- 1Genome Science and Technology Program, University of British Columbia, Vancouver, BC, Canada
- 2Department of Microbiology and Immunology, University of British Columbia, Vancouver, BC, Canada
- 3Life Sciences Institute, University of British Columbia, Vancouver, BC, Canada
- 4School of Biomedical Engineering, University of British Columbia, Vancouver, BC, Canada
- 5Graduate Program in Bioinformatics, University of British Columbia, Vancouver, BC, Canada
- 6Department of Biochemistry, Chulalongkorn University, Bangkok, Thailand
- 7Bradshaw Research Institute for Minerals and Mining (BRIMM), University of British Columbia, Vancouver, BC, Canada
- 8ECOSCOPE Training Program, University of British Columbia, Vancouver, BC, Canada
Volatile organic compounds (VOCs) are carbon-containing molecules with high vapor pressure and low water solubility that are released from biotic and abiotic matrices. Because they are in the gaseous phase, these compounds tend to remain undetected when using conventional metabolomic profiling methods. Despite this omission, efforts to profile VOCs can provide useful information related to metabolic status and identify potential signaling pathways or toxicological impacts in natural or engineered environments. Over the past several decades mass spectrometry (MS) platform innovation has instigated new opportunities for VOC detection from previously intractable matrices. In parallel, volatilome research linking VOC profiles to other forms of multi-omic information (DNA, RNA, protein, and other metabolites) has gained prominence in resolving genotype/phenotype relationships at different levels of biological organization. This review explores both on-line and off-line methods used in VOC profiling with MS from different matrices. On-line methods involve direct sample injection into the MS platform without any prior compound separation, while off-line methods involve chromatographic separation prior to sample injection and analyte detection. Attention is given to the technical evolution of platforms needed for increasingly resolved VOC profiles, tracing technical progress over time with particular emphasis on emerging microbiome and diagnostic applications.
1 Introduction
Volatile organic compounds (VOCs) are gaseous carbon-containing compounds released from biotic and abiotic matrices, manifesting both high vapor pressure and low water solubility (US EPA, 2022). High vapor pressure is correlated with low boiling point and serves as a measure of compound volatility. In some cases, VOCs are associated with adverse health effects depending on concentration and exposure time. The United States Environmental Protection Agency (US EPA) has established a classification system for VOCs that recognizes three primary categories including very volatile organic compounds (VVOCs) < 0°C to 50–100°C, volatile organic compounds (VOCs) 50–100°C to 240–260°C, and semi-volatile organic compounds (SVOCs) 240–260°C to 380–400°C (US EPA, 2022). Volatilome research arises in part from an awareness that both biotic and abiotic matrices emit VOCs, and that VOC profiles obtained using mass spectrometry (MS) platforms can provide useful information about the metabolic status of biological systems as well as potential signaling pathways or toxicological impacts in natural or engineered environments. For example, researchers have identified VOCs in plant (D'Alessandro and Turlings, 2006; Majchrzak et al., 2020), human (Amann et al., 2014; Drabińska et al., 2021; Bauermeister et al., 2022; Ferrandino et al., 2023; Fu et al., 2023), microbial (Boots et al., 2014; Drabińska et al., 2022), food (Cao et al., 2016; Carraturo et al., 2020; Tiwari et al., 2020), and environmental (Liu and Phillips, 1991; Higashikawa et al., 2013; Ullah et al., 2014) matrices. Moreover, several studies have evaluated the role of VOCs in mediating regulatory and metabolic interactions at the population and community levels of biological organization (Audrain et al., 2015; Weisskopf et al., 2021).
In plants, VOCs including terpenoids, fatty acids, and benzenoids are released under normal growth conditions (Dudareva et al., 2004), or in response to environmental stressors such as increased temperature and salinity or herbivory (Sharkey and Yeh, 2001; Mumm et al., 2003; Schröder et al., 2005). Plant-derived VOCs have gained increasing attention in relation to food security and climate change due to potential applications in promoting crop stress responses, pathogen defense, and enhanced biomass production connected to carbon capture and conversion processes (Materić et al., 2015). For example, gas chromatography-mass spectrometry (GC-MS) investigation of Xanthomonas c. pv. vesicatoria 85–10 resolved over 50 VOC compounds including several plant growth promoting ketone and methylketone compounds, and one compound linked to growth inhibition of the necrotrophic fungus Rhizoctonia solani (Weise et al., 2012). From an environmental perspective, VOCs associated with 48 Actinobacteria species isolated from soil and airborne-dust were profiled using GC-MS, resolving 126 predominantly C1 to C5 compounds, including alcohols, ketones, esters with the potential to mediate metabolic interactions among and between microbes and plants (Choudoir et al., 2019). In humans, VOCs have emerged as biomarkers for diagnostic screening and monitoring disease progression (Janssens et al., 2020; Berenguer et al., 2022), as well as detection of pathogens and antimicrobial resistance (AMR) phenotypes (Dixon et al., 2022). For example, VOC detection has been used for biomarker discovery in pulmonary tuberculosis (Fu et al., 2023), cystic fibrosis (Kaeslin et al., 2021), asthma, chronic obstructive pulmonary disease (Ratiu et al., 2020), lung (Ratiu et al., 2020; Temerdashev et al., 2023), prostate (Berenguer et al., 2022) and other type of cancers (Le and Priefer, 2023), urinary tract (Drabińska et al., 2022) and intestinal infections (John et al., 2021), irritable bowel syndrome (Zhang et al., 2023), as well as neurological disorders (Khan et al., 2021). In addition, several studies using isolated Escherichia coli have detected VOCs using solid-phase microextraction-gas chromatography coupled with mass spectrometry (SPME-GC-MS) on liquid cultures (Drabińska et al., 2022), or in strains cultivated on blood agar plates using thermal desorption gas chromatography coupled with time-of-flight MS (TD-GC-TOF-MS) (Boots et al., 2014). Similarly, from an industrial perspective, GC-MS analysis of Chinese milk fan (cheese) containing bacteria affiliated with Lactococcus, Lactobacillus, Raoultella and fungi affiliated with Rhodotorula, Torulaspora, and Candida fungi species identified 60 VOCs, including alcohols, aldehydes, ketones, esters, and aromatic compounds contributing to milk fan aroma (Chen et al., 2021).
The emergence of volatilome research is closely coupled with MS platform innovation instigating new opportunities for VOC detection from previously intractable matrices. MS platforms can use either on-line or off-line VOC detection methods that are closely coupled with increasing throughput and resolving power, respectively. On-line methods utilize direct sample introduction to the MS without upstream sample cleanup and compound separation protocols, while off-line techniques employ various analyte separation methodologies prior to MS detection. Previous reviews have discussed the on-line versus off-line methods for VOC detection within specific biological systems (Lindinger et al., 1998; Smith and Španěl, 2005; Materić et al., 2015; Ahmed et al., 2017; Lawal et al., 2017; Gould et al., 2021; Westphal et al., 2023). Here we expand on these accounts by presenting a matrix-centered review of volatilome research in relation to platform innovation over time, providing a practical guide for both practitioners and potential end-users with particular emphasis on emerging microbiome and diagnostic applications.
2 VOC detection platforms
Numerous contemporary reviews on analytical methods for detecting VOCs from different matrices are available (Materić et al., 2015; Ahmed et al., 2017; Lough et al., 2017; Lubes and Goodarzi, 2017; Majchrzak et al., 2018; Gould et al., 2021). Figure 1 provides a graphical overview of MS platforms used for VOC detection over time and Table 1 summarizes key scientific literature in relation to different matrix categories and cognate detection platforms. Emphasis is placed on differentiating between on-line methods in which sample preparation is directly coupled to sample injection and analysis, and off-line methods in which the process of sample preparation and analysis are uncoupled. Table 1 includes an overarching selection of MS platform innovation from the early stage of introducing ion molecule reaction MS in 1993 to the latest technological advancements in GCxGC-TOF-MS, in 2023. Review articles are also included in the table to complement research articles with the aim to offer a comprehensive view on VOC analysis combined with MS techniques, in a wide range of matrices.
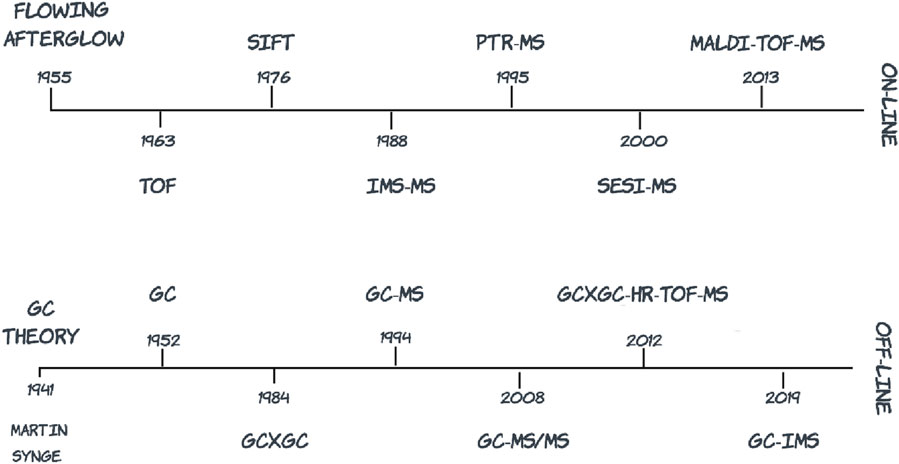
Figure 1. Summary of mass spectrometry platform innovation over the past 60 years in relation to volatile organic compound detection (TOF, time-of-flight; SIFT, selected ion flow tube; IMS-MS, ion mobility spectrometry mass spectrometry; PTR-MS, proton transfer reaction mass spectrometry; SESI-MS, secondary electrospray ionization mass spectrometry; MALDI, matrix-assisted laser desorption ionization; GC, gas chromatography; GCxGC, comprehensive two-dimensional gas chromatography; GC-MS, gas chromatography mass spectrometry; GC-MS/MS, gas chromatography triple quadrupole mass spectrometry; HR-TOF-MS, high resolution time-of-flight mass spectrometry).

Table 1. Summary of analytical techniques and applications used in the analysis of volatile organic compounds with mass spectrometry*.
2.1 On-line methods
On-line methods associated with real-time detection of analytes require minimal preparation, are compatible with both portable and high-throughput platform integration and provide relatively rapid results with reduced price point per sample. However, complex samples containing multiple structurally related analytes present a particular challenge for on-line detection.
2.1.1 Flowing afterglow and SIFT-MS
The flowing afterglow method was developed over 60 years ago to quantitatively measure ion-molecule reaction rate constants. This method utilized a microwave discharge to ionize a primary gas, with the resulting luminous glow migrating to the reaction area, where an ion-molecule reaction transfers charge to neutral species introduced into the buffer gas (Ferguson et al., 1969). In the 1970s flowing afterglow was implemented in a selected ion flow tube (SIFT) where a positively charged single species low energy primary beam was used to ionize neutral components present in a carrier gas, and the reaction was detected using quadrupole MS (Adams and Smith, 1976). The low energy ionization associated with SIFT-MS resolves fewer analyte fragments with less complex mass spectra formation in gas mixtures and alternative precursor ions can be matched with different matrices to obtain representative product ions. For example, SIFT-MS platforms have been used to quantify trace amounts of gas, even in the parts per billion (ppb) range (Smith and Spanel, 1996) down to parts per trillion volume (pptv) (Bierbaum, 2015; Smith et al., 2022) in both air and human breath using primary ion beams composed of dioxygenyl (O2+), hydronium (H3O+), or nitrosonium (NO+) (Španěl et al., 1999; Smith and Španěl, 2005) ions. SIFT-MS has also been used to monitor bacterial growth (Allardyce et al., 2006a; Allardyce et al., 2006b; Scotter et al., 2006) and to explore ion-molecule reaction kinetics in breath collection bags compared to other methods (Malásková et al., 2019).
2.1.2 IMS-MS
First developed in the 1970s, ion mobility spectrometry (IMS) initially known as plasma chromatography (Cohen and Karasek, 1970), typically uses a radioactive source to ionize analyte gasses that are then separated in a drift tube at atmospheric pressure under an electric field and the counterflow of an inert drift gas (Karasek et al., 1976; Karpas et al., 1988). Due to collisions with the drift gas molecules and electric field acceleration, charged species attain an ion mobility proportional to their shape, charge, size, etc., and their different arrival times at the Faraday plate detector enables selective and sensitive measurements (Hill et al., 1990; Fernández Maestre, 2012). IMS has branched into different forms including drift-tube ion mobility spectrometry, traveling-wave ion mobility spectrometry, trapped ion mobility spectrometry, and field-asymmetric waveform ion mobility spectrometry (Dodds et al., 2020). IMS has been used to measure VOCs in environmental samples (Takaya et al., 2022), and for more complex matrices, IMS can be interfaced with an upstream multicapillary column (MCC) containing up to 1,000 parallel capillaries to separate VOCs prior to ionization (Vautz et al., 2006; Handa et al., 2014). More recent integration of IMS with MS detection (Mukhopadhyay, 2008) enables compound detection based on drift time and mass-to-charge (m/z) ratio (Collins and Lee, 2002). Collision cross-section calculations using IMS-MS spectra can also be used to estimate the gas-phase size of ions useful for untargeted analysis (Collins and Lee, 2002; Lapthorn et al., 2013; Dodds and Baker, 2019).
