- 1Laboratory of Experimental Psychology and Neuroscience (LPEN), Institute of Cognitive and Translational Neuroscience (INCyT), INECO Foundation, Favaloro University, Buenos Aires, Argentina
- 2National Scientific and Technical Research Council (CONICET), Buenos Aires, Argentina
- 3Universidad Autónoma del Caribe, Barranquilla, Colombia
- 4Center for Social and Cognitive Neuroscience (CSCN), School of Psychology, Universidad Adolfo Ibañez, Santiago de Chile, Chile
- 5Centre of Excellence in Cognition and its Disorders, Australian Research Council (ARC), Sydney, NSW, Australia
- 6Faculty of Education, National University of Cuyo (UNCuyo), Mendoza, Argentina
Non-invasive brain stimulation (NIBS) techniques can significantly modulate cognitive functions in healthy subjects and patients with neuropsychiatric disorders. Recently, they have been applied in patients with mild cognitive impairment (MCI) and subjective cognitive impairment (SCI) to prevent or delay the development of Alzheimer’s disease (AD). Here we review this emerging empirical corpus and discuss therapeutic effects of NIBS on several target functions (e.g., memory for face-name associations and non-verbal recognition, attention, psychomotor speed, everyday memory). Available studies have yielded mixed results, possibly due to differences among their tasks, designs, and samples, let alone the latter’s small sizes. Thus, the impact of NIBS on cognitive performance in MCI and SCI remains to be determined. To foster progress in this direction, we outline methodological approaches that could improve the efficacy and specificity of NIBS in both conditions. Furthermore, we discuss the need for multicenter studies, accurate diagnosis, and longitudinal approaches combining NIBS with specific training regimes. These tenets could cement biomedical developments supporting new treatments for MCI and preventive therapies for AD.
Introduction
In the face of an aging society, an urgent need has emerged to prevent and treat dementia, in general, and Alzheimer’s disease (AD), in particular (Ferri et al., 2006). Despite wide-ranging research efforts to elucidate the putative pathological mechanisms of dementia, available treatments remain limited (Alzheimer’s Association, 2012). Promisingly, however, various factors have been shown to boost the dynamics of relevant neural networks, delaying and even reducing clinical symptoms (Fregni and Pascual-Leone, 2007). Therefore, interventions that enhance neuroplastic responses in early disease stages might be particularly useful to attenuate the rate of cognitive decline in AD patients.
A key model to assess this possibility is afforded by patients with mild cognitive impairment (MCI). This construct has evolved over the past two decades to denote a cognitive state intermediate between normal aging and very early dementia (Petersen and Negash, 2008). Patients with this condition feature objective memory impairment and other cognitive deficits, but their dysfunction is not so severe as to compromise daily activities (Petersen and Negash, 2008). MCI comprises four clinical subtypes: (i) single-domain amnestic MCI (aMCI); (ii) multiple-domain aMCI; (iii) single-domain non-amnestic MCI; and (iv) multiple-domain non-amnestic MCI (Winblad et al., 2004; Anstey et al., 2008; Albert et al., 2011). These subtypes differ in etiology and outcome: whereas both forms of aMCI imply a high chance of progression to AD, non-amnestic varieties involve considerable risk of conversion to non-AD dementia (Winblad et al., 2004; Anstey et al., 2008; Albert et al., 2011).
A second relevant model can be found in individuals featuring subjective cognitive impairment (SCI). This notion encompasses people who report everyday concerns about their cognitive functioning, even in the absence of objectively established impairments. Given its association with subsequent cognitive decline, SCI represents a potential prodromal state of AD occurring even before MCI (Reisberg and Gauthier, 2008).
In sum, aMCI and SCI are likely to anticipate incipient dementia. Despite the high between-patient variability and the uncertainty about their causative physiopathological mechanisms, both conditions offer highly relevant models to implement interventions aimed to boost cognitive performance through modulations of neuronal activity and connectivity. In this sense, non-invasive brain stimulation (NIBS) techniques have emerged as a potentially useful alternative. These tools can selectively enhance adaptive activity patterns, suppress maladaptive patterns, and influence learning and skill acquisition processes by inducing synaptic plasticity and network reorganization (Hummel and Cohen, 2006; Nitsche et al., 2008). In particular, NIBS can have a direct impact on memory mechanisms (including working, episodic, and associative memory) in young adults, elderly adults and patients with neurological dysfunctions (Manenti et al., 2012; Elder and Taylor, 2014).
Building on these antecedentes, a number of studies have assessed the impact of NIBS on MCI patients. Here we aim to critically review such emerging literature. While other reviews have addressed the impact of NIBS in cognitively impaired patients (Freitas et al., 2011; Nardone et al., 2014), here we focus on the key questions and difficulties of setting a treatment protocol. To this end, first we characterize the main features of NIBS. Thereupon, we review available studies examining the impact of NIBS on learning and memory in MCI and SCI. In particular, we describe and discuss in detail the studies’ protocols and parameters, highlight the advantages of combining NIBS with cognitive training. Finally, we outline methodological strategies that could promote major developments in this promising research area.
NIBS: Features and Mechanisms
With NIBS, researchers can transiently influence behavior by altering neural activity (Miniussi et al., 2013). A considerable body of evidence has been forged through the two most commonly used techniques in humans: repetitive transcranial magnetic stimulation (rTMS) and transcranial direct current stimulation (tDCS). Although they differ in several aspects (Table 1), both tools can induce long-term after effects on cortical excitability and neuronal plasticity. Typically, this brings about facilitatory or inhibitory effects (Nitsche and Paulus, 2000), broadly mirroring the workings of long-term potentiation (LTP) and long-term depression (LTD; Dayan et al., 2013). Since such effects can considerably outlast the actual stimulation period, NIBS techniques open valuable avenues for rehabilitation, especially when stimulation protocols are combined with adequate training-based interventions (Zimerman et al., 2013).
TMS has been recently approved in some countries to treat depression and other disorders, subject to approval by local ethical review boards (Rossi et al., 2009). These reservations largely follow from the suboptimal understanding of the putative mechanisms underlying NIBS-related effects. However, a number of contributing factors have been recently identified. A critical one concerns modulation of neurotransmitter levels (Ridding and Ziemann, 2010). Transcranial stimulation may alter the dynamics of excitatory/inhibitory neurotransmitter systems (e.g., GABA and glutamate) affecting the regulation of cortical neuronal activity. For example, rTMS effects decrease if the subjects consume a drug that interferes with NMDA receptors (Reis et al., 2006). Likewise, post-tDCS effects can be blocked by an NMDA-antagonist, whereas a partial NMDA-agonist (d-cycloserine) can selectively extend the duration of motor cortical excitability induced by anodal tDCS (Nitsche et al., 2004). Moreover, MR spectroscopy research (Stagg et al., 2009) shows that anodal tDCS decreases GABAergic transmission, while cathodal tDCS yields similar effects on glutamate concentrations over the motor cortex (MC).
NIBS may also exert long-lasting plastic changes through gene induction. Research based on rTMS protocols (Fritsch et al., 2010) has shown significant enhancements of brain-derived neurotrophic factor (BDNF) mRNA in the hippocampal, parietal, and piriform cortices. For instance, LTP-like effects in adult mice are abolished for specimens with deletion of the BDNF gene, suggesting that this factor could be a critical mediator of direct stimulation effects (Fritsch et al., 2010). Importantly, as a member of the neurotrophin family, BDNF plays an important role in synaptogenesis and synaptic mechanisms related to learning and memory processes.
Additionally, TMS has been successfully used in AD and MCI to track and modulate abnormal mechanisms, including glutamatergic dysfunctions, disruption of synaptic plasticity, and inhibition of LTP (Nardone et al., 2014). For example, TMS research consistently shows that such patients feature a decreased resting motor threshold, which has been interpreted as the electrophysiological correlate of cortical glutamatergic dysfunctions and as a marker of reduced cortical hyperexcitability in AD (Di Lazzaro et al., 2003, 2004; Inghilleri et al., 2006; Trebbastoni et al., 2016). Furthermore, rTMS reduces facilitation of the motor-evoked potential (MEP) in aMCI patients (Trebbastoni et al., 2016), revealing an initial impairment of the mechanisms that underlie glutamate-induced synaptic potentiation (Trebbastoni et al., 2016). On the other hand, a TMS-MRI study indicates that hyperexcitability of the sensorimotor cortex might represent a protective mechanism counteracting the prominent loss of cortical volume in AD and MCI. However, this supposed protective mechanism was found neither on the precuneus or cuneus of AD patients, nor in an MCI group (Niskanen et al., 2011). Furthermore, an assessment of navigated TMS-evoked EEG responses in AD patients showed prominent changes in functional connectivity and reactivity (Julkunen et al., 2008, 2011). In particular, TMS-evoked responses at 30–50 ms decreased significantly in AD over widespread brain regions, suggesting dysfunctions of a large-scale sensorimotor network. Compatibly, a TMS-EEG co-registration study in AD patients offered direct evidence of MC hyperexcitability, extending to the whole sensorimotor system (Ferreri et al., 2016). Although speculative, these changes could be interpreted as a compensatory mechanism allowing for the preservation of sensorimotor programming and execution over a long period, regardless of the disease’s progression. Additional evidence on cortical plasticity in relevant patient samples comes from paired associative stimulation (PAS) and cortical responses to rTMS. Null PAS effects in MCI patients have been reported by Lahr et al. (2016). However, this result should be considered with caution, since the study mixed patients with single- and multiple-domain aMCI which may have clouded effects on a specific subsample. Indeed, previous evidence from Nardone et al. (2012) demonstrated that short-latency afferent inhibition is significantly reduced in patients with multiple-domain amnestic MCI relative to controls, but not in patients with single-domain amnestic MCI or with non-amnestic MCI. Taken together, this incipient evidence suggests that NIBS can directly impinge on the pathological mechanisms underlying some forms of AD and MCI.
Finally, note that the effects of rTMS and tDCS are not restricted to the stimulation site. Depending on stimulation parameters, they can induce changes at distant points through effective modulations of remote, interconnected networks (Plewnia et al., 2003; Hummel and Cohen, 2006). This particular property of NIBS techniques has been assessed in proof-of-principle studies aimed to enhance and decrease excitability of critical brain regions involved in the recovery process (for details see Hummel and Cohen, 2006; Nowak et al., 2010; Schulz et al., 2013). All these tenets illuminate the evidence gathered so far in NIBS studies on MCI patients, which we review below.
Does NIBS Improve Learning and Formation of Novel Memories in MCI?
Growing evidence indicates that NIBS techniques can significantly modulate various cognitive processes in both elderly neurotypicals and patients with AD. While the impact of NIBS on the latter population has been widely discussed (Hsu et al., 2015), much less attention has been paid to NIBS research on MCI and SCI. Below we offer a joint assessment of this empirical corpus, discussing available results in terms of stimulated brain regions. We deal with TMS studies first, and then consider those employing tDCS (for full details of each study see Table 2).
TMS Studies with MCI Patients
Prefrontal Cortex
In a study with SCI patients, Solé-Padullés et al. (2006) reported improvements of face-name associative memory following up-regulation of the bilateral prefrontal cortex (PFC) through high-frequency (5 Hz) rTMS. Specifically, the behavioral enhancement positively correlated with the recruitment of the right PFC and posterior bilateral cortices, as shown by fMRI. In another study, Turriziani et al. (2012) found improvements of non-verbal recognition memory in MCI patients after low-frequency (1 Hz) stimulation and subsequent down-regulation of the right but not the left dorsolateral prefrontal cortex (DLPFC). Morever, a double-blind randomized sham-controlled study using 10-Hz rTMS in MCI patients (Drumond Marra et al., 2015) showed that up-regulation of the DLPFC led to improvements in everyday memory. Interestingly, this enhancement remained for at least a month. However, stimulation of prefrontal structures has also yielded null results. In particular, a blind study with a crossover design (Sedlackova et al., 2008) failed to induce positive or negative behavioral effects following either low- or high-frequency rTMSof the DLPFC in seven patients with vascular MCI.
Parietal Cortex
In a single-case study with an aMCI patient, Cotelli et al. (2012) assessed whether up-regulation of the left parietal cortex could lead to long-term improvements in face-name associations. The 10-day protocol consisted in daily 25-min-long stimulation sessions with high-frequency (20 Hz) rTMS. Behavioral enhancements suggested a putative role of the target region in associative memory.
Inferior Frontal Gyrus and Superior Temporal Gyrus
Two related studies Eliasova et al. (2014) and Anderkova et al. (2015) found that rTMS significantly improved Stroop-task performance in aMCI and AD patients. The stimulation protocol comprised three randomized 22-min-long sessions, during which 10-Hz rTMS was applied over the IFG, the right superior temporal gyrus (STG), and the vertex (VTX, a control stimulation site). As compared to the VTX, both IFG and STG simulation improved the patients’ behavioral outcome. In particular, through a combination of rTMS and MRI, Anderkova et al. (2015) found that MCI/AD subjects had significant atrophy in the inferior temporal and fusiform gyri, putamen and cerebellum relative to healthy controls. Moreover, the level of atrophy correlated with the change in Stroop-task performance after rTMS of the STG.
Interim Summary
Taken together, these studies indicate that rTMS can have significant effects on cognitive functions in patients with SCI and MCI. Specifically, this form of stimulation appears to enhance face-name association memory, non-verbal recognition memory, attention, psychomotor speed, and everyday memory. However, inconsistencies among comparable studies and null results in some of them cast doubts on the robustness of this technique to restore cognitive function in MCI. A number of caveats can be identified. First, rTMS might not directly stimulate deep brain areas critically compromised in MCI and AD (e.g., the hippocampus). Second, discrepancies in stimulation parameters (frequency, intensity, duration, number of sessions, stimulation site) and experimental design across studies make it difficult to determine which configuration could systematically yield effective results. Finally, there is only one double-blind randomized sham-controlled rTMS study with MCI patients. Thus, further work and more randomized placebo-controlled studies are needed to establish the optimal stimulation parameters for potential treatments in this population. Critically, a more through characterization is needed of how results are influenced by multiple interrelated factors, such as the patients’ clinical profile, specific target areas, stimulation frequency, number of treatment sessions, sessionduration and specific cognitive domains tapped through selected behavioral tasks.
tDCS Studies with MCI Patients
To date, only two studies have explored the potential neurocognitive benefits of anodal tDCS in MCI patients. The target regions in them were the IFG and the PFC.
Inferior Frontal Gyrus
Meinzer et al. (2015) performed a double-blind, crossover, sham-controlled stimulation study with simultaneous task-related and resting-state fMRI recordings. An MRI-compatible stimulator was used to administer a constant direct current (1 mA, for 20 min) over the IFG. During sham stimulation, MCI patients produced fewer correct responses than healthy controls in a semantic fluency task. Interestingly, the degree of correct responses was associated with hyperactivity in bilateral prefrontal regions. Anodal tDCS significantly improved performance in MCI patients to healthy-control levels. Furthermore, such stimulation reduced task-related prefrontal hyperactivity and normalized altered network configurations at during resting state. This study provides evidence that anodal tDCS could ameliorate cognitive dysfunction in MCI patients and temporarily reverse abnormalities in relevant brain activity.
Prefrontal Cortex
A recent study assessing MCI patients with Parkinson’s disease (PD; Manenti et al., 2016) evaluated the impact of up-regulating the right or left DLPFC via anodal tDCS combined with physical therapies. For 2 weeks, patients underwent daily administration of 2-mA stimulation during 25 min. Scores on the PD Cognitive Rating Scale and a verbal fluency test increased only in the real tDCS group (compared with the sham group). Notably, the effect remained stable for up to 3 months after the intervention.
Interim Discussion
These extremely preliminary results suggest that anodal tDCS can ameliorate cognitive dysfunction in different subtypes of MCI. In particular, long-term results obtained by combining stimulation with physical training open interesting avenues for further research.
Challenges and Future Directions
So far, NIBS studies seeking to improve cognitive function in MCI/SCI patients have yielded inconclusive findings. Although some studies showed favorable results, statistically significant differences in an experimental setting do not necessarily imply clinical applicability. Consequently, the evidence gathered so far, though promising, must be considered extremely preliminary. No definite answer can yet be provided for the ultimate question underlying the field: can NIBS-induced cognitive improvements persist beyond treatment and translate into daily-life benefits? The methodological considerations listed below emerge as critical milestones to properly address this issue.
Stimulation Parameters
A number of methodological considerations and caveats must be addressed before claiming that a genuine, reliable and replicable effect has been found. TMS stimulation protocols involve several parameters, such as stimulation frequency, intensity, coil shape, pulse and stimulation site. While neuroimaging tools have improved selection of target areas for stimulation, the latter are typically anatomically ill-defined and hugely variable among individual brains. This heterogeneity is reduced by using neuronavigation procedures and factoring individual anatomy into the protocol, thus maximizing the chances that one specific target node is being systematically stimulated within a critical network (Danner et al., 2008). Regarding stimulation frequency and intensity, a general finding is that TMS increases neuronal excitability above a 5-Hz frequency, whereas it yields the opposite effect below 1 Hz (Hallett, 2000; Sparing et al., 2001; Knoch et al., 2006). Stimulation intensity in TMS protocols is usually defined as a percentage of a subject’s individual motor threshold, namely, the minimal stimulation intensity that induces a reliable motor evoked potential of minimal amplitude in the targeted muscle (Rossini et al., 2015). This measure is useful to determine the intensity needed to comparably stimulate primary motor regions across subjects. However, the relevance of this parameter remains unknown for the stimulation of other cortical regions. Future research should examine the possibility of designing subject- and area-specific simulation protocols (Klooster et al., 2016), together with region-specific stimulation-tuning.
In the case of tDCS, key parameters include electrode patch positioning, inter-electrode distance, stimulation duration, and stimulation intensity (Klooster et al., 2016). With this technique, anodal and cathodal currents increase and inhibit neural-network activity, respectively. In this sense, one important difference with TMS is that tDCS allows stimulating and inhibiting different target regions at the same time (Ferrucci et al., 2009; Lindenberg et al., 2010; Mahmoudi et al., 2011), which increases the range of methodological decisions on the part of researchers. Another factor known to modulate tDCS results is age. Indeed, montages inducing effects in young adults do not necessary yield the same results in older adults (Zimerman et al., 2013). This highlights the importance of considering information about age-associated brain reorganization when aiming to induce durable neuroplastic effects in aging subjects (Perceval et al., 2016), including MCI patients.
Behavioral Effects of Stimulation in Combination with Cognitive Training
Typically, neuro-rehabilitation protocols aim to stimulate the whole spatially-distributed network subserving a target cognitive function. To guarantee that the right mechanisms are being stimulated, brain enhancement must be combined with training of key relevant functions. Theoretically, targeting a dysfunctional neural circuit while it is actively engaged by a behavioral task should bring about better therapeutic effects than mere resting-state stimulation of the same area (Miniussi et al., 2013). Given that both cognitive training and NIBS can enhance adaptive plastic mechanisms, their combination could produce synergistic positive effects on behavior (Ditye et al., 2012). This approach offers a chance to stimulate very precise neurocognitive mechanisms, implement experimental designs with strict control of stimulus- and task-related factors and increase protocol viability through short-term longitudinal or pre/post models.
To date, this combined approach has been used in only one study (Park et al., 2014). After 10 daily sessions of tDCS with cognitive training, healthy elderly subjects increased working memory skills for up to 28 days. However, as this study lacked a control group without training, no further conclusions can be drawn regarding the potential benefits of combined NIBS-training interventions. Also relevant is the study protocol proposed by Cheng et al. (2015), aimed to improve working memory in aMCI patients. The design consists of a 4-week, double-blind, randomized controlled trial involving anodal tDCS or sham intervention combined with a working memory (n-back) paradigm or a control cognitive training condition. They hypothesize that this joint approach could significantly improve cognitive performance relative to isolated NIBS or n-back training. Furthermore, one of the studies reviewed above Manenti et al. (2016) reported cognitive enhancements in PD patients by combining tDCS with physical exercise cognitive enhancements. Taken together, this incipient evidence suggests that significant and long-lasting cognitive improvements could be induced through a combination of NIBS and specific cognitive training in MCI/SCI patients. Future research in the field should test this hypothesis by comparing the impact of joint and single-method interventions in both populations.
The Critical Questions
Where to Stimulate?
Most of the reviewed studies targeted the DLPFC, a region implicated in working memory, everyday memory and automatic processing of learned tasks. These functions, indeed, were enhanced by NIBS in MCI patients and healthy subjects (Turriziani et al., 2012; Iuculano and Cohen Kadosh, 2013; Drumond Marra et al., 2015). Another region targeted within the frontal lobe has been the IFG. Stimulation over this structure significantly improved word retrieval and decreased hyperactivity in the bilateral PFC, the right middle temporal gyrus, the left basal ganglia, and the thalamus (Meinzer et al., 2015). Furthermore, rTMS over the STG has been observed to improve processing speed (Anderkova et al., 2015). Encouraging results have also been obtained through stimulation of the inferior parietal lobe, which enhanced associative and long-term memory in MCI patients (Cotelli et al., 2012). In this sense, note that other portions of the parietal cortex are known to increase blood oxygen level-dependent signals during working memory storage (Linden et al., 2003; Xu and Chun, 2006). The relevance of parietal structures as promising target areas for NIBS in MCI samples is highlighted by its role in cognitive impairments during early stage AD (Reiman et al., 2012; Weintraub et al., 2012).
In sum, available evidence highlights the relevance of specific prefrontal, frontal, temporal, and parietal areas as key targets to develop more robust stimulation protocols in MCI. The main findings emerging from their stimulation in MCI patients are summarized in Figure 1.
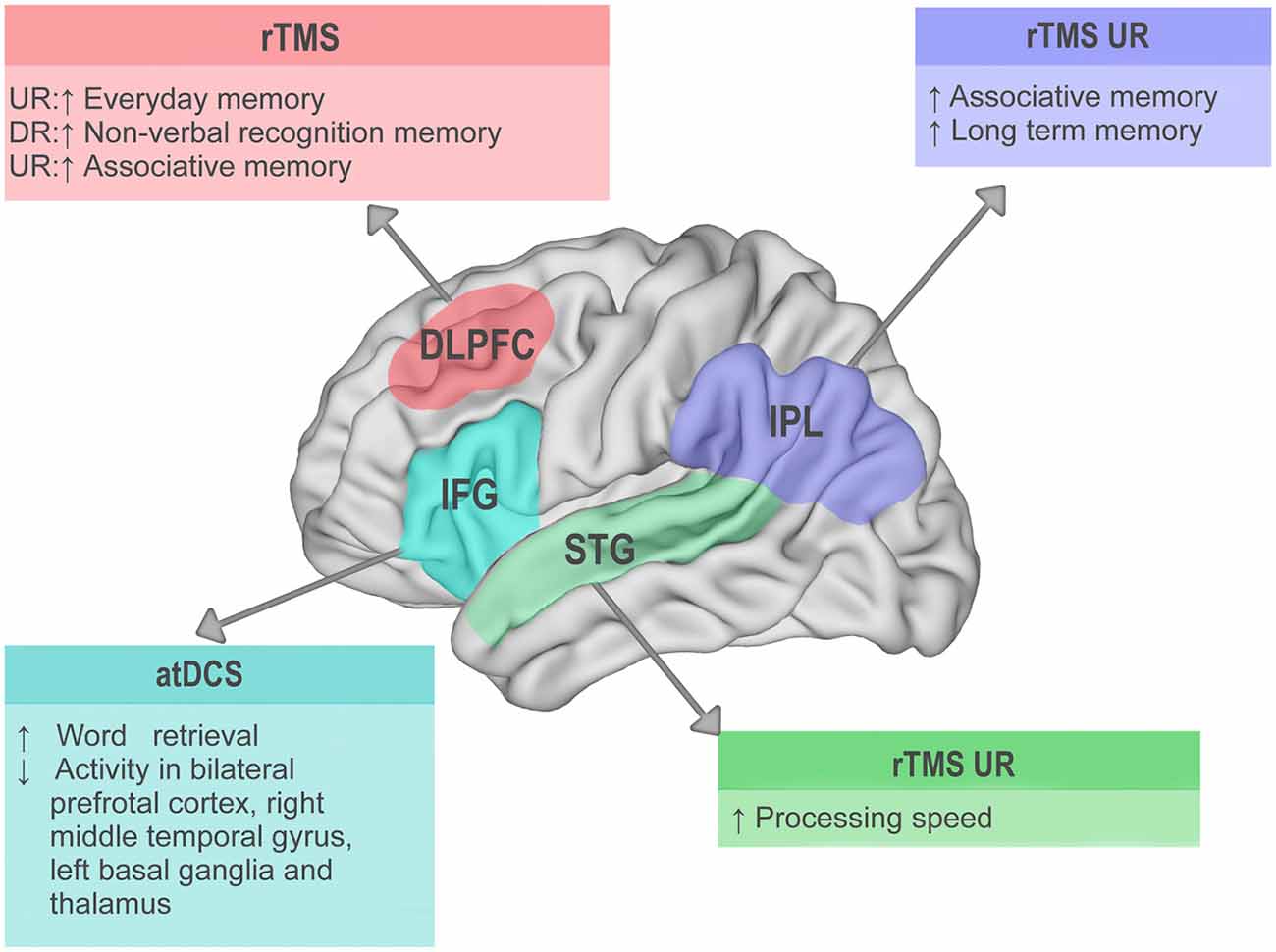
Figure 1. Visual summary of main results from non-invasive brain stimulation (NIBS) studies with mild cognitive impairment (MCI) patients. Lateral view of the left hemisphere. Target areas stimulated in the reviewed studies are highlighted in different colors. DLPFC, dorsolateral prefrontal cortex; IFG, inferior frontal gyrus; STG, superior temporal gyrus; IPL, inferior parietal lobule; atDCS, anodal transcranial direct current stimulation; rTMS, repetitive transcranial magnetic stimulation; ↑ behavioral improvement; ↓ behavioral decrement; UR, up-regulation; DR, down-regulation.
How to Stimulate?
In general, rTMS protocols follow an offline approach, testing hypothesized cognitive changes roughly 30–60 min after stimulation (Ziemann et al., 2008). The use of patterned rTMS protocols, like theta burst stimulation, allows delivering 600 pulses with sub-threshold intensity in less than a minute. This induces longer-lasting effects in a shorter delivery time (Suppa et al., 2016). The impact of theta burst stimulation has been examined in several psychiatric conditions, like schizophrenia and obsessive-compulsive disorder (Suppa et al., 2016). However, studies in patients with memory impairment are still lacking.
On the other hand, tDCS is a smaller, portable tool which allows designing more ecological paradigms and obtaining online measurements. Thus, tDCS can be used to stimulate a particular neural network during behavioral intervention. Thanks to new developments, tDCS protocols can now employ more than two electrodes, enabling multifocal stimulation of brain networks to increase neurofunctional precision (Alam et al., 2016).
For increased effectiveness, NIBS can be applied in multi-session designs. Cognitive improvement in MCI patients is increased over time by repetitive, daily rTMS sessions (Cotelli et al., 2012; Drumond Marra et al., 2015). An alternative approach could rely on the use of spaced stimulation patterns, with multiple daily sessions. This might potentially lead to prolonged after effects via late-phase LTP/LTD-like neuroplastic mechanisms (Goldsworthy et al., 2015). Regarding tDCS, Manenti et al. (2016) worked with PD patients and found cognitive improvements lasting at least 3 months, after 10 daily sessions (Manenti et al., 2016). The only study employing tDCS in MCI patients employed a single-session protocol (Meinzer et al., 2015), so that no evidence is available on whether multiple-session tDCS protocols can induce long-term beneficial effects in this population.
Another important challenge lies in combining NIBS with daily life activities. This includes home-based training focused on specific cognitive skills. Self-delivered NIBS by the patient in a household environment, ideally according to a patient-specific stimulation protocol, may be a crucial step to hone the applicability of tDCS as a therapeutic tool (Klooster et al., 2016). To be effective, home-based portable devices should incorporate features which guarantee that stimulation is applied over the right brain regions, such as patient-specific cap-positioning tDCS electrodes. This may result in more efficient and less expensive cognitive intervention. In consequence, future research should develop new paradigms and technologies specific to each patient and relevant target areas.
Whom to Stimulate?
The use of NIBS to enhance memory in the elderly represents a promising avenue for both basic and translational research. However, the effects of NIBS likely depend on inter-individual differences in the degree of age-related cognitive impairment and brain reorganization (Craik, 1994; Mungas et al., 2010; Reuter-Lorenz and Park, 2014), under the influence of various genetic and lifestyle factors (Bishop et al., 2010; Grady, 2012). Moreover, different subtypes of MCI may respond in different ways to the same stimulation protocols. This calls for further research on the multiple subject-related variables that can modulate results within and across studies.
Correct diagnosis of MCI subtypes proves critical to discern stimulation effects. Approximately 50% of patients with aMCI convert to AD within 5 years (Gauthier et al., 2006). Also, different genetic influences impact on the development of AD. For instance, the presence of one or two ε4 alleles in the apolipoprotein E (APOE) gene confers risk of AD. An individual who meets the clinical, cognitive and etiologic criteria for MCI, and is also APOE ε4 positive, is more likely to progress to AD dementia within a few years than an individual without this genetic trait (Winblad et al., 2004; Anstey et al., 2008; Albert et al., 2011). Conversely, other familial versions will progress to AD with a 100% of probability (e.g., presenilin 1, PS1). Moreover, despite well-established risk genes (e.g., APOE, SORL1) or causative genes (e.g., APP, PSEN1, PSEN2) of AD, more than 20 loci have been associated with disease risk (Karch et al., 2014). In this sense, the epigenetic approach to clinical phenotypes offers a promissory agenda (Bennett et al., 2015). The need thus arises for more research on the interaction between NIBS and previous genetic risk in the MCI-AD spectrum.
On the other hand, fewer prognostic data are available about patients with non-memory-related impairments, who may develop non-AD dementia (Winblad et al., 2004; Anstey et al., 2008; Albert et al., 2011). Three out of the nine articles reviewed in “Does NIBS Improve Learning and Formation of Novel Memories in MCI?” Section targeted aMCI patients (Cotelli et al., 2012; Eliasova et al., 2014; Anderkova et al., 2015), one focused on vascular MCI (Sedlackova et al., 2008), and another one assessed MCI linked to PD (Manenti et al., 2016). The rest did not specify the MCI subtype. This distinction is crucial to assess the efficacy and extent of the stimulation protocol. For example, no effects were observed in vascular MCI when stimulating the DLPFC with rTMS (Sedlackova et al., 2008). It seems that each subtype of MCI could respond different to stimulation, although it is not clear which ones would profit the most from intervention. In consequence, it is necessary to stratify MCI subtypes to recognize condition-specific stimulation effects and develop more fine-grained protocols for each patient, according to his or her diagnosis.
Finally, given that aMCI patients may be at increased risk of developing AD, future interventions might focus on familial-MCI patients with high probability to progress into AD. Early-onset AD patients present MCI, rapidly followed by severe deficits in cortical functions (aphasia, apraxia, and agnosia), whereas late-onset AD patients show a slower overall decline and pronounced memory deficits, particularly in the semantic domain (Joubert et al., 2016). Since the progression of early-onset AD is faster than in late-onset AD, the latter variety may profit more from NIBS as a tool to attenuate the rate of cognitive decline. The identification of causative genetic mutations leading to early-onset AD (APP, PSEN1, PSEN2) and susceptibility markers associated with later disease onset (e.g., APOE ε4 carriers) is crucial to determine specific patterns of cognitive impairment in younger patients with a family history of AD or other neurodegenerative diseases (Rocchi et al., 2003; Winblad et al., 2004; Albert et al., 2011), and to better screen candidates for different stimulation protocols targeting specific neurofunctional mechanisms.
A Call for Multicenter Studies
A thorough understanding of potential neurocognitive changes induced by NIBS requires protocols to be complemented with other functional techniques, like electroencephalography (EEG), magnetoelectroencephalography (MEG), positron-emission tomography (PET), fMRI, and magnetic resonance spectroscopy (MRS). Therefore, the direct comparison of neuro-anatomical changes before and after stimulation could shed light on the potentially specific effects of NIBS. Also, the establishment of NIBS techniques as therapeutic tools for MCI requires systematic assessment of multiple subject variables (e.g., cortical thinning, white matter volume and integrity, functional and structural connectivity, genetic variants, learning capacity, age at disease onset and cognitive reserve). In order to gain multidimensional insights into the impact of NIBS in MCI, above and beyond between-subject variability, longer multicenter studies are urgently needed. By revealing common patterns despite differences in diagnosis, sociodemographic factors, neurobiological features, brain recording parameters, measurements, and analyses, such joint protocols will help identify the most consistent changes induced by NIBS, while revealing the most effective stimulation parameters.
In this context, the implementation of normative databases based on neuroscientific techniques might help to recognize abnormal patterns, detect the disease at early stages, and define treatment protocol strategies (Gordon and Konopka, 2005). Available EEG neuromarkers of MCI and AD are critical to such ends (Ibanez and Parra, 2014). Quantitative EEG (qEEG) shows that AD and MCI are characterized by increased theta power, decreased alpha and beta power and decreased coherence in the alpha and theta band in posterior regions (Jelic et al., 2000). These abnormalities are thought to be associated with functional disconnections among cortical areas, loss of cortical neurons, axonal degeneration, and cholinergic deficits. Furthermore, EEG/MEG techniques have been useful to characterize AD and to detect changes in preclinical familial AD and MCI (Jackson and Snyder, 2008; Stam, 2010; Pietto et al., 2016). For example, source EEG functional network disruption in AD and MCI is associated with cognitive decline (Gianotti et al., 2007; Kurimoto et al., 2008; Ishii et al., 2010; Hsiao et al., 2013) and APOE genotype (Canuet et al., 2012). Moreover, relative to normative samples, subjects with SCI present elevated alpha power and increased number of spatiotemporal wave events (Alexander et al., 2006). Furthermore, event-related potential (ERP) measures have shown utility in predicting the conversion to dementia among elderly individuals at risk for AD (Gironell et al., 2005). Beyond its to diagnose functional impairment, EEG is also useful to set stimulation parameters and assess the effect of NIBS in patients (Kropotov, 2016). Combining NIBS with EEG would help to identify stimulus intensity, frequency of the stimulation, location, and duration needed to normalize EEG activity (Jin et al., 2012). This is critical since most rTMS protocols use the same parameters for all patients. With this approach, a customized treatment would be available for each individual based on analysis of resting EEG. In this sense, Marceglia et al. (2016) applied tDCS to change cortical activity as represented by qEEG. Despite working on a small sample, the authors found that the abnormal pattern of EEG activity in AD during memory processing is partially reversed by anodal transcranial direct current stimulation (atDCS) and this reversion correlated with an improvement in word recognition, suggesting that such benefits are supported by the modulation of neuronal cortical activity. In conclusion, normative databases should be systematically considered as a reference to establish the efficacy of individualized stimulation protocols.
Conclusions
The present review aimed to summarize research on the effects of NIBS in MCI and to outline the approaches that may improve the efficacy of stimulation protocols. Despite major advances over the past few decades, extant MCI/AD treatments are not completely effective. Although NIBS have not yet been massively applied in SCI/MCI patients, available results hold considerable promise. First, TMS and tDCS are well-suited to explore brain plasticity across the lifespan (Heise et al., 2014). Second, they provide valuable physiologic biomarkers of the state of cortical reactivity, brain network connectivity, dynamics, and mechanisms of brain plasticity. Third, NIBS techniques avoid systemic side-effects and allow for temporally and spatially precise neural interventions that escape the possibilities of pharmacological or complementary therapies. Moreover, discrepancies among available studies may be largely due to differences among tasks, designs, and samples, as opposed to limitations inherent to NIBS. However, many issues remain to be solved and large longitudinal studies are needed to more stringently assess the robustness of available positive results. Furthermore, even if NIBS techniques proved irrelevant for clinical purposes, they could remain highly useful for experimental research. While no definitive conclusions on the efficacy and specificity of NIBS can be advanced at this stage, our review delineates the major challenges to be faced in future studies. In this sense, pre-post neuroimaging designs could reveal putative mechanisms underlying NIBS effects. Also, concurrent use of NIBS techniques with specific tasks targeting impaired domains in individual patients could potentially enhance LTP-like after-effects. In particular, applying NIBS over a dysfunctional neural network while the latter is engaged by a particular behavioral activity could significantly enhance learning- and memory-related effects. Furthermore, the application of NIBS across multiple sessions could render such effects longer lasting. All these considerations should be accompanied by very precise diagnosis of each patient’s MCI subtype, so that subject-specific protocols can be designed and assessed with the implementation of normative databases. Moreover, further studies are required to identify the optimal stimulation parameters yielding robust outcomes for different MCI subtypes as well as early- and late-onset AD. Finally, we emphasize the need to conduct longer longitudinal studies to establish the duration of NIBS-induced benefits. By contemplating these issues, future NIBS studies could shed unprecedented light on functional restoration options for MCI patients, paving the way for new treatments aimed to delay (or event prevent) the development of AD and other dementias.
Author Contributions
AB did the literature research and wrote the manuscript. AI and AMG provided insightful thoughts to study concepts, and revised the manuscript. LS and JF assisted with the literature review and revised the final version. MZ wrote, revised critically and approved the final version.
Conflict of Interest Statement
The authors declare that the research was conducted in the absence of any commercial or financial relationships that could be construed as a potential conflict of interest.
Acknowledgments
This work was partially supported by grants from Consejo Nacional de Investigaciones Científicas y Técnicas (CONICET), Comisión Nacional de Investigación Científica y Tecnológica (CONICYT)/Fondo Nacional de Desarrollo Científico y Tecnológico (FONDECYT) Regular (1130920), Fondo para la Investigación Científica y Tecnológica (FONCyT-PICT; Grant no. 2012-0412, 2012-1309), Fondo de Financiamiento de Centros de Investigación en Áreas Prioritarias (FONDAP; 15150012) and the Instituto de Neurología Cognitiva (INECO) Foundation.
References
Alam, M., Truong, D. Q., Khadka, N., and Bikson, M. (2016). Spatial and polarity precision of concentric high-definition transcranial direct current stimulation (HD-tDCS). Phys. Med. Biol. 61, 4506–4521. doi: 10.1088/0031-9155/61/12/4506
Albert, M. S., DeKosky, S. T., Dickson, D., Dubois, B., Feldman, H. H., Fox, N. C., et al. (2011). The diagnosis of mild cognitive impairment due to Alzheimer’s disease: recommendations from the national institute on aging-Alzheimer’s association workgroups on diagnostic guidelines for Alzheimer’s disease. Alzheimers Dement. 7, 270–279. doi: 10.1016/j.jalz.2011.03.008
Alexander, D. M., Arns, M. W., Paul, R. H., Rowe, D. L., Cooper, N., Esser, A. H., et al. (2006). EEG markers for cognitive decline in elderly subjects with subjective memory complaints. J. Integr. Neurosci. 5, 49–74. doi: 10.1142/s0219635206001021
Anderkova, L., Eliasova, I., Marecek, R., Janousova, E., and Rektorova, I. (2015). Distinct pattern of gray matter atrophy in mild Alzheimer’s disease impacts on cognitive outcomes of noninvasive brain stimulation. J. Alzheimers Dis. 48, 251–260. doi: 10.3233/JAD-150067
Anstey, K. J., Cherbuin, N., Christensen, H., Burns, R., Reglade-Meslin, C., Salim, A., et al. (2008). Follow-up of mild cognitive impairment and related disorders over four years in adults in their sixties: the PATH through life study. Dement. Geriatr. Cogn. Disord. 26, 226–233. doi: 10.1159/000154646
Alzheimer’s Association. (2012). 2012 Alzheimer’s disease facts and figures. Alzheimers Dement. 8, 131–168. doi: 10.1016/j.jalz.2012.02.001
Bennett, D. A., Yu, L., Yang, J., Srivastava, G. P., Aubin, C., and De Jager, P. L. (2015). Epigenomics of Alzheimer’s disease. Transl Res. 165, 200–220. doi: 10.1016/j.trsl.2014.05.006
Bishop, N. A., Lu, T., and Yankner, B. A. (2010). Neural mechanisms of ageing and cognitive decline. Nature 464, 529–535. doi: 10.1038/nature08983
Canuet, L., Tellado, I., Couceiro, V., Fraile, C., Fernandez-Novoa, L., Ishii, R., et al. (2012). Resting-state network disruption and APOE genotype in Alzheimer’s disease: a lagged functional connectivity study. PLoS One 7:e46289. doi: 10.1371/journal.pone.0046289
Cheng, C. P., Chan, S. S., Mak, A. D., Chan, W. C., Cheng, S. T., Shi, L., et al. (2015). Would transcranial direct current stimulation (tDCS) enhance the effects of working memory training in older adults with mild neurocognitive disorder due to Alzheimer’s disease: study protocol for a randomized controlled trial. Trials 16:479. doi: 10.1186/s13063-015-0999-0
Cotelli, M., Calabria, M., Manenti, R., Rosini, S., Maioli, C., Zanetti, O., et al. (2012). Brain stimulation improves associative memory in an individual with amnestic mild cognitive impairment. Neurocase 18, 217–223. doi: 10.1080/13554794.2011.588176
Craik, F. I. M. (1994). Memory changes in normal aging. Curr. Dir. Psychol. Sci. 3, 155–158. doi: 10.1111/1467-8721.ep10770653
Danner, N., Julkunen, P., Könönen, M., Säisänen, L., Nurkkala, J., and Karhu, J. (2008). Navigated transcranial magnetic stimulation and computed electric field strength reduce stimulator-dependent differences in the motor threshold. J. Neurosci. Methods 174, 116–122. doi: 10.1016/j.jneumeth.2008.06.032
Dayan, E., Censor, N., Buch, E. R., Sandrini, M., and Cohen, L. G. (2013). Noninvasive brain stimulation: from physiology to network dynamics and back. Nat. Neurosci. 16, 838–844. doi: 10.1038/nn.3422
Ditye, T., Jacobson, L., Walsh, V., and Lavidor, M. (2012). Modulating behavioral inhibition by tDCS combined with cognitive training. Exp. Brain Res. 219, 363–368. doi: 10.1007/s00221-012-3098-4
Drumond Marra, H. L., Myczkowski, M. L., Maia Memória, C., Arnaut, D., Leite Ribeiro, P., Sardinha Mansur, C. G., et al. (2015). Transcranial magnetic stimulation to address mild cognitive impairment in the elderly: a randomized controlled study. Behav. Neurol. 501:287843. doi: 10.1155/2015/287843
Elder, G. J., and Taylor, J. P. (2014). Transcranial magnetic stimulation and transcranial direct current stimulation: treatments for cognitive and neuropsychiatric symptoms in the neurodegenerative dementias? Alzheimers Res. Ther. 6:74. doi: 10.1186/s13195-014-0074-1
Eliasova, I., Anderkova, L., Marecek, R., and Rektorova, I. (2014). Non-invasive brain stimulation of the right inferior frontal gyrus may improve attention in early Alzheimer’s disease: a pilot study. J. Neurol. Sci. 346, 318–322. doi: 10.1016/j.jns.2014.08.036
Ferreri, F., Vecchio, F., Vollero, L., Guerra, A., Petrichella, S., Ponzo, D., et al. (2016). Sensorimotor cortex excitability and connectivity in Alzheimer’s disease: a TMS-EEG Co-registration study. Hum. Brain Mapp. 37, 2083–2096. doi: 10.1002/hbm.23158
Ferri, C. P., Prince, M., Brayne, C., Brodaty, H., Fratiglioni, L., Ganguli, M., et al. (2006). Global prevalence of dementia: a Delphi consensus study. Lancet 366, 2112–2117. doi: 10.1016/S0140-6736(05)67889-0
Ferrucci, R., Bortolomasi, M., Vergari, M., Tadini, L., Salvoro, B., Giacopuzzi, M., et al. (2009). Transcranial direct current stimulation in severe, drug-resistant major depression. J. Affect. Disord. 118, 215–219. doi: 10.1016/j.jad.2009.02.015
Fregni, F., and Pascual-Leone, A. (2007). Technology insight: noninvasive brain stimulation in neurology—perspectives on the therapeutic potential of rTMS and tDCS. Nat. Clin. Pract. Neurol. 3, 383–393. doi: 10.1038/ncpneuro0530
Freitas, C., Mondragón-Llorca, H., and Pascual-Leone, A. (2011). Noninvasive brain stimulation in Alzheimer’s disease: systematic review and perspectives for the future. Exp. Gerontol. 46, 611–627. doi: 10.1016/j.exger.2011.04.001
Fritsch, B., Reis, J., Martinowich, K., Schambra, H. M., Ji, Y., Cohen, L. G., et al. (2010). Direct current stimulation promotes BDNF-dependent synaptic plasticity: potential implications for motor learning. Neuron 66, 198–204. doi: 10.1016/j.neuron.2010.03.035
Gauthier, S., Reisberg, B., Zaudig, M., Petersen, R. C., Ritchie, K., Broich, K., et al. (2006). Mild cognitive impairment. Lancet 367, 1262–1270. doi: 10.1016/S0140-6736(06)68542-5
Gianotti, L. R., Künig, G., Lehmann, D., Faber, P. L., Pascual-Marqui, R. D., Kochi, K., et al. (2007). Correlation between disease severity and brain electric LORETA tomography in Alzheimer’s disease. Clin. Neurophysiol. 118, 186–196. doi: 10.1016/j.clinph.2006.09.007
Gironell, A., García-Sánchez, C., Estévez-González, A., Boltes, A., and Kulisevsky, J. (2005). Usefulness of p300 in subjective memory complaints: a prospective study. J. Clin. Neurophysiol. 22, 279–284. doi: 10.1097/01.wnp.0000173559.60113.ab
Goldsworthy, M. R., Pitcher, J. B., and Ridding, M. C. (2015). Spaced noninvasive brain stimulation: prospects for inducing long-lasting human cortical plasticity. Neurorehabil. Neural Repair 29, 714–721. doi: 10.1177/1545968314562649
Gordon, E., and Konopka, L. M. (2005). Special issue: EEG databases in research and clinical practice: current status and future directions. Clin. EEG Neurosci. 36, 53–54. doi: 10.1177/155005940503600203
Grady, C. (2012). The cognitive neuroscience of ageing. Nat. Rev. Neurosci. 13, 491–505. doi: 10.1038/nrn3256
Hallett, M. (2000). Transcranial magnetic stimulation and the human brain. Nature 406, 147–150. doi: 10.1038/35018000
Heise, K. F., Niehoff, M., Feldheim, J. F., Liuzzi, G., Gerloff, C., and Hummel, F. C. (2014). Differential behavioural and physiological effects of anodal transcranial direct current stimulation in healthy adults of younger and older age. Front. Aging Neurosci. 6:146. doi: 10.3389/fnagi.2014.00146
Hsiao, F.-J., Wang, Y. J., Yan, S. H., Chen, W. T., and Lin, Y. Y. (2013). Altered oscillation and synchronization of default-mode network activity in mild Alzheimer’s disease compared to mild cognitive impairment: an electrophysiological study. PLoS One 8:e68792. doi: 10.1371/journal.pone.0068792
Hsu, W.-Y., Ku, Y., Zanto, T. P., and Gazzaley, A. (2015). Effects of noninvasive brain stimulation on cognitive function in healthy aging and Alzheimer’s disease: a systematic review and meta-analysis. Neurobiol. Aging 36, 2348–2359. doi: 10.1016/j.neurobiolaging.2015.04.016
Hummel, F. C., and Cohen, L. G. (2006). Non-invasive brain stimulation: a new strategy to improve neurorehabilitation after stroke? Lancet Neurol. 5, 708–712. doi: 10.1016/s1474-4422(06)70525-7
Ibanez, A., and Parra, M. A. (2014). Mapping memory binding onto the connectome’s temporal dynamics: toward a combined biomarker for Alzheimer’s disease. Front. Hum. Neurosci. 8:237. doi: 10.3389/fnhum.2014.00237
Inghilleri, M., Conte, A., Frasca, V., Scaldaferri, N., Gilio, F., Santini, M., et al. (2006). Altered response to rTMS in patients with Alzheimer’s disease. Clin. Neurophysiol. 117, 103–109. doi: 10.1016/j.clinph.2005.09.016
Ishii, R., Canuet, L., Kurimoto, R., Ikezawa, K., Aoki, Y., Azechi, M., et al. (2010). Frontal shift of posterior alpha activity is correlated with cognitive impairment in early Alzheimer’s disease: a magnetoencephalography-beamformer study. Psychogeriatrics 10, 138–143. doi: 10.1111/j.1479-8301.2010.00326.x
Iuculano, T., and Cohen Kadosh, R. (2013). The mental cost of cognitive enhancement. J. Neurosci. 33, 4482–4486. doi: 10.1523/JNEUROSCI.4927-12.2013
Jackson, C. E., and Snyder, P. J. (2008). Electroencephalography and event-related potentials as biomarkers of mild cognitive impairment and mild Alzheimer’s disease. Alzheimers Dement. 4, S137–S143. doi: 10.1016/j.jalz.2007.10.008
Jelic, V., Johansson, S., Almkvist, O., Shigeta, M., Julin, P., Nordberg, A., et al. (2000). Quantitative electroencephalography in mild cognitive impairment: longitudinal changes and possible prediction of Alzheimer’s disease. Neurobiol. Aging 21, 533–540. doi: 10.1016/s0197-4580(00)00153-6
Jin, Y., Kemp, A. S., Huang, Y., Thai, T. M., Liu, Z., Xu, W., et al. (2012). Alpha EEG guided TMS in schizophrenia. Brain Stimul. 5, 560–568. doi: 10.1016/j.brs.2011.09.005
Joubert, S., Gour, N., Guedj, E., Didic, M., Guériot, C., Koric, L., et al. (2016). Early-onset and late-onset Alzheimer’s disease are associated with distinct patterns of memory impairment. Cortex 74, 217–232. doi: 10.1016/j.cortex.2015.10.014
Julkunen, P., Jauhiainen, A. M., Könönen, M., Pääkkönen, A., Karhu, J., and Soininen, H. (2011). Combining transcranial magnetic stimulation and electroencephalography may contribute to assess the severity of Alzheimer’s disease. Int. J. Alzheimers Dis. 2011:654794. doi: 10.4061/2011/654794
Julkunen, P., Jauhiainen, A. M., Westerén-Punnonen, S., Pirinen, E., Soininen, H., Könönen, M., et al. (2008). Navigated TMS combined with EEG in mild cognitive impairment and Alzheimer’s disease: a pilot study. J. Neurosci. Methods 172, 270–276. doi: 10.1016/j.jneumeth.2008.04.021
Karch, C. M., Cruchaga, C., and Goate, A. M. (2014). Alzheimer’s disease genetics: from the bench to the clinic. Neuron 83, 11–26. doi: 10.1016/j.neuron.2014.05.041
Klooster, D., de Louw, A., Aldenkamp, A. P., Besseling, R. M., Mestrom, R. M., Carrette, S., et al. (2016). Technical aspects of neurostimulation: focus on equipment, electric field modeling and stimulation protocols. Neurosci. Biobehav. Rev. 65, 113–141. doi: 10.1016/j.neubiorev.2016.02.016
Knoch, D., Gianotti, L. R., Pascual-Leone, A., Treyer, V., Regard, M., Hohmann, M., et al. (2006). Disruption of right prefrontal cortex by low-frequency repetitive transcranial magnetic stimulation induces risk-taking behavior. J. Neurosci. 26, 6469–6472. doi: 10.1523/JNEUROSCI.0804-06.2006
Kropotov, J. D. (2016). Functional Neuromarkers for Psychiatry: Applications for Diagnosis and Treatment. Cambridge, MA: Academic Press.
Kurimoto, R., Ishii, R., Canuet, L., Ikezawa, K., Azechi, M., Iwase, M., et al. (2008). Event-related synchronization of alpha activity in early Alzheimer’s disease and mild cognitive impairment: an MEG study combining beamformer and group comparison. Neurosci. Lett. 443, 86–89. doi: 10.1016/j.neulet.2008.07.015
Lahr, J., Peter, J., Minkova, L., Lauer, E., Reis, J., Heimbach, B., et al. (2016). No difference in paired associative stimulation induced cortical neuroplasticity between patients with mild cognitive impairment and elderly controls. Clin. Neurophysiol. 127, 1254–1260. doi: 10.1016/j.clinph.2015.08.010
Di Lazzaro, V., Oliviero, A., Pilato, F., Saturno, E., Dileone, M., Marra, C., et al. (2004). Motor cortex hyperexcitability to transcranial magnetic stimulation in Alzheimer’s disease. J. Neurol. Neurosurg. Psychiatry 75, 555–559. doi: 10.1136/jnnp.2003.018127
Di Lazzaro, V., Oliviero, A., Tonali, P. A., Mazzone, P., Insola, A., Pilato, F., et al. (2003). Direct demonstration of reduction of the output of the human motor cortex induced by a fatiguing muscle contraction. Exp. Brain Res. 149, 535–538. doi: 10.1007/s00221-003-1408-6
Linden, D. E., Bittner, R. A., Muckli, L., Waltz, J. A., Kriegeskorte, N., Goebel, R., et al. (2003). Cortical capacity constraints for visual working memory: dissociation of fMRI load effects in a fronto-parietal network. Neuroimage 20, 1518–1530. doi: 10.1016/j.neuroimage.2003.07.021
Lindenberg, R., Renga, V., Zhu, L. L., Nair, D., and Schlaug, G. (2010). Bihemispheric brain stimulation facilitates motor recovery in chronic stroke patients. Neurology 75, 2176–2184. doi: 10.1212/WNL.0b013e318202013a
Mahmoudi, H., Borhani Haghighi, A., Petramfar, P., Jahanshahi, S., Salehi, Z., and Fregni, F. (2011). Transcranial direct current stimulation: electrode montage in stroke. Disabil. Rehabil. 33, 1383–1388. doi: 10.3109/09638288.2010.532283
Manenti, R., Brambilla, M., Benussi, A., Rosini, S., Cobelli, C., Ferrari, C., et al. (2016). Mild cognitive impairment in Parkinson’s disease is improved by transcranial direct current stimulation combined with physical therapy. Mov. Disord. 31, 715–724. doi: 10.1002/mds.26561
Manenti, R., Cotelli, M., Robertson, I. H., and Miniussi, C. (2012). Transcranial brain stimulation studies of episodic memory in young adults, elderly adults and individuals with memory dysfunction: a review. Brain Stimul. 5, 103–109. doi: 10.1016/j.brs.2012.03.004
Marceglia, S., Mrakic-Sposta, S., Rosa, M., Ferrucci, R., Mameli, F., Vergari, M., et al. (2016). Transcranial direct current stimulation modulates cortical neuronal activity in Alzheimer’s disease. Front. Neurosci. 10:134. doi: 10.3389/fnins.2016.00134
Meinzer, M., Lindenberg, R., Phan, M. T., Ulm, L., Volk, C., and Flöel, A. (2015). Transcranial direct current stimulation in mild cognitive impairment: behavioral effects and neural mechanisms. Alzheimers Dement. 11, 1032–1040. doi: 10.1016/j.jalz.2014.07.159
Miniussi, C., Harris, J. A., and Ruzzoli, M. (2013). Modelling non-invasive brain stimulation in cognitive neuroscience. Neurosci. Biobehav. Rev. 37, 1702–1712. doi: 10.1016/j.neubiorev.2013.06.014
Mungas, D., Beckett, L., Harvey, D., Farias, S. T., Reed, B., Carmichael, O., et al. (2010). Heterogeneity of cognitive trajectories in diverse older persons. Psychol. Aging 25, 606–619. doi: 10.1037/a0019502
Nardone, R., Bergmann, J., Christova, M., Caleri, F., Tezzon, F., Ladurner, G., et al. (2012). Short latency afferent inhibition differs among the subtypes of mild cognitive impairment. J. Neural Transm. 119, 463–471. doi: 10.1007/s00702-011-0725-3
Nardone, F. T., Tezzon, F., Höller, Y., Golaszewski, S., Trinka, E., and Brigo, F. (2014). Transcranial magnetic stimulation (TMS)/repetitive TMS in mild cognitive impairment and Alzheimer’s disease. Acta Neurol. Scand. 129, 351–366. doi: 10.1111/ane.12223
Niskanen, E., Könönen, M., Määttä, S., Hallikainen, M., Kivipelto, M., Casarotto, S., et al. (2011). New insights into Alzheimer’s disease progression: a combined TMS and structural MRI study. PLoS One 6:e26113. doi: 10.1371/journal.pone.0026113
Nitsche, M. A., Cohen, L. G., Wassermann, E. M., Priori, A., Lang, N., Antal, A., et al. (2008). Transcranial direct current stimulation: state of the art 2008. Brain Stimul. 1, 206–223. doi: 10.1016/j.brs.2008.06.004
Nitsche, M. A., Jaussi, W., Liebetanz, D., Lang, N., Tergau, F., and Paulus, W. (2004). Consolidation of human motor cortical neuroplasticity by D-cycloserine. Neuropsychopharmacology 29, 1573–1578. doi: 10.1038/sj.npp.1300517
Nitsche, M., and Paulus, W. (2000). Excitability changes induced in the human motor cortex by weak transcranial direct current stimulation. J. Physiol. 527, 633–639. doi: 10.1111/j.1469-7793.2000.t01-1-00633.x
Nowak, D. A., Bösl, K., Podubeckà, J., and Carey, J. R. (2010). Noninvasive brain stimulation and motor recovery after stroke. Restor. Neurol. Neurosci. 28, 531–544. doi: 10.3233/RNN-2010-0552
Park, S.-H., Seo, J.-H., Kim, Y. H., and Ko, M. H. (2014). Long-term effects of transcranial direct current stimulation combined with computer-assisted cognitive training in healthy older adults. Neuroreport 25, 122–126. doi: 10.1097/WNR.0000000000000080
Perceval, G., Flöel, A., and Meinzer, M. (2016). Can transcranial direct current stimulation counteract age-associated functional impairment? Neurosci. Biobehav. Rev. 65, 157–172. doi: 10.1016/j.neubiorev.2016.03.028
Petersen, R. C., and Negash, S. (2008). Mild cognitive impairment: an overview. CNS Spectr. 13, 45–53. doi: 10.1017/s1092852900016151
Pietto, M., Parra, M. A., Trujillo, N., Flores, F., García, A. M., Bustin, J., et al. (2016). Behavioral and electrophysiological correlates of memory binding deficits in patients at different risk levels for Alzheimer’s disease. J. Alzheimers Dis. 53, 1325–1340. doi: 10.3233/JAD-160056
Plewnia, C., Lotze, M., and Gerloff, C. (2003). Disinhibition of the contralateral motor cortex by low-frequency rTMS. Neuroreport 14, 609–612. doi: 10.1097/00001756-200303240-00017
Reiman, E. M., Quiroz, Y. T., Fleisher, A. S., Chen, K., Velez-Pardo, C., Jimenez-Del-Rio, M., et al. (2012). Brain imaging and fluid biomarker analysis in young adults at genetic risk for autosomal dominant Alzheimer’s disease in the presenilin 1 E280A kindred: a case-control study. Lancet Neurol. 11, 1048–1056. doi: 10.1016/S1474-4422(12)70228-4
Reis, J., John, D., Heimeroth, A., Mueller, H. H., Oertel, W. H., Arndt, T., et al. (2006). Modulation of human motor cortex excitability by single doses of amantadine. Neuropsychopharmacology 31, 2758–2766. doi: 10.1038/sj.npp.1301122
Reisberg, B., and Gauthier, S. (2008). Current evidence for subjective cognitive impairment (SCI) as the pre-mild cognitive impairment (MCI) stage of subsequently manifest Alzheimer’s disease. Int. Psychogeriatr. 20, 1–16. doi: 10.1017/S1041610207006412
Reuter-Lorenz, P. A., and Park, D. C. (2014). How does it STAC up? Revisiting the scaffolding theory of aging and cognition. Neuropsychol. Rev. 24, 355–370. doi: 10.1007/s11065-014-9270-9
Ridding, M. C., and Ziemann, U. (2010). Determinants of the induction of cortical plasticity by non-invasive brain stimulation in healthy subjects. J. Physiol. 588, 2291–2304. doi: 10.1113/jphysiol.2010.190314
Rocchi, A., Pellegrini, S., Siciliano, G., and Murri, L. (2003). Causative and susceptibility genes for Alzheimer’s disease: a review. Brain Res. Bull. 61, 1–24. doi: 10.1016/s0361-9230(03)00067-4
Rossi, S., Hallett, M., Rossini, P. M., Pascual-Leone, A., and Safety of TMS Consensus Group. (2009). Safety, ethical considerations and application guidelines for the use of transcranial magnetic stimulation in clinical practice and research. Clin. Neurophysiol. 120, 2008–2039. doi: 10.1016/j.clinph.2009.08.016
Rossini, P., Burke, D., Chen, R., Cohen, L. G., Daskalakis, Z., Di Iorio, R., et al. (2015). Non-invasive electrical and magnetic stimulation of the brain, spinal cord, roots and peripheral nerves: basic principles and procedures for routine clinical and research application. An updated report from an IFCN Committee. Clin. Neurophysiol. 126, 1071–1107. doi: 10.1016/j.clinph.2015.02.001
Schulz, R., Gerloff, C., and Hummel, F. C. (2013). Non-invasive brain stimulation in neurological diseases. Neuropharmacology 64, 579–587. doi: 10.1016/j.neuropharm.2012.05.016
Sedlackova, S., Rektorova, I., Fanfrdlova, Z., and Rektor, I. (2008). Neurocognitive effects of repetitive transcranial magnetic stimulation in patients with cerebrovascular disease without dementia. J. Psychophysiol. 22, 14–19. doi: 10.1027/0269-8803.22.1.14
Solé-Padullés, C., Bartrés-Faz, D., Junqué, C., Clemente, I. C., Molinuevo, J. L., Bargalló, N., et al. (2006). Repetitive transcranial magnetic stimulation effects on brain function and cognition among elders with memory dysfunction. A randomized sham-controlled study. Cereb. Cortex 16, 1487–1493. doi: 10.1093/cercor/bhj083
Sparing, R., Mottaghy, F. M., Hungs, M., Brügmann, M., Foltys, H., Huber, W., et al. (2001). Repetitive transcranial magnetic stimulation effects on language function depend on the stimulation parameters. J. Clin. Neurophysiol. 18, 326–330. doi: 10.1097/00004691-200107000-00004
Stagg, C. J., Best, J. G., Stephenson, M. C., O’Shea, J., Wylezinska, M., Kincses, Z. T., et al. (2009). Polarity-sensitive modulation of cortical neurotransmitters by transcranial stimulation. J. Neurosci. 29, 5202–5206. doi: 10.1523/JNEUROSCI.4432-08.2009
Stam, C. J. (2010). Use of magnetoencephalography (MEG) to study functional brain networks in neurodegenerative disorders. J. Neurol. Sci. 289, 128–134. doi: 10.1016/j.jns.2009.08.028
Suppa, A., Huang, Y.-Z., Funke, K., Ridding, M. C., Cheeran, B., Di Lazzaro, V., et al. (2016). Ten years of theta burst stimulation in humans: established knowledge, unknowns and prospects. Brain Stimul. 9, 323–335. doi: 10.1016/j.brs.2016.01.006
Trebbastoni, A., Pichiorri, F., D’Antonio, F., Campanelli, A., Onesti, E., Ceccanti, M., et al. (2016). Altered cortical synaptic plasticity in response to 5-Hz repetitive transcranial magnetic stimulation as a new electrophysiological finding in amnestic mild cognitive impairment converting to Alzheimer’s disease: results from a 4-year prospective cohort study. Front. Aging Neurosci. 7:253. doi: 10.3389/fnagi.2015.00253
Turriziani, P., Smirni, D., Zappalà, G., Mangano, G. R., Oliveri, M., and Cipolotti, L. (2012). Enhancing memory performance with rTMS in healthy subjects and individuals with Mild Cognitive Impairment: the role of the right dorsolateral prefrontal cortex. Front. Hum. Neurosci. 6:62. doi: 10.3389/fnhum.2012.00062
Weintraub, D., Dietz, N., Duda, J. E., Wolk, D. A., Doshi, J., Xie, S. X., et al. (2012). Alzheimer’s disease pattern of brain atrophy predicts cognitive decline in Parkinson’s disease. Brain 135, 170–180. doi: 10.1093/brain/awr277
Winblad, B., Palmer, K., Kivipelto, M., Jelic, V., Fratiglioni, L., Wahlund, L. O., et al. (2004). Mild cognitive impairment–beyond controversies, towards a consensus: report of the international working group on mild cognitive impairment. J. Intern. Med. 256, 240–246. doi: 10.1111/j.1365-2796.2004.01380.x
Xu, Y., and Chun, M. M. (2006). Dissociable neural mechanisms supporting visual short-term memory for objects. Nature 440, 91–95. doi: 10.1038/nature04262
Ziemann, U., Paulus, W., Nitsche, M. A., Pascual-Leone, A., Byblow, W. D., Berardelli, A., et al. (2008). Consensus: motor cortex plasticity protocols. Brain Stimul. 1, 164–182. doi: 10.1016/j.brs.2008.06.006
Zimerman, M., and Hummel, F. C. (2010). Non-invasive brain stimulation: enhancing motor and cognitive functions in healthy old subjects. Front. Aging Neurosci. 2:149. doi: 10.3389/fnagi.2010.00149
Keywords: mild cognitive impairment, non-invasive brain stimulation, neuroenhancement, transcranial magnetic stimulation, transcranial direct current stimulation
Citation: Birba A, Ibáñez A, Sedeño L, Ferrari J, García AM and Zimerman M (2017) Non-Invasive Brain Stimulation: A New Strategy in Mild Cognitive Impairment? Front. Aging Neurosci. 9:16. doi: 10.3389/fnagi.2017.00016
Received: 27 October 2016; Accepted: 20 January 2017;
Published: 13 February 2017.
Edited by:
Catarina Oliveira, University of Coimbra, PortugalReviewed by:
Yury (Juri) Kropotov, Institute of the Human Brain of Russian Academy of Sciences, RussiaPanteleimon Giannakopoulos, University of Geneva, Switzerland
Copyright © 2017 Birba, Ibáñez, Sedeño, Ferrari, García and Zimerman. This is an open-access article distributed under the terms of the Creative Commons Attribution License (CC BY). The use, distribution and reproduction in other forums is permitted, provided the original author(s) or licensor are credited and that the original publication in this journal is cited, in accordance with accepted academic practice. No use, distribution or reproduction is permitted which does not comply with these terms.
*Correspondence: Máximo Zimerman, bXppbWVybWFuQGluZWNvLm9yZy5hcg==