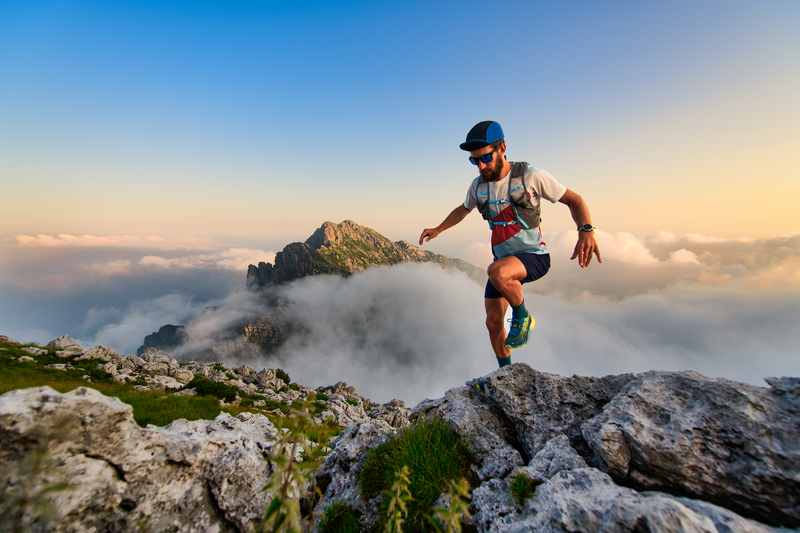
95% of researchers rate our articles as excellent or good
Learn more about the work of our research integrity team to safeguard the quality of each article we publish.
Find out more
REVIEW article
Front. Aging Neurosci. , 06 October 2020
Sec. Alzheimer's Disease and Related Dementias
Volume 12 - 2020 | https://doi.org/10.3389/fnagi.2020.544235
This article is part of the Research Topic Age-Related Neuroimmunology of Degeneration and Repair View all 15 articles
Alzheimer’s disease (AD) is commonly an age-associated dementia with neurodegeneration. The pathogenesis of AD is complex and still remains unclear. The inflammation, amyloid β (Aβ), and neurofibrillary tangles as well misfolded tau protein in the brain may contribute to the occurrence and development of AD. Compared with tau protein, Aβ is less toxic. So far, all efforts made in the treatments of AD with targeting these pathogenic factors were unsuccessful over the past decades. Recently, many studies demonstrated that changes of the intestinal environment and gut microbiota via gut–brain axis pathway can cause neurological disorders, such as AD, which may be involved in the pathogenesis of AD. Thus, remodeling the gut microbiota by various ways to maintain their balance might be a novel therapeutic strategy for AD. In the review article, we analyzed the characteristics of gut microbiota and its dysbiosis in AD and its animal models and investigated the possibility of targeting the gut microbiota in the treatment of the patients with AD in the future.
- The gut microbes communicate with the brain by several regulating pathways via the gut–brain axis involved in the physiological activities to maintain homeostasis of the human body. The imbalance of gut microbiota is associated with AD.
- The gut dysbiosis caused by several factors may aggravate neuroinflammation and other pathologies promoting the development and progression of AD.
- Targeting the gut dysbiosis or remodeling the gut microbiota might be a novel strategy for AD therapy.
Alzheimer’s disease (AD) is a chronic progressive neurodegenerative disorder and the most common form of age-associated dementia. In year 2017, it has been reported that about 40 million people suffered from AD in the world (Alzheimer’s Association, 2017; Esquerda-Canals et al., 2017). Despite a lot of previous intensive studies, the pathogenesis of AD remains insufficiently understood. Pathologic changes in the brain of AD include amyloid β (Aβ) plaque deposits and neurofibrillary tangles formed by intracellular accumulation of hyperphosphorylated tau protein and neuroinflammation. The pathological characteristics are current major theory of the pathogenesis of AD (Angelucci et al., 2019). However, the great efforts in therapeutic AD basis on the pathogenesis of AD with pathogenic Aβ or tau over the past decades have witnessed continuous failure, indicating that the pathogenesis of AD should be multifactorial and is more complex than a simple pathogenic Aβ or tau would suggest. With aging of human beings, the incidence of AD is rising continuously in the world, which has become a major public health problem (Angelucci et al., 2019). In order to develop the effective treatment, we need further a better understanding of the pathogenesis of AD.
Over the past 10 years, the researchers have been very concerned and interested in the role of the gut microbiome in modulating brain function, although the results were obtained mainly from animal models (Long-Smith et al., 2020). Microbiota may be a crucial predisposing factor for AD and other neurological disorders, which has been proven by a growing number of studies (Zhuang et al., 2018; Sochocka et al., 2019; Cryan et al., 2020; Long-Smith et al., 2020). AD has been considered as a systemic disease related to inflammation, and the inflammatory–infectious hypothesis of its pathogenesis becomes more significant (Bronzuoli et al., 2016). It has been evidenced that microbes and their products from the periphery infiltrating into the brain causing chronic inflammation are an important predisposing factor of neuroinflammation and neurodegenerative changes observed in AD (Cattaneo et al., 2017; Ashraf et al., 2019). AD and cognitive decline, as well as other neurodegenerative diseases, are often associated with gastrointestinal (GI) dysfunction (Zhuang et al., 2018; Sochocka et al., 2019; Ticinesi et al., 2019). It is postulated that AD may begin in the gut and is related to the imbalance of gut microbiota, while the intestinal inflammation and infections caused by various pathogens may control the changes of the gut microbiota first, and then other factors, as described below, are also involved in controlling these changes.
Throughout the course of these diseases, the GI disturbances may occur in the different stage of the diseases as a clinical manifestation. The alteration of enteric neuroimmune system (ENIS) and dysbiosis of the gut microbiota may lead to the occurrence of GI dysfunction and neurologic disorders (Pellegrini et al., 2018; Sochocka et al., 2019). Therefore, it has been hypothesized that AD is closely related to gut microbial alteration, and it is consistent with the pivotal role of inflammation in the pathogenesis of AD (Calsolaro and Edison, 2016; Haran et al., 2019). In the review article, we clarify the characteristics of the gut microbiota, analyze the role of dysbiosis of the gut microbes in the pathogenesis of AD, and discuss the possibility whether targeting the dysbiosis of gut microbes can be as a future therapeutic manipulation in AD.
Normal microorganisms in human being consist of bacteria, fungi, viruses, etc. and 95% of them are located in the large intestine (Swidsinski et al., 2005; Galland, 2014). The microbiota refers to bacteria, fungi, viruses, etc., existing in an ecosystem/habitat, and the intestinal microbial community is named gut microbiota (Shahi et al., 2017). The amount of microorganisms is 1014 with a total weight of approximately 2 kg (Picca et al., 2018). In human, distribution and species of the bacteria residing in the intestinal tract are always changing and uncertain, which depend on the physiological condition of the GI tract. So far, the exact species of microbe populations are unclear.
However, the comprehensive view of human-connected microbes has been offered by the Metagenomics of the Human Intestinal Tract and the Human Microbiome Project. There are a total of 2,172 species of microbes classified into 12 phyla in human, and Proteobacteria, Firmicutes, Actinobacteria, and Bacteroidetes phyla possess 93.5% of total microbes (Li et al., 2014; Hugon et al., 2015; Bilen et al., 2018). The phyla Firmicutes and Bacteroidetes are the majority, containing the genera Prevotella, Bacteroides, and Ruminococcus, as well as Verrucomicrobia and Actinobacteria, but Proteobacteria phyla members have a small quantity (Mowry and Glenn, 2018). Because many factors impact on gut microbiota, for example, genetic factors, sex, diet, and others, such as place of residence, smoking, etc., therefore, different ethnicities have different gut microbiomes (Blum, 2017).
The microbiota is involved in important homeostatic processes and essential for the homeostasis of intestinal intraepithelial lymphocytes (Liu L. et al., 2019). Besides the role of microbiota associated with GI function, the microbiota also contributes to inflammation and immune response, central and peripheral (enteric) neurotransmission, glucose metabolism, etc. (Liu et al., 2020). Therefore, the gut microbes play a beneficial role in maintaining homeostasis of immune systems of the host. The necessary vitamins and other substances involved in the development of the central nervous system (CNS) and immune regulation are produced by gut microbiota (Blum, 2017; Picca et al., 2018).
In a healthy organism, microbiota can also create a protective barrier against the infectious agents in the gut (Angelucci et al., 2019; Liu L. et al., 2019). Furthermore, a dynamic network is formed via the interaction among intestinal epithelial barrier, intestinal immune system, gut microbiota, and enteric nervous system (ENS) to coordinate the GI physiology and maintain homeostasis of gut (Pellegrini et al., 2018). The association of gut microbiota and its interaction with intestinal mucosal barrier and immune system in maintaining brain homeostasis have been demonstrated by more and more evidence (Foster et al., 2017; Fung et al., 2017).
In addition to destabilizing the intestinal environment, the dysbiosis of gut microbes can affect behavior, learning, and memory, as well as neurogenesis, etc. (Fang et al., 2016; Luczynski et al., 2016; Minato et al., 2017; Tremlett et al., 2017). Therefore, the gut microbiome plays a key role in maintaining the body healthy. So far, several review articles summarized well the role of gut microbiota in the maintenance of brain homeostasis (Fung et al., 2017; Tognini, 2017; Tremlett et al., 2017; Askarova et al., 2020). Here, we describe concisely the most important results about the role of gut microbiota in the regulation of brain physiological processes.
The relationship between the gut microbiota and the senescent brain is unclear, and until now, it is still an unanswerable question. As several neurodegenerative diseases occur in the elderly, it has attracted attention to the relationship between the gut microbiota and aging. However, at present, not many clinical and experimental studies evaluated this in the field. Claesson et al. (2012) studied the composition of the gut microbiota from 16 older than 65 years in the Ireland and showed more diverse gut microbiota with better health outcomes, indicating that the composition of the gut microbiota is closely related to health condition and immune function, and a diet rich in fruits and vegetables has a greater diversity of gut microbiota. Thus, one of the features of healthy aging may be the diversity of the gut microbiota (Claesson et al., 2012). Unfortunately, the study did not provide the information regarding the relationship between the gut microbiota and the senescence. The field is nascent, and so far, not many studies have been published.
The evidence concerning the relationship between gut microbiota and aging in mice showed that age-associated behavioral impairments were consistent with alterations of the microbiota (Scott et al., 2017), which is a direct evidence to confirm the close correlation between microbiota and aging. In the process of aging, the gut microbiota’s composition is altered, accompanied by increasing proteobacteria and decreasing probiotics, such as bifidobacteria, and neuroprotective molecules (Lambert et al., 2009; Caracciolo et al., 2014). The probiotic bacteria called “good” microbes play an advantageous role in human health and produce the essential substances to inhibit inflammation (Mukherjee et al., 2018).
The age-associated neuroinflammation, a crucial pathogenic factor in the development of AD and the cause or consequence of most neurodegenerative diseases, was ameliorated by administration with prebiotic inulin that targets the microbiota (Games et al., 1995). Microglial activation as an inflammatory hallmark in the pathology of AD is regulated by the microbiota, which plays a key role in aging and neurodegeneration (Abbas et al., 2002; Lambert et al., 2009). Moreover, high levels of proinflammatory cytokines in healthy elderly subjects were related to the disorder of microbiome function, particularly the genes encoding short-chain fatty acids (SCFAs; Claesson et al., 2012), which is a basic characteristic for the extensive age-related pathologies, such as age-related dysbiosis of gut microbes and neurological decline (Franceschi et al., 2000). Although lots of studies demonstrated this correlation, unfortunately a direct cause effect has not yet been established (Sun et al., 2019b; Kim et al., 2020). Thus, more studies are needed to show evidence of the relationship.
The gut microbiome is involved in bidirectional communication between the gut and brain, which is a significant scientific discovery recently (Erny et al., 2017; Fung, 2020). It has been suggested that human gut microbiome may be considered as the “second brain” and contributes to AD and other neurodegenerative disorders (Gershon, 1999; Schneider et al., 2019; Sochocka et al., 2019).
