- Biological Sciences, University of Southampton, Southampton General Hospital, Southampton, United Kingdom
Inflammation is a major component of neurodegenerative diseases. Microglia are the innate immune cells in the central nervous system (CNS). In the healthy brain, microglia contribute to tissue homeostasis and regulation of synaptic plasticity. Under disease conditions, they play a key role in the development and maintenance of the neuroinflammatory response, by showing enhanced proliferation and activation. Prion diseases are progressive chronic neurodegenerative disorders associated with the accumulation of the scrapie prion protein PrPSc, a misfolded conformer of the cellular prion protein PrPC. This review article provides the current knowledge on the role of microglia in the pathogenesis of prion disease. A large body of evidence shows that microglia can trigger neurotoxic pathways contributing to progressive degeneration. Yet, microglia are also crucial for controlling inflammatory, repair and regenerative processes. This dual role of microglia is regulated by multiple pathways and evidences the ability of these cells to polarize into distinct phenotypes with characteristic functions. The awareness that the neuroinflammatory response is inextricably involved in producing tissue damage as well as repair in neurodegenerative disorders, opens new perspectives for the modulation of the immune system. A better understanding of this complex process will be essential for developing effective therapies for neurodegenerative diseases, in order to improve the quality of life of patients and mitigating the personal, economic and social consequences derived from these diseases.
Microglia in The Healthy Brain
Origin and Turnover of Microglia
Since the initial description of microglial cells by Pio Del Rio Hortega (del Río-Hortega, 1920, 1932; del Río-Hortega and Penfield, 1927), their origin has been a source of debate. However, it has been recently established that tissue resident macrophages as microglia originate from erythroid-myeloid progenitors (EMPs) emerging from the yolk sac (YS) during primitive hemeatopoiesis at embryonic stages 7.0 (E7.0) to E9.5 (Cuadros et al., 1993; Alliot et al., 1999; Schulz et al., 2012; Gomez Perdiguero et al., 2015; Sheng et al., 2015; Wang et al., 2015). A pioneering study by Ginhoux et al. (2010) allowed finding the earliest microglial progenitors in the YS during mouse development, thanks to fate-mapping experiments that allowed tagging early YS blood-island cells and then follow the emergence of microglial cells into the central nervous system (CNS). Fate mapping of YS progenitors from E6.5 to E7.0 produced tagging of less than 4% of adult microglia, whereas mapping from E7.0 to E7.25 produced 29% microglia being labeled, allowing the definition that primitive EMPs that arise before E7.5 are the main contributors to the adult microglial population (Ginhoux et al., 2010). Then, several studies using tamoxifen-inducible Cre lines in which the Cre-ER-T2/Mer-Cre-Mer protein was expressed under the control of different genes such as Colony stimulating factor receptor 1 (Csf1r; Schulz et al., 2012), C-kit (Sheng et al., 2015), TEK Receptor Tyrosine Kinase (Tie2; Gomez Perdiguero et al., 2015) corroborated that microglial progenitors have a YS origin. A recent study by Mass et al. (2016) has allowed a more precise definition of the sequence of differentiation steps leading to the adult microglial population. In the YS, uncommitted EMPs (Kit+ CD45lo Csf1r+ AA4.1+) differentiate into pre-macrophage (pMac; kit− CD45hi F4/80−) that do not yet have a microglial phenotype. From E9.5, as they initiate a core macrophage transcriptional program, those pMacs colonize the whole embryo in a C-X3-C motif chemokine receptor 1 (CX3CR1)-dependent manner. Indeed, at E9.5 and E10.5, CX3CR1-deficient embryos exhibit a delay in the colonization of progenitors and a decrease of pMacs and macrophages population in the head while they display an accumulation of pMacs in the YS and fetal liver (Mass et al., 2016). Immediately following colonization of the embryonic brain, a tissue specific transcriptional program is triggered and leads to the production of postnatal microglia, including a downregulation of T-cell immunoglobulin and mucin domain containing 4 (Timd4) and mannose receptor (Cd206) and an upregulation of Sal-like (Sall)1 and Sall3 (Lavin et al., 2014; Mass et al., 2016). As the embryo develops, microglia progenitors mature in a Interferon regulatory factor 8 (IRF8) and PU.1-dependant manner by expressing a set of different markers including CSF1R, Runt-related transcription factor (Runx1), ionized calcium-binding adaptor molecule 1 (Iba1), C-X3-C Motif Chemokine Receptor (CX3CR1), Tie2, the cluster of differentiation 45 (CD45) or C-kit (Kierdorf et al., 2013; Mass et al., 2016).
In humans, microglial cells are identified in the extracerebral mesenchyme around 4.5 gestational weeks. At 5 gestational weeks, they invade the parenchyma by entering the brain primordium via the developing meninges, ventricular zone and choroid plexus (Monier et al., 2006; Verney et al., 2010) and they only exhibit a ramified morphology around the 35th week (Hutchins et al., 1990; Esiri et al., 1991; Rezaie and Male, 1999).
In the adult, the microglial population is maintained by a self-renewal process (Lawson et al., 1992; Askew et al., 2017; Figure 1). A foundational study by Lawson et al. (1992) defined a remarkably slow turnover rate of adult murine microglia, by means of analyzing short-term 3H tymidine incorporation. However, more recent insight arising from repopulation paradigms suggested that microglia could have a higher turnover capacity in the steady state. The pharmacological depletion of microglia, by using a potent CSF1R inhibitor, is followed by the rapid reconstitution of the microglia population by proliferation of resident microglia, without the contribution of circulating monocytes (Elmore et al., 2014). A transgenic paradigm, allowing the depletion of microglia by diphtheria toxin injection, validated this repopulating capacity, independent of circulating monocytes (Bruttger et al., 2015). Although these repopulation paradigms are distal from modeling a homeostatic system, they suggested a latent potential for microglial cells to proliferate more rapidly than thought before, allowing the colonization of an empty niche. In this line, a recent study from our group showed that in mice and humans the turnover of microglia in the steady state is remarkably fast, allowing the whole population to be renewed several times during a lifetime (Askew et al., 2017). Indeed, whereas the previous study in the healthy brain, using 3H thymidine and immunohistochemistry for F4/80, had shown that, at a given time, only 0.05% of the microglia were proliferating (Lawson et al., 1992), Askew et al. (2017) recently demonstrated that 0.69% of the microglial population is proliferating by using more sensitive techniques (BrdU incorporation detected in Iba1+ cells and 2-photon live imaging). Microglial proliferation is balanced by microglial apoptosis, with these two mechanisms being synchronized in time and space, allowing for a rapid remodeling of the microglial landscape during a lifetime, without the contribution of circulating monocytes (Askew et al., 2017).
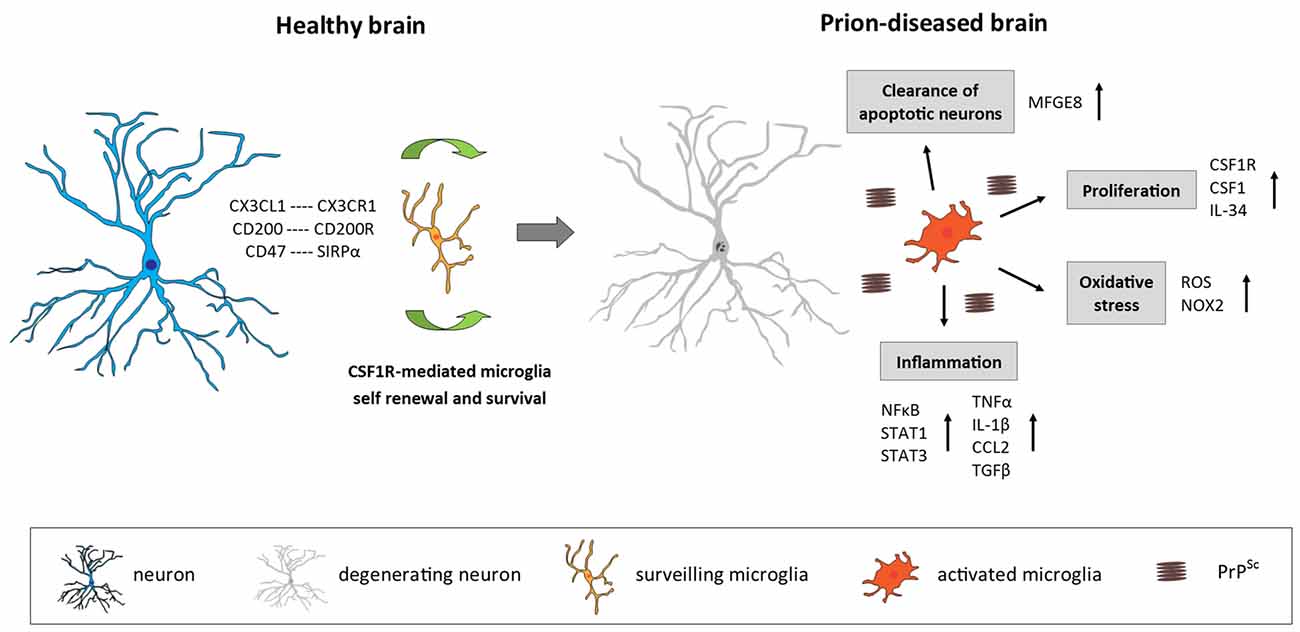
Figure 1. Microglia in the prion-diseased brain. In the healthy brain, microglia constantly surveil their microenvironment for any disturbance of brain homeostasis and are kept in this surveiling state by signals mainly originating from healthy neurons. The microglial population is maintained by local self-renewal mediated via CSF1R and its ligands CSF1 and IL-34. In the context of neurodegeneration and protein accumulation present in the prion-diseased brain, the microglial response is characterized by functional changes involving increased proliferation, an inflammatory activation and the removal of apoptotic neurons. CX3CL1, C-X3-C motif chemokine ligand 1; CX3CR1, C-X3-C motif chemokine receptor 1; CD200, Cluster of differentiation 200; CD47, Cluster of differentiation 47; SIRPα, Signal regulatory protein α; CSF1R, Colony stimulating factor 1 receptor; CSF1, Colony stimulating factor; IL-34, Interleukin 34; MFGE8, Milk fat globule-EGF factor 8 protein; ROS, Reactive oxygen species; NOX2, Nicotinamide adenine dinucleotide phosphate-oxidase (NADPH) oxidase 2; NFκB, Nuclear factor kappa-light-chain-enhancer of activated B cells; STAT1/3, Signal transducer and activator of transcription 1/3; TNFα, Tumor nerosis factor α; IL-1β, Interleukin 1β; CCL2, C-C motif chemokine ligand 2; TGFβ, Transforming growth factor β; PrPSc misfolded prion protein (scrapie).
Distribution and Function of Microglia in the Adult Brain
The density and morphology of microglia varies considerably across the healthy adult brain. In the mouse brain, there are an estimated total number of 3.5 × 106 microglial cells, however their distribution varies from 5% in the cortex and corpus callosum, to 12% in the substantia nigra (Lawson et al., 1990). In mice, microglia are more numerous in gray matter than white matter and areas as the hippocampus, basal ganglia and substantia nigra are particularly densely populated in microglia (Lawson et al., 1990). In comparison, the less densely populated areas include fiber tracts, cerebellum and much of the brainstem whereas the cerebral cortex, thalamus and hypothalamus have average cell densities (Lawson et al., 1990). This cell density remains constant from early postnatal development to aging, thanks to the constant turnover of the population (Askew et al., 2017). In the human brain, microglia has been estimated to make up 6%–18% of neocortical cells (Mittelbronn et al., 2001; Pelvig et al., 2008; Lyck et al., 2009). Similarly, microglial morphology varies considerably depending on specific regional properties. Whereas they are usually more ramified in gray matter, in white matter they display elongated somata and less ramified processes preferentially oriented along fiber tracts (Lawson et al., 1990; Mittelbronn et al., 2001; Torres-Platas et al., 2014). The molecular determinants of those anatomical differences in diversity and morphology are not clearly defined, however recent studies showed that microglia have a distinct region-dependent and age-dependent transcriptomic signature corroborating the existence of the regional heterogeneity of microglial phenotypes (Hickman et al., 2013; Grabert et al., 2016; Soreq et al., 2017).
