- 1The Russell H. Morgan Department of Radiology and Radiological Science, School of Medicine, Johns Hopkins University, Baltimore, MD, USA
- 2Department of Psychiatry and Behavioral Sciences, Johns Hopkins Bayview and Johns Hopkins Medicine, Baltimore, MD, USA
Alzheimer’s disease (AD) is the most common form of neurodegenerative dementia. Researchers have long been focused on the cortical pathology of AD, since the most important pathologic features are the senile plaques found in the cortex, and the neurofibrillary tangles and neuronal loss that begin in the entorhinal cortex and the hippocampus. In addition to these gray matter (GM) structures, histopathological studies indicate that the white matter (WM) is also a good target for both the early diagnosis of AD and for monitoring disease progression. The fornix is a WM bundle that constitutes a core element of the limbic circuits, and is one of the most important anatomical structures related to memory. Functional and anatomical features of the fornix have naturally captured researchers’ attention as possible diagnostic and prognostic markers of AD. Indeed, neurodegeneration of the fornix has been histologically observed in AD, and growing evidence indicates that the alterations seen in the fornix are potentially a good marker to predict future conversion from mild cognitive impairment (MCI) to AD, and even from cognitively normal individuals to AD. The degree of alteration is correlated with the degree of memory impairment, indicating the potential for the use of the fornix as a functional marker. Moreover, there have been attempts to stimulate the fornix using deep brain stimulation (DBS) to augment cognitive function in AD, and ongoing research has suggested positive effects of DBS on brain glucose metabolism in AD patients. On the other hand, disease specificity for fornix degeneration, methodologies to evaluate fornix degeneration, and the clinical significance of the fornix DBS, especially for the long-term impact on the quality of life, are mostly unknown and need to be elucidated.
Introduction
Alzheimer’s disease (AD) research has targeted beta-amyloid and tau pathologies as diagnostic or progression markers. White matter (WM) shows less pathologic evidence of beta-amyloid or tau protein; nonetheless, alterations are seen in WM as well. Among WM bundles reportedly abnormal in AD, the fornix is especially interesting because robust and consistent alterations have been demonstrated. These could predict future gray matter (GM) atrophy and conversion from mild cognitive impairment (MCI) to AD, and even from cognitively normalcy to AD. Moreover, there are ongoing efforts to impact the biologic processes of AD by stimulating the fornix using deep brain stimulation (DBS). This review focuses on the fornix as a potential alternative diagnostic and prognostic marker, as well as a potential therapeutic target in AD.
WM Alterations Seen in AD
Histopathological studies have revealed various types of WM alterations in AD. Symmetrical WM changes compatible with incomplete infarction, independent of the GM changes, have been reported. These WM changes are partly explained by co-existing small vessel disease (Brun and Englund, 1986; Englund et al., 1988). WM changes are also related to cerebral amyloid angiopathy of AD, supporting a vascular contribution to the WM alterations (Haglund and Englund, 2002). Chronic hypoxia caused by vascular alterations might induce oxidative stress that could damage axonal membranes and myelin, and the reactivity of oligodendroglia to such damage (Back et al., 2011). However, in preclinical AD, WM alterations are independent of vascular alterations (de la Monte, 1989). In addition, degeneration of the WM was more obvious than that of the GM in early stages (de la Monte, 1989), suggesting that WM alteration precedes GM pathology. Indeed, the earliest disease-related anatomical changes have been observed in the axons and dendrites of AD animal model (Gunawardena and Goldstein, 2001; Pigino et al., 2003; Stokin et al., 2005; Chevalier-Larsen and Holzbaur, 2006). Therefore, mechanisms that cause the early pathological changes, aside from vascular alterations, might exist. According to the updated amyloid cascade hypothesis (Hardy and Selkoe, 2002), one of the high upstream events is production of soluble beta-amyloid oligomers, been discovered to be toxic to WM structure and function (Lue et al., 1999; Xu et al., 2001; Lee et al., 2004). This could be a major cause of the primary WM alterations in AD (Roher et al., 2002; Chalmers et al., 2005) at least in early stages.
In addition to widespread WM alterations, structure-specific WM alterations have been observed, especially in limbic structures (Damoiseaux et al., 2009; Acosta-Cabronero et al., 2010; Smith et al., 2010; Liu et al., 2011). This might at least partly be explained by aberrant axonal transport due to misregulation of tau seen in early disease stage (Alonso et al., 1994; Ebneth et al., 1998; Dawson et al., 2010), with resultant tau aggregation that is closely related to neurodegeneration (Braak and Braak, 1995). Since the neurodegeneration of AD usually propagates systematically from the transentorhinal area to limbic structures, to neocortex at the most advanced stage, associated Wallerian-like degeneration (Bossy-Wetzel et al., 2004; Coleman, 2005) likely follows the same order, which might explain structure-specific WM alterations in AD (Bramblett et al., 1992).
Congruent with histopathological findings, WM alteration has been observed in vivo using various imaging modalities. Among these, diffusion tensor imaging (DTI) is one of the most effective modalities for the investigation of the WM anatomy since DTI has the ability to visualize WM fibers, based on directionality, and has the capability to provide detailed information about microscopic organization of the fibers. A meta-analysis of DTI studies indicated that WM alterations in AD are widespread throughout the brain (Sexton et al., 2011), particularly in limbic fibers, direct connections to the medial temporal lobe and the most vulnerable WM structures (Rose et al., 2000; Kantarci et al., 2001; Medina et al., 2006; Ringman et al., 2007; Stahl et al., 2007; Zhang et al., 2007; Zhou et al., 2008; Damoiseaux et al., 2009; Mielke et al., 2009; Salat et al., 2010). WM damage quantified by DTI has been correlated with atrophy in anatomically connected GM areas in AD patients, but the correlation was not robust in patients with amnestic MCI (Agosta et al., 2011). These findings support the histopathological observation that primary WM alterations may precede both GM degeneration, as well as secondary degenerative processes of the WM after neuronal loss. Based on the evidence about WM pathology in AD, Sachdev et al. proposed incorporating WM alterations into the model (Jack et al., 2010) of the pathophysiological cascade in AD (Sachdev et al., 2013).
Why is the Fornix Important?
The fornices are WM bundles that originate from the bilateral hippocampi, merge at the midline of the brain, again divide into the left and right side, then into precommissural and postcommissural fibers, terminating at the septal nuclei, nucleus accumbens (precommissural fornix), and hypothalamus (postcommissural fornix). The fornix constitutes a core element of the limbic circuit that is vulnerable in AD, and one of the most important anatomical structures related to episodic memory, impairment of which is an initial symptom of AD (Hopper and Vogel, 1976; Mehraein and Rothemund, 1976). The fornix is also related to the cholinergic dysfunction characteristic of AD (Milner and Amaral, 1984; Wenk et al., 1987; Ransmayr et al., 1989; Sara, 1989; Schegg et al., 1989; Bunce et al., 2003; Colom et al., 2010). These functional and anatomical features have naturally captured researcher attention seeking diagnostic and prognostic markers of AD. Indeed, alterations of the fornix, such as demyelination or axonal loss, were reported as early as in 1976 (Hopper and Vogel, 1976) and have been consistently identified in AD (Ringman et al., 2007; Teipel et al., 2007; Mielke et al., 2009; Acosta-Cabronero et al., 2010; Salat et al., 2010; Liu et al., 2011; Douaud et al., 2013).
