- 1Department of Neurology, New York University Grossman School of Medicine, New York, NY, United States
- 2Department of Ophthalmology, New York University Grossman School of Medicine, New York, NY, United States
- 3Department of Population Health, New York University Grossman School of Medicine, New York, NY, United States
Vision, which requires extensive neural involvement, is often impaired in Alzheimer’s disease (AD). Over the last few decades, accumulating evidence has shown that various visual functions and structures are compromised in Alzheimer’s dementia and when measured can detect those with dementia from those with normal aging. These visual changes involve both the afferent and efferent parts of the visual system, which correspond to the sensory and eye movement aspects of vision, respectively. There are fewer, but a growing number of studies, that focus on the detection of predementia stages. Visual biomarkers that detect these stages are paramount in the development of successful disease-modifying therapies by identifying appropriate research participants and in identifying those who would receive future therapies. This review provides a summary and update on common afferent and efferent visual markers of AD with a focus on mild cognitive impairment (MCI) and preclinical disease detection. We further propose future directions in this area. Given the ease of performing visual tests, the accessibility of the eye, and advances in ocular technology, visual measures have the potential to be effective, practical, and non-invasive biomarkers of AD.
Introduction
Alzheimer’s disease (AD), the most common cause of dementia worldwide (McKhann et al., 2011), is a growing public health issue estimated to affect 13.8 million Americans by 2050 (Alzheimer’s Disease Facts and Figures, 2019). The pathophysiology of AD involves brain deposition of extracellular amyloid-beta plaques and intracellular tau neurofibrillary tangles, which lead to neuronal degeneration and subsequent cognitive impairment (Murphy and LeVine, 2010). AD is diagnosed by clinical and neuropsychiatric assessments complemented by neuroimaging (McKhann et al., 2011). Since it is a clinical diagnosis, individuals are often diagnosed at later stages when symptomatic. However, biomarkers of AD pathophysiology can precede clinical symptoms by many years (Fagan et al., 2007; Jack et al., 2009; Roe et al., 2013; Petersen et al., 2016; Betthauser et al., 2020), making it possible for earlier detection in predementia stages. The progression to AD dementia is divided into three broad stages. In the preclinical stage, individuals are cognitively and functionally normal on a standardized assessment but have biomarkers of AD pathology on a molecular or imaging level, and may be at-risk for future decline. As such, subjective cognitive decline in normal individuals has been proposed to be preclinical AD given its association with imaging and cerebrospinal fluid AD biomarkers and higher rates of future objective decline compared to those without subjective concern (Jack et al., 2018; Jessen et al., 2020). Mild cognitive impairment (MCI) due to AD is considered the prodromal stage, which includes individuals with objectively measurable cognitive deficits but with intact or only minimally impaired function. The third and final stage is dementia due to AD, when cognition and function are compromised, and is stratified into mild, moderate, or severe (Albert et al., 2011; Sperling et al., 2011). Early detection is key in identifying at-risk individuals who would benefit from future disease-modifying therapies. Biomarkers that accurately reflect disease status and severity may also facilitate the success of future trials by identifying appropriate research participants and helping monitor treatment efficacy. Current widely-accepted biomarkers for AD include cerebrospinal fluid levels of tau and amyloid-beta and amyloid positron emission tomography imaging, but these involve expensive and invasive procedures and are not routinely measured or accessible for patients (El Kadmiri et al., 2018).
In the past few decades, visual biomarkers have become an area of interest with studies showing that AD pathophysiology affects vision-related structures. In 1986, Hinton et al. (1986) were the first to report histological evidence of visual structure degeneration in AD. Post-mortem histological samples showed stark axonal degeneration of optic nerves and retinal ganglion cell loss in AD patients compared to controls (Hinton et al., 1986). Amyloid and tau deposition have also been reported in various vision-related subcortical and cortical structures of the brain. Amyloid plaques and neurofibrillary tangles have been found in the lateral geniculate nucleus (LGN) and primary visual cortex, structures important in relaying and processing of visual information, as well as the superior colliculus and pulvinar nucleus, which are involved in eye movement control (Kusne et al., 2017). Classically, it was thought that AD pathophysiology initially spared these structures, with involvement only in late-stage disease (Albers et al., 2015).
However, more studies suggest that AD pathophysiology may be present in vision-related brain structures earlier on. Neurofibrillary tangles and neuritic plaques have been found in the visual association cortex Brodmann area 19 of patients with preclinical disease and MCI (McKee et al., 2006). More recently, amyloid plaques have been discovered in post-mortem retinas of early AD patients but not controls. Retinal plaques correlated with cerebral and visual cortex amyloid burden and in vivo experiments suggest that amyloid plaques in the retina may precede those in the brain (Koronyo-Hamaoui et al., 2011). Moreover, retinal tau pathology in the form of neurofibrillary tangles and hyperphosphorylated tau have been reported in AD patients (Kusne et al., 2017; den Haan et al., 2018). These findings provide histopathological evidence that the visual system may be disrupted much earlier in the AD disease process than previously thought, and thus corroborates the use of visual biomarkers in detecting early disease.
Further, AD patients commonly present with visual symptoms (Katz and Rimmer, 1989; Brewer and Barton, 2014), which is consistent with the fact that roughly half of the cerebral cortex is involved in visual processing (Felleman and Van Essen, 1991). Many studies have also reported significant differences in structural and functional visual measures between AD dementia patients and those with normal cognition (Javaid et al., 2016; Polo et al., 2017; Chan et al., 2019), with some tests capable of detecting MCI (Galetta et al., 2017). Vision is unique because unlike psychometric measures and testing of other senses such as smell, it is often education and culture-invariant and can be more objectively measured. As vision tests are also non-invasive and quick to perform, visual measures have the potential to be practical, cost-effective, and sensitive biomarkers for AD. More studies are now exploring the capacity of visual biomarkers for MCI and preclinical disease detection.
Search Criteria
A PubMed literature search was conducted to identify all relevant studies within the last 5 years. Our search included Mesh terms or keywords for visual measures such as “visual dysfunction,” “contrast sensitivity,” “color vision,” “color perception,” “optical coherence tomography (OCT),” “eye movements,” “saccades,” “eye tracking,” “anti-saccades,” “pupillary responses, “pupil dilation,” “pupillometry,” “motion processing,” “motion perception,” “visuospatial function” “object identification” “picture naming” “higher visual function” and those related to AD such as “AD,” “cognitive dysfunction,” “MCI,” and “mild neurocognitive disorder.” Only clinical research articles related to AD published from January 2015 to March 2020 and references of these articles were included after a manual curation based on relevance. There are two main reasons for the selection of this time window. Visual markers of AD have been studied for many decades. This topic has also been the focus of review articles published before 2015 (Kirby et al., 2010; Valenti, 2010; Chang et al., 2014; Tzekov and Mullan, 2014). One of our aims was to provide an update by summarizing relevant articles published within the last 5 years. Secondly, modern research diagnostic criteria for MCI and AD dementia, including the use of imaging/cerebrospinal fluid analysis of amyloid-beta and tau, was established in 2011 (Albert et al., 2011; McKhann et al., 2011). Our selected time window ensures that we are including studies using this most recent diagnostic criterion.
Afferent and Efferent Visual Systems
The visual system is composed of the afferent and efferent visual systems (Figure 1), which are both affected in AD. The afferent visual pathway is related to the sensory aspect of vision and involves all the structures responsible for receiving, transmitting, and processing visual information. Visual information is initially captured by light-sensitive photoreceptors in the retina and channeled through various synaptic connections to reach the retinal ganglion cells in the inner retina. Retinal ganglion cell axons form the optic nerve and tract and synapse on the LGN of the thalamus. From the LGN, the optic radiations carry information to the primary visual cortex in the occipital lobe for initial visual processing and then multiple extrastriate cortices for higher-level processing including visual recognition and visuospatial processing (Figure 1A; Prasad and Galetta, 2011). The efferent visual pathways are related to the oculomotor part of vision and facilitate eye movements that allow for an in-focus view of objects to capture visual information. Saccades, fast eye movements between various fixation points, are the most common type of eye movement and are generated by a network of cortical and brain stem structures. Excitatory input from cortical regions such as the frontal eye field, parietal eye field, and supplementary eye field and inhibitory input from the basal ganglia feed into the superior colliculus, and signals from the superior colliculus are then sent to the saccade burst generator in the reticular formation to initiate a specific type of saccade. Another important cortical region is the dorsolateral prefrontal cortex (DLPFC), which is particularly important in modulating anti-saccades (Figure 1B; Pierrot-Deseilligny et al., 1995; Girard and Berthoz, 2005).
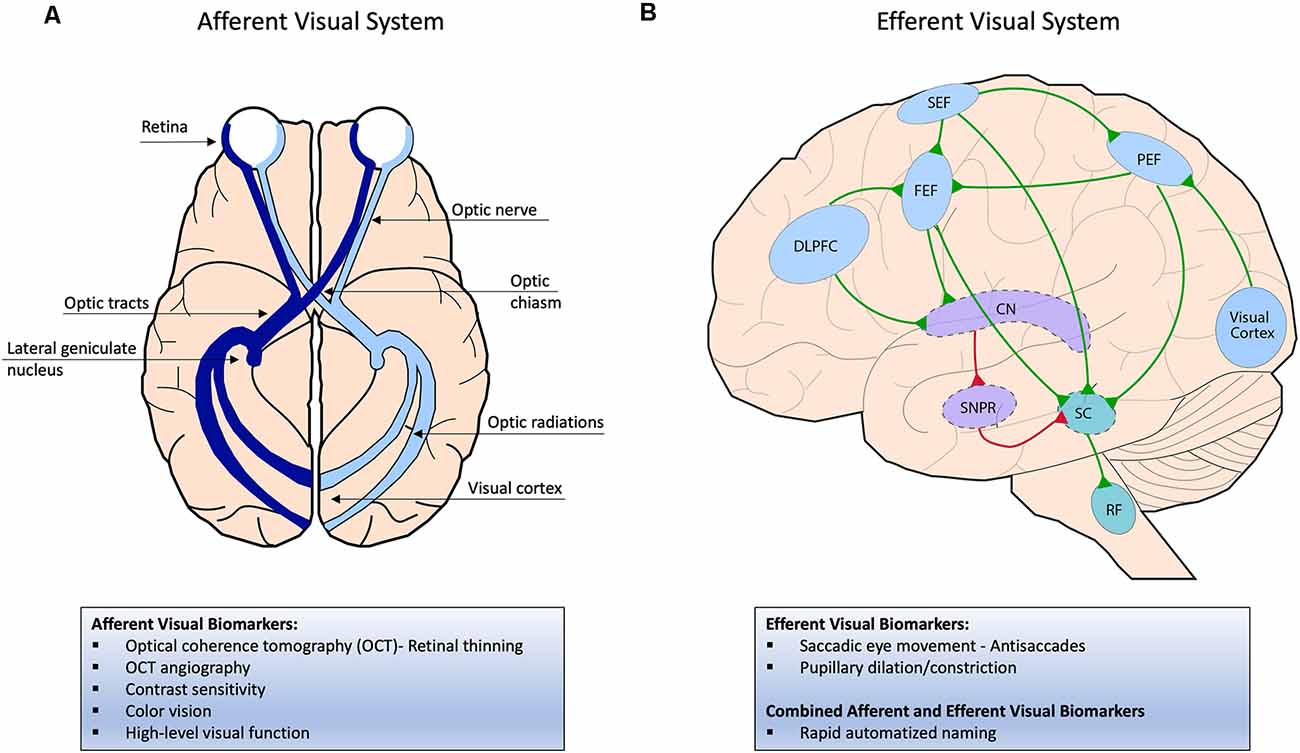
Figure 1. (A) Afferent visual system; visual information is initially captured by light-sensitive photoreceptors in the retina and transmitted through the optic nerve and then optic tract, which directly synapses on the lateral geniculate nucleus (LGN) of the thalamus. From the LGN, the optic radiations carry information to the primary visual cortex in the occipital lobe for initial visual processing and then multiple extra-striate cortices for higher-level processing (not pictured here). (B) Efferent visual system; To initiate a saccade, excitatory signals from cortical regions such as the frontal, parietal, and supplementary eye fields (FEF, PEF, SEF) are sent to the superior colliculus (SC) in the brainstem, which then projects to the saccade burst generator in the reticular formation. The FEF initiates voluntary and memory-guided saccades, the PEF initiates reflexive saccades, and the SEF initiates saccades that correlate with body movement. In the indirect pathway, the substantia nigra pars reticulata (SNPR) in the basal ganglia sends inhibitory signals to the SC to inhibit a saccade. To override the indirect pathway, the FEF is activated before the saccade generation, which inhibits the SNPR through the caudate nucleus (CN). The dorsolateral prefrontal cortex (DLPFC) helps modulate anti-saccades by inhibiting reflexive saccades and generating subsequent voluntary saccades away from a presented stimulus. Excitatory signals are shown in green and inhibitory signals in red.
Afferent Visual Biomarkers
Retinal Structure
Advances in ocular imaging modalities now allow for detailed in vivo analysis of the optic nerve head and specific layers within the retina, which are affected by AD pathophysiological processes. OCT, a non-invasive imaging technique utilizing infrared light, is capable of unparalleled quantification of retinal thickness and vasculature and has become one of the most studied visual biomarkers in AD.