In the healthy brain, microglia display a “surveilling” phenotype characterized by a small cell body and long branching processes serving to continuously sense the microenvironment to detect any alteration of CNS homeostasis (Kettenmann et al., 2013; Figure 1). Microglia show invariant soma positions but continually and rapidly moving processes (average velocity around 2.5 μm/min; Davalos et al., 2005; Nimmerjahn et al., 2005; Wake et al., 2009). Microglias rapidly change their surveilling phenotype into a diversity of “activated” phenotypes after the alteration of the CNS homeostasis or presence of a threat to neuronal integrity. They adopt a more amoeboid and less ramified phenotype with a large soma and rapidly trigger appropriate responses, which could range from upregulation or de novo synthesis of cell-surface molecules such as CD68 and major histocompatibility complex (MHC) class II to phagocytosis or the release of molecular mediators, including immune and non-immune factors (Kettenmann et al., 2011; Figure 1). Consequently, this activated phenotype is a generalized term that fails to take into account that microglia can adopt numerous functionally different phenotypes, depending on the exact nature of the stimulus (Perry et al., 2010).
In the healthy brain, microglia is involved in several mechanisms regulating CNS physiology. Microglia are in direct contact with dendritic spines, axons and synapses, suggesting that they participate in the regulation of synaptic structure and function (Wake et al., 2009; Paolicelli et al., 2011; Squarzoni et al., 2014). One of these mechanisms is synaptic pruning, a process by which excess synapses formed in the developing brain are eliminated to thereby increase the efficiency of the neural network. In this process, microglia has been shown in direct contact with the synapses and removing unwanted elements by phagocytosis (Paolicelli et al., 2011). Electron microscopy and high resolution in vivo engulfment assays have showed also the presence of presynaptic and postsynaptic elements inside microglial lysosomes (Berbel and Innocenti, 1988; Tremblay et al., 2010; Schafer et al., 2012). Some proteins as complement receptor 3 (CR3/CD11b), CX3CR1 or the adaptor protein DNAX-activating protein of 12-kDa (DAP12), highly expressed by microglia, are involved in the process of synaptic pruning. Indeed it has been shown that disruption of one of those proteins resulted in synaptic abnormalities in both prenatal and postnatal brain development (Stevens et al., 2007; Paolicelli et al., 2011; Squarzoni et al., 2014).
As mentioned above, the interactions between microglia and their surrounding cells have a major role in the determination of the microglial phenotype. Healthy neurons maintain microglia in their surveilling state via secreted and membrane bound signals. The interaction of neuronal CD200 with microglial CD200R leads to inactivation of microglia and plays a critical role in neuroprotection (Lyons et al., 2007). In CD200-deficient mice, microglia are more numerous, form more aggregate-like structures, display less ramifications with shorter processes and show an upregulation of CD45 and CD11b, which are markers of activation (Hoek et al., 2000). The bidirectional signaling between SIRPα and CD47 that can be co-expressed by both neurons and microglia maintains microglia in their surveilling state by inhibiting phagocytosis and inducing the synthesis of the anti-inflammatory cytokines (Zhang et al., 2015). The interaction of neuronal CX3CL1 with microglial CX3CR1 constrains microglial activation (Lyons et al., 2009). In models for Parkinson’s disease (PD) under a CX3CR1-deficient background, microglia exhibit an over-activated phenotype and neuronal cell death is enhanced (Bhaskar et al., 2010; Cho et al., 2011). The neurotoxicity induced by activated microglia in neurodegenerative diseases seems to be worsened in CX3CR1-deficient mice, suggesting that the signaling through CX3CL1/CX3CR1 regulates the phenotype of microglia (Cardona et al., 2006). Another receptor involved in the maintenance of the microglial surveilling state is the triggering receptor expressed on myeloid cells 2 (TREM2) associated with the adaptor protein DAP12. TREM2 is essential for phagocytosis process by microglia (Takahashi et al., 2007) and has recently been shown to interact with specific lipids to promote microglial survival (Wang et al., 2015). Mutations leading to a loss of function in TREM2 or DAP12 underlie the Nasu–Hakola disease, in which patients display progressive presenile dementia (Paloneva et al., 2000, 2002).
Microglia also express receptors that trigger essential cellular survival and developmental signals. CSF1R plays a major role in microglial development and survival. Expressed by microglia (Akiyama et al., 1994; Raivich et al., 1998), CSF1R is activated by two homodimeric glycoprotein ligands, CSF1 (Stanley and Heard, 1977) and Interleukin 34 (IL-34; Lin et al., 2008). In the brain, IL-34 is primarily expressed by neurons (Mizuno et al., 2011; Wang et al., 2012) whereas CSF1 is mainly expressed by microglia (Chitu et al., 2016). These two ligands present different patterns of regional expression in the prenatal and postnatal brain. CSF1 is highly expressed in the neocortex, corpus callosum, cerebellum and spinal cord, whereas IL-34 is highly expressed in the forebrain (neocortex, olfactory bulb and striatum; Wei et al., 2010; Nandi et al., 2012). The binding of CSF1 or IL-34 to CSF1R leads to the oligomerization and transphosphorylation of CSF1R followed by the phosphorylation and activation of downstream cytoplasmic mediators that promote microglia development, survival and proliferation (Ségaliny et al., 2015). As described previously, the development of tissue-resident macrophages including microglia is dependent on Csf1r expression from the first stages of development (Mass et al., 2016). Moreover, IL-34-deficient and CSF1-deficient mice display fewer microglia in various regions of the brain while Csf1r-deficient mice are completely devoid of them (Dai et al., 2002; Ginhoux et al., 2010).
In addition to their manifold functions in maintaining homeostasis in the healthy brain, microglia have been shown to play a major role in driving innate inflammatory responses in many neurodegenerative diseases. Prion disease is characterized by progressive neurodegeneration, which is accompanied by a pronounced microglia-mediated immune response, therefore being an extraordinary model to study the role of microglia in chronic neurodegenerative diseases. In this review, we will explore the molecular determinants of the contribution of microglia to the pathogenic cascade in prion disease, aiming to address some of the most relevant remaining unknowns of the role of microglia in prion disease: is microglial activation merely a bystander effect of prion pathology, or what aspects of microglia-mediated immune response are contributing to disease outcome in a beneficial or detrimental manner? Can the microglia-derived inflammatory response directly harm neurons and lead to neuronal degeneration, or is neuronal loss a consequence of misfolded prion protein (scrapie) (PrPsc) accumulation, or a combination of both? In the following sections, we will provide a comprehensive picture defining many aspects of microglial biology in prion disease.
Prion Pathology
Transmissible spongiform encephalopathies or prion diseases, such as Creutzfeldt-Jakob disease (CJD), are fatal neurodegenerative disorders that affect humans and many other mammals (Aguzzi and Calella, 2009). The infectious agent consists of PrPSc. PrPSc can aggregate, recruit and convert benign cellular prion protein (PrPC) into abnormal pathological isoforms (Aguzzi and Calella, 2009). Thereby, prions act as “seeds” that trigger a chain reaction of PrP misfolding and aggregation (Jarrett and Lansbury, 1993). Prion diseases have a heterogeneous etiology, as they can be genetic, infectious or sporadic. Infectivity requires the transfer of prion seeds from affected individuals into healthy hosts, whereas in genetic and idiopathic cases prion protein undergoes a spontaneous misfolding of PrP molecules into self-propagating seeds. In humans, sporadic CJD (sCJD) is the most common prion disease, followed by genetic CJD (gCJD) and transmitted CJD (iatrogenic CJD and variant CJD; Aguzzi and Calella, 2009). CJD has been also shown to be transmitted through blood or blood derivatives (Llewelyn et al., 2004; Bishop et al., 2013).
The prion neuropathology is characterized by spongiform degeneration, synaptic and neuronal loss, gliosis and the accumulation of aggregated PrPSC (DeArmond and Prusiner, 1995; Cunningham et al., 2003; Wadsworth and Collinge, 2011; Hilton et al., 2013). Prion disease typically has long incubation times and a rapid disease progression, and can be manifested in different ways, with behavioral and pathological differences within and across species (Prusiner, 1998; Tanaka et al., 2006; Collinge and Clarke, 2007; Colby and Prusiner, 2011). Interestingly, different strains of prion (e.g., ME7, 79A, 22L, 22A) preferentially affect specific regions of the brain in mice (Cunningham et al., 2005a). The simplest explanation for such regional selectivity would be a differential tropism of prion strains and thereby a regional aggregation and toxicity. However, recent experiments assessing prion misfolding using highly sensitive techniques showed that prion protein seeds accumulate in all brain regions irrespective of neurodegeneration (Alibhai et al., 2016).
Prions disease also provides an interesting experimental approach to model many aspects of neurodegenerative diseases associated with protein misfolding. In mouse models of prion disease, microglia become activated early in the disease process thereby representing a valuable tool for elucidating the impact of neuroinflammation in chronic neurodegenerative disorders.
Microglial Proliferation and Activation in Prion Disease
Prion disease is characterized by an increase in the number of microglia, associated with an activated and phagocytic phenotype (Perry et al., 2002; Perry and O’Connor, 2010; Figure 1). The relative contribution of local proliferation of microglia vs. the infiltration of bone-marrow derived progenitors to this increase has been a source of debate during recent years (Gomez-Nicola and Perry, 2015). However, a recent study demonstrated that, in a murine model of prion disease, local proliferation of resident microglial cells is a major component in the evolution of chronic neurodegeneration (Gómez-Nicola et al., 2013). The increase in microglial density and proliferative activity varies across different regions such as the hippocampus (CA1) and the thalamus, the later showing the biggest increase in cell numbers (Gómez-Nicola et al., 2013). This increase in microglial numbers is independent of the recruitment of circulating monocytes, evidenced by comparing microglial density in prion diseased mice with a C-C chemokine receptor type 2 (CCR2)−/− background with WT mice (Gómez-Nicola et al., 2014).
The proliferation of microglia in prion disease is regulated by the activation of CSF1R and the transcription factors PU.1 and CCAAT/enhancer-binding protein alpha (C/EBPα, being this system also active in human variant CJD and Alzheimer’s disease (AD; Gómez-Nicola et al., 2013; Olmos-Alonso et al., 2016). The inhibition of CSF1R blocks the proliferation of microglia, leading to a decrease in neuronal death in the hippocampus (Gómez-Nicola et al., 2013). A recent study showed that prolonged inhibition of CSF1R in APPswe/PSEN1dE9 (APP/PS1) mice, a model of AD-like pathology, blocks microglial proliferation and leads to the prevention of synaptic degeneration and to an improvement of performance in memory and exploratory tasks (Olmos-Alonso et al., 2016). CSF1R blockade also showed positive effect in mutant superoxide dismutase 1 (SOD1) models of Amyotrophic Lateral Sclerosis (ALS) by reducing microglial proliferation in the spinal cord and macrophage infiltration into peripheral nerves (Martínez-Muriana et al., 2016). The studies focused to targeting CSF1R suggest that microglial proliferation in prion disease, AD and ALS has a net detrimental contribution to the disease progression.
Targeting microglial proliferation by the specific inhibition of CSF1R renders a different experimental outcome than the unspecific removal of microglia. Some studies have aimed at eliminating microglial cells in prion disease, either by the transgenic expression of thymidine kinase (TK) and “suicide” of proliferating CD11b+ cells (Zhu et al., 2016), or by the non-specific blocker of mitosis cytosine arabinoside (Ara-C; Gómez-Nicola et al., 2013). These approaches indicated a neutral or beneficial role of microglia, as their elimination did not change the trajectory of the disease. However, the technical limitations of these targeting approaches difficult the interpretation. For example, the use of CD11b-TK mice leads to a prominent and uncontrolled death of microglia in the context of on-going neurodegeneration, not providing a physiologically silent way to address the contribution of the cells. Also, the TK transgene in CD11b-TK mice is activated by the administration of ganciclovir, an agent recently identified to have a potent anti-proliferative impact on microglia during brain pathology (Ding et al., 2014). Similarly, the use of Ara-C causes a shift in the microglial phenotype towards a detrimental pro-inflammatory profile, independent from its effects on cell proliferation, accelerating neuronal death (Gómez-Nicola et al., 2013). Together, these findings suggest that the specific and selective targeting of microglial proliferation, instead to their elimination, is an optimal approach to understand the contribution of these cells to the pathology.
