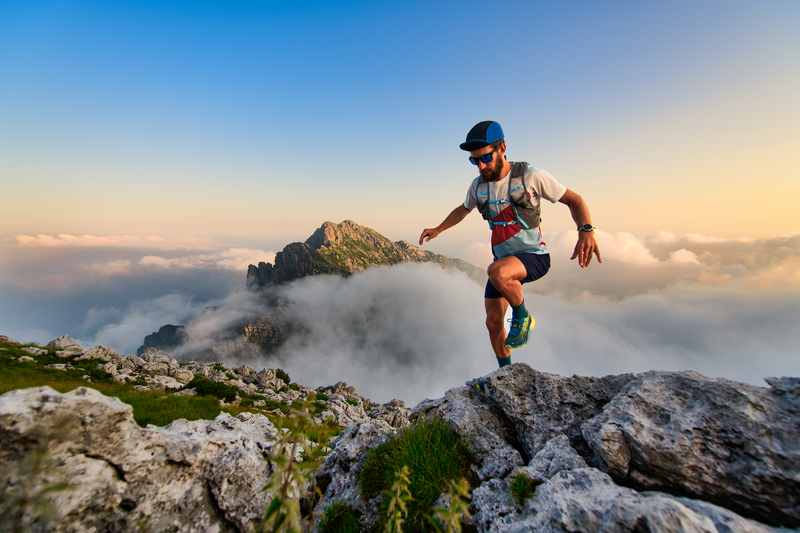
94% of researchers rate our articles as excellent or good
Learn more about the work of our research integrity team to safeguard the quality of each article we publish.
Find out more
MINI REVIEW article
Front. Aging Neurosci. , 23 July 2018
Sec. Cellular and Molecular Mechanisms of Brain-aging
Volume 10 - 2018 | https://doi.org/10.3389/fnagi.2018.00220
Prion diseases are transmissible progressive neurodegenerative conditions characterized by rapid neuronal loss accompanied by a heterogeneous neuropathology, including spongiform degeneration, gliosis and protein aggregation. The pathogenic mechanisms and the origins of prion diseases remain unclear on the molecular level. Even though neurodegenerative diseases, including prion diseases, represent distinct entities, their pathogenesis shares a number of features including disturbed protein homeostasis, an overload of protein clearance pathways, the aggregation of pathological altered proteins, and the dysfunction and/or loss of specific neuronal populations. Recently, direct links have been established between neurodegenerative diseases and miRNA dysregulated patterns. miRNAs are a class of small non-coding RNAs involved in the fundamental post-transcriptional regulation of gene expression. Studies of miRNA alterations in the brain and body fluids in human prion diseases provide important insights into potential miRNA-associated disease mechanisms and biomarker candidates. miRNA alterations in prion disease models represent a unique tool to investigate the cause-consequence relationships of miRNA dysregulation in prion disease pathology, and to evaluate the use of miRNAs in diagnosis as biomarkers. Here, we provide an overview of studies on miRNA alterations in human prion diseases and relevant disease models, in relation to pertinent studies on other neurodegenerative diseases.
Prion diseases are rapidly progressive, fatal neurodegenerative disorders, characterized by widespread neuronal loss, gliosis, spongiform change and deposition of the pathological PrPSc protein in the Central Nervous System (CNS) (Appleby et al., 2009). Similarly to other neurodegenerative disorders (NDs), dysfunctional proteostasis is a key feature in prion diseases. Changes in the cellular prion protein (PrPC) metabolism and its conversion into an aberrant isoform (PrPSc) in a self-propagating manner are attributed to prion diseases pathogenesis (Colby and Prusiner, 2011). However, precise molecular pathogenic mechanisms remain unclear. Prion disease model systems, including animal and cell culture models, recapitulate different aspects of disease mechanisms and are valuable for exploring underlying pathogenesis (Zou and Gambetti, 2004; Watts and Prusiner, 2014; Brandner and Jaunmuktane, 2017).
miRNA dysregulation has been linked to several NDs (Hébert and De Strooper, 2009; Abe and Bonini, 2013; Tan et al., 2015), including prion diseases. miRNAs are a class of short, non-coding RNAs that regulate gene expression post-transcriptionally through translational inhibition and/or mRNA degradation. They are involved in several biological processes, including neuronal function and survival (Zhang et al., 2007). miRNA biogenesis includes several processing steps mediated by multiple miRNA maturating proteins in the nucleus and cytoplasm (Ha and Kim, 2014). miRNAs are usually transcribed by RNA polymerase II as long, primary miRNA transcripts and undergo a series of cleavage events by the ribonucleases Drosha and Dicer, as well as post-transcriptional modifications, such as splicing and editing (Slezak-Prochazka et al., 2010). Mature miRNAs interact with Argonaute proteins to form the miRNA-induced silencing complex (RISC), which mediates translational repression or target degradation through binding to mRNAs (Bartel, 2009; Hammond, 2015). Various regulatory mechanisms affect miRNAs processing efficiency and activity (Finnegan and Pasquinelli, 2013; Ha and Kim, 2014).
A collective view of miRNA alterations in prion disease patients and/or relevant disease models is currently unavailable. We review experimentally validated miRNA alterations in the brain and body fluids of human prion diseases and/or disease models. We compare these deregulated miRNAs with other NDs; we comment on possible outcomes of miRNA dysregulation in prion diseases and discuss potential underlying mechanisms of this deregulation. We further discuss the diagnostic and therapeutic potential of miRNAs. Finally, we present future perspectives in the prion diseases miRNA research field.
Studies on miRNA alterations were conducted in the brain of patients afflicted with sporadic Creutzfeldt-Jakob disease (sCJD) (Montag et al., 2009; Lukiw et al., 2011; Llorens et al., 2018), Fatal Familial Insomnia (FFI) (Llorens et al., 2018) and Gerstmann–Sträussler–Scheinker syndrome (GSS) (Lukiw et al., 2011).
Altered miRNA expression has been investigated in animal and cell culture models including: (a) a sCJD mouse model that recapitulates CJD brain neuropathology (Padilla et al., 2011; Llorens et al., 2014, 2017, 2018), (b) BSE-infected macaques (Montag et al., 2009) (c) murine models intracerebrally inoculated with various scrapie strains [RML (Majer et al., 2012; Boese et al., 2016), 139A (Gao et al., 2016), Me7 (Gao et al., 2016) and 22A (Saba et al., 2008)] or scrapie infected SMB-S15 cells (Gao et al., 2016), (d) murine hypothalamic GT1-7 cells infected with a mouse adapted human GSS strain (Bellingham et al., 2012; Bellingham and Hill, 2017) and (e) murine neuroblastoma cells propagating the 22L scrapie strain (Montag et al., 2012).
We detected limited overlap among the altered miRNAs reported by these studies. This is likely explained by the multitude of factors that vary between analyzed prion models and/or strains, different miRNA profiling platforms and heterogeneity of analyzed tissues (bulk tissue, microdissected neurons, isolated synaptoneurosomes, etc.). When comparing different miRNA profiling platforms, interplatform reproducibility is often not fully achieved and even variations within the same platform have been described (Chugh and Dittmer, 2013). Methodological advantages, drawbacks and features, possibly contributing to limited results overlap are briefly discussed below.
