- 1National Engineering and Technology Research Center of Chirality Pharmaceutical, Lunan Pharmaceutical Group Co., Ltd., Linyi, China
- 2State Key Laboratory of Experimental Hematology, Tianjin Key Laboratory on Technologies Enabling Development of Clinical Therapeutics and Diagnostics, The School of Pharmacy, Tianjin Medical University, Tianjin, China
- 3Department of Nuclear Medicine, Tianjin Medical University General Hospital, Tianjin, China
- 4Department of Chemistry and Biochemistry, University of Wisconsin-Milwaukee, Milwaukee, WI, United States
Proteolysis-targeting chimeras (PROTACs) offer a groundbreaking approach to selectively degrade disease-related proteins by utilizing the ubiquitin-proteasome system. While this strategy shows great potential in preclinical and clinical settings, off-tissue effects remain a major challenge, leading to toxicity in healthy tissues. This review explores recent advancements aimed at improving PROTAC specificity, including tumor-specific ligand-directed PROTACs, pro-PROTACs activated in tumor environments, and E3 ligase overexpression strategies. Innovations such as PEGylation and nanotechnology also play a role in optimizing PROTAC efficacy. These developments hold promise for safer, more effective cancer therapies, though challenges remain for clinical translation.
1 Introduction
Proteolysis-targeting chimeras (PROTACs) represent a breakthrough in targeted protein degradation, leveraging the ubiquitin-proteasome system (UPS) to eliminate disease-causing proteins, many of which are considered “undruggable” by conventional therapies (Chirnomas et al., 2023; Diehl and Ciulli, 2022). Composed of a target protein ligand, an E3 ligase ligand, and a linker, PROTACs promote the ubiquitination and degradation of disease-related proteins by bringing them into proximity with an E3 ligase (Yan et al., 2024; Li et al., 2022).
Introduced in the early 2000s by Craig Crews, the first generation of PROTACs was peptidic, targeting proteins like METAP2 (Sakamoto et al., 2001) (Figure 1). While promising, these early PROTACs were large, lacked cell permeability, and were rapidly degraded by proteases, limiting their bioavailability. The introduction of small-molecule PROTACs, such as SARM-nutlin in 2008, addressed many of these limitations by improving pharmacokinetics, cell permeability, and overall efficacy, broadening the scope of therapeutic applications to diseases like cancer (Schneekloth et al., 2008). The development of VHL-based and CRBN-based PROTACs marked significant milestones in this technology. The first VHL-based PROTAC, targeting the androgen receptor (AR) in prostate cancer, was developed in 2012, while the first CRBN-based PROTAC, targeting BRD4, followed in 2015 (Bondeson et al., 2015; Lu et al., 2015). These innovations demonstrated the ability of PROTACs to selectively degrade target proteins, setting the stage for the rapid advancement of the field. ARV-110 and ARV-471 are notable examples of PROTACs that have progressed to clinical trials, focusing on targeting the androgen receptor and estrogen receptor, respectively (Liu Z. et al., 2022) (Figure 1). These drugs hold the potential to overcome resistance mechanisms seen in traditional therapies, marking a significant step forward in cancer treatment and expanding the horizon for drugging previously “undruggable” proteins.
PROTACs have shown considerable promise in both preclinical and clinical settings (Békés et al., 2022; Hu and Crews, 2022; Shi et al., 2024). However, challenges remain, and a key concern is off-tissue degradation, where PROTACs may induce unwanted protein degradation in non-target tissues, leading to toxicity (Wang et al., 2024b; Zhang et al., 2024; Chen et al., 2023; Li et al., 2024). This risk is heightened by the ubiquitous expression of E3 ligases, which can ubiquitinate target proteins in both diseased and healthy tissues (Kannt and Dikic, 2021; Yu et al., 2024; Jiang et al., 2023). For instance, ARV-110, developed for prostate cancer, has shown efficacy but underscored the need for greater precision to avoid degradation in normal tissues and reduce side effects (Chen et al., 2024; Huang et al., 2022). Additionally, neutropenia was observed as a side effect during the clinical trial of CFT7455 (Li K. et al., 2022).
To address these challenges, several strategies are being developed, including tumor-specific ligand-directed PROTACs, pro-PROTACs that are selectively activated in target tissues, and exploiting overexpressed E3 ligases in certain cancers (Yim et al., 2024) (Figure 2). Additionally, approaches like PEGylation and nanotechnology are being employed to improve pharmacokinetics, reduce non-specific binding, and enhance the selectivity of protein degradation, thereby expanding the therapeutic window (Li D. et al., 2022; Zhang et al., 2023; Zhang et al., 2022) (Figure 2). These efforts reflect the evolving landscape of PROTAC technology as researchers strive to balance potency with safety through chemically engineering, aiming for more precise and effective cancer therapies.
2 Precision-engineered PROTACs
2.1 Tumor-specific ligand-directed PROTACs
To minimize off-target toxicity, a key strategy is the chemical conjugation of tumor-specific targeting ligands, which direct PROTACs to specific tumors, concentrating therapeutic effects while reducing adverse reactions elsewhere (Zhao et al., 2020) (Figure 3). Antibody-conjugated PROTACs (Ab-PROTACs), for example, target overexpressed cell surface antigens in cancer cells (Chang et al., 2023; Guo et al., 2024). After binding, the PROTAC moiety is internalized and degrades intracellular proteins (Figure 3A). HER2-targeted Ab-PROTACs selectively degrade proteins in HER2-positive cancer cells, sparing healthy tissues in cancer therapy (Hu et al., 2022; Tsuchikama et al., 2024). Despite their potential, the large size of antibodies poses challenges like reduced stability, immunogenicity, and difficulty in internalization, all of which must be addressed to enhance their therapeutic viability (Elbakri et al., 2010). Beyond Ab-PROTACs, small molecule-based PROTACs conjugated with tumor-specific ligands, such as folate, show promising results (Figure 3B). Folate conjugation, for instance, selectively targets folate receptor-overexpressing cancer cells, allowing localized protein degradation and minimizing systemic toxicity (Liu et al., 2021). RGD (Arg-Gly-Asp) peptides are known to bind α(v)β(3) integrin, which is overexpressed in many tumors and play a crucial role in tumor angiogenesis and metastasis (Figure 3C). Similarly, RGD peptide-conjugated PROTACs target integrin-expressing tumors, selectively degrading proteins like BCL-xL in these cancer cells (Zanella et al., 2019; Danhier et al., 2012).