Despite the presence of negative studies (Pillai et al., 2016; Poroy and Yucel, 2018; Sánchez et al., 2018, 2020; den Haan et al., 2019), there is compelling evidence that significant retinal thinning occurs in AD dementia. AD patients are found to have significant thinning in the peripapillary retinal nerve fiber layer and macular sections containing ganglion cells such as the ganglion cell-inner plexiform layer, ganglion cell layer, and ganglion cell complex (Bambo et al., 2015; Cunha et al., 2016; Garcia-Martin et al., 2016; Ferrari et al., 2017; Kim and Kang, 2019). While the ganglion cell- inner plexiform layer is composed of macular ganglion cell bodies and their dendrites, the ganglion cell layer contains solely the cell bodies, and ganglion cell complex includes the ganglion cell- inner plexiform layer and the macular retinal nerve fiber layer, which represent the ganglion cell axons present on the macula. The peripapillary retinal nerve fiber layer is comprised of ganglion cell axons about to enter the optic nerve. The superior and inferior quadrants of the peripapillary retinal nerve fiber layer often exhibit the most significant reduction (Cunha et al., 2016; Kwon et al., 2017; Kim and Kang, 2019); however, thinning in the temporal region such as the superior temporal and inferior temporal regions have also been reported (Garcia-Martin et al., 2016). Recent meta-analyses support these findings. Mean and quadrant-specific peripapillary retinal nerve fiber layer thickness was significantly reduced in AD patients with the most significant reduction in the superior quadrant (Coppola et al., 2015; Thomson et al., 2015; Chan et al., 2019). A significant reduction of ganglion cell- inner plexiform layer and macular volume or thickness were also seen in AD patients (den Haan et al., 2017; Chan et al., 2019). These structural changes are consistent with post-mortem histology findings of amyloid deposits concentrated in the superior quadrant of the retina and within macular retinal ganglion cells, which may cause anterograde degeneration of the superior quadrant of the optic disc and macular degeneration, respectively (La Morgia et al., 2016; Koronyo et al., 2017). Interestingly, when the retina is examined at pixel resolution, AD participants were found to have a non-uniform distribution of retinal thickening interspersed with thinning compared to controls (Lad et al., 2018; Janez-Escalada et al., 2019). Some studies have also reported macular thickening in moderate stage AD (Salobrar-García et al., 2019). These findings may be a result of inflammatory changes triggered by amyloid deposition or development of granular membranes secondary to retinal gliosis (Zhang et al., 2019a). Although the timeline of these changes is unclear, the proposed dynamic nature of the retina may partially explain negative OCT studies, which examine retinal thickness across pre-set areas. Negative studies may also be explained by non-psychometrically characterized controls (Poroy and Yucel, 2018; den Haan et al., 2019), comparatively younger AD cohorts in the context of no retinal differences in early-onset AD (Pillai et al., 2016; Haan et al., 2019a), and use of different OCT devices (Poroy and Yucel, 2018; Sánchez et al., 2018, 2020).
OCT studies with MCI or subjective cognitive decline are fewer and less congruent (Table 1). The discrepancy in results may be explained by small sample sizes, variable diagnostic criteria, or use of various OCT devices (Oktem et al., 2015; Ferrari et al., 2017; Almeida et al., 2019; Kim and Kang, 2019). While some report no significant differences in the peripapillary retinal nerve fiber layer (Knoll et al., 2016; Lad et al., 2018; Sánchez et al., 2018; Kim and Kang, 2019), others have shown a significant decrease in mean or quadrant-specific peripapillary retinal nerve fiber layer thicknesses in MCI compared to controls (Oktem et al., 2015; Ferrari et al., 2017; Lopez-de-Eguileta et al., 2019; Tao et al., 2019). Even more compelling, a study with amyloid positive- MCI subjects exhibited thinning in the peripapillary retinal nerve fiber layer (Lopez-de-Eguileta et al., 2019). MCI subjects also have reduced thicknesses of the total macula, ganglion cell- inner plexiform layer, and ganglion cell complex, particularly in regions close to the fovea (Gimenez Castejon et al., 2016; Shao et al., 2018; Wu et al., 2018; Almeida et al., 2019). Yet, some studies do not report a difference in either average ganglion cell- inner plexiform layer or macular thickness between MCI and controls (Choi et al., 2016; Pillai et al., 2016; Kwon et al., 2017; Sánchez et al., 2020). Relevant meta-analyses also demonstrate conflicting results. While one meta-analysis found a significant reduction in the peripapillary retinal nerve fiber layer in MCI patients compared to controls (Coppola et al., 2015), a more recent meta-analysis including only spectral-domain-OCT studies found no significant difference in both the peripapillary retinal nerve fiber layer and ganglion cell- inner plexiform layer between these groups (Chan et al., 2019). Interestingly, in these meta-analyses, significant reductions in macular measures including the ganglion cell- inner plexiform layer and macular thickness and volume were only reported in those with Alzheimer’s dementia and not in MCI, while peripapillary retinal nerve fiber layer thinning has been implicated in MCI (den Haan et al., 2017; Chan et al., 2019). This may suggest that the optic nerve head may be affected earlier in disease and serve as a more sensitive site to measure. However, individuals with subjective cognitive decline have been reported to have significantly reduced macular thickness compared to controls (Gimenez Castejon et al., 2016), without having a difference in the peripapillary retinal nerve fiber layer (Santos et al., 2018). In another study, despite no baseline difference in retinal thickness, preclinical AD vs. control participants had significantly decreased macular retinal nerve fiber layer volumes over 27 months (p = 0.05; Santos et al., 2018). These results support the macula as the initial site of degeneration, but more studies are needed to determine which layers are affected first.
Ultimately, to be a good biomarker, retinal changes should parallel brain processes. Studies have investigated the relationship between retinal measures and brain imaging in individuals who may be healthy or cognitively impaired without dementia. One of these studies shows retinal nerve fiber layer and ganglion cell layer thinning associated with global gray and white-matter volume loss and compromised white-matter microstructure, even when controlling for age, sex, and cardiovascular risk factors (Mutlu et al., 2017). Retinal parameters have also been associated with changes in vision-related brain structures and the temporal lobe. Reduced retinal nerve fiber layer and ganglion cell layer thickness have been associated with lower gray matter density in the visual cortex and lingual gyrus and reduced white matter integrity of the optic radiations on MRI (Méndez-Gómez et al., 2018; Mutlu et al., 2018b; Shi et al., 2020b). It is proposed that this relationship may be explained by either retrograde degeneration from visual cortex damage, anterograde degeneration from retinal ganglion cell loss, or simultaneous retinal and brain changes from AD pathophysiology- which may be more likely as retinal thinning seems to also correlate with other brain changes (Mutlu et al., 2018a). Decreased gray matter volume in the temporal lobes and hippocampal volumes correlated with thinner retinal nerve fiber and ganglion cell- inner plexiform layers (Méndez-Gómez et al., 2018; Shi et al., 2020b). In one study with cognitively normal older adults, thinning of these layers was associated with reduced entorhinal volume in the medial temporal lobe, but not regions associated with aging (bilateral midfrontal cortex) or with later stages of AD (temporoparietal cortex, precuneus, or posterior cingulate region; Casaletto et al., 2017). Since degeneration of the entorhinal cortex is often a hallmark feature of early AD (Albert et al., 2011), perhaps this suggests the potential for the retina to parallel preclinical or prodromal disease. Notably, these retinal measures were not significantly associated with verbal or visual episodic memory performance supporting the notion that changes in the retina and associated medial temporal lobe volumes may precede cognitive change. This is often seen in the case when substantial medial temporal lobe degeneration may occur before any cognitive dysfunction (Jack et al., 2013).
Retinal parameters are also found to correlate with cognition, functioning, and future disease progression. Average and inferior peripapillary retinal nerve fiber layer, ganglion cell- inner plexiform layer, ganglion cell complex, and inner macula thicknesses are reported to moderately correlate with mini-mental status exam scores of AD patients (Cunha et al., 2016). However, not all studies report a correlation (Kim and Kang, 2019). Since mini-mental status exam performance is affected by education level and often not a reliable measure of impairment in mild disease (Arevalo-Rodriguez et al., 2015; Tsoi et al., 2015), Global Deterioration Scale and Clinical Dementia Rating scores are alternatives that better reflect cognition and daily functioning. Using these parameters, one study found that total macula and ganglion cell- inner plexiform layer thicknesses were negatively correlated with the Global Deterioration Scale and Clinical Dementia Rating scores (Kim and Kang, 2019). Clinical Dementia Rating-Sum of box scores, which represent a more granular cognitive scale compared to the normal score, had a negative relationship with ganglion cell- inner plexiform layer thickness and change in this score over 2 years was negatively associated with baseline ganglion cell- inner plexiform layer thickness. Temporal peripapillary retinal nerve fiber layer and average and minimum ganglion cell- inner plexiform layer thicknesses at baseline were also significantly thinner in MCI individuals that progressed to dementia (Choi et al., 2016). Recent longitudinal studies further support retinal parameters as important prognosticators of cognitive decline. One study in roughly 32,000 healthy adults from the United Kingdom showed that those with the thinnest retinal nerve fiber layers were more likely to fail at least one baseline cognitive test and have worse cognition at follow-up 3 years later (Ko et al., 2018). Healthy adults with thinner retinal nerve fiber layer at baseline were also associated with a greater risk of developing AD or any type of dementia after 3–8 years, even with adjustment for cardiovascular risk factors (Mutlu et al., 2018b). Retinal changes perhaps precede cognitive decline and support the potential of retinal parameters as biomarkers even of preclinical disease.
Retinal Vasculature
Consistent with cerebral vascular dysfunction in AD (Patton et al., 2005), retinal vasculature changes have been reported in AD. With laser doppler and retinal function imager technology, AD patients were found to have decreased blood flow and velocity of retinal veins, venules, and arterioles and reduced retinal tissue perfusion (a function of blood flow and tissue volume) compared to controls. Retinal vein blood flow was also significantly decreased in MCI individuals compared to controls (Table 1, Feke et al., 2015; Jiang et al., 2018a).
The majority of studies investigating retinal microvasculature utilize OCT angiography, a high-resolution, non-invasive imaging technique that can visualize retinal capillaries in specific retinal layers (Kashani et al., 2017). The retinal vasculature of the macula is primarily comprised of two interconnected plexuses: the superficial vascular plexus found in the ganglion cell layer and the deep vascular plexus below the inner nuclear layer. In the fovea, these two vascular plexuses create a region without capillaries known as the foveal avascular zone, which enlarges secondary to retinal ischemia (Conrath et al., 2005). The radial peripapillary capillary plexus is found around the optic nerve head and supplies the retinal nerve fiber layer in this region (Campbell et al., 2017). AD individuals compared to controls are found to have decreased vessel density in both the superficial and deep vascular plexuses mainly in the parafoveal region (2.5 mm from the fovea), but also in outer parts of the macula (Bulut et al., 2018; Jiang et al., 2018b; Yoon et al., 2019a). Parafoveal vessel density has been shown to correlate with mini-mental status exam scores of AD participants. Foveal avascular zone enlargement, often a result of capillary drop out from ischemia, has also been reported in individuals with AD, those with MCI, and those with biomarker-proven preclinical AD (Bulut et al., 2018; O’Bryhim et al., 2018; Wu et al., 2020), but not all have reported significant change from controls (Table 1, Lahme et al., 2018; Yoon et al., 2019a; Zhang et al., 2019b). This discrepancy may be explained by normal variations of this zone due to gender or central retinal thicknesses or unknown confounding factors that may mask a positive finding. Thus, changes in the foveal avascular zone need further investigation (Yoon et al., 2019a). In terms of vascular changes in the optic nerve head, the radial peripapillary capillary plexus was decreased in those with AD dementia, but not those with amnestic MCI (Table 1, Lahme et al., 2018; Zhang et al., 2019b). MCI subjects compared to controls are found to have decreased vessel density specific to the parafoveal deep vascular plexus (Jiang et al., 2018b; Wu et al., 2020). Although, one study reported changes in the parafoveal superficial vascular plexus when considering a group of both MCI and mild AD dementia participants (Zhang et al., 2019b). And another study detected no significant difference between MCI and controls in either plexus, but MCI subjects had a trend of lower density in only the deep vascular plexus (Querques et al., 2019; Zhang et al., 2019b). These results suggest the parafoveal deep vascular plexus may be the more sensitive vascular biomarker. It is proposed that the deep vascular plexus is affected earlier in the disease process because it is composed of smaller vessels than the superficial plexus and therefore more prone to damage from pathophysiologic factors in AD. Perhaps vascular dysfunction starts at the deep vascular plexus closest to the fovea, spreads outward and to other layers of the retina, and then involves the other plexuses. In the presence of vascular changes, some of these studies found corresponding retinal thinning in the peripapillary retinal nerve fiber and ganglion cell- inner plexiform layers (Yoon et al., 2019a), while others did not (Jiang et al., 2018b), suggesting that vascular abnormalities may precede structural change.
These retinal microvascular changes have also been linked to volumetric changes in MRI. Yoon et al. (2019b) performed OCT angiography and volumetric MRI analysis on a small sample of seven MCI individuals and nine AD individuals and found that reduction of the parafoveal vessel and perfusion density correlated with increased inferolateral ventricle volume, a finding secondary to medial temporal lobe atrophy. A few studies have also reported correlations between retinal vasculature parameters and cerebral vascular disease in those with AD. In a study with 48 amyloid-positive AD subjects, vessel density in the perifovea (3–6 mm away from the fovea) was seen to inversely correlate with Fazekas score, a measure of periventricular and subcortical white matter hyperintensity lesions (Haan et al., 2019b). A similar relationship was seen in a group of subjects with amyloid-positive AD, subcortical vascular cognitive impairment, and subjective cognitive decline, where retinal arteriolar fractal dimension negatively correlated with white matter hyperintensity burden (Jung et al., 2019). Interestingly, in a separate study, blood flow density within the superficial vascular plexus in AD subjects was inversely associated with Fazekas scores, but not with amyloid-beta or tau levels in the cerebrospinal fluid (Lahme et al., 2018). These correlations suggest that alterations in retinal microcirculation may parallel that in the brain and cause neurodegeneration. At the same time, there is a possibility that retinal vascular changes are not specific to AD pathology and may reflect comorbid vascular disease. Further studies are needed to assess the specificity of OCT angiography as an AD biomarker.