Microarray technology, quantitative real-time reverse transcription PCR (qRT-PCR) and/or Northern Blot analyses were used for targeted miRNA profiling. Microarrays enabled simultaneous quantification of several miRNAs at the expense of specificity, due to the homogeneous hybridization conditions used. Individual miRNA qRT-PCR assays, based on either stem-loop or locked nucleic acid-modified (LNA) primers, enhanced specificity and enabled differentiation of mature miRNAs from precursors (Pritchard et al., 2012; Chugh and Dittmer, 2013). Even though highly sensitive, Northern Blot analysis, is time-consuming and laborious for large scale miRNA analysis (Koshiol et al., 2010).
Application of small RNA sequencing (RNAseq) allowed high-throughput analysis on single-nucleotide resolution, offering comprehensive and unbiased miRNA quantifications. However, method-dependent distortions in miRNA quantification have been reported. Multiple factors, such as RNA G/C-content or secondary RNA structures, can influence cDNA synthesis (Raabe et al., 2014). Overall, platform-specific biases affect the consistency and accuracy of miRNA profiling, which may contribute to variability in miRNA quantification (Chugh and Dittmer, 2013).
Despite the limited overlap, some miRNAs presented similar deregulation in at least two independent disease contexts. Table 1 summarizes experimentally validated miRNAs found to display similar deregulation patterns in prion affected brain tissue, at clinical disease.
Upregulation of miR-146a-5p was demonstrated in six independent studies, including sCJD and GSS (Lukiw et al., 2011), sCJD and the corresponding mice model (Llorens et al., 2018), mice scrapie models [139A (Lukiw et al., 2011; Gao et al., 2016), Me7, S15 (Gao et al., 2016), 22A (Saba et al., 2008)], and CA1 (Cornu Ammonis region 1) neurons (Majer et al., 2012), as well as forebrain synaptoneuroso (Montag et al., 2009). Analogous deregulation, also extending to 22A-scrapie mice, was observed for miR-342-3p (Saba et al., 2008). Upregulation of miRNA-195-5p was reported in FFI, sCJD and in sCJD-mice (Llorens et al., 2018). Increased levels of miR-16-5p, miR-29b-3p, let-7i-3p, miR-378a-3p, miR-449a, and miR-154-5p were observed in sCJD patients and in the sCJD mice model brain, suggesting disease-specific miRNA profiles (Llorens et al., 2018). Further, upregulation of miR-341-3p, miR-3470a, miR-3473a, miR-879-5p, and miR-200b-5p in the cortex of mice infected with several scrapie strains (Gao et al., 2016) suggests common responses at the miRNA level elicited by different scrapie strains.
Among downregulated miRNAs, miR-124-3p was reduced in the frontal cortex and cerebellum of both sCJD patients and sCJD mice (Llorens et al., 2018) and in RML-infected mice CA1 neurons (Majer et al., 2012). Other miRNAs downregulated in the cortex of mice infected with different scrapie strains were miR-182-5p, miR-200a-3p, miR-96-5p, and miR-200b-3p (Gao et al., 2016). A similar pattern was observed for miRNA-141-3p, which was also downregulated in RML-mice forebrain synaptoneurosomes (Boese et al., 2016).
Temporal miRNA analyses in: (a) RML mice CA1 microdissected neurons (Majer et al., 2012; Burak et al., 2018), (b) RML mice forebrain and hippocampus synaptoneurosomes (Boese et al., 2016) and (c) sCJD mice cortex and cerebellum (Llorens et al., 2018), suggest distinct and dynamic miRNA signatures in disease progression. A trend toward upregulation for miR-124-3p, miR-16-5p, miR-26a-5p, miR-29a-3p, miR-132-3p, and miR-140-5p at pre-clinical stages, followed by downregulation to basal levels during disease progression and a further reduction throughout the clinical disease stage was identified in RML mice CA1 neurons; miR-146a-5p displayed increased levels throughout disease progression, reaching its highest levels at preclinical stages (Majer et al., 2012). Upregulation of miR-16-5p was also observed during early prion disease in RML mice neurons by a follow-up study (Burak et al., 2018). Upregulation of miR-124-3p was also detected in pre-clinical RML mice synaptoneurosomes, along with miR-32-5p, miR-136-5p, miR-150-5p, miR-345-5p, and miR-361-5p, while miR-141-3p, miR-183-5p, and miR-200c-3p were reduced in the same preparations (Boese et al., 2016).
Interestingly, among a pool of miRNAs specifically dysregulated in clinical sCJD disease, miR-124-3p and miR-16-5p change their expression during early symptomatic phase in sCJD mice (Llorens et al., 2018), suggesting their contribution in pre- and early clinical disease mechanisms.
A schematic illustration of temporal miRNA alterations during disease progression is shown in Figure 1.
FIGURE 1. Correlation between miRNA alterations and disease progression in prion diseases. Temporal miRNA profilng during prion disease progression in CA1 neurons (Majer et al., 2012), synaptoneurosomes (Boese et al., 2016) and brain regions (Llorens et al., 2017) revealed distinct miRNA alterations with disease progression. Different disease stages are featured by specific miRNA signatures. Upregulation of miR-146a-5p, miR-16-5p and miR-26a-5p appeared in two prion disease models across disease progression. Downregulation of miR-124-3p was specifically observed during clinical prion disease stages, while the same miRNA (miR-124-3p) was found upregulated in CA1 neurons and synaptoneurosomes at pre-clinical stages in two prion disease models.
Some miRNAs dysregulated in prion diseases are also altered in the brain of other NDs, displaying a similar or contrasting trend. The upregulated in prion diseases miR-146a-5p has been found overexpressed in active Multiple Sclerosis (MS) lesions (Junker et al., 2009), and in Alzheimer’s disease (AD) brain (Sethi and Lukiw, 2009; Müller et al., 2014) associated with different cellular contexts, including neurons (Wang et al., 2016) and astrocytes (Cui et al., 2010; Arena et al., 2017). Differential miR-146a-5p expression is suggested by in vitro studies in brain cells contributing to inflammatory cell type-specific functions during neurodegeneration (Li et al., 2011). Similar to miR-146a-5p, miR-195-5p is upregulated in prion disease brain and in inactive MS lesions (Junker et al., 2009); miR-16-5p is upregulated in both prion diseases and AD brain (Müller et al., 2014) and miR-26a-5p is increased in prion diseases and in dopaminergic midbrain Parkinson’s disease (PD) neurons (Briggs et al., 2015).
On the contrary, miR-29b-3p displays opposite regulation in MS and AD compared to prion diseases, as it is downregulated in normal appearing white matter (NAWM) (Noorbakhsh et al., 2011) and in chronic MS lesions (Lau et al., 2013); the same miRNA is decreased in the anterior temporal cortex and cerebellum of AD patients with increased BACE1 expression (Hebert et al., 2008).
Similar miRNA alterations in prion diseases and other NDs may indicate common miRNA-regulated molecular pathways. Identification of prion-disease-specific miRNA alterations in human brain, followed by robust confirmation in appropriate models, are required to gain insights into the specific role of miRNAs deregulation in prion diseases, and/or therapeutic potential.
One outstanding question is to what extent miRNA alterations (Table 1) reflect prion disease processes. Since miRNAs are involved in the regulation of complex gene networks, reported miRNA alterations in prion disease have the potential to be involved in virtually every aspect of disease pathophysiological mechanisms.