The other important anatomical feature of the fornix, compared to other WM areas, is its location. The body of the fornix is readily identifiable in the mid-sagittal plane of a brain MRI, without other WM structures adjacent to it. For research using brain MRI, this feature is particularly important. The thickness of the fornix can be easily measured on structural MRI, using T1- and T2-weighted images. Generally, identification of the boundaries of WM structures on structural MRI is not easy because the boundaries between adjacent WM structures are often invisible: the fornix is one of several exceptions. DTI is a good choice with which to identify the boundaries of WM bundles. Although a drawback of DTI is susceptibility to B0 distortion, especially for areas close to air cavities (e.g., nasal and oral cavities, frontal, ethmoid, sphenoid, and maxillary sinuses, and mastoid antrum), the fornix is less affected because there is a sufficient distance from the fornix to these air cavities. The anatomical location is also attractive from a neurosurgical point of view, since the fornix is surgically accessible for implanting electrodes for DBS (see Section Treatment), while other limbic structures are not easily accessible.
Imaging Study of the Fornix in AD
Region-of-Interest Studies
Atrophy of the fornix measured using T1-weighted MRI has often been reported in AD (Callen et al., 2001; Copenhaver et al., 2006), although the onset of this atrophy has been a subject of debate. Fletcher et al. (2013) reported that atrophy of the fornix is a strong predictor of conversion from normal cognition to MCI or AD, suggesting that atrophy is present in the fornix at an early disease stage, before clinical manifestations. Abnormalities in the fornix have also been identified using DTI. A reduction of fractional anisotropy (FA) and an increase in diffusivity in the fornix is a robust and consistent finding in AD as demonstrated with manual ROI- analysis (Ringman et al., 2007; Mielke et al., 2009; Oishi et al., 2012), and the extent of the changes in DTI-derived parameters have been correlated with cognitive decline (Mielke et al., 2009; Oishi et al., 2012). Notably, FA reduction was already present in an asymptomatic gene carrier of familial AD who went on to develop AD in the future (Ringman et al., 2007). Longitudinal observation of cognitively normal elderly or individuals with amnestic MCI indicated that reduced fornix FA predicts conversion from normal cognition to amnestic MCI and from amnestic MCI to AD (Oishi et al., 2012). Moreover, lower fornix FA predicted later cognitive decline and hippocampal atrophy (Mielke et al., 2012). These studies suggest that volume loss and FA reduction of the fornix is one of the earliest anatomical changes in AD that happens before clinical manifestations.
Whole-Brain Studies
While region-of-interest analysis is a good choice when there are specific hypotheses (e.g., the fornix is affected in AD), the drawback is the hypothesis dependency. To evaluate the spatial specificity of these findings, whole-brain analysis is a good choice. Voxel-based analysis is one of the most widely used approaches for whole-brain analysis, and has been used to evaluate regional abnormalities in WM volume (Li et al., 2008; Balthazar et al., 2009; Guo et al., 2010; Serra et al., 2010; Yoon et al., 2011) or in DTI-derived parameters, such as anisotropy and diffusivity (Head et al., 2004; Medina et al., 2006; Xie et al., 2006; Teipel et al., 2007; Zhang et al., 2009). Although cross-sectional group comparisons have identified widespread WM abnormalities in AD, the above studies failed to identify reduced volume or FA in the fornix, except for a study that used multivariate analysis (Teipel et al., 2007). The main explanation is probably inaccuracy in image normalization: the accuracy of automated non-linear registration used in these studies was limited for thin structures like the fornix, in which only a few pixels of misregistration cause significant loss of sensitivity to detect differences between groups (Oishi et al., 2009). A common approach to solve this problem is to apply Tract-Based Spatial Statistics (TBSS; Smith et al., 2006), in which WM tracts are “skeletonized” to summarize the anatomy of WM structures, from which statistics are calculated. Since the scalar values perpendicular to the skeleton are projected onto the skeleton, the effect of WM misregistration is largely ameliorated. Most of the whole brain TBSS studies applied to AD have, indeed, identified an FA reduction in the fornix, as well as in other limbic fibers, even in early-symptomatic patients or in individuals at high risk for developing AD (Damoiseaux et al., 2009; Honea et al., 2009; Stricker et al., 2009; Zarei et al., 2009; Acosta-Cabronero et al., 2010; Bosch et al., 2010; Smith et al., 2010; Liu et al., 2011; Douaud et al., 2013).
Application of large deformation diffeomorphic metric mapping (LDDMM) to DTI is another way to increase the sensitivity of whole-brain analysis, by which registration accuracy of the WM bundles could be increased. By using LDDMM, significant volume loss, FA reduction, and increases in mean diffusivity (MD) and radial diffusivity were detected in the fornix of individuals with AD (Oishi et al., 2011a,b). Figure 1 is an example of such an analysis, designed to find brain areas with AD-specific WM alterations. This analysis indicates significant FA reduction in the fornix, the splenium of the corpus callosum, as well as in several small areas in superficial WM in the frontal lobes. To further increase sensitivity, multivariate models (Ashburner and Kloppel, 2011), such as principal component analysis (PCA; Teipel et al., 2007), or to apply voxel-grouping methods, such as atlas-based analysis (ABA; Oishi et al., 2009), were applied: all of these detected abnormalities in the fornix in AD. These whole-brain studies using sophisticated approaches consistently indicate the fornix as the “hotspot”, in which the earliest anatomical changes begin (“Fornix First” (Landhuis, 2013)), and where the most robust changes are seen in AD.
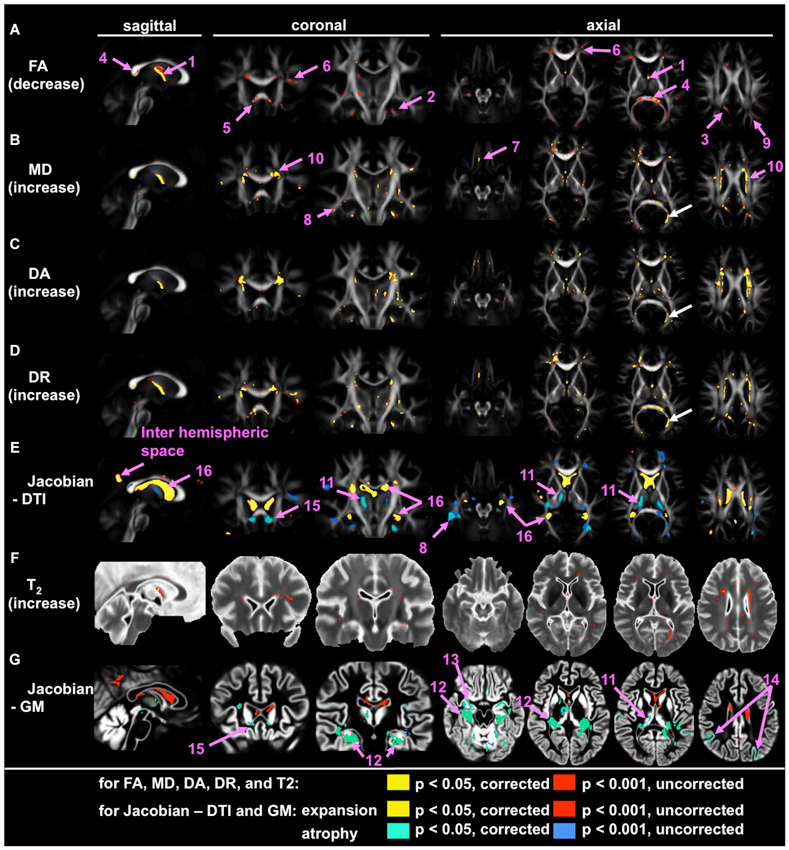
Figure 1. Voxel-based group comparison between 19 AD and 22 cognitively normal age-matched control participants (NC), indicating the fornix as the hotspot. Areas with signal or volume alterations in AD compared to NC are shown as colored maps, overlaid on an averaged FA map (A–E), an averaged T2 map (F), and an averaged GM segmentation map (G). (A) Areas with reduced FA. (B) Areas with increased MD. (C) Areas with increased λ‖. (D) Areas with increased λ⊥. (E) Areas with an increased and decreased Jacobian, which was calculated from a transformation matrix obtained from the normalization of DTI. (F) Area with increased T2. (G) Areas with increased and decreased Jacobian, which were calculated from a transformation matrix obtained from the normalization of a GM segmentation map. Pink arrows with numbers indicate: 1 = the fornix; 2 = the cingulum; 3 = the posterior cingulate gyrus white matter; 4 = splenium of the corpus callosum; 5 = genu of the corpus callosum; 6 = the prefrontal white matter; 7 = the orbitofrontal white matter; the temporal white matter; 9 = the parietal white matter; 10 = the periventricular area; 11 = the thalamus; 12 = the hippocampus; 13 = the entorhinal area; 14 = the parietal cortex; 15 = the area between the caudate head and the gyrus rectus; 16 = the lateral ventricle. White arrows show the misregistration seen in the left posterior horn of the lateral ventricle (From Oishi et al., 2011a; with permission).