Activation of microglia is detectable from early stages of prion disease pathogenesis (Betmouni et al., 1996) and becomes more widespread as the disease progresses, closely associated with the spread of neurodegeneration (Perry, 2016). Microglial activation appears simultaneously with first behavioral deficits (Guenther et al., 2001), at a time point when synapses start to degenerate in the stratum radiatum of the hippocampus, but no neuronal loss occurs yet (Cunningham et al., 2003; Gray et al., 2009). Whether microglia activation is directly caused by accumulating misfolded PrPsc or as a response to synaptic damage cannot be reliably concluded. While studies in vitro have demonstrated that microglia can be activated directly by PrP and subsequently damage neurons (Brown et al., 1996; Giese et al., 1998), there is limited evidence of a direct response to PrPSc aggregates in vivo. In contrast, the mere presence of misfolded prion protein, as detected in various brain regions using high sensitivity techniques, might not be sufficient to induce a microglia-mediated immune response in all brain regions (Alibhai et al., 2016). However, it is widely accepted that microglia activation precedes neuronal degeneration and the onset of clinical disease (Williams et al., 1997; Giese et al., 1998).
The cytokine profile in the prion-diseased brain is associated with the expression of both pro- and anti-inflammatory molecules (Perry, 2016; Figure 1). While a number of studies demonstrated a profile shifted to the anti-inflammatory spectrum, dominated by the expression of transforming growth factor β (TGFβ, C-C Motif Chemokine Ligand 2 (CCL2) and prostaglandin E2 (PGE2) with a limited pro-inflammatory response characterized by IL-1β and tumor necrosis factor α (TNFa; Minghetti et al., 2000; Walsh et al., 2001; Cunningham et al., 2002, 2005b; Perry et al., 2002), other studies have reported that also pro-inflammatory factors are up-regulated in the prion brain (Campbell et al., 1994; Williams et al., 1994; Kordek et al., 1996), suggestive of a mixed inflammatory profile. The lack of consensus regarding the inflammatory profile in the prion brain may arise from the fact that different prion strains, stages of disease and techniques of detection were used. Recent studies using a broader panel of markers support the hypothesis that both pro-and anti-inflammatory factors contribute to the immune response in prion disease. Vincenti et al. (2015) re-analyzed a large transcriptomic database of brains from multiple mouse strains exposed to various prion strains and collected at different stages of disease progression (Hwang et al., 2009) and proposed that most of the differentially expressed genes in the prion brain were of microglial origin and associated with the inflammatory response. Microglia isolated from 79A-infected mice showed increased expression of IL-1β, TNFα and CSF1, but not IL-6, IL-10 or TGFβ, which correlates with disease progression and indicates a classical activation phenotype of microglia in this prion model (Vincenti et al., 2015). A recent longitudinal study reported new inflammatory genes upregulated early in the prion brain, including genes involved in inflammation, monocyte recruitment and growth regulation (Carroll et al., 2015). Concerning signal transduction pathways, an early activation predominantly of the Signal transducer and activator of transcription (STAT)- and Nuclear factor kappa-light-chain-enhancer of activated B cells (NFkB) pathways has been observed in prion disease models, determined by the up-regulation of STAT- and NFkB-responsive genes, including many cytokines and chemokines, as well as by the detection of increased phosphorylation of STAT1 and STAT3 specifically (Llorens et al., 2014; Carroll et al., 2015). The inflammatory response seems to be quite consistent between different murine prion strains. Although prion strains 22L, RML and ME7 revealed cellular and regional differences in PrPSc accumulation, they showed a similar up-regulation mainly of pro-inflammatory genes and chemokines which correlated with the deposition of PrPSc and the onset of glial activation (Carroll et al., 2016).
Targeting individual immune pathways which are dysregulated in prion disease have shown differential effects in modifying pathology. The absence of CCL2 does not drastically affect the disease course (Felton et al., 2005; O’Shea et al., 2008) and similarly, reducing PGE2 levels using dapsone or Nonsteroidal anti-inflammatory drugs (NSAIDs) did not impact disease progression (Guenther et al., 2001; Perry, 2010). On the contrary, inhibition of TGFβ enhanced neurodegeneration, indicating that this immune mediator is critically involved in regulation of the innate immune response in prion disease (Boche et al., 2006). TNFα as well as TNF receptor 1 knockout mice show normal prion disease progression after intracerebral injection of the prion protein (Klein et al., 1997; Mabbott et al., 2000), whereas deficiency of IL-1 receptor type 1 prolongs prion incubation time (Tamgüney et al., 2008) and delays disease onset and protein aggregation and increases survival of diseased mice (Schultz et al., 2004). PrP fibrils have been found to induce IL-1β secretion by microglia in vitro, dependent on components of the Nod-like receptor family pyrin domain containing 3 (NLRP3) inflammasome, is sufficient to induce neuronal toxicity (Hafner-Bratkovič et al., 2012; Shi et al., 2012). However, an in vivo study using mice deficient in NLRP3 or the adaptor protein apoptosis-associated speck-like protein containing a caspase recruitment domain (ASC) and thereby lacking functional NLRP3 inflammasome, failed to demonstrate a significant impact on prion pathogenesis, indicating that inflammasomes do not contribute to prion progression (Nuvolone et al., 2015).
Numerous studies have reported signs of oxidative stress in brains of CJD patients as well as in murine models of prion disease (Guentchev et al., 2002; Van Everbroeck et al., 2004; Yun et al., 2006), which might play a role in disease progression by contributing to neurotoxicity and neurodegeneration. Stimulation of microglia with PrP fragments in vitro has been shown to induce growth arrest and the release of nitric oxide (NO) and PGE2 (Villa et al., 2016). Furthermore, in patients with CJD as well as in a mouse model of prion, production of NADPH oxidase 2 (NOX2) was up-regulated specifically by microglia in the affected brain regions (Sorce et al., 2014). Prion-induced mice deficient in NOX2 demonstrated decreased production of reactive oxygen species (ROS), a delayed onset of motor deficits and an increased survival time, indicating that microglia-specific NOX2 production leads to the release of ROS and affects prion pathology (Sorce et al., 2014).
It has been shown that microglia are able to engulf and clear apoptotic neurons (Hughes et al., 2010; Kranich et al., 2010). The phagocytic function of microglia is increased in the prion-diseased brain and associated with enhanced expression of scavenger receptors, cathepsins, and proteins of the respiratory burst, while phagocytic microglia were characterized by a lack of IL-1β expression (Hughes et al., 2010). While microglia were efficient in the uptake of injected latex beads and apoptotic cells, they were unable to remove prion protein aggregates, even upon additional stimulation with lipopolysaccharide (LPS; Hughes et al., 2010). Phagocytosis of apoptotic neurons by microglia has been shown to be dependent on milk fat globule epidermal growth factor 8 (MFGE8). Ablation of mfge8 resulted in accelerated prion pathology, with reduced clearance of apoptotic bodies and increased prion protein accumulation, indicating that microglia phagocytosis via MFGE8 is a protective mechanism in prion disease (Kranich et al., 2010). While in vitro studies using microglia cells or organotypic brain slices proposed that microglia can clear PrP and thereby decrease prion titers (McHattie et al., 1999; Falsig et al., 2009; Kranich et al., 2010; Zhu et al., 2016), evidence that they do so in vivo is still missing. It is possible that the removal of misfolded prion protein is simply inefficient or that PrPsc is not a sufficient trigger to induce phagocytosis, a similar concept to that proposed for phagocytosis of amyloid β (Aβ in AD (Guillot-Sestier and Town, 2013; Prokop et al., 2013). Furthermore, while the phagocytic function of microglia in prion disease is mostly considered to be beneficial and protective, it is also conceivable that microglia, by taking up cell debris from prion infected cells or possibly PrP aggregates, might even contribute to the spreading of the pathogenic protein (Baker et al., 2002).
TREM2 has been implicated in several neurodegenerative diseases such as AD (Jonsson et al., 2012; Guerreiro R. J. et al., 2013), frontotemporal dementia (Guerreiro R. et al., 2013) and ALS (Cady et al., 2014). TREM2 is an innate immune cell receptor expressed on microglia and other myeloid cells is thought to be involved in phagocytosis of apoptotic neurons and promoting an anti-inflammatory phenotype (Takahashi et al., 2005, 2007; Hsieh et al., 2009). In the context of prion disease, TREM2 has been demonstrated to be up-regulated after prion infection, but the depletion of TREM2 did neither change incubation time and survival, nor microglia immune phenotype during prion disease (Zhu et al., 2015).
Recently, there has been evidence of the involvement of non-coding microRNAs (miR) in regulating the microglia inflammatory response in prion disease. A number of miRs implicated in the regulation of gliosis, glial cell proliferation, the innate-immune response, inflammatory signaling, deficits in neurotrophic signaling and synaptogenesis have been found to be upregulated in human prion disease cases (Zhao et al., 2016). Among them, miR-146a was observed to influence immune response and activation state of microglia (Saba et al., 2012).
While increasing evidence indicates that microglia activation seems to contribute to prion pathogenesis, not much is known about the mechanistic underpinnings. We have shown recently that microglial expansion negatively affects prion disease pathology (Gómez-Nicola et al., 2013), pointing towards a net detrimental role of microglia during chronic neurodegeneration. Another recent study emphasized a protective role of microglia in prion disease, demonstrating accelerated disease pathology upon microglia ablation in the brain (Zhu et al., 2016). This dual role of microglia during prion disease provides further proof that microglia function is highly dynamic and versatile, by promoting neurotoxic effects through facilitating a potentially aberrant and harmful inflammation in prion disease, but also by provoking a protective response through tissue maintenance and repair.
Systemic Inflammation and Microglial Priming
The study of immune to brain communication is gaining interest, with the immune system as a transducer of both endogenous and exogenous challenges to the host. It is known that systemic infection and inflammation are able to produce behavioral changes, known as “sickness behaviors”, that include fever, malaise, anorexia, lethargy and depression (Dantzer et al., 1998), demonstrating an important influence of the immune system on CNS processes. LPS challenge in healthy humans has been shown to produce sickness behavior with fever and neuropsychological symptoms, including reduced declarative memory performance (Krabbe et al., 2005) and increased symptoms of depression (DellaGioia et al., 2012). Systemic inflammation communicates with the brain by several neural and humoral pathways. Receptors for inflammatory mediators present on vagus nerve fibers can respond to inflammatory signals and inform the nucleus of the solitary tract and other regions of the brain (Wang et al., 2003). Macrophages in the circumventricular organs (CVOs), which are regions of the CNS without a tight blood-brain barrier (BBB), are also able to communicate with systemic inflammatory mediators. Signals generated in the CVOs subsequently travel to other regions of the brain (Lacroix et al., 1998). A third route of communication involves cerebral endothelial cells of the BBB, which can transduce signals from blood to the CNS (Laflamme and Rivest, 1999). The molecular pathways by which systemic inflammation alters brain function leading to sickness behavior are not completely elucidated. It has been suggested that the link between the systemic immune system and the CNS is located at the level of the hypothalamus, the main brain region controlling the neuroendocrine system. Systemic inflammation or ageing can modify the hypothalamic function though NFkB activation and microglial-neuronal crosstalk (Li et al., 2012; Zhang et al., 2013). Further studies are needed to elucidate the exact mechanism underpinning the alteration of neuronal function by systemic inflammation.
