.3389/fnmol.2018.00343
Hall, J., Thomas, K. L., and Everitt, B. J. (2000). Rapid and selective induction of BDNF expression in the hippocampus during contextual learning. Nat. Neurosci. 3, 533–535. doi: 10.1038/75698
Han, K. H., Arlian, B. M., Macauley, M. S., Paulson, J. C., and Lerner, R. A. (2018). Migration-based selections of antibodies that convert bone marrow into trafficking microglia-like cells that reduce brain amyloid β. Proc. Natl. Acad. Sci. U S A 115, E372–E381. doi: 10.1073/pnas.1719259115
Han, Y., You, X., Xing, W., Zhang, Z., and Zou, W. (2018). Paracrine and endocrine actions of bone-the functions of secretory proteins from osteoblasts, osteocytes, and osteoclasts. Bone Res. 6:16. doi: 10.1038/s41413-018-0019-6
Harach, T., Jammes, F., Muller, C., Duthilleul, N., Cheatham, V., Zufferey, V., et al. (2017). Administrations of human adult ischemia-tolerant mesenchymal stem cells and factors reduce amyloid β pathology in a mouse model of Alzheimer’s disease. Neurobiol. Aging 51, 83–96. doi: 10.1016/j.neurobiolaging.2016.11.009
Harty, J. T., Tvinnereim, A. R., and White, D. W. (2000). CD8+ T cell effector mechanisms in resistance to infection. Annu. Rev. Immunol. 18, 275–308. doi: 10.1146/annurev.immunol.18.1.275
Hauschka, P. V., Lian, J. B., and Gallop, P. M. (1975). Direct identification of the calcium-binding amino acid, γ-carboxyglutamate, in mineralized tissue. Proc. Natl. Acad. Sci. U S A 72, 3925–3929. doi: 10.1073/pnas.72.10.3925
He, B., Yao, Q., Liang, Z., Lin, J., Xie, Y., Li, S., et al. (2016). The dose of intravenously transplanted bone marrow stromal cells determines the therapeutic effect on vascular remodeling in a rat model of ischemic stroke. Cell Transplant. 25, 2173–2185. doi: 10.3727/096368916X692627
Hemmings, B. A., and Restuccia, D. F. (2012). PI3K-PKB/Akt pathway. Cold Spring Harb. Perspect. Biol. 4:a011189. doi: 10.1101/cshperspect.a011189
Hofbauer, L. C., Cepok, S., and Hemmer, B. (2004). Osteoprotegerin is highly expressed in the spinal cord and cerebrospinal fluid. Acta Neuropathol. 107, 575–577, author reply 578. doi: 10.1007/s00401-004-0854-y
Huang, X., Jiang, Y., and Xia, W. (2013). FGF23 and phosphate wasting disorders. Bone Res. 1, 120–132. doi: 10.4248/BR201302002
Huang, Y., Liu, L., and Liu, A. (2018). Dickkopf-1: current knowledge and related diseases. Life Sci. 209, 249–254. doi: 10.1016/j.lfs.2018.08.019
Hur, E. M., Youssef, S., Haws, M. E., Zhang, S. Y., Sobel, R. A., and Steinman, L. (2007). Osteopontin-induced relapse and progression of autoimmune brain disease through enhanced survival of activated T cells. Nat. Immunol. 8, 74–83. doi: 10.1038/ni1415
Huseby, E. S., Liggitt, D., Brabb, T., Schnabel, B., Ohlen, C., and Goverman, J. (2001). A pathogenic role for myelin-specific CD8(+) T cells in a model for multiple sclerosis. J. Exp. Med. 194, 669–676. doi: 10.1084/jem.194.5.669
Hwang, S. M., Wilson, P. D., Laskin, J. D., and Denhardt, D. T. (1994). Age and development-related changes in osteopontin and nitric oxide synthase mRNA levels in human kidney proximal tubule epithelial cells: contrasting responses to hypoxia and reoxygenation. J. Cell. Physiol. 160, 61–68. doi: 10.1002/jcp.1041600108
Iczkiewicz, J., Broom, L., Cooper, J. D., Wong, A. M., Rose, S., and Jenner, P. (2010). The RGD-containing peptide fragment of osteopontin protects tyrosine hydroxylase positive cells against toxic insult in primary ventral mesencephalic cultures and in the rat substantia nigra. J. Neurochem. 114, 1792–1804. doi: 10.1111/j.1471-4159.2010.06896.x
Iczkiewicz, J., Rose, S., and Jenner, P. (2004). Osteopontin (Eta-1) is present in the rat basal ganglia. Mol. Brain Res. 132, 64–72. doi: 10.1016/j.molbrainres.2004.09.013
Inestrosa, N. C., and Varela-Nallar, L. (2014). Wnt signaling in the nervous system and in Alzheimer’s disease. J. Mol. Cell Biol. 6, 64–74. doi: 10.1093/jmcb/mjt051
Ip, J. P. K., Nocon, A. L., Hofer, M. J., Lim, S. L., Muller, M., and Campbell, I. L. (2011). Lipocalin 2 in the central nervous system host response to systemic lipopolysaccharide administration. J. Neuroinflammation 8:124. doi: 10.1186/1742-2094-8-124
Iyer, A., Zurolo, E., Prabowo, A., Fluiter, K., Spliet, W. G., van Rijen, P. C., et al. (2012). MicroRNA-146a: a key regulator of astrocyte-mediated inflammatory response. PLoS One 7:e44789. doi: 10.1371/journal.pone.0044789
Jamshidian, A., Shaygannejad, V., Pourazar, A., Zarkesh-Esfahani, S. H., and Gharagozloo, M. (2013). Biased Treg/Th17 balance away from regulatory toward inflammatory phenotype in relapsed multiple sclerosis and its correlation with severity of symptoms. J. Neuroimmunol. 262, 106–112. doi: 10.1016/j.jneuroim.2013.06.007
Jansson, M., Panoutsakopoulou, V., Baker, J., Klein, L., and Cantor, H. (2002). Cutting edge: attenuated experimental autoimmune encephalomyelitis in eta-1/osteopontin-deficient mice. J. Immunol. 168, 2096–2099. doi: 10.4049/jimmunol.168.5.2096
