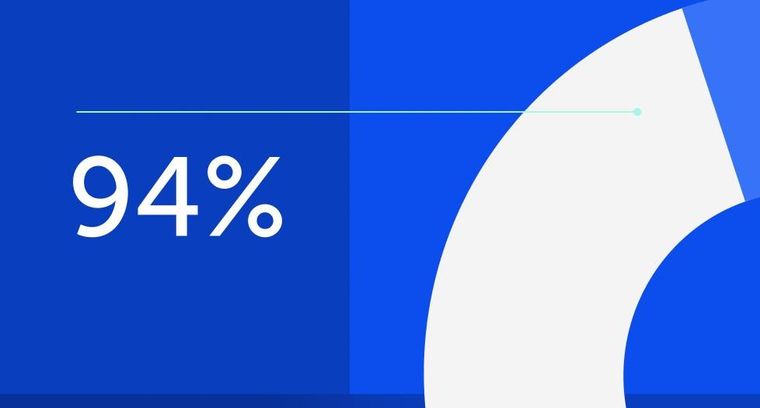
94% of researchers rate our articles as excellent or good
Learn more about the work of our research integrity team to safeguard the quality of each article we publish.
Find out more
MINI REVIEW article
Front. Aging Neurosci., 27 September 2019
Sec. Alzheimer's Disease and Related Dementias
Volume 11 - 2019 | https://doi.org/10.3389/fnagi.2019.00269
This article is part of the Research TopicIdentification of Multiple Targets in the Fight against Alzheimer’s DiseaseView all 21 articles
Alzheimer’s disease (AD) is a progressive neurodegenerative disorder that has important health and economic impacts in the elderly. Despite a better understanding of the molecular mechanisms leading to the appearance of major pathological hallmarks (senile plaques and neurofibrillary tangles), effective treatments are still lacking. Sporadic AD forms (98% of all cases) are multifactorial, and a panoply of risk factors have been identified. While the major risk factor is aging, growing evidence suggests that chronic stress or stress-related disorders increase the probability to develop AD. An early dysregulation of the hypothalamic-pituitary-adrenal axis (HPA axis or stress axis) has been observed in patients. The direct consequence of such perturbation is an oversecretion of glucocorticoids (GC) associated with an impairment of its receptors (glucocorticoid receptors, GR). These steroids hormones easily penetrate the brain and act in synergy with excitatory amino acids. An overexposure could be highly toxic in limbic structures (prefrontal cortex and hippocampus) and contribute in the cognitive decline occurring in AD. GC and GR dysregulations seem to be involved in lots of functions disturbed in AD and a vicious cycle appears, where AD induces HPA axis dysregulation, which in turn potentiates the pathology. This review article presents some preclinical and clinical studies focusing on the HPA axis hormones and their receptors to fight AD. Due to its primordial role in the maintenance of homeostasis, the HPA axis appears as a key-actor in the etiology of AD and a prime target to tackle AD by offering multiple angles of action.
Sporadic Alzheimer’s disease (AD; 98% cases) is a progressive neurodegenerative pathology, and its complexity could be explained by a wide range of risk factors. Growing evidence suggests that lifetime events such as chronic stress or stress-related disorders, like major depression disorder (MDD) or anxiety, may increase the probability to develop AD (Heininger, 2000; Blennow et al., 2006; Querfurth and LaFerla, 2010; Canet et al., 2019). In patients, an early dysregulation of the hypothalamic-pituitary-adrenal (HPA) axis was observed (Hartmann et al., 1997; Csernansky et al., 2006). The direct consequence of such perturbation is a glucocorticoids (GC) over-secretion associated with GC receptors (GR) signaling impairment. These steroid hormones easily penetrate the brain tissue and act in synergy with excitatory amino acids. A GC overexposure is highly toxic in limbic structures (McEwen, 2008), especially in prefrontal cortex and hippocampus, which could participate to the cognitive decline occurring in AD. Furthermore, GC and GR dysregulations are involved in lots of functions disturbed in AD: i.e., dysregulation of the amyloid precursor protein (APP) processing, Tau phosphorylation, neuroinflammation, oxidative stress and excitotoxicity (Sapolsky, 1996; McEwen, 2008; Bengoetxea et al., 2016). Thus, a vicious cycle between AD and the HPA axis seems to occur, where AD induces the dysregulation of the HPA axis, which in turn potentiates the pathology (Brureau et al., 2013; Pineau et al., 2016; Canet et al., 2019).
This article reviews some preclinical and clinical studies focusing on the HPA axis hormones and receptors as potential targets for AD. Promising results are obtained by targeting corticotropin releasing hormone (CRH), arginine vasopressin (AVP) and GR, or by inhibiting the 11β-hydroxysteroid dehydrogenase-1 (11β-HSD1), involved in GC synthesis. Finally, this review intends to show that the HPA axis, due to its essential role in the maintenance of homeostasis, could be a key factor in the etiology of AD and a prime target to tackle AD by offering multiple druggable opportunities.
The HPA axis is required to provide appropriate adaptation to external or internal challenges called stress, which initiates a cascade of hormonal processes (Figure 1A). This cascade starts in the hypothalamic paraventricular nucleus (PVN) with a release of CRH and AVP at the median eminence level. AVP acts in synergy with CRH to induce pituitary adrenocorticotropin (ACTH) release in blood (Whitnall et al., 1987; Mouri et al., 1993; Raff, 1993; Torner et al., 2017). Then, ACTH triggers the adrenal cortex to release GC (cortisol in human, corticosterone in rodents). These hormones act widely throughout the body and brain to mobilize energy resources in order to fight stress and maintain homeostasis (Carroll et al., 2011). To avoid an overactivation of the HPA axis, GC exert an inhibitory feedback at all stages of the axis (Figure 1A; Tasker and Herman, 2011). These steroid hormones bind to low affinity GR and high affinity mineralocorticoid receptors (MR; Reul and de Kloet, 1985). These nuclear receptors are necessary for normal cellular activity and crucial for many central nervous system functions, including learning and memory (Roozendaal, 2000). The GC circadian regulation is under the control of MR, while under stress conditions, GC rise significantly causing substantial activation of GR (Reul and de Kloet, 1985; Thomas, 2015).