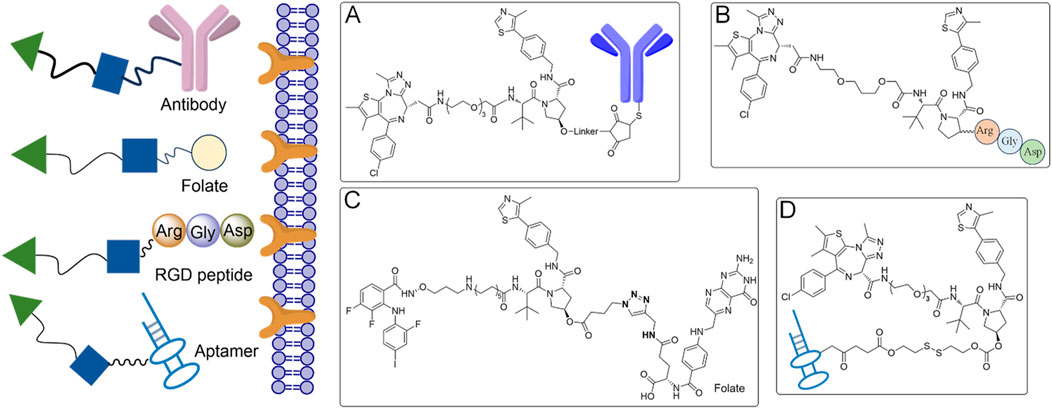
Figure 3. Tumor-specific ligand-directed PROTACs by antibody, folate, RGD peptide and aptamer. (A) The PROTACs achieve targeted delivery to cancer cells via antibody-mediated recognition. (B) Folate receptors on cancer cells are exploited by the PROTACs for selective targeting. (C) The PROTACs utilize RGD peptides to specifically bind to integrins on cancer cells. (D) Aptamers are employed by the PROTACs for precise cancer cell targeting.
Aptamer-based PROTACs offer another innovative approach (Liu et al., 2023a) (Figure 3D). Aptamers, single-stranded nucleic acids with high specificity for targets, can guide PROTACs to specific tissues. An aptamer-conjugated PROTAC designed to degrade HER2 protein has been shown to selectively target HER2-positive breast cancer cells, enhancing treatment precision and reducing off-target effects (He et al., 2021; Li et al., 2023). Beyond cancer, aptamer-based PROTACs could be further explored in other diseases where tumor-specific protein degradation is critical.
These advancements in tumor-specific ligand-directed PROTACs could make PROTAC therapies more precise and safer, paving the way for broader applications in personalized medicine. Further optimization of bio-stability, conjugation techniques, and POI degradation efficiency will be crucial to fully realizing their potential in clinical settings (Joubert et al., 2017).
2.2 Pro-PROTAC approaches
Pro-PROTAC approach offers a promising strategy to minimize systemic toxicity by ensuring activation primarily within the target tissue (Chen et al., 2023) (Figure 4). These inactive PROTAC precursors remain inert until selectively activated by specific environmental triggers, such as light, X-ray, or the unique enzymatic (e.g., NQO1) and chemical (e.g., redox state) conditions in tumor cells (An et al., 2023; Saxon and Peng, 2022). This is especially advantageous in cancer therapy.
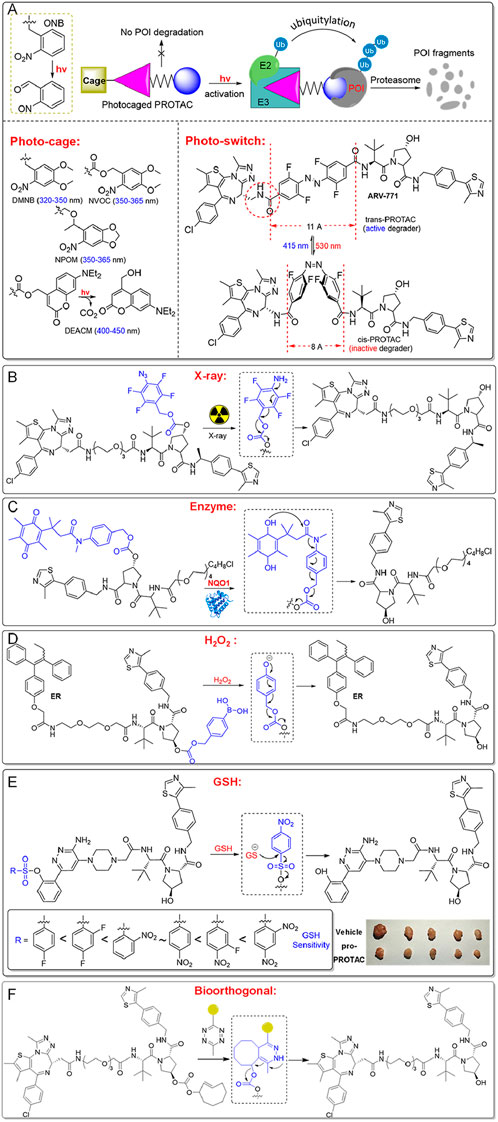
Figure 4. Pro-PROTACs allow for selective activation in cancer cells. (A) Pro-PROTACs can be activated specifically through photolysis. (B) Pro-PROTACs can be activated by exposure to X-ray irradiation. (C) Pro-PROTACs can undergo activation via targeted enzymatic reactions. (D) Pro-PROTACs can be activated by elevated hydrogen peroxide in cancer cells. (E) Pro-PROTACs can be activated by intracellular GSH, exploiting its high levels in cancer cells. (F) Pro-PROTACs can be triggered through bioorthogonal chemical reactions.
Photo-PROTACs provide a straightforward method to achieve spatial and temporal control over protein degradation. Photo-cage PROTACs typically feature a photolabile “cage” that blocks the active site of the PROTAC (Xue et al., 2019) (Figure 4A). Upon exposure to a specific wavelength of light, the photocage is removed, releasing the active PROTAC to degrade target proteins. Common photolabile groups include Ortho-Nitrobenzyl (ONB, 300–365 nm), Nitroveratryloxycarbonyl (NVOC, 350–365 nm), 6-Nitropiperonyloxymethyl (NPOM, 350–365 nm), 4,5-Dimethoxy-2-nitrobenzyl (DMNB, 320–350 nm), and Diethylaminocoumarin (DEACM, 400–450 nm) (Klán et al., 2013; Liu et al., 2020; Naro et al., 2020). These photo-cage PROTACs remain inactive until illuminated, enabling precise control over when and where protein degradation occurs.
Additionally, azobenzene-based photo-switch PROTACs (Azo-PROTACs) offer reversible control of protein degradation (Jin et al., 2020; Pfaff et al., 2019). These PROTACs incorporate azobenzene moieties between ligands targeting the E3 ligase and the protein of interest (Figure 4A). Light induces conformational changes in the azobenzene, switching the PROTAC between active and inactive states. For instance, the trans-isomer (415 nm) enables effective protein degradation, while the cis-isomer (530 nm) is inactive due to a shorter linker distance that prevents the necessary protein-protein interactions. Photo-switch PROTACs have shown efficacy in degrading target proteins like BCR-ABL in leukemia cells, underscoring their potential for precise therapeutic applications.