The gut microbiota can modulate brain signals and activity via the microbiome–gut–brain axis through the nervous, endocrine, and immune systems proven by many animal and preclinical experiments. Also the chemical substances produced by themselves (monoamines and amino acids) can cross the blood–brain barrier (BBB) reaching the CNS (Collins et al., 2012; Crane et al., 2015; Yano et al., 2015) and influence brain activity with possible repercussions on behavior (Wekerle, 2016; Kowalski and Mulak, 2019). The gut microbiota can also receive signals from the brain in the form of neurotransmitters, including acetylcholine, the modified amino acids glutamate and γ-aminobutyric acid (GABA), and the biogenic amines dopamine (DA), serotonin (5-HT), and histamine, interacting with the brain (Briguglio et al., 2018). Furthermore, the concept of the microbiome–gut–brain axis has been supported by the current research data; thus, the gut microbiome can communicate with the brain and is responsible for some neurodegenerative disorders (Haran et al., 2019). The new perspective makes us realize that the gut microbiota may play an important role in this mutual relationship between brain and gut communication, as well as physiological regulation. Figure 1 presents the microbiome–gut–brain axis containing several molecular pathways and their interactions. However, the microbiome–gut–brain axis is a complex multidirectional system between the gut microbiota, ENS, and the brain, which is still poorly understood.
Figure 1. The role of the gut microbiota and communication with the brain. A healthy gut contains large fractions of the phyla Firmicutes and Bacteroidetes, including the genera Prevotella, Bacteroides, and Ruminococcus followed by Verrucomicrobia and Actinobacteria, but contains a low number of Proteobacteria phyla members (Mowry and Glenn, 2018). The gut microbiota is related to GI function, but also involved in several complex modulatory processes, inflammation and immune response, and peripheral (enteric) and central neurotransmission, as well as synthetize and secrete essential substances. The gut microbiota contributes to important homeostatic processes and is essential for the homeostasis of intestinal intraepithelial lymphocytes (Liu L. et al., 2019). The gut microbiota can affect brain function and bioactivity through gut–brain axis via several pathways: (1) the neural regulating pathway, in which vagus nerve links between the gut and the spinal cord (autonomic nervous system; Bonaz et al., 2018). The gut microbiota can secrete and regulate neurotransmitters of the CNS. (2) The endocrine pathway. The HPA axis can release glucocorticoids, etc., after stimulations by stress or other factors, which can alter gut microbiota composition and increase gut epithelium permeability and immune responses in gut (Ait-Belgnaoui et al., 2012; Park et al., 2013; Bellavance and Rivest, 2014). (3) The immune-regulating pathway via lymphocyte, cytokines, chemokines, and antigen-presenting effect of SCFAs communicating with the brain. (4) The blood circulation pathway (Logsdon et al., 2018). The immune and endocrine molecules, such as cytokines and hormones, can pass BBB and intestinal mucosa to influence both gut and brain functions (Zac-Varghese et al., 2010). BBB, blood–brain barrier; CNS, central nervous system; HPA axis, hypothalamic–pituitary–adrenal axis; SCFA, short-chain fatty acids; APC, antigen-presenting cells.
The pathways of communication between the gut and brain have been reported (Dinan and Cryan, 2017). The first pathway is neural regulation pathway, in which the vagus nerve links between the gut and the spinal cord (autonomic nervous system; Bonaz et al., 2018). The ended vagus nerve of brain stem nuclei receiving and giving afferent and efferent fibers may regulate the gut functions and send messages to other regions of CNS (Bonaz et al., 2018). The catecholamines or acetylcholine secreted from the brain affecting ENS circuits can modulate the gut functions (Mayer et al., 2015; Weinstein et al., 2015). It is also through the gut bacteria to exchange signals between ENS and CNS (Carabotti et al., 2015).
On the other hand, the gut microbiota is able to produce and modulate neurotransmitters in both CNS and peripheral nervous system, and intestinal environmental changes can affect lymphocytes of the gut to produce more cytokines and chemokines, such as interleukin 1 (IL-1), IL-6, IL-17, IL-22, tumor necrosis factor-α (TNF-α; Thaiss et al., 2016; Sochocka et al., 2019), and transforming growth factor β (Ma et al., 2017), as well as chemokine, fractalkine, and its receptor (CX3CR1; Merino et al., 2011), affecting the CNS through activating the endocrine or paracrine systems. The proinflammatory cytokines, IL-1, IL-6, IL-17, and TNF-α, are potentially harmful to the brain (Angelucci et al., 2019). The gut microbes secrete several important substances such as GABA, histamine, 5-HT, and DA, which contribute to neuroactive and immune regulation (Barrett et al., 2012; Lyte, 2013), and also produce toxic substances to the brain, such as ammonia and others (Galland, 2014). In addition, the microbiome–gut–brain axis can be affected by microbiota via immunological, neuroendocrine, and direct neural mechanisms (Logsdon et al., 2018), which insulted the brain to cause memory impairment, anxiety, and other cognitive dysfunctions (Gareau et al., 2011; Galland, 2014; Johnson and Foster, 2018) and resulted in several diseases, such as anxiety and depression (Lach et al., 2018; Capuco et al., 2020), neurodegenerative diseases, and drug-resistant epilepsy (Braakman and van Ingen, 2018).
Endocrine regulating pathway is the second pathway of communication between the gut and brain. The hypothalamic–pituitary–adrenal (HPA) axis can release glucocorticoids, mineralocorticoids, or catecholamines after stimulations by stress or other factors, which result in the changes of intestinal microbiota components and the intestinal epithelium permeability, as well as immune responses in the gut (Ait-Belgnaoui et al., 2012; Park et al., 2013; Bellavance and Rivest, 2014). Enhanced genus Clostridium and declined Bacteroides as the feature of the gut dysbiosis were caused by high corticosterone levels in the stressed mice (Bailey et al., 2011). The glucocorticoids have both proinflammatory and anti-inflammatory roles; however, inflammations are related to damaged HPA axis functionality in AD and other neurodegenerative disorders (Silverman and Sternberg, 2012; Bellavance and Rivest, 2014; Hueston and Deak, 2014).
Immunoregulating pathway as the third pathway also participates in this communication between the gut and brain via gut microbes, which could affect antigen presentations and regulate cytokines production and lymphocyte function, as well as the development of two types of immune system through the gut–brain axis (Olszak et al., 2012; Fung et al., 2017). The gut microbiota also impacts on productions of SCFAs that can activate immune response and trigger inflammation in the brain, resulting in a series of neurological symptoms. Additionally, SCFAs are related to G-protein-coupled receptor 43 (GPR43) to lead a strong anti-inflammatory reaction (Maslowski et al., 2009). The gut microbes are necessary for host immunity generation in the GI tract. The data obtained from germ-free (GF) mice have shown that the maturations of the immune, endocrine, and nervous systems are affected by gut bacteria, which is a strong evidence for the gut microbiota linking with the brain via microbiome–gut–brain axis (Wang and Wang, 2016; Kowalski and Mulak, 2019).
Communication between the gut and brain is also through the blood circulation (Logsdon et al., 2018). The cytokines and hormones as well as some molecules can pass BBB and intestinal mucosa to influence both gut and brain functions (Zac-Varghese et al., 2010). Furthermore, the central, peripheral, immune, and endocrine systems are involved in the communication between gut and brain in a multifunctional network formed by the microbiome–gut–brain axis (Borre et al., 2014).
However, the mechanisms that mediate gut–brain communication remain in its infancy. There are still many questions to explore, such as the molecular and cellular mechanisms underlying the microbiome–gut–brain axis in health and under pathological conditions, etc.
Generally, the gut microbial communities in human are stable; however, they can be altered in the different conditions by the effects of various factors through their direct action (microbial infection) or indirect actions (antimicrobial protection hypothesis, hygiene hypothesis; DiSabato et al., 2016; Ashraf et al., 2019; Kong et al., 2020). Recently, the studies of several groups have been demonstrated that various diseases, including intestinal diseases and more systemic diseases such as diabetes, metabolic syndrome, and neurodegenerative disorders, including AD and others, are related to the imbalance of gut microbiota called “dysbiosis” (Del Tredici et al., 2002; Murono et al., 2015; Hu et al., 2016; Jiang et al., 2017). Occurrence and development of AD and other neurodegenerative disorders may be accompanied by the gut microbiome dysbiosis, inflammation, and dysfunction of the gut–brain axis. It has been speculated that AD may appear during the aging of immune system based on the theory of age-related dysbiosis derived from the association between gut microbiota and AD, which has been evidenced by clinical and experimental studies (Cattaneo et al., 2017; Pellegrini et al., 2018).
Generally, the traditional ecological measures are used to characterize the composition of the gut microbiome, including richness [the number of unique operational taxonomic units (OTUs) present in a participant], alpha diversity (the richness and abundance of OTUs within each participant), and beta diversity (the similarity or difference in composition between participants). Declined microbial richness and diversity as well as a distinct composition of the gut microbiome were found in AD patients. The levels of differentially abundant genera were correlated with cerebrospinal fluid (CSF) biomarkers of AD pathology (Vogt et al., 2017). In short, definite genera as more abundant in AD were related to greater AD pathology, whereas genera as less abundant in AD were associated with less AD pathology (Vogt et al., 2017).
As mentioned previously, immune response system participates in this communication between the gut microbes and brain. There is also a close interaction between gut microbes and the local as well as systemic immune system. In general, the gut dysbiosis could lead to dysfunctions of both innate and adoptive immune through several ways, such as changing antigen presentations, cytokines production, and lymphocyte functions, as well as increasing inflammation, etc., also can cause the gut–brain axis malfunction (Levy et al., 2017). In AD patients, the molecular and cellular alterations involving immune cells, such as T cells, B cells, microglia, etc., as well as immune mediators, occur not only in the peripheral blood, but also in the brain and the CSF, which may be associated with triggering immune response by the gut dysbiosis. The gut dysbiosis impacts on innate and adoptive immune response in AD patients obviously via activating immune/inflammatory cells, shifting them into inflammatory type to enhance immune mediated inflammatory response, and promoting neurodegeneration in the brain. The gut dysbiosis in AD was obviously correlated with more T helper 1 (TH1) cell infiltration into the brain (Togo et al., 2002; Monsonego et al., 2003), and increased T-cell infiltration in the brain parenchyma and peripheral T-cell responses to Aβ have been found in AD patients (Rogers et al., 1988; Monsonego et al., 2003).
Pathologically, deposition of Aβ plaques in the brain is a major character, and it has been considered as one of the important pathogenic factors in AD (Salter and Stevens, 2017; Angelucci et al., 2019). Reactive gliosis and neuroinflammation are the histological hallmarks and key factors in the pathogenesis of AD (Salter and Stevens, 2017; Yeh et al., 2017; Zhang et al., 2020). Microglial activation in the CNS is heterogeneous and categorized into two types: proinflammatory and anti-inflammatory microglia (Tang and Le, 2016; Yu et al., 2019). Microglia plays either a cytotoxic or neuroprotective role, depending on the types activated, which can be changed in the different stages of AD. The anti-inflammatory microglia phagocytizes Aβ plaques by the Fc receptors and promotes the cleaning and degradation of Aβ by possibly increased phagocytic and lysosomal activity, as well as restriction of the inflammatory response (Kamphuis et al., 2016; Dubbelaar et al., 2018). Oppositely, proinflammatory microglia leads to Aβ accumulation, inducing cell death and worsening disease (Fakhoury, 2018). We speculated that microglial malfunction may be the basis of AD pathogenesis and precede and accelerate the onset of AD.
Table 1 compares the gut microbiota between healthy subjects and AD patients, in which alterations of the gut microbiota can be seen in the AD patients; thus, dysbiosis of gut microbes may be involved in AD-related impairments.
Table 1. The comparisons of the gut microbiota between healthy subjects and Alzheimer’s disease (AD) patients.