Despite reports of retinal vascular changes in AD, the underlying pathophysiology remains unclear. However, some histopathological studies have discovered pathogenic forms of amyloid deposits within and along retinal vessels of AD subjects (La Morgia et al., 2016; Koronyo et al., 2017). These findings suggest that vasculature changes may be secondary to amyloid deposition, which may disrupt the blood-retina barrier. This is similar to how cerebral amyloid angiopathy plays a role in blood-brain barrier dysfunction (del Valle et al., 2011). A recent study has explored the integrity of the blood-retina barrier in post-mortem retinas from 56 AD subjects. They report that there is an early and progressive loss of retinal pericytes and vascular platelet-derived growth factor receptor β expression in MCI and AD dementia subjects. Within the brain, platelet-derived growth factor receptor β signaling is proposed to play a role in preserving pericyte count and thus maintaining the blood-brain barrier (Nikolakopoulou et al., 2017). In the study, reduced platelet-derived growth factor receptor β expression was associated with increased amyloid deposits within the retinal vasculature and, more importantly, with cerebral amyloid angiopathy severity, cerebral amyloid plaques, and cognitive status (Shi et al., 2020a). This study suggests that retinal vascular amyloidosis and pericyte loss are present in early AD, mirror brain pathophysiology, and serve as potential visual biomarkers. Given in vivo studies using hyperspectral imaging to detect retinal amyloid (Hadoux et al., 2019) and adaptive optics to image retinal pericytes (Schallek et al., 2013), future studies should work towards developing non-invasive technology to visualize these structures in humans. These markers of blood-retina barrier integrity would be promising for early AD detection.
Contrast Sensitivity
Contrast sensitivity is defined as the ability to differentiate an object from its surroundings and is often considered more sensitive at detecting subclinical visual impairment compared to normal visual acuity (Velten et al., 1999). It is also a measure that correlates with daily function, which makes it a particularly useful measure in AD where deficits in daily function may represent disease progression and increased caretaker needs (Elliott et al., 1990; Dargent-Molina et al., 1996). Different tests that measure low contrast letter acuity (Pelli-Robson), contrast sensitivity across spatial frequencies (CSV-1000E), and contrast sensitivity across a visual field (Frequency doubling technology) have shown deficits in AD. These impairments exist across both low and high spatial frequencies and have been associated with mini-mental status exam and memory test performance (Risacher et al., 2013; Salobrar-García et al., 2019). Interestingly, poor contrast sensitivity correlates with decreased macular thickness and average and superior quadrant retinal nerve fiber layer thickness in AD patients, with a stronger correlation than other functional visual measures such as color vision. A proposed theory is that loss of retinal ganglion cells may at least partially contribute to alterations in visual pathways for contrast sensitivity (Polo et al., 2017). However, the extent of peripheral or central involvement in altered contrast sensitivity remains unclear.
Only one study has investigated contrast sensitivity in individuals with predementia stages of AD. Using frequency doubling technology to assess contrast sensitivity, Risacher et al. (2020) reported significantly reduced general contrast sensitivity and variability of contrast sensitivity across the retina in those with amnestic MCI. This impairment was less pronounced in those with subjective cognitive decline (Table 1, Risacher et al., 2013). These deficits in MCI and subjective cognitive decline have been associated with increased amyloid and tau cortical deposition in temporal, parietal, and occipital lobes, and are significant predictors of amyloid and tau presence on imaging (Risacher et al., 2020). Since contrast sensitivity deficits have been shown to precede the clinical onset of dementia (Ward et al., 2018), these results suggest that contrast sensitivity may be a good biomarker of preclinical and early AD pathophysiology.
Color Vision
Historically, the ability of color vision tests to reliably differentiate those with and without AD has been controversial with some studies reporting impairment (Pache et al., 2003), while others do not (Wood et al., 1997; Massoud et al., 2002). In recent years, the few new studies examining color vision in AD have reported a difference in test performance from controls. AD patients had impaired chromatic vision based on scores from the Farnsworth D15 and L’Anthony D15, color arrangement tests that detect severe and mild color defects, respectively. Impaired color vision in these participants had a significant, but mild association with retinal thinning, particularly macular thickness. As the parvocellular retinal ganglion cells are responsible for visual pathways that help discern color and pattern (Polo et al., 2017), general retinal ganglion cell loss within the macula may lead to damage to this pathway and subsequent chromatic impairment. However, another study using the Roth 28-Hue test, a similar test to the Farnsworth D15, showed that mild and moderate AD did not exhibit significant dyschromatopsia compared to controls. Yet performance between the groups differed as AD patients had significantly more total errors and non-specific errors in the Tritan (blue spectrum) and deutan (red-green spectrum) regions of the test, with errors correlating inversely with mini-mental status exam scores (Salobrar-García et al., 2019). More studies, preferentially those implementing similar types of chromatic tests, are needed to clarify the degree of chromatic impairment in AD.
High-Level Visual Function
Higher-order visual processing is also affected by AD. From the primary visual cortex, visual information is proposed to follow one of two streams for higher-level processing. The ventral stream (or “vision for perception” pathway) consists of projections from the primary visual cortex to the occipitotemporal association cortex and is involved in object/form recognition, object discrimination, and facial recognition. The dorsal stream (or “vision for action” pathway) relays information from the primary visual cortex to the parieto-occipital association cortex and determines the spatial layout of a scene, by analyzing motion and spatial relationships between objects and between the body and surrounding visual stimuli (Milner and Goodale, 1995).
In recent years, motion perception deficits have been well studied in AD. A significant finding is that the perception of complex motion is more impaired than simple translational motion in AD and may serve to distinguish AD dementia patients from controls. AD dementia patients performed significantly worse than MCI and similar-aged subjects when detecting rotational motion stimuli displayed with increasing levels of coherence (percentage of background noise disrupting the presented stimuli). In the same study, the authors provided evidence that tasks involving complex motion detection were more effective at differentiating AD severity than those with complex form detection. In addition to motion stimuli, participants were presented with concentric form stimuli of equivalent difficulty. AD dementia patients performed significantly worse on the motion than form task (Porter et al., 2017). In another study, AD dementia patients had significant impairment when detecting motion and direction of optic flow compared to age-matched controls, but direction deficits were less. Conversely, controls had greater impairment of direction than motion detection in the same task, suggesting that complex motion detection rather than directionality may best differentiate AD dementia from normal aging (Liu et al., 2019). This is supported by a study demonstrating that AD dementia patients and controls perform similarly on a horizontal motion discrimination task (Landy et al., 2015). Although there are limited recent studies on motion in MCI, past studies have shown similar deficits in higher-level motion tasks. MCI subjects had significant trouble identifying 3D moving spheres (structure-from-motion) compared to controls, demonstrated by an increased coherence threshold (Table 2, Lemos et al., 2012). In another study, MCI subjects had comparable detection of optic flow motion compared to controls but exhibited significantly delayed EEG responses, particularly in the inferior parietal lobule, during this task. This suggests early dysfunction in high-level dorsal stream processing in AD, which can be mapped to a certain brain region (Table 2, Yamasaki et al., 2012).
AD subjects are also reported to have abnormalities in normal, perceptual illusions involving motion. Normal illusions of direction repulsion, motion-induced position shift, and center-surround suppression of large, high-contrast stimuli have all been significantly changed in AD dementia patients compared to controls (Li et al., 2017; Zhuang et al., 2017; Ye et al., 2018). Direction repulsion is the perception that two stimuli are moving away at a greater angle than reality. Motion-induced position shift is the phenomenon where a moving object is perceived to be displaced in the direction of motion, and center-surround suppression is a perceptual phenomenon that causes reduced motion sensitivity of larger stimuli. Increased irregularity on direction repulsion and center-surround suppression tasks correlated with decreased cognitive function as measured by the mini-mental status exam (Li et al., 2017; Zhuang et al., 2017).
The dorsal stream is not the only visual processing pathway impaired in AD. Tests that primarily recruit the ventral stream are also affected. The multilingual naming test, which was recently added to the National Alzheimer’s Coordinating Center’s neuropsychological test battery, is an untimed picture naming test composed of 32 black and white drawings of objects. Performance on this test was significantly different between different stages of AD including MCI vs. controls, MCI vs. mild AD, and mild AD vs. moderate AD. However, good diagnostic accuracy was only achieved between AD dementia and controls (Table 2, Stasenko et al., 2019).
Many tasks involving both visual processing streams are also affected in AD. AD dementia patients performed significantly worse than controls on the Hooper Visual Organization Test, which requires timely identification of 30 line drawings of objects that have been fragmented into pieces (Mitolo et al., 2016). This task requires mental reorganization of the parts for identification of a whole object and has been shown to stimulate dorsal and ventral stream cortical regions on functional MRI (Moritz et al., 2004). MCI subjects have similar trouble with mental rotation and object identification (Table 2). The object recognition and discrimination task is a test wherein each trial, participants are shown four presented stimuli [three of which are the same object (non-target), and one that is different (target)], and asked to identify the target stimulus as quickly and accurately as possible. During “difficult” trials, a visuospatial component is added where non-target stimuli are rotated. Performance on difficult trials was able to distinguish controls from AD dementia, amnestic MCI, and even pre-MCI (defined as clinical dementia rating score of 0.5 with no memory impairment on psychometric testing or clinical dementia rating of 0 with memory impairment on testing; Gaynor et al., 2019). These results are consistent with early AD pathology in the form of tau deposition affecting the perirhinal cortex, which is crucial for feature integration for object identification and discrimination (Devlin and Price, 2007; Sone et al., 2017). Detection of moving faces and chairs (structure-from-motion perception) was also worse in MCI patients compared to controls, but less so for chairs (Table 2). On functional MRI, performance on this task was positively correlated with the cortical thickness of occipital lobe regions and ventral fusiform areas involved in visual face processing (Lemos et al., 2016). These studies taken together suggest widespread cortical dysfunction involving both streams in AD.
Interestingly, impairment of the ventral and dorsal streams in MCI is selective for high-level pathways. Low-level ventral and dorsal stream pathways are thought to exist between the retina and the primary visual cortex. Low-level ventral pathways process high spatial resolution, low temporal resolution, low contrast sensitivity, and color sensitivity, while low-level dorsal pathways process low spatial resolution, high temporal resolution, high contrast sensitivity, and color insensitivity. In a small study, MCI patients had significantly prolonged visual evoked potentials in response to higher-level ventral (faces), and dorsal (optic flow motion) stimuli, but not to lower-level stimuli (Table 2). In contrast, similar-aged controls had relatively preserved VEPs in response to higher-level, but not lower-level ventral and dorsal stimuli (Yamasaki et al., 2016). Amyloid-beta deposits, the earliest indicator of AD pathology, is thought to first originate in the cortex, and proceed to subcortical regions of the brain (Hardy and Selkoe, 2002). Although primary sensory cortices such as the primary visual cortex are initially spared by this deposition, the temporal, occipital, and parietal lobe can be involved earlier during this process (Braak and Braak, 1991). Amyloid-beta deposition in these areas may disrupt neuronal connections in high-level visual processing areas, and thus cause for impairment in prodromal disease. This theory is supported by findings of gray matter atrophy in three dorsal-stream-related visual cortices in late MCI and extensive atrophy of cortices related to dorsal and ventral stream processing in AD dementia individuals (Deng et al., 2016).
Efferent Biomarkers
Saccadic Eye Movement
In the last few decades, saccadic eye movement abnormalities have become one of the most common types of oculomotor dysfunction documented in AD. With high-quality video-based eye trackers, these eye movements are also easily captured. Saccades are quick eye movements that allow for the eyes to fixate on various spatial locations, placing the object of interest onto the fovea, the region of the retina responsible for the highest visual acuity. The majority of AD studies have focused on prosaccades, eye movements towards a presented stimulus, and antisaccades, eye movements away from a stimulus. AD patients are noted to have longer latencies in initiating prosaccades and antisaccades and have increased errors on antisaccade tasks with more errors left uncorrected compared to controls (Crawford et al., 2005, 2013; Yang et al., 2011, 2013; Noiret et al., 2018). Errors on the antisaccade task occur when the participant makes an eye movement toward rather than away from a presented stimulus. Self-corrected errors occur when a participant makes an error, but quickly corrects by looking away from the presented stimulus. Other eye movements that are affected in AD include smooth pursuit, the action of continuously following and maintaining gaze on a moving target, and microsaccades, which are less than one-degree shifts in the gaze that occur naturally during fixation. AD patients often have more non-horizontal, or oblique, microsaccades compared to controls (Kapoula et al., 2014), and during smooth pursuits tasks, AD patients struggle to initiate saccades and often make either compensatory saccades to keep up with the target or premature, anticipatory saccades leading the target (Hutton et al., 1984; Garbutt et al., 2008).
Studies within the last 5 years support previous eye movement findings in Alzheimer’s dementia patients and provide more evidence for saccadic eye movements as biomarkers for earlier-stage disease. Most studies that include MCI include participants with amnestic MCI, a subgroup characterized by memory impairment and most likely to progress to AD dementia (Fischer et al., 2007). Unlike studies with AD dementia participants, prosaccade and antisaccade latencies do not reliably distinguish between amnestic MCI and controls (Kahana Levy et al., 2018). However, reduced saccadic accuracy during prosaccade and antisaccade tasks has been reported in amnestic MCI patients compared to controls (Table 3, Holden et al., 2018; Chehrehnegar et al., 2019), and previously reported in AD (Yang et al., 2011). Saccadic accuracy refers to the amplitude of the eye movement compared to that of the target stimulus. However, the most robust changes in oculomotor function seen in amnestic MCI seem to be in antisaccade tasks. A recent meta-analysis reported that 87% of the 20 studies examined showed AD or MCI patients with increased antisaccade error rates compared to controls (Kahana Levy et al., 2018). Increased antisaccade errors in amnestic MCI participants compared to controls were also found in a study using a commercial, automated eye video tracker, with antisaccade errors inversely correlating with mini-mental status exam score. Number of self-corrected errors differentiated mild AD dementia, MCI, and cognitively normal individuals from each other (Table 3, Holden et al., 2018).