Jha, N. K., Jha, S. K., Kumar, D., Kejriwal, N., Sharma, R., Ambasta, R. K., et al. (2015). Impact of insulin degrading enzyme and neprilysin in Alzheimer’s disease biology: characterization of putative cognates for therapeutic applications. J. Alzheimers Dis. 48, 891–917. doi: 10.3233/JAD-150379
Joshi, P., Turola, E., Ruiz, A., Bergami, A., Libera, D. D., Benussi, L., et al. (2014). Microglia convert aggregated amyloid-β into neurotoxic forms through the shedding of microvesicles. Cell Death Differ. 21, 582–593. doi: 10.1038/cdd.2013.180
Kanamaru, T., Kamimura, N., Yokota, T., Nishimaki, K., Iuchi, K., Lee, H., et al. (2015). Intravenous transplantation of bone marrow-derived mononuclear cells prevents memory impairment in transgenic mouse models of Alzheimer’s disease. Brain Res. 1605, 49–58. doi: 10.1016/j.brainres.2015.02.011
Kang, H. G., Park, H. Y., Ryu, H. U., and Suk, S. H. (2018). Bone mineral loss and cognitive impairment: the PRESENT project. Medicine 97:e12755. doi: 10.1097/MD.0000000000012755
Karsenty, G., and Ferron, M. (2012). The contribution of bone to whole-organism physiology. Nature 481, 314–320. doi: 10.1038/nature10763
Karsenty, G., and Olson, E. N. (2016). Bone and muscle endocrine functions: unexpected paradigms of inter-organ communication. Cell 164, 1248–1256. doi: 10.1016/j.cell.2016.02.043
Katila, N., Bhurtel, S., Shadfar, S., Srivastav, S., Neupane, S., Ojha, U., et al. (2017). Metformin lowers α-synuclein phosphorylation and upregulates neurotrophic factor in the MPTP mouse model of Parkinson’s disease. Neuropharmacology 125, 396–407. doi: 10.1016/j.neuropharm.2017.08.015
Kawanishi, S., Takata, K., Itezono, S., Nagayama, H., Konoya, S., Chisaki, Y., et al. (2018). Bone-marrow-derived microglia-like cells ameliorate brain amyloid pathology and cognitive impairment in a mouse model of Alzheimer’s disease. J. Alzheimers Dis. 64, 563–585. doi: 10.3233/JAD-170994
Ke, H. Z., Richards, W. G., Li, X., and Ominsky, M. S. (2012). Sclerostin and Dickkopf-1 as therapeutic targets in bone diseases. Endocr. Rev. 33, 747–783. doi: 10.1210/er.2011-1060
Kebir, H., Kreymborg, K., Ifergan, I., Dodelet-Devillers, A., Cayrol, R., Bernard, M., et al. (2007). Human TH17 lymphocytes promote blood-brain barrier disruption and central nervous system inflammation. Nat. Med. 13, 1173–1175. doi: 10.1038/nm1651
Khan, S. A., Lopez-Chua, C. A., Zhang, J., Fisher, L. W., Sorensen, E. S., and Denhardt, D. T. (2002). Soluble osteopontin inhibits apoptosis of adherent endothelial cells deprived of growth factors. J. Cell Biochem. 85, 728–736. doi: 10.1002/jcb.10170
Khrimian, L., Obri, A., Ramos-Brossier, M., Rousseaud, A., Moriceau, S., Nicot, A. S., et al. (2017). Gpr158 mediates osteocalcin’s regulation of cognition. J. Exp. Med. 214, 2859–2873. doi: 10.1084/jem.20171320
Kim, B.-W., Jeong, K. H., Kim, J.-H., Jin, M., Kim, J.-H., Lee, M.-G., et al. (2016). Pathogenic upregulation of glial lipocalin-2 in the Parkinsonian dopaminergic System. J. Neurosci. 36, 5608–5622. doi: 10.1523/JNEUROSCI.4261-15.2016
Kim, J.-H., Ko, P.-W., Lee, H.-W., Jeong, J.-Y., Lee, M.-G., Kim, J.-H., et al. (2017). Astrocyte-derived lipocalin-2 mediates hippocampal damage and cognitive deficits in experimental models of vascular dementia. Glia 65, 1471–1490. doi: 10.1002/glia.23174
Kobilo, T., Guerrieri, D., Zhang, Y., Collica, S. C., Becker, K. G., and van Praag, H. (2014). AMPK agonist AICAR improves cognition and motor coordination in young and aged mice. Learn. Mem. 21, 119–126. doi: 10.1101/lm.033332.113
Koduah, P., Paul, F., and Dorr, J.-M. (2017). Vitamin D in the prevention, prediction and treatment of neurodegenerative and neuroinflammatory diseases. EPMA J. 8, 313–325. doi: 10.1007/s13167-017-0120-8
Kokovay, E., and Cunningham, L. A. (2005). Bone marrow-derived microglia contribute to the neuroinflammatory response and express iNOS in the MPTP mouse model of Parkinson’s disease. Neurobiol. Dis. 19, 471–478. doi: 10.1016/j.nbd.2005.01.023
Koronyo, Y., Salumbides, B. C., Sheyn, J., Pelissier, L., Li, S., Ljubimov, V., et al. (2015). Therapeutic effects of glatiramer acetate and grafted CD115(+) monocytes in a mouse model of Alzheimer’s disease. Brain 138, 2399–2422. doi: 10.1093/brain/awv150
Kubota, K., Nakano, M., Kobayashi, E., Mizue, Y., Chikenji, T., Otani, M., et al. (2018). An enriched environment prevents diabetes-induced cognitive impairment in rats by enhancing exosomal miR-146a secretion from endogenous bone marrow-derived mesenchymal stem cells. PLoS One 13:e0204252. doi: 10.1371/journal.pone.0204252
Kunert, S. K., Hartmann, H., Haffner, D., and Leifheit-Nestler, M. (2017). Klotho and fibroblast growth factor 23 in cerebrospinal fluid in children. J. Bone Miner. Metab. 35, 215–226. doi: 10.1007/s00774-016-0746-y
