- 1School of Fundamental Sciences, China Medical University, Shenyang, China
- 2College of Life and Health Sciences, Northeastern University, Shenyang, China
- 3Institute of Health Science, China Medical University, Shenyang, China
The microtubule-associated protein tau is closely correlated with hypometabolism in Alzheimer’s disease (AD). α-lipoic acid (LA), which is a naturally occurring cofactor in mitochondrial, has been shown to have properties that can inhibit the tau pathology and neuronal damage in our previous research. However, if LA affects glucose metabolism when it reverses tau pathology remains unclear, especially concerning the potential mechanism. Therefore, we make a further study using the P301S mouse model (a tauopathy and AD mouse model which overexpressing fibrillary tau) to gain a clear idea of the aforementioned problems. Here, we found chronic LA administration significantly increased glucose availability by elevating glucose transporter 3 (GLUT3), GLUT4, vascular endothelial growth factor (VEGF) protein and mRNA level, and heme oxygenase-1 (HO-1) protein level in P301S mouse brains. Meanwhile, we found that LA also promoted glycolysis by directly upregulating hexokinase (HK) activity, indirectly by increasing proliferator-activated receptor gamma coactivator 1-alpha (PGC-1α) and DNA repair enzymes (OGG1/2 and MTH1). Further, we found the underlying mechanism of restored glucose metabolism might involve in the activation of brain-derived neurotrophic factor (BDNF)/tyrosine Kinase receptor B (TrkB)/hypoxia-inducible factor-1α (HIF-1α) signaling pathway by LA treatment.
Introduction
Alzheimer’s disease (AD) is a growing health burden worldwide. Extracellular amyloid plaques, intracellular neurofibrillary tangles (NFTs), and decreased glucose metabolism are the main characteristics of AD brains (Scheltens et al., 2016; Butterfield and Halliwell, 2019). Recent studies have reported a spatial association between tau and glucose metabolism, the brain region where has the decreased glucose metabolism level is strongly associated with increased tau level in AD patients (Adams et al., 2019; Baghel et al., 2019). Glucose transporters (GLUTs) deficiency and glucose deprivation have been shown to trigger tau hyperphosphorylation and cognitive impairment (Liu et al., 2008; Lauretti and Pratico, 2015; Lauretti et al., 2017), which suggests a strong relationship between hyperphosphorylated tau levels and glucose metabolism in AD progression.
The brain mostly uses glucose for energy, but glucose metabolism is dramatically decreased in AD. Glucose uptake and glycolysis are major factors of glucose metabolism in neurons. However, not only the glucose uptake but also glycolysis is dysregulated in AD (Winkler et al., 2015). Glucose uptake occurs mainly by facilitated diffusion through specific GLUTs (Jais et al., 2016). To date, 14 GLUTs have been detected in mammals, and GLUT1, GLUT3, and GLUT4 play major roles in facilitating brain glucose uptake (McEwen and Reagan, 2004; Simpson et al., 2008). GLUT1 is primarily found in microvascular endothelial and glial cells (Simpson et al., 1999), GLUT3 and GLUT4 are primarily present in neurons (Maher et al., 1994). Postmortem investigations showed decreased GLUT1 and GLUT3 protein levels in AD brains (Simpson et al., 1994). Therefore, reductions in GLUT1 and GLUT3 levels might contribute to neuronal dysfunction and neurodegeneration (Winkler et al., 2015). Also, vascular endothelial growth factor (VEGF), has been proved tightly associated with glucose uptake. The ablation of VEGF reduces brain glucose uptake and cognitive function (Jais et al., 2016). However, increased VEGF expression can enhance GLUTs expression and cerebral glucose metabolism (Yeh et al., 2008; Jais et al., 2016; Wang et al., 2018).
Neurons process glucose directly through glycolysis. Hexokinase 1 (HK1) and Hexokinase 2 (HK2) are the ubiquitously expressed phosphofructokinase, which catalyzes the rate-limiting and first irreversible step of glycolysis (Zheng et al., 2016). HK1 and HK2 may be causally linked to neurodegenerative disorders, the activity of HK declines quickly in the elderly and AD patients (Harris et al., 2014; An et al., 2018). Besides, the transcriptional coactivator proliferator-activated receptor gamma coactivator 1-alpha (PGC1-α), which plays a key role in HK activity regulation, has also decreased in AD (Qin et al., 2009).
DNA repair deficiency contributes to mitochondrial dysfunction and deteriorates the glucose metabolism (Demarest et al., 2020). MutY homolog (MUTYH), MutT Homolog 1 (MTH1) and Oxoguanine DNA glycosylase 1 and 2 (OGG1/2) are crucial DNA repair enzymes, the oxidative DNA damage can be minimized by the functions of MUTYH with the mispaired bases, MTH1 with oxidized dNTPs and OGG1/2 with the 7,8-dihydro-8-oxoguanine (8oxoG). Increasing evidence indicates a decreased level of MTH1 and OGG1/2 in AD brains (Sliwinska et al., 2016; Abolhassani et al., 2017).
Recent studies have identified HIF-1α as an important transcription factor in glucose metabolism via regulating key factors involved in glucose uptake and glycolysis (Ferrara and Davis-Smyth, 1997; Harris et al., 2014). GLUT1 and GLUT3 are two important HIF-1α-regulated genes (Chen et al., 2001; Hayashi et al., 2004). Liu et al. (2008) revealed that decreased GLUT1 and GLUT3 may result from downregulated HIF-1α levels in AD brains, and it has strong connections with abnormal hyperphosphorylation of tau. HIF-1α also regulates GLUT4, and correlative research reported that HIF-1α reduction impaired GLUT4 translocation and glucose uptake (Sakagami et al., 2014). VEGF, which is the main factor involved in cerebral blood flow, plays a critical compensatory role to reinstate brain glucose metabolism (Jais et al., 2016). Interestingly, emerging evidence shows that the infusion of brain-derived neurotrophic factor (BDNF) improves glucose metabolism and cognitive function (Kuroda et al., 2003; Li et al., 2012; Kuipers et al., 2016). BDNF increased VEGF mRNA and protein expression via a HIF-1α signal transduction pathway (Lin et al., 2014), and the underlying mechanism was the activation of the BDNF/tyrosine Kinase receptor B (TrkB) pathway, which stimulate increases in HIF-1α (Nakamura et al., 2006; Helan et al., 2014; Jin et al., 2019).
Agents, such as iron chelators, effectively maintain HIF-1α levels in neurons (Guo et al., 2017). Therefore, we hypothesized that the maintenance of HIF-1α levels via the BDNF/TrkB pathway using iron chelators would be an effective way to attenuate glucose metabolism and abnormal tau pathology. α-Lipoic acid (LA) is a potent iron chelator and antioxidant that was found to be beneficial in the mitigation of oxidative stress, inflammation, ferroptosis, and tauopathy in our previous study (Zhang et al., 2018). Vassilopoulos and Papazafiri (2005) reported that LA maintained cardiomyocytes function and survival via stabilization of HIF-1α. However, no evidence of an association between LA and HIF-1α in AD has been reported. The present study first reported that LA supplementation effectively induced HIF-1α expression and alleviated cognitive deficits in Tau P301S mice (an AD mouse model) by reinstating glucose metabolism impairment via the BDNF/TrkB/HIF-1α signaling pathway.
Materials and Methods
Animals and Pharmacological Treatments
The P301S transgenic mice [B6C3-Tg (Prnp-MAPT*P301S) PS19 Vle/J] were originally obtained from the Jackson Laboratory (Bar Harbor, ME, USA), and housed in controlled temperature and natural light-cycle room. Twenty-one female mice were raised in filtered cages with free food and water until 5 months, then they were randomly assigned to three treatment groups (7 mice/group) corresponding to vehicle control, 3 mg/kg LA, and 10 mg/kg LA (T5625, Sigma–Adrich, St. Louis, MO, USA). LA was administered by intraperitoneal injection once per day, and the vehicle control mice received an identical volume of physiological saline. After continuously injected for 3 days, the animals got 1 day off, and treatment was stopped when cumulative injection add up to 2 months. The dosage and treatment protocol of LA was determined according to previous studies (Maritim et al., 2003; Zhao et al., 2015; Mahboob et al., 2016). During chronic treatment with LA, the body weight and health conditions were monitored daily. The wild type (WT) mice used as control were purchased from HFK bioscience company (Beijing, China) on a C57BL/6 background. All experiment procedures were accordance with the National Institutes of Health guidelines and approved by the Laboratory Animal Ethical Committee of China Medical University.
Tissue Preparation
When all the treatment finished, mice (8-month-old) were euthanized by carbon dioxide euthanasia and perfused by physiological saline. The brain tissues were immediately taken from mice and cut into separate hemispheres on ice. The cerebral cortex was dissected from left hemisphere and stored in −80°C refrigerator for Western blot, RT-PCR, and hexokinase activity analysis. The right hemisphere was fixed in 4% paraformaldehyde at 4°C overnight and dehydrated in 30% sucrose until sinking to the bottom, then cut into frozen coronal sections (10 μm) for immunohistochemistry.
Quantification of Hexokinase Activity
Hexokinase (HK) activity assay was performed using a hexokinase activity colorimetric kit (Solarbio, BC0740, China) according to the manufacturer’s protocol. In brief, the cortex of brain tissues was homogenized in extraction buffer on ice. Then samples were centrifuged at 8,000× g for 10 min at 4°C to obtain a supernatant. Forty microliter supernatant was loaded with the preheated reaction mix (37°C) and read the initial absorbance (340 nm) at 20 s with a microplate reader (BioTek, Cytation5, USA), then the mixture was incubated in 37°C water bath for 5 min and read the absorbance (340 nm) at 5 min 20 s. The activity of HK was calculated according to the formula in the manufacturer’s protocol.
Western Blotting Analysis
Protein extracts from WT and P301S transgenic mouse cortex tissues, and the Western blots, were prepared as described previously (Zhang et al., 2018). In brief, samples were sonicated in a well-mixed buffer which adds phosphatase inhibitor cocktail, protease inhibitor cocktail, sodium fluoride, and phenylmethanesulfonyl fluoride to RIPA lysis buffer in the proportion of 1:100. After incubated 1 h on ice, all samples were centrifuged at 13,684× g for 25 min at 4°C and the supernatants were collected. The nuclear and cytosolic protein fractions were separated using a Nuclear Protein Extraction Kit according to the manufacturer’s instructions (Solarbio, R0050, China). The protein concentrations of all samples were determined by BCA kits (Beyotime, P0010S, China).