So far, the evidence obtained about the role of dysbiosis of gut microbes in AD pathophysiology is mainly from its animal models. A significant decrease in the Aβ pathology was observed in GF mice, and after the control mice were exposed to the gut microbiota, the Aβ pathology occurred again (Harach et al., 2017). In addition, an obvious absence of amyloid plaque deposit and neuroinflammation were seen in GF mice when microbes were not present (Harach et al., 2017). Of course, the pathological manifestations in GF mice may not be completely attributed to gut dysbiosis, because GF mice also exhibited defects in the immune system and difficulties with energy acquisition, etc., which also impact on the pathological changes in GF mice. The changes of gut microbiota promoted Aβ protein accumulations in the gut. Evidently, a thoroughly changed gut microbiome was found in APP transgenic (Tg) mice (AβAPP) [a genetic model of AD; the mice overexpress mutated forms of human amyloid precursor protein (APP) linked to familial AD] when compared to wild-type mice (WT; Wang X.-L. et al., 2015). The removal of gut microbiome was related to central Aβ levels in AD mice; however, increased amyloid accumulation was found in the brain after transplantation by microbiota from AD mice (Harach et al., 2017). Similarly, high levels of Aβ protein of brain and related behavioral alterations were associated with the gut dysbiosis in APP/PS1 mice [a genetic model of AD; APP/PS1 mice are double Tg mice expressing a chimeric mouse/human APP (Mo/HuAPP695swe) and a mutant human presenilin 1 (PS1-dE9); Shen et al., 2017]. Moreover, both enhanced Aβ protein precursor (AβPP) accumulation in the gut and Firmicutes/Bacteroidetes ratio were found in 5xFAD mice (a transgenetic model of AD; 5xFAD mice express human APP and PSEN1 transgenes with a total of five AD-linked mutations: APP KM670/671NL, APP I716V, APP V7171, and PSEN1M146L and PSEN1L286V) following the change of the gut microbiota composition in these mice since the earliest phase of the diseases (Brandscheid et al., 2017). These data suggest that changing of gut microbiome in the animal models of AD promotes deposit of Aβ protein in the brain.
A significant study indicated that calorie restriction decreased Aβ deposition in the brain of AD mouse model. During aging process, calorie restriction could change the gut microbiome, including an increase in Bacteroides, which was found obviously in female Tg2576 mouse model when compared to WT mice. It has been demonstrated that the specific gut microbiota change was related to Aβ levels, and the change had a greater impact on females than males. Furthermore, long-term calorie restriction can change the gut environment and prevent the expansion of microbes that promotes age-related cognitive decline (Cox et al., 2019).
Interestingly, there was activation of immune/inflammatory cells and high expressions of Aβ and phosphorylated tau (p-tau) protein, as well as neuronal coding rearrangements in the gut of APP/PS1 mice, which feature is accompanied by lower levels of neuronal nitric oxide synthase and choline acetyltransferase, suggesting that Aβ and p-tau protein deposits in the gut can influence local and peripheral neurogenic/inflammatory responses and promote inflammation and neurodegeneration in the brain of AD models (Haghikia et al., 2016; Feng et al., 2018). It has been shown that enhanced Aβ protein expression in the gut precedes inflammation in the brain of TgCRND8 mice (a genetic model of AD; TgCRND8 mice overexpress mutant human APP KM670/671NL and APP V717F; Semar et al., 2013). Aβ protein can also be transmitted to the CNS through myenteric neurons and nerve gut–brain axis involved in the pathogenesis of AD directly (Zhao and Lukiw, 2015; Pistollato et al., 2016). However, the causal relationship between these possible pathogenic factors is unclear; therefore, further studies are needed to investigate.
It is beyond doubt and has been evidenced that inflammation is a crucial factor in the pathogenesis of AD. Recent studies have shown that a strong correlation between NLRP3 inflammasomes, one of the multiprotein complexes, and initiation of inflammation and neurological diseases, is identified (Pellegrini et al., 2019). NLRP3 inflammasomes are key molecules in neuroinflammation and Aβ caused AD pathology in AD models (Heneka et al., 2013; Ising et al., 2019). Conversely, impaired NLRP3 inflammasome function lowered tau hyperphosphorylation by regulating tau kinases and phosphatases (Ising et al., 2019; Tejera et al., 2019). NLRP3 knockout (KO) mice exhibited significant difference of the composition of gut microbiota and behaviors compared with WT mice, suggesting that NLRP3 inflammasome deficiency affected the gut microbiota composition (Zhang et al., 2019). Transplantation of the gut microbiota of NLRP3 KO mice or using NLRP3’s inhibitor ameliorated depressive-like behaviors via remodeling gut microbiota (Zhang et al., 2019). The cognitive function of AD mice was repaired by using NLRP3 inhibitor, which may be associated with altering gut microbiota (Daniels et al., 2016; Ising et al., 2019; Tejera et al., 2019). Inflammation playing a central role in AD is linked to the closed relationship between gut microbiota and AD (Calsolaro and Edison, 2016).
In AD patients, the proportion and prevalence of bacteria synthesizing butyrate were low, and the abundances of taxa were high that lead to inflammation compared to healthy or other dementia types, which evidenced that the nexus between the gut microbiome and an altered epithelial homeostasis could have an effect on AD (Daniels et al., 2016) by increases in inflammatory and decreases in anti-inflammatory microbial metabolism (Haran et al., 2019).
Several studies on dysbiosis of gut microbes in AD patients have exhibited that AD’s main pathological features in the brain, such as amyloidosis and inflammation, are linked to inflammatory bacteria and their neurotoxic products, like lipopolysaccharides (Bester et al., 2015; Cattaneo et al., 2017). In AD patients, increased Bacteroides and Blautia and decreased relative abundance of the genera SMB53 and Dialister were a feature of the changes of gut microbiota, which was associated with high levels of chitinase-3-like protein 1 and p-tau, accompanied by a low Aβ42/Aβ40 ratio in CSF (Vogt et al., 2017). Intestinal inflammation in AD patients was positively correlated with a high level of fecal calprotectin (Leblhuber et al., 2015). However, the clinical evidence on accumulations of Aβ protein, AβPP, and p-tau in the gut of AD patients is rare, and the data obtained are contradictory (Joachim et al., 1989; Puig et al., 2015). Meanwhile, studies with no matched healthy controls are also unable to make such conclusion; i.e., there is a causal relationship between intestinal Aβ and p-tau deposition, inflammation and gut dysbiosis.
The chronic Helicobacter pylori infection can trigger the release of inflammatory mediators and is associated with low Mini-Mental State Examination score in AD patients when compared with patients without infections (Roubaud-Baudron et al., 2012).
Infections by H. pylori, Borrelia burgdorferi, and Chlamydia pneumoniae, and so on, increased levels of Aβ40 and Aβ42 in serum of AD patients (Bu et al., 2015). In vitro, the neuroblastoma cell cultures treated by H. pylori filtrate induced tau hyperphosphorylation, which was similar to AD tau pathological changes (Wang X.-L. et al., 2015). Furthermore, the inflammatory disorders are also linked to gut dysbiosis caused by viruses, such as herpes simplex virus type 1, which can be one of the crucial risk factors for AD. Maintaining the homeostasis of intestinal intraepithelial lymphocytes required these commensal viruses; however, the sustaining intestinal homeostasis can be also destroyed by infections with bacteria and virus (Harris and Harris, 2015). Collectively, the gut dysbiosis could be a risk factor for AD due to lacking or reducing immune defenses in the seniors (Angelucci et al., 2019).
Although gut dysbiosis contributes to the pathogenesis of many neurological and neurodegenerative diseases generally, the types of microbiota changes in the gut of AD are different from those of other neurodegenerative diseases, when compared with multiple sclerosis (MS), Parkinson disease (PD), and amyotrophic lateral sclerosis as shown in Table 2. We speculated that some microbial changes are relatively specific to AD due to causing AD pathology different from other diseases. Increased Bacteroides was found in the AD patients, and Bacteroides colonization aggravated Aβ deposition, which is speculated to be a mechanism whereby the gut impacts AD pathogenesis (Cox et al., 2019). However, there are too many factors affecting the gut microbiota; it is difficult to determine a causal relationship and needs to be further explored.
Table 2. The gut microbes in central nervous system (CNS) disorders and treatments by microbes or microbial products.
Up to now, no study confirmed clearly that Aβ deposition or tau accumulation is related to alter a particular microbe in the gut of AD patients. However, the specific microbes resulting in inflammation may promote Aβ and p-tau protein deposits in the gut indirectly, and the two promote each other. We considered that the infections by H. pylori and B. burgdorferi and enhanced Bacteroides may promote Aβ deposition or tau accumulation in the gut of AD patients, which needed to be evidenced in the future studies.
The factors that disturbed the gut microbiota to lead gut dysbiosis could target AD (Stenman et al., 2013; Vogt et al., 2018; MahmoudianDehkordi et al., 2019). There was an interaction between gut microbiome and bile acid (BA) levels. The bacteria containing the abundant bile salt hydrolase can easily change BA pattern to modulate the commensal bacteria and protect the integrity of the intestinal barrier (Stenman et al., 2013; Shapiro et al., 2014; MahmoudianDehkordi et al., 2019). In the CSF of mild cognitive impairment (MCI) and AD patients, the high level of trimethylamine n-oxide (TMAO), a metabolite derived from gut microbiota, was related to the biomarkers of AD in CSF (Vogt et al., 2018).
A pivotal pathogenic factor, oxidative stress (OS) has been shown to contribute to the development of AD. In the CNS, the reactive oxygen species (ROS) levels and inflammation can be enhanced by a microbiota type to favors abnormal aggregation of Aβ, which speculated that high levels of CNS OS may be due to gut dysbiosis or its consequence (Jones et al., 2012; Dumitrescu et al., 2018). In short, it can be seen from the above results and summarized as follows: (1) chronic bacterial infections as a possible etiology linking AD pathogenesis; (2) obvious alteration in the compositions of gut microbiome in AD; and (3) rising proinflammatory and lowering anti-inflammatory bacteria in the gut related to systemic inflammation in the patients suffering from the brain amyloidosis and cognitive impairment, which changes might impact brain functions. Thus, microbial dysbiosis or imbalance may potentially contribute to the pathogenesis of AD.
The gut microbiota may impact on AD development and progress as descripted above. Dysbiosis of gut microbes supposes to be involved in the pathogenesis of AD. Despite much disappointment in anti-AD drug discovery previously, it is still promising and possible to find new treatments basis on gut microbe impacting on AD. Modifying the microbiota composition or remodeling gut microbes using the substances or manipulations that are able to change their composition or balance gut microbes, such as antibiotics and others, may affect or provide therapy for AD and other neurological diseases (Chu et al., 2018).
Usually, eliminating and avoiding bacterial colonization are the main effects of antibiotics on human, rather than targeting the specific types of bacteria (Angelucci et al., 2019). After treatments by the broad-spectrum antibiotics, the composition of the gut microbiota was markedly altered, and its biodiversity was declined, as well as the colonization was temporized (Angelucci et al., 2019). Therapies with antibiotic could change the gut microbiota during the different length of time (Ianiro et al., 2016) and alter behavior we know well as brain chemistry in both humans and animals (Jernberg et al., 2007; Fröhlich et al., 2016). However, the evidences from two studies displayed that the antibiotic treatment caused also neuropsychiatric symptoms such as anxiety, psychosis, and delirium in AD patients who received antibiotic as a cocktail therapy (Loeb et al., 2004; Molloy et al., 2013), which is associated with antibiotic treatment of H. pylori infections, but these neuropsychiatric symptoms as side effects were not found in the general population (Neufeld et al., 2017). The effects of antibiotics on AD may be extensive or even opposite, depending on the antibiotics applied and on the role of targeted gut microbiome in the pathogenesis of AD. The antibiotic therapy was effective in the animal models of AD, but it has not yet been widely investigated in AD patients, because it is not clear which microbiomes dominate in the gut of AD patients and whether there are safe antibiotics available (Panza et al., 2019). Besides, there is a lack of such study on the effect of different antibiotics on AD pathology; further study is needed.