Using light as a trigger for PROTACs allows precise control over the timing and location of protein degradation, but it faces limitations such as poor tissue penetration of UV/visible light and potential phototoxicity (Min et al., 2015). Near-infrared (NIR) and two-photon techniques offer alternatives, but their efficiency remains too low for practical clinical use (Shaw et al., 2022). X-ray radiation-responsive PROTACs, on the other hand, take advantage of X-ray’s deep tissue penetration (Geng et al., 2021). These PROTACs typically contain radiation-cleavable groups, such as phenyl azide, which block the interaction between the PROTAC and its target or the E3 ligase (Figure 4B). Upon exposure to X-ray during radiotherapy, the phenyl azide group is reduced, releasing the active PROTAC. This approach enhances specificity and shows synergistic antitumor effects, as demonstrated in MCF-7 xenograft models (Yang et al., 2023).
Pro-PROTACs, designed to respond to the unique enzymatic or chemical environment of tumor cells, are another promising strategy (Liang et al., 2022; Fan et al., 2023) (Figure 4). For example, NQO1, an enzyme overexpressed in certain cancers, can activate NQO1-PROTACs by cleaving their caging groups (Figure 4C). This releases the active PROTAC, enabling it to bind target proteins like HDAC6 and recruit an E3 ligase for degradation (Jia et al., 2023b). This strategy ensures that the PROTAC remains inactive in normal cells, reducing systemic toxicity and off-target effects, offering high selectivity for cancer cells.
Redox-sensitive PROTACs offer a sophisticated and selective strategy for cancer treatment by exploiting the distinct redox imbalance in tumor cells (Figure 4D). Cancer cells often exhibit elevated levels of hydrogen peroxide (H2O2) and glutathione (GSH), which are essential for maintaining their altered redox homeostasis (Yu et al., 2023a; Ali et al., 2024; Wang et al., 2017; Chen et al., 2018; Xue et al., 2023; Jia et al., 2023a). This disparity between cancerous and normal tissues provides a unique opportunity for targeted therapeutic intervention (Saxon et al., 2024; Cao et al., 2023; Sun et al., 2024). Typically, H2O2-sensitive PROTACs are designed with H2O2-responsive linkers or protective groups, such as aryboronates/boronic acids (converted into phenol group), or phenyl selenide (converted into benzeneselenenic acid), which act as cage units to mask the active PROTAC moiety (Yu et al., 2023b; Yu et al., 2022). Upon reacting with elevated levels of hydrogen peroxide (H2O2) in tumor cells, these groups are de-caged, releasing active PROTACs. For instance, H2O2-inducible PROTAC precursors release active PROTACs in cancer cells, such as A549 and H1299, leading to selective degradation of BRD4 or the estrogen receptor, inducing cytotoxicity in cancer cells while sparing H2O2-deficient normal cells, such as WI38. This mechanism enhances therapeutic precision by minimizing off-target effects in healthy tissues.
Encouraging results have also been observed with GSH-responsive PROTACs, which utilize benzenesulfonyl cage groups to block PROTAC activity (Xue et al., 2022; Chai et al., 2024). These groups are activated in the glutathione (GSH)-rich environment of cancer cells, particularly in lung cancer, restoring PROTAC functionality (Figure 4E). The reactivity of these precursors can be fine-tuned by introducing electron-withdrawing substituents, such as -F or -NO2, on the benzenesulfonyl unit to increase sensitivity to GSH (Fan et al., 2023; Sun et al., 2024; Zhou et al., 2023). For example, a GSH-inducible SMARCA2/4-targeting PROTAC demonstrates selective degradation of SMARCA2/4 in cancer cells, promoting DNA damage and apoptosis while inhibiting tumor growth in xenograft models without adverse effects on normal cells (Ji et al., 2024). This redox-inducible strategy marks a significant advancement in PROTAC design, providing a versatile and effective approach for improving therapeutic precision in cancer treatment (Figure 4E). By enhancing selectivity and reducing off-target toxicity, redox-sensitive PROTACs represent a promising avenue for the development of safer, more effective cancer therapies.
Bioorthogonal pro-PROTACs are another cutting-edge method in targeted cancer therapy, enabling the precise activation of PROTACs within cancer cells via bioorthogonal chemical reactions with exogenous chemical agents (Bi et al., 2023; Huang et al., 2023) (Figure 4F). For instance, inactive pro-PROTACs like TCO-ARV-771 and TCO-DT2216, which are conjugated with a bioorthogonal trans-cyclooctene (TCO) group, can be selectively activated by tetrazine (Tz)-modified RGD peptide, c(RGDyK)-Tz, in cancer cells expressing the integrin αvβ3 biomarker. Once activated, the prodrugs release active PROTACs, promoting targeted protein degradation within cancer cells while minimizing off-target effects on healthy cells (Chang et al., 2023). This approach allows for spatially and temporally controlled PROTAC activation, thereby improving therapeutic specificity and reducing unintended toxicity. These advancements pave the way for the development of more selective and efficient cancer treatments, offering significant potential for future biomedical applications.
2.3 PROTACs targeting overexpressed E3 ligases
E3 ubiquitin ligases are critical components of the ubiquitin-proteasome system (UPS), responsible for ubiquitination and subsequent degradation of target proteins. PROTACs that exploit overexpressed E3 ubiquitin ligases in cancer cells have emerged as a novel therapeutic strategy with improved selectivity (Figure 5). Overexpression of specific E3 ligases, such as MDM2 and IAPs, makes them attractive targets for selective degradation of oncogenic proteins through PROTAC technology (Liu et al., 2023b).