Kuroda, E., Nishimura, K., Kawanishi, S., Sueyoshi, M., Ueno, F., Toji, Y., et al. (2020a). Mouse bone marrow-derived microglia-like cells secrete transforming growth factor-β1 and promote microglial aβ phagocytosis and reduction of brain aβ. Neuroscience 438, 217–228. doi: 10.1016/j.neuroscience.2020.05.004
Kuroda, E., Takata, K., Nishimura, K., Oka, H., Sueyoshi, M., Aitani, M., et al. (2020b). Peripheral blood-derived microglia-like cells decrease amyloid-β burden and ameliorate cognitive impairment in a mouse model of Alzheimer’s disease. J. Alzheimers Dis. 73, 413–429. doi: 10.3233/JAD-190974
Lampron, A., Gosselin, D., and Rivest, S. (2011). Targeting the hematopoietic system for the treatment of Alzheimer’s disease. Brain Behav. Immun. 25, S71–79. doi: 10.1016/j.bbi.2010.12.018
Laszczyk, A. M., Nettles, D., Pollock, T. A., Fox, S., Garcia, M. L., Wang, J., et al. (2019). FGF-23 deficiency impairs hippocampal-dependent cognitive function. eNeuro 6:ENEURO.0469-18.2019. doi: 10.1523/ENEURO.0469-18.2019
Lebson, L., Nash, K., Kamath, S., Herber, D., Carty, N., Lee, D. C., et al. (2010). Trafficking CD11b-positive blood cells deliver therapeutic genes to the brain of amyloid-depositing transgenic mice. J. Neurosci. 30, 9651–9658. doi: 10.1523/JNEUROSCI.0329-10.2010
Lee, J. S., Hong, J. M., Moon, G. J., Lee, P. H., Ahn, Y. H., Bang, O. Y., et al. (2010). A long-term follow-up study of intravenous autologous mesenchymal stem cell transplantation in patients with ischemic stroke. Stem Cells 28, 1099–1106. doi: 10.1002/stem.430
Lee, J. K., Jin, H. K., and Bae, J.-S. (2009). Bone marrow-derived mesenchymal stem cells reduce brain amyloid-β deposition and accelerate the activation of microglia in an acutely induced Alzheimer’s disease mouse model. Neurosci. Lett. 450, 136–141. doi: 10.1016/j.neulet.2008.11.059
Lee, S., Lee, W.-H., Lee, M.-S., Mori, K., and Suk, K. (2012). Regulation by lipocalin-2 of neuronal cell death, migration, and morphology. J. Neurosci. Res. 90, 540–550. doi: 10.1002/jnr.22779
Lee, J. K., Schuchman, E. H., Jin, H. K., and Bae, J. S. (2012). Soluble CCL5 derived from bone marrow-derived mesenchymal stem cells and activated by amyloid β ameliorates Alzheimer’s disease in mice by recruiting bone marrow-induced microglia immune responses. Stem Cells 30, 1544–1555. doi: 10.1002/stem.1125
Le Goff, B., Bouvard, B., Lequerre, T., Lespessailles, E., Marotte, H., Pers, Y. M., et al. (2019). Implication of IL-17 in bone loss and structural damage in inflammatory rheumatic diseases. Mediators Inflamm. 2019:8659302. doi: 10.1155/2019/8659302
Lei, Q., Liu, T., Gao, F., Xie, H., Sun, L., Zhao, A., et al. (2017). Microvesicles as potential biomarkers for the identification of senescence in human mesenchymal stem cells. Theranostics 7, 2673–2689. doi: 10.7150/thno.18915
Levy, E. (2017). Exosomes in the diseased brain: first insights from in vivo studies. Front. Neurosci. 11:142. doi: 10.3389/fnins.2017.00142
Li, Y., Yang, Y.-Y., Ren, J.-L., Xu, F., Chen, F.-M., and Li, A. (2017). Exosomes secreted by stem cells from human exfoliated deciduous teeth contribute to functional recovery after traumatic brain injury by shifting microglia M1/M2 polarization in rats. Stem Cell Res. Ther. 8:198. doi: 10.1186/s13287-017-0648-5
Li, Z., Ye, H., Cai, X., Sun, W., He, B., Yang, Z., et al. (2019). Bone marrow-mesenchymal stem cells modulate microglial activation in the peri-infarct area in rats during the acute phase of stroke. Brain Res. Bull. 153, 324–333. doi: 10.1016/j.brainresbull.2019.10.001
Liang, Z., Zhao, Y., Ruan, L., Zhu, L., Jin, K., Zhuge, Q., et al. (2017). Impact of aging immune system on neurodegeneration and potential immunotherapies. Prog. Neurobiol. 157, 2–28. doi: 10.1016/j.pneurobio.2017.07.006
Lin, Y. H., and Yang-Yen, H. F. (2001). The osteopontin-CD44 survival signal involves activation of the phosphatidylinositol 3-kinase/Akt signaling pathway. J. Biol. Chem. 276, 46024–46030. doi: 10.1074/jbc.M105132200
Liu, P., Chen, L., Bai, X., Karaplis, A., Miao, D., and Gu, N. (2011). Impairment of spatial learning and memory in transgenic mice overexpressing human fibroblast growth factor-23. Brain Res. 1412, 9–17. doi: 10.1016/j.brainres.2011.07.028
Liu, Q., Xu, X., Yang, Z., Liu, Y., Wu, X., Huang, Z., et al. (2019). Metformin alleviates the bone loss induced by ketogenic diet: an in vivo study in mice. Calcif. Tissue Int. 104, 59–69. doi: 10.1007/s00223-018-0468-3
Livingston, G., Sommerlad, A., Orgeta, V., Costafreda, S. G., Huntley, J., Ames, D., et al. (2017). Dementia prevention, intervention, and care. Lancet 390, 2673–2734. doi: 10.1016/S0140-6736(17)31363-6
Lueg, G., Gross, C. C., Lohmann, H., Johnen, A., Kemmling, A., Deppe, M., et al. (2015). Clinical relevance of specific T-cell activation in the blood and cerebrospinal fluid of patients with mild Alzheimer’s disease. Neurobiol. Aging 36, 81–89. doi: 10.1016/j.neurobiolaging.2014.08.008