Proteins (30 μg) were separated using 10% or 12% SDS-PAGE as previously described (Zhang et al., 2018) and transferred onto polyvinylidene difluoride (PVDF) membranes (Millipore, IPVH00010, Darmstadt, Germany). Membranes were blocked with 5% skim milk and incubated overnight at 4°C with the follwing primary antibodies: rabbit anti-HIF-1α (1:2,000; Thermo Fisher Scientific, PA316521, USA), rabbit anti-BDNF (1:1,000; Santa Cruz Biotechnology, sc-546, USA), rabbit anti-p-TrkA/B (1:2,000; Thermo Fisher Scientific, MA5-14926, USA), rabbit anti-TrkB (1:2,000; Cell Signaling Technology, 4638T, USA), rabbit anti-GLUT1 (1:2,000, BIOSS, bs-20173R, China), mouse anti-GLUT3 (1:1,000; Santa Cruz Biotechnology, sc-74497, USA), rabbit anti-GLUT4 (1:2,000, BIOSS, bs-0384R, China), rabbit anti-hexokinase II (1:2,000; Cell Signaling Technology, C35C4, USA), rabbit anti-hexokinase II (1:2,000; Cell Signaling Technology, C64G5, USA), rabbit anti-HO-1 (1:2,000; Thermo Fisher Scientific, PA5-27338, USA), rabbit anti-VEGF (1:2,000; Cell Signaling Technology, 2463S, USA), rabbit anti-PGC-1α (1:1,000; Cell Signaling Technology, 2178S, USA), mouse anti-MUTYH (1:1,000; Santa Cruz Biotechnology, sc-374571, USA), mouse anti-OGG1/2 (1:1,000; Santa Cruz Biotechnology, sc-376935, USA), rabbit anti-MTH1 (1:1,000; Santa Cruz Biotechnology, sc-67291, USA), mouse anti-β-actin (1:10,000; Sigma–Aldrich, A1978, USA). After rinsed with TBST (leagene, pw0020, Beijing, China), the membranes were incubated with horseradish peroxidase-labeled secondary antibodies (1:10,000; Thermo Fisher Scientific, A27035 and A27022, USA) for 1 h at room temperature. Membranes were incubated with enhanced chemiluminescence (ECL) kits (Tanon, 180-5001B, China) for blot signal detection and visualized using Chem Doc XRS with Quantity One software (Tanon, 5500, China). Protein levels were normalized to actin, and phosphorylated proteins were normalized to their respective total protein amounts. Quantification and analyses were performed using ImageJ and Prism software.
Immunohistochemistry and Confocal Microscopy
The sections were repaired in L.A.B solution (Polyscience, 24310-500, USA) and blocked with goat serum for 1 h at room temperature. After incubating with primary antibodies overnight at 4°C, the sections were rinsed with PBS, incubated with a secondary fluorescent-labeled antibody, and stained with DAPI (Solarbio, C0065, China). Then the sections were sealed in an anti-fade mounting medium. Immunohistochemical detection was performed using a fluorescence microscope (Leica, SP8, Germany). The following primary antibodies were used in the present study: rabbit-anti-BDNF (1:200; Sigma–Aldrich, SAB2108004, USA), mouse-anti-NeuN (1:200; Abcam, ab104224, UK), rabbit-anti-TrkB (1:50; Bioss, bs-0175R, China) and rabbit-anti-HIF-1α (1:100; Novus, NB100-479, Japan).
Real-Time PCR
Total RNA of the cortex was extracted using Trizol (Thermo Scientific, 10296010, USA), and the RNA concentration was quantified using a Nanodrop at 260/280 nm (Thermo Scientific, ND1000, USA). One microgram total RNA was reverse transcribed to cDNA according to the manufacturer’s recommendations using GoTaqR 2-Step RT-qPCR System (Promega, A5001, USA). The generated cDNA was used for subsequent PCR reactions. All PCR reactions performed in a total volume of 20 μl: DNA polymerase activation for 10 min at 95°C, then 40 cycles of denaturing for 30 s at 95°C and annealing and extension for 30 s at 58°C. All applied primer pairs were listed in Supplementary Table S1. The mRNA expression levels were calculated using ΔΔCt (threshold cycle, Ct) values normalized to GAPDH.
Statistical Analysis
The difference between groups was analyzed by the unpaired two-tailed Student’s t-test for two data sets, or the one-way analysis of variance (ANOVA) for the three data sets by Graphpad Prism 5.0 (Graphpad Software, USA). Before applying the one-way ANOVA analysis, the Gaussian distribution of data was analyzed by the Kolmogorov–Smirnov method. All data are presented as mean ± SEM. Significance was set at p < 0.05.
Results
LA Treatment Improves Glucose Metabolism Deficiency in P301S Mice Brains
To see whether glucose metabolism was altered in P301S mice brains, we monitored glucose uptake and metabolism-related protein expression in P301S and WT mice. As a result, we found that GLUT1, GLUT3, GLUT4 expression level, and HK activity were decreased in P301S mice brains by ~19%, 25%, 22%, and 20%, respectively (Figures 1A–G). Whereas no significant alteration was observed in HK1 and HK2 protein level (Figures 1A–G). These data suggested glucose metabolism deficiency might exist in P301S mice.
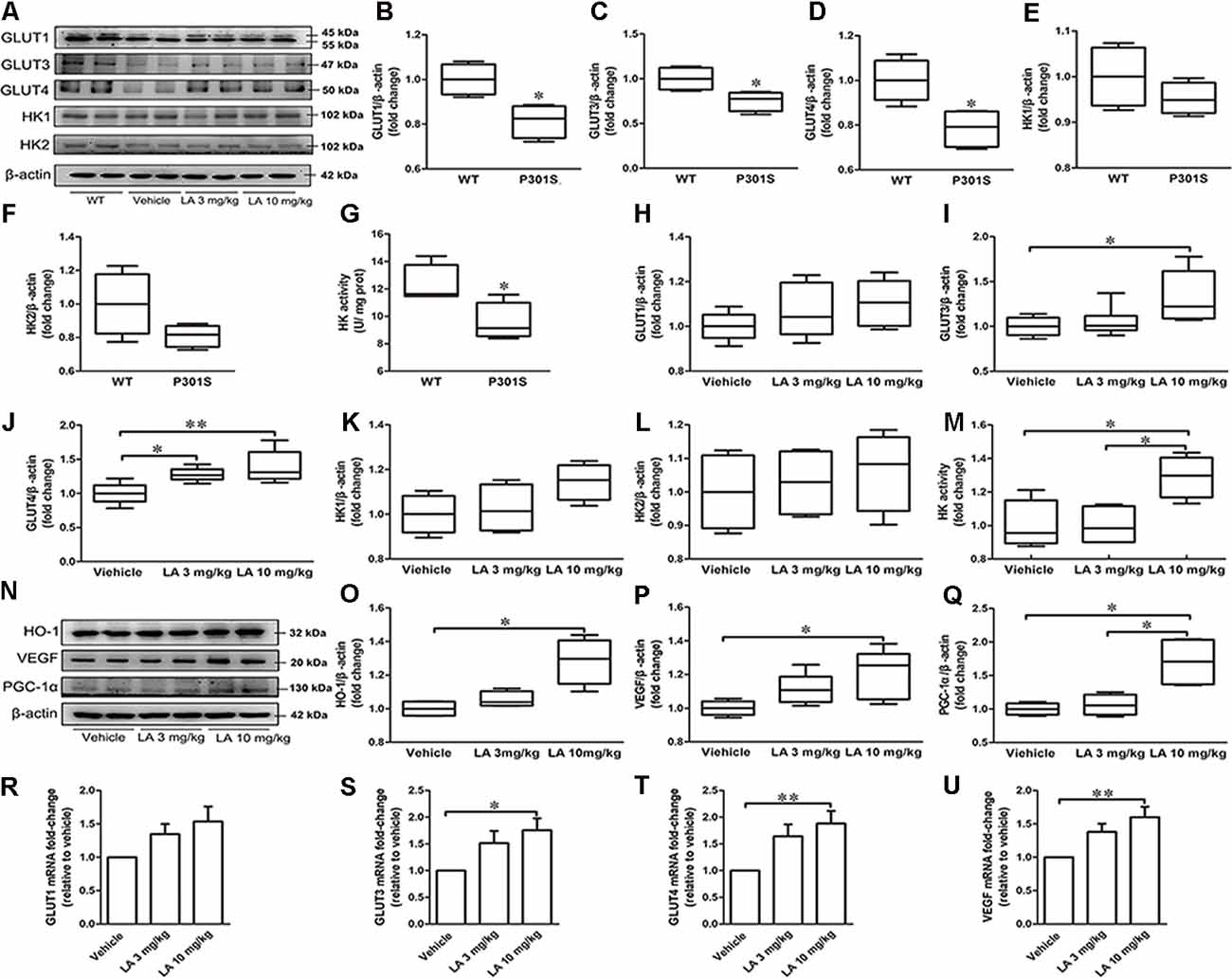
Figure 1. α-Lipoic acid (LA) improved glucose metabolism deficiency in P301S mice. (A) Representative western blots showed the expression levels of glucose transporter 1 (GLUT1), GLUT3, GLUT4, hexokinase-1 (HK-1), and HK2. (B–F) Quantified results of GLUT1, GLUT3, GLUT4, HK1, and HK2 levels between wild type (WT) and P301S mice. β-actin served as an internal loading control. (G) HK activity between WT and P301S mice. (H–L) Quantified results of the levels of GLUT1, GLUT3, GLUT4, HK-1, and HK2 among vehicle, LA 3 mg/kg, and 10 mg/kg groups. β-actin served as an internal loading control. (M) HK activity among vehicles, LA 3 mg/kg, and 10 mg/kg groups. (N–Q) Representative western blots and quantified results of the levels of heme oxygenase-1 (HO-1), vascular endothelial growth factor (VEGF), and proliferator-activated receptor gamma coactivator 1-alpha (PGC-1α). β-actin served as an internal loading control. (R–U) mRNA level of GLUT1, GLUT3, GLUT4, and VEGF. Values are represented as the means ± SEM (n = 7). *p < 0.05, **p < 0.01.