Interesting functional implications for potentially disease-associated miRNAs have been observed. The brain-enriched, NF-κB-sensitive miR-146a-5p has been suggested as a mediator of inflammatory microglial responses in prion disease (Lukiw et al., 2011; Saba et al., 2012). Additionally, compelling evidences revealed significant miR-146a-5p overexpression in prion disease neurons (Majer et al., 2012; Boese et al., 2016; Llorens et al., 2018), suggesting that neuronal miR-146a-5p induction is triggered by pathological stimuli. Further, miR-146a-5p overexpression in a neuronal cell culture model resulted in MAP1B downregulation, indicating miRNA-mediated mechanisms involved in microtubules networks regulation, with extensions to synaptic plasticity (Chen and Shen, 2013). Moreover, miR-146a-5p has been linked with regulation of reactive oxygen species generation (redoximiR), through NOX4 repression (Cheng et al., 2013).
The neuron-enriched miR-124-3p has been associated with neurite outgrowth (Maiorano and Mallamaci, 2009), dendritic complexity (Sanuki et al., 2011) and cholinergic anti-inflammatory responses (Sun et al., 2013), suggesting that its pre-clinical upregulation may reflect compensating mechanisms to overcome synaptic dysfunction (Cunningham et al., 2003; Hilton et al., 2013) and inflammatory processes (Carroll et al., 2015) during early disease responses.
In vitro studies revealed that lentiviral-based miR-16-5p overexpression, mimicking miR-16-5p upregulation observed in disease condition, induce reduced neurite length and branching of cultured hippocampal neurons (Burak et al., 2018). Also neuroprotective roles were suggested for miR-16-5p in the aging brain (Parsi et al., 2015), indicating multivariable effects of single miRNAs on cellular processes (Wilczynska and Bushell, 2015).
All the above highlight the complexity of miRNA-mediated regulation of cellular processes, stressing the need for further studies to globally identify miRNA-mRNA interactions within the disease context.
Expression and cellular miRNA abundance is influenced by mechanisms including genetic and epigenetic factors, RNA editing, alteration of transcription factors targeting miRNA, altered miRNA biogenesis, miRNA turnover and/or miRNA sorting in specific cellular compartments (Schanen and Li, 2011; Bronevetsky and Ansel, 2013; Tomaselli et al., 2013; Gulyaeva and Kushlinskiy, 2016).
Genetic variations, such as single nucleotide polymorphisms (SNPs) in miRNA genes or their target sites affecting miRNA expression, biogenesis and target binding (Jin and Lee, 2013; Saba and Booth, 2013) have been associated with NDs (Saba et al., 2014; Zhang et al., 2015; Moszyńska et al., 2017). A miR-146a promoter SNP (rs57095329) is associated with susceptibility to FFI and correlated with appearance of mutism and detection of the 14-3-3 protein in the Cerebrospinal Fluid (CSF) of sCJD patients (Gao et al., 2017). The same SNP has been suggested as a risk factor for AD (Cui et al., 2014), while another SNP (rs2910164) residing in pri-miR-146a, has been associated with AD (Zhang et al., 2015) and MS (Kiselev et al., 2015; Li et al., 2015; Park et al., 2016; Zhou et al., 2017).
Dysfunctional regulatory pathways involving transcription factors may underlie miRNA alterations in prion diseases. A pool of miRNAs upregulated in sCJD brain has been observed to be under the control of STAT3 (Llorens et al., 2018), which is upregulated and activated in prion diseased brain (Llorens et al., 2014; Carroll et al., 2015), suggesting a STAT3-dependent mechanism of miRNA deregulation in sCJD.
Disruption of miRNA biogenesis may contribute to miRNA dysregulation in prion diseases. Reduced levels of the essential miRNA maturing proteins Drosha, its cofactor DGCR8 and Dicer have been reported in sCJD patients brain (Llorens et al., 2018). Argonaute proteins, essential components of the miRNA-guided gene regulation, show altered subcellular distribution in sCJD brain (Llorens et al., 2018). Interestingly, PrPC has been shown to interact with Argonaute proteins under physiological conditions, promoting and stabilizing RISC complexes (Gibbings et al., 2012). Whether the presence of PrPSc or the lack of PrPC functionality directly contributes to miRNA dysregulation in prion disease deserves investigation. Figure 2 summarizes potential mechanisms of miRNA deregulation in prion diseases.
FIGURE 2. Schematic representation of cellular mechanisms possibly implicated in miRNA dysregulation in prion diseases. The canonical miRNA biogenesis and function pathway is indicated in green boxes. Mechanisms possibly contributing to miRNA dyregulation in prion diseases are indicated with dashed red lines. miRNA genes, residing in exonic, intronic or intergenic regions, are transcribed by RNA polymerase II or III (1). Altered miRNA gene transcription rate may impact miRNA levels. This can be associated to altered (≠) abundance of Transciption Factors (TF) inducing miRNA gene expression (e.g., STAT3), and/or to Single Nucleotide Variants (SNPs) residing within miRNA-gene promoter regions (e.g., rs57095329, illustrated as solid red line). Upon transcription, pri-miRNAs’ flanking sequences are removed by the microprossesor complex, containing Drosha and DGCR8 (2). This processing generates pre-miRNA stem-loop structures (3). Pre-miRNAs are exported into the cytoplasm through Exportin5 (EXPO5). Dicer removes the pre-miRNA loop, generating a miRNA duplex (4). SNPs in miRNA genes may interfere with pri- and/or pre-miRNAs processing by Drosha/DGCR8 (2) and Dicer (4) respectively. RNA editing, mediated by ADARs and/or APOBEC1, may occur in pri- and/or pre-miRNAs, introducing Single Nucleotide Variants (SNVs) with the potential to affect miRNA maturation (2, 4). Reduced Drosha, DGCR8 and Dicer levels, reported in human prion diseases, may impact miRNA maturation (2, 4). Following Dicer processing, the miRNA duplex is loaded from the RISC-loading complex (RLC) onto RISC. Ago proteins, are core components of RISC, forming the active functional unit of the miRNA-mediated gene regulation (5). Abnormal subcellular localization of Ago proteins, reported in sCJD, may affect miRNA machinery (5). PrPC-Ago interactions, which stabilize RISC, may be affected by PrPSc presence. Similarly, PrPSc could possibly impact PrPC-Dicer interactions and thus miRNA maturation and function. Incorporated into the RISC complex, miRNAs promote either translational repression or mRNA degradation, according to sequence complementary (5). SNPs in miRNA seed sequences and/or RNA editing events possibly occurring within miRNA seed sequences may impact miRNA-target mRNA interactions and even redirect miRNAs in new targets (5).
Other NDs also display deregulated miRNA biogenesis (Tan et al., 2015); upregulation of Drosha, DGCR8 and Dicer has been observed in MS patients brain (Jafari et al., 2015); further, abnormal cellular distribution of Drosha (Porta et al., 2015) and Dicer dysfunction (Emde et al., 2015) have been reported in ALS patients brains.
Peripheral circulating miRNA profiles corresponding to miRNAs from peripheral blood mononuclear cells (PBMCs), blood plasma/serum and CSF, either as free-circulating molecules or as exosome contents, may provide important indicators of pathophysiological processes in the brain (Sheinerman and Umansky, 2013; Grasso et al., 2015).
In contrast to other NDs such as AD (Kiko et al., 2013; Müller et al., 2014; Lusardi et al., 2016) and MS (Quintana et al., 2017), very limited information on experimentally validated miRNA alterations in CSF from healthy and prion diseases affected individuals is available. A preliminary analysis including a small number of miRNAs selected based on their deregulation in sCJD human brain, indicated low correspondence between altered miRNAs in sCJD brain and CSF. Several miRNAs displaying increased levels in sCJD brain, such as miR-26a-5p, miR-195-5p, let-7i-3p, miR-378a-3p, miR-449a, miR-124-3p, and miR-154-5p, did not show significant differences in sCJD CSF samples compared to healthy individuals (Llorens et al., 2018). However, identification of miR-204-5p differential levels in the CSF of controls and sCJD patients, indicates the potential use of CSF miRNAs as biomarkers for prion diseases diagnosis.