It is known that microglia play an important role in immune-to-brain communication (Perry and Teeling, 2013). Within this context, the concept of microglial priming has been proposed. Microglial priming occurs as a result of damage associated with chronic neurodegenerative conditions (e.g., misfolded protein, neuronal debris or vascular changes), or several other stressors affecting the nervous system, such as maternal separation, acute injury or aging. It consists of an exaggerated or heightened microglial response—much stronger than that observed in stimulus-naïve microglia—to a second inflammatory stimulus. Most of the evidence supporting the hypothesis of microglial priming in chronic neurodegenerative conditions arises from the study of prion models. In fact, microglial priming was first described in prion brains subjected to a systemic challenge mimicking systemic infection (Combrinck et al., 2002). Cunningham et al. (2005b) showed how intracerebral LPS injection in prion mice results in exacerbated IL-1β expression, neutrophil infiltration and NO expression, accompanied with increased cell death. Systemic injection of LPS (Cunningham et al., 2009), Polyinosinic-polycytidylic acid (poly I:C; Cunningham et al., 2002, 2005b, 2007; Field et al., 2010) or TNFα (Hennessy et al., 2017) in prion mice also leads to exacerbated in the brain, with increased expression of pro-inflammatory cytokines and chemokines including IL-1β TNFα and CCL2. Interestingly, the increased inflammation triggered by LPS in ME7 prion brain is independent on circulating IL-1β and IL-6 (Murray et al., 2011; Hennessy et al., 2017). Although the exact mechanism by which microglia is primed is still unknown, it has been suggested that cyclooxygenase 1 (COX-1) expression in microglia mediates the systemic effects of LPS in the prion brain (Griffin et al., 2013). More recently, Hennessy et al. (2017) reported that astrocytes could be also primed in the prion brain, and generate exaggerated levels of cytokines and chemokines after systemic LPS challenge. Although these findings suggest that microglia in the prion diseased brain are primed by the ongoing pathology and that a secondary stimulus switches these cells to an aggressive phenotype, there is still no defining criteria to classify this state, and it remains unclear whether different patterns of priming result from different forms of neurodegeneration or different systemic inflammatory stimuli.
Interestingly, priming observations and the impact of the immune system on the CNS are not restricted to prion disease (Perry, 2010; Cunningham, 2013). Systemic LPS injection can induce degeneration of cells in the substantia nigra (Gao et al., 2002; Qin et al., 2007) and systemic challenge with IL-1β in an animal model of PD leads to enhanced degeneration of substantia nigra neurons and pro-inflammatory cytokine production (Pott-Godoy et al., 2008). Systemic challenge with LPS or other bacterial toxins in an experimental model of multiple sclerosis, Experimental autoimmune encephalomyelitis (EAE), can also exacerbate neurological symptoms (Schiffenbauer et al., 1993). Systemic LPS challenge caused increased synthesis of pro-inflammatory cytokines in the CNS of transgenic mouse models of AD-like pathology (Sly et al., 2001), and tau phosphorylation (Roe et al., 2011). Chronic systemic inflammation in the form of osteoarthritis results in accelerated neuroinflammation and Aβ pathology in APP/PS1/Col1-IL1βXAT mice (Kyrkanides et al., 2011). There is also evidence about the impact of the immune system upon human neurodegenerative diseases. Systemic infection and increased systemic inflammation have been associated to an enhanced cognitive decline in AD patients (Holmes et al., 2003, 2009, 2011), and systemic infections are associated with relapses in multiple sclerosis in patients (Buljevac et al., 2002).
Overall, evidence suggests that systemic inflammation contributes to the pathology during chronic neurodegenerative conditions, with microglia acting as a hub of communication between the systemic and CNS compartments. According to this view, it is likely that a correct treatment and management of systemic inflammation could potentially delay the progression of neurodegenerative disorders.
Role of Microglia in Human Prion Diseases
Evidence from the literature supports a role of microglia-mediated inflammation in human prion diseases. Activated microglia are found in the brains of CJD patients (Sasaki et al., 1993; Szpak et al., 2006) where they appear to be closely associated with PrPSc deposits (Miyazono et al., 1991; Guiroy et al., 1994; Muhleisen et al., 1995). However the degree of microglial reactivity seems to depend on the subtype of prion disease and the type of biochemical PrPSc (Puoti et al., 2005; Shi et al., 2013). Increased levels of inflammatory cytokines such as IL-8, CCL2, TGFβ, TNFα and IL-1β have been found in the cerebrospinal fluid (CSF) of sporadic CJD cases (Sharief et al., 1999; Stoeck et al., 2006, 2014) and the inflammatory response seems to correlate with the severity of lesions (Van Everbroeck et al., 2002). A recent study demonstrated subtype-specific and region-specific changes in glia activation and the expression of inflammatory mediators in CJD, with inflammation being pre-dominant in the cerebral cortex in the CJD subtype PRNP codon 129 Met/Met type 1 (MM1) and in the cerebellum in PRNP codon 129 Val/Val type 2 (VV2) cases (Llorens et al., 2014). Microglia markers such as CD11b, Iba-1 and CD68 were found to be up-regulated in a region- and subtype-specific manner which correlated with the up-regulation of pro- and anti-inflammatory cytokines, members of the complement system, the integrin family and C-type lectin/C-type lectin-like domain (CTL/CTLD) superfamily, toll-like receptors, colony-stimulating factors and cathepsins (Llorens et al., 2014). Additionally, regulatory proteins IL-34, PU.1 and C/EBPα involved in microglial proliferation are increased in variant CJD (Gómez-Nicola et al., 2013). The increased inflammatory response observed in the two subtypes of CJD was further associated with an activation of NFkB and STAT1, 3 signaling pathways (Llorens et al., 2014). The finding that key regulators of inflammationCOX-1/2 and PGE2, are elevated in brains of CJD (Minghetti et al., 2000; Deininger et al., 2003; Llorens et al., 2014) further speaks for a crucial role of inflammatory processes in human prion disease, however the exact role of microglia-mediated inflammation during the course of the fatal disease remains to be elucidated.
Conclusion
Increasing evidence highlights the major contribution of the expansion and activation of microglia to the pathogenesis of prion disease. Activated microglia adopts a variety of functionally diverse phenotypes depending on the disease stage and systemic influences. While their response is manifold during prion disease, targeting the microglia-mediated immune response appears to be a useful approach to modify the disease course. Determining the exact mechanistic underpinnings of the neuroinflammatory processes in prion disease is an informative step in order to develop novel treatment strategies targeting neurodegenerative disease.
Author Contributions
JO, ES and RM jointly wrote the manuscript and contributed to the drafting. DG-N designed the contents of the revision and drafted the manuscript.
Funding
We would like to acknowledge the Medical Research Council, Wellcome Trust and Alzheimer’s Research UK for funding the experimental work of the lab.
Conflict of Interest Statement
The authors declare that the research was conducted in the absence of any commercial or financial relationships that could be construed as a potential conflict of interest.
Abbreviations
Aβ, amyloid-β; AD, Alzheimer’s disease; ALS, amyotrophic lateral sclerosis; APP/PS1, APPswe/PSEN1dE9; Ara-C, cytosine arabinoside; ASC, apoptosis-associated speck-like protein containing a caspase recruitment domain; BBB, blood-brain barrier; CCL2, C-C motif chemokine ligand 2; CCR2, C-C chemokine receptor type 2; CD, Cluster of differentiation; C/EBPα, CCAAT/enhancer-binding protein alpha; COX, cyclooxygenase; CVO, circumventricular organs; CJD, Creutzfeldt-Jakob disease; CNS, Central nervous system; CR3, complement receptor 3; CSF, cerebrospinal fluid; CSF1, Colony stimulating factor; CSF1R, Colony stimulating factor 1 receptor; CTL/CTLD, C-type lectin/C-type lectin-like domain; CX3CL1, C-X3-C motif chemokine ligand 1; CX3CR1, C-X3-C motif chemokine receptor 1; DAP12, DNAX-activating protein of 12-kDa; EAE, Experimental autoimmune encephalomyelitis; EMP, Erythroid-myeloid progenitors; Iba1, Ionized calcium-binding adaptor molecule 1; IL-1β, Interleukin 1β; IL-34, Interleukin 34; IRF8, Interferon regulatory factor 8; LPS, lipopolysaccharide; MFGE8, Milk fβt globule-EGF factor 8 protein; MHC, Major histocompatibility complex; miR, microRNA; NFκB, Nuclear factor kappa-light-chain-enhancer of activated B cells; NLRP3, Nod-like receptor family pyrin domain containing 3; NSAID, Nonsteroidal anti-inflammatory drug; NO, nitric oxide; NOX2, Nicotinamide adenine dinucleotide phosphate-oxidase (NADPH) oxidase 2; PD, Parkinson’s disease; PGE2, prostaglandin E2; pMac, pre-macrophage; poly I:C, Polyinosinic-polycytidylic acid; MM1, PRNP codon 129 Met/Met type 1; PrPC, cellular prion protein; PrPSc, misfolded prion protein (scrapie); ROS, Reactive oxygen species; Runt1, Runt-related transcription factor 1; Sall1/3, Sal-like 1/3; SIRPα, Signal regulatory protein α; SOD1, superoxide dismutase 1; STAT1/3, Signal transducer and activator of transcription 1/3; Tie2, TEK receptor tyrosine kinase; TGFβ, Transforming growth factor β; TK, thymidine kinase; TIMD4, T-cell immunoglobulin and mucin domain containing 4; TNFα, Tumor nerosis factor α; TREM2, triggering receptor expressed on myeloid cells 2; VV2, PRNP codon 129 Val/Val type 2; YS, yolk sac.
References
Aguzzi, A., and Calella, A. M. (2009). Prions: protein aggregation and infectious diseases. Physiol. Rev. 89, 1105–1152. doi: 10.1152/physrev.00006.2009
Akiyama, H., Nishimura, T., Kondo, H., Ikeda, K., Hayashi, Y., and McGeer, P. L. (1994). Expression of the receptor for macrophage colony stimulating factor by brain microglia and its upregulation in brains of patients with Alzheimer’s disease and amyotrophic lateral sclerosis. Brain Res. 639, 171–174. doi: 10.1016/0006-8993(94)91779-5
Alibhai, J., Blanco, R. A., Barria, M. A., Piccardo, P., Caughey, B., Perry, V. H., et al. (2016). Distribution of misfolded prion protein seeding activity alone does not predict regions of neurodegeneration. PLoS Biol. 14:e1002579. doi: 10.1371/journal.pbio.1002579
Alliot, F., Godin, I., and Pessac, B. (1999). Microglia derive from progenitors, originating from the yolk sac and which proliferate in the brain. Dev. Brain Res. 117, 145–152. doi: 10.1016/s0165-3806(99)00113-3
Askew, K., Li, K., Olmos-Alonso, A., Garcia-Moreno, F., Liang, Y., Richardson, P., et al. (2017). Coupled proliferation and apoptosis maintain the rapid turnover of microglia in the adult brain. Cell Rep. 18, 391–405. doi: 10.1016/j.celrep.2016.12.041
Baker, C. A., Martin, D., and Manuelidis, L. (2002). Microglia from Creutzfeldt-Jakob disease-infected brains are infectious and show specific mRNA activation profiles. J. Virol. 76, 10905–10913. doi: 10.1128/jvi.76.21.10905-10913.2002
Berbel, P., and Innocenti, G. M. (1988). The development of the corpus callosum in cats: a light- and electron-microscopic study. J. Comp. Neurol. 276, 132–156. doi: 10.1002/cne.902760109
Betmouni, S., Perry, V. H., and Gordon, J. L. (1996). Evidence for an early inflammatory response in the central nervous system of mice with scrapie. Neuroscience 74, 1–5. doi: 10.1016/0306-4522(96)00212-6
Bhaskar, K., Konerth, M., Kokiko-Cochran, O. N., Cardona, A., Ransohoff, R. M., and Lamb, B. T. (2010). Regulation of tau pathology by the microglial fractalkine receptor. Neuron 68, 19–31. doi: 10.1016/j.neuron.2010.08.023
Bishop, M. T., Bishop, M. T., Diack, A. B., Ritchie, D. L., Ironside, J. W., Will, R. G., et al. (2013). Prion infectivity in the spleen of aPRNP heterozygous individual with subclinical variant Creutzfeldt-Jakob disease. Brain 136, 1139–1145. doi: 10.1093/brain/awt032
Boche, D., Cunningham, C., Docagne, F., Scott, H., and Perry, V. H. (2006). TGFβ1 regulates the inflammatory response during chronic neurodegeneration. Neurobiol. Dis. 22, 638–650. doi: 10.1016/j.nbd.2006.01.004
Brown, D. R., Schmidt, B., and Kretzschmar, H. A. (1996). Role of microglia and host prion protein in neurotoxicity of a prion protein fragment. Nature 380, 345–347. doi: 10.1038/380345a0
Bruttger, J., Karram, K., Wortge, S., Regen, T., Marini, F., Hoppmann, N., et al. (2015). Genetic cell ablation reveals clusters of local self-renewing microglia in the mammalian central nervous system. Immunity 43, 92–106. doi: 10.1016/j.immuni.2015.06.012
Buljevac, D., Flach, H. Z., Hop, W. C., Hijdra, D., Laman, J. D., Savelkoul, H. F., et al. (2002). Prospective study on the relationship between infections and multiple sclerosis exacerbations. Brain 125, 952–960. doi: 10.1093/brain/awf098
Cady, J., Koval, E. D., Benitez, B. A., Zaidman, C., Jockel-Balsarotti, J., Allred, P., et al. (2014). TREM2 variant p.R47H as a risk factor for sporadic amyotrophic lateral sclerosis. JAMA Neurol. 71, 449–453. doi: 10.1001/jamaneurol.2013.6237
Campbell, I. L., Eddleston, M., Kemper, P., Oldstone, M. B., and Hobbs, M. V. (1994). Activation of cerebral cytokine gene expression and its correlation with onset of reactive astrocyte and acute-phase response gene expression in scrapie. J. Virol. 68, 2383–2387.