To test if the dysregulated glucose metabolism can be restored by LA treatment, we first examined the glucose uptake related protein level. As shown in Figure 1A, the protein expression level of GLUT3 was significantly increased by ~32% in 10 mg/kg LA treated group when compared to the vehicle group (Figures 1A,I). The protein expression level of GLUT4 was significantly increased in both 3 mg/kg (~28% of vehicle control) and 10 mg/kg (~39% of vehicle control) LA treated groups (Figures 1A,J). In line with this, the mRNA level of GLUT3 (Figure 1S, ~72% of vehicle control) and GLUT4 (Figure 1T, ~37% of vehicle control) are also increased in 10 mg/kg LA treated group. However, there were no significant differences in GLUT1 protein or mRNA levels among the three groups (Figures 1A,H,R).
VEGF is an effective compensatory factor that facilitates glucose uptake and metabolism. To investigate whether LA treatment altered VEGF expression, Western blotting and RT-PCR were used. Our data showed a significant elevation in VEGF protein (Figures 1N,P, ~20% of vehicle control) and mRNA levels (Figure 1U, ~60% of vehicle control) in the 10 mg/kg LA-treated group. VEGF is regulated by HO-1. We further examined HO-1 expression using Western blotting, and the HO-1 expression level was significantly increased by ~28% in P301S mouse brains after 10 mg/kg LA treatment (Figures 1N,O).
Next, we evaluated the HK expression level and activity in the cortex of P301S mice after LA treatment. We found LA treatment did not change the HK1 and HK2 expression levels (Figures 1A,K,L). However, the HK activity was significantly increased in 10 mg/kg LA treated group (Figure 1M, ~29% of vehicle control). Additionally, we also examined the PGC-1α level, which involved in the regulation of HK activity. 10 mg/kg LA treatment dramatically upregulated the expression of PGC-1α (Figures 1N,Q, ~70% of vehicle control, ~64% of 3 mg/kg LA group). Collectively, the glucose metabolism deficiency in P301S mice was improved by LA treatment in a dose-dependent manner.
LA Treatment Induces the Expression of DNA Repair Enzymes in P301S Tau Mice
DNA repair deficiency is tightly correlated with glucose metabolism. Therefore, we measured MTH1, OGG1/2, and MUTYH expression levels in the brain, which are the three main enzymes involved in the repairing of DNA oxidative damage (Nakabeppu, 2014). No significant differences in the MUTYH level were observed between LA-treated and vehicle groups (Figures 2A,B). However, OGG1, OGG2 and MTH1 levels were increased by ~47%, 73%, and 41%, respectively, in 10 mg/kg LA treated group (Figures 2A,C–E). The results indicated upregulated DNA repairment by 10 mg/kg LA administration thus contributing to the glucose metabolism restoration.
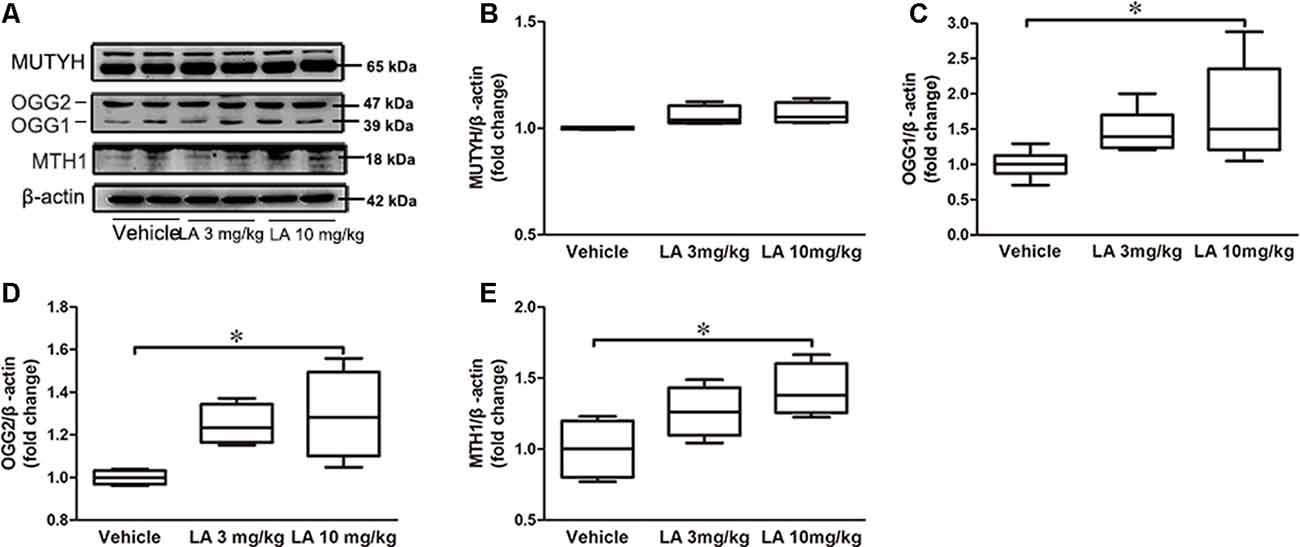
Figure 2. LA treatment promotes DNA repair by upregulating MutT H homolog (MTH1) and Oxoguanine DNA glycosylase 1 and 2 (OGG1/2). (A) Representative western blots showed the expression levels of MutY homolog (MUTYH), OGG1/2, MTH1. (B–E) Quantified results of the levels of MUTYH, OGG1/2, MTH1. β-Actin served as an internal loading control. Values are represented as the means ± SEM (n = 7). *p < 0.05.
LA Treatment Upregulates HIF-1α Expression via the BDNF/TrkB Pathway
To assess the effects of LA treatment on HIF-1α, we used Western blotting, immunohistochemistry, and RT-PCR to evaluate HIF-1α expression levels. 10 mg/kg LA treatment promoted HIF-1α translocation from the cytosol into nuclear and dramatically upregulated the nuclear HIF-1α expression levels (Figures 3A,B, ~95% of vehicle control, ~75% of 3 mg/kg LA group). Also, we observed an increasing trend of cytosol HIF-1α after LA treatment but without significant change (Figures 3A,C). The mRNA levels of HIF-1α were also dramatically upregulated by 10 mg/kg LA treatment (Figure 3G, ~94% of vehicle control). Likewise, we also observed that 10 mg/kg LA treatment significantly increased HIF-1α expression and nucleus translocation in the cortex of P301S mice by immunofluorescence staining (Figure 4).
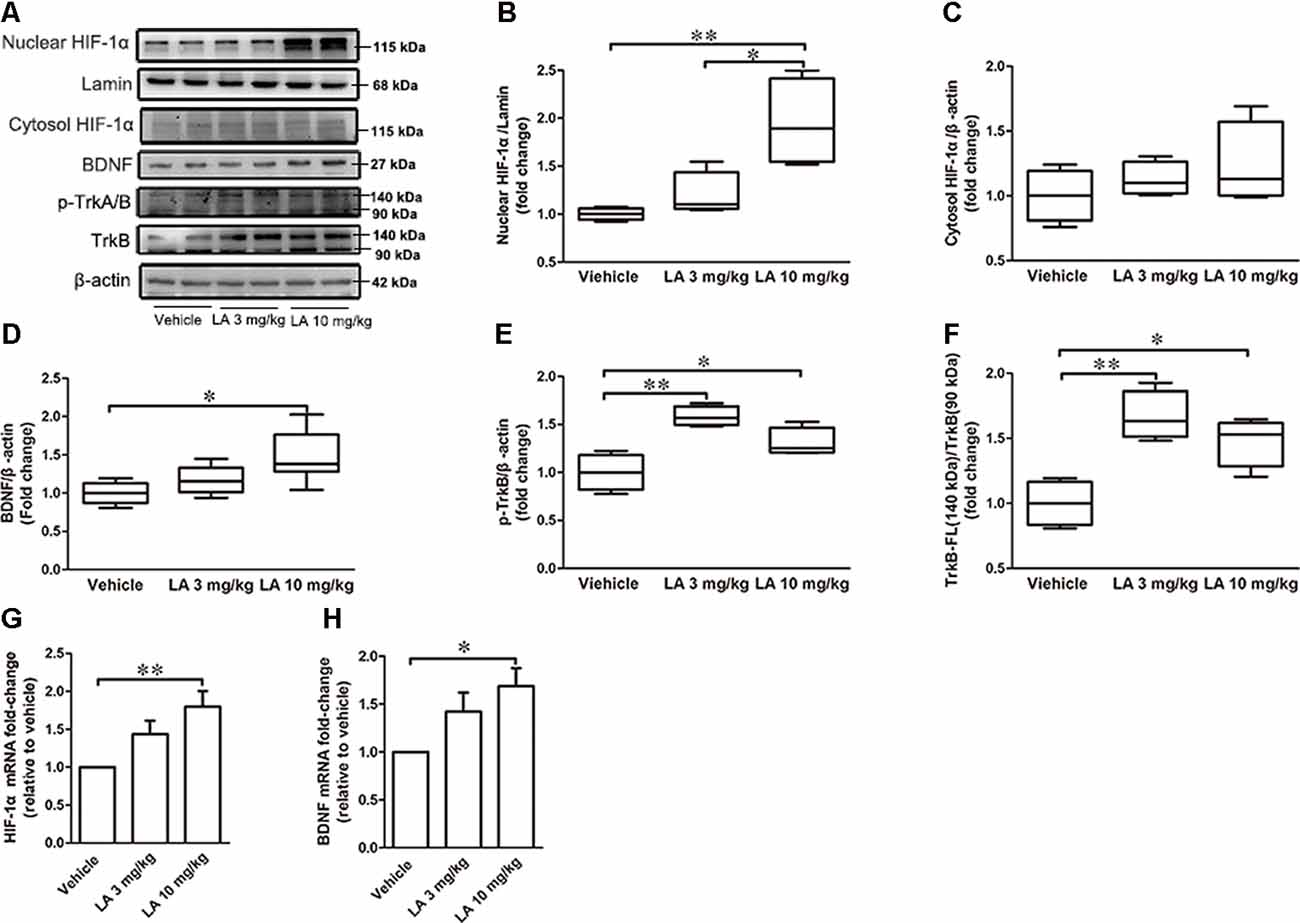
Figure 3. LA mediated the upregulation of hypoxia-inducible factor-1α (HIF-1α) expression via brain-derived neurotrophic factor/tyrosine Kinase receptor B (BDNF/TrkB) pathway. (A) Western blot results showed the protein expression levels of nuclear HIF-1α, Lamin, and cytosol HIF-1α, BDNF, p-TrkA/B, TrkB. (B) Quantified results of nuclear HIF-1α levels. Lamin served as an internal loading control. (C–F) Relative protein levels of cytosol HIF-1α, BDNF, p-TrkB, TrkB-FL which were established after normalization to β-actin. (G,H) mRNA levels of HIF-1α and BDNF. Values are represented as the means ± SEM (n = 7). *p < 0.05, **p < 0.01 compared with the vehicle group.