Regarding the diagnostic potential of miRNAs in prion diseases utilizing less invasive sampling methods, a recent study reported increased levels of circulating miR-342-3p and miR-21-5p in the plasma of sheep naturally affected by scrapie (Rubio et al., 2017), encouraging high-throughput analyses of plasma miRNAs in animal and human prion disease cases in relation to control samples and further validation of the most potent targets.
Interestingly, increased levels of miR-342-3p and miR-21-5p were also reported in exosomes released from murine hypothalamic cells infected with the mouse-adapted M1000 strain of human GSS (GSS-GT1-7) (Bellingham et al., 2012). Exosomes are lipid vesicles derived from most cells, including neurons and glia; they carry specific enriched subsets of nucleic acids, including miRNAs and mRNAs, various proteins and lipids. Exosomal pathways are considered as contributors to inter-cellular communication, and to several neurodegenerative states by spreading pathological proteins and other disease-associated molecules (Hartmann et al., 2017; Soria et al., 2017). In prion diseases, the exosomal miRNA provides an appealing approach for disease-specific diagnostic signatures. Since exosomes may be detected in CSF, blood plasma or serum, it is tempting to speculate that exosomes isolated from body fluids of prion affected individuals would present specific miRNA patterns of potential diagnostic value. Toward this goal, miR-21-5p and miR-322-5p (also identified as upregulated in exosomes from GSS infected cells, Bellingham et al., 2012), not previously suggested as biomarkers in blood serum/plasma of other NDs such as AD (Geekiyanage et al., 2012; Kiko et al., 2013; Dong et al., 2015; Guo et al., 2017; Nagaraj et al., 2017; Zeng et al., 2017), MS (Vistbakka et al., 2017), PD (Ding et al., 2016; Dong et al., 2016; Ma et al., 2016), and ALS (Freischmidt et al., 2013), emerge as prion-specific biomarker candidates. Other prion infected exosomal miRNAs with diagnostic potential include let-7b-5p, miR-29b-3p, miR-222-3p, and miR-342-3p; these miRNAs display inverse deregulation in prion infected exosomes (upregulation, Bellingham et al., 2012) compared to ALS (let-7b-5p in ALS patients serum, Freischmidt et al., 2013), or AD [miR-29b-ep in AD patients serum (Geekiyanage et al., 2012) and/or PBMCs (Villa et al., 2013), miR-222-3p in AD patients serum (Zeng et al., 2017) and miR-342-3p in AD serum (Tan et al., 2014)]. Other upregulated in prion infected exosomes miRNAs, such as let-7i-5p and miR-128-3p, and the downregulated miR-146a-5p, display similar deregulation patterns in MS patients exosomes (let-7i-5p, Kimura et al., 2018), Primary Progressive (PPMS) MS patients serum (miR-128-3p, Vistbakka et al., 2017) and AD (miR-146a-5p, Kiko et al., 2013; Dong et al., 2015) or PD patients serum (miR-146a-5p, Ma et al., 2016).
Further high throughput studies and subsequent validation are required to identify effective prion specific miRNA biomarkers in body fluids.
To explore the functional role of dysregulated miRNA networks in prion disease pathology it is crucial to understand the contribution of specific miRNA-mRNA target interactions involved in disease mechanisms in vivo. Transcriptomic-wide studies, such as high-throughput sequencing of RNA isolated by RISC immunoprecipitation could allow the global identification of miRNAs and their targets in a tissue- and cell-specific manner in prion diseases. The first approach toward profiling active miRNAs in prion disease was provided by (Llorens et al., 2018), through identification of disease-relevant miRNAs bound to Argonaute proteins in sCJD brain. In order to gain a complete picture of the role of miRNA dysregulation in disease, reported miRNA alterations need to be integrated into the complex cellular context of the brain and temporal disease evolution. Temporal and cellular resolution can be achieved by single-cell RNAseq, miRNA in situ hybridization techniques and a broad range of experimental methods that are able to reflect in vivo disease processes.
miRNAs hold potential to restore dysregulated pathways within critical time periods in prion disease progression, due to their ability to simultaneously control a large number of genes (Ouellet et al., 2006). The use of artificial miRNAs targeting PrPC has been demonstrated to reduce PrPC expression, inhibiting prion propagation in neuroblastoma cells (Kang et al., 2011) and in primary neuronal cultures (Pfeifer et al., 2006; Kang et al., 2017). Lentivector-mediated RNAi reduction of endogenous PrPC in scrapie-infected mice renders them resistant to prion infection, demonstrating the therapeutic potential of RNAi based therapy in prion diseases (Pfeifer et al., 2006; Ridolfi and Abdel-Haq, 2018). In this context, recent studies revealed exosomes as promising delivery systems, crossing the blood brain barrier (Ha et al., 2016; Chen et al., 2017; Yang et al., 2017).
Studying miRNA dysregulation in prion diseases mouse models is an appealing approach to dissect the causative involvement and consequential effects of miRNA alterations in prion disease pathology. Especially, the sCJD mouse model recapitulates disease hallmarks (Padilla et al., 2011) and resembles dysregulated gene expression networks during disease progression, including the brain region-specific miRNA alterations observed in sCJD (Llorens et al., 2014, 2018). This model provides an attractive tool to study the specific role of miRNA-regulated pathways in prion disease progression and a unique platform to assess biomarker candidates and/or therapeutic targets.
We summarized the current knowledge on miRNA alterations and underlying cellular mechanisms in human prion diseases, highlighting potential links between impaired miRNA regulatory pathways and disease etiology. Gaining a complete picture of disease-associated miRNA signatures in a cell-type dependent manner will be the first step to new research lines in developing therapeutic strategies for prion diseases.
EK, KT, and FL conceived the idea. EK and KT drafted the review manuscript. KX, IF, DD, IZ, TS, and FL critically reviewed the article. All the authors contributed to literature review, and revised and approved the manuscript.
This study was funded by IKY (State Scholarships Foundation, Greece) Postdoc Fellowships of Excellence – Siemens to EK, the Spanish Ministry of Health, Instituto Carlos III-Fondo Social Europeo (CP16/00041) to FL, and the Interreg POCTEFA – FEDER project to IF.
The authors declare that the research was conducted in the absence of any commercial or financial relationships that could be construed as a potential conflict of interest.
We acknowledge support by the German Research Foundation and the Open Access Publication Funds of the Göttingen University. We would like to thank Mrs. Aikaterini Kerezoglou for providing administrative assistance.