Most interestingly, Wilcockson et al. (2019) were the first group to study saccadic eye movement within MCI subtypes. They found that the antisaccade error rate is significantly higher in those with amnestic MCI compared to those with non-amnestic MCI and was negatively associated with memory score as measured by the free and cued selective reminding test free recall (Table 3, Wilcockson et al., 2019). Different subtypes of MCI are often determined by thorough neuropsychological testing with amnestic MCI defined as an impairment of memory alone or with other cognitive domains and non-amnestic MCI defined as an impairment of at least one non-memory cognitive domain (Kelley and Petersen, 2007). Results from this study are important because they introduce an easier test that could potentially differentiate MCI subtypes. As amnestic MCI is associated with a higher risk of progression to AD dementia, performance on the antisaccade task may help identify those truly at risk of Alzheimer’s dementia.
A theory for why antisaccades are more sensitive in detecting earlier AD than prosaccades is that antisaccades require more higher-level executive processing. Performing an antisaccade task requires controlled inhibition of a reflexive saccade towards a visual stimulus and then intact working memory to make a saccade away from the stimulus, which are both functions that are associated with the DLPFC, an area found to be active during antisaccade tasks (Hutton and Ettinger, 2006). Consistent with this explanation are results that show the frequency of antisaccade errors correlating with various neuropsychological tests that relate to executive function but with strongest correlation to the Stroop test, which measures inhibitory control (Heuer et al., 2013; Holden et al., 2018). Also, spatial working memory was highly correlated to the frequency of uncorrected antisaccade errors (Crawford et al., 2013). Spatial working memory is thought to be important in actively maintaining the task goal when presented with a visual stimulus in the antisaccade task. Slight lapses in working memory would allow for loss of inhibition of the presented stimulus and could result in uncorrected errors (Unsworth et al., 2004). Meanwhile, performance on prosaccades requires automatic processing and saccade initiation (Peltsch et al., 2014), which may require less cognitive burden and thus be relatively spared in early-stage disease.
Pupillometry
Task-evoked pupillary dilation is a promising visual biomarker in AD. Pupil dilation has been of interest because it is linked to activity in the locus coeruleus, which undergoes degenerative changes in early AD (Grudzien et al., 2007; Braak et al., 2011). The relationship between the pupil and locus coeruleus is particularly evident during cognitive tasks, where pupil dilation and neuronal activation in the locus coeruleus increase in parallel with cognitive effort. A functional MRI study has also shown an association between locus coeruleus-generated cortical activity and impaired task-related pupil dilation (Elman et al., 2017). The locus coeruleus is important in AD because studies suggest that this region may be the earliest site of tau pathology, and tau neurofibrillary tangles and volume loss in the locus coeruleus are associated with AD duration and severity (Bondareff et al., 1987; Grudzien et al., 2007; Braak and Del Tredici, 2011). During cognitive tasks, pupil dilation is a measure of compensatory cognitive effort. Thus, someone with reduced cognitive ability at baseline would exhibit increased pupil dilation, until the task at hand requires a cognitive load that exceeds baseline ability and compensatory effort, in which pupil size would decrease and performance declines. In one study, single-domain amnestic MCI participants exhibited greater pupil dilation than single-domain non-amnestic MCI and controls when asked to perform digit-span recall tasks with lower cognitive load. However, task performance did not differ between groups (Table 3, Granholm et al., 2017). These results suggest that pupillary changes may capture subtle cognitive abnormalities not yet manifest in cognitive performance measures and that pupil dilation can differentiate between MCI subtypes. Interestingly, impaired task-evoked pupil dilation in cognitively normal adults correlated with a higher risk of progression to MCI (Kremen et al., 2019). This supports the use of task-evoked pupillary measures in identifying at-risk individuals for AD. However, more studies are needed to explore their potential as a visual biomarker.
Light-induced pupillary responses have also been studied in AD. Pupillary size and response are controlled by opposing sympathetic and parasympathetic systems. In the presence of light, pupillary constriction is driven by the parasympathetic or cholinergic system, which requires acetylcholine to induce iris sphincter contraction (Spector, 1990). This response can be measured by a pupillometer with a white flash stimulus and is called the pupil flash response. Past studies show that AD dementia patients have abnormal, less reactive pupil flash responses (Fotiou et al., 2000, 2007; Granholm et al., 2003). It is thought that impairment in this response may be due to cholinergic deficiency in AD patients. More recent literature supports these findings. AD dementia patients were found to have significantly reduced pupil constriction acceleration, velocity, and amplitude and significantly increased latencies to constriction compared to controls (Fotiou et al., 2015; Frost et al., 2017). These pupillary responses were correlated with mini-mental status exam and Wechsler Memory Scale scores (Fotiou et al., 2015). Only two studies have explored light-induced pupillary responses in preclinical AD. In a small study comparing biomarker-positive preclinical AD to controls, there was no significant difference in pupil constriction velocity, percent constriction, or constriction latency between groups (Van Stavern et al., 2019). Another study found reduced maximum constriction velocity in preclinical AD compared to controls but no other differences in pupil flash response (Frost et al., 2017). These findings suggest that abnormalities in light-induced pupil responses are found in later stage AD, but are smaller and less measurable in preclinical disease.
Combined Visual Tests
Performance on vision-based tests that incorporate both the afferent and efferent visual system is also compromised in AD. The King-Devick Test is a timed rapid number naming test that requires participants to read out loud numbers arranged in different configurations and spacing. Performance on this test requires intact lower-order and higher-order visual processing, visual tracking, and saccadic eye movements. In one study, the King-Devick test was shown to be a highly sensitive screening test for both MCI and AD dementia, as it was able to differentiate these groups from controls (Table 3, Galetta et al., 2017). Other vision-based rapid automatizing naming tests exist but have not yet been tested in those with AD. The Mobile Universal Lexicon Evaluation System, a rapid picture naming test, however, has been shown to reliably detect concussion and other neurodegenerative diseases such as Parkinson’s disease and multiple sclerosis (Akhand et al., 2018; Seay et al., 2018; Conway et al., 2020). These tests are unique because they assess various dimensions of vision, but also those for cognitive processes such as attention, concentration, processing speed, and language. Since all these areas can be affected by AD, these tests may serve as a more robust screening tool.
Discussion
There is growing evidence that visual measures can differentiate early-stage AD, particularly MCI, from those without disease. These visual measures and potential biomarkers fall under both the afferent and efferent visual systems with some correlating with cognition, brain neurodegeneration, and AD pathophysiology. It is important to note that in recent years, literature in this area has focused predominantly on the afferent limb of the visual system. There has been a particular focus on examining visual pathway structure in the retina with OCT and OCT angiography techniques. This may be due in part to the novelty of some of these techniques and also their accessibility, as OCT has been successfully integrated into ophthalmic clinical practice. This trend highlights where the field is going, but also the need for further investigation of efferent markers, especially those that may show more promise than afferent markers. Further, although we discuss many promising visual measures in this review, there are still many important questions that need to be addressed.
A strong biomarker is one that has high sensitivity. Currently, it remains unclear which visual measures are most sensitive at detecting the earliest disease given the small number of studies with MCI and preclinical disease (subjective cognitive decline or no symptoms), if any. To best assess sensitivity, future studies should focus on individuals with MCI or preclinical disease. These individuals should ideally be well-characterized with accepted AD biomarkers to guarantee AD etiology, especially since MCI can have multiple etiologies. Controls should also be psychometrically characterized as normal to best detect differences from those with early disease, as well as have their amyloid and tau status documented. Currently, few existing studies have biomarker-positive participants and some use healthy volunteers as controls or mini-mental status exam score cutoffs, which do not reliably separate those that are normal from MCI (Trzepacz et al., 2015). Investigating more vision-based cognitive tests may also increase sensitivity because these tests involve more neural circuits that may be impaired.
Another attribute of a good biomarker is specificity. A known challenge of visual biomarkers is that visual changes can be shared between AD and other dementias or age-related neurodegenerative and ophthalmic diseases. It is hard to assess which visual biomarkers are most specific because few studies directly compare disease entities. However, some visual measures have been shown to differentiate between etiologies. For example, retinal vasculature is impaired in different regions in glaucoma vs. AD and increased horizontal saccade latency differentiates AD from frontotemporal dementia (Boxer et al., 2012; Zabel et al., 2019). More studies are needed to evaluate individual biomarker specificity. Although machine learning requires big data, implementing these techniques on quantitative forms of data such as retinal structural and vasculature data may help differentiate AD from other common etiologies. Machine learning techniques have been successful at differentiating individuals with glaucomatous damage from those without using OCT-derived parameters (Burgansky-Eliash et al., 2005). Multimodal visual testing may also increase specificity by creating distinct visual profiles for AD.
Based on current literature, there are a few visual measures that seem most promising as strong, practical biomarkers. Saccadic eye movement, particularly errors on the antisaccade task can differentiate amnestic MCI from controls, and even memory from non-memory impaired MCI subtypes. Performance on a combination of prosaccade and antisaccade tasks can further distinguish AD from Lewy body dementia, Parkinson’s disease dementia, and frontotemporal dementia (Mosimann et al., 2005; Antoniades and Kennard, 2015). Eye movements can be easily captured by a commercial, automated video-tracking system and with proper software, eye movements can be auto-coded and analyzed. A relatively new biomarker, which deserves future exploration, is task-evoked pupillary dilation, which differentiates MCI subtypes and has been associated with future risk of MCI. This test may be especially sensitive and specific to AD because performance parallels the activity of the locus coeruleus, which is an initial site of subcortical tau deposition in early-stage AD (Braak et al., 2011). All these visual tests often require access to a single, commercially sold machine, which is less expensive than current techniques to obtain AD biomarkers. Rapid automatized naming tests may also be sensitive and cost-effective. These tests simultaneously assess multiple aspects of vision and cognition such as visual processing, saccadic eye movement, object-related memory, color vision, and language, which may be impaired in AD. Usually presented in a physical or digital format, they do not require any expensive equipment. These tests are not specific but may be combined with another test to enhance specificity. Similarly, tests evaluating motion perception, visuospatial function, and object identification/discrimination may be particularly sensitive for early disease, as these often involve multiple cortical regions that may be affected by amyloid deposition. Retinal thinning has been the most studied biomarker in recent years and has been associated with cognition, brain changes, and future dementia. However, lack of specificity and the ability to consistently identify early-stage disease make it difficult to use as a diagnostic biomarker.
Despite the number of studies in this field, many unexplored areas remain. With most studies focused on typical AD, there is a paucity of studies examining visual biomarkers in atypical presentations including logopenic primary progressive aphasia, a frontal variant of AD, and posterior cortical atrophy. Clinically, these forms of AD overlap with other non-AD dementias and often rely on AD biomarkers for a more definitive diagnosis (Wolk, 2013). Since all have similar distributions of cerebral amyloid pathology compared to typical AD (Wolk, 2013), visual measures may also be affected and serve as diagnostic markers in these presentations. Another area to explore is visual biomarkers in early-onset AD characterized by autosomal dominant mutations in amyloid precursor protein and presenilin genes and late-onset AD associated with the apolipoprotein epsilon 4 allele. These genes play crucial roles in amyloid plaque accumulation in AD (Selkoe and Hardy, 2016). Since amyloid deposition tomography differs in early and late-onset AD (Cho et al., 2013), these gene mutations may be associated with differential amyloid deposition in the brain and in vision-related structures. It would be interesting to investigate if visual biomarkers can also detect early onset-AD and if these various gene mutations influence the type of visual impairment experienced.
The ability of visual biomarkers to differentiate between dementia types and MCI types has also been largely unexplored. However, there is much clinical value in finding a visual measure that can distinguish between dementia types, particularly AD from Lewy body dementia, which is often hard to clinically differentiate in early disease (McKeith et al., 2005). Lewy body dementia subjects have more impairment in retinal structure, contrast sensitivity, saccadic latencies, and color vision than their AD counterparts (Mosimann et al., 2005; Moreno-Ramos et al., 2013; Oishi et al., 2018). In particular, color vision impairment is a strong predictor of Lewy body dementia more so than in AD where color vision defects are less frequent and perhaps less pronounced (Postuma et al., 2015; Matar et al., 2019). Regarding high-level visual processing, Lewy body dementia patients also exhibit worse motion discrimination and performance on tests requiring spatial processing and object identification (Landy et al., 2015; Mitolo et al., 2016). Future studies should assess for differences in color test performance, motion perception, and visuospatial function between these two groups of patients. Antisaccade performance should also be assessed in these subject groups since this task has been successful in differentiating amnestic MCI individuals (most likely to progress to AD) from non-amnestic MCI (most likely to progress to Lewy body or frontotemporal dementia; Kelley and Petersen, 2007).
Finally, longitudinal studies examining multiple biomarkers together should be performed to establish temporality and causality. Retinal structure, vasculature, visual function, and brain changes are interlinked, but thus far have only been shown to correlate at different stages of disease. Longitudinal assessment would clarify visual changes that occur earliest in the disease process and identify those that can be used to assess disease progression. Longitudinal studies would also assess whether changes in visual measures over time can serve as biomarkers. AD participants are reported to have a faster decline in retinal thickness compared to controls (Trebbastoni et al., 2016; Santos et al., 2018). The degree of decline in visual measures may confer differential risk of disease progression and should be further investigated.