Obviously, lower amounts of microglia and astrocyte accumulation around amyloid plaques in the hippocampus and reduced insoluble Aβ plaques in aged APPSWE/PS1DeltaE9 Tg mice of AD model (which overexpress the Swedish mutation of APP KM670/671NL together with PS1 deleted in exon 9) after treatment with an antibiotic cocktail (Minter et al., 2017) were found, which is only circumstantial evidence that antibiotic interfered with microglial activation through reducing the amounts of microglia. However, in APP/PS1 Tg mice, the treatments with cocktail of antibiotics resulted in enhanced neuroinflammation and proinflammatory cytokine levels, and the disease itself was deteriorated (Minter et al., 2016). The harmful effects of antibiotics may break down the balance of gut bacteria, as streptozotocin and ampicillin did, which favors AD or worsens its course (Zarrinpar et al., 2018). Ampicillin increased rat serum corticosterone related to memory dysfunctions and decreased brain-derived neurotrophic factor in hippocampus, the features of AD pathology. Also, ampicillin deteriorated the anxiety-like behavior and impaired spatial memory in rats (Fröhlich et al., 2016). Surprisingly, the disorder of physiological and psychological function caused by ampicillin in rats was turned down by administration with probiotics (Wang T. et al., 2015). The clinical and experimental studies highlight that the results using antibiotics targeting and remodeling gut microbes in AD patients are controversial. Also there were some adverse consequences after antibiotic treatment, such as gut microbes coming back with their same features. According to the results of the current studies, it might be difficult to determine the effect of antibiotics in the treatment of AD. Attention should be paid to protection of the new beneficial and specific microbes and to the focus of future therapeutic trials by antibiotics in AD.
A new therapeutic method with fecal microbiota transplantation (FMT) has been applied in the neurodegenerative disorders and their animal models, as well as other diseases recently (Allegretti et al., 2018). FMT consists of obtaining a fecal specimen from a healthy donor and administering a sample through either the mouth or the rectum of the ill person. The obtained results from FMT were encouraging and remarkably good in patients with recurrent Clostridium difficile infection, and FMT has become an important care option. FMT improved clinical symptoms obviously and fecal microbiome in the dog model with inflammatory bowel disease (Niina et al., 2019). In this respect, most clinical and experimental studies have been done in the patients with PD and its animal model. The exciting results with slowing down clinical progress in PD patients were obtained after reconstruction of the gut microbiome by FMT (Dutta et al., 2019). A study reported that constipation in a PD patient was clearly relieved after FMT through reconstruction of gut microbiota (Huang et al., 2019). The mechanisms behind the therapeutic effects of FMT are related to significant reduction of gut microbial dysbiosis and fecal SCFAs, as well as increment of levels of striatal DA and 5-HT, which has been evidenced in PD mice model. Furthermore, the activation of glial cells in the substantia nigra and TLR4/TNF-α signaling pathway molecules was inhibited by FMT in both gut and brain, which further evidences that gut microbial dysbiosis contributes to PD development, and FMT is beneficial to PD models (Sun et al., 2018). Therefore, the gut microbiota reconstruction may have therapeutic effects on PD patients and is a new therapeutic option (Fang, 2019). However, the study on treatment with FMT in AD and its animal models is scarce.
Recently, DeFilipp et al. (2019) reported a patient death treated with FMT due to extended-spectrum beta-lactamase-producing Escherichia coli bacteremia. To avoid similar accidents, it is necessary to enhance donor screening in order to reduce the transmission of microorganisms when treating patients with FMT and to properly evaluate the benefits and risks of FMT in different patient populations (DeFilipp et al., 2019), which should improve the new approaches for treatments in AD patients in the future (Blaser, 2019).
Moreover, prebiotic fructooligosaccharides (FOSs) as dietary supplements ameliorated cognitive deficits and pathological changes in the APPSWE/PS1DeltaE9 Tg mice and increased the levels of synapsin I and synaptic plasticity markers, postsynaptic density protein 95, and decreased the phosphorylated level of c-Jun N-terminal kinase, indicating that FOS can modulate the gut microbiota-glucagon-like peptide-1 (GLP-1)/GLP-1 receptor (GLP-1R) pathway to play a beneficial role in AD (Sun et al., 2019a).
However, the results obtained from a double blind clinical trial, which was carried out in AD patients treated by probiotic supplementation (PS) and placebo, respectively, for 12 weeks, were negative, indicating that treatment with PS was ineffective in the severe AD patients, and the curative effect with PS was related to severity of AD at least (Agahi et al., 2018). In another clinical trial, treatment with multispecies probiotic for 4 weeks changed gut microbiota composition and tryptophan metabolism in serum of AD patients. Furthermore, a correlation between kynurenine/tryptophan and neopterin levels was observed indicating activation of macrophages and/or dendritic cells in AD patients (Leblhuber et al., 2018).
An exciting new drug for treatment of AD named GV-971 (sodium oligomannate) is discovered more recently (Wang et al., 2019). Polysaccharides or oligosaccharides are able to regulate gut microbiota (Thomson et al., 2018). Main therapeutic effects of GV-971 on AD are: (1) restoring the balance of gut microbiota via targeting and remodeling gut microbiota; and (2) inhibiting the neuroinflammation caused by gut bacterial amino acids to slow down AD progression (Wang et al., 2019). The enhanced levels of phenylalanine and isoleucine in periphery caused by dysbiosis of the gut microbiota can induce activation of proinflammatory microglia and proliferation of infiltrated inflammatory TH1 cells from blood into the brain of AD mice deteriorating inflammation. Meanwhile, there were high levels of phenylalanine, isoleucine, and TH1 cell in blood of MCI patients. The oligomannate sodium GV-971 is a carbohydrate-based anti-AD drug that markedly improved cognition in Chinese patients by targeting gut dysbiosis, dropping phenylalanine/isoleucine in the feces and blood, and inhibiting TH1-associated neuroinflammation in the brain to reverse the cognition impairment (Wang et al., 2019).
In the study, the authors focused on the gut microbiota associated with neuroinflammation in AD patients and animal models through observing TH1 and proinflammatory microglia activities. They showed that alterations of gut microbiota composition in AD were obviously correlated with more TH1 cell infiltration into the brain. Also removing the gut microbiota by administrating antibiotic in AD mice can block TH1 cell infiltration and proinflammatory microglia activation. Additionally, strengthened TH1 cell infiltration and proinflammatory microglia activation in WT mice can be caused by FMT from AD mice and prolonged contact with fecal bacteria (Wang et al., 2019). By contrary, less TH1 cell infiltration can be seen in Tg mice receiving FMT from WT mice (Wang et al., 2019). The new discoveries emphasize the abnormal phenylalanine and isoleucine induced by gut microbiota worsening TH1 cell–mediated inflammation in AD and its models and effectively remodeling the gut microbiota is a novel strategy for AD therapy (Figure 2). The therapeutic strategies targeting the gut microbiota in AD patients and animal models are summarized in Table 3. Unfortunately, it is still difficult to determine which microbes are special targets for AD therapy currently, because many factors, including diet, place of residence, smoking, ethnicity, etc., can also influence the changes of the gut microbiota in AD.
Figure 2. Dysbiosis of gut microbes in AD and the intervention strategy. The communications between the gut microbes and brain is through gut–brain axis to involve in the modulatory processes, inflammation, and immune response to maintain homeostasis of body. The gut microbiota can affect brain function and bioactivity (Liu L. et al., 2019). Several factors including inflammation (such as NLPR3 inflammasomes), chronic infections by bacteria or viruses, aging, and increased inflammatory molecules (OS, ROS, and TMAO) production, etc., can cause the changes of microbiota composition, microbial dysbiosis, and ENIS alterations, which contribute to AD pathology in the brain, including neuroinflammation due to glial cell and M1 microglia activations and increased inflammatory molecules production and enhanced Aβ deposition and tau tangles in the brain, these being the classical pathological features in AD (Angelucci et al., 2019). The microbial dysbiosis also leads to the peripheral accumulation of phenylalanine and isoleucine, which promotes the differentiation and proliferation of proinflammatory T helper 1 cells that infiltrated into the brain of AD mice via blood circulation, associated with the M1 microglia activation, contributing to neuroinflammation in AD (Liu P. et al., 2019). AD pathological changes result in a series of clinical symptoms, as cognitive impairment, anxiety, depression, and others. The novel intervention strategies contain applying probiotics, antibiotics, and FMT, as well as using a compound, such as GV-971 and others, which are targeting and remodeling gut microbiota and suppressing gut bacterial amino acids-shaping neuroinflammation to inhibit AD progression. AD, Alzheimer disease; OS, oxidative stress; ROS, reactive oxygen species; TMAO, trimethylamine n-oxide; ENIS, enteric neuroimmune system; FMT, fecal microbiota transplantation.
Targeting dysbiosis of gut microbes as a future therapeutic manipulation in many diseases including AD is a promising therapeutic strategy. The advantages of this manipulation are effective to treat or alleviate diseases. It prevented the recurrence of MS by adding microbes to the daily diet for long-term use (Tremlett et al., 2016) and slowing down the clinical progress in PD patients (Dutta et al., 2019), as well as treated refractory constipation in a PD patient (Huang et al., 2019), etc., in which the conventional treatments were helpless. This therapeutic manipulation overcomes the disadvantages of conventional treatments that either overlook the microbes in the mechanism of action or remove vast populations of microbes via antibiotics. It aims at rebalancing the gut microbiota balance, preventing dysbiosis, and keeping the internal environment stable. Thus, the therapeutic manipulation is exciting and challenging. However, there are still many problems and disadvantages in microbial treatment. First, the obtained results treated by microbes or microbial products in human disorders are contradictory. Also the sample size treated by microbes is too small, which causes doubt to the effectiveness of the treatments. Second, it is difficult to select the correct therapeutic scheme. Third, although the side effects of the microbial treatment were reported sporadically, the exact side effects were not found because of the small sample size. It is unclear whether microbial therapy can cause other diseases.
There are many ways to balance the microbiota in the gut, such as reducing or inhibiting infections and inflammation of the system and gut, eating a healthy diet, quitting smoking, increasing immunity, and exercise, etc.; thus, balancing the microbiota can be maintained for a long time.
The gut microbiome may contribute to the pathogenesis of AD and neurodegenerative disorders through the microbiota–gut–brain axis pathway. The dysbiosis of the gut microbiome and resulting inflammation may be important pathogenic factors in development and progression of AD. Targeting and remodeling the gut microbiome open a potential new door to an effective therapeutic strategy in AD patients. However, the field is nascent, and the data obtained are controversial, as well as many factors influence the gut microbiome. It is still difficult to establish the relationship between the gut microbiome and brain bioactivity in any specific disorder of humans via the microbiota–gut–brain axis pathway. Therefore, the longitudinal study and randomized controlled trials in humans are essential to determine the role of the gut microbiota in AD and other neurological diseases. Finding potent drugs targeting the microbiome may be more promising for future clinical therapeutic strategies.
FZ prepared the manuscript. CL provided views and revised the manuscript. FC and XT helped to correct the manuscript and prepared figures. JZ designed the framework of manuscript, prepared and finalized the manuscript. All authors contributed to the article and approved the submitted version.
This work was supported by Sanming Project of Medicine in Shenzhen city (SZSM201801014), Guangdong Province of China and by The First Hospital of Jilin University, Changchun, China.
The authors declare that the research was conducted in the absence of any commercial or financial relationships that could be construed as a potential conflict of interest.