2.1.3 IMR-MS and PTR-MS
In the 1980s ion-molecule reaction mass spectrometry (IMR-MS) emerged as an alternative to SIFT-MS. In contrast to SIFT-MS, IMR-MS employs a two-stage ionization process with Krypton (Kr+) or Xeon (Xe+) primary beams (Lindinger et al., 1993). During the first stage, primary reagent gas is generated through electron impact ionization, and in the second stage, reagent gas enters a reaction chamber through a lens system where charge is then transferred (Lindinger et al., 1993). Initially, IMR-MS systems were employed to analyze industrial gas mixtures, such as emission from furnaces and motors that required the use of Kr+ or Xe+ for efficient ionization (Lindinger et al., 1993). In later manifestations, IMR-MS systems capable of alternating between different primary ion beams, including Kr+, Xe+ or Mercury (Hg+) were developed and used to differentiate VOCs produced by Gram-negative and Gram-positive bacteria in headspace analysis of anaerobic blood samples (Dolch et al., 2012a; Dolch et al., 2012b). IMR-MS was also used to analyze VOCs in exhaled breath (Dolch et al., 2015; Meidert et al., 2021).
Proton transfer reaction MS (PTR-MS) introduced in the 1990s is similar to IMR-MS, but uses an ion beam composed of H3O+ with high proton affinity (Lindinger et al., 1993; Hansel et al., 1995). Use of H3O+ results in low energy ionization resolving fewer analyte fragments with less complex mass spectra formation in gas mixtures. From a VOC detection standpoint, H3O+ is effective in detecting trace gas components in exhaled breath (Lindinger et al., 1993; Hansel et al., 1995; Smith and Spanel, 1996). Additionally, the high proton affinity of H3O+ reduces ion-molecule reactions with major air components including nitrogen (N2), oxygen (O2), carbon dioxide (CO2), and water (H2O) (Lagg et al., 1994), reducing potential matrix effects (Bruderer et al., 2020). The singular use of a H3O+ makes PTR-MS less versatile than SIFT-MS with respect to matching precursor ions with different matrices to obtain representative product ions (Smith and Španěl, 2005). Despite this limitation, PTR-MS has diversified into several forms including PTR-single quadrupole MS (PTR-QMS) (Smith et al., 2014), PTR-triple-quadrupole MS (PTR-QqQ-MS) (Kohl et al., 2013), and PTR-TOF-MS (Smith et al., 2014).
PTR-QMS is a scanning MS platform with a relatively slow data acquisition rate and nominal mass resolution. However, it is effective for targeted analyses to quantitate known VOCs with accuracy and precision (Smith et al., 2014). Combined use of PTR-MS and solid phase microextraction gas chromatography time-of-flight mass spectrometry (SPME-GC-TOF-MS) has been used for monitoring applications (King et al., 2010; Majchrzak et al., 2018) including real-time detection of VOCs released from thermoplastics during 3D printing (Wojnowski et al., 2022). In addition, direct infusion (DI) PTR-MS has been used for real-time detection of VOCs released from plants in combination with multi-omic sequencing to establish a monitoring network to refine global emission budgets and observe plant metabolism at different levels of biological organization (Majchrzak et al., 2020). PTR-QqQ-MS is an extension of PTR-QMS providing higher specificity and sensitivity (Kohl et al., 2013). PTR-TOF-MS platforms collect full mass spectra with high mass resolution (Dodonov et al., 2000; Cooper-Shepherd et al., 2023) making them effective in separating isobaric compounds with high sensitivity and potentially accurate mass for untargeted analyses of complex matrices with many analyte fragments (Smith et al., 2014). Biomarkers from exhaled breath of patients with kidney dysfunction have been identified using a combination of PTR-MS, PTR-TOF-MS with structural elucidation using PTR-QqQ-MS (Kohl et al., 2013).
2.1.4 SESI-MS and Orbitrap-MS
First introduced in the late 1980s, electrospray ionization (ESI) made it efficient to ionize liquid phase polar molecules using a sensitive and low energy process (Whitehouse et al., 1986; Fenn et al., 1989; Fenn, 2002). In a variation of ESI called secondary electrospray ionization (SESI) developed in the 2000s, neutral compounds in gas phase were introduced into the nebulized spray of an ESI stream, where ionized droplets transfer charge to the gaseous species followed by MS detection (Wu et al., 2000; Vidal-de-Miguel and Herrero, 2012). SESI-MS has been used to analyze volatile fatty acids (VFAs) in exhaled breath (Martínez-Lozano et al., 2011) and has been used in combination with high-resolution MS (HRMS) time-of-flight (TOF) and Orbitrap instruments. The first commercial Orbitrap platform was introduced in 2005 for high-resolution mass spectrometry. Produced ions enter a curved trap, where they are collisionally cooled and enriched. The concentrated ion packets are injected orthogonally into the orbitrap where they go into an axial oscillation along a central electrode with a frequency that is proportional to their m/z ratio. The central electrode has an opposing electrical charge, and ion stability is achieved by high velocity oscillation that prevents the ions from crashing into the electrode. The resulting resonance signal undergoes a mathematical treatment with Fourier transform, where the oscillation signal is converted to a mass spectrum (Makarov, 2000; Zubarev and Makarov, 2013).
Both SESI and Orbitrap are typically combined with off-line chromatography methods to improve compound identification and mass resolution. SESI-HRMS with TOF has been employed to identify biomarkers for cystic fibrosis in bacterial cultures (Kaeslin et al., 2021), to benchmark PTR-HRMS results (Bruderer et al., 2020), and to monitor VOC production in plants over light-dark cycles (Barrios-Collado et al., 2016). More recently SESI has developed into so-called super SESI, an advanced, electrode-free design with less background noise and memory effects (Blanco and Vidal-de-Miguel, 2021). Super SESI-HRMS with TOF and Orbitrap has been used to profile VOCs in exhaled breath (Blanco and Vidal-de-Miguel, 2021; Weber et al., 2023a; Weber et al., 2023b). Orbitrap-HRMS has also been used to detect VOCs associated with cometary ice analogs (Javelle et al., 2021), as well as SVOCs emanating from environmental dust samples (Pourasil et al., 2022), and plant volatilome (Majchrzak et al., 2020).
2.1.5 TOF-MS
Time-of-flight MS (TOF-MS) introduced in the mid-1950s accelerates ions in an electric field within a flight tube. Ions with a smaller m/z ratio travel faster, while those with a higher m/z travel slower. Ions are simultaneously detected at a microchannel plate detector, where cascades of electrons are converted into photons amplified by a photomultiplier to generate signals for measurement (Cotter, 1989). The difference between the arrival time of ions at the detector decreases as their m/z values increase, and ions with the same m/z ratio arrive with a time distribution that can overlap with adjacent ions, reducing mass resolution. Linear TOF-MS instruments, particularly matrix-assisted laser desorption ionization TOF MS (MALDI-TOF-MS) systems are now routinely used in analytical labs with applications as varied as proteomics, biomarker discovery, imaging, materials and environmental monitoring (Clark et al., 2013). A more intricate TOF-MS design is the orthogonal acceleration TOF-MS (oaTOF-MS), where ions are accelerated in the drift region perpendicular to their original direction. Except for the MALDI-TOF-MS, most contemporary TOF-MS platforms use orthogonal acceleration and are commonly referred to as TOF-MS.
High resolution TOF-MS instruments emerged in the 1990s with improved mass resolution using collisional focusing (Douglas and French, 1992) and orthogonal acceleration (Guilhaus et al., 2000), or reflectrons (Mamyrin et al., 1973; Wang et al., 1994; Weggler et al., 2019; Cooper-Shepherd et al., 2023) to increase resolution and the flight path of the ions. A typical drift length in earlier linear oaTOF systems was around 1.5 m (Guilhaus et al., 2000), and this length could be significantly extended by employing different geometric designs. However, increasing the number of passes across mirror grids in reflectrons reduces sensitivity and duty cycle (Cooper-Shepherd et al., 2023). Duty cycle (DuC) describes the proportion of time that ions can enter the TOF for analysis. As mass resolution increases, the duty cycle decreases, with the highest duty cycle attained for the highest m/z ion and diminishing for smaller ions (Chernushevich et al., 2017; Willis et al., 2021). The introduction of a linear ion trap/release setup also referred to as “Zeno pulsing” has enabled nearly 100% DuC over a wide m/z range using V- (Loboda and Chernushevich, 2009) and W-geometry systems (Chernushevich et al., 2017) in TOF-MS configurations with 20,000 and 90,000 resolving power, respectively. More recently, high resolution, accurate mass, quadrupole-multi-reflecting time-of-flight (Q-MRT) MS instruments featuring open-loop, planar, gridless ion mirrors with fourth-order energy focusing and a 48 m flight path have reached over 200,000 resolving power with sub-ppm mass accuracy (Cooper-Shepherd et al., 2023).
In conventional TOF-MS systems, the push cycle in the accelerator region is followed by a pause period until the largest m/z ion arrives at the detector. With longer flight paths, the waiting period may become excessively long, rendering the DuC impractical. Diminishing DuC in instruments with extended flight times can be mitigated by multiplexing using Encoded Frequent Pushing (EFP) technology, where the flight tube is continually filled with ions from subsequent pulses, and the time offset of the pulse frequency is encoded in a sequence that can be accurately decoded to represent individual signals in Q-MRT systems (Willis et al., 2021; Cooper-Shepherd et al., 2023). For example, high resolution multi-reflecting time-of-flight mass spectrometry with folded 20 m flight path (HR-MR-TOF-MS FFP) combined with EFP technology reaches a 50,000 resolving power. Analysis of Egyptian mummy bandage extracts using a GCxGC-HR-MR-TOF-MS FFP with EFP system indicated improvements in signal intensity, dynamic mass range, accurate mass data, and limit of detection confirming the reliable operation of decoding algorithms and hardware with increased transient length in HR-TOF-MS instruments (Willis et al., 2021; LECO Corporation White paper, 2021).
TOF-MS can be combined with numerous off-line methods for VOC detection. For instance, a PTR-TOF-MS method was developed for improved detection of aldehydes, fatty acids, and phenols in humid air (Romano and Hanna, 2018). The same method was used to profile VOCs produced by Porphyromonas gingivalis, a common constituent of the human oral microbiome identifying biomarkers in exhaled breath and saliva samples (Roslund et al., 2022). Advances in high-speed electronics, collisional cooling and orthogonal acceleration have increased the resolution of TOF instruments (Douglas and French, 1992; Guilhaus et al., 2000; Chernushevich and Thomson, 2004). In such instruments, ions pass through a multi-pole lens system filled with a low-pressure inert gas and applied radio frequency (RF) resulting in collisional cooling that forms a narrow and dense ion beam. The beam enters an acceleration region, where it is further enriched and the ions are pushed as concentrated packets into the flight tube in an orthogonal direction (Dodonov et al., 1987; Dodonov et al., 1993; Dodonov et al., 2000). High resolution TOF-MS (HR-TOF-MS) has been used to profile VOCs in exhaled breath (Weber et al., 2023a; Weber et al., 2023b), to identify biomarkers for cystic fibrosis in bacterial cultures (Kaeslin et al., 2021), and to benchmark SESI and PTR-HRMS results (Bruderer et al., 2020).
2.2 Off-line methods
Off-line or hyphenated methods integrate chromatographic separation processes upstream of analyte detection. For example, gas chromatography to separate sample components prior to MS can improve resolution, allowing for more precise identification and quantification of the analytes. Gas chromatography (GC), formerly known as gas-liquid chromatography, evolved from liquid chromatography (LC) in 1941, when Martin and Synge proposed during their research on liquid-liquid partition chromatography that the mobile liquid phase could be replaced with a gas phase (Martin and Synge, 1941). The first application with GC was published in 1952 reporting the separation of VFAs from other acidic components and it can be considered the first publication on VOC analysis using GC (James and Martin, 1952). Nowadays, VOC profiling involves various types of GC utilizing open tubular capillary columns with diverse stationary phases connected to a wide range of detectors. These methods require more intensive sample preparation and more time to implement with respect to method development and sample analysis. Although the process of sample preparation can be semi-automated, the throughput of off-line methods tends to be much lower than on-line methods. The primary advantage of using off-line methods is the increased resolution of analytes with less deconvolution needed to interpret mass spectra resulting in improved identification within more complex matrices.
2.2.1 GC-MS
First introduced in the 1950s, GC-MS instruments employed packed columns connected to a magnetic sector mass spectrometer (Grayson, 2016). The first GC with a single quadrupole MS (QMS) became commercially available in 1961 (Finnigan, 2016) and has become one of the most widely used platforms for small molecule detection in liquid or gaseous phase samples across diverse matrices. Currently, most contemporary GC-MS platforms use GCs with capillary columns coupled with a QMS and are commonly referred to as GC-MS. GC-MS instruments are recognized for their robustness, user-friendly configurations, affordability, and high chromatographic resolution with low mass resolution, enabling detection and quantitation of analytes with nominal mass (Rey-Stolle et al., 2022).