Figure 1. Mechanisms linking HPA axis dysregulation and AD. Following acute stress (A), hypothalamic PVN releases CRH and AVP in the blood portal of median eminence. In response to CRH and AVP, corticotropic cells of anterior pituitary release ACTH in the peripheral circulation to induce GC secretion in blood by adrenal cortex. Succinctly, (1) GC mobilize energy resources and increase cardiovascular function to fight stress. Besides, GC inhibit unnecessary functions in the early phase of stress response, such as immunity, growth, digestion and reproduction. Then, (2) to avoid runaway of the system, GC exert an inhibitory feedback at all stages of HPA axis (hypothalamus and pituitary). In addition, as they easily penetrate in the brain, GC also act on several regions involved in the control of HPA axis activity, such as hippocampus and prefrontal cortex (tonic inhibition) or amygdala (tonic stimulation, Canet et al., 2018). However, chronic stress leads to a sustained activation of HPA axis and could induce stress-related disorders, as for instance MMD and AD (B, Canet et al., 2018). In this context, GC over-secretion is associated with GC resistance and GR signaling impairment (Chrousos et al., 1993). Homeostasis maintenance is compromised, leading to insulin resistance, dyslipidemia, atherosclerosis, hypertension and a massive peripheral inflammation (Vitellius et al., 2018; Maslov et al., 2019). In limbic structures (hippocampus, prefrontal cortex), it was shown that GC overexposure induces hippocampal and cortical atrophy (McEwen, 2008) and amygdala hypertrophy (Vyas et al., 2003, 2004), that could be related to learning and memory deficits, emotional impairment, excitotoxicity, neuroinflammation and oxidative stress (Sapolsky, 1996; McEwen, 2008; Bengoetxea et al., 2016). In the AD context, high levels of GC, and the dysregulation of the HPA axis activity observed in patients (Hartmann et al., 1997; Swanwick et al., 1998), seems to be particularly involved in the induction of amyloidogenic pathway and the abnormal phosphorylation of Tau (Green et al., 2006; Pineau et al., 2016; Sotiropoulos and Sousa, 2016; Vyas et al., 2016; Canet et al., 2019). Thus, it appears that the rise of circulating GC increases AD pathology, resulting in a vicious cycle by which pathology induces HPA axis dysregulation, GC overexposure and GR signaling impairment, which in turn potentiates the pathology. Due to its primordial role in the maintenance of homeostasis, targeting HPA axis offers multiple angles of action to break this vicious cycle and pave the way to new therapeutic strategies (C). Abbreviations: 11-βHSD1, 11β-hydroxysteroid dehydrogenase-1; Aβ, amyloid-β protein; APP, amyloid precursor protein; ACTH, adrenocorticotropin; AD, Alzheimer’s disease; AVP, arginine-vasopressin; CRH, corticotropin releasing hormone; CRH-R1, CRH receptor type 1; GC, glucocorticoids; GR, glucocorticoid receptors; HPA axis, Hypothalamic-pituitary adrenal axis; MDD, Major depressive disorder; PVN, paraventricular nucleus; sGRm, Selective GR modulator; V1b, Arginine-vasopressin receptor sub-type 1b.
It is interesting to note that AVP and CRH are also locally synthesized within the brain, where they are important neuromodulators involved in the central organization of various brain-mediated stress responses (Buijs, 1990; Tilders et al., 1993).
In human, chronic stress and HPA axis dysregulation with chronic GC hypersecretion appear to exert detrimental effects in normal aging and AD (Hartmann et al., 1997; Lupien et al., 1998; Notarianni, 2013; Givalois, 2014; Canet et al., 2018), but also in Parkinson’s disease (Wu et al., 2016), Cushing’s syndrome (Kroon et al., 2018), and MDD (Canet et al., 2018). A prolonged exposure to GC appears to damage particularly limbic structures (hippocampus and prefrontal cortex) by inducing a state of vulnerability in these neurons through the disruption of various cellular mechanisms (Sapolsky, 1996; McEwen, 2008). This review will present several current strategies in AD, aiming to restore HPA axis function and to limit GC production and toxicity.
CRH acts via G-protein-coupled receptor type 1 or 2 (CRH-R1 and CRH-R2) with widespread brain expression to orchestrate the stress response (Bale and Vale, 2004). In AD patients, investigators reported a reduction of immunoreactive cells for CRH in the cortex, associated with an increase of postsynaptic CRH receptors density (Whitehouse et al., 1987; Pomara et al., 1989; Behan et al., 1995). Besides clinical evidence, it was shown that CRH and CRH-R1 play critical roles in the regulation of stress-induced neuropathogenesis and behavioral deficits in mice models of AD (Dong et al., 2008; Carroll et al., 2011; Zhang et al., 2016; Table 1). CRH overexpressing mice display increased Tau hyperphosphorylation and aggregation in the hippocampus (Campbell et al., 2014). In Tg2576-AD mice, chronic isolation stress increases the expression of CRH-R1 in cortex and hippocampus (Dong et al., 2008). These authors also showed that chronic administration of antalarmin (a CRH-R1 antagonist) significantly decreased plasma corticosterone levels, tissue Aβ1–42 levels and Aβ plaques deposition in the brain, and blocked the effects of isolation stress on anxiety levels and memory (Dong et al., 2014). A few years later, a 3 month-treatment in food with CRH-R1 antagonists (Antalarmin and R121919) was shown to prevent stress-induced behavioral changes (anxiety and memory) and synaptic loss in aged rats, perhaps by reversing HPA axis dysfunction (Dong et al., 2018). Moreover, in another mouse model of AD (APP/PS1) a 5 month-treatment with R121919 prevented the onset of cognitive impairment, reduced cellular and synaptic deficits and Aβ levels (Zhang et al., 2016). Similarly, pre-treatment with another CRH-R1 antagonist (NBI27914) in PS19-AD mice (P301S mutation) before restraint/isolation stress prevented Tau hyperphosphorylation and aggregation, neurodegeneration and fear-memory impairment (Carroll et al., 2011).
Table 1. Preclinical and clinical studies targeting corticotropin releasing hormone (CRH), arginine vasopressin (AVP), glucocorticoids (GC) or glucocorticoid receptors (GR).
Such molecules have already been used in clinical trials for mood disorders (Zorrilla and Koob, 2004, 2010) and MDD (Nielsen, 2006) for many years, with disappointing results. However, all these preclinical studies highlight a promising therapeutic potential of CRH-R1 antagonists as treatments for neurodegenerative disorders such as AD. New CRH-R1 antagonists are in development to improve their bioavailability and safety profiles (Spierling and Zorrilla, 2017).
Hypothalamic neurons of PVN and supra-optic nucleus are the major source of AVP (Swaab, 1998). This hormone is especially involved in the regulation of water, electrolyte balance and in the stress response (Swaab, 1995; Twist et al., 2000). AVP is also implicated in many central processes including learning and memory (de Wied et al., 1993; Caldwell et al., 2008; Lee et al., 2009), anxiety (Caldwell et al., 2008; Lee et al., 2009), and processing of social information (Cilz et al., 2018). The effects of AVP are mediated by different receptor subtypes: V1a, V1b, and V2, which are G-protein coupled receptors (Cilz et al., 2018). However, the limbic action of AVP seems to be mediated by the V1b subtype, enriched in the hippocampus (Young et al., 2006). In rodents, its pharmacological activation enhances excitatory post-synaptic currents (EPSCs) that are absent in V1b−/− mice. V1b potentiation of EPSCs is dependent of N-methyl-D-aspartate (NMDA) receptors activation and intracellular Ca2+ signaling (Pagani et al., 2015; Cilz et al., 2018).