Lund, S. A., Giachelli, C. M., and Scatena, M. (2009). The role of osteopontin in inflammatory processes. J. Cell Commun. Signal. 3, 311–322. doi: 10.1007/s12079-009-0068-0
Lv, L., Tan, X., Peng, X., Bai, R., Xiao, Q., Zou, T., et al. (2020). The relationships of vitamin D, vitamin D receptor gene polymorphisms and vitamin D supplementation with Parkinson’s disease. Transl. Neurodegener. 9:34. doi: 10.1186/s40035-020-00213-2
Maetzler, W., Berg, D., Schalamberidze, N., Melms, A., Schott, K., Mueller, J. C., et al. (2007). Osteopontin is elevated in Parkinson’s disease and its absence leads to reduced neurodegeneration in the MPTP model. Neurobiol. Dis. 25, 473–482. doi: 10.1016/j.nbd.2006.10.020
Maiti, P., Manna, J., and Dunbar, G. L. (2017). Current understanding of the molecular mechanisms in Parkinson’s disease: targets for potential treatments. Transl. Neurodegener. 6:28. doi: 10.1186/s40035-017-0099-z
Malm, T., Loppi, S., and Kanninen, K. M. (2016). Exosomes in Alzheimer’s disease. Neurochem. Int. 97, 193–199. doi: 10.1016/j.neuint.2016.04.011
Marebwa, B. K., Adams, R. J., Magwood, G. S., Kindy, M., Wilmskoetter, J., Wolf, M., et al. (2018). Fibroblast growth factor23 is associated with axonal integrity and neural network architecture in the human frontal lobes. PLoS One 13:e0203460. doi: 10.1371/journal.pone.0203460
Maryanovich, M., Takeishi, S., and Frenette, P. S. (2018). Neural regulation of bone and bone marrow. Cold Spring Harb. Perspect. Med. 8:a031344. doi: 10.1101/cshperspect.a031344
Marzo, A., Galli, S., Lopes, D., McLeod, F., Podpolny, M., Segovia-Roldan, M., et al. (2016). Reversal of synapse degeneration by restoring wnt signaling in the adult hippocampus. Curr. Biol. 26, 2551–2561. doi: 10.1016/j.cub.2016.07.024
Matrisciano, F., Busceti, C. L., Bucci, D., Orlando, R., Caruso, A., Molinaro, G., et al. (2011). Induction of the Wnt antagonist Dickkopf-1 is involved in stress-induced hippocampal damage. PLoS One 6:e16447. doi: 10.1371/journal.pone.0016447
McGrath, E. R., Himali, J. J., Levy, D., Conner, S. C., Pase, M. P., Abraham, C. R., et al. (2019). Circulating fibroblast growth factor 23 levels and incident dementia: the Framingham heart study. PLoS One 14:e0213321. doi: 10.1371/journal.pone.0213321
Mera, P., Laue, K., Ferron, M., Confavreux, C., Wei, J., Galan-Diez, M., et al. (2016). Osteocalcin signaling in myofibers is necessary and sufficient for optimum adaptation to exercise. Cell Metab. 23, 1078–1092. doi: 10.1016/j.cmet.2016.05.004
Mesquita, S. D., Ferreira, A. C., Falcao, A. M., Sousa, J. C., Oliveira, T. G., Correia-Neves, M., et al. (2014). Lipocalin 2 modulates the cellular response to amyloid β. Cell Death Differ. 21, 1588–1599. doi: 10.1038/cdd.2014.68
Mezey, E., and Chandross, K. J. (2000). Bone marrow: a possible alternative source of cells in the adult nervous system. Eur. J. Pharmacol. 405, 297–302. doi: 10.1016/s0014-2999(00)00561-6
Mezey, E., Chandross, K. J., Harta, G., Maki, R. A., and McKercher, S. R. (2000). Turning blood into brain: cells bearing neuronal antigens generated in vivo from bone marrow. Science 290, 1779–1782. doi: 10.1126/science.290.5497.1779
Mildner, A., Schmidt, H., Nitsche, M., Merkler, D., Hanisch, U. K., Mack, M., et al. (2007). Microglia in the adult brain arise from Ly-6ChiCCR2+ monocytes only under defined host conditions. Nat. Neurosci. 10, 1544–1553. doi: 10.1038/nn2015
Mizokami, A., Kawakubo-Yasukochi, T., and Hirata, M. (2017). Osteocalcin and its endocrine functions. Biochem. Pharmacol. 132, 1–8. doi: 10.1016/j.bcp.2017.02.001
Monroe, D. G., McGee-Lawrence, M. E., Oursler, M. J., and Westendorf, J. J. (2012). Update on Wnt signaling in bone cell biology and bone disease. Gene 492, 1–18. doi: 10.1016/j.gene.2011.10.044
Morishita, K., Shirai, A., Kubota, M., Katakura, Y., Nabeshima, Y., Takeshige, K., et al. (2001). The progression of aging in klotho mutant mice can be modified by dietary phosphorus and zinc. J. Nutr. 131, 3182–3188. doi: 10.1093/jn/131.12.3182
Mosialou, I., Shikhel, S., Liu, J.-M., Maurizi, A., Luo, N., He, Z., et al. (2017). MC4R-dependent suppression of appetite by bone-derived lipocalin 2. Nature 543, 385–390. doi: 10.1038/nature21697
Mourya, A., Akhtar, A., Ahuja, S., Sah, S. P., and Kumar, A. (2018). Synergistic action of ursolic acid and metformin in experimental model of insulin resistance and related behavioral alterations. Eur. J. Pharmacol. 835, 31–40. doi: 10.1016/j.ejphar.2018.07.056
Naaldijk, Y., Jäger, C., Fabian, C., Leovsky, C., Bluher, A., Rudolph, L., et al. (2017). Effect of systemic transplantation of bone marrow-derived mesenchymal stem cells on neuropathology markers in APP/PS1 Alzheimer mice. Neuropathol. Appl. Neurobiol. 43, 299–314. doi: 10.1111/nan.12319