Liquid samples can be introduced directly into the injection port, where they are vaporized, focused, and carried to the column by the carrier gas in either a split or splitless injection mode. Gas-phase samples are injected in a similar way except the analytes are captured from the headspace (HS) of the sample container using static HS (SHS), dynamic HS (DHS), SPME, thermal desorption (TD), purge-trap, needle trap, etc., methods (Sugita and Sato, 2021) followed by sample introduction to the injection port. Thermally labile compounds, instead of being vaporized, are injected via cool on-column injection. Analytes are separated based on their physico-chemical properties in relation to the column’s stationary phase, carrier gas, oven temperature programming, and other experimental parameters. Analytes eluted from the column are ionized, typically using electron impact (EI) ionization, where an electron beam emitted by a tungsten filament bombards the molecules, fragmenting them into smaller pieces. This process produces radical cations where the molecular ion is typically not observed. While hard ionization EI is useful for quantitation in targeted analysis, it is less optimal when conducting compound characterization, structural elucidation, or untargeted analysis. Alternatively, analytes can be ionized with chemical ionization, where the electron beam ionizes a reagent gas (e.g., methane, ammonia, etc.) first, then the ionized reagent gas transfers charge to the analyte in a soft ionization process that produces negatively or positively charged ions with the molecular ion and less fragmentation (González et al., 1995; Lubes and Goodarzi, 2017). The ionized species enter a single quadrupole MS, where a constant ratio of direct current (DC)/RF is applied and ramped on the rods of the quadrupole, resulting in ions following either an unstable or stable trajectory. The trajectory of an ion is determined by its m/z ratio with unstable ions hitting the rods, discharging, and venting from the system, while stable ions travel through the quadrupole, generating a signal at the detector (Pedder, 2001).
From a VOC detection standpoint, TD-GC-MS has been used to develop standards for exhaled breath (Henderson et al., 2020), and HS-SPME-GC-MS has been used to identify biomarkers of meat spoilage associated with Salmonella Typhimurium and Campylobacter jejuni reducing the time required for regulatory compliance (Carraturo et al., 2020). Similarly, HS-SPME-GC-MS has been used to profile metabolites associated with the Candida spp. volatilome identifying sesquiterpene indicators for Candida albicans infection (Fitzgerald et al., 2022). In special cases, GC ionization has been achieved using ESI where the column effluent was introduced into an electrospray plume using a multiple channel ESI MS technique. This method was applied to study the chemical reactions of VOCs with solid catalysts, such as the dehydration of dimethylhydrazine in the presence of mercury oxide (Lee and Shiea, 1998). More recently, GC-IMS has been developed to analyze VOCs in the headspace of both aerobic and anaerobic human blood culture samples, identifying species-specific volatiles that enabled the identification of bacterial strains in bloodstream infections after a 6-h incubations (Drees et al., 2019). Similar to TD-GC-MS, HS-GC-IMS has been used to benchmark VOC detection standards for exhaled breath (Ruszkiewicz et al., 2022) and to profile clinical samples including HS-MCC-GC-IMS system used to detect VOCs associated with Listeria spp. infections (Taylor et al., 2017). Anishchenko and colleagues developed a modular and reconfigurable system combined with differential mobility spectrometry, a variant form of IMS, to detect VOCs associated with Phytophthora ramorum, a protistan plant pathogen (Anishchenko et al., 2018). Combining GC-MS methods with various collection and detection modules provides a versatile and customizable framework for detecting VOCs from diverse matrices.
2.2.2 GC-MS/MS
The first commercially available tandem GC-MS/MS platform appeared on the market in 2008 incorporating a primary quadrupole (MS1), collision cell, and secondary quadrupole (MS2) with ion optics and focusing lenses. Precursor ions exiting MS1 undergo fragmentation in the collision cell by colliding with an inert, pressurized collision gas (such as helium or nitrogen), and the resulting product ions enter MS2, where they are filtered by applying and ramping a constant DC/RF ratio on the quadrupole rods, as previously explained. The primary advantage of GC-MS/MS lies in its selective and sensitive quantification of targeted analytes in complex matrices, achieved through a multiple reaction monitoring (MRM) mode of operation. In MRM mode, the most abundant precursor and product ions obtained from MS1 and MS2 are paired into a joint MRM signal enabling the targeted detection of the compound with high selectivity and sensitivity. In MRM, unlike ramping voltages, specific DC/RF ratios are applied to MS1 and MS2, ensuring stable trajectories for the precursor and product ions of selected analytes while reducing matrix effects. High-speed electronics enable the rapid interchange of many DC/RF ratios on the quadrupoles in microseconds, allowing simultaneous detection and quantification of numerous analytes in complex matrices (Lubes and Goodarzi, 2017). For example, HS-SPME-GC-MS/MS was used to profile microbial VOCs in human urine and blood as potential biomarkers for indoor mold exposure, resolving 21 analytes as potential occupational health risks (Tabbal et al., 2022b). A modified version of this method was employed to determine urine/air, blood/air, and plasma/air partition coefficients of microbial VOCs in relation to indoor mold exposure (Berkane et al., 2023). In a related study conducted by the Canadian Government, SPME-GC-MS/MS was used to monitor volatile halogenated and BTEX compounds in human blood to determine differences between indoor and outdoor exposure risks (Aranda-Rodriguez et al., 2015). A similar method using HS-GC-MS/MS profiled BTEX and other VOCs in sewage sludge samples from various wastewater treatment plants to better constrain odor control management practices (Byliński et al., 2019). From a biomarker discovery perspective, triple-bed needle trap micro-extraction with GC-MS/MS was used to profile VOCs in exhaled breath of patients with congestive heart failure to identify indicators of disease progression (Biagini et al., 2017; Bellagambi et al., 2021), while the use of a derivatizing agent such as pentafluorobenzyl hydroxylamine pre-loaded on Tenax GR sorbent tubes has been used in conjunction with TD-GC-MS/MS analysis to profile VOCs in exhaled breath from similar patients to monitor clinical improvement over time (Lomonaco et al., 2018).
2.2.3 Multi-dimensional GC (2DGC, GCxGC)
In complex matrices, analytes may persistently coelute even after chromatographic separation using long columns with advanced column chemistry. Efficiently separating multiple coeluting peaks and achieving positive compound identification using deconvolution algorithms can be difficult, especially when working with poorly characterized sample types. Multi-dimensional GC attempts to address these challenges by separating analytes across different phases (Seeley and Seeley, 2013). For example, in two-dimensional GC (2DGC) effluent from the first-dimension column is diverted onto a second-dimension column with a different stationary phase for added separation. The two columns have separate detectors, generating distinct data files. Another option is comprehensive two-dimensional GC (GCxGC) that employs two ovens and columns featuring different stationary phases, along with a modulator for peak manipulation and a single detector (Giddings, 1984; Liu and Phillips, 1991). Modulation is achieved using rapid flow or thermal separation methods to improve analyte detection (Amaral et al., 2020), e.g., thermal modulation using GCxGC with dual-stage quad-jet thermal modulation and cryogenic cooling. During thermal modulation, coeluting peaks arriving from the first-dimension column undergo added separation by entering the modulator, where the peaks are segmented into slices, focused, injected, and subsequently separated in the second-dimension column, where the sliced sections of the peaks represent analytes resolved from previously coeluting peaks in the first dimension (Sarbach et al., 2013). TD-GCxGC-TOF-MS and PTR-TOF-MS have been used to establish standards for profiling VOCs in exhaled breath using the ReCIVA breath biopsy device including assessment of analyte loss resulting and false positive detection (Pham et al., 2023). Similarly, TD-GCxGC-TOF-MS was used to profile VOCs from the exhaled breath of febrile children infected with the malaria causing parasite Plasmodium falciparum resulting in the identification of six potential biomarkers associated with infection status (Schaber et al., 2018). Interestingly, these VOCS were related to terpenes known to attract mosquito vectors involved in malaria transmission. From an industrial perspective, tri-bed SPME-GCxGC-TOF-MS has been used to identify 241 VOCs including esters, alcohols, aldehydes, and alkenes involved in determining pear aroma that could be correlated with genetic differences between cultivars (Wang et al., 2019).
3 Conclusion
This review explores both on-line and off-line methods used in VOC profiling with MS from different matrices. Attention is given to the technical evolution of on-line and off-line methods needed for increasingly resolved VOC profiles, tracing technical progress over time with particular emphasis on emerging microbiome and diagnostic applications (summarized in Table 2). VOC profiling has grown expansively over the past 2 decades across multiple different platforms. It is expected that this trend will continue as more scientists and clinicians turn to increasingly sensitive MS detection platforms including different forms of GCxGC-TOF-MS and PTR-TOF-MS for development of diagnostic and monitoring solutions. At the same time emerging bioinformatics workflows enabling integration of multi-omic data sets (DNA, RNA, proteins, metabolites) promise to invigorate and inform volatilome research across increasingly diverse matrices (Figure 2). For example, Guo and colleagues developed an automated cuvette system in conjunction with on-line PTR-ToF-MS and off-line GC-MS to evaluate fungal VOCs from 43 individual fungal isolates and used the resulting spectra to identify patterns of covariation that informed a machine learning model for biomarker detection within higher level taxonomic groups or functional guilds (Guo et al., 2021).
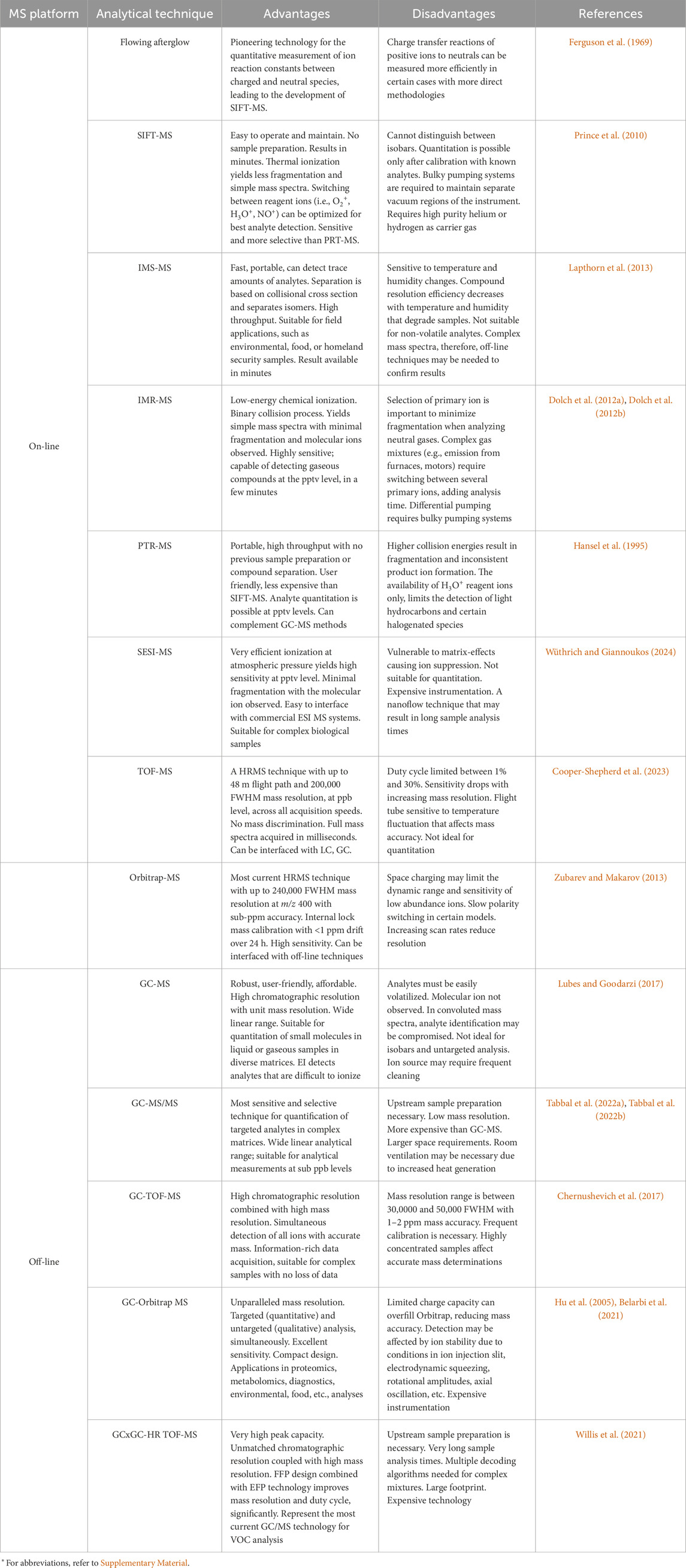
Table 2. Summary of advantages and disadvantages of on-line and off-line mass spectrometry techniques*.
