- Department of Pharmaceutical Sciences, University of Connecticut, Storrs, CT, United States
Introduction: The plasma membrane-bound protein, multi-drug resistance-associated protein 4 (MRP4/ABCC4), has gained attention for its pivotal role in facilitating the efflux of a wide range of endogenous and xenobiotic molecules. Its significance in adipogenesis and fatty acid metabolism has been brought to light by recent studies. Notably, research on ABCC4 knockout (ABCC4−/−) mice has established a link between the absence of ABCC4 and the development of obesity and diabetes. Nevertheless, the specific contribution of ABCC4 within adipose tissue remains largely unexplored.
Methods: To address this gap, we conducted a study to elucidate the role of the ABCC4 transporter in mature adipocytes, using siRNA constructs to silence its gene function.
Results: The successful knockdown of ABCC4 significantly altered lipid status and adipogenic gene expression in mature 3T3-L1 adipocytes. Intriguingly, this knockdown also altered the gene expression patterns of other ABCC transporter family members in 3T3-L1 cells. The downregulation of ABCC5 expression was particularly noteworthy, suggesting potential crosstalk between ABCC transporters in mature adipocytes. Additionally, knocking down ABCC5 resulted in significantly higher adipogenic and lipogenic gene expression levels. Oil Red O staining confirmed increased lipid accumulation following the knockdown of ABCC4 and ABCC5. Surprisingly, the simultaneous knockdown of both transporters did not show a cumulative effect on adipogenesis, rather it led to higher levels of intracellular cAMP and extracellular prostaglandin metabolite, both of which are essential signaling molecules in adipogenesis.
Conclusion: These results highlight the complex interplay between ABCC4 and ABCC5 transporters in adipocyte function and suggest their individual contributions toward obesity and related disorders.
1 Introduction
Obesity and diabetes have become increasingly prevalent worldwide over the past 3 decades (Tiwari and Balasundaram, 2023). Since 1975, global obesity rates have nearly tripled, presenting a significant risk factor for cardiovascular disease due to increased insulin resistance and inflammation (Bhupathiraju and Hu, 2016; Ortega et al., 2016). Overall, obesity has garnered considerable attention as a significant health hazard, underscoring the urgent need to identify novel factors involved in its progression. Our laboratory has recently made important contributions in identifying a novel genetic factor associated with the development of obesity and establishing its plausible molecular mechanism(s) (Donepudi et al., 2021).
Obesity is characterized by increased adipose tissue mass that results from hypertrophy and hyperplasia of adipocytes (Hajer et al., 2008). Adipocytes are specialized cells that play an important role in energy homeostasis (Luo and Liu, 2016). ABCC4, an ATP-binding cassette class of plasma membrane efflux transporter, has been reported to play an important role in adipose tissue physiology by regulating adipogenesis. Our laboratory has established that ABCC4 knockout mice exhibited a noteworthy increase in adipose tissue weight and adipocyte hypertrophy compared to wild-type mice (Donepudi et al., 2021). Blocking ABCC4 function in mice embryonic fibroblasts or pre-adipocyte cells using pharmacological inhibitors promotes adipogenesis by upregulating various signaling molecules involved in this process. However, there are concerns regarding the substrate selectivity and specificity of pharmacological inhibitors of ABCC4. MK-571, developed initially as a cysteinyl leukotriene receptor 1 antagonist, is a broad-spectrum ABCC inhibitor and thus does not display selectivity towards ABCC4 (Bertollotto et al., 2018). Moreover, there have been reports that other ABCC transporters, such as ABCC1 and ABCC5, which transport similar substrates as ABCC4, also play an important role in adipogenesis (Cheung et al., 2014).
To investigate the specific role of ABCC4 in adipose tissue physiology, we employed a loss of gene function approach utilizing siRNA. Our study utilized 3T3-L1 cells, derived from mouse embryonic fibroblasts, as a model system. Prior studies have reported difficulties in silencing genes using siRNA in differentiated 3T3-L1 cells with a standard lipid-based transfection system (Kilroy et al., 2009). In addition, the timing of siRNA treatment in 3T3-L1 cells impacts the degree of adipogenesis, as these cells require 8–10 days to differentiate fully.
To address these challenges, we have used the non-liposomal polymeric system TransIT-TKO (MirusBio, Madison, WI) and multiple siRNA treatments during the differentiation of 3T3-L1 cells. Following ABCC4 silencing, we examined the compensatory regulation of other ABCC family transporters and their involvement in adipogenesis. This study highlights the selectivity of ABCC4 silencing and its impact on the broader transport network within adipocytes.
2 Materials and methods
2.1 3T3-L1 cell differentiation
Murine 3T3-L1 cells (2 × 105 cells) were cultured in 12 well plates using Dulbecco’s Modified Eagle’s Medium (DMEM) with high glucose (Gibco). The media was supplemented with 10% Fetal Bovine Serum, Qualified (Gibco), and 1% penicillin and streptomycin (Gibco). The cells were grown in complete media until they reached 100% confluency. Differentiation was initiated 2 days post-confluency using a MDI cocktail containing 0.5 mM isobutyl methylxanthine (IBMX), 1 μM dexamethasone, and 10 μg/mL insulin. After 48 h of induction, the media was changed to post-differentiation media (complete growth media with 10 μg/mL insulin). After an additional 48 h, the medium was again changed to complete growth media. The cells were maintained in complete media for 72–96 h. Eight days after adding the differentiation media, cells were fully matured to differentiated adipocytes.
2.2 Quantitative RT-qPCR
Total RNA was purified from the cultured cells using the phenol-chloroform standard isolation method. The total RNA was measured using NANODROP 2000c Spectrophotometer (Thermo Fisher Scientific). Complementary DNA was made using the High-Capacity cDNA Reverse Transcription Kit (Applied Biosystems, 4368814) according to the manufacturer’s protocol. Real-time PCR was performed using Universal Master Mix II, no UNG. (Catalog: 4440040) with TaqMan™ probe-based assay and measured using 7,500 Fast Real-Time PCR System (Applied Biosystems) and the 7,500 v.2.3 software. Expression of each gene transcript was determined relative to the reference gene transcript (GAPDH) and normalized to the expression of the target gene using 2−ΔΔCT method. Gene specific primers of ABCC4 (Assay ID: Mm01226372_m1), ABCC1 (Assay ID: Mm00456156_m1), ABCC2 (Assay ID: Mm00496899_m1), ABCC5 (Assay ID: Mm01343626_m1), GAPDH (Assay ID: Mm99999915_g1), PPARγ (Assay ID: Mm01184321_m1), LPL (Assay ID: Mm00434764_m1), FABP4 (Assay ID: Mm00445878_m1), C/EBPα (Assay ID: Mm07294206_s1), PGC-1α (Assay ID: Mm01208835_m1), ATGL (Assay ID: Mm00503040_m1), GLUT4 (Assay ID: Mm00436615_m1) and CD-36 (Assay ID: Mm00432403_m1) were all procured from Thermo Fisher Scientific. Data were compared with the control group treated with Silencer™ Select Negative Control No. 1 siRNA.