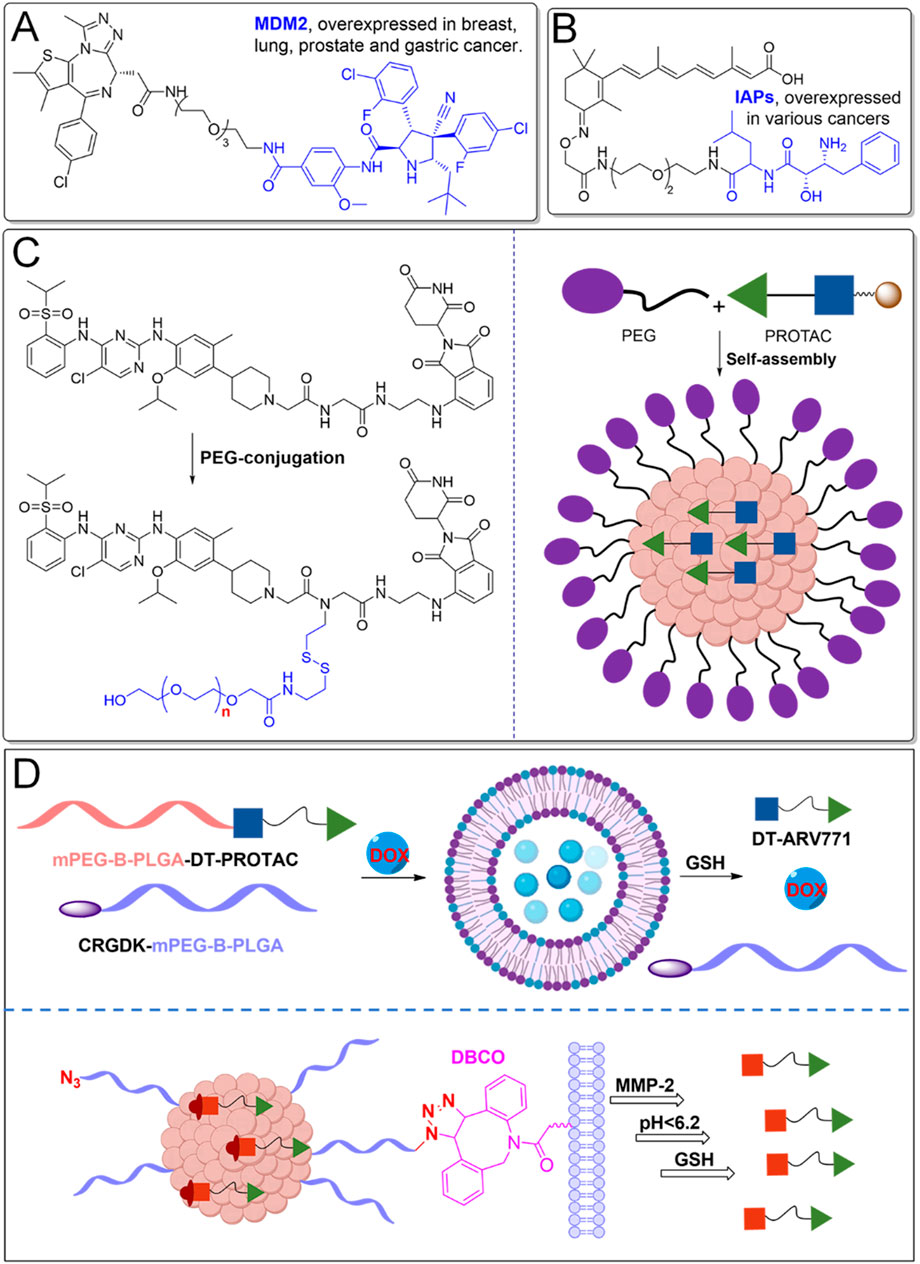
Figure 5. PROTACs leveraging overexpressed E3 ligases and nanotechnology. (A) PROTACs leverage overexpressed E3 ligases, such as MDM2, in certain cancers to enhance their targeted protein degradation. (B) PROTACs utilize overexpressed IAPs in specific cancers for therapeutic purposes. (C) PEGylation significantly enhances the pharmacokinetic properties of PROTACs. (D) Nanotechnology facilitates better delivery and bioavailability.
MDM2-targeting PROTACs exploit the overexpression of the MDM2 ligase, common in cancers such as sarcomas and gliomas, which typically degrade the tumor suppressor protein p53 (Shangary and Wang, 2008) (Figure 5A). In these cancers, p53’s tumor-suppressive function is impaired, contributing to unchecked proliferation (Traweek et al., 2022). MDM2-based PROTACs redirect MDM2 to degrade oncogenic proteins like BRD4, while simultaneously restoring p53’s activity. This dual action is particularly beneficial in cancers with intact but functionally inactive p53, offering a promising route for therapeutic intervention (Hines et al., 2019).
IAPs, including cIAP1, cIAP2, and XIAP, also serve as E3 ligases and regulators of apoptosis (Liu X. et al., 2022) (Figure 5B). Overexpressed in many cancers, they contribute to resistance against apoptosis. IAP-based PROTACs, designed to target oncogenic proteins such as BCL-XL, provide a more selective alternative to VHL- or CRBN-based PROTACs (Zhang et al., 2020). This approach reduces the risk of platelet toxicity, a common side effect with other ligases, making IAP-based PROTACs a promising option for targeting proteins like BCL-XL in cancer therapies, while minimizing toxicity (Ma et al., 2021). It should be noted that only a limited number of E3 ligases have been effectively utilized in PROTAC designs, restricting their applicability to some cancers, as these ligases are not universally overexpressed across all cancer types. Expanding the pool of E3 ligases, particularly those with tumor-specific expression, could significantly enhance the precision and therapeutic scope of PROTACs. Moreover, advanced delivery systems, such as nanoparticle carriers, may further improve the tumor-targeting efficiency of PROTACs by increasing their selective accumulation in cancerous tissues, thus reducing systemic toxicity and enhancing overall therapeutic efficacy.
2.4 PEGylation and nanotechnology-based PROTACs
Optimizing the pharmacokinetics of PROTACs through PEGylation and nanotechnology can effectively reduce off-target accumulation, minimizing unintended effects (Cecchini et al., 2022) (Figure 5C). These modifications enhance hydrophilicity, decrease non-specific binding, and extend circulation time, allowing for more selective tissue uptake (Chen et al., 2022). PEGylation improves the solubility and stability of PROTACs by enabling them to self-assemble into micelles, which remain stable in circulation and release the active PROTAC in response to elevated glutathione (GSH) levels in tumor environments. Preclinical studies have shown superior bioavailability (84.8%) compared to unmodified versions, significantly reducing tumor size in xenograft models, indicating strong potential for cancer therapy (Wang et al., 2024a).
Nano-PROTACs further enhance tumor specificity by leveraging nanotechnology (Zhang et al., 2023; Song et al., 2024). For example, SPNpro, a self-assembling nano-PROTAC, incorporates a semiconductor polymer core that generates singlet oxygen upon light activation, killing tumor cells (Figure 5C). A cancer biomarker-responsive peptide cleaves SPNpro, releasing active PROTACs to degrade immunosuppressive targets such as IDO, reversing immune suppression and promoting antitumor immunity (Zhang et al., 2021). This strategy also mitigates issues like the “hook effect” by enabling dose-dependent protein degradation. In preclinical models, Nano-PROTACs demonstrated a 95% degradation rate with long-lasting potency and significant tumor inhibition. Additionally, phototherapeutic nano-PROTACs, like those targeting COX-1/2 and activated by tumor-overexpressed cathepsin B, enhance tumor-specific protein degradation, reprogram the tumor microenvironment (TME), and stimulate immune responses, further suppressing tumor growth (Gao et al., 2024).
To further improve selectivity in cancer therapy, advanced nano-PROTAC combination therapy approaches and multiple stimuli-responsive nano-PROTAC systems have been developed (Figure 5D). Nano-PROTAC combination therapy enables the co-delivery of a PROTAC degrader alongside conventional small-molecule drugs, allowing for synergistic effects (Zou et al., 2024). Self-assembling pro-PROTAC nanoparticles are engineered to specifically target tumor cells, where they can be activated to release the free PROTACs, facilitating precise protein degradation. Additionally, multiple stimuli-responsive nano-PROTAC systems allow for enhanced precision in protein degradation. For example, an azide-modified nano-PROTAC utilizes bioorthogonal click chemistry to amplify PROTAC delivery selectively to tumor tissues (Gao et al., 2022). These nano-PROTACs can sequentially respond to extracellular matrix metalloproteinase-2 (MMP-2), acidic intracellular environments (pH < 6.2), and the reductive tumor microenvironment (GSH) to release the active PROTACs. This targeted release facilitates tumor-specific BRD4 degradation, offering a highly selective and efficient approach to PROTAC-based cancer therapy.