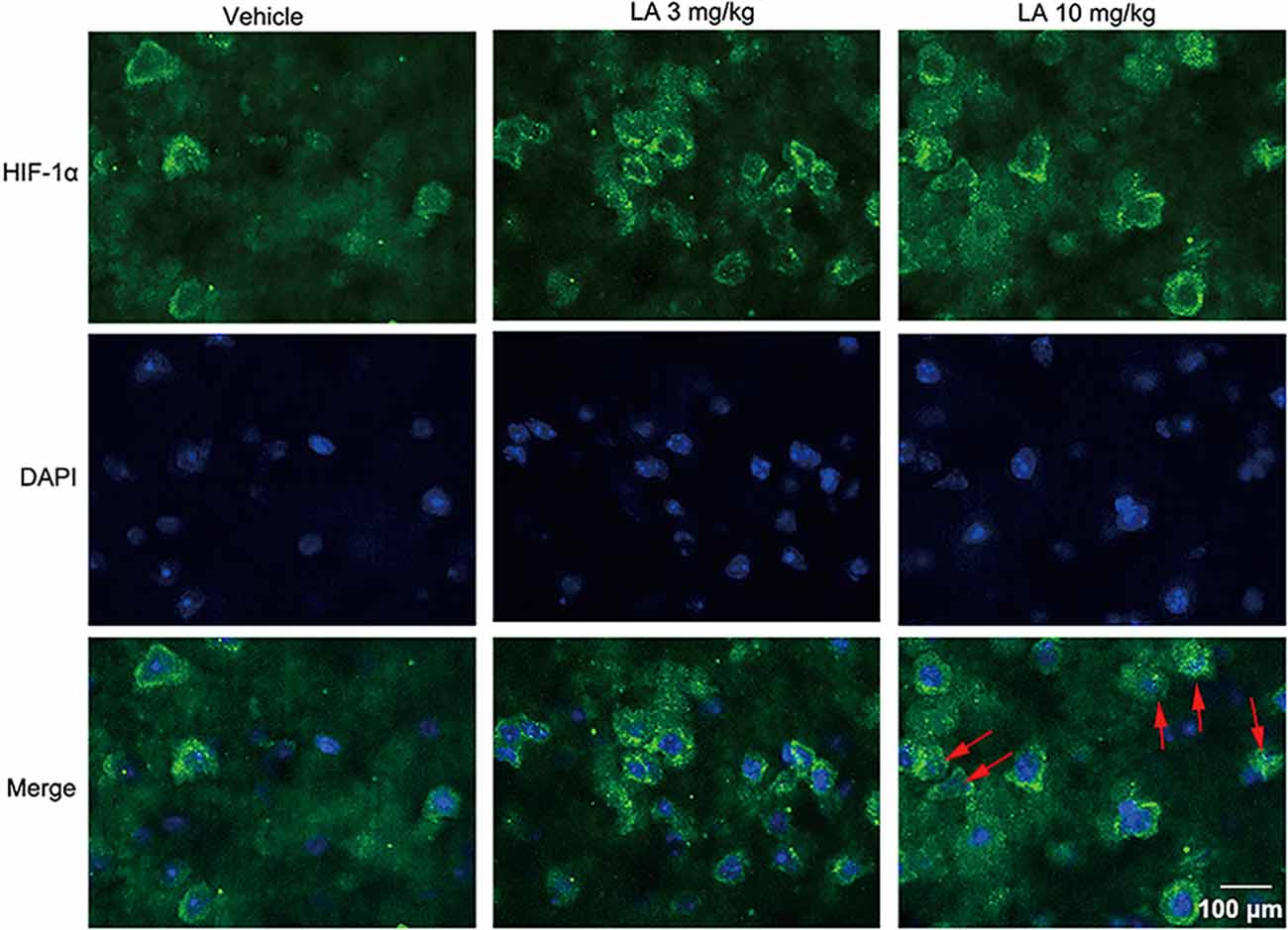
Figure 4. LA induces HIF-1α expression and nuclear translocation in cortex neurons. HIF-1α activity and its cellular localization in the cortex was determined by immunofluorescence staining. HIF-1α was predominantly found in the cytosol and nucleus of cortex neurons in 10 mg/kg LA-treated P301S mice. The red arrowheads point out the translocation of HIF-1α into the nucleus. Scale bar equals 100 μm.
To gain insight into the mechanisms underlying the elevated HIF-1α expression level, the BDNF/TrkB pathway was analyzed. 10 mg/kg LA administration significantly enhanced the protein and mRNA levels of BDNF by ~48% and 69%, respectively, in P301S mice brains (Figures 3A,D,H). BDNF levels were also measured using immunofluorescence staining in the cortex of P301S mice, and the results showed a dramatic increase in BDNF expression of 10 mg/kg LA-treated group (Figure 5). Notably, we also observed astrocyte-derived BDNF in 10 mg/kg LA-treated group (Figure 5, indicated by the red arrows), but these changes were not observed in the vehicle group (Figure 5). These results suggested that LA upregulates neuron- and astrocyte-derived BDNF expression levels in a dose-dependent manner.
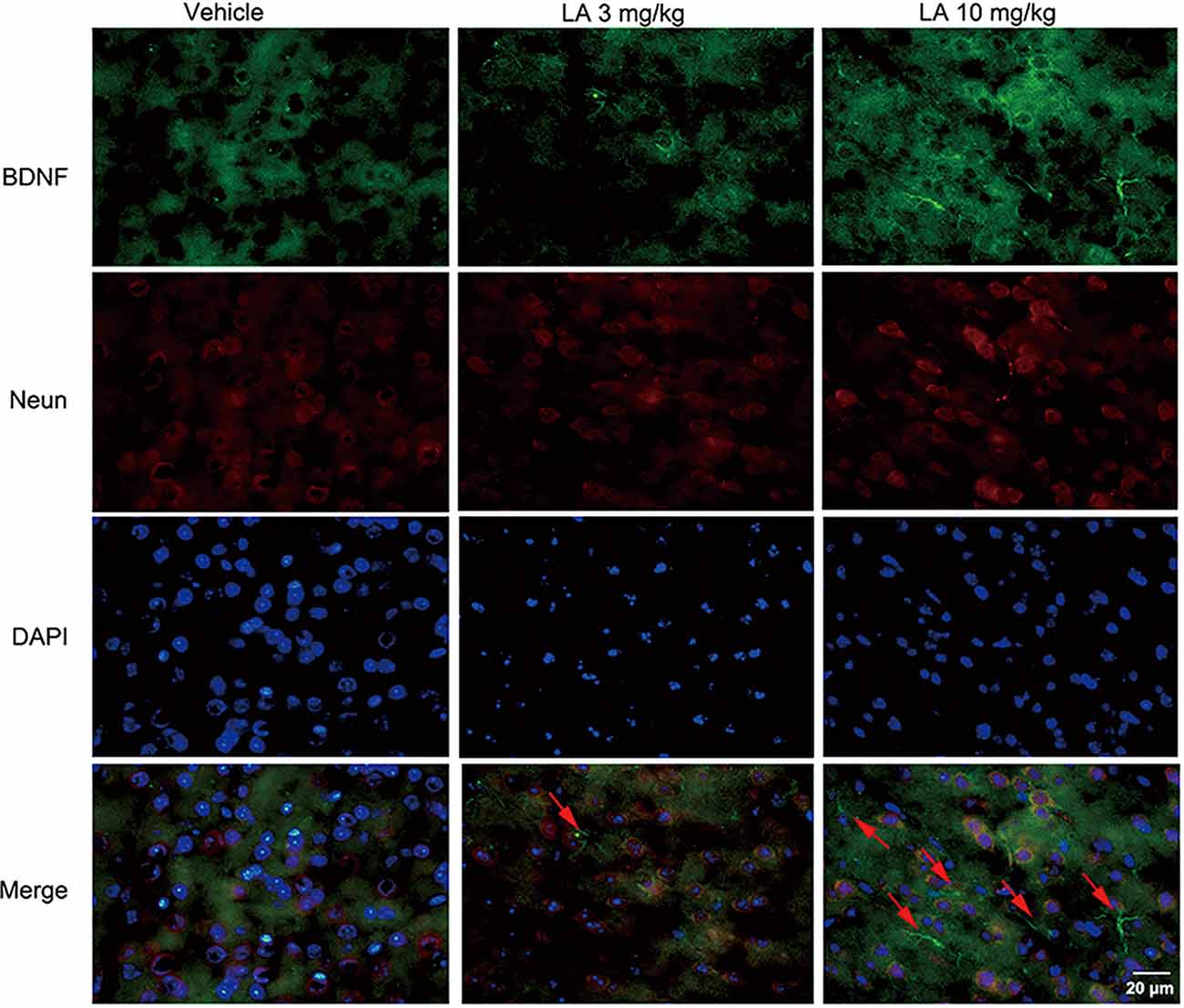
Figure 5. Effect of LA on BDNF expression in the P301S mice cortex. Double immunofluorescence of frozen brain slices using BDNF (green) and Neun (red). A stronger BDNF immunoreactivity was observed in both 3 mg/kg and 10 mg/kg LA-treated group. The red arrowheads point out the astrocytes-derived BDNF. Scale bar represents 20 μm.
TrkB is the main receptor for BDNF, which has two main isoforms, the full-length form (TrkB-FL, 140 kDa) and the truncated form (tTrkB, 90 kDa). Binding of BDNF to TrkB-FL triggers BDNF dimerization and TrkB activation (autophosphorylation). Therefore, we analyzed the expression levels of TrkB-FL and tTrkB using Western blotting. Significantly increased levels of TrkB-FL were observed in the 3 mg/kg (~67% of vehicle control) and 10 mg/kg (~48% of vehicle control) LA-treated group (Figures 3A,F). We also examined phosphorylated TrkB (p-TrkB, Tyr516) expression levels in P301S mouse brains. The significant upregulation of p-TrkB (Tyr516) level was observed in 3 mg/kg (~58% of vehicle control) and 10 mg/kg (~31% of vehicle control) LA-treated mice (Figures 3A,E). Besides, TrkB levels were also determined using immunofluorescence staining in the cortex of P301S mice, and the results showed a significant increase in TrkB expression of both 3 mg/kg and 10 mg/kg LA-treated group (Figure 6), which was consistent with the results of WB.