Through this review, we highlight potential afferent and efferent visual biomarkers of AD and explore their ability to detect prodromal and preclinical disease. Although more studies are needed, visual measures are promising, objective, practical, and sensitive markers of AD.
Author Contributions
All authors contributed to the article and approved the submitted version. SW was responsible for literature review, manuscript drafting, and table and figure design. AM and LB were involved in manuscript editing. SW, AM, and LB were involved in revisions. AM and LB were involved in providing oversight of this project.
Funding
This research was supported in part by the NYU CTSA grant UL1TR001445 from the National Center for Advancing Translational Sciences, National Institutes of Health as well as the NYU Alzheimer’s Disease Research Center grant P30AG066512 from the National Institute on Aging, National Institutes of Health.
Conflict of Interest
The authors declare that the research was conducted in the absence of any commercial or financial relationships that could be construed as a potential conflict of interest.
The reviewer Z-LL declared a shared affiliation, with no collaboration, with the authors to the handling editor at the time of review.
References
Akhand, O., Galetta, M. S., Cobbs, L., Hasanaj, L., Webb, N., Drattell, J., et al. (2018). The new mobile universal lexicon evaluation system (MULES): a test of rapid picture naming for concussion sized for the sidelines. J. Neurol. Sci. 387, 199–204. doi: 10.1016/j.jns.2018.02.031
Albers, M. W., Gilmore, G. C., Kaye, J., Murphy, C., Wingfield, A., Bennett, D. A., et al. (2015). At the interface of sensory and motor dysfunctions and Alzheimer’s disease. Alzheimers Dement. 11, 70–98. doi: 10.1016/j.jalz.2014.04.514
Albert, M. S., DeKosky, S. T., Dickson, D., Dubois, B., Feldman, H. H., Fox, N. C., et al. (2011). The diagnosis of mild cognitive impairment due to Alzheimer’s disease: recommendations from the national institute on aging-Alzheimer’s association workgroups on diagnostic guidelines for Alzheimer’s disease. Alzheimers Dement. 7, 270–279. doi: 10.1016/j.jalz.2011.03.008
Almeida, A. L. M., Pires, L. A., Figueiredo, E. A., Costa-Cunha, L. V. F., Zacharias, L. C., Preti, R. C., et al. (2019). Correlation between cognitive impairment and retinal neural loss assessed by swept-source optical coherence tomography in patients with mild cognitive impairment. Alzheimers Dement. 11, 659–669. doi: 10.1016/j.dadm.2019.08.006
Alzheimer’s Disease Facts and Figures (2019). Alzheimer’s Association. Available online at: www.alz.org/facts/. Accessed April 9, 2019.
Antoniades, C. A., and Kennard, C. (2015). Ocular motor abnormalities in neurodegenerative disorders. Eye 29, 200–207. doi: 10.1038/eye.2014.276
Arevalo-Rodriguez, I., Smailagic, N., Roqué, I. F. M., Ciapponi, A., Sanchez-Perez, E., Giannakou, A., et al. (2015). Mini-mental state examination (MMSE) for the detection of Alzheimer’s disease and other dementias in people with mild cognitive impairment (MCI). Cochrane Database Syst. Rev. 2015:CD010783. doi: 10.1002/14651858.CD010783.pub2
Bambo, M. P., Garcia-Martin, E., Otin, S., Pinilla, J., Larrosa, J. M., Polo, V., et al. (2015). Visual function and retinal nerve fibre layer degeneration in patients with Alzheimer disease: correlations with severity of dementia. Acta Ophthalmol. 93, e507–e508. doi: 10.1111/aos.12635
Betthauser, T. J., Koscik, R. L., Jonaitis, E. M., Allison, S. L., Cody, K. A., Erickson, C. M., et al. (2020). Amyloid and tau imaging biomarkers explain cognitive decline from late middle-age. Brain 143, 320–335. doi: 10.1093/brain/awz378
Bondareff, W., Mountjoy, C. Q., Roth, M., Rossor, M. N., Iversen, L. L., Reynolds, G. P., et al. (1987). Neuronal degeneration in locus ceruleus and cortical correlates of Alzheimer disease. Alzheimer Dis. Assoc. Disord. 1, 256–262. doi: 10.1097/00002093-198701040-00005
Boxer, A. L., Garbutt, S., Seeley, W. W., Jafari, A., Heuer, H. W., Mirsky, J., et al. (2012). Saccade abnormalities in autopsy-confirmed frontotemporal lobar degeneration and Alzheimer disease. Arch. Neurol. 69, 509–517. doi: 10.1001/archneurol.2011.1021
Braak, H., and Braak, E. (1991). Neuropathological stageing of Alzheimer-related changes. Acta Neuropathol. 82, 239–259. doi: 10.1007/bf00308809
Braak, H., and Del Tredici, K. (2011). The pathological process underlying Alzheimer’s disease in individuals under thirty. Acta Neuropathol. 121, 171–181. doi: 10.1007/s00401-010-0789-4
Braak, H., Thal, D. R., Ghebremedhin, E., and Del Tredici, K. (2011). Stages of the pathologic process in Alzheimer disease: age categories from 1 to 100 years. J. Neuropathol. Exp. Neurol. 70, 960–969. doi: 10.1097/NEN.0b013e318232a379
Brewer, A. A., and Barton, B. (2014). Visual cortex in aging and Alzheimer’s disease: changes in visual field maps and population receptive fields. Front. Psychol. 5:74. doi: 10.3389/fpsyg.2014.00074
Bulut, M., Kurtulus, F., Gozkaya, O., Erol, M. K., Cengiz, A., Akidan, M., et al. (2018). Evaluation of optical coherence tomography angiographic findings in Alzheimer’s type dementia. Br. J. Ophthalmol. 102, 233–237. doi: 10.1136/bjophthalmol-2017-310476
Burgansky-Eliash, Z., Wollstein, G., Chu, T., Ramsey, J. D., Glymour, C., Noecker, R. J., et al. (2005). Optical coherence tomography machine learning classifiers for glaucoma detection: a preliminary study. Invest. Ophthalmol. Vis. Sci. 46, 4147–4152. doi: 10.1167/iovs.05-0366
Campbell, J. P., Zhang, M., Hwang, T. S., Bailey, S. T., Wilson, D. J., Jia, Y., et al. (2017). Detailed vascular anatomy of the human retina by projection-resolved optical coherence tomography angiography. Sci. Rep. 7:42201. doi: 10.1038/srep42201
Casaletto, K. B., Ward, M. E., Baker, N. S., Bettcher, B. M., Gelfand, J. M., Li, Y., et al. (2017). Retinal thinning is uniquely associated with medial temporal lobe atrophy in neurologically normal older adults. Neurobiol. Aging 51, 141–147. doi: 10.1016/j.neurobiolaging.2016.12.011
Chan, V. T. T., Sun, Z., Tang, S., Chen, L. J., Wong, A., Tham, C. C., et al. (2019). Spectral-domain OCT measurements in Alzheimer’s disease: a systematic review and meta-analysis. Ophthalmology 126, 497–510. doi: 10.1016/j.ophtha.2018.08.009
Chang, L. Y. L., Lowe, J., Ardiles, A., Lim, J., Grey, A. C., Robertson, K., et al. (2014). Alzheimer’s disease in the human eye. Clinical tests that identify ocular and visual information processing deficit as biomarkers. Alzheimers Dement. 10, 251–261. doi: 10.1016/j.jalz.2013.06.004
Chehrehnegar, N., Nejati, V., Shati, M., Esmaeili, M., Rezvani, Z., Haghi, M., et al. (2019). Behavioral and cognitive markers of mild cognitive impairment: diagnostic value of saccadic eye movements and simon task. Aging Clin. Exp. Res. 31, 1591–1600. doi: 10.1007/s40520-019-01121-w
Cho, H., Seo, S. W., Kim, J.-H., Suh, M. K., Lee, J.-H., Choe, Y. S., et al. (2013). Amyloid deposition in early onset versus late onset alzheimer’s disease. J. Alzheimers Dis. 35, 813–821. doi: 10.3233/jad-121927
Choi, S. H., Park, S. J., and Kim, N. R. (2016). Macular ganglion cell -inner plexiform layer thickness is associated with clinical progression in mild cognitive impairment and Alzheimers disease. PLoS One 11:e0162202. doi: 10.1371/journal.pone.0162202
Conrath, J., Giorgi, R., Raccah, D., and Ridings, B. (2005). Foveal avascular zone in diabetic retinopathy: quantitative vs. qualitative assessment. Eye 19, 322–326. doi: 10.1038/sj.eye.6701456
Conway, J., Ilardi, M., Gonzalez, C., Dahan, N., Fallon, S., Moehringer, N., et al. (2020). Rapid picture naming in Parkinson’s disease using the mobile universal lexicon evaluation system (MULES). J. Neurol. Sci. 410:116680. doi: 10.1016/j.jns.2020.116680
Coppola, G., Di Renzo, A., Ziccardi, L., Martelli, F., Fadda, A., Manni, G., et al. (2015). Optical coherence tomography in Alzheimer’s disease: a meta-analysis. PLoS One 10:e0134750. doi: 10.1371/journal.pone.0134750
Crawford, T. J., Higham, S., Mayes, J., Dale, M., Shaunak, S., and Lekwuwa, G. (2013). The role of working memory and attentional disengagement on inhibitory control: effects of aging and Alzheimer’s disease. Age 35, 1637–1650. doi: 10.1007/s11357-012-9466-y
Crawford, T. J., Higham, S., Renvoize, T., Patel, J., Dale, M., Suriya, A., et al. (2005). Inhibitory control of saccadic eye movements and cognitive impairment in Alzheimer’s disease. Biol. Psychiatry 57, 1052–1060. doi: 10.1016/j.biopsych.2005.01.017
Cunha, L. P., Lopes, L. C., Costa-Cunha, L. V., Costa, C. F., Pires, L. A., Almeida, A. L., et al. (2016). Macular thickness measurements with frequency domain-OCT for quantification of retinal neural loss and its correlation with cognitive impairment in Alzheimer’s disease. PLoS One 11:e0153830. doi: 10.1371/journal.pone.0153830
Dargent-Molina, P., Hays, M., and Bréart, G. (1996). Sensory impairments and physical disability in aged women living at home. Int. J. Epidemiol. 25, 621–629. doi: 10.1093/ije/25.3.621
del Valle, J., Duran-Vilaregut, J., Manich, G., Pallàs, M., Camins, A., Vilaplana, J., et al. (2011). Cerebral amyloid angiopathy, blood-brain barrier disruption and amyloid accumulation in SAMP8 mice. Neurodegener. Dis. 8, 421–429. doi: 10.1159/000324757
den Haan, J., Csinscik, L., Parker, T., Paterson, R. W., Slattery, C. F., Foulkes, A., et al. (2019). Retinal thickness as potential biomarker in posterior cortical atrophy and typical Alzheimer’s disease. Alzheimers Res. Ther. 11:62. doi: 10.1186/s13195-019-0516-x
den Haan, J., Morrema, T. H. J., Verbraak, F. D., de Boer, J. F., Scheltens, P., Rozemuller, A. J., et al. (2018). Amyloid-beta and phosphorylated tau in post-mortem Alzheimer’s disease retinas. Acta Neuropathol. Commun. 6:147. doi: 10.1186/s40478-018-0650-x
den Haan, J., Verbraak, F. D., Visser, P. J., and Bouwman, F. H. (2017). Retinal thickness in Alzheimer’s disease: a systematic review and meta-analysis. Alzheimers Dement. 6, 162–170. doi: 10.1016/j.dadm.2016.12.014
Deng, Y., Shi, L., Lei, Y., Liang, P., Li, K., Chu, W. C., et al. (2016). Mapping the “what” and “where” visual cortices and their atrophy in Alzheimer’s disease: combined activation likelihood estimation with voxel-based morphometry. Front. Hum. Neurosci. 10:333. doi: 10.3389/fnhum.2016.00333
Devlin, J. T., and Price, C. J. (2007). Perirhinal contributions to human visual perception. Curr. Biol. 17, 1484–1488. doi: 10.1016/j.cub.2007.07.066
El Kadmiri, N., Said, N., Slassi, I., El Moutawakil, B., and Nadifi, S. (2018). Biomarkers for Alzheimer Disease: classical and novel candidates’ review. Neuroscience 370, 181–190. doi: 10.1016/j.neuroscience.2017.07.017
Elliott, D. B., Hurst, M. A., and Weatherill, J. (1990). Comparing clinical tests of visual function in cataract with the patient’s perceived visual disability. Eye 4, 712–717. doi: 10.1038/eye.1990.100
Elman, J. A., Panizzon, M. S., Hagler, D. J. Jr., Eyler, L. T., Granholm, E. L., Fennema-Notestine, C., et al. (2017). Task-evoked pupil dilation and BOLD variance as indicators of locus coeruleus dysfunction. Cortex 97, 60–69. doi: 10.1016/j.cortex.2017.09.025
Fagan, A. M., Roe, C. M., Xiong, C., Mintun, M. A., Morris, J. C., and Holtzman, D. M. (2007). Cerebrospinal fluid tau/beta-amyloid(42) ratio as a prediction of cognitive decline in nondemented older adults. Arch. Neurol. 64, 343–349. doi: 10.1001/archneur.64.3.noc60123
Feke, G. T., Hyman, B. T., Stern, R. A., and Pasquale, L. R. (2015). Retinal blood flow in mild cognitive impairment and Alzheimer’s disease. Alzheimers Dement. 1, 144–151. doi: 10.1016/j.dadm.2015.01.004
Felleman, D. J., and Van Essen, D. C. (1991). Distributed hierarchical processing in the primate cerebral cortex. Cereb. Cortex 1, 1–47. doi: 10.1093/cercor/1.1.1
Ferrari, L., Huang, S. C., Magnani, G., Ambrosi, A., Comi, G., and Leocani, L. (2017). Optical coherence tomography reveals retinal neuroaxonal thinning in frontotemporal dementia as in Alzheimer’s disease. J. Alzheimers Dis. 56, 1101–1107. doi: 10.3233/jad-160886
Fischer, P., Jungwirth, S., Zehetmayer, S., Weissgram, S., Hoenigschnabl, S., Gelpi, E., et al. (2007). Conversion from subtypes of mild cognitive impairment to Alzheimer dementia. Neurology 68, 288–291. doi: 10.1212/01.wnl.0000252358.03285.9d