Abe, M., and Bonini, N. M. (2013). MicroRNAs and neurodegeneration: role and impact. Trends Cell Biol. 23, 30–36. doi: 10.1016/j.tcb.2012.08.013
Appleby, B. S., Appleby, K. K., Crain, B. J., Onyike, C. U., Wallin, M. T., and Rabins, P. V. (2009). Characteristics of established and proposed sporadic Creutzfeldt-Jakob disease variants. Arch. Neurol. 66, 208–215. doi: 10.1001/archneurol.2008.533
Arena, A., Iyer, A. M., Milenkovic, I., Kovács, G. G., Ferrer, I., Perluigi, M., et al. (2017). Developmental expression and dysregulation of miR-146a and miR-155 in down’s syndrome and mouse models of down’s syndrome and Alzheimer’s disease. Curr. Alzheimer Res. 14, 1305–1317. doi: 10.2174/1567205014666170706112701
Bartel, D. P. (2009). MicroRNAs: target recognition and regulatory functions. Cell 136, 215–233. doi: 10.1016/j.cell.2009.01.002
Bellingham, S. A., Coleman, B. M., and Hill, A. F. (2012). Small RNA deep sequencing reveals a distinct miRNA signature released in exosomes from prion-infected neuronal cells. Nucleic Acids Res. 40, 10937–10949. doi: 10.1093/nar/gks832
Bellingham, S. A., and Hill, A. F. (2017). Analysis of miRNA signatures in neurodegenerative prion disease. Methods Mol. Biol. 1658, 67–80. doi: 10.1007/978-1-4939-7244-9_6
Boese, A. S., Saba, R., Campbell, K., Majer, A., Medina, S., Burton, L., et al. (2016). MicroRNA abundance is altered in synaptoneurosomes during prion disease. Mol. Cell. Neurosci. 71, 13–24. doi: 10.1016/j.mcn.2015.12.001
Brandner, S., and Jaunmuktane, Z. (2017). Prion disease: experimental models and reality. Acta Neuropathol. 133, 197–222. doi: 10.1007/s00401-017-1670-1675
Briggs, C. E., Wang, Y., Kong, B., Woo, T. U., Iyer, L. K., and Sonntag, K. C. (2015). Midbrain dopamine neurons in Parkinson’s disease exhibit a dysregulated miRNA and target-gene network. Brain Res. 1618, 111–121. doi: 10.1016/j.brainres.2015.05.021
Bronevetsky, Y., and Ansel, K. M. (2013). Regulation of miRNA biogenesis and turnover in the immune system. Immunol. Rev. 253, 304–316. doi: 10.1111/imr.12059
Burak, K., Lamoureux, L., Boese, A., Majer, A., Saba, R., and Niu, Y. (2018). MicroRNA-16 targets mRNA involved in neurite extension and branching in hippocampal neurons during presymptomatic prion disease. Neurobiol. Dis. 112, 1–13. doi: 10.1016/j.nbd.2017.12.011
Carroll, J. A., Striebel, J. F., Race, B., Phillips, K., and Chesebro, B. (2015). Prion infection of mouse brain reveals multiple new upregulated genes involved in neuroinflammation or signal transduction. J. Virol. 89, 2388–2404. doi: 10.1128/JVI.02952-2914
Chen, J., Zhao, J. B., Zhao, J., and Li, S. (2017). Potential roles of exosomal MicroRNAs as diagnostic biomarkers and therapeutic application in Alzheimer’s disease. Neural Plast. 2017:7027380. doi: 10.1155/2017/7027380
Chen, Y.-L., and Shen, C.-K. (2013). Modulation of mGluR-dependent MAP1B translation and AMPA receptor endocytosis by MicroRNA miR-146a-5p. J. Neurosci. 33, 9013–9020. doi: 10.1523/JNEUROSCI.5210-12.2013
Cheng, X., Ku, C. H., and Siow, R. C. (2013). Regulation of the Nrf2 antioxidant pathway by microRNAs: new players in micromanaging redox homeostasis. Free Radic. Biol. Med. 64, 4–11. doi: 10.1016/j.freeradbiomed.2013.07.025
Chugh, P., and Dittmer, D. P. (2013). Potential pitfalls in microRNA profiling. Wiley Interdiscip. Rev. RNA 3, 17–36. doi: 10.1002/wrna.1120.Potential
Colby, D. W., and Prusiner, S. B. (2011). Prions. Cold Spring Harb. Perspect. Med. 3:a006833. doi: 10.1101/cshperspect.a006833
Cui, J. G., Li, Y. Y., Zhao, Y., Bhattacharjee, S., and Lukiw, W. J. (2010). Differential regulation of interleukin-1 receptor-associated kinase-1 (IRAK-1) and IRAK-2 by microRNA-146a and NF-κB in stressed human astroglial cells and in Alzheimer disease. J. Biol. Chem. 285, 38951–38960. doi: 10.1074/jbc.M110.178848
Cui, L., Li, Y., Ma, G., Wang, Y., Cai, Y., Liu, S., et al. (2014). A functional polymorphism in the promoter region of microRNA-146a is associated with the risk of Alzheimer disease and the rate of cognitive decline in patients. PLoS One 9:e89019. doi: 10.1371/journal.pone.0089019
Cunningham, C., Deacon, R., Wells, H., Boche, D., Waters, S., and Picanco Diniz, C. (2003). Synaptic changes characterize early behavioural signs in the ME7 model of murine prion disease. Eur. J. Neurosci. 17, 2147–2155. doi: 10.1046/j.1460-9568.2003.02662.x
Ding, H., Huang, Z., Chen, M., Wang, C., Chen, X., Chen, J., et al. (2016). Identification of a panel of five serum miRNAs as a biomarker for Parkinson’s disease. Parkinsonism Relat. Disord. 22, 68–73. doi: 10.1016/j.parkreldis.2015.11.014
Dong, H., Li, J., Huang, L., Chen, X., Li, D., Wang, T., et al. (2015). Serum MicroRNA profiles serve as novel biomarkers for the diagnosis of Alzheimer’s disease. Dis. Markers 2015:625659. doi: 10.1155/2015/625659
Dong, H., Wang, C., Lu, S., Yu, C., Huang, L., Feng, W., et al. (2016). A panel of four decreased serum microRNAs as a novel biomarker for early Parkinson’s disease. Biomarkers 21, 129–137. doi: 10.3109/1354750X.2015.1118544
Emde, A., Eitan, C., Liou, L.-L., Libby, R. T., Rivkin, N., Magen, I., et al. (2015). Dysregulated miRNA biogenesis downstream of cellular stress and ALS-causing mutations: a new mechanism for ALS. EMBO J. 34, 2633–2651. doi: 10.15252/embj.201490493
Finnegan, E. F., and Pasquinelli, A. E. (2013). MicroRNA biogenesis- regulating the regulators. Crit. Rev. Biochem. Mol. Biol. 48, 51–68. doi: 10.3109/10409238.2012.738643
Freischmidt, A., Müller, K., Ludolph, A. C., and Weishaupt, J. H. (2013). Systemic dysregulation of TDP-43 binding microRNAs in amyotrophic lateral sclerosis. Acta Neuropathol. Commun. 1:42. doi: 10.1186/2051-5960-1-42
Gao, C., Shi, Q., Wei, J., Zhou, W., Xiao, K., Wang, J., et al. (2017). The Associations of two SNPs in miRNA-146a and one SNP in ZBTB38-RASA2 with the disease susceptibility and the clinical features of the Chinese patients of sCJD and FFI. Prion 12, 34–41. doi: 10.1080/19336896.2017.1405885
Gao, C., Wei, J., Zhang, B. Y., Shi, Q., Chen, C., Wang, J., et al. (2016). MiRNA expression profiles in the brains of mice infected with scrapie agents 139A, ME7 and S15. Emerg. Microbes Infect. 5:e115. doi: 10.1038/emi.2016.120