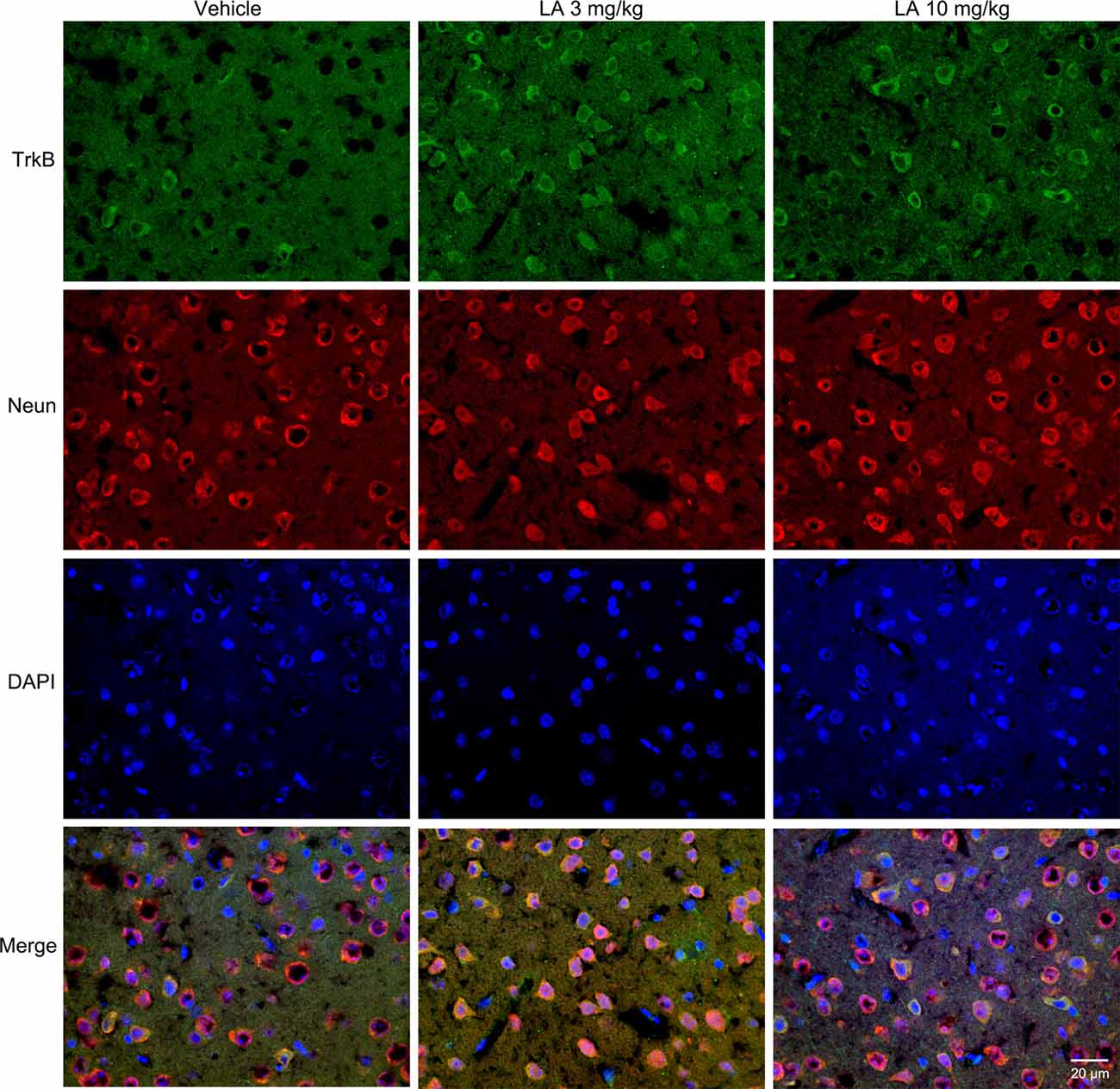
Figure 6. Effect of LA on TrkB expression in the P301S mice cortex. Double immunofluorescence of frozen brain slices using TrkB (green) and Neun (red). A stronger TrkB immunoreactivity was observed in both 3 mg/kg and 10 mg/kg LA-treated group. Scale bar represents 20 μm.
Discussion
It is widely known that LA has a variety of properties that can interfere with the pathogenesis or progression of AD. The efficacy of LA on AD has been approved by several clinical trials (Hager et al., 2001, 2007; Salinthone et al., 2010; Fava et al., 2013; Shinto et al., 2014). Now, a growing body of evidence suggests that glucose hypometabolism may be a key player in AD (Kuehn, 2020). The anti-AD properties of LA include stimulating glucose uptake and utilization (Maczurek et al., 2008), however, the underlying mechanism remains unclear. In the present study, we showed that LA treatment could restore the glucose uptake and glycolysis in P301S mice by upregulating BDNF/TrkB/HIF-1α pathway.
Glucose uptake and utilization will significantly influence the survival and normal function of neurons. GLUT1, GLUT3, and GLUT4 are highly expressed in the brain (Marko et al., 2020) and correlates positively with cerebral glucose utilization due to a high affinity for glucose (Jin et al., 2013). Researches showed the lower levels of these GLUTs are associated with more severe AD pathology (An et al., 2018), and the ablation of GLUT3 and GLUT4 in mouse brain triggered glucose uptake deficits and synaptic deficits (Ashrafi et al., 2017; Reno et al., 2017). Glycolysis is the first step of glucose metabolism and provides metabolic precursors for neuronal energy production. HK1 and HK2 are the main rate-limiting enzymes in glycolysis and the decreased HK activity has been reported in AD brains (Harris et al., 2014). In the present study, we assessed the glucose metabolism in the P301S transgenic mouse, which expressed 5-fold higher human tau protein than the endogenous mouse tau protein in the brain. We found altered glucose metabolism in P301S mice brains accompanied downregulated glucose transporters (GLUT1, GLUT3, and GLUT4) and HK enzyme activity, which indicated the glucose metabolism deficiency in P301S mice.
Interestingly, several studies suggest LA could improve glucose metabolism disorder in several metabolic syndromes (Agathos et al., 2018; Aslfalah et al., 2019; Gosselin et al., 2019). However, researches on the effect of LA in AD glucose metabolism is still rarely reported at present. In the present study, we found 10 mg/kg LA could significantly increase both protein and mRNA level of the neuronal glucose transporter (GLUT3 and GLUT4) in the cortex of P301S mice, which suggested that LA reinstated glucose uptake in neurons of P301S mice, which were consistent with previous studies (Bitar et al., 2004; Maczurek et al., 2008). Besides directly upregulating GLUTs, our data also suggest that LA treatment indirectly contributes to glucose uptake via VEGF induction in a dose-dependent manner. VEGF is required to maintain brain glucose uptake across the blood-brain barrier (Schüler et al., 2018). We further analyzed the mechanisms underlying the upregulated effect of LA on VEGF by examining the expression of the main regulator, HO-1, in P301S mouse brains. We found that LA treatment effectively induced HO-1 expression, which suggested that LA compensated glucose uptake deficiency via VEGF by upregulating HO-1.
Glucose uptake is upstream of fuel supplement for neurons, and the subsequent glycolysis is the major pathway for neuronal energy production. Researches showed glycolysis upregulation is neuroprotective as a compensatory mechanism in neurodegenerative disorders (Manzo et al., 2019). Our results indicated that the activity of HK was significantly increased after 10 mg/kg LA treatment. Consistent with the results, the level of PGC-1α was upregulated in LA treated mice, which involved in the regulation of HK activity. This is following another study that shows LA improves glycolysis deficiency might include PGC-1α-mediated increases in HK expression and activity (Fu et al., 2014; Yang et al., 2014).
DNA repair deficiency is believed to deteriorate the glucose metabolism by driving mitochondrial dysfunction (Demarest et al., 2020). Herein, we evaluated the expression levels of three important DNA repair enzymes, MTH1, OGG1/2, and MUTYH (Nakabeppu, 2014). We observed increased OGG1/2 and MTH1 expression in the LA-treated group, but there were no significant changes in the MUTYH level. MTH1 and OGG1/2 can suppress neurodegeneration by preventing 8-oxoG accumulation in cellular DNA, and mutant mice lacking MTH1 and OGG1 exhibit severe neurodegeneration (Sheng et al., 2012). The OGG1 splice variant (39 kDa) is the most prevalent form, and it localizes to the nucleus. The OGG2 splice variant (47 kDa) is targeted to the mitochondria. Our results indicated an upregulation of DNA repair in the nucleus and mitochondria via the induction of OGG1/2 and MTH1 expression, which suggested the restoration effect of LA on glucose metabolism.
LA treatment increased glucose availability in the cortex of P301S mice, which may be involved in the regulation of HIF-1α. HIF-1α is an important transcription factor that regulates glucose uptake and glycolysis at the transcriptional level (Huang et al., 2012; Harris et al., 2014; Masoud and Li, 2015). Several studies have shown that HIF-1α upregulates glucose transporters (GLUT1, GLUT3, GLUT4; Toberer et al., 2020), glycolysis enzyme (HK2; Cao et al., 2020; Shen et al., 2020), pro-angiogenic factors (HO-1, VEGF; Choi et al., 2006). Whereas, downregulation of HIF-1α suppressed the target genes and protein expressions, such as GLUT1, GLUT3, and HK2 (Liu et al., 2008; Lee et al., 2019). In the present study, LA treatment effectively triggered the upregulation and nuclear translocation of HIF-1α, which activated the transcription of several downstream target genes (Morand et al., 2019). As expected, we observed an enhanced level of GLUT3, GLUT4, VEGF, HO-1, HK activity in 10 mg/kg LA treatment group.