Cardona, A. E., Pioro, E. P., Sasse, M. E., Kostenko, V., Cardona, S. M., Dijkstra, I. M., et al. (2006). Control of microglial neurotoxicity by the fractalkine receptor. Nat. Neurosci. 9, 917–924. doi: 10.1038/nn1715
Carroll, J. A., Striebel, J. F., Race, B., Phillips, K., and Chesebro, B. (2015). Prion infection of mouse brain reveals multiple new upregulated genes involved in neuroinflammation or signal transduction. J. Virol. 89, 2388–2404. doi: 10.1128/jvi.02952-14
Carroll, J. A., Striebel, J. F., Rangel, A., Woods, T., Phillips, K., Peterson, K. E., et al. (2016). Prion strain differences in accumulation of PrPSc on neurons and glia are associated with similar expression profiles of neuroinflammatory genes: comparison of three prion strains. PLoS Pathog. 12:e1005551. doi: 10.1371/journal.ppat.1005551
Chitu, V., Gokhan, S., Nandi, S., Mehler, M. F., and Stanley, E. R. (2016). Emerging roles for CSF-1 receptor and its ligands in the nervous system. Trends Neurosci. 39, 378–393. doi: 10.1016/j.tins.2016.03.005
Cho, S.-H., Sun, B., Zhou, Y., Kauppinen, T. M., Halabisky, B., Wes, P., et al. (2011). CX3CR1 protein signaling modulates microglial activation and protects against plaque-independent cognitive deficits in a mouse model of Alzheimer disease. J. Biol. Chem. 286, 32713–32722. doi: 10.1074/jbc.m111.254268
Colby, D. W., and Prusiner, S. B. (2011). Prions. Cold Spring Harb. Perspect. Biol. 3:a006833. doi: 10.1101/cshperspect.a006833
Collinge, J., and Clarke, A. R. (2007). A general model of prion strains and their pathogenicity. Science 318, 930–936. doi: 10.1126/science.1138718
Combrinck, M. I., Perry, V. H., and Cunningham, C. (2002). Peripheral infection evokes exaggerated sickness behaviour in pre-clinical murine prion disease. Neuroscience 112, 7–11. doi: 10.1016/s0306-4522(02)00030-1
Cuadros, M. A., Martin, C., Coltey, P., Almendros, A., and Navascués, J. (1993). First appearance, distribution and origin of macrophages in the early development of the avian central nervous system. J. Comp. Neurol. 330, 113–129. doi: 10.1002/cne.903300110
Cunningham, C. (2013). Microglia and neurodegeneration: the role of systemic inflammation. Glia 61, 71–90. doi: 10.1002/glia.22350
Cunningham, C., Boche, D., and Perry, V. H. (2002). Transforming growth factor β1, the dominant cytokine in murine prion disease: influence on inflammatory cytokine synthesis and alteration of vascular extracellular matrix. Neuropathol. Appl. Neurobiol. 28, 107–119. doi: 10.1046/j.1365-2990.2002.00383.x
Cunningham, C., Campion, S., Lunnon, K., Murray, C. L., Woods, J. F., Deacon, R. M., et al. (2009). Systemic inflammation induces acute behavioral and cognitive changes and accelerates neurodegenerative disease. Biol. Psychiatry 65, 304–312. doi: 10.1016/j.biopsych.2008.07.024
Cunningham, C., Campion, S., Teeling, J., Felton, L., and Perry, V. H. (2007). The sickness behaviour and CNS inflammatory mediator profile induced by systemic challenge of mice with synthetic double-stranded RNA (poly I:C). Brain Behav. Immun. 24, 590–602. doi: 10.1016/j.bbi.2006.12.007
Cunningham, C., Deacon, R. M., Chan, K., Boche, D., Rawlins, J. N., and Perry, V. H. (2005a). Neuropathologically disctint prion strains give rise to similar temporal profiles of behavioral deficits. Neurobiol. Dis. 18, 258–269. doi: 10.1016/j.nbd.2004.08.015
Cunningham, C., Wilcockson, D. C., Campion, S., Lunnon, K., and Perry, V. H. (2005b). Central and systemic endotoxin challenges exacerbate the local inflammatory response and increase neuronal death during chronic neurodegeneration. J. Neurosci. 25, 9275–9284. doi: 10.1523/jneurosci.2614-05.2005
Cunningham, C., Deacon, R., Wells, H., Boche, D., Waters, S., Diniz, C. P., et al. (2003). Synaptic changes characterize early behavioural signs in the ME7 model of murine prion disease. Eur. J. Neurosci. 17, 2147–2155. doi: 10.1046/j.1460-9568.2003.02662.x
Dai, X. M., Ryan, G. R., Hapel, A. J., Dominguez, M. G., Russell, R. G., Kapp, S., et al. (2002). Targeted disruption of the mouse colony-stimulating factor 1 receptor gene results in osteopetrosis, mononuclear phagocyte deficiency, increased primitive progenitor cell frequencies and reproductive defects. Blood 99, 111–120. doi: 10.1182/blood.v99.1.111
Dantzer, R., Bluthé, R. M., Layé, S., Bret-Dibat, J. L., Parnet, P., and Kelley, K. W. (1998). Cytokines and sickness behavior. Ann. N Y Acad. Sci. 840, 586–590. doi: 10.1111/j.1749-6632.1998.tb09597.x
Davalos, D., Grutzendler, J., Yang, G., Kim, J. V., Zuo, Y., Jung, S., et al. (2005). ATP mediates rapid microglial response to local brain injury in vivo. Nat. Neurosci. 8, 752–758. doi: 10.1038/nn1472
DeArmond, S. J., and Prusiner, S. B. (1995). Etiology and pathogenesis of prion diseases. Am. J. Pathol. 146, 785–811.
Deininger, M. H., Bekure-Nemariam, K., Trautmann, K., Morgalla, M., Meyermann, R., and Schluesener, H. J. (2003). Cyclooxygenase-1 and -2 in brains of patients who died with sporadic Creutzfeldt-Jakob disease. J. Mol. Neurosci. 20, 25–30. doi: 10.1385/jmn:20:1:25
del Río-Hortega, P. (1920). Estudios sobre la neuroglía. La microglia y su transformación en células en bastoncito y cuerpos gránulo-adiposos. Trab. Lab Inv. Biol. 18, 37–82.
del Río-Hortega, P. (1932). “Microglia,” in Cytology and Cellular Pathology of the Nervous System (Vol. 2), ed. W. Penfield (New York, NY: P.B. Hoeber, Inc.), 483–534.
del Río-Hortega, P., and Penfield, W. G. (1927). Cerebral cicatrix: the reaction of neuroglia and microglia to brain wounds. Bull. John Hopkins. Hosp. 41, 278–303.
DellaGioia, N., Devine, L., Pittman, B., and Hannestad, J. (2012). Bupropion pre-treatment of endotoxin-induced depressive symptoms. Brain Behav. Immun. 31, 197–204. doi: 10.1016/j.bbi.2012.10.008
Ding, Z., Mathur, V., Ho, P. P., James, M. L., Lucin, K. M., Hoehne, A., et al. (2014). Antiviral drug ganciclovir is a potent inhibitor of microglial proliferation and neuroinflammation. J. Exp. Med. 211, 189–198. doi: 10.1084/jem.20120696
Elmore, M. R., Najafi, A. R., Koike, M. A., Dagher, N. N., Spangenberg, E. E., Rice, R. A., et al. (2014). Colony-stimulating factor 1 receptor signaling is necessary for microglia viability, unmasking a microglia progenitor cell in the adult brain. Neuron 82, 380–397. doi: 10.1016/j.neuron.2014.02.040
Esiri, M. M., al Izzi, M. S., and Reading, M. C. (1991). Macrophages, microglial cells and HLA-DR antigens in fetal and infant brain. J. Clin. Pathol. 44, 102–106. doi: 10.1136/jcp.44.2.102
Falsig, J., Julius, C., Margalith, I., Schwarz, P., Heppner, F. L., and Aguzzi, A. (2009). A versatile prion replication assay in organotypic brain slices. Nat. Neurosci. 11, 109–117. doi: 10.1038/nn2028
Felton, L. M., Cunningham, C., Rankine, E. L., Waters, S., Boche, D., and Perry, V. H. (2005). MCP-1 and murine prion disease: Separation of early behavioural dysfunction from overt clinical disease. Neurobiol. Dis. 20, 283–295. doi: 10.1016/j.nbd.2005.03.008
Field, R., Campion, S., Warren, C., Murray, C., and Cunningham, C. (2010). Systemic challenge with the TLR3 agonist poly I:C induces amplified IFNα/β and IL-1β responses in the diseased brain and exacerbates chronic neurodegeneration. Brain Behav. Immun. 24, 996–1007. doi: 10.1016/j.bbi.2010.04.004
Gao, H. M., Jiang, J., Wilson, B., Zhang, W., Hong, J. S., and Liu, B. (2002). Microglial activation-mediated delayed and progressive degeneration of rat nigral dopaminergic neurons: relevance to Parkinson’s disease. J. Neurochem. 81, 1285–1297. doi: 10.1046/j.1471-4159.2002.00928.x
Giese, A., Brown, D. R., Groschup, M. H., Feldmann, C., Haist, I., and Kretzschmar, H. A. (1998). Role of microglia in neuronal cell death in prion disease. Brain Pathol. 8, 449–457. doi: 10.1111/j.1750-3639.1998.tb00167.x
Ginhoux, F., Greter, M., Leboeuf, M., Nandi, S., See, P., Gokhan, S., et al. (2010). Fate mapping analysis reveals that adult microglia derive from primitive macrophages. Science 330, 841–845. doi: 10.1126/science.1194637
Gomez Perdiguero, E., Klapproth, K., Schulz, C., Busch, K., Azzoni, E., Crozet, L., et al. (2015). Tissue-resident macrophages originate from yolk-sac-derived erythro-myeloid progenitors. Nature 518, 547–551. doi: 10.1038/nature13989
Gómez-Nicola, D., Fransen, N. L., Suzzi, S., and Perry, V. H. (2013). Regulation of microglial proliferation during chronic neurodegeneration. J. Neurosci. 33, 2481–2493. doi: 10.1523/JNEUROSCI.4440-12.2013
Gomez-Nicola, D., and Perry, V. H. (2015). Microglial dynamics and role in the healthy and diseased brain: a paradigm of functional plasticity. Neuroscientist 21, 169–184. doi: 10.1177/1073858414530512
Gómez-Nicola, D., Schetters, S. T., and Perry, V. H. (2014). Differential role of CCR2 in the dynamics of microglia and perivascular macrophages during prion disease. Glia 62, 1041–1052. doi: 10.1002/glia.22660
Grabert, K., Michoel, T., Karavolos, M. H., Clohisey, S., Baillie, J. K., Stevens, M. P., et al. (2016). Microglial brain region-dependent diversity and selective regional sensitivities to aging. Nat. Neurosci. 19, 504–516. doi: 10.1038/nn.4222
Gray, B. C., Siskova, Z., Perry, V. H., and O’Connor, V. (2009). Selective presynaptic degeneration in the synaptopathy associated with ME7-induced hippocampal pathology. Neurobiol. Dis. 35, 63–74. doi: 10.1016/j.nbd.2009.04.001
Griffin, É. W., Skelly, D. T., Murray, C. L., and Cunningham, C. (2013). Cyclooxygenase-1-dependent prostaglandins mediate susceptibility to systemic inflammation-induced acute cognitive dysfunction. J. Neurosci. 38, 15248–15258. doi: 10.1523/JNEUROSCI.6361-11.2013
Guentchev, M., Siedlak, S. L., Jarius, C., Tagliavini, F., Castellani, R. J., Perry, G., et al. (2002). Oxidative damage to nucleic acids in human prion disease. Neurobiol. Dis. 9, 275–281. doi: 10.1006/nbdi.2002.0477
Guenther, K., Deacon, R. M., Perry, V. H., and Rawlins, J. N. (2001). Early behavioural changes in scrapie-affected mice and the influence of dapsone. Eur. J. Neurosci. 14, 401–409. doi: 10.1046/j.0953-816x.2001.01645.x
Guerreiro, R. J., Lohmann, E., Brás, J. M., Gibbs, J. R., Rohrer, J. D., Gurunlian, N., et al. (2013). Using exome sequencing to reveal mutations in TREM2 presenting as a frontotemporal dementia-like syndrome without bone involvement. JAMA Neurol. 70, 78–84. doi: 10.1001/jamaneurol.2013.579
Guerreiro, R., Wojtas, A., Bras, J., Carrasquillo, M., Rogaeva, E., Majounie, E., et al. (2013). TREM2 variants in Alzheimer’s disease. N. Engl. J. Med. 368, 117–127. doi: 10.1056/NEJMoa1211851