Geekiyanage, H., Jicha, G. A., Nelson, P. T., and Chan, C. (2012). Blood serum miRNA: non-invasive biomarkers for Alzheimer’s disease. Exp. Neurol. 235, 491–496. doi: 10.1016/j.expneurol.2011.11.026
Gibbings, D., Leblanc, P., Jay, F., Pontier, D., Michel, F., Schwab, Y., et al. (2012). Human prion protein binds argonaute and promotes accumulation of microRNA effector complexes. Nat. Struct. Mol. Biol. 19, 517–524. doi: 10.1038/nsmb.2273
Grasso, M., Piscopo, P., Crestini, A., Confaloni, A., and Denti, M. A. (2015). Circulating microRNAs in neurodegenerative diseases. EXS 106, 151–169. doi: 10.1007/978-3-0348-0955-9_7
Gulyaeva, L. F., and Kushlinskiy, N. E. (2016). Regulatory mechanisms of microRNA expression. J. Trans. Med. 14:143. doi: 10.1186/s12967-016-0893-x
Guo, R., Fan, G., Zhang, J., Wu, C., Du, Y., Ye, H., et al. (2017). A 9-microRNA signature in serum serves as a noninvasive biomarker in early diagnosis of Alzheimer’s disease. J. Alzheimers Dis. 60, 1365–1377. doi: 10.3233/JAD-170343
Ha, D., Yang, N., and Nadithe, V. (2016). Exosomes as therapeutic drug carriers and delivery vehicles across biological membranes: current perspectives and future challenges. Acta Pharm. Sin. B 6, 287–296. doi: 10.1016/j.apsb.2016.02.001
Ha, M., and Kim, V. N. (2014). Regulation of microRNA biogenesis. Nat. Rev. Mol. Cell Biol. 15, 509–524. doi: 10.1038/nrm3838
Hammond, S. M. (2015). An overview of microRNAs. Adv. Drug Deliv. Rev. 87, 3–14. doi: 10.1016/j.addr.2015.05.001
Hartmann, A., Muth, C., Dabrowski, O., Krasemann, S., and Glatzel, M. (2017). Exosomes and the prion protein: more than one truth. Front. Neurosci. 11:194. doi: 10.3389/fnins.2017.00194
Hébert, S. S., and De Strooper, B. (2009). Alterations of the microRNA network cause neurodegenerative disease. Trends Neurosci. 32, 199–206. doi: 10.1016/j.tins.2008.12.003
Hebert, S. S., Horre, K., Nicolai, L., Papadopoulou, A. S., Mandemakers, W., and Silahtaroglu, A. N. (2008). Loss of microRNA cluster miR-29a/b-1 in sporadic Alzheimer’s disease correlates with increased BACE1/-secretase expression. Proc. Natl. Acad. Sci. U.S.A. 105, 6415–6420. doi: 10.1073/pnas.0710263105
Hilton, K. J., Cunningham, C., Reynolds, R. A., and Perry, V. H. (2013). Early hippocampal synaptic loss precedes neuronal loss and associates with early behavioural deficits in three distinct strains of prion disease. PLoS One 8:e68062. doi: 10.1371/journal.pone.0068062
Jafari, N., Shaghaghi, H., Mahmoodi, D., Shirzad, Z., Alibeiki, F., and Bohlooli, S. (2015). Overexpression of microRNA biogenesis machinery: drosha, DGCR8 and dicer in multiple sclerosis patients. J. Clin. Neurosci. 22, 200–203. doi: 10.1016/j.jocn.2014.06.106
Jin, Y., and Lee, C. (2013). Single nucleotide polymorphisms associated with MicroRNA regulation. Biomolecules 3, 287–302. doi: 10.3390/biom3020287
Junker, A., Krumbholz, M., Eisele, S., Mohan, H., Augstein, F., and Bittner, R. (2009). MicroRNA profiling of multiple sclerosis lesions identifies modulators of the regulatory protein CD47. Brain 132, 3342–3352. doi: 10.1093/brain/awp300
Kang, S. G., Kim, C., Aiken, J., Yoo, H. S., and McKenzie, D. (2017). Dual MicroRNA to cellular prion protein inhibits propagation of pathogenic prion protein in cultured cells. Mol. Neurobiol. Mol. Neurobiol. 55, 2384–2396. doi: 10.1007/s12035-017-0495-495
Kang, S. G., Mi Roh, Y., Lau, A., Westaway, D., McKenzie, D., and Aiken, J. (2011). Establishment and characterization of PRNP knock-down neuroblastoma cells using dual microRNA-mediated RNA interference. Prion 5, 93–102. doi: 10.4161/pri.5.2.15621
Kiko, T., Nakagawa, K., Tsuduki, T., Furukawa, K., Arai, H., and Miyazawa, T. (2013). MicroRNAs in plasma and cerebrospinal fluid as potential markers for Alzheimer’s disease. J. Alzheimers Dis.? 39, 253–259. doi: 10.3233/JAD-130932
Kimura, K., Hohjoh, H., Fukuoka, M., Sato, W., Oki, S., and Tomi, C. (2018). Circulating exosomes suppress the induction of regulatory T cells via let-7i in multiple sclerosis. Nat. Commun. 9:17. doi: 10.1038/s41467-017-02406-2402
Kiselev, I., Bashinskaya, V., Kulakova, O., Baulina, N., Popova, E., and Boyko, A. (2015). Variants of microRNA genes: gender-specific associations with multiple sclerosis risk and severity. Int. J. Mol. Sci. 16, 20067–20081. doi: 10.3390/ijmsl60820067
Koshiol, J., Wang, E., Zhao, Y., Marincola, F., and Landi, M. T. (2010). Strengths and limitations of laboratory procedures for microRNA detection. Cancer Epidemiol. Biomark. Prev.? 19, 907–911. doi: 10.1158/1055-9965.EPI-10-0071
Lau, P., Bossers, K., Janky, R., Salta, E., Frigerio, C. S., Barbash, S., et al. (2013). Alteration of the microRNA network during the progression of Alzheimer’s disease. EMBO Mol. Med. 5, 1613–1634. doi: 10.1002/emmm.201201974
Li, Y., Du, C., Wang, W., Ma, G., Cui, L., Zhou, H., et al. (2015). Genetic association of MiR-146a with multiple sclerosis susceptibility in the Chinese population. Cell Physiol. Biochem. 35, 281–291. doi: 10.1159/000369695
Li, Y. Y., Cui, J. G., Dua, P., Pogue, A. I., Bhattacharjee, S., and Lukiw, W. J. (2011). Differential expression of miRNA-146a-regulated inflammatory genes in human primary neural, astroglial and microglial cells. Neurosci. Lett. 499, 109–113. doi: 10.1016/j.neulet.2011.05.044
Llorens, F., Lopez-Gonzalez, I., Thune, K., Carmona, M., Zafar, S., and Andeoletti, O. (2014). Subtype and regional-specific neuroinflammation in sporadic Creutzfeldt-Jakob disease. Front. Aging Neurosci. 6:198. doi: 10.3389/fnagi.2014.00198
Llorens, F., Thüne, K., Martí, E., Kanata, E., Dafou, D., Díaz-Lucena, D., et al. (2018). Regional and subtype-dependent miRNA signatures in sporadic Creutzfeldt-Jakob disease are accompanied by alterations in miRNA silencing machinery and biogenesis. PLoS Pathog. 14:e1006802. doi: 10.1371/journal.ppat.1006802
Llorens, F., Thüne, K., Sikorska, B., Schmitz, M., Tahir, W., Fernández-Borges, N., et al. (2017). Altered Ca(2+) homeostasis induces calpain-cathepsin axis activation in sporadic Creutzfeldt-Jakob disease. Acta Neuropathol. Commun. 5:35. doi: 10.1186/s40478-017-0431-y