Pitfalls and Issues in Fornix Image Analysis
Misregistration
As mentioned previously, TBSS is currently a standard method by which to ameliorate WM tract misregistration. However, in patients with ventricular enlargement, like AD, the fornix is strongly deformed by the elevated and stretched corpus callosum, which results in uncorrectable misregistration, even with TBSS (Hattori et al., 2012). Therefore, careful interpretation is needed for AD studies. An inverse-projection of TBSS results onto original images is a simple method with which to identify invalid results caused by misregistration.
One solution to reduce the impact of erroneous mapping of voxels, and the consequent mis-parcellation of target structures, is to adopt multi-atlas approaches. Rather than using a single atlas for anatomical labeling, this approach uses multiple atlases to compute the likelihoods of labels, which are then fused to create an anatomical parcellation map for each image. This approach, which accounts for variations in brain anatomy, has been adapted to parcellate WM structures in DTI (Tang et al., 2014) with high accuracy even for individuals with ventricular enlargement. Applicability of the multi-atlas label fusion method to accurately define the fornix is a promising approach, which needs to be developed in the future with automated image analysis of AD.
Contamination of signal from cerebrospinal fluid
The fornix is prone to partial volume effects caused by contamination of signal from the cerebrospinal fluid (CSF), since it is a thin bundle that runs through the lateral ventricles that are filled with CSF. The effect is especially seen in individuals with AD because of atrophy, and thus, increased CSF signal in each voxel (Pfefferbaum and Sullivan, 2003; Metzler-Baddeley et al., 2012; Berlot et al., 2014). The greater diffusivity in CSF relative to the WM structures leads to false elevation of diffusivity values and a false reduction in FA values (Alexander et al., 2001; Vos et al., 2011). Therefore, reduced FA or increased diffusivity identified in the DTI studies of AD probably reflects pathological alterations of the fornix itself plus the partial volume effects caused by atrophy. Although this dual effect (atrophy + microstructural change) on the DTI parameters might make DTI a sensitive tool with which to detect early anatomical changes related to AD, it complicates inferences about the underlying anatomical changes. There have been several attempts to ameliorate the effects of CSF contamination in order to draw inferences about the microstructural changes in the WM structures, such as CSF-suppressed diffusion imaging (Kwong et al., 1991), or the free water elimination and mapping method that is applicable at the post-acquisition stage (Pasternak et al., 2009), both of which could detect alteration of the fornix in AD (Kantarci et al., 2010; Fletcher et al., 2014). Investigations of underlying changes in WM structures seem to suggest a good indication for the use these advanced methods (Metzler-Baddeley et al., 2012).
Cause of fornix degeneration and DTI parameters of choice
Since the contribution of each factor (see WM alterations seen in AD) to the degeneration of the fornix might vary depending on the degree of disease progression, longitudinal observation of the histopathology, from the preclinical to the advanced stages, is especially important. Use of the AD model in animals is an option for such longitudinal observation. A reduced size of the fornix is seen in the PDAPP mice (Gonzalez-Lima et al., 2001) and transgenic APP/PS1 mice (Delatour et al., 2006), and immunohistochemistry shows the presence of increasing amyloidosis and related microgliosis and astrogliosis (Maheswaran et al., 2009). However, longitudinal changes in the size of the fornix differ depending on the type of AD model mice. Since type and variations in gene mutation are relevant to the pathophysiology of AD model animals, and considerable differences are seen between human AD and AD model animals, human in vivo neuroimaging studies are important to understand the pathophysiological underpinnings. For early AD, diffusion measures (MD and axial and radial diffusivities) could detect the widespread pathology of AD more sensitively than FA (Acosta-Cabronero et al., 2010; Oishi et al., 2011a). However, for the fornix, there seems to be a tendency that a decrease in FA is more sensitive than an increase in diffusivity measures. Whether there is a difference in pathophysiology between the fornix and other WM areas remains to be elucidated.
Clinical Applications of the Findings Seen in the Fornix
Diagnosis and Prediction
There are several hurdles when applying research findings to clinical practice. Research studies are based on selected participants with strict inclusion and exclusion criteria, and usually age- and gender-matched participants are recruited as controls. However, routine image-based diagnosis is almost exclusively performed by qualitative visual inspection, which is applied to each individual for clinical decision-making. Although numerous publications link AD to degeneration in the fornix, volume or the diffusivity are not quantified in daily practice because of the time-consuming nature of this process, and the inconsistent quantification methods used in research. The fornix sign, a discoloration of the fornix observed on color-coded FA maps of AD (Oishi et al., 2012), is one attempt to define qualitative image signs applicable to clinical images (Figure 2). Although sensitivity was limited, specificity was high. The positive likelihood ratio differentiating AD from controls and hence the ability to predict conversion of cognitively normal individuals to amnestic MCI, or from amnestic MCI to AD, was strong. However, further efforts are needed to identify appropriate scan parameters, cut-off values for the diagnosis and prediction, as well as to investigate degeneration in the fornix seen in other types of dementia.