Nakano, M., Nagaishi, K., Konari, N., Saito, Y., Chikenji, T., Mizue, Y., et al. (2016). Bone marrow-derived mesenchymal stem cells improve diabetes-induced cognitive impairment by exosome transfer into damaged neurons and astrocytes. Sci. Rep. 6:24805. doi: 10.1038/srep24805
Nakazawa, K., Quirk, M. C., Chitwood, R. A., Watanabe, M., Yeckel, M. F., Sun, L. D., et al. (2002). Requirement for hippocampal CA3 NMDA receptors in associative memory recall. Science 297, 211–218. doi: 10.1126/science.1071795
Naudé, P. J., Nyakas, C., Eiden, L. E., Ait-Ali, D., van der Heide, R., Engelborghs, S., et al. (2012). Lipocalin 2: novel component of proinflammatory signaling in Alzheimer’s disease. FASEB J. 26, 2811–2823. doi: 10.1096/fj.11-202457
Obri, A., Khrimian, L., Karsenty, G., and Oury, F. (2018). Osteocalcin in the brain: from embryonic development to age-related decline in cognition. Nat. Rev. Endocrinol. 14, 174–182. doi: 10.1038/nrendo.2017.181
Ohsawa, I., Nishimaki, K., Murakami, Y., Suzuki, Y., Ishikawa, M., and Ohta, S. (2008). Age-dependent neurodegeneration accompanying memory loss in transgenic mice defective in mitochondrial aldehyde dehydrogenase 2 activity. J. Neurosci. 28, 6239–6249. doi: 10.1523/JNEUROSCI.4956-07.2008
Ortiz-Matamoros, A., and Arias, C. (2018). Chronic infusion of Wnt7a, Wnt5a and Dkk-1 in the adult hippocampus induces structural synaptic changes and modifies anxiety and memory performance. Brain Res. Bull. 139, 243–255. doi: 10.1016/j.brainresbull.2018.03.008
Oury, F., Khrimian, L., Denny, C. A., Gardin, A., Chamouni, A., Goeden, N., et al. (2013). Maternal and offspring pools of osteocalcin influence brain development and functions. Cell 155, 228–241. doi: 10.1016/j.cell.2013.08.042
Pellicano, M., Larbi, A., Goldeck, D., Colonna-Romano, G., Buffa, S., Bulati, M., et al. (2012). Immune profiling of Alzheimer patients. J. Neuroimmunol. 242, 52–59. doi: 10.1016/j.jneuroim.2011.11.005
Perry, E. K., Johnson, M., Ekonomou, A., Perry, R. H., Ballard, C., and Attems, J. (2012). Neurogenic abnormalities in Alzheimer’s disease differ between stages of neurogenesis and are partly related to cholinergic pathology. Neurobiol. Dis. 47, 155–162. doi: 10.1016/j.nbd.2012.03.033
Pinyopornpanish, K., Chattipakorn, N., and Chattipakorn, S. C. (2019). Lipocalin-2: its perspectives in brain pathology and possible roles in cognition. J. Neuroendocrinol. 31:e12779. doi: 10.1111/jne.12779
Poewe, W., Seppi, K., Tanner, C. M., Halliday, G. M., Brundin, P., Volkmann, J., et al. (2017). Parkinson disease. Nat. Rev. Dis. Primers 3:17013. doi: 10.1038/nrdp.2017.13
Poole, K. E., van Bezooijen, R. L., Loveridge, N., Hamersma, H., Papapoulos, S. E., Lowik, C. W., et al. (2005). Sclerostin is a delayed secreted product of osteocytes that inhibits bone formation. FASEB J. 19, 1842–1844. doi: 10.1096/fj.05-4221fje
Price, P. A., Otsuka, A. A., Poser, J. W., Kristaponis, J., and Raman, N. (1976). Characterization of a γ-carboxyglutamic acid-containing protein from bone. Proc. Natl. Acad. Sci. U S A 73, 1447–1451. doi: 10.1073/pnas.73.5.1447
Priller, J., Flügel, A., Wehner, T., Boentert, M., Haas, C. A., Prinz, M., et al. (2001a). Targeting gene-modified hematopoietic cells to the central nervous system: use of green fluorescent protein uncovers microglial engraftment. Nat. Med. 7, 1356–1361. doi: 10.1038/nm1201-1356
Priller, J., Persons, D. A., Klett, F. F., Kempermann, G., Kreutzberg, G. W., and Dirnagl, U. (2001b). Neogenesis of cerebellar Purkinje neurons from gene-marked bone marrow cells in vivo. J. Cell Biol. 155, 733–738. doi: 10.1083/jcb.200105103
Purro, S. A., Dickins, E. M., and Salinas, P. C. (2012). The secreted Wnt antagonist Dickkopf-1 is required for amyloid β-mediated synaptic loss. J. Neurosci. 32, 3492–3498. doi: 10.1523/JNEUROSCI.4562-11.2012
Purro, S. A., Galli, S., and Salinas, P. C. (2014). Dysfunction of Wnt signaling and synaptic disassembly in neurodegenerative diseases. J. Mol. Cell Biol. 6, 75–80. doi: 10.1093/jmcb/mjt049
Qin, C., Lu, Y., Wang, K., Bai, L., Shi, G., Huang, Y., et al. (2020). Transplantation of bone marrow mesenchymal stem cells improves cognitive deficits and alleviates neuropathology in animal models of Alzheimer’s disease: a meta-analytic review on potential mechanisms. Transl. Neurodegener. 9:20. doi: 10.1186/s40035-020-00199-x
Querfurth, H. W., and LaFerla, F. M. (2010). Alzheimer’s disease. N. Engl. J. Med. 362, 329–344. doi: 10.1056/NEJMra0909142
Rajendran, L., Honsho, M., Zahn, T. R., Keller, P., Geiger, K. D., Verkade, P., et al. (2006). Alzheimer’s disease β-amyloid peptides are released in association with exosomes. Proc. Natl. Acad. Sci. U S A 103, 11172–11177. doi: 10.1073/pnas.0603838103
Reinholt, F. P., Hultenby, K., Oldberg, A., and Heinegard, D. (1990). Osteopontin—a possible anchor of osteoclasts to bone. Proc. Natl. Acad. Sci. U S A 87, 4473–4475. doi: 10.1073/pnas.87.12.4473