Fotiou, D., Kaltsatou, A., Tsiptsios, D., and Nakou, M. (2015). Evaluation of the cholinergic hypothesis in Alzheimer’s disease with neuropsychological methods. Aging Clin. Exp. Res. 27, 727–733. doi: 10.1007/s40520-015-0321-8
Fotiou, D. F., Brozou, C. G., Haidich, A. B., Tsiptsios, D., Nakou, M., Kabitsi, A., et al. (2007). Pupil reaction to light in Alzheimer’s disease: evaluation of pupil size changes and mobility. Aging Clin. Exp. Res. 19, 364–371. doi: 10.1007/bf03324716
Fotiou, F., Fountoulakis, K. N., Tsolaki, M., Goulas, A., and Palikaras, A. (2000). Changes in pupil reaction to light in Alzheimer’s disease patients: a preliminary report. Int. J. Psychophysiol. 37, 111–120. doi: 10.1016/s0167-8760(00)00099-4
Frost, S., Robinson, L., Rowe, C. C., Ames, D., Masters, C. L., Taddei, K., et al. (2017). Evaluation of cholinergic deficiency in preclinical Alzheimer’s disease using pupillometry. J. Ophthalmol. 2017:7935406. doi: 10.1155/2017/7935406
Galetta, K. M., Chapman, K. R., Essis, M. D., Alosco, M. L., Gillard, D., Steinberg, E., et al. (2017). Screening utility of the king-devick test in mild cognitive impairment and Alzheimer Disease dementia. Alzheimer Dis. Assoc. Disord. 31, 152–158. doi: 10.1097/wad.0000000000000157
Garbutt, S., Matlin, A., Hellmuth, J., Schenk, A. K., Johnson, J. K., Rosen, H., et al. (2008). Oculomotor function in frontotemporal lobar degeneration, related disorders and Alzheimer’s disease. Brain 131, 1268–1281. doi: 10.1093/brain/awn047
Garcia-Martin, E., Bambo, M. P., Marques, M. L., Satue, M., Otin, S., Larrosa, J. M., et al. (2016). Ganglion cell layer measurements correlate with disease severity in patients with Alzheimer’s disease. Acta Ophthalmol. 94, e454–e459. doi: 10.1111/aos.12977
Gaynor, L. S., CurielCid, R. E., Penate, A., Rosselli, M., Burke, S. N., Wicklund, M., et al. (2019). Visual object discrimination impairment as an early predictor of mild cognitive impairment and Alzheimer’s disease. J. Int. Neuropsychol. Soc. 25, 688–698. doi: 10.1017/s1355617719000316
Gimenez Castejon, D., Dudekova, M., Gomez Gallego, M., and Lajara Blesa, J. (2016). Macular thickness in subjective memory complaints and mild cognitive impairment: a non-invasive biomarker. Neuroophthalmology 40, 16–22. doi: 10.3109/01658107.2015.1118516
Girard, B., and Berthoz, A. (2005). From brainstem to cortex: computational models of saccade generation circuitry. Prog. Neurobiol. 77, 215–251. doi: 10.1016/j.pneurobio.2005.11.001
Granholm, E., Morris, S., Galasko, D., Shults, C., Rogers, E., and Vukov, B. (2003). Tropicamide effects on pupil size and pupillary light reflexes in Alzheimer’s and Parkinson’s disease. Int. J. Psychophysiol. 47, 95–115. doi: 10.1016/s0167-8760(02)00122-8
Granholm, E. L., Panizzon, M. S., Elman, J. A., Jak, A. J., Hauger, R. L., Bondi, M. W., et al. (2017). Pupillary responses as a biomarker of early risk for Alzheimer’s disease. J. Alzheimers Dis. 56, 1419–1428. doi: 10.3233/jad-161078
Grudzien, A., Shaw, P., Weintraub, S., Bigio, E., Mash, D. C., and Mesulam, M. M. (2007). Locus coeruleus neurofibrillary degeneration in aging, mild cognitive impairment and early Alzheimer’s disease. Neurobiol. Aging 28, 327–335. doi: 10.1016/j.neurobiolaging.2006.02.007
Haan, J. D., van de Kreeke, J. A., Konijnenberg, E., Kate, M. T., Braber, A. D., Barkhof, F., et al. (2019a). Retinal thickness as a potential biomarker in patients with amyloid-proven early- and late-onset Alzheimer’s disease. Alzheimers Dement. 11, 463–471. doi: 10.1016/j.dadm.2019.05.002
Haan, J. D., van de Kreeke, J. A., van Berckel, B. N., Barkhof, F., Teunissen, C. E., Scheltens, P., et al. (2019b). Is retinal vasculature a biomarker in amyloid proven Alzheimer’s disease? Alzheimers Dement. 11, 383–391. doi: 10.1016/j.dadm.2019.03.006
Hadoux, X., Hui, F., Lim, J. K. H., Masters, C. L., Pébay, A., Chevalier, S., et al. (2019). Non-invasive in vivo hyperspectral imaging of the retina for potential biomarker use in Alzheimer’s disease. Nat. Commun. 10:4227. doi: 10.1038/s41467-019-12242-1
Hardy, J., and Selkoe, D. J. (2002). The amyloid hypothesis of Alzheimer’s disease: progress and problems on the road to therapeutics. Science 297, 353–356. doi: 10.1126/science.1072994
Heuer, H. W., Mirsky, J. B., Kong, E. L., Dickerson, B. C., Miller, B. L., Kramer, J. H., et al. (2013). Antisaccade task reflects cortical involvement in mild cognitive impairment. Neurology 81, 1235–1243. doi: 10.1212/WNL.0b013e3182a6cbfe
Hinton, D. R., Sadun, A. A., Blanks, J. C., and Miller, C. A. (1986). Optic-nerve degeneration in Alzheimer’s disease. N. Engl. J. Med. 315, 485–487. doi: 10.1056/nejm198608213150804
Holden, J. G., Cosnard, A., Laurens, B., Asselineau, J., Biotti, D., Cubizolle, S., et al. (2018). Prodromal Alzheimer’s disease demonstrates increased errors at a simple and automated anti-saccade task. J. Alzheimers Dis. 65, 1209–1223. doi: 10.3233/jad-180082
Hutton, J. T., Nagel, J. A., and Loewenson, R. B. (1984). Eye tracking dysfunction in Alzheimer-type dementia. Neurology 34, 99–102. doi: 10.1212/wnl.34.1.99
Hutton, S. B., and Ettinger, U. (2006). The antisaccade task as a research tool in psychopathology: a critical review. Psychophysiology 43, 302–313. doi: 10.1111/j.1469-8986.2006.00403.x
Jack, C. R. Jr., Bennett, D. A., Blennow, K., Carrillo, M. C., Dunn, B., Haeberlein, S. B., et al. (2018). NIA-AA research framework: toward a biological definition of Alzheimer’s disease. Alzheimers Dement. 14, 535–562. doi: 10.1016/j.jalz.2018.02.018
Jack, C. R. Jr., Knopman, D. S., Jagust, W. J., Petersen, R. C., Weiner, M. W., Aisen, P. S., et al. (2013). Tracking pathophysiological processes in Alzheimer’s disease: an updated hypothetical model of dynamic biomarkers. Lancet Neurol. 12, 207–216. doi: 10.1016/s1474-4422(12)70291-0
Jack, C. R. Jr., Lowe, V. J., Weigand, S. D., Wiste, H. J., Senjem, M. L., Knopman, D. S., et al. (2009). Serial PIB and MRI in normal, mild cognitive impairment and Alzheimer’s disease: implications for sequence of pathological events in Alzheimer’s disease. Brain 132, 1355–1365. doi: 10.1093/brain/awp062
Janez-Escalada, L., Janez-Garcia, L., Salobrar-Garcia, E., Santos-Mayo, A., de Hoz, R., Yubero, R., et al. (2019). Spatial analysis of thickness changes in ten retinal layers of Alzheimer’s disease patients based on optical coherence tomography. Sci. Rep. 9:13000. doi: 10.1038/s41598-019-49353-0
Javaid, F. Z., Brenton, J., Guo, L., and Cordeiro, M. F. (2016). Visual and ocular manifestations of Alzheimer’s disease and their use as biomarkers for diagnosis and progression. Front. Neurol. 7:55. doi: 10.3389/fneur.2016.00055
Jessen, F., Amariglio, R. E., Buckley, R. F., van der Flier, W. M., Han, Y., Molinuevo, J. L., et al. (2020). The characterisation of subjective cognitive decline. Lancet Neurol. 19, 271–278. doi: 10.1016/s1474-4422(19)30368-0
Jiang, H., Liu, Y., Wei, Y., Shi, Y., Wright, C. B., Sun, X., et al. (2018a). Impaired retinal microcirculation in patients with Alzheimer’s disease. PLoS One 13:e0192154. doi: 10.1371/journal.pone.0192154
Jiang, H., Wei, Y., Shi, Y., Wright, C. B., Sun, X., Gregori, G., et al. (2018b). Altered macular microvasculature in mild cognitive impairment and Alzheimer disease. J. Neuroophthalmol. 38, 292–298. doi: 10.1097/wno.0000000000000580
Jung, N. Y., Han, J. C., Ong, Y. T., Cheung, C. Y., Chen, C. P., Wong, T. Y., et al. (2019). Retinal microvasculature changes in amyloid-negative subcortical vascular cognitive impairment compared to amyloid-positive Alzheimer’s disease. J. Neurol. Sci. 396, 94–101. doi: 10.1016/j.jns.2018.10.025
Kahana Levy, N., Lavidor, M., and Vakil, E. (2018). Prosaccade and antisaccade paradigms in persons with Alzheimer’s disease: a meta-analytic review. Neuropsychol. Rev. 28, 16–31. doi: 10.1007/s11065-017-9362-4
Kapoula, Z., Yang, Q., Otero-Millan, J., Xiao, S., Macknik, S. L., Lang, A., et al. (2014). Distinctive features of microsaccades in Alzheimer’s disease and in mild cognitive impairment. Age 36, 535–543. doi: 10.1007/s11357-013-9582-3
Kashani, A. H., Chen, C. L., Gahm, J. K., Zheng, F., Richter, G. M., Rosenfeld, P. J., et al. (2017). Optical coherence tomography angiography: a comprehensive review of current methods and clinical applications. Prog. Retin. Eye Res. 60, 66–100. doi: 10.1016/j.preteyeres.2017.07.002
Katz, B., and Rimmer, S. (1989). Ophthalmologic manifestations of Alzheimer’s disease. Surv. Ophthalmol. 34, 31–43. doi: 10.1016/0039-6257(89)90127-6
Kelley, B. J., and Petersen, R. C. (2007). Alzheimer’s disease and mild cognitive impairment. Neurol. Clin. 25, 577–609. doi: 10.1016/j.ncl.2007.03.008
Kim, J. I., and Kang, B. H. (2019). Decreased retinal thickness in patients with Alzheimer’s disease is correlated with disease severity. PLoS One 14:e0224180. doi: 10.1371/journal.pone.0224180
Kirby, E., Bandelow, S., and Hogervorst, E. (2010). Visual impairment in Alzheimer’s disease: a critical review. J. Alzheimers Dis. 21, 15–34. doi: 10.3233/jad-2010-080785
Knoll, B., Simonett, J., Volpe, N. J., Farsiu, S., Ward, M., Rademaker, A., et al. (2016). Retinal nerve fiber layer thickness in amnestic mild cognitive impairment: case-control study and meta-analysis. Alzheimers Dement. 4, 85–93. doi: 10.1016/j.dadm.2016.07.004
Ko, F., Muthy, Z. A., Gallacher, J., Sudlow, C., Rees, G., Yang, Q., et al. (2018). Association of retinal nerve fiber layer thinning with current and future cognitive decline: a study using optical coherence tomography. JAMA Neurol. 75, 1198–1205. doi: 10.1001/jamaneurol.2018.1578
Koronyo, Y., Biggs, D., Barron, E., Boyer, D. S., Pearlman, J. A., Au, W. J., et al. (2017). Retinal amyloid pathology and proof-of-concept imaging trial in Alzheimer’s disease. JCI Insight 2:e93621. doi: 10.1172/jci.insight.93621
Koronyo-Hamaoui, M., Koronyo, Y., Ljubimov, A. V., Miller, C. A., Ko, M. K., Black, K. L., et al. (2011). Identification of amyloid plaques in retinas from Alzheimer’s patients and noninvasive in vivo optical imaging of retinal plaques in a mouse model. NeuroImage 54, S204–S217. doi: 10.1016/j.neuroimage.2010.06.020
Kremen, W. S., Panizzon, M. S., Elman, J. A., Granholm, E. L., Andreassen, O. A., Dale, A. M., et al. (2019). Pupillary dilation responses as a midlife indicator of risk for Alzheimer’s disease: association with Alzheimer’s disease polygenic risk. Neurobiol. Aging 83, 114–121. doi: 10.1016/j.neurobiolaging.2019.09.001
Kusne, Y., Wolf, A. B., Townley, K., Conway, M., and Peyman, G. A. (2017). Visual system manifestations of Alzheimer’s disease. Acta Ophthalmol. 95, e668–e676. doi: 10.1111/aos.13319
Kwon, J. Y., Yang, J. H., Han, J. S., and Kim, D. G. (2017). Analysis of the retinal nerve fiber layer thickness in Alzheimer disease and mild cognitive impairment. Korean J. Ophthalmol. 31, 548–556. doi: 10.3341/kjo.2016.0118
La Morgia, C., Ross-Cisneros, F. N., Koronyo, Y., Hannibal, J., Gallassi, R., Cantalupo, G., et al. (2016). Melanopsin retinal ganglion cell loss in Alzheimer disease. Ann. Neurol. 79, 90–109. doi: 10.1002/ana.24548
Lad, E. M., Mukherjee, D., Stinnett, S. S., Cousins, S. W., Potter, G. G., Burke, J. R., et al. (2018). Evaluation of inner retinal layers as biomarkers in mild cognitive impairment to moderate Alzheimer’s disease. PLoS One 13:e0192646. doi: 10.1371/journal.pone.0192646
Lahme, L., Esser, E. L., Mihailovic, N., Schubert, F., Lauermann, J., Johnen, A., et al. (2018). Evaluation of ocular perfusion in Alzheimer’s disease using optical coherence tomography angiography. J. Alzheimers Dis. 66, 1745–1752. doi: 10.3233/jad-180738
Landy, K. M., Salmon, D. P., Galasko, D., Filoteo, J. V., Festa, E. K., Heindel, W. C., et al. (2015). Motion discrimination in dementia with Lewy bodies and Alzheimer disease. Neurology 85, 1376–1382. doi: 10.1212/wnl.0000000000002028
Lemos, R., Figueiredo, P., Santana, I., Simões, M. R., and Castelo-Branco, M. (2012). Temporal integration of 3D coherent motion cues defining visual objects of unknown orientation is impaired in amnestic mild cognitive impairment and Alzheimer’s disease. J. Alzheimers Dis. 28, 885–896. doi: 10.3233/jad-2011-110719
Lemos, R., Santana, I., Caetano, G., Bernardino, I., Morais, R., Farivar, R., et al. (2016). Three-dimensional face recognition in mild cognitive impairment: a psychophysical and structural MR study. J. Int. Neuropsychol. Soc. 22, 744–754. doi: 10.1017/s135561771600059X
Li, Y., Guo, S., Wang, Y., and Chen, H. (2017). Altered motion repulsion in Alzheimer’s disease. Sci. Rep. 7:40946. doi: 10.1038/srep40946