Alzheimer’s Association. (2017). 2017 Alzheimer’s disease facts and figures. Alzheimers Dement. 13, 325–373. doi: 10.1016/j.jalz.2017.02.001
Abbas, N., Bednar, I., Mix, E., Marie, S., Paterson, D., Ljungberg, A., et al. (2002). Up-regulation of the inflammatory cytokines IFN-γ and IL-12 and down-regulation of IL-4 in cerebral cortex regions of APP(SWE) transgenic mice. J. Neuroimmunol. 126, 50–57. doi: 10.1016/s0165-5728(02)00050-4
Abraham, D., Feher, J., Scuderi, G. L., Szabo, D., Dobolyi, A., Cservenak, M., et al. (2019). Exercise and probiotics attenuate the development of Alzheimer’s disease in transgenic mice: role of microbiome. Exp. Gerontol. 115, 122–131. doi: 10.1016/j.exger.2018.12.005
Agahi, A., Hamidi, G. A., Daneshvar, R., Hamdieh, M., Soheili, M., Alinaghipour, A., et al. (2018). Does severity of Alzheimer’s disease contribute to its responsiveness to modifying gut microbiota? A double blind clinical trial. Front. Neurol. 9:662. doi: 10.3389/fneur.2019.00667
Ait-Belgnaoui, A., Durand, H., Cartier, C., Chaumaz, G., Eutamene, H., Ferrier, L., et al. (2012). Prevention of gut leakiness by a probiotic treatment leads to attenuated HPA response to an acute psychological stress in rats. Psychoneuroendocrinology 37, 1885–1895. doi: 10.1016/j.psyneuen.2012.03.024
Akbari, E., Asemi, Z., Daneshvar Kakhaki, R., Bahmani, F., Kouchaki, E., Tamtaji, O. R., et al. (2016). Effect of probiotic supplementation on cognitive function and metabolic status in Alzheimer’s disease: a randomized, double-blind and controlled trial. Front. Aging Neurosci. 8:256. doi: 10.3389/fnagi.2018.00054
Allegretti, J. R., Kassam, Z., Osman, M., Budree, S., Fischer, M., and Kelly, C. R. (2018). The 5D framework: a clinical primer for fecal microbiota transplantation to treat Clostridium difficile infection. Gastrointest. Endosc. 87, 18–29. doi: 10.1016/j.gie.2017.05.036
Angelucci, F., Cechova, K., Amlerova, J., and Hort, J. (2019). Antibiotics, gut microbiota, and Alzheimer’s disease. J. Neuroinflammation 16:108. doi: 10.1186/s12974-019-1494-4
Ashraf, G. M., Tarasov, V. V., Makhmutova, A., Chubarev, V. N., Avila-Rodriguez, M., Bachurin, S. O., et al. (2019). The possibility of an infectious etiology of Alzheimer disease. Mol. Neurobiol. 56, 4479–4491. doi: 10.1007/s12035-018-1388-y
Askarova, S., Umbayev, B., Masoud, A. R., Kaiyrlykyzy, A., Safarova, Y., Tsoy, A., et al. (2020). The links between the gut microbiome, aging, modern lifestyle and Alzheimer’s disease. Front. Cell. Infect. Microbiol. 10:104. doi: 10.3389/fcimb.2020.00104
Athari Nik Azm, S., Djazayeri, A., Safa, M., Azami, K., Ahmadvand, B., Sabbaghziarani, F., et al. (2018). Lactobacilli and bifidobacteria ameliorate memory and learning deficits and oxidative stress in β-amyloid (1–42) injected rats. Appl. Physiol. Nutr. Metab. 43, 718–726. doi: 10.1139/apnm-2017-0648
Bailey, M. T., Dowd, S. E., Galley, J. D., Hufnagle, A. R., Allen, R. G., and Lyte, M. (2011). Exposure to a social stressor alters the structure of the intestinal microbiota: implications for stressor-induced immunomodulation. Brain Behav. Immun. 25, 397–407. doi: 10.1016/j.bbi.2010.10.023
Barrett, E., Ross, R. P., O’Toole, P. W., Fitzgerald, G. F., and Stanton, C. (2012). γ-aminobutyric acid production by culturable bacteria from the human intestine. J. Appl. Microbiol. 113, 411–417. doi: 10.1111/j.1365-2672.2012.05344.x
Bellavance, M.-A., and Rivest, S. (2014). The HPA—immune axis and the immunomodulatory actions of glucocorticoids in the brain. Front. Immunol. 5:136. doi: 10.3389/fimmu.2014.00136
Bester, J., Soma, P., Kell, D. B., and Pretorius, E. (2015). Viscoelastic and ultrastructural characteristics of whole blood and plasma in Alzheimer-type dementia and the possible role of bacterial lipopolysaccharides (LPS). Oncotarget 6, 35284–35303. doi: 10.18632/oncotarget.6074
Bilen, M., Dufour, J.-C., Lagier, J.-C., Cadoret, F., Daoud, Z., Dubourg, G., et al. (2018). The contribution of culturomics to the repertoire of isolated human bacterial and archaeal species. Microbiome 6:94. doi: 10.1186/s40168-018-0485-5
Blaser, M. J. (2019). Fecal microbiota transplantation for dysbiosis—predictable risks. N. Engl. J. Med. 381, 2064–2066. doi: 10.1056/nejme1913807
Blum, H. E. (2017). The human microbiome. Adv. Med. Sci. 62, 414–420. doi: 10.1016/j.advms.2017.04.005
Bonaz, B., Bazin, T., and Pellissier, S. (2018). The vagus nerve at the interface of the microbiota-gut-brain axis. Front. Neurosci. 12:49. doi: 10.3389/fnins.2018.00049
Borre, Y. E., Moloney, R. D., Clarke, G., Dinan, T. G., and Cryan, J. F. (2014). The impact of microbiota on brain and behavior: mechanisms and therapeutic potential. Adv. Exp. Med. Biol. 817, 373–403. doi: 10.1007/978-1-4939-0897-4_17
Braakman, H. M. H., and van Ingen, J. (2018). Can epilepsy be treated by antibiotics? J. Neurol. 265, 1934–1936. doi: 10.1007/s00415-018-8943-3
Brandscheid, C., Schuck, F., Reinhardt, S., Schäfer, K.-H., Pietrzik, C. U., Grimm, M., et al. (2017). Altered gut microbiome composition and tryptic activity of the 5xFAD Alzheimer’s mouse model. J. Alzheimers Dis. 56, 775–788. doi: 10.3233/jad-160926
Briguglio, M., Dell’Osso, B., Panzica, G., Malgaroli, A., Banfi, G., Zanaboni Dina, C., et al. (2018). Dietary neurotransmitters: a narrative review on current knowledge. Nutrients 10:591. doi: 10.3390/nu10050591
Bronzuoli, M. R., Iacomino, A., Steardo, L., and Scuderi, C. (2016). Targeting neuroinflammation in Alzheimer’s disease. J. Inflamm. Res. 9, 199–208. doi: 10.2147/jir.s86958
Bu, X.-L., Yao, X.-Q., Jiao, S.-S., Zeng, F., Liu, Y.-H., Xiang, Y., et al. (2015). A study on the association between infectious burden and Alzheimer’s disease. Eur. J. Neurol. 22, 1519–1525. doi: 10.1111/ene.12477
Calsolaro, V., and Edison, P. (2016). Neuroinflammation in Alzheimer’s disease: current evidence and future directions. Alzheimers Dement. 12, 719–732. doi: 10.1016/j.jalz.2016.02.010
Cantarel, B. L., Waubant, E., Chehoud, C., Kuczynski, J., DeSantis, T. Z., Warrington, J., et al. (2015). Gut microbiota in multiple sclerosis. J. Investig. Med. 63, 729–734. doi: 10.1097/JIM.0000000000000192
Capuco, A., Urits, I., Hasoon, J., Chun, R., Gerald, B., Wang, J. K., et al. (2020). Current perspectives on gut microbiome dysbiosis and depression. Adv. Ther. 37, 1328–1346. doi: 10.1007/s12325-020-01272-7
Carabotti, M., Scirocco, A., Maselli, M. A., and Severi, C. (2015). The gut-brain axis: interactions between enteric microbiota, central and enteric nervous systems. Ann. Gastroenterol. 28, 203–209.
Caracciolo, B., Xu, W., Collins, S., and Fratiglioni, L. (2014). Cognitive decline, dietary factors and gut-brain interactions. Mech. Ageing Dev. 136–137, 59–69. doi: 10.1016/j.mad.2013.11.011
Cattaneo, A., Cattane, N., Galluzzi, S., Provasi, S., Lopizzo, N., Festari, C., et al. (2017). Association of brain amyloidosis with pro-inflammatory gut bacterial taxa and peripheral inflammation markers in cognitively impaired elderly. Neurobiol. Aging 49, 60–68. doi: 10.1016/j.neurobiolaging.2016.08.019
Chen, D., Yang, X., Yang, J., Lai, G., Yong, T., Tang, X., et al. (2017). Prebiotic effect of fructooligosaccharides from Morinda officinalis on Alzheimer’s disease in rodent models by targeting the microbiota-gut-brain axis. Front. Aging Neurosci. 9:403. doi: 10.3389/fnagi.2017.00403
Chu, F., Shi, M., Lang, Y., Shen, D., Jin, T., Zhu, J., et al. (2018). Gut microbiota in multiple sclerosis and experimental autoimmune encephalomyelitis: current applications and future perspectives. Mediators Inflamm. 2018:8168717. doi: 10.3389/fmicb.2019.00740
Claesson, M. J., Jeffery, I. B., Conde, S., Power, S. E., O’Connor, E. M., Cusack, S., et al. (2012). Gut microbiota composition correlates with diet and health in the elderly. Nature 488, 178–184. doi: 10.1038/nature11319
Collins, S. M., Surette, M., and Bercik, P. (2012). The interplay between the intestinal microbiota and the brain. Nat. Rev. Microbiol. 10, 735–742. doi: 10.1038/nrmicro2876
Cox, L. M., Schafer, M. J., Sohn, J., Vincentini, J., Weiner, H. L., Ginsberg, S. D., et al. (2019). Calorie restriction slows age-related microbiota changes in an Alzheimer’s disease model in female mice. Sci. Rep. 9:17904. doi: 10.1038/s41598-019-54187-x
Crane, J. D., Palanivel, R., Mottillo, E. P., Bujak, A. L., Wang, H., Ford, R. J., et al. (2015). Inhibiting peripheral serotonin synthesis reduces obesity and metabolic dysfunction by promoting brown adipose tissue thermogenesis. Nat. Med. 21, 166–172. doi: 10.1038/nm.3766
Cryan, J. F., O’Riordan, K. J., Sandhu, K., Peterson, V., and Dinan, T. G. (2020). The gut microbiome in neurological disorders. Lancet Neurol. 19, 179–194. doi: 10.1016/S1474-4422(19)30356-4
Daniels, M. J. D., Rivers-Auty, J., Schilling, T., Spencer, N. G., Watremez, W., Fasolino, V., et al. (2016). Fenamate NSAIDs inhibit the NLRP3 inflammasome and protect against Alzheimer’s disease in rodent models. Nat. Commun. 7:12504. doi: 10.1038/ncomms12504
DeFilipp, Z., Bloom, P. P., Torres Soto, M., Mansour, M. K., Sater, M. R. A., Huntley, M. H., et al. (2019). Drug-resistant E. coli bacteremia transmitted by fecal microbiota transplant. N. Engl. J. Med. 381, 2043–2050. doi: 10.1056/NEJMoa1910437
Del Tredici, K., Rüb, U., De Vos, R. A. I., Bohl, J. R. E., and Braak, H. (2002). Where does parkinson disease pathology begin in the brain? J. Neuropathol. Exp. Neurol. 61, 413–426. doi: 10.1093/jnen/61.5.413
Dinan, T. G., and Cryan, J. F. (2017). The microbiome-gut-brain axis in health and disease. Gastroenterol. Clin. North Am. 46, 77–89. doi: 10.1016/j.gtc.2016.09.007
DiSabato, D. J., Quan, N., and Godbout, J. P. (2016). Neuroinflammation: the devil is in the details. J. Neurochem. 139, 136–153. doi: 10.1111/jnc.13607
Dubbelaar, M., Kracht, L., Eggen, B. J., and Boddeke, E. W. G. M. (2018). The kaleidoscope of microglial phenotypes. Front. Immunol. 9:1753. doi: 10.3389/fimmu.2018.01753
Duffy, S. S., Lees, J. G., and Moalem-Taylor, G. (2014). The contribution of immune and glial cell types in experimental autoimmune encephalomyelitis and multiple sclerosis. Mult. Scler. Int. 2014:285245. doi: 10.1155/2014/285245
Dumitrescu, L., Popescu-Olaru, I., Cozma, L., Tulbă, D., Hinescu, M. E., Ceafalan, L. C., et al. (2018). Oxidative stress and the microbiota-gut-brain axis. Oxid. Med. Cell. Longev. 2018:2406594. doi: 10.1155/2018/2406594
Dutta, S. K., Verma, S., Jain, V., Surapaneni, B. K., Vinayek, R., Phillips, L., et al. (2019). Parkinson’s disease: the emerging role of gut dysbiosis, antibiotics, probiotics and fecal microbiota transplantation. J. Neurogastroenterol. Motil. 25, 363–376. doi: 10.5056/jnm19044
Erny, D., Hrabě de Angelis, A. L., and Prinz, M. (2017). Communicating systems in the body: how microbiota and microglia cooperate. Immunology 150, 7–15. doi: 10.1111/imm.12645
Esquerda-Canals, G., Montoliu-Gaya, L., Güell-Bosch, J., and Villegas, S. (2017). Mouse models of Alzheimer’s disease. J. Alzheimers Dis. 57, 1171–1183. doi: 10.3233/JAD-170045
Fakhoury, M. (2018). Microglia and astrocytes in Alzheimer’s disease: implications for therapy. Curr. Neuropharmacol. 16, 508–518. doi: 10.2174/1570159x15666170720095240
Fang, X. (2019). Microbial treatment: the potential application for Parkinson’s disease. Neurol. Sci. 40, 51–58. doi: 10.1007/s10072-018-3641-6
Fang, X., Wang, X., Yang, S., Meng, F., Wang, X., Wei, H., et al. (2016). Evaluation of the microbial diversity in amyotrophic lateral sclerosis using high-throughput sequencing. Front. Microbiol. 7:1479. doi: 10.3389/fmicb.2016.01479