In a depressive and/or anxiety context, the therapeutic interest of V1b antagonists has been known for many years (Griebel et al., 2003). This target is particularly studied in pathologies associated with HPA axis dysregulation (Griebel et al., 2003; Katz et al., 2016; Table 1). The first selective and orally active V1b antagonist developed was SSR149415. In rodent models of anxiety and depression, SSR149415 produced a clear-cut anxiolytic-like activity in traumatic stress models (social defeat paradigm) and antidepressant-like effects (Griebel et al., 2002). More recently, in the same paradigm, other V1b antagonists (TASP0390325 and TASP0233278) elicited antidepressant-like effects (Iijima et al., 2014). These findings evidenced that V1b receptor blockade is particularly interesting for the treatment of affective and emotional disorders. For instance, several clinical trials are in progress with the V1b antagonist ABT-436, which is in phase 1b for depression treatment (Katz et al., 2016, 2017), and in phase 2 for alcohol dependence treatment (Ryan et al., 2017).
Thus, while evidences about a direct involvement of hypothalamic AVP in AD patients are not yet available (Goudsmit et al., 1990; Lucassen et al., 1994; Ishunina et al., 2002), many studies showed a crucial role played by AVP in stress and stress-related disorders such as MDD or anxiety. These findings are interesting as MDD could be a prodromal feature of AD and dementia (Herbert and Lucassen, 2016; Ishijima et al., 2017; Canet et al., 2018), justifying further investigations.
11β-HSD1 is a key enzyme that mediates the intracellular conversion of inactive cortisone to cortisol in humans (11β-dehydrocorticosterone to corticosterone in rodent), the active form of GC, thus amplifying steroid action. An haplotype in the 11β-HSD1 gene that increases 6-fold the risk to develop AD was reported (de Quervain et al., 2004).
Lowering GC exposure in the brain via intracellular inhibition of 11β-HSD1 has emerged as a therapeutic strategy to treat cognitive impairment in AD (Table 1). Pharmacological inhibition of 11β-HSD1 (UE1961) in aged mice improved spatial memory performance (Sooy et al., 2010). More recently, authors also demonstrated using another inhibitor (UE2316), a reduction of Aβ plaques in the cortex of old Tg2576-AD mice associated with an increase of IDE levels and memory improvements (Sooy et al., 2015). Moreover, in aged rodents, modulation of 11β-HSD1 by genetic knockdown or pharmacological inhibition improved memory. In Molher’s study, the authors tested an acute treatment with two novels inhibitors (A-918446 and A-801195, Abbott Laboratories). These compounds were able to improve memory consolidation, short-term memory, and recall in inhibitory avoidance (Mohler et al., 2011).
It is also known that aged mice develop elevated plasma corticosterone levels that correlate with learning deficits in the water-maze. This poor performance in a long-term memory task can be ameliorated by an 11β-HSD1 knockout, implicating lower intraneuronal corticosterone levels (Yau et al., 2001, 2015). In senescence-accelerated SAMP8 mice, pre-treatment with metyrapone, which inhibits the 11β-hydroxylase, completely normalized corticosterone levels and restored spatial memory (Iinuma et al., 2008).
These promising data led to a clinical trial with a novel brain-penetrant 11β-HSD1 inhibitor (UE2343, XanamemTM) for AD treatment. The Phase 1 clinical studies showed that UE2343 is safe, well tolerated and is able to moderately penetrate the brain (Webster et al., 2017). In addition, it is interesting to note that treatment of cognitively impaired elderly male patients with carbenoxolone (a non-selective 11β-HSD1 inhibitor) improved verbal fluency and memory (Sandeep et al., 2004).
The ubiquitous expression of GR and their involvement in a broad range of biological processes make them a prime target in many diseases. On the other hand, the global inhibition or activation of these receptors can lead to many potential side effects. For instance, it was demonstrated that a blockade of brain GR with Mifepristone (RU486, the reference non-selective GR antagonist) impaired spatial learning and memory in rodent (Roozendaal and McGaugh, 1997; Oitzl et al., 1998). A more recent study showed that central or peripheral administration of RU486, immediately following memory reactivation, induced a deficit in post-retrieval long-term memory, and memory did not re-emerge after a footshock reminder (Nikzad et al., 2011). Indeed, GC, GR and associated signaling pathways are crucial for memory consolidation. Acute stress and GR activation were shown to enhance memory consolidation and to inhibit working memory at the same time (Barsegyan et al., 2010). Many factors should be taken into consideration to understand and predict these GC contradictory effects. Depending on the brain area and on acute vs. chronic stress conditions, GC/GR can exert opposite effects (Roozendaal, 2002; McEwen, 2008; Barsegyan et al., 2010; Figure 1).
It is also important to mention the key role of GR in inflammatory processes. GR are necessary to maintain homeostasis since they control the expression of a large part of anti- and pro-inflammatory genes (Barnes, 1998; Van Bogaert et al., 2011; Coutinho and Chapman, 2011). An antagonist can prevent a crucial action of GR and lead to severe side effects associated with aberrant inflammatory processes. For example, it was shown that RU486 blocked the anti-inflammatory effects of exercise in a murine model of allergen-induced pulmonary inflammation (Pastva et al., 2005).
In AD, it was reported that patients treated with prednisone (a GC used for its anti-inflammatory properties) presented a more pronounced behavioral decline compared to the placebo-treated cohort (Aisen, 2000). Similarly, it was shown that GC administration (dexamethasone) in 3xTg-AD mice increases Aβ pathology and subsequent Tau accumulation and hyperphosphorylation (Green et al., 2006). It results inexorably in a vicious cycle whereby the pathology increases the secretion of GC, which further enhances the pathology (Baglietto-Vargas et al., 2013; Brureau et al., 2013; Pineau et al., 2016; Canet et al., 2018, 2019). All these findings tend to prove that in AD, but also in all pathologies implying GC, both GR agonists or antagonists should be used with caution.
Recently, in a search to abrogate potential side effects of GR antagonism, new compounds were developed and act as highly selective GR modulators (sGRm; Clark et al., 2008; Beaudry et al., 2014; Hunt et al., 2015; Pineau et al., 2016; Meijer et al., 2018; Viho et al., 2019). The use of sGRm is an attractive approach to separate wanted from unwanted outcomes. Different sGRm were developed and vary substantially in their biological activities. These molecules have the ability to act as agonist, as well as antagonist depending on the tissue or gene targets, but also on the physiological context. However, one thing to keep in mind is that the prediction of exact sGRm action is really challenging considering the numerous factors to take into account. Meijer et al. (2018) recently stated about the complexity of sGRm mechanisms: “even if we know the coactivators that will be recruited by a sGRm-GR complex, in most cases it is unknown which signaling pathways are involved in which transcriptional process. Given the large number of coactivators and their highly gene- and tissue-specific regulation, such analyses are very time consuming, if informative”.
In a direct application, sGRm offered promising results (Table 1). One of them, and the first to be developed by Corcept Therapeutics (Menlo Park, San Mateo, CA, USA), is CORT108297. It was first described as an antagonist (Belanoff et al., 2010), but its modulatory properties were discovered few years later (Zalachoras et al., 2013). In fact, the authors surprisingly observed that CORT108297 induced a unique interaction profile between GR and its coregulators compared with the full agonist dexamethasone and the non-selective antagonist, RU486. This atypical profile could explain the paradoxical effects of CORT108297 according to the brain region (Zalachoras et al., 2013; Viho et al., 2019). In rats, CORT108297 attenuates electroconvulsive shock-induced retrograde amnesia (Andrade et al., 2012). This molecule also presents antidepressant properties and reduces neuroendocrine stress responses and immobility in the forced-swimming test (FST), alike imipramine, a classic treatment for MDD (Solomon et al., 2014). By contrast, treatment with another member of this family, CORT118335, which has the particularity of being a GR modulator but also a MR antagonist, did not affect immobility in the FST (Nguyen et al., 2018), confirming a differential specificity and efficacy of each molecule.