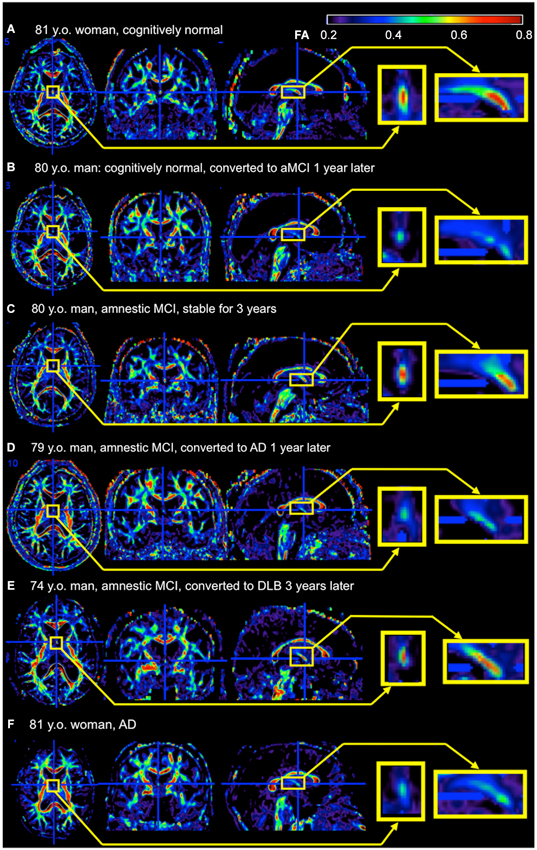
Figure 2. Example of the fornix sign. The axial (left), coronal (middle), and sagittal (right) slices of the color-scaled FA map are shown with the magnified view of the fornix (yellow rectangle). (A) A cognitively normal 81-year-old woman without the fornix sign. The core part of the fornix appears yellow to red (FA 0.5–0.8). (B) A cognitively normal 80-year-old man with the fornix sign. The fornix appears green (FA < 0.5). He converted to amnestic MCI (aMCI) 1 year after the scan. (C) An 80-year-old man with aMCI without the fornix sign. He was stable during 3 years observation period after the scan. (D) A 79-year-old man with aMCI with the fornix sign. He converted to AD 1 year after the scan. (E) A 74-year-old man with aMCI without the fornix sign. He converted to dementia with Lewy bodies 3 years after the scan. (F) An 81-year-old woman with AD with the fornix sign. FA, fractional anisotropy.
Given that individuals with different types of memory complaints visit clinics, and physicians need to identify the etiology of the memory complaint for medical decision-making, the value of imaging signs that can separate AD from individuals with normal cognition are limited. Rather, clinically relevant imaging signs must provide clues that can be used to separate AD from other diseases that cause memory impairment. Indeed, the fornix might be affected by tumors, infections, inflammations (Yamamoto et al., 1990), metabolic abnormalities, vascular diseases (Molino et al., 2014), malformations, trauma, and hydrocephalus (Hattori et al., 2012) (for review, Thomas et al., 2011). Psychiatric diseases, such as schizophrenia and bipolar affective disorder (Oertel-Knöchel et al., 2014), or medical conditions, such as heart failure (Wu et al., 2007), also cause damage in the fornix. Less is known about the involvement of the fornix in other neurodegenerative dementias, such as frontotemporal dementia (FTD), dementia with Lewy bodies (DLB), and corticobasal degeneration (CBD). A study indicated substantial FA reduction in the fornix of the behavioral variant FTD that correlated with memory decline (Hornberger et al., 2012), and other studies have indicated that the fornix is affected in Pick’s disease, FTD with tau mutation, or TAR-DNA-binding protein-43 type C pathology, but not for FTD caused by a progranulin mutation or with fused-in-sarcoma protein accumulation (Rohrer et al., 2011; Hornberger et al., 2012). Changes in the fornix were not detected by group comparison between DLB and control groups (Kantarci et al., 2010), or between CBD and control groups (Rohrer et al., 2011), but other research has reported reduced FA in the fornix of some DLB patients, especially for those with hippocampal atrophy (Firbank et al., 2011). The appropriate clinical use of imaging modalities for the evaluation of the fornix is, therefore, an important future direction.
Treatment
Inspired by neuroimaging evidence about early impairment of the limbic circuit seen in AD, and an unexpected augmentation of recollection during the hypothalamic/fornix DBS performed on a patient with obesity (Hamani et al., 2008), DBS of the fornix has been proposed as a novel therapy for AD to improve the neuronal circuitry involved in memory (Laxton et al., 2010). Based on the hypothesis that an important aspect of AD is that it is a system-level disorder affecting several integrated pathways related to memory and cognition (Lyketsos et al., 2012), and supported by rodent studies indicating that DBS improved memory and produced hippocampal neurogenesis (Toda et al., 2008; Hamani et al., 2011), a clinical trial evaluating the effect of DBS targeting the fornix is ongoing. Electrodes are placed adjacent to the fornix for high frequency electronic stimulation, with the hope of an immediate effect (direct effects of stimulating the fornix) and a disease-modifying effect (neurogenesis). Although its number of participants was small, the earlier Phase 1 trial suggested improved glucose metabolism in the posterior cortical areas, especially in cognitively improved participants after fornix DBS (Laxton et al., 2010; Smith et al., 2012). The assessment of long-term impact on cognitive function, activities of daily living, quality of life, and mortality is an important future direction.
Conflict of Interest Statement
Constantine G. Lyketsos has received support from the following organizations: Associated Jewish Federation of Baltimore, Weinberg Foundation, Forest, Glaxo-Smith-Kline, Eisai, Pfizer, Astra-Zeneca, Lilly, Ortho-McNeil, Bristol-Myers, and Novartis. Constantine G. Lyketsos has served as a consultant/advisor for Astra-Zeneca, Glaxo-Smith-Kline, Eisai, Novartis, Forest, Supernus, Adlyfe, Takeda, Wyeth, Lundbeck, Merz, Lilly, and Genentech. Constantine G. Lyketsos has received honorarium or travel support from Pfizer, Forest, Glaxo-Smith-Kline, and Health Monitor. This arrangement has been approved by the Johns Hopkins University in accordance with its conflict of interest policies.
Acknowledgments
The authors thank Ms. Mary McAllister for help with manuscript editing. This study was supported by NIH grants, R01AG031348, R01AG042165 (Constantine G. Lyketsos), R21AG033774, P50AG005146, and R01HD065955 (Kenichi Oishi). Its contents are solely the responsibility of the authors and do not necessarily represent the official view of NIA, NICHD, or NIH.