Guillot-Sestier, M.-V., and Town, T. (2013). Innate immunity in Alzheimer’s disease: a complex affair. CNS Neurol. Disord. Drug Targets 12, 593–607. doi: 10.2174/1871527311312050008
Guiroy, D. C., Wakayama, I., Liberski, P. P., and Gajdusek, D. C. (1994). Relationship of microglia and scrapie amyloid-immunoreactive plaques in kuru, Creutzfeldt-Jakob disease and Gerstmann-Sträussler syndrome. Acta Neuropathol. 87, 526–530. doi: 10.1007/s004010050119
Hafner-Bratkovič, I., Benčina, M., Fitzgerald, K. A., Golenbock, D., and Jerala, R. (2012). NLRP3 inflammasome activation in macrophage cell lines by prion protein fibrils as the source of IL-1β and neuronal toxicity. Cell. Mol. Life Sci. 69, 4215–4228. doi: 10.1007/s00018-012-1140-0
Hennessy, E., Gormley, S., Lopez-Rodriguez, A. B., Murray, C., Murray, C., and Cunningham, C. (2017). Systemic TNF-α produces acute cognitive dysfunction and exaggerated sickness behavior when superimposed upon progressive neurodegeneration. Brain. Behav. Immun. 59, 233–244. doi: 10.1016/j.bbi.2016.09.011
Hickman, S. E., Kingery, N. D., Ohsumi, T. K., Borowsky, M. L., Wang, L. C., Means, T. K., et al. (2013). The microglial sensome revealed by direct RNA sequencing. Nat. Neurosci. 16, 1896–1905. doi: 10.1038/nn.3554
Hilton, K. J., Cunningham, C., Reynolds, R. A., and Perry, V. H. (2013). Early hippocampal synaptic loss precedes neuronal loss and associates with early behavioral deficits in three distinct strains of prion disease. PLoS One 8:e68062. doi: 10.1371/journal.pone.0068062
Hoek, R. M., Ruuls, S. R., Murphy, C. A., Wright, G. J., Goddard, R., Zurawski, S. M., et al. (2000). Down-regulation of the macrophage lineage through interaction with OX2 (CD200). Science 290, 1768–1771. doi: 10.1126/science.290.5497.1768
Holmes, C., Arranz, M., Collier, D., Powell, J., and Lovestone, S. (2003). Depression in Alzheimer’s disease: the effect of serotonin receptor gene variation. Am. J. Med. Genet. B Neuropsychiatr. Genet. 119B, 40–43. doi: 10.1002/ajmg.b.10068
Holmes, C., Cunningham, C., Zotova, E., Culliford, D., and Perry, V. H. (2011). Proinflammatory cytokines, sickness behavior, and Alzheimer disease. Neurology 77, 212–218. doi: 10.1212/WNL.0b013e318225ae07
Holmes, C., Cunningham, C., Zotova, E., Woolford, J., Dean, C., Kerr, S., et al. (2009). Systemic inflammation and disease progression in Alzheimer disease. Neurology 73, 768–774. doi: 10.1212/WNL.0b013e3181b6bb95
Hsieh, C. L., Koike, M., Spusta, S. C., Niemi, E. C., Yenari, M., Nakamura, M. C., et al. (2009). A role for TREM2 ligands in the phagocytosis of apoptotic neuronal cells by microglia. J. Neurochem. 109, 1144–1156. doi: 10.1111/j.1471-4159.2009.06042.x
Hughes, M. M., Field, R. H., Perry, V. H., Murray, C. L., and Cunningham, C. (2010). Microglia in the degenerating brain are capable of phagocytosis of beads asncd of apoptotic cells, but do not efficiently remove PrPSc, even upon LPS stimulation. Glia 58, 2017–2030. doi: 10.1002/glia.21070
Hutchins, K. D., Dickson, D. W., Rashbaum, W. K., and Lyman, W. D. (1990). Localization of morphologically distinct microglial populations in the developing human fetal brain: implications for ontogeny. Dev. Brain Res. 55, 95–102. doi: 10.1016/0165-3806(90)90109-c
Hwang, D., Lee, I. Y., Yoo, H., Gehlenborg, N., Cho, J.-H., Petritis, B., et al. (2009). A systems approach to prion disease. Mol. Syst. Biol. 5:252. doi: 10.1038/msb.2009.10
Jarrett, J. T., and Lansbury, P. T. Jr. (1993). Seeding “one-dimensional crystallization” of amyloid: a pathogenic mechanism in Alzheimer’s disease and scrapie? Cell 73, 1055–1058. doi: 10.1016/0092-8674(93)90635-4
Jonsson, T., Atwal, J. K., Steinberg, S., Snaedal, J., Jonsson, P. V., Bjornsson, S., et al. (2012). A mutation in APP protects against Alzheimer’s disease and age-related cognitive decline. Nature 488, 96–99. doi: 10.1038/nature11283
Kettenmann, H., Hanisch, U. K., Noda, M., and Verkhratsky, A. (2011). Physiology of microglia. Physiol. Rev. 91, 461–553. doi: 10.1152/physrev.00011.2010
Kettenmann, H., Kirchhoff, F., and Verkhratsky, A. (2013). Microglia: new roles for the synaptic stripper. Neuron 77, 10–18. doi: 10.1016/j.neuron.2012.12.023
Kierdorf, K., Erny, D., Goldmann, T., Sander, V., Schulz, C., Perdiguero, E. G., et al. (2013). Microglia emerge from erythromyeloid precursors via Pu.1- and Irf8-dependent pathways. Nat. Neurosci. 16, 273–280. doi: 10.1038/nn.3318
Klein, M. A., Frigg, R., Flechsig, E., Raeber, A. J., Kalinke, U., Bluethmann, H., et al. (1997). A crucial role for B cells in neuroinvasive scrapie. Nature 390, 687–690.
Kordek, R., Nerurkar, V. R., Liberski, P. P., Isaacson, S., Yanagihara, R., and Gajdusek, D. C. (1996). Heightened expression of tumor necrosis factor α, interleukin 1 α and glial fibrillary acidic protein in experimental Creutzfeldt-Jakob disease in mice. Proc. Natl. Acad. Sci. U S A 93, 9754–9758. doi: 10.1073/pnas.93.18.9754
Krabbe, K. S., Reichenberg, A., Yirmiya, R., Smed, A., Pedersen, B. K., and Bruunsgaard, H. (2005). Low-dose endotoxemia and human neuropsychological functions. Brain Behav. Immun. 19, 454–460. doi: 10.1016/j.bbi.2005.04.010
Kranich, J., Krautler, N. J., Falsig, J., Ballmer, B., Li, S., Hutter, G., et al. (2010). Engulfment of cerebral apoptotic bodies controls the course of prion disease in a mouse strain-dependent manner. J. Exp. Med. 207, 2271–2281. doi: 10.1084/jem.20092401
Kyrkanides, S., Tallents, R. H., Miller, J.-N., Olschowka, M. E., Johnson, R., Yang, M., et al. (2011). Osteoarthritis accelerates and exacerbates Alzheimer’s disease pathology in mice. J. Neuroinflammation 8:112. doi: 10.1186/1742-2094-8-112
Lacroix, S., Feinstein, D., and Rivest, S. (1998). The bacterial endotoxin lipopolysaccharide has the ability to target the brain in upregulating its membrane CD14 receptor within specific cellular populations. Brain Pathol. 8, 625–640. doi: 10.1111/j.1750-3639.1998.tb00189.x
Laflamme, N., and Rivest, S. (1999). Effects of systemic immunogenic insults and circulating proinflammatory cytokines on the transcription of the inhibitory factor κBα within specific cellular populations of the rat brain. J. Neurochem. 73, 309–321. doi: 10.1046/j.1471-4159.1999.0730309.x
Lavin, Y., Winter, D., Blecher-Gonen, R., David, E., Keren-Shaul, H., Merad, M., et al. (2014). Tissue-resident macrophage enhancer landscapes are shaped by the local microenvironment. Cell 159, 1312–1326. doi: 10.1016/j.cell.2014.11.018
Lawson, L. J., Perry, V. H., and Gordon, S. (1992). Turnover of resident microglia in the normal adult mouse brain. Neuroscience 48, 405–415. doi: 10.1016/0306-4522(92)90500-2
Lawson, L. J., Perry, V. H., Dri, P., and Gordon, S. (1990). Heterogeneity in the distribution and morphology of microglia in the normal adult mouse brain. Neuroscience 39, 151–170. doi: 10.1016/0306-4522(90)90229-w
Li, J., Tang, Y., and Cai, D. (2012). IKKβ/NF-κB disrupts adult hypothalamic neural stem cells to mediate a neurodegenerative mechanism of dietary obesity and pre-diabetes. Nat. Cell Biol. 14, 999–1012. doi: 10.1038/ncb2562
Lin, H., Lee, E., Hestir, K., Leo, C., Huang, M., Bosch, E., et al. (2008). Discovery of a cytokine and its receptor by functional screening of the extracellular proteome. Science 320, 807–811. doi: 10.1126/science.1154370
Llewelyn, C. A., Hewitt, P. E., Knight, R. S., Amar, K., Cousens, S., Mackenzie, J., et al. (2004). Possible transmission of variant Creutzfeldt-Jakob disease by blood transfusion. Lancet 363, 417–421. doi: 10.1016/s0140-6736(04)15486-x
Llorens, F., López-González, I., Thüne, K., Carmona, M., Zafar, S., Andéoletti, O., et al. (2014). Subtype and regional-specific neuroinflammation in sporadic creutzfeldt-jakob disease. Front. Aging Neurosci. 6:198. doi: 10.3389/fnagi.2014.00198
Lyck, L., Santamaria, I. D., Pakkenberg, B., Chemnitz, J., Schrøder, H. D., Finsen, B., et al. (2009). An empirical analysis of the precision of estimating the numbers of neurons and glia in human neocortex using a fractionator-design with sub-sampling. J. Neurosci. Methods 182, 143–156. doi: 10.1016/j.jneumeth.2009.06.003
Lyons, A., Downer, E. J., Crotty, S., Nolan, Y. M., Mills, K. H., and Lynch, M. A. (2007). CD200 ligand receptor interaction modulates microglial activation in vivo and in vitro: a role for IL-4. J. Neurosci. 27, 8309–8313. doi: 10.1523/JNEUROSCI.1781-07.2007
Lyons, A., Lynch, A. M., Downer, E. J., Hanley, R., O’Sullivan, J. B., Smith, A., et al. (2009). Fractalkine-induced activation of the phosphatidylinositol-3 kinase pathway attentuates microglial activation in vivo and in vitro. J. Neurochem. 110, 1547–1556. doi: 10.1111/j.1471-4159.2009.06253.x
Mabbott, N. A., Williams, A., Farquhar, C. F., Pasparakis, M., Kollias, G., and Bruce, M. E. (2000). Tumor necrosis factor α-deficient, but not interleukin-6-deficient, mice resist peripheral infection with scrapie. J. Virol. 74, 3338–3344. doi: 10.1128/jvi.74.7.3338-3344.2000
Martínez-Muriana, A., Mancuso, R., Francos-Quijorna, I., Olmos-Alonso, A., Osta, R., Perry, V. H., et al. (2016). CSF1R blockade slows the progression of amyotrophic lateral sclerosis by reducing microgliosis and invasion of macrophages into peripheral nerves. Sci. Rep. 6:25663. doi: 10.1038/srep25663
Mass, E., Ballesteros, I., Farlik, M., Halbritter, F., Günther, P., Crozet, L., et al. (2016). Specification of tissue-resident macrophages during organogenesis. Science 353:aaf4238. doi: 10.1126/science.aaf4238
McHattie, S. J., Brown, D. R., and Bird, M. M. (1999). Cellular uptake of the prion protein fragment PrP106–126 in vitro. J. Neurocytol. 28, 149–159. doi: 10.1023/A:1007028323666
Minghetti, L., Greco, A., Cardone, F., Puopolo, M., Ladogana, A., Almonti, S., et al. (2000). Increased brain synthesis of prostaglandin E2 and F2-isoprostane in human and experimental transmissible spongiform encephalopathies. J. Neuropathol. Exp. Neurol. 59, 866–871. doi: 10.1093/jnen/59.10.866
Mittelbronn, M., Dietz, K., Schluesener, H. J., and Meyermann, R. (2001). Local distribution of microglia in the normal adult human central nervous system differs by up to one order of magnitude. Acta Neuropathol. 101, 249–255. doi: 10.1007/s004010000284
Miyazono, M., Iwaki, T., Kitamoto, T., Kaneko, Y., Doh-ura, K., and Tateishi, J. (1991). A comparative immunohistochemical study of Kuru and senile plaques with a special reference to glial reactions at various stages of amyloid plaque formation. Am. J. Pathol. 139, 589–598.