In the 3xTg-AD mice, a 21 days treatment with CORT108297 reduced APP C-terminal fragments and p25 levels (Baglietto-Vargas et al., 2013), which is the activator of cyclin dependent kinase 5 (Cdk5), involved in AD pathophysiology (Patrick et al., 1999). In an acute rat model of AD (the oAβ25–35 model), we tested the therapeutic potential of CORT108297 and CORT113176 (Pineau et al., 2016). CORT113176 is also a sGRm, but displays a better affinity for GR than CORT108297 (Beaudry et al., 2014; Pineau et al., 2016). In this acute model, we previously evidenced a strong, long-lasting activation of the HPA axis, associated with a modification of GR and MR expression in brain regions involved in the control of GC secretion (hippocampus, amygdala and hypothalamus; Brureau et al., 2013). We found that both compounds (CORT113176 at a lower dose than CORT108297) were able to reverse neuroinflammatory and apoptotic processes, cognitive and synaptic deficits, and APP misprocessing (Pineau et al., 2016). To confirm the interest of sGRm, we compared these compounds with RU486. Interestingly, we observed that this non-selective antagonist only partially reversed previously observed pathological impairments (Pineau et al., 2016). In the same line of evidence, CORT113176 showed promising effects in a mouse model of amyotrophic lateral sclerosis by reducing neuronal injury and neuroinflammation in the spinal cord (Meyer et al., 2014). Thus, even if these compounds seemed to exhibit predominantly antagonistic actions in limbic structures in pathological conditions (Andrade et al., 2012; Atucha et al., 2015; Pineau et al., 2016), they could avoid side effects of GR blockade by some agonistic properties.
If we postulate that AD is a stress-related disorder, targeting HPA axis hormones and receptors could be an attractive approach and pave the way for new therapeutic strategies. Indeed, due to its primordial role in the maintenance of homeostasis, the HPA axis seems to be a key-actor in the etiology of AD and a prime target to tackle the pathology by offering multiple angles of action. Several strategies aiming to restore a normal functionality and activity of the HPA axis are in development with promising results. Some of these molecules are already in clinical studies, not necessarily in an AD context. Since some of them already obtained a safe and tolerated profile, testing them in AD patients could be a really encouraging challenge in the near future. Finally, this review wishes also to alert about the risk in prescribing GC-based therapies in the elderly or in early AD patients.
GC, CH, CZ, NC, CD and LG equally contributed to the definition of the scope and to writing of the manuscript.
LG is supported by “France Alzheimer” and “Fédération pour la Recherche sur le Cerveau” (Grant AAP SM2016#1512). CD and CZ are supported by the “Agence Nationale de la Recherche” (ANR) under the program “Investissements d’Avenir” (ANR-11-LABEX-0021-LipSTIC). GC and CH are supported by a PhD fellowship from the University of Montpellier, France (CBS2 PhD program).
The authors declare that the research was conducted in the absence of any commercial or financial relationships that could be construed as a potential conflict of interest.
We thank Pr. Emmanuel Planel (CHUQ, Québec, Canada) for his advices and pertinent corrections.
Aisen, P. S. (2000). Anti-inflammatory therapy for Alzheimer’s disease: implications of the prednisone trial. Acta Neurol. Scand. Suppl. 176, 85–89. doi: 10.1034/j.1600-0404.2000.00312.x
Andrade, C., Shaikh, S. A., Narayan, L., Blasey, C., and Belanoff, J. (2012). Administration of a selective glucocorticoid antagonist attenuates electroconvulsive shock-induced retrograde amnesia. J. Neural Transm. 119, 337–344. doi: 10.1007/s00702-011-0712-8
Atucha, E., Zalachoras, I., van den Heuvel, J. K., van Weert, L. T. C. M., Melchers, D., Mol, I. M., et al. (2015). A mixed glucocorticoid/mineralocorticoid selective modulator with dominant antagonism in the male rat brain. Endocrinology 156, 4105–4114. doi: 10.1210/en.2015-1390
Baglietto-Vargas, D., Medeiros, R., Martinez-Coria, H., LaFerla, F. M., and Green, K. N. (2013). Mifepristone alters amyloid precursor protein processing to preclude amyloid β and also reduces tau pathology. Biol. Psychiatry 74, 357–366. doi: 10.1016/j.biopsych.2012.12.003
Bale, T. L., and Vale, W. W. (2004). CRF and CRF receptors: role in stress responsivity and other behaviors. Annu. Rev. Pharmacol. Toxicol. 44, 525–557. doi: 10.1146/annurev.pharmtox.44.101802.121410
Barnes, P. J. (1998). Anti-inflammatory actions of glucocorticoids: molecular mechanisms. Clin. Sci. 94, 557–572. doi: 10.1042/cs0940557
Barsegyan, A., Mackenzie, S. M., Kurose, B. D., McGaugh, J. L., and Roozendaal, B. (2010). Glucocorticoids in the prefrontal cortex enhance memory consolidation and impair working memory by a common neural mechanism. Proc. Natl. Acad. Sci. U S A 107, 16655–16660. doi: 10.1073/pnas.1011975107
Beaudry, J. L., Dunford, E. C., Teich, T., Zaharieva, D., Hunt, H., Belanoff, J. K., et al. (2014). Effects of selective and non-selective glucocorticoid receptor: II antagonists on rapid-onset diabetes in young rats. PLoS One 9:e91248. doi: 10.1371/journal.pone.0091248
Behan, D. P., Heinrichs, S. C., Troncoso, J. C., Liu, X. J., Kawas, C. H., Ling, N., et al. (1995). Displacement of corticotropin releasing factor from its binding protein as a possible treatment for Alzheimer’s disease. Nature 378, 284–287. doi: 10.1038/378284a0
Belanoff, J. K., Blasey, C. M., Clark, R. D., and Roe, R. L. (2010). Selective glucocorticoid receptor (type II) antagonist prevents and reverses olanzapine-induced weight gain. Diabetes Obes. Metab. 12, 545–547. doi: 10.1111/j.1463-1326.2009.01185.x
Bengoetxea, X., de Cerain, A. L., Azqueta, A., and Ramirez, M. J. (2016). Purported interactions of amyloid-β and glucocorticoids in cytotoxicity and genotoxicity: implications in Alzheimer’s disease. J. Alzheimers Dis. 54, 1085–1094. doi: 10.3233/jad-160636
Blennow, K., de Leon, M. J., and Zetterberg, H. (2006). Alzheimer’s disease. Lancet 368, 387–403. doi: 10.1016/S0140-6736(06)69113-7
Brureau, A., Zussy, C., Delair, B., Ogier, C., Ixart, G., Maurice, T., et al. (2013). Deregulation of hypothalamic-pituitary-adrenal axis functions in an Alzheimer’s disease rat model. Neurobiol. Aging 34, 1426–1439. doi: 10.1016/j.neurobiolaging.2012.11.015
Buijs, R. M. (1990). Vasopressin and oxytocin localization and putative functions in the brain. Acta Neurochir. Suppl. 47, 86–89. doi: 10.1007/978-3-7091-9062-3_10
Caldwell, H. K., Lee, H.-J., Macbeth, A. H., and Young, W. S. III. (2008). Vasopressin: behavioral roles of an “original” neuropeptide. Prog. Neurobiol. 84, 1–24. doi: 10.1016/j.pneurobio.2007.10.007
Campbell, S. N., Zhang, C., Monte, L., Roe, A. D., Rice, K. C., Taché, Y., et al. (2014). Increased tau phosphorylation and aggregation in the hippocampus of mice overexpressing corticotropin-releasing factor. J. Alzheimers Dis. 43, 967–976. doi: 10.3233/jad-141281
Canet, G., Chevallier, N., Perrier, V., Desrumaux, C., and Givalois, L. (2019). “Targeting glucocorticoid receptors: a new avenue for Alzheimer’s disease therapy,” in Pathology, Prevention and Therapeutics of Neurodegenerative Disease, eds S. Singh and N. Joshi (Singapore: Springer Singapore), 173–183.