Lukiw, W. J., Dua, P., Pogue, A. I., Eicken, C., and Hill, J. M. (2011). Upregulation of micro RNA-146a (miRNA-146a), a marker for inflammatory neurodegeneration, in sporadic Creutzfeldt-Jakob disease (sCJD) and Gerstmann-Straussler-Scheinker (GSS) syndrome. J. Toxicol. Environ. Health A 74, 1460–1468. doi: 10.1080/15287394.2011.618973
Lusardi, T. A., Phillips, J. I., Wiedrick, J. T., Harrington, C. A., Lind, B., and Lapidus, J. A. (2016). MicroRNAs in human cerebrospinal fluid as biomarkers for Alzheimer’s disease. J. Alzheimers Dis? 55, 1223–1233. doi: 10.3233/JAD-160835
Ma, W., Li, Y., Wang, C., Xu, F., Wang, M., and Liu, Y. (2016). Serum miR-221 serves as a biomarker for Parkinson’s disease: miR-221 as biomarker for PD. Cell Biochem. Funct. 34, 511–515. doi: 10.1002/cbf.3224
Maiorano, N. A., and Mallamaci, A. (2009). Promotion of embryonic cortico-cerebral neuronogenesis by miR-124. Neural Dev. 4:40. doi: 10.1186/1749-8104-4-40
Majer, A., Medina, S. J., Niu, Y., Abrenica, B., Manguiat, K. J., and Frost, K. L. (2012). Early mechanisms of pathobiology are revealed by transcriptional temporal dynamics in hippocampal CA1 neurons of prion infected mice. PLoS Pathog. 8:e1003002. doi: 10.1371/journal.ppat.1003002
Montag, J., Brameier, M., Schmädicke, C., Gilch, S., Schätzl, H. M., and Motzkus, D. (2012). A genome-wide survey for prion-regulated miRNAs associated with cholesterol homeostasis. BMC Genomics 13:486. doi: 10.1186/1471-2164-13-486
Montag, J., Hitt, R., Opitz, L., Schulz-Schaeffer, W. J., Hunsmann, G., and Motzkus, D. (2009). Upregulation of miRNA hsa-miR-342-3p in experimental and idiopathic prion disease. Mol. Neurodegener. 4:36. doi: 10.1186/1750-1326-4-36
Moszyńska, A., Gebert, M., Collawn, J. F., and Bartoszewski, R. (2017). SNPs in microRNA target sites and their potential role in human disease. Open Biol. 7:170019. doi: 10.1098/rsob.170019
Müller, M., Bea Kuiperij, H., Claassen, J. A., Küsters, B., and Verbeek, M. M. (2014). MicroRNAs in Alzheimer’s disease: differential expression in hippocampus and cell-free cerebrospinal fluid. Neurobiol. Aging 35, 152–158. doi: 10.1016/j.neurobiolaging.2013.07.005
Nagaraj, S., Laskowska-Kaszub, K., Dêbski, K. J., Wojsiat, J., Debrowski, M., and Gabryelewicz, T. (2017). Profile of 6 microRNA in blood plasma distinguish early stage Alzheimer’s disease patients from non-demented subjects. Oncotarget 8, 16122–16143. doi: 10.18632/oncotarget.15109
Noorbakhsh, F., Ellestad, K. K., Maingat, F., Warren, K. G., and Han, M. H. (2011). Impaired neurosteroid synthesis in multiple sclerosis. Brain 134, 2703–2721. doi: 10.1093/brain/awr200
Ouellet, D. L., Perron, M. P., Gobeil, L. A., Plante, P., and Provost, P. (2006). MicroRNAs in gene regulation: when the smallest governs it all. J. Biomed. Biotechnol. 2006:69616. doi: 10.1155/JBB/2006/69616
Padilla, D., Beringue, V., Espinosa, C., Andreoletti, O., Jaumain, E., Reine, F., et al. (2011). Sheep and goat BSE propagate more efficiently than cattle BSE in human PrP transgenic mice. PLoS Pathog. 7:e1001319. doi: 10.1371/journal.ppat.1001319
Park, R., Lee, W. J., and Ji, J. D. (2016). Association between the three functional miR-146a single-nucleotide polymorphisms, rs2910164, rs57095329, and rs2431697, and autoimmune disease susceptibility: a meta-analysis. Autoimmunity 49, 451–458. doi: 10.3109/08916934.2016.1171854
Parsi, S., Smith, P. Y., Goupil, C., Dorval, V., and Hébert, S. (2015). Preclinical evaluation of miR-15/107 family members as multifactorial drug targets for Alzheimer’s disease. Mol. Ther. Nucleic Acids 4:e256. doi: 10.1038/mtna.2015.33
Pfeifer, A., Eigenbrod, S., Al-Khadra, S., Hofmann, A., Mitteregger, G., and Moser, M. (2006). Lentivector-mediated RNAi efficiently suppresses prion protein and prolongs survival of scrapie-infected mice. J. Clin. Investig. 116, 3204–3210. doi: 10.1172/JCI29236
Porta, S., Kwong, L. K., Trojanowski, J. Q., and Lee, V. M. (2015). Drosha inclusions are new components of dipeptide-repeat protein aggregates in FTLD-TDP and ALS C9orf72 expansion cases. J. Neuropathol. Exp. Neurol. 74, 380–387. doi: 10.1097/NEN.0000000000000182
Pritchard, C. C., Cheng, H. H., and Tewari, M. (2012). MicroRNA profiling: approaches and considerations. Nat. Rev. Genet. 13, 358–369. doi: 10.1038/nrg3198
Quintana, E., José Ortega, F., Robles-Cedeńo, M., Luisa Villar, R., Buxó, M., Maria Mercader, J., et al. (2017). miRNAs in cerebrospinal fluid identify patients with MS and specifically those with lipid-specific oligoclonal IgM bands. Mult. Scler. 23, 1716–1726. doi: 10.1177/1352458516684213
Raabe, C. A., Tang, T. H., Brosius, J., and Rozhdestvensky, T. S. (2014). Biases in small RNA deep sequencing data. Nucleic Acids Res. 42, 1414–1426. doi: 10.1093/nar/gkt1021
Ridolfi, B., and Abdel-Haq, H. (2018). Neurodegenerative disorders treatment: the MicroRNA role. Curr. Gene Ther. 18, 327–363. doi: 10.2174/1566523218666180119120726
Rubio, D. S., López-Pérez,Ó., De Andrés Pablo,Á., Bolea, R., Osta, R., Badiola, J. J., et al. (2017). Increased circulating microRNAs miR-342-3p and miR-21-5p in natural sheep prion disease. J. Gen. Virol. 98, 305–310. doi: 10.1099/jgv.0.000685
Saba, R., and Booth, S. A. (2013). Polymorphisms affecting miRNA regulation: a new level of genetic variation affecting disorders and diseases of the human CNS. Future Neurol. 8, 411–431. doi: 10.2217/fnl.13.25
Saba, R., Goodman, C. D., Rhiannon, L. C. H., Robertson, C., and Booth, S. A. (2008). A miRNA signature of prion induced neurodegeneration. PLoS One 3:e3652. doi: 10.1371/journal.pone.0003652
Saba, R., Gushue, S., Rhiannon, L. C. H., Manguiat, K., Medina, S., and Robertson, C. (2012). MicroRNA 146a (miR-146a) is over-expressed during prion disease and modulates the innate immune response and the microglial activation state. PLoS One 7:e30832. doi: 10.1371/journal.pone.0030832
Saba, R., Medina, S. J., and Booth, S. A. (2014). A functional SNP catalog of overlapping miRNA-binding sites in genes implicated in prion disease and other neurodegenerative disorders. Hum. Mutat. 35, 1233–1248. doi: 10.1002/humu.22627