Fasano, A., Visanji, N. P., Liu, L. W., Lang, A. E., and Pfeiffer, R. F. (2015). Gastrointestinal dysfunction in Parkinson’s disease. Lancet Neurol. 14, 625–639. doi: 10.1016/S1474-4422(15)00007-1
Feng, J., Dong, L., Zhang, J., Han, X., Tang, S., Song, L., et al. (2018). Unique expression pattern of KIBRA in the enteric nervous system of APP/PS1 mice. Neurosci. Lett. 675, 41–47. doi: 10.1016/j.neulet.2018.03.014
Foster, J. A., Rinaman, L., and Cryan, J. F. (2017). Stress and the gut-brain axis: regulation by the microbiome. Neurobiol. Stress 7, 124–136. doi: 10.1016/j.ynstr.2017.03.001
Franceschi, C., Bonafè, M., Valensin, S., Olivieri, F., De Luca, M., Ottaviani, E., et al. (2000). Inflamm-aging. An evolutionary perspective on immunosenescence. Ann. N Y Acad. Sci. 908, 244–254. doi: 10.1111/j.1749-6632.2000.tb06651.x
Fröhlich, E. E., Farzi, A., Mayerhofer, R., Reichmann, F., Jačan, A., Wagner, B., et al. (2016). Cognitive impairment by antibiotic-induced gut dysbiosis: analysis of gut microbiota-brain communication. Brain Behav. Immun. 56, 140–155. doi: 10.1016/j.bbi.2016.02.020
Fung, T. C. (2020). The microbiota-immune axis as a central mediator of gut-brain communication. Neurobiol. Dis. 136:104714. doi: 10.1016/j.nbd.2019.104714
Fung, T. C., Olson, C. A., and Hsiao, E. Y. (2017). Interactions between the microbiota, immune and nervous systems in health and disease. Nat. Neurosci. 20, 145–155. doi: 10.1038/nn.4476
Galland, L. (2014). The gut microbiome and the brain. J. Med. Food 17, 1261–1272. doi: 10.1089/jmf.2014.7000
Games, D., Adams, D., Alessandrini, R., Barbour, R., Berthelette, P., Blackwell, C., et al. (1995). Alzheimer-type neuropathology in transgenic mice overexpressing V717F β-amyloid precursor protein. Nature 373, 523–527. doi: 10.1038/373523a0
Gareau, M. G., Wine, E., Rodrigues, D. M., Cho, J. H., Whary, M. T., Philpott, D. J., et al. (2011). Bacterial infection causes stress-induced memory dysfunction in mice. Gut 60, 307–317. doi: 10.1136/gut.2009.202515
Gershon, M. D. (1999). The enteric nervous system: a second brain. Hosp. Pract. 34, 31–32, 35–38, 41–32passim. doi: 10.3810/hp.1999.07.153
Haghikia, A., Jörg, S., Duscha, A., Berg, J., Manzel, A., Waschbisch, A., et al. (2016). Dietary fatty acids directly impact central nervous system autoimmunity via the small intestine. Immunity 44, 951–953. doi: 10.1016/j.immuni.2015.09.007
Harach, T., Marungruang, N., Duthilleul, N., Cheatham, V., Mc Coy, K. D., Frisoni, G., et al. (2017). Reduction of Aβ amyloid pathology in APPPS1 transgenic mice in the absence of gut microbiota. Sci. Rep. 7:41802. doi: 10.1038/srep46856
Haran, J. P., Bhattarai, S. K., Foley, S. E., Dutta, P., Ward, D. V., Bucci, V., et al. (2019). Alzheimer’s disease microbiome is associated with dysregulation of the anti-inflammatory P-glycoprotein pathway. mBio 10:e00632-19. doi: 10.1128/mbio.00632-19
Harris, S. A., and Harris, E. A. (2015). Herpes simplex virus type 1 and other pathogens are key causative factors in sporadic Alzheimer’s disease. J. Alzheimers Dis. 48, 319–353. doi: 10.3233/jad-142853
Heneka, M. T., Kummer, M. P., Stutz, A., Delekate, A., Schwartz, S., Vieira-Saecker, A., et al. (2013). NLRP3 is activated in Alzheimer’s disease and contributes to pathology in APP/PS1 mice. Nature 493, 674–678. doi: 10.1038/nature11729
Hu, X., Wang, T., and Jin, F. (2016). Alzheimer’s disease and gut microbiota. Sci. China Life Sci. 59, 1006–1023. doi: 10.1007/s11427-016-5083-9
Huang, H., Xu, H., Luo, Q., He, J., Li, M., Chen, H., et al. (2019). Fecal microbiota transplantation to treat Parkinson’s disease with constipation: a case report. Medicine 98:e16163. doi: 10.1097/md.0000000000016163
Hueston, C. M., and Deak, T. (2014). The inflamed axis: the interaction between stress, hormones and the expression of inflammatory-related genes within key structures comprising the hypothalamic-pituitary-adrenal axis. Physiol. Behav. 124, 77–91. doi: 10.1016/j.physbeh.2013.10.035
Hugon, P., Dufour, J.-C., Colson, P., Fournier, P.-E., Sallah, K., and Raoult, D. (2015). A comprehensive repertoire of prokaryotic species identified in human beings. Lancet Infect. Dis. 15, 1211–1219. doi: 10.1016/s1473-3099(15)00293-5
Ianiro, G., Tilg, H., and Gasbarrini, A. (2016). Antibiotics as deep modulators of gut microbiota: between good and evil. Gut 65, 1906–1915. doi: 10.1136/gutjnl-2016-312297
Ising, C., Venegas, C., Zhang, S., Scheiblich, H., Schmidt, S. V., Vieira-Saecker, A., et al. (2019). NLRP3 inflammasome activation drives tau pathology. Nature 575, 669–673. doi: 10.1038/s41586-019-1769-z
Jangi, S., Gandhi, R., Cox, L. M., Li, N., von Glehn, F., Yan, R., et al. (2016). Alterations of the human gut microbiome in multiple sclerosis. Nat. Commun. 7:12015. doi: 10.1038/ncomms12015
Jernberg, C., Löfmark, S., Edlund, C., and Jansson, J. K. (2007). Long-term ecological impacts of antibiotic administration on the human intestinal microbiota. ISME J. 1, 56–66. doi: 10.1038/ismej.2007.3
Jiang, C., Li, G., Huang, P., Liu, Z., and Zhao, B. (2017). The gut microbiota and Alzheimer’s disease. J. Alzheimers Dis. 58, 1–15. doi: 10.3233/JAD-161141
Joachim, C. L., Mori, H., and Selkoe, D. J. (1989). Amyloid β-protein deposition in tissues other than brain in Alzheimer’s disease. Nature 341, 226–230. doi: 10.1038/341226a0
Johnson, K. V. A., and Foster, K. R. (2018). Why does the microbiome affect behaviour? Nat. Rev. Microbiol. 16, 647–655. doi: 10.1038/s41579-018-0014-3
Jones, R. M., Mercante, J. W., and Neish, A. S. (2012). Reactive oxygen production induced by the gut microbiota: pharmacotherapeutic implications. Curr. Med. Chem. 19, 1519–1529. doi: 10.2174/092986712799828283
Kamphuis, W., Kooijman, L., Schetters, S., Orre, M., and Hol, E. M. (2016). Transcriptional profiling of CD11c-positive microglia accumulating around amyloid plaques in a mouse model for Alzheimer’s disease. Biochim. Biophys. Acta 1862, 1847–1860. doi: 10.1016/j.bbadis.2016.07.007
Kowalski, K., and Mulak, A. (2019). Brain-gut-microbiota axis in Alzheimer’s disease. J. Neurogastroenterol. Motil. 25, 48–60. doi: 10.5056/jnm18087
Kim, M.-S., Kim, Y., Choi, H., Kim, W., Park, S., Lee, D., et al. (2020). Transfer of a healthy microbiota reduces amyloid and tau pathology in an Alzheimer’s disease animal model. Gut 69, 283–294. doi: 10.1136/gutjnl-2018-317431
Kobayashi, Y., Sugahara, H., Shimada, K., Mitsuyama, E., Kuhara, T., Yasuoka, A., et al. (2017). Therapeutic potential of Bifidobacterium breve strain A1 for preventing cognitive impairment in Alzheimer’s disease. Sci. Rep. 7:13510. doi: 10.1038/s41598-017-13368-2
Kong, G., Cao, K.-A. L., Judd, L. M., Li, S., Renoir, T., and Hannan, A. J. (2020). Microbiome profiling reveals gut dysbiosis in a transgenic mouse model of Huntington’s disease. Neurobiol. Dis. 135:104268. doi: 10.1016/j.nbd.2018.09.001
Kountouras, J., Boziki, M., Gavalas, E., Zavos, C., Grigoriadis, N., Deretzi, G., et al. (2009). Eradication of Helicobacter pylori may be beneficial in the management of Alzheimer’s disease. J. Neurol. 256, 758–767. doi: 10.1007/s00415-009-5011-z
Lach, G., Schellekens, H., Dinan, T. G., and Cryan, J. F. (2018). Anxiety, depression, and the microbiome: a role for gut peptides. Neurotherapeutics 15, 36–59. doi: 10.1007/s13311-017-0585-0
Lambert, J.-C., Heath, S., Even, G., Campion, D., Sleegers, K., Hiltunen, M., et al. (2009). Genome-wide association study identifies variants at CLU and CR1 associated with Alzheimer’s disease. Nat. Genet. 41, 1094–1099. doi: 10.1038/ng.439
Leblhuber, F., Geisler, S., Steiner, K., Fuchs, D., and Schütz, B. (2015). Elevated fecal calprotectin in patients with Alzheimer’s dementia indicates leaky gut. J. Neural Transm. 122, 1319–1322. doi: 10.1007/s00702-015-1381-9
Leblhuber, F., Steiner, K., Schuetz, B., Fuchs, D., and Gostner, J. M. (2018). Probiotic supplementation in patients with Alzheimer’s dementia—an explorative intervention study. Curr. Alzheimer Res. 15, 1106–1113. doi: 10.2174/1389200219666180813144834
Levy, M., Kolodziejczyk, A. A., Thaiss, C. A., and Elinav, E. (2017). Dysbiosis and the immune system. Nat. Rev. Immunol. 17, 219–232. doi: 10.1038/nri.2017.7
Li, J., Jia, H., Cai, X., Zhong, H., Feng, Q., Sunagawa, S., et al. (2014). An integrated catalog of reference genes in the human gut microbiome. Nat. Biotechnol. 32, 834–841. doi: 10.1038/nbt.2942
Liu, L., Gong, T., Tao, W., Lin, B., Li, C., Zheng, X., et al. (2019). Commensal viruses maintain intestinal intraepithelial lymphocytes via noncanonical RIG-I signaling. Nat. Immunol. 20, 1681–1691. doi: 10.1038/s41590-019-0513-z
Liu, J., He, Z., Ma, N., and Chen, Z.-Y. (2020). Beneficial effects of dietary polyphenols on high-fat diet-induced obesity linking with modulation of gut microbiota. J. Agric. Food Chem. 68, 33–47. doi: 10.1021/acs.jafc.9b06817
Liu, P., Wu, L., Peng, G., Han, Y., Tang, R., Ge, J., et al. (2019). Altered microbiomes distinguish Alzheimer’s disease from amnestic mild cognitive impairment and health in a Chinese cohort. Brain Behav. Immun. 80, 633–643. doi: 10.1016/j.bbi.2019.05.008
Loeb, M. B., Molloy, D. W., Smieja, M., Standish, T., Goldsmith, C. H., Mahony, J., et al. (2004). A randomized, controlled trial of doxycycline and rifampin for patients with Alzheimer’s disease. J. Am. Geriatr. Soc. 52, 381–387. doi: 10.1111/j.1532-5415.2004.52109.x
Logsdon, A. F., Erickson, M. A., Rhea, E. M., Salameh, T. S., and Banks, W. A. (2018). Gut reactions: how the blood-brain barrier connects the microbiome and the brain. Exp. Biol. Med. 243, 159–165. doi: 10.1177/1535370217743766
Long-Smith, C., O’Riordan, K. J., Clarke, G., Stanton, C., Dinan, T. G., and Cryan, J. F. (2020). Microbiota-gut-brain axis: new therapeutic opportunities. Annu. Rev. Pharmacol. Toxicol. 60, 477–502. doi: 10.1146/annurev-pharmtox-010919-023628
Luczynski, P., McVey Neufeld, K.-A., Oriach, C. S., Clarke, G., Dinan, T. G., and Cryan, J. F. (2016). Growing up in a bubble: using germ-free animals to assess the influence of the gut microbiota on brain and behavior. Int. J. Neuropsychopharmacol. 19:pyw020. doi: 10.1093/ijnp/pyw020
Lyte, M. (2013). Microbial endocrinology in the microbiome-gut-brain axis: how bacterial production and utilization of neurochemicals influence behavior. PLoS Pathog. 9:e1003726. doi: 10.1371/journal.ppat.1003726
Ma, S., Santhosh, D., Kumar, T. P., and Huang, Z. (2017). A brain-region-specific neural pathway regulating germinal matrix angiogenesis. Dev. Cell 41, 366.e4–381.e4. doi: 10.1016/j.devcel.2017.04.014
MahmoudianDehkordi, S., Arnold, M., Nho, K., Ahmad, S., Jia, W., Xie, G., et al. (2019). Altered bile acid profile associates with cognitive impairment in Alzheimer’s disease-An emerging role for gut microbiome. Alzheimers Dement. 15, 76–92. doi: 10.1016/j.jalz.2019.03.002
Maslowski, K. M., Vieira, A. T., Ng, A., Kranich, J., Sierro, F., Yu, D., et al. (2009). Regulation of inflammatory responses by gut microbiota and chemoattractant receptor GPR43. Nature 461, 1282–1286. doi: 10.1038/nature08530
Mayer, E. A., Tillisch, K., and Gupta, A. (2015). Gut/brain axis and the microbiota. J. Clin. Invest. 125, 926–938. doi: 10.1172/JCI76304
Mazzini, L., Mogna, L., De Marchi, F., Amoruso, A., Pane, M., et al. (2018). “Potential role of gut microbiota in als pathogenesis and possible novel therapeutic strategies. J. Clin. Gastroenterol 52 Suppl 1,” in Proceedings from the 9th Probiotics, Prebiotics and New Foods, Nutraceuticals and Botanicals for Nutrition and Human and Microbiota Health Meeting, Held in Rome, Italy from September 10 to 12, 2017, S68–S70.