Canet, G., Chevallier, N., Zussy, C., Desrumaux, C., and Givalois, L. (2018). Central role of glucocorticoid receptors in Alzheimer’s disease and depression. Front. Neurosci. 12:739. doi: 10.3389/fnins.2018.00739
Carroll, J. C., Iba, M., Bangasser, D. A., Valentino, R. J., James, M. J., Brunden, K. R., et al. (2011). Chronic stress exacerbates tau pathology, neurodegeneration, and cognitive performance through a corticotropin-releasing factor receptor-dependent mechanism in a transgenic mouse model of tauopathy. J. Neurosci. 31, 14436–14449. doi: 10.1523/JNEUROSCI.3836-11.2011
Chrousos, G. P., Detera-Wadleigh, S. D., and Karl, M. (1993). Syndromes of glucocorticoid resistance. Ann. Intern. Med. 119, 1113–1124. doi: 10.7326/0003-4819-119-11-199312010-00009
Cilz, N. I., Cymerblit-Sabba, A., and Young, W. S. (2018). Oxytocin and vasopressin in the rodent hippocampus. Genes Brain Behav. 18:e12535. doi: 10.1111/gbb.12535
Clark, R. D., Ray, N. C., Williams, K., Blaney, P., Ward, S., Crackett, P. H., et al. (2008). 1H-Pyrazolo[3,4-g]hexahydro-isoquinolines as selective glucocorticoid receptor antagonists with high functional activity. Bioorg. Med. Chem. Lett. 18, 1312–1317. doi: 10.1016/j.bmcl.2008.01.027
Coutinho, A. E., and Chapman, K. E. (2011). The anti-inflammatory and immunosuppressive effects of glucocorticoids, recent developments and mechanistic insights. Mol. Cell. Endocrinol. 335, 2–13. doi: 10.1016/j.mce.2010.04.005
Csernansky, J. G., Dong, H., Fagan, A. M., Wang, L., Xiong, C., Holtzman, D. M., et al. (2006). Plasma cortisol and progression of dementia in subjects with Alzheimer-type dementia. Am. J. Psychiatry 163, 2164–2169. doi: 10.1176/appi.ajp.163.12.2164
de Quervain, D. J.-F., Poirier, R., Wollmer, M. A., Grimaldi, L. M. E., Tsolaki, M., Streffer, J. R., et al. (2004). Glucocorticoid-related genetic susceptibility for Alzheimer’s disease. Hum. Mol. Genet. 13, 47–52. doi: 10.1093/hmg/ddg361
de Wied, D., Diamant, M., and Fodor, M. (1993). Central nervous system effects of the neurohypophyseal hormones and related peptides. Front. Neuroendocrinol. 14, 251–302. doi: 10.1006/frne.1993.1009
Dong, H., Keegan, J. M., Hong, E., Gallardo, C., Montalvo-Ortiz, J., Wang, B., et al. (2018). Corticotrophin releasing factor receptor 1 antagonists prevent chronic stress-induced behavioral changes and synapse loss in aged rats. Psychoneuroendocrinology 90, 92–101. doi: 10.1016/j.psyneuen.2018.02.013
Dong, H., Wang, S., Zeng, Z., Li, F., Montalvo-Ortiz, J., Tucker, C., et al. (2014). Effects of corticotrophin-releasing factor receptor 1 antagonists on amyloid-β and behavior in Tg2576 mice. Psychopharmacology 231, 4711–4722. doi: 10.1007/s00213-014-3629-8
Dong, H., Yuede, C. M., Yoo, H.-S., Martin, M. V., Deal, C., Mace, A. G., et al. (2008). Corticosterone and related receptor expression are associated with increased β-amyloid plaques in isolated Tg2576 mice. Neuroscience 155, 154–163. doi: 10.1016/j.neuroscience.2008.05.017
Givalois, L. (2014). The glucocorticoid receptors regulation in Alzheimer’s disease. Neurobiol. Aging 35, e17–e18. doi: 10.1016/j.neurobiolaging.2013.12.012
Goudsmit, E., Hofman, M. A., Fliers, E., and Swaab, D. F. (1990). The supraoptic and paraventricular nuclei of the human hypothalamus in relation to sex, age and Alzheimer’s disease. Neurobiol. Aging 11, 529–536. doi: 10.1016/0197-4580(90)90114-f
Green, K. N., Billings, L. M., Roozendaal, B., McGaugh, J. L., and LaFerla, F. M. (2006). Glucocorticoids increase amyloid-β and tau pathology in a mouse model of Alzheimer’s disease. J. Neurosci. 26, 9047–9056. doi: 10.1523/JNEUROSCI.2797-06.2006
Griebel, G., Simiand, J., Serradeil-Le Gal, C., Wagnon, J., Pascal, M., Scatton, B., et al. (2002). Anxiolytic- and antidepressant-like effects of the non-peptide vasopressin V1b receptor antagonist, SSR149415, suggest an innovative approach for the treatment of stress-related disorders. Proc. Natl. Acad. Sci. U S A 99, 6370–6375. doi: 10.1073/pnas.092012099
Griebel, G., Simiand, J., Stemmelin, J., Gal, C. S.-L., and Steinberg, R. (2003). The vasopressin V1b receptor as a therapeutic target in stress-related disorders. Curr. Drug Targets CNS Neurol. Disord. 2, 191–200. doi: 10.2174/1568007033482850
Hartmann, A., Veldhuis, J. D., Deuschle, M., Standhardt, H., and Heuser, I. (1997). Twenty-four hour cortisol release profiles in patients with Alzheimer’s and Parkinson’s disease compared to normal controls: ultradian secretory pulsatility and diurnal variation. Neurobiol. Aging 18, 285–289. doi: 10.1016/s0197-4580(97)80309-0
Heininger, K. (2000). A unifying hypothesis of Alzheimer’s disease. IV. Causation and sequence of events. Rev. Neurosci. 11, 213–328. doi: 10.1515/REVNEURO.2000.11.S1.213
Herbert, J., and Lucassen, P. J. (2016). Depression as a risk factor for Alzheimer’s disease: genes, steroids, cytokines and neurogenesis—what do we need to know? Front. Neuroendocrinol. 41, 153–171. doi: 10.1016/j.yfrne.2015.12.001
Hunt, H. J., Belanoff, J. K., Golding, E., Gourdet, B., Phillips, T., Swift, D., et al. (2015). 1H-Pyrazolo[3,4-g]hexahydro-isoquinolines as potent GR antagonists with reduced hERG inhibition and an improved pharmacokinetic profile. Bioorg. Med. Chem. Lett. 25, 5720–5725. doi: 10.1016/j.bmcl.2015.10.097
Iijima, M., Yoshimizu, T., Shimazaki, T., Tokugawa, K., Fukumoto, K., Kurosu, S., et al. (2014). Antidepressant and anxiolytic profiles of newly synthesized arginine vasopressin V1B receptor antagonists: TASP0233278 and TASP0390325: pharmacological profiles of V1B receptor antagonists. Br. J. Pharmacol. 171, 3511–3525. doi: 10.1111/bph.12699