These findings highlight the potential of PEGylation and nano-PROTACs in overcoming the traditional limitations of PROTACs, such as poor bioavailability and solubility, while enhancing therapeutic efficacy. However, introducing PEGylation and nanoplatforms raises concerns about biocompatibility, immune response, and safety, which may complicate their clinical application (Ernst et al., 2021). Balancing efficacy with these potential risks is crucial for the future of these innovative therapies.
3 Conclusion
PROTACs offer a revolutionary approach to targeted protein degradation, showing great promise in both preclinical and clinical studies. However, challenges such as off-tissue effects and systemic toxicity remain obstacles to clinical success. Recent strategies, such as tumor-specific ligand-directed PROTACs, E3 ligase-specific designs, pro-PROTACs, and nanotechnology are helping to mitigate these issues by enhancing selectivity and reducing unwanted degradation. Despite encouraging progress, more advancements are necessary, particularly in developing biocompatible linkers, novel ligands, and exploring new E3 ligases to improve specificity and reduce toxicity, essential for clinical translation.
Author contributions
HS: Conceptualization, Funding acquisition, Investigation, Supervision, Writing–original draft, Writing–review and editing. JS: Conceptualization, Data curation, Formal Analysis, Methodology, Software, Writing–original draft. LW: Data curation, Formal Analysis, Methodology, Visualization, Writing–original draft. XZ: Data curation, Methodology, Writing–original draft. CX: Data curation, Software, Funding acquisition, Writing–original draft. ZM: Formal Analysis, Investigation, Writing–original draft. AC: Conceptualization, Visualization, Writing–original draft. LW: Conceptualization, Formal Analysis, Writing–original draft. HF: Conceptualization, Funding acquisition, Investigation, Methodology, Supervision, Writing–original draft, Writing–review and editing.
Funding
The author(s) declare that financial support was received for the research, authorship, and/or publication of this article. HF and HS acknowledge financial support from the Natural Science Foundation of Tianjin (22JCYBJC00020) and State Key Laboratory of Experimental Hematology. CX acknowledges financial support from the Natural Science Foundation of Tianjin (22JCYBJC00020).
Acknowledgments
We are grateful to the core Facility of Research Center of Basic Medical Sciences at Tianjin Medical University for technical assistance.
Conflict of interest
Author JS was employed by Lunan Pharmaceutical Group Co., Ltd.
The remaining authors declare that the research was conducted in the absence of any commercial or financial relationships that could be construed as a potential conflict of interest.
Generative AI statement
The author(s) declare that Generative AI was used in the creation of this manuscript. ChatGPT was used to correct grammar and language errors in the manuscript.
Publisher’s note
All claims expressed in this article are solely those of the authors and do not necessarily represent those of their affiliated organizations, or those of the publisher, the editors and the reviewers. Any product that may be evaluated in this article, or claim that may be made by its manufacturer, is not guaranteed or endorsed by the publisher.
References
Ali, T., Li, D., Ponnamperumage, T. N. F., Peterson, A. K., Pandey, J., Fatima, K., et al. (2024). Generation of hydrogen peroxide in cancer cells: advancing therapeutic approaches for cancer treatment. Cancers (Basel) 16, 2171. doi:10.3390/cancers16122171
An, K., Deng, X., Chi, H., Zhang, Y., Li, Y., Cheng, M., et al. (2023). Stimuli-responsive PROTACs for controlled protein degradation. Angew. Chem. Int. Ed. Engl. 62, e202306824. doi:10.1002/anie.202306824
Békés, M., Langley, D. R., and Crews, C. M. (2022). PROTAC targeted protein degraders: the past is prologue. Nat. Rev. Drug Discov. 21, 181–200. doi:10.1038/s41573-021-00371-6
Bi, T., Liang, P., Zhou, Y., Wang, H., Huang, R., Sun, Q., et al. (2023). Rational design of bioorthogonally activatable PROTAC for tumor-targeted protein degradation. J. Med. Chem. 66, 14843–14852. doi:10.1021/acs.jmedchem.3c01423
Bondeson, D. P., Mares, A., Smith, I. E., Ko, E., Campos, S., Miah, A. H., et al. (2015). Catalytic in vivo protein knockdown by small-molecule PROTACs. Nat. Chem. Biol. 11, 611–617. doi:10.1038/nchembio.1858
Cao, S., Wang, Y., Li, D., and Peng, X. (2023). H(2) O(2) -inducible DNA cross-linking agents capable of releasing multiple DNA alkylators as anticancer prodrugs. ChemMedChem 18, e202300273. doi:10.1002/cmdc.202300273
Cecchini, C., Tardy, S., and Scapozza, L. (2022). Linkers as game-changers in PROTAC technology: emphasizing general trends in PROTAC pharmacokinetics for their rational design. Chim. (Aarau). 76, 341–345. doi:10.2533/chimia.2022.341
Chai, J., Su, M., Zhang, R., Li, N., Jia, Y., Zheng, W., et al. (2024). Selective anti-tumor activity of glutathione-responsive abasic site trapping agent in anaplastic thyroid carcinoma. BMC Cancer 24, 816. doi:10.1186/s12885-024-12511-3
Chang, M., Gao, F., Pontigon, D., Gnawali, G., Xu, H., and Wang, W. (2023). Bioorthogonal PROTAC prodrugs enabled by on-target activation. J. Am. Chem. Soc. 145, 14155–14163. doi:10.1021/jacs.3c05159
Chen, C., Yang, Y., Wang, Z., Li, H., Dong, C., and Zhang, X. (2023). Recent advances in pro-PROTAC development to address on-target off-tumor toxicity. J. Med. Chem. 66, 8428–8440. doi:10.1021/acs.jmedchem.3c00302
Chen, Q. H., Munoz, E., and Ashong, D. (2024). Insight into recent advances in degrading androgen receptor for castration-resistant prostate cancer. Cancers (Basel) 16, 663. doi:10.3390/cancers16030663