Merino, J., Aller, M. A., Rubio, S., Arias, N., Nava, M. P., Loscertales, M., et al. (2011). Gut-brain chemokine changes in portal hypertensive rats. Dig. Dis. Sci. 56, 2309–2317. doi: 10.1007/s10620-011-1625-y
Minato, T., Maeda, T., Fujisawa, Y., Tsuji, H., Nomoto, K., Ohno, K., et al. (2017). Progression of Parkinson’s disease is associated with gut dysbiosis: two-year follow-up study. PLoS One 12:e0187307. doi: 10.1371/journal.pone.0187307
Minter, M. R., Hinterleitner, R., Meisel, M., Zhang, C., Leone, V., Zhang, X., et al. (2017). Antibiotic-induced perturbations in microbial diversity during post-natal development alters amyloid pathology in an aged APP/PS1 murine model of Alzheimer’s disease. Sci. Rep. 7:10411. doi: 10.1038/s41598-017-11047-w
Minter, M. R., Zhang, C., Leone, V., Ringus, D. L., Zhang, X., Oyler-Castrillo, P., et al. (2016). Antibiotic-induced perturbations in gut microbial diversity influences neuro-inflammation and amyloidosis in a murine model of Alzheimer’s disease. Sci. Rep. 6:30028. doi: 10.1038/srep30028
Miyake, S., Kim, S., Suda, W., Oshima, K., Nakamura, M., Matsuoka, T., et al. (2015). Dysbiosis in the gut microbiota of patients with multiple sclerosis, with a striking depletion of species belonging to clostridia XIVa and IV clusters. PLoS One 10:e0137429. doi: 10.1371/journal.pone.0137429
Molloy, D. W., Standish, T. I., Zhou, Q., and Guyatt, G. (2013). A multicenter, blinded, randomized, factorial controlled trial of doxycycline and rifampin for treatment of Alzheimer’s disease: the DARAD trial. Int. J. Geriatr. Psychiatry 28, 463–470. doi: 10.1002/gps.3846
Monsonego, A., Zota, V., Karni, A., Krieger, J. I., Bar-Or, A., Bitan, G., et al. (2003). Increased T cell reactivity to amyloid β protein in older humans and patients with Alzheimer disease. J. Clin. Invest. 112, 415–422. doi: 10.1172/jci200318104
Mowry, E. M., and Glenn, J. D. (2018). The dynamics of the gut microbiome in multiple sclerosis in relation to disease. Neurol. Clin. 36, 185–196. doi: 10.1016/j.ncl.2017.08.008
Mukherjee, S., Joardar, N., Sengupta, S., and Sinha Babu, S. P. (2018). Gut microbes as future therapeutics in treating inflammatory and infectious diseases: lessons from recent findings. J. Nutr. Biochem. 61, 111–128. doi: 10.1016/j.jnutbio.2018.07.010
Murono, S., Hamaguchi, T., Yoshida, H., Nakanishi, Y., Tsuji, A., Endo, K., et al. (2015). Evaluation of dysphagia at the initial diagnosis of amyotrophic lateral sclerosis. Auris Nasus Larynx 42, 213–217. doi: 10.1016/j.anl.2014.10.012
Neufeld, N. H., Mohamed, N. S., Grujich, N., and Shulman, K. (2017). Acute neuropsychiatric symptoms associated with antibiotic treatment of Helicobacter pylori infections: a review. J. Psychiatr. Pract. 23, 25–35. doi: 10.1097/pra.0000000000000205
Niina, A., Kibe, R., Suzuki, R., Yuchi, Y., Teshima, T., Matsumoto, H., et al. (2019). Improvement in clinical symptoms and fecal microbiome after fecal microbiota transplantation in a dog with inflammatory bowel disease. Vet. Med. 10, 197–201. doi: 10.2147/vmrr.s230862
Nimgampalle, M., and Kuna, Y. (2017). Anti-alzheimer properties of probiotic, lactobacillus plantarum MTCC 1325 in Alzheimer’s disease induced albino rats. J. Clin. Diagn. Res. 11, KC01–KC05. doi: 10.7860/jcdr/2017/26106.10428
Olszak, T., An, D., Zeissig, S., Vera, M. P., Richter, J., Franke, A., et al. (2012). Microbial exposure during early life has persistent effects on natural killer T cell function. Science 336, 489–493. doi: 10.1126/science.1219328
Panza, F., Lozupone, M., Solfrizzi, V., Watling, M., and Imbimbo, B. P. (2019). Time to test antibacterial therapy in Alzheimer’s disease. Brain 142, 2905–2929. doi: 10.1093/brain/awz244
Park, A. J., Collins, J., Blennerhassett, P. A., Ghia, J. E., Verdu, E. F., Bercik, P., et al. (2013). Altered colonic function and microbiota profile in a mouse model of chronic depression. Neurogastroenterol. Motil. 25:733-e575. doi: 10.1111/nmo.12153
Pellegrini, C., Antonioli, L., Colucci, R., Blandizzi, C., and Fornai, M. (2018). Interplay among gut microbiota, intestinal mucosal barrier and enteric neuro-immune system: a common path to neurodegenerative diseases? Acta Neuropathol. 136, 345–361. doi: 10.1007/s00401-018-1856-5
Pellegrini, C., Fornai, M., Antonioli, L., Blandizzi, C., and Calderone, V. (2019). Phytochemicals as novel therapeutic strategies for NLRP3 inflammasome-related neurological, metabolic, and inflammatory diseases. Int. J. Mol. Sci. 20:2876. doi: 10.3390/ijms20122876
Picca, A., Fanelli, F., Calvani, R., Mulè, G., Pesce, V., Sisto, A., et al. (2018). Gut dysbiosis and muscle aging: searching for novel targets against sarcopenia. Mediators Inflamm. 2018:7026198. doi: 10.1155/2018/7026198
Pistollato, F., Sumalla Cano, S., Elio, I., Masias Vergara, M., Giampieri, F., and Battino, M. (2016). Role of gut microbiota and nutrients in amyloid formation and pathogenesis of Alzheimer disease. Nutr. Rev. 74, 624–634. doi: 10.1093/nutrit/nuw023
Puig, K. L., Lutz, B. M., Urquhart, S. A., Rebel, A. A., Zhou, X., Manocha, G. D., et al. (2015). Overexpression of mutant amyloid-β protein precursor and presenilin 1 modulates enteric nervous system. J. Alzheimers Dis. 44, 1263–1278. doi: 10.3233/JAD-142259
Rogers, J., Luber-Narod, J., Styren, S. D., and Civin, W. H. (1988). Expression of immune system-associated antigens by cells of the human central nervous system: relationship to the pathology of Alzheimer’s disease. Neurobiol. Aging 9, 339–349. doi: 10.1016/s0197-4580(88)80079-4
Roubaud-Baudron, C., Krolak-Salmon, P., Quadrio, I., Mégraud, F., and Salles, N. (2012). Impact of chronic Helicobacter pylori infection on Alzheimer’s disease: preliminary results. Neurobiol. Aging 33, 1009.e11–1009.e19. doi: 10.1016/j.neurobiolaging.2011.10.021
Rumah, K. R., Vartanian, T. K., and Fischetti, V. A. (2017). Oral multiple sclerosis drugs inhibit the in vitro growth of epsilon toxin producing gut bacterium, Clostridium perfringens. Front. Cell. Infect. Microbiol. 7:11. doi: 10.3389/fcimb.2017.00011
Salter, M. W., and Stevens, B. (2017). Microglia emerge as central players in brain disease. Nat. Med. 23, 1018–1027. doi: 10.1038/nm.4397
Scheperjans, F., Aho, V., Pereira, P. A., Koskinen, K., Paulin, L., Pekkonen, E., et al. (2015). Gut microbiota are related to Parkinson’s disease and clinical phenotype. Mov. Disord. 30, 350–358. doi: 10.1002/mds.26069
Schneider, S., Wright, C. M., and Heuckeroth, R. O. (2019). Unexpected roles for the second brain: enteric nervous system as master regulator of bowel function. Annu. Rev. Physiol. 81, 235–259. doi: 10.1146/annurev-physiol-021317-121515
Scott, K. A., Ida, M., Peterson, V. L., Prenderville, J. A., Moloney, G. M., Izumo, T., et al. (2017). Revisiting Metchnikoff: age-related alterations in microbiota-gut-brain axis in the mouse. Brain Behav. Immun. 65, 20–32. doi: 10.1016/j.bbi.2017.02.004
Semar, S., Klotz, M., Letiembre, M., Van Ginneken, C., Braun, A., Jost, V., et al. (2013). Changes of the enteric nervous system in amyloid-β protein precursor transgenic mice correlate with disease progression. J. Alzheimers Dis. 36, 7–20. doi: 10.1002/9780470988756.ch1
Shahi, S. K., Freedman, S. N., and Mangalam, A. K. (2017). Gut microbiome in multiple sclerosis: the players involved and the roles they play. Gut Microbes 8, 607–615. doi: 10.1080/19490976.2017.1349041
Shapiro, H., Thaiss, C. A., Levy, M., and Elinav, E. (2014). The cross talk between microbiota and the immune system: metabolites take center stage. Curr. Opin. Immunol. 30, 54–62. doi: 10.1016/j.coi.2014.07.003
Shen, L., Liu, L., and Ji, H.-F. (2017). Alzheimer’s disease histological and behavioral manifestations in transgenic mice correlate with specific gut microbiome state. J. Alzheimers Dis. 56, 385–390. doi: 10.3233/jad-160884
Silverman, M. N., and Sternberg, E. M. (2012). Glucocorticoid regulation of inflammation and its functional correlates: from HPA axis to glucocorticoid receptor dysfunction. Ann. N Y Acad. Sci. 1261, 55–63. doi: 10.1111/j.1749-6632.2012.06633.x
Sochocka, M., Donskow-Lysoniewska, K., Diniz, B. S., Kurpas, D., Brzozowska, E., and Leszek, J. (2019). The gut microbiome alterations and inflammation-driven pathogenesis of Alzheimer’s disease-a critical review. Mol. Neurobiol. 56, 1841–1851. doi: 10.1007/s12035-018-1188-4
Stenman, L. K., Holma, R., Eggert, A., and Korpela, R. (2013). A novel mechanism for gut barrier dysfunction by dietary fat: epithelial disruption by hydrophobic bile acids. Am. J. Physiol. Gastrointest. Liver Physiol. 304, G227–G234. doi: 10.1152/ajpgi.00267.2012
Sun, J., Liu, S., Ling, Z., Wang, F., Ling, Y., Gong, T., et al. (2019a). Fructooligosaccharides ameliorating cognitive deficits and neurodegeneration in APP/PS1 transgenic mice through modulating gut microbiota. J. Agric. Food Chem. 67, 3006–3017. doi: 10.1021/acs.jafc.8b07313