Iinuma, M., Ichihashi, Y., Hioki, Y., Kurata, C., Tamura, Y., and Kubo, K. (2008). Malocclusion induces chronic stress. Okajimas Folia Anat. Jpn. 85, 35–42. doi: 10.2535/ofaj.85.35
Ishijima, S., Baba, H., Maeshima, H., Shimano, T., Inoue, M., Suzuki, T., et al. (2017). Glucocorticoid may influence amyloid β metabolism in patients with depression. Psychiatry Res. 259, 191–196. doi: 10.1016/j.psychres.2017.10.008
Ishunina, T. A., Wouda, J., Fisser, B., and Swaab, D. F. (2002). Sex differences in estrogen receptor α and β expression in vasopressin neurons of the supraoptic nucleus in elderly and Alzheimer’s disease patients: no relationship with cytoskeletal alterations. Brain Res. 951, 322–329. doi: 10.1016/s0006-8993(02)03269-9
Katz, D. A., Liu, W., Locke, C., Dutta, S., and Tracy, K. A. (2016). Clinical safety and hypothalamic-pituitary-adrenal axis effects of the arginine vasopressin type 1B receptor antagonist ABT-436. Psychopharmacology 233, 71–81. doi: 10.1007/s00213-015-4089-5
Katz, D. A., Locke, C., Greco, N., Liu, W., and Tracy, K. A. (2017). Hypothalamic-pituitary-adrenal axis and depression symptom effects of an arginine vasopressin type 1B receptor antagonist in a one-week randomized Phase 1b trial. Brain Behav. 7:e00628. doi: 10.1002/brb3.628
Kroon, J., Koorneef, L. L., van den Heuvel, J. K., Verzijl, C. R. C., van de Velde, N. M., Mol, I. M., et al. (2018). Selective glucocorticoid receptor antagonist CORT125281 activates brown adipose tissue and alters lipid distribution in male mice. Endocrinology 159, 535–546. doi: 10.1210/en.2017-00512
Lee, H.-J., Macbeth, A. H., Pagani, J., and Young, W. S. (2009). Oxytocin: the great facilitator of life. Prog. Neurobiol. 88, 127–151. doi: 10.1016/j.pneurobio.2009.04.001
Lucassen, P. J., Salehi, A., Pool, C. W., Gonatas, N. K., and Swaab, D. F. (1994). Activation of vasopressin neurons in aging and Alzheimer’s disease. J. Neuroendocrinol. 6, 673–679. doi: 10.1111/j.1365-2826.1994.tb00634.x
Lupien, S. J., de Leon, M., de Santi, S., Convit, A., Tarshish, C., Nair, N. P., et al. (1998). Cortisol levels during human aging predict hippocampal atrophy and memory deficits. Nat. Neurosci. 1, 69–73. doi: 10.1038/271
Maslov, L. N., Naryzhnaya, N. V., Boshchenko, A. A., Popov, S. V., Ivanov, V. V., and Oeltgen, P. R. (2019). Is oxidative stress of adipocytes a cause or a consequence of the metabolic syndrome? J. Clin. Transl. Endocrinol. 15, 1–5. doi: 10.1016/j.jcte.2018.11.001
McEwen, B. S. (2008). Central effects of stress hormones in health and disease: understanding the protective and damaging effects of stress and stress mediators. Eur. J. Pharmacol. 583, 174–185. doi: 10.1016/j.ejphar.2007.11.071
Meijer, O. C., Koorneef, L. L., and Kroon, J. (2018). Glucocorticoid receptor modulators. Ann. Endocrinol. 79, 107–111. doi: 10.1016/j.ando.2018.03.004
Meyer, M., Gonzalez Deniselle, M. C., Hunt, H., de Kloet, E. R., and De Nicola, A. F. (2014). The selective glucocorticoid receptor modulator CORT108297 restores faulty hippocampal parameters in Wobbler and corticosterone-treated mice. J. Steroid Biochem. Mol. Biol. 143, 40–48. doi: 10.1016/j.jsbmb.2014.02.007
Mohler, E. G., Browman, K. E., Roderwald, V. A., Cronin, E. A., Markosyan, S., Scott Bitner, R., et al. (2011). Acute inhibition of 11 -hydroxysteroid dehydrogenase type-1 improves memory in rodent models of cognition. J. Neurosci. 31, 5406–5413. doi: 10.1523/JNEUROSCI.4046-10.2011
Mouri, T., Itoi, K., Takahashi, K., Suda, T., Murakami, O., Yoshinaga, K., et al. (1993). Colocalization of corticotropin-releasing factor and vasopressin in the paraventricular nucleus of the human hypothalamus. Neuroendocrinology 57, 34–39. doi: 10.1210/me.7.10.1357
Nguyen, E. T., Caldwell, J. L., Streicher, J., Ghisays, V., Balmer, N. J., Estrada, C. M., et al. (2018). Differential effects of imipramine and CORT118335 (Glucocorticoid receptor modulator/mineralocorticoid receptor antagonist) on brain-endocrine stress responses and depression-like behavior in female rats. Behav. Brain Res. 336, 99–110. doi: 10.1016/j.bbr.2017.08.045
Nielsen, D. M. (2006). Corticotropin-releasing factor type-1 receptor antagonists: the next class of antidepressants? Life Sci. 78, 909–919. doi: 10.1016/j.lfs.2005.06.003
Nikzad, S., Vafaei, A. A., Rashidy-Pour, A., and Haghighi, S. (2011). Systemic and intrahippocampal administrations of the glucocorticoid receptor antagonist RU38486 impairs fear memory reconsolidation in rats. Stress 14, 459–464. doi: 10.3109/10253890.2010.548171
Notarianni, E. (2013). Hypercortisolemia and glucocorticoid receptor-signaling insufficiency in Alzheimer’s disease initiation and development. Curr. Alzheimer Res. 10, 714–731. doi: 10.2174/15672050113109990137
Oitzl, M. S., Fluttert, M., Sutanto, W., and de Kloet, E. R. (1998). Continuous blockade of brain glucocorticoid receptors facilitates spatial learning and memory in rats. Eur. J. Neurosci. 10, 3759–3766. doi: 10.1046/j.1460-9568.1998.00381.x
Pagani, J. H., Zhao, M., Cui, Z., Williams Avram, S. K., Caruana, D. A., Dudek, S. M., et al. (2015). Role of the vasopressin 1b receptor in rodent aggressive behavior and synaptic plasticity in hippocampal area CA2. Mol. Psychiatry 20, 490–499. doi: 10.1038/mp.2014.47
Pastva, A., Estell, K., Schoeb, T. R., and Schwiebert, L. M. (2005). RU486 blocks the anti-inflammatory effects of exercise in a murine model of allergen-induced pulmonary inflammation. Brain Behav. Immun. 19, 413–422. doi: 10.1016/j.bbi.2005.04.004