Chen, W., Fan, H., Balakrishnan, K., Wang, Y., Sun, H., Fan, Y., et al. (2018). Discovery and optimization of novel hydrogen peroxide activated aromatic nitrogen mustard derivatives as highly potent anticancer agents. J. Med. Chem. 61, 9132–9145. doi:10.1021/acs.jmedchem.8b00559
Chen, Y., Tandon, I., Heelan, W., Wang, Y., Tang, W., and Hu, Q. (2022). Proteolysis-targeting chimera (PROTAC) delivery system: advancing protein degraders towards clinical translation. Chem. Soc. Rev. 51, 5330–5350. doi:10.1039/d1cs00762a
Chirnomas, D., Hornberger, K. R., and Crews, C. M. (2023). Protein degraders enter the clinic - a new approach to cancer therapy. Nat. Rev. Clin. Oncol. 20, 265–278. doi:10.1038/s41571-023-00736-3
Danhier, F., Le Breton, A., and Préat, V. (2012). RGD-based strategies to target alpha(v) beta(3) integrin in cancer therapy and diagnosis. Mol. Pharm. 9, 2961–2973. doi:10.1021/mp3002733
Diehl, C. J., and Ciulli, A. (2022). Discovery of small molecule ligands for the von Hippel-Lindau (VHL) E3 ligase and their use as inhibitors and PROTAC degraders. Chem. Soc. Rev. 51, 8216–8257. doi:10.1039/d2cs00387b
Elbakri, A., Nelson, P. N., and Abu Odeh, R. O. (2010). The state of antibody therapy. Hum. Immunol. 71, 1243–1250. doi:10.1016/j.humimm.2010.09.007
Ernst, L. M., Casals, E., Italiani, P., Boraschi, D., and Puntes, V. (2021). The interactions between nanoparticles and the innate immune system from a nanotechnologist perspective. Nanomater. (Basel) 11, 2991. doi:10.3390/nano11112991
Fan, H., Zhou, Z., Yu, D., Sun, J., Wang, L., Jia, Y., et al. (2023). Selective degradation of BRD4 suppresses lung cancer cell proliferation using GSH-responsive PROTAC precursors. Bioorg Chem. 140, 106793. doi:10.1016/j.bioorg.2023.106793
Gao, J., Hou, B., Zhu, Q., Yang, L., Jiang, X., Zou, Z., et al. (2022). Engineered bioorthogonal POLY-PROTAC nanoparticles for tumour-specific protein degradation and precise cancer therapy. Nat. Commun. 13, 4318. doi:10.1038/s41467-022-32050-4
Gao, J., Jiang, X., Lei, S., Cheng, W., Lai, Y., Li, M., et al. (2024). A region-confined PROTAC nanoplatform for spatiotemporally tunable protein degradation and enhanced cancer therapy. Nat. Commun. 15, 6608. doi:10.1038/s41467-024-50735-w
Geng, J., Zhang, Y., Gao, Q., Neumann, K., Dong, H., Porter, H., et al. (2021). Switching on prodrugs using radiotherapy. Nat. Chem. 13, 805–810. doi:10.1038/s41557-021-00711-4
Guo, Y., Li, X., Xie, Y., and Wang, Y. (2024). What influences the activity of Degrader-Antibody conjugates (DACs). Eur. J. Med. Chem. 268, 116216. doi:10.1016/j.ejmech.2024.116216
He, S., Gao, F., Ma, J., Ma, H., Dong, G., and Sheng, C. (2021). Aptamer-PROTAC conjugates (APCs) for tumor-specific targeting in breast cancer. Angew. Chem. Int. Ed. Engl. 60, 23299–23305. doi:10.1002/anie.202107347
Hines, J., Lartigue, S., Dong, H., Qian, Y., and Crews, C. M. (2019). MDM2-Recruiting PROTAC offers superior, synergistic antiproliferative activity via simultaneous degradation of BRD4 and stabilization of p53. Cancer Res. 79, 251–262. doi:10.1158/0008-5472.Can-18-2918
Hu, M., Li, Y., Li, J., Zhou, H., Liu, C., Liu, Z., et al. (2022). Discovery of potent and selective HER2 PROTAC degrader based Tucatinib with improved efficacy against HER2 positive cancers. Eur. J. Med. Chem. 244, 114775. doi:10.1016/j.ejmech.2022.114775
Hu, Z., and Crews, C. M. (2022). Recent developments in PROTAC-mediated protein degradation: from bench to clinic. Chembiochem 23, e202100270. doi:10.1002/cbic.202100270
Huang, J., Lin, B., and Li, B. (2022). Anti-androgen receptor therapies in prostate cancer: a brief update and perspective. Front. Oncol. 12, 865350. doi:10.3389/fonc.2022.865350
Huang, J., Yao, Z., Li, B., and Ping, Y. (2023). Targeted delivery of PROTAC-based prodrug activated by bond-cleavage bioorthogonal chemistry for microneedle-assisted cancer therapy. J. Control Release 361, 270–279. doi:10.1016/j.jconrel.2023.07.062
Ji, M., Yu, D., Liu, X., Wang, L., Zhang, D., Yang, Z., et al. (2024). Glutathione-dependent degradation of SMARCA2/4 for targeted lung cancer therapy with improved selectivity. Eur. J. Med. Chem. 277, 116751. doi:10.1016/j.ejmech.2024.116751
Jia, Q., Zhang, Y., Liu, F., Dong, W., Zhu, L., Wang, F., et al. (2023a). Cell-specific degradation of histone deacetylase using warhead-caged proteolysis targeting chimeras. Anal. Chem. 95, 16474–16480. doi:10.1021/acs.analchem.3c01236
Jia, Y., Sun, J., Yu, D., Wang, L., Campbell, A., Fan, H., et al. (2023b). Light and hydrogen peroxide dual-responsive DNA interstrand crosslink precursors with potent cytotoxicity. Bioorg Chem. 130, 106270. doi:10.1016/j.bioorg.2022.106270
Jiang, H., Xiong, H., Gu, S. X., and Wang, M. (2023). E3 ligase ligand optimization of Clinical PROTACs. Front. Chem. 11, 1098331. doi:10.3389/fchem.2023.1098331
Jin, Y. H., Lu, M. C., Wang, Y., Shan, W. X., Wang, X. Y., You, Q. D., et al. (2020). Azo-PROTAC: novel light-controlled small-molecule tool for protein knockdown. J. Med. Chem. 63, 4644–4654. doi:10.1021/acs.jmedchem.9b02058
Joubert, N., Denevault-Sabourin, C., Bryden, F., and Viaud-Massuard, M. C. (2017). Towards antibody-drug conjugates and prodrug strategies with extracellular stimuli-responsive drug delivery in the tumor microenvironment for cancer therapy. Eur. J. Med. Chem. 142, 393–415. doi:10.1016/j.ejmech.2017.08.049
Kannt, A., and Đikić, I. (2021). Expanding the arsenal of E3 ubiquitin ligases for proximity-induced protein degradation. Cell Chem. Biol. 28, 1014–1031. doi:10.1016/j.chembiol.2021.04.007
Klán, P., Šolomek, T., Bochet, C. G., Blanc, A., Givens, R., Rubina, M., et al. (2013). Photoremovable protecting groups in chemistry and biology: reaction mechanisms and efficacy. Chem. Rev. 113, 119–191. doi:10.1021/cr300177k