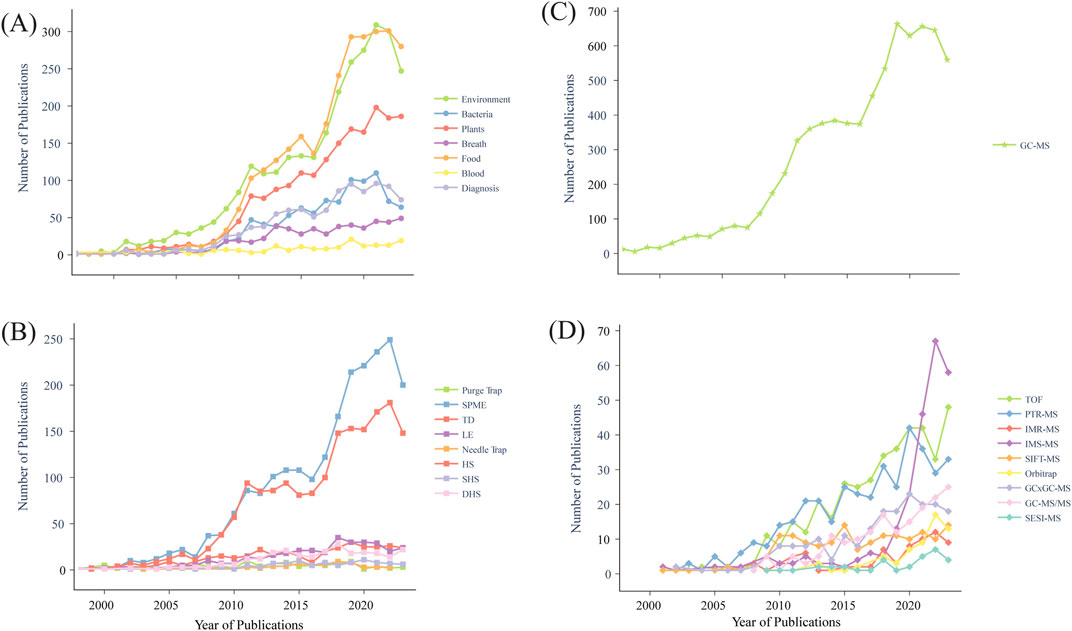
Figure 2. Increasing number of publications related to volatile organic compound detection over the past 20 years for different matrices, methods, and platforms based on PubMed searches. (A) various matrices, (B) extraction methods (SPME, solid phase microextraction; TD, thermal desorption; LE, liquid extraction; HS, headspace; SHS, static headspace; DHS, dynamic headspace), (C) GC-MS (gas chromatography mass spectrometry), (D) other mass spectrometry (MS) platforms (TOF, time-of-flight; PTR-MS, proton transfer reaction MS; IMR-MS, ion molecule reaction MS; IMS-MS, ion mobility spectrometry MS; SIFT-MS, selected ion flow tube MS; GCxGC-MS, comprehensive two-dimensional gas chromatography MS; GC-MS/MS, gas chromatography triple quadrupole MS; SESI-MS, secondary electrospray ionization MS).
On-line platforms offer benefits in clinical laboratories due to their quick analysis times, lack of sample preparation, user-friendly software interfaces, and portability to point-of-care (Majchrzak et al., 2018; Gould et al., 2021). Quantitation is possible for targeted analytes with prior calibration of the machine using standards (Španěl et al., 1999; Smith and Španěl, 2005; Fernández Maestre, 2012), while non-target analytes are eliminated from the measurement. SIFT-MS instruments provide the flexibility to choose from various precursor ions, including O2+, H3O+, and NO+, which allows users to select the primary ionization agents most suitable for the analyzed matrix. In contrast, PTR-MS employs H3O+ as its sole ionizing agent, limiting its versatility but yielding less fragmentation (Smith and Španěl, 2005). On-line techniques are less ideal when conducting untargeted analysis of complex samples where overlapping m/z values can limit analyte identification (Kohl et al., 2013; Smith et al., 2014; Lubes and Goodarzi, 2017). Mass resolution can be improved using HRMS systems that reduce the error between accurate mass spectra and predicted mass listed in regulatory-compliant repositories, such as the National Institute of Standards and Technology (NIST) (Ausloos, et al., 1999), Wiley (McLafferty and Sttauffer, 1989), Fiehn (Kind et al., 2009), Golm (Kopka et al., 2004), or other reference libraries. Such repositories utilize information-rich databases and powerful search functions, such as vocBinBase (Skogerson et al., 2011), BinVestigate (Lai et al., 2018) with deconvolution and annotation tools, including MS-DIAL and MS-FINDER (Tsugawa et al., 2015; Tsugawa et al., 2016; Lai et al., 2018), as well as advanced database queries (Kind and Fiehn, 2006). For example, the Wiley Registry/NIST Mass Spectral Library was used to identify various metabolites including terpenoids, saponins, flavonoids, and alkaloids produced by six Nigella sativa (black cumin) species (Farag et al., 2014). In a separate integrative study, changes in gene expression and terpenoid production were used to annotate genes and pathways responsible for berry maturation processes (Wang et al., 2017). Despite this potential for multi-omics integration, in the absence of analyte separation even the most accurate mass spectral libraries are confounded by overlapping m/z values (Bruderer et al., 2020; Kaeslin et al., 2021).
Off-line platforms, although more labor intensive to operate, offer more detailed chromatographic separations supporting analyte identification, quantification, and structural elucidation (Ahmed et al., 2017; Gould et al., 2021). While development and validation of GC-MS/MS methods requires investment of time and expertise, they remain optimal for profiling VOCs across diverse matrices (Tabbal et al., 2022a; Tabbal et al., 2022b; Berkane et al., 2023). In the analysis of complex samples containing potentially hundreds of analytes, GCxGC-HRMS technology presents several advantages in resolution and analyte identification. The GCxGC module enhances chromatographic peak separation, potentially achieving baseline resolution, and the in-line HRMS system further improves selective spectral identification through high resolution mass measurement of the separated analytes. While low-resolution MS (LRMS) instruments typically measure m/z ratios to one decimal place, which suffices for targeted quantification, untargeted analyses require HRMS (Rey-Stolle et al., 2022). HRMS ensures measurement of unknowns with accurate mass where, for example, compounds with nominal mass m/z 28.0 have four possible chemical formulas, as shown in Table 3. LRMS is incapable of distinguishing between these possibilities. HRMS compares the measured accurate mass spectra with predicted mass listed in regulatory-compliant repositories and the analyte with the lowest mass error is selected as the most likely hit; in this case m/z 28.00562 (Table 3). The chromatographic separation power and accurate mass measurement of GCxGC-HRMS places these platforms at the forefront of VOC detection with the best analyte separation and accurate mass identification (Weggler et al., 2019; Willis et al., 2021; Cooper-Shepherd et al., 2023; LECO Corporation White paper, 2021).
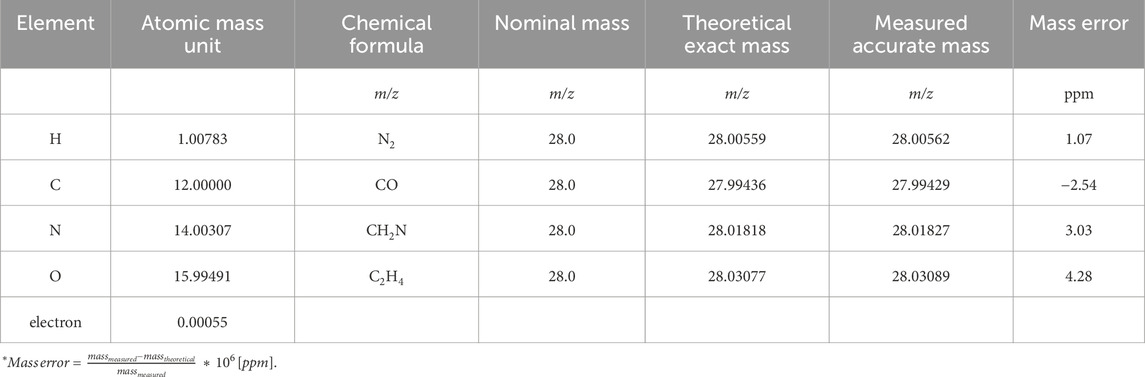
Table 3. Determination of compounds, m/z 28.0 with accurate mass and the possible chemical formulas.
Living cells and cell systems produce diverse VOC profiles that can be differentially detected using on-line and off-line methods. For example, VOCs produced by plants include terpenoids, phenylpropanoids, benzenoids, and fatty acid derivatives (Picazo-Aragonés et al., 2020; Liu et al., 2023); VOCs detected in blood include alcohols, aldehydes, acids, acetone, hydrogen sulfide, methanethiol, dimethyl sulfide, dimethyldisulfide, trimethylamine, indole, aminoacetophenone (Allardyce et al., 2006a; Allardyce et al., 2006b); VOCs detected in breath include hydrocarbons, alcohols, ketones, aldehydes, carboxylic acids, esters, isoprenoids, furan, nitrogen- and sulfur-containing compounds, aromatics, cyclic hydrocarbons (Issitt et al., 2022; Moura et al., 2023) (https://neomeditec.com/VOCdatabase/); VOCs produced by microorganisms include hydrocarbons, alcohols, aldehydes, acids, ketones, esters, aromatics, phenols, nitrogen- and sulfur-containing compounds (Ratiu et al., 2017); VOCs detected in food include alcohols, aldehydes, acids, esters, terpenes, furans, and pyrazines (Starowicz, 2021); and VOCs detected in environmental samples include alkanes, alkenes, alkynes, alcohols, aldehydes, aromatic compounds, ketones, esters, ethers, haloalkanes, nitriles, organic acids, and acrylamide (Lü et al., 2022). While many of these compound classes are shared between sources, more granular analysis reveals a complex array of molecular forms with potential to serve specific signaling or regulatory roles.
In this regard there is increasing interest in VOC profiling to develop new metrics that improve understanding of microbial interactions and trait-based contributions to functions and services in natural and engineered environments including our own bodies (Figure 3). For example, microbial VOCs are increasingly being linked back to antimicrobial properties and plant host interactions including defense mechanisms and root growth (Weisskopf et al., 2021; Razo-Belmán et al., 2023; Schmidt et al., 2023). From a diagnostic or biotechnology innovation perspective this not only applies to the detection of VOCs involved in community-level interactions and host associations, but to development of non-invasive point of care diagnostics in health and disease. For example, lung cancer breath analysis has become a promising method of screening, with ∼500 exhaled compounds currently associated with lung cancer status (Schmidt et al., 2023). Identifying correlations between lung microbiome and VOCs in relation to lung cancer status is an active area of research that requires increased throughput, mass resolution and methods standardization. These requirements also represent common challenges to scaling VOC detection in relation to community level interactions as well as in development of screening paradigms to recover genes or gene cassettes producing VOCs from environmental genomes. Despite these challenges, the trajectory of MS platform innovation and continuous improvement in integrative methods of data analysis and statistical modeling provides an exciting opportunity for researchers to ask fundamental questions with real world implications.
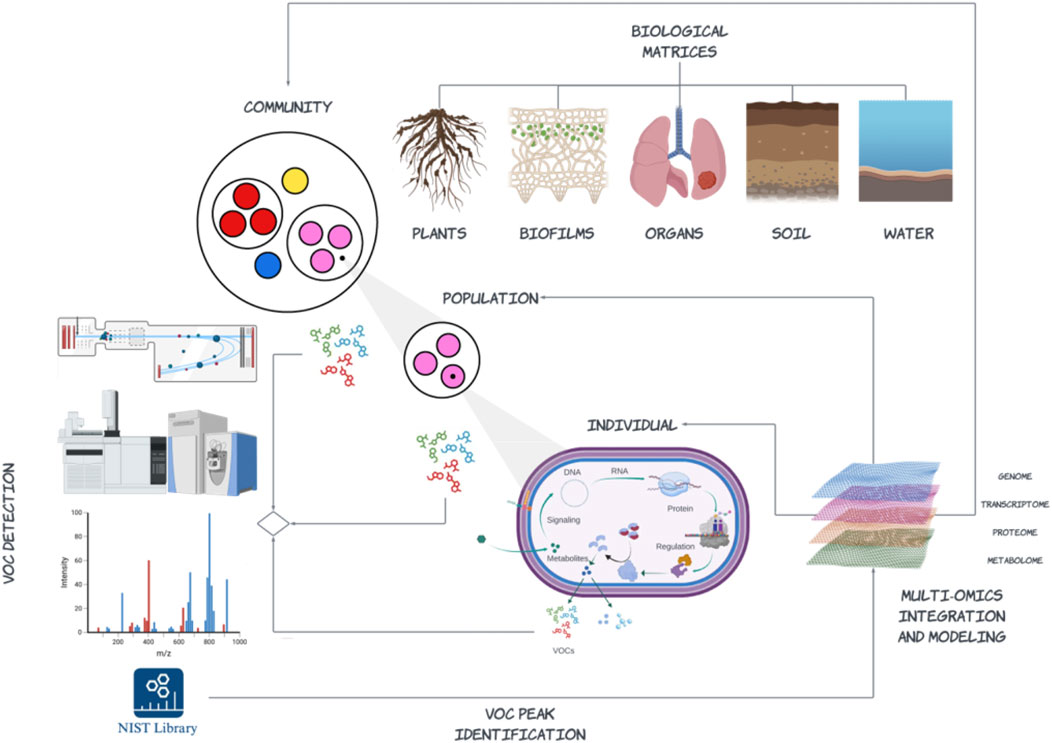
Figure 3. An emerging paradigm for volatilome research integrating multi-omic data (DNA, RNA, protein, and metabolites) spanning different levels of biological organization to resolve volatile organic compound (VOC) profiles emerging from biological matrices sourced from natural or engineered environments including our own bodies. This paradigm includes enrichment and isolation methods to more closely link specific VOCs with specific individuals or populations of microorganisms as well as community-level analysis that define emergent patterns of VOC production and signaling among and between different taxonomic lineages. The development of more extensive regulatory-compliant repositories, e.g., Wiley Registry/National Institute of Standards and Technology (NIST) Mass Spectral Library compound reference libraries and integrative methods linking identified VOCs onto the background metabolic network at scale remain ongoing challenges (Created with BioRender.com).