Mizuno, T., Doi, Y., Mizoguchi, H., Jin, S., Noda, M., Sonobe, Y., et al. (2011). Interleukin-34 selectively enhances the neuroprotective effects of microglia to attenuate oligomeric amyloid-β neurotoxicity. Am. J. Pathol. 179, 2016–2027. doi: 10.1016/j.ajpath.2011.06.011
Monier, A., Evrard, P., Gressens, P., and Verney, C. (2006). Distribution and differentiation of microglia in the human encephalon during the first two trimesters of gestation. J. Comp. Neurol. 499, 565–582. doi: 10.1002/cne.21123
Muhleisen, H., Gehrmann, J., and Meyermann, R. (1995). Reactive microglia in Creutzfeldt-Jakob disease. Neuropathol. Appl. Neurobiol. 21, 505–517. doi: 10.1111/j.1365-2990.1995.tb01097.x
Murray, C. L., Skelly, D. T., and Cunningham, C. (2011). Exacerbation of CNS inflammation and neurodegeneration by systemic LPS treatment is independent of circulating IL-1β and IL-6. J. Neuroinflammation 8:50. doi: 10.1186/1742-2094-8-50
Nandi, S., Gokhan, S., Dai, X. M., Wei, S., Enikolopov, G., Lin, H., et al. (2012). The CSF-1 receptor ligands IL-34 and CSF-1 exhibit distinct developmental brain expression patterns and regulate neural progenitor cell maintenance and maturation. Dev. Biol. 367, 100–113. doi: 10.1016/j.ydbio.2012.03.026
Nimmerjahn, A., Kirchhoff, F., and Helmchen, F. (2005). Resting microglial cells are highly dynamic surveillants of brain parenchyma in vivo. Science 308, 1314–1318. doi: 10.1126/science.1110647
Nuvolone, M., Sorce, S., Schwarz, P., and Aguzzi, A. (2015). Prion pathogenesis in the absence of NLRP3/ASC inflammasomes. PLoS One 10:e0117208. doi: 10.1371/journal.pone.0117208
O’Shea, M., Maytham, E. G., Linehan, J. M., Brandner, S., Collinge, J., and Lloyd, S. E. (2008). Investigation of mcp1 as a quantitative trait gene for prion disease incubation time in mouse. Genetics 180, 559–566. doi: 10.1534/genetics.108.090894
Olmos-Alonso, A., Schetters, S. T., Sri, S., Askew, K., Mancuso, R., Vargas-Caballero, M., et al. (2016). Pharmacological targeting of CSF1R inhibits microglial proliferation and prevents the progression of Alzheimer’s-like pathology. Brain 139, 891–907. doi: 10.1093/brain/awv379
Paloneva, J., Kestilä, M., Wu, J., Salminen, A., Böhling, T., Ruotsalainen, V., et al. (2000). Loss-of-function mutations in TYROBP (DAP12) result in a presenile dementia with bone cysts. Nat. Genet. 25, 357–361. doi: 10.1038/77153
Paloneva, J., Manninen, T., Christman, G., Hovanes, K., Mandelin, J., Adolfsson, R., et al. (2002). Mutations in two genes encoding different subunits of a receptor signaling complex result in an identical disease phenotype. Am. J. Hum. Genet. 71, 656–662. doi: 10.1086/342259
Paolicelli, R. C., Bolasco, G., Pagani, F., Maggi, L., Scianni, M., Panzanelli, P., et al. (2011). Synaptic pruning by microglia is necessary for normal brain development. Science 333, 1456–1458. doi: 10.1126/science.1202529
Pelvig, D. P., Pakkenberg, H., Stark, A. K., and Pakkenberg, B. (2008). Neocortical glial cell numbers in human brains. Neurobiol Aging 29, 1754–1762. doi: 10.1016/j.neurobiolaging.2007.04.013
Perry, V. H. (2010). Contribution of systemic inflammation to chronic neurodegeneration. Acta Neuropathol. 120, 277–286. doi: 10.1007/s00401-010-0722-x
Perry, V. H., Cunningham, C., and Boche, D. (2002). Atypical inflammation in the central nervous system in prion disease. Curr. Opin. Neurol. 15, 349–354. doi: 10.1097/00019052-200206000-00020
Perry, V. H., Nicoll, J. A., and Holmes, C. (2010). Microglia in neurodegenerative disease. Nat. Rev. Neurol. 6, 193–201. doi: 10.1038/nrneurol.2010.17
Perry, V. H., and O’Connor, V. (2010). The role of microglia in synaptic stripping and synaptic degeneration: a revised perspective. ASN Neuro 2:e00047. doi: 10.1042/AN20100024
Perry, V. H., and Teeling, J. (2013). Microglia and macrophages of the central nervous system: the contribution of microglia priming and systemic inflammation to chronic neurodegeneration. Semin. Immunopathol. 35, 601–612. doi: 10.1007/s00281-013-0382-8
Pott-Godoy, M. C., Tarelli, R., Ferrari, C. C., Sarchi, M. I., and Pitossi, F. J. (2008). Central and systemic IL-1 exacerbates neurodegeneration and motor symptoms in a model of Parkinson’s disease. Brain 131, 1880–1894. doi: 10.1093/brain/awn101
Prokop, S., Miller, K. R., and Heppner, F. L. (2013). Microglia actions in Alzheimer’s disease. Acta Neuropathol. 126, 461–477. doi: 10.1007/s00401-013-1182-x
Prusiner, S. B. (1998). Prions. Proc. Natl. Acad. Sci. U S A 95, 13363–13383. doi: 10.1073/pnas.95.23.13363
Puoti, G., Giaccone, G., Mangieri, M., Limido, L., Fociani, P., Zerbi, P., et al. (2005). Sporadic Creutzfeldt-Jakob disease: the extent of microglia activation is dependent on the biochemical type of PrPSc. J. Neuropathol. Exp. Neurol. 64, 902–909. doi: 10.1097/01.jnen.0000183346.19447.55
Qin, L., Wu, X., Block, M. L., Liu, Y., Breese, G. R., Hong, J. S., et al. (2007). Systemic LPS causes chronic neuroinflammation and progressive neurodegeneration. Glia 55, 453–462. doi: 10.1002/glia.20467
Raivich, G., Haas, S., Werner, A., Klein, M. A., Kloss, C., and Kreutzberg, G. W. (1998). Regulation of MCSF receptors on microglia in the normal and injured mouse central nervous system: a quantitative immunofluorescence study using confocal laser microscopy. J. Comp. Neurol. 395, 342–358. doi: 10.1002/(SICI)1096-9861(19980808)395:3<342::aid-cne6>3.0.co;2-2
Rezaie, P., and Male, D. (1999). Colonisation of the developing human brain and spinal cord by microglia: a review. Microsc. Res. Tech. 45, 359–382. doi: 10.1002/(sici)1097-0029(19990615)45:6<359::aid-jemt4>3.3.co;2-4
Roe, A. D., Staup, M. A., Serrats, J., Sawchenko, P. E., and Rissman, R. A. (2011). Lipopolysaccharide-induced tau phosphorylation and kinase activity—modulation, but not mediation, by corticotropin-releasing factor receptors. Eur. J. Neurosci. 34, 448–456. doi: 10.1111/j.1460-9568.2011.07764.x
Saba, R., Gushue, S., Huzarewich, R. L., Manguiat, K., Medina, S., Robertson, C., et al. (2012). MicroRNA 146a (miR-146a) is over-expressed during prion disease and modulates the innate immune response and the microglial activation state. PLoS One 7:e30832. doi: 10.1371/journal.pone.0030832
Sasaki, A., Hirato, J., and Nakazato, Y. (1993). Immunohistochemical study of microglia in the Creutzfeldt-Jakob diseased brain. Acta Neuropathol. 86, 337–344. doi: 10.1007/bf00369445
Schafer, D. P., Lehrman, E. K., Kautzman, A. G., Koyama, R., Mardinly, A. R., Yamasaki, R., et al. (2012). Microglia sculpt postnatal neural circuits in an activity and complement-dependent manner. Neuron 74, 691–705. doi: 10.1016/j.neuron.2012.03.026
Schiffenbauer, J., Johnson, H. M., Butfiloski, E. J., Wegrzyn, L., and Soos, J. M. (1993). Staphylococcal enterotoxins can reactivate experimental allergic encephalomyelitis. Proc. Natl. Acad. Sci. U S A 90, 8543–8854. doi: 10.1073/pnas.90.18.8543
Schultz, J., Schwarz, A., Neidhold, S., Burwinkel, M., Riemer, C., Simon, D., et al. (2004). Role of interleukin-1 in prion disease-associated astrocyte activation. Am. J. Pathol. 165, 671–678. doi: 10.1016/s0002-9440(10)63331-7
Schulz, C., Gomez Perdiguero, E., Chorro, L., Szabo-Rogers, H., Cagnard, N., Kierdorf, K., et al. (2012). A lineage of myeloid cells independent of Myb and hematopoietic stem cells. Science 336, 86–90. doi: 10.1126/science.1219179
Ségaliny, A. I., Brion, R., Brulin, B., Maillasson, M., Charrier, C., Téletchéa, S., et al. (2015). IL-34 and M-CSF form a novel heteromeric cytokine and regulate the M-CSF receptor activation and localization. Cytokine 76, 170–181. doi: 10.1016/j.cyto.2015.05.029
Sharief, M. K., Green, A., Dick, J. P., Gawler, J., and Thompson, E. J. (1999). Heightened intrathecal release of proinflammatory cytokines in Creutzfeldt-Jakob disease. Neurology 52, 1289–1291. doi: 10.1212/wnl.52.6.1289
Sheng, J., Ruedl, C., and Karjalainen, K. (2015). Most tissue-resident macrophages except microglia are derived from fetal hematopoietic stem cells. Immunity 43, 382–393. doi: 10.1016/j.immuni.2015.07.016
Shi, Q., Xie, W.-L., Zhang, B., Chen, L.-N., Xu, Y., Wang, K., et al. (2013). Brain microglia were activated in sporadic CJD but almost unchanged in fatal familial insomnia and G114V genetic CJD. Virol. J. 10:216. doi: 10.1186/1743-422x-10-216
Shi, F., Yang, L., Kouadir, M., Yang, Y., Wang, J., Zhou, X., et al. (2012). The NALP3 inflammasome is involved in neurotoxic prion peptide-induced microglial activation. J. Neuroinflammation 9:73. doi: 10.1186/1742-2094-9-73
Sly, L. M., Krzesicki, R. F., Brashler, J. R., Buhl, A. E., McKinley, D. D., Carter, D. B., et al. (2001). Endogenous brain cytokine mRNA and inflammatory responses to lipopolysaccharide are elevated in the Tg2576 transgenic mouse model of Alzheimer’s disease. Brain Res. Bull. 56, 581–588. doi: 10.1016/s0361-9230(01)00730-4
Sorce, S., Nuvolone, M., Keller, A., Falsig, J., Varol, A., Schwarz, P., et al. (2014). The role of the NADPH oxidase NOX2 in prion pathogenesis. PLoS Pathog. 10:e1004531. doi: 10.1371/journal.ppat.1004531
Soreq, L., UK Brain Expression Consortium, North American Brain Expression Consortium, Rose, J., Soreq, E., Hardy, J., et al. (2017). Major shifts in glial regional identity are a transcriptional hallmark of human brain aging. Cell Rep. 18, 557–570. doi: 10.1016/j.celrep.2016.12.011
Squarzoni, P., Oller, G., Hoeffel, G., Pont-Lezica, L., Rostaing, P., Low, D., et al. (2014). Microglia modulate wiring of the embryonic forebrain. Cell Rep. 8, 1271–1279. doi: 10.1016/j.celrep.2014.07.042
Stanley, E. R., and Heard, P. M. (1977). Factors regulating macrophage production and growth. Purification and some properties of the colony stimulating factor from medium conditioned by mouse L cells. J. Biol. Chem. 252, 4305–4312.