Li, D., Yu, D., Li, Y., and Yang, R. (2022). A bibliometric analysis of PROTAC from 2001 to 2021. Eur. J. Med. Chem. 244, 114838. doi:10.1016/j.ejmech.2022.114838
Li, K., and Crews, C. M. (2022). PROTACs: past, present and future. Chem. Soc. Rev. 51, 5214–5236. doi:10.1039/d2cs00193d
Li, X., Zhang, Z., Gao, F., Ma, Y., Wei, D., Lu, Z., et al. (2023). c-Myc-Targeting PROTAC based on a TNA-DNA bivalent binder for combination therapy of triple-negative breast cancer. J. Am. Chem. Soc. 145, 9334–9342. doi:10.1021/jacs.3c02619
Li, Z., Jiang, T., Yuan, X., Li, B., Wu, C., Li, Y., et al. (2024). Controlled bioorthogonal activation of Bromodomain-containing protein 4 degrader by co-delivery of PROTAC and Pd-catalyst for tumor-specific therapy. J. Control Release 374, 441–453. doi:10.1016/j.jconrel.2024.08.032
Liang, C., Zheng, Q., Luo, T., Cai, W., Mao, L., and Wang, M. (2022). Enzyme-catalyzed activation of pro-PROTAC for cell-selective protein degradation. CCS Chem. 4, 3809–3819. doi:10.31635/ccschem.022.202101529
Liu, J., Chen, H., Liu, Y., Shen, Y., Meng, F., Kaniskan, H., et al. (2021). Cancer selective target degradation by folate-caged PROTACs. J. Am. Chem. Soc. 143, 7380–7387. doi:10.1021/jacs.1c00451
Liu, J., Chen, H., Ma, L., He, Z., Wang, D., Liu, Y., et al. (2020). Light-induced control of protein destruction by opto-PROTAC. Sci. Adv. 6, eaay5154. doi:10.1126/sciadv.aay5154
Liu, X., Yao, J. J., Chen, Z., Lei, W., Duan, R., and Yao, Z. (2022). Lipopolysaccharide sensitizes the therapeutic response of breast cancer to IAP antagonist. Front. Immunol. 13, 906357. doi:10.3389/fimmu.2022.906357
Liu, Y., Qian, X., Ran, C., Li, L., Fu, T., Su, D., et al. (2023a). Aptamer-based targeted protein degradation. ACS Nano 17, 6150–6164. doi:10.1021/acsnano.2c10379
Liu, Y., Yang, J., Wang, T., Luo, M., Chen, Y., Chen, C., et al. (2023b). Expanding PROTACtable genome universe of E3 ligases. Nat. Commun. 14, 6509. doi:10.1038/s41467-023-42233-2
Liu, Z., Hu, M., Yang, Y., Du, C., Zhou, H., Liu, C., et al. (2022). An overview of PROTACs: a promising drug discovery paradigm. Mol. Biomed. 3, 46. doi:10.1186/s43556-022-00112-0
Lu, J., Qian, Y., Altieri, M., Dong, H., Wang, J., Raina, K., et al. (2015). Hijacking the E3 ubiquitin ligase cereblon to efficiently target BRD4. Chem. Biol. 22, 755–763. doi:10.1016/j.chembiol.2015.05.009
Ma, Z., Ji, Y., Yu, Y., and Liang, D. (2021). Specific non-genetic IAP-based protein erasers (SNIPERs) as a potential therapeutic strategy. Eur. J. Med. Chem. 216, 113247. doi:10.1016/j.ejmech.2021.113247
Min, D., Jeong, D., Choi, M. G., and Na, K. (2015). Photochemical tissue penetration via photosensitizer for effective drug penetration in a non-vascular tumor. Biomaterials 52, 484–493. doi:10.1016/j.biomaterials.2015.02.060
Naro, Y., Darrah, K., and Deiters, A. (2020). Optical control of small molecule-induced protein degradation. J. Am. Chem. Soc. 142, 2193–2197. doi:10.1021/jacs.9b12718
Pfaff, P., Samarasinghe, K. T. G., Crews, C. M., and Carreira, E. M. (2019). Reversible spatiotemporal control of induced protein degradation by bistable PhotoPROTACs. ACS Cent. Sci. 5, 1682–1690. doi:10.1021/acscentsci.9b00713
Sakamoto, K. M., Kim, K. B., Kumagai, A., Mercurio, F., Crews, C. M., and Deshaies, R. J. (2001). Protacs: chimeric molecules that target proteins to the Skp1-Cullin-F box complex for ubiquitination and degradation. Proc. Natl. Acad. Sci. U. S. A. 98, 8554–8559. doi:10.1073/pnas.141230798
Saxon, E., Ali, T., and Peng, X. (2024). Hydrogen peroxide responsive theranostics for cancer-selective activation of DNA alkylators and real-time fluorescence monitoring in living cells. Eur. J. Med. Chem. 276, 116695. doi:10.1016/j.ejmech.2024.116695
Saxon, E., and Peng, X. (2022). Recent advances in hydrogen peroxide responsive organoborons for biological and biomedical applications. Chembiochem 23, e202100366. doi:10.1002/cbic.202100366
Schneekloth, A. R., Pucheault, M., Tae, H. S., and Crews, C. M. (2008). Targeted intracellular protein degradation induced by a small molecule: en route to chemical proteomics. Bioorg Med. Chem. Lett. 18, 5904–5908. doi:10.1016/j.bmcl.2008.07.114
Shangary, S., and Wang, S. (2008). Targeting the MDM2-p53 interaction for cancer therapy. Clin. Cancer Res. 14, 5318–5324. doi:10.1158/1078-0432.Ccr-07-5136
Shaw, P. A., Forsyth, E., Haseeb, F., Yang, S., Bradley, M., and Klausen, M. (2022). Two-photon absorption: an open door to the NIR-II biological window? Front. Chem. 10, 921354. doi:10.3389/fchem.2022.921354
Shi, Y. Y., Fan, G., Tan, R., Li, S., Sun, H. B., Li, R., et al. (2024). Treating ICB-resistant cancer by inhibiting PD-L1 via DHHC3 degradation induced by cell penetrating peptide-induced chimera conjugates. Cell Death Dis. 15, 701. doi:10.1038/s41419-024-07073-y
Song, Y., Dong, Q. Q., Ni, Y. K., Xu, X. L., Chen, C. X., and Chen, W. (2024). Nano-proteolysis targeting chimeras (Nano-PROTACs) in cancer therapy. Int. J. Nanomedicine 19, 5739–5761. doi:10.2147/ijn.S448684
Sun, H., Fan, H., Wang, L., Zeng, X., Xiong, C., Yu, D., et al. (2024). Redox-inducible radiomimetic photosensitizers selectively suppress cancer cell proliferation by damaging DNA through radical cation chemistry. Angew. Chem. Int. Ed. Engl., e202413352. doi:10.1002/anie.202413352
Traweek, R. S., Cope, B. M., Roland, C. L., Keung, E. Z., Nassif, E. F., and Erstad, D. J. (2022). Targeting the MDM2-p53 pathway in dedifferentiated liposarcoma. Front. Oncol. 12, 1006959. doi:10.3389/fonc.2022.1006959
Tsuchikama, K., Anami, Y., Ha, S. Y. Y., and Yamazaki, C. M. (2024). Exploring the next generation of antibody-drug conjugates. Nat. Rev. Clin. Oncol. 21, 203–223. doi:10.1038/s41571-023-00850-2
Wang, C., Zhang, Y., Chen, W., Wu, Y., and Xing, D. (2024a). New-generation advanced PROTACs as potential therapeutic agents in cancer therapy. Mol. Cancer 23, 110. doi:10.1186/s12943-024-02024-9
Wang, S., Feng, Z., Qu, C., Yu, S., Zhang, H., Deng, R., et al. (2024b). Novel amphiphilic PROTAC with enhanced pharmacokinetic properties for ALK protein degradation. J. Med. Chem. 67, 9842–9856. doi:10.1021/acs.jmedchem.3c02340
Wang, Y., Fan, H., Balakrishnan, K., Lin, Z., Cao, S., Chen, W., et al. (2017). Hydrogen peroxide activated quinone methide precursors with enhanced DNA cross-linking capability and cytotoxicity towards cancer cells. Eur. J. Med. Chem. 133, 197–207. doi:10.1016/j.ejmech.2017.03.041
Xue, G., Wang, K., Zhou, D., Zhong, H., and Pan, Z. (2019). Light-Induced protein degradation with photocaged PROTACs. J. Am. Chem. Soc. 141, 18370–18374. doi:10.1021/jacs.9b06422
Xue, L., Yu, D., Sun, J., Guan, L., Xie, C., Wang, L., et al. (2023). Rapid GSH detection and versatile peptide/protein labelling to track cell penetration using coumarin-based probes. Analyst 148, 532–538. doi:10.1039/d2an01510b
Xue, L., Yu, D., Wang, L., Sun, J., Song, Y., Jia, Y., et al. (2022). Selective antitumor activity and photocytotoxicity of glutathione-activated abasic site trapping agents. ACS Chem. Biol. 17, 797–803. doi:10.1021/acschembio.2c00061
Yan, S., Zhang, G., Luo, W., Xu, M., Peng, R., Du, Z., et al. (2024). PROTAC technology: from drug development to probe technology for target deconvolution. Eur. J. Med. Chem. 276, 116725. doi:10.1016/j.ejmech.2024.116725
Yang, C., Yang, Y., Li, Y., Ni, Q., and Li, J. (2023). Radiotherapy-triggered proteolysis targeting chimera prodrug activation in tumors. J. Am. Chem. Soc. 145, 385–391. doi:10.1021/jacs.2c10177
Yim, J., Park, J., Kim, G., Jo, A., Koh, M., Park, J., et al. (2024). Conditional PROTAC: recent strategies for modulating targeted protein degradation. ChemMedChem, e202400326. doi:10.1002/cmdc.202400326
Yu, D., Fan, H., Sun, J., Xue, L., Wang, L., Jia, Y., et al. (2022). Phenyl selenide-based precursors as hydrogen peroxide inducible DNA interstrand cross-linkers. Chembiochem 23, e202200086. doi:10.1002/cbic.202200086
Yu, D., Fan, H., Zhou, Z., Zhang, Y., Sun, J., Wang, L., et al. (2023a). Hydrogen peroxide-inducible PROTACs for targeted protein degradation in cancer cells. Chembiochem 24, e202300422. doi:10.1002/cbic.202300422
Yu, D., Wang, L., Li, J., Zeng, X., Jia, Y., Tian, J., et al. (2023b). Dual-responsive probe and DNA interstrand crosslink precursor target the unique redox status of cancer cells. Chem. Commun. (Camb) 59, 14705–14708. doi:10.1039/d3cc05175g
Yu, J., Zhao, Y., and Xie, Y. (2024). Advances of E3 ligases in lung cancer. Biochem. Biophys. Rep. 38, 101740. doi:10.1016/j.bbrep.2024.101740
Zanella, S., Bocchinfuso, G., De Zotti, M., Arosio, D., Marino, F., Raniolo, S., et al. (2019). Rational design of antiangiogenic helical oligopeptides targeting the vascular endothelial growth factor receptors. Front. Chem. 7, 170. doi:10.3389/fchem.2019.00170
Zhang, C., He, S., Zeng, Z., Cheng, P., and Pu, K. (2022). Smart nano-PROTACs reprogram tumor microenvironment for activatable photo-metabolic cancer immunotherapy. Angew. Chem. Int. Ed. Engl. 61, e202114957. doi:10.1002/anie.202114957
Zhang, C., Zeng, Z., Cui, D., He, S., Jiang, Y., Li, J., et al. (2021). Semiconducting polymer nano-PROTACs for activatable photo-immunometabolic cancer therapy. Nat. Commun. 12, 2934. doi:10.1038/s41467-021-23194-w
Zhang, N. Y., Hou, D. Y., Hu, X. J., Liang, J. X., Wang, M. D., Song, Z. Z., et al. (2023). Nano proteolysis targeting chimeras (PROTACs) with anti-hook effect for tumor therapy. Angew. Chem. Int. Ed. Engl. 62, e202308049. doi:10.1002/anie.202308049
Zhang, R., Xie, S., Ran, J., and Li, T. (2024). Restraining the power of Proteolysis Targeting Chimeras in the cage: a necessary and important refinement for therapeutic safety. J. Cell Physiol. 239, e31255. doi:10.1002/jcp.31255
Zhang, X., He, Y., Zhang, P., Budamagunta, V., Lv, D., Thummuri, D., et al. (2020). Discovery of IAP-recruiting BCL-X(L) PROTACs as potent degraders across multiple cancer cell lines. Eur. J. Med. Chem. 199, 112397. doi:10.1016/j.ejmech.2020.112397
Zhao, Z., Ukidve, A., Kim, J., and Mitragotri, S. (2020). Targeting strategies for tissue-specific drug delivery. Cell 181, 151–167. doi:10.1016/j.cell.2020.02.001
Zhou, Z., Fan, H., Yu, D., Shi, F., Li, Q., Zhang, Z., et al. (2023). Glutathione-responsive PROTAC for targeted degradation of ERα in breast cancer cells. Bioorg Med. Chem. 96, 117526. doi:10.1016/j.bmc.2023.117526
Keywords: PROTACs, off-tissue effects, precision-engineered, targeted protein degradation, PRO-PROTAC, redox-inducible
Citation: Shi J, Wang L, Zeng X, Xie C, Meng Z, Campbell A, Wang L, Fan H and Sun H (2024) Precision-engineered PROTACs minimize off-tissue effects in cancer therapy. Front. Mol. Biosci. 11:1505255. doi: 10.3389/fmolb.2024.1505255
Received: 02 October 2024; Accepted: 28 October 2024;
Published: 22 November 2024.
Edited by:
Ponti Donatella, Sapienza University of Rome, ItalyReviewed by:
Si Si Liew, National University of Singapore, SingaporeGong Zhang, Chongqing University, China
Shubo Du, Dalian University of Technology, China
Copyright © 2024 Shi, Wang, Zeng, Xie, Meng, Campbell, Wang, Fan and Sun. This is an open-access article distributed under the terms of the Creative Commons Attribution License (CC BY). The use, distribution or reproduction in other forums is permitted, provided the original author(s) and the copyright owner(s) are credited and that the original publication in this journal is cited, in accordance with accepted academic practice. No use, distribution or reproduction is permitted which does not comply with these terms.
*Correspondence: Heli Fan, aGVsaWZhbkB0bXUuZWR1LmNu; Huabing Sun, c3VuaHVhYmluZ0B1bmkuZWR1
†These authors have contributed equally to this work