Several pieces of research indicate that activation of the BDNF/TrkB pathway upregulates HIF-1α expression even under normoxic conditions (Nakamura et al., 2006; Helan et al., 2014; Lin et al., 2014; Jin et al., 2019). BDNF is essential for the maintenance of cortical neurons, whose downregulation contributes to the initial loss of short-term memory in AD (Giuffrida et al., 2018). Our data showed that 10 mg/kg LA administration significantly elevated BDNF expression levels both in neurons and astrocytes. The neurotrophic function of BDNF is mainly regulated by the TrkB. TrkB has two main isoforms: the full-length form (TrkB-FL) and the truncated form (tTrkB). The binding of BDNF to TrkB-FL is necessary for the receptor homodimerization, which initiates tyrosine residue phosphorylation and translocation of the BDNF/p-TrkB complex and regulation of the downstream signaling pathways. The results showed a significant increase of TrkB-FL expression level after 10 mg/kg LA administration. Ca2+-dependent calpain is the major mechanism of TrkB-FL cleavage (Tejeda et al., 2019). Our previous study found that LA treatment significantly decreased Ca2+ content and calpain expression levels (Zhang et al., 2018), which is consistent with the decreased TrkB-FL cleavage in the present study. The phosphorylation of tyrosine sites of TrkB contributes to BDNF signal transduction (Huang and Reichardt, 2003), and we observed significantly increased levels of p-TrkB (Tyr 516) in 10 mg/kg LA-treated group. Collectively, our results suggest that chronic LA administration might enhance HIF-1α expression via the BDNF-TrkB signaling pathway.
Conclusion
LA is used clinically to treat AD (Hager et al., 2007, 2010; Fava et al., 2013), but the growing knowledge we provide on glucose metabolism restoration by LA via the BDNF/TrkB/HIF-1α pathway in AD may support a novel therapy target for neurodegenerative diseases (Nakamura et al., 2006; Li et al., 2012; Lin et al., 2014; Jiao et al., 2016). Our study supports LA as a critical supplement to reinstate brain glucose metabolism, which is involved in the upregulated expression of GLUT3, GLUT4, VEGF, OGG1/2, MTH1, and HK activity. A schematic diagram of the role of LA in maintaining brain glucose metabolism is shown in Figure 7. The present study establishes LA as a therapeutic option in AD in the future.
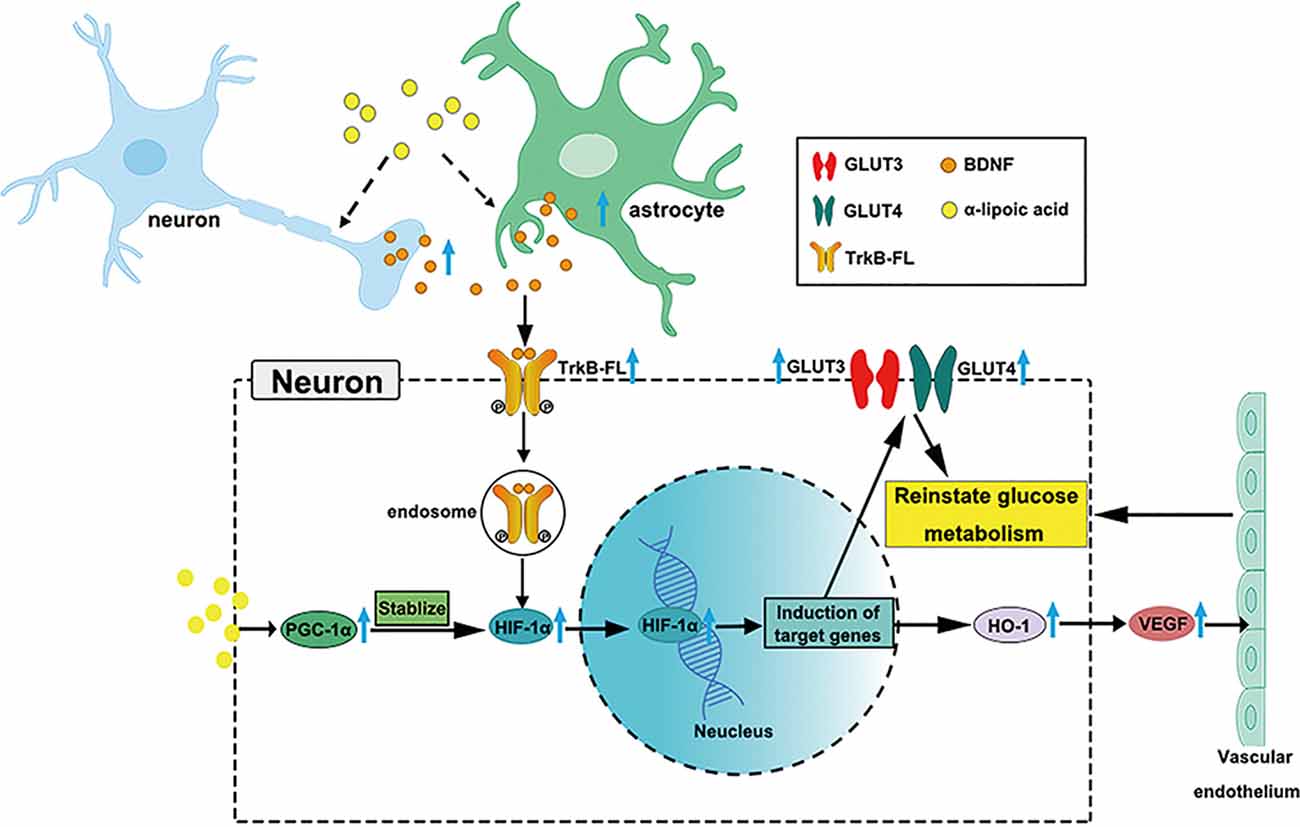
Figure 7. Schematic model of the possible mechanisms of BDNF/TrkB/HIF-1α involvement in maintaining brain glucose metabolism in P301S mice. Chronic LA treatment induces increased BDNF expression level in neurons and astrocytes in P301S mice brain, then BDNF binding to TrkB-FL induces the activation of TrkB-FL (autophosphorylation of tyrosine sites), BDNF and p-TrkB-FL complex translocate from the cellular membrane through forming endosomes. The complex upregulates HIF-1α protein level and contributes to HIF-1α nucleus translocation, inducing the downstream target genes expression, such as GLUT3, GLUT4, and HO-1, VEGF. The increased glucose transporters and vascular endothelium reinstate glucose metabolism in P301S mice. Also, LA administration upregulates PGC-1α expression and promotes the stabilization of HIF-1α protein level, couple with HIF-1α to induce the downstream target genes.
Data Availability Statement
All datasets generated for this study are included in the article/Supplementary Material.
Ethics Statement
The animal study was reviewed and approved in accordance with the National Institutes of Health guidelines, and the experimental procedures were approved by Laboratory Animal Ethical Committee of China Medical University.
Author Contributions
QA and CG conceived and designed the experiments. Y-HZ performed most of the experiments and wrote the manuscript. X-ZY, S-FX, Z-QP, L-BL, YY, Y-GF, ZW and XY contributed to generating and validating the mouse model. All authors contributed to the article and approved the submitted version.
Funding
This work was supported by the National Key R&D Program of China (2017YFA0105802), National Natural Science Foundation of China (Nos. 81771351 and 31900836), China Postdoctoral Science Foundation (No. 3200219029), Liaoning Education Department Foundation (Department of Education of Liaoning Province; No. QN2019036), and China Medical University Foundation (No. QGZ2018091).
Conflict of Interest
The authors declare that the research was conducted in the absence of any commercial or financial relationships that could be construed as a potential conflict of interest.
Supplementary Material
The Supplementary Material for this article can be found online at: https://www.frontiersin.org/articles/10.3389/fnagi.2020.00262/full#supplementary-material.
References
Abolhassani, N., Leon, J., Sheng, Z., Oka, S., Hamasaki, H., Iwaki, T., et al. (2017). Molecular pathophysiology of impaired glucose metabolism, mitochondrial dysfunction, and oxidative DNA damage in Alzheimer’s disease brain. Mech. Ageing Dev. 161, 95–104. doi: 10.1016/j.mad.2016.05.005
Adams, J. N., Lockhart, S. N., Li, L., and Jagust, W. J. (2019). Relationships between tau and glucose metabolism reflect Alzheimer’s disease pathology in cognitively normal older adults. Cereb. Cortex 29, 1997–2009. doi: 10.1093/cercor/bhy078
Agathos, E., Tentolouris, A., Eleftheriadou, I., Katsaouni, P., Nemtzas, I., Petrou, A., et al. (2018). Effect of α-lipoic acid on symptoms and quality of life in patients with painful diabetic neuropathy. J. Int. Med. Res. 46, 1779–1790. doi: 10.1177/0300060518756540
An, Y., Varma, V. R., Varma, S., Casanova, R., Dammer, E., Pletnikova, O., et al. (2018). Evidence for brain glucose dysregulation in Alzheimer’s disease. Alzheimers Dement. 14, 318–329. doi: 10.1016/j.jalz.2017.09.011
Ashrafi, G., Wu, Z., Farrell, R. J., and Ryan, T. A. (2017). GLUT4 mobilization supports energetic demands of active synapses. Neuron 93, 606.e3–615.e3. doi: 10.1016/j.neuron.2016.12.020
Aslfalah, H., Jamilian, M., Rafiei, F., and Khosrowbeygi, A. (2019). Reduction in maternal serum values of glucose and γ-glutamyltransferase after supplementation with α-lipoic acid in women with gestational diabetes mellitus. J. Obstet. Gynaecol. Res. 45, 313–317. doi: 10.1111/jog.13842
Baghel, V., Tripathi, M., Parida, G., Gupta, R., Yadav, S., Kumar, P., et al. (2019). In vivo assessment of tau deposition in Alzheimer disease and assessing its relationship to regional brain glucose metabolism and cognition. Clin. Nucl. Med. 44, e597–e601. doi: 10.1097/rlu.0000000000002791
Bitar, M. S., Wahid, S., Pilcher, C. W., Al-Saleh, E., and Al-Mulla, F. (2004). α-lipoic acid mitigates insulin resistance in Goto-Kakizaki rats. Horm. Metab. Res. 36, 542–549. doi: 10.1055/s-2004-825760
Butterfield, D. A., and Halliwell, B. (2019). Oxidative stress, dysfunctional glucose metabolism and Alzheimer disease. Nat. Rev. Neurosci. 20, 148–160. doi: 10.1038/s41583-019-0132-6
Cao, L., Wang, M., Dong, Y., Xu, B., Chen, J., Ding, Y., et al. (2020). Circular RNA circRNF20 promotes breast cancer tumorigenesis and Warburg effect through miR-487a/HIF-1α/HK2. Cell Death Dis. 11:145. doi: 10.1038/s41419-020-2336-0
Chen, C., Pore, N., Behrooz, A., Ismail-Beigi, F., and Maity, A. (2001). Regulation of glut1 mRNA by hypoxia-inducible factor-1. Interaction between H-ras and hypoxia. J. Biol. Chem. 276, 9519–9525. doi: 10.1074/jbc.m010144200
Choi, S. M., Choi, K. O., Park, Y. K., Cho, H., Yang, E. G., and Park, H. (2006). Clioquinol, a Cu(II)/Zn(II) chelator, inhibits both ubiquitination and asparagine hydroxylation of hypoxia-inducible factor-1α, leading to expression of vascular endothelial growth factor and erythropoietin in normoxic cells. J. Biol. Chem. 281, 34056–34063. doi: 10.1074/jbc.m603913200
Demarest, T. G., Varma, V. R., Estrada, D., Babbar, M., Basu, S., Mahajan, U. V., et al. (2020). Biological sex and DNA repair deficiency drive Alzheimer’s disease via systemic metabolic remodeling and brain mitochondrial dysfunction. Acta Neuropathol. 140, 25–47. doi: 10.1007/s00401-020-02152-8
Fava, A., Pirritano, D., Plastino, M., Cristiano, D., Puccio, G., Colica, C., et al. (2013). The effect of lipoic acid therapy on cognitive functioning in patients with Alzheimer’s disease. J. Neurodegener. Dis. 2013:454253. doi: 10.1155/2013/454253
Ferrara, N., and Davis-Smyth, T. (1997). The biology of vascular endothelial growth factor. Endocr. Rev. 18, 4–25. doi: 10.1210/edrv.18.1.0287
Fu, B., Zhang, J., Zhang, X., Zhang, C., Li, Y., Zhang, Y., et al. (2014). α-lipoic acid upregulates SIRT1-dependent PGC-1α expression and protects mouse brain against focal ischemia. Neuroscience 281, 251–257. doi: 10.1016/j.neuroscience.2014.09.058
Giuffrida, M. L., Copani, A., and Rizzarelli, E. (2018). A promising connection between BDNF and Alzheimer’s disease. Aging 10, 1791–1792. doi: 10.18632/aging.101518
Gosselin, L. E., Chrapowitzky, L., and Rideout, T. C. (2019). Metabolic effects of α-lipoic acid supplementation in pre-diabetics: a randomized, placebo-controlled pilot study. Food Funct. 10, 5732–5738. doi: 10.1039/c9fo00390h
Guo, C., Yang, Z. H., Zhang, S., Chai, R., Xue, H., Zhang, Y. H., et al. (2017). Intranasal lactoferrin enhances α-secretase-dependent amyloid precursor protein processing via the ERK1/2-CREB and HIF-1α pathways in an Alzheimer’s disease mouse model. Neuropsychopharmacology 42, 2504–2515. doi: 10.1038/npp.2017.8
Hager, K., Kenklies, M., McAfoose, J., Engel, J., and Münch, G. (2007). α-lipoic acid as a new treatment option for Alzheimer’s disease—a 48 months follow-up analysis. Neuropsychiatric Disorders An Integrative Approach. Journal of Neural Transmission., eds M. Gerlach, J. Deckert, K. Double and E. Koutsilieri (Vienna: Springer), 189–193.