4 Scope statement
Volatile organic compounds (VOCs) are gas-phase small molecules released from biotic and abiotic matrices into the environment. Because they are volatile, VOCs are typically not detected during conventional metabolite analysis and require specific profiling methods and platforms to measure. One of the most popular platforms is gas chromatography-mass spectrometry that is commonly used for detecting VOCs and can be automated with headspace solid-phase microextraction, etc., methods. The study of VOCs in relation to other forms of biological information, e.g., DNA, RNA, protein, and other metabolites encompasses volatilome research. Emerging lines of evidence suggest that the volatilome plays an integral role in signaling and metabolite exchange within natural and engineered environments including our own bodies where the interplay between microorganisms and host cells defines a complex adaptive network. In this review we trace the evolution of mass spectrometry platforms used in the detection of VOCs in relation to different biological matrices and provide contemporary insight into how VOC profiling is becoming increasingly used to develop non-invasive diagnostic tests across a range of application areas in health, industry, and the environment. Since this review examines VOC analysis using various mass spectrometry platforms with a multi-omics approach, it will fit well in this Research Topic.
Author contributions
ASz: Conceptualization, Data curation, Investigation, Methodology, Project administration, Resources, Supervision, Visualization, Writing–original draft, Writing–review and editing. ASu: Data curation, Investigation, Visualization, Writing–original draft, Writing–review and editing. SH: Conceptualization, Data curation, Formal Analysis, Funding acquisition, Investigation, Methodology, Project administration, Writing–original draft, Writing–review and editing.
Funding
The author(s) declare that financial support was received for the research, authorship, and/or publication of this article. This work was performed under the auspices of the Natural Sciences and Engineering Research Council (NSERC) of Canada, Genome British Columbia, the Terry Fox New Frontiers Program, and the Canada Foundation for Innovation (CFI).
Acknowledgments
We would like to thank Cody Foley in the Biofactorial automation core facility at UBC, and Jasmeen Kaur for insightful conversations regarding multi-omic approaches and VOC detection, as well as Tony Liu and Juan Camilo Santana Martinez in the Hallam lab for useful discussions related to integrating VOC profiling with multi-omic data sets.
Conflict of interest
The authors declare that the research was conducted in the absence of any commercial or financial relationships that could be construed as a potential conflict of interest.
The author(s) declared that they were an editorial board member of Frontiers, at the time of submission. This had no impact on the peer review process and the final decision.
Publisher’s note
All claims expressed in this article are solely those of the authors and do not necessarily represent those of their affiliated organizations, or those of the publisher, the editors and the reviewers. Any product that may be evaluated in this article, or claim that may be made by its manufacturer, is not guaranteed or endorsed by the publisher.
Supplementary material
The Supplementary Material for this article can be found online at: https://www.frontiersin.org/articles/10.3389/fmolb.2024.1421330/full#supplementary-material
References
Adams, N. G., and Smith, D. (1976). The selected ion flow tube (SIFT); A technique for studying ion-neutral reactions. Int. J. Mass Spectrom. Ion. Phys. 21 (3), 349–359. doi:10.1016/0020-7381(76)80133-7
Ahmed, W. M., Lawal, O., Nijsen, T. M., Goodacre, R., and Fowler, S. J. (2017). Exhaled volatile organic compounds of infection: a systematic review. ACS Infect. Dis. 3 (10), 695–710. doi:10.1021/acsinfecdis.7b00088
Allardyce, R. A., Hill, A. L., and Murdoch, D. R. (2006a). The rapid evaluation of bacterial growth and antibiotic susceptibility in blood cultures by selected ion flow tube mass spectrometry. Diagn. Microbiol. Infect. Dis. 55 (4), 255–261. doi:10.1016/j.diagmicrobio.2006.01.031
Allardyce, R. A., Langford, V. S., Hill, A. L., and Murdoch, D. R. (2006b). Detection of volatile metabolites produced by bacterial growth in blood culture media by selected ion flow tube mass spectrometry (SIFT-MS). J. Microbiol. Methods 65 (2), 361–365. doi:10.1016/j.mimet.2005.09.003
Amann, A., Costello Bde, L., Miekisch, W., Schubert, J., Buszewski, B., Pleil, J., et al. (2014). The human volatilome: volatile organic compounds (VOCs) in exhaled breath, skin emanations, urine, feces and saliva. J. Breath. Res. 8 (3), 034001. doi:10.1088/1752-7155/8/3/034001
Amaral, M. S. S., Nolvachai, Y., and Marriott, P. J. (2020). Comprehensive two-dimensional gas chromatography advances in technology and applications: biennial update. Anal. Chem. 92 (1), 85–104. doi:10.1021/acs.analchem.9b05412
Anishchenko, I. M., McCartney, M. M., Fung, A. G., Peirano, D. J., Schirle, M. J., Kenyon, N. J., et al. (2018). Modular and reconfigurable gas chromatography/differential mobility spectrometry (GC/DMS) package for detection of volatile organic compounds (VOCs). Int. J. Ion. Mobil. Spectrom. 21 (4), 125–136. doi:10.1007/s12127-018-0240-4
Aranda-Rodriguez, R., Cabecinha, A., Harvie, J., Jin, Z., Marchand, A., Tardif, R., et al. (2015). A method for quantification of volatile organic compounds in blood by SPME-GC–MS/MS with broader application: from non-occupational exposure population to exposure studies. J. Chromatogr. B 992, 76–85. doi:10.1016/j.jchromb.2015.04.020
Audrain, B., Farag, M. A., Ryu, C.-M., and Ghigo, J.-M. (2015). Role of bacterial volatile compounds in bacterial biology. FEMS Microbiol. Rev. 39 (2), 222–233. doi:10.1093/femsre/fuu013
Ausloos, P., Clifton, C. L., Lias, S. G., Mikaya, A. I., Stein, S. E., Tchekhovskoi, D. V., et al. (1999). The critical evaluation of a comprehensive mass spectral library. J. Am. Soc. Mass Spectrom. 10 (4), 287–299. doi:10.1016/S1044-0305(98)00159-7
Barrios-Collado, C., García-Gómez, D., Zenobi, R., Vidal-de-Miguel, G., Ibáñez, A. J., and Martinez-Lozano Sinues, P. (2016). Capturing in vivo plant metabolism by real-time analysis of low to high molecular weight volatiles. Anal. Chem. 88 (4), 2406–2412. doi:10.1021/acs.analchem.5b04452
Bauermeister, A., Mannochio-Russo, H., Costa-Lotufo, L. V., Jarmusch, A. K., and Dorrestein, P. C. (2022). Mass spectrometry-based metabolomics in microbiome investigations. Nat. Rev. Microbiol. 20 (3), 143–160. doi:10.1038/s41579-021-00621-9
Belarbi, S., Vivier, M., Zaghouani, W., Sloovere, A. D., Agasse-Peulon, V., and Cardinael, P. (2021). Comparison of new approach of GC-HRMS (Q-Orbitrap) to GC–MS/MS (triple-quadrupole) in analyzing the pesticide residues and contaminants in complex food matrices. Food Chem. 359, 129932. doi:10.1016/j.foodchem.2021.129932
Belizário, J. E., Faintuch, J., and Malpartida, M. G. (2021). Breath biopsy and discovery of exclusive volatile organic compounds for diagnosis of infectious diseases. Front. Cell. Infect. Microbiol. 10. doi:10.3389/fcimb.2020.564194
Bellagambi, F. G., Lomonaco, T., Ghimenti, S., Biagini, D., Fuoco, R., and Francesco, F. D. (2021). Determination of peppermint compounds in breath by needle trap micro-extraction coupled with gas chromatography–tandem mass spectrometry. J. Breath. Res. 15 (1), 016014. doi:10.1088/1752-7163/abcdec
Berenguer, C. V., Pereira, F., Pereira, J. A. M., and Câmara, J. S. (2022). Volatilomics: an emerging and promising avenue for the detection of potential prostate cancer biomarkers. Cancers (Basel) 14 (16), 3982. doi:10.3390/cancers14163982
Berkane, W., El Aroussi, B., Bouchard, M., Marchand, G., and Haddad, S. (2023). Determination of blood:air, urine:air and plasma:air partition coefficients of selected microbial volatile organic compounds. Chemosphere 343, 140305. doi:10.1016/j.chemosphere.2023.140305
Biagini, D., Lomonaco, T., Ghimenti, S., Bellagambi, F. G., Onor, M., Scali, M. C., et al. (2017). Determination of volatile organic compounds in exhaled breath of heart failure patients by needle trap micro-extraction coupled with gas chromatography-tandem mass spectrometry. J. Breath. Res. 11 (4), 047110. doi:10.1088/1752-7163/aa94e7
Bierbaum, V. M. (2015). Go with the flow: fifty years of innovation and ion chemistry using the flowing afterglow. Int. J. Mass Spectrom. 377, 456–466. doi:10.1016/j.ijms.2014.07.021
Blanco, F. G., and Vidal-de-Miguel, G. (2021). Breath analysis by secondary electro-spray ionization - mass spectrometry to interrogate biologically significant metabolites non-invasively. Crit. Rev. Anal. Chem. 53, 825–837. doi:10.1080/10408347.2021.1981226
Boots, A. W., Smolinska, A., van Berkel, J. J. B. N., Fijten, R. R. R., Stobberingh, E. E., Boumans, M. L. L., et al. (2014). Identification of microorganisms based on headspace analysis of volatile organic compounds by gas chromatography–mass spectrometry. J. Breath. Res. 8 (2), 027106. doi:10.1088/1752-7155/8/2/027106
Bruderer, T., Gaugg, M. T., Cappellin, L., Lopez-Hilfiker, F., Hutterli, M., Perkins, N., et al. (2020). Detection of volatile organic compounds with secondary electrospray ionization and proton transfer reaction high-resolution mass spectrometry: a feature comparison. J. Am. Soc. Mass Spectrom. 31 (8), 1632–1640. doi:10.1021/jasms.0c00059
Byliński, H., Aszyk, J., Kubica, P., Szopińska, M., Fudala-Książek, S., and Namieśnik, J. (2019). Differences between selected volatile aromatic compound concentrations in sludge samples in various steps of wastewater treatment plant operations. J. Environ. Manage. 249, 109426. doi:10.1016/j.jenvman.2019.109426
Cao, X.-L., Sparling, M., and Dabeka, R. (2016). Occurrence of 13 volatile organic compounds in foods from the Canadian total diet study. Food Addit. Contam. Part A 33 (2), 373–382. doi:10.1080/19440049.2015.1129072
Carraturo, F., Libralato, G., Esposito, R., Galdiero, E., Aliberti, F., Amoresano, A., et al. (2020). Metabolomic profiling of food matrices: preliminary identification of potential markers of microbial contamination. J. Food Sci. 85 (10), 3467–3477. doi:10.1111/1750-3841.15418
Chen, C., Huang, K., Yu, H., and Tian, H. (2021). The diversity of microbial communities in Chinese milk fan and their effects on volatile organic compound profiles. J. Dairy Sci. 104 (3), 2581–2593. doi:10.3168/jds.2020-19053
Chernushevich, I. V., Merenbloom, S. I., Liu, S., and Bloomfield, N. (2017). A W-geometry ortho-TOF MS with high resolution and up to 100% duty cycle for MS/MS. J. Am. Soc. Mass Spectrom. 28 (10), 2143–2150. doi:10.1007/s13361-017-1742-8
Chernushevich, I. V., and Thomson, B. A. (2004). Collisional cooling of large ions in electrospray mass spectrometry. Anal. Chem. 76 (6), 1754–1760. doi:10.1021/ac035406j
Choudoir, M., Rossabi, S., Gebert, M., Helmig, D., and Fierer, N. (2019). A phylogenetic and functional perspective on volatile organic compound production by Actinobacteria. mSystems 4 (2). doi:10.1128/mSystems.00295-18
Clark, A. E., Kaleta, E. J., Arora, A., and Wolk, D. M. (2013). Matrix-assisted laser desorption ionization–time of flight mass spectrometry: a fundamental shift in the routine practice of clinical microbiology. Clin. Microbiol. Rev. 26(3), 547–603. doi:10.1128/cmr.00072-12
Cohen, M. J., and Karasek, F. W. (1970). Plasma chromatography™—a new dimension for gas chromatography and mass spectrometry. J. Chromatogr. Sci. 8 (6), 330–337. doi:10.1093/chromsci/8.6.330
Collins, D., and Lee, M. (2002). Developments in ion mobility spectrometry–mass spectrometry. Anal. Bioanal. Chem. 372 (1), 66–73. doi:10.1007/s00216-001-1195-5
Cooper-Shepherd, D. A., Wildgoose, J., Kozlov, B., Johnson, W. J., Tyldesley-Worster, R., Palmer, M. E., et al. (2023). Novel hybrid quadrupole-multireflecting time-of-flight mass spectrometry system. J. Am. Soc. Mass Spectrom. 34 (2), 264–272. doi:10.1021/jasms.2c00281
Cotter, R. J. (1989). Time-of-flight mass spectrometry: an increasing role in the life sciences. Biomed. Environ. Mass Spectrom. 18 (8), 513–532. doi:10.1002/bms.1200180803
D'Alessandro, M., and Turlings, T. C. (2006). Advances and challenges in the identification of volatiles that mediate interactions among plants and arthropods. Analyst 131 (1), 24–32. doi:10.1039/b507589k
Dhandapani, S., Jin, J., Sridhar, V., Sarojam, R., Chua, N.-H., and Jang, I.-C. (2017). Integrated metabolome and transcriptome analysis of Magnolia champaca identifies biosynthetic pathways for floral volatile organic compounds. BMC Genomics 18 (1), 463. doi:10.1186/s12864-017-3846-8
Dixon, B., Ahmed, W. M., Felton, T., and Fowler, S. J. (2022). Molecular phenotyping approaches for the detection and monitoring of carbapenem-resistant Enterobacteriaceae by mass spectrometry. J. Mass Spectrom. Adv. Clin. Lab. 26, 9–19. doi:10.1016/j.jmsacl.2022.09.001
Dodds, J. N., and Baker, E. S. (2019). Ion mobility spectrometry: fundamental concepts, instrumentation, applications, and the road ahead. J. Am. Soc. Mass Spectrom. 30 (11), 2185–2195. doi:10.1007/s13361-019-02288-2
Dodds, J. N., Hopkins, Z. R., Knappe, D. R. U., and Baker, E. S. (2020). Rapid characterization of per- and polyfluoroalkyl substances (PFAS) by ion mobility spectrometry–mass spectrometry (IMS-MS). Anal. Chem. 92 (6), 4427–4435. doi:10.1021/acs.analchem.9b05364
Dodonov, A. F., Chernushevich, I. V., and Laiko, V. V. (1993). “Electrospray ionization on a reflecting time-of-flight mass spectrometer,” in Time-of-Flight mass spectrometry (American Chemical Society), 108–123.