Patrick, G. N., Zukerberg, L., Nikolic, M., de la Monte, S., Dikkes, P., and Tsai, L.-H. (1999). Conversion of p35 to p25 deregulates Cdk5 activity and promotes neurodegeneration. Nature 402, 615–622. doi: 10.1038/45159
Pineau, F., Canet, G., Desrumaux, C., Hunt, H., Chevallier, N., Ollivier, M., et al. (2016). New selective glucocorticoid receptor modulators reverse amyloid-β peptide-induced hippocampus toxicity. Neurobiol. Aging 45, 109–122. doi: 10.1016/j.neurobiolaging.2016.05.018
Pomara, N., Singh, R. R., Deptula, D., LeWitt, P. A., Bissette, G., Stanley, M., et al. (1989). CSF corticotropin-releasing factor (CRF) in Alzheimer’s disease: its relationship to severity of dementia and monoamine metabolites. Biol. Psychiatry 26, 500–504. doi: 10.1016/0006-3223(89)90071-1
Querfurth, H. W., and LaFerla, F. M. (2010). Alzheimer’s disease. N. Engl. J. Med. 362, 329–344. doi: 10.1056/NEJMra0909142
Raff, H. (1993). Interactions between neurohypophysial hormones and the ACTH-adrenocortical axis. Ann. N Y Acad. Sci. 689, 411–425. doi: 10.1111/j.1749-6632.1993.tb55564.x
Reul, J. M., and de Kloet, E. R. (1985). Two receptor systems for corticosterone in rat brain: microdistribution and differential occupation. Endocrinology 117, 2505–2511. doi: 10.1210/endo-117-6-2505
Roozendaal, B. (2000). 1999 Curt P. Richter award. Glucocorticoids and the regulation of memory consolidation. Psychoneuroendocrinology 25, 213–238. doi: 10.1016/s0306-4530(99)00058-x
Roozendaal, B. (2002). Stress and memory: opposing effects of glucocorticoids on memory consolidation and memory retrieval. Neurobiol. Learn. Mem. 78, 578–595. doi: 10.1006/nlme.2002.4080
Roozendaal, B., and McGaugh, J. L. (1997). Glucocorticoid receptor agonist and antagonist administration into the basolateral but not central amygdala modulates memory storage. Neurobiol. Learn. Mem. 67, 176–179. doi: 10.1006/nlme.1996.3765
Ryan, M. L., Falk, D. E., Fertig, J. B., Rendenbach-Mueller, B., Katz, D. A., Tracy, K. A., et al. (2017). A phase 2, double-blind, placebo-controlled randomized trial assessing the efficacy of ABT-436, a novel V1b receptor antagonist, for alcohol dependence. Neuropsychopharmacology 42, 1012–1023. doi: 10.1038/npp.2016.214
Sandeep, T. C., Yau, J. L. W., MacLullich, A. M. J., Noble, J., Deary, I. J., Walker, B. R., et al. (2004). 11β-hydroxysteroid dehydrogenase inhibition improves cognitive function in healthy elderly men and type 2 diabetics. Proc. Natl. Acad. Sci. U S A 101, 6734–6739. doi: 10.1073/pnas.0306996101
Sapolsky, R. M. (1996). Stress, glucocorticoids, and damage to the nervous system: the current state of confusion. Stress 1, 1–19. doi: 10.3109/10253899609001092
Solomon, M. B., Wulsin, A. C., Rice, T., Wick, D., Myers, B., McKlveen, J., et al. (2014). The selective glucocorticoid receptor antagonist CORT 108297 decreases neuroendocrine stress responses and immobility in the forced swim test. Horm. Behav. 65, 363–371. doi: 10.1016/j.yhbeh.2014.02.002
Sooy, K., Noble, J., McBride, A., Binnie, M., Yau, J. L. W., Seckl, J. R., et al. (2015). Cognitive and disease-modifying effects of 11β-hydroxysteroid dehydrogenase type 1 inhibition in male Tg2576 mice, a model of Alzheimer’s disease. Endocrinology 156, 4592–4603. doi: 10.1210/en.2015-1395
Sooy, K., Webster, S. P., Noble, J., Binnie, M., Walker, B. R., Seckl, J. R., et al. (2010). Partial deficiency or short-term inhibition of 11 -hydroxysteroid dehydrogenase type 1 improves cognitive function in aging mice. J. Neurosci. 30, 13867–13872. doi: 10.1523/JNEUROSCI.2783-10.2010
Sotiropoulos, I., and Sousa, N. (2016). Tau as the converging protein between chronic stress and Alzheimer’s disease synaptic pathology. Neurodegener. Dis. 16, 22–25. doi: 10.1159/000440844
Spierling, S. R., and Zorrilla, E. P. (2017). Don’t stress about CRF: assessing the translational failures of CRF1antagonists. Psychopharmacology 234, 1467–1481. doi: 10.1007/s00213-017-4556-2
Swaab, D. F. (1998). The human hypothalamo-neurohypophysial system in health and disease. Prog. Brain Res. 119, 577–618. doi: 10.1016/s0079-6123(08)61594-0
Swanwick, G. R., Kirby, M., Bruce, I., Buggy, F., Coen, R. F., Coakley, D., et al. (1998). Hypothalamic-pituitary-adrenal axis dysfunction in Alzheimer’s disease: lack of association between longitudinal and cross-sectional findings. Am. J. Psychiatry 155, 286–289. doi: 10.1176/ajp.155.2.286
Tasker, J. G., and Herman, J. P. (2011). Mechanisms of rapid glucocorticoid feedback inhibition of the hypothalamic-pituitary-adrenal axis. Stress 14, 398–406. doi: 10.3109/10253890.2011.586446
Thomas, S. A. (2015). Neuromodulatory signaling in hippocampus-dependent memory retrieval. Hippocampus 25, 415–431. doi: 10.1002/hipo.22394
Tilders, F. J., Schmidt, E. D., and de Goeij, D. C. (1993). Phenotypic plasticity of CRF neurons during stress. Ann. N Y Acad. Sci. 697, 39–52. doi: 10.1111/j.1749-6632.1993.tb49921.x
Torner, L., Plotsky, P. M., Neumann, I. D., and de Jong, T. R. (2017). Forced swimming-induced oxytocin release into blood and brain: effects of adrenalectomy and corticosterone treatment. Psychoneuroendocrinology 77, 165–174. doi: 10.1016/j.psyneuen.2016.12.006
Twist, S. J., Taylor, G. A., Weddell, A., Weightman, D. R., Edwardson, J. A., and Morris, C. M. (2000). Brain oestradiol and testosterone levels in Alzheimer’s disease. Neurosci. Lett. 286, 1–4. doi: 10.1016/s0304-3940(00)01078-8
Van Bogaert, T., Vandevyver, S., Dejager, L., Hauwermeiren, F. V., Pinheiro, I., Petta, I., et al. (2011). Tumor necrosis factor inhibits glucocorticoid receptor function in mice. J. Biol. Chem. 286, 26555–26567. doi: 10.1074/jbc.M110.212365