Sanuki, R., Onishi, A., Koike, C., Muramatsu, R., Watanabe, S., Muranishi, Y., et al. (2011). miR-124a is required for hippocampal axogenesis and retinal cone survival through Lhx2 suppression. Nat. Neurosci. 14, 1125–1134. doi: 10.1038/nn.2897
Schanen, B. C., and Li, X. (2011). Transcriptional regulation of mammalian miRNA genes. Genomics 97, 1–6. doi: 10.1016/j.ygeno.2010.10.005
Sethi, P., and Lukiw, W. J. (2009). Micro-RNA abundance and stability in human brain: specific alterations in Alzheimer’s disease temporal lobe neocortex. Neurosci. Lett. 459, 100–104. doi: 10.1016/j.neulet.2009.04.052
Sheinerman, K. S., and Umansky, S. R. (2013). Circulating cell-free microRNA as biomarkers for screening, diagnosis and monitoring of neurodegenerative diseases and other neurologic pathologies. Front. Cell. Neurosci. 7:150. doi: 10.3389/fncel.2013.00150
Slezak-Prochazka, I., Durmus, S., Kroesen, B. J., and van den Berg, A. (2010). MicroRNAs, macrocontrol: regulation of miRNA processing. RNA 16, 1087–1095. doi: 10.1261/rna.1804410
Soria, F. N., Pampliega, O., Bourdenx, M., Meissner, W. G., Bezard, E., and Dehay, B. (2017). Exosomes, an unmasked culprit in neurodegenerative diseases. Front. Neurosci. 11:26. doi: 10.3389/fnins.2017.00026
Sun, Y., Li, Q., Gui, H., Xu, P., Yang, L., Su, F., et al. (2013). MicroRNA-124 mediates the cholinergic anti-inflammatory action through inhibiting the production of pro-inflammatory cytokines. Cell Res. 23, 1270–1283. doi: 10.1038/cr.2013.116
Tan, L., Yu, J. T., Tan, M. S., Liu, Y., Wang, F., Zhang, W., et al. (2014). Genome-wide serum microRNA expression profiling identifies serum biomarkers for Alzheimer’s disease. J. Alzheimers Dis. 40, 1017–1027. doi: 10.3233/JAD-132144
Tan, L., Yu, T., and Tan, L. (2015). Causes and consequences of MicroRNA dysregulation in neurodegenerative diseases. Mol. Neurobiol. 51, 1249–1262. doi: 10.1007/s12035-014-8803-8809
Tomaselli, S., Bonamassa, B., Alisi, A., Locatelli, N. F., and Gallo, A. (2013). ADAR enzyme and miRNA story: a nucleotide that can make the difference. Int. J. Mol. Sci. 14, 22796–22816. doi: 10.3390/ijms141122796
Villa, C., Ridolfi, E., Fenoglio, C., Ghezzi, L., Vimercati, R., Clerici, F., et al. (2013). Expression of the transcription factor Sp1 and its regulatory Hsa-miR-29b in peripheral blood mononuclear cells from patients with Alzheimer’s disease. J. Alzheimers Dis. 35, 487–494. doi: 10.3233/JAD-122263
Vistbakka, J., Elovaara, I., Lehtimäki, T., and Hagman, S. (2017). Circulating microRNAs as biomarkers in progressive multiple sclerosis. Mult. Scler. J. 23, 403–412. doi: 10.1177/1352458516651141
Wang, G., Huang, Y., Wang, L., Zhang, Y. F., Xu, J., Zhou, Y., et al. (2016). MicroRNA-146a suppresses ROCK1 allowing hyperphosphorylation of Tau in Alzheimer’s disease. Sci. Rep. 6:26697. doi: 10.1038/srep26697
Watts, J. C., and Prusiner, S. B. (2014). Mouse models for studying the formation and propagation of prions. J. Biol. Chem. 289, 19841–19849. doi: 10.1074/jbc.R114.550707
Wilczynska, A., and Bushell, M. (2015). The complexity of miRNA-mediated repression. Cell Death Differ. 22, 22–33. doi: 10.1038/cdd.2014.112
Yang, J., Zhang, X., Chen, X., Wang, L., and Yang, G. (2017). Exosome mediated delivery of miR-124 promotes neurogenesis after ischemia. Mol. Ther. Nucleic Acids 7, 278–287. doi: 10.1016/j.omtn.2017.04.010
Zeng, Q., Zou, L., Qian, L., Zhou, F., Nie, H., Yu, S., et al. (2017). Expression of microRNA-222 in serum of patients with Alzheimer’s disease. Mol. Med. Rep. 16, 5575–5579. doi: 10.3892/mmr.2017.7301
Zhang, B., Wang, A., Xia, C., Lin, Q., and Che, C. (2015). A single nucleotide polymorphism in primary-microRNA-146a reduces the expression of mature microRNA-146a in patients with Alzheimer’s disease and is associated with the pathogenesis of Alzheimer’s disease. Mol. Med. Rep. 12, 4037–4042. doi: 10.3892/mmr.2015.3968
Zhang, Q., Wang, Q., and Pan, X. (2007). MicroRNAs and their regulatory roles in animals and plants. J. Cell. Physiol. 210, 279–289. doi: 10.1002/jcp.20869
Zhou, Y., Chen, M., Simpson, S., Lucas, R. M., Charlesworth, J. C., Blackburn, N., et al. (2017). Common genetic variation within miR-146a predicts disease onset and relapse in multiple sclerosis. Neurol. Sci. 39, 297–304. doi: 10.1007/s10072-017-3177-3171
Keywords: microRNAs, prion diseases, sCJD, prion diseases animal models, brain, CSF, blood, neurodegenerative disorders
Citation: Kanata E, Thüne K, Xanthopoulos K, Ferrer I, Dafou D, Zerr I, Sklaviadis T and Llorens F (2018) MicroRNA Alterations in the Brain and Body Fluids of Humans and Animal Prion Disease Models: Current Status and Perspectives. Front. Aging Neurosci. 10:220. doi: 10.3389/fnagi.2018.00220
Received: 10 April 2018; Accepted: 28 June 2018;
Published: 23 July 2018.
Edited by:
Ashok Kumar, University of Florida, United StatesReviewed by:
Harshini Sarojini, University of Louisville, United StatesCopyright © 2018 Kanata, Thüne, Xanthopoulos, Ferrer, Dafou, Zerr, Sklaviadis and Llorens. This is an open-access article distributed under the terms of the Creative Commons Attribution License (CC BY). The use, distribution or reproduction in other forums is permitted, provided the original author(s) and the copyright owner(s) are credited and that the original publication in this journal is cited, in accordance with accepted academic practice. No use, distribution or reproduction is permitted which does not comply with these terms.
*Correspondence: Eirini Kanata, ZWthbmF0YUBiaW8uYXV0aC5ncg== Katrin Thüne, ay50aHVlbmVAaG90bWFpbC5jb20=
†These authors have contributed equally to this work.
‡These authors share senior authorship.
Disclaimer: All claims expressed in this article are solely those of the authors and do not necessarily represent those of their affiliated organizations, or those of the publisher, the editors and the reviewers. Any product that may be evaluated in this article or claim that may be made by its manufacturer is not guaranteed or endorsed by the publisher.
Research integrity at Frontiers
Learn more about the work of our research integrity team to safeguard the quality of each article we publish.