References
Acosta-Cabronero, J., Williams, G. B., Pengas, G., and Nestor, P. J. (2010). Absolute diffusivities define the landscape of white matter degeneration in Alzheimer’s disease. Brain 133, 529–539. doi: 10.1093/brain/awp257
Agosta, F., Pievani, M., Sala, S., Geroldi, C., Galluzzi, S., Frisoni, G. B., et al. (2011). White matter damage in Alzheimer disease and its relationship to gray matter atrophy. Radiology 258, 853–863. doi: 10.1148/radiol.10101284
Alexander, A. L., Hasan, K. M., Lazar, M., Tsuruda, J. S., and Parker, D. L. (2001). Analysis of partial volume effects in diffusion-tensor MRI. Magn. Reson. Med. 45, 770–780. doi: 10.1002/mrm.1105
Alonso, A. C., Zaidi, T., Grundke-Iqbal, I., and Iqbal, K. (1994). Role of abnormally phosphorylated tau in the breakdown of microtubules in Alzheimer disease. Proc. Natl. Acad. Sci. U S A 91, 5562–5566. doi: 10.1016/0197-4580(94)92603-4
Ashburner, J., and Kloppel, S. (2011). Multivariate models of inter-subject anatomical variability. Neuroimage. 56, 422–439. doi: 10.1016/j.neuroimage.2010.03.059
Back, S. A., Kroenke, C. D., Sherman, L. S., Lawrence, G., Gong, X., Taber, E. N., et al. (2011). White matter lesions defined by diffusion tensor imaging in older adults. Ann. Neurol. 70, 465–476. doi: 10.1002/ana.22484
Balthazar, M. L., Yasuda, C. L., Pereira, F. R., Pedro, T., Damasceno, B. P., and Cendes, F. (2009). Differences in grey and white matter atrophy in amnestic mild cognitive impairment and mild Alzheimer’s disease. Eur. J. Neurol. 16, 468–474. doi: 10.1111/j.1468-1331.2008.02408.x
Berlot, R., Metzler-Baddeley, C., Jones, D. K., and O’sullivan, M. J. (2014). CSF contamination contributes to apparent microstructural alterations in mild cognitive impairment. Neuroimage 92, 27–35. doi: 10.1016/j.neuroimage.2014.01.031
Bosch, B., Arenaza-Urquijo, E. M., Rami, L., Sala-Llonch, R., Junqué, C., Solé-Padullés, C., et al. (2010). Multiple DTI index analysis in normal aging, amnestic MCI and AD. Relationship with neuropsychological performance. Neurobiol. Aging 33, 61–74. doi: 10.1016/j.neurobiolaging.2010.02.004
Bossy-Wetzel, E., Schwarzenbacher, R., and Lipton, S. A. (2004). Molecular pathways to neurodegeneration. Nat. Med. 10(Suppl.), S2–S9. doi: 10.1038/nm1067
Braak, H., and Braak, E. (1995). Staging of Alzheimer’s disease-related neurofibrillary changes. Neurobiol. Aging 16, 271–278; discussion 278–284. doi: 10.1016/0197-4580(95)00021-6
Bramblett, G. T., Trojanowski, J. Q., and Lee, V. M. (1992). Regions with abundant neurofibrillary pathology in human brain exhibit a selective reduction in levels of binding-competent tau and accumulation of abnormal tau-isoforms (A68 proteins). Lab. Invest. 66, 212–222.
Brun, A., and Englund, E. (1986). A white matter disorder in dementia of the Alzheimer type: a pathoanatomical study. Ann. Neurol. 19, 253–262. doi: 10.1002/ana.410190306
Bunce, J. G., Sabolek, H. R., and Chrobak, J. J. (2003). Intraseptal infusion of oxotremorine impairs memory in a delayed-non-match-to-sample radial maze task. Neuroscience 121, 259–267. doi: 10.1016/s0306-4522(03)00462-7
Callen, D. J., Black, S. E., Gao, F., Caldwell, C. B., and Szalai, J. P. (2001). Beyond the hippocampus: MRI volumetry confirms widespread limbic atrophy in AD. Neurology 57, 1669–1674. doi: 10.1212/wnl.57.9.1669
Chalmers, K., Wilcock, G., and Love, S. (2005). Contributors to white matter damage in the frontal lobe in Alzheimer’s disease. Neuropathol. Appl. Neurobiol. 31, 623–631. doi: 10.1111/j.1365-2990.2005.00678.x
Chevalier-Larsen, E., and Holzbaur, E. L. (2006). Axonal transport and neurodegenerative disease. Biochim. Biophys. Acta 1762, 1094–1108. doi: 10.1016/j.bbadis.2006.04.002
Coleman, M. (2005). Axon degeneration mechanisms: commonality amid diversity. Nat. Rev. Neurosci. 6, 889–898. doi: 10.1038/nrn1788
Colom, L. V., Castañeda, M. T., Bañuelos, C., Puras, G., García-Hernández, A., Hernandez, S., et al. (2010). Medial septal beta-amyloid 1-40 injections alter septo-hippocampal anatomy and function. Neurobiol. Aging 31, 46–57. doi: 10.1016/j.neurobiolaging.2008.05.006
Copenhaver, B. R., Rabin, L. A., Saykin, A. J., Roth, R. M., Wishart, H. A., Flashman, L. A., et al. (2006). The fornix and mammillary bodies in older adults with Alzheimer’s disease, mild cognitive impairment and cognitive complaints: a volumetric MRI study. Psychiatry Res. 147, 93–103. doi: 10.1016/j.pscychresns.2006.01.015
Damoiseaux, J. S., Smith, S. M., Witter, M. P., Sanz-Arigita, E. J., Barkhof, F., Scheltens, P., et al. (2009). White matter tract integrity in aging and Alzheimer’s disease. Hum. Brain Mapp. 30, 1051–1059. doi: 10.1002/hbm.20563
Dawson, H. N., Cantillana, V., Jansen, M., Wang, H., Vitek, M. P., Wilcock, D. M., et al. (2010). Loss of tau elicits axonal degeneration in a mouse model of Alzheimer’s disease. Neuroscience 169, 516–531. doi: 10.1016/j.neuroscience.2010.04.037
de la Monte, S. M. (1989). Quantitation of cerebral atrophy in preclinical and end-stage Alzheimer’s disease. Ann. Neurol. 25, 450–459. doi: 10.1002/ana.410250506
Delatour, B., Guégan, M., Volk, A., and Dhenain, M. (2006). In vivo MRI and histological evaluation of brain atrophy in APP/PS1 transgenic mice. Neurobiol. Aging 27, 835–847. doi: 10.1016/j.neurobiolaging.2005.04.011
Douaud, G., Menke, R. A., Gass, A., Monsch, A. U., Rao, A., Whitcher, B., et al. (2013). Brain microstructure reveals early abnormalities more than two years prior to clinical progression from mild cognitive impairment to Alzheimer’s disease. J. Neurosci. 33, 2147–2155. doi: 10.1523/jneurosci.4437-12.2013
Ebneth, A., Godemann, R., Stamer, K., Illenberger, S., Trinczek, B., and Mandelkow, E. (1998). Overexpression of tau protein inhibits kinesin-dependent trafficking of vesicles, mitochondria and endoplasmic reticulum: implications for Alzheimer’s disease. J. Cell Biol. 143, 777–794. doi: 10.1083/jcb.143.3.777
Englund, E., Brun, A., and Alling, C. (1988). White matter changes in dementia of Alzheimer’s type. Biochemical and neuropathological correlates. Brain 111(Pt. 6), 1425–1439. doi: 10.1093/brain/111.6.1425
Firbank, M. J., Blamire, A. M., Teodorczuk, A., Teper, E., Mitra, D., and O’brien, J. T. (2011). Diffusion tensor imaging in Alzheimer’s disease and dementia with Lewy bodies. Psychiatry Res. 194, 176–183. doi: 10.1016/j.pscychresns.2011.08.002