Hager, K., Marahrens, A., Kenklies, M., Riederer, P., and Münch, G. (2001). α-lipoic acid as a new treatment option for Alzheimer [corrected] type dementia. Arch. Gerontol. Geriatr. 32, 275–282. doi: 10.1016/s0167-4943(01)00104-2
Hager, K., Marahrens, A., Kenklies, M., Riederer, P., and Münch, G. (2010). α-lipoic acid as a new treatment option for Alzheimer type dementia (vol 32, pg 275, 2001). Arch. Gerontol. Geriatr. 51, 110–110. doi: 10.1016/j.archger.2009.08.001
Harris, R. A., Tindale, L., and Cumming, R. C. (2014). Age-dependent metabolic dysregulation in cancer and Alzheimer’s disease. Biogerontology 15, 559–577. doi: 10.1007/s10522-014-9534-z
Hayashi, M., Sakata, M., Takeda, T., Yamamoto, T., Okamoto, Y., Sawada, K., et al. (2004). Induction of glucose transporter 1 expression through hypoxia-inducible factor 1α under hypoxic conditions in trophoblast-derived cells. J. Endocrinol. 183, 145–154. doi: 10.1677/joe.1.05599
Helan, M., Aravamudan, B., Hartman, W. R., Thompson, M. A., Johnson, B. D., Pabelick, C. M., et al. (2014). BDNF secretion by human pulmonary artery endothelial cells in response to hypoxia. J. Mol. Cell. Cardiol. 68, 89–97. doi: 10.1016/j.yjmcc.2014.01.006
Huang, Y., Lei, L., Liu, D., Jovin, I., Russell, R., Johnson, R. S., et al. (2012). Normal glucose uptake in the brain and heart requires an endothelial cell-specific HIF-1α-dependent function. Proc. Natl. Acad. Sci. U S A 109, 17478–17483. doi: 10.1073/pnas.1209281109
Huang, E. J., and Reichardt, L. F. (2003). Trk receptors: roles in neuronal signal transduction. Annu. Rev. Biochem. 72, 609–642. doi: 10.1146/annurev.biochem.72.121801.161629
Jais, A., Solas, M., Backes, H., Chaurasia, B., Kleinridders, A., Theurich, S., et al. (2016). Myeloid-cell-derived VEGF maintains brain glucose uptake and limits cognitive impairment in obesity. Cell 166, 1338–1340. doi: 10.1016/j.cell.2016.03.033
Jiao, S. S., Shen, L. L., Zhu, C., Bu, X. L., Liu, Y. H., Liu, C. H., et al. (2016). Brain-derived neurotrophic factor protects against tau-related neurodegeneration of Alzheimer’s disease. Transl. Psychiatry 6:e907. doi: 10.1038/tp.2016.186
Jin, N., Qian, W., Yin, X., Zhang, L., Iqbal, K., Grundke-Iqbal, I., et al. (2013). CREB regulates the expression of neuronal glucose transporter 3: a possible mechanism related to impaired brain glucose uptake in Alzheimer’s disease. Nucleic Acids Res. 41, 3240–3256. doi: 10.1093/nar/gks1227
Jin, H., Zhu, Y., Li, Y., Ding, X., Ma, W., Han, X., et al. (2019). BDNF-mediated mitophagy alleviates high-glucose-induced brain microvascular endothelial cell injury. Apoptosis 24, 511–528. doi: 10.1007/s10495-019-01535-x
Kuehn, B. M. (2020). In Alzheimer research, glucose metabolism moves to center stage. JAMA 323, 297–299. doi: 10.1001/jama.2019.20939
Kuipers, S. D., Trentani, A., Tiron, A., Mao, X., Kuhl, D., and Bramham, C. R. (2016). BDNF-induced LTP is associated with rapid Arc/Arg3.1-dependent enhancement in adult hippocampal neurogenesis. Sci. Rep. 6:21222. doi: 10.1038/srep21222
Kuroda, A., Yamasaki, Y., Matsuhisa, M., Kubota, M., Nakahara, I., Nakatani, Y., et al. (2003). Brain-derived neurotrophic factor ameliorates hepatic insulin resistance in Zucker fatty rats. Metabolism 52, 203–208. doi: 10.1053/meta.2003.50026
Lauretti, E., Li, J.-G., Di Meco, A., and Praticò, D. (2017). Glucose deficit triggers tau pathology and synaptic dysfunction in a tauopathy mouse model. Transl. Psychiatry 7:e1020. doi: 10.1038/tp.2016.296
Lauretti, E., and Pratico, D. (2015). Glucose deprivation increases tau phosphorylation via P38 mitogen-activated protein kinase. Aging Cell 14, 1067–1074. doi: 10.1111/acel.12381
Lee, S., Hallis, S. P., Jung, K.-A., Ryu, D., and Kwak, M.-K. (2019). Impairment of HIF-1α-mediated metabolic adaption by NRF2-silencing in breast cancer cells. Redox Biol. 24:101210. doi: 10.1016/j.redox.2019.101210
Li, M., Dai, F.-R., Du, X.-P., Yang, Q.-D., Zhang, X., and Chen, Y. (2012). Infusion of BDNF into the nucleus accumbens of aged rats improves cognition and structural synaptic plasticity through PI3K-ILK-Akt signaling. Behav. Brain Res. 231, 146–153. doi: 10.1016/j.bbr.2012.03.010
Lin, C.-Y., Hung, S.-Y., Chen, H.-T., Tsou, H.-K., Fong, Y.-C., Wang, S.-W., et al. (2014). Brain-derived neurotrophic factor increases vascular endothelial growth factor expression and enhances angiogenesis in human chondrosarcoma cells. Biochem. Pharmacol. 91, 522–533. doi: 10.1016/j.bcp.2014.08.008
Liu, Y., Liu, F., Iqbal, K., Grundke-Iqbal, I., and Gong, C. X. (2008). Decreased glucose transporters correlate to abnormal hyperphosphorylation of tau in Alzheimer disease. FEBS Lett. 582, 359–364. doi: 10.1016/j.febslet.2007.12.035
Maczurek, A., Hager, K., Kenklies, M., Sharman, M., Martins, R., Engel, J., et al. (2008). Lipoic acid as an anti-inflammatory and neuroprotective treatment for Alzheimer’s disease. Adv. Drug Deliv. Rev. 60, 1463–1470. doi: 10.1016/j.addr.2008.04.015
Mahboob, A., Farhat, S. M., Iqbal, G., Babar, M. M., Zaidi, N. U., Nabavi, S. M., et al. (2016). α-lipoic acid-mediated activation of muscarinic receptors improves hippocampus- and amygdala-dependent memory. Brain Res. Bull. 122, 19–28. doi: 10.1016/j.brainresbull.2016.02.014
Maher, F., Vannucci, S. J., and Simpson, I. A. (1994). Glucose transporter proteins in brain. FASEB J. 8, 1003–1011. doi: 10.1096/fasebj.8.13.7926364
Manzo, E., Lorenzini, I., Barrameda, D., O’Conner, A. G., Barrows, J. M., Starr, A., et al. (2019). Glycolysis upregulation is neuroprotective as a compensatory mechanism in ALS. Elife 8:e45114. doi: 10.7554/eLife.45114
Maritim, A. C., Sanders, R. A., and Watkins, J. B. III. (2003). Effects of α-lipoic acid on biomarkers of oxidative stress in streptozotocin-induced diabetic rats. J. Nutr. Biochem. 14, 288–294. doi: 10.1016/s0955-2863(03)00036-6
Marko, D. M., Foran, G., Vlavcheski, F., Baron, D. C., Hayward, G. C., Baranowski, B. J., et al. (2020). Interleukin-6 treatment results in GLUT4 translocation and AMPK phosphorylation in neuronal SH-SY5Y cells. Cells 9:1114. doi: 10.3390/cells9051114
Masoud, G. N., and Li, W. (2015). HIF-1α pathway: role, regulation and intervention for cancer therapy. Acta Pharm. Sin. B 5, 378–389. doi: 10.1016/j.apsb.2015.05.007
McEwen, B. S., and Reagan, L. P. (2004). Glucose transporter expression in the central nervous system: relationship to synaptic function. Eur. J. Pharmacol. 490, 13–24. doi: 10.1016/j.ejphar.2004.02.041
Morand, J., Briancon-Marjollet, A., Lemarie, E., Gonthier, B., Arnaud, J., Korichneva, I., et al. (2019). Zinc deficiency up-regulates ET-1 mediated secretion and endothelial cell migration through nuclear HIF-1 translocation. Am. J. Physiol. Cell Physiol. 317, C270–C276. doi: 10.1152/ajpcell.00460.2018
Nakabeppu, Y. (2014). Cellular levels of 8-oxoguanine in either DNA or the nucleotide pool play pivotal roles in carcinogenesis and survival of cancer cells. Int. J. Mol. Sci. 15, 12543–12557. doi: 10.3390/ijms150712543
Nakamura, K., Martin, K. C., Jackson, J. K., Beppu, K., Woo, C. W., and Thiele, C. J. (2006). Brain-derived neurotrophic factor activation of TrkB induces vascular endothelial growth factor expression via hypoxia-inducible factor-1α in neuroblastoma cells. Cancer Res. 66, 4249–4255. doi: 10.1158/0008-5472.can-05-2789