Stevens, B., Allen, N. J., Vazquez, L. E., Howell, G. R., Christopherson, K. S., Nouri, N., et al. (2007). The classical complement cascade mediates CNS synapse elimination. Cell 131, 1164–1178. doi: 10.1016/j.cell.2007.10.036
Stoeck, K., Bodemer, M., and Zerr, I. (2006). Pro- and anti-inflammatory cytokines in the CSF of patients with Creutzfeldt-Jakob disease. J. Neuroimmunol. 172, 175–181. doi: 10.1016/j.jneuroim.2005.10.008
Stoeck, K., Schmitz, M., Ebert, E., Schmidt, C., and Zerr, I. (2014). Immune responses in rapidly progressive dementia: a comparative study of neuroinflammatory markers in Creutzfeldt-Jakob disease, Alzheimer’s disease and multiple sclerosis. J. Neuroinflammation 11:170. doi: 10.1186/s12974-014-0170-y
Szpak, G. M., Lewandowska, E., Lechowicz, W., Wierzba-Bobrowicz, T., Kulczycki, J., Bertrand, E., et al. (2006). The brain immune response in human prion diseases. Microglial activation and microglial disease. I. Sporadic Creutzfeldt-Jakob disease. Folia Neuropathol. 44, 202–213.
Takahashi, K., Prinz, M., Stagi, M., Chechneva, O., and Neumann, H. (2007). TREM2-transduced myeloid precursors mediate nervous tissue debris clearance and facilitate recovery in an animal model of multiple sclerosis. PLoS Med. 4:e124. doi: 10.1371/journal.pmed.0040124
Takahashi, K., Rochford, C. D. P., and Neumann, H. (2005). Clearance of apoptotic neurons without inflammation by microglial triggering receptor expressed on myeloid cells-2. J. Exp. Med. 201, 647–657. doi: 10.1084/jem.20041611
Tamgüney, G., Giles, K., Glidden, D. V., Lessard, P., Wille, H., Tremblay, P., et al. (2008). Genes contributing to prion pathogenesis. J. Gen. Virol. 89, 1777–1788. doi: 10.1099/vir.0.2008/001255-0
Tanaka, M., Collins, S. R., Toyama, B. H., and Weissman, J. S. (2006). The physical basis of how prion conformations determine strain phenotypes. Nature 442, 585–589. doi: 10.1038/nature04922
Torres-Platas, S. G., Comeau, S., Rachalski, A., Bo, G. D., Cruceanu, C., Turecki, G., et al. (2014). Morphometric characterization of microglial phenotypes in human cerebral cortex. J. Neuroinflammation 11:12. doi: 10.1186/1742-2094-11-12
Tremblay, M. E., Lowery, R. L., and Majewska, A. K. (2010). Microglial interactions with synapses are modulated by visual experience. PLoS Biol. 8:e1000527. doi: 10.1371/journal.pbio.1000527
Van Everbroeck, B., Dewulf, E., Pals, P., Lübke, U., Martin, J.-J., and Cras, P. (2002). The role of cytokines, astrocytes, microglia and apoptosis in Creutzfeldt-Jakob disease. Neurobiol. Aging 23, 59–64. doi: 10.1016/s0197-4580(01)00236-6
Van Everbroeck, B., Dobbeleir, I., De Waele, M., De Leenheir, E., Lübke, U., Martin, J.-J., et al. (2004). Extracellular protein deposition correlates with glial activation and oxidative stress in Creutzfeldt-Jakob and Alzheimer’s disease. Acta Neuropathol. 108, 194–200. doi: 10.1007/s00401-004-0879-2
Verney, C., Monier, A., Fallet-Bianco, C., and Gressens, P. (2010). Early microglial colonization of the human forebrain and possible involvement in periventricular white-matter injury of preterm infants. J. Anat. 217, 436–448. doi: 10.1111/j.1469-7580.2010.01245.x
Villa, V., Thellung, S., Corsaro, A., Novelli, F., Tasso, B., Colucci-D’Amato, L., et al. (2016). Celecoxib inhibits prion protein 90-231-mediated pro-inflammatory responses in microglial cells. Mol. Neurobiol. 53, 57–72. doi: 10.1007/s12035-014-8982-4
Vincenti, J. E., Murphy, L., Grabert, K., McColl, B. W., Cancellotti, E., Freeman, T. C., et al. (2015). Defining the microglia response during the time course of chronic neurodegeneration. J. Virol. 90, 3003–3017. doi: 10.1128/jvi.02613-15
Wadsworth, J. D., and Collinge, J. (2011). Molecular pathology of human prion disease. Acta Neuropathol. 121, 69–77. doi: 10.1007/s00401-010-0735-5
Wake, H., Moorhouse, A. J., Jinno, S., Kohsaka, S., and Nabekura, J. (2009). Resting microglia directly monitor the functional state of synapses in vivo and determine the fate of ischemic terminals. J. Neurosci. 29, 3974–3980. doi: 10.1523/JNEUROSCI.4363-08.2009
Walsh, D. T., Betmouni, S., and Perry, V. H. (2001). Absence of detectable IL-1β production in murine prion disease: a model of chronic neurodegeneration. J. Neuropathol. Exp. Neurol. 60, 173–182. doi: 10.1093/jnen/60.2.173
Wang, Y., Cella, M., Mallinson, K., Ulrich, J. D., Young, K. L., Robinette, M. L., et al. (2015). TREM2 lipid sensing sustains the microglial response in an Alzheimer’s disease model. Cell 160, 1061–1071. doi: 10.1016/j.cell.2015.01.049
Wang, Y., Szretter, K. J., Vermi, W., Gilfillan, S., Rossini, C., Cella, M., et al. (2012). IL-34 is a tissue-restricted ligand of CSF1R required for the development of Langerhans cells and microglia. Nat. Immunol. 13, 753–760. doi: 10.1038/ni.2360
Wang, H., Yu, M., Ochani, M., Amella, C. A., Tanovic, M., Susarla, S., et al. (2003). Nicotinic acetylcholine receptor α7 subunit is an essential regulator of inflammation. Nature 421, 384–388. doi: 10.1038/nature01339
Wei, S., Nandi, S., Chitu, V., Yeung, Y.-G., Yu, W., Huang, M., et al. (2010). Functional overlap but differential expression of CSF-1 and IL-34 in their CSF-1 receptor-mediated regulation of myeloid cells. J. Leukoc. Biol. 88, 495–505. doi: 10.1189/jlb.1209822
Williams, A., Lucassen, P. J., Ritchie, D., and Bruce, M. (1997). PrP deposition, microglial activation, and neuronal apoptosis in murine scrapie. Exp. Neurol. 144, 433–438. doi: 10.1006/exnr.1997.6424
Williams, A. E., van Dam, A. M., Man-A-Hing, W. K., Berkenbosch, F., Eikelenboom, P., and Fraser, H. (1994). Cytokines, prostaglandins and lipocortin-1 are present in the brains of scrapie-infected mice. Brain Res. 654, 200–206. doi: 10.1016/0197-4580(94)92818-5
Yun, S.-W., Gerlach, M., Riederer, P., and Klein, M. A. (2006). Oxidative stress in the brain at early preclinical stages of mouse scrapie. Exp. Neurol. 201, 90–98. doi: 10.1016/j.expneurol.2006.03.025
Zhang, G., Li, J., Purkayastha, S., Tang, Y., Zhang, H., Yin, Y., et al. (2013). Hypothalamic programming of systemic ageing involving IKK-β, NF-κB and GnRH. Nature 497, 211–216. doi: 10.1038/nature12143
Zhang, H., Li, F., Yang, Y., Chen, J., and Hu, X. (2015). SIRP/CD47 signaling in neurological disorders. Brain Res. 1623, 74–80. doi: 10.1016/j.brainres.2015.03.012
Zhao, Y., Jaber, V., and Lukiw, W. J. (2016). Over-expressed pathogenic miRNAs in Alzheimer’s disease (AD) and prion disease (PrD) drive deficits in TREM2-mediated Aβ42 peptide clearance. Front. Aging Neurosci. 8:140. doi: 10.3389/fnagi.2016.00140
Zhu, C., Herrmann, U. S., Falsig, J., Abakumova, I., Nuvolone, M., Schwarz, P., et al. (2016). A neuroprotective role for microglia in prion diseases. J. Exp. Med. 213, 1047–1059. doi: 10.1084/jem.20151000
Zhu, C., Herrmann, U. S., Li, B., Abakumova, I., Moos, R., Schwarz, P., et al. (2015). Triggering receptor expressed on myeloid cells-2 is involved in prion-induced microglial activation but does not contribute to prion pathogenesis in mouse brains. Neurobiol. Aging 36, 1994–2003. doi: 10.1016/j.neurobiolaging.2015.02.019
Keywords: microglia, Csf1r, proliferation, neuroinflammation, neurodegnerative diseases
Citation: Obst J, Simon E, Mancuso R and Gomez-Nicola D (2017) The Role of Microglia in Prion Diseases: A Paradigm of Functional Diversity. Front. Aging Neurosci. 9:207. doi: 10.3389/fnagi.2017.00207
Received: 21 March 2017; Accepted: 09 June 2017;
Published: 23 June 2017.
Edited by:
Yu Tang, University of Texas Southwestern Medical Center, United StatesReviewed by:
Walter J. Lukiw, LSU Health Sciences Center New Orleans, United StatesRafael M. Mariante, Oswaldo Cruz Foundation, Brazil
Copyright © 2017 Obst, Simon, Mancuso and Gomez-Nicola. This is an open-access article distributed under the terms of the Creative Commons Attribution License (CC BY). The use, distribution or reproduction in other forums is permitted, provided the original author(s) or licensor are credited and that the original publication in this journal is cited, in accordance with accepted academic practice. No use, distribution or reproduction is permitted which does not comply with these terms.
*Correspondence: Diego Gomez-Nicola, ZC5nb21lei1uaWNvbGFAc290b24uYWMudWs=
† These authors have contributed equally to this work.