Ren, C., Gu, X., Li, H., Lei, S., Wang, Z., Wang, J., et al. (2019). The role of DKK1 in Alzheimer’s disease: a potential intervention point of brain damage prevention? Pharmacol. Res. 144, 331–335. doi: 10.1016/j.phrs.2019.04.033
Rentsendorj, A., Sheyn, J., Fuchs, D. T., Daley, D., Salumbides, B. C., Schubloom, H. E., et al. (2018). A novel role for osteopontin in macrophage-mediated amyloid-β clearance in Alzheimer’s models. Brain Behav. Immun. 67, 163–180. doi: 10.1016/j.bbi.2017.08.019
Reza-Zaldivar, E. E., Hernandez-Sapiens, M. A., Minjarez, B., Gutierrez-Mercado, Y. K., Marquez-Aguirre, A. L., and Canales-Aguirre, A. A. (2018). Potential effects of MSC-derived exosomes in neuroplasticity in Alzheimer’s disease. Front. Cell. Neurosci. 12:317. doi: 10.3389/fncel.2018.00317
Rittling, S. R., and Singh, R. (2015). Osteopontin in Immune-mediated Diseases. J. Dent. Res. 94, 1638–1645. doi: 10.1177/0022034515605270
Rochette, L., Meloux, A., Rigal, E., Zeller, M., Malka, G., Cottin, Y., et al. (2019). The role of osteoprotegerin in vascular calcification and bone metabolism: the basis for developing new therapeutics. Calcif. Tissue Int. 105, 239–251. doi: 10.1007/s00223-019-00573-6
Romagnani, S. (2014). T cell subpopulations. Chem. Immunol. Allergy 100, 155–164. doi: 10.1159/000358622
Román, F., Urra, C., Porras, O., Pino, A. M., Rosen, C. J., and Rodriguez, J. P. (2017). Real-time H2 O2 measurements in bone marrow mesenchymal stem cells (MSCs) show increased antioxidant capacity in cells from osteoporotic women. J. Cell Biochem. 118, 585–593. doi: 10.1002/jcb.25739
Rosi, M. C., Luccarini, I., Grossi, C., Fiorentini, A., Spillantini, M. G., Prisco, A., et al. (2010). Increased Dickkopf-1 expression in transgenic mouse models of neurodegenerative disease. J. Neurochem. 112, 1539–1551. doi: 10.1111/j.1471-4159.2009.06566.x
Ross, R. D., Shah, R. C., Leurgans, S., Bottiglieri, T., Wilson, R. S., and Sumner, D. R. (2018). Circulating Dkk1 and TRAIL are associated with cognitive decline in community-dwelling, older adults with cognitive concerns. J. Gerontol. A Biol. Sci. Med. Sci. 73, 1688–1694. doi: 10.1093/gerona/glx252
Roy, A., Liu, X., and Pahan, K. (2007). Myelin basic protein-primed T cells induce neurotrophins in glial cells via αvβ3 [corrected] integrin. J. Biol. Chem. 282, 32222–32232. doi: 10.1074/jbc.M702899200
Roy, A., and Pahan, K. (2013). Myelin basic protein-primed T helper 2 cells suppress microglial activation via αVβ3 integrin: implications for multiple sclerosis. J. Clin. Cell. Immunol. 7:158. doi: 10.4172/2155-9899.1000158
Safar, M. M., Arab, H. H., Rizk, S. M., and El-Maraghy, S. A. (2016). Bone marrow-derived endothelial progenitor cells protect against scopolamine-induced alzheimer-like pathological aberrations. Mol. Neurobiol. 53, 1403–1418. doi: 10.1007/s12035-014-9051-8
Salem, A. M., Ahmed, H. H., Atta, H. M., Ghazy, M. A., and Aglan, H. A. (2014). Potential of bone marrow mesenchymal stem cells in management of Alzheimer’s disease in female rats. Cell Biol. Int. 38, 1367–1383. doi: 10.1002/cbin.10331
Salminen, A., Hyttinen, J. M., and Kaarniranta, K. (2011). AMP-activated protein kinase inhibits NF-kappaB signaling and inflammation: impact on healthspan and lifespan. J. Mol. Med. 89, 667–676. doi: 10.1007/s00109-011-0748-0
Saman, S., Kim, W., Raya, M., Visnick, Y., Miro, S., Saman, S., et al. (2012). Exosome-associated tau is secreted in tauopathy models and is selectively phosphorylated in cerebrospinal fluid in early Alzheimer disease. J. Biol. Chem. 287, 3842–3849. doi: 10.1074/jbc.M111.277061
Saman, S., Lee, N. C., Inoyo, I., Jin, J., Li, Z., Doyle, T., et al. (2014). Proteins recruited to exosomes by tau overexpression implicate novel cellular mechanisms linking tau secretion with Alzheimer’s disease. J. Alzheimers Dis. 40, S47–70. doi: 10.3233/JAD-132135
Sanchez-Guajardo, V., Barnum, C. J., Tansey, M. G., and Romero-Ramos, M. (2013). Neuroimmunological processes in Parkinson’s disease and their relation to α-synuclein: microglia as the referee between neuronal processes and peripheral immunity. ASN Neuro 5, 113–139. doi: 10.1042/AN20120066
Sanchez-Ramos, J., Song, S., Cardozo-Pelaez, F., Hazzi, C., Stedeford, T., Willing, A., et al. (2000). Adult bone marrow stromal cells differentiate into neural cells in vitro. Exp. Neurol. 164, 247–256. doi: 10.1006/exnr.2000.7389
Saresella, M., Calabrese, E., Marventano, I., Piancone, F., Gatti, A., Alberoni, M., et al. (2011). Increased activity of Th-17 and Th-9 lymphocytes and a skewing of the post-thymic differentiation pathway are seen in Alzheimer’s disease. Brain Behav. Immun. 25, 539–547. doi: 10.1016/j.bbi.2010.12.004
Schäfer, S., Calas, A. G., Vergouts, M., and Hermans, E. (2012). Immunomodulatory influence of bone marrow-derived mesenchymal stem cells on neuroinflammation in astrocyte cultures. J. Neuroimmunol. 249, 40–48. doi: 10.1016/j.jneuroim.2012.04.018