2.3 siRNA transfection
Gene silencing of ABCC4, ABCC1, and ABCC5 was carried out by siRNA procured from Thermo Fisher Scientific. 3T3-L1 cells were transfected with Silencer™ Select Negative Control No. 1 siRNA, ABCC4 Silencer™ Select (Assay ID: s108961), ABCC1 Silencer™ Select (Assay ID: s69747) and ABCC5 Silencer™ Select (Assay ID: s203177) using TransIT-TKO (MirusBio, Madison, WI), a non-liposomal-based polymeric transfection reagent. TransIT-TKO was diluted with Reduced Serum Medium OPTI-MEM (Gibco). The sequence of siABCC4 is sense, 5′- CGAAUGGAAAUAUAACGGAtt-3′, guide strand 5′- UCCGUUAUAUUUCCAUUCGca-3′, siABCC1 sense 5′-GGCUUAACACCAUAAUGGAtt-3′, guide strand 5′-UCCAUUAUGGUGUUAAGCCga-3′ and siABCC5 sense 5′- CCCGAGUGGUUCACAAGAAtt-3′, guide strand 5′-UUCUUGUGAACCACUGGGGcc-3’. For single treatment, 3T3-L cells were seeded at a density of 2 × 105 per well and were treated with 50 nM siRNA on day 6 of the differentiation process. After 48 h, the transfected cells were analyzed by RT-qPCR. For multiple siRNA treatments, cells were treated with 25 nM siRNA on day 0, day 3, and day 6 during the differentiation process, and the cells were analyzed 48 h after the last treatment.
2.4 Western blotting analysis
2 × 105 3T3-L1 cells were seeded per well in 12 well plates and differentiated into mature adipocytes. Total protein was isolated from mature cells using RIPA buffer and was quantified using Pierce™ BCA Protein Assay kit. Isolated proteins (30 µg/well) were electrophoretically resolved using 8%–10% polyacrylamide gels which were then transferred onto the PVDF membrane. Immunochemical detection of protein was performed using MRP4 (M4I-10, 1:500) antibody (Abcam, Cambridge, MA), and GAPDH (14C10, 1:3000) antibody (Cell Signaling Technology, Danvers, MA). Protein-antibody complexes were detected using peroxidase-conjugated affinipure Goat Anti-Rat IgG (H + L) secondary antibody. Protein bands were visualized by Chemidoc (Bio-Rad) using luminol: peroxide (1:1) solution. Data were analyzed using ImageJ software.
2.5 Lipid content assays
Lipid accumulation and adipogenesis of 3T3-L1 cells were determined using Oil Red O (ORO) staining. 2 × 105 cells were seeded and differentiated into mature adipocytes. On the day of staining (day 8), cell culture media was removed, and cells were washed with PBS twice. After washing, cells were fixed with 10% neutral buffered formalin for 15 min. Cells were further washed with water and air-dried for 2–3 min. Post drying, 60% isopropyl alcohol was added in each well for 5 min and then cells were stained with 60% ORO working solution for 30 min. The staining reagent was removed from the wells and cells were washed twice with water. Stained lipids inside the cells were visualized under the microscope. Quantification of ORO accumulation in the cells was carried out by eluting the accumulated dye in 100% isopropyl alcohol. The optical density of untreated and treated cells was measured at 540 nm using a BioTek multi-plate reader.
2.6 Determination of intracellular cAMP levels
Intracellular cAMP levels were analyzed using a cAMP ELISA kit (Catalog: 581001, Cayman Chemical, Ann Arbor, MI). 2 × 105 cells were seeded in 12 well plates. After 48 h of the last siRNA treatment, cells were treated with forskolin (10 µM) for 30 min in the serum-free media. After 30 min, the cell supernatant was removed, and the cells were washed with PBS. After washing, cells were incubated for 20 min in 0.1 N HCl. Cell lysate was collected, and intracellular cAMP levels were determined using standard manufacturer protocol.
2.7 Determination of extracellular prostaglandin E levels
Extracellular prostaglandin E2 (PGE2) levels in the cell culture media were determined using PGE2 ELISA kit-Monoclonal (Catalog: 514010, Cayman Chemical, Ann Arbor, MI) as per the standard manufacturer’s protocol. 3T3-L1 cells (2 × 105) were seeded in 12 well plates and were differentiated into mature adipocytes. Cell culture media in each well were collected 48 h after the last treatment of siRNA and used for the analysis.
2.8 Statistical analysis
Statistical analysis was performed using GraphPad Prism 8 software. One-way ANOVA followed by multiple comparison tests were carried out. For statistical analysis, a 95% confidence interval and p-value <0.05 were considered significant.
3 Results
3.1 3T3-L1 cell differentiation and expression of ATP-binding cassette transporters
To gain insights into the expression profile of the ABCC4 transporter throughout 3T3-L1 cell differentiation, we conducted assessments of both mRNA and protein levels of ABCC4 at different stages of the process. Isobutyl methylxanthine (IBMX), dexamethasone, and insulin (MDI) hormone treatment stimulates adipogenesis in 3T3-L1 fibroblasts wherein the long elliptical pre-adipocytes get converted into oval-shaped cells associated with lipid droplets in the cytoplasm. We noted that adding an MDI cocktail in the media after 48 h post-confluency of pre-adipocytes showed optimal differentiation with significant lipid deposition compared to differentiation when initiated at pre-confluency (Supplementary Figure S1).
Next, post-addition of the MDI cocktail, cells showed a 1.74-fold high expression of the ABCC4 gene compared to the growth phase (in pre-adipocytes) and a gradual decrease in ABCC4 expression as cells matured into adipocytes (on day 8). The protein levels of ABCC4 were much higher (0.8-fold) in pre-adipocytes than in mature adipocytes (Figures 1A, B).