Liu, Y., Feng, D., and Wang, H. (2019). Distinct forms of motion sensitivity impairments in Alzheimer’s disease. Sci. Rep. 9:12931. doi: 10.1038/s41598-019-48942-3
Lopez-de-Eguileta, A., Lage, C., Lopez-Garcia, S., Pozueta, A., Garcia-Martinez, M., Kazimierczak, M., et al. (2019). Ganglion cell layer thinning in prodromal Alzheimer’s disease defined by amyloid PET. Alzheimers Dement. 5, 570–578. doi: 10.1016/j.trci.2019.08.008
Massoud, F., Chertkow, H., Whitehead, V., Overbury, O., and Bergman, H. (2002). Word-reading thresholds in Alzheimer disease and mild memory loss: a pilot study. Alzheimer Dis. Assoc. Disord. 16, 31–39. doi: 10.1097/00002093-200201000-00005
Matar, E., Phillips, J. R., Martens, K. A. E., Halliday, G. M., and Lewis, S. J. G. (2019). Impaired color discrimination-a specific marker of hallucinations in lewy body disorders. J. Geriatr. Psychiatry Neurol. 32, 257–264. doi: 10.1177/0891988719845501
McKee, A. C., Au, R., Cabral, H. J., Kowall, N. W., Seshadri, S., Kubilus, C. A., et al. (2006). Visual association pathology in preclinical Alzheimer disease. J. Neuropathol. Exp. Neurol. 65, 621–630. doi: 10.1097/00005072-200606000-00010
McKeith, I. G., Dickson, D. W., Lowe, J., Emre, M., O’Brien, J. T., Feldman, H., et al. (2005). Diagnosis and management of dementia with lewy bodies: third report of the DLB Consortium. Neurology 65, 1863–1872. doi: 10.1212/01.wnl.0000187889.17253.b1
McKhann, G. M., Knopman, D. S., Chertkow, H., Hyman, B. T., Jack, C. R. Jr., Kawas, C. H., et al. (2011). The diagnosis of dementia due to Alzheimer’s disease: recommendations from the national institute on aging-Alzheimer’s association workgroups on diagnostic guidelines for Alzheimer’s disease. Alzheimers Dement. 7, 263–269. doi: 10.1016/j.jalz.2011.03.005
Méndez-Gómez, J. L., Pelletier, A., Rougier, M.-B., Korobelnik, J.-F., Schweitzer, C., Delyfer, M. N., et al. (2018). Association of retinal nerve fiber layer thickness with brain alterations in the visual and limbic networks in elderly adults without dementia. JAMA Netw. Open 1:e184406. doi: 10.1001/jamanetworkopen.2018.4406
Milner, A. D., and Goodale, M. (1995). The Visual Brain in Action. Oxford, UK: Oxford University Press.
Mitolo, M., Hamilton, J. M., Landy, K. M., Hansen, L. A., Galasko, D., Pazzaglia, F., et al. (2016). Visual perceptual organization ability in autopsy-verified dementia with lewy bodies and Alzheimer’s disease. J. Int. Neuropsychol. Soc. 22, 609–619. doi: 10.1017/s1355617716000436
Moreno-Ramos, T., Benito-León, J., Villarejo, A., and Bermejo-Pareja, F. (2013). Retinal nerve fiber layer thinning in dementia associated with Parkinson’s disease, dementia with Lewy bodies and Alzheimer’s disease. J. Alzheimers Dis. 34, 659–664. doi: 10.3233/jad-121975
Moritz, C. H., Johnson, S. C., McMillan, K. M., Haughton, V. M., and Meyerand, M. E. (2004). Functional MRI neuroanatomic correlates of the hooper visual organization test. J. Int. Neuropsychol. Soc. 10, 939–947. doi: 10.1017/s1355617704107042
Mosimann, U. P., Müri, R. M., Burn, D. J., Felblinger, J., O’Brien, J. T., and McKeith, I. G. (2005). Saccadic eye movement changes in Parkinson’s disease dementia and dementia with lewy bodies. Brain 128, 1267–1276. doi: 10.1093/brain/awh484
Murphy, M. P., and LeVine, H. III. (2010). Alzheimer’s disease and the amyloid-beta peptide. J. Alzheimers Dis. 19, 311–323. doi: 10.3233/jad-2010-1221
Mutlu, U., Bonnemaijer, P. W. M., Ikram, M. A., Colijn, J. M., Cremers, L. G. M., Buitendijk, G. H. S., et al. (2017). Retinal neurodegeneration and brain MRI markers: the rotterdam study. Neurobiol. Aging 60, 183–191. doi: 10.1016/j.neurobiolaging.2017.09.003
Mutlu, U., Colijn, J. M., Ikram, M. A., Bonnemaijer, P. W. M., Licher, S., Wolters, F. J., et al. (2018a). Association of retinal neurodegeneration on optical coherence tomography with dementia: a population-based study. JAMA Neurol. 75, 1256–1263. doi: 10.1001/jamaneurol.2018.1563
Mutlu, U., Ikram, M. K., Roshchupkin, G. V., Bonnemaijer, P. W. M., Colijn, J. M., Vingerling, J. R., et al. (2018b). Thinner retinal layers are associated with changes in the visual pathway: a population-based study. Hum. Brain Mapp. 39, 4290–4301. doi: 10.1002/hbm.24246
Nikolakopoulou, A. M., Zhao, Z., Montagne, A., and Zlokovic, B. V. (2017). Regional early and progressive loss of brain pericytes but not vascular smooth muscle cells in adult mice with disrupted platelet-derived growth factor receptor-β signaling. PLoS One 12:e0176225. doi: 10.1371/journal.pone.0176225
Noiret, N., Carvalho, N., Laurent, É., Chopard, G., Binetruy, M., Nicolier, M., et al. (2018). Saccadic eye movements and attentional control in Alzheimer’s disease. Arch. Clin. Neuropsychol. 33, 1–13. doi: 10.1093/arclin/acx044
O’Bryhim, B. E., Apte, R. S., Kung, N., Coble, D., and Van Stavern, G. P. (2018). Association of preclinical Alzheimer disease with optical coherence tomographic angiography findings. JAMA Ophthalmol. 136, 1242–1248. doi: 10.1001/jamaophthalmol.2018.3556
Oishi, Y., Imamura, T., Shimomura, T., and Suzuki, K. (2018). Visual texture agnosia in dementia with lewy bodies and Alzheimer’s disease. Cortex 103, 277–290. doi: 10.1016/j.cortex.2018.03.018
Oktem, E. O., Derle, E., Kibaroglu, S., Oktem, C., Akkoyun, I., and Can, U. (2015). The relationship between the degree of cognitive impairment and retinal nerve fiber layer thickness. Neurol. Sci. 36, 1141–1146. doi: 10.1007/s10072-014-2055-3
Pache, M., Smeets, C. H., Gasio, P. F., Savaskan, E., Flammer, J., Wirz-Justice, A., et al. (2003). Colour vision deficiencies in Alzheimer’s disease. Age Ageing 32, 422–426. doi: 10.1093/ageing/32.4.422
Patton, N., Aslam, T., Macgillivray, T., Pattie, A., Deary, I. J., and Dhillon, B. (2005). Retinal vascular image analysis as a potential screening tool for cerebrovascular disease: a rationale based on homology between cerebral and retinal microvasculatures. J. Anat. 206, 319–348. doi: 10.1111/j.1469-7580.2005.00395.x
Peltsch, A., Hemraj, A., Garcia, A., and Munoz, D. P. (2014). Saccade deficits in amnestic mild cognitive impairment resemble mild Alzheimer’s disease. Eur. J. Neurosci. 39, 2000–2013. doi: 10.1111/ejn.12617
Petersen, R. C., Wiste, H. J., Weigand, S. D., Rocca, W. A., Roberts, R. O., Mielke, M. M., et al. (2016). Association of elevated amyloid levels with cognition and biomarkers in cognitively normal people from the community. JAMA Neurol. 73, 85–92. doi: 10.1001/jamaneurol.2015.3098
Pierrot-Deseilligny, C., Rivaud, S., Gaymard, B., Müri, R., and Vermersch, A. I. (1995). Cortical control of saccades. Ann. Neurol. 37, 557–567. doi: 10.1002/ana.410370504
Pillai, J. A., Bermel, R., Bonner-Jackson, A., Rae-Grant, A., Fernandez, H., Bena, J., et al. (2016). Retinal nerve fiber layer thinning in Alzheimer’s disease: a case-control study in comparison to normal aging, Parkinson’s disease and non-Alzheimer’s dementia. Am. J. Alzheimers Dis. Other Demen. 31, 430–436. doi: 10.1177/1533317515628053
Polo, V., Rodrigo, M. J., Garcia-Martin, E., Otin, S., Larrosa, J. M., Fuertes, M. I., et al. (2017). Visual dysfunction and its correlation with retinal changes in patients with Alzheimer’s disease. Eye 31, 1034–1041. doi: 10.1038/eye.2017.23
Poroy, C., and Yucel, A. A. (2018). Optical coherence tomography: is really a new biomarker for Alzheimer’s disease?. Ann. Indian Acad. Neurol. 21, 119–125. doi: 10.4103/aian.AIAN_368_17
Porter, G., Wattam-Bell, J., Bayer, A., Haworth, J., Braddick, O., Atkinson, J., et al. (2017). Different trajectories of decline for global form and global motion processing in aging, mild cognitive impairment and Alzheimer’s disease. Neurobiol. Aging 56, 17–24. doi: 10.1016/j.neurobiolaging.2017.03.004
Postuma, R. B., Gagnon, J. F., Bertrand, J. A., Génier Marchand, D., and Montplaisir, J. Y. (2015). Parkinson risk in idiopathic REM sleep behavior disorder: preparing for neuroprotective trials. Neurology 84, 1104–1113. doi: 10.1212/wnl.0000000000001364
Prasad, S., and Galetta, S. L. (2011). Anatomy and physiology of the afferent visual system. Handb. Clin. Neurol. 102, 3–19. doi: 10.1016/b978-0-444-52903-9.00007-8
Querques, G., Borrelli, E., Sacconi, R., De Vitis, L., Leocani, L., Santangelo, R., et al. (2019). Functional and morphological changes of the retinal vessels in Alzheimer’s disease and mild cognitive impairment. Sci. Rep. 9:63. doi: 10.1038/s41598-018-37271-6
Risacher, S. L., Wudunn, D., Pepin, S. M., MaGee, T. R., McDonald, B. C., Flashman, L. A., et al. (2013). Visual contrast sensitivity in Alzheimer’s disease, mild cognitive impairment and older adults with cognitive complaints. Neurobiol. Aging 34, 1133–1144. doi: 10.1016/j.neurobiolaging.2012.08.007
Risacher, S. L., WuDunn, D., Tallman, E. F., West, J. D., Gao, S., Farlow, M. R., et al. (2020). Visual contrast sensitivity is associated with the presence of cerebral amyloid and tau deposition. Brain Commun. 2:fcaa019. doi: 10.1093/braincomms/fcaa019
Roe, C. M., Fagan, A. M., Grant, E. A., Hassenstab, J., Moulder, K. L., Maue Dreyfus, D., et al. (2013). Amyloid imaging and CSF biomarkers in predicting cognitive impairment up to 7.5 years later. Neurology 80, 1784–1791. doi: 10.1212/WNL.0b013e3182918ca6
Salobrar-García, E., de Hoz, R., Ramirez, A. I., López-Cuenca, I., Rojas, P., Vazirani, R., et al. (2019). Changes in visual function and retinal structure in the progression of Alzheimer’s disease. PLoS One 14:e0220535. doi: 10.1371/journal.pone.0220535
Sánchez, D., Castilla-Marti, M., Marquié, M., Valero, S., Moreno-Grau, S., Rodríguez-Gómez, O., et al. (2020). Evaluation of macular thickness and volume tested by optical coherence tomography as biomarkers for Alzheimer’s disease in a memory clinic. Sci. Rep. 10:1580. doi: 10.1038/s41598-020-58399-4
Sánchez, D., Castilla-Marti, M., Rodríguez-Gómez, O., Valero, S., Piferrer, A., Martínez, G., et al. (2018). Usefulness of peripapillary nerve fiber layer thickness assessed by optical coherence tomography as a biomarker for Alzheimer’s disease. Sci. Rep. 8:16345. doi: 10.1038/s41598-018-34577-3
Santos, C. Y., Johnson, L. N., Sinoff, S. E., Festa, E. K., Heindel, W. C., and Snyder, P. J. (2018). Change in retinal structural anatomy during the preclinical stage of Alzheimer’s disease. Alzheimers Dement. 10, 196–209. doi: 10.1016/j.dadm.2018.01.003
Schallek, J., Geng, Y., Nguyen, H., and Williams, D. R. (2013). Morphology and topography of retinal pericytes in the living mouse retina using in vivo adaptive optics imaging and ex vivo characterization. Invest. Ophthalmol. Vis. Sci. 54, 8237–8250. doi: 10.1167/iovs.13-12581
Seay, M., Akhand, O., Galetta, M. S., Cobbs, L., Hasanaj, L., Amorapanth, P., et al. (2018). Mobile universal lexicon evaluation system (MULES) in MS: evaluation of a new visual test of rapid picture naming. J. Neurol. Sci. 394, 1–5. doi: 10.1016/j.jns.2018.08.019
Selkoe, D. J., and Hardy, J. (2016). The amyloid hypothesis of Alzheimer’s disease at 25 years. EMBO Mol. Med. 8, 595–608. doi: 10.15252/emmm.201606210
Shao, Y., Jiang, H., Wei, Y., Shi, Y., Shi, C., Wright, C. B., et al. (2018). Visualization of focal thinning of the ganglion cell-inner plexiform layer in patients with mild cognitive impairment and Alzheimer’s disease. J. Alzheimers Dis. 64, 1261–1273. doi: 10.3233/jad-180070
Shi, H., Koronyo, Y., Rentsendorj, A., Regis, G. C., Sheyn, J., Fuchs, D. T., et al. (2020a). Identification of early pericyte loss and vascular amyloidosis in Alzheimer’s disease retina. Acta Neuropathol. 139, 813–836. doi: 10.1007/s00401-020-02134-w
Shi, Z., Cao, X., Hu, J., Jiang, L., Mei, X., Zheng, H., et al. (2020b). Retinal nerve fiber layer thickness is associated with hippocampus and lingual gyrus volumes in nondemented older adults. Prog. Neuropsychopharmacol. Biol. Psychiatry 99:109824. doi: 10.1016/j.pnpbp.2019.109824
Sone, D., Imabayashi, E., Maikusa, N., Okamura, N., Furumoto, S., Kudo, Y., et al. (2017). Regional tau deposition and subregion atrophy of medial temporal structures in early Alzheimer’s disease: a combined positron emission tomography/magnetic resonance imaging study. Alzheimers Dement. 9, 35–40. doi: 10.1016/j.dadm.2017.07.001
Spector, R. (1990). “The Pupils,” in Clinical Methods: The History, Physical and Laboratory Examinations, eds H. K. Walker, W. D. Hall, J. W. Hurst (Boston: Butterworths).