Fletcher, E., Carmichael, O., Pasternak, O., Maier-Hein, K. H., and Decarli, C. (2014). Early brain loss in circuits affected by Alzheimer’s disease is predicted by fornix microstructure but may be independent of gray matter. Front. Aging Neurosci. 6:106. doi: 10.3389/fnagi.2014.00106
Fletcher, E., Raman, M., Huebner, P., Liu, A., Mungas, D., Carmichael, O., et al. (2013). Loss of fornix white matter volume as a predictor of cognitive impairment in cognitively normal elderly individuals. JAMA Neurol. 70, 1389–1395. doi: 10.1001/jamaneurol.2013.3263
Gonzalez-Lima, F., Berndt, J. D., Valla, J. E., Games, D., and Reiman, E. M. (2001). Reduced corpus callosum, fornix and hippocampus in PDAPP transgenic mouse model of Alzheimer’s disease. Neuroreport 12, 2375–2379. doi: 10.1097/00001756-200108080-00018
Gunawardena, S., and Goldstein, L. S. (2001). Disruption of axonal transport and neuronal viability by amyloid precursor protein mutations in Drosophila. Neuron 32, 389–401. doi: 10.1016/s0896-6273(01)00496-2
Guo, X., Wang, Z., Li, K., Li, Z., Qi, Z., Jin, Z., et al. (2010). Voxel-based assessment of gray and white matter volumes in Alzheimer’s disease. Neurosci. Lett. 468, 146–150. doi: 10.1016/j.neulet.2009.10.086
Haglund, M., and Englund, E. (2002). Cerebral amyloid angiopathy, white matter lesions and Alzheimer encephalopathy - a histopathological assessment. Dement. Geriatr. Cogn. Disord. 14, 161–166. doi: 10.1159/000063606
Hamani, C., Mcandrews, M. P., Cohn, M., Oh, M., Zumsteg, D., Shapiro, C. M., et al. (2008). Memory enhancement induced by hypothalamic/fornix deep brain stimulation. Ann. Neurol. 63, 119–123. doi: 10.1002/ana.21295
Hamani, C., Stone, S. S., Garten, A., Lozano, A. M., and Winocur, G. (2011). Memory rescue and enhanced neurogenesis following electrical stimulation of the anterior thalamus in rats treated with corticosterone. Exp. Neurol. 232, 100–104. doi: 10.1016/j.expneurol.2011.08.023
Hardy, J., and Selkoe, D. J. (2002). The amyloid hypothesis of Alzheimer’s disease: progress and problems on the road to therapeutics. Science 297, 353–356. doi: 10.1126/science.1072994
Hattori, T., Ito, K., Aoki, S., Yuasa, T., Sato, R., Ishikawa, M., et al. (2012). White matter alteration in idiopathic normal pressure hydrocephalus: tract-based spatial statistics study. AJNR Am. J. Neuroradiol. 33, 97–103. doi: 10.3174/ajnr.a2706
Head, D., Buckner, R. L., Shimony, J. S., Williams, L. E., Akbudak, E., Conturo, T. E., et al. (2004). Differential vulnerability of anterior white matter in nondemented aging with minimal acceleration in dementia of the Alzheimer type: evidence from diffusion tensor imaging. Cereb. Cortex 14, 410–423. doi: 10.1093/cercor/bhh003
Honea, R. A., Vidoni, E., Harsha, A., and Burns, J. M. (2009). Impact of APOE on the healthy aging brain: a voxel-based MRI and DTI study. J. Alzheimers Dis. 18, 553–564. doi: 10.3233/JAD-2009-1163
Hopper, M. W., and Vogel, F. S. (1976). The limbic system in Alzheimer’s disease. A neuropathologic investigation. Am. J. Pathol. 85, 1–20.
Hornberger, M., Wong, S., Tan, R., Irish, M., Piguet, O., Kril, J., et al. (2012). In vivo and post-mortem memory circuit integrity in frontotemporal dementia and Alzheimer’s disease. Brain 135, 3015–3025. doi: 10.1093/brain/aws239
Jack, C. R. Jr., Knopman, D. S., Jagust, W. J., Shaw, L. M., Aisen, P. S., Weiner, M. W., et al. (2010). Hypothetical model of dynamic biomarkers of the Alzheimer’s pathological cascade. Lancet Neurol. 9, 119–128. doi: 10.1016/s1474-4422(09)70299-6
Kantarci, K., Avula, R., Senjem, M. L., Samikoglu, A. R., Zhang, B., Weigand, S. D., et al. (2010). Dementia with Lewy bodies and Alzheimer disease: neurodegenerative patterns characterized by DTI. Neurology 74, 1814–1821. doi: 10.1212/wnl.0b013e3181e0f7cf
Kantarci, K., Jack, C. R. Jr., Xu, Y. C., Campeau, N. G., O’brien, P. C., Smith, G. E., et al. (2001). Mild cognitive impairment and Alzheimer disease: regional diffusivity of water. Radiology 219, 101–107. doi: 10.1148/radiology.219.1.r01ap14101
Kwong, K. K., Mckinstry, R. C., Chien, D., Crawley, A. P., Pearlman, J. D., and Rosen, B. R. (1991). CSF-suppressed quantitative single-shot diffusion imaging. Magn. Reson. Med. 21, 157–163. doi: 10.1002/mrm.1910210120
Landhuis, E. (2013). Fornix First—Atrophy Foreshadows Decline in Normal Aging? Available online at: http://www.alzforum.org/news/research-news/fornix-first-atrophy-foreshadows-decline-normal-aging. Accessed on June 19 2014.
Laxton, A. W., Tang-Wai, D. F., Mcandrews, M. P., Zumsteg, D., Wennberg, R., Keren, R., et al. (2010). A phase I trial of deep brain stimulation of memory circuits in Alzheimer’s disease. Ann. Neurol. 68, 521–534. doi: 10.1002/ana.22089
Lee, J. T., Xu, J., Lee, J. M., Ku, G., Han, X., Yang, D. I., et al. (2004). Amyloid-beta peptide induces oligodendrocyte death by activating the neutral sphingomyelinase-ceramide pathway. J. Cell Biol. 164, 123–131. doi: 10.1083/jcb.200307017
Li, S., Pu, F., Shi, F., Xie, S., Wang, Y., and Jiang, T. (2008). Regional white matter decreases in Alzheimer’s disease using optimized voxel-based morphometry. Acta Radiol. 49, 84–90. doi: 10.1080/02841850701627181
Liu, Y., Spulber, G., Lehtimäki, K. K., Könönen, M., Hallikainen, I., Gröhn, H., et al. (2011). Diffusion tensor imaging and tract-based spatial statistics in Alzheimer’s disease and mild cognitive impairment. Neurobiol. Aging 32, 1558–1571. doi: 10.1016/j.neurobiolaging.2009.10.006
Lue, L. F., Kuo, Y. M., Roher, A. E., Brachova, L., Shen, Y., Sue, L., et al. (1999). Soluble amyloid beta peptide concentration as a predictor of synaptic change in Alzheimer’s disease. Am. J. Pathol. 155, 853–862. doi: 10.1016/s0002-9440(10)65184-x
Lyketsos, C. G., Targum, S. D., Pendergrass, J. C., and Lozano, A. M. (2012). Deep brain stimulation: a novel strategy for treating Alzheimer’s disease. Innov. Clin. Neurosci. 9, 10–17.
Maheswaran, S., Barjat, H., Rueckert, D., Bate, S. T., Howlett, D. R., Tilling, L., et al. (2009). Longitudinal regional brain volume changes quantified in normal aging and Alzheimer’s APP x PS1 mice using MRI. Brain Res. 1270, 19–32. doi: 10.1016/j.brainres.2009.02.045
Medina, D., Detoledo-Morrell, L., Urresta, F., Gabrieli, J. D., Moseley, M., Fleischman, D., et al. (2006). White matter changes in mild cognitive impairment and AD: a diffusion tensor imaging study. Neurobiol. Aging 27, 663–672. doi: 10.1016/j.neurobiolaging.2005.03.026
Mehraein, P., and Rothemund, E. (1976). [Neuroanatomical correlates of the amnestic syndrome (author’s transl)]. Arch. Psychiatr. Nervenkr. 222, 153–176.