Schneider-Hohendorf, T., Stenner, M. P., Weidenfeller, C., Zozulya, A. L., Simon, O. J., Schwab, N., et al. (2010). Regulatory T cells exhibit enhanced migratory characteristics, a feature impaired in patients with multiple sclerosis. Eur. J. Immunol. 40, 3581–3590. doi: 10.1002/eji.201040558
Schoppet, M., Preissner, K. T., and Hofbauer, L. C. (2002). RANK ligand osteoprotegerin: paracrine regulators of bone metabolism and vascular function. Arterioscler. Thromb. Vasc. Biol. 22, 549–553. doi: 10.1161/01.atv.0000012303.37971.da
Scott, E. L., and Brann, D. W. (2013). Estrogen regulation of Dkk1 and Wnt/β-Catenin signaling in neurodegenerative disease. Brain Res. 1514, 63–74. doi: 10.1016/j.brainres.2012.12.015
Shan, C., Ghosh, A., Guo, X.-Z., Wang, S.-M., Hou, Y.-F., Li, S.-T., et al. (2019). Roles for osteocalcin in brain signalling: implications in cognition- and motor-related disorders. Mol. Brain 12:23. doi: 10.1186/s13041-019-0444-5
Shimamura, M., Nakagami, H., Osako, M. K., Kurinami, H., Koriyama, H., Zhengda, P., et al. (2014). OPG/RANKL/RANK axis is a critical inflammatory signaling system in ischemic brain in mice. Proc. Natl. Acad. Sci. U S A 111, 8191–8196. doi: 10.1073/pnas.1400544111
Simard, A. R., Soulet, D., Gowing, G., Julien, J. P., and Rivest, S. (2006). Bone marrow-derived microglia play a critical role in restricting senile plaque formation in Alzheimer’s disease. Neuron 49, 489–502. doi: 10.1016/j.neuron.2006.01.022
Smieszek, A., Tomaszewski, K. A., Kornicka, K., and Marycz, K. (2018). Metformin promotes osteogenic differentiation of adipose-derived stromal cells and exerts pro-osteogenic effect stimulating bone regeneration. J. Clin. Med. 7:482. doi: 10.3390/jcm7120482
Smith, S. M., Giedzinski, E., Angulo, M. C., Lui, T., Lu, C., Park, A. L., et al. (2020). Functional equivalence of stem cell and stem cell-derived extracellular vesicle transplantation to repair the irradiated brain. Stem Cells Transl. Med. 9, 93–105. doi: 10.1002/sctm.18-0227
Soulet, D., and Rivest, S. (2008). Bone-marrow-derived microglia: myth or reality? Curr. Opin. Pharmacol. 8, 508–518. doi: 10.1016/j.coph.2008.04.002
Steinman, L. (2009). A molecular trio in relapse and remission in multiple sclerosis. Nat. Rev. Immunol. 9, 440–447. doi: 10.1038/nri2548
Uede, T. (2011). Osteopontin, intrinsic tissue regulator of intractable inflammatory diseases. Pathol. Int. 61, 265–280. doi: 10.1111/j.1440-1827.2011.02649.x
Urakawa, I., Yamazaki, Y., Shimada, T., Iijima, K., Hasegawa, H., Okawa, K., et al. (2006). Klotho converts canonical FGF receptor into a specific receptor for FGF23. Nature 444, 770–774. doi: 10.1038/nature05315
Vervloet, M. G., Massy, Z. A., Brandenburg, V. M., Mazzaferro, S., Cozzolino, M., Ureña-Torres, P., et al. (2014). Bone: a new endocrine organ at the heart of chronic kidney disease and mineral and bone disorders. Lancet Diabetes Endocrinol. 2, 427–436. doi: 10.1016/S2213-8587(14)70059-2
Viswambharan, V., Thanseem, I., Vasu, M. M., Poovathinal, S. A., and Anitha, A. (2017). miRNAs as biomarkers of neurodegenerative disorders. Biomark. Med. 11, 151–167. doi: 10.2217/bmm-2016-0242
Walker, J. A., and McKenzie, A. N. J. (2018). TH2 cell development and function. Nat. Rev. Immunol. 18, 121–133. doi: 10.1038/nri.2017.118
Wang, F., Yasuhara, T., Shingo, T., Kameda, M., Tajiri, N., Yuan, W. J., et al. (2010). Intravenous administration of mesenchymal stem cells exerts therapeutic effects on parkinsonian model of rats: focusing on neuroprotective effects of stromal cell-derived factor-1α. BMC Neurosci. 11:52. doi: 10.1186/1471-2202-11-52
Watanabe, S., Kawamoto, S., Ohtani, N., and Hara, E. (2017). Impact of senescence-associated secretory phenotype and its potential as a therapeutic target for senescence-associated diseases. Cancer Sci. 108, 563–569. doi: 10.1111/cas.13184
Wei, J., Hanna, T., Suda, N., Karsenty, G., and Ducy, P. (2014). Osteocalcin promotes β-cell proliferation during development and adulthood through Gprc6a. Diabetes 63, 1021–1031. doi: 10.2337/db13-0887
Wu, M., Rementer, C., and Giachelli, C. M. (2013). Vascular calcification: an update on mechanisms and challenges in treatment. Calcif. Tissue Int. 93, 365–373. doi: 10.1007/s00223-013-9712-z
Xie, L., Choudhury, G. R., Winters, A., Yang, S. H., and Jin, K. (2015). Cerebral regulatory T cells restrain microglia/macrophage-mediated inflammatory responses via IL-10. Eur. J. Immunol. 45, 180–191. doi: 10.1002/eji.201444823
Xin, H., Li, Y., Buller, B., Katakowski, M., Zhang, Y., Wang, X., et al. (2012). Exosome-mediated transfer of miR-133b from multipotent mesenchymal stromal cells to neural cells contributes to neurite outgrowth. Stem Cells 30, 1556–1564. doi: 10.1002/stem.1129