Qin, W., Haroutunian, V., Katsel, P., Cardozo, C. P., Ho, L., Buxbaum, J. D., et al. (2009). PGC-1α expression decreases in the Alzheimer disease brain as a function of dementia. Arch. Neurol. 66, 352–361. doi: 10.1001/archneurol.2008.588
Reno, C. M., Puente, E. C., Sheng, Z., Daphna-Iken, D., Bree, A. J., Routh, V. H., et al. (2017). Brain GLUT4 knockout mice have impaired glucose tolerance, decreased insulin sensitivity, and impaired hypoglycemic counterregulation. Diabetes 66, 587–597. doi: 10.2337/db16-0917
Sakagami, H., Makino, Y., Mizumoto, K., Isoe, T., Takeda, Y., Watanabe, J., et al. (2014). Loss of HIF-1α impairs GLUT4 translocation and glucose uptake by the skeletal muscle cells. Am. J. Physiol. Endocrinol. Metab. 306, E1065–E1076. doi: 10.1152/ajpendo.00597.2012
Salinthone, S., Yadav, V., Schillace, R. V., Bourdette, D. N., and Carr, D. W. (2010). Lipoic acid attenuates inflammation via cAMP and protein kinase A signaling. PLoS One 5:e13058. doi: 10.1371/journal.pone.0013058
Scheltens, P., Blennow, K., Breteler, M. M., de Strooper, B., Frisoni, G. B., Salloway, S., et al. (2016). Alzheimer’s disease. Lancet 388, 505–517. doi: 10.1016/S0140-6736(15)01124-1
Schüler, R., Seebeck, N., Osterhoff, M. A., Witte, V., Flöel, A., Busjahn, A., et al. (2018). VEGF and GLUT1 are highly heritable, inversely correlated and affected by dietary fat intake: consequences for cognitive function in humans. Mol. Metab. 11, 129–136. doi: 10.1016/j.molmet.2018.02.004
Shen, N., Wang, Y., Sun, X., Bai, X., He, J., Cui, Q., et al. (2020). Expression of hypoxia-inducible factor 1α, glucose transporter 1, and hexokinase 2 in primary central nervous system lymphoma and the correlation with the biological behaviors. Brain Behav. doi: 10.1002/brb3.1718 [Epub ahead of print].
Sheng, Z., Oka, S., Tsuchimoto, D., Abolhassani, N., Nomaru, H., Sakumi, K., et al. (2012). 8-Oxoguanine causes neurodegeneration during MUTYH-mediated DNA base excision repair. J. Clin. Invest. 122, 4344–4361. doi: 10.1172/jci65053
Shinto, L., Quinn, J., Montine, T., Dodge, H. H., Woodward, W., Baldauf-Wagner, S., et al. (2014). A randomized placebo-controlled pilot trial of omega-3 fatty acids and α lipoic acid in Alzheimer’s disease. J. Alzheimers Dis. 38, 111–120. doi: 10.3233/jad-130722
Simpson, I. A., Appel, N. M., Hokari, M., Oki, J., Holman, G. D., Maher, F., et al. (1999). Blood-brain barrier glucose transporter: effects of hypo- and hyperglycemia revisited. J. Neurochem. 72, 238–247. doi: 10.1046/j.1471-4159.1999.0720238.x
Simpson, I. A., Chundu, K. R., Davies-Hill, T., Honer, W. G., and Davies, P. (1994). Decreased concentrations of GLUT1 and GLUT3 glucose transporters in the brains of patients with Alzheimer’s disease. Ann. Neurol. 35, 546–551. doi: 10.1002/ana.410350507
Simpson, I. A., Dwyer, D., Malide, D., Moley, K. H., Travis, A., and Vannucci, S. J. (2008). The facilitative glucose transporter GLUT3: 20 years of distinction. Am. J. Physiol. Endocrinol. Metab. 295, E242–E253. doi: 10.1152/ajpendo.90388.2008
Sliwinska, A., Kwiatkowski, D., Czarny, P., Toma, M., Wigner, P., Drzewoski, J., et al. (2016). The levels of 7,8-dihydrodeoxyguanosine (8-oxoG) and 8-oxoguanine DNA glycosylase 1 (OGG1)—a potential diagnostic biomarkers of Alzheimer’s disease. J. Neurol. Sci. 368, 155–159. doi: 10.1016/j.jns.2016.07.008
Tejeda, G. S., Esteban-Ortega, G. M., San Antonio, E., Vidaurre, O. G., and Diaz-Guerra, M. (2019). Prevention of excitotoxicity-induced processing of BDNF receptor TrkB-FL leads to stroke neuroprotection. EMBO Mol. Med. 11:e9950. doi: 10.15252/emmm.201809950
Toberer, F., Haenssle, H. A., Heinzel-Gutenbrunner, M., Enk, A., Hartschuh, W., Helmbold, P., et al. (2020). Metabolic reprogramming and angiogenesis in primary cutaneous Merkel cell carcinoma: expression of hypoxia-inducible factor-1α and its central downstream factors. J. Eur. Acad. Dermatol. Venereol. doi: 10.1111/jdv.16600 [Epub ahead of print].
Vassilopoulos, A., and Papazafiri, P. (2005). Attenuation of oxidative stress in HL-1 cardiomyocytes improves mitochondrial function and stabilizes Hif-1α. Free Radic. Res. 39, 1273–1284. doi: 10.1080/10715760500166685
Wang, Z., Zhou, W., Zhou, B., Zhang, J., and for Alzheimer’s Disease Neuroimaging Initiative. (2018). Association of vascular endothelial growth factor levels in CSF and cerebral glucose metabolism across the Alzheimer’s disease spectrum. Neurosci. Lett. 687, 276–279. doi: 10.1016/j.neulet.2018.09.055
Winkler, E. A., Nishida, Y., Sagare, A. P., Rege, S. V., Bell, R. D., Perlmutter, D., et al. (2015). GLUT1 reductions exacerbate Alzheimer’s disease vasculo-neuronal dysfunction and degeneration. Nat. Neurosci. 18, 521–530. doi: 10.1038/nn.3966
Yang, Y., Li, W., Liu, Y., Li, Y., Gao, L., and Zhao, J. J. (2014). α-lipoic acid attenuates insulin resistance and improves glucose metabolism in high fat diet-fed mice. Acta Pharmacol. Sin. 35, 1285–1292. doi: 10.1038/aps.2014.64
Yeh, W.-L., Lin, C.-J., and Fu, W.-M. (2008). Enhancement of glucose transporter expression of brain endothelial cells by vascular endothelial growth factor derived from glioma exposed to hypoxia. Mol. Pharmacol. 73, 170–177. doi: 10.1124/mol.107.038851
Zhang, Y. H., Wang, D. W., Xu, S. F., Zhang, S., Fan, Y. G., Yang, Y. Y., et al. (2018). α-Lipoic acid improves abnormal behavior by mitigation of oxidative stress, inflammation, ferroptosis, and tauopathy in P301S Tau transgenic mice. Redox Biol. 14, 535–548. doi: 10.1016/j.redox.2017.11.001
Zhao, R. R., Xu, F., Xu, X. C., Tan, G. J., Liu, L. M., Wu, N., et al. (2015). Effects of α-lipoic acid on spatial learning and memory, oxidative stress, and central cholinergic system in a rat model of vascular dementia. Neurosci. Lett. 587, 113–119. doi: 10.1016/j.neulet.2014.12.037
Keywords: Alzheimer’s disease, α-lipoic acid, hypoxia-inducible factor-1α, glucose metabolism, tau phosphorylation
Citation: Zhang Y, Yan X, Xu S, Pang Z, Li L, Yang Y, Fan Y, Wang Z, Yu X, Guo C and Ao Q (2020) α-Lipoic Acid Maintains Brain Glucose Metabolism via BDNF/TrkB/HIF-1α Signaling Pathway in P301S Mice. Front. Aging Neurosci. 12:262. doi: 10.3389/fnagi.2020.00262
Received: 06 March 2020; Accepted: 31 July 2020;
Published: 21 August 2020.
Edited by:
Ignacio Torres-Aleman, Consejo Superior de Investigaciones Científicas (CSIC), SpainReviewed by:
Ana I. Duarte, University of Coimbra, PortugalJulien Rossignol, Central Michigan University, United States
Copyright © 2020 Zhang, Yan, Xu, Pang, Li, Yang, Fan, Wang, Yu, Guo and Ao. This is an open-access article distributed under the terms of the Creative Commons Attribution License (CC BY). The use, distribution or reproduction in other forums is permitted, provided the original author(s) and the copyright owner(s) are credited and that the original publication in this journal is cited, in accordance with accepted academic practice. No use, distribution or reproduction is permitted which does not comply with these terms.
*Correspondence: Chuang Guo, guoc@mail.neu.edu.cn; Qiang Ao, aoqiang@mail.tsinghua.edu.cn