Metzler-Baddeley, C., O’sullivan, M. J., Bells, S., Pasternak, O., and Jones, D. K. (2012). How and how not to correct for CSF-contamination in diffusion MRI. Neuroimage 59, 1394–1403. doi: 10.1016/j.neuroimage.2011.08.043
Mielke, M. M., Kozauer, N. A., Chan, K. C., George, M., Toroney, J., Zerrate, M., et al. (2009). Regionally-specific diffusion tensor imaging in mild cognitive impairment and Alzheimer’s disease. Neuroimage 46, 47–55. doi: 10.1016/j.neuroimage.2009.01.054
Mielke, M. M., Okonkwo, O. C., Oishi, K., Mori, S., Tighe, S., Miller, M. I., et al. (2012). Fornix integrity and hippocampal volume predict memory decline and progression to Alzheimer’s disease. Alzheimers Dement. 8, 105–113. doi: 10.1016/j.jalz.2011.05.2416
Milner, T. A., and Amaral, D. G. (1984). Evidence for a ventral septal projection to the hippocampal formation of the rat. Exp. Brain Res. 55, 579–585. doi: 10.1007/bf00235290
Molino, I., Cavaliere, C., Salvatore, E., Quarantelli, M., Colucci, L., and Fasanaro, A. M. (2014). Is anterior communicating artery syndrome related to fornix lesions? J. Alzheimers Dis. 42, S199–S204. doi: 10.3233/JAD-132648
Oertel-Knöchel, V., Reinke, B., Alves, G., Jurcoane, A., Wenzler, S., Prvulovic, D., et al. (2014). Frontal white matter alterations are associated with executive cognitive function in euthymic bipolar patients. J. Affect. Disord. 155, 223–233. doi: 10.1016/j.jad.2013.11.004
Oishi, K., Akhter, K., Mielke, M., Ceritoglu, C., Zhang, J., Jiang, H., et al. (2011a). Multi-modal MRI analysis with disease-specific spatial filtering: initial testing to predict mild cognitive impairment patients who convert to Alzheimer’s disease. Front. Neurol. 2:54. doi: 10.3389/fneur.2011.00054
Oishi, K., Faria, A., Jiang, H., Li, X., Akhter, K., Zhang, J., et al. (2009). Atlas-based whole brain white matter analysis using large deformation diffeomorphic metric mapping: application to normal elderly and Alzheimer’s disease participantstlas. Neuroimage 46, 486–499. doi: 10.1016/j.neuroimage.2009.01.002
Oishi, K., Mielke, M. M., Albert, M., Lyketsos, C. G., and Mori, S. (2011b). DTI analyses and clinical applications in Alzheimer’s disease. J. Alzheimers Dis. 26(Suppl. 3), 287–296. doi: 10.3233/JAD-2011-0007
Oishi, K., Mielke, M. M., Albert, M., Lyketsos, C. G., and Mori, S. (2012). The fornix sign: a potential sign for Alzheimer’s disease based on diffusion tensor imaging. J. Neuroimaging 22, 365–374. doi: 10.1111/j.1552-6569.2011.00633.x
Pasternak, O., Sochen, N., Gur, Y., Intrator, N., and Assaf, Y. (2009). Free water elimination and mapping from diffusion MRI. Magn. Reson. Med. 62, 717–730. doi: 10.1002/mrm.22055
Pfefferbaum, A., and Sullivan, E. V. (2003). Increased brain white matter diffusivity in normal adult aging: relationship to anisotropy and partial voluming. Magn. Reson. Med. 49, 953–961. doi: 10.1002/mrm.10452
Pigino, G., Morfini, G., Pelsman, A., Mattson, M. P., Brady, S. T., and Busciglio, J. (2003). Alzheimer’s presenilin 1 mutations impair kinesin-based axonal transport. J. Neurosci. 23, 4499–4508.
Ransmayr, G., Cervera, P., Hirsch, E., Ruberg, M., Hersh, L. B., Duyckaerts, C., et al. (1989). Choline acetyltransferase-like immunoreactivity in the hippocampal formation of control subjects and patients with Alzheimer’s disease. Neuroscience 32, 701–714. doi: 10.1016/0306-4522(89)90291-1
Ringman, J. M., O’neill, J., Geschwind, D., Medina, L., Apostolova, L. G., Rodriguez, Y., et al. (2007). Diffusion tensor imaging in preclinical and presymptomatic carriers of familial Alzheimer’s disease mutations. Brain 130, 1767–1776. doi: 10.1093/brain/awm102
Roher, A. E., Weiss, N., Kokjohn, T. A., Kuo, Y. M., Kalback, W., Anthony, J., et al. (2002). Increased A beta peptides and reduced cholesterol and myelin proteins characterize white matter degeneration in Alzheimer’s disease. Biochemistry 41, 11080–11090. doi: 10.1021/bi026173d
Rohrer, J. D., Lashley, T., Schott, J. M., Warren, J. E., Mead, S., Isaacs, A. M., et al. (2011). Clinical and neuroanatomical signatures of tissue pathology in frontotemporal lobar degeneration. Brain 134, 2565–2581. doi: 10.1093/brain/awr198
Rose, S. E., Chen, F., Chalk, J. B., Zelaya, F. O., Strugnell, W. E., Benson, M., et al. (2000). Loss of connectivity in Alzheimer’s disease: an evaluation of white matter tract integrity with colour coded MR diffusion tensor imaging. J. Neurol. Neurosurg. Psychiatry 69, 528–530. doi: 10.1136/jnnp.69.4.528
Sachdev, P. S., Zhuang, L., Braidy, N., and Wen, W. (2013). Is Alzheimer’s a disease of the white matter? Curr. Opin. Psychiatry 26, 244–251. doi: 10.1097/YCO.0b013e32835ed6e8
Salat, D. H., Tuch, D. S., Van Der Kouwe, A. J., Greve, D. N., Pappu, V., Lee, S. Y., et al. (2010). White matter pathology isolates the hippocampal formation in Alzheimer’s disease. Neurobiol. Aging 31, 244–256. doi: 10.1016/j.neurobiolaging.2008.03.013
Sara, S. J. (1989). Noradrenergic-cholinergic interaction: its possible role in memory dysfunction associated with senile dementia. Arch. Gerontol. Geriatr. Suppl. 1, 99–108.
Schegg, K. M., Nielsen, S., Zweig, R. M., and Peacock, J. H. (1989). Decrease in membrane-bound G4 form of acetylcholinesterase in postmortem Alzheimer brain. Prog. Clin. Biol. Res. 317, 437–452.