Xin, H., Li, Y., Liu, Z., Wang, X., Shang, X., Cui, Y., et al. (2013). MiR-133b promotes neural plasticity and functional recovery after treatment of stroke with multipotent mesenchymal stromal cells in rats via transfer of exosome-enriched extracellular particles. Stem Cells 31, 2737–2746. doi: 10.1002/stem.1409
Xin, H., Wang, F., Li, Y., Lu, Q. E., Cheung, W. L., Zhang, Y., et al. (2017). Secondary release of exosomes from astrocytes contributes to the increase in neural plasticity and improvement of functional recovery after stroke in rats treated with exosomes harvested from microrna 133b-overexpressing multipotent mesenchymal stromal cells. Cell Transplant. 26, 243–257. doi: 10.3727/096368916X693031
Xiong, Y., Mahmood, A., and Chopp, M. (2017). Emerging potential of exosomes for treatment of traumatic brain injury. Neural Regen. Res. 12, 19–22. doi: 10.4103/1673-5374.198966
Yan, Q. W., Yang, Q., Mody, N., Graham, T. E., Hsu, C. H., Xu, Z., et al. (2007). The adipokine lipocalin 2 is regulated by obesity and promotes insulin resistance. Diabetes 56, 2533–2540. doi: 10.2337/db07-0007
Yang, Y., Ye, Y., Su, X., He, J., Bai, W., and He, X. (2017). MSCs-derived exosomes and neuroinflammation, neurogenesis and therapy of traumatic brain injury. Front. Cell. Neurosci. 11:55. doi: 10.3389/fncel.2017.00055
Yano, K., Tsuda, E., Washida, N., Kobayashi, F., Goto, M., Harada, A., et al. (1999). Immunological characterization of circulating osteoprotegerin/osteoclastogenesis inhibitory factor: increased serum concentrations in postmenopausal women with osteoporosis. J. Bone Miner. Res. 14, 518–527. doi: 10.1359/jbmr.1999.14.4.518
Yokokawa, K., Iwahara, N., Hisahara, S., Emoto, M. C., Saito, T., Suzuki, H., et al. (2019). Transplantation of mesenchymal stem cells improves amyloid-β pathology by modifying microglial function and suppressing oxidative stress. J. Alzheimers Dis. 72, 867–884. doi: 10.3233/JAD-190817
Yu, H., Liu, X., and Zhong, Y. (2017). The effect of osteopontin on microglia. Biomed. Res. Int. 2017:1879437. doi: 10.1155/2017/1879437
Yuan, J., Meloni, B. P., Shi, T., Bonser, A., Papadimitriou, J. M., Mastaglia, F. L., et al. (2019). The potential influence of bone-derived modulators on the progression of Alzheimer’s disease. J. Alzheimers Dis. 69, 59–70. doi: 10.3233/JAD-181249
Zhang, L., Dong, Z. F., and Zhang, J. Y. (2020). Immunomodulatory role of mesenchymal stem cells in Alzheimer’s disease. Life Sci. 246:117405. doi: 10.1016/j.lfs.2020.117405
Zhang, J., Fu, Q., Ren, Z., Wang, Y., Wang, C., Shen, T., et al. (2015). Changes of serum cytokines-related Th1/Th2/Th17 concentration in patients with postmenopausal osteoporosis. Gynecol. Endocrinol. 31, 183–190. doi: 10.3109/09513590.2014.975683
Zhang, J., Zhang, Z. G., Lu, M., Wang, X., Shang, X., Elias, S. B., et al. (2017). MiR-146a promotes remyelination in a cuprizone model of demyelinating injury. Neuroscience 348, 252–263. doi: 10.1016/j.neuroscience.2017.02.029
Zhao, Y., Shen, L., and Ji, H. F. (2012). Alzheimer’s disease and risk of hip fracture: a meta-analysis study. Sci. World J. 2012:872173. doi: 10.1100/2012/872173
Zhao, E., Xu, H., Wang, L., Kryczek, I., Wu, K., Hu, Y., et al. (2012). Bone marrow and the control of immunity. Cell. Mol. Immunol. 9, 11–19. doi: 10.1038/cmi.2011.47
Zhou, R., Deng, J., Zhang, M., Zhou, H. D., and Wang, Y. J. (2011). Association between bone mineral density and the risk of Alzheimer’s disease. J. Alzheimers Dis. 24, 101–108. doi: 10.3233/JAD-2010-101467
Zoch, M. L., Clemens, T. L., and Riddle, R. C. (2016). New insights into the biology of osteocalcin. Bone 82, 42–49. doi: 10.1016/j.bone.2015.05.046
Keywords: bone, bone marrow, neurodegenerative diseases, mesenchymal stem cells, immune, osteokines
Citation: Yu Z, Ling Z, Lu L, Zhao J, Chen X, Xu P and Zou X (2020) Regulatory Roles of Bone in Neurodegenerative Diseases. Front. Aging Neurosci. 12:610581. doi: 10.3389/fnagi.2020.610581
Received: 26 September 2020; Accepted: 24 November 2020;
Published: 21 December 2020.
Edited by:
Daniel Ortuño-Sahagún, University of Guadalajara, MexicoReviewed by:
Michele D’Angelo, University of L’Aquila, ItalyVanessa Castelli, University of L’Aquila, Italy
Copyright © 2020 Yu, Ling, Lu, Zhao, Chen, Xu and Zou. This is an open-access article distributed under the terms of the Creative Commons Attribution License (CC BY). The use, distribution or reproduction in other forums is permitted, provided the original author(s) and the copyright owner(s) are credited and that the original publication in this journal is cited, in accordance with accepted academic practice. No use, distribution or reproduction is permitted which does not comply with these terms.
*Correspondence: Xuenong Zou, enhub25nQGhvdG1haWwuY29t; em91eHVlbkBtYWlsLnN5c3UuZWR1LmNu; Pingyi Xu, cGluZ3lpeHVAc2luYS5jb20=
† These authors have contributed equally to this work