Dodonov, A. F., Chernushevich, I. V., Dodonova, T. F., Raznikov, V. V., and Talrose, V. L. (1987). Mass reflectron. Moscow, USSR, Soviet Patent no. 1681340A1 (filed February 1987).
Dodonov, A. F., Kozlovski, V. I., Soulimenkov, I. V., Raznikov, V. V., Loboda, A. V., Zhen, Z., et al. (2000). High-resolution electrospray ionization orthogonal-injection time-of-flight mass spectrometer. Eur. J. Mass Spectrom. 6 (6), 481–490. doi:10.1255/ejms.378
Dolch, M. E., Choukèr, A., Hornuss, C., Frey, L., Irlbeck, M., Praun, S., et al. (2015). Quantification of propionaldehyde in breath of patients after lung transplantation. Free Radic. Biol. Med. 85, 157–164. doi:10.1016/j.freeradbiomed.2015.04.003
Dolch, M. E., Hornuss, C., Klocke, C., Praun, S., Villinger, J., Denzer, W., et al. (2012a). Volatile compound profiling for the identification of Gram-negative bacteria by ion-molecule reaction–mass spectrometry. J. Appl. Microbiol. 113 (5), 1097–1105. doi:10.1111/j.1365-2672.2012.05414.x
Dolch, M. E., Hornuss, C., Klocke, C., Praun, S., Villinger, J., Denzer, W., et al. (2012b). Volatile organic compound analysis by ion molecule reaction mass spectrometry for Gram-positive bacteria differentiation. Eur. J. Clin. Microbiol. Infect. Dis. 31 (11), 3007–3013. doi:10.1007/s10096-012-1654-2
Douglas, D. J., and French, J. B. (1992). Collisional focusing effects in radio frequency quadrupoles. J. Am. Soc. Mass Spectrom. 3 (4), 398–408. doi:10.1016/1044-0305(92)87067-9
Drabińska, N., Flynn, C., Ratcliffe, N., Belluomo, I., Myridakis, A., Gould, O., et al. (2021). A literature survey of all volatiles from healthy human breath and bodily fluids: the human volatilome. J. Breath. Res. 15 (3), 034001. doi:10.1088/1752-7163/abf1d0
Drabińska, N., Hewett, K., White, P., Avison, M. B., Persad, R., Ratcliffe, N. M., et al. (2022). Application of a solid-phase microextraction-gas chromatography-mass spectrometry/metal oxide sensor system for detection of antibiotic susceptibility in urinary tract infection-causing Escherichia coli – a proof of principle study. Adv. Med. Sci. 67 (1), 1–9. doi:10.1016/j.advms.2021.09.001
Drees, C., Vautz, W., Liedtke, S., Rosin, C., Althoff, K., Lippmann, M., et al. (2019). GC-IMS headspace analyses allow early recognition of bacterial growth and rapid pathogen differentiation in standard blood cultures. Appl. Microbiol. Biotechnol. 103 (21-22), 9091–9101. doi:10.1007/s00253-019-10181-x
Dudareva, N., Pichersky, E., and Gershenzon, J. (2004). Biochemistry of plant volatiles. Plant Physiol. 135 (4), 1893–1902. doi:10.1104/pp.104.049981
Farag, M. A., Gad, H. A., Heiss, A. G., and Wessjohann, L. A. (2014). Metabolomics driven analysis of six Nigella species seeds via UPLC-qTOF-MS and GC–MS coupled to chemometrics. Food Chem. 151, 333–342. doi:10.1016/j.foodchem.2013.11.032
Fenn, J. B. (2002). Electrospray ionization mass spectrometry: how it all began. J. Biomol. Tech. 13 (3), 101–118.
Fenn, J. B., Mann, M., Meng, C. K., Wong, S. F., and Whitehouse, C. M. (1989). Electrospray ionization for mass spectrometry of large biomolecules. Science 246 (4926), 64–71. doi:10.1126/science.2675315
Ferguson, E. E., Fehsenfeld, F. C., and Schmeltekopf, A. L. (1969). “Flowing afterglow measurements of ion-neutral reactions,” in Advances in atomic and molecular physics. Editors D. R. Bates, and I. Estermann (Academic Press), 1–56.
Fernández Maestre, R. (2012). Ion mobility spectrometry: history, characteristics and applications. Rev. U.D.C.A Act. and Div. Cient. 15 (2), 467–479. doi:10.31910/rudca.v15.n2.2012.848
Ferrandino, G., De Palo, G., Murgia, A., Birch, O., Tawfike, A., Smith, R., et al. (2023). Breath Biopsy® to identify exhaled volatile organic compounds biomarkers for liver cirrhosis detection. J. Clin. Transl. Hepatol. 11 (3), 000–648. doi:10.14218/jcth.2022.00309
Finnigan, R. (2016). “Development of the business of mass spectrometry (1960–75),” in The encyclopedia of mass spectrometry. Editors M. L. Gross, and R. M. Caprioli (Boston: Elsevier), 315–317.
Fitzgerald, S., Furlong, C., Holland, L., and Morrin, A. (2022). Multi-strain and -species investigation of volatile metabolites emitted from planktonic and biofilm Candida cultures. Metabolites 12 (5), 432. doi:10.3390/metabo12050432
Fu, L., Wang, L., Wang, H., Yang, M., Yang, Q., Lin, Y., et al. (2023). A cross-sectional study: a breathomics based pulmonary tuberculosis detection method. BMC Infect. Dis. 23 (1), 148. doi:10.1186/s12879-023-08112-3
Giddings, J. C. (1984). Two-dimensional separations: concept and promise. Anal. Chem. 56 (12), 1258A-1260A, 1262A, 1264A passim–1260A. 1262A, 1264A passim. doi:10.1021/ac00276a003
González, M. L., Carnicero, M., de la Torre, R., Ortuño, J., and Segura, J. (1995). Influence of the injection technique on the thermal degradation of cocaine and its metabolites in gas chromatography. J. Chromatogr. B Biomed. Sci. Appl. 664 (2), 317–327. doi:10.1016/0378-4347(94)00484-m
Gould, O., Drabińska, N., Ratcliffe, N., and de Lacy Costello, B. (2021). Hyphenated mass spectrometry versus real-time mass spectrometry techniques for the detection of volatile compounds from the human body. Molecules 26 (23), 7185. doi:10.3390/molecules26237185
Grayson, M. A. (2016). “A history of gas chromatography mass spectrometry (GC/MS),” in The encyclopedia of mass spectrometry. Editors M. L. Gross, and R. M. Caprioli (Boston: Elsevier), 152–158.
Guilhaus, M., Selby, D., and Mlynski, V. (2000). Orthogonal acceleration time-of-flight mass spectrometry. Mass Spectrom. Rev. 19 (2), 65–107. doi:10.1002/(SICI)1098-2787(2000)19:2<65::AID-MAS1>3.0.CO;2-E
Guo, Y., Jud, W., Weikl, F., Ghirardo, A., Junker, R. R., Polle, A., et al. (2021). Volatile organic compound patterns predict fungal trophic mode and lifestyle. Commun. Biol. 4 (1), 673. doi:10.1038/s42003-021-02198-8
Handa, H., Usuba, A., Maddula, S., Baumbach, J. I., Mineshita, M., and Miyazawa, T. (2014). Exhaled breath analysis for lung cancer detection using ion mobility spectrometry. PLoS One 9 (12), e114555. doi:10.1371/journal.pone.0114555
Hansel, A., Jordan, A., Holzinger, R., Prazeller, P., Vogel, W., and Lindinger, W. (1995). Proton transfer reaction mass spectrometry: on-line trace gas analysis at the ppb level. Int. J. Mass Spectrom. Ion. Process. 149-150, 609–619. doi:10.1016/0168-1176(95)04294-U
Henderson, B., Ruszkiewicz, D. M., Wilkinson, M., Beauchamp, J. D., Cristescu, S. M., Fowler, S. J., et al. (2020). A benchmarking protocol for breath analysis: the peppermint experiment. J. Breath. Res. 14 (4), 046008. doi:10.1088/1752-7163/aba130
Higashikawa, F. S., Cayuela, M. L., Roig, A., Silva, C. A., and Sánchez-Monedero, M. A. (2013). Matrix effect on the performance of headspace solid phase microextraction method for the analysis of target volatile organic compounds (VOCs) in environmental samples. Chemosphere 93 (10), 2311–2318. doi:10.1016/j.chemosphere.2013.08.023
Hill, H. H., Siems, W. F., and St. Louis, R. H. (1990). Ion mobility spectrometry. Anal. Chem. 62 (23), 1201A–1209A. doi:10.1021/ac00222a001
Hu, Q., Noll, R. J., Li, H., Makarov, A., Hardman, M., and Graham Cooks, R. (2005). The Orbitrap: a new mass spectrometer. J. Mass Spectrom. 40 (4), 430–443. doi:10.1002/jms.856
Issitt, T., Wiggins, L., Veysey, M., Sweeney, S. T., Brackenbury, W. J., and Redeker, K. (2022). Volatile compounds in human breath: critical review and meta-analysis. J. Breath. Res. 16 (2), 024001. doi:10.1088/1752-7163/ac5230
James, A. T., and Martin, A. J. P. (1952). Gas-liquid partition chromatography: the separation and micro-estimation of volatile fatty acids from formic acid to dodecanoic acid. Biochem. J. 50 (5), 679–690. doi:10.1042/bj0500679
Janssens, E., van Meerbeeck, J. P., and Lamote, K. (2020). Volatile organic compounds in human matrices as lung cancer biomarkers: a systematic review. Crit. Rev. Oncol. Hematol. 153, 103037. doi:10.1016/j.critrevonc.2020.103037
Javelle, T., Righezza, M., and Danger, G. (2021). Identify low mass volatile organic compounds from cometary ice analogs using gas chromatography coupled to an Orbitrap mass spectrometer associated to electron and chemical ionizations. J. Chromatogr. A 1652, 462343. doi:10.1016/j.chroma.2021.462343
John, T. M., Shrestha, N. K., Procop, G. W., Grove, D., Leal, S. M., Jacob, C. N., et al. (2021). Diagnosis of Clostridioides difficile infection by analysis of volatile organic compounds in breath, plasma, and stool: a cross-sectional proof-of-concept study. PLoS One 16 (8), e0256259. doi:10.1371/journal.pone.0256259
Kaeslin, J., Micic, S., Weber, R., Müller, S., Perkins, N., Berger, C., et al. (2021). Differentiation of cystic fibrosis-related pathogens by volatile organic compound analysis with secondary electrospray ionization mass spectrometry. Metabolites 11 (11), 773. doi:10.3390/metabo11110773
Karasek, F. W., Kim, S. H., and Hill, H. H. (1976). Mass identified mobility spectra of p-nitrophenol and reactant ions in plasma chromatography. Anal. Chem. 48 (8), 1133–1137. doi:10.1021/ac50002a017
Karpas, Z., Stimac, R. M., and Rappoport, Z. (1988). Differentiating between large isomers and derivation of structural information by ion mobility spectrometry/mass spectrometry techniques. Int. J. Mass Spectrom. Ion. Process. 83(1), 163–175. doi:10.1016/0168-1176(88)80093-4
Khan, A., Kanwal, H., Bibi, S., Mushtaq, S., Khan, A., Khan, Y. H., et al. (2021). “Volatile organic compounds and neurological disorders: from exposure to preventive interventions,” in Environmental contaminants and neurological disorders. Editors M. S. H. Akash, and K. Rehman (Cham: Springer International Publishing), 201–230.