Sperling, R. A., Aisen, P. S., Beckett, L. A., Bennett, D. A., Craft, S., Fagan, A. M., et al. (2011). Toward defining the preclinical stages of Alzheimer’s disease: recommendations from the national institute on aging-Alzheimer’s association workgroups on diagnostic guidelines for Alzheimer’s disease. Alzheimers Dement. 7, 280–292. doi: 10.1016/j.jalz.2011.03.003
Stasenko, A., Jacobs, D. M., Salmon, D. P., and Gollan, T. H. (2019). The multilingual naming test (MINT) as a measure of picture naming ability in Alzheimer’s disease. J. Int. Neuropsychol. Soc. 25, 821–833. doi: 10.1017/s1355617719000560
Tao, R., Lu, Z., Ding, D., Fu, S., Hong, Z., Liang, X., et al. (2019). Perifovea retinal thickness as an ophthalmic biomarker for mild cognitive impairment and early Alzheimer’s disease. Alzheimers Dement. 11, 405–414. doi: 10.1016/j.dadm.2019.04.003
Thomson, K. L., Yeo, J. M., Waddell, B., Cameron, J. R., and Pal, S. (2015). A systematic review and meta-analysis of retinal nerve fiber layer change in dementia, using optical coherence tomography. Alzheimers Dement. 1, 136–143. doi: 10.1016/j.dadm.2015.03.001
Trebbastoni, A., D’Antonio, F., Bruscolini, A., Marcelli, M., Cecere, M., Campanelli, A., et al. (2016). Retinal nerve fibre layer thickness changes in Alzheimer’s disease: results from a 12-month prospective case series. Neurosci. Lett. 629, 165–170. doi: 10.1016/j.neulet.2016.07.006
Trzepacz, P. T., Hochstetler, H., Wang, S., Walker, B., and Saykin, A. J. (2015). Relationship between the montreal cognitive assessment and mini-mental state examination for assessment of mild cognitive impairment in older adults. BMC Geriatr 15:107. doi: 10.1186/s12877-015-0103-3
Tsoi, K. K. F., Chan, J. Y. C., Hirai, H. W., Wong, S. Y. S., and Kwok, T. C. Y. (2015). Cognitive tests to detect dementia: a systematic review and meta-analysis. JAMA Intern. Med. 175, 1450–1458. doi: 10.1001/jamainternmed.2015.2152
Tzekov, R., and Mullan, M. (2014). Vision function abnormalities in Alzheimer disease. Surv. Ophthalmol. 59, 414–433. doi: 10.1016/j.survophthal.2013.10.002
Unsworth, N., Schrock, J. C., and Engle, R. W. (2004). Working memory capacity and the antisaccade task: individual differences in voluntary saccade control. J. Exp. Psychol. Learn. Mem. Cogn. 30, 1302–1321. doi: 10.1037/0278-7393.30.6.1302
Valenti, D. A. (2010). Alzheimer’s disease: visual system review. Optometry 81, 12–21. doi: 10.1016/j.optm.2009.04.101
Van Stavern, G. P., Bei, L., Shui, Y. B., Huecker, J., and Gordon, M. (2019). Pupillary light reaction in preclinical Alzheimer’s disease subjects compared with normal ageing controls. Br. J. Ophthalmol. 103, 971–975. doi: 10.1136/bjophthalmol-2018-312425
Velten, I. M., Korth, M., Horn, F. K., and Budde, W. M. (1999). Temporal contrast sensitivity with peripheral and central stimulation in glaucoma diagnosis. Br. J. Ophthalmol. 83, 199–205. doi: 10.1136/bjo.83.2.199
Ward, M. E., Gelfand, J. M., Lui, L.-Y., Ou, Y., Green, A. J., Stone, K., et al. (2018). Reduced contrast sensitivity among older women is associated with increased risk of cognitive impairment. Ann. Neurol. 83, 730–738. doi: 10.1002/ana.25196
Wilcockson, T. D. W., Mardanbegi, D., Xia, B., Taylor, S., Sawyer, P., Gellersen, H. W., et al. (2019). Abnormalities of saccadic eye movements in dementia due to Alzheimer’s disease and mild cognitive impairment. Aging 11, 5389–5398. doi: 10.18632/aging.102118
Wolk, D. A. (2013). Amyloid imaging in atypical presentations of Alzheimer’s disease. Curr. Neurol. Neurosci. Rep. 13:412. doi: 10.1007/s11910-013-0412-x
Wood, S., Mortel, K. F., Hiscock, M., Breitmeyer, B. G., and Caroselli, J. S. (1997). Adaptive and maladaptive utilization of color cues by patients with mild to moderate Alzheimer’s disease. Arch. Clin. Neuropsychol. 12, 483–489.
Wu, J., Wang, N., Wang, X. N., Han, Y., Ma, D., and Lu, Y. (2018). Regularity changes of the retinal nerve fiber layer and macular ganglion cell complex in patients with the amnestic mild cognitive impairment. Int. J. Neurosci. 128, 849–853. doi: 10.1080/00207454.2018.1438428
Wu, J., Zhang, X., Azhati, G., Li, T., Xu, G., and Liu, F. (2020). Retinal microvascular attenuation in mental cognitive impairment and Alzheimer’s disease by optical coherence tomography angiography. Acta Ophthalmol. 98, e781–e787. doi: 10.1111/aos.14381
Yamasaki, T., Goto, Y., Ohyagi, Y., Monji, A., Munetsuna, S., Minohara, M., et al. (2012). Selective impairment of optic flow perception in amnestic mild cognitive impairment: evidence from event-related potentials. J. Alzheimers Dis. 28, 695–708. doi: 10.3233/jad-2011-110167
Yamasaki, T., Horie, S., Ohyagi, Y., Tanaka, E., Nakamura, N., Goto, Y., et al. (2016). A potential VEP biomarker for mild cognitive impairment: evidence from selective visual deficit of higher-level dorsal pathway. J. Alzheimers Dis. 53, 661–676. doi: 10.3233/jad-150939
Yang, Q., Wang, T., Su, N., Liu, Y., Xiao, S., and Kapoula, Z. (2011). Long latency and high variability in accuracy-speed of prosaccades in Alzheimer’s disease at mild to moderate stage. Dement. Geriatr. Cogn. Dis. Extra. 1, 318–329. doi: 10.1159/000333080
Yang, Q., Wang, T., Su, N., Xiao, S., and Kapoula, Z. (2013). Specific saccade deficits in patients with Alzheimer’s disease at mild to moderate stage and in patients with amnestic mild cognitive impairment. Age 35, 1287–1298. doi: 10.1007/s11357-012-9420-z
Ye, F., Ye, M., An, J., Wang, D., Wang, Q., Chen, Y., et al. (2018). Motion-induced position shift in early Alzheimer’s disease. Sci. Rep. 8:9833. doi: 10.1038/s41598-018-27991-0
Yoon, S. P., Grewal, D. S., Thompson, A. C., Polascik, B. W., Dunn, C., Burke, J. R., et al. (2019a). Retinal microvascular and neurodegenerative changes in Alzheimer’s disease and mild cognitive impairment compared with control participants. Ophthalmol. Retina 3, 489–499. doi: 10.1016/j.oret.2019.02.002
Yoon, S. P., Thompson, A. C., Polascik, B. W., Calixte, C., Burke, J. R., Petrella, J. R., et al. (2019b). Correlation of OCTA and volumetric MRI in mild cognitive impairment and Alzheimer’s disease. Ophthalmic Surg. Lasers Imaging Retina 50, 709–718. doi: 10.3928/23258160-20191031-06
Zabel, P., Kaluzny, J. J., Wilkosc-Debczynska, M., Gebska-Toloczko, M., Suwala, K., Kucharski, R., et al. (2019). Peripapillary retinal nerve fiber layer thickness in patients with Alzheimer’s disease: a comparison of eyes of patients with Alzheimer’s disease, primary open-angle glaucoma and preperimetric glaucoma and healthy controls. Med. Sci. Monit. 25, 1001–1008. doi: 10.12659/msm.914889
Zhang, Y. S., Onishi, A. C., Zhou, N., Song, J., Samra, S., Weintraub, S., et al. (2019a). Characterization of inner retinal hyperreflective alterations in early cognitive impairment on adaptive optics scanning laser ophthalmoscopy. Invest. Ophthalmol. Vis. Sci. 60, 3527–3536. doi: 10.1167/iovs.19-27135
Zhang, Y. S., Zhou, N., Knoll, B. M., Samra, S., Ward, M. R., Weintraub, S., et al. (2019b). Parafoveal vessel loss and correlation between peripapillary vessel density and cognitive performance in amnestic mild cognitive impairment and early Alzheimer’s disease on optical coherence tomography angiography. PLoS One 14:e0214685. doi: 10.1371/journal.pone.0214685
Keywords: Alzheimer’s disease, mild cognitive impairment, visual biomarkers, afferent visual system, efferent visual system, optical coherence tomography, saccadic eye movement, pupillometry
Citation: Wu SZ, Masurkar AV and Balcer LJ (2020) Afferent and Efferent Visual Markers of Alzheimer’s Disease: A Review and Update in Early Stage Disease. Front. Aging Neurosci. 12:572337. doi: 10.3389/fnagi.2020.572337
Received: 13 June 2020; Accepted: 20 August 2020;
Published: 11 September 2020.
Edited by:
Taher Darreh-Shori, Karolinska Institutet (KI), SwedenReviewed by:
Zhong-Lin Lu, New York University, United StatesFang Hou, Wenzhou Medical University, China
Copyright © 2020 Wu, Masurkar and Balcer. This is an open-access article distributed under the terms of the Creative Commons Attribution License (CC BY). The use, distribution or reproduction in other forums is permitted, provided the original author(s) and the copyright owner(s) are credited and that the original publication in this journal is cited, in accordance with accepted academic practice. No use, distribution or reproduction is permitted which does not comply with these terms.
*Correspondence: Laura J. Balcer, bGF1cmEuYmFsY2VyQG55dWxhbmdvbmUub3Jn