Sun, J., Xu, J., Ling, Y., Wang, F., Gong, T., Yang, C., et al. (2019b). Fecal microbiota transplantation alleviated Alzheimer’s disease-like pathogenesis in APP/PS1 transgenic mice. Transl. Psychiatry 9:189. doi: 10.1038/s41398-019-0525-3
Sun, M.-F., Zhu, Y.-L., Zhou, Z.-L., Jia, X.-B., Xu, Y.-D., Yang, Q., et al. (2018). Neuroprotective effects of fecal microbiota transplantation on MPTP-induced Parkinson’s disease mice: gut microbiota, glial reaction and TLR4/TNF-α signaling pathway. Brain Behav. Immun. 70, 48–60. doi: 10.1016/j.bbi.2018.02.005
Swidsinski, A., Loening-Baucke, V., Lochs, H., and Hale, L.-P. (2005). Spatial organization of bacterial flora in normal and inflamed intestine: a fluorescence in situ hybridization study in mice. World J. Gastroenterol. 11, 1131–1140. doi: 10.3748/wjg.v11.i8.1131
Tamtaji, O. R., Heidari-Soureshjani, R., Mirhosseini, N., Kouchaki, E., Bahmani, F., Aghadavod, E., et al. (2019a). Probiotic and selenium co-supplementation and the effects on clinical, metabolic and genetic status in Alzheimer’s disease: a randomized, double-blind, controlled trial. Clin. Nutr. 38, 2569–2575. doi: 10.1016/j.clnu.2018.11.034
Tamtaji, O. R., Taghizadeh, M., Daneshvar Kakhaki, R., Kouchaki, E., Bahmani, F., Borzabadi, S., et al. (2019b). Clinical and metabolic response to probiotic administration in people with Parkinson’s disease: a randomized, double-blind, placebo-controlled trial. Clin. Nutr. 38, 1031–1035. doi: 10.1016/j.clnu.2018.05.018
Tang, Y., and Le, W. (2016). Differential roles of M1 and M2 microglia in neurodegenerative diseases. Mol. Neurobiol. 53, 1181–1194. doi: 10.1007/s12035-014-9070-5
Tejera, D., Mercan, D., Sanchez-Caro, J. M., Hanan, M., Greenberg, D., Soreq, H., et al. (2019). Systemic inflammation impairs microglial Aβ clearance through NLRP3 inflammasome. EMBO J. 38:e101064. doi: 10.15252/embj.2018101064
Thaiss, C. A., Levy, M., Korem, T., Dohnalová, L., Shapiro, H., Jaitin, D. A., et al. (2016). Microbiota diurnal rhythmicity programs host transcriptome oscillations. Cell 167, 1495.e12–1510.e12. doi: 10.1016/j.cell.2016.11.003
Thomson, P., Medina, D. A., and Garrido, D. (2018). Human milk oligosaccharides and infant gut bifidobacteria: molecular strategies for their utilization. Food Microbiol. 75, 37–46. doi: 10.1016/j.fm.2017.09.001
Ticinesi, A., Nouvenne, A., Tana, C., Prati, B., and Meschi, T. (2019). Gut microbiota and microbiota-related metabolites as possible biomarkers of cognitive aging. Adv. Exp. Med. Biol. 1178, 129–154. doi: 10.1007/978-3-030-25650-0_8
Tognini, P. (2017). Gut microbiota: a potential regulator of neurodevelopment. Front. Cell. Neurosci. 11:25. doi: 10.3389/fncel.2017.00025
Togo, T., Akiyama, H., Iseki, E., Kondo, H., Ikeda, K., Kato, M., et al. (2002). Occurrence of T cells in the brain of Alzheimer’s disease and other neurological diseases. J. Neuroimmunol. 124, 83–92. doi: 10.1016/s0165-5728(01)00496-9
Tremlett, H., Fadrosh, D. W., Faruqi, A. A., Hart, J., Roalstad, S., Graves, J., et al. (2016). Gut microbiota composition and relapse risk in pediatric MS: a pilot study. J. Neurol. Sci. 363, 153–157. doi: 10.1016/j.jns.2016.02.042
Tremlett, H., Bauer, K. C., Appel-Cresswell, S., Finlay, B. B., and Waubant, E. (2017). The gut microbiome in human neurological disease: a review. Ann. Neurol. 81, 369–382. doi: 10.1002/ana.24901
Vogt, N. M., Kerby, R. L., Dill-McFarland, K. A., Harding, S. J., Merluzzi, A. P., Johnson, S. C., et al. (2017). Gut microbiome alterations in Alzheimer’s disease. Sci. Rep. 7:13537. doi: 10.1038/s41598-017-13601-y
Vogt, N. M., Romano, K. A., Darst, B. F., Engelman, C. D., Johnson, S. C., Carlsson, C. M., et al. (2018). The gut microbiota-derived metabolite trimethylamine N-oxide is elevated in Alzheimer’s disease. Alzheimers Res. Ther. 10:124. doi: 10.1186/s13195-018-0451-2
Wang, T., Hu, X., Liang, S., Li, W., Wu, X., Wang, L., et al. (2015). Lactobacillus fermentum NS9 restores the antibiotic induced physiological and psychological abnormalities in rats. Benef. Microbes 6, 707–717. doi: 10.3920/bm2014.0177
Wang, X., Sun, G., Feng, T., Zhang, J., Huang, X., Wang, T., et al. (2019). Sodium oligomannate therapeutically remodels gut microbiota and suppresses gut bacterial amino acids-shaped neuroinflammation to inhibit Alzheimer’s disease progression. Cell Res. 29, 787–803. doi: 10.1038/s41422-019-0216-x
Wang, H.-X., and Wang, Y.-P. (2016). Gut microbiota-brain Axis. Chin. Med. J. 129, 2373–2380. doi: 10.4103/0366-6999.190667
Wang, X.-L., Zeng, J., Yang, Y., Xiong, Y., Zhang, Z.-H., Qiu, M., et al. (2015). Helicobacter pylori filtrate induces Alzheimer-like tau hyperphosphorylation by activating glycogen synthase kinase-3β. J. Alzheimers Dis. 43, 153–165. doi: 10.3233/jad-140198
Weinstein, L. I., Revuelta, A., and Pando, R. H. (2015). Catecholamines and acetylcholine are key regulators of the interaction between microbes and the immune system. Ann. N Y Acad. Sci. 1351, 39–51. doi: 10.1111/nyas.12792
Wekerle, H. (2016). The gut-brain connection: triggering of brain autoimmune disease by commensal gut bacteria. Rheumatology 55, ii68–ii75. doi: 10.1093/rheumatology/kew353
Xin, Y., Diling, C., Jian, Y., Ting, L., Guoyan, H., Hualun, L., et al. (2018). Effects of oligosaccharides from morinda officinalis on gut microbiota and metabolome of APP/PS1 transgenic mice. Front. Neurol. 9:412. doi: 10.3389/fneur.2018.00412
Yano, J. M., Yu, K., Donaldson, G. P., Shastri, G. G., Ann, P., Ma, L., et al. (2015). Indigenous bacteria from the gut microbiota regulate host serotonin biosynthesis. Cell 161, 264–276. doi: 10.1016/j.cell.2015.02.047
Yeh, F. L., Hansen, D. V., and Sheng, M. (2017). TREM2, microglia and neurodegenerative diseases. Trends Mol. Med. 23, 512–533. doi: 10.1016/j.molmed.2017.03.008
Yu, T., Hong, Y., Bo, Z., Dan, W., Bo, L., Jie, Z., et al. (2019). Promising neuroprotective function for M2 microglia in Kainic Acid-induced neurotoxicity via the down-regulation of NF-κB and caspase 3 signaling pathways. Neuroscience 406, 86–96. doi: 10.1016/j.neuroscience.2019.03.002
Zac-Varghese, S., Tan, T., and Bloom, S. R. (2010). Hormonal interactions between gut and brain. Discov. Med. 10, 543–552.
Zarrinpar, A., Chaix, A., Xu, Z. Z., Chang, M. W., Marotz, C. A., Saghatelian, A., et al. (2018). Antibiotic-induced microbiome depletion alters metabolic homeostasis by affecting gut signaling and colonic metabolism. Nat. Commun. 9:2872. doi: 10.1038/s41467-018-05336-9
Zhang, X., Dan, W., Bo, Z., Jie, Z., Zhulin, Z., and Li, C. (2020). Regulation of microglia by glutamate and its signal pathway in the neurodegenerative diseases. Drug Discov. Today 6, 1074–1085. doi: 10.1016/j.drudis.2020.04.001
Zhang, Y., Huang, R., Cheng, M., Wang, L., Chao, J., Li, J., et al. (2019). Gut microbiota from NLRP3-deficient mice ameliorates depressive-like behaviors by regulating astrocyte dysfunction via circHIPK2. Microbiome 7:116. doi: 10.1186/s40168-019-0733-3
Zhao, Y., and Lukiw, W. J. (2015). Microbiome-generated amyloid and potential impact on amyloidogenesis in Alzheimer’s disease (AD). J. Nat. Sci. 1:e138.
Zhu, S., Jiang, Y., Xu, K., Cui, M., Ye, W., Zhao, G., et al. (2020). The progress of gut microbiome research related to brain disorders. J. Neuroinflammation 17:25. doi: 10.1186/s12974-020-1705-z
Keywords: Alzheimer’s disease, gut microbiota, dysbiosis, microbiota-gut-brain axis, treatment
Citation: Zhu F, Li C, Chu F, Tian X and Zhu J (2020) Target Dysbiosis of Gut Microbes as a Future Therapeutic Manipulation in Alzheimer’s Disease. Front. Aging Neurosci. 12:544235. doi: 10.3389/fnagi.2020.544235
Received: 20 March 2020; Accepted: 24 August 2020;
Published: 06 October 2020.
Edited by:
Khalil Sherali Rawji, University of Cambridge, United KingdomReviewed by:
Deepak Kumar Kaushik, University of Calgary, CanadaCopyright © 2020 Zhu, Li, Chu, Tian and Zhu. This is an open-access article distributed under the terms of the Creative Commons Attribution License (CC BY). The use, distribution or reproduction in other forums is permitted, provided the original author(s) and the copyright owner(s) are credited and that the original publication in this journal is cited, in accordance with accepted academic practice. No use, distribution or reproduction is permitted which does not comply with these terms.
*Correspondence: Jie Zhu, anpodWhzQHlhaG9vLmNvbQ==
Disclaimer: All claims expressed in this article are solely those of the authors and do not necessarily represent those of their affiliated organizations, or those of the publisher, the editors and the reviewers. Any product that may be evaluated in this article or claim that may be made by its manufacturer is not guaranteed or endorsed by the publisher.
Research integrity at Frontiers
Learn more about the work of our research integrity team to safeguard the quality of each article we publish.