Kind, T., and Fiehn, O. (2006). Metabolomic database annotations via query of elemental compositions: mass accuracy is insufficient even at less than 1 ppm. BMC Bioinforma. 7 (1), 234. doi:10.1186/1471-2105-7-234
Kind, T., Wohlgemuth, G., Lee, D. Y., Lu, Y., Palazoglu, M., Shahbaz, S., et al. (2009). FiehnLib: mass spectral and retention index libraries for metabolomics based on quadrupole and time-of-flight gas chromatography/mass spectrometry. Anal. Chem. 81 (24), 10038–10048. doi:10.1021/ac9019522
King, J., Mochalski, P., Kupferthaler, A., Unterkofler, K., Koc, H., Filipiak, W., et al. (2010). Dynamic profiles of volatile organic compounds in exhaled breath as determined by a coupled PTR-MS/GC-MS study. Physiol. Meas. 31 (9), 1169–1184. doi:10.1088/0967-3334/31/9/008
Kohl, I., Beauchamp, J., Cakar-Beck, F., Herbig, J., Dunkl, J., Tietje, O., et al. (2013). First observation of a potential non-invasive breath gas biomarker for kidney function. J. Breath. Res. 7 (1), 017110. doi:10.1088/1752-7155/7/1/017110
Kopka, J., Schauer, N., Krueger, S., Birkemeyer, C., Usadel, B., Bergmüller, E., et al. (2004).R01EQENTQi5EQg==: the Golm metabolome database. Bioinformatics 21 (8), 1635–1638. doi:10.1093/bioinformatics/bti236
Lagg, A., Taucher, J., Hansel, A., and Lindinger, W. (1994). Applications of proton transfer reactions to gas analysis. Int. J. Mass Spectrom. Ion. Process. 134 (1), 55–66. doi:10.1016/0168-1176(94)03965-8
Lai, Z., Tsugawa, H., Wohlgemuth, G., Mehta, S., Mueller, M., Zheng, Y., et al. (2018). Identifying metabolites by integrating metabolome databases with mass spectrometry cheminformatics. Nat. Methods 15 (1), 53–56. doi:10.1038/nmeth.4512
Lapthorn, C., Pullen, F., and Chowdhry, B. Z. (2013). Ion mobility spectrometry-mass spectrometry (IMS-MS) of small molecules: separating and assigning structures to ions. Mass Spectrom. Rev. 32 (1), 43–71. doi:10.1002/mas.21349
Lawal, O., Ahmed, W. M., Nijsen, T. M. E., Goodacre, R., and Fowler, S. J. (2017). Exhaled breath analysis: a review of “breathtaking” methods for off-line analysis. Metabolomics 13, 110. doi:10.1007/s11306-017-1241-8
Le, T., and Priefer, R. (2023). Detection technologies of volatile organic compounds in the breath for cancer diagnoses. Talanta 265, 124767. doi:10.1016/j.talanta.2023.124767
LECO Corporation White paper (2021). High resolution multi-reflecting time-of-Flight mass analyzer with folded flight Path® HR MR-TOFMS FFP®. St. Joseph, MI, United States: LECO Corporation White paper, 1–19. 209-281-008 - 6/21, 1-19. https://www.leco.com/documents/articles/209-281-008/.
Lee, C. Y., and Shiea, J. (1998). Gas chromatography connected to multiple channel electrospray ionization mass spectrometry for the detection of volatile organic compounds. Anal. Chem. 70 (13), 2757–2761. doi:10.1021/ac971325+
Lindinger, W., Hansel, A., and Jordan, A. (1998). On-line monitoring of volatile organic compounds at pptv levels by means of proton-transfer-reaction mass spectrometry (PTR-MS) medical applications, food control and environmental research. Int. J. Mass Spectrom. Ion. Process. 173 (3), 191–241. doi:10.1016/S0168-1176(97)00281-4
Lindinger, W., Hirber, J., and Paretzke, H. (1993). An ion/molecule-reaction mass spectrometer used for on-line trace gas analysis. Int. J. Mass Spectrom. Ion. Process. 129, 79–88. doi:10.1016/0168-1176(93)87031-M
Liu, Z., and Phillips, J. B. (1991). Comprehensive two-dimensional gas chromatography using an on-column thermal modulator interface. J. Chromatogr. Sci. 29 (6), 227–231. doi:10.1093/chromsci/29.6.227
Liu, Z., Wang, M., Wu, M., Li, X., Liu, H., Niu, N., et al. (2023). Volatile organic compounds (VOCs) from plants: from release to detection. Trends Anal. Chem. 158, 116872. doi:10.1016/j.trac.2022.116872
Loboda, A. V., and Chernushevich, I. V. (2009). A novel ion trap that enables high duty cycle and wide m/z range on an orthogonal injection TOF mass spectrometer. J. Am. Soc. Mass Spectrom. 20 (7), 1342–1348. doi:10.1016/j.jasms.2009.03.018
Lomonaco, T., Romani, A., Ghimenti, S., Biagini, D., Bellagambi, F. G., Onor, M., et al. (2018). Determination of carbonyl compounds in exhaled breath by on-sorbent derivatization coupled with thermal desorption and gas chromatography-tandem mass spectrometry. J. Breath. Res. 12 (4), 046004. doi:10.1088/1752-7163/aad202
Lough, F., Perry, J. D., Stanforth, S. P., and Dean, J. R. (2017). Detection of exogenous VOCs as a novel in vitro diagnostic technique for the detection of pathogenic bacteria. Trends Anal. Chem. 87, 71–81. doi:10.1016/j.trac.2016.12.004
Lü, F., Chen, W., Duan, H., Zhang, H., Shao, L., and He, P. (2022). Monitor process state of batch anaerobic digestion in reliance on volatile and semi-volatile metabolome. Bioresour. Technol. 351, 126953. doi:10.1016/j.biortech.2022.126953
Lubes, G., and Goodarzi, M. (2017). Analysis of volatile compounds by advanced analytical techniques and multivariate chemometrics. Chem. Rev. 117 (9), 6399–6422. doi:10.1021/acs.chemrev.6b00698
Majchrzak, T., Wojnowski, W., Lubinska-Szczygeł, M., Różańska, A., Namieśnik, J., and Dymerski, T. (2018). PTR-MS and GC-MS as complementary techniques for analysis of volatiles: a tutorial review. Anal. Chim. Acta 1035, 1–13. doi:10.1016/j.aca.2018.06.056
Majchrzak, T., Wojnowski, W., Rutkowska, M., and Wasik, A. (2020). Real-time volatilomics: a novel approach for analyzing biological samples. Trends Plant Sci. 25 (3), 302–312. doi:10.1016/j.tplants.2019.12.005
Makarov, A. (2000). Electrostatic axially harmonic orbital trapping: a high-performance technique of mass analysis. Anal. Chem. 72 (6), 1156–1162. doi:10.1021/ac991131p
Malásková, M., Henderson, B., Chellayah, P. D., Ruzsanyi, V., Mochalski, P., Cristescu, S. M., et al. (2019). Proton transfer reaction time-of-flight mass spectrometric measurements of volatile compounds contained in peppermint oil capsules of relevance to real-time pharmacokinetic breath studies. J. Breath. Res. 13 (4), 046009. doi:10.1088/1752-7163/ab26e2
Mamyrin, B. A., Karataev, V. I., Shmikk, D. V., and Zagulin, V. A. (1973). Mass reflection: a new nonmagnetic time-of-flight high resolution mass-spectrometer. Sov. Phys. JETP 37, 45–48.
Martin, A. J., and Synge, R. L. (1941). A new form of chromatogram employing two liquid phases: a theory of chromatography. 2. Application to the micro-determination of the higher monoamino-acids in proteins. Biochem. J. 35 (12), 1358–1368. doi:10.1042/bj0351358
Martínez-Lozano, P., Zingaro, L., Finiguerra, A., and Cristoni, S. (2011). Secondary electrospray ionization-mass spectrometry: breath study on a control group. J. Breath. Res. 5 (1), 016002. doi:10.1088/1752-7155/5/1/016002
Materić, D., Bruhn, D., Turner, C., Morgan, G., Mason, N., and Gauci, V. (2015). Methods in plant foliar volatile organic compounds research. Appl. Plant Sci. 3 (12). doi:10.3732/apps.1500044
McLafferty, F. W., and Sttauffer, D. (1989). Wiley/NBS Registry of mass spectral data (Accessed July 17, 2024).
Meidert, A. S., Choukèr, A., Praun, S., Schelling, G., and Dolch, M. E. (2021). Exhaled breath and oxygenator sweep gas propionaldehyde in acute respiratory distress syndrome. Molecules 26 (1), 145. doi:10.3390/molecules26010145
Moura, P. C., Raposo, M., and Vassilenko, V. (2023). Breath volatile organic compounds (VOCs) as biomarkers for the diagnosis of pathological conditions: a review. Biomed. J. 46 (4), 100623. doi:10.1016/j.bj.2023.100623
Mukhopadhyay, R. (2008). IMS/MS: its time has come. Anal. Chem. 80 (21), 7918–7920. doi:10.1021/ac8018608
Mumm, R., Schrank, K., Wegener, R., Schulz, S., and Hilker, M. (2003). Chemical analysis of volatiles emitted by Pinus svlvestris after induction by insect oviposition. J. Chem. Ecol. 29 (5), 1235–1252. doi:10.1023/a:1023841909199
Pedder, R. E. (2001). “Practical quadrupole theory: graphical theory,” in Extrel application note RA_2010A poster presented at the 49th ASMS conference on mass spectrometry and allied topics.
Pham, Y. L., Holz, O., and Beauchamp, J. (2023). Emissions and uptake of volatiles by sampling components in breath analysis. J. Breath. Res. 17 (3), 037102. doi:10.1088/1752-7163/acce34
Picazo-Aragonés, J., Terrab, A., and Balao, F. (2020). Plant volatile organic compounds evolution: transcriptional regulation, epigenetics and polyploidy. Int. J. Mol. Sci. 21 (23), 8956. doi:10.3390/ijms21238956
Pourasil, R. S. M., Cristale, J., Lacorte, S., and Tauler, R. (2022). Non-targeted Gas Chromatography Orbitrap Mass Spectrometry qualitative and quantitative analysis of semi-volatile organic compounds in indoor dust using the Regions of Interest Multivariate Curve Resolution chemometrics procedure. J. Chromatogr. A 1668, 462907. doi:10.1016/j.chroma.2022.462907
Prince, B. J., Milligan, D. B., and McEwan, M. J. (2010). Application of selected ion flow tube mass spectrometry to real-time atmospheric monitoring. Rapid Commun. Mass Spectrom. 24 (12), 1763–1769. doi:10.1002/rcm.4574
Ratiu, I.-A., Ligor, T., Bocos-Bintintan, V., and Buszewski, B. (2017). Mass spectrometric techniques for the analysis of volatile organic compounds emitted from bacteria. Bioanalysis 9 (14), 1069–1092. doi:10.4155/bio-2017-0051
Ratiu, I. A., Ligor, T., Bocos-Bintintan, V., Mayhew, C. A., and Buszewski, B. (2020). Volatile organic compounds in exhaled breath as fingerprints of lung cancer, asthma and COPD. J. Clin. Med. 10 (1), 32. doi:10.3390/jcm10010032
Razo-Belmán, R., Ángeles-López, Y. I., García-Ortega, L. F., León-Ramírez, C. G., Ortiz-Castellanos, L., Yu, H., et al. (2023). Fungal volatile organic compounds: mechanisms involved in their sensing and dynamic communication with plants. Front. Plant Sci. 14. doi:10.3389/fpls.2023.1257098
Rey-Stolle, F., Dudzik, D., Gonzalez-Riano, C., Fernández-García, M., Alonso-Herranz, V., Rojo, D., et al. (2022). Low and high resolution gas chromatography-mass spectrometry for untargeted metabolomics: a tutorial. Anal. Chim. Acta 1210, 339043. doi:10.1016/j.aca.2021.339043
Romano, A., and Hanna, G. B. (2018). Identification and quantification of VOCs by proton transfer reaction time of flight mass spectrometry: an experimental workflow for the optimization of specificity, sensitivity, and accuracy. J. Mass Spectrom. 53 (4), 287–295. doi:10.1002/jms.4063
Roslund, K., Uosukainen, M., Järvik, K., Hartonen, K., Lehto, M., Pussinen, P., et al. (2022). Antibiotic treatment and supplemental hemin availability affect the volatile organic compounds produced by P. gingivalis in vitro. Sci. Rep. 12 (1), 22534. doi:10.1038/s41598-022-26497-0
Ruszkiewicz, D. M., Myers, R., Henderson, B., Yusof, H., Meister, A., Moreno, S., et al. (2022). Peppermint protocol: first results for gas chromatography-ion mobility spectrometry. J. Breath. Res. 16 (3), 036004. doi:10.1088/1752-7163/ac6ca0
Sarbach, C., Stevens, P., Whiting, J., Puget, P., Humbert, M., Cohen-Kaminsky, S., et al. (2013). Evidence of endogenous volatile organic compounds as biomarkers of diseases in alveolar breath. Ann. Pharm. Fr. 71 (4), 203–215. doi:10.1016/j.pharma.2013.05.002
Schaber, C. L., Katta, N., Bollinger, L. B., Mwale, M., Mlotha-Mitole, R., Trehan, I., et al. (2018). Breathprinting reveals malaria-associated biomarkers and mosquito attractants. J. Infect. Dis. 217 (10), 1553–1560. doi:10.1093/infdis/jiy072
Schmidt, F., Kohlbrenner, D., Malesevic, S., Huang, A., Klein, S. D., Puhan, M. A., et al. (2023). Mapping the landscape of lung cancer breath analysis: a scoping review (ELCABA). Lung Cancer 175, 131–140. doi:10.1016/j.lungcan.2022.12.003
Schröder, R., Forstreuter, M., and Hilker, M. (2005). A plant notices insect egg deposition and changes its rate of photosynthesis. Plant Physiol. 138 (1), 470–477. doi:10.1104/pp.105.059915
Scotter, J. M., Allardyce, R. A., Langford, V. S., Hill, A., and Murdoch, D. R. (2006). The rapid evaluation of bacterial growth in blood cultures by selected ion flow tube–mass spectrometry (SIFT-MS) and comparison with the BacT/ALERT automated blood culture system. J. Microbiol. Methods 65(3), 628–631. doi:10.1016/j.mimet.2005.09.016
Seeley, J. V., and Seeley, S. K. (2013). Multidimensional gas chromatography: fundamental advances and new applications. Anal. Chem. 85 (2), 557–578. doi:10.1021/ac303195u
Sharkey, T. D., and Yeh, S. (2001). Isoprene emission from plants. Annu. Rev. Plant Physiol. Plant Mol. Biol. 52, 407–436. doi:10.1146/annurev.arplant.52.1.407
Skogerson, K., Wohlgemuth, G., Barupal, D. K., and Fiehn, O. (2011). The volatile compound BinBase mass spectral database. BMC Bioinforma. 12, 321. doi:10.1186/1471-2105-12-321
Smith, D., and Spanel, P. (1996). The novel selected-ion flow tube approach to trace gas analysis of air and breath. Rapid Commun. Mass Spectrom. 10 (10), 1183–1198. doi:10.1002/(SICI)1097-0231(19960731)10:10<1183::AID-RCM641>3.0.CO;2-3
Smith, D., and Španěl, P. (2005). Selected ion flow tube mass spectrometry (SIFT-MS) for on-line trace gas analysis. Mass Spectrom. Rev. 24 (5), 661–700. doi:10.1002/mas.20033
Smith, D., Španěl, P., Demarais, N., Langford, V. S., and McEwan, M. J. (2022). Recent developments and applications of selected ion flow tube mass spectrometry (SIFT-MS). Mass Spectrom. Rev. n/a, e21835. doi:10.1002/mas.21835
Smith, D., Španěl, P., Herbig, J., and Beauchamp, J. (2014). Mass spectrometry for real-time quantitative breath analysis. J. Breath. Res. 8 (2), 027101. doi:10.1088/1752-7155/8/2/027101
Španěl, P., Davies, S., and Smith, D. (1999). Quantification of breath isoprene using the selected ion flow tube mass spectrometric analytical method. Rapid Commun. Mass Spectrom. 13 (17), 1733–1738. doi:10.1002/(SICI)1097-0231(19990915)13:17<1733::AID-RCM707>3.0.CO;2-S
Starowicz, M. (2021). Analysis of volatiles in food products. Separations 8 (9), 157. doi:10.3390/separations8090157
Sugita, K., and Sato, H. (2021). Sample introduction method in gas chromatography. Anal. Sci. 37 (1), 159–165. doi:10.2116/analsci.20SAR19
Tabbal, S., El Aroussi, B., Bouchard, M., Marchand, G., and Haddad, S. (2022a). Development and validation of a method for the simultaneous quantification of 21 microbial volatile organic compounds in ambient and exhaled air by thermal desorption and gas chromatography–mass spectrometry. Mass Spectrom. Atmos. 13 (9), 1432. doi:10.3390/atmos13091432
Tabbal, S., El Aroussi, B., Bouchard, M., Marchand, G., and Haddad, S. (2022b). A new headspace solid-phase microextraction coupled with gas chromatography-tandem mass spectrometry method for the simultaneous quantification of 21 microbial volatile organic compounds in urine and blood. Chemosphere 296, 133901. doi:10.1016/j.chemosphere.2022.133901
Takaya, K., Hagiwara, M., Matoba, S., Takaya, M., and Shibata, N. (2022). A real-time gas monitoring system based on ion mobility spectrometry for workplace environmental measurements. Ind. Health 60 (1), 40–46. doi:10.2486/indhealth.2021-0037
Taylor, C., Lough, F., Stanforth, S. P., Schwalbe, E. C., Fowlis, I. A., and Dean, J. R. (2017). Analysis of Listeria using exogenous volatile organic compound metabolites and their detection by static headspace–multi-capillary column–gas chromatography–ion mobility spectrometry (SHS–MCC–GC–IMS). Anal. Bioanal. Chem. 409 (17), 4247–4256. doi:10.1007/s00216-017-0375-x
Temerdashev, A. Z., Gashimova, E. M., Porkhanov, V. A., Polyakov, I. S., Perunov, D. V., and Dmitrieva, E. V. (2023). Non-invasive lung cancer diagnostics through metabolites in exhaled breath: influence of the disease variability and comorbidities. Metabolites 13 (2), 203. doi:10.3390/metabo13020203
Tiwari, S., Kate, A., Mohapatra, D., Tripathi, M. K., Ray, H., Akuli, A., et al. (2020). Volatile organic compounds (VOCs): biomarkers for quality management of horticultural commodities during storage through e-sensing. Trends Food Sci. Technol. 106, 417–433. doi:10.1016/j.tifs.2020.10.039
Tsugawa, H., Cajka, T., Kind, T., Ma, Y., Higgins, B., Ikeda, K., et al. (2015). MS-DIAL: data-independent MS/MS deconvolution for comprehensive metabolome analysis. Nat. Methods 12 (6), 523–526. doi:10.1038/nmeth.3393
Tsugawa, H., Kind, T., Nakabayashi, R., Yukihira, D., Tanaka, W., Cajka, T., et al. (2016). Hydrogen rearrangement rules: computational MS/MS fragmentation and structure elucidation using MS-FINDER software. Anal. Chem. 88 (16), 7946–7958. doi:10.1021/acs.analchem.6b00770
Ullah, M. A., Kim, K.-H., Szulejko, J. E., and Cho, J. (2014). The gas chromatographic determination of volatile fatty acids in wastewater samples: evaluation of experimental biases in direct injection method against thermal desorption method. Anal. Chim. Acta 820, 159–167. doi:10.1016/j.aca.2014.02.012
US EPA (2022). “Technical overview of volatile organic compounds,” in Indoor air quality (IAQ) (Washington, D.C., United States: United States Environmental Protection Agency). Available at: https://www.epa.gov/indoor-air-quality-iaq/technical-overview-volatile-organic-compounds.
Vautz, W., Zimmermann, D., Hartmann, M., Baumbach, J. I., Nolte, J., and Jung, J. (2006). Ion mobility spectrometry for food quality and safety. Food Addit. Contam. 23 (11), 1064–1073. doi:10.1080/02652030600889590
Vidal-de-Miguel, G., and Herrero, A. (2012). Secondary electrospray ionization of complex vapor mixtures. Theoretical and experimental approach. J. Am. Soc. Mass Spectrom. 23 (6), 1085–1096. doi:10.1007/s13361-012-0369-z
Wang, C., Zhang, W., Li, H., Mao, J., Guo, C., Ding, R., et al. (2019). Analysis of volatile compounds in pears by HS-SPME-GC×GC-TOFMS. Molecules 24 (9), 1795. doi:10.3390/molecules24091795
Wang, T. I., Chu, C. W., Hung, H. M., Kuo, G. S., and Han, C. C. (1994). Design parameters of dual-stage ion reflectrons. Rev. Sci. Instrum. 65 (5), 1585–1589. doi:10.1063/1.1144896
Wang, W., Khalil-Ur-Rehman, M., Feng, J., and Tao, J. (2017). RNA-seq based transcriptomic analysis of CPPU treated grape berries and emission of volatile compounds. J. Plant Physiol. 218, 155–166. doi:10.1016/j.jplph.2017.08.004
Weber, R., Kaeslin, J., Moeller, S., Perkins, N., Micic, S., and Moeller, A. (2023a). Effects of a volatile organic compound filter on breath profiles measured by secondary electrospray high-resolution mass spectrometry. Molecules 28 (1), 45. doi:10.3390/molecules28010045
Weber, R., Streckenbach, B., Welti, L., Inci, D., Kohler, M., Perkins, N., et al. (2023b). Online breath analysis with SESI/HRMS for metabolic signatures in children with allergic asthma. Front. Mol. Biosci. 10. doi:10.3389/fmolb.2023.1154536
Weggler, B. A., Gruber, B., and Dorman, F. L. (2019). Rapid screening of complex matrices: utilizing kendrick mass defect to enhance knowledge-based group type evaluation of multidimensional gas chromatography–high-resolution time-of-flight mass spectrometry data. Anal. Chem. 91 (17), 10949–10954. doi:10.1021/acs.analchem.9b01750
Weise, T., Kai, M., Gummesson, A., Troeger, A., von Reuß, S., Piepenborn, S., et al. (2012). Volatile organic compounds produced by the phytopathogenic bacterium Xanthomonas campestris pv. vesicatoria 85-10. Beilstein J. Org. Chem. 8, 579–596. doi:10.3762/bjoc.8.65
Weisskopf, L., Schulz, S., and Garbeva, P. (2021). Microbial volatile organic compounds in intra-kingdom and inter-kingdom interactions. Nat. Rev. Microbiol. 19 (6), 391–404. doi:10.1038/s41579-020-00508-1
Westphal, K., Dudzik, D., Waszczuk-Jankowska, M., Graff, B., Narkiewicz, K., and Markuszewski, M. J. (2023). Common strategies and factors affecting off-line breath sampling and volatile organic compounds analysis using thermal desorption-gas chromatography-mass spectrometry (TD-GC-MS). Metabolites 13 (1), 8. doi:10.3390/metabo13010008
Whitehouse, C. M., Meng, C. K., and Fenn, J. B. (1986). Proceedings of the 34th ASMS conference on mass spectrometry and allied topics. Denver, CO, 507.
Willis, P., Jaloszynski, J., and Artaev, V. (2021). Improving duty cycle in the Folded Flight Path high-resolution time-of-flight mass spectrometer. Int. J. Mass Spectrom. 459, 116467. doi:10.1016/j.ijms.2020.116467
Wojnowski, W., Kalinowska, K., Majchrzak, T., and Zabiegała, B. (2022). Real-time monitoring of the emission of volatile organic compounds from polylactide 3D printing filaments. Sci. Total Environ. 805, 150181. doi:10.1016/j.scitotenv.2021.150181
Wu, C., Siems, W. F., and Hill, H. H. (2000). Secondary electrospray ionization ion mobility spectrometry/mass spectrometry of illicit drugs. Anal. Chem. 72 (2), 396–403. doi:10.1021/ac9907235
Wüthrich, C., and Giannoukos, S. (2024). Advances in secondary electrospray ionization for breath analysis and volatilomics. Int. J. Mass Spectrom. 498, 117213. doi:10.1016/j.ijms.2024.117213
Zhang, V. R., Ramachandran, G. K., Loo, E. X. L., Soh, A. Y. S., Yong, W. P., and Siah, K. T. H. (2023). Volatile organic compounds as potential biomarkers of irritable bowel syndrome: a systematic review. Neurogastroenterol. Motil. 35 (7), e14536. doi:10.1111/nmo.14536
Zheng, Y., Wang, P., Chen, X., Sun, Y., Yue, C., and Ye, N. (2019). Transcriptome and metabolite profiling reveal novel insights into volatile heterosis in the tea plant (camellia sinensis). Molecules 24 (18), 3380. doi:10.3390/molecules24183380
Keywords: volatile organic compounds, selected ion flow tube-mass spectrometry, ion mobility spectrometry-mass spectrometry, proton transfer reaction-mass spectrometry, secondary electrospray ionization-mass spectrometry, time-of-flight mass spectrometry, comprehensive two-dimensional gas chromatography, high resolution multi-reflecting time-of-flight mass spectrometry
Citation: Szeitz A, Sutton AG and Hallam SJ (2024) A matrix-centered view of mass spectrometry platform innovation for volatilome research. Front. Mol. Biosci. 11:1421330. doi: 10.3389/fmolb.2024.1421330
Received: 22 April 2024; Accepted: 15 October 2024;
Published: 30 October 2024.
Edited by:
Joana Pinto, University of Porto, PortugalReviewed by:
Paula Guedes De Pinho, University of Porto, PortugalMiyako Kusano, University of Tsukuba, Japan
Copyright © 2024 Szeitz, Sutton and Hallam. This is an open-access article distributed under the terms of the Creative Commons Attribution License (CC BY). The use, distribution or reproduction in other forums is permitted, provided the original author(s) and the copyright owner(s) are credited and that the original publication in this journal is cited, in accordance with accepted academic practice. No use, distribution or reproduction is permitted which does not comply with these terms.
*Correspondence: Steven J. Hallam, c2hhbGxhbUBtYWlsLnViYy5jYQ==