Viho, E., Buurstede, J. C., Mahfouz, A., Koorneef, L. L., van Weert, L. T. C. M., Houtman, R., et al. (2019). Corticosteroid action in the brain: the potential of selective receptor modulation. Neuroendocrinology 109, 266–276. doi: 10.1159/000499659
Vitellius, G., Trabado, S., Bouligand, J., Delemer, B., and Lombès, M. (2018). Pathophysiology of glucocorticoid signaling. Ann. Endocrinol. 79, 98–106. doi: 10.1016/j.ando.2018.03.001
Vyas, A., Bernal, S., and Chattarji, S. (2003). Effects of chronic stress on dendritic arborization in the central and extended amygdala. Brain Res. 965, 290–294. doi: 10.1016/s0006-8993(02)04162-8
Vyas, A., Pillai, A. G., and Chattarji, S. (2004). Recovery after chronic stress fails to reverse amygdaloid neuronal hypertrophy and enhanced anxiety-like behavior. Neuroscience 128, 667–673. doi: 10.1016/j.neuroscience.2004.07.013
Vyas, S., Rodrigues, A. J., Silva, J. M., Tronche, F., Almeida, O. F. X., Sousa, N., et al. (2016). Chronic stress and glucocorticoids: from neuronal plasticity to neurodegeneration. Neural Plast. 2016:6391686. doi: 10.1155/2016/6391686
Webster, S. P., McBride, A., Binnie, M., Sooy, K., Seckl, J. R., Andrew, R., et al. (2017). Selection and early clinical evaluation of the brain-penetrant 11β-hydroxysteroid dehydrogenase type 1 (11β-HSD1) inhibitor UE2343 (XanamemTM). Br. J. Pharmacol. 174, 396–408. doi: 10.1111/bph.13699
Whitehouse, P. J., Vale, W. W., Zweig, R. M., Singer, H. S., Mayeux, R., Kuhar, M. J., et al. (1987). Reductions in corticotropin releasing factor-like immunoreactivity in cerebral cortex in Alzheimer’s disease, Parkinson’s disease, and progressive supranuclear palsy. Neurology 37, 905–909. doi: 10.1212/wnl.37.6.905
Whitnall, M. H., Smyth, D., and Gainer, H. (1987). Vasopressin coexists in half of the corticotropin-releasing factor axons present in the external zone of the median eminence in normal rats. Neuroendocrinology 45, 420–424. doi: 10.1159/000124768
Wu, Q., Yang, X., Zhang, Y., Zhang, L., and Feng, L. (2016). Chronic mild stress accelerates the progression of Parkinson’s disease in A53T α-synuclein transgenic mice. Exp. Neurol. 285, 61–71. doi: 10.1016/j.expneurol.2016.09.004
Yau, J. L. W., Noble, J., Kenyon, C. J., Hibberd, C., Kotelevtsev, Y., Mullins, J. J., et al. (2001). Lack of tissue glucocorticoid reactivation in 11-hydroxysteroid dehydrogenase type 1 knockout mice ameliorates age-related learning impairments. Proc. Natl. Acad. Sci. U S A 98, 4716–4721. doi: 10.1073/pnas.071562698
Yau, J. L. W., Wheelan, N., Noble, J., Walker, B. R., Webster, S. P., Kenyon, C. J., et al. (2015). Intrahippocampal glucocorticoids generated by β-HSD1 affect memory in aged mice. Neurobiol. Aging 36, 334–343. doi: 10.1016/j.neurobiolaging.2014.07.007
Young, W. S., Li, J., Wersinger, S. R., and Palkovits, M. (2006). The vasopressin 1b receptor is prominent in the hippocampal area CA2 where it is unaffected by restraint stress or adrenalectomy. Neuroscience 143, 1031–1039. doi: 10.1016/j.neuroscience.2006.08.040
Zalachoras, I., Houtman, R., Atucha, E., Devos, R., Tijssen, A. M. I., Hu, P., et al. (2013). Differential targeting of brain stress circuits with a selective glucocorticoid receptor modulator. Proc. Natl. Acad. Sci. U S A 110, 7910–7915. doi: 10.1073/pnas.1219411110
Zhang, C., Kuo, C.-C., Moghadam, S. H., Monte, L., Campbell, S. N., Rice, K. C., et al. (2016). Corticotropin-releasing factor receptor-1 antagonism mitigates β amyloid pathology and cognitive and synaptic deficits in a mouse model of Alzheimer’s disease. Alzheimers Dement. 12, 527–537. doi: 10.1016/j.jalz.2015.09.007
Zorrilla, E. P., and Koob, G. F. (2004). The therapeutic potential of CRF1 antagonists for anxiety. Expert Opin. Investig. Drugs 13, 799–828. doi: 10.1517/13543784.13.7.799
Keywords: Alzheimer’s disease, glucococorticoids, stress-related disorder, CRH (corticotropin-releasing hormone), HPA axis (hypothalamus-pituitary-adrenal), AVP (arginine vasopressin), 11β hydroxysteroid dehydrogenase
Citation: Canet G, Hernandez C, Zussy C, Chevallier N, Desrumaux C and Givalois L (2019) Is AD a Stress-Related Disorder? Focus on the HPA Axis and Its Promising Therapeutic Targets. Front. Aging Neurosci. 11:269. doi: 10.3389/fnagi.2019.00269
Received: 03 June 2019; Accepted: 18 September 2019;
Published: 27 September 2019.
Edited by:
Patrizia Giannoni, University of Nîmes, FranceReviewed by:
Xiao-Xin Yan, Central South University, ChinaCopyright © 2019 Canet, Hernandez, Zussy, Chevallier, Desrumaux and Givalois. This is an open-access article distributed under the terms of the Creative Commons Attribution License (CC BY). The use, distribution or reproduction in other forums is permitted, provided the original author(s) and the copyright owner(s) are credited and that the original publication in this journal is cited, in accordance with accepted academic practice. No use, distribution or reproduction is permitted which does not comply with these terms.
*Correspondence: Laurent Givalois, bGF1cmVudC5naXZhbG9pc0B1bW9udHBlbGxpZXIuZnI=
Disclaimer: All claims expressed in this article are solely those of the authors and do not necessarily represent those of their affiliated organizations, or those of the publisher, the editors and the reviewers. Any product that may be evaluated in this article or claim that may be made by its manufacturer is not guaranteed or endorsed by the publisher.
Research integrity at Frontiers
Learn more about the work of our research integrity team to safeguard the quality of each article we publish.