Serra, L., Cercignani, M., Lenzi, D., Perri, R., Fadda, L., Caltagirone, C., et al. (2010). Grey and white matter changes at different stages of Alzheimer’s disease. J. Alzheimers Dis. 19, 147–159. doi: 10.3233/JAD-2010-1223
Sexton, C. E., Kalu, U. G., Filippini, N., Mackay, C. E., and Ebmeier, K. P. (2011). A meta-analysis of diffusion tensor imaging in mild cognitive impairment and Alzheimer’s disease. Neurobiol. Aging 32, 2322.e5–2322.e18. doi: 10.1016/j.neurobiolaging.2010.05.019
Smith, C. D., Chebrolu, H., Andersen, A. H., Powell, D. A., Lovell, M. A., Xiong, S., et al. (2010). White matter diffusion alterations in normal women at risk of Alzheimer’s disease. Neurobiol. Aging 31, 1122–1131. doi: 10.1016/j.neurobiolaging.2008.08.006
Smith, S. M., Jenkinson, M., Johansen-Berg, H., Rueckert, D., Nichols, T. E., Mackay, C. E., et al. (2006). Tract-based spatial statistics: voxelwise analysis of multi-subject diffusion data. Neuroimage 31, 1487–1505. doi: 10.1016/j.neuroimage.2006.02.024
Smith, G. S., Laxton, A. W., Tang-Wai, D. F., Mcandrews, M. P., Diaconescu, A. O., Workman, C. I., et al. (2012). Increased cerebral metabolism after 1 year of deep brain stimulation in Alzheimer disease. Arch. Neurol. 69, 1141–1148. doi: 10.1001/archneurol.2012.590
Stahl, R., Dietrich, O., Teipel, S. J., Hampel, H., Reiser, M. F., and Schoenberg, S. O. (2007). White matter damage in Alzheimer disease and mild cognitive impairment: assessment with diffusion-tensor MR imaging and parallel imaging techniques. Radiology 243, 483–492. doi: 10.1148/radiol.2432051714
Stokin, G. B., Lillo, C., Falzone, T. L., Brusch, R. G., Rockenstein, E., Mount, S. L., et al. (2005). Axonopathy and transport deficits early in the pathogenesis of Alzheimer’s disease. Science 307, 1282–1288. doi: 10.1126/science.1105681
Stricker, N. H., Schweinsburg, B. C., Delano-Wood, L., Wierenga, C. E., Bangen, K. J., Haaland, K. Y., et al. (2009). Decreased white matter integrity in late-myelinating fiber pathways in Alzheimer’s disease supports retrogenesis. Neuroimage 45, 10–16. doi: 10.1016/j.neuroimage.2008.11.027
Tang, X., Yoshida, S., Hsu, J., Huisman, T. A., Faria, A. V., Oishi, K., et al. (2014). Multi-contrast multi-atlas parcellation of diffusion tensor imaging of the human brain. PLoS One 9:e96985. doi: 10.1371/journal.pone.0096985
Teipel, S. J., Stahl, R., Dietrich, O., Schoenberg, S. O., Perneczky, R., Bokde, A. L., et al. (2007). Multivariate network analysis of fiber tract integrity in Alzheimer’s disease. Neuroimage 34, 985–995. doi: 10.1016/j.neuroimage.2006.07.047
Thomas, A. G., Koumellis, P., and Dineen, R. A. (2011). The fornix in health and disease: an imaging review. Radiographics 31, 1107–1121. doi: 10.1148/rg.314105729
Toda, H., Hamani, C., Fawcett, A. P., Hutchison, W. D., and Lozano, A. M. (2008). The regulation of adult rodent hippocampal neurogenesis by deep brain stimulation. J. Neurosurg. 108, 132–138. doi: 10.3171/jns/2008/108/01/0132
Vos, S. B., Jones, D. K., Viergever, M. A., and Leemans, A. (2011). Partial volume effect as a hidden covariate in DTI analyses. Neuroimage 55, 1566–1576. doi: 10.1016/j.neuroimage.2011.01.048
Wenk, G., Hughey, D., Boundy, V., Kim, A., Walker, L., and Olton, D. (1987). Neurotransmitters and memory: role of cholinergic, serotonergic and noradrenergic systems. Behav. Neurosci. 101, 325–332. doi: 10.1037//0735-7044.101.3.325
Wu, M., Rosano, C., Lopez-Garcia, P., Carter, C. S., and Aizenstein, H. J. (2007). Optimum template selection for atlas-based segmentation. Neuroimage 34, 1612–1618. doi: 10.1016/j.neuroimage.2006.07.050
Xie, S., Xiao, J. X., Gong, G. L., Zang, Y. F., Wang, Y. H., Wu, H. K., et al. (2006). Voxel-based detection of white matter abnormalities in mild Alzheimer disease. Neurology 66, 1845–1849. doi: 10.1212/01.wnl.0000219625.77625.aa
Xu, J., Chen, S., Ahmed, S. H., Chen, H., Ku, G., Goldberg, M. P., et al. (2001). Amyloid-beta peptides are cytotoxic to oligodendrocytes. J. Neurosci. 21:RC118.
Yamamoto, T., Kurobe, H., Kawamura, J., Hashimoto, S., and Nakamura, M. (1990). Subacute dementia with necrotising encephalitis selectively involving the fornix and splenium. Retrograde development of Alzheimer’s neurofibrillar tangles in the subiculum. J. Neurol. Sci. 96, 159–172. doi: 10.1016/0022-510x(90)90129-b
Yoon, B., Shim, Y. S., Hong, Y. J., Koo, B. B., Kim, Y. D., Lee, K. O., et al. (2011). Comparison of diffusion tensor imaging and voxel-based morphometry to detect white matter damage in Alzheimer’s disease. J. Neurol. Sci. 302, 89–95. doi: 10.1016/j.jns.2010.11.012
Zarei, M., Damoiseaux, J. S., Morgese, C., Beckmann, C. F., Smith, S. M., Matthews, P. M., et al. (2009). Regional white matter integrity differentiates between vascular dementia and Alzheimer disease. Stroke 40, 773–779. doi: 10.1161/strokeaha.108.530832
Zhang, Y., Schuff, N., Du, A. T., Rosen, H. J., Kramer, J. H., Gorno-Tempini, M. L., et al. (2009). White matter damage in frontotemporal dementia and Alzheimer’s disease measured by diffusion MRI. Brain 132, 2579–2592. doi: 10.1093/brain/awp071
Zhang, Y., Schuff, N., Jahng, G. H., Bayne, W., Mori, S., Schad, L., et al. (2007). Diffusion tensor imaging of cingulum fibers in mild cognitive impairment and Alzheimer disease. Neurology 68, 13–19. doi: 10.1212/01.wnl.0000250326.77323.01
Keywords: Alzheimer’s disease, mild cognitive impairment, diffusion tensor imaging, deep brain stimulation, fornix, limbic system, magnetic resonance imaging, white matter
Citation: Oishi K and Lyketsos CG (2014) Alzheimer’s disease and the fornix. Front. Aging Neurosci. 6:241. doi: 10.3389/fnagi.2014.00241
Received: 02 July 2014; Accepted: 22 August 2014;
Published online: 11 September 2014.
Edited by:
P. Hemachandra Reddy, Oregen Health and Science University, USAReviewed by:
Mario Parra, University of Edinburgh, UKRussell H. Swerdlow, University of Kansas Medical Center, USA
Copyright © 2014 Oishi and Lyketsos. This is an open-access article distributed under the terms of the Creative Commons Attribution License (CC BY). The use, distribution or reproduction in other forums is permitted, provided the original author(s) or licensor are credited and that the original publication in this journal is cited, in accordance with accepted academic practice. No use, distribution or reproduction is permitted which does not comply with these terms.
*Correspondence: Kenichi Oishi, The Russell H. Morgan Department of Radiology and Radiological Science, School of Medicine, Johns Hopkins University, 217 Traylor Building, 720 Rutland Avenue, Baltimore, MD 21205, USA e-mail: koishi@mri.jhu.edu