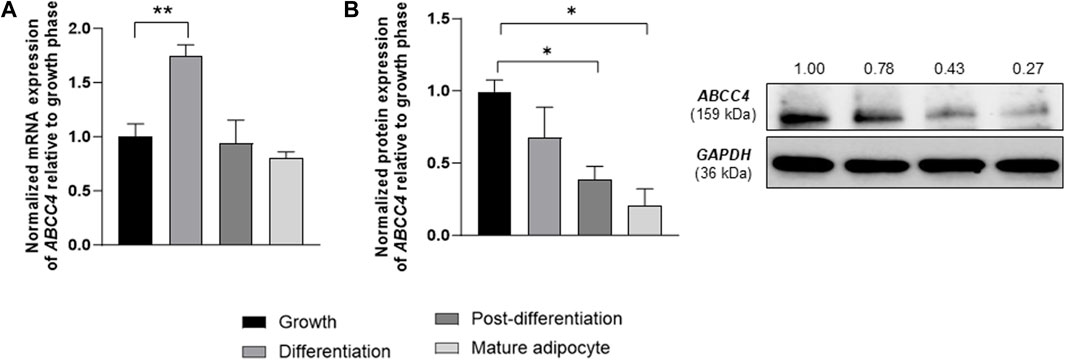
Figure 1. 3T3-L1 cell differentiation and expression of ATP-binding cassette transporters. (A) ABCC4 gene expression levels in 3T3-L1 cells at indicated phases of differentiation. Gene expression levels were normalized with GAPDH as control. Data are presented as mean + SD (n = 3). (B) ABCC4 protein levels in 3T3-L1 cells at indicated phases of differentiation. Protein levels were normalized relative to GAPDH as control. Data are presented as mean + SD (n = 3). One-way ANOVA followed by the Dunnett test was performed. Asterisks represent significant p values. *p ≤ 0.05 and **p ≤ 0.01 were considered statistically significant.
3.2 ABCC4 gene silencing by siRNA
Following confirmation of ABCC4 expression in 3T3-L1 cells across various differentiation stages, we employed siRNA to investigate ABCC4 transporter function on 3T3-L1 adipocyte physiology. Initially, siRNA treatment on day 6 of differentiation on adherent 3T3-L1 cells (forward transfection) did not result in ABCC4 downregulation. However, siRNA treatment by reverse transfection on day 6 reduced ABCC4 gene expression by 50%, though no significant change in protein expression was observed. Furthermore, reverse transfection altered cell morphology and led to a notable reduction in entrapped lipids (Data not shown).
We employed the non-liposomal lipid/polymeric TransIT-TKO (MirusBio, Madison, WI) to enhance silencing efficiency as a transfection agent. Treatment with siRNA on day 6 of 3T3-L1 cell differentiation resulted in a 0.34-fold change in ABCC4 gene expression. Interestingly, this silencing effect did not induce changes in cell morphology, and ABCC4 protein expression levels were unchanged (Supplementary Figure S2). Furthermore, the knockdown of ABCC4 did not demonstrate any observable effects on downstream targets associated with adipogenesis and lipogenesis (Data not shown).
Subsequently, we conducted multiple siRNA treatments on days 0, 3, and 6 during differentiation (Figure 2A). These multiple treatments significantly impacted gene and protein expression levels around 48 h after the last siRNA treatment. We observed approximately a 0.41-fold reduction in ABCC4 gene expression and a 0.49-fold change in protein levels (Figure 2B).
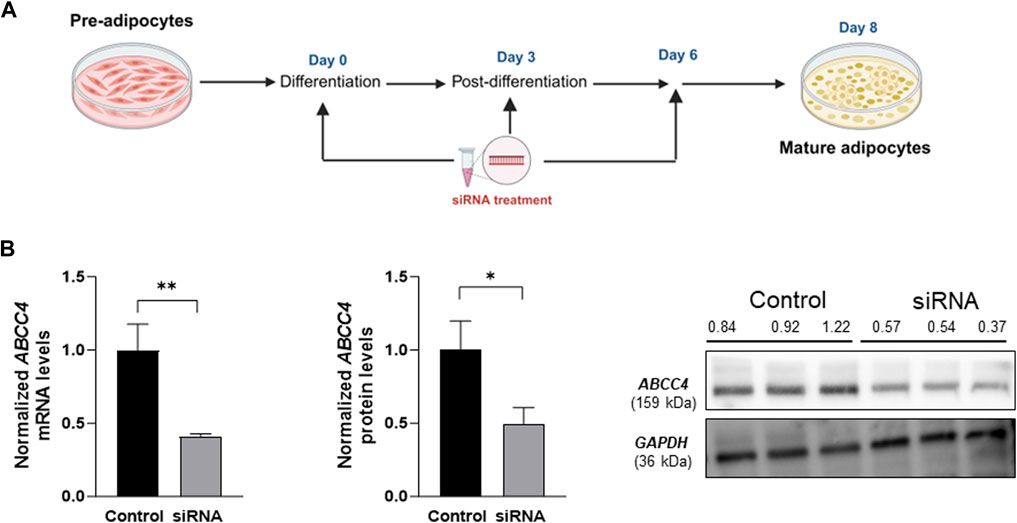
Figure 2. ABCC4 gene silencing by siRNA. (A) Workflow for multiple siRNA treatments at 25 nM each during 3T3-L1 cell differentiation. (B) Normalized ABCC4 gene and protein expression levels post multiple siRNA treatment. Both gene and protein level expression data sets were normalized against GAPDH as control. Data are presented as mean + SD (n = 3). An unpaired t-test was performed. Asterisks represent significant p values. *p ≤ 0.05 and **p ≤ 0.01 were considered statistically significant.
3.3 Crosstalk between ATP-binding cassette transporters in 3T3-L1 mature adipocytes
Among the ATP-binding cassette transporter family ‘C' genes, ABCC4, ABCC1, ABCC2, and ABCC5 were detected in 3T3-L1 mature adipocytes. Notably, ABCC2 levels were the lowest amongst other transporters and ABCC5 expression was found to be 1.24-fold higher as compared to ABCC4 (Figure 3). Through siRNA-mediated knockdown of ABCC4 gene function, significant alterations in the gene expression patterns of ABCC1 and ABCC5 were observed. Specifically, ABCC1 expression increased by 1.67-fold, while ABCC5 expression decreased by 0.60-fold, indicating potential crosstalk among ABCC transporters in mature adipocytes (Figure 4A).
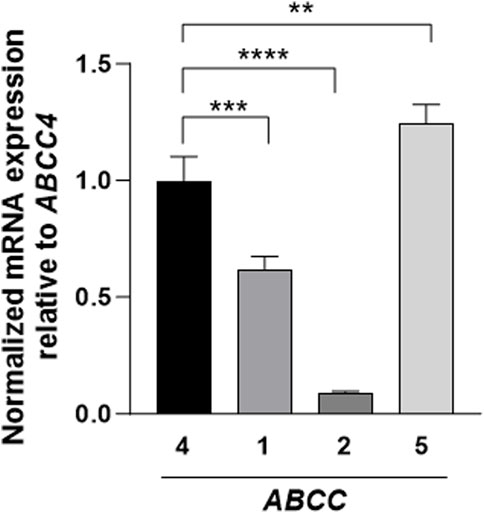
Figure 3. Basal expression of ABCC transporters in mature 3T3-L1 cells. Basal gene expression levels of ABCC transporters in comparison with ABCC4. Gene expressions were normalized against GAPDH as control. Data are presented as mean + SD (n = 3). One-way ANOVA followed by the Dunnett test was performed. Asterisks represent significant p values. **p ≤ 0.01, ***p ≤ 0.001 and ****p ≤ 0.0001 were considered statistically significant.
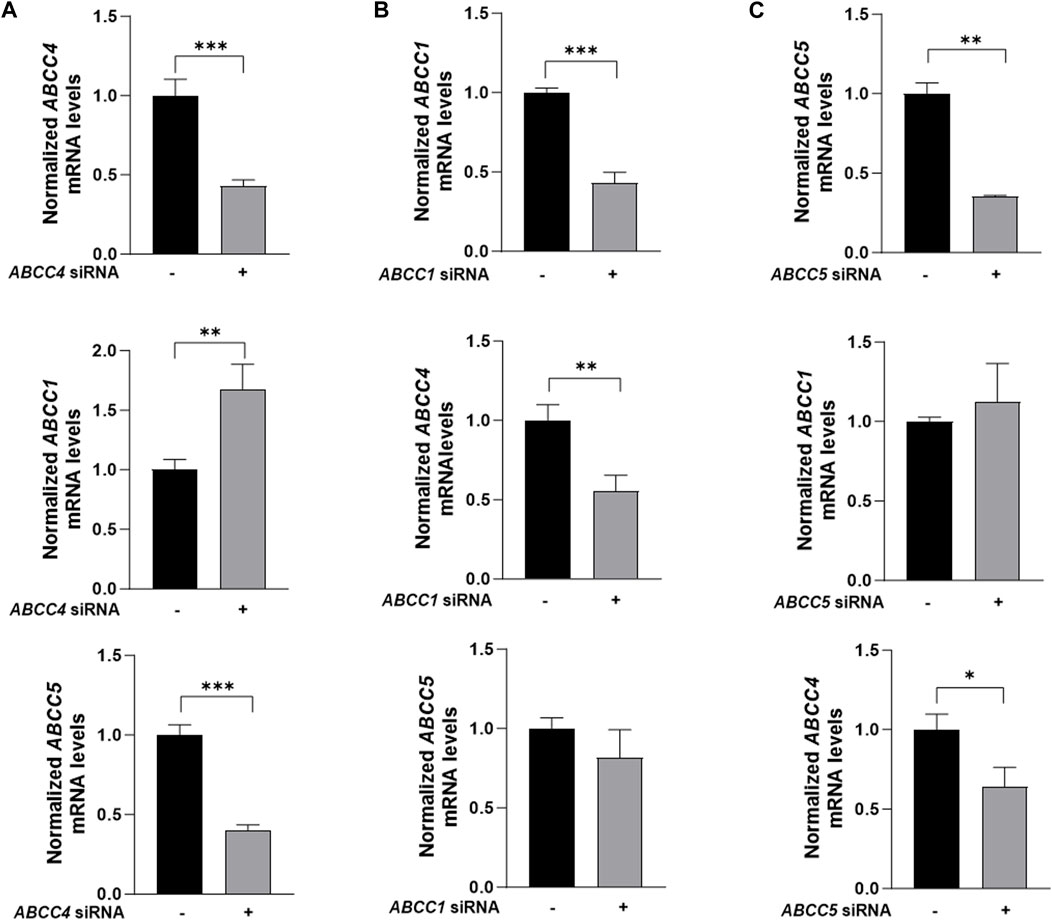
Figure 4. Silencing effect of ABCC transporters in 3T3-L1 mature adipocytes. (A) Effect of ABCC4 silencing on ABCC1 and ABCC5. (B) Effect of ABCC1 silencing on ABCC4 and ABCC5. (C) Effect of ABCC5 silencing on ABCC1 and ABCC4. Gene expression levels were normalized with GAPDH as control. Data are presented as mean + SD (n = 3). An unpaired t-test was performed. Asterisks represent significant p values. *p ≤ 0.05, ** p ≤ 0.01 and ***p ≤ 0.001 were considered statistically significant.
To further investigate this crosstalk, individual gene knockdown experiments were conducted, and their effects on other transporters were evaluated. ABCC1 gene knockdown using siRNA resulted in a 0.45-fold reduction in ABCC4 expression, while no significant effect was observed on ABCC5 levels (Figure 4B). Conversely, ABCC5 siRNA knockdown led to a 0.36-fold change in ABCC4 gene levels, without impacting ABCC1 expression (Figure 4C).
Given the high expression levels of ABCC4 and ABCC5 in mature adipocytes, we focused on these transporters to investigate crosstalk in 3T3-L1 mature cells.
3.4 ABCC4 and ABCC5 knockdown promotes lipid recruitment
Silencing ABCC4 function in 3T3-L1 cells resulted in a reduction in the expression level of the ABCC5 gene, and interestingly, we observed that this effect was bidirectional. However, their combined effect did not demonstrate synergy when ABCC4 and ABCC5 were simultaneously knocked down. This was evident from the lipid recruitment in 3T3-L1 cells post-maturation. Upon staining with Oil Red O, fully differentiated 3T3-L1 cells treated with siRNA exhibited significantly higher lipid accumulation than those treated with a control (Figure 5A). Upon quantification of lipid accumulation, we noticed that there was no significant difference between the lipid accumulation levels observed in individual gene knockdown versus the combination treatment (Figure 5B).
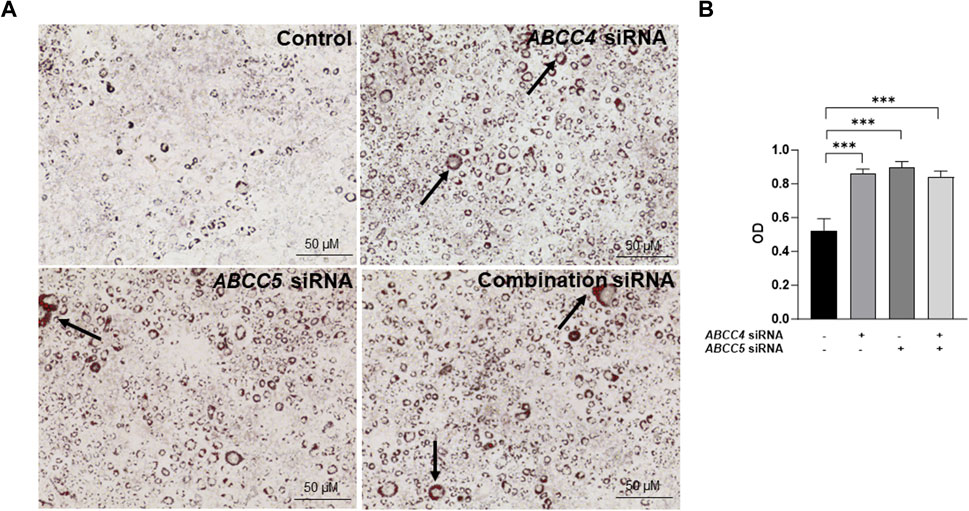
Figure 5. ABCC4 and ABCC5 knockdown promotes lipid recruitment. (A) Brightfield images of Oil red O staining of 3T3-L1 cells post silencing (Images taken at ×100 magnification). The arrow indicates the lipid droplets. The scale bar represents 50 μM. (B) Data represent lipid droplets’ optical density (OD) values following Oil red O staining. Data are presented as mean + SD (n = 3). One-way ANOVA followed by the Dunnett test was performed. Asterisks represent significant p values. ***p ≤ 0.001 was considered statistically significant.
3.5 ABCC4 and ABCC5 knockdown promotes adipogenesis in 3T3-L1 differentiated cells
After evaluating lipid recruitment following ABCC4 and ABCC5 knockdown, we investigated whether ABCC4/ABCC5 silencing alters adipose physiology by assessing the gene expression of adipogenic markers. Gene expression analysis in completely differentiated cells showed that knocking down ABCC4 and ABCC5 function increased the expression of adipogenic genes such as peroxisome proliferator-activated receptor gamma (PPARγ), CCAAT/enhancer-binding protein-alpha (C/EBPα), fatty acid binding protein 4 (FABP4), lipoprotein lipase (LPL) and glucose transporter type 4 (GLUT4) (Figure 6). ABCC4 and ABCC5 knockdown significantly increased FABP4 expression by 3.99 and 4.19-fold, respectively. For FABP4 expression, combined knockdown of ABCC4 and ABCC5 showed a more significant effect, with approximately a 5.5-fold upregulation compared to the control. Likewise, PPARγ, a transcription factor involved in regulating FABP4 expression and adipogenesis, was upregulated by 2.99-fold following ABCC4 silencing and by 4.26 after ABCC5 individual treatment, compared to the control. However, PPARγ did not show a synergistic effect with the combination treatment, though the impact of ABCC5 knockdown was more pronounced than that of ABCC4 knockdown. C/EBPα, reported to be involved in the regulation of PPARγ expression and considered an early regulator of adipogenesis (Guo et al., 2015), was found to be upregulated significantly more by ABCC5 knockdown more considerably than by ABCC4 siRNA or the combined knockdown (3.88-fold). LPL plays a critical role in lipid metabolism by breaking down triglyceride into free fatty acids, which adipose tissue can take up. ABCC4 and ABCC5 silencing upregulated LPL expression by 2.8 and 5.2-fold respectively. A combination of ABCC4 and ABCC5 silencing showed a 5.1-fold increased expression of LPL.
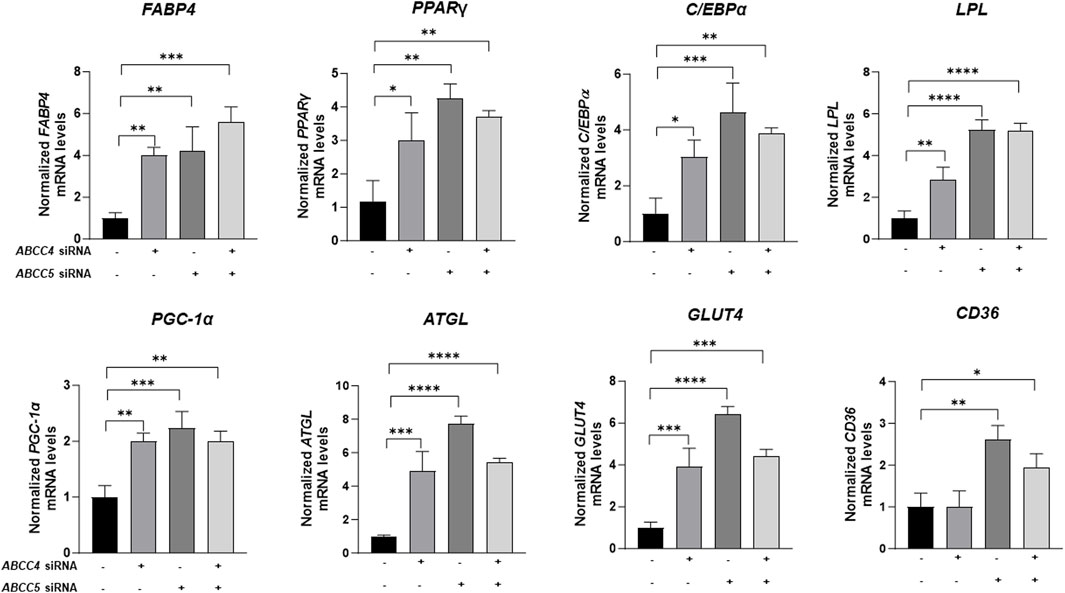
Figure 6. Effect of ABCC4 and ABCC5 siRNA knockdown on genes associated with adipogenesis. PPARγ-Peroxisome proliferator-activated receptor gamma, LPL-Lipoprotein lipase FABP4-Fatty acid binding protein 4, C/EBPα-CCAAT/enhancer-binding protein-alpha, PGC-1α-Peroxisome proliferator-activated receptor-gamma coactivator 1 alpha, ATGL- Adipose triglyceride lipase, GLUT4- Glucose transporter type 4, CD36− Cluster of differentiation 36. Effect ABCC silencing on adipogenic genes. Gene expression levels were normalized with GAPDH as control. Data are presented as mean + SD (n = 3). One-way ANOVA followed by the Dunnett test was performed. Asterisks represent significant p values. *p ≤ 0.05, **p ≤ 0.01, ***p ≤ 0.001 and ****p ≤ 0.0001 were considered as statistically significant.
PGC-1α is a transcription co-activator that interacts with a broad range of transcription factors involved in fatty acid metabolism (Liang and Ward, 2006). Increased expression of PGC-1α promotes transcriptional activation of PPARγ. siRNA silencing of ABCC4 and ABCC5 upregulated the expression of PGC-1α by 2 and 2.23-fold, respectively. The combination treatment also upregulated the expression of PGC-1α, but at a lesser magnitude than ABCC5. ATGL, an enzyme that catalyzes the first reaction of lipolysis and releases free fatty acids from triglyceride (Cerk et al., 2018) was also found to be upregulated in siRNA treatment groups. ABCC5 silencing had a prominent effect on ATGL levels compared to ABCC4 silencing and combined siRNA knockdown. Increased expression of GLUT4 is an indicator of impaired glucose uptake and energy expenditure that leads to adipose cell hypertrophy due to excessive lipid storage (Wang et al., 2020). Significant upregulation of GLUT4 after ABCC4 and ABCC5 siRNA treatment indicates its role in promoting adipogenesis. ABCC5 siRNA treatment showed a 6.40-fold increased expression of GLUT4, significantly higher than ABCC4 siRNA and siRNA combination treatment. In addition, the gene involved in fatty acid translocation, CD36, is also upregulated at the highest level after ABCC5 siRNA treatment compared to ABCC4 and combination. ABCC4 siRNA treatment did not change the CD36 gene as compared to negative control siRNA treatment.
3.6 ABCC4 and ABCC5 mediate the transport of cAMP and prostaglandins (PGE2) in differentiated 3T3-L1 cells
Cyclic nucleotides, bile acids, and prostaglandins are important signaling molecules transported by ABCC4 and ABCC5 (Russel et al., 2008). Specifically, cAMP and prostaglandins are recognized as substrates of both ABCC4 and ABCC5, contributing significantly to regulating adipogenesis (Petersen et al., 2008; Fujimori, 2012) Knockdown of ABCC4 and ABCC5 led to increased intracellular cAMP levels (Figure 7A), underscoring their role in promoting adipogenesis. Interestingly, simultaneous knockdown resulted in a slightly more pronounced increase in cAMP levels, although the difference was not statistically significant compared to individual treatments. Additionally, we assessed prostaglandin E (PGE2), a known inhibitor of adipogenesis (Coetzee et al., 2005) and observed decreased levels of PGE2 in the extracellular media (Figure 7B). This finding further confirms the role of ABCC4 and ABCC5 in promoting adipogenesis in differentiated 3T3-L1 cells.
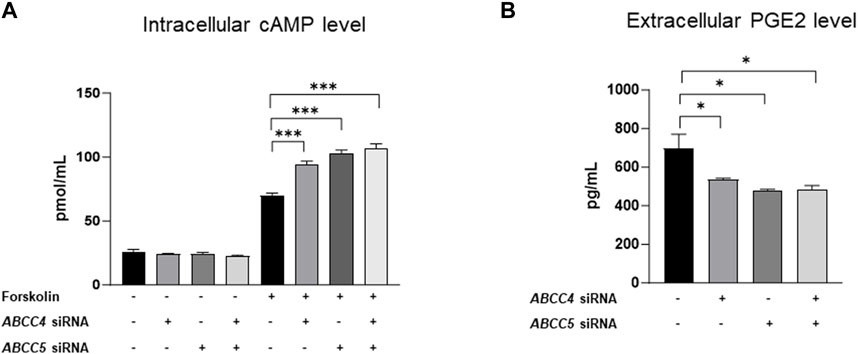
Figure 7. ABCC4 and ABCC5 silencing promotes adipogenesis in 3T3-L1 differentiated cells. The deficiency of ABCC4 and ABCC5 function alters cAMP and PGE2 levels in intra- and extracellular compartments, respectively. (A) Intracellular cAMP levels in 3T3-L1 cells treated with negative control or forskolin or ABCC4 and ABCC5 siRNA. (B) Intracellular PGE2 levels in 3T3-L1 cells treated with negative control or ABCC4 and ABCC5 siRNA. Data are presented as mean + SD (n = 3). One-way ANOVA followed by the Bonferroni (for cAMP) and Dunnett (for PGE2) were performed. Asterisks represent significant p values. *p ≤ 0.05 and ***p ≤ 0.001 were considered as statistically significant.
4 Discussion
Adipogenesis is a multi-step process regulated by complex signaling steps, resulting in significant changes in cell morphology (Jakab et al., 2021). After adipogenesis, pre-adipocytes are occupied by large lipid droplets and considered mature adipocytes, which play a crucial role in the regulation of nutrient metabolism (Wang et al., 2013). Past few studies provide evidence of the involvement of transporter protein in regulating nutrient balance. ABCC4 protein has gained a lot of interest for its role in adipogenesis. Employing a loss-of-function approach, we investigated the specific impact of ABCC4 on lipid homeostasis. Mice embryonic fibroblast, 3T3-L1 cells were used in this study, which can be considered an ideal model for studying adipogenesis (Ruiz-Ojeda et al., 2016).
Gene silencing in mature adipocytes is associated with challenges that arise due to lipids in the cells, particularly in fully differentiated 3T3-L1 cells. Previous reports also highlighted the hindrance posed by intracellular neutral lipid content in mature 3T3-L1 cells for the entry of positively charged lipid-based transfection reagents (Kilroy et al., 2009). Our approach of utilizing a polymeric non-liposomal transfection reagent successfully overcame this difficulty, ensuring effective silencing of ABCC4 in differentiated adipocytes. Moreover, the timing of siRNA treatment during the differentiation process significantly influences transfection efficiency and downstream effects on adipogenesis-related targets. Our experiments demonstrated that ABCC4 silencing on day 6 of differentiation achieved robust knockdown of the ABCC4 gene. However, the lack of significant impact on adipogenesis suggests that by day 6, the cells are already extensively differentiated into adipocytes, exhibiting minimal morphological changes in response to gene silencing. Multiple siRNA treatments on day 0, day 3, and day 6 showed a silencing effect at both gene and protein levels and affected downstream genes related to adipogenesis.
Various studies have highlighted the outcome of altered expression of ABCC transporters. Reports have highlighted failure in the treatment of pediatric patients with acute lymphoblastic leukemia due to altered expression of the ABCC transporter gene. Their finding revealed that expression of ABCC2-6 was elevated and ABCC1 and 10 were downregulated which is associated with the progression of acute lymphoblastic leukemia (Mehrvar et al., 2019).
Our observations also indicate that silencing the function of ABCC4 triggers alterations in the levels of other ABCC family transporters, suggesting the presence of positive feedback regulation among these transporters. Particularly important was the identified crosstalk between ABCC4 and ABCC5, wherein silencing ABCC4 led to a subsequent reduction in ABCC5 expression. This phenomenon underscores the interconnected nature of transporter networks and implies potential regulatory interactions between ABCC4 and ABCC5 in adipocyte biology. In addition, another reason behind ABCC4 and ABCC5 mutual regulation is shared signaling pathways or common transcription factors. ABCC4 and ABCC5 are also involved in nucleotide transport and drug resistance and are reported to be co-regulated by stress response mechanisms (Ritter et al., 2005). ABCC1 silencing did not show any effect on ABCC5, which could be due to the higher expression level of ABCC5 compared to ABCC1 in adipocytes, which diminishes any observable regulatory impact of ABCC1 on ABCC5.
The present study also provides proof of concept, demonstrating that silencing ABCC4 and ABCC5 disrupts the transport of cAMP and PEG2, pivotal players in the process of adipogenesis (Donepudi et al., 2021). Specifically, inhibition of ABCC4 function leads to an increase in intracellular cAMP levels. Elevated cAMP levels trigger various downstream signaling pathways crucial for promoting adipogenesis, including upregulation of C/EBPα and PPARγ expression (Fox et al., 2006). At the basal level, it is difficult to measure the levels of cAMP due to its low abundance and high phosphodiesterase activity in mature adipocytes. Forskolin challenge helps in amplifying the cAMP levels by directly stimulating adenylate cyclase, bringing them to a detectable range (Alasbahi and Melzig, 2012; Cero et al., 2023). The transcriptional activity of PPARγ is regulated synergistically by cAMP, which is an important factor for adipogenesis and glucose metabolism (Petersen et al., 2008). In our experiments with ABCC4 and ABCC5 knockdown of 3T3-L1 cells, we observed an increase in the expression of C/EBPα and PPARγ, further supporting this pathway’s role in adipogenesis.
ABCC4’s role in transporting prostaglandin E metabolites is also significant in adipogenesis. Research has shown that prostaglandin E2 (PGE2) downregulates the expression of PPARγ, a pivotal regulator of adipogenesis (Fujimori, 2012). This anti-adipogenic effect of PGE2 is mediated through activating its cell surface receptors, E-type prostanoid receptors (EP3 and EP4) (Gu et al., 2016). Inhibition of ABCC4 function reduces the levels of PGE2 in the extracellular space, potentially leading to decreased activation of EP3 and EP4 receptors. Consequently, this reduction in receptor activation could promote adipogenesis by alleviating the anti-adipogenic effects mediated by PGE2. Interestingly, our study also revealed that ABCC5 influences PGE2 transport, contributing to the upregulation of adipogenesis. The resulting changes in cAMP and PGE2 levels collectively promote adipogenesis in ABCC4 and ABCC5 silenced cells.
In conclusion, our study highlights the intricate interplay between ABCC transporters and their impact on adipocyte biology. Understanding the functional roles of specific transporters such as ABCC4 and ABCC5 enhances our knowledge of adipogenesis regulation and holds promise for potential therapeutic interventions targeting adipocyte function and metabolism.
5 Future direction
Considering the findings from our present study, our future investigations aim to explore the response of 3T3-L1 cells under ABCC4 overexpression conditions and its impact on the degree of adipogenesis. Additionally, during the differentiation phase, we observed an increase in ABCC4 mRNA levels, which returned to the same level as preadipocyte levels by the time the cells fully matured. However, at the protein level, ABCC4 expression showed a gradual reduction. This discrepancy could be attributed to the highly differentiating state of 3T3-L1 cells, which may lead to post-translational modifications affecting protein stability or degradation. While we did not explore these mechanisms in the current study, this is an important area for future research better to understand the regulation of ABCC4 protein expression during adipogenesis. We are also interested in the potential crosstalk among ABCC family transporters and plan to delve deeper into understanding its underlying mechanisms. Furthermore, differential metabolomics could help identify ABCC4 transported molecules that play functional roles in adipogenesis, offering insights into the metabolic pathways influenced by ABCC4 activity.
Data availability statement
The original contributions presented in the study are included in the article/Supplementary Material, further inquiries can be directed to the corresponding author.
Ethics statement
Ethical approval was not required for the studies on animals in accordance with the local legislation and institutional requirements because only commercially available established cell lines were used.
Author contributions
AL: Data curation, Formal Analysis, Methodology, Project administration, Writing–original draft. AW: Data curation, Formal Analysis, Methodology, Project administration, Writing–original draft. RB: Data curation, Funding acquisition, Investigation, Resources, Supervision, Validation, Visualization, Writing–review and editing. JM: Conceptualization, Data curation, Funding acquisition, Investigation, Resources, Supervision, Validation, Visualization, Writing–review and editing.
Funding
The author(s) declare that financial support was received for the research, authorship, and/or publication of this article. This work was supported by NIH R01 (1R01CA241194-01A1) grant to RB.
Conflict of interest
Author JM is the Boehringer Ingelheim Pharmaceuticals, Inc. Endowed Chair in Mechanistic Toxicology and is supported by an Endowment Fund.
The remaining authors declare that the research was conducted in the absence of any commercial or financial relationships that could be construed as a potential conflict of interest.
Publisher’s note
All claims expressed in this article are solely those of the authors and do not necessarily represent those of their affiliated organizations, or those of the publisher, the editors and the reviewers. Any product that may be evaluated in this article, or claim that may be made by its manufacturer, is not guaranteed or endorsed by the publisher.
Supplementary material
The Supplementary Material for this article can be found online at: https://www.frontiersin.org/articles/10.3389/fmolb.2024.1498946/full#supplementary-material
Abbreviations
ABC, ATP-binding cassette transporters; ATGL, Adipose triglyceride lipase; C/EBPα, CCAAT/enhancer-binding protein alpha; cAMP, Cyclic adenosine monophosphate; CD36, Cluster of differentiation 36; EP, E-type prostanoid receptors; FABP4, Fatty acid binding protein 4; GLUT4, Glucose transporter type 4; IBMX, Isobutyl methylxanthine; LPL, Lipoprotein lipase; MDI, Isobutyl methylxanthine, dexamethasone, and insulin; PGC-1α, Peroxisome proliferator-activated receptor-gamma coactivator 1 alpha; PGE2, prostaglandin E2; PPARγ, Peroxisome proliferator-activated receptor gamma.
References
Alasbahi, R. H., and Melzig, M. F. (2012). Forskolin and derivatives as tools for studying the role of cAMP. Pharmazie 67, 5–13. doi:10.1691/PH.2012.1642
Bertollotto, G. M., De Oliveira, M. G., Alexandre, E. C., Calmasini, F. B., Passos, G. R., Antunes, E., et al. (2018). Inhibition of multidrug resistance proteins by MK 571 enhances bladder, prostate, and urethra relaxation through cAMP or cGMP accumulation. J. Pharmacol. Exp. Ther. 367, 138–146. doi:10.1124/JPET.118.250076
Bhupathiraju, S. N., and Hu, F. B. (2016). Epidemiology of obesity and diabetes and their cardiovascular complications. Circ. Res. 118, 1723–1735. doi:10.1161/CIRCRESAHA.115.306825
Cerk, I. K., Wechselberger, L., and Oberer, M. (2018). Adipose triglyceride lipase regulation: an overview. Curr. Protein Pept. Sci. 19, 221–233. doi:10.2174/1389203718666170918160110
Cero, C., Shu, W., Reese, A. L., Douglas, D., Maddox, M., Singh, A. P., et al. (2023). Standardized in vitro models of human adipose tissue reveal metabolic flexibility in Brown adipocyte thermogenesis. Endocrinology 164, bqad161. doi:10.1210/ENDOCR/BQAD161
Cheung, L., Flemming, C. L., Watt, F., Masada, N., Yu, D. M. T., Huynh, T., et al. (2014). High-throughput screening identifies Ceefourin 1 and Ceefourin 2 as highly selective inhibitors of multidrug resistance protein 4 (MRP4). Biochem. Pharmacol. 91, 97–108. doi:10.1016/J.BCP.2014.05.023
Coetzee, M., Haag, M., Claassen, N., and Kruger, M. C. (2005). Stimulation of prostaglandin E2 (PGE2) production by arachidonic acid, oestrogen and parathyroid hormone in MG-63 and MC3T3-E1 osteoblast-like cells. Prostagl. Leukot. Essent. Fat. Acids 73, 423–430. doi:10.1016/J.PLEFA.2005.08.005
Donepudi, A. C., Lee, Y., Lee, J. Y., Schuetz, J. D., and Manautou, J. E. (2021). Multidrug resistance-associated protein 4 (Mrp4) is a novel genetic factor in the pathogenesis of obesity and diabetes. FASEB J. 35, e21304. doi:10.1096/FJ.202001299RR
Fox, K. E., Fankell, D. M., Erickson, P. F., Majka, S. M., Crossno, J. T., and Klemm, D. J. (2006). Depletion of cAMP-response element-binding protein/ATF1 inhibits adipogenic conversion of 3T3-L1 cells ectopically expressing CCAAT/enhancer-binding protein (C/EBP) alpha, C/EBP beta, or PPAR gamma 2. J. Biol. Chem. 281, 40341–40353. doi:10.1074/JBC.M605077200
Fujimori, K. (2012). Prostaglandins as PPARγ modulators in adipogenesis. PPAR Res. 2012, 527607. doi:10.1155/2012/527607
Gu, X., Xu, J., Zhu, L., Bryson, T., Yang, X. P., Peterson, E., et al. (2016). Prostaglandin E2 reduces cardiac contractility via EP3 receptor. Circ. Heart Fail 9. doi:10.1161/CIRCHEARTFAILURE.116.003291
Guo, L., Li, X., and Tang, Q. Q. (2015). Transcriptional regulation of adipocyte differentiation: a central role for CCAAT/enhancer-binding protein (C/EBP) β. J. Biol. Chem. 290, 755–761. doi:10.1074/JBC.R114.619957
Hajer, G. R., Van Haeften, T. W., and Visseren, F. L. J. (2008). Adipose tissue dysfunction in obesity, diabetes, and vascular diseases. Eur. Heart J. 29, 2959–2971. doi:10.1093/EURHEARTJ/EHN387
Jakab, J., Miškić, B., Mikšić, Š., Juranić, B., Ćosić, V., Schwarz, D., et al. (2021). Adipogenesis as a potential anti-obesity target: a review of pharmacological treatment and natural products. Diabetes Metab. Syndr. Obes. 14, 67–83. doi:10.2147/DMSO.S281186
Kilroy, G., Burk, D. H., and Floyd, Z. E. (2009). High efficiency lipid-based siRNA transfection of adipocytes in suspension. PLoS One 4, e6940. doi:10.1371/JOURNAL.PONE.0006940
Liang, H., and Ward, W. F. (2006). PGC-1alpha: a key regulator of energy metabolism. Am. J. Physiology. Adv. Physiology Educ. 30, 145–151. doi:10.1152/advan.00052.2006
Luo, L., and Liu, M. (2016). Adipose tissue in control of metabolism. J. Endocrinol. 231, R77–R99. doi:10.1530/JOE-16-0211
Mehrvar, N., Abolghasemi, H., Rezvany, M. R., Akbari, M. E., Saberynejad, J., Mehrvar, A., et al. (2019). Pattern of ABCC transporter gene expression in pediatric patients with relapsed acute lymphoblastic leukemia. Rep. Biochem. Mol. Biol. 8, 184. 193.
Ortega, F. B., Lavie, C. J., and Blair, S. N. (2016). Obesity and cardiovascular disease. Circ. Res. 118, 1752–1770. doi:10.1161/CIRCRESAHA.115.306883
Petersen, R. K., Madsen, L., Pedersen, L. M., Hallenborg, P., Hagland, H., Viste, K., et al. (2008). Cyclic AMP (cAMP)-mediated stimulation of adipocyte differentiation requires the synergistic action of Epac- and cAMP-dependent protein kinase-dependent processes. Mol. Cell. Biol. 28, 3804–3816. doi:10.1128/MCB.00709-07
Ritter, C. A., Jedlitschky, G., Meyer zu Schwabedissen, H., Grube, M., Köck, K., and Kroemer, H. K. (2005). Cellular export of drugs and signaling molecules by the ATP-binding cassette transporters MRP4 (ABCC4) and MRP5 (ABCC5). Drug Metab. Rev. 37, 253–278. doi:10.1081/DMR-200047984
Ruiz-Ojeda, F. J., Rupérez, A. I., Gomez-Llorente, C., Gil, A., and Aguilera, C. M. (2016). Cell models and their application for studying adipogenic differentiation in relation to obesity: a review. Int. J. Mol. Sci. 17, 1040. doi:10.3390/IJMS17071040
Russel, F. G. M., Koenderink, J. B., and Masereeuw, R. (2008). Multidrug resistance protein 4 (MRP4/ABCC4): a versatile efflux transporter for drugs and signalling molecules. Trends Pharmacol. Sci. 29, 200–207. doi:10.1016/J.TIPS.2008.01.006
Tiwari, A., and Balasundaram, P. (2023). Public health considerations regarding obesity. StatPearls. Available at: https://www.ncbi.nlm.nih.gov/books/NBK572122/(Accessed December 21, 2023).
Wang, Q. A., Tao, C., Gupta, R. K., and Scherer, P. E. (2013). Tracking adipogenesis during white adipose tissue development, expansion and regeneration. Nat. Med. 19, 1338–1344. doi:10.1038/nm.3324
Keywords: 3T3-L1 cells, ABCC transporter, adipogenesis, lipids, siRNA, cAMP
Citation: Laddha AP, Wahane A, Bahal R and Manautou JE (2024) Investigating the crosstalk between ABCC4 and ABCC5 in 3T3-L1 adipocyte differentiation. Front. Mol. Biosci. 11:1498946. doi: 10.3389/fmolb.2024.1498946
Received: 23 September 2024; Accepted: 18 November 2024;
Published: 09 December 2024.
Edited by:
Paola Palestini, University of Milano-Bicocca, ItalyReviewed by:
Takehito Uruno, Kyushu University, JapanKartick Patra, National Institute of Diabetes and Digestive and Kidney Diseases (NIH), United States
Copyright © 2024 Laddha, Wahane, Bahal and Manautou. This is an open-access article distributed under the terms of the Creative Commons Attribution License (CC BY). The use, distribution or reproduction in other forums is permitted, provided the original author(s) and the copyright owner(s) are credited and that the original publication in this journal is cited, in accordance with accepted academic practice. No use, distribution or reproduction is permitted which does not comply with these terms.
*Correspondence: José E. Manautou, am9zZS5tYW5hdXRvdUB1Y29ubi5lZHU=