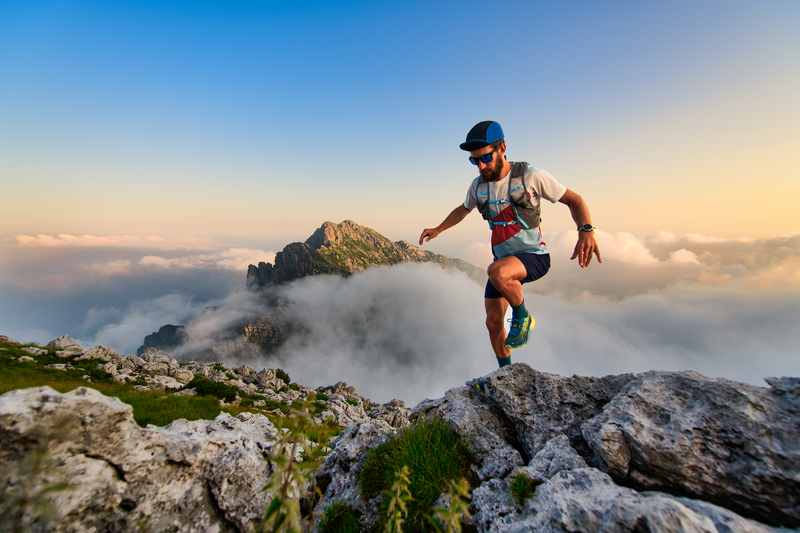
94% of researchers rate our articles as excellent or good
Learn more about the work of our research integrity team to safeguard the quality of each article we publish.
Find out more
REVIEW article
Front. Aging Neurosci. , 23 April 2015
Sec. Cellular and Molecular Mechanisms of Brain-aging
Volume 7 - 2015 | https://doi.org/10.3389/fnagi.2015.00060
This article is part of the Research Topic Aging, neurogenesis and neuroinflammation in hearing loss and protection View all 13 articles
Hearing loss is one of the most prevalent conditions around the world, in particular among people over 60 years old. Thus, an increase of this affection is predicted as result of the aging process in our population. In this context, it is important to further explore the function of molecular targets involved in the biology of inner ear sensory cells to better individuate new candidates for therapeutic application. One of the main causes of deafness resides into the premature death of hair cells and auditory neurons. In this regard, neurotrophins and growth factors such as insulin like growth factor are known to be beneficial by favoring the survival of these cells. An elevated number of published data in the last 20 years have individuated sphingolipids not only as structural components of biological membranes but also as critical regulators of key biological processes, including cell survival. Ceramide, formed by catabolism of sphingomyelin (SM) and other complex sphingolipids, is a strong inducer of apoptotic pathway, whereas sphingosine 1-phosphate (S1P), generated by cleavage of ceramide to sphingosine and phosphorylation catalyzed by two distinct sphingosine kinase (SK) enzymes, stimulates cell survival. Interestingly S1P, by acting as intracellular mediator or as ligand of a family of five distinct S1P receptors (S1P1–S1P5), is a very powerful bioactive sphingolipid, capable of triggering also other diverse cellular responses such as cell migration, proliferation and differentiation, and is critically involved in the development and homeostasis of several organs and tissues. Although new interesting data have become available, the information on S1P pathway and other sphingolipids in the biology of the inner ear is limited. Nonetheless, there are several lines of evidence implicating these signaling molecules during neurogenesis in other cell populations. In this review, we discuss the role of S1P during inner ear development, also as guidance for future studies.
Sphingolipids are a fascinating subclass of complex lipids known since a long time as key players in the correct structural organization of biological membranes. Subsequently to the understanding of their fundamental structural properties, it has been made clear that intermediates in their biosynthesis and breakdown are indeed critical signaling molecules implicated in the regulation of essential biological events. In this regard, it is presently well accepted that the vast majority of the extracellular cues involved in the regulation of cell functioning exploits at least in part sphingolipid metabolism for the accomplishment of a variety of specific biological responses.
Among the various types of sphingolipids, sphingomyelin (SM) is of paramount relevance as potential source of bioactive sphingoid molecules. Numerous reviews focus on the intricate regulation of the sphingolipid pathway (Huwiler et al., 2000; Hannun and Obeid, 2008; Figure 1). Its breakdown, catalyzed by a small family of SMases, gives rise to the formation of ceramide, an intracellular mediator per se, as well as a central hub for the production of other critical bioactive compounds. Ceramide is composed by a sphingoid base, named sphingosine, linked to a fatty acid via an amide bond, thus, depending on the length of the fatty acid acyl chain, various types of ceramide do exist. Another metabolic pathway that can produce ceramide is represented by its de novo synthesis that begins with the condensation of palmitoyl-CoA and serine catalyzed by serine palmitoyl transferase to give 3-keto-dihydrosphingosine, then reduced to dihydrosphingosine, followed by acylation reaction performed by a family of six distinct ceramide synthases, several of which are co-expressed in many different cell systems (Stiban et al., 2010; Mullen et al., 2012). The last step involves the oxidation of dihydroceramide to ceramide which is dependent on the action of a specific desaturase. Accumulation of ceramide within the cell is associated with a number of biological responses including cell growth arrest, apoptotic cell death, cell senescence, stress response making the regulation of its intracellular content critical for the fate of a given cell type (Hannun and Obeid, 2011). Once produced, ceramide can be utilized in various distinct biosynthetic pathways. The most abundant sphingolipid in plasma membrane, named SM, is generated by SM synthases in a reaction that, via transfer of phosphocholine from phosphatidylcholine onto ceramide, yields also diacylglycerol (Taniguchi and Okazaki, 2014). Alternatively, ceramide can serve as backbone in the building of glycosphingolipids, the first step being catalyzed by glucosylceramide synthase (GCS), which produces glucosylceramide, the simplest member of this family (Messner and Cabot, 2010). In turn, by addition of a galactose moiety glucosylceramide is transformed into lactosylceramide, which, by addition of one or more monosaccharides, gives raise to individual ganglioside species, recognized as vital components of membrane microdomains with a role in cell-cell recognition, adhesion, and signal transduction (D’Angelo et al., 2013). Moreover, selective phosphorylation of ceramide brought about by ceramide kinase (CK) generates ceramide 1-phosphate, a bioactive sphingolipid regarded as a powerful pro-inflammatory mediator (Gomez-Muñoz et al., 2013).
Figure 1. Metabolism of sphingolipids. Ceramide is considered the hearth of sphingolipid metabolism. It can be formed by de novo synthesis and then converted to other bioactive lipids. Sphingosine 1-phosphate lyase (S1P lyase) catalyzes the irreversible exit from the pathway. Abbreviations: serine palmitoyl-CoA-acyltransferase (SPT), 3-ketosphinganine reductase (KSR), (dihydro)-ceramide synthase (CerS), ceramide desaturase (DES), ceramide kinase (CK), glucosylceramide synthase (GCS), glucosyl ceramidase (GCase), ceramidase (CDase), sphingosine-1-phosphate lyase (S1P lyase), sphingosine kinase (SK), sphingosine 1-phosphate phosphatase (S1PPase), sphingomyelin (SM) synthase (SMS), sphingomyelinase (SMase).
Finally, it is worth noticing that also the catabolic route by which ceramide is degraded is responsible for the production of other bioactive sphingoid compounds, among which sphingosine 1-phosphate (S1P) plays a prominent role. S1P is produced by ceramide via two specific enzymatic reactions: at first ceramidases (CDase) catalyze ceramide deacylation to sphingosine (Mao and Obeid, 2008; Ito et al., 2014), then the sphingoid base is phosphorylated to S1P by sphingosine kinase (SK). Two distinct isoforms of SK exist, designated SK1 and SK2 (Takabe et al., 2008). They are ubiquitously expressed, each contributing to intracellular S1P production. Although in some instances SK1 and SK2 can have overlapping functions, in certain cell types they have been found to differ for intracellular localization, as well as for their biological role (Maceyka et al., 2012; Neubauer and Pitson, 2013). Catabolism of S1P is also under the control of multiple enzymes. S1P lyase catalyzes the irreversible breakdown of S1P to hexadecenal and phosphoethanolamine (Kumar and Saba, 2009), while two distinct specific S1P phosphatases by removing the phosphate moiety generate sphingosine, that can be further phosphorylated to S1P or employed to feed into the so called sphingolipid salvage pathway, responsible for ceramide biosynthesis from sphingolipid breakdown (Le Stunff et al., 2002).
S1P was discovered as an intracellular mediator more than 20 years ago, even though some of its intracellular targets have been identified only recently (Maceyka et al., 2012). Meanwhile, a large body of experimental evidence has been accumulated in favor of the critical role played by S1P as ligand of a family of five specific, high affinity, G protein coupled receptors named S1P1–5 (Ishii et al., 2004; Meyer zu Heringdorf and Jakobs, 2007). The majority of these receptors are able to activate multiple heterotrimeric G proteins, making thus possible the triggering of a wide variety of signaling pathways as well as various biological responses. Notably, S1P1, S1P2 and S1P3 are almost ubiquitous, while S1P4 and S1P5 expression appears to be limited to specific cell types such as those belonging to lymphoid and nervous tissue (Im et al., 2000; Kluk and Hla, 2002). Thus, taking into consideration the selective signaling downstream of individual S1P receptor subtypes, the final biological response evoked by S1P in a given cellular setting is specific, being often strictly dependent on the specific pattern of S1P receptor expressed. Exogenous S1P, mainly via ligation to one or more S1P receptors, is capable of regulating key biological processes, including cell proliferation, survival, motility as well as cell differentiation (Mendelson et al., 2014). Since ceramide and S1P are readily interconvertible lipids exerting opposite biological actions, the balance of their intracellular content is regarded as a biostat determining cell fate (Maceyka et al., 2002). Circulating plasma contains high nanomolar levels of S1P, whereas S1P availability within tissues is much lower (Schwab et al., 2005). The occurrence of such a gradient between blood and tissues is of utmost importance for the correct driving of lymphocyte and hematopoietic cell trafficking (Cyster and Schwab, 2012). Specific cell types such as erythrocytes, platelets and endothelial cells are responsible for the maintaining of the relatively high concentration of S1P in plasma (Pappu et al., 2007), although in principle every cell system can release endogenous S1P in the extracellular environment via the functioning of the widely expressed selective S1P transporter spinster-2 (SPNS2; Kawahara et al., 2009), as well as other less specific transporters such as several members of the ATP binding cassette (ABC) family (Nishi et al., 2014). Indeed, release of S1P by a given cell is regarded as a crucial step in the general mechanism by which this sphingolipid can act as paracrine or autocrine cue in the so called inside-out signaling (Figure 2). In this regard it has been clearly established that in a wide variety of cellular contexts intracellular S1P metabolism is tightly regulated by multiple extracellular agents including growth factors, hormones, cytokines and neurotransmitters and the subsequent functional interaction with S1P receptors is integral to their final biological actions (Takabe et al., 2008; Xia and Wadham, 2011; Maceyka et al., 2012). Interestingly, extensive experimental evidence has been provided for the occurrence of a complex cross-talk between S1P signaling axis and numerous different extracellular agents including: epithelial growth factor (EGF), platelet derived growth factor (PDGF), vascular endothelial growth factor (VEGF), IGF1, as well as tumor necrosis factor alpha (TNFα) and transforming growth factor beta (TGFβ), that comprises expression changes of S1P receptors and/or enzymes of S1P metabolism and/or S1P-dependent transactivation of enzyme-linked membrane receptors (Pyne et al., 2003; Takabe et al., 2008; Xia and Wadham, 2011; Maceyka et al., 2012; Donati et al., 2013a,b).
Figure 2. Schematic diagram describing the inside-out signaling of S1P. Sphingosine kinase (SK) activation by different extracellular agents leads to S1P production that both can act via intracellular targets or is extracellularly released to bind specific S1P receptors (S1P1–5). Transporters have been involved in S1P export such as the ATP binding cassette (ABC), and the specific spinster 2 (SPNS2).
In keeping with the essential role of S1P metabolism and signaling for correct vertebrate development, S1P biosynthesis was found to be necessary for embryonal neurogenesis. Double knockout (KO) mice for SK1 and SK2 exhibited a severely disturbed neurogenesis, including neural tube closure, defects in angiogenesis and caused embryonic lethality (Mizugishi et al., 2005). The observed neural tube defect was ascribed to the absence of S1P and the consequent increased apoptosis of neuroepithelium. The biological action of S1P was mediated at least in part by S1P1, given that S1pr1 KO mice were found to display similar, even though milder, neural defects (Liu et al., 2000). A number of different studies have implicated S1P in the control of multiple biological events that occur in the various cell types present in central nervous tissue. Neural progenitor cells express S1P receptors and proliferate in response to S1P challenge (Harada et al., 2004); moreover, they are recruited by this bioactive sphingoid molecule toward a pathological area of the central nervous system (CNS) in a S1P1-dependent manner (Kimura et al., 2007). In this regard, S1P-mediated migration of neural progenitor cells toward an area of brain injury was found to be enhanced by antagonizing S1P2 (Kimura et al., 2008).
S1P is also efficacious in regulating proliferation of astrocytes (Sorensen et al., 2003; Bassi et al., 2006), specialized glial cells that by interacting with blood vessels and synapses regulate multiple aspects of brain homeostasis and functioning. Importantly, S1P was found to be secreted in response to fibroblast growth factor 2 (FGF2) identifying an autocrine/paracrine mechanism of action of this sphingolipid in the proliferation of astrocytes, with a role in the FGF2-induced cell growth signaling (Bassi et al., 2006). Since a pilot study reported that S1P induces the expression of Fgf2 mRNA in rat astrocytes (Sato et al., 2000), it is tempting to speculate that a positive feedback loop takes place between FGF2 and S1P signaling pathway in astrocytes.
The finding that S1P5 is abundantly expressed in the white matter of brain (Im et al., 2001) led to the identification of this receptor subtype as predominantly present in oligodendrocytes, the myelinating cells of the CNS. In pre-oligodendrocytes S1P acting via S1P5 elicited process retraction, whereas it promoted survival of mature cells (Jaillard et al., 2005). However, S1P5 is not the unique transducer of S1P action in this cell type since S1P1 was reported to be up regulated by PDGF in oligodendrocyte progenitors and implicated in the growth factor-induced mitogenesis (Jung et al., 2007).
A wealth of experimental data mainly performed in hippocampal neurons support an important role of S1P receptors in the modulation of neuronal excitability as well as synapse plasticity and transmission (Kajimoto et al., 2007; Sim-Selley et al., 2009; Kanno et al., 2010; Norman et al., 2010; Kempf et al., 2014). In agreement, aberrant S1P levels and S1P receptor signaling have been reported in a range of diseases of CNS. In multiple sclerosis patients S1P concentration in cerebrospinal fluid was augmented, in accordance with the chronic inflammation status associated with this degenerative disease (Kulakowska et al., 2010). S1P concentrations were selectively decreased in the cerebrospinal fluid of adult rats in an acute and an inflammatory pain model (Coste et al., 2008). Finally, region specific S1P content decline was recently observed during the course of Alzheimer’s disease, primarily attributed to a loss of SK1 and SK2 in the hippocampus (Couttas et al., 2014).
It is important to underline that the recently developed fingolimod (FTY720) as oral therapy of multiple sclerosis directly targets S1P signaling pathway (Brinkmann et al., 2010). This compound is converted in vivo by SK2 into p-FTY720, that acts as high affinity agonist for all S1P receptors, except S1P2, and results in sequestration of lymphocytes into secondary lymphoid tissues. Besides this key mechanism of action, nonimmunological CNS mechanisms for fingolimod efficacy in multiple sclerosis therapy were identified that implicate S1P1 signaling in astrocytes as a key mediator, thus highlighting S1P signaling pathways within the CNS as targets for multiple sclerosis therapies (Choi et al., 2011). Intense recent studies aimed at exploring additional therapeutic applications of fingolimod, reviewed exhaustively in Brunkhorst et al. (2014), have produced very promising results that hopefully will enable the translation of some of these experimental findings into the clinics opening new avenues for the treatment of Alzheimer’s disease, cerebral malaria, neuroblastoma and neuroprotection in cranial irradiation, among others.
The mature inner ear of mammals is a remarkable structure composed by a myriad of exquisitely arranged cell types within a complex set of ducts and chambers: the vestibular system composed of the semicircular canals, the saccule and the utricle, and the auditory system formed by the cochlea, a convoluted structure where the organ of Corti (OC) resides. In this review we will emphasize the auditory system, in particular the spiral ganglion neurons (SGN), the innervation that transports the electrical stimulus from OC to the CNS. Although many questions are still open, the molecular events occurring from the initial formation of the otic placode, to the final complex tonotopic arrangement of mature SGN are starting to be understood. Several signaling pathways govern every stage in inner ear development, for example members of the FGF and WNT families are between the earliest signals triggering otic development. Later on, retinoic acid (RA) and IGF1 play an important role in the regionalization of the otic vesicle and the survival of auditory neuroblasts. Perhaps some of the most studied proteins involved in the development of SGN are neurotrophins, in particular brain-derived neurotrophic factor (BDNF) and neurotrophin 3 (NT3). The study of these neurotrophins and other signaling molecules involved in the formation and survival of SGN is also relevant from the point of view of therapeutic applications, since SGN are critical in the effectiveness of the cochlear implant.
In addition, in recent years several stem cell-based differentiation protocols have been proposed to create hair cells and sensory neurons that could be used in cell-replacement strategies (Oshima et al., 2010; Koehler and Hashino, 2014). Indeed it was recently shown that human embryonic stem cell-derived inner ear progenitors are able to restore hearing in deafened gerbils (Chen et al., 2012). It is worth mentioning that this and other protocols are developmentally-informed approaches, highlighting that a better understanding of inner ear development will be translated in more efficient differentiation protocols.
As previously mentioned, S1P signaling is functionally ubiquitous, and our group and others have shown that several cytokines, growth factors and morphogenetic cues modulate and are modulated by S1P receptors, S1P lyase and SKs.
We will briefly revisit the development of the inner and the main morphogenetic cues involved in the process and present the current knowledge on the interaction of such cues with S1P pathway in other systems. The evidence presented suggest that apart from the essential role of S1P signaling in hearing, it is worth looking at S1P pathway at earlier developmental stages.
Preceding the formation of the otic placode, the border region between the neural plate and the lateral ectoderm acquires the competence to respond to otic inducing signals. This region surrounding the head ectoderm called the pre-placodal domain (PPD) is established by the cooperation of bone morphogenetic protein (BMP), WNT and FGF signals emanating from the mesenchyme and the neural plate.
In X.laevis, the PPD marker Six1 is expressed in animal caps when the BMP inhibitors noggin and cerberus are overexpressed (Brugmann et al., 2004). In line with this, BMP blocks the expression of Six1 in its endogenous domain while noggin expands it (Ahrens and Schlosser, 2005).
In the chick Wnt8a and BMPs are expressed posteriorly and laterally to the PPD and when these pathways are blocked, the expression of the PPD markers SIX4 and EYA2 expands beyond its endogenous territory (Litsiou et al., 2005). In both works it was also demonstrated that FGF signaling was necessary to fully induce the expression of the PPD markers ectopically (Ahrens and Schlosser, 2005; Litsiou et al., 2005).
The PPD is the common ground of all cranial placodes, characterized by the expression of members of DLX and FOX families of transcription factors in several species (Quint et al., 2000; McLarren et al., 2003; Hans et al., 2004). Experiments in the chick have shown that FGF2 is able to induce the early otic marker PAX2 in explants derived from any region within the PPD but not in lateral or trunk ectoderm (Martin and Groves, 2006). Similarly in the Zebrafish fgf8 misexpression can enlarge the size of the otic vesicle only in the regions where PPD markers have been previously expressed (Hans et al., 2004).
FGF family are also important signals that initiate inner ear development across vertebrates. In particular, the role of FGF3 during otic induction is conserved in different species. However, other FGFs such as FGF8, FGF10, FGF19 are also involved depending on the species. For example, zebrafish embryos treated with the FGF receptor inhibitor SU5402, or fgf3/fgf8 morpholino injected do not form otic vesicles neither express the placodal marker Pax8 (Phillips et al., 2001; Maroon et al., 2002). In the chick FGF19 and FGF3 have a synergistic effect during otic placode induction (Ladher et al., 2000), although FGF2 has also been shown to induce the expression of the otic markers PAX2 and DlX3 (Martin and Groves, 2006). In mice, FGF3 together with FGF10 play a redundant role during otocyst formation, double KO animals are completely devoid of otocyst (Alvarez et al., 2003; Wright and Mansour, 2003; Zelarayan et al., 2007). In line with this, FGF receptor 2 isoform IIIb (Fgfr2IIIb) KO mice, the receptor isoform for FGF3 and FGF10, present gross morphological defects in the inner ear (Xu et al., 1998; Pirvola et al., 2000).
In addition to the FGF signals, WNT plays also important role during the induction and formation of the otic placode. For example, it has been reported that WNT8A induces the expression of FGF3 before the appearance of otic placode markers in the chick (Ladher et al., 2000). In murine models, conditional expression and deletion of β-catenin (CTNNB1) within the PAX2 territory increases or diminishes respectively the size of the otic domain (Ohyama et al., 2006). In agreement, overexpression of Dkk1 gene, a WNT signaling inhibitor, impairs the development of the otic placode (Freter et al., 2008).
In summary, FGFs together with a specific level of BMP and WNT signaling inhibition first establish the PPD. Then local FGFs (e.g., FGF3 and FGF10) restrict the formation of the otic placode next to the hindbrain, which is then compartmentalized into otic and epidermis fates by the action of WNT signals.
To our knowledge, only one study has evaluated the direct involvement of S1P signaling during inner ear development (Hu et al., 2013), however there are reports indicating that S1P axis is involved in the signaling pathways that are required for the proper development of the inner ear. For example, in PC12 cells nerve growth factor (NGF) and FGF2, both important differentiation signals acting via tyrosine kinase receptors, increase the extracellular levels of S1P (Rius et al., 1997), suggesting that even different signaling molecules share in common the modulation of the S1P metabolism. Likewise, in astrocytes FGF2 can increase the activity of SK and the secretion of S1P, while S1P also induces the expression of FGF2 and phosphorylate ERK (Sato et al., 1999; Bassi et al., 2006), supporting the existence of a positive feedback regulatory loop between both signaling pathways in these cells. Analogous interaction of S1P and FGF signaling has been observed in human umbilical vein endothelial cells (HUVECs), where S1P induces the expression of VEGF, an important angiogenic factor. In these cells it was observed that the S1P-induced expression of FGF1 and its specific receptor FGFR1 preceded that of VEGF, since in cells treated with the FGFR inhibitor SU5402 or transfected with FGF1 and FGFR1 siRNA, S1P was unable to induce the VEGF expression (Chang et al., 2013). As observed in astrocytes, in HUVECs a mutual regulation between FGF and S1P signaling pathways occurs. For instance, it has been recently shown that FGF signaling is capable of regulating S1P metabolism by inducing the expression of SK1. In this system, the transcription factor KLF14, induced by FGF2, directly binds to the promoter region of SK1 gene (de Assuncao et al., 2014), establishing more clearly the mechanism of interaction between FGF and the S1P axis.
S1P plays also an important role in osteoblast differentiation. Osteoclast-conditioned medium induced the differentiation of human mesenchymal stem cells (hMSCs) towards osteoblasts. SK1, BMP6, WNT10B genes were highly expressed by osteoclasts and indeed when S1P signaling was blocked in hMSCs, differentiation and chemokinesis were reduced (Pederson et al., 2008). In addition, in osteoblasts it has been recently found that S1P axis increases BMP2-induced differentiation through the phosphorylation of ERK and Smad1/5/8 at a level higher than that observed following BMP2 treatment alone (Sato et al., 2012). Thus in this system S1P works as enhancer of the biological effect exerted by BMP signaling. Also in osteoblasts, S1P has been shown to interact with WNT signaling pathway by activating AKT, which inhibits GSK3β and lead to the nuclear traslocation of β-Catenin, downstream effector of WNT signaling. Indeed WNT3a-induced differentiation was impaired by the use AKT pharmacological inhibitors (Matsuzaki et al., 2013). These results and the known effects of BMP and WNT signaling in differentiation suggest that S1P could also behave as an enhancer of WNT signaling in bone formation similarly to its interaction with the BMP pathway.
Although morphologically uniform, the otocyst is compartmentalized by the expression of otic markers allocated at different regions within the otocyst. Members of Notch, FGF, RA and SHH signaling pathways are involved in this process.
Although Fgf3/Fgf10 double KO mice lack completely otic vesicle, the effect of single mutation affects only the expression patter of individual markers, such as DLX5 and PAX2, within the vesicle (Wright and Mansour, 2003). Indeed FGF3 and FGF10 are also expressed at different territories in the otocyst (Pirvola et al., 2000), suggesting that FGFs are important in otocyst regionalization.
RA is a morphogen with a known role in otic vesicle regionalization. In chick and mouse it was found that RA synthesis and degradation are allocated to the posterior and anterior ectoderm respectively, creating in this manner a RA gradient in the otic vesicle. Indeed exposure to RA posteriorise the entire otocyst, reducing the size of the prosensory domain, the area where sensory cells arise (Blentic et al., 2003; Bok et al., 2011). In the zebrafish RA expands anteriorly the expression of the transcription factor Tbx1 normally restricted to the posterior otic vesicle. This leads to increased Notch activation and reduction of the sensory domain (Radosevic et al., 2011).
RA also controls the expression pattern of FGF3 and FGF10, which may underlie the development of the sensory domain (Cadot et al., 2012; Economou et al., 2013). In this regard, it has been shown that FGF10 expression precedes the proneural genes Neurog1 and Neurod1 in the otocyst, and FGF10 overexpression increases the number of neuroblasts that delaminate (Alsina et al., 2004).
Sonic hedgehog (SHH) is another signaling molecule that participates in the formation of the otocyst ventromedial domain. Shh KO mice fail to maintain the expression of PAX2, OTX2, in the otocyst neurogenic region. As a consequence, ventral structures such as the cochlea and the cochlear ganglion never form (Riccomagno et al., 2002; Bok et al., 2007).
As early as E9 in the mouse, sensory neuroblast start to delaminate from the ventral neurogenic domain of the otocyst, the process continues until E14. Notch, neurotrophins and IGF1 are important in the differentiation and survival of neuroblast. Notch pathway has several functions, it restricts the non-neuronal domain of the otic vesicle posteriorly, and in the other hand, by mechanism of lateral inhibition limits the number of otic neuroblast that delaminate (NEUROD1+ cells) (Haddon et al., 1998; Abelló et al., 2007). In general, it has been observed that Notch signaling activation is necessary to the formation of sensory cells (Kiernan et al., 2006; Daudet et al., 2007). Indeed, the cell decision between neurogenic vs. sensory is regulated by Notch as NEUROG1+ cells restrict neighbor cells of adopting a neural fate but maintains them as sensory precursors (Raft et al., 2007). As neuroblasts (NEUROG1+) continue their way out of the otocyst, another transcription factor NEUROD1 is switched on. These genes are considered to be essential in the formation of the spiral ganglion. Neurog1 and Neurod1 KO mice lack completely innervation to the cochlea which appears morphologically normal (Ma et al., 2000; Kim et al., 2001). However, Neurod1 mutants form sensory neurons but these do not mature and undergo apoptosis. The expression of the neurotrophin receptors TRKB and TRKC failed in these animals, explaining the increased cell death.
Neurotrophins are important survival factors for many neuronal populations. The inner ear expresses two of them, BDNF and NT3 which are essential for the development of the SGNs. Double KO mice for either Bdnf/Nt3 or their receptors TrckB/TrckC are devoid of cochlear innervation (Ernfors et al., 1995; Silos-Santiago et al., 1997). Their expression pattern forms a gradient for the topographic organization of the sensory neurons (Pirvola et al., 1992; Fariñas et al., 2001). For example BDNF expression starts at the apex of the cochlea at E12.5 and progresses towards the base as the hair cells mature while NT3 has an inverse base-to-apex expression pattern (Schimmang et al., 2003; Sugawara et al., 2007). In fact, exposure to one or the other changes the behavior of SGN towards apical or basal neurons regardless of their position (Adamson et al., 2002). It has been observed that BDNF and NT3 are able to differentially regulate important electrophysiological proteins such as AMPA receptors and synaptophisin (Flores-Otero et al., 2007).
There are other cues involved in the formation of the spiral ganglion. FGFs have been shown to influence the migration and neurite outgrowth of inner ear neuroblasts. In chick otocyst explants, the expression of the BDNF receptor, TRKB is induced by the treatment with FGF2, together with this change, neural progenitor migration and the axon outgrowth accelerates (Brumwell et al., 2000).
IGF1 also plays a role in the formation of mature spiral ganglion cells. Igf1 KO mice are profoundly deaf by the third postnatal week. Although the cochlea seems normal at birth in these mice, the maturation of several structures of the inner ear such as the tectorial membrane and the spiral ganglion is impaired during the second and third postnatal week (Camarero et al., 2001). A marked apoptosis of SGNs, decreased soma size and an immature synapsis with the OC were observed in Igf1 KO mouse. Thus IGF1 is prescindible for normal development but it is later required for the maturation and survival of spiral ganglion cells at postnatal stages. Nonetheless examination of the inner ear of Igf1 KO at earlier developmental stages is still missing. In chick, IGF1 is necessary for the proliferation and further differentiation of auditory neuroblasts (Camarero et al., 2003). The biological effect of IGF1 in this model has been shown to be mediated by the activation of PI3K-AKT signaling pathway (Aburto et al., 2012).
Compelling evidence in several cellular settings models highlights the involvement of S1P axis in the signaling pathways discussed before. In human breast cancer cell lines for example, RA has shown an anti-proliferative effect mediated upon binding to the RA receptor RARα, leading to overproduction of ceramide and downregualtion of SK1. On the contrary, in cells transfected with a dominant-negative form of RARα, RA-induced growth inhibition is hampered, being accompanied by the increased expression and activity of SK1 (Somenzi et al., 2007). On the other hand S1P metabolism affects RA signaling: for example in colon cancer cells, S1P down-regulates the receptor RARβ, thus making cells refractory to RA-induced growth inhibition (Sun et al., 2012).
The cross-talk between neurotrophins and S1P metabolism is also well established. In PC12 and dorsal root ganglion cells, neuritogenesis induced by NGF depends in the membrane translocation of SK1 and the activation of S1P1, while S1P2 and S1P5 have an inhibitory role in neurite formation (Toman et al., 2004). This underlines the importance of understanding the function of this pathway in a particular cell type, since different S1P receptors can trigger disparate biological functions.
In a mouse model of Rett syndrome, Mecp2 KO, the S1P agonist p-FTY720 showed effectiveness in improving the symptoms of the disease, correlated with increased BDNF levels in several neuronal populations (Deogracias et al., 2012). In another study using cortical neurons p-FTY720 also induced the expression BDNF which protected cells against oligomeric amyloid-β toxicity in an in vitro model of Alzheimer disease (Doi et al., 2013). In oligodendrocytes, NT3-induced survival was found to be dependent upon SK expression and translocation (Saini et al., 2005).
Apart from differentiation and survival, it was demonstrated that NGF increased the number action potentials in sensory neurons through a SK1-dependent mechanism (Zhang et al., 2006), even S1P treatment alone was enough augment the number of action potentials in these cells. Thus, it seems that the modulation of S1P metabolism is common molecular mechanism to transmit several of the biological effects elicited by neurotrophins in diverse neuronal populations.
As regards the interaction of S1P with IGF1 signaling, it is important to recall that our laboratory recently found an involvement of S1P axis in IGF1 signaling. We proved that the myogenic effect of IGF1 in skeletal muscle cells is dependent on the activity of SK1 and SK2; if these kinases are blocked by pharmacological inhibitors or siRNA down-regulation, the expression of the myogenic markers myogenin and caveolin-3 is reduced (Bernacchioni et al., 2012). In the ear, IGF1 is currently being studied as a potential polypeptide to be used in the treatment of sensorineural hearing loss and the first results of a clinical trial involving 25 patients with sudden sensorineural hearing loss are very promising (Nakagawa et al., 2014; Yamamoto et al., 2014). Thus, from the point of view of medical application, it would be very interesting to investigate the potential involvement of S1P axis in IGF1 signaling in the context of the inner ear, and explore the possibility of using S1P or S1P receptor agonists/antagonists to prevent hearing loss.
In summary, the formation of the inner ear is a complex and dynamic process that involves the integration of signals such as WNTs, FGFs, RA, SHH and Notch ligands. The recognized interaction between these extracellular cues and S1P in other cell systems should prompt to study its possible involvement in the inner ear. This would shed light not only in the understanding of basic development of the inner ear, but also in the translation of more robust differentiation protocols to generate sensory cells in vitro from stem/progenitor cells.
It is still not clear the precise role of different sphingolipids in the biology of the inner ear, but there are several reports that highlight their importance and make them attractive targets that deserve further investigation.
For example it was recently found that the simplest ganglioside named GM3, generated by addition of a sialic acid to lactosylceramide in a reaction catalyzed by GM3 synthase, plays an important role in inner ear physiology. GM3 synthase (St3gal5) KO mice have been shown to be profoundly deaf due to specific deterioration of the OC (Yoshikawa et al., 2009). The hearing loss started as early as P14, progressing rapidly and by P17 KO mice were profoundly deaf with a substantial loss of hair cells. The molecular mechanism responsible for hearing loss was not determined. Although gangliosides GM3 and GT1b were expressed in the OC, SGN and stria vascularis (SV), no changes in K+ and endocochlear potential (EP) were observed in the KO mice (Yoshikawa et al., 2009). Noteworthy, in rat organotipic cultures gangliosides GM1 and GM3 were shown to diminish gentamicin-induced hair cell death (Nishimura et al., 2010). In addition, in the St3gal5 mutants there was an accumulation of other ganglioside species, whether these can induce apoptosis is unknown. In this regard, GM3 synthase deficient human fibroblasts showed increased apoptosis and accumulation of these ganglioside species (Liu et al., 2008). An alternative explanation could be that GM3-derived gangliosides could modulate growth factor response necessary for the maturation and survival. Indeed, in the work of Liu et al. fibroblasts of such patients have reduced EGF-induced proliferation and migration (Liu et al., 2008). It is important to mention that one of these patients was deaf (Fragaki et al., 2013).
Sphingomyelin, the most abundant sphingolipid in biological membranes, has also been demonstrated to be involved in hearing and maintenance of inner ear structures such as SV and hair cells and the EP. The SM synthase-1 (Sms-1) KO mouse presents hearing loss although this is milder than the one observed in St3gal5 KO mouse and other mutant mouse strains discussed later, Spns2 and Sp1r2 KOs, and affects mainly the low and middle frequency hearing (Lu et al., 2012). These mice have a decreased EP compared with wild type (WT) of the same age, but the drop was not as robust as the ones observed in the St3gal5KO for example, perhaps accounting for the milder phenotype. Together with the SV defects and the decreased EP, there was an aberrant expression of the K+ channel KCNQ1 in KO mice. However this was probably a secondary consequence, as its expression in the SV was only assessed at 3 months of age and the K+ concentration was found to be equal at 1 month between KO and WT littermates.
Finally, only recently S1P signaling emerged as an important pathway in the development of the inner ear and there are only few reports describing its role. For instance, explants cultures of rat cochlea have shown that gentamicin-induced hair cell death can be reduced upon the addition of S1P or increased by the presence of ceramide in the culture media, suggesting that hair cells could respond to these sphingolipids (Nishimura et al., 2010). Moreover, a recent work provides some details on the molecular mechanism that is responsible for the protective effect of S1P on hair cells. Indeed, S1P1–3 were found to be expressed in rat cochlea, both in spiral ganglion and OC, by non quantitative RT-PCR analysis and more interestingly an S1P2 antagonist was capable to augment gentamicin-induced hair cell loss, pointing at a role of this receptor in inner ear cell survival (Nakayama et al., 2014). Therefore it is not clear if the effect of ceramide and S1P may also occur indirectly by a secreted signal released from supporting cells, where for example S1P2 has been observed (Herr et al., 2007). Nonetheless, the insights could have an important implication for the use of these metabolites as otoprotective agents. In addition, it remains to be addressed if S1P and ceramide content change after ototoxic damage in vivo in the cochlea, this could shed new light into the role of these sphingolipids during hair cell degeneration.
Single S1pr2 and double S1pr2/S1pr3 KO have been shown to be profoundly deaf by the third postnatal week (MacLennan et al., 2006; Herr et al., 2007; Kono et al., 2007). Histological examination of the mutants at 6 weeks after birth shows a normal cochlear structure devoid of hair cells and auditory neurons. Kono et al. suggested that the loss of auditory function was caused by defects in the SV, a highly vascularized structure that maintains the EP. Indeed, well before the loss of hair cell and neurons, at postnatal week 2, a thickened SV with aberrant capillaries morphology and defects in the marginal cell boundaries could be observed (Kono et al., 2007). However, K+ concentration and the EP were not measured in these mutant mice. Thus, the loss of hair cells could not be completely attributed to an altered EP. Recently, the S1P specific transporter SPNS2 was found to be involved in audition, as mutant mice showed a gradual and profound hearing loss starting from the second postnatal week (Chen et al., 2014). Similarly to the S1pr2 KO mice, Spns2 mutants showed defects in the SV characterized by thicker capillaries with increased branching, as well as abnormal marginal cell borders. These defects and a decrease in the EP were the earliest abnormalities observed in the Spns2 KO (P14). Thus, it was concluded that SPNS2 is necessary to maintain the function of the SV and the EP, while the loss of hair cells and the downregulation of KCNQ1, KCNJ10, GJB2 and GJB6, known to maintain the EP, was secondary. This work is in agreement with the results obtained in the S1pr2 KO mice (Kono et al., 2007), and suggests an involvement of the S1P-SPNS2-S1P2 axis in the maintenance of the structure of the SV. However, how the S1P signaling maintains the EP is not clear, indeed, in the Spns2 KO mice no permeability between perylimph and endolymph compartments, neither leakage from the capillaries of the SV was observed in the mutants (Chen et al., 2014). In this regard, S1P has shown to increase the excitability of cardiac fibroblast by activating the K+ channel KIR6.1 in a S1P3-dependent manner (Benamer et al., 2011) or increase the firing rate of rat hippocampal neurons (Norman et al., 2010), suggesting that this pathway can regulate electrophysiological properties in diverse cell types. Another open question that deserves further investigation is the possible involvement of other S1P transporters in the inner ear. For instance in the S1pr2 KO mice, a vestibular phenotype was observed while in the Spns2 mutant only hearing function was disrupted. In this regard, it is unknown if in the vestibular system other S1P transporters may substitute the function of SPNS2. Only the MRP1 transporter has been found in the auditory and vestibular system and although it is known to transport S1P in other systems (Mitra et al., 2006), no vestibular phenotypes has been reported in the KO mice (Zhang et al., 2000). Other non-specific S1P transporters have been found in the mice sensory epithelia such as ABCG2 (Savary et al., 2007), but it is not known if it has role in regulating the extracellular levels of S1P. In fact, it is also unknown if the local release of S1P is necessary to maintain the EP as S1P has not been measured in the cochlea of WT or in Spns2 KO mice.
In addition, a direct involvement of the S1P signaling in the maintenance of hair cells separate from the EP cannot be ruled out until cell type specific mutants become available. In this regard there are some indications that S1P signaling may have a direct role in sensory cells, evidenced for example by the localized expression of SPNS2 in outer hair cells (Chen et al., 2014), S1P2 expression in supporting cells and S1P3 in that area of SGN (Herr et al., 2007). Even more, the zebrafish ortholog of mammal S1pr2, miles-apart (mil) has been expressed during developmental stages at positions where the neuromast develop. In addition mil morphants have a reduced number of neuromasts in the lateral line while the mRNA transfected embryos have an excess of neuromasts. Equally, morphants and mRNA transfected animals presented patterning defects in the otic vesicles (Hu et al., 2013). In summary, at least in the zebrafish S1P2 is involved in the formation of the otic vesicle and hair cells. If it has a similar role in the mouse has not been studied yet although the gross morphology of the cochlea and the hair cells is normal at birth in the S1pr2 KO mice.
Altogether these works indicate that sphingolipids are essential for normal hearing function, but their molecular targets are still to be discovered either in the sensory epithelium itself, the spiral ganglion and importantly, in the SV, that shows some of the earliest defects in the KO models. The results described before are summarized and presented in Table 1. Another important aspect to be explored in the future will be the relationship between different categories of sphingolipids, using cell lines derived from these animal models. Those studies will help us to pinpoint if there is a common mechanism involving hearing loss in the models described before. Such a task will be accomplished further with the help of cell lines with selective deletion of enzymes and receptors involved in S1P signaling axis, and possibly by using iPS-derived cells to uncover the role of S1P signaling during the differentiation of inner ear sensory cells.
Table 1. Knockout models for genes involved in sphingolipid metabolism and signaling have been found to present inner ear defects.
Overall, the here reported experimental findings highlight the crucial role of S1P signaling axis in inner ear biology. However there are several remaining questions that deserve further investigation. The different animal models discussed here support that S1P signaling is necessary during inner ear maturation and hearing at postnatal age in mice. Nonetheless, to our knowledge only one study in the zebrafish has assessed the involvement of this pathway during development (Hu et al., 2013). Thus, it remains to be seen if deletion of S1P receptors or S1P-metabolizing enzymes in mice affect gene expression in the inner ear at early developmental stages.
Importantly, it is still necessary to investigate in more detail the molecular mechanism by which S1P axis is regulating inner ear function in postnatal age, such as the modulation of growth factor receptors, the expression of K+ channels, critical for the EP, as well as the activation of prosurvival pathway in hair cells and sensory neurons. This is important and consistent with the variety of biological effects elicited by S1P in diverse cell types.
Future studies will confidently clarify the molecular mechanisms that regulate S1P metabolism and S1P receptor expression in the various aspects of inner ear sensory cell biology and hopefully they will disclose the therapeutic potential of S1P signaling pathway in hearing loss.
The authors declare that the research was conducted in the absence of any commercial or financial relationships that could be construed as a potential conflict of interest.
This work was supported by funds from FP7-PEOPLE-2013-IAPP-TARGEAR to PB.
Abelló, G., Khatri, S., Giráldez, F., and Alsina, B. (2007). Early regionalization of the otic placode and its regulation by the Notch signaling pathway. Mech. Dev. 124, 631–645. doi: 10.1016/j.mod.2007.04.002
PubMed Abstract | Full Text | CrossRef Full Text | Google Scholar
Aburto, M. R., Magariños, M., Leon, Y., Varela-Nieto, I., and Sanchez-Calderon, H. (2012). AKT signaling mediates IGF-I survival actions on otic neural progenitors. PLoS One 7:e30790. doi: 10.1371/journal.pone.0030790
PubMed Abstract | Full Text | CrossRef Full Text | Google Scholar
Adamson, C. L., Reid, M. A., and Davis, R. L. (2002). Opposite actions of brain-derived neurotrophic factor and neurotrophin-3 on firing features and ion channel composition of murine spiral ganglion neurons. J. Neurosci. 22, 1385–1396.
Ahrens, K., and Schlosser, G. (2005). Tissues and signals involved in the induction of placodal Six1 expression in Xenopus laevis. Dev. Biol. 288, 40–59. doi: 10.1016/j.ydbio.2005.07.022
PubMed Abstract | Full Text | CrossRef Full Text | Google Scholar
Alsina, B., Abelló, G., Ulloa, E., Henrique, D., Pujades, C., and Giraldez, F. (2004). FGF signaling is required for determination of otic neuroblasts in the chick embryo. Dev. Biol. 267, 119–134. doi: 10.1016/j.ydbio.2003.11.012
PubMed Abstract | Full Text | CrossRef Full Text | Google Scholar
Alvarez, Y., Alonso, M. T., Vendrell, V., Zelarayan, L. C., Chamero, P., Theil, T., et al. (2003). Requirements for FGF3 and FGF10 during inner ear formation. Development 130, 6329–6338. doi: 10.1242/dev.00881
PubMed Abstract | Full Text | CrossRef Full Text | Google Scholar
Bassi, R., Anelli, V., Giussani, P., Tettamanti, G., Viani, P., and Riboni, L. (2006). Sphingosine-1-phosphate is released by cerebellar astrocytes in response to bFGF and induces astrocyte proliferation through Gi-protein-coupled receptors. Glia 53, 621–630. doi: 10.1002/glia.20324
PubMed Abstract | Full Text | CrossRef Full Text | Google Scholar
Benamer, N., Fares, N., Bois, P., and Faivre, J. F. (2011). Electrophysiological and functional effects of sphingosine-1-phosphate in mouse ventricular fibroblasts. Biochem. Biophys. Res. Commun. 408, 6–11. doi: 10.1016/j.bbrc.2011.03.072
PubMed Abstract | Full Text | CrossRef Full Text | Google Scholar
Bernacchioni, C., Cencetti, F., Blescia, S., Donati, C., and Bruni, P. (2012). Sphingosine kinase/sphingosine 1-phosphate axis: a new player for insulin-like growth factor-1-induced myoblast differentiation. Skelet Muscle 2:15. doi: 10.1186/2044-5040-2-15
PubMed Abstract | Full Text | CrossRef Full Text | Google Scholar
Blentic, A., Gale, E., and Maden, M. (2003). Retinoic acid signalling centres in the avian embryo identified by sites of expression of synthesising and catabolising enzymes. Dev. Dyn. 227, 114–127. doi: 10.1002/dvdy.10292
PubMed Abstract | Full Text | CrossRef Full Text | Google Scholar
Bok, J., Dolson, D. K., Hill, P., Rüther, U., Epstein, D. J., and Wu, D. K. (2007). Opposing gradients of Gli repressor and activators mediate Shh signaling along the dorsoventral axis of the inner ear. Development 134, 1713–1722. doi: 10.1242/dev.000760
PubMed Abstract | Full Text | CrossRef Full Text | Google Scholar
Bok, J., Raft, S., Kong, K. A., Koo, S. K., Dräger, U. C., and Wu, D. K. (2011). Transient retinoic acid signaling confers anterior-posterior polarity to the inner ear. Proc. Natl. Acad. Sci. U S A 108, 161–166. doi: 10.1073/pnas.1010547108
PubMed Abstract | Full Text | CrossRef Full Text | Google Scholar
Brinkmann, V., Billich, A., Baumruker, T., Heining, P., Schmouder, R., Francis, G., et al. (2010). Fingolimod (FTY720): discovery and development of an oral drug to treat multiple sclerosis. Nat. Rev. Drug Discov. 9, 883–897. doi: 10.1038/nrd3248
PubMed Abstract | Full Text | CrossRef Full Text | Google Scholar
Brugmann, S. A., Pandur, P. D., Kenyon, K. L., Pignoni, F., and Moody, S. A. (2004). Six1 promotes a placodal fate within the lateral neurogenic ectoderm by functioning as both a transcriptional activator and repressor. Development 131, 5871–5881. doi: 10.1242/dev.01516
PubMed Abstract | Full Text | CrossRef Full Text | Google Scholar
Brumwell, C. L., Hossain, W. A., Morest, D. K., and Bernd, P. (2000). Role for basic fibroblast growth factor (FGF-2) in tyrosine kinase (TrkB) expression in the early development and innervation of the auditory receptor: in vitro and in situ studies. Exp. Neurol. 162, 121–145. doi: 10.1006/exnr.2000.7317
PubMed Abstract | Full Text | CrossRef Full Text | Google Scholar
Brunkhorst, R., Vutukuri, R., and Pfeilschifter, W. (2014). Fingolimod for the treatment of neurological diseases-state of play and future perspectives. Front. Cell. Neurosci. 8:283. doi: 10.3389/fncel.2014.00283
PubMed Abstract | Full Text | CrossRef Full Text | Google Scholar
Cadot, S., Frenz, D., and Maconochie, M. (2012). A novel method for retinoic acid administration reveals differential and dose-dependent downregulation of Fgf3 in the developing inner ear and anterior CNS. Dev. Dyn. 241, 741–758. doi: 10.1002/dvdy.23748
PubMed Abstract | Full Text | CrossRef Full Text | Google Scholar
Camarero, G., Avendano, C., Fernandez-Moreno, C., Villar, A., Contreras, J., de Pablo, F., et al. (2001). Delayed inner ear maturation and neuronal loss in postnatal Igf-1-deficient mice. J. Neurosci. 21, 7630–7641.
Camarero, G., Leon, Y., Gorospe, I., De Pablo, F., Alsina, B., Giraldez, F., et al. (2003). Insulin-like growth factor 1 is required for survival of transit-amplifying neuroblasts and differentiation of otic neurons. Dev. Biol. 262, 242–253. doi: 10.1016/s0012-1606(03)00387-7
PubMed Abstract | Full Text | CrossRef Full Text | Google Scholar
Chang, C. H., Huang, Y. L., Shyu, M. K., Chen, S. U., Lin, C. H., Ju, T. K., et al. (2013). Sphingosine-1-phosphate induces VEGF-C expression through a MMP-2/FGF-1/FGFR-1-dependent pathway in endothelial cells in vitro. Acta Pharmacol. Sin. 34, 360–366. doi: 10.1038/aps.2012.186
PubMed Abstract | Full Text | CrossRef Full Text | Google Scholar
Chen, J., Ingham, N., Kelly, J., Jadeja, S., Goulding, D., Pass, J., et al. (2014). Spinster homolog 2 (spns2) deficiency causes early onset progressive hearing loss. PLoS Genet. 10:e1004688. doi: 10.1371/journal.pgen.1004688
PubMed Abstract | Full Text | CrossRef Full Text | Google Scholar
Chen, W., Jongkamonwiwat, N., Abbas, L., Eshtan, S. J., Johnson, S. L., Kuhn, S., et al. (2012). Restoration of auditory evoked responses by human ES-cell-derived otic progenitors. Nature 490, 278–282. doi: 10.1038/nature11415
PubMed Abstract | Full Text | CrossRef Full Text | Google Scholar
Choi, J. W., Gardell, S. E., Herr, D. R., Rivera, R., Lee, C. W., Noguchi, K., et al. (2011). FTY720 (fingolimod) efficacy in an animal model of multiple sclerosis requires astrocyte sphingosine 1-phosphate receptor 1 (S1P1) modulation. Proc. Natl. Acad. Sci. U S A 108, 751–756. doi: 10.1073/pnas.1014154108
PubMed Abstract | Full Text | CrossRef Full Text | Google Scholar
Coste, O., Brenneis, C., Linke, B., Pierre, S., Maeurer, C., Becker, W., et al. (2008). Sphingosine 1-phosphate modulates spinal nociceptive processing. J. Biol. Chem. 283, 32442–32451. doi: 10.1074/jbc.m806410200
PubMed Abstract | Full Text | CrossRef Full Text | Google Scholar
Couttas, T. A., Kain, N., Daniels, B., Lim, X. Y., Shepherd, C., Kril, J., et al. (2014). Loss of the neuroprotective factor Sphingosine 1-phosphate early in Alzheimer’s disease pathogenesis. Acta Neuropathol. Commun. 2:9. doi: 10.1186/2051-5960-2-9
PubMed Abstract | Full Text | CrossRef Full Text | Google Scholar
Cyster, J. G., and Schwab, S. R. (2012). Sphingosine-1-phosphate and lymphocyte egress from lymphoid organs. Annu. Rev. Immunol. 30, 69–94. doi: 10.1146/annurev-immunol-020711-075011
PubMed Abstract | Full Text | CrossRef Full Text | Google Scholar
D’Angelo, G., Capasso, S., Sticco, L., and Russo, D. (2013). Glycosphingolipids: synthesis and functions. Febs J. 280, 6338–6353. doi: 10.1111/febs.12559
PubMed Abstract | Full Text | CrossRef Full Text | Google Scholar
Daudet, N., Ariza-Mcnaughton, L., and Lewis, J. (2007). Notch signalling is needed to maintain, but not to initiate, the formation of prosensory patches in the chick inner ear. Development 134, 2369–2378. doi: 10.1242/dev.001842
PubMed Abstract | Full Text | CrossRef Full Text | Google Scholar
de Assuncao, T. M., Lomberk, G., Cao, S., Yaqoob, U., Mathison, A., Simonetto, D. A., et al. (2014). New role for Kruppel-like factor 14 as a transcriptional activator involved in the generation of signaling lipids. J. Biol. Chem. 289, 15798–15809. doi: 10.1074/jbc.m113.544346
PubMed Abstract | Full Text | CrossRef Full Text | Google Scholar
Deogracias, R., Yazdani, M., Dekkers, M. P., Guy, J., Ionescu, M. C., Vogt, K. E., et al. (2012). Fingolimod, a sphingosine-1 phosphate receptor modulator, increases BDNF levels and improves symptoms of a mouse model of Rett syndrome. Proc. Natl. Acad. Sci. U S A 109, 14230–14235. doi: 10.1073/pnas.1206093109
PubMed Abstract | Full Text | CrossRef Full Text | Google Scholar
Doi, Y., Takeuchi, H., Horiuchi, H., Hanyu, T., Kawanokuchi, J., Jin, S., et al. (2013). Fingolimod phosphate attenuates oligomeric amyloid beta-induced neurotoxicity via increased brain-derived neurotrophic factor expression in neurons. PLoS One 8:e61988. doi: 10.1371/journal.pone.0061988
PubMed Abstract | Full Text | CrossRef Full Text | Google Scholar
Donati, C., Cencetti, F., and Bruni, P. (2013a). New insights into the role of sphingosine 1-phosphate and lysophosphatidic acid in the regulation of skeletal muscle cell biology. Biochim. Biophys. Acta 1831, 176–184. doi: 10.1016/j.bbalip.2012.06.013
PubMed Abstract | Full Text | CrossRef Full Text | Google Scholar
Donati, C., Cencetti, F., and Bruni, P. (2013b). Sphingosine 1-phosphate axis: a new leader actor in skeletal muscle biology. Front. Physiol. 4:338. doi: 10.3389/fphys.2013.00338
PubMed Abstract | Full Text | CrossRef Full Text | Google Scholar
Economou, A., Datta, P., Georgiadis, V., Cadot, S., Frenz, D., and Maconochie, M. (2013). Gata3 directly regulates early inner ear expression of Fgf10. Dev. Biol. 374, 210–222. doi: 10.1016/j.ydbio.2012.11.028
PubMed Abstract | Full Text | CrossRef Full Text | Google Scholar
Ernfors, P., Van De Water, T., Loring, J., and Jaenisch, R. (1995). Complementary roles of BDNF and NT-3 in vestibular and auditory development. Neuron 14, 1153–1164. doi: 10.1016/0896-6273(95)90263-5
PubMed Abstract | Full Text | CrossRef Full Text | Google Scholar
Fariñas, I., Jones, K. R., Tessarollo, L., Vigers, A. J., Huang, E., Kirstein, M., et al. (2001). Spatial shaping of cochlear innervation by temporally regulated neurotrophin expression. J. Neurosci. 21, 6170–6180.
Flores-Otero, J., Xue, H. Z., and Davis, R. L. (2007). Reciprocal regulation of presynaptic and postsynaptic proteins in bipolar spiral ganglion neurons by neurotrophins. J. Neurosci. 27, 14023–14034. doi: 10.1523/jneurosci.3219-07.2007
PubMed Abstract | Full Text | CrossRef Full Text | Google Scholar
Fragaki, K., Ait-El-Mkadem, S., Chaussenot, A., Gire, C., Mengual, R., Bonesso, L., et al. (2013). Refractory epilepsy and mitochondrial dysfunction due to GM3 synthase deficiency. Eur. J. Hum. Genet. 21, 528–534. doi: 10.1038/ejhg.2012.202
PubMed Abstract | Full Text | CrossRef Full Text | Google Scholar
Freter, S., Muta, Y., Mak, S. S., Rinkwitz, S., and Ladher, R. K. (2008). Progressive restriction of otic fate: the role of FGF and Wnt in resolving inner ear potential. Development 135, 3415–3424. doi: 10.1242/dev.026674
PubMed Abstract | Full Text | CrossRef Full Text | Google Scholar
Gomez-Muñoz, A., Gangoiti, P., Arana, L., Ouro, A., Rivera, I. G., Ordonez, M., et al. (2013). New insights on the role of ceramide 1-phosphate in inflammation. Biochim. Biophys. Acta 1831, 1060–1066. doi: 10.1016/j.bbalip.2013.02.001
PubMed Abstract | Full Text | CrossRef Full Text | Google Scholar
Haddon, C., Jiang, Y. J., Smithers, L., and Lewis, J. (1998). Delta-Notch signalling and the patterning of sensory cell differentiation in the zebrafish ear: evidence from the mind bomb mutant. Development 125, 4637–4644.
Hannun, Y. A., and Obeid, L. M. (2008). Principles of bioactive lipid signalling: lessons from sphingolipids. Nat. Rev. Mol. Cell Biol. 9, 139–150. doi: 10.1038/nrm2329
PubMed Abstract | Full Text | CrossRef Full Text | Google Scholar
Hannun, Y. A., and Obeid, L. M. (2011). Many ceramides. J. Biol. Chem. 286, 27855–27862. doi: 10.1074/jbc.r111.254359
PubMed Abstract | Full Text | CrossRef Full Text | Google Scholar
Hans, S., Liu, D., and Westerfield, M. (2004). Pax8 and Pax2a function synergistically in otic specification, downstream of the Foxi1 and Dlx3b transcription factors. Development 131, 5091–5102. doi: 10.1242/dev.01346
PubMed Abstract | Full Text | CrossRef Full Text | Google Scholar
Harada, J., Foley, M., Moskowitz, M. A., and Waeber, C. (2004). Sphingosine-1-phosphate induces proliferation and morphological changes of neural progenitor cells. J. Neurochem. 88, 1026–1039. doi: 10.1046/j.1471-4159.2003.02219.x
PubMed Abstract | Full Text | CrossRef Full Text | Google Scholar
Herr, D. R., Grillet, N., Schwander, M., Rivera, R., Müller, U., and Chun, J. (2007). Sphingosine 1-phosphate (S1P) signaling is required for maintenance of hair cells mainly via activation of S1P2. J. Neurosci. 27, 1474–1478. doi: 10.1523/jneurosci.4245-06.2007
PubMed Abstract | Full Text | CrossRef Full Text | Google Scholar
Hu, Z. Y., Zhang, Q. Y., Qin, W., Tong, J. W., Zhao, Q., Han, Y., et al. (2013). Gene miles-apart is required for formation of otic vesicle and hair cells in zebrafish. Cell Death Dis. 4:e900. doi: 10.1038/cddis.2013.432
PubMed Abstract | Full Text | CrossRef Full Text | Google Scholar
Huwiler, A., Kolter, T., Pfeilschifter, J., and Sandhoff, K. (2000). Physiology and pathophysiology of sphingolipid metabolism and signaling. Biochim. Biophys. Acta 1485, 63–99. doi: 10.1016/s1388-1981(00)00042-1
PubMed Abstract | Full Text | CrossRef Full Text | Google Scholar
Im, D. S., Clemens, J., Macdonald, T. L., and Lynch, K. R. (2001). Characterization of the human and mouse sphingosine 1-phosphate receptor, S1P5 (Edg-8): structure-activity relationship of sphingosine1-phosphate receptors. Biochemistry 40, 14053–14060. doi: 10.1021/bi011606i
PubMed Abstract | Full Text | CrossRef Full Text | Google Scholar
Im, D. S., Heise, C. E., Ancellin, N., O’Dowd, B. F., Shei, G. J., Heavens, R. P., et al. (2000). Characterization of a novel sphingosine 1-phosphate receptor, Edg-8. J. Biol. Chem. 275, 14281–14286. doi: 10.1074/jbc.275.19.14281
PubMed Abstract | Full Text | CrossRef Full Text | Google Scholar
Ishii, I., Fukushima, N., Ye, X., and Chun, J. (2004). Lysophospholipid receptors: signaling and biology. Annu. Rev. Biochem. 73, 321–354. doi: 10.1146/annurev.biochem.73.011303.073731
PubMed Abstract | Full Text | CrossRef Full Text | Google Scholar
Ito, M., Okino, N., and Tani, M. (2014). New insight into the structure, reaction mechanism and biological functions of neutral ceramidase. Biochim. Biophys. Acta 1841, 682–691. doi: 10.1016/j.bbalip.2013.09.008
PubMed Abstract | Full Text | CrossRef Full Text | Google Scholar
Jaillard, C., Harrison, S., Stankoff, B., Aigrot, M. S., Calver, A. R., Duddy, G., et al. (2005). Edg8/S1P5: an oligodendroglial receptor with dual function on process retraction and cell survival. J. Neurosci. 25, 1459–1469. doi: 10.1523/jneurosci.4645-04.2005
PubMed Abstract | Full Text | CrossRef Full Text | Google Scholar
Jung, C. G., Kim, H. J., Miron, V. E., Cook, S., Kennedy, T. E., Foster, C. A., et al. (2007). Functional consequences of S1P receptor modulation in rat oligodendroglial lineage cells. Glia 55, 1656–1667. doi: 10.1002/glia.20576
PubMed Abstract | Full Text | CrossRef Full Text | Google Scholar
Kajimoto, T., Okada, T., Yu, H., Goparaju, S. K., Jahangeer, S., and Nakamura, S. (2007). Involvement of sphingosine-1-phosphate in glutamate secretion in hippocampal neurons. Mol. Cell. Biol. 27, 3429–3440. doi: 10.1128/mcb.01465-06
PubMed Abstract | Full Text | CrossRef Full Text | Google Scholar
Kanno, T., Nishizaki, T., Proia, R. L., Kajimoto, T., Jahangeer, S., Okada, T., et al. (2010). Regulation of synaptic strength by sphingosine 1-phosphate in the hippocampus. Neuroscience 171, 973–980. doi: 10.1016/j.neuroscience.2010.10.021
PubMed Abstract | Full Text | CrossRef Full Text | Google Scholar
Kawahara, A., Nishi, T., Hisano, Y., Fukui, H., Yamaguchi, A., and Mochizuki, N. (2009). The sphingolipid transporter spns2 functions in migration of zebrafish myocardial precursors. Science 323, 524–527. doi: 10.1126/science.1167449
PubMed Abstract | Full Text | CrossRef Full Text | Google Scholar
Kempf, A., Tews, B., Arzt, M. E., Weinmann, O., Obermair, F. J., Pernet, V., et al. (2014). The sphingolipid receptor S1PR2 is a receptor for Nogo-a repressing synaptic plasticity. PLoS Biol. 12:e1001763. doi: 10.1371/journal.pbio.1001763
PubMed Abstract | Full Text | CrossRef Full Text | Google Scholar
Kiernan, A. E., Xu, J., and Gridley, T. (2006). The Notch ligand JAG1 is required for sensory progenitor development in the mammalian inner ear. PLoS Genet. 2:e4. doi: 10.1371/journal.pgen.0020004
PubMed Abstract | Full Text | CrossRef Full Text | Google Scholar
Kim, W. Y., Fritzsch, B., Serls, A., Bakel, L. A., Huang, E. J., Reichardt, L. F., et al. (2001). NeuroD-null mice are deaf due to a severe loss of the inner ear sensory neurons during development. Development 128, 417–426.
Kimura, A., Ohmori, T., Kashiwakura, Y., Ohkawa, R., Madoiwa, S., Mimuro, J., et al. (2008). Antagonism of sphingosine 1-phosphate receptor-2 enhances migration of neural progenitor cells toward an area of brain. Stroke 39, 3411–3417. doi: 10.1161/STROKEAHA.108.514612
PubMed Abstract | Full Text | CrossRef Full Text | Google Scholar
Kimura, A., Ohmori, T., Ohkawa, R., Madoiwa, S., Mimuro, J., Murakami, T., et al. (2007). Essential roles of sphingosine 1-phosphate/S1P1 receptor axis in the migration of neural stem cells toward a site of spinal cord injury. Stem Cells 25, 115–124. doi: 10.1634/stemcells.2006-0223
PubMed Abstract | Full Text | CrossRef Full Text | Google Scholar
Kluk, M. J., and Hla, T. (2002). Signaling of sphingosine-1-phosphate via the S1P/EDG-family of G-protein-coupled receptors. Biochim. Biophys. Acta 1582, 72–80. doi: 10.1016/s1388-1981(02)00139-7
PubMed Abstract | Full Text | CrossRef Full Text | Google Scholar
Koehler, K. R., and Hashino, E. (2014). 3D mouse embryonic stem cell culture for generating inner ear organoids. Nat. Protoc. 9, 1229–1244. doi: 10.1038/nprot.2014.100
PubMed Abstract | Full Text | CrossRef Full Text | Google Scholar
Kono, M., Belyantseva, I. A., Skoura, A., Frolenkov, G. I., Starost, M. F., Dreier, J. L., et al. (2007). Deafness and stria vascularis defects in S1P2 receptor-null mice. J. Biol. Chem. 282, 10690–10696. doi: 10.1074/jbc.m700370200
PubMed Abstract | Full Text | CrossRef Full Text | Google Scholar
Kulakowska, A., Zendzian-Piotrowska, M., Baranowski, M., Konończuk, T., Drozdowski, W., Górski, J., et al. (2010). Intrathecal increase of sphingosine 1-phosphate at early stage multiple sclerosis. Neurosci. Lett. 477, 149–152. doi: 10.1016/j.neulet.2010.04.052
PubMed Abstract | Full Text | CrossRef Full Text | Google Scholar
Kumar, A., and Saba, J. D. (2009). Lyase to live by: sphingosine phosphate lyase as a therapeutic target. Expert Opin. Ther. Targets 13, 1013–1025. doi: 10.1517/14728220903039722
PubMed Abstract | Full Text | CrossRef Full Text | Google Scholar
Ladher, R. K., Anakwe, K. U., Gurney, A. L., Schoenwolf, G. C., and Francis-West, P. H. (2000). Identification of synergistic signals initiating inner ear development. Science 290, 1965–1967. doi: 10.1126/science.290.5498.1965
PubMed Abstract | Full Text | CrossRef Full Text | Google Scholar
Le Stunff, H., Galve-Roperh, I., Peterson, C., Milstien, S., and Spiegel, S. (2002). Sphingosine-1-phosphate phosphohydrolase in regulation of sphingolipid metabolism and apoptosis. J. Cell Biol. 158, 1039–1049. doi: 10.1083/jcb.200203123
PubMed Abstract | Full Text | CrossRef Full Text | Google Scholar
Litsiou, A., Hanson, S., and Streit, A. (2005). A balance of FGF, BMP and WNT signalling positions the future placode territory in the head. Development 132, 4051–4062. doi: 10.1242/dev.01964
PubMed Abstract | Full Text | CrossRef Full Text | Google Scholar
Liu, Y., Su, Y., Wiznitzer, M., Epifano, O., and Ladisch, S. (2008). Ganglioside depletion and EGF responses of human GM3 synthase-deficient fibroblasts. Glycobiology 18, 593–601. doi: 10.1093/glycob/cwn039
PubMed Abstract | Full Text | CrossRef Full Text | Google Scholar
Liu, Y., Wada, R., Yamashita, T., Mi, Y., Deng, C. X., Hobson, J. P., et al. (2000). Edg-1, the G protein-coupled receptor for sphingosine-1-phosphate, is essential for vascular maturation. J. Clin. Invest. 106, 951–961. doi: 10.1172/jci10905
PubMed Abstract | Full Text | CrossRef Full Text | Google Scholar
Lu, M. H., Takemoto, M., Watanabe, K., Luo, H., Nishimura, M., Yano, M., et al. (2012). Deficiency of sphingomyelin synthase-1 but not sphingomyelin synthase-2 causes hearing impairments in mice. J. Physiol. 590, 4029–4044. doi: 10.1113/jphysiol.2012.235846
PubMed Abstract | Full Text | CrossRef Full Text | Google Scholar
Ma, Q., Anderson, D. J., and Fritzsch, B. (2000). Neurogenin 1 null mutant ears develop fewer, morphologically normal hair cells in smaller sensory epithelia devoid of innervation. J. Assoc. Res. Otolaryngol. 1, 129–143. doi: 10.1007/s101620010017
PubMed Abstract | Full Text | CrossRef Full Text | Google Scholar
Maceyka, M., Harikumar, K. B., Milstien, S., and Spiegel, S. (2012). Sphingosine-1-phosphate signaling and its role in disease. Trends Cell Biol. 22, 50–60. doi: 10.1016/j.tcb.2011.09.003
PubMed Abstract | Full Text | CrossRef Full Text | Google Scholar
Maceyka, M., Payne, S. G., Milstien, S., and Spiegel, S. (2002). Sphingosine kinase, sphingosine-1-phosphate and apoptosis. Biochim. Biophys. Acta 1585, 193–201. doi: 10.1016/S1388-1981(02)00341-4
PubMed Abstract | Full Text | CrossRef Full Text | Google Scholar
MacLennan, A. J., Benner, S. J., Andringa, A., Chaves, A. H., Rosing, J. L., Vesey, R., et al. (2006). The S1P2 sphingosine 1-phosphate receptor is essential for auditory and vestibular function. Hear. Res. 220, 38–48. doi: 10.1016/j.heares.2006.06.016
PubMed Abstract | Full Text | CrossRef Full Text | Google Scholar
Mao, C., and Obeid, L. M. (2008). Ceramidases: regulators of cellular responses mediated by ceramide, sphingosine and sphingosine-1-phosphate. Biochim. Biophys. Acta 1781, 424–434. doi: 10.1016/j.bbalip.2008.06.002
PubMed Abstract | Full Text | CrossRef Full Text | Google Scholar
Maroon, H., Walshe, J., Mahmood, R., Kiefer, P., Dickson, C., and Mason, I. (2002). Fgf3 and Fgf8 are required together for formation of the otic placode and vesicle. Development 129, 2099–2108.
Martin, K., and Groves, A. K. (2006). Competence of cranial ectoderm to respond to Fgf signaling suggests a two-step model of otic placode induction. Development 133, 877–887. doi: 10.1242/dev.02267
PubMed Abstract | Full Text | CrossRef Full Text | Google Scholar
Matsuzaki, E., Hiratsuka, S., Hamachi, T., Takahashi-Yanaga, F., Hashimoto, Y., Higashi, K., et al. (2013). Sphingosine-1-phosphate promotes the nuclear translocation of beta-catenin and thereby induces osteoprotegerin gene expression in osteoblast-like cell lines. Bone 55, 315–324. doi: 10.1016/j.bone.2013.04.008
PubMed Abstract | Full Text | CrossRef Full Text | Google Scholar
McLarren, K. W., Litsiou, A., and Streit, A. (2003). DLX5 positions the neural crest and preplacode region at the border of the neural plate. Dev. Biol. 259, 34–47. doi: 10.1016/s0012-1606(03)00177-5
PubMed Abstract | Full Text | CrossRef Full Text | Google Scholar
Mendelson, K., Evans, T., and Hla, T. (2014). Sphingosine 1-phosphate signalling. Development 141, 5–9. doi: 10.1242/dev.094805
PubMed Abstract | Full Text | CrossRef Full Text | Google Scholar
Messner, M. C., and Cabot, M. C. (2010). Glucosylceramide in humans. Adv. Exp. Med. Biol. 688, 156–164. doi: 10.1007/978-1-4419-6741-1_11
PubMed Abstract | Full Text | CrossRef Full Text | Google Scholar
Meyer zu Heringdorf, D., and Jakobs, K. H. (2007). Lysophospholipid receptors: signalling, pharmacology and regulation by lysophospholipid metabolism. Biochim. Biophys. Acta 1768, 923–940. doi: 10.1016/j.bbamem.2006.09.026
PubMed Abstract | Full Text | CrossRef Full Text | Google Scholar
Mitra, P., Oskeritzian, C. A., Payne, S. G., Beaven, M. A., Milstien, S., and Spiegel, S. (2006). Role of ABCC1 in export of sphingosine-1-phosphate from mast cells. Proc. Natl. Acad. Sci. U S A 103, 16394–16399. doi: 10.1073/pnas.0603734103
PubMed Abstract | Full Text | CrossRef Full Text | Google Scholar
Mizugishi, K., Yamashita, T., Olivera, A., Miller, G. F., Spiegel, S., and Proia, R. L. (2005). Essential role for sphingosine kinases in neural and vascular development. Mol. Cell. Biol. 25, 11113–11121. doi: 10.1128/mcb.25.24.11113-11121.2005
PubMed Abstract | Full Text | CrossRef Full Text | Google Scholar
Mullen, T. D., Hannun, Y. A., and Obeid, L. M. (2012). Ceramide synthases at the centre of sphingolipid metabolism and biology. Biochem. J. 441, 789–802. doi: 10.1042/bj20111626
Nakagawa, T., Kumakawa, K., Usami, S., Hato, N., Tabuchi, K., Takahashi, M., et al. (2014). A randomized controlled clinical trial of topical insulin-like growth factor-1 therapy for sudden deafness refractory to systemic corticosteroid treatment. BMC Med. 12:219. doi: 10.1186/s12916-014-0219-x
PubMed Abstract | Full Text | CrossRef Full Text | Google Scholar
Nakayama, M., Tabuchi, K., Hoshino, T., Nakamagoe, M., Nishimura, B., and Hara, A. (2014). The influence of sphingosine-1-phosphate receptor antagonists on gentamicin-induced hair cell loss of the rat cochlea. Neurosci. Lett. 561, 91–95. doi: 10.1016/j.neulet.2013.12.063
PubMed Abstract | Full Text | CrossRef Full Text | Google Scholar
Neubauer, H. A., and Pitson, S. M. (2013). Roles, regulation and inhibitors of sphingosine kinase 2. FEBS J. 280, 5317–5336. doi: 10.1111/febs.12314
PubMed Abstract | Full Text | CrossRef Full Text | Google Scholar
Nishi, T., Kobayashi, N., Hisano, Y., Kawahara, A., and Yamaguchi, A. (2014). Molecular and physiological functions of sphingosine 1-phosphate transporters. Biochim. Biophys. Acta 1841, 759–765. doi: 10.1016/j.bbalip.2013.07.012
PubMed Abstract | Full Text | CrossRef Full Text | Google Scholar
Nishimura, B., Tabuchi, K., Nakamagoe, M., and Hara, A. (2010). The influences of sphingolipid metabolites on gentamicin-induced hair cell loss of the rat cochlea. Neurosci. Lett. 485, 1–5. doi: 10.1016/j.neulet.2010.08.014
PubMed Abstract | Full Text | CrossRef Full Text | Google Scholar
Norman, E., Cutler, R. G., Flannery, R., Wang, Y., and Mattson, M. P. (2010). Plasma membrane sphingomyelin hydrolysis increases hippocampal neuron excitability by sphingosine-1-phosphate mediated mechanisms. J. Neurochem. 114, 430–439. doi: 10.1111/j.1471-4159.2010.06779.x
PubMed Abstract | Full Text | CrossRef Full Text | Google Scholar
Ohyama, T., Mohamed, O. A., Taketo, M. M., Dufort, D., and Groves, A. K. (2006). Wnt signals mediate a fate decision between otic placode and epidermis. Development 133, 865–875. doi: 10.1242/dev.02271
PubMed Abstract | Full Text | CrossRef Full Text | Google Scholar
Oshima, K., Shin, K., Diensthuber, M., Peng, A. W., Ricci, A. J., and Heller, S. (2010). Mechanosensitive hair cell-like cells from embryonic and induced pluripotent stem cells. Cell 141, 704–716. doi: 10.1016/j.cell.2010.03.035
PubMed Abstract | Full Text | CrossRef Full Text | Google Scholar
Pappu, R., Schwab, S. R., Cornelissen, I., Pereira, J. P., Regard, J. B., Xu, Y., et al. (2007). Promotion of lymphocyte egress into blood and lymph by distinct sources of sphingosine-1-phosphate. Science 316, 295–298. doi: 10.1126/science.1139221
PubMed Abstract | Full Text | CrossRef Full Text | Google Scholar
Pederson, L., Ruan, M., Westendorf, J. J., Khosla, S., and Oursler, M. J. (2008). Regulation of bone formation by osteoclasts involves Wnt/BMP signaling and the chemokine sphingosine-1-phosphate. Proc. Natl. Acad. Sci. U S A 105, 20764–20769. doi: 10.1073/pnas.0805133106
PubMed Abstract | Full Text | CrossRef Full Text | Google Scholar
Phillips, B. T., Bolding, K., and Riley, B. B. (2001). Zebrafish fgf3 and fgf8 encode redundant functions required for otic placode induction. Dev. Biol. 235, 351–365. doi: 10.1006/dbio.2001.0297
PubMed Abstract | Full Text | CrossRef Full Text | Google Scholar
Pirvola, U., Spencer-Dene, B., Xing-Qun, L., Kettunen, P., Thesleff, I., Fritzsch, B., et al. (2000). FGF/FGFR-2(IIIb) signaling is essential for inner ear morphogenesis. J. Neurosci. 20, 6125–6134.
Pirvola, U., Ylikoski, J., Palgi, J., Lehtonen, E., Arumäe, U., and Saarma, M. (1992). Brain-derived neurotrophic factor and neurotrophin 3 mRNAs in the peripheral target fields of developing inner ear ganglia. Proc. Natl. Acad. Sci. U S A 89, 9915–9919. doi: 10.1073/pnas.89.20.9915
PubMed Abstract | Full Text | CrossRef Full Text | Google Scholar
Pyne, N. J., Waters, C., Moughal, N. A., Sambi, B. S., and Pyne, S. (2003). Receptor tyrosine kinase-GPCR signal complexes. Biochem. Soc. Trans. 31, 1220–1225. doi: 10.1042/bst0311220
PubMed Abstract | Full Text | CrossRef Full Text | Google Scholar
Quint, E., Zerucha, T., and Ekker, M. (2000). Differential expression of orthologous Dlx genes in zebrafish and mice: implications for the evolution of the Dlx homeobox gene family. J. Exp. Zool. 288, 235–241. doi: 10.1002/1097-010x(20001015)288:3<235::aid-jez4>3.0.co;2-j
PubMed Abstract | Full Text | CrossRef Full Text | Google Scholar
Radosevic, M., Robert-Moreno, A., Coolen, M., Bally-Cuif, L., and Alsina, B. (2011). Her9 represses neurogenic fate downstream of Tbx1 and retinoic acid signaling in the inner ear. Development 138, 397–408. doi: 10.1242/dev.056093
PubMed Abstract | Full Text | CrossRef Full Text | Google Scholar
Raft, S., Koundakjian, E. J., Quinones, H., Jayasena, C. S., Goodrich, L. V., Johnson, J. E., et al. (2007). Cross-regulation of Ngn1 and Math1 coordinates the production of neurons and sensory hair cells during inner ear development. Development 134, 4405–4415. doi: 10.1242/dev.009118
PubMed Abstract | Full Text | CrossRef Full Text | Google Scholar
Riccomagno, M. M., Martinu, L., Mulheisen, M., Wu, D. K., and Epstein, D. J. (2002). Specification of the mammalian cochlea is dependent on Sonic hedgehog. Genes Dev. 16, 2365–2378. doi: 10.1101/gad.1013302
PubMed Abstract | Full Text | CrossRef Full Text | Google Scholar
Rius, R. A., Edsall, L. C., and Spiegel, S. (1997). Activation of sphingosine kinase in pheochromocytoma PC12 neuronal cells in response to trophic factors. FEBS Lett. 417, 173–176. doi: 10.1016/s0014-5793(97)01277-5
PubMed Abstract | Full Text | CrossRef Full Text | Google Scholar
Saini, H. S., Coelho, R. P., Goparaju, S. K., Jolly, P. S., Maceyka, M., Spiegel, S., et al. (2005). Novel role of sphingosine kinase 1 as a mediator of neurotrophin-3 action in oligodendrocyte progenitors. J. Neurochem. 95, 1298–1310. doi: 10.1111/j.1471-4159.2005.03451.x
PubMed Abstract | Full Text | CrossRef Full Text | Google Scholar
Sato, K., Ishikawa, K., Ui, M., and Okajima, F. (1999). Sphingosine 1-phosphate induces expression of early growth response-1 and fibroblast growth factor-2 through mechanism involving extracellular signal-regulated kinase in astroglial cells. Brain Res. Mol. Brain Res. 74, 182–189. doi: 10.1016/s0169-328x(99)00279-x
PubMed Abstract | Full Text | CrossRef Full Text | Google Scholar
Sato, C., Iwasaki, T., Kitano, S., Tsunemi, S., and Sano, H. (2012). Sphingosine 1-phosphate receptor activation enhances BMP-2-induced osteoblast differentiation. Biochem. Biophys. Res. Commun. 423, 200–205. doi: 10.1016/j.bbrc.2012.05.130
PubMed Abstract | Full Text | CrossRef Full Text | Google Scholar
Sato, K., Ui, M., and Okajima, F. (2000). Differential roles of Edg-1 and Edg-5, sphingosine 1-phosphate receptors, in the signaling pathways in C6 glioma cells. Brain Res. Mol. Brain Res. 85, 151–160. doi: 10.1016/s0169-328x(00)00262-x
PubMed Abstract | Full Text | CrossRef Full Text | Google Scholar
Savary, E., Hugnot, J. P., Chassigneux, Y., Travo, C., Duperray, C., Van De Water, T., et al. (2007). Distinct population of hair cell progenitors can be isolated from the postnatal mouse cochlea using side population analysis. Stem Cells 25, 332–339. doi: 10.1634/stemcells.2006-0303
PubMed Abstract | Full Text | CrossRef Full Text | Google Scholar
Schimmang, T., Tan, J., Müller, M., Zimmermann, U., Rohbock, K., Kôpschall, I., et al. (2003). Lack of Bdnf and TrkB signalling in the postnatal cochlea leads to a spatial reshaping of innervation along the tonotopic axis and hearing loss. Development 130, 4741–4750. doi: 10.1242/dev.00676
PubMed Abstract | Full Text | CrossRef Full Text | Google Scholar
Schwab, S. R., Pereira, J. P., Matloubian, M., Xu, Y., Huang, Y., and Cyster, J. G. (2005). Lymphocyte sequestration through S1P lyase inhibition and disruption of S1P gradients. Science 309, 1735–1739. doi: 10.1126/science.1113640
PubMed Abstract | Full Text | CrossRef Full Text | Google Scholar
Silos-Santiago, I., Fagan, A. M., Garber, M., Fritzsch, B., and Barbacid, M. (1997). Severe sensory deficits but normal CNS development in newborn mice lacking TrkB and TrkC tyrosine protein kinase receptors. Eur. J. Neurosci. 9, 2045–2056. doi: 10.1111/j.1460-9568.1997.tb01372.x
PubMed Abstract | Full Text | CrossRef Full Text | Google Scholar
Sim-Selley, L. J., Goforth, P. B., Mba, M. U., Macdonald, T. L., Lynch, K. R., Milstien, S., et al. (2009). Sphingosine-1-phosphate receptors mediate neuromodulatory functions in the CNS. J. Neurochem. 110, 1191–1202. doi: 10.1111/j.1471-4159.2009.06202.x
PubMed Abstract | Full Text | CrossRef Full Text | Google Scholar
Somenzi, G., Sala, G., Rossetti, S., Ren, M., Ghidoni, R., and Sacchi, N. (2007). Disruption of retinoic acid receptor alpha reveals the growth promoter face of retinoic acid. PLoS One 2:e836. doi: 10.1371/journal.pone.0000836
PubMed Abstract | Full Text | CrossRef Full Text | Google Scholar
Sorensen, S. D., Nicole, O., Peavy, R. D., Montoya, L. M., Lee, C. J., Murphy, T. J., et al. (2003). Common signaling pathways link activation of murine PAR-1, LPA and S1P receptors to proliferation of astrocytes. Mol. Pharmacol. 64, 1199–1209. doi: 10.1124/mol.64.5.1199
PubMed Abstract | Full Text | CrossRef Full Text | Google Scholar
Stiban, J., Tidhar, R., and Futerman, A. H. (2010). Ceramide synthases: roles in cell physiology and signaling. Adv. Exp. Med. Biol. 688, 60–71. doi: 10.1007/978-1-4419-6741-1_4
PubMed Abstract | Full Text | CrossRef Full Text | Google Scholar
Sugawara, M., Murtie, J. C., Stankovic, K. M., Liberman, M. C., and Corfas, G. (2007). Dynamic patterns of neurotrophin 3 expression in the postnatal mouse inner ear. J. Comp. Neurol. 501, 30–37. doi: 10.1002/cne.21227
PubMed Abstract | Full Text | CrossRef Full Text | Google Scholar
Sun, D. F., Gao, Z. H., Liu, H. P., Yuan, Y., and Qu, X. J. (2012). Sphingosine 1-phosphate antagonizes the effect of all-trans retinoic acid (ATRA) in a human colon cancer cell line by modulation of RARbeta expression. Cancer Lett. 319, 182–189. doi: 10.1016/j.canlet.2012.01.012
PubMed Abstract | Full Text | CrossRef Full Text | Google Scholar
Takabe, K., Paugh, S. W., Milstien, S., and Spiegel, S. (2008). “Inside-out” signaling of sphingosine-1-phosphate: therapeutic targets. Pharmacol. Rev. 60, 181–195. doi: 10.1124/pr.107.07113
PubMed Abstract | Full Text | CrossRef Full Text | Google Scholar
Taniguchi, M., and Okazaki, T. (2014). The role of sphingomyelin and sphingomyelin synthases in cell death, proliferation and migration-from cell and animal models to human disorders. Biochim. Biophys. Acta 1841, 692–703. doi: 10.1016/j.bbalip.2013.12.003
PubMed Abstract | Full Text | CrossRef Full Text | Google Scholar
Toman, R. E., Payne, S. G., Watterson, K. R., Maceyka, M., Lee, N. H., Milstien, S., et al. (2004). Differential transactivation of sphingosine-1-phosphate receptors modulates NGF-induced neurite extension. J. Cell Biol. 166, 381–392. doi: 10.1083/jcb.200402016
PubMed Abstract | Full Text | CrossRef Full Text | Google Scholar
Wright, T. J., and Mansour, S. L. (2003). Fgf3 and Fgf10 are required for mouse otic placode induction. Development 130, 3379–3390. doi: 10.1242/dev.00555
PubMed Abstract | Full Text | CrossRef Full Text | Google Scholar
Xia, P., and Wadham, C. (2011). Sphingosine 1-phosphate, a key mediator of the cytokine network: juxtacrine signaling. Cytokine Growth Factor Rev. 22, 45–53. doi: 10.1016/j.cytogfr.2010.09.004
PubMed Abstract | Full Text | CrossRef Full Text | Google Scholar
Xu, X., Weinstein, M., Li, C., Naski, M., Cohen, R. I., Ornitz, D. M., et al. (1998). Fibroblast growth factor receptor 2 (FGFR2)-mediated reciprocal regulation loop between FGF8 and FGF10 is essential for limb induction. Development 125, 753–765.
Yamamoto, N., Nakagawa, T., and Ito, J. (2014). Application of insulin-like growth factor-1 in the treatment of inner ear disorders. Front. Pharmacol. 5:208. doi: 10.3389/fphar.2014.00208
PubMed Abstract | Full Text | CrossRef Full Text | Google Scholar
Yoshikawa, M., Go, S., Takasaki, K., Kakazu, Y., Ohashi, M., Nagafuku, M., et al. (2009). Mice lacking ganglioside GM3 synthase exhibit complete hearing loss due to selective degeneration of the organ of Corti. Proc. Natl. Acad. Sci. U S A 106, 9483–9488. doi: 10.1073/pnas.0903279106
PubMed Abstract | Full Text | CrossRef Full Text | Google Scholar
Zelarayan, L. C., Vendrell, V., Alvarez, Y., Domínguez-Frutos, E., Theil, T., Alonso, M. T., et al. (2007). Differential requirements for FGF3, FGF8 and FGF10 during inner ear development. Dev. Biol. 308, 379–391. doi: 10.1016/j.ydbio.2007.05.033
PubMed Abstract | Full Text | CrossRef Full Text | Google Scholar
Zhang, Z. J., Saito, T., Kimura, Y., Sugimoto, C., Ohtsubo, T., and Saito, H. (2000). Disruption of mdr1a p-glycoprotein gene results in dysfunction of blood-inner ear barrier in mice. Brain Res. 852, 116–126. doi: 10.1016/s0006-8993(99)02223-4
PubMed Abstract | Full Text | CrossRef Full Text | Google Scholar
Zhang, Y. H., Vasko, M. R., and Nicol, G. D. (2006). Intracellular sphingosine 1-phosphate mediates the increased excitability produced by nerve growth factor in rat sensory neurons. J. Physiol. 575, 101–113. doi: 10.1113/jphysiol.2006.111575
PubMed Abstract | Full Text | CrossRef Full Text | Google Scholar
Keywords: sphingosine 1-phosphate, sensory hair-cells, inner ear neurogenesis, hearing loss, auditory neurons, neurotrophins, sphingolipids, growth factors
Citation: Romero-Guevara R, Cencetti F, Donati C and Bruni P (2015) Sphingosine 1-phosphate signaling pathway in inner ear biology. New therapeutic strategies for hearing loss? Front. Aging Neurosci. 7:60. doi: 10.3389/fnagi.2015.00060
Received: 23 January 2015; Accepted: 08 April 2015;
Published online: 23 April 2015.
Edited by:
Marta Magarinos, Universidad Autonoma de Madrid, SpainCopyright © 2015 Romero-Guevara, Cencetti, Donati and Bruni. This is an open-access article distributed under the terms of the Creative Commons Attribution License (CC BY). The use, distribution and reproduction in other forums is permitted, provided the original author(s) or licensor are credited and that the original publication in this journal is cited, in accordance with accepted academic practice. No use, distribution or reproduction is permitted which does not comply with these terms.
*Correspondence: Paola Bruni, Department Scienze Biomediche Sperimentali e Cliniche “Mario Serio”, University of Florence, Viale GB Morgagni 50, 50134 Firenze, Italy Tel: +39 055 2751204, Fax: +39 055 7830303cGFvbGEuYnJ1bmlAdW5pZmkuaXQ=
Disclaimer: All claims expressed in this article are solely those of the authors and do not necessarily represent those of their affiliated organizations, or those of the publisher, the editors and the reviewers. Any product that may be evaluated in this article or claim that may be made by its manufacturer is not guaranteed or endorsed by the publisher.
Research integrity at Frontiers
Learn more about the work of our research integrity team to safeguard the quality of each article we publish.