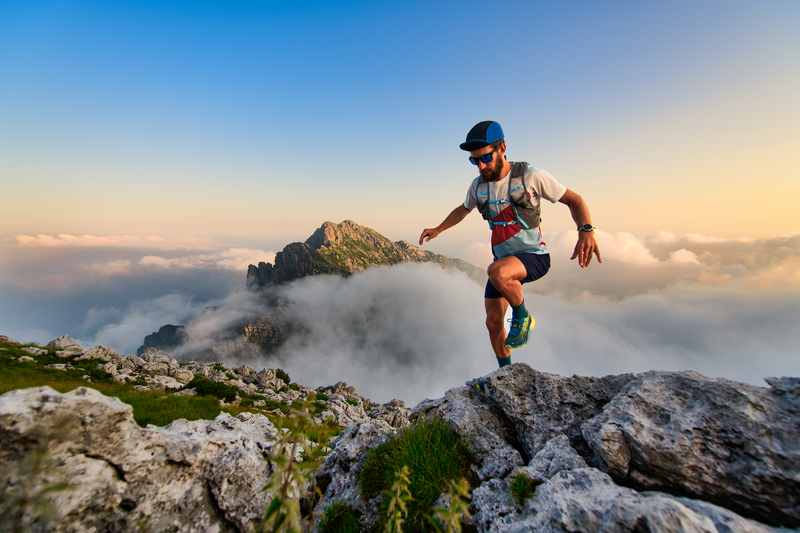
95% of researchers rate our articles as excellent or good
Learn more about the work of our research integrity team to safeguard the quality of each article we publish.
Find out more
ORIGINAL RESEARCH article
Front. Aging Neurosci. , 30 August 2018
Sec. Neurocognitive Aging and Behavior
Volume 10 - 2018 | https://doi.org/10.3389/fnagi.2018.00242
The aging brain undergoes remodeling processes because of biological and environmental factors. To counteract brain aging, neuronal plasticity should be preserved. The aim of this study was to test if the capacity of generating short-time synaptic plasticity in older adults may be related to either physical activity, nutritional status, cognition, or neurophysiological activity. Thirty-six participants (mean age 73.3 ± 5.9 years) received transcranial magnetic stimulation in combination with peripheral nerve stimulation to experimentally induce short-time synaptic plasticity by paired associative stimulation (PAS). Adaptations in neuronal excitability were assessed by motor-evoked potential (MEP) in the right m. tibialis anterior before and after PAS. The Physical Activity Questionnaire 50+ and the StepWatchTM captured physical activity levels. Nutritional status was assessed by the Mini Nutritional Assessment. Cognition was assessed by reaction time for a divided attention test and with the Montreal Cognitive Assessment. Neurophysiological activity was assessed by electroencephalography during the divided attention test. MEPs of the highest stimulation intensity resulted significantly different comparing before, 5 min, or 30 min after PAS (p < 0.05). Data-driven automatic hierarchical classification of the individual recruitment curve slopes over the three-time points indicated four different response types, however, response groups did not significantly differ based on physical activity, nutritional status, cognition, or neurophysiological activity. In a second-level analysis, participants having an increased slope showed a significant higher energy expenditure (z = -2.165, p = 0.030, r = 0.36) and revealed a significant higher power activity in the alpha frequency band (z = -2.008, p = 0.046, r = 0.37) at the prefrontal-located EEG electrodes, compared to the participants having a decreased slope. This study hints toward older adults differing in their neuronal excitability which is strongly associated to their short-time synaptic plasticity levels. Furthermore, a physically active lifestyle and higher EEG power in the alpha frequency band seem to be connected to the capacity of generating long-term potentiation-like synaptic plasticity in older adults. Future studies should consider more sensitive assessments and bigger sample sizes to get a broad scope of the older adults’ population.
Aging is a naturally occurring physiological process that develops without concurrent diseases (Mora, 2013). In humans, the central nervous system, in general, is affected by aging- and behavior-related alterations. This alteration process includes changes in brain neurochemistry as well as in neuroanatomical structures including gray and white matter (Park and Reuter-Lorenz, 2009; Seidler et al., 2010). Especially, gray and white matter in (pre)frontal and parietal cortices are susceptible to atrophy (Colcombe et al., 2003; Resnick et al., 2003; Seidler et al., 2010). White matter integrity decreases in an anterior-to-posterior gradient illustrated by diffusion tensor imaging (Head et al., 2004). Frontal and parietal cortices, including the motor and sensory cortical regions, are important for the realization of motor performance (Seidler et al., 2010; Blumen et al., 2018). Atrophy of the motor cortical regions may coincide with motor performance declines such as movement slowing, disorders in balance, and instable gait leading to fall events and fragility that, in turn, affect activity of daily living and maintenance of older adults’ independence (Seidler et al., 2010). One reason for successful (brain) aging might be to retain considerable neuronal plasticity, which leaves room for brain function adaptations triggered by lifestyle factors; e.g., physical activity and/or nutrition (Meeusen and De Meirleir, 1995; Carvalho et al., 2014). Thus, behavioral factors that might support successful (brain) aging and that might keep the aging brain adaptable to slow down the brain aging process need to be identified. Better understanding about brain plasticity might help older adults to counteract weakness, to remain independent and to get a balanced life (Taube et al., 2008).
Regarding brain aging, physical activity can strengthen neuronal structures, synaptic plasticity, and neuronal transmission (Cai et al., 2014). Physical activity seems to be essential in stimulating the transcription of neurotrophins, especially BDNF (Neeper et al., 1995; Flöel et al., 2010; Gomez-Pinilla et al., 2011; Sleiman et al., 2016). BDNF’s neurotrophic and neuroprotective properties include neuronal survival, neurite expression, and axonal as well as dendritic growth and remodeling (Lu and Figurov, 1997; Cowansage et al., 2010; Mora, 2013; Meeusen, 2014) and, therefore, BDNF modifies neuronal excitability (Figurov et al., 1996; Lu and Figurov, 1997). The maintenance of basal BDNF levels is needed for hippocampal neurogenesis (Yau et al., 2014). Older participants showed an increased hippocampal volume after chronic exercise (Erickson et al., 2009). Moreover, physically active older adults are better able to recruit additional brain resources to improve on various motor tasks than their sedentary counterparts (Seidler et al., 2010). Furthermore, older adults with a high aerobic fitness level have greater brain volumes in brain areas that are vulnerable to behavior-related effects (Colcombe et al., 2003). Thus, physical activity seems to be important for triggering molecular and cellular mechanisms in order to counteract age- and behavior-related decline in the brain (Cotman and Berchtold, 2002; Voss et al., 2013; Phillips et al., 2015).
Next to physical activity, also nutrition is believed to influence neurogenesis and synapse formation by altering neurotrophin levels, inflammation, and energy metabolism (Dauncey, 2009; Mora, 2013). Specific nutrients (e.g., uridine, docosahexaenoic acid, and choline) are needed for the formation of neuronal membranes and, thus, of synapses (Wurtman et al., 2009). Furthermore, several nutritional supplementations and/or dietary modifications may be able to prevent or postpone cognitive decline, e.g., omega-3 polyunsaturated fatty acids, amino acids, and polyphenols (Miquel et al., 2017; Phillips, 2017). Nevertheless, one should keep in mind that not a single nutrient seems to be essential, but a combination of several nutrients that interact to evoke beneficial and synergistic effects on the brain (von Arnim et al., 2010; Miquel et al., 2017). Mediterranean dietary including high consumption of fruits, vegetables, legumes, cereals, fish, and olive oil and low consumption of meat and dairy products could play a major role in cognitive health (Aridi et al., 2017). A recent study showed that a diet based on plant foods and fish was associated with better cognitive functions in older Japanese people (Okubo et al., 2017). Furthermore, the combination of a diet including health-supporting nutrients and physical activity could build a synergy effect on important molecular and cellular pathways for neurogenesis, cell survival, synaptic plasticity, and vascular function (van Praag, 2009). A recent review illustrated the importance of a combinatory approach including physical activity and nutrition in older adults (Schättin et al., 2016).
Cognitive status is a further factor that plays an important role in terms of aging. Older adults who engage in intellectually stimulating activities experience less hippocampal atrophy (Valenzuela et al., 2008). Enriched and/or stimulating environments upregulate BDNF and increase neurogenesis contributing to neuronal plasticity (van Praag et al., 1999, van Praag et al., 2000; Kempermann et al., 2002; Brown et al., 2003). Therefore, cognitive stimulation should be added to physical activity to promote the survival of newly born structures (Phillips, 2017). In older adults, cognitive dysfunction, especially disorders in executive functioning, plays a role in causing gait disturbance and fall events (Rapport et al., 1998; Scherder et al., 2011). Strengthened EFs, especially divided attention, might contribute to gait performance (Pichierri et al., 2012) and might reduce fall events as EFs performance predicted the risk for future falls (Mirelman et al., 2012). Moreover, performance in EFs was associated with larger prefrontal volume and greater PFC thickness in healthy adults (Yuan and Raz, 2014). During aging, the PFC is vulnerable to degenerative processes (Pfefferbaum et al., 2013; Fjell et al., 2014).
Regarding neurophysiology, previous research findings by means of EEG showed that aging is linked with a lowering of the individual peak of the EEG power within the alpha frequency band as well as a decrease of the latter and usually an increase of slow waves < ∼7 Hz (i.e., delta and theta frequency bands), so-called age-related “slowing” (Rossini et al., 2007; Ho et al., 2012). A reversal of this age-related process observed by means of EEG might be linked to ameliorated cognitive functioning, improved motor performance, and enhanced sensory processing (Rossini et al., 2007).
Indirect measures of strengthening the activity between neuronal populations may be used as a biomarker of synaptic plasticity (Voss et al., 2013). TMS can be used, in combination with PNS, to experimentally induce short-time synaptic plasticity by PAS (Cirillo et al., 2009) and can then be used to record adaptations by changes in neuronal excitability. With the use of TMS methodology, greater synaptic plasticity in the left abductor pollicis brevis muscle motor circuit could be related to more active young adults (age 18–38 years) (Cirillo et al., 2009). Information on the influence of physical activity, nutritional status, and cognition on synaptic plasticity in older adults focusing on the lower extremities is, however, lacking. This study, therefore, explores how either physical activity, nutritional status, cognition or neurophysiological activity by means of EEG might be related to synaptic functionality in elderly. A recent review summarized that several factors including increased physical activity, improved nutrition, and the performance of mental exercise can attenuate cognitive decline (Miquel et al., 2017). The aim of this study was to test whether the capacity of generating short-time synaptic plasticity in older adults may be related to either physical activity, nutritional status, cognition, or neurophysiological activity. We hypothesized that the capacity of generating short-time synaptic plasticity of the motor cortex is heterogeneous and this heterogeneity can be related to either physical activity, nutrition, cognition, or neurophysiological activity.
This is a cross-sectional study that tested whether the capacity of generating short-time synaptic plasticity in older adults may be related to either physical activity, nutritional status, cognition, or neurophysiological activity by means of EEG. Potential participants were recruited through public advertisement on the homepage of the Senior University Zürich (Switzerland), in local senior residency dwellings (Zürich, Switzerland), and through study presentations in local elderly sports or leisure time groups (Zürich, Switzerland). The study was performed in the laboratory at the sport center ETH Hönggerberg (Zürich, Switzerland). Recruitment and measurements were executed within July 2015 and February 2016. The ethics committee of ETH Zürich, Switzerland (EK 2015-N-10) approved the study protocol. Before any measurements were performed, all eligible participants had to sign written informed consent according to the Declaration of Helsinki.
The potential participants had to be able to provide written informed consent and understand the study procedure and instructions. Moreover, the participants were screened for depression using the GDS (Yesavage et al., 1982; Yesavage and Sheikh, 1986) and the short EHI to determine the handedness of the participants (Oldfield, 1971; Veale, 2014). Furthermore, the participants completed a health questionnaire including questions about physical impairments, medical history, anthropometric data, and physical activity level. Participants fulfilling all of the following inclusion criteria were eligible for the study: (1) age ≥ 65 years, (2) live independently or in a residency dwelling, (3) non-smoker, (4) healthy (self-reported), (5) right-handed. Participants were excluded from the study, if they exhibit one of the following exclusion criteria: (1) mobility or cognitive impairments, (2) severe health problems (e.g., recent cardiac infarction, uncontrolled diabetes, or uncontrolled hypertension), (3) signs of an upcoming depression, (4) Alzheimer’s disease or another dementia, (5) rapidly progressive or terminal illness, (6) acute or chronic illness, (7) history of stroke or epilepsy, (8) history of seizure or individuals with a recent head injury, (9) medication that acts on neuronal level (psychotropic medications), (10) electronic or metallic head implants.
Figure 1 presents the study flow diagram that included screening, assessments to measure neuronal excitability, physical activity, nutritional status, cognition, neurophysiological activity by means of EEG, and PAS to elicit short-time synaptic plasticity.
On an adjustable chair, participants were seated comfortably with hip, knee, and ankle joint angles of 100, 120, and 90°, respectively. Only the dominant side, in this case the right side, was assessed because of the symmetrical nature of TMS-related measurements of the lower limb (Brouwer and Ashby, 1990). The right m. tibialis anterior was activated by stimulation over the motor cortex using a “figure-of-eight” coil by means of a TMS stimulator (Magstim Company, Ltd., Whitland, Dyfed, United Kingdom), using a previously established reliable TMS-related measurement protocol involving TA and healthy participants (Cacchio et al., 2009).
First, the participants got a bathing cap that fitted tight at the head. On the top of the cap, a grid was drawn using the vertex as initial position. The vertex was defined as half distance from nasion to inion and half distance from right to left pre-tragus. Detailed distance recordings were made from the nasion, inion, and bilateral pre-tragus to the vertex to maintain consistent coil positioning across sessions. The optimal activation of the TA seems to be obtained if the coil is placed parallel to and approximately 0.5–1.0 cm lateral to the midline and its mid-point is aligned anterior-posteriorly against the vertex (Cz) (Devanne et al., 1997). Second, the ideal stimulation point was assessed (hotspot). On the grid, the hotspot is where the threshold is lowest to evoke a MEP response on the TA (Rossini et al., 1994). Third, RMT was determined. RMT was the lowest intensity of magnetic stimulation required to evoke MEPs at TA with a peak-to-peak amplitude of minimum 50 μV, in at least 6 of 10 consecutive trials (Rossini et al., 1994).
Muscle activity of TA was recorded by Telemyo DTS (Noraxon, AZ, United States). For preparation, the skin of the shank was shaved (if needed) and prepared with an abrasive paste (OneStep AbrasivPlus, H+H Medizinprodukte, Münster, Germany). Afterward, two Ag/AgCl electrodes (Ambu Blue Sensor N, Cambridgeshire, United Kingdom) were placed on the muscle belly of the right TA (inter-electrodes distance of ∼2 cm). The EMG signals were high-pass filtered at 30 Hz. EMG data analysis was performed using MATLAB R2016a (The Mathworks, Natick, MA, United States). A time window encompassing the TMS impulse occurrence was used to identify the peak-to-peak amplitude of MEPs. For each stimulation intensity, the MEP was normalized using the baseline 140% RMT value of each individual participant.
At the level of synapse, no human neuroscience methods exist that can assess synaptic remodeling non-invasively. However, biomarkers of synaptic excitability/plasticity may represent indirect measures of acquisition and strengthening of activity between neuronal populations (Voss et al., 2013). PAS is a non-invasive approach to generate bidirectional changes in the excitability of the cortical projections to the muscles. PAS is a protocol involving TMS in combination with PNS to experimentally induce short-term synaptic plasticity (Cirillo et al., 2009). The PAS-based plastic changes of the cortical synaptic activity shows properties that seem to be similar to LTP (Bi and Poo, 1998) as rapid onset, associativity, duration, specificity, and NMDA-receptor dependence (Cooke and Bliss, 2006; Ziemann et al., 2008).
Within the PAS protocol of this study, PNS consisted of a percutaneous single electrical stimulation of the right common peroneal nerve delivered at 300% of the perceptual threshold followed by a single TMS pulse (120% RMT) 55 ms later over the motor cortex. The ISI was determined based on a previous study where an ISI of 55 ms was found to be optimal for inducing the greatest increase in the TA MEP amplitude for PAS during rest (Mrachacz-Kersting et al., 2007). A total of 90 stimuli were applied at a rate of 0.05 Hz lasting about 30 min. A constant current stimulator (DS7A stimulator, Digitimer, Ltd., Welwyn Garden City, United Kingdom) was used to apply electrical stimuli as squared wave pulses with a pulse width of 200 μs. Bipolar surface electrodes, separated by 30 mm, were placed on the right common peroneal nerve. As PAS induced plasticity on hand muscles seems to be connected to attention-dependent activation of the ascending cholinergic system (Stefan et al., 2004; Ziemann et al., 2008), our participants had to count the sensory stimuli during the PAS protocol to minimize the influence of this factor.
Recruitment curve was performed before PAS, 5 min after PAS, and 30 min after PAS. RC was obtained from 90% RMT to 140% RMT by increasing intensities in 10% steps. The intensities were applied in a random order (Möller et al., 2009). Each stimulation intensity contained 10 stimuli including a stimulus interval of 7 s with a 20% variance to avoid familiarization. The slope of the RC was calculated as the slope of the linear regression line through a supplied set of x-values (normalized MEP values) and y-values (stimulation intensities). An a priori data-driven strategy was used in order to subgroup the participants based on the PAS-response. That is, subgrouping was performed by an automatic hierarchical two-steps classification, taking into account: (1) the increase or decrease of the RC slope 5 min after PAS compared to baseline; (2) the increase or decrease of the RC slope 30 min after PAS compared to 5 min after PAS.
The PAQ 50+ retrospectively quantifies the physical activity of older adults performed per week during the last month. The German PAQ 50+ shows good reliability for the total time of physical activity (r = 0.60) and for the total energy expenditure (r = 0.52) (Huy and Schneider, 2008). The questionnaire is based on the Yale Physical Activity Surveys and the Physical Activity Scale for the Elderly, which both show good test validity (Dipietro et al., 1993; Washburn et al., 1993; Montoye et al., 1996; Harada et al., 2001). The 37 questions cover activities including the household, gardening, leisure activities, sports, activity during work, and anthropometrical information (age, sex, height, and weight). Each activity is assessed by determining a certain number of MET-values. Through an equation involving the specific activity/MET-value, the duration of activity performance, and the weight of the person, the energy expenditure (kcal per week) was calculated. The sum of all activities equals the total score of the PAQ 50+ in kilocalories per week.
The StepWatch (modus health IIc, Washington, DC, United States) is an accelerometer attached to the ankle measuring the daily physical activity range. The StepWatch is a valid device to assess physical activity in community-dwelling older adults (Bergman et al., 2008). At slow walking speed, the StepWatch was more accurate than other activity monitors (Storti et al., 2008). The accuracy of the StepWatch is high with 96.06% for indoor use and 99.58% for outdoor use, respectively. Reliability was reported with an intra-class correlation coefficient of 0.91 (Mudge et al., 2010). Each participant wore the accelerometer for 1 week after the measurement procedure. StepWatch software was used to discern the average amount of steps per day for each participant.
The MNA is a questionnaire evaluating the nutritional status (Guigoz et al., 1996). In total, 24 to 30 points are equivalent to a normal nutritional status, 17 to 23.5 points to being at risk of malnutrition, and less than 17 points means to be malnourished. The MNA is well-validated and correlates highly with objective indicators of nutritional status and clinical assessment (Guigoz et al., 2002). The scale has been used to assess the nutritional risk of older adults (Guigoz et al., 2002).
The D-TAP 2.3 VL (PSYTEST, Psychologische Testsysteme, Herzogenrath) is a valid test battery to assess deficits in attention and the subtests measure independent attentional functions (Zimmermann and Fimm, 2002). In this study, the focus was on the divided attention subtest lasting about 3 min. The test consists of concurrent appearance of visual and acoustic signals resulting in reaction time for key stimuli.
The MoCa assesses multiple cognitive domains including memory, language, EFs, visuospatial skills, calculation, abstraction, attention, concentration, and orientation (Nasreddine et al., 2005; Julayanont et al., 2013). The participants can get a maximum of 30 points while 22 points are a cutoff for mild cognitive impairment. The MoCa is a reliable (r = 0.75) and valid quantitative estimate of overall cognitive abilities (Koski et al., 2009).
Electroencephalography measurements were performed during the divided attention subtest of the TAP. After proper skin cleansing and preparation by applying an abrasive skin paste (NuPrep, Weaver and Company, Aurora, CO, United States), EEG activity was recorded using two Ag/AgCl sensors (Ambu White Sensors, Ambu A/S, Denmark) placed over the left and right PFC, respectively Fp1 and Fp2, according to the international 10/20 electrodes placement system and sampled at 128 Hz (Alpha-Active, Ltd., Devon, United Kingdom). The reference and ground were settled up by placing a passive CMS electrode at Fpz and two active DRL over the left and right mastoid, respectively. EEG data analysis was performed using custom scripts written in MATLAB R2017b (The Mathworks, Natick, MA, United States) and jointly using functions from EEGLAB 14.1.0b (Delorme and Makeig, 2004), ERPLAB (Lopez-Calderon and Luck, 2014) and Fieldtrip (Oostenveld et al., 2011) open source toolboxes. First, raw EEG data were high-pass filtered [zero-phase Hamming windowed sinc FIR, order: 425, cut-off frequency (-6 dB): 0.5 Hz, passband edge: 1 Hz, transition bandwidth: 1 Hz] and then low-pass filtered [zero-phase Hamming windowed sinc FIR, order: 59, cut-off frequency (-6 dB): 33.75 Hz, passband edge: 30 Hz, transition bandwidth: 7.5 Hz]. Then, EEG data were epoched in segments of 1 s duration time locked from 0.25 s before to 0.75 s after either auditory or visual stimulus onset. The total number of EEG data segments for each participant was 29 (15 relative to auditory stimuli and 14 relative to visual stimuli). Each entire EEG epoch, separately for auditory and visual stimuli segments and in each participant, was automatically checked for the presence of artifacts (e.g., eye blinks, ocular movements) by means of an absolute peak-to-peak voltage threshold criterion detection of ± 100 μV within a 200 ms width sliding window (100 ms steps). Subsequently, the accepted data segments were further checked and rejected when they exceeded both locally and globally the average probability of ±5 standard deviation (SD). For each participant, if a data segment relative to either Fp1 or Fp2 electrode was marked for rejection, the EEG epochs relative to both channels were rejected. Additionally, a rule of 50% maximum of rejected epochs was applied for each subject, removing the entire participant from further analysis in case this threshold was reached. Six participants were removed using these criteria (in both auditory and visual EEG epochs) and, on average, ∼13 (∼84% of each participant; SD: ∼15%; range: ∼53–100%) and ∼12 (∼84% of each participant; SD: ∼13%; range: ∼50–100%) total epochs remained for further analysis of the auditory and visual EEG data segments, respectively. For spectral EEG analysis, a multitaper Fast Fourier Transform was then employed in order to obtain the theta (4–8 Hz) and alpha (9–13 Hz) power spectrum by using a single Hanning taper with a frequency resolution of 1 Hz. The achieved individual theta and alpha power spectrum was log-transformed and averaged.
Due to non-normality of data, non-parametric tests were used. Outliers were replaced with a value that was the mean and two times standard deviation. Friedman test was used to compare the peak-to-peak amplitudes of the same stimulation intensities and the slopes of the RCs over the three measurement time points. Dunn–Bonferroni test was used for post hoc comparisons. Comparisons for physical activity, nutritional status, cognition, or neurophysiological activity between different subgroups obtained from the abovementioned data-driven automatic hierarchical classification were performed using Kruskal–Wallis test or Mann–Whitney U-test. Effect sizes were calculated and expressed as r = Z/√N. An effect size of r = 0.1 is considered a “small” effect, around 0.3 a “medium” effect, and 0.5 and above a “large” effect. A probability level of α = 0.05 was considered to be statistically significant. All analyses were conducted using SPSS Version 24.0 for Windows (SPSS, Inc., Chicago, IL, United States).
The recruitment phase resulted in 75 potentially interested elderly who received further study information. 36 older adults were included while 39 either declined participation or exhibited exclusion criteria. Table 1 summarizes characteristics of the included participants.
Three TMS stimulation intensities spread over two participants were not well-recorded and, thus, were not considered for analysis. The RC of normalized MEP peak-to-peak amplitudes over all three-time points is presented in Figure 2. Comparing MEP peak-to-peak amplitudes over all three-time points, significant differences were found for 130% RMT [χ2(2) = 6.000, p = 0.050, n = 36] and 140% RMT [χ2(2) = 13.556, p = 0.001, n = 36] (Supplementary Table 1). Post hoc test showed no significant differences for 130% RMT. Post hoc test for 140% RMT showed significant higher MEP peak-to-peak amplitudes for PAS30 minafter compared to PASbefore (z = -3.064, padapted = 0.007, r = 0.39) and compared to PAS5 minafter (z = -3.300, pdapted = 0.003, r = 0.36).
FIGURE 2. Recruitment curve of normalized MEP peak-to-peak amplitudes for before, 5 min after, and 30 min after PAS. Data points are median values. For each stimulation intensity, the comparison of before, 5 min after, and 30 min after PAS was performed using Friedman test. Dunn–Bonferroni post hoc test was performed when a significant comparison was analyzed. ∗p < 0.05. PAS, paired associative stimulation; RMT, resting motor threshold.
Comparing the slope of the RCs over all three-time points, no significant difference was found [χ2(2) = 4.408, p = 0.110, n = 36] (Table 2). The data-driven automatic hierarchical classification of the individual RC slopes over the three-time points indicated four different response types to the PAS protocol (Figure 3). The results of the RC slope within group comparisons are presented in Table 2.
TABLE 2. Comparison of recruitment curve slopes for before, 5 min after, and 30 min after paired associative stimulation over all the participants and for the four different response groups.
FIGURE 3. Slope of recruitment curves (RCs) for before, 5 min after, and 30 min after PAS divided into four different response groups. (A) The participants (N = 8) showed an increase of the slope from before to 5 min after PAS and to 30 min after PAS. (B) The participants (N = 8) showed an increase of the slope from before to 5 min after PAS followed by a decrease of the slope from 5 to 30 min after PAS. (C) The participants (N = 15) showed a decrease of the slope from before to 5 min after PAS followed by an increase of the slope from 5 to 30 min after PAS. (D) The participants (N = 5) showed a decrease of the slope from before to 5 min after PAS and to 30 min after PAS. Data are median values (interquartile range) as indicated. The slope of the RC was calculated as the slope of the linear regression line through a supplied set of x-values (normalized motor-evoked potential values) and y-values (stimulation intensities). For each group, the slope comparisons of before, 5 min after, and 30 min after PAS were performed using Friedman test. Dunn–Bonferroni post hoc test was performed when a significant comparison was analyzed. ∗p < 0.05.
Due to technical problems, StepWatchTM data of one participant and EEG data of one participant were not recorded. The results of the assessments for each response group are listed in Tables 3, 4. The comparison of each assessment between the four response groups revealed no significant differences (Tables 3, 4). Considering an additional sub-analysis, the comparison of the two groups (Groups A and B) classified by their increased RC slope with the two groups (Groups C and D) classified by their decreased RC slope, showed a significant difference for PAQ 50+ (z = -2.165, p = 0.030, r = 0.36). The groups having an increased slope [median (IQR): 8834.5 (5107; 11661) kcal/week] showed a higher energy expenditure than the groups having a decreased RC slope [median (IQR): 5345.5 (4365.5; 7207) kcal/week]. Furthermore, the log-transformed alpha power at Fp1 during auditory stimuli in the divided attention test led to a significant difference between the same abovementioned groups (z = -2.008, p = 0.046, r = 0.37). The groups having an increased RC slope showed a higher power in the alpha frequency band [median (IQR): 0.53 (0.36; 0.70) log-transformed power] compared to the groups having a decreased RC slope [median (IQR): 0.33 (0.25; 0.56) log-transformed power].
TABLE 3. Comparison of physical activity, nutritional status, and cognition between the different response groups.
TABLE 4. Comparison of prefrontal cortex log-transformed power in theta and alpha frequency bands during the divided attention test between the different response groups.
The aim of this study was to test whether the capacity of generating short-time synaptic plasticity in older adults may be related to either physical activity, nutritional status, cognition, or neurophysiological activity. The results showed a significant increase of the TA MEP peak-to-peak amplitudes for the highest stimulation intensity comparing 30 min after PAS to before PAS as well as to 5 min after PAS. The data-driven automatic hierarchical classification of the individual slopes over the three-time points led to the identification of four different response types to the PAS protocol. The comparisons of the assessments including physical activity, nutritional status, cognition, and neurophysiological activity between the response groups, however, revealed no significant differences between these groups. Nevertheless, the two groups (Groups A and B) having an increased RC slope showed a significantly higher energy expenditure and power activity in the alpha frequency band at the prefrontal-located EEG electrodes compared to the two groups (Groups C and D) having a decreased RC slope. Possible explanations for these findings will be discussed in the following part.
Over all the participants, the RC showed significant increased MEP peak-to-peak amplitudes after PAS (>30 min) visible in the highest stimulation intensity (140% RMT). These enlarged MEP amplitudes indicate an increase in the neuronal excitability induced by increased activation of populations of either cortico-cortical, corticospinal, or spinal motor neurons (or summation of all or some of these). PAS induced short-term neuronal adaptations in form of short-term neuronal plasticity. A reversible increase in the excitability of cortical structures is achieved when a sub-threshold stimulus arrives at the post-synaptic cell before a second supra-threshold stimulus (Mrachacz-Kersting et al., 2007) (Hebb’s law of coincident summation 1949). TMS can be used, in combination with PNS, to experimentally induce short-term synaptic plasticity by PAS, also called Hebbian plasticity (Cirillo et al., 2009). The PAS-based plastic changes of the synaptic structures shows properties that seem to be similar to LTP (Bi and Poo, 1998) as rapid onset, associativity, duration, specificity, and NMDA-receptor dependence (Cooke and Bliss, 2006; Ziemann et al., 2008). PAS as well as motor learning share common neuronal mechanism and, therefore, PAS tests functionally relevant neuronal circuits (Ziemann et al., 2004; Stefan et al., 2006; Rosenkranz et al., 2007; Jung and Ziemann, 2009). Although PAS is one of the most used method, different strategies can be used to induce cortico-cortical and/or corticospinal plasticity, for example, repetitive-TMS and paired-pulse TMS can be also adopted for similar purposes, and, probably, different individual responses can be obtained (Rossini et al., 2007). To sum up, the stimulation of the motor cortex in our older participants and its post-synaptic environment seem to still adapt up to a certain amount to external stimuli. This plastic adaptation is in line with previous studies that showed the possibility of cortical plasticity in older adults triggered by different kind of interventions, e.g., physical and cognitive exercise (Bamidis et al., 2014).
As an increased MEP peak-to-peak amplitude, also an increased RC slope can be used to indicate PAS-induced LTP-like plasticity (Meunier et al., 2007; Rosenkranz et al., 2007). The data-driven automatic hierarchical classification of the individual RC slope led to four different response groups following PAS. Interindividual variability might explain the formation of different response groups after PAS. Several studies focused on interindividual variability following PAS and discussed possible reasons why participants showed different reaction patterns. In our study, the four response groups differ mainly for two factors: RC slope and response duration. For the RC slope, one group (Group A) showed a significant increase after 30 min, which may well indicate PAS-induced LTP-like plasticity (Meunier et al., 2007; Rosenkranz et al., 2007). While another group (Group D) showed a significant decrease of the RC slope after 30 min, which may well indicate PAS-induced LTD-like adaptations. LTD can be generated when the sub-threshold stimulus arrives at the post-synaptic cell after a second supra-threshold stimulus (Hebb’s law of coincident summation 1949) (Ziemann et al., 2008). It might be that the ISI time was too short for those participants as a shorter ISI time can be accompanied by decreased MEP amplitudes (Mrachacz-Kersting et al., 2007). Different heights of the participants and possibly different conduction times for the peripheral nerve and top–down volley are factors that might have contributed to this circumstance (Mrachacz-Kersting et al., 2007) in our older adults. However, no significant height differences were present in this study. Furthermore, the two other groups showed indications for LTP-like (Group B) or LDP-like (Group C) plasticity though the effects appeared already after 5 min and receded 30 min after PAS. Explanations for the short response duration might be that the stimuli were not strong enough to generate a long-lasting effect or the post-synaptic properties of the stimulated motor cortical area were restricted in reacting with minimal plastic adaptations as the magnitude of PAS-induced LTP-like plasticity in human primary motor cortex declines with age (Müller-Dahlhaus et al., 2008; Tecchio et al., 2008). However, no significant age differences were present in this study. In addition to the aforementioned factors, several other factors might be important for interindividual variability including attention stage, time of the day, neuronal motor cortical activity before or during PAS, and endophenotype characteristics (Ziemann et al., 2008). Furthermore, most sources describing PAS approaches have used paradigms in which upper extremities muscles were stimulated as opposed to our paradigm using the TA. Besides, studies showed that lifestyle-based characteristics, e.g., physical activity, influence PAS-induced LTP-like plasticity in the motor cortex (Cirillo et al., 2009; Kumpulainen et al., 2015).
The comparisons of the assessments including physical activity, nutritional status, cognition, and neurophysiological activity between the four response groups revealed no significant differences. General reasons as well as specific reasons might explain why the assessed factors were not related to the appearance of four different response groups. A reason might be that the used measurement methods were not sensitive enough or their acquisition spectrum was limited to measure their influence on neuronal excitability. For physical activity assessments, the PAQ 50+ is a questionnaire that includes subjective responses that might differ from the real activity behavior. However, the objective assessment using the StepWatch also did not lead to any differences. Motor test batteries (e.g., testing strength or balance) or fitness tests might get a broader scope as several aspects of motor functioning are tested. A previous study showed a differential motor cortical plasticity in skilled and endurance trained athletes that might be because of different training-induced adaptations (Kumpulainen et al., 2015). Another study demonstrated that an association exists between motor skill learning and PAS-induced LTP-like plasticity (Frantseva et al., 2008). For the nutritional assessment, the rationale behind this was that a normal nutritional status should be present to ensure brain plasticity. However, with the exceptions of three participants (21, 21, and 22.5 points; 17 to 23.5 points to being at risk of malnutrition), the participants showed a normal nutritional status. In addition, we have to keep in mind that the questionnaire is not an objective assessment of the nutritional status. The recording of the nutritional status using the questionnaire might be too superficial. Future studies could consider a detailed recording of nutritional intake over a certain period (e.g., by using a diet weekly diary) or blood samples to determine the status of nutrients that are important for the brain, e.g., omega-3 fatty acids. For the cognitive assessment, the direct relation to the primary motor cortex plasticity might be minor to act as a distinctive feature, given that cognitive tests, such as the divided attention task, are well-known to involve mostly the PFC, which is related to executive functioning (Yuan and Raz, 2014). Another reason why no differences for the assessments were observed could be that the grouping was too strict leading to a smaller number of participants in each group and this, in turn, might have limited the detection of any differences. Finally, previous reports indicated that variability in responses to TMS-based neuroplasticity interventions are not uncommon (Fratello et al., 2006; Müller-Dahlhaus et al., 2008; Sale et al., 2008; Japyassu and Malange, 2014; López-Alonso et al., 2014) and controlling all possible contributing factors to this variability is not straightforward (see Ridding and Ziemann, 2010, for an overview). For example, sleep behavior in older adults can be mentioned as a potentially important factor. Maintenance of synapse homeostasis is influenced by slow waves during sleep (Tononi and Cirelli, 2006), which is known to be affected in many older adults (Varga et al., 2016). This may have altered the potentiation capacity of synapses in response to the neuroplasticity intervention (Dickins et al., 2017). Investigation of and trying to control additional factors that influence responses to PAS in older adults might help in reducing the variability in plasticity responses in future studies.
Taking the groups together according to the criteria of RC slope increase or decrease, the two groups (Groups A and B) having a RC slope increase showed a significantly higher energy expenditure than the groups having a decreased RC slope (Groups C and D). The participants with a higher energy expenditure showed an increase of the RC slope that in turn indicates PAS-induced LTP-like plasticity (Meunier et al., 2007; Rosenkranz et al., 2007). However, it has to be remarked that one of the four sub-groups of participants, obtained after the data-driven automatic hierarchical classification, just showed an increase of the RC slope at 5 min after PAS compared to baseline, which receded at 30 min after PAS compared to the first increase. For PAQ 50+ questionnaire, a higher energy expenditure goes hand in hand with a more active lifestyle. Furthermore, the PAQ 50+ might have captured more accurately the physical activity level, within a broader range of lifestyle tasks and exercises of the elderly participants, including housework, gardening, leisure time, sports, and work. The findings of our study seem to be in line with a previous study that demonstrated enhanced corticospinal excitability/plasticity in physically active individuals (determined by a questionnaire) measured for a small hand muscle in young subjects (Cirillo et al., 2009). Based on the results, the researchers suggested that physical activity is an additional factor that can contribute to increased neuroplasticity after PAS and they concluded that regular physical exercise may offer benefits to primary motor cortex functions. Physical exercise can provide a supportive neural environment by increasing the blood flow to the primary motor cortex and this, in turn, is accompanied by increased neurotrophic factors (Klintsova et al., 2004; Vaynman and Gomez-Pinilla, 2005; Adkins et al., 2006). For the questionnaire, however, we should still consider that the energy expenditure was calculated in terms of subjective responses of the participants and we recommend a more objective assessment of energy expenditure and physical activity for future studies.
Furthermore, the older adults with a RC slope increase (Groups A and B) showed significant higher EEG alpha power at Fp1 channel and a (non-significant) upward trend at the contralateral channel compared to the two groups (Groups C and D) having a RC slope decrease. Physiological aging is usually linked to a general decrease in EEG power within the alpha frequency band, which is most pronounced on the occipital area. Nevertheless, in aging the distribution of EEG alpha power seems to shift toward most frontal area as well (Niedermeyer, 1997; Babiloni et al., 2006; Rossini et al., 2007). Interestingly, next to alterations in cortical oscillatory activity, studies showed that getting older can be accompanied by physiological cortical atrophy, especially for the frontal lobe (Raz et al., 2005), and this, in turn, might also affect neuronal excitability because of the increased distance to the excitable elements (Rossini et al., 2007). As mentioned before, the older adults who were more physically active showed an increase of the RC slope. Thus, it might be that both neuronal excitability and activity benefit from the physically active lifestyle by possibly counteracting the physiological aging processes occurring at cortical level. Regarding brain aging, physical activity can strengthen neuronal structures, synaptic plasticity, and neuronal transmission (Cai et al., 2014) and, thus, being important for triggering molecular and cellular mechanisms in order to counteract age- and behavior-related neuroanatomical and neurophysiological decline (Cotman and Berchtold, 2002; Voss et al., 2013; Phillips et al., 2015). EEG power in the alpha frequency band increased during the auditory stimuli of the divided attention task. This might be due to the fact that perceptual operations of acoustic inputs are encoded by working memory and these processes are affected by age-limited processing resources (Wingfield et al., 2005). The older adults of our study might still have good working memory abilities so that sufficient processing resources are available for acoustic inputs. A study showed that frontal alpha peak frequency was positively associated with working memory performance independent of age (Richard Clark et al., 2004). In addition, a larger enhancement of frontal alpha in response to auditory stimuli in older adults has been reported (Yordanova et al., 1998). To sum up, a connection might exist between a physically active lifestyle, the capacity of generating LTP-like synaptic plasticity, and the levels of EEG power in the alpha frequency band in healthy older adults. Future studies might examine the behavior of EEG power in similar settings involving nutrition, cognition, and corticospinal excitability/plasticity, but in older adults showing either a sedentary or a physically active lifestyle or after a training intervention involving only motor, cognitive, or combined motor-cognitive components.
Several factors might have limited this study. The measurements were just a snapshot of the participants’ characteristics/life style factors and their corticospinal functioning. Consideration of specific predispositions not taken into account by us (e.g., previous physical training, educational status, etc.), a longer observation period and qualitative assessment of both physical activity and nutrition might have provided a more accurate specification regarding to physical activity, nutritional status, and cognition. Another limitation might be that the TMS measurements of the individual participants were not performed at the same time of day (Ziemann et al., 2008). Furthermore, it cannot be excluded that the measured adaptations were generated on cortical level, as PAS protocols may well-induce changes on either supra-spinal and sub-cortical level (or both), although, the used ISI was determined by a previous study to be long enough to reach the cortical structures (Kumpulainen et al., 2012). Individual ISI time determination is possible by measuring the somatosensory-evoked potential and the central processing time resulting in P32 + 18 ms for the leg area (Kumpulainen et al., 2012). Thus, future studies should consider the inter-individual variability that can affect the response to the PAS protocol. Moreover, also different strategies to induce plasticity at cortical level should be considered (i.e., repetitive TMS, paired-pulse TMS). On the neurophysiological assessment, it should be noted that in this study a low-density EEG device was used. Future studies should consider employing a high-density EEG device (i.e., 64 or more channels), which can allow a broader coverage of the cortical activity as well as additional analyses on source space.
This study is one of the first that considered exploration of several factors that may be related to PAS-induced plasticity of the motor cortex in older adults. The results of this study indicated that older adults differ in the capacity to generate short-time synaptic plasticity using the PAS protocol. Some participants were able to generate short-time synaptic plasticity as demonstrated by increased MEP peak-to-peak amplitudes and steeper RC slopes. A connection might exist between a physically active lifestyle, EEG power in the alpha frequency band over the PFC and the capacity of generating LTP-like plasticity in the motor cortex in healthy older adults. A plastic brain and a supportive neuronal environment, especially for the primary motor cortex, is important for proper motor functioning. Intact motor functioning is crucial to preserve an active lifestyle in older adults and, to close the circle, physical activity influences molecular and cellular mechanisms counteracting age- and behavior-related neuroanatomical and neurophysiological decline (Cotman and Berchtold, 2002; Voss et al., 2013; Phillips et al., 2015). Future studies should consider different assessments and approaches that might be more sensitive. Moreover, future studies should consider bigger sample sizes to get a broader scope of the older adults’ population to identify differences according to the capacity of synaptic plasticity.
AS, ME, and SV developed the research question under the lead of EDB. The concept and design part was established by AS, ME, and SV while EDB acted as methodological council. AS, ME, and SV did data acquisition, analysis and interpretation of the results, which was edited and improved by EDB. FG performed the EEG data analysis and interpretation of the results. AS, FG, and EDB substantially revised the manuscript to bring it to its current version. All authors have read and approved the final manuscript.
This article was supported by the ETH Foundation through ETH Research (Grant No. ETH-17 13-2).
The authors declare that the research was conducted in the absence of any commercial or financial relationships that could be construed as a potential conflict of interest.
The authors would like to thank Stephan Häfliger for supporting the analysis.
The Supplementary Material for this article can be found online at: https://www.frontiersin.org/articles/10.3389/fnagi.2018.00242/full#supplementary-material
BDNF, brain-derived neurotrophic factor; CMS, common mode sense; DRL, driven right leg; EEG, electroencephalography; EF, executive function; EHI, Edinburgh Handedness Inventory; EMG, electromyography; GDS, Geriatric Depression Scale; ISI, interstimulus interval; LTD, long-term depression; LTP, long-term potentiation; MEP, motor-evoked potential; MET, metabolic equivalents of task; MNA, Mini Nutritional Assessment; MoCA, Montreal Cognitive Assessment; NMDA, N-methyl-D-aspartate; PAQ, Physical Activity Questionnaire; PAS, paired associative stimulation; PFC, prefrontal cortex; PNS, peripheral nerve stimulation; RC, recruitment curve; RMT, resting motor threshold; SD, standard deviation; TA, tibialis anterior; TAP, Test of Attentional Performance; TMS, transcranial magnetic stimulation.
Adkins, D. L., Boychuk, J., Remple, M. S., and Kleim, J. A. (2006). Motor training induces experience-specific patterns of plasticity across motor cortex and spinal cord. J. Appl. Physiol. 101, 1776–1782. doi: 10.1152/japplphysiol.00515.2006
Aridi, Y. S., Walker, J. L., and Wright, O. R. L. (2017). The association between the mediterranean dietary pattern and cognitive health: a systematic review. Nutrients 9:E674. doi: 10.3390/nu9070674
Babiloni, C., Binetti, G., Cassetta, E., Dal Forno, G., Del Percio, C., Ferreri, F., et al. (2006). Sources of cortical rhythms change as a function of cognitive impairment in pathological aging: a multicenter study. Clin. Neurophysiol. 117, 252–268. doi: 10.1016/j.clinph.2005.09.019
Bamidis, P., Vivas, A. B., Styliadis, C., Frantzidis, C., Klados, M., Schlee, W., et al. (2014). A review of physical and cognitive interventions in aging. Neurosci. Biobehav. Rev. 44, 206–220. doi: 10.1016/j.neubiorev.2014.03.019
Bergman, R. J., Bassett, D., and Klein, D. A. (2008). Validity of 2 devices for measuring steps taken by older adults in assisted-living facilities. J. Phys. Activ. Health 5, S166. doi: 10.1123/jpah.5.s1.s166
Bi, G.-Q., and Poo, M.-M. (1998). Synaptic modifications in cultured hippocampal neurons: dependence on spike timing, synaptic strength, and postsynaptic cell type. J. Neurosci. 18, 10464–10472. doi: 10.1523/JNEUROSCI.18-24-10464.1998
Blumen, H. M., Allali, G., Beauchet, O., Lipton, R. B., and Verghese, J. (2018). A gray matter volume covariance network associated with the motoric cognitive risk syndrome: a multi-cohort MRI study. J. Gerontol A Biol. Sci. Med. Sci. doi: 10.1093/gerona/gly158 [Epub ahead of print].
Brouwer, B., and Ashby, P. (1990). Corticospinal projections to upper and lower limb spinal motoneurons in man. Electroencephalogr. Clin. Neurophysiol. 76, 509–519. doi: 10.1016/0013-4694(90)90002-2
Brown, J., Cooper-Kuhn, C. M., Kempermann, G., Van Praag, H., Winkler, J., Gage, F. H., et al. (2003). Enriched environment and physical activity stimulate hippocampal but not olfactory bulb neurogenesis. Eur. J. Neurosci. 17, 2042–2046. doi: 10.1046/j.1460-9568.2003.02647.x
Cacchio, A., Cimini, N., Alosi, P., Santilli, V., and Marrelli, A. (2009). Reliability of transcranial magnetic stimulation-related measurements of tibialis anterior muscle in healthy subjects. Clin. Neurophysiol. 120, 414–419. doi: 10.1016/j.clinph.2008.11.019
Cai, L., Chan, J. S., Yan, J. H., and Peng, K. (2014). Brain plasticity and motor practice in cognitive aging. Front. Aging Neurosci. 6:31. doi: 10.3389/fnagi.2014.00031
Carvalho, A., Rea, I. M., Parimon, T., and Cusack, B. J. (2014). Physical activity and cognitive function in individuals over 60 years of age: a systematic review. Clin. Interv. Aging 9, 661–682. doi: 10.2147/CIA.S55520
Cirillo, J., Lavender, A. P., Ridding, M. C., and Semmler, J. G. (2009). Motor cortex plasticity induced by paired associative stimulation is enhanced in physically active individuals. J. Physiol. 587, 5831–5842. doi: 10.1113/jphysiol.2009.181834
Colcombe, S. J., Erickson, K. I., Raz, N., Webb, A. G., Cohen, N. J., McAuley, E., et al. (2003). Aerobic fitness reduces brain tissue loss in aging humans. J. Gerontol. A Biol. Sci. Med. Sci. 58, 176–180. doi: 10.1093/gerona/58.2.M176
Cooke, S. F., and Bliss, T. V. (2006). Plasticity in the human central nervous system. Brain 129(Pt 7), 1659–1673. doi: 10.1093/brain/awl082
Cotman, C. W., and Berchtold, N. C. (2002). Exercise: a behavioral intervention to enhance brain health and plasticity. Trends Neurosci. 25, 295–301. doi: 10.1016/S0166-2236(02)02143-4
Cowansage, K. K., LeDoux, J. E., and Monfils, M. H. (2010). Brain-derived neurotrophic factor: a dynamic gatekeeper of neural plasticity. Curr. Mol. Pharmacol. 3, 12–29. doi: 10.2174/1874467211003010012
Dauncey, M. J. (2009). New insights into nutrition and cognitive neuroscience. Proc. Nutr. Soc. 68, 408–415. doi: 10.1017/S0029665109990188
Delorme, A., and Makeig, S. (2004). EEGLAB: an open source toolbox for analysis of single-trial EEG dynamics including independent component analysis. J. Neurosci. Methods 134, 9–21. doi: 10.1016/j.jneumeth.2003.10.009
Devanne, H., Lavoie, B. A., and Capaday, C. (1997). Input-output properties and gain changes in the human corticospinal pathway. Exp. Brain Res. 114, 329–338. doi: 10.1007/PL00005641
Dickins, D. S. E., Kamke, M. R., and Sale, M. V. (2017). Corticospinal plasticity in bilateral primary motor cortices induced by paired associative stimulation to the dominant hemisphere does not differ between young and older adults. Neural Plast. 2017:8319049. doi: 10.1155/2017/8319049
Dipietro, L., Caspersen, C. J., Ostfeld, A. M., and Nadel, E. R. (1993). A survey for assessing physical activity among older adults. Med. Sci. Sports Exer. 25, 624–642. doi: 10.1249/00005768-199305000-00016
Erickson, K. I., Prakash, R. S., Voss, M. W., Chaddock, L., Hu, L., Morris, K. S., et al. (2009). Aerobic fitness is associated with hippocampal volume in elderly humans. Hippocampus 19, 1030–1039. doi: 10.1002/hipo.20547
Figurov, A., Pozzo-Miller, L. D., Olafsson, P., Wang, T., and Lu, B. (1996). Regulation of synaptic responses to high-frequency stimulation and LTP by neurotrophins in the hippocampus. Nature 381, 706–709. doi: 10.1038/381706a0
Fjell, A. M., McEvoy, L., Holland, D., Dale, A. M., Walhovd, K. B., and Alzheimer’s Disease Neuroimaging Initiative (2014). What is normal in normal aging? Effects of aging, amyloid and Alzheimer’s disease on the cerebral cortex and the hippocampus. Prog. Neurobiol. 117, 20–40. doi: 10.1016/j.pneurobio.2014.02.004
Flöel, A., Ruscheweyh, R., Krüger, K., Willemer, C., Winter, B., Völker, K., et al. (2010). Physical activity and memory functions: are neurotrophins and cerebral gray matter volume the missing link? Neuroimage 49, 2756–2763. doi: 10.1016/j.neuroimage.2009.10.043
Frantseva, M. V., Fitzgerald, P. B., Chen, R., Möller, B., Daigle, M., and Daskalakis, Z. J. (2008). Evidence for impaired long-term potentiation in schizophrenia and its relationship to motor skill learning. Cereb. Cortex 18, 990–996. doi: 10.1093/cercor/bhm151
Fratello, F., Veniero, D., Curcio, G., Ferrara, M., Marzano, C., Moroni, F., et al. (2006). Modulation of corticospinal excitability by paired associative stimulation: reproducibility of effects and intraindividual reliability. Clin. Neurophysiol. 117, 2667–2674. doi: 10.1016/j.clinph.2006.07.315
Gomez-Pinilla, F., Zhuang, Y., Feng, J., Ying, Z., and Fan, G. (2011). Exercise impacts brain-derived neurotrophic factor plasticity by engaging mechanisms of epigenetic regulation. Eur. J. Neurosci. 33, 383–390. doi: 10.1111/j.1460-9568.2010.07508.x
Guigoz, Y., Lauque, S., and Vellas, B. J. (2002). Identifying the elderly at risk for malnutrition: the Mini Nutritional Assessment. Clin. Geriat. Med. 18, 737–757. doi: 10.1016/S0749-0690(02)00059-9
Guigoz, Y., Vellas, B., and Garry, P. J. (1996). Assessing the nutritional status of the elderly: the Mini Nutritional Assessment as part of the geriatric evaluation. Nutr. Rev. 54, S59–S65. doi: 10.1111/j.1753-4887.1996.tb03793.x
Harada, N. D., Chiu, V., King, A. C., and Stewart, A. L. (2001). An evaluation of three self-report physical activity instruments for older adults. Med. Sci. Sports Exerc. 33, 962–970. doi: 10.1097/00005768-200106000-00016
Head, D., Buckner, R. L., Shimony, J. S., Williams, L. E., Akbudak, E., Conturo, T. E., et al. (2004). Differential vulnerability of anterior white matter in nondemented aging with minimal acceleration in dementia of the Alzheimer type: evidence from diffusion tensor imaging. Cereb. Cortex 14, 410–423. doi: 10.1093/cercor/bhh003
Ho, M. C., Chou, C. Y., Huang, C. F., Lin, Y. T., Shih, C. S., Han, S. Y., et al. (2012). Age-related changes of task-specific brain activity in normal aging. Neurosci. Lett. 507, 78–83. doi: 10.1016/j.neulet.2011.11.057
Huy, C., and Schneider, S. (2008). Instrument für die Erfassung der physischen Aktivität bei Personen im mittleren und höheren Erwachsenenalter. Z. Gerontol. Geriat. 41, 208–216. doi: 10.1007/s00391-007-0474-y
Japyassu, H. F., and Malange, J. (2014). Plasticity, stereotypy, intra-individual variability and personality: handle with care. Behav. Process. 109(Pt A), 40–47. doi: 10.1016/j.beproc.2014.09.016
Julayanont, P., et al. (2013). “Montreal Cognitive Assessment (MoCA): concept and clinical review,” in Cognitive Screening Instruments, ed. A. Larner (Berlin: Springer), 111–151. doi: 10.1007/978-1-4471-2452-8_6
Jung, P., and Ziemann, U. (2009). Homeostatic and nonhomeostatic modulation of learning in human motor cortex. J. Neurosci. 29, 5597–5604. doi: 10.1523/JNEUROSCI.0222-09.2009
Kempermann, G., Gast, D., and Gage, F. H. (2002). Neuroplasticity in old age: sustained fivefold induction of hippocampal neurogenesis by long-term environmental enrichment. Ann. Neurol. 52, 135–143. doi: 10.1002/ana.10262
Klintsova, A. Y., Dickson, E., Yoshida, R., and Greenough, W. T. (2004). Altered expression of BDNF and its high-affinity receptor TrkB in response to complex motor learning and moderate exercise. Brain Res. 1028, 92–104. doi: 10.1016/j.brainres.2004.09.003
Koski, L., Xie, H., and Finch, L. (2009). Measuring cognition in a geriatric outpatient clinic: rasch analysis of the Montreal Cognitive Assessment. J. Geriat. Psychiatry Neurol. 22, 151–160. doi: 10.1177/0891988709332944
Kumpulainen, S., Avela, J., Gruber, M., Bergmann, J., Voigt, M., Linnamo, V., et al. (2015). Differential modulation of motor cortex plasticity in skill- and endurance-trained athletes. Eur. J. Appl. Physiol. 115, 1107–1115. doi: 10.1007/s00421-014-3092-6
Kumpulainen, S., Mrachacz-Kersting, N., Peltonen, J., Voigt, M., and Avela, J. (2012). The optimal interstimulus interval and repeatability of paired associative stimulation when the soleus muscle is targeted. Exp. Brain Res. 221, 241–249. doi: 10.1007/s00221-012-3165-x
López-Alonso, V., Cheeran, B., Río-Rodríguez, D., and Fernández-Del-Olmo, M. (2014). Inter-individual variability in response to non-invasive brain stimulation paradigms. Brain Stimul. 7, 372–380. doi: 10.1016/j.brs.2014.02.004
Lopez-Calderon, J., and Luck, S. J. (2014). ERPLAB: an open-source toolbox for the analysis of event-related potentials. Front. Hum. Neurosci. 8:213. doi: 10.3389/fnhum.2014.00213
Lu, B., and Figurov, A. (1997). Role of neurotrophins in synapse development and plasticity. Rev. Neurosci. 8, 1–12. doi: 10.1515/REVNEURO.1997.8.1.1
Meeusen, R. (2014). Exercise, nutrition and the brain. Sports Med. 44(Suppl. 1), S47–S56. doi: 10.1007/s40279-014-0150-5
Meeusen, R., and De Meirleir, K. (1995). Exercise and brain neurotransmission. Sports Med. 20, 160–188. doi: 10.2165/00007256-199520030-00004
Meunier, S., Russmann, H., Simonetta-Moreau, M., and Hallett, M. (2007). Changes in spinal excitability after PAS. J. Neurophysiol. 97, 3131–3135. doi: 10.1152/jn.01086.2006
Miquel, S., Champ, C., Day, J., Aarts, E., Bahr, B. A., Bakker, M., et al. (2017). Poor cognitive ageing: vulnerabilities, mechanisms and the impact of nutritional interventions. Ageing Res. Rev. 42, 40–55. doi: 10.1016/j.arr.2017.12.004
Mirelman, A., Herman, T., Brozgol, M., Dorfman, M., Sprecher, E., Schweiger, A., et al. (2012). Executive function and falls in older adults: new findings from a five-year prospective study link fall risk to cognition. PLoS One. 7:e40297. doi: 10.1371/journal.pone.0040297
Möller, C., Arai, N., Lücke, J., and Ziemann, U. (2009). Hysteresis effects on the input–output curve of motor evoked potentials. Clin. Neurophysiol. 120, 1003–1008. doi: 10.1016/j.clinph.2009.03.001
Montoye, H. J., et al. (1996). Measuring Physical Activity and Energy Expenditure. Champaign, IL: Human Kinetics.
Mora, F. (2013). Successful brain aging: plasticity, environmental enrichment, and lifestyle. Dialogues Clin. Neurosci. 15, 45–52.
Mrachacz-Kersting, N., Fong, M., Murphy, B. A., and Sinkjaer, T. (2007). Changes in excitability of the cortical projections to the human tibialis anterior after paired associative stimulation. J. Neurophysiol. 97, 1951–1958. doi: 10.1152/jn.01176.2006
Mudge, S., Taylor, D., Chang, O., and Wong, R. (2010). Test-retest reliability of the StepWatch activity monitor outputs in healthy adults. J. Phys. Act. Health 7, 671–676. doi: 10.1123/jpah.7.5.671
Müller-Dahlhaus, J. F., Orekhov, Y., Liu, Y., and Ziemann, U. (2008). Interindividual variability and age-dependency of motor cortical plasticity induced by paired associative stimulation. Exp. Brain Res. 187, 467–475. doi: 10.1007/s00221-008-1319-7
Nasreddine, Z. S., Phillips, N. A., Bédirian, V., Charbonneau, S., Whitehead, V., Collin, I., et al. (2005). The Montreal Cognitive Assessment, MoCA: a brief screening tool for mild cognitive impairment. J. Am. Geriat. Soc. 53, 695–699. doi: 10.1111/j.1532-5415.2005.53221.x
Neeper, S. A., Gómez-Pinilla, F., Choi, J., and Cotman, C. (1995). Exercise and brain neurotrophins. Nature 373:109. doi: 10.1038/373109a0
Niedermeyer, E. (1997). Alpha rhythms as physiological and abnormal phenomena. Int. J. Psychophysiol. 26, 31–49. doi: 10.1016/S0167-8760(97)00754-X
Okubo, H., Inagaki, H., Gondo, Y., Kamide, K., Ikebe, K., Masui, Y., et al. (2017). Association between dietary patterns and cognitive function among 70-year-old Japanese elderly: a cross-sectional analysis of the SONIC study. Nutr. J. 16:56. doi: 10.1186/s12937-017-0273-2
Oldfield, R. C. (1971). The assessment and analysis of handedness: the Edinburgh inventory. Neuropsychologia 9, 97–113. doi: 10.1016/0028-3932(71)90067-4
Oostenveld, R., Fries, P., Maris, E., and Schoffelen, J. M. (2011). FieldTrip: open source software for advanced analysis of MEG, EEG, and invasive electrophysiological data. Comput. Intell. Neurosci. 2011:156869. doi: 10.1155/2011/156869
Park, D. C., and Reuter-Lorenz, P. (2009). The adaptive brain: aging and neurocognitive scaffolding. Annu. Rev. Psychol. 60, 173–196. doi: 10.1146/annurev.psych.59.103006.093656
Pfefferbaum, A., Rohlfing, T., Rosenbloom, M. J., Chu, W., Colrain, I. M., and Sullivan, E. V. (2013). Variation in longitudinal trajectories of regional brain volumes of healthy men and women (ages 10–85 years) measured with atlas-based parcellation of MRI. Neuroimage 65, 176–193. doi: 10.1016/j.neuroimage.2012.10.008
Phillips, C. (2017). Lifestyle modulators of neuroplasticity: how physical activity, mental engagement, and diet promote cognitive health during aging. Neural Plast. 2017:3589271. doi: 10.1155/2017/3589271
Phillips, C., Baktir, M. A., Das, D., Lin, B., and Salehi, A. (2015). The link between physical activity and cognitive dysfunction in alzheimer disease. Phys. Ther. 95, 1046–1060. doi: 10.2522/ptj.20140212
Pichierri, G., Murer, K., and de Bruin, E. D. (2012). A cognitive-motor intervention using a dance video game to enhance foot placement accuracy and gait under dual task conditions in older adults: a randomized controlled trial. BMC Geriatr. 12:74. doi: 10.1186/1471-2318-12-74
Rapport, L. J., Hanks, R. A., Millis, S. R., and Deshpande, S. A. (1998). Executive functioning and predictors of falls in the rehabilitation setting. Arch. Phys. Med. Rehabil. 79, 629–633. doi: 10.1016/S0003-9993(98)90035-1
Raz, N., Lindenberger, U., Rodrigue, K. M., Kennedy, K. M., Head, D., Williamson, A., et al. (2005). Regional brain changes in aging healthy adults: general trends, individual differences and modifiers. Cereb. Cortex 15, 1676–1689. doi: 10.1093/cercor/bhi044
Resnick, S. M., Pham, D. L., Kraut, M. A., Zonderman, A. B., and Davatzikos, C. (2003). Longitudinal magnetic resonance imaging studies of older adults: a shrinking brain. J. Neurosci. 23, 3295–3301. doi: 10.1523/JNEUROSCI.23-08-03295.2003
Richard Clark C, Veltmeyer, M. D., Hamilton, R. J., Simms, E., Paul, R., Hermens, D., et al. (2004). Spontaneous alpha peak frequency predicts working memory performance across the age span. Int. J. Psychophysiol. 53, 1–9. doi: 10.1016/j.ijpsycho.2003.12.011
Ridding, M. C., and Ziemann, U. (2010). Determinants of the induction of cortical plasticity by non-invasive brain stimulation in healthy subjects. J. Physiol. 588(Pt 13), 2291–2304. doi: 10.1113/jphysiol.2010.190314
Rosenkranz, K., Kacar, A., and Rothwell, J. C. (2007). Differential modulation of motor cortical plasticity and excitability in early and late phases of human motor learning. J. Neurosci. 27, 12058–12066. doi: 10.1523/JNEUROSCI.2663-07.2007
Rossini, P. M., Burke, D., Chen, R., Cohen, L. G., Daskalakis, Z., Di Iorio, R., et al. (1994). Non-invasive electrical and magnetic stimulation of the brain, spinal cord and roots: basic principles and procedures for routine clinical application. Report of an IFCN committee. Electroencephalogr. Clin. Neurophysiol. 91, 79–92. doi: 10.1016/0013-4694(94)90029-9
Rossini, P. M., Rossi, S., Babiloni, C., and Polich, J. (2007). Clinical neurophysiology of aging brain: from normal aging to neurodegeneration. Prog. Neurobiol. 83, 375–400. doi: 10.1016/j.pneurobio.2007.07.010
Sale, M. V., Ridding, M. C., and Nordstrom, M. A. (2008). Cortisol inhibits neuroplasticity induction in human motor cortex. J. Neurosci. 28, 8285–8293. doi: 10.1523/JNEUROSCI.1963-08.2008
Schättin, A., Baur, K., Stutz, J., Wolf, P., and de Bruin, E. D. (2016). Effects of physical exercise combined with nutritional supplements on aging brain related structures and functions: a systematic review. Front. Aging Neurosci. 8:161. doi: 10.3389/fnagi.2016.00161
Scherder, E., Eggermont, L., Visscher, C., Scheltens, P., and Swaab, D. (2011). Understanding higher level gait disturbances in mild dementia in order to improve rehabilitation: ’last in-first out’. Neurosci. Biobehav. Rev. 35, 699–714. doi: 10.1016/j.neubiorev.2010.08.009
Seidler, R. D., Bernard, J. A., Burutolu, T. B., Fling, B. W., Gordon, M. T., Gwin, J. T., et al. (2010). Motor control and aging: links to age-related brain structural, functional, and biochemical effects. Neurosci. Biobehav. Rev. 34, 721–733. doi: 10.1016/j.neubiorev.2009.10.005
Sleiman, S. F., Henry, J., Al-Haddad, R., El, Hayek L, Abou, Haidar E, Stringer, T., et al. (2016). Exercise promotes the expression of brain derived neurotrophic factor (BDNF) through the action of the ketone body beta-hydroxybutyrate. Elife 5:e15092. doi: 10.7554/eLife.15092
Stefan, K., Wycislo, M., and Classen, J. (2004). Modulation of associative human motor cortical plasticity by attention. J. Neurophysiol. 92, 66–72. doi: 10.1152/jn.00383.2003
Stefan, K., Wycislo, M., Gentner, R., Schramm, A., Naumann, M., Reiners, K., et al. (2006). Temporary occlusion of associative motor cortical plasticity by prior dynamic motor training. Cereb. Cortex 16, 376–385. doi: 10.1093/cercor/bhi116
Storti, K. L., Pettee, K. K., Brach, J. S., Talkowski, J. B., Richardson, C. R., and Kriska, A. M. (2008). Gait speed and step-count monitor accuracy in community-dwelling older adults. Med. Sci. Sports Exerc. 40:59. doi: 10.1249/mss.0b013e318158b504
Taube, W., Gruber, M., and Gollhofer, A. (2008). Spinal and supraspinal adaptations associated with balance training and their functional relevance. Acta Physiol. (Oxf.) 193, 101–116. doi: 10.1111/j.1748-1716.2008.01850.x
Tecchio, F., Zappasodi, F., Pasqualetti, P., Gennaro, L., Pellicciari, M. C., Ercolani, M., et al. (2008). Age dependence of primary motor cortex plasticity induced by paired associative stimulation. Clin. Neurophysiol. 119, 675–682. doi: 10.1016/j.clinph.2007.10.023
Tononi, G., and Cirelli, C. (2006). Sleep function and synaptic homeostasis. Sleep Med. Rev. 10, 49–62. doi: 10.1016/j.smrv.2005.05.002
Valenzuela, M. J., Sachdev, P., Wen, W., Chen, X., and Brodaty, H. (2008). Lifespan mental activity predicts diminished rate of hippocampal atrophy. PLoS One 3:e2598. doi: 10.1371/journal.pone.0002598
van Praag H, Christie, B. R., Sejnowski, T. J., and Gage, F. H. (1999). Running enhances neurogenesis, learning, and long-term potentiation in mice. Proc. Natl. Acad. Sci. U.S.A. 96, 13427–13431. doi: 10.1073/pnas.96.23.13427
van Praag, H. (2009). Exercise and the brain: something to chew on. Trends Neurosci. 32, 283–290. doi: 10.1016/j.tins.2008.12.007
van Praag, H., Kempermann, G., and Gage, F. H. (2000). Neural consequences of environmental enrichment. Nat. Rev. Neurosci. 1, 191–198. doi: 10.1038/35044558
Varga, A. W., Ducca, E. L., Kishi, A., Fischer, E., Parekh, A., Koushyk, V., et al. (2016). Effects of aging on slow-wave sleep dynamics and human spatial navigational memory consolidation. Neurobiol. Aging. 42, 142–149. doi: 10.1016/j.neurobiolaging.2016.03.008
Vaynman, S., and Gomez-Pinilla, F. (2005). License to run: exercise impacts functional plasticity in the intact and injured central nervous system by using neurotrophins. Neurorehabil. Neural Repair. 19, 283–295. doi: 10.1177/1545968305280753
Veale, J. F. (2014). Edinburgh Handedness Inventory–Short Form: a revised version based on confirmatory factor analysis. Laterality 19, 164–177. doi: 10.1080/1357650X.2013.783045
von Arnim, C. A., Gola, U., and Biesalski, H. K. (2010). More than the sum of its parts? Nutr. Alzheimer’s Dis. Nutr. 26, 694–700. doi: 10.1016/j.nut.2009.11.009
Voss, M. W., Vivar, C., Kramer, A. F., and van, Praag H (2013). Bridging animal and human models of exercise-induced brain plasticity. Trends Cogn. Sci. 17, 525–544. doi: 10.1016/j.tics.2013.08.001
Washburn, R. A., Smith, K. W., Jette, A. M., and Janney, C. A. (1993). The physical activity scale for the elderly (PASE): development and evaluation. J. Clin. Epidemiol. 46, 153–162. doi: 10.1016/0895-4356(93)90053-4
Wingfield, A., Tun, P. A., and McCoy, S. L. (2005). Hearing loss in older adulthood: what it is and how it interacts with cognitive performance. Curr. Direct. Psychol. Sci. 14, 144–148. doi: 10.1111/j.0963-7214.2005.00356.x
Wurtman, R. J., Cansev, M., Sakamoto, T., and Ulus, I. H. (2009). Use of phosphatide precursors to promote synaptogenesis. Annu. Rev. Nutr. 29, 59–87. doi: 10.1146/annurev-nutr-080508-141059
Yau, S. Y., Gil-Mohapel, J., Christie, B. R., and So, K. F. (2014). Physical exercise-induced adult neurogenesis: a good strategy to prevent cognitive decline in neurodegenerative diseases? Biomed. Res. Int. 2014:403120. doi: 10.1155/2014/403120
Yesavage, J. A., Brink, T. L., Rose, T. L., Lum, O., Huang, V., Adey, M., et al. (1982). Development and validation of a geriatric depression screening scale: a preliminary report. J. Psychiatry Res. 17, 37–49. doi: 10.1016/0022-3956(82)90033-4
Yesavage, J. A., and Sheikh, J. I. (1986). 9/Geriatric depression scale (GDS) recent evidence and development of a shorter violence. Clin. Gerontol. 5, 165–173. doi: 10.3109/09638288.2010.503835
Yordanova, J. Y., Kolev, V. N., and Basar, E. (1998). EEG theta and frontal alpha oscillations during auditory processing change with aging. Electroencephalogr. Clin. Neurophysiol. 108, 497–505.
Yuan, P., and Raz, N. (2014). Prefrontal cortex and executive functions in healthy adults: a meta-analysis of structural neuroimaging studies. Neurosci. Biobehav. Rev. 42, 180–192. doi: 10.1016/j.neubiorev.2014.02.005
Ziemann, U., Iliæ, T. V., Pauli, C., Meintzschel, F., and Ruge, D. (2004). Learning modifies subsequent induction of long-term potentiation-like and long-term depression-like plasticity in human motor cortex. J. Neurosci. 24, 1666–1672. doi: 10.1523/JNEUROSCI.5016-03.2004
Ziemann, U., Paulus, W., Nitsche, M. A., Pascual-Leone, A., Byblow, W. D., Berardelli, A., et al. (2008). Consensus: motor cortex plasticity protocols. Brain Stimul. 1, 164–182. doi: 10.1016/j.brs.2008.06.006
Keywords: older adults, synaptic plasticity, physical activity, nutrition, cognition, paired associative stimulation, transcranial magnetic stimulation, neurophysiology
Citation: Schättin A, Gennaro F, Egloff M, Vogt S and de Bruin ED (2018) Physical Activity, Nutrition, Cognition, Neurophysiology, and Short-Time Synaptic Plasticity in Healthy Older Adults: A Cross-Sectional Study. Front. Aging Neurosci. 10:242. doi: 10.3389/fnagi.2018.00242
Received: 17 May 2018; Accepted: 24 July 2018;
Published: 30 August 2018.
Edited by:
Ashok Kumar, University of Florida, United StatesReviewed by:
Mihai Moldovan, University of Copenhagen, DenmarkCopyright © 2018 Schättin, Gennaro, Egloff, Vogt and de Bruin. This is an open-access article distributed under the terms of the Creative Commons Attribution License (CC BY). The use, distribution or reproduction in other forums is permitted, provided the original author(s) and the copyright owner(s) are credited and that the original publication in this journal is cited, in accordance with accepted academic practice. No use, distribution or reproduction is permitted which does not comply with these terms.
*Correspondence: Alexandra Schättin, c2NoYWV0dGFAaGVzdC5ldGh6LmNo; c2NoYWV0dGFAZXRoei5jaA==
Disclaimer: All claims expressed in this article are solely those of the authors and do not necessarily represent those of their affiliated organizations, or those of the publisher, the editors and the reviewers. Any product that may be evaluated in this article or claim that may be made by its manufacturer is not guaranteed or endorsed by the publisher.
Research integrity at Frontiers
Learn more about the work of our research integrity team to safeguard